- 1Amity Institute of Microbial Technology, Amity University Rajasthan, Jaipur, India
- 2Translational Health Science and Technology Institute, National Capital Region (NCR) Biotech Science Cluster, Faridabad, India
Advances in understanding the genetic basis of cancer have driven alternative treatment approaches. Recent findings have demonstrated the potential of bacteria and it’s components to serve as robust theranostic agents for cancer eradication. Compared to traditional cancer therapies like surgery, chemotherapy, radiotherapy, bacteria mediated tumor therapy has exhibited superior cancer suppressing property which is attributed a lot to it’s tumor proliferating and accumulating characteristics. Genetically modified bacteria has reduced inherent toxicity and enhanced specificity towards tumor microenvironment. This anti- tumor activity of bacteria is attributed to its toxins and other active components from the cell membrane, cell wall and spores. Furthermore, bacterial genes can be regulated to express and deliver cytokines, antibodies and cancer therapeutics. Although there is less clinical data available, the pre- clinical research clearly indicates the feasibility and potential of bacteria- mediated cancer therapy.
Introduction
Cancer brings about psychological and physical anguish to the patient and family. Cancer related deaths are one of the leading cause of demise globally with cardiovascular related deaths at the forefront (1–3). Colon, lung, liver, pancreas, stomach, breast and bowel continue to be the most common organs impacted by the disease (3, 4). Tumorous condition begins with uncontrolled growth of cells and formation of a mass within an organ or tissue. It can be either benign, and grow but do not spread to distant tissues or it can be malignant and spread to other tissues of the individual. Uncontrolled and invasive growth of self- cells beyond the tissue of origination and spread to otherwise healthy tissues leads to metastatic phase of cancer. Genetic and epigenetic alterations resulting from ageing, prolonged exposure to different mutagens, adverse epigenetic factors, and chronic infections results in an increased proliferation, affecting cell cycle through gene functioning in proto- onco and tumor suppressor genes (6–9). Advancements in the field of cancer therapy and diagnostics have led to the development of surgery, chemotherapy, and radiotherapy resulting in increased survival rates of cancer patient world- wide (10). However, due to a diversity of anatomic location of the cancer cells, histologic origin, intertumoral heterogeneity due to genomic alterations and diverse immunological characteristics within the tumor micro- environment have shown the need for more advanced cancer prognosis and therapeutics (11). These inherent properties of tumor cells along with non- specific toxicity of cancer therapy, surgically unremovable and probable drug resistance have prompted the need for development of alternative approaches. Targeted therapy and immunotherapy are two of the promising strategies in the fight against cancer. Targeted therapy aims at abnormalities associated with cancerous cells to increase tumor specificity and reduce toxicity in healthy tissues. Solid tumors are characterized by poor oxygen, low pH, elevated interstitial fluid which supports tumor proliferation, immunosuppression and resistance against conventional tumor therapy (12, 13). Targeted therapy aims to target these abnormalities to increase specificity of anti- cancer therapeutics and reduce non- specific cytotoxicity. Immunotherapy harnesses a host’s immune system to eliminate tumor by activating the immune cells and interacting with the tumor antigens. Recent years have seen profound development with immune checkpoint blockade therapy (monoclonal antibodies against programmed death protein 1; PD1 and cytotoxic T-lymphocyte associated protein 4; CTLA4) (14–17) and chimeric antigen receptor T cell (CAR- T) therapy proving themselves to be powerful anti- cancer therapeutics (18, 19). The sensitivity and efficacy of these therapies however depend on the innate tumor micro- environment and specific ligands expressed by the tumor cells which requires developing personalized treatment strategies. Major efforts are being made to develop alternative approaches that can circumvent the obstacles of the current cancer treatment regimen. Application of therapeutic bacteria as anti- cancer therapeutic has fascinated researchers globally. The application of bacteria and bacterial toxins as anti- cancer agents is more than a century old with initial findings of German physician Wilhelm Busch in 1868, where he observed tumor regression in cancer patients after accidental infection by Streptococcus pyogenes (20). His findings were reproduced by Friedrich Fehleisen in 1882 who identified Streptococcus pyogenes as the causative agent of erysipelas and shrinkage of malignancy (20). However, the noteworthy reports of bacteria mediated tumor therapy gained momentum after William Coley’s well documented report of bacteria mediated regression of inoperable skin cancer. In late 1800’s, his trials utilizing inactivated bacterial species Streptococcus pyogenes and Serratia marcescens could successfully treat patients with carcinoma, lymphoma, melanoma and myeloma (21, 22). The contribution of William Bradley Coley in the field of cancer immunotherapy using bacterial toxins made researchers realize the potential of the bacteria in tumor treatment (23). Furthermore, pediatric vaccinations have been correlated with decreased incidences of childhood cancer. By utilizing population data and meta- analysis, various research groups have attributed that exposure to bacterial or viral vaccines during infancy serves as immunomodulators leading to a reduction in cancer incidences (24–30). These reports further strengthen the potential of bacteria and bacterial mediated therapeutics to treat cancer.
Coley’s revolutionary work opened the doors for new experimental candidates with bacterial species ranging from Salmonella, Clostridium, Listeria, Lactobacillus, Escherichia, Pseudomonas and Caulobacter with some bacterial species going to clinical trials (Figure 1). The initial application of bacteria mediated cancer therapy was met with criticism due to the adverse effects ranging from bacteria mediated septic shock and death due to immune- suppression. However, genetic engineering enabled researchers to manipulate the microorganism and knockout/knockdown genes which contributed to reduction of cytotoxic proteins, or virulence factors but enhanced penetration of tumor microenvironment and activate the host’s antitumor immune system (31–33). Bacilli Calmette- Guerin (BCG) has been a success story of bacteria mediated cancer immunotherapy and approved by FDA for treatment of superficial bladder cancer (34). Bacterial cell wall components like lipopolysaccharide and peptidoglycan can act as pathogen associated molecular patterns (PAMPs) and potentiate the host immune system. Intratumoral delivery of these bacterial components can mimic an in- situ vaccine and activate antigen presenting cell’s (APC’s) like dendritic cells which can phagocytose tumor antigen’s and migrate to the draining lymph nodes where they can prime cytotoxic T cells (35). In an article by Chowdhury et al., the researchers have reported quorum sensing genetically modified bacteria encoding for a single- chain antibody fragment targeting the phagocyte inhibitory ligand CD47 in macrophages. This strategy led to development of a systemic anti- tumor immunity resulting in tumor regression, metastasis prevention and prolonging survival of mice (36). Chemotactic mediated bacterial targeting and accumulation within tumor’s has been associated with tumor hypoxic micro- environment, pre- dominant expression of clusterin, serglycin, TGF- β2 etc (37). Furthermore, research groups have reported bacteria as a vector to deliver anti- cancer therapeutics (38–40). Various mechanism hypothesizing the role of bacterial enzymes like bacteriocin, bacterial biofilms and activation of host inflammasome pathway in antitumor immunity have been reported (41–43).
In this article, we have summarized the recent progress in the field of bacterial mediated cancer immunotherapy and it’s future as one of the potential weapons in the arsenal in the fight against cancer. We also discussed the mechanism associated with tumor microenvironement mediated egression of bacteria and the challenges associated with this strategy.
Cancer progression, the human body, and therapeutics
The ability of the human immune system to distinguish between self and non-self enables it to defend the body against both foreign and endogenous illnesses. The human immune system is a complex network comprising of white blood cells as well as lymphatic organs such as thymus, spleen, tonsils, and lymph. The immune system recognizes multiple dangers and removes them to maintain homeostasis in the nodes, lymphatic vessels, and blood. Cancer is a “developmental disorder” which arises when cells divide uncontrollably and spread into surrounding or distant tissues. Host immune system acts as a double- edged sword in cancer metastasis. CD4+ Th1 cells, CD8+ T cells, NK cells, IFN-γ, M1 macrophages uninterruptedly function to be anti- tumor, while regulatory T cells (Treg), M2 macrophages, Th17 cells, overexpression of TGF- β, and IL- 10 facilitates immune evasion of the tumor cells (5, 44). Understanding the relationship between cancer cells and the immune system is necessary to comprehend how immunotherapy has developed into a mainstay of cancer treatment. While there are many distinct types of immunotherapies with diverse mechanisms of action, they primarily rely on the host immune system to destroy tumor cells, unlike chemotherapy, which kills cancer through cytotoxic qualities (45).
Cancer development and progression can be initiated by genetic and functional abnormalities. Epigenetic modifications such as DNA methylation, histone acetylation, and genetic mutations can lead to an altered gene expression and abnormal cell functionality (46–48). Cancer and its treatment negatively affect the physiology the patient, such as the removal of body parts, colostomies, hair loss, and other things. Several of the modifications are apparent to others, like hair loss, while others, like colostomy, are less obvious. For a cancer survivor, the physical changes brought on by the disease or treatment are frequently profound. Psychosocial cancer research has long recognized the significance of a modified physical appearance following treatment when patients evaluate their quality of life (49).
The most common cause and hallmark of cancer-related mortality is metastasis, and more than 90% of cancer related death occur due to metastasis. “Metastasis” refers to the growth of secondary tumors in an area of the body that is remote from the main site of tumor growth. Understanding the molecular mechanisms underlying the metastatic process needs to be established in order to identify therapeutic windows for effective interventions. Some of the molecular underpinnings of this dispersion process have been revealed as a result of the ongoing evolution of cancer biology research and the introduction of new paradigms in the study of metastasis which are influenced by genetic and epigenetic changes inside the host’s tumor cell and its surroundings. Large quantities of cancer cells are discharged into the bloodstream every day in cancer patients, although melanoma research in animal models suggests that only 0.1% of tumor cells spread to cause metastasis. Targeting metastatic seeding and colonization remains a difficulty despite substantial studies. The notion that metastatic cancer cells dynamically and selectively change their metabolism at every stage of the metastatic cascade has just recently come to light. In addition, many metastases differ metabolically from the primary tumors from which they arise, allowing for survival and expansion in the new environment. Knowing the kinetics of this process as well as targeting new metabolic qualities that emerge in metastases may enable their elimination or offer molecular therapeutics that may slow or even stop the spread of cancer (50, 51).
Early efforts to treat cancer by Paul Ehrlich in 1900’s using aniline dyes and alkylating agents were not very encouraging (as reviewed by DeVita and Chu in (52)). Research by Yale pharmacologists, Alfred Gilman and Louis Goodman on mice bearing a lymphoid tumor demonstrated a marked regression of the tumor when it was treated with a compound, nitrogen mustard (53). The same regression was observed when a patient with non- Hodgkin’s lymphoma was treated with the compound (53). These results brought a ray of hope to cancer treatment in the mid 1940’s (54, 55). However, remissions turned out to be brief and incomplete which created an air of pessimism in that period (56). However, discovery of fluoropyrimidine 5- fluorouracil (5- FU) by Charles Heidelberger at the University of Wisconsin for treatment of nonhematologic cancers can be stated as a pathbreaker in chemotherapy. 5- FU still remains the cornerstone for treatment of colorectal cancer. 1960’s and 1970’s witnessed the advent of combination chemotherapy with a combination of 5- FU, cyclophosphamide and methotrexate against metastatic cancer which demonstrated promising results (57). Despite enhancing survival, anti- cancer chemotherapy still remains a concern for both patients and clinicians. Chemotherapy induced nausea and vomiting (CINV) are amongst the most common side- effects with patients undergoing chemotherapy. Other severe side effects include oral and gastrointestinal mucositis leading to anorexia, weight loss, anemia, and fatigue. Hypersensitivity, cardiovascular toxicity and nephrotoxicity have been reported to be associated with platinum chemotherapy. Chemotherapy induced peripheral neuropathy (CIPN) like depression, ataxia, insomnia are other side effects related to anti- cancer drugs like platinum- based, vinca alkaloids, taxanes, and proteosome and angiogenesis inhibitors (58). Hence, new approaches to improve tolerance and reduce sequlae of chemotherapy is an urgent need of the hour (59).
Cancer and bacterial relationship within the host
The normal microflora present on the human body consists of different bacteria, protozoa, fungi and viruses. This microflora interacts with each other and helps in regulating the human metabolism and immunity. Recent research on the gut and resident microflora have been found to affect the host’s antitumor immunity (60). The gut microbiota is frequently cited as one of the most crucial elements in maintaining a healthy homeostasis. In some studies, probiotic bacteria have been demonstrated to exhibit antitumor activities and to play a substantial role in immunomodulation. Short-chain fatty acids, which influence cell death and proliferation are known signaling molecules in the immune system, can be produced by bacteria and can lead to the detection and destruction of potential carcinogens. Due to their influence on immunomodulation, lactic acid bacteria found in the gut have been shown to play a part in the reversal of carcinogenesis. This finding supports the notion that bacterial metabolites interact with immunological and epithelial cells (61). Furthermore, probiotic bacteria can influence the production of anti-inflammatory cytokines, which are crucial in the elimination of tumor cells. Probiotic bacteria can also influence and activate phagocytes to get rid of cancerous cells. Synergistic application of radiation and probiotic bacteria have improved the immune system’s ability to recognize cancer cells. The immunity of mice to carcinogens is directly correlated to the presence or absence of an active microbiome. The idea of employing probiotic bacteria as medication delivery vehicles has recently gained traction as a result of multiple articles revealing promising outcomes. Probiotic bacteria and gut microbiota are likely to play a significant role in cancer prevention and therapy over the next several years (61).
Human tumors are known to act as host for bacteria; however, it is unknown whether these bacteria exhibit a commensal or symbiotic relation with the tumors. Bacterial makeup varies with the type of tumor. For instance, bacteria that make monothiol, which can detoxify reactive oxygen species, were more prevalent in breast cancer subtypes that were characterized by elevated oxidative stress (62). In some instances, bacteria can promote tumor development in specific organs. Until recently tumors were supposed to be sterile, but Fu et al., demonstrated that bacteria can colonize tumor and promotes host cell survival to circulating tumor cells by reorganizing actin cytoskeleton and enhancing resistance (63). Moreover modern genotyping techniques involving immunohistochemistry, and fluorescence in- situ hybridization (FISH) and 16S ribosomal RNA sequencing have revealed distinct microbiota across 1526 tumors across seven cancer types including breast, lung, ovary, pancreas, melanoma, bone and brain cancer (62).
Mechanism of action of bacteria as anti- cancer therapeutic
Cancer is considered one of the deadliest diseases and a definitive cure for this disease is still the need of the hour. The disease involves proliferation of abnormal cells that invade normal tissues and organs. Along with the traditional cancer treatment approaches like surgery, radiation and chemotherapy, monoclonal antibody-based immunotherapy has been recognized as a milestone in the treatment of cancer. Monoclonal antibodies target tumor cell receptors and induces long lasting antitumor responses (64) . The very first attempt of involving the host immune system for cancer treatment was done by William B. Coley is recognized as the father of immunotherapy (65). Traditional treatment strategies like chemotherapy and radiotherapy resulted in unwanted cytotoxicity, poor drug adsorption, ineffective tumor clearance and developing resistance against anti- cancer drugs. Bacterial immunotherapy has been shown to help in overcoming the problems faced during conventional treatment method. Bacteria and archaea contain numerous bioactive compounds which effectively work against the tumor by inhibiting the growth of cancer cells (66, 67). Injecting tumor targeting bacteria enables the bacteria to colonize the tumor and orchestrate a plethora of immune which results in destructing the tumor cells. Bacteria has been used alone or in combination with conventional methods including radiotherapy, chemotherapy and have shown effective results in inhibiting cancer cell metastasis as well as reduction of tumor. Bacterial species like Salmonella, Clostridium, Bifidobacterium, Lactobacillus, Escherichia, Pseudomonas, Caulobacter, Listeria, Proteus, and Streptococcus have been explored as potential anti- cancer agents by various research groups (68). Bacterial mediated regression of tumor can be attributed to various mechanisms, likely bacterial species, their intrinsic properties like toxin secretion or pathogen associated molecular pattern (PAMP), genetic modification, delivery vector, synergism with host immune system etc (Figure 2).
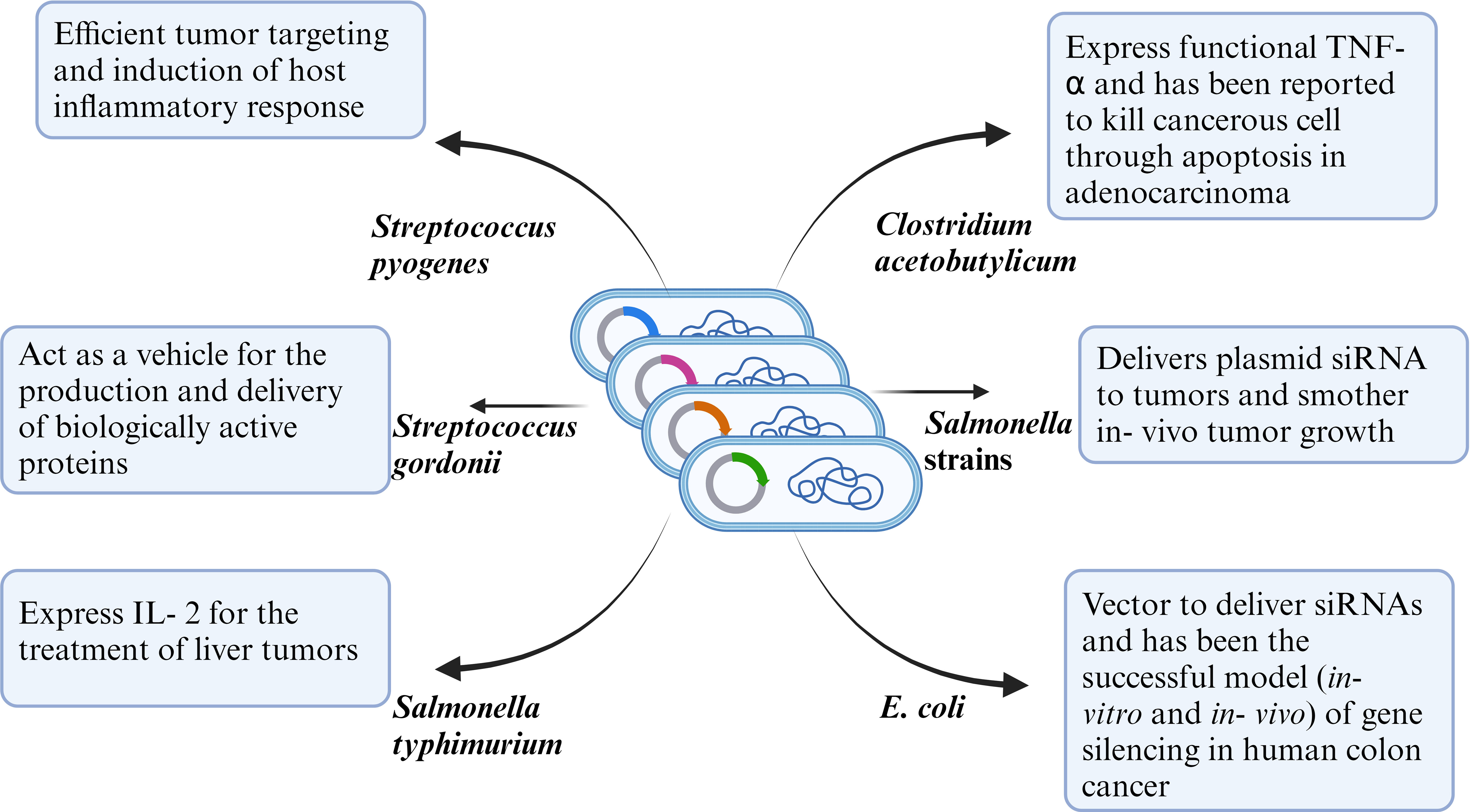
Figure 2 Representative figure of mechanism of anti-tumor efficacy of WT or genetically modified bacterial strains.
Toxin mediated anti- tumor efficacy: Coley’s toxins consisted of exotoxins secreted by Streptococcus pyogenes and Seratia marcescens. Additionally, S. pyogenes also secretes some pyrogenic exotoxins such as SpeA, SpeB and SpeC. These pyrogenic toxins stimulate CD4+ lymphocytes resulting in a rapid release of cytokines (69). Prodigiosin, a low molecular toxin produced by Serratia marcescens, Serratia plymuthica, Hahella chejuensis, Pseudomonas magnesiorubra and Vibrio psychroerythreus has demonstrated antitumor activity in drug resistant cancer cells like A2780RCIS (MRP1,2 overexpressing human epithelial ovarian cancer cell line), EPG85- 257RNOV (BCRP overexpressing human gastric carcinoma cell line), EPG85- 257RDB (MDR1 overexpressing human gastric carcinoma cell line) (70). On the other hand, Pseudomonas spp secretes exotoxins which binds to tumor cell receptors and induces cell death (71). Clostridium perfringens produce enterotoxins, an anticancer agent which works by binding to receptors (CLDN3 and CLDN4) highly expressed on colon carcinoma and epithelial tumors resulting in a multi- protein membrane pore complex thereby causing lysis of tumor cells (72). Similarly Pseudomonas aeruginosa produce exotoxin T which reduces the growth of B16 melanoma (67). Modified forms of toxins have often resulted in superior efficacy as compared to its natural counterpart. Diphtheria toxin produced by Corynebacterium diphtheria is not efficient in cancer therapeutics, but it’s modified form DT modified with the amino terminal (AT) fragment of urokinase- type plasminogen activator (DTAT) has been tested on cell lines and murine model and reported to target vascular endothelial of the tumor, reduced tumor size (73) and resulted in significant regression of human U118MG tumor induced in nude mice (74, 75).
Enzymatic action: Different bacterial enzymes have also been reported to act as anti- cancer therapeutic agents. For instance, L- asparginase isolated from E. coli have been reported to activate aspargine hydrolysis which resulted in cell death of different tumor cell lines like MCF- 7, HepG2 and SK-LU-1. Moreover, asparginase also acted as anti- neoplastic drug in lymphoblastic leukemia (76). Arginine deaminase isolated from Streptococcus pyogenes reduced proliferation of arginine deficient tumor glioblastoma multiforme (77). Likewise, purified pyocin S2, secreted by Pseudomonas aeruginosa has been demonstrated to exhibit cytotoxic effects on Im9 (a human immunoglobulin- secreting cell line derived from multiple myeloma) and no effect on normal human cells (78). Microcin E492 secreted by Klebsiella pneumoniae, pediocin from Pediococcus acidilactici K2a2- 3, nisin from Lactobacillus lactis have been reported to exert cytotoxic effects in human malignant cell lines like Jurkat (T cell derived from acute T cell leukemia), RJ2.25 (variant of Burkitt’s lymphoma), HT29 (human colon adenocarcinoma), MCF-7 (human breast adenocarcinoma), HepG2 and head and neck squamous cell carcinoma, but non- toxic on normal human cells (79–83).
Tumor colonization and regression: Facultative anaerobic bacterial species also exhibit the property to colonize tumor and replicate within the hypoxic tumor which results in the inhibition of cancerous growth (84). Salmonella typhi a flagellated, Gram-negative bacilli is very well known to cause typhoid fever and other food borne diseases. Although pathogenic, it also works as a promising cancer therapeutic agent because of its facultatively anaerobic nature i.e. it can survive in both anaerobic as well as aerobic environment and can occupy both the hypoxic as well as non-hypoxic areas of the tumor (40, 85). Salmonella directly infects the tumor cell and induce caspase 1 by inflammasomes and destroy them by apoptosis or regulating autophagy via AKT/mTOR pathway (23).Salmonella also mediates T cell and innate immune cell infiltration to tumor site which enhanced killing of Salmonella localized tumor (86). Study on Listeria spp. infection generates reactive oxygen species by activating nicotinamide adenine dinucleotide phosphate (NADP+) oxidase or elevating intracellular Ca2+ levels (87). These ROS induces the immunogenic death of tumor cells and activate CD8+ T cells (88).
PAMP’s and their anti- tumor potential: Bacterial cell wall consists of pathogen-associated molecular patterns (PAMPs) which have the ability to trigger the immune cells even in the tumor immunosuppressive microenvironment which enhances specific immune recognition and help in the elimination of tumor cells (23). Along with bacteria-based cancer therapy, pathogen-associated molecular patterns (PAMPs) of bacterial membranes has also attracted the world’s attention in generating anti- tumor response. PAMP’s are acknowledged by the antigen presenting cells leading to activation of the T cell mediated immunity. Along with the activation of T cell mediated immunity, toll-like receptors (TLR’s) are also activated, and they are responsible for the production of cytokines like IL-12 and other molecules like CD40. Furthermore, a strong immune response is generated against the cancer cells by the Th-1 dependent immune response and CD8+ T effector cells (73).
Cell wall components as vectors: Outer membrane vesicle (OMV) of bacteria has also attracted researchers as potential drug delivery vehicle. Outer membrane vesicles from Gram positive and Gram negative bacteria have been coated on polymeric micelles that contained drugs to create an inventive nanomedicine for efficient cancer immunotherapy and metastasis prevention using bioengineering technique (23, 89). The loaded drug within polymeric micelles can exert both chemotherapeutic and immunomodulatory activities to sensitize cancer cells to cytotoxic T lymphocytes (CTLs) and to directly kill cancer cells, whereas OMVs might trigger the host immune response for cancer immunotherapy. Systemic injection of such a bioinspired immunotherapeutic drug would not only greatly limit tumor growth in vivo and increase the survival rate of melanoma mice but would also give excellent protective immunity against the development of melanoma. In addition, nanomedicine could successfully prevent lung metastasis of the tumor. The bacterial-based cancer immunotherapy formulation is repurposed by the bioinspired immunomodulatory nanomedicine, which also provides a helpful bioengineering technique to enhance the current cancer immunotherapeutic drugs and delivery systems (89). An alternative approach in cancer treatment apart from OMV’s are bacterial ghosts (BGs) and archaeosomes which have been used as a nano formulation as drug delivery system to deliver anti- cancer therapeutics. Combination of different drugs are incorporated into these biological nanocarriers which are present inside the bacteria and used to kill the cancerous cells (67). These biological nanocarriers work as an efficient drug delivery system because of their certain properties as they are biodegradable, they fuse with the target cells, circulate longer in the body by escaping the immune system and enhance the cellular uptake (67). Implication of doxorubicin loaded OMV from attenuated Klebsiella pneumoniae prevented A549 tumor growth in BALB/c nude mice. The formulation was well tolerable and OMV resulted in recruitment of macrophages in the TME (90). In yet another approach, Kim et al., designed E. coli protoplast derived vesicles and epidermal growth factor (EGF) tagged protoplast derived vesicles encapsulating doxorubicin/idarubicin. Tagging of EGF on these vesicles resulted in higher accumulation of the drug within the tumor sites and resulted in significant decrease in tumor size as compared to free drug (91). OMV’s stimulate anti- tumor response by secreting cytokines and chemokines like IFN-γ, IL-12p40, CXCL10, TNF- α, IL-6 and have been predicted to be self- sufficient for less destructive tumors like CT26 or MC38 adenocarcinoma (92).
Genetic engineering of bacteria: With the advent of recombinant DNA technology, researchers have designed genetically modified bacteria with inherent ability to colonize the tumor. These bacterial therapies not only increase the efficacy and efficiency but also minimizes the toxic effects which normally occur during cancer treatments. A major limitation in developing an efficient cancer therapeutics is overcoming the immunosuppressive tumor microenvironment (TME) which consists of M2 type macrophages, myeloid derived suppressor cells etc (93–95). This TME can be effectively utilized to deliver genetically modified bacteria to be protected from the host’s immune system and exhibit its anti- cancer efficacy. The tumor apoptosis is initiated by the bacteria due to the different interactions that take place between the cancer cells, bacteria, cytokines and chemokines (66). Manipulation of S. typhi to express either the proapoptotic Fas ligand or CCL21(chemokine with anti- tumor properties) as a delivery vehicle carrying anti- cancer therapeutics has demonstrated primary tumor inhibition (23). In another study, Sedighi and group utilized and demonstrated Clostridia, Bifidiobacteria, and Salmonellae species as vectors to deliver suicide genes, tumor associated antigens or for expressing tumor suppressor genes. Salmonella typhimurium and Clostridium butyricum have been utilized for their inherent capability to selectively colonize the tumor and are used as delivery vectors in mouse tumor models and do not exert any adverse side effects. Sedighi et al. have demonstrated the ability of genetically modified bacteria as opposed to normal bacteria to multiply significantly more in tumorous cells (68). Furthermore, they have also reported rapid tumor regression & regression of cancer has been observed by the help of immunotherapeutic agents such as Streptococcus pyogenes infecting the patient with erysipelas (68). Certain bacteria like Staphylococcus epidermis colonize the skin and can induce a highly specific systemic immune response. Utilizing this concept, in a most recent research, Chen et al., engineered S. epidermis to express melanoma tumor antigens. Skin colonization of the bacteria led to development of tumor specific T cells which were circulatory, could infiltrate local and metastatic lesions and induced tumor regression (96).
With the help of genetic engineering, synthetic biology is ushering in a new age in medicine. The development of designed systems that can intelligently perceive and react to various environments is made possible by this innovative method, which eventually adds specificity and efficacy that go beyond the capabilities of molecular-based treatments. Chowdhury and group designed a non-pathogenic strain of Escherichia coli to specifically lyse within the tumor microenvironment and release an encoded nanobody antagonist of CD47 (CD47nb), an anti-phagocytic receptor that is frequently overexpressed in a number of human cancer types (36). In a syngeneic tumor model in mice, CD47nb delivery by tumor-colonizing bacteria boosted activation of tumor-infiltrating T cells, drove rapid tumor regression, inhibited metastasis, and promoted long-term survival. Furthermore, local injection of CD47nb-expressing bacteria triggered systemic tumor-antigen-specific immune responses that inhibited the growth of untreated tumors, demonstrating the feasibility of an abscopal effect brought on by an engineered bacterial immunotherapy. Hence, a safe and local administration of immunotherapeutic payloads that induce systemic antitumor immunity may be accomplished using modified microorganisms (36). In another approach, Salmonella typhimurium was engineered to deliver shRNA- expressing vectors targeting Bcl2 or indolamine 2,3 – dioxygenase 1 (IDO1) significantly silenced the gene in a murine melanoma model, enhanced tumor cell death and prolonged survival (97, 98).
Exploiting trained immunity: Along with injecting patients with bacteria which has the ability to express tumor antigens, another alternative way to activate the immune system by utilizing immunodominant T cell antigens from pathogens such as tetanus toxoid, poliovirus or measles. Childhood vaccination against these pathogens generate memory cells which can be utilized for the destruction of tumor cells. This process involves the presentation of immunodominant T cell antigens on the surface of tumor cell infected by the tumor targeting bacteria carrying the expression cassettes for these antigens (99).
Limitations of bacteria mediated cancer therapy
Although bacteria- mediated cancer therapy has managed to garner attention of the scientific community and have demonstrated its efficacy in in- vitro and in- vivo models, there are still some unsolved limitations related to potential host immune responses, efficacy and accuracy of targeted delivery and impeded self- reproduction. Innate bacterial toxicity leading to sepsis, chronic inflammation, lymphatic proliferation, potential of DNA mutations thereby resulting in loss of functionality or exaggerated infection, induction of hormones upregulating tumor cell proliferation, production of carcinogenic metabolites which interrupts regulation of cell growth or directly effecting oncogenesis are major concerns associated with the therapy (33, 100, 101). Another major problem reported by Patyar et al., is the incomplete lysis of tumor thereby necessiting the combination therapy with chemotherapeutic treatments (33). This therapy also lacks relevant clinical trials and a reproducibility issue among patients has been a matter of concern. Even the attenuated Mycobacterium bovis (Bacilli Calmette- Guerin), the only clinically approved anti- cancer bacterial therapy developed tissue sepsis and high rates of tumor relapse (102).
Discussion
Although methods such as chemotherapy and radiotherapy are considered the cornerstone of cancer treatment, their results are associated with severe weakness in cancer patients. Moreover, pathophysiology of solid tumors imposes barriers which prevent penetration and efficacy of anti- tumor chemotherapy drugs. With advances in medical technology, conventional cancer treatment strategies have undergone significant improvement. Newer therapies like checkpoint blockade inhibitors and CAR- T therapy have revolutionized anti- cancer treatments. Bacteria can be considered as a promising therapeutic to treat cancer. Their intrinsic properties like hypoxia tropism, self- propelled motility, ability to genetically insert dene or drug make them excellent candidates. Genetically engineered bacteria with reduced virulence but retained tumor targeting have been developed. Several bacteria ranging from Salmonella sp, Streptococcus sp, E. coli, Clostridium sp have been described. Intravenous administration of members of Clostridium sp, C. sporongenes, C. novyi- NT, C. acetobutylicum, and C. beijerinckii have shown to result in tumor colonization and extensive oncolysis. Attenuated Salmonella have demonstrated high penetrability into solid tumors which are otherwise unreachable by conventional therapeutic strategies (103). Genetically attenuated Salmonella typhimurium, obtained by chromosomal deletion of purI and msbB genes, resulted in low pathogenicity, strong tumor accumulation and anti- tumor activity. This double mutant strain, VNP20009 have demonstrated its anti- tumor efficacy in different tumor models like B16- F10 murine melanoma, LOX human melanoma, DLD-1 human colon carcinoma (104). In another instance, Salmonella mutant strain ΔppGpp enhanced production of TNF- α and IL-1β by macrophages and dendritic cells within tumor cells and induced tumor cell apoptosis (43, 105). Genetically engineered S. typhimurium and C. novyi- NT have been tested in clinical trials and activated a plethora of host cytokines and chemokines like IL-2, IL- 18, CCL- 21 (106–108). S. Typhimurium VNP20009 entered the human clinical trials in 1999 as the 1st bacteria mediated cancer immunotherapeutic agent (109). The efficacy of the strain was poor as it failed to reduce tumor size in 24 patients with metastatic melanoma and in 1 patient with metastatic renal carcinoma which was attributed to a difference in tumor structure and growth rates that effects bacterial penetration, proliferation and clearance within tumors (109). Another factor which was attributed was TLR4- mediated signaling which could be important for tumor colonization and anti- tumor efficacy. S. typhimurium strain lacked efficient recognition by TLR4 and failed to colonize tumors sufficiently to suppress tumor growth (110). Clinical trials based on C. novyi- NT spores demonstrated extensive tumor destruction when immunized via either intravenous or intratumoral route. However, the strain failed to eradicate all the tumorous cells resulti.ng in tumor relapse (109, 111, 112). Currently, a combinatorial therapeutic based on C. novyi- NT strain alongwith anti- PD1 antibody is in phase 1b clinical trials (111) (Table 1). Lastly, genetic modifications of bacterial strains can enhance bacteria to respond to stimuli like pH, ultrasound, chemical and thermal which can increase its accumulation at the tumor site. For instance, Qin et al., placed cytolysin A under the control of the acid sensitive promoter adiA resulting in the release of ClyA and inhibiting CT26 tumor progression and metastasis (113). Ahmedi et al., demonstrated that utilizing temperature- dependent transcriptional repressors, TlpA39 and Tcl42 in the clinically approved E. coli Nissle 1917 (EcN) could lead to a temperature regulated release of anti CTLA-4 and anti PD-L1 antibodies resulting in A20 tumor retardation in a murine model (114). Tumor cells rapidly utilizes glucose via glycolysis resulting in glucose depletion (115). Panteli et al., demonstrated a glucose concentration dependent fusion protein Trz1 transformed in E. coli to sense glucose concentration and induce expression of GFP which was under the osmoporin promoter POmpc, which could be a strategy to develop tumor environment specific therapeutics (116).
Host microbiome has recently been studied as an important contributor to tumorigenesis and tumor progression (62, 117) and can even negatively affect chemotherapy (118–121). The mechanism by which bacterium like Bacteriodes fragilis, Escherichia coli, Campylobacter jejuni, Fusobacterium nucleatum, Salmonella typhi, Helicobacter pylori contributes to tumorigenesis and progression has been reported and attributed to factors like (a) Secretion of proteins, secondary metabolites like toxins and reactive oxygen species which contribute to DNA damage and genomic instability and accelerates tumorigenesis (122–129), (b) altering host immune regulation pathways and upregulates pro- oncogene like cyclin D and c- Myc (130, 131), (c) promoting exosome secretion enriched in miR- 1246/92b-3p/27a-3p which promotes tumor metastasis (132, 133). Understanding the genotypic makeup of these bacterium which enables them to localize within the tumor can help us further fine tune targeted bacterial therapy in the future.
Apart from genetically modified bacteria, functionalized bacteria has been designed to achieve accurate delivery and controlled release of drugs and maintaining excellent biocompatibility (134). Although bacteria mediated regression of tumors has been established in experimental models and have been demonstrated to be well tolerated by the host, there are unanswered questions related to the technique. Firstly, like traditional approaches, most of the administered bacteria can be unavoidably eliminated by reticuloendothelial system before arrival at the site of tumor. Secondly, bacterial propagation would not lead to proliferation of therapeutic material resulting in its dilution. Lastly, bacteria induced systemic infection is another potential hazard and carries a significant risk. Bacterial mediated cancer therapy is in its infancy, but holds immense potential to change the current strategies of cancer treatment. As compared to treatment methods like Fecal microbial transplant (FMT), genetically modified bacteria holds certain advantage. FMT involves transfer of fecal microbiota from healthy individuals has showed encouraging results in patients with clostridiodes difficile infection, inflammatory bowel disease, irritable bowel syndrome, multiple sclerosis, hepatic encephalopathy, Parkinson’s disease and diabetic neuropathy (135–141). The efficacy of FMT as an anti- cancer therapy still under studies in conjugation with immune checkpoint blockade inhibitors like anti- PD1/PD-L1 or anti- CTLA4 antibodies (142–145) and are in different phases of clinical trials (NCT03353402, NCT03341143, NCT03772899, NCT03819296, NCT04577729, NCT04116775, NCT04758507, NCT04951583, 04988841, NCT05286294, NCT05279677, NCT0438619, NCT05008861, NCT04521075, NCT03819296, NCT05251389). However, presence of drug- resistant microbes in the microbiota of otherwise healthy patient have been reported to cause clinically life- threatening complications undergoing cancer treatment (146). Furthermore, microbiome varies from individual to individual which can effect the efficacy of the treatment (147, 148). Bacteria based cancer therapy on the other hand involves genetic engineering which alter the genetic makeup of the bacteria to nullify it’s virulence and safe for clinical applications. Further combinatorial therapy involving bacteria and conventional therapy involving radio and chemotherapy have also been implemented by research groups in animal models and human patients which have yielded promising results (Table 2).
Conclusion
Owing to properties like hypoxia targeting, motility, immunogenicity, and ability to deliver oncolytic genes or drugs, bacteria mediated cancer immunotherapy can be regarded as a promising approach. Several genetically engineered bacteria are already in the clinical trials and their outcome will provide a much-awaited proof of concept for the utility of this approach as anticancer therapeutics. These milestones will enable researchers to establish safety dosage, administration, feasibility of combining bacterial immunotherapy with conventional therapy, and upstream processing. Optimizing these parameters will open up a new avenue of cancer therapeutics and address the unmet needs of patients.
Author contributions
HG: Conceptualization, Funding acquisition, Methodology, Software, Writing – review & editing. SS: Writing – original draft, Reviewing. HS: Writing – original draft, Writing – review & editing.
Funding
The author(s) declare financial support was received for the research, authorship, and/or publication of this article. DST- FIST(AIMT) (Project no: SR/FST/LS-1/2019/502), DST- PURSE and SERB- Ramanujan (RJF/2022/000040): sponsoring the facilities for data collection towards preparation of the manuscript.
Conflict of interest
The authors declare that the research was conducted in the absence of any commercial or financial relationships that could be construed as a potential conflict of interest.
Publisher’s note
All claims expressed in this article are solely those of the authors and do not necessarily represent those of their affiliated organizations, or those of the publisher, the editors and the reviewers. Any product that may be evaluated in this article, or claim that may be made by its manufacturer, is not guaranteed or endorsed by the publisher.
References
1. ReFaey K, Tripathi S, Grewal SS, Bhargav AG, Quinones DJ, Chaichana KL, et al. Cancer mortality rates increasing vs cardiovascular disease mortality decreasing in the world: future implications. Mayo Clinic Proceedings: Innovations Qual Outcomes (2021) 5:645–53. doi: 10.1016/j.mayocpiqo.2021.05.005
2. Nagai H, Kim YH. Cancer prevention from the perspective of global cancer burden patterns. J Thorac Dis (2017) 9:448. doi: 10.21037/jtd.2017.02.75
3. Siegel RL, Miller KD. Cancer statistics. CA: A Cancer J Clinic (20232023) 73:17–48. doi: 10.3322/caac.21763
4. Mattiuzzi C, Lippi G. Current cancer epidemiology. J Epidemiol Global Health (2019) 9:217. doi: 10.2991/jegh.k.191008.001
5. Goldszmid RS, Dzutsev A, Trinchieri G. Host immune response to infection and cancer: unexpected commonalities. Cell Host Microbe (2014) 15:295–305. doi: 10.1016/j.chom.2014.02.003
6. Takeshima H, Ushijima T. Accumulation of genetic and epigenetic alterations in normal cells and cancer risk. NPJ Precis Oncol (2019) 3:7. doi: 10.1038/s41698-019-0079-0
7. Kumari S, Sharma S, Advani D, Khosla A, Kumar P, Ambasta RK. Unboxing the molecular modalities of mutagens in cancer. Environ Sci pollut Res (2021) 29:1–49. doi: 10.1007/s11356-021-16726-w
8. Rosendahl Huber A, Van Hoeck A, Van Boxtel R. The mutagenic impact of environmental exposures in human cells and cancer: imprints through time. Front Genet (2021) 2065. doi: 10.3389/fgene.2021.760039
9. Basu AK. DNA damage, mutagenesis and cancer. Int J Mol Sci (2018) 19:970. doi: 10.3390/ijms19040970
10. Miller KD, Nogueira L, Devasia T, Mariotto AB, Yabroff KR, Jemal A, et al. Cancer treatment and survivorship statistics, 2022. CA: Cancer J Clin (2022) 72:409–36. doi: 10.3322/caac.21731
11. Diaz-Cano SJ. Tumor heterogeneity: mechanisms and bases for a reliable application of molecular marker design. Int J Mol Sci (2012) 13:1951–2011. doi: 10.3390/ijms13021951
12. Jing X, Yang F, Shao C, Wei K, Xie M, Shen H, et al. Role of hypoxia in cancer therapy by regulating the tumor microenvironment. Mol Cancer (2019) 18:1–15. doi: 10.1186/s12943-019-1089-9
13. Baghban R, Roshangar L, Jahanban-Esfahlan R, Seidi K, Ebrahimi-Kalan A, Jaymand M, et al. Tumor microenvironment complexity and therapeutic implications at a glance. Cell Communication Signaling (2020) 18:1–19. doi: 10.1186/s12964-020-0530-4
14. Wieder T, Eigentler T, Brenner E, Röcken M. Immune checkpoint blockade therapy. J Allergy Clin Immunol (2018) 142:1403–14. doi: 10.1016/j.jaci.2018.02.042
15. Wei SC, Duffy CR, Allison JP. Fundamental mechanisms of immune checkpoint blockade TherapyFundamental mechanisms of immune checkpoint blockade therapy. Cancer Discovery (2018) 8:1069–86. doi: 10.1158/2159-8290.CD-18-0367
16. Ghahremanloo A, Soltani A, Modaresi SMS, Hashemy SI. Recent advances in the clinical development of immune checkpoint blockade therapy. Cell Oncol (2019) 42:609–26. doi: 10.1007/s13402-019-00456-w
17. Petitprez F, Meylan M, de Reyniès A, Sautès-Fridman C, Fridman WH. The tumor microenvironment in the response to immune checkpoint blockade therapies. Front Immunol (2020) 11:784. doi: 10.3389/fimmu.2020.00784
18. Almåsbak H, Aarvak T, Vemuri MC. CAR T cell therapy: a game changer in cancer treatment. J Immunol Res (2016) 2016. doi: 10.1155/2016/5474602
19. Castelli S, Young RM, June CH. Off-the-shelf CAR T cells to treat cancer. Cell Res (2022) 32:1–2. doi: 10.1038/s41422-022-00745-4
20. Oelschlaeger TA. Bacteria as tumor therapeutics? Bioengineered bugs (2010) 1:146–7. doi: 10.4161/bbug.1.2.11248
21. Coley WB. The treatment of Malignant tumors by repeated inoculations of erysipelas: With a report of ten original cases. 1. Am J Med Sci (1827-1924) (1893) 105:487. doi: 10.1097/00000441-189305000-00001
22. Coley WB. The treatment of inoperable sarcoma with the'mixed toxins of erysipelas and bacillus prodigiosus: immediate and final results in one hundred and forty cases. J Am Med Assoc (1898) 31:456–65. doi: 10.1001/jama.1898.92450090022001g
23. Huang X, Pan J, Xu F, Shao B, Wang Y, Guo X, et al. Bacteria-based cancer immunotherapy. Advanced Sci (2021) 8:2003572. doi: 10.1002/advs.202003572
24. Rosenthal SR, Crispen RG, Thorne MG, Piekarski N, Raisys N, Rettig PG. BCG vaccination and leukemia mortality. Jama (1972) 222:1543–4. doi: 10.1001/jama.1972.03210120041010
25. Grange J, Stanford J. BCG vaccination and cancer. Tubercle (1990) 71:61–4. doi: 10.1016/0041-3879(90)90063-E
26. Grange JM, Stanford JL, Stanford CA, Kölmel KF. Vaccination strategies to reduce the risk of leukaemia and melanoma. J R Soc Med (2003) 96:389–92. doi: 10.1177/014107680309600806
27. Morra ME, Kien ND, Elmaraezy A, Abdelaziz OAM, Elsayed AL, Halhouli O, et al. Early vaccination protects against childhood leukemia: a systematic review and meta-analysis. Sci Rep (2017) 7:15986. doi: 10.1038/s41598-017-16067-0
28. Hauer J, Fischer U, Borkhardt A. Toward prevention of childhood ALL by early-life immune training. Blood J Am Soc Hematol (2021) 138:1412–28. doi: 10.1182/blood.2020009895
29. Singh S, Kishore D, Singh RK. “Trained Immunity” from Mycobacterium spp. exposure (BCG vaccination and environmental) may have an impact on the incidence of early childhood leukemia. Front Immunol (2023) 14:1193859. doi: 10.3389/fimmu.2023.1193859
30. Singh S, Diwakar A, Singh RK. BCG vaccination policy, natural boosting and pediatric brain and CNS tumor incidences. Front Immunol (2023) 14:1174006. doi: 10.3389/fimmu.2023.1174006
31. Forbes NS. Engineering the perfect (bacterial) cancer therapy. Nat Rev Cancer (2010) 10:785–94. doi: 10.1038/nrc2934
32. Fan J-X, Niu M-T, Qin Y-T, Sun Y-X, Zhang X-Z. Progress of engineered bacteria for tumor therapy. Advanced Drug Delivery Rev (2022) 114296. doi: 10.1016/j.addr.2022.114296
33. Patyar S, Joshi R, Byrav Prasad DS, Prakash A, Medhi B, Das BK, et al. Bacteria in cancer therapy: a novel experimental strategy. J Biomed Sci (2010) 17:1–9. doi: 10.1186/1423-0127-17-21
34. Guallar-Garrido S, Julián E. Bacillus Calmette-Guérin (BCG) therapy for bladder cancer: an update. ImmunoTargets Ther (2020) 9:1–11. doi: 10.2147/ITT.S202006
35. Dougan M, Dougan SK. Programmable bacteria as cancer therapy. Nat Med (2019) 25:1030–1. doi: 10.1038/s41591-019-0513-4
36. Chowdhury S, Castro S, Coker C, Hinchliffe TE, Arpaia N, Danino T, et al. Programmable bacteria induce durable tumor regression and systemic antitumor immunity. Nat Med (2019) 25:1057–63. doi: 10.1038/s41591-019-0498-z
37. Song J, Zhang Y, Zhang C, Du X, Guo Z, Kuang Y, et al. A microfluidic device for studying chemotaxis mechanism of bacterial cancer targeting. Sci Rep (2018) 8(1):6394. doi: 10.1038/s41598-018-24748-7
38. Cao Z, Liu J. Bacteria and bacterial derivatives as drug carriers for cancer therapy. J Controlled Release (2020) 326:396–407. doi: 10.1016/j.jconrel.2020.07.009
39. Bermudes D, Zheng L, King IC. Live bacteria as anticancer agents and tumor-selective protein delivery vectors. Curr Opin Drug Discovery Dev (2002) 5:194–9.
40. Badie F, Ghandali M, Tabatabaei SA, Safari M, Khorshidi A, Shayestehpou M, et al. Use of Salmonella Bacteria in Cancer therapy: direct, drug delivery and combination approaches. Front Oncol (2021) 11:624759. doi: 10.3389/fonc.2021.624759
41. Miyake K, Yamamoto S, Iijima S. Blocking adhesion of cancer cells to endothelial cell types by S. agalactiae type-specific polysaccharides. Cytotechnology (1996) 22:205–9. doi: 10.1007/BF00353940
42. Kunda NK. Antimicrobial peptides as novel therapeutics for non-small cell lung cancer. Drug Discovery Today (2020) 25:238–47. doi: 10.1016/j.drudis.2019.11.012
43. Kim J-E, Phan TX, Nguyen VH, Dinh-Vu H-V, Zheng JH, Yun M, et al. Salmonella typhimurium Suppresses Tumor Growth via the Pro-Infammatory Cytokine Interleukin-1 beta. Theranostics (2015) 5:1328–42. doi: 10.7150/thno.11432
44. Lakshmi Narendra B, Eshvendar Reddy K, Shantikumar S, Ramakrishna S. Immune system: a double-edged sword in cancer. Inflammation Res (2013) 62:823–34. doi: 10.1007/s00011-013-0645-9
46. Baylin SB, Jones PA. Epigenetic determinants of cancer. Cold Spring Harbor Perspect Biol (2016) 8:a019505. doi: 10.1101/cshperspect.a019505
47. Kanwal R, Gupta S. Epigenetic modifications in cancer. Clin Genet (2012) 81:303–11. doi: 10.1111/j.1399-0004.2011.01809.x
48. Cheng Y, He C, Wang M, Ma X, Mo F, Yang S, et al. Targeting epigenetic regulators for cancer therapy: mechanisms and advances in clinical trials. Signal transduction targeted Ther (2019) 4:62. doi: 10.1038/s41392-019-0095-0
49. Rasmussen DM, Hansen HP, Elverdam B. How cancer survivors experience their changed body encountering others. Eur J Oncol Nurs (2010) 14:154–9. doi: 10.1016/j.ejon.2009.10.001
50. Fares J, Fares MY, Khachfe HH, Salhab HA, Fares Y. Molecular principles of metastasis: a hallmark of cancer revisited. Signal transduction targeted Ther (2020) 5:28. doi: 10.1038/s41392-020-0134-x
51. Bergers G, Fendt S-M. The metabolism of cancer cells during metastasis. Nat Rev Cancer (2021) 21:162–80. doi: 10.1038/s41568-020-00320-2
52. DeVita VT Jr. & Chu, E. A history of cancer chemotherapy. Cancer Res (2008) 68:8643–53. doi: 10.1158/0008-5472.can-07-6611
53. Goodman LS, Wintrobe MM, Dameshek W, Goodman MJ, Gilman A, McLennan MT. Nitrogen mustard therapy; use of methyl-bis (beta-chloroethyl) amine hydrochloride and tris (beta-chloroethyl) amine hydrochloride for Hodgkin's disease, lymphosarcoma, leukemia and certain allied and miscellaneous disorders. J Am Med Assoc (1946) 132:126–32. doi: 10.1001/jama.1946.02870380008004
54. Blattner RJ. Nitrogen mustard as a therapeutic agent. J Pediatr (1950) 37:126–32. doi: 10.1016/s0022-3476(50)80015-x
55. Gilman A, Philips FS. The biological actions and therapeutic applications of the B-chloroethyl amines and sulfides. Sci (New York N.Y.) (1946) 103:409–36. doi: 10.1126/science.103.2675.409
56. Gilman A. The initial clinical trial of nitrogen mustard. Am J Surg (1963) 105:574–8. doi: 10.1016/0002-9610(63)90232-0
57. DeVita VT Jr. & Chu, E. A history of cancer chemotherapy. Cancer Res (2008) 68:8643–53. doi: 10.1158/0008-5472.CAN-07-6611
58. Kerckhove N, Collin A, Condé S, Chaleteix C, Pezet D, Balayssac D. Long-term effects, pathophysiological mechanisms, and risk factors of chemotherapy-induced peripheral neuropathies: A comprehensive literature review. Front Pharmacol (2017) 8:86. doi: 10.3389/fphar.2017.00086
59. Nurgali K, Jagoe RT, Abalo R. Frontiers Media SA (Frontiers), Vol. 9 245. (2018). doi: 10.3389/fphar.2018.00245
60. Yu Z-K, Xie R-L, You R, Liu Y-P, Chen X-Y, Chen M-Y, et al. The role of the bacterial microbiome in the treatment of cancer. BMC Cancer (2021) 21:1–14. doi: 10.1186/s12885-021-08664-0
61. Górska A, Przystupski D, Niemczura MJ, Kulbacka J. Probiotic bacteria: a promising tool in cancer prevention and therapy. Curr Microbiol (2019) 76:939–49. doi: 10.1007/s00284-019-01679-8
62. Nejman D, Livyatan I, Fuks G, Gavert N, Zwang Y, Geller LT, et al. The human tumor microbiome is composed of tumor type–specific intracellular bacteria. Sci (New York N.Y.) (2020) 368:973–80. doi: 10.1126/science.aay9189
63. Fu A, Yao B, Dong T, Chen Y, Yao J, Liu Y, et al. Tumor-resident intracellular microbiota promotes metastatic colonization in breast cancer. Cell (2022) 185:1356–72.e1326. doi: 10.1016/j.cell.2022.02.027
64. Zahavi D, Weiner L. Monoclonal antibodies in cancer therapy. Antibodies (2020) 9:34. doi: 10.3390/antib9030034
65. Esfahani K, Roudaia L, Buhlaiga N, Del Rincon SV, Papneja N, Miller WH Jr. A review of cancer immunotherapy: from the past, to the present, to the future. Curr Oncol (2020) 27:87–97. doi: 10.3747/co.27.5223
66. Fan JY, Huang Y, Li Y, Muluh TA, Fu SZ, Wu JB. Bacteria in cancer therapy: A new generation of weapons. Cancer Med (2022) 11:4457–68. doi: 10.1002/cam4.4799
67. Moghimipour E, Abedishirehjin S, Baghbadorani MA, Handali S. Bacteria and Archaea: A new era of cancer therapy. J Controlled Release (2021) 338:1–7. doi: 10.1016/j.jconrel.2021.08.019
68. Sedighi M, Bialvaei AZ, Hamblin MR, Ohadi E, Asadi A, Halajzadeh M, et al. Therapeutic bacteria to combat cancer; current advances, challenges, and opportunities. Cancer Med (2019) 8:3167–81. doi: 10.1002/cam4.2148
70. Elahian F, Moghimi B, Dinmohammadi F, Ghamghami M, Hamidi M, Mirzaei SA. The anticancer agent prodigiosin is not a multidrug resistance protein substrate. DNA Cell Biol (2013) 32:90–7. doi: 10.1089/dna.2012.1902
71. Goldufsky J, Wood S, Hajihossainlou B, Rehman T, Majdobeh O, Kaufman HL, et al. Pseudomonas aeruginosa exotoxin T induces potent cytotoxicity against a variety of murine and human cancer cell lines. J Med Microbiol (2015) 64:164. doi: 10.1099/jmm.0.000003
72. Weerakkody LR, Witharana C. The role of bacterial toxins and spores in cancer therapy. Life Sci (2019) 235:116839. doi: 10.1016/j.lfs.2019.116839
73. Wei X, Du M, Chen Z, Yuan Z. Recent advances in bacteria-based cancer treatment. Cancers (2022) 14:4945. doi: 10.3390/cancers14194945
74. Vallera DA, Li C, Jin N, Panoskaltsis-Mortari A, Hall WA. Targeting urokinase-type plasminogen activator receptor on human glioblastoma tumors with diphtheria toxin fusion protein DTAT. J Natl Cancer Institute (2002) 94:597–606. doi: 10.1093/jnci/94.8.597
75. Frankel AE, Beran M, Hogge DE, Powell BL, Thorburn A, Chen YQ, et al. Malignant progenitors from patients with CD87+ acute myelogenous leukemia are sensitive to a diphtheria toxin-urokinase fusion protein. Exp Hematol (2002) 30:1316–23. doi: 10.1016/S0301-472X(02)00925-6
76. Priya K. Bacterial cancer therapy: A turning point for new paradigms. Feature Drug Discovery Today (2022) 27:2043–50. doi: 10.1016/j.drudis.2022.03.007
77. Fiedler T, Strauss M, Hering S, Redanz U, William D, Rosche Y, et al. Arginine deprivation by arginine deiminase of Streptococcus pyogenes controls primary glioblastoma growth in vitro and in vivo. Cancer Biol Ther (2015) 16:1047–55. doi: 10.1080/15384047.2015.1026478
78. Abdi-Ali A, Worobec EA, Deezagi A, Malekzadeh F. Cytotoxic effects of pyocin S2 produced by Pseudomonas aeruginosa on the growth of three human cell lines. Can J Microbiol (2004) 50:375–81. doi: 10.1139/w04-019
79. Chang J, Liu Y, Han B, Zhou C, Bai C, Li J. Pseudomonas aeruginosa preparation plus chemotherapy for advanced non-small-cell lung cancer: a randomized, multicenter, double-blind phase III study. Med Oncol (Northwood London England) (2015) 32:139. doi: 10.1007/s12032-015-0583-1
80. Villarante KI, Elegado FB, Iwatani S, Zendo T, Sonomoto K, Guzman EE. Purification, characterization and in vitro cytotoxicity of the bacteriocin from Pediococcus acidilactici K2a2-3 against human colon adenocarcinoma (HT29) and human cervical carcinoma (HeLa) cells. World J Microbiol Biotechnol (2011) 27:975–80. doi: 10.1007/s11274-010-0541-1
81. Tagg JR, Dajani AS, Wannamaker LW. Bacteriocins of gram-positive bacteria. Bacteriological Rev (1976) 40:722–56. doi: 10.1128/br.40.3.722-756.1976
82. Paiva AD, Oliveira MD, Paula SO, Baracat-Pereira MC, Breukink E, Mantovani HC. Toxicity of bovicin HC5 against mammalian cell lines and the role of cholesterol in bacteriocin activity. Microbiology (2012) 158:2851–8. doi: 10.1099/mic.0.062190-0
83. Joo NE, Ritchie K, Kamarajan P, Miao D, Kapila YL. Nisin, an apoptogenic bacteriocin and food preservative, attenuates HNSCC tumorigenesis via CHAC 1. Cancer Med (2012) 1:295–305. doi: 10.1002/cam4.35
84. Sarotra P, Medhi B. Use of bacteria in cancer therapy. Curr Strategies Cancer Gene Ther (2016) 209:111–21. doi: 10.1007/978-3-319-42934-2_8
85. Aganja RP, Sivasankar C, Senevirathne A, Lee JH. Salmonella as a promising curative tool against cancer. Pharmaceutics (2022) 14:2100. doi: 10.3390/pharmaceutics14102100
86. Avogadri F, Martinoli C, Petrovska L, Chiodoni C, Transidico P, Bronte V, et al. Cancer immunotherapy based on killing of Salmonella-infected tumor cells. Cancer Res (2005) 65:3920–7. doi: 10.1158/0008-5472.CAN-04-3002
87. Kim SH, Castro F, Paterson Y, Gravekamp C. High efficacy of a Listeria-based vaccine against metastatic breast cancer reveals a dual mode of action. Cancer Res (2009) 69:5860–6. doi: 10.1158/0008-5472.can-08-4855
88. Jahangir A, Chandra D, Quispe-Tintaya W, Singh M, Selvanesan BC, Gravekamp C. Immunotherapy with Listeria reduces metastatic breast cancer in young and old mice through different mechanisms. Oncoimmunology (2017) 6:e1342025. doi: 10.1080/2162402x.2017.1342025
89. Chen Q, Bai H, Wu W, Huang G, Li Y, Wu M, et al. Bioengineering bacterial vesicle-coated polymeric nanomedicine for enhanced cancer immunotherapy and metastasis prevention. Nano Lett (2019) 20:11–21. doi: 10.1021/acs.nanolett.9b02182
90. Kuerban K, Gao X, Zhang H, Liu J, Dong M, Wu L, et al. Doxorubicin-loaded bacterial outer-membrane vesicles exert enhanced anti-tumor efficacy in non-small-cell lung cancer. Acta Pharm Sin B (2020) 10:1534–48. doi: 10.1016/j.apsb.2020.02.002
91. Kim OY, Dinh NTH, Park HT, Choi SJ, Hong K, Gho YS. Bacterial protoplast-derived nanovesicles for tumor targeted delivery of chemotherapeutics. Biomaterials (2017) 113:68–79. doi: 10.1016/j.biomaterials.2016.10.037
92. Kim OY, Park HT, Dinh NTH, Choi SJ, Lee J, Kim JH, et al. Bacterial outer membrane vesicles suppress tumor by interferon-γ-mediated antitumor response. Nat Commun (2017) 8:626. doi: 10.1038/s41467-017-00729-8
93. Vasievich EA, Huang L. The suppressive tumor microenvironment: a challenge in cancer immunotherapy. Mol pharmaceutics (2011) 8:635–41. doi: 10.1021/mp1004228
94. Li K, Shi H, Zhang B, Ou X, Ma Q, Chen Y, et al. Myeloid-derived suppressor cells as immunosuppressive regulators and therapeutic targets in cancer. Signal Transduction Targeted Ther (2021) 6:362. doi: 10.1038/s41392-021-00670-9
95. Labani-Motlagh A, Ashja-Mahdavi M, Loskog A. The tumor microenvironment: a milieu hindering and obstructing antitumor immune responses. Front Immunol (2020) 11:940. doi: 10.3389/fimmu.2020.00940
96. Chen YE, Bousbaine D, Veinbachs A, Atabakhsh K, Dimas A, Yu VK, et al. Engineered skin bacteria induce antitumor T cell responses against melanoma. Science (2023) 380:203–10. doi: 10.1126/science.abp9563
97. Yang N, Zhu X, Chen L, Li S, Ren D. Oral administration of attenuated S. typhimurium carrying shRNA-expressing vectors as a cancer therapeutic. Cancer Biol Ther (2008) 7:145–51. doi: 10.4161/cbt.7.1.5195
98. Blache CA, Manuel ER, Kaltcheva TI, Wong AN, Ellenhorn JDI, Blazar BR, et al. Systemic delivery of Salmonella typhimurium transformed with IDO shRNA enhances intratumoral vector colonization and suppresses tumor growth. Cancer Res (2012) 72:6447–56. doi: 10.1158/0008-5472.CAN-12-0193
99. Zhou S, Gravekamp C, Bermudes D, Liu K. Tumour-targeting bacteria engineered to fight cancer. Nat Rev Cancer (2018) 18:727–43. doi: 10.1038/s41568-018-0070-z
100. Laliani G, Sorboni SG, Lari R, Yaghoubi A, Soleimanpour S, Khazaei M, et al. Bacteria and cancer: Different sides of the same coin. Life Sci (2020) 246:117398. doi: 10.1016/j.lfs.2020.117398
101. Yaghoubi A, Khazaei M, Jalili S, Hasanian SM, Avan A, Soleimanpour S, et al. Bacteria as a double-action sword in cancer. Biochim Biophys Acta (BBA)-Reviews Cancer (2020) 1874:188388. doi: 10.1016/j.bbcan.2020.188388
102. Sieow BF-L, Wun KS, Yong WP, Hwang IY, Chang MW. Tweak to treat: reprograming bacteria for cancer treatment. Trends Cancer (2021) 7:447–64. doi: 10.1016/j.trecan.2020.11.004
103. Li T, Gao L, Zhang B, Nie G, Xie Z, Zhang H, et al. Material-based engineering of bacteria for cancer diagnosis and therapy. Appl Materials Today (2021) 25:101212. doi: 10.1016/j.apmt.2021.101212
104. Clairmont C, Lee KC, Pike J, Ittensohn M, Low KB, Pawelek J, et al. Biodistribution and genetic stability of the novel antitumor agent VNP20009, a genetically modified strain of Salmonella typhimuvium. J Infect Dis (2000) 181:1996–2002. doi: 10.1086/315497
105. Lim D, Kim KS, Kim H, Ko K-C, Song JJ, Choi JH, et al. Anti-tumor activity of an immunotoxin (TGFα-PE38) delivered by attenuated Salmonella typhimurium. Oncotarget (2017) 8:37550. doi: 10.18632/oncotarget.17197
106. Nallar SC, Xu D-Q, Kalvakolanu DV. Bacteria and genetically modified bacteria as cancer therapeutics: Current advances and challenges. Cytokine (2017) 89:160–72. doi: 10.1016/j.cyto.2016.01.002
107. Nguyen VH, Kim H-S, Ha J-M, Hong Y, Choy HE, Min JJ. Genetically engineered Salmonella typhimurium as an imageable therapeutic probe for cancer. Cancer Res (2010) 70:18–23. doi: 10.1158/0008-5472.CAN-09-3453
108. Flentie K, Kocher B, Gammon ST, Novack DV, McKinney JS, Piwnica-Worms D. A bioluminescent transposon reporter-trap identifies tumor-specific microenvironment-induced promoters in salmonella for conditional bacterial-based tumor therapyCancer cell-induced transcriptional response of salmonella. Cancer Discovery (2012) 2:624–37. doi: 10.1158/2159-8290.CD-11-0201
109. Toso JF, Gill VJ, Hwu P, Marincola FM, Restifo NP, Schwartzentruber DJ, et al. Phase I study of the intravenous administration of attenuated Salmonella typhimurium to patients with metastatic melanoma. J Clin oncology: Off J Am Soc Clin Oncol (2002) 20:142. doi: 10.1200/JCO.2002.20.1.142
110. Teghanemt A, Zhang D, Levis EN, Weiss JP, Gioannini TL. Molecular basis of reduced potency of underacylated endotoxins. J Immunol (2005) 175:4669–76. doi: 10.4049/jimmunol.175.7.4669
111. Duong MT-Q, Qin Y, You S-H, Min J-J. Bacteria-cancer interactions: bacteria-based cancer therapy. Exp Mol Med (2019) 51:1–15. doi: 10.1038/s12276-019-0297-0
112. Heimann DM, Rosenberg SA. Continuous intravenous administration of live genetically modified salmonella typhimurium in patients with metastatic melanoma. J Immunotherapy (2003) 26:179–80. doi: 10.1097/00002371-200303000-00011
113. Qin W, Xu W, Wang L, Ren D, Cheng Y, Song W, et al. Bacteria-elicited specific thrombosis utilizing acid-induced cytolysin A expression to enable potent tumor therapy. Advanced Sci (2022) 9:2105086. doi: 10.1002/advs.202105086
114. Abedi MH, Yao MS, Mittelstein DR, Bar-Zion A, Swift MB, Lee-Gosselin A, et al. Ultrasound-controllable engineered bacteria for cancer immunotherapy. Nat Commun (2022) 13:1585. doi: 10.1038/s41467-022-29065-2
115. Anderson KG, Stromnes IM, Greenberg PD. Obstacles posed by the tumor microenvironment to T cell activity: a case for synergistic therapies. Cancer Cell (2017) 31:311–25. doi: 10.1016/j.ccell.2017.02.008
116. Panteli JT, Forbes NS. Engineered bacteria detect spatial profiles in glucose concentration within solid tumor cell masses. Biotechnol bioengineering (2016) 113:2474–84. doi: 10.1002/bit.26006
117. Sfanos KS, Sauvageot J, Fedor HL, Dick JD, Marzo De AM, Isaacs WB. A molecular analysis of prokaryotic and viral DNA sequences in prostate tissue from patients with prostate cancer indicates the presence of multiple and diverse microorganisms. Prostate (2008) 68:306–20. doi: 10.1002/pros.20680
118. Geller LT, Straussman R. Intratumoral bacteria may elicit chemoresistance by metabolizing anticancer agents. Mol Cell Oncol (2018) 5:e1405139. doi: 10.1080/23723556.2017.1405139
119. Geller LT, Barzily-Rokni M, Danino T, Jonas OH, Shental N, Nejman D, et al. Potential role of intratumor bacteria in mediating tumor resistance to the chemotherapeutic drug gemcitabine. Sci (New York N.Y.) (2017) 357:1156–60. doi: 10.1126/science.aah5043
120. Yu T, Guo F, Yu Y, Sun T, Ma D, Han J, et al. Fusobacterium nucleatum promotes chemoresistance to colorectal cancer by modulating autophagy. Cell (2017) 170:548–63.e516. doi: 10.1016/j.cell.2017.07.008
121. Zhang S, Yang Y, Weng W, Guo B, Cai G, Ma Y, et al. Fusobacterium nucleatum promotes chemoresistance to 5-fluorouracil by upregulation of BIRC3 expression in colorectal cancer. J Exp Clin Cancer Res (2019) 38:1–13. doi: 10.1186/s13046-018-0985-y
122. Goodwin AC, Shields CED, Wu S, Huso DL, Wu X, Murray-Stewart TR, et al. Polyamine catabolism contributes to enterotoxigenic Bacteroides fragilis-induced colon tumorigenesis. Proc Natl Acad Sci (2011) 108:15354–9. doi: 10.1073/pnas.1010203108
123. Dejea CM, Fathi P, Craig JM, Boleij A, Taddese R, Geis AL, et al. Patients with familial adenomatous polyposis harbor colonic biofilms containing tumorigenic bacteria. Sci (New York N.Y.) (2018) 359:592–7. doi: 10.1126/science.aah3648
124. He Z, Gharaibeh RZ, Newsome RC, Pope JL, Dougherty MW, Tomkovich S, et al. Campylobacter jejuni promotes colorectal tumorigenesis through the action of cytolethal distending toxin. Gut (2019) 68:289–300. doi: 10.1136/gutjnl-2018-317200
125. Bezine E, Malaisé Y, Loeuillet A, Chevalier M, Boutet-Robinet E, Salles B, et al. Cell resistance to the Cytolethal Distending Toxin involves an association of DNA repair mechanisms. Sci Rep (2016) 6:36022. doi: 10.1038/srep36022
126. Fedor Y, Vignard J, Nicolau-Travers M-L, Boutet-Robinet E, Watrin C, Salles B, et al. From single-strand breaks to double-strand breaks during S-phase: a new mode of action of the E scherichia coli C ytolethal D istending T oxin. Cell Microbiol (2013) 15:1–15. doi: 10.1111/cmi.12028
127. Putze J, Hennequin C, Nougayrède J-P, Zhang W, Homburg S, Karch H, et al. Genetic structure and distribution of the colibactin genomic island among members of the family Enterobacteriaceae. Infection Immun (2009) 77:4696–703. doi: 10.1128/IAI.00522-09
128. Nougayrède J-P, Homburg S, Taieb F, Boury M, Brzuszkiewicz E, Gottschalk G, et al. Escherichia coli induces DNA double-strand breaks in eukaryotic cells. Sci (New York N.Y.) (2006) 313:848–51. doi: 10.1126/science.1127059
129. Barrett M, Hand CK, Shanahan F, Murphy T, O’Toole PW. Mutagenesis by microbe: The role of the microbiota in shaping the cancer genome. Trends Cancer (2020) 6:277–87. doi: 10.1016/j.trecan.2020.01.019
130. Rubinstein MR, Wang X, Liu W, Hao Y, Cai G, Han YW. Fusobacterium nucleatum promotes colorectal carcinogenesis by modulating E-cadherin/β-catenin signaling via its FadA adhesin. Cell Host Microbe (2013) 14:195–206. doi: 10.1016/j.chom.2013.07.012
131. Wu S, Morin PJ, Maouyo D, Sears CL. Bacteroides fragilis enterotoxin induces c-Myc expression and cellular proliferation. Gastroenterology (2003) 124:392–400. doi: 10.1053/gast.2003.50047
132. Cao Y, Wang Z, Yan Y, Ji L, He J, Xuan B, et al. Enterotoxigenic Bacteroides fragilis promotes intestinal inflammation and Malignancy by inhibiting exosome-packaged miR-149-3p. Gastroenterology (2021) 161:1552–66.e1512. doi: 10.1053/j.gastro.2021.08.003
133. Guo S, Chen J, Chen F, Zeng Q, Liu W-L, Zhang G. Exosomes derived from Fusobacterium nucleatum-infected colorectal cancer cells facilitate tumour metastasis by selectively carrying miR-1246/92b-3p/27a-3p and CXCL16. Gut (2021) 70:1507–19. doi: 10.1136/gutjnl-2020-321187
134. Yin T, Diao Z, Blum NT, Qiu L, Ma A, Huang P. Engineering bacteria and bionic bacterial derivatives with nanoparticles for cancer therapy. Small (2022) 18:2104643. doi: 10.1002/smll.202104643
135. Baunwall SMD, Lee MM, Eriksen MK, Mullish BH, Marchesi JR, Dahlerup JF, et al. Faecal microbiota transplantation for recurrent Clostridioides difficile infection: an updated systematic review and meta-analysis. EClinicalMedicine (2020) 29. doi: 10.1016/j.eclinm.2020.100642
136. Kelly CR, Yen EF, Grinspan AM, Kahn SA, Atreja A, Lewis JD, et al. Fecal microbiota transplantation is highly effective in real-world practice: initial results from the FMT national registry. Gastroenterology (2021) 160:183–92.e183. doi: 10.1053/j.gastro.2020.09.038
137. El-Salhy M, Hatlebakk JG, Gilja OH, Kristoffersen AB, Hausken T. Efficacy of faecal microbiota transplantation for patients with irritable bowel syndrome in a randomised, double-blind, placebo-controlled study. Gut (2020) 69:859–67. doi: 10.1136/gutjnl-2019-319630
138. Ghani R, Mullish BH, McDonald JAK, Ghazy A, Williams HRT, Brannigan ET, et al. Disease prevention not decolonization: a model for fecal microbiota transplantation in patients colonized with multidrug-resistant organisms. Clin Infect Dis (2021) 72:1444–7. doi: 10.1093/cid/ciaa948
139. Makkawi S, Camara-Lemarroy C, Metz L. Fecal microbiota transplantation associated with 10 years of stability in a patient with SPMS. Neurology-Neuroimmunology Neuroinflamm (2018) 5. doi: 10.1212/NXI.0000000000000459
140. Mullish BH, Quraishi MN, Segal JP, McCune VL, Baxter M, Marsden GL, et al. The use of faecal microbiota transplant as treatment for recurrent or refractory Clostridium difficile infection and other potential indications: joint British Society of Gastroenterology (BSG) and Healthcare Infection Society (HIS) guidelines. Gut (2018) 67:1920–41. doi: 10.1136/gutjnl-2018-316818
141. Vendrik KEW, Ooijevaar RE, Jong PRC, Laman JD, Oosten van BW, Hilten van JJ, et al. Fecal microbiota transplantation in neurological disorders. Front Cell infection Microbiol (2020) 10:98. doi: 10.3389/fcimb.2020.00098
142. Andrews MC, Duong CPM, Gopalakrishnan V, Iebba V, Chen W-S, Derosa L, et al. Gut microbiota signatures are associated with toxicity to combined CTLA-4 and PD-1 blockade. Nat Med (2021) 27:1432–41. doi: 10.1038/s41591-021-01406-6
143. Alexander JL, Wilson ID, Teare J, Marchesi JR, Nicholson JK, Kinross JM. Gut microbiota modulation of chemotherapy efficacy and toxicity. Nat Rev Gastroenterol Hepatol (2017) 14:356–65. doi: 10.1038/nrgastro.2017.20
144. York A. Gut microbiota sways response to cancer immunotherapy. Nat Rev Microbiol (2018) 16:121–1. doi: 10.1038/nrmicro.2018.12
145. Sivan A, Corrales L, Hubert N, Williams JB, Aquino-Michaels K, Earley ZM, et al. Commensal Bifidobacterium promotes antitumor immunity and facilitates anti–PD-L1 efficacy. Sci (New York N.Y.) (2015) 350:1084–9. doi: 10.1126/science.aac4255
146. DeFilipp Z, Bloom PP, Soto MT, Mansour MK, Sater MRA, Huntley MH, et al. Drug-resistant E. coli bacteremia transmitted by fecal microbiota transplant. New Engl J Med (2019) 381:2043–50. doi: 10.1056/NEJMoa1910437
147. Ng SC, Kamm MA, Yeoh YK, Chan PKS, Zuo T, Tang W, et al. Scientific frontiers in faecal microbiota transplantation: joint document of Asia-Pacific Association of Gastroenterology (APAGE) and Asia-Pacific Society for Digestive Endoscopy (APSDE). Gut (2020) 69:83–91. doi: 10.1136/gutjnl-2019-319407
148. Wen X, Wang H-G, Zhang M-N, Zhang M-H, Wang H, Yang X-Z. Fecal microbiota transplantation ameliorates experimental colitis via gut microbiota and T-cell modulation. World J Gastroenterol (2021) 27:2834. doi: 10.3748/wjg.v27.i21.2834
149. Liu X, Jiang S, Piao L, Yuan F. Radiotherapy combined with an engineered Salmonella typhimurium inhibits tumor growth in a mouse model of colon cancer. Exp Anim (2016) 65:413–8. doi: 10.1538/expanim.16-0033
150. Platt J, Sodi S, Kelley M, Rockwell S, Bermudes D, Low KB, et al. Antitumour effects of genetically engineered Salmonella in combination with radiation. Eur J Cancer (2000) 36:2397–402. doi: 10.1016/S0959-8049(00)00336-1
151. Jiang S-N, Phan TX, Nam T-K, Nguyen VH, Kim H-S, Bom H-S, et al. Inhibition of tumor growth and metastasis by a combination of Escherichia coli–mediated cytolytic therapy and radiotherapy. Mol Ther (2010) 18:635–42. doi: 10.1038/mt.2009.295
152. Dietzel F, Gericke D, König W. Tumor hyperthermia using high frequency for increase of oncolysis by Clostridium butyricum (M 55). Strahlentherapie (1976) 152:537–41.
153. Dietzel F, Gericke D. Intensification of the oncolysis by clostridia by means of radio-frequency hyperthermy in experiments on animals–dependence on dosage and on intervals (author's transl). Strahlentherapie (1977) 153:263–6.
154. Gericke D, Dietzel F, König W, Rüster I, Schumacher L. Further progress with oncolysis due to apathogenic clostridia. Zentralblatt fur Bakteriologie Parasitenkunde Infektionskrankheiten und Hygiene. Erste Abteilung Originale. Reihe A: Medizinische Mikrobiologie und Parasitologie (1979) 243:102–12.
155. Bettegowda C, Dang LH, Abrams R, Huso DL, Dillehay L, Cheong I, et al. Overcoming the hypoxic barrier to radiation therapy with anaerobic bacteria. Proc Natl Acad Sci (2003) 100:15083–8. doi: 10.1073/pnas.2036598100
156. Nemunaitis J, Cunningham C, Senzer N, Kuhn J, Cramm J, Litz C, et al. Pilot trial of genetically modified, attenuated Salmonella expressing the E. coli cytosine deaminase gene in refractory cancer patients. Cancer Gene Ther (2003) 10:737–44. doi: 10.1038/sj.cgt.7700634
Keywords: bacteria, cancer, immunotherapy, tumor-microenvironment, vaccine
Citation: Sharma S, Sharma H and Gogoi H (2023) Bacterial immunotherapy: is it a weapon in our arsenal in the fight against cancer? Front. Immunol. 14:1277677. doi: 10.3389/fimmu.2023.1277677
Received: 15 August 2023; Accepted: 03 November 2023;
Published: 27 November 2023.
Edited by:
Tetsuya Mitsudomi, Aichi Cancer Center, JapanReviewed by:
Sudhir Kumar, Medical College of Wisconsin, United StatesSuojun Zhang, Huazhong University of Science and Technology, China
Rohit Mahar, Hemwati Nandan Bahuguna Garhwal University, India
Copyright © 2023 Sharma, Sharma and Gogoi. This is an open-access article distributed under the terms of the Creative Commons Attribution License (CC BY). The use, distribution or reproduction in other forums is permitted, provided the original author(s) and the copyright owner(s) are credited and that the original publication in this journal is cited, in accordance with accepted academic practice. No use, distribution or reproduction is permitted which does not comply with these terms.
*Correspondence: Himanshu Gogoi, aGltYW5zaHVocmMyMDEwQGdtYWlsLmNvbQ==