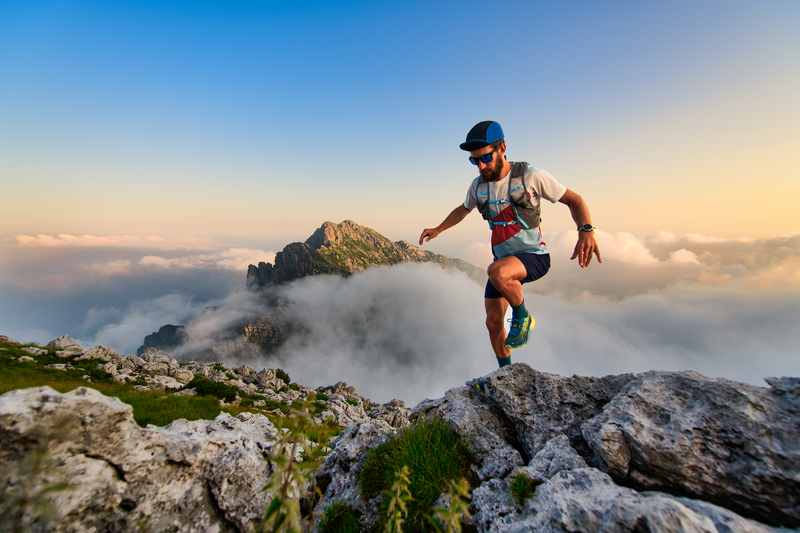
95% of researchers rate our articles as excellent or good
Learn more about the work of our research integrity team to safeguard the quality of each article we publish.
Find out more
REVIEW article
Front. Immunol. , 08 November 2023
Sec. Molecular Innate Immunity
Volume 14 - 2023 | https://doi.org/10.3389/fimmu.2023.1273570
This article is part of the Research Topic Crosstalk between Cell Death, Oxidative Stress, and Immune Regulation View all 11 articles
Life expectancy is increasing throughout the world and coincides with a rise in non-communicable diseases (NCDs), especially for metabolic disease that includes diabetes mellitus (DM) and neurodegenerative disorders. The debilitating effects of metabolic disorders influence the entire body and significantly affect the nervous system impacting greater than one billion people with disability in the peripheral nervous system as well as with cognitive loss, now the seventh leading cause of death worldwide. Metabolic disorders, such as DM, and neurologic disease remain a significant challenge for the treatment and care of individuals since present therapies may limit symptoms but do not halt overall disease progression. These clinical challenges to address the interplay between metabolic and neurodegenerative disorders warrant innovative strategies that can focus upon the underlying mechanisms of aging-related disorders, oxidative stress, cell senescence, and cell death. Programmed cell death pathways that involve autophagy, apoptosis, ferroptosis, and pyroptosis can play a critical role in metabolic and neurodegenerative disorders and oversee processes that include insulin resistance, β-cell function, mitochondrial integrity, reactive oxygen species release, and inflammatory cell activation. The silent mating type information regulation 2 homolog 1 (Saccharomyces cerevisiae) (SIRT1), AMP activated protein kinase (AMPK), and Wnt1 inducible signaling pathway protein 1 (WISP1) are novel targets that can oversee programmed cell death pathways tied to β-nicotinamide adenine dinucleotide (NAD+), nicotinamide, apolipoprotein E (APOE), severe acute respiratory syndrome (SARS-CoV-2) exposure with coronavirus disease 2019 (COVID-19), and trophic factors, such as erythropoietin (EPO). The pathways of programmed cell death, SIRT1, AMPK, and WISP1 offer exciting prospects for maintaining metabolic homeostasis and nervous system function that can be compromised during aging-related disorders and lead to cognitive impairment, but these pathways have dual roles in determining the ultimate fate of cells and organ systems that warrant thoughtful insight into complex autofeedback mechanisms.
Disorders such as diabetes mellitus (DM) and cellular metabolic disease are increasing in prevalence throughout the world. Over a thirty-five year course from the year 1980, the number of individuals with DM increased from one hundred eight million to over four hundred twenty-two million individuals (1, 2). By the year 2045, seven hundred million individuals may have DM (3, 4). From the years 2013 to 2016, the prevalence of DM has risen from over nine percent (5). DM is a chronic disorder that affects all organs of the body leading to cardiac disease, retinal disease, hepatic injury, cerebral ischemia, limb amputation, and renal failure (5–18). Almost one half billion individuals have DM and at least half of the four million deaths that occur per year with DM impact individuals less than seventy years of age (1, 7, 19–22). Ten percent of the population in the United States (US) are currently reported to suffer from DM (23, 24). However, it is believed that many additional individuals have disorders of metabolism or have elevated risk to develop DM, but remain undiagnosed at present (3, 16, 21, 25–37). It is estimated that in individuals greater than eighteen years old, seven million may not be correctly diagnosed as having DM and more than thirty-five percent of US adults may have prediabetes due to elevations in their fasting glucose and hemoglobin A1c (HbA1c) parameters (7, 38). Globally, four hundred million individuals are estimated to have metabolic disease or be at risk for developing DM (3, 39–41).
Metabolic disease affects low and middle income countries more than high income developed countries with approximately eighty percent of people residing in low-income nations (3, 42). This may be a result of the prevalence of DM being affected by a number of parameters that include socioeconomic status, comorbidities such as infection with the severe acute respiratory syndrome coronavirus (SARS-CoV-2), and level of education (43–54). In regard to education level, those individuals with less than a high school education represent thirteen percent of DM patients, individuals with a high school education equal ten percent of DM patients, and those individuals with more than a high school education represent approximately seven percent of DM patients (2). Other factors that can contribute to the development and progression of DM include limited exercise, tobacco consumption, high serum cholesterol, hypertension, and obesity (8, 13, 48, 55–57). Obesity alters a number of pathways in the body and can impact oxidative stress cell injury, stem cell survival, inflammation, aging processes, and the maintenance of mitochondrial function (21, 33, 40, 51, 56, 58–73). As a result, the additional body weight fosters insulin insensitivity and glucose intolerance that progresses to DM (19, 24, 28, 36, 74–80) (Table 1).
Table 1 Highlights The Impact of Aging and Oxidative Stress in Metabolic and Nervous System Disorders: Programmed Cell Death and Molecular Signal Transduction Crosstalk.
Additional challenges for the care of individuals with DM involve financial expenditures. At least twenty thousand United States Dollars (USD) on an annual basis is necessary for the basic care of people with DM that can involve the maintenance of glucose homeostasis, wound care, and nutritional education (6, 9, 19, 20, 28, 41, 74, 81–87). Yet, the required resources for DM care is growing and is greater than seven hundred sixty billion USD with an additional seventy billion USD necessary for those with severe disability and loss of function (3). Greater than seventeen percent of the Gross Domestic Product in the US is consumed for the care of people with DM (88).
The debilitating nature of DM affects the entire body and leads to the degeneration of all organ systems (6, 7, 12, 16, 19, 21, 24, 27, 31, 34, 36, 46, 60, 85, 89–94). In particular, the metabolic disorders affect the nervous system and can lead to cognitive impairment, peripheral neuropathies, demyelinating disorders, and risk for developing infection as well as memory loss (Figure 1). An additional risk that includes metabolic disease for the development of neurodegenerative disorders is the observed rise in lifespan (95–100). Life expectancy is increasing especially in developed nations (101) and over the past fifty years the number of people greater than the age of sixty-five has increased greater than one hundred percent (4, 68, 96, 97, 102–113). Neurodegenerative disorders comprise a portion of non-communicable diseases (NCDs) and over seventy to seventy-five percent of the deaths that occur each year are due to NCDs (8, 22, 56, 60, 114–116). The increase in lifespan coincides with the rise of NCDs (91, 111, 117–129). As a result, the increase in lifespan for the world’s population has resulted in an increased prevalence for diseases of the nervous system (117, 130–133). Nervous system disorders comprise greater than six hundred disease entities, lead to the death of over seven million people annually, and can impact greater than one billion people (111, 117, 134–146). In relation to financial exposure, more than eight hundred billion USD in the US is required annually to care for multiple neurological disorders that include stroke, trauma, epilepsy, back pain, Parkinson’s disease (PD), Huntington’s disease (HD), amyotrophic lateral sclerosis (ALS), and dementia (116). Cognitive loss can be the most significant burden to the financial system and these cost considerations do not include the expenses required for companion care, social health programs, and senior daily care with the additional greater than seventy million clinicians and social workers needed to fill these unmet needs (22, 116, 147). These additional services will reach 2 trillion USD annually in the US and more than four million people will need over four billion USD for treatment each year. The market for dementia could exceed eleven billion USD (34, 141, 148). Furthermore, additional significant costs involve other neurological disorders, such as PD with greater than fifty-five billion USD necessary for care in the US annually. In the year 2030, the number of those affected with PD is predicted to double. Present expenses are currently at a large annual cost per individual of approximately twenty-five thousand USD per year (5, 97, 137, 144, 146, 149–165).
Figure 1 The Clinical Implications of Metabolic Dysfunction and Neurodegenerative Disease. Loss of metabolic homeostasis can lead to multiple disorders. Metabolic disorders affect both the peripheral and central nervous systems and can be affected by several risk factors. These disorders include diabetes mellitus that affects all systems of the body, cognitive impairment, Alzheimer’s disease, Multiple Sclerosis, and peripheral neuropathies. Additional entities such as the apolipoprotein E (APOE-ε4) gene, severe acute respiratory syndrome coronavirus (SARS-CoV-2), and coronavirus disease 2019 (COVID-19) can lead to memory loss, cognitive failure, and cortical vascular disease.
Metabolic disorders, such as DM, increase the risk for the onset and progression of neurodegenerative disorders through multiple pathways. DM is a primary mechanism for the onset of cardiovascular disease that can ultimately lead to disorders of the nervous system (8, 53, 166–170). When compared to people that do not have DM, individuals with DM can have two times the risk of developing cardiac disability or cerebral ischemia (40, 43, 91, 108, 111). DM results in insulin resistance (9, 19, 24, 60, 80, 90, 171–174), vascular injury (16, 19, 26, 27, 29, 30, 32, 33, 61, 74, 86, 87, 170, 175–182), alterations in cerebral blood flow (2, 7, 9, 53, 91, 176, 183), endothelial dysfunction (19, 27, 40, 83, 94, 184), mitochondrial injury (13, 28, 53, 60, 115, 172, 178, 185, 186), retinal disease (30, 66, 94, 187–189), stem cell loss (35, 38, 54, 66, 72, 77, 84, 111, 190), susceptibility to infections (34, 46, 48–51, 54, 92, 191, 192), and immune system dysfunction (30, 54, 64, 68, 84, 173, 178, 193–199).
DM and cellular metabolism also play a significant role in the processes of oxidative stress and aging (7, 16, 47, 64, 75, 89–91, 108, 178, 196, 200). DM can lead to changes in transcriptional networks, loss of mitochondrial homeostasis, inflammation, production of reactive oxygen species (ROS), and cell senescence (7, 16, 20, 21, 30, 32, 66, 78, 79, 90, 91, 108, 174, 178, 193, 194, 201–203). ROS that are generated during oxidative stress include hydrogen peroxide, superoxide free radicals, nitric oxide, singlet oxygen, and peroxynitrite (36, 37, 65, 90, 96, 113, 120, 137, 154, 180, 186, 204–218). During conditions that oversee the detrimental effects of ROS, antioxidant systems are in play that involve glutathione peroxidase, catalase, superoxide dismutase, and the nutrient vitamins B, K, E, D, and C (20, 34, 42, 47, 53, 100, 132, 186, 202, 217, 219–225). If these systems are overwhelmed or unable to limit excessive ROS production, mitochondrial injury, loss of DNA integrity, and shortened lifespan can occur (8, 34, 69, 78, 96, 104, 120, 122, 162, 207, 209, 218, 226–229). Oxidative stress can result in vascular endothelial cell injury (9, 40, 83, 230–234), neuronal cell compromise (24, 66, 122, 123, 152, 156, 162, 233, 235–245), alterations in neurotransmitters (69, 246, 247), myelin degradation (79, 224, 248–252), cell senescence (8, 33, 55, 112, 253–255), loss of stem cell proliferation (34, 66, 168, 228, 241, 251, 256–259), and cognitive impairment (7, 10, 120, 121, 200, 223, 243, 245, 260–266).
In regard to aging and cellular metabolism, the shortening of telomeres (TLs), complexes of deoxyribonucleic acid (DNA), can lead to the increased risk for the development of DM (8, 267) and greater cell senescence with the loss metabolic homeostasis (16, 33, 178, 190, 268). Changes in TL length can promote aging processes, cellular senescence, and neurodegeneration as well (70, 112, 113, 118, 154, 255, 269–275). TLs are positioned on chromosome ends and oversee replication of cells, preservation of the genomic DNA, and cell survival (8, 16, 274, 276–279). More than two thousand repetitions of double-stranded non-coding DNA with the ‘TTAGGG” sequence that is finalized with guanine rich single-stranded DNA compose TLs (8, 70, 139, 265, 279). Complexes of proteins that include CTC1-STN1-TEN1 (CST), shelterin, and telosome are part of the TL family (7, 139, 252). To oversee the division of cells, these proteins control function and stability of TLs that can lose twenty-five to over two hundred base pairs during the process of dividing cells. Telomerase protein can prevent the loss of base pairs in TLs by providing tandem repeat ribonucleic acid (RNA) templates (274, 276, 280). However, cell senescence ultimately ensues when TLs become very short with less than five hundred base pairs and telomerase function is impaired (33, 95, 102, 118, 134, 154, 221, 253, 255, 269, 272, 275, 281). At this point, tissues and organs cannot undergo repair, the immune system is less viable, and age-related disorders can progress (7, 73, 139, 164, 282–286). These events with the shortening of TLs and cellular senescence also promote oxidative stress and the release of ROS that impairs cell organelles, such as mitochondria and cellular energy homeostasis (7, 32, 104, 121, 122, 196, 282, 287, 288).
Given the ability of DM to lead to aging processes tied to oxidative stress, neuronal and vascular injury, mitochondrial dysfunction, stem cell loss, and immune system disorders, it becomes evident that loss of metabolic homeostasis with DM can result in multiple neurodegenerative disorders. Metabolic disorders can affect both the peripheral and central nervous systems (Figure 1). In the peripheral nervous system in the presence of DM, autonomic dysfunction (289–291) and neuropathies can be common and affect more than seventy-five percent of individuals (5, 38, 78, 79, 83, 290, 292).
In the central nervous system, cognitive loss with DM is a significant co-morbidity. DM results in memory impairment (7, 10, 55, 115, 200, 263, 293–296) and can lead to the onset and progression of Alzheimer’s disease (AD) (2, 6, 28, 44, 89, 153, 201, 266, 297–302) (Figure 1). Dementia is present in all nations throughout the world and is now considered to be the seventh primary reason for death (109, 116, 131, 141, 144, 161, 164, 223, 252, 266, 303–308). At least five percent of the world’s population has dementia and by the year 2050 it is believed that over one hundred fifty-five million people will have cognitive loss (2, 73, 97, 115, 126, 205, 226, 275, 309, 310). Of those individuals with dementia, approximately sixty percent of people have the sporadic form of AD and greater than ten percent are over the age of sixty-five (5, 110, 139, 148, 277, 311–313). Diagnosis for dementia can fall significantly behind the onset of the disorder and may not be recognized until twelve to twenty-four months after the initial clinical presentation (38, 252, 314). Currently, more than six million people in the US have the sporadic form of AD (5, 309, 315–318), but this is expected to increase to thirty million people during the next two decades (6, 7, 110, 205, 226, 245, 282, 319, 320). In contrast, familial AD (FAD) is present in about two hundred families in the world (6, 73, 110, 121, 134, 282, 311). FAD represents an autosomal dominant version of amyloid precursor protein (APP) gene that is mutated, occurs in variable single-gene mutations on chromosomes 1, 14, and 21, and is usually clinical present prior to fifty-five years of age (115, 321, 322).
Risk factors also exist for dementia that can have a metabolic basis as well. In experimental models, insulin signaling can be associated with AD pathology (71). Late-onset AD can result in the presence of the ϵ4 allele of the apolipoprotein E (APOE-ε4) gene (5, 7, 148, 161, 252, 323–325) (Figure 1). The risk for developing AD is more than twenty times greater in those individuals with two APOE-ϵ4 alleles. APOE is produced in liver cells and is vital for metabolic cellular function to oversee the homeostasis of lipids through the transport of triglycerides, phospholipids, and cholesterol (7, 127, 161, 325–328). In the brain, astrocytes produce APOE to modulate the transfer of cholesterol to neurons through APOE receptors (7, 127, 252, 326, 328, 329). Interestingly, β-amyloid (Aβ) can be removed and destroyed by APOE through apoptosis and the exposure of phosphatidylserine (PS) membranes that are a part of the apoptotic cell death process (330, 331). Other forms of APOE that do not involve APOE-ε4 may inhibit Aβ aggregation during PS membrane exposure (332). However, Aβ aggregation is not believed to be blocked by APOE-ϵ4 which can therefore allow amyloid deposition to proceed and potentially foster the development of AD (44, 161, 323, 332–334). APOE-ϵ4 also may assist with the infection of viral antigens and lead to cerebral microhemorrhages during severe acute respiratory syndrome (SARS-CoV-2) exposure with coronavirus disease 2019 (COVID-19) (326) (Figure 1). SARS-CoV-2, a β-coronavirus family virion, has resulted in a global pandemic (34, 46, 49, 50, 335, 336) and can attach to nasal epithelial cells (337) and neurovascular cells in the brain (54). These processes subsequently result in hyperactivation of the immune system (335, 336, 338–340). Following SARS-CoV-2 infection, memory loss and cognitive failure can develop and lead to long-COVID, also termed long-haul COVID, chronic COVID-19, or post-acute COVID (34, 49, 50, 325, 336, 341–345). The combination of APOE-ϵ4 and SARS-CoV-2 infection can lead to cognitive loss and cortical vascular disease (57, 97, 181, 226, 326, 346–350).
Multiple sclerosis (MS), an additional significant neurodegenerative disorder, also may develop as a result of metabolic disease, pathways of APOE-ϵ4, and Aβ deposition (125, 252, 350–359) (Figure 1). MS impacts large portions of the global population, affects greater than two and one-half million people, and is a primary disease of myelin and myelin producing cells that is immune system mediated (159, 351, 358, 360–364). MS appears to lead to disease in more women than men (357) and can markedly impair cognitive function (252, 365, 366). The memory loss can be progressive in nature and affect both women and men (367). At least sixty-five percent of patients with MS have difficulty with memory recall and executive ability (252). Cognitive loss in MS patients may be tied to metabolic pathways and APOE-ϵ4 since individuals with MS can demonstrate lower cognitive function and delayed responses to stimuli (368). APOE serum levels are elevated in individuals with demyelinating optic neuritis and the genotype of APOE ϵ3/ϵ3 may lead to the male onset of optic neuritis (327). In the setting of APOE risk factors and metabolic dysfunction similar to AD that can increase susceptibility to viral infections, MS patients may experience higher rates of death during SARS-CoV-2 with COVID-19 (369). Treatment with metformin, commonly used during DM, can reduce the degree of functional impairment in obese individuals or those with DM during COVID-19 (52, 370). MS also may have common pathways with AD and Aβ (140, 252, 371). Tau seeding, also present in AD (7, 123, 311, 372–376), has been reported in the brains of MS patients (140) and this tau deposition may produce demyelination through injury to oligodendrocytes (128). Alterations in Aβ deposition similar to those observed in AD may also indicate early memory impairment in people with MS (371).
Multiple factors can impact the role of metabolic disorders that can lead to the onset and progression of neurodegenerative disease. If one focuses upon metabolic disease and DM, therapies that improve nutritional intake that can be complemented by pharmaceutical agents to assist with serum glucose homeostasis and insulin resistance may limit periods of hyperglycemia and the complications of hypoglycemia (6, 8, 16, 19–21, 25, 27–29, 49, 167, 175, 176, 370, 377–379). Yet, progression of DM even at a less marked pace will ensue and can be affected by off-target treatment effects that result in cellular injury, neuronal and vascular cell loss, and the atrophy of organs (33, 87, 172, 380). In the nervous system, a number of diverse pathways that involve inflammation, infection, circadian rhythm, excitotoxicity, metabotropic receptors, tau, Aβ, mitochondrial injury, acetylcholine loss, heavy metal toxicity, and oxidative stress can lead to cognitive loss (24, 33, 34, 42, 50, 63, 65, 66, 96, 106, 119, 121, 131, 165, 168, 175, 205, 223, 226, 261, 282, 286, 303, 330, 338, 346, 381–398). Current therapies for AD that employ cholinesterase inhibitors may limit memory loss but these treatments do not stop the progression of disease (115, 164, 299, 399, 400). Recent developments for AD to use immunotherapy to decrease Aβ load in the brain also may reduce memory loss, but these therapies are currently limited to a small group of patients that are not at risk for cerebral microhemorrhages and also such treatments do not prevent overall disease progression (119, 126, 401). In regard to MS, disease modifying therapies (DMTs) can reduce the frequency of relapses in relapsing–remitting MS, but disease progression can continue (252, 362, 371). For example, despite the reduction in brain volume loss with DMTs, cognitive impairment can continue unabated (402). These treatment considerations that rest on the side of metabolic disorders as well as neurodegenerative disease warrant new avenues of inquiry for the development of innovative therapeutic strategies that may address the onset and progression of these disorders. Novel pathways that may offer new insights into these disorders involve programmed cell death regulation, the silent mating type information regulation 2 homolog 1 (Saccharomyces cerevisiae) (SIRT1), AMP activated protein kinase (AMPK), and Wnt1 inducible signaling pathway protein 1 (WISP1) (Figure 2).
Figure 2 Innovative Avenues to Address Metabolic and Neurodegenerative Disease. Current therapies for metabolic and neurodegenerative disorders are unable to prevent the onset and progression of these disorders. The clinical course of these disorders is closely tied to intracellular process that are linked to aging-related disorders, telomere dysfunction, cellular senescence, and oxidative stress. Novel and innovative therapeutic strategies are needed to address metabolic and neurodegenerative diseases. Pathways that may offer new insights into these disorders involve the silent mating type information regulation 2 homolog 1 (Saccharomyces cerevisiae) (SIRT1), AMP activated protein kinase (AMPK), and Wnt1 inducible signaling pathway protein 1 (WISP1). These pathways are closely interconnected, can form complexes, and involve ß-nicotinamide adenine dinucleotide (NAD+), nicotinamide (NIC), and trophic factors such as erythropoietin (EPO). Ultimately these pathways serve to provide oversight of programmed cell death mechanisms that involve autophagy, apoptosis, pyroptosis, and ferroptosis as well as mechanisms that can lead to mitochondrial stress such as with nicotinamide adenine dinucleotide phosphate (NADPH) depletion.
Programmed cell death that involves autophagy, apoptosis, ferroptosis, and pyroptosis has a vital role in the determination of both metabolic and neurodegenerative disorders (Figure 2). Recent studies on exome sequence analysis indicate that metabolic cellular dysfunction directly affects neuronal cell death through DNA and apoptosis (403). In metabolic disorders, programmed cell death can affect neuronal survival (24, 122, 310, 404–411), vascular integrity (8, 40, 61, 87, 182, 188, 412–414), mitochondrial function (42, 61, 172, 186), and inflammation (36, 50, 87, 186, 415–418). In a similar manner, programmed cell death in the nervous system can lead to neuronal and non-neuronal cell injury (65, 121, 132–134, 143, 148, 155, 224, 240, 262, 376, 405, 419–428), cerebral ischemia (91, 122, 182, 405, 423, 427, 429–433), microglial cell loss (120, 125, 133, 152, 356, 421, 430, 434, 435), and dysfunction of pathways for cognitive function (100, 120, 121, 141, 143, 148, 260–262, 275, 301, 421, 436–440).
During autophagy, organelles in the cytoplasm as well as other subunits in the cell are recycled for future remodeling of tissues (5, 121, 376, 408, 409, 428, 441, 442). Of the different forms of autophagy, macroautophagy is the prominent type of autophagy that is usually described and consists of sequestering proteins and organelles in the cytoplasm of cells into autophagosomes that will be merged into lysosomes that can be degraded and recycled (117, 136, 141, 275, 443). During microautophagy, components of the cytoplasm are sequestered for eventual digestion through invagination of lysosomal membranes (97). In chaperone-mediated autophagy, protein “chaperones” are created in the cytoplasm to carry components of the cytoplasm over the membranes of lysosomes (134, 136, 444, 445).
Autophagy can foster beneficial outcomes during metabolic and neurodegenerative disorders. Activation of autophagy can be necessary for fatty acid metabolism during obesity (62) and for the oversight of muscle tissue generation (441). Autophagy can oversee the proliferation and size of pancreatic β-cells (446), may limit insulin resistance during inflammation with high serum lipids in obesity models of autophagy Atg7 gene deletion (447), may prevent diabetic nephropathy with maintenance of Atg7, Atg5, and LC3 autophagy proteins (448), and can prevent DM progression through promoting β-cell function and eliminating misfolded proteins and dysfunctional mitochondria (449). Increased physical activity in murine models helps control glucose serum regulation through autophagy pathways (450) that are tied to greater insulin efficacy (451) and improved function of microglial cells (416). In the brain, loss of autophagy activation can lead to memory impairment in AD with the progression of DM (44). The pathways that lead to the activation of autophagy may require inhibition of the mechanistic target of rapamycin (mTOR) with agents such as rapamycin or metformin (7, 49, 121, 284, 335, 359, 376, 393, 442, 452–457). In addition, activation of mTOR can inhibit autophagy induction through the phosphorylation of the autophagic related gene (Atg) protein Atg13 and UNC-51 like kinases (ULKs) such as UNC-51 like kinase 1 (ULK1) to prevent formation of the ULK-Atg13-FIP200 complex (97, 458). During mTOR inhibition and autophagy activation, reduction in ROS release occurs (459), dopamine cell survival is increased (460), neuronal demise is blocked through pathways of glutamine (461), and mitochondrial integrity is preserved (462). Autophagy activation can limit tau deposition (463) and reduce Aß accumulation with improved memory function and metabolic homeostasis (464). Control of blood mononuclear cells in MS during inflammation can be mediated by autophagy activation (465), induction of autophagy can improve the clinical outcome of relapsing-remitting and experimental autoimmune encephalomyelitis (466) and reduce retinal MS-induced degeneration (189), cytokine release and microglial activation is limited during autophagy activation in experimental autoimmune encephalomyelitis (358), and the risk for developing MS may be lessened during mTOR blockade and autophagy induction (359). Autophagy activity with metformin treatment also may lead to myelin repair with oligodendrocytes (356) and assist with the reduction of viral susceptibility during DM in over-weight individuals exposed to COVID-19 (52, 370).
However, there exists another side to autophagy that suggests careful modulation of activity is required for clinical disease. Cardiomyopathy (467), atherosclerosis (468), and endoplasmic reticulum stress (469) can ensue with advanced glycation end products (AGEs), elevated glucose exposure, and autophagy activation. Induction of autophagy can lead to the reduction in cardiac and liver tissue mass during treatments to improve glucose regulation (380), promote neuronal cell death under some conditions (470–472), prevent cerebral interneuron progenitor cell growth (473), result in mitochondrial injury (61, 145, 172, 216, 306, 379, 474–479), reduce numbers of progenitor endothelial cells (480), limit angiogenesis in the presence of elevated glucose (480), and lead to cognitive loss (141, 148, 275, 361, 393, 437). In addition, growth factor cell protection, such as with the trophic factor erythropoietin (EPO) (114, 304, 385, 481–484), requires decreases in autophagy activation in conjunction with modulation of mTOR, protein kinase B (Akt), the proline rich Akt substrate 40 kDa (PRAS40), and mammalian forkhead transcription factors to promote neuronal and vascular survival (145, 485–489).
Apoptotic cell injury can be initiated through pathways of oxidative stress (40, 68, 96, 120, 201, 216, 244, 245, 256, 260, 261, 303, 490–495) and inflammation (7, 36, 120, 143, 169, 212, 225, 256, 260, 275, 303, 310, 425, 430, 496–501) as part of metabolic and neurodegenerative disorders. Apoptotic cell death has early and late components that can occur in this process (97, 122, 408, 409, 426). The loss of PS membrane asymmetry is the early phase of apoptotic cell death (502–506). Once cells become injured, the PS residues become externalized on the cell membrane that attracts inflammatory microglia to recognize these injured cells and remove them from the central and peripheral nervous systems (502, 507–510). However, injured cells may recover if they are not engulfed by microglia. Treatments directed to restore PS membrane asymmetry for injured cells can then prevent microglial attraction and preserve the function of these necessary cells in the nervous system (66, 511–513). In contrast, the later phase of apoptotic cell death that consists of the destruction of nuclear deoxyribonucleic acid (DNA) (18, 96, 113, 229, 483, 514–518) and a cascade of caspase activation (65, 96, 130, 143, 347, 348, 405, 425, 483, 519) is not reversible.
Reductions in apoptosis activation can prevent cell injury during glial cell excessive activity and oxidative stress (120), limit dopaminergic cell demise during inflammatory cell activation (152), protect retinal cells during ischemia exposure (516), and increase neuronal cell survival during Aß toxicity (320, 520–522). Controlling apoptotic cell death also reduces inflammatory cell pathways (50, 186, 212, 225, 256, 260, 261, 418, 496, 497, 499, 500, 523–525) and can limit memory loss (7, 100, 143, 262, 421, 439). These pathways are significantly tied to microglial activity. Microglia account for about fifteen percent of the cells in the central nervous system and as noted can remove injured cells during apoptosis (97, 120, 133, 134, 152, 155, 425, 430, 502, 503). These inflammatory cells can release ROS to generate oxidative stress (7, 18, 62, 121, 282, 526–528) through pathways that involve Wnt signaling (2, 6, 97, 122, 181, 529–531), mammalian forkhead transcription factors (17, 67, 68, 117, 426, 519, 532), and growth factors with EPO (6, 145, 159, 482, 533–536). During cognitive dysfunction, microglial activity may lead to increased risk for the development of AD (141, 537) as well as endothelial dysfunction (119).
Ferroptosis is a process in the programmed cell death pathway that leads to the storage of iron in the cell that results in the inability to maintain glutathione homeostasis (225, 538, 539). Once oxidative defenses that require glutathione are lost, lipid peroxidation can ensue to result in the demise of cells (224, 229, 540). Ferroptosis can lead to cell death in multiple systems such as the musculoskeletal system (225), cardiovascular system (8, 540), and breast tissue (229). In the nervous system, ferroptosis may lead to cognitive impairment (7, 224, 274) and produce pathogenic T lymphocytes that lead to dysfunction in neuronal and glial cells (252, 538).
Given the associations of autophagy, apoptosis, and ferroptosis with inflammatory cell pathways, it is of interest to note that pyroptosis is part of the programmed cell death pathway that can specifically modulate inflammatory cell activity (5, 178, 410, 500, 541). The inflammasome, also known as the pyroptosome, is a supramolecular entity that initiates the pyroptotic cell death process. The inflammasome family of nucleotide-binding oligomerization domain and leucine-rich repeat-containing receptors (NLRs) has the members NLRP1, NLRP3, NLRP6, and NLRC4. Pattern recognition receptors responding to damage associated molecular pattern (DAMP) in host cells and pathogen-associated molecular pattern (PAMP) in families of microbes lead to the activation of inflammasomes and caspase 4, caspase 1, and caspase 5 (7, 303, 358, 515, 541–545). DAMP molecules with DNA and adenosine triphosphate (ATP) traverse through open cell membranes and lead to NLRP3 canonical inflammasome activation while caspase 5 and caspase 4 can result in noncanonical inflammasome activation with lipopolysaccharide proteins in infections with Gram-negative bacteria. For membranes to open, pores are formed through the degradation of N-terminal domain with the C-terminal domains as part of gasdermin proteins. Cytokines such as interleukin-1 family members are released to generate inflammatory reactions and require gasdermin since these cytokines cannot alone result in pore formation (2, 212, 515). Pyroptosis can result in cell injury as a result of cytokine release (410, 411) and lead to neuronal and vascular cell dysfunction that results in loss of memory and executive function (11, 60, 252, 263, 275, 298, 301, 344, 546). In addition, elevated cytokine release during pyroptosis can affect immune cell activity in the body (358) and lead to failed clinical outcomes, such as in MS patients, with elevated inflammasome levels (541).
The silent mating type information regulation 2 homolog 1 (Saccharomyces cerevisiae) (SIRT1) controls both cellular metabolism (11, 60, 111, 263, 275, 294, 298, 344, 400, 546–549) and neurodegeneration (98, 102, 117, 156, 210, 232, 240, 400, 438, 550–555). There exist mammalian homologues of Sir2 that are SIRT1, SIRT2, SIRT3, SIRT4, SIRT5, SIRT6, and SIRT7 (6, 12, 13, 97, 134, 255, 333, 556). SIRT1 exists in the brain, liver, heart, skeletal muscle, pancreas, adipose tissue, and spleen (16, 179, 210, 223, 440, 550, 557). SIRT1 is a histone deacetylase that controls transcription of DNA that involves acetyl group transfer from ϵ-N-acetyl lysine amino acids to DNA histones (6, 17, 70, 78, 106, 117, 223, 259, 309, 335, 558, 559) (Figure 2). Histone deacetylases oversee multiple cellular processes such as aging, wound healing, neuronal function, oxidative stress, transcription factor activity, cardiovascular function, and cancer (8, 78, 98, 175, 550, 560–563). One substrate for SIRT1 is the coenzyme ß-nicotinamide adenine dinucleotide (NAD+) (24, 42, 65, 70, 78, 96, 98, 111, 196, 330, 475, 550, 564).
SIRT1 regulation of cellular metabolic homeostasis can be critical to the onset and progression of disorders in the nervous system (13, 16, 17, 38, 98, 223, 259, 333, 544, 549, 565, 566). SIRT1 is dependent upon NAD+ and nicotinamide (24, 98, 210, 255, 417, 475, 514, 550). As a precursor for NAD+, nicotinamide is the amide form of vitamin B3 (niacin) (8, 34, 42, 330, 378, 567–570). Nicotinamide can be produced through SIRT1 transferring of the acetyl residue of the histone acetyllysine residue to the ADP-ribose moiety of NAD+. As part of a feedback pathway, nicotinamide can limit the activity of SIRT1 through the interception of an ADP-ribosyl-enzyme-acetyl peptide intermediate with the regeneration of NAD+ (571). As a result, nicotinamide can bind to sirtuins through NAD+ in the C pocket of sirtuins (572) and can noncompetitively inhibit SIRT1 (560) and prevent anti-inflammatory gene expression (573). In addition, SIRT1 activation through nicotinamide phosphoribosyltransferase (NAMPT) can occur with periods of glucose restriction. This leads to increases in NAD+ and reduction in nicotinamide levels that become ineffective to block SIRT1 (574). Replenishment of NAD+ can assist with cardiovascular health (53) with SIRT1 activation limiting inflammation, metabolic dysfunction, and cell injury (111, 113, 203). As an example during hyperglycemia, SIRT1 can increase vascular cell survival (575).
SIRT1 can oversee insulin sensitivity (8, 61, 78, 417, 576–578) and mitochondrial function (13, 34, 70, 240, 475, 558). SIRT1 expression is reduced in the liver and pancreas during high fat diets that can lead to insulin resistance (579). Elevated SIRT1 activity can modulate glucose and hepatic lipid processing to prevent metabolic syndrome dysfunction (580). SIRT1 controls insulin sensitivity via protein tyrosine phosphatase (PTP) (335, 581). SIRT1 also is a positive feedback system for insulin signaling through Akt and can lead to the activity of Akt through phosphotidylinositide 3-kinase (PI 3-K) (532, 581).
In the nervous system, SIRT1 activity can lead to neurite outgrowth and enhance neuronal survival in environments that limit nutrients (582). SIRT1 can foster survival for photoreceptor cells (583), prevent the senescence of endothelial cells (584), and enhance the function of mitochondria in embryonic stem cells during oxidative stress (585). The absence of SIRT1 activity may lead to dysregulation in the immune system such as during MS (406). SIRT1 activity may be required for limiting the toxicity of oxidative stress and preserving memory (262), fostering Aβ degradation (586), increase lifespan in higher level organisms (587), and protecting neuronal and vascular cells against oxidative stress (98, 134, 232, 240, 438, 502, 510, 550, 551, 554, 555, 588).
SIRT1 also functions through trophic factor regulation in metabolic and neurological disorders that is linked to NAD+ activity (34, 145, 203, 499, 533–535, 589). The trophic factor EPO employs SIRT1 to block depolarization of mitochondrial, release of cytochrome c, induction of BCL2 associated agonist of cell death (Bad) activation, and caspase cleavage (510). EPO through SIRT1 can protect neurons (590) that may be responsible for SIRT1 synaptic memory improvement (223). As a result of SIRT1 activity, EPO prevents mitochondrial injury (483, 521, 536, 591–593), increases microglial survival (594), blocks caspase activity (520), protects human cardiomyocytes (592), and oversees cellular metabolism (304, 482, 595, 596).
AMP-activated protein kinase (AMPK) is an important component of the mTOR pathway, is intimately associated with SIRT1, and is a significant target for metabolic and neurodegenerative disorders (2, 30, 38, 112, 113, 118, 218, 229, 452, 558, 559, 597–600) (Figure 2). AMPK can lead to the generation of adenosine triphosphate (ATP), improve insulin sensitivity, oversee the oxidation of fatty acids, and reduce levels of oxidative stress (30, 34, 229, 230, 234, 288, 309). Increased AMPK activity is present in diets high in fish oil that can prevent endothelial cell injury (601) and improve insulin sensitivity (451). In the nervous system, AMPK can limit stroke damage in animal models of DM (602), reduce tau deposition (463), regulate neuroinflammation (134, 226, 603), reduce Aß brain accumulation (604), block Aß toxicity (605), oversee mitophagy with ULK1 (606, 607) and improve cognition in experimental models with DM and AD (608). Pain sensation that can become problematic with peripheral neuropathies in DM can be attenuated in experimental models with AMPK (292).
A number of agents that are involved in metabolic homeostasis also rely upon AMPK. Nicotinamide can protect mitochondria with the activation of AMPK (288). In addition, metformin and biguanides control autophagy through AMPK. DM cardiac cell injury through the activation of autophagy with AMPK is reduced during treatment with metformin (609). As previously noted, activation of autophagy under some circumstances can reduce toxicity from oxidative stress (33, 121, 145, 189, 216, 226, 306, 309, 540) and may shift to beneficial oxidative metabolism (610). In addition, other pathways such as Wnt family members may require AMPK activity to reduce neuronal brain injury (611). AMPK signaling is necessary with inhibition of mTOR pathways for the maintenance of electrical activity of the brain for control of behavior (597), for the acceleration of myelin brain recovery during treatment with metformin (356), and for mitochondrial preservation during ferroptotic cell death (229). Without AMPK activity, cell death, cell senescence, and mitochondrial loss can result (16, 113).
Bidirectional pathways of modulation of activity also exist for SIRT1 and AMPK. SIRT1 expression leads to deacetylation of serine-threonine liver kinase B1 (LKB1) that may be through indirect or direct means and can result in AMPK activation (612). Although AMPK does not directly activate SIRT1, SIRT1 activity can increase with AMPK either by elevating the cellular NAD+/NADH ratio that leads to deacetylation and downstream SIRT1 target activity changes to involve peroxisome proliferator-activated receptor-gamma coactivator-1α (PGC-1α) and forkhead transcription factors (613) or through increasing NAMPT to raise NAD+ and lower SIRT1 inhibitors such as nicotinamide (574). Resveratrol, an activator of SIRT1, can elevate AMPK activity through SIRT1 dependent or independent pathways (613, 614). Through combined pathways, AMPK and SIRT1 block mitochondrial loss (61) and limit endothelial cell death during elevated glucose exposure (575).
Closely coordinated with the pathways AMPK in metabolic and neurodegenerative disease is the Wnt1 inducible signaling pathway protein 1 (WISP1) (6, 75, 529, 615–618). WISP1 is a downstream target of the wingless pathway of Wnt proteins. Wnt proteins are cysteine-rich glycosylated proteins that can control cellular metabolism, stem cell proliferation, new vascular cell growth, musculoskeletal disease, nervous system sensation, and neuronal cell development (57, 83, 122, 181, 203, 346, 349, 350, 530, 531, 619, 620). Wnt signaling that includes Wnt1 can control autophagy (621–624), prevent endothelial cell death in experimental models of DM (412), limit dopaminergic neuron cell loss in PD (625), oversee repair of wounds during DM (626), assist with human β-cell proliferation (627), foster growth in the musculoskeletal system (57, 497, 628), and inhibit cognitive loss with DM and aging (629).
WISP1 is a CCN family member that consists of six secreted extracellular matrix associated proteins that are termed by the first three members of the family that include Cysteine-rich protein 61, Connective tissue growth factor, and Nephroblastoma over-expressed gene (130, 630) (Figure 2). WISP1 can be influenced by increased weight in humans, becomes elevated with insulin resistance in children and adolescents (616, 631), and is elevated during gestational DM (632). WISP1 may be vital for glucose homeostasis since it is over-expressed during regeneration of the pancreas (633), controls β-cell proliferation (75), and can control cellular senescence (634). WISP1 can stabilize atherosclerotic plaques (347) that can ultimately lead to cerebrovascular disease, can limit lipopolysaccharide-induced cell injury through pathways of Akt (348), can attenuate blood-brain barrier disruption (635), and decrease toxicity of oxidative stress and Aß exposure (97, 520, 522). However, it should be noted that WISP1, a trophic agent, also can promote tumorigenesis (281, 529, 636–642).
WISP1 is dependent upon the pathways of AMPK for glucose homeostasis and the ability to affect neuronal survival. WISP1 can oversee AMPK post-translational phosphorylation during cellular metabolism (6, 33, 455, 643–645). WISP1 controls the activation of AMPK activation by differentially decreasing phosphorylation of tuberous sclerosis 2 (TSC2) at serine1387, an AMPK target, and increasing phosphorylation of TSC2 at threonine1462, an Akt target (522). This process allows WISP1 to provide a minimal level of TSC2 and AMPK activity to offer a proper biological balance for optimum metabolic homeostasis and survival of cells. This balance in AMPK activation and levels is critical. Although AMPK activation can limit insulin resistance and oxidative stress (451) and assist with differentiation of adipocytes during lipid accumulation in obesity (646), under other conditions AMPK through autophagy may lead to cell injury. A fine balance of AMPK activity is necessary to increase basal autophagy activity and maintain neuronal and endothelial cell survival during periods of metabolic homeostasis loss (230, 234, 575, 584). AMPK can modulate apoptosis and autophagy during coronary artery disease (647) and ROS release (234, 648). This is also seen with the growth factor EPO. EPO controls AMPK during oxidative stress (522), inflammation (114, 385, 649), angiogenesis (650, 651), and modulation of endothelial nitric oxide synthase (652). The concentration of EPO and duration of treatment can influence a specific level of AMPK activity, as well as the activity of mTOR (114, 653–655). If this activity is not balanced, elevated EPO and AMK activity can lead to cell injury (656).
With the increase in lifespan and NCDs, disorders of cellular metabolism that include DM and neurodegenerative disorders are increasing in prevalence throughout the world. These disorders may be significantly under diagnosed with estimates of at least thirty-five percent of individuals in developed countries not receiving appropriate care to slow the progression of metabolic and neurodegenerative disease. Metabolic and neurodegenerative disorders also impose a significant financial challenge for the treatment and care of individuals. It is expected that additional care costs for current unmet clinical and staffing needs will exceed over two trillion USD per year.
The clinical onset and progression of metabolic and neurodegenerative disorders is closely tied to aging, dysfunction in telomere processing, and the processes of cellular senescence and oxidative stress (Table 1). These underlying processes not only lead to neuronal and vascular death, mitochondrial loss, stem cell injury, and immune system dysfunction, but also result in significant co-morbidities in the nervous system leading autonomic dysfunction and peripheral neuropathies as well as cognitive loss. In fact, cognitive loss is now the seventh cause throughout the world for death and it is estimated that close to two hundred million individuals may have dementia by the year 2050. Although dementia may present in multiple neurological disorders, AD encompasses almost sixty percent of individuals with cognitive loss and it is estimated that more than sixty-five percent of people with MS have cognitive impairment. The cognitive loss in neurological disorders has an important metabolic basis and involves risk factors with APOE that can lead to the cognitive loss present in AD and MS as well as increase susceptibility to viral infections, such as during SARS-CoV-2 with COVID-19.
Metabolic disorders, such as DM, and neurologic disease remain a significant challenge for the treatment and care of individuals. Although strategies that address nutritional intake and the use of pharmaceutical agents to control glucose homeostasis can slow disease progression of DM, clinical off-target effects can lead to progressive neuronal and vascular cell loss and the atrophy of organs in the body. To a similar degree, present therapies for AD assist with symptoms of memory loss, but do not halt disease progression. In addition, recently approved therapies and DMTs for AD and MS that involve immunotherapies may be suited for a small subset of people, such as with AD, and can achieve some decrease in disease progression but the overall course of cognitive loss in these disorders will continue unabated. These clinical challenges to address the interplay between metabolic and neurodegenerative disorders require innovative strategies that can focus upon the underlying mechanisms of aging, oxidative stress, cell senescence, and cell death.
Programmed cell death pathways that involve autophagy, apoptosis, ferroptosis, and pyroptosis can play an important role in DM and neurodegenerative disorders. Autophagy can regulate the proliferation and size of pancreatic β-cells, reduce insulin resistance, prevent diabetic nephropathy, and limit progression of DM through promoting β-cell function and eliminating misfolded proteins and dysfunctional mitochondria. Autophagy activation in conjunction with mTOR inhibition can reduce ROS release, protect dopamine cells, preserve mitochondrial integrity, reduce inflammatory processes that may lead to clinical relapses, limit Aß and tau brain deposition, reduce cytokine release and microglial activity, and repair myelin in the nervous system. In regard to apoptotic cell death, strategies that can limit apoptosis activation can prevent cell injury during excessive glial activity during oxidative stress, limit ischemic toxicity to retinal cells, preserve dopaminergic cells during inflammation, and block cell demise during Aß exposure. Similar to apoptotic cell injury but involving iron accumulation in the cell with the loss of glutathione homeostasis, ferroptosis leads to cognitive impairment, immune dysfunction in T lymphocytes that can injure neuronal and glial cells, and cell death in the musculoskeletal system, cardiovascular system, and breast tissue. Pyroptosis cell death involves inflammasome activation that yields cytokine release, cell injury, inflammatory cell dysfunction, and neurovascular injury with cognitive loss.
Given that pathways of programmed cell death play a dual role in determining the fate of cellular survival and organ systems, it is important to recognize that a balance among these pathways is essential to optimize clinical outcome. For example, activation of autophagy can lead to cardiac disease, atherosclerosis, block interneuron progenitor cell growth, foster neuronal death, lead to memory impairment, and prevent neurovascular protection with growth factors. As a result, the basal activity of autophagy should be considered since changes in the autophagic flux have been shown to limit the induction of cell senescence. With apoptosis, it can be equally as crucial to control early apoptotic pathways that involve PS membrane asymmetry in an effort to block the later phase progression of the apoptotic cascade and prevent nuclear DNA degradation that leads to cell death. In addition, apoptotic pathways are tightly linked to inflammatory cell activity. Although increased glial cell activity may contribute to oxidative stress and apoptosis, microglial cells can be beneficial at times with autophagy induction to maintain cholesterol homeostasis (133), provide protection during amyotrophic lateral sclerosis (657), and for the clearance of amyloid in the brain (598). Triggering receptor expressed on myeloid cells 2 (TREM2) that can foster microglial survival can prevent inflammation during AD through forkhead transcription factors (FoxOs), Wnt signaling, and microglial activation (323, 658).
Pathways with SIRT1, AMPK, and WISP1 also provide us with clues for maintaining a proper environmental homeostasis for cells especially during aging processes that can lead to cognitive loss. SIRT1 is dependent upon NAD+ and nicotinamide and can control insulin sensitivity, prevent cell senescence, modulate immune system activity, promote Aβ degradation, and increase lifespan in higher organisms. In addition, mitochondrial oxidative stress can be dependent upon nicotinamide adenine dinucleotide phosphate (NADPH) depletion and increase of aldose reductase. These pathways are important in the pentose phosphate pathway that involves transaldolase, which is encoded by the TALDO1 gene (659) and has been linked to Parkinson’s disease (660). Such mitochondrial dysfunction can be mediated through mTOR pathways that lead to antiphospholipid antibodies (661). Yet, feedback pathways are required at times through nicotinamide and Akt for replenishment of NAD+ and effective modulation of cellular pathways, such as those involving insulin signaling and inflammation. AMPK activation can improve insulin sensitivity, reduce oxidative stress toxicity, limit tau and Aß cell injury, and lead to cognitive improvement in conjunction with autophagy activation. Yet, during periods when autophagy activation can be detrimental, AMPK may require modulation to limit its activity. This loss of AMPK activity to alter autophagy pathways may negatively impact other pathways such as with SIRT1. There exist conditions when SIRT1 in conjunction with AMPK is required to prevent mitochondrial loss and vascular cell death. WISP1 also requires AMPK pathways to offer glucose homeostasis and protection of neuronal and vascular cells. The ability for WISP1 to effectively promote metabolic stability and cell survival is dependent upon WISP1 providing a proper biological balance of AMPK activity to foster cell survival, control inflammatory pathways, assist with growth factor protection, and prevent cell injury that can occur through autophagic and apoptotic mechanisms. The pathways of programmed cell death, SIRT1, AMPK, and WISP1 offer vital insights and are extremely attractive for identifying processes that can contribute to the onset and progression of metabolic and neurodegenerative diseases that can be linked to multiple entities such as APOE, SARS-CoV-2, NAD+, nicotinamide, and trophic factors, such as EPO, but will require further insight into the elaborate relationship of these pathways for effective clinical translation.
KM: Conceptualization, Writing – original draft, Writing – review & editing.
The author(s) declare financial support was received for the research, authorship, and/or publication of this article. This research was supported by the following grants to KM: American Diabetes Association, American Heart Association, NIH NIEHS, NIH NIA, NIH NINDS, NS053956, and NIH ARRA.
The author declares that the research was conducted in the absence of any commercial or financial relationships that could be construed as a potential conflict of interest.
All claims expressed in this article are solely those of the authors and do not necessarily represent those of their affiliated organizations, or those of the publisher, the editors and the reviewers. Any product that may be evaluated in this article, or claim that may be made by its manufacturer, is not guaranteed or endorsed by the publisher.
2. Maiese K. Dysregulation of Metabolic flexibility: the impact of mtor on autophagy in neurodegenerative disease. Int Rev Neurobiol (2020) 155:1–35. doi: 10.1016/bs.irn.2020.01.009
3. International Diabetes Federation. Diabetes. 9th Edition. Brussels, Belgium: IDF Diabetes Atlas (2019).
4. Maiese K, Chong ZZ, Shang YC, Hou J. Novel avenues of drug discovery and biomarkers for diabetes mellitus. J Clin Pharmacol (2011) 51(2):128–52. doi: 10.1177/0091270010362904
5. Maiese K. The metabolic basis for nervous system dysfunction in alzheimer’s disease, parkinson’s disease, and huntington’s disease. Curr Neurovasc Res (2023) 20(3):314–33. doi: 10.2174/1567202620666230721122957
6. Maiese K. Cognitive impairment with diabetes mellitus and metabolic disease: innovative insights with the mechanistic target of rapamycin and circadian clock gene pathways. Expert Rev Clin Pharmacol (2020) 13(1):23–34. doi: 10.1080/17512433.2020.1698288
7. Maiese K. Cellular metabolism: A fundamental component of degeneration in the nervous system. Biomolecules (2023) 13(5):816. doi: 10.3390/biom13050816
8. Maiese K. Innovative therapeutic strategies for cardiovascular disease. EXCLI J (2023) 22:690–715. doi: 10.17179/excli2023-6306
9. Papachristoforou E, Lambadiari V, Maratou E, Makrilakis K. Association of glycemic indices (Hyperglycemia, glucose variability, and hypoglycemia) with oxidative stress and diabetic complications. J Diabetes Res (2020) 2020:7489795. doi: 10.1155/2020/7489795
10. Min AY, Yoo JM, Sok DE, Kim MR. Mulberry fruit prevents diabetes and diabetic dementia by regulation of blood glucose through upregulation of antioxidative activities and creb/bdnf pathway in alloxan-induced diabetic mice. Oxid Med Cell Longev (2020) 2020:1298691. doi: 10.1155/2020/1298691
11. Xu T, Liu J, Li XR, Yu Y, Luo X, Zheng X, et al. The mtor/nf-κb pathway mediates neuroinflammation and synaptic plasticity in diabetic encephalopathy. Mol Neurobiol (2021) 58(8):3848–62. doi: 10.1007/s12035-021-02390-1
12. Wang Z, Wu Q, Wang H, Gao Y, Nie K, Tang Y, et al. Diosgenin Protects against Podocyte Injury in Early Phase of Diabetic Nephropathy through Regulating Sirt6. Phytomedicine: Int J phytotherapy phytopharmacology (2022) 104:154276. doi: 10.1016/j.phymed.2022.154276
13. Wasserfurth P, Nebl J, Rühling MR, Shammas H, Bednarczyk J, Koehler K, et al. Impact of dietary modifications on plasma sirtuins 1, 3 and 5 in older overweight individuals undergoing 12-weeks of circuit training. Nutrients (2021) 13(11). doi: 10.3390/nu13113824
14. Miranda LMO, Agostini LDC, Lima WG, Camini FC, Costa DC. Silymarin attenuates hepatic and pancreatic redox imbalance independent of glycemic regulation in the alloxan-induced diabetic rat model. BioMed Environ Sci (2020) 33(9):690–700. doi: 10.3967/bes2020.090
15. Wang H, Zhang R, Wu X, Chen Y, Ji W, Wang J, et al. The wnt signaling pathway in diabetic nephropathy. Front Cell Dev Biol (2021) 9:701547. doi: 10.3389/fcell.2021.701547
16. Barcena ML, Tonini G, Haritonow N, Breiter P, Milting H, Baczko I, et al. Sex and age differences in ampk phosphorylation, mitochondrial homeostasis, and inflammation in hearts from inflammatory cardiomyopathy patients. Aging Cell (2023) 22(8):e13894. doi: 10.1111/acel.13894
17. Kostić M, Korićanac G, Tepavčević S, Stanišić J, Romić S, Ćulafić T, et al. Low-intensity exercise affects cardiac fatty acid oxidation by increasing the nuclear content of pparα, foxo1, and lipin1 in fructose-fed rats. Metab Syndr Relat Disord (2023) 21(2):122–31. doi: 10.1089/met.2022.0078
18. Stojanovic D, Stojanovic M, Milenkovic J, Velickov A, Ignjatovic A, Milojkovic M. The multi-faceted nature of renalase for mitochondrial dysfunction improvement in cardiac disease. Cells (2023) 12(12):1607. doi: 10.3390/cells12121607
19. Jiang W, Ding K, Yue R, Lei M. Therapeutic effects of icariin and icariside ii on diabetes mellitus and its complications. Crit Rev Food Sci Nutr (2023) 1–26. doi: 10.1080/10408398.2022.2159317
20. Raghuvanshi DS, Chakole S, Kumar M. Relationship between vitamins and diabetes. Cureus (2023) 15(3):e36815. doi: 10.7759/cureus.36815
21. Raut SK, Khullar M. Oxidative stress in metabolic diseases: current scenario and therapeutic relevance. Mol Cell Biochem (2023) 478(1):185–96. doi: 10.1007/s11010-022-04496-z
22. World health organization. Description of the global burden of ncds, their risk factors and determinants. Global Status Rep noncommunicable Dis 2010 (2011), 1–176.
23. Centers for disease control and prevention Vol. 314227-A. Atlanta, GA, USA: National Diabetes Statistics Report (2020) p. 1–30.
24. Maiese K. Nicotinamide as a foundation for treating neurodegenerative disease and metabolic disorders. Curr Neurovasc Res (2021) 18(1):134–49. doi: 10.2174/1567202617999210104220334
25. Ajiboye BO, Shonibare MT, Oyinloye BE. Antidiabetic activity of watermelon (Citrullus lanatus) juice in alloxan-induced diabetic rats. J Diabetes Metab Disord (2020) 19(1):343–52. doi: 10.1007/s40200-020-00515-2
26. Arildsen L, Andersen JV, Waagepetersen HS, Nissen JBD, Sheykhzade M. Hypermetabolism and impaired endothelium-dependent vasodilation in mesenteric arteries of type 2 diabetes mellitus db/db mice. Diabetes Vasc Dis Res (2019) 16(6):1479164119865885. doi: 10.1177/1479164119865885
27. Bayaraa O, Inman CK, Thomas SA, Al Jallaf F, Alshaikh M, Idaghdour Y, et al. Hyperglycemic conditions induce rapid cell dysfunction-promoting transcriptional alterations in human aortic endothelial cells. Sci Rep (2022) 12(1):20912. doi: 10.1038/s41598-022-24999-5
28. Burillo J, Marqués P, Jiménez B, González-Blanco C, Benito M, Guillén C. Insulin resistance and diabetes mellitus in alzheimer’s disease. Cells (2021) 10(5):1236. doi: 10.3390/cells10051236
29. Chen Y, Huang C, Zhu SY, Zou HC, Xu CY, Chen YX. Overexpression of hotair attenuates pi-induced vascular calcification by inhibiting wnt/β-catenin through regulating mir-126/klotho/sirt1 axis. Mol Cell Biochem (2021) 476(10):3551–61. doi: 10.1007/s11010-021-04164-8
30. Gong Q, Wang H, Yu P, Qian T, Xu X. Protective or harmful: the dual roles of autophagy in diabetic retinopathy. Front Med (Lausanne) (2021) 8:644121. doi: 10.3389/fmed.2021.644121
31. Karamzad N, Faraji E, Adeli S, Sullman MJM, Pourghassem Gargari B. The effect of menaquinone-7 supplementation on dp-ucmgp, pivkaii, inflammatory markers, and body composition in type 2 diabetes patients: A randomized clinical trial. Nutr Diabetes (2022) 12(1):15. doi: 10.1038/s41387-022-00192-5
32. Maiese K. New insights for oxidative stress and diabetes mellitus. Oxid Med Cell Longev (2015) 2015:875961. doi: 10.1155/2015/875961
33. Maiese K. Prospects and perspectives for wisp1 (Ccn4) in diabetes mellitus. Curr Neurovasc Res (2020) 17(3):327–31. doi: 10.2174/1567202617666200327125257
34. Maiese K. Nicotinamide: oversight of metabolic dysfunction through sirt1, mtor, and clock genes. Curr Neurovasc Res (2020) 17(5):765–83. doi: 10.2174/1567202617999201111195232
35. O’Donnell BT, Monjure TA, Al-Ghadban S, Ives CJ, L’Ecuyer MP, Rhee C, et al. Aberrant expression of cox-2 and foxg1 in infrapatellar fat pad-derived ascs from pre-diabetic donors. Cells (2022) 11(15):2367. doi: 10.3390/cells11152367
36. Ren L. Circular rna pip5k1a act as microrna-552-3p sponge to regulates inflammation, oxidative damage in glucolipotoxicity-induced pancreatic ins-1 β-cells via janus kinase 1. Bioengineered (2022) 13(3):5724–36. doi: 10.1080/21655979.2021.2022076
37. Zuo J, Zhang Z, Luo M, Zhou L, Nice EC, Zhang W, et al. Redox Signaling at the Crossroads of Human Health And disease. MedComm (2020) (2022) 3(2):e127. doi: 10.1002/mco2.127
38. Maiese K. Novel nervous and multi-system regenerative therapeutic strategies for diabetes mellitus with mtor. Neural regeneration Res (2016) 11(3):372–85. doi: 10.4103/1673-5374.179032
39. Harris MI, Eastman RC. Early detection of undiagnosed diabetes mellitus: A us perspective. Diabetes Metab Res Rev (2000) 16(4):230–6. doi: 10.1002/1520-7560(2000)9999:9999<::aid-dmrr122>3.0.co;2-w
40. Maiese K. Mtor: driving apoptosis and autophagy for neurocardiac complications of diabetes mellitus. World J Diabetes (2015) 6(2):217–24. doi: 10.4239/wjd.v6.i2.217
41. Maiese K, Chong ZZ, Shang YC. Mechanistic insights into diabetes mellitus and oxidative stress. Curr Med Chem (2007) 14(16):1729–38. doi: 10.2174/092986707781058968
42. Maiese K. New insights for nicotinamide: metabolic disease, autophagy, and mtor. Front bioscience (Landmark edition) (2020) 25:1925–73. doi: 10.2741/4886
43. Bahorik A, Bobrow K, Hoang T, Yaffe K. Increased risk of dementia in older female us veterans with alcohol use disorder. Addiction (2021) 116(8):2049–55. doi: 10.1111/add.15416
44. Caberlotto L, Nguyen TP, Lauria M, Priami C, Rimondini R, Maioli S, et al. Cross-disease analysis of alzheimer’s disease and type-2 diabetes highlights the role of autophagy in the pathophysiology of two highly comorbid diseases. Sci Rep (2019) 9(1):3965. doi: 10.1038/s41598-019-39828-5
45. Karamzad N, Maleki V, Carson-Chahhoud K, Azizi S, Sahebkar A, Gargari BP. A systematic review on the mechanisms of vitamin K effects on the complications of diabetes and pre-diabetes. BioFactors (Oxford England) (2019) 46(1):21–37. doi: 10.1002/biof.1569
46. Pinchera B, Scotto R, Buonomo AR, Zappulo E, Stagnaro F, Gallicchio A, et al. Diabetes and covid-19: the potential role of mtor. Diabetes Res Clin Pract (2022) 186:109813. doi: 10.1016/j.diabres.2022.109813
47. Orkaby AR, Dushkes R, Ward R, Djousse L, Buring JE, Lee IM, et al. Effect of vitamin D3 and omega-3 fatty acid supplementation on risk of frailty: an ancillary study of a randomized clinical trial. JAMA Network Open (2022) 5(9):e2231206. doi: 10.1001/jamanetworkopen.2022.31206
48. Alves HR, Lomba GSB, Gonçalves-de-Albuquerque CF, Burth P. Irisin, exercise, and covid-19. Front Endocrinol (2022) 13:879066. doi: 10.3389/fendo.2022.879066
49. Bramante CT, Beckman KB, Mehta T, Karger AB, Odde DJ, Tignanelli CJ, et al. Metformin reduces sars-cov-2 in a phase 3 randomized placebo controlled clinical trial. medRxiv (2023). doi: 10.1101/2023.06.06.23290989
50. Maiese K. The mechanistic target of rapamycin (Mtor): novel considerations as an antiviral treatment. Curr Neurovasc Res (2020) 17(3):332–7. doi: 10.2174/1567202617666200425205122
51. Miller R, Wentzel AR, Richards GA. Covid-19: nad(+) deficiency may predispose the aged, obese and type2 diabetics to mortality through its effect on sirt1 activity. Med Hypotheses (2020) 144:110044. doi: 10.1016/j.mehy.2020.110044
52. Ong AN, Tan CC, Cañete MT, Lim BA, Robles J. Association between metformin use and mortality among patients with type 2 diabetes mellitus hospitalized for covid-19 infection. J ASEAN Fed Endocr Soc (2021) 36(2):133–41. doi: 10.15605/jafes.036.02.20
53. Rotllan N, Camacho M, Tondo M, Diarte-Añazco EMG, Canyelles M, Méndez-Lara KA, et al. Therapeutic potential of emerging nad+-increasing strategies for cardiovascular diseases. Antioxidants (Basel Switzerland) (2021) 10(12):1939. doi: 10.3390/antiox10121939
54. Swain O, Romano SK, Miryala R, Tsai J, Parikh V, Umanah GKE. Sars-cov-2 neuronal invasion and complications: potential mechanisms and therapeutic approaches. J Neurosci (2021) 41(25):5338–49. doi: 10.1523/jneurosci.3188-20.2021
55. Prattichizzo F, De Nigris V, Spiga R, Mancuso E, La Sala L, Antonicelli R, et al. Inflammageing and metaflammation: the yin and yang of type 2 diabetes. Ageing Res Rev (2018) 41:1–17. doi: 10.1016/j.arr.2017.10.003
56. Speer H, D’Cunha NM, Alexopoulos NI, McKune AJ, Naumovski N. Anthocyanins and human health-a focus on oxidative stress, inflammation and disease. Antioxidants (Basel Switzerland) (2020) 9(5):366. doi: 10.3390/antiox9050366
57. Maiese K. Picking a bone with wisp1 (Ccn4): new strategies against degenerative joint disease. J Transl Sci (2016) 1(3):83–5. doi: 10.15761/JTS.1000120
58. Liu Z, Gan L, Zhang T, Ren Q, Sun C. Melatonin alleviates adipose inflammation through elevating alpha-ketoglutarate and diverting adipose-derived exosomes to macrophages in mice. J Pineal Res (2018) 64(1):12455. doi: 10.1111/jpi.12455
59. Quesada I, de Paola M, Torres-Palazzolo C, Camargo A, Ferder L, Manucha W, et al. Effect of garlic’s active constituents in inflammation, obesity and cardiovascular disease. Curr Hypertens Rep (2020) 22(1):6. doi: 10.1007/s11906-019-1009-9
60. Schell M, Wardelmann K, Kleinridders A. Untangling the effect of insulin action on brain mitochondria and metabolism. J Neuroendocrinol (2021) 33(4):e12932. doi: 10.1111/jne.12932
61. Yang J, Suo H, Song J. Protective role of mitoquinone against impaired mitochondrial homeostasis in metabolic syndrome. Crit Rev Food Sci Nutr (2021) 61(22):3857–387. doi: 10.1080/10408398.2020.1809344
62. Ciesielska K, Gajewska M. Fatty acids as potent modulators of autophagy activity in white adipose tissue. Biomolecules (2023) 13(2):255. doi: 10.3390/biom13020255
63. du Toit WL, Kruger R, Gafane-Matemane LF, Schutte AE, Louw R, Mels CMC. Markers of arterial stiffness and urinary metabolomics in young adults with early cardiovascular risk: the african-predict study. Metabolomics (2023) 19(4):28. doi: 10.1007/s11306-023-01987-y
64. Kahmini FR, Ghaleh HD, Shahgaldi S. Sirtuins: subtle regulators involved in convoluted mechanisms of pregnancy. Cell Physiol Biochem (2022) 56(6):644–62. doi: 10.33594/000000588
65. Chong ZZ, Li F, Maiese K. Oxidative stress in the brain: novel cellular targets that govern survival during neurodegenerative disease. Prog Neurobiol (2005) 75(3):207–46. doi: 10.1016/j.pneurobio.2005.02.004
66. Maiese K. Novel applications of trophic factors, wnt and wisp for neuronal repair and regeneration in metabolic disease. Neural regeneration Res (2015) 10(4):518–28. doi: 10.4103/1673-5374.155427
67. Maiese K, Chong ZZ, Hou J, Shang YC. Oxidative stress: biomarkers and novel therapeutic pathways. Exp Gerontol (2010) 45(3):217–34. doi: 10.1016/j.exger.2010.01.004
68. Maiese K, Chong ZZ, Shang YC. Outfoxoing disease and disability: the therapeutic potential of targeting foxo proteins. Trends Mol Med (2008) 14(5):219–27. doi: 10.1016/j.molmed.2008.03.002
69. Fernandes J, Uppal K, Liu KH, Hu X, Orr M, Tran V, et al. Antagonistic interactions in mitochondria ros signaling responses to manganese. Antioxidants (Basel Switzerland) (2023) 12(4):804. doi: 10.3390/antiox12040804
70. Sun C, Bai S, Liang Y, Liu D, Liao J, Chen Y, et al. The role of sirtuin 1 and its activators in age-related lung disease. BioMed Pharmacother (2023) 162:114573. doi: 10.1016/j.biopha.2023.114573
71. Tramutola A, Lanzillotta S, Aceto G, Pagnotta S, Ruffolo G, Cifelli P, et al. Intranasal administration of kyccsrk peptide rescues brain insulin signaling activation and reduces alzheimer’s disease-like neuropathology in a mouse model for down syndrome. Antioxidants (Basel Switzerland) (2023) 12(1):111. doi: 10.3390/antiox12010111
72. Muthu S, Jeyaraman M, Jeyaraman N, Rajendran RL, Gangadaran P. Where do we stand in stem cell therapy for the management of diabetes mellitus?-a scientometric research trend analysis from 1990 to 2020. Bioengineering (Basel) (2021) 8(11):159. doi: 10.3390/bioengineering8110159
73. Wang R, Zhu Y, Qin LF, Xu ZG, Gao XR, Liu CB, et al. Comprehensive bibliometric analysis of stem cell research in alzheimer’s disease from 2004 to 2022. Dement Geriatr Cognit Disord (2023) 52(2):41–73. doi: 10.1159/000528886
74. Feng J, Wang H, Jing Z, Wang Y, Cheng Y, Wang W, et al. Role of magnesium in type 2 diabetes mellitus. Biol Trace element Res (2019) 196(1):74–85. doi: 10.1007/s12011-019-01922-0
75. Fernandez-Ruiz R, García-Alamán A, Esteban Y, Mir-Coll J, Serra-Navarro B, Fontcuberta-PiSunyer M, et al. Wisp1 is a circulating factor that stimulates proliferation of adult mouse and human beta cells. Nat Commun (2020) 11(1):5982. doi: 10.1038/s41467-020-19657-1
76. Guo T, Liu T, Sun Y, Liu X, Xiong R, Li H, et al. Sonodynamic therapy inhibits palmitate-induced beta cell dysfunction via pink1/parkin-dependent mitophagy. Cell Death Dis (2019) 10(6):457. doi: 10.1038/s41419-019-1695-x
77. Hu R, Zhu X, Yuan M, Ho KH, Kaverina I, Gu G. Microtubules and Gαo-signaling modulate the preferential secretion of young insulin secretory granules in islet β Cells via independent pathways. PloS One (2021) 16(7):e0241939. doi: 10.1371/journal.pone.0241939
78. Jalgaonkar MP, Parmar UM, Kulkarni YA, Oza MJ. Sirt1-foxos activity regulates diabetic complications. Pharmacol Res (2022) 175:106014. doi: 10.1016/j.phrs.2021.106014
79. Li R, Wang B, Wu C, Li D, Wu Y, Ye L, et al. Acidic fibroblast growth factor attenuates type 2 diabetes-induced demyelination via suppressing oxidative stress damage. Cell Death Dis (2021) 12(1):107. doi: 10.1038/s41419-021-03407-2
80. Quintana-Pérez JC, García-Dolores F, Valdez-Guerrero AS, Alemán-González-Duhart D, Arellano-Mendoza MG, Rojas Hernández S, et al. Modeling type 2 diabetes in rats by administering tacrolimus. Islets (2022) 14(1):114–27. doi: 10.1080/19382014.2022.2051991
81. Hill JH, Solt C, Foster MT. Obesity associated disease risk: the role of inherent differences and location of adipose depots. Hormone Mol Biol Clin Invest (2018) 33(2):20180012. doi: 10.1515/hmbci-2018-0012
82. Nie X, Wei X, Ma H, Fan L, Chen WD. The complex role of wnt ligands in type 2 diabetes mellitus and related complications. J Cell Mol Med (2021) 25(14):6479–95. doi: 10.1111/jcmm.16663
83. Sanabria-de la Torre R, García-Fontana C, González-Salvatierra S, Andújar-Vera F, Martínez-Heredia L, García-Fontana B, et al. The contribution of wnt signaling to vascular complications in type 2 diabetes mellitus. Int J Mol Sci (2022) 23(13):6995. doi: 10.3390/ijms23136995
84. Sun ZY, Yu TY, Jiang FX, Wang W. Functional maturation of immature β Cells: A roadblock for stem cell therapy for type 1 diabetes. World J Stem Cells (2021) 13(3):193–207. doi: 10.4252/wjsc.v13.i3.193
85. Tan S, Zang G, Wang Y, Sun Z, Li Y, Lu C, et al. Differences of angiogenesis factors in tumor and diabetes mellitus. Diabetes Metab Syndr Obes (2021) 14:3375–88. doi: 10.2147/dmso.S315362
86. Zaiou M. Circrnas signature as potential diagnostic and prognostic biomarker for diabetes mellitus and related cardiovascular complications. Cells (2020) 9(3):659. doi: 10.3390/cells9030659
87. Zarneshan SN, Fakhri S, Farzaei MH, Khan H, Saso L. Astaxanthin targets pi3k/akt signaling pathway toward potential therapeutic applications. Food Chem Toxicol (2020) 145:111714. doi: 10.1016/j.fct.2020.111714
88. Centers for Medicare and Medicaid Services. National health expenditure projections 2018-2027 (Baltimore, Maryland, USA). (2019).
89. Sonsalla MM, Lamming DW. Geroprotective interventions in the 3xtg mouse model of alzheimer’s disease. Geroscience (2023) 45(3):1343–81. doi: 10.1007/s11357-023-00782-w
90. Singh A, Kukreti R, Saso L, Kukreti S. Mechanistic insight into oxidative stress-triggered signaling pathways and type 2 diabetes. Molecules (2022) 27(3):950. doi: 10.3390/molecules27030950
91. Liu L, Cao Q, Gao W, Li BY, Zeng C, Xia Z, et al. Melatonin ameliorates cerebral ischemia-reperfusion injury in diabetic mice by enhancing autophagy via the sirt1-bmal1 pathway. FASEB J (2021) 35(12):e22040. doi: 10.1096/fj.202002718RR
92. Heer CD, Sanderson DJ, Voth LS, Alhammad YMO, Schmidt MS, Trammell SAJ, et al. Coronavirus infection and parp expression dysregulate the nad metabolome: an actionable component of innate immunity. J Biol Chem (2020) 295(52):17986–96. doi: 10.1074/jbc.RA120.015138
93. Li J, Lin FH, Zhu XM, Lv ZM. Impact of diabetic hyperglycaemia and insulin therapy on autophagy and impairment in rat epididymis. Andrologia (2020) 52(11):e13889. doi: 10.1111/and.13889
94. Tomita Y, Lee D, Tsubota K, Kurihara T. Pparα Agonist oral therapy in diabetic retinopathy. Biomedicines (2020) 8(10):433. doi: 10.3390/biomedicines8100433
95. Maiese K. Driving neural regeneration through the mammalian target of rapamycin. Neural regeneration Res (2014) 9(15):1413–7. doi: 10.4103/1673-5374.139453
96. Maiese K. The bright side of reactive oxygen species: lifespan extension without cellular demise. J Transl Sci (2016) 2(3):185–7. doi: 10.15761/jts.1000138
97. Maiese K. The mechanistic target of rapamycin (Mtor) and the silent mating-type information regulation 2 homolog 1 (Sirt1): oversight for neurodegenerative disorders. Biochem Soc Trans (2018) 46(2):351–60. doi: 10.1042/bst20170121
98. Ministrini S, Puspitasari YM, Beer G, Liberale L, Montecucco F, Camici GG. Sirtuin 1 in endothelial dysfunction and cardiovascular aging. Front Physiol (2021) 12:733696. doi: 10.3389/fphys.2021.733696
99. Olejniczak I, Pilorz V, Oster H. Circle(S) of life: the circadian clock from birth to death. Biol (Basel) (2023) 12(3):383. doi: 10.3390/biology12030383
100. Yamamoto H, Shimomura N, Oura K, Hasegawa Y. Nacre extract from pearl oyster shell prevents D-galactose-induced brain and skin aging. Mar Biotechnol (NY) (2023) 25(4):503–18. doi: 10.1007/s10126-022-10192-2
101. National Center for Health Statistics. National vital statisitcs system. Hyattsville, Maryland, USA: National Center for Health Statistics Fact Sheet (2019) p. 1–2.
102. Begum MK, Konja D, Singh S, Chlopicki S, Wang Y. Endothelial sirt1 as a target for the prevention of arterial aging: promises and challenges. J Cardiovasc Pharmacol (2021) 78(Suppl 6):S63–s77. doi: 10.1097/fjc.0000000000001154
103. Braidy N, Liu Y. Nad+ Therapy in age-related degenerative disorders: A benefit/risk analysis. Exp Gerontol (2020) 132:110831. doi: 10.1016/j.exger.2020.110831
104. Castro-Portuguez R, Sutphin GL. Kynurenine pathway, nad(+) synthesis, and mitochondrial function: targeting tryptophan metabolism to promote longevity and healthspan. Exp Gerontol (2020) 132:110841. doi: 10.1016/j.exger.2020.110841
105. Chong ZZ, Maiese K. Mammalian target of rapamycin signaling in diabetic cardiovascular disease. Cardiovasc Diabetol (2012) 11(1):45. doi: 10.1186/1475-2840-11-45
106. Chong ZZ, Shang YC, Wang S, Maiese K. Sirt1: new avenues of discovery for disorders of oxidative stress. Expert Opin Ther Targets (2012) 16(2):167–78. doi: 10.1517/14728222.2012.648926
107. De Nobrega AK, Luz KV, Lyons LC. Resetting the aging clock: implications for managing age-related diseases. Adv Exp Med Biol (2020) 1260:193–265. doi: 10.1007/978-3-030-42667-5_9
108. Fischer F, Grigolon G, Benner C, Ristow M. Evolutionarily conserved transcription factors as regulators of longevity and targets for geroprotection. Physiol Rev (2022) 102(3):1449–94. doi: 10.1152/physrev.00017.2021
109. Ji JS, Liu L, Zeng Y, Yan LL. Effect of foxo3 and air pollution on cognitive function: A longitudinal cohort study of older adults in China from 2000 to 2014. J Gerontol A Biol Sci Med Sci (2022) 77(8):1534–41. doi: 10.1093/gerona/glac016
110. Lathe R, St Clair D. Programmed ageing: decline of stem cell renewal, immunosenescence, and alzheimer’s disease. Biol Rev Cambridge Philos Soc (2023) 98(4):1424–58. doi: 10.1111/brv.12959
111. Maiese K. Sirt1 and stem cells: in the forefront with cardiovascular disease, neurodegeneration and cancer. World J Stem Cells (2015) 7(2):235–42. doi: 10.4252/wjsc.v7.i2.235
112. Tabibzadeh S. Signaling pathways and effectors of aging. Front bioscience (Landmark edition) (2021) 26:50–96. doi: 10.2741/4889
113. Watroba M, Szukiewicz D. Sirtuins at the service of healthy longevity. Front Physiol (2021) 12:724506. doi: 10.3389/fphys.2021.724506
114. Maiese K. Erythropoietin and mtor: A “One-two punch” for aging-related disorders accompanied by enhanced life expectancy. Curr Neurovasc Res (2016) 13(4):329–40. doi: 10.2174/1567202613666160729164900
115. Maiese K. Sirtuins: developing innovative treatments for aged-related memory loss and alzheimer’s disease. Curr Neurovasc Res (2018) 15(4):367–71. doi: 10.2174/1567202616666181128120003
116. World Health Organization. Global action plan on the Public Health Response to Dementia 2017-2025 (Geneva, Switzerland). (2017). pp. 1–44.
117. Maiese K. Targeting the core of neurodegeneration: foxo, mtor, and sirt1. Neural regeneration Res (2021) 16(3):448–55. doi: 10.4103/1673-5374.291382
118. Yu M, Zhang H, Wang B, Zhang Y, Zheng X, Shao B, et al. Key signaling pathways in aging and potential interventions for healthy aging. Cells (2021) 10(3):660. doi: 10.3390/cells10030660
119. Adhikari UK, Khan R, Mikhael M, Balez R, David MA, Mahns D, et al. Therapeutic Anti-Amyloid β Antibodies Cause Neuronal Disturbances. Alzheimer’s & dementia: the journal of the Alzheimer’s Association (2022). doi: 10.1002/alz.12833
120. Ahmad R, Khan A, Rehman IU, Lee HJ, Khan I, Kim MO. Lupeol Treatment Attenuates activation of glial cells and oxidative-stress-mediated neuropathology in mouse model of traumatic brain injury. Int J Mol Sci (2022) 23(11):6086. doi: 10.3390/ijms23116086
121. Amini J, Sanchooli N, Milajerdi MH, Baeeri M, Haddadi M, Sanadgol N. The interplay between tauopathy and aging through interruption of upr/nrf2/autophagy crosstalk in the alzheimer’s disease transgenic experimental models. Int J Neurosci (2023), 1–27. doi: 10.1080/00207454.2023.2210409
122. Guo T, Chen M, Liu J, Wei Z, Yuan J, Wu W, et al. Neuropilin-1 promotes mitochondrial structural repair and functional recovery in rats with cerebral ischemia. J Trans Med (2023) 21(1):297. doi: 10.1186/s12967-023-04125-3
123. Jarero-Basulto J, Rivera-Cervantes M, Gasca-Martínez D, García-Sierra F, Gasca-Martínez Y, Beas-Zárate C. Current evidence on the protective effects of recombinant human erythropoietin and its molecular variants against pathological hallmarks of alzheimer’s disease. Pharm (Basel Switzerland) (2020) 13(424):1–22. doi: 10.3390/ph13120424
124. Liu B, Zhao G, Jin L, Shi J. Nicotinamide improves cognitive function in mice with chronic cerebral hypoperfusion. Front Neurol (2021) 12:596641. doi: 10.3389/fneur.2021.596641
125. Maiese K. The many facets of cell injury: angiogenesis to autophagy. Curr Neurovasc Res (2012) 9(2):1–2. doi: 10.2174/156720212800410911
126. Mavroidi B, Kaminari A, Matiadis D, Hadjipavlou-Litina D, Pelecanou M, Tzinia A, et al. The prophylactic and multimodal activity of two isatin thiosemicarbazones against alzheimer’s disease in vitro. Brain Sci (2022) 12(6):806. doi: 10.3390/brainsci12060806
127. Pontifex MG, Martinsen A, Saleh RNM, Harden G, Tejera N, Müller M, et al. Apoe4 genotype exacerbates the impact of menopause on cognition and synaptic plasticity in apoe-tr mice. FASEB J (2021) 35(5):e21583. doi: 10.1096/fj.202002621RR
128. Torii T, Miyamoto Y, Nakata R, Higashi Y, Shinmyo Y, Kawasaki H, et al. Identification of tau protein as a novel marker for maturation and pathological changes of oligodendrocytes. Glia (2023) 71(4):1002–17. doi: 10.1002/glia.24322
129. Yalçin M, Mundorf A, Thiel F, Amatriain-Fernández S, Kalthoff IS, Beucke JC, et al. It’s about time: the circadian network as time-keeper for cognitive functioning, locomotor activity and mental health. Front Physiol (2022) 13:873237. doi: 10.3389/fphys.2022.873237
130. Maiese K. Wisp1: clinical insights for a proliferative and restorative member of the ccn family. Curr Neurovasc Res (2014) 11(4):378–89. doi: 10.2174/1567202611666140912115107
131. Levine KS, Leonard HL, Blauwendraat C, Iwaki H, Johnson N, Bandres-Ciga S, et al. Virus exposure and neurodegenerative disease risk across national biobanks. Neuron (2023) 111(7):1086–93. doi: 10.1016/j.neuron.2022.12.029
132. Pradhan SS, Rao KR, Manjunath M, Saiswaroop R, Patnana DP, Phalguna KS, et al. Vitamin B(6), B(12) and folate modulate deregulated pathways and protein aggregation in yeast model of huntington disease. 3 Biotech (2023) 13(3):96. doi: 10.1007/s13205-023-03525-y
133. Zhang WB, Huang Y, Guo XR, Zhang MQ, Yuan XS, Zu HB. Dhcr24 reverses alzheimer’s disease-related pathology and cognitive impairment via increasing hippocampal cholesterol levels in 5xfad mice. Acta neuropathologica Commun (2023) 11(1):102. doi: 10.1186/s40478-023-01593-y
134. Maiese K. Targeting molecules to medicine with mtor, autophagy and neurodegenerative disorders. Br J Clin Pharmacol (2016) 82(5):1245–66. doi: 10.1111/bcp.12804
135. Borowicz-Reutt KK, Czuczwar SJ. Role of oxidative stress in epileptogenesis and potential implications for therapy. Pharmacol Rep (2020) 72(5):1218–26. doi: 10.1007/s43440-020-00143-w
136. Corti O, Blomgren K, Poletti A, Beart PM. Autophagy in neurodegeneration: new insights underpinning therapy for neurological diseases. J Neurochem (2020) 154(4):e15002. doi: 10.1111/jnc.15002
137. Duitama M, Vargas-López V, Casas Z, Albarracin SL, Sutachan JJ, Torres YP. Trp channels role in pain associated with neurodegenerative diseases. Front Neurosci (2020) 14:782. doi: 10.3389/fnins.2020.00782
138. Kaur D, Behl T, Sehgal A, Singh S, Sharma N, Badavath VN, et al. Unravelling the potential neuroprotective facets of erythropoietin for the treatment of alzheimer’s disease. Metab Brain Dis (2021) 37(1):1–16. doi: 10.1007/s11011-021-00820-6
139. Kuan XY, Fauzi NSA, Ng KY, Bakhtiar A. Exploring the causal relationship between telomere biology and alzheimer’s disease. Mol Neurobiol (2023) 60(8):4169–83. doi: 10.1007/s12035-023-03337-4
140. LaCroix MS, Mirbaha H, Shang P, Zandee S, Foong C, Prat A, et al. Tau seeding in cases of multiple sclerosis. Acta neuropathologica Commun (2022) 10(1):146. doi: 10.1186/s40478-022-01444-2
141. Maiese K. Neurodegeneration, memory loss, and dementia: the impact of biological clocks and circadian rhythm. Front bioscience (Landmark edition) (2021) 26(9):614–27. doi: 10.52586/4971
142. Mishra P, Davies DA, Albensi BC. The interaction between nf-κb and estrogen in alzheimer’s disease. Mol Neurobiol (2022) 60(3):1515–26. doi: 10.1007/s12035-022-03152-3
143. Sabzali M, Eidi A, Khaksari M, Khastar H. Anti-inflammatory, antioxidant, and antiapoptotic action of metformin attenuates ethanol neurotoxicity in the animal model of fetal alcohol spectrum disorders. Neurotox Res (2022) 40(2):605–13. doi: 10.1007/s12640-022-00499-2
144. Salemi M, Mogavero MP, Lanza G, Mongioì LM, Calogero AE, Ferri R. Examples of inverse comorbidity between cancer and neurodegenerative diseases: A possible role for noncoding rna. Cells (2022) 11(12):1930. doi: 10.3390/cells11121930
145. Senousy MA, Hanafy ME, Shehata N, Rizk SM. Erythropoietin and bacillus calmette-guérin vaccination mitigate 3-nitropropionic acid-induced huntington-like disease in rats by modulating the pi3k/akt/mtor/P70s6k pathway and enhancing the autophagy. ACS Chem Neurosci (2022) 13(6):721–32. doi: 10.1021/acschemneuro.1c00523
146. Su LD, Wang N, Han J, Shen Y. Group 1 metabotropic glutamate receptors in neurological and psychiatric diseases: mechanisms and prospective. Neuroscientist (2021) 28(5):10738584211021018. doi: 10.1177/10738584211021018
147. World Health Organization. Dementia: A public health priority. Geneva: World Health Organization (2012) p. 1–4.
148. Maiese K. Taking Aim at Alzheimer’s Disease through the Mammalian Target of Rapamycin. Ann Med (2014) 46(8):587–96. doi: 10.3109/07853890.2014.941921
149. Farmer K, Abd-Elrahman KS, Derksen A, Rowe EM, Thompson AM, Rudyk CA, et al. Mglur5 allosteric modulation promotes neurorecovery in a 6-ohda-toxicant model of parkinson’s disease. Mol Neurobiol (2020) 57(3):1418–31. doi: 10.1007/s12035-019-01818-z
150. Galzigna L, De Iuliis A, Zanatta L. Enzymatic dopamine peroxidation in substantia nigra of human brain. Clin Chim Acta (2000) 300(1-2):131–8. doi: 10.1016/s0009-8981(00)00313-2
151. Gonzalo-Gobernado R, Perucho J, Vallejo-Muñoz M, Casarejos MJ, Reimers D, Jiménez-Escrig A, et al. Liver growth factor “Lgf” as a therapeutic agent for alzheimer’s disease. Int J Mol Sci (2020) 21(3):9201. doi: 10.3390/ijms21239201
152. Jayaraj RL, Beiram R, Azimullah S, Mf NM, Ojha SK, Adem A, et al. Valeric acid protects dopaminergic neurons by suppressing oxidative stress, neuroinflammation and modulating autophagy pathways. Int J Mol Sci (2020) 21(20):7670. doi: 10.3390/ijms21207670
153. Khan H, Tundis R, Ullah H, Aschner M, Belwal T, Mirzaei H, et al. Flavonoids targeting nrf2 in neurodegenerative disorders. Food Chem Toxicol (2020) 146:111817. doi: 10.1016/j.fct.2020.111817
154. Kowalska M, Piekut T, Prendecki M, Sodel A, Kozubski W, Dorszewska J. Mitochondrial and nuclear DNA oxidative damage in physiological and pathological aging. DNA Cell Biol (2020) 39(8):1410–20. doi: 10.1089/dna.2019.5347
155. Lei Q, Wu T, Wu J, Hu X, Guan Y, Wang Y, et al. Roles of α−Synuclein in gastrointestinal microbiome dysbiosis−Related parkinson’s disease progression (Review). Mol Med Rep (2021) 24(4):734. doi: 10.3892/mmr.2021.12374
156. Li X, Feng Y, Wang XX, Truong D, Wu YC. The critical role of sirt1 in parkinson’s disease: mechanism and therapeutic considerations. Aging Dis (2020) 11(6):1608–22. doi: 10.14336/ad.2020.0216
157. Liu J, Liu H, Zhao Z, Wang J, Guo D, Liu Y. Regulation of actg1 and gsta2 is possible mechanism by which capsaicin alleviates apoptosis in cell model of 6-ohda-induced parkinson’s disease. Bioscience Rep (2020) 40(6):BSR20191796. doi: 10.1042/bsr20191796
158. Maiese K. Biomarkers for parkinson’s disease and neurodegenerative disorders: A role for non-coding rnas. Curr Neurovasc Res (2022) 19(2):127–30. doi: 10.2174/1567202619666220602125806
159. Maiese K, Chong ZZ, Shang YC, Wang S. Erythropoietin: new directions for the nervous system. Int J Mol Sci (2012) 13(9):11102–29. doi: 10.3390/ijms130911102
160. Marchetti B. Nrf2/wnt resilience orchestrates rejuvenation of glia-neuron dialogue in parkinson’s disease. Redox Biol (2020) 36:101664. doi: 10.1016/j.redox.2020.101664
161. Margrett JA, Schofield T, Martin P, Poon LW, Masaki K, Donlon TA, et al. Novel functional, health, and genetic determinants of cognitive terminal decline: kuakini honolulu heart program/honolulu-asia aging study. J Gerontol A Biol Sci Med Sci (2021) 77(8):1525–33. doi: 10.1093/gerona/glab327
162. Odnokoz O, Nakatsuka K, Wright C, Castellanos J, Klichko VI, Kretzschmar D, et al. Mitochondrial redox signaling is critical to the normal functioning of the neuronal system. Front Cell Dev Biol (2021) 9:613036. doi: 10.3389/fcell.2021.613036
163. Querfurth H, Lee HK. Mammalian/mechanistic target of rapamycin (Mtor) complexes in neurodegeneration. Mol neurodegeneration (2021) 16(1):44. doi: 10.1186/s13024-021-00428-5
164. Ullah H, Hussain A, Asif M, Nawaz F, Rasool M. Natural products as bioactive agents in the prevention of dementia. CNS Neurol Disord Drug Targets (2023) 22(4):466–76. doi: 10.2174/1871527321666220422085835
165. Wang Q, Zheng J, Pettersson S, Reynolds R, Tan EK. The link between neuroinflammation and the neurovascular unit in synucleinopathies. Sci Adv (2023) 9(7):eabq1141. doi: 10.1126/sciadv.abq1141
166. Liu JJ, Shentu LM, Ma N, Wang LY, Zhang GM, Sun Y, et al. Inhibition of nf-kappab and wnt/beta-catenin/gsk3beta signaling pathways ameliorates cardiomyocyte hypertrophy and fibrosis in streptozotocin (Stz)-induced type 1 diabetic rats. Curr Med Sci (2020) 40(1):35–47. doi: 10.1007/s11596-020-2144-x
167. Maiese K. Erythropoietin and diabetes mellitus. World J Diabetes (2015) 6(14):1259–73. doi: 10.4239/wjd.v6.i14.1259
168. Maiese K, Chong ZZ, Shang YC, Hou J. Foxo proteins: cunning concepts and considerations for the cardiovascular system. Clin Sci (Lond) (2009) 116(3):191–203. doi: 10.1042/CS20080113
169. Maiese K, Hou J, Chong ZZ, Shang YC. A fork in the path: developing therapeutic inroads with foxo proteins. Oxid Med Cell Longev (2009) 2(3):119–29. doi: 10.4161/oxim.2.3.8916
170. Pabel S, Hamdani N, Luedde M, Sossalla S. Sglt2 inhibitors and their mode of action in heart failure-has the mystery been unravelled? Curr Heart Fail Rep (2021) 18(5):315–28. doi: 10.1007/s11897-021-00529-8
171. Ciardullo S, Muraca E, Bianconi E, Cannistraci R, Perra S, Zerbini F, et al. Diabetes mellitus is associated with higher serum neurofilament light chain levels in the general us population. J Clin Endocrinol Metab (2023) 108(2):361–7. doi: 10.1210/clinem/dgac580
172. Maiese K. Programming apoptosis and autophagy with novel approaches for diabetes mellitus. Curr Neurovasc Res (2015) 12(2):173–88. doi: 10.2174/1567202612666150305110929
173. Maiese K. Foxo transcription factors and regenerative pathways in diabetes mellitus. Curr Neurovasc Res (2015) 12(4):404–13. doi: 10.2174/1567202612666150807112524
174. Pouresmaeil V, Al Abudi AH, Mahimid AH, Sarafraz Yazdi M, Es-Haghi A. Evaluation of serum selenium and copper levels with inflammatory cytokines and indices of oxidative stress in type 2 diabetes. Biol Trace element Res (2022) 201(2):617–26. doi: 10.1007/s12011-022-03191-w
175. Beegum F, Anunrajana PV, George KT, Divya KP, Begum F, Krishnadas N, et al. Sirtuins as therapeutic targets for improving delayed wound healing in diabetes. J Drug Target (2022) 30(9):911–926. doi: 10.1080/1061186x.2022.2085729
176. Chiareli RA, Carvalho GA, Marques BL, Mota LS, Oliveira-Lima OC, Gomes RM, et al. The role of astrocytes in the neurorepair process. Front Cell Dev Biol (2021) 9:665795. doi: 10.3389/fcell.2021.665795
177. Ding S, Zhu Y, Liang Y, Huang H, Xu Y, Zhong C. Circular rnas in vascular functions and diseases. Adv Exp Med Biol (2018) 1087:287–97. doi: 10.1007/978-981-13-1426-1_23
178. Geng K, Ma X, Jiang Z, Huang W, Gao C, Pu Y, et al. Innate immunity in diabetic wound healing: focus on the mastermind hidden in chronic inflammatory. Front Pharmacol (2021) 12:653940. doi: 10.3389/fphar.2021.653940
179. Hajibabaie F, Abedpoor N, Safavi K, Taghian F. Natural remedies medicine derived from flaxseed (Secoisolariciresinol diglucoside, lignans, and α-linolenic acid) improve network targeting efficiency of diabetic heart conditions based on computational chemistry techniques and pharmacophore modeling. J Food Biochem (2022) 46(12):e14480. doi: 10.1111/jfbc.14480
180. Liu P, Liu J, Wu Y, Xi W, Wei Y, Yuan Z, et al. Zinc supplementation protects against diabetic endothelial dysfunction via gtp cyclohydrolase 1 restoration. Biochem Biophys Res Commun (2020) 521(4):1049–54. doi: 10.1016/j.bbrc.2019.11.046
181. Maiese K, Li F, Chong ZZ, Shang YC. The wnt signaling pathway: aging gracefully as a protectionist? Pharmacol Ther (2008) 118(1):58–81. doi: 10.1016/j.pharmthera.2008.01.004
182. Zhou Q, Tang S, Zhang X, Chen L. Targeting pras40: A novel therapeutic strategy for human diseases. J Drug Target (2021) 29(7):703–715. doi: 10.1080/1061186x.2021.1882470
183. El-Marasy SA, Abdel-Rahman RF, Abd-Elsalam RM. Neuroprotective effect of vildagliptin against cerebral ischemia in rats. Naunyn Schmiedebergs Arch Pharmacol (2018) 391(10):1133–45. doi: 10.1007/s00210-018-1537-x
184. Januszewski AS, Watson CJ, O’Neill V, McDonald K, Ledwidge M, Robson T, et al. Fkbpl is associated with metabolic parameters and is a novel determinant of cardiovascular disease. Sci Rep (2020) 10(1):21655. doi: 10.1038/s41598-020-78676-6
185. Esterline RL, Vaag A, Oscarsson J, Vora J. Mechanisms in endocrinology: sglt2 inhibitors; clinical benefits by restoration of normal diurnal metabolism? Eur J Endocrinol (2018) 178(4):R113–25. doi: 10.1530/eje-17-0832
186. Mocayar Marón FJ, Ferder L, Reiter RJ, Manucha W. Daily and seasonal mitochondrial protection: unraveling common possible mechanisms involving vitamin D and melatonin. J Steroid Biochem Mol Biol (2020) 199:105595. doi: 10.1016/j.jsbmb.2020.105595
187. Mishra M, Duraisamy AJ, Kowluru RA. Sirt1- a guardian of the development of diabetic retinopathy. Diabetes (2018) 67(4):745–54. doi: 10.2337/db17-0996
188. Qi X, Mitter SK, Yan Y, Busik JV, Grant MB, Boulton ME. Diurnal rhythmicity of autophagy is impaired in the diabetic retina. Cells (2020) 9(4):905. doi: 10.3390/cells9040905
189. Casciano F, Zauli E, Rimondi E, Mura M, Previati M, Busin M, et al. The role of the mtor pathway in diabetic retinopathy. Front Med (Lausanne) (2022) 9:973856. doi: 10.3389/fmed.2022.973856
190. Kita A, Saito Y, Miura N, Miyajima M, Yamamoto S, Sato T, et al. Altered regulation of mesenchymal cell senescence in adipose tissue promotes pathological changes associated with diabetic wound healing. Commun Biol (2022) 5(1):310. doi: 10.1038/s42003-022-03266-3
191. Fadini GP, Morieri ML, Longato E, Avogaro A. Prevalence and impact of diabetes among people infected with sars-cov-2. J endocrinological Invest (2020) 43(6):867–9. doi: 10.1007/s40618-020-01236-2
192. Lally MA, Tsoukas P, Halladay CW, O’Neill E, Gravenstein S, Rudolph JL. Metformin is associated with decreased 30-day mortality among nursing home residents infected with sars-cov2. J Am Med Dir Assoc (2021) 22(1):193–8. doi: 10.1016/j.jamda.2020.10.031
193. Gutiérrez-Pliego LE, Martínez-Carrillo BE, Reséndiz-Albor AA, Valdés-Ramos R. Effect on adipose tissue of diabetic mice supplemented with N-3 fatty acids extracted from microalgae. Endocr Metab Immune Disord Drug Targets (2020) 20(5):728–35. doi: 10.2174/1871530320666200213111452
194. Li S, Vaziri ND, Swentek L, Takasu C, Vo K, Stamos MJ, et al. Prevention of autoimmune diabetes in nod mice by dimethyl fumarate. Antioxidants (Basel Switzerland) (2021) 10(2):193. doi: 10.3390/antiox10020193
195. Maiese K, Chong ZZ, Hou J, Shang YC. The “O” Class: crafting clinical care with foxo transcription factors. Adv Exp Med Biol (2009) 665:242–60. doi: 10.1007/978-1-4419-1599-3_18
196. Maiese K, Chong ZZ, Hou J, Shang YC. The vitamin nicotinamide: translating nutrition into clinical care. Molecules (2009) 14(9):3446–85. doi: 10.3390/molecules14093446
197. Rashidi S, Mansouri R, Ali-Hassanzadeh M, Mojtahedi Z, Shafiei R, Savardashtaki A, et al. The host mtor pathway and parasitic diseases pathogenesis. Parasitol Res (2021) 120(4):1151–66. doi: 10.1007/s00436-021-07070-6
198. Wen S, Jiang W, Zhou L. Islet autoantibodies in the patients with sjogren’s syndrome and thyroid disease and risk of progression to latent autoimmune diabetes in adults: A case series. Diabetes Metab Syndr Obes (2021) 14:1025–33. doi: 10.2147/dmso.S295847
199. Zhuang X, Magri A, Hill M, Lai AG, Kumar A, Rambhatla SB, et al. The circadian clock components bmal1 and rev-erbα Regulate flavivirus replication. Nat Commun (2019) 10(1):377. doi: 10.1038/s41467-019-08299-7
200. Zhang Y, Yuan Y, Zhang J, Zhao Y, Zhang Y, Fu J. Astragaloside iv supplementation attenuates cognitive impairment by inhibiting neuroinflammation and oxidative stress in type 2 diabetic mice. Front Aging Neurosci (2022) 14:1004557. doi: 10.3389/fnagi.2022.1004557
201. Maiese K, Chong ZZ, Wang S, Shang YC. Oxidant stress and signal transduction in the nervous system with the pi 3-K, akt, and mtor cascade. Int J Mol Sci (2013) 13(11):13830–66. doi: 10.3390/ijms131113830
202. Liu C, Zhong C, Chen R, Zhou X, Wu J, Han J, et al. Higher dietary vitamin C intake is associated with a lower risk of gestational diabetes mellitus: A longitudinal cohort study. Clin Nutr (2020) 39(1):198–203. doi: 10.1016/j.clnu.2019.01.015
203. Maiese K. Triple play: promoting neurovascular longevity with nicotinamide, wnt, and erythropoietin in diabetes mellitus. BioMed Pharmacother (2008) 62(4):218–32. doi: 10.1016/j.biopha.2008.01.009
204. Akila Parvathy Dharshini S, Taguchi YH, Michael Gromiha M. Exploring the selective vulnerability in alzheimer disease using tissue specific variant analysis. Genomics (2019) 111(4):936–49. doi: 10.1016/j.ygeno.2018.05.024
205. Amanollahi M, Jameie M, Heidari A, Rezaei N. The dialogue between neuroinflammation and adult neurogenesis: mechanisms involved and alterations in neurological diseases. Mol Neurobiol (2022) 60(2):923–59. doi: 10.1007/s12035-022-03102-z
206. Barnett JA, Bandy ML, Gibson DL. Is the use of glyphosate in modern agriculture resulting in increased neuropsychiatric conditions through modulation of the gut-brain-microbiome axis? Front Nutr (2022) 9:827384. doi: 10.3389/fnut.2022.827384
207. Chen Z, He Y, Hu F, Li M, Yao Y. Genkwanin alleviates mitochondrial dysfunction and oxidative stress in a murine model of experimental colitis: the participation of sirt1. Ann Clin Lab Sci (2022) 52(2):301–13.
208. Lee SH, Lee JJ, Kim GH, Kim JA, Cho HS. Role of reactive oxygen species at reperfusion stage in isoflurane preconditioning-induced neuroprotection. Brain Res (2019) 1723:146405. doi: 10.1016/j.brainres.2019.146405
209. Ponzetti M, Rucci N, Falone S. Rna methylation and cellular response to oxidative stress-promoting anticancer agents. Cell Cycle (2023) 22(8):870–905. doi: 10.1080/15384101.2023.2165632
210. Teertam SK, Prakash Babu P. Differential role of sirt1/mapk pathway during cerebral ischemia in rats and humans. Sci Rep (2021) 11(1):6339. doi: 10.1038/s41598-021-85577-9
211. Wawi MJ, Mahler C, Inguimbert N, Marder TB, Ribou AC. A new mitochondrial probe combining pyrene and a triphenylphosphonium salt for cellular oxygen and free radical detection via fluorescence lifetime measurements. Free Radic Res (2022) 56(3-4):258–272. doi: 10.1080/10715762.2022.2077202
212. Wu L, Xiong X, Wu X, Ye Y, Jian Z, Zhi Z, et al. Targeting oxidative stress and inflammation to prevent ischemia-reperfusion injury. Front Mol Neurosci (2020) 13:28. doi: 10.3389/fnmol.2020.00028
213. Xiong J, Bonney S, Gonçalves RV, Esposito D. Brassinosteroids control the inflammation, oxidative stress and cell migration through the control of mitochondrial function on skin regeneration. Life Sci (2022) 307:120887. doi: 10.1016/j.lfs.2022.120887
214. Zadeh-Haghighi H, Simon C. Magnetic field effects in biology from the perspective of the radical pair mechanism. J R Soc Interface (2022) 19(193):20220325. doi: 10.1098/rsif.2022.0325
215. Zhang Y, Zhu X, Wang G, Chen L, Yang H, He F, et al. Melatonin rescues the ti particle-impaired osteogenic potential of bone marrow mesenchymal stem cells via the sirt1/sod2 signaling pathway. Calcif Tissue Int (2020) 107(5):474–88. doi: 10.1007/s00223-020-00741-z
216. Zhao HY, Li HY, Jin J, Jin JZ, Zhang LY, Xuan MY, et al. L-carnitine treatment attenuates renal tubulointerstitial fibrosis induced by unilateral ureteral obstruction. Korean J Intern Med (2020) 36(Suppl 1):S180–95. doi: 10.3904/kjim.2019.413
217. Zheng Z, Xie J, Ma L, Hao Z, Zhang W, Li L. Vitamin D receptor activation targets ros-mediated crosstalk between autophagy and apoptosis in hepatocytes in cholestasis mice. Cell Mol Gastroenterol Hepatol (2022) 15(4):887–901. doi: 10.1016/j.jcmgh.2022.10.011
218. Zhuang X, Ma J, Xu G, Sun Z. Shp-1 knockdown suppresses mitochondrial biogenesis and aggravates mitochondria-dependent apoptosis induced by all trans retinal through the sting/ampk pathways. Mol Med (2022) 28(1):125. doi: 10.1186/s10020-022-00554-w
219. Nikooyeh B, Zahedirad M, Kalayi A, Shariatzadeh N, Hollis BW, Neyestani TR. Improvement of vitamin D status through consumption of either fortified food products or supplement pills increased hemoglobin concentration in adult subjects: analysis of pooled data from two randomized clinical trials. Nutr Health (2023) 29(3):567–74. doi: 10.1177/02601060221085351
220. Zheng Y, Chen ZY, Ma WJ, Wang QZ, Liang H, Ma AG. B vitamins supplementation can improve cognitive functions and may relate to the enhancement of transketolase activity in a rat model of cognitive impairment associated with high-fat diets. Curr Med Sci (2021) 41(5):847–56. doi: 10.1007/s11596-021-2456-5
221. Zhou J, Chen H, Wang Q, Chen S, Wang R, Wang Z, et al. Sirt1 overexpression improves senescence-associated pulmonary fibrosis induced by vitamin D deficiency through downregulating il-11 transcription. Aging Cell (2022) 21(8):e13680. doi: 10.1111/acel.13680
222. Doroftei B, Ilie OD, Cojocariu RO, Ciobica A, Maftei R, Grab D, et al. Minireview exploring the biological cycle of vitamin B3 and its influence on oxidative stress: further molecular and clinical aspects. Molecules (2020) 25(15):3323. doi: 10.3390/molecules25153323
223. Jahan R, Yousaf M, Khan H, Shah SA, Khan AA, Bibi N, et al. Zinc ortho methyl carbonodithioate improved pre and post-synapse memory impairment via sirt1/P-jnk pathway against scopolamine in adult mice. J neuroimmune Pharmacol (2023) 18(1-2):183–94. doi: 10.1007/s11481-023-10067-w
224. Qin D, Li D, Wang C, Guo S. Ferroptosis and central nervous system demyelinating diseases. J Neurochem (2023) 165(6):759–71. doi: 10.1111/jnc.15831
225. Zhao C, Sun G, Li Y, Kong K, Li X, Kan T, et al. Forkhead box O3 attenuates osteoarthritis by suppressing ferroptosis through inactivation of nf-κb/mapk signaling. J Orthop Translat (2023) 39:147–62. doi: 10.1016/j.jot.2023.02.005
226. Maiese K. Moving to the rhythm with clock (Circadian) genes, autophagy, mtor, and sirt1 in degenerative disease and cancer. Curr Neurovasc Res (2017) 14(3):299–304. doi: 10.2174/1567202614666170718092010
227. Mladenovic Djordjevic A, Loncarevic-Vasiljkovic N, Gonos ES. Dietary restriction and oxidative stress: friends or enemies? Antioxid Redox Signal (2020) 34(5):421–38. doi: 10.1089/ars.2019.7959
228. Oyefeso FA, Muotri AR, Wilson CG, Pecaut MJ. Brain organoids: A promising model to assess oxidative stress-induced central nervous system damage. Dev Neurobiol (2021) 81(5):653–70. doi: 10.1002/dneu.22828
229. Zhong S, Chen W, Wang B, Gao C, Liu X, Song Y, et al. Energy stress modulation of ampk/foxo3 signaling inhibits mitochondria-associated ferroptosis. Redox Biol (2023) 63:102760. doi: 10.1016/j.redox.2023.102760
230. Maiese K. Warming up to new possibilities with the capsaicin receptor trpv1: mtor, ampk, and erythropoietin. Curr Neurovasc Res (2017) 14(2):184–9. doi: 10.2174/1567202614666170313105337
231. Meng J, Chen Y, Wang J, Qiu J, Chang C, Bi F, et al. Egcg protects vascular endothelial cells from oxidative stress-induced damage by targeting the autophagy-dependent pi3k-akt-mtor pathway. Ann Transl Med (2020) 8(5):200. doi: 10.21037/atm.2020.01.92
232. Piao S, Lee I, Jin SA, Kim S, Nagar H, Choi SJ, et al. Sirt1 activation attenuates the cardiac dysfunction induced by endothelial cell-specific deletion of crif1. Biomedicines (2021) 9(1):52. doi: 10.3390/biomedicines9010052
233. Wang F, Cao Y, Ma L, Pei H, Rausch WD, Li H. Dysfunction of cerebrovascular endothelial cells: prelude to vascular dementia. Front Aging Neurosci (2018) 10:376. doi: 10.3389/fnagi.2018.00376
234. Zhao D, Sun X, Lv S, Sun M, Guo H, Zhai Y, et al. Salidroside attenuates oxidized lowdensity lipoproteininduced endothelial cell injury via promotion of the ampk/sirt1 pathway. Int J Mol Med (2019) 43(6):2279–90. doi: 10.3892/ijmm.2019.4153
235. Aksenov MY, Aksenova MV, Butterfield DA, Geddes JW, Markesbery WR. Protein oxidation in the brain in alzheimer’s disease. Neuroscience (2001) 103(2):373–83. doi: 10.1016/s0306-4522(00)00580-7
236. Chen GJ, Xu J, Lahousse SA, Caggiano NL, de la Monte SM. Transient hypoxia causes alzheimer-type molecular and biochemical abnormalities in cortical neurons: potential strategies for neuroprotection. J Alzheimers Dis (2003) 5(3):209–28. doi: 10.3233/jad-2003-5305
237. Dai C, Xiao X, Li J, Ciccotosto GD, Cappai R, Tang S, et al. Molecular mechanisms of neurotoxicity induced by polymyxins and chemo-prevention. ACS Chem Neurosci (2018) 10(1):120–31. doi: 10.1021/acschemneuro.8b00300
238. Fanoudi S, Hosseini M, Alavi MS, Boroushaki MT, Hosseini A, Sadeghnia HR. Everolimus, a mammalian target of rapamycin inhibitor, ameliorated streptozotocin-induced learning and memory deficits via neurochemical alterations in male rats. Excli J (2018) 17:999–1017. doi: 10.17179/excli2018-1626
239. Feng HX, Li CP, Shu SJ, Liu H, Zhang HY. A11, a novel diaryl acylhydrazone derivative, exerts neuroprotection against ischemic injury in vitro and in vivo. Acta Pharmacol Sin (2018) 40(2):160–9. doi: 10.1038/s41401-018-0028-4
240. Groen CM, Podratz JL, Pathoulas J, Staff N, Windebank AJ. Genetic reduction of mitochondria complex I subunits is protective against cisplatin-induced neurotoxicity in drosophila. J Neurosci (2021) 42(5):922–37. doi: 10.1523/jneurosci.1479-20.2021
241. Jaganjac M, Milkovic L, Zarkovic N, Zarkovic K. Oxidative stress and regeneration. Free Radic Biol Med (2022) 181:154–65. doi: 10.1016/j.freeradbiomed.2022.02.004
242. Li P, Liu H, Shi X, Prokosch V. Hydrogen sulfide: novel endogenous and exogenous modulator of oxidative stress in retinal degeneration diseases. Molecules (2021) 26(9):2411. doi: 10.3390/molecules26092411
243. Maiese K, Chong ZZ. Insights into oxidative stress and potential novel therapeutic targets for alzheimer disease. Restor Neurol Neurosci (2004) 22(2):87–104.
244. Samaiya PK, Krishnamurthy S, Kumar A. Mitochondrial dysfunction in perinatal asphyxia: role in pathogenesis and potential therapeutic interventions. Mol Cell Biochem (2021) 476(12):4421–34. doi: 10.1007/s11010-021-04253-8
245. Sharma VK, Singh TG, Singh S, Garg N, Dhiman S. Apoptotic pathways and alzheimer’s disease: probing therapeutic potential. Neurochem Res (2021) 46(12):3103–22. doi: 10.1007/s11064-021-03418-7
246. El-Missiry MA, Othman AI, Amer MA, Sedki M, Ali SM, El-Sherbiny IM. Nanoformulated ellagic acid ameliorates pentylenetetrazol-induced experimental epileptic seizures by modulating oxidative stress, inflammatory cytokines and apoptosis in the brains of male mice. Metab Brain Dis (2019) 35(2):385–99. doi: 10.1007/s11011-019-00502-4
247. Motawi TK, Darwish HA, Hamed MA, El-Rigal NS, Naser AF. A therapeutic insight of niacin and coenzyme Q10 against diabetic encephalopathy in rats. Mol Neurobiol (2017) 54(5):3924. doi: 10.1007/s12035-016-9765-x
248. Dąbrowska-Bouta B, Strużyńska L, Sidoryk-Węgrzynowicz M, Sulkowski G. Memantine modulates oxidative stress in the rat brain following experimental autoimmune encephalomyelitis. Int J Mol Sci (2021) 22(21):11330. doi: 10.3390/ijms222111330
249. Dai C, Tang S, Biao X, Xiao X, Chen C, Li J. Colistin induced peripheral neurotoxicity involves mitochondrial dysfunction and oxidative stress in mice. Mol Biol Rep (2019) 46(2):1963–72. doi: 10.1007/s11033-019-04646-5
250. Govindappa PK, Talukder MAH, Gurjar AA, Hegarty JP, Elfar JC. An effective erythropoietin dose regimen protects against severe nerve injury-induced pathophysiological changes with improved neural gene expression and enhances functional recovery. Int Immunopharmacol (2020) 82:106330. doi: 10.1016/j.intimp.2020.106330
251. Oliveira ALL, Santos GGL, Espirito-Santo RF, Silva GSA, Evangelista AF, Silva DN, et al. Reestablishment of redox homeostasis in the nociceptive primary afferent as a mechanism of antinociception promoted by mesenchymal stem/stromal cells in oxaliplatin-induced chronic peripheral neuropathy. Stem Cells Int (2021) 2021:8815206. doi: 10.1155/2021/8815206
252. Maiese K. Cognitive impairment in multiple sclerosis. Bioengineering (Basel) (2023) 10(7):871. doi: 10.3390/bioengineering10070871
253. Liu W, Li Y, Luo B. Current perspective on the regulation of foxo4 and its role in disease progression. Cell Mol Life Sci (2020) 77(4):651–63. doi: 10.1007/s00018-019-03297-w
254. Puigoriol-Illamola D, Grinan-Ferre C, Vasilopoulou F, Leiva R, Vazquez S, Pallas M. 11beta-hsd1 inhibition by rl-118 promotes autophagy and correlates with reduced oxidative stress and inflammation, enhancing cognitive performance in samp8 mouse model. Mol Neurobiol (2018) 55(12):8904–15. doi: 10.1007/s12035-018-1026-8
255. Zhang GZ, Deng YJ, Xie QQ, Ren EH, Ma ZJ, He XG, et al. Sirtuins and intervertebral disc degeneration: roles in inflammation, oxidative stress, and mitochondrial function. Clin Chim Acta (2020) 508:33–42. doi: 10.1016/j.cca.2020.04.016
256. Bahrampour Juybari K, Pourhanifeh MH, Hosseinzadeh A, Hemati K, Mehrzadi S. Melatonin potentials against viral infections including covid-19: current evidence and new findings. Virus Res (2020) 287:198108. doi: 10.1016/j.virusres.2020.198108
257. Jarosz-Griffiths HH, Corbett NJ, Rowland HA, Fisher K, Jones AC, Baron J, et al. Proteolytic shedding of the prion protein via activation of metallopeptidase adam10 reduces cellular binding and toxicity of amyloid-beta oligomers. J Biol Chem (2019) 294(17):7085–97. doi: 10.1074/jbc.RA118.005364
258. Kuscu N, Gungor-Ordueri NE, Sozen B, Adiguzel D, Celik-Ozenci C. Foxo transcription factors regulate mouse preimplantation embryo development. J Assist Reprod Genet (2019) 36(12):2605. doi: 10.1007/s10815-019-01555-1
259. Maiese K. Harnessing the power of sirt1 and non-coding rnas in vascular disease. Curr Neurovasc Res (2017) 14(1):82–8. doi: 10.2174/1567202613666161129112822
260. Cheema PS, Nandi D, Nag A. Exploring the therapeutic potential of forkhead box O for outfoxing covid-19. Open Biol (2021) 11(6):210069. doi: 10.1098/rsob.210069
261. Fang Y, Lu L, Liang Y, Peng D, Aschner M, Jiang Y. Signal transduction associated with lead-induced neurological disorders: A review. Food Chem Toxicol (2021) 150:112063. doi: 10.1016/j.fct.2021.112063
262. Khan M, Ullah R, Rehman SU, Shah SA, Saeed K, Muhammad T, et al. 17beta-estradiol modulates sirt1 and halts oxidative stress-mediated cognitive impairment in a male aging mouse model. Cells (2019) 8(8):928. doi: 10.3390/cells8080928
263. Mahmoudi N, Kiasalari Z, Rahmani T, Sanaierad A, Afshin-Majd S, Naderi G, et al. Diosgenin attenuates cognitive impairment in streptozotocin-induced diabetic rats: underlying mechanisms. Neuropsychobiology (2021) 11:25–35. doi: 10.1159/000507398
264. Ruhal P, Dhingra D. Inosine improves cognitive function and decreases aging-induced oxidative stress and neuroinflammation in aged female rats. Inflammopharmacology (2018) 26(5):1317–29. doi: 10.1007/s10787-018-0476-y
265. Dhakal S, Kushairi N, Phan CW, Adhikari B, Sabaratnam V, Macreadie I. Dietary polyphenols: A multifactorial strategy to target alzheimer’s disease. Int J Mol Sci (2019) 20(20):5090. doi: 10.3390/ijms20205090
266. Engin AB, Engin A. Alzheimer’s disease and protein kinases. Adv Exp Med Biol (2021) 1275:285–321. doi: 10.1007/978-3-030-49844-3_11
267. Zhao J, Miao K, Wang H, Ding H, Wang DW. Association between telomere length and type 2 diabetes mellitus: A meta-analysis. PloS One (2013) 8(11):e79993. doi: 10.1371/journal.pone.0079993
268. Lamoke F, Shaw S, Yuan J, Ananth S, Duncan M, Martin P, et al. Increased oxidative and nitrative stress accelerates aging of the retinal vasculature in the diabetic retina. PloS One (2015) 10(10):e0139664. doi: 10.1371/journal.pone.0139664
269. Cai J, Qi H, Yao K, Yao Y, Jing D, Liao W, et al. Non-coding rnas steering the senescence-related progress, properties, and application of mesenchymal stem cells. Front Cell Dev Biol (2021) 9:650431. doi: 10.3389/fcell.2021.650431
270. Chung CL, Lawrence I, Hoffman M, Elgindi D, Nadhan K, Potnis M, et al. Topical rapamycin reduces markers of senescence and aging in human skin: an exploratory, prospective, randomized trial. Geroscience (2019) 41:6. doi: 10.1007/s11357-019-00113-y
271. Csicsar A, Tarantini S, Yabluchanskiy A, Balasubramanian P, Kiss T, Farkas E, et al. Role of endothelial nad+ Deficiency in age-related vascular dysfunction. Am J Physiol Heart Circ Physiol (2019) 316(6):H1253–H66. doi: 10.1152/ajpheart.00039.2019
272. Dorvash M, Farahmandnia M, Tavassoly I. A systems biology roadmap to decode mtor control system in cancer. Interdiscip Sci (2020) 12(1):1–11. doi: 10.1007/s12539-019-00347-6
273. Maiese K. Disease onset and aging in the world of circular rnas. J Transl Sci (2016) 2(6):327–9. doi: 10.15761/jts.1000158
274. Maiese K. The implications of telomere length: advanced aging, cell senescence, mri phenotypes, stem cells and alzheimer’s disease. Curr Neurovasc Res (2023) 20(2):171–4. doi: 10.2174/1567202620666230510150337
275. Rapaka D, Bitra VR, Challa SR, Adiukwu PC. Mtor signaling as a molecular target for the alleviation of alzheimer’s disease pathogenesis. Neurochem Int (2022) 155:105311. doi: 10.1016/j.neuint.2022.105311
276. Ferrara-Romeo I, Martinez P, Saraswati S, Whittemore K, Graña-Castro O, Thelma Poluha L, et al. The mtor pathway is necessary for survival of mice with short telomeres. Nat Commun (2020) 11(1):1168. doi: 10.1038/s41467-020-14962-1
277. Lai KY, Webster C, Kumari S, Gallacher JEJ, Sarkar C. The associations of socioeconomic status with incident dementia and alzheimer’s disease are modified by leucocyte telomere length: A population-based cohort study. Sci Rep (2023) 13(1):6163. doi: 10.1038/s41598-023-32974-x
278. Okada M, Kim HW, Matsu-Ura K, Wang YG, Xu M, Ashraf M. Abrogation of age-induced microrna-195 rejuvenates the senescent mesenchymal stem cells by reactivating telomerase. Stem Cells (2015) 34(1):148–59. doi: 10.1002/stem.2211
279. Topiwala A, Nichols TE, Williams LZJ, Robinson EC, Alfaro-Almagro F, Taschler B, et al. Telomere length and brain imaging phenotypes in uk biobank. PloS One (2023) 18(3):e0282363. doi: 10.1371/journal.pone.0282363
280. Cardoso S, López IP, Piñeiro-Hermida S, Pichel JG, Moreira PI. Igf1r deficiency modulates brain signaling pathways and disturbs mitochondria and redox homeostasis. Biomedicines (2021) 9(2):158. doi: 10.3390/biomedicines9020158
281. Maiese K. Stem cell guidance through the mechanistic target of rapamycin. World J Stem Cells (2015) 7(7):999–1009. doi: 10.4252/wjsc.v7.i7.999
282. Amidfar M, Garcez ML, Kim YK. The shared molecular mechanisms underlying aging of the brain, major depressive disorder, and alzheimer’s disease: the role of circadian rhythm disturbances. Prog Neuropsychopharmacol Biol Psychiatry (2023) 123:110721. doi: 10.1016/j.pnpbp.2023.110721
283. Chen YL, Hsieh CC, Chu PM, Chen JY, Huang YC, Chen CY. Roles of protein tyrosine phosphatases in hepatocellular carcinoma progression (Review). Oncol Rep (2023) 49(3):48. doi: 10.3892/or.2023.8485
284. Hafez N, Refaat L, ElGebaly OK, Elhariry HM, Ghareeb M, Fathalla LA. Prognostic value of rgs1 and mtor immunohistochemical expression in Egyptian multiple myeloma patients; a single center study. PloS One (2023) 18(7):e0288357. doi: 10.1371/journal.pone.0288357
285. Meng JJ, Shen JW, Li G, Ouyang CJ, Hu JX, Li ZS, et al. Light modulates glucose metabolism by a retina-hypothalamus-brown adipose tissue axis. Cell (2023) 186(2):398–412.e17. doi: 10.1016/j.cell.2022.12.024
286. Xu Y, Wang Y, Jiang Y, Liu M, Zhong W, Ge Z, et al. Relationship between cognitive dysfunction and the promoter methylation of per1 and cry1 in patients with cerebral small vessel disease. Front Aging Neurosci (2023) 15:1174541. doi: 10.3389/fnagi.2023.1174541
287. Fakouri NB, Hou Y, Demarest TG, Christiansen LS, Okur MN, Mohanty JG, et al. Towards understanding genomic instability, mitochondrial dysfunction and aging. FEBS J (2018) 286(6):1058–73. doi: 10.1111/febs.14663
288. Lai YF, Wang L, Liu WY. Nicotinamide pretreatment alleviates mitochondrial stress and protects hypoxic myocardial cells via ampk pathway. Eur Rev Med Pharmacol Sci (2019) 23(4):1797–806. doi: 10.26355/eurrev_201902_17143
289. Albiero M, Poncina N, Tjwa M, Ciciliot S, Menegazzo L, Ceolotto G, et al. Diabetes causes bone marrow autonomic neuropathy and impairs stem cell mobilization via dysregulated P66shc and sirt1. Diabetes (2014) 63(4):1353–65. doi: 10.2337/db13-0894
290. Gomes MB, Negrato CA. Alpha-lipoic acid as a pleiotropic compound with potential therapeutic use in diabetes and other chronic diseases. Diabetol Metab syndrome (2014) 6(1):80. doi: 10.1186/1758-5996-6-80
291. Khoshdel A, Carney S, Gillies A, Mourad A, Jones B, Nanra R, et al. Potential roles of erythropoietin in the management of anaemia and other complications diabetes. Diabetes Obes Metab (2008) 10(1):1–9. doi: 10.1111/j.1463-1326.2007.00711.x
292. Atef MM, El-Sayed NM, Ahmed AAM, Mostafa YM. Donepezil improves neuropathy through activation of ampk signalling pathway in streptozotocin-induced diabetic mice. Biochem Pharmacol (2019) 159:1–10. doi: 10.1016/j.bcp.2018.11.006
293. Jenwitheesuk A, Nopparat C, Mukda S, Wongchitrat P, Govitrapong P. Melatonin regulates aging and neurodegeneration through energy metabolism, epigenetics, autophagy and circadian rhythm pathways. Int J Mol Sci (2014) 15(9):16848–84. doi: 10.3390/ijms150916848
294. Lee HJ, Yang SJ. Supplementation with nicotinamide riboside reduces brain inflammation and improves cognitive function in diabetic mice. Int J Mol Sci (2019) 20(17):4196. doi: 10.3390/ijms20174196
295. Ong WY, Wu YJ, Farooqui T, Farooqui AA. Qi fu yin-a ming dynasty prescription for the treatment of dementia. Mol Neurobiol (2018) 55(9):7389–400. doi: 10.1007/s12035-018-0908-0
296. Othman MAM, Rajab E, AlMubarak A, AlNaisar M, Bahzad N, Kamal A. Erythropoietin protects against cognitive impairment and hippocampal neurodegeneration in diabetic mice. Behav Sci (Basel Switzerland) (2018) 9(1):4. doi: 10.3390/bs9010004
297. Crespo MC, Tome-Carneiro J, Pintado C, Davalos A, Visioli F, Burgos-Ramos E. Hydroxytyrosol restores proper insulin signaling in an astrocytic model of alzheimer’s disease. BioFactors (Oxford England) (2017) 43(4):540–8. doi: 10.1002/biof.1356
298. Fan X, Zhao Z, Wang D, Xiao J. Glycogen synthase kinase-3 as a key regulator of cognitive function. Acta Biochim Biophys Sin (2020) 52(3):219–30. doi: 10.1093/abbs/gmz156
299. Hu Z, Jiao R, Wang P, Zhu Y, Zhao J, De Jager P, et al. Shared causal paths underlying alzheimer’s dementia and type 2 diabetes. Sci Rep (2020) 10(1):4107. doi: 10.1038/s41598-020-60682-3
300. Li F, Chong ZZ, Maiese K. Cell life versus cell longevity: the mysteries surrounding the nad(+) precursor nicotinamide. Curr Med Chem (2006) 13(8):883–95. doi: 10.2174/092986706776361058
301. Su M, Naderi K, Samson N, Youssef I, Fulop L, Bozso Z, et al. Mechanisms associated with type 2 diabetes as a risk factor for alzheimer-related pathology. Mol Neurobiol (2019) 56(8):5815–34. doi: 10.1007/s12035-019-1475-8
302. Yamashima T, Ota T, Mizukoshi E, Nakamura H, Yamamoto Y, Kikuchi M, et al. Intake of Ω-6 polyunsaturated fatty acid-rich vegetable oils and risk of lifestyle diseases. Adv Nutr (2020) 11(6):1489–509. doi: 10.1093/advances/nmaa072
303. Feng H, Xue M, Deng H, Cheng S, Hu Y, Zhou C. Ginsenoside and its therapeutic potential for cognitive impairment. Biomolecules (2022) 12(9):1310. doi: 10.3390/biom12091310
304. Fessel J. Cure of alzheimer’s dementia requires addressing all of the affected brain cell types. J Clin Med (2023) 12(2049):1–14. doi: 10.3390/jcm12052049
305. Maiese K. Cognitive impairment and dementia: gaining insight through circadian clock gene pathways. Biomolecules (2021) 11(7):1–18. doi: 10.3390/biom11071002
306. Perluigi M, Di Domenico F, Barone E, Butterfield DA. Mtor in alzheimer disease and its earlier stages: links to oxidative damage in the progression of this dementing disorder. Free Radic Biol Med (2021) 169:382–96. doi: 10.1016/j.freeradbiomed.2021.04.025
307. Rehman IU, Khan A, Ahmad R, Choe K, Park HY, Lee HJ, et al. Neuroprotective effects of nicotinamide against mptp-induced parkinson’s disease in mice: impact on oxidative stress, neuroinflammation, nrf2/ho-1 and tlr4 signaling pathways. Biomedicines (2022) 10(11):2929. doi: 10.3390/biomedicines10112929
308. Zhu G, Tong Q, Ye X, Li J, Zhou L, Sun P, et al. Phototherapy for cognitive function in patients with dementia: A systematic review and meta-analysis. Front Aging Neurosci (2022) 14:936489. doi: 10.3389/fnagi.2022.936489
309. Ding MR, Qu YJ, Hu B, An HM. Signal pathways in the treatment of alzheimer’s disease with traditional chinese medicine. BioMed Pharmacother (2022) 152:113208. doi: 10.1016/j.biopha.2022.113208
310. Jayaraman A, Reynolds R. Diverse pathways to neuronal necroptosis in alzheimer’s disease. Eur J Neurosci (2022) 56(9):5428–41. doi: 10.1111/ejn.15662
311. Rani S, Dhar SB, Khajuria A, Gupta D, Jaiswal PK, Singla N, et al. Advanced overview of biomarkers and techniques for early diagnosis of alzheimer’s disease. Cell Mol Neurobiol (2023) 43(6):2491–523. doi: 10.1007/s10571-023-01330-y
312. Tong Z, Chu G, Wan C, Wang Q, Yang J, Meng Z, et al. Multiple metabolites derived from mushrooms and their beneficial effect on alzheimer’s diseases. Nutrients (2023) 15(12):2758. doi: 10.3390/nu15122758
313. Wang MD, Zhang S, Liu XY, Wang PP, Zhu YF, Zhu JR, et al. Salvianolic acid B ameliorates retinal deficits in an early-stage alzheimer’s disease mouse model through downregulating bace1 and Aβ Generation. Acta Pharmacol Sin (2023). doi: 10.1038/s41401-023-01125-3
314. Maiese K. Impacting dementia and cognitive loss with innovative strategies: mechanistic target of rapamycin, clock genes, circular non-coding ribonucleic acids, and rho/rock. Neural regeneration Res (2019) 14(5):773–4. doi: 10.4103/1673-5374.249224
315. Rantanen LM, Bitar M, Lampinen R, Stewart R, Quek H, Oikari LE, et al. An alzheimer’s disease patient-derived olfactory stem cell model identifies gene expression changes associated with cognition. Cells (2022) 11(20):3258. doi: 10.3390/cells11203258
316. Schubert CR, Paulsen AJ, Pinto AA, Merten N, Cruickshanks KJ. Effect of long-term storage on the reliability of blood biomarkers for alzheimer’s disease and neurodegeneration. J Alzheimers Dis (2022) 85(3):1021–9. doi: 10.3233/jad-215096
317. Shiravandi A, Yari F, Tofigh N, Kazemi Ashtiani M, Shahpasand K, Ghanian MH, et al. Earlier detection of alzheimer’s disease based on a novel biomarker cis P-tau by a label-free electrochemical immunosensor. Biosensors (Basel) (2022) 12(10):879. doi: 10.3390/bios12100879
318. Tang B, Zeng W, Song LL, Wang HM, Qu LQ, Lo HH, et al. Extracellular vesicle delivery of neferine for the attenuation of neurodegenerative disease proteins and motor deficit in an alzheimer’s disease mouse model. Pharm (Basel Switzerland) (2022) 15(1):83. doi: 10.3390/ph15010083
319. Agarwal D, Kumari R, Ilyas A, Tyagi S, Kumar R, Poddar NK. Crosstalk between epigenetics and mtor as a gateway to new insights in pathophysiology and treatment of alzheimer’s disease. Int J Biol macromolecules (2021) 192:895–903. doi: 10.1016/j.ijbiomac.2021.10.026
320. Xu P, Wu Z, Peng Y, Gao J, Zheng F, Tan J, et al. Neuroprotection of triptolide against amyloid-beta1-42-induced toxicity via the akt/mtor/P70s6k-mediated autophagy pathway. Acad Bras Cienc (2022) 94(2):e20210938. doi: 10.1590/0001-3765202220210938
321. Filley CM, Rollins YD, Anderson CA, Arciniegas DB, Howard KL, Murrell JR, et al. The genetics of very early onset alzheimer disease. Cogn Behav Neurol (2007) 20(3):149–56. doi: 10.1097/WNN.0b013e318145a8c8
322. Agis-Torres A, Solhuber M, Fernandez M, Sanchez-Montero JM. Multi-target-directed ligands and other therapeutic strategies in the search of a real solution for alzheimer’s disease. Curr neuropharmacology (2014) 12(1):2–36. doi: 10.2174/1570159x113116660047
323. Morris G, Berk M, Maes M, Puri BK. Could alzheimer’s disease originate in the periphery and if so how so? Mol Neurobiol (2019) 56(1):406–34. doi: 10.1007/s12035-018-1092-y
324. Safdari Lord J, Soltani Rezaiezadeh J, Yekaninejad MS, Izadi P. The association of apoe genotype with covid-19 disease severity. Sci Rep (2022) 12(1):13483. doi: 10.1038/s41598-022-17262-4
325. Maiese K. Apolipoprotein-E4 allele (Apoe-E4) as a mediator of cognitive loss and dementia in long covid-19. Curr Neurovasc Res (2022) 19(5):435–9. doi: 10.2174/156720261905221227114624
326. Kurki SN, Kantonen J, Kaivola K, Hokkanen L, Mäyränpää MI, Puttonen H, et al. Apoe E4 associates with increased risk of severe covid-19, cerebral microhaemorrhages and post-covid mental fatigue: A finnish biobank, autopsy and clinical study. Acta neuropathologica Commun (2021) 9(1):199. doi: 10.1186/s40478-021-01302-7
327. Momkute L, Vilkeviciute A, Gedvilaite G, Dubinskaite G, Kriauciuniene L, Liutkeviciene R. Association of apoe serum levels and apoe E2, E3, and E4 alleles with optic neuritis. Genes (Basel) (2022) 13(7):1188. doi: 10.3390/genes13071188
328. Ojo JO, Reed JM, Crynen G, Vallabhaneni P, Evans J, Shackleton B, et al. Apoe genotype dependent molecular abnormalities in the cerebrovasculature of alzheimer’s disease and age-matched non-demented brains. Mol Brain (2021) 14(1):110. doi: 10.1186/s13041-021-00803-9
329. Di Patre PL, Read SL, Cummings JL, Tomiyasu U, Vartavarian LM, Secor DL, et al. Progression of clinical deterioration and pathological changes in patients with alzheimer disease evaluated at biopsy and autopsy. Arch Neurol (1999) 56(10):1254–61. doi: 10.1001/archneur.56.10.1254
330. Maiese K, Chong ZZ. Nicotinamide: necessary nutrient emerges as a novel cytoprotectant for the brain. Trends Pharmacol Sci (2003) 24(5):228–32. doi: 10.1016/S0165-6147(03)00078-6
331. Maiese K, Vincent AM. Membrane asymmetry and DNA degradation: functionally distinct determinants of neuronal programmed cell death. J Neurosci Res (2000) 59(4):568–80. doi: 10.1002/(SICI)1097-4547(20000215)59:4<568::AID-JNR13>3.0.CO;2-R
332. Lee G, Pollard HB, Arispe N. Annexin 5 and Apolipoprotein E2 Protect against Alzheimer’s Amyloid-Beta-Peptide Cytotoxicity by Competitive Inhibition at a Common Phosphatidylserine Interaction Site. Peptides (2002) 23(7):1249–63. doi: 10.1016/s0196-9781(02)00060-8
333. Cacabelos R, Carril JC, Cacabelos N, Kazantsev AG, Vostrov AV, Corzo L, et al. Sirtuins in alzheimer’s disease: sirt2-related genophenotypes and implications for pharmacoepigenetics. Int J Mol Sci (2019) 20(5):1249. doi: 10.3390/ijms20051249
334. Zheng H, Jia L, Liu CC, Li Zhong ZR, Yang L, Chen XF, et al. Trem2 promotes microglial survival by activating wnt/beta-catenin pathway. J Neurosci (2017) 37(7):1771–84. doi: 10.1523/jneurosci.2459-16.2017
335. Liu Z, Huang H, Yu Y, Jia Y, Li L, Shi X, et al. Exploring the potential mechanism of action of ursolic acid against gastric cancer and covid-19 using network pharmacology and bioinformatics analysis. Curr Pharm Des (2023) 29(16):1274–92. doi: 10.2174/1381612829666230510124716
336. Theoharides TC. Could sars-cov-2 spike protein be responsible for long-covid syndrome? Mol Neurobiol (2022) 59(3):1850–61. doi: 10.1007/s12035-021-02696-0
337. Sungnak W, Huang N, Becavin C, Berg M, Queen R, Litvinukova M, et al. Sars-cov-2 entry factors are highly expressed in nasal epithelial cells together with innate immune genes. Nat Med (2020) 26(5):681–7. doi: 10.1038/s41591-020-0868-6
338. Al-Qahtani AA, Pantazi I, Alhamlan FS, Alothaid H, Matou-Nasri S, Sourvinos G, et al. Sars-cov-2 modulates inflammatory responses of alveolar epithelial type ii cells via pi3k/akt pathway. Front Immunol (2022) 13:1020624. doi: 10.3389/fimmu.2022.1020624
339. Geier C, Perl A. Therapeutic mtor blockade in systemic autoimmunity: implications for antiviral immunity and extension of lifespan. Autoimmun Rev (2021) 20(12):102984. doi: 10.1016/j.autrev.2021.102984
340. Shirzad M, Nourigorji M, Sajedi A, Ranjbar M, Rasti F, Sourani Z, et al. Targeted therapy in coronavirus disease 2019 (Covid-19): implication from cell and gene therapy to immunotherapy and vaccine. Int Immunopharmacol (2022) 111:109161. doi: 10.1016/j.intimp.2022.109161
341. Jansen van Vuren E, Steyn SF, Brink CB, Möller M, Viljoen FP, Harvey BH. The neuropsychiatric manifestations of covid-19: interactions with psychiatric illness and pharmacological treatment. BioMed Pharmacother (2021) 135:111200. doi: 10.1016/j.biopha.2020.111200
342. Blagosklonny MV. From causes of aging to death from covid-19. Aging (Albany NY) (2020) 12(11):10004–21. doi: 10.18632/aging.103493
343. Borges do Nascimento IJ, Cacic N, Abdulazeem HM, von Groote TC, Jayarajah U, Weerasekara I, et al. Novel coronavirus infection (Covid-19) in humans: A scoping review and meta-analysis. J Clin Med (2020) 9(4):941. doi: 10.3390/jcm9040941
344. Gusev E, Sarapultsev A, Hu D, Chereshnev V. Problems of pathogenesis and pathogenetic therapy of covid-19 from the perspective of the general theory of pathological systems (General pathological processes). Int J Mol Sci (2021) 22(14):7582. doi: 10.3390/ijms22147582
345. Cavalli E, Bramanti A, Ciurleo R, Tchorbanov AI, Giordano A, Fagone P, et al. Entangling covid-19 associated thrombosis into a secondary antiphospholipid antibody syndrome: diagnostic and therapeutic perspectives (Review). Int J Mol Med (2020) 46(3):903–12. doi: 10.3892/ijmm.2020.4659
346. González-Fernández C, González P, González-Pérez F, Rodríguez F. Characterization of ex vivo and in vitro wnt transcriptome induced by spinal cord injury in rat microglial cells. Brain Sci (2022) 12(708):708. doi: 10.3390/brainsci12060708
347. Liu D, Zhang M, Tian J, Gao M, Liu M, Fu X, et al. Wnt1-inducible signalling pathway protein 1 stabilizes atherosclerotic plaques in apolipoprotein-E-deficient mice via the focal adhesion kinase/mitogen-activated extracellular signal-regulated kinase/extracellular signal-regulated kinase pathway. J hypertension (2022) 40(9):1666–81. doi: 10.1097/hjh.0000000000003195
348. Liu L, Xu S, Li P, Li L. A novel adipokine wisp1 attenuates lipopolysaccharide-induced cell injury in 3t3-L1 adipocytes by regulating the pi3k/akt pathway. Obes Res Clin Pract (2022) 16(2):122–9. doi: 10.1016/j.orcp.2022.03.001
349. Ren LL, Zhou JY, Liang SJ, Wang XQ. Impaired intestinal stem cell activity in etec infection: enterotoxins, cyclic nucleotides, and wnt signaling. Arch Toxicol (2022) 96(5):1213–25. doi: 10.1007/s00204-021-03213-x
350. Tang Y, Chen Y, Liu R, Li W, Hua B, Bao Y. Wnt signaling pathways: A role in pain processing. Neuromolecular Med (2022) 24(3):233–49. doi: 10.1007/s12017-021-08700-z
351. Marinelli C, Bertalot T, Zusso M, Skaper SD, Giusti P. Systematic review of pharmacological properties of the oligodendrocyte lineage. Front Cell Neurosci (2016) 10:27. doi: 10.1007/s13311-020-00923-5
352. Gökdoğan Edgünlü T, Ünal Y, Karakaş Çelik S, Genç Ö, Emre U, Kutlu G. The effect of foxo gene family variants and global DNA metylation on rrms disease. Gene (2020) 726:144172. doi: 10.1016/j.gene.2019.144172
353. Kell DB, Pretorius E. No effects without causes: the iron dysregulation and dormant microbes hypothesis for chronic, inflammatory diseases. Biol Rev Cambridge Philos Soc (2018) 93(3):1518–57. doi: 10.1111/brv.12407
354. Maiese K. Novel insights for multiple sclerosis and demyelinating disorders with apoptosis, autophagy, foxo, and mtor. Curr Neurovasc Res (2021) 18(2):1–4. doi: 10.2174/1567202618999210505124235
355. Oktelik FB, Yilmaz V, Turkoglu R, Akbayir E, Tuzun E, Deniz G, et al. Expression of akt1 and P-akt1 in peripheral T cell subsets of multiple sclerosis patients. Acta Neurol Belg (2020) 121(6):1777–82. doi: 10.1007/s13760-020-01518-9
356. Sanadgol N, Barati M, Houshmand F, Hassani S, Clarner T, Shahlaei M, et al. Metformin accelerates myelin recovery and ameliorates behavioral deficits in the animal model of multiple sclerosis via adjustment of ampk/nrf2/mtor signaling and maintenance of endogenous oligodendrogenesis during brain self-repairing period. Pharmacol Rep (2020) 72(3):641–58. doi: 10.1007/s43440-019-00019-8
357. Wallin MT, Culpepper WJ, Campbell JD, Nelson LM, Langer-Gould A, Marrie RA, et al. The prevalence of ms in the United States: A population-based estimate using health claims data. Neurology (2019) 92(10):e1029–e40. doi: 10.1212/wnl.0000000000007035
358. Xu L, Zhang C, Jiang N, He D, Bai Y, Xin Y. Rapamycin combined with mcc950 to treat multiple sclerosis in experimental autoimmune encephalomyelitis. J Cell Biochem (2019) 120(4):5160–8. doi: 10.1002/jcb.27792
359. Zhang YC, Fan KY, Wang Q, Hu JX, Wang Q, Zhang HY, et al. Genetically determined levels of mtor-dependent circulating proteins and risk of multiple sclerosis. Neurol Ther (2023) 12(3):751–62. doi: 10.1007/s40120-023-00455-y
360. Hemmer B, Cepok S, Zhou D, Sommer N. Multiple sclerosis - a coordinated immune attack across the blood brain barrier. Curr Neurovasc Res (2004) 1(2):141–50. doi: 10.2174/1567202043480152
361. Maiese K. The challenges for drug development: cytokines, genes, and stem cells. Curr Neurovasc Res (2012) 9(4):231–2. doi: 10.2174/156720212803530690
362. Martin A, Tegla CA, Cudrici CD, Kruszewski AM, Azimzadeh P, Boodhoo D, et al. Role of sirt1 in autoimmune demyelination and neurodegeneration. Immunologic Res (2015) 61(3):187–97. doi: 10.1007/s12026-014-8557-5
363. Mouzaki A, Rodi M, Dimisianos N, Emmanuil A, Kalavrizioti D, Lagoudaki R, et al. Immune parameters that distinguish multiple sclerosis patients from patients with other neurological disorders at presentation. PloS One (2015) 10(8):e0135434. doi: 10.1371/journal.pone.0135434
364. Rodi M, Dimisianos N, de Lastic AL, Sakellaraki P, Deraos G, Matsoukas J, et al. Regulatory cell populations in relapsing-remitting multiple sclerosis (Rrms) patients: effect of disease activity and treatment regimens. Int J Mol Sci (2016) 17(9):1398. doi: 10.3390/ijms17091398
365. Sun JJ, Ren QG, Xu L, Zhang ZJ. Lingo-1 antibody ameliorates myelin impairment and spatial memory deficits in experimental autoimmune encephalomyelitis mice. Sci Rep (2015) 5:14235. doi: 10.1038/srep14235
366. Yap SM, Davenport L, Cogley C, Craddock F, Kennedy A, Gaughan M, et al. Word finding, prosody and social cognition in multiple sclerosis. J Neuropsychol (2023) 17(1):32–62. doi: 10.1111/jnp.12285
367. Staff NP, Lucchinetti CF, Keegan BM. Multiple sclerosis with predominant, severe cognitive impairment. Arch Neurol (2009) 66(9):1139–43. doi: 10.1001/archneurol.2009.190
368. Naseri A, Baghernezhad K, Seyedi-Sahebari S, Alhoseini SA, Gholipour-Khalili E, Zafarani F, et al. (Apoe) genotype and cognitive outcomes in multiple sclerosis; a systematic review and meta-analysis. Mult Scler Relat Disord (2022) 65:104011. doi: 10.1016/j.msard.2022.104011
369. Fedeli U, Amidei CB, Avossa F, Schievano E, Kingwell E. Association of multiple sclerosis-related mortality with covid-19 and other common infections: A multiple causes of death analysis. Eur J Neurol (2023) 30(9):2870–3. doi: 10.1111/ene.15912
370. Bramante C, Ingraham N, Murray T, Marmor S, Hoversten S, Gronski J, et al. Observational study of metformin and risk of mortality in patients hospitalized with covid-19. medRxiv (2020). doi: 10.1101/2020.06.19.20135095
371. Tiu VE, Popescu BO, Enache II, Tiu C, Terecoasa E, Panea CA. Serum and csf biomarkers predict active early cognitive decline rather than established cognitive impairment at the moment of rrms diagnosis. Diagnostics (Basel) (2022) 12(11):2571. doi: 10.3390/diagnostics12112571
372. Diallo AB, Gay L, Coiffard B, Leone M, Mezouar S, Mege JL. Daytime variation in sars-cov-2 infection and cytokine production. Microb Pathog (2021) 158:105067. doi: 10.1016/j.micpath.2021.105067
373. Gu X, Zhu J. Roles of exosomes and exosomal micrornas in postoperative sleep disturbance. Nat Sci Sleep (2021) 13:1363–75. doi: 10.2147/nss.S310351
374. Lio CT, Kacprowski T, Klaedtke M, Jensen LR, Bouter Y, Bayer TA, et al. Small rna sequencing in the tg4-42 mouse model suggests the involvement of snornas in the etiology of alzheimer’s disease. J Alzheimers Dis (2022) 87(4):1671–81. doi: 10.3233/jad-220110
375. Zhang W, Bai S, Yang J, Zhang Y, Liu Y, Nie J, et al. Foxo1 overexpression reduces Aβ Production and tau phosphorylation in vitro. Neurosci Lett (2020) 738:135322. doi: 10.1016/j.neulet.2020.135322
376. Zhao W, Xie C, Zhang X, Liu J, Liu J, Xia Z. Advances in the mtor signaling pathway and its inhibitor rapamycin in epilepsy. Brain Behav (2023) 13(6):e2995. doi: 10.1002/brb3.2995
377. El-Beltagy A, Saleh AMB, Attaallah A, Gahnem RA. Therapeutic role of azadirachta indica leaves ethanolic extract against diabetic nephropathy in rats neonatally induced by streptozotocin. Ultrastruct Pathol (2021) 46(6):391–406. doi: 10.1080/01913123.2021.1988015
378. Espinoza SE, Khosla S, Baur JA, de Cabo R, Musi N. Drugs targeting mechanisms of aging to delay age-related disease and promote healthspan: proceedings of a national institute on aging workshop. J Gerontol A Biol Sci Med Sci (2023) 78(Supplement_1):53–60. doi: 10.1093/gerona/glad034
379. McCoin CS, Franczak E, Deng F, Pei D, Ding WX, Thyfault JP. Acute exercise rapidly activates hepatic mitophagic flux. J Appl Physiol (2022) 132(3):862–73. doi: 10.1152/japplphysiol.00704.2021
380. Lee JH, Lee JH, Jin M, Han SD, Chon GR, Kim IH, et al. Diet control to achieve euglycemia induces significant loss of heart and liver weight via increased autophagy compared with ad libitum diet in diabetic rats. Exp Mol Med (2014) 46:e111. doi: 10.1038/emm.2014.52
381. Al-Kuraishy HM, Al-Buhadily AK, Al-Gareeb AI, Alorabi M, Hadi Al-Harcan NA, El-Bouseary MM, et al. Citicoline and covid-19: vis-À-vis conjectured. Naunyn Schmiedebergs Arch Pharmacol (2022) 395(12):1463–75. doi: 10.1007/s00210-022-02284-6
382. Al-Kuraishy HM, Al-Gareeb AI, Al-Maiahy TJ, Alexiou A, Mukerjee N, Batiha GE. Prostaglandins and non-steroidal anti-inflammatory drugs in covid-19. Biotechnol Genet Eng Rev (2022), 1–21. doi: 10.1080/02648725.2022.2122290
383. Assogna M, Di Lorenzo F, Martorana A, Koch G. Synaptic effects of palmitoylethanolamide in neurodegenerative disorders. Biomolecules (2022) 12(8):1161. doi: 10.3390/biom12081161
384. Braun S, Zaucke F, Brenneis M, Rapp AE, Pollinger P, Sohn R, et al. The corpus adiposum infrapatellare (Hoffa’s fat pad)-the role of the infrapatellar fat pad in osteoarthritis pathogenesis. Biomedicines (2022) 10(5):1071. doi: 10.3390/biomedicines10051071
385. Maiese K, Li F, Chong ZZ. New avenues of exploration for erythropoietin. Jama (2005) 293(1):90–5. doi: 10.1001/jama.293.1.90
386. Martins B, Vieira M, Delerue-Matos C, Grosso C, Soares C. Biological potential, gastrointestinal digestion, absorption, and bioavailability of algae-derived compounds with neuroprotective activity: A comprehensive review. Mar Drugs (2022) 20(6):362. doi: 10.3390/md20060362
387. Pan J, Zhou L, Zhang C, Xu Q, Sun Y. Targeting protein phosphatases for the treatment of inflammation-related diseases: from signaling to therapy. Signal Transduct Target Ther (2022) 7(1):177. doi: 10.1038/s41392-022-01038-3
388. Pantazi P, Clements T, Venø M, Abrahams VM, Holder B. Distinct non-coding rna cargo of extracellular vesicles from M1 and M2 human primary macrophages. J Extracell Vesicles (2022) 11(12):e12293. doi: 10.1002/jev2.12293
389. Liu M, Jiang L, Cao W, Wu J, Chen X. Identification of inhibitors and drug targets for human adenovirus infections. Viruses (2022) 14(5):959. doi: 10.3390/v14050959
390. Zhou Y, Xu J, Hou Y, Leverenz JB, Kallianpur A, Mehra R, et al. Network medicine links sars-cov-2/covid-19 infection to brain microvascular injury and neuroinflammation in dementia-like cognitive impairment. Alzheimers Res Ther (2021) 13(1):110. doi: 10.1186/s13195-021-00850-3
391. Zhuang X, Tsukuda S, Wrensch F, Wing PA, Schilling M, Harris JM, et al. The circadian clock component bmal1 regulates sars-cov-2 entry and replication in lung epithelial cells. iScience (2021) 24(10):103144. doi: 10.1101/2021.03.20.436163
392. Birnie MT, Claydon MDB, Troy O, Flynn BP, Yoshimura M, Kershaw YM, et al. Circadian regulation of hippocampal function is disrupted with corticosteroid treatment. Proc Natl Acad Sci U.S.A. (2023) 120(15):e2211996120. doi: 10.1073/pnas.2211996120
393. Li JB, Hu XY, Chen MW, Xiong CH, Zhao N, Ge YH, et al. P85s6k sustains synaptic glua1 to ameliorate cognitive deficits in alzheimer’s disease. Trans neurodegeneration (2023) 12(1):1. doi: 10.1186/s40035-022-00334-w
394. Felten M, Dame C, Lachmann G, Spies C, Rubarth K, Balzer F, et al. Circadian rhythm disruption in critically ill patients. Acta Physiol (Oxf) (2023) 238(1):e13962. doi: 10.1111/apha.13962
395. Huang C, Zhang C, Cao Y, Li J, Bi F. Major roles of the circadian clock in cancer. Cancer Biol Med (2023) 20(1):1–24. doi: 10.20892/j.issn.2095-3941.2022.0474
396. Kalam F, James DL, Li YR, Coleman MF, Kiesel VA, Cespedes Feliciano EM, et al. Intermittent fasting interventions to leverage metabolic and circadian mechanisms for cancer treatment and supportive care outcomes. J Natl Cancer Inst Monogr (2023) 2023(61):84–103. doi: 10.1093/jncimonographs/lgad008
397. Chong ZZ, Kang JQ, Maiese K. Metabotropic glutamate receptors promote neuronal and vascular plasticity through novel intracellular pathways. Histol Histopathol (2003) 18(1):173–89. doi: 10.14670/HH-18.173
398. Lin SH, Maiese K. The metabotropic glutamate receptor system protects against ischemic free radical programmed cell death in rat brain endothelial cells. J Cereb Blood Flow Metab (2001) 21(3):262–75. doi: 10.1097/00004647-200103000-00010
399. Cheng X, Song C, Du Y, Gaur U, Yang M. Pharmacological treatment of alzheimer’s disease: insights from drosophila melanogaster. Int J Mol Sci (2020) 21(13):4621. doi: 10.3390/ijms21134621
400. Maiese K. Foxo proteins in the nervous system. Anal Cell Pathol (Amst) (2015) 2015:569392. doi: 10.1155/2015/569392
401. van Dyck CH, Swanson CJ, Aisen P, Bateman RJ, Chen C, Gee M, et al. Lecanemab in early alzheimer’s disease. N Engl J Med (2023) 388(1):9–21. doi: 10.1056/NEJMoa2212948
402. Slezáková D, Kadlic P, Jezberová M, Boleková V, Valkovič P, Minar M. Brain volume loss in multiple sclerosis is independent of disease activity and might be prevented by early disease-modifying therapy. Neurol Neurochir Pol (2023) 57(3):282–8. doi: 10.5603/PJNNS.a2023.0031
403. Kaisinger LR, Kentistou KA, Stankovic S, Gardner EJ, Day FR, Zhao Y, et al. Large-scale exome sequence analysis identifies sex- and age-specific determinants of obesity. Cell Genomics (2023) 3(8):100362. doi: 10.1016/j.xgen.2023.100362
404. Dong J, Li H, Bai Y, Wu C. Muscone ameliorates diabetic peripheral neuropathy through activating akt/mtor signalling pathway. J Pharm Pharmacol (2019) 71(11):1706–13. doi: 10.1111/jphp.13157
405. Lan T, Xu Y, Li S, Li N, Zhang S, Zhu H. Cornin protects against cerebral ischemia/reperfusion injury by preventing autophagy via the pi3k/akt/mtor pathway. BMC Pharmacol Toxicol (2022) 23(1):82. doi: 10.1186/s40360-022-00620-3
406. Sharma N, Shandilya A, Kumar N, Mehan S. Dysregulation of sirt-1 signaling in multiple sclerosis and neuroimmune disorders: A systematic review of sirtuin activators as potential immunomodulators and their influences on other dysfunctions. Endocr Metab Immune Disord Drug Targets (2021) 21(10):1845–68. doi: 10.2174/1871530321666210309112234
407. Yang X, Huo F, Liu B, Liu J, Chen T, Li J, et al. Crocin inhibits oxidative stress and pro-inflammatory response of microglial cells associated with diabetic retinopathy through the activation of pi3k/akt signaling pathway. J Mol Neurosci (2017) 61(4):581–9. doi: 10.1007/s12031-017-0899-8
408. Klionsky DJ, Abdel-Aziz AK, Abdelfatah S, Abdellatif M, Abdoli A, Abel S, et al. Guidelines for the use and interpretation of assays for monitoring autophagy (4th edition). Autophagy (2021) 17(1):1–382. doi: 10.1080/15548627.2020.1797280
409. Maiese K, Chong ZZ, Shang YC, Wang S. Targeting disease through novel pathways of apoptosis and autophagy. Expert Opin Ther Targets (2012) 16(12):1203–14. doi: 10.1517/14728222.2012.719499
410. Farahani M, Niknam Z, Mohammadi Amirabad L, Amiri-Dashatan N, Koushki M, Nemati M, et al. Molecular pathways involved in covid-19 and potential pathway-based therapeutic targets. BioMed Pharmacother (2021) 145:112420. doi: 10.1016/j.biopha.2021.112420
411. Yan WT, Lu S, Yang YD, Ning WY, Cai Y, Hu XM, et al. Research trends, hot spots and prospects for necroptosis in the field of neuroscience. Neural regeneration Res (2021) 16(8):1628–37. doi: 10.4103/1673-5374.303032
412. Chong ZZ, Shang YC, Maiese K. Vascular injury during elevated glucose can be mitigated by erythropoietin and wnt signaling. Curr Neurovasc Res (2007) 4(3):194–204. doi: 10.2174/156720207781387150
413. Kandula V, Kosuru R, Li H, Yan D, Zhu Q, Lian Q, et al. Forkhead box transcription factor 1: role in the pathogenesis of diabetic cardiomyopathy. Cardiovasc Diabetol (2016) 15(1):44. doi: 10.1186/s12933-016-0361-1
414. Weikel KA, Cacicedo JM, Ruderman NB, Ido Y. Knockdown of gsk3beta increases basal autophagy and ampk signaling in nutrient-laden human aortic endothelial cells. Bioscience Rep (2016) 36(5):e00382. doi: 10.1042/bsr20160174
415. Barchetta I, Cimini FA, Ciccarelli G, Baroni MG, Cavallo MG. Sick fat: the good and the bad of old and new circulating markers of adipose tissue inflammation. J endocrinological Invest (2019) 42(11):1257–72. doi: 10.1007/s40618-019-01052-3
416. Hsieh CF, Liu CK, Lee CT, Yu LE, Wang JY. Acute glucose fluctuation impacts microglial activity, leading to inflammatory activation or self-degradation. Sci Rep (2019) 9(1):840. doi: 10.1038/s41598-018-37215-0
417. Maiese K, Chong ZZ, Shang YC, Wang S. Novel directions for diabetes mellitus drug discovery. Expert Opin Drug Discovery (2013) 8(1):35–48. doi: 10.1517/17460441.2013.736485
418. Ran D, Hong W, Yan W, Mengdie W. Properties and molecular mechanisms underlying geniposide-mediated therapeutic effects in chronic inflammatory diseases. J Ethnopharmacol (2021) 273:113958. doi: 10.1016/j.jep.2021.113958
419. Adle-Biassette H, Levy Y, Colombel M, Poron F, Natchev S, Keohane C, et al. Neuronal apoptosis in hiv infection in adults. Neuropathol Appl Neurobiol (1995) 21(3):218–27. doi: 10.1111/j.1365-2990.1995.tb01053.x
420. Chong ZZ, Shang YC, Hou J, Maiese K. Wnt1 Neuroprotection Translates into Improved Neurological Function During Oxidant Stress and Cerebral Ischemia through Akt1 and Mitochondrial Apoptotic Pathways. Oxid Med Cell Longev (2010) 3(2):153–65. doi: 10.4161/oxim.3.2.11758
421. Fu L, Liu C, Chen L, Lv Y, Meng G, Hu M, et al. Protective effects of 1-methylnicotinamide on abeta1-42-induced cognitive deficits, neuroinflammation and apoptosis in mice. J neuroimmune Pharmacol (2019) 14(3):401–2. doi: 10.1007/s11481-018-09830-1
422. Groc L, Bezin L, Foster JA, Jiang H, Jackson TS, Weissmann D, et al. Lipid peroxidation-mediated oxidative stress and dopamine neuronal apoptosis in the substantia nigra during development. Neurochem Int (2001) 39(2):127–33. doi: 10.1016/s0197-0186(01)00013-4
423. He Z, Zhao Y, Zhu Y, Wang W, Liu X, Lu F. Interfering tug1 attenuates cerebrovascular endothelial apoptosis and inflammatory injury after cerebral ischemia/reperfusion via tug1/mir-410/foxo3 cerna axis. Neurotox Res (2021) 40(1):1–13. doi: 10.1007/s12640-021-00446-7
424. Ko HW, Han KS, Kim EY, Ryu BR, Yoon WJ, Jung YK, et al. Synergetic Activation of P38 Mitogen-Activated Protein Kinase and Caspase-3-Like Proteases for Execution of Calyculin a-Induced Apoptosis but Not N-Methyl-D-Aspartate-Induced Necrosis in Mouse Cortical Neurons. J Neurochem (2000) 74(6):2455–61. doi: 10.1046/j.1471-4159.2000.0742455.x
425. Mansour RM, El Sayed NS, Ahmed MAE, El-Sahar AE. Addressing peroxisome proliferator-activated receptor-gamma in 3-nitropropionic acid-induced striatal neurotoxicity in rats. Mol Neurobiol (2022) 59(7):4368–83. doi: 10.1007/s12035-022-02856-w
426. Zhao T, Miao H, Song Z, Li Y, Xia N, Zhang Z, et al. Metformin alleviates the cognitive impairment induced by benzo[a]Pyrene via glucolipid metabolism regulated by fto/foxo6 pathway in mice. Environ Sci pollut Res Int (2023) 30(26):69192–204. doi: 10.1007/s11356-023-27303-8
427. Zhao Y, Lützen U, Gohlke P, Jiang P, Herdegen T, Culman J. Neuroprotective and antioxidative effects of pioglitazone in brain tissue adjacent to the ischemic core are mediated by pi3k/akt and nrf2/are pathways. J Mol Med (Berlin Germany) (2021) 99(8):1073–83. doi: 10.1007/s00109-021-02065-3
428. Mastrapasqua M, Rossi R, De Cosmo L, Resta A, Errede M, Bizzoca A, et al. Autophagy increase in merosin-deficient congenital muscular dystrophy type 1a. Eur J Transl Myol (2023) 33(3):11501. doi: 10.4081/ejtm.2023.11501
429. Chong ZZ, Li F, Maiese K. Cellular demise and inflammatory microglial activation during beta-amyloid toxicity are governed by wnt1 and canonical signaling pathways. Cell Signal (2007) 19(6):1150–62. doi: 10.1016/j.cellsig.2006.12.009
430. Dehghanian F, Soltani Z, Khaksari M. Can mesenchymal stem cells act multipotential in traumatic brain injury? J Mol Neurosci (2020) 70(5):677–88. doi: 10.1007/s12031-019-01475-w
431. Liu A, Wu J, Yang C, Wu Y, Zhang Y, Zhao F, et al. Trpm7 in chbp-induced renoprotection upon ischemia reperfusion-related injury. Sci Rep (2018) 8(1):5510. doi: 10.1038/s41598-018-22852-2
432. Simon F, Floros N, Ibing W, Schelzig H, Knapsis A. Neurotherapeutic potential of erythropoietin after ischemic injury of the central nervous system. Neural regeneration Res (2019) 14(8):1309–12. doi: 10.4103/1673-5374.253507
433. Zhang Z, Yang JL, Zhang LL, Chen ZZ, Chen JO, Cao YG, et al. 2-(2-Benzofuranyl)-2-Imidazoline Treatment within 5 Hours after Cerebral Ischemia/Reperfusion Protects the Brain. Neural regeneration Res (2018) 13(12):2111–8. doi: 10.4103/1673-5374.241461
434. Sun F, Li SG, Zhang HW, Hua FW, Sun GZ, Huang Z. Mirna-411 attenuates inflammatory damage and apoptosis following spinal cord injury. Eur Rev Med Pharmacol Sci (2020) 24(2):491–8. doi: 10.26355/eurrev_202001_20022
435. Yue J, Liang C, Wu K, Hou Z, Wang L, Zhang C, et al. Upregulated shp-2 expression in the epileptogenic zone of temporal lobe epilepsy and various effects of shp099 treatment on a pilocarpine model. Brain Pathol (2019) 30(2):373–85. doi: 10.1111/bpa.12777
436. Li Y, Liu L, Tian Y, Zhang J. Rapamycin improves sevoflurane−Induced cognitive dysfunction in aged rats by mediating autophagy through the tlr4/myd88/nf−κb signaling pathway. Mol Med Rep (2019) 20(4):3085–94. doi: 10.3892/mmr.2019.10541
437. Sun XL, Zhang JB, Guo YX, Xia TS, Xu LC, Rahmand K, et al. Xanthohumol ameliorates memory impairment and reduces the deposition of β-amyloid in app/ps1 mice via regulating the mtor/lc3ii and bax/bcl-2 signalling pathways. J Pharm Pharmacol (2021) 73(9):1230–9. doi: 10.1093/jpp/rgab052
438. Wang Y, Gao S, Zheng V, Chen L, Ma M, Shen S, et al. A novel pde4d inhibitor bpn14770 reverses scopolamine-induced cognitive deficits via camp/sirt1/akt/bcl-2 pathway. Front Cell Dev Biol (2020) 8:599389. doi: 10.3389/fcell.2020.599389
439. Zhang SH, Liu D, Hu Q, Zhu J, Wang S, Zhou S. Ferulic acid ameliorates pentylenetetrazol-induced seizures by reducing neuron cell death. Epilepsy Res (2019) 156:106183. doi: 10.1016/j.eplepsyres.2019.106183
440. Maiese K. Forkhead transcription factors: new considerations for alzheimer’s disease and dementia. J Transl Sci (2016) 2(4):241–7. doi: 10.15761/JTS.1000146
441. Guo Y, Zeng Q, Brooks D, Geisbrecht ER. A conserved stripak complex is required for autophagy in muscle tissue. Mol Biol Cell (2023) 34(9):ar91. doi: 10.1091/mbc.E23-01-0006
442. Thomas SD, Jha NK, Ojha S, Sadek B. Mtor signaling disruption and its association with the development of autism spectrum disorder. Molecules (2023) 28(4):1889. doi: 10.3390/molecules28041889
443. Eshraghi M, Ahmadi M, Afshar S, Lorzadeh S, Adlimoghaddam A, Rezvani Jalal N, et al. Enhancing autophagy in alzheimer’s disease through drug repositioning. Pharmacol Ther (2022) 237:108171. doi: 10.1016/j.pharmthera.2022.108171
444. Moors TE, Hoozemans JJ, Ingrassia A, Beccari T, Parnetti L, Chartier-Harlin MC, et al. Therapeutic potential of autophagy-enhancing agents in parkinson’s disease. Mol neurodegeneration (2017) 12(1):11. doi: 10.1186/s13024-017-0154-3
445. Zhou ZD, Selvaratnam T, Lee JCT, Chao YX, Tan EK. Molecular targets for modulating the protein translation vital to proteostasis and neuron degeneration in parkinson’s disease. Trans neurodegeneration (2019) 8:6. doi: 10.1186/s40035-019-0145-0
446. Gu Y, Lindner J, Kumar A, Yuan W, Magnuson MA. Rictor/mtorc2 is essential for maintaining a balance between beta-cell proliferation and cell size. Diabetes (2011) 60(3):827–37. doi: 10.2337/db10-1194
447. Lim YM, Lim H, Hur KY, Quan W, Lee HY, Cheon H, et al. Systemic autophagy insufficiency compromises adaptation to metabolic stress and facilitates progression from obesity to diabetes. Nat Commun (2014) 5:4934. doi: 10.1038/ncomms5934
448. Ma L, Fu R, Duan Z, Lu J, Gao J, Tian L, et al. Sirt1 is essential for resveratrol enhancement of hypoxia-induced autophagy in the type 2 diabetic nephropathy rat. Pathology Res Pract (2016) 212(4):310–8. doi: 10.1016/j.prp.2016.02.001
449. Liu Z, Stanojevic V, Brindamour LJ, Habener JF. Glp1-derived nonapeptide glp1(28-36)Amide protects pancreatic beta-cells from glucolipotoxicity. J Endocrinol (2012) 213(2):143–54. doi: 10.1530/joe-11-0328
450. He C, Bassik MC, Moresi V, Sun K, Wei Y, Zou Z, et al. Exercise-induced bcl2-regulated autophagy is required for muscle glucose homeostasis. Nature (2012) 481(7382):511–5. doi: 10.1038/nature10758
451. Liu Y, Palanivel R, Rai E, Park M, Gabor TV, Scheid MP, et al. Adiponectin stimulates autophagy and reduces oxidative stress to enhance insulin sensitivity during high fat diet feeding in mice. Diabetes (2014) 64(1):36–48. doi: 10.2337/db14-0267
452. Hua K, Li T, He Y, Guan A, Chen L, Gao Y, et al. Resistin secreted by porcine alveolar macrophages leads to endothelial cell dysfunction during haemophilus parasuis infection. Virulence (2023) 14(1):2171636. doi: 10.1080/21505594.2023.2171636
453. Tan C, Ai J, Zhu Y. Mtorc1-dependent protein and parkinson’s disease: A mendelian randomization study. Brain Sci (2023) 13(4):536. doi: 10.3390/brainsci13040536
454. Chong ZZ, Shang YC, Maiese K. Cardiovascular disease and mtor signaling. Trends Cardiovasc Med (2011) 21(5):151–5. doi: 10.1016/j.tcm.2012.04.005
455. Maiese K, Chong ZZ, Shang YC, Wang S. Mtor: on target for novel therapeutic strategies in the nervous system. Trends Mol Med (2013) 19(1):51–60. doi: 10.1016/j.molmed.2012.11.001
456. Radulovic J, Gabbay V. Pfc mtor signaling as a biological signature for cognitive deficits in bipolar disorder without psychosis. Cell Rep Med (2021) 2(5):100282. doi: 10.1016/j.xcrm.2021.100282
457. Cappoli N, Mezzogori D, Tabolacci E, Coletta I, Navarra P, Pani G, et al. The mtor kinase inhibitor rapamycin enhances the expression and release of pro-inflammatory cytokine interleukin 6 modulating the activation of human microglial cells. Excli J (2019) 18:779–98. doi: 10.17179/excli2019-1715
458. Jung CH, Jun CB, Ro SH, Kim YM, Otto NM, Cao J, et al. Ulk-atg13-fip200 complexes mediate mtor signaling to the autophagy machinery. Mol Biol Cell (2009) 20(7):1992–2003. doi: 10.1091/mbc.e08-12-1249
459. Javdan N, Ayatollahi SA, Choudhary MI, Al-Hasani S, Kobarfard F, Athar A, et al. Capsaicin Protects against Testicular Torsion Injury through Mtor-Dependent Mechanism. Theriogenology (2018) 113:247–52. doi: 10.1016/j.theriogenology.2018.03.012
460. Park A, Koh HC. Nf-kappab/mtor-mediated autophagy can regulate diquat-induced apoptosis. Arch Toxicol (2019) 93(5):1239–53. doi: 10.1007/s00204-019-02424-7
461. Zhao Y, Wang Q, Wang Y, Li J, Lu G, Liu Z. Glutamine Protects against Oxidative Stress Injury through Inhibiting the Activation of Pi3k/Akt Signaling Pathway in Parkinsonian Cell Model. Environ Health Prev Med (2019) 24(1):4. doi: 10.1186/s12199-018-0757-5
462. Dai C, Ciccotosto GD, Cappai R, Wang Y, Tang S, Hoyer D, et al. Rapamycin Confers Neuroprotection against Colistin-Induced Oxidative Stress, Mitochondria Dysfunction and Apoptosis through the Activation of Autophagy and Mtor/Akt/Creb Signaling Pathways. ACS Chem Neurosci (2017) 9(4):824–37. doi: 10.1021/acschemneuro.7b00323
463. Zhang ZH, Wu QY, Zheng R, Chen C, Chen Y, Liu Q, et al. Selenomethionine mitigates cognitive decline by targeting both tau hyperphosphorylation and autophagic clearance in an alzheimer’s disease mouse model. J Neurosci (2017) 37(9):2449–62. doi: 10.1523/jneurosci.3229-16.2017
464. Han K, Jia N, Zhong Y, Shang X. S14g-humanin alleviates insulin resistance and increases autophagy in neurons of app/ps1 transgenic mouse. J Cell Biochem (2017) 19(4):3111–7. doi: 10.1002/jcb.26452
465. Damstra-Oddy JL, Warren EC, Perry CJ, Desfougères Y, Fitzpatrick JK, Schaf J, et al. Phytocannabinoid-dependent mtorc1 regulation is dependent upon inositol polyphosphate multikinase activity. Br J Pharmacol (2021) 178(5):1149–63. doi: 10.1111/bph.15351
466. Dello Russo C, Lisi L, Feinstein DL, Navarra P. Mtor kinase, a key player in the regulation of glial functions: relevance for the therapy of multiple sclerosis. Glia (2013) 61(3):301–11. doi: 10.1002/glia.22433
467. Lee Y, Hong Y, Lee SR, Chang KT. Autophagy contributes to retardation of cardiac growth in diabetic rats. Lab Anim Res (2012) 28(2):99–107. doi: 10.5625/lar.2012.28.2.99
468. Hu P, Lai D, Lu P, Gao J, He H. Erk and akt signaling pathways are involved in advanced glycation end product-induced autophagy in rat vascular smooth muscle cells. Int J Mol Med (2012) 29(4):613–8. doi: 10.3892/ijmm.2012.891
469. Martino L, Masini M, Novelli M, Beffy P, Bugliani M, Marselli L, et al. Palmitate activates autophagy in ins-1e beta-cells and in isolated rat and human pancreatic islets. PloS One (2012) 7(5):e36188. doi: 10.1371/journal.pone.0036188
470. Saleem S, Biswas SC. Tribbles pseudokinase 3 induces both apoptosis and autophagy in amyloid-beta-induced neuronal death. J Biol Chem (2017) 292(7):2571–85. doi: 10.1074/jbc.M116.744730
471. Li Q, Han Y, Du J, Jin H, Zhang J, Niu M, et al. Recombinant human erythropoietin protects against hippocampal damage in developing rats with seizures by modulating autophagy via the S6 protein in a time-dependent manner. Neurochem Res (2017) 43(2):465–76. doi: 10.1007/s11064-017-2443-1
472. Ding C, Zhang J, Li B, Ding Z, Cheng W, Gao F, et al. Cornin Protects Shsy5y Cells against Oxygen and Glucose Deprivationinduced Autophagy through the Pi3k/Akt/Mtor Pathway. Mol Med Rep (2017) 17(1):87–92. doi: 10.3892/mmr.2017.7864
473. Ka M, Smith AL, Kim WY. Mtor controls genesis and autophagy of gabaergic interneurons during brain development. Autophagy (2017) 13(8):1348–63. doi: 10.1080/15548627.2017.1327927
474. Fields CR, Bengoa-Vergniory N, Wade-Martins R. Targeting alpha-synuclein as a therapy for parkinson’s disease. Front Mol Neurosci (2019) 12:299. doi: 10.3389/fnmol.2019.00299
475. Jobst M, Kiss E, Gerner C, Marko D, Del Favero G. Activation of autophagy triggers mitochondrial loss and changes acetylation profile relevant for mechanotransduction in bladder cancer cells. Arch Toxicol (2022) 97(1):217–33. doi: 10.1007/s00204-022-03375-2
476. Wang N, Luo Z, Jin M, Sheng W, Wang HT, Long X, et al. Exploration of age-related mitochondrial dysfunction and the anti-aging effects of resveratrol in zebrafish retina. Aging (Albany NY) (2019) 11(10):3117–37. doi: 10.18632/aging.101966
477. Xu G, Shen H, Nibona E, Wu K, Ke X, Al Hafiz MA, et al. Fundc1 is necessary for proper body axis formation during embryogenesis in zebrafish. Sci Rep (2019) 9(1):18910. doi: 10.1038/s41598-019-55415-0
478. Zeng Z, Liang J, Wu L, Zhang H, Lv J, Chen N. Exercise-induced autophagy suppresses sarcopenia through akt/mtor and akt/foxo3a signal pathways and ampk-mediated mitochondrial quality control. Front Physiol (2020) 11:583478. doi: 10.3389/fphys.2020.583478
479. Dechandt CRP, Ferrari GD, Dos Santos JR, de Oliveira JAC, da Silva-Jr RMP, Cunha AOS, et al. Energy metabolism and redox state in brains of wistar audiogenic rats, a genetic model of epilepsy. Front Neurol (2019) 10:1007. doi: 10.3389/fneur.2019.01007
480. Kim KA, Shin YJ, Akram M, Kim ES, Choi KW, Suh H, et al. High glucose condition induces autophagy in endothelial progenitor cells contributing to angiogenic impairment. Biol Pharm Bull (2014) 37(7):1248–52. doi: 10.1248/bpb.b14-00172
481. Fessel J. Supplementary pharmacotherapy for the behavioral abnormalities caused by stressors in humans, focused on post-traumatic stress disorder (Ptsd). J Clin Med (2023) 12(4):1680. doi: 10.3390/jcm12041680
482. Yang K, Zhang L, Chen W, Cheng J, Zhao X, Zhang Y, et al. Expression of epo and related factors in the liver and kidney of plain and tibetan sheep. Histol Histopathol (2023), 18592. doi: 10.14670/hh-18-592
483. Chong ZZ, Kang JQ, Maiese K. Erythropoietin is a novel vascular protectant through activation of akt1 and mitochondrial modulation of cysteine proteases. Circulation (2002) 106(23):2973–9. doi: 10.1161/01.cir.0000039103.58920.1f
484. García-Llano M, Pedroso-Ibáñez I, Morales-Chacón L, Rodríguez-Obaya T, Pérez-Ruiz L, Sosa-Testé I, et al. Short-term tolerance of nasally-administered neuroepo in patients with parkinson disease. MEDICC Rev (2021) 23(1):49–54. doi: 10.37757/mr2021.V23.N1.10
485. Chong ZZ, Maiese K. Erythropoietin involves the phosphatidylinositol 3-kinase pathway, 14-3-3 protein and foxo3a nuclear trafficking to preserve endothelial cell integrity. Br J Pharmacol (2007) 150(7):839–50. doi: 10.1038/sj.bjp.0707161
486. Maiese K, Hou J, Chong ZZ, Shang YC. Erythropoietin, forkhead proteins, and oxidative injury: biomarkers and biology. ScientificWorldJournal (2009) 9:1072–104. doi: 10.1100/tsw.2009.121
487. Chamorro ME, Wenker SD, Vota DM, Vittori DC, Nesse AB. Signaling pathways of cell proliferation are involved in the differential effect of erythropoietin and its carbamylated derivative. Biochim Biophys Acta (2013) 1833(8):1960–8. doi: 10.1016/j.bbamcr.2013.04.006
488. Chong ZZ, Shang YC, Wang S, Maiese K. Pras40 is an integral regulatory component of erythropoietin mtor signaling and cytoprotection. PloS One (2012) 7(9):e45456. doi: 10.1371/journal.pone.0045456
489. Wang GB, Ni YL, Zhou XP, Zhang WF. The akt/mtor pathway mediates neuronal protective effects of erythropoietin in sepsis. Mol Cell Biochem (2014) 385(1-2):125–32. doi: 10.1007/s11010-013-1821-5
490. BinMowyna MN, AlFaris NA. Kaempferol suppresses acetaminophen-induced liver damage by upregulation/activation of sirt1. Pharm Biol (2021) 59(1):146–56. doi: 10.1080/13880209.2021.1877734
491. Dai C, Xiao X, Zhang Y, Xiang B, Hoyer D, Shen J, et al. Curcumin attenuates colistin-induced peripheral neurotoxicity in mice. ACS Infect Dis (2020) 6(4):715–24. doi: 10.1021/acsinfecdis.9b00341
492. De Giorgi F, Lartigue L, Bauer MK, Schubert A, Grimm S, Hanson GT, et al. The permeability transition pore signals apoptosis by directing bax translocation and multimerization. FASEB J (2002) 16(6):607–9. doi: 10.1096/fj.01-0269fje
493. Deng D, Yan J, Wu Y, Wu K, Li W. Morroniside suppresses hydrogen peroxide-stimulated autophagy and apoptosis in rat ovarian granulosa cells through the pi3k/akt/mtor pathway. Hum Exp Toxicol (2020) 40(4):577–86. doi: 10.1177/0960327120960768
494. Hacioglu C, Kar F, Kanbak G. Reproductive effects of nicotinamide on testicular function and structure in old male rats: oxidative, apoptotic, hormonal, and morphological analyses. Reprod Sci (2021) 28(12):3352–60. doi: 10.1007/s43032-021-00647-7
495. Hajializadeh Z, Khaksari M. The protective effects of 17-β Estradiol and sirt1 against cardiac hypertrophy: A review. Heart failure Rev (2021) 27(2):725–38. doi: 10.1007/s10741-021-10171-0
496. Alloza I, Salegi A, Mena J, Navarro RT, Martin C, Aspichueta P, et al. Birc6 is associated with vulnerability of carotid atherosclerotic plaque. Int J Mol Sci (2020) 21(24):9387. doi: 10.3390/ijms21249387
497. Chen S, Li B. Mir-128-3p post-transcriptionally inhibits wisp1 to suppress apoptosis and inflammation in human articular chondrocytes via the pi3k/akt/nf-κb signaling pathway. Cell Transplant (2020) 29:963689720939131. doi: 10.1177/0963689720939131
498. Gallyas F Jr., Sumegi B, Szabo C. Role of akt activation in parp inhibitor resistance in cancer. Cancers (2020) 12(3):532. doi: 10.3390/cancers12030532
499. Govindappa PK, Elfar JC. Erythropoietin promotes M2 macrophage phagocytosis of schwann cells in peripheral nerve injury. Cell Death Dis (2022) 13(3):245. doi: 10.1038/s41419-022-04671-6
500. Ye M, Zhao Y, Wang Y, Xie R, Tong Y, Sauer JD, et al. Nad(H)-loaded nanoparticles for efficient sepsis therapy via modulating immune and vascular homeostasis. Nat Nanotechnol (2022) 17(8):880–90. doi: 10.1038/s41565-022-01137-w
501. Yang L, Cheng CF, Li ZF, Huang XJ, Cai SQ, Ye SY, et al. Berberine blocks inflammasome activation and alleviates diabetic cardiomyopathy via the miR-18a-3p/Gsdmd pathway. Int J Mol Med (2023) 51(6):49. doi: 10.1002/biof.1607
502. Hou J, Chong ZZ, Shang YC, Maiese K. Early apoptotic vascular signaling is determined by sirt1 through nuclear shuttling, forkhead trafficking, bad, and mitochondrial caspase activation. Curr Neurovasc Res (2010) 7(2):95–112. doi: 10.2174/156720210791184899
503. Shang YC, Chong ZZ, Hou J, Maiese K. Wnt1, foxo3a, and nf-kappab oversee microglial integrity and activation during oxidant stress. Cell Signal (2010) 22(9):1317–29. doi: 10.1016/j.cellsig.2010.04.009
504. Taveira GB, Mello EO, Souza SB, Monteiro RM, Ramos AC, Carvalho AO, et al. Programmed cell death in yeast by thionin-like peptide from capsicum annuum fruits involving activation of capases and extracelullar H(+) flux. Bioscience Rep (2018) 38(2):BSR20180119. doi: 10.1042/bsr20180119
505. Almasieh M, Catrinescu MM, Binan L, Costantino S, Levin LA. Axonal degeneration in retinal ganglion cells is associated with a membrane polarity-sensitive redox process. J Neurosci (2017) 37(14):3824–39. doi: 10.1523/jneurosci.3882-16.2017
506. Viola G, Bortolozzi R, Hamel E, Moro S, Brun P, Castagliuolo I, et al. Mg-2477, a new tubulin inhibitor, induces autophagy through inhibition of the akt/mtor pathway and delayed apoptosis in A549 cells. Biochem Pharmacol (2012) 83(1):16–26. doi: 10.1016/j.bcp.2011.09.017
507. Bailey TJ, Fossum SL, Fimbel SM, Montgomery JE, Hyde DR. The inhibitor of phagocytosis, O-phospho-L-serine, suppresses muller glia proliferation and cone cell regeneration in the light-damaged zebrafish retina. Exp Eye Res (2010) 91(5):601–12. doi: 10.1016/j.exer.2010.07.017
508. Shang YC, Chong ZZ, Hou J, Maiese K. Foxo3a governs early microglial proliferation and employs mitochondrial depolarization with caspase 3, 8, and 9 cleavage during oxidant induced apoptosis. Curr Neurovasc Res (2009) 6(4):223–38. doi: 10.2174/156720209789630302
509. Wei L, Sun C, Lei M, Li G, Yi L, Luo F, et al. Activation of wnt/beta-catenin pathway by exogenous wnt1 protects sh-sy5y cells against 6-hydroxydopamine toxicity. J Mol Neurosci (2013) 49(1):105–15. doi: 10.1007/s12031-012-9900-8
510. Hou J, Wang S, Shang YC, Chong ZZ, Maiese K. Erythropoietin employs cell longevity pathways of sirt1 to foster endothelial vascular integrity during oxidant stress. Curr Neurovasc Res (2011) 8(3):220–35. doi: 10.2174/156720211796558069
511. Kim S, Kang IH, Nam JB, Cho Y, Chung DY, Kim SH, et al. Ameliorating the effect of astragaloside iv on learning and memory deficit after chronic cerebral hypoperfusion in rats. Molecules (2015) 20(2):1904–21. doi: 10.3390/molecules20021904
512. Xin YJ, Yuan B, Yu B, Wang YQ, Wu JJ, Zhou WH, et al. Tet1-mediated DNA demethylation regulates neuronal cell death induced by oxidative stress. Sci Rep (2015) 5:7645. doi: 10.1038/srep07645
513. Yu T, Li L, Chen T, Liu Z, Liu H, Li Z. Erythropoietin attenuates advanced glycation endproducts-induced toxicity of schwann cells in vitro. Neurochem Res (2015) 40(4):698–712. doi: 10.1007/s11064-015-1516-2
514. Yousafzai NA, Jin H, Ullah M, Wang X. Recent advances of sirt1 and implications in chemotherapeutics resistance in cancer. Am J Cancer Res (2021) 11(11):5233–48.
515. Maiese K. Pyroptosis, apoptosis, and autophagy: critical players of inflammation and cell demise in the nervous system. Curr Neurovasc Res (2022) 19:241–4. doi: 10.2174/1567202619666220729093449
516. Pang Y, Qin M, Hu P, Ji K, Xiao R, Sun N, et al. Resveratrol protects retinal ganglion cells against ischemia induced damage by increasing opa1 expression. Int J Mol Med (2020) 46(5):1707–20. doi: 10.3892/ijmm.2020.4711
517. Cui L, Weiyao J, Chenghong S, Limei L, Xinghua Z, Bo Y, et al. Rheumatoid arthritis and mitochondrial homeostasis: the crossroads of metabolism and immunity. Front Med (Lausanne) (2022) 9:1017650. doi: 10.3389/fmed.2022.1017650
518. Maiese K, Vincent AM. Critical temporal modulation of neuronal programmed cell injury. Cell Mol Neurobiol (2000) 20(3):383–400. doi: 10.1023/A:1007070311203
519. Razzaghi A, Choobineh S, Gaeini A, Soori R. Interaction of exercise training with taurine attenuates infarct size and cardiac dysfunction via akt-foxo3a-caspase-8 signaling pathway. Amino Acids (2023) 55(7):869–80. doi: 10.1007/s00726-023-03275-4
520. Shang YC, Chong ZZ, Wang S, Maiese K. Wnt1 inducible signaling pathway protein 1 (Wisp1) targets pras40 to govern beta-amyloid apoptotic injury of microglia. Curr Neurovasc Res (2012) 9(4):239–49. doi: 10.2174/156720212803530618
521. Shang YC, Chong ZZ, Wang S, Maiese K. Prevention of beta-amyloid degeneration of microglia by erythropoietin depends on wnt1, the pi 3-K/mtor pathway, bad, and bcl-xl. Aging (Albany NY) (2012) 4(3):187–201. doi: 10.18632/aging.100440
522. Shang YC, Chong ZZ, Wang S, Maiese K. Tuberous sclerosis protein 2 (Tsc2) modulates ccn4 cytoprotection during apoptotic amyloid toxicity in microglia. Curr Neurovasc Res (2013) 10(1):29–38. doi: 10.2174/156720213804806007
523. Bhowmick S, D’Mello V, Caruso D, Abdul-Muneer PM. Traumatic brain injury-induced downregulation of nrf2 activates inflammatory response and apoptotic cell death. J Mol Med (Berlin Germany) (2019) 97(12):1627–41. doi: 10.1007/s00109-019-01851-4
524. Chong ZZ, Li F, Maiese K. Stress in the brain: novel cellular mechanisms of injury linked to alzheimer’s disease. Brain Res Brain Res Rev (2005) 49(1):1–21. doi: 10.1016/j.brainresrev.2004.11.005
525. Najjar RS, Turner CG, Wong BJ, Feresin RG. Berry-derived polyphenols in cardiovascular pathologies: mechanisms of disease and the role of diet and sex. Nutrients (2021) 13(2):387. doi: 10.3390/nu13020387
526. Liang H, Liu Q. The role of non-coding rna in lupus nephritis. Hum Cell (2023) 36(3):923–36. doi: 10.1007/s13577-023-00883-w
527. Scrimieri R, Locatelli L, Cazzaniga A, Cazzola R, Malucelli E, Sorrentino A, et al. Ultrastructural features mirror metabolic derangement in human endothelial cells exposed to high glucose. Sci Rep (2023) 13(1):15133. doi: 10.1038/s41598-023-42333-5
528. Wang J, Chen S, Zhao X, Guo Q, Yang R, Zhang C, et al. Effect of pparγ on oxidative stress in diabetes-related dry eye. Exp Eye Res (2023) 231:109498. doi: 10.1016/j.exer.2023.109498
529. Yeger H. Ccn proteins: opportunities for clinical studies-a personal perspective. J Cell Commun Signal (2023) 17(2):333–52. doi: 10.1007/s12079-023-00761-y
530. Sierra-Pagan JE, Dsouza N, Das S, Larson TA, Sorensen JR, Ma X, et al. Foxk1 regulates wnt signaling to promote cardiogenesis. Cardiovasc Res (2023) 119(8):1728–39. doi: 10.1093/cvr/cvad054
531. Zhang Y, Zhou H, Ding C. The ameliorative effect of cangfu daotan decoction on polycystic ovary syndrome of rodent model is associated with M6a methylation and wnt/β-catenin pathway. Gynecol Endocrinol (2023) 39(1):2181637. doi: 10.1080/09513590.2023.2181637
532. Farid HA, Sayed RH, El-Shamarka ME, Abdel-Salam OME, El Sayed NS. Pi3k/akt signaling activation by roflumilast ameliorates rotenone-induced parkinson’s disease in rats. Inflammopharmacology (2023). doi: 10.1007/s10787-023-01305-x
533. Hu G, Wang T, Ma C. Epo activates pi3k-ikkα-cdk1 signaling pathway to promote the proliferation of glial cells under hypoxia environment. Genet Mol Biol (2022) 45(1):e20210249. doi: 10.1590/1678-4685-gmb-2021-0249
534. Liu H, Wang C, Sun X, Zhan C, Li Z, Qiu L, et al. Silk fibroin/collagen/hydroxyapatite scaffolds obtained by 3d printing technology and loaded with recombinant human erythropoietin in the reconstruction of alveolar bone defects. ACS Biomater Sci Eng (2022) 8(12):5245–56. doi: 10.1021/acsbiomaterials.2c00690
535. Sergio CM, Rolando CA. Erythropoietin regulates signaling pathways associated with neuroprotective events. Exp Brain Res (2022) 240(5):1303–15. doi: 10.1007/s00221-022-06331-9
536. Maiese K. Regeneration in the nervous system with erythropoietin. Front bioscience (Landmark edition) (2016) 21:561–96. doi: 10.2741/4408
537. Sun N, Victor MB, Park YP, Xiong X, Scannail AN, Leary N, et al. Human microglial state dynamics in alzheimer’s disease progression. Cell (2023) 186(20):4386–403.e29. doi: 10.1016/j.cell.2023.08.037
538. Duarte-Silva E, Meuth SG, Peixoto CA. The role of iron metabolism in the pathogenesis and treatment of multiple sclerosis. Front Immunol (2023) 14:1137635. doi: 10.3389/fimmu.2023.1137635
539. Maiese K. Ferroptosis, iron metabolism, and forkhead transcription factors (Foxos). Curr Neurovasc Res (2023) 20(3):291–5. doi: 10.2174/1567202620666230706160056
540. He L, Yang Y, Chen J, Zou P, Li J. Transcriptional activation of enpp2 by foxo4 protects cardiomyocytes from doxorubicin−Induced toxicity. Mol Med Rep (2021) 24(3):668. doi: 10.3892/mmr.2021.12307
541. Malhotra S, Hurtado-Navarro L, Pappolla A, Villar LMM, Río J, Montalban X, et al. Increased nlrp3 inflammasome activation and pyroptosis in patients with multiple sclerosis with fingolimod treatment failure. Neurol Neuroimmunol Neuroinflamm (2023) 10(3):e200100. doi: 10.1212/nxi.0000000000200100
542. Conti P, Ronconi G, Caraffa A, Gallenga CE, Ross R, Frydas I, et al. Induction of pro-inflammatory cytokines (Il-1 and il-6) and lung inflammation by coronavirus-19 (Covi-19 or sars-cov-2): anti-inflammatory strategies. J Biol Regul Homeost Agents (2020) 34(2):327–31. doi: 10.23812/conti-e
543. Crespo I, Fernández-Palanca P, San-Miguel B, Álvarez M, González-Gallego J, Tuñón MJ. Melatonin modulates mitophagy, innate immunity and circadian clocks in a model of viral-induced fulminant hepatic failure. J Cell Mol Med (2020) 24(13):7625–36. doi: 10.1111/jcmm.15398
544. Park MH, Gutierrez-Garcia AK, Choudhury M. Mono-(2-ethylhexyl) phthalate aggravates inflammatory response via sirtuin regulation and inflammasome activation in raw 264.7 cells. Chem Res Toxicol (2019) 32(5):935–42. doi: 10.1021/acs.chemrestox.9b00101
545. Vaamonde-Garcia C, Lopez-Armada MJ. Role of mitochondrial dysfunction on rheumatic diseases. Biochem Pharmacol (2019) 165:181–95. doi: 10.1016/j.bcp.2019.03.008
546. Qian D, Dai S, Sun Y, Yuan Y, Wang L. Mir-128-3p attenuates the neurotoxicity in rats induced by isoflurane anesthesia. Neurotox Res (2022) 40(3):714–20. doi: 10.1007/s12640-022-00512-8
547. Toniolo S, Scarioni M, Di Lorenzo F, Hort J, Georges J, Tomic S, et al. Dementia and covid-19, a bidirectional liaison: risk factors, biomarkers, and optimal health care. J Alzheimers Dis (2021) 82(3):883–98. doi: 10.3233/jad-210335
548. Hassanein EHM, Saleh FM, Ali FEM, Rashwan EK, Atwa AM, Abd El-Ghafar OAM. Neuroprotective effect of canagliflozin against cisplatin-induced cerebral cortex injury is mediated by regulation of ho-1/ppar-Γ, sirt1/foxo-3, jnk/ap-1, tlr4/inos, and ang ii/ang 1-7 signals. Immunopharmacol Immunotoxicol (2023) 45(3):304–16. doi: 10.1080/08923973.2022.2143371
549. Maiese K. Sirtuins in metabolic disease: Innovative Therapeutic Strategies with Sirt1, Ampk, Mtor, and Nicotinamide. In: Maiese K, editor. Sirtuin Biology in Cancer and Metabolic Disease: Cellular Pathways for Clinical Discovery. Academic Press, Elsevier (2021), Cambridge, MA, USA; Elsevier, Amsterdam, The Netherlands: ISBN.
550. Fangma Y, Wan H, Shao C, Jin L, He Y. Research progress on the role of sirtuin 1 in cerebral ischemia. Cell Mol Neurobiol (2022) 43(5):1769–83. doi: 10.1007/s10571-022-01288-3
551. Kang X, Li C, Xie X, Zhan KB, Yang SQ, Tang YY, et al. Hydrogen sulfide inhibits homocysteine-induced neuronal senescence by up-regulation of sirt1. Int J Med Sci (2020) 17(3):310–9. doi: 10.7150/ijms.38602
552. Li H, Peng D, Zhang SJ, Zhang Y, Wang Q, Guan L. Buyang huanwu decoction promotes neurogenesis via sirtuin 1/autophagy pathway in a cerebral ischemia model. Mol Med Rep (2021) 24(5):791. doi: 10.3892/mmr.2021.12431
553. Maiese K. Novel treatment strategies for the nervous system: circadian clock genes, non-coding rnas, and forkhead transcription factors. Curr Neurovasc Res (2018) 15(1):81–91. doi: 10.2174/1567202615666180319151244
554. Yin Q, Wang JF, Xu XH, Xie H. Effect of lycopene on pain facilitation and the sirt1/mtor pathway in the dorsal horn of burn injury rats. Eur J Pharmacol (2020) 889:173365. doi: 10.1016/j.ejphar.2020.173365
555. Lee Y, Im E. Regulation of mirnas by natural antioxidants in cardiovascular diseases: focus on sirt1 and enos. Antioxidants (Basel Switzerland) (2021) 10(3):377. doi: 10.3390/antiox10030377
556. Mori M, Cazzaniga G, Meneghetti F, Villa S, Gelain A. Insights on the modulation of sirt5 activity: A challenging balance. Molecules (2022) 27(14):4449. doi: 10.3390/molecules27144449
557. Rong Y, Ren J, Song W, Xiang R, Ge Y, Lu W, et al. Resveratrol suppresses severe acute pancreatitis-induced microcirculation disturbance through targeting sirt1-foxo1 axis. Oxid Med Cell Longev (2021) 2021:8891544. doi: 10.1155/2021/8891544
558. Guimera AM, Clark P, Wordsworth J, Anugula S, Rasmussen LJ, Shanley DP. Systems modelling predicts chronic inflammation and genomic instability prevent effective mitochondrial regulation during biological ageing. Exp Gerontol (2022) 166:111889. doi: 10.1016/j.exger.2022.111889
559. Sadria M, Seo D, Layton AT. The mixed blessing of ampk signaling in cancer treatments. BMC Cancer (2022) 22(1):105. doi: 10.1186/s12885-022-09211-1
560. Bitterman KJ, Anderson RM, Cohen HY, Latorre-Esteves M, Sinclair DA. Inhibition of silencing and accelerated aging by nicotinamide, a putative negative regulator of yeast sir2 and human sirt1. J Biol Chem (2002) 277(47):45099–107. doi: 10.1074/jbc.M205670200
561. Guerra J, Devesa J. Usefulness of melatonin and other compounds as antioxidants and epidrugs in the treatment of head and neck cancer. Antioxidants (Basel Switzerland) (2021) 11(1):35. doi: 10.3390/antiox11010035
562. Maiese K, Chong ZZ, Shang YC, Wang S. Translating cell survival and cell longevity into treatment strategies with sirt1. Rom J Morphol Embryol (2011) 52(4):1173–85.
563. Penteado AB, Hassanie H, Gomes RA, Silva Emery FD, Goulart Trossini GH. Human sirtuin 2 inhibitors, their mechanisms and binding modes. Future Med Chem (2023) 15(3):291–311. doi: 10.4155/fmc-2022-0253
564. Giacalone S, Spigariolo CB, Bortoluzzi P, Nazzaro G. Oral nicotinamide: the role in skin cancer chemoprevention. Dermatol Ther (2021) 34(3):e14892. doi: 10.1111/dth.14892
565. Chong MC, Silva A, James PF, Wu SSX, Howitt J. Exercise increases the release of nampt in extracellular vesicles and alters nad(+) activity in recipient cells. Aging Cell (2022) 21(7):e13647. doi: 10.1111/acel.13647
566. Potthast AB, Nebl J, Wasserfurth P, Haufe S, Eigendorf J, Hahn A, et al. Impact of nutrition on short-term exercise-induced sirtuin regulation: vegans differ from omnivores and lacto-ovo vegetarians. Nutrients (2020) 12(4):1004. doi: 10.3390/nu12041004
567. AlSaleh A, Shahid M, Farid E, Bindayna K. The effect of ascorbic acid and nicotinamide on panton-valentine leukocidin cytotoxicity: an ex vivo study. Toxins (Basel) (2023) 15(1):38. doi: 10.3390/toxins15010038
568. Kumar A, Ou Y. From bench to behaviour: the role of lifestyle factors on intraocular pressure, neuroprotection, and disease progression in glaucoma. Clin Exp Ophthalmol (2023) 51(4):380–94. doi: 10.1111/ceo.14218
569. Li M, Zhang L, Pan L, Zhou P, Yu R, Zhang Z, et al. Nicotinamide efficiently suppresses porcine epidemic diarrhea virus and porcine deltacoronavirus replication. Viruses (2023) 15(7):1591. doi: 10.3390/v15071591
570. Tai S-H, Chao L-C, Huang S-Y, Lin H-W, Lee A-H, Chen Y-Y, et al. Nicotinamide deteriorates post-stroke immunodepression following cerebral ischemia–reperfusion injury in mice. Biomedicines (2023) 11(8):2145. doi: 10.3390/biomedicines11082145
571. Jackson MD, Schmidt MT, Oppenheimer NJ, Denu JM. Mechanism of nicotinamide inhibition and transglycosidation by sir2 histone/protein deacetylases. J Biol Chem (2003) 278(51):50985–98. doi: 10.1074/jbc.M306552200
572. Avalos JL, Bever KM, Wolberger C. Mechanism of sirtuin inhibition by nicotinamide: altering the nad(+) cosubstrate specificity of a sir2 enzyme. Mol Cell (2005) 17(6):855–68. doi: 10.1016/j.molcel.2005.02.022
573. Zhang XM, Jing YP, Jia MY, Zhang L. Negative transcriptional regulation of inflammatory genes by group B3 vitamin nicotinamide. Mol Biol Rep (2012) 39(12):10367–71. doi: 10.1007/s11033-012-1915-2
574. Fulco M, Cen Y, Zhao P, Hoffman EP, McBurney MW, Sauve AA, et al. Glucose restriction inhibits skeletal myoblast differentiation by activating sirt1 through ampk-mediated regulation of nampt. Dev Cell (2008) 14(5):661–73. doi: 10.1016/j.devcel.2008.02.004
575. Pal PB, Sonowal H, Shukla K, Srivastava SK, Ramana KV. Aldose reductase regulates hyperglycemia-induced huvec death via sirt1/ampk-alpha1/mtor pathway. J Mol Endocrinol (2019) 63(1):11–25. doi: 10.1530/jme-19-0080
576. Geng C, Xu H, Zhang Y, Gao Y, Li M, Liu X, et al. Retinoic acid ameliorates high-fat diet-induced liver steatosis through sirt1. Sci China Life Sci (2017) 60(11):1234–41. doi: 10.1007/s11427-016-9027-6
577. Ghiasi R, Naderi R, Sheervalilou R, Alipour MR. Swimming training by affecting the pancreatic sirtuin1 (Sirt1) and oxidative stress, improves insulin sensitivity in diabetic male rats. Hormone Mol Biol Clin Invest (2019) 40(3). doi: 10.1515/hmbci-2019-0011
578. Xue P, Zhao J, Zheng A, Li L, Chen H, Tu W, et al. Chrysophanol alleviates myocardial injury in diabetic db/db mice by regulating the sirt1/hmgb1/nf-kappab signaling pathway. Exp Ther Med (2019) 18(6):4406–12. doi: 10.3892/etm.2019.8083
579. Chen YR, Fang SR, Fu YC, Zhou XH, Xu MY, Xu WC. Calorie restriction on insulin resistance and expression of sirt1 and sirt4 in rats. Biochem Cell Biol (2010) 88(4):715–22. doi: 10.1139/O10-010
580. Caron AZ, He X, Mottawea W, Seifert EL, Jardine K, Dewar-Darch D, et al. The sirt1 deacetylase protects mice against the symptoms of metabolic syndrome. FASEB J (2014) 28(3):1306–16. doi: 10.1096/fj.13-243568
581. Frojdo S, Durand C, Molin L, Carey AL, El-Osta A, Kingwell BA, et al. Phosphoinositide 3-kinase as a novel functional target for the regulation of the insulin signaling pathway by sirt1. Mol Cell Endocrinol (2011) 335(2):166–76. doi: 10.1016/j.mce.2011.01.008
582. Guo W, Qian L, Zhang J, Zhang W, Morrison A, Hayes P, et al. Sirt1 overexpression in neurons promotes neurite outgrowth and cell survival through inhibition of the mtor signaling. J Neurosci Res (2011) 89(11):1723–36. doi: 10.1002/jnr.22725
583. Pan YR, Song JY, Fan B, Wang Y, Che L, Zhang SM, et al. Mtor may interact with parp-1 to regulate visible light-induced parthanatos in photoreceptors. Cell Commun Signal (2020) 18(1):27. doi: 10.1186/s12964-019-0498-0
584. Zhang H, Yang X, Pang X, Zhao Z, Yu H, Zhou H. Genistein Protects against Ox-Ldl-Induced Senescence through Enhancing Sirt1/Lkb1/Ampk-Mediated Autophagy Flux in Huvecs. Mol Cell Biochem (2019) 455((1-2):127–34. doi: 10.1007/s11010-018-3476-8
585. Ou X, Lee MR, Huang X, Messina-Graham S, Broxmeyer HE. Sirt1 positively regulates autophagy and mitochondria function in embryonic stem cells under oxidative stress. Stem Cells (2014) 32(5):1183–94. doi: 10.1002/stem.1641
586. Li MZ, Zheng LJ, Shen J, Li XY, Zhang Q, Bai X, et al. Sirt1 facilitates amyloid beta peptide degradation by upregulating lysosome number in primary astrocytes. Neural regeneration Res (2018) 13(11):2005–13. doi: 10.4103/1673-5374.239449
587. Balan V, Miller GS, Kaplun L, Balan K, Chong ZZ, Li F, et al. Life span extension and neuronal cell protection by drosophila nicotinamidase. J Biol Chem (2008) 283(41):27810–9. doi: 10.1074/jbc.M804681200
588. Wang S, Chong ZZ, Shang YC, Maiese K. Wisp1 neuroprotection requires foxo3a post-translational modulation with autoregulatory control of sirt1. Curr Neurovasc Res (2013) 10(1):54–60. doi: 10.2174/156720213804805945
589. Maiese K, Shang YC, Chong ZZ, Hou J. Diabetes mellitus: channeling care through cellular discovery. Curr Neurovasc Res (2010) 7(1):59–64. doi: 10.2174/156720210790820217
590. Wu H, Wang H, Zhang W, Wei X, Zhao J, Yan P, et al. Rhepo affects apoptosis in hippocampus of aging rats by upregulating sirt1. Int J Clin Exp Pathol (2015) 8(6):6870–80.
591. Chong ZZ, Kang JQ, Maiese K. Erythropoietin fosters both intrinsic and extrinsic neuronal protection through modulation of microglia, akt1, bad, and caspase-mediated pathways. Br J Pharmacol (2003) 138(6):1107–18. doi: 10.1038/sj.bjp.0705161
592. Cui L, Guo J, Zhang Q, Yin J, Li J, Zhou W, et al. Erythropoietin activates sirt1 to protect human cardiomyocytes against doxorubicin-induced mitochondrial dysfunction and toxicity. Toxicol Lett (2017) 275:28–38. doi: 10.1016/j.toxlet.2017.04.018
593. Rey F, Ottolenghi S, Giallongo T, Balsari A, Martinelli C, Rey R, et al. Mitochondrial metabolism as target of the neuroprotective role of erythropoietin in parkinson’s disease. Antioxidants (Basel Switzerland) (2021) 10(1):121. doi: 10.3390/antiox10010121
594. Shang YC, Chong ZZ, Wang S, Maiese K. Erythropoietin and wnt1 govern pathways of mtor, apaf-1, and xiap in inflammatory microglia. Curr Neurovasc Res (2011) 8(4):270–85. doi: 10.2174/156720211798120990
595. Entezari M, Flavarjani ZK, Ramezani A, Nikkhah H, Karimi S, Moghadam HF, et al. Combination of intravitreal bevacizumab and erythropoietin versus intravitreal bevacizumab alone for refractory diabetic macular edema: A randomized double-blind clinical trial. Graefes Arch Clin Exp Ophthalmol (2019) 257(11):2375–80. doi: 10.1007/s00417-019-04383-2
596. Montesano A, Bonfigli AR, De Luca M, Crocco P, Garagnani P, Marasco E, et al. Erythropoietin (Epo) haplotype associated with all-cause mortality in a cohort of italian patients with type-2 diabetes. Sci Rep (2019) 9(1):10395. doi: 10.1038/s41598-019-46894-2
597. Kim SH, Yu HS, Huh S, Kang UG, Kim YS. Electroconvulsive seizure inhibits the mtor signaling pathway via ampk in the rat frontal cortex. Psychopharmacology (2022) 239(2):443–54. doi: 10.1007/s00213-021-06015-2
598. Li X, Li K, Chu F, Huang J, Yang Z. Graphene oxide enhances β-amyloid clearance by inducing autophagy of microglia and neurons. Chem Biol Interact (2020) 325:109126. doi: 10.1016/j.cbi.2020.109126
599. Nejabati HR, Samadi N, Shahnazi V, Mihanfar A, Fattahi A, Latifi Z, et al. Nicotinamide and its metabolite N1-methylnicotinamide alleviate endocrine and metabolic abnormalities in adipose and ovarian tissues in rat model of polycystic ovary syndrome. Chem Biol Interact (2020) 324:109093. doi: 10.1016/j.cbi.2020.109093
600. Chong ZZ, Maiese K. The src homology 2 domain tyrosine phosphatases shp-1 and shp-2: diversified control of cell growth, inflammation, and injury. Histol Histopathol (2007) 22(11):1251–67. doi: 10.14670/HH-22.1251
601. Chiu SC, Chao CY, Chiang EI, Syu JN, Rodriguez RL, Tang FY. N-3 polyunsaturated fatty acids alleviate high glucose-mediated dysfunction of endothelial progenitor cells and prevent ischemic injuries both in vitro and in vivo. J Nutr Biochem (2017) 42:172–81. doi: 10.1016/j.jnutbio.2017.01.009
602. Liu P, Yang X, Hei C, Meli Y, Niu J, Sun T, et al. Rapamycin reduced ischemic brain damage in diabetic animals is associated with suppressions of mtor and erk1/2 signaling. Int J Biol Sci (2016) 12(8):1032–40. doi: 10.7150/ijbs.15624
603. Peixoto CA, de Oliveira WH, da Rocha Araujo SM, Nunes AKS. Ampk activation: role in the signaling pathways of neuroinflammation and neurodegeneration. Exp Neurol (2017) 298(PtA):31–41. doi: 10.1016/j.expneurol.2017.08.013
604. Zhao H, Wang ZC, Wang KF, Chen XY. Abeta peptide secretion is reduced by radix polygalaeinduced autophagy via activation of the ampk/mtor pathway. Mol Med Rep (2015) 12(2):2771–6. doi: 10.3892/mmr.2015.3781
605. Lin CL, Huang WN, Li HH, Huang CN, Hsieh S, Lai C, et al. Hydrogen-rich water attenuates amyloid beta-induced cytotoxicity through upregulation of sirt1-foxo3a by stimulation of amp-activated protein kinase in sk-N-mc cells. Chem Biol Interact (2015) 240:12–21. doi: 10.1016/j.cbi.2015.07.013
606. An T, Zhang X, Li H, Dou L, Huang X, Man Y, et al. Gpr120 facilitates cholesterol efflux in macrophages through activation of ampk signaling pathway. FEBS J (2020) 287(23):5080–95. doi: 10.1111/febs.15310
607. Hung CM, Lombardo PS, Malik N, Brun SN, Hellberg K, Van Nostrand JL, et al. Ampk/ulk1-mediated phosphorylation of parkin act domain mediates an early step in mitophagy. Sci Adv (2021) 7(15):eabg4544. doi: 10.1126/sciadv.abg4544
608. Du LL, Chai DM, Zhao LN, Li XH, Zhang FC, Zhang HB, et al. Ampk activation ameliorates alzheimer’s disease-like pathology and spatial memory impairment in a streptozotocin-induced alzheimer’s disease model in rats. J Alzheimers Dis (2015) 43(3):775–84. doi: 10.3233/jad-140564
609. He C, Zhu H, Li H, Zou MH, Xie Z. Dissociation of bcl-2-beclin1 complex by activated ampk enhances cardiac autophagy and protects against cardiomyocyte apoptosis in diabetes. Diabetes (2013) 62(4):1270–81. doi: 10.2337/db12-0533
610. Moroz N, Carmona JJ, Anderson E, Hart AC, Sinclair DA, Blackwell TK. Dietary restriction involves nad -dependent mechanisms and a shift toward oxidative metabolism. Aging Cell (2014) 13(6):1075–85. doi: 10.1111/acel.12273
611. Gao J, Yao M, Chang D, Liu J. Mtor (Mammalian target of rapamycin): hitting the bull’s eye for enhancing neurogenesis after cerebral ischemia? Stroke (2022) 54(1):279–85. doi: 10.1161/strokeaha.122.040376
612. Lan F, Cacicedo JM, Ruderman N, Ido Y. Sirt1 modulation of the acetylation status, cytosolic localization, and activity of lkb1. Possible role in amp-activated protein kinase activation. J Biol Chem (2008) 283(41):27628–35. doi: 10.1074/jbc.M805711200
613. Canto C, Auwerx J. Caloric restriction, sirt1 and longevity. Trends Endocrinol Metab (2009) 20(7):325–31. doi: 10.1016/j.tem.2009.03.008
614. Herranz D, Serrano M. Sirt1: recent lessons from mouse models. Nat Rev Cancer (2010) 10(12):819–23. doi: 10.1038/nrc2962
615. Barchetta I, Cimini FA, Capoccia D, De Gioannis R, Porzia A, Mainiero F, et al. Wisp1 is a marker of systemic and adipose tissue inflammation in dysmetabolic subjects with or without type 2 diabetes. J Endocr Soc (2017) 1(6):660–70. doi: 10.1210/js.2017-00108
616. Liu L, Hu J, Yang L, Wang N, Liu Y, Wei X, et al. Association of wisp1/ccn4 with risk of overweight and gestational diabetes mellitus in chinese pregnant women. Dis Markers (2020) 2020:4934206. doi: 10.1155/2020/4934206
617. Murahovschi V, Pivovarova O, Ilkavets I, Dmitrieva RM, Docke S, Keyhani-Nejad F, et al. Wisp1 is a novel adipokine linked to inflammation in obesity. Diabetes (2015) 64(3):856–66. doi: 10.2337/db14-0444
618. Maiese K. Wnt1 inducible Signaling Pathway Protein 1 (Wisp1). In: Encyclopedia of Signaling Molecules (2017) (Cham, Switzerland). doi: 10.1007/978-1-4614-6438-9_101926-1
619. Gao J, Xu H, Rong Z, Chen L. Wnt family member 1 (Wnt1) overexpression-induced M2 polarization of microglia alleviates inflammation-sensitized neonatal brain injuries. Bioengineered (2022) 13(5):12409–20. doi: 10.1080/21655979.2022.2074767
620. Li P, Wu C, Guo X, Wen Y, Liu L, Liang X, et al. Integrative analysis of genome-wide association studies and DNA methylation profile identified genetic control genes of DNA methylation for kashin-beck disease. Cartilage (2021) 13(1_suppl):780s–8s. doi: 10.1177/1947603519858748
621. Fu Y, Chang H, Peng X, Bai Q, Yi L, Zhou Y, et al. Resveratrol inhibits breast cancer stem-like cells and induces autophagy via suppressing wnt/beta-catenin signaling pathway. PloS One (2014) 9(7):e102535. doi: 10.1371/journal.pone.0102535
622. Geng Y, Ju Y, Ren F, Qiu Y, Tomita Y, Tomoeda M, et al. Insulin receptor substrate 1/2 (Irs1/2) regulates wnt/beta-catenin signaling through blocking autophagic degradation of dishevelled2. J Biol Chem (2014) 289(16):11230–41. doi: 10.1074/jbc.M113.544999
623. Ortiz-Masia D, Cosin-Roger J, Calatayud S, Hernandez C, Alos R, Hinojosa J, et al. Hypoxic macrophages impair autophagy in epithelial cells through wnt1: relevance in ibd. Mucosal Immunol (2014) 7(4):929–38. doi: 10.1038/mi.2013.108
624. Wang S, Chong ZZ, Shang YC, Maiese K. Wisp1 (Ccn4) autoregulates its expression and nuclear trafficking of beta-catenin during oxidant stress with limited effects upon neuronal autophagy. Curr Neurovasc Res (2012) 9(2):91–101. doi: 10.2174/156720212800410858
625. L’Episcopo F, Tirolo C, Testa N, Caniglia S, Morale MC, Deleidi M, et al. Plasticity of subventricular zone neuroprogenitors in mptp (1-methyl-4-phenyl-1,2,3,6-tetrahydropyridine) mouse model of parkinson’s disease involves cross talk between inflammatory and wnt/beta-catenin signaling pathways: functional consequences for neuroprotection and repair. J Neurosci (2012) 32(6):2062–85. doi: 10.1523/jneurosci.5259-11.2012
626. Sun TJ, Tao R, Han YQ, Xu G, Liu J, Han YF. Therapeutic potential of umbilical cord mesenchymal stem cells with wnt/beta-catenin signaling pathway pre-activated for the treatment of diabetic wounds. Eur Rev Med Pharmacol Sci (2014) 18(17):2460–4.
627. Aly H, Rohatgi N, Marshall CA, Grossenheider TC, Miyoshi H, Stappenbeck TS, et al. A novel strategy to increase the proliferative potential of adult human beta-cells while maintaining their differentiated phenotype. PloS One (2013) 8(6):e66131. doi: 10.1371/journal.pone.0066131
628. Cai D, Hong S, Yang J, San P. The effects of microrna-515-5p on the toll-like receptor 4 (Tlr4)/jnk signaling pathway and wnt1-inducible-signaling pathway protein 1 (Wisp-1) expression in rheumatoid arthritis fibroblast-like synovial (Rafls) cells following treatment with receptor activator of nuclear factor-kappa-B ligand (Rankl). Med Sci Monit (2020) 26:e920611. doi: 10.12659/msm.920611
629. Bayod S, Felice P, Andres P, Rosa P, Camins A, Pallas M, et al. Downregulation of canonical wnt signaling in hippocampus of samp8 mice. Neurobiol Aging (2015) 36(2):720–39. doi: 10.1016/j.neurobiolaging.2014.09.017
630. Krupska I, Bruford EA, Chaqour B. Eyeing the cyr61/ctgf/nov (Ccn) group of genes in development and diseases: highlights of their structural likenesses and functional dissimilarities. Hum Genomics (2015) 9(1):24. doi: 10.1186/s40246-015-0046-y
631. Wang AR, Yan XQ, Zhang C, Du CQ, Long WJ, Zhan D, et al. Characterization of wnt1-inducible signaling pathway protein-1 in obese children and adolescents. Curr Med Sci (2018) 38(5):868–74. doi: 10.1007/s11596-018-1955-5
632. Sahin Ersoy G, Altun Ensari T, Subas S, Giray B, Simsek EE, Cevik O. Wisp1 is a novel adipokine linked to metabolic parameters in gestational diabetes mellitus. J Matern Fetal Neonatal Med (2016) 30(8):942–6. doi: 10.1080/14767058.2016.1192118
633. Lim HW, Lee JE, Shin SJ, Lee YE, Oh SH, Park JY, et al. Identification of differentially expressed mrna during pancreas regeneration of rat by mrna differential display. Biochem Biophys Res Commun (2002) 299(5):806–12. doi: 10.1016/s0006-291x(02)02741-9
634. Du J, Klein JD, Hassounah F, Zhang J, Zhang C, Wang XH. Aging increases ccn1 expression leading to muscle senescence. Am J Physiol Cell Physiol (2014) 306(1):C28–36. doi: 10.1152/ajpcell.00066.2013
635. He W, Lu Q, Sherchan P, Huang L, Hu X, Zhang JH, et al. Activation of Frizzled-7 Attenuates Blood-Brain Barrier Disruption through Dvl/β-Catenin/Wisp1 Signaling Pathway after Intracerebral Hemorrhage in Mice. Fluids Barriers CNS (2021) 18(1):44. doi: 10.1186/s12987-021-00278-9
636. Gaudreau PO, Clairefond S, Class CA, Boulay PL, Chrobak P, Allard B, et al. Wisp1 is associated to advanced disease, emt and an inflamed tumor microenvironment in multiple solid tumors. Oncoimmunology (2019) 8(5):e1581545. doi: 10.1080/2162402x.2019.1581545
637. Li Y, Wang F, Liu T, Lv N, Yuan X, Li P. Wisp1 induces ovarian cancer via the igf1/αvβ3/wnt axis. J Ovarian Res (2022) 15(1):94. doi: 10.1186/s13048-022-01016-x
638. Li Y, Zhu Z, Hou X, Sun Y. Lncrna afap1-as1 promotes the progression of colorectal cancer through mir-195-5p and wisp1. J Oncol (2021) 2021:6242798. doi: 10.1155/2021/6242798
639. Liu Y, Qin W, Zhang F, Wang J, Li X, Li S, et al. Association between wnt-1-inducible signaling pathway protein-1 (Wisp1) genetic polymorphisms and the risk of gastric cancer in guangxi chinese. Cancer Cell Int (2021) 21(1):405. doi: 10.1186/s12935-021-02116-2
640. Wang QY, Feng YJ, Ji R. High expression of wisp1 promotes metastasis and predicts poor prognosis in hepatocellular carcinoma. Eur Rev Med Pharmacol Sci (2020) 24(20):10445–51. doi: 10.26355/eurrev_202010_23396
641. Wang Y, Yang SH, Hsu PW, Chien SY, Wang CQ, Su CM, et al. Impact of wnt1-inducible signaling pathway protein-1 (Wisp-1) genetic polymorphisms and clinical aspects of breast cancer. Medicine (2019) 98(44):e17854. doi: 10.1097/md.0000000000017854
642. Zhu Y, Li W, Yang Y, Li Y, Zhao Y. Wisp1 indicates poor prognosis and regulates cell proliferation and apoptosis in gastric cancer via targeting akt/mtor signaling pathway. Am J Transl Res (2020) 12(11):7297–311.
643. Chong ZZ, Shang YC, Zhang L, Wang S, Maiese K. Mammalian target of rapamycin: hitting the bull’s-eye for neurological disorders. Oxid Med Cell Longev (2010) 3(6):374–91. doi: 10.4161/oxim.3.6.14787
644. Kopp C, Hosseini A, Singh SP, Regenhard P, Khalilvandi-Behroozyar H, Sauerwein H, et al. Nicotinic acid increases adiponectin secretion from differentiated bovine preadipocytes through G-protein coupled receptor signaling. Int J Mol Sci (2014) 15(11):21401–18. doi: 10.3390/ijms151121401
645. Martinez de Morentin PB, Martinez-Sanchez N, Roa J, Ferno J, Nogueiras R, Tena-Sempere M, et al. Hypothalamic mtor: the rookie energy sensor. Curr Mol Med (2014) 14(1):3–21. doi: 10.2174/1566524013666131118103706
646. Lai CS, Tsai ML, Badmaev V, Jimenez M, Ho CT, Pan MH. Xanthigen suppresses preadipocyte differentiation and adipogenesis through down-regulation of ppargamma and C/ebps and modulation of sirt-1, ampk, and foxo pathways. J Agric Food Chem (2012) 60(4):1094–101. doi: 10.1021/jf204862d
647. Dong Y, Chen H, Gao J, Liu Y, Li J, Wang J. Molecular machinery and interplay of apoptosis and autophagy in coronary heart disease. J Mol Cell Cardiol (2019) 136:27–41. doi: 10.1016/j.yjmcc.2019.09.001
648. Shokri Afra H, Zangooei M, Meshkani R, Ghahremani MH, Ilbeigi D, Khedri A, et al. Hesperetin is a potent bioactivator that activates sirt1-ampk signaling pathway in hepg2 cells. J Physiol Biochem (2019) 75(2):125–33. doi: 10.1007/s13105-019-00678-4
649. Tsai CF, Kuo YH, Yeh WL, Wu CY, Lin HY, Lai SW, et al. Regulatory effects of caffeic acid phenethyl ester on neuroinflammation in microglial cells. Int J Mol Sci (2015) 16(3):5572–89. doi: 10.3390/ijms16035572
650. Chen GH, Li XL, Deng YQ, Zhou FM, Zou WQ, Jiang WX, et al. The Molecular Mechanism of Epo Regulates the Angiogenesis after Cerebral Ischemia through Ampk-Klf2 Signaling Pathway. Crit Rev Eukaryot Gene Expr (2019) 29(2):105–12. doi: 10.1615/CritRevEukaryotGeneExpr.2019029018
651. Wang D, Song Y, Zhang J, Pang W, Wang X, Zhu Y, et al. Ampk-klf2 signaling pathway mediates the proangiogenic effect of erythropoietin in endothelial colony-forming cells. Am J Physiol Cell Physiol (2017) 313(6):C674–c85. doi: 10.1152/ajpcell.00257.2016
652. Su KH, Yu YB, Hou HH, Zhao JF, Kou YR, Cheng LC, et al. Amp-activated protein kinase mediates erythropoietin-induced activation of endothelial nitric oxide synthase. J Cell Physiol (2012) 227(8):3053–62. doi: 10.1002/jcp.23052
653. Jang W, Kim HJ, Li H, Jo KD, Lee MK, Yang HO. The neuroprotective effect of erythropoietin on rotenone-induced neurotoxicity in sh-sy5y cells through the induction of autophagy. Mol Neurobiol (2015) 53(6):3812–21. doi: 10.1007/s12035-015-9316-x
654. Maiese K. Charting a course for erythropoietin in traumatic brain injury. J Transl Sci (2016) 2(2):140–4. doi: 10.15761/JTS.1000131
655. Wang L, Di L, Noguchi CT. Ampk is involved in mediation of erythropoietin influence on metabolic activity and reactive oxygen species production in white adipocytes. Int J Biochem Cell Biol (2014) 54:1–9. doi: 10.1016/j.biocel.2014.06.008
656. Andreucci M, Fuiano G, Presta P, Lucisano G, Leone F, Fuiano L, et al. Downregulation of cell survival signalling pathways and increased cell damage in hydrogen peroxide-treated human renal proximal tubular cells by alpha-erythropoietin. Cell Prolif (2009) 42(4):554–61. doi: 10.1111/j.1365-2184.2009.00617.x
657. Kubat Oktem E, Aydin B, Yazar M, Arga KY. Integrative analysis of motor neuron and microglial transcriptomes from sod1(G93a) mice models uncover potential drug treatments for als. J Mol Neurosci (2022) 72(11):2360–76. doi: 10.1007/s12031-022-02071-1
658. Wang Y, Lin Y, Wang L, Zhan H, Luo X, Zeng Y, et al. Trem2 ameliorates neuroinflammatory response and cognitive impairment via pi3k/akt/foxo3a signaling pathway in alzheimer’s disease mice. Aging (Albany NY) (2020) 12:20862–79. doi: 10.18632/aging.104104
659. Oaks Z, Patel A, Huang N, Choudhary G, Winans T, Faludi T, et al. Cytosolic aldose metabolism contributes to progression from cirrhosis to hepatocarcinogenesis. Nat Metab (2023) 5(1):41–60. doi: 10.1038/s42255-022-00711-9
660. Monti C, Colugnat I, Lopiano L, Chiò A, Alberio T. Network analysis identifies disease-specific pathways for parkinson’s disease. Mol Neurobiol (2018) 55(1):370–81. doi: 10.1007/s12035-016-0326-0
Keywords: AMPK, APOE-ε4, autophagy, diabetes mellitus, ferroptosis, pyroptosis, SIRT1, WISP1
Citation: Maiese K (2023) The impact of aging and oxidative stress in metabolic and nervous system disorders: programmed cell death and molecular signal transduction crosstalk. Front. Immunol. 14:1273570. doi: 10.3389/fimmu.2023.1273570
Received: 06 August 2023; Accepted: 23 October 2023;
Published: 08 November 2023.
Edited by:
Chung Nga Ko, C-MER International Eye Research Center, ChinaReviewed by:
Adriana Sumoza-Toledo, Universidad Veracruzana, MexicoCopyright © 2023 Maiese. This is an open-access article distributed under the terms of the Creative Commons Attribution License (CC BY). The use, distribution or reproduction in other forums is permitted, provided the original author(s) and the copyright owner(s) are credited and that the original publication in this journal is cited, in accordance with accepted academic practice. No use, distribution or reproduction is permitted which does not comply with these terms.
*Correspondence: Kenneth Maiese, d250aW43NUB5YWhvby5jb20=
Disclaimer: All claims expressed in this article are solely those of the authors and do not necessarily represent those of their affiliated organizations, or those of the publisher, the editors and the reviewers. Any product that may be evaluated in this article or claim that may be made by its manufacturer is not guaranteed or endorsed by the publisher.
Research integrity at Frontiers
Learn more about the work of our research integrity team to safeguard the quality of each article we publish.