- 1Laboratory of Metabolic Disorders, School of Applied Sciences, University of Campinas, Limeira, Brazil
- 2Laboratory of Nutrients and Tissue Repair, School of Applied Sciences, University of Campinas, Limeira, Brazil
- 3Obesity and Comorbidities Research Center, University of Campinas, Campinas, Brazil
- 4Department of Obstetrics and Gynecology, David Geffen School of Medicine, University of California Los Angeles at Harbor-UCLA, Torrance, CA, United States
Introduction: High-fat diet (HFD) consumption is associated with various metabolic disorders and diseases. Both pre-pregnancy and maternal obesity can have long-term consequences on offspring health. Furthermore, consuming an HFD in adulthood significantly increases the risk of obesity and metabolic disorders. However, an intriguing phenomenon known as the obesity paradox suggests that obesity may confer a protective effect on mortality outcomes in sepsis. In sepsis, activation of the cholinergic anti-inflammatory pathway (CAP) can help mitigate systemic inflammation. We employed a metabolic programming model to explore the relationship between maternal HFD consumption and offspring response to sepsis.
Methods: We fed female mice either a standard diet (SC) or an HFD during the pre-pregnancy, pregnancy, and lactation periods. Subsequently, we evaluated 28-day-old male offspring.
Results: Notably, we discovered that offspring from HFD-fed dams (HFD-O) exhibited a higher survival rate compared with offspring from SC-fed dams (SC-O). Importantly, inhibition of the m1 muscarinic acetylcholine receptor (m1mAChR), involved in the CAP, in the hypothalamus abolished this protection. The expression of m1mAChR in the hypothalamus was higher in HFD-O at different ages, peaking on day 28. Treatment with an m1mAChR agonist could modulate the inflammatory response in peripheral tissues. Specifically, CAP activation was greater in the liver of HFD-O following agonist treatment. Interestingly, lipopolysaccharide (LPS) challenge failed to induce a more inflammatory state in HFD-O, in contrast to SC-O, and agonist treatment had no additional effect. Analysis of spleen immune cells revealed a distinct phenotype in HFD-O, characterized by elevated levels of CD4+ lymphocytes rather than CD8+ lymphocytes. Moreover, basal Il17 messenger RNA (mRNA) levels were lower while Il22 mRNA levels were higher in HFD-O, and we observed the same pattern after LPS challenge.
Discussion: Further examination of myeloid cells isolated from bone marrow and allowed to differentiate showed that HFD-O macrophages displayed an anti-inflammatory phenotype. Additionally, treatment with the m1mAChR agonist contributed to reducing inflammatory marker levels in both groups. In summary, our findings demonstrate that HFD-O are protected against LPS-induced sepsis, and this protection is mediated by the central m1mAChR. Moreover, the inflammatory response in the liver, spleen, and bone marrow-differentiated macrophages is diminished. However, more extensive analysis is necessary to elucidate the specific mechanisms by which m1mAChR modulates the immune response during sepsis.
1 Introduction
Obesity paradoxically exhibits an association with improved mortality outcomes in sepsis when compared with leaner patients (1, 2). This phenomenon, known as the obesity paradox, has been discussed previously (3, 4). However, it is important to note that the protective effect of obesity in sepsis remains a topic of debate (5). Some studies have demonstrated the beneficial impact of obesity on sepsis outcomes (6, 7). Conversely, other studies have found that after adjusting for comorbidities, the effect of obesity on sepsis outcomes becomes statistically insignificant (8–10). Furthermore, the precise mechanisms underlying the protective function of overweight and obesity in sepsis remain poorly understood, although some studies have proposed that energy stores in adipose tissue and differential inflammatory responses in individuals with obesity may play an important role (3, 11, 12).
The central nervous system plays a critical role in communicating with the immune system, with the vagus nerve being particularly important (13). The regulation of inflammatory responses mediated by the vagus nerve is referred to as the cholinergic anti-inflammatory pathway (CAP) with participation of cholinergic receptors, the α7 nicotinic acetylcholine receptor (α7nAChR) and the m1 muscarinic acetylcholine receptor (m1mAChR) (14–16).
Stimulation of the CAP can attenuate inflammatory responses in sepsis (17). The JAK2/STAT3 pathway plays a crucial role in the anti-inflammatory effects associated with α7nAChR activation (18, 19), downregulating NF-κB binding to DNA, subsequently reducing cytokine expression (20). In a previous study from our research group, we observed that short-term consumption of a high-fat diet (HFD) for 3 days resulted in reduced expression of hypothalamic α7nAChR and increased mortality in C57/BL6 mice following sepsis induced by administration of lipopolysaccharide (LPS) or caecal ligation and puncture (CLP). Moreover, HFD consumption impaired the ability of PNU (a specific agonist of α7nAChR) to reduce inflammatory markers after LPS injection, thereby contributing to a higher probability of death in sepsis (21).
The global increase in obesity has contributed to a rise in pre-pregnant and maternal obesity, which has long-term implications for the health of both mothers and their offspring (22–24). Animal studies utilizing rodent and non-human primate models have demonstrated that maternal obesity induced through dietary interventions leads to various health issues in the offspring, including obesity, diabetes, hypertension, fatty liver, and behavioural changes (25–29). However, there are few studies available regarding the impact of maternal obesity on the inflammatory response in offspring. Studies conducted on both rodent models and humans have uncovered that maternal obesity can instigate significant modifications in the immune response, microbiota, and the development of the immune system (30–33).
Therefore, our objective was to investigate the impact of maternal HFD consumption on the systemic inflammatory response and sepsis susceptibility in the offspring.
2 Materials and methods
2.1 Animals
Five-week-old Swiss female mice were obtained from the Multidisciplinary Center for Biological Research at the University of Campinas (Campinas, Brazil). The mice were kept in a temperature-controlled environment with a 12-h photoperiod. Experiments were performed in accordance with the ethical guidelines and regulations for use of laboratory animals. Ethics approval for this study, including the design for sepsis development and mortality analysis presented in this paper, was obtained from the State University of Campinas Ethics Committee (Protocol 5733-1). Importantly animals did not experience any form of suffering throughout the study. The female mice were randomly separated into two groups (25 dams per group), fed with either a HFD or a standard diet (SC) (NUVILAB® Cr-1, Nuvital, PR, Brazil) (Table 1) for 4 weeks ad libitum before mating. Dams continued with the diet during pregnancy and lactation. After birth, the litter size was culled to eight mice per litter. Male offspring were weaned on postnatal day 18 (P18) and fed with standard chow until P28 (Figure 1). Each experimental protocol, such as surgery and survival tests, was conducted using one pup from each dam to constitute the respective group. Offspring at different ages were utilized for the receptor expression experiment, specifically at birth (P0), P56, and P82. The HFD was prepared in our laboratory according to the AIN-93G but modified for high-fat content (45%) as described previously (34).
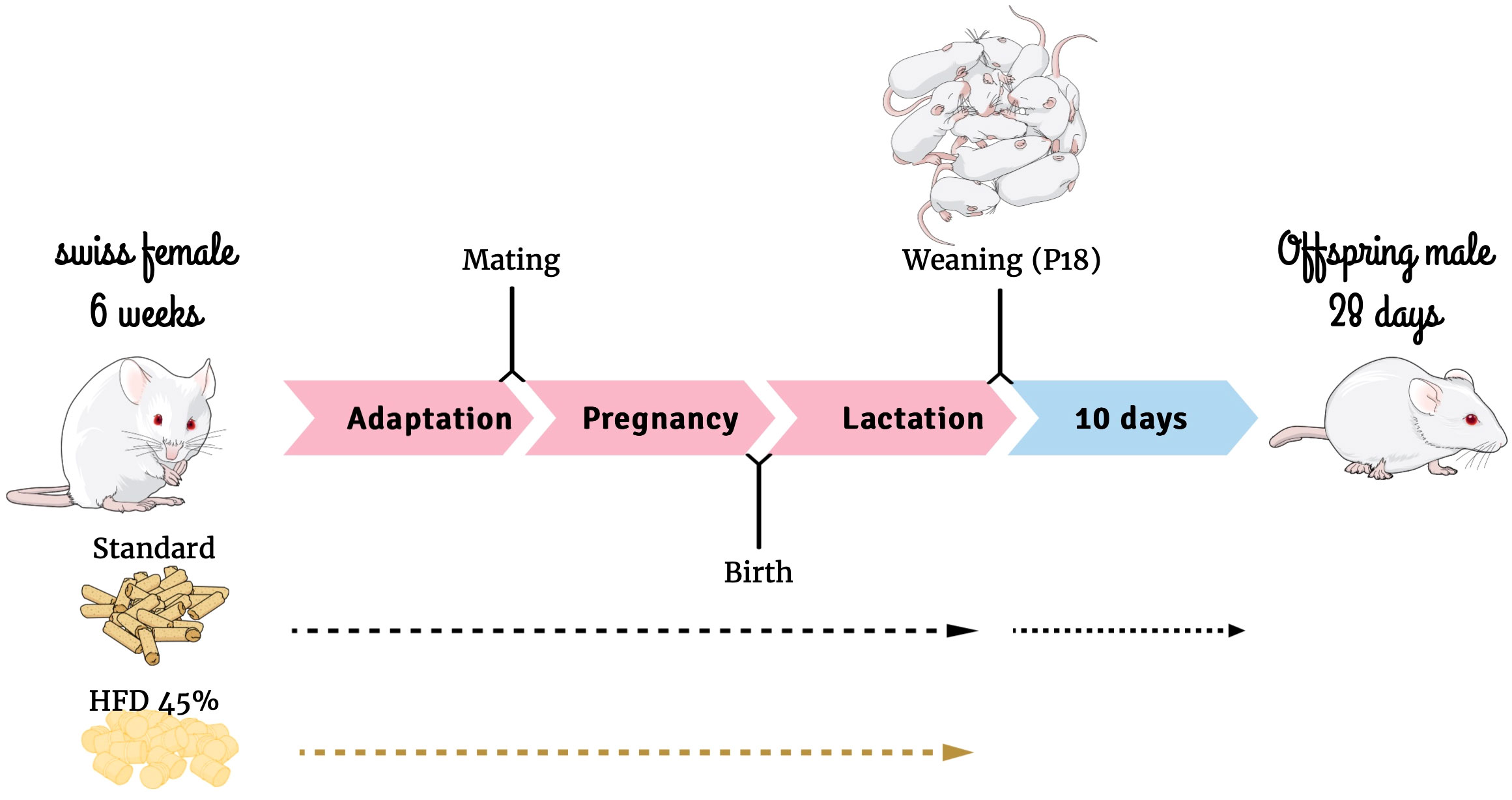
Figure 1 Experimental design. Mind the Graph website was used to prepare Figure 1.
2.2 Anaesthesia and tissue extraction
Mice were anaesthetized with a mixture containing ketamine (139.2 mg kg-1 body weight [bw]), diazepam (4 mg kg-1 bw), and xylazine (18.4 mg kg-1bw) and subsequently euthanized by decapitation for tissue collection. Tissue samples were frozen in liquid nitrogen and stored at -80°C until processing. Isoflurane, an inhalational anaesthetic, was used for induction and maintenance of general anaesthesia during stereotaxic surgery. It was used at 3%–4% for induction and reduced to 2% during surgery.
2.3 Inflammatory response
The offspring were separated as described below to evaluate the inflammatory response.
Design 1
An LPS-induced sepsis mouse model was used. Mice were treated with a lethal dose of LPS diluted in sterile saline and administered intraperitoneally (IP) at 30 mg kg-1 bw. The offspring were observed for 72 h. The survival rate was recorded every 1 h. In the survival study, mice were allowed ad libitum access to food and water. The experiment was replicated twice to validate the obtained results.
In a separate study, mice were treated intracerebroventricularly (ICV) with benztropine (mesylate) 20 min before the LPS challenge. Benztropine, an m1mAChR antagonist, or phosphate-buffered saline (PBS) was injected at 40 µg kg-1 bw. The mice were sacrificed 10 h after LPS administration; the serum was collected for analysis. The time was defined based on the survival rate.
Design 2
To explore the underlying mechanisms of central m1mAChR-mediated anti-inflammatory effects in mice, McN-A-343, an m1mAChR agonist, was administered ICV at 5 ng kg-1 bw. The mice were euthanized 2 h after injection. In a separate study, the mice were treated with an agonist (ICV) 20 min before LPS challenge (1 mg kg-1 bw IP). The mice were euthanized 2 h after LPS challenge.
2.4 Immunofluorescence analysis
At P28, offspring of both groups (SC-O and HFD-O) were perfused with 4% paraformaldehyde (PFA). Then, the brains were extracted and fixed in 4% PFA. Subsequently, the brains were embedded in Tissue-Tek (Sakura, Torrance, CA, USA), frozen, and cut into 15-µm thick coronal sections, following The Rat Brain in Stereotaxic Coordinates by Charles Watson and George Paxinos. The slides were incubated in blocking solution (3% bovine albumin; Sigma-Aldrich, St. Louis, MO, USA) for 90 min, followed by incubation with specific primary antibodies overnight at 4°C. The primary antibodies used were anti-Chrm1 diluted 1:500 (sc-365966, Santa Cruz Biotechnology, Inc, California) and anti-F480 diluted 1:500 (ab6640, Abcam, Inc, Boston). The slides were washed and incubated with appropriate secondary antibodies for 120 min. The secondary antibodies used were Donkey anti-rabbit conjugated to Alexa 488 diluted 1:500 (A-21206, Thermo Fisher Scientific, Inc, Waltham, MA) and goat anti-Rat conjugated to Cy3 diluted 1:1000 (ab6953, Abcam). TO-PRO-3 Iodide was used for nuclear labelling (1:1000; Life Technologies Inc, Carlsbad, CA). The slides were visualized and images were captured using a TCS SP5II Leica confocal microscope (Leica Microsystems, Wetzlar, Germany).
2.5 Serum measurements
The offspring were sacrificed by decapitation on P28 after overnight fasting, and blood was collected. The samples were centrifuged at 1300 rpm for 15 min at room temperature. The serum was collected and stored at -80°C until processing. The cytokine levels were measured using DuoSet enzyme-linked immunosorbent assay kits, DY410-05 mouse TNF; DY401-05 mouse IL1-β/IL1-F2; and DY417-05 mouse IL-10 (R&D Systems, Minneapolis, MN, USA). CD14 levels were measured using Mouse CD14 Quantikine ELISA Kit, #MC140 (R&D Systems, Minneapolis, MN, USA. The C-reactive protein (CRP- K059-8.1) and albumin levels (K040-1) were obtained from biochemical analysis provided by BIOCLIN (Quimica Basica LTDA, Belo Horizonte, Brazil).
2.6 Stereotaxic surgery
The offspring from control and HFD dams received 3%–4% isoflurane inhalational anaesthesia and were placed in a stereotaxic instrument (Stoelting Co. Wood Dale, Illinois). Isoflurane was reduced to 2% during surgery for cannula implantation. Afterwards, a 26G needle was used for the cannula and inserted into the lateral ventricle through a cranial incision. The following coordinates relative to the bregma were used to access the lateral ventricle: anterior/posterior axis, 0.34 mm from bregma to the rear; lateral, 1 mm from the midline; dorsoventral, 2.2 mm from the surface of the skull. Dental acrylic glue was added to secure the cannula following correct positioning. After surgery, the animals were allowed to recover from anaesthesia on a warm pad. Carprofen, an analgesic, was administered for postoperative pain (5 mg kg-1 bw, IP). The cannula placement was tested 6 days after the surgery by measuring the dipsogenic response to angiotensin II injection (2 µL of a 1 × 10-6M solution, ICV) (Sigma-Aldrich Inc, MERK, St Louis, MO). The time and dose of McN-A-343 (agonist) and benztropine (antagonist) treatment were standardized from time-course and dose-response experiments (data not shown).
2.7 Isolation of bone marrow cells
Bone marrow cells were isolated as described previously (21). The long bones (femur and tibia) were removed from the offspring and placed in 0.5 mL perforated tubes inside 1.5 mL tubes, which were then centrifuged at 1200 g for 15 s at 4°C. The cell pellet was resuspended in Roswell Park Memorial Institute (RPMI) 1640 culture medium (Invitrogen, Carlsbad, CA, USA) supplemented with 10% foetal bovine serum (FBS; Invitrogen) and 1% penicillin (100 U/mL)/streptomycin (100 μg/mL) (Invitrogen). The cells were counted with a Neubauer chamber and placed on 60 mm culture dishes. The cells were cultivated for 7 days at 37°C in an atmosphere containing 5% CO2 and 95% humidity. After this period, photos were taken of the culture, and cells were trypsinized and collected for western blotting, RT-PCR, and flow cytometry analysis.
2.8 Western blotting analysis
Tissues were homogenized in freshly prepared ice-cold buffer (1% v/v Triton X-100, 0.1 M Tris, pH 7.4, 0.1 M sodium pyrophosphate, 0.1 M sodium fluoride, 0.01 M EDTA, 0.01 M sodium vanadate, 0.002 M PMSF (Phenylmethylsufonyl fluoride), and 0.01 mg mL-1 aprotinin). The samples were centrifuged at 12,000 rpm for 30 min at 4°C. The supernatant was removed and the protein concentration was determined using the Bradford dye-bleeding method. The samples were resuspended in Laemmli sample buffer and boiled for 5 min before separation by SDS-PAGE using a miniature slab gel apparatus (Bio-Rad, Richmond, CA, USA). The separated proteins were electrotransferred from the gel to a nitrocellulose membrane for 30 min in a transfer buffer that contained methanol and SDS. These membranes were incubated overnight at 4°C with specific antibodies: α7nAChR (bs-1049R, Bioss Antibodies Inc, Woburn, MA), phosphorylated JNK (#9255; Cell Signaling Technology Inc, Danvers, MA), phosphorylated STAT3 (#9145, Cell Signaling), GAPDH (sc-32233, Santa Cruz Biotechnology, Inc., California), and phosphorylated NF-κB (#30335, Cell Signaling Technology Inc, Danvers, MA). Then, after washing with Tris-buffered saline (TBS)-Tween 20 (TTBS; 10 mM Tris, 150 mM NaCl, 0.5% Tween 20), the nitrocellulose membranes were probed with peroxidase-conjugated secondary antibodies (KPL, Gaithersburg, MD, USA) for 90 min at room temperature. Proteins were detected by a chemiluminescence kit (SuperSignal West Pico Chemiluminescent Substrate, Thermo Fisher Scientific Inc) and bands were evaluated by densitometry using Scion Image software (ScionCorp, MD, USA). The intensities of the bands were normalized to the loading control (GAPDH).
2.9 RT-PCR analysis
Frozen tissues were homogenized in TRIzol reagent (Life Technologies) for RNA extraction according to the manufacturer’s instructions. After incubation for 5 min at room temperature for complete dissociation, chloroform was added to the homogenate. Following centrifugation, the RNA phase was precipitated with isopropyl alcohol and the pellet was washed with 75% and 100% ethanol. After drying, the pellet was resuspended in ultra-pure water and stored at -80°C. RNA was quantified with a Nanodrop ND-2000 (Thermo Fisher Scientific). Reverse transcription was performed with 3 µg of total RNA using the High-Capacity cDNA Reverse Transcription kit (Life Technologies). Relative expression was determined using TaqMan Gene Expression Assays (Thermo Fisher Scientific) and SYBR Green Master Mix (Bio-Rad). The following TaqMan Gene Expression Assays were used: Chrna7 (Mm01312230_m1), Il6 (Mm01312230_m1), Tnf (Mm00443258_m1), Il1b (Mm00434228_m1), Socs3 (Mm00545913_s1), and Il10 (Mm01288386_m1). Gapdh (4351309; Applied Biosystems, USA) was used as endogenous control.
Quantitative PCR was performed with the SYBR Green Master Mix (Bio-Rad). The primers used are listed in Table 1. Real-time PCR was performed on an AB/Prism 7500 fast platform. The data were analysed using the Sequence Detection System 2.0.5 software.
2.10 Flow cytometry analysis
Cells isolated from the spleen and bone marrow were submitted to flow cytometry analysis. The spleen and bone marrow cells were evaluated with a macrophage panel, which determined the number of CD45+, F480+, CD11c+, and CD206+ cells. Spleen cells were also evaluated with a lymphocyte panel, which determined the number of CD3+, CD4+, CD8+, and Ly6 G+ cells.
Spleens were collected, and then gently dissociated by a needle and rinsed with PBS. Single-cell suspensions (1 × 106) were then suspended in DMEM/FBS). The cells were treated with specific antibodies conjugated to FITC (CD45; CD206), PECy7 (CD45), APC (F480; CD4), PE (CD11c; Ly6G; CD8), and Alexa 488 (CD3). The incubation was at room temperature for 15 min protected from light. The analysis was performed in BD-FACS Accuri Cytometry (Becton Dickinson, MD, USA) and 10,000 events were acquired. The data were analysed using the FlowJo 7.6 software.
Bone marrow cells were collected after 7 days of spontaneous differentiation. The cells were treated with specific antibodies conjugated to FITC (CD206), PECy7 (CD45), APC (F480), and PE (CD11c). The incubation was at 4°C for 15 min protected from light. Analysis was performed in BD-FACS Accuri Cytometry (Becton Dickinson) and 10,000 events were acquired. The data were analysed using the FlowJo 7.6 software.
2.11 Data presentation and statistical analysis
The results are presented as the mean ± standard error. The data were evaluated with the Kolmogorov–Smirnov test to determine whether they were normally distributed. After confirming a normal distribution, Student’s t-test for unpaired samples or analysis of variance (ANOVA) was used. ANOVA was followed by the Bonferroni post hoc test to determine differences between more than two groups. The log-rank test was used to analyse the survival rate. Statistical significance for all analyses was set at p < 0.05. All statistical comparisons were performed using GraphPad Prism 9.5.3 (GraphPad Software, San Diego, CA, USA).
3 Results
3.1 Maternal HFD consumption protects HFD-O against sepsis mortality
In the study, we administered a lethal dose of LPS to SC-O and HFD-O to induce sepsis and then recorded their survival rates. First, we assessed whether the LPS challenge was effective in inducing sepsis in both groups. We measured biomarkers of sepsis and the inflammatory response in the serum of the offspring 10 h after the LPS challenge.
The CRP levels were higher after LPS treatment, with no significant difference between SC-O and HFD-O. Albumin levels did not show any significant differences between the groups. CD14 levels were elevated in all offspring treated with LPS and exceeded the highest point of the standard curve (Table 2). TNF, IL1-β, and IL-10 were undetectable in SC-O and HFD-O that were not challenged with LPS. TNF and IL-10 levels were lower in HFD-O compared with SC-O after LPS injection. However, this decrease was prevented by treatment with an m1mAChR antagonist. IL1-β was only detected in SC-O after LPS injection and in HFD-O treated with the m1mAChR antagonist (Table 2). We also evaluated the spleen weight. LPS significantly increased the spleen weight in SC-O compared with SC-O that were not treated with LPS (Table 2). However, there was no difference in spleen weight in HFD-O treated with LPS.
Considering that LPS was sufficient to activate the inflammatory response, we assessed the SC-O and HFD-O survival curves. The mortality rate was significantly higher in SC-O compared with HFD-O (Figure 2). However, prior ICV treatment with benztropine (mesylate), a pharmacological antagonist of m1mAChR, increased the mortality of HFD-O to levels comparable to SC-O (Figure 2). This finding suggests that central m1mAChR is involved in protecting HFD-O against sepsis.
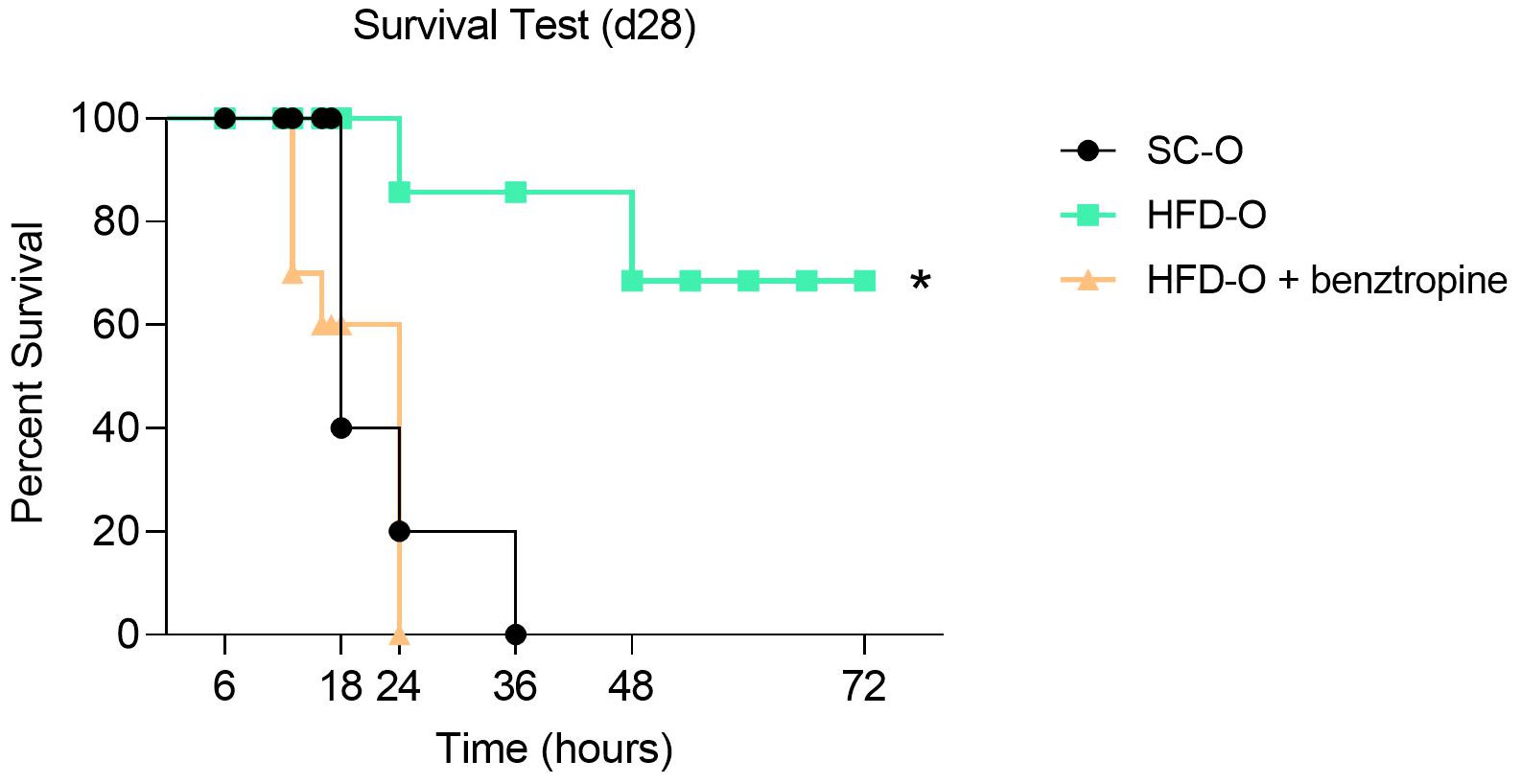
Figure 2 Survival analysis of the offspring. Sepsis was induced with a lethal dose of lipopolysaccharide (LPS; 30 mg LPS kg-1 body weight, intraperitoneal) administered to control offspring (SC-O, n = 10) and high-fat diet offspring (HFD-O, n = 10) at postnatal day 28. Some HFD-O were pretreated for 20 min with the m1mAChR antagonist benztropine (intracerebroventricular) (HFD-O + Benztropine, n = 10). The mice were observed for 72 h, and the survival rate was recorded every 1 h. The data represent the mean ± standard error of the mean. *p < 0.05 HFD-O versus SC-O and HFD-O + Benztropine versus HFD-O (log-rank test).
3.2 Maternal HFD consumption leads to higher central m1mAChR expression in HFD-O
We evaluated the distribution and expression of cholinergic receptors (α7nAChR and m1mAChR) in the hypothalamus (Figure 3). First, we evaluated the mRNA levels of both receptors at P0, P28, P56, and P82. While m1mAChR mRNA expression was higher in HFD-O at P0, P28, and P82 compared with SC-O (Figure 3A), α7nAChR mRNA expression in HFD-O was reduced at P0 and increased at P82 compared with SC-O (Figure 3B). Additionally, we evaluated m1mAChR protein expression by western blot at P28, P56, and P82. We noted increased hypothalamic m1mAChR protein expression in HFD-O at P28 and P56 compared with SC-O (Figures 3C, D). Considering the higher hypothalamic expression of m1mAChR, we evaluated he distribution of m1mAChR expression with immunofluorescence at P28 (Figure 3E). There were more m1mAChR+ cells in the median eminence of HFD-O compared with SC-O (Figure 3E). Nevertheless, m1mAChR expression seems to be higher in the arcuate nucleus compared with the median eminence of SC-O (Figure 3E).
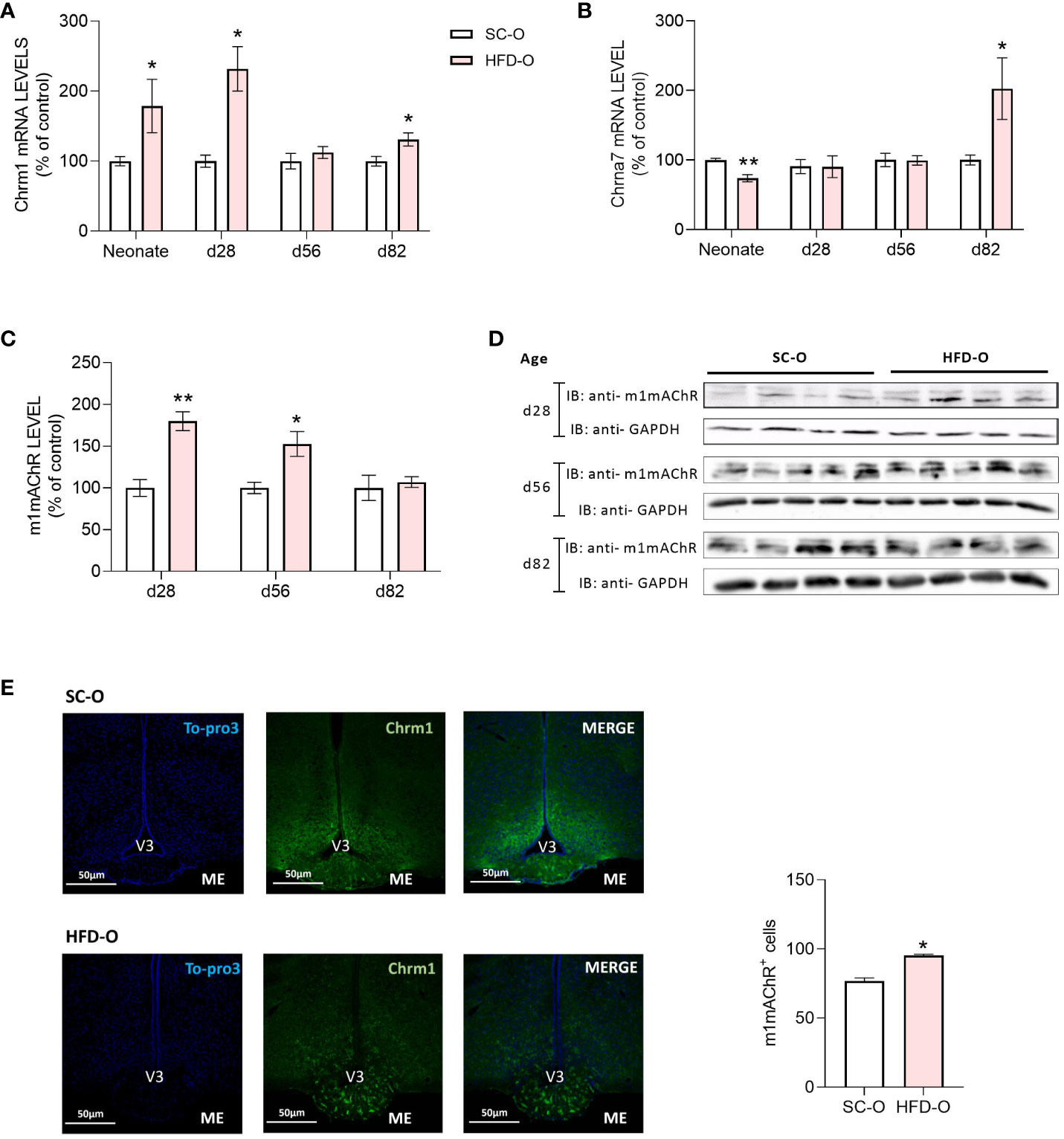
Figure 3 Cholinergic anti-inflammatory pathway receptor expression in the hypothalamus of the offspring. Hypothalamic m1mAChR and α7nAChR messenger RNA (mRNA) (A, B) and protein (C, D) levels were evaluated by RT-PCR and western blot, respectively, in control and high-fat diet offspring (SC-O and HFD-O, respectively). The mRNA and protein levels were evaluated at birth (neonate) and postnatal days 28, 56, and 82. Molecular weight of proteins: m1mAChR – 52KDa; α7nAChR – 55KDa; and GAPDH – 35KDa. The percent expression of control (GAPDH) is shown (mean ± standard error of the mean, n = 5 pups per group). Asterisks indicate significant differences determined by Student’s t-test (*p < 0.05 and **p < 0.01). Confocal images illustrating m1mAChR+ cells (green) and nuclear labelling with TO-PRO-3 (blue) in coronal brain sections (15 µm thick) from 28-day-old offspring (E). Scale of images 50 µm. The number of m1mAChR+ cells in the median eminence (ME) of SD-O and HFD-O (n = 3 per group) (E). V3: third ventricle.
3.3 m1mAChR reduces inflammatory pathway activation in the liver of HFD-O
To assess the impact of central m1mAChR activation on liver signalling pathways and α7nAChR expression in offspring at P28, we administered McN-A-343 (ICV), a pharmacological agonist of m1mAChR (Figure 4A). Figures 4B, C indicate that hypothalamic activation of m1mAChR increased α7nAChR and phosphorylated STAT3 (pSTAT3) protein expression in the liver of SC-O and HFD-O. Notably, pSTAT3 expression was higher in the liver of HFD-O than SC-O. Additionally, m1mAChR activation was accompanied by a reduction in phosphorylated JNK (pJNK) expression in the liver (Figures 4B, C).
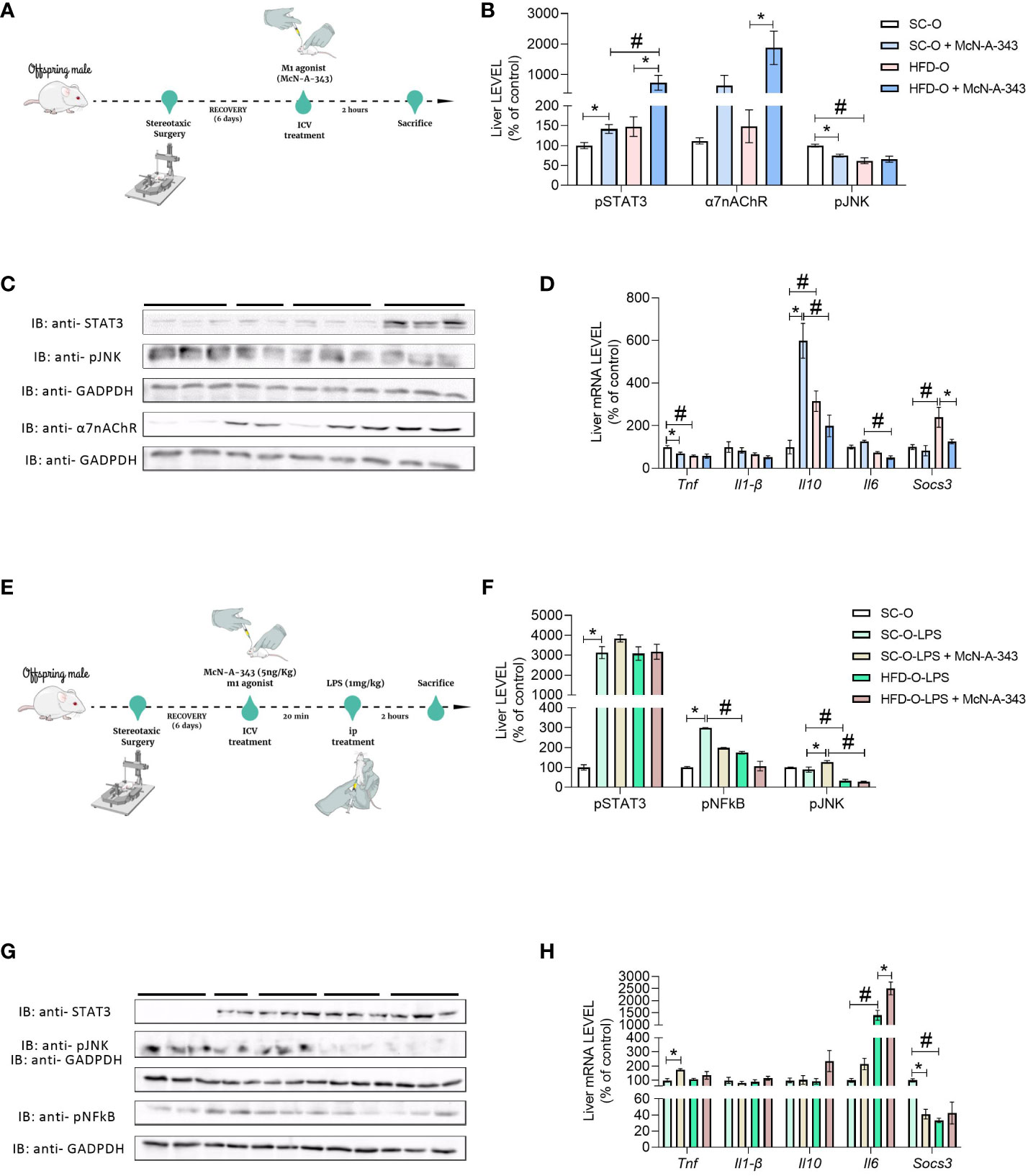
Figure 4 Inflammatory markers in the liver of the offspring. Experimental design for pharmacological treatment with the m1mAChR agonist McN-A-343 (delivered intracerebroventricularly [ICV]) (A). Hepatic pSTAT3, α7nAChR, and pJNK protein expression (B, C) and Tnf, Il1b, Il10, Il6, and Socs3 messenger RNA (mRNA) expression (D) were evaluated by western blot and RT-PCR, respectively, in 28-day-old standard and high-fat diet offspring (SC-O and HFD-O, respectively). The mice were treated with the m1mAChR agonist McN-A-343 (5 ng kg-1, ICV). Experimental design for simultaneous administration of lipopolysaccharide (LPS; 1 mg kg-1, intraperitoneal) and the m1mAChR agonist (ICV) (E). Hepatic pSTAT3, pNF-κB, and pJNK protein expression (F, G) and Tnf, Il1b, Il10, Il6, and Socs3 mRNA expression (H) were evaluated by western blot and RT-PCR in 28-day-old SC-O and HFD-O. Molecular weight of proteins: pSTAT3 – 90KDa; α7nAChR – 55KDa; pJNK – 55KDa; pNFkB – 65KDa; and GAPDH – 35KDa. The data represent the mean ± standard error of the mean (n = 5 per group). The data were analysed with analysis of variance. *Significant difference (p < 0.05) between basal and agonist or LPS treatment. #Significant difference (p < 0.05) between HFD-O and SC-O. Mind the Graph website was used to prepare Figures 4A, E.
We also evaluated liver cytokine mRNA levels. Il1b expression was not different between the groups, indicating similar levels of this cytokine. Interestingly, Tnf expression in HFD-O liver was lower compared with SC-O liver. However, treatment with the m1mAChR agonist (McN-A-343) via ICV administration reduced Tnf mRNA expression in SC-O liver, but there was no additional effect in HFD-O liver (Figure 4D). Il10 mRNA expression increased in HFD-O liver compared with SC-O liver, but ICV administration of McN-A-343 significantly increased Il10 mRNA expression in SC-O liver compared with HFD-O liver. Furthermore, McN-A-343 delivered via ICV administration demonstrated a greater inhibitory effect on liver Il6 mRNA expression in HFD-O compared with SC-O. Similarly, the mRNA levels of Socs3, an important regulatory molecule in inflammation, were higher in HFD-O liver (Figure 4D). This indicates that maternal HFD consumption may lead to increased SOCS3 expression in HFD-O liver, potentially impacting the regulation of inflammatory responses.
As shown in the Figure 4E, we investigated the hepatic inflammatory response in SC-O and HFD-O after LPS treatment. We simultaneously administered SC-O and HFD-O LPS (1 mg kg-1, IP) and an m1mAChR agonist. Liver pSTAT3, a component of the anti-inflammatory pathway, was increased in all LPS-challenged mice. This suggests activation of the anti-inflammatory response in both SC-O and HFD-O following LPS treatment. Moreover, phosphorylated NF-κB (pNF-κB) and pJNK expression was decreased in the liver of LPS-treated HFD-O compared with LPS-treated SC-O. This indicates that maternal HFD consumption may have modulated the hepatic inflammatory response in the offspring, resulting in reduced activation of these pro-inflammatory signalling pathways. Interestingly, when we administered LPS-treated HFD-O with an m1mAChR agonist, there was a further decrease in pNF-κB and pJNK expression in the liver (Figures 4F, G). This suggests that activation of m1mAChR can enhance the anti-inflammatory response and attenuate the activation of pro-inflammatory signaling pathways in the liver of HFD-O following LPS challenge, as depicted in Figure 4H. These findings indicate that maternal HFD consumption and m1mAChR activation can influence the hepatic inflammatory response in offspring, potentially leading to a more pronounced anti-inflammatory state and reduced activation of pro-inflammatory pathways in HFD-O following LPS treatment.
3.4 m1mAChR activates the lymphocyte response in the spleen of HFD-O
The inflammatory and immune response to pathogens depends on the spleen. We investigated the levels of inflammatory cytokines in the spleen of SC-O and HFD-O following ICV treatment with the m1mAChR agonist. However, there were no significant differences in the inflammatory markers between the groups (data not shown).
Next, we performed flow cytometry using macrophage and lymphocyte panels to evaluate the inflammatory response of the spleen. In the macrophage panel, there was a decrease in CD45+CD11c+ cells in HFD-O compared with SC-O, and an increase in CD45+CD206+ cells in HFD-O (Figure 5A). These findings were supported by the differential expression of macrophage markers in the spleen. Similarly, Nos2 mRNA expression was decreased in HFD-O compared with SC-O (Figure 5C), whereas Arg1 mRNA expression appeared to be increased in HFD-O (Figure 5C). Furthermore, treatment with the m1mAChR agonist decreased Nos2 mRNA expression and increased Arg1 expression only in SC-O after LPS challenge (Figure 5D). Taken together, these observations confirm that the macrophages present in the spleen exhibit an anti-inflammatory profile.
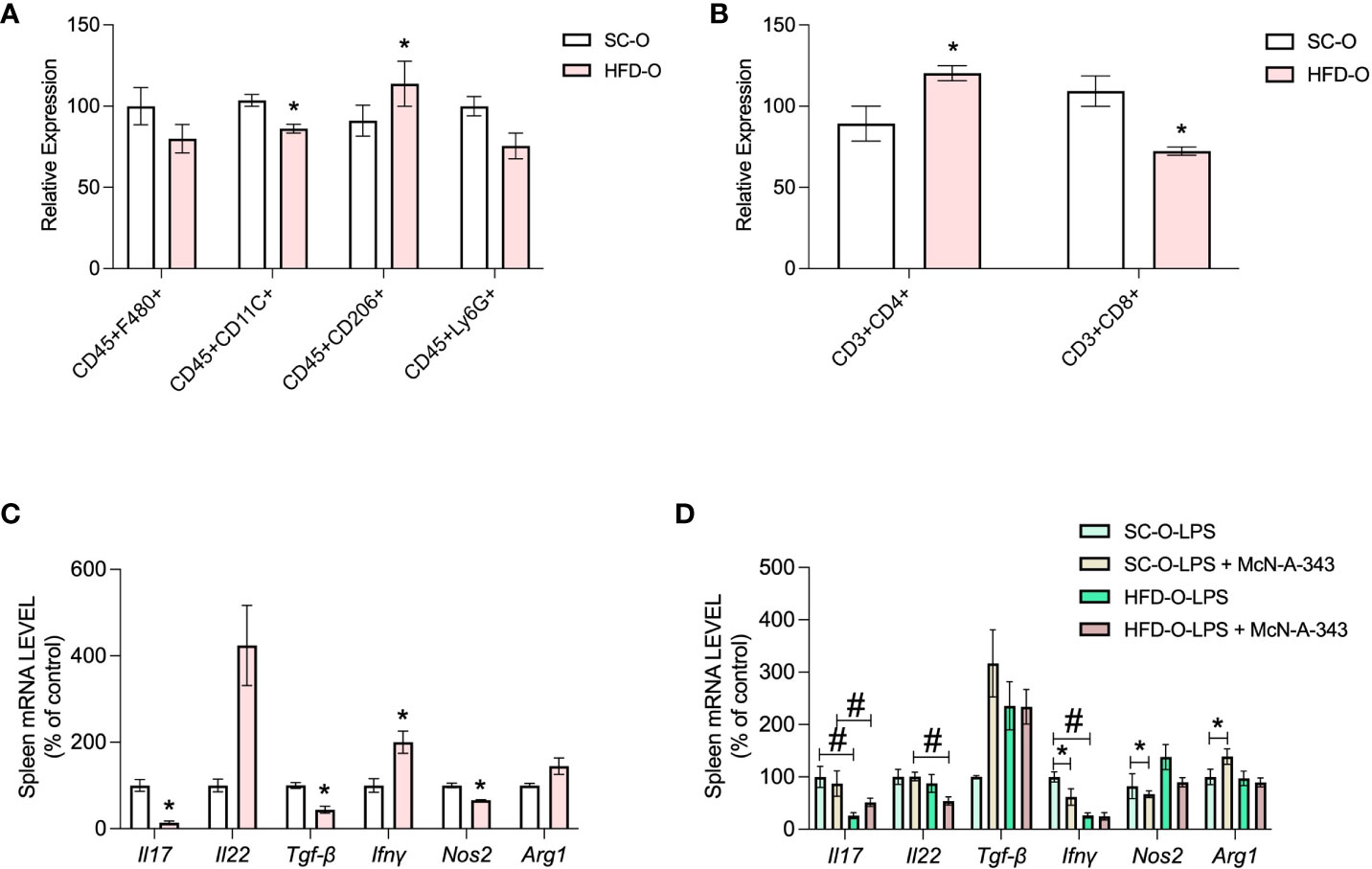
Figure 5 Immune response markers in the spleen of the offspring. Splenic CD45+F480+, CD45+CD11c+, CD45+CD206+, and CD45+Ly6G+ cells were evaluated with the macrophage panel (A) and CD3+CD4+ and CD3+ CD8+ cells were evaluated with the lymphocyte panel (B) by flow cytometry. The data represent expression of high-fat diet offspring (HFD-O) relative to the control offspring (SC-O). Splenic Il17, Il22, Tgfb, Ifng, Nos2, and Arg1 messenger RNA (mRNA) expression (C) was evaluated by RT-PCR in 28-day-old SC-O and HFD-O. Splenic Il17, Il22, Tgfb, Ifng, Nos2, and Arg1 mRNA expression (D) was evaluated in the offspring following lipopolysaccharide (LPS) challenge (1 mg kg-1, intraperitoneal) and treatment with the m1mAChR agonist McN-A-343 (5 ng kg-1, intracerebroventricular). The data represent the mean ± standard error of the mean. The data were analysed with analysis of variance. *Significant difference (p < 0.05) between basal and agonist or LPS treatment. #Significant difference (p < 0.05) between HFD-O and SC-O.
The lymphocyte panel revealed an increase in CD3+CD4+ cells in the spleen of HFD-O compared with SC-O, and a reduction in CD3+CD8+ cells in HFD-O (Figure 5B). These findings were accompanied by a decrease in Il17 and Tgfb mRNA expression in the spleen of HFD-O compared with SC-O (Figure 5C). To investigate the increase in T-helper lymphocytes, we determined the specific type of T-helper lymphocytes present in the spleen of HFD-O after LPS challenge and treatment with an m1mAChR agonist. Interestingly, we observed a reduction in Il17 mRNA expression in the presence of LPS (Figure 5D), while Il22 mRNA expression appeared to be increased in HFD-O (Figure 5C). These observations highlight the presence of distinct lymphocyte profiles in HFD-O. Additionally, Ifng mRNA expression was decreased in HFD-O following LPS challenge, and the m1mAChR agonist reduced Ifng mRNA expression in LPS-treated SC-O (Figure 5D). Overall, these findings suggest reduced differentiation of lymphocytes towards the Th17 profile in HFD-O in the basal state and after LPS challenge.
3.5 m1mAchR activates bone marrow cell differentiation in anti-inflammatory macrophages profile in HFD-O
To investigate the relationship between central muscarinic receptor and macrophage activation, we isolated bone marrow cells from the offspring. We cultured these cells and after 7 days of differentiation, we submitted them for further analysis. We counted the number of cells with a Neubauer chamber immediately after isolation (Figure 6A). There were fewer cells isolated from HFD-O compared with SC-O (Figure 6B). Additionally, a representative image shows that after 7 days of differentiation, the number of macrophages appears to be reduced in HFD-O compared with SC-O (Figure 6C). However, when evaluating the macrophage panel with flow cytometry, there were no significant differences between the groups in terms of the number of positive cells for either inflammatory or anti-inflammatory markers (Figure 6D).
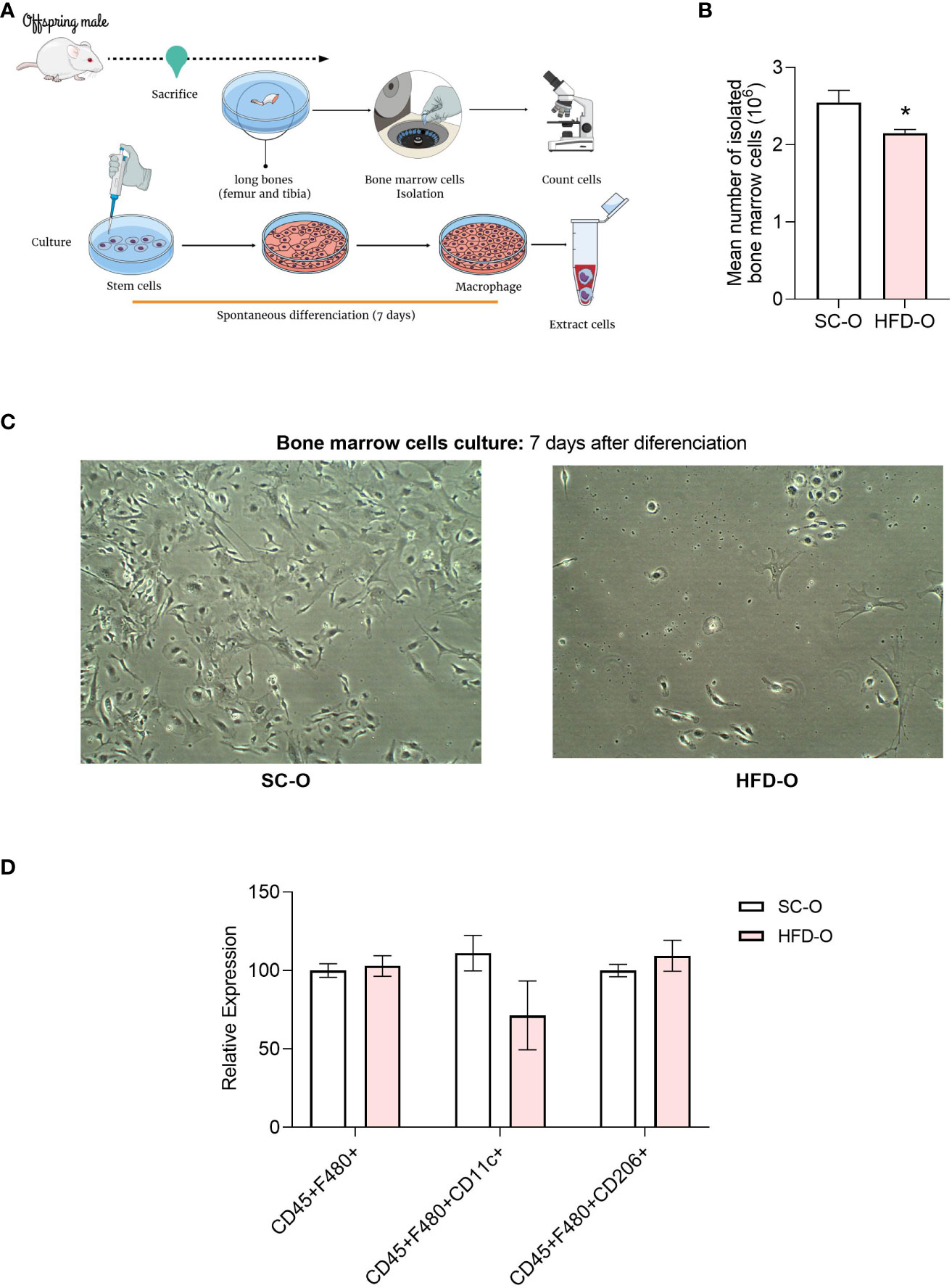
Figure 6 Profile of bone marrow cells after differentiation. Bone marrow cells were isolated from the long bones of 28-day-old control and high-fat diet offspring (SC-O and HFD-O, respectively) (A). The cells were counted before the culture (B). After 7 days of spontaneous differentiation, pictures were taken of the culture (C) to confirm that the cells had differentiated into macrophages. CD45+F480+, CD45+CD11c+, and CD45+CD206+ cells were evaluated with the macrophage panel by flow cytometry (D). The data represent the mean ± standard error of the mean. *Significant difference (p < 0.05) based on Student’s t-test. Mind the Graph website was used to prepare Figure 6A.
Next, we evaluated the impact of central m1mAChR activation with McN-A-343 on the macrophage profile of isolated bone marrow cells (Figure 7). We noted a decrease in IL1-β protein expression in the culture medium in both groups following treatment with the m1mAChR agonist (Figure 7A). We also assessed Tnf, Il1b, Il10, Il6, Nos2, and Arg1 mRNA expression (Figure 7B). Specifically, Il1b, Il6, and Nos2 mRNA expression was significantly reduced upon administration of the agonist McN-A-343. Conversely, McN-A-343 administration increased Il10 mRNA expression. Interestingly, Il10 mRNA expression increased in both groups upon agonist treatment. Furthermore, there was decreased Tnf and Il6 mRNA in HFD-O compared with SC-O. Nos2 mRNA expression, indicative of the inflammatory profile, was decreased in HFD-O, and the agonist treatment further decreased Nos2 mRNA expression in both groups. Conversely, Arg1 mRNA expression, a marker of the anti-inflammatory profile, was increased in HFD-O (Figure 7B). Notably, phosphorylation of STAT3, a protein downstream in the cholinergic anti-inflammatory pathway, was increased in both groups following treatment with the m1mAChR agonist (Figures 7C, D).
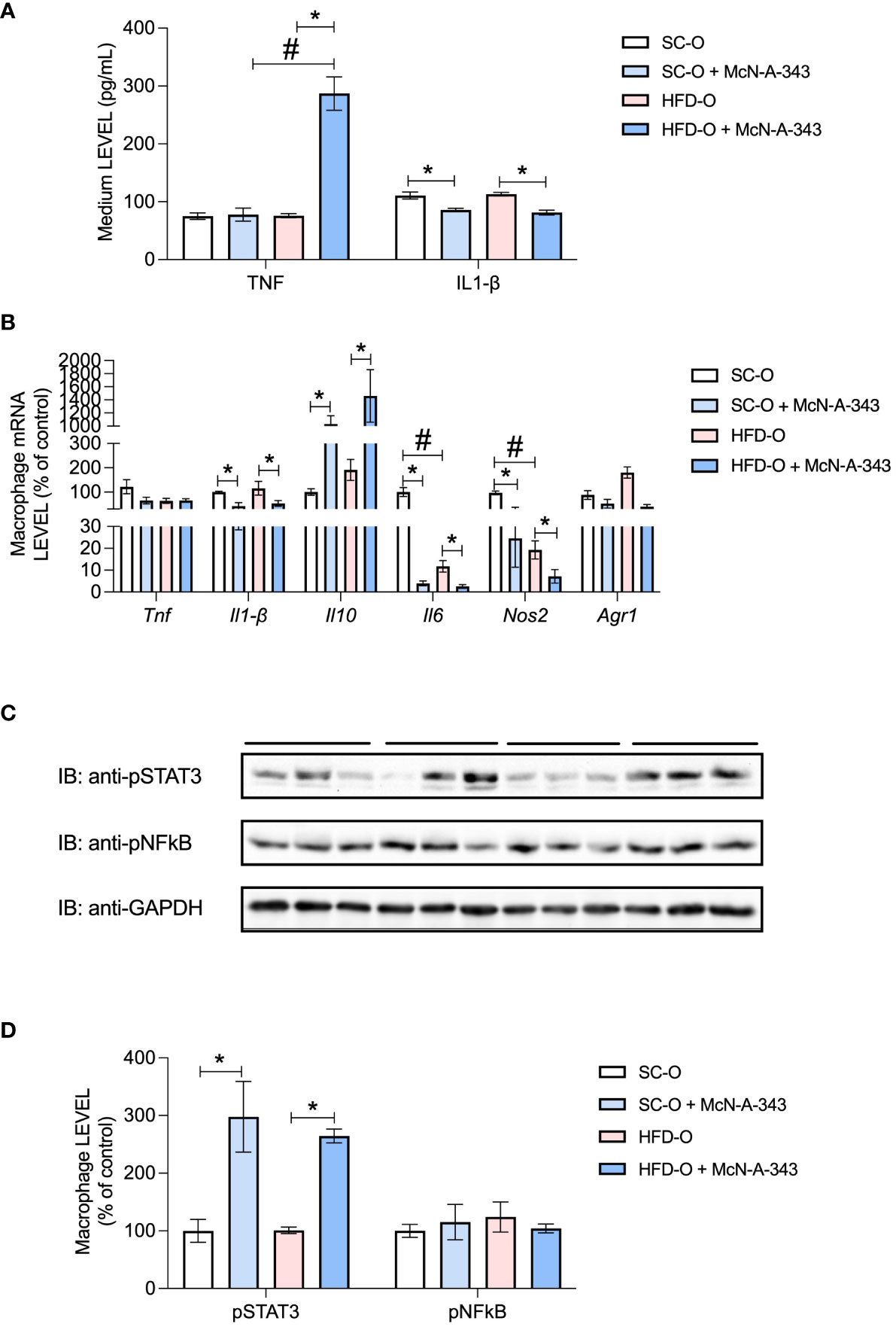
Figure 7 Inflammatory markers in bone marrow cells after differentiation. TNF and IL-1β protein expression was evaluated with enzyme-linked immunosorbent assays (A) in the culture medium after 7 days of spontaneous differentiation. Tnf, Il1b, Il10, Il6, Nos2, and Arg1 messenger RNA (mRNA) expression (B) and pSTAT3 and pNF-κB protein expression (C, D) were evaluated by RT-PCR and western blot, respectively, in macrophages differentiated from control and high-fat diet offspring (SC-O and HFD-O, respectively) bone marrow. The mice were treated with the m1mAChR agonist McN-A-343 (5 ng kg-1, intracerebroventricular). The bars at the top of blots represent the groups. The data represent the mean ± standard error of the mean. Molecular weight of proteins: pSTAT3 – 90KDa; pNFkB – 65KDa; and GAPDH – 35KDa. The data were analysed with analysis of variance. *Significant difference (p < 0.05) between basal and agonist or LPS treatment. #Significant difference (p < 0.05) between HFD-O and SC-O.
4 Discussion
Obesity is widely acknowledged for its association with numerous comorbidities (35–37). Surprisingly, it seems to bestow a certain degree of protection against sepsis. Epidemiological data indicate that individuals with obesity exhibit a heightened likelihood of survival during clinical systemic inflammatory responses (1, 2). This intriguing phenomenon is commonly referred to as the ‘obesity paradox’ (3, 4, 6, 38), yet the actual protective mechanisms in the context of sepsis continue to be a subject of debate (5). An essential factor to consider in this analysis is the programming process during the intrauterine and lactation period, as per the Developmental Origins of Health and Disease (DOHaD) concept. However, the precise impact of maternal obesity on the inflammatory response of offspring during sepsis remains an unresolved question. What role does maternal obesity play in shaping the inflammatory response in the context of sepsis?
Several studies have demonstrated that offspring of obese dams exhibit metabolic impairments following inflammatory challenges (39–41). Sepsis is a complex disorder that is widely recognized as a leading cause of high mortality rates (42–44). It is characterized by an initial systemic inflammatory response syndrome (SIRS), followed by a counter-regulatory anti-inflammatory response syndrome (CARS) (45, 46). The prognosis for sepsis is closely related to the balance between pro- and anti-inflammatory responses.
We found that HFD-O exhibit enhanced resistance to death in LPS-induced sepsis. They also demonstrate increased expression of hypothalamic m1mAChR and reduced levels of inflammatory markers in the serum following LPS administration. Notably, inhibition of central m1mAChR completely abolishes the protective effect against sepsis mortality in HFD-O. Both HFD-O and SC-O show the onset of sepsis pathogenesis following LPS administration. However, HFD-O displays a quicker recovery compared to SC-O, indicating a more effective counter-regulatory anti-inflammatory response. Central m1mAChR appears to play a protective role against sepsis in HFD-O, potentially associated with attenuated sepsis-induced immune and metabolic dysregulation, as suggested by previous studies investigating the role of this receptor in the prevention of endotoxemia (21, 47). In contrast, a previous study demonstrated that knockout mice lacking m1mAChR exhibit higher perioperative mortality following invasive surgery to remove the adrenal glands (48).
The expression of cholinergic receptors appears to be regulated through post-transcriptional mechanisms. Zaghloul and colleagues (49) demonstrated a decrease in m1mAChR expression in the central nervous system of mice with CLP-induced sepsis. Additionally, a previous study from our group revealed that short-term HFD consumption reduces hypothalamic α7nAChR expression and increases mortality in a model of sepsis induced by CLP surgery (21). Interestingly, HFD-O did not show any modifications in hypothalamic α7nAChR expression but exhibited a significant increase in m1mAChR expression. These studies suggest that modulation of cholinergic receptor expression is a dynamic process that can be influenced by inflammatory conditions.
Epigenetic mechanisms during developmental phases, such as pregnancy and lactation, have been associated with improved prognostics in sepsis (50). Epigenetics plays a significant role in various stages of sepsis, including pathogen–host interactions, immunosuppression, and the inflammatory response (51, 52). In the later stages of sepsis, anti-inflammatory cytokines are produced, contributing to the immune tolerance of the host. This phenomenon, referred to as immunologic memory, may be associated with protection against future infections (50, 53).
The liver plays a crucial role in both inflammatory and innate immune responses (54, 55). Furthermore, studies have demonstrated the significant role of the liver in the response to sepsis stages (56, 57). The liver is responsible for the secretion of acute phase proteins (APP), which are regulated by IL-6 levels and STAT3 activation (54, 58, 59). Sander et al. (55) demonstrated that the activation of APP, such as amyloid A, along with elevated CXCL1 levels, promote the mobilization, accumulation, and survival of myeloid cells in the liver. Interestingly, our study revealed that pharmacological activation of hypothalamic m1mAChR in HFD-O leads to increased phosphorylation of liver STAT3 and expression of α7nAChR compared with SC-O. Furthermore, HFD-O exhibit higher liver IL-6 expression in response to LPS compared with SC-O. It is worth noting that the JAK2/STAT3 pathway, as demonstrated by De Jonge and Ulloa (60), operates downstream of α7nAChR and can reduce the inflammatory response by inhibiting NF-κB and TNFα expression. Consequently, there is an enhanced anti-inflammatory response in HFD-O due to downregulation of inflammatory cytokine expression and the stimulation of APP secretion. This effect is achieved through activation of liver α7nAChR as well as STAT3 via IL-6 signalling.
During the acute phase of sepsis, immune cells such as macrophages, T and B lymphocytes, and neutrophils in lymphoid tissues, including the spleen and thymus, undergo activation. However, in the late phase of sepsis, these cells experience substantial apoptosis (61, 62). We found an increase in CD4+ T lymphocytes and a decrease in CD8+ cells in HFD-O compared with SC-O. Similarly, a study examining sepsis induced by CLP also reported reduced activity of CD4+ T lymphocytes. However, activation of the CAP significantly reverses the immunosuppressive state of CD4+ T lymphocytes (63). Given that immune cells undergo apoptosis as sepsis progresses, the elevated levels of lymphocytes in the spleen prior to sepsis infection could potentially confer protection against the pathogenesis of sepsis.
CD4+ lymphocytes have the capacity to differentiate into various phenotypes, including Th1, Th2, Th17, and Th22. Th17 differentiation is initiated by the secretion of TGF-β, IL6, and IL1β, which induce the activation of RORγt, a transcription factor associated with Th17 differentiation (64). Lymphocyte CD4+ Th17 cells can exhibit pathogenic characteristics and induce an inflammatory response (64–66). On the other hand, the differentiation of lymphocyte CD4+ Th22 cells can be regulated by Th17 cells and the levels of IL-17 and IL-22. Studies have indicated that elevated levels of IL-6 contribute to the differentiation of naive CD4+ T cells into Th22 cells (67, 68). Our findings indicate that TGF-β and IL-17 levels are reduced in the spleen of HFD-O, while IL-22 and IL-6 levels are increased. As a result, lymphocytes in the spleen appear to differentiate into the Th22 phenotype rather than the Th17 phenotype. Th22 cells are associated with anti-inflammatory responses and play a role in promoting the innate immune defence against infections (69, 70). Furthermore, spleen macrophages and bone marrow–derived macrophages in HFD-O appear to exhibit an anti-inflammatory phenotype, even when confronted with an inflammatory challenge such as LPS. Boomer et al. (71) highlighted the crucial role of spleen macrophages in the anti-inflammatory response during sepsis. These macrophages are responsible for reducing cytokine levels, including IFNγ, TNF, IL-6, and IL-10, in patients with sepsis. Moreover, in sepsis survivors, there is an increase in myeloid progenitor cells, and trained immunity leads to the reprogramming of naïve bone marrow monocytes (72, 73).
In conclusion, our data provide evidence that HFD-O exhibit partial protection against LPS-induced sepsis. This protective effect appears to be mediated through upregulation of central m1mAChR. While we have identified this central muscarinic receptor as the primary activator of the CAP, we did not investigate the role of central α7nAChR in this process, as suggested by Ren and colleagues (63). However, we did not observe an increase in central α7nAChR expression in HFD-O compared with SC-O. Additional studies are required to fully understand the mechanism underlying the modulation of m1mAChR expression and its role in protecting offspring against sepsis mortality.
Although the results presented in this study suggest that maternal obesity brought advantages to the offspring in terms of the anti-inflammatory response, we need to be cautious in assimilating this information. It is widely known that other physiological processes are affected by maternal obesity. Therefore, it is essential to monitor the development of these offspring and the maintenance of this characteristic of the immune system. The use of anti-inflammatory therapies, although promising in these cases, needs to be used in the correct stages of sepsis evolution.
As limitations of study, we utilized an LPS model for inducing sepsis instead of the recommended CLP surgery. Our decision was influenced by the age of the involved offspring, which were 28 days old. We were concerned about the size of mice for conducting this highly impactful surgery, as it might potentially yield false positive results. Furthermore, while we evaluated IL17 and IL22 levels using qPCR, performing cytometry analysis of lymphocytes in the spleen might have provided more comprehensive insights. Our study assessed changes in the immune response in the offspring at 28 days of age. At this age, we cannot dismiss the contribution of maternal milk to the results shown. A study involving adult mice could provide us with information regarding the persistence of these alterations in the inflammatory response.
Furthermore, new studies need to be conducted to identify nutritional components and/or inflammatory factors that may act in the development of the immune system. Additionally, in our study, we did not investigate whether the programming of the inflammatory response occurred during the gestation or lactation period. However, this study demonstrates that the inflammatory response can be programmed in such a way as to provide individuals with greater protection in situations of exposure to infectious agents and may have been an important mechanism of evolutionary adaptation for many species.
Data availability statement
The original contributions presented in the study are included in the article/supplementary material. Further inquiries can be directed to the corresponding author.
Ethics statement
The animal study was approved by State University of Campinas Ethics Committee (Protocol 5733-1). The study was conducted in accordance with the local legislation and institutional requirements.
Author contributions
SC: Conceptualization, Formal analysis, Investigation, Methodology, Validation, Writing – original draft. WC: Formal analysis, Investigation, Methodology, Validation, Writing – original draft. PL: Formal analysis, Investigation, Methodology, Validation, Writing – original draft. IS: Formal analysis, Investigation, Methodology, Validation, Writing – original draft. BB: Formal analysis, Investigation, Methodology, Writing – original draft. LI-S: Data curation, Formal analysis, Resources, Supervision, Writing – review & editing. AT: Funding acquisition, Methodology, Resources, Supervision, Writing – review & editing. MM: Methodology, Resources, Writing – review & editing. HR: Formal analysis, Investigation, Methodology, Resources, Writing – review & editing. MD: Funding acquisition, Resources, Writing – review & editing. MR: Funding acquisition, Resources, Writing – review & editing. MT: Conceptualization, Funding acquisition, Project administration, Resources, Supervision, Writing – review & editing.
Funding
The author(s) declare financial support was received for the research, authorship, and/or publication of this article. This work was supported by grants from Coordenação de Aperfeiçoamento de Pessoal de Nivel Superior – Brasil (CAPES, Finance Code 001), the National Council for Scientific and Technological Development (CNPq), the São Paulo Research Foundation – FAPESP (grant # 16/23484-1; # 13/07607-8; #19/07615-7, and # 21/11772-0), and the National Institutes of Health (grant 1R01HD099813-01). The funders had no role in the study design, data collection or analysis, decision to publish, or preparation of the manuscript.
Acknowledgments
Mind the Graph was the platform used to create and design figures.
Conflict of interest
The authors declare that the research was conducted in the absence of any commercial or financial relationships that could be construed as a potential conflict of interest.
Publisher’s note
All claims expressed in this article are solely those of the authors and do not necessarily represent those of their affiliated organizations, or those of the publisher, the editors and the reviewers. Any product that may be evaluated in this article, or claim that may be made by its manufacturer, is not guaranteed or endorsed by the publisher.
Abbreviations
APP, Acute Phase Proteins; Benztropine, selective antagonist of m1mAChR; CAP, Cholinergic Anti-Inflammatory Pathway; CARS, Counter-regulatory Anti-inflammatory Response Syndrome; Chrm1, name of the gene of m1mAChR; Chrna7, name of the gene of α7nAChR; CLP, caecal ligation and puncture; CRP, C-Reactive Protein; HFD, High Fat Diet; HFD-O, offspring of HFD dams; ICV, intracerebroventricular injection; IP, intraperitoneal injection; LPS, lipopolysaccharide; m1mAChR, m1 muscarinic acetylcholine receptor; McN-A-343, selective agonist of m1mAChR; SC- Standard Chow diet; SC-O, offspring of SC dams; SIRS, Systemic Inflammatory Response Syndrome; α7nAChR, α7 nicotinic acetylcholine receptor.
References
1. Alsiö Å, Nasic S, Ljungström L, Jacobsson G. Impact of obesity on outcome of severe bacterial infections. PloS One (2021) 16:e0251887. doi: 10.1371/journal.pone.0251887
2. Yeo HJ, Kim TH, Jang JH, Jeon K, Oh DK, Park MH, et al. Obesity paradox and functional outcomes in sepsis: A multicenter prospective study. Crit Care Med (2023) 51(6):1–11. doi: 10.1097/CCM.0000000000005801
3. Cichon I, Ortmann W, Kolaczkowska E. Metabolic pathways involved in formation of spontaneous and lipopolysaccharide-induced neutrophil extracellular traps (NETs) differ in obesity and systemic inflammation. Int J Mol Sci (2021) 22:7718. doi: 10.3390/ijms22147718
4. Wang Q, Huang J, Chen X, Wang J, Fang F. Transcriptomic markers in pediatric septic shock prognosis: An integrative analysis of gene expression profiles. Braz J Med Biol Res (2021) 54:1–8. doi: 10.1590/1414-431X202010152
5. Kalani C, Venigalla T, Bailey J, Udeani G, Surani S. Sepsis patients in critical care units with obesity: is obesity protective? Cureus (2020) 12. doi: 10.7759/cureus.6929
6. Danninger T, Rezar R, Mamandipoor B, Dankl D, Koköfer A, Jung C, et al. Underweight but not overweight is associated with excess mortality in septic ICU patients. Wien Klin Wochenschr (2022) 134:139–47. doi: 10.1007/s00508-021-01912-0
7. Pepper DJ, Demirkale CY, Sun J, Rhee C, Fram D, Eichacker P, et al. Does obesity protect against death in sepsis? A retrospective cohort study of 55,038 adult patients*. Crit Care Med (2019) 47:643–50. doi: 10.1097/CCM.0000000000003692
8. Arabi YM, Dara SI, Tamim HM, Rishu AH, Bouchama A, Khedr MK, et al. Clinical characteristics, sepsis interventions and outcomes in the obese patients with septic shock: An international multicenter cohort study. Crit Care (2013) 17:1–13. doi: 10.1186/cc12680
9. Kuperman EF, Showalter JW, Lehman EB, Leib AE, Kraschnewski JL. The impact of obesity on sepsis mortality: A retrospective review. BMC Infect Dis (2013) 13. doi: 10.1186/1471-2334-13-377
10. Paulsen J, Askim A, Mohus RM, Mehl A, Dewan A, SolligArd E, et al. Associations of obesity and lifestyle with the risk and mortality of bloodstream infection in a general population: A 15-year follow-up of 64 027 individuals in the HUNT Study. Int J Epidemiol (2017) 46:1573–81. doi: 10.1093/ije/dyx091
11. Harris K, Zhou J, Liu X, Hassan E, Badawi O. The obesity paradox is not observed in critically III patients on early enteral nutrition. Crit Care Med (2017) 45:828–34. doi: 10.1097/CCM.0000000000002326
12. Robinson MK, Mogensen KM, Casey JD, McKane CK, Moromizato T, Rawn JD, et al. The relationship among obesity, nutritional status, and mortality in the critically ill*. Crit Care Med (2015) 43:87–100. doi: 10.1097/CCM.0000000000000602
13. Chang EH, Chavan SS, Pavlov VA. Cholinergic control of inflammation, metabolic dysfunction, and cognitive impairment in obesity-associated disorders: Mechanisms and novel therapeutic opportunities. Front Neurosci (2019) 13:263. doi: 10.3389/fnins.2019.00263
14. Alen NV. The cholinergic anti-inflammatory pathway in humans: State-of-the-art review and future directions. Neurosci Biobehav Rev (2022) 136. doi: 10.1016/j.neubiorev.2022.104622
15. Pavlov VA, Tracey KJ. The vagus nerve and the inflammatory reflex—linking immunity and metabolism. Nat Rev Endocrinol (2012) 8:743–54. doi: 10.1038/nrendo.2012.189
16. Zila I, Mokra D, Kopi a J, Kolomaznik M, Javorka M, Calkovska A. Vagal-immune interactions involved in cholinergic anti-inflammatory pathway. Physiol Res (2017) 66:S139–45. doi: 10.33549/physiolres.933671
17. Kim TH, Kim SJ, Lee SM. Stimulation of the α7 nicotinic acetylcholine receptor protects against sepsis by inhibiting toll-like receptor via phosphoinositide 3-kinase activation. J Infect Dis (2014) 209:1668–77. doi: 10.1093/infdis/jit669
18. Physiology C. JAK2/STAT3 pathway is required for α7nAChR-dependent expression of POMC and AGRP neuropeptides in male mice. Cell Physiol Biochem. (2019) 3:701–12. doi: 10.33594/000000166
19. de Jonge WJ, van der Zanden EP, The FO, Bijlsma MF, van Westerloo DJ, Bennink RJ, et al. Stimulation of the vagus nerve attenuates macrophage activation by activating the Jak2-STAT3 signaling pathway. Nat Immunol (2005) 6:844–51. doi: 10.1038/ni1229
20. Pavlov VA, Tracey KJ. Neural regulation of immunity: Molecular mechanisms and clinical translation. Nat Neurosci (2017) 20:156–66. doi: 10.1038/nn.4477
21. Souza ACP, Souza CM, Amaral CL, Lemes SF, Santucci LF, Milanski M, et al. Short-term high-fat diet consumption reduces hypothalamic expression of the nicotinic acetylcholine receptor α7 subunit (α7nAChR) and affects the anti-inflammatory response in a mouse model of sepsis. Front Immunol (2019) 10:565. doi: 10.3389/fimmu.2019.00565
22. Drake AJ, Reynolds RM. Impact of maternal obesity on offspring obesity and cardiometabolic disease risk. Reproduction (2010) 140:387–98. doi: 10.1530/REP-10-0077
23. Patel N, Pasupathy D, Poston L. Determining the consequences of maternal obesity for offspring health. Exp Physiol (2015) 100:1421–8. doi: 10.1113/EP085132
24. Poston L, Caleyachetty R, Cnattingius S, Corvalán C, Uauy R, Herring S, et al. Preconceptional and maternal obesity: epidemiology and health consequences. Lancet Diabetes Endocrinol (2016) 4:1025–36. doi: 10.1016/S2213-8587(16)30217-0
25. Costa SO, Souza CM, Lanza PG, Sartori JO, Ignacio-Souza LM, Candreva T, et al. Maternal high fat diet consumption reduces liver α7 nicotinic cholinergic receptor expression and impairs insulin signalling in the offspring. Sci Rep (2020) 10:1–10. doi: 10.1038/s41598-019-56880-3
26. Dunn GA, Mitchell AJ, Selby M, Fair DA, Gustafsson HC, Sullivan EL. Maternal diet and obesity shape offspring central and peripheral inflammatory outcomes in juvenile non-human primates. Brain Behav Immun (2022) 102:224–36. doi: 10.1016/j.bbi.2022.02.024
27. Godfrey KM, Reynolds RM, Prescott SL, Nyirenda M, Jaddoe VWV, Eriksson JG, et al. Influence of maternal obesity on the long-term health of offspring. Lancet Diabetes Endocrinol (2017) 5:53–64. doi: 10.1016/S2213-8587(16)30107-3
28. Kulhanek D, Abrahante Llorens JE, Buckley L, Tkac I, Rao R, Paulsen ME. Female and male C57BL/6J offspring exposed to maternal obesogenic diet develop altered hypothalamic energy metabolism in adulthood. Am J Physiol-Endocrinol Metab (2022) 323:E448–66. doi: 10.1152/ajpendo.00100.2022
29. Seki Y, Suzuki M, Guo X, Glenn AS, Vuguin PM, Fiallo A, et al. In utero exposure to a high-fat diet programs hepatic hypermethylation and gene dysregulation and development of metabolic syndrome in male mice. Endocrinology (2017) 158:2860–72. doi: 10.1210/en.2017-00334
30. e-Lacerda RR, CJ T, Bordin S, Antunes E, Anhê GF. Maternal obesity in mice exacerbates the allergic inflammatory response in the airways of male offspring. Nutrients (2019) 11:2902. doi: 10.3390/nu11122902
31. Myles IA, Fontecilla NM, Janelsins BM, Vithayathil PJ, Segre JA, Datta SK. Parental dietary fat intake alters offspring microbiome and immunity. J Immunol (2013) 191:3200–9. doi: 10.4049/jimmunol.1301057
32. Odaka Y, Nakano M, Tanaka T, Kaburagi T, Yoshino H, Sato-Mito N, et al. The influence of a high-fat dietary environment in the fetal period on postnatal metabolic and immune function. Obesity (2010) 18:1688–94. doi: 10.1038/oby.2009.513
33. Wilson RM, Marshall NE, Jeske DR, Purnell JQ, Thornburg K, Messaoudi I. Maternal obesity alters immune cell frequencies and responses in umbilical cord blood samples. Pediatr Allergy Immunol (2015) 26:344–51. doi: 10.1111/pai.12387
34. Melo AM, Benatti RO, Ignacio-Souza LM, Okino C, Torsoni AS, Milanski M, et al. Hypothalamic endoplasmic reticulum stress and insulin resistance in offspring of mice dams fed high-fat diet during pregnancy and lactation. Metabolism (2014) 63:682–92. doi: 10.1016/j.metabol.2014.02.002
35. Dobson R, Burgess MI, Sprung VS, Irwin A, Hamer M, Jones J, et al. Metabolically healthy and unhealthy obesity: Differential effects on myocardial function according to metabolic syndrome, rather than obesity. Int J Obes (2016) 40:153–61. doi: 10.1038/ijo.2015.151
36. Gregory JW. Prevention of obesity and metabolic syndrome in children. Front Endocrinol (Lausanne) (2019) 10:669. doi: 10.3389/fendo.2019.00669
37. Ying M, Hu X, Li Q, Dong H, Zhou Y, Chen Z. Long-term trajectories of BMI and cumulative incident metabolic syndrome: A cohort study. Front Endocrinol (Lausanne) (2022) 13:915394. doi: 10.3389/fendo.2022.915394
38. Cichon I, Ortmann W, Santocki M, Opydo-Chanek M, Kolaczkowska E. Scrutinizing mechanisms of the ‘Obesity paradox in sepsis’: obesity is accompanied by diminished formation of neutrophil extracellular traps (NETs) due to restricted neutrophil–platelet interactions. Cells (2021) 10:384. doi: 10.3390/cells10020384
39. Challier JC, Basu S, Bintein T, Minium J, Hotmire K, Catalano PM, et al. Obesity in pregnancy stimulates macrophage accumulation and inflammation in the placenta. Placenta (2008) 29:274–81. doi: 10.1016/j.placenta.2007.12.010
40. Linabery AM, Nahhas RW, Johnson W, Choh AC, Towne B, Odegaard AO, et al. Stronger influence of maternal than paternal obesity on infant and early childhood body mass index: The Fels Longitudinal Study. Pediatr Obes (2013) 8:159–69. doi: 10.1111/j.I2047T-6310.201Y
41. Yeung EH, Sundaram R, Ghassabian A, Xie Y, Buck Louis G. Parental obesity and early childhood development. Pediatrics (2017) 139:e20161459. doi: 10.1542/peds.2016-1459
42. Evans L, Rhodes A, Alhazzani W, Antonelli M, Coopersmith CM, French C, et al. Executive summary: surviving sepsis campaign: international guidelines for the management of sepsis and septic shock 2021. Crit Care Med (2021) 49:1974–82. doi: 10.1097/CCM.0000000000005357
43. Howell MD, Davis AM. Management of sepsis and septic shock. JAMA J Am Med Assoc (2017) 317:847–8. doi: 10.1001/jama.2017.0131
44. Singer M, Deutschman CS, Seymour C, Shankar-Hari M, Annane D, Bauer M, et al. The third international consensus definitions for sepsis and septic shock (sepsis-3). JAMA J Am Med Assoc (2016) 315:801–10. doi: 10.1001/jama.2016.0287
45. Lonsdale DO, Shah RV, Lipman J. Infection, sepsis and the inflammatory response: mechanisms and therapy. Front Med (Lausanne) (2020) 7:588863. doi: 10.3389/fmed.2020.588863
46. Zetoune FS, Ward PA. Role of complement and histones in sepsis. Front Med (Lausanne) (2020) 7:616957. doi: 10.3389/fmed.2020.616957
47. Martins ICA, Contieri LS, Amaral CL, Costa SO, Souza ACP, Ignacio-Souza LM, et al. Omega-3 supplementation prevents short-term high-fat diet effects on the α 7 nicotinic cholinergic receptor expression and inflammatory response. Mediators Inflammation (2021) 2021. doi: 10.1155/2021/5526940
48. Pierce H, Zhang D, Magnon C, Lucas D, Christin JR, Huggins M, et al. Cholinergic signals from the CNS regulate G-CSF-mediated HSC mobilization from bone marrow via a glucocorticoid signaling relay. Cell Stem Cell (2017) 20:648–658.e4. doi: 10.1016/j.stem.2017.01.002
49. Zaghloul N, Addorisio ME, Silverman HA, Patel HL, Valdés-Ferrer SI, Ayasolla KR, et al. Forebrain cholinergic dysfunction and systemic and brain inflammation in murine sepsis survivors. Front Immunol (2017) 8:1673. doi: 10.3389/fimmu.2017.01673
50. Córneo E da S, Michels M, Dal-Pizzol F. Sepsis, immunosuppression and the role of epigenetic mechanisms. Expert Rev Clin Immunol (2021) 17:169–76. doi: 10.1080/1744666X.2021.1875820
51. Sherwood ER, Burelbach KR, McBride MA, Stothers CL, Owen AM, Hernandez A, et al. Innate immune memory and the host response to infection. J Immunol (2022) 208:785–92. doi: 10.4049/jimmunol.2101058
52. Vachharajani V, McCall CE. Epigenetic and metabolic programming of innate immunity in sepsis. Innate Immun (2019) 25:267–79. doi: 10.1177/1753425919842320
53. Carson WF IV, Cavassani KA, Dou Y, Kunkel SL. Epigenetic regulation of immune cell functions during post-septic immunosuppression. Epigenetics (2011) 6:273–83. doi: 10.4161/epi.6.3.14017
54. Bode JG, Albrecht U, Häussinger D, Heinrich PC, Schaper F. Hepatic acute phase proteins - Regulation by IL-6- and IL-1-type cytokines involving STAT3 and its crosstalk with NF-κB-dependent signaling. Eur J Cell Biol (2012) 91:496–505. doi: 10.1016/j.ejcb.2011.09.008
55. Sander LE, Sackett SD, Dierssen U, Beraza N, Linke RP, Müller M, et al. Hepatic acute-phase proteins control innate immune responses during infection by promoting myeloid-derived suppressor cell function. J Exp Med (2010) 207:1453–64. doi: 10.1084/jem.20091474
56. Kaplan JM, Nowell M, Lahni P, Shen H, Shanmukhappa SK, Zingarelli B. Obesity enhances sepsis-induced liver inflammation and injury in mice. Obesity (2016) 24:1480–8. doi: 10.1002/oby.21504
57. Williamson L, Ayalon I, Shen H, Kaplan J. Hepatic STAT3 inhibition amplifies the inflammatory response in obese mice during sepsis. Am J Physiol Endocrinol Metab (2019) 316:E286–92. doi: 10.1152/ajpendo.00341.2018
58. Cray C. Acute phase proteins in animals. In: Progress in Molecular Biology and Translational Science. Elsevier Inc (2012). p. 113–50. doi: 10.1016/B978-0-12-394596-9.00005-6
59. Nakata K, Saitoh R, Amano J, Koshiyama A, Ichibangase T, Murao N, et al. Alteration of intracellular secretory acute phase response proteins expressed in human hepatocyte induced by exposure with interleukin-6. Cytokine (2012) 59:317–23. doi: 10.1016/j.cyto.2012.04.025
60. De Jonge WJ, Ulloa L. The α7 nicotinic acetylcholine receptor as a pharmacological target for inflammation. Br J Pharmacol (2007) 151:915–29. doi: 10.1038/sj.bjp.0707264
61. Lee Y, Awasthi A, Yosef N, Quintana FJ, Xiao S, Peters A, et al. Induction and molecular signature of pathogenic T H 17 cells. Nat Immunol (2012) 13:991–9. doi: 10.1038/ni.2416
62. Rendon JL, Choudhry MA. Th17 cells: critical mediators of host responses to burn injury and sepsis. J Leukoc Biol. (2012) 92:1–10. doi: 10.1189/jlb.0212083
63. Ren C, Li X, Wang S, Wang L, Dong N, Wu Y, et al. Activation of central α 7 nicotinic acetylcholine receptor reverses suppressed immune function of T lymphocytes and protects against sepsis lethality. Int J Biol Sci (2018) 14:748–59. doi: 10.7150/ijbs.24576
64. Mills KHG. IL-17 and IL-17-producing cells in protection versus pathology. Nat Rev Immunol (2023) 23:38–54. doi: 10.1038/s41577-022-00746-9
65. Munyaka P, Rabbi MF, Pavlov VA, Tracey KJ, Khafipour E, Ghia JE. Central muscarinic cholinergic activation alters interaction between splenic dendritic cell and CD4+CD25- T cells in experimental colitis. PloS One (2014) 9:1–12. doi: 10.1371/journal.pone.0109272
66. Venet F, Demaret J, Gossez M, Monneret G. Myeloid cells in sepsis-acquired immunodeficiency. Ann N Y Acad Sci (2021) 1499:3–17. doi: 10.1111/nyas.14333
67. Duhen T, Geiger R, Jarrossay D, Lanzavecchia A, Sallusto F. Production of interleukin 22 but not interleukin 17 by a subset of human skin-homing memory T cells. Nat Immunol (2009) 10:857–63. doi: 10.1038/ni.1767
68. Pavlidis P, Tsakmaki A, Pantazi E, Li K, Cozzetto D, Digby- Bell J, et al. Interleukin-22 regulates neutrophil recruitment in ulcerative colitis and is associated with resistance to ustekinumab therapy. Nat Commun (2022) 13:5820. doi: 10.1038/s41467-022-33331-8
69. Ouyang W, O’Garra A. IL-10 family cytokines IL-10 and IL-22: from basic science to clinical translation. Immunity (2019) 50:871–91. doi: 10.1016/j.immuni.2019.03.020
70. Song Q, Wang X, Wu X, Kang TH, Qin H, Zhao D, et al. IL-22-dependent dysbiosis and mononuclear phagocyte depletion contribute to steroid-resistant gut graft-versus-host disease in mice. Nat Commun (2021) 12:805. doi: 10.1038/s41467-021-21133-3
71. Boomer JS, To K, Chang KC, Takasu O, Osborne DF, Walton AH, et al. Immunosuppression in patients who die of sepsis and multiple organ failure. JAMA (2011) 306:2594–605. doi: 10.1001/jama.2011.1829
72. Bomans K, Schenz J, Sztwiertnia I, Schaack D, Weigand MA, Uhle F. Sepsis induces a long-lasting state of trained immunity in bone marrow monocytes. Front Immunol (2018) 9:2685. doi: 10.3389/fimmu.2018.02685
Keywords: high fat diet (HFD), cholinergic, hypothalamus, obesity, muscarinic 1 acetylcholine receptors, DOHaD (Developmental origins of health and disease), maternal programming
Citation: Costa SO, Chaves WF, Lopes PKF, Silva IM, Burguer B, Ignácio-Souza LM, Torsoni AS, Milanski M, Rodrigues HG, Desai M, Ross MG and Torsoni MA (2023) Maternal consumption of a high-fat diet modulates the inflammatory response in their offspring, mediated by the M1 muscarinic receptor. Front. Immunol. 14:1273556. doi: 10.3389/fimmu.2023.1273556
Received: 06 August 2023; Accepted: 27 November 2023;
Published: 18 December 2023.
Edited by:
Robert J. Lee, University of Pennsylvania, United StatesReviewed by:
Mourad Aribi, University of Abou Bekr Belkaïd, AlgeriaXue Jiang, Changchun University of Science and Technology, China
Copyright © 2023 Costa, Chaves, Lopes, Silva, Burguer, Ignácio-Souza, Torsoni, Milanski, Rodrigues, Desai, Ross and Torsoni. This is an open-access article distributed under the terms of the Creative Commons Attribution License (CC BY). The use, distribution or reproduction in other forums is permitted, provided the original author(s) and the copyright owner(s) are credited and that the original publication in this journal is cited, in accordance with accepted academic practice. No use, distribution or reproduction is permitted which does not comply with these terms.
*Correspondence: Marcio Alberto Torsoni, bWFyY2lvLnRvcnNvbmlAZmNhLnVuaWNhbXAuYnI=