- 1Collaborative Innovation Center of Aquatic Sciences, Guangdong Key Laboratory of Animal Breeding and Nutrition, Institute of Animal Science, Guangdong Academy of Agricultural Sciences, Guangzhou, China
- 2College of Fisheries, Guangdong Ocean University, Zhanjiang, China
The aim of this study was to investigate the effects of sodium butyrate (SB) supplementation on growth performance, antioxidant enzyme activities, inflammatory factors, and hypoxic stress in largemouth bass (Micropterus salmoides). Diets were supplemented with different doses of SB at 0 (SB0), 0.5 (SB1), 1.0 (SB2) and 2.0 (SB3) g/kg. The hypoxic stress experiment was performed after 56 days of culture. The results showed that compared with the SB0 group, the final body weight, weight gain rate and protein deposition rate of the SB3 group were significantly increased (P<0.05), while FCR was significantly decreased (P<0.05). The contents of dry matter, crude lipids, and ash in the SB2 group were significantly higher than those in the SB0 group (P<0.05). The urea level was significantly decreased (P<0.05), and the glucose content was significantly increased (P<0.05) in the SB supplement group. Compared with the SB0 group, the SB2 group had significant reductions in the levels of serum triglyceride, cholesterol, elevated-density lipoprotein cholesterol, and low-density lipoprotein (P<0.05), and significant reductions in the levels of liver alkaline phosphatase and malondialdehyde (P<0.05). The total antioxidant capacity of the SB1 group was higher than that of other groups (P<0.05). Compared with the SB0 group, the mRNA expression of TLR22, MyD88, TGF-β1, IL-1β and IL-8 in the SB2 group significantly decreased (P<0.05). The cumulative mortality rate was significantly decreased in the SB2 and SB3 groups in comparison with that in the SB0 group after three hours of hypoxic stress (P<0.05). In a 56-day feeding trial, SB enhanced largemouth bass growth by increasing antioxidant enzyme activity and inhibiting TLR22-MyD88 signaling, therefore increasing cumulative mortality from hypoxic stress in largemouth bass.
1 Introduction
Largemouth bass (Micropterus salmoides) is extremely popular in China because of its delicious meat, quick growth, and great economic value (1). With large-scale intensive farming, high stocking density, excessive feed, imbalanced dietary composition, exogenous pathogenic bacteria, and viruses may result in oxidative stress (2–4). Hypoxic stress and other environmental stress responses are common problems that threaten largemouth bass culture. Sustained prolonged-term stress will considerably affect the growth and energy metabolism of fish, making them more susceptible to infection by various pathogens, resulting in the continuous spread of fish diseases (5). At the same time, it will also weaken its immune function and disease resistance, and may even lead to death, which will cause serious damage to economic interests and ecological balance (6). In aquaculture, factors such as water eutrophication, elevated culture density and abnormal weather can lead to decreased dissolved oxygen concentration in water, increasing the risk of anoxic stress (7). Hypoxic stress leads to excessive accumulation of reactive oxygen species in fish, resulting in protein denaturation, lipid peroxidation, cell damage, and eventually apoptosis (8, 9). Oxidative stress can damage immune cells and trigger inflammatory response, which is the cause of numerous diseases (10, 11). The antioxidant system can prevent the generation of reactive oxygen species and avoid immune cell damage (12). When fish are confronted with hypoxic stress, the body resists the stress through a complex series of physiological and biochemical processes such as lowering metabolic rate, increasing aeration and anaerobic respiration, and increasing the oxygen affinity of hemoglobin (13, 14). Hypoxic conditions have been demonstrated to negatively affect growth performance and immunity in Nile tilapia (Oreochromis niloticus) (15). In mammals, hypoxia enhances the production of lactate and glucose, affecting nutrient metabolism (16). Chronic hypoxia activates anaerobic glycolysis, reduces glycogen and lactate concentrations in the hepatic, and increases the expression of phosphoenolpyruvate carboxykinase in juvenile European perch (Linnaeus) (17). Hypoxic stress limits the sustainability of largemouth bass farming and highlights the urgent need for solutions to this problem in the aquaculture industry.
Over the years, studies have found that organic acids and their salts positively affect animal nutrition and health (18). Short-chain fatty acids (SCFAs) including formic acid, acetic acid, and butyric acid (19), can regulate body metabolism through actions such as chelation with certain elements, modulation of intestinal acidity, and promotion of proliferation of gastrointestinal mucosal epithelial cells (20–22). Anaerobic bacteria produce butyric acid by digesting carbohydrates, which can provide energy for intestinal cells, promote intestinal cell differentiation and proliferation, and enhance the intestinal environment (23, 24). Sodium butyrate (SB), a chelated form of butyric acid, is a popular ingredient in animal feed for its improved stability and palatability (25). The solid form of SB provides better handling and storage properties, making it a practical addition to animal feed formulations (26). SB has emerged as a highly regarded additive in aquatic animal nutrition, with growing evidence supporting its effectiveness in improving growth performance and promoting intestinal health (27), such as Nile tilapia (25, 28, 29), hybrid grouper (Epinephelus fuscoguttatus ♀ × E. lanceolatus ♂) (19), largemouth bass (Micropterus salmoides) (30), turbot (Scophthalmus maximus L.) (31), yellow catfish (Pelteobagrus fulvidraco) (32), golden pompano (Trachinotus ovatus) (33). Several recent studies have found that SB has a role in improving immunity and antioxidant capacity (34). Transcription factor NF-E2-related factor 2 (Nrf2), as a protective transcription molecule, is considered to be the main regulator of cellular antioxidant defense response against environmental stress, and regulates the expression of cellular protection genes (35, 36). SB can regulate the activity of Nrf2 to promote the release of antioxidant enzymes and reduce cell apoptosis (37, 38). Sodium butyrate supplementation increased superoxide dismutase (SOD), catalase (CAT), and glutathione peroxidase (GSH-Px) activities in heat-stressed Nile tilapia (39). Dietary supplementation of 2.5 g/kg sodium butyrate increased the activity of lysozyme, decreased the expression of tumor necrosis factor α (TNF-α), and up-regulated the expression level of SOD in rainbow trout (Oncorhynchus mykiss) (40). In addition, previous studies in our laboratory have shown that an appropriate dose of sodium butyrate can enhance the resistance of juvenile yellow catfish to ammonia stress by improving antioxidant and immune responses (41). Based on these previous studies, SB offers a possible solution to hypoxic stress in aquatic animals. The effect of SB on improving antioxidants and immunity in aquatic animals, including largemouth bass, is unknown and deserves further exploration.
Largemouth bass, as a commercially valuable species, is one of the most economically significant freshwater fish in China with a production of more than 470,000 tons in 2019 (42, 43). It frequently experiences oxidative stress reactions such as hypoxia in pond culture, which threatens the health of the fish (43). The study aimed to address the health risks of oxidative stress in largemouth bass and to evaluate the effects of dietary sodium butyrate on blood metabolism, liver antioxidant status, immune response, and resistance to hypoxic stress in largemouth bass. This trial will elucidate the mechanism of action of sodium butyrate in protecting the health of largemouth bass. It will provide valuable insights to support the well-being of these aquatic organisms.
2 Materials and methods
All experimental procedures were performed in accordance with the Guide for Laboratory Animals of the Guangdong Academy of Agricultural Sciences, China. Animal use and care protocols were reviewed and approved by the Animal care and Use Committee of the Guangdong Academy of Agricultural Sciences (SC-GDAAS-2021-032).
2.1 Experimental diets
The formula and proximate composition of the experimental diet were shown in Table 1. Fish meal and soybean meal are the main proteins, and fish oil and soybean oil are the main lipids. In the study of yellow catfish supplemented with 0.25-2.0 g/kg sodium butyrate in the diet of our laboratory, it was found that the optimal supplemental amount of sodium butyrate was between 0.5-1.0 g/kg according to the growth, digestive enzyme activity and antioxidant indexes (32, 41). According to the results of previous experiments, the dosage of sodium butyrate in this experiment was determined to be 0.5-2.0 g/kg. Four isonitrogenous (50%) and isolipidic (9%) diets containing 0 (control, SB0), 0.5 g/kg (SB1), 1.0 g/kg (SB2), and 2.0 g/kg (SB3) SB were prepared. Eighty percent of the SB was purchased from Shanghai Yuanye Bio-Technology (Co., Ltd, China). All the ingredients were ground (AHZC1265 Hammer Mill, Buhler Machinery Co., LTD., Guangzhou, China) and, passed through a 60-mesh sieve, weighed accurately according to the formula. The blended feed ingredients were well mixed with lipid and water (30%) (AHML2000 Mixer, Buhler Machinery Co., LTD., Changzhou, China) before being structured into 2-mm pellet feed (pressure 1.83 MPa, SLX-80 Twin-screw Extruder, South China University of Technology Machinery Factory), dried at 55°C (HMO-205 Oven Dryer, Dongguan Haiming Electronic Technology Co., LTD., China), and kept at -20°C until use. The exact SB contents in the four diets were 0.0 g/kg, 0.38 g/kg, 0.75 g/kg, and 1.78 g/kg, which were measured by high-performance liquid chromatography (HPLC) (44).
2.2 Feeding management
The Institute of Animal Science, Guangdong Academy of Agricultural Sciences (Guangzhou, China), conducted this experiment in an indoor recirculating aquaculture system. Largemouth bass was obtained from Guangzhou Jinglong Fishery (Guangzhou, China). Acclimate to the experimental settings for 14 days, during which the fish were fed the control food twice daily. After that, 480 healthy fish of similar weight (5.02 ± 0.01 g) were randomly assigned to 12 fiberglass tanks (300 liters) with 40 fish per tank in triplicate for each treatment. Fish were fed two times daily at 8:30 and 16:30 to apparent satiation. Half an hour after the end of each feeding, check the bottom of the cylinder for the presence of excess feed, if any, siphon the residual feed out. We collected any unconsumed feed to calculate overall consumption. The daily food intake of fish in each tank was recorded and used to calculate adjusted intake based on the previous day’s consumption. During the 56-day feeding trial, the water temperature was between 28 and 30°C, pH 7.3–7.7, ammonia ≤ 0.02 mg/L, nitrite ≤ 0.2 mg/L, and dissolved oxygen ≥ 6.0 mg/L, and the photoperiod regime was 12 h light and 12 h dark.
2.3 Sample collections and analysis
Fish were fasted for 24 hours after the terminal of the feeding trial and anesthetized with 40 mg/L MS-222 (Sigma, USA) for weighing and counting. The number and weight of fish in each tank were recorded to determine the final body weight (FBW), weight gain rate (WGR) and feed conversion ratio (FCR). The condition factor (CF) was determined by weighing and measuring the body length of six fish from each tank. Three fish were picked at random from each tank and held at -20°C for analysis of the approximate makeup of their whole bodies. The protein deposition rate (PDR) was calculated based on body composition and feed intake. Blood samples were collected from the tail vein of the fish using a 1 mL syringe and processed for coagulation at − 4°C. We centrifuged the collected blood samples at 3500 rpm for ten min in a centrifuge and then extracted the serum and stored it at − 80°C for subsequent examination. Fish intact livers were placed in tubes, temporarily frozen in liquid nitrogen, and stored at − 80°C after the essay for quantitative real-time PCR of antioxidant enzyme activity and expression of inflammation-related genes.
Crude protein, crude lipid and ash content in fish feed and whole fish were determined according to the Association of Official Analytical Chemists (45). Dry matter (DM) was measured by drying the samples to a constant weight at 105°C (AOAC, 1999; #930.15). Crude protein (N × 6.25) was determined by semi-automatic Kjeldahl nitrogen determination system after acid digestion (AOAC, 1999; #2001.11). Crude lipid content was determined by weight after lipid extraction by Soxhlet extraction using ether (36680 analyzer, Buchi, Switzerland) (AOAC, 1999; #920.39). Ash content was measured after six h combustion in a muffle furnace at 550°C (AOAC, 1999; #942.05). Serum metabolic parameters such as glucose (GLU), triglycerides (TG), urea nitrogen (UREA), cholesterol (CHO), high-density lipoprotein cholesterol (HDL), and low-density lipoprotein cholesterol (LDL) were examined using an automated chemical analyzer (Hitachi 7180, Tokyo, Japan).
Hepatic samples were homogenized and centrifuged at 2500 rpm for 15 minutes at 4°C according to the instructions of the kit, and the supernatant was separated for analysis. Superoxide dismutase (SOD), catalase (CAT), total antioxidant capacity (T-AOC), glutathione peroxidase (GSH-Px), alkaline phosphatase (AKP), and malondialdehyde (MDA) were determined using commercial kits (Nanjing Jiancheng Institute of Biological Engineering, Nanjing, China) in accordance with the manufacturer’s instructions (46).
2.4 Hypoxia stress experiment
At the end of the 56-day experiment, 16 fish from each tank were selected for the hypoxic stress test. The amount of water in each tank was maintained at approximately 40 L, the water surface was covered with a transparent plastic film, and the water circulation of the farming system was turned off (35). The dissolved oxygen meter was inserted into the gas cylinder and the cumulative number of dissolved oxygen and fish deaths was recorded every half hour. Dissolved oxygen in the water was maintained at 0 mg/L after 2 h of the test. At 3 h, fish mortality in the SB0 group reached 50%, at which point the experiment was stopped and the number of dead fish in each test pool was recorded. Fish were considered dead when there was no respiratory activity and no response to mechanical stimuli.
The cumulative mortality rate (CMR) was calculated as follows: CMR (%) = 100×the number of dead fish after the stress test/the number of fish before the stress test.
2.5 RNA extraction and real-time quantitative PCR analysis
The hepatic’s total RNA was extracted using the Trizol (Invitrogen, USA) method, and its quality and quantity were evaluated using 1% agarose gel electrophoresis and spectrophotometric analysis (A 260:280 nm ratio). The RNA was subsequently transcribed into cDNA using the HiScript® III RT SuperMix kit (Vazyme, Nanjing, China). Primer sets were designed to quantify gene mRNA levels based on the sequences of immune and antioxidant-related genes of largemouth bass in GenBank (Table 2). The selected primers were synthesized by Shanghai Bioengineering Technology Co., Ltd., and real-time quantitative PCR (RT-qPCR) was performed on an ABI 7500 real-time PCR machine (Applied Biosystems, USA). β-actin was used as a non-regulatory reference gene in largemouth bass studies. Gene sequence amplification efficiency was close to 100%. In our present study, β-actin gene expression in the intestinal was also stable and not significantly affected by dietary SB. For all reactions, mRNA expression samples were assayed in triplicate, and gene expression levels were finally analyzed by the 2−ΔΔCT method.
2.6 Calculation formula
2.7 Statistical analysis
All data analyzes were performed in the statistical software SPSS 25.0 (Chicago, USA) and reported as mean ± SEM. To test for changes in growth performance, enzyme activity, and gene mRNA expression among groups, data were processed as one-way ANOVA with both linear and quadratic significance tests followed by Duncan’s test to determine significant differences between treatments, with P<0.05 considered significant and 0.05≤P<0.10 considered trend effects. The final graph of this experiment was created using the GraphPad Prism 5 software (GraphPad Software U.S.A).
3 Results
3.1 Growth performance
Table 3 presents information about the growth performance of experimental fish. Fish feed intake (FI) increased significantly with SB supplementation, and at the same time, FI decreased linearly and quadratic (P<0.05). The FBW and WGR of the basal diet and SB3-supplemented fish presented the lowest and highest values, respectively (P<0.05); that is, all experimental diets had higher FBW and WGR indices than the control diet. In addition, all diets containing the supplement reduced FCR, with SB2 and SB3 being significantly lower than the control (P<0.05). The SB3 group had a substantially greater PDR than the SB0 group (P<0.05). FBW, WGR and PDR increased linearly with the increase of SB (P<0.05). On the contrary, FCR decreased linearly with the increase of SB (P<0.05). The SB3 group had significantly lower hepatosomatic index (HSI) than the other groups (P<0.05). The visceralosomatic index (VSI) of largemouth bass-fed SB was not significantly different from that of the SB0 group (P>0.05). Compared to the SB0 group, dietary supplementation with SB showed no impact on CF in largemouth bass (P>0.05).
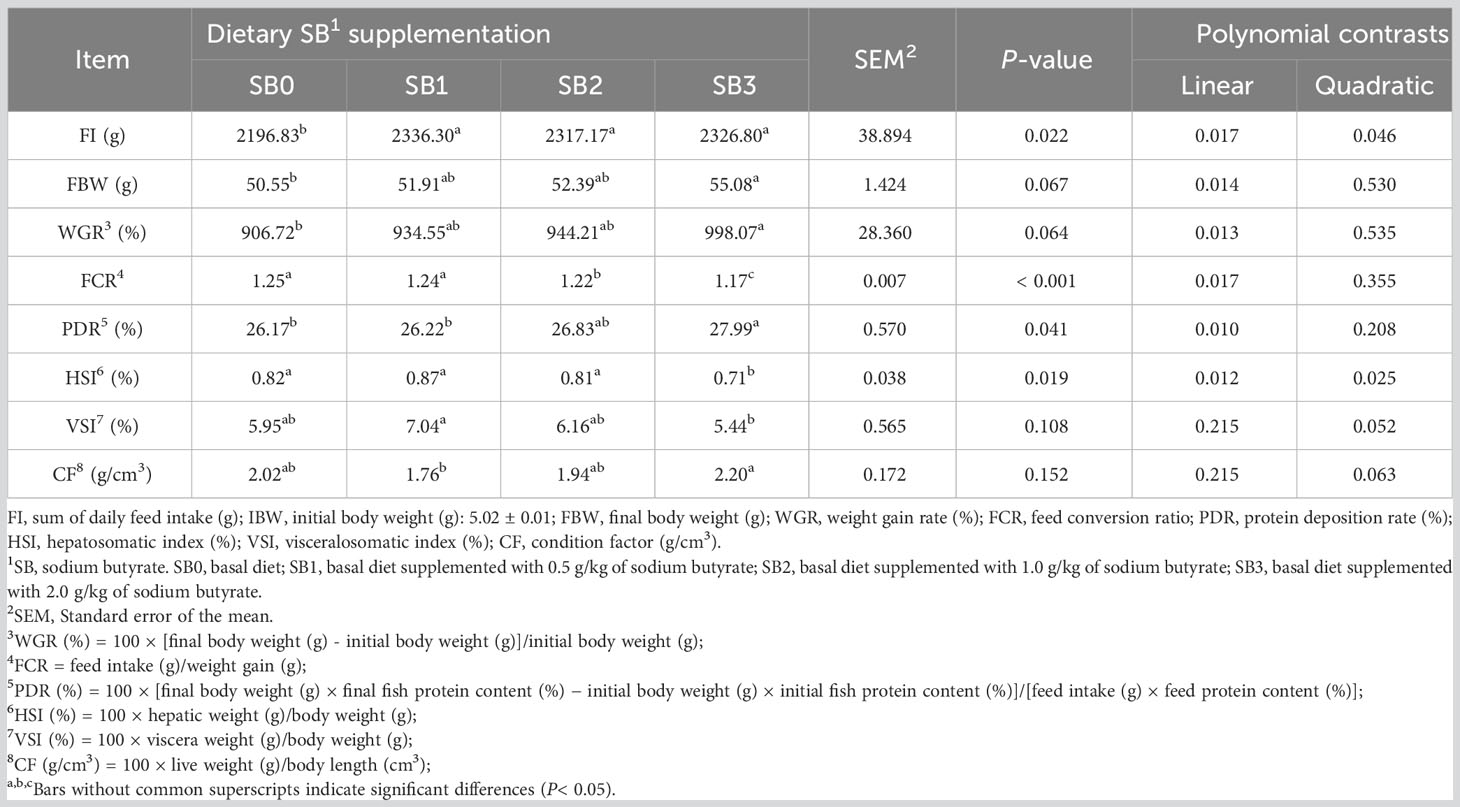
Table 3 Growth performance of largemouth bass fed a diet supplemented with sodium butyrate for 8 weeks.
3.2 Whole body composition
The effects of SB feeding on body composition of largemouth bass after 56 days are shown in Table 4. Compared with SB0 group, the contents of dry matter and crude lipid in SB2 group were significantly increased (P<0.05). Dry matter increased linearly and quadratic (P<0.05), and crude lipid increased linearly (P<0.05). Ash content in SB1 and SB2 groups was significantly increased (P<0.05). There was no significant difference in crude protein and water content among all groups (P>0.05). However, crude protein showed a secondary increase trend (P=0.077, 0.05<P<0.1).
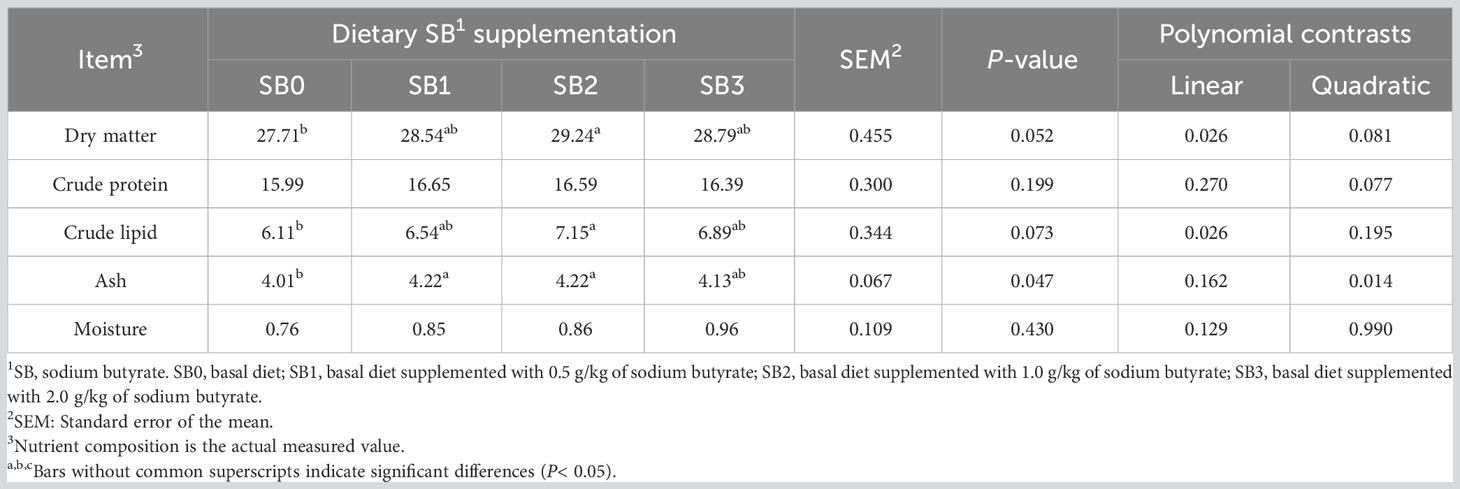
Table 4 Effects of feeding supplemented Sodium butyrate diet for 56 days on body composition of largemouth bass (% wet weight).
3.3 Serum biochemistry
The effects of SB on serum biochemistry of largemouth bass are summarized in Table 5. Compared with the SB0 group, fish fed the SB diet had lower urea levels with a linear and quadratic decrease (P<0.05), while GLU was significantly higher with a linear and quadratic increase (P<0.05). The levels of TG, CHO, HDL and LDL of fish fed SB2 diet were lower than those of fish fed SB0 diet (P<0.05). There was a linear decrease in TG, HDL, and LDL, and a linear and quadratic decrease in CHO. Compared with the SB0 group, the AKP level in the SB2 group was significantly decreased, showing a linear and quadratic decrease (P<0.05).
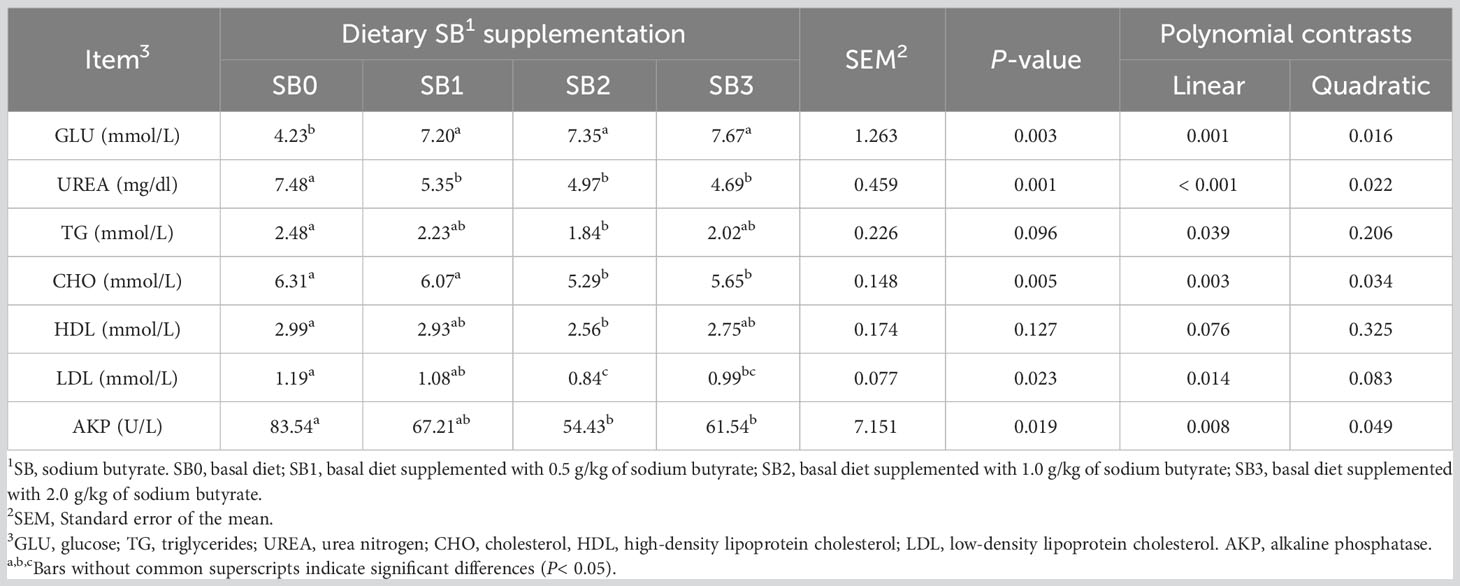
Table 5 Serum biochemical correlation indices of largemouth bass fed dietary supplementation with different levels of sodium butyrate diets for 56 days.
3.4 Hepatic antioxidant enzyme activities
The effects of dietary SB on AKP, CAT, MAD, TAOC, SOD, and GSH-Px in the liver are shown in Table 6. MDA in SB supplementation group was significantly lower than that in SB0 group, showing a linear and quadratic decrease (P<0.05). The T-AOC of fish fed SB1 was higher than that of other groups (P<0.05). The levels of AKP, CAT, SOD and GSH-Px in each experimental group were similar (P>0.05).
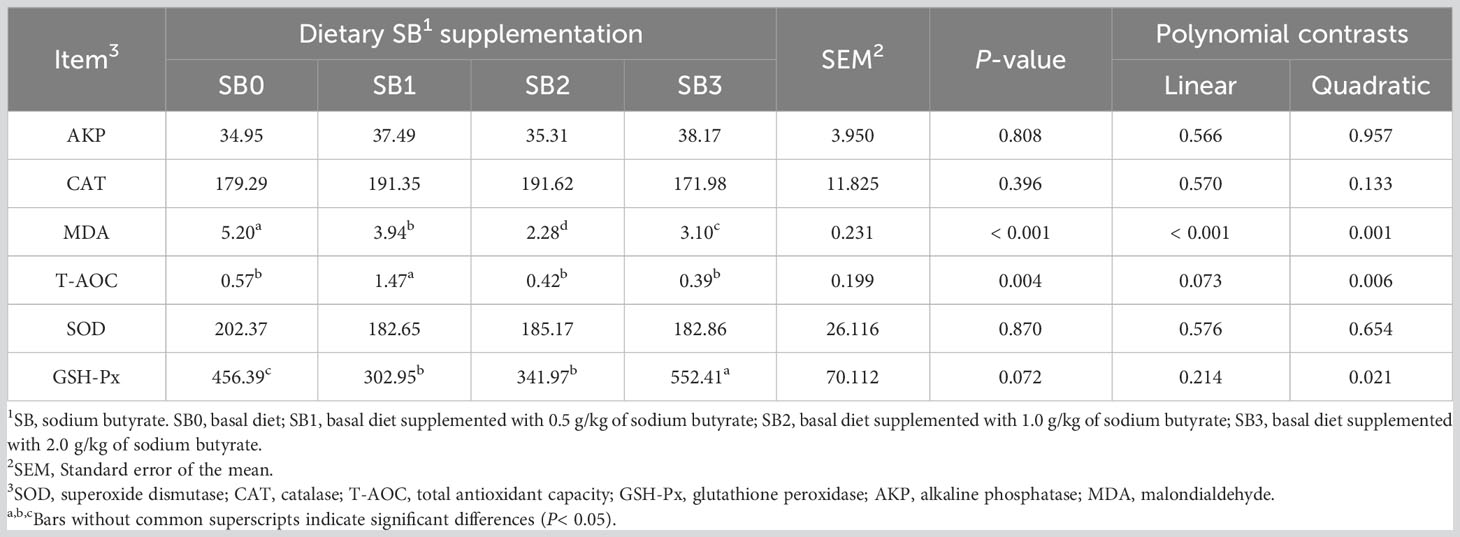
Table 6 Antioxidant enzyme related indices of largemouth bass fed dietary supplementation with different levels of sodium butyrate diets for 56 days.
3.5 Gene expression
3.5.1 Hepatic signaling pathway related gene expression
The effects of dietary SB on liver immune and growth-related genes are shown in Table 7. The mRNA expression of TLR22 and MyD88 in the liver of juvenile largemouth bass fed with SB1, SB2 and SB3 diets was significantly lower than that in the SB0 group, with linear and quadratic reductions (P<0.05). The mRNA expression of TLR22 in the liver of the SB2 group was significantly lower than that in the SB0 group (P<0.05). Compared with the control group, the expression of TGF-β1 mRNA decreased linearly with the increase of SB supplementation (P<0.05).
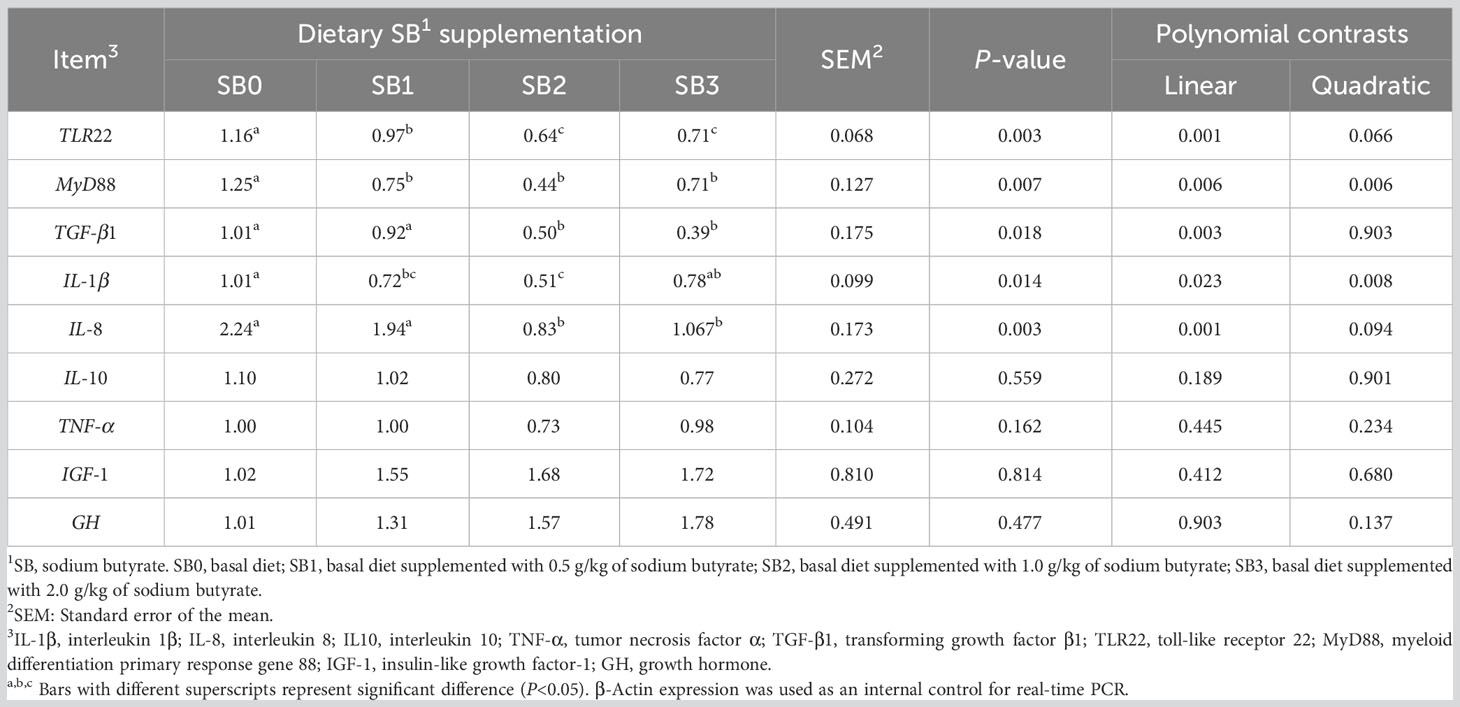
Table 7 Expression of inflammatory factor in largemouth bass fed diets supplemented with different levels of sodium butyrate for 56 days.
3.5.2 Hepatic inflammation-related gene expression
In the diet supplemented with SB, IL-1β was linearly and quadratic decreased (P<0.05), and the relative expression of IL-8 was linearly decreased (P<0.05). The expression levels of TNF-α and IL-10 in fish diets supplemented with SB tended to decrease, but there was no statistical significance between groups (P>0.05).
3.5.3 Hepatic growth-related gene expression
Dietary SB supplementation increased GH and IGF-1 mRNA expression in the liver of largemouth bass, but none of the groups showed statistical significance (P>0.05).
3.6 hypoxic stress
Table 8 shows the cumulative mortality of largemouth bass juvenile exposed to hypoxic stress for 3 hours. After 2 h of hypoxic stress, the cumulative mortality in SB2 and SB3 groups was significantly lower than that in SB0 group (P<0.05). After 3 hours of hypoxic stress, the cumulative mortality rates in the SB0, SB1, SB2 and SB3 groups were 68.8%, 58.3%, 35.4% and 37.5%, respectively. The cumulative mortality in SB2 and SB3 groups was significantly lower than that in SB0 group (P<0.05). The cumulative mortality decreased linearly at 3 h (P< 0.05). By quadratic regression analysis, we found that the mortality from hypoxic stress was minimal when the supplemental dose of SB was 1.62g/kg (Figure 1).
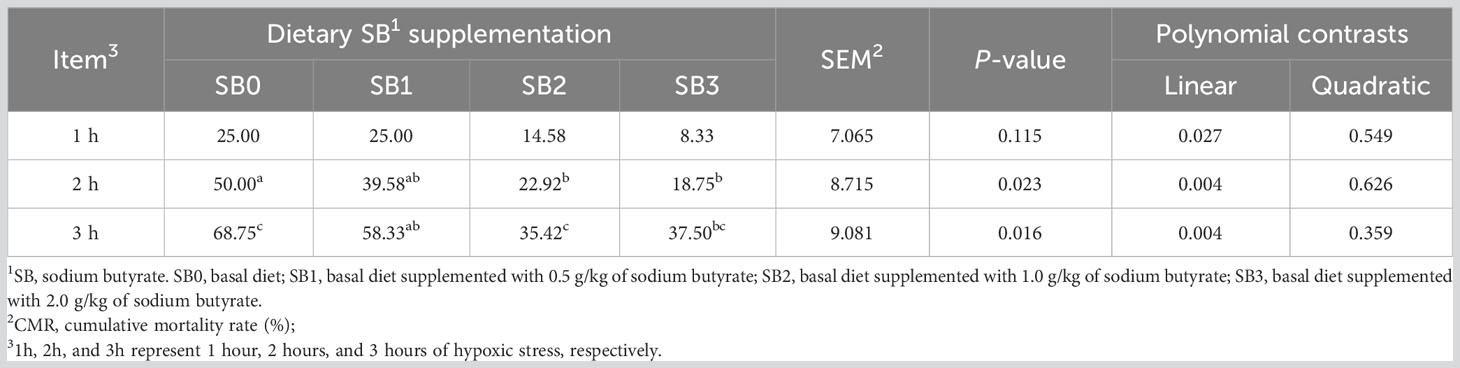
Table 8 Cumulative mortality rate (%) of largemouth bass under hypoxic stress at the end of the 56-day test. a,b,c Bars without common superscripts indicate significant differences (P<0.05).
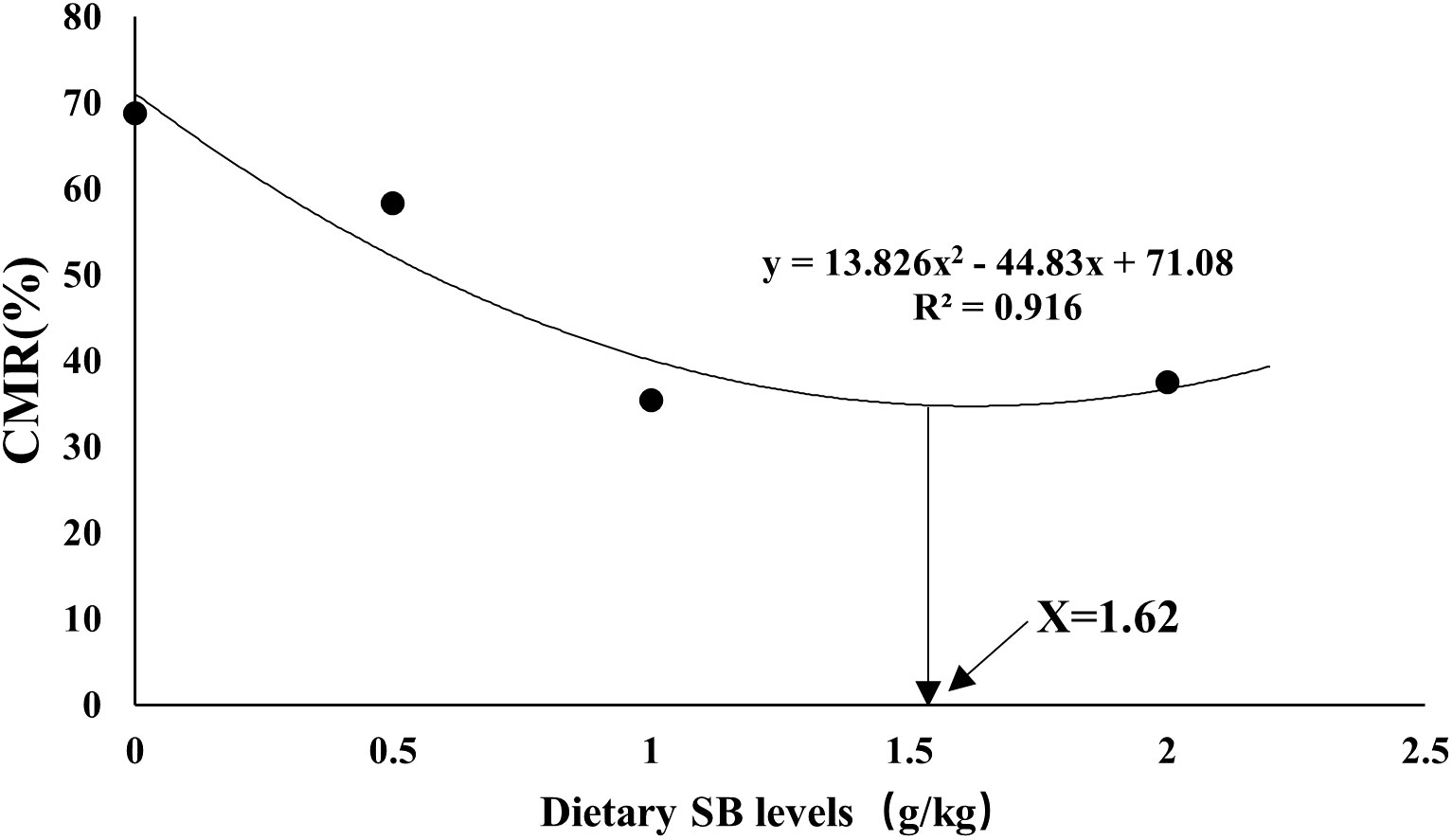
Figure 1 Quadratic regression analysis of cumulative mortality (CMR, %) and dietary SB level of largemouth sea bass after 56 days of hypoxia stress.
4 Discussion
In this study, the effects of SB on growth performance, serum biochemistry, immuno-antioxidant and hypoxic stress of largemouth bass were investigated. In this study, SB was found to improve the growth performance of largemouth bass, which was confirmed by analyzing terminal weight gain, weight gain rate, feed efficiency and protein deposition rate. Studies have shown that dietary sodium butyrate improves the growth of fish such as Nile tilapia (47), turbot (Scophthalmus maximus) (31), Asian sea bass (Lates calcarifer) (48), carp (Cyprinus carpio) (23), tilapia (28), crucian carp (Carassius auratus tripl) (49), and sea bream (Sparus aurata) (50). SB has been shown to improve fish growth and intestinal health. First, butyrate promotes the growth and development of intestinal villi and increases the contact area with nutrients (23). Secondly, butyrate can provide essential amino acids and vitamins for fish growth, or increase the activity of digestive enzymes and improve the intestinal flora to promote the digestion and absorption of nutrients (50, 51). Finally, butyrate can improve cellular immunity and antioxidant capacity to improve intestinal health (52). The present study found that dietary SB supplementation increased the expression of growth hormone (GH) and insulin-like growth factor 1 (IGF-1) in the liver. Growth hormone (GH), mediated by insulin-like growth factor (IGF), plays an important role in the regulation of fish growth, and growth hormone levels affect the growth rate of fish (53, 54). Similarly, dietary granule sodium butyrate was found to significantly increase GH and IGF-1 mRNA expression in Nile tilapia (55). However, the results of this study are inconsistent with the literature, as no differences in SB on body weight gain were observed in Atlantic salmon [0.5-2%, (56)], rainbow trout [0.5-2%, (57)], red hybrid tilapia [200-2000 mg/kg, (58)] and African catfish trout [200-2 000 mg/kg, (52)]. Feed supplementation with low doses (0.1-0.2%) of butyric acid promoted fish growth, and feeding 0.3% sodium butyrate to juvenile grass carp had no significant effect on their growth performance (59). The study found 0.2% to 0.3% sodium butyrate to be the appropriate dose for European seabass (60). The study found that there is a dose-effect relationship between SB and fish growth (32, 59), with low doses of butyric acid promoting fish growth, and 0.3% sodium butyrate having no significant effect on the growth performance of juvenile grass carp. Interestingly, in this experiment, the addition of different doses of SB (max. 2%) all promoted the growth of largemouth bass, which is consistent with the conclusion of a previous study in juvenile yellow catfish, and the reason for this may be related to the dosage of SB and the species of fish (32). Differential responses to SB addition on growth performance may be related to diet composition, intestinal maturation status, and SB dose (61).
Short-chain fatty acids play an important role in nutrient metabolism. Butyrate can enhance the ability of the intestine to produce SCFAs by increasing the Lactobacillus flora in the intestine, and acetate produced in the intestine can be converted into acetyl-coA, which can be used as high-energy substrates in the citric acid cycle or for lipid synthesis (29, 62, 63). This study examined the effects of dietary supplementation with SB to improve nutrient retention in juvenile largemouth bass. It has been reported that crude protein and ash levels of juvenile yellow catfish supplemented with 1 000 mg/kg SB diet were significantly increased, and crude lipid levels of juvenile yellow catfish supplemented with 2 000 mg/kg SB diet were significantly increased (32). A diet supplemented with sodium butyrate led to an increase in crude lipid and crude protein levels in Pacific white shrimp, Litopenaeus vannamei (64). However, dietary sodium butyrate supplementation did not significantly affect crude protein, crude fat, crude ash, and water content in rainbow trout (65), golden trout (Trachinotus ovatus) (33), and grass carp (59). Therefore, the differences in aquatic animal species may also be one of the reasons for the differences in results.
Multiple components of serum are dynamically balanced in response to environmental and dietary changes, and serum biochemical indices reflect the nutritional metabolism and health status of animals (66). Reduced serum urea nitrogen concentration is associated with nitrogen utilization efficiency (67, 68), and in the present study, dietary supplementation with SB significantly reduced serum urea concentration, suggesting that improved nitrogen utilization by SB was confirmed. Consistent with the results of the present study, SB reduced serum urea nitrogen concentration in pigs (69, 70). GLU is a major source of energy for body tissues and cells, and serum levels of GLU are closely related to digestion and absorption (71). In the present study, serum GLU levels were found to be significantly higher in largemouth bass fed SB. Ebrahimi et al. (2017a) reported a trend of elevated plasma glucose in red hybrid tilapia treated with dietary butyrate as compared to the control group. Similarly, feeding 5.0 g/kg butyrate was found to increase GLU levels in triploid carps compared to controls (72). In addition, butyrate increased the transcript levels of genes related to energy metabolism and fatty acid metabolism in the human colon (73). Previous studies have shown that butyrate inhibits fat accumulation and reduces obesity induced by a high-fat diet in mice (74, 75). In this study, SB reduced serum TG, CHO, HDL, and LDL levels in largemouth bass. Studies in large yellow croaker have shown that appropriate amounts of butyrate can regulate the expression of genes involved in lipid synthesis and lipid oxidation, thereby reducing lipid content in large yellow croaker (76). Oral administration of short-chain fatty acids, including butyrate, may reduce lipid deposition by decreasing lipogenesis and enhancing lipolysis in different tissues (77). Fang et al. (78) and Ullah et al. (82) found that SB reduced the HSI, which is consistent with the results of the present study, and may be related to the reduction of lipid content in the livers of largemouth bass by sodium butyrate. As in the present study, SB reduced TG content in the liver of largemouth bass (79), and additional SB supplementation reduced TG in grass carp on a high-fat diet (80). However, the underlying mechanism of sodium butyrate’s effects on lipid metabolism in aquatic animals remains to be elucidated.
ROS originate mainly from energy metabolism, and ROS production is associated with homeostasis of mitochondrial activity and cellular redox reactions (81). Antioxidant enzymes are crucial for scavenging free radicals and preventing the production of reactive oxygen species (39, 82–84). Lipid peroxidation is a common result of oxidative stress, leading to the production of the toxic by-product malondialdehyde (MDA), which can cause mutations and atherosclerosis (85). Supplementation of organic acids, such as SB, has been shown to improve the antioxidant capacity and protect the health of aquatic animals (86). T-AOC is an indicator for assessing the overall efficacy of the antioxidant defense system in an organism, which encompasses the performance of both enzymatic and non-enzymatic systems in the fight against free radical metabolism and compensatory capacity (87). This study showed that total antioxidant capacity (T-AOC) level was significantly increased and MDA level was significantly decreased in largemouth bass fed SB diet. SB inhibited glycogen synthase kinase 3β (GSK-3β) and increased the activity of nuclear factor erythroid 2-related factor 2 (Nrf2), thereby increasing the expression of downstream antioxidant enzymes and reducing oxidative stress in cells (37). Similar to our study, under heat stress conditions, sodium butyrate supplementation improved the activity of antioxidant enzymes (SOD, CAT, and GSH-Px) in Nile tilapia while reducing the MDA content, effectively improving the antioxidant capacity of the fish (39). The findings in yellow catfish were the same as the results of this experiment, and SB up-regulated the expression levels of SOD, CAT, and GSH-Px mRNAs under ammonia stress, which improved the resistance (41). Overall, the results suggest that SB has a positive effect on activating the antioxidant defense mechanism of largemouth bass, enhancing the activity of antioxidant enzymes, and improving their stress resistance.
Stimulation of the host immune response enhances the protection of fish against bacterial and viral diseases; therefore, this experiment evaluated the changes of SB on the expression levels of immune-related genes in the liver of largemouth bass. Transforming growth factor beta (TGF-β) has been identified as a chemokine that can trigger inflammation; it is associated with the malignancy of many cancers and the defective response to growth inhibition, and it has a significant impact on immunosuppression (88). SB can inhibit cancer cell invasion and metastasis by inhibiting transforming growth factor-β1 (TGF-β1) (89). Butyrate blocked the signal transduction of TGF-β1 by inhibiting the expression of TGF-β1 mRNA (90). The results of the present study showed that SB decreased TGF-β1 expression water, indicating that SB alleviated the immunosuppressive effect of TGF-β1 on largemouth bass. It is interesting that opposite results of SB were observed in yellow drum (Nibea albiflora) (91) and grass carp (51), and the specific reasons need to be further studied. When an inflammatory response occurs, cells reduce damage from the inflammatory response by upregulating mRNA levels of anti-inflammatory factors (e.g., IL-10) or downregulating pro-inflammatory factors (e.g., TNF-α, IL-8, IL-1β) (92). Toll-like receptors (TLR) recognize and bind pathogen-associated molecular patterns (PAMP), and upon recognition, TLR interacts with myeloid differentiation factor 88 (MyD88) to activate NF-κB and induce the production of cytosolic pro-inflammatory factors (93). Nuclear factor kappa B (nuclear factor kappa B, NF-κB) proteins are protein complexes that control transcription of DNA, cytokine production and cell survival (36, 94). Toll-like receptor 22 (TLR22) and MyD88 have been shown to play important roles in the innate immune response of grass carp (95) and weanling piglets (70). Butyric acid has been found to inhibit the release of NF-κB and prevent its translocation from the cytoplasm to the nucleus, ultimately inhibiting the expression of pro-inflammatory cytokines (96). In this study, dietary SB downregulated the expression levels of the pro-inflammatory factors TNF-α, IL-1β, IL-8, and TLR22, MYD88 mRNA, with 1% supplementation with SB showing the best effect. The results showed that dietary sodium butyrate could effectively improve the immunity of largemouth bass and could down-regulate the mRNA expression level of pro-inflammatory cytokines regulated by the TLR22-MyD88-NF-κB signaling pathway. The results of previous studies were consistent with the present study in that SB inhibited the NF-κB p65 signaling pathway, improved 2,4,6-trinitrobenzene sulfonic acid-induced intestinal inflammation in mice, and maintained intestinal integrity (97). This study suggests that this anti-inflammatory potential of butyrate may be related to the inhibition of the NF-κB-P65 pathway. Another study in grass carp showed that cytokine regulation might be involved in the NF-kB-p65 signaling pathway and confirmed that SB supplementation improved resistance to enteritis in grass carp (98). In conclusion, butyrate reduces inflammatory damage by activating TLR22-MyD88 signaling pathway to reduce the expression of inflammatory factors and enhance resistance to inflammatory responses.
Fish stressors include fish transport, high or low body temperature, hypoxic conditions, high or low salinity, poor nutrition, and various types of contaminants. These environmental changes can disrupt the dynamic balance of ROS in fish and thus produce a stress response that affects the normal morphology and physiology of fish (99, 100). Effective levels of dissolved oxygen (DO) are required for aerobic fish metabolism, and in intensive, high-density culture environments, DO in water is a major limiting factor in fish farming (101). If a portion of the fish’s energy is used to respond to stressors, less energy is available for other biological functions, including immunity (102, 103). Hypoxia may cause damage to the immune system of fish and reduce resistance to pathogenic infections (104). Hypoxic stress reduces antioxidant enzyme activity and increases malondialdehyde levels (101). In previous studies, it has been demonstrated that dietary supplementation with short-chain fatty acids (e.g., sodium propionate) can increase the expression levels of antioxidant enzyme genes in carp (105). Furthermore, there have been numerous studies evaluating the effect of SB addition to the diet on the antioxidant activity of different fish species (40, 106–108). In this study, placing largemouth bass under low oxygen emergency conditions, dietary supplementation with SB was effective in reducing the cumulative number of fish mortality, with 1.0 g/kg SB supplementation having the lowest cumulative mortality, which is consistent with the analysis of MDA, indicating that fish in the 1.0 g/kg supplementation group had the strongest antioxidant capacity and showed the best resistance to low oxygen stress. Similar to our study, the addition of SB to the diet up-regulated the expression levels of antioxidant enzymes (e.g., SOD, CAT, and GSH-Px) in rainbow trout (40) and increased activities of antioxidant enzymes (T-AOC, SOD, and GSH-Px) and reduced MDA levels were also found in the intestine of grass carp juvenile (109). SB decreased the expression of tumor suppressor gene P53 and pro-apoptotic gene Bax and inhibited cell apoptosis under ammonia stress (41). In conclusion, dietary supplementation of SB can effectively reduce the cumulative mortality of largemouth bass under anoxic stress. The reason may be that SB can increase the activity of antioxidant enzymes and reduce inflammatory factor production and inhibit cell damage and apoptosis by decreasing TLR22, MyD88, and TGF-β1 expression levels, thus reducing mortality under hypoxic stress conditions, but the mechanism of effect still needs to be further explored.
5 Conclusions
The data obtained in this study detailed that SB could better improve the growth performance of largemouth bass by increasing antioxidant enzyme activity, while activating TLR22-MyD88 signaling pathway and down-regulating inflammatory factor expression levels. In the hypoxic stress test, SB significantly increased the survival rate and showed good antioxidant capacity.
Data availability statement
The original contributions presented in the study are included in the article/supplementary files. Further inquiries can be directed to the corresponding author.
Ethics statement
The animal study was approved by Animal Care and Use Committee of the Guangdong Academy of Agricultural Sciences. The study was conducted in accordance with the local legislation and institutional requirements.
Author contributions
DH: Data curation, Methodology, Writing – original draft. ML: Investigation, Writing – original draft. PL: Methodology, Supervision, Writing – review & editing. BC: Formal Analysis, Project administration, Writing – review & editing. WH: Supervision, Writing – review & editing. HG: Validation, Writing – review & editing. JC: Methodology, Resources, Supervision, Writing – review & editing. HZ: Formal Analysis, Methodology, Supervision, Writing – review & editing.
Funding
The author(s) declare financial support was received for the research, authorship, and/or publication of this article. This research was supported by the National Natural Science Foundation of China (31402307), the Guangdong Science and Technology Program Project (KTP20210322) and the Foshan Municipal Financial Special Fund - the Project of Jointly Building Guangdong Agricultural Science and Technology Demonstration City in 2021 (Key Technology Research and Application of Standardization Cultivation of largemouth bass seed factory).
Conflict of interest
The authors declare that the research was conducted in the absence of any commercial or financial relationships that could be construed as a potential conflict of interest.
Publisher’s note
All claims expressed in this article are solely those of the authors and do not necessarily represent those of their affiliated organizations, or those of the publisher, the editors and the reviewers. Any product that may be evaluated in this article, or claim that may be made by its manufacturer, is not guaranteed or endorsed by the publisher.
References
1. Yuan J, Ni M, Liu M, Wang H, Zhang C, Mi G, et al. Analysis of the growth performances, muscle quality, blood biochemistry and antioxidant status of Micropterus salmoides farmed in in-pond raceway systems versus usual-pond systems. Aquaculture (2019) 511:734241. doi: 10.1016/j.aquaculture.2019.734241
2. Gao J, Koshio S, Ishikawa M, Yokoyama S, Ren T, Komilus CF, et al. Effects of dietary palm oil supplements with oxidized and non-oxidized fish oil on growth performances and fatty acid compositions of juvenile Japanese sea bass, Lateolabrax japonicus. Aquaculture (2012) 324:97–103. doi: 10.1016/j.aquaculture.2011.10.031
3. Blier P. Fish health: an oxidative stress perspective. Fisheries Aquaculture J (2014) 5:2150–3508. doi: 10.4172/2150-3508.1000e105
4. Sandoval-Vargas L, Silva Jimenez M, Risopatron Gonzalez J, Villalobos EF, Cabrita E, Valdebenito Isler I. Oxidative stress and use of antioxidants in fish semen cryopreservation. Rev Aquaculture (2021) 13(1):365–87. doi: 10.1111/raq.12479
5. Petitjean Q, Jean S, Gandar A, Côte J, Laffaille P, Jacquin L. Stress responses in fish: From molecular to evolutionary processes. Sci Total Environ (2019) 684:371–80. doi: 10.1016/j.scitotenv.2019.05.357
6. Yu Z, Zhao Y-Y, Jiang N, Zhang A-Z, Li M-Y. Bioflocs attenuates lipopolysaccharide-induced inflammation, immunosuppression and oxidative stress in Channa argus. Fish Shellfish Immunol (2021) 114:218–28. doi: 10.1016/j.fsi.2021.05.006
7. Jia Y, Wang F, Gao Y, Qin H, Guan C. Hypoxia stress induces hepatic antioxidant activity and apoptosis, but stimulates immunity and immune-related gene expression in black rockfish Sebastes schlegelii. Aquat Toxicol (2023) 258:106502. doi: 10.1016/j.aquatox.2023.106502
8. Peng K, Lv X, Zhao H, Chen B, Chen X, Huang W. Antioxidant and intestinal recovery function of condensed tannins in Lateolabrax maculatus responded to in vivo and in vitro oxidative stress. Aquaculture (2022) 547:737399. doi: 10.1016/j.aquaculture.2021.737399
9. Martínez-Álvarez RM, Morales AE, Sanz A. Antioxidant defenses in fish: biotic and abiotic factors. Rev Fish Biol fisheries (2005) 15:75–88. doi: 10.1007/s11160-005-7846-4
10. Khadim RM, Al-Fartusie FS. Antioxidant vitamins and their effect on immune system. J Physics: Conf Ser (2021), 012065. doi: 10.1088/1742-6596/1853/1/012065
11. Zhao L, Yuan B-D, Zhao J-L, Jiang N, Zhang A-Z, Wang G-Q, et al. Amelioration of hexavalent chromium-induced bioaccumulation, oxidative stress, tight junction proteins and immune-related signaling factors by Allium mongolicum Regel flavonoids in Ctenopharyngodon idella. Fish & Shellfish Immunol (2020) 106:993–1003. doi: 10.1016/j.fsi.2020.09.005
12. Hughes DA. Effects of dietary antioxidants on the immune function of middle-aged adults. Proc Nutr Soc (1999) 58(1):79–84. doi: 10.1079/PNS19990012
13. Rahman MS, Thomas P. Molecular cloning, characterization and expression of two hypoxia-inducible factor alpha subunits, HIF-1α and HIF-2α, in a hypoxia-tolerant marine teleost, Atlantic croaker (Micropogonias undulatus). Gene (2007) 396:273–82. doi: 10.1016/j.gene.2007.03.009
14. Terova G, Rimoldi S, Corà S, Bernardini G, Gornati R, Saroglia M. Acute and chronic hypoxia affects HIF-1α mRNA levels in sea bass (Dicentrarchus labrax). Aquaculture (2008) 279:150–9. doi: 10.1016/j.aquaculture.2008.03.041
15. Abdel-Tawwab M, Hagras AE, Elbaghdady HAM, Monier MN. Effects of dissolved oxygen and fish size on Nile tilapia, Oreochromis niloticus (L.): growth performance, whole-body composition, and innate immunity. Aquaculture Int (2015) 23:1261–74. doi: 10.1007/s10499-015-9882-y
16. Cuninghame S, Jackson R, Lees SJ, Zehbe I. Two common variants of human papillomavirus type 16 E6 differentially deregulate sugar metabolism and hypoxia signalling in permissive human keratinocytes. J Gen Virol (2017) 98(9):2310–9. doi: 10.1099/jgv.0.000905
17. Cadiz L, Zambonino-Infante J-L, Quazuguel P, Madec L, le Delliou H, Mazurais D. Metabolic response to hypoxia in European sea bass (Dicentrarchus labrax) displays developmental plasticity. Comp Biochem Physiol B: Biochem Mol Biol (2018) 215:1–9. doi: 10.1016/j.cbpb.2017.09.005
18. Ng W, Koh C. The utilization and mode of action of organic acids in the feeds of cultured aquatic animals. Rev Aquac (2017) 9(4):342–68. doi: 10.1111/raq.12141
19. Yin B, Liu H, Tan B, Dong X, Chi S, Yang Q, et al. Dietary supplementation of β-conglycinin, with or without sodium butyrate on the growth, immune response and intestinal health of hybrid grouper. Sci Rep (2021) 11(1):17298. doi: 10.1038/s41598-021-96693-x
20. Cross HS, Debiec H, Peterlik M. Mechanism and regulation of intestinal phosphate absorption. Miner Electrolyte Metab (1990) 16(2-3):115–24.
21. Sugiura SH, Dong FM, Hardy RW. Effects of dietary supplements on the availability of minerals in fish meal; preliminary observations. Aquaculture (1998) 160(3-4):283–303. doi: 10.1016/S0044-8486(97)00302-5
22. Baruah K, Sahu NP, Pal AK, Jain KK, Debnath D, Mukherjee SC. Dietary microbial phytase and citric acid synergistically enhances nutrient digestibility and growth performance of Labeo rohita (Hamilton) juveniles at sub-optimal protein level. Aquac Res (2007) 38(2):109–20. doi: 10.1111/j.1365-2109.2006.01624.x
23. Liu W, Yang Y, Zhang J, Gatlin DM, Ringø E, Zhou Z. Effects of dietary microencapsulated sodium butyrate on growth, intestinal mucosal morphology, immune response and adhesive bacteria in juvenile common carp (Cyprinus carpio) pre-fed with or without oxidised oil. Br J Nutr (2014) 112(1):15–29. doi: 10.1017/S0007114514000610
24. Kaiko GE, Ryu SH, Koues OI, Collins PL, Solnica-Krezel L, Pearce EJ, et al. The colonic crypt protects stem cells from microbiota-derived metabolites. Cell (2016) 165(7):1708–20. doi: 10.1016/j.cell.2016.05.018
25. Jesus GFA, Owatari MS, Dutra SAP, Silva BC, Martins M. Effects of sodium butyrate and Lippia origanoides essential oil blend on growth, intestinal microbiota, histology, and haemato-immunological response of Nile tilapia. Fish Shellfish Immunol (2021) 117:62–9. doi: 10.1016/j.fsi.2021.07.008
26. Silva BC, Nolasco-Soria H, Magallón-Barajas F, Civera-Cerecedo R, Casillas-Hernández R. Improved digestion and initial performance of whiteleg shrimp using organic salt supplements. Aquac Nutr (2015) 22(5):997–1005.
27. Abdel-Latif HMR, Abdel-Tawwab M, Dawood MAO, Menanteau-Ledouble S, El-Matbouli M. Benefits of dietary butyric acid, sodium butyrate, and their protected forms in aquafeeds: A review. Rev Fisheries Sci Aquaculture (2020) 28(4):421–48. doi: 10.1080/23308249.2020.1758899
28. Ahmed H, Sadek K. Impact of dietary supplementation of sodium butyrate and/or protexin on the growth performance, some blood parameters, and immune response of Oreochromis niloticus. Int J Agric Innov Res (2015) 3(4):985–91.
29. Jesus GFA, Pereira SA, Owatari MS, Addam K, Silva BC, Sterzelecki FC, et al. Use of protected forms of sodium butyrate benefit the development and intestinal health of Nile tilapia during the sexual reversion period. Aquaculture (2019) 504:326–33. doi: 10.1016/j.aquaculture.2019.02.018
30. Chen W, Chang K, Chen J, Zhao X, Gao S. Dietary sodium butyrate supplementation attenuates intestinal inflammatory response and improves gut microbiota composition in largemouth bass (Micropterus salmoides) fed with a high soybean meal diet. Fish Physiol Biochem (2021) 47:1805–1819. doi: 10.1007/s10695-021-01004-w
31. Liu Y, Dai J, Yang P, Xu W, Ai Q, Zhang W, et al. Sodium butyrate supplementation in high-soybean meal diets for turbot (Scophthalmus maximus L.): Effects on inflammatory status, mucosal barriers and microbiota in the intestine. Fish Shellfish Immunol (2019) 88:65–75. doi: 10.1016/j.fsi.2019.02.064
32. Zhao H, Wang G, Wang H, Mo W, Huang Y, Cao J, et al. Effects of dietary sodium butyrate on growth, digestive enzymes, body composition and nutrient retention-related gene expression of juvenile yellow catfish (Pelteobagrus fulvidraco). Anim Nutr (2021) 7(2):539–47. doi: 10.1016/j.aninu.2020.12.007
33. Zhou C, Lin H, Huang Z, Wang J, Wang Y, Yu W. Effect of dietary sodium butyrate on growth performance, enzyme activities and intestinal proliferation-related gene expression of juvenile golden pompano Trachinotus ovatus. Aquac Nutr (2019) 25(6):1261–71. doi: 10.1111/anu.12940
34. Mehrgan MS, Shekarabi SPH, Azari A, Yilmaz S, Lückstädt C, Rajabi Islami H. Synergistic effects of sodium butyrate and sodium propionate on the growth performance, blood biochemistry, immunity, and immune-related gene expression of goldfish (Carassius auratus). Aquaculture Int (2022) 30(6):3179–93. doi: 10.1007/s10499-022-00954-z
35. Peng K, Wang G, Wang Y, Chen B, Sun Y, Mo W, et al. Condensed tannins enhanced antioxidant capacity and hypoxic stress survivability but not growth performance and fatty acid profile of juvenile Japanese seabass (Lateolabrax japonicus). Anim Feed Sci Technol (2020) 269:114671. doi: 10.1016/j.anifeedsci.2020.114671
36. Yu Z, Zhao L, Zhao J-L, Xu W, Guo Z, Zhang A-Z, et al. Dietary Taraxacum mongolicum polysaccharide ameliorates the growth, immune response, and antioxidant status in association with NF-κB, Nrf2 and TOR in Jian carp (Cyprinus carpio var. Jian) Aquaculture (2022) 547:737522. doi: 10.1016/j.aquaculture.2021.737522
37. Xing X, Jiang Z, Tang X, Wang P, Li Y, Sun Y, et al. Sodium butyrate protects against oxidative stress in HepG2 cells through modulating Nrf2 pathway and mitochondrial function. J Physiol Biochem (2016) 73:405–14. doi: 10.1007/s13105-017-0568-y
38. Bayazid AB, Jang YA, Kim YM, Kim JG, Lim BO. Neuroprotective effects of sodium butyrate through suppressing neuroinflammation and modulating antioxidant enzymes. Neurochem Res (2021) 46(9):2348–58. doi: 10.1007/s11064-021-03369-z
39. Dawood M, Eweedah NM, Elbialy ZI, Abdelhamid AI. Dietary sodium butyrate ameliorated the blood stress biomarkers, heat shock proteins, and immune response of Nile tilapia (Oreochromis niloticus) exposed to heat stress. J Therm Biol (2020) 88:102500–. doi: 10.1016/j.jtherbio.2019.102500
40. Atm A, Py A, Ms A, Hp B, Smh C. Dietary sodium butyrate (Butirex C4) supplementation modulates intestinal transcriptomic responses and augments disease resistance of rainbow trout (Oncorhynchus mykiss). Fish Shellfish Immunol (2019) 92:621–8. doi: 10.1016/j.fsi.2019.06.046
41. Zhao H, Kai P, Gw A, Wm A, Yh A, Jca B. Metabolic changes, antioxidant status, immune response and resistance to ammonia stress in juvenile yellow catfish (Pelteobagrus fulvidraco) fed diet supplemented with sodium butyrate. Aquaculture (2021) 536:736441. doi: 10.1016/j.aquaculture.2021.736441
42. Xie S, Yin P, Tian L, Yu Y, Liu Y, Niu J. Dietary supplementation of astaxanthin improved the growth performance, antioxidant ability and immune response of juvenile largemouth bass (Micropterus salmoides) fed high-fat diet. Mar Drugs (2020) 18(12):642. doi: 10.3390/md18120642
43. Tao J, Wang S, Qiu H, Xie R, Zhang H, Chen N, et al. Modulation of growth performance, antioxidant capacity, non-specific immunity and disease resistance in largemouth bass (Micropterus salmoides) upon compound probiotic cultures inclusion. Fish Shellfish Immunol (2022) 127:804–12. doi: 10.1016/j.fsi.2022.07.031
44. Liu W, Yang Y, Zhang J, Gatlin DM, Ringø E, Zhou Z. Effects of dietary microencapsulated sodium butyrate on growth, intestinal mucosal morphology, immune response and adhesive bacteria in juvenile common carp (Cyprinus carpio) pre-fed with or without oxidised oil. Br J Nutr (2014) 112(1):15–29. doi: 10.1017/S0007114514000610
45. AOAC. Official methods of analysis of the Association of Official Aericultural Chemists. 16th ed. Gaithersburg, MD, USA: AOAC lnternational (1999).
46. Du J-H, Xu M-Y, Wang Y, Lei Z, Yu Z, Li M-Y. Evaluation of Taraxacum mongolicum flavonoids in diets for Channa argus based on growth performance, immune responses, apoptosis and antioxidant defense system under lipopolysaccharide stress. Fish Shellfish Immunol (2022) 131:1224–33. doi: 10.1016/j.fsi.2022.11.034
47. Hu ED, Chen DZ, Wu JL, Lu FB, Chen L, Zheng MH, et al. High fiber dietary and sodium butyrate attenuate experimental autoimmune hepatitis through regulation of immune regulatory cells and intestinal barrier. Cell Immunol (2018) S0008874918301126. doi: 10.1016/j.cellimm.2018.03.003
48. Alamifar H, Soltanian S, Vazirzadeh A, Akhlaghi M, Torfi M. Dietary butyric acid improved growth, digestive enzymes activities and humoral immune parameters in Barramundi (Lates calcarifer). Aquac Nutr (2020) 26(1):156–64. doi: 10.1111/anu.12977
49. Sun L, Liu Z, Hao G, Zhou L, Lu SQ, Zhang JS, et al. Effects of sodium butyrate on growth and intestinal cell proliferation of Carassius auratus. J Fishery Sci China (2013) 20(4):893–901. doi: 10.3724/SP.J.1118.2013.00893
50. Robles R, Lozano AB, Sevilla A, Márquez L, Nuez-Ortín W. Effect of partially protected butyrate used as feed additive on growth and intestinal metabolism in sea bream (Sparus aurata). Fish Physiol Biochem (2013) 39:1567–80. doi: 10.1007/s10695-013-9809-3
51. Tian L, Zhou X-Q, Jiang W-D, Liu Y, Wu P, Jiang J, et al. Sodium butyrate improved intestinal immune function associated with NF-κB and p38MAPK signalling pathways in young grass carp (Ctenopharyngodon idella). Fish Shellfish Immunol (2017) 66:548–63. doi: 10.1016/j.fsi.2017.05.049
52. Ebrahimi M, Daeman NH, Chong CM, Karami A, Kumar V, Hoseinifar SH, et al. Comparing the effects of different dietary organic acids on the growth, intestinal short-chain fatty acids, and liver histopathology of red hybrid tilapia (Oreochromis sp.) and potential use of these as preservatives. Fish Physiol Biochem (2017) 43:1195–207. doi: 10.1007/s10695-017-0365-0
53. Reindl KM, Kittilson JD, Bergan HE, Sheridan MA. Growth hormone-stimulated insulin-like growth factor-1 expression in rainbow trout (Oncorhynchus mykiss) hepatocytes is mediated by ERK, PI3K-AKT, and JAK-STAT. Am J Physiology-Regulatory Integr Comp Physiol (2011) 301(1):R236–43. doi: 10.1152/ajpregu.00414.2010
54. Picha ME, Turano MJ, Beckman BR, Borski RJ. Endocrine biomarkers of growth and applications to aquaculture: a minireview of growth hormone, insulin-like growth factor (IGF)-I, and IGF-binding proteins as potential growth indicators in fish. N Am J Aquac (2008) 70(2):196–211. doi: 10.1577/A07-038.1
55. Abdel-Tawwab M, Shukry M, Farrag FA, El-Shafai NM, Dawood MAO, Abdel-Latif HMR. Dietary sodium butyrate nanoparticles enhanced growth, digestive enzyme activities, intestinal histomorphometry, and transcription of growth-related genes in Nile tilapia juveniles. Aquaculture (2021) 536:736467. doi: 10.1016/j.aquaculture.2021.736467
56. Bjerkeng B. Cholesterol and short-chain fatty acids in diets for Atlantic salmon Salmo salar (L.): effects on growth, organ indices, macronutrient digestibility, and fatty acid composition. Aquac Nutr (1999) 5(3):181–191.
57. Gao Y, Storebakken T, Shearer KD, Penn M, Øverland M. Supplementation of fishmeal and plant protein-based diets for rainbow trout with a mixture of sodium formate and butyrate. Aquaculture (2011) 311(1-4):233–40. doi: 10.1016/j.aquaculture.2010.11.048
58. Owen MAG, Waines P, Bradley G, Davies S. The effect of dietary supplementation of sodium butyrate on the growth and microflora of Clarias gariepinus (Burchell 1822). Proc XII Int symposium fish Nutr feeding (2006), 147.
59. Liu M, Guo W, Wu F, Qu Q, Tan Q, Gong W. Dietary supplementation of sodium butyrate may benefit growth performance and intestinal function in juvenile grass carp (Ctenopharyngodon idellus) Aquac Res (2017) 48(8):4102–11. doi: 10.1111/are.13230
60. Abdel-Mohsen HH, Wassef EA, Saleh NE, Barakat K, Al E. Advantageous effects of dietary butyrate on growth, immunity response, intestinal microbiota and histomorphology of European Seabass (Dicentrarchus labrax) fry. Egypt J Aquat Biol Fisheries (2018) 22(4):93–110. doi: 10.21608/ejabf.2018.12055
61. Partanen KH, Mroz Z. Organic acids for performance enhancement in pig diets. Nutr Res Rev (1999) 12(1):117–45. doi: 10.1079/095442299108728884
62. Clements KD, Gleeson VP, Slaytor M. Short-chain fatty acid metabolism in temperate marine herbivorous fish. J Comp Physiol B (1994) 164:372–7. doi: 10.1007/BF00302552
63. Ballard FJ. Supply and utilization of acetate in mammals. Am J Clin Nutr (1972) 25(8):773–9. doi: 10.1093/ajcn/25.8.773
64. Yarahmadi P, Mirghaed AT, Shekarabi SPH. Zootechnical performance, immune response, and resistance to hypoxia stress and Vibrio harveyi infection in Pacific white shrimp (Litopeneaus vannamei) fed different fishmeal diets with and without addition of sodium butyrate. Aquac Rep (2022) 26:101319. doi: 10.1016/j.aqrep.2022.101319
65. Lin X, Zhang C, Cao K, Li Z, Zhao Z, Li X, et al. Dietary sodium butyrate changed intestinal histology and microbiota of rainbow trout (Oncorhynchus mykiss), but did not promote growth and nutrient utilization. Aquac Nutr (2023) 2023). doi: 10.1155/2023/3706109
66. Vicari T, van den Borne J, Gerrits WJJ, Zbinden Y, Blum JW. Postprandial blood hormone and metabolite concentrations influenced by feeding frequency and feeding level in veal calves. Domest Anim Endocrinol (2008) 34(1):74–88. doi: 10.1016/j.domaniend.2006.11.002
67. Miller LF. Characterization of a species homologous bioassay for recombinantly derived porcine somatotropin (rpSt) based upon linear depression of blood urea nitrogen. J Anim Sci (1989) 67(2):105.
68. Rosell VL, Zimmerman DR. Threonine requirement of pigs weighing 5 to 15 kg and the effect of excess methionine in diets marginal in threonine. J Anim Sci (1985) 60(2):480–6. doi: 10.2527/jas1985.602480x
69. Close WH, Berschauer F, Heavens RP. The influence of protein: energy value of the ration and level of feed intake on the energy and nitrogen metabolism of the growing pig: 1. Energy metabolism. Br J Nutr (1983) 49(2):255–69.
70. Fang CL, Sun H, Wu J, Niu HH, Feng J. Effects of sodium butyrate on growth performance, haematological and immunological characteristics of weanling piglets. J Anim Physiol Anim Nutr (Berl) (2014) 98(4):680–5. doi: 10.1111/jpn.12122
71. Zhen R, Liu C, Wei C, Luo Y, Hu X, Liu G, et al. Effect of different dosages of sodium butyrate and niacin on growth, faecal microbiota and Vitamin B metabolism in weaned piglets. J Appl Microbiol (2022) 132(6):4466–75. doi: 10.1111/jam.15545
72. Sun L, Liu Z, Hao G, Wang S, Zhou L, Feng J, et al. Effects of sodium butyrate on protein metabolism and its related gene expression of triploid crucian carp (Carassius auratus tripl). Chin J Anim Nutr (2013) 25(11):2775–82.
73. Vanhoutvin SALW, Troost FJ, Hamer HM, Lindsey PJ, Koek GH, Jonkers DMAE, et al. Butyrate-induced transcriptional changes in human colonic mucosa. PloS One (2009) 4(8):e6759. doi: 10.1371/journal.pone.0006759
74. Gao Z, Yin J, Zhang J, Ward RE, Martin RJ, Lefevre M, et al. Butyrate improves insulin sensitivity and increases energy expenditure in mice. Diabetes (2009) 58(7):1509–17. doi: 10.2337/db08-1637
75. Jin CJ, Engstler AJ, Sellmann C, Ziegenhardt D, Landmann M, Kanuri G, et al. Sodium butyrate protects mice from the development of the early signs of non-alcoholic fatty liver disease: role of melatonin and lipid peroxidation. Br J Nutr (2016) 116:1682–93. doi: 10.1017/S0007114516004025
76. Xu N, Ding T, Liu Y, Zheng W, Liu Q, Yin Z, et al. Effects of dietary tributyrin on growth performance, body composition, serum biochemical indexes and lipid metabolism-related genes expression of juvenile large yellow croaker (Larimichthys crocea) fed with high level soybean oil diets. Aquac Nutr (2021) 27(2):395–406. doi: 10.1111/anu.13192
77. Jiao AR, Diao H, Yu B, He J, Yu J, Zheng P, et al. Oral administration of short chain fatty acids could attenuate fat deposition of pigs. PloS One (2018) 13(5):e0196867. doi: 10.1371/journal.pone.0196867
78. Fang L, Wang Q, Guo X, Pan X, Li X. Effects of dietary sodium butyrate on growth performance, antioxidant capacity, intestinal histomorphology and immune response in juvenile Pengze crucian carp (Carassius auratus Pengze). Aquac Rep (2021) 21:100828. doi: 10.1016/j.aqrep.2021.100828
79. Chen W, Gao S, Chang K, Zhao X, Niu B. Dietary sodium butyrate supplementation improves fish growth, intestinal microbiota composition, and liver health in largemouth bass (Micropterus salmoides) fed high-fat diets. Aquaculture (2023) 564:739040. doi: 10.1016/j.aquaculture.2022.739040
80. Gao S, Zhao X, Niu B, Chang K, Chen W. Dietary sodium butyrate supplementation attenuates the detrimental effects of high-fat diets on growth performance, liver health, and disease resistance in grass carp. N Am J Aquac (2022) 84(3):392–401. doi: 10.1002/naaq.10250
81. Dikalov S. Cross talk between mitochondria and NADPH oxidases. Free Radic Biol Med (2011) 51(7):1289–301. doi: 10.1016/j.freeradbiomed.2011.06.033
82. Ullah S, Zhang G, Zhang J, Shengli T, Wang L, Kalhoro H, et al. Effects of microencapsulated sodium butyrate supplementation on growth performance, intestinal development and antioxidative capacity of juvenile black sea bream (Acanthopagrus schlegelii). Aquac Res (2020) 51(12):4893–904. doi: 10.1111/are.14824
83. Mourente G, Dıaz-Salvago E, Bell JG, Tocher DR. Increased activities of hepatic antioxidant defence enzymes in juvenile gilthead sea bream (Sparus aurata L.) fed dietary oxidised oil: attenuation by dietary vitamin E. Aquaculture (2002) 214(1-4):343–61.
84. Zhao L, Zhao J-L, Bai Z, Du J, Shi Y, Wang Y, et al. Polysaccharide from dandelion enriched nutritional composition, antioxidant capacity, and inhibited bioaccumulation and inflammation in Channa asiatica under hexavalent chromium exposure. Int J Biol Macromol (2022) 201:557–68. doi: 10.1016/j.ijbiomac.2021.12.117
85. del Rio D, Stewart AJ, Pellegrini N. A review of recent studies on malondialdehyde as toxic molecule and biological marker of oxidative stress. Nutrition Metab Cardiovasc Dis (2005) 15(4):316–28.
86. Hoseinifar SH, Sun Y, Caipang CM. Short-chain fatty acids as feed supplements for sustainable aquaculture: an updated view. Aquac Res (2017) 48(4):1380–91. doi: 10.1111/are.13239
87. Yu Z, Dai Z, Li L, Qin G, Wu L. Dietary supplementation with biofloc promotes growth, improves immune and antioxidant status, and upregulates NF-κB/Nrf2 signalling molecules and stress resistance in Rhynchocypris lagowskii Dybowski. Aquac Nutr (2021) 27(1):225–39. doi: 10.1111/anu.13180
88. Han G, Li F, Singh TP, Wolf P, Wang X-J. The pro-inflammatory role of TGFβ1: a paradox? Int J Biol Sci (2012) 8:228. doi: 10.7150/ijbs.8.228
89. Wang H-G, Huang X-D, Shen P, Li L-R, Xue H-T, Ji G-Z. Anticancer effects of sodium butyrate on hepatocellular carcinoma cells. vitro Int J Mol Med (2013) 31(4):967–74. doi: 10.3892/ijmm.2013.1285
90. Torii K, Maeshige N, Aoyama-Ishikawa M, Miyoshi M, Terashi H, Usami M. Combination therapy with butyrate and docosahexaenoic acid for keloid fibrogenesis: an in vitro study. Anais Brasileiros de Dermatol (2017) 92:184–90. doi: 10.1590/abd1806-4841.20176198
91. Wu X, Wang L, Xie Q, Tan P. Effects of dietary sodium butyrate on growth, diet conversion, body chemical compositions and distal intestinal health in yellow drum (Nibea albiflora, Richardson). Aquac Res (2020) 51(1):69–79. doi: 10.1111/are.14348
92. Wang T, Secombes CJ. The cytokine networks of adaptive immunity in fish. Fish Shellfish Immunol (2013) 35(6):1703–18. doi: 10.1016/j.fsi.2013.08.030
93. Thompson A, Locarnini SA. Toll-like receptors, RIG-I-like RNA helicases and the antiviral innate immune response. Immunol Cell Biol (2007) 85(6):435–45. doi: 10.1038/sj.icb.7100100
94. Li M, Chen L, Zhao Y, Sun H, Zhao L. Research on the mechanism of HRP relieving IPEC-J2 cells immunological stress based on transcriptome sequencing analysis. Front Nutr (2022) 9:944390. doi: 10.3389/fnut.2022.944390
95. Zhou JS, Guo P, Yu HB, Ji H, Lai ZW, Chen YA. Growth performance, lipid metabolism, and health status of grass carp (Ctenopharyngodon idella) fed three different forms of sodium butyrate. Fish Physiol Biochem (2019) 45:287–98. doi: 10.1007/s10695-018-0561-6
96. Chang G, Liu X, Ma N, Yan J, Dai H. Dietary addition of sodium butyrate contributes to attenuated feeding-induced hepatocyte apoptosis in dairy goats. J Agric Food Chem (2018) 66(38):9995–10002. doi: 10.1021/acs.jafc.8b03526
97. Chen G, Ran X, Li B, Li Y, He D, Huang B, et al. Sodium butyrate inhibits inflammation and maintains epithelium barrier integrity in a TNBS-induced inflammatory bowel disease mice model. EBioMedicine (2018) 30:317–325. doi: 10.1016/j.ebiom.2018.03.030
98. Tian L, Zhou XQ, Jiang WD, Liu Y, Wu P, Jiang J, et al. Sodium butyrate improved intestinal immune function associated with NF-kappa B and p38MAPK signalling pathways in young grass carp (Ctenopharyngodon idella). Fish Shellfish Immunol (2017) 66:548. doi: 10.1016/j.fsi.2017.05.049
99. Harper C, Wolf JC. Morphologic effects of the stress response in fish. ILAR J (2009) 50(4):387–96. doi: 10.1093/ilar.50.4.387
100. Chowdhury S, Saikia SK. Oxidative stress in fish: a review. J Sci Res (2020) 12:145–60. doi: 10.3329/jsr.v12i1.41716
101. Abdel-Tawwab M, Monier MN, Hoseinifar SH, Faggio C. Fish response to hypoxia stress: growth, physiological, and immunological biomarkers. Fish Physiol Biochem (2019) 45:997–1013. doi: 10.1007/s10695-019-00614-9
102. Segner H, Sundh H, Buchmann K, Douxfils J, Sundell KS, Mathieu C, et al. Health of farmed fish: its relation to fish welfare and its utility as welfare indicator. Fish Physiol Biochem (2012) 38:85–105. doi: 10.1007/s10695-011-9517-9
103. Douxfils J, Deprez M, Mandiki SNM, Milla S, Henrotte E, Mathieu C, et al. Physiological and proteomic responses to single and repeated hypoxia in juvenile Eurasian perch under domestication–clues to physiological acclimation and humoral immune modulations. Fish Shellfish Immunol (2012) 33(5):1112–22. doi: 10.1016/j.fsi.2012.08.013
104. Di Marco P, Priori A, Finoia MG, Massari A, Mandich A, Marino G. Physiological responses of European sea bass Dicentrarchus labrax to different stocking densities and acute stress challenge. Aquaculture (2008) 275(1-4):319–28. doi: 10.1016/j.aquaculture.2007.12.012
105. Safari R, Hoseinifar SH, Nejadmoghadam S, Khalili M. Non-specific immune parameters, immune, antioxidant and growth-related genes expression of common carp (Cyprinus carpio L.) fed sodium propionate. Aquac Res (2017) 48(8):4470–4478. doi: 10.1111/are.13272
106. Pei W, Tian L, Zhou XQ, Jiang WD, Feng L. Sodium butyrate enhanced physical barrier function referring to Nrf2, JNK and MLCK signaling pathways in the intestine of young grass carp (Ctenopharyngodon idella) Fish Shellfish Immunol. (2018) 73:121–132. doi: 10.1016/j.fsi.2017.12.009
107. Liu M, Guo W, Wu F, Qu Q, Tan Q. Dietary supplementation of sodium butyrate may benefit growth performance and intestinal function in juvenile grass carp (Ctenopharyngodon idellus). Aquac Res (2017) 48(8):4102–4111. doi: 10.1111/are.13230
108. Song-Lin Z, Jian-Bo C, Ji-Dan YE, Chun-Xiao Z. Effects of sodium butyrate on feeding,Growth performance and antioxidant capacity of Anguilla rostrata. Fujian J Agric ences (2011) 26(4): 549–551.
109. Wu P, Tian LI, Zhou X-Q, Jiang W-D, Liu Y, Jiang J, et al. Sodium butyrate enhanced physical barrier function referring to Nrf2, JNK and MLCK signaling pathways in the intestine of young grass carp (Ctenopharyngodon idella). Fish Shellfish Immunol (2018) 73:121–32. doi: 10.1016/j.fsi.2017.12.009
Keywords: sodium butyrate, largemouth bass, antioxidant system, inflammatory response, hypoxic stress
Citation: Hou D, Li M, Li P, Chen B, Huang W, Guo H, Cao J and Zhao H (2023) Effects of sodium butyrate on growth performance, antioxidant status, inflammatory response and resistance to hypoxic stress in juvenile largemouth bass (Micropterus salmoides). Front. Immunol. 14:1265963. doi: 10.3389/fimmu.2023.1265963
Received: 24 July 2023; Accepted: 05 October 2023;
Published: 03 November 2023.
Edited by:
Weicheng Hu, Yangzhou University, ChinaReviewed by:
Zirui Wang, Jiangxi Agricultural University, ChinaKenneth Prudence Abasubong, Nanjing Agricultural University, China
Dongming Zhang, Jilin Agriculture University, China
Ngoc Tuan Tran, Shantou University, China
Copyright © 2023 Hou, Li, Li, Chen, Huang, Guo, Cao and Zhao. This is an open-access article distributed under the terms of the Creative Commons Attribution License (CC BY). The use, distribution or reproduction in other forums is permitted, provided the original author(s) and the copyright owner(s) are credited and that the original publication in this journal is cited, in accordance with accepted academic practice. No use, distribution or reproduction is permitted which does not comply with these terms.
*Correspondence: Hongxia Zhao, emhhb2hvbmd4aWE4ODY2QDE2My5jb20=
†These authors have contributed equally to this work