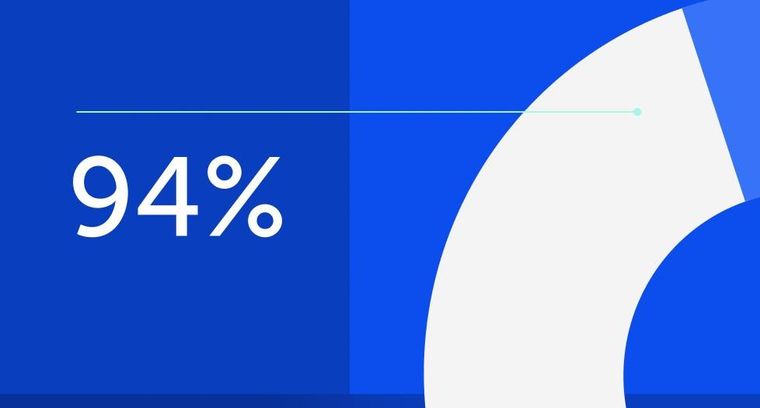
94% of researchers rate our articles as excellent or good
Learn more about the work of our research integrity team to safeguard the quality of each article we publish.
Find out more
HYPOTHESIS AND THEORY article
Front. Immunol., 23 October 2023
Sec. Microbial Immunology
Volume 14 - 2023 | https://doi.org/10.3389/fimmu.2023.1265511
Epidemiological studies have shown high tuberculosis (TB) prevalence among chronic opioid users. Opioid receptors are found on multiple immune cells and immunomodulatory properties of opioids could be a contributory factor for ensuing immunosuppression and development or reactivation of TB. Toll-like receptors (TLR) mediate an immune response against microbial pathogens, including Mycobacterium tuberculosis. Mycobacterial antigens and opioids co-stimulate TLRs 2/4/9 in immune cells, with resulting receptor cross-talk via multiple cytosolic secondary messengers, leading to significant immunomodulatory downstream effects. Blockade of specific immune pathways involved in the host defence against TB by morphine may play a critical role in causing tuberculosis among chronic morphine users despite multiple confounding factors such as socioeconomic deprivation, Human immunodeficiency virus co-infection and malnutrition. In this review, we map out immune pathways involved when immune cells are co-stimulated with mycobacterial antigens and morphine to explore a potential immunopathological basis for TB amongst long-term opioid users.
Tuberculosis (TB) remains a longstanding global health challenge, claiming 1.5 million lives in 2020. Two-thirds of new cases come from the eight highest-burdened nations, with six of them in South and Southeast Asia. (1)population is latently infected with TB. The risk of reactivation of latent Mycobacterium tuberculosis (MTB) is rising due to international travel, migration, immunosuppressive co-morbidities, and medication use, affecting both developed and developing countries. (2) In order to achieve the World Health Organization goal of reducing 90% of new TB cases by 2035, it is vital to better understand the key causes of reactivation of latent TB. Multiple risk factors for TB are clustered in different subpopulations. (3–5) Epidemiological data have shown that long-term opioid users are more susceptible to TB than the rest. (6) As per a comprehensive community-based case-control study, higher TB risk was independently associated with tobacco smoking, drug use (especially injectable drugs OR = 5.67; 95%CI: 2.68, 11.98), homelessness and area-level deprivation. The strongest risk factor among the intermediate social determinants was misuse of class A injectable drugs (e.g., Ecstasy, Cocaine, Crack Cocaine, Heroin), with five times higher TB odds (OR = 5.67; 95%CI = 2·68, 11.98) compared to those who never misused class A drugs (adjusted for age, sex, BCG vaccination status and long stays in high TB area). (7) A multivariate analysis has shown that drug use was associated with smear-positive TB (OR 2.2, CI 311 – 401, p<0.001). (8) Even accounting for genetic, environmental, socio-economic, and culture-related risk factors, raises the possibility of an underlying immunopathological basis leading to immune suppression. (6, 9–11) TB is often the most common opportunistic infection in endemic areas. (11) This highlights the importance of exploring independent pathophysiological mechanisms causing immune impairment in chronic drug use separately in different infections despite the multiple confounding factors causing generalised immune suppression.
The World Drug Report of 2021 has reported that around 275 million people used illicit substances in 2020 globally, highlighting another growing health challenge. Opioids are the most heavily used substance. Over 75% of substance users live in developing countries, where the prevalence of TB is also highest. (12) Long-term use takes different forms, including misuse of prescription opioids, habitual use without dependence, and increased use as a long-term analgesic. (13) A recent review published in the Lancet has highlighted the TB burden among vulnerable groups worldwide with variable prevalence. (14) Table 1 further summarises studies assessing a link between drug use and TB. Except for one study, others point towards a strong link between drug use and TB risk. As multiple confounders co-exist in these vulnerable populations, causality cannot be independently assessed. Epidemiological data assessing TB infection among drug users taking it as an independent variable is a future need. More specific details of the nature of drug use and the immune status will further help fill the missing data gap.
Immunomodulatory properties of opioids vary in the presence of different microbial antigens. Detailed characterisation of immune pathways affected by opioids in the presence of specific microbial pathogens is essential to characterise mechanisms of immunopathogenesis further. (20, 21) Due to the widespread testing of morphine in multiple previous pre-clinical studies (22), this review collates the immunomodulatory properties of morphine, and it suggests a potential role for opioids during chronic use in the immune pathogenesis of TB.
Opioids act on their receptor (OR), a G protein-coupled receptor (GPCR). They are categorised into two distinct groups: classical OR, which includes morphine (mu) MOR, ketocyclazocine (kappa) KOR, and vas deferens (delta) DOR, and non-classical OR, which includes nociceptin Orphanin FQ peptide receptor (NOR). (23, 24) ORs are located in multiple immune cells, including macrophages and lymphocytes, and are widely distributed in the human body. Both endogenous opioid peptides and exogenous synthetic opioids with different molecular properties act on the same receptors, giving rise to variable downstream effects. (21, 25)
Chronic morphine administration is known to cause impairment of both innate and adaptive arms of the immune response. (26) The concept of direct and indirect morphine action was on the immune system first introduced through work in preclinical studies, which indicated that the MOR mediates morphine-induced immunosuppression and that although some functions are amplified in the presence of cortisol or sympathetic activation. (27) The activation of KOR has also been shown to reduce antibody production, inhibit phagocytic cell activity, inhibit T cell development and alter the production of various pro-inflammatory cytokines, chemokines, and the receptors for these mediators. (28) In vitro experiments have shown that the DOR agonist KNT-127 causes immune suppression in rat models with colitis. (29) It has further shown the functional differentiation of OR subtypes located on the immune cells responding differently to endogenous and exogenous opioids. The novel M3OR subtype has been characterised as an opioid peptide-insensitive and opiate alkaloid-selective GPCR that is functionally linked to constitutive nitric oxide synthase activation. Opioid peptides stimulate granulocyte and immunocyte activation and chemotaxis via the activation of a novel leukocyte D2OR subtype. However, opiate alkaloid M3OR agonists inhibit these same cellular activities. (30)
Chemokine receptors (CCR), which mediate chemokine response, belong to the same class of GPCR as OR and possibly share a common evolutionary origin. The evidence suggests that these receptors cross-desensitise each other, whereas morphine that binds to OR can block CCR signalling and vice versa. (31, 32) The mechanisms underlying heterogenous desensitisation could be the formation of receptor heterodimers and protein kinase C-mediated phosphorylation of Serine, Threonine and Tyrosine moieties. Heterogenous desensitisation may be one mechanism of immune suppression by opioids with high doses and long-term exposure. (33, 34) Bivalent molecules can be tested to modify the complex and its cellular effects. Different immune effects mediated by OR in the presence of mycobacterial antigens and morphine are discussed in the subsequent sections. Bivalent molecules that selectively modulate the OR-CCR complex have therapeutic potential, such as VZMC013, which targets the MOR-CCR5 heterodimer to inhibit opioid-exacerbated HIV1 entry into the immune cells. Similar molecules may have applications in managing opioid-induced immune suppression, including the potential TB risk (35).
A focused literature search in Google Scholar, PubMed and Medline was carried out to extract the studies conducted to find the effects of ‘morphine’ on the ‘immune system’. Tables 2, 3 comprehensively summarise the immune cells and mediators influenced by morphine administration. Morphine suppresses multiple immune cells, including macrophages, which play a crucial role in the immunopathogenesis of TB. The consequences of morphine exposure extend to inhibiting chemotaxis and multiple cellular functions in macrophages, including respiratory burst activity, phagocytosis, and colony formation (Table 2).
Suppression of the critical immune mediators such as tumour necrosis factor (TNF α), interferon (IFN γ), and nitrous oxide (NO) produced by macrophages was also reported in multiple studies (Table 3). Figure 1 illustrates the immune pathways affected during the co-stimulation of macrophages by mycobacterial antigens and morphine. Suppression of the neutral killer (NK) cell by morphine is modulated via direct and centralised mechanisms. Lymphocytes are inhibited by multiple means, including reduced cytotoxicity and altered CD4/CD8 cell ratios.
Figure 1 Illustration of the co-binding of M. tuberculosis (MTB) antigens and opioids with TLR4. Illustration of the co-binding of MTB antigens and opioids with TLR4. Co-stimulation of the TLR4 leads to MyD88-dependent and independent activation, leading to NFkB-dependent and independent mechanisms affecting chemical mediators downstream. [TLR, toll-like receptor; NF-κB, Nuclear factor-κB; TIRAP, Toll-interleukin-1 Receptor (TIR) domain-containing adaptor protein; TRIF, TIR-domain-containing adaptor-inducing interferon-β; TRAM, TRIF-related adaptor molecule].
TLRs are found in various immune cells and play a critical role in recognising molecular patterns of pathogens to trigger the immune system. Multiple TLRs, including TLR2, TLR4, TLR8, and TLR9, interact with various mycobacterial antigens. Morphine interacts with TLR2, TLR4, and TLR9, and co-stimulation by morphine and mycobacterial antigens may lead to complex immune effects downstream (49, 101–105).
Multiple mycobacterial antigens interact with immune cells via TLR-dependent and independent mechanisms. Figure 1 illustrates the co-binding of mycobacterial antigen and opioids with the TLR4 and its adaptor proteins. Rapidly growing, non-pathogenic mycobacteria containing AraLAM in their cell walls activate CD14 cells expressing TLR2 and macrophages. In contrast, slow-growing pathogenic MTB containing ManLAM has shown a relative inability to activate macrophages independent manner, potentially contributing to their virulence (Figure 2). However, other soluble and cell wall-associated mycobacterial antigens distinct from LAM can mediate immune cell activation via TLR. For example, a soluble heat-stable and protease-resistant factor mediates TLR2-dependent activation of immune cells, whereas a heat-sensitive cell-associated mycobacterial factor mediates TLR4-dependent activation of them. (106–112) Interestingly, induction of adaptive T cell response in TB does not require TLR2/4/9. In TLR2/4/9-deficient mice, mycobacterial replication is controlled by TLR-independent mechanisms to induce an adaptive T-cell response (113).
Figure 2 Illustration of the interactions by tuberculous antigens and morphine with the cell surface and intracellular receptors of a macrophage. The interaction between tuberculous antigens and morphine within macrophages. Morphine (M) and Mycobacterial antigens engage with TLR 2/4/9, while Mycobacterial antigens interact with NLR, MR, and Dectin-1. M enhances Mycobacterial antigen virulence by inhibiting TLR 2/4 and OR. Morphine influences various macrophage functions: (1) Desensitizing multiple CCRs via MOR and DOR. (2) Inhibiting FcR-mediated apoptosis via MOR, DOR, and KOR. (3) Enhancing TGF-induced apoptosis. (4) Impairing NO and SO synthesis, respiratory burst activity, and bacilli killing. NLR2 augments TLR 2/4 actions through cross-talk. (6) M inhibits NFκB-mediated cytokine and chemokine synthesis via OR-TLR cross-talk. TLR9 elicits a proinflammatory response with Mycobacterial antigens and M, while TLR2/4 induces an anti-inflammatory response. (7) MR, present in AM, regulates protective macrophage responses. MTB or ManLAM upregulates PPARγ via MR, increasing IL8, COX2, and PGE2. ManLAM generates an anti-inflammatory response, inhibiting proinflammatory TNF and IL12 while inducing immunosuppressive IL10 and TGF β. ManLAM signalling via TLR2 and TLR4 triggers chemokine secretion in monocytes, with M exerting inhibitory effects via TLR 2/4 (10). Dectin-1, in combination with TLR2, induces TNF production in macrophages, particularly in attenuated MTB strains (11). LM blocks TLR2-induced TNF biosynthesis, permitting MTB to evade the host immune response. Antigen-specific variations are noted, with M-induced immune suppression amplifying antigen virulence mechanisms. (A more detailed version of this figure legend is provided in the Online Data Supplement).
Morphine interacts with TLR2, TLR4, and CD14 cells, causing inhibitory effects. (Figures 2, 3) These effects of morphine are exerted on different immune cell types interrupting their functions, which are vital for the immune defence against TB. Further, endomorphin-1, the endogenous form of opioids, has been shown to down-regulate TLR expression as a part of the negative feedback control. Consequently, external opioids, when strongly influencing the same pathway, may contribute to impaired and delayed antigen processing. (114) Consequently, the cells’ capacity to interact with mycobacterial antigens and trigger a protective immune response is ultimately reduced.
Figure 3 Co-stimulation of OR, TLR, and NLR with mycobacterial antigens and morphine and their intracellular cross-talking. Interactions between Mycobacterial antigens, morphine (M), and various receptors within macrophages. Both M and Mycobacterial antigens engage TLR 2/4/9. Specific antigens (LTA, HSP, PE6) interact with TLR4, inducing proinflammatory cytokines and apoptosis. Several interactions and pathways are highlighted: (1) M and bacterial antigens induce cross-talk between TLR2 and NLR2, inhibiting IL23 synthesis. (2) PE6 triggers proinflammatory cytokines via TLR4, possibly blocked by M. (3) M indirectly induces HMGB1, promoting TLR4 upregulation. (4) TLR4/OR pathways activate MAPK with proinflammatory effects in the CNS. (5) Intracellular TLR4/OR cross-talk affects cytokine secretion. (6) M inhibits LPS-induced NFκB activity. (7) M and M. tuberculosis induce TLR9 expression with pro-inflammatory consequences. (8) TLR9 regulates mycobacteria-induced Th1 responses. (9) NLR2 activates NFκB and cross-talks with TLR9. (10) M influences apoptosis-related molecules. (11) TLR2 plays a role in cytokine production regulation. (12) β-arrestin-2 negatively regulates TLR2-mediated apoptosis. (13) TLR2 activates PI3K/Akt signalling with M. (14) AraLAM induces cell activation and MTB killing. (15) TLR2-dependent inhibition of TLR9-dependent IFN α/β expression leads to decreased MHC-I cross-processing. (16) β-arrestin-2 regulates GPCR desensitization. These interactions reveal intricate immune responses in tuberculosis and opioid co-stimulation. (A more detailed version of this figure legend is provided in the Online Data Supplement).
Figure 3 summarises the cross-talk between OR, TLR, and nucleotide-binding and oligomerisation domain-like receptors (NLR) 2 via multiple cytosolic second messengers during the interaction with morphine and mycobacterial antigens. In the central nervous system, cross-taking between OR and TLR share common cytosolic molecules such as MAPK, β-arrestin-2/TRAF6 complex, and the DNA-binding protein HMGB1. (115) NLR2 on the immune cells interacts directly with mycobacterial antigens and cross-talks with TLR 2/9 to modulate the immune response. (61) Cross-talk between MOR and TLR in cancer models has shown decreased NK cytotoxicity, decreased leucocyte migration, suppression of mast cell recruitment, and the induction of M2 cell polarisation, which may contribute to the immune impairment in TB (116). Further exploration of the role of these compounds within the immune system in modulating cellular function is required.
Co-stimulation of TLRs with antigenic material of a pathogen, together with morphine, has shown entirely different effects than the binding of either alone. Extensive in vitro and in vivo studies have consistently shown that morphine binding to TLR4 triggers a proinflammatory cytokine response downstream. In contrast, its binding to OR (opioid receptors) elicits an anti-inflammatory response. (104, 117) We hypothesise that opioid compounds interact with TLR as other natural compounds, modulating the host immune response, and it needs direct testing of this in pre-clinical models in the presence of tuberculosis antigens. (Figure 3).
Morphine causes an antiinflammatory response in dendritic cells (DC) cells via TLR2 and NLR2 when co-stimulated with S. pneumoniae, in contrast to the proinflammatory response induced by S. pneumoniae alone (Figure 3). (49) Morphine has also been shown to inhibit the TLR9 pathway when co-binding with HIV, promoting its replication in macrophages. (105) Similar variations of the immune effects have been observed with morphine and mycobacterial antigens in preclinical studies. Plasmacytoid DC expresses TLR9 in both humans and mice. (118–120) M. tuberculosis and morphine cotreatment have significantly upregulated TLR9 expression in mice. Its role is more proinflammatory, enhancing the levels of critical cytokines including TNF α, (interleukin) IL1β, and IL6, which contrasts with the antiinflammatory response exerted by TLR2/4 when co-stimulated by the same. (20, 121) This contracting proinflammatory action of TLR9 compared to other TLRs indicates the downstream receptor action heterogeneity, possibly explained by the unique binding of TB antigens with TLR9.
In the immunopathogenesis of tuberculosis, morphine exerts immunomodulatory effects, as depicted in Figure 4. The acquisition of TB bacilli occurs through the inhalation of respiratory droplets containing the organism. Morphine’s influence leads to the suppression of NK cells and DC, as highlighted in Table 1. Consequently, this suppression can impair the initial defence against TB bacilli, including nonspecific killing and antigen presentation by these cells, ultimately increasing the host’s susceptibility to TB infection.
Figure 4 Effects due to the interactions of mycobacterial antigens and morphine with the immune cells and their mediators in the context of TB immunopathogenesis. The impact of mycobacterial antigens and morphine (M) on immune responses in tuberculosis (TB) immunopathogenesis. (1) TB bacilli virulence factors trigger a robust immune response. (2) ESX-1 induces MMP9 and MMP1 secretion; M reduces MMP9 and elevates MMP1 levels. (3) Neutrophils reduce initial Mycobacterial load. (4) M enhances TGFβ, lowering reactive oxygen intermediates. (5) M modulates CCR and CCL levels, affecting IFNγ-positive CD4 T cell migration. (6) M inhibits IL23 by dendritic cells. (7) M inhibits IL8 from neutrophils. (8) M suppresses NK cells. (9) M suppresses B cell activity. (10) M inhibits IL1, TNFα, and IL12, impacting IFNγ-induced T cell responses. (11) M inhibits IFNγ and TNFα from Th1 cells. (12) M inhibits IL2 from T cells. (13) M induces Th2 cell differentiation. (14) M promotes M2 macrophage switch via IL4. (15) MMP9 inhibition leads to M2 macrophage transformation. (16) Methadone increases CTLA-4 expression with variable PD-1 expression, impacting immune regulation in TB. (A more detailed version of this figure legend is provided in the Online Data Supplement).
A TB granuloma is a unique pathological entity comprising activated macrophages, monocytes, DC, neutrophils, and T lymphocytes (Figure 4). An established granuloma contains infected activated macrophages and epithelioid cells forming a central necrotic core and activated macrophages and layers of CD4+ and CD8+ T cells defining a dense cellular wall encircling the necrotic core. (122, 123) It is a dynamic structure that controls two processes: the induction of apoptosis of infected macrophages and the recruitment of uninfected macrophages by creating a chemotactic gradient (123).
Granuloma formation is triggered by the mycobacterial virulence factor ESX-1 (124) It triggers matrix metallopeptidase (MMP)9 secretion by the epithelial cell matrix surrounding a granuloma (Figure 4). (125) It potentially induces the chemotaxis of macrophages via (chemokine ligand (CCL)7, a substrate for MMP9 produced by macrophages. (126–129) Both well-coordinated processes of new macrophage recruitment and infected macrophage apoptosis are essential to maintain the immune integrity of TB granulomas. Morphine decreases the levels of MMP9 and increases the tissue inhibitor of MMP1, dysregulating this process (Figure 4) (108).
Macrophages, crucial in granuloma formation, face inhibition through various mechanisms induced by morphine (Figure 2). When infected with M. tuberculosis, macrophages experience heightened caspase-8-dependent apoptosis due to TLR2 signalling. However, mycobacteria take advantage of this situation during the initial stages of infection, as they depend on macrophages to penetrate deeper tissues and subsequently undergo apoptosis to expand within the granuloma. (123, 130) Morphine causes enhancement of TLR9-induced apoptosis of macrophages by stimulating TLR9 signalling, and multiple chemical mediators also potentiate apoptosis (Figures 1, 2). (20, 59) The impact of the induction of apoptosis by morphine depends on the exact stage of the infection.
MOR located on macrophages inhibits chemotaxis, which supports the notion of an antiinflammatory role of MOR. (131) Morphine’s effects on phagocytosis were variable, with inhibition observed through a naloxone-reversible mechanism. Mycobacteria employ the cell wall-associated lipid Phthiocerol dimycocerosate (PDIM) to conceal underlying pathogen-associated molecular patterns (PAMPs), effectively evading the recruitment of microbicidal macrophages via TLR-dependent pathways. Additionally, a structurally related molecule called Surface-associated Phenolic glycolipid induces the expression of CCL2, leading to the recruitment and infection of CCR2-expressing macrophages. Morphine suppresses CCL2, counteracting this pathway and suppresses multiple chemokines important in chemotaxis (Table 3).
Studies have shown that the reduction in total T cell counts and altered CD4/CD8 cell ratios are caused by morphine. Suppression of IL2 levels by morphine leads to a drop in T cell count (Table 2). CTLA-4 and PD-1 are two members of the CD28 family of receptors involved in T-cell inhibition by morphine. (132–134) In murine studies, MOR agonists have been shown to upregulate the expression of MOR, DOR, CD28, CTLA-4, and PD-1, which suppresses T-cell response. However, chronic opioid use has led to increased expression of CTLA-4, with unchanged PD-1 expression favouring an anti-inflammatory response among humans. (135) Morphine further triggers the Th2 switch, which may impair the cytotoxic potential of T cells against TB bacilli (Figure 4). Further studies are required to explore variable immune effects caused by different opioids on T cells.
Neutrophils are abundant in both early granulomas and late cavitary granulomas. (32, 136) They exhibit reduced NADPH oxidase-dependent mycobacterial killing when they ingest mycobacteria. However, their role in mediating the clearance of infected, dying macrophages appears to be host-protective. This mechanism lowers the mycobacterial load and reduces intercellular spread into uninfected macrophages. (123) The inhibition of neutrophils and IL8 secretion by morphine may lead to reduced neutrophil-mediated killing, increasing the risk of TB bacilli dissemination (Table 3).
IL17 recruits Th1 cells that secrete antigen-specific IFN γ, inhibiting MTB growth. Th1-mediated IFN γ is the critical chemical controller in granuloma formation. M. tuberculosis induces an IFN γ response through TLR9’s action. (21) Once stimulated by TB antigens via TLRs, macrophages and DCs secrete cytokines, including IL-12 and IL23, to induce IFN-γ production by T and NK cells. IFN-γ increases phagocytosis, phagolysosomal fusion, oxidative burst, and other nonoxidative mechanisms. (137) For an effective T helper 1 (Th1, IFN-γ producer cells) differentiation, costimulation (e.g., CD40L-CD40 and CD28-CD80/CD86 interactions) and NF-κB dependent signalling are essential. (138) IFN γ deficiency leads to a failure in granuloma formation, with subsequent infiltration of neutrophils leading to cellular necrosis. (122, 123) Bloom et al. have shown that macrophage-induced NO is the primary bactericidal mechanism of macrophages. It is established that IFN γ is an inducer of macrophage inducible NO synthase that leads to the production of NO (Figure 2). (139) IL1β is another mediator induced by mycobacterial antigens, which upregulate iNOS and subsequent NO production. NO-mediated killing by macrophages is the primary mechanism for controlling mycobacterial replication. A hypothesis can be proposed that the inhibitory effects of morphine on IFN γ, NO, and IL1β may lead to a dysregulation of this process, ultimately exerting negative impacts on granuloma formation (Figure 4; Table 2). Therefore, the suppression of INF-γ by morphine induces multiple significant negative implications on the immune defence against TB.
The role of IFN α/β on TB immunity is highly variable in contrast to the protective role of IFN-γ. Type I IFNs (IFN α/β) are potent inhibitors of IL-12 production by macrophages, which induces IFN-γ. (140) Conversely, they induce IFN-γ production by T and NK cells in an IL-12-independent way. (141) IFNα/β is shown to reduce monocyte viability. compromises their bacteriostatic activity and antigen presentation ability. (142) Type I IFNs have been used as an adjunctive therapeutic agent for PTB patients harbouring multi-drug resistant MTB strains. (143, 144)Multiple studies have reported that the induction of Type I IFNs precedes the onset of clinical tuberculosis. (145, 146) MTB inhibits the production of IFN α/β in response to TLR9 signalling. Morphine further suppresses this, producing complex effects requiring further characterisation in controlled studies (Table 2; Figure 3).
M. tuberculosis–induced TNF α production appears to be controlled via TLR2. (122) Both TNF α deficiency and excess can lead to granuloma necrosis. (147, 148) The TNF α signalling deficiency in mice produced disorganised tuberculous granulomas. (149, 150) Deficient TNF α signalling increases intra-macrophage mycobacterial load and accelerates the formation of disorganised granulomas, ultimately leading to granuloma necrosis (130, 151). Morphine has been shown to suppress TNFα levels in many studies, which may enhance the progression locally (Tables 2, 3). It may further affect disorganised secondary granuloma formation in distal organs, leading to disseminated disease.
Morphine upregulates CCR expression while downregulating CCL levels, causing a net deficiency of CCL. (Figure 2) (21) IL6 stimulates macrophage and cytotoxic T-cell differentiation. At the same time, IL10 inhibits proinflammatory cytokines, blocks the generation of ROI and NOI, blocks antigen processing and presentation in different APCs, and diminishes T-cell responses. IL12 is a crucial cytokine in developing and maintaining type 1 cellular response in MTB infection. IL12 binds to its receptor IL12R-β2 and activates the JAK-STAT pathway, inducing IFN γ to differentiate CD4+ T cells into Th1 effectors. Preclinical evidence has shown that IL-12 p40−/− deficient mice could not control bacterial growth, which appeared to be linked to the absence of both innate and acquired sources of IFN-γ. (152) This shows the central role played by IL12 in the defence against TB infection. IL23 induces IL17 production by memory T cells, creating an inflammatory response by Th17 cells, and it generates protective cellular responses. Morphine blocks the synthesis of all these vital mediators and damages the chemical coordination in the immune defence against TB. Moreover, IL12 induces inflammation by suppressing TGF ß and stimulating NK cells, contributing to increased CCL2 and CCL3 levels. (153) However, this effect may be counteracted by overall anti-inflammatory actions caused by morphine and the virulence mechanisms of mycobacterial antigens. (Table 3, Figure 4).
Chronic morphine administration causes suppression of multiple protective immune pathways vital in the defence against MTB. Multiple cellular receptors in immune cells, including OR, TLR2, and NLR 2, play critical roles in immunosuppression via complex intracellular cross-talk. Various cell types and their mediators involved in granuloma formation are inhibited by morphine via multiple mechanisms. This leads to a state of immunodeficiency that likely contributes to the reactivation, progression, and dissemination of MTB. Further studies are required to characterise potential therapeutic immunomodulatory targets in chronic opioid users at risk of infection with/reactivation of MTB.
In vitro and in vivo preclinical studies assessing the immunomodulatory properties of opioids have been mostly limited to the testing of morphine. Considering the wide structural diversity and functional variation of opioids, direct testing of other categories of opioids and their antagonists is needed to delineate further the postulated mechanisms of the immunopathogenesis of TB potentiated by chronic opioid use. The duration and dosage of morphine use in patients may vary widely, with the added effect of the landscape of genetic heterogeneity across cultures, the impact of this review may be biased towards findings reported from the majority of studies originating from the Western, further studies are desperately needed from third-world nations investigating this phenomenon associating opioid use and the predisposition to TB. Chronic opioid use is associated with confounding factors, socioeconomic deprivation, malnutrition and infections associated with IVDU which may contribute to immune suppression.
The original contributions presented in the study are included in the article/Supplementary Material. Further inquiries can be directed to the corresponding author.
VB: Conceptualization, Data curation, Methodology, Software, Visualization, Writing – original draft. HS: Data curation, Formal Analysis, Writing – review & editing. NR: Supervision, Writing – review & editing. LD: Supervision, Writing – review & editing.
The author(s) declare that no financial support was received for the research, authorship, and/or publication of this article.
I acknowledge the guidance and encouragement from Professor Anoop Chauhan and my colleagues from the Research Department, Portsmouth University Hospital, UK. Professor Francis Drobniewski (Professor of Global Health and Tuberculosis Infectious Diseases and Immunity) and Dr Brian Robertson from the Imperial College London, UK, provided valuable feedback.
The authors declare that the research was conducted in the absence of any commercial or financial relationships that could be construed as a potential conflict of interest.
All claims expressed in this article are solely those of the authors and do not necessarily represent those of their affiliated organizations, or those of the publisher, the editors and the reviewers. Any product that may be evaluated in this article, or claim that may be made by its manufacturer, is not guaranteed or endorsed by the publisher.
The Supplementary Material for this article can be found online at: https://www.frontiersin.org/articles/10.3389/fimmu.2023.1265511/full#supplementary-material
APC, Antigen presenting cell; AraLAM, Abinofuranosyl-capped lipoarabinomannan; BCG, Bacillus Calmette–Guérin; CTLA-4, Cytotoxic T lymphocyte-associated antigen-4; DNA, Deoxyribonucleic acid; ESX-1, ESAT-6 secretion system 1; HIV, Human immunodeficiency virus; HMGB1, High mobility group box 1; JAK-STAT, Janus kinase/signal transducers and activators of transcription; ManLAM, Mannosylated lipoarabinomannan; MAPK, Mitogen-activated protein kinase; NADPH, Nicotinamide adenine dinucleotide phosphate; NOI, Negative oxygen Ion; PAMP, Pathogen associated molecular patterns; PD-1, Programmed cell death protein 1; PDIM, Phthiocerol dimycocerosate; ROI, Reactive oxygen intermediates; TGF, Transferrin growth factor; TRAF6, Tumour Necrosis Factor Receptor Associated Factor 6; WHO, World Health Organization.
1. Global Tuberculosis Report 2021. Available at: https://www.who.int/teams/global-tuberculosis-programme/tb-reports/global-tuberculosis-report-2021 (Accessed November 13, 2021).
2. Castañeda-Hernández DM, Rodriguez-Morales AJ. Epidemiological burden of tuberculosis in developing countries. Curr Topics Public Health (2013). doi: 10.5772/53363
3. Pescarini JM, Rodrigues LC, Gomes MGM, Waldman EA. Migration to middle-income countries and tuberculosis-global policies for global economies. Global Health (2017) 13. doi: 10.1186/S12992-017-0236-6
4. Hayward S, Harding RM, McShane H, Tanner R. Factors influencing the higher incidence of tuberculosis among migrants and ethnic minorities in the UK. F1000Res (2018) 7:461. doi: 10.12688/F1000RESEARCH.14476.2
5. Hannah HA, Miramontes R, Gandhi NR. Sociodemographic and clinical risk factors associated with tuberculosis mortality in the United States, 2009-2013. Public Health Rep (2017) 132:366. doi: 10.1177/0033354917698117
6. Durante AJ, Selwyn PA, O’Connor PG. Risk factors for and knowledge of Mycobacterium tuberculosis infection among drug users in substance abuse treatment. Addict (Abingdon England) (1998) 93:1393–401. doi: 10.1046/J.1360-0443.1998.939139310.X
7. Nguipdop-Djomo P, Rodrigues LC, Smith PG, Abubakar I, Mangtani P. Drug misuse, tobacco smoking, alcohol and other social determinants of tuberculosis in UK-born adults in England: a community-based case-control study. Sci Rep (2020) 10:5639. doi: 10.1038/s41598-020-62667-8
8. Story A, Murad S, Roberts W, Verheyen M, Hayward AC. Tuberculosis in London: The importance of homelessness. problem Drug Use prison Thorax (2007) 62:667–671. doi: 10.1136/thx.2006.065409
9. Quaglio G, Lugoboni F, Talamini G, Lechi A, Mezzelani P. Prevalence of tuberculosis infection and comparison of multiple-puncture liquid tuberculin test and Mantoux test among drug users. Scand J Infect Dis (2002) 34:574–6. doi: 10.1080/00365540110080791
10. Rubinstien EM, Madden GM, Lyons RW. Active tuberculosis in HIV-infected injecting drug users from a low-rate tuberculosis area. J Acquir Immune Defic Syndr Hum Retrovirol (1996) 11:448–54. doi: 10.1097/00042560-199604150-00004
11. Deiss RG, Rodwell TC, Garfein RS. Tuberculosis and illicit drug use: Review and update. Clin Infect Dis (2009) 48:72–82. doi: 10.1086/594126
12. Aceijas C, Stimson GV, Hickman M, Rhodes T. Global overview of injecting drug use and HIV infection among injecting drug users. AIDS (2004) 18:2295–303. doi: 10.1097/00002030-200411190-00010
13. UNODC World Drug Report 2021: pandemic effects ramp up drug risks, as youth underestimate cannabis dangers. Available at: https://www.unodc.org/unodc/press/releases/2021/June/unodc-world-drug-report-2021_-pandemic-effects-ramp-up-drug-risks–as-youth-underestimate-cannabis-dangers.html (Accessed November 13, 2021).
14. Ranzani OT, Rodrigues LC, Bombarda S, Minto CM, Waldman EA, Carvalho CRR. Long-term survival and cause-specific mortality of patients newly diagnosed with tuberculosis in São Paulo state, Brazil, 2010–15: a population-based, longitudinal study. Lancet Infect Dis (2020) 20:123–132. doi: 10.1016/S1473-3099(19)30518-3
15. Kamenska N, Nabirova D, Davtyan K, Davtyan H, Zachariah R, Aslanyan G. Strategies for active detection of tuberculosis in Ukraine: Comparative effectiveness amongst key populations (2014-2018). J Infect Dev Ctries (2019) 13:89S–94S. doi: 10.3855/jidc.11294
16. Dolan K, Wirtz AL, Moazen B, Ndeffo-mbah M, Galvani A, Kinner SA, et al. Global burden of HIV, viral hepatitis, and tuberculosis in prisoners and detainees. Lancet (2016) 388:1089–1102. doi: 10.1016/S0140-6736(16)30466-4
17. Nagot N, Hai VV, Dong TTT, Hai OKT, Rapoud D, Hoang GT, et al. Alarming tuberculosis rate among people who inject drugs in Vietnam. Open Forum Infect Dis (2022) 9:ofab548. doi: 10.1093/ofid/ofab548
18. Bernier A, Perrineau S, Reques L, Kouamé A, N’Guessan R, N’Zi L, et al. Prevalence and management of tuberculosis among people who use drugs in Abidjan, Ivory Coast. Int J Drug Policy (2020) 83:102862. doi: 10.1016/j.drugpo.2020.102862
19. Minja LT, Hella J, Mbwambo J, Nyandindi C, Omary US, Levira F, et al. High burden of tuberculosis infection and disease among people receiving medication-assisted treatment for substance use disorder in Tanzania. PloS One (2021) 16:e0250038. doi: 10.1371/journal.pone.0250038
20. Chen L, Shi W, Li H, Sun X, Fan X, LeSage G, et al. Critical role of toll-like receptor 9 in morphine and mycobacterium tuberculosis–induced apoptosis in mice. PloS One (2010) 5:e9205. doi: 10.1371/JOURNAL.PONE.0009205
21. Eisenstein TK. The role of opioid receptors in immune system function. Front Immunol (2019) 10:2904/BIBTEX. doi: 10.3389/FIMMU.2019.02904/BIBTEX
22. Khosrow-Khavar F, Kurteva S, Cui Y, Filion KB, Douros A. Opioids and the risk of infection: A critical appraisal of the pharmacologic and clinical evidence. Expert Opin Drug Metab Toxicol (2019) 15:565–75. doi: 10.1080/17425255.2019.1634053
24. McDonald J, Lambert DG. Opioid receptors. Continuing Educ Anaesthesia Crit Care Pain (2005) 5:22–5. doi: 10.1093/BJACEACCP/MKI004
25. Brejchova J, Holan V, Svoboda P. Expression of opioid receptors in cells of the immune system. Int J Mol Sci (2021) 22:1–13. doi: 10.3390/IJMS22010315
26. Vallejo R, de Leon-Casasola O, Benyamin R. Opioid therapy and immunosuppression: a review. Am J Ther (2004) 11:354–65. doi: 10.1097/01.MJT.0000132250.95650.85
27. Ninković J, Roy S. Role of the mu-opioid receptor in opioid modulation of immune function. Amino Acids (2013) 45:9–24. doi: 10.1007/s00726-011-1163-0
28. Rogers TJ. Kappa opioid receptor expression and function in cells of the immune system. Handb Exp Pharmacol (2022) 271:419–433. doi: 10.1007/164_2021_441
29. Nagata K, Nagase H, Okuzumi A, Nishiyama C. Delta opioid receptor agonists ameliorate colonic inflammation by modulating immune responses. Front Immunol (2021) 12:730706/BIBTEX. doi: 10.3389/FIMMU.2021.730706/BIBTEX
30. Esch T, Kream RM, Stefano GB. Emerging regulatory roles of opioid peptides, endogenous morphine, and opioid receptor subtypes in immunomodulatory processes: Metabolic, behavioral, and evolutionary perspectives. Immunol Lett (2020) 227:28–33. doi: 10.1016/J.IMLET.2020.08.007
31. Rivat C, Sebaihi S, van Steenwinckel J, Fouquet S, Kitabgi P, Pohl M, et al. Src family kinases involved in CXCL12-induced loss of acute morphine analgesia. Brain Behav Immun (2014) 38:38–52. doi: 10.1016/J.BBI.2013.11.010
32. Makman MH, Bilfinger T v, Stefano GB. Human granulocytes contain an opiate alkaloid-selective receptor mediating inhibition of cytokine-induced activation and chemotaxis. J Immunol (1995) 154:e0250038.
33. Barnes PJ. Receptor heterodimerization: a new level of cross-talk. J Clin Invest (2006) 116:1210–2. doi: 10.1172/JCI28535
34. Rogers TJ. Bidirectional regulation of opioid and chemokine function. Front Immunol (2020) 11:94. doi: 10.3389/FIMMU.2020.00094
35. Huang B, Wang H, Zheng Y, Li M, Kang G, Barreto-De-Souza V, et al. Structure-based design and development of chemical probes targeting putative MOR-CCR5 heterodimers to inhibit opioid exacerbated HIV-1 infectivity. J Med Chem (2021) 64:7702–23. doi: 10.1021/ACS.JMEDCHEM.1C00408/SUPPL_FILE/JM1C00408_SI_002.PDF
36. Shavit Y, Depaulis A, Martin FC, Terman GW, Pechnick RN, Zane CJ, et al. Involvement of brain opiate receptors in the immune-suppressive effect of morphine. Proc Natl Acad Sci U.S.A. (1986) 83:7114–7. doi: 10.1073/PNAS.83.18.7114
37. Shavit Y, Terman GW, Lewis JW, Zane CJ, Gale RP, Liebeskind JC. Effects of footshock stress and morphine on natural killer lymphocytes in rats: studies of tolerance and cross-tolerance. Brain Res (1986) 372:382–5. doi: 10.1016/0006-8993(86)91149-2
38. Shavit Y, Martin FC, Yirmiya R, Ben-Eliyahu S, Terman GW, Weiner H, et al. Effects of a single administration of morphine or footshock stress on natural killer cell cytotoxicity. Brain Behav Immun (1987) 1:318–28. doi: 10.1016/0889-1591(87)90034-1
39. Carr DJ, Gerak LR, France CP. Naltrexone antagonizes the analgesic and immunosuppressive effects of morphine in mice. J Pharmacol Exp Ther (1994) 269:693–698.
40. Saurer TB, Carrigan KA, Ijames SG, Lysle DT. Suppression of natural killer cell activity by morphine is mediated by the nucleus accumbens shell. J Neuroimmunol (2006) 173:3–11. doi: 10.1016/J.JNEUROIM.2005.11.009
41. Saurer TB, Ijames SG, Lysle DT. Neuropeptide Y Y1 receptors mediate morphine-induced reductions of natural killer cell activity. J Neuroimmunol (2006) 177:18–26. doi: 10.1016/J.JNEUROIM.2006.05.002
42. Evidence for sympathetic and adrenal involvement in the immunomodulatory effects of acute morphine treatment in rats. | Journal of Pharmacology and Experimental Therapeutics. Available at: https://jpet.aspetjournals.org/content/277/2/633.long (Accessed November 15, 2021).
43. Freier DO, Fuchs BA. A mechanism of action for morphine-induced immunosuppression: corticosterone mediates morphine-induced suppression of natural killer cell activity. J Pharmacol Exp Ther (1994) 270:1127–1133.
44. Weber RJ, Pert A. The periaqueductal gray matter mediates opiate-induced immunosuppression. Science (1989) 245:188–90. doi: 10.1126/SCIENCE.2749256
45. Suo JL, Weber RJ. Immunomodulation mediated by microinjection of morphine into the periaqueductal gray matter of the mesencephalon. Adv Exp Med Biol (1998) 437:177–82. doi: 10.1007/978-1-4615-5347-2_19
46. Yokota T, Uehara K, Nomoto Y. Addition of noradrenaline to intrathecal morphine augments the postoperative suppression of natural killer cell activity. J Anesth (2004) 18:190–5. doi: 10.1007/S00540-004-0247-3
47. Börner C, Stumm R, Höllt V, Kraus J. Comparative analysis of mu-opioid receptor expression in immune and neuronal cells. J Neuroimmunol (2007) 188:56–63. doi: 10.1016/J.JNEUROIM.2007.05.007
48. Maher DP, Walia D, Heller NM. Suppression of human natural killer cells by different classes of opioids. Anesth Analg (2019) 128:1013–21. doi: 10.1213/ANE.0000000000004058
49. Wang J, Ma J, Charboneau R, Barke R, Roy S. Morphine inhibits murine dendritic cell IL-23 production by modulating Toll-like receptor 2 and Nod2 signaling. J Biol Chem (2011) 286:10225–32. doi: 10.1074/JBC.M110.188680
50. Bryant HU, Bernton EW, Holaday JW. Immunosuppressive effects of chronic morphine treatment in mice. Life Sci (1987) 41:1731–8. doi: 10.1016/0024-3205(87)90601-1
51. Bryant HU, Bernton EW, Holaday JW. Morphine pellet-induced immunomodulation in mice: temporal relationships. J Pharmacol Exp Ther (1988) 245:913–920.
52. Arora PK, Fride E, Petitto J, Waggie K, Skolnick P. Morphine-induced immune alterations in vivo. Cell Immunol (1990) 126:343–53. doi: 10.1016/0008-8749(90)90326-M
53. Carr DJJ, France CP. Immune alterations in chronic morphine-treated rhesus monkeys. Adv Exp Med Biol (1993) 335:35–9. doi: 10.1007/978-1-4615-2980-4_6
54. Zhang EY, Xiong J, Parker BL, Chen AY, Fields PE, Ma X, et al. Depletion and recovery of lymphoid subsets following morphine administration. Br J Pharmacol (2011) 164:1829. doi: 10.1111/J.1476-5381.2011.01475.X
55. Sacerdote P, Manfredi B, Mantegazza P, Panerai AE. Antinociceptive and immunosuppressive effects of opiate drugs: a structure-related activity study. Br J Pharmacol (1997) 121:834–40. doi: 10.1038/SJ.BJP.0701138
56. Yin D, Mufson RA, Wang R, Shi Y. Fas-mediated cell death promoted by opioids. Nature (1999) 397:218–8. doi: 10.1038/16612
57. Carr DJJ, Carpenter GW, Garza HH, France CP, Prakash O. Chronic & infrequent opioid exposure suppresses IL-2r expression on rhesus monkey peripheral blood mononuclear cells following stimulation with pokeweed mttogen. Int J Neurosci (1995) 81:137–48. doi: 10.3109/00207459509015305
58. Nair MPN, Schwartz SA, Polasani R, Hou J, Sweet A, Chadha KC. Immunoregulatory effects of morphine on human lymphocytes. Clin Diagn Lab Immunol (1997) 4:127–32. doi: 10.1128/CDLI.4.2.127-132.1997
59. Singhal PC, Sharma P, Kapasi AA, Reddy K, Franki N, Gibbons N. Morphine enhances macrophage apoptosis. J Immunol (1998) 160:1886–93.
60. Roy S, Balasubramanian S, Sumandeep S, Charboneau R, Wang J, Melnyk D, et al. Morphine directs T cells toward T(H2) differentiation. Surgery (2001) 130:304–9. doi: 10.1067/MSY.2001.116033
61. Grimm MC, Ben-Baruch A, Taub DD, Howard OMZ, Wang JM, Oppenheim JJ. Opiate inhibition of chemokine-induced chemotaxis. Ann N Y Acad Sci (1998) 840:9–20. doi: 10.1111/J.1749-6632.1998.TB09544.X
62. Casellas AM, Guardiola H, Renaud FL. Inhibition by opioids of phagocytosis in peritoneal macrophages. Neuropeptides (1991) 18:35–40. doi: 10.1016/0143-4179(91)90161-B
63. Szabo I, Rojavin M, Bussiere JL, Eisenstein TK, Adler MW, Rogers TJ. Suppression of peritoneal macrophage phagocytosis of Candida albicans by opioids. J Pharmacol Exp Ther (1993) 267.
64. Tubaro E, Avico U, Santiangeli C, Zuccaro P, Cavallo G, Pacifici R, et al. Morphine and methadone impact on human phagocytic physiology. Int J Immunopharmacol (1985) 7:865–74. doi: 10.1016/0192-0561(85)90049-9
65. Rojavin M, Szabo I, Bussiere JL, Rogers TJ, Adler MW, Eisenstein TK. Morphine treatment in vitro or in vivo decreases phagocytic functions of murine macrophages. Life Sci (1993) 53:997–1006. doi: 10.1016/0024-3205(93)90122-J
66. Wang J, Barke RA, Charboneau R, Roy S. Morphine impairs host innate immune response and increases susceptibility to Streptococcus pneumoniae lung infection. J Immunol (2005) 174:426–34. doi: 10.4049/JIMMUNOL.174.1.426
67. Welters ID, Menzebach A, Goumon Y, Langefeld TW, Teschemacher H, Hempelmann G, et al. Morphine suppresses complement receptor expression, phagocytosis, and respiratory burst in neutrophils by a nitric oxide and μ3 opiate receptor-dependent mechanism. J Neuroimmunol (2000) 111:139–45. doi: 10.1016/S0165-5728(00)00401-X
68. Menzebach A, Hirsch J, Nöst R, Mogk M, Hempelmann G, Welters ID. [Morphine inhibits complement receptor expression, phagocytosis and oxidative burst by a nitric oxide dependent mechanism]. Anasthesiol Intensivmed Notfallmed Schmerzther (2004) 39:204–11. doi: 10.1055/S-2004-814389
69. Roy S, Ramakrishnan S, Loh HH, Lee NM. Chronic morphine treatment selectively suppresses macrophage colony formation in bone marrow. Eur J Pharmacol (1991) 195:359–63. doi: 10.1016/0014-2999(91)90476-7
70. Ni X, Gritman KR, Eisenstein TK, Adler MW, Arfors KE, Tuma RF. Morphine attenuates leukocyte/endothelial interactions. Microvasc Res (2000) 60:121–30. doi: 10.1006/MVRE.2000.2253
71. Bryant HU, Bernton EW, Kenner JR, Holaday JW, Bryant HU. Role of adrenal cortical activation in the immunosuppressive effects of chronic morphine treatment. Endocrinology (1991) 128:3253–8. doi: 10.1210/ENDO-128-6-3253
72. Thomas PT, Bhargava HN, House R v.. Immunomodulatory effects of in vitro exposure to morphine and its metabolites. Pharmacology (1995) 50:51–62. doi: 10.1159/000139266
73. Sei Y, McIntyre T, Fride E, Yoshimoto K, Skolnick P, Arora PK. Inhibition of calcium mobilization is an early event in opiate-induced immunosuppression. FASEB J (1991) 5:2194–9. doi: 10.1096/fasebj.5.8.2022315
74. Bayer BM, Daussin S, Hernandez M, Irvin L. Morphine inhibition of lymphocyte activity is mediated by an opioid dependent mechanism. Neuropharmacology (1990) 29:369–74. doi: 10.1016/0028-3908(90)90096-A
75. Hernandez MC, Flores LR, Bayer BM. Immunosuppression by morphine is mediated by central pathways. J Pharmacol Exp Ther (1993) 267.
76. Flores LR, Hernandez MC, Bayer BM. Acute immunosuppressive effects of morphine: lack of involvement of pituitary and adrenal factors. J Pharmacol Exp Ther (1994) 268.
77. Sharp BM, Keane WF, Suh HJ, Gekker G, Tsukayama D, Peterson PK. Opioid peptides rapidly stimulate superoxide production by human polymorphonuclear leukocytes and macrophages. Endocrinology (1985) 117:793–5. doi: 10.1210/ENDO-117-2-793
78. Bussiere JL, Adler MW, Rogers TJ, Eisenstein TK. Cytokine reversal of morphine-induced suppression of the antibody response. J Pharmacol Exp Ther (1993) 264.
79. Rogers TJ, Peterson PK. Opioid G protein-coupled receptors: signals at the crossroads of inflammation. Trends Immunol (2003) 24:116–21. doi: 10.1016/S1471-4906(03)00003-6
80. Khabbazi S, Goumon Y, Parat MO. Morphine Modulates Interleukin-4- or Breast Cancer Cell-induced Pro-metastatic Activation of Macrophages. Sci Rep 2015 5:1 (2015) 5:1–12. doi: 10.1038/srep11389
81. Merighi S, Gessi S, Varani K, Fazzi D, Stefanelli A, Borea PA. Morphine mediates a proinflammatory phenotype via μ-opioid receptor-PKCε-Akt-ERK1/2 signaling pathway in activated microglial cells. Biochem Pharmacol (2013) 86:487–96. doi: 10.1016/J.BCP.2013.05.027
82. Gessi S, Borea PA, Bencivenni S, Fazzi D, Varani K, Merighi S. The activation of μ-opioid receptor potentiates LPS-induced NF-kB promoting an inflammatory phenotype in microglia. FEBS Lett (2016) 590:2813–26. doi: 10.1002/1873-3468.12313
83. Limiroli E, Gaspani L, Panerai AE, Sacerdote P. Differential morphine tolerance development in the modulation of macrophage cytokine production in mice. J Leukoc Biol (2002) 72:43–8. doi: 10.1189/JLB.72.1.43
84. Martucci C, Franchi S, Lattuada D, Panerai AE, Sacerdote P. Differential involvement of RelB in morphine-induced modulation of chemotaxis, NO, and cytokine production in murine macrophages and lymphocytes. J Leukoc Biol (2007) 81:344–54. doi: 10.1189/JLB.0406237
85. Roy S, Cain KJ, Chapin RB, Charboneau RG, Barke RA. Morphine modulates NF kappa B activation in macrophages. Biochem Biophys Res Commun (1998) 245:392–6. doi: 10.1006/BBRC.1998.8415
86. Morphine enhances interleukin-12 and the production of other pro-inflammatory cytokines in mouse peritoneal macrophages . Available at: https://pubmed.ncbi.nlm.nih.gov/11073113/ (Accessed January 15, 2022).
87. Roy S, Cain KJ, Chapin RB, Charboneau RG, Barke RA. Morphine Modulates NFκB Activation in Macrophages. Biochem Biophys Res Commun (1998) 245:392–6. doi: 10.1006/BBRC.1998.8415
88. Peterson PK, Sharp B, Gekker G, Brummitt C, Keane WF. Opioid-mediated suppression of interferon-γ production by cultured peripheral blood mononuclear cells. J Clin Invest (1987) 80:824–31. doi: 10.1172/JCI113140
89. Lysle DT, Coussons ME, Watts VJ, Bennett EH, Dykstra LA. Morphine-induced alterations of immune status: dose dependency, compartment specificity and antagonism by naltrexone. J Pharmacol Exp Ther (1993) 265.
90. Happel C, Kutzler M, Rogers TJ. Opioid-induced chemokine expression requires NF-κB activity: the role of PKCζ. J Leukoc Biol (2011) 89:301–9. doi: 10.1189/JLB.0710402
91. Happel C, Steele AD, Finley MJ, Kutzler MA, Rogers TJ. DAMGO-induced expression of chemokines and chemokine receptors: the role of TGF-β1. J Leukoc Biol (2008) 83:956–63. doi: 10.1189/JLB.1007685
92. Jing M, Wang J, Wan J, Charboneau R, Chang Y, Barke RA, et al. Morphine disrupts interleukin-23 (IL-23)/IL-17-mediated pulmonary mucosal host defense against Streptococcus pneumoniae infection. Infect Immun (2010) 78:830–7. doi: 10.1128/IAI.00914-09
93. Jessop JJ, Taplits MS. Effect of high doses of morphine on Con-A induced lymphokine production in vitro. Immunopharmacology (1991) 22:175–84. doi: 10.1016/0162-3109(91)90042-W
94. Philippe D, Dubuquoy L, Groux H, Brun V, van Chuoï-Mariot MT, Gaveriaux-Ruff C, et al. Anti-inflammatory properties of the μ opioid receptor support its use in the treatment of colon inflammation. J Clin Invest (2003) 111:1329–38. doi: 10.1172/JCI16750
95. Nyssen P, Mouithys-Mickalad A, Minguet G, Sauvage E, Wouters J, Franck T, et al. Morphine, a potential inhibitor of myeloperoxidase activity. Biochim Biophys Acta Gen Subj (2018) 1862:2236–44. doi: 10.1016/J.BBAGEN.2018.07.007
96. Mahajan SD, Schwartz SA, Aalinkeel R, Chawda RP, Sykes DE, Nair MPN. Morphine modulates chemokine gene regulation in normal human astrocytes. Clin Immunol (2005) 115:323–32. doi: 10.1016/J.CLIM.2005.02.004
97. El-Hage N, Gurwell JA, Singh IN, Knapp PE, Nath A, Hauser KF. Synergistic increases in intracellular Ca2+, and the release of MCP-1, RANTES, and IL-6 by astrocytes treated with opiates and HIV-1 Tat. Glia (2005) 50:91–106. doi: 10.1002/GLIA.20148
98. Davis RL, Das S, Thomas Curtis J, Stevens CW. The opioid antagonist, β-funaltrexamine, inhibits NF-κB signaling and chemokine expression in human astrocytes and in mice. Eur J Pharmacol (2015) 762:193. doi: 10.1016/J.EJPHAR.2015.05.040
99. Neudeck BL, Loeb J, Buck J. Activation of the κ-opioid receptor in Caco-2 cells decreases interleukin-8 secretion. Eur J Pharmacol (2003) 467:81–4. doi: 10.1016/S0014-2999(03)01633-9
100. Neudeck BL, Loeb JM. Endomorphin-1 alters interleukin-8 secretion in Caco-2 cells via a receptor mediated process. Immunol Lett (2002) 84:217–21. doi: 10.1016/S0165-2478(02)00198-0
101. Faridgohar M, Nikoueinejad H. New findings of Toll-like receptors involved in Mycobacterium tuberculosis infection. Pathog Glob Health (2017) 111:256. doi: 10.1080/20477724.2017.1351080
102. Shah M, Choi S. Toll-like Receptor-Dependent Negative Effects of Opioids: A Battle between Analgesia and Hyperalgesia. Front Immunol (2017) 8:642. doi: 10.3389/FIMMU.2017.00642
103. Cavallone LF, Montana MC. Pain, the immune system and opioids are cross-talking: are we just listening in, or can we shape the conversation? Minerva Anestesiol (2021) 87:150–2. doi: 10.23736/S0375-9393.20.15384-7
104. Gabr MM, Saeed I, Miles JA, Ross BP, Shaw PN, Hollmann MW, et al. Interaction of Opioids with TLR4—Mechanisms and Ramifications. Cancers (Basel) (2021) 13. doi: 10.3390/CANCERS13215274
105. Liao Y, Jiang J, Liang B, Wei F, Huang J, Pan P, et al. Opiate use inhibits TLR9 signaling pathway in vivo: possible role in pathogenesis of HIV-1 infection. Sci Rep 2017 7:1 (2017) 7:1–13. doi: 10.1038/s41598-017-12066-3
106. Yoshimura DT, Golenbock MJ, Fenton TK, Means S, Wang E, Lien A. Mycobacterium tuberculosis Activation by Human Toll-Like Receptors Mediate Cellular(1999). Available at: http://www.jimmunol.org/content/163/7/http://www.jimmunol.org/content/163/7/3920.full#ref-list-1 (Accessed December 19, 2021).
107. Prinzis S, Chatterjee D, Brennan PJ. Structure and antigenicity of lipoarabinomannan from Mycobacterium bovis BCG. J Gen Microbiol (1993) 139:2649–58. doi: 10.1099/00221287-139-11-2649
108. Savedra R, Delude R1, Ingalls RR, Fenton MJ, Golenblock DT. Mycobacterial lipoarabinomannan recognition requires a receptor that shares components of the endotoxin signaling system. J Immunol (1996) 157:2549–54. doi: 10.4049/JIMMUNOL.157.6.2549
109. Strohmeier GR, Fenton MJ. Roles of lipoarabinomannan in the pathogenesis of tuberculosis. Microbes Infect (1999) 1:709–17. doi: 10.1016/S1286-4579(99)80072-0
110. Vercellone A, Nigou J, Puzo G. Relationships between the structure and the roles of lipoarabinomannans and related glycoconjugates in tuberculosis pathogenesis. Front Biosci (1998) 3. doi: 10.2741/a372
111. Chatterjee D, Roberts AD, Lowell K, Brennan PJ, Orme IM. Structural basis of capacity of lipoarabinomannan to induce secretion of tumor necrosis factor. Infect Immun (1992) 60:1249–53. doi: 10.1128/IAI.60.3.1249-1253.1992
112. Roach TI, Barton CH, Chatterjee D, Blackwell JM. Macrophage activation: lipoarabinomannan from avirulent and virulent strains of Mycobacterium tuberculosis differentially induces the early genes c-fosand tumor necrosis factor-alpha(1993). Available at: https://europepmc.org/article/MED/8436823 (Accessed December 25, 2022).
113. Hölscher C, Reiling N, Schaible UE, Hölscher A, Bathmann C, Korbel D, et al. Containment of aerogenic Mycobacterium tuberculosis infection in mice does not require MyD88 adaptor function for TLR2, -4 and -9. Eur J Immunol (2008) 38:680–94. doi: 10.1002/EJI.200736458
114. Liu CM, Yang TH, Huang M, Zhou C, Li YH, Li ZH. [Effect of Endomorphin-1 on Maturation and Expression of TLR4 in Peripheral Blood Dendritic Cells Induced by High Glucose]. Zhongguo Shi Yan Xue Ye Xue Za Zhi (2018) 26:886–93. doi: 10.7534/J.ISSN.1009-2137.2018.03.043
115. Zhang P, Yang M, Chen C, Liu L, Wei X, Zeng S. Toll-Like Receptor 4 (TLR4)/Opioid Receptor Pathway Crosstalk and Impact on Opioid Analgesia, Immune Function, and Gastrointestinal Motility. Front Immunol (2020) 11:1455/BIBTEX. doi: 10.3389/FIMMU.2020.01455/BIBTEX
116. Carli M, Donnini S, Pellegrini C, Coppi E, Bocci G. Opioid receptors beyond pain control: The role in cancer pathology and the debated importance of their pharmacological modulation. Pharmacol Res (2020) 159. doi: 10.1016/j.phrs.2020.104938
117. Ellis A, Grace PM, Wieseler J, Favret J, Springer K, Skarda B, et al. Morphine amplifies mechanical allodynia via TLR4 in a rat model of spinal cord injury. Brain Behav Immun (2016) 58:348–56. doi: 10.1016/J.BBI.2016.08.004
118. Iwasaki A, Medzhitov R. Toll-like receptor control of the adaptive immune responses. Nat Immunol 2004 5:10 (2004) 5:987–95. doi: 10.1038/ni1112
119. Rehli M. Of mice and men: species variations of Toll-like receptor expression. Trends Immunol (2002) 23:375–8. doi: 10.1016/S1471-4906(02)02259-7
120. An H, Xu H, Yu Y, Zhang M, Qi R, Yan X, et al. Up-regulation of TLR9 gene expression by LPS in mouse macrophages via activation of NF-kappaB, ERK and p38 MAPK signal pathways. Immunol Lett (2002) 81:165–9. doi: 10.1016/S0165-2478(02)00010-X
121. Dunne A, O’Neill LAJ. Adaptor usage and Toll-like receptor signaling specificity. FEBS Lett (2005) 579:3330–5. doi: 10.1016/J.FEBSLET.2005.04.024
122. Bafica A, Scanga CA, Feng CG, Leifer C, Cheever A, Sher A. TLR9 regulates Th1 responses and cooperates with TLR2 in mediating optimal resistance to Mycobacterium tuberculosis. J Exp Med (2005) 202:1715. doi: 10.1084/JEM.20051782
123. Pagán AJ, Ramakrishnan L. Immunity and Immunopathology in the Tuberculous Granuloma. Cold Spring Harb Perspect Med (2015) 5. doi: 10.1101/CSHPERSPECT.A018499
124. Chirakos AE, Balaram A, Conrad W, Champion PA. Modeling Tubercular ESX-1 Secretion Using Mycobacterium marinum. Microbiol Mol Biol Rev (2020) 84. doi: 10.1128/MMBR.00082-19
125. Elkington PT, Green JA, Emerson JE, Lopez-Pascua LD, Boyle JJ, et al. Synergistic up-regulation of epithelial cell matrix metalloproteinase-9 secretion in tuberculosis. Am J Respir Cell Mol Biol (2007) 37:431–7. doi: 10.1165/RCMB.2007-0011OC
126. Parks WC, Wilson CL, López-Boado YS. Matrix metalloproteinases as modulators of inflammation and innate immunity. Nat Rev Immunol 2004 4:8 (2004) 4:617–29. doi: 10.1038/nri1418
127. Greenlee KJ, Corry DB, Engler DA, Matsunami RK, Tessier P, Cook RG, et al. Proteomic Identification of In Vivo Substrates for Matrix Metalloproteinases 2 and 9 Reveals a Mechanism for Resolution of Inflammation. J Immunol (2006) 177:7312. doi: 10.4049/JIMMUNOL.177.10.7312
128. Soehnlein O, Lindbom L. Phagocyte partnership during the onset and resolution of inflammation. Nat Rev Immunol 2010 10:6 (2010) 10:427–39. doi: 10.1038/nri2779
129. Scott HM, Flynn JAL. Mycobacterium tuberculosis in chemokine receptor 2-deficient mice: influence of dose on disease progression. Infect Immun (2002) 70:5946–54. doi: 10.1128/IAI.70.11.5946-5954.2002
130. Volkman HE, Pozos TC, Zheng J, Davis JM, Rawls JF, Ramakrishnan L. Tuberculous granuloma induction via interaction of a bacterial secreted protein with host epithelium. Science (2010) 327:466–9. doi: 10.1126/SCIENCE.1179663
131. Activation of Mu Opioid Receptors Inhibits Microglial Cell Chemotaxis. Available at: https://jpet.aspetjournals.org/content/281/2/998 (Accessed January 25, 2023).
132. Sweany HC. The tubercle bacillus in the pulmonary lesion of man: histobacteriology and its bearing on the therapy of pulmonary tuberculosis. Dis Chest (1955) 28:699–701. doi: 10.1016/S0096-0217(15)32386-4
133. Barber DL, Mayer-Barber KD, Feng CG, Sharpe AH, Sher A. CD4 T cells promote rather than control tuberculosis in the absence of PD-1-mediated inhibition. J Immunol (2011) 186:1598–607. doi: 10.4049/JIMMUNOL.1003304
134. Lázár-Molnár E, Chen B, Sweeney KA, Wang EJ, Liu W, Lin J, et al. Programmed death-1 (PD-1)-deficient mice are extraordinarily sensitive to tuberculosis. Proc Natl Acad Sci U.S.A. (2010) 107:13402–7. doi: 10.1073/PNAS.1007394107
135. Mazahery C, Benson BL, Cruz-Lebrón A, Levine AD. Chronic methadone use alters the cd8 + t cell phenotype in vivo and modulates its responsiveness ex vivo to opioid receptor and tcr stimuli. J Immunol (2020) 204:1188–200. doi: 10.4049/JIMMUNOL.1900862/-/DCSUPPLEMENTAL
136. Fratazzi C, Arbeit RD, Carini C, Remold HG. Programmed cell death of Mycobacterium avium serovar 4-infected human macrophages prevents the mycobacteria from spreading and induces mycobacterial growth inhibition by freshly added, uninfected macrophages. J Immunol (1997) 158.
137. Errante P, Frazao J, Condino-Neto A. The use of interferon-gamma therapy in chronic granulomatous disease. Recent Pat Antiinfect Drug Discovery (2008) 3. doi: 10.2174/157489108786242378
138. Alcaïs A, Abel L, Casanova JL. Human genetics of infectious diseases: Between proof of principle and paradigm. J Clin Invest (2009) 119. doi: 10.1172/JCI38111
139. Chan J, Xing Y, Magliozzo RS, Bloom BR. Killing of virulent mycobacterium tuberculosis by reactive nitrogen intermediates produced by activated murine macrophages. Available at: http://rupress.org/jem/article-pdf/175/4/1111/1102432/1111.pdf (Accessed April 5, 2023).
140. Byrnes AA, Xiaojing M, Cuomo P, Park K, Wahl L, Wolf SF, et al. Type I interferons and IL-12: Convergence and cross-regulation among mediators of cellular immunity. Eur J Immunol (2001) 31. doi: 10.1002/1521-4141(200107)31:7<2026::AID-IMMU2026>3.0.CO;2-U
141. Freudenberg MA, Merlin T, Kalis C, Chvatchko Y, Stübig H, Galanos C. Cutting edge: A murine, IL-12-independent pathway of IFN-γ induction by gram-negative bacteria based on stat4 activation by type I IFN and IL-18 signaling. J Immunol (2002) 169. doi: 10.4049/jimmunol.169.4.1665
142. Bouchonnet F, Boechat N, Bonay M, Hance AJ. Alpha/beta interferon impairs the ability of human macrophages to control growth of Mycobacterium bovis BCG. Infect Immun (2002) 70. doi: 10.1128/IAI.70.6.3020-3025.2002
143. Giosuè S, Casarini M, Alemanno L, Galluccio G, Mattia P, Pedicelli G, et al. Effects of aerosolized interferon-α in patients with pulmonary tuberculosis. Am J Respir Crit Care Med (1998) 158. doi: 10.1164/ajrccm.158.4.9803065
144. Palmero DJ, Eiguchi K, Rendo P, Castro Zorrilla L, Abbate E, González Montaner LJ. Phase II trial of recombinant interferon-α2b in patients with advanced intractable multidrug-resistant pulmonary tuberculosis: Long-term follow-up. Int J Tuberculosis Lung Dis (1999) 3.
145. Remoli ME, Giacomini E, Lutfalla G, Dondi E, Orefici G, Battistini A, et al. Selective Expression of Type I IFN Genes in Human Dendritic Cells Infected with Mycobacterium tuberculosis. J Immunol (2002) 169. doi: 10.4049/jimmunol.169.1.366
146. Travar M, Petkovic M, Verhaz A. Type I, II, and III interferons: regulating immunity to mycobacterium tuberculosis infection. Arch Immunol Ther Exp (Warsz) (2016) 64. doi: 10.1007/s00005-015-0365-7
147. Ramakrishnan L. Revisiting the role of the granuloma in tuberculosis. Nat Rev Immunol (2012) 12:352–66. doi: 10.1038/NRI3211
148. Clay H, Davis JM, Beery D, Huttenlocher A, Lyons SE, Ramakrishnan L. Dichotomous role of the macrophage in early Mycobacterium marinum infection of the zebrafish. Cell Host Microbe (2007) 2:29–39. doi: 10.1016/J.CHOM.2007.06.004
149. Flynn JAL, Goldstein MM, Chan J, Triebold KJ, Pfeffer K, Lowenstein CJ, et al. Tumor necrosis factor-alpha is required in the protective immune response against Mycobacterium tuberculosis in mice. Immunity (1995) 2:561–72. doi: 10.1016/1074-7613(95)90001-2
150. Lin PL, Myers A, Smith L, Bigbee C, Bigbee M, Fuhrman C, et al. Tumor necrosis factor neutralization results in disseminated disease in acute and latent Mycobacterium tuberculosis infection with normal granuloma structure in a cynomolgus macaque model. Arthritis Rheum (2010) 62:340–50. doi: 10.1002/ART.27271
151. Clay H, Volkman HE, Ramakrishnan L. Tumor necrosis factor signaling mediates resistance to mycobacteria by inhibiting bacterial growth and macrophage death. Immunity (2008) 29:283–94. doi: 10.1016/J.IMMUNI.2008.06.011
152. Cooper AM, Magram J, Ferrante J, Orme IM. Interleukin 12 (IL-12) is crucial to the development of protective immunity in mice intravenously infected with mycobacterium tuberculosis. J Exp Med (1997) 186. doi: 10.1084/jem.186.1.39
Keywords: immunopathogenesis of tuberculosis, morphine, opioids, opioid receptors, toll-like receptors, immunosuppression
Citation: Bataduwaarachchi VR, Hansanie SMN, Rockwood N and D'Cruz LG (2023) Immunomodulatory properties of morphine and the hypothesised role of long-term opioid use in the immunopathogenesis of tuberculosis. Front. Immunol. 14:1265511. doi: 10.3389/fimmu.2023.1265511
Received: 23 July 2023; Accepted: 25 September 2023;
Published: 23 October 2023.
Edited by:
Aleksander M. Grabiec, Jagiellonian University, PolandReviewed by:
Anu Goenka, University of Bristol, United KingdomCopyright © 2023 Bataduwaarachchi, Hansanie, Rockwood and D'Cruz. This is an open-access article distributed under the terms of the Creative Commons Attribution License (CC BY). The use, distribution or reproduction in other forums is permitted, provided the original author(s) and the copyright owner(s) are credited and that the original publication in this journal is cited, in accordance with accepted academic practice. No use, distribution or reproduction is permitted which does not comply with these terms.
*Correspondence: Vipula R. Bataduwaarachchi, dmlwdWxhQHBoYXJtLmNtYi5hYy5saw==
Disclaimer: All claims expressed in this article are solely those of the authors and do not necessarily represent those of their affiliated organizations, or those of the publisher, the editors and the reviewers. Any product that may be evaluated in this article or claim that may be made by its manufacturer is not guaranteed or endorsed by the publisher.
Research integrity at Frontiers
Learn more about the work of our research integrity team to safeguard the quality of each article we publish.