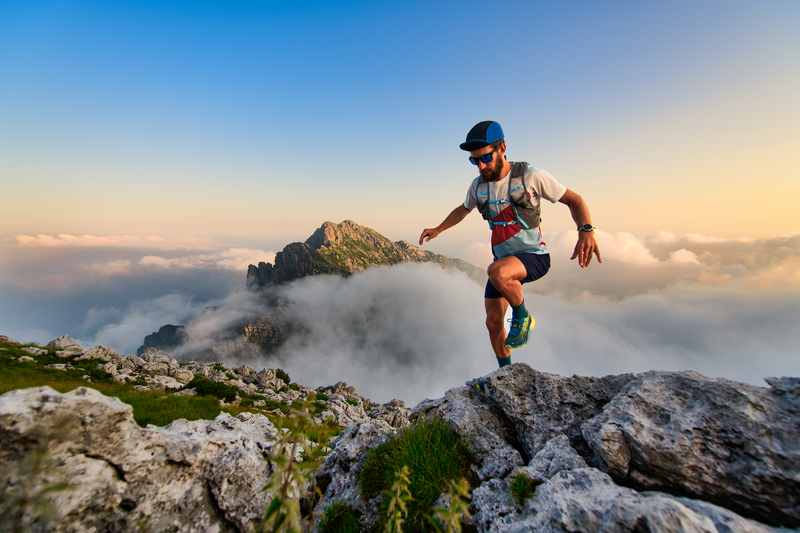
95% of researchers rate our articles as excellent or good
Learn more about the work of our research integrity team to safeguard the quality of each article we publish.
Find out more
REVIEW article
Front. Immunol. , 23 October 2023
Sec. Vaccines and Molecular Therapeutics
Volume 14 - 2023 | https://doi.org/10.3389/fimmu.2023.1261243
This article is part of the Research Topic Emerging Talents in Vaccines and Molecular Therapeutics: 2022 View all 9 articles
Cancer vaccines drive the activation and proliferation of tumor-reactive immune cells, thereby eliciting tumor-specific immunity that kills tumor cells. Accordingly, they possess immense potential in cancer treatment. However, such vaccines are also faced with challenges related to their design and considerable differences among individual tumors. The success of messenger RNA (mRNA) vaccines against coronavirus disease 2019 has prompted the application of mRNA vaccine technology platforms to the field of oncotherapy. These platforms include linear, circular, and amplifying mRNA vaccines. In particular, amplifying mRNA vaccines are characterized by high-level and prolonged antigen gene expression at low doses. They can also stimulate specific cellular immunity, making them highly promising in cancer vaccine research. In this review, we summarize the research progress in amplifying mRNA vaccines and provide an outlook of their prospects and future directions in oncotherapy.
Tumors pose a severe threat to human health. Both the suppression and reprogramming of the immune system play key roles in the onset and progression of tumors. Immuno-oncotherapy refers to a series of methods involving the activation of the immune system for cancer treatment. It includes immune checkpoint inhibitors, therapeutic antibodies, adoptive cell transfer, small molecule immunosuppressants, and cancer vaccines (1). Among these methods, cancer vaccines serve as a form of active immunotherapy. Upon vaccination, they activate the specific antitumor immune responses of patients to eradicate tumor cells. In clinical studies, cancer vaccines have demonstrated positive effects in the active immunotherapy of tumors, including the eradication of tumor cells and prevention of tumor metastasis and recurrence (2, 3). However, cancer vaccines, such as peptide vaccines and cell vaccines, have a single antigen, poor antitumor immune activation effect, and high production cost. mRNA vaccines can deliver multiple antigens, induce stronger T cell responses, and have a relatively lower production cost.
In 1995, Conry et al. first proposed the concept of using messenger RNAs (mRNAs) for immuno-oncotherapy (4). The coronavirus disease (COVID-19) mRNA vaccine Comirnaty was approved for marketing by the United States Food and Drug Administration (US FDA) in 2021, making it the first-ever approved mRNA vaccine (5). With the rapid development, manufacturing, and application of mRNA vaccines against COVID-19, increasing attention has been focused on mRNA vaccines over the last few years. Compared with conventional vaccines, mRNA vaccines provide the advantages of rapid editability, low biosafety risk, T-cell activation, and induction of stronger immunogenicity (1, 6) Two mRNA cancer vaccines, mRNA-4157 and BNT122, have recently exhibited good therapeutic effects in clinical studies on melanoma and pancreatic ductal adenocarcinoma, respectively. These findings have led to widespread interest in the use of mRNA vaccines for cancer treatment (7).
In particular, mRNA vaccines include linear mRNA, circular mRNA, and amplifying mRNA vaccines (8). The characteristics of amplifying mRNA vaccines are as follows (1): They are dependent on self-amplifying RNAs (saRNAs) that are capable of synthesizing RNA within host cells using an RNA-dependent RNA polymerase (RdRp). This enables the high-level and prolonged expression of the tumor antigen genes carried by vectors, thereby achieving levels of protein expression similar to those of linear mRNA vaccines but at lower doses and a relatively lower production cost (2); Amplifying mRNAs possess the potential for stimulating immune responses as they can form double-stranded RNAs during the amplification process. This is highly similar to the viral RNA replication process, which can activate the innate immune response of the host and further enhance the effects of the vaccine (3); Amplifying mRNAs can simultaneously express multiple antigens, thereby inducing humoral and cellular immune responses targeted toward different antigens (9, 10). Therefore, amplifying mRNA vaccines offer promising prospects for the research and application of vaccines against infectious diseases and cancer. At present, there are 43 COVID-19 mRNA vaccines in the clinical stage, of which 14 are amplifying mRNA vaccines. Among the various amplifying mRNA-based cancer vaccines, dozens are in the preclinical stage and five are in the clinical stage (11–13).
In this study, we present a review of the research progress and working principles of amplifying mRNA vaccines and highlight the considerations and outstanding issues in the quality control of amplifying mRNA vaccines, so as to provide a reference for the future development of amplifying mRNA cancer vaccines.
Research on amplifying mRNA vaccines dates back to the investigation of the characteristics of RNA viruses in the 1950s and 1960s. When RNA extracted from ascites tumor cells infected with Mengo encephalitis virus was introduced into cells, it was found that complete infectious viral particles could be synthesized (14). In 1989, Malone et al. conceived the concept of mRNA-based drugs (15). Zhou et al. in 1994 reported the generation of humoral responses with high antibody titers in mice through the expression of the nucleoprotein of influenza virus using the Semliki Forest virus (SFV) replicon, and first proposed the concept of using synthesized amplifying mRNAs as vaccines (16). In 1999, Ying et al. proposed that naked, noninfectious saRNAs may be used for the development of cancer vaccines (17). In 2003, in a phase I clinical trial of an mRNA vaccine encoding prostate-specific antigen (PSA), it was found that the vaccine effectively evoked T-cell-mediated antitumor immune responses in vivo (18). The first amplifying mRNA-based therapeutic cancer vaccine AVX701 entered phase I clinical testing in 2007 (19). In 2019, Beissert et al. designed a novel bipartite amplifying mRNA vector system using trans-amplifying RNA (taRNA). Compared with conventional self-amplifying mRNA (samRNA) vaccines, this method has key advantages in the aspects of safety, production, and ease of optimization (20). During the same year, Samsa et al. pioneered the use of lipid nanoparticles (LNPs) for the delivery of a live-attenuated Venezuelan equine encephalitis virus (VEEV) RNA vaccine (21). In 2021, Imperial College London reported the outcomes of a clinical trial of the first amplifying mRNA COVID-19 vaccine worldwide. Among 23 participants who received the vaccine at doses of 5.0 and 10.0 μg, 20 developed immune responses (22). At present, the amplifying mRNA vaccine technology platform has been applied in the clinical research of vaccines for infectious viruses such as influenza, respiratory syncytial virus (RSV), rabies, Ebola, and HIV-1 and for cancers such as melanoma and has exhibited immense potential in the fields of infectious disease prevention and oncotherapy (12, 23) (Figure 1).
Amplifying mRNAs differ from nonamplifying mRNAs in that they can serve as templates for amplification. Besides conventional structural elements such as the cap, 5′-UTR, 3′-UTR, and poly(A) tail, amplifying mRNAs also contain a sequence within the 5′ open reading frame that encodes an RNA-dependent RNA polymerase (RdRp) complex (replicase) and a subgenomic promoter (24). The gene sequences encoding viral structural proteins are usually located downstream of the subgenomic promoter. During the design of amplifying mRNA vaccines, this sequence is replaced by a gene sequence encoding the vaccine antigen of interest (25). The genomes of alphaviruses such as the Venezuelan equine encephalitis virus (VEEV) (26), Sindbis virus (SINV) (27), and Semliki forest virus (SFV) (28) are commonly used in the design of amplifying mRNA vaccines. Other genomes used include those of the tick-borne encephalitis virus (TBEV) (29), yellow fever virus (YFV) (30), and Kunjin virus (KUNV) (31). For instance, the replicase encoded by an alphavirus vector is composed of four nonstructural proteins (NSPs). NSP1 is responsible for the connection between the replicase complex and cell membrane and the 5′-end capping of the viral RNA (32); NSP2 serves as a RNA helicase and protease for polyprotein processing (33, 34); NSP3 mediates various virus-host protein-protein interactions (35); while NSP4 is the RdRp (36). Aggregation of these four NSPs through a complex multistep process causes the formation of an replicase, which provides amplifying mRNA with the ability to self-replicate (37).
Following its entrance in the host cell, the amplifying mRNA is translated in a host ribosome-dependent manner and then undergoes processing within the host cell to form replicase. As the sequence of the amplifying mRNA is a sense (+)-strand RNA, it is used by replicase as a template for amplification, thereby forming the antisense (–)-strand amplifying mRNA. Subsequently, amplification of the (–)-strand amplifying mRNA leads to the synthesis of two different types of (+)-strand RNA. One is a copy of the original full-length genomic RNA, whereas the other is a subgenomic RNA encoding the target gene. Given the presence of a subgenomic promoter and the gene of interest (GOI) in the amplifying mRNA sequence, the viral replicase recognizes the subgenomic promoter in the (–)-strand amplifying mRNA. Consequently, a large amount of (+)-strand mRNA containing the target gene sequence is synthesized, and the required target product is then obtained through translation (23). Hence, amplifying mRNA results in the high-level and prolonged expression of the target protein at low doses. Figure 2A shows a schematic diagram of the working principle of a self-amplifying mRNA.
Figure 2 Alphaviruses-based amplifying mRNA expression process. (A) Mechanism of self-amplifying mRNA. (B) Mechanism of Trans-amplifying mRNA. Green rectangular, Non-structural proteins (NSP) RNA sequence; green oval, RNA-dependent RNA polymerase; orange rectangular, gene of interest RNA sequence; orange cloud, antigen; grey oval, N7-methylguanosine RNA sequence.
Compared with nonamplifying linear mRNA, amplifying mRNA contains an additional sequence that encodes the replicase. For instance, the replicase sequence in the VEEV-encoded RNA has a length of up to 7 kb (38). As RNA stability is negatively correlated with sequence length, this has resulted in strict requirements for RNA storage and transportation. To address this issue, a split-vector trans-amplifying RNA system can be adopted, that is, the sequence encoding the RdRp complex and that encoding the target gene can be split into two independent transcripts for delivery This contributes to the reduction of mRNA size, thereby enhancing overall mRNA stability (20). The working principle of a trans-amplifying mRNA is shown in Figure 2B.
Amplifying mRNAs, which serve as a novel vaccine technology and treatment method, have been increasingly adopted in the fields of infectious disease prevention and oncotherapy. This section summarizes the applications of amplifying mRNA cancer vaccines in preclinical (Table 1) and clinical studies (Table 2).
At present, amplifying mRNA cancer vaccines are used for the high-level expression of tumor antigens. These vaccines have demonstrated the ability to elicit strong cellular and humoral immune responses in animal models (12). Preclinical studies in this area have primarily focused on tumor-associated antigens (TAAs), tumor-specific antigens (TSAs), and immunomodulatory molecules and have achieved promising therapeutic effects in various mouse tumor models.
Differences in the levels of expression of TAAs between tumors and normal cells serve as possible targets for cancer vaccine therapy. Currently, the design of amplifying mRNA cancer vaccines in the preclinical stage mainly involves the targeting of TAAs, including tyrosinase, pMEL17/gp100, gp75/tyrosinase-related protein (TRP)-1, MART-1/melan-A, and dopachrome tautomase/TRP-2 which are preferentially expressed in melanoma cells. Avogadri et al.’s research suggested that amplifying mRNA cancer vaccine based on VEEV vector expressing TRP-2 induced Humoral immunity against TRP-2, played a role in immunotherapy of Melanoma, and cooperated with tumor specific CD8 T cell reaction (45). Six-transmembrane epithelial antigen of the prostate (STEAP) is highly overexpressed in human prostate cancer tissue. Garcia-Hernandez et al. used VEE virus-like replicon particles (VEE VRPs) for vaccination in prophylactic and therapeutic mouse prostate tumor models, and found that vaccination increased the overall survival rate of prostate tumor-bearing mice (48).
TSAs, also known as neoantigens, originate from random somatic cell mutations within tumor cells. These antigens do not exist in normal cells and are thus ideal targets for cancer vaccines. More than 99% of cervical cancers express the E6 and E7 oncogenes, which are necessary for the malignant phenotype. Daemen et al. used a SFV vector to carry an E6E7 fusion protein that possessed higher stability than the individual E6 and E7 proteins. Compared with mice vaccinated with SFV vectors independently expressing E6 and E7 proteins, mice immunized with the SFV vector expressing the E6E7 fusion protein exhibited stronger cytotoxic T lymphocyte (CTL) responses. Immunization of tumor-bearing mice led to tumor regression and eradication, indicating good oncotherapeutic effects (39).
IL-12 can induce the differentiation of naïve T-cells into T helper type 1 (Th1) cells, which are essential for cellular immunity-mediated antitumor responses. The antitumor effects of IL-12 are exerted through the activation of CTLs and natural killer (NK) cells and the induction of interferon-gamma (IFN-γ) production. Rodriguez Madoz et al. used SFV vector expressing IL-12 to treat MC38 cell line colon cancer mice, which significantly enhanced the anti-tumor immune response of mice, leading to tumor regression and complete eradication (42). IL-18 can increase NK cell viability and T-cell proliferation, promote the secretion of IFN-γ and granulocyte-macrophage colony-stimulating factor (GM-CSF) by NK and T-cells, and enhance the production of Th1-type cytokines, which are associated with antitumor CTL responses. Yamanaka et al. combined intratumoral injection of IL-18 bound by a genetically engineered SFV vector with systemic administration of IL-12 to induce responses from antibrain tumor-specific CD4+ and CD8+ T-cells and NK cells. This strategy led to a significant enhancement in antitumor effects (41).
Preclinical studies have shown that amplifying mRNA cancer vaccines can evoke strong humoral and cellular immune responses, effectively inhibiting f tumors growth such as melanoma, cervical cancer, and prostate cancer. These results may serve as valuable reference data for subsequent clinical studies.
Presently, many candidate amplifying mRNA cancer vaccines have entered clinical testing. Various clinical trials have revealed that amplifying mRNA cancer vaccines enhance T-cell immune responses and improve patient outcomes and survival. Therefore, these vaccines provide new tools and support for oncotherapy. On November 9, 2022, JCXH-211 was approved for phase I clinical testing by the National Medical Products Administration (NMPA) of China. This is a novel samRNA-based therapeutic encoding human IL-12 for the treatment of various solid tumors that is presently in the subject recruitment phase (55). Vvax001, a therapeutic cancer vaccine based on a self-amplifying SFV vector, is currently undergoing phase I clinical trial testing for the evaluation of its immunogenicity, safety, and tolerability in the treatment of human papillomavirus (HPV)-induced cancers (52). AVX701 is a self-amplifying VEEV vector-based cancer vaccine expressing a modified carcinoembryonic antigen gene (CEA (6D)). Preliminary results of a phase I/II clinical trial in patients with metastatic colorectal cancer revealed that vaccinated patients exhibited stronger T-cell immune responses and longer survival (19, 51). AVX901, an amplifying mRNA vaccine based on a self-amplifying VEEV vector encoding HER2, has completed phase I clinical trial in the treatment of HER2+ breast cancer, and the phase II clinical trial for its concomitant use with pembrolizumab is currently in the recruitment stage (50). In tumors that carry nonsynonymous DNA mutations, human leukocyte antigens on tumor cell surfaces express peptides containing these mutations as nonself antigens. A subset of these mutated peptides serve as neoantigens that are capable of generating T-cell responses targeting tumor cells. GRANITE is a vaccine based on the self-amplifying VEEV vector GRT-R902 and adenoviral vector GRT-R901 that expresses (54) neoantigens. It is used in combination with checkpoint inhibitors for the treatment of advanced metastatic solid tumors and is currently undergoing phase II/III clinical testing.
Notably, there has been a rapid rise in the number of clinical trials of mRNA cancer vaccines. Although clinical trials of mRNA cancer vaccines are still in the early stages, swift advancements achieved in this field suggest that the prospects of amplifying mRNA cancer vaccines remain promising.
The length of amplifying mRNAs exceeds that of conventional nonamplifying linear mRNAs by 7,000 bp, leading to disadvantages such as higher tendency for mRNA degradation; increased difficulty in the preparation of in vitro transcription (IVT)systems; higher complexity in chemistry, manufacture, and control (CMC) processes; low LNP encapsulation efficiency; and low delivery efficiency. In recent years, amplifying RNA cancer vaccines have achieved a series of breakthroughs in stability, translation efficiency, and delivery efficiency, with advancements made mainly in the following areas (1): Design and optimization of amplifying mRNA carrier backbone sequences to enhance the intensity and stability of mRNA expression while reducing the innate immune responses of the host (2); Selection and combination of tumor antigens (3); Optimization of IVT systems (4); Design of novel delivery systems suitable for amplifying mRNAs.
Amplifying mRNAs activate intracellular pattern recognition receptors during in vivo transcription and synthesis, which can inhibit the overall protein translation process within cells. Inhibition of the protein kinase R (PKR) and IFN pathways significantly improves the translation efficiency of amplifying mRNAs in vivo and in vitro (56, 57). Notably, amplifying mRNAs, which contain a self-replicase sequence with a length of 7–8 kb, have a much longer length than that of conventional linear mRNAs, increasing the demands on mRNA stability. Blakney et al. developed a split replicon (splitzicon) system that demonstrated the self-amplifying characteristics of replicon RNA. The splitzicon system was also used for encoding multiple antigens, providing a novel approach for the design of multivalent RNA vaccines (58). Beissert et al. developed a bipartite taRNA system that demonstrated high-efficiency levels of protein expression similar to those achieved by amplifying mRNAs (20). In recent years, artificial intelligence (AI) tools have been employed for the optimization of mRNA vaccine sequences, leading to significant increases in mRNA half-life, protein expression levels, and thermal stability of mRNAs (59). Li et al. developed an in vitro evolution strategy based on the VEEV replicon system and screened two effective mutant replicons, achieving significant increases in the intensity and duration of luciferase expression within mice compared with that of wild-type replicons (60). Conclusively, the optimization of amplifying mRNA vector sequences for reducing the host innate immune responses can significantly enhance the stability and expression efficiency of these vectors.
Antigen selection is crucial for the development of cancer vaccines. Two important features that cancer vaccines should possess are tumor specificity and the ability to induce high-level and controllable antitumor immune responses. Tumor antigens, including TSAs and TAAs, are antigenic molecules that emerge or are overexpressed during the onset and progression of tumors. TSAs, which include HPV E6, E7, CMV pp65, and neoantigens, are newly formed antigens that are either specific to tumor cells or exist only in certain types of tumor but not in normal cells. By contrast, TAAs are not specific to tumor cells and also exist in normal cells and tissues. However, their expression is significantly increased during carcinogenesis. Examples of TAAs include MUC1, which is abnormally overexpressed in many tumor cells, as well as gp100 and MART1, which are respectively overexpressed and abnormally expressed in most melanoma cancer cells. Immunomodulatory molecules such as IL-7, IL-12, IL-15, GM-CSF, and IFN-a and tumor suppressor genes such as p53 and PTEN have also been applied in the antigen design of cancer vaccines. Table 3 lists the antigens of cancer vaccines currently used in clinical and preclinical studies.
Multiple mutation sites usually exist in the antigenic epitopes of tumor cells, with the suppressive tumor microenvironment also exerting certain negative effects on cancer vaccines. In cancer immunotherapy, combination therapy is commonly adopted for enhancing the effects of cancer vaccines. An effective strategy is the simultaneous expression of multiple antigens. Compared with single-antigen OVA1 mRNA, the dual antigens OVA1 and OVA2 mRNAs caused a 30% increase in the antigen-specific activation of T-cells and a two-fold increase in their proliferation. Cotransfection with a combination of CD40L, CD70, and constitutively active TLR4 encoding mRNA was reported to enhance the human dendritic cell-induced activation of T-cells. Interestingly, the adoption of a combination therapy strategy for the treatment of pancreatic ductal adenocarcinoma (PDAC) not only inhibited the expression of PD-1 but also simultaneously activated IL-2Rβγ and suppressed IL-2α signaling, thereby providing excellent therapeutic effects to patients with PDAC (102). Besides tumor antigen targets, mutation sites were also introduced into the nonstructural proteins of the VEE replicon backbone by Li et al. Compared with wild-type VEE, the saRNA modified with nonstructural proteins led to a significant increase in the levels of expression of the target protein in model animals. In addition, the infiltration of CD8+ T-cells was enhanced and tumor growth was delayed (60).
The characteristics of amplifying mRNA vaccines should be considered during the formulation of suitable antigen combination strategies targeted toward different tumor types in order to enhance the therapeutic effects of active immunotherapy on tumors.
Compared with conventional mRNAs, amplifying mRNAs are longer and contain more secondary structures. Therefore, the optimization of conventional mRNA IVT synthesis systems is necessary. With the application of the Quality by Design (QbD) concept to IVT system optimization, the effects of critical process parameters on critical quality attributes (CQAs) can be rapidly determined. This will enable the development and continuous production of safe and effective RNA vaccines. Samnuan et al. adopted a design of experiment approach to investigate the various factors affecting the yield of amplifying mRNAs produced by the IVT reaction. Subsequently, the optimum component ratios for IVT were determined and used for the synthesis of amplifying mRNAs with high yield and quality (103). Moderna developed a modified T7 RNA polymerase that reduced dsRNA impurities during transcription reactions. This eased the subsequent purification and enhanced the production efficiency (104). Other researchers have found that a novel psychrophilic phage VSW-3 RNA polymerase is capable of reducing terminal and full-length dsRNA byproducts during IVT, which is beneficial for the production of RNAs for in vivo use (105). The development of stable IVT systems with high yield and low byproduct formation remains a key area for future research.
Delivery systems are a key aspect of mRNA vaccines. Initially, the direct delivery of naked saRNA into cells was the simplest strategy used. For example, injection of 50 mg of saRNA carrying the influenza virus nucleoprotein was found to induce specific humoral responses in mice. Subsequently, various delivery methods were developed to enhance the stability and delivery efficiency of mRNAs (16). These methods include electroporation, protamine, LNPs, cationic nanoemulsions, polyethylenimine, polymer-based nanoparticles, exosomes, extracellular vesicles, mesoporous silica, and calcium phosphate (9, 106). LNPs can also function as an adjuvant (107). Miao et al. used LNPs containing cyclic lipid components that stimulated the stimulator of interferon genes pathway for the encapsulation of an OVA mRNA vaccine and observed a significant enhancement in its antitumor effects (108). However, the design of novel delivery systems for amplifying mRNA cancer vaccines still holds many key challenges and opportunities. These include the improvement of stability and delivery efficiency of amplifying mRNAs, delivery targeting, and controllability of the activation of the immune system.
Regarding mRNA vaccines, which are a novel type of vaccine, quality control is of utmost importance. The assurance of good quality, safety, and efficacy will contribute to sufficient trust and confidence among the public in mRNA vaccines and other innovative products and therapies. The World Health Organization, United States Pharmacopeial Convention, and Center for Drug Evaluation of the NMPA of China have published relevant guidelines and documents, thereby providing regulatory agencies with considerations regarding quality control of mRNA vaccines and methods for the analysis of mRNA quality (109–111). In particular, mRNA sequence and integrity, content and purity, capping efficiency, and encapsulation efficiency are key quality parameters specific to mRNA vaccines that determine both their effectiveness and safety.
Currently, nine COVID-19 mRNA vaccines have been approved or granted emergency use authorizations. However, details such as test items, analysis methods, and acceptance criteria of many types of mRNA vaccines have not yet been publicly disclosed (112). Although COVID-19 mRNA vaccines have demonstrated high safety and efficacy, rare serious adverse events such as myocarditis and hypersensitivity reactions have also been reported, suggesting that the quality control of mRNAs requires further research (60, 113, 114) The application of the QbD concept enables the accurate determination of CQAs, key process parameters, and operational spaces of mRNA vaccines, contributing to the establishment of rational control strategies for the production process, which is of utmost importance for the quality control of mRNA vaccines. As mentioned before, mRNA stability is affected by the length of the mRNA fragment. Previous studies have found that the length of mRNA in yeast is negatively correlated with its half-life (115). Amplifying mRNAs carry an additional coding sequence for replicase, which enables their amplification. Consequently, the length of amplifying mRNAs exceeds that of conventional linear mRNAs by 7,000 bp, resulting in poorer stability. Therefore, to achieve the quality control of amplifying mRNA vaccines, further research should be performed on key CQAs such as their stability and in vivo replicase activity. In view of the prolonged expression time of amplifying mRNAs, the duration of antigen expression should be calculated for the evaluation of their in vitro and in vivo activities. Regulatory agencies and vaccine manufacturers should also consider establishing reference standards for the measurement of nucleic acid content, purity, and biological activity of amplifying mRNAs for use in the quality control testing of mRNA vaccines.
Amplifying mRNA cancer vaccines are a highly promising technological platform in the field of cancer treatment. A large number of biopharmaceutical companies and research teams worldwide have made considerable efforts to the research and development of such vaccines, achieving significant progress. To date, five types of amplifying mRNA cancer vaccines have entered clinical testing. However, mRNA vaccines face obstacles such as higher tendency for mRNA degradation, antigen selection and optimization and targeted delivery to tumor. And many issues regarding the research and development, production, quality control, and safety remain to be resolved for amplifying mRNA cancer vaccines. Recently, researchers have proposed the concept of Quality by Digital Design concept as an extension of the QbD approach. Efforts should be made to accelerate the integration of such a concept into the research and production of amplifying mRNA vaccines (116).
Currently, RdRp used for constructing amplifying mRNA cancer vaccines mainly originates from alphaviruses and have a gene sequence length of up to 7 kb. The use of longer gene sequences hinders the production and quality control of mRNA vaccines and may lead to their poorer stability. Therefore, research should be focused on the exploration of RdRp with a shorter gene sequence or the design and optimization of various component elements to reduce the mRNA length in vaccines. Delivery systems are of critical importance for mRNA vaccines. Given the relatively long nucleotide sequences of amplifying mRNA vaccines, existing LNPs may not be suitable for encapsulating longer mRNAs. The optimization of currently available LNPs or development of new delivery systems suited to the encapsulation of longer mRNAs is therefore required. Targeted therapy not only enables precise killing of tumors but also reduces the side-effects of medications. Therefore, the exploration and development of delivery systems with higher targeting capabilities for use with amplifying mRNA cancer vaccines is highly necessary. Many studies have indicated that tumor tissues are generally in a state of hypoxia. Recent studies have also demonstrated that hypoxia within cells or tissues can lead to reduced transfection and expression efficiency of LNP-mRNA. Therefore, further design and optimization of amplifying mRNA cancer vaccines should be performed to enhance their transfection and expression efficiencies within the body.
The relatively long sequences of amplifying mRNA vaccines may also affect the efficiency of IVT. Therefore, further optimization of the reaction conditions and systems of IVT and the development of more efficient IVT enzymes should be carried out. The use of cap analogs from different sources and optimization of the cap analog-guanosine ratio may enhance IVT efficiency. In addition, longer mRNA sequences may also cause premature termination of IVT, resulting in decreased integrity of the generated mRNA sequences. Therefore, optimization of the IVT reaction is required to achieve the generation of complete target sequences. The production of mRNA vaccines also requires a purification step. Considering that the IVT products of amplifying mRNA vaccines may contain a considerable proportion of incomplete mRNAs, it is necessary to develop and optimize suitable purification processes to enhance purification efficiency.
The establishment and validation of quality control methods for amplifying mRNA vaccines should be performed based on specific requirements stipulated in the General Chapter <1220> “The Analytical Procedure Lifecycle” recently released by the USP and the ICH Q2 “Validation of Analytical Procedures” and Q14 “Analytical Procedure Development” draft guidelines published by the International Council for Harmonization of Technical Requirements for Pharmaceuticals for Human Use (ICH) for which public comment is currently being sought (117, 118). This includes the establishment of an analytical target profile, risk analysis and identification, confirmation of CQAs, experimental design, process control, and in-use monitoring. The most prominent characteristic of amplifying mRNA vaccines is their ability to replicate within cells. Therefore, it is important to focus on the quality control of their replicating properties. Given the lack of research on relevant quality control methods, there is a need to develop pertinent methods and establish standards to facilitate the research and development of such vaccines. The relatively long sequences of amplifying mRNAs also cause lower stability of the resultant vaccines. Therefore, it is also crucial to focus on quality control of the markers of integrity of vaccine sequences.
In terms of safety, given that amplifying mRNA vaccines can continuously generate new mRNAs within the body, attention should be paid to the possibility of evoking strong systemic or local inflammatory reactions. The fact that such vaccines encode RdRp signifies that the body will express RdRp after immunization. However, in the case of viral infection in the body post-immunization, RdRp may bind to the RdRp binding site in the viral genome, raising concerns about the promotion of viral genome replication. Further investigation is also needed to determine whether this system might cause the replication of the host mRNA, thereby affecting gene expression in the host.
CH: Writing – original draft, Writing – review & editing. JL: Writing – original draft, Writing – review & editing. FC: Writing – original draft, Writing – review & editing. YB: Writing – review & editing. QM: Conceptualization, Funding acquisition, Writing – review & editing. MX: Conceptualization, Funding acquisition, Writing – review & editing. ZL: Conceptualization, Funding acquisition, Writing – review & editing.
The author(s) declare financial support was received for the research, authorship, and/or publication of this article. This review study was supported by the Emergency Key Program (NO. EKPG21-30-1) of Guangzhou Laboratory, China.
The authors declare that the research was conducted in the absence of any commercial or financial relationships that could be construed as a potential conflict of interest.
All claims expressed in this article are solely those of the authors and do not necessarily represent those of their affiliated organizations, or those of the publisher, the editors and the reviewers. Any product that may be evaluated in this article, or claim that may be made by its manufacturer, is not guaranteed or endorsed by the publisher.
1. Waldman AD, Fritz JM, Lenardo MJ. A guide to cancer immunotherapy: from T cell basic science to clinical practice. Nat Rev Immunol (2020) 20(11):651–68. doi: 10.1038/s41577-020-0306-5
2. Rojas LA, Sethna Z, Soares KC, Olcese C, Pang N, Patterson E, et al. Personalized RNA neoantigen vaccines stimulate T cells in pancreatic cancer. Nature (2023) 618(7963):144–50. doi: 10.1038/s41586-023-06063-y
3. Lorentzen CL, Haanen JB, Met O, Svane IM. Clinical advances and ongoing trials on mRNA vaccines for cancer treatment. Lancet Oncol (2022) 23(10):e450–e8. doi: 10.1016/S1470-2045(22)00372-2
4. Conry RM, LoBuglio AF, Wright M, Sumerel L, Pike MJ, Johanning F, et al. Characterization of a messenger RNA polynucleotide vaccine vector. Cancer Res (1995) 55(7):1397–400.
5. Food and Drug Administration. (2023). Available at: https://www.fda.gov/news-events/press-announcements/fda-approves-first-covid-19-vaccine (Accessed July 13, 2023).
6. Pardi N, Hogan MJ, Porter FW, Weissman D. mRNA vaccines - a new era in vaccinology. Nat Rev Drug Discovery (2018) 17(4):261–79. doi: 10.1038/nrd.2017.243
7. Moderna. (2023). Available at: https://www.modernatx.com/media-center/all-media/blogs/fight-against-cancer (Accessed July 13, 2023).
8. Bai Y, Liu D, He Q, Liu J, Mao Q, Liang Z. Research progress on circular RNA vaccines. Front Immunol (2022) 13:1091797. doi: 10.3389/fimmu.2022.1091797
9. Ballesteros-Briones MC, Silva-Pilipich N, Herrador-Canete G, Vanrell L, Smerdou C. A new generation of vaccines based on alphavirus self-amplifying RNA. Curr Opin Virol (2020) 44:145–53. doi: 10.1016/j.coviro.2020.08.003
10. Erasmus JH, Khandhar AP, O'Connor MA, Walls AC, Hemann EA, Murapa P, et al. An Alphavirus-derived replicon RNA vaccine induces SARS-CoV-2 neutralizing antibody and T cell responses in mice and nonhuman primates. Sci Transl Med (2020) 12(555):eabc9396. doi: 10.1126/scitranslmed.abc9396
11. World Health Organization. (2023). Available at: https://www.who.int/publications/m/item/draft-landscape-of-covid-19-candidate-vaccines (Accessed July 13, 2023).
12. Blakney AK, Ip S, Geall AJ. An update on self-amplifying mRNA vaccine development. Vaccines (Basel). (2021) 9(2):97. doi: 10.3390/vaccines9020097
13. Bai Y, He Q, Yang J, Lu S, Mao Q, Gao F, et al. B.1.351 SARS-CoV-2 Variant Exhibits Higher Virulence but Less Viral Shedding than That of the Ancestral Strain in Young Nonhuman Primates. Microbiol Spectr (2022) 10(5):e0226322. doi: 10.1128/spectrum.02263-22
14. Colter JS, Bird HH, Brown RA. Infectivity of ribonucleic acid from Ehrlich ascites tumour cells infected with Mengo encephalitis. Nature (1957) 179(4565):859–60. doi: 10.1038/179859a0
15. Malone RW, Felgner PL, Verma IM. Cationic liposome-mediated RNA transfection. Proc Natl Acad Sci U S A. (1989) 86(16):6077–81. doi: 10.1073/pnas.86.16.6077
16. Zhou X, Berglund P, Rhodes G, Parker SE, Jondal M, Liljestrom P. Self-replicating Semliki Forest virus RNA as recombinant vaccine. Vaccine (1994) 12(16):1510–4. doi: 10.1016/0264-410X(94)90074-4
17. Ying H, Zaks TZ, Wang RF, Irvine KR, Kammula US, Marincola FM, et al. Cancer therapy using a self-replicating RNA vaccine. Nat Med (1999) 5(7):823–7. doi: 10.1038/10548
18. Heiser A, Coleman D, Dannull J, Yancey D, Maurice MA, Lallas CD, et al. Autologous dendritic cells transfected with prostate-specific antigen RNA stimulate CTL responses against metastatic prostate tumors. J Clin Invest (2002) 109(3):409–17. doi: 10.1172/JCI0214364
19. Crosby EJ, Hobeika AC, Niedzwiecki D, Rushing C, Hsu D, Berglund P, et al. Long-term survival of patients with stage III colon cancer treated with VRP-CEA(6D), an alphavirus vector that increases the CD8+ effector memory T cell to Treg ratio. J Immunother Cancer (2020) 8(2):e001662. doi: 10.1136/jitc-2020-001662
20. Beissert T, Perkovic M, Vogel A, Erbar S, Walzer KC, Hempel T, et al. A trans-amplifying RNA vaccine strategy for induction of potent protective immunity. Mol Ther (2020) 28(1):119–28. doi: 10.1016/j.ymthe.2019.09.009
21. Samsa MM, Dupuy LC, Beard CW, Six CM, Schmaljohn CS, Mason PW, et al. Self-amplifying RNA vaccines for Venezuelan equine encephalitis virus induce robust protective immunogenicity in mice. Mol Ther (2019) 27(4):850–65. doi: 10.1016/j.ymthe.2018.12.013
22. Pollock KM, Cheeseman HM, Szubert AJ, Libri V, Boffito M, Owen D, et al. Safety and immunogenicity of a self-amplifying RNA vaccine against COVID-19: COVAC1, a phase I, dose-ranging trial. EClinicalMedicine (2022) 44:101262. doi: 10.1016/j.eclinm.2021.101262
23. Maruggi G, Ulmer JB, Rappuoli R, Yu D. Self-amplifying mRNA-based vaccine technology and its mode of action. Curr Top Microbiol Immunol (2022) 440:31–70. doi: 10.1007/82_2021_233
24. Xu S, Yang K, Li R, Zhang L. mRNA vaccine era-mechanisms, drug platform and clinical prospection. Int J Mol Sci (2020) 21(18):6582. doi: 10.3390/ijms21186582
25. Rodriguez-Gascon A, del Pozo-Rodriguez A, Solinis MA. Development of nucleic acid vaccines: use of self-amplifying RNA in lipid nanoparticles. Int J Nanomed (2014) 9:1833–43. doi: 10.2147/IJN.S39810
26. Davis NL, Willis LV, Smith JF, Johnston RE. In vitro synthesis of infectious Venezuelan equine encephalitis virus RNA from a cDNA clone: analysis of a viable deletion mutant. Virology (1989) 171(1):189–204. doi: 10.1016/0042-6822(89)90526-6
27. Xiong C, Levis R, Shen P, Schlesinger S, Rice CM, Huang HV. Sindbis virus: an efficient, broad host range vector for gene expression in animal cells. Science (1989) 243(4895):1188–91. doi: 10.1126/science.2922607
28. Liljestrom P, Garoff H. A new generation of animal cell expression vectors based on the Semliki Forest virus replicon. Biotechnol (N Y). (1991) 9(12):1356–61. doi: 10.1038/nbt1291-1356
29. Aberle JH, Aberle SW, Kofler RM, Mandl CW. Humoral and cellular immune response to RNA immunization with flavivirus replicons derived from tick-borne encephalitis virus. J Virol (2005) 79(24):15107–13. doi: 10.1128/JVI.79.24.15107-15113.2005
30. Molenkamp R, Kooi EA, Lucassen MA, Greve S, Thijssen JC, Spaan WJ, et al. Yellow fever virus replicons as an expression system for hepatitis C virus structural proteins. J Virol (2003) 77(2):1644–8. doi: 10.1128/JVI.77.2.1644-1648.2003
31. Pijlman GP, Suhrbier A, Khromykh AA. Kunjin virus replicons: an RNA-based, non-cytopathic viral vector system for protein production, vaccine and gene therapy applications. Expert Opin Biol Ther (2006) 6(2):135–45. doi: 10.1517/14712598.6.2.135
32. Ahola T, Kaariainen L. Reaction in alphavirus mRNA capping: formation of a covalent complex of nonstructural protein nsP1 with 7-methyl-GMP. Proc Natl Acad Sci U S A. (1995) 92(2):507–11. doi: 10.1073/pnas.92.2.507
33. Das PK, Merits A, Lulla A. Functional cross-talk between distant domains of chikungunya virus non-structural protein 2 is decisive for its RNA-modulating activity. J Biol Chem (2014) 289(9):5635–53. doi: 10.1074/jbc.M113.503433
34. Vasiljeva L, Valmu L, Kaariainen L, Merits A. Site-specific protease activity of the carboxyl-terminal domain of Semliki Forest virus replicase protein nsP2. J Biol Chem (2001) 276(33):30786–93. doi: 10.1074/jbc.M104786200
35. Gotte B, Liu L, McInerney GM. The enigmatic alphavirus non-structural protein 3 (nsP3) revealing its secrets at last. Viruses (2018) 10(3):105. doi: 10.3390/v10030105
36. Rubach JK, Wasik BR, Rupp JC, Kuhn RJ, Hardy RW, Smith JL. Characterization of purified Sindbis virus nsP4 RNA-dependent RNA polymerase activity in vitro. Virology (2009) 384(1):201–8. doi: 10.1016/j.virol.2008.10.030
37. Pietila MK, Hellstrom K, Ahola T. Alphavirus polymerase and RNA replication. Virus Res (2017) 234:44–57. doi: 10.1016/j.virusres.2017.01.007
38. Brito LA, Kommareddy S, Maione D, Uematsu Y, Giovani C, Berlanda Scorza F, et al. Self-amplifying mRNA vaccines. Adv Genet (2015) 89:179–233. doi: 10.1016/bs.adgen.2014.10.005
39. Daemen T, Riezebos-Brilman A, Bungener L, Regts J, Dontje B, Wilschut J. Eradication of established HPV16-transformed tumours after immunisation with recombinant Semliki Forest virus expressing a fusion protein of E6 and E7. Vaccine (2003) 21(11-12):1082–8. doi: 10.1016/S0264-410X(02)00558-3
40. Lyons JA, Sheahan BJ, Galbraith SE, Mehra R, Atkins GJ, Fleeton MN. Inhibition of angiogenesis by a Semliki Forest virus vector expressing VEGFR-2 reduces tumour growth and metastasis in mice. Gene Ther (2007) 14(6):503–13. doi: 10.1038/sj.gt.3302889
41. Yamanaka R, Tsuchiya N, Yajima N, Honma J, Hasegawa H, Tanaka R, et al. Induction of an antitumor immunological response by an intratumoral injection of dendritic cells pulsed with genetically engineered Semliki Forest virus to produce interleukin-18 combined with the systemic administration of interleukin-12. J Neurosurg (2003) 99(4):746–53. doi: 10.3171/jns.2003.99.4.0746
42. Rodriguez-Madoz JR, Prieto J, Smerdou C. Semliki forest virus vectors engineered to express higher IL-12 levels induce efficient elimination of murine colon adenocarcinomas. Mol Ther (2005) 12(1):153–63. doi: 10.1016/j.ymthe.2005.02.011
43. Kramer MG, Masner M, Casales E, Moreno M, Smerdou C, Chabalgoity JA. Neoadjuvant administration of Semliki Forest virus expressing interleukin-12 combined with attenuated Salmonella eradicates breast cancer metastasis and achieves long-term survival in immunocompetent mice. BMC Cancer. (2015) 15:620. doi: 10.1186/s12885-015-1618-x
44. Velders MP, McElhiney S, Cassetti MC, Eiben GL, Higgins T, Kovacs GR, et al. Eradication of established tumors by vaccination with Venezuelan equine encephalitis virus replicon particles delivering human papillomavirus 16 E7 RNA. Cancer Res (2001) 61(21):7861–7.
45. Avogadri F, Merghoub T, Maughan MF, Hirschhorn-Cymerman D, Morris J, Ritter E, et al. Alphavirus replicon particles expressing TRP-2 provide potent therapeutic effect on melanoma through activation of humoral and cellular immunity. PloS One (2010) 5(9):e12670. doi: 10.1371/journal.pone.0012670
46. Avogadri F, Zappasodi R, Yang A, Budhu S, Malandro N, Hirschhorn-Cymerman D, et al. Combination of alphavirus replicon particle-based vaccination with immunomodulatory antibodies: therapeutic activity in the B16 melanoma mouse model and immune correlates. Cancer Immunol Res (2014) 2(5):448–58. doi: 10.1158/2326-6066.CIR-13-0220
47. Durso RJ, Andjelic S, Gardner JP, Margitich DJ, Donovan GP, Arrigale RR, et al. A novel alphavirus vaccine encoding prostate-specific membrane antigen elicits potent cellular and humoral immune responses. Clin Cancer Res (2007) 13(13):3999–4008. doi: 10.1158/1078-0432.CCR-06-2202
48. Garcia-Hernandez Mde L, Gray A, Hubby B, Kast WM. In vivo effects of vaccination with six-transmembrane epithelial antigen of the prostate: a candidate antigen for treating prostate cancer. Cancer Res (2007) 67(3):1344–51. doi: 10.1158/0008-5472.CAN-06-2996
49. Crosby EJ, Gwin W, Blackwell K, Marcom PK, Chang S, Maecker HT, et al. Vaccine-induced memory CD8(+) T cells provide clinical benefit in HER2 expressing breast cancer: A mouse to human translational study. Clin Cancer Res (2019) 25(9):2725–36. doi: 10.1158/1078-0432.CCR-18-3102
50. Crosby EJ, Acharya CR, Haddad AF, Rabiola CA, Lei G, Wei JP, et al. Stimulation of Oncogene-Specific Tumor-Infiltrating T Cells through Combined Vaccine and alphaPD-1 Enable Sustained Antitumor Responses against Established HER2 Breast Cancer. Clin Cancer Res (2020) 26(17):4670–81. doi: 10.1158/1078-0432.CCR-20-0389
51. Morse MA, Hobeika AC, Osada T, Berglund P, Hubby B, Negri S, et al. An alphavirus vector overcomes the presence of neutralizing antibodies and elevated numbers of Tregs to induce immune responses in humans with advanced cancer. J Clin Invest. (2010) 120(9):3234–41. doi: 10.1172/JCI42672
52. Draghiciu O, Boerma A, Hoogeboom BN, Nijman HW, Daemen T. A rationally designed combined treatment with an alphavirus-based cancer vaccine, sunitinib and low-dose tumor irradiation completely blocks tumor development. Oncoimmunology (2015) 4(10):e1029699. doi: 10.1080/2162402X.2015.1029699
53. Chawla SP, Van Tine BA, Pollack SM, Ganjoo KN, Elias AD, Riedel RF, et al. Phase II randomized study of CMB305 and atezolizumab compared with atezolizumab alone in soft-tissue sarcomas expressing NY-ESO-1. J Clin Oncol (2022) 40(12):1291–300. doi: 10.1200/JCO.20.03452
54. Palmer CD, Rappaport AR, Davis MJ, Hart MG, Scallan CD, Hong SJ, et al. Individualized, heterologous chimpanzee adenovirus and self-amplifying mRNA neoantigen vaccine for advanced metastatic solid tumors: phase 1 trial interim results. Nat Med (2022) 28(8):1619–29. doi: 10.1038/s41591-022-01937-6
55. ClinicalTrials.gov. (2023). Available at: https://classic.clinicaltrials.gov/ct2/show/NCT05579275?term=JCXH-212&draw=2&rank=1 (Accessed July 03, 2023).
56. Beissert T, Koste L, Perkovic M, Walzer KC, Erbar S, Selmi A, et al. Improvement of in vivo expression of genes delivered by self-amplifying RNA using vaccinia virus immune evasion proteins. Hum Gene Ther (2017) 28(12):1138–46. doi: 10.1089/hum.2017.121
57. Pepini T, Pulichino AM, Carsillo T, Carlson AL, Sari-Sarraf F, Ramsauer K, et al. Induction of an IFN-mediated antiviral response by a self-amplifying RNA vaccine: implications for vaccine design. J Immunol (2017) 198(10):4012–24. doi: 10.4049/jimmunol.1601877
58. Blakney AK, McKay PF, Shattock RJ. Structural components for amplification of positive and negative strand VEEV splitzicons. Front Mol Biosci (2018) 5:71. doi: 10.3389/fmolb.2018.00071
59. Zhang H, Zhang L, Lin A, Xu C, Li Z, Liu K, et al. Algorithm for optimized mRNA design improves stability and immunogenicity. Nature (2023) 621(7978):396–403. doi: 10.1038/s41586-023-06127-z
60. Li Y, Teague B, Zhang Y, Su Z, Porter E, Dobosh B, et al. In vitro evolution of enhanced RNA replicons for immunotherapy. Sci Rep (2019) 9(1):6932. doi: 10.1038/s41598-019-43422-0
61. Dariushnejad H, Ghorbanzadeh V, Akbari S, Hashemzadeh P. Design of a novel recombinant multi-epitope vaccine against triple-negative breast cancer. Iran BioMed J (2022) 26(2):160–74. doi: 10.52547/ibj.26.2.160
62. Wach MM, Subjeck JR, Wang XY, Repasky E, Matsuzaki J, Yu H, et al. Recombinant human Hsp110-gp100 chaperone complex vaccine is nontoxic and induces response in advanced stage melanoma patients. Melanoma Res (2022) 32(2):88–97. doi: 10.1097/CMR.0000000000000796
63. Borobova EA, Antonets DV, Starostina EV, Karpenko LI, Ilyichev AA, Bazhan SI. Design of artificial immunogens containing melanoma-associated T-cell epitopes. Curr Gene Ther (2018) 18(6):375–85. doi: 10.2174/1566523218666181113112829
64. Sun QF, Zhao XN, Peng CL, Hao YT, Zhao YP, Jiang N, et al. Immunotherapy for Lewis lung carcinoma utilizing dendritic cells infected with CK19 gene recombinant adenoviral vectors. Oncol Rep (2015) 34(5):2289–95. doi: 10.3892/or.2015.4231
65. Cao S, Wang JR, Ji S, Yang P, Dai Y, Guo S, et al. Estimation of tumor cell total mRNA expression in 15 cancer types predicts disease progression. Nat Biotechnol (2022) 40(11):1624–33. doi: 10.1038/s41587-022-01342-x
66. Wang F, Li Z, Feng X, Yang D, Lin M. Advances in PSMA-targeted therapy for prostate cancer. Prostate Cancer Prostatic Dis (2022) 25(1):11–26. doi: 10.1038/s41391-021-00394-5
67. Rocha SM, Barroca-Ferreira J, Passarinha LA, Socorro S, Maia CJ. The Usefulness of STEAP Proteins in Prostate Cancer Clinical Practice. In: Bott SRJ, Ng KL, editors. Prostate Cancer. Brisbane, Australia: Brisbane (AU) (2021).
68. Kurupati RK, Zhou X, Xiang Z, Keller LH, Ertl HCJ. Safety and immunogenicity of a potential checkpoint blockade vaccine for canine melanoma. Cancer Immunol Immunother. (2018) 67(10):1533–44. doi: 10.1007/s00262-018-2201-5
69. Salomon N, Vascotto F, Selmi A, Vormehr M, Quinkhardt J, Bukur T, et al. A liposomal RNA vaccine inducing neoantigen-specific CD4(+) T cells augments the antitumor activity of local radiotherapy in mice. Oncoimmunology (2020) 9(1):1771925. doi: 10.1080/2162402X.2020.1771925
70. Gao T, Cen Q, Lei H. A review on development of MUC1-based cancer vaccine. BioMed Pharmacother. (2020) 132:110888. doi: 10.1016/j.biopha.2020.110888
71. Ji Z, Tan Z, Li M, Tao J, Guan E, Du J, et al. Multi-functional nanocomplex codelivery of Trp2 and R837 to activate melanoma-specific immunity. Int J Pharm (2020) 582:119310. doi: 10.1016/j.ijpharm.2020.119310
72. Berois N, Pittini A, Osinaga E. Targeting tumor glycans for cancer therapy: successes, limitations, and perspectives. Cancers (Basel). (2022) 14(3):645. doi: 10.3390/cancers14030645
73. Madan RA, Bilusic M, Heery C, Schlom J, Gulley JL. Clinical evaluation of TRICOM vector therapeutic cancer vaccines. Semin Oncol (2012) 39(3):296–304. doi: 10.1053/j.seminoncol.2012.02.010
74. Chung DJ, Carvajal RD, Postow MA, Sharma S, Pronschinske KB, Shyer JA, et al. Langerhans-type dendritic cells electroporated with TRP-2 mRNA stimulate cellular immunity against melanoma: Results of a phase I vaccine trial. Oncoimmunology (2017) 7(1):e1372081. doi: 10.1080/2162402X.2017.1372081
75. Gulley JL, Borre M, Vogelzang NJ, Ng S, Agarwal N, Parker CC, et al. Phase III trial of PROSTVAC in asymptomatic or minimally symptomatic metastatic castration-resistant prostate cancer. J Clin Oncol (2019) 37(13):1051–61. doi: 10.1200/JCO.18.02031
76. Fotin-Mleczek M, Duchardt KM, Lorenz C, Pfeiffer R, Ojkic-Zrna S, Probst J, et al. Messenger RNA-based vaccines with dual activity induce balanced TLR-7 dependent adaptive immune responses and provide antitumor activity. J Immunother. (2011) 34(1):1–15. doi: 10.1097/CJI.0b013e3181f7dbe8
77. Sahin U, Oehm P, Derhovanessian E, Jabulowsky RA, Vormehr M, Gold M, et al. An RNA vaccine drives immunity in checkpoint-inhibitor-treated melanoma. Nature (2020) 585(7823):107–12. doi: 10.1038/s41586-020-2537-9
78. Tan TH, Sridhar S, Angell H, Jones E, Raymond A, Willis S, et al. 269 Tumoral and peripheral landscape of Viral- versus Carcinogen-Driven Head and Neck Cancer. Regular young investigator award abstracts (2020) 8:A165–A6. doi: 10.1136/jitc-2020-SITC2020.0269
79. Miles D, Roche H, Martin M, Perren TJ, Cameron DA, Glaspy J, et al. Phase III multicenter clinical trial of the sialyl-TN (STn)-keyhole limpet hemocyanin (KLH) vaccine for metastatic breast cancer. Oncologist (2011) 16(8):1092–100. doi: 10.1634/theoncologist.2010-0307
80. Giaccone G, Debruyne C, Felip E, Chapman PB, Grant SC, Millward M, et al. Phase III study of adjuvant vaccination with Bec2/bacille Calmette-Guerin in responding patients with limited-disease small-cell lung cancer (European Organisation for Research and Treatment of Cancer 08971-08971B; Silva Study). J Clin Oncol (2005) 23(28):6854–64. doi: 10.1200/JCO.2005.17.186
81. Sabbatini P, Harter P, Scambia G, Sehouli J, Meier W, Wimberger P, et al. Abagovomab as maintenance therapy in patients with epithelial ovarian cancer: a phase III trial of the AGO OVAR, COGI, GINECO, and GEICO–the MIMOSA study. J Clin Oncol (2013) 31(12):1554–61. doi: 10.1200/JCO.2012.46.4057
82. Yamaue H, Tsunoda T, Tani M, Miyazawa M, Yamao K, Mizuno N, et al. Randomized phase II/III clinical trial of elpamotide for patients with advanced pancreatic cancer: PEGASUS-PC Study. Cancer Sci (2015) 106(7):883–90. doi: 10.1111/cas.12674
83. Weller M, Butowski N, Tran DD, Recht LD, Lim M, Hirte H, et al. Rindopepimut with temozolomide for patients with newly diagnosed, EGFRvIII-expressing glioblastoma (ACT IV): a randomised, double-blind, international phase 3 trial. Lancet Oncol (2017) 18(10):1373–85. doi: 10.1016/S1470-2045(17)30517-X
84. Le Moignic A, Malard V, Benvegnu T, Lemiegre L, Berchel M, Jaffres PA, et al. Preclinical evaluation of mRNA trimannosylated lipopolyplexes as therapeutic cancer vaccines targeting dendritic cells. J Control Release. (2018) 278:110–21. doi: 10.1016/j.jconrel.2018.03.035
85. Haabeth OAW, Blake TR, McKinlay CJ, Waymouth RM, Wender PA, Levy R. mRNA vaccination with charge-altering releasable transporters elicits human T cell responses and cures established tumors in mice. Proc Natl Acad Sci U S A. (2018) 115(39):E9153–E61. doi: 10.1073/pnas.1810002115
86. Pal A, Kundu R. Human papillomavirus E6 and E7: the cervical cancer hallmarks and targets for therapy. Front Microbiol (2019) 10:3116. doi: 10.3389/fmicb.2019.03116
87. Sahin U, Derhovanessian E, Miller M, Kloke BP, Simon P, Lower M, et al. Personalized RNA mutanome vaccines mobilize poly-specific therapeutic immunity against cancer. Nature (2017) 547(7662):222–6. doi: 10.1038/nature23003
88. Lang F, Schrors B, Lower M, Tureci O, Sahin U. Identification of neoantigens for individualized therapeutic cancer vaccines. Nat Rev Drug Discovery (2022) 21(4):261–82. doi: 10.1038/s41573-021-00387-y
89. Cheng EM, Tsarovsky NW, Sondel PM, Rakhmilevich AL. Interleukin-12 as an in situ cancer vaccine component: a review. Cancer Immunol Immunother. (2022) 71(9):2057–65. doi: 10.1007/s00262-022-03144-1
90. Xu X, Sun Q, Mei Y, Liu Y, Zhao L. Newcastle disease virus co-expressing interleukin 7 and interleukin 15 modified tumor cells as a vaccine for cancer immunotherapy. Cancer Sci (2018) 109(2):279–88. doi: 10.1111/cas.13468
91. Lian T, Hao X, Li J, Wang H, Li C. B7-1 and GM-CSF enhance the anti-tumor immune effect of DC-tumor fusion vaccine in the treatment of prostate cancer. Med Oncol (2020) 37(11):107. doi: 10.1007/s12032-020-01433-2
92. Lapenta C, Gabriele L, Santini SM. IFN-alpha-mediated differentiation of dendritic cells for cancer immunotherapy: advances and perspectives. Vaccines (Basel). (2020) 8(4):617. doi: 10.3390/vaccines8040617
93. Deng Z, Yang H, Tian Y, Liu Z, Sun F, Yang P. An OX40L mRNA vaccine inhibits the growth of hepatocellular carcinoma. Front Oncol (2022) 12:975408. doi: 10.3389/fonc.2022.975408
94. Oniki S, Nagai H, Horikawa T, Furukawa J, Belladonna ML, Yoshimoto T, et al. Interleukin-23 and interleukin-27 exert quite different antitumor and vaccine effects on poorly immunogenic melanoma. Cancer Res (2006) 66(12):6395–404. doi: 10.1158/0008-5472.CAN-05-4087
95. Le N, Luk I, Chisanga D, Shi W, Pang L, Scholz G, et al. IL-36G promotes cancer-cell intrinsic hallmarks in human gastric cancer cells. Cytokine (2022) 155:155887. doi: 10.1016/j.cyto.2022.155887
96. Lee SE, Shin AR, Sohn HJ, Cho HI, Kim TG. T cells modified with CD70 as an alternative cellular vaccine for antitumor immunity. Cancer Res Treat (2020) 52(3):747–63. doi: 10.4143/crt.2019.721
97. Shetab Boushehri MA, Lamprecht A. TLR4-based immunotherapeutics in cancer: A review of the achievements and shortcomings. Mol Pharm (2018) 15(11):4777–800. doi: 10.1021/acs.molpharmaceut.8b00691
98. Bauer TM, Jimeno A, Wang D, LoRusso P, Tu Do K, Stemmer SM. Patel MReaApIsom-, a lipid nanoparticle encapsulating mRNAs encoding human OX40L, IL-23, and IL-36γ, for intratumoral (iTu) injection alone and in combination with durvalumab. J Clin Oncol (2020) 38:3092–2. doi: 10.1200/JCO.2020.38.15_suppl.3092
99. Turnham DJ, Bullock N, Dass MS, Staffurth JN, Pearson HB. The PTEN conundrum: how to target PTEN-deficient prostate cancer. Cells (2020) 9(11):2342. doi: 10.3390/cells9112342
100. Hu J, Cao J, Topatana W, Juengpanich S, Li S, Zhang B, et al. Targeting mutant p53 for cancer therapy: direct and indirect strategies. J Hematol Oncol (2021) 14(1):157. doi: 10.1186/s13045-021-01169-0
101. Berd D, Mastrangelo MJ. Effect of low dose cyclophosphamide on the immune system of cancer patients: depletion of CD4+, 2H4+ suppressor-inducer T-cells. Cancer Res (1988) 48(6):1671–5.
102. Piper M, Hoen M, Darragh LB, Knitz MW, Nguyen D, Gadwa J, et al. Simultaneous targeting of PD-1 and IL-2Rbetagamma with radiation therapy inhibits pancreatic cancer growth and metastasis. Cancer Cell (2023) 41(5):950–69 e6. doi: 10.1016/j.ccell.2023.04.001
103. Samnuan K, Blakney AK, McKay PF, Shattock RJ. Design-of-experiments in vitro transcription yield optimization of selfAmplifying RNA. BioRxiv (2021). doi: 10.1101/2021.01.08.425833v1
104. Dousis A, Ravichandran K, Hobert EM, Moore MJ, Rabideau AE. An engineered T7 RNA polymerase that produces mRNA free of immunostimulatory byproducts. Nat Biotechnol (2023) 41(4):560–8. doi: 10.1038/s41587-022-01525-6
105. Xia H, Yu B, Jiang Y, Cheng R, Lu X, Wu H, et al. Psychrophilic phage VSW-3 RNA polymerase reduces both terminal and full-length dsRNA byproducts in in vitro transcription. RNA Biol (2022) 19(1):1130–42. doi: 10.1080/15476286.2022.2139113
106. Qin S, Tang X, Chen Y, Chen K, Fan N, Xiao W, et al. mRNA-based therapeutics: powerful and versatile tools to combat diseases. Signal Transduct Target Ther (2022) 7(1):166. doi: 10.1038/s41392-022-01007-w
107. Liu D, An C, Bai Y, Li K, Liu J, Wang Q, et al. A novel single-stranded RNA-based adjuvant improves the immunogenicity of the SARS-coV-2 recombinant protein vaccine. Viruses (2022) 14(9):1854. doi: 10.3390/v14091854
108. Miao L, Li L, Huang Y, Delcassian D, Chahal J, Han J, et al. Delivery of mRNA vaccines with heterocyclic lipids increases anti-tumor efficacy by STING-mediated immune cell activation. Nat Biotechnol (2019) 37(10):1174–85. doi: 10.1038/s41587-019-0247-3
109. National Medical Products Administration. (2023). Available at: https://www.nmpa.gov.cn/yaopin/ypggtg/20200814230916157.html (Accessed July 13, 2023).
110. US Pharmacopeia. (2023). Available at: https://www.uspnf.com/sites/default/files/usp_pdf/EN/USPNF/usp-nfnotices/gc-xxx-analytical-procedures-mRNA-vaccines.pdf (Accessed July 13, 2023).
111. World Health Organization . Available at: https://www.who.int/docs/default-source/biologicals/ecbs/reg-considerations-on-rna-vaccines_1st-draft_pc_tz_22122020.pdf?sfvrsn=c13e1e20_3 (Accessed July 13, 2023).
112. COVID-19 Vaccine Tracker (2023). Available at: https://covid19.trackvaccines.org/ (Accessed July 13, 2023).
113. World Health Organization. (2023). Available at: https://www.who.int/news/item/09-07-2021-gacvs-guidance-myocarditis-pericarditis-covid-19-mrna-vaccines (Accessed July 13, 2023).
114. Science (2023). Available at: https://www.science.org/content/article/suspicions-grow-nanoparticles-pfizer-s-covid-19-vaccine-trigger-rare-allergic-reactions (Accessed July 13, 2023).
115. Santiago TC, Purvis IJ, Bettany AJ, Brown AJ. The relationship between mRNA stability and length in Saccharomyces cerevisiae. Nucleic Acids Res (1986) 14(21):8347–60. doi: 10.1093/nar/14.21.8347
116. Daniel S, Kis Z, Kontoravdi C, Shah N. Quality by Design for enabling RNA platform production processes. Trends Biotechnol (2022) 40(10):1213–28. doi: 10.1016/j.tibtech.2022.03.012
117. U.S. Pharmacopeia / National Formulary (2023). Available at: https://www.uspnf.com/sites/default/files/usp_pdf/EN/USPNF/usp-nf-notices/gc-1220-pre-post-20210924.pdf (Accessed July 13, 2023).
118. International Conference on Harmonization (2023). Available at: https://database.ich.org/sites/default/files/ICH_Q14_Document_Step2_Guideline_2022_0324.pdf (Accessed July 13, 2023).
Keywords: cancer vaccine, amplifying mRNA, tumor-specific antigen, tumor associated antigen, in vitro transcription
Citation: Hu C, Liu J, Cheng F, Bai Y, Mao Q, Xu M and Liang Z (2023) Amplifying mRNA vaccines: potential versatile magicians for oncotherapy. Front. Immunol. 14:1261243. doi: 10.3389/fimmu.2023.1261243
Received: 19 July 2023; Accepted: 10 October 2023;
Published: 23 October 2023.
Edited by:
Susu M. Zughaier, Qatar University, QatarReviewed by:
Sharon Vijayanand, Mercer University, United StatesCopyright © 2023 Hu, Liu, Cheng, Bai, Mao, Xu and Liang. This is an open-access article distributed under the terms of the Creative Commons Attribution License (CC BY). The use, distribution or reproduction in other forums is permitted, provided the original author(s) and the copyright owner(s) are credited and that the original publication in this journal is cited, in accordance with accepted academic practice. No use, distribution or reproduction is permitted which does not comply with these terms.
*Correspondence: Qunying Mao, bWFvcXVueWluZ0AxMjYuY29t; Miao Xu, eHVtaWFvYmpAMTI2LmNvbQ==; Zhenglun Liang, bHpoZW5nbHVuQDEyNi5jb20=
†These authors have contributed equally to this work and share first authorship
Disclaimer: All claims expressed in this article are solely those of the authors and do not necessarily represent those of their affiliated organizations, or those of the publisher, the editors and the reviewers. Any product that may be evaluated in this article or claim that may be made by its manufacturer is not guaranteed or endorsed by the publisher.
Research integrity at Frontiers
Learn more about the work of our research integrity team to safeguard the quality of each article we publish.