- 1Zunyi Medical University, Zunyi, China
- 2Department of Burn Surgery, The First People’s Hospital of Foshan, Foshan, China
- 3Department of Burn Surgery, The First Affiliated Hospital of Sun Yat-Sen University, Guangzhou, China
- 4Department of Burn and Plastic Surgery, Guangzhou First People’s Hospital, South China University of Technology, Guangzhou, China
Wound repair is a complex problem for both clinical practitioners and scientific investigators. Conventional approaches to wound repair have been associated with several limitations, including prolonged treatment duration, high treatment expenses, and significant economic and psychological strain on patients. Consequently, there is a pressing demand for more efficacious and secure treatment modalities to enhance the existing treatment landscapes. In the field of wound repair, cell-free therapy, particularly the use of mesenchymal stem cell-derived exosomes (MSC-Exos), has made notable advancements in recent years. Exosomes, which are small lipid bilayer vesicles discharged by MSCs, harbor bioactive constituents such as proteins, lipids, microRNA (miRNA), and messenger RNA (mRNA). These constituents facilitate material transfer and information exchange between the cells, thereby regulating their biological functions. This article presents a comprehensive survey of the function and mechanisms of MSC-Exos in the context of wound healing, emphasizing their beneficial impact on each phase of the process, including the regulation of the immune response, inhibition of inflammation, promotion of angiogenesis, advancement of cell proliferation and migration, and reduction of scar formation.
1 Introduction
The skin is the largest multifunctional organ of the human body and serves as a protective barrier against pathogenic invasion as well as chemical and physical assault from the external environment (1–3). Skin trauma resulting from thermal injury or radiation exposure can disrupt structural integrity and internal homeostasis of the skin, leading to wound development. Although the skin possesses inherent self-repair capabilities (4), the wound healing process may occasionally be aberrant, leading to the development of chronic wounds or hyperplastic scars/keloids. Conventional wound management techniques include the use of antibiotics, dressing alterations, negative-pressure wound therapy, debridement, skin grafting and flap transplantation, laser therapy, biological scaffolds, topical administration of specific growth factors, and gene therapy. However, these interventions are associated with potential adverse effects, such as atrophic scarring, abnormal pigmentation, prolonged healing duration, immune rejection, elevated expenses, and increased susceptibility to infection (5).
Recently, stem cell therapy has garnered significant interest because of the pluripotency, self-renewal ability, and capacity of stem cells to stimulate the secretion of regenerative cytokines. Mesenchymal stem cells (MSCs) are pluripotent stem cells derived from the bone marrow and other tissues. As important members of the stem cell family, MSCs can differentiate into various lineages, such as bone, cartilage, and fat. Its positive effects on wound healing have been widely demonstrated. However, in the practical application of stem cell therapy, several limitations have surfaced, including ethical dilemmas, issues pertaining to the source of stem cells, heterogeneity, long-term survival, directional differentiation and proliferation, low migration rates, and chemotaxis rates of stem cells toward the site of injury (6). Furthermore, concerns have been raised regarding abnormal differentiation, immune rejection, teratogenesis, tumorigenesis, and other biosafety issues (7, 8) as well as challenges related to the storage and transportation of stem cells (9).
Interestingly, certain studies have indicated that the biological effects of MSCs can be replicated in conditioned media (10). Subsequent research validated that the modus operandi of MSC therapy encompasses paracrine effects facilitated by MSCs (11–13). Exosomes, which are nanoparticles ranging 30–120nm in diameter enveloped by a bilayer phospholipid membrane, are secreted by biological cells. Initially perceived as mere carriers of cellular waste, numerous studies have established their crucial role in intercellular communication (14). In contrast to stem cell therapy, exosomes offer distinct advantages, including multifaceted biotherapeutic effects, efficient delivery of exogenous cargo, superior biocompatibility, and enhanced safety (15). Furthermore, exosomes exhibit stability and convenience in storage, do not elicit immune system rejection, possess homing ability, and offer ease of dosage regulation (16, 17). Most importantly, extensive studies have shown that mesenchymal stem cell-derived exosomes (MSC-Exos) exert positive effects at all stages of wound healing.
Therefore, this article aimed to review the mechanism of action of the three main types of MSC-Exos in each step of wound healing. Additionally, we discuss the current status of the clinical application of MSC-Exos in wound healing.
2 Overview of exosomes
2.1 Exosomes and extracellular vesicles
The term “exosome” is commonly employed to refer to all vesicles discharged into the Extracellular milieu by diverse cells; however, it is important to note that exosomes are exclusively a subset of EVs. EVs are membrane vesicles that are released from both the interior and exterior of cells, characterized by a phospholipid bilayer structure and a diameter ranging 50 nm to 5 μm (18). EVs exhibit a high degree of heterogeneity and are capable of being secreted by a diverse range of cell types, including T cells, B cells, dendritic cells, platelets, mast cells, epithelial cells, endothelial cells, neurons, cancer cells, oligodendrocytes, Schwann cells, embryonic cells, and MSCs (19). EVs were first discovered in 1983 during the maturation of reticulocytes (20), and are significant indicators of intercellular communication within multicellular organisms (19). They play crucial roles in both normal physiological processes and in the development of diseases (21), including but not limited to material transmission, signal transduction, cell survival, apoptosis, and cell proliferation. Based on their source and dimensions, generalized EVs can be classified into three categories: Apoptotic bodies (with a diameter ranging 100 nm to 5 μm), Microvesicles (with a diameter ranging 50 nm to 1 μm), and Exosomes (with a diameter ranging 50–150 nm) (22). Apoptotic bodies emerge from the plasma membrane of apoptotic cells and typically encompass organelles and DNA fragments derived from parent cells (23). Microvesicles are extruded from the cell membrane and discharged into the extracellular milieu predominantly through a “budding” mechanism (24). Exosomes are vesicles that are secreted by the plasma membrane and undergo invagination and inward budding (25), ultimately displaying a distinctive cup-shaped morphology characterized by the expression of CD9, CD63, CD81, ALG-2-interacting protein X (ALIX), tumor susceptibility gene 101 (TSG101), heat shock cognate 71 kDa protein (Hsc70), and major histocompatibility complex (MHC) class II markers (26, 27). However, in numerous studies, no clear distinction is made between “exosomes” and “EVs,” and the two terms are often used interchangeably.
2.2 Composition of exosomes
The term “exosome” was coined by Johnstone et al. in 1987 (28). The exosomes were cup-shaped, with a suspension density ranged 1.10–1.21 g/ml (29). Various specific proteins such as membrane transport and fusion proteins (guanosine triphosphatase [GTPases], annexins, and flotillin) are used as markers on the surface of exosomes. Proteins (ALIX and TSG101) are involved in the biogenesis of multivesicular bodies (MVBs), four-molecule transmembrane proteins (CD9, CD63, and CD81), and heat shock proteins (Hsps, Hsp70, and Hsp90) (30–32).
Exosomes contain a diverse array of microRNAs (miRNAs), proteins, cytokines, and lipids that facilitate intercellular communication, enabling the transmission of vital biological information between neighboring and remote cells (33, 34). The lipids present in the samples consisted of cholesterol, sphingomyelin, ganglioside GM3, and externalized phosphatidylserine. The nucleic acids detected included messenger RNA (mRNAs), transfer RNA (tRNA), long non-coding RNA (lncRNA), mitochondrial DNA (mtDNA), and miRNA (35, 36).
2.3 Biogenesis of exosomes
The generation of exosomes consists of three main stages: (1) invagination of the plasma membrane to form endocytic vesicles and (2) inward germination of the endosomal membrane to form (MVBs) or early exosomes, leading to a gradual accumulation of luminal vesicles (ILVs) that engulf cytoplasmic components within MVBs. In addition, proteins are incorporated into invaginated membranes. During this process, proteins, mRNAs, and ncRNAs are encapsulated within luminal ILVs (37, 38). (3) In addition to some MVBS entering the lysosome and being degraded, most MVBS fuse with the plasma membrane together with early exosomes and secrete cystic contents to form exosomes (39–43). Among these, the soluble N-ethylmaleimide-sensitive factor attachment protein receptor (SNAREs) complex and endosomal sorting complex (ESCRT) are involved in exosome formation (43–45). The ESCRT consists of ALIX, ESCRT-II, charged multivesicular protein 2A (CHMP2A), CHMP4A/B/C, and vacuolar protein sorting 4 (VPS4) (46). The anterograde and retrograde protein sorting steps between the Golgi and plasma membranes are implemented by v-SNAREs and t-SNAREs (47). Three distinct mechanisms of intercellular communication are mediated by exosomes: (1) upon phagocytosis, exosomes release “messenger substances” into the cytoplasm and subsequently reform vesicles within the recipient cells; (2) following fusion with the plasma membrane, “messenger substances” are released into the cytoplasm; and (3) the ligand on the exosome binds to the receptor located on the target cell membrane (48–50). Exosomes or EVs undergo endocytosis and are internalized by recipient cells, subsequently releasing their contents through reverse fusion. The endocytic pathways available for this process are varied and include clathrin-mediated endocytosis and clathrin-independent pathways, such as caveolin-mediated uptake, macroendocytosis, phagocytosis, and lipid raft-mediated internalization (51) (Figure 1).
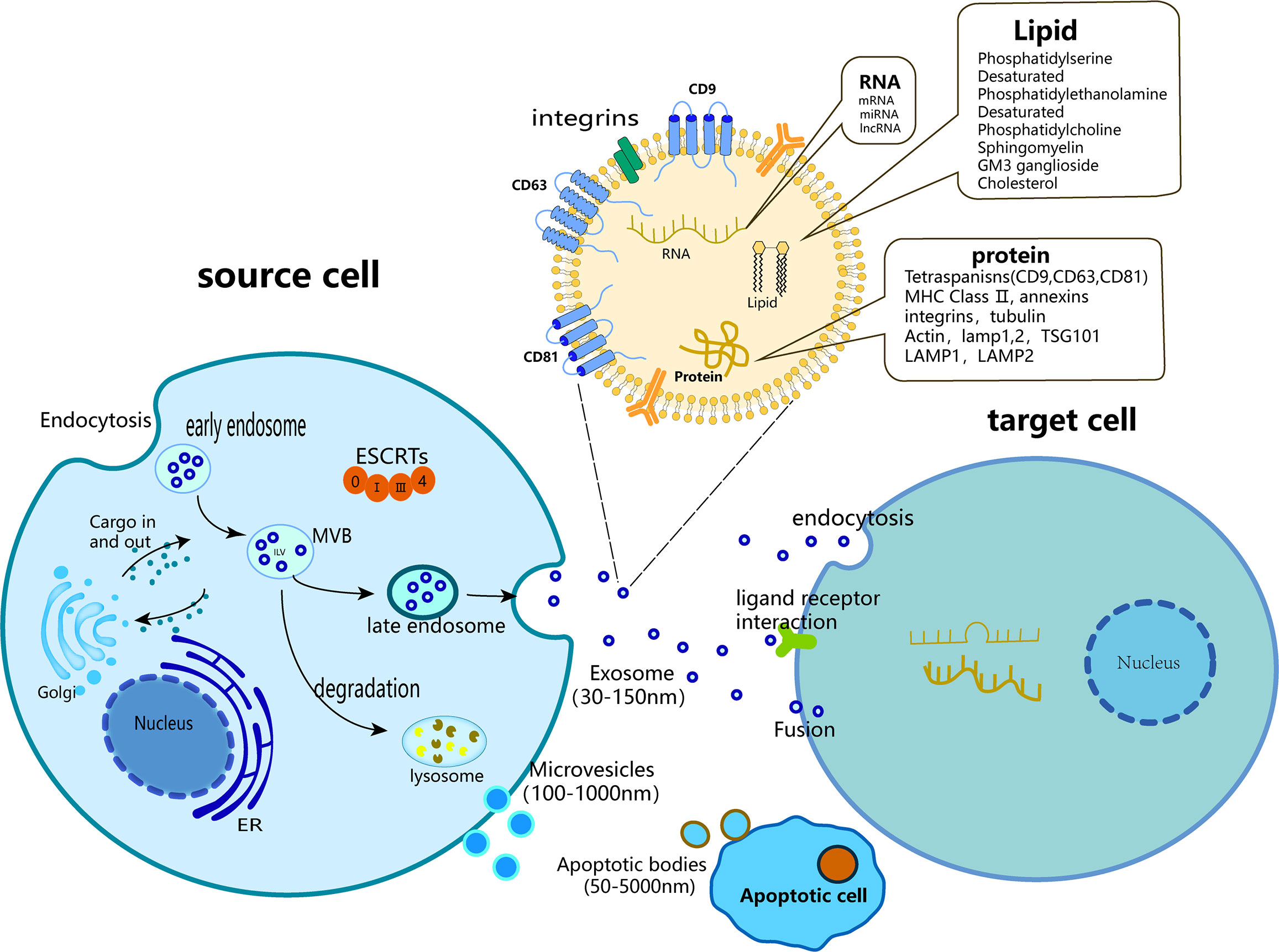
Figure 1 Biogenesis of three major subtypes of EVs including exosomes, microvesicles and apoptotic bodies.
2.4 Functions of exosomes
Exosomes, an emerging cell-free modality for wound management, possess several noteworthy attributes including resemblance to their cellular source, prolonged efficacy, facile transportability, minimal immunogenicity, controllable concentration, and adaptability to the surrounding microenvironment (39, 52–55). Exosomes represent a novel avenue for wound healing because they possess the ability to impede inflammation, stimulate angiogenesis, and facilitate cellular proliferation and migration (56–58).
During the initial stages of exploration, exosomes were believed to be the mechanism by which cells expel waste materials. Subsequent investigations revealed that exosomes play a crucial role in sustaining regular physiological functions, such as preserving progenitor cell function (59), upholding immune tolerance within the body (60), and regulating cell apoptosis through diverse signaling pathways (61). As research has progressed, it has become evident that exosomes are implicated in nearly all pathological and disease processes. Exosomes have demonstrated significant diagnostic and therapeutic potential in various clinical conditions, with a considerable body of research concentrating on their application in tumor treatment. Tumor cells secrete a substantial number of exosomes harboring miRNAs that can serve as noninvasive tumor markers for diagnosis. For instance, miRNAs present in exosomes, such as miR-21, miR-141, and miR-200, can be used as tumor markers for the detection of ovarian cancer (62). The use of miR-125a-3p as a diagnostic indicator for the detection of early-stage colorectal cancer is also feasible (63). The identification of specific biomarkers such as epidermal growth factor receptor (EGFR), epithelial cell adhesion molecule (EpCAM), mucin1 (MUC1), glypican (GPC1), and Wnt2 within exosomes has demonstrated their potential for the diagnosis and prognosis of pancreatic ductal adenocarcinoma (64). Exosomes have demonstrated significant potential for targeted drug delivery and as gene carriers in regenerative medicine (65, 66). They possess a robust loading capacity, are capable of accommodating biological macromolecules, short peptides, and small-molecule drugs (67), and can be used as natural carriers of mRNA, miRNA, and intercellular proteins (68). The modified exosomes also delivered the let-7 miR to EGFR-expressing breast cancer cells, thereby reducing the tumor burden in mice (69). Rabies virus glycoprotein peptide (RVG)-modified exosomes can deliver small interfering RNAs (siRNAs) to the neurons of the central nervous system to knock down α-Syn siRNA in the brain, thereby delaying the characteristic pathological process of Parkinson’s disease (PD) (70).
Wound healing encompasses a sequence of molecular and cellular events including angiogenesis, proliferation, cell migration, tissue remodeling, and extracellular matrix (ECM) deposition. Exosomes facilitate this process through intricate mechanisms. Owing to the protection of their lipid layer from proteolytic enzymes, exosomes can effectively transmit signals to target cells such as vascular endothelial cells, fibroblasts, and keratinocytes (71). MSC-Exos can activate signaling pathways in target cells, including transducer and activator of transcription 3 (STAT3), AKT, Wnt/β-catenin, and extracellular regulated protein kinase (ERK), which play important roles in wound healing (72). The activation of signaling pathways has been observed to augment the expression of various cytokines in target cells, including, but not limited to, interleukin-6 (IL-6), STAT3, hepatocyte growth factor (HGF), insulin-like growth factor-1 (IGF-1), and stromal cell-derived factor-1 (SDF-1). These cytokines have been reported to facilitate processes such as angiogenesis, cell migration, cell proliferation, and re-epithelialization (73).
The biological functions of distinct MSC-Exos in wound repair are similar, owing to the presence of comparable bioactive factors. Nonetheless, their unique biological characteristics are contingent on the expression of specific molecules (74). Bone marrow MSC-derived-exosomes (BMSC-Exos) exhibit biological stability, low immunogenicity, and robust proliferation and viability after transplantation, primarily targeting the promotion of proliferation. Umbilical cord-derived MSC-derived exosomes (UCMSC-Exos) can be noninvasively isolated, possess low immunogenicity, and demonstrate strong self-renewal and proliferation capabilities. However, limitations exist in maintaining biological activity and delivering clinical treatment. Adipose-derived MSC-derived exosomes (ADSC-Exos) are an abundant source that can be obtained with minimal invasiveness without inducing pain. These exosomes possess pluripotent and plastic properties, are amenable to storage, and are stable in blood or body fluids. Their primary effect is on angiogenesis during wound healing.
3 Wound healing
Skin wound healing is a continuous and intertwined physiological process that is also the response of the body to injuries such as trauma, burns, or diabetic ulcers (75). It includes hemostatic, inflammatory, proliferative, and remodeling phases (76). Inaccuracies in any of these procedures may impede wound healing, resulting in the development of chronic wounds or scarring (Figure 2).
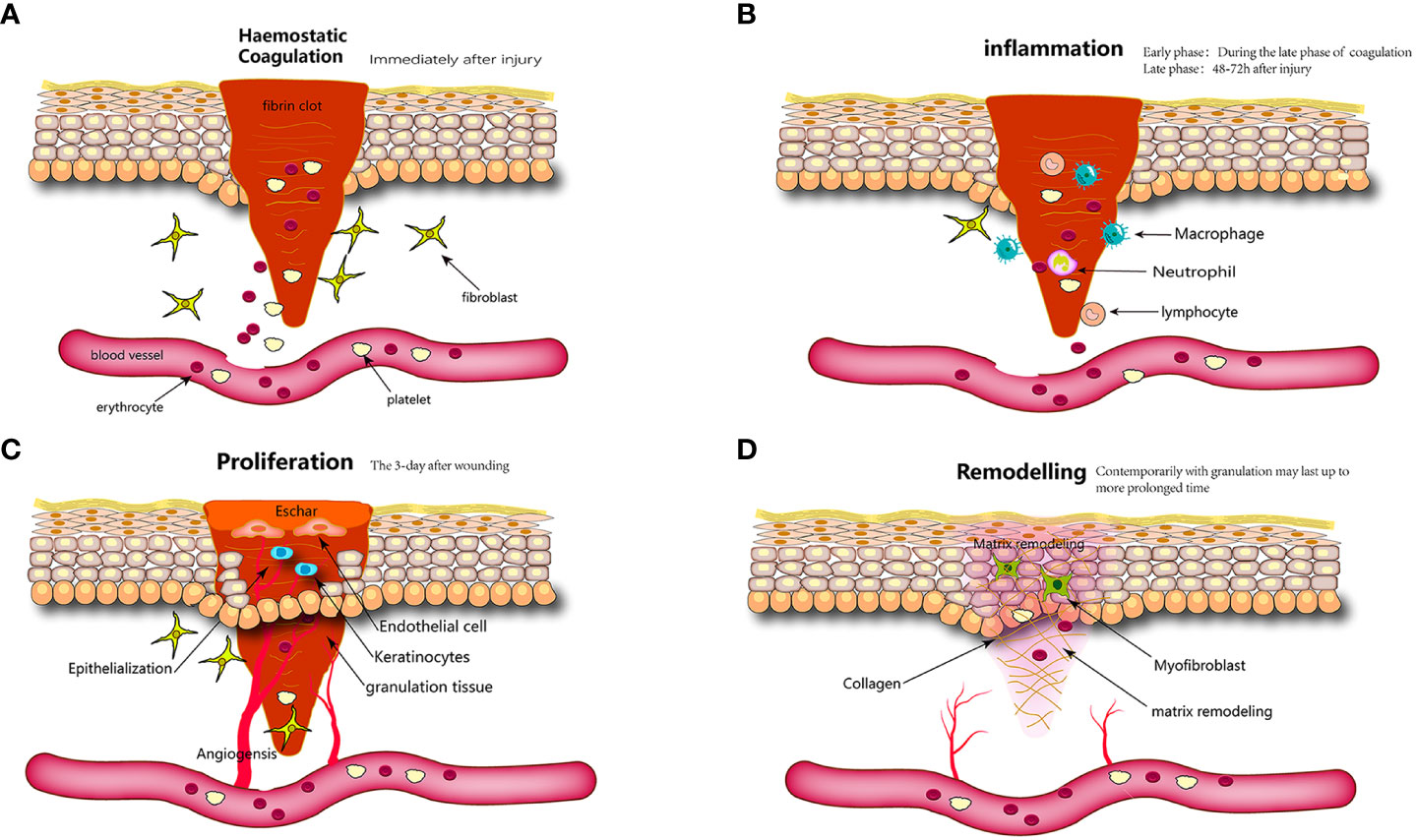
Figure 2 Wound healing is classically divided into four stages: (A) hemostasis, (B) inflammation, (C) proliferation, and (D) remodeling. Each stage is characterized by key molecular and cellular events and is coordinated by a host of secreted factors that are recognized and released by the cells of the wounding response.
3.1 Hemostatic phase
The initial stage of wound healing is hemostasis, which commences promptly following skin trauma and involves nearly simultaneous vasoconstriction and platelet aggregation (77, 78). Concurrently, injury elicits a direct response in the form of thromboxane and prostaglandin release from the cellular membrane (79). The latter induces the contraction of vascular smooth muscle cells in the local region, resulting in a reduction in blood flow through blood vessels (76). Trauma induces pathophysiological changes that result in endothelial disruption; basement membrane exposure; and subsequent contact between flowing blood, collagen, and tissue factors, ultimately leading to thrombosis (80). Platelets are integral to this process, as they secrete various biological cytokines, including chemokines that activate immune cells to migrate toward the site of injury, and growth factors that promote the proliferation of resident fibroblasts and keratinocytes. Furthermore, platelet-derived growth factor (PdGF) stimulate the repair of vascular walls by inducing the proliferation of smooth muscle and endothelial cells. Platelet toll-like receptors (TLRs) exhibit antibacterial properties. Tissue factors function as coagulation factors that initiate a cascade of coagulation events culminating in thrombin production. Thrombin facilitates the transformation of fibrinogen into fibrin, thereby promoting the formation of durable platelet clot (81). The clot, composed of collagen and platelets, is enmeshed within cross-linked fibrin fibers, thrombospondin, and fibronectin (FN). These clots serve the dual purposes of wound coverage and hemostasis, while also serving as substrates for the migration of resident skin and immune cells (82, 83). Upon completion of its hemostatic function, the clot formed within the body undergoes resolution through a fibrinolytic system (84).
3.2 Inflammatory phase
The second stage of wound healing involves the initiation of the inflammatory phase, which is primarily induced by chemokines/cytokines, bacterial byproducts, and platelet-derived mediators (77). This process eradicates deteriorating bacteria, eliminate debris and damaged matrix proteins, and facilitate the creation of a wound bed that supports the growth of new tissue (85–87). Immune cells play a central role in this process by controlling bleeding and stopping infection by direct activity or by releasing a range of soluble mediators (88).
3.2.1 Neutrophiles
Neutrophils represent the initial cellular responders that emerge at the site of injury and serve as the primary defense mechanism against bacterial pathogens (89). By the second day post injury, neutrophils comprise over 50% of all cells present in the wound (90). Additionally, activated neutrophils release cytokines to prolong and amplify neutrophil infiltration, thereby creating a positive feedback loop (91). Neutrophils release toxic particles, generate oxidative bursts, initiate phagocytosis, and produce neutrophil extracellular traps (NETs) to suppress infectious threats (92).
3.2.2 Monocytes/Macrophages
During the initial stages of inflammation, local and systemic defense responses are triggered at the wound site. Within a timeframe of 48–96 h post-injury, monocytes originating from nearby tissues and circulating in the bloodstream are recruited to the affected area and differentiate into macrophages. These activated macrophages play a crucial role in the synthesis of nitric oxide, facilitation of angiogenesis and fibroplasia, and transition from the inflammatory phase to the proliferative phase (93). Under physiological circumstances, circulating monocytes continuously survey the endothelial wall of the vascular lumen and detect potential damage. During the inflammatory phase of wound healing, macrophages have the ability to impede infection by discharging proinflammatory cytokines, namely IL-6, tumor necrosis factor (TNF)-α, and IL-1β. Simultaneously, the initial macrophages secrete monocyte chemoattractant protein (MCP)-1 to entice additional monocytes from the bone marrow and intensify the macrophage response. Macrophages perform crucial functions during the various stages of wound healing. During the proliferative phase, macrophages facilitate the delivery of cytokines, including vascular endothelial growth factor (VEGF) and platelet-derived growth factor (PDGF), to activate endothelial cells and promote angiogenesis. Furthermore, during the remodeling phase, macrophages aid in the clearance of cell debris and excessive ECM, thereby expediting skin wound healing (94). In summary, the significance of monocytes/macrophages in wound healing has been well established. Numerous studies have shown that macrophage deficiency in mice results in delayed wound healing (95, 96).
3.2.3 Lymphocytes
Lymphocytes are the cells that arrive at the site of injury. Within this population, γδ+ T cells have the capacity to generate various mediators, including IGF-1, fibroblast growth factors (FGFs), and KGFs, which facilitate the proliferation and viability of fibroblasts, immune cells, and keratinocytes. Furthermore, αβ+ T cells, a distinct subset of T cells, are also present during the inflammatory phase and contribute to the protection against pathogenic microorganisms (87, 97, 98). The inflammatory phase of wound healing involves three crucial types of inflammatory cells. However, their excessive presence can impede wound healing. Numerous studies have demonstrated that chronic wounds frequently exhibit a prolonged inflammatory phase compared to typical wounds (99–101). Subsequent investigations demonstrated that persistent inflammatory conditions impede the progression of wound repair (102). Several studies have indicated that the predominant inflammatory cells in chronic wounds are neutrophils and macrophages (103, 104).
3.3 Phase of proliferation
The onset of the proliferative phase commences on the third day post-injury and persists for approximately 2 weeks. During this phase, vascular endothelial cells, keratinocytes, and fibroblasts proliferate and migrate, leading to reepithelialization, angiogenesis, granulation tissue, ECM, and collagen deposition.
3.3.1 Re-epithelialization
Re-epithelialization is initiated in response to the activation of inflammatory cytokines (IL-1 and TNF-), which upregulate KGF gene expression in fibroblasts shortly after skin injury. This process is dependent on the migration of epithelial cells at the wound edge and accessory structures in the dermis. The migration and proliferation of epithelial cells persist until complete coverage of the wound is achieved and an intact epithelial barrier (105). Macrophages are significant contributors to epithelialization as they regulate the proliferation and migration of keratinocytes, fibroblasts, and endothelial cells, thereby promoting tissue regeneration and the production of substantial quantities of ECM (106).
3.3.2 Granulation tissue
The initial characterization and subsequent refinement of granulation tissue formation and evolution were attributed to English surgeon John Hunter and French surgeon Alexis Carrel, respectively. The ECM is composed of proteoglycans, hyaluronic acid, collagen, and elastin, which gradually form granulation tissue rather than a thrombus. Numerous cytokines and growth factors participate in this process, including the transforming growth factor-B family (TGF-b, encompassing TGF-b1, TGF-b2, and TGF-b3), IL family, and angiogenic factors. This phase can persist for several days or weeks (107). Activated fibroblasts are the primary contributors to the synthesis of a new ECM, wound contraction, and the provision of a scaffold for other cellular and component constituents, including newly synthesized ECM, neovascularization, and inflammatory cells. Ultimately, the process of wound remodeling culminates in the replacement of granulation tissue with normal connective tissue (87).
3.3.3 Angiogenesis
Upon the stimulation of TNF-β, the angiogenic process focuses on the migration of endothelial cells and the formation of capillaries, as well as the accumulation of a large number of cells and a large amount of connective tissue. Neovascularization is essential for maintaining the delivery of nutrients and a physiological steady state of oxygen (87, 108). The process of angiogenesis is intricately linked to activation of microvascular endothelial cells (ECs) in the local environment. In response to hypoxia-responsive growth factors such as VEGF and PDGF, ECs in the wound environment undergo a series of cellular events, including degradation of the ECM in granulation tissue, proliferation, migration, formation of new cell-cell junctions, and branching to generate new capillaries (109). The granulation and tissue deposition require capillaries to deliver nutrients, and capillary dysfunction is likely to lead to the formation of chronic wounds.
3.4 Remodeling
Remodeling, also known as maturation, is the ultimate phase of wound healing and is characterized by a gradual reduction in cellular and vascular components (87). Typically, this stage commences after the culmination of granulation tissue development and persists for an extended duration, typically ranging 21 d to 1 year post-injury (110). The remodeling phase is characterized by the deposition of collagen and the gradual formation of an organized organic network. Any deviation from this process, such as excessive collagen synthesis, can have a detrimental effect on the healing strength and may result in the formation of scars or keloids (111). The remodeling phase encompasses the degradation of new blood vessels, periodic deposition of ECM, and reconstruction of granulation tissue. Vascular remodeling is a key aspect of this phase, which involves cessation of capillary growth, decreased blood flow to the wound site, and reduction in the metabolic rate at the site of injury (112–114). Ultimately, most blood vessels, fibroblasts, and inflammatory cells disappear from the wound during the remodeling phase due to cell migration, apoptosis, or other unknown mechanisms of cell death (115).
3.4.1 Remodeling of the ECM
During fibrosis, myofibroblasts predominantly synthesize the ECM. These cells replace the initial fibrin clots with hyaluronic acid, FN, and proteoglycans, ultimately resulting in the formation of mature collagen fibers during the later stages of tissue repair (112, 116, 117). The ECM undergoes reorganization, degradation, and resynthesis to attain optimal tensile strength. Compared to native tissue, collagen fibers can restore approximately 80% of their initial reparative strength, with the duration of this process being a critical determinant of the ultimate strength achieved. Notably, the original strength of the tissue cannot be fully regained (113, 118).
3.4.2 Granulation tissue remodeling
Wound healing involves the gradual transformation of granulation tissue into scar tissue, which is characterized by decreased cellular and vascular densities and a significant increase in collagen fiber concentration. The initial composition of granulation tissue is primarily collagen type III (COL-3); however, during the remodeling phase, a portion of the tissue is replaced by COL-1, resulting in a marked improvement in the tensile strength of the resulting scar (119). Subsequently, the concurrent processes of collagen type I and type III (COL-1 and COL-3) breakdown take place (87, 116). In subsequent phases, fibroblasts present in the granulation tissue undergo phenotypic transformation into myofibroblasts, which is accompanied by the temporary expression of smooth muscle actin (120).
4 Mechanisms of the effect of MSC-Exos on wound healing
Skin wound healing is a multifaceted and systematic reparative process that encompasses inflammation, cellular proliferation and migration, angiogenesis, and ECM deposition and remodeling. Numerous studies have demonstrated beneficial effects of MSC-Exos on all facets of wound healing. This review aimed to elucidate the mechanisms underlying the effects of three primary exosomes derived from MSCs on wound healing.
4.1 BMSC-Exos
BMSCs were the earliest isolated MSCs, and have been extensively studied and used in stem cell therapy. Recent studies have highlighted the positive effect of BMSC-Exos on all facets of wound healing, resulting in accelerated healing (Figure 3).
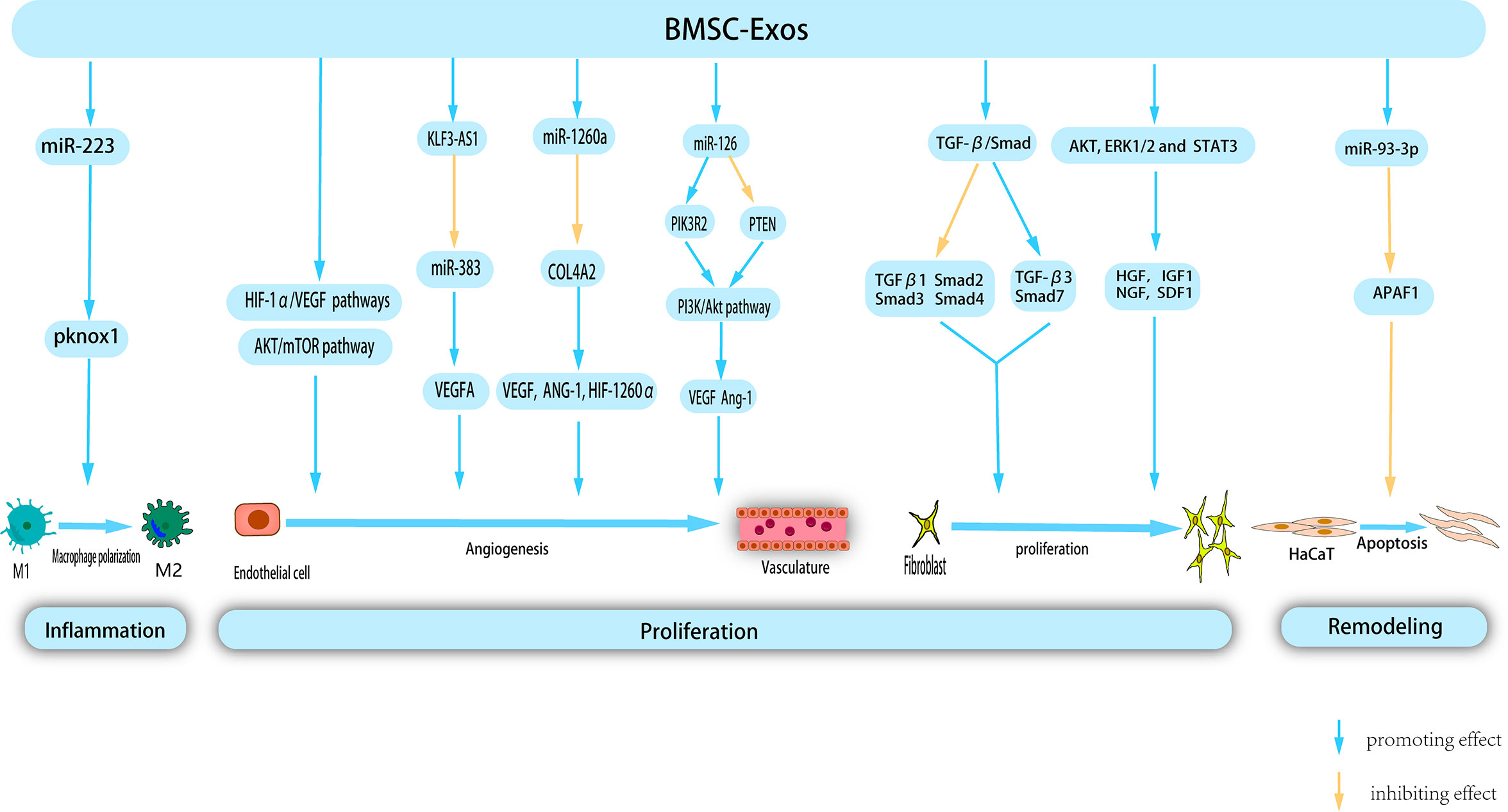
Figure 3 BMSC-Exos exert therapeutic effects on wounds through multiple mechanism. BMSC-Exos possess certain nucleic acids, which enable them to modulate macrophage polarization, thereby inhibiting inflammation. Additionally, during the proliferation phase, BMSC-Exos promote angiogenesis, and proliferation and migration of fibroblast. Morover, BMSC-Exos inhibit Apoptosis of HaCat in remodeling phase, thereby providing a novel approach to the treatment of cutaneous wounds.
4.1.1 Anti-inflammatory effect
Administration of BMSC-Exos expedites polarization of M2 macrophages during the inflammatory phase of wound healing, resulting in a reduced duration of the inflammatory process. Luo et al. conducted a study wherein they observed that intramuscular injection of BMSC-Exos following muscle contusion led to a decrease in inflammation levels. This has been attributed to the promotion of M2 macrophage polarization and anti-inflammatory factor expression, as well as a decrease in the production of inflammatory cytokines within the inflammatory microenvironment (121). Another study proposed that systemic administration of BMSC can facilitate their migration to the wound site and induce the polarization of macrophages toward the M2 phenotype, consequently enhancing the process of wound healing. Subsequent research has shown that miR-223 derived from BMSC-Exos targets pknox1 to regulate macrophage polarization (122). The etiology of chronic diabetic wound injury is primarily attributed to persistent inflammatory response, weakened angiogenesis, low immune function, and bacterial proliferation. Geng et al. developed a novel hydrogel (MSC-Exos@CEC-DCMC HG) composed of carboxyethyl chitosan (CEC) and dialdehyde carboxymethyl cellulose (DCMC) that incorporated BMSC-Exos to treat chronic diabetic wounds. A previous study revealed that BMSC-Exos facilitate the conversion of M1 to M2 macrophages, mitigate the adverse effects of inflammation, and consequently regulate the inflammatory microenvironment of the wound (123). In a study conducted by Shi et al., the group treated with BMSC-Exos exhibited a higher count of M2 macrophages and a greater presence of anti-inflammatory factors in the wound area. Conversely, the occurrence of apoptosis, M1 macrophages, and associated pro-inflammatory factors was shown to be diminished compared to the control group (124).
4.1.2 Promoting angiogenesis
Under hypoxic stimulation, BMSC released a significant quantity of BMSC-EXOs. These exosomes can be internalized by venous endothelial cells, subsequently stimulating in vitro proliferation, migration, and tube formation (125). Hypoxia-inducible factor-1α (HIF-1α) is a key regulator of angiogenesis (126). Zhang et al. (127) confirmed that the activation of the HIF-1α/VEGF signaling pathway by BMSC-Exos promotes angiogenesis and facilitates wound healing. VEGFA, a crucial regulator of angiogenesis, plays a significant role in this process. Furthermore, lncRNAs, which do not encode proteins, but regulate cellular behavior, are essential components of BMSC-derived exosomes. Evidence has shown that KLF3-AS1, carried by BMSC-Exos, promotes VEGFA signaling and wound healing in diabetes by suppressing miR-383 (128). VEGF promotes angiogenesis. microRNA-126 (miR-126), an endothelium-specific miRNA derived from BMSC-Exos that contributes to vascular homeostasis and angiogenesis. Zhang et al. demonstrated that Exo-miR-126 facilitates angiogenesis in HUVECs by activating the PI3K/AKT signaling pathway by targeting phosphatidylinositol 3 kinase regulatory subunit 2 (PIK3R2) and upregulating the gene expression of angiogenesis-related VEGF and Ang-1. Furthermore, in vivo experiments confirmed that Exo-miR-126 application significantly enhanced the formation of new capillaries at the wound site and promoted wound healing (129).
BMSC-Exos have been widely shown to activate multiple signaling pathways to stimulate angiogenesis. Liang et al. demonstrated that low-dose dimethylglyoxyglycine-pretreated BMSC-Exos (DMOG-MSC-Exos) exerted advanced proangiogenic properties by activating the AKT/mTOR pathway in HUVECs (130). Ding et al. (131) demonstrated that exosomes from bone marrow MSCs pretreated with deferoxamine (DFO-Exos) significantly enhanced the angiogenic potential of human umbilical vein endothelial cells (HUVECs) by activating the PI3K/AKT signaling pathway through miR-126-mediated PTEN downregulation in diabetic rats. These findings suggest that DFO-Exos may serve as a promising therapeutic agent for promoting wound healing and angiogenesis in rats with STZ-induced diabetes. Additional research has demonstrated that pretreatment may augment the pro-angiogenic potential of BMSC-Exos. Wu et al. demonstrated that the use of magnetic nanoparticles and a static magnetic field (SMF) as a pretreatment method can augment the tissue regeneration potential of BMSC-Exos. Compared with BMSC-Exos, the administration of low-dose Fe3O4 nanoparticles in conjunction with SMF (BMSC-Fe3O4-SMF-Exos) resulted in an amplified angiogenic capacity. This effect is attributed to the presence of miR-1260a, which is highly concentrated in exosomes, and its ability to suppress collagen type IV A2 (COL-4A2) while concurrently upregulating the expression of genes associated with angiogenesis (VEGF, ANG-1, and HIF-1260α) (132).
4.1.3 Promoting proliferation
BMSC-Exos can effectively stimulate the proliferation of human keratinocytes (HaCaT) and human dermal fibroblasts (HDFs) by downregulating the expression of TGFβ1, suppressor of mothers against decapentaplegic 2 (Smad2), Smad3, and Smad4 and upregulating the expression of TGF-β3 and Smad7 in the TGF-β/Smad signaling pathway. Therefore, it promoted skin wound healing (133). The role of Apoptosis Peptidase Activating Factor 1 (APAF1) is primarily associated with mitochondrial apoptosis pathway signal transduction. Overexpression of APAF1 has been observed to significantly decreased HaCaT cell viability and migration. Shen et al. demonstrated that miR-93-3p derived from BMSC-Exos can restore cellular function and inhibit apoptosis in epithelial HaCaT cells by deactivating APAF1 (134). Shabbir et al. (135)provided evidence that BMSC-Exos activate significant signaling cascades (AKT, ERK1/2, and STAT3) in recipient cells, thereby inducing the expression of growth factors, including HGF, IGF1, nerve growth factor (NGF), and SDF1, which ultimately facilitates wound healing.
These findings provide a theoretical foundation for the practical implementation of BMSC-Exos in clinical settings. It is postulated that through additional investigation of its mechanism, BMSC-Exos may emerge as a novel therapeutic intervention for wound healing.
4.2 UCMSC-Exos
Umbilical cord MSCs (UCMSCs) are a versatile type of stem cell that are present in the neonatal umbilical cord tissue and have significant potential for clinical applications owing to their abundant availability. Unlike other MSC-EXOs, UCMSC-Exos exhibit low immunogenicity and are devoid of tumorigenicity. Notably, UCMSC-Exos have been extensively employed in regenerative medicine and the management of diverse ailments owing to their noninvasive procurement, robust proliferative capacity, and low immunogenicity (136) (Figure 4).
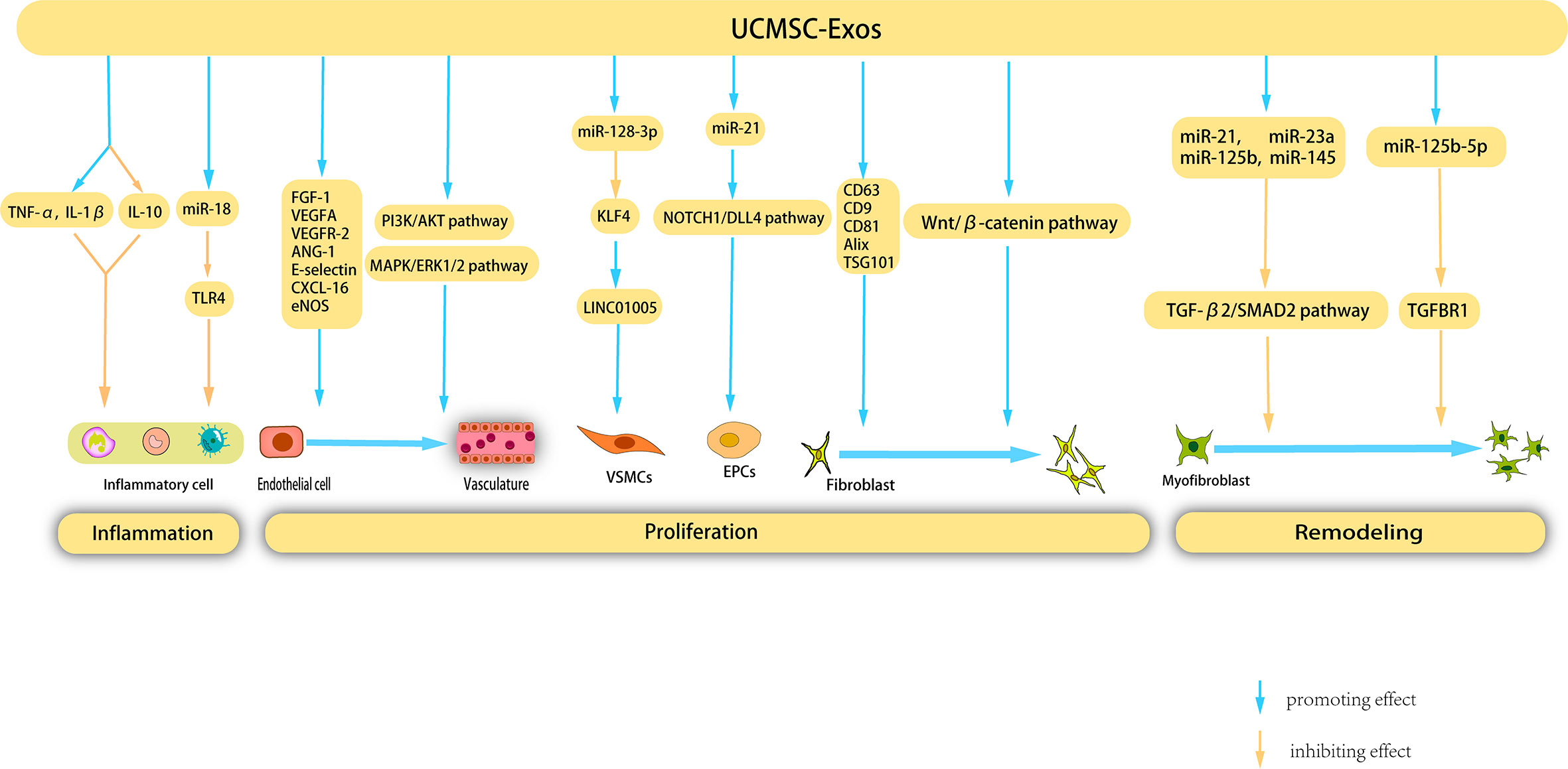
Figure 4 Mechanisms underlying the therapeutic effects of UCMSC-Exos on cutaneous wound healing. UCMSC-Exos contain specific cytokines and cucleic acids that facilitate the suppression of inflammatory cells, ultimately inhibiting inflammation. Furthermore, during the proliferation stage, UCMSC-Exos stimulate angiogenesis, as well as the proliferation and migration of fibroblasts, VSMCs, and EPCs. Additionally, UCMSC-Exos impede the proliferation of myofibroblast during the remodeling phase, presenting a novel therapeutic strategy for the management of cutaneous wounds.
4.2.1 Anti-inflammatory effect
Previous studies have shown that UCMSC-Exos inhibit inflammation through various pathways, thereby promoting wound healing. Li et al. showed that burn significantly increased the inflammatory response of rats or macrophages exposed to lipopolysaccharide (LPS), and UCMSC-Exos reversed this response by increasing the levels of TNF-α and IL-1β and decreasing the level of IL-10. Further studies have shown that miR-181c in UCMSC-Exos reduces burn-induced inflammation by downregulating the TLR4 signaling pathway, thereby accelerating wound healing (137). Wang et al. investigated the therapeutic potential of UCMSC-Exos in the treatment of eczema using a mouse model. These findings revealed that UCMSC-Exos inhibit the proliferation of peripheral blood mononuclear cells, facilitate the differentiation of Regulatory T cells (Tregs), reduce the infiltration of lymphocytes, and significantly enhance the rate of wound closure (138).
4.2.2 Angiogenesis promotion
UCMSC-Exos facilitate angiogenesis during wound healing through various mechanisms. Similar to BMSC-derived exosomes, UCMSC-Exos upregulate VEGF expression. Evidence has shown that UCMSC-Exos induce upregulation of VEGF and HIF-1α expression, thereby promoting angiogenesis in a rat model. The efficacy of HIF-1α in enhancing UCMSC-Exo-induced VEGF expression and promoting angiogenesis was confirmed using specific RNA inhibitors or siRNAs (139). Qu et al. injected UCMSC-Exos into a vein transplantation rat model and confirmed that UCMSC-Exos can accelerate re-endothelialization of the vein graft, reduce intimal hyperplasia of the vein graft, and enhance the proliferation and migration of cells in vitro by activating the PI3K/AKT and MAPK/ERK1/2 signaling pathways, in which VEGF plays an important role (140).
Vascular smooth muscle cells (VSMCs) are a crucial cell population involved in angiogenesis and their phenotypic modulation plays a significant role in vascular development. Exosomes derived from human umbilical vein endothelial cells (HUVECs) treated with oxidized low-density lipoprotein (ox-LDL) (ox-LDL-exo) showed high LINC01005 expression. LINC01005 functions as a sponge for miR-128-3p, leading to decrease in the expression of Kruppel-like factor 4 (KLF4). This regulatory mechanism promotes VSMC phenotype modulation, proliferation, and migration by modulating the miR-128-3p–KLF4 axis, thereby facilitating angiogenesis (141). Vascular endothelial cells are another type of cell involved in angiogenesis, and a substantial body of research has demonstrated that UCMSC-Exos possess the capacity to promote the proliferation of vascular endothelial cells. Zhang et al. (142) demonstrated that UCMSC-Exos possess the ability to enhance the proliferation and migration of vascular endothelial cells, as evidenced by cell counting kit-8 (CCK-8), scratch wound healing, transport, and tube formation assays. Li et al. corroborated that UCMSC-Exos can expedite skin wound healing in diabetic rats by stimulating endothelial cells and augmenting the expression of angiogenesis-related molecules, such as FGF-1, VEGFA, VEGFR-2, ANG-1, E-selectin, CXCL-16, and eNOS (143). Liu et al. corroborated that UCMSC-Exos expedited angiogenesis and elicited an increase in the rate of wound closure in a rat model of deep partial-thickness burns. This effect was primarily ascribed to the capacity of angiopoietin-2 (Ang-2) present in UCMSC-Exos to stimulate proliferation, migration, and tube formation of vascular endothelial cells (144). AKT, a protein kinase B, is a crucial component of several pivotal signaling pathways that facilitate cell proliferation and impede cell apoptosis. In a study by Ma et al., an adenovirus transfection system was employed to transfect AKT into UCMSC, which resulted in the acquisition of AKT-Exos. The authors confirmed that AKT-Exos augmented the proliferation, migration, and tube formation of vascular endothelial cells in vitro and increased angiogenesis in vivo. This process was significantly influenced by the high concentration of platelet-derived growth factor-D (PDGF-D) carried by AKT-Exos (145). Endothelial progenitor cells (EPCs) are precursors of vascular endothelial cells that participate in the formation of blood vessels. Zhang et al. showed that miR-21 in UCMSC-Exos serves as a potential intercellular messenger that enhances the proliferation, migration, and angiogenesis of EPCs by upregulating the NOTCH1/DLL4 pathway (146).
4.2.3 Promotion of proliferation
UCMSC-Exos stimulated fibroblast proliferation and collagen synthesis through various mechanisms. Kim et al. (147) validated the ability of UCMSC-Exos to enhance HDF proliferation and collagen synthesis. Moreover, the application of UCMSC-Exos in the treatment of human skin wounds resulted in increased expression of COL-I and elastin. Another study suggested that UCMSC-Exos express typical exosome markers such as CD63, CD9, CD81, Alix, and TSG101, which significantly stimulate the migration and proliferation of mouse fibroblasts in vitro (148). UCMSC-Exos stimulated HaCaT cell proliferation. Liu et al. demonstrated that UCMSC-Exos can augment the proliferation and migration of normal HaCaT cells while concurrently mitigating apoptosis and senescence in these cells. Additionally, UCMSC-Exos elevated COL-I (149). UCMSC-Exos also promoted the synthesis of ECM. Using proteomic analysis, Zhang et al. determined that 382 proteins exclusive to UCMSC-Exos participate in the composition of the ECM and extracellular structure (142). UCMSC-Exos have been observed to facilitate re-epithelialization, as evidenced by Zhang et al.’s findings of accelerated re-epithelialization in rat skin burn wounds following UCMSC-Exos treatment, along with increased in vivo expression of CK19, PCNA, and COL-I. The presence of Wnt4 in UCMSC-Exos has been shown to promote β-catenin nuclear translocation and activity, ultimately activating the-catenin pathway, which plays a pivotal role in wound re-epithelialization and cell proliferation (150).
The role of UCMSC-Exos in inhibiting scar formation was also demonstrated. Myofibroblast aggregation is a key factor involved in scar formation. Fang et al. (151) used high-throughput RNA sequencing and functional analysis to validate that certain miRNAs (miR-21, -23a, -125b, and -145) present in UCMSC-Exos effectively hindered myofibroblast aggregation and minimized scar formation, both in vitro and in vivo, by impeding the TGF-β2/SMAD2 pathway. Similarly, Zhang et al. (152) also confirmed that UCMSC-Exos inhibit myofibroblast differentiation. miR-21-5p and miR-125b-5p are highly enriched in UCMSC-Exos. These two miRNAs inhibit TGF-β receptor type II (TGFBR2) and TGF-β receptor type I (TGFBR1) respectively, thereby inhibiting TGF-β-induced myofibroblast differentiation in HDFS.
Although there is still a lack of high-quality evidence regarding the specific mechanism of UCMSC-Exos in wound healing, these results provide a new perspective and therapeutic strategy for the application of UCMSC-Exos in skin wound repair.
4.3 ADSC-Exos
ADSC-Exos have garnered significant attention in recent years due to their widespread distribution, ease of isolation and acquisition, and low cost, making them the most extensively researched MSC-Exos. Several studies have demonstrated that ADSC-Exos exhibit superior wound-healing properties compared to other MSC-Exos. Pelizzo et al. used a skin injury model to compare the efficacy of BMSC-Exos- and ADSC-Exos in promoting wound healing. The findings revealed that ADSC-Exos treatment exhibited a significantly superior wound healing effect compared with BMSC-Exos (153). According to transmission electron microscopy (TEM) and nanoparticle tracking analysis (NTA), ADSC-Exos were circular, cup-shaped, and uniform in size, with diameters ranging 30–150 nm. ADSC-Exos secrete numerous special protein markers, including CD63, CD81, CD9, ALIX, ANXA5, and LAMP1 (48, 55). In this section, we summarize the most recent advancements in our understanding of the mechanism of action of ADSC-Exos in the context of wound healing (Figure 5).
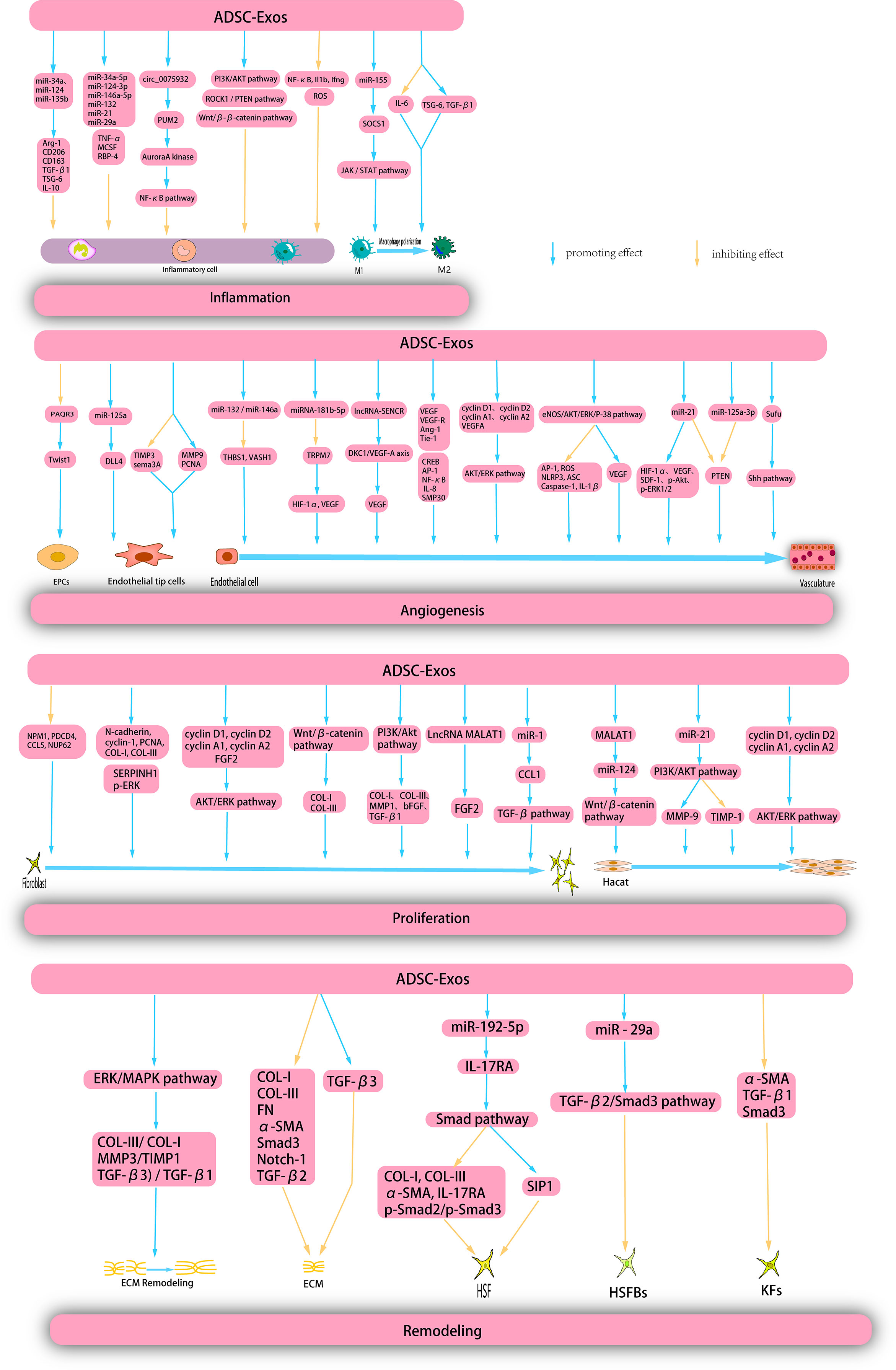
Figure 5 ADSC-Exos exert therapeutic effects on wounds through multiple mechanisms. ADSC-Exos exert a direct inhibitory effects on inflammatory cells and facilitate the polarization of M1 macrophages through diverse mechanisms, thereby impeding the progression of inflammation. Moreover, ADSC-Exos elicit the proliferation and migration of endothelial progenitor cells (EPCs), endothelial tip cells, and endothelial cells, thereby promoting angiogenesis. Additionally, ADSC-Exos enhance the proliferation and migration of hacat and fibroblasts during the proliferation phase. Furthermore, during the remodeling phase, ADSC-Exos facilitate extracellular matrix (ECM) remodeling, suppress the proliferation of ECM, human skin fibroblasts (HSFs), human skin fribroblasts cells (HSFBs), and keratinocytes (KFs), and inhibit scar formation.
4.3.1 Anti-inflammatory action
The presence of inflammation at the base level is a significant determinant of the risk of wound healing and frequently results in persistent wound-related complications. ADSC-Exos are characterized by a substantial concentration of long non-coding RNA growth arrest specific-5 (GAS5), which can effectively govern inflammatory pathways and genes in the inflammatory microenvironment that are commonly associated with chronic ailments such as diabetes and obesity, thereby facilitating wound healing (154). Furthermore, ADSC-Exos reduced inflammatory cytokine levels. Li et al. conducted a study which revealed that ADSC-Exos, which overexpress nuclear factor erythroid2-related factor2 (Nrf2), effectively decreased the levels of reactive oxygen species (ROS) and inflammatory cytokines. This intervention was shown to be effective in promoting the healing of foot wounds and reducing the ulcer area in diabetic rats (155).
Numerous studies have provided evidence that ADSC-Exos possess the ability to modulate macrophages to suppress inflammation. Specifically, ADSC-Exos may directly interact with macrophages, resulting in the inhibition of macrophage nuclear factor kappa-B (NF-κB) activity and particular pro-inflammatory genes, ultimately leading to the alleviation of macrophage-mediated inflammatory responses (156). Furthermore, some studies have confirmed that the administration of ADSC-Exos results in a reduction of the inflammatory response in mouse RAW264.7, a decrease in macrophage infiltration and pro-inflammatory cytokine levels, and an increase in the secretion of anti-inflammatory cytokines (157). ADSC-Exos can induce the polarization of M1 macrophages and expedite the inflammatory process. miR-155 produced by ADSC-Exos can be transported to macrophages, where it targets SOCS1, a confirmed miR-155 target, and modulates JAK/STAT signaling to facilitate M1 macrophage polarization and mitigate chronic inflammation (158). Heo et al. reported that ADSC-Exos down-regulate the expression of IL-6 and up-regulate the expression of tumor necrosis factor α-stimulated gene-6 (TSG-6) and TGF-β1 in an inflammatory environment, thereby promoting the polarization of M1 macrophages (159). Heo et al. revealed that ADSC-Exos secrete distinct miRNAs that play crucial roles in the anti-inflammatory processes. Specifically, ADSC-Exos exhibited high expression of miR-34a-5p, miR-124-3p, miR-146a-5p, miR-132, miR-21, and miR-29a, which have been shown to effectively mitigated inflammation and promoted wound healing (159). The following year, Heo et al. showed that ADSC-Exos can significantly up-regulate the expression of anti-inflammatory mRNA related to M2 macrophages in the inflammatory environment treated with interferon-γ and tumor necrosis factor-α. The study also showed that some miRNAs (miR-34a, miR-124, and miR-135b) were upregulated in melatonin-stimulated ADSC-Exos compared to ordinary ADSC-Exos. The up-regulation of these miRNAs contributed to gene expression of Arg-1, CD206, CD163, TGF-β1, TSG-6, and IL-10 genes, which contribute to the promotion of anti-inflammatory microenvironment (160). Blazquez et al. conducted experiments wherein they analyzed the phenotypic characteristics and functional properties of cytotoxic and helper T cells. The results indicated that ADSC-Exos exhibited an inhibitory effect on T cell differentiation and activation as well as on the proliferation of T cells and the release of IFN-γ from stimulated cells in vitro. Consequently, ADSC-Exos have the potential to suppress inflammation (161). Furthermore, ADSC-Exos secrete numerous immunomodulatory proteins, including TNF-α, macrophage colony-stimulating factor (MCSF), and retinol-binding protein 4 (RBP-4), which play crucial roles in the regulation of anti-inflammatory responses (162).
ADSC-Exos modulate multiple signaling pathways, resulting in the inhibition of inflammation. Heo et al. showed that ADSC-exos specifically target the ROCK1/PTEN pathway to exert their anti-inflammatory effects. ROCK1, a Rho-associated coil-containing protein kinase 1, regulates inflammation by modulating the phosphatase tensin homologue (PTEN), which in turn inhibits inflammation through the Wnt/β-catenin pathway (163). Circular RNA has_circ_0075932, is a single-exonic molecule that is expressed in normal human adipose tissue and is overexpressed in the burned skin of obese individuals. Overexpression of circ_0075932 in ADSC-Exos modulates the inflammatory response of dermal keratinocytes. This regulatory mechanism is attributed to the direct binding of circ_0075932 to the RNA-binding protein PUM2, which results in the upregulation of Aurora A kinase and subsequent activation of the NF-κB pathway (164). Wang et al. reported that hypoxia-induced adipose-derived stem cell exosomes (HypADSC-Exos) can impede the inflammatory response and enhance the healing of diabetic wounds through the PI3K/AKT signaling pathway (165).
4.3.2 Angiogenesis
Numerous studies have demonstrated the ability of ADSC-Exos to facilitate angiogenesis during the repair of diabetic foot ulcers (DFUs). The presence of a high-glucose environment has been shown to impede the proliferation, migration, and angiogenesis of endothelial progenitor cells (EPCs), while increasing the expression of PAQR3 and inhibiting the expression of Twist1. Qiu et al. reported that ADSC-Exos overexpressing linc00511 can enhance angiogenesis and ameliorate DFUs by suppressing PAQR3-induced Twist1 ubiquitination and degradation and elevating Twist1 protein levels in EPCs (166). SMP30, a 34-kDa cytosolic protein commonly referred to as regucalcin, regulates intracellular Ca2+ homeostasis, ascorbate biosynthesis, and oxidative stress, and serves as a senescence marker. The absence of SMP30 exacerbates oxidative stress and impedes angiogenic activity. In patients with diabetes, the phosphorylation levels of SMP30 and VEGFR2 are significantly reduced. Li et al. demonstrated that the overexpression of Nrf2 in ADSC-Exos elevated the levels of SMP30 and VEGF and enhanced the phosphorylation of VEGFR2 to expedite angiogenesis (155).
Endothelial tip cells (ETCs) are another group of critical cells involved in angiogenesis. ADSC-Exos decreased the expression of tissue inhibitor of metalloproteinase 3 (TIMP3) and increased the expression of matrix metalloproteinase 9 (MMP9) and proliferating cell nuclear antigen (PCNA) in endothelial tip cells. Furthermore, miR-199-3p-modified ADSC-Exos targeted sema3A and downregulated it to promote proliferation and migration of endothelial tip cells (167). The enrichment of miR-125a in ADSCs-Exos results in the inhibition of Delta-like protein 4 (DLL4), an angiogenesis inhibitor, through the targeting of its 3’ untranslated region. This process ultimately promotes angiogenesis in endothelial cells by facilitating the formation of endothelial tip cells (168).
ADSC-Exos modulate angiogenic genes to facilitate angiogenesis. Previous studies indicated that miR-132 and miR-146a act as angiogenic enhancers and target the anti-angiogenic genes THBS1 and VASH1, respectively. Heo et al. showed that elevated levels of miR-132/miR-146a in ADSC-Exos regulate angiogenesis in HUVEC by suppressing the expression of antiangiogenic genes (163). Another study showed that hypoxia-treated ADSC-Exos upregulate angiogenesis-stimulating genes, deregulate angiogenesis-inhibiting genes, increase VEGF expression, and activate the protein kinase A (PKA) signaling pathway (169). VEGF expression was upregulated by ADSC-Exos. This was corroborated by Yang et al., who demonstrated that miRNA-181b-5p in ADSC-Exos targeted TRPM7 directly, leading to a decrease in TRPM7 mRNA and protein levels and an increase in the protein expression of HIF-1α and VEGF (170). Sun et al. demonstrated that ADSC-Exos elicit upregulation of enriched migration/differentiation-related long non-coding RNAs (lncRNA-sencrr) in smooth muscle and endothelial cells, activate the dyskerin pseudouridine synthase 1 (DKC1)/VEGF-A axis, and subsequently upregulate VEGF expression (171). In addition, ADSC-exos induced the activation of numerous growth factors. HypoADSC-Exos significantly increase the expression of VEGF and VEGF-R, EGF, FGF, angiopoietin-1 (Ang-1), and tyrosine kinase with immunoglobulin-like and EGF-like domains 1(Tie-1) (172).
ADSC-Exos can also stimulate angiogenesis through various signaling pathways. Evidence showed that ADSC-Exos are capable of modulating the cAMP response element binding protein (CREB), activator protein 1 (AP-1), nuclear factor-κb (NF-κB) signaling pathways, and IL-8 production in receptor HUVEC, thereby promoting angiogenesis, migration, and tube formation of HUVECs. Furthermore, proteomic analysis of ADSC-LPS-exos identified eukaryotic translation initiation factor 4E, amyloid βA4 protein, integrin β-1, and RAS-associated C3 botulinum toxin substrate 1 (RAC1) as potential candidates for enhanced exosome-mediated angiogenesis (173). This was followed by activation of the AKT and ERK signaling pathways. ADSC-Exos activated the AKT/ERK signaling pathway through the upregulation of cell proliferation markers (cyclins D1, D2, A1, and A2) and VEGFA gene expression. This phenomenon significantly enhanced the proliferation of HUVECs both in vitro and in vivo (174). Zhang et al. used an ischemic lower limb model in diabetic mice to investigate the effects of in vivo transplantation of ADSC-derived exosomes overexpressing glyoxalase 1 (GLO-1) (G-ADSC-Exos) on the laser Doppler perfusion index and microvessel density. A previous study revealed that G-ADSC-Exos can activate the eNOS/AKT/ERK/P-38 signaling pathway, promote VEGF secretion, and inhibit AP-1/ROS/NLRP3/ASC/Caspase-1/IL-1β secretion, thereby improving the aforementioned parameters (175). Recent studies have demonstrated that ADSC-Exos can downregulate the PTEN pathway and that miR-21 is a crucial miRNA involved in angiogenesis. An et al. used quantitative real-time reverse transcription-polymerase chain reaction (qRT-PCR) and western blotting (WB) to establish that ADSC-Exos overexpressing miR-21 up-regulated HIF-1α, VEGF, SDF-1, p-AKT, and p-ERK1/2 while down-regulating PTEN. These findings suggest that miR-21-enriched ADSC-Exos promote angiogenesis through the activation of AKT and ERK and the expression of HIF-1α and SDF-1 (176). miR-125a-3p in ADSC-Exos can also promote wound healing and angiogenesis in mice by inhibiting PTEN in the granulation tissue of wounds (177). Finally, the fusion suppressor factor (Sufu) and activation of the Sonic Hedgehog (Shh) signaling pathway were studied. Shh signalling may be a potential target in regenerative skin wound healing (178). miR-378 in ADSC-Exos can negatively regulate Sufu and activate the Shh signaling pathway, thus promoting angiogenesis (179). These results suggest that ADSC-Exos may be promising candidates for wound angiogenesis; however, further studies are needed to elucidate their angiogenic capacity.
4.3.3 Promote proliferation
Numerous studies have demonstrated that ADSC-Exos facilitate fibroblast proliferation and augment collagen production. Specifically, ADSC-Exos can be internalized by fibroblasts, thereby inducing cell migration, proliferation, and collagen synthesis in a dose-dependent manner, while upregulating the expression of N-cadherin, cyclin-1, PCNA, and COL-I and -III genes. Additionally, histological analysis revealed that systemic administration of ADSC-Exos led to an increased production of COL-I and COL-III during the initial phases of wound healing (73). Choi et al. (55)demonstrated that ADSC-Exos harbor distinct miRNAs that impede the activity of proteins linked to aging and growth dysregulation, namely nuclear phosphate (NPM1), programmed cell death 4 (PDCD4), C-C motif chemokine ligand 5 (CCL5), and nucleotide 62 kDa (NUP62), thereby promoting the proliferation of dermal fibroblasts. Wang et al. injected ADSC-Exos into diabetic wounds of mice. Compared to the control group (phosphate-buffered saline [PBS] group), ADSC-Exos significantly promoted wound healing, and more collagen fibers were deposited in the late healing stage (180). ADSC-Exos stimulate fibroblasts by upregulating certain growth factors. Pi et al. reported that the long non-coding RNA metastasis-associated lung adenocarcinoma transcript 1 (MALAT1), derived from ADSC-Exos, upregulates FGF2, thereby enhancing the viability and migration of human skin fibroblasts (181). Other studies have demonstrated that the miR-19b present in ADSC-Exos stimulates collagen synthesis and fibroblast migration during wound healing. This effect is achieved by targeting CCL1 and subsequently regulating the TGF-β pathway (182).
In addition to growth factors, ADSC-Exos stimulated fibroblast formation by upregulating the expression of cell proliferation markers. A study conducted by Ren et al. revealed that ADSC-Exos induced the activation of the AKT/ERK signaling pathway through the upregulation of gene expression related to cell proliferation markers (cyclin D1, cyclin D2, cyclin A1, and cyclin A2) and FGF2. Consequently, this intervention significantly enhances the proliferation and migration of fibroblasts both in vitro and in vivo (174). In addition, ADSC-Exos have been shown to activate associated enzymes, thereby facilitating fibroblast proliferation. Studies have reported that miRNA-146a-modified ADSC-Exos upregulate serine protease inhibitor family H member 1 (SERPINH1) and phosphorylated ERK (p-ERK), which in turn promote fibroblast migration and proliferation, ultimately leading to wound healing (183). Li et al. validated that the activation of the WNT/β-catenin signaling pathway by ADSC-Exos resulted in increased expression levels of COL-I and COL-III in fibroblasts, as well as dose-dependent stimulation of fibroblast migration and proliferation (184). Zhang et al. demonstrated that ADSC-Exos can upregulate the mRNA and protein expression of COL-I, COL-III, MMP1, bFGF, and TGF-β1 in fibroblasts by mediating the PI3K/AKT signaling pathway (185).
Numerous studies have demonstrated that ADSC-Exos possess the ability to enhance the proliferation of HaCaT cells. He et al. demonstrated that MALAT1 present in ADSC-Exos facilitates wound healing in response to H2O2 by targeting miR-124 and activating the-catenin pathway. This process promotes the proliferation and migration of HaCaT cells while simultaneously inhibiting apoptosis (186). ADSC-Exos also promote the proliferation of HaCaT cells and accelerate the re-epithelialization process by upregulating cell proliferation markers (cyclin D1, cyclin D2, cyclin A1, and cyclin A2) and stimulating the activation of the AKT/ERK signaling pathway (174). MMP have the potential to attenuate adhesion between HaCaT cells and the substrate, thereby facilitating cellular migration. Conversely, TIMP, a natural inhibitor of MMP, impedes the biological activity of MMP. Yang et al. reported that miR-21 expression is markedly elevated in ADSC-Exos, which effectively augments the proliferation and migration of HaCaT cells. This phenomenon is likely mediated by the activation of AKT phosphorylation, which, in turn, enhances MMP-9 and reduces TIMP-1 expression through the PI3K/AKT signaling pathway (187). The promotion of ECM (ECM) production. We used mass spectrometry to identify MMP6, COL-1A, CTSD, and TN-C within ADSC-Exos, which are integral to ECM homeostasis and participate in multiple signaling pathways associated with ECM repair and regeneration (188). The cytoskeletal protein vimentin plays an active role in wound healing. Parvania et al. indicated that vimentin present in ADSC-Exos facilitates fibroblast proliferation, migration, and ECM secretion, thereby reducing the duration of wound healing (189). Furthermore, pre-treatment significantly augmented the efficacy of ADSC-Exos in promoting cell proliferation. Wang et al. (165) confirmed that HypADSC-Exos significantly upregulated miR-21-3p/miR-126-5p/miR-31-5p and downregulated miR-99b/miR-146-a compared to ADSC-Exos. These miRNAs significantly promote fibroblast proliferation and migration. Further studies are required to elucidate the specific molecular mechanisms by which ADSC-Exos promote cell proliferation during wound healing and skin regeneration.
4.3.4 Inhibition of scar formation
Scar formation often arises from the excessive proliferation of fibroblasts, synthesis of collagen, and deposition of ECM. However, ADSC-Exos may inhibit fibroblast proliferation, excessive collagen synthesis (73), and ECM deposition in specific cases, thereby impeding scar formation. First, miR-192-5p, which is present in ADSC-Exos, regulates the Smad pathway in hypertrophic scar fibrosis by targeting IL-17RA. This results in a decrease in the expression of key fibrotic markers such as COL-I, COL-III, α-smooth muscle actin (α-SMA), IL-17RA, and p-Smad2/p-Smad3, while increasing Smad interacting protein 1 (SIP1) expression. Consequently, miR-192-5p effectively inhibits the proliferation and migration of HSFS, collagen deposition, transdifferentiation of fibroblasts into myofibroblasts, and, ultimately, the formation of hypertrophic scars (190). Furthermore, the presence of miR-29a within ADSCs-Exos has been observed to impede fibrosis and scar hyperplasia in human hypertrophic scar fibroblasts (HSFBs) subsequent to scald injury, through the targeting of the TGF-β2/Smad3 signaling pathway, ultimately leading to a reduction in the formation of pathological scars (191). Wang et al. reported that ADSC-Exos impeded the differentiation of fibroblasts into myofibroblasts by activating the ERK/MAPK pathway. This process results in an elevated COL-3/COL-1 ratio, TGF-β3/TGF-β1 ratio, and MMP3/TIMP1 ratio, which facilitate ECM reconstruction during skin wound healing and diminish scar formation (192). Keloid fibroblasts (KFs) represent a distinctive subset of cells whose inhibition of the apoptotic program results in keloid formation. Administration of ADSC-Exos promotes KFs apoptosis and inhibits keloid formation. Research has indicated that ADSC-Exos may impede KFs proliferation and migration, while promoting apoptosis by suppressing the mRNA and protein expression of α-SMA, TGF-β1, and Smad3 (193, 194). Finally, the administration of ADSC-Exos resulted in the suppression of COL-I, COL-III, FN, α-SMA, Smad3, Notch-1, and TGF-β2 gene and protein expression in KFs, while simultaneously upregulating TGF-β3 expression. Consequently, the production of ECM is inhibited, leading to reduced keloid formation (195).
These data provided strong in vitro and ex vivo evidence that ADSC-Exos have potential clinical applications in wound healing. However, several unresolved issues remain regarding the practical applications of ADSC-Exos. For instance, the extant research on ADSC-Exos and wounds is predominantly short-term, necessitating further investigation of the long-term efficacy of ADSC-Exos through in vivo studies with large sample sizes. Furthermore, the specific therapeutic dose of ADSC-Exos that is both effective and safe remains unclear. Future research should focus on determining the biological safety range of ADSC-Exos.
5 Clinical applications of MSC-Exos in wound healing
As mentioned above, a large amount of evidence has shown that MSC-Exos have positive effects on skin wound healing in animal studies and preclinical tests; however, clinical studies on exosomes in skin wound healing are still insufficient. However, it is worth mentioning that some researchers have conducted systematic reviews and meta-analyses of preclinical studies of MSC-Exos, confirming their efficacy in wound healing. A systematic review of MSC-Exos by Bailey et al. suggested that administration of MSC-Exos improved diabetic wound closure compared with control, with a large observed effect (standardized mean difference [SMD] 5.48, 95% confidence interval [CI] 3.55–8.13). MSC-Exos enriched in non-coding RNAs or microRNAs further enhanced healing compared with control (SMD 9.89, 95%CI 7.32–12.46). Other outcomes, such as vessel density and number, scar width, and re-epithelialization improved with MSC-Exos administration with significant effect (196). This suggests that MSC-Exos are a promising treatment for diabetic wounds and warrants further investigation. In addition, a double-blind, randomized, first-in-human clinical trial by Kwon et al. showed that ADSC-Exos and fractional CO2 laser treatment of acne scars resulted in better improvement than the control, which broadly suggests that ADSC-Exos can provide synergy in the efficacy and safety of atrophic acne scar treatment (197). Therefore, MSC-Exos have good prospects for clinical applications.
6 Exploration of the combined effects of exosomes and biomaterials and Engineered exosomes
In most contemporary studies, MSC-Exos are administered through subcutaneous or intravenous injection onto the wound surface. Nonetheless, this method of delivery poses the potential hazard of sudden discharge and swift elimination due to the movement of bodily fluids. The injected exosomes exhibited a limited ability to accumulate at the intended site, resulting in a brief duration of exposure to the wound and suboptimal use of the therapeutic benefits of MSC-Exos. Researchers are exploring the potential of combining MSC-Exos with biomaterials to achieve synergistic therapeutic effects. Controlled release is a necessary attribute of biological materials, with hydrogels being the most extensively researched. Hydrogels serve as three-dimensional porous scaffolds that facilitate the accumulation of exosomes, sustain their functionality at the wound site, create a conducive milieu for cellular proliferation and ECM remodeling, enhance efficacy and safety, and synergistically expedite wound healing. Alternatively, the properties of a hydrogel allow it to fill the wound area, thereby creating an environment suitable for granulation growth (198). In general, hydrogel-based exosomes can be released continuously for a long time and exert long-lasting therapeutic effects, thereby achieving better wound healing. The use of chitosan (CS) hydrogel as a carrier for the sustained release of nanoparticles, including exosomes, has been identified as an optimal approach because of its hemostatic, antibacterial, biodegradable, and biocompatible properties. This approach ensures the sustained release of MSC-Exos, making them an ideal carrier for this purpose (199, 200). Tao et al. (201) demonstrated that the use of chitosan hydrogels loaded with MSC-Exos leads to a significant extension of exosome delivery, thereby prolonging the exposure of MSC-Exos to the wound site. Wang et al. (202)have confirmed that an exosome-loaded natural methylcellulose-chitosan hydrogel exhibited appropriate strength, a straightforward preparation process, efficient self-healing, biocompatibility, and an integrated structure that was suitable for the treatment of severe diabetic wounds. Shi et al. (203) employed a freeze-drying method to fabricate a chitosan/silk hydrogel sponge as an exosome scaffold, which was subsequently used in conjunction with MSC-Exos for wound treatment in diabetic rats. This study revealed a significant increase in the number of microvessels, thereby facilitating skin wound healing. Shafei et al. (204) designed alginate-based hydrogels loaded with MSC-Exos. The biodegradability and biocompatibility of this bioactive scaffold were evaluated, and it was demonstrated to effectively retain exosomes at the wound site in an animal model. Another investigator created a peptide-based FHE hydrogel (F127/OHA-EPL) that was injectable, self-healing, and antimicrobial, and incorporated stimuli-responsive MSC-Exos to enhance chronic wound healing and complete skin regeneration in a synergistic manner. The combination of the FHE hydrogel and exosomes demonstrated superior healing outcomes compared to exosomes alone, including enhanced wound closure rate, accelerated angiogenesis, expedited epithelialization, and increased collagen deposition at the wound site (205).
In addition to hydrogels, other biomaterials have exhibited promising outcomes in facilitating the use of exosomes for the advancement of wound healing. Graphene-Based Nanomaterials (GBNs) have emerged as a focus of biomaterial research. The substantial specific surface area and drug-loading capacity of GBNs contribute to augmentation of the efficacious dose of MSC-Exos at the wound site, whereas the robust sustained release capability of GBNs enables MSC-Exos to attain prolonged action duration, retention rate, and stability (206). The combination of exosomes and dressings increases their efficacy. Shiekh et al. (207)devised and assessed a wound dressing, OxOB, which was enriched with exosomes that released oxygen and antioxidants. This dressing exhibits remarkable potential for mitigating oxidative stress, promoting angiogenesis, and augmenting collagen remodeling, all of which are advantageous for the healing of diabetic wounds. Xiao et al. (208) have developed a scaffold dressing for a human acellular amniotic membrane (hAAM) loaded with MSC-Exos. The hAAM exhibited good swelling and moisturizing properties. A moist environment is essential for wound reepithelialization (209). The hAAM scaffold dressing was deemed highly appropriate for the delivery of exosomes. hAAM-Exos exhibit inflammatory regulatory functions, induce angiogenesis, and enhance ECM production, thereby expediting the healing of diabetic wounds. The exosome-carrier complex demonstrated superior healing outcomes compared with exosomes alone, indicating the synergistic impact of the sustained release of MSC-Exos.
The efficacy of natural exosomes is hindered by several limitations including low yield, impurities, and lack of targeting (210, 211). Bioengineered exosomes may be a promising solution to this problem. There are many methods for engineering exosomes, such as modifying parental cells and directly processing stem cell-derived exosomes. These methods include co-incubation, genetic engineering, electroporation, ultrasound, parent cell surface modification, and artificial synthetic engineering. Engineered stem cell exosomes can be loaded with nucleic acids, proteins, or other small molecules (212). Engineered MSC-Exos have the potential to enhance various aspects of exosomes, including yield, purity, targeting, drug delivery, and therapeutic efficacy (213, 214). The collective use of these strategies may ultimately improve the therapeutic efficacy of exosomes for skin wound healing and regeneration (Figure 6).
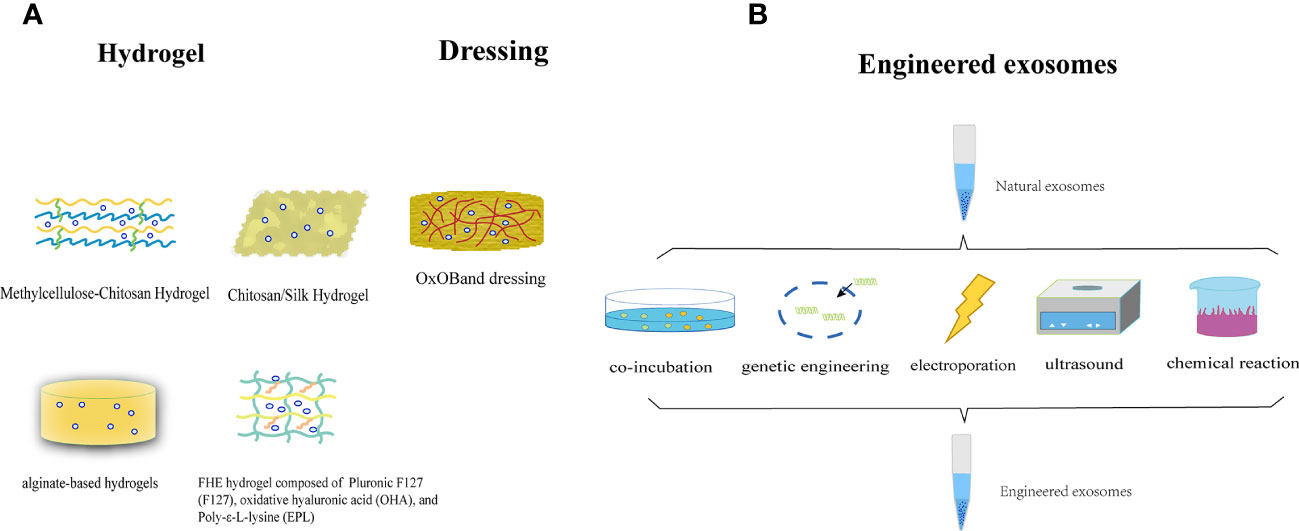
Figure 6 (A) The utilization of MSC-Exos in conjunction with biomaterials to attain a mutually reinforcing therapeutic outcome. (B) Illustration of the synthesis of engineered stem cell exosomes.
7 Summary and prospects
Recently, researchers conducted in-depth studies on the role of MSC-Exos in wound repair. MSC-Exos-based therapy is emerging as a promising technology for promoting wound healing with minimal scarring. MSC-Exos represent a potential solution to the limitations of stem cell therapy, including immune rejection, ethical concerns, tumorigenicity, and malformation. Exosomes offer numerous advantages such as accessibility, ample supply, convenient storage and transport, high stability, sustained viability, small size, efficient delivery, non-proliferation, ease of quantification, and targeted recruitment to the site of injury. Furthermore, MSC-Exos contain a diverse array of proteins, lipids, and RNA molecules that confer enhanced safety and greater potential for tissue regeneration than single-cytokine therapies.
Consequently, MSC-Exos are superior to other wound therapies. In summary, despite the distinct differentiation capabilities of exosomes originating from various MSCs, they exhibit greater uniformity in fostering wound healing. Presently, most of the mechanisms elucidating the impact of MSC-Exos on wound healing have been investigated in rodents, and the extrapolation of animal physiology to humans is not direct. Therefore, it is imperative to conduct additional clinical trials using exosomes derived from human sources to establish the therapeutic potential of MSC-Exos for wound repair in a larger patient population. Furthermore, most current investigations into the mechanism of MSC-Exos rely on the in vitro isolation of these exosomes in a standard medium, thereby disregarding the interplay between the paracrine effect of MSC and their microenvironment. MSC-Exos produced under various microenvironmental conditions, such as hypoxia, exhibit distinct components and diverse biological effects. Most studies have failed to consider this particular aspect. Furthermore, the extraction and purification techniques used for exosomes are considerably intricate, and there is currently no universally accepted methodology appropriate for large-scale pharmaceutical production. This may impede the reproducibility of the research findings. Prompt isolation of the required quantity of exosomes to satisfy our requirements was not feasible. In addition, there is a lack of established criteria for the identification, quantity, size, purity, and content of exosome-based therapeutic agents. However, a complete understanding of the biological safety, efficacy, reproducibility, potency, formation mechanism, and biological function of exosomes in promoting wound healing remains elusive. The resolution of these issues would have a substantial impact on wound repair and skin regeneration through the use of MSC-Exos as a cell-free therapy.
Author contributions
XQ: Conceptualization, Writing – original draft, Writing – review & editing. JH: Writing – original draft, Writing – review & editing. XW: Conceptualization, Writing – review & editing. JW: Funding acquisition, Writing – review & editing. RY: Funding acquisition, Writing – review & editing. XC: Funding acquisition, Writing – review & editing.
Funding
This study was supported by National Natural Science Foundation of China (No. 82172205, No. 82272276), GuangDong Basic and Applied Basic Research Foundation (2021A1515011453, 2022A1515012160), The Medical Clinical Key Specialty Construction Project for 14th Five-Year Plan of Guangdong Province, The Medical High level - Key Specialty Construction Project for 14th Five-Year Plan of Foshan City.
Conflict of interest
The authors declare that the research was conducted in the absence of any commercial or financial relationships that could be construed as a potential conflict of interest.
Publisher’s note
All claims expressed in this article are solely those of the authors and do not necessarily represent those of their affiliated organizations, or those of the publisher, the editors and the reviewers. Any product that may be evaluated in this article, or claim that may be made by its manufacturer, is not guaranteed or endorsed by the publisher.
References
1. Proksch E, Brandner JM, Jensen J-M. The skin: an indispensable barrier. Exp Dermatol (2008) 17(12):1063–72. doi: 10.1111/j.1600-0625.2008.00786.x
2. Yang R, Liu F, Wang J, Chen X, Xie J, Xiong K. Epidermal stem cells in wound healing and their clinical applications. Stem Cell Res Ther (2019) 10(1):229. doi: 10.1186/s13287-019-1312-z
3. Wang X, Liu Y, He J, Wang J, Chen X, Yang R. Regulation of signaling pathways in hair follicle stem cells. Burns Trauma (2022) 10:tkac022. doi: 10.1093/burnst/tkac022
4. Singer AJ, Clark RA. Cutaneous wound healing. New Engl J Med (1999) 341(10):738–46. doi: 10.1056/NEJM199909023411006
5. Vu NB, Nguyen HT, Palumbo R, Pellicano R, Fagoonee S, Pham PV, et al. Stem cell-derived exosomes for wound healing: current status and promising directions. Minerva Med (2021) 112(3):384–400. doi: 10.23736/S0026-4806.20.07205-5
6. Caplan H, Olson SD, Kumar A, George M, Prabhakara KS, Wenzel P, et al. Mesenchymal stromal cell therapeutic delivery: Translational challenges to clinical application. Front Immunol (2019) 10:1645. doi: 10.3389/fimmu.2019.01645
7. Wang Z, Gagliardi M, Mohamadi RM, Ahmed SU, Labib M, Zhang L, et al. Ultrasensitive and rapid quantification of rare tumorigenic stem cells in hPSC-derived cardiomyocyte populations. Sci Adv (2020) 6(12):eaay7629. doi: 10.1126/sciadv.aay7629
8. Sissung TM, Figg WD. Stem cell clinics: risk of proliferation. Lancet Oncol (2020) 21(2):205–6. doi: 10.1016/S1470-2045(19)30787-9
9. Slack JMW. What is a stem cell? Wiley Interdisciplinary Reviews. Dev Biol (2018) 7(5):e323. doi: 10.1002/9781119235293.ch1
10. Tsatsaronis JA, Franch-Arroyo S, Resch U, Charpentier E. Extracellular vesicle RNA: A universal mediator of microbial communication? Trends Microbiol (2018) 26(5):401–10. doi: 10.1016/j.tim.2018.02.009
11. Timmers L, Lim SK, Arslan F, Armstrong JS, Hoefer IE, Doevendans PA, et al. Reduction of myocardial infarct size by human mesenchymal stem cell conditioned medium. Stem Cell Res (2007) 1(2):129–37. doi: 10.1016/j.scr.2008.02.002
12. Liang X, Ding Y, Zhang Y, Tse HF, Lian Q. Paracrine mechanisms of mesenchymal stem cell-based therapy: current status and perspectives. Cell Transplant (2014) 23(9):1045–59. doi: 10.3727/096368913X667709
13. Yang J, Chen Z, Pan D, Li H, Shen J. Umbilical cord-derived mesenchymal stem cell-derived exosomes combined pluronic F127 hydrogel promote chronic diabetic wound healing and complete skin regeneration. Int J Nanomedicine (2020) 15:5911–26. doi: 10.2147/IJN.S249129
14. Pan BT, Johnstone RM. Fate of the transferrin receptor during maturation of sheep reticulocytes in vitro: selective externalization of the receptor. Cell (1983) 33(3):967–78. doi: 10.1016/0092-8674(83)90040-5
15. Brennan MÁ, Layrolle P, Mooney DJ. Biomaterials functionalized with MSC secreted extracellular vesicles and soluble factors for tissue regeneration. Advanced Funct Materials (2020) 30(37):1909125. doi: 10.1002/adfm.201909125
16. Baglio SR, Pegtel DM, Baldini N. Mesenchymal stem cell secreted vesicles provide novel opportunities in (stem) cell-free therapy. Front Physiol (2012) 3:359. doi: 10.3389/fphys.2012.00359
17. Stoorvogel W, Kleijmeer MJ, Geuze HJ, Raposo G. The biogenesis and functions of exosomes. Traffic (Copenhagen Denmark) (2002) 3(5):321–30. doi: 10.1034/j.1600-0854.2002.30502.x
18. György B, Szabó TG, Pásztói M, Pál Z, Misják P, Aradi B, et al. Membrane vesicles, current state-of-the-art: emerging role of extracellular vesicles. Cell Mol Life sciences: CMLS (2011) 68(16):2667–88. doi: 10.1007/s00018-011-0689-3
19. Raposo G, Stoorvogel W. Extracellular vesicles: exosomes, microvesicles, and friends. J Cell Biol (2013) 200(4):373–83. doi: 10.1083/jcb.201211138
20. Konala VBR, Mamidi MK, Bhonde R, Das AK, Pochampally R, Pal R. The current landscape of the mesenchymal stromal cell secretome: A new paradigm for cell-free regeneration. Cytotherapy (2016) 18(1):13–24. doi: 10.1016/j.jcyt.2015.10.008
21. Kalluri R, LeBleu VS. The biology, function, and biomedical applications of exosomes. Sci (New York N.Y.) (2020) 367(6478):eaau6977. doi: 10.1126/science.aau6977
22. Coumans FAW, Brisson AR, Buzas EI, Dignat-George F, Drees EEE, El-Andaloussi S, et al. Methodological guidelines to study extracellular vesicles. Circ Res (2017) 120(10):1632–48. doi: 10.1161/CIRCRESAHA.117.309417
23. Akers JC, Gonda D, Kim R, Carter BS, Chen CC. Biogenesis of extracellular vesicles (EV): exosomes, microvesicles, retrovirus-like vesicles, and apoptotic bodies. J Neuro-Oncology (2013) 113(1):1–11. doi: 10.1007/s11060-013-1084-8
24. Cunnane EM, Weinbaum JS, O’Brien FJ, Vorp DA. Future perspectives on the role of stem cells and extracellular vesicles in vascular tissue regeneration. Front Cardiovasc Med (2018) 5:86. doi: 10.3389/fcvm.2018.00086
25. Corso G, Mäger I, Lee Y, Görgens A, Bultema J, Giebel B, et al. Reproducible and scalable purification of extracellular vesicles using combined bind-elute and size exclusion chromatography. Sci Rep (2017) 7(1):11561. doi: 10.1038/s41598-017-10646-x
26. Koniusz S, Andrzejewska A, Muraca M, Srivastava AK, Janowski M, Lukomska B, et al. Extracellular vesicles in physiology, pathology, and therapy of the immune and central nervous system, with focus on extracellular vesicles derived from mesenchymal stem cells as therapeutic tools. Front Cell Neurosci (2016) 10:109. doi: 10.3389/fncel.2016.00109
27. Hong P, Yang H, Wu Y, Li K, Tang Z. The functions and clinical application potential of exosomes derived from adipose mesenchymal stem cells: a comprehensive review. Stem Cell Res Ther (2019) 10(1):242. doi: 10.1186/s13287-019-1358-y
28. Johnstone RM, Adam M, Hammond JR, Orr L, Turbide C. Vesicle formation during reticulocyte maturation. Association of plasma membrane activities with released vesicles (exosomes). J Biol Chem (1987) 262(19):9412–20.
29. Mathivanan S, Fahner CJ, Reid GE, Simpson RJ. ExoCarta 2012: database of exosomal proteins, RNA and lipids. Nucleic Acids Res (2012) 40(Database issue):D1241–1244. doi: 10.1093/nar/gkr828
30. Escola JM, Kleijmeer MJ, Stoorvogel W, Griffith JM, Yoshie O, Geuze HJ, et al. Selective enrichment of tetraspan proteins on the internal vesicles of multivesicular endosomes and on exosomes secreted by human B-lymphocytes. J Biol Chem (1998) 273(32):20121–7. doi: 10.1074/jbc.273.32.20121
31. Conde-Vancells J, Rodriguez-Suarez E, Embade N, Gil D, Matthiesen R, Valle M, et al. Characterization and comprehensive proteome profiling of exosomes secreted by hepatocytes. J Proteome Res (2008) 7(12):5157–66. doi: 10.1021/pr8004887
32. Segura MF, Hanniford D, Menendez S, Reavie L, Zou X, Alvarez-Diaz S, et al. Aberrant miR-182 expression promotes melanoma metastasis by repressing FOXO3 and microphthalmia-associated transcription factor. Proc Natl Acad Sci United States America (2009) 106(6):1814–9. doi: 10.1073/pnas.0808263106
33. New SE, Alvarez-Gonzalez C, Vagaska B, Gomez SG, Bulstrode NW, Madrigal A, et al. A matter of identity—Phenotype and differentiation potential of human somatic stem cells. Stem Cell Res (2015) 15(1):1–13. doi: 10.1016/j.scr.2015.04.003
34. Chevillet JR, Kang Q, Ruf IK, Briggs HA, Vojtech LN, Hughes SM, et al. Quantitative and stoichiometric analysis of the microRNA content of exosomes. Proc Natl Acad Sci United States America (2014) 111(41):14888–93. doi: 10.1073/pnas.1408301111
35. Jeppesen DK, Fenix AM, Franklin JL, Higginbotham JN, Zhang Q, Zimmerman LJ, et al. Reassessment of exosome composition. Cell (2019) 177(2):428–445.e18. doi: 10.1016/j.cell.2019.02.029
36. Kowal J, Tkach M, Théry C. Biogenesis and secretion of exosomes. Curr Opin Cell Biol (2014) 29:116–25. doi: 10.1016/j.ceb.2014.05.004
37. Anand S, Samuel M, Kumar S, Mathivanan S. Ticket to a bubble ride: Cargo sorting into exosomes and extracellular vesicles. Biochim Et Biophys Acta Proteins Proteomics (2019) 1867(12):140203. doi: 10.1016/j.bbapap.2019.02.005
38. Van Niel G, Porto-Carreiro I, Simoes S, Raposo G. Exosomes: a common pathway for a specialized function. J Biochem (2006) 140(1):13–21. doi: 10.1093/jb/mvj128
39. Moghadasi S, Elveny M, Rahman HS, Suksatan W, Jalil AT, Abdelbasset WK, et al. A paradigm shift in cell-free approach: the emerging role of MSCs-derived exosomes in regenerative medicine. J Trans Med (2021) 19(1):302. doi: 10.1186/s12967-021-02980-6
40. Keller S, Sanderson MP, Stoeck A, Altevogt P. Exosomes: from biogenesis and secretion to biological function. Immunol Lett (2006) 107(2):102–8. doi: 10.1016/j.imlet.2006.09.005
41. Hassanzadeh A, Rahman HS, Markov A, Endjun JJ, Zekiy AO, Chartrand MS, et al. Mesenchymal stem/stromal cell-derived exosomes in regenerative medicine and cancer; overview of development, challenges, and opportunities. Stem Cell Res Ther (2021) 12(1):297. doi: 10.1186/s13287-021-02378-7
42. Zhang Y, Liu Y, Liu H, Tang WH. Exosomes: biogenesis, biologic function and clinical potential. Cell Bioscience (2019) 9:19. doi: 10.1186/s13578-019-0282-2
43. Hessvik NP, Llorente A. Current knowledge on exosome biogenesis and release. Cell Mol Life sciences: CMLS (2018) 75(2):193–208. doi: 10.1007/s00018-017-2595-9
44. Jadli AS, Ballasy N, Edalat P, Patel VB. Inside(sight) of tiny communicator: exosome biogenesis, secretion, and uptake. Mol Cell Biochem (2020) 467(1–2):77–94. doi: 10.1007/s11010-020-03703-z
46. McCullough J, Colf LA, Sundquist WI. Membrane fission reactions of the mamMalian ESCRT pathway. Annu Rev Biochem (2013) 82:663–92. doi: 10.1146/annurev-biochem-072909-101058
47. Gurunathan S, Kang MH, Jeyaraj M, Qasim M, Kim JH. Review of the isolation, characterization, biological function, and multifarious therapeutic approaches of exosomes. Cells (2019) 8(4):E307. doi: 10.3390/cells8040307
48. Qiu H, Liu S, Wu K, Zhao R, Cao L, Wang H, et al. Prospective application of exosomes derived from adipose-derived stem cells in skin wound healing: A review. J Cosmetic Dermatol (2020) 19(3):574–81. doi: 10.1111/jocd.13215
49. Tkach M, Théry C. Communication by extracellular vesicles: Where we are and where we need to go. Cell (2016) 164(6):1226–32. doi: 10.1016/j.cell.2016.01.043
50. O’Loughlin AJ, Woffindale CA, Wood MJA. Exosomes and the emerging field of exosome-based gene therapy. Curr Gene Ther (2012) 12(4):262–74. doi: 10.2174/156652312802083594
51. Mulcahy LA, Pink RC, Carter DRF. Routes and mechanisms of extracellular vesicle uptake. J Extracellular Vesicles (2014), 3. doi: 10.3402/jev.v3.24641
52. Aryani A, Denecke B. Exosomes as a nanodelivery system: A key to the future of neuromedicine? Mol Neurobiol (2016) 53(2):818–34. doi: 10.1007/s12035-014-9054-5
53. Lopez-Verrilli MA, Caviedes A, Cabrera A, Sandoval S, Wyneken U, Khoury M. Mesenchymal stem cell-derived exosomes from different sources selectively promote neuritic outgrowth. Neuroscience (2016) 320:129–39. doi: 10.1016/j.neuroscience.2016.01.061
54. Baglio SR, Rooijers K, Koppers-Lalic D, Verweij FJ, Pérez Lanzón M, Zini N, et al. Human bone marrow- and adipose-mesenchymal stem cells secrete exosomes enriched in distinctive miRNA and tRNA species. Stem Cell Res Ther (2015) 6:127. doi: 10.1186/s13287-015-0116-z
55. Choi EW, Seo MK, Woo EY, Kim SH, Park EJ, Kim S, et al. Exosomes from human adipose-derived stem cells promote proliferation and migration of skin fibroblasts. Exp Dermatol (2018) 27(10):1170–2. doi: 10.1111/exd.13451
56. Chen CY, Rao SS, Ren L, Hu XK, Tan YJ, Hu Y, et al. Exosomal DMBT1 from human urine-derived stem cells facilitates diabetic wound repair by promoting angiogenesis. Theranostics (2018) 8(6):1607–23. doi: 10.7150/thno.22958
57. Keshtkar S, Azarpira N, Ghahremani MH. Mesenchymal stem cell-derived extracellular vesicles: novel frontiers in regenerative medicine. Stem Cell Res Ther (2018) 9(1):63. doi: 10.1186/s13287-018-0791-7
58. Goodarzi P, Larijani B, Alavi-Moghadam S, Tayanloo-Beik A, Mohamadi-Jahani F, Ranjbaran N, et al. Mesenchymal stem cells-derived exosomes for wound regeneration. Adv Exp Med Biol (2018) 1119:119–31. doi: 10.1007/5584_2018_251
59. Mistry DS, Chen Y, Sen GL. Progenitor function in self-renewing human epidermis is maintained by the exosome. Cell Stem Cell (2012) 11(1):127–35. doi: 10.1016/j.stem.2012.04.022
60. Lindenbergh MFS, Wubbolts R, Borg EGF, van 't Veld EM, Boes M, Stoorvogel W, et al. Dendritic cells release exosomes together with phagocytosed pathogen; potential implications for the role of exosomes in antigen presentation. J Extracellular Vesicles (2020) 9(1):1798606. doi: 10.1080/20013078.2020.1798606
61. Li W, Wang Q. Recent progress in the research of exosomes and Dscam regulated crab antiviral immunity. Dev Comp Immunol (2021) 116:103925. doi: 10.1016/j.dci.2020.103925
62. Taylor DD, Gercel-Taylor C. MicroRNA signatures of tumor-derived exosomes as diagnostic biomarkers of ovarian cancer. Gynecologic Oncol (2008) 110(1):13–21. doi: 10.1016/j.ygyno.2008.04.033
63. Wang J, Yan F, Zhao Q, Zhan F, Wang R, Wang L, et al. Circulating exosomal miR-125a-3p as a novel biomarker for early-stage colon cancer. Sci Rep (2017) 7(1):4150. doi: 10.1038/s41598-017-04386-1
64. Yang KS, Im H, Hong S, Pergolini I, Del Castillo AF, Wang R, et al. Multiparametric plasma EV profiling facilitates diagnosis of pancreatic Malignancy. Sci Trans Med (2017) 9(391):4. doi: 10.1126/scitranslmed.aal3226
65. Kalluri R. The biology and function of exosomes in cancer. J Clin Invest (2016) 126(4):1208–15. doi: 10.1172/JCI81135
66. Lamichhane TN, Sokic S, Schardt JS, Raiker RS, Lin JW, Jay SM, et al. Emerging roles for extracellular vesicles in tissue engineering and regenerative medicine. Tissue Engineering. Part B Rev (2015) 21(1):45–54. doi: 10.1089/ten.teb.2014.0300
67. Zhang J, Li S, Li L, Li M, Guo C, Yao J, et al. Exosome and exosomal microRNA: trafficking, sorting, and function. Genomics Proteomics Bioinf (2015) 13(1):17–24. doi: 10.1016/j.gpb.2015.02.001
68. Valadi H, Ekstrom K, Bossios A, Sjöstrand M, Lee JJ, Lötvall JO, et al. Exosome-mediated transfer of mRNAs and microRNAs is a novel mechanism of genetic exchange between cells. Nat Cell Biol (2007) 9(6):657–8. doi: 10.1038/ncb1596
69. Ohno S, Takanashi M, Sudo K, Ueda S, Ishikawa A, Matsuyama N, et al. Systemically injected exosomes targeted to EGFR deliver antitumor microRNA to breast cancer cells. Mol Therapy: J Am Soc Gene Ther (2013) 21(1):185–91. doi: 10.1038/mt.2012.180
70. Cooper JM, Wiklander PB, Nordin JZ, Al-Shawi R, Wood MJ, Vithlani M, et al. Systemic exosomal siRNA delivery reduced alpha-synuclein aggregates in brains of transgenic mice. Movement Disord (2014) 29(12):1476–85. doi: 10.1002/mds.25978
71. Schwab A, Meyering SS, Lepene B, Iordanskiy S, van Hoek ML, Hakami RM, et al. Extracellular vesicles from infected cells: potential for direct pathogenesis. Front Microbiol (2015) 6:1132. doi: 10.3389/fmicb.2015.01132
72. Rani S, Ritter T. The exosome—A naturally secreted nanoparticle and its application to wound healing. Advanced Materials (Deerfield Beach Fla.) (2016) 28(27):5542–52. doi: 10.1002/adma.201504009
73. Hu L, Wang J, Zhou X, Xiong Z, Zhao J, Yu R, et al. Exosomes derived from human adipose mensenchymal stem cells accelerates cutaneous wound healing via optimizing the characteristics of fibroblasts. Sci Rep (2016) 6:32993. doi: 10.1038/srep32993
74. Pomatto M, Gai C, Negro F, Cedrino M, Grange C, Ceccotti E, et al. Differential therapeutic effect of extracellular vesicles derived by bone marrow and adipose mesenchymal stem cells on wound healing of diabetic ulcers and correlation to their cargoes. Int J Mol Sci (2021) 22(8):3851. doi: 10.3390/ijms22083851
75. Blakytny R, Jude E. The molecular biology of chronic wounds and delayed healing in diabetes. Diabetic Medicine: A J Br Diabetic Assoc (2006) 23(6):594–608. doi: 10.1111/j.1464-5491.2006.01773.x
76. Sun BK, Siprashvili Z, Khavari PA. Advances in skin grafting and treatment of cutaneous wounds. Sci (New York N.Y.) (2014) 346(6212):941–5. doi: 10.1126/science.1253836
77. Zeng Q-L, Liu D-W. Mesenchymal stem cell-derived exosomes: An emerging therapeutic strategy for normal and chronic wound healing. World J Clin cases (2021) 9(22):6218–33. doi: 10.12998/wjcc.v9.i22.6218
78. Clark RAF. Fibrin is a many splendored thing. J Invest Dermatol (2003) 121(5):xxi–xxii. doi: 10.1046/j.1523-1747.2003.12575.x
79. An Y, Lin S, Tan X, Zhu S, Nie F, Zhen Y, et al. Exosomes from adipose-derived stem cells and application to skin wound healing. Cell Proliferation (2021) 54(3):e12993. doi: 10.1111/cpr.12993
80. Furie B, Furie BC. Mechanisms of thrombus formation. New Engl J Med (2008) 359(9):938–49. doi: 10.1056/NEJMra0801082
81. Marofi F, Alexandrovna KI, Margiana R, Bahramali M, Suksatan W, Abdelbasset WK, et al. MSCs and their exosomes: a rapidly evolving approach in the context of cutaneous wounds therapy. Stem Cell Res Ther (2021) 12(1):597. doi: 10.1186/s13287-021-02662-6
82. Reish RG, Eriksson E. Scars: a review of emerging and currently available therapies. Plast Reconstructive Surg (2008) 122(4):1068–78. doi: 10.1097/PRS.0b013e318185d38f
83. Martin P. Wound healing—aiming for perfect skin regeneration. Sci (New York N.Y.) (1997) 276(5309):75–81. doi: 10.1126/science.276.5309.75
84. Weisel JW, Litvinov RI. Fibrin formation, structure and properties. Sub-Cellular Biochem (2017) 82:405–56. doi: 10.1007/978-3-319-49674-0_13
85. Shah A, Amini-Nik S. The role of phytochemicals in the inflammatory phase of wound healing. Int J Mol Sci (2017) 18(5):E1068. doi: 10.3390/ijms18051068
86. Ellis S, Lin EJ, Tartar D. Immunology of wound healing. Curr Dermatol Rep (2018) 7(4):350–8. doi: 10.1007/s13671-018-0234-9
87. Gurtner GC, Werner S, Barrandon Y, Longaker MT. Wound repair and regeneration. Nature (2008) 453(7193):314–21. doi: 10.1038/nature07039
88. Flanagan M. The physiology of wound healing. J Wound Care (2000) 9(6):299–300. doi: 10.12968/jowc.2000.9.6.25994
89. Wilgus TA, Roy S, McDaniel JC. Neutrophils and wound repair: Positive actions and negative reactions. Adv Wound Care (2013) 2(7):379–88. doi: 10.1089/wound.2012.0383
90. Gillitzer R, Goebeler M. Chemokines in cutaneous wound healing. J Leukocyte Biol (2001) 69(4):513–21. doi: 10.1189/jlb.69.4.513
91. Su Y, Richmond A. Chemokine regulation of neutrophil infiltration of skin wounds. Adv Wound Care (2015) 4(11):631–40. doi: 10.1089/wound.2014.0559
92. Rodrigues M, Kosaric N, Bonham CA, Gurtner GC. Wound healing: A cellular perspective. Physiol Rev (2019) 99(1):665–706. doi: 10.1152/physrev.00067.2017
93. Witte MB, Barbul A. Role of nitric oxide in wound repair. Am J Surg (2002) 183(4):406–12. doi: 10.1016/S0002-9610(02)00815-2
94. Englander HR. Fluoridation protects occlusal areas. J Am Dental Assoc (1939) (1979) 98(1):11. doi: 10.14219/jada.archive.1979.0021
95. Goren I, Allmann N, Yogev N, Schürmann C, Linke A, Holdener M, et al. A transgenic mouse model of inducible macrophage depletion: effects of diphtheria toxin-driven lysozyme M-specific cell lineage ablation on wound inflammatory, angiogenic, and contractive processes. Am J Pathol (2009) 175(1):132–47. doi: 10.2353/ajpath.2009.081002
96. Mirza R, DiPietro LA, Koh TJ. Selective and specific macrophage ablation is detrimental to wound healing in mice. Am J Pathol (2009) 175(6):2454–62. doi: 10.2353/ajpath.2009.090248
97. Eming SA, Martin P, Tomic-Canic M. Wound repair and regeneration: mechanisms, signaling, and translation. Sci Trans Med (2014) 6(265):265sr6. doi: 10.1126/scitranslmed.3009337
98. Havran WL, Jameson JM. Epidermal T cells and wound healing. J Immunol (Baltimore Md.: 1950) (2010) 184(10):5423–8. doi: 10.4049/jimmunol.0902733
99. Ferreira A da F, Gomes DA. Stem cell extracellular vesicles in skin repair. Bioengineering (Basel Switzerland) (2018) 6(1):E4. doi: 10.3390/bioengineering6010004
100. Eming SA, Krieg T, Davidson JM. Inflammation in wound repair: molecular and cellular mechanisms. J Invest Dermatol (2007) 127(3):514–25. doi: 10.1038/sj.jid.5700701
101. Xue M, Jackson CJ. Extracellular matrix reorganization during wound healing and its impact on abnormal scarring. Adv Wound Care (2015) 4(3):119–36. doi: 10.1089/wound.2013.0485
102. Eming SA, Hammerschmidt M, Krieg T, Roers A. Interrelation of immunity and tissue repair or regeneration. Semin Cell Dev Biol (2009) 20(5):517–27. doi: 10.1016/j.semcdb.2009.04.009
103. Sindrilaru A, Peters T, Wieschalka S, Baican C, Baican A, Peter H, et al. An unrestrained proinflammatory M1 macrophage population induced by iron impairs wound healing in humans and mice. J Clin Invest (2011) 121(3):985–97. doi: 10.1172/JCI44490
104. Loots MA, Lamme EN, Zeegelaar J, Mekkes JR, Bos JD, Middelkoop E, et al. Differences in cellular infiltrate and extracellular matrix of chronic diabetic and venous ulcers versus acute wounds. J Invest Dermatol (1998) 111(5):850–7. doi: 10.1046/j.1523-1747.1998.00381.x
105. Grotendorst GR, Soma Y, Takehara K, Charette M. EGF and TGF-alpha are potent chemoattractants for endothelial cells and EGF-like peptides are present at sites of tissue regeneration. J Cell Physiol (1989) 139(3):617–23. doi: 10.1002/jcp.1041390323
106. Velnar T, Bailey T, Smrkolj V. The wound healing process: an overview of the cellular and molecular mechanisms. J Int Med Res (2009) 37(5):1528–42. doi: 10.1177/147323000903700531
107. Su WH, Cheng MH, Lee WL, Tsou TS, Chang WH, Chen CS, et al. Nonsteroidal anti-inflammatory drugs for wounds: pain relief or excessive scar formation? Mediators Inflammation (2010) 2010:413238. doi: 10.1155/2010/413238
108. Alhajj M, Goyal A. Physiology. In: Granulation tissue. Treasure Island, FL, USA: StatPearls Publishing (2022).
109. Eilken HM, Adams RH. Dynamics of endothelial cell behavior in sprouting angiogenesis. Curr Opin Cell Biol (2010) 22(5):617–25. doi: 10.1016/j.ceb.2010.08.010
110. Landén NX, Li D, Ståhle M. Transition from inflammation to proliferation: a critical step during wound healing. Cell Mol Life sciences: CMLS (2016) 73(20):3861–85. doi: 10.1007/s00018-016-2268-0
111. Wang X, Liang B, Li J, Pi X, Zhang P, Zhou X, et al. Identification and characterization of four immune-related signatures in keloid. Front Immunol (2022) 13:942446. doi: 10.3389/fimmu.2022.942446
112. Baum CL, Arpey CJ. Normal cutaneous wound healing: clinical correlation with cellular and molecular events. Dermatologic Surgery: Off Publ Am Soc Dermatologic Surg (2005) 31(6):674–86. doi: 10.1097/00042728-200506000-00011
113. Clark RA. Regulation of fibroplasia in cutaneous wound repair. Am J Med Sci (1993) 306(1):42–8. doi: 10.1097/00000441-199307000-00011
115. Gonzalez AC, Costa TF, Andrade ZA, Medrado AR. Wound healing—A literature review. Anais Brasileiros Dermatologia (2016) 91(5):614–20. doi: 10.1590/abd1806-4841.20164741
116. Darby IA, Laverdet B, Bonté F, Desmoulière A. Fibroblasts and myofibroblasts in wound healing. Clinical Cosmetic Investigational Dermatol (2014) 7:301–11. doi: 10.2147/CCID.S50046
117. Hunt TK. The physiology of wound healing. Ann Emergency Med (1988) 17(12):1265–73. doi: 10.1016/S0196-0644(88)80351-2
118. Robson MC, Steed DL, Franz MG. Wound healing: biologic features and approaches to maximize healing trajectories. Curr Problems Surg (2001) 38(2):72–140. doi: 10.1067/msg.2001.111167
119. Diegelmann RF, Evans MC. Wound healing: an overview of acute, fibrotic and delayed healing. Front Bioscience: A J Virtual Library (2004) 9:283–9. doi: 10.2741/1184
120. Medrado AR, Pugliese LS, Reis SR, Andrade ZA. Influence of low level laser therapy on wound healing and its biological action upon myofibroblasts. Lasers Surg Med (2003) 32(3):239–44. doi: 10.1002/lsm.10126
121. Luo Z, Lin J, Sun Y, Wang C, Chen J. Bone marrow stromal cell-derived exosomes promote muscle healing following contusion through macrophage polarization. Stem Cells Dev (2021) 30(3):135–48. doi: 10.1089/scd.2020.0167
122. He X, Dong Z, Cao Y, Wang H, Liu S, Liao L, et al. MSC-derived exosome promotes M2 polarization and enhances cutaneous wound healing. Stem Cells Int (2019) 2019:7132708. doi: 10.1155/2019/7132708
123. Geng X, Qi Y, Liu X, Shi Y, Li H, Zhao L, et al. A multifunctional antibacterial and self-healing hydrogel laden with bone marrow mesenchymal stem cell-derived exosomes for accelerating diabetic wound healing. Biomaterials Adv (2022) 133:112613. doi: 10.1016/j.msec.2021.112613
124. Shi Y, Kang X, Wang Y, Bian X, He G, Zhou M, et al. Exosomes derived from bone marrow stromal cells (BMSCs) enhance tendon-bone healing by regulating macrophage polarization. Med Sci Monitor: Int Med J Exp Clin Res (2020) 26:e923328. doi: 10.12659/MSM.923328
125. Bian S, Zhang L, Duan L, Wang X, Min Y, Yu H, et al. Extracellular vesicles derived from human bone marrow mesenchymal stem cells promote angiogenesis in a rat myocardial infarction model. J Mol Med (Berlin Germany) (2014) 92(4):387–97. doi: 10.1007/s00109-013-1110-5
126. Sang X, Wang Z, Qin T, Li Y. Elevated concentrations of hypoxia-inducible factor-1α in patients with fracture and concomitant traumatic brain injury. Ann Clin Biochem (2017) 54(5):584–92. doi: 10.1177/0004563216673087
127. Zhang L, Jiao G, Ren S, Zhang X, Li C, Wu W, et al. Exosomes from bone marrow mesenchymal stem cells enhance fracture healing through the promotion of osteogenesis and angiogenesis in a rat model of nonunion. Stem Cell Res Ther (2020) 11(1):38. doi: 10.1186/s13287-020-1562-9
128. Han ZF, Cao JH, Liu ZY, Yang Z, Qi RX, Xu HL, et al. Exosomal lncRNA KLF3-AS1 derived from bone marrow mesenchymal stem cells stimulates angiogenesis to promote diabetic cutaneous wound healing. Diabetes Res Clin Pract (2022) 183:109126. doi: 10.1016/j.diabres.2021.109126
129. Zhang L, Ouyang P, He G, Wang X, Song D, Yang Y, et al. Exosomes from microRNA-126 overexpressing mesenchymal stem cells promote angiogenesis by targeting the PIK3R2-mediated PI3K/Akt signalling pathway. J Cell Mol Med (2021) 25(4):2148–62. doi: 10.1111/jcmm.16192
130. Liang B, Liang JM, Ding JN, Xu J, Xu JG, Chai YM, et al. Dimethyloxaloylglycine-stimulated human bone marrow mesenchymal stem cell-derived exosomes enhance bone regeneration through angiogenesis by targeting the AKT/mTOR pathway. Stem Cell Res Ther (2019) 10(1):335. doi: 10.1186/s13287-019-1410-y
131. Ding J, Wang X, Chen B, Zhang J, Xu J. Exosomes derived from human bone marrow mesenchymal stem cells stimulated by deferoxamine accelerate cutaneous wound healing by promoting angiogenesis. BioMed Res Int (2019) 2019:9742765. doi: 10.1155/2019/9742765
132. Wu D, Chang X, Tian J, Kang L, Wu Y, Liu J, et al. Bone mesenchymal stem cells stimulation by magnetic nanoparticles and a static magnetic field: release of exosomal miR-1260a improves osteogenesis and angiogenesis. J Nanobiotechnology (2021) 19(1):209. doi: 10.1186/s12951-021-00958-6
133. Jiang T, Wang Z, Sun J. Human bone marrow mesenchymal stem cell-derived exosomes stimulate cutaneous wound healing mediates through TGF-β/Smad signaling pathway. Stem Cell Res Ther (2020) 11(1):198. doi: 10.1186/s13287-020-01723-6
134. Shen C, Tao C, Zhang A, Li X, Guo Y, Wei H, et al. Exosomal microRNA⁃93⁃3p secreted by bone marrow mesenchymal stem cells downregulates apoptotic peptidase activating factor 1 to promote wound healing. Bioengineered (2022) 13(1):27–37. doi: 10.1080/21655979.2021.1997077
135. Shabbir A, Cox A, Rodriguez-Menocal L, Salgado M, Van Badiavas E. Mesenchymal stem cell exosomes induce proliferation and migration of normal and chronic wound fibroblasts, and enhance angiogenesis in vitro. Stem Cells Dev (2015) 24(14):1635–47. doi: 10.1089/scd.2014.0316
136. Yaghoubi Y, Movassaghpour A, Zamani M, Talebi M, Mehdizadeh A, Yousefi M, et al. Human umbilical cord mesenchymal stem cells derived-exosomes in diseases treatment. Life Sci (2019) 233:116733. doi: 10.1016/j.lfs.2019.116733
137. Li X, Liu L, Yang J, Yu Y, Chai J, Wang L, et al. Exosome derived from human umbilical cord mesenchymal stem cell mediates miR-181c attenuating burn-induced excessive inflammation. EBioMedicine (2016) 8:72–82. doi: 10.1016/j.ebiom.2016.04.030
138. Wang M, Zhao Y, Zhang Q. Human mesenchymal stem cell-derived exosomes accelerate wound healing of mice eczema. J Dermatol Treat (2022) 33(3):1401–5. doi: 10.1080/09546634.2020.1820935
139. Zhang Y, Hao Z, Wang P, Xia Y, Wu J, Xia D, et al. Exosomes from human umbilical cord mesenchymal stem cells enhance fracture healing through HIF-1α-mediated promotion of angiogenesis in a rat model of stabilized fracture. Cell Proliferation (2019) 52(2):e12570. doi: 10.1111/cpr.12570
140. Qu Q, Pang Y, Zhang C, Liu L, Bi Y. Exosomes derived from human umbilical cord mesenchymal stem cells inhibit vein graft intimal hyperplasia and accelerate reendothelialization by enhancing endothelial function. Stem Cell Res Ther (2020) 11(1):133. doi: 10.1186/s13287-020-01639-1
141. Zhang Z, Yi D, Zhou J, Zheng Y, Gao Z, Hu X, et al. Exosomal LINC01005 derived from oxidized low-density lipoprotein-treated endothelial cells regulates vascular smooth muscle cell phenotypic switch. BioFactors (Oxford England) (2020) 46(5):743–53. doi: 10.1002/biof.1665
142. Zhang Z, Mi T, Jin L, Li M, Zhanghuang C, Wang J, et al. Comprehensive proteomic analysis of exosome mimetic vesicles and exosomes derived from human umbilical cord mesenchymal stem cells. Stem Cell Res Ther (2022) 13(1):312. doi: 10.1186/s13287-022-03008-6
143. Li X, Jiang C, Zhao J. Human endothelial progenitor cells-derived exosomes accelerate cutaneous wound healing in diabetic rats by promoting endothelial function. J Diabetes Its Complications (2016) 30(6):986–92. doi: 10.1016/j.jdiacomp.2016.05.009
144. Liu J, Yan Z, Yang F, Huang Y, Yu Y, Zhou L, et al. Exosomes derived from human umbilical cord mesenchymal stem cells accelerate cutaneous wound healing by enhancing angiogenesis through delivering angiopoietin-2. Stem Cell Rev Rep (2021) 17(2):305–17. doi: 10.1007/s12015-020-09992-7
145. Ma J, Zhao Y, Sun L, Sun X, Zhao X, Sun X, et al. Exosomes derived from akt-modified human umbilical cord mesenchymal stem cells improve cardiac regeneration and promote angiogenesis via activating platelet-derived growth factor D. Stem Cells Trans Med (2017) 6(1):51–9. doi: 10.5966/sctm.2016-0038
146. Zhang Y, Xie Y, Hao Z, Zhou P, Wang P, Fang S, et al. Umbilical mesenchymal stem cell-derived exosome-encapsulated hydrogels accelerate bone repair by enhancing angiogenesis. ACS Appl materials interfaces (2021) 13(16):18472–87. doi: 10.1021/acsami.0c22671
147. Kim YJ, Yoo SM, Park HH, Lim HJ, Kim YL, Lee S, et al. Exosomes derived from human umbilical cord blood mesenchymal stem cells stimulates rejuvenation of human skin. Biochem Biophys Res Commun (2017) 493(2):1102–8. doi: 10.1016/j.bbrc.2017.09.056
148. Faruqu FN, Liam-Or R, Zhou S, Nip R, Al-Jamal KT. Defined serum-free three-dimensional culture of umbilical cord-derived mesenchymal stem cells yields exosomes that promote fibroblast proliferation and migration in vitro. FASEB journal: Off Publ Fed Am Societies Exp Biol (2021) 35(1):e21206. doi: 10.1096/fj.202001768RR
149. Liu SJ, Meng MY, Han S, Gao H, Zhao YY, Yang Y, et al. Umbilical cord mesenchymal stem cell-derived exosomes ameliorate haCaT cell photo-aging. Rejuvenation Res (2021) 24(4):283–93. doi: 10.1089/rej.2020.2313
150. Zhang B, Wang M, Gong A, Zhang X, Wu X, Zhu Y, et al. HucMSC-exosome mediated-wnt4 signaling is required for cutaneous wound healing. Stem Cells (Dayton Ohio) (2015) 33(7):2158–68. doi: 10.1002/stem.1771
151. Fang S, Xu C, Zhang Y, Xue C, Yang C, Bi H, et al. Umbilical cord-derived mesenchymal stem cell-derived exosomal microRNAs suppress myofibroblast differentiation by inhibiting the transforming growth factor-β/SMAD2 pathway during wound healing. Stem Cells Trans Med (2016) 5(10):1425–39. doi: 10.5966/sctm.2015-0367
152. Zhang Y, Pan Y, Liu Y, Li X, Tang L, Duan M, et al. Exosomes derived from human umbilical cord blood mesenchymal stem cells stimulate regenerative wound healing via transforming growth factor-β receptor inhibition. Stem Cell Res Ther (2021) 12(1):434. doi: 10.1186/s13287-021-02517-0
153. Pelizzo G, Avanzini MA, Icaro Cornaglia A, De Silvestri A, Mantelli M, Travaglino P, et al. Extracellular vesicles derived from mesenchymal cells: perspective treatment for cutaneous wound healing in pediatrics. Regenerative Med (2018) 13(4):385–94. doi: 10.2217/rme-2018-0001
154. Patel RS, Impreso S, Lui A, Vidyarthi G, Albear P, Patel NA, et al. Long noncoding RNA GAS5 contained in exosomes derived from human adipose stem cells promotes repair and modulates inflammation in a chronic dermal wound healing model. Biology (2022) 11(3):426. doi: 10.3390/biology11030426
155. Li X, Xie X, Lian W, Shi R, Han S, Zhang H, et al. Exosomes from adipose-derived stem cells overexpressing Nrf2 accelerate cutaneous wound healing by promoting vascularization in a diabetic foot ulcer rat model. Exp Mol Med (2018) 50(4):1–14. doi: 10.1038/s12276-018-0058-5
156. Shen H, Yoneda S, Abu-Amer Y, Guilak F, Gelberman RH. Stem cell-derived extracellular vesicles attenuate the early inflammatory response after tendon injury and repair. J Orthopaedic Research: Off Publ Orthopaedic Res Soc (2020) 38(1):117–27. doi: 10.1002/jor.24406
157. Shen K, Wang XJ, Liu KT, Li SH, Li J, Zhang JX, et al. [Effects of exosomes from human adipose-derived mesenchymal stem cells on inflammatory response of mouse RAW264.7 cells and wound healing of full-thickness skin defects in mice]. Zhonghua Shao Shang Za Zhi = Zhonghua Shaoshang Zazhi = Chin J Burns (2022) 38(3):215–26. doi: 10.3760/cma.j.cn501120-20201116-00477
158. Zhang Y, Mei H, Chang X, Chen F, Zhu Y, Han X, et al. Adipocyte-derived microvesicles from obese mice induce M1 macrophage phenotype through secreted miR-155. J Mol Cell Biol (2016) 8(6):505–17. doi: 10.1093/jmcb/mjw040
159. Heo JS, Kim S, Yang CE, Choi Y, Song SY, Kim HO, et al. Human adipose mesenchymal stem cell-derived exosomes: A key player in wound healing. Tissue Eng Regenerative Med (2021) 18(4):537–48. doi: 10.1007/s13770-020-00316-x
160. Heo JS, Lim JY, Yoon DW, Pyo S, Kim J. Exosome and Melatonin Additively Attenuates Inflammation by Transferring miR-34a, miR-124, and miR-135b. BioMed Res Int (2020) 2020:1621394. doi: 10.1155/2020/1621394
161. Blazquez R, Sanchez-Margallo FM, de la Rosa O, Dalemans W, Alvarez V, Tarazona R, et al. Immunomodulatory potential of human adipose mesenchymal stem cells derived exosomes on in vitro stimulated T cells. Front Immunol (2014) 5:556. doi: 10.3389/fimmu.2014.00556
162. Kranendonk ME, Visseren FL, Van Balkom BW, Nolte-'t Hoen EN, van Herwaarden JA, de Jager W, et al. Human adipocyte extracellular vesicles in reciprocal signaling between adipocytes and macrophages. Obes (Silver Spring Md.) (2014) 22(5):1296–308. doi: 10.1002/oby.20679
163. Heo JS, Kim S. Human adipose mesenchymal stem cells modulate inflammation and angiogenesis through exosomes. Sci Rep (2022) 12(1):2776. doi: 10.1038/s41598-022-06824-1
164. Zhang X, Chen L, Xiao B, Liu H, Su Y. Circ_0075932 in adipocyte-derived exosomes induces inflammation and apoptosis in human dermal keratinocytes by directly binding with PUM2 and promoting PUM2-mediated activation of AuroraA/NF-κB pathway. Biochem Biophys Res Commun (2019) 511(3):551–8. doi: 10.1016/j.bbrc.2019.02.082
165. Wang J, Wu H, Peng Y, Zhao Y, Qin Y, Zhang Y, et al. Hypoxia adipose stem cell-derived exosomes promote high-quality healing of diabetic wound involves activation of PI3K/Akt pathways. J Nanobiotechnology (2021) 19(1):202. doi: 10.1186/s12951-021-00942-0
166. Qiu J, Shu C, Li X, Ye C, Zhang WC. Exosomes from linc00511-overexpressing ADSCs accelerates angiogenesis in diabetic foot ulcers healing by suppressing PAQR3-induced Twist1 degradation. Diabetes Res Clin Pract (2021) 180:109032. doi: 10.1016/j.diabres.2021.109032
167. Du L, Li G, Yang Y, Yang G, Wan J, Ma Z, et al. Exosomes from microRNA-199-3p-modified adipose-derived stem cells promote proliferation and migration of endothelial tip cells by downregulation of semaphorin 3A. Int J Clin Exp Pathol (2018) 11(10):4879–88.
168. Liang X, Zhang L, Wang S, Han Q, Zhao RC. Exosomes secreted by mesenchymal stem cells promote endothelial cell angiogenesis by transferring miR-125a. J Cell Sci (2016) 129(11):2182–9. doi: 10.1242/jcs.170373
169. Xue C, Shen Y, Li X, Li B, Zhao S, Gu J, et al. Exosomes derived from hypoxia-treated human adipose mesenchymal stem cells enhance angiogenesis through the PKA signaling pathway. Stem Cells Dev (2018) 27(7):456–65. doi: 10.1089/scd.2017.0296
170. Yang Y, Cai Y, Zhang Y, Liu J, Xu Z. Exosomes secreted by adipose-derived stem cells contribute to angiogenesis of brain microvascular endothelial cells following oxygen-glucose deprivation in vitro through microRNA-181b/TRPM7 axis. J Mol neuroscience: MN (2018) 65(1):74–83. doi: 10.1007/s12031-018-1071-9
171. Sun Y, Ju Y, Fang B. Exosomes from human adipose-derived mesenchymal stromal/stem cells accelerate angiogenesis in wound healing: implication of the EGR-1/lncRNA-SENCR/DKC1/VEGF-A axis. Hum Cell (2022) 35(5):1375–90. doi: 10.1007/s13577-022-00732-2
172. Han Y, Ren J, Bai Y, Pei X, Han Y. Exosomes from hypoxia-treated human adipose-derived mesenchymal stem cells enhance angiogenesis through VEGF/VEGF-R. Int J Biochem Cell Biol (2019) 109:59–68. doi: 10.1016/j.biocel.2019.01.017
173. Wu SC, Kuo PJ, Rau CS, Huang LH, Lin CW, Wu YC, et al. Increased angiogenesis by exosomes secreted by adipose-derived stem cells upon lipopolysaccharide stimulation. Int J Mol Sci (2021) 22(16):8877. doi: 10.3390/ijms22168877
174. Ren S, Chen J, Duscher D, Liu Y, Guo G, Kang Y, et al. Microvesicles from human adipose stem cells promote wound healing by optimizing cellular functions via AKT and ERK signaling pathways. Stem Cell Res Ther (2019) 10(1):47. doi: 10.1186/s13287-019-1152-x
175. Zhang X, Jiang Y, Huang Q, Wu Z, Pu H, Xu Z, et al. Exosomes derived from adipose-derived stem cells overexpressing glyoxalase-1 protect endothelial cells and enhance angiogenesis in type 2 diabetic mice with limb ischemia. Stem Cell Res Ther (2021) 12(1):403. doi: 10.1186/s13287-020-02086-8
176. An Y, Zhao J, Nie F, Qin Z, Xue H, Wang G, et al. Exosomes from adipose-derived stem cells (ADSCs) overexpressing miR-21 promote vascularization of endothelial cells. Sci Rep (2019) 9(1):12861. doi: 10.1038/s41598-019-49339-y
177. Pi L, Yang L, Fang BR, Meng XX, Qian L. Exosomal microRNA-125a-3p from human adipose-derived mesenchymal stem cells promotes angiogenesis of wound healing through inhibiting PTEN. Mol Cell Biochem (2022) 477(1):115–27. doi: 10.1007/s11010-021-04251-w
178. Hu XM, Li ZX, Zhang DY, Yang YC, Fu SA, Zhang ZQ, et al. A systematic summary of survival and death signalling during the life of hair follicle stem cells. Stem Cell Res Ther (2021) 12(1):453. doi: 10.1186/s13287-021-02527-y
179. Nan K, Zhang Y, Zhang X, Li D, Zhao Y, Jing Z, et al. Exosomes from miRNA-378-modified adipose-derived stem cells prevent glucocorticoid-induced osteonecrosis of the femoral head by enhancing angiogenesis and osteogenesis via targeting miR-378 negatively regulated suppressor of fused (Sufu). Stem Cell Res Ther (2021) 12(1):331. doi: 10.1186/s13287-021-02390-x
180. Wang J, Yi Y, Zhu Y, Wang Z, Wu S, Zhang J, et al. [Effects of adipose-derived stem cell released exosomes on wound healing in diabetic mice]. Zhongguo Xiu Fu Chong Jian Wai Ke Za Zhi = Zhongguo Xiufu Chongjian Waike Zazhi = Chin J Reparative Reconstructive Surg (2020) 34(1):124–31. doi: 10.7507/1002-1892.201903058
181. Pi L, Yang L, Fang BR, Meng XX, Qian L. LncRNA MALAT1 from human adipose-derived stem cell exosomes accelerates wound healing via miR-378a/FGF2 axis. Regenerative Med (2022) 17(9):627–41. doi: 10.2217/rme-2021-0170
182. Cao G, Chen B, Zhang X, Chen H. Human adipose-derived mesenchymal stem cells-derived exosomal microRNA-19b promotes the healing of skin wounds through modulation of the CCL1/TGF-β Signaling axis. Clinical Cosmetic Investigational Dermatol (2020) 13:957–71. doi: 10.2147/CCID.S274370
183. Chen Md G, Wu Md Y, Zou Md L, Zeng Md Y. Effect of microRNA-146a modified adipose-derived stem cell exosomes on rat back wound healing. Int J Lower Extremity Wounds (2021) 15347346211038092. doi: 10.1177/15347346211038092
184. Li C, An Y, Sun Y, Yang F, Xu Q, Wang Z. Adipose mesenchymal stem cell-derived exosomes promote wound healing through the WNT/β-catenin signaling pathway in dermal fibroblasts. Stem Cell Rev Rep (2022) 18(6):2059–73. doi: 10.1007/s12015-022-10378-0
185. Zhang W, Bai X, Zhao B, Li Y, Zhang Y, Li Z, et al. Cell-free therapy based on adipose tissue stem cell-derived exosomes promotes wound healing via the PI3K/Akt signaling pathway. Exp Cell Res (2018) 370(2):333–42. doi: 10.1016/j.yexcr.2018.06.035
186. He L, Zhu C, Jia J, Hao XY, Yu XY, Liu XY, et al. ADSC-Exos containing MALAT1 promotes wound healing by targeting miR-124 through activating Wnt/β-catenin pathway. Bioscience Rep (2020) 40(5):BSR20192549. doi: 10.1042/BSR20192549
187. Yang C, Luo L, Bai X, Shen K, Liu K, Wang J, et al. Highly-expressed micoRNA-21 in adipose derived stem cell exosomes can enhance the migration and proliferation of the HaCaT cells by increasing the MMP-9 expression through the PI3K/AKT pathway. Arch Biochem Biophysics (2020) 681:108259. doi: 10.1016/j.abb.2020.108259
188. Thankam FG, Chandra I, Diaz C, Dilisio MF, Fleegel J, Gross RM, et al. Matrix regeneration proteins in the hypoxia-triggered exosomes of shoulder tenocytes and adipose-derived mesenchymal stem cells. Mol Cell Biochem (2020) 465(1–2):75–87. doi: 10.1007/s11010-019-03669-7
189. Parvanian S, Yan F, Su D, Coelho-Rato LS, Venu AP, Yang P, et al. Exosomal vimentin from adipocyte progenitors accelerates wound healing. Cytoskeleton (Hoboken N.J.) (2020) 77(10):399–413. doi: 10.1002/cm.21634
190. Li Y, Zhang J, Shi J, Liu K, Wang X, Jia Y, et al. Exosomes derived from human adipose mesenchymal stem cells attenuate hypertrophic scar fibrosis by miR-192-5p/IL-17RA/Smad axis. Stem Cell Res Ther (2021) 12(1):221. doi: 10.1186/s13287-021-02290-0
191. Yuan R, Dai X, Li Y, Li C, Liu L. Exosomes from miR-29a-modified adipose-derived mesenchymal stem cells reduce excessive scar formation by inhibiting TGF-β2/Smad3 signaling. Mol Med Rep (2021) 24(5):758. doi: 10.3892/mmr.2021.12398
192. Wang L, Hu L, Zhou X, Xiong Z, Zhang C, Shehada HMA, et al. Exosomes secreted by human adipose mesenchymal stem cells promote scarless cutaneous repair by regulating extracellular matrix remodelling. Sci Rep (2017) 7(1):13321. doi: 10.1038/s41598-017-12919-x
193. Wu ZY, Zhang HJ, Zhou ZH, Li ZP, Liao SM, Wu ZY, et al. The effect of inhibiting exosomes derived from adipose-derived stem cells via the TGF-β1/Smad pathway on the fibrosis of keloid fibroblasts. Gland Surg (2021) 10(3):1046–56. doi: 10.21037/gs-21-4
194. Ma T, Fu B, Yang X, Xiao Y, Pan M. Adipose mesenchymal stem cell-derived exosomes promote cell proliferation, migration, and inhibit cell apoptosis via Wnt/β-catenin signaling in cutaneous wound healing. J Cell Biochem (2019) 120(6):10847–54. doi: 10.1002/jcb.28376
195. Li J, Li Z, Wang S, Bi J, Huo R. Exosomes from human adipose-derived mesenchymal stem cells inhibit production of extracellular matrix in keloid fibroblasts via downregulating transforming growth factor-β2 and Notch-1 expression. Bioengineered (2022) 13(4):8515–25. doi: 10.1080/21655979.2022.2051838
196. Bailey AJM, Li H, Kirkham AM, Tieu A, Maganti HB, Shorr R, et al. MSC-derived extracellular vesicles to heal diabetic wounds: A systematic review and meta-analysis of preclinical animal studies. Stem Cell Rev Rep (2022) 18(3):968–79. doi: 10.1007/s12015-021-10164-4
197. Kwon HH, Yang SH, Lee J, Park BC, Park KY, Jung JY, et al. Combination treatment with human adipose tissue stem cell-derived exosomes and fractional CO2 laser for acne scars: A 12-week prospective, double-blind, randomized, split-face study. Acta Dermato-Venereologica (2020) 100(18):adv00310. doi: 10.2340/00015555-3666
198. Yang R, Yang S, Zhao J, Hu X, Chen X, Wang J, et al. Progress in studies of epidermal stem cells and their application in skin tissue engineering. Stem Cell Res Ther (2020) 11(1):303. doi: 10.1186/s13287-020-01796-3
199. Wu N, Zhang X, Li J, Gan Y. Targeting exosomal miRNA with pH-sensitive liposome coated chitosan-siRNA nanoparticles for inhibition of hepatocellular carcinoma metastasis. J Controlled Release: Off J Controlled Release Soc (2015) 213:e82. doi: 10.1016/j.jconrel.2015.05.136
200. Guo Y, Chen Y, Han P, Liu Y, Li W, Zhu F, et al. Biocompatible chitosan-carbon nanocage hybrids for sustained drug release and highly efficient laser and microwave co-irradiation induced cancer therapy. Acta Biomaterialia (2020) 103:237–46. doi: 10.1016/j.actbio.2019.12.010
201. Tao SC, Guo SC, Li M, Ke QF, Guo YP, Zhang CQ, et al. Chitosan wound dressings incorporating exosomes derived from microRNA-126-overexpressing synovium mesenchymal stem cells provide sustained release of exosomes and heal full-thickness skin defects in a diabetic rat model. Stem Cells Trans Med (2017) 6(3):736–47. doi: 10.5966/sctm.2016-0275
202. Wang C, Liang C, Wang R, Yao X, Guo P, Yuan W, et al. The fabrication of a highly efficient self-healing hydrogel from natural biopolymers loaded with exosomes for the synergistic promotion of severe wound healing. Biomaterials Sci (2019) 8(1):313–24. doi: 10.1039/c9bm01207a
203. Shi Q, Qian Z, Liu D, Sun J, Wang X, Liu H, et al. GMSC-derived exosomes combined with a chitosan/silk hydrogel sponge accelerates wound healing in a diabetic rat skin defect model. Front Physiol (2017) 8:904. doi: 10.3389/fphys.2017.00904
204. Shafei S, Khanmohammadi M, Heidari R, Ghanbari H, Taghdiri Nooshabadi V, Farzamfar S, et al. Exosome loaded alginate hydrogel promotes tissue regeneration in full-thickness skin wounds: An in vivo study. J Biomed Materials Res Part A (2020) 108(3):545–56. doi: 10.1002/jbm.a.36835
205. Wang C, Wang M, Xu T, Zhang X, Lin C, Gao W, et al. Engineering bioactive self-healing antibacterial exosomes hydrogel for promoting chronic diabetic wound healing and complete skin regeneration. Theranostics (2019) 9(1):65–76. doi: 10.7150/thno.29766
206. Zhao M, Shi J, Cai W, Liu K, Shen K, Li Z, et al. Advances on graphene-based nanomaterials and mesenchymal stem cell-derived exosomes applied in cutaneous wound healing. Int J Nanomedicine (2021) 16:2647–65. doi: 10.2147/IJN.S300326
207. Shiekh PA, Singh A, Kumar A. Exosome laden oxygen releasing antioxidant and antibacterial cryogel wound dressing OxOBand alleviate diabetic and infectious wound healing. Biomaterials (2020) 249:120020. doi: 10.1016/j.biomaterials.2020.120020
208. Xiao S, Xiao C, Miao Y, Wang J, Chen R, Fan Z, et al. Human acellular amniotic membrane incorporating exosomes from adipose-derived mesenchymal stem cells promotes diabetic wound healing. Stem Cell Res Ther (2021) 12(1):255. doi: 10.1186/s13287-021-02333-6
209. Korting HC, Schöllmann C, White RJ. Management of minor acute cutaneous wounds: importance of wound healing in a moist environment. J Eur Acad Dermatol Venereology: JEADV (2011) 25(2):130–7. doi: 10.1111/j.1468-3083.2010.03775.x
210. Shao H, Im H, Castro CM, Breakefield X, Weissleder R, Lee H, et al. New technologies for analysis of extracellular vesicles. Chem Rev (2018) 118(4):1917–50. doi: 10.1021/acs.chemrev.7b00534
211. Théry C, Witwer KW, Aikawa E, Alcaraz MJ, Anderson JD, Andriantsitohaina R, et al. Minimal information for studies of extracellular vesicles 2018 (MISEV2018): A position statement of the International Society for Extracellular Vesicles and update of the MISEV2014 guidelines. J Extracellular Vesicles (2018) 7(1):1535750. doi: 10.1080/20013078.2018.1535750
212. Hao M, Duan M, Yang Z, Zhou H, Li S, Xiang J, et al. Engineered stem cell exosomes for oral and maxillofacial wound healing. Front Bioengineering Biotechnol (2022) 10:1038261. doi: 10.3389/fbioe.2022.1038261
213. Kučuk N, Primožič M, Knez Ž, Leitgeb M. Exosomes engineering and their roles as therapy delivery tools, therapeutic targets, and biomarkers. Int J Mol Sci (2021) 22(17):9543. doi: 10.3390/ijms22179543
Keywords: mesenchymal stem cell-derived exosomes, wound repair, hemostasis, inflammation, immune, proliferation, remodeling
Citation: Qin X, He J, Wang X, Wang J, Yang R and Chen X (2023) The functions and clinical application potential of exosomes derived from mesenchymal stem cells on wound repair: a review of recent research advances. Front. Immunol. 14:1256687. doi: 10.3389/fimmu.2023.1256687
Received: 11 July 2023; Accepted: 07 August 2023;
Published: 25 August 2023.
Edited by:
Takashi Karako, National Center for Global Health and Medicine, JapanReviewed by:
Lei Yang, Southern Medical University, ChinaWang Yunchuan, Fourth Military Medical University, China
Copyright © 2023 Qin, He, Wang, Wang, Yang and Chen. This is an open-access article distributed under the terms of the Creative Commons Attribution License (CC BY). The use, distribution or reproduction in other forums is permitted, provided the original author(s) and the copyright owner(s) are credited and that the original publication in this journal is cited, in accordance with accepted academic practice. No use, distribution or reproduction is permitted which does not comply with these terms.
*Correspondence: Jingru Wang, bGxsaXR0bGVqaW5nQDE2My5jb20=; Ronghua Yang, MjE3MjAwOTFAcXEuY29t; Xiaodong Chen, Y3hkMjM0QDE2My5jb20=
†These authors have contributed equally to this work