- 1Department of General Surgery, Affiliated Hospital of Zunyi Medical University, Zunyi, Guizhou, China
- 2The First People's Hospital of Zunyi, The Third Affiliated Hospital of Zunyi Medical University, Zunyi, Guizhou, China
Ferroptosis is an iron-dependent, novel form of programmed cell death characterized by lipid peroxidation and glutathione depletion and is widespread in a variety of diseases. CD8+ T cells are the most important effector cells of cytotoxic T cells, capable of specifically recognizing and killing cancer cells. Traditionally, CD8+ T cells are thought to induce cancer cell death mainly through perforin and granzyme, and Fas-L/Fas binding. In recent years, CD8+ T cell-derived IFN-γ was found to promote cancer cell ferroptosis by multiple mechanisms, including upregulation of IRF1 and IRF8, and downregulation of the system XC-, while cancer cells ferroptosis was shown to enhance the anti-tumor effects of CD8+ T cell by heating the tumor immune microenvironment through the exposure and release of tumor-associated specific antigens, which results in a positive feedback pathway. Unfortunately, the intra-tumoral CD8+ T cells are more sensitive to ferroptosis than cancer cells, which limits the application of ferroptosis inducers in cancer. In addition, CD8+ T cells are susceptible to being regulated by other immune cell ferroptosis in the TME, such as tumor-associated macrophages, dendritic cells, Treg, and bone marrow-derived immunosuppressive cells. Together, these factors build a complex network of CD8+ T cells and ferroptosis in cancer. Therefore, we aim to integrate relevant studies to reveal the potential mechanisms of crosstalk between CD8+ T cells and ferroptosis, and to summarize preclinical models in cancer therapy to find new therapeutic strategies in this review.
1 Introduction
CD8+ T cells can resist tumor growth and metastasis through various mechanisms (1–3). Firstly, when normal cells transform into cancer cells, they express specific antigens. CD8+ T cells directly attack cancer cells by recognizing these specific antigens and inhibit or slow down tumor growth (4, 5). Secondly, the exposure and release of tumor antigens can activate other immune cells to directly or indirectly promote the anti-tumor effects of CD8+ T cells (6, 7). However, cancer cells can also evade the attack of CD8+ T cells through various strategies, such as reducing the expression of tumor-specific antigens, increasing infiltration of immune suppressor cells, blocking the activation of CD8+ T cells, inducing the exhaustion and death of CD8+ T cells (8–13). Therefore, the study is a hot topic about how to enhance the immune response of CD8+ T cells against tumors and overcome the escape mechanisms of cancer cells in the field of cancer immunotherapy.
Ferroptosis was proposed in 2012, referring to a distinct form of programmed cell death triggered by iron-dependent and lipid peroxidation pathways, which is widely present in various diseases, especially cancer (14–18). In recent years, the mechanisms of ferroptosis have been rapidly elucidated, including system XC- inhibition (SLC7A11/SLC3A2), upregulation of glutathione peroxidase 4 (GPX4), iron homeostasis imbalance, and phospholipid peroxidation (14, 19–22). Multiple factors can also regulate the sensitivity of cell ferroptosis in pathological conditions to mediate disease progression (23). The system XC- is a reverse transporter of cystine and glutamate, which can increase cellular uptake of cystine and convert it to glutathione (GSH) under the action of thioredoxin reductase 1 (TXNRD1), and GPX4 relies on GSH as a substrate to increase its activity and promote the conversion of lipid peroxide (PL-OOH) to lipid alcohol (PL-OH). Thus, It prevents cells ferroptosis by decreasing the accumulation of PL-OOH. Erastin and RSL3 act as inhibitors of the system XC- and GPX4, respectively, to promote cell ferroptosis (14, 19, 23). Iron homeostasis imbalance is another classical pathway in ferroptosis, regulated mainly by the network of transferrin receptor 1 (TfR1), iron regulatory protein 1 (IRP1), and iron regulatory protein 2 (IRP2) to influence cellular iron uptake, storage, and release. The production of reactive oxygen species (ROS) and phospholipid peroxidation require a large amount of metabolic enzyme involvement and iron acts as a catalyst and essential element for these enzymes. Iron-dependent Fenton reaction rapidly amplifies PL-OOHs and produces various reactive free radicals to induce cancer cell ferroptosis (24–26). Infinite lipid peroxidation is a hallmark of ferroptosis, and acyl-CoA synthetase long-chain family member 4 (ACSL4) is a key enzyme in the conversion of polyunsaturated fatty acids (PUFAs) to PUFA-PE. PUFA-PE promotes the intracellular accumulation of lipid peroxides under the action of various enzymes. The cell membrane contains abundant PUFA-PL, and phospholipid peroxidation is considered the direct executor of cell ferroptosis (27–29).
In recent years, it has been found that CD8+ T cells- derived IFN-γ promotes cancer cells ferroptosis, which can release multiple tumor antigens and further activate CD8+ T cells through the role of antigen-presenting cells (APCs) to enhance anti-cancer immunity. In addition, CD8+ T cells and other immune cells can undergo ferroptosis in the tumor microenvironment (TME), thereby altering the immune function of CD8+ T cells in tumors. Therefore, this review introduces mainly the network of CD8+ T cells and ferroptosis in cancer and reveals the underlying mechanisms.
2 CD8+ T cells ferroptosis causes immune escape of cancer cells
CD8+ T cells are the most effective immune cells in anti-cancer immunity and can directly kill cancer cells in multiple ways, earning them the nickname “executioner” of the tumor immune system (30). Perforin and granzyme released by CD8+ T cells are effector factors of cancer cell apoptosis, which can lead to cancer cell protein degradation and destruction, causing cell apoptosis (31). Another way is through CD8+T cells Fas-L binding to target cell Fas, sequentially activating caspase 8 and caspase 3 proteases to promote protein degradation, causing lethal damage to cancer cells (32–34). CD8+ T cells can also indirectly kill cancer cells by releasing cytokines such as tumor necrosis factor (TNF) (35). In recent years, it has been found that CD8+ T cells-derived IFN-γ can induce cancer cells ferroptosis by binding to the surface IFN- γ receptor (IFNγR) on cancer cells, which enriches the mechanisms of CD8+ T cell killing cancer cells (36–39). Ferroptosis plays a crucial role in CD8+ T cell-mediated anti-tumor immunity. However, CD8+ T cells are also vulnerable in the TME. Cancer cells upregulate molecules such as PD-L1 and Fas-L to cause the dysfunction and exhaustion of CD8+ T cells and promote immune evasion of cancer cells (9, 40–43). New evidence also suggests that cancer cells induce CD8+ T cell ferroptosis by interfering with the TME, weakening their anti-cancer immune function (44).
Recent studies have shown that CD8+ T cells are more sensitive to ferroptosis than cancer cells and are susceptible to spontaneous ferroptosis influenced by the TME, depriving survival opportunities of CD8+ T cells. This leads to a decrease in the abundance of intra-tumoral CD8+ T cells and induces functional impairment, promoting immune evasion of cancer cells. Drijvers et al. demonstrated that activated CD8+ T cells were significantly more sensitive to RSL3-induced ferroptosis than cancer cells when CD8+ T cells were co-cultured with cancer cells, resulting in ferroptosis of CD8+ T cells and immune regression against cancer. Lack of ACSL4 protected CD8+ T cells from the threat of high-dose RSL3-induced ferroptosis but also resulted in functional defects of CD8+ T cells, enabling cancer cells to evade the specific killing of CD8+ T cells. Therefore, the key enzyme ACSL4, which regulates lipid peroxidation during cell ferroptosis, is necessary to maintain the function of CD8+ T cells (44). In addition, the sensitivity of CD8+ T cell ferroptosis is also influenced by the metabolic state of the TME. Ma et al. found that cholesterol is rich in the TME and gradually increases with tumor progression. Cholesterol induces the expression of CD36 on the intra-tumoral CD8+ T cells. CD36 can increase intracellular fatty acid uptake and lipid accumulation to trigger lipid peroxidation-induced ferroptosis and weaken the anti-cancer ability of CD8+ T cells. This explains why the gradual upregulation of CD36 expression reduces the infiltration of intra-tumoral CD8+ T cells through ferroptosis during tumor progression, leading to a gradual decline in anti-tumor immune function (45, 46). Conversely, CD8+ T cells can also evade ferroptosis by adjusting the expression of ferroptosis-related genes. Tc1 is a typical subset of CD8+T cells, and Tc9 is a subset of CD8+T cells that secrete IL-9 (47). Xiao et al. found Tc9 cells transferred into tumor-bearing mice exhibit longer lifespan and anti-tumor activity than Tc1 cells, thanks to the high expression of IL-9 in Tc9 cells. STAT3 is a downstream target of IL-9 and can bind to the CPT1A promoter to induce transcription. CPT1A, as a key enzyme in fatty acid oxidation, can increase mitochondrial activity and reduce lipid peroxidation, thereby protecting Tc9 cells from tumor-induced ferroptosis. In melanoma, the IL-9 expression is lower and the expression of genes related to lipid peroxidation and ferroptosis is higher on the tumor Infiltrating CD8+ T cells, compared with circulating CD8+ T cells, which may be related to increased sensitivity of the intra-tumoral CD8+ T cells to ferroptosis (48). It is worth noting that IL-9 has been shown to activate adaptive immune to suppressive tumor growth in various tumors (49), but it has a tumor-promoting effect in T cell-derived hematological cancers (50, 51). It needs further investigation whether high expression of IL-9 is involved in protecting T cell-derived hematological cancer cells from ferroptosis.
The high sensitivity of CD8+ T cells to ferroptosis in the TME limits the application of ferroptosis inducers in cancer and increases the immune evasion of cancer cells. By further studying CD8+ T cells and identifying protective factors that prevent them from undergoing ferroptosis, it may be possible to enhance the effectiveness of anti-tumor immune therapy.
3 The interaction of CD8+ T cells and cancer cells ferroptosis enhances tumor suppression
The interaction between CD8+ T cells and cancer cell ferroptosis is mutually promoting. The CD8+ T cells-derived IFN-γ induces cancer cell ferroptosis by binding to IFNγR and activating multiple pathways. Cancer cell ferroptosis releases various tumor antigens that activate CD8+ T cells through APCs, forming a “positive closed-loop pathway” that significantly enhances tumor suppression (Figure 1).
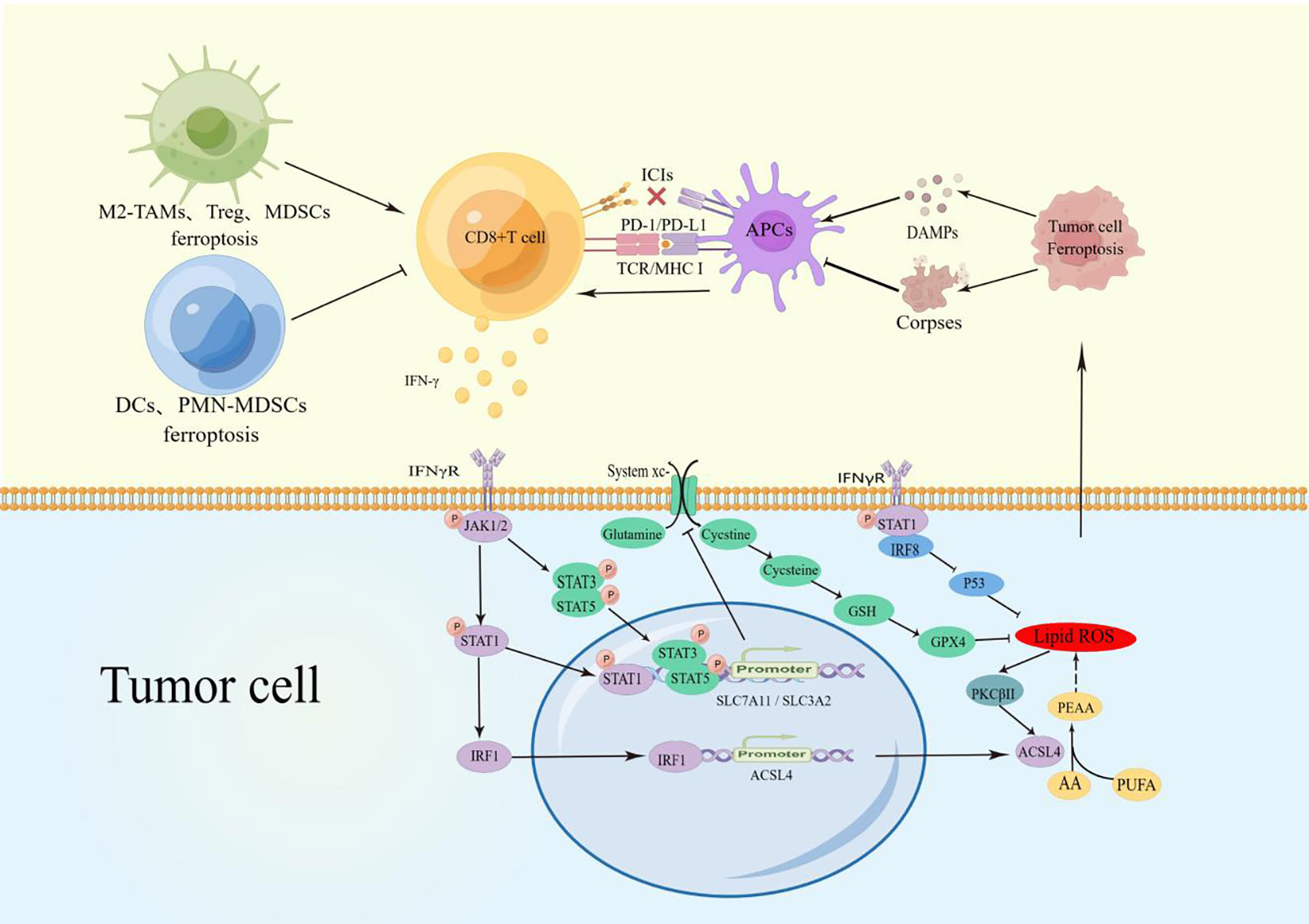
Figure 1 TAMs Ferroptosis reduced M2-macrophage infiltration and increased polarization to M1 macrophages to promote CD8+ T cell activity. Treg, MDSCs, and TINs ferroptosis reduced the inhibition of CD8+ T cells. PMN-MDSCs and DCs blocked the CD8+ T-cell activation pathway.CD8+ T cell-derived IFN-γ binds to IFNγR on the surface of cancer cells and promotes phosphorylation of STATs via JAK1/2. P-STAT1 promotes ACSL4 expression through upregulation of IRF1, and the combination of ACSL4 and AA can induce cell ferroptosis. During ferroptosis, the increased lipid ROS can activate PKCβII and ACSL4 to Significantly magnify this effect. P-STAT1, p-STAT3, and p-STAT5 inhibits SLC7A11/SLCA2 expression to block the uptake of cystine and reduce the activity of GPX4, which also promotes cells ferroptosis. In addition, IFNγR can up-regulate the expression of IRF8. These factors together amply tumor cell ferroptosis. The release of DAMPs during cancer cell ferroptosis induces the maturation of APCs and activation of CD8+ T cells, establishing a positive recycling pathway. Due to engulfing ferroptosis cell corpses, APCs lose their antigen-presenting ability and have difficulty activating CD8+T cells.
3.1 CD8+ T cells promote cancer cell ferroptosis
IFN-γ is one of the effector factors secreted by activated CD8+ T cells and exerts its function by binding to IFNγR on cancer cells. STATs and interferon regulatory factors (IRF) family are mainly downstream genes of IFN-γ (52). In CD8+ T cell-induced cancer cell ferroptosis, IFN-γ regulates the phosphorylation of STATs (P- STATs) and changes the transcriptional activation of target genes. P-STATs mediate the expression of IRF1 and IRF8 in cancer cells (53, 54), which act as the transcriptional activation factors of ACSL4 and the transcriptional inhibitory factors of P53, respectively, to participate in the induction of cancer cell ferroptosis (36, 55). The low expression of IRF8 in hepatocellular carcinoma and breast cancer has been associated with a low response to immune therapy (IFN-γ and ICIs). In addition, IFN-γ is involved in mitochondrial damage and cell cycle arrest, further promoting cancer cell ferroptosis.
With the action of IFN-γ, the activation of JAK1/2 and phosphorylation of STAT1 increase the expression of IRF1 on cancer cells. IRF1 acts as a transcriptional activation factor on the ACSL4 promoter. ACSL4 is a key enzyme for fatty acid oxidation and reshapes the lipid spectrum in cancer cells under the action of arachidonic acid (AA), increasing lipid peroxidation levels and ferroptosis. Therefore, IFN-γ combined with AA is thought as the endogenous triggering factor for ACSL4-mediated cancer cell ferroptosis, and immune checkpoint inhibitors (ICIs) can significantly enhance the anti-tumor effect (36). Interestingly, recent studies have shown that the accumulation of lipid peroxides induces the activation of PKCβII in cancer cells, and PKCβII can also interact with the Thr328 site of ACSL4 to activate ACSL4. Therefore, the combined use of IFN-γ and fatty acids may promote the rapid amplification of lipid peroxides through positive feedback between PKCβII and ACSL4, reaching a lethal level of cancer cell ferroptosis and enhancing the ability of IFN-γ to induce ferroptosis (56). In another study, Wang et al. found that IFN-γ-mediated P-STAT1 can directly bind to the promoter region to inhibit the expression of SLC7A11 and increase significantly intracellular GSH depletion, lipid peroxidation, and ferroptosis in cancer (37). Consistent with the above studies, Kong et al. verified that IFN-γ treatment induces cancer cell ferroptosis through the STAT1/IRF1/ACSL4 axis in hepatocellular carcinoma. In addition, they found that IFN-γ increases the phosphorylation of STAT3 and inhibits the transcription of SLC7A11, further reducing cystine uptake, and disrupting intracellular redox balance. They also found that the combination of IFN-γ and Erastin enhances mitochondrial oxidation and the loss of mitochondrial membrane potential (MMP), further triggering ferroptosis. Moreover, the combination of IFN-γ and ferroptosis inducers can inhibit the expression of CyclinD1, CDK4, and CDK6 to mediate more cell cycle arrest (39). It has been reported that phosphorylated STAT5 can also bind to the promoter region of SLC7A11 to inhibit its expression, participating in cystine deprivation and disrupting intracellular redox balance, which may trigger ferroptosis (57). IRF8 is another target gene of IFN-γ. Poschel et al. found that the expression level of IRF8 is significantly higher in responders to nivolumab (PD-1 inhibitor) treatment in melanoma patients compared to non-responders. IRF8 can inhibit the expression of P53 protein, increase lipid peroxidation, and promote ferroptosis in melanoma cells (55). Similarly, the IFN-γ and ICIs immune therapies signaling pathway is significantly inhibited with low levels of IRF8 in hepatocellular carcinoma and is associated with poor prognosis (58), while high expression of IRF8 in human breast cancer is associated with better response to immune therapy and chemotherapy (59). Whether IRF8 regulated by immune therapy interferes with ferroptosis is worth further investigation.
In summary, activated CD8+ T cells secrete IFN-γ and act on the key targets of cancer cell ferroptosis. This leads to a decrease in the oxidative buffering capacity of GPX4 and other GSH-dependent enzymes. IFN-γ also promotes lipid peroxidation through ACSL4 using fatty acids and results in selective enrichment of PUFAs to induce cells ferroptosis (38). Ferroptosis is usually induced by exogenous chemical molecules, including erastin, RSL3, and Sorafenib. CD8+ T cell-derived IFN-γ is an important component of anti-cancer immunity, and AA exists in plasma and cells, jointly opening the door to endogenous ferroptosis (36).
3.2 Cancer cell ferroptosis influences the antitumor ability of CD8+ T cell
The Cell Death Naming Committee has defined immunogenic cell death (ICD) as “a regulated form of cell death that is capable of activating adaptive immune responses in immunocompetent hosts of the same genotype” (60). Many studies have shown that various factors are exposed or released during cell death processes such as necrosis, pyroptosis, autophagy, and apoptosis, which present “find me” and “eat me” signals to mediate immune responses. These factors are collectively referred to as damage-associated molecular patterns (DAMPs) (61). Consistent with other death-related ICDs, Ferroptosis, as a novel form of programmed cell death, can also release DAMPs such as HMGB1, calreticulin, ATP, HSP70, and HSP90 during the death process. These factors are often simultaneously released by cancer cells and act as immune adjuvants to attract and stimulate antigen-presenting cells (APCs). The activated APCs can engulf and process tumor-associated antigens. This ultimately leads to the clonal expansion of tumor-specific CD8+ T cells and elicit an immune response. However, inconsistent with other ICDs, ferroptosis can also inhibit the activation of CD8 T cells due to lipids peroxidation, which causes lipid droplet accumulation and loss of antigen function after APCs engulf ferroptosis corpses (Table 1).
High mobility group box 1 (HMGB1) is a non-histone nuclear protein that has different functions depending on its subcellular localization. Extracellular HMGB1 regulates immune responses by binding to immune cell receptors (70, 71). In various diseases, HMGB1 acts as a redox protein that increases the accumulation of reactive oxygen species (ROS). ROS is considered a stress factor for ferroptosis, and the migration and release of HMGB1 are associated with ROS. Therefore, HMGB1 is often released during ferroptosis and participates in the regulation of CD8+ T cells as tumor antigens (72–77). Song et al. found that overexpression of OTUD1 inhibits the ubiquitination and degradation of iron-responsive element-binding protein 2 (IREB2) in colorectal cancer cells. IREB2, as an iron sensor, participates in the regulation of iron transport proteins and promotes iron uptake and ferroptosis in cancer cells, which significantly increases the release of HMGB1 in the tumor stroma and promotes the CD8+ T cells infiltration in cancer (62). In addition, Efimova et al. treated fibrosarcoma cells and glioblastoma cells with RSL3 and detected the immunogenic characteristics of ferroptosis cells at different time points. The release of HMGB1 reached its maximum level only in the late stage of ferroptosis (24h). The level of HMGB1 in the supernatant of early-stage ferroptotic cells (3h) showed no statistically significant difference compared to that in surviving cancer cells. However, when fibrosarcoma cells and glioblastoma cells were co-cultured with bone marrow-derived dendritic cells (BMDCs), the level of HMGB1 reached its maximum value in the early stage (3h). Whether BMDCs alter the spatiotemporal release of HMGB1 from ferroptotic cells is still unclear. However, early-stage ferroptotic cells can induce BMDCs maturation and active CD8+ T cells to suppress tumor growth by involving. Late-stage ferroptosis cells, on the other hand, can be cleared, but lack immunogenic characteristics (63).
It is worth noting that the role of HMGB1 in immunity is not solely to promote antigen presentation and activate CD8+ T cells. Some studies have shown that HMGB1 can increase the infiltration of immune suppressive cells to weaken the anti-cancer effects of CD8+ T cells (78). Conche et al. found that GPX4 deficiency induces lipid peroxidation and ferroptosis in hepatocellular carcinoma. During this process, it can recruit CD8+ T cells by increasing the expression of CXCL10 and PD-L1 in cancer cells. However, the increase of HMGB1 during ferroptosis leads to the infiltration of myeloid-derived suppressor cells (MDSCs), which are immune suppressive cells in the TME. Blocking the inhibitory effect of MDSCs on CD8+ T cells through ICIs can enhance the anti-cancer effects. The same treatment did not inhibit tumor growth in mice with colon cancer but reduced liver metastasis, indicating the complexity of ferroptosis-induced immunity in the different TMEs (64).
Adenosine triphosphate (ATP) is an essential nucleotide for metabolism and is released into the TME during cell death through lysosomal secretion and vesicle formation. It binds to immune cell receptors, such as DCs’ P2X7R receptor, to initiate tumor immune responses (79). In contrast to HMGB1, ATP is released by fibrosarcoma and glioma cells after 3 hours of RSL3 treatment, reaching a peak at 6 hours. However, the released ATP is depleted by 24 hours. This suggests that ATP induces DCs maturation and initiates CD8+ T cell immunity in the early stages of ferroptosis, but late-stage ferroptosis cells cannot acquire adaptive immunity. In esophageal cancer, inhibiting SCD1 under radiotherapy prevents the conversion of saturated fatty acids to unsaturated fatty acids, increases intracellular lipid peroxidation and ferroptosis, and activates DCs through recognition and activation of ATP released by cancer cells (65). Shikonin enhances ferroptosis and ATP release in multiple myeloma by inhibiting GOTI and promoting iron phagocytosis, thereby activating adaptive immunity (66).
Calreticulin (CRT) is an endoplasmic reticulum luminal Ca2+ buffering protein that is involved in regulating Ca2+ homeostasis and endoplasmic reticulum Ca2+ capacity (80). The damage of cancer cells triggers the gradual translocation and exposure of CRT as a “find me” signal. APCs, especially immature dendritic cells (DCs), can bind to CRT through the CD91 receptor to increase the activation of tumor-specific naive CD8+ T cells (81–84). Therefore, CRT is an important DAMP in the process of cell death. Cancer cell ferroptosis has also been shown to induce tumor immune responses through the exposure of CRT. Zhao et al. found a significant negative correlation between the expression of GPX4 and calreticulin in HNSCC. Inhibition of GPX4 by RSL3 significantly increased cancer cell ferroptosis, promoted the translocation of CRT to the cell surface, downregulated myeloid-derived suppressor cells and M2-like macrophages, increased the number of CD4+ T cells and CD8+ T cells, improved the immunosuppressive microenvironment of head and neck squamous cell carcinoma (HNSCC), and inhibited tumor progression (67). Similarly, Yu et al. used nanopolymer-mediated intracellular Fenton reaction and oxidative stress in cancer cells, which resulted in the exposure of CRT on ferroptotic cancer cells, leading to DCs maturation and infiltration of cytotoxic T lymphocytes (68).
Other DAMPs Cancer cell death is accompanied by the release of a large number of tumor antigenic molecules. In addition to HMGB1, CRT, and ATP mentioned above, there are many other antigenic factors involved in the process of ferroptosis, such as HSP70 and HSP90 (77). These factors usually accumulate in the TME, including but not limited to inducing the maturation and activation of M1-like macrophages or DCs, to amplify the immune response and enhance the anti-tumor ability of CD8+ T cells.
Contradictorily, recent reports have shown that cancer cell ferroptosis impedes DCs-mediated anti-tumor immunity, which challenges previous studies. ML266-induced cancer cell ferroptosis can release ATP and HMGB1, as well as expose CRT. However, when bone marrow-derived dendritic cells (BMDCs) were co-cultured with ferroptotic cancer cells, it was found that the maturation of BMDCs was negatively correlated with early-stage (1-2h) ferroptotic cancer cells. Although the maturation marker molecules of BMDCs increased in the mid-stage (3-4h) and late-stage (5-8h), the maturation of BMDCs was not sufficient to induce an immune response due to the loss of antigen presentation ability after BMDCs engulf ferroptosis cell corpse. this is manifested by the decrease of antigen presentation-related gene expression and the accumulation of lipid droplets. Thus, ferroptotic cancer cells weaken the ability to activate CD8+ T cells. The inoculation of ferroptotic cancer cells cannot induce tumor immunogenic protection against newly formed tumors in vivo, regardless of the stage of cell death (early, mid, or late stage) (69). This may explain the concept that ferroptotic cancer cells are associated with poor prognosis in various cancer patients (85–87).
In a word, CD8+T cells have a higher sensitivity to ferroptosis than cancer cells, and ferroptosis cell corpses can block the activation of CD8+T cells. In addition, ferroptosis is associated with poor prognosis of various tumors (85–87). This may pose a risk to the use of ferroptosis in cancer. On the other hand, it has been shown to be effective that building polymeric drugs were specifically ingested by cancer cells to interfere with ferroptosis and boost immunity (detailed in a later chapter). Therefore, targeting cancer cells ferroptosis should be more cautious.
4 CD8+ T cells are susceptible to being regulated by other immune cell ferroptosis in the TME
The TME is a dynamic system composed of cancer cells, cytokines, extracellular matrix, and immune cell subsets. The intercellular interactions affect the survival and function of cancer cells, stromal cells, T cells, and other immune cells. As the ultimate executors of tumor immunity, CD8+ T cells are also influenced by other immune cells. Therefore, other immune cell ferroptosis can alter the immune activity of CD8+ T cells in cancer (Figure 1; Table 2).
4.1 DCs ferroptosis
DCs are the main pathway for the activation of CD8+ T cells. DCs can recognize tumor-specific antigens to activate CD8+ T cells by TCR receptors (97). Therefore, DCs ferroptosis blocks the activation pathway of CD8+ T cells and downregulates anti-cancer immunity. Han et al. found that PPARG/PPARγ, a nuclear receptor involved in regulating lipid metabolism, promotes RSL3-induced ferroptosis of DCs. Genetic depletion of PPARG restores the maturation and function of DCs, activates cytotoxic T cells through signal transduction, and enhances CD8+ T cell-mediated anti-cancer immunity (88).
4.2 Macrophages ferroptosis
Tumor-associated macrophages (TAMs) play a “double-edged sword” role in the development of cancer. This may be related to TAMs subsets. Although M1-like macrophages and M2-like macrophages are TAMs subsets, M1-like macrophages showed anti-tumor immunity while M2 macrophages showed pro-tumor effect (98). Iron overload and ROS accumulation have been shown to promote the polarization of macrophages toward M1-like macrophages during ferroptosis. This may lead to partial polarization of TAMs toward M1-like macrophages and reduce the number of intra-tumoral M2-like macrophages to increase CD8+ T cell infiltration in the TME (99–103). Li et al. demonstrated that Dihydroartemisinin (DHA) increases intracellular iron levels by upregulating TFR1 and, in combination with GPX4 inhibition, initiates ferroptosis. In turn, lipid peroxidation during ferroptosis induces DNA damage response and further activates NF-kB, promoting polarization of macrophages toward the M1 phenotype (89). Similarly, Hao et al. found that inhibition of AOPCI promotes the upregulation of the multiple ferroptosis genes and enhances M2 macrophage polarization toward to M1 phenotype through the ferroptosis pathway, thereby activating CD8+ T cells and promoting anti-cancer immunity in hepatocellular carcinoma (90). Consistently, Tang et al. found that TAMs manifested xCT upregulation, ferroptosis Reduction, and M2-like polarization in hepatocellular carcinoma. Targeted xCT-mediated ferroptosis and protumoral polarization of Macrophages is effective. Inhibition or elimination of xCT combined with PD-1 can significantly reduce TAMs infiltration and M2-macrophage polarization, and enhance CD8+T cell activity (91).
4.3 MDSCs ferroptosis
Myeloid-derived suppressor cells (MDSCs) are immunosuppressive components of the TME. They can suppress the anti-cancer effects of CD8+ T cells. Promoting the polarization of MDSCs and reducing their infiltration can improve cancer immunotherapy (104). Li et al. found that TLR2 agonists promote the polarization of MDSCs and the production of ROS in hepatocellular carcinoma, which may be related to Runx1 in MDSCs. RNA sequencing of MDSCs after TLR2 agonist treatment revealed differential gene expression concentrating in the ferroptosis pathway, suggesting a link between MDSCs polarization and ferroptosis. Polarized MDSCs can increase CD8+ T cell activity and suppress tumor growth (92). Zhu et al. also found that ASAH2 is highly expressed in MDSCs and inhibits ferroptosis mediated by the p53 protein pathway. Therefore, inhibiting ASAH2 promotes ferroptosis in MDSCs, reducing their immunosuppressive ability and improving prognosis through CD8+ T cell infiltration and IFN-γ secretion (93). It is worth noting that the decrease in immunosuppressive cells is generally believed to alleviate the immunosuppressive effects of the TME. However, pathologically activated neutrophils, known as polymorphonuclear myeloid-derived suppressor cells (PMN-MDSCs), have more immunosuppressive effects due to spontaneous ferroptosis mediated by low oxygen and FATP2. Although ferroptosis reduces the presence of PMN-MDSCs, the release of oxygenated lipids and prostaglandin E2 (PGE2) limits the activity of CD8+ T cells in humans and mice. In immunocompetent mice, genetic or pharmacological inhibition of ferroptosis can prevent PMN-MDSCs ferroptosis and reduce their suppressive activity, slowing tumor progression. The synergistic effect with ICIs can further enhance anti-tumor immunity, opening up new perspectives on the role of ferroptosis in immunosuppressive cells (94). Previous reports verified the equivalent identity between PMN-MDSCs and tumor-infiltrating neutrophils (TINs) (105, 106). Zhao et al. identified aconitate decarboxylase 1 (Acod1) as the most upregulated metabolic enzyme in TINs. Acod1 produces itaconate through the GM-CSF-JAK/STAT5-C/EBPb pathway to defend against ferroptosis and upholds the persistence of TINs. Acod1 ablation bolsters antitumor T cell immunity and boosts the efficacy of immune checkpoint blockade by inducing TINs ferroptosis (95).
4.4 Treg ferroptosis
Regulatory T cell (Treg) is another important component of the immune suppression TME, which can induce CD8+ T cell exhaustion and cause the immune escape of cancer cells (107, 108). Treg evades ferroptosis by upregulating Gpx4 in the TME. TCR/CD28 co-stimulation leads to excessive accumulation of lipid peroxides and subsequent ferroptosis in Gpx4-deficient Treg, reducing the suppressive effect of Treg in the TME. In addition, IL-1B is expressed during the ferroptosis process of GPX4-deficient Treg, promoting the activation of DCs and CD8+ T cells, and inhibiting tumor growth (96).
5 The applications of CD8+ T cells and ferroptosis in cancer therapy
ICIs have achieved great success in the clinical application of tumor immunotherapy. However, they are ineffective in “cold tumors” lacking T cell infiltration. The strategy of converting “cold tumors” into “hot tumors” is an immunotherapy approach (Figure 2) (109). As mentioned above, there is a positive feedback loop promoting the relationship between CD8+ T cells and ferroptosis in cancer. Cancer cells ferroptosis can heat the TME and enhance the anti-tumor immune ability, the increased CD8+T cells can further inhibit tumor growth by promoting cancer cells ferroptosis, which may provide new hopes for cancer therapy (Table 3).
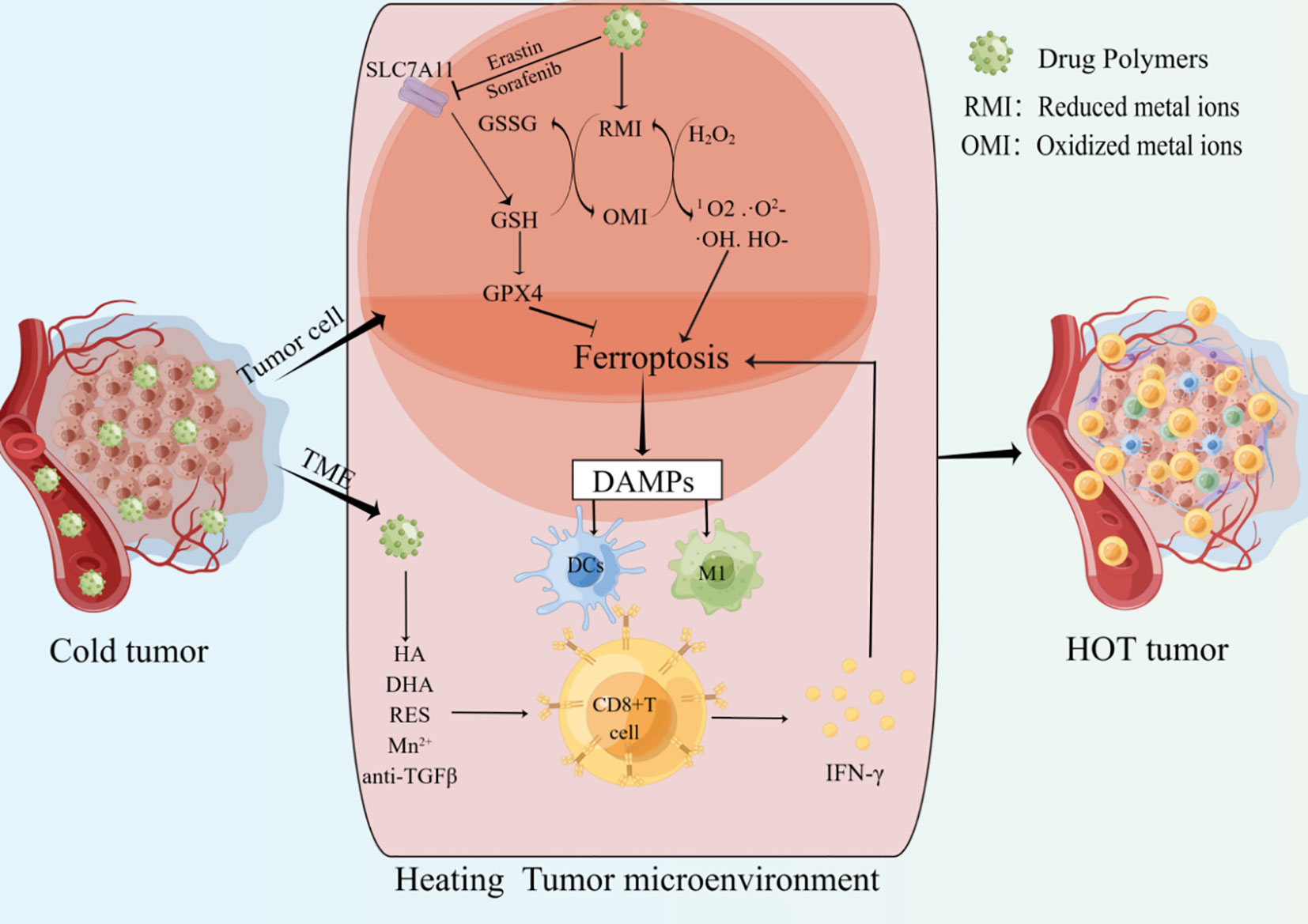
Figure 2 Drug polymers enter cancer cells and release metal ions or ferroptosis inducers. the shift of Metal ions between oxidized and reduced states can deplete GSH, generate highly toxic free radicals, and increase the accumulation of ROS. Ferroptosis inducers can inhibit SLC7A11 and other targets to induce tumor cells ferroptosis. The release of ferroptosis-related DAMPs promotes the infiltration and activation of CD8+ T cells. IFN-γ derived from CD8+ T cells can further promote tumor cells ferroptosis. In addition, drug polymers can also directly release drugs to promote the infiltration and activation of CD8+ T cells in the tumor microenvironment. Multiple mechanisms together heat the tumor microenvironment and improve the anti-tumor ability.
5.1 Promoting CD8+ T cell-mediated cancer cells ferroptosis significantly inhibits tumor growth
Cytotoxic T-lymphocyte-associated protein 4 (CTLA-4) and programmed cell death protein 1 (PD-1) are important inhibitors of T-cell responses (127, 128). Maintaining the activation of CD8+ T cells and protecting them from exhaustion and death is an effective approach in cancer immunotherapy (129, 130). Anti-CTLA-4 antibodies can attenuate the inhibition of CD8+ T cell activity, and PD-1/PD-L1 checkpoint blockade can reduce CD8+ T cell exhaustion. Therefore, blocking CTLA-4 and PD-1/PD-L1 can inhibit CD8+ T cell exhaustion and death to promote cancer cells ferroptosis, and suppress tumor growth (131, 132).
Graphene oxide (GO) is a good drug delivery carrier, and PEI-PEG is a water-soluble polymer with high-density amine and a solubilizer that can maintain stable circulation of drug molecules in the blood (133–135). Zhao et al. developed a polymeric drug molecule (GO-PEI-PEG/PD-L1 siRNA) that carries PD-L1 siRNA and can be specifically taken up by cancer tissue. The PD-L1 siRNA is released under lysosomal action, reducing the abundance of cancer cells PD-L1 and preventing PD-L1/PD-1-mediated CD8+ T cell exhaustion. Combined therapy with sorafenib significantly improved the intra-tumoral CD8+ T cell infiltration and upregulated IFN-γ expression, promoting hepatocellular carcinoma ferroptosis (110). Besides, Huang et al. designed a porous and hollow carrier using attenuated Burkholderia pseudomallei as a vehicle, loading with tumor cells lysate and adjuvant CpG to serve as a tumor vaccine(SB-LC). Tumor-associated-antigens promoted the maturation of DCs by binding to PAR1 and MR on the surface of DCs, leading to the activation of CD8+ T cells. Enhancing the anti-cancer effect of CD8+ T cells inhibits tumor growth through the ferroptosis pathway. the positive feedback loop significantly suppressed tumor growth in various mouse tumor models (111).
5.2 Targeting cancer cells ferroptosis promotes the transition from “cold tumors” to “hot tumors”
High ferroptosis sensitivity of immune cells limits the non-targeted application of ferroptosis inducers in cancer (44). Therefore, it is crucial to construct targeted drugs that can be specifically taken up by cancer cells and induce ferroptosis. Multiple molecules have been shown to play important roles in the process of ferroptosis, and targeting key nodes can induce ferroptosis (136, 137). “Cold tumors” are described as lacking immunogenicity and low T cell infiltration. Cancer cell ferroptosis has been shown to promote the transition from “cold tumors” to “hot tumors” and enhance adaptive immune response (Figure 2) (138). One study showed that ferroptosis was a kind of ICD and promoted the maturation of BMDCs, then continuously activated the adaptive immune system and inhibited tumor growth in vivo (139). In addition, Incorporating ferroptosis and ultrasound-triggered sonodynamic therapy (SDT) synergistically elicited strong antitumor immunity by increasing the numbers of mature DCs and activated CD8+ cells and decreasing the number of MDSCs in the TME (140). Currently, preclinical studies about ferroptosis mainly focus on the construction of drug polymers that can be specifically taken up by cancer cells. These drug polymers release metal elements in cancer cells, and the transition between the reduced and oxidized states of these metal ions consumes GSH, including iron (Fe), manganese (Mn), copper (Cu), and iridium (Ir). Depletion of GSH increases lipid peroxidation, and this transition can also use H2O2 to generate highly toxic free radicals (Fenton reaction), such as 1O2, ·O2-, ·OH, HO-, amplify the ROS storm, promote lipid peroxidation and ferroptosis. In addition, drug polymers can also carry ferroptosis inducers, such as oxaliplatin prodrug, Erastin, and sorafenib, to promote cancer cell ferroptosis by inhibiting the intracellular system XC- and depleting GSH. Overall, regardless of how drug-polymer molecules induce cancer cell ferroptosis, the DAMPs released from cancer cell ferroptosis can activate APCs and CD8+ T cells to heat the TME and further enhance tumor suppression.
Based on the role of iron in ferroptosis, Ling et al. prepared a photosensitizer containing Fe2+ (IrFc1). IrFc1 can entered triple-negative breast cancer cells (TNBC) through transferrin, caused oxidative stress and lipid peroxidation when activated by light. This can lead to cancer cells ferroptosis and induce CD8+ T cell infiltration to enhance anti-tumor immunity (112). Jeong et al. created a polymer carrying Fe3+ (TA-Fe3- DOX-DSPE-PEG), which increased intracellular ROS and LPO through the Fenton reaction to mediate cell ferroptosis and enhance CD8+ cell-mediated anti-tumor immunity in cancer cells (113). Hu et al. prepared a polymer that not only carries Fe3+ but also adds oxaliplatin prodrug (PCN-Oxpt/PEG). The generation of oxaliplatin consumes GSH accompanied by the production of highly toxic -OH. Fe3+ further induces cancer cell ferroptosis through the Fenton reaction, and ferroptosis-related DAMPs increase CD8+ T cells derived IFN-γ to further enhance cell ferroptosis (114). Other metal ions can also promote ferroptosis in cancer cells through redox reactions. Li et al. prepared a polymer-drug molecule carrying erastin and Cu2+(Cu2-xSe/ZIF- 8@Era-PEG-FA). Erastin is released to inhibit SLC7A11/SLC3A2, and Cu2+ further enhances cancer cell ferroptosis through the mutual conversion between Cu+ and Cu2+. Reducing the miR301 in cancer cell-derived exosomes promotes the polarization of TAMs the M1 phenotype. These factors increase CD8+T cell infiltration to enhance tumor suppression (115). Ir is another metal element. Wang et al. synthesized a complex containing Ir3+ (Ir-pbt-Bpa). The intracellular Ir-pbt-Bpa can generate singlet oxygen and superoxide anion radicals under light irradiation, inducing ferroptosis and releasing DAMPs to induce CD8+ T cell immune responses. Even if only one site is irradiated, Tumors in the other site are significantly inhibited, indicating that Ir-pbt-Bpa can increase the number of effector memory T cells and achieve long-term anti-tumor immunity (116). Sorafenib is a drug approved by the FDA for the treatment of various advanced solid tumors. Zhou et al. prepared a cinnamaldehyde dimer carrying sorafenib (CDC@SRF), which rapidly ruptures in the cytoplasm after reaching the tumor, releasing drugs. SRF significantly enhances ferroptosis by inhibiting the system XC- and directly consuming GSH, and it also promotes DCs maturation and CD8+ T cell activation, triggering a strong immune response in vivo. After multiple doses of injection, CDC@SRF cured all mice with breast cancer (117).
5.3 Targeting both CD8+ T cells and cancer cell ferroptosis amplifies tumor suppression
Given the potent anti-tumor abilities of both CD8+ T cells and cancer cell ferroptosis, constructing nanopolymer drugs containing CD8+ T cell activators and ferroptosis targets can simultaneously activate CD8+ T cells and promote cancer cell ferroptosis, thereby amplifying the tumor therapeutic effects. ZVI-NP prepared by Hsieh et al. exhibits dual anti-tumor effects in cancer. The first mechanism involves the activation of the AMPK/mTOR signaling pathway, enhancingGSK3/-TrCP-dependent NRF2 degradation, thereby triggering ferroptosis in lung cancer cells. The second mechanism involves regulating TAMs to polarize toward the M1 phenotype and enhancing the immune activity of CD8+ T cells, fully exploiting the roles of ferroptosis and CD8+ T cells in cancer (118). Similarly, Chin et al. prepared CPBA-modified Fe3O4@Chl/Fe-CNPs to be ingested by targeting glycoprotein on bladder cancer cells, depleting GSH through the Fenton reaction, and promoting lipid peroxide-mediated ferroptosis with photodynamic therapy (PDT) and chemical dynamic therapy (CDT). The nanopolymer also reprograms the tumor immune microenvironment by reducing PD-L1, IDO-1, and TGF-β and increasing CD8+ T cells, M1-like macrophages, which unleashes the potential of transforming the tumor from cold tumor to hot tumor, greatly inhibiting tumor growth, and improving the survival rate of bladder cancer mice (119). Gold nanoparticles (AuNp) are excellent drug-delivery tools. Singh et al. applied AuNp loading miR-21-3p to directly target TXNRD1 in cancer cells, disrupting the redox balance and triggering ferroptosis, enhancing the sensitivity to anti-PD-1 antibodies. Therefore, the combination of miR-21-3p-AuNp and ICIs significantly enhances tumor suppression (120, 121). Liu et al. constructed a composite nano-platform that co-expresses six enzymes(FeCo/Fe-Co DAzyme/PL). The nano-platform can induce ROS storms, depleting GSH, inactivating GPX4, and catalyzing LOX to promote irreversible cancer cell immunogenic ferroptosis. IFN-γ from CD8+ T cells can interact with AA generated during the oxidative storm, further enhancing ferroptosis and overcoming current immunotherapy limitations (122). It has been demonstrated that hyaluronic acid (HA) molecules binding to cancer cells can guide lymphocytes to migrate deep into tumors to enhance the efficacy of immunotherapy (141). Therefore, Zhang et al. constructed a nanopolymer (Fe3+-TA@HA) that specifically targets CD44 overexpressed in squamous cell carcinoma (SCC), inducing cancer cell ferroptosis through peroxidase activity and GSH depletion. Fe3+-TA@HA also promotes the recruitment of CD4+ and CD8+ T cells in mouse tumors and suppresses tumor growth through cytokine secretion. ICIs further enhance the tumor suppression effect (123).
Some natural molecules have been shown to directly trigger ferroptosis and tumor immunity in cancer, and their combination with ICIs further enhances tumor suppression. Resveratrol (RES) can inhibit the interaction of HMMR and SLC7A11 to mediate cancer cell ferroptosis. RES also enhances the cytotoxicity of CD8+ T cells co-cultured with cancer cells and modulates the tumor immune microenvironment (124). Another natural molecule, Dihydroartemisinin (DHA), induces pancreatic cancer cell ferroptosis through upregulation of P53 and ALOX12-dependent mechanisms. DHA treatment also increases CD8+ T cells in mouse cancer tissues (125). Previous research has shown that local Mn2+ in the TME enhances cGAS-STING activity to promote the accumulation of CD8+ T cells and IFN-γ secretion, while Mn2+ entering cancer cells consumes GSH in the process of hydroxyl radical (·OH) generation during oxidative reactions, leading to ferroptosis (142). Therefore, Huang et al. prepared a nanopolymer Fe-MnO2/DHA loaded with Mn and DHA, which can simultaneously promote cancer cell ferroptosis and CD8+ T cell immunity in cancer, amplifying the anti-tumor effects and further inhibiting tumor progression (126).
6 Summary and prospect
Based on the powerful anti-cancer effects of CD8+ T cells, immune therapies represented by ICIs have greatly improved the clinical efficacy of malignant tumor treatment. However, primary or acquired resistance limits the application of these drugs in tumors (143). In recent years, we have discovered a new form of CD8+ T cell-mediated tumor growth and metastasis called ferroptosis. Although there is variability in the sensitivity of CD8+ T cells and cancer cells to ferroptosis, activating CD8+ T cells and promoting cancer cell ferroptosis synergistically amplify the tumor-suppressive effect. Furthermore, the positive feedback loop between CD8+ T cells and cancer cell ferroptosis can expand the intra-tumoral CD8+ T cells, which brings new hope for CD8+ T cells and ferroptosis in cancer immunotherapy. The intra-tumoral CD8+ T cells are also regulated by other immune cells ferroptosis, such as macrophages and Tregs, which enhance the anti-cancer effect of CD8+ T cells. In contrast, DCs’ ferroptosis inhibits the activation of CD8+ T cells. It is worth noting that the ferroptosis of MDSCs, an immune-suppressive component in the TME, does not always activate CD8+ T cells. During the ferroptosis process of PMN-MDSCs, the release of oxygen-containing lipids and PGE2 limits the activity of CD8+ T cells. New evidence also suggests that cancer cell ferroptosis is not always immunogenic cell death, which may impair the antigen presentation function of DCs and weaken the CD8+ T cell-centered anti-cancer immune response (69). Therefore, CD8+ T cells and ferroptosis form a complex network in cancer. Currently, nanotechnology is mainly used to construct polymeric molecules containing multiple drugs, which inhibit tumor growth by targeting cancer cell ferroptosis and obtaining adaptive immunity. However, the complex tumor-immunity network may bring uncertainties, requiring more comprehensive and in-depth research to clarify the communication between ferroptosis and CD8+ T cells in the TME and pave the way for clinical treatment.
Author contributions
ZL: Writing – original draft. SZ: Writing – review & editing. KW: Writing – review & editing.
Funding
The present review was supported by the National Natural Science Foundation of China (grant no. 82160575) and the Outstanding Young Technological and Innovative Talent Cultivation Project of Zunyi Municipal Science and Technology Bureau, 2021 (no. 10).
Acknowledgments
Figures are drawn by Figdraw.
Conflict of interest
The authors declare that the research was conducted in the absence of any commercial or financial relationships that could be construed as a potential conflict of interest.
Publisher’s note
All claims expressed in this article are solely those of the authors and do not necessarily represent those of their affiliated organizations, or those of the publisher, the editors and the reviewers. Any product that may be evaluated in this article, or claim that may be made by its manufacturer, is not guaranteed or endorsed by the publisher.
Glossary
References
1. St Paul M, Ohashi PS. The roles of CD8(+) T cell subsets in antitumor immunity. Trends Cell Biol (2020) 30(9):695–704. doi: 10.1016/j.tcb.2020.06.003
2. Tang R, Xu J, Zhang B, Liu J, Liang C, Hua J, et al. Ferroptosis, necroptosis, and pyroptosis in anticancer immunity. J Hematol Oncol (2020) 13(1):110. doi: 10.1186/s13045-020-00946-7
3. Han J, Khatwani N, Searles TG, Turk MJ, Angeles CV. Memory CD8(+) T cell responses to cancer. Semin Immunol (2020) 49:101435. doi: 10.1016/j.smim.2020.101435
4. Jhunjhunwala S, Hammer C, Delamarre L. Antigen presentation in cancer: insights into tumour immunogenicity and immune evasion. Nat Rev Cancer (2021) 21(5):298–312. doi: 10.1038/s41568-021-00339-z
5. Fotakis G, Trajanoski Z, Rieder D. Computational cancer neoantigen prediction: current status and recent advances. Immunooncol Technol (2021) 12:100052. doi: 10.1016/j.iotech.2021.100052
6. He J, Xiong X, Yang H, Li D, Liu X, Li S, et al. Defined tumor antigen-specific T cells potentiate personalized TCR-T cell therapy and prediction of immunotherapy response. Cell Res (2022) 32(6):530–42. doi: 10.1038/s41422-022-00627-9
7. Schumacher TN, Schreiber RD. Neoantigens in cancer immunotherapy. Science (2015) 348(6230):69–74. doi: 10.1126/science.aaa4971
8. Farhood B, Najafi M, Mortezaee K. CD8(+) cytotoxic T lymphocytes in cancer immunotherapy: A review. J Cell Physiol (2019) 234(6):8509–21. doi: 10.1002/jcp.27782
9. Dolina JS, Van Braeckel-Budimir N, Thomas GD, Salek-Ardakani S. CD8(+) T cell exhaustion in cancer. Front Immunol (2021) 12:715234. doi: 10.3389/fimmu.2021.715234
10. Marcovecchio PM, Thomas G, Salek-Ardakani S. CXCL9-expressing tumor-associated macrophages: new players in the fight against cancer. J Immunother Cancer (2021) 9(2):e002045. doi: 10.1136/jitc-2020-002045
11. Wang X, Xiong H, Ning Z. Implications of NKG2A in immunity and immune-mediated diseases. Front Immunol (2022) 13:960852.doi: 10.3389/fimmu.2022.960852
12. Reeves E, James E. Antigen processing and immune regulation in the response to tumours. Immunology (2017) 150(1):16–24. doi: 10.1111/imm.12675
13. Kumar S, Singh SK, Rana B, Rana A. Tumor-infiltrating CD8(+) T cell antitumor efficacy and exhaustion: molecular insights. Drug Discov Today (2021) 26(4):951–67. doi: 10.1016/j.drudis.2021.01.002
14. Dixon SJ, Lemberg KM, Lamprecht MR, Skouta R, Zaitsev EM, Gleason CE, et al. Ferroptosis: an iron-dependent form of nonapoptotic cell death. Cell (2012) 149(5):1060–72. doi: 10.1016/j.cell.2012.03.042
15. Martin-Sanchez D, Fontecha-Barriuso M, Martinez-Moreno JM, Ramos AM, Sanchez-Niño MD, Guerrero-Hue M, et al. Ferroptosis and kidney disease. Nefrologia (Engl Ed) (2020) 40(4):384–94. doi: 10.1016/j.nefroe.2020.09.006
16. Chen X, Kang R, Kroemer G, Tang D. Ferroptosis in infection, inflammation, and immunity. J Exp Med (2021) 218(6):e20210518. doi: 10.1084/jem.20210518
17. Xie LH, Fefelova N, Pamarthi SH, Gwathmey JK. Molecular mechanisms of ferroptosis and relevance to cardiovascular disease. Cells (2022) 11(17):2726. doi: 10.3390/cells11172726
18. Zhao L, Zhou X, Xie F, Zhang L, Yan H, Huang J, et al. Ferroptosis in cancer and cancer immunotherapy. Cancer Commun (Lond) (2022) 42(2):88–116. doi: 10.1002/cac2.12250
19. Friedmann Angeli JP, Schneider M, Proneth B, Tyurina YY, Tyurin VA, Hammond VJ, et al. Inactivation of the ferroptosis regulator Gpx4 triggers acute renal failure in mice. Nat Cell Biol (2014) 16(12):1180–91. doi: 10.1038/ncb3064
20. Dixon SJ, Stockwell BR. The role of iron and reactive oxygen species in cell death. Nat Chem Biol (2014) 10(1):9–17. doi: 10.1038/nchembio.1416
21. Liu MR, Zhu WT, Pei DS. System Xc(-): a key regulatory target of ferroptosis in cancer. Invest New Drugs (2021) 39(4):1123–31. doi: 10.1007/s10637-021-01070-0
22. Ursini F, Maiorino M. Lipid peroxidation and ferroptosis: The role of GSH and GPx4. Free Radic Biol Med (2020) 152:175–85. doi: 10.1016/j.freeradbiomed.2020.02.027
23. Stockwell BR. Ferroptosis turns 10: Emerging mechanisms, physiological functions, and therapeutic applications. Cell (2022) 185(14):2401–21. doi: 10.1016/j.cell.2022.06.003
24. Galaris D, Barbouti A, Pantopoulos K. Iron homeostasis and oxidative stress: An intimate relationship. Biochim Biophys Acta Mol Cell Res (2019) 1866(12):118535. doi: 10.1016/j.bbamcr.2019.118535
25. Fang X, Ardehali H, Min J, Wang F. The molecular and metabolic landscape of iron and ferroptosis in cardiovascular disease. Nat Rev Cardiol (2023) 20(1):7–23. doi: 10.1038/s41569-022-00735-4
26. Chen S, Chen Y, Zhang Y, Kuang X, Liu Y, Guo M, et al. Iron metabolism and ferroptosis in epilepsy. Front Neurosci (2020) 14:601193. doi: 10.3389/fnins.2020.601193
27. Doll S, Proneth B, Tyurina YY, Panzilius E, Kobayashi S, Ingold I, et al. ACSL4 dictates ferroptosis sensitivity by shaping cellular lipid composition. Nat Chem Biol (2017) 13(1):91–8. doi: 10.1038/nchembio.2239
28. Kuwata H, Hara S. Role of acyl-CoA synthetase ACSL4 in arachidonic acid metabolism. Prostaglandins Other Lipid Mediat (2019) 144:106363. doi: 10.1016/j.prostaglandins.2019.106363
29. Li D, Li Y. The interaction between ferroptosis and lipid metabolism in cancer. Signal Transduct Target Ther (2020) 5(1):108. doi: 10.1038/s41392-020-00216-5
30. Durgeau A, Virk Y, Corgnac S, Mami-Chouaib F. Recent advances in targeting CD8 T-cell immunity for more effective cancer immunotherapy. Front Immunol (2018) 9:14. doi: 10.3389/fimmu.2018.00014
31. Voskoboinik I, Whisstock JC, Trapani JA. Perforin and granzymes: function, dysfunction and human pathology. Nat Rev Immunol (2015) 15(6):388–400. doi: 10.1038/nri3839
32. Martínez-Lorenzo MJ, Anel A, Gamen S, Monleón I, Lasierra P, Larrad L, et al. Activated Human T Cells Release Bioa Source. J Immunol (1999) 163(3):1274–81. doi: 10.4049/jimmunol.163.3.1274
33. Walsh CM, Glass AA, Chiu V, Clark WR. The role of the Fas lytic pathway in a perforin-less CTL hybridoma. J Immunol (1994) 153(6):2506–14. doi: 10.4049/jimmunol.153.6.2506
34. Krammer PH, Igney FH. Immune Escape Of Tumors Apoptosis R Source. J Leukoc Biol (2002) 71(6):907. doi: 10.1189/jlb.71.6.907
35. Chen SW, Zhu SQ, Pei X, Qiu BQ, Xiong D, Long X, et al. Cancer cell-derived exosomal circUSP7 induces CD8(+) T cell dysfunction and anti-PD1 resistance by regulating the miR-934/SHP2 axis in NSCLC. Mol Cancer (2021) 20(1):144. doi: 10.1186/s12943-020-01292-5
36. Liao P, Wang W, Wang W, Kryczek I, Li X, Bian Y, et al. CD8(+) T cells and fatty acids orchestrate tumor ferroptosis and immunity via ACSL4. Cancer Cell (2022) 40(4):365–78. doi: 10.1016/j.ccell.2022.02.003
37. Wang W, Green M, Choi JE, Gijón M, Kennedy PD, Johnson JK, et al. CD8(+) T cells regulate tumour ferroptosis during cancer immunotherapy. Nature (2019) 569(7755):270–4. doi: 10.1038/s41586-019-1170-y
38. Friedmann Angeli JP, Xavier da Silva TN, Schilling B. CD8(+) T cells PUF(A)ing the flames of cancer ferroptotic cell death. Cancer Cell (2022) 40(4):346–8. doi: 10.1016/j.ccell.2022.03.003
39. Kong R, Wang N, Han W, Bao W, Lu J. IIFNγ-mediated repression of system xc(-) drives vulnerability to induced ferroptosis in hepatocellular carcinoma cells. J Leukoc Biol (2021) 110(2):301–14. doi: 10.1002/JLB.3MA1220-815RRR
40. Ge W, Yue M, Lin R, Zhou T, Xu H, Wang Y, et al. PLA2G2A(+) cancer-associated fibroblasts mediate pancreatic cancer immune escape via impeding antitumor immune response of CD8(+) cytotoxic T cells. Cancer Lett (2023) 558:216095. doi: 10.1016/j.canlet.2023.216095
41. Walker PR, Saas P, Dietrich PY. Role of Fas ligand (CD95L) in immune escape: the tumor cell strikes back. J Immunol (1997) 158(10):4521–4. doi: 10.4049/jimmunol.158.10.4521
42. Ioachim HL, Decuseara R, Giancotti F, Dorsett BH. FAS and FAS-L expression by tumor cells and lymphocytes in breast carcinomas and their lymph node metastases. Pathol Res Pract (2005) 200(11-12):743–51. doi: 10.1016/j.prp.2004.09.006
43. Zhu J, Petit PF, Van den Eynde BJ. Apoptosis of tumor-infiltrating T lymphocytes: a new immune checkpoint mechanism. Cancer Immunol Immunother (2019) 68(5):835–47. doi: 10.1007/s00262-018-2269-y
44. Drijvers JM, Gillis JE, Muijlwijk T, Nguyen TH, Gaudiano EF, Harris IS, et al. Pharmacologic screening identifies metabolic vulnerabilities of CD8(+) T cells. Cancer Immunol Res (2021) 9(2):184–99. doi: 10.1158/2326-6066.CIR-20-0384
45. Ma X, Xiao L, Liu L, Ye L, Su P, Bi E, et al. CD36-mediated ferroptosis dampens intratumoral CD8(+) T cell effector function and impairs their antitumor ability. Cell Metab (2021) 33(5):1001–12.015. doi: 10.1016/j.cmet.2021.02.015
46. Ma X, Bi E, Lu Y, Su P, Huang C, Liu L, et al. Cholesterol induces CD8(+) T cell exhaustion in the TME. Cell Metab (2019) 30(1):143–156.e5. doi: 10.1016/j.cmet.2019.04.002
47. St. Paul M, Ohashi PS. The roles of CD8+ T cell subsets in antitumor immunity. Trends Cell Biol (2020) 30(9):695–704. doi: 10.1016/j.tcb.2020.06.003
48. Xiao L, Ma X, Ye L, Su P, Xiong W, Bi E, et al. IL-9/STAT3/fatty acid oxidation-mediated lipid peroxidation contributes to Tc9 cell longevity and enhanced antitumor activity. J Clin Invest (2022) 132(7):e153247. doi: 10.1172/JCI153247
49. Zheng N, Lu Y. Targeting the IL-9 pathway in cancer immunotherapy. Hum Vaccin Immunother (2020) 16(10):2333–40. doi: 10.1080/21645515.2019.1710413
50. Lavorgna A, Matsuoka M, Harhaj EW. A critical role for IL-17RB signaling in HTLV-1 tax-induced NF-κB activation and T-cell transformation. PloS Pathog (2014) 10(10):e1004418. doi: 10.1371/journal.ppat.1004418
51. Qiu L, Lai R, Lin Q, Lau E, Thomazy DM, Calame D, et al. Autocrine release of interleukin-9 promotes Jak3-dependent survival of ALK+ anaplastic large-cell lymphoma cells. Blood (2006) 108(7):2407–15. doi: 10.1182/blood-2006-04-020305
52. Ivashkiv LB. IFNgamma: signalling, epigenetics and roles in immunity, metabolism, disease and cancer immunotherapy. Nat Rev Immunol (2018) 18(9):545–58. doi: 10.1038/s41577-018-0029-z
53. Zhang Q, Zhang L, Li L, Wang Z, Ying J, Fan Y, et al. Interferon regulatory factor 8 functions as a tumor suppressor in renal cell carcinoma and its promoter methylation is associated with patient poor prognosis. Cancer Lett (2014) 354(2):227–34. doi: 10.1016/j.canlet.2014.07.040
54. Chen Q, Zhuang S, Hong Y, Yang L, Guo P, Mo P, et al. Demethylase JMJD2D induces PD-L1 expression to promote colorectal cancer immune escape by enhancing IFNGR1-STAT3-IRF1 signaling. Oncogene (2022) 41(10):1421–33. doi: 10.1038/s41388-021-02173-x
55. Poschel DB, Kehinde-Ige M, Klement JD, Yang D, Merting AD, Savage NM, et al. IRF8 Regulates Intrinsic Ferroptosis through Repressing p53 Expression to Maintain Tumor Cell Sensitivity to Cytotoxic T Lymphocytes. Cells (2023) 12(2):310. doi: 10.3390/cells12020310
56. Zhang HL, Hu BX, Li ZL, Du T, Shan JL, Ye ZP, et al. PKCβII phosphorylates ACSL4 to amplify lipid peroxidation to induce ferroptosis. Nat Cell Biol (2022) 24(1):88–98. doi: 10.1038/s41556-021-00818-3
57. Linher-Melville K, Haftchenary S, Gunning P, Singh G. Signal transducer and activator of transcription 3 and 5 regulate system Xc- and redox balance in human breast cancer cells. Mol Cell Biochem (2015) 405(1-2):205–21. doi: 10.1007/s11010-015-2412-4
58. Wu H, Li Y, Shi G, Du S, Wang X, Ye W, et al. Hepatic interferon regulatory factor 8 expression suppresses hepatocellular carcinoma progression and enhances the response to anti-programmed cell death protein-1 therapy. Hepatology (2022) 76(6):1602–16. doi: 10.1002/hep.32316
59. Gatti G, Betts C, Rocha D, Nicola M, Grupe V, Ditada C, et al. High IRF8 expression correlates with CD8 T cell infiltration and is a predictive biomarker of therapy response in ER-negative breast cancer. Breast Cancer Res (2021) 23(1):40. doi: 10.1186/s13058-021-01418-7
60. Galluzzi L, Vitale I, Warren S, Adjemian S, Agostinis P, Martinez AB, et al. Consensus guidelines for the definition, detection and interpretation of immunogenic cell death. J Immunother Cancer (2020) 8(1):e000337. doi: 10.1136/jitc-2019-000337corr1
61. Zhou J, Wang G, Chen Y, Wang H, Hua Y, Cai Z. Immunogenic cell death in cancer therapy: Present and emerging inducers. J Cell Mol Med (2019) 23(8):4854–65. doi: 10.1111/jcmm.14356
62. Song J, Liu T, Yin Y, Zhao W, Lin Z, Yin Y, et al. The deubiquitinase OTUD1 enhances iron transport and potentiates host antitumor immunity. EMBO Rep (2021) 22(2):e51162. doi: 10.15252/embr.202051162
63. Efimova I, Catanzaro E, Van der Meeren L, Turubanova VD, Hammad H, Mishchenko TA, et al. Vaccination with early ferroptotic cancer cells induces efficient antitumor immunity. J Immunother Cancer (2020) 8(2):e001369. doi: 10.1136/jitc-2020-001369
64. Conche C, Finkelmeier F, Pešić M, Nicolas AM, Böttger TW, Kennel KB, et al. Combining ferroptosis induction with MDSC blockade renders primary tumours and metastases in liver sensitive to immune checkpoint blockade. Gut (2023) 72(9):1774–82. doi: 10.1136/gutjnl-2022-327909
65. Luo H, Wang X, Song S, Wang Y, Dan Q, Ge H. Targeting stearoyl-coa desaturase enhances radiation induced ferroptosis and immunogenic cell death in esophageal squamous cell carcinoma. Oncoimmunology (2022) 11(1):2101769. doi: 10.1080/2162402X.2022.2101769
66. Li W, Fu H, Fang L, Chai H, Gao T, Chen Z, et al. Shikonin induces ferroptosis in multiple myeloma via GOT1-mediated ferritinophagy. Front Oncol (2022) 12:1025067. doi: 10.3389/fonc.2022.1025067
67. Zhao YY, Lian JX, Lan Z, Zou KL, Wang WM, Yu GT. Ferroptosis promotes anti-tumor immune response by inducing immunogenic exposure in HNSCC. Oral Dis (2023) 29(3):933–41. doi: 10.1111/odi.14077
68. Yu B, Choi B, Li W, Kim DH. Magnetic field boosted ferroptosis-like cell death and responsive MRI using hybrid vesicles for cancer immunotherapy. Nat Commun (2020) 11(1):3637. doi: 10.1038/s41467-020-17380-5
69. Wiernicki B, Maschalidi S, Pinney J, Adjemian S, Vanden Berghe T, Ravichandran KS, et al. Cancer cells dying from ferroptosis impede dendritic cell-mediated anti-tumor immunity. Nat Commun (2022) 13(1):3676. doi: 10.1038/s41467-022-31218-2
70. Deng M, Scott MJ, Fan J, Billiar TR. Location is the key to function: HMGB1 in sepsis and trauma-induced inflammation. J Leukoc Biol (2019) 106(1):161–9. doi: 10.1002/JLB.3MIR1218-497R
71. Gao Q, Wang S, Chen X, Cheng S, Zhang Z, Li F, et al. Cancer-cell-secreted CXCL11 promoted CD8(+) T cells infiltration through docetaxel-induced-release of HMGB1 in NSCLC. J Immunother Cancer (2019) 7(1):42. doi: 10.1186/s40425-019-0511-6
72. Chen R, Zou J, Kang R, Tang D. The redox protein HMGB1 in cell death and cancer. Antioxid Redox Signal (2023). doi: 10.1089/ars.2023.0007
73. Ye F, Chai W, Xie M, Yang M, Yu Y, Cao L, et al. HMGB1 regulates erastin-induced ferroptosis via RAS-JNK/p38 signaling in HL-60/NRAS(Q61L) cells. Am J Cancer Res (2019) 9(4):730–9.
74. Wang X, Simayi A, Fu J, Zhao X, Xu G. Resveratrol mediates the miR-149/HMGB1 axis and regulates the ferroptosis pathway to protect myocardium in endotoxemia mice. Am J Physiol Endocrinol Metab (2022) 323(1):E21–32. doi: 10.1152/ajpendo.00227.2021
75. Zhu K, Zhu X, Liu S, Yu J, Wu S, Hei M. Glycyrrhizin attenuates hypoxic-ischemic brain damage by inhibiting ferroptosis and neuroinflammation in neonatal rats via the HMGB1/GPX4 pathway. Oxid Med Cell Longev (2022) 2022:8438528. doi: 10.1155/2022/8438528
76. Wu Y, Zhao Y, Yang HZ, Wang YJ, Chen Y. HMGB1 regulates ferroptosis through Nrf2 pathway in mesangial cells in response to high glucose. Biosci Rep (2021) 41(2):BSR20202924. doi: 10.1042/BSR20202924
77. Wen Q, Liu J, Kang R, Zhou B, Tang D. The release and activity of HMGB1 in ferroptosis. Biochem Biophys Res Commun (2019) 510(2):278–83. doi: 10.1016/j.bbrc.2019.01.090
78. Hubert P, Roncarati P, Demoulin S, Pilard C, Ancion M, Reynders C, et al. Extracellular HMGB1 blockade inhibits tumor growth through profoundly remodeling immune microenvironment and enhances checkpoint inhibitor-based immunotherapy. J Immunother Cancer (2021) 9(3):e001966. doi: 10.1136/jitc-2020-001966
79. Ahmed A, Tait SWG. Targeting immunogenic cell death in cancer. Mol Oncol (2020) 14(12):2994–3006. doi: 10.1002/1878-0261.12851
80. Michalak M, Groenendyk J, Szabo E, Gold LI, Opas M. Calreticulin, a multi-process calcium-buffering chaperone of the endoplasmic reticulum. Biochem J (2009) 417(3):651–66. doi: 10.1042/BJ20081847
81. Fucikova J, Spisek R, Kroemer G, Galluzzi L. Calreticulin and cancer. Cell Res (2021) 31(1):5–16. doi: 10.1038/s41422-020-0383-9
82. Tesniere A, Schlemmer F, Boige V, Kepp O, Martins I, Ghiringhelli F, et al. Immunogenic death of colon cancer cells treated with oxaliplatin. Oncogene (2010) 29(4):482–91. doi: 10.1038/onc.2009.356
83. Obeid M, Tesniere A, Ghiringhelli F, Fimia GM, Apetoh L, Perfettini JL, et al. Calreticulin exposure dictates the immunogenicity of cancer cell death. Nat Med (2007) 13(1):54–61. doi: 10.1038/nm1523
84. Phadatare P, Debnath J. Lysosomal lipid peroxidation mediates immunogenic cell death. J Clin Invest (2023) 133(8):e169240. doi: 10.1172/JCI169240
85. Zhu L, Yang F, Wang L, Dong L, Huang Z, Wang G, et al. Identification the ferroptosis-related gene signature in patients with esophageal adenocarcinoma. Cancer Cell Int (2021) 21(1):124. doi: 10.1186/s12935-021-01821-2
86. Chen J, Zhan Y, Zhang R, Chen B, Huang J, Li C, et al. A new prognostic risk signature of eight ferroptosis-related genes in the clear cell renal cell carcinoma. Front Oncol (2021) 11:700084. doi: 10.3389/fonc.2021.700084
87. Jiang X, Yan Q, Xie L, Xu S, Jiang K, Huang J, et al. Construction and validation of a ferroptosis-related prognostic model for gastric cancer. J Oncol (2021) 2021:6635526. doi: 10.1155/2021/6635526
88. Han L, Bai L, Qu C, Dai E, Liu J, Kang R, et al. PPARG-mediated ferroptosis in dendritic cells limits antitumor immunity. Biochem Biophys Res Commun (2021) 576:33–9. doi: 10.1016/j.bbrc.2021.08.082
89. Li LG, Peng XC, Yu TT, Xu HZ, Han N, Yang XX, et al. Dihydroartemisinin remodels macrophage into an M1 phenotype via ferroptosis-mediated DNA damage. Front Pharmacol (2022) 13:949835. doi: 10.3389/fphar.2022.949835
90. Hao X, Zheng Z, Liu H, Zhang Y, Kang J, Kong X, et al. Inhibition of APOC1 promotes the transformation of M2 into M1 macrophages via the ferroptosis pathway and enhances anti-PD1 immunotherapy in hepatocellular carcinoma based on single-cell RNA sequencing. Redox Biol (2022) 56:102463. doi: 10.1016/j.redox.2022.102463
91. Tang B, Zhu J, Wang Y, Chen W, Fang S, Mao W, et al. Targeted xCT-mediated Ferroptosis and Protumoral Polarization of Macrophages Is Effective against HCC and Enhances the Efficacy of the Anti-PD-1/L1 Response. Adv Sci (Weinh) (2023) 10(2):e2203973. doi: 10.1002/advs.202203973
92. Li S, Li F, Xu L, Liu X, Zhu X, Gao W, et al. TLR2 agonist promotes myeloid-derived suppressor cell polarization via Runx1 in hepatocellular carcinoma. Int Immunopharmacol (2022) 111:109168. doi: 10.1016/j.intimp.2022.109168
93. Zhu H, Klement JD, Lu C, Redd PS, Yang D, Smith AD, et al. Asah2 represses the p53-hmox1 axis to protect myeloid-derived suppressor cells from ferroptosis. J Immunol (2021) 206(6):1395–404. doi: 10.4049/jimmunol.2000500
94. Kim R, Hashimoto A, Markosyan N, Tyurin VA, Tyurina YY, Kar G, et al. Ferroptosis of tumour neutrophils causes immune suppression in cancer. Nature (2022) 612(7939):338–46. doi: 10.1038/s41586-022-05443-0
95. Zhao Y, Liu Z, Liu G, Zhang Y, Liu S, Gan D, et al. Neutrophils resist ferroptosis and promote breast cancer metastasis through aconitate decarboxylase 1. Cell Metab (2023) 35(10):1688–1703.e10. doi: 10.1016/j.cmet.2023.09.004
96. Xu C, Sun S, Johnson T, Qi R, Zhang S, Zhang J, et al. The glutathione peroxidase Gpx4 prevents lipid peroxidation and ferroptosis to sustain Treg cell activation and suppression of antitumor immunity. Cell Rep (2021) 35(11):109235. doi: 10.1016/j.celrep.2021.109235
97. Wang Y, Xiang Y, Xin VW, Wang XW, Peng XC, Liu XQ, et al. Dendritic cell biology and its role in tumor immunotherapy. J Hematol Oncol (2020) 13(1):107. doi: 10.1186/s13045-020-00939-6
98. Pan Y, Yu Y, Wang X, Zhang T. Tumor-associated macrophages in tumor immunity. Front Immunol (2020) 11:583084. doi: 10.3389/fimmu.2020.583084
99. Yang Y, Wang Y, Guo L, Gao W, Tang TL, Yan M. Interaction between macrophages and ferroptosis. Cell Death Dis (2022) 13(4):355. doi: 10.1038/s41419-022-04775-z
100. Liu Z, Ma Y, Cui Q, Xu J, Tang Z, Wang Y, et al. Toll-like receptor 4 plays a key role in advanced glycation end products-induced M1 macrophage polarization. Biochem Biophys Res Commun (2020) 531(4):602–8. doi: 10.1016/j.bbrc.2020.08.014
101. Zhou Y, Que KT, Zhang Z, Yi ZJ, Zhao PX, You Y, et al. Iron overloaded polarizes macrophage to proinflammation phenotype through ROS/acetyl-p53 pathway. Cancer Med (2018) 7(8):4012–22. doi: 10.1002/cam4.1670
102. Hu X, Cai X, Ma R, Fu W, Zhang C, Du X. Iron-load exacerbates the severity of atherosclerosis via inducing inflammation and enhancing the glycolysis in macrophages. J Cell Physiol (2019) 234(10):18792–800. doi: 10.1002/jcp.28518
103. Handa P, Thomas S, Morgan-Stevenson V, Maliken BD, Gochanour E, Boukhar S, et al. Iron alters macrophage polarization status and leads to steatohepatitis and fibrogenesis. J Leukoc Biol (2019) 105(5):1015–26. doi: 10.1002/JLB.3A0318-108R
104. Dysthe M, Parihar R. Myeloid-derived suppressor cells in the tumor microenvironment. Adv Exp Med Biol (2020) 1224:117–40. doi: 10.1007/978-3-030-35723-8_8
105. Li P, Lu M, Shi J, Hua L, Gong Z, Li Q, et al. Dual roles of neutrophils in metastatic colonization are governed by the host NK cell status. Nat Commun (2020) 11(1):4387. doi: 10.1038/s41467-020-18125-0
106. Veglia F, Sanseviero E, Gabrilovich DI. Myeloid-derived suppressor cells in the era of increasing myeloid cell diversity. Nat Rev Immunol (2021) 21:485–98. doi: 10.1038/s41577-020-00490-y
107. Noyes D, Bag A, Oseni S, Semidey-Hurtado J, Cen L, Sarnaik AA, et al. Tumor-associated Tregs obstruct antitumor immunity by promoting T cell dysfunction and restricting clonal diversity in tumor-infiltrating CD8+ T cells. J Immunother Cancer (2022) 10(5):e004605. doi: 10.1136/jitc-2022-004605
108. Khazaie K, von Boehmer H. The impact of CD4+CD25+ Treg on tumor specific CD8+ T cell cytotoxicity and cancer. Semin Cancer Biol (2006) 16(2):124–36. doi: 10.1016/j.semcancer.2005.11.006
109. Liu YT, Sun ZJ. Turning cold tumors into hot tumors by improving T-cell infiltration. Theranostics (2021) 11(11):5365–86. doi: 10.7150/thno.58390
110. Li Z, Bu J, Zhu X, Zhou H, Ren K, Chu PK, et al. Anti-tumor immunity and ferroptosis of hepatocellular carcinoma are enhanced by combined therapy of sorafenib and delivering modified GO-based PD-L1 siRNAs. Biomater Adv (2022) 136:212761. doi: 10.1016/j.bioadv.2022.212761
111. Huang FY, Dai SZ, Wang JY, Lin YY, Wang CC, Zheng WP, et al. Engineered porous/hollow Burkholderia pseudomallei loading tumor lysate as a vaccine. Biomaterials (2021) 278:121141. doi: 10.1016/j.biomaterials.2021.121141
112. Ling YY, Wang WJ, Hao L, Wu XW, Liang JH, Zhang H, et al. Self-amplifying iridium(III) photosensitizer for ferroptosis-mediated immunotherapy against transferrin receptor-overexpressing cancer. Small (2022) 18(49):e2203659. doi: 10.1002/smll.202203659
113. Jeong SD, Jung BK, Lee D, Ha J, Chang HG, Lee J, et al. Enhanced immunogenic cell death by apoptosis/ferroptosis hybrid pathway potentiates PD-L1 blockade cancer immunotherapy. ACS Biomater Sci Eng (2022) 8(12):5188–98. doi: 10.1021/acsbiomaterials.2c00950
114. Hu X, Li R, Wu W, Fang K, Zhu Z, Wang Y, et al. A Fe(III)-porphyrin-oxaliplatin(IV) nanoplatform for enhanced ferroptosis and combined therapy. J Control Release (2022) 348:660–71. doi: 10.1016/j.jconrel.2022.06.019
115. Li K, Xu K, He Y, Yang Y, Tan M, Mao Y, et al. Oxygen self-generating nanoreactor mediated ferroptosis activation and immunotherapy in triple-negative breast cancer. ACS Nano (2023) 17(5):4667–87. doi: 10.1021/acsnano.2c10893
116. Wang L, Karges J, Wei F, Xie L, Chen Z, Gasser G, et al. A mitochondria-localized iridium(iii) photosensitizer for two-photon photodynamic immunotherapy against melanoma. Chem Sci (2023) 14(6):1461–71. doi: 10.1039/D2SC06675K
117. Zhou Z, Liang H, Yang R, Yang Y, Dong J, Di Y, et al. Glutathione depletion-induced activation of dimersomes for potentiating the ferroptosis and immunotherapy of "Cold" Tumor. Angew Chem Int Ed Engl (2022) 61(22):e202202843. doi: 10.1002/anie.202202843
118. Hsieh CH, Hsieh HC, Shih FS, Wang PW, Yang LX, Shieh DB, et al. An innovative NRF2 nano-modulator induces lung cancer ferroptosis and elicits an immunostimulatory tumor microenvironment. Theranostics (2021) 11(14):7072–91. doi: 10.7150/thno.57803
119. Chin YC, Yang LX, Hsu FT, Hsu CW, Chang TW, Chen HY, et al. Iron oxide@chlorophyll clustered nanoparticles eliminate bladder cancer by photodynamic immunotherapy-initiated ferroptosis and immunostimulation. J Nanobiotechnol (2022) 20(1):373. doi: 10.1186/s12951-022-01575-7
120. Singh P, Pandit S, Mokkapati V, Garg A, Ravikumar V, Mijakovic I. Gold nanoparticles in diagnostics and therapeutics for human cancer. Int J Mol Sci (2018) 19(7):1979. doi: 10.3390/ijms19071979
121. Guo W, Wu Z, Chen J, Guo S, You W, Wang S, et al. Nanoparticle delivery of miR-21-3p sensitizes melanoma to anti-PD-1 immunotherapy by promoting ferroptosis. J Immunother Cancer (2022) 10(6):e004381. doi: 10.1136/jitc-2021-004381
122. Liu Y, Niu R, Deng R, Song S, Wang Y, Zhang H. Multi-enzyme co-expressed dual-atom nanozymes induce cascade immunogenic ferroptosis via activating interferon-γ and targeting arachidonic acid metabolism. J Am Chem Soc (2023) 145(16):8965–78. doi: 10.1021/jacs.2c13689
123. Zhang P, Cui Y, Wang J, Cheng J, Zhu L, Liu C, et al. Dual-stimuli responsive smart nanoprobe for precise diagnosis and synergistic multi-modalities therapy of superficial squamous cell carcinoma. J Nanobiotechnol (2023) 21(1):4. doi: 10.1186/s12951-022-01759-1
124. Shan G, Minchao K, Jizhao W, Rui Z, Guangjian Z, Jin Z, et al. Resveratrol improves the cytotoxic effect of CD8 +T cells in the TME by regulating HMMR/Ferroptosis in lung squamous cell carcinoma. J Pharm BioMed Anal (2023) 229:115346. doi: 10.1016/j.jpba.2023.115346
125. Zhang H, Zhuo Y, Li D, Zhang L, Gao Q, Yang L, et al. Dihydroartemisinin inhibits the growth of pancreatic cells by inducing ferroptosis and activating antitumor immunity. Eur J Pharmacol (2022) 926:175028. doi: 10.1016/j.ejphar.2022.175028
126. Huang D, Xu D, Chen W, Wu R, Wen Y, Liu A, et al. Fe-MnO(2) nanosheets loading dihydroartemisinin for ferroptosis and immunotherapy. BioMed Pharmacother (2023) 161:114431. doi: 10.1016/j.biopha.2023.114431
127. Hosseini A, Gharibi T, Marofi F, Babaloo Z, Baradaran B. CTLA-4: From mechanism to autoimmune therapy. Int Immunopharmacol (2020) 80:106221. doi: 10.1016/j.intimp.2020.106221
128. Sharpe AH, Pauken KE. The diverse functions of the PD1 inhibitory pathway. Nat Rev Immunol (2018) 18(3):153–67. doi: 10.1038/nri.2017.108
129. Bonaventura P, Shekarian T, Alcazer V, Valladeau-Guilemond J, Valsesia-Wittmann S, Amigorena S, et al. Cold tumors: A therapeutic challenge for immunotherapy. Front Immunol (2019) 10:168. doi: 10.3389/fimmu.2019.00168
130. Budimir N, Thomas GD, Dolina JS, Salek-Ardakani S. Reversing T-cell exhaustion in cancer: lessons learned from PD-1/PD-L1 immune checkpoint blockade. Cancer Immunol Res (2022) 10(2):146–53. doi: 10.1158/2326-6066.CIR-21-0515
131. Li B, Chan HL, Chen P. Immune checkpoint inhibitors: basics and challenges. Curr Med Chem (2019) 26(17):3009–25. doi: 10.2174/0929867324666170804143706
132. Kubli SP, Berger T, Araujo DV, Siu LL, Mak TW. Beyond immune checkpoint blockade: emerging immunological strategies. Nat Rev Drug Discov (2021) 20(12):899–919. doi: 10.1038/s41573-021-00155-y
133. Daniyal M, Liu B, Wang W. Comprehensive review on graphene oxide for use in drug delivery system. Curr Med Chem (2020) 27(22):3665–85. doi: 10.2174/13816128256661902011296290
134. Zhao C, Zhou B. Polyethyleneimine-based drug delivery systems for cancer theranostics. J Funct Biomater (2022) 14(1):12. doi: 10.3390/jfb14010012
135. D'Souza A A, Shegokar R. Polyethylene glycol (PEG): a versatile polymer for pharmaceutical applications. Expert Opin Drug Deliv (2016) 13(9):1257–75. doi: 10.1080/17425247.2016.1182485
136. Jiang X, Stockwell BR, Conrad M. Ferroptosis: mechanisms, biology and role in disease. Nat Rev Mol Cell Biol (2021) 22(4):266–82. doi: 10.1038/s41580-020-00324-8
137. Hassannia B, Vandenabeele P, Vanden Berghe T. Targeting ferroptosis to iron out cancer. Cancer Cell (2019) 35(6):830–49. doi: 10.1016/j.ccell.2019.04.002
138. Qi D, Peng M. Ferroptosis-mediated immune responses in cancer. Front Immunol (2023) 14. doi: 10.3389/fimmu.2023.1188365
139. Turubanova VD, Balalaeva IV, Mishchenko TA, Catanzaro E, Alzeibak R, Peskova NN, et al. Immunogenic cell death induced by a new photodynamic therapy based on photosens and photodithazine. J Immunother Cancer (2019) 7(1):350. doi: 10.1186/s40425-019-0826-3
140. Xu Q, Zhan G, Zhang Z, Yong T, Yang X, Gan L. Manganese porphyrin-based metal-organic framework for synergistic sonodynamic therapy and ferroptosis in hypoxic tumors. Theranostics (2021) 11(4):1937–52. doi: 10.7150/thno.45511
141. Sun W, Yang J, Hou M, Xie S, Xiong L, Li B, et al. A nano “Immune-guide” Recruiting lymphocytes and modulating the ratio of macrophages from different origins to enhance cancer immunotherapy. Adv Funct Mater (2021) 31(23). doi: 10.1002/adfm.202009116
142. He H, Du L, Xue H, An Y, Zeng K, Huang H, et al. Triple tumor microenvironment-responsive ferroptosis pathways induced by manganese-based imageable nanoenzymes for enhanced breast cancer theranostics. Small Methods (2023) 7(7):e2300230. doi: 10.1002/smtd.202300230
Keywords: CD8+ T cells, IFN-γ, ferroptosis, DAMPs, immunotherapy
Citation: Lin Z, Zou S and Wen K (2024) The crosstalk of CD8+ T cells and ferroptosis in cancer. Front. Immunol. 14:1255443. doi: 10.3389/fimmu.2023.1255443
Received: 08 July 2023; Accepted: 28 December 2023;
Published: 15 January 2024.
Edited by:
Hongwei Liang, China Pharmaceutical University, ChinaReviewed by:
Yuanliang Xia, First Affiliated Hospital of Jilin University, ChinaLongchao Liu, Chinese Academy of Sciences (CAS), China
Copyright © 2024 Lin, Zou and Wen. This is an open-access article distributed under the terms of the Creative Commons Attribution License (CC BY). The use, distribution or reproduction in other forums is permitted, provided the original author(s) and the copyright owner(s) are credited and that the original publication in this journal is cited, in accordance with accepted academic practice. No use, distribution or reproduction is permitted which does not comply with these terms.
*Correspondence: Kunming Wen, MzgxMjI0NjE5QHFxLmNvbQ==