- 1Department of Otolaryngology Head and Neck Surgery Surgery, Sichuan Provincial People’s Hospital, School of Medicine, University of Electronic Science and Technology of China, Chengdu, China
- 2Department of Emergency Medicine, Laboratory of Emergency Medicine, West China Hospital, and Disaster Medical Center, Sichuan University, Chengdu, Sichuan, China
- 3National Clinical Research Center for Infectious Disease, Shenzhen Third People’s Hospital, Shenzhen, China
- 4Department of Thoracic Surgery, Sichuan Provincial People’s Hospital, University of Electronic Science and Technology of China, Chengdu, China
Previously, it was believed that type III interferon (IFN-III) has functions similar to those of type I interferon (IFN-I). However, recently, emerging findings have increasingly indicated the non-redundant role of IFN-III in innate antiviral immune responses. Still, the regulatory activity of IFN-III in adaptive immune response has not been clearly reported yet due to the low expression of IFN-III receptors on most immune cells. In the present study, we reviewed the adjuvant, antiviral, antitumor, and disease-moderating activities of IFN-III in adaptive immunity; moreover, we further elucidated the mechanisms of IFN-III in mediating the adaptive antiviral immune response in a thymic stromal lymphopoietin (TSLP)-dependent manner, a pleiotropic cytokine involved in mucosal adaptive immunity. Research has shown that IFN-III can enhance the antiviral immunogenic response in mouse species by activating germinal center B (GC B) cell responses after stimulating TSLP production by microfold (M) cells, while in human species, TSLP exerts OX40L for regulating GC B cell immune responses, which may also depend on IFN-III. In conclusion, our review highlights the unique role of the IFN-III/TSLP axis in mediating host adaptive immunity, which is mechanically different from IFN-I. Therefore, the IFN-III/TSLP axis may provide novel insights for clinical immunotherapy.
1 Introduction
The interferon (IFN) response is a critical host defense mechanism against viral or microbial infections. According to sequence homology, IFNs can be subdivided into type I (IFN-I), type II (IFN-II), and type III IFNs (IFN-III). In humans and most mammals, IFN-I mainly include IFN-α, IFN-β, IFN-ϵ, IFN-κ, and IFN-ω; IFN-II group has only a single member, IFN-γ; IFN-III are the most recently discovered IFN family in 2003, which are composed of four isoforms including IFN-λ1 (IL-29), IFN-λ2 (IL-28A), IFN-λ3 (IL-28B), and IFN-λ4 (1). Humans have all IFN-III genes, while in mice, only the genes coding for IFN-λ2 and IFN-λ3 are present and IFN-λ1 is a pseudogene (2, 3). IFN-λ4 gene is the last to be discovered in human hepatocytes in 2013, but the genomic region encoding IFN-λ4 is absent in mice (4). Although IFN-II is a key cytokine for establishing adaptive immunity and mediating pro-inflammatory and immunomodulatory features (5), IFN-I and IFN-III are more essential for innate immune responses, which is initiated by the recognition of pathogen-associated molecular patterns (PAMPs) via pattern recognition receptors (PRRs). In the context of virus invasion, viral DNA and RNA, as well as viral protein are sensed by PRRs, thus triggering the downstream signaling cascades to activate the production of IFNs from immune and non-immune cells (6, 7). Distinctly, IFN-I binds to cell surface receptors composed of two subunits IFNAR1 and IFNAR2, while IFN-III binds to the heterodimeric IFN-lambda receptor (IFNLR) composed of IFNLR1 and IL-10Rβ. After IFNs binding to their receptors, the Janus kinase/signal transducer and activator of transcription (JAK/STAT) signaling pathway is activated, which provides innate antiviral immune responses that depend on the expression of IFN-stimulated genes (ISGs). Ubiquitin-specific protease 18 (USP18) and suppressor of cytokine signaling 1 (SOCS1) are antagonisms of IFN-I, while IFN-III is less influenced by these negative regulatory mechanisms (8–12). Unlike IFN-I that is primarily produced by most immune cells, IFN-III is mostly secreted by epithelial cells and few immune cells like dendritic cells (DCs) (13). Additionally, although IFN-I receptors are ubiquitously expressed, IFNLR1 is preferentially expressed on the mucosal surface of epithelial cells rather than immune cells, except for neutrophils, which have recently been characterized as IFN-III responders (14–16).
Primarily, accumulating studies have provided evidence for the dominant role of IFN-I in mediating innate and adaptive immunity (17–21). Although IFN-λ has been discovered as a part of innate immune cytokines, its function in immune regulation remains elusive. The non-redundant role of IFN-λ in antiviral innate immunity has been widely summarized, such as host defense in the respiratory and gastrointestinal tracts, which can not be compensated by IFN-I (22–29). However, currently, little is known about the role of IFN-III in adaptive immune response. Over the past decade, the relevant research about the effect of IFN-III on adaptive antiviral immunity is limited, which may be due to the low expression of IFNLR in most immune cells (30), resulting in a limited understanding of the antiviral activity of IFN-III. With the further deepening of research, numerous reports regarding the responsibility of IFN-III in stimulating adaptive antiviral immunity continue to emerge (2, 16, 30–32). Particularly, the revealed mechanism of thymic stromal lymphopoietin (TSLP) unveils the mysterious role of IFN-III in orchestrating adaptive immune response (33–35). The assistance of TSLP in IFN-III-mediated immunoregulatory faculties triggers our interest in further research. Hence, this review will focus on the antiviral mechanisms of IFN-III in adaptive immune regulation, especially the IFN-III/TSLP axis in adaptive antiviral immunoregulation, as well as the differences between IFN-III and IFN-I in this antiviral immune system. Additionally, the inconsistent research conclusions between mouse and human species will also be discussed, yearning to provide novel viewpoints for vaccine development and clinical antiviral therapeutics.
2 IFN-III in adaptive immunity
2.1 Adjuvant activity
Adjuvants are substances added to vaccines to enhance the host’s antigen-specific immune response (36). The commonly used adjuvants (such as aluminum salts) could enhance immune response thought stimulating the NLRP3-inflammasomes and promoting the release of cytokines (such as IL-1β) (37). Other adjuvants (such as MF59 and AS03) exert functions by activating dendritic cells and other antigen-presenting cells (38). Additionally, some adjuvants interact with toll-like receptors (TLRs) or directly target specific immune cell populations. Cytokines, as important immunomodulatory agents, are available to affect any aspect of immune responses in a more or less specific manner (39, 40). As a newly described IFN family, the potential ability of IFN-III as a vaccine adjuvant to affect adaptive immune response has not been fully evaluated until an in vivo study shows that plasmid-encoded IL-28B can increase the virus-specific IgG2a titer in serum. This study reveals the significant potential benefits of IFN-III as an adjuvant in adaptive immune regulation. Moreover, IFN-III has been reported to further enhance splenic CD8+ T cell-mediated immunity by reducing regulatory T cell population during DNA vaccination (41). In another study, IL-28B adjuvant can remarkably elevate the antibody levels and neutralization titers of herpes simplex virus 2 in mice by DNA immunization. The IL-28B adjuvant-regulated immune response is significantly more effective than immunization with antigen DNA vaccine alone. Due to the involvement of IL-28B, T helper 1/2 (Th1/Th2) cytokines secreted by activated immune cells are crucial for controlling viral infection and disease progression (42). In addition, IFN-III subtype IFN-λ2 can also influence the production of antibodies against the influenza subunit vaccines. As a result, the influenza virus hemagglutinin (HA)-specific total serum IgG levels and its subclass IgG1 levels are about 15-fold enhanced. Besides, IFN-λ2 vaccination results in a significant increase in IgA levels in the bronchoalveolar lavage (BAL) fluids. Similar phenomenon can also be observed in another influenza vaccine CTA1-3M2e-DD (designated M2e) applied with IFN-λ2 (33). Obviously, IFN-λ2 and IFN-λ3 in mice can potentiate both cellular and humoral immune response in mice, representing promising molecular adjuvants. However, since the potential protection of IFN-III upon virus vaccination exhibits specificity on the mucosal surface, different immune approaches may affect the phenotype of immune responses (16, 22, 33). The characteristics of IFNLR enable IFN-III to serve as a significant front-line defense against viral infections at mucosal sites.
2.2 Adaptive antiviral effects
In addition to adjuvant activity, the IFN-III signaling also directly contributes to adaptive immunity against virus infection, which is mediated by direct stimulation of DCs or indirect effects on other immune cells like natural killer (NK) cells, monocytes/macrophages, T cells, and B cells to upregulate cytokines and chemokines (2, 8, 32). IFN-III can indirectly modulate the activation of NK cells and the inflammatory response of monocytes/macrophages, thus exerting auxiliary benefits in the antiviral activity of adaptive immunity against chronic hepatitis C virus (HCV) (43). The common single-nucleotide polymorphisms (SNPs) in the IFN-III signaling are strongly associated with the treatment of chronic HCV infection (31, 32). In the context of yellow fever virus infection, IFN-III deficiency leads to impaired T cell activation and severe perturbation of pro-inflammatory cytokines in mice (44). In response to lymphocytic choriomeningitis virus (LCMV) infection, IFN-III increases CD8+ T cell expansion and diminishes weight loss in the chronic pathological stage instead of the acute infective phase, indicating that virus-specific host interactions affect the regulatory mode of IFN-III in the adaptive immune response (45). Further researches investigate the critical role of the IFN-III signaling in combating different subtypes of influenza A virus (IAV) strains (PR8 and X31), which is orchestrated through indirect adaptive immune mechanisms. The adaptive immunomodulation does not rely on the direct attachment of IFN-III to CD8+ T cells, but rather on IFN-III triggering DCs, particularly manipulating the migration and function of CD103+ DCs, which is essential for optimal antigen uptake and CD8+ T cell activation, thus providing permanent IAV protection (46). The activation program of DCs is probably regulated by TSLP, but it has not been confirmed yet.
2.3 Anti-tumor effects
IFN-III has been demonstrated to possess additional benefits in suppressing tumors in mice by directly reducing the tumorigenicity of tumor cells, inhibiting cell proliferation, and inducing apoptosis (47–49). IFN-III also mediates indirect anti-tumor mechanisms by regulating the tumor microenvironment (50). NK cells and CD8+ T cells are recruited into the microenvironment to participate in the anti-tumor mechanism of IFN-III in a murine model of melanoma (51). A murine model of fibrosarcoma further confirms the indirect anti-tumor mechanism of IFN-III. In vivo, antibody depletion of NK cells, neutrophils, and CD8+ T cells, but not CD4+ T cells, induces IL-28-mediated inhibition of tumor growth. Meanwhile, the inoculation of IL-28-encoded fibrosarcoma cell line in mice advances the production of IFN-γ and evokes the activity of cytotoxic T cells, indicating that the anti-tumor action of IL-28 also partly depends on IFN-γ (52). The IFN-III signaling can target mammary epithelial cells and promote the chemokine CXCL10 production, thereby recruiting CD4+ T cells into the tumor microenvironment. Besides, the immune balance between Th1 and Th2 altered by IFN-III may take advantages in the treatment of cancer (53). In addition, IFNLR-deficient (Ifnlr-/-) mice are more sensitive to experimental tumor metastasis and carcinogen-induced tumor formation and are also more susceptible to the growth of NK cell-sensitive lymphoma. Administration of IFN-III postpones the fatality in the B16F10 melanoma model mice with adoptive cell transfer and diminishes tumor growth in methylcholanthrene-treated mice (54). Therefore, IFN-III may be utilized as an adjuvant anti-tumor therapy with its ability to modulate tumorigenesis directly and indirectly. Due to the lower expression of IFNLR1 in most cell types, IFN-III offers a more selective targeted anti-tumor ability with fewer side effects compared to IFN-I (55).
2.4 IFN-III in hypersensitivity disorder
2.4.1 Autoimmune disease
IFN-III was initially described as a specialized system that inhibits viral replication on the surface of the epithelial barrier while limiting inflammatory damage. However, although IFN-III has complex effects on both innate and adaptive immunity, it is also associated with systemic autoimmune diseases (56). IFN-III exerts protective activity in chronic skin diseases such as psoriasis by secreting CXCL10 and CXCL11, to attract NK cells, CD4+ and CD8+ T cells. Elevated IFN-III in human keratinocytes contributes to relieving psoriatic lesions (57). Importantly, IFN-III demonstrates its heterogeneous characteristics in autoimmune rheumatic diseases. Compared with healthy controls, patients with systemic lupus erythematosus (SLE) have higher serum levels of IFN-III (58), which is also correlated with the upregulation of inflammatory cytokines including IL-6, IL-27, Th17, and B cell activating factor (BAFF) (59–61). Data from TLR7-induced lupus Ifnlr-/- mouse models support the mechanistic role of IFN-III in SLE. The infiltration of CD4+ and CD8+ effector memory T cells, B cells, macrophages, and neutrophils in Ifnlr-/- mice are less than that in wild-type (WT) mice, which contributes to weakening the over-activated immunity and diminishing inflammation in the skin and kidneys (62). These data suggest that IFN-III may promote the autoimmune pathology of SLE. The serum level of IFN-λ1 has also been found to increase in rheumatoid arthritis (RA) patients (63), and the pro-inflammatory cytokines like IL-6 and IL-8 are further increased in synovial fibroblasts after IFN-λ1 application (64). However, another research demonstrates the protective effect of IFN-λ2 in mice with collagen-induced arthritis (65). Treatment with IL-28A effectively reverses the development of arthritis by reducing IL-17 levels in joints and inguinal lymph nodes after restricting the recruitment of IL-1β-expressing neutrophils (65). Therefore, whether IFN-III is the culprit of RA remains unclear. Furthermore, IFN-III also contributes to the pathogenesis of autoimmune disease in the central nervous system (CNS). In an experimental autoimmune encephalomyelitis (EAE) murine model of multiple sclerosis (MS), Ifnlr-/- mice are associated with diminished Th1 cells and decreased pro-inflammatory cytokines compared with WT animals. The neutralization of IFN-III signaling also promotes the recovery of autoimmunity in the CNS (66). Taken together, these data highlight the intimate relationship between IFN-III and autoimmune diseases.
2.4.2 Asthma
For hosts with asthma, IFN-III has been reported to hamper hypersensitized immunity. In experimental allergic models, the application of IFN-III can not only reduce granulocyte recruitment in BAL and inflammation in lung tissues (67) but further modulate TH1 and TH2 responses in a dose-dependent manner (68). Additionally, most patients suffer from asthma exacerbation after virus infection. For that, IFN-III production stimulated by the virus in the lung exerts antiviral effects without triggering inflammation (69). IFN-III has been evidenced to decrease IL-4, IL-5, and IL-13 production in asthma, which also provides protective advantages in hypersensitivity disorder (70). However, the role of IFN-III varies in patients of different ages and with different disease stages; for instance, IFN-III level is generally stable in stable asthma in adults, which represents more effective disease control (71).
3 TSLP in mucosal adaptive immunity
TSLP is a short-chain hematopoietic cytokine that signals through TSLP receptor (TSLPR), a high-affinity heterodimeric receptor composed of IL-7α-chain and a unique TSLP chain (72). TSLP is predominantly produced by epithelial cells in mucosal tissue (73). Additionally, TSLP can also be produced by other immune cells (such as fibroblasts, DCs, monocytes, and mast cells) following stimulation (74). In most studies, TSLP is described as a pathogenic cytokine that can trigger allergic diseases (such as allergic dermatitis and asthma) (75) and induce pro-inflammatory factors in autoimmune diseases (76–78), particularly in barrier tissues (such as skin, lungs, and intestines) (79). TSLP can activate DCs to promote a Th2-type immune response associated with allergy disease. Moreover, TSLP also affects regulatory T cells (Tregs) development, contributing to immune tolerance (80, 81).
However, TSLP also performs a non-redundant role in antiviral, antibacterial, and antitumor regulation to some extent. TSLP interacts with TSLPR on CD11b+ DCs to participate in immune responses and promote the long-term antiviral immunity of CD8+ T cells following influenza infection in mucosal tissues, especially in the respiratory tract (82, 83). The TSLP-TSLPR pathway in CD11c+ cells is also essential for inducing respiratory antigen-specific IgA in response to pneumococcal infection (84). Moreover, TSLP can potently induce adaptive immunity mediated by CD4+ Th2 cells to block the early carcinogenesis of breast cancer without causing inflammatory responses in normal breast tissues (85). However, recent researches have also suggested that TSLP might have both pro-tumorigenic and antitumor effects, and its activity depends on the tumor microenvironment context and the cancer type (74, 86).
As a B cell-associated cytokine, TSLP not only performs immune activity via direct ingestion but also serves as a vaccine adjuvant (87). With the participation of TSLP as an enhancer, TSLP-activated DCs can induce much robust CD8+ T cell expansion, thus providing more efficient anti-tumor immunotherapies than historically used adjuvant Bacillus Calmette–Guérin (88). As an adjuvant of the human immunodeficiency virus (HIV) vaccine CN54gp140, intranasal inoculation of TSLP, rather than intradermal inoculation, triggers potent and sustained serum and mucosal immune responses without inducing additional inflammatory responses. With the aid of TSLP, DCs located in the nasal mucosa are activated and Th2-cell-skewed immune responses are strongly promoted, followed by B cell differentiation and antigen-specific IgA and IgG1 secretion (89). The combination of TSLP and IAV-M2e vaccine can damper the weight loss of mice, and all animals can survive during the challenge infection period. Furthermore, TSLP stimulates an increase in serum M2e-specific IgG1 levels. The same results can be observed when TSLP is given together with another IAV vaccine haemagglutinin (HA), a commercial influenza vaccine containing particle-derived hemagglutinin. In TSLPR deficient (Tslpr-/-) mice, intranasal immunization with the IAV vaccine fails to boost neither antigen-specific IgG1 nor nucleoprotein-specific CD8+ T cells compared with WT mice (33, 35). Taken together, these data verify the non-redundant role of TSLP in mucosal adaptive immunity, whether administrated alone or combined with vaccination.
4 IFN-III mediates adaptive immunity via TSLP
4.1 Importance of the IFN-III/TSLP axis in adaptive immunity
Considering that both IFN-III and TSLP are produced by epithelial cells and both play roles in immune response as described above, it is of great significance to investigate the relationship between these two cytokines. Current studies have shown that intranasal inoculation of influenza virus subunit vaccine containing TSLP can effectively reinforce the serum IgG1 levels to a similar extent as IFN-λ2 triggered. However, in Tslpr-/- mice, immunization with M2e or HA-based Influsplit Tetra IAV vaccine combined with IFN-III as an adjuvant inhibits the enhancing effect of IgG1, indicating that TSLP can replace the enhancing activity of IFN-III, and TSLP is required for IFN-III-induced immune protection. Simultaneously, the losing function of TSLP in the IAV vaccine in Tslpr-/- mice can’t be compensated even in the presence of IFN-λ2 (33). These results indicate that the positive effects of IFN-III on the production of IgG1 and IgA depend mechanistically on TSLP. Interestingly, thus TSLP-mediated protective antiviral immunity of the IFN-III can similarly be realized through rectal vaccine inoculation. Whereas the stimulating effect of IFN-III as an adjuvant in influenza vaccine is canceled if administered intraperitoneally or subcutaneously, implying that these non-mucosal sites do not express significant IFN-III-responsive cells to produce TSLP-dependent antibodies (35). Taken together, we proved that TSLP was responsible for the IFN-III-related adaptive immune system. The IFN-III/TSLP axis (refer to some immune interactions between TSLP and IFN-III) participated in antiviral adaptive immune regulation at mucosal sites, particularly in the respiratory and gastrointestinal tracts (Figure 1).
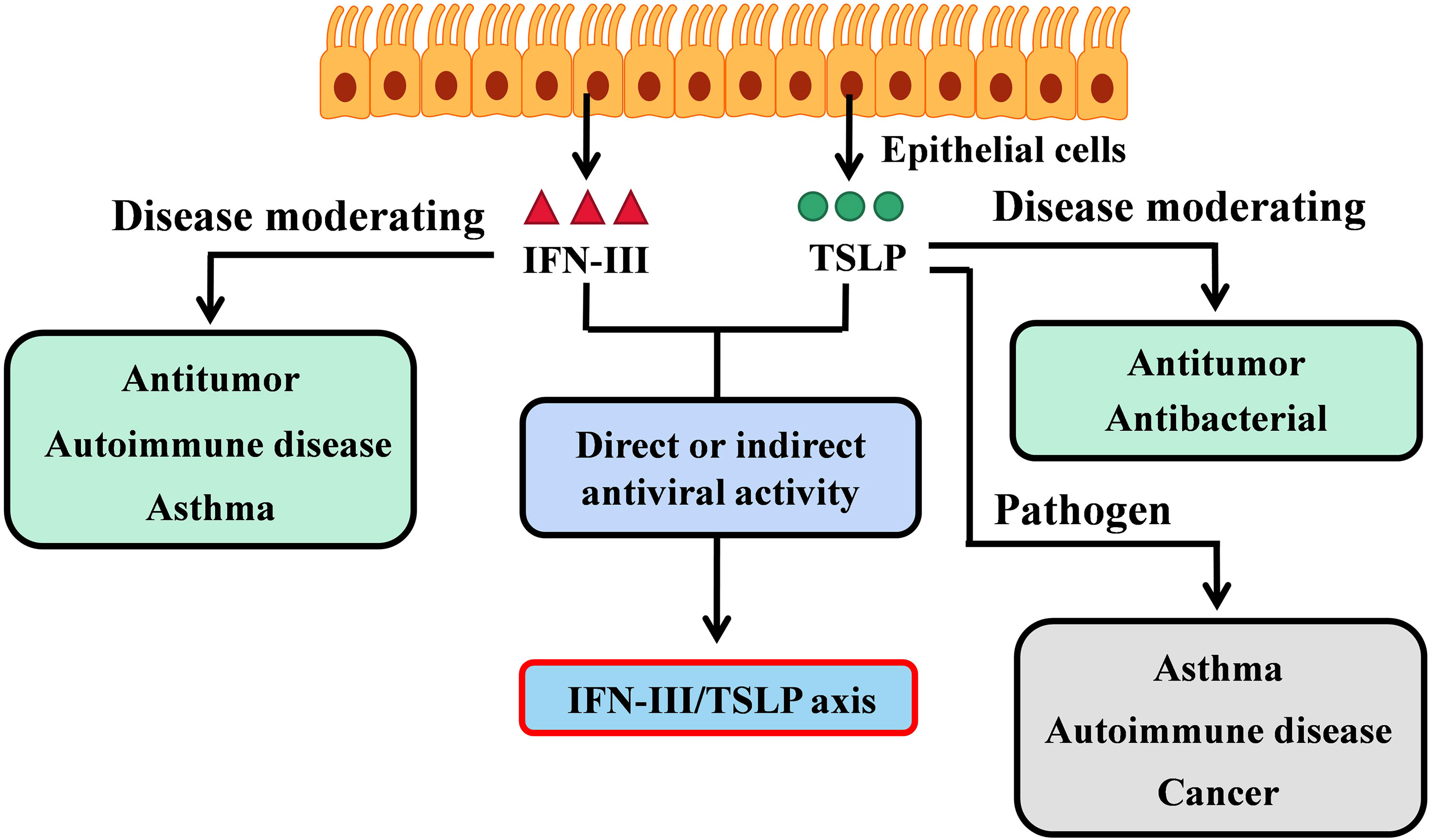
Figure 1 The IFN-III/TSLP axis in adaptive immunity. IFN-III and TSLP are both produce by epithelial cells, but perform different biological activities. IFN-III is critical in modulating tumor invasion or hypersensitivity disorders (such as autoimmune disease and asthma); TSLP is the pathogen of asthma and autoimmune disease but contradicts in cancer. Interestingly, both IFN-III and TSLP show direct or indirect antiviral activities on mucosal sites. The interaction between IFN-III and TSLP has also been revealed by the latest researches (33, 35). Therefore, the IFN-III/TSLP axis is responsible for adaptive antiviral immunity but is unclear in the context of cancer or hypersensitivity disorder.
Although IFN-III and TSLP are both produced by epithelial cells and exert effects on antiviral and antitumor immune regulation, a direct or well-defined interaction between the two in moderating cancer or hypersensitivity disorder is still lacking. Nevertheless, based on adaptive immunity exerted by the IFN-III/TSLP axis in the context of virus infection, there remains an area of ongoing research.
4.2 The IFN-III/TSLP axis is mechanistically different in mouse and human
IFNLR is expressed in human B cells while lacking in mice, which suggests that the IFN-III/TSLP axis may have different immunoregulation functions in mice and humans (14). As mentioned previously, TSLP is a cytokine modulating antibody production towards IgA and IgG via the intranasal route (89). IFN-III vaccination in mice infected with the influenza virus can strongly trigger microfold (M) cells on the upper respiratory tract, which induces TSLP production, CD103+ DCs migration, and promotes the production of follicular helper T (Tfh) cells in lymph nodes. Later, TSLP-dependent germinal center (GC) B cells in lymph nodes are activated and thus numerous high-affinity IgA and IgG antibodies are secreted (33, 90). Besides, apart from the critical role of IFN-III in mucosal homeostasis (91), TSLP has also been found highly expressed in antigen-stimulated colonic epithelial cells, which in turn activates Tregs through the mTORC1 pathway to maintain mucosal homeostasis (92). Hence, the IFN-III/TSLP axis may also participate in modulating mucosal homeostasis to some extent (Figure 2, left).
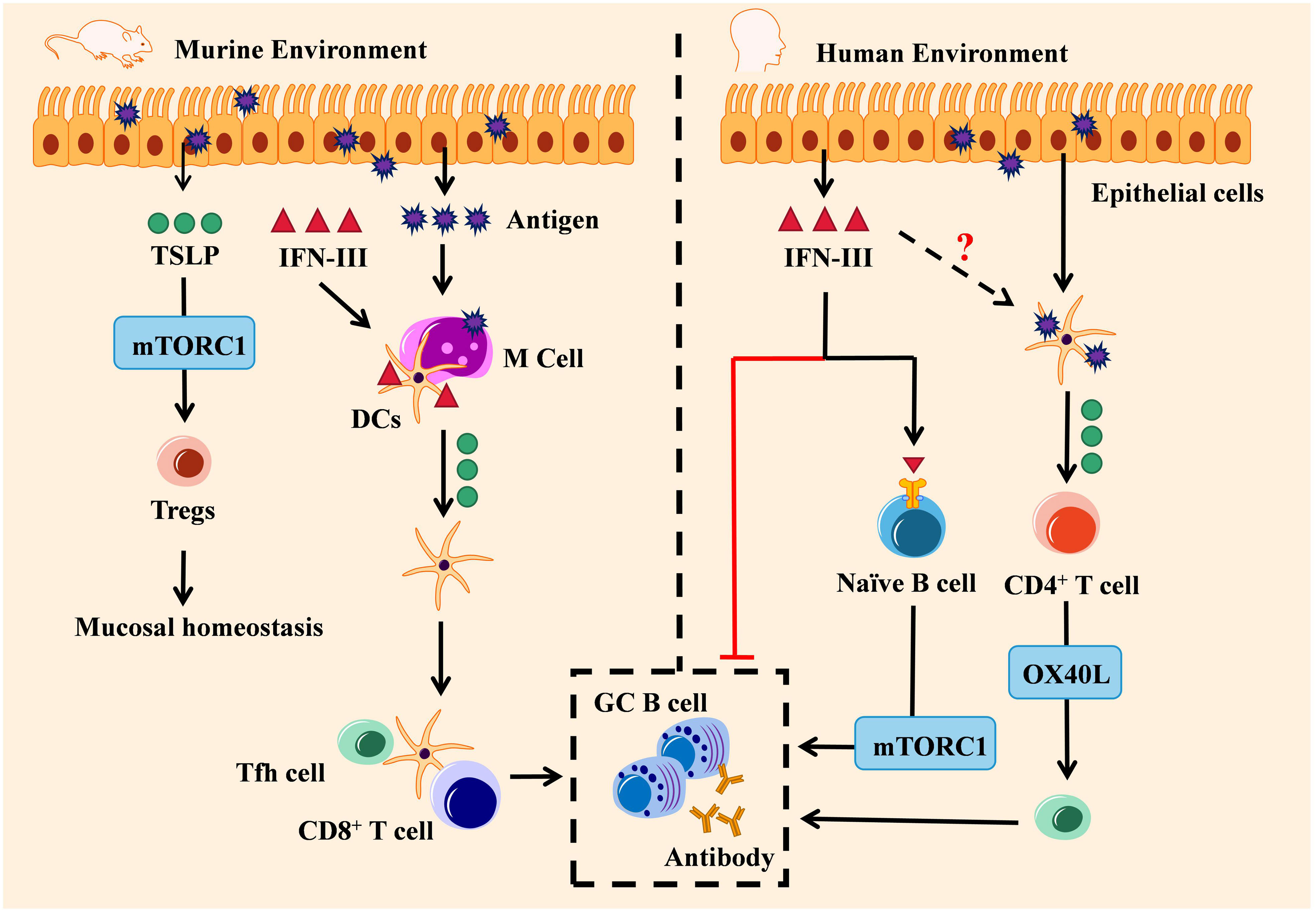
Figure 2 Engagement of the IFN-III/TSLP axis in adaptive antiviral activity: environment decided. The IFN-III/TSLP axis exerts distinct adaptive antiviral activities in mice and human. In the murine environment (left), IFN-III induces TSLP production by virus-infected M cells and promotes the migration of CD103+ DCs, thereby promoting TSLP-dependent GC B cell response by Tfh cells and fostering high-affinity antibody production. Antigen-stimulated TSLP also regulates mucosal homeostasis by activating Tregs through the mTORC1 pathway. In the human environment (right), on the one hand, IFN-III can either inhibit antibody production or enhance GC B cell response by naïve B cells through the mTORC1 pathway; on the other hand, TSLP-activated DCs are capable of inducing naïve CD4+ T cells to differentiate into Th2 via the OX40L pathway, and this process may also depend on IFN-III.
In the human species, the IFN-III/TSLP axis seems to show inconsistent roles. A previously in-vitro study has indicated that IFN-III is associated with a decrease in Th2-cytokines, B-cell proliferation, and IgG production in IAV-infected patients (93). By contrast, another study has shown that human naïve B cells interact with IFN-III stimulation and then differentiate into plasmablasts through the mTORC1 pathway (94). In respiratory epithelial cells, human Tslp genes are expressed during respiratory syncytial virus infection (95). TSLP-activated DC cells can induce Tfh cells to differentiate from naïve CD4+ T cells into a Th2-skewed environment, mechanistically depending on OX40L, a ligand of OX40 (96). Since previous in vitro studies have demonstrated that human TSLP can modulate GC-B cells’ immune response and B cells from humans directly respond to IFN-III (94, 97), it is currently unknown whether the coordination of the IFN-III/TSLP axis in adaptive antiviral immunity can also be replicated in humans, as observed in mice (Figure 2, right).
The reason why IFN-III-stimulated B cells show completely different functions between humans and mice is currently unclear. However, there are indications that the IFNLRI gene in various cell types is correlated with epigenetic changes, including DNA methylation and histone modifications. As has been evidenced previously, the transcriptional regulation of the IFNLR1 gene is dynamic, which may influence IFNLR1 expression and result in multiple functions. Indeed, histone deacetylase inhibitors can cancel the silencing of the IFNLR1 gene and confer IFN-III responsibility in previously inactivated human cell lines. The dynamic expression of IFNLR may explain the conflicting role of IFN-III in adaptive viral protection (98).
Considering that the in-vivo mouse models and in-vitro human studies cannot completely demonstrate the exact operation of IFN-III in the human body, a more relevant model on non-human primates may exhibit closer effects. Interestingly, under IFN-λ3 stimulation, macaques exhibit an adaptive immune response consistent with the immune activity in mice. In rhesus macaques, DNA vaccination encoding IFN-III exerts a positive effect on enhancing the antiviral activity of antigen-specific CD8+ T cells. Moreover, IFN-λ3 adjuvant can further induce continuous Th1 cell-biased immune responses (99, 100).
4.3 IFN-I orchestrates adaptive immunity via a TSLP-independent mechanism
IFN-I can promote adaptive immune response or possess adjuvant activity against virus infection, bacterial burden, and tumor invasion (18, 101–105). The role of IFN-I in autoimmune diseases is bifacial. Though IFN-I is correlated with severe SLE progression, it may also play a positive role in the pathological improvement of RA (106, 107). Here we focus on the adaptive antiviral activity of IFN-I.
IFN-I and IFN-III can commonly be secreted by most nucleated cells, while IFN-III is particularly secreted by epithelial cells of mucosal organs. Although the signaling pathways triggered by these two types of IFNs are similar, their adaptive antiviral immune responses display functionally different (8–12). IFN-I modulates adaptive immunity via direct or indirect mechanism that depends on its impact on B and T cells involved in the adaptive immune response. In indirect mechanism, IFN-I firstly binds to DCs expressing IFNAR (108), thereby stimulating DCs to produce chemokines and cytokines that enhance the survival and proliferation of T cells including CD4+ T cells and CD8+ T cells, as well as induce the differentiation of T helper cell subsets including Th1 and Tfh cells (109–111). The activated Tfh cells migrate to B cell follicles and participate in GC reactions to generate high-affinity antibodies. Th1 cells on the other way express the master transcription factor T-bet and produce high levels of IFN-γ, which promotes macrophage activation and enhances CD8+ T cell responses. However, the effect of IFN-I on CD4+ T cell differentiating into Tfh and Th1 may disparate, which depends on the cells sensing these cytokines, the nature of the pathogen, and the stage of the infection (112–115). IFN-I signaling also regulates B cell response indirectly by inducing DCs to secret B cell stimulatory cytokines, thereby promoting immunoglobulin (Ig) isotype switching (116). This pathway acts on the mediation of IFN-I between CD4+-differentiated Th1 and Tfh to prosper GC B cell response (117). In the lungs, IFN-I-induced CXCL13 can initiate CXCR5-dependent recruitment of B cells and support ectopic GC formation (118). In direct adaptive immune regulation, IFN-I signaling immediately activates T cell receptor, thus promoting T cell proliferation and differentiation (110). IFNAR-deficient B cells induce an impaired antibody response and subclass switching when treated with IFN-α, indicating a direct effect of IFN-I on B cells (119). Indeed, IFN-I is required for different stages of B cell response, including early B cell activation and GC formation (120). Unlike IFNAR that is extensively expressed on most hematopoietic and non-hematopoietic cells, IFNLR is absent on the surface of mouse B and T cells (30), but specifically activated on the epithelial surface (16). Hence, IFN-I exhibits an adaptive immune response directly by interacting with immune cells, instead of mucosal epithelial cells, which is completely different from IFN-III (Figure 3).
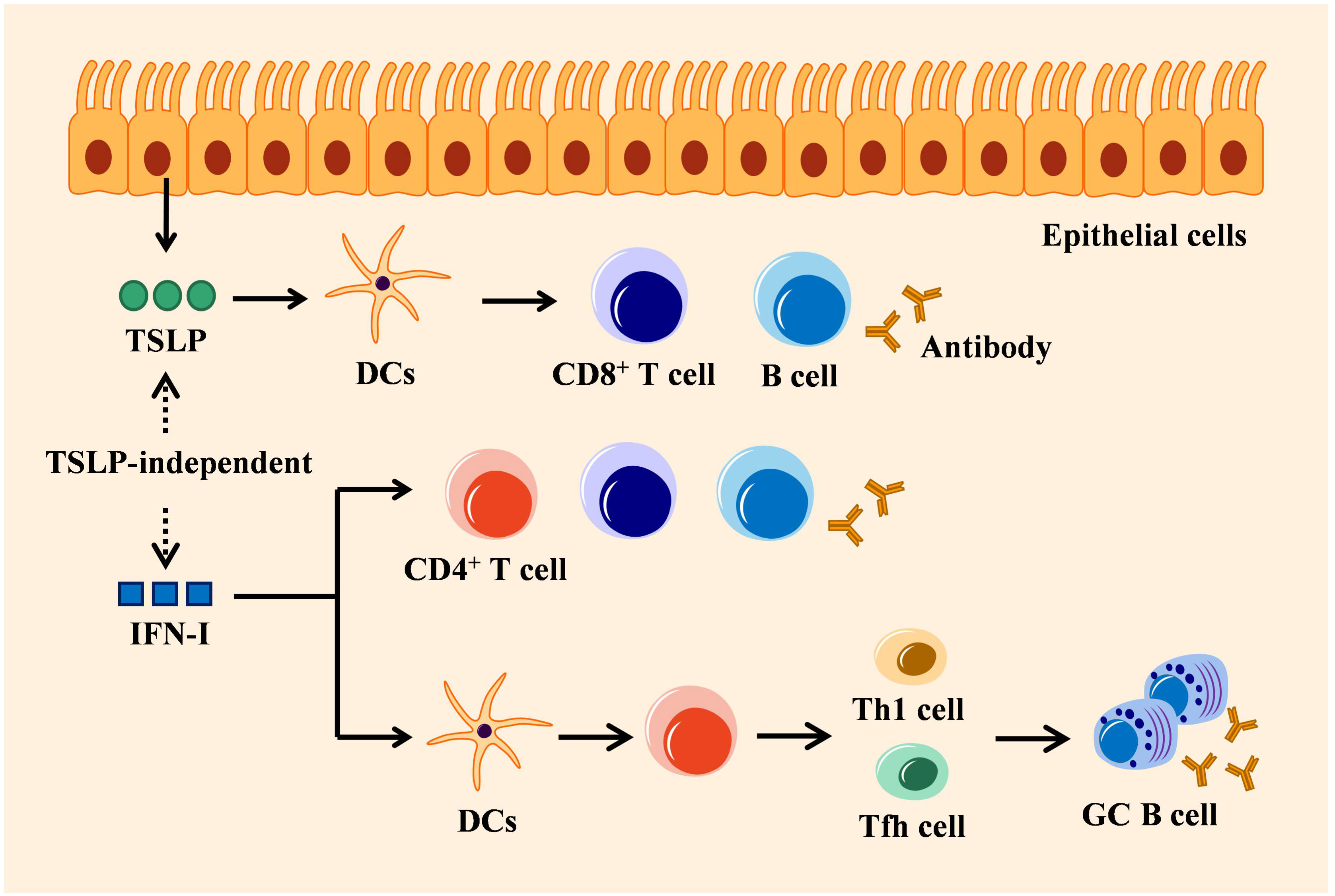
Figure 3 Mechanisms of IFN-I in adaptive immunity: TSLP-independent. Epithelial cells-derived TSLP exerts adaptive antiviral activity by activating DCs that trigger CD8+ T cell amplification, B cell differentiation, and antigen-specific antibody secretion. IFN-I regulates adaptive immune response directly by stimulating immune cells (such as T cells or B cells), which is independent of TSLP, or indirectly by stimulating DCs to induce CD4+ T cells to differentiate into Tfh or Th1 cells that can activate antibody production by GC B cells.
Although IFN-I and IFN-III are engaged in almost the same intracellular signaling pathway, it is of interest that, the functions of IFN-I and IFN-III in their adjuvant activities for influenza vaccines are different. On the one hand, when the influenza vaccine is applied with IFN-αB/D intranasally, both the antigen-specific IgG1 and IgG2c levels in serum and the IgA levels in BAL fluids are elevated. IFN-I can also improve vaccine-induced serum antibodies after intraperitoneal immunization. In contrast, IFN-λ2-enriched influenza vaccine promotes the IgA levels in BAL fluids and IgG1 levels in serum but not IgG2c. Thus IFN-III-induced vaccine immune responses are not observed either through intraperitoneal or subcutaneous routes. On the other hand, while the immune activity of IFN-λ2 plus vaccine is dampened in Tslpr-/- mice, antibodies in both serum and BAL fluids remain unaffected in TSLP-deficient mice administrated with IFN-αB/D-involved influenza vaccine (33, 35). In summary, the adjuvant activity of IFN-I is not restricted to mucosal tissue. The vaccine-induced influenza virus resistance mediated by IFN-I does not rely on TSLP immune regulation. On the contrary, TSLP is important for IFN-III-mediated adaptive immune response (34). Hence, IFNs can be selectively used in the application of clinical adjuvants. IFN-III may be more suitable for mucosal immune regulation and epithelial surface protection, whereas IFN-I provides more benefits in systematical immune responses (121). Therefore, the selective use of IFNs contributes to efficient and targeted immunotherapy.
5 Discussion
The studies we mentioned above have revealed the non-redundant role of IFN-III and TSLP in adaptive immune modulation respectively. Moreover, the vital role of the IFN-III/TSLP axis in adaptive response was further highlighted. As described, the adaptive immune regulatory faculty of IFN-III is indirect and depends on TSLP. IFN-III triggers TSLP synthesis from M cells specialized in the upper respiratory tract; TSLP then promotes the maturation and migration of CD103+ DCs to draining lymph nodes, where DCs stimulate CD8+ T cells from naïve state and activated GC B cells by affecting Tfh cells, thus producing virus-specific IgG1 and IgA (33, 35). With the participation of adjuvants, vaccine-induced protective immunity can achieve stronger immune responses than antigens alone (122). In the mouse models of influenza virus vaccination, adaptive antiviral immune responses are markedly dependent on the previously unknown IFN-III/TSLP axis, which is not found in IFN-I adjuvant activity (34). Cytokines, as natural peptides closely related to the host immune response, have unique advantages as potential vaccine adjuvants (123). We herein highlighted the crucial function of IFN-III and TSLP cytokines, as well as the IFN-III/TSLP axis in modulating adaptive immune responses.
Nevertheless, the potential function of the IFN-III/TSLP axis in modulating adaptive immunity has not been fully explained. On the one hand, limited by the genetically different IFN-III system between humans and mice, which IFN-III subtypes confer immune activity in the IFN-III/TSLP system in both humans and mice remains unclear. On the other hand, our review shows that IFN-III modulates mucosal adaptive immunity via TSLP in mice; however, considering the second TSLP mRNA isoform (referred to as ‘short-form TSLP’) identified from normal human bronchial epithelial cells, whether two isoforms of TSLP (short-form and long-form TSLP) provide the identical function in the IFN-III/TSLP axis remains to be clarified (124). Moreover, when it comes to clinical application, it is currently unknown whether the IFN-III/TSLP axis is available to stimulate vaccine-induced antibody production in the human upper respiratory tract, as observed in mice. In addition, since IFNLR is specifically expressed on epithelial cells, the protective effect of the IFN-III/TSLP axis is exhibited particularly on mucosal sites through the intranasal and rectal vaccination way rather than the intraperitoneal or subcutaneous way. Therefore, mucosal vaccination seems to be the optimal choice for children and the elderly, as this vaccination method produces less pain and high compliance. Besides, in vivo studies have shown that IFN-III induces fewer inflammatory responses than IFN-I in the respiratory and gastrointestinal tracts (12, 14, 125, 126), while TSLPR has also been reported to regulate intestinal inflammation (127), the IFN-III/TSLP axis thereby exhibits many benefits when coming into application. Although there are currently no approved IFN-III drugs for use in humans, pre-clinical experiments have suggested the bright prospects of IFN-III for its fewer side effects compared with IFN-I. A phase IIb study has shown that pegylated IFN-λ1 treatment can produce a similar or improved immune response against chronic hepatitis C virus infection with fewer side effects when compared to IFN-I (128). Another phase IIb trial has indicated the benefits of pegylated IFN-III therapy in chronic hepatitis B patients by enhancing NK and CD8+ T cell response, which therefore impeded viral replication and antigen levels, while the treatment of IFN-I induced detrimental outcomes (129). Hence, IFN-III vaccination and its regulated IFN-III/TSLP axis are undoubtedly more efficient in future applications. Additionally, although the immunity benefits of IFN-III have been revealed, it is not yet clear whether the IFN-III/TSLP axis functions in defeating severe acute respiratory syndrome coronavirus 2 (SARS-CoV-2) in the context of coronavirus disease 2019 (COVID-19) pandemic (130–135). To date, various SARS-CoV-2 vaccines and recombinant vaccines are consecutively developed and applied in clinical practice (136–138). However, there still exist multiple challenges. On the one hand, the adjuvants of SARS-CoV-2 vaccines are limited and only contain aluminum salt-based adjuvants, emulsion adjuvants (MF9 and AS03), and toll-like receptor agonists. On the other hand, vaccines-induced immune response is restricted, usually leading to TH1 activation instead of TH2. Besides, current vaccines are inoculated via the intramuscular route, which may lack the protection of mucosal immune responses. In this review, we illuminate that IFN-III can target mucosal surfaces and provide adaptive immunoregulatory functions in vaccine administration via the IFN-III/TSLP axis. Therefore, IFN-III is a potential candidate for the SARS-CoV-2 vaccine adjuvant, which can promote mucosal immunity and help to establish a stronger immune defense line by supplementing adaptive immune responses, especially by activating mucosal immunity, thereby better combating coronavirus-caused respiratory infections. Obviously, IFN-III has great values in clinical research and application. Further experimental studies and clinical trials are required to explore the characteristics of IFN-III in the field of COVID-19 vaccines.
Author contributions
Conceptualization, LC and SX; methodology, LC; software, SX and ZM; validation, LC, ZM and WQ; formal analysis, SX; investigation, WQ; resources, LC; data curation, WQ and SX; writing—original draft preparation, LC; writing—review and editing, WQ and WL; visualization, SX; supervision, WQ; project administration, LC; funding acquisition, ZM. All authors contributed to the article and approved the submitted version.
Conflict of interest
The authors declare that the research was conducted in the absence of any commercial or financial relationships that could be construed as a potential conflict of interest.
Publisher’s note
All claims expressed in this article are solely those of the authors and do not necessarily represent those of their affiliated organizations, or those of the publisher, the editors and the reviewers. Any product that may be evaluated in this article, or claim that may be made by its manufacturer, is not guaranteed or endorsed by the publisher.
References
1. Sheppard P, Kindsvogel W, Xu W, Henderson K, Schlutsmeyer S, Whitmore TE, et al. IL-28, IL-29 and their class II cytokine receptor IL-28R. Nat Immunol (2003) 4:63–8. doi: 10.1038/ni873
2. Zanoni I, Granucci F, Broggi A. Interferon (IFN)-lambda takes the helm: immunomodulatory roles of type III IFNs. Front Immunol (2017) 8:1661. doi: 10.3389/fimmu.2017.01661
3. Kotenko SV, Gallagher G, Baurin VV, Lewis-Antes A, Shen M, Shah NK, et al. IFN-lambdas mediate antiviral protection through a distinct class II cytokine receptor complex. Nat Immunol (2003) 4:69–77. doi: 10.1038/ni875
4. Prokunina-Olsson L, Muchmore B, Tang W, Pfeiffer RM, Park H, Dickensheets H, et al. A variant upstream of IFNL3 (IL28B) creating a new interferon gene IFNL4 is associated with impaired clearance of hepatitis C virus. Nat Genet (2013) 45:164–71. doi: 10.1038/ng.2521
5. Alspach E, Lussier DM, Schreiber RD. Interferon gamma and its important roles in promoting and inhibiting spontaneous and therapeutic cancer immunity. Cold Spring Harb Perspect Biol (2019) 11(3):a028480. doi: 10.1101/cshperspect.a028480
6. Danastas K, Miranda-Saksena M, Cunningham AL. Herpes simplex virus type 1 interactions with the interferon system. Int J Mol Sci (2020) 21(14):5150. doi: 10.3390/ijms21145150
7. Yin Y, Favoreel HW. Herpesviruses and the type III interferon system. Virol Sin (2021) 36:577–87. doi: 10.1007/s12250-020-00330-2
8. Levy DE, Marie IJ, Durbin JE. Induction and function of type I and III interferon in response to viral infection. Curr Opin Virol (2011) 1:476–86. doi: 10.1016/j.coviro.2011.11.001
9. Lazear HM, Schoggins JW, Diamond MS. Shared and distinct functions of type I and type III interferons. Immunity (2019) 50:907–23. doi: 10.1016/j.immuni.2019.03.025
10. Mesev EV, LeDesma RA, Ploss A. Decoding type I and III interferon signalling during viral infection. Nat Microbiol (2019) 4:914–24. doi: 10.1038/s41564-019-0421-x
11. Stanifer ML, Pervolaraki K, Boulant S. Differential regulation of type I and type III interferon signaling. Int J Mol Sci (2019) 20(6):1445. doi: 10.3390/ijms20061445
12. Ingle H, Peterson ST, Baldridge MT. Distinct effects of type I and III interferons on enteric viruses. Viruses (2018) 10(1):46. doi: 10.3390/v10010046
13. MacDuff DA, Baldridge MT, Qaqish AM, Nice TJ, Darbandi AD, Hartley VL, et al. HOIL1 is essential for the induction of type I and III interferons by MDA5 and regulates persistent murine norovirus infection. J Virol (2018) 92(23):e01368-18. doi: 10.1128/JVI.01368-18
14. Broggi A, Tan Y, Granucci F, Zanoni I. IFN-lambda suppresses intestinal inflammation by non-translational regulation of neutrophil function. Nat Immunol (2017) 18:1084–93. doi: 10.1038/ni.3821
15. Wack A, Terczynska-Dyla E, Hartmann R. Guarding the frontiers: the biology of type III interferons. Nat Immunol (2015) 16:802–9. doi: 10.1038/ni.3212
16. Lazear HM, Nice TJ, Diamond MS. Interferon-lambda: immune functions at barrier surfaces and beyond. Immunity (2015) 43:15–28. doi: 10.1016/j.immuni.2015.07.001
17. Ivashkiv LB, Donlin LT. Regulation of type I interferon responses. Nat Rev Immunol (2014) 14:36–49. doi: 10.1038/nri3581
18. Teijaro JR. Type I interferons in viral control and immune regulation. Curr Opin Virol (2016) 16:31–40. doi: 10.1016/j.coviro.2016.01.001
19. Hervas-Stubbs S, Perez-Gracia JL, Rouzaut A, Sanmamed MF, Le Bon A, Melero I. Direct effects of type I interferons on cells of the immune system. Clin Cancer Res (2011) 17:2619–27. doi: 10.1158/1078-0432.CCR-10-1114
20. Sa Ribero M, Jouvenet N, Dreux M, Nisole S. Interplay between SARS-CoV-2 and the type I interferon response. PloS Pathog (2020) 16:e1008737. doi: 10.1371/journal.ppat.1008737
21. Takaoka A, Yanai H. Interferon signalling network in innate defence. Cell Microbiol (2006) 8:907–22. doi: 10.1111/j.1462-5822.2006.00716.x
22. Mordstein M, Neugebauer E, Ditt V, Jessen B, Rieger T, Falcone V, et al. Lambda interferon renders epithelial cells of the respiratory and gastrointestinal tracts resistant to viral infections. J Virol (2010) 84:5670–7. doi: 10.1128/JVI.00272-10
23. Pott J, Mahlakoiv T, Mordstein M, Duerr CU, Michiels T, Stockinger S, et al. IFN-lambda determines the intestinal epithelial antiviral host defense. Proc Natl Acad Sci U.S.A. (2011) 108:7944–9. doi: 10.1073/pnas.1100552108
24. Mahlakoiv T, Hernandez P, Gronke K, Diefenbach A, Staeheli P. Leukocyte-derived IFN-alpha/beta and epithelial IFN-lambda constitute a compartmentalized mucosal defense system that restricts enteric virus infections. PloS Pathog (2015) 11:e1004782. doi: 10.1371/journal.ppat.1004782
25. Espinosa V, Dutta O, McElrath C, Du P, Chang YJ, Cicciarelli B, et al. Type III interferon is a critical regulator of innate antifungal immunity. Sci Immunol (2017) 2(16):eaan5357. doi: 10.1126/sciimmunol.aan5357
26. Klinkhammer J, Schnepf D, Ye L, Schwaderlapp M, Gad HH, Hartmann R, et al. IFN-lambda prevents influenza virus spread from the upper airways to the lungs and limits virus transmission. Elife (2018) 7:e33354. doi: 10.7554/eLife.33354
27. Lee S, Baldridge MT. Interferon-lambda: A potent regulator of intestinal viral infections. Front Immunol (2017) 8:749. doi: 10.3389/fimmu.2017.00749
28. Nice TJ, Baldridge MT, McCune BT, Norman JM, Lazear HM, Artyomov M, et al. Interferon-lambda cures persistent murine norovirus infection in the absence of adaptive immunity. Science (2015) 347:269–73. doi: 10.1126/science.1258100
29. Hemann EA, Gale M Jr., Savan R. Interferon lambda genetics and biology in regulation of viral control. Front Immunol (2017) 8:1707. doi: 10.3389/fimmu.2017.01707
30. Ye L, Schnepf D, Staeheli P. Interferon-lambda orchestrates innate and adaptive mucosal immune responses. Nat Rev Immunol (2019) 19:614–25. doi: 10.1038/s41577-019-0182-z
31. Egli A, Santer DM, O'Shea D, Tyrrell DL, Houghton M. The impact of the interferon-lambda family on the innate and adaptive immune response to viral infections. Emerg Microbes Infect (2014) 3:e51. doi: 10.1038/emi.2014.51
32. Syedbasha M, Egli A. Interferon lambda: modulating immunity in infectious diseases. Front Immunol (2017) 8:119. doi: 10.3389/fimmu.2017.00119
33. Ye L, Schnepf D, Becker J, Ebert K, Tanriver Y, Bernasconi V, et al. Interferon-λ enhances adaptive mucosal immunity by boosting release of thymic stromal lymphopoietin. Nat Immunol (2019) 20(5):593–601. doi: 10.1038/s41590-019-0345-x
34. Ye L, Ohnemus A, Ong LC, Gad HH, Hartmann R, Lycke N, et al. Type I and type III interferons differ in their adjuvant activities for influenza vaccines. J Virol (2019) 93(23):e01262–19. doi: 10.1128/jvi.01262-19
35. Ye L, Schnepf D, Ohnemus A, Ong LC, Gad HH, Hartmann R, et al. Interferon-lambda improves the efficacy of intranasally or rectally administered influenza subunit vaccines by a thymic stromal lymphopoietin-dependent mechanism. Front Immunol (2021) 12:749325. doi: 10.3389/fimmu.2021.749325
36. Pulendran B, SA P, O'Hagan DT. Emerging concepts in the science of vaccine adjuvants. Nat Rev Drug Discovery (2021) 20:454–75. doi: 10.1038/s41573-021-00163-y
37. Hogenesch H. Mechanism of immunopotentiation and safety of aluminum adjuvants. Front Immunol (2012) 3:406. doi: 10.3389/fimmu.2012.00406
38. Wilkins AL, Kazmin D, Napolitani G, Clutterbuck EA, Pulendran B, Siegrist CA, et al. AS03- and MF59-adjuvanted influenza vaccines in children. Front Immunol (2017) 8:1760. doi: 10.3389/fimmu.2017.01760
39. Steinhagen F, Kinjo T, Bode C, Klinman DM. TLR-based immune adjuvants. Vaccine (2011) 29:3341–55. doi: 10.1016/j.vaccine.2010.08.002
40. Kawai T, Akira S. The role of pattern-recognition receptors in innate immunity: update on Toll-like receptors. Nat Immunol (2010) 11:373–84. doi: 10.1038/ni.1863
41. Morrow MP, Pankhong P, Laddy DJ, Schoenly KA, Yan J, Cisper N, et al. Comparative ability of IL-12 and IL-28B to regulate Treg populations and enhance adaptive cellular immunity. Blood (2009) 113:5868–77. doi: 10.1182/blood-2008-11-190520
42. Zhou Y, Wang Z, Xu Y, Zhang Z, Hua R, Liu W, et al. Optimized DNA vaccine enhanced by adjuvant IL28B induces protective immune responses against herpes simplex virus type 2 in mice. Viral Immunol (2017) 30:601–14. doi: 10.1089/vim.2017.0033
43. Boisvert M, Shoukry NH. Type III interferons in hepatitis C virus infection. Front Immunol (2016) 7:628. doi: 10.3389/fimmu.2016.00628
44. Douam F, Soto Albrecht YE, Hrebikova G, Sadimin E, Davidson C, Kotenko SV, et al. Type III interferon-mediated signaling is critical for controlling live attenuated yellow fever virus infection in vivo. mBio (2017) 8. doi: 10.1128/mBio.00819-17
45. Misumi I, Whitmire JK. IFN-lambda exerts opposing effects on T cell responses depending on the chronicity of the virus infection. J Immunol (2014) 192:3596–606. doi: 10.4049/jimmunol.1301705
46. Hemann EA, Green R, Turnbull JB, Langlois RA, Savan R, Gale M Jr. Interferon-lambda modulates dendritic cells to facilitate T cell immunity during infection with influenza A virus. Nat Immunol (2019) 20:1035–45. doi: 10.1038/s41590-019-0408-z
47. Lasfar A, Zloza A, de la Torre A, Cohen-Solal KA. IFN-lambda: A new inducer of local immunity against cancer and infections. Front Immunol (2016) 7:598. doi: 10.3389/fimmu.2016.00598
48. Stiff A, Carson W III. Investigations of interferon-lambda for the treatment of cancer. J Innate Immun (2015) 7:243–50. doi: 10.1159/000370113
49. Sato A, Ohtsuki M, Hata M, Kobayashi E, Murakami T. Antitumor activity of IFN-lambda in murine tumor models. J Immunol (2006) 176:7686–94. doi: 10.4049/jimmunol.176.12.7686
50. Lasfar A, Lewis-Antes A, Smirnov SV, Anantha S, Abushahba W, Tian B, et al. Characterization of the mouse IFN-lambda ligand-receptor system: IFN-lambdas exhibit antitumor activity against B16 melanoma. Cancer Res (2006) 66:4468–77. doi: 10.1158/0008-5472.CAN-05-3653
51. Lasfar A, de laTorre A, Abushahba W, Cohen-Solal KA, Castaneda I, Yuan Y, et al. Concerted action of IFN-α and IFN-λ induces local NK cell immunity and halts cancer growth. Oncotarget (2016) 7:49259–67. doi: 10.18632/oncotarget.10272
52. Numasaki M, Tagawa M, Iwata F, Suzuki T, Nakamura A, Okada M, et al. IL-28 elicits antitumor responses against murine fibrosarcoma. J Immunol (2007) 178:5086–98. doi: 10.4049/jimmunol.178.8.5086
53. Burkart C, Arimoto K, Tang T, Cong X, Xiao N, Liu YC, et al. Usp18 deficient mammary epithelial cells create an antitumour environment driven by hypersensitivity to IFN-λ and elevated secretion of Cxcl10. EMBO Mol Med (2013) 5:1035–50. doi: 10.1002/emmm.201201864
54. Souza-Fonseca-Guimaraes F, Young A, Mittal D, Martinet L, Bruedigam C, Takeda K, et al. NK cells require IL-28R for optimal in vivo activity. Proc Natl Acad Sci U.S.A. (2015) 112:E2376–84. doi: 10.1073/pnas.1424241112
55. Lasfar A, Gogas H, Zloza A, Kaufman HL, Kirkwood JM. IFN-λ cancer immunotherapy: new kid on the block. Immunotherapy (2016) 8:877–88. doi: 10.2217/imt-2015-0021
56. Manivasagam S, Klein RS. Type III interferons: emerging roles in autoimmunity. Front Immunol (2021) 12:764062. doi: 10.3389/fimmu.2021.764062
57. Witte E, Kokolakis G, Witte K, Warszawska K, Friedrich M, Christou D, et al. Interleukin-29 induces epithelial production of CXCR3A ligands and T-cell infiltration. J Mol Med (Berl) (2016) 94:391–400. doi: 10.1007/s00109-015-1367-y
58. Lin SC, Kuo CC, Tsao JT, Lin LJ. Profiling the expression of interleukin (IL)-28 and IL-28 receptor α in systemic lupus erythematosus patients. Eur J Clin Invest (2012) 42:61–9. doi: 10.1111/j.1365-2362.2011.02557.x
59. Goel RR, Kotenko SV, Kaplan MJ. Interferon lambda in inflammation and autoimmune rheumatic diseases. Nat Rev Rheumatol (2021) 17:349–62. doi: 10.1038/s41584-021-00606-1
60. Oke V, Brauner S, Larsson A, Gustafsson J, Zickert A, Gunnarsson I, et al. IFN-λ1 with Th17 axis cytokines and IFN-α define different subsets in systemic lupus erythematosus (SLE). Arthritis Res Ther (2017) 19:139. doi: 10.1186/s13075-017-1344-7
61. Parodis I, Stockfelt M, Sjowall C. B cell therapy in systemic lupus erythematosus: from rationale to clinical practice. Front Med (Lausanne) (2020) 7:316. doi: 10.3389/fmed.2020.00316
62. Goel RR, Wang X, O'Neil LJ, Nakabo S, Hasneen K, Gupta S, et al. Interferon lambda promotes immune dysregulation and tissue inflammation in TLR7-induced lupus. Proc Natl Acad Sci U.S.A. (2020) 117:5409–19. doi: 10.1073/pnas.1916897117
63. Wu Q, Yang Q, Sun H, Li M, Zhang Y, La Cava A. Serum IFN-lambda1 is abnormally elevated in rheumatoid arthritis patients. Autoimmunity (2013) 46:40–3. doi: 10.3109/08916934.2012.730587
64. Wang F, Xu L, Feng X, Guo D, Tan W, Zhang M. Interleukin-29 modulates proinflammatory cytokine production in synovial inflammation of rheumatoid arthritis. Arthritis Res Ther (2012) 14:R228. doi: 10.1186/ar4067
65. Blazek K, Eames HL, Weiss M, Byrne AJ, Perocheau D, Pease JE, et al. IFN-lambda resolves inflammation via suppression of neutrophil infiltration and IL-1beta production. J Exp Med (2015) 212:845–53. doi: 10.1084/jem.20140995
66. Manivasagam S, Williams JL, Vollmer LL, Bollman B, Bartleson JM, Ai S, et al. Targeting IFN-λ Signaling promotes recovery from central nervous system autoimmunity. J Immunol (2022) 208:1341–51. doi: 10.4049/jimmunol.2101041
67. Koltsida O, Hausding M, Stavropoulos A, Koch S, Tzelepis G, Ubel C, et al. IL-28A (IFN-λ2) modulates lung DC function to promote Th1 immune skewing and suppress allergic airway disease. EMBO Mol Med (2011) 3:348–61. doi: 10.1002/emmm.201100142
68. Jordan WJ, Eskdale J, Srinivas S, Pekarek V, Kelner D, Rodia M, et al. Human interferon lambda-1 (IFN-lambda1/IL-29) modulates the Th1/Th2 response. Genes Immun (2007) 8:254–61. doi: 10.1038/sj.gene.6364382
69. Galani IE, Triantafyllia V, Eleminiadou EE, Koltsida O, Stavropoulos A, Manioudaki M, et al. Interferon-λ Mediates Non-redundant Front-Line Antiviral Protection against Influenza Virus Infection without Compromising Host Fitness. Immunity (2017) 46:875–90.e6. doi: 10.1016/j.immuni.2017.04.025
70. Lambrecht BN, Hammad H, Fahy JV. The cytokines of asthma. Immunity (2019) 50:975–91. doi: 10.1016/j.immuni.2019.03.018
71. Krammer S, Sicorschi Gutu C, Grund JC, Chiriac MT, Zirlik S, Finotto S. Regulation and function of interferon-lambda (IFNλ) and its receptor in asthma. Front Immunol (2021) 12:731807. doi: 10.3389/fimmu.2021.731807
72. Pandey A, Ozaki K, Baumann H, Levin SD, Puel A, Farr AG, et al. Cloning of a receptor subunit required for signaling by thymic stromal lymphopoietin. Nat Immunol (2000) 1:59–64. doi: 10.1038/76923
73. Soumelis V, Reche PA, Kanzler H, Yuan W, Edward G, Homey B, et al. Human epithelial cells trigger dendritic cell mediated allergic inflammation by producing TSLP. Nat Immunol (2002) 3:673–80. doi: 10.1038/ni805
74. Ebina-Shibuya R, Leonard WJ. Role of thymic stromal lymphopoietin in allergy and beyond. Nat Rev Immunol (2023) 23:24–37. doi: 10.1038/s41577-022-00735-y
75. Parnes JR, Molfino NA, Colice G, Martin U, Corren J, Menzies-Gow A. Targeting TSLP in asthma. J Asthma Allergy (2022) 15:749–65. doi: 10.2147/jaa.S275039
76. Varricchi G, Pecoraro A, Marone G, Criscuolo G, Spadaro G, Genovese A, et al. Thymic stromal lymphopoietin isoforms, inflammatory disorders, and cancer. Front Immunol (2018) 9:1595. doi: 10.3389/fimmu.2018.01595
77. Marković I, Savvides SN. Modulation of signaling mediated by TSLP and IL-7 in inflammation, autoimmune diseases, and cancer. Front Immunol (2020) 11:1557. doi: 10.3389/fimmu.2020.01557
78. Li W, Liao C, Du J, Hu J, Wang L, Song X, et al. Increased expression of long-isoform thymic stromal lymphopoietin is associated with rheumatoid arthritis and fosters inflammatory responses. Front Immunol (2022) 13:1079415. doi: 10.3389/fimmu.2022.1079415
79. Zhang Y, Zhou B. Functions of thymic stromal lymphopoietin in immunity and disease. Immunol Res (2012) 52:211–23. doi: 10.1007/s12026-012-8264-z
80. Ziegler SF, Roan F, Bell BD, Stoklasek TA, Kitajima M, Han H. The biology of thymic stromal lymphopoietin (TSLP). Adv Pharmacol (2013) 66:129–55. doi: 10.1016/b978-0-12-404717-4.00004-4
81. Seshasayee D, Lee WP, Zhou M, Shu J, Suto E, Zhang J, et al. In vivo blockade of OX40 ligand inhibits thymic stromal lymphopoietin driven atopic inflammation. J Clin Invest (2007) 117:3868–78. doi: 10.1172/jci33559
82. Yadava K, Sichelstiel A, Luescher IF, Nicod LP, Harris NL, Marsland BJ. TSLP promotes influenza-specific CD8+ T-cell responses by augmenting local inflammatory dendritic cell function. Mucosal Immunol (2013) 6:83–92. doi: 10.1038/mi.2012.50
83. Shane HL, Klonowski KD. A direct and nonredundant role for thymic stromal lymphopoietin on antiviral CD8 T cell responses in the respiratory mucosa. J Immunol (2014) 192:2261–70. doi: 10.4049/jimmunol.1302085
84. Joo S, Fukuyama Y, Park EJ, Yuki Y, Kurashima Y, Ouchida R, et al. Critical role of TSLP-responsive mucosal dendritic cells in the induction of nasal antigen-specific IgA response. Mucosal Immunol (2017) 10:901–11. doi: 10.1038/mi.2016.103
85. Demehri S, Cunningham TJ, Manivasagam S, Ngo KH, Moradi Tuchayi S, Reddy R, et al. Thymic stromal lymphopoietin blocks early stages of breast carcinogenesis. J Clin Invest (2016) 126:1458–70. doi: 10.1172/jci83724
86. Corren J, Ziegler SF. TSLP: from allergy to cancer. Nat Immunol (2019) 20:1603–9. doi: 10.1038/s41590-019-0524-9
87. Soumelis V. TSLP: from allergy to vaccine adjuvant. Eur J Immunol (2012) 42:293–5. doi: 10.1002/eji.201142337
88. Sugimoto H, Ito T, Torii Y, Amuro H, Yokoi T, Tanijiri T, et al. Thymic stromal lymphopoietin plays an adjuvant role in BCG-mediated CD8(+) cytotoxic T cell responses through dendritic cell activation. Clin Immunol (2010) 136:205–16. doi: 10.1016/j.clim.2010.04.006
89. Van Roey GA, Arias MA, Tregoning JS, Rowe G, Shattock RJ. Thymic stromal lymphopoietin (TSLP) acts as a potent mucosal adjuvant for HIV-1 gp140 vaccination in mice. Eur J Immunol (2012) 42:353–63. doi: 10.1002/eji.201141787
90. Wieland SF, Heim MH. The IFN-lambda pony express. Nat Immunol (2019) 20:522–4. doi: 10.1038/s41590-019-0362-9
91. Ye L, Schnepf D, Staeheli P. Interferon-λ orchestrates innate and adaptive mucosal immune responses. Nat Rev Immunol (2019) 19:614–25. doi: 10.1038/s41577-019-0182-z
92. Kasuya T, Tanaka S, Tamura J, Etori K, Shoda J, Hattori K, et al. Epithelial cell-derived cytokine TSLP activates regulatory T cells by enhancing fatty acid uptake. Sci Rep (2023) 13:1653. doi: 10.1038/s41598-023-28987-1
93. Egli A, Santer DM, O'Shea D, Barakat K, Syedbasha M, Vollmer M, et al. IL-28B is a key regulator of B- and T-cell vaccine responses against influenza. PloS Pathog (2014) 10:e1004556. doi: 10.1371/journal.ppat.1004556
94. Syedbasha M, Bonfiglio F, Linnik J, Stuehler C, Wuthrich D, Egli A. Interferon-lambda Enhances the Differentiation of Naive B Cells into Plasmablasts via the mTORC1 Pathway. Cell Rep (2020) 33:108211. doi: 10.1016/j.celrep.2020.108211
95. Lee HC, Headley MB, Loo YM, Berlin A, Gale M Jr., Debley JS, et al. Thymic stromal lymphopoietin is induced by respiratory syncytial virus-infected airway epithelial cells and promotes a type 2 response to infection. J Allergy Clin Immunol (2012) 130:1187–96 e5. doi: 10.1016/j.jaci.2012.07.031
96. Pattarini L, Trichot C, Bogiatzi S, Grandclaudon M, Meller S, Keuylian Z, et al. TSLP-activated dendritic cells induce human T follicular helper cell differentiation through OX40-ligand. J Exp Med (2017) 214:1529–46. doi: 10.1084/jem.20150402
97. de Groen RA, Groothuismink ZM, Liu BS, Boonstra A. IFN-lambda is able to augment TLR-mediated activation and subsequent function of primary human B cells. J Leukoc Biol (2015) 98:623–30. doi: 10.1189/jlb.3A0215-041RR
98. Ding S, Khoury-Hanold W, Iwasaki A, Robek MD. Epigenetic reprogramming of the type III interferon response potentiates antiviral activity and suppresses tumor growth. PloS Biol (2014) 12:e1001758. doi: 10.1371/journal.pbio.1001758
99. Morrow MP, Yan J, Pankhong P, Ferraro B, Lewis MG, Khan AS, et al. Unique Th1/Th2 phenotypes induced during priming and memory phases by use of interleukin-12 (IL-12) or IL-28B vaccine adjuvants in rhesus macaques. Clin Vaccine Immunol (2010) 17:1493–9. doi: 10.1128/CVI.00181-10
100. Morrow MP, Yan J, Pankhong P, Shedlock DJ, Lewis MG, Talbott K, et al. IL-28B/IFN-lambda 3 drives granzyme B loading and significantly increases CTL killing activity in macaques. Mol Ther (2010) 18:1714–23. doi: 10.1038/mt.2010.118
101. Gonzalez-Navajas JM, Lee J, David M, Raz E. Immunomodulatory functions of type I interferons. Nat Rev Immunol (2012) 12:125–35. doi: 10.1038/nri3133
102. Arico E, Belardelli F. Interferon-alpha as antiviral and antitumor vaccine adjuvants: mechanisms of action and response signature. J Interferon Cytokine Res (2012) 32:235–47. doi: 10.1089/jir.2011.0077
103. Snell LM, McGaha TL, Brooks DG. Type I interferon in chronic virus infection and cancer. Trends Immunol (2017) 38:542–57. doi: 10.1016/j.it.2017.05.005
104. Borden EC. Interferons α and β in cancer: therapeutic opportunities from new insights. Nat Rev Drug Discovery (2019) 18:219–34. doi: 10.1038/s41573-018-0011-2
105. Gommerman JL, Browning JL, Ware CF. The Lymphotoxin Network: orchestrating a type I interferon response to optimize adaptive immunity. Cytokine Growth Factor Rev (2014) 25:139–45. doi: 10.1016/j.cytogfr.2014.02.002
106. Psarras A, Emery P, Vital EM. Type I interferon-mediated autoimmune diseases: pathogenesis, diagnosis and targeted therapy. Rheumatol (Oxford) (2017) 56:1662–75. doi: 10.1093/rheumatology/kew431
107. Chasset F, Dayer JM, Chizzolini C. Type I interferons in systemic autoimmune diseases: distinguishing between afferent and efferent functions for precision medicine and individualized treatment. Front Pharmacol (2021) 12:633821. doi: 10.3389/fphar.2021.633821
108. Montoya M, Schiavoni G, Mattei F, Gresser I, Belardelli F, Borrow P, et al. Type I interferons produced by dendritic cells promote their phenotypic and functional activation. Blood (2002) 99:3263–71. doi: 10.1182/blood.v99.9.3263
109. Le Bon A, Etchart N, Rossmann C, Ashton M, Hou S, Gewert D, et al. Cross-priming of CD8+ T cells stimulated by virus-induced type I interferon. Nat Immunol (2003) 4:1009–15. doi: 10.1038/ni978
110. Crouse J, Kalinke U, Oxenius A. Regulation of antiviral T cell responses by type I interferons. Nat Rev Immunol (2015) 15:231–42. doi: 10.1038/nri3806
111. Cucak H, Yrlid U, Reizis B, Kalinke U, Johansson-Lindbom B. Type I interferon signaling in dendritic cells stimulates the development of lymph-node-resident T follicular helper cells. Immunity (2009) 31:491–501. doi: 10.1016/j.immuni.2009.07.005
112. Crotty S. T follicular helper cell biology: A decade of discovery and diseases. Immunity (2019) 50:1132–48. doi: 10.1016/j.immuni.2019.04.011
113. Kuka M, De Giovanni M, Iannacone M. The role of type I interferons in CD4(+) T cell differentiation. Immunol Lett (2019) 215:19–23. doi: 10.1016/j.imlet.2019.01.013
114. Vitale S, Russo V, Dettori B, Palombi C, Baev D, Proietti E, et al. Type I interferons induce peripheral T regulatory cell differentiation under tolerogenic conditions. Int Immunol (2020) 33(2):59–77. doi: 10.1093/intimm/dxaa058
115. Ray JP, Marshall HD, Laidlaw BJ, Staron MM, Kaech SM, Craft J. Transcription factor STAT3 and type I interferons are corepressive insulators for differentiation of follicular helper and T helper 1 cells. Immunity (2014) 40:367–77. doi: 10.1016/j.immuni.2014.02.005
116. Le Bon A, Schiavoni G, D'Agostino G, Gresser I, Belardelli F, Tough DF. Type i interferons potently enhance humoral immunity and can promote isotype switching by stimulating dendritic cells. vivo. Immun (2001) 14:461–70. doi: 10.1016/s1074-7613(01)00126-1
117. Dahlgren MW, Plumb AW, Niss K, Lahl K, Brunak S, Johansson-Lindbom B. Type I interferons promote germinal centers through B cell intrinsic signaling and dendritic cell dependent Th1 and Tfh cell lineages. Front Immunol (2020) 13:932388. doi: 10.3389/fimmu.2022.932388
118. Denton AE, Innocentin S, Carr EJ, Bradford BM, Lafouresse F, Mabbott NA, et al. Type I interferon induces CXCL13 to support ectopic germinal center formation. J Exp Med (2019) 216:621–37. doi: 10.1084/jem.20181216
119. Le Bon A, Thompson C, Kamphuis E, Durand V, Rossmann C, Kalinke U, et al. Cutting edge: enhancement of antibody responses through direct stimulation of B and T cells by type I IFN. J Immunol (2006) 176:2074–8. doi: 10.4049/jimmunol.176.4.2074
120. Zhu J, Huang X, Yang Y. Type I IFN signaling on both B and CD4 T cells is required for protective antibody response to adenovirus. J Immunol (2007) 178:3505–10. doi: 10.4049/jimmunol.178.6.3505
121. Broggi A, Granucci F, Zanoni I. Type III interferons: Balancing tissue tolerance and resistance to pathogen invasion. J Exp Med (2020) 217(1):e20190295. doi: 10.1084/jem.20190295
122. Awate S, Babiuk LA, Mutwiri G. Mechanisms of action of adjuvants. Front Immunol (2013) 4:114. doi: 10.3389/fimmu.2013.00114
123. Nohria A, Rubin RH. Cytokines as potential vaccine adjuvants. Biotherapy (1994) 7:261–9. doi: 10.1007/bf01878491
124. Harada M, Hirota T, Jodo AI, Doi S, Kameda M, Fujita K, et al. Functional analysis of the thymic stromal lymphopoietin variants in human bronchial epithelial cells. Am J Respir Cell Mol Biol (2009) 40:368–74. doi: 10.1165/rcmb.2008-0041OC
125. Galani IE, Triantafyllia V, Eleminiadou EE, Koltsida O, Stavropoulos A, Manioudaki M, et al. Interferon-lambda Mediates Non-redundant Front-Line Antiviral Protection against Influenza Virus Infection without Compromising Host Fitness. Immunity (2017) 46:875–90 e6. doi: 10.1016/j.immuni.2017.04.025
126. Davidson S, McCabe TM, Crotta S, Gad HH, Hessel EM, Beinke S, et al. IFNλ is a potent anti-influenza therapeutic without the inflammatory side effects of IFNα treatment. EMBO Mol Med (2016) 8:1099–112. doi: 10.15252/emmm.201606413
127. Taylor BC, Zaph C, Troy AE, Du Y, Guild KJ, Comeau MR, et al. TSLP regulates intestinal immunity and inflammation in mouse models of helminth infection and colitis. J Exp Med (2009) 206:655–67. doi: 10.1084/jem.20081499
128. Muir AJ, Arora S, Everson G, Flisiak R, George J, Ghalib R, et al. A randomized phase 2b study of peginterferon lambda-1a for the treatment of chronic HCV infection. J Hepatol (2014) 61:1238–46. doi: 10.1016/j.jhep.2014.07.022
129. Phillips S, Mistry S, Riva A, Cooksley H, Hadzhiolova-Lebeau T, Plavova S, et al. Peg-interferon lambda treatment induces robust innate and adaptive immunity in chronic hepatitis B patients. Front Immunol (2017) 8:621. doi: 10.3389/fimmu.2017.00621
130. Stanifer ML, Kee C, Cortese M, Zumaran CM, Triana S, Mukenhirn M, et al. Critical role of type III interferon in controlling SARS-coV-2 infection in human intestinal epithelial cells. Cell Rep (2020) 32:107863. doi: 10.1016/j.celrep.2020.107863
131. Kim YM, Shin EC. Type I and III interferon responses in SARS-CoV-2 infection. Exp Mol Med (2021) 53:750–60. doi: 10.1038/s12276-021-00592-0
132. Vanderheiden A, Ralfs P, Chirkova T, Upadhyay AA, Zimmerman MG, Bedoya S, et al. Type I and type III interferons restrict SARS-coV-2 infection of human airway epithelial cultures. J Virol (2020) 94(19):e00985-20. doi: 10.1128/jvi.00985-20
133. Park A, Iwasaki A. Type I and type III interferons - induction, signaling, evasion, and application to combat COVID-19. Cell Host Microbe (2020) 27:870–8. doi: 10.1016/j.chom.2020.05.008
134. Felgenhauer U, Schoen A, Gad HH, Hartmann R, Schaubmar AR, Failing K, et al. Inhibition of SARS-CoV-2 by type I and type III interferons. J Biol Chem (2020) 295:13958–64. doi: 10.1074/jbc.AC120.013788
135. Prokunina-Olsson L, Alphonse N, Dickenson RE, Durbin JE, Glenn JS, Hartmann R, et al. COVID-19 and emerging viral infections: The case for interferon lambda. J Exp Med (2020) 217(5):e20200653. doi: 10.1084/jem.20200653
136. Awadasseid A, Wu Y, Tanaka Y, Zhang W. Current advances in the development of SARS-CoV-2 vaccines. Int J Biol Sci (2021) 17:8–19. doi: 10.7150/ijbs.52569
137. Grigoryan L, Pulendran B. The immunology of SARS-CoV-2 infections and vaccines. Semin Immunol (2020) 50:101422. doi: 10.1016/j.smim.2020.101422
Keywords: type III interferon, thymic stromal lymphopoietin, IFN-III/TSLP axis, adaptive immunity, adaptive antiviral activity
Citation: Cao L, Qian W, Li W, Ma Z and Xie S (2023) Type III interferon exerts thymic stromal lymphopoietin in mediating adaptive antiviral immune response. Front. Immunol. 14:1250541. doi: 10.3389/fimmu.2023.1250541
Received: 30 June 2023; Accepted: 07 September 2023;
Published: 22 September 2023.
Edited by:
Xulin Chen, Jinan University, ChinaReviewed by:
Juandy Jo, University of Pelita Harapan, IndonesiaPeter Staeheli, University of Freiburg, Germany
Copyright © 2023 Cao, Qian, Li, Ma and Xie. This is an open-access article distributed under the terms of the Creative Commons Attribution License (CC BY). The use, distribution or reproduction in other forums is permitted, provided the original author(s) and the copyright owner(s) are credited and that the original publication in this journal is cited, in accordance with accepted academic practice. No use, distribution or reproduction is permitted which does not comply with these terms.
*Correspondence: Zhiyue Ma, bWF6aGl5dWUyMTQyQDE2My5jb20=; Shenglong Xie, eGllc2hlbmdsb25nQG1lZC51ZXN0Yy5lZHUuY24=
†These authors share first authorship