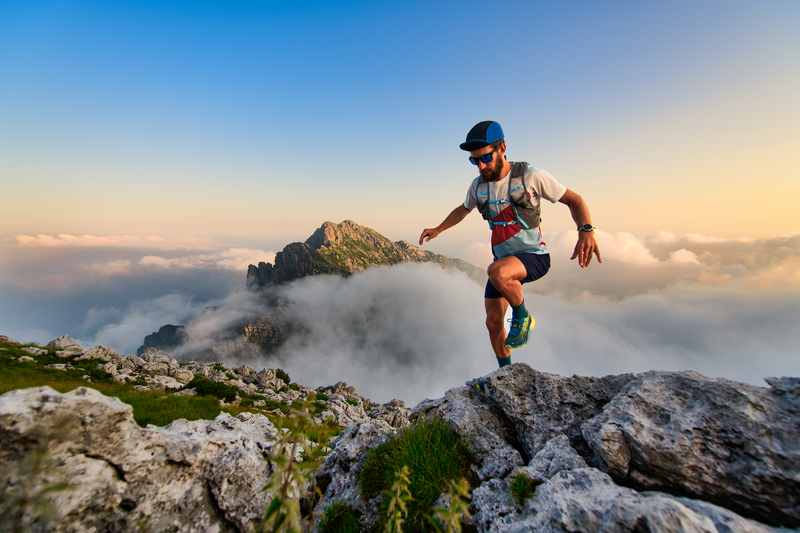
95% of researchers rate our articles as excellent or good
Learn more about the work of our research integrity team to safeguard the quality of each article we publish.
Find out more
REVIEW article
Front. Immunol. , 04 September 2023
Sec. Cancer Immunity and Immunotherapy
Volume 14 - 2023 | https://doi.org/10.3389/fimmu.2023.1241791
This article is part of the Research Topic Reactive Oxygen Species (ROS): Key Components in Cancer Immunotherapy View all 11 articles
Glioma is the most common primary intracranial tumor in adults with poor prognosis. Current clinical treatment for glioma includes surgical resection along with chemoradiotherapy. However, the therapeutic efficacy is still unsatisfactory. The invasive nature of the glioma makes it impossible to completely resect it. The presence of blood-brain barrier (BBB) blocks chemotherapeutic drugs access to brain parenchyma for glioma treatment. Besides, tumor heterogeneity and hypoxic tumor microenvironment remarkably limit the efficacy of radiotherapy. With rapid advances of nanotechnology, the emergence of a new treatment approach, namely, reactive oxygen species (ROS)-based nanotherapy, provides an effective approach for eliminating glioma via generating large amounts of ROS in glioma cells. In addition, the emerging nanotechnology also provides BBB-crossing strategies, which allows effective ROS-based nanotherapy of glioma. In this review, we summarized ROS-based nanomedicine and their application in glioma treatment, including photodynamic therapy (PDT), photothermal therapy (PTT), chemodynamic therapy (CDT), sonodynamic therapy (SDT), radiation therapy, etc. Moreover, the current challenges and future prospects of ROS-based nanomedicine are also elucidated with the intention to accelerate its clinical translation.
Glioma is the most common primary intracranial tumor in adults, with an annual incidence of 3-6.4 per 100,000, accounting for 23.3% of all central nervous system (CNS) tumors and 78.3% of CNS malignant tumors (1). Among them, the WHO grade IV glioblastoma has the highest annual incidence rate (4.03 per 100,000), accounting for 48.6% of all primary CNS malignancies (1). Recent studies have shown that glioblastoma has surpassed liver cancer and pancreatic cancer to become the first refractory tumor (2). Current clinical treatment for glioma includes maximally surgical resection, radiotherapy and chemotherapy. However, most gliomas deeply infiltrate into the surrounding normal cerebral tissue, making it impossible to resect completely (3). With unlimited tissue penetration depth, radiotherapy can damage DNA and kill tumor cells, but its efficiency is often limited by several factors, such as hypoxic tumor microenvironment, low radiation absorption of tumor tissues (4). Chemotherapy, as the most commonly used anti-cancer approach, has a limited therapeutic effect on glioma. The existence of the blood-brain barrier (BBB) allows only 1-2% of the chemotherapeutic drugs enter the glioma sites, and the rest of the chemotherapeutic drugs are distributed in other normal tissues and organs, inducing serious toxic and side effects (5). Additionally, the high heterogeneity, hypoxia, and presence of tumor stem cells in glioma cause drug resistance in tumor tissue (6, 7). Therefore, it is urgent to explore and develop new treatment approach for glioma.
The BBB was first proposed by Edwin Goldman in 1913, but the definite structure was only known to scientists when the scanning electron microscope appeared in 1937 (8). Specifically, BBB is a highly selective semipermeable barrier between blood plasma and neural cells, which can selectively prevent certain substances in blood circulation from entering the brain (5). The BBB is important for the homeostasis of the CNS. The abnormal disruption of BBB might lead to nervous dysfunction. The BBB mainly contains brain capillary endothelial cells (BCECs), end-feet of astrocytes, and pericytes (9). Among them, there are tight junctions between BCECs, which can strictly control the diffusion of small water-soluble molecules through the paracellular pathway. As a biochemical and physical barrier, the intact BBB can effectively restrict more than 98% of small molecule drugs and almost all macromolecular drugs from entering the CNS (10). Small fat-soluble molecules smaller than 400–500 Da (such as oxygen, CO2, steroid hormones) can freely enter and exit via transcellular pathways. Some essential nutrients (such as glucose, electrolytes, etc.) can enter the brain via carrier-mediated transcytosis (CMT) pathway. For example, the glucose transport receptor 1 (GLUT1) can facilitate the transport of glucose (11). Substances such as transferrin (Tf) need to enter the brain through the receptor-mediated transcytosis (RMT) pathway, and they can be absorbed by specific receptors on the BBB (such as low-density lipoprotein receptor, transferrin receptor). Cationic proteins can electrostatically adsorb to anionic sites on the surface of BCEC membranes and enter the brain through adsorption-mediated transcytosis (AMT). In addition, current studies have found that immune cells and stem cells can span the BBB through cell-mediated transport pathways (12, 13). Previous studies have shown that the BBB in glioma could be partly disrupted during rapid tumor growth (14, 15). However, the BBB in glioma remains a hindrance for most chemotherapeutic drugs. The presence of BBB can limit the entry of chemotherapeutic drugs into the CNS, thereby restricting effective treatment of glioma. Notably, recent development of nanotechnology have produced a large number of nanoparticles capable of crossing or bypassing the BBB, enabling effective theranostics of glioma (5). For example, surface modifications of BBB/glioma-targeting peptides or cell-penetration peptides allow the nanoparticles to effectively span the BBB and further precisely target tumor cells (16–19). Besides, GLUT1, the most common transporter in CMT pathway, which is widely expressed on BCECs, and its expression level is significantly higher than that of other transporters. Anraku et al. constructed a glucosylated nanocarrier and promoted the its efficiency of crossing BBB by regulating blood glucose level (20, 21). In addition, the several cell types or their nanovesicles, such as neural stem cells, mesenchymal stem cells, and macrophages, etc, could effectively cross the BBB (22–25). Furthermore, the microbubble-mediated focused ultrasound could also open the BBB with the acoustic cavitation effect (26–28).
Generally, reactive oxygen species (ROS) is a series of chemicals with a high degree of oxidative activity, including hydrogen peroxide (H2O2), singlet oxygen (1O2), superoxide anion (O2•−), and hydroxyl radical (•OH) (29). The overexpressed ROS in tumor cells could induce imbalance of oxidative stress, resulting in severe damage to tumor cells. The tumor cells could generate ROS at various subcellular locations. For instance, the electrons escaped from respiratory chain in mitochondria can convert oxygen into O2•−, which can further react to produce other ROS (e.g., H2O2, •OH, etc.) through different reactions (30). High reactivity is the major feature of the ROS, resulting in various ROS-mediated reaction in tumor cells, including reduction, oxidation, and dismutation. According to the reaction principles, the ROS is divided into two types: one-electron oxidants (e.g., O2•−, •OH, etc.) and two-electron oxidants (H2O2) (31). In addition, compared with normal cells, the ROS content is significantly increased in tumor cells, which was associated with the tumorigenesis of malignancies (32, 33). However, the excessive level of ROS can result in oxidative injury of cells. With intracellular antioxidant defense mechanisms, the antioxidants, such as glutathione (GSH), are also highly expressed in tumor cells, which keeps the redox homeostasis in tumor cells (34, 35). In recent years, with the rapid development of nanotechnology, the ROS-based nanomedicine has been widely applied in cancer treatment, including photodynamic therapy (PDT), photothermal therapy (PTT), chemodynamic therapy (CDT), sonodynamic therapy (SDT), radiation therapy, etc (36–38). These treatment approaches utilize nanosensitizers and exo/endogenous stimulus (e.g., light, ultrasound, X-ray, H2O2, etc.) to generate ROS in tumor cells, thereby disrupting the redox balance and effectively kill the tumor cells.
PDT, the first ROS-based treatment for cancer regression, was mentioned in Raab’s introduction of light-related cytotoxicity in 1990. There are three key components for PDT: light, photosensitizer (PS), and intratumoral oxygen. The laser could activate the PSs to convert their excited-state energy to surrounding oxygen to produce ROS, which augments the oxidative stress in cancer cells and further kill the cancer cells (39). Since the PSs could only induce ROS generation upon light irradiation, the PDT is highly selective in eradicating tumors with little effect on healthy organs (e.g., heart, spleen, liver, kidney, etc.). In addition, PDT could be divided into two main subtypes according to photochemical reaction mechanisms: type I PDT and type II PDT. Upon activation of laser, the PSs convert the ground singlet state to the excited triple state and then trigger the photochemical reaction through two pathways (type I and type II). For type I PDT, the PSs catalyze biological substrate to produce radical intermediates which further interact with water and triplet oxygen (3O2) to produce hydroxyl radicals (·OH) and superoxide anions (O2·-). PSs transfer directly the energy to the surrounding 3O2 into singlet oxygen (1O2) for the type II PDT. Thus, type I PDT is oxygen-independent, while the type II PDT can only occur in well-oxygenated microenvironment.
Nowadays, PDT has been widely used in cancer treatments in clinics, including lung cancer, esophageal cancer, skin cancer, and glioma, etc. The 5-aminolevulinic acid (5-ALA), an intermediate metabolite in hemoglobin pathway, has been approved by United States Food and Drug Administration (FDA) for fluorescence imaging-guided surgical resection of solid tumors. In recent years, the 5-ALA has been gradually utilized as a PS for PDT of glioma. 5-ALA could accumulate in glioma cells after oral administration, which is transformed into protoporphyrin (PpIX) in the mitochondria. Upon light irradiation, the PpIX could induce large amount of ROS in glioma cells, which could trigger imbalance of oxidative stress and cause cell death. However, there are several challenges for the 5-ALA-based PDT, including lacking of active targeting ability, short wavelength of excitation wavelength, insufficient tissue penetration depth, and inefficient in hypoxic environments, etc.
With rapid development of nanotechnology, the nanomedicine-mediated PDT has widely applied in treatment of glioma (Table 1) (42, 43, 52–58). For example, Cai group developed a disulfide bond-conjugated polymer for targeted PDT and chemotherapy of orthotopic glioma (40). The camptothecin (CPT)-S-S-PEG-COOH was able to assemble into polymeric micelles which were further loaded with IR780 as PS and modified with iRGD peptide for BBB penetration and tumor targeting (Figures 1A, B). Due to the conjugation of iRGD peptide, the CPT-S-S-PEG-iRGD@IR780 micelles could effectively span the BBB and actively target tumor cells through interaction between iRGD peptide and αvβ integrin (Figure 1C). The in vivo experiments showed that these micelles could effectively deliver the IR780 into the orthotopic glioma region, leading to excellent anti-tumor effect upon laser irradiation. Overall, the CPT-S-S-PEG-iRGD@IR780 micelles were an ideal delivery nano carrier of PS (IR780) and chemotherapeutic drug (CPT), and the micelle-mediated PDT is a promising treatment for glioma. In addition, to enhance the tumor accumulation of PSs, Chen group proposed a novel drug delivery strategy in which the platelet was used as carrier of PSs (41). They loaded the chlorine e6 (Ce6) into boron nitride nanoparticles and further coated with doxorubicin and polyglycerol (BNPD-Ce6). Then, the BNPD-Ce6 nanoparticles were loaded into mouse platelets to form the BNPD-Ce6@Plt nanocarriers. The in vivo experiment showed that the BNPD-Ce6@Plt could rapidly accumulated in both subcutaneous and orthotopic GL261 tumors after intravenous administration. Upon light irradiation at a wavelength of 808 nm, the BNPD-Ce6@Plt nanoparticles could produce amount of ROS, inducing DNA damage and tumor cell death. In vivo therapeutic efficacy evaluation revealed that the BNPD-Ce6@Plt-mediated PDT remarkably regress the orthotopic glioma and prolong the survival time of mouse.
Figure 1 (A) Chemical structure of the prepared CPT-S-S-PEG-iRGD nanopolymer. (B) Schematic illustration of BBB penetration and tumor cell targeting of the prepared polymers. (C) The mechanism of iRGD for BBB penetration and tumor cell targeting. Reproduced with permission from ref (40). Copyright 2020, Elsevier.
The principle of PTT is that photothermal agents (PTAs) convert light into heat energy when irradiated by near-infrared light to increase the temperature of the surrounding environment, thereby killing the tumor cells (59, 60). The advantages of PTT include the energy of the external light source, high efficiency and non-invasiveness (61). High enrichment in tumor tissue is one of the necessary conditions for PTAs to produce great PTT effects (62, 63).
At present, PTAs are mainly divided into two categories: inorganic materials and organic materials. Among them, inorganic materials include noble metal materials (64, 65), transition metal materials (66), carbon-based nanomaterials (such as carbon nanotubes and graphene) (67), and other two-dimensional materials (such as black phosphorus nanosheets, boron nitride, and graphitic carbon nitride) (68, 69); organic materials include near-infrared responsive small molecules and semiconducting polymers, etc (70, 71). Inorganic PTAs have higher photothermal conversion efficiency and better photothermal stability, while organic PTAs have better biocompatibility and biodegradability.
The nanomedicine-mediated PTT has been widely applied in glioma treatment. For example, Jia et al. fabricated indocyanine green (ICG)-loaded liposomes and further embedded the glioma cell membrane protein into the liposomes (BLIPO-ICG nanoparticles). With the glioma cell membrane protein, the BLIPO-ICG nanoparticles could cross the BBB and target the orthotopic glioma cells. The ICG in BLIPO-ICG nanoparticles could be used for in vivo fluorescence imaging. Besides, the photothermal effect of BLIPO-ICG nanoparticles could rapidly heat up under the laser irradiation, which could significantly suppress the glioma growth with an inhibition rate of 94.2%. these results. Overall, the PTT has shown great application prospect in glioma treatment.
CDT uses metal ion-based Fenton or Fenton-like reactions to convert less reactive H2O2 into more toxic ·OH, killing tumor cells (44, 72–75). Compared with normal cells, tumor cells can produce higher levels of H2O2. Utilizing the over-generated H2O2 in tumor as an endogenous prodrug, CDT enables tumor specific ·OH production to induce intracellular oxidative stress imbalance and reduce damage to normal tissues (76, 77). Moreover, several types of metal ions have exhibited Fenton-like activity, such as Mn2+, Cu2+, Co2+, Pt2+, etc (74, 78–81). However, the efficiency of CDT in tumor cells might be limited by various factors, such as limited H2O2 content in tumor cells, high level of intratumoral GSH that is able to neutralize the produced ·OH, intracellular pH and temperature, etc (72, 73, 82). Researchers have designed various nanoparticles to overcome these obstacles.
According to the Warburg effect, cancer cells are more dependent on glucose for nutrition than normal cells, and the glucose level in tumor cells is significantly higher than that in normal cells (83). To improve the H2O2 level in tumor cells, several studies added glucose oxidase (GOD) into the nanomedicines for enhancement of CDT efficiency (84–87). GOD can catalyze the oxidation of intratumoral glucose and generate H2O2 (88). The supplementary H2O2 can serve as substrate for the Fenton reaction, augmenting the CDT effect. In addition, hyperthermia in tumor site via PTT could also enhance CDT efficiency (89). Compared with near infrared I (NIR-I), near infrared II (NIR-II) lasers have deeper tissue penetration and higher maximum permissible exposure (MPE) (90, 91). Usually, the MPE of 808 nm (NIR-I) laser is 0.33 W/cm2, but the MPE of 1064 nm (NIR-II) laser is 1W/cm2. Human skin will burn when exposed to lasers with power higher than MPE (92). Therefore, nanomaterials with higher NIR-II absorption were chosen for PTT-enhanced CDT. Besides, GSH plays an important role in protecting cells against external damage (93). However, high levels of GSH in tumor cells can neutralize high reactive ROS, such as ·OH, thereby weakening the effect of CDT (94). Therefore, reducing the level of GSH in tumor cells can improve CDT efficiency.
In addition, Pan et al. proposed a localized NIR-II laser mediated CDT strategy for treatment of glioblastoma (44). They first prepared ultrasmall CuFeSe2 nanocrystals as CDT agent. Then, the CuFeSe2 nanocrystals were conjugated with lactate oxidase (LOD) and further coated with biomimetic fused liposome membrane (CuFeSe2-LOD@Lipo-CM, CLLC, Figure 2). The in vivo fluorescence imaging showed that CLLC nanocatalysts could penetrate the BBB and actively target orthotopic glioblastoma. After entering the glioblastoma cells, the Fenton-like reaction mediated by Cu+ in CLLC nanocatalysts could convert H2O2 into ·OH for CDT (Figure 2). The level of lactic acid in tumor is significantly higher than that in normal tissues. The LOD in CLLC nanocatalysts was able to catalyze oxidation of lactic acid to H2O2, which could be utilized to augment localized Fenton-like reaction. Furthermore, the photothermal conversion rate of CLLC nanocatalysts was 49.7%, indicating that the NIR-II laser mediated PTT could remarkably increase in situ temperature of tumor site and further enhance CDT. In addition, the mouse skulls severely block laser from entering the brain. Thus, the skull above orthotopic glioblastoma was resected to allow the laser to reach the glioblastoma site. Besides, the photoacoustic (PA) imaging could guide NIR II laser irradiation and monitor the CLLC-based CDT process. The in vivo therapeutic evaluation revealed that CLLC+NIR-II laser could significantly inhibit glioblastoma growth and prolong the median survival time (43 d). In addition, biological safety evaluation revealed that laser irradiation showed negligible neural toxicity on healthy mice. These results suggested that the CLLC-mediated localized CDT could be a promising treatment for glioblastoma.
Figure 2 Schematic illustration of CLLC-mediated CDT of glioma. Reproduced with permission from ref (44). Copyright 2022, Elsevier.
Besides, Tan et al. utilized Mn2+-mediated Fenton-like reaction for CDT of glioma (95). They loaded temozolomide (TMZ) and manganese oxide (MnO) into an iRGD-modified polymeric micelle (iRPPA@TMZ/MnO). Due to the modification of iRGD, the iRPPA@TMZ/MnO micelles could efficiently cross the BBB and further precisely target the glioma tissues. The MnO in iRPPA@TMZ/MnO micelles could responsively degrade into Mn2+ and oxygen after reaching the tumor site. Simultaneously, the TMZ was rapidly released for chemotherapy of glioma cells. The Mn2+-mediated Fenton-like reaction could produce a large number of ·OH, inducing intracellular oxidative stress and kill the glioma cells. In addition, the generated oxygen could alleviate hypoxic tumor microenvironment, which could enhance the therapeutic efficiency of CDT and chemotherapy. Furthermore, the Mn2+ could also be used for T1-weighted magnetic resonance imaging (MRI) that could monitor the treatment process. In vivo therapeutic effect evaluation revealed that the median survival time of mice with orthotopic gliomas in iRPPA@TMZ/MnO group was 35 days, significantly longer than that in PBS group (15 days). These results suggested that iRPPA@TMZ/MnO-mediated CDT/chemotherapy could be an alternative strategy for glioma treatment.
Ultrasound (US), which uses mechanical vibration waves (frequency > 16 kHz) with a deep tissue penetration depth to treat and diagnose diseases, has been widely applied in clinical applications, including US imaging and high-intensity focused US (HIFU) (96). In 2004, Rosenthal et al. first define the SDT as a treatment method consisting of the synergistic effect of US and a chemical compound (sonosensitizer) (97). SDT is an emerging therapeutic modality that utilized low-intensity US to stimulate the sonosensitizers within specific tissues for generation of ROS and cavitation bubbles, thereby eliminate tumor tissues (98, 99). Due to low invasiveness and strong tissue penetration ability, SDT has gradually used to treat glioma. SDT was first used to treat glioma in 2008 (100). Until now, the mechanism of SDT for cancer therapy has not been totally uncovered. At present, it is generally believed that there are mainly two mechanisms for SDT: ROS production and ultrasonic cavitation effect (101, 102). Traditional sonosensitizers, including porphyrin and hematoporphyrin, were utilized to treat the glioma under US irradiation. However, the therapeutic efficiency was unsatisfactory, since these traditional sonosensitizers could not effectively across the BBB and accumulate in tumor regions. Moreover, the hypoxic tumor microenvironment significantly limits the SDT efficiency. Notably, with the rapid development of nanotechnology, several nanosonosensitizers were constructed to improve the antitumor efficacy of SDT (103–110).
Wang group constructed an intelligent nanosonosensitizer for efficient SDT of glioblastoma (47). First, the MnO2 nanocrystals were grown onto the holo-transferrin (holo-Tf) via reformative biomineralization. Then, the protoporphyrin (ppIX) as a sonosensitizer was conjugated with the holo-Tf to prepare the MnO2@Tf-ppIX nanocomposites (TMP). The TEM results displayed that TMP nanocomposites showed uniform morphology. The zeta potential of TMP nanocomposites was -20 ± 0.2 mV, which was suitable to blood circulation and BBB crossing. Due to transferrin receptors of the BBB, the TMP nanocomposites could efficiently cross the BBB and enrich in the glioblastoma sites. Furthermore, the MnO2 could responsively degrade to Mn2+ and oxygen under the conditions of glutathione (GSH) and acid tumor microenvironment (Figure 3). The Mn2 could be utilized for T1-weighted magnetic resonance imaging (MRI) which could guide the SDT of tumor. Besides, the generated oxygen could alleviate hypoxic tumor microenvironment, which could enhance the SDT efficiency. In addition, the intratumoral TMP nanocomposites could produce a large number of 1O2, thereby induced apoptosis of tumor cells. Antitumor evaluation results showed that the tumors in tumor-bearing mice in TMP + US group (1.0 MHz, 1.5 W/cm2, 3 min) were fully eliminated, however, the tumor inhibition rates in other groups were unsatisfactory. Overall, the TMP-mediated SDT could be an alternative treatment approach for glioblastoma.
Figure 3 Schematic illustration of application of TMP NPs for SDT of glioblastoma. Reproduced with permission from ref (47). Copyright 2020, Wiley.
Indocyanine green (ICG) as a sonosensitizer has been approved by the US Food and Drug Administration (FDA) for clinical applications. Liu group loaded catalase (CAT) and ICG into silica nanoparticles (defined as CSI) for hypoxia alleviation and SDT of glioblastoma (49). Besides, the CSI nanoparticles were coated with AS1411 aptamer-conjugated macrophage exosomes (CSI@Ex-A) for enhancing BBB crossing ability. The CSI@Ex-A nanoparticles could effectively cross the BBB and target the glioblastoma cells. After entering the glioblastoma cells, the CSI@Ex-A nanoparticles could be degraded responsively in the presence of GSH, resulting in release of CAT and ICG. The CAT could catalyze (H2O2) to generate oxygen, thereby alleviating hypoxic tumor microenvironment. The ICG could produce amount of ROS under US irradiation and induce tumor cell death. In addition, both GSH resuming and supplementary oxygen could augment the SDT efficiency. The combined treatment (CSI@Ex-A + US irradiation) could remarkably suppress tumor growth and prolong the median survival time of orthotopic glioblastoma-bearing mice to 35 days, much longer than 23 days of PBS group. In addition, the CSI@Ex-A nanoparticles showed quite low toxicity to normal tissues and organs. These results suggested that the CSI@Ex-A nanoagents could be a promising sonosensitizer for glioblastoma treatment. US-targeted microbubble destruction (UTMD) is a US technique that can reversibly open the BBB and facilitate drug accumulation in glioma sites. Qu et al. fabricated an all-in-one nanosonosensitizer to enhance SDT of glioma (48). They loaded sonosensitizers chlorin e6 (Ce6) and hydroxychloroquine (HCQ, an autophagy inhibitor) into liposomes, which were further modified with targeting peptide angiopep-2 (defined as ACHL). UTMD-based BBB opening allowed ACHL to accumulate in glioma sites. The Ce6 could generate ROS under US irradiation and further induce apoptosis and MAPK/p38-PINK1-PRKN-dependent mitophagy. Besides, the released HCQ was able to inhibit autophagosome degradation, thereby amplifying intratumoral ROS generation. In vivo treatment evaluation revealed that ACHL-SDT could significantly suppress tumor growth and prolong median survival time of tumor-bearing mice. Therefore, the ACHL-mediated SDT might achieve precision treatment for glioma by integrating SDT-induced apoptosis and mitophagy inhibition. Overall, the SDT could be an emerging ROS-generation treatment approach for glioma.
In addition, temozolomide, the most commonly used chemotherapeutic drug of glioma could produce ROS under US irradiation and induce necroptosis in glioma, indicating that temozolomide plus US could be a promising treatment for glioma (111).
Radiation therapy (radiotherapy) as a crucial treatment modality which is applied to more than half of all cancer patients (112, 113). Furthermore, conventional treatment for glioma includes surgery, radiotherapy and chemotherapy. Almost most glioma patients will receive radiotherapy. In general, radiotherapy works through ionizing radiation, which includes photon radiation, including X-rays and gamma rays, but also particle radiation, such as α particles, carbon ions, β particles, electrons, neutrons, etc (114–116). Radiotherapy kills tumor cells through direct and indirect effects. Direct action is biomolecular damage, particularly DNA double strand breaks, leading to necrosis or apoptosis. The indirect effect refers to the promotion of apoptosis by ROS generated by the radiolysis of water molecules (116–121). Rapid development of nanotechnology remarkably improves the radiotherapy efficiency via alleviation of hypoxic tumor microenvironment, X-ray absorbance of high-Z elements, increase of tumor accumulation of radiosensitizers, etc (122). In addition, the nanotechnology-mediated radiosensitization has been widely utilized to treat glioma and has achieved excellent outcomes (123–125).
For example, Zhao et al. conjugated the silver nanoparticles (AgNPs) with polyethylene glycol (PEG) and As1411 to fabricate AsNPs for radiosensitization of glioma (50). In vitro experiments revealed that the AsNPs could actively target glioma cells and penetrate deeply into tumor spheroids. In vivo fluorescence imaging showed that the Cy5-AsNPs were able to effectively accumulate at the glioma region, and the fluorescence intensity of Cy5-AsNPs in glioma sites was much higher than that of PEGylated AgNPs. In vivo treatment efficacy evaluation revealed that the tumor inhibition rate of AsNPs + X-ray irradiation was highest and that the median survival time of glioma-bearing mice in this group was significantly extended. In addition, the AGuIX nanoparticles has been utilized in clinical trials for radiosensitization of brain metastases (NCT02820454, NCT04094077, NCT04899908, and NCT03818386). Verry et al. utilized gadolinium-based AGuIX nanoparticles for MRI-guided radiotherapy of glioma (126). The in vivo MRI results showed that the tumor regions could showed T1-weighted enhancement even 1 day after intravenous injection of AGuIX nanoparticles. Furthermore, the tumor growth was significantly inhibited when the tumor-bearing mice received X-ray irradiation (6 mV). Moreover, the pharmacokinetics and toxicology evaluations revealed that the AGuIX nanoparticles exhibited excellent biosafety. These results indicated that the AGuIX nanoparticles were a promising type of nanoradiosensitizers for glioma treatment.
The radioresistance leads to poor radiotherapy efficacy of glioblastoma. The resistance of tumor cells is mainly associated with their ability of damaged DNA repairing. Besides, the protective autophagy was reported to play a key role in clearance and degradation of damage organelles and DNA, thereby rescuing the damaged tumor cells (127). Thus, effective inhibition of the damaged DNA repair and protective autophagy was important for improvement of radiotherapy efficacy. Xu et al. coated gold nanoparticles with core-shell copper selenide to construct Au@Cu2-xSe NPs for enhancing radiotherapy of glioblastoma (Figure 4) (51). The prepared Au@Cu2-xSe NPs could alkalize lysosomes and further suppress the autophagy flux. In addition, they also found that Au@Cu2-xSe NPs could enhance the degradation of DNA repair protein Rad51 and decrease the DNA repair. Both suppression of DNA repair and protective autophagy could remarkably kill the glioblastoma cells via combination of Au@Cu2-xSe NPs and radiation. This work suggested that suppression of protective autophagy flux and DNA repair of cancer cells via well-designed nanoradiosensitizers is a promising strategy for improvement of radiotherapy efficacy of glioblastoma.
Figure 4 Schematic illustration of application of Au@Cu2-xSe NPs for glioblastoma radiotherapy. Reproduced with permission from ref (51). Copyright 2022, Elsevier.
Combination of different ROS-based nanomedicine strategies has been shown great synergistic therapeutic effects on glioma. For example, Zhang group prepared a nanocomposite with upconversion nanoparticle, NH2-MIL-53 (Fe) and DOX for PDT/CDT/chemotherapy of glioma (45). The prepared nanocomposites were further modified with lactoferrin, allowing them to cross the BBB and target the tumor cells. The upconversion nanoparticles were able to transfer the 808 nm laser into UV light to irradiate NH2-MIL-53 for PDT. Besides, the Fe ions in the nanocomposites could catalyze the H2O2 into ·OH for CDT. In addition, the DOX could be released under the acid tumor microenvironment for chemotherapy of glioma. The in vivo therapeutic evaluation showed that the synergistic CDT/PDT/chemotherapy could significantly inhibit the growth of glioma. Thus, the ROS-based multimodal therapies could be a promising treatment for glioma.
ROS-based nanomedicine provides an alternative strategy for the treatment of glioma. Nanosensitizers could catalyze the production of ROS under irradiation of light, radiation, or US as well as intracellular H2O2. In this review, we summarize the recent advances of ROS-based nanomedicine in their BBB crossing ability and application against glioma. Although promising, there are still several limitations need to be solved in future research.
Emerging BBB-crossing nanotechnology provides great opportunities to deliver chemotherapeutic drugs and sensitizers to glioma for effective treatments. These nanosensitizers could cross the BBB through AMT, CMT, RMT, etc. However, there are still several challenges need to be overcome to translate these nanosensitizers from bench to bedsides. The nanoparticles can absorb proteins or small molecules onto their surface after intravenous administration, leading to formation of “protein corona”. The “protein corona” might alter the surface chemistry of the nanoparticles, weakening their BBB-crossing ability and the therapeutic efficacy. Besides, although the nanosensitizers could cross the BBB and produce ROS in tumor sites, the drug concentration in the glioma is still limited. Thus, other injection methods might be considered to treat the glioma, such as intracerebral injection, intranasal administration and intrathecal injection.
The hypoxic tumor microenvironment was a major limitation for PDT. In recent years, type I PDT has been gradually reported to treat tumors, especially hypoxic solid tumor, due to its oxygen independence property (128). Therefore, the type I PDT might provide a promising treatment strategy for glioma in future. CDT is driven by endogenous factors, such as H2O2. On the one hand, the efficacy of CDT is still limited by H2O2 content in tumor cells. Thus, recent studies proposed various H2O2-supplementary strategy, such as GOD, LOD, etc (44, 129, 130). The GOD or LOD is able to catalyze oxidation of glucose or lactic acid to produce H2O2, thereby provide reaction material to CDT. On the other hand, the catalytic efficiency can be further enhanced by increasing intratumoral temperature and application of single atom nanozymes. SDT as a novel treatment approach has shown excellent therapy efficiency and biosafety. However, there are still some obstacles that need to be further resolved in the future. First, the potential mechanisms of antitumor effect of SDT still need to be explored in future research. Second, traditional sonosensitizers are mainly small organic molecules. There are some shortcomings to these sonosentizers, including low stability, poor BBB crossing ability, poor tumor selectivity, etc. Thus, future studies might focus on development of inorganic sonosentizers, such as Ti-based sonosentizers, black phosphorus, etc. Third, the optimal parameters of frequency and power of US for SDT need to be further studied.
Besides, the mouse glioma models are quite important for efficacy evaluation of ROS-based nanomedicine. First, the glioma models in most studies were built with homogenous glioma cell lines, which is difficult to stimulate the tumor heterogeneity in clinical patients. Second, several studies used the subcutaneous glioma models which cannot be used to assess the ability to cross the BBB. Third, most glioma models were established into immunodeficiency animals, which making it difficult to evaluate the systemic immunity induced by ROS-based nanomedicine. Thus, more suitable glioma model for ROS therapy evaluation should be developed in future research.
DW conceived the study and drafted the manuscript. XC, SZ, and BL revised the manuscript critically for important intellectual content. All authors approved the final manuscript. All authors contributed to the article and approved the submitted version.
The authors declare that the research was conducted in the absence of any commercial or financial relationships that could be construed as a potential conflict of interest.
All claims expressed in this article are solely those of the authors and do not necessarily represent those of their affiliated organizations, or those of the publisher, the editors and the reviewers. Any product that may be evaluated in this article, or claim that may be made by its manufacturer, is not guaranteed or endorsed by the publisher.
BBB, Blood-brain barrier; ROS, Reactive oxygen species; PDT, Photodynamic therapy; CDT, Chemodynamic therapy; PTT, Photothermal therapy; SDT, Sonodynamic therapy; CNS, Central nervous system; bcecs, Brain capillary endothelial cells; CMT, Carrier-mediated transcytosis; GLUT1, Glucose transport receptor 1; Tf, Transferrin; RMT, Receptor-mediated transcytosis; AMT, Adsorption-mediated transcytosis; H2O2, Hydrogen peroxide; 1O2, Singlet oxygen; O2•−, Superoxide anion; •OH, Hydroxyl radical; GSH, Glutathione; PS, Photosensitizer; FDA, Food and Drug Administration; ppix, Protoporphyrin; CPT, Camptothecin; Ce6, Chlorine e6; GOD, Glucose oxidase; NIR-I, Near infrared I; NIR-II, Near infrared II; MPE, Maximum permissible exposure; TMZ, Temozolomide; mno, Manganese oxide; HIFU, High-intensity focused US; MRI, Magnetic resonance imaging; CAT, Catalase; PEG, Polyethylene glycol; LOD, Lactate oxidase.
1. Sung H, Ferlay J, Siegel RL, Laversanne M, Soerjomataram I, Jemal A, et al. Global cancer statistics 2020: globocan estimates of incidence and mortality worldwide for 36 cancers in 185 countries. CA: Cancer J Clin (2021) 71(3):209–49. doi: 10.3322/caac.21660
2. Chen F, Wendl MC, Wyczalkowski MA, Bailey MH, Li Y, Ding L. Moving pan-cancer studies from basic research toward the clinic. Nat Cancer (2021) 2(9):879–90. doi: 10.1038/s43018-021-00250-4
3. Bucci MK, Maity A, Janss AJ, Belasco JB, Fisher MJ, Tochner ZA, et al. Near complete surgical resection predicts a favorable outcome in pediatric patients with nonbrainstem, Malignant gliomas: results from a single center in the magnetic resonance imaging era. Cancer (2004) 101(4):817–24. doi: 10.1002/cncr.20422
4. Fan W, Yung B, Huang P, Chen X. Nanotechnology for multimodal synergistic cancer therapy. Chem Rev (2017) 117(22):13566–638. doi: 10.1021/acs.chemrev.7b00258
5. Tang W, Fan W, Lau J, Deng L, Shen Z, Chen X. Emerging blood–brain-barrier-crossing nanotechnology for brain cancer theranostics. Chem Soc Rev (2019) 48(11):2967–3014. doi: 10.1039/C8CS00805A
6. Auvergne RM, Sim FJ, Wang S, Chandler-Militello D, Burch J, Al Fanek Y, et al. Transcriptional differences between normal and glioma-derived glial progenitor cells identify a core set of dysregulated genes. Cell Rep (2013) 3(6):2127–41. doi: 10.1016/j.celrep.2013.04.035
7. Modrek AS, Bayin NS, Placantonakis DG. Brain stem cells as the cell of origin in glioma. World J Stem Cells (2014) 6(1):43. doi: 10.4252/wjsc.v6.i1.43
8. Ribatti D, Nico B, Crivellato E, Artico M. Development of the blood-brain barrier: A historical point of view. Anatomical Rec Part B: New Anatomist: Off Publ Am Assoc Anatomists (2006) 289(1):3–8. doi: 10.1002/ar.b.20087
9. Abbott NJ, Rönnbäck L, Hansson E. Astrocyte–endothelial interactions at the blood–brain barrier. Nat Rev Neurosci (2006) 7(1):41–53. doi: 10.1038/nrn1824
10. Pardridge WM. The blood-brain barrier: bottleneck in brain drug development. NeuroRx (2005) 2:3–14. doi: 10.1602/neurorx.2.1.3
11. Banks WA. From blood–brain barrier to blood–brain interface: new opportunities for cns drug delivery. Nat Rev Drug Discovery (2016) 15(4):275–92. doi: 10.1038/nrd.2015.21
12. Ali IU, Chen X. Penetrating the blood–brain barrier: promise of novel nanoplatforms and delivery vehicles. ACS nano (2015) 9(10):9470–4. doi: 10.1021/acsnano.5b05341
13. Chen Y, Liu L. Modern methods for delivery of drugs across the blood–brain barrier. Advanced Drug delivery Rev (2012) 64(7):640–65. doi: 10.1016/j.addr.2011.11.010
14. Zhan C, Lu W. The blood-brain/tumor barriers: challenges and chances for Malignant gliomas targeted drug delivery. Curr Pharm Biotechnol (2012) 13(12):2380–7. doi: 10.2174/138920112803341798
15. De Vries NA, Beijnen JH, Boogerd W, Van Tellingen O. Blood–brain barrier and chemotherapeutic treatment of brain tumors. Expert Rev Neurother (2006) 6(8):1199–209. doi: 10.1586/14737175.6.8.1199
16. Habib S, Singh M. Angiopep-2-modified nanoparticles for brain-directed delivery of therapeutics: A review. Polymers (2022) 14(4):712. doi: 10.3390/polym14040712
17. Lin T, Zhao P, Jiang Y, Tang Y, Jin H, Pan Z, et al. Blood–brain-barrier-penetrating albumin nanoparticles for biomimetic drug delivery via albumin-binding protein pathways for antiglioma therapy. ACS Nano (2016) 10(11):9999–10012. doi: 10.1021/acsnano.6b04268
18. Mehdipour G, Wintrasiri MN, Ghasemi S. Cpp-based bioactive drug delivery to penetrate the blood-brain barrier: A potential therapy for glioblastoma multiforme. Curr Drug Targets (2022) 23(7):719–28. doi: 10.2174/1389450123666220207143750
19. Ruan S, Yuan M, Zhang L, Hu G, Chen J, Cun X, et al. Tumor microenvironment sensitive doxorubicin delivery and release to glioma using angiopep-2 decorated gold nanoparticles. Biomaterials (2015) 37:425–35. doi: 10.1016/j.biomaterials.2014.10.007
20. Anraku Y, Kuwahara H, Fukusato Y, Mizoguchi A, Ishii T, Nitta K, et al. Glycaemic control boosts glucosylated nanocarrier crossing the bbb into the brain. Nat Commun (2017) 8(1):1001. doi: 10.1038/s41467-017-00952-3
21. Uchida Y, Ohtsuki S, Katsukura Y, Ikeda C, Suzuki T, Kamiie J, et al. Quantitative targeted absolute proteomics of human blood–brain barrier transporters and receptors. J neurochemistry (2011) 117(2):333–45. doi: 10.1111/j.1471-4159.2011.07208.x
22. Cheng Y, Morshed R, Cheng SH, Tobias A, Auffinger B, Wainwright DA, et al. Nanoparticle-programmed self-destructive neural stem cells for glioblastoma targeting and therapy. Small (2013) 9(24):4123–9. doi: 10.1002/smll.201301111
23. Huang X, Zhang F, Wang H, Niu G, Choi KY, Swierczewska M, et al. Mesenchymal stem cell-based cell engineering with multifunctional mesoporous silica nanoparticles for tumor delivery. Biomaterials (2013) 34(7):1772–80. doi: 10.1016/j.biomaterials.2012.11.032
24. Jia G, Han Y, An Y, Ding Y, He C, Wang X, et al. Nrp-1 targeted and cargo-loaded exosomes facilitate simultaneous imaging and therapy of glioma in vitro and in vivo. Biomaterials (2018) 178:302–16. doi: 10.1016/j.biomaterials.2018.06.029
25. Li T-F, Li K, Wang C, Liu X, Wen Y, Xu Y-H, et al. Harnessing the cross-talk between tumor cells and tumor-associated macrophages with a nano-drug for modulation of glioblastoma immune microenvironment. J Controlled Release (2017) 268:128–46. doi: 10.1016/j.jconrel.2017.10.024
26. Burgess M, Apostolakis I, Konofagou E. Power cavitation-guided blood-brain barrier opening with focused ultrasound and microbubbles. Phys Med Biol (2018) 63(6):065009. doi: 10.1088/1361-6560/aab05c
27. McDannold N, Zhang Y, Vykhodtseva N. Blood-brain barrier disruption and vascular damage induced by ultrasound bursts combined with microbubbles can be influenced by choice of anesthesia protocol. Ultrasound Med Biol (2011) 37(8):1259–70. doi: 10.1016/j.ultrasmedbio.2011.04.019
28. Pouliopoulos AN, Burgess MT, Konofagou EE. Pulse inversion enhances the passive mapping of microbubble-based ultrasound therapy. Appl Phys Lett (2018) 113(4). doi: 10.1063/1.5036516
29. Zhang Q, Luo Q, Liu Z, Sun M, Dong X. Nano-ros-generating approaches to cancer dynamic therapy: lessons from nanoparticles. Chem Eng J (2023) 457:141225. doi: 10.1016/j.cej.2022.141225
30. Sabharwal SS, Schumacker PT. Mitochondrial ros in cancer: initiators, amplifiers or an achilles' Heel? Nat Rev Cancer (2014) 14(11):709–21. doi: 10.1038/nrc3803
31. Yang B, Chen Y, Shi J. Reactive oxygen species (Ros)-based nanomedicine. Chem Rev (2019) 119(8):4881–985. doi: 10.1021/acs.chemrev.8b00626
32. Oshi M, Gandhi S, Yan L, Tokumaru Y, Wu R, Yamada A, et al. Abundance of reactive oxygen species (Ros) is associated with tumor aggressiveness, immune response, and worse survival in breast cancer. Breast Cancer Res Treat (2022) 194(2):231–41. doi: 10.1007/s10549-022-06633-0
33. Weinberg F, Ramnath N, Nagrath D. Reactive oxygen species in the tumor microenvironment: an overview. Cancers (2019) 11(8):1191. doi: 10.3390/cancers11081191
34. de Sá Junior PL, Câmara DAD, Porcacchia AS, Fonseca PMM, Jorge SD, Araldi RP, et al. The roles of ros in cancer heterogeneity and therapy. Oxid Med Cell Longevity (2017) 2017. doi: 10.1155/2017/2467940
35. Yao J, Cheng Y, Zhou M, Zhao S, Lin S, Wang X, et al. Ros scavenging mn 3 O 4 nanozymes for in vivo anti-inflammation. Chem Sci (2018) 9(11):2927–33. doi: 10.1039/C7SC05476A
36. Zhu Y, Wang W, Gong P, Zhao Y, Pan Y, Zou J, et al. Enhancing catalytic activity of a nickel single atom enzyme by polynary heteroatom doping for ferroptosis-based tumor therapy. ACS Nano (2023) 17(3):3064–76. doi: 10.1021/acsnano.2c11923
37. Lyu M, Chen M, Liu L, Zhu D, Wu X, Li Y, et al. A platelet-mimicking theranostic platform for cancer interstitial brachytherapy. Theranostics (2021) 11(15):7589–99. doi: 10.7150/thno.61259
38. Shi Y, Zeng L, Pan Y, Zhang H, Wang Z, Shi Y, et al. Endo/exo-genous dual-stimuli responsive gold nanotetrapod-base D nanoprob E for magnetic resonance imaging and enhanced multimodal therapeutics by amplifying (Vol 154, pg 549, 2022). Acta BIOMATERIALIA (2023) 161:327–. doi: 10.1016/j.actbio.2023.02.008
39. Cheng K, Guo Q, Shen Z, Yang W, Wang Y, Sun Z, et al. Bibliometric analysis of global research on cancer photodynamic therapy: focus on nano-related research. Front Pharmacol (2022) 13:927219. doi: 10.3389/fphar.2022.927219
40. Lu L, Zhao X, Fu T, Li K, He Y, Luo Z, et al. An irgd-conjugated prodrug micelle with blood-brain-barrier penetrability for anti-glioma therapy. Biomaterials (2020) 230:119666. doi: 10.1016/j.biomaterials.2019.119666
41. Xu HZ, Li TF, Ma Y, Li K, Zhang Q, Xu YH, et al. Targeted photodynamic therapy of glioblastoma mediated by platelets with photo-controlled release property. Biomaterials (2022) 290:121833. doi: 10.1016/j.biomaterials.2022.121833
42. Tang XL, Jing F, Lin BL, Cui S, Yu RT, Shen XD, et al. Ph-responsive magnetic mesoporous silica-based nanoplatform for synergistic photodynamic therapy/chemotherapy. ACS Appl Mater Interfaces (2018) 10(17):15001–11. doi: 10.1021/acsami.7b19797
43. Wang R, Wang X, Li J, Di L, Zhou J, Ding Y. Lipoprotein-biomimetic nanostructure enables tumor-targeted penetration delivery for enhanced photo-gene therapy towards glioma. Bioact Mater (2022) 13:286–99. doi: 10.1016/j.bioactmat.2021.10.039
44. Pan Y, Xu C, Deng H, You Q, Zhao C, Li Y, et al. Localized nir-ii laser mediated chemodynamic therapy of glioblastoma. Nano Today (2022) 43:101435. doi: 10.1016/j.nantod.2022.101435
45. Lv Z, Cao Y, Xue D, Zhang H, Zhou S, Yin N, et al. A multiphoton transition activated iron based metal organic framework for synergistic therapy of photodynamic therapy/chemodynamic therapy/chemotherapy for orthotopic gliomas. J Mater Chem B (2023) 11(5):1100–7. doi: 10.1039/d2tb02273g
46. Pan Y, Zhu Y, Xu C, Pan C, Shi Y, Zou J, et al. Biomimetic yolk-shell nanocatalysts for activatable dual-modal-image-guided triple-augmented chemodynamic therapy of cancer. ACS Nano (2022) 16(11):19038–52. doi: 10.1021/acsnano.2c08077
47. Liang K, Li Z, Luo Y, Zhang Q, Yin F, Xu L, et al. Intelligent nanocomposites with intrinsic blood-brain-barrier crossing ability designed for highly specific mr imaging and sonodynamic therapy of glioblastoma. Small (2020) 16(8):e1906985. doi: 10.1002/smll.201906985
48. Qu F, Wang P, Zhang K, Shi Y, Li Y, Li C, et al. Manipulation of mitophagy by "All-in-one" Nanosensitizer augments sonodynamic glioma therapy. Autophagy (2020) 16(8):1413–35. doi: 10.1080/15548627.2019.1687210
49. Wu T, Liu Y, Cao Y, Liu Z. Engineering macrophage exosome disguised biodegradable nanoplatform for enhanced sonodynamic therapy of glioblastoma. Adv Mater (2022) 34(15):e2110364. doi: 10.1002/adma.202110364
50. Zhao J, Liu P, Ma J, Li D, Yang H, Chen W, et al. Enhancement of radiosensitization by silver nanoparticles functionalized with polyethylene glycol and aptamer as1411 for glioma irradiation therapy. Int J Nanomedicine (2019) 14:9483–96. doi: 10.2147/ijn.S224160
51. Xu Q, Zhang H, Liu H, Han Y, Qiu W, Li Z. Inhibiting autophagy flux and DNA repair of tumor cells to boost radiotherapy of orthotopic glioblastoma. Biomaterials (2022) 280:121287. doi: 10.1016/j.biomaterials.2021.121287
52. An R, Liu L, Wei S, Huang Z, Qiu L, Lin J, et al. Controlling disassembly of paramagnetic prodrug and photosensitizer nanoassemblies for on-demand orthotopic glioma theranostics. ACS Nano (2022) 16(12):20607–21. doi: 10.1021/acsnano.2c07491
53. Cao X, Li S, Chen W, Lu H, Ye L, Min Z, et al. Multifunctional hybrid hydrogel system enhanced the therapeutic efficacy of treatments for postoperative glioma. ACS Appl Mater Interfaces (2022) 14(24):27623–33. doi: 10.1021/acsami.2c05147
54. Chibh S, Katoch V, Singh M, Prakash B, Panda JJ. Miniatured fluidics-mediated modular self-assembly of anticancer drug-amino acid composite microbowls for combined chemo-photodynamic therapy in glioma. ACS Biomater Sci Eng (2021) 7(12):5654–65. doi: 10.1021/acsbiomaterials.1c01023
55. Lin X, Chen F, Yu X, Wang H, Qiu H, Li Y, et al. Phenylthiol-bodipy-based supramolecular metallacycles for synergistic tumor chemo-photodynamic therapy. Proc Natl Acad Sci U.S.A. (2022) 119(29):e2203994119. doi: 10.1073/pnas.2203994119
56. Lv Z, Jin L, Gao W, Cao Y, Zhang H, Xue D, et al. Novel yof-based theranostic agents with a cascade effect for nir-ii fluorescence imaging and synergistic starvation/photodynamic therapy of orthotopic gliomas. ACS Appl Mater Interfaces (2022) 14(27):30523–32. doi: 10.1021/acsami.2c05354
57. Tang XL, Wu J, Lin BL, Cui S, Liu HM, Yu RT, et al. Near-infrared light-activated red-emitting upconverting nanoplatform for T(1)-weighted magnetic resonance imaging and photodynamic therapy. Acta Biomater (2018) 74:360–73. doi: 10.1016/j.actbio.2018.05.017
58. Zhu X, Zhou H, Liu Y, Wen Y, Wei C, Yu Q, et al. Transferrin/aptamer conjugated mesoporous ruthenium nanosystem for redox-controlled and targeted chemo-photodynamic therapy of glioma. Acta Biomater (2018) 82:143–57. doi: 10.1016/j.actbio.2018.10.012
59. Abadeer NS, Murphy CJ. Recent progress in cancer thermal therapy using gold nanoparticles. Nanomaterials Neoplasms (2021) 120:143–217. doi: 10.1201/9780429027819-3
60. Beik J, Abed Z, Ghoreishi FS, Hosseini-Nami S, Mehrzadi S, Shakeri-Zadeh A, et al. Nanotechnology in hyperthermia cancer therapy: from fundamental principles to advanced applications. J Controlled Release (2016) 235:205–21. doi: 10.1016/j.jconrel.2016.05.062
61. Gai S, Yang G, Yang P, He F, Lin J, Jin D, et al. Recent advances in functional nanomaterials for light–triggered cancer therapy. Nano Today (2018) 19:146–87. doi: 10.1016/j.nantod.2018.02.010
62. Cheng L, Wang C, Feng L, Yang K, Liu Z. Functional nanomaterials for phototherapies of cancer. Chem Rev (2014) 114(21):10869–939. doi: 10.1021/cr400532z
63. Jung HS, Verwilst P, Sharma A, Shin J, Sessler JL, Kim JS. Organic molecule-based photothermal agents: an expanding photothermal therapy universe. Chem Soc Rev (2018) 47(7):2280–97. doi: 10.1039/C7CS00522A
64. Dreaden EC, Alkilany AM, Huang X, Murphy CJ, El-Sayed MA. The golden age: gold nanoparticles for biomedicine. Chem Soc Rev (2012) 41(7):2740–79. doi: 10.1039/C1CS15237H
65. Yang T, Wang Q, Lv X, Song X, Ke H, Guo Z, et al. Albumin-coordinated assembly of clearable platinum nanodots for photo-induced cancer theranostics. Biomaterials (2018) 154:248–60. doi: 10.1016/j.biomaterials.2017.10.030
66. Li X, Shan J, Zhang W, Su S, Yuwen L, Wang L. Recent advances in synthesis and biomedical applications of two-dimensional transition metal dichalcogenide nanosheets. Small (2017) 13(5):1602660. doi: 10.1002/smll.201602660
67. Cheng L, Shen S, Shi S, Yi Y, Wang X, Song G, et al. Fese(2)-decorated bi(2)Se(3) nanosheets fabricated via cation exchange for chelator-free (64)Cu-labeling and multimodal image-guided photothermal-radiation therapy. Adv Funct Mater (2016) 26(13):2185–97. doi: 10.1002/adfm.201504810
68. Huang K, Li Z, Lin J, Han G, Huang P. Two-dimensional transition metal carbides and nitrides (Mxenes) for biomedical applications. Chem Soc Rev (2018) 47(14):5109–24. doi: 10.1039/C7CS00838D
69. Qian X, Xie K, Guo S, Liang Q, Zhang S, Xiong Z, et al. Beneficial restacking of 2d nanomaterials for electrocatalysis: A case of mos2 membranes. Chem Commun (2020) 56(51):7005–8. doi: 10.1039/D0CC02139C
70. Sun Y, Miao H, Ma S, Zhang L, You C, Tang F, et al. Fept-Cys Nanoparticles Induce Ros-Dependent Cell Toxicity, and Enhance Chemo-Radiation Sensitivity of Nsclc Cells In vivo and In vitro. Cancer Lett (2018) 418:27–40. doi: 10.1016/j.canlet.2018.01.024
71. Song X, Chen Q, Liu Z. Recent advances in the development of organic photothermal nano-agents. Nano Res (2015) 8(2):340–54. doi: 10.1007/s12274-014-0620-y
72. Lin LS, Song J, Song L, Ke K, Liu Y, Zhou Z, et al. Simultaneous fenton-like ion delivery and glutathione depletion by mno2 -based nanoagent to enhance chemodynamic therapy. Angew Chem Int Ed Engl (2018) 57(18):4902–6. doi: 10.1002/anie.201712027
73. Dong X, Cheng R, Zhu S, Liu H, Zhou R, Zhang C, et al. A heterojunction structured wo(2.9)-wse(2) nanoradiosensitizer increases local tumor ablation and checkpoint blockade immunotherapy upon low radiation dose. ACS Nano (2020) 14(5):5400–16. doi: 10.1021/acsnano.9b08962
74. Xu A, Li X, Ye S, Yin G, Zeng Q. Catalyzed oxidative degradation of methylene blue by in situ generated cobalt (Ii)-bicarbonate complexes with hydrogen peroxide. Appl Catalysis B: Environ (2011) 102(1-2):37–43. doi: 10.1016/j.apcatb.2010.11.022
75. Zhu Y, Wang W, Cheng J, Qu Y, Dai Y, Liu M, et al. Stimuli-responsive manganese single-atom nanozyme for tumor therapy via integrated cascade reactions. Angew Chem Int Ed Engl (2021) 60(17):9480–8. doi: 10.1002/anie.202017152
76. Zhu Y, Liao Y, Zou J, Cheng J, Pan Y, Lin L, et al. Engineering single-atom nanozymes for catalytic biomedical applications. Small (2023) 19:2300750. doi: 10.1002/smll.202300750
77. Zhu Y, Pan Y, Guo Z, Jin D, Wang W, Liu M, et al. Photothermal enhanced and tumor microenvironment responsive nanozyme for amplified cascade enzyme catalytic therapy. Advanced Healthcare Materials (2023) 12(7):2202198. doi: 10.1002/adhm.202202198
78. Ember E, Rothbart S, Puchta R, van Eldik R. Metal ion-catalyzed oxidative degradation of orange ii by H 2 O 2. High Catalytic Activity Simple Manganese Salts. New J Chem (2009) 33(1):34–49. doi: 10.1039/B813725K
79. Poyton MF, Sendecki AM, Cong X, Cremer PS. Cu2+ Binds to phosphatidylethanolamine and increases oxidation in lipid membranes. J Am Chem Soc (2016) 138(5):1584–90. doi: 10.1021/jacs.5b11561
80. Pan Y, Zhu Y, Xu C, Pan C, Shi Y, Zou J, et al. Biomimetic yolk–shell nanocatalysts for activatable dual-modal-image-guided triple-augmented chemodynamic therapy of cancer. ACS Nano (2022) 16(11):19038–52. doi: 10.1021/acsnano.2c08077
81. Shi Y, Zeng L, Pan Y, Zhang H, Wang Z, Shi Y, et al. Endo/exo-genous dual-stimuli responsive gold nanotetrapod-based nanoprobe for magnetic resonance imaging and enhanced multimodal therapeutics by amplifying· Oh generation. Acta Biomaterialia (2022) 154:549–58. doi: 10.1016/j.actbio.2022.10.014
82. Feng W, Han X, Wang R, Gao X, Hu P, Yue W, et al. Nanocatalysts-augmented and photothermal-enhanced tumor-specific sequential nanocatalytic therapy in both nir-I and nir-ii biowindows. Advanced materials (Deerfield Beach Fla) (2019) 31(5):e1805919. doi: 10.1002/adma.201805919
83. Nascimento RA, Oüzel RE, Mak WH, Mulato M, Singaram B, Pourmand N. Single cell “Glucose nanosensor” Verifies elevated glucose levels in individual cancer cells. Nano Lett (2016) 16(2):1194–200. doi: 10.1021/acs.nanolett.5b04495
84. Wang M, Wang D, Chen Q, Li C, Li Z, Lin J. Recent advances in glucose-oxidase-based nanocomposites for tumor therapy. Small (2019) 15(51):1903895. doi: 10.1002/smll.201903895
85. Yang C, Younis MR, Zhang J, Qu J, Lin J, Huang P. Programmable nir-ii photothermal-enhanced starvation-primed chemodynamic therapy using glucose oxidase-functionalized ancient pigment nanosheets. Small (2020) 16(25):2001518. doi: 10.1002/smll.202001518
86. Zhong Z, Liu C, Xu Y, Si W, Wang W, Zhong L, et al. Γ-fe2o3 loading mitoxantrone and glucose oxidase for ph-responsive chemo/chemodynamic/photothermal synergistic cancer therapy. Advanced Healthcare Materials (2022) 11(11):2102632. doi: 10.1002/adhm.202102632
87. Zhu L, Gui T, Song P, Li W, Wang J, Hu C, et al. Metal–organic framework pcn-224 integrated with manganese dioxide, platinum nanoparticles, and glucose oxidase for enhanced cancer chemodynamic therapy. ACS Appl Nano Materials (2023) 6(9):7446–55. doi: 10.1021/acsanm.3c00610
88. Peng F, Tu Y, Van Hest JC, Wilson DA. Self-guided supramolecular cargo-loaded nanomotors with chemotactic behavior towards cells. Angewandte Chemie Int Edition (2015) 54(40):11662–5. doi: 10.1002/anie.201504186
89. Pidamaimaiti G, Huang X, Pang K, Su Z, Wang F. A microenvironment-mediated cu2o–mos2 nanoplatform with enhanced fenton-like reaction activity for tumor chemodynamic/photothermal therapy. New J Chem (2021) 45(23):10296–302. doi: 10.1039/D1NJ01272J
90. Antaris AL, Robinson JT, Yaghi OK, Hong G, Diao S, Luong R, et al. Ultra-low doses of chirality sorted (6, 5) carbon nanotubes for simultaneous tumor imaging and photothermal therapy. ACS nano (2013) 7(4):3644–52. doi: 10.1021/nn4006472
91. Lin H, Gao S, Dai C, Chen Y, Shi J. A two-dimensional biodegradable niobium carbide (Mxene) for photothermal tumor eradication in nir-I and nir-ii biowindows. J Am Chem Soc (2017) 139(45):16235–47. doi: 10.1021/jacs.7b07818
92. Wang X, Ma Y, Sheng X, Wang Y, Xu H. Ultrathin polypyrrole nanosheets via space-confined synthesis for efficient photothermal therapy in the second near-infrared window. Nano Lett (2018) 18(4):2217–25. doi: 10.1021/acs.nanolett.7b04675
93. Arrick BA, Nathan CF. Glutathione metabolism as a determinant of therapeutic efficacy: A review. Cancer Res (1984) 44(10):4224–32.
94. Lin LS, Song J, Song L, Ke K, Liu Y, Zhou Z, et al. Simultaneous fenton-like ion delivery and glutathione depletion by mno2-based nanoagent to enhance chemodynamic therapy. Angewandte Chemie (2018) 130(18):4996–5000. doi: 10.1002/ange.201712027
95. Tan J, Duan X, Zhang F, Ban X, Mao J, Cao M, et al. Theranostic nanomedicine for synergistic chemodynamic therapy and chemotherapy of orthotopic glioma. Adv Sci (Weinh) (2020) 7(24):2003036. doi: 10.1002/advs.202003036
96. Guo QL, Dai XL, Yin MY, Cheng HW, Qian HS, Wang H, et al. Nanosensitizers for sonodynamic therapy for glioblastoma multiforme: current progress and future perspectives. Mil Med Res (2022) 9(1):26. doi: 10.1186/s40779-022-00386-z
97. Rosenthal I, Sostaric JZ, Riesz P. Sonodynamic therapy—-a review of the synergistic effects of drugs and ultrasound. Ultrasonics sonochemistry (2004) 11(6):349–63. doi: 10.1016/j.ultsonch.2004.03.004
98. Lin X, Song J, Chen X, Yang H. Ultrasound-activated sensitizers and applications. Angewandte Chemie Int Edition (2020) 59(34):14212–33. doi: 10.1002/anie.201906823
99. Wu Z, Cheng K, Shen Z, Lu Y, Wang H, Wang G, et al. Mapping knowledge landscapes and emerging trends of sonodynamic therapy: A bibliometric and visualized study. Front Pharmacol (2022) 13:1048211. doi: 10.3389/fphar.2022.1048211
100. Li J-H, Song D-Y, Xu Y-G, Huang Z, Yue W. In vitro study of haematoporphyrin monomethyl ether-mediated sonodynamic effects on C6 glioma cells. Neurological Sci (2008) 29:229–35. doi: 10.1007/s10072-008-0972-8
101. Li H, Shi W, Huang W, Yao E-P, Han J, Chen Z, et al. Carbon quantum dots/tio X electron transport layer boosts efficiency of planar heterojunction perovskite solar cells to 19%. Nano Lett (2017) 17(4):2328–35. doi: 10.1021/acs.nanolett.6b05177
102. McHale AP, Callan JF, Nomikou N, Fowley C, Callan B. Sonodynamic therapy: concept, mechanism and application to cancer treatment. Ther Ultrasound (2016) 880:429–50. doi: 10.1007/978-3-319-22536-4_22
103. Bilmin K, Kujawska T, Grieb P. Sonodynamic therapy for gliomas. Perspectives and prospects of selective sonosensitization of glioma cells. Cells (2019) 8(11). doi: 10.3390/cells8111428
104. D'Ammando A, Raspagliesi L, Gionso M, Franzini A, Porto E, Di Meco F, et al. Sonodynamic therapy for the treatment of intracranial gliomas. J Clin Med (2021) 10(5). doi: 10.3390/jcm10051101
105. Lv Z, Jin L, Cao Y, Zhang H, Xue D, Yin N, et al. A nanotheranostic agent based on nd(3+)-doped yvo(4) with blood-brain-barrier permeability for nir-ii fluorescence imaging/magnetic resonance imaging and boosted sonodynamic therapy of orthotopic glioma. Light Sci Appl (2022) 11(1):116. doi: 10.1038/s41377-022-00794-9
106. Prada F, Sheybani N, Franzini A, Moore D, Cordeiro D, Sheehan J, et al. Fluorescein-mediated sonodynamic therapy in a rat glioma model. J Neurooncol (2020) 148(3):445–54. doi: 10.1007/s11060-020-03536-2
107. Sun Y, Wang H, Zhang K, Liu J, Wang P, Wang X, et al. Sonodynamic therapy induces oxidative stress, DNA damage and apoptosis in glioma cells. RSC Adv (2018) 8(63):36245–56. doi: 10.1039/c8ra07099g
108. Wu H, Gao X, Luo Y, Yu J, Long G, Jiang Z, et al. Targeted delivery of chemo-sonodynamic therapy via brain targeting, glutathione-consumable polymeric nanoparticles for effective brain cancer treatment. Adv Sci (Weinh) (2022) 9(28):e2203894. doi: 10.1002/advs.202203894
109. Zhu M, Wu P, Li Y, Zhang L, Zong Y, Wan M. Synergistic therapy for orthotopic gliomas via biomimetic nanosonosensitizer-mediated sonodynamic therapy and ferroptosis. Biomater Sci (2022) 10(14):3911–23. doi: 10.1039/d2bm00562j
110. Liu H, Zhou M, Sheng Z, Chen Y, Yeh CK, Chen W, et al. Theranostic nanosensitizers for highly efficient mr/fluorescence imaging-guided sonodynamic therapy of gliomas. J Cell Mol Med (2018) 22(11):5394–405. doi: 10.1111/jcmm.13811
111. Wang F, Xu L, Wen B, Song S, Zhou Y, Wu H, et al. Ultrasound-excited temozolomide sonosensitization induces necroptosis in glioblastoma. Cancer Lett (2023) 554:216033. doi: 10.1016/j.canlet.2022.216033
112. Petroni G, Cantley LC, Santambrogio L, Formenti SC, Galluzzi L. Radiotherapy as a tool to elicit clinically actionable signalling pathways in cancer. Nat Rev Clin Oncol (2022) 19(2):114–31. doi: 10.1038/s41571-021-00579-w
113. Chandra RA, Keane FK, Voncken FEM, Thomas CR Jr. Contemporary radiotherapy: present and future. Lancet (2021) 398(10295):171–84. doi: 10.1016/s0140-6736(21)00233-6
114. Chinnaiyan AM, Prasad U, Shankar S, Hamstra DA, Shanaiah M, Chenevert TL, et al. Combined effect of tumor necrosis factor-related apoptosis-inducing ligand and ionizing radiation in breast cancer therapy. Proc Natl Acad Sci USA (2000) 97(4):1754–9. doi: 10.1073/pnas.030545097
115. Giulietti A. Laser-Driven Particle Acceleration for Radiobiology and Radiotherapy: Where We Are and Where We Are Going: SPIE. (Bellingham: Conference on medical applications of laser-generated beams of particles IV) (2017).
116. Imai R, Kamada T, Araki N, Abe S, Iwamoto Y, Ozaki T, et al. Carbon ion radiation therapy for unresectable sacral chordoma: an analysis of 188 cases. Int J Radiat Oncol Biol Phys (2016) 95(1):322–7. doi: 10.1016/j.ijrobp.2016.02.012
117. Hall S, Rudrawar S, Zunk M, Bernaitis N, Arora D, McDermott CM, et al. Protection against radiotherapy-induced toxicity. Antioxidants (2016) 5(3):22. doi: 10.3390/antiox5030022
118. Kusumoto T, Ogawara R, Igawa K, Baba K, Konishi T, Furusawa Y, et al. Scaling parameter of the lethal effect of mamMalian cells based on radiation-induced oh radicals: effectiveness of direct action in radiation therapy. J Radiat Res (2020) 62(1):86–93. doi: 10.1093/jrr/rraa111
119. Liew H, Mein S, Debus J, Dokic I, MaIrani A. Modeling Direct and Indirect Action on Cell Survival after Photon Irradiation under Normoxia and Hypoxia. Int J Mol Sci (2020) 21(10):3471. doi: 10.3390/ijms21103471
120. Borek C. Antioxidants and radiation therapy. J Nutr (2004) 134(11):3207S–9S. doi: 10.1093/jn/134.11.3207S
121. Wang H, Mu X, He H, Zhang X-D. Cancer radiosensitizers. Trends Pharmacol Sci (2018) 39(1):24–48. doi: 10.1016/j.tips.2017.11.003
122. Pan Y, Tang W, Fan W, Zhang J, Chen X. Development of nanotechnology-mediated precision radiotherapy for anti-metastasis and radioprotection. Chem Soc Rev (2022) 51:9759–9830. doi: 10.1039/D1CS01145F
123. Erel-Akbaba G, Carvalho LA, Tian T, Zinter M, Akbaba H, Obeid PJ, et al. Radiation-induced targeted nanoparticle-based gene delivery for brain tumor therapy. ACS Nano (2019) 13(4):4028–40. doi: 10.1021/acsnano.8b08177
124. Li R, Wang H, Liang Q, Chen L, Ren J. Radiotherapy for glioblastoma: clinical issues and nanotechnology strategies. Biomater Sci (2022) 10(4):892–908. doi: 10.1039/d1bm01401c
125. Liang HT, Lai XS, Wei MF, Lu SH, Wen WF, Kuo SH, et al. Intratumoral injection of thermogelling and sustained-release carboplatin-loaded hydrogel simplifies the administration and remains the synergistic effect with radiotherapy for mice gliomas. Biomaterials (2018) 151:38–52. doi: 10.1016/j.biomaterials.2017.10.015
126. Verry C, Dufort S, Barbier EL, Montigon O, Peoc'h M, Chartier P, et al. Mri-guided clinical 6-mv radiosensitization of glioma using a unique gadolinium-based nanoparticles injection. Nanomedicine (Lond) (2016) 11(18):2405–17. doi: 10.2217/nnm-2016-0203
127. Ambrosio S, Majello B. Autophagy roles in genome maintenance. Cancers (2020) 12(7):1793. doi: 10.3390/cancers12071793
128. Fan W, Huang P, Chen X. Overcoming the achilles' Heel of photodynamic therapy. Chem Soc Rev (2016) 45(23):6488–519. doi: 10.1039/C6CS00616G
129. Liu Y, Chen K, Yang Y, Shi P. Glucose oxidase-modified metal–organic framework for starving-enhanced chemodynamic therapy. ACS Appl Bio Materials (2023) 6(2):857–64. doi: 10.1021/acsabm.2c01004
Keywords: reactive oxygen species (ROS), nanomedicine, glioma, blood-brain barrier (BBB), photodynamic therapy
Citation: Wu D, Chen X, Zhou S and Li B (2023) Reactive oxidative species (ROS)-based nanomedicine for BBB crossing and glioma treatment: current status and future directions. Front. Immunol. 14:1241791. doi: 10.3389/fimmu.2023.1241791
Received: 17 June 2023; Accepted: 21 August 2023;
Published: 04 September 2023.
Edited by:
Yu-Hang Zhang, Channing Division of Network Medicine and Brigham and Women’s Hospital, United StatesReviewed by:
Haiyang Wu, Tianjin Medical University, ChinaCopyright © 2023 Wu, Chen, Zhou and Li. This is an open-access article distributed under the terms of the Creative Commons Attribution License (CC BY). The use, distribution or reproduction in other forums is permitted, provided the original author(s) and the copyright owner(s) are credited and that the original publication in this journal is cited, in accordance with accepted academic practice. No use, distribution or reproduction is permitted which does not comply with these terms.
*Correspondence: Bin Li, bGliaW55dWhhbmdAMTYzLmNvbQ==
Disclaimer: All claims expressed in this article are solely those of the authors and do not necessarily represent those of their affiliated organizations, or those of the publisher, the editors and the reviewers. Any product that may be evaluated in this article or claim that may be made by its manufacturer is not guaranteed or endorsed by the publisher.
Research integrity at Frontiers
Learn more about the work of our research integrity team to safeguard the quality of each article we publish.