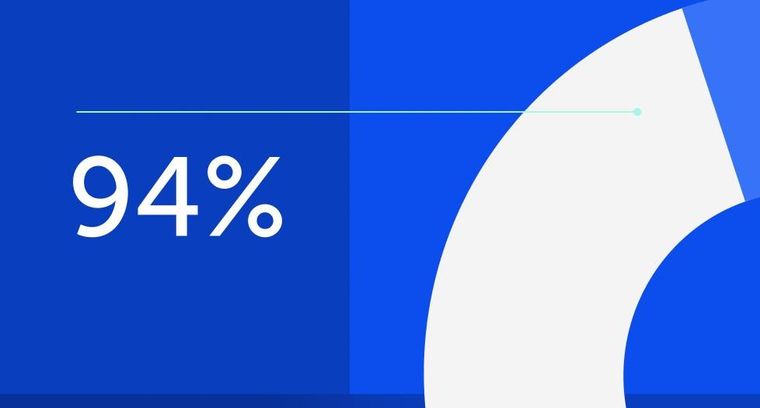
94% of researchers rate our articles as excellent or good
Learn more about the work of our research integrity team to safeguard the quality of each article we publish.
Find out more
REVIEW article
Front. Immunol., 23 October 2023
Sec. Molecular Innate Immunity
Volume 14 - 2023 | https://doi.org/10.3389/fimmu.2023.1227833
This article is part of the Research TopicThe Role of Toll-like Receptors and their Related Signaling Pathways in Viral Infection and InflammationView all 14 articles
Toll-like receptors (TLRs) are pattern recognition receptors (PRRs) expressed in various immune cell types and perform multiple purposes and duties involved in the induction of innate and adaptive immunity. Their capability to propagate immunity makes them attractive targets for the expansion of numerous immunotherapeutic approaches targeting cancer. These immunotherapeutic strategies include using TLR ligands/agonists as monotherapy or combined therapeutic strategies. Several TLR agonists have demonstrated significant efficacy in advanced clinical trials. In recent years, multiple reports established the applicability of TLR agonists as adjuvants to chemotherapeutic drugs, radiation, and immunotherapies, including cancer vaccines. Cancer vaccines are a relatively novel approach in the field of cancer immunotherapy and are currently under extensive evaluation for treating different cancers. In the present review, we tried to deliver an inclusive discussion of the significant TLR agonists and discussed their application and challenges to their incorporation into cancer immunotherapy approaches, particularly highlighting the usage of TLR agonists as functional adjuvants to cancer vaccines. Finally, we present the translational potential of rWTC-MBTA vaccination [irradiated whole tumor cells (rWTC) pulsed with phagocytic agonists Mannan-BAM, TLR ligands, and anti-CD40 agonisticAntibody], an autologous cancer vaccine leveraging membrane-bound Mannan-BAM, and the immune-inducing prowess of TLR agonists as a probable immunotherapy in multiple cancer types.
Emerging strategies in cancer immunotherapy, including immune checkpoint inhibitors (ICIs), cancer vaccines, and chimeric antigen receptor-T cells (CAR-T), have shown exceptional promise in clinical trials, giving rise to a plethora of ongoing research and development in this field (1). ICIs, including antibodies targeting anti-CTLA4 and anti-PD-1/PDL-1, have significantly progressed in various clinical trials to treat diverse cancer types (1). Meanwhile, adoptive cell therapies like CAR-T have shown promising results in treating multiple hematopoietic malignancies (2). Additionally, regulatory agencies have approved several preventive and therapeutic cancer vaccines for treating different cancers with numerous other vaccines in various development stages (3–7). These immunotherapies have underscored the importance of stimulating a robust anti-tumor immune response in cancer patients as a potential avenue for combating cancer. Exploring and harnessing appropriate immunostimulatory mechanisms is crucial in developing new cancer immunotherapy approaches. Toll-like receptors (TLRs) are a particular group of membrane receptor molecules that play the above-mentioned immunostimulatory functions in several innate immunity pathways (8). Consequently, TLRs are some of the most sought-after molecules used as vaccine adjuvants and in several immunotherapeutic approaches related to preventing and treating several infectious diseases, including cancer (8).
The inception of cancer immunotherapy dates back over a century, with the initial attempts involving using bacteria or bacterial products to activate the immune system (9–11). In 1891, William Coley pioneered the field of immunotherapy by administering a blend of heat-inactivated Streptococcus pyogenes (a Gram-positive bacteria) and Serratia marcescens (a Gram-negative bacteria) through intratumoral injections (9–11). This bacterial mixture became known as Coley’s toxin later (11, 12). This approach by Coley achieved a robust immune response against sarcomas, resulting in reduced tumor growth and, in some cases, tumor elimination even though the inherent mechanism remained unclear at that time (10–12). Subsequent research elucidated this therapeutic response, unveiling the significance of unique signaling molecules like pattern recognition receptors (PRRs) and pathogen-associated molecular patterns (PAMPs), which serve as ligands or activators for PRRs (11, 13–15).
TLRs are a subclass of receptors from the PRR family and serve as central players in innate immune responses (16). TLRs are transmembrane domain proteins (type I) with tripartite motifs (16, 17). TLRs feature three distinct functional domains: an leucine-rich repeats (LRRs) containing amino (N)-terminal responsible for ligand binding (folded into a typical horseshoe-like structure), a transmembrane spanning region, and a carboxyl (C)-terminal cytoplasmic domain resembling the cytoplasmic region of globular Toll/interleukin-1 (IL-1) receptor (TIR) (16, 17). To date, ten human and thirteen murine TLRs have been identified (18). Based on their subcellular localization, TLRs are categorized into extracellular and intracellular groups (16, 19). TLRs such as TLR1, TLR2, TLR5, TLR6, and TLR10 are exclusively expressed on the plasma membrane and belong to the extracellular group; while TLR3, TLR7, TLR8, and TLR9 fall within the intracellular group, are expressed on the endosome and endoplasmic reticulum (16, 19). Only TLR4 is present in both intracellular components and the plasma membrane (16, 19). For each of the TLRs, there is a specific ligand(s) (Figure 1, Table 1). Every TLR with its ligand activates specific downstream signaling pathways either through myeloid differentiation primary response protein 88 (MyD88) and/or TIR-domain-containing adapter-inducing IFNβ (TRIF) (16, 20, 39–41). TLR-mediated signaling initiates the secretion of multiple cytokines that enhance the immune system’s ability to combat external pathogens and infectious agents (16, 17, 39). Moreover, TLRs play a crucial role in activating and maturing various immune cells involved in innate and adaptive immune responses (16–18). We have listed the location of all the TLRs and their agonists in Figure 1. Moreover, we have provided a detailed classification, localization, and involved ligands of the TLRs in Table 1.
Figure 1 Location and agonists of different TLRs. TLRs 1/2, 2, 2/6, 4, and 5 are present in the extracellular region of the plasma membrane. In contrast, TLRs 3, 7, 8(only in humans), and 9 are localized on the endosomal membrane. The TLRs are stimulated by their specific ligand or agonists. The extracellular TLRs are mainly activated by exogenous agonists of bacterial, viral or pathogenic origin. The major exogenous ligands or microbial agonists are flagellin protein from bacterial flagella, lipoteichoic acid (LTA) and peptidoglycan (PGN) from Gram-positive bacteria, LPS from Gram-negative bacteria, lipoarabinomannan (LAM), lipopeptides, lipoglycans, and lipomannans from mycobacteria, zymosan from yeast (20, 21). There are synthetic TLR agonists like Pam3CSK4 or recombinant flagellin to stimulate the extracellular TLRs (20, 21). In contrast, nucleic acid ligands stimulate the endosomal TLRs 3, 7, 8, and 9. For example, TLR3 is stimulated by viral double-stranded RNA (dsRNA); TLR7 and 8 are triggered by viral and bacterial single-stranded RNA (ssRNA), and TLR9 recognizes CpG DNA from viruses and bacteria (20–25). There are also some endogenous ligands resulting from cellular injury, cell death, extracellular matrix components (e.g., hyaluronan, fibronectin, and fibrinogen), plasma membrane constituents, nuclear and cytosolic proteins and heat shock proteins, and elements of damaged/fragmented organelles such as mitochondrial DNA (mtDNA) (26, 27). TLRs 2 and 4 bind to most of the endogenous ligands resulting from cellular injury, cell death, and extracellular matrix components (27). The endogenous nucleic acid ligands like RNA or mtDNA bind with the endosomal ligands TLRs 3, 7, 8, and 9 (26, 27).
This review aims to consolidate the current strategies involving TLR agonists as potential therapeutics for cancer, either as standalone treatments, in combination therapies or as adjuvants for cancer vaccines. We also explore the supporting evidence for TLR agonists as adjuvants in cancer vaccines, promoting innate and adaptive immune responses against cancer cells, specifically focusing on the rWTC-MBTA autologous vaccine developed by our research group.
It’s crucial to note that TLRs are present in various types of cells, encompassing innate immune system components such as macrophages, neutrophils, dendritic cells (DCs), natural killer (NK) cells, and mast cells. They are also found in adaptive immune system elements like T and B lymphocytes, stromal cells, and various tumor cells. When TLRs engage with a ligand, they can significantly boost the expression of multiple costimulatory molecules on the cell membranes, activating cytokines and T-cell activation (16, 17). The following section briefly discusses how TLRs stimulate different immune cells and their role in regulating cancer immunity.
Dendritic cells (DCs) are widely recognized as the immune system’s most efficient professional antigen-presenting cells (APCs) (42). DCs are also the most studied cells among all the TLR-expressing immune cells in the milieu of instigation of adaptive immunity (42). When faced with an infection or inflammation, immature DCs undergo a process of activation and transformation into mature DCs. These mature DCs are responsible for activating adaptive immune cells such as B and T lymphocytes (43). The maturation of DCs involves a series of complex stages, including changes in the composition of receptors involved in endocytosis and phagocytosis, increased expression of co-stimulatory molecules like CD40, CD58, and CD86, alterations in morphology, and reorganization of lysosomal and MHC compartments (44). It’s important to note that the DC population is highly diverse, consisting of various subtypes that exhibit differences in their functions, phenotypes, and distribution within the body (45). The two central populations of DCs found in the human immune system are lymphoid‐derived plasmacytoid dendritic cells (pDCs) and myeloid‐derived dendritic cells (mDCs) (45). Both pDCs and mDCs can activate the CD4+ and CD8+ T cells as well as facilitate the process of antigen cross-presentation to initiate the proliferation of CD8+ T cells (46–48). Other phenotypes of DCs are monocyte-derived DCs (moDCs) and CD34+ cell–derived DCs (49). The activation status of dendritic cells is critical in determining how the immune system responds to a particular threat. All the subsets of DCs express distinctive TLRs, permitting themselves to generate a dedicated response against different pathogens (44). The major TLRs involved in DC maturation and function are TLR2, 3, 4, 5, 7/8, and 9 (50). TLR signaling is the key to DC-mediated cytotoxic T-cell activation (50). Previous studies demonstrated that TLR-mediated stimulation augments maturation and antigen presentation of murine DCs followed by induction of cytotoxic T cells (51, 52). Maturation of DC sub-populations and onset of cytotoxic CD8+ T cells through IL-27-mediated signaling were reported after ligand mediated activation of TLR3 and TLR7 (51, 52). As suggested by the distinct Toll-like receptor (TLR) expression profiles in different DC subsets, the pDCs are primarily activated by viral pathogens, while mDCs primarily respond to fungal and bacterial antigens, and these functional characteristics are exploited in DC-mediated vaccination and immunotherapy (53, 54). Similarly, the anti-tumor outcome of TLR7 activation was evident in central nervous system tumors, increasing maturation of DCs and activating tumor specific cytotoxic CD8+ T cells (55). Another report demonstrated TLR mediated enrichment of the Th1 microenvironment and promoted activation of cytotoxic T-cells via IFN-λ-induced IL-12 released by breast cancer-associated dendritic cells (56). TLR4 activation also induced anti-colorectal cancer T cell response in vitro through DC maturation (57). Likewise, activation of TLR-4 and processing of tumor antigens stimulate DC maturation to markedly increasing in vivo CD8+IFNγ+ cytotoxic T cells (58). Interestingly, activating TLR7 and 8 was a crucial step in promoting the maturation of DCs isolated from AML patients and subsequently activating cytotoxic T cells in vitro (59). Interestingly, TLR7 signaling activated plasmacytoid dendritic cells (pDCs) leading to killing of murine melanoma cells through stimulation of NK cells and activating CD8+ cytotoxic T-cells (60). Similarly, in ALL patients, activation of pDCs vial TLR9 molecules led to increased IFN production, which stimulated NK cells through TRAIL and CD69-mediated signaling (61). An earlier report about DC-targeted vaccines, demonstrated CD8+ T cell response and better therapeutic efficacy after the activation of DCs through TLR7/8 and TLR3 mediated signaling (62). The immunosuppression present in the tumor microenvironment (TME) impedes the success of cancer immunotherapy, and DCs are extremely important in generating anti-tumor immunity inside the TME (42). Research has shown that the tumor microenvironment (TME) can hinder the growth and differentiation of mDCs (63). However, multiple studies demonstrated that TLR-mediated signaling has the potential to reactivate the immune functions of these inhibited dendritic cells, and this method could be beneficial to effectively counteract immunosuppression in the TME, offering a new arrow in the quiver of immunotherapy (64, 65).
Macrophages were the first immune cells identified to uphold tissue homeostasis, facilitate tissue repair, orchestrate immune responses, and combat pathogens (66, 67). Subsequently, it became evident that they also infiltrate and inhabit tumor sites and influence tumor development (66, 67). In addition, macrophages can alter their transcriptional profile, display remarkable cellular plasticity, and modify their functions in response to various inflammatory, tissue-specific, external pathogenic, and environmental stimuli, leading to anti-tumor and pro-tumor effects (67). In inflammatory conditions, classifying the tumor-associated macrophages (TAM) is still a complex task (66, 67). Classically, the macrophages were mainly categorized into two polarization states: M1, with pro-inflammatory traits, and M2, with anti-inflammatory characteristics (66, 67). M2 macrophages were considered to support tissue remodeling, tumor growth, and cancer-related processes, including cell proliferation, invasion, metastasis, and immune suppression; whereas, M1 macrophages were designated to drive immune responses, cause tissue damage, and inhibit tumor growth by enhancing anti-tumor responses of T cells and natural killer cells (66, 67). Macrophages are one of the most influential players in the TME, rendering them as a vital point for cancer immunotherapy (68). The anti-inflammatory M2 subtype of macrophages supports tumor growth and maintenance, but the pro-inflammatory M1 subtype promotes inflammation and tumoricidal properties (68). Switching the M2 subtypes to M1 in the tumor microenvironment by stimulants can promote tumoricidal activity (69). Regrettably, this simplified classification of macrophages into just the M1 or M2 category failed to define the diverse range of macrophage polarization states present within tumors or the TME (70), leading to a modern classification of TAMs where M1 and M2 represent the extremes of a spectrum with numerous intermediate subsets (66, 67). In relation to the complex interactions between different cell types within the TME, the TAMs are now considered into two main subtypes, M1-like (pro-inflammatory macrophages) and M2-like (anti-inflammatory macrophages) (67). Stimulation of Toll-like receptors (TLRs) in macrophages has been long recognized as a mechanism that drives macrophages toward a pro-inflammatory phenotype, and this renders TLR agonists particularly attractive in the context of cancer immunotherapy (71). It’s noteworthy that since 2015, over 60 clinical trials have been initiated to assess the therapeutic potential of TLR agonists in treating various cancers (4). Activation of TLR3, recruits in vitro and in vivo IFN signaling cascade resulting in switching to M1 phenotype from M2 phenotype (69). The switching of M2 to M1 involves signaling associated with CD86, CD80, CD40, IL-12, IL-6, and TNF-α ensuing in enhanced antigen uptake by the macrophages and activation of T cells-mediated mice tumor growth regulation (72). Comparable anti-tumor results were detected in mice models of Lewis lung carcinoma and sarcoma following induction of TLR3 and TLR4 mediated signaling, respectively (73, 74). TLR4 was also suspected to promote the migration of macrophages through the upregulation of proinflammatory molecules like TNF-α, NF-κB, and VEGF (75). In a similar study, TLR-mediated signaling promoted the antitumor M1 phenotypes along with the upregulation of immunostimulatory cytokines like IL-18 (76). These immunostimulatory cytokines directed an antitumor collaboration between macrophages and NK cells ex vivo in ovarian cancer to stimulate IFN-γ secretion and Th1-type immune responses via NK cells (76). It is worth noting that TLR7/8 activation has been found to influence the differentiation of myeloid-derived suppressor cells (MDSCs) towards M1 phenotype within the tumor microenvironment, ultimately resulting in a regression of colorectal tumors in mice and a decrease in resistance to oxaliplatin (77). Oxaliplatin hindered the transformation of MDSCs into M1-like macrophages, but in combination with TLRs 7/8 agonist R-848, this hindrance was overcome (77). The addition of R-848 augmented the polarization of MDSCs to M1-like pro-inflammatory macrophages leading to increased apoptosis of the colorectal tumor cells (77). Furthermore, the stimulation of macrophages by TLR2/6 led to the activation of the NK cells and cytotoxic CD8+-T cells in several tumors, including metastasis mice models and pancreatic cancer (78, 79). This was accompanied by increased immune surveillance in tumors with concomitant increase of COX-2 expression in macrophages (78). COX-2 is the rate limiting enzyme of Prostaglandin E2 (PGE2) biosynthesis, and PGE2 is a strong suppressor of NK cells in the TME (78). Macrophage-activating lipopeptide-2 (MALP-2), a TLR2/6 agonist, enhances NK cell cytotoxicity towards the tumor cells, while the PGE2 mediated immunosuppression was blocked by COX-2 inhibitor (78).
NK or natural killer cells are a group of lymphocyte, an indispensable component of the innate immune system, and they are best recognized for killing pathogen or virus infected cells and also responsible to detect and regulating initial signs of cancerous tissues (80). NK cells are termed as the first rank of defense against cancer cells, with the capability to kill the cancer cells without any prior activation or priming. That is why they are named “natural killers” (80). Multiple reports documented that depending upon originating population, NK cells express almost all types of TLRs (81). Amid all the TLR ligands TLR3, 7, 8, and 9 mediated signaling demonstrated a significant role in cancer biology. Human NK cell lines for instance YTC12, YTS, and NK92 expressed high amounts of activated TLR3, causing cytotoxic killing effects on K562 cancer cells (82). Furthermore, head and neck squamous cell carcinoma (HNSCC) cells are killed via IFNγ secreting NK cells activated through TLR3 (82, 83). TLRs like TLR7, 8, and 9 can sense foreign nucleic acids, and their subsequent activation on NK cells empowers anti-tumor immune responses (84, 85). Though the activation of this nucleic acid-sensing, NK cell-associated TLRs are mostly dependent on the signaling induced by other cells present in the associated tumor microenvironment (84, 85). While there is some argument concerning the expression of TLR7 and 8 on NK cells (84, 85), several reports depicted the activation and proliferation of NK cells by the cytokines secreted from neighboring cells of the tumor microenvironment (84, 85). In addition to TLR9 stimulated cytotoxicity of NK cells on B16 melanoma cells, the secretion of inflammatory cytokines as IFNγ and IL-12 was promoted via TLR7/8 activation, which in order aided the NK cells to eliminate the HNSCC cells and B16-F10 melanoma cancer cells (86–88). Interestingly, another report showed an acceleration of antitumor activity of HER2(human epidermal growth factor receptor 2)-targeting monoclonal antibodies both in vivo and in vitro after TLR2-mediated activation of NK cells (89).
B cells are the production house of antigen-specific antibodies and considered as the epicenter of the adaptive immune system (90). To date, several distinct B-cell subsets have been identified performing diverse functions in both adaptive and innate immune responses (90). B cells express an array of TLRs, whose signaling is collaborated with the B cell receptor signaling (91). Signaling from TLRs like TLR7 and TLR8 are well documented to augment the antibody and cytokine production from B cells (90). This TLR-mediated stimulation of B cells depicted increased expression of B7 costimulatory molecules and amplified survival as like B cell activation by CD40 (90). Activated B cells were reported to secrete multiple chemokines and cytokines after stimulation of TLR1/2, TLR7, and TLR9 (92). TLR-mediated signaling enhances cytokine secretion, promotes better antigen presentation from B cells along with overexpression of the costimulatory molecules, and, which sequentially augments the activation of helper T cells (91). Moreover, multiple reports demonstrated the activation of effector functions of B cells by TLR signaling, e.g., proliferation, antibody production, and immunoglobulin class switching (93–95). While B cells are known for their ample Toll-like receptor (TLR) expression and their critical role in humoral immunity and the adaptive immune response, their potential for TLR-mediated utilization in cancer immunotherapy remains relatively unexplored (91–93). Nevertheless, there have been a few instances where TLR-mediated activation of B cells has been applied in the context of cancer immunotherapy. B cells use TLRs to coordinate antibody responses during infection and autoimmune diseases, where the B cell receptors (BCR) and TLR7 or 9 are activated in response to self-antigens complexed with nucleic acids, such as RNA or DNA-containing immune complexes (96). This TLR-mediated stimulation can also be harnessed to generate tumor-specific antigen (TSA)-specific responses (44, 50). When high levels of antibodies are required for protection, be it infection or anti-tumor immunity, targeting TLRs on B cells can prove to be an effective strategy to enhance antibody production (97). The presence of tumor-specific antigens (TSAs) is essential for activating T cell and B cell immunity (98). Notably, B cells are the sole immune cells that consistently express TLR9 (97). Several studies have demonstrated that TLR9 agonists can induce significant anti-tumor immunity by activating B cells. TLR9 agonists endorse the differentiation of B cells into plasma cells and enhance antibody-dependent cellular cytotoxicity (ADCC) (99, 100). Brody et al. (101) reported clinically significant anti-B cell lymphoma responses following in-situ tumor vaccination with a TLR9 agonist. These studies underscore the efficacy and advantages of administering TLR agonists directly at the tumor site rather than systemically. TLR9 ligands like CpG-ODNs have shown great potential in stimulating B cell-mediated adaptive immunity (102–104). CpG-ODNs strongly induce B cell proliferation, activate plasmacytoid dendritic cells (pDCs) and monocyte maturation, stimulate natural killer (NK) cell activation, and trigger the production of inflammatory cytokines (102). B cell stimulation by CpG-ODNs increases their sensitivity to antigen stimulation and promotes their differentiation into antibody-secreting plasma cells, resulting in increased production of antigen-specific antibodies (103). TAC-001, an antibody-ODN conjugate consisting of a specialized TLR9 agonist (T-CpG) linked to an antibody against CD22 (a receptor restricted to B cells), is designed to deliver potent and targeted immune activation through systemic administration (104). In vitro stimulation of B cells with TAC-001 leads to increased expression of co-stimulatory molecules, immunoglobulin secretion, and cross-presentation, ultimately leading to T cell proliferation (104). TAC-001 has demonstrated efficient and durable single-agent anti-tumor activity in checkpoint inhibitor-resistant and refractory murine tumor models (104). Systemic administration of TAC-001 in mice has resulted in increased B cell infiltration, enhanced T cell effector functions, modulation of myeloid-derived suppressor cells (MDSCs), and a significant decrease in IL-10+ regulatory B cells within the tumor microenvironment (104). Intravenous administration of TAC-001 in monkeys has shown favorable tolerability, pharmacokinetics, and pharmacodynamic profiles (104). Additionally, TLR9 activation in B cells leads to the expression of co-stimulatory molecules, enhancing cross-presentation, and allowing for the activation and proliferation of T cells, as well as the secretion of chemokines, cytokines, and immunoglobulins (3). Among other TLR9 ligands, Lefitolimod (MGN1703) has been utilized in several preclinical studies to assess B cell-mediated immunity. Multiple studies have demonstrated that MGN1703 significantly activates both innate and adaptive immune cells, including B cells, and induces the secretion of various inflammatory cytokines (IL-6, IL-8, IFN-α, and IFN-γ) and chemokines (CD40, CD69, CD86, CD169, and IP-10) from activated immune cells (105, 106). The combination therapy of TLR3 agonist with a TLR9 agonist (CpG: 5’-cytosine-phosphate-guanine-3’) along with adoptive T cell transfer (ACT) has shown promise in increasing the abundance of various immune cell types, including B cells with CD4+ and CD8+ T cells, macrophages, neutrophils, and NK cells in tumor-draining lymph nodes (107). This combination therapy has augmented the elimination of murine melanoma cells and improved the survival of tumor-bearing mice, doubling their survival compared to untreated mice (107). Combination therapy involving TLR9 agonists and immune checkpoint inhibitors (ICIs) has also shown promising effects in clinical studies (108). For instance, Ribas et al. evaluated the safety and anti-tumor activity of co-treatment with intratumoral SD-101, a synthetic CpG oligonucleotide ligand for TLR9, and pembrolizumab in patients with melanoma (108). This combination therapy was well tolerated and improved overall survival, accompanied by a significant increase in B cells within the tumor microenvironment (TME), as well as other immune cell populations (108). These results indicate that combining SD-101 administration with PD-1 blockade potentially enhances clinical efficacy and reduces PD-1 blockade-related toxicity (108). Apart from TLR9, monophosphoryl lipid A (MPLA), a TLR4 ligand derived from the lipopolysaccharide (LPS) of Salmonella Minnesota, is used as an adjuvant in a prophylactic vaccine against human papillomavirus types 16 and 18, which are common causes of cervical cancer (109). As an adjuvant, MPLA enhances the antigen-presenting capabilities of macrophages and B cells, primes naive T cells, induces the maturation of dendritic cells, and stimulates antibody production (109).
The effector T cells carry out multiple functions of the immune responses, like cytotoxicity, helper, and regulatory (110). Diverse types of effector T-cells express different TLRs, which can consequently regulate associated T-cell functions and antitumor immune activities (111). TLR1, 2, 5, 7, and 8 mediated signaling is identified to activate the proliferation of CD4+ memory T-cells and upregulate accompanying cytokine secretion (112). For example, the presence of a higher amount of TLR5 agonists amplified CD4+ T-cell population and concomitant expression of the cytokine IL2 (112). Activation of multiple TLRs like TLR2, 3, and 9 in purified B6 expressing CD4+ T cells provides costimulatory signals aimed at T cell receptor (TCR) activation. NF-κB signaling through TLR2-mediated signaling in CD8+ T cells and TLR9 activation in CD4+ T cells inhibits apoptosis and promotes survival (113). In a similar study, activation of TLR7 along with TLR8 on CD4+ T-cells enhanced the secretion of IFNγ, IL-2, and IL-10; in addition to proliferation of T cells (114). Interestingly, the antitumor commotion of CD8+ T-cells was promoted via glucose-uptake-dependent MyD88 and AKT-mTOR pathway through activation of TLR7 (115). It is well established that stimulation of immune cells like NK cells, DCs, and Tregs can control the CD8+ effector T cell functions (51, 52, 82); similarly, multiple TLRs can control multiple functional characteristics of CD8+ T-cells directly inside the tumor microenvironment (116). Activation of TLR1/2 promotes the effector activity of CD8+ T cells in B16 melanoma cells both in vivo and in vitro through upregulation of perforin, Granzyme B, IFNγ and TNF-α (116). In addition, effector CD8+ T cell functions along with increased IFNγ expression as a functional coactivator was also reported to be regulated by activation of TLR3 (117). Besides, transgenic OT-1 (CD8+) T cells were stimulated through an antigen-independent manner after activation of TLR3, as measured by in vitro assays, leading to increased expression and robust expansion of immune-activation markers in vivo (118).
The immunosuppressive activity of both human and murine regulatory T-cells (Tregs) can be modulated by some of the TLRs, and it is documented in multiple reports. TLR4 activation on Tregs enhanced their viability and immunosuppressive commotion (112). Also, a slight ligand-mediated activation of TLR5 increased the Treg marker FOXP3 on CD4+CD25+ Treg cells and increases their immunosuppressive aptitude slightly (112). There is also a contrasting report of reverting Treg’s suppressive properties in vivo through the TLR8-MyD88-IRAK4 signaling cascade (119). There are some controversies regarding the reversion of suppressive activity of Tregs after TLR2 activation even though multiple reports depicted an increase in Treg proliferation after TLR2 activation (120, 121). For instance, activation of TLR2, inhibited the immunosuppressive activity of Tregs though it increased proliferation of Treg cells in vivo (122). In the tumor microenvironment (TME), activation of immunosuppressive cells like tolerogenic dendritic cells (DCs) and Treg cells is vital for establishing immunosuppression (123). Using antibodies to inhibit Treg cell function is an initial approach to enhance the effectiveness of cancer vaccines by reducing TME’s immunosuppressive effects and boosting effector T cell function (124). Consistently, administering a DC vaccine with the TLR4 agonist LPS resulted in a notable increase in NK cells and a significant reduction in Treg cells within the tumor microenvironment in an ovarian cancer mouse model (125). TLR ligands can also trigger Th1 inflammatory cytokines like IL-12, which facilitate the transition of CD4+ T cells from Th2 to Th1 subtype, boost CD8+ T cell responses, and suppress Treg cell function (126). TLR3 ligands were also reported to overturn the immunosuppressive TME towards anti-tumor immunity through modulation of the Treg cells (127, 128). TLR3 ligand poly A:U shifts the immunosuppressive tumor microenvironment towards anti-tumor immunity by altering the composition in favor of antigen-specific CD8+ granzyme B+ T cells, resulting in a lower Treg/CD8+ cell ratio (127). Salazar et al. reported that administration of poly-ICLC induced in situ vaccination in a rhabdomyosarcoma patient induced local tumor inflammation and a systemic immune response, leading to a significant reduction in a facial tumor (128). Their research revealed that tumor regression was a result of the activation of both local and systemic anti-cancer immunity triggered by intratumoral and intramuscular poly-ICLC injections. Their findings suggest that intramuscular poly-ICLC maintenance therapy contributes to a systemic anti-tumor immune response through the induction of chemokines, co-stimulatory molecules, inflammasome formation, and an increase in the Teff/Treg cell ratio (128). In addition to TLR3, TLR7 agonists also inhibit Treg cell function, and activate NK cells, promoting anti-cancer immune responses (87, 129). Topical imiquimod application in a melanoma mouse model reduces Treg cell-related chemokine mRNA expression and increases cytotoxic molecules like granzyme B and perforin within tumors (130). Imiquimod also decreases Tregs and boosts CD8+ cells in the tumor microenvironment (TME) (130). Intratumoral administration of SZU101(another TLR7 agonist) triggers a systemic anti-tumor response and alters the TME by increasing CD4+ and CD8+ cells while reducing Treg cells in a murine breast tumor model (131). Intraperitoneal injection of the TLR7 agonist resiquimod in mice with pancreatic ductal adenocarcinoma (PDAC) tumors reduces Tregs in the TME, enhances activation, infiltration, and cytotoxicity of CD8+ T cells, suppressing tumor growth and improving survival (65). Combination therapy of radiation and imiquimod decreases Treg cells and MDSCs while increasing CD4+ and CD8+ T cell recruitment in the TME, commencing systemic anti-cancer responses and potentially limiting metastasis ultimately leading to increased survival (132). Administrating imiquimod as adjuvant preceding HPV vaccination enhances intratumoral CD4+ and CD8+ T cell infiltration while reducing Treg cells in the TME (133). Importantly, the effectiveness of the vaccination correlates with pre-existing and post-treatment (with imiquimod) Treg cell levels (133). Combining PD-L1 blockade with resiquimod reduces tumor size, activates DCs, diminishes Treg cells, and boosts the CD8+ T cell/Treg cell ratio in the TME in mice tumor models (134). Furthermore, in the case of mice PDAC derived tumors, resiquimod elicited a robust immune response characterized by heightened immune complexity, reduced growth, enhanced infiltration of CD8+ T cells, and a lowered frequency of intratumoral CD4+CD25+FOXP3+ Treg cells (135). TLR9 ligands were also reported to modulate the TME via the suppression of Treg cells. CpG-A, the TLR9 ligand, induces IFNα and IFNβ production, promoting effector CD4+ T cell proliferation by counteracting Treg cell suppression (119). In a mouse tumor model, adoptive transfer of Treg cells after pretreatment with poly-G10 (a TLR8 ligand) enhances antitumor activity and reduces Treg cell suppression (119). Remarkably, this study also suggests that Treg cells express TLR9 and can recognize CpG DNA molecules (119). Furthermore, CpG ODNs reduce Treg cell population in the draining lymph node (136). Local administration of CpG enhances OX40 expression, a TNF receptor, on both effector T and Treg cells in the tumor microenvironment (TME) (137). PF-3512676, one of the earlier synthetic TLR9 agonists to treat melanoma patients, increases pDC and mDC frequency, along with the release of inflammatory cytokines, while markedly reducing Treg cell population in the sentinel lymph nodes (SLN) (138). In addition, SD-101 (TLR9 agonist) treatment also reduces numbers of Treg cells and T follicular helper cells within tumors (139).
With their ability to activate several innate immunity pathways, TLR ligands or agonists are considered compelling immunomodulators (44, 140, 141). Upon stimulation of TLRs by agonists, the downstream signaling also initiates enduring adaptive immune responses including cytotoxic NK cells, T-cells and maturation of DCs (140). Several TLR agonists demonstrated significant therapeutic efficacy against multiple ailments, including cancer (44, 141). Recent reports depicted improved efficacy of current immunotherapy approaches in cancer patients, such as cell-based immunotherapy combined with TLR agonists (19). TLR agonists are also known to sensitize cancer cells to conventional cancer therapies like radiation and chemotherapy (4, 140). Apart from combined therapy, TLR agonists are also administered as monotherapy in several malignancies (19). With growing information about several TLRs and involved TLR-agonists along with their downstream signaling pathways, several natural (resourced from microbes) or chemically synthesized TLR agonists, are being involved in cancer immunotherapy approaches (4, 140). But there are some early clinical setbacks in using TLR agonists as cancer therapeutics (142) because of the pro-tumorigenic nature of some TLRs in certain cancer types (44), and the activation of some of the TLRs led to an increase in tumor growth and metastasis (143). For this reason, it is necessary to determine the right tumor type and appropriate TLRs to be targeted and involved combinatorial approaches before treating any tumor with TLR-based immunotherapy. For the last two decades, TLR agonists have been used to stimulate and activate DCs in cancer immunotherapy (123). Moreover, in multiple vaccination strategies and immunotherapy approaches, TLRs can modify T-cell responses, which is revealed to be an excellent means to control and direct adaptive immunity (144, 145). One of the reasons for the growing popularity of TLR agonists for treating tumors is their aptitude to reinstate the activity of immunosuppressed DCs, which can be extremely useful for reversing the immunosuppressive atmosphere inside the TME (64, 65). TLR ligands have been widely utilized as adjuvants of anti-cancer vaccines, or in combination with other traditional standard of cares for cancer (19, 44). Some TLR agonists have shown great promise at the clinical scenarios (19, 44). In recent years, against poorly immunogenic tumors, synthetic TLR agonists performed markedly better as adjuvants of cancer vaccines by enhancing the Th1 or Th2-mediated immune response (146). In Table 2, we have listed all the current clinical trials where TLR agonists are used as adjuvant(s) to cancer vaccines (3, 4, 6, 7, 90).
At the next section of this review, we are trying to present and discuss multiple clinical and preclinical pieces of evidence which demonstrates that TLR agonists can significantly improve the therapeutic outcome in different types of cancer, either in combined immunotherapy or as a cancer vaccine adjuvant.
Bacillus Calmette-Guerin (BCG) is the first successful TLR ligand/agonist approved for cancer treatment (147, 148). More than 40 years ago, BCG was first approved by the US Food and Drug Administration (FDA) to treat bladder cancer (147, 148). BCG wields its anti-cancer effect by the dual activation of TLR2, and TLR4 (147, 148). BCG as an adjuvant with whole cell vaccines also has been widely assessed in melanoma and colorectal cancer (CRC) (149, 150). In a randomized Phase III trial, Canvaxin, an allogeneic melanoma vaccine utilizing BCG as an adjuvant, failed to improve both overall and disease-free survival, despite showing promise in phase II (149). Interestingly, in the same trial with resected stage-III and stage-IV melanoma, monotherapy of BCG in patients demonstrated improved efficacy (149, 150). OncoVAX, an autologous colon cancer vaccine with BCG as an adjuvant, in a phase II study showed significant improvement in disease-free and overall survival (150, 151). Similarly, anti-tumor effect generated through activation of TLR2 and 4, by OM-174 (CXR-526), a lipid A (Escherichia coli origin) derivative, is currently being evaluated as a vaccine adjuvant for the treatment of melanoma in phase I/II trials, in addition to a phase I trial against solid tumors (152). The observed results from a few preclinical studies depict the increase of TNF-α, IFN-γ, and iNOS behind the therapeutic activity of OM-174 administration (152, 153). Another lipid A derivative, monophosphoryl lipid A (MPL), also an activator of TLR4, is also used in several vaccines as an adjuvant (153, 154). Stimuvax, the liposomal cancer vaccine against the MUC1 tumor antigen, uses MPL as an adjuvant (153). Stimuvax underwent phase III evaluation against advanced NSCLC but failed to add any marked therapeutic advantage (153). A cancer vaccine targeting the MAGE A3 tumor antigen utilizes a MPL containing special mixed adjuvant system (AS15, AS02b) (155). Other TLR4 activators like AS04 (MPL derivative, cervical cancer) and GLA-SE (lymphoma Merkel cell carcinoma) are also studied via preclinical and clinical studies (156–158). Meanwhile, lipoteichoic acids (LTA) from Gram-negative bacterial cell walls, responsible for the “endotoxin” of bacteria, can also act as an agonist of TLR2 receptors and trigger anti-tumor immune responses (11, 14).
The activation of TLR3-mediated signaling by sense double-stranded RNA was first discovered by Alexopoulou et al. (159). Currently, the synthetic polynucleotide polyinosinic-polycytidylic acid or poly(I:C) is being used as a TLR3 agonist, and is known as a powerful activator of the innate immune responses (160). Poly(I:C) promoted the activation of DCs and subsequently enhanced antigen presentation for CD8+ cytotoxic T cells (161). Additionally, after stimulation via poly(I:C), DCs can indirectly activate NK cells and T-cells, generating robust antitumor immune responses, and for this reason poly(I:C) is often employed in cancer vaccines (162). Because of the associated toxic effects and hasty degradation of poly(I:C) in the body, several stable derivatives or variants of poly(I:C) are established through experimental studies (163, 164). For example, Hiltonol or poly-ICLC is stabilized through the addition of poly-lysine and was assessed in several clinical trials involving different types of solid tumors (165, 166). Though these clinical studies didn’t demonstrate significant anti-tumor efficacy but still it managed to be physiologically safe without any adverse side effects (165, 166). Additionally, rintatolimod or poly(I:C12U) (Ampligen), another poly(I:C) derivative, is stabilized by the substitution of cytidine with uridine and is approved for the treatment of pancreatic, triple-negative breast cancer, and brain tumors in combinatorial therapy with some vaccines demonstrated substantial efficacy (167–169). Poly(I:C) and all of its derivatives induce maturation of DCs, as well as intensify the expression of Th1-related cytokine, and currently evaluated in multiple clinical trials as potent vaccine adjuvants (170). In addition, poly-ICLC was moderately successful as combinatorial therapy with peptide or DC vaccines in various advanced malignancies, including malignant glioma (136).
TLR5 is activated by bacterial flagellum protein flagellin, and flagellin derivatives are evaluated for anticancer efficacy (171–173). The clinical efficacy of CBLB502 (natural flagellin/entolimod derived from natural Salmonella flagellin) is evaluated against squamous cell head and neck cancer and solid tumors in phase I clinical trials (174). Mobilan, a recombinant nonreplicating adenovirus encoding flagellin which is commercially available as M-VM3 is also has been studied for anti-cancer effects in prostate cancer (175). Treatment of breast cancer cells with the TLR5 agonist flagellin also reported to suppress cell proliferation and inhibiting anchorage-independent tumor growth (171). In another interesting in vivo study, the researcher demonstrated contrasting effects on tumor growth after TLR5 activation by flagellin. Activation of TLR5 by flagellin reduces the growth of strongly immunogenic tumors, but it failed to do the same for weakly immunogenic variants (172). These conflicting results were caused by the disproportionation between IFN-γ:IL-4 ratio and the concomitant number of CD4+CD25+ T regulatory cells (172). Also in the same study, early combinatory treatment of flagellin and CpG-containing oligodeoxynucleotides (CpG ODNs) completely inhibited tumor growth (172).
Among all the TLRs, ligands or agonists of TLR7 and 8 have shown the most promising immunomodulatory and anticancer effects, and many of them transitioned to the clinic (176). TLR7 and 8, both recognize ssRNA as ligands, and this property has been exploited to synthesize several types of TLR7/8 agonists that could achieve stimulation of these receptors simultaneously (176). Based on chemical structures, TLR7/8 agonists are organized into guanosine and adenosine analogs or imidazoquinoline derivatives with modified RNA sequences (176, 177). Dual agonists of TLR7/TLR8, resiquimod (R-848), and loxoribine (178) failed to enter phase III trials because they lacked significant efficacies (179). Initial studies attributed the lack of local immune activation by resiquimod to its property of solubility in body fluid and subsequent dispersion from the injection site (178). Resiquimod was thereafter administered as a dermal cream to counteract this problem (180). Resiquimod induces the expression of TNF-α, IFN-α, and other proinflammatory cytokines, via the initiation of the TLR7-MyD88-dependent pathway (22). Imiquimod, another imidazoquinoline marketed as Aldara (5% imiquimod cream), was approved by the European Medicines Agency and FDA in 1997 for treating human papillomavirus (HPV) induced genital warts (181). Later in 2004, imiquimod was also approved as therapeutics of primary skin malignancies like superficial basal cell carcinoma and premalignant actinic keratosis (182). Topical ointment of imiquimod is also used for the treatment of other local cutaneous tumors, including melanoma, and interestingly imiquimod exerts an impressive over 85% success rate while treating lentigo maligna melanoma (183, 184). Imiquimod also demonstrated significant efficacy in combinatorial therapies with other traditional anticancer therapies like chemotherapy or radiotherapy in multiple cancer types (180). Imiquimod has also shown promise in developing DC-based vaccines (6, 185). Imiquimod promoted the stimulation and maturation of immature DCs and their consequent migration to draining lymph nodes in cancer patients (185). Topical imiquimod ointment amplified the immunogenicity of the peptide vaccine for melanoma (185). In patients with resected melanoma, imiquimod as an adjuvant also augmented the immunogenicity of the NY-ESO-1 peptide vaccine, recruiting and activating pDCs and mDCs subcutaneously and in inflammatory infiltrates (6). In contrast to imiquimod’s topical application, 852A (a TLR7 agonist) and VTX-2337 (a TLR8 agonist) are dispensed systemically, and their efficacy are under evaluation through multiple phase I/II clinical trials involving malignant breast, ovarian, endometrial, cervical, and head and neck cancers (186). 852A activates APCs and stimulate NK cells with increased secretion of IFN-α from plasmacytoid DCs in cancer patients (179, 187). In a phase II trial, 852A demonstrated safety and systemic immune activation in metastatic melanoma patients who had failed chemotherapy, leading to prolonged disease stabilization (188). While treating patients suffering from chronic hepatitis C virus (HCV) infection and cancer, ANA773- the orally administered TLR7/8 agonist, induced IFN-α, activated NK cells, and reduced serum HCV RNA levels (189). Lastly, 3M-052 (a lipid-modified imidazoquinoline derivative), was assessed as a cancer vaccine adjuvant demonstrating marked synergistic efficacy in tandem with checkpoint-blocking antibodies for CTLA4 and PDL-1 (190). This result highlighted the potential on the aspect of utilizing TLR7/8 agonists in combinatorial therapies with other immunotherapeutic agents like immune-checkpoint inhibitors (ICIs).
Unmethylated cytidine phosphate guanosine (CpG) and oligonucleotides (CpG ODNs) are the main ligands or agonists for TLR9 receptors (191). Several CpG oligodeoxynucleotides are verified for their anticancer effects both in vitro and in vivo models of multiple cancers (192–194) and clinical trials (195). Some of the significant examples of TLR9 agonists undergoing clinical trials are IMO2055 (a CpG ODN-based oligonucleotide tested in advanced NSCLC), dSLIM (two single-stranded oligodeoxynucleotide loops connected with double-stranded oligodeoxynucleotide stem, currently tested in advanced colorectal cancer), MGN1703 (a natural DNA molecule assessed in small cell lung cancer and advanced solid tumors), CpG-7909 (a single-stranded CpG ODN, currently evaluated in melanoma, renal cell carcinoma, non-Hodgkin’s lymphoma, glioblastoma, cutaneous T cell lymphoma, and NSCLC), KSK-CpG (phosphorothioated derivative of CpG ODNs, being evaluated in melanoma), SD-101 (tested in follicular lymphoma), ODN M362 (tested in hepatocarcinoma), and CpG-1826 (demonstrated amplified antitumor effect in glioma xenografts) (106, 191, 196, 197). Even after promising preclinical studies, the administration of IMO2055 in combination with platinum-based drugs against recurrent and metastatic head and neck cancer, raised some safety issues in a phase II trial (142). Similar safety concerns were raised against CPG7909, in a phase III trial involving NSCLC (142). CpG ODNs blended (emulsified) with Montanide ISA 51, being used as an adjuvant with vaccines targeting cancer-testis antigens, demonstrated promising results by promoting pDC-mediated infiltration of lymphocytes at the site of vaccination (198). Therefore, the application of CpG ODN as an adjuvant of cancer vaccines or intratumoral injection could be a potential opportunity to direct effector lymphocyte-mediated response.
Till now in the previous sections, we discussed the classification, the immunomodulatory functions of TLRs, and the administration of TLR agonists in immunotherapeutic strategies and cancer vaccines. We concisely described how different TLR agonists are employed as adjuvants to cancer vaccines in several preclinical and clinical studies. Most of the current immunotherapy trends are heavily oriented toward the onset of adaptive immunity via immunomodulators like TLR agonists. Here we present a new immunotherapeutic approach developed by our research group based on autologous tumor neoantigens and TLR agonists that are proficient in triggering both the innate and adaptive immune responses (199, 200). It’s an autologous vaccine strategy that leverages the PRR assets of TLRs as well as fungal polysaccharides, and it has been tested in diverse types of mouse tumor models (11, 14, 199–201).
This vaccine is called rWTC-MBTA, comprising of irradiated autologous tumor cells (rWTC) mixed with mannan-BAM, TLR agonists, and anti-CD40 antibody (MBTA) (199–202). This vaccination strategy was initiated with the utilization of TLR agonists accompanied by concurrent labeling of tumor cells with phagocytosis-inducing ligands leading to enhanced recognition of the tumor cells by the different immune cells (203, 204). Mannan, a branched polysaccharide from the yeast Saccharomyces cerevisiae, is affixed to the cancer cell membranes through linkage with the hydrophobic BAM (Biocompatible Anchor for Membrane) anchor and serves as a PAMP, which in turn is recognized by the mannan-binding lectin complex (MBL) (11, 14) (Figure 2). Recognition of mannan-BAM by MBL activates the lectin pathway of complement activation and ultimately leading to iC3b-mediated opsonization and phagocytosis of tumor cells (11, 14, 201). To augment the vaccine-induced innate immune responses through the initiation of multiple inflammatory pathways, along with mannan-BAM, we use TLR ligands lipoteichoic acid (LTA)(agonist of TLR2), polyinosinic-polycytidylic acid (poly(I:C))(agonist of TLR3), and resiquimod (R-848)(agonist of TLR7/8) (11, 199–201) (Figure 2). Several previous reports from our group demonstrated the applicability of TLR ligands as adjuvants in rWTC-MBTA vaccines (11, 199–201). This vaccine strategy plays on stimulating the immune system on various stages, starting from primary activation of innate immunity trailed by concomitant activation of adaptive immunity (11, 14, 201). Each TLR ligands as adjuvants in the vaccine as unique function. The strongly immunogenic LTA, which is sourced from the Gram-Positive bacteria Bacillus subtilis, stimulates the TLR2-mediated inflammatory pathway, resulting in elevated secretion of TNF-alpha and heightened inflammatory response (205, 206). Poly(I:C) triggers TLR3-mediated signaling, leading to activation of antigen-presenting cells (APCs) and activates tumor associated macrophages (206–208). R-848 or resiquimod induces the activation of innate immune cells and the promotes Th1 cell-mediated immune responses (209, 210). In addition, anti-CD40 monoclonal antibodies in the vaccine preparation binds with the CD40L ligand to activate the CD4+ T lymphocytes, permitting the dendritic cells to mediate the adaptive immune responses (199, 200) (Figure 2). To prepare rWTC-MBTA-Vax, (i) autologous or syngeneic cancer cells are irradiated so that it remains alive but non-replicative, (ii) irradiated cells are combined with mannan-BAM and TLR agonists, along with anti-CD40 antibody, to produce the effective vaccine, and (iii) the prepared rWTC-MBTA is injected peripherally over four weeks to propagate a tumor-specific immune response to inhibit tumor or cancerous growth, metastasis, and prevent recurrence (199, 200).
Figure 2 Mechanism of action for MBTA vaccine therapy. (A). The MBTA vaccine consists of mannan-BAM tagged irradiated cancer cells mixed with TLR ligands resiquimod, poly(I:C), and LTA along with anti-CD40 antibody. The polysaccharide mannan is chemically linked with the hydrophobic lipid tail biocompatible anchor for membrane (BAM). The hydrophobic lipid tail enables the attachment of mannan to the plasma membrane of irradiated tumor cells. Mannan-BAM acts as a PAMP and exploits the pattern recognition properties of Mannose-binding lectin (MBL). This recognition of Mannan-BAM by MBL culminates into the activation of the lectin pathway of complement activation through the proteolytic cleavage of complement protein C3, and iC3b, the inactive cleaved form of C3 initiate the opsonization of the tumor cells. Concurrently, the three TLR ligands (resiquimod/R-848, poly(I:C), LTA) and anti-CD40-antibody act as adjuvants facilitate recruitment of the innate immune cells like macrophages, dendritic cells, neutrophils, and monocytes into the tumor (199–202). (B). The TLR agonists activate the innate immune cells with augmented expression of inflammatory cytokines and chemokines that endorse maturation of APCs. The activated APCs opsonize and phagocytose the tumor cells and process tumor neoantigens. These activated APCs further internalize tumor antigens and display them to T cells in lymph nodes. This leads to the initiation of adaptive immune cells like effector T cells along with generation of immunologic memory through memory T cells (199–202).
One of the foremost advantages of the MBTA vaccines or rWTC-MBTA is that it can affect the immunogenic status of the TME to facilitate the outcome of the immunotherapy. The “cold” TME of some solid tumors is a major barrier to cancer immunotherapy (211–213). The immunogenically cold TME status is associated with a lack of inflammatory T-cell infiltration and lower neoantigen presentation (214). TLR agonists can promote Th1(T helper) mediated inflammatory responses and activate APCs in the TME, facilitating tumor infiltration as well as improved functioning of the effector immune cells, like CD8+ T cells and NK cells (212). When used as adjuvants, the TLR agonists augment the antigen presentation capacity of APCs and initiate the expressions of Th1 inflammatory cytokines along with increasing the expression of several co-stimulatory factors (126, 212, 215). The Th1 family of inflammatory cytokines endorse the switching of CD4+ T cells from Th2 subtype to Th1 subtype, increase CD8+ effector T-cell responses, and impede the immunosuppressive activity of Treg cells (126). The MBTA vaccine approach manipulates the TME, by the application of TLR agonists to turn the cold TME to hot, and it was evident by the efficacy of rWTC-MBTA on curbing immunogenically cold tumors like glioblastoma multiforme (GBM) (200) or triple-negative breast cancer (202).
Previous investigations involving intratumoral MBTA injections sustained a profound antitumor response, but patients are prone to secondary inflammatory damage or mass effect due to the in situ delivery of the vaccine (151, 216). Earlier studies of the MBTA anti-cancer therapeutics involved a unique combination of two different types of PAMPs, mannan-BAM serving as a tag for phagocytosis and soluble TLR agonist ligands as triggers of innate immunity (11, 14). This unique amalgamation of mannan-BAM and TLR agonists generates a robust infiltration of inflammatory cells toward the tumor. This led to reduction of tumor burden and even, in some experimental mice models, complete remission of the tumor (11, 14, 203, 204). In our preceding study, in a colon carcinoma preclinical mouse model, compared to control or injecting irradiated whole tumor cells alone, we found that subcutaneous injection of the rWTC-MBTA vaccine (irradiated whole tumor cells mixed with MBTA) caused a substantial decrease in tumor volumes and improved overall survival (199). In one of our recently published manuscripts, we demonstrated that the rWTC-MBTA vaccine effectively inhibited the metastasis and impeded the growth of tumors in animal models of both breast cancer and melanoma (202). Additionally, in a therapeutically mimicking postoperative model of breast cancer, it prevented the metastasis of residual tumors and extended the survival (202). Our results also demonstrated that the rWTC-MBTA vaccine effectively prevented the growth of autologous tumors but rendered ineffective against allogeneic tumors (202). Mechanistic studies regarding the rWTC-MBTA vaccination revealed enhanced activation of APCs, heightened CD4+ and CD8+ T-cell mediated response, generation of immune memory along with tumor specific cytotoxicity (202). Moreover, we also proved rWTC-MBTA vaccine efficacy was T-cell dependent through T-cell depletion assay (202). With the surmounting preclinical evidence, we want to translate rWTC-MBTA to the clinic for further investigations, and altogether the efficacy of rWTC-MBTA is dependent on TLR agonists, and this can direct toward a new track of cancer immunotherapy.
TLR activation sparks immune responses against pathogens, making TLR agonists promising cancer immunotherapy. Targeting TLRs, alone or combined with other methods, offers a potential pathway to enhance the immune system and eradicate cancer cells. TLRs are crucial components of the immune system, playing a significant role in both innate and adaptive immunity. It’s exciting to know that there are currently various TLR agonists being evaluated in both preclinical and clinical settings worldwide. However, there are some foremost expected roadblocks with the implementation of TLR agonists as therapeutic options. Toll-like receptors in cancer have a dual role; on one side, they activate innate immunity, recruiting immune cells to eliminate invasive pathogens like tumor cells, but they can also contribute to chronic inflammation, driven by TLRs, resulting in anti-apoptotic effects through NF-κB and promoting tumor growth (217, 218). As TLRs regulate the stimulation of several immune cells of the human body, any improper tuning of the TLR agonists can trigger autoimmune diseases. There are also chances of the onset of chronic inflammatory side effects through uncalled activation of cytokines. Multiple examples of inflammatory side effects exist while using TLR ligands in immunotherapy (142, 217, 219, 220). Therefore, choosing the right TLR agonist for the treatment of a specific type of cancer is a vital issue to diminish the chances of post-therapeutic complications.
Both pre-clinical and clinical findings suggest that combining TLR agonists with antigens, immune modulators, or other treatments can enhance their effectiveness (19, 221). Therapies like chemotherapy or phototherapy release tumor antigens and cellular factors from dying cells, further activating dendritic cells and promoting cross-presentation to T cells (221). Current research highlights the potential of TLR-targeted drugs in cancer treatment. However, it’s crucial to acknowledge that factors like tumor characteristics and the microenvironment can impact the clinical success of TLR-targeting immunotherapies. These variables should be carefully addressed, especially in preclinical animal studies. Recent data suggests that combining TLR antagonists with other immunotherapy approaches, like checkpoint inhibitors and cell-based treatments, could improve overall immunotherapy effectiveness (19). Current trends of using TLR agonists in clinical trials project them as a compelling booster of immune responses in combination with cancer vaccines or other therapeutic approaches, suggesting a more promising strategy (Table 2). Another challenge is the difficulty of translating many TLR agonists from animal studies to human applications due to significant species-specific differences in TLRs (97). For instance, murine TLR8 reacts differently to imiquimod and R848 compared to human TLR8 (97). This highlights the importance of assessing potential TLR agonists in appropriate animal models and considering species-specific variations when interpreting results. Despite the potential of TLR agonists to activate the immune system for anti-tumor effects, they face persistent limitations. For instance, small molecule TLR ligands often fail to accumulate adequately in lymph nodes to activate immune cells effectively, leading to drug resistance. Rapid dispersion of TLR ligands can also trigger the production of immunosuppressive factors and undesirable immune responses. Additionally, these agonists/ligands have a short in vivo lifespan, especially endosomal TLR ligands, which are vulnerable to nucleases (222). As a result, innovative and efficient delivery platforms like dendrimers, stimuli‐responsive polymeric particles, liposomes, hydrogels, lipoprotein‐based scaffolds, and complexes have been devised to address these challenges (222).
Here in this review article, we presented the immense therapeutic potential, background aspects, and key investigations of using TLR ligands or agonists as an emerging component of immunotherapy. We also review the current landscape of using TLR agonists in cancer immunotherapy, including ongoing clinical trials and their drawbacks. Moreover, we explored how TLR agonists can induce diverse components of the immune system and how they are or could be applied as adjuvants of cancer vaccines. Last but not the least, our research group implemented TLR agonists as adjuvants augmented the immunogenicity of the rWTC-MBTA whole-cell autologous cancer vaccine. By continuing further studies, we have confidence in TLR agonist-based immunotherapy, in combination with other conventional therapies like surgery, chemotherapy, and radiotherapy, can shift the much-needed paradigm in the treatment of cancer and confirm an improved quality of life (QoL) for cancer patients.
SC did the literature survey and wrote the article. JY, HW, MS, YZ, and XS contributed valuable inputs on the scientific contents. ZZ planned the sections of the article with SC. and gave scientific inputs. All authors have revised the manuscript and approved its submission.
This study was supported by the Intramural Research Program of the National Institute of Neurological Disorders and Stroke and the National Cancer Institute of the National Institutes of Health. The figures are created with BioRender.com.
Author SC is employed by NE1 Inc.
The remaining authors declare that the research was conducted in the absence of any commercial or financial relationships that could be construed as a potential conflict of interest.
All claims expressed in this article are solely those of the authors and do not necessarily represent those of their affiliated organizations, or those of the publisher, the editors and the reviewers. Any product that may be evaluated in this article, or claim that may be made by its manufacturer, is not guaranteed or endorsed by the publisher.
1. Ribas A, Wolchok JD. Cancer immunotherapy using checkpoint blockade. Science (2018) 359(6382):1350–5. doi: 10.1126/science.aar4060
2. Han D, Xu Z, Zhuang Y, Ye Z, Qian Q. Current progress in CAR-T cell therapy for hematological Malignancies. J Cancer. (2021) 12(2):326–34. doi: 10.7150/jca.48976
3. Le Naour J, Kroemer G. Trial watch: Toll-like receptor ligands in cancer therapy. Oncoimmunology (2023) 12(1):2180237. doi: 10.1080/2162402X.2023.2180237
4. Smith M, García-Martínez E, Pitter MR, Fucikova J, Spisek R, Zitvogel L, et al. Trial Watch: Toll-like receptor agonists in cancer immunotherapy. Oncoimmunology (2018) 7(12):e1526250. doi: 10.1080/2162402X.2018.1526250
5. Humeau J, Le Naour J, Galluzzi L, Kroemer G, Pol JG. Trial watch: intratumoral immunotherapy. OncoImmunology (2021) 10(1):1984677. doi: 10.1080/2162402X.2021.1984677
6. Iribarren K, Bloy N, Buqué A, Cremer I, Eggermont A, Fridman WH, et al. Trial Watch: Immunostimulation with Toll-like receptor agonists in cancer therapy. Oncoimmunology (2016) 5(3):e1088631. doi: 10.1080/2162402X.2015.1088631
7. Frega G, Wu Q, Le Naour J, Vacchelli E, Galluzzi L, Kroemer G, et al. Trial Watch: experimental TLR7/TLR8 agonists for oncological indications. OncoImmunology (2020) 9(1):1796002. doi: 10.1080/2162402X.2020.1796002
8. Iwasaki A, Medzhitov R. Toll-like receptor control of the adaptive immune responses. Nat Immunol (2004) 5(10):987–95. doi: 10.1038/ni1112
9. Coley WB. The treatment of Malignant tumors by repeated inoculations of erysipelas. With a report of ten original cases. 1893. Clin Orthop Relat Res (1991) 262):3–11.
10. Mellman I, Coukos G, Dranoff G. Cancer immunotherapy comes of age. Nature (2011) 480(7378):480–9. doi: 10.1038/nature10673
11. Uher O, Caisova V, Hansen P, Kopecky J, Chmelar J, Zhuang Z, et al. Coley's immunotherapy revived: Innate immunity as a link in priming cancer cells for an attack by adaptive immunity. Semin Oncol (2019) 46(4-5):385–92. doi: 10.1053/j.seminoncol.2019.10.004
12. Felgner S, Kocijancic D, Frahm M, Weiss S. Bacteria in cancer therapy: renaissance of an old concept. Int J Microbiol (2016) 2016:8451728. doi: 10.1155/2016/8451728
13. Akira S, Uematsu S, Takeuchi O. Pathogen recognition and innate immunity. Cell (2006) 124(4):783–801. doi: 10.1016/j.cell.2006.02.015
14. Caisová V, Uher O, Nedbalová P, Jochmanová I, Kvardová K, Masáková K, et al. Effective cancer immunotherapy based on combination of TLR agonists with stimulation of phagocytosis. Int Immunopharmacol (2018) 59:86–96. doi: 10.1016/j.intimp.2018.03.038
15. Chen GY, Nuñez G. Sterile inflammation: sensing and reacting to damage. Nat Rev Immunol (2010) 10(12):826–37. doi: 10.1038/nri2873
16. Lim KH, Staudt LM. Toll-like receptor signaling. Cold Spring Harb Perspect Biol (2013) 5(1):a011247. doi: 10.1101/cshperspect.a011247
17. Bowie A, O'Neill LA. The interleukin-1 receptor/Toll-like receptor superfamily: signal generators for pro-inflammatory interleukins and microbial products. J Leukoc Biol (2000) 67(4):508–14. doi: 10.1002/jlb.67.4.508
18. Wang Y, Song E, Bai B, Vanhoutte PM. Toll-like receptors mediating vascular malfunction: Lessons from receptor subtypes. Pharmacol Ther (2016) 158:91–100. doi: 10.1016/j.pharmthera.2015.12.005
19. Pahlavanneshan S, Sayadmanesh A, Ebrahimiyan H, Basiri M. Toll-like receptor-based strategies for cancer immunotherapy. J Immunol Res (2021) 2021:9912188. doi: 10.1155/2021/9912188
20. De Nardo D. Toll-like receptors: Activation, signalling and transcriptional modulation. Cytokine (2015) 74(2):181–9. doi: 10.1016/j.cyto.2015.02.025
21. Fitzgerald KA, Kagan JC. Toll-like receptors and the control of immunity. Cell (2020) 180(6):1044–66. doi: 10.1016/j.cell.2020.02.041
22. Hemmi H, Kaisho T, Takeuchi O, Sato S, Sanjo H, Hoshino K, et al. Small anti-viral compounds activate immune cells via the TLR7 MyD88-dependent signaling pathway. Nat Immunol (2002) 3(2):196–200. doi: 10.1038/ni758
23. Ayres JS, Schneider DS. Tolerance of infections. Annu Rev Immunol (2012) 30:271–94. doi: 10.1146/annurev-immunol-020711-075030
24. Hemmi H, Takeuchi O, Kawai T, Kaisho T, Sato S, Sanjo H, et al. A Toll-like receptor recognizes bacterial DNA. Nature (2000) 408(6813):740–5. doi: 10.1038/35047123
25. Hoebe K, Du X, Georgel P, Janssen E, Tabeta K, Kim SO, et al. Identification of Lps2 as a key transducer of MyD88-independent TIR signalling. Nature (2003) 424(6950):743–8. doi: 10.1038/nature01889
26. Murad S. Toll-like receptor 4 in inflammation and angiogenesis: a double-edged sword. Front Immunol (2014) 5:313. doi: 10.3389/fimmu.2014.00313
27. Yu L, Wang L, Chen S. Endogenous toll-like receptor ligands and their biological significance. J Cell Mol Med (2010) 14(11):2592–603. doi: 10.1111/j.1582-4934.2010.01127.x
28. Takeuchi O, Sato S, Horiuchi T, Hoshino K, Takeda K, Dong Z, et al. Cutting edge: role of toll-like receptor 1 in mediating immune response to microbial lipoproteins1. J Immunol (2002) 169(1):10–4. doi: 10.4049/jimmunol.169.1.10
29. Kang JY, Nan X, Jin MS, Youn S-J, Ryu YH, Mah S, et al. Recognition of lipopeptide patterns by toll-like receptor 2-toll-like receptor 6 heterodimer. Immunity (2009) 31(6):873–84. doi: 10.1016/j.immuni.2009.09.018
30. Borrello S, Nicolò C, Delogu G, Pandolfi F, Ria F. TLR2: A crossroads between infections and autoimmunity? Int J Immunopathol Pharmacol (2011) 24(3):549–56. doi: 10.1177/039463201102400301
31. Leoni V, Gianni T, Salvioli S, Campadelli-Fiume G. Herpes simplex virus glycoproteins gH/gL and gB bind Toll-like receptor 2, and soluble gH/gL is sufficient to activate NF-κB. J Virol (2012) 86(12):6555–62. doi: 10.1128/JVI.00295-12
32. Moore CE, Segal S, Berendt AR, Hill AV, Day NP. Lack of association between Toll-like receptor 2 polymorphisms and susceptibility to severe disease caused by Staphylococcus aureus. Clin Diagn Lab Immunol (2004) 11(6):1194–7. doi: 10.1128/CDLI.11.6.1194-1197.2004
33. Jackson Hoffman BA, Pumford EA, Enueme AI, Fetah KL, Friedl OM, Kasko AM. Engineered macromolecular Toll-like receptor agents and assemblies. Trends Biotechnol (2023) 41(9):1139–54. doi: 10.1016/j.tibtech.2023.03.008
34. Ciesielska A, Matyjek M, Kwiatkowska K. TLR4 and CD14 trafficking and its influence on LPS-induced pro-inflammatory signaling. Cell Mol Life Sci (2021) 78(4):1233–61. doi: 10.1007/s00018-020-03656-y
35. Heil F, Hemmi H, Hochrein H, Ampenberger F, Kirschning C, Akira S, et al. Species-specific recognition of single-stranded RNA via toll-like receptor 7 and 8. Science (2004) 303(5663):1526–9. doi: 10.1126/science.1093620
36. Zhang Y, El-Far M, Dupuy FP, Abdel-Hakeem MS, He Z, Procopio FA, et al. HCV RNA activates APCs via TLR7/TLR8 while virus selectively stimulates macrophages without inducing antiviral responses. Sci Rep (2016) 6:29447. doi: 10.1038/srep29447
37. Martínez-Campos C, Burguete-García AI, Madrid-Marina V. Role of TLR9 in oncogenic virus-produced cancer. Viral Immunol (2017) 30(2):98–105. doi: 10.1089/vim.2016.0103
38. Yarovinsky F, Hieny S, Sher A. Recognition of Toxoplasma gondii by TLR11 prevents parasite-induced immunopathology. J Immunol (2008) 181(12):8478–84. doi: 10.4049/jimmunol.181.12.8478
39. Akira S, Takeda K. Toll-like receptor signalling. Nat Rev Immunol (2004) 4(7):499–511. doi: 10.1038/nri1391
40. Burns K, Janssens S, Brissoni B, Olivos N, Beyaert R, Tschopp J. Inhibition of interleukin 1 receptor/Toll-like receptor signaling through the alternatively spliced, short form of MyD88 is due to its failure to recruit IRAK-4. J Exp Med (2003) 197(2):263–8. doi: 10.1084/jem.20021790
41. Cusson-Hermance N, Khurana S, Lee TH, Fitzgerald KA, Kelliher MA. Rip1 mediates the Trif-dependent toll-like receptor 3- and 4-induced NF-{kappa}B activation but does not contribute to interferon regulatory factor 3 activation. J Biol Chem (2005) 280(44):36560–6. doi: 10.1074/jbc.M506831200
42. Amon L, Hatscher L, Heger L, Dudziak D, Lehmann CHK. Harnessing the complete repertoire of conventional dendritic cell functions for cancer immunotherapy. Pharmaceutics (2020) 12(7). doi: 10.3390/pharmaceutics12070663
43. Banchereau J, Steinman RM. Dendritic cells and the control of immunity. Nature (1998) 392(6673):245–52. doi: 10.1038/32588
44. Javaid N, Choi S. Toll-like receptors from the perspective of cancer treatment. Cancers (Basel). (2020) 12(2). doi: 10.3390/cancers12020297
45. Chiang CL, Kandalaft LE. In vivo cancer vaccination: Which dendritic cells to target and how? Cancer Treat Rev (2018) 71:88–101. doi: 10.1016/j.ctrv.2018.10.012
46. Fu C, Peng P, Loschko J, Feng L, Pham P, Cui W, et al. Plasmacytoid dendritic cells cross-prime naive CD8 T cells by transferring antigen to conventional dendritic cells through exosomes. Proc Natl Acad Sci U S A. (2020) 117(38):23730–41. doi: 10.1073/pnas.2002345117
47. Gutiérrez-Martínez E, Planès R, Anselmi G, Reynolds M, Menezes S, Adiko AC, et al. Cross-presentation of cell-associated antigens by MHC class I in dendritic cell subsets. Front Immunol (2015) 6. doi: 10.3389/fimmu.2015.00363
48. Tel J, Schreibelt G, Sittig SP, Mathan TSM, Buschow SI, Cruz LJ, et al. Human plasmacytoid dendritic cells efficiently cross-present exogenous Ags to CD8+ T cells despite lower Ag uptake than myeloid dendritic cell subsets. Blood (2013) 121(3):459–67. doi: 10.1182/blood-2012-06-435644
49. Shortman K, Liu Y-J. Mouse and human dendritic cell subtypes. Nat Rev Immunol (2002) 2(3):151–61. doi: 10.1038/nri746
50. Chen X, Zhang Y, Fu Y. The critical role of Toll-like receptor-mediated signaling in cancer immunotherapy. Med Drug Discov (2022) 14:100122. doi: 10.1016/j.medidd.2022.100122
51. Desch AN, Gibbings SL, Clambey ET, Janssen WJ, Slansky JE, Kedl RM, et al. Dendritic cell subsets require cis-activation for cytotoxic CD8 T-cell induction. Nat Commun (2014) 5:4674. doi: 10.1038/ncomms5674
52. Pantel A, Teixeira A, Haddad E, Wood EG, Steinman RM, Longhi MP. Direct type I IFN but not MDA5/TLR3 activation of dendritic cells is required for maturation and metabolic shift to glycolysis after poly IC stimulation. PloS Biol (2014) 12(1):e1001759. doi: 10.1371/journal.pbio.1001759
53. Boonstra A, Asselin-Paturel C, Gilliet M, Crain C, Trinchieri G, Liu Y-J, et al. Flexibility of mouse classical and plasmacytoid-derived dendritic cells in directing T helper type 1 and 2 cell development : dependency on antigen dose and differential toll-like receptor ligation. J Exp Med (2003) 197(1):101–9. doi: 10.1084/jem.20021908
54. Ito T, Amakawa R, Kaisho T, Hemmi H, Tajima K, Uehira K, et al. Interferon-alpha and interleukin-12 are induced differentially by Toll-like receptor 7 ligands in human blood dendritic cell subsets. J Exp Med (2002) 195(11):1507–12. doi: 10.1084/jem.20020207
55. Prins RM, Craft N, Bruhn KW, Khan-Farooqi H, Koya RC, Stripecke R, et al. The TLR-7 agonist, imiquimod, enhances dendritic cell survival and promotes tumor antigen-specific T cell priming: relation to central nervous system antitumor immunity. J Immunol (2006) 176(1):157–64. doi: 10.4049/jimmunol.176.1.157
56. Hubert M, Gobbini E, Couillault C, Manh TV, Doffin AC, Berthet J, et al. IFN-III is selectively produced by cDC1 and predicts good clinical outcome in breast cancer. Sci Immunol (2020) 5(46). doi: 10.1126/sciimmunol.aav3942
57. Fang H, Ang B, Xu X, Huang X, Wu Y, Sun Y, et al. TLR4 is essential for dendritic cell activation and anti-tumor T-cell response enhancement by DAMPs released from chemically stressed cancer cells. Cell Mol Immunol (2014) 11(2):150–9. doi: 10.1038/cmi.2013.59
58. Park HJ, Jang GY, Kim YS, Park JH, Lee SE, Vo MC, et al. A novel TLR4 binding protein, 40S ribosomal protein S3, has potential utility as an adjuvant in a dendritic cell-based vaccine. J Immunother Cancer. (2019) 7(1):60. doi: 10.1186/s40425-019-0539-7
59. Nourizadeh M, Masoumi F, Memarian A, Alimoghaddam K, Moazzeni SM, Yaghmaie M, et al. In vitro induction of potent tumor-specific cytotoxic T lymphocytes using TLR agonist-activated AML-DC. Target Oncol (2014) 9(3):225–37. doi: 10.1007/s11523-013-0285-6
60. Drobits B, Holcmann M, Amberg N, Swiecki M, Grundtner R, Hammer M, et al. Imiquimod clears tumors in mice independent of adaptive immunity by converting pDCs into tumor-killing effector cells. J Clin Invest (2012) 122(2):575–85. doi: 10.1172/JCI61034
61. Lelaidier M, Dìaz-Rodriguez Y, Cordeau M, Cordeiro P, Haddad E, Herblot S, et al. TRAIL-mediated killing of acute lymphoblastic leukemia by plasmacytoid dendritic cell-activated natural killer cells. Oncotarget (2015) 6(30):29440–55. doi: 10.18632/oncotarget.4984
62. Ramakrishna V, Vasilakos JP, Tario JD Jr., Berger MA, Wallace PK, Keler T. Toll-like receptor activation enhances cell-mediated immunity induced by an antibody vaccine targeting human dendritic cells. J Transl Med (2007) 5:5. doi: 10.1186/1479-5876-5-5
63. Zhong H, Gutkin DW, Han B, Ma Y, Keskinov AA, Shurin MR, et al. Origin and pharmacological modulation of tumor-associated regulatory dendritic cells. Int J Cancer. (2014) 134(11):2633–45. doi: 10.1002/ijc.28590
64. Mende I, Engleman EG. Breaking self-tolerance to tumor-associated antigens by in vivo manipulation of dendritic cells. Methods Mol Biol (2007) 380:457–68. doi: 10.1007/978-1-59745-395-0_29
65. Michaelis KA, Norgard MA, Zhu X, Levasseur PR, Sivagnanam S, Liudahl SM, et al. The TLR7/8 agonist R848 remodels tumor and host responses to promote survival in pancreatic cancer. Nat Commun (2019) 10(1):4682. doi: 10.1038/s41467-019-12657-w
66. Cassetta L, Pollard JW. A timeline of tumour-associated macrophage biology. Nat Rev Cancer. (2023) 23(4):238–57. doi: 10.1038/s41568-022-00547-1
67. Kerneur C, Cano CE, Olive D. Major pathways involved in macrophage polarization in cancer. Front Immunol (2022) 13. doi: 10.3389/fimmu.2022.1026954
68. Zeng Q, Jewell CM. Directing toll-like receptor signaling in macrophages to enhance tumor immunotherapy. Curr Opin Biotechnol (2019) 60:138–45. doi: 10.1016/j.copbio.2019.01.010
69. Sica A, Mantovani A. Macrophage plasticity and polarization: in vivo veritas. J Clin Invest (2012) 122(3):787–95. doi: 10.1172/JCI59643
70. Qian BZ, Pollard JW. Macrophage diversity enhances tumor progression and metastasis. Cell (2010) 141(1):39–51. doi: 10.1016/j.cell.2010.03.014
71. Cao X, Lai SWT, Chen S, Wang S, Feng M. Chapter Three - Targeting tumor-associated macrophages for cancer immunotherapy. In: Mariani SA, Cassetta L, Galluzzi L, editors. International Review of Cell and Molecular Biology, vol. 368. Academic Press (2022) 368 p. 61–108. doi: 10.1016/bs.ircmb.2022.02.002
72. Vidyarthi A, Khan N, Agnihotri T, Negi S, Das DK, Aqdas M, et al. TLR-3 stimulation skews M2 macrophages to M1 through IFN-αβ Signaling and restricts tumor progression. Front Immunol (2018) 9:1650. doi: 10.3389/fimmu.2018.01650
73. Huang Z, Yang Y, Jiang Y, Shao J, Sun X, Chen J, et al. Anti-tumor immune responses of tumor-associated macrophages via toll-like receptor 4 triggered by cationic polymers. Biomaterials (2013) 34(3):746–55. doi: 10.1016/j.biomaterials.2012.09.062
74. Müller E, Speth M, Christopoulos PF, Lunde A, Avdagic A, Øynebråten I, et al. Both type I and type II interferons can activate antitumor M1 macrophages when combined with TLR stimulation. Front Immunol (2018) 9:2520. doi: 10.3389/fimmu.2018.02520
75. Lee CH, Wu CL, Shiau AL. Toll-like receptor 4 signaling promotes tumor growth. J Immunother (2010) 33(1):73–82. doi: 10.1097/CJI.0b013e3181b7a0a4
76. Bellora F, Castriconi R, Dondero A, Pessino A, Nencioni A, Liggieri G, et al. TLR activation of tumor-associated macrophages from ovarian cancer patients triggers cytolytic activity of NK cells. Eur J Immunol (2014) 44(6):1814–22. doi: 10.1002/eji.201344130
77. Liu Z, Xie Y, Xiong Y, Liu S, Qiu C, Zhu Z, et al. TLR 7/8 agonist reverses oxaliplatin resistance in colorectal cancer via directing the myeloid-derived suppressor cells to tumoricidal M1-macrophages. Cancer Lett (2020) 469:173–85. doi: 10.1016/j.canlet.2019.10.020
78. Müller C, Tufa DM, Chatterjee D, Mühlradt PF, Schmidt RE, Jacobs R. The TLR-2/TLR-6 agonist macrophage-activating lipopeptide-2 augments human NK cell cytotoxicity when PGE2 production by monocytes is inhibited by a COX-2 blocker. Cancer Immunol Immunother (2015) 64(9):1175–84. doi: 10.1007/s00262-015-1723-3
79. Schmidt J, Welsch T, Jäger D, Mühlradt PF, Büchler MW, Märten A. Intratumoural injection of the toll-like receptor-2/6 agonist 'macrophage-activating lipopeptide-2' in patients with pancreatic carcinoma: a phase I/II trial. Br J Cancer. (2007) 97(5):598–604. doi: 10.1038/sj.bjc.6603903
80. Gang M, Wong P, Berrien-Elliott MM, Fehniger TA. Memory-like natural killer cells for cancer immunotherapy. Semin Hematol (2020) 57(4):185–93. doi: 10.1053/j.seminhematol.2020.11.003
81. Noh JY, Yoon SR, Kim TD, Choi I, Jung H. Toll-like receptors in natural killer cells and their application for immunotherapy. J Immunol Res (2020) 2020:2045860. doi: 10.1155/2020/2045860
82. Schmidt KN, Leung B, Kwong M, Zarember KA, Satyal S, Navas TA, et al. APC-independent activation of NK cells by the Toll-like receptor 3 agonist double-stranded RNA. J Immunol (2004) 172(1):138–43. doi: 10.4049/jimmunol.172.1.138
83. Xie L, Pries R, Kesselring R, Wulff S, Wollenberg B. Head and neck cancer triggers the internalization of TLR3 in natural killer cells. Int J Mol Med (2007) 20(4):493–9. doi: 10.3892/ijmm.20.4.493
84. Gorski KS, Waller EL, Bjornton-Severson J, Hanten JA, Riter CL, Kieper WC, et al. Distinct indirect pathways govern human NK-cell activation by TLR-7 and TLR-8 agonists. Int Immunol (2006) 18(7):1115–26. doi: 10.1093/intimm/dxl046
85. Hart OM, Athie-Morales V, O'Connor GM, Gardiner CM. TLR7/8-mediated activation of human NK cells results in accessory cell-dependent IFN-gamma production. J Immunol (2005) 175(3):1636–42. doi: 10.4049/jimmunol.175.3.1636
86. Lester RT, Yao XD, Ball TB, McKinnon LR, Kaul R, Wachihi C, et al. Toll-like receptor expression and responsiveness are increased in viraemic HIV-1 infection. Aids (2008) 22(6):685–94. doi: 10.1097/QAD.0b013e3282f4de35
87. Ma F, Zhang J, Zhang J, Zhang C. The TLR7 agonists imiquimod and gardiquimod improve DC-based immunotherapy for melanoma in mice. Cell Mol Immunol (2010) 7(5):381–8. doi: 10.1038/cmi.2010.30
88. Sabel MS, Sondak VK. Pros and cons of adjuvant interferon in the treatment of melanoma. Oncologist (2003) 8(5):451–8. doi: 10.1634/theoncologist.8-5-451
89. Lu H, Yang Y, Gad E, Inatsuka C, Wenner CA, Disis ML, et al. TLR2 agonist PSK activates human NK cells and enhances the antitumor effect of HER2-targeted monoclonal antibody therapy. Clin Cancer Res (2011) 17(21):6742–53. doi: 10.1158/1078-0432.CCR-11-1142
90. Bishop GA, Hsing Y, Hostager BS, Jalukar SV, Ramirez LM, Tomai MA. Molecular mechanisms of B lymphocyte activation by the immune response modifier R-848. J Immunol (2000) 165(10):5552–7. doi: 10.4049/jimmunol.165.10.5552
91. Buchta CM, Bishop GA. Toll-like receptors and B cells: functions and mechanisms. Immunol Res (2014) 59(1-3):12–22. doi: 10.1007/s12026-014-8523-2
92. Agrawal S, Gupta S. TLR1/2, TLR7, and TLR9 signals directly activate human peripheral blood naive and memory B cell subsets to produce cytokines, chemokines, and hematopoietic growth factors. J Clin Immunol (2011) 31(1):89–98. doi: 10.1007/s10875-010-9456-8
93. Genestier L, Taillardet M, Mondiere P, Gheit H, Bella C, DeFrance T. TLR agonists selectively promote terminal plasma cell differentiation of B cell subsets specialized in thymus-independent responses. J Immunol (2007) 178(12):7779–86. doi: 10.4049/jimmunol.178.12.7779
94. Jiang W, Lederman MM, Harding CV, Rodriguez B, Mohner RJ, Sieg SF. TLR9 stimulation drives naïve B cells to proliferate and to attain enhanced antigen presenting function. Eur J Immunol (2007) 37(8):2205–13. doi: 10.1002/eji.200636984
95. Lin L, Gerth AJ, Peng SL. CpG DNA redirects class-switching towards "Th1-like" Ig isotype production via TLR9 and MyD88. Eur J Immunol (2004) 34(5):1483–7. doi: 10.1002/eji.200324736
96. Leadbetter EA, Rifkin IR, Hohlbaum AM, Beaudette BC, Shlomchik MJ, Marshak-Rothstein A. Chromatin-IgG complexes activate B cells by dual engagement of IgM and Toll-like receptors. Nature (2002) 416(6881):603–7. doi: 10.1038/416603a
97. Kaur A, Baldwin J, Brar D, Salunke DB, Petrovsky N. Toll-like receptor (TLR) agonists as a driving force behind next-generation vaccine adjuvants and cancer therapeutics. Curr Opin Chem Biol (2022) 70:102172. doi: 10.1016/j.cbpa.2022.102172
98. Wu H, Fu X, Zhai Y, Gao S, Yang X, Zhai G. Development of effective tumor vaccine strategies based on immune response cascade reactions. Adv Healthc Mater (2021) 10(13):2100299. doi: 10.1002/adhm.202100299
99. Hofmann MA, Kors C, Audring H, Walden P, Sterry W, Trefzer U. Phase 1 evaluation of intralesionally injected TLR9-agonist PF-3512676 in patients with basal cell carcinoma or metastatic melanoma. J Immunother (2008) 31(5):520–7. doi: 10.1097/CJI.0b013e318174a4df
100. Krieg AM. Toll-like receptor 9 (TLR9) agonists in the treatment of cancer. Oncogene (2008) 27(2):161–7. doi: 10.1038/sj.onc.1210911
101. Brody JD, Ai WZ, Czerwinski DK, Torchia JA, Levy M, Advani RH, et al. In situ vaccination with a TLR9 agonist induces systemic lymphoma regression: a phase I/II study. J Clin Oncol (2010) 28(28):4324–32. doi: 10.1200/JCO.2010.28.9793
102. Bode C, Zhao G, Steinhagen F, Kinjo T, Klinman DM. CpG DNA as a vaccine adjuvant. Expert Rev Vaccines (2011) 10(4):499–511. doi: 10.1586/erv.10.174
103. Bernasconi NL, Traggiai E, Lanzavecchia A. Maintenance of serological memory by polyclonal activation of human memory B cells. Science (2002) 298(5601):2199–202. doi: 10.1126/science.1076071
104. Kuo TC, Harrabi O, Chen A, Sangalang ER, Doyle L, Fontaine D, et al. Abstract 1721: TAC-001, a toll-like receptor 9 (TLR9) agonist antibody conjugate targeting B cells, promotes anti-tumor immunity and favorable safety profile following systemic administration in preclinical models. Cancer Res (2021) 81(13_Supplement):1721. doi: 10.1158/1538-7445.AM2021-1721
105. Kapp K, Volz B, Oswald D, Wittig B, Baumann M, Schmidt M. Beneficial modulation of the tumor microenvironment and generation of anti-tumor responses by TLR9 agonist lefitolimod alone and in combination with checkpoint inhibitors. Oncoimmunology (2019) 8(12):e1659096. doi: 10.1080/2162402X.2019.1659096
106. Wittig B, Schmidt M, Scheithauer W, Schmoll H-J. MGN1703, an immunomodulator and toll-like receptor 9 (TLR-9) agonist: From bench to bedside. Crit Rev Oncology/Hematol (2015) 94(1):31–44. doi: 10.1016/j.critrevonc.2014.12.002
107. Amos SM, Pegram HJ, Westwood JA, John LB, Devaud C, Clarke CJ, et al. Adoptive immunotherapy combined with intratumoral TLR agonist delivery eradicates established melanoma in mice. Cancer Immunol Immunother (2011) 60(5):671–83. doi: 10.1007/s00262-011-0984-8
108. Ribas A, Medina T, Kummar S, Amin A, Kalbasi A, Drabick JJ, et al. SD-101 in combination with pembrolizumab in advanced melanoma: results of a phase ib, multicenter study. Cancer Discov (2018) 8(10):1250–7. doi: 10.1158/2159-8290.CD-18-0280
109. Fuge O, Vasdev N, Allchorne P, Green JS. Immunotherapy for bladder cancer. Res Rep Urol (2015) 7:65–79. doi: 10.2147/rru.S63447
110. Kaech SM, Wherry EJ, Ahmed R. Effector and memory T-cell differentiation: implications for vaccine development. Nat Rev Immunol (2002) 2(4):251–62. doi: 10.1038/nri778
111. Rahman AH, Taylor DK, Turka LA. The contribution of direct TLR signaling to T cell responses. Immunol Res (2009) 45(1):25–36. doi: 10.1007/s12026-009-8113-x
112. Crellin NK, Garcia RV, Hadisfar O, Allan SE, Steiner TS, Levings MK. Human CD4+ T cells express TLR5 and its ligand flagellin enhances the suppressive capacity and expression of FOXP3 in CD4+CD25+ T regulatory cells. J Immunol (2005) 175(12):8051–9. doi: 10.4049/jimmunol.175.12.8051
113. Cottalorda A, Verschelde C, Marçais A, Tomkowiak M, Musette P, Uematsu S, et al. TLR2 engagement on CD8 T cells lowers the threshold for optimal antigen-induced T cell activation. Eur J Immunol (2006) 36(7):1684–93. doi: 10.1002/eji.200636181
114. Caron G, Duluc D, Frémaux I, Jeannin P, David C, Gascan H, et al. Direct stimulation of human T cells via TLR5 and TLR7/8: flagellin and R-848 up-regulate proliferation and IFN-gamma production by memory CD4+ T cells. J Immunol (2005) 175(3):1551–7. doi: 10.4049/jimmunol.175.3.1551
115. Li Q, Yan Y, Liu J, Huang X, Zhang X, Kirschning C, et al. Toll-like receptor 7 activation enhances CD8+ T cell effector functions by promoting cellular glycolysis. Front Immunol (2019) 10:2191. doi: 10.3389/fimmu.2019.02191
116. Asprodites N, Zheng L, Geng D, Velasco-Gonzalez C, Sanchez-Perez L, Davila E. Engagement of Toll-like receptor-2 on cytotoxic T-lymphocytes occurs in vivo and augments antitumor activity. FASEB J (2008) 22(10):3628–37. doi: 10.1096/fj.08-108274
117. Tabiasco J, Devêvre E, Rufer N, Salaun B, Cerottini JC, Speiser D, et al. Human effector CD8+ T lymphocytes express TLR3 as a functional coreceptor. J Immunol (2006) 177(12):8708–13. doi: 10.4049/jimmunol.177.12.8708
118. Salem ML, Diaz-Montero CM, El-Naggar SA, Chen Y, Moussa O, Cole DJ. The TLR3 agonist poly(I:C) targets CD8+ T cells and augments their antigen-specific responses upon their adoptive transfer into naïve recipient mice. Vaccine (2009) 27(4):549–57. doi: 10.1016/j.vaccine.2008.11.013
119. Peng G, Guo Z, Kiniwa Y, Voo KS, Peng W, Fu T, et al. Toll-like receptor 8-mediated reversal of CD4+ regulatory T cell function. Science (2005) 309(5739):1380–4. doi: 10.1126/science.1113401
120. Oberg HH, Ly TT, Ussat S, Meyer T, Kabelitz D, Wesch D. Differential but direct abolishment of human regulatory T cell suppressive capacity by various TLR2 ligands. J Immunol (2010) 184(9):4733–40. doi: 10.4049/jimmunol.0804279
121. Sutmuller RP, den Brok MH, Kramer M, Bennink EJ, Toonen LW, Kullberg BJ, et al. Toll-like receptor 2 controls expansion and function of regulatory T cells. J Clin Invest (2006) 116(2):485–94. doi: 10.1172/JCI25439
122. Liu H, Komai-Koma M, Xu D, Liew FY. Toll-like receptor 2 signaling modulates the functions of CD4+ CD25+ regulatory T cells. Proc Natl Acad Sci U S A. (2006) 103(18):7048–53. doi: 10.1073/pnas.0601554103
123. Li K, Qu S, Chen X, Wu Q, Shi M. Promising targets for cancer immunotherapy: TLRs, RLRs, and STING-mediated innate immune pathways. Int J Mol Sci (2017) 18(2). doi: 10.3390/ijms18020404
124. Tang H, Qiao J, Fu Y-X. Immunotherapy and tumor microenvironment. Cancer Lett (2016) 370(1):85–90. doi: 10.1016/j.canlet.2015.10.009
125. Vindevogel E, Baert T, Hoylandt Av, Verbist G, Velde Gv, Garg Ad, et al. The use of toll-like receptor 4 agonist to reshape the immune signature in ovarian cancer. Anticancer Res (2016) 36(11):5781–92. doi: 10.21873/anticanres.11162
126. Mullins SR, Vasilakos JP, Deschler K, Grigsby I, Gillis P, John J, et al. Intratumoral immunotherapy with TLR7/8 agonist MEDI9197 modulates the tumor microenvironment leading to enhanced activity when combined with other immunotherapies. J Immunother Cancer. (2019) 7(1):244. doi: 10.1186/s40425-019-0724-8
127. Roselli E, Araya P, Núñez NG, Gatti G, Graziano F, Sedlik C, et al. TLR3 activation of intratumoral CD103+ Dendritic cells modifies the tumor infiltrate conferring anti-tumor immunity. Front Immunol (2019) 10. doi: 10.3389/fimmu.2019.00503
128. Salazar AM, Erlich RB, Mark A, Bhardwaj N, Herberman RB. Therapeutic in situ autovaccination against solid cancers with intratumoral poly-ICLC: case report, hypothesis, and clinical trial. Cancer Immunol Res (2014) 2(8):720–4. doi: 10.1158/2326-6066.CIR-14-0024
129. Hotz C, Bourquin C. Systemic cancer immunotherapy with Toll-like receptor 7 agonists. OncoImmunology (2012) 1(2):227–8. doi: 10.4161/onci.1.2.18169
130. Furudate S, Fujimura T, Kambayashi Y, Kakizaki A, Hidaka T, Aiba S. Immunomodulatory effect of imiquimod through CCL22 produced by tumor-associated macrophages in B16F10 melanomas. Anticancer Res (2017) 37(7):3461–71. doi: 10.21873/anticanres.11714
131. Diao Y, Wang X, Wan Y, Zhong J, Gao D, Liu Y, et al. Antitumor activity of a novel small molecule TLR7 agonist via immune response induction and tumor microenvironment modulation. Oncol Rep (2016) 35(2):793–800. doi: 10.3892/or.2015.4436
132. Cho JH, Lee HJ, Ko HJ, Yoon BI, Choe J, Kim KC, et al. The TLR7 agonist imiquimod induces anti-cancer effects via autophagic cell death and enhances anti-tumoral and systemic immunity during radiotherapy for melanoma. Oncotarget (2017) 8(15):24932–48. doi: 10.18632/oncotarget.15326
133. Daayana S, Elkord E, Winters U, Pawlita M, Roden R, Stern PL, et al. Phase II trial of imiquimod and HPV therapeutic vaccination in patients with vulval intraepithelial neoplasia. Br J Cancer. (2010) 102(7):1129–36. doi: 10.1038/sj.bjc.6605611
134. Nishii N, TaChinami H, Kondo Y, Xia Y, Kashima Y, Ohno T, et al. Systemic administration of a TLR7 agonist attenuates regulatory T cells by dendritic cell modification and overcomes resistance to PD-L1 blockade therapy. Oncotarget (2018) 9(17):13301–12. doi: 10.18632/oncotarget.24327
135. Lu R, Groer C, Kleindl PA, Moulder KR, Huang A, Hunt JR, et al. Formulation and preclinical evaluation of a toll-like receptor 7/8 agonist as an anti-tumoral immunomodulator. J Controlled Release (2019) 306:165–76. doi: 10.1016/j.jconrel.2019.06.003
136. Adams S. Toll-like receptor agonists in cancer therapy. Immunotherapy (2009) 1(6):949–64. doi: 10.2217/imt.09.70
137. Gallotta M, Assi H, Degagné É, Kannan SK, Coffman RL, Guiducci C. Inhaled TLR9 agonist renders lung tumors permissive to PD-1 blockade by promoting optimal CD4+ and CD8+ T-cell interplay. Cancer Res (2018) 78(17):4943–56. doi: 10.1158/0008-5472.CAN-18-0729
138. Molenkamp BG, van Leeuwen PAM, Meijer S, Sluijter BJR, Wijnands PGJTB, Baars A, et al. Intradermal cpG-B activates both plasmacytoid and myeloid dendritic cells in the sentinel lymph node of melanoma patients. Clin Cancer Res (2007) 13(10):2961–9. doi: 10.1158/1078-0432.CCR-07-0050
139. Frank MJ, Reagan PM, Bartlett NL, Gordon LI, Friedberg JW, Czerwinski DK, et al. In situ vaccination with a TLR9 agonist and local low-dose radiation induces systemic responses in untreated indolent lymphoma. Cancer Discov (2018) 8(10):1258–69. doi: 10.1158/2159-8290.CD-18-0743
140. Keshavarz A, Pourbagheri-Sigaroodi A, Zafari P, Bagheri N, Ghaffari SH, Bashash D. Toll-like receptors (TLRs) in cancer; with an extensive focus on TLR agonists and antagonists. IUBMB Life (2021) 73(1):10–25. doi: 10.1002/iub.2412
141. Rostamizadeh L, Molavi O, Rashid M, Ramazani F, Baradaran B, Lavasanaifar A, et al. Recent advances in cancer immunotherapy: Modulation of tumor microenvironment by Toll-like receptor ligands. Bioimpacts (2022) 12(3):261–90. doi: 10.34172/bi.2022.23896
142. Guha M. Anticancer TLR agonists on the ropes. Nat Rev Drug Discovery (2012) 11(7):503–5. doi: 10.1038/nrd3775
143. Basith S, Manavalan B, Yoo TH, Kim SG, Choi S. Roles of toll-like receptors in cancer: a double-edged sword for defense and offense. Arch Pharm Res (2012) 35(8):1297–316. doi: 10.1007/s12272-012-0802-7
144. Netea MG, van der Meer JW, Sutmuller RP, Adema GJ, Kullberg BJ. From the Th1/Th2 paradigm towards a Toll-like receptor/T-helper bias. Antimicrob Agents Chemother (2005) 49(10):3991–6. doi: 10.1128/AAC.49.10.3991-3996.2005
145. Re F, Strominger JL. IL-10 released by concomitant TLR2 stimulation blocks the induction of a subset of Th1 cytokines that are specifically induced by TLR4 or TLR3 in human dendritic cells. J Immunol (2004) 173(12):7548–55. doi: 10.4049/jimmunol.173.12.7548
146. Vaure C, Liu Y. A comparative review of toll-like receptor 4 expression and functionality in different animal species. Front Immunol (2014) 5:316. doi: 10.3389/fimmu.2014.00316
147. Morton DL, Eilber FR, Holmes EC, Hunt JS, Ketcham AS, Silverstein MJ, et al. BCG immunotherapy of Malignant melanoma: summary of a seven-year experience. Ann Surg (1974) 180(4):635–43. doi: 10.1097/00000658-197410000-00029
148. Silverstein MJ, DeKernion J, Morton DL. Malignant melanoma metastatic to the bladder. Regression following intratumor injection of BCG vaccine. Jama (1974) 229(6):688. doi: 10.1001/jama.1974.03230440046032
149. Morton DL, Mozzillo N, Thompson JF, Kelley MC, Faries M, Wagner J, et al. An international, randomized, phase III trial of bacillus Calmette-Guerin (BCG) plus allogeneic melanoma vaccine (MCV) or placebo after complete resection of melanoma metastatic to regional or distant sites. J Clin Oncol (2007) 25(18_suppl):8508. doi: 10.1200/jco.2007.25.18_suppl.8508
150. Vermorken JB, Claessen AM, van Tinteren H, Gall HE, Ezinga R, Meijer S, et al. Active specific immunotherapy for stage II and stage III human colon cancer: a randomised trial. Lancet (1999) 353(9150):345–50. doi: 10.1016/S0140-6736(98)07186-4
151. Uyl-de Groot CA, Vermorken JB, Hanna MG Jr., Verboom P, Groot MT, Bonsel GJ, et al. Immunotherapy with autologous tumor cell-BCG vaccine in patients with colon cancer: a prospective study of medical and economic benefits. Vaccine (2005) 23(17-18):2379–87. doi: 10.1016/j.vaccine.2005.01.015
152. D'Agostini C, Pica F, Febbraro G, Grelli S, Chiavaroli C, Garaci E. Antitumour effect of OM-174 and cyclophosphamide on murine B16 melanoma in different experimental conditions. Int Immunopharmacol (2005) 5(7-8):1205–12. doi: 10.1016/j.intimp.2005.02.013
153. Kroemer G, Zitvogel L, Galluzzi L. Victories and deceptions in tumor immunology: Stimuvax(®). Oncoimmunology (2013) 2(1):e23687. doi: 10.4161/onci.23687
154. MacLean GD, Reddish M, Koganty RR, Wong T, Gandhi S, Smolenski M, et al. Immunization of breast cancer patients using a synthetic sialyl-Tn glycoconjugate plus Detox adjuvant. Cancer Immunol Immunother (1993) 36(4):215–22. doi: 10.1007/BF01740902
155. Brichard VG, Lejeune D. GSK's antigen-specific cancer immunotherapy programme: pilot results leading to Phase III clinical development. Vaccine (2007) 25 Suppl 2:B61–71. doi: 10.1016/j.vaccine.2007.06.038
156. Bhatia S, Miller NJ, Lu H, Longino NV, Ibrani D, Shinohara MM, et al. Intratumoral G100, a TLR4 agonist, induces antitumor immune responses and tumor regression in patients with merkel cell carcinoma. Clin Cancer Res (2019) 25(4):1185–95. doi: 10.1158/1078-0432.CCR-18-0469
157. Lambert SL, Yang CF, Liu Z, Sweetwood R, Zhao J, Cheng L, et al. Molecular and cellular response profiles induced by the TLR4 agonist-based adjuvant Glucopyranosyl Lipid A. PloS One (2012) 7(12):e51618. doi: 10.1371/journal.pone.0051618
158. Lu H, Hewitt J, Meulen Jt. Abstract 4885: Intratumoral injection of G100 (TLR4 agonist glycopyranosyl lipid A) modulates tumor microenvironment and induces CD8 T cell-dependent, systemic anti-tumor immunity. Cancer Res (2016) 76(14_Supplement):4885. doi: 10.1158/1538-7445.AM2016-4885
159. Alexopoulou L, Holt AC, Medzhitov R, Flavell RA. Recognition of double-stranded RNA and activation of NF-kappaB by Toll-like receptor 3. Nature (2001) 413(6857):732–8. doi: 10.1038/35099560
160. Kato H, Takeuchi O, Mikamo-Satoh E, Hirai R, Kawai T, Matsushita K, et al. Length-dependent recognition of double-stranded ribonucleic acids by retinoic acid-inducible gene-I and melanoma differentiation-associated gene 5. J Exp Med (2008) 205(7):1601–10. doi: 10.1084/jem.20080091
161. Datta SK, Redecke V, Prilliman KR, Takabayashi K, Corr M, Tallant T, et al. A subset of toll-like receptor ligands induces cross-presentation by bone marrow-derived dendritic cells1. J Immunol (2003) 170(8):4102–10. doi: 10.4049/jimmunol.170.8.4102
162. Perrot I, Deauvieau F, Massacrier C, Hughes N, Garrone P, Durand I, et al. TLR3 and rig-like receptor on myeloid dendritic cells and rig-like receptor on human NK cells are both mandatory for production of IFN-γ in response to double-stranded RNA. J Immunol (2010) 185(4):2080–8. doi: 10.4049/jimmunol.1000532
163. Jasani B, Navabi H, Adams M. Ampligen: a potential toll-like 3 receptor adjuvant for immunotherapy of cancer. Vaccine (2009) 27(25-26):3401–4. doi: 10.1016/j.vaccine.2009.01.071
164. Levy HB, Baer G, Baron S, Buckler CE, Gibbs CJ, Iadarola MJ, et al. A modified polyriboinosinic-polyribocytidylic acid complex that induces interferon in primates. J Infect Dis (1975) 132(4):434–9. doi: 10.1093/infdis/132.4.434
165. Isakoff SJ, Tolaney SM, Tung NM, Adams S, Soliman HH, Brachtel EF, et al. A phase 1b study of safety and immune response to PVX-410 vaccine alone and in combination with durvalumab (MEDI4736) in HLA-A2+ patients following adjuvant therapy for stage 2/3 triple negative breast cancer. J Clin Oncol (2017) 35(15_suppl):TPS1126–TPS. doi: 10.1200/JCO.2017.35.15_suppl.TPS1126
166. Karki NR, Bano K, Ramses S, Nayak A. A phase II trial of pembrolizumab and poly-ICLC in patients with metastatic mismatch repair-proficient colon cancer. J Clin Oncol (2021) 39(15_suppl):e15552–e. doi: 10.1200/JCO.2021.39.15_suppl.e15552
167. Matijevic Glavan T, Pavelic J. The exploitation of toll-like receptor 3 signaling in cancer therapy. Curr Pharm Design (2014) 20(42):6555–64. doi: 10.2174/1381612820666140826153347
168. Haddaoui H, Brood R, Latifi D, Oostvogels AA, Klaver Y, Moskie M, et al. Rintatolimod (Ampligen®) enhances numbers of peripheral B cells and is associated with longer survival in patients with locally advanced and metastasized pancreatic cancer pre-treated with FOLFIRINOX: A single-center named patient program. Cancers (2022) 14(6):1377. doi: 10.3390/cancers14061377
169. Gandhi S, Opyrchal M, Grimm M, Slomba R, Kokolus K, Battaglia S, et al. Abstract CT145: Systemic rintatolimod and interferon-α2b selectively reprogram local tumor microenvironment in patients with metastatic triple negative breast cancer for enhanced influx of cytotoxic T-lymphocytes but not regulatory T-cells. Cancer Res (2022) 82(12_Supplement):CT145–CT. doi: 10.1158/1538-7445.AM2022-CT145
170. Navabi H, Jasani B, Reece A, Clayton A, Tabi Z, Donninger C, et al. A clinical grade poly I:C-analogue (Ampligen) promotes optimal DC maturation and Th1-type T cell responses of healthy donors and cancer patients in vitro. Vaccine (2009) 27(1):107–15. doi: 10.1016/j.vaccine.2008.10.024
171. Cai Z, Sanchez A, Shi Z, Zhang T, Liu M, Zhang D. Activation of Toll-like receptor 5 on breast cancer cells by flagellin suppresses cell proliferation and tumor growth. Cancer Res (2011) 71(7):2466–75. doi: 10.1158/0008-5472.CAN-10-1993
172. Sfondrini L, Rossini A, Besusso D, Merlo A, Tagliabue E, Mènard S, et al. Antitumor activity of the TLR-5 ligand flagellin in mouse models of cancer. J Immunol (2006) 176(11):6624–30. doi: 10.4049/jimmunol.176.11.6624
173. Garaude J, Kent A, van Rooijen N, Blander JM. Simultaneous targeting of toll- and nod-like receptors induces effective tumor-specific immune responses. Sci Transl Med (2012) 4(120):120ra16. doi: 10.1126/scitranslmed.3002868
174. Burdelya LG, Krivokrysenko VI, Tallant TC, Strom E, Gleiberman AS, Gupta D, et al. An agonist of toll-like receptor 5 has radioprotective activity in mouse and primate models. Science (2008) 320(5873):226–30. doi: 10.1126/science.1154986
175. Mett V, Komarova EA, Greene K, Bespalov I, Brackett C, Gillard B, et al. Mobilan: a recombinant adenovirus carrying Toll-like receptor 5 self-activating cassette for cancer immunotherapy. Oncogene (2018) 37(4):439–49. doi: 10.1038/onc.2017.346
176. McGowan DC. Latest advances in small molecule TLR 7/8 agonist drug research. Curr Top Med Chem (2019) 19(24):2228–38. doi: 10.2174/1568026619666191009165418
177. Lan T, Kandimalla ER, Yu D, Bhagat L, Li Y, Wang D, et al. Stabilized immune modulatory RNA compounds as agonists of Toll-like receptors 7 and 8. Proc Natl Acad Sci U S A. (2007) 104(34):13750–5. doi: 10.1073/pnas.0706059104
178. Rodell CB, Ahmed MS, Garris CS, Pittet MJ, Weissleder R. Development of adamantane-conjugated TLR7/8 agonists for supramolecular delivery and cancer immunotherapy. Theranostics (2019) 9(26):8426–36. doi: 10.7150/thno.35434
179. Dudek AZ, Yunis C, Harrison LI, Kumar S, Hawkinson R, Cooley S, et al. First in human phase I trial of 852A, a novel systemic toll-like receptor 7 agonist, to activate innate immune responses in patients with advanced cancer. Clin Cancer Res (2007) 13(23):7119–25. doi: 10.1158/1078-0432.Ccr-07-1443
180. Vasilakos JP, Tomai MA. The use of Toll-like receptor 7/8 agonists as vaccine adjuvants. Expert Rev Vaccines (2013) 12(7):809–19. doi: 10.1586/14760584.2013.811208
181. AG A, Tyring SK, Rosen T. Beyond a decade of 5% imiquimod topical therapy. J Drugs Dermatol (2009) 8(5):467–74.
182. Geisse J, Caro I, Lindholm J, Golitz L, Stampone P, Owens M, et al. Imiquimod 5% cream for the treatment of superficial basal cell carcinoma: results from two phase III, randomized, vehicle-controlled studies. J Am Acad Dermatol (2004) 50(5):722–33. doi: 10.1016/j.jaad.2003.11.066
183. Bong AB, Bonnekoh B, Franke I, Schön M, Ulrich J, Gollnick H. Imiquimod, a topical immune response modifier, in the treatment of cutaneous metastases of Malignant melanoma. Dermatology (2002) 205(2):135–8. doi: 10.1159/000063904
184. Wolf IH, Smolle J, Binder B, Cerroni L, Richtig E, Kerl H. Topical imiquimod in the treatment of metastatic melanoma to skin. Arch Dermatol (2003) 139(3):273–6. doi: 10.1001/archderm.139.3.273
185. Nair S, McLaughlin C, Weizer A, Su Z, Boczkowski D, Dannull J, et al. Injection of immature dendritic cells into adjuvant-treated skin obviates the need for ex vivo maturation. J Immunol (2003) 171(11):6275–82. doi: 10.4049/jimmunol.171.11.6275
186. Chi H, Li C, Zhao FS, Zhang L, Ng TB, Jin G, et al. Anti-tumor activity of toll-like receptor 7 agonists. Front Pharmacol (2017) 8. doi: 10.3389/fphar.2017.00304
187. Harrison LI, Astry C, Kumar S, Yunis C. Pharmacokinetics of 852A, an imidazoquinoline Toll-like receptor 7-specific agonist, following intravenous, subcutaneous, and oral administrations in humans. J Clin Pharmacol (2007) 47(8):962–9. doi: 10.1177/0091270007303766
188. Dummer R, Hauschild A, Becker JC, Grob JJ, SChadendorf D, Tebbs V, et al. An exploratory study of systemic administration of the toll-like receptor-7 agonist 852A in patients with refractory metastatic melanoma. Clin Cancer Res (2008) 14(3):856–64. doi: 10.1158/1078-0432.CCR-07-1938
189. Bergmann JF, de Bruijne J, Hotho DM, de Knegt RJ, Boonstra A, Weegink CJ, et al. Randomised clinical trial: anti-viral activity of ANA773, an oral inducer of endogenous interferons acting via TLR7, in chronic HCV. Aliment Pharmacol Ther (2011) 34(4):443–53. doi: 10.1111/j.1365-2036.2011.04745.x
190. Singh M, Khong H, Dai Z, Huang XF, Wargo JA, Cooper ZA, et al. Effective innate and adaptive antimelanoma immunity through localized TLR7/8 activation. J Immunol (2014) 193(9):4722–31. doi: 10.4049/jimmunol.1401160
191. Karapetyan L, Luke JJ, Davar D. Toll-like receptor 9 agonists in cancer. Onco Targets Ther (2020) 13:10039–60. doi: 10.2147/OTT.S247050
192. Baines J, Celis E. Immune-mediated tumor regression induced by CpG-containing oligodeoxynucleotides. Clin Cancer Res (2003) 9(7):2693–700.
193. Krieg AM. Antiinfective applications of toll-like receptor 9 agonists. Proc Am Thorac Soc (2007) 4(3):289–94. doi: 10.1513/pats.200701-021AW
194. Heckelsmiller K, Rall K, Beck S, Schlamp A, Seiderer J, Jahrsdörfer B, et al. Peritumoral CpG DNA elicits a coordinated response of CD8 T cells and innate effectors to cure established tumors in a murine colon carcinoma model. J Immunol (2002) 169(7):3892–9. doi: 10.4049/jimmunol.169.7.3892
195. Goutagny N, Estornes Y, Hasan U, Lebecque S, Caux C. Targeting pattern recognition receptors in cancer immunotherapy. Target Oncol (2012) 7(1):29–54. doi: 10.1007/s11523-012-0213-1
196. Cunningham D, Zurlo A, Salazar R, Ducreux M, Waddell TS, Stein A, et al. IMPALA, a randomized phase III study in patients with metastatic colorectal carcinoma: Immunomodulatory maintenance therapy with TLR-9 agonist MGN1703. J Clin Oncol (2015) 33(3_suppl):TPS791–TPS. doi: 10.1200/jco.2015.33.3_suppl.tps791
197. Houot R, Levy R. T-cell modulation combined with intratumoral CpG cures lymphoma in a mouse model without the need for chemotherapy. Blood (2009) 113(15):3546–52. doi: 10.1182/blood-2008-07-170274
198. Valmori D, Souleimanian NE, Tosello V, Bhardwaj N, Adams S, O'Neill D, et al. Vaccination with NY-ESO-1 protein and CpG in Montanide induces integrated antibody/Th1 responses and CD8 T cells through cross-priming. Proc Natl Acad Sci U S A. (2007) 104(21):8947–52. doi: 10.1073/pnas.0703395104
199. Medina R, Wang H, Caisová V, Cui J, Indig IH, Uher O, et al. Induction of immune response against metastatic tumors via vaccination of mannan-BAM, TLR ligands and anti-CD40 antibody (MBTA). Adv Ther (Weinh) (2020) 3(9). doi: 10.1002/adtp.202000044
200. Lookian PP, Zhao D, Medina R, Wang H, Zenka J, Gilbert MR, et al. Mannan-BAM, TLR ligands, anti-CD40 antibody (MBTA) vaccine immunotherapy: A review of current evidence and applications in glioblastoma. Int J Mol Sci (2021) 22(7). doi: 10.3390/ijms22073455
201. Caisova V, Li L, Gupta G, Jochmanova I, Jha A, Uher O, et al. The significant reduction or complete eradication of subcutaneous and metastatic lesions in a pheochromocytoma mouse model after immunotherapy using mannan-BAM, TLR ligands, and anti-CD40. Cancers (Basel) (2019) 11(5). doi: 10.3390/cancers11050654
202. Ye J, Wang H, Medina R, Chakraborty S, Sun M, Valenzuela A, et al. rWTC-MBTA: autologous vaccine prevents metastases via antitumor immune responses. J Exp Clin Cancer Res (2023) 42(1):163. doi: 10.1186/s13046-023-02744-8
203. Janotová T, Jalovecká M, Auerová M, Švecová I, Bruzlová P, Maierová V, et al. The use of anchored agonists of phagocytic receptors for cancer immunotherapy: B16-F10 murine melanoma model. PloS One (2014) 9(1):e85222. doi: 10.1371/journal.pone.0085222
204. Waldmannová E, Caisová V, Fáberová J, Sváčková P, Kovářová M, Sváčková D, et al. The use of Zymosan A and bacteria anchored to tumor cells for effective cancer immunotherapy: B16-F10 murine melanoma model. Int Immunopharmacol (2016) 39:295–306. doi: 10.1016/j.intimp.2016.08.004
205. Seo HS, Michalek SM, Nahm MH. Lipoteichoic acid is important in innate immune responses to gram-positive bacteria. Infect Immun (2008) 76(1):206–13. doi: 10.1128/IAI.01140-07
206. Urban-Wojciuk Z, Khan MM, Oyler BL, Fåhraeus R, Marek-Trzonkowska N, Nita-Lazar A, et al. The role of TLRs in anti-cancer immunity and tumor rejection. Front Immunol (2019) 10. doi: 10.3389/fimmu.2019.02388
207. Bianchi F, Pretto S, Tagliabue E, Balsari A, Sfondrini L. Exploiting poly(I:C) to induce cancer cell apoptosis. Cancer Biol Ther (2017) 18(10):747–56. doi: 10.1080/15384047.2017.1373220
208. Steinhagen F, Kinjo T, Bode C, Klinman DM. TLR-based immune adjuvants. Vaccine (2011) 29(17):3341–55. doi: 10.1016/j.vaccine.2010.08.002
209. Rook AH, Gelfand JM, Wysocka M, Troxel AB, Benoit B, Surber C, et al. Topical resiquimod can induce disease regression and enhance T-cell effector functions in cutaneous T-cell lymphoma. Blood (2015) 126(12):1452–61. doi: 10.1182/blood-2015-02-630335
210. Wu JJ, Huang DB, Tyring SK. Resiquimod: a new immune response modifier with potential as a vaccine adjuvant for Th1 immune responses. Antiviral Res (2004) 64(2):79–83. doi: 10.1016/j.antiviral.2004.07.002
211. Binnewies M, Roberts EW, Kersten K, Chan V, Fearon DF, Merad M, et al. Understanding the tumor immune microenvironment (TIME) for effective therapy. Nat Med (2018) 24(5):541–50. doi: 10.1038/s41591-018-0014-x
212. Duan Q, Zhang H, Zheng J, Zhang L. Turning Cold into Hot: Firing up the Tumor Microenvironment. Trends Cancer. (2020) 6(7):605–18. doi: 10.1016/j.trecan.2020.02.022
213. Liu Y, Guo J, Huang L. Modulation of tumor microenvironment for immunotherapy: focus on nanomaterial-based strategies. Theranostics (2020) 10(7):3099–117. doi: 10.7150/thno.42998
214. Agrawal S, Kandimalla ER. Intratumoural immunotherapy: activation of nucleic acid sensing pattern recognition receptors. Immunooncol Technol (2019) 3:15–23. doi: 10.1016/j.iotech.2019.10.001
215. Wang D, Jiang W, Zhu F, Mao X, Agrawal S. Modulation of the tumor microenvironment by intratumoral administration of IMO-2125, a novel TLR9 agonist, for cancer immunotherapy. Int J Oncol (2018) 53(3):1193–203. doi: 10.3892/ijo.2018.4456
216. Koster BD, Santegoets SJAM, Harting J, Baars A, van Ham SM, Scheper RJ, et al. Autologous tumor cell vaccination combined with systemic CpG-B and IFN-α promotes immune activation and induces clinical responses in patients with metastatic renal cell carcinoma: a phase II trial. Cancer Immunol Immunother (2019) 68(6):1025–35. doi: 10.1007/s00262-019-02320-0
217. Gilliet M, Conrad C, Geiges M, Cozzio A, Thürlimann W, Burg G, et al. Psoriasis triggered by toll-like receptor 7 agonist imiquimod in the presence of dermal plasmacytoid dendritic cell precursors. Arch Dermatol (2004) 140(12):1490–5. doi: 10.1001/archderm.140.12.1490
218. Pradere JP, Dapito DH, Schwabe RF. The Yin and Yang of Toll-like receptors in cancer. Oncogene (2014) 33(27):3485–95. doi: 10.1038/onc.2013.302
219. Gearing AJ. Targeting toll-like receptors for drug development: a summary of commercial approaches. Immunol Cell Biol (2007) 85(6):490–4. doi: 10.1038/sj.icb.7100102
220. Tse K, Horner AA. Update on toll-like receptor-directed therapies for human disease. Ann Rheum Dis (2007) 66 Suppl 3(Suppl 3):iii77–80. doi: 10.1136/ard.2007.078998
221. Tran TH, Tran TTP, Truong DH, Nguyen HT, Pham TT, Yong CS, et al. Toll-like receptor-targeted particles: A paradigm to manipulate the tumor microenvironment for cancer immunotherapy. Acta Biomater (2019) 94:82–96. doi: 10.1016/j.actbio.2019.05.043
Keywords: TLR - toll-like receptor, TLR agonists, cancer vaccine, immunotherapy, adjuvant, cancer immuno therapy
Citation: Chakraborty S, Ye J, Wang H, Sun M, Zhang Y, Sang X and Zhuang Z (2023) Application of toll-like receptors (TLRs) and their agonists in cancer vaccines and immunotherapy. Front. Immunol. 14:1227833. doi: 10.3389/fimmu.2023.1227833
Received: 23 May 2023; Accepted: 10 October 2023;
Published: 23 October 2023.
Edited by:
Ralf Kircheis, Syntacoll GmbH, GermanyReviewed by:
Hiroaki Shime, Nagoya City University, JapanCopyright © 2023 Chakraborty, Ye, Wang, Sun, Zhang, Sang and Zhuang. This is an open-access article distributed under the terms of the Creative Commons Attribution License (CC BY). The use, distribution or reproduction in other forums is permitted, provided the original author(s) and the copyright owner(s) are credited and that the original publication in this journal is cited, in accordance with accepted academic practice. No use, distribution or reproduction is permitted which does not comply with these terms.
*Correspondence: Zhengping Zhuang, emhlbmdwaW5nLnpodWFuZ0BuaWguZ292
†These authors share senior authorship
Disclaimer: All claims expressed in this article are solely those of the authors and do not necessarily represent those of their affiliated organizations, or those of the publisher, the editors and the reviewers. Any product that may be evaluated in this article or claim that may be made by its manufacturer is not guaranteed or endorsed by the publisher.
Research integrity at Frontiers
Learn more about the work of our research integrity team to safeguard the quality of each article we publish.