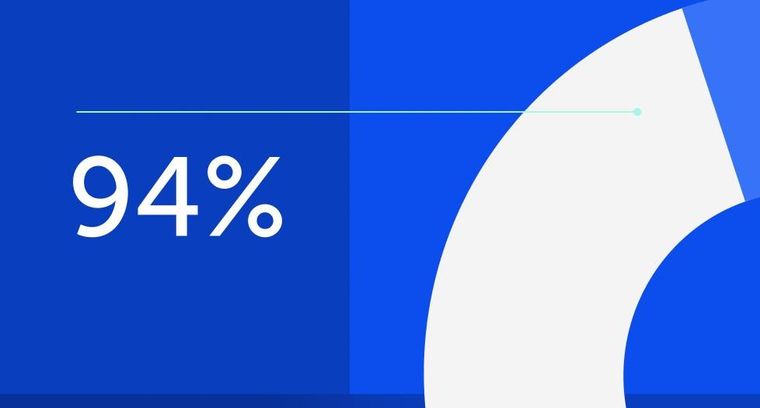
94% of researchers rate our articles as excellent or good
Learn more about the work of our research integrity team to safeguard the quality of each article we publish.
Find out more
REVIEW article
Front. Immunol., 14 August 2023
Sec. Cancer Immunity and Immunotherapy
Volume 14 - 2023 | https://doi.org/10.3389/fimmu.2023.1226443
This article is part of the Research TopicTherapeutic Targeting Of MDSC In The Tumor And Immune MicroenvironmentView all 10 articles
Myeloid-derived suppressor cells (MDSCs) are a heterogeneous myeloid cell population and serve as a vital contributor to the tumor microenvironment. Reactive oxygen species (ROS) are byproducts of aerobic respiration and are involved in regulating normal biological activities and disease progression. MDSCs can produce ROS to fulfill their immunosuppressive activity and eliminate excessive ROS to survive comfily through the redox system. This review focuses on how MDSCs survive and function in high levels of ROS and summarizes immunotherapy targeting ROS in MDSCs. The distinctive role of ROS in MDSCs will inspire us to widely apply the blocked oxidative stress strategy in targeting MDSC therapy to future clinical therapeutics.
Myeloid-derived suppressor cells (MDSCs) are a heterogeneous population of myeloid cells with immunosuppressive activity. MDSCs play a crucial role in tumorigenesis and inhibit antitumor immune responses to promote tumor development (1, 2). In addition to cancer, MDSCs are also involved in autoimmune diseases, sepsis, bone marrow transplantation and infection diseases (1, 3).Reactive oxygen species (ROS) have miscellaneous effects and are involved in both cell biological activities and oxidative stress disease (4). Notably, ROS are one of the dominant immunosuppressive functional effector molecules of MDSCs, and MDSCs can also adjust the ROS level to a proper level to maintain the state of MDSCs. Currently, immunotherapy that targets MDSCs has achieved significant results, but targeting ROS in MDSCs has not yet become a therapeutic focus that will be worth further investigation.
This paper summarizes the distinctive regulation, scavenging and effects of ROS in MDSCs. In addition, we generalize immunotherapy that targets ROS in MDSCs. This will provide novel potential insight for targeting MDSC immunotherapy.
Myeloid-derived suppressor cells (MDSCs) are a heterogeneous population composed of immature myeloid cells (IMCs). In pathological conditions such as cancer, infectious diseases, trauma, and some autoimmune disorders, IMCs cannot differentiate into mature myeloid cells, which causes the activation and expansion of MDSCs (1, 5). At present, we can identify MDSCs by phenotype and immunosuppressive function. The phenotype of mouse MDSCs is CD11b+Gr-1+. According to different epitopes (Ly6G and Ly6C) in Gr-1, mouse MDSCs can be further divided into two subgroups: CD11b+Gr-1+Ly6GhighLy6Clowgranulocyte/polymorphonuclear MDSCs (G-MDSCs/PMN-MDSCs) and CD11b+Gr-1+Ly6GlowLy6Chighmonocytic MDSCs (M-MDSCs) (6). More importantly, we can use different antiapoptotic molecules to discriminate PMN-MDSCs and M-MDSCs. The antiapoptotic molecule MCL-1 is required for the development of PMN-MDSCs, while M-MDSCs require another antiapoptotic molecule, c-FLIP (7). The phenotype of human MDSCs and their subsets is different from that of mice. Human MDSCs express CD11b+CD33+HLA-DR-/low. Human PMN-MDSCs express CD15, while human M-MDSCs express CD14 (8). In most types of cancer, PMN-MDSCs are the predominant population, while M-MDSCs have stronger immunosuppressive activity than PMN-MDSCs (1). Except for phenotypic identification, many original methods are being exploited to identify MDSCs. Single-cell RNA sequencing (scRNAseq) technology could describe MDSCs by novel surface markers (CD84, JAML) and definite PMN-MDSCs with enrichment genes (Ngp, Ltf, Anxa1, Mmp8 and Cybb) (9, 10). IHC staining analysis showed that MDSCs are located in the tumor epithelial border (11). Moreover, metabolite and lipid analyses of MDSCs also demonstrated that MDSCs have a specific response to high glucose concentrations (12).
The immunosuppressive activity of MDSCs relies on the expansion and activation of MDSCs. There are a variety of factors accounting for the expansion of the MDSCs, such as cyclooxygenase 2 (COX2), prostaglandin, stem-cell factor (SCF), macrophage CSF (M-CSF), granulocyte/macrophage CSF (GM-CSF), vascular endothelial growth factor (VEGF), TNF-α, polyunsaturated fatty acids, MyD88 and HIF-1α (13–15). Most of these factors advance the expansion of the MDSCs by triggering the STAT3, IRF8, C/EBP-β and NOTCH signaling (16). Among them, STAT3 is a vital regulator of the expansion of MDSCs, which can also upregulate the proinflammatory protein S100A8/9 expression and induce the expression of the downstream targets of STAT3 including survivin, BCL-XL and cyclin-D1 (13, 14). Endoplasmic reticulum (ER) stress can promote the accumulation of MDSCs by activating TNF-related ligand receptors which induce the apoptosis (15). Last but not least, the metabolites adenosine, IDO and lactic acid accumulated in the TME can also contribute to the expansion of MDSCs (17). Other factors, such as IFN-γ, IL-1β, IL-4, IL-6, IL-13, TNF and high mobility group Box 1(HMGB1), could influence the MDSCs suppressive activity by activating STAT1, STAT3, STAT6 and NF-κB signaling pathways (18).
Several major molecules contribute to MDSC-mediated immune suppression, including arginase 1 (Arg-1), inducible nitric oxide synthase (iNOS), COX2, TGF-β, IL-10 and ROS. Many factors, such as STAT3, C/EBPβ, p50 NF-κB, and IDO1, play a critical role in MDSC function by regulating these functional effector molecules (16, 19, 20). Arg-1, which converts L-arginine to urea and L-ornithine, inhibits T-cell function by decreasing the expression of the CD3ζ chain and impairing the expression of cyclin D3 and cyclin-dependent kinase 4 (cdk4) (21). ROS are the characteristic molecules of PMN-MDSCs, while M-MDSCs mainly produce NO (8). NO produced by MDSCs leads to the suppression of T-cell responses by reducing the tyrosine phosphorylation of JAK3 and STAT5, preventing MHC II transcription and triggering T-cell apoptosis (22). The interaction between ROS and NO can promote the formation of peroxynitrite, which leads to the desensitization of T-cell receptors and T-cell tolerance. Treatment of cancer with AT38 ([3-(aminocarbonyl)furoxan-4-yl] methyl salicylate) could increase antitumor immunity by interfering with the expression of ARG1 and NOS2 enzymes in myeloid cells (23).
In addition, MDSCs can recruit and expand Treg cells via the immunosuppressive cytokines IL-10 and TGF-β. MDSCs can also reduce the secretion of IL-6 and TNF-α by macrophages and shape them into the M2-type phenotype, which promotes tumor progression (24). In turn, Treg cells induce the expression of B7 homolog 1 (B7-H1), B7-H3 and B7-H4 on the cell surface of MDSCs, which causes an increase in IL-10 production and immunosuppressive activity of MDSCs (25). In addition, MDSCs can produce adenosine due to the high expression of CD73 and CD39, which hydrolyze ATP into adenosine, and adenosine can inhibit the immune responses of both T cells and NK cells in the tumor microenvironment (26).
Reactive oxygen species (ROS), oxygen-containing derivatives, include a range of species such as superoxide (O2.-), hydrogen peroxide (H2O2), nitric oxide, peroxynitrite, hypochlorous acid, singlet oxygen and hydroxyl radicals (27). Among them, the three most common forms of ROS are superoxide, H2O2 and hydroxyl. Different forms of ROS can have different targets. To illustrate, H2O2 takes effect through the modification of specific cysteine, selenocysteine, methionine and histidine residues in targeted proteins (28, 29), but O2.-, hydroxyl radicals and peroxynitrite can irreversibly undermine intracellular proteins, DNA and lipids (30). In cancer, the most studied ROS components are O2.-and H2O2 (31). However, the main increased pool of ROS released by MDSCs is primarily H2O2 under pathological conditions (32).
ROS are byproducts of aerobic respiration that can be produced by many cells, including hematopoietic stem cells (HSCs), tumor cells, cancer stem cells (CSCs) and immune cells (33). The production of ROS relies on cell type. Tumor cells, MDSCs and professional phagocytes can produce abundant ROS. However, HSCs and CSCs have low ROS content (34, 35).
ROS are short-lived, strong-effect and short reaction distance compounds that serve as a double-edged sword that elicits both beneficial and harmful effects in cells. The most common influence is the toxic side effects of ROS. Elevated levels of ROS can damage cells and intracellular components, cause DNA hydroxylation, protein denaturation and tissue damage, and ultimately lead to cell cycle G2/M arrest, apoptosis, senescence and death, and ROS can also participate in mitochondria, death receptors, and endoplasmic reticulum-mediated apoptosis (36). However, ROS also serve as the second messenger of cell signal transmission to play a regulatory role in many crucial biological activities of normal cells (4).
NADPH oxidase (NOX) enzymes and mitochondria are major sources of endogenous ROS. In addition, there are numerous cellular sources of ROS, including xanthine oxidase, cyclooxygenases, cytochrome p450 enzymes, lipoxygenases and the endoplasmic reticulum (28).
Two major sources of ROS in MDSCs are NOX2 and mitochondria. Compared with MDSCs, cancer cells and macrophages also utilize mitochondria and NADPH oxidase to produce ROS. However, T cells express no or very low levels of NADPH oxidase (37).
Mitochondria have ten sites to generate O2.-, particularly those derived from mitochondrial electron transport chain (ETC) complexes. Complex I and III of the ETC generate O2.-, which is rapidly converted to H2O2 via mitochondrial SOD2, while the O2.- from the complex can be converted into H2O2 by cytosolic SOD1 (38). Mitochondrial ROS are implicated in diverse diseases, including cancer, diabetes and inflammatory disorders, and regulate healthy cell physiological function (39).
The NOX family has seven members: NOX1–5, DUOX1 and DUOX2 (40). NOX2 is a multicomponent complex that is made up of a transmembrane heterodimer that contains NOX2 and p22phox. Other components are cytosolic protein factors, including p47phox, p67phox, p40phox and small GTP-binding proteins such as G proteins RAC1 or RAC2. Under basal conditions, gp91phox and p22phox are transmembrane proteins, while the cytosolic subunits p47phox, p67phox and p40phox are connected together, and RAC combined with GDP forms a complex with its inhibitor Rho-GDI and does not interact with the other three cytosolic subunits. When exposed to stimulus, NOX2 is activated. Upon activation, p47phox is phosphorylated and then migrates to the membrane, where it combines with gp91phox and p22phox. Rho-GDI separates from the complex, and then RAC binding with GDP combines with gp91phox to form a multicomponent complex (41). NOX2 catalyzes the conversion of oxygen molecules into superoxide anions, which generates H2O2 by SOD. Deficiency or dysfunction of NOX2 in phagocytes may reduce ROS production, resulting in chronic granulomatous disease (CGD) (42). Comparably, MDSCs in NOX2-deficient mice produced less ROS, which lose the ability to inhibit the CD8+ T-cell immune response (43). Rats and mice with decreased ROS caused by allelic polymorphisms of p47phox were more susceptible to developing severe arthritis (44).
Many factors can regulate ROS production, such as GM-CSF, interleukin, TGF, TNF, FGF, platelet-derived growth factor, TLR agonists, protease, nucleotide receptors, TCR stimulation and peroxynitrite (32, 45, 46).
Various types of cells and survival environments possess different ROS regulatory mechanisms. In terms of MDSCs, it has been proven that multiple molecules can govern intracellular ROS, such as STAT3, fatty acid transport protein 2 (FATP2) and noncoding RNAs. STAT3 is an important transcription factor related to the expansion, differentiation and function of MDSCs. STAT3 directly increases the expression of p47phox, which belongs to the NOX2 complex, by binding to the promoter region of p47phox. Blocking STAT3 could downregulate the expression of gp91phox and p47phox to decrease ROS production (43, 47, 48). In addition, tumor-derived GM-CSF activated STAT3 signaling to induce the expression of FATP2 in MDSCs. Subsequently, FATP2 in MDSCs can take up abundant lipids that cause elevated ROS levels (49). Furthermore, noncoding RNAs (lincRNAs and miRNAs) that have been upregulated during bacterial and viral infection are reported to influence ROS generation in MDSCs (50). During virus infection, lncRNA RUNXOR and HOTAIRM1 are upregulated and are responsible for elevated levels of ROS, Arg-1 and iNOS in MDSCs (51, 52). MiRNA-10a and miRNA-21, which are also upregulated in hypoxia-induced glioma-derived exosomes, could strengthen ROS and NO production in MDSCs with the potential to enhance the suppressive activity of MDSCs (53).
In addition, cancer-associated fibroblasts (CAFs) can polarize monocytes to MDSCs, which suppress CD8+ T-cell proliferation and function by generating ROS (54). Murine olfactory ecto-mesenchymal stem cell-derived exosomes could also enhance the suppressive activity of MDSCs by upregulating ROS and NO levels (55).
In contrast to MDSCs, other myeloid cells, such as macrophages, can stimulate NADPH oxidase expression and activity to elevate the level of ROS by other disparate factors, such as P2X7, brain-specific angiogenesis inhibitor 1 (BAI1), beryllium, myocardin-related transcription Factor A (MRTF-A) and TLRs (56–60). However, mitochondrial uncoupling protein 2 (UCP2), paraoxonase 1 (PON1) and IL-10 negatively regulate the ROS level in macrophages (61–63).
In general, the cell needs an appropriate level of ROS to maintain normal physiological function. Either too few or too many ROS are harmful. Normally, ROS production is controlled in a safe range, and superfluous ROS can be neutralized by the antioxidant system to maintain cell homeostasis. The antioxidant system contains antioxidant enzymes and nonenzymatic molecules. Common antioxidant enzymes include superoxide dismutase (SODs), catalase, peroxidase (PRDXs), peroxiredoxins (Prxs) and glutathione peroxidase (GPXs) (64). Other nonenzymatic antioxidant molecules are glutathione, flavonoids, thioredoxin, and vitamins A, C and E (65, 66). If the redox system is out of balance, the rising ROS will lead to oxidative stress. Oxidative stress is considered a vital inducer of many pathological diseases, such as cancer, atherosclerosis, multiple sclerosis, ischemia and reperfusion injury, Alzheimer’s disease, cardiovascular diseases and traumatic brain injury (67–71).
For example, the antioxidant system of tumor cells can cope with the production of ROS properly via antioxidant enzymes and autophagy (72). The overproduction of ROS in tumor cells could maintain the pro-tumourigenic signaling, which results from the upregulation of SOD expression, local inactivation of a H2O2-degrading enzyme, oxidative inactivation of phosphatase and tension homolog (PTEN) and mutations in Nrf2 and P53 transcription factors (4, 73–75).
Surprisingly, MDSCs could still survive and function excellently by producing high levels of ROS. How can MDSCs scavenge superfluous ROS? This can be ascribed to some essential factors, such as Nrf2, HMGB1, IDO1, calcium-calmodulin kinase 2 (CaMKK2), HIF-1α, pyruvate dehydrogenase kinase 1 (PDK1) and phosphoenolpyruvate (PEP) (Figure 1)
The Nrf2 transcription factor plays a crucial role in regulating the antioxidative response and inducing the expression of antioxidant and detoxification enzyme genes, including heme oxygenase-1 (HO-1), NAD(P)H:quinone oxidoreductase 1 (NQO1), catalase and SOD (76). Under normal circumstances, Nrf2 combined with Kelch-like ECH-associated protein 1 (Keap1) is limited to degradation in the cytoplasm. However, under oxidative stress conditions, Keap1 is modified at a specific cysteine position to disable its E3 ligase adaptor and release Nrf2. The released Nrf2 translocates into the nucleus and binds to the small musculoaponeurotic fibrosarcoma (sMaf) protein to form active heterodimers that transactivate downstream antioxidant response elements (AREs) and induce their transcription to exert antioxidant effects (77). Nrf2 is greatly applied to reduce intracellular oxidative stress and apoptosis. Compared to wild-type MDSCs, Nrf2-deficient MDSCs display a greater accumulation of intracellular ROS and attenuated antioxidant enzyme induction (78). MDSCs in the host expressing Nrf2 reduce oxidative stress and cell apoptosis; thus, MDSCs can survive longer (79, 80).
With the exception of Nrf2, the existence of HMGB1 in MDSCs cannot be underestimated. HMGB1, a damage-associated molecular pattern (DAMP) molecule, is a vital driver of MDSC accumulation and immunosuppressive function, as reported in early studies. In the tumor microenvironment, elevated ROS can increase cytoplasmic translocation and release HMGB1 (81). Subsequently, HMGB1 promotes the survival and viability of MDSCs by inducing autophagy (80, 82).
MDSCs also express some enzymes, such as IDO1 and CaMKK2, to negatively modulate the generation of ROS. IDO1, a heme-binding metabolic enzyme, consumes superoxide anion radicals and peroxides to catalyze tryptophan (Trp) into kynurenine (Kyn) (83). CD11b+Gr1+ MDSCs from IDO-KO hosts enhanced ROS generation and downregulated the expression of ROS scavenging genes (84, 85). Moreover, CaMKK2 could upregulate the transcription level of Nrf2, not NOX1 and NOX2, to decrease the ROS level by phosphorylating and activating its downstream target AMPK (86, 87).
In addition, hypoxia plays a crucial role in regulating the function of tumor derived MDSCs. HIF-1α could decrease NOX2 expression and excessive ROS production, which may give rise to the preferable survival of MDSCs in the tumor microenvironment (88). In turn, ROS could facilitate HIF-1α accumulation, and then HIF-1α activates PDK1, which could prevent the persistence of potentially harmful and superfluous mitochondrial ROS by inhibiting pyruvate dehydrogenase to restrain the conversion of pyruvate to acetyl-CoA, resulting in a lessened tricarboxylic acid (TCA) cycle (89, 90). Apart from hypoxia, tumor cells can increase the glycolysis of MDSCs in the tumor microenvironment. Tumor derived MDSCs displayed higher glycolysis to prevent the cell apoptosis by restraining excess ROS production. Most importantly, the glycolytic metabolite phosphoenolpyruvate (PEP) is a crucial antioxidant agent that averts MDSC apoptosis and contributes to MDSC survival by hindering excessive ROS production (91).
In contrast to MDSCs, HSCs and CSCs have low ROS content. Several signaling molecules, such as ataxia telangiectasia mutated (TAM), PI3K/Akt, FoxO3 (FoxO transcription Factors 3), phosphatase and tensin homology (PTEN), p53, Prdm16 (PR domain-containing 16), HIF-1α, p38MAPK, and Nrf2, account for the low ROS level to maintain stemness and quiescence in HSCs (33). For example, neural stem cells have a high level of ROS (92). CSC cells also have reduced levels of ROS, which may be attributed to the variant isoform CD44v of the adhesion molecules CD44 and CD13 that boosts the activity of the free radical scavenging system (93, 94).
MDSCs can produce ROS by mitochondria and NOX2. MDSCs can take up lipids through FATP2 to promote mitochondrial ROS production. Moreover, the transcription factor STAT3 can increase NOX2 expression to upregulate ROS levels in MDSCs. Then, the elevated ROS level can activate the antioxidant system to eliminate excessive ROS. Nrf2 could be transcriptionally activated to initiate the expression of its downstream antioxidant genes. The high level of ROS can also induce nuclear heterotopic HMGB1 to promote the survival of MDSCs by autophagy. In addition, HIF-1α can activate PDK1 to inhibit mitochondrial ROS production. IDO1 can scavenge ROS with its metabolic characteristics. Another enzyme, CaMKK2, can activate AMPK to decrease ROS production. The glycolytic metabolite PEP could also prevent massive ROS production to keep the ROS level in a suitable range.
ROS signaling can activate cellular signaling pathways, such as NF-κB, mitogen-activated protein kinase (MAPK), JAK/STAT and phosphoinositide 3-kinase (PI3K)/AKT (4, 95). Furthermore, ROS also enhance the activity of activator protein-1 (AP-1) by stimulating MAPK cascades to dominate a wide range of cellular processes and trigger P53 transactivation that mediates apoptosis, and ROS can induce the expression of redox factor-1 (Ref-1), leading to the transcriptional activity of HIF-1α (96, 97). Generally, ROS are considered to have proinflammatory effects, but it has also been reported that ROS derived from NOX2 have anti-inflammatory functions (45). In the murine arthritis (CIA) model, NADPH-deficient dendritic cells can produce more proinflammatory cytokines and induce both Th1 and Th17 responses to promote autoimmune arthritis (98). In addition, ROS derived from NOX2 could inhibit the NLRP3 inflammasome via the PI3K/Akt/NF-κB pathway at 3 days after stroke (99).
ROS produced by MDSCs could have distinct impacts on different cells (Figure 2). In the tumor microenvironment, PMN-MDSCs release ROS into the extracellular space to directly and indirectly support tumor progression. ROS produced by PMN-MDSCs inhibited T-cell responses through p-STAT3 signaling. ROS have an impact on the activation, proliferation and effect of T cells by regulating cell surface thiol levels (44, 100). Specifically, peroxynitrite could nitrate the TCR/CD8 complex, which prevented it from combining with pMHC, and H2O2 reduced the TCRζ chain and IFN-γ secretion of T cells to destroy T-cell function (43, 48). In addition, when encountering circulating tumor cells (CTCs), PMN-MDSCs can produce excessive levels of ROS to upregulate Notch1 expression in CTCs via the Nrf-2-ARE axis. Notch1 could bind to the ligand jagged on the surface of PMN-MDSCs. In addition, Nodal, the downstream target gene of Notch1 in CTCs, can bind to Noda1 recptor cripto in PMN-MDSCs in turn, and the interaction between these signals eventually promotes the survival and proliferation of CTCs (101). Likewise, ROS derived from macrophages and granulocytes can inhibit the activation, proliferation and effect of T cells, and macrophages and activated T cells produce ROS to induce regulatory T cells (102–105).
In addition to inhibiting T cells, ROS released by MDSCs have immunosuppressive activities on B cells and NK cells under infection pathological conditions. During virus infection, two subsets of MDSCs rapidly accumulate at the infected site. In detail, PMN-MDSCs inhibit the activation, proliferation and function of NK cells and reduce the secretion of IFN-γ and granzyme B via ROS (106, 107), while M-MDSCs release ROS, including superoxide, peroxynitrite, and nitric oxide, but not H2O2, to suppress B-cell responses (108). Similarly, human PMN-MDSCs isolated from buffy coats could also produce ROS and other soluble mediators to suppress B-cell proliferation and antibody production (109).
However, professional phagocytes, tumor cells and CSCs are distinct from MDSCs. Professional phagocytes generate ROS to effectively jeopardize pathogens by interacting with microbial components to impair bacterial metabolism (110). ROS in tumor cells have dualistic impacts on the initiation, promotion, progression and metastasis of tumor cells (111). Increased ROS levels in tumor cells could facilitate tumorigenicity by enhancing the proliferation, growth, survival, invasion and metastasis of tumor cells. In contrast to these effects, ROS can suppress tumor growth by inducing apoptosis, autophagy, necrosis and ferroptosis. Both normal stem cells and CSCs exhibit low levels of intracellular ROS content to maintain stemness (112).
In summary, MDSCs and ROS are interactive and mutually beneficial. MDSCs can produce ROS to inhibit antigen-specific T cells (32, 47). In turn, ROS could regulate the differentiation and immunosuppressive activity of MDSCs. In the absence of ROS, the function of MDSCs could be lost to suppress adaptive T-cell responses (43). Additionally, ROS can affect the differentiation of myeloid cells by regulating related gene expression. High levels of ROS can prevent MDSCs from differentiating into mature myeloid cells, while low levels of ROS resulting from catalase and a lack of NOX2 activity enable MDSCs to differentiate into TAMs and DCs (113). How to control ROS levels in MDSCs is a priority and needs further investigation.
ROS produced by MDSCs can have diverse effects on different kinds of cells. MDSCs-derived ROS can promote the proliferation and metastasis of circulating tumor cells by Nrf2/Notch1/Nodal signaling. MDSCs-derived ROS have an inhibitory effect on other immune cells, such as T, B and NK cells, and promote disease progression by inhibiting their function.
Currently, a variety of immune therapies to target MDSCs are being exploited to improve the efficacy of cancer immunotherapy. MDSCs mediated immuno- suppressive function could be abrogated when ROS production is inhibited (114). Remarkable achievements have been made in strategies to lessen the ROS production and block the induction of oxidative stress in MDSCs (115) (Table 1).
The most representative molecules of anti-inflammatory and antitumor drugs are bardoxolone methyl (CDDO-Me), nitroaspirin and Embelin. On account of its capacity to upregulate several antioxidant genes, including NAD(P)H: quinone oxidoreductase 1 (NQO1), thioredoxin, catalase, superoxide dismutase and heme oxygenase, CDDO-Me could efficiently abrogate the immune suppressive effect of MDSCs and enhance T-cell function by activating the target gene NQO1 to decrease MDSC-mediated ROS production, while CDDO-Me did not affect the NO level in MDSCs (116). Nitroaspirin has also been proven to inhibit ROS production and limit the activity of Arg-1 and iNOS in MDSCs (18). Treatment combining vaccination against gp70 with nitroaspirin could inhibit MDSC function and enhance antitumor activity (117). Embelin has anti-inflammatory and antitumor effects in previous studies. It could impair the immunosuppressive activity of MDSCs by reducing the generation of ROS through STAT3 signaling to improve the antitumor immune response in colitis-associated cancer mice (129).
In addition, many inhibitors are being exploited to reduce ROS level such as N-acetylcysteine (NAC), L-NIL, histamine dihydrochloride (HDC), celecoxib, alisertib, SAHA and sildenafil. NAC, a well-established antioxidant that had the ability to reduce ROS and increase the extracellular pool of cysteine. Many animal models have verified its antitumor efficacy. NAC could stimulate the degradation of HIF-1 and inhibit its activity by neutralizing ROS (131). Moreover, the iNOS inhibitor L-NIL, NOX2 inhibitor HDC and COX-2 inhibitor celecoxib could weaken MDSCs function by downregulating ROS production, resulting in enhanced antigen-specific cytotoxicity of CTL (124–126).
Along with inhibitors that target the effector molecules of the immunosuppressive activity of MDSCs, enzyme inhibitors can achieve similar outcomes. The selective Aurora-A kinase inhibitor alisertib directly weakened the immunosuppressive function of MDSCs by notably downregulating the mRNA expression levels of associated genes, such as NOS2, S100A8, S100A9, CYBB and NCF1, and compromising ROS production by inhibiting the JAK2-STAT3 pathway (128). Phosphodiesterase-5 (PDE-5) inhibitors reversed surgery-induced PMN-MDSC immunosuppression by downregulating the level of ROS (130).
Out of the ordinary, the histone deacetylase inhibitor SAHA could augment the intracellular ROS to induce apoptosis in MDSCs. That might be a promising and novel MDSCs-targeted therapy (127). In addition, TLR2 agonist Pam3CSK4 could attenuate hepatocellular carcinoma progression by decreasing ROS content and promoting MDSCs polarization (132).
Natural products are increasingly being discovered and researched in tumor therapy. With the deeper comprehension of natural products, many plant extracts have antioxidant impacts. Among them, withaferin A (WA), a component of the root extract of the plant Withania somnifera Dunal (WRE), could decrease ROS production in MDSCs through a STAT3-dependent mechanism (135). The polysaccharide nCKAP-2 contained in native Curcumae Rhizoma (CR) could induce MDSC apoptosis in a dose-dependent manner through the TLR/NF-κB pathway. In addition, nCKAP-2 can significantly relieve the inhibitory effect of MDSCs on T cells by reducing the ROS level (136). Moreover, curcumin has been reported to lessen the production of ROS and the Arg-1 expression level in MDSCs, which not only inhibited the accumulation of MDSCs in spleen and tumor tissue but also weakened the immunosuppressive function of MDSCs (134). Swertianolin isolated from Swertia and sanguinarine (SNG) derived from Sanguinaria canadensis could prominently decrease the secretion of ROS to inhibit the immunosuppressive effect of MDSCs (118, 133).
Traditional Chinese medicines have made enormous achievements in antioxidant activity. Baicalein is a traditional Chinese herbal medicine. Baicalein prevented the expansion and function of MDSCs in lupus mice, which can be attributed to decreased ROS levels and enhanced Nrf2 activation (119). Jianpi Huayu decoction (JHD), another traditional Chinese medicine, is an experienced prescription for tumor therapy. When MDSCs were treated with JHD, MDSCs could differentiate into macrophages and dendritic cells, and ROS levels were reduced (120).
Furthermore, endostatin (ES) derived from collagen XVIII has the potential to target PMN-MDSCs selectively, resulting in obviously reduced ROS production (122). Doxorubicin (Dox), the conventional chemotherapy to reduce the number of MDSCs in tumor tissues and promote antitumor responses, is converted into a liposomal formulation to improve the efficacy of therapy, as well as the peptide named the E75 epitope (Pep) originating from human epidermal growth factor receptor 2 (HER2/neu). Combination therapy with liposomal nonliposomal Dox and liposomal Pep was the best treatment compared to other single therapies, which decreased ROS generation and downregulated multiple genes related to immunosuppressive function, such as S100A8, S100A9, Arg-1 and iNOS (137). 1a,25-Dihydroxyvitamin D3 (calcitriol) supplementation could reverse the increased level of ROS in IL-6-induced MDSCs (121). In the same way, iron supplementation with ferumoxytol could attenuate MDSC function by significantly downregulating ROS production and inhibiting the expansion of MDSCs in LPS-induced septic mice (123). In addition, GMI is a fungal immunomodulatory protein isolated from Ganoderma microsporum that reduces MDSC expansion in bone marrow cells (BMCs) stimulated by S. aureus biofilms, which was attributed to increased cytokine expression and a reduction in ROS levels (138, 140, 141). L-4F, an apolipoprotein A-I (ApoA-I) mimetic peptide, inhibited the immunosuppressive function of PMN-MDSCs but not M-MDSCs by decreasing ROS and H2O2 production (139).
Based on a previously published review, this paper further updated and listed the new molecules found in recent years that can regulate ROS levels in MDSCs and comprehensively summarized the therapeutic drugs that can target ROS levels in MDSCs. This provides a treatment strategy for cancer immunotherapy.
Compared to other myeloid cells, such as macrophages or tumor cells, ROS play an irreplaceable and distinctive role in MDSCs. On the one hand, MDSCs are required to produce ROS to suppress the antitumor immune response. In turn, excessive ROS can be removed to promote MDSC survival comfortably by activating factors, such as Nrf2, HMGB1, HIF-1α, IDO1, CaMKK2 and PEP. On the other hand, an appropriate level of ROS can prevent further differentiation of MDSCs to better maintain their state and nature. However, other myeloid cells, such as macrophages, have the same sources of ROS as MDSCs and regulate intracellular ROS levels by different factors, such as P2X7 and BAI1. Tumor cells can also induce autophagy to scavenge excessive ROS.
It is widely believed that ROS can promote the development of tumors, but a large number of studies have shown that ROS can promote tumor cell apoptosis and death. At present, studies are emerging that tend to exploit immunotherapies that utilize the ability of ROS to kill tumor cells. Therapy targeting ROS in MDSCs can be therapeutic by impairing MDSC differentiation and function. How to combine targeted ROS therapy in MDSCs and tumor cells requires further consideration.
JH drafted the manuscript. YZ, KZ and KY discussed and revised the manuscript. SW designed the study and revised the manuscript. All authors contributed to the article and approved the submitted version.
This work was supported by the Research Project of the Jiangsu Commission of Health (Grant No. K2019019) and Jiangsu Provincial Medical Key Discipline Cultivation Unit (Grant No. JSDW202241).
The authors declare that the research was conducted in the absence of any commercial or financial relationships that could be construed as a potential conflict of interest.
All claims expressed in this article are solely those of the authors and do not necessarily represent those of their affiliated organizations, or those of the publisher, the editors and the reviewers. Any product that may be evaluated in this article, or claim that may be made by its manufacturer, is not guaranteed or endorsed by the publisher.
1. Gabrilovich DI. Myeloid-derived suppressor cells. Cancer Immunol Res (2017) 5:3–8. doi: 10.1158/2326-6066.Cir-16-0297
2. Xia X, Mao Z, Wang W, Ma J, Tian J, Wang S, et al. Netrin-1 promotes the immunosuppressive activity of MDSCs in colorectal cancer. Cancer Immunol Res (2023) 11:600–13. doi: 10.1158/2326-6066.CIR-22-0658
3. Tian J, Rui K, Hong Y, Wang X, Xiao F, Lin X, et al. Increased GITRL impairs the function of myeloid-derived suppressor cells and exacerbates primary Sjögren's syndrome. J Immunol (2019) 202:1693–703. doi: 10.4049/jimmunol.1801051
4. Leslie NR, Bennett D, Lindsay YE, Stewart H, Gray A, Downes CP. Redox regulation of PI 3-kinase signalling via inactivation of PTEN. EMBO J (2003) 22:5501–10. doi: 10.1093/emboj/cdg513
5. Wang W, Xia X, Mao L, Wang S. The CCAAT/enhancer-binding protein family: its roles in MDSC expansion and function. Front Immunol (2019) 10:1804. doi: 10.3389/fimmu.2019.01804
6. Dolcetti L, Peranzoni E, Ugel S, Marigo I, Fernandez Gomez A, Mesa C, et al. Hierarchy of immunosuppressive strength among myeloid-derived suppressor cell subsets is determined by GM-CSF. Eur J Immunol (2010) 40:22–35. doi: 10.1002/eji.200939903
7. Haverkamp JM, Smith AM, Weinlich R, Dillon CP, Qualls JE, Neale G, et al. Myeloid-derived suppressor activity is mediated by monocytic lineages maintained by continuous inhibition of extrinsic and intrinsic death pathways. Immunity (2014) 41:947–59. doi: 10.1016/j.immuni.2014.10.020
8. Ostrand-Rosenberg S, Fenselau C. Myeloid-derived suppressor cells: immune-suppressive cells that impair antitumor immunity and are sculpted by their environment. J Immunol (2018) 200:422–31. doi: 10.4049/jimmunol.1701019
9. Alshetaiwi H, Pervolarakis N, McIntyre LL, Ma D, Nguyen Q, Rath JA, et al. Defining the emergence of myeloid-derived suppressor cells in breast cancer using single-cell transcriptomics. Sci Immunol (2020) 5:eaay6017. doi: 10.1126/sciimmunol.aay6017
10. Veglia F, Hashimoto A, Dweep H, Sanseviero E, De Leo A, Tcyganov E, et al. Analysis of classical neutrophils and polymorphonuclear myeloid-derived suppressor cells in cancer patients and tumor-bearing mice. J Exp Med (2021) 218:e20201803. doi: 10.1084/jem.20201803
11. Zwing N, Failmezger H, Ooi CH, Hibar DP, Cañamero M, Gomes B, et al. Analysis of spatial organization of suppressive myeloid cells and effector T cells in colorectal cancer-A potential tool for discovering prognostic biomarkers in clinical research. Front Immunol (2020) 11:550250. doi: 10.3389/fimmu.2020.550250
12. Kim J, Lee H, Choi HK, Min H. Discovery of myeloid-derived suppressor cell-specific metabolism by metabolomic and lipidomic profiling. Metabolites (2023) 13:477. doi: 10.3390/metabo13040477
13. Law AMK, Valdes-Mora F, Gallego-Ortega D. Myeloid-derived suppressor cells as a therapeutic target for cancer. Cells (2020) 9:561. doi: 10.3390/cells9030561
14. Sinha P, Okoro C, Foell D, Freeze HH, Ostrand-Rosenberg S, Srikrishna G. Proinflammatory S100 proteins regulate the accumulation of myeloid-derived suppressor cells. J Immunol (Baltimore Md. 1950) (2008) 181:4666–75. doi: 10.4049/jimmunol.181.7.4666
15. Condamine T, Kumar V, Ramachandran IR, Youn JI, Celis E, Finnberg N, et al. ER stress regulates myeloid-derived suppressor cell fate through TRAIL-R-mediated apoptosis. J Clin Invest (2014) 124:2626–39. doi: 10.1172/jci74056
16. Marigo I, Bosio E, Solito S, Mesa C, Fernandez A, Dolcetti L, et al. Tumor-induced tolerance and immune suppression depend on the C/EBPbeta transcription factor. Immunity (2010) 32:790–802. doi: 10.1016/j.immuni.2010.05.010
17. Li N, Kang Y, Wang L, Huff S, Tang R, Hui H, et al. ALKBH5 regulates anti-PD-1 therapy response by modulating lactate and suppressive immune cell accumulation in tumor microenvironment. Proc Natl Acad Sci USA (2020) 117:20159–70. doi: 10.1073/pnas.1918986117
18. Gabrilovich DI, Nagaraj S. Myeloid-derived suppressor cells as regulators of the immune system. Nat Rev Immunol (2009) 9:162–74. doi: 10.1038/nri2506
19. Porta C, Consonni FM, Morlacchi S, Sangaletti S, Bleve A, Totaro MG, et al. Tumor-derived prostaglandin E2 promotes p50 NF-κB-dependent differentiation of monocytic MDSCs. Cancer Res (2020) 80:2874–88. doi: 10.1158/0008-5472.Can-19-2843
20. Mondanelli G, Bianchi R, Pallotta MT, Orabona C, Albini E, Iacono A, et al. A relay pathway between arginine and tryptophan metabolism confers immunosuppressive properties on dendritic cells. Immunity (2017) 46:233–44. doi: 10.1016/j.immuni.2017.01.005
21. Rodriguez PC, Quiceno DG, Ochoa AC. L-arginine availability regulates T-lymphocyte cell-cycle progression. Blood (2007) 109:1568–73. doi: 10.1182/blood-2006-06-031856
22. Harari O, Liao JK. Inhibition of MHC II gene transcription by nitric oxide and antioxidants. Curr Pharm Des (2004) 10:893–8. doi: 10.2174/1381612043452893
23. De Sanctis F, Lamolinara A, Boschi F, Musiu C, Caligola S, Trovato R, et al. Interrupting the nitrosative stress fuels tumor-specific cytotoxic T lymphocytes in pancreatic cancer. J immunother Cancer (2022) 10:e003549. doi: 10.1136/jitc-2021-003549
24. Beury DW, Parker KH, Nyandjo M, Sinha P, Carter KA, Ostrand-Rosenberg S. Cross-talk among myeloid-derived suppressor cells, macrophages, and tumor cells impacts the inflammatory milieu of solid tumors. J leukocyte Biol (2014) 96:1109–18. doi: 10.1189/jlb.3A0414-210R
25. Fujimura T, Ring S, Umansky V, Mahnke K, Enk AH. Regulatory T cells stimulate B7-H1 expression in myeloid-derived suppressor cells in ret melanomas. J Invest Dermatol (2012) 132:1239–46. doi: 10.1038/jid.2011.416
26. Hoskin DW, Mader JS, Furlong SJ, Conrad DM, Blay J. Inhibition of T cell and natural killer cell function by adenosine and its contribution to immune evasion by tumor cells (Review). Int J Oncol (2008) 32:527–35. doi: 10.3892/ijo.32.3.527
27. Murphy MP, Holmgren A, Larsson NG, Halliwell B, Chang CJ, Kalyanaraman B, et al. Unraveling the biological roles of reactive oxygen species. Cell Metab (2011) 13:361–6. doi: 10.1016/j.cmet.2011.03.010
28. Finkel T. Signal transduction by reactive oxygen species. J Cell Biol (2011) 194:7–15. doi: 10.1083/jcb.201102095
29. Hawkes WC, Alkan Z. Regulation of redox signaling by selenoproteins. Biol Trace element Res (2010) 134:235–51. doi: 10.1007/s12011-010-8656-7
30. Winterbourn CC. Hydrogen peroxide reactivity and specificity in thiol-based cell signalling. Biochem Soc Trans (2020) 48:745–54. doi: 10.1042/bst20190049
31. Reczek CR, Chandel NS. ROS-dependent signal transduction. Curr Opin Cell Biol (2015) 33:8–13. doi: 10.1016/j.ceb.2014.09.010
32. Kusmartsev S, Nefedova Y, Yoder D, Gabrilovich DI. Antigen-specific inhibition of CD8+ T cell response by immature myeloid cells in cancer is mediated by reactive oxygen species. J Immunol (Baltimore Md. 1950) (2004) 172:989–99. doi: 10.4049/jimmunol.172.2.989
33. Shi X, Zhang Y, Zheng J, Pan J. Reactive oxygen species in cancer stem cells. Antioxid Redox Signal (2012) 16:1215–28. doi: 10.1089/ars.2012.4529
34. Jang YY, Sharkis SJ. A low level of reactive oxygen species selects for primitive hematopoietic stem cells that may reside in the low-oxygenic niche. Blood (2007) 110:3056–63. doi: 10.1182/blood-2007-05-087759
35. Ye XQ, Li Q, Wang GH, Sun FF, Huang GJ, Bian XW, et al. Mitochondrial and energy metabolism-related properties as novel indicators of lung cancer stem cells. Int J Cancer (2011) 129:820–31. doi: 10.1002/ijc.25944
36. Moon DO, Kim MO, Choi YH, Hyun JW, Chang WY, Kim GY. Butein induces G(2)/M phase arrest and apoptosis in human hepatoma cancer cells through ROS generation. Cancer Lett (2010) 288:204–13. doi: 10.1016/j.canlet.2009.07.002
37. Cheung EC, Vousden KH. The role of ROS in tumour development and progression. Nat Rev Cancer (2022) 22:280–97. doi: 10.1038/s41568-021-00435-0
38. Diebold L, Chandel NS. Mitochondrial ROS regulation of proliferating cells. Free Radic Biol Med (2016) 100:86–93. doi: 10.1016/j.freeradbiomed.2016.04.198
39. Sena LA, Chandel NS. Physiological roles of mitochondrial reactive oxygen species. Mol Cell (2012) 48:158–67. doi: 10.1016/j.molcel.2012.09.025
40. Bedard K, Krause KH. The NOX family of ROS-generating NADPH oxidases: physiology and pathophysiology. Physiol Rev (2007) 87:245–313. doi: 10.1152/physrev.00044.2005
41. Vermot A, Petit-Härtlein I, Smith SME, Fieschi F. NADPH oxidases (NOX): an overview from discovery, molecular mechanisms to physiology and pathology. Antioxidants (Basel Switzerland) (2021) 10:890. doi: 10.3390/antiox10060890
42. O'Neill S, Brault J, Stasia MJ, Knaus UG. Genetic disorders coupled to ROS deficiency. Redox Biol (2015) 6:135–56. doi: 10.1016/j.redox.2015.07.009
43. Corzo CA, Cotter MJ, Cheng P, Cheng F, Kusmartsev S, Sotomayor E, et al. Mechanism regulating reactive oxygen species in tumor-induced myeloid-derived suppressor cells. J Immunol (Baltimore Md. 1950) (2009) 182:5693–701. doi: 10.4049/jimmunol.0900092
44. Gelderman KA, Hultqvist M, Holmberg J, Olofsson P, Holmdahl R. T cell surface redox levels determine T cell reactivity and arthritis susceptibility. Proc Natl Acad Sci USA (2006) 103:12831–6. doi: 10.1073/pnas.0604571103
45. Sareila O, Kelkka T, Pizzolla A, Hultqvist M, Holmdahl R. NOX2 complex-derived ROS as immune regulators. Antioxid Redox Signal (2011) 15:2197–208. doi: 10.1089/ars.2010.3635
46. Guerra AN, Gavala ML, Chung HS, Bertics PJ. Nucleotide receptor signalling and the generation of reactive oxygen species. Purinergic Signal (2007) 3:39–51. doi: 10.1007/s11302-006-9035-x
47. Ohl K, Tenbrock K. Reactive oxygen species as regulators of MDSC-mediated immune suppression. Front Immunol (2018) 9:2499. doi: 10.3389/fimmu.2018.02499
48. Zhong LM, Liu ZG, Zhou X, Song SH, Weng GY, Wen Y, et al. Expansion of PMN-myeloid derived suppressor cells and their clinical relevance in patients with oral squamous cell carcinoma. Oral Oncol (2019) 95:157–63. doi: 10.1016/j.oraloncology.2019.06.004
49. Adeshakin AO, Liu W, Adeshakin FO, Afolabi LO, Zhang M, Zhang G, et al. Regulation of ROS in myeloid-derived suppressor cells through targeting fatty acid transport protein 2 enhanced anti-PD-L1 tumor immunotherapy. Cell Immunol (2021) 362:104286. doi: 10.1016/j.cellimm.2021.104286
50. Qiu XM, Li X, Liu R. [Non-coding RNA and innate immune signal regulation]. Sichuan Da Xue Xue Bao Yi Xue Ban (2022) 53:20–7. doi: 10.12182/20220160202
51. Thakuri BKC, Zhang J, Zhao J, Nguyen LN, Nguyen LNT, Schank M, et al. HCV-associated exosomes upregulate RUNXOR and RUNX1 expressions to promote MDSC expansion and suppressive functions through STAT3-miR124 axis. Cells (2020) 9:2715. doi: 10.3390/cells9122715
52. Zhang J, Thakuri BKC, Zhao J, Nguyen LN, Nguyen LNT, Cao D, et al. Long noncoding RNA HOTAIRM1 promotes myeloid-derived suppressor cell expansion and suppressive functions through up-regulating HOXA1 expression during latent HIV infection. Aids (2020) 34:2211–21. doi: 10.1097/qad.0000000000002700
53. Guo X, Qiu W, Liu Q, Qian M, Wang S, Zhang Z, et al. Immunosuppressive effects of hypoxia-induced glioma exosomes through myeloid-derived suppressor cells via the miR-10a/Rora and miR-21/Pten Pathways. Oncogene (2018) 37:4239–59. doi: 10.1038/s41388-018-0261-9
54. Xiang H, Ramil CP, Hai J, Zhang C, Wang H, Watkins AA, et al. Cancer-associated fibroblasts promote immunosuppression by inducing ROS-generating monocytic MDSCs in lung squamous cell carcinoma. Cancer Immunol Res (2020) 8:436–50. doi: 10.1158/2326-6066.Cir-19-0507
55. Rui K, Hong Y, Zhu Q, Shi X, Xiao F, Fu H, et al. Olfactory ecto-mesenchymal stem cell-derived exosomes ameliorate murine Sjögren's syndrome by modulating the function of myeloid-derived suppressor cells. Cell Mol Immunol (2021) 18:440–51. doi: 10.1038/s41423-020-00587-3
56. Pfeiffer ZA, Guerra AN, Hill LM, Gavala ML, Prabhu U, Aga M, et al. Nucleotide receptor signaling in murine macrophages is linked to reactive oxygen species generation. Free Radic Biol Med (2007) 42:1506–16. doi: 10.1016/j.freeradbiomed.2007.02.010
57. Billings EA, Lee CS, Owen KA, D'Souza RS, Ravichandran KS, Casanova JE. The adhesion GPCR BAI1 mediates macrophage ROS production and microbicidal activity against Gram-negative bacteria. Sci Signal (2016) 9:ra14. doi: 10.1126/scisignal.aac6250
58. Liu L, Wu X, Xu H, Yu L, Zhang X, Li L, et al. Myocardin-related transcription factor A (MRTF-A) contributes to acute kidney injury by regulating macrophage ROS production. Biochim Biophys Acta Mol Basis Dis (2018) 1864:3109–21. doi: 10.1016/j.bbadis.2018.05.026
59. West AP, Brodsky IE, Rahner C, Woo DK, Erdjument-Bromage H, Tempst P, et al. TLR signalling augments macrophage bactericidal activity through mitochondrial ROS. Nature (2011) 472:476–80. doi: 10.1038/nature09973
60. Sawyer RT, Dobis DR, Goldstein M, Velsor L, Maier LA, Fontenot AP, et al. Beryllium-stimulated reactive oxygen species and macrophage apoptosis. Free Radic Biol Med (2005) 38:928–37. doi: 10.1016/j.freeradbiomed.2004.12.014
61. Gupta AK, Ghosh K, Palit S, Barua J, Das PK, Ukil A. Leishmania donovani inhibits inflammasome-dependent macrophage activation by exploiting the negative regulatory proteins A20 and UCP2. FASEB J (2017) 31:5087–101. doi: 10.1096/fj.201700407R
62. Li B, Alli R, Vogel P, Geiger TL. IL-10 modulates DSS-induced colitis through a macrophage-ROS-NO axis. Mucosal Immunol (2014) 7:869–78. doi: 10.1038/mi.2013.103
63. Tavori H, Aviram M, Khatib S, Musa R, Mannheim D, Karmeli R, et al. Paraoxonase 1 protects macrophages from atherogenicity of a specific triglyceride isolated from human carotid lesion. Free Radic Biol Med (2011) 51:234–42. doi: 10.1016/j.freeradbiomed.2011.03.041
64. D'Autréaux B, Toledano MB. ROS as signalling molecules: mechanisms that generate specificity in ROS homeostasis. Nat Rev Mol Cell Biol (2007) 8:813–24. doi: 10.1038/nrm2256
65. He L, He T, Farrar S, Ji L, Liu T, Ma X. Antioxidants maintain cellular redox homeostasis by elimination of reactive oxygen species. Cell Physiol Biochem (2017) 44:532–53. doi: 10.1159/000485089
66. Irato P, Santovito G. Enzymatic and non-enzymatic molecules with antioxidant function. Antioxidants (Basel Switzerland) (2021) 10:579. doi: 10.3390/antiox10040579
67. Gilgun-Sherki Y, Melamed E, Offen D. The role of oxidative stress in the pathogenesis of multiple sclerosis: the need for effective antioxidant therapy. J Neurol (2004) 251:261–8. doi: 10.1007/s00415-004-0348-9
68. Kattoor AJ, Pothineni NVK, Palagiri D, Mehta JL. Oxidative stress in atherosclerosis. Curr Atheroscler Rep (2017) 19:42. doi: 10.1007/s11883-017-0678-6
69. Chen Z, Zhong C. Oxidative stress in Alzheimer's disease. Neurosci Bull (2014) 30:271–81. doi: 10.1007/s12264-013-1423-y
70. Steven S, Frenis K, Oelze M, Kalinovic S, Kuntic M, Bayo Jimenez MT, et al. Vascular inflammation and oxidative stress: major triggers for cardiovascular disease. Oxid Med Cell Longev (2019) 2019:7092151. doi: 10.1155/2019/7092151
71. Khatri N, Thakur M, Pareek V, Kumar S, Sharma S, Datusalia AK. Oxidative stress: major threat in traumatic brain injury. CNS Neurol Disord Drug Targets (2018) 17:689–95. doi: 10.2174/1871527317666180627120501
72. Nazio F, Bordi M, Cianfanelli V, Locatelli F, Cecconi F. Autophagy and cancer stem cells: molecular mechanisms and therapeutic applications. Cell Death Differ (2019) 26:690–702. doi: 10.1038/s41418-019-0292-y
73. Toledano MB, Planson AG, Delaunay-Moisan A. Reining in H(2)O(2) for safe signaling. Cell (2010) 140:454–6. doi: 10.1016/j.cell.2010.02.003
74. Yoo NJ, Kim HR, Kim YR, An CH, Lee SH. Somatic mutations of the KEAP1 gene in common solid cancers. Histopathology (2012) 60:943–52. doi: 10.1111/j.1365-2559.2012.04178.x
75. Kruiswijk F, Labuschagne CF, Vousden KH. p53 in survival, death and metabolic health: a lifeguard with a licence to kill. Nat Rev Mol Cell Biol (2015) 16:393–405. doi: 10.1038/nrm4007
76. Jiang T, Harder B, Rojo de la Vega M, Wong PK, Chapman E, Zhang DD. p62 links autophagy and Nrf2 signaling. Free Radic Biol Med (2015) 88:199–204. doi: 10.1016/j.freeradbiomed.2015.06.014
77. Liu S, Pi J, Zhang Q. Signal amplification in the KEAP1-NRF2-ARE antioxidant response pathway. Redox Biol (2022) 54:102389. doi: 10.1016/j.redox.2022.102389
78. Satoh H, Moriguchi T, Taguchi K, Takai J, Maher JM, Suzuki T, et al. Nrf2-deficiency creates a responsive microenvironment for metastasis to the lung. Carcinogenesis (2010) 31:1833–43. doi: 10.1093/carcin/bgq105
79. Beury DW, Carter KA, Nelson C, Sinha P, Hanson E, Nyandjo M, et al. Myeloid-derived suppressor cell survival and function are regulated by the transcription factor nrf2. J Immunol (Baltimore Md. 1950) (2016) 196:3470–8. doi: 10.4049/jimmunol.1501785
80. Ostrand-Rosenberg S, Beury DW, Parker KH, Horn LA. Survival of the fittest: how myeloid-derived suppressor cells survive in the inhospitable tumor microenvironment. Cancer Immunol Immunother (2020) 69:215–21. doi: 10.1007/s00262-019-02388-8
81. Tang D, Kang R, Livesey KM, Zeh HJ 3rd, Lotze MT. High mobility group box 1 (HMGB1) activates an autophagic response to oxidative stress. Antioxid Redox Signal (2011) 15:2185–95. doi: 10.1089/ars.2010.3666
82. Parker KH, Horn LA, Ostrand-Rosenberg S. High-mobility group box protein 1 promotes the survival of myeloid-derived suppressor cells by inducing autophagy. J leukocyte Biol (2016) 100:463–70. doi: 10.1189/jlb.3HI0715-305R
83. Kuo HH, Mauk AG. Indole peroxygenase activity of indoleamine 2,3-dioxygenase. Proc Natl Acad Sci USA (2012) 109:13966–71. doi: 10.1073/pnas.1207191109
84. Munn DH, Mellor AL. Indoleamine 2,3 dioxygenase and metabolic control of immune responses. Trends Immunol (2013) 34:137–43. doi: 10.1016/j.it.2012.10.001
85. Ju JM, Nam G, Lee YK, Jung M, Chang H, Kim W, et al. IDO1 scavenges reactive oxygen species in myeloid-derived suppressor cells to prevent graft-versus-host disease. Proc Natl Acad Sci USA (2021) 118:e2011170118. doi: 10.1073/pnas.2011170118
86. Woods A, Dickerson K, Heath R, Hong SP, Momcilovic M, Johnstone SR, et al. Ca2+/calmodulin-dependent protein kinase kinase-beta acts upstream of AMP-activated protein kinase in mamMalian cells. Cell Metab (2005) 2:21–33. doi: 10.1016/j.cmet.2005.06.005
87. Huang W, Liu Y, Luz A, Berrong M, Meyer JN, Zou Y, et al. Calcium/calmodulin dependent protein kinase kinase 2 regulates the expansion of tumor-induced myeloid-derived suppressor cells. Front Immunol (2021) 12:754083. doi: 10.3389/fimmu.2021.754083
88. Corzo CA, Condamine T, Lu L, Cotter MJ, Youn JI, Cheng P, et al. HIF-1α regulates function and differentiation of myeloid-derived suppressor cells in the tumor microenvironment. J Exp Med (2010) 207:2439–53. doi: 10.1084/jem.20100587
89. Simon MC. Coming up for air: HIF-1 and mitochondrial oxygen consumption. Cell Metab (2006) 3:150–1. doi: 10.1016/j.cmet.2006.02.007
90. Kim JW, Tchernyshyov I, Semenza GL, Dang CV. HIF-1-mediated expression of pyruvate dehydrogenase kinase: a metabolic switch required for cellular adaptation to hypoxia. Cell Metab (2006) 3:177–85. doi: 10.1016/j.cmet.2006.02.002
91. Jian SL, Chen WW, Su YC, Su YW, Chuang TH, Hsu SC, et al. Glycolysis regulates the expansion of myeloid-derived suppressor cells in tumor-bearing hosts through prevention of ROS-mediated apoptosis. Cell Death Dis (2017) 8:e2779. doi: 10.1038/cddis.2017.192
92. Le Belle JE, Orozco NM, Paucar AA, Saxe JP, Mottahedeh J, Pyle AD, et al. Proliferative neural stem cells have high endogenous ROS levels that regulate self-renewal and neurogenesis in a PI3K/Akt-dependant manner. Cell Stem Cell (2011) 8:59–71. doi: 10.1016/j.stem.2010.11.028
93. Haraguchi N, Ishii H, Mimori K, Tanaka F, Ohkuma M, Kim HM, et al. CD13 is a therapeutic target in human liver cancer stem cells. J Clin Invest (2010) 120:3326–39. doi: 10.1172/jci42550
94. Ishimoto T, Nagano O, Yae T, Tamada M, Motohara T, Oshima H, et al. CD44 variant regulates redox status in cancer cells by stabilizing the xCT subunit of system xc(-) and thereby promotes tumor growth. Cancer Cell (2011) 19:387–400. doi: 10.1016/j.ccr.2011.01.038
95. Simon AR, Rai U, Fanburg BL, Cochran BH. Activation of the JAK-STAT pathway by reactive oxygen species. Am J Physiol (1998) 275:C1640–1652. doi: 10.1152/ajpcell.1998.275.6.C1640
96. Liu B, Chen Y, St Clair DK. ROS and p53: a versatile partnership. Free Radic Biol Med (2008) 44:1529–35. doi: 10.1016/j.freeradbiomed.2008.01.011
97. Kobayashi Y, Oguro A, Imaoka S. Feedback of hypoxia-inducible factor-1alpha (HIF-1alpha) transcriptional activity via redox factor-1 (Ref-1) induction by reactive oxygen species (ROS). Free Radic Res (2021) 55:154–64. doi: 10.1080/10715762.2020.1870685
98. George-Chandy A, Nordström I, Nygren E, Jonsson IM, Postigo J, Collins LV, et al. Th17 development and autoimmune arthritis in the absence of reactive oxygen species. Eur J Immunol (2008) 38:1118–26. doi: 10.1002/eji.200737348
99. Yingze Y, Zhihong J, Tong J, Yina L, Zhi Z, Xu Z, et al. NOX2-mediated reactive oxygen species are double-edged swords in focal cerebral ischemia in mice. J Neuroinflamm (2022) 19:184. doi: 10.1186/s12974-022-02551-6
100. Jackson SH, Devadas S, Kwon J, Pinto LA, Williams MS. T cells express a phagocyte-type NADPH oxidase that is activated after T cell receptor stimulation. Nat Immunol (2004) 5:818–27. doi: 10.1038/ni1096
101. Sprouse ML, Welte T, Boral D, Liu HN, Yin W, Vishnoi M, et al. PMN-MDSCs Enhance CTC Metastatic Properties through Reciprocal Interactions via ROS/Notch/Nodal Signaling. Int J Mol Sci (2019) 20:1916. doi: 10.3390/ijms20081916
102. Gelderman KA, Hultqvist M, Pizzolla A, Zhao M, Nandakumar KS, Mattsson R, et al. Macrophages suppress T cell responses and arthritis development in mice by producing reactive oxygen species. J Clin Invest (2007) 117:3020–8. doi: 10.1172/jci31935
103. Schmielau J, Finn OJ. Activated granulocytes and granulocyte-derived hydrogen peroxide are the underlying mechanism of suppression of t-cell function in advanced cancer patients. Cancer Res (2001) 61:4756–60. doi: 10.1371/journal.pone.0031524
104. Kraaij MD, Savage ND, van der Kooij SW, Koekkoek K, Wang J, van den Berg JM, et al. Induction of regulatory T cells by macrophages is dependent on production of reactive oxygen species. Proc Natl Acad Sci USA (2010) 107:17686–91. doi: 10.1073/pnas.1012016107
105. Amarnath S, Dong L, Li J, Wu Y, Chen W. Endogenous TGF-beta activation by reactive oxygen species is key to Foxp3 induction in TCR-stimulated and HIV-1-infected human CD4+CD25- T cells. Retrovirology (2007) 4:57. doi: 10.1186/1742-4690-4-57
106. Fortin C, Huang X, Yang Y. NK cell response to vaccinia virus is regulated by myeloid-derived suppressor cells. J Immunol (Baltimore Md. 1950) (2012) 189:1843–9. doi: 10.4049/jimmunol.1200584
107. Zhu J, Huang X, Yang Y. Myeloid-derived suppressor cells regulate natural killer cell response to adenovirus-mediated gene transfer. J Virol (2012) 86:13689–96. doi: 10.1128/jvi.01595-12
108. Rastad JL, Green WR. Myeloid-derived suppressor cells in murine AIDS inhibit B-cell responses in part via soluble mediators including reactive oxygen and nitrogen species, and TGF-β. Virology (2016) 499:9–22. doi: 10.1016/j.virol.2016.08.031
109. Lelis FJN, Jaufmann J, Singh A, Fromm K, Teschner AC, Pöschel S, et al. Myeloid-derived suppressor cells modulate B-cell responses. Immunol Lett (2017) 188:108–15. doi: 10.1016/j.imlet.2017.07.003
110. Flannagan RS, Cosío G, Grinstein S. Antimicrobial mechanisms of phagocytes and bacterial evasion strategies. Nat Rev Microbiol (2009) 7:355–66. doi: 10.1038/nrmicro2128
111. Reuter S, Gupta SC, Chaturvedi MM, Aggarwal BB. Oxidative stress, inflammation, and cancer: how are they linked? Free Radic Biol Med (2010) 49:1603–16. doi: 10.1016/j.freeradbiomed.2010.09.006
112. Khan SU, Rayees S, Sharma P, Malik F. Targeting redox regulation and autophagy systems in cancer stem cells. Clin Exp Med (2022). doi: 10.1007/s10238-022-00955-5
113. Kusmartsev S, Gabrilovich DI. Inhibition of myeloid cell differentiation in cancer: the role of reactive oxygen species. J leukocyte Biol (2003) 74:186–96. doi: 10.1189/jlb.0103010
114. Waldron TJ, Quatromoni JG, Karakasheva TA, Singhal S, Rustgi AK. Myeloid derived suppressor cells: Targets for therapy. Oncoimmunology (2013) 2:e24117. doi: 10.4161/onci.24117
115. Draghiciu O, Lubbers J, Nijman HW, Daemen T. Myeloid derived suppressor cells-An overview of combat strategies to increase immunotherapy efficacy. Oncoimmunology (2015) 4:e954829. doi: 10.4161/21624011.2014.954829
116. Nagaraj S, Youn JI, Weber H, Iclozan C, Lu L, Cotter MJ, et al. Anti-inflammatory triterpenoid blocks immune suppressive function of MDSCs and improves immune response in cancer. Clin Cancer Res an Off J Am Assoc Cancer Res (2010) 16:1812–23. doi: 10.1158/1078-0432.Ccr-09-3272
117. De Santo C, Serafini P, Marigo I, Dolcetti L, Bolla M, Del Soldato P, et al. Nitroaspirin corrects immune dysfunction in tumor-bearing hosts and promotes tumor eradication by cancer vaccination. Proc Natl Acad Sci USA (2005) 102:4185–90. doi: 10.1073/pnas.0409783102
118. Li B, Luo Y, Zhou Y, Wu J, Fang Z, Li Y. Role of sanguinarine in regulating immunosuppression in a Lewis lung cancer mouse model. Int Immunopharmacol (2022) 110:108964. doi: 10.1016/j.intimp.2022.108964
119. Li D, Shi G, Wang J, Zhang D, Pan Y, Dou H, et al. Baicalein ameliorates pristane-induced lupus nephritis via activating Nrf2/HO-1 in myeloid-derived suppressor cells. Arthritis Res Ther (2019) 21:105. doi: 10.1186/s13075-019-1876-0
120. Xie Y, Zhang Y, Wei X, Zhou C, Huang Y, Zhu X, et al. Jianpi huayu decoction attenuates the immunosuppressive status of H(22) hepatocellular carcinoma-bearing mice: by targeting myeloid-derived suppressor cells. Front Pharmacol (2020) 11:16. doi: 10.3389/fphar.2020.00016
121. Chen PT, Hsieh CC, Wu CT, Yen TC, Lin PY, Chen WC, et al. 1α,25-dihydroxyvitamin D3 inhibits esophageal squamous cell carcinoma progression by reducing IL6 signaling. Mol Cancer Ther (2015) 14:1365–75. doi: 10.1158/1535-7163.Mct-14-0952
122. Chaves KC, Costa EM, Teixeira LF, Bellini MH. Impact of endostatin gene therapy on myeloid-derived suppressor cells from a metastatic renal cell carcinoma. Exp Oncol (2018) 40:24–32. doi: 10.31768/2312-8852.2018.40(1):24-32
123. Xue Y, Xu Y, Liu X, Sun Z, Pan Y, Lu X, et al. Ferumoxytol attenuates the function of MDSCs to ameliorate LPS-induced immunosuppression in sepsis. Nanoscale Res Lett (2019) 14:379. doi: 10.1186/s11671-019-3209-2
124. Jayaraman P, Parikh F, Lopez-Rivera E, Hailemichael Y, Clark A, Ma G, et al. Tumor-expressed inducible nitric oxide synthase controls induction of functional myeloid-derived suppressor cells through modulation of vascular endothelial growth factor release. J Immunol (Baltimore Md. 1950) (2012) 188:5365–76. doi: 10.4049/jimmunol.1103553
125. Grauers Wiktorin H, Nilsson MS, Kiffin R, Sander FE, Lenox B, Rydström A, et al. Histamine targets myeloid-derived suppressor cells and improves the anti-tumor efficacy of PD-1/PD-L1 checkpoint blockade. Cancer Immunol Immunother (2019) 68:163–74. doi: 10.1007/s00262-018-2253-6
126. Veltman JD, Lambers ME, van Nimwegen M, Hendriks RW, Hoogsteden HC, Aerts JG, et al. COX-2 inhibition improves immunotherapy and is associated with decreased numbers of myeloid-derived suppressor cells in mesothelioma. Celecoxib influences MDSC function. BMC Cancer (2010) 10:464. doi: 10.1186/1471-2407-10-464
127. Wang HF, Ning F, Liu ZC, Wu L, Li ZQ, Qi YF, et al. Histone deacetylase inhibitors deplete myeloid-derived suppressor cells induced by 4T1 mammary tumors in vivo and in vitro. Cancer Immunol Immunother (2017) 66:355–66. doi: 10.1007/s00262-016-1935-1
128. Yin T, Zhao ZB, Guo J, Wang T, Yang JB, Wang C, et al. Aurora A inhibition eliminates myeloid cell-mediated immunosuppression and enhances the efficacy of anti-PD-L1 therapy in breast cancer. Cancer Res (2019) 79:3431–44. doi: 10.1158/0008-5472.Can-18-3397
129. Wu T, Wang C, Wang W, Hui Y, Zhang R, Qiao L, et al. Embelin impairs the accumulation and activation of MDSCs in colitis-associated tumorigenesis. Oncoimmunology (2018) 7:e1498437. doi: 10.1080/2162402x.2018.1498437
130. Tai LH, Alkayyal AA, Leslie AL, Sahi S, Bennett S, Tanese de Souza C, et al. Phosphodiesterase-5 inhibition reduces postoperative metastatic disease by targeting surgery-induced myeloid derived suppressor cell-dependent inhibition of Natural Killer cell cytotoxicity. Oncoimmunology (2018) 7:e1431082. doi: 10.1080/2162402x.2018.1431082
131. Gao P, Zhang H, Dinavahi R, Li F, Xiang Y, Raman V, et al. HIF-dependent antitumorigenic effect of antioxidants in vivo. Cancer Cell (2007) 12:230–8. doi: 10.1016/j.ccr.2007.08.004
132. Li S, Li F, Xu L, Liu X, Zhu X, Gao W, et al. TLR2 agonist promotes myeloid-derived suppressor cell polarization via Runx1 in hepatocellular carcinoma. Int Immunopharmacol (2022) 111:109168. doi: 10.1016/j.intimp.2022.109168
133. Ren Z, Tang H, Wan L, Liu X, Tang N, Wang L, et al. Swertianolin ameliorates immune dysfunction in sepsis via blocking the immunosuppressive function of myeloid- derived suppressor cells. Eur J Histochem (2021) 65:3292. doi: 10.4081/ejh.2021.3292
134. Liu D, You M, Xu Y, Li F, Zhang D, Li X, et al. Inhibition of curcumin on myeloid-derived suppressor cells is requisite for controlling lung cancer. Int Immunopharmacol (2016) 39:265–72. doi: 10.1016/j.intimp.2016.07.035
135. Sinha P, Ostrand-Rosenberg S. Myeloid-derived suppressor cell function is reduced by Withaferin A, a potent and abundant component of Withania somnifera root extract. Cancer Immunol Immunother (2013) 62:1663–73. doi: 10.1007/s00262-013-1470-2
136. Jiang S, Ma J, Li Y, Lu B, Du J, Xu J, et al. A polysaccharide from native Curcuma kwangsiensis and its mechanism of reversing MDSC-induced suppressive function. Carbohydr Polym (2022) 297:120020. doi: 10.1016/j.carbpol.2022.120020
137. Zamani P, Navashenaq JG, Teymouri M, Karimi M, Mashreghi M, Jaafari MR. Combination therapy with liposomal doxorubicin and liposomal vaccine containing E75, an HER-2/neu-derived peptide, reduces myeloid-derived suppressor cells and improved tumor therapy. Life Sci (2020) 252:117646. doi: 10.1016/j.lfs.2020.117646
138. Peng KT, Chen JL, Kuo LT, Yu PA, Hsu WH, Lee CW, et al. GMI, an Immunomodulatory Peptide from Ganoderma microsporum, Restrains Periprosthetic Joint Infections via Modulating the Functions of Myeloid-Derived Suppressor Cells and Effector T Cells. Int J Mol Sci (2021) 22:6854. doi: 10.3390/ijms22136854
139. Peng M, Zhang Q, Liu Y, Guo X, Ju J, Xu L, et al. Apolipoprotein A-I mimetic peptide L-4F suppresses granulocytic-myeloid-derived suppressor cells in mouse pancreatic cancer. Front Pharmacol (2020) 11:576. doi: 10.3389/fphar.2020.00576
140. Wang TY, Yu CC, Hsieh PL, Liao YW, Yu CH, Chou MY. GMI ablates cancer stemness and cisplatin resistance in oral carcinomas stem cells through IL-6/Stat3 signaling inhibition. Oncotarget (2017) 8:70422–30. doi: 10.18632/oncotarget.19711
141. Tseng AJ, Tu TH, Hua WJ, Yeh H, Chen CJ, Lin ZH, et al. GMI, Ganoderma microsporum protein, suppresses cell mobility and increases temozolomide sensitivity through induction of Slug degradation in glioblastoma multiforme cells. Int J Biol Macromol (2022) 219:940–8. doi: 10.1016/j.ijbiomac.2022.08.024
Keywords: MDSCs, ROS, immunotherapy, tumor, tumor micro environment (TME)
Citation: Huang J, Zhao Y, Zhao K, Yin K and Wang S (2023) Function of reactive oxygen species in myeloid-derived suppressor cells. Front. Immunol. 14:1226443. doi: 10.3389/fimmu.2023.1226443
Received: 21 May 2023; Accepted: 26 July 2023;
Published: 14 August 2023.
Edited by:
Alessandra Romano, University of Catania, ItalyReviewed by:
Stefano Ugel, University of Verona, ItalyCopyright © 2023 Huang, Zhao, Zhao, Yin and Wang. This is an open-access article distributed under the terms of the Creative Commons Attribution License (CC BY). The use, distribution or reproduction in other forums is permitted, provided the original author(s) and the copyright owner(s) are credited and that the original publication in this journal is cited, in accordance with accepted academic practice. No use, distribution or reproduction is permitted which does not comply with these terms.
*Correspondence: Shengjun Wang, c2p3anNAdWpzLmVkdS5jbg==
Disclaimer: All claims expressed in this article are solely those of the authors and do not necessarily represent those of their affiliated organizations, or those of the publisher, the editors and the reviewers. Any product that may be evaluated in this article or claim that may be made by its manufacturer is not guaranteed or endorsed by the publisher.
Research integrity at Frontiers
Learn more about the work of our research integrity team to safeguard the quality of each article we publish.