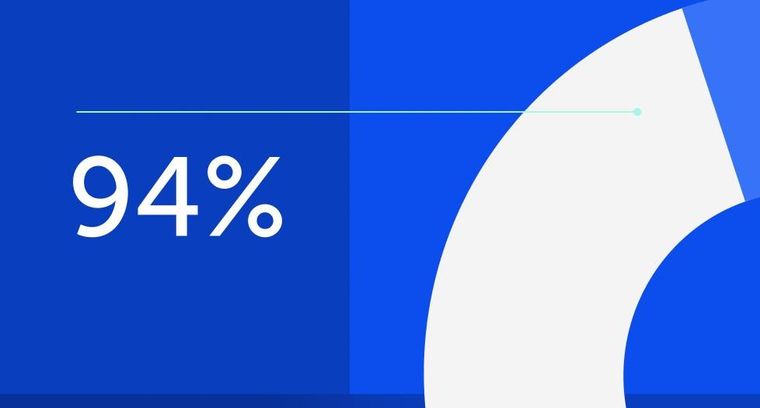
94% of researchers rate our articles as excellent or good
Learn more about the work of our research integrity team to safeguard the quality of each article we publish.
Find out more
OPINION article
Front. Immunol., 16 June 2023
Sec. Multiple Sclerosis and Neuroimmunology
Volume 14 - 2023 | https://doi.org/10.3389/fimmu.2023.1217176
Multiple sclerosis (MS) is a chronic inflammatory disease whose cause is unknown; however, a viral infection is thought to be involved. Current theory favors Epstein-Barr virus (EBV); yet EBV’s ubiquitous presence and ease of transmission are inconsistent with low MS concordance across genetically identical twins. Further, causality has not been demonstrated and the mechanism of disease induction is unknown. As an alternative hypothesis, MS may be triggered when myeloid dendritic cells (mDCs) become infected by lymphocytic choriomeningitis virus (LCMV). As mDCs are critical to thymic development of regulatory T cells (1), LCMV infection could hypothetically suggest a mechanism for disease initiation. Elucidating the mechanism of MS disease initiation is critical to our ability to prevent this debilitating disease.
MS is a chronic inflammatory disease in which self-antigens such as myelin proteins are attacked by autoreactive T cells (2). Normally, immunologic attacks on self-antigens are suppressed by a specialized subset of CD4+ cells called regulatory T cells (Tregs). However, the suppressive capacity of Tregs from relapsing-remitting MS (RRMS) patients is diminished. Peripheral Tregs from RRMS patients contain relatively few recent thymic emigrants, suggesting a defect in thymic Treg neogenesis (3).
Normal Treg development in the thymus requires strong stimulation from CD11c+ myeloid dendritic cells (mDCs). Such stimulation requires that mDCs upregulate HLA-DR and the costimulatory molecules CD40, CD80, and CD86 in response to thymic stromal lymphopoietin (TSLP) (4, 5). However, TSLP fails to induce upregulation of these costimulatory molecules in mDCs taken from RRMS patients (6). This reduced potency of TSLP is in part due to downregulation of one subunit of the TSLP receptor, IL-7Rα, independent of the IL7RA gene polymorphism (rs6897932) associated with MS (1, 6). Thus it appears that failure of self-tolerance in RRMS can be attributed, at least in part, to reduced surface molecule expression and subsequent impairment of mDCs (1).
The reason for mDC impairment is unknown but may be initiated by a viral infection. Several lines of evidence support a role for viral infection in MS. For example, MS clusters have appeared as epidemics (7) or regional hotspots (8, 9). MS relapses have appeared after respiratory infections (10). MS pathology is similar to an ongoing infectious process (11) and has been simulated experimentally through viral infection (12). And MS symptoms are relieved by administration of the antiviral cytokine, beta-interferon (IFN-β) (13).
The efficacy of IFN-β in a subset of patients points to a possible dysregulation of antiviral defense in RRMS. Normally, viral genetic material is recognized by specialized proteins called “toll-like receptors” (TLRs), which signal the release of antiviral cytokines such as type I interferons (IFN-α/β) and IL-12. This process appears to be altered in MS. Genes in the interferon pathway, such as IRF3, IRF7, and IFN, are downregulated in a subset of patients (14–16). Further, release of IL-12 in response to stimulation of TLR8, but not the other endosomal TLRs, is reduced in RRMS (17), suggesting an impairment of TLR8 signaling. Because TLR8 is expressed primarily by CD11c+ mDCs (18), the poor response to TLR8 signaling in RRMS patients may be further evidence of mDC impairment.
While the above findings are suggestive of viral involvement in RRMS, they do not tell us which virus(es) may initiate disease, nor the mechanism by which they do it. One highly researched candidate is Epstein-Barr virus (EBV). An association between EBV and MS was hypothesized based on several factors, such as higher seropositivity against EBV among MS patients (19–21); higher presence of EBV in MS brain (19, 22, 23); an increase in EBV-specific CD8+ cells during MS relapses (19); and EBV-specific oligoclonal bands in MS CSF (24). However, a causal relationship between EBV and MS has not been demonstrated. It is unclear how EBV, which infects B cells, could cause the impairment of mDCs or the diminished response to TLR8 stimulation observed in RRMS patients. Nor does EBV explain the geographic distribution of MS. For example, EBV’s high overall prevalence (~95%) and ease of person-to-person transmission is inconsistent with the low MS concordance across monozygotic twin pairs (25–30). Further, MS prevalence follows a latitudinal gradient, with increasing risk farther from the equator (31); in contrast, exposure to EBV is delayed in countries of higher latitude (32), forming a reverse latitudinal gradient. This inconsistency has been rationalized by assuming the “hygiene hypothesis,” which proposes that delayed exposure to EBV increases risk of MS. However, the hygiene hypothesis would lead to the untenable conclusion that EBV-negative individuals incur the highest MS risk (33). Recent evidence suggests that EBV may be a marker of chronic inflammation, indicating T cell exhaustion and an inability to clear the virus (34, 35), rather than a causative agent per se.
Given the downregulation of costimulatory molecules and receptors on mDCs in RRMS, impairing their ability to stimulate Treg development in the thymus, it is reasonable to hypothesize that a trigger virus for MS may impair mDCs. One such virus is lymphocytic choriomeningitis virus (LCMV).
LCMV is a zoonotic ssRNA virus whose natural host is the common house mouse. Transmission to humans occurs primarily by inhalation of aerosolized rodent excreta, by bites, or by contact with rodent urine, feces, or saliva (36). LCMV infection in humans is usually mild or asymptomatic, but may occasionally lead to aseptic meningitis (37).
LCMV strains differ with respect to tropism and pathogenicity. While the wild-type strain induces acute infection that is rapidly cleared, strains carrying the F260L mutation in the GP1 glycoprotein gene suppress the immune response and establish persistent infection (38). The F260L variant infects humans1 as well as mice. This variant preferentially infects CD11c+ mDCs (38), the cell type that is impaired in RRMS.
Any pathogen proposed as an instigator of MS should explain how mDCs become impaired. LCMV may provide some answers in this respect. LCMV persistent strains preferentially infect CD11c+ mDCs, resulting in downregulation of key cell surface molecules involved in antigen presentation and T cell maturation (39). Specifically, expression of MHC (HLA in humans), CD40, CD80, and CD86 is reduced in LCMV-infected mDCs. As a consequence, infected mDCs bind developing T cells less tightly and fail to stimulate their proliferation (39). Such persistent viral infection mimics the impairments observed in RRMS patients (Table 1). Contributing to the Treg failure in RRMS is downregulation of the IL-7Rα subunit on both T cells and mDCs (6). While the IL-7Rα subunit was not explicitly studied in mDCs from persistently infected mice, LCMV infection reduced expression of IL-7R on T cells (41, 42). The similarities between LCMV infection and RRMS with respect to cell surface molecule expression on mDCs and, possibly, T cells is intriguing and worthy of further investigation.
A pathogen involved in initiating MS should also explain the observed dysregulation of the innate immune system. Two aspects of innate immunity altered in RRMS are relevant here. First, TLR8 is less effective than other endosomal TLRs in signaling the release of IL-12 from PBMCs of RRMS patients (17). Second, a subset of RRMS patients who benefit from exogenous IFN-β treatment show downregulation of genes in the interferon pathway (14, 54). Both these aspects of innate immunity can be impacted by LCMV. TLR8 senses ssRNA and is expressed primarily on mDCs, the cell type infected by LCMV persistent strains. This contrasts with TLR7, which also senses ssRNA but is expressed by a different cell type (plasmacytoid DCs). The difference between TLR8 and TLR7 signaling in RRMS patients suggests a defect in mDCs, which could hypothetically be a consequence of LCMV infection. LCMV infection does inhibit IL-12 secretion (40, 43–45), although the involvement of TLR8 has not been tested directly. Further, LCMV inhibits IFN production by blocking activation of the interferon transcription factor IRF3 (46, 47). The predilection of LCMV to infect the primary cell type expressing TLR8, along with its ability to suppress IL-12 and IFN release, are consistent with similar observations from RRMS patients (Table 1). LCMV-induced inhibition of cytokine release and evasion of host recognition should be investigated further to determine whether they contribute to immune dysfunction in MS.
Finally, a pathogen involved in MS initiation should be consistent with epidemiologic observations about MS. Epidemiology was one of the earliest tools used to study MS. While MS research has now moved more toward molecular and genetic epidemiology, some of the old population-based findings still hold. MS continues to be more prevalent in the temperate zone geographically, with a latitudinal gradient (31). MS prevalence is still under 1% (48, 55), and concordance across monozygotic twin pairs remains around 1 in 4 (25–30). These characteristics cannot easily be explained by EBV, which is highly prevalent, easily spread person-to-person, and shows a reverse latitudinal gradient of childhood exposure. In contrast, LCMV is most prevalent in the temperate zone with a latitudinal gradient (49) and overall prevalence on the order of 2–5% (49, 50). The virus is transmitted directly from rodents and their excreta, without human-to-human spread (50), consistent with the low disease concordance reported by MS twin studies. Aside from these global measures, a few regional hotspots of MS are approximately colocalized with areas of high LCMV prevalence (Table 1). For example, the country with the highest MS incidence during the reporting period 2005–2007 was Croatia (48); at the same time, Croatia’s Vir Island reported that 36% of residents were positive for anti-LCMV antibodies (51). While the evidence is circumstantial, the low prevalence, temperate zone distribution, and lack of human-to-human transmission that characterize LCMV are consistent with the low twin concordance and geographic distribution observed for MS.
The infectious pathogen that induces MS has not yet been identified with certainty. LCMV is a viable candidate due to its ability to impair mDCs, whose function is required for thymic development of regulatory T cells. However, the evidence in favor of LCMV, such as immune evasion or geographic distribution, is largely circumstantial and does not constitute proof. We believe that rigorous scientific evidence, either for or against the LCMV hypothesis, is important and feasible to obtain.
A preliminary assessment can be both simple and cost-effective. Since seroprevalence of anti-LCMV antibodies in the US and Western Europe is low, on the order of 5%, testing for increased seroprevalence among MS patients could be accomplished with a very small sample of subjects.
A study evaluating the association between a pathogen and MS should consider the impact of gene-environment interactions. The genetic influence most likely relevant to pathogen-induced autoimmunity is HLA type. A pathogenic virus may initiate inflammation by mimicking an endogenous peptide when bound to HLA; however, the orientation of any given peptide will vary by HLA type (56–58). For example, an immunodominant peptide from myelin basic protein (MBP) binds to the high-risk HLA DRB1*1501 in a different orientation than it does to other HLA types (57), where it may not bind at all. A peptide from LCMV predicted to mimic this MBP peptide (49) meets criteria (53) for binding to HLA DRB1*1501, but would likely not match criteria for binding to another HLA type. It is plausible, perhaps likely, that any specific virus operating through molecular mimicry may be successful in only a subset of the population. For these reasons, clinical investigation of a proposed trigger virus should control for relevant risk genes such as HLA in the study design or analysis.
If even a small subset of MS cases is associated with LCMV infection, further exploration of this subset may enhance our understanding of autoimmunity and provide new options for therapeutic interventions. We urge clinical investigators to consider the potential benefits of exploring LCMV seroprevalence among RRMS patients.
The author confirms being the sole contributor of this work and has approved it for publication.
The author declares that the research was conducted in the absence of any commercial or financial relationships that could be construed as a potential conflict of interest.
All claims expressed in this article are solely those of the authors and do not necessarily represent those of their affiliated organizations, or those of the publisher, the editors and the reviewers. Any product that may be evaluated in this article, or claim that may be made by its manufacturer, is not guaranteed or endorsed by the publisher.
CSF, cerebrospinal fluid; EBV, Epstein-Barr virus; HLA, human leukocyte antigen; IFN, interferon; IRF3, interferon regulatory factor 3; IRF7, interferon regulatory factor 7; LCMV, lymphocytic choriomeningitis virus; MBP, myelin basic protein; mDC, myeloid dendritic cell; MHC, major histocompatibility complex; MS, multiple sclerosis; PBMC, peripheral blood mononuclear cell; RRMS, relapsing-remitting multiple sclerosis; ssRNA, single-stranded RNA; TLR, toll-like receptor; Treg, regulatory T cell; TSLP, thymic stromal lymphopoietin.
1. Haas J, Schwarz A, Korporal-Kuhnke M, Jarius S, Wildemann B. Myeloid dendritic cells exhibit defects in activation and function in patients with multiple sclerosis. J Neuroimmunol (2016) 301:53–60. doi: 10.1016/j.jneuroim.2016.10.007
2. Costantino CM, Hutton J, Baecher-Allan C, Hafler DA. Multiple sclerosis and regulatory T cells. J Clin Immunol (2008) 28(6):697–706. doi: 10.1007/s10875-008-9236-x
3. Haas J, Fritzsching B, Trübswetter P, Korporal M, Milkova L, Fritz B, et al. Prevalence of newly generated naive regulatory T cells (Treg) is critical for Treg suppressive function and determines Treg dysfunction in multiple sclerosis. J Immunol (2007) 179:1322–30. doi: 10.4049/jimmunol.179.2.1322
4. Watanabe N, Wang Y-H, Lee HK, Ito T, Wang Y-H, Cao W, et al. Hassall’s corpuscles instruct dendritic cells to induce CD4+CD25+ regulatory T cells in human thymus. Nature (2005) 436(7054):1181–5. doi: 10.1038/nature03886
5. Soumelis V, Reche PA, Kanzler H, Yuan W, Edward G, Homey B, et al. Human epithelial cells trigger dendritic cell-mediated allergic inflammation by producing TSLP. Nat Immunol (2002) 3(7):673–80. doi: 10.1038/ni805
6. Haas J, Korporal M, Schwarz A, Balint B, Wildemann B. The interleukin-7 receptor α chain contributes to altered homeostasis of regulatory T cells in multiple sclerosis. Eur J Immunol (2011) 41:845–53. doi: 10.1002/eji.201041139
7. Kurtzke JF. Epidemiologic evidence for multiple sclerosis as an infection. Clin Microbiol Rev (1993) 6(4):382–427. doi: 10.1128/CMR.6.4.382
8. Peterlin B, Ristić S, Sepčić J, Vračko BK, Rako A, Lovrečić L, et al. Region with persistent high frequency of multiple sclerosis in Croatia and Slovenia. J Neurol Sci (2006) 247:169–72. doi: 10.1016/j.jns.2006.04.002
9. Granieri E, Economou N-T, de Gennaro R, Tola MR, Caniatti L, Govoni V, et al. Multiple sclerosis in the province of Ferrara: evidence for an increasing trend. J Neurol (2007) 254:1642–8. doi: 10.1007/s00415-007-0560-5
10. Edwards S, Zvartau M, Clarke H, Irving W, Blumhardt LD. Clinical relapses and disease activity on magnetic resonance imaging associated with viral upper respiratory tract infections in multiple sclerosis. J Neurol Neurosurg Psychiatry (1998) 64:736–41. doi: 10.1136/jnnp.64.6.736
11. Steiner I, Nisipianu P, Wirguin I. Infection and the etiology and pathogenesis of multiple sclerosis. Curr Neurol Neurosci Rep (2001) 1(3):271–6. doi: 10.1007/s11910-001-0030-x
12. Pike SC, Welsh N, Linzey M, Gilli F. Theiler’s virus-induced demyelinating disease as an infectious model of progressive multiple sclerosis. Front Mol Neurosci (2022) 15:1019799. doi: 10.3389/fnmol.2022.1019799
13. Madsen C. The innovative development in interferon beta treatments of relapsing-remitting multiple sclerosis. Brain Behav (2017) 7(6):e00696. doi: 10.1002/brb3.696
14. van Baarsen LGM, Vosslamber S, Tijssen M, Baggen JMC, van der Voort LF, Killestein J, et al. Pharmacogenomics of interferon-β therapy in multiple sclerosis: baseline IFN signature determines pharmacological differences between patients. PloS One (2008) 3(4):e1927. doi: 10.1371/journal.pone.0001927
15. Comabella M, Lünemann JD, Río J, Sánchez A, López C, Julià E, et al. A type I interferon signature in monocytes is associated with poor response to interferon-β in multiple sclerosis. Brain (2009) 132:3353–65. doi: 10.1093/brain/awp228
16. Börnsen L, Christensen JR, Ratzer R, Hedegaard C, Søndergaard HB, Krakauer M, et al. Endogenous interferon-β-inducible gene expression and interferon-β-treatment are associated with reduced T cell responses to myelin basic protein in multiple sclerosis. PloS One (2015) 10(3):e0118830. doi: 10.1371/journal.pone.0118830
17. Johnson TP, Tyagi R, Patel K, Schiess N, Calabresi PA, Nath A. Impaired toll-like receptor 8 signaling in multiple sclerosis. J Neuroinflamm (2013) 10:74. doi: 10.1186/1742-2094-10-74
18. Cervantes JL, Weinerman B, Basole C, Salazar JC. TLR8: the forgotten relative revindicated. Cell Mol Immunol (2012) 9(6):434–38. doi: 10.1038/cmi.2012.38
19. Angelini DF, Serafini B, Piras E, Severa M, Coccia EM, Rosicarelli B, et al. Increased CD8+ T cell response to Epstein-Barr virus lytic antigens in the active phase of multiple sclerosis. PloS Pathog (2013) 9(4):e1003220. doi: 10.1371/journal.ppat.1003220
20. Yea C, Tellier R, Chong P, Westmacott G, Marrie RA, Bar-Or A, et al. Epstein-Barr Virus in oral shedding of children with multiple sclerosis. Neurology (2013) 81(16):1392–99. doi: 10.1212/WNL.0b013e3182a841e4
21. Bjornevik K, Cortese M, Healy BC, Kuhle J, Mina MJ, Leng Y, et al. Longitudinal analysis reveals high prevalence of Epstein-Barr virus associated with multiple sclerosis. Science (2022) 375:296–301. doi: 10.1126/science.abj8222
22. Serafini B, Rosicarelli B, Franciotta D, Magliozzi R, Reynolds R, Cinque P, et al. Dysregulated Epstein-Barr virus infection in the multiple sclerosis brain. J Exp Med (2007) 204(12):2899–912. doi: 10.1084/jem.20071030
23. Hassani A, Corboy JR, Al-Salam S, Khan G. Epstein-Barr virus is present in the brain of most cases of multiple sclerosis and may engage more than just B cells. PloS One (2018) 13(2):e0192109. doi: 10.1371/journal.pone.0192109
24. Virtanen JO, Wohler J, Fenton K, Reich DS, Jacobson S. Oligoclonal bands in multiple sclerosis reactive against two herpesviruses and association with magnetic resonance imaging findings. Mult Scler (2014) 20(1):27–34. doi: 10.1177/1352458513490545
25. Willer CJ, Dyment DA, Risch NJ, Sadovnick AD, Ebers GC. Twin concordance and sibling recurrence rates in multiple sclerosis. PNAS (2003) 100(22):12877–82. doi: 10.1073/pnas.1932604100
26. Islam T, Gauderman WJ, Cozen W, Hamilton AS, Burnett ME, Mack TM. Differential twin concordance for multiple sclerosis by latitude of birthplace. Ann Neurol (2006) 60:56–64. doi: 10.1002/ana.20871
27. Mumford CJ, Wood NW, Kellar-Wood H, Thorpe JW, Miller DH, Compston DA. The British Isles survey of multiple sclerosis in twins. Neurology (1994) 44(1):11–5. doi: 10.1212/wnl.44.1.11
28. Kuusisto H, Kaprio J, Kinnunen E, Luukkaala T, Koskenvuo M, Elovaara I. Concordance and heritability of multiple sclerosis in Finland: study on a nationwide series of twins. Eur J Neurol (2008) 15:1106–10. doi: 10.1111/j.1468-1331.2008.02262.x
29. Ristori G, Cannoni S, Stazi MA, Vanacore N, Cotichini R, Alfò M, et al. Multiple sclerosis in twins from continental Italy and Sardinia: a nationwide study. Ann Neurol (2006) 59(1):27–34. doi: 10.1002/ana.20683
30. Sadovnick AD, Armstrong H, Rice GP, Bulman D, Hashimoto L, Paty DW, et al. A population-based study of multiple sclerosis in twins: update. Ann Neurol (1993) 33:281–5. doi: 10.1002/ana.410330309
31. Simpson S, Blizzard L, Otahal P, van der Mei I, Taylor B. Latitude is significantly associated with the prevalence of multiple sclerosis: a meta-analysis. J Neurol Neurosurg Psychiatry (2011) 82:1132–41. doi: 10.1136/jnnp.2011.240432
32. Hjalgrim H, Friborg J, Melbye M. The epidemiology of EBV and its association with malignant disease, in: Human herpesviruses: biology, therapy, and immunoprophylaxis (2007). Cambridge: Cambridge University Press. Available at: http://www.ncbi.nlm.nih.gov/books/NBK47424/ (Accessed 7/5/2019).
33. Ascherio A, Munger KL. 99th Dahlem conference on infection, inflammation and chronic inflammatory disorders: Epstein-Barr virus and multiple sclerosis: epidemiological evidence. Clin Exp Immunol (2010) 160(1):120–4. doi: 10.1111/j.1365-2249.2010.04121.x
34. Pender MP, Csurhes PA, Burrows JM, Burrows SR. Defective T-cell control of Epstein-Barr virus infection in multiple sclerosis. Clin Transl Immunol (2017) 6(1):e126. doi: 10.1038/cti.2016.87
35. Castellazi M, Contini C, Tamborino C, Fasolo F, Roversi G, Seraceni S, et al. Epstein-Barr virus-specific intrathecal oligoclonal IgG production in relapsing-remitting multiple sclerosis is limited to a subset of patients and is composed of low-affinity antibodies. J Neuroinflamm (2014) 11:188. doi: 10.1186/s12974-014-0188-1
36. Charrel RN, de Lamballerie X. Zoonotic aspects of arenavirus infections. Vet Microbiol (2010) 140(3–4):213–20. doi: 10.1016/j.vetmic.2009.08.027
37. Bonthius DJ. Lymphocytic choriomeningitis virus: an under-recognized cause of neurologic disease in the fetus, child, and adult. Semin Pediatr Neurol (2012) 19(3):89–95. doi: 10.1016/j.spen.2012.02.002
38. Sevilla N, Kunz S, Holz A, Lewicki H, Homann D, Yamada H, et al. Immunosuppression and resultant viral persistence by specific viral targeting of dendritic cells. J Exp Med (2000) 192(9):1249–60. doi: 10.1084/jem.192.9.1249
39. Sevilla N, McGavern DB, Teng C, Kunz S, Oldstone MBA. Viral targeting of hematopoietic progenitors and inhibition of DC maturation as a dual strategy for immune subversion. J Clin Invest (2004) 113(5):737–45. doi: 10.1172/JCI20243
40. Sevilla N, Kunz S, McGavern D, Oldstone MBA. Infection of dendritic cells by lymphocytic choriomeningitis virus. Curr Top Microbiol Immunol (2003) 276:125–44. doi: 10.1007/978-3-662-06508-2_6
41. Che JW, Kraft ARM, Selin LK, Welsh RM. Regulatory T cells resist virus infection-induced apoptosis. J Virol (2015) 89(4):2112–20. doi: 10.1128/JVI.02245-14
42. Lang KS, Recher M, Navarini AA, Harris NL, Löhning M, Junt T, et al. Inverse correlation between IL-7 receptor expression and CD8 T cell exhaustion during persistent antigen stimulation. Eur J Immunol (2005) 35:738–45. doi: 10.1002/eji.200425828
43. Ozato K, Tsujimura H, Tamura T. Toll-like receptor signaling and regulation of cytokine gene expression in the immune system. Biotechniques (2002) Suppl:66–68:70, 72 passim. doi: 10.2144/Oct0208
44. Mat NFC, Siddiqui S, Mehta D, Seaver K, Banete A, Alothaimeen T, et al. Lymphocytic choriomeningitis virus infection of dendritic cells interferes with TLR-induced IL-12/IL-23 cytokine production in an IL-10 independent manner. Cytokine (2018) 108:105–14. doi: 10.1016/j.cyto.2018.03.017
45. Dalod M, Salazar-Mather TP, Malmgaard L, Lewis C, Asselin-Paturel C, Brière F, et al. Interferon α/β and interleukin 12 responses to viral infections: pathways regulating dendritic cell cytokine expression in vivo. J Exp Med (2002) 195(4):517–28. doi: 10.1084/jem.20011672
46. Martínez-Sobrido L, Zúñiga EI, Rosario D, García-Sastre A, de la Torre JC. Inhibition of the type I interferon response by the nucleoprotein of the prototypic arenavirus lymphocytic choriomeningitis virus. J Virol (2006) 80(18):9192–9. doi: 10.1128/JVI.00555-06
47. Pythoud C, Rodrigo WWSI, Pasqual G, Rothenberger S, Martínez-Sobrido L, de la Torre JC, et al. Arenavirus nucleoprotein targets interferon regulatory factor-activating kinase IKKε. J Virol (2012) 86(15):7728–38. doi: 10.1128/JVI.00187-12
48. World Health Organization (WHO). Atlas: multiple sclerosis resources in the world 2008. Geneva: WHO Press (2008)2008.
49. Hogeboom C. Peptide motif analysis predicts lymphocytic choriomeningitis virus as trigger for multiple sclerosis. Mol Immunol (2015) 67:625–35. doi: 10.1016/j.molimm.2015.07.041
50. Centers for Disease Control and Prevention. Lymphocytic choriomeningitis (LCM) (2021). Available at: https://www.cdc.gov/vhf/lcm/pdf/factsheet.pdf (Accessed April 28, 2023).
51. Dobec M, Dzelalija B, Punda-Polic V, Zoric I. High prevalence of antibodies to lymphocytic choriomeningitis virus in a murine typhus endemic region in Croatia. J Med Virol (2006) 78:1643–7. doi: 10.1002/jmv.20749
52. Duh D, Varljen-Buzan E, Hasic S, Charrel R. Increased seroprevalence of lymphocytic choriomeningitis virus infection in mice sampled in illegal waste sites. Parasit Vectors (2014) 7(Suppl 1):O31. doi: 10.1186/1756-3305-7-S1-O31
53. Hausmann S, Martin M, Gauthier L, Wucherpfennig KW. Structural features of autoreactive TCR that determine the degree of degeneracy in peptide recognition. J Immunol (1999) 162:338–44. doi: 10.4049/jimmunol.162.1.338
54. van Baarsen LGM, van der Pouw Kraan TCTM, Kragt JJ, Baggen JMC, Rustenburg F, Hooper T, et al. A subtype of multiple sclerosis defined by an activated immune defense program. Genes Immun (2006) 7:522–31. doi: 10.1038/sj.gene.6364324
55. Browne P, Chandraratna D, Angood C, Tremlett H, Baker C, Taylor BV, et al. Atlas of multiple sclerosis 2013: a growing global problem with widespread inequity. Neurology (2014) 83(11):1022–4. doi: 10.1212/WNL.0000000000000768
56. Smith KJ, Pyrdol J, Gauthier L, Wiley DC, Wucherpfennig KW. Crystal structure of HLA-DR2 (DRA*0101, DRB1*1501) complexed with a protein from human myelin basic protein. J Exp Med (1998) 188(8):1511–20. doi: 10.1084/jem.188.8.1511
57. Li Y, Li H, Martin R, Mariuzza RA. Structural basis for the binding of an immunodominant peptide from myelin basic protein in different registers by two HLA-DR2 proteins. J Mol Biol (2000) 304:177–88. doi: 10.1006/jmbi.2000.4198
Keywords: multiple sclerosis, lymphocytic choriomeningitis virus, myeloid dendritic cells, regulatory T cells, toll-like receptor 8 (TLR8)
Citation: Hogeboom C (2023) Does multiple sclerosis have a zoonotic origin? Correlations with lymphocytic choriomeningitis virus infection. Front. Immunol. 14:1217176. doi: 10.3389/fimmu.2023.1217176
Received: 04 May 2023; Accepted: 06 June 2023;
Published: 16 June 2023.
Edited by:
Judith M. Greer, The University of Queensland, AustraliaReviewed by:
Raymond Sobel, Stanford University, United StatesCopyright © 2023 Hogeboom. This is an open-access article distributed under the terms of the Creative Commons Attribution License (CC BY). The use, distribution or reproduction in other forums is permitted, provided the original author(s) and the copyright owner(s) are credited and that the original publication in this journal is cited, in accordance with accepted academic practice. No use, distribution or reproduction is permitted which does not comply with these terms.
*Correspondence: Charissa Hogeboom, Q2hhcmlzc2EuSG9nZWJvb21Ab3V0bG9vay5jb20=
Disclaimer: All claims expressed in this article are solely those of the authors and do not necessarily represent those of their affiliated organizations, or those of the publisher, the editors and the reviewers. Any product that may be evaluated in this article or claim that may be made by its manufacturer is not guaranteed or endorsed by the publisher.
Research integrity at Frontiers
Learn more about the work of our research integrity team to safeguard the quality of each article we publish.