- 1Division of Pulmonary, Critical Care and Allergy, Drexel University College of Medicine, Philadelphia, PA, United States
- 2Department of Pharmacology, Jikei University School of Medicine, Tokyo, Japan
- 3Danmir Therapeutics, LLC, Haverford, PA, United States
Since the late 1970s, there has been an alarming increase in the incidence of asthma and its morbidity and mortality. Acute obstruction and inflammation of allergic asthmatic airways are frequently caused by inhalation of exogenous substances such as allergens cross-linking IgE receptors expressed on the surface of the human lung mast cells (HLMC). The degree of constriction of human airways produced by identical amounts of inhaled allergens may vary from day to day and even hour to hour. Endogenous factors in the human mast cell (HMC)’s microenvironment during allergen exposure may markedly modulate the degranulation response. An increase in allergic responsiveness may significantly enhance bronchoconstriction and breathlessness. This review focuses on the role that the ubiquitous endogenous purine nucleotide, extracellular adenosine 5’-triphosphate (ATP), which is a component of the damage-associated molecular patterns, plays in mast cells’ physiology. ATP activates P2 purinergic cell-surface receptors (P2R) to trigger signaling cascades resulting in heightened inflammatory responses. ATP is the most potent enhancer of IgE-mediated HLMC degranulation described to date. Current knowledge of ATP as it relates to targeted receptor(s) on HMC along with most recent studies exploring HMC post-receptor activation pathways are discussed. In addition, the reviewed studies may explain why brief, minimal exposures to allergens (e.g., dust, cat, mouse, and grass) can unpredictably lead to intense clinical reactions. Furthermore, potential therapeutic approaches targeting ATP-related enhancement of allergic reactions are presented.
Introduction
Within minutes of allergic activation of IgE receptors expressed by HMC, the cells release histamine and a spectrum of other pro-inflammatory mediators to induce airway bronchoconstriction and contribute to acute and chronic lung inflammation (1–3). Preformed cytoplasmic granular mediators are released over seconds (e.g., histamine and TNF alpha), select chemicals are newly formed over minutes (e.g., leukotrienes and other lipids), and other mediators including cytokines over hours (e.g., Interleukin (IL) -5, IL-13, IL-8, and GM-CSF) (1, 4). Depending on the strength of the stimulation, the localized inflammatory milieu, and the organs involved, mast cell (MC) activation can result in multiple responses including edema, hives, bronchoconstriction, or systemic anaphylaxis, which can not only decrease the quality of life but may also be life-threatening. Many publications have described studies of “human mast cells.” Most of these studies have used neoplastic MC (5–7) or cells cultured in vitro derived from differing precursor cells (e.g., cord blood, peripheral blood, and fetal liver), using culture media that include various combinations of cytokines (8–19). Relatively few studies used freshly isolated and purified human lung mast cells (HLMC) as a model of the organ-specific responses of the human lung (20). Also, there is increasing appreciation that MC are heterogeneous, and their biology can differ markedly not only between species (e.g., mouse vs. human) but also between MC isolated from different human organ sources (e.g., skin vs. lung), within the same organ (e.g., gut), and freshly isolated organ-derived MC vs. in vitro-derived MC (8, 21–26).
Progress in understanding HLMC biology has been extremely slow because of difficulties in procuring freshly resected human specimens, time-consuming and technical challenges associated with the isolation and purification of these cells, and their limited survival in vitro (i.e., 2-4 days). Our seminal report on the methods of isolation and purification of HLMC (27) facilitated many subsequent studies on their biology including ultrastructure (28–32), heterogeneity (33–35), mediators’ release biochemistry (36, 37), secretagogues (38–45), mediators (46–55), and pharmacological modulation of mediators’ release (40–42, 45, 56).
The text below focuses on the progress made in recent years in our understanding of the critical interaction of the extracellular purine nucleotide adenosine 5′-triphosphate (ATP), with IgE-mediated activation of the HLMC (57, 58) and intracellular signal transduction pathway associated with IgE receptor activation in LAD2 cell line (6, 59, 60). Our own interest in this regard mostly relates to allergic asthma, but other important aspects of ATP’s role in pulmonary pathophysiology have been investigated including cystic fibrosis, pulmonary embolism, cough, bronchoconstriction, pulmonary fibrosis, lung cancer, mechanical ventilation-induced lung injury, and pulmonary hypertension (61–68).
Intracellular ATP
Intracellular ATP plays a critical role in cellular metabolism and energetics (69). ATP is found at a concentration of 5–10 mM in every cell, except for platelets, in which its concentration is far higher. The concentration of ATP in chromaffin cells’ secretory granules approaches 100 mM; platelets’ ATP level is up to 500 mM. However, the concentration of extracellular ATP is only approximately 10 nM (70)(see below).
Extracellular ATP
Release of ATP from cells
In 1929, Drury and Szent-Gyorgyi described the effects of a simple extract of heart muscle and other tissues on the cardiovascular system. The active ingredient in this extract was identified as adenylic acid (71). Subsequent studies have shown that adenosine and ATP were the most active vasodilators and bradycardic ingredients of these extracts (72–74). ATP is released from cells by various mechanisms under physiologic and pathophysiologic conditions in response to different stimuli or micro-environmental conditions. These include exocytosis, large membrane pores, and specific trans-cell membrane ionic channels (75, 76). There are several sources of extracellular ATP (23, 77). Large amounts of ATP are found in platelets and ATP is released during platelet activation. Upon platelet aggregation, the serum concentration of ATP and adenosine diphosphate (ADP) reaches 50 uM but is much higher at the cell surface (78–80). ATP is also stored in red blood cells (RBC) from which it is released under conditions of imbalance between O2 supply and O2 demand (81–84). In addition, several biologic substances as well as increased blood flow can induce the release of ATP from vascular endothelial cells (77, 85–88) and smooth muscle cells (89, 90). Other ATPs release stimuli and cellular sources including mechanical deformation of cells, ischemic cells, immune cells, and necrotic/apoptotic cells (23, 91–94). Pannexin channel and connexin hemichannel play a critical role in this release (95). ATP is also released from neural elements as a co-transmitter and from exercising skeletal muscles (96). In the heart, ATP is released into the extracellular fluid under various conditions. Specifically, ATP release is evoked by sympathetic nerve stimulation and by catecholamines (97–101). In addition, ATP is released in the heart during acute myocardial ischemia (102) and from cardiac myocytes in response to hypoxia (103, 104).
Importantly, during inflammation, ATP is released from inflammatory cells. For example, elevated extracellular levels of ATP have been found in the lungs of COPD patients (105, 106). Similarly, pulmonary levels of ATP were increased in a mouse model of smoke-induced acute lung inflammation and emphysema (107–109).
Degradation of extracellular ATP
Extracellular ATP is rapidly and sequentially degraded by ectonucleotidases including ectonucleoside triphosphate diphosphorylase-1 (CD39), and ecto-5’-nucleotidase (CD73). CD39 hydrolyzes extracellular ATP and ADP to AMP. CD73 catalyzes the hydrolysis of AMP, releasing inorganic phosphate and adenosine, which exerts its own effects by activating P1 purinergic receptors (A1, A2a, A2b, and A3) (110–113).
CD73 is widely expressed in a variety of tissues, including the colon, kidney, brain, liver, heart, lung, spleen, and bone marrow (114). CD39 is expressed by multiple cell types including epithelial, endothelial, and immune cells. It is highly expressed in different human tumor types (115). Adenosine is rapidly eliminated from the extracellular space by ectoadenosine deaminase and actively transported into cells (109). Therefore, the levels of CD39 and CD73 and their enzymatic activities play a critical role in controlling the duration and magnitude of autocrine and paracrine effects of ATP and adenosine. Multiple studies have shown that the level of these enzymes is altered during pathophysiologic conditions. For example, increased expression of CD39 and CD73 by pulmonary epithelial and endothelial cells was observed during high inspiratory pressure level-induced lung injury (116). Also, upregulation of CD39/CD73 expression has been observed in patients with small-cell lung cancer and patients with a broad spectrum of solid cancers (117).
ATP: A paracrine and autocrine agent
Extracellular ATP acts as a paracrine and autocrine agent (23, 91, 118), the actions of which are mediated by cell surface purinergic receptors (P2R) (109). The latter are divided into two families: P2Y: seven trans-cell membrane domain G-protein coupled receptors (metabotropic), and P2X: trans-cell membrane cationic channels (ionotropic). Eight P2YR and seven homotrimeric P2X receptors (P2X1-7) have been cloned heretofore (Figure 1). Multiple heteromeric assemblies comprising P2X subunits have been described including P2X2/P2X3, P2X4/P2X6, P2X2/P2X6, and P2X1/P2X5, but not all have been detected in native tissues (119).
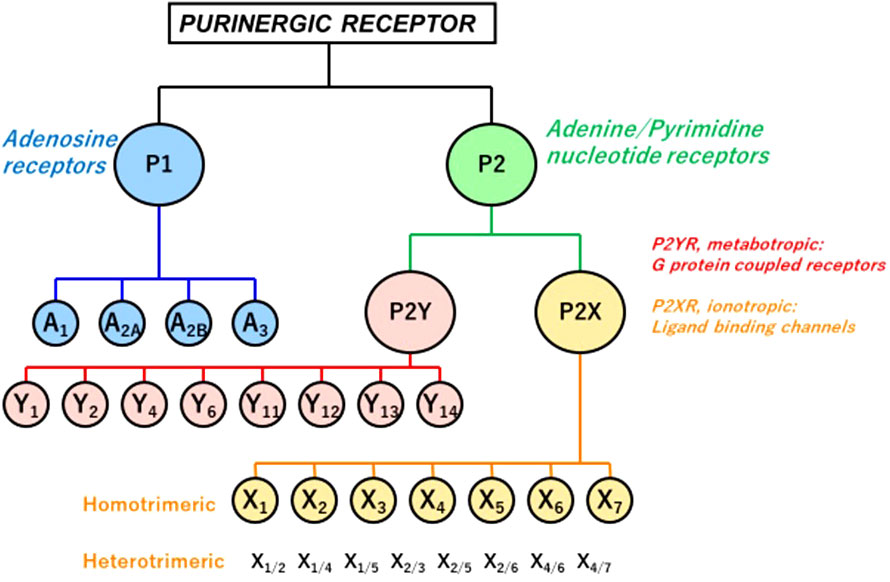
Figure 1 P1 and P2 Purinergic Receptors. Extracellular signals triggered by ATP, its hydrolytic products ADP and adenosine, and other nucleotides and nucleotide sugars (UTP, UDP, and UDP-glucose) regulate physiological and pathophysiological processes. Two families of purinoceptors are shown. P1R consists of four subtypes of adenosine-activated receptors. P2R is divided into P2XR ionotropic ligand-gated ion channel receptors, principally activated by ATP, P2YR, and G-protein-coupled (metabotropic) receptors. Also shown are multiple heteromeric assemblies comprising P2X subunits, but not all have been detected in native tissues (119).
In contrast to P2XR, which is principally activated by ATP, P2YR shows striking differential sensitivity for varying nucleotides. Single cells commonly express more than one P2YR, for which a given nucleotide has an affinity. Pharmacologically, P2YR are subdivided into adenine-nucleotides sensitive group responding mainly to adenosine diphosphate (P2Y1R, P2Y12R, and P2Y13R: ADP), adenosine triphosphate (P2Y11R: ATP), uridine triphosphate (P2Y4R: UTP), uridine diphosphate (P2Y6R: UDP), UDP and sugar derivatives sensitive receptor (P2Y14R: UDP-glucose and UDP-galactose), and receptors manifesting mixed agonist affinity (P2Y2R: ATP=UTP) (120) (Table 1). ATP is also an antagonist or partial agonist at the P2Y1 receptor (121). Furthermore, whereas ATP activates human P2Y11 receptors, ADP is a canine P2Y11 receptor agonist (122). P2Y1, -2, -4, -6, and -11 receptors belong to the P2Y1-like subfamily and couple to Gq/11, Go, G12/13, and Gs protein, whereas P2Y12, -13, and -14 receptors are categorized as P2Y12-like and couple to Gi/o protein. ATP is the only agonist that can activate both P2XR and P2YR (23, 123) (Table 1).
Inhalation of aerosolized ATP triggered bronchoconstriction in healthy and more so in asthmatic human subjects; in the latter, ATP was 50-fold more potent than methacholine and 87-fold more potent than histamine in producing a 15% decrease in FEV1 (124). In 2002, based on multiple studies in both canine and human models (124–126), a mechanistic role of ATP in pulmonary disorders was proposed for the first time in a seminal review, and termed “Adenosine 5′-triphosphate axis in obstructive airway diseases” (127, 128).
ATP enhances IgE-mediated HLMC degranulation
Early experiments in HLMC suggested that ATP plays an important modulatory role in degranulation as measured by the percentage of total cellular histamine released within minutes following IgE-mediated challenge (58). In these experiments, freshly purified HLMC (10-50 x 103/tube) were incubated with or without purine nucleotides for 15 min prior to a 20 min challenge with anti-IgE (3 ug/ml), calcium ionophore A23187 (0.1 ug/ml), or buffer control. Cells were exposed to ATP, UTP, the stable ATP analogs α, β methylene-ATP (α, β-MeATP), (β, γ methylene-ATP (β, γ-MeATP), and 2-methylthio-ATP (2-MeSATP), as well as adenosine, the product of ATP’s degradation.
Neither the nucleotides (10-7-10-3 M) nor adenosine (10−6–10−3 M) directly triggered degranulation, contrary to results obtained using murine mast cell models (129–133). In HLMC, adenosine exhibited a bimodal effect on anti-IgE-induced histamine release, enhancing it at 10−6 to 10−4 M (P > 0.05, NS) and inhibiting it at 10−3 M (P < 0.05) (58), in congruence with prior reports on HLMC (41, 134, 135). ATP (10−4 M) enhanced anti-IgE–induced histamine release (10.9 ± 2.7% to 19.2 ± 2.9%, n = 20, P < 0.01). Importantly, ATP’s effects were most striking in cells manifesting low (< 3%) IgE-mediated net histamine release termed a weak allergic stimulation (WAS). When WAS-dependent histamine release was “low”, i.e., 1.8 ± 0.4%; range: 0.5–2.9%; n=6), ATP (10-4 M) enhanced histamine release to 13.5 ± 2.7% (750% enhancement). In contrast, ATP had no effects on the ionophore A23187-induced histamine release (n = 10). All adenine nucleotides enhanced IgE-mediated HLMC histamine release, but ATP was the most potent followed by 2-MeSATP, α, β-MeATP, and β, γ-MeATP. This potency order strongly suggests that the action of ATP is mediated by a P2YR subtype (136). Further evidence against the involvement of a P2XR, especially P2X7R, was the failure of the selective P2XR antagonist pyridoxalphosphate-6-azophenyl-2′,4′-disulfonic acid (PPADS) (137) to affect ATP’s enhancement.
The P2YR- agonist UTP also significantly enhanced anti-IgE–induced histamine release, which is most consistent with a P2YR and not P2XR mediation. However, UTP was less potent than ATP. Control anti-IgE–induced histamine release of 14.9 ± 3.9% was enhanced by ATP (10-4 M) to 23.0 ± 4.7% (p < 0.05), compared with 19.2 ± 5.0% (p < 0.05) in the presence of an equimolar concentration of UTP. This result did not support sole mediation by P2Y2R, of which UTP is the preferred agonist over ATP (138).
HLMCs fail to exhibit functional ecto-ATPase activities
Over the course of 15-minute incubations with ATP, HLMC’s media did not contain breakdown products of ATP, indicating that the histamine-release enhancement by ATP was not mediated by ADP, AMP, or adenosine. It should be noted that under identical experimental conditions, products of ATP’s degradation were found in media containing whole fragments of the human lung (58).
Expression of P2Rs in HLMC
Further support for a P2YR mediating the effects of ATP on IgE-mediated degranulation enhancement from HLMC was the constitutive expression of P2Y1R and P2Y2R and weak expression of P2Y4 demonstrated by reverse transcription–polymerase chain reaction (RT-PCR) of freshly purified HLMC (58). A receptor, previously known as P2Y7, was weakly manifested in the RT-PCR assay in one of five individual fresh isolations of HLMC. P2Y7 was later identified as the receptor for leukotriene B4 (B-LTR) (139). Feng et al. reported the expression of P2Y1, P2Y2, P2Y11, P2Y12, and P2Y13 by RT-PCR in cord blood-derived human MC and highlighted the potential roles of different P2YR in complementary or opposing functions in cell activation (140). Wareham et al. reported P2X7 expression by RT-PCR in HLMC that were cultured in a cytokine mix pre-experimentation. In addition, using a whole-cell patch-clamp technique, they described a P2X7-like non-desensitizing current in response to high concentrations of ATP (1–5 mM). P2X1 and P2X4 transcripts were also found in their HLMC preparations (141).
Stimulation of the P2YRs can result in the activation of two different pathways: one that involves pertussis toxin (PTX)-sensitive G protein(s) and adenylyl cyclase (Gi-coupled; P2Y12, P2Y13, and P2Y14), and the other that involves PTX-insensitive G protein and phospholipase C (Gq-coupled; P2Y1, P2Y2, P2Y4, P2Y6, and Gq/s-coupled; P2Y11) (142). Because PTX failed to modify the enhancing effect of ATP on IgE-mediated histamine release, it seems likely that the latter pathway mediates the effect of ATP (58). However, the exact post-P2YR mechanism(s) by which this pathway(s) operates to enhance IgE-mediated degranulation in HLMC remains to be elucidated.
ATP enhances IgE-mediated LAD2 HMC degranulation
Though P2YRs are expressed by HMC of different sources (58, 140, 143), until recently, only one receptor (i.e., P2Y14R) in LAD2 cells was proposed to be linked to allergen/IgE-induced degranulation (60). To further elucidate the specific receptor(s) and, importantly, post-receptor pathways accountable for nucleotide-P2YR mediated effects on IgE-mediated degranulation, sufficient cell numbers were needed beyond what the HLMC model could provide. The LAD2 human-derived MC line was developed at the NIH from a patient with systemic mastocytosis (5, 6, 10). As recently reported, LAD2 cells express five P2YRs (P2Y1, P2Y6, P2Y11, P2Y12, and P2Y14) along with three P1Rs (A2aR, A2bR, and A3R), and two P2XRs (P2X1 and P2X7) (59). LAD2 cells express CD39 but not CD73, suggesting extracellular nucleotides could be degraded to AMP but not to adenosine (59).
In non-sensitized LAD2 cells challenged with P1R and P2R agonists [adenosine, ATP, ADP, UTP, UDP, 2-methylthio-ATP (2-MeSATP)], and the P2Y11R non-hydrolysable agonist [adenosine 5’-(3-thio) triphosphate (ATPγS)] at physiological concentrations (1–100 μM), none of the agonists alone triggered degranulation as measured by histamine or beta-hexosaminidase release. High concentrations (1mM) of BzATP, a P2X7R agonist, triggered degranulation in non-sensitized LAD2 cells. Sensitized LAD2 cells were then challenged in the absence and presence of P2YR agonists, using a predetermined low concentration of antigen to produce a weak allergic stimulation (WAS) and a low level of degranulation (<10% release). As noted above for HLMC, ATP’s enhancement was most pronounced under WAS conditions; only the P2Y11R non-hydrolyzable agonist, ATPγS (144–147), mimicked the effects of ATP and produced a net 7-fold increase in release (n = 4; p < 0.01). The failure of UTP and ADP to enhance the release of histamine excluded the involvement of their respective receptors, P2Y1R, P2Y2, P2Y4R, P2Y12R, and P2Y13R. P2Y11R protein expression by LAD2 cells was confirmed by Western blot. The effects of ATPγS were dose-dependently inhibited by NF157, a P2Y11R antagonist (148, 149). Further evidence for P2Y11 as the receptor mediating the degranulation-enhancing effect of ATP in LAD2 cells is its known coupling to both Gq/11 and Gs proteins that are known to be linked to induction and suppression of MC degranulation, respectively.
None of the P2YR agonists tested in LAD2 cells, including high concentrations of ATPγS (1000 μM) enhanced the WAS-induced intracellular Ca2+ mobilization, which is an essential component of activated FcϵRI-induced degranulation. The effects of ATPγS on the phosphorylation of key kinases related to intracellular PI3K(δ)’s activation cascades showed that both PI3K(δ) and Akt were phosphorylated by ATPγS and are further upregulated by WAS, especially in the case of Akt. However, PDK-1, which is known to be a link between PI3K(δ) and Akt, was not phosphorylated. These data indicated that the P2YR-mediated enhancement effect on IgE-mediated degranulation in an HMC is via the PI3K/protein kinase B pathway (Figure 2). In further experiments, a shRNA directed against PI3K(δ) and a PI3K(δ) inhibitor, compound 15e (150, 151), suppressed ATPγS’s effect on WAS-induced degranulation enhancement, the latter in a concentration-dependent manner. In additional experiments, an antagonist of the P2Y11R and NF157 significantly inhibited the enhancing effects of ATPγS (100 μM) on WAS-induced degranulation and experiments using siRNA knockdown of the P2Y11R, which itself abolished the enhancing effects (59).
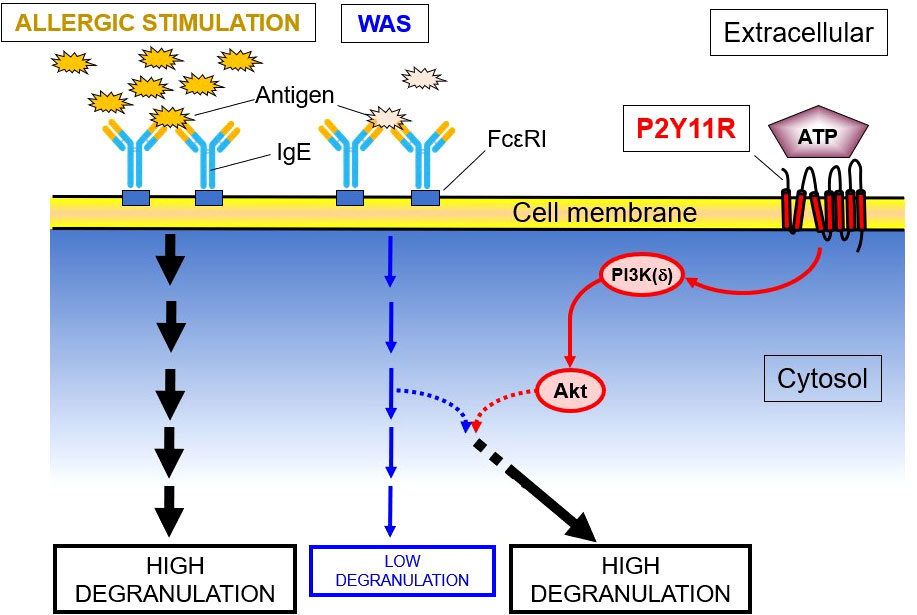
Figure 2 P2YR11 Modifies a WAS in LAD2 Human MC. This schematic shows that P2Y11R, an endogenous purine (ATP) receptor, modulates the enzymatic cascade between PI3K(δ) and Akt, leading to the enhancement of a weak allergic stimulus (WAS). PDK-1, though a known link between PI3K and Akt, is not phosphorylated and is not involved in the degranulation enhancement.
Discussion
Historical perspective
Over the past four decades, adenosine, but not adenine nucleotides, has received considerable attention for a potential role in human allergies and asthma (41, 57, 130, 134, 135, 152–156). In 1996, Pelleg and Hurt showed for the first time that extracellular ATP stimulates vagal sensory nerve terminals in the canine lungs by activating P2XR (125). Subsequent studies have indicated that this action of ATP leads to bronchoconstriction and cough and is probably also pro-inflammatory due to the localized release of neuropeptides via the axon reflex (62, 157, 158). The suggestion that this latter pathway could be mechanistically involved in asthma (159) prompted us to investigate the potential effects of extracellular ATP on HLMC. Our early findings indicated that ATP potentiates IgE-mediated degranulation from HLMC (58). Subsequent studies have shown a similar phenomenon in LAD2 cells, which was not mediated by adenosine, which is the product of ATP’s degradation by ecto-enzymes. Since then, additional studies have investigated the role of extracellular and intra-MC ATP in MC’s role in allergic inflammation (160).
Mechanisms of actions of ATP
Mediation by P2R
ATP is unimodal, only enhancing degranulation, whereas adenosine is bimodal; at high concentrations, it inhibits degranulation and at low concentrations, it potentiates degranulation. At equimolar concentrations, ATP is more potent than adenosine in enhancing HMC degranulation (57–59). ATP’s enhancing effects on IgE-mediated degranulation in freshly isolated HLMC, without exposure to varied cytokine mixtures over days to weeks in cultures, is substantial and reproducible. The specific P2R subtype (s) mediating this degranulation-enhancing effect in HLMC remain unknown, but several lines of evidence strongly suggest the involvement of P2YR and not P2XR. It is also possible that two or more P2YRs are activated in concert during different physiologic conditions. Along these lines, Feng et al. reported the expression of multiple P2YRs in human cord blood-derived MC, including P2Y1R, P2Y2R, P2Y11R, P2Y12R, and P2Y13R, that could play complementary and opposing functions during mast cell activation (140). Regrettably, the difficulties involved in procuring specimens of human lungs and isolating and purifying HLMC stymied efforts to fully elucidate the relevant mechanisms of ATP’s enhancement of the immune reaction-dependent MC degranulation.
More recently, insights into pathways involving P2YR expressed by HMC were obtained by quantifying the effects of ATP on LAD2 cells, which are proliferating neoplastic HMC lines. However, LAD2 cells are more analogous to MCTC-type HMC which predominate in skin and other organs and represent a distinct minority population in the human lung where MCT predominates (25, 161–163). That notwithstanding, LAD2 cells do show similarity to HLMC: ATP does not trigger degranulation of these cells by itself as it does in murine MCs (130, 132, 141, 164), and ATP-induced enhancement of IgE-mediated degranulation is observed in both HLMC and LAD2 cells.
There is no evidence for P2X7R expression in freshly isolated HLMC which contrasts with the positive RT-PCR for P2X7R and functional activity under whole-cell patch clamp conditions reported in cultured HLMC by Wareham et al. (141). The latter also reported that HLMC express P2X1R and P2X4R and suggested that the differential findings related to P2X7R could be the result of different cell isolation techniques and/or differences in HLMC receptor expression at the time of fresh isolation vs. the pre-experimentation culture of the cells in cytokine-containing mixtures. However, we have performed long-term HLMC culture (2 weeks to 4 months) in a medium containing a combination of Stem Cell Factor and IL-4 (56) and still failed to identify the expression of P2X7R using RT-PCR. By contrast, RT-PCR assays showed that the HMC-1 mast cell leukemia line (58) and the LAD2 cell line do robustly express P2X7R, and in the latter’s case, when challenged with BzATP triethylammonium salt, the prototypic P2X7R agonist will lead to degranulation. This agonist failed to trigger degranulation in HLMC (58).
Matsuoka et al. (165) and Yoshida et al. (166) clearly demonstrated that P2X4R activation potentiated Mas-related G protein-coupled receptor X2 (MrgprB2)-mediated pseudo-allergic responses in murine MC. MrgprB2 is the mouse ortholog of human MRGPRX2. However, few reports have demonstrated potentiating effects on P2X4R-stimulated allergic responses in HMC. Bonvini et al. reported that a Transient receptor potential cation channel, subfamily V, member 4 (TRPV4) agonist will cause ATP release from, but not contraction of, isolated airway smooth muscle. Smooth muscle contraction required co-incubation with HLMC. TRPV4-mediated ATP release by the airway smooth muscle-stimulated HLMC to release cysteinyl leukotrienes via a P2X4R-dependent mechanism which subsequently induced smooth muscle contractions in an IgE-independent fashion (167). In any case, these reports suggest that P2X4R, but not P2X7, expressed on MC may also be associated with pathological effects.
Additional evidence for a P2YR subtype(s) and not a P2XR subtype mediating the effects of ATP on HLMC goes beyond transcriptional data. Specifically, the pattern of pharmacologic responsiveness to stable analogs of ATP (i.e., ATP > 2-MeSATP > α, β -MeATP > β, γ-MeATP) is consistent with mediation by P2YR and not P2XR (23, 91, 118, 136). Second, we examined the putative P2XR-selective antagonist PPADS (137) on ATP’s effects on enhancing IgE-mediated HLMC degranulation. The failure of this agent to influence ATP-induced enhancement of degranulation along with the previously stated failure of BzATP triethylammonium salt to stimulate HLMC release further argues against the involvement of P2XR.
ATP-induced enhancement of IgE-mediated degranulation in LAD2 cells shows responses linked to P2Y11R activation. This conclusion was based on (i) the robust expression of P2Y11R, (ii) the lack of enhancing effect of ADP and UTP, which excluded P2Y1R, P2Y2R, P2Y4R, P2Y12R, and P2Y13R, (iii) strong enhancing effects of ATPγS, which acts as an agonist at the P2Y11R (145, 168, 169), (iv) the marked inhibition of ATPγS’ effects by NF157, which is a selective P2Y11R antagonist (148, 149), and (v) the fact that P2Y11R is coupled to both Gq/11 and Gs proteins (118), the former mediating enhancement of allergen-induced degranulation.
Gao et al. (60) reported that P2Y11R is the most robustly expressed among P2YRs in LAD2 cells, and P2Y11R agonists, including ATPγS, do not directly trigger degranulation. Gao et al. also investigated the role of P2YR in ATP’s enhancement of allergic degranulation in LAD2 cells. They failed to find an effect of ATPγS. However, their test dose was at 10 μM, whereas ours was 100 μM. In addition, those investigators studied the effects on maximal but not minimal antigen-induced degranulation; they also proposed that multiple P2Rs including UDP-glucose-sensitive P2Y14R may play a role in this action of ATP.
Intracellular signal transduction pathways
P2Y11R activation in LAD2 cells leads to activation of intracellular pathways involving PI3K/Akt (Figure 2). As previously reported, the PI3K/Akt pathway can operate without the induction of intracellular Ca2+ mobilization (170, 171). It is well known that IgE receptor (FcϵRI) activation itself, leading to degranulation, is associated with intracellular Ca2+ mobilization (172). Intracellular Ca2+-independent steps in MC degranulation have previously been reported (173–176). This suggests that the potentiating effect of extracellular ATP on anti-IgE–induced degranulation is due to a complex interaction between the two relevant signal-transduction pathways, rather than merely an increase in Ca2+ influx induced by ATP.
That PI3Kδ plays a critical role in ATP enhancement is shown by a dose-dependent inhibition of the ATPγS’ enhancing effects by compound 15e, a PI3K inhibitor (150, 151), and marked diminution of enhancing effects in PI3Kδ knockdown LAD2 cells (59). PDK-1 is a key element of the PI3K/Akt pathway (177–179), but the lack of PDK-1 phosphorylation does not support its involvement in ATPγS’ enhancement of WAS in LAD2 cells.
Modulation by ecto-enzymes
The enhancement of WAS by ATPγS in LAD2 cells was unrelated to its rate of breakdown by ectonucleotidases. However, expressions of multiple purinergic receptors, and/or up- or down-regulation of ectonucleotidases in vivo may affect cell responses to extracellular nucleotides. Experiments showed that LAD2 cells’ exposures to ATPγS did not affect extracellular concentrations of ATP for at least 60 minutes. However, under experimental conditions, ATP could be released from LAD2 cells.
We have previously reported that the half-life of extracellular ATP/ADP (1 mM) was 14.88 min in another human cell line expressing ectonucleotidases CD39, CD73, and alkaline phosphatase (180). Studies have emphasized the role of ectonucleotidases in the magnitude of ATP’s effects in pulmonary and other organ disorders (68, 181). Tsai and Takeda (160) have recently shown in mice that E-NPP3, an activation marker induced in IgE-mediated reactions, hydrolyzes extracellular ATP on basophil and MC surfaces to prevent ATP-dependent excess activation. In the absence of E-NPP3, basophils and MC become overactivated and the mice experience severe chronic allergic inflammation (160). This work suggests a potential therapeutic role for ATP hydrolysis strategies to control MC-mediated allergic responses. It increasingly appears that a complex of physiological factors interacts to either maintain or degrade extracellular ATP in vivo, depending on localized physiological conditions such as pH.
Concluding remarks
Extracellular ATP is a potent modulator of immune reaction-induced HLMC degranulation and thereby release of inflammatory mediators. Since extracellular ATP is rapidly degraded to ADP and adenosine by ecto-enzymes, and adenosine exerts its own effects on inflammatory cells, the variable levels of these enzymes under physiologic and pathophysiologic conditions are critical modulators of the effects of extracellular ATP and adenosine in this setting.
Although the exact mechanism of ATP’s effect on HLMC degranulation has not been delineated heretofore, the voluminous relevant data strongly suggest that extracellular ATP plays an important mechanistic role in HLMC allergic reactions. Thus, because of the exponential research work in this arena over the past two decades since the original hypothesis of the “ATP axis in the Lungs” was put forward (127), we are now on the cusp of developing novel therapeutic approaches for allergic disorders in general and asthma in particular. This view is supported by the recent developments in a parallel field of ATP-chronic cough, in which pre-clinical studies and clinical trials with P2X3R antagonists are being evaluated as novel anti-tussive agents (182).
The effects of ATP are most pronounced with a concurrent WAS. It is plausible that the cellular ATP environment may change over short periods (e.g., exercise, irritants, etc.), while antigen exposure may not vary and/or remain weak (e.g., dust, cat, mouse, and grass), yet a full-blown asthmatic exacerbation or urticarial episode may ensue. The identity of the receptor(s) mediating the effects of ATP on HMC and the ectoenzymes that hydrolyze ATP in different tissues must be further explored so that novel therapeutic approaches can be tested in the clinical setting.
Author contributions
The potential role of purine nucleotides in the function of human lung MCs under physiologic and pathophysiologic conditions was originally conceived by AP and ES. The HLMC experiments were supervised by ES. HN conducted all LAD2 experiments. ES wrote the first draft of the manuscript, and it was edited by HN and AP. All authors have read the final draft of the manuscript and approved its submission for publication.
Funding
This work was supported by the Margaret Wolf Research Endowment (ESS), the LIXIL Jyukankyo Foundation, and The Jikei University Research Fund (HN).
Acknowledgments
We thank Prof. Arnold Kirshenbaum (NIH) for supplying the LAD2 cells.
Conflict of interest
AP is the CEO and CSO of Danmir Therapeutics, LLC, a biopharmaceutical company that focuses on P2 receptors’ signal transductions.
The remaining authors declare that the research was conducted in the absence of any commercial or financial relationships that could be construed as a potential conflict of interest.
Publisher’s note
All claims expressed in this article are solely those of the authors and do not necessarily represent those of their affiliated organizations, or those of the publisher, the editors and the reviewers. Any product that may be evaluated in this article, or claim that may be made by its manufacturer, is not guaranteed or endorsed by the publisher.
Abbreviations
eATP, extracellular adenosine 5’-triphosphate; ATP; 2-MeSATP, 2-methylthio-ATP; ATPγS, adenosine 5’-(3-thio)triphosphate; HMC, human mast cell; HLMC, human lung mast cell; PDK-1, phosphoinositide-dependent kinase-1; PI3K(δ), phosphoinositide 3-kinase type δ; P2XR, purinergic receptor P2X ligand-gated ion channel; P2YR, metabotropic purinergic P2Y receptor; P2Y11R, metabotropic purinergic P2Y11 receptor; CD39, ectonucleoside triphosphate diphosphorylase-1; CD73, ecto-5’-nucleotidase; WAS, weak allergic stimulation.
References
1. Schulman ES, Samarasinghe A, Sporn P. Mast Cells and Eosinophils. In: Grippi M A-OD, Dela Cruz CS, Kotloff R, Kotton CN, Pack A, editors. Fishman's Pulmonary Diseases and Disorders, 6th ed. New York: McGraw-Hill Medical (2022). p. 28–50.
2. Blank U, Huang H, Kawakami T. The high affinity IgE receptor: a signaling update. Curr Opin Immunol (2021) 72:51–8. doi: 10.1016/j.coi.2021.03.015
3. Levi-Schaffer F, Gibbs BF, Hallgren J, Pucillo C, Redegeld F, Siebenhaar F, et al. Selected recent advances in understanding the role of human mast cells in health and disease. J Allergy Clin Immunol (2022) 149(6):1833–44. doi: 10.1016/j.jaci.2022.01.030
4. Schulman ES. The role of mast cells in inflammatory responses in the lung. Crit Rev Immunol (1993) 13(1):35–70.
5. Kirshenbaum AS, Akin C, Wu Y, Rottem M, Goff JP, Beaven MA, et al. Characterization of novel stem cell factor responsive human mast cell lines LAD 1 and 2 established from a patient with mast cell sarcoma/leukemia; activation following aggregation of FcepsilonRI or FcgammaRI. Leuk Res (2003) 27(8):677–82. doi: 10.1016/S0145-2126(02)00343-0
6. Kirshenbaum AS, Petrik A, Walsh R, Kirby TL, Vepa S, Wangsa D, et al. A ten-year retrospective analysis of the distribution, use and phenotypic characteristics of the LAD2 human mast cell line. Int Arch Allergy Immunol (2014) 164(4):265–70. doi: 10.1159/000365729
7. Kirshenbaum AS, Yin Y, Sundstrom JB, Bandara G, Metcalfe DD. Description and characterization of a novel human mast cell line for scientific study. Int J Mol Sci (2019) 20(22). doi: 10.3390/ijms20225520
8. Valent P, Akin C, Hartmann K, Nilsson G, Reiter A, Hermine O, et al. Mast cells as a unique hematopoietic lineage and cell system: From Paul Ehrlich's visions to precision medicine concepts. Theranostics (2020) 10(23):10743–68. doi: 10.7150/thno.46719
9. Bandara G, Metcalfe DD, Kirshenbaum AS. Growth of human mast cells from bone marrow and peripheral blood-derived CD34(+) pluripotent hematopoietic cells. Methods Mol Biol (2015) 1220:155–62. doi: 10.1007/978-1-4939-1568-2_10
10. Kirshenbaum AS, Metcalfe DD. Growth of human mast cells from bone marrow and peripheral blood-derived CD34+ pluripotent progenitor cells. Methods Mol Biol (2006) 315:105–12. doi: 10.1385/1-59259-967-2:105
11. Shimizu Y, Sakai K, Miura T, Narita T, Tsukagoshi H, Satoh Y, et al. Characterization of 'adult-type' mast cells derived from human bone marrow CD34(+) cells cultured in the presence of stem cell factor and interleukin-6. Interleukin-4 is not required for constitutive expression of CD54, Fc epsilon RI alpha and chymase, and CD13 expression is reduced during differentiation. Clin Exp Allergy (2002) 32(6):872–80. doi: 10.1046/j.1365-2222.2002.01373.x
12. Kambe M, Kambe N, Oskeritzian CA, Schechter N, Schwartz LB. IL-6 attenuates apoptosis, while neither IL-6 nor IL-10 affect the numbers or protease phenotype of fetal liver-derived human mast cells. Clin Exp Allergy (2001) 31(7):1077–85. doi: 10.1046/j.1365-2222.2001.01126.x
13. Oskeritzian CA, Zhao W, Pozez AL, Cohen NM, Grimes M, Schwartz LB. Neutralizing endogenous IL-6 renders mast cells of the MCT type from lung, but not the MCTC type from skin and lung, susceptible to human recombinant IL-4-induced apoptosis. J Immunol (2004) 172(1):593–600. doi: 10.4049/jimmunol.172.1.593
14. Igarashi Y, Kurosawa M, Ishikawa O, Miyachi Y, Saito H, Ebisawa M, et al. Characteristics of histamine release from cultured human mast cells. Clin Exp Allergy (1996) 26(5):597–602. doi: 10.1111/j.1365-2222.1996.tb00582.x
15. Inomata N, Tomita H, Ikezawa Z, Saito H. Differential gene expression profile between cord blood progenitor-derived and adult progenitor-derived human mast cells. Immunol Lett (2005) 98(2):265–71. doi: 10.1016/j.imlet.2004.12.001
16. Okayama Y, Okumura S, Sagara H, Yuki K, Sasaki T, Watanabe N, et al. FcepsilonRI-mediated thymic stromal lymphopoietin production by interleukin-4-primed human mast cells. Eur Respir J (2009) 34(2):425–35. doi: 10.1183/09031936.00121008
17. Saito H, Ebisawa M, Sakaguchi N, Onda T, Iikura Y, Yanagida M, et al. Characterization of cord-blood-derived human mast cells cultured in the presence of Steel factor and interleukin-6. Int Arch Allergy Immunol (1995) 107(1-3):63–5. doi: 10.1159/000236932
18. Saito H, Kato A, Matsumoto K, Okayama Y. Culture of human mast cells from peripheral blood progenitors. Nat Protoc (2006) 1(4):2178–83. doi: 10.1038/nprot.2006.344
19. Yanagida M, Fukamachi H, Takei M, Hagiwara T, Uzumaki H, Tokiwa T, et al. Interferon-gamma promotes the survival and Fc epsilon RI-mediated histamine release in cultured human mast cells. Immunology (1996) 89(4):547–52. doi: 10.1046/j.1365-2567.1996.d01-768.x
20. Ravindran A, Rönnberg E, Dahlin JS, Mazzurana L, Säfholm J, Orre AC, et al. An optimized protocol for the isolation and functional analysis of human lung mast cells. Front Immunol (2018) 9:2193. doi: 10.3389/fimmu.2018.02193
21. Andersen HB, Holm M, Hetland TE, Dahl C, Junker S, Schiøtz PO, et al. Comparison of short term in vitro cultured human mast cells from different progenitors - Peripheral blood-derived progenitors generate highly mature and functional mast cells. J Immunol Methods (2008) 336(2):166–74. doi: 10.1016/j.jim.2008.04.016
22. Derakhshan T, Bhowmick R, Ritchey JW, Gappa-Fahlenkamp H. Development of human mast cells from hematopoietic stem cells within a 3D collagen matrix: effect of stem cell media on mast cell generation. Stem Cells Int (2018) 2018:2136193. doi: 10.1155/2018/2136193
23. Bodin P, Burnstock G. Purinergic signalling: ATP release. Neurochem Res (2001) 26(8-9):959–69. doi: 10.1023/A:1012388618693
24. Falduto GH, Pfeiffer A, Luker A, Metcalfe DD, Olivera A. Emerging mechanisms contributing to mast cell-mediated pathophysiology with therapeutic implications. Pharmacol Ther (2021) 220:107718. doi: 10.1016/j.pharmthera.2020.107718
25. West PW, Bulfone-Paus S. Mast cell tissue heterogeneity and specificity of immune cell recruitment. Front Immunol (2022) 13:932090. doi: 10.3389/fimmu.2022.932090
26. Frossi B, Mion F, Sibilano R, Danelli L, Pucillo CEM. Is it time for a new classification of mast cells? What do we know about mast cell heterogeneity? Immunol Rev (2018) 282(1):35–46. doi: 10.1111/imr.12636
27. Schulman ES, MacGlashan DW Jr., Peters SP, Schleimer RP, Newball HH, Lichtenstein LM. Human lung mast cells: purification and characterization. J Immunol (1982) 129(6):2662–7. doi: 10.4049/jimmunol.129.6.2662
28. Dvorak AM, Galli SJ, Schulman ES, Lichtenstein LM, Dvorak HF. Basophil and mast cell degranulation: ultrastructural analysis of mechanisms of mediator release. Fed Proc (1983) 42(8):2510–5.
29. Dvorak AM, Hammel I, Schulman ES, Peters SP, MacGlashan DW Jr., Schleimer RP, et al. Differences in the behavior of cytoplasmic granules and lipid bodies during human lung mast cell degranulation. J Cell Biol (1984) 99(5):1678–87. doi: 10.1083/jcb.99.5.1678
30. Dvorak AM, Schleimer RP, Schulman ES, Lichtenstein LM. Human mast cells use conservation and condensation mechanisms during recovery from degranulation. In vitro studies with mast cells purified from human lungs. Lab Invest (1986) 54(6):663–78.
31. Dvorak AM, Schulman ES, Peters SP, MacGlashan DW Jr., Newball HH, Schleimer RP, et al. Immunoglobulin E-mediated degranulation of isolated human lung mast cells. Lab Invest (1985) 53(1):45–56.
32. Hammel I, Dvorak AM, Peters SP, Schulman ES, Dvorak HF, Lichtenstein LM, et al. Differences in the volume distributions of human lung mast cell granules and lipid bodies: evidence that the size of these organelles is regulated by distinct mechanisms. J Cell Biol (1985) 100(5):1488–92. doi: 10.1083/jcb.100.5.1488
33. Schulman ES, Kagey-Sobotka A, MacGlashan DW Jr., Adkinson NF Jr., Peters SP, Schleimer RP, et al. Heterogeneity of human mast cells. J Immunol (1983) 131(4):1936–41. doi: 10.4049/jimmunol.131.4.1936
34. Schulman ES, Pollack RB, Post TJ, Peters SP. Histochemical heterogeneity of dispersed human lung mast cells. J Immunol (1990) 144(11):4195–201. doi: 10.4049/jimmunol.144.11.4195
35. Schulman ES, Post TJ, Vigderman RJ. Density heterogeneity of human lung mast cells. J Allergy Clin Immunol (1988) 82(1):78–86. doi: 10.1016/0091-6749(88)90055-3
36. Ishizaka T, Conrad DH, Schulman ES, Sterk AR, Ishizaka K. Biochemical analysis of initial triggering events of IgE-mediated histamine release from human lung mast cells. J Immunol (1983) 130(5):2357–62. doi: 10.4049/jimmunol.130.5.2357
37. Ishizaka T, Conrad DH, Schulman ES, Sterk AR, Ko CG, Ishizaka K. IgE-mediated triggering signals for mediator release from human mast cells and basophils. Fed Proc (1984) 43(13):2840–5.
38. Gonen B, O'Donnell P, Post TJ, Quinn TJ, Schulman ES. Very low density lipoproteins (VLDL) trigger the release of histamine from human basophils. Biochim Biophys Acta (1987) 917(3):418–24. doi: 10.1016/0005-2760(87)90121-4
39. Kelly SJ, Uri AJ, Freeland HS, Woods EJ, Schulman ES, Peters SP, et al. Effects of colchicine on IgE-mediated early and late airway reactions. Chest (1995) 107(4):985–91. doi: 10.1378/chest.107.4.985
40. MacGlashan DW Jr., Schleimer RP, Peters SP, Schulman ES, Adams GK, Sobotka AK, et al. Comparative studies of human basophils and mast cells. Fed Proc (1983) 42(8):2504–9.
41. Peters SP, Schulman ES, Schleimer RP, MacGlashan DW Jr., Newball HH, Lichtenstein LM. Dispersed human lung mast cells. Pharmacologic aspects and comparison with human lung tissue fragments. Am Rev Respir Dis (1982) 126(6):1034–9. doi: 10.1164/arrd.1982.126.6.1034
42. Schleimer RP, MacGlashan DW Jr., Schulman ES, Peters SP, Adams GK 3rd, Adkinson NF Jr., et al. Human mast cells and basophils–structure, function, pharmacology, and biochemistry. Clin Rev Allergy (1983) 1(3):327–41. doi: 10.1007/BF02991224
43. Schulman ES, Liu MC, Proud D, MacGlashan DW Jr., Lichtenstein LM, Plaut M. Human lung macrophages induce histamine release from basophils and mast cells. Am Rev Respir Dis (1985) 131(2):230–5. doi: 10.1164/arrd.1985.131.2.230
44. Schulman ES, Post TJ, Henson PM, Giclas PC. Differential effects of the complement peptides, C5a and C5a des Arg on human basophil and lung mast cell histamine release. J Clin Invest (1988) 81(3):918–23. doi: 10.1172/JCI113403
45. Schulman ES, Quinn TJ, Post TJ, O'Donnell P, Rodriguez A, Gonen B. Low density lipoprotein (LDL) inhibits histamine release from human mast cells. Biochem Biophys Res Commun (1987) 148(2):553–9. doi: 10.1016/0006-291X(87)90912-0
46. Glaum MC, Jaffe JS, Gillespie DH, Raible DG, Post TJ, Wang Y, et al. IgE-dependent expression of interleukin-5 mRNA and protein in human lung: modulation by dexamethasone. Clin Immunol Immunopathol (1995) 75(2):171–8. doi: 10.1006/clin.1995.1068
47. Jaffe JS, Glaum MC, Raible DG, Post TJ, Dimitry E, Govindarao D, et al. Human lung mast cell IL-5 gene and protein expression: temporal analysis of upregulation following IgE-mediated activation. Am J Respir Cell Mol Biol (1995) 13(6):665–75. doi: 10.1165/ajrcmb.13.6.7576704
48. Jaffe JS, Raible DG, Post TJ, Wang Y, Glaum MC, Butterfield JH, et al. Human lung mast cell activation leads to IL-13 mRNA expression and protein release. Am J Respir Cell Mol Biol (1996) 15(4):473–81. doi: 10.1165/ajrcmb.15.4.8879181
49. MacGlashan DW Jr., Schleimer RP, Peters SP, Schulman ES, Adams GK 3rd, Newball HH, et al. Generation of leukotrienes by purified human lung mast cells. J Clin Invest (1982) 70(4):747–51. doi: 10.1172/JCI110670
50. Meier HL, Heck LW, Schulman ES, MacGlashan DW Jr. Purified human mast cells and basophils release human elastase and cathepsin G by an IgE-mediated mechanism. Int Arch Allergy Appl Immunol (1985) 77(1-2):179–83. doi: 10.1159/000233779
51. Meier HL, Schulman ES, Heck LW, MacGlashan D, Newball HH, Kaplan AP. Release of elastase from purified human lung mast cells and basophils. Identification as a Hageman factor cleaving enzyme. Inflammation (1989) 13(3):295–308. doi: 10.1007/BF00914396
52. Peters SP, MacGlashan DW Jr., Schulman ES, Schleimer RP, Hayes EC, Rokach J, et al. Arachidonic acid metabolism in purified human lung mast cells. J Immunol (1984) 132(4):1972–9. doi: 10.4049/jimmunol.132.4.1972
53. Peters SP, Schulman ES, Liu MC, Hayes EC, Lichtenstein LM. Separation of major prostaglandins, leukotrienes, and monoHETEs by high performance liquid chromatography. J Immunol Methods (1983) 64(3):335–43. doi: 10.1016/0022-1759(83)90441-6
54. Proud D, MacGlashan DW Jr., Newball HH, Schulman ES, Lichtenstein LM. Immunoglobulin E-mediated release of a kininogenase from purified human lung mast cells. Am Rev Respir Dis (1985) 132(2):405–8. doi: 10.1164/arrd.1985.132.2.405
55. Thompson HL, Schulman ES, Metcalfe DD. Identification of chondroitin sulfate E in human lung mast cells. J Immunol (1988) 140(8):2708–13. doi: 10.4049/jimmunol.140.8.2708
56. Schleimer RP, Schulman ES, MacGlashan DW Jr., Peters SP, Hayes EC, Adams GK 3rd, et al. Effects of dexamethasone on mediator release from human lung fragments and purified human lung mast cells. J Clin Invest (1983) 71(6):1830–5. doi: 10.1172/JCI110938
57. Nishi H, Pelleg A, Schulman ES. IgE receptor-mediated histamine release in human lung mast cells: modulation by purinergic receptor ligands. Ann Clin Lab Sci (2016) 46(5):463–9.
58. Schulman ES, Glaum MC, Post T, Wang Y, Raible DG, Mohanty J, et al. ATP modulates anti-IgE-induced release of histamine from human lung mast cells. Am J Respir Cell Mol Biol (1999) 20(3):530–7. doi: 10.1165/ajrcmb.20.3.3387
59. Nishi H, Niyonsaba F, Pelleg A, Schulman ES. Enhancement of mast cell degranulation mediated by purinergic receptors' Activation and PI3K type delta. J Immunol (2021) 207(4):1001–8. doi: 10.4049/jimmunol.2001002
60. Gao ZG, Wei Q, Jayasekara MP, Jacobson KA. The role of P2Y(14) and other P2Y receptors in degranulation of human LAD2 mast cells. Purinergic Signal (2013) 9(1):31–40. doi: 10.1007/s11302-012-9325-4
61. Pelleg A. Extracellular adenosine 5'-triphosphate in pulmonary disorders. Biochem Pharmacol (2021) 187:114319. doi: 10.1016/j.bcp.2020.114319
62. Pelleg A, Schulman ES, Barnes PJ. Extracellular adenosine 5'-triphosphate in obstructive airway diseases. Chest (2016) 150(4):908–15. doi: 10.1016/j.chest.2016.06.045
63. Pelleg A, Schulman ES, Barnes PJ. Adenosine 5'-triphosphate's role in bradycardia and syncope associated with pulmonary embolism. Respir Res (2018) 19(1):142. doi: 10.1186/s12931-018-0848-2
64. Pelleg A, Sirtori E, Rolland J-F, Mahadevan A. DT-0111: a novel P2X3 receptor antagonist. Purinergic Signalling (2023) 19(3):469–79. doi: 10.1007/s11302-023-09930-5
65. Pelleg A, Xu F, Zhuang J, Undem B, Burnstock G. DT-0111: a novel drug-candidate for the treatment of COPD and chronic cough. Ther Adv Respir Dis (2019) 13:1753466619877960. doi: 10.1177/1753466619877960
66. Lazarowski ER, Boucher RC. Purinergic receptors in airway hydration. Biochem Pharmacol (2021) 187:114387. doi: 10.1016/j.bcp.2020.114387
67. Okada SF, Ribeiro CM, Sesma JI, Seminario-Vidal L, Abdullah LH, van Heusden C, et al. Inflammation promotes airway epithelial ATP release via calcium-dependent vesicular pathways. Am J Respir Cell Mol Biol (2013) 49(5):814–20. doi: 10.1165/rcmb.2012-0493OC
68. van Heusden C, Button B, Anderson WH, Ceppe A, Morton LC, O'Neal WK, et al. Inhibition of ATP hydrolysis restores airway surface liquid production in cystic fibrosis airway epithelia. Am J Physiol Lung Cell Mol Physiol (2020) 318(2):L356–l65. doi: 10.1152/ajplung.00449.2019
69. Nirody JA, Budin I, Rangamani P. ATP synthase: Evolution, energetics, and membrane interactions. J Gen Physiol (2020) 152(11). doi: 10.1085/jgp.201912475
70. Trautmann A. Extracellular ATP in the immune system: more than just a "danger signal". Sci Signal (2009) 2(56):pe6. doi: 10.1126/scisignal.256pe6
71. Drury AN, Szent-Györgyi A. The physiological activity of adenine compounds with especial reference to their action upon the mammalian heart. J Physiol (1929) 68(3):213–37. doi: 10.1113/jphysiol.1929.sp002608
72. Hopkins SV. The action of ATP in the Guinea-pig heart. Biochem Pharmacol (1973) 22(3):335–9. doi: 10.1016/0006-2952(73)90414-0
73. Schrader J, Gerlach E. Compartmentation of cardiac adenine nucleotides and formation of adenosine. Pflugers Arch (1976) 367(2):129–35. doi: 10.1007/BF00585148
74. Cheek DJ, McHugh JM, Blood-Siegfried J, McFetridge JF, Turner BS. A historical perspective on the discovery of adenyl purines. Biol Res Nurs (2000) 1(4):265–75. doi: 10.1177/109980040000100403
76. Vultaggio-Poma V, Sarti AC, Di Virgilio F. Extracellular ATP: A feasible target for cancer therapy. Cells (2020) 9(11). doi: 10.3390/cells9112496
77. Bodin P, Bailey D, Burnstock G. Increased flow-induced ATP release from isolated vascular endothelial cells but not smooth muscle cells. Br J Pharmacol (1991) 103(1):1203–5. doi: 10.1111/j.1476-5381.1991.tb12324.x
78. Mills DC, Robb IA, Roberts GC. The release of nucleotides, 5-hydroxytryptamine and enzymes from human blood platelets during aggregation. J Physiol (1968) 195(3):715–29. doi: 10.1113/jphysiol.1968.sp008484
79. Day HJ, Holmsen H. Concepts of the blood platelet release reaction. Ser Haematol (1971) 4(1):3–27.
81. Dean BM, Perrett D. Studies on adenine and adenosine metabolism by intact human erythrocytes using high performance liquid chromatography. Biochim Biophys Acta (1976) 437(1):1–5. doi: 10.1016/0304-4165(76)90342-1
82. Bergfeld GR, Forrester T. Release of ATP from human erythrocytes in response to a brief period of hypoxia and hypercapnia. Cardiovasc Res (1992) 26(1):40–7. doi: 10.1093/cvr/26.1.40
83. Ellsworth ML, Forrester T, Ellis CG, Dietrich HH. The erythrocyte as a regulator of vascular tone. Am J Physiol (1995) 269(6 Pt 2):H2155–61. doi: 10.1152/ajpheart.1995.269.6.H2155
84. Zhang H, Shen Z, Hogan B, Barakat AI, Misbah C. ATP release by red blood cells under flow: model and simulations. Biophys J (2018) 115(11):2218–29. doi: 10.1016/j.bpj.2018.09.033
85. Pearson JD, Gordon JL. Vascular endothelial and smooth muscle cells in culture selectively release adenine nucleotides. Nature (1979) 281(5730):384–6. doi: 10.1038/281384a0
86. Ralevic V, Milner P, Kirkpatrick KA, Burnstock G. Flow-induced release of adenosine 5'-triphosphate from endothelial cells of the rat mesenteric arterial bed. Experientia (1992) 48(1):31–4. doi: 10.1007/BF01923600
87. Yang S, Cheek DJ, Westfall DP, Buxton IL. Purinergic axis in cardiac blood vessels. Agonist-mediated release of ATP from cardiac endothelial cells. Circ Res (1994) 74(3):401–7. doi: 10.1161/01.res.74.3.401
88. Bodin P, Burnstock G. ATP-stimulated release of ATP by human endothelial cells. J Cardiovasc Pharmacol (1996) 27(6):872–5. doi: 10.1097/00005344-199606000-00015
89. Katsuragi T, Tokunaga T, Ogawa S, Soejima O, Sato C, Furukawa T. Existence of ATP-evoked ATP release system in smooth muscles. J Pharmacol Exp Ther (1991) 259(2):513–8.
90. Katsuragi T, Soejima O, Tokunaga T, Furukawa T. Evidence for postjunctional release of ATP evoked by stimulation of muscarinic receptors in ileal longitudinal muscles of Guinea pig. J Pharmacol Exp Ther (1992) 260(3):1309–13.
91. Burnstock G, Boeynaems JM. Purinergic signalling and immune cells. Purinergic Signal (2014) 10(4):529–64. doi: 10.1007/s11302-014-9427-2
92. Junger WG. Immune cell regulation by autocrine purinergic signalling. Nat Rev Immunol (2011) 11(3):201–12. doi: 10.1038/nri2938
93. Di Virgilio F, Vuerich M. Purinergic signaling in the immune system. Auton Neurosci (2015) 191:117–23. doi: 10.1016/j.autneu.2015.04.011
94. Gov NS, Safran SA. Red blood cell membrane fluctuations and shape controlled by ATP-induced cytoskeletal defects. Biophys J (2005) 88(3):1859–74. doi: 10.1529/biophysj.104.045328
95. Begandt D, Good ME, Keller AS, DeLalio LJ, Rowley C, Isakson BE, et al. Pannexin channel and connexin hemichannel expression in vascular function and inflammation. BMC Cell Biol (2017) 18(Suppl 1):2. doi: 10.1186/s12860-016-0119-3
96. Forrester T. An estimate of adenosine triphosphate release into the venous effluent from exercising human forearm muscle. J Physiol (1972) 224(3):611–28. doi: 10.1113/jphysiol.1972.sp009915
97. Fredholm BB, Hedqvist P, Lindström K, Wennmalm M. Release of nucleosides and nucleotides from the rabbit heart by sympathetic nerve stimulation. Acta Physiol Scand (1982) 116(3):285–95. doi: 10.1111/j.1748-1716.1982.tb07142.x
98. Darius H, Stahl GL, Lefer AM. Pharmacologic modulation of ATP release from isolated rat hearts in response to vasoconstrictor stimuli using a continuous flow technique. J Pharmacol Exp Ther (1987) 240(2):542–7.
99. Katsuragi T, Tokunaga T, Ohba M, Sato C, Furukawa T. Implication of ATP released from atrial, but not papillary, muscle segments of Guinea pig by isoproterenol and forskolin. Life Sci (1993) 53(11):961–7. doi: 10.1016/0024-3205(93)90449-D
100. Tokunaga T, Katsuragi T, Sato C, Furukawa T. ATP release evoked by isoprenaline from adrenergic nerves of Guinea pig atrium. Neurosci Lett (1995) 186(2-3):95–8. doi: 10.1016/0304-3940(95)11290-D
101. Pelleg A, Katchanov G, Xu J. Purinergic modulation of neural control of cardiac function. J Auton Pharmacol (1996) 16(6):401–5. doi: 10.1111/j.1474-8673.1996.tb00063.x
102. Paddle BM, Burnstock G. Release of ATP from perfused heart during coronary vasodilatation. Blood Vessels (1974) 11(3):110–9. doi: 10.1159/000158005
103. Forrester T, Williams CA. Release of adenosine triphosphate from isolated adult heart cells in response to hypoxia. J Physiol (1977) 268(2):371–90. doi: 10.1113/jphysiol.1977.sp011862
104. Williams CA, Forrester T. Possible source of adenosine triphosphate released from rat myocytes in response to hypoxia and acidosis. Cardiovasc Res (1983) 17(5):301–12. doi: 10.1093/cvr/17.5.301
105. Baxter M, Eltom S, Dekkak B, Yew-Booth L, Dubuis ED, Maher SA, et al. Role of transient receptor potential and pannexin channels in cigarette smoke-triggered ATP release in the lung. Thorax (2014) 69(12):1080–9. doi: 10.1136/thoraxjnl-2014-205467
106. Bucchioni E, Csoma Z, Polkey M, Collins JV, Cramer D, Allegra L, et al. Adenosine 50-triphosphate (ATP) is increased in exhaled breath condensate in COPD. Am J Respir Crit Care Med (2002) 165:A599.
107. Mortaz E, Braber S, Nazary M, Givi ME, Nijkamp FP, Folkerts G. ATP in the pathogenesis of lung emphysema. Eur J Pharmacol (2009) 619(1-3):92–6. doi: 10.1016/j.ejphar.2009.07.022
108. Cicko S, Lucattelli M, Müller T, Lommatzsch M, De Cunto G, Cardini S, et al. Purinergic receptor inhibition prevents the development of smoke-induced lung injury and emphysema. J Immunol (2010) 185(1):688–97. doi: 10.4049/jimmunol.0904042
109. Jacobson KA, Pradhan B, Wen Z, Pramanik A. New paradigms in purinergic receptor ligand discovery. Neuropharmacology (2023) 230:109503. doi: 10.1016/j.neuropharm.2023.109503
110. Colgan SP, Eltzschig HK, Eckle T, Thompson LF. Physiological roles for ecto-5'-nucleotidase (CD73). Purinergic Signal (2006) 2(2):351–60. doi: 10.1007/s11302-005-5302-5
111. Knapp K, Zebisch M, Pippel J, El-Tayeb A, Müller CE, Sträter N. Crystal structure of the human ecto-5'-nucleotidase (CD73): insights into the regulation of purinergic signaling. Structure (2012) 20(12):2161–73. doi: 10.1016/j.str.2012.10.001
112. Schetinger MR, Morsch VM, Bonan CD, Wyse AT. NTPDase and 5'-nucleotidase activities in physiological and disease conditions: new perspectives for human health. Biofactors (2007) 31(2):77–98. doi: 10.1002/biof.5520310205
113. Zhao H, Bo C, Kang Y, Li H. What else can CD39 tell us? Front Immunol (2017) 8. doi: 10.3389/fimmu.2017.00727
114. Antonioli L, Pacher P, Vizi ES, Haskó G. CD39 and CD73 in immunity and inflammation. Trends Mol Med (2013) 19(6):355–67. doi: 10.1016/j.molmed.2013.03.005
115. Timperi E, Barnaba V. CD39 regulation and functions in T cells. Int J Mol Sci (2021) 22(15). doi: 10.3390/ijms22158068
116. Eckle T, Füllbier L, Wehrmann M, Khoury J, Mittelbronn M, Ibla J, et al. Identification of ectonucleotidases CD39 and CD73 in innate protection during acute lung injury. J Immunol (2007) 178(12):8127–37. doi: 10.4049/jimmunol.178.12.8127
117. Li J, Wang L, Chen X, Li L, Li Y, Ping Y, et al. CD39/CD73 upregulation on myeloid-derived suppressor cells via TGF-β-mTOR-HIF-1 signaling in patients with non-small cell lung cancer. Oncoimmunology (2017) 6(6):e1320011. doi: 10.1080/2162402X.2017.1320011
118. Burnstock G, Brouns I, Adriaensen D, Timmermans JP. Purinergic signaling in the airways. Pharmacol Rev (2012) 64(4):834–68. doi: 10.1124/pr.111.005389
119. Saul A, Hausmann R, Kless A, Nicke A. Heteromeric assembly of P2X subunits. Front Cell Neurosci (2013) 7:250. doi: 10.3389/fncel.2013.00250
120. Abbracchio MP, Burnstock G, Boeynaems JM, Barnard EA, Boyer JL, Kennedy C, et al. International Union of Pharmacology LVIII: update on the P2Y G protein-coupled nucleotide receptors: from molecular mechanisms and pathophysiology to therapy. Pharmacol Rev (2006) 58(3):281–341. doi: 10.1124/pr.58.3.3
121. Waldo GL, Harden TK. Agonist binding and Gq-stimulating activities of the purified human P2Y1 receptor. Mol Pharmacol (2004) 65(2):426–36. doi: 10.1124/mol.65.2.426
122. Alexander SPH, Christopoulos A, Davenport AP, Kelly E, Mathie A, Peters JA, et al. THE CONCISE GUIDE TO PHARMACOLOGY 2019/20: G protein-coupled receptors. Br J Pharmacol (2019) 176 Suppl 1(Suppl 1):S21–s141. doi: 10.1111/bph.14748
123. Burnstock G. Introduction and perspective, historical note. Front Cell Neurosci (2013) 7:227. doi: 10.3389/fncel.2013.00227
124. Pellegrino R, Wilson O, Jenouri G, Rodarte JR. Lung mechanics during induced bronchoconstriction. J Appl Physiol (1985) (1996) 81(2):964–75. doi: 10.1152/jappl.1996.81.2.964
125. Pelleg A, Hurt CM. Mechanism of action of ATP on canine pulmonary vagal C fibre nerve terminals. J Physiol (1996) 490(Pt 1):265–75. doi: 10.1113/jphysiol.1996.sp021142
126. Katchanov G, Xu J, Schulman ES, Pelleg A. ATP causes neurogenic bronchoconstriction in the dog. Drug Dev Res (1998) 45(3-4):342–9. doi: 10.1002/(SICI)1098-2299(199811/12)45:3/4<342::AID-DDR34>3.0.CO;2-P
127. Pelleg A, Schulman ES. Adenosine 5'-triphosphate axis in obstructive airway diseases. Am J Ther (2002) 9(5):454–64. doi: 10.1097/00045391-200209000-00014
128. Arzola-Martínez L, Benavente R, Vega G, Ríos M, Fonseca W, Rasky AJ, et al. Blocking ATP-releasing channels prevents high extracellular ATP levels and airway hyperreactivity in an asthmatic mouse model. Am J Physiol Lung Cell Mol Physiol (2021) 321(2):L466–l76. doi: 10.1152/ajplung.00450.2020
129. Straus DB, Pryor D, Haque TT, Kee SA, Dailey JM, Jackson KG, et al. IL-33 priming amplifies ATP-mediated mast cell cytokine production. Cell Immunol (2022) 371:104470. doi: 10.1016/j.cellimm.2021.104470
130. Bennett JP, Cockcroft S, Gomperts BD. Rat mast cells permeabilized with ATP secrete histamine in response to calcium ions buffered in the micromolar range. J Physiol (1981) 317:335–45. doi: 10.1113/jphysiol.1981.sp013828
131. Osipchuk Y, Cahalan M. Cell-to-cell spread of calcium signals mediated by ATP receptors in mast cells. Nature (1992) 359(6392):241–4. doi: 10.1038/359241a0
132. Tatham PE, Cusack NJ, Gomperts BD. Characterisation of the ATP4- receptor that mediates permeabilisation of rat mast cells. Eur J Pharmacol (1988) 147(1):13–21. doi: 10.1016/0014-2999(88)90628-0
133. Ye F, Lv J, Shen X, Zhang J, Zong Y, Zhu C, et al. Rutin ameliorates inflammatory pain by inhibiting P2X7 receptor in mast cells. J Physiol Biochem (2022) 79(2):287–95. doi: 10.1007/s13105-022-00938-w
134. Peachell PT, Columbo M, Kagey-Sobotka A, Lichtenstein LM, Marone G. Adenosine potentiates mediator release from human lung mast cells. Am Rev Respir Dis (1988) 138(5):1143–51. doi: 10.1164/ajrccm/138.5.1143
135. Hughes PJ, Holgate ST, Church MK. Adenosine inhibits and potentiates IgE-dependent histamine release from human lung mast cells by an A2-purinoceptor mediated mechanism. Biochem Pharmacol (1984) 33(23):3847–52. doi: 10.1016/0006-2952(84)90050-9
136. Abbracchio MP, Burnstock G. Purinoceptors: are there families of P2X and P2Y purinoceptors? Pharmacol Ther (1994) 64(3):445–75. doi: 10.1016/0163-7258(94)00048-4
137. Lambrecht G, Friebe T, Grimm U, Windscheif U, Bungardt E, Hildebrandt C, et al. PPADS, a novel functionally selective antagonist of P2 purinoceptor-mediated responses. Eur J Pharmacol (1992) 217(2-3):217–9. doi: 10.1016/0014-2999(92)90877-7
138. Di Virgilio F, Ferrari D, Falzoni S, Chiozzi P, Munerati M, Steinberg TH, et al. P2 purinoceptors in the immune system. Ciba Found Symp (1996) 198:290–302; discussion -5. doi: 10.1002/9780470514900.ch17
139. Dasari VR, Jin J, Kunapuli SP. Distribution of leukotriene B4 receptors in human hematopoietic cells. Immunopharmacology (2000) 48(2):157–63. doi: 10.1016/S0162-3109(00)00201-0
140. Feng C, Mery AG, Beller EM, Favot C, Boyce JA. Adenine nucleotides inhibit cytokine generation by human mast cells through a Gs-coupled receptor. J Immunol (2004) 173(12):7539–47. doi: 10.4049/jimmunol.173.12.7539
141. Wareham K, Vial C, Wykes RC, Bradding P, Seward EP. Functional evidence for the expression of P2X1, P2X4 and P2X7 receptors in human lung mast cells. Br J Pharmacol (2009) 157(7):1215–24. doi: 10.1111/j.1476-5381.2009.00287.x
142. von Kügelgen I. Molecular pharmacology of P2Y receptor subtypes. Biochem Pharmacol (2021) 187:114361. doi: 10.1016/j.bcp.2020.114361
143. Mellor EA, Maekawa A, Austen KF, Boyce JA. Cysteinyl leukotriene receptor 1 is also a pyrimidinergic receptor and is expressed by human mast cells. Proc Natl Acad Sci U S A. (2001) 98(14):7964–9. doi: 10.1073/pnas.141221498
144. Communi D, Janssens R, Robaye B, Zeelis N, Boeynaems JM. Rapid up-regulation of P2Y messengers during granulocytic differentiation of HL-60 cells. FEBS Lett (2000) 475(1):39–42. doi: 10.1016/S0014-5793(00)01618-5
145. Communi D, Robaye B, Boeynaems JM. Pharmacological characterization of the human P2Y11 receptor. Br J Pharmacol (1999) 128(6):1199–206. doi: 10.1038/sj.bjp.0702909
146. Inoue K, Hosoi J, Denda M. Extracellular ATP has stimulatory effects on the expression and release of IL-6 via purinergic receptors in normal human epidermal keratinocytes. J Invest Dermatol (2007) 127(2):362–71. doi: 10.1038/sj.jid.5700526
147. van der Weyden L, Adams DJ, Luttrell BM, Conigrave AD, Morris MB. Pharmacological characterisation of the P2Y11 receptor in stably transfected haematological cell lines. Mol Cell Biochem (2000) 213(1-2):75–81. doi: 10.1023/A:1007168215748
148. Kuang Y, Liu H, Guo S, Wang Y, Zhang H, Qiao Y. The antagonist of P2Y11 receptor NF157 ameliorates oxidized LDL-induced vascular endothelial inflammation. Artif Cells Nanomed Biotechnol (2019) 47(1):1839–45. doi: 10.1080/21691401.2019.1610412
149. Ullmann H, Meis S, Hongwiset D, Marzian C, Wiese M, Nickel P, et al. Synthesis and structure-activity relationships of suramin-derived P2Y11 receptor antagonists with nanomolar potency. J Med Chem (2005) 48(22):7040–8. doi: 10.1021/jm050301p
150. Bekhite MM, Finkensieper A, Binas S, Müller J, Wetzker R, Figulla HR, et al. VEGF-mediated PI3K class IA and PKC signaling in cardiomyogenesis and vasculogenesis of mouse embryonic stem cells. J Cell Sci (2011) 124(Pt 11):1819–30. doi: 10.1242/jcs.077594
151. Pritchard RA, Falk L, Larsson M, Leinders M, Sorkin LS. Different phosphoinositide 3-kinase isoforms mediate carrageenan nociception and inflammation. Pain (2016) 157(1):137–46. doi: 10.1097/j.pain.0000000000000341
152. Antonioli L, Fornai M, Blandizzi C, Pacher P, Haskó G. Adenosine signaling and the immune system: When a lot could be too much. Immunol Letters (2019) 205:9–15. doi: 10.1016/j.imlet.2018.04.006
153. Church MK, Holgate ST, Hughes PJ. Adenosine inhibits and potentiates IgE-dependent histamine release from human basophils by an A2-receptor mediated mechanism. Br J Pharmacol (1983) 80(4):719–26. doi: 10.1111/j.1476-5381.1983.tb10063.x
154. Feoktistov I, Polosa R, Holgate ST, Biaggioni I. Adenosine A2B receptors: a novel therapeutic target in asthma? Trends Pharmacol Sci (1998) 19(4):148–53. doi: 10.1016/S0165-6147(98)01179-1
155. Ott I, Lohse MJ, Klotz KN, Vogt-Moykopf I, Schwabe U. Effects of adenosine on histamine release from human lung fragments. Int Arch Allergy Immunol (1992) 98(1):50–6. doi: 10.1159/000236163
156. Ryzhov S, Zaynagetdinov R, Goldstein AE, Novitskiy SV, Dikov MM, Blackburn MR, et al. Effect of A2B adenosine receptor gene ablation on proinflammatory adenosine signaling in mast cells. J Immunol (2008) 180(11):7212–20. doi: 10.4049/jimmunol.180.11.7212
157. Barnes PJ. Asthma as an axon reflex. Lancet (1986) 1(8475):242–5. doi: 10.1016/S0140-6736(86)90777-4
158. Basoglu OK, Pelleg A, Kharitonov SA, Barnes PJ. Contrasting effects of ATP and adenosine on capsaicin challenge in asthmatic patients. Pulm Pharmacol Ther (2017) 45:13–8. doi: 10.1016/j.pupt.2017.04.004
159. Barnes PJ. Neuropeptides and asthma. Am Rev Respir Dis (1991) 143(3 Pt 2):S28–32. doi: 10.1164/ajrccm/143.3_Pt_2.S28
160. Tsai SH, Takeda K. Regulation of allergic inflammation by the ectoenzyme E-NPP3 (CD203c) on basophils and mast cells. Semin Immunopathol (2016) 38(5):571–9. doi: 10.1007/s00281-016-0564-2
161. Niyonsaba F, Ushio H, Hara M, Yokoi H, Tominaga M, Takamori K, et al. Antimicrobial peptides human beta-defensins and cathelicidin LL-37 induce the secretion of a pruritogenic cytokine IL-31 by human mast cells. J Immunol (2010) 184(7):3526–34. doi: 10.4049/jimmunol.0900712
162. Irani AM, Goldstein SM, Wintroub BU, Bradford T, Schwartz LB. Human mast cell carboxypeptidase. Selective localization to MCTC cells. J Immunol (1991) 147(1):247–53.
163. Irani AM, Schwartz LB. Human mast cell heterogeneity. Allergy Proc (1994) 15(6):303–8. doi: 10.2500/108854194778816472
164. Müller T, Vieira RP, Grimm M, Dürk T, Cicko S, Zeiser R, et al. A potential role for P2X7R in allergic airway inflammation in mice and humans. Am J Respir Cell Mol Biol (2011) 44(4):456–64. doi: 10.1165/rcmb.2010-0129OC
165. Matsuoka I, Yoshida K, Ito MA. Purinergic regulation of mast cell function: P2X4 receptor-mediated enhancement of allergic responses. J Pharmacol Sci (2022) 150(2):94–9. doi: 10.1016/j.jphs.2022.07.005
166. Yoshida K, Tanihara S, Miyashita Y, Obayashi K, Ito MA, Yamamoto K, et al. P2X4 receptor stimulation enhances MrgprB2-mediated mast cell activation and pseudoallergic reactions in mice. Sci Rep (2022) 12(1):18613. doi: 10.1038/s41598-022-21667-6
167. Bonvini SJ, Birrell MA, Dubuis E, Adcock JJ, Wortley MA, Flajolet P, et al. Novel airway smooth muscle-mast cell interactions and a role for the TRPV4-ATP axis in non-atopic asthma. Eur Respir J (2020) 56(1). doi: 10.1183/13993003.01458-2019
168. Alberto AV, Faria RX, de Menezes JR, Surrage A, da Rocha NC, Ferreira LG, et al. Role of P2 receptors as modulators of rat eosinophil recruitment in allergic inflammation. PloS One (2016) 11(1):e0145392. doi: 10.1371/journal.pone.0145392
169. Gruenbacher G, Gander H, Rahm A, Dobler G, Drasche A, Troppmair J, et al. The human G protein-coupled ATP receptor P2Y(11) is associated with IL-10 driven macrophage differentiation. Front Immunol (2019) 10:1870. doi: 10.3389/fimmu.2019.01870
170. Alsaleh NB, Persaud I, Brown JM. Silver nanoparticle-directed mast cell degranulation is mediated through calcium and PI3K signaling independent of the high affinity igE receptor. PloS One (2016) 11(12):e0167366. doi: 10.1371/journal.pone.0167366
171. Huber M, Cato ACB, Ainooson GK, Freichel M, Tsvilovskyy V, Jessberger R, et al. Regulation of the pleiotropic effects of tissue-resident mast cells. J Allergy Clin Immunol (2019) 144(4s):S31–s45. doi: 10.1016/j.jaci.2019.02.004
172. Munoz I, Danelli L, Claver J, Goudin N, Kurowska M, Madera-Salcedo IK, et al. Kinesin-1 controls mast cell degranulation and anaphylaxis through PI3K-dependent recruitment to the granular Slp3/Rab27b complex. J Cell Biol (2016) 215(2):203–16. doi: 10.1083/jcb.201605073
173. Nishida K, Yamasaki S, Hasegawa A, Iwamatsu A, Koseki H, Hirano T. Gab2, via PI-3K, regulates ARF1 in FcϵRI-mediated granule translocation and mast cell degranulation. J Immunol (2011) 187(2):932–41. doi: 10.4049/jimmunol.1100360
174. Santos Mde S, Naal RM, Baird B, Holowka D. Inhibitors of PI(4,5)P2 synthesis reveal dynamic regulation of IgE receptor signaling by phosphoinositides in RBL mast cells. Mol Pharmacol (2013) 83(4):793–804. doi: 10.1124/mol.112.082834
175. Nishida K, Wang L, Morii E, Park SJ, Narimatsu M, Itoh S, et al. Requirement of Gab2 for mast cell development and KitL/c-Kit signaling. Blood (2002) 99(5):1866–9. doi: 10.1182/blood.V99.5.1866
176. Siraganian RP, Hazard KA. Mechanisms of mouse mast cell activation and inactivation for IgE-mediated histamine release. J Immunol (1979) 122(5):1719–25. doi: 10.4049/jimmunol.122.5.1719
177. Chalhoub N, Zhu G, Zhu X, Baker SJ. Cell type specificity of PI3K signaling in Pdk1- and Pten-deficient brains. Genes Dev (2009) 23(14):1619–24. doi: 10.1101/gad.1799609
178. Lee-Rivera I, López E, Parrales A, Alvarez-Arce A, López-Colomé AM. Thrombin promotes the expression of Ccnd1 gene in RPE cells through the activation of converging signaling pathways. Exp Eye Res (2015) 139:81–9. doi: 10.1016/j.exer.2015.08.001
179. Tsuchiya A, Kanno T, Nishizaki T. PI3 kinase directly phosphorylates Akt1/2 at Ser473/474 in the insulin signal transduction pathway. J Endocrinol (2014) 220(1):49–59. doi: 10.1530/JOE-13-0172
180. Nishi H, Arai H, Momiyama T. NCI-H295R, a human adrenal cortex-derived cell line, expresses purinergic receptors linked to Ca²+-mobilization/influx and cortisol secretion. PloS One (2013) 8(8):e71022. doi: 10.1371/journal.pone.0071022
181. Le TT, Berg NK, Harting MT, Li X, Eltzschig HK, Yuan X. Purinergic signaling in pulmonary inflammation. Front Immunol (2019) 10:1633. doi: 10.3389/fimmu.2019.01633
Keywords: human lung mast cells, LAD2 cell line, P2 purinergic receptors, allergic degranulation, FcϵRI, PI3K(δ), P2Y11R
Citation: Schulman ES, Nishi H and Pelleg A (2023) Degranulation of human mast cells: modulation by P2 receptors’ agonists. Front. Immunol. 14:1216580. doi: 10.3389/fimmu.2023.1216580
Received: 04 May 2023; Accepted: 04 September 2023;
Published: 05 October 2023.
Edited by:
Ronald Sluyter, University of Wollongong, AustraliaReviewed by:
Li-Na Wang, Shanghai University of Traditional Chinese Medicine, ChinaStephen Fuller, The University of Sydney, Australia
Copyright © 2023 Schulman, Nishi and Pelleg. This is an open-access article distributed under the terms of the Creative Commons Attribution License (CC BY). The use, distribution or reproduction in other forums is permitted, provided the original author(s) and the copyright owner(s) are credited and that the original publication in this journal is cited, in accordance with accepted academic practice. No use, distribution or reproduction is permitted which does not comply with these terms.
*Correspondence: Edward S. Schulman, ZXMyOUBkcmV4ZWwuZWR1
†ORCID: Edward S. Schulman, orcid.org/0000-0003-2928-2665