- 1Centre International de Recherche en Infectiologie, Team Groupe sur l'immunité des muqueuses et agents pathogènes (GIMAP), Université Jean Monnet, Université Claude Bernard Lyon, Inserm, Saint-Etienne, France
- 2Centre d'investigation clinique (CIC) 1408 Inserm Vaccinology, University Hospital of Saint-Etienne, Saint-Etienne, France
- 3Immunology Department, iBiothera Reference Center, University Hospital of Saint-Etienne, Saint-Etienne, France
Introduction
Tissue-resident memory lymphocytes at mucosal surfaces have been shown to be critical in long-term protection following mucosal infection. Tissue-resident memory T cells (Trm) have been well characterized in animal models and in humans, while knowledge about tissue-resident memory B cells (Brm) is currently more limited. Due to specific features of tissue-resident memory lymphocytes such as cross-reactivity and polyfunctionality, Trm and Brm have been demonstrated to be an ideal target for vaccine strategies aiming to induce protection at mucosal surfaces. The route of vaccine administration, the choice of antigenic epitopes and the impact of microenvironment appeared to be crucial parameters in the development of vaccine-induced mucosal tissue-resident memory responses in animal models. However, it remains significant gaps in understanding systemic and local signals needed to establish and maintain protective mucosal Trm subsets without inducing pathogenic populations. In addition, the development of Brm is currently not well understood. Discovery of innovative recombinant antigens and identification of safe mucosal adjuvants will be crucial in the development of vaccine formulations efficient to induce Trm and Brm at mucosal surfaces. This Opinion article will describe current knowledge about mucosal Trm and Brm and vaccine approaches already tested to induce tissue-resident memory lymphocytes at mucosal surfaces. It will pinpoint gaps in knowledge. It will suggest research avenues and highlight considerations to design vaccine strategies inducing mucosal tissue-resident memory lymphocytes and it will provide suggestions to improve methodology to quantify mucosal tissue-resident memory responses.
Characterization and features of Trm at mucosal surfaces
Three memory T cell subsets have been characterized: circulating effector memory T cells are abundant in non-lymphoid tissues, circulating central memory T cells are predominant in secondary lymphoid organs and non-circulating tissue-resident memory T cells (Trm) are able to persist in non-lymphoid tissues. Trm subset was discovered in parabiosis and tissue transplantation animal studies more than 15 years ago (1) and it is the most abundant memory T cell population. Trm have the ability to reside in mucosal tissues such as lung (2), nasal (3), gut (4), skin (5–8) and reproductive tract tissues (9), following infection (10). Trm were characterized in mice, non-human primates (NHP) (11) and humans (12–14). They were mostly defined by the high expression of the adhesion molecule CD69 which can be associated with an upregulation of the αE integrin CD103 (1), and by the downregulation of molecules preventing tissue exit such as CCR7 and CD62L (10). Other markers were associated with Trm such as CXCR3, CD49a (15) (16) or CD44 (1) in specific tissues. Regarding the functions of Trm, antigen-specific CD8 and CD4 Trm in the respiratory tract were shown to be associated with a better control of viral and bacterial infections (e.g. reduction of viral load (12), limitation of intracellular replication of bacteria (17)). In addition, several animal and human studies described the polyfunctionality of influenza- (18, 19) or respiratory syncytial virus (RSV)-specific CD8 Trm responses (20) as a key feature of protective Trm. The cross-reactivity of human Trm via recognition of conserved regions was also reported in the context of influenza (21, 22) and Severe Acute Respiratory Syndrome Coronavirus 2 (SARS-CoV-2) (3) infections. Another interesting feature of Trm is their potential innate-like functions helping in mucosal protection. It was demonstrated that CD8 Trm located in lung parenchyma of mice intranasally infected with influenza virus engineered to express ovalbumin as an antigen, could reduce the severity of a subsequent pulmonary bacterial infection through neutrophil recruitment (23). A similar bystander activation of T cells was observed in a Herpes Simplex Virus (HSV) murine challenge model. Indeed, it was shown that a subset of vaginal CD8 T cells which were unspecific to the HSV antigen used for immunization, could play a partial role in genital protection. In this HSV challenge model, both peripheral CD8 T cells able to migrate to inflamed vaginal tissue and vaginal CD8 Trm of irrelevant antigen specificity were involved in this innate-like function (24).
Discovery of mucosal Brm
The development of tissue-resident memory B cells (Brm) following infection has been discovered in murine parabiosis (25), adoptive transfer (26) and depletion studies (27). The presence of lung Brm early post-infection was reported in mouse models of influenza (25) and pneumococcal pneumonia (28). It was shown that the induction of Brm required local encounter with antigen (25) and that Brm contributed to early plasmablast responses leading to the secretion of cross-neutralizing antibodies against viruses and bacteria (27, 28). Compared to Trm, Brm have been less characterized and specific markers have not been fully defined. However, it was shown that Brm expressed CD69, the hallmark of tissue-resident lymphocytes, in mice (25), NHP and humans (14). Other markers were also associated with Brm in mice such as CXCR3 and CD44 (25, 26, 28). In addition, antigen-specific CD73 positive and negative pulmonary Brm subsets were described in a mouse influenza model (25). In animal studies, the analysis of Brm is based on the discrimination of resident and circulatory B cells by intravenous labelling. In animal models and humans, gating strategies based on characterized memory B cell markers and similarities with Trm transcriptional profiles are also used to describe Brm subsets (29). Interestingly, a study found a potential intestinal Brm subset. Indeed, most CD19+CD27+ B cells in human intestine were CD45RB+CD69+ B cells. In addition, sets of gene expressed in lung Trm were enriched in this gut B cell subset suggesting that it could be an intestinal-resident population (30). The presence of Brm in other mucosal tissues such as skin or reproductive tract is currently unclear.
Vaccine approaches to induce tissue-resident memory responses at mucosal sites
For more than two centuries, vaccination has been a successful global strategy to reduce the burden of several infectious diseases (31). Historically, the immunogenicity induced by vaccines was associated with systemic humoral response which can be easily measured in blood using antibody assays (32). However, for several decades, efforts have been put into the understanding of vaccine-induced cellular and local immune responses. Could the protective capacities of mucosal Trm and Brm be harnessed to improve immunity at mucosal barriers?
A range of vaccine approaches have especially been tested in mouse models to improve mucosal Trm development (33). The route of vaccine administration has been shown to play a crucial role. Growing evidences suggest that mucosal vaccines on their own or combined with systemic vaccines could be a promising strategy to enhance the development of mucosal Trm. For example, a study found that intranasal administration of live-attenuated influenza virus induced the development of CD4 and CD8 Trm in lungs, whereas systemic immunisation with live-attenuated influenza virus did not generate similar Trm response in mice (34). Similarly, intranasal immunization of mice with a chimpanzee adenoviral-based SARS-CoV-2 vaccine was shown to induce CD103+CD69+CD8 T cells in lungs, while vaccination by intramuscular route failed to generate pulmonary Trm cells (35). Another strategy named ‘prime and pull’ was tested to generate Trm in vaginal tract. Mice were subcutaneously immunized with an attenuated strain of HSV-2 (prime). Then, pro-inflammatory chemokines were applied to the vagina of mice in order to recruit HSV-specific CD8 T cells to this mucosal site (pull). Compared to the other experimental groups which were primed and boosted by intravaginal or subcutaneous routes only, the prime and pull strategy was the only one leading to the establishment of CD8 Trm in the genital mucosa. This study suggested that inflammation on its own could lead to the recruitment of Trm to genital mucosa and that a persistent antigen stimulation was not needed for the establishment of Trm (36). Similar findings were reported in nasal, upper respiratory tract (2) and skin (6). However, it was demonstrated that a local antigen encounter was needed to establish CD8 Trm in lungs (37–39). For instance, mice immunized by intraperitoneal route with influenza virus (prime) could exclusively generate pulmonary Trm following an intranasal immunization (pull) with CpG oligodeoxynucleotides combined with the antigen. The authors of this study suggested that circulating antigen-specific CD8 T cells could cause local tissue damage, which could play a role in Trm conversion (39). Interestingly, the importance of vaccine epitopes was pinpointed in a study describing the sequence design and immunogenicity of a CD8 T cell peptide Coronavirus Disease 19 (COVID-19) vaccine. This COVID-19 vaccine candidate was based on a range of 11 structural and non-structural SARS-CoV-2 proteins including conserved regions. The sequence was designed using SARS-CoV-2 immuno-dominant epitopes determined by screening and SARS-CoV-2 neoepitopes selected using a computational multi-neoepitopes based peptide vaccine approach, which had been shown to be safe and efficient in clinical trials evaluating a vaccine candidate against lung cancer. Following one subcutaneous vaccination in a mouse model, the COVID-19 vaccine candidate could induce a significant number of peripheral viral-specific CD8 T cells expressing Trm markers such as CD103 and CD49a, in spleen and draining lymph nodes. Even though the authors did not analyse Trm in lungs, this study highlights the importance of epitope selection to induce lymphocytes with a tissue-residency signature (40). Another study compared the functionality of T cells and Trm in lung biopsies collected for cancer suspicion in SARS-CoV-2 infected patients or individuals vaccinated with COVID-19 mRNA vaccines. The current spike-based COVID-19 mRNA vaccines were shown to induce similar SARS-CoV-2 spike-specific IFNγ CD4 T cell responses in lungs of vaccinees and convalescents, while antigen-specific CD8 T cell responses were not induced, neither in convalescents, nor in vaccinated individuals. Regarding tissue-resident memory responses in lungs, polyfunctional CD4 and CD8 Trm induction was shown to be limited post-vaccination compared to post-infection. A selection of SARS-CoV-2 epitopes, as previously described, could help to improve tissue-resident memory responses generated by the current COVID-19 mRNA vaccines (41). Determining the role of local antigen stimulation, mucosal inflammation and antigenic epitopes in specific vaccine strategies to induce tissue-resident memory lymphocytes is fundamental. These parameters may impact on the choice of the antigen, the administration route and the vector used to deliver the vaccine (10).
Regarding Brm, their induction has especially been evaluated in mouse pulmonary tissues (25). As it was determined that the establishment of pulmonary Brm post-infection required a local antigen encounter (25), it could be hypothesized that mucosal vaccination might also be beneficial to generate Brm in lung tissues at least. However, this hypothesis remains to be demonstrated. Interestingly, evidences revealed that the establishment of Brm pool did not correlate with the presence of Trm in the reproductive tract of female mice immunized with HSV (42). These data suggest that Trm and Brm responses could be induced in an independent manner at least in the reproductive tract. Trm and Brm development might have different kinetics and/or might require different local microenvironments. This correlation needs to be studied in other tissues given a lack of correlation might significantly impact on the development of vaccines targeting mucosal tissue-resident memory responses. Do specific vaccine approaches need to be developed to induce either Trm or Brm? It is currently unknown.
Research avenues and considerations to design vaccines inducing protective tissue-resident memory lymphocytes at mucosal surfaces
Understanding mucosal tissue-resident memory lymphocyte generation
A better understanding of Trm and Brm establishment and maintenance at mucosal surfaces is needed to tailor efficient vaccine strategies. Based on animal studies, two general models have been developed to explain Trm formation. The local divergence model suggests that Trm differentiate within tissues from pluripotent circulating effector T cells. The systemic divergence model proposes that there is a subset of circulating Trm precursors intended for migrating into tissues where they finish their differentiation into mature Trm (32). Regarding Brm formation, their origin has not been fully characterized and has been mainly based on lung Brm studies in mouse models. Influenza virus infection models suggest that Brm could originate from germinal centres in mediastinal lymph nodes or from germinal centre-like structures in the inducible bronchus-associated lymphoid tissues (25, 43). Identification of circulating Trm and Brm precursors by flow cytometry and transcriptomics using known tissue-resident memory markers and genes could be a way to find novel circulating lymphocyte populations sharing residency-promoting signature with Trm. This type of study could be performed before and after infection or vaccination in animal models. It would help to validate these models or hypotheses even though they might be non-exclusive (32), tissue- and context-dependent. Recently, some evidences have suggested the presence of precursor CD8+ Trm within circulation (44). In addition, some studies have demonstrated that Trm were able to egress and migrate to lymph nodes (45) or to distant mucosal sites (46). Understanding potential movements of tissue-resident memory lymphocytes can be crucial in order to optimize the routes of administration and to determine whether mucosal vaccination on its own or whether mucosal vaccination after a systemic prime is the best strategy. The influence of the priming route should be studied using parabiosis mouse models.
Some studies suggested that specific cytokines or metabolites could impact on Trm formation. Indeed the role of TGFβ has been described in the generation of lung (47), nasal/upper respiratory tract (2), gut (48) and skin (7, 49) CD103+ Trm. Modulation of TGFβ might be a key parameter to optimize vaccine strategies aiming to induce Trm including lung CD8 Trm (50, 51). A role of other cytokines such as IL-10 (52), IL-21 (53), IL-15 (54, 55) and IL-1/IL-2 (56) was also described in Trm formation in lungs or other tissues. Recently, it has been demonstrated that a prime of T cells in the mesenteric lymph node of mice infected with Listeria monocytogenes by oral route, licensed T cells to differentiate into CD103+ T cells in intestine and that this licensing was regulated by retinoic acid (57). Systemic and mucosal signals required to generate tissue-resident memory lymphocytes need to be further elucidated in order to design vaccine formulations leading to an appropriate microenvironment. The use of knockout mice for specific cytokines or their receptors, mouse Cre-LoxP system, as well as reporter systems may help to understand the role of systemic and mucosal signalling pathways and microenvironments involved in the expansion and responsiveness of tissue-resident memory lymphocytes post-vaccination at different mucosal sites. Gene-based systems such as transcription factor profiling of historical activity in specific tissues (58) or novel DNA-based memory system (59) could help to define the epigenetic state of potential precursor populations but also early signals linked to tissue-resident memory lymphocyte formation.
Complicating the picture of tissue-resident memory responses, sub-populations of CD4 Trm have been described based on specific cytokine profiles. Indeed, lung Trm1, Trm2, Trh, Trm17 have been characterized following different respiratory infections (53) (60). However, it is unclear whether their differentiation requires different signalling pathways, specific microenvironments and whether their persistence is similar in mucosal tissues. It is also important to consider that some particular Trm subsets have been associated with persistent immunopathology after viral infection or with chronic diseases (60). For instance, an expansion and activation of Trm17 was identified in bronchoalveolar lavage fluid from severe COVID-19 patients following SARS-CoV-2 clearance. The characterization of this subset showed a pathogenic cytokine profile associated with severe disease and lung damage (61). It was also observed that an enrichment of CD69+CD103- Trm population in bronchoalveolar lavage fluid collected from patients with post-COVID-19 acute sequelae negatively correlated with their lung function (62). A specific CD103+CD161+CCR5+CD4+Trm sub-population was also reported to be predominant in the intestine of Crohn’s disease patients (63). These examples of pathogenic Trm profiles pinpoint the importance to clearly define the parameters leading to the establishment of protective tissue-resident memory lymphocytes at mucosal surfaces following vaccination. The development of protective or exuberant tissue-resident memory lymphocytes might be related to the type of stimulus, the persistence of stimulation, the local environment and the type of activated signalling pathways. Qualitative and quantitative differences between protective and pathological tissue-resident memory responses need to be elucidated. During the development of vaccine candidates aiming to induce mucosal Trm or Brm, it will be crucial to determine the cytokine profile of tissue-resident memory lymphocytes generated at mucosal surfaces after vaccination in order to evaluate the maintenance of mucosal homeostasis even though limited inflammation can transiently be induced by vaccination. Spatial transcriptomics in situ may be a critical approach to detect inflammation associated with tissue-resident memory lymphocyte populations (64).
Optimizing vaccine formulations
Given the ability of tissue-resident memory lymphocytes to generate cross-reactive immune responses specific to conserved epitopes, designing recombinant antigens which include conserved epitopes might be of great interest. Systems vaccinology and artificial intelligence could be approaches which should be explored to predict epitopes able to induce tissue-resident memory responses. In addition, the role of adjuvants may be essential to enhance and tailor tissue-resident memory responses at mucosal sites. Some promising adjuvants administered by mucosal route have been already identified in preclinical models. Marinaik et al. showed that acrylic-acid-based adjuvant associated with a Toll-like receptor agonist glucopyranosyl lipid adjuvant was the most effective vaccine formulation to induce influenza-specific CD103+ CD8 Trm in lungs of mice immunized by intranasal route (65). Using the same administration route, it was also shown that influenza antigens associated with IL-1β enhanced the number of antigen-specific CD103+CD69+ Trm in lungs of mice (66). Interestingly, some adjuvants administered by systemic route have shown to be able to enhance the induction of mucosal cellular responses. Indeed, it was reported that all-trans-retinoic acid administered by intraperitoneal route could enhance the frequency of antigen-specific memory T cells in murine intestine (67). Woodworth et al. demonstrated that CAF®10b, a liposomal adjuvant administered by intramuscular route, could prime T cells in order to recall them in the lungs or skin using the antigen only administered by intratracheal and intradermal routes in NHP (68). If all-trans-retinoic acid or CAF®10b adjuvants are beneficial to induce mucosal tissue-resident memory responses, it remains to be confirmed. The main challenge to design vaccine formulations able to induce mucosal responses including mucosal Trm/Brm is the current lack of adjuvants licensed for mucosal administration in humans (69). Identifying effective and safe mucosal adjuvants is a key factor to pursue the development of vaccine strategies to generate mucosal tissue-resident memory responses. Unfortunately, the lack of in vitro predictive assays for adjuvants does not help and in vivo models remain the gold standard for these analyses (70).
Finally, evidences have shown that sex (71) and age (60) (72) could impact on tissue-resident memory response profile and functionality. These parameters need to be further evaluated in the context of vaccine development. Vaccine strategies used to generate Trm/Brm should be tested in both female and male animals, as well as aged animals at some stages of development. It will help to tailor vaccine strategies aiming to induce protective Trm and Brm responses in human populations with different pre-existing chronic mucosal conditions. In addition, the role of microbiota or microbiota-derived metabolites in the development, maintenance, metabolism and modulation of tissue-resident memory lymphocytes also have to be considered (73) especially in the context of mucosal vaccination.
Evaluating tissue-resident memory responses
The gold standard to analyze mucosal Trm and Brm responses remains animal models and the type of animal models is a crucial parameter. Inbred mice are commonly used in the studies. However, they may not be the best models to analyze tissue-resident memory responses at mucosal surfaces. Even though it remains challenging to mimic multiple mucosal exposures to a range of pathogens impacting on polyfunctional and polyreactive Trm and Brm in animal models, the use of outbred mice could better recapitulate observations found in humans as developing more tissue-resident memory lymphocytes in non-lymphoid tissues (74).
To evaluate the efficacy of vaccine strategies able to enhance Trm and Brm responses in humans, robust sampling and quantification methods are required to analyze mucosal tissue-resident memory responses. Analysis of post-mortem tissues or tissues after resection surgery is currently the best way to study tissue-resident memory responses at mucosal surfaces by flow cytometry or histological staining (75). Following SARS-CoV-2 infection, human nasal tissue-resident memory T cells have been recently analyzed using specific device for nasal sampling (76). However, isolation of human Trm and Brm from mucosal surfaces remains challenging. Consequently, defining correlations between peripheral markers and mucosal tissue-resident memory responses is crucial to include the analysis of mucosal tissue-resident memory responses in human vaccine trials. Peripheral markers could be based on mucosal homing markers expressed on circulating lymphocyte populations (77) or the circulation of tissue-resident memory precursors.
Conclusion
Vaccines able to induce long-term mucosal responses are needed to improve protection against infection at mucosal surfaces. Expanding polyfunctional and cross-reactive tissue-resident memory responses using mucosal vaccination on its own or combined to systemic vaccination looks a promising way to reach this goal. An ideal vaccine would induce controlled and balanced Trm and/or Brm responses at mucosal sites (Figure 1). For this purpose, a better knowledge is needed to understand the formation of effective tissue-resident memory responses at mucosal surfaces and to determine the specific environment needed in each mucosal tissue to generate protective Trm and Brm subsets. Identifying effective mucosal adjuvants able to induce mucosal tissue-resident memory responses is a key parameter to optimize vaccine formulations (Figure 2). However, it will be challenging to move vaccine candidates into clinical trials if there are not any standard procedures to quantify Trm and Brm responses in human mucosal tissues. Identification of peripheral markers correlating with Trm and/or Brm responses could be an easy way to evaluate mucosal tissue-resident memory responses post-vaccination in humans.
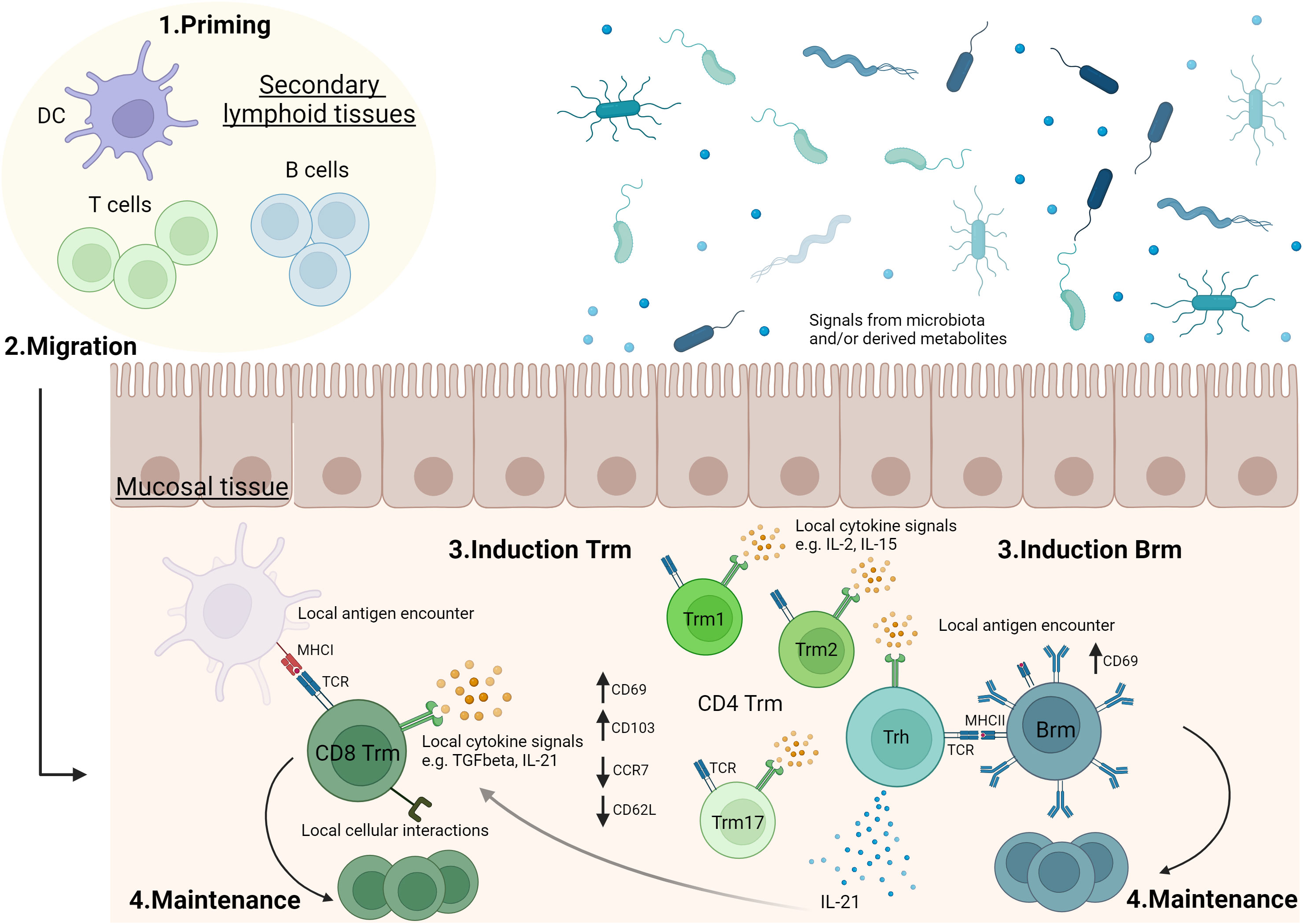
Figure 1 Generation of tissue-resident memory lymphocytes in mucosal tissues.1. T and B cells are activated in secondary lymphoid organs (e.g. lymph nodes, mucosa-associated lymphoid tissues). 2. Following activation, lymphocytes can migrate to mucosal tissues where they will convert into tissue-resident memory T cells (Trm) and B cells (Brm). 3 & 4. In mucosal tissue, antigen stimulation, cytokine signals and/or cellular interactions can drive mucosal tissue-resident memory lymphocyte induction and play a role in their maintenance. The role of these parameters may differ according to the type of mucosal tissue and there are still significant gaps in the current knowledge. For example, the role of local antigen encounter (37–39), TGFβ (50, 51), IL-2 (56), IL-15 (54) or IL-21 (53) in Trm induction, as well as the formation of sub-populations of CD4 Trm have been described in lungs (53, 60). Evidences showing a role of microbiota or microbiota-derived metabolites in Trm modulation have been reported, especially in intestine (73). Vaccination may impact on priming, migration, generation and maintenance of Trm and Brm in mucosal tissues. Trm1: tissue-resident memory CD4 T cells secreting Th1 cytokines. Trm2: tissue-resident memory CD4 T cells secreting Th2 cytokines. Trm17: tissue-resident memory CD4 T cells secreting Th17 cytokines. Trh: tissue-resident memory CD4 T helper cells. The figure was created with BioRender.com.
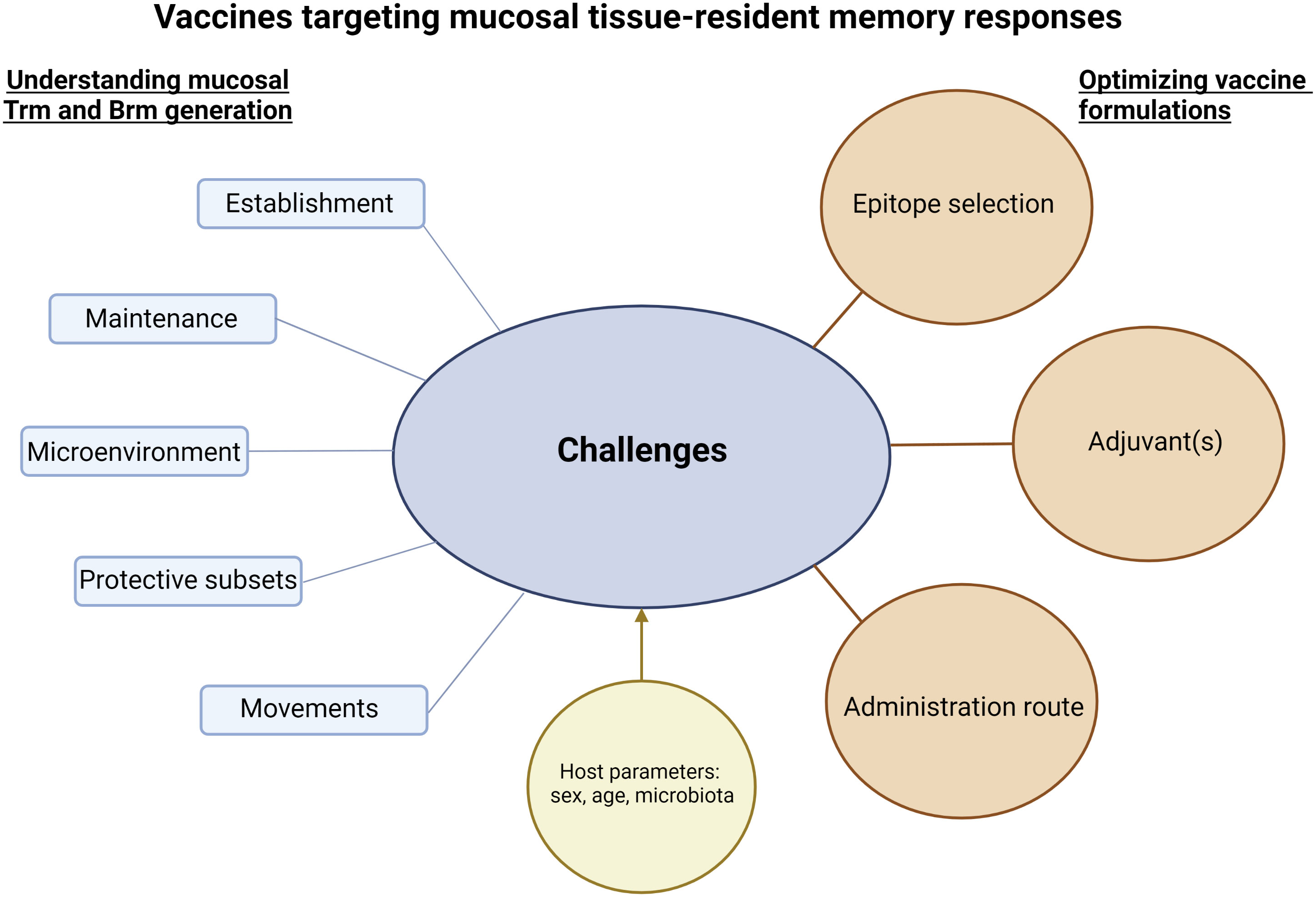
Figure 2 Challenges and considerations to develop vaccines targeting mucosal tissue-resident memory responses. Successful vaccine strategies to induce mucosal tissue-resident memory responses will be based on a better knowledge of the establishment and maintenance of protective Trm and Brm in mucosal tissues. In addition, understanding their potential movements to specific mucosal tissues and/or the movements of their precursors, as well as microenvironments needed to lead to functional Trm and Brm will be crucial. Interactions between Trm and Brm responses and host parameters such as sex, age or microbiota should be assessed. The sum of this knowledge will be essential to optimize vaccine formulations. The figure was created with BioRender.com.
Author contributions
SP and SL have written the manuscript. All authors contributedto the article and approved the submitted version.
Funding
This study is supported by Agence Nationale de Recherche SIgCOVIV, MSD Avenir, Université Jean Monnet Nosivax Mucovid.
Conflict of interest
The authors declare that the research was conducted in the absence of any commercial or financial relationships that could be construed as a potential conflict of interest.
Publisher’s note
All claims expressed in this article are solely those of the authors and do not necessarily represent those of their affiliated organizations, or those of the publisher, the editors and the reviewers. Any product that may be evaluated in this article, or claim that may be made by its manufacturer, is not guaranteed or endorsed by the publisher.
References
1. Mueller SN, Mackay LK. Tissue-resident memory T cells: local specialists in immune defence. Nat Rev Immunol (2016) 16(2):79–89. doi: 10.1038/nri.2015.3
2. Pizzolla A, Nguyen THO, Smith JM, Brooks AG, Kedzieska K, Heath WR, et al. Resident memory CD8+ T cells in the upper respiratory tract prevent pulmonary influenza virus infection. Sci Immunol (2017) 2(12):eaam6970. doi: 10.1126/sciimmunol.aam6970
3. Diniz MO, Mitsi E, Swadling L, Rylance J, Johnson M, Goldblatt D, et al. Airway-resident T cells from unexposed individuals cross-recognize SARS-CoV-2. Nat Immunol (2022) 23(9):1324–9. doi: 10.1038/s41590-022-01292-1
4. Masopust D, Choo D, Vezys V, Wherry EJ, Duraiswamy J, Akondy R, et al. Dynamic T cell migration program provides resident memory within intestinal epithelium. J Exp Med (2010) 207(3):553–64. doi: 10.1084/jem.20090858
5. Gebhardt T, Wakim LM, Eidsmo L, Reading PC, Heath WR, Carbone FR. Memory T cells in nonlymphoid tissue that provide enhanced local immunity during infection with herpes simplex virus. Nat Immunol (2009) 10(5):524–30. doi: 10.1038/ni.1718
6. Mackay LK, Stock AT, Ma JZ, Jones CM, Kent SJ, Mueller SN, et al. Long-lived epithelial immunity by tissue-resident memory T (TRM) cells in the absence of persisting local antigen presentation. Proc Natl Acad Sci U S A. (2012) 109(18):7037–42. doi: 10.1073/pnas.1202288109
7. Mackay LK, Rahimpour A, Ma JZ, Collins N, Stock AT, Hafon ML, et al. The developmental pathway for CD103(+)CD8+ tissue-resident memory T cells of skin. Nat Immunol (2013) 14(12):1294–301. doi: 10.1038/ni.2744
8. Glennie ND, Yeramilli VA, Beiting DP, Volk SW, Weaver CT, Scott P. Skin-resident memory CD4+ T cells enhance protection against Leishmania major infection. J Exp Med (2015) 212(9):1405–14. doi: 10.1084/jem.20142101
9. Çuburu N, Graham BS, Buck CB, Kines RC, Pang YYS, Day PM, et al. Intravaginal immunization with HPV vectors induces tissue-resident CD8+ T cell responses. J Clin Invest. (2012) 122(12):4606–20. doi: 10.1172/JCI63287
10. Zheng MZM, Wakim LM. Tissue resident memory T cells in the respiratory tract. Mucosal Immunol (2022) 15(3):379–88. doi: 10.1038/s41385-021-00461-z
11. Darrah PA, Zeppa JJ, Maiello P, Hackney JA, Wadsworth MH, Hughes TK, et al. Prevention of tuberculosis in macaques after intravenous BCG immunization. Nature (2020) 577(7788):95–102. doi: 10.1038/s41586-019-1817-8
12. Jozwik A, Habibi MS, Paras A, Zhu J, Guvenel A, Dhariwal J, et al. RSV-specific airway resident memory CD8+ T cells and differential disease severity after experimental human infection. Nat Commun (2015) 6:10224. doi: 10.1038/ncomms10224
13. Ogongo P, Tezera LB, Ardain A, Nhamoyebonde S, Ramsuran D, Singh A, et al. Tissue-resident-like CD4+ T cells secreting IL-17 control Mycobacterium tuberculosis in the human lung. J Clin Invest. (2021) 131(10):e142014. doi: 10.1172/JCI142014
14. Humphries DC, O’Connor RA, Stewart HL, Quinn TM, Gaughan EE, Mills B, et al. Specific in situ immuno-imaging of pulmonary-resident memory lymphocytes in human lungs. Front Immunol (2023) 14:1100161. doi: 10.3389/fimmu.2023.1100161
15. Topham DJ, Reilly EC. Tissue-resident memory CD8+ T cells: from phenotype to function. Front Immunol (2018) 9:515. doi: 10.3389/fimmu.2018.00515
16. Reilly EC, Lambert Emo K, Buckley PM, Reilly NS, Smith I, Chaves FA, et al. TRM integrins CD103 and CD49a differentially support adherence and motility after resolution of influenza virus infection. Proc Natl Acad Sci U S A. (2020) 117(22):12306–14. doi: 10.1073/pnas.1915681117
17. Yang Q, Zhang M, Chen Q, Chen W, Wei C, Qiao K, et al. Cutting edge: characterization of human tissue-resident memory T cells at different infection sites in patients with tuberculosis. J Immunol (2020) 204(9):2331–6. doi: 10.4049/jimmunol.1901326
18. Pizzolla A, Nguyen TH, Sant S, Jaffar J, Loudovaris T, Mannering SI, et al. Influenza-specific lung-resident memory T cells are proliferative and polyfunctional and maintain diverse TCR profiles. J Clin Invest. (2018) 128(2):721–33. doi: 10.1172/JCI96957
19. Pichyangkul S, Yongvanitchit K, Limsalakpetch A, Kum-Arb U, Im-Erbsin R, Boonnak K, et al. Tissue distribution of memory T and B cells in rhesus monkeys following influenza A infection. J Immunol (2015) 195(9):4378–86. doi: 10.4049/jimmunol.1501702
20. Li H, Callahan C, Citron M, Wen Z, Touch S, Monslow MA, et al. Respiratory syncytial virus elicits enriched CD8+ T lymphocyte responses in lung compared with blood in African green monkeys. PloS One (2017) 12(11):e0187642. doi: 10.1371/journal.pone.0187642
21. Koutsakos M, Illing PT, Nguyen THO, Mifsud NA, Crawford JC, Rizzetto S, et al. Human CD8+ T cell cross-reactivity across influenza A, B and C viruses. Nat Immunol (2019) 20(5):613–25. doi: 10.1038/s41590-019-0320-6
22. Pizzolla A, Wakim LM. Memory T cell dynamics in the lung during influenza virus infection. J Immunol (2019) 202(2):374–81. doi: 10.4049/jimmunol.1800979
23. Ge C, Monk IR, Pizzolla A, Wang N, Bedford JG, Stinear TP, et al. Bystander activation of pulmonary trm cells attenuates the severity of bacterial pneumonia by enhancing neutrophil recruitment. Cell Rep (2019) 29(13):4236–44. doi: 10.1016/j.celrep.2019.11.103
24. Arkatkar T, Davé VA, Cruz Talavera I, Graham JB, Swarts JL, Hughes SM, et al. Memory T cells possess an innate-like function in local protection from mucosal infection. J Clin Invest. (2023) 133(10):e162800. doi: 10.1172/JCI162800
25. Allie SR, Bradley JE, Mudunuru U, Schultz MD, Graf BA, Lund FE, et al. The establishment of resident memory B cells in the lung requires local antigen encounter. Nat Immunol (2019) 20(1):97–108. doi: 10.1038/s41590-018-0260-6
26. Onodera T, Takahashi Y, Yokoi Y, Ato M, Kodama Y, Hachimura S, et al. Memory B cells in the lung participate in protective humoral immune responses to pulmonary influenza virus reinfection. Proc Natl Acad Sci U S A. (2012) 109(7):2485–90. doi: 10.1073/pnas.1115369109
27. Rangel-Moreno J, Carragher DM, Misra RS, Kusser K, Hartson L, Moquin A, et al. B cells promote resistance to heterosubtypic strains of influenza via multiple mechanisms. J Immunol (2008) 180(1):454–63. doi: 10.4049/jimmunol.180.1.454
28. Barker KA, Etesami NS, Shenoy AT, Arafa EI, Lyon de Ana C, Smith NM, et al. Lung-resident memory B cells protect against bacterial pneumonia. J Clin Invest. (2021) 131(11):e141810. doi: 10.1172/JCI141810
29. Lee CM, Oh JE. Resident memory B cells in barrier tissues. Front Immunol (2022) 13:953088. doi: 10.3389/fimmu.2022.953088
30. Weisel NM, Weisel FJ, Farber DL, Borghesi LA, Shen Y, Ma W, et al. Comprehensive analyses of B-cell compartments across the human body reveal novel subsets and a gut-resident memory phenotype. Blood (2020) 136(24):2774–85. doi: 10.1182/blood.2019002782
31. Pollard AJ, Bijker EM. A guide to vaccinology: from basic principles to new developments. Nat Rev Immunol (2021) 21(2):83–100. doi: 10.1038/s41577-020-00479-7
32. Rotrosen E, Kupper TS. Assessing the generation of tissue resident memory T cells by vaccines. Nat Rev Immunol (2023) 1–11. doi: 10.1038/s41577-023-00853-1
33. Knight FC, Wilson JT. Engineering vaccines for tissue-resident memory T cells. Adv Ther (Weinh). (2021) 4(4):2000230. doi: 10.1002/adtp.202000230
34. Zens KD, Chen JK, Farber DL. Vaccine-generated lung tissue-resident memory T cells provide heterosubtypic protection to influenza infection. JCI Insight (2016) 1(10):e85832. doi: 10.1172/jci.insight.85832
35. Hassan AO, Kafai NM, Dmitriev IP, Fox JM, Smith BK, Harvey IB, et al. A single-dose intranasal chAd vaccine protects upper and lower respiratory tracts against SARS-coV-2. Cell (2020) 183(1):169–184.e13. doi: 10.1016/j.cell.2020.08.026
36. Shin H, Iwasaki A. A vaccine strategy that protects against genital herpes by establishing local memory T cells. Nature (2012) 491(7424):463–7. doi: 10.1038/nature11522
37. McMaster SR, Wein AN, Dunbar PR, Hayward SL, Cartwright EK, Denning TL, et al. Pulmonary antigen encounter regulates the establishment of tissue-resident CD8 memory T cells in the lung airways and parenchyma. Mucosal Immunol (2018) 11(4):1071–8. doi: 10.1038/s41385-018-0003-x
38. Wang Z, Wang S, Goplen NP, Li C, Cheon IS, Dai Q, et al. PD-1hi CD8+ resident memory T cells balance immunity and fibrotic sequelae. Sci Immunol (2019) 4(36):eaaw1217. doi: 10.1126/sciimmunol.aaw121
39. Takamura S, Yagi H, Hakata Y, Motozono C, McMaster SR, Masumoto T, et al. Specific niches for lung-resident memory CD8+ T cells at the site of tissue regeneration enable CD69-independent maintenance. J Exp Med (2016) 213(13):3057–73. doi: 10.1084/jem.20160938
40. Gauttier V, Morello A, Girault I, Mary C, Belarif L, Desselle A, et al. Tissue-resident memory CD8 T-cell responses elicited by a single injection of a multi-target COVID-19 vaccine. bioRxiv (2020) 240093. doi: 10.1101/2020.08.14.240093
41. Pieren DKJ, Kuguel SG, Rosado J, Robles AG, Rey-Cano J, Mancebo C, et al. Limited induction of polyfunctional lung-resident memory T cells against SARS-CoV-2 by mRNA vaccination compared to infection. Nat Commun (2023) 14(1):1887. doi: 10.1038/s41467-023-37559-w
42. Oh JE, Iijima N, Song E, Lu P, Klein J, Jiang R, et al. Migrant memory B cells secrete luminal antibody in the vagina. Nature (2019) 571(7763):122–6. doi: 10.1038/s41586-019-1285-1
43. Adachi Y, Onodera T, Yamada Y, Daio R, Tsuiji M, Inoue T, et al. Distinct germinal center selection at local sites shapes memory B cell response to viral escape. J Exp Med (2015) 212(10):1709–23. doi: 10.1084/jem.20142284
44. Kok L, Masopust D, Schumacher TN. The precursors of CD8+ tissue resident memory T cells: from lymphoid organs to infected tissues. Nat Rev Immunol (2022) 22(5):283–93. doi: 10.1038/s41577-021-00590-3
45. Stolley JM, Johnston TS, Soerens AG, Beura LK, Rosato PC, Joag V, et al. Retrograde migration supplies resident memory T cells to lung-draining LN after influenza infection. J Exp Med (2020) 217(8):e20192197. doi: 10.1084/jem.20192197
46. Klicznik MM, Morawski PA, Höllbacher B, Varkhande SR, Motley SJ, Kuri-Cervantes L, et al. Human CD4+CD103+ cutaneous resident memory T cells are found in the circulation of healthy individuals. Sci Immunol (2019) 4(37):eaav8995. doi: 10.1126/sciimmunol.aav8995
47. Zhang M, Li N, He Y, Shi T, Jie Z. Pulmonary resident memory T cells in respiratory virus infection and their inspiration on therapeutic strategies. Front Immunol (2022) 13:943331. doi: 10.3389/fimmu.2022.943331
48. Zhang N, Bevan MJ. Transforming growth factor-β signaling controls the formation and maintenance of gut-resident memory T cells by regulating migration and retention. Immunity (2013) 39(4):687–96. doi: 10.1016/j.immuni.2013.08.019
49. Mackay LK, Wynne-Jones E, Freestone D, Pellicci DG, Mielke LA, Newman DM, et al. T-box transcription factors combine with the cytokines TGF-β and IL-15 to control tissue-resident memory T cell fate. Immunity (2015) 43(6):1101–11. doi: 10.1016/j.immuni.2015.11.008
50. Wakim LM, Smith J, Caminschi I, Lahoud MH, Villadangos JA. Antibody-targeted vaccination to lung dendritic cells generates tissue-resident memory CD8 T cells that are highly protective against influenza virus infection. Mucosal Immunol (2015) 8(5):1060–71. doi: 10.1038/mi.2014.133
51. Goplen NP, Wu Y, Son YM, Li C, Wang Z, Cheon IS, et al. Tissue-resident CD8+ T cells drive age-associated chronic lung sequelae after viral pneumonia. Sci Immunol (2020) 5(53):eabc4557. doi: 10.1126/sciimmunol.abc4557
52. Nelson CE, Foreman TW, Kauffman KD, Sakai S, Gould ST, Fleegle JD, et al. IL-10 suppresses T cell expansion while promoting tissue-resident memory cell formation during SARS-CoV-2 infection in rhesus macaques. bioRxiv (2022) 507852. doi: 10.1101/2022.09.13.507852
53. Son YM, Cheon IS, Wu Y, Li C, Wang Z, Gao X, et al. Tissue-resident CD4+ T helper cells assist the development of protective respiratory B and CD8+ T cell memory responses. Sci Immunol (2021) 6(55):eabb6852. doi: 10.1126/sciimmunol.abb6852
54. Jarjour NN, Wanhainen KM, Peng C, Gavil NV, Maurice NJ, Borges da Silva H, et al. Responsiveness to interleukin-15 therapy is shared between tissue-resident and circulating memory CD8+ T cell subsets. Proc Natl Acad Sci U S A. (2022) 119(43):e2209021119. doi: 10.1073/pnas.2209021119
55. Tieu R, Zeng Q, Zhao D, Zhang G, Feizi N, Manandhar P, et al. Tissue-resident memory T cell maintenance during antigen persistence requires both cognate antigen and interleukin-15. Sci Immunol (2023) 8(82):eadd8454. doi: 10.1126/sciimmunol.add8454
56. Depew CE, Rixon JA, McSorley SJ. Optimal generation of hepatic tissue-resident memory CD4 T cells requires IL-1 and IL-2. Proc Natl Acad Sci U S A. (2023) 120(16):e2214699120. doi: 10.1073/pnas.2214699120
57. Qiu Z, Khairallah C, Chu TH, Imperato JN, Lei X, ROmanov G, et al. Retinoic acid signaling during priming licenses intestinal CD103+ CD8 TRM cell differentiation. J Exp Med (2023) 220(5):e20210923. doi: 10.1084/jem.20210923
58. Cammack AJ, Moudgil A, Chen J, Vasek MJ, Shabsovich M, McCullough K, et al. A viral toolkit for recording transcription factor-DNA interactions in live mouse tissues. Proc Natl Acad Sci U S A. (2020) 117(18):10003–14. doi: 10.1073/pnas.1918241117
59. Sheth RU, Wang HH. DNA-based memory devices for recording cellular events. Nat Rev Genet (2018) 19(11):718–32. doi: 10.1038/s41576-018-0052-8
60. Cheon IS, Son YM, Sun J. Tissue-resident memory T cells and lung immunopathology. Immunol Rev (2023) 316(1):63–83. doi: 10.1111/imr.13201
61. Zhao Y, Kilian C, Turner JE, Bosurgi L, Roedl K, Bartsch P, et al. Clonal expansion and activation of tissue-resident memory-like Th17 cells expressing GM-CSF in the lungs of severe COVID-19 patients. Sci Immunol (2021) 6(56):eabf6692. doi: 10.1126/sciimmunol.abf6692
62. Cheon IS, Li C, Son YM, Goplen NP, Wu Y, Cassmann T, et al. Immune signatures underlying post-acute COVID-19 lung sequelae. Sci Immunol (2021) 6(65):eabk1741. doi: 10.1126/sciimmunol.abk1741
63. Yokoi T, Murakami M, Kihara T, Seno S, Arase M, Wing JB, et al. Identification of a unique subset of tissue-resident memory CD4+ T cells in Crohn’s disease. Proc Natl Acad Sci U S A. (2023) 120(1):e2204269120. doi: 10.1073/pnas.2204269120
64. Lund JM, Hladik F, Prlic M. Advances and challenges in studying the tissue-resident T cell compartment in the human female reproductive tract. Immunol Rev (2023) 316(1):52–62. doi: 10.1111/imr.13212
65. Marinaik CB, Kingstad-Bakke B, Lee W, Hatta M, Sonsalla M, Larsen A, et al. Programming multifaceted pulmonary T cell immunity by combination adjuvants. Cell Rep Med (2020) 1(6):100095. doi: 10.1016/j.xcrm.2020.100095
66. Lapuente D, Storcksdieck Genannt Bonsmann M, Maaske A, Stab V, Heinecke V, Watzstedt K, et al. IL-1β as mucosal vaccine adjuvant: the specific induction of tissue-resident memory T cells improves the heterosubtypic immunity against influenza A viruses. Mucosal Immunol (2018) 11(4):1265–78. doi: 10.1038/s41385-018-0017-4
67. Tan X, Sande JL, Pufnock JS, Blattman JN, Greenberg PD. Retinoic acid as a vaccine adjuvant enhances CD8+ T cell response and mucosal protection from viral challenge. J Virol (2011) 85(16):8316–27. doi: 10.1128/JVI.00781-11
68. Woodworth JS, Contreras V, Christensen D, Naninck T, Kahlaoui N, Gallouët AS, et al. A novel adjuvant formulation induces robust Th1/Th17 memory and mucosal recall responses in Non-Human Primates. bioRxiv (2023) 529651. doi: 10.1101/2023.02.23.529651
69. Lavelle EC, Ward RW. Mucosal vaccines - fortifying the frontiers. Nat Rev Immunol (2022) 22(4):236–50. doi: 10.1038/s41577-021-00583-2
70. Turley JL, Lavelle EC. Resolving adjuvant mode of action to enhance vaccine efficacy. Curr Opin Immunol (2022) 77:102229. doi: 10.1016/j.coi.2022.102229
71. Bachnak L, Godwin M, McLachlan JB. Assessing how biological sex effects tissue-resident memory T cell responses to influenza infection. J Immunol (2022) 208(1_Supplement):182.06. doi: 10.4049/jimmunol.208.Supp.182.06
72. Booth JS, Goldberg E, Patil SA, Barnes RS, Greenwald BD, Sztein MB. Age-dependency of terminal ileum tissue resident memory T cell responsiveness profiles to S. Typhi following oral Ty21a immunization in humans. Immun Ageing. (2021) 18(1):19. doi: 10.1186/s12979-021-00227-y
73. Overacre-Delgoffe AE, Hand TW. Regulation of tissue-resident memory T cells by the Microbiota. Mucosal Immunol (2022) 15(3):408–17. doi: 10.1038/s41385-022-00491-1
74. Szabo PA, Miron M, Farber DL. Location, location, location: Tissue resident memory T cells in mice and humans. Sci Immunol (2019) 4(34):eaas9673. doi: 10.1126/sciimmunol.aas9673
75. Humphries DC, O’Connor RA, Larocque D, Chabaud-Riou M, Dhaliwal K, Pavot V. Pulmonary-resident memory lymphocytes: pivotal orchestrators of local immunity against respiratory infections. Front Immunol (2021) 12:738955. doi: 10.3389/fimmu.2021.738955
76. Lim JME, Tan AT, Le Bert N, Hang SK, Low JGH, Bertoletti A. SARS-CoV-2 breakthrough infection in vaccinees induces virus-specific nasal-resident CD8+ and CD4+ T cells of broad specificity. J Exp Med (2022) 219(10):e20220780. doi: 10.1084/jem.20220780
Keywords: mucosal immunity, tissue-resident memory immune responses, infectious diseases, protection, vaccination
Citation: Longet S and Paul S (2023) Pivotal role of tissue-resident memory lymphocytes in the control of mucosal infections: can mucosal vaccination induce protective tissue-resident memory T and B cells? Front. Immunol. 14:1216402. doi: 10.3389/fimmu.2023.1216402
Received: 03 May 2023; Accepted: 28 August 2023;
Published: 11 September 2023.
Edited by:
Anukul T. Shenoy, University of Michigan, United StatesReviewed by:
Sophie Hillion, INSERM U1227 Lymphocytes B et Autoimmunite (LBAI), FranceCopyright © 2023 Longet and Paul. This is an open-access article distributed under the terms of the Creative Commons Attribution License (CC BY). The use, distribution or reproduction in other forums is permitted, provided the original author(s) and the copyright owner(s) are credited and that the original publication in this journal is cited, in accordance with accepted academic practice. No use, distribution or reproduction is permitted which does not comply with these terms.
*Correspondence: Stephane Paul, c3RlcGhhbmUucGF1bEBjaHUtc3QtZXRpZW5uZS5mcg==