- 1The First School of Clinical Medicine, Gannan Medical University, Ganzhou, China
- 2Department of Laboratory Medicine, First Affiliated Hospital of Gannan Medical University, Ganzhou, China
- 3Department of Medical School, Kunming University of Science and Technology, Kunming, China
- 4Department of Nephrology, First Affiliated Hospital of Gannan Medical University, Ganzhou, China
Renal cell carcinoma (RCC) represents an extremely challenging disease in terms of both diagnosis and treatment. It poses a significant threat to human health, with incidence rates increasing at a yearly rate of roughly 2%. Extracellular vesicles (EVs) are lipid-based bilayer structures of membranes that are essential for intercellular interaction and have been linked to the advancement of RCC. This review provides an overview of recent studies on the role of EVs in RCC progression, including involvement in the interaction of tumor cells with M2 macrophages, mediating the generation of immune tolerance, and assuming the role of communication messengers in the tumor microenvironment leading to disease progression. Finally, the “ troika “ of EVs in RCC therapy is presented, including engineered sEVs’ or EVs tumor vaccines, mesenchymal stem cell EVs therapy, and reduction of tumor-derived EVs secretion. In this context, we highlight the limitations and challenges of EV-based research and the prospects for future developments in this field. Overall, this review provides a comprehensive summary of the role of EVs in RCC and their potential as a viable pathway for the future treatment of this complex disease.
1 Introduction
Renal Cell Carcinoma (RCC) is among the most prevalent kind of kidney cancer, which accounts for approximately 90% of all occurrences. The American Cancer Society estimates that there will be roughly 76,080 cases reported and 13,780 deaths from RCC in the USA in the year 2021 (1). The prevalence of RCC has been progressively growing over the last several decades, and the level is predicted to continue to climb as a result of causes such as population aging and changes in lifestyle and environmental exposure. and it is expected to continue to rise due to factors such as population aging and changes in lifestyle and environmental exposure. Clear cell renal cell carcinomas (ccRCC) are the more frequent subtype, accounting for approximately 80-90% of all occurrences (2). In the early stage, many RCC patients have no obvious clinical symptoms and tumor markers are not sensitive to the early diagnosis of RCC, so about 16% of RCC patients already have metastasis at the time of diagnosis (3). The 5-year survival rate for early-stage RCC typically exceeds 90%, indicating a high proportion of patients survive within 5 years of treatment initiation. Surgical resection represents the most efficacious treatment modality for early-stage RCC, particularly when the disease is detected early. In contrast, the 5-year survival rate for advanced RCC is usually low, ranging between 30 and 40 percent (4). The choice of treatment and prognosis for advanced RCC is dictated by several factors, including tumor size, location, pathological type, and grade. However, the outlook for advanced RCC remained bleak, having only a 12% five-year survival rate for stage IV cancer (5). Moreover, existing therapies often cause significant side effects and are associated with high costs and risks of treatment resistance and disease relapse. Therefore, research on the diagnosis, disease progression, and treatment of RCC is crucial to save patients’ lives and improve their quality of life.
Extracellular vesicles (EVs) are small, membrane-encased nanoparticles that are purposely released into the extracellular milieu by cells (6). Initially, EV secretion is found in reticulocytes and is thought to be the mechanism for the removal of excess membrane proteins, therefore, corresponding studies were scarce; nonetheless, the varied and significant potential for EVs was brought to the fore when sEVs were discovered to carry potent biological molecules and play critical functions in communication between cells (7). According to MISEV2018, EVs have broadly classified into two types according to their sizes: sizes < 200 nm are called small extracellular vesicles (sEVs), and sizes > 200 nm are large extracellular vesicles (lEVs) (8). This review will be named using sEVs or LEVs according to the extracellular vesicle size of the original authors, and EVs will be used uniformly for cases where the size of the vesicles is not specified in the original text, or when some concepts are being described. EVs include a diverse range of bioactive compounds, such as proteins, nucleic acids, and lipids, which may be ingested by various cells and bestow a variety of regulatory effects. These particles are recognized as essential mediators of interaction between cells and continue to be a focus of study into their potential utility in diagnostic and therapeutic contexts for a variety of disorders, including cancer (9).
In RCC, EVs have been shown to have a vital function in tumor growth and metastases through their capacity to stimulate angiogenesis, evade detection by the immune system, and bolster drug resistance mechanisms (10). The accessibility of EVs from a multiplexity of biological fluids positions them as an appealing avenue for the non-invasive detection and monitoring of RCC (11). And because EVs, as lipid bilayer vesicles, can effectively protect their internal RNA from hydrolysis, their use as anti-cancer drug carriers, tumor vaccines, and therapeutic targets has received increasing attention from a wide range of researchers (12).
2 EVs in RCC deterioration
The tumor microenvironment is a complex environment made up of many kinds of cells, including stromal cells, fibroblasts, vascular endothelial cells, immune cells, and the extracellular matrix, all of which play an important role in tumor genesis and development (13). EVs, which are high in proteins, lncRNAs, and microRNAs, enable communication between cells in the tumor microenvironment and these EVs indirectly or directly contribute to the deterioration of RCC (Figure 1).
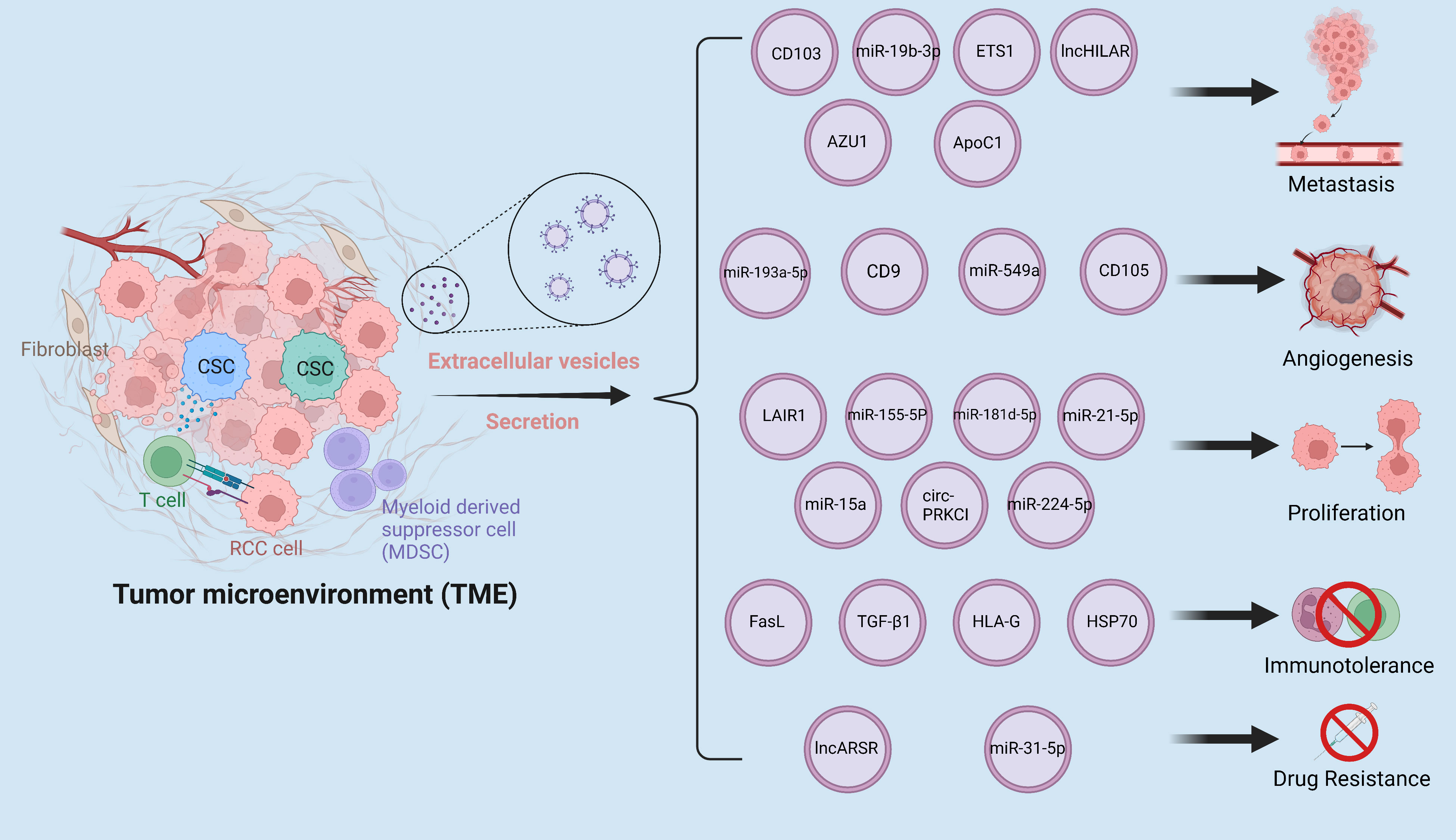
Figure 1 The tumor microenvironment is a complex milieu composed of various components that interact with one another through the secretion of EVs. These EVs facilitate the dissemination of pro-cancer cargoes, leading to enhanced metastasis, angiogenesis, proliferation, drug resistance, and immune evasion in RCC. The interplay of these components within the tumor microenvironment has been implicated in the progression and aggressiveness of RCC, highlighting the critical role of EVs in modulating tumor behavior.
2.1 RCC-Derived EVs in the immune system
2.1.1 EVs in the vicious circle of M2 macrophages and RCC cells
Tumor-associated macrophage (TAM) are macrophages that infiltrate tumor tissues and are derived from circulating peripheral blood mononuclear cells (14). Tumor cells secrete a plethora of chemokines, including colony-stimulating factor-1 and interleukins, to attract monocytes from the peripheral blood circulation into the tumor microenvironment (TME), ultimately inducing monocytes to differentiate into macrophages (15). Broadly speaking, TAMs primarily consist of classically activated macrophages (M1) and alternatively activated macrophages (M2), with M1 macrophages exhibiting anti-tumor actions and M2 macrophages supporting tumor development, invasion, as well as metastasis (16). Macrophages infiltrating in the periphery of malignant tumor tissues are mostly of M2 type, so many places define M2 form macrophages as TAM in a narrow sense. M0 macrophages are in an unactivated, immature state. M0 macrophages have the function of phagocytosis and removal of cellular debris, as well as the initiation of the inflammatory response. m1 and m2 macrophages have different roles in the immune response and inflammatory process, whereas m0 macrophages are in a neutral state and have undifferentiated characteristics. The switch from the M0/1 to M2 phenotype of macrophages may contribute to cancer development by boosting cell proliferation, metastasis, drug dependency, and immune evasion (17). Recent studies have shed light on the role of EVs in mediating this communication. Specifically, EVs secreted by RCC cells have been shown to promote a malignant cycle that drives tumorigenesis and metastasis. These EVs are taken up by TAMs, leading to changes in gene expression and altered cellular behavior. The next sections will mostly investigate the close interaction between M2 macrophages and RCC cells.
Huang et al. (18) reported that sEVs secreted by RCC cells contain markedly elevated levels of circSAFB2. These sEVs were observed to be internalized by macrophages, where they targeted miR-620 in spongy macrophages. This led to a significant increase in JAK1 and STAT3 protein expression, ultimately resulting in the activation of the JAK1-STAT3 pathway. Consequently, M2 polarization was promoted in macrophages. Importantly, the authors demonstrated through both in vivo and in vitro experiments that macrophages with elevated expression of circSAFB2 can, in turn, stimulate the proliferation, invasion, and in vivo metastatic ability of RCC cells. These findings provide novel insights into the intricate interplay between sEVs, macrophages, and RCC cells, highlighting the potential of circSAFB2 as a viable therapeutic target in the management of RCC. Moreover, RCC-derived sEVs exhibited high levels of long non-coding RNAs (lncRNAs) with pro-cancer effects, including lncARSR and AP000439.2. Notably, macrophages that received lncARSR delivered by tumor cells demonstrated a significant increase in CD163 and CD206 expression, along with a rise in anti-inflammatory cytokines TGFβ-1 and IL-10 secreted by macrophages. Conversely, the levels of pro-inflammatory cytokines IL-6, IL-12, and IL-1β decreased, indicating that macrophages underwent M2 polarization, a process thought to be mediated through the activation of the STAT3 signaling pathway (19). However, this study did not reveal the specific mechanism by which lncARSR exerts its role in promoting M2 polarization. In a separate study on lncARSR-mediated sunitinib resistance in RCC, lncARSR was found to act through the competitive binding of miR-34 and miR-449 (20). Nevertheless, the downstream molecular mechanisms of lncARSR during the occurrence of M2 polarization in macrophages require further research. Likewise, AP000439.2 was observed to induce M2 polarization by stimulating STAT3 phosphorylation in macrophages, activating the NF-κB signaling pathway, and subsequently triggering M2 polarization in macrophages upon exposure to AP000439.2. Ultimately, this process accelerated ccRCC migration (21).
The aforementioned studies have underscored the significance of RCC-derived EVs in driving M2 polarization of macrophages within the tumor microenvironment. We will now discuss several investigations that have explored the ability of M2 macrophages, activated by EVs, to promote RCC progression. In the hypoxic microenvironment, TAMs demonstrate a significant enrichment of miR-155-5P, which may be transmitted to RCC cells via sEVs. miR-155-5P plays a crucial role in promoting the activation of the PI3K/AKT signaling cascade by enhancing the mRNA stability of insulin-like growth factor 1 receptor (IGF1R) through the human antigen R (HuR) pathway. Ultimately, this contributes to the malignant transformation of RCC cells. Remarkably, this represents a rare example of a miRNA exerting a positive regulatory effect on its target gene (22). In a study by Zhang et al. (23), M2 macrophages were found to transmit miR-21-5p to RCC cells through sEVs. This miRNA targets the 3’ untranslated regions (3’UTRs) of PTEN, an oncogene encoding PIP3 phosphatase, which dephosphorylates PIP3 to PIP2, thereby exerting negative regulation over the PI3K/Akt signaling pathway. Upon PTEN downregulation, Akt signaling is overactivated, ultimately promoting the proliferation, invasion, and metastasis of RCC cells. Liu et al. (24) revealed that hypoxia-inducible factor 1A (HIF1A) was markedly upregulated in TAMs. HIF1A binds to hypoxia-response element 2 (HRE2) in the miR-193a promoter region, thereby initiating the transcription of miR-193a-5p in TAMs. Subsequently, the upregulated miR-193a-5p is sorted into sEVs by TAMs. Upon uptake by tumor cells, the highly expressed miR-193a-5p promotes tumor angiogenesis, invasion, and proliferation by sponge-adsorbing tissue inhibitor of metalloproteinases 2 (TIMP2).
The intricate crosstalk between RCC cells and TAMs, mediated by EVs, creates a vicious cycle that fuels tumorigenesis and fosters malignant transformations within the tumor microenvironment. RCC cells secrete EVs that activate TAMs, which in turn secrete EVs containing oncogenic factors that promote the proliferation, invasion, and metastasis of RCC cells. This reciprocal communication between RCC cells and TAMs not only exacerbates the malignant phenotype of RCC but also contributes to the formation of an immunosuppressive tumor microenvironment, thereby facilitating tumor immune evasion and therapy resistance (Figure 2).
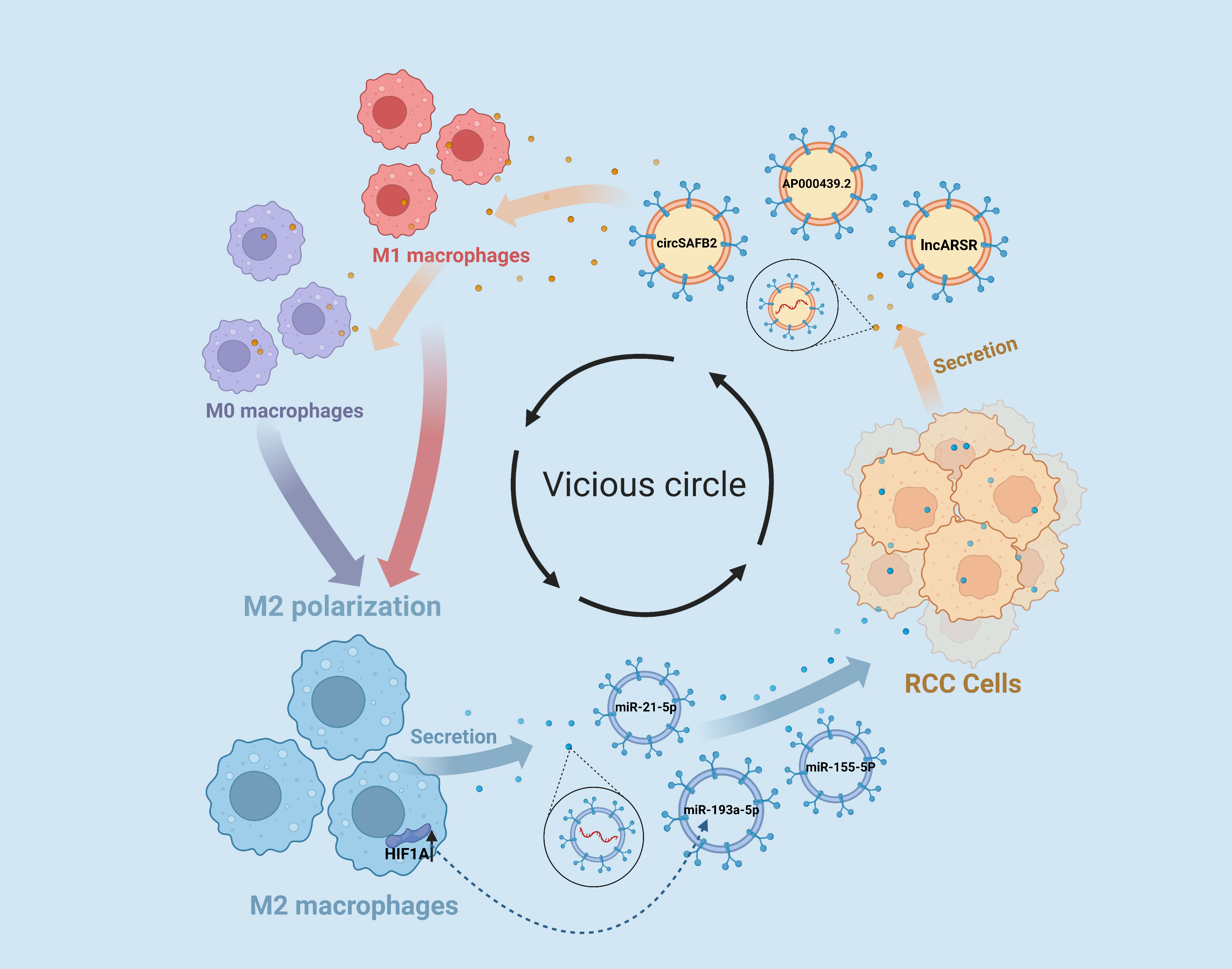
Figure 2 EVs Contribute to the Vicious Cycle of RCC-M2 Macrophage Interactions. RCC cells release extracellular vesicle EVs enriched with the lncARSR and AP000439.2, as well as the circular RNA circSAFB2, which play a significant role in promoting M2 macrophage polarization within the tumor microenvironment. Furthermore, M2 macrophages can reciprocate by releasing EVs containing microRNAs miR-193a-5p, miR-21-5p, and miR-155-5p that promote malignant transformations in RCC cells.
2.1.2 Involvement in immune escape
Tumor cells can present tumor-specific antigens, which are recognized by various immune cells, allowing the immune system of the body to recognize and remove cancerous cells. Tumor cells, on the other hand, can modify their surface antigens to avoid immune detection, which enables tumor progression and metastasis (25). In addition, recent studies have shown that tumor-derived EVs can exert immunosuppressive effects by inhibiting the immune system, thereby promoting tumor immune evasion and facilitating tumor progression (26). These findings highlight the critical role of tumor-derived EVs in shaping the tumor microenvironment and regulating the immune response and suggest that targeting EVs-mediated immunosuppression may represent a promising therapeutic strategy for cancer treatment.
Wang et al. have demonstrated that tumor cell-derived sEVs are pivotal in driving immune escape and drug resistance in ccRCC via a complex interplay of signaling pathways, including mTOR, ERK, STAT, and NF-κB. However, the specific cargo molecules within these sEVs that are responsible for this biological effect remain unknown (27). It was found that RCC cell-derived sEVs can significantly suppress the secretion of cytokines, such as interleukin-2 and interferon-gamma, by T cells, and in addition, the presence of Fas ligands within these sEVs can induce T cell apoptosis through binding with Fas, thereby promoting immune evasion by tumor cells (28). Despite the crucial role of NK cells in tumor immunity, their anti-tumor activity can be impeded by impaired degranulation and cytokine secretion in TGF-β1-enriched tumor microenvironments, which Xia et al. (29) demonstrated can be induced by TGF-β1 transported by RCC cell-derived sEVs through the phosphorylation of Smad2 and Smad3, resulting in defective NK cell function. CD105+ renal cancer stems cells-derived sEVs serve as crucial communication molecules that impede the differentiation and maturation of monocytes into dendritic cells (DCs). This inhibition of DC differentiation has been shown to suppress the proliferation of CD3+ T lymphocytes in the TME, resulting in immunological escape. The identification of human leukocyte antigen-G (HLA-G) as a key factor in this process suggests that targeted therapies aimed at disrupting the sEV-mediated delivery of HLA-G may hold promise for enhancing the effectiveness of anti-tumor immune responses in the context of RCC (30). Further studies are needed to elucidate the precise mechanisms underlying the immunosuppressive effects of sEVs and to identify additional targets for therapeutic intervention. Gao (31) and Diao (32) independently reported that RCC cell-derived sEVs had high expression of heat shock protein 70 (HSP70), which is important in the formation of immunological tolerance. In their studies, HSP70 was found to act through two distinct mechanisms. The first mechanism involves initiating antigen-specific immunosuppression of myeloid-derived suppressor cells (MDSCs) in a toll-like receptor 2 (TLR2)-dependent manner, leading to antigen-specific immunosuppression of cytotoxic T lymphocytes (CTLs). The second mechanism involves stimulating the STAT3 signaling pathway to promote MDSCs expression, eventually culminating in immune escape. These findings highlight the critical role of RCC cell-derived sEVs in modulating the immune microenvironment and suggest that HSP70 may be a promising therapeutic target for the development of novel RCC immunotherapies.
2.2 Mediating epithelial-mesenchymal transition
The epithelial-mesenchymal transition (EMT) is a crucial phase in cancer cell metastasis in which epithelial traits are lost and mesenchymal qualities are acquired, ultimately conferring stronger invasive capabilities, stem-cell properties, immunosuppressive abilities, and drug resistance upon cancer cells (33). Several studies have established that the dissemination of cancer-derived EVs is critical in inducing EMT (34).
Wang et al. (35) found that RCC stem cells secrete more CD103+ sEVs, and these CD103+ sEVs fulfill a “pathfinder” role, leading RCC cells to diverse metastatic sites. Notably, CD103+ deficiency severely impacts the RCC cells’ capacity to colonize distant organs. Concurrently, these sEVs serve as carriers of miR-19b-3p to target recipient cells and orchestrate EMT in ccRCC by targeting PTEN, thus heightening RCC cells’ metastatic capacity. Li et al. (36) observed an abnormal increase in the level of apolipoprotein C1 (ApoC1) in ccRCC, with survival rate negatively correlated to ApoC1 levels. They further showed that ApoC1 induces EMT in ccRCC via massively stimulating STAT3 activation. Importantly, in this process, sEVs carry ApoC1 from ccRCC cells to vascular endothelial cells and induce EMT via STAT3 activation, consequently boosting the proliferative, invasive, and metastatic abilities of ccRCC cells. Additionally, Jin et al. (37) discovered that sEVs derived from RCC cells transmit metastasis-associated lung adenocarcinoma transcript 1 (MALAT1), which orchestrates TFCP2L1 expression by modulating the transcription factor ETS1, ultimately promoting EMT in RCC cells.
In conclusion, we have summarized the important role of EVs in regulating EMT in RCC. However, it is vital to acknowledge that the regulation of EMT is a multifaceted process that is intricately linked to the entire process of tumor development, involving a complex interplay of diverse factors and signaling pathways (38). Notably, despite the growing recognition of the importance of EVs in EMT induction in RCC, the number of studies exploring this phenomenon remains limited. Therefore, future research endeavors should aim to broaden our understanding of the complex interactions between EVs and other factors involved in EMT regulation, rather than limiting the focus to a single cargo of EVs. Such a holistic approach would provide a more comprehensive understanding of RCC progression and metastasis, ultimately paving the way for the development of novel therapeutic strategies to curtail RCC metastasis and improve patient outcomes.
2.3 Promotes proliferation, invasion, and migration
In the TME, EVs of cancer-associated fibroblasts (CAFs) play a significant part in the development of RCC. Fu et al. (39) demonstrated that sEVs released by CAFs are effectively internalized by RCC cells. These sEVs were found to enhance the invasive properties of RCC cells by upregulating proteins implicated in metastasis, including fibronectin, N-cadherin, vimentin, MMP9, and MMP2. Furthermore, these sEVs were shown to modulate cell cycle progression, enhancing the proliferative and anti-apoptotic capacities of RCC cells unfortunately, they did not specify which cargoes in sEVs mediated these functions. Ding et al. (40) found that sEVs delivered by CAFs were enriched with miR-181d-5p. These CAF-derived sEVs were effectively internalized by RCC cells, where the high levels of miR-181d-5p led to the activation of the Wnt/β-catenin signaling pathway. Specifically, miR-181d-5p was found to bind to the 3′-UTR of the tumor suppressor RNF43, resulting in the downregulation of its protein expression. This event ultimately resulted in enhanced cancer stemness and tumor growth. Additionally, sEVs derived from CAFs are capable of delivering miR-224-5p to ccRCC cells, thus contributing to their malignant changes (41). These findings underscore the critical role played by CAF-derived EVs in RCC pathogenesis. However, further studies are warranted to unravel the intricate mechanisms underlying the functional interplay between CAF-derived EVs and RCC cells and to identify specific cargo molecules that can serve as potential therapeutic targets.
In RCC, Cancer-derived sEVs have been demonstrated to downregulate hepatocyte cell adhesion molecule (hepaCAM) expression in RCC in a p-AKT-dependent way (42). According to a previous study by Zhang et al. (43), hepaCAM was discovered to arrest RCC cells in the G1 stage of the cell cycle, resulting in cell proliferation suppression, and thus RCC-derived sEVs-mediated downregulation of hepaCAM may be associated with excessive proliferation of RCC cells (42). Moreover, highly malignant RCC cells can pass circ-PRKCI to less malignant RCC cells via sEVs, and transferred circ-PRKCI acts as a sponge for miR-545-3p, increasing the expression of Cyclin D1 (CCND1), a cell cycle regulator associated with cell proliferation in a variety of tumor cells, and overexpression of CCND1 ultimately increased proliferation, migration and invasion of RCC cells (44). Cell-secreted EVs in the tumor microenvironment have been shown to play an important role in the PI3k/AKT signaling pathway, promoting the malignant progression of RCC (Figure 3). For instance, Li et al. (45) discovered that sEVs generated by RCC cells can regulate the activity of RCC cells via miR-15a. B-cell translocation gene 2 (BTG2), a miR-15a downstream gene, had a negative connection with miR-15a expression. RCC cells release sEVs containing miR-15a, which stimulates the PI3K/AKT signaling pathway by inhibiting BTG2 expression, hence speeding RCC proliferation. In another insightful study conducted by Jingushi et al. (46) immunoglobulin-like receptor 1 (LAIR1) was significantly enhanced in tumor-derived EVs and capable of increasing RCC cell proliferation and development of tumors via AKT phosphorylation.
2.4 EVs in hypoxic microenvironments
Many solid tumors, including renal cell carcinoma (RCC), are characterized by a hypoxic microenvironment, which is a hallmark of the tumor microenvironment and is closely associated with cancer progression and poor prognosis (47). Hypoxia in the tumor microenvironment triggers a complex network of molecular pathways, such as hypoxia-inducible factor signaling, to promote tumor growth, angiogenesis, and metastasis (48). In RCC, the hypoxic microenvironment is particularly notable due to the high metabolic demands of the tumor and the limited vascularization in the surrounding tissue (49). In this context, EVs derived from hypoxic cancer cells have been implicated in promoting RCC progression and metastasis by transferring oncogenic cargoes, including miRNAs and proteins, to recipient cells. Notably, these hypoxia-induced EVs have been shown to modulate key biological processes, including angiogenesis, immune evasion, and metastasis, with significant implications for RCC pathogenesis.
As previously mentioned, in the hypoxic tumor microenvironment, TAMs have been shown to deliver miR-155-5p to RCC cells via sEVs. This miRNA has been shown to enhance the stability of IGF1R mRNA via the RNA-binding protein HuR, thereby promoting the activation of the PI3K/AKT signaling cascade (22). There have been additional reports concerning miR-155 and its role in response to hypoxic conditions. In RCC, sEVs secreted by hypoxic RCC cells were found to be 2.03-2.37 times higher than those secreted under normoxic conditions. Furthermore, sEVs secreted by RCC cells were found to contain higher levels of miR-155. This miRNA was shown to downregulate the expression of tumor suppressor genes p15, p21, and Gadd45, as well as apoptosis-related genes Bim and TRAIL, by targeting the phosphorylation of Forkhead Box O3 (FOXO3) in normoxic RCC cells (50). This mechanism inhibited cell apoptosis while promoting cell migration and proliferation. Samoylenko et al. (51) employed time-gated Raman spectroscopy (TG-RS) to study the sEVs generated by RCC cells in a hypoxic tumor microenvironment. This innovative technique enabled the authors to achieve improved signal-to-noise ratios, allowing for a more robust analysis of the sEVs ‘ protein content. They reported a significant upregulation of cell adhesion and CD9 proteins in these sEVs, suggesting their potential involvement in RCC tumor progression. These findings highlight the utility of advanced spectroscopic techniques in characterizing the complex molecular landscape of sEVs and their role in modulating RCC pathogenesis and can be applied to other cancers as well. RCC cells residing in hypoxic microenvironments secrete sEVs containing hypoxia-induced LncRNA associated with RCC (lncHILAR). Upregulation of lncHILAR in RCC cells is largely attributed to aberrant methylation and acetylation following hypoxic exposure. The uptake of these sEVs by normoxic RCC cells leads to the upregulation of their target gene CXCR4. This process occurs not only by sponging miR-613/206/1-1-3p but also indirectly via the activation of the target Jagged1/Notch pathway. The elevated CXCR4 levels ultimately promote the metastatic capacity of RCC cells (52). Moreover, in response to the hypoxia-induced by rapid proliferation, hypoxic RCC cells were found to secrete increased levels of small extracellular vesicles (sEVs) containing carbonic anhydrase 9 (CA9) (53). CA9 is known for its high expression in the hypoxic microenvironment of many solid tumors and is believed to play a crucial role in promoting angiogenesis. In RCC, these sEVs are thought to facilitate angiogenesis by transporting CA9 in response to nutrient deprivation in the hypoxic microenvironment (54). In summary, EVs hold immense potential as therapeutic targets for RCC, especially in the context of the hypoxic tumor microenvironment. Further investigations are warranted to elucidate the precise mechanisms underlying the functional interplay between hypoxia-induced EVs and recipient cells, with a focus on identifying specific cargo molecules that can be targeted to curtail RCC progression and improve patient outcomes.
2.5 Pro-angiogenic effects or altered vascular permeability in tumor development
The formidable proliferative capacity of tumor cells necessitates an extensive amount of oxygen and nutrients which can initially rely on diffusion when the tumor volume is less than 2 mm3. However, as the tumor size increases, a hypoxic microenvironment will form, and the tumor must produce new blood vessels to obtain sufficient oxygen (55). This angiogenesis process optimizes the supply of nutrients to support tumor growth, while the accompanying altered vascular permeability establishes a pre-metastatic ecological niche, thus promoting cancer’s metastatic spread (56).
Lindoso et al. (57) revealed that mesenchymal stem cells (MSCs), upon uptake of EVs derived from RCC stem cells, exhibited expression elevated of MMP1, MMP3, and CXCR4, promoting angiogenesis and facilitating tumor growth in vivo. Nevertheless, the precise substance responsible for this effect remains unknown. Subsequently, sEVs secreted from tyrosine kinase inhibitor (TKI) -resistant RCC cells were found to have a low expression of miR-549a, leading to elevated expression of hypoxia-inducible factor-1α (HIF1α) in vascular endothelial cells. The resultant increase in tumor angiogenesis and enhanced vascular permeability contributes to the creation of a pre-transfer ecological position, facilitating tumor cells to initiate metastasis post-acquiring TKI resistance in ccRCC (58). CD105+ sEVs secreted by RCC stem cells contain numerous pro-angiogenic factors including VEGF, FGF2, ephrin A3, and angiopoietin 1, as well as mRNAs associated with stimulating angiogenesis like MMP2 and MMP9. CD105+ sEVs have a pivotal role in promoting tumor angiogenesis and establishing pre-metastatic ecological niches by delivering these mRNAs (59). Earlier, Jingushi et al. (60) extracted EVs from RCC tissue samples and compared them to EVs from adjacent normal kidney tissue. They found that tumor tissue-derived sEVs were rich in azurocidin 1 (AZU1) and effectively altered vascular endothelial cell layer permeability. In a follow-up study (61), it was established that AZU1 is packaged into sEVs via N-linked glycosylation, which alters vascular endothelial cell layer permeability by elevating intracellular Ca2+ concentrations. These findings suggest that AZU1 glycosylation inhibition could be a promising RCC treatment strategy.
2.6 Induces development of therapeutic resistance
Tumor drug resistance has been a longstanding challenge in the treatment of various malignancies. With the increasing focus on EVs, increasing data shows that they exert a significant part in transferring drug resistance among tumor cells (62). Advanced RCC, in particular, is highly vulnerable to drug resistance, which significantly impacts patient survival quality. Around twenty percent of terminal RCC patients are unresponsive to TKI treatment (e.g., sunitinib and sorafenib), while patients who display early treatment response often develop drug resistance, resulting in tumor development after 15 months of treatment (63).
The propagation of drug resistance between resistant and sensitive cells is a complex process governed by intercellular communication mechanisms, with EVs playing a critical role. These EVs serve as vehicles for the transfer of bioactive molecules, including proteins, lipids, and nucleic acids, between cells in the tumor microenvironment, facilitating the spread of drug resistance. In our previous discussion, we highlighted the ability of lncARSR to mediate the M2 polarization of macrophages via sEVs in RCC. In this section, we will discuss the research conducted by Qu et al. on the association between lncARSR and sunitinib resistance in RCC. Qu et al. (20) observed that activated AKT in sunitinib-resistant RCC cells downregulates FOXO1 and FOXO3a, which subsequently activates the transcription of lncARSR, resulting in its high expression in drug-resistant cells. The 5’ end of lncARSR is sorted into sEVs by binding to heterogeneous nuclear ribonucleoprotein A2B1 (hnRNPA2B1). Upon uptake by sunitinib-sensitive RCC cells, these sEVs upregulate the levels of lncARSR, which acts as a sponge for miR-34 and miR-449 in target cells, thereby blocking their biological functions. Qu et al. found that miR-34 and miR-449 can target and inhibit AXL and c-MET. Consequently, the upregulation of lncARSR ultimately upregulates AXL and c-MET in sunitinib-sensitive cells, leading to the development of sunitinib resistance. Furthermore, the overactivation of AXL and c-MET is known to activate the STAT3, AKT, and ERK signaling pathways, which are also activated by the AKT signaling pathway that upregulates lncARSR in drug-resistant RCC cells. This creates a positive feedback loop, resulting in continuous transcription of lncARSR and its sorting into sEVs, leading to the widespread propagation of sunitinib resistance. Moreover, it has been observed that RCC cells treated with sunitinib and axitinib secrete a higher number of sEVs, including both large and small vesicles. In contrast, RCC cells treated with tyrosine kinase inhibitors display high levels of glucose transporter 1 (GLUT1) sorted into sEVs, which leads to increased uptake of glucose and enhanced glycolytic activity (64). This phenomenon may contribute to the development of drug resistance mechanisms in RCC. In a recent study, He et al. (65) discovered that sorafenib-resistant RCC cells secrete sEVs that contain high levels of miR-31-5p. They further demonstrated in vitro and in vivo that miR-31-5p plays a pivotal role in inducing sorafenib resistance. Upon uptake by target cells, the overexpression of miR-31-5p within the EVs promotes drug resistance by targeting the expression of MutL homolog 1 (MLH1).
Understanding the molecular mechanisms underlying EVs-mediated drug resistance and metabolic reprogramming is crucial for developing effective therapies for RCC. Targeting EVs and their cargo molecules, including lncARSR and miR-31-5p, may represent promising therapeutic strategies to overcome drug resistance and improve the efficacy of RCC treatments. Further studies are warranted to elucidate the precise mechanisms involved in EVs-mediated drug resistance and metabolic reprogramming in RCC and other cancers.
3 Advancements in the diagnosis of RCC via EVs
Non-invasive or minimally invasive diagnosis methods of RCC are highly desirable, and an increasing number of studies have identified cargo-containing EVs as promising biomarkers for the early identification of RCC. For example, Horie et al. (66) conducted a study to investigate the clinical relevance of sEVs’ γ-glutamyl transpeptidase (GGT) activity as a possible biomarker for RCC diagnosis and prognosis. The authors discovered that serum sEVs’ GGT expression was considerably more elevated in patients with advanced RCC and microvascular infiltration, implying that it may be used as a clinical marker for advanced clinical and pathological features in RCC patients. Furthermore, the preoperative serum sEVs’ GGT assay combined with pathological examination of resected specimens during surgery could predict the possibility of tumor invasion of microvessels, opening up new possibilities for RCC evaluation and long-term monitoring. Xp11.2 translocation renal cell carcinoma (Xp11 tRCC) is an uncommon sporadic child RCC for which early detection methods are still lacking. Kurahashi et al. (67) constructed a mouse model of Xp11 tRCC and found that miR-204-5p levels were significantly higher in urinary sEVs of the Xp11 tRCC mouse model than in controls. They thus proposed that miR-204-5p in urine sEVs might be a promising preliminary diagnostic biomarker for Xp11 tRCC. Niu et al. (68) developed a novel sEVs-associated lncRNA model based on the Bayesian spike-and-slab lasso method, prognostic levels of ccRCC patients were successfully predicted by several lncRNAs and highlighting its potential as a prognostic marker for RCC. Nevertheless, the utilization of sEVs-contained cargoes as biomarkers for RCC diagnosis and prognosis poses significant challenges compared to traditional tumor markers, imaging, and pathological diagnosis. The current limitations of sEVs extraction technology and extraction cost further complicate the clinical application of sEVs-carried cargo as tumor markers. Therefore, urgently needed are the development of techniques for large-scale and rapid extraction and purification of sEVs from human fluids, and the establishment of uniform standards for sEVs cargo analysis. Given these challenges, there is still a long way to go before EVs cargoes can be used as reliable markers for RCC. To summarize some of the promising EVs cargoes that could potentially serve as candidate RCC markers, we have provided a table (Table 1) instead of going into further details.
4 The “Troika” of EVs in the treatment of RCC
Recent research has demonstrated that EVs have the potential to be used as a cell-free therapy in the treatment of RCC. EVs can be engineered to deliver drugs or nucleic acids to target cells or to modulate immune responses against tumor cells. Furthermore, In preclinical investigations, EVs produced from anti-tumor cells, including mesenchymal stem cells, showed promising outcomes in battling RCC development and metastasis. Finally, targeting the secretion of tumor-derived EVs represents a promising strategy for slowing RCC development (Figure 4).
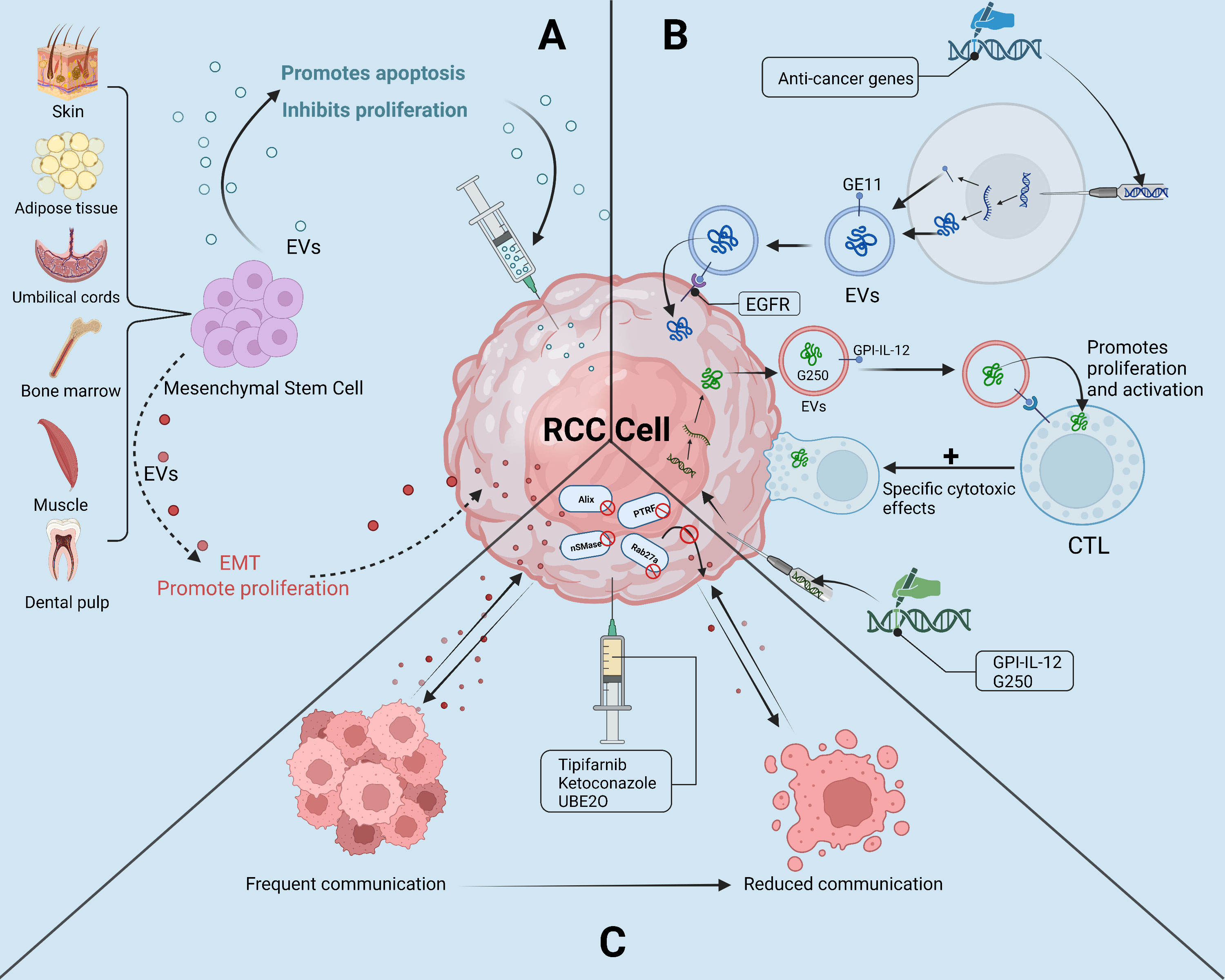
Figure 4 The “troika” of EVs in the treatment of RCC. (A): Mesenchymal Stem Cell-derived EVs as a “double-edged sword” in the treatment of RCC; (B): Potential of engineered EVs and EVs tumor vaccines in the treatment of RCC; (C): By selectively reducing the secretion of tumor-derived EVs, the reduction of RCC cells with tumor microenvironment or the ability of other tumor cells to communicate with.
4.1 Engineered sEVs and tumor vaccines
The engineering of sEVs offers a versatile platform for tailoring the properties of sEVs to enhance their targeting specificity for tissues and cells, and to load them with therapeutic cargoes for improving disease treatments (77). Engineering sEVs has emerged as a promising approach to improve the safety and effectiveness of sEVs-based therapeutics in cancer and other diseases (78–80). Through surface modifications or the incorporation of therapeutic moieties, engineered sEVs can achieve precise delivery of therapeutics, thus enhancing their selective accumulation at the tumor site, while reducing non-specific dissemination throughout the body (81). These advancements have highlighted the enormous potential of engineered sEVs for safe, targeted, and effective drug delivery, paving the way for the development of more advanced and personalized therapies (82).
Adem et al. (83) performed experiments with engineered sEVs in ccRCC. Specifically, the authors incorporated a peptide named GE11 onto sEVs that were being secreted by 293T cells to specifically target the abnormally high levels of EGFR in ccRCC cells. Remarkably, the engineered sEVs overexpressing GE11 were shown to be preferentially absorbed by ccRCC cells rather than normal kidney cells, thus providing a specific delivery system for anticancer drugs. Although unfortunately, this article does not document more experimental results and detailed information, it is still a very interesting point about engineered sEVs that may be an excellent cell-free strategy for the therapy of many diseases, including RCC. Further research is necessary to fully elucidate the potential of this approach and determine its clinical feasibility.
In addition, it has been shown that by combining the immunomodulatory function of sEVs with their drug carrier properties, engineered sEVs can serve as potent tumor vaccines that can stimulate anti-tumor immune responses alongside chemotherapy (84). For example, Zhang et al. (85) constructed a mammalian co-expression plasmid of glycolipid-anchored-IL-12 (GPI-IL-12) and transfected GPI-IL-12 into RCC cells so that its secreted sEVs expressed GPI-IL-12 and renal cell carcinoma-associated antigen G250, this sEVs exhibited excellent antitumor effects, and its strong immunogenicity can exacerbate the cytotoxic effect on tumor cells by inducing the generation of antigen-specific cytotoxic T lymphocytes. In addition, RenCa cells secreted sEVs (RDE) can stimulate an increase in the proportion of CD8+ T cells by activating the FasL/Fas signaling pathway, and the cytotoxic effect of CD8+ T cells is enhanced after ingestion of RDE, which has specific cytotoxicity to RCC cells, this experimental result is expected to promote the development of RCC vaccine (86).
Although there are still few studies on engineered sEVs and sEVs tumor vaccines in RCC, such efforts offer great potential for advancing the field of personalized medicine for RCC treatment. By highlighting these critical aspects of research, we aim to promote further breakthroughs in engineered sEVs and sEVs tumor vaccine development for RCC.
4.2 Mesenchymal stem cell-derived EVs therapy
Because of the anti-inflammatory and immunomodulatory effects exhibited in preclinical and clinical research, mesenchymal stem cell-derived EVs (MSCs-EVs) have emerged as a viable strategy for cell-free therapy in a variety of disorders (87). In comparison with traditional MSCs-based therapies, the advantages of MSCs-EVs in terms of safety, stability, and preservation have provided a strong impetus for the development of MSCs-EVs-based treatments for a variety of disorders, including cancer (88), Covid-19 (89), neurological disorders (90), spinal cord injuries (91), and another kidney disease (92). These investigations have emphasized the promise of MSCs-EVs as a cell-free alternative to MSCs-based treatments, offering efficient and safer effects, and pointing to an exciting new avenue for regenerative medicine (93).
Li et al. (94) reported that intravenous administration of human umbilical cord mesenchymal stem cell sEVs (hucMSC-sEVs) in an animal model of RCC showed enhanced T cell-mediated immune responses against ccRCC via upregulation of miR-182, which inhibited VEGFA expression and delayed ccRCC progression. Similarly, Fonsato et al. (95) demonstrated that co-treatment with sEVs secreted from human liver stem cells (HLSC-sEVs) and TKI enhanced the anticancer efficacy of TKI by inhibiting the mTOR signaling pathway. Moreover, further studies revealed that HLSC-derived sEVs harbored abundant anticancer miRNAs, including miR-Let7b, miR-200b, miR-200c, and miR-223, which greatly suppressed the proliferation, invasiveness, and promoted apoptosis of RCC stem cells in vitro. Treatment with HLSC-sEVs was found to effectively inhibit tumor growth and angiogenesis by delivering miR-145, which downregulates EGFR and MMP1. The treatment also demonstrated the ability to attenuate tumor metastasis to the lung and significantly improve survival in a mouse model of RCC stem cell induction (96). These studies highlight the curative value of EVs produced from diverse stem cells as innovative RCC therapy techniques and offer new avenues for the development of cell-free therapies that target RCC.
However, stem cell EVs therapy is also a double-edged sword, as MSCs-EVs have also been found to have pro-tumor effects in many tumors. This may be caused by the source of the EVs cargoes or MSCs. For instance, the miR-21-5p carried by MSCs-sEVs induces the production of M2 polarization by targeting PTEN in macrophages, leading to the suppression of immune function in lung cancer (97). miR-301b-3p in MSCs-EVs has been shown to contribute to multidrug resistance in gastric cancer cells (98), and miR-193a-3p, miR-210-3p, and miR-5100 carried by bone marrow mesenchymal stem cells-derived sEVs can contribute to the progression of lung cancer by promoting EMT (99). Although the precise mechanisms underlying the dual role of MSCs-EVs remain unclear, reducing the potential risks associated with MSCs-EVs therapies in cancer will be a critical focus of future research.
Due to the potential risk of MSCs-EVs promoting cancer, which may be associated with the cargo they carry, current research aims to reduce the potential risks associated with using MSCs-EVs as a cancer treatment. One approach to achieving this is to artificially package drugs into MSCs-EVs, thereby using them as drug delivery systems (100). Studies have shown that MSCs-EVs have excellent tumor-targeting capabilities, and due to their low immunogenicity, they are not cleared by the immune cells. For example, Bagheri et al. (101) used electroporation to package doxorubicin into MSCs-sEVs and found that the drug-carrying MSCs-sEVs could effectively and safely target and kill colon cancer cells in mice. Melzer et al. (102) pretreated MSCs with paclitaxel before extracting MSCs-sEVs, allowing paclitaxel to be encapsulated into sEVs. Although the concentration of paclitaxel in MSCs-EVs was not ideal, compared with untreated MSCs-sEVs, paclitaxel-containing MSCs-EVs showed effective tumor-targeting properties and cytotoxicity in breast, lung, and ovarian cancer.
To enhance the tumor-specific targeting ability of MSCs-sEVs, it is necessary to combine MSCs-sEVs with engineered EVs technology. Using genetic engineering techniques, highly expressed factors in different tumors can be fixed on the membrane structure of MSCs-sEVs, and different targeting molecules can be selected according to different types of cancer (103). By artificially packaging anticancer drugs into MSCs-sEVs, it is theoretically possible to achieve a safe and effective MSCs-EVs cell-free anti-tumor therapy. However, it is still necessary to further understand the complex interactions between tumor cells and MSCs-EVs, which is critical for developing safer and more effective treatment strategies.
4.3 Reduced secretion of tumor-derived EVs
Tsuruda et al. (104) observed RAB27B overexpression in sunitinib-resistant RCC cells, suggesting its role in conferring drug resistance. Additionally, RAB27B is essential in the biogenesis of sEVs. Although the knockdown of RAB27B reduced the viability of RCC cells, it had little effect on sEVs secretion. However, this observation raises significant implications for a potential therapeutic target in RCC through two distinctive pathways. First, as mentioned earlier, the knockdown of RAB27B directly inhibits the aggressive and proliferative properties of RCC cells. Secondly, previous studies have demonstrated that tumor-secreted sEVs significantly contribute to the progression of RCC. By suppressing the production of sEVs through the inhibition of RAB27B or other sEVs-related proteins, it is possible to weaken or disrupt the communication between RCC cells and neighboring cells in the TAM. Experiments performed by other researchers have quickly validated this perspective.
Greenberg et al. (105) have conducted several studies on drug combination therapy for sEVs-associated RCC, and their findings reveal that the combination of ketoconazole and sunitinib increases the anticancer effect of sunitinib. Ketoconazole selectively reduces sEVs formation and transport in RCC cells by targeting the ERK signaling pathway to inhibit proteins associated with sEVs production and secretion in RCC cells, thereby inhibiting the proliferation of RCC cells and enhancing the anticancer efficacy of sunitinib. Another study found that sunitinib-resistant RCC cell-derived sEVs had greater amounts of PD-L1, which promoted the death of T cells by PD-1 and PD-L1 interactions and led to immunological tolerance. Similarly, tipifarnib suppressed sEVs formation and release via ESCRT-dependent along with non-dependent routes, while also exhibiting synergistic interactions with sunitinib to combat drug resistance and cause death in resistant cells (106). Taken together, these studies suggest that ketoconazole or tipifenib has the potential to act as an adjuvant to reduce the secretion of tumor-derived sEVs during the treatment of RCC in combination with sunitinib. Furthermore, the increased expression of Polymerase I and Transcript Release Factor (PTRF) associated with ccRCC cells and the sEVs they secrete, so the tumors secreted more sEVs compared to normal tissue, and Cen et al. (107) have demonstrated that Ubiquitin-conjugating enzyme E2O (UBE2O) can reduce the number of sEVs secreted by ccRCC cells by ubiquitinating PTRF, thus reducing caveolae formation which is involved in sEVs biogenesis. By suppressing the release of EVs by cancer cells, downstream signaling pathways that promote RCC growth, metastasis, drug resistance, and immune evasion may be disrupted. Therefore, this approach holds great potential for developing effective therapies for RCC patients.
5 Summary
EVs are found in nearly all body fluids and play a significant part in numerous biological functions in humans. This study gives a review of the developments achieved in the knowledge of EVs in the etiology and therapy of RCC. Tumor cells can exchange substances with the microenvironment and neighboring cells through their secreted EVs. These sEVs can significantly affect the microenvironment, suppress the immune system while promoting tumor growth and metastasis, and also confer drug resistance to neighboring tumor cells. Overall, the study of EVs in RCC presents an exciting avenue of research with significant potential for improving the diagnosis and therapy of this disease. More research is required to completely understand the molecular pathways behind the participation of EVs in RCC and to maximize their diagnostic and therapeutic applications. Nonetheless, the rapid progress in this field suggests that EV-based approaches may soon alter the way we treat RCC.
Nonetheless, there remain considerable challenges that must be surmounted before these promising findings can be translated into clinical practice. Firstly, researchers must optimize existing methods for the isolation and preparation of EVs and establish uniform standards to ensure the reproducibility of results across different laboratories. Second, extensive testing is required to determine the security and effectiveness of EV-based therapeutics, as well as to assess any potential synergistic benefits with existing treatment modalities. Importantly, a thorough understanding of the molecular mechanisms underpinning EVs’ function in RCC is also required. Only with a comprehensive understanding of these mechanisms can EV-based strategies be optimized for personalized and effective therapies.
Looking toward the future, the field of EVs in RCC research is expected to grow rapidly, propelled by enhancements in EVs biology, nucleic acid sequencing, and gene editing technologies. It is hoped that these advances will facilitate the development of personalized treatments that take into consideration the distinct molecular and genetic characteristics of individual patients. Overall, the study of EVs in RCC represents an intriguing area of research that holds great promise for better understanding and treating this deadly disease.
Author contributions
YL finished collecting and analyzing relevant material, writing the first draft of the paper, and designing diagrams. MZ and JZ assisted in the initial writing, graph design, and data gathering. The investigation and categorization of the literature, the creation of the diagrams, and the review of the semantics were all done by XL and LW The graphs were designed by YM and the papers were collected by XH, RG wrote and reviewed the articles in the table to make grammatical corrections. ZC chose the topic, directed the drafting of the paper, gave final approval of the published version, chose the journal to which the paper would be submitted, and agreed to be accountable for all elements of the work. All authors contributed to the article and approved the submitted version.
Funding
This research was funded by Natural Science Foundation of Jiangxi Province (No.20202BABL206119), Science and Technology Plan of Jiangxi Health and Family Planning Commission (202310839, 202210860), Guiding Science and Technology Plan of Ganzhou (GZ2021ZSF004), Science and Technology Plan of Gannan Medical University First Affiliated Hospita (YJYB202110).
Acknowledgments
All participant’s help is much appreciated. The images in this article were created by BioRender.com.
Conflict of interest
The authors declare that the research was conducted in the absence of any commercial or financial relationships that could be construed as a potential conflict of interest.
Publisher’s note
All claims expressed in this article are solely those of the authors and do not necessarily represent those of their affiliated organizations, or those of the publisher, the editors and the reviewers. Any product that may be evaluated in this article, or claim that may be made by its manufacturer, is not guaranteed or endorsed by the publisher.
Glossary
References
1. Siegel RL, Miller KD, Fuchs HE, Jemal A. Cancer statistics, 2021. CA Cancer J Clin (2021) 71(1):7–33. doi: 10.3322/caac.21654
2. Xue YJ, Chen SN, Chen WG, Wu GQ, Liao YF, Xu JB, et al. Cripto-1 expression in patients with clear cell renal cell carcinoma is associated with poor disease outcome. J Exp Clin Cancer Res (2019) 38(1):378. doi: 10.1186/s13046-019-1386-6
3. Turajlic S, Swanton C, Boshoff C. Kidney cancer: the next decade. J Exp Med (2018) 215(10):2477–9. doi: 10.1084/jem.20181617
4. Heng DY, Xie W, Regan MM, Warren MA, Golshayan AR, Sahi C, et al. Prognostic factors for overall survival in patients with metastatic renal cell carcinoma treated with vascular endothelial growth factor-targeted agents: results from a large, multicenter study. J Clin Oncol (2009) 27(34):5794–9. doi: 10.1200/JCO.2008.21.4809
5. Motzer RJ, Jonasch E, Agarwal N, Alva A, Baine M, Beckermann K, et al. Kidney cancer, version 3.2022, NCCN clinical practice guidelines in oncology. J Natl Compr Canc Netw (2022) 20(1):71–90. doi: 10.6004/jnccn.2022.0001
6. Zhao S, Mi Y, Zheng B, Wei P, Gu Y, Zhang Z, et al. Highly-metastatic colorectal cancer cell released miR-181a-5p-rich extracellular vesicles promote liver metastasis by activating hepatic stellate cells and remodelling the tumour microenvironment. J Extracell Vesicles (2022) 11(1):e12186. doi: 10.1002/jev2.12186
7. Valadi H, Ekstrom K, Bossios A, Sjostrand M, Lee JJ, Lotvall JO. Exosome-mediated transfer of mRNAs and microRNAs is a novel mechanism of genetic exchange between cells. Nat Cell Biol (2007) 9(6):654–9. doi: 10.1038/ncb1596
8. Thery C, Witwer KW, Aikawa E, Alcaraz MJ, Anderson JD, Andriantsitohaina R, et al. Minimal information for studies of extracellular vesicles 2018 (MISEV2018): a position statement of the international society for extracellular vesicles and update of the MISEV2014 guidelines. J Extracell Vesicles (2018) 7(1):1535750. doi: 10.1080/20013078.2018.1535750
9. Tey SK, Wong SWK, Chan JYT, Mao X, Ng TH, Yeung CLS, et al. Patient pIgR-enriched extracellular vesicles drive cancer stemness, tumorigenesis and metastasis in hepatocellular carcinoma. J Hepatol (2022) 76(4):883–95. doi: 10.1016/j.jhep.2021.12.005
10. Qin Z, Xu Q, Hu H, Yu L, Zeng S. Extracellular vesicles in renal cell carcinoma: multifaceted roles and potential applications identified by experimental and computational methods. Front Oncol (2020) 10:724. doi: 10.3389/fonc.2020.00724
11. He X, Tian F, Guo F, Zhang F, Zhang H, Ji J, et al. Circulating exosomal mRNA signatures for the early diagnosis of clear cell renal cell carcinoma. BMC Med (2022) 20(1):270. doi: 10.1186/s12916-022-02467-1
12. Huang D, Chen J, Hu D, Xie F, Yang T, Li Z, et al. Advances in biological function and clinical application of small extracellular vesicle membrane proteins. Front Oncol (2021) 11:675940. doi: 10.3389/fonc.2021.675940
13. de Visser KE, Joyce JA. The evolving tumor microenvironment: from cancer initiation to metastatic outgrowth. Cancer Cell (2023) 41(3):374–403. doi: 10.1016/j.ccell.2023.02.016
14. Wang X, Huang D, Wu J, Li Z, Yi X, Zhong T. The biological effect of small extracellular vesicles on colorectal cancer metastasis. Cells (2022) 11(24):4071. doi: 10.3390/cells11244071
15. Gao J, Liang Y, Wang L. Shaping polarization of tumor-associated macrophages in cancer immunotherapy. Front Immunol (2022) 13:888713. doi: 10.3389/fimmu.2022.888713
16. Sun J, Park C, Guenthner N, Gurley S, Zhang L, Lubben B, et al. Tumor-associated macrophages in multiple myeloma: advances in biology and therapy. J Immunother Cancer (2022) 10(4):e003975. doi: 10.1136/jitc-2021-003975
17. Xu Z, Chen Y, Ma L, Chen Y, Liu J, Guo Y, et al. Role of exosomal non-coding RNAs from tumor cells and tumor-associated macrophages in the tumor microenvironment. Mol Ther (2022) 30(10):3133–54. doi: 10.1016/j.ymthe.2022.01.046
18. Huang X, Wang J, Guan J, Zheng Z, Hao J, Sheng Z, et al. Exosomal Circsafb2 reshaping tumor environment to promote renal cell carcinoma progression by mediating M2 macrophage polarization. Front Oncol (2022) 12:808888. doi: 10.3389/fonc.2022.808888
19. Zhang W, Zheng X, Yu Y, Zheng L, Lan J, Wu Y, et al. Renal cell carcinoma-derived exosomes deliver lncARSR to induce macrophage polarization and promote tumor progression via STAT3 pathway. Int J Biol Sci (2022) 18(8):3209–22. doi: 10.7150/ijbs.70289
20. Qu L, Ding J, Chen C, Wu ZJ, Liu B, Gao Y, et al. Exosome-transmitted lncARSR promotes sunitinib resistance in renal cancer by acting as a competing endogenous RNA. Cancer Cell (2016) 29(5):653–68. doi: 10.1016/j.ccell.2016.03.004
21. Shen T, Miao S, Zhou Y, Yi X, Xue S, Du B, et al. Exosomal AP000439.2 from clear cell renal cell carcinoma induces M2 macrophage polarization to promote tumor progression through activation of STAT3. Cell Commun Signal (2022) 20(1):152. doi: 10.1186/s12964-022-00957-6
22. Gu W, Gong L, Wu X, Yao X. Hypoxic TAM-derived exosomal miR-155-5p promotes RCC progression through HuR-dependent IGF1R/AKT/PI3K pathway. Cell Death Discovery (2021) 7(1):147. doi: 10.1038/s41420-021-00525-w
23. Zhang Z, Hu J, Ishihara M, Sharrow AC, Flora K, He Y, et al. The miRNA-21-5p payload in exosomes from M2 macrophages drives tumor cell aggression via PTEN/Akt signaling in renal cell carcinoma. Int J Mol Sci (2022) 23(6):3005. doi: 10.3390/ijms23063005
24. Liu Q, Zhao E, Geng B, Gao S, Yu H, He X, et al. Tumor-associated macrophage-derived exosomes transmitting miR-193a-5p promote the progression of renal cell carcinoma via TIMP2-dependent vasculogenic mimicry. Cell Death Dis (2022) 13(4):382. doi: 10.1038/s41419-022-04814-9
25. Jhunjhunwala S, Hammer C, Delamarre L. Antigen presentation in cancer: insights into tumour immunogenicity and immune evasion. Nat Rev Cancer (2021) 21(5):298–312. doi: 10.1038/s41568-021-00339-z
26. Graner MW, Schnell S, Olin MR. Tumor-derived exosomes, microRNAs, and cancer immune suppression. Semin Immunopathol (2018) 40(5):505–15. doi: 10.1007/s00281-018-0689-6
27. Wang X, Shi Q, Cui L, Wang K, Gong P, He X, et al. Tumor-derived exosomes facilitate tumor cells escape from drug therapy in clear cell renal cell carcinoma. Transl Cancer Res (2020) 9(5):3416–25. doi: 10.21037/tcr-19-2246
28. Yang L, Wu X, Wang D, Luo C, Chen L. Renal carcinoma cell-derived exosomes induce human immortalized line of jurkat T lymphocyte apoptosis in vitro. Urol Int (2013) 91(3):363–9. doi: 10.1159/000348747
29. Xia Y, Zhang Q, Zhen Q, Zhao Y, Liu N, Li T, et al. Negative regulation of tumor-infiltrating NK cell in clear cell renal cell carcinoma patients through the exosomal pathway. Oncotarget (2017) 8(23):37783–95. doi: 10.18632/oncotarget.16354
30. Grange C, Tapparo M, Tritta S, Deregibus MC, Battaglia A, Gontero P, et al. Role of HLA-G and extracellular vesicles in renal cancer stem cell-induced inhibition of dendritic cell differentiation. BMC Cancer (2015) 15:1009. doi: 10.1186/s12885-015-2025-z
31. Gao Y, Xu H, Li N, Wang H, Ma L, Chen S, et al. Renal cancer-derived exosomes induce tumor immune tolerance by MDSCs-mediated antigen-specific immunosuppression. Cell Commun Signal (2020) 18(1):106. doi: 10.1186/s12964-020-00611-z
32. Diao J, Yang X, Song X, Chen S, He Y, Wang Q, et al. Exosomal Hsp70 mediates immunosuppressive activity of the myeloid-derived suppressor cells via phosphorylation of Stat3. Med Oncol (2015) 32(2):453. doi: 10.1007/s12032-014-0453-2
33. Bao Z, Zeng W, Zhang D, Wang L, Deng X, Lai J, et al. SNAIL induces EMT and lung metastasis of tumours secreting CXCL2 to promote the invasion of M2-type immunosuppressed macrophages in colorectal cancer. Int J Biol Sci (2022) 18(7):2867–81. doi: 10.7150/ijbs.66854
34. Liu J, Ren L, Li S, Li W, Zheng X, Yang Y, et al. The biology, function, and applications of exosomes in cancer. Acta Pharm Sin B (2021) 11(9):2783–97. doi: 10.1016/j.apsb.2021.01.001
35. Wang L, Yang G, Zhao D, Wang J, Bai Y, Peng Q, et al. CD103-positive CSC exosome promotes EMT of clear cell renal cell carcinoma: role of remote MiR-19b-3p. Mol Cancer (2019) 18(1):86. doi: 10.1186/s12943-019-0997-z
36. Li YL, Wu LW, Zeng LH, Zhang ZY, Wang W, Zhang C, et al. ApoC1 promotes the metastasis of clear cell renal cell carcinoma via activation of STAT3. Oncogene (2020) 39(39):6203–17. doi: 10.1038/s41388-020-01428-3
37. Jin C, Shi L, Li K, Liu W, Qiu Y, Zhao Y, et al. Mechanism of tumor−derived extracellular vesicles in regulating renal cell carcinoma progression by the delivery of MALAT1. Oncol Rep (2021) 46(3):187. doi: 10.3892/or.2021.8138
38. Amicone L, Marchetti A, Cicchini C. Exosome-associated circRNAs as key regulators of EMT in cancer. Cells (2022) 11(10):1716. doi: 10.3390/cells11101716
39. Fu W, Zhao H, Liu Y, Nie H, Gao B, Yin F, et al. Exosomes derived from cancer-associated fibroblasts regulate cell progression in clear-cell renal-cell carcinoma. Nephron (2022) 146(4):383–92. doi: 10.1159/000520304
40. Ding M, Zhao X, Chen X, Diao W, Kan Y, Cao W, et al. Cancer-associated fibroblasts promote the stemness and progression of renal cell carcinoma via exosomal miR-181d-5p. Cell Death Discovery (2022) 8(1):439. doi: 10.1038/s41420-022-01219-7
41. Liu Y, Fu W, Cao X, Li S, Xiong T, Zhang X, et al. Delivery of miR-224-5p by exosomes from cancer-associated fibroblasts potentiates progression of clear cell renal cell carcinoma. Comput Math Methods Med (2021) 2021:5517747. doi: 10.1155/2021/5517747
42. Jiang XL, Zhang Y, Tan B, Luo CL, Wu XH. Renal tumor-derived exosomes inhibit hepaCAM expression of renal carcinoma cells in a p-AKT-dependent manner. Neoplasma (2014) 61(4):416–23. doi: 10.4149/neo_2014_051
43. Zhang QL, Luo CL, Wu XH, Wang CY, Xu X, Zhang YY, et al. HepaCAM induces G1 phase arrest and promotes c-myc degradation in human renal cell carcinoma. J Cell Biochem (2011) 112(10):2910–9. doi: 10.1002/jcb.23207
44. Qian Y, Li Y, Xu L, Chen K, Liu N, Yang X, et al. Tumor cell-derived exosomal circ-PRKCI promotes proliferation of renal cell carcinoma via regulating miR-545-3p/CCND1 axis. Cancers (Basel) (2022) 15(1):123. doi: 10.3390/cancers15010123
45. Li DY, Lin FF, Li GP, Zeng FC. Exosomal microRNA-15a from ACHN cells aggravates clear cell renal cell carcinoma via the BTG2/PI3K/AKT axis. Kaohsiung J Med Sci (2021) 37(11):973–82. doi: 10.1002/kjm2.12428
46. Jingushi K, Uemura M, Nakano K, Hayashi Y, Wang C, Ishizuya Y, et al. Leukocyte−associated immunoglobulin−like receptor 1 promotes tumorigenesis in RCC. Oncol Rep (2019) 41(2):1293–303. doi: 10.3892/or.2018.6875
47. Angel CZ, Stafford MYC, McNally CJ, Nesbitt H, McKenna DJ. MiR-21 is induced by hypoxia and down-regulates RHOB in prostate cancer. Cancers (Basel) (2023) 15(4):1291. doi: 10.3390/cancers15041291
48. Cowman SJ, Koh MY. Revisiting the HIF switch in the tumor and its immune microenvironment. Trends Cancer (2022) 8(1):28–42. doi: 10.1016/j.trecan.2021.10.004
49. Schodel J, Grampp S, Maher ER, Moch H, Ratcliffe PJ, Russo P, et al. Hypoxia, hypoxia-inducible transcription factors, and renal cancer. Eur Urol (2016) 69(4):646–57. doi: 10.1016/j.eururo.2015.08.007
50. Meng L, Xing Z, Guo Z, Qiu Y, Liu Z. Hypoxia-induced microRNA-155 overexpression in extracellular vesicles promotes renal cell carcinoma progression by targeting FOXO3. Aging (Albany NY) (2021) 13(7):9613–26. doi: 10.18632/aging.202706
51. Samoylenko A, Kogler M, Zhyvolozhnyi A, Makieieva O, Bart G, Andoh SS, et al. Time-gated raman spectroscopy and proteomics analyses of hypoxic and normoxic renal carcinoma extracellular vesicles. Sci Rep (2021) 11(1):19594. doi: 10.1038/s41598-021-99004-6
52. Hu G, Ma J, Zhang J, Chen Y, Liu H, Huang Y, et al. Hypoxia-induced lncHILAR promotes renal cancer metastasis via ceRNA for the miR-613/206/ 1-1-3p/Jagged-1/Notch/CXCR4 signaling pathway. Mol Ther (2021) 29(10):2979–94. doi: 10.1016/j.ymthe.2021.05.020
53. Horie K, Kawakami K, Fujita Y, Sugaya M, Kameyama K, Mizutani K, et al. Exosomes expressing carbonic anhydrase 9 promote angiogenesis. Biochem Biophys Res Commun (2017) 492(3):356–61. doi: 10.1016/j.bbrc.2017.08.107
54. Pastorek J, Pastorekova S. Hypoxia-induced carbonic anhydrase IX as a target for cancer therapy: from biology to clinical use. Semin Cancer Biol (2015) 31:52–64. doi: 10.1016/j.semcancer.2014.08.002
55. Xu X, Shen L, Li W, Liu X, Yang P, Cai J. ITGA5 promotes tumor angiogenesis in cervical cancer. Cancer Med (2023) 12(10):11983–99. doi: 10.1002/cam4.5873
56. Dou R, Liu K, Yang C, Zheng J, Shi D, Lin X, et al. EMT-cancer cells-derived exosomal miR-27b-3p promotes circulating tumour cells-mediated metastasis by modulating vascular permeability in colorectal cancer. Clin Transl Med (2021) 11(12):e595. doi: 10.1002/ctm2.595
57. Lindoso RS, Collino F, Camussi G. Extracellular vesicles derived from renal cancer stem cells induce a pro-tumorigenic phenotype in mesenchymal stromal cells. Oncotarget (2015) 6(10):7959–69. doi: 10.18632/oncotarget.3503
58. Xuan Z, Chen C, Tang W, Ye S, Zheng J, Zhao Y, et al. TKI-resistant renal cancer secretes low-level exosomal miR-549a to induce vascular permeability and angiogenesis to promote tumor metastasis. Front Cell Dev Biol (2021) 9:689947. doi: 10.3389/fcell.2021.689947
59. Grange C, Tapparo M, Collino F, Vitillo L, Damasco C, Deregibus MC, et al. Microvesicles released from human renal cancer stem cells stimulate angiogenesis and formation of lung premetastatic niche. Cancer Res (2011) 71(15):5346–56. doi: 10.1158/0008-5472.CAN-11-0241
60. Jingushi K, Uemura M, Ohnishi N, Nakata W, Fujita K, Naito T, et al. Extracellular vesicles isolated from human renal cell carcinoma tissues disrupt vascular endothelial cell morphology via azurocidin. Int J Cancer (2018) 142(3):607–17. doi: 10.1002/ijc.31080
61. Naito T, Jingushi K, Ueda K, Tsujikawa K. Azurocidin is loaded into small extracellular vesicles via its n-linked glycosylation and promotes intravasation of renal cell carcinoma cells. FEBS Lett (2021) 595(19):2522–32. doi: 10.1002/1873-3468.14183
62. Xavier CPR, Belisario DC, Rebelo R, Assaraf YG, Giovannetti E, Kopecka J, et al. The role of extracellular vesicles in the transfer of drug resistance competences to cancer cells. Drug Resist Updat (2022) 62:100833. doi: 10.1016/j.drup.2022.100833
63. Chen Y, Lu Z, Qi C, Yu C, Li Y, Huan W, et al. N(6)-methyladenosine-modified TRAF1 promotes sunitinib resistance by regulating apoptosis and angiogenesis in a METTL14-dependent manner in renal cell carcinoma. Mol Cancer (2022) 21(1):111. doi: 10.1186/s12943-022-01549-1
64. Lim AR, Vincent BG, Weaver AM, Rathmell WK. Sunitinib and axitinib increase secretion and glycolytic activity of small extracellular vesicles in renal cell carcinoma. Cancer Gene Ther (2022) 29(6):683–96. doi: 10.1038/s41417-021-00345-1
65. He J, He J, Min L, He Y, Guan H, Wang J, et al. Extracellular vesicles transmitted miR-31-5p promotes sorafenib resistance by targeting MLH1 in renal cell carcinoma. Int J Cancer (2020) 146(4):1052–63. doi: 10.1002/ijc.32543
66. Horie K, Kawakami K, Fujita Y, Matsuda Y, Arai T, Suzui N, et al. Serum exosomal gamma-glutamyltransferase activity increased in patients with renal cell carcinoma with advanced clinicopathological features. Oncology (2020) 98(10):734–42. doi: 10.1159/000508688
67. Kurahashi R, Kadomatsu T, Baba M, Hara C, Itoh H, Miyata K, et al. MicroRNA-204-5p: a novel candidate urinary biomarker of Xp11.2 translocation renal cell carcinoma. Cancer Sci (2019) 110(6):1897–908. doi: 10.1111/cas.14026
68. Niu Y, Jia X, Wang N, Yuan M, Dong A, Yang Y, et al. Identification of exosomes-related lncRNAs in clear cell renal cell carcinoma based on Bayesian spike-and-slab lasso approach. Funct Integr Genomics (2023) 23(1):62. doi: 10.1007/s10142-023-00985-6
69. Himbert D, Zeuschner P, Ayoubian H, Heinzelmann J, Stockle M, Junker K. Characterization of CD147, CA9, and CD70 as tumor-specific markers on extracellular vesicles in clear cell renal cell carcinoma. Diagn (Basel) (2020) 10(12):1034. doi: 10.3390/diagnostics10121034
70. Zhao Y, Wang Y, Zhao E, Tan Y, Geng B, Kang C, et al. PTRF/CAVIN1, regulated by SHC1 through the EGFR pathway, is found in urine exosomes as a potential biomarker of ccRCC. Carcinogenesis (2020) 41(3):274–83. doi: 10.1093/carcin/bgz147
71. Dias F, Teixeira AL, Nogueira I, Morais M, Maia J, Bodo C, et al. Plasma extracellular vesicle-derived TIMP-1 mRNA as a prognostic biomarker in clear cell renal cell carcinoma: a pilot study. Int J Mol Sci (2020) 21(13):4624. doi: 10.3390/ijms21134624
72. Yoshino H, Tatarano S, Tamai M, Tsuruda M, Iizasa S, Arima J, et al. Exosomal microRNA-1 and MYO15A as a target for therapy and diagnosis in renal cell carcinoma. Biochem Biophys Res Commun (2022) 630:71–6. doi: 10.1016/j.bbrc.2022.09.056
73. De Palma G, Sallustio F, Curci C, Galleggiante V, Rutigliano M, Serino G, et al. The three-gene signature in urinary extracellular vesicles from patients with clear cell renal cell carcinoma. J Cancer (2016) 7(14):1960–7. doi: 10.7150/jca.16123
74. Dias F, Teixeira AL, Nogueira I, Morais M, Maia J, Bodo C, et al. Extracellular vesicles enriched in hsa-miR-301a-3p and hsa-miR-1293 dynamics in clear cell renal cell carcinoma patients: potential biomarkers of metastatic disease. Cancers (Basel) (2020) 12(6):1450. doi: 10.3390/cancers12061450
75. Muramatsu-Maekawa Y, Kawakami K, Fujita Y, Takai M, Kato D, Nakane K, et al. Profiling of serum extracellular vesicles reveals miRNA-4525 as a potential biomarker for advanced renal cell carcinoma. Cancer Genomics Proteomics (2021) 18(3):253–9. doi: 10.21873/cgp.20256
76. Song S, Long M, Yu G, Cheng Y, Yang Q, Liu J, et al. Urinary exosome miR-30c-5p as a biomarker of clear cell renal cell carcinoma that inhibits progression by targeting HSPA5. J Cell Mol Med (2019) 23(10):6755–65. doi: 10.1111/jcmm.14553
77. Mondal J, Pillarisetti S, Junnuthula V, Saha M, Hwang SR, Park IK, et al. Hybrid exosomes, exosome-like nanovesicles and engineered exosomes for therapeutic applications. J Control Release (2023) 353:1127–49. doi: 10.1016/j.jconrel.2022.12.027
78. Wu T, Liu Y, Cao Y, Liu Z. Engineering macrophage exosome disguised biodegradable nanoplatform for enhanced sonodynamic therapy of glioblastoma. Adv Mater (2022) 34(15):e2110364. doi: 10.1002/adma.202110364
79. Cheng J, Sun Y, Ma Y, Ao Y, Hu X, Meng Q. Engineering of MSC-derived exosomes: a promising cell-free therapy for osteoarthritis. Membranes (Basel) (2022) 12(8):739. doi: 10.3390/membranes12080739
80. Huang L, Rong Y, Tang X, Yi K, Qi P, Hou J, et al. Engineered exosomes as an in situ DC-primed vaccine to boost antitumor immunity in breast cancer. Mol Cancer (2022) 21(1):45. doi: 10.1186/s12943-022-01515-x
81. Zhang M, Hu S, Liu L, Dang P, Liu Y, Sun Z, et al. Engineered exosomes from different sources for cancer-targeted therapy. Signal Transduct Target Ther (2023) 8(1):124. doi: 10.1038/s41392-023-01382-y
82. Liu W, Huang Y, Li Z, Li L, Zhao Y, Li M. Multivalent engineering of exosomes with activatable aptamer probes for specific regulation and monitoring of cell targeting. Anal Chem (2022) 94(9):3840–8. doi: 10.1021/acs.analchem.1c04741
83. Adem B, Teixeira AL, Dias F, Ruivo C, Medeiros R, Melo SA. GE11 positive exosomes as a potential RNAi delivery system in clear cell renal cell carcinoma: PS081. Porto BioMed J (2017) 2(5):181. doi: 10.1016/j.pbj.2017.07.016
84. Wang S, Shi Y. Exosomes derived from immune cells: the new role of tumor immune microenvironment and tumor therapy. Int J Nanomed (2022) 17:6527–50. doi: 10.2147/IJN.S388604
85. Zhang Y, Luo CL, He BC, Zhang JM, Cheng G, Wu XH. Exosomes derived from IL-12-anchored renal cancer cells increase induction of specific antitumor response in vitro: a novel vaccine for renal cell carcinoma. Int J Oncol (2010) 36(1):133–40. doi: 10.3892/ijo_00000484
86. Xu HY, Li N, Yao N, Xu XF, Wang HX, Liu XY, et al. CD8+ T cells stimulated by exosomes derived from RenCa cells mediate specific immune responses through the FasL/Fas signaling pathway and, combined with GM−CSF and IL−12, enhance the anti−renal cortical adenocarcinoma effect. Oncol Rep (2019) 42(2):866–79. doi: 10.3892/or.2019.7208
87. Wang C, Zhou H, Wu R, Guo Y, Gong L, Fu K, et al. Mesenchymal stem cell-derived exosomes and non-coding RNAs: regulatory and therapeutic role in liver diseases. BioMed Pharmacother (2023) 157:114040. doi: 10.1016/j.biopha.2022.114040
88. Liu XN, Zhang CB, Lin H, Tang XY, Zhou R, Wen HL, et al. microRNA-204 shuttled by mesenchymal stem cell-derived exosomes inhibits the migration and invasion of non-small-cell lung cancer cells via the KLF7/AKT/HIF-1alpha axis. Neoplasma (2021) 68(4):719–31. doi: 10.4149/neo_2021_201208N1328
89. Akbari A, Rezaie J. Potential therapeutic application of mesenchymal stem cell-derived exosomes in SARS-CoV-2 pneumonia. Stem Cell Res Ther (2020) 11(1):356. doi: 10.1186/s13287-020-01866-6
90. Hu Z, Yuan Y, Zhang X, Lu Y, Dong N, Jiang X, et al. Human umbilical cord mesenchymal stem cell-derived exosomes attenuate oxygen-glucose Deprivation/Reperfusion-induced microglial pyroptosis by promoting FOXO3a-dependent mitophagy. Oxid Med Cell Longev (2021) 2021:6219715. doi: 10.1155/2021/6219715
91. Guo S, Perets N, Betzer O, Ben-Shaul S, Sheinin A, Michaelevski I, et al. Intranasal delivery of mesenchymal stem cell derived exosomes loaded with phosphatase and tensin homolog siRNA repairs complete spinal cord injury. ACS Nano (2019) 13(9):10015–28. doi: 10.1021/acsnano.9b01892
92. Xie X, Yang X, Wu J, Tang S, Yang L, Fei X, et al. Exosome from indoleamine 2,3-dioxygenase-overexpressing bone marrow mesenchymal stem cells accelerates repair process of ischemia/reperfusion-induced acute kidney injury by regulating macrophages polarization. Stem Cell Res Ther (2022) 13(1):367. doi: 10.1186/s13287-022-03075-9
93. Xin D, Li T, Chu X, Ke H, Liu D, Wang Z. MSCs-extracellular vesicles attenuated neuroinflammation, synapse damage and microglial phagocytosis after hypoxia-ischemia injury by preventing osteopontin expression. Pharmacol Res (2021) 164:105322. doi: 10.1016/j.phrs.2020.105322
94. Li D, Lin F, Li G, Zeng F. Exosomes derived from mesenchymal stem cells curbs the progression of clear cell renal cell carcinoma through T-cell immune response. Cytotechnology (2021) 73(4):593–604. doi: 10.1007/s10616-021-00480-5
95. Fonsato V, De Lena M, Tritta S, Brossa A, Calvetti R, Tetta C, et al. Human liver stem cell-derived extracellular vesicles enhance cancer stem cell sensitivity to tyrosine kinase inhibitors through Akt/mTOR/PTEN combined modulation. Oncotarget (2018) 9(90):36151–65. doi: 10.18632/oncotarget.26319
96. Brossa A, Fonsato V, Grange C, Tapparo M, Calvetti R, Calvetti R, et al. Extracellular vesicles from human liver stem cells inhibit renal cancer stem cell-derived tumor growth in vitro and in vivo. Int J Cancer (2020) 147(6):1694–706. doi: 10.1002/ijc.32925
97. Ren W, Hou J, Yang C, Wang H, Wu S, Wu Y, et al. Extracellular vesicles secreted by hypoxia pre-challenged mesenchymal stem cells promote non-small cell lung cancer cell growth and mobility as well as macrophage M2 polarization via miR-21-5p delivery. J Exp Clin Cancer Res (2019) 38(1):62. doi: 10.1186/s13046-019-1027-0
98. Zhu T, Hu Z, Wang Z, Ding H, Li R, Wang J, et al. microRNA-301b-3p from mesenchymal stem cells-derived extracellular vesicles inhibits TXNIP to promote multidrug resistance of gastric cancer cells. Cell Biol Toxicol (2022) doi: 10.1007/s10565-021-09675-0
99. Zhang X, Sai B, Wang F, Wang L, Wang Y, Zheng L, et al. Hypoxic BMSC-derived exosomal miRNAs promote metastasis of lung cancer cells via STAT3-induced EMT. Mol Cancer (2019) 18(1):40. doi: 10.1186/s12943-019-0959-5
100. Zhang F, Guo J, Zhang Z, Qian Y, Wang G, Duan M, et al. Mesenchymal stem cell-derived exosome: a tumor regulator and carrier for targeted tumor therapy. Cancer Lett (2022) 526:29–40. doi: 10.1016/j.canlet.2021.11.015
101. Bagheri E, Abnous K, Farzad SA, Taghdisi SM, Ramezani M, Alibolandi M. Targeted doxorubicin-loaded mesenchymal stem cells-derived exosomes as a versatile platform for fighting against colorectal cancer. Life Sci (2020) 261:118369. doi: 10.1016/j.lfs.2020.118369
102. Melzer C, Rehn V, Yang Y, Bahre H, von der Ohe J, Hass R. Taxol-loaded MSC-derived exosomes provide a therapeutic vehicle to target metastatic breast cancer and other carcinoma cells. Cancers (Basel) (2019) 11(6):798. doi: 10.3390/cancers11060798
103. Ahmadi M, Mahmoodi M, Shoaran M, Nazari-Khanamiri F, Rezaie J. Harnessing normal and engineered mesenchymal stem cells derived exosomes for cancer therapy: opportunity and challenges. Int J Mol Sci (2022) 23(22):13974. doi: 10.3390/ijms232213974
104. Tsuruda M, Yoshino H, Okamura S, Kuroshima K, Osako Y, Sakaguchi T, et al. Oncogenic effects of RAB27B through exosome independent function in renal cell carcinoma including sunitinib-resistant. PloS One (2020) 15(5):e0232545. doi: 10.1371/journal.pone.0232545
105. Greenberg JW, Kim H, Moustafa AA, Datta A, Barata PC, Boulares AH, et al. Repurposing ketoconazole as an exosome directed adjunct to sunitinib in treating renal cell carcinoma. Sci Rep (2021) 11(1):10200. doi: 10.1038/s41598-021-89655-w
106. Greenberg JW, Kim H, Ahn M, Moustafa AA, Zhou H, Barata PC, et al. Combination of tipifarnib and sunitinib overcomes renal cell carcinoma resistance to tyrosine kinase inhibitors via tumor-derived exosome and T cell modulation. Cancers (Basel) (2022) 14(4):903. doi: 10.3390/cancers14040903
Keywords: extracellular vesicles, renal cell carcinoma, tumor microenvironment, engineered extracellular vesicles, tumor vaccine
Citation: Lu Y, Zhang M, Zhou J, Liu X, Wang L, Hu X, Mao Y, Gan R and Chen Z (2023) Extracellular vesicles in renal cell carcinoma: challenges and opportunities coexist. Front. Immunol. 14:1212101. doi: 10.3389/fimmu.2023.1212101
Received: 25 April 2023; Accepted: 19 June 2023;
Published: 04 July 2023.
Edited by:
Shenglong Li, China Medical University, ChinaReviewed by:
Juan Antonio Fafian Labora, University of A Coruña, SpainZijun Wang, The Rockefeller University, United States
Copyright © 2023 Lu, Zhang, Zhou, Liu, Wang, Hu, Mao, Gan and Chen. This is an open-access article distributed under the terms of the Creative Commons Attribution License (CC BY). The use, distribution or reproduction in other forums is permitted, provided the original author(s) and the copyright owner(s) are credited and that the original publication in this journal is cited, in accordance with accepted academic practice. No use, distribution or reproduction is permitted which does not comply with these terms.
*Correspondence: Zhiping Chen, MTUwODM3MzcyODBAZ211LmVkdS5jbg==