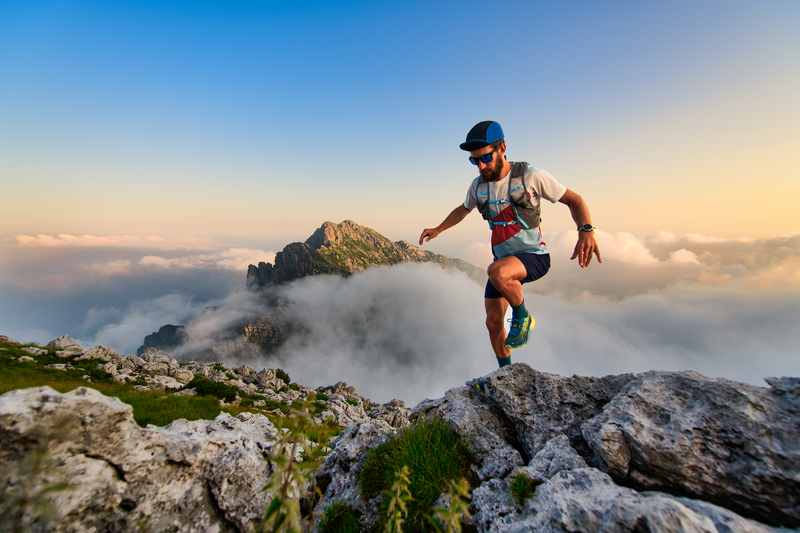
95% of researchers rate our articles as excellent or good
Learn more about the work of our research integrity team to safeguard the quality of each article we publish.
Find out more
REVIEW article
Front. Immunol. , 22 May 2023
Sec. Inflammation
Volume 14 - 2023 | https://doi.org/10.3389/fimmu.2023.1197422
This article is part of the Research Topic Crosstalk between Peripheral and Local Immune Response in the Pathophysiology of Stroke and Neurodegeneration Diseases, Volume II View all 10 articles
Stroke is a leading cause of mortality and disability worldwide, with most survivors reporting dysfunctions of motor, sensation, deglutition, cognition, emotion, and speech, etc. Repetitive transcranial magnetic stimulation (rTMS), one of noninvasive brain stimulation (NIBS) techniques, is able to modulate neural excitability of brain regions and has been utilized in neurological and psychiatric diseases. Moreover, a large number of studies have shown that the rTMS presents positive effects on function recovery of stroke patients. In this review, we would like to summarized the clinical benefits of rTMS for stroke rehabilitation, including improvements of motor impairment, dysphagia, depression, cognitive function, and central post-stroke pain. In addition, this review will also discuss the molecular and cellular mechanisms underlying rTMS-mediated stroke rehabilitation, especially immune regulatory mechanisms, such as regulation of immune cells and inflammatory cytokines. Moreover, the neuroimaging technique as an important tool in rTMS-mediated stroke rehabilitation has been discussed, to better understanding the mechanisms underlying the effects of rTMS. Finally, the current challenges and future prospects of rTMS-mediated stroke rehabilitation are also elucidated with the intention to accelerate its widespread clinical application.
Stroke is one of the leading causes of mortality and disability worldwide, with most survivors reporting a decrease in quality of life, placing heavy financial burden on patients’ families and society (1, 2). The stroke can lead to an imbalance in blood supply and thereby induce severe brain damages, result in various dysfunctions, such as motor impairment, dysphagia, cognition impairment, post-stroke pain, depression, etc (3). Current best stroke treatment is to minimize initial brain damage and prevent subsequent complications, and then improve function through rehabilitation training (4). Moreover, most stroke miss the best therapeutic window for emergency treatment, such as thrombolysis or thrombectomy, therefore, the rehabilitation is quite important for stroke patients to improve motor, swallowing, and cognitive functions (5, 6). Currently, lots of rehabilitation programs have been applied by rehabilitation specialist to recovery the impaired functions and improve the quality of life. Commonly used rehabilitation programs in clinical practice include: physical therapy, occupational therapy, speech therapy, hyperbaric oxygenation, acupuncture, etc (7), which can improve post-stroke dysfunction by stimulating central nervous system (CNS) input through sensorimotor system or by promoting CNS remodeling through intensive training of motor patterns. At present, these traditional rehabilitation programs remain the main methods for post-stroke recovery, which can promote the recovery of various dysfunctions after stroke to a certain extent. However, there are also some shortcomings for these rehabilitation programs, such as slow onset, time-consuming, and poor patient compliance (8). Due to the certain shortcomings of traditional rehabilitation programs, various novel techniques have been utilized for stroke rehabilitation, such as repetitive transcranial magnetic stimulation (rTMS), transcranial direct current stimulation (tDCS), virtual reality, augmented reality, brain-computer interface, and rehabilitation robot, which have shown great stroke rehabilitation effects (9–12).
rTMS, as a noninvasive brain stimulation (NIBS), is a neurostimulation technique that uses a pulsed magnetic field to regulate the membrane potential of neurons, thereby selectively modulating the neural excitability of brain regions (13). rTMS has been applied in neurological and psychiatric diseases, including cognitive impairment, depression, mental disease, and Alzheimer’s disease (AD) (14–17). It is painless and easy to operate, making it a valuable tool for providing more precise brain area regulation and prognostic assessment in stroke rehabilitation. The interhemispheric inhibition (IHI) model is the primary theoretical basis for the application of rTMS in stroke rehabilitation (18). This model suggests that the two brain hemispheres are connected by the corpus callosum, which not only transmits information between the hemispheres but also regulates their interactions. Under healthy condition, the excitatory or inhibitory activity between the two hemispheres is balanced through the corpus callosum. However, stroke disrupts the balance of mutual inhibition between the hemispheres, resulting in weakened inhibition of the affected hemisphere on the unaffected hemisphere, and increased inhibition of the unaffected hemisphere on the affected hemisphere (4). Another model is called compensation, which suggests that the neural conduction in the damaged area is disrupted after stroke (19). The neurons and astrocytes in the unaffected hemisphere can form new circuits to compensate for the damaged area, allowing for the recovery of motor function in stroke patients. However, the compensation model cannot be applied when a patient experiences a bilateral stroke (20). A lager number of clinical trials have been conducted to evaluate the potential of rTMS to promote rehabilitation of limb, swallowing, and cognitive functions post-stroke, which showed satisfactory recovery outcomes (21–24).
In this review, the clinical benefits of rTMS for stroke rehabilitation are summarized, including improvements of motor impairment, dysphagia, depression, cognitive function, and central post-stroke pain. Besides, this review will also focus on the molecular and cellular mechanisms underlying rTMS-mediated stroke rehabilitation, especially those related to immune regulation.
rTMS have been shown to promote effectively rehabilitation of neurological sequelae post-stroke, including motor impairment, dysphagia, cognitive impairment, mental diseases, and neuropathic pain, which will be summarized and discussed in this section (Table 1).
Most stroke patients experience upper limb motor impairment, with only 5%-20% of them being able to fully recover their upper limb function (35). Upper limb impairment can significantly affect stoke patients’ abilities, leading to a negative impact on their quality of life (36). For example, The loss of upper limb function can severely affect tasks such as eating, dressing, and personal hygiene care, and result in a loss of independence (37). Stroke disrupts the balance between the brain hemispheres, which is an important cause of upper limb motor impairment after stroke (38, 39). Repetitive transcranial magnetic stimulation (rTMS) can modulate cortical excitability and thus recovery the balance post-stroke (40, 41). According to IHI model, there are two main options to using rTMS to promote functional recovery post-stroke: one is to use low-frequency (≤1Hz) rTMS (LF-rTMS) to stimulate the unaffected hemisphere and reduce its excitability, thus decreasing its inhibitory effect on the affected hemisphere; the other is to use high-frequency (≥3Hz) rTMS (HF-rTMS) to stimulate the affected hemisphere and increase its excitability, thereby restoring balance between the hemispheres (42–44). Numerous studies have revealed that rTMS over primary motor cortex (M1) can improve upper limb motor function post-stroke (25, 26, 28, 43, 45, 46). A meta-analysis of Hsu et al. included 392 stroke patients from 18 studies, which suggest that rTMS could promote upper limb motor recovery in stroke patients, especially those with subcortical stroke (47). And they found that applying LF-rTMS on the unaffected hemisphere might be safer and more effective than HF-rTMS on the affected hemisphere in improving upper limb motor function after stroke. The evidence-based guidelines on the therapeutic use of rTMS, updated by International Federation of Clinical Neurophysiology in 2019, recommended that LF-rTMS over M1 of unaffected hemisphere at the subacute stage of stroke as level A evidence (definite efficacy) could recover hand motor effectively (48). HF-rTMS over the M1 of affected hemisphere at the subacute stage as well as LF-rTMS over M1 of unaffected hemisphere at the chronic stage of stroke were recommended as level B (probable efficacy) and C (possible efficacy) evidences. Consistent with the guideline, a meta-analysis of Mu group including 904 stroke patients from 34 studies indicated that the effectiveness of rTMS on stroke patient present timing-dependent manner: the acute phase > the subacute phase > the chronic phase (49). In addition, rTMS can be combined with other therapies to improve upper limb function after stroke, including occupational therapy (22), virtual reality training (50), action observation (51), and upper-limb training (52), etc.
Motor impairment increases the risk of falling due to gait impairments, resulting in limitations in activities of daily living and a lower quality of life (46, 53). Clinicians prioritize the improvement of lower limb motor function and walking ability when treating stroke patients (54, 55). Therefore, the study of rTMS on lower limb motor function also has significant clinical implications. Tung et al. performed a meta-analysis based on 169 stroke patients and found that rTMS could remarkably improve walking speed, lower limb activity, and Fugl-Meyer Assessment lower limb scores (56). Another meta-analysis from Li group indicated that rTMS, especially HF-rTMS over unaffected hemisphere, could significantly improve walking speed of stroke patients (57). In addition, the meta-analysis conducted by Vaz et al. revealed that either HF-rTMS or LF-rTMS combined with other rehabilitation therapies could significantly improve gait speed in both acute/subacute and chronic stages of stroke (58). In addition to improving walking speed, Choi et al. found that HF-rTMS [10 Hz, 90% resting motor threshold (RMT)] over the trunk motor cortex could remarkably improve the balance function of chronic stroke patients without any side effects (30).
Post-stroke lower limb spasticity impairs gait and balance to reduce speed of walking, thereby increasing the need of wheelchair and caregiver (59). A meta-analysis of Liu et al., including 554 stroke patients from 9 studies, aimed to evaluate the efficacy of rTMS in improving post-stroke lower limb spasticity (60). They revealed that rTMS could decrease Modified Ashworth Scale (MAS) score and elevate Modified Barthel Index (MBI) score, compared with the control group. Further subgroup analysis concluded that LF-rTMS showed a positive effect on lower limb spasticity after stroke, while the HF-rTMS showed no significant effect on the lower limb motor function. Due to the limited studies included in this meta-analysis, the recovery effect of HF-rTMS on lower limb spasticity remains to be studied. Besides, the mechanism by which rTMS improves lower limb spasticity is still unclear and needs to be further explored in future studies.
Dysphagia is a common complication in stroke patients, with a prevalence of ~53%, which can result in aspiration pneumonia, malnutrition, electrolyte imbalances, and even death (61). Dysphagia is associated with prolonged hospital stay, poor life quality, elevated mortality, make it imperative to prioritize early intervention for improving swallowing function (62, 63). Current treatments include postural interventions, surgery, botulinum toxin injections and exercise, but these are less effective (64–66). Recently, noninvasive neurostimulation therapies have been found to improve the dysphagia in stroke patients (67). Among the noninvasive neurostimulation therapies, rTMS might be the most effective treatment for dysphagia after stroke, compared to tDCS, pharyngeal electrical stimulation (PES), and surface neuromuscular electrical stimulation (sNMES) (67). For instance, Khedr et al. evaluated the recovery effect of rTMS on the swallowing performance in 26 patients with dysphagia at the acute stage of stroke (31). In rTMS-treated group, the esophageal cortex of the affected hemisphere was received HF-rTMS daily (3 Hz and 120% of RMT) for 5-10 days. The results showed that the rTMS significantly improved the dysphagia of stroke patients for several months. In another study, Verin et al. applied LF-rTMS (1 Hz) on the mylohyoid cortical area of the unaffected hemisphere in patients with dysphagia at the chronic stage of stroke, resulting in a greater improvement in swallowing function and a remarkable decrease in the aspiration score for liquids (68). Besides, HF-rTMS (5 Hz) applied over the tongue region of the motor cortex of the unaffected hemisphere has been shown to improve swallowing performance of stroke patients with chronic dysphagia (24). Although the rTMS has been shown to improve swallowing function in a large number of studies, there is no unified treatment standard for its stimulation site, intensity and treatment duration (69–71). Therefore, it is necessary to conduct large-scale multi-center clinical studies on rTMS for dysphagia, and further develop standardized treatment guideline in the future.
Post-stroke depression (PSD) is the most common neuropsychological complication of stroke with an incidence rate of ~33% (72). PSD has been shown to reduce quality of life, affect rehabilitation outcome, and increase mortality rate (32, 73, 74). Current therapies for PSD include pharmacotherapy, psychotherapy and physical therapy, however, some patients do not benefit from these first-line treatments (75–77). Fortunately, several studies indicated that rTMS was expected to improve the neuropsychological disorder (78, 79). And the U.S. Food and Drug Administration (FDA) approved rTMS over left dorsolateral prefrontal cortex for treatment of the major depressive disorder (MDD) in 2008 (80). Thus, a large number of clinical trials have explored the therapeutic efficacy of rTMS on the PSD (81, 82). However, the results from these clinical trials were inconsistent. Thus, a meta-analysis of Shen et al. included 1764 PSD patients from 22 randomized controlled trials (RCTs) studies to explore the therapeutic efficacy of rTMS for PSD (83). The results showed that rTMS could remarkably improve the PSD measured by Hamilton Depression Rating Scale (HAMD). Further safety evaluation showed no statistical difference in withdrawals owing to adverse events. However, most of these results are from single studies with varying degrees of limitations. Therefore, definitive conclusions about the treatment of PSD with rTMS need to be further confirmed by multicenter RCTs. The traditional rTMS protocol requires treatment 5 days per week for more than 4 weeks. Frey et al. proposed an accelerated rTMS strategy to reduce the number of days needed to complete treatment, which can bring convenience to the patients and increase compliancy. They applied HF-rTMS (20 Hz) at 110% RMT over the left dorsolateral prefrontal cortex of the PSD patients 5 sessions per day for 4 days. The results revealed that HAMD of PSD patients remarkably reduced after the accelerated rTMS, which maintained for 3 months. Besides, no significant adverse events associated with rTMS treatment were observed in the study. This study suggested that the accelerated rTMS protocol could be a more convenient adjuvant treatment option for PSD patients.
Post-stroke cognitive impairment (PSCI) occurred in nearly 75% of stroke patients (84). Only half of them are able to recovery the cognitive function, whereas the others might develop vascular dementia (85). Since stroke patients with PSCI may experience impaired judgment and memory problems, PSCI can hinder physical recovery (86). Furthermore, prolonged cognitive impairment can significantly affect activities of daily living (ADL), quality of life, and reintegration into the community (87–89). Thus, effective intervention to improve PSCI is a very important part of stroke rehabilitation. The therapies of PSCI included pharmacological therapy (e.g., acetylcholinesterase inhibitor, memantine, traditional Chinese medicines, etc.), cognitive training, risk factor prevention and intervention (90, 91). In recent years, rTMS has been applied to treat cognitive impairment induced by several CNS diseases, including Alzheimer’s disease, depression, Parkinson’s disease, and bipolar disorder (14, 15, 92, 93). Notably, several studies have found that rTMS also showed positive therapeutic effects on PSCI (23, 34, 94–96). For example, Yin et al. applied 20 sessions of rTMS (10 Hz, 80% RMT) over the left dorsal lateral prefrontal cortex of PSCI patients, which revealed that rTMS could improve cognitive function and ADLs of PSCI patients (34). Moreover, the functional MRI (fMRI) of rTMS-treated patients indicated that the rTMS might activate left medial prefrontal cortex and augmented the functional connectivity to right medial prefrontal cortex and ventral anterior cingulate cortex, resulting in the improvements of cognitive function. These studies suggested that the rTMS can be an important and effective treatment to rescue the cognitive function in PSCI patients, and that functional connectivity (FC) and neural activity in cognition-related brain regions could be crucial indicators to clarify the effect of rTMS on PSCI. In addition, rTMS has exhibited a superior modulating effect in cognitive function compared to other non-invasive stimulation techniques (96). Intermittent theta-burst stimulation (iTBS) as a type of TMS has also been applied to improve the PSCI. Tsai et al. evaluated the therapeutic effect of rTMS (5 Hz, 80% RMT) and iTBS on the PSCI patients, suggesting that both rTMS and iTBS could effectively improve cognitive impairment, including global cognitive function, memory function, and attention (96). Compared with the iTBS group, the rTMS group showed better improvement in attention.
Central post-stroke pain (CPSP) is a neuropathic pain syndrome that can occur after a cerebrovascular accident, with an incidence rate of 1~12% (97, 98). The main treatment for CPSP is pharmacology (99). However, the pharmacology has limited pain control and unpleasant side effects. Recently, several studies found that HF-rTMS could relieve CPSP to some extent (100–102). Leung et al. found that rTMS showed great analgesic effect on CPSP (16.7% of visual analog scale score reduction), and rTMS exhibited better improvement in CPSP than peripheral neuropathic pain (101), suggesting that rTMS might be a promising therapeutic tool for CPSP.
FMA, Fugl–Meyer Assessment; BBT, Box and Blocks Test; FIM, Functional Independence Measurement; FAS, Functional Ambulation Scale; rTMS, repetitive transcranial magnetic stimulation; PMd, dorsal premotor cortex; RMT, resting motor threshold; MT, motor threshold; JTT, Jebsen–Taylor Hand Function Test; MEP, motor evoked potential; CSP, cortical silent period; ISP, ipsilateral silent period; NMES, neuromuscular electrical stimulation; fMRI, Functional magnetic resonance imaging; BRS, brunnstrom recovery stage; UE-MI, Upper extremity motricity index; BI, barthel index; MAS, Modified ashworth scale; BS, Brunnstrom stages; NIHSS, National Institutes of Health Stroke Scale; ABMS II, Ability for Basic Movement Scale Revised; ipsi, ipsilateral; contra, contralateral; BBS, Berg Balance Scale; CDP, Computerized dynamic posturogphy; SAPP, Swallowing Activity and Participation Profile; TPA, tongue pressure assessment; VFSS, Videofluoroscopic swallowing study; QIDS, Quick Inventory of Depressive Symptomatology; AS, Apathy Scale; dACC, dorsal anterior cingulate cortex; mPFC, medial PFC; mBI, modified Barthel Index; HAMD, Hamilton Depression Scale; mRS, modified Rankin Scale; DLPFC, dorsolateral prefrontal cortex; BDI, Beck Depression Inventory; CPT, Continuous Performance Test; ALFF, amplitude of low-frequency fluctuation; FC, functional connectivity.
Despite the confirmed value, the regulatory mechanism via which TMS shows the beneficial effects on stroke rehabilitation remain unclear. Recent studies have shown that the basic mechanisms might be involved in regulation of neurotransmitters release, immune cells, and cytokines (Figure 1) (102–105).
Neurotransmitters as chemical messengers are released from a neuron to excite or inhibit other neurons (106). Neurotransmitters, including glutamate, gamma-aminobutyric acid (GABA), glycine (Gly), and acetylcholine (ACh), play an important role in chemical synapses of the CNS. Thus, the abnormal metabolism or release of the neurotransmitters can result in synaptic dysfunction, impaired neurogenesis, impairment of cognitive function, depression, memory deficits and CPSP, etc (107–109). Numerous studies have shown that stroke could induce abnormal release of neurotransmitters (107, 110). Therefore, modulation the neurotransmitters might be a rational approach to promote the stroke rehabilitation.
A number of studies have shown that rTMS could alter the release and expression of neurotransmitters in the CNS, which might be the underlying mechanisms of rTMS-based stroke rehabilitation (102, 105, 111–114). As an excitatory neurotransmitter, glutamate-mediated excitotoxicity is a crucial mechanism resulting in post-stroke injury (115). GABA and glycine are major inhibitory neurotransmitters in the brain (116). Ikeda et al. revealed that application of rTMS (20 Hz) were able to regulate the mRNA expression levels of several neurotransmitter-related genes, including GABAergic, glutamatergic, and glycinergic neurotransmission systems in mouse cerebellum and brain stem, suggesting that rTMS can modulate the activity of neurons and synaptic plasticity via regulating the levels of neurotransmitters (111, 114). Zangen et al. revealed that TMS over the frontal or caudal cortex of the healthy rat brain elevated the extracellular dopamine and glutamate levels in the nucleus accumbens (117). However, LF-rTMS (1 Hz) on the primary motor cortex of healthy human could not influence the excitatory (glutamate) neurotransmitter but decrease the inhibitory (GABA) neurotransmitter in both ipsilateral and contralateral motor cortices (112). Moreover, Chen et al. reported that rTMS (10 Hz) at the affected M1 and rTMS (1 Hz) at the unaffected M1 could reduce the GABA content in M1 of ischemic stroke patients, which was associated with the improvement of motor function (105). Several studies have shown that decreased GABAergic neurotransmission in the CNS might be a main cause of chronic neuropathic pain, such as CPSP (118). Decrease of inhibitory GABAergic tone was found at the level of dorsal spinal cord, somatosensory cortex, and thalamus sensory nuclei, which led to neuronal hyperactivity in the sensorimotor cortex (119). Besides, the neuronal hyperactivity related to deafferentation pain was caused by abnormal recruitment of N-methyl-D-aspartate (NMDA) receptors (120). Lefaucheur et al. found that HF-rTMS (10 Hz) on CPSP patients could significantly increase intracortical inhibition (ICI) which could reflect GABAergic neurotransmission function (113). Overall, the CPSP was associated with the imbalance between GABAergic and glutamatergic transmission in the CNS, which could be restored through rTMS-mediated GABAergic neurotransmission regulation.
Cholinergic neurons can synthesize and release the Ach when they are excited. Ach is an excitatory neurotransmitter and plays a key role in learning and memory processes (121). Li group treated the vascular dementia (VD) rats with rTMS (0.5 Hz, 1.33T) for 30 days and found that the rTMS treatment could remarkably enhance the acetylcholinesterase (AChE) and choline acetyltransferase (ChAT) activities, and increase the density of cholinergic neurons (122). The learning and memory deficits of VD rats was also significantly improved, the underlying mechanism of which might be associated with recovery of cholinergic nervous system activity in CA1 region of the hippocampus. Furthermore, brain-derived neurotrophic factor (BDNF), a neurotransmitter modulator, can promote the migration and proliferation of neural stem cells, and further enhance stroke recovery. Luo et al. revealed that HF-rTMS could activate BDNF/tropomyosin-related kinase B (TrkB) signaling pathway and antiapoptotic pathways to increase number of BrdU+ NESTIN+ cells, decrease Bcl-2 expression and elevate Bax expression, leading to the improvement of the cognitive impairment of rats with ischemic stroke (123). In addition, Cambiaghi et al. found that HF-rTMS was able to enhance dendritic complexity and spine number of neurons through BDNF and calcium-dependent signaling pathways (124).
Previous researches on the mechanism of rTMS were mainly concentrated on the effects on neurons. The HF-rTMS enhances neuronal excitatory at the affected hemisphere, while LF-rTMS decreases neuronal excitatory at the unaffected hemisphere (125). Nevertheless, little is known about the influence of rTMS on the other neural cells, such as astrocyte and microglia.
Astrocytes are the most abundant glial cells and play an important role in the maintenance of the blood-brain barrier (BBB) and CNS homeostasis (126). The astrocytes are activated after stroke and further polarized into two distinct phenotypes: neurotoxic (pro-inflammatory) type A1 and neuroprotective (anti- inflammatory) type A2. A1 astrocytes can secrete inflammatory cytokines and neurotoxic mediators to induce neuroinflammation and further aggravate brain damage, while A2 astrocytes mainly secrete anti-inflammatory cytokines and nerve growth factor to promote neuroregeneration and exert neuroprotective functions. In addition, astrocytes have neurotransmitter receptors and ion channels, which play a crucial role in synaptic neurotransmission (127). Therefore, rTMS might be able to alter cell membrane potential and further regulate the function of astrocytes (128, 129).
rTMS with different frequencies has been shown to regulate the expression of GFAP (a cytoskeletal marker of astrocytic reactivity) and the density of GFAP-positive astrocytes in various in vivo brain injury or disease model, such as cortical stab injury, Parkinson’s disease, etc (130–132). Moreover, Clarke et al. found that rTMS (1 or 10 Hz) over the culture mouse cortical astrocytes could alter the expression of genes and proteins related to calcium signaling and inflammation, such as intercellular adhesion molecule 1 (Icam1), stromal interaction molecule 1 (Stim1), and ORAI calcium release-activated calcium modulator 3 (Orai3), etc (133).
Astrocyte modulation has also been indicated as one mechanism for rTMS-mediated stroke rehabilitation. Hong et al. evaluated the effects of rTMS on astrocyte polarization in in vitro and in vivo cerebral ischemic/reperfusion injury model (134). They revealed that the rTMS (10 Hz) could decrease the concentration of pro-inflammatory cytokine TNF-α, increase anti-inflammatory cytokine IL-10, and regulate astrocytic polarization (from A1 to A2 astrocytes) after ischemic/reperfusion injury. Besides, the astrocyte culture medium collected from rTMS-treated astrocytes could remarkably reduce ischemic/reperfusion injury-induced neuronal apoptosis. In addition, the rTMS could suppress the excessive astrocyte-vessel interactions and promote the vasculature-associated A1 to A2 astrocyte switch in the peri-infarct cortex after stroke, which was beneficial to vessel and BBB protection post-stroke (103). Further mechanistic studies displayed that rTMS significantly upregulated the expression level of platelet-derived grow factor receptor beta (PDGFRβ) which mediated the interactions of A2 astrocytes and their adjacent vessels, and that the angiogenesis-associated factors (TGFβ and VEGF) in A2 astrocytes were remarkably increased. Overall, these results suggested that rTMS could reduce neuroinflammation, inhibit neuronal apoptosis, and protect the vasculature by regulating astrocyte polarization, thus promoting stroke rehabilitation.
Microglia are the resident immune cells of the CNS, which are distributed in the gray matter and white matter, accounting for ~10% of the number of cells in the brain (135). Microglia maintain a resting state with certain migration and swallowing ability when not activated. They are able to monitor the CNS microenvironment and remove the necrotic neurons timely, thereby maintaining the CNS homeostasis (136). Once activated, microglia can polarize into two distinct phenotypes: the pro-inflammatory M1 and the anti-inflammatory M2 (137). In addition, microglia are early participants in post-stroke neuroinflammation and play an important role in the post-stroke recovery stage (138, 139). Shifting the balance of microglial polarization towards the anti-inflammatory M2 phenotype has been shown to exhibit neuroprotective effects in cerebral stroke (140–142). However, the influence of rTMS on the microglia has been largely unexplored. The number or state of microglia in motor cortex of healthy rats was not changed following application of chronic LF-rTMS (1 Hz) (143). Nevertheless, application of HF-rTMS on the Mongolian gerbils with cerebral ischemia has been shown to significantly increase the number of activated microglia and the expression of Iba1 in the hippocampus (144). Zong et al. applied HF-rTMS (50 Hz) over the affected hemisphere of rat photothrombotic (PT) stroke model, which significantly improved behavioral functions and infarct volume post-stroke (104). Microglial over-activation has been shown to affect neuroinflammation, leading to neuronal death. The immunofluorescence results displayed that Iba1 immunoactivity was obviously increased in the peri-infarct region of PT stroke rat, which could be remarkably rescued by rTMS treatment. Besides, they found that rTMS could effectively induce a M1 to M2 switch in microglial phenotypes, as evidenced by downregulation and upregulation of proteins related to M1 activation (CD74, CD32, and CD86) and the M2 phenotype (CD206, IL-10, and IL-4), respectively. Furthermore, the reactive microglia can release signals to promote astrocytic activation and polarization (145). The A1 to A2 switch in astrocytic phenotypes was also found in rTMS-treated PT stroke rat (103). Luo et al. revealed that rTMS could induce the anti-inflammatory M2 phenotype of microglia and facilitate the generation of anti-inflammatory cytokines (146). Moreover, they found that neural stem cells cultured with medium from rTMS-treated microglia presented decreased apoptosis and increased neuronal differentiation. In addition, Chen et al. revealed that HF-rTMS could regulate the Janus kinase 2 (JAK2)-signal transducer and transcription 3 (STAT3) pathways, and further inhibit microglial activation as well as promote the switch of microglia toward the neuroprotective M2 phenotype, resulting in alleviation of ischemic white matter damage and improvement of cognitive impairment (147). Taken together, rTMS may regulate the microglial polarization and further modulate the inflammatory microenvironment, thereby promoting neurogenesis and improving stroke rehabilitation.
Previous studies have shown that the expression levels of inflammatory cytokine, including interleukin (IL)-1β, IL-2, IL-6, IL-10, IL-17a, interferon (IFN)-γ, transforming growth factor beta (TGF-β), and tumor necrosis factor alpha (TNF-α), in brain tissues and peripheral blood were significantly changed after stroke (148, 149). Among these cytokines, IL-1β, IL-2, IL-6, TNF-α, and IFN-γ are pro-inflammatory, while the IL-10 and TGF-β belong to anti-inflammatory cytokines. IL-10 were reported to be secreted by several cell types after stroke, including M2 microglia, macrophages, regulatory T cells, and B lymphocytes. TGF-β, a factor secreted by A2 astrocytes which regulates cell growth and differentiation, can inhibit the activation of inflammatory/immune cells and the release of various inflammatory mediators, thereby promoting tissue repair after injury (150). Inflammatory cells, including microglia, astrocytes, and infiltrating peripheral immune cells (neutrophils, monocytes and macrophages, etc.), in the ischemic lesions after acute ischemic stroke can release a large number of pro-inflammatory cytokines, including TNF-α, IL-1β, and IL-6, etc, which can cause neuronal damage and upregulate the levels of selectin and ICAM-1 to increase the permeability of cerebral vascular endothelium (151, 152). Moreover, these pro-inflammatory cytokines can also recruit peripheral neutrophils, macrophages, and lymphocytes, which further amplifies the neuroinflammation, eventually causing a vicious cycle of pro-inflammatory. Additionally, the occurrence of PSD is closely related to the imbalance of serum levels of inflammatory cytokines, such as IL-2, IL-10, IL-17a and IFN-γ (153). Among various mechanisms of rTMS-mediated stroke rehabilitation, regulation of inflammatory cytokine may be one of the important mechanisms. Cha et al. revealed that HF-rTMS on the affected dorsolateral prefrontal cortex (DLPFC) of stroke patients could significantly reduce the mRNA level of pro-inflammatory cytokines (IL-1β, IL-6, TGF-β, TNF-α) in blood samples, indicating the anti-inflammatory effect of rTMS (154). Besides, the reduction of IL-6 was strongly correlated with increase of auditory verbal learning test (AVLT, r=-0.928) and complex figure copy test (CFT, r=-0.886). Another study on the ischemic stroke also found that the rTMS were able to decrease the serum levels of IL-6, IL-8, and TNF-α (155). Furthermore, the rTMS treatment could suppress the M1 microglia polarization via let-7b-5p/HMGA2/NF-κB pathway, and further decrease the TNF-α level but elevate the IL-10 concentration, promoting the anti-inflammatory effect (155). iTBS treatment could remarkably reduce the high concentrations of IL-1β, IFN-γ, TNF-α, and IL-17A as well as increase the IL-10 level in brain tissues of cerebral ischemic mice (156). In addition, the pro-inflammatory cytokines could affect the synaptic plasticity, which might further influence cognitive function and behavior (157–159). For example, the TNF-α, produced by microglia and peripheral immune cells, could affect long-term potentiation (LTP) of excitatory neurotransmission at higher concentration (160, 161). Therefore, the regulation of cytokine concentrations in serum and brain tissues is an important mechanism of rTMS-mediated rehabilitation, which could also be used as indicators to evaluate the effect of stroke rehabilitation.
Imaging techniques play an important role in understanding the mechanisms underlying the effects of rTMS and in guiding the optimization of rTMS protocols. First, these imaging techniques could be applied to identify the optimal target. Specifically, imaging techniques such as fMRI and TMS mapping can help identify the regions of the brain that are affected by the stroke and the regions that need to be targeted by rTMS for optimal recovery (162). Secondly, the imaging techniques can be used to assess the treatment effects of rTMS on brain function and connectivity of stroke patients. For example, fMRI can be utilized to measure changes in brain activity, while diffusion tensor imaging (DTI) can be used to detect changes in white matter integrity (163, 164).Li et al. utilized resting-state fMRI (rs-fMRI) to explore the effect and mechanism of rTMS on PSCI patients (95). They revealed that the rTMS (5 Hz, 100% RMT) could significantly improve cognitive functions measured by Minimum Mental State Examination (MMSE) and Montreal cognitive assessment (MoCA). They also found that FC and neural activity in several cognition-related brain regions were significantly regulated by rTMS therapy. These imaging results indicated that the rTMS showed effective impact on the PSCI patients to improve their cognitive function. Besides, the imaging techniques can be applied to personalize rTMS treatment for individual patients. For instance, fMRI can be used to identify regions of the brain that are still functionally active after stroke, and then rTMS can be targeted to these regions to enhance recovery (165). In addition, the imaging techniques can be also utilized to predict the outcomes of rTMS-mediated stroke rehabilitation. The diffusion MRI can be used to predict motor function recovery after stroke, which could also guide rTMS treatment (166). Overall, imaging techniques are an important tool in rTMS-mediated stroke rehabilitation, providing valuable information on the underlying mechanisms of stroke recovery and helping to optimize rTMS protocols for individual patients.
rTMS is a novel technique that can regulate neurotransmitters, activation/polarization of immune cells (astrocytes and microglia), and inflammatory cytokines in the brain, thereby affecting brain function and improving post-stroke dysfunctions. Moreover, rTMS combined with other traditional rehabilitation programs can present a synergistic effect and further enhance the rehabilitation effect of stroke patients.
According to previous safety evaluation, although the incidence rate is low, rTMS could induce several side effects, including epilepsy, syncope, short-term hearing loss, headache pain, dizziness, toothache, and paresthesia, etc (167–172). Thus, the rTMS should be applied within the range of treatment parameters according to the guideline. There are many stimulation parameters of rTMS, including coil type, coil position, stimulation frequency, stimulation time, stimulation interval, and total stimulation volume, etc, which might be the main reason for the heterogeneity of clinical treatment effects and basic research results. Due to the obvious differences in different rTMS stimulations and clinical outcome evaluation indicators, it has brought great difficulties to the study of optimal stimulation parameters. In some clinical studies, patients in control group did not receive reliable sham stimulation, therefore, it is difficult to determine the relative efficacy of placebo in rTMS. Generally, the sample size of current clinical studies of rTMS-mediated stroke rehabilitation is still insufficient. Therefore, randomized, double-blind, large sample size, and placebo-controlled clinical studies should be performed in the future to further clarify the clinical value of TMS. In addition, LF-rTMS and HF-rTMS are more clinically applied at present. TBS has fewer clinical applications, but it requires lower stimulation intensity, fewer pulses, and produces longer-lasting cortical excitability compared to the formers. Thus, TBS might be a promising approach for stroke rehabilitation, which needs to further explored in the future study.
Recent study has shown that LF-rTMS on the frontal cortex could reduce the local inhibition and disrupt feedforward and feedback connections, whereas, the LF-rTMS on the occipital cortex could enhance the local inhibition and increase forward signaling. A more thorough understanding of the mechanism underlying the rTMS-mediated CNS regulation allow us to better apply rTMS to clinical treatment. However, the current mechanism research is still in the preliminary stage, thus, future studies need to further explore the regulatory mechanism of rTMS on the CNS, especially the regulation of immune inflammation.
RS wrote the manuscript. PY drew the pictures. CC and HC revised the manuscript. RS and PY collected the literature. All authors contributed to the article and approved the submitted version.
The authors declare that the research was conducted in the absence of any commercial or financial relationships that could be construed as a potential conflict of interest.
All claims expressed in this article are solely those of the authors and do not necessarily represent those of their affiliated organizations, or those of the publisher, the editors and the reviewers. Any product that may be evaluated in this article, or claim that may be made by its manufacturer, is not guaranteed or endorsed by the publisher.
1. Kolominsky-Rabas PL, Weber M, Gefeller O, Neundoerfer B, Heuschmann PU. Epidemiology of ischemic stroke subtypes according to TOAST criteria: incidence, recurrence, and long-term survival in ischemic stroke subtypes: a population-based study. Stroke (2001) 32(12):2735–40. doi: 10.1161/hs1201.100209
2. Roger VL, Go AS, Lloyd-Jones DM, Adams RJ, Berry JD, Brown TM, et al. Heart disease and stroke statistics–2011 update: a report from the American heart association. Circulation (2011) 123(4):e18–e209. doi: 10.1161/CIR.0b013e3182009701
3. Starosta M, Cichon N, Saluk -Bijak J, Miller E. Benefits from repetitive transcranial magnetic stimulation in post-stroke rehabilitation. J Clin Med (2022) 11(8). doi: 10.3390/jcm11082149
4. Di Pino G, Pellegrino G, Assenza G, Capone F, Ferreri F, Formica D, et al. Modulation of brain plasticity in stroke: a novel model for neurorehabilitation. Nat Rev Neurol (2014) 10(10):597–608. doi: 10.1038/nrneurol.2014.162
5. Rusu L, Paun E, Marin MI, Hemanth J, Rusu MR, Calina ML, et al. Plantar pressure and contact area measurement of foot abnormalities in stroke rehabilitation. Brain Sci (2021) 11(9). doi: 10.3390/brainsci11091213
6. Hebert D, Lindsay MP, McIntyre A, Kirton A, Rumney PG, Bagg S, et al. Canadian Stroke best practice recommendations: stroke rehabilitation practice guidelines, update 2015. Int J Stroke (2016) 11(4):459–84. doi: 10.1177/1747493016643553
7. Nair K, Taly A. Stroke rehabilitation: traditional and modern approaches. Neurol India (2002) 50(50):85–93.
8. Ying W, Aimin W. Augmented reality based upper limb rehabilitation system. in: 2017 13th IEEE International Conference on Electronic Measurement & Instruments (ICEMI), (Yangzhou: The Institute of Electrical and Electronics Engineers). (2017).
9. Chang WH, Kim YH. Robot-assisted therapy in stroke rehabilitation. J Stroke (2013) 15(3):174–81. doi: 10.5853/jos.2013.15.3.174
10. Laver KE, Lange B, George S, Deutsch JE, Saposnik G, Crotty M. Virtual reality for stroke rehabilitation. Cochrane Database Syst Rev (2017) 11(11):CD008349. doi: 10.1002/14651858.CD008349.pub4
11. Ramos-Murguialday A, Broetz D, Rea M, Läer L, Yilmaz O, Brasil FL, et al. Brain-machine interface in chronic stroke rehabilitation: a controlled study. Ann Neurol (2013) 74(1):100–8. doi: 10.1002/ana.23879
12. Schlaug G, Renga V, Nair D. Transcranial direct current stimulation in stroke recovery. Arch Neurol (2008) 65(12):1571–6. doi: 10.1001/archneur.65.12.1571
13. Dimyan MA, Cohen LG. Contribution of transcranial magnetic stimulation to the understanding of functional recovery mechanisms after stroke. Neurorehabil Neural Repair (2010) 24(2):125–35. doi: 10.1177/1545968309345270
14. Nardone R, Tezzon F, Höller Y, Golaszewski S, Trinka E, Brigo F. Transcranial magnetic stimulation (TMS)/repetitive TMS in mild cognitive impairment and alzheimer’s disease. Acta Neurol Scand (2014) 129(6):351–66. doi: 10.1111/ane.12223
15. Martin DM, McClintock SM, Forster JJ, Lo TY, Loo CK. Cognitive enhancing effects of rTMS administered to the prefrontal cortex in patients with depression: a systematic review and meta-analysis of individual task effects. Depress Anxiety (2017) 34(11):1029–39. doi: 10.1002/da.22658
16. McIntyre A, Thompson S, Burhan A, Mehta S, Teasell R. Repetitive transcranial magnetic stimulation for depression due to cerebrovascular disease: a systematic review. J Stroke Cerebrovasc Dis (2016) 25(12):2792–800. doi: 10.1016/j.jstrokecerebrovasdis.2016.08.043
17. Lanza G, Bella R, Cantone M, Pennisi G, Ferri R, Pennisi M. Cognitive impairment and celiac disease: is transcranial magnetic stimulation a trait d’Union between gut and brain? Int J Mol Sci (2018) 19(8). doi: 10.3390/ijms19082243
18. Boddington LJ, Reynolds JNJ. Targeting interhemispheric inhibition with neuromodulation to enhance stroke rehabilitation. Brain Stimul (2017) 10(2):214–22. doi: 10.1016/j.brs.2017.01.006
19. Rogalewski A, Schäbitz WR. Stroke recovery enhancing therapies: lessons from recent clinical trials. Neural Regener Res (2022) 17(4):717–20. doi: 10.4103/1673-5374.314287
20. Sankarasubramanian V, Machado AG, Conforto AB, Potter-Baker KA, Cunningham DA, Varnerin NM, et al. Inhibition versus facilitation of contralesional motor cortices in stroke: deriving a model to tailor brain stimulation. Clin Neurophysiol (2017) 128(6):892–902. doi: 10.1016/j.clinph.2017.03.030
21. Lüdemann-Podubecká J, Bösl K, Rothhardt S, Verheyden G, Nowak DA. Transcranial direct current stimulation for motor recovery of upper limb function after stroke. Neurosci Biobehav Rev (2014) 47:245–59. doi: 10.1016/j.neubiorev.2014.07.022
22. Kinoshita S, Kakuda W, Yamada N, Momosaki R, Okuma R, Watanabe S, et al. Therapeutic administration of atomoxetine combined with rTMS and occupational therapy for upper limb hemiparesis after stroke: a case series study of three patients. Acta Neurol Belg (2016) 116(1):31–7. doi: 10.1007/s13760-015-0503-3
23. Kim BR, Kim DY, Chun MH, Yi JH, Kwon JS. Effect of repetitive transcranial magnetic stimulation on cognition and mood in stroke patients: a double-blind, sham-controlled trial. Am J Phys Med Rehabil (2010) 89(5):362–8. doi: 10.1097/PHM.0b013e3181d8a5b1
24. Cheng IK, Chan KM, Wong CS, Cheung RT. Preliminary evidence of the effects of high-frequency repetitive transcranial magnetic stimulation (rTMS) on swallowing functions in post-stroke individuals with chronic dysphagia. Int J Lang Commun Disord (2015) 50(3):389–96. doi: 10.1111/1460-6984.12144
25. Aşkın A, Tosun A, Demirdal Ü S. Effects of low-frequency repetitive transcranial magnetic stimulation on upper extremity motor recovery and functional outcomes in chronic stroke patients: a randomized controlled trial. Somatosens Mot Res (2017) 34(2):102–7. doi: 10.1080/08990220.2017.1316254
26. Lüdemann-Podubecká J, Bösl K, Nowak DA. Inhibition of the contralesional dorsal premotor cortex improves motor function of the affected hand following stroke. Eur J Neurol (2016) 23(4):823–30. doi: 10.1111/ene.12949
27. Tosun A, Türe S, Askin A, Yardimci EU, Demirdal SU, Kurt Incesu T, et al. Effects of low-frequency repetitive transcranial magnetic stimulation and neuromuscular electrical stimulation on upper extremity motor recovery in the early period after stroke: a preliminary study. Top Stroke Rehabil (2017) 24(5):361–7. doi: 10.1080/10749357.2017.1305644
28. Hosomi K, Morris S, Sakamoto T, Taguchi J, Maruo T, Kageyama Y, et al. Daily repetitive transcranial magnetic stimulation for poststroke upper limb paresis in the subacute period. J Stroke Cerebrovasc Dis (2016) 25(7):1655–64. doi: 10.1016/j.jstrokecerebrovasdis.2016.02.024
29. Sasaki N, Abo M, Hara T, Yamada N, Niimi M, Kakuda W. High-frequency rTMS on leg motor area in the early phase of stroke. Acta Neurol Belg (2017) 117(1):189–94. doi: 10.1007/s13760-016-0687-1
30. Choi CM, Kim JH, Lee JK, Lee BY, Kee HS, Jung KI, et al. Effects of repetitive transcranial magnetic stimulation over trunk motor spot on balance function in stroke patients. Ann Rehabil Med (2016) 40(5):826–34. doi: 10.5535/arm.2016.40.5.826
31. Khedr EM, Abo-Elfetoh N, Rothwell JC. Treatment of post-stroke dysphagia with repetitive transcranial magnetic stimulation. Acta Neurol Scand (2009) 119(3):155–61. doi: 10.1111/j.1600-0404.2008.01093.x
32. Sasaki N, Hara T, Yamada N, Niimi M, Kakuda W, Abo M. The efficacy of high-frequency repetitive transcranial magnetic stimulation for improving apathy in chronic stroke patients. Eur Neurol (2017) 78(1-2):28–32. doi: 10.1159/000477440
33. Sharma H, Vishnu VY, Kumar N, Sreenivas V, Rajeswari MR, Bhatia R, et al. Efficacy of low-frequency repetitive transcranial magnetic stimulation in ischemic stroke: a double-blind randomized controlled trial. Arch Rehabil Res Clin Transl (2020) 2(1):100039. doi: 10.1016/j.arrct.2020.100039
34. Yin M, Liu Y, Zhang L, Zheng H, Peng L, Ai Y, et al. Effects of rTMS treatment on cognitive impairment and resting-state brain activity in stroke patients: a randomized clinical trial. Front Neural Circuits (2020) 14:563777. doi: 10.3389/fncir.2020.563777
35. Kwah LK, Harvey LA, Diong J, Herbert RD. Models containing age and NIHSS predict recovery of ambulation and upper limb function six months after stroke: an observational study. J Physiother (2013) 59(3):189–97. doi: 10.1016/s1836-9553(13)70183-8
36. Raghavan P. Upper limb motor impairment after stroke. Phys Med Rehabil Clin N Am (2015) 26(4):599–610. doi: 10.1016/j.pmr.2015.06.008
37. Cordella F, Ciancio AL, Sacchetti R, Davalli A, Cutti AG, Guglielmelli E, et al. Literature review on needs of upper limb prosthesis users. Front Neurosci (2016) 10:209. doi: 10.3389/fnins.2016.00209
38. Rehme AK, Eickhoff SB, Rottschy C, Fink GR, Grefkes C. Activation likelihood estimation meta-analysis of motor-related neural activity after stroke. Neuroimage (2012) 59(3):2771–82. doi: 10.1016/j.neuroimage.2011.10.023
39. Grefkes C, Fink GR. Connectivity-based approaches in stroke and recovery of function. Lancet Neurol (2014) 13(2):206–16. doi: 10.1016/s1474-4422(13)70264-3
40. van Lieshout ECC, Visser-Meily JMA, Neggers SFW, van der Worp HB, Dijkhuizen RM. Brain stimulation for arm recovery after stroke (B-STARS): protocol for a randomised controlled trial in subacute stroke patients. BMJ Open (2017) 7(8):e016566. doi: 10.1136/bmjopen-2017-016566
41. Blesneag AV, Slă voacă DF, Popa L, Stan AD, Jemna N, Isai Moldovan F, et al. Low-frequency rTMS in patients with subacute ischemic stroke: clinical evaluation of short and long-term outcomes and neurophysiological assessment of cortical excitability. J Med Life (2015) 8(3):378–87.
42. Kubis N. Non-invasive brain stimulation to enhance post-stroke recovery. Front Neural Circuits (2016) 10:56. doi: 10.3389/fncir.2016.00056
43. Harvey RL, Edwards D, Dunning K, Fregni F, Stein J, Laine J, et al. Randomized sham-controlled trial of navigated repetitive transcranial magnetic stimulation for motor recovery in stroke. Stroke (2018) 49(9):2138–46. doi: 10.1161/strokeaha.117.020607
44. Corti M, Patten C, Triggs W. Repetitive transcranial magnetic stimulation of motor cortex after stroke: a focused review. Am J Phys Med Rehabil (2012) 91(3):254–70. doi: 10.1097/PHM.0b013e318228bf0c
45. Lüdemann-Podubecká J, Bösl K, Theilig S, Wiederer R, Nowak DA. The effectiveness of 1 Hz rTMS over the primary motor area of the unaffected hemisphere to improve hand function after stroke depends on hemispheric dominance. Brain Stimul (2015) 8(4):823–30. doi: 10.1016/j.brs.2015.02.004
46. Kim H, Lee G, Song C. Effect of functional electrical stimulation with mirror therapy on upper extremity motor function in poststroke patients. J Stroke Cerebrovasc Dis (2014) 23(4):655–61. doi: 10.1016/j.jstrokecerebrovasdis.2013.06.017
47. Hsu WY, Cheng CH, Liao KK, Lee IH, Lin YY. Effects of repetitive transcranial magnetic stimulation on motor functions in patients with stroke: a meta-analysis. Stroke (2012) 43(7):1849–57. doi: 10.1161/strokeaha.111.649756
48. Lefaucheur JP, Aleman A, Baeken C, Benninger DH, Brunelin J, Di Lazzaro V, et al. Evidence-based guidelines on the therapeutic use of repetitive transcranial magnetic stimulation (rTMS): an update (2014-2018). Clin Neurophysiol (2020) 131(2):474–528. doi: 10.1016/j.clinph.2019.11.002
49. Zhang L, Xing G, Fan Y, Guo Z, Chen H, Mu Q. Short- and long-term effects of repetitive transcranial magnetic stimulation on upper limb motor function after stroke: a systematic review and meta-analysis. Clin Rehabil (2017) 31(9):1137–53. doi: 10.1177/0269215517692386
50. Zheng CJ, Liao WJ, Xia WG. Effect of combined low-frequency repetitive transcranial magnetic stimulation and virtual reality training on upper limb function in subacute stroke: a double-blind randomized controlled trail. J Huazhong Univ Sci Technolog Med Sci (2015) 35(2):248–54. doi: 10.1007/s11596-015-1419-0
51. Noh JS, Lim JH, Choi TW, Jang SG, Pyun SB. Effects and safety of combined rTMS and action observation for recovery of function in the upper extremities in stroke patients: a randomized controlled trial. Restor Neurol Neurosci (2019) 37(3):219–30. doi: 10.3233/rnn-180883
52. Graef P, Dadalt MLR, Rodrigués D, Stein C, Pagnussat AS. Transcranial magnetic stimulation combined with upper-limb training for improving function after stroke: a systematic review and meta-analysis. J Neurol Sci (2016) 369:149–58. doi: 10.1016/j.jns.2016.08.016
53. Robinson CA, Shumway-Cook A, Matsuda PN, Ciol MA. Understanding physical factors associated with participation in community ambulation following stroke. Disabil Rehabil (2011) 33(12):1033–42. doi: 10.3109/09638288.2010.520803
54. Winstein CJ, Stein J, Arena R, Bates B, Cherney LR, Cramer SC, et al. Guidelines for adult stroke rehabilitation and recovery: a guideline for healthcare professionals from the American heart Association/American stroke association. Stroke (2016) 47(6):e98–e169. doi: 10.1161/str.0000000000000098
56. Tung YC, Lai CH, Liao CD, Huang SW, Liou TH, Chen HC. Repetitive transcranial magnetic stimulation of lower limb motor function in patients with stroke: a systematic review and meta-analysis of randomized controlled trials. Clin Rehabil (2019) 33(7):1102–12. doi: 10.1177/0269215519835889
57. Li Y, Fan J, Yang J, He C, Li S. Effects of repetitive transcranial magnetic stimulation on walking and balance function after stroke: a systematic review and meta-analysis. Am J Phys Med Rehabil (2018) 97(11):773–81. doi: 10.1097/phm.0000000000000948
58. Vaz PG, Salazar A, Stein C, Marchese RR, Lukrafka JL, Plentz RDM, et al. Noninvasive brain stimulation combined with other therapies improves gait speed after stroke: a systematic review and meta-analysis. Top Stroke Rehabil (2019) 26(3):201–13. doi: 10.1080/10749357.2019.1565696
59. Santamato A, Cinone N, Panza F, Letizia S, Santoro L, Lozupone M, et al. Botulinum toxin type a for the treatment of lower limb spasticity after stroke. Drugs (2019) 79(2):143–60. doi: 10.1007/s40265-018-1042-z
60. Liu Y, Li H, Zhang J, Zhao QQ, Mei HN, Ma J. A meta-analysis: whether repetitive transcranial magnetic stimulation improves dysfunction caused by stroke with lower limb spasticity. Evid Based Complement Alternat Med (2021), 7219293. doi: 10.1155/2021/7219293
61. Yang G, Wang Y, Zeng Y, Gao GF, Liang X, Zhou M, et al. Rapid health transition in China, 1990-2010: findings from the global burden of disease study 2010. Lancet (2013) 381(9882):1987–2015. doi: 10.1016/s0140-6736(13)61097-1
62. Suntrup S, Kemmling A, Warnecke T, Hamacher C, Oelenberg S, Niederstadt T, et al. The impact of lesion location on dysphagia incidence, pattern and complications in acute stroke. part 1: dysphagia incidence, severity and aspiration. Eur J Neurol (2015) 22(5):832–8. doi: 10.1111/ene.12670
63. Martino R, Foley N, Bhogal S, Diamant N, Speechley M, Teasell R. Dysphagia after stroke: incidence, diagnosis, and pulmonary complications. Stroke (2005) 36(12):2756–63. doi: 10.1161/01.STR.0000190056.76543.eb
64. Castelão M, Marques RE, Duarte GS, Rodrigues FB, Ferreira J, Sampaio C, et al. Botulinum toxin type a therapy for cervical dystonia. Cochrane Database Syst Rev (2017) 12(12). doi: 10.1002/14651858.CD003633.pub3
65. Geeganage C, Beavan J, Ellender S, Bath PM. Interventions for dysphagia and nutritional support in acute and subacute stroke. Cochrane Database Syst Rev (2012) 10:Cd000323. doi: 10.1002/14651858.CD000323.pub2
66. Ashford J, McCabe D, Wheeler-Hegland K, Frymark T, Mullen R, Musson N, et al. Evidence-based systematic review: oropharyngeal dysphagia behavioral treatments. part III–impact of dysphagia treatments on populations with neurological disorders. J Rehabil Res Dev (2009) 46(2):195–204. doi: 10.1682/JRRD.2008.08.0091
67. Chiang CF, Lin MT, Hsiao MY, Yeh YC, Liang YC, Wang TG. Comparative efficacy of noninvasive neurostimulation therapies for acute and subacute poststroke dysphagia: a systematic review and network meta-analysis. Arch Phys Med Rehabil (2019) 100(4):739–750.e4. doi: 10.1016/j.apmr.2018.09.117
68. Verin E, Leroi AM. Poststroke dysphagia rehabilitation by repetitive transcranial magnetic stimulation: a noncontrolled pilot study. Dysphagia (2009) 24(2):204–10. doi: 10.1007/s00455-008-9195-7
69. Lee JH, Kim SB, Lee KW, Lee SJ, Lee JU. Effect of repetitive transcranial magnetic stimulation according to the stimulation site in stroke patients with dysphagia. Ann Rehabil Med (2015) 39(3):432–9. doi: 10.5535/arm.2015.39.3.432
70. Park E, Kim MS, Chang WH, Oh SM, Kim YK, Lee A, et al. Effects of bilateral repetitive transcranial magnetic stimulation on post-stroke dysphagia. Brain Stimul (2017) 10(1):75–82. doi: 10.1016/j.brs.2016.08.005
71. Yang SN, Pyun SB, Kim HJ, Ahn HS, Rhyu BJ. Effectiveness of non-invasive brain stimulation in dysphagia subsequent to stroke: a systemic review and meta-analysis. Dysphagia (2015) 30(4):383–91. doi: 10.1007/s00455-015-9619-0
72. Carnes-Vendrell A, Deus-Yela J, Molina-Seguin J, Pifarre-Paredero J, Purroy F. Update on post-stroke depression: posing new challenges in patients with a minor stroke or transient ischaemic attack. Rev Neurol (2016) 62(10):460–7. doi: 10.33588/rn.6210.2015473
73. Bartoli F, Lillia N, Lax A, Crocamo C, Mantero V, Carrà G, et al. Depression after stroke and risk of mortality: a systematic review and meta-analysis. Stroke Res Treat (2013), 862978. doi: 10.1155/2013/862978
74. Espárrago Llorca G, Castilla-Guerra L, Fernández Moreno MC, Ruiz Doblado S, Jiménez Hernández MD. Post-stroke depression: an update. Neurologia (2015) 30(1):23–31. doi: 10.1016/j.nrl.2012.06.008
75. Kim JS. Post-stroke mood and emotional disturbances: pharmacological therapy based on mechanisms. J Stroke (2016) 18(3):244–55. doi: 10.5853/jos.2016.01144
76. Al-Harbi KS. Treatment-resistant depression: therapeutic trends, challenges, and future directions. Patient Prefer Adherence (2012) 6:369–88. doi: 10.2147/ppa.S29716
77. Xu X-M, Zou D-Z, Shen L, Liu Y, Zhou X, Pu J, et al. Efficacy and feasibility of antidepressant treatment in patients with post-stroke depression. Medicine (2016) 95. doi: 10.1097/MD.0000000000005349
78. Blumberger DM, Vila-Rodriguez F, Thorpe KE, Feffer K, Noda Y, Giacobbe P, et al. Effectiveness of theta burst versus high-frequency repetitive transcranial magnetic stimulation in patients with depression (THREE-d): a randomised non-inferiority trial. Lancet (2018) 391(10131):1683–92. doi: 10.1016/s0140-6736(18)30295-2
79. Cash RFH, Cocchi L, Lv J, Fitzgerald PB, Zalesky A. Functional magnetic resonance imaging-guided personalization of transcranial magnetic stimulation treatment for depression. JAMA Psychiatry (2021) 78(3):337–9. doi: 10.1001/jamapsychiatry.2020.3794
80. O’Reardon JP, Solvason HB, Janicak PG, Sampson S, Isenberg KE, Nahas Z, et al. Efficacy and safety of transcranial magnetic stimulation in the acute treatment of major depression: a multisite randomized controlled trial. Biol Psychiatry (2007) 62(11):1208–16. doi: 10.1016/j.biopsych.2007.01.018
81. Jorge RE, Robinson RG, Tateno A, Narushima K, Acion L, Moser D, et al. Repetitive transcranial magnetic stimulation as treatment of poststroke depression: a preliminary study. Biol Psychiatry (2004) 55(4):398–405. doi: 10.1016/j.biopsych.2003.08.017
82. Hordacre B, Comacchio K, Williams L, Hillier S. Repetitive transcranial magnetic stimulation for post-stroke depression: a randomised trial with neurophysiological insight. J Neurol (2021) 268(4):1474–84. doi: 10.1007/s00415-020-10315-6
83. Shen X, Liu M, Cheng Y, Jia C, Pan X, Gou Q, et al. Repetitive transcranial magnetic stimulation for the treatment of post-stroke depression: a systematic review and meta-analysis of randomized controlled clinical trials. J Affect Disord (2017) 211:65–74. doi: 10.1016/j.jad.2016.12.058
84. Aben HP, Reijmer YD, Visser-Meily JM, Spikman JM, de Bresser J, Biessels GJ, et al. A role for new brain magnetic resonance imaging modalities in daily clinical practice: protocol of the prediction of cognitive recovery after stroke (PROCRAS) study. JMIR Res Protoc (2018) 7(5):e127. doi: 10.2196/resprot.9431
85. Portegies ML, Wolters FJ, Hofman A, Ikram MK, Koudstaal PJ, Ikram MA. Prestroke vascular pathology and the risk of recurrent stroke and poststroke dementia. Stroke (2016) 47(8):2119–22. doi: 10.1161/strokeaha.116.014094
86. Mole JA, Demeyere N. The relationship between early post-stroke cognition and longer term activities and participation: a systematic review. Neuropsychol Rehabil (2020) 30(2):346–70. doi: 10.1080/09602011.2018.1464934
87. Barker-Collo S, Feigin VL, Parag V, Lawes CM, Senior H. Auckland Stroke outcomes study. part 2: cognition and functional outcomes 5 years poststroke. Neurology (2010) 75(18):1608–16. doi: 10.1212/WNL.0b013e3181fb44c8
88. Pinter MM, Brainin M. Rehabilitation after stroke in older people. Maturitas (2012) 71(2):104–8. doi: 10.1016/j.maturitas.2011.11.011
89. Planton M, Peiffer S, Albucher JF, Barbeau EJ, Tardy J, Pastor J, et al. Neuropsychological outcome after a first symptomatic ischaemic stroke with ‘good recovery’. Eur J Neurol (2012) 19(2):212–9. doi: 10.1111/j.1468-1331.2011.03450.x
90. Beristain X, Golombievski E. Pharmacotherapy to enhance cognitive and motor recovery following stroke. Drugs Aging (2015) 32(10):765–72. doi: 10.1007/s40266-015-0299-0
91. Iadecola C, Duering M, Hachinski V, Joutel A, Pendlebury ST, Schneider JA, et al. Vascular cognitive impairment and dementia: JACC scientific expert panel. J Am Coll Cardiol (2019) 73(25):3326–44. doi: 10.1016/j.jacc.2019.04.034
92. Trung J, Hanganu A, Jobert S, Degroot C, Mejia-Constain B, Kibreab M, et al. Transcranial magnetic stimulation improves cognition over time in parkinson’s disease. Parkinsonism Relat Disord (2019) 66:3–8. doi: 10.1016/j.parkreldis.2019.07.006
93. McIntyre RS, Lee Y, Rodrigues NB, Nasri F, Lao G, Zeng W, et al. Repetitive transcranial magnetic stimulation for cognitive function in adults with bipolar disorder: a pilot study. J Affect Disord (2021) 293:73–7. doi: 10.1016/j.jad.2021.05.075
94. Hara T, Abo M, Kakita K, Masuda T, Yamazaki R. Does a combined intervention program of repetitive transcranial magnetic stimulation and intensive occupational therapy affect cognitive function in patients with post-stroke upper limb hemiparesis? Neural Regener Res (2016) 11(12):1932–9. doi: 10.4103/1673-5374.197134
95. Li Y, Luo H, Yu Q, Yin L, Li K, Li Y, et al. Cerebral functional manipulation of repetitive transcranial magnetic stimulation in cognitive impairment patients after stroke: an fMRI study. Front Neurol (2020) 11:977. doi: 10.3389/fneur.2020.00977
96. Tsai PY, Lin WS, Tsai KT, Kuo CY, Lin PH. High-frequency versus theta burst transcranial magnetic stimulation for the treatment of poststroke cognitive impairment in humans. J Psychiatry Neurosci (2020) 45(4):262–70. doi: 10.1503/jpn.190060
97. Boivie J, Leijon G, Johansson I. Central post-stroke pain {{/amp]]mdash; a study of the mechanisms through analyses of the sensory abnormalities. PAIN (1989) 37(2):173–85. doi: 10.1016/0304-3959(89)90128-0
98. Jang SH, Seo JP, Lee SJ. Diffusion tensor tractography studies of central post-stroke pain due to the spinothalamic tract injury: a mini-review. Front Neurol (2019) 10:787. doi: 10.3389/fneur.2019.00787
99. Kim JS. Pharmacological management of central post-stroke pain: a practical guide. CNS Drugs (2014) 28(9):787–97. doi: 10.1007/s40263-014-0194-y
100. Chen CC, Chuang YF, Huang AC, Chen CK, Chang YJ. The antalgic effects of non-invasive physical modalities on central post-stroke pain: a systematic review. J Phys Ther Sci (2016) 28(4):1368–73. doi: 10.1589/jpts.28.1368
101. Leung A, Donohue M, Xu R, Lee R, Lefaucheur JP, Khedr EM, et al. rTMS for suppressing neuropathic pain: a meta-analysis. J Pain (2009) 10(12):1205–16. doi: 10.1016/j.jpain.2009.03.010
102. Pan LJ, Zhu HQ, Zhang XA, Wang XQ. The mechanism and effect of repetitive transcranial magnetic stimulation for post-stroke pain. Front Mol Neurosci (2022) 15:1091402. doi: 10.3389/fnmol.2022.1091402
103. Zong X, Li Y, Liu C, Qi W, Han D, Tucker L, et al. Theta-burst transcranial magnetic stimulation promotes stroke recovery by vascular protection and neovascularization. Theranostics (2020) 10(26):12090–110. doi: 10.7150/thno.51573
104. Zong X, Dong Y, Li Y, Yang L, Li Y, Yang B, et al. Beneficial effects of theta-burst transcranial magnetic stimulation on stroke injury via improving neuronal microenvironment and mitochondrial integrity. Transl Stroke Res (2020) 11(3):450–67. doi: 10.1007/s12975-019-00731-w
105. Chen QM, Yao FR, Sun HW, Chen ZG, Ke J, Liao J, et al. Combining inhibitory and facilitatory repetitive transcranial magnetic stimulation (rTMS) treatment improves motor function by modulating GABA in acute ischemic stroke patients. Restor Neurol Neurosci (2021) 39(6):419–34. doi: 10.3233/rnn-211195
106. Snyder SH. Neurotransmitters and CNS disease. schizophrenia. Lancet (1982) 2(8305):970–4. doi: 10.1016/s0140-6736(82)90167-2
107. Villa RF, Ferrari F, Moretti A. Post-stroke depression: mechanisms and pharmacological treatment. Pharmacol Ther (2018) 184:131–44. doi: 10.1016/j.pharmthera.2017.11.005
108. Mouro FM, Köfalvi A, André LA, Baqi Y, Müller CE, Ribeiro JA, et al. Memory deficits induced by chronic cannabinoid exposure are prevented by adenosine A(2A)R receptor antagonism. Neuropharmacology (2019) 155:10–21. doi: 10.1016/j.neuropharm.2019.05.003
109. Nutt DJ. Relationship of neurotransmitters to the symptoms of major depressive disorder. J Clin Psychiatry (2008) 69 Suppl E1:4–7.
110. Sánchez-Mendoza E, Bellver-Landete V, Merino JJ, González MP, Martínez-Murillo R, Oset-Gasque MJ. Review: could neurotransmitters influence neurogenesis and neurorepair after stroke? Neuropathology Appl Neurobiol (2013) 39(7):722–35. doi: 10.1111/nan.12082
111. Ikeda T, Kobayashi S, Morimoto C. Effects of repetitive transcranial magnetic stimulation on ER stress-related genes and glutamate, γ-aminobutyric acid and glycine transporter genes in mouse brain. Biochem Biophys Rep (2019) 17:10–6. doi: 10.1016/j.bbrep.2018.10.015
112. Gröhn H, Gillick BT, Tkáč I, Bednař ík P, Mascali D, Deelchand DK, et al. Influence of repetitive transcranial magnetic stimulation on human neurochemistry and functional connectivity: a pilot MRI/MRS study at 7 T. Front Neurosci (2019) 13:1260. doi: 10.3389/fnins.2019.01260
113. Lefaucheur JP, Drouot X, Ménard-Lefaucheur I, Keravel Y, Nguyen JP. Motor cortex rTMS restores defective intracortical inhibition in chronic neuropathic pain. Neurology (2006) 67(9):1568–74. doi: 10.1212/01.wnl.0000242731.10074.3c
114. Ikeda T, Kobayashi S, Morimoto C. Gene expression microarray data from mouse CBS treated with rTMS for 30 days, mouse cerebrum and CBS treated with rTMS for 40 days. Data Brief (2018) 17:1078–81. doi: 10.1016/j.dib.2018.01.079
115. Shen Z, Xiang M, Chen C, Ding F, Wang Y, Shang C, et al. Glutamate excitotoxicity: potential therapeutic target for ischemic stroke. BioMed Pharmacother (2022) 151:113125. doi: 10.1016/j.biopha.2022.113125
116. Ito S. GABA and glycine in the developing brain. J Physiol Sci (2016) 66(5):375–9. doi: 10.1007/s12576-016-0442-7
117. Zangen A, Hyodo K. Transcranial magnetic stimulation induces increases in extracellular levels of dopamine and glutamate in the nucleus accumbens. Neuroreport (2002) 13(18):2401–5. doi: 10.1097/00001756-200212200-00005
118. Canavero S, Bonicalzi V. The neurochemistry of central pain: evidence from clinical studies, hypothesis and therapeutic implications. Pain (1998) 74(2-3):109–14. doi: 10.1016/s0304-3959(97)00089-4
119. Guilbaud G, Benoist JM, Levante A, Gautron M, Willer JC. Primary somatosensory cortex in rats with pain-related behaviours due to a peripheral mononeuropathy after moderate ligation of one sciatic nerve: neuronal responsivity to somatic stimulation. Exp Brain Res (1992) 92(2):227–45. doi: 10.1007/bf00227967
120. Koyama S, Katayama Y, Maejima S, Hirayama T, Fujii M, Tsubokawa T. Thalamic neuronal hyperactivity following transection of the spinothalamic tract in the cat: involvement of n-methyl-D-aspartate receptor. Brain Res (1993) 612(1-2):345–50. doi: 10.1016/0006-8993(93)91684-k
121. Bonnì S, Ponzo V, Di Lorenzo F, Caltagirone C, Koch G. Real-time activation of central cholinergic circuits during recognition memory. Eur J Neurosci (2017) 45(11):1485–9. doi: 10.1111/ejn.13588
122. Zhang XQ, Li L, Huo JT, Cheng M, Li LH. Effects of repetitive transcranial magnetic stimulation on cognitive function and cholinergic activity in the rat hippocampus after vascular dementia. Neural Regener Res (2018) 13(8):1384–9. doi: 10.4103/1673-5374.235251
123. Luo J, Zheng H, Zhang L, Zhang Q, Li L, Pei Z, et al. High-frequency repetitive transcranial magnetic stimulation (rTMS) improves functional recovery by enhancing neurogenesis and activating BDNF/TrkB signaling in ischemic rats. Int J Mol Sci (2017) 18(2). doi: 10.3390/ijms18020455
124. Cambiaghi M, Cherchi L, Masin L, Infortuna C, Briski N, Caviasco C, et al. High-frequency repetitive transcranial magnetic stimulation enhances layer II/III morphological dendritic plasticity in mouse primary motor cortex. Behav Brain Res (2021) 410:113352. doi: 10.1016/j.bbr.2021.113352
125. Houdayer E, Degardin A, Cassim F, Bocquillon P, Derambure P, Devanne H. The effects of low- and high-frequency repetitive TMS on the input/output properties of the human corticospinal pathway. Exp Brain Res (2008) 187(2):207–17. doi: 10.1007/s00221-008-1294-z
126. Patabendige A, Singh A, Jenkins S, Sen J, Chen R. Astrocyte activation in neurovascular damage and repair following ischaemic stroke. Int J Mol Sci (2021) 22(8). doi: 10.3390/ijms22084280
127. Verkhratsky A, Nedergaard M. Physiology of astroglia. Physiol Rev (2018) 98(1):239–389. doi: 10.1152/physrev.00042.2016
128. Ruohonen J, Karhu J. tDCS possibly stimulates glial cells. Clin Neurophysiol (2012) 123(10):2006–9. doi: 10.1016/j.clinph.2012.02.082
129. Medina-Fernández FJ, Luque E, Aguilar-Luque M, Agüera E, Feijóo M, García-Maceira FI, et al. Transcranial magnetic stimulation modifies astrocytosis, cell density and lipopolysaccharide levels in experimental autoimmune encephalomyelitis. Life Sci (2017) 169:20–6. doi: 10.1016/j.lfs.2016.11.011
130. Cacace F, Mineo D, Viscomi MT, Latagliata EC, Mancini M, Sasso V, et al. Intermittent theta-burst stimulation rescues dopamine-dependent corticostriatal synaptic plasticity and motor behavior in experimental parkinsonism: possible role of glial activity. Mov Disord (2017) 32(7):1035–46. doi: 10.1002/mds.26982
131. Sasso V, Bisicchia E, Latini L, Ghiglieri V, Cacace F, Carola V, et al. Repetitive transcranial magnetic stimulation reduces remote apoptotic cell death and inflammation after focal brain injury. J Neuroinflamm (2016) 13(1):150. doi: 10.1186/s12974-016-0616-5
132. Clarke D, Penrose MA, Harvey AR, Rodger J, Bates KA. Low intensity rTMS has sex-dependent effects on the local response of glia following a penetrating cortical stab injury. Exp Neurol (2017) 295:233–42. doi: 10.1016/j.expneurol.2017.06.019
133. Clarke D, Beros J, Bates KA, Harvey AR, Tang AD, Rodger J. Low intensity repetitive magnetic stimulation reduces expression of genes related to inflammation and calcium signalling in cultured mouse cortical astrocytes. Brain Stimul (2021) 14(1):183–91. doi: 10.1016/j.brs.2020.12.007
134. Hong Y, Liu Q, Peng M, Bai M, Li J, Sun R, et al. High-frequency repetitive transcranial magnetic stimulation improves functional recovery by inhibiting neurotoxic polarization of astrocytes in ischemic rats. J Neuroinflamm (2020) 17(1):150. doi: 10.1186/s12974-020-01747-y
135. Sousa C, Biber K, Michelucci A. Cellular and molecular characterization of microglia: a unique immune cell population. Front Immunol (2017) 8:198. doi: 10.3389/fimmu.2017.00198
136. Li Q, Barres BA. Microglia and macrophages in brain homeostasis and disease. Nat Rev Immunol (2018) 18(4):225–42. doi: 10.1038/nri.2017.125
137. Zhao SC, Ma LS, Chu ZH, Xu H, Wu WQ, Liu F. Regulation of microglial activation in stroke. Acta Pharmacol Sin (2017) 38(4):445–58. doi: 10.1038/aps.2016.162
138. Dheen ST, Kaur C, Ling EA. Microglial activation and its implications in the brain diseases. Curr Med Chem (2007) 14(11):1189–97. doi: 10.2174/092986707780597961
139. Gülke E, Gelderblom M, Magnus T. Danger signals in stroke and their role on microglia activation after ischemia. Ther Adv Neurol Disord (2018) 11:1756286418774254. doi: 10.1177/1756286418774254
140. He C, Liu R, Fan Z, Li Y, Yang M, Wugang H, et al. Microglia in the pathophysiology of hemorrhagic stroke and the relationship between microglia and pain after stroke: a narrative review. Pain Ther (2021) 10(2):927–39. doi: 10.1007/s40122-021-00288-3
141. Li Q, Dai Z, Cao Y, Wang L. Caspase-1 inhibition mediates neuroprotection in experimental stroke by polarizing M2 microglia/macrophage and suppressing NF-κB activation. Biochem Biophys Res Commun (2019) 513(2):479–85. doi: 10.1016/j.bbrc.2019.03.202
142. Yang L, Tucker D, Dong Y, Wu C, Lu Y, Li Y, et al. Photobiomodulation therapy promotes neurogenesis by improving post-stroke local microenvironment and stimulating neuroprogenitor cells. Exp Neurol (2018) 299(Pt A):86–96. doi: 10.1016/j.expneurol.2017.10.013
143. Liebetanz D, Fauser S, Michaelis T, Czéh B, Watanabe T, Paulus W, et al. Safety aspects of chronic low-frequency transcranial magnetic stimulation based on localized proton magnetic resonance spectroscopy and histology of the rat brain. J Psychiatr Res (2003) 37(4):277–86. doi: 10.1016/s0022-3956(03)00017-7
144. Rauš S, Selakovič V, Manojlovič -Stojanoski M, Radenovič L, Prolič Z, Janač B. Response of hippocampal neurons and glial cells to alternating magnetic field in gerbils submitted to global cerebral ischemia. Neurotox Res (2013) 23(1):79–91. doi: 10.1007/s12640-012-9333-8
145. Liddelow SA, Guttenplan KA, Clarke LE, Bennett FC, Bohlen CJ, Schirmer L, et al. Neurotoxic reactive astrocytes are induced by activated microglia. Nature (2017) 541(7638):481–7. doi: 10.1038/nature21029
146. Luo J, Feng Y, Li M, Yin M, Qin F, Hu X. Repetitive transcranial magnetic stimulation improves neurological function and promotes the anti-inflammatory polarization of microglia in ischemic rats. Front Cell Neurosci (2022) 16:878345. doi: 10.3389/fncel.2022.878345
147. Chen J, Zeng Y, Hong J, Li C, Zhang X, Wen H. Effects of HF-rTMS on microglial polarization and white matter integrity in rats with poststroke cognitive impairment. Behav Brain Res (2023) 439:114242. doi: 10.1016/j.bbr.2022.114242
148. Tuttolomondo A, Di Raimondo D, di Sciacca R, Pinto A, Licata G. Inflammatory cytokines in acute ischemic stroke. Curr Pharm design (2008) 14(33):3574–89. doi: 10.2174/138161208786848739
149. Vila N, Castillo J, Dávalos A, Esteve A, Planas AM, Chamorro Á. Levels of anti-inflammatory cytokines and neurological worsening in acute ischemic stroke. Stroke (2003) 34(3):671–5. doi: 10.1161/01.STR.0000057976.53301.69
150. Roedel F, Kley N, Beuscher HU, Hildebrandt G, Keilholz L, Kern P, et al. Anti-inflammatory effect of low-dose X-irradiation and the involvement of a TGF-β 1 -induced down-regulation of leukocyte/endothelial cell adhesion. Int J Radiat Biol (2002) 78(8):711–9. doi: 10.1080/09553000210137671
151. Vogelgesang A, Becker KJ, Dressel A. Immunological consequences of ischemic stroke. Acta Neurologica Scandinavica (2014) 129(1):1–12. doi: 10.1111/ane.12165
152. Winklewski PJ, Radkowski M, Demkow U. Cross-talk between the inflammatory response, sympathetic activation and pulmonary infection in the ischemic stroke. J Neuroinflamm (2014) 11(1):213. doi: 10.1186/s12974-014-0213-4
153. Majd M, Saunders EFH, Engeland CG. Inflammation and the dimensions of depression: a review. Front Neuroendocrinol (2020) 56:100800. doi: 10.1016/j.yfrne.2019.100800
154. Cha B, et al. Therapeutic effect of repetitive transcranial magnetic stimulation for post-stroke vascular cognitive impairment: a prospective pilot study. Front Neurol (2022) 13:813597. doi: 10.3389/fneur.2022.813597
155. Ge L, Zhao Y-X, Chang Y-X, Cui W-S, Zhai X-Z, Ma Q-Y. Effect of low-rTMS in combined with edaravone on the inflammatory cytokines and cerebral metabolites in patients with cerebral infarction and aphasia. J Hainan Med Univ (2017) 5:132–5.
156. Luo L, Liu M, Fan Y, Zhang J, Liu L, Li Y, et al. Intermittent theta-burst stimulation improves motor function by inhibiting neuronal pyroptosis and regulating microglial polarization via TLR4/NFκB/NLRP3 signaling pathway in cerebral ischemic mice. J Neuroinflamm (2022) 19(1):141. doi: 10.1186/s12974-022-02501-2
157. Pascual O, Ben Achour S, Rostaing P, Triller A, Bessis A. Microglia activation triggers astrocyte-mediated modulation of excitatory neurotransmission. Proc Natl Acad Sci (2012) 109(4):E197–205. doi: 10.1073/pnas.1111098109
158. Salter MW, Stevens B. Microglia emerge as central players in brain disease. Nat Med (2017) 23(9):1018–27. doi: 10.1038/nm.4397
159. Wu Y, Dissing-Olesen L, MacVicar BA, Stevens B. Microglia: dynamic mediators of synapse development and plasticity. Trends Immunol (2015) 36(10):605–13. doi: 10.1016/j.it.2015.08.008
160. Maggio N, Vlachos A. Tumor necrosis factor (TNF) modulates synaptic plasticity in a concentration-dependent manner through intracellular calcium stores. J Mol Med (2018) 96:1039–47. doi: 10.1007/s00109-018-1674-1
161. Sawada M, et al. Production of tumor necrosis factor-alpha by microglia and astrocytes in culture. Brain Res (1989) 491(2):394–7. doi: 10.1016/0006-8993(89)90078-4
162. Takeuchi N, Izumi S-I. Rehabilitation with poststroke motor recovery: a review with a focus on neural plasticity. Stroke Res Treat (2013) 2013. doi: 10.1155/2013/128641
163. Voelcker-Rehage C, Godde B, Staudinger U. Cardiovascular and coordination training differentially improve cognitive performance and neural processing in older adults. Front Hum Neurosci (2011) 5:26. doi: 10.3389/fnhum.2011.00026
164. Stoeckel LE, Garrison KA, Ghosh SS, Wighton P, Hanlon CA, Gilman JM, et al. Optimizing real time fMRI neurofeedback for therapeutic discovery and development. NeuroImage: Clin (2014) 5:245–55. doi: 10.1016/j.nicl.2014.07.002
165. Kim YH, You SH, Ko MH, Park JW, Lee KH, Jang SH, et al. Repetitive transcranial magnetic stimulation-induced corticomotor excitability and associated motor skill acquisition in chronic stroke. Stroke (2006) 37(6):1471–6. doi: 10.1161/01.Str.0000221233.55497.51
166. Grefkes C, Ward NS. Cortical reorganization after stroke: how much and how functional? Neuroscientist (2014) 20(1):56–70. doi: 10.1177/1073858413491147
167. Croarkin PE, Wall CA, McClintock SM, Kozel FA, Husain MM, Sampson SM. The emerging role for repetitive transcranial magnetic stimulation in optimizing the treatment of adolescent depression. J ect (2010) 26(4):323–9. doi: 10.1097/YCT.0b013e3181dd17eb
168. Hett D, Rogers J, Humpston C, Marwaha S. Repetitive transcranial magnetic stimulation (rTMS) for the treatment of depression in adolescence: a systematic review. J Affect Disord (2021) 278:460–9. doi: 10.1016/j.jad.2020.09.058
169. O’Reardon JP, Fontecha JF, Cristancho MA, Newman S. Unexpected reduction in migraine and psychogenic headaches following rTMS treatment for major depression: a report of two cases. CNS Spectrums (2007) 12(12):921–5. doi: 10.1017/S1092852900015716
170. Rouwhorst R, van Oostrom I, Dijkstra E, Zwienenberg L, van Dijk H, Arns M. Vasovagal syncope as a specific side effect of DLPFC-rTMS: a frontal-vagal dose-finding study. Brain Stimulation: Basic Translational Clin Res Neuromodulation (2022) 15(5):1233–5. doi: 10.1016/j.brs.2022.08.015
171. Prikryl R, Kucerova H. Occurrence of epileptic paroxysm during repetitive transcranial magnetic stimulation treatment. J Psychopharmacol (2005) 19(3):313. doi: 10.1177/0269881105051545
Keywords: transcranial magnetic stimulation, stroke, rehabilitation, neuroinflammation, microglia, neurotransmitter, neuroimaging technique
Citation: Sheng R, Chen C, Chen H and Yu P (2023) Repetitive transcranial magnetic stimulation for stroke rehabilitation: insights into the molecular and cellular mechanisms of neuroinflammation. Front. Immunol. 14:1197422. doi: 10.3389/fimmu.2023.1197422
Received: 31 March 2023; Accepted: 10 May 2023;
Published: 22 May 2023.
Edited by:
Anwen Shao, Zhejiang University, ChinaReviewed by:
Rui-zhe Zheng, Fudan University, ChinaCopyright © 2023 Sheng, Chen, Chen and Yu. This is an open-access article distributed under the terms of the Creative Commons Attribution License (CC BY). The use, distribution or reproduction in other forums is permitted, provided the original author(s) and the copyright owner(s) are credited and that the original publication in this journal is cited, in accordance with accepted academic practice. No use, distribution or reproduction is permitted which does not comply with these terms.
*Correspondence: Peipei Yu, eXVwZWlwZWlAempzbXl5LmNvbQ==
Disclaimer: All claims expressed in this article are solely those of the authors and do not necessarily represent those of their affiliated organizations, or those of the publisher, the editors and the reviewers. Any product that may be evaluated in this article or claim that may be made by its manufacturer is not guaranteed or endorsed by the publisher.
Research integrity at Frontiers
Learn more about the work of our research integrity team to safeguard the quality of each article we publish.