- 1Department of Microbiology, Institute for Immunology and Immunological Diseases, Graduate School of Medical Science, Brain Korea 21 Project, Yonsei University College of Medicine, Seoul, Republic of Korea
- 2Department of Microbiology and Medical Science, College of Medicine, Chungnam National University, Daejeon, Republic of Korea
Although tuberculosis (TB) remains one of the leading causes of death from an infectious disease worldwide, the development of vaccines more effective than bacille Calmette-Guérin (BCG), the only licensed TB vaccine, has progressed slowly even in the context of the tremendous global impact of TB. Most vaccine candidates have been developed to strongly induce interferon-γ (IFN-γ)-producing T-helper type 1 (Th1) cell responses; however, accumulating evidence has suggested that other immune factors are required for optimal protection against Mycobacterium tuberculosis (Mtb) infection. In this review, we briefly describe the five hurdles that must be overcome to develop more effective TB vaccines, including those with various purposes and tested in recent promising clinical trials. In addition, we discuss the current knowledge gaps between preclinical experiments and clinical studies regarding peripheral versus tissue-specific immune responses, different underlying conditions of individuals, and newly emerging immune correlates of protection. Moreover, we propose how recently discovered TB risk or susceptibility factors can be better utilized as novel biomarkers for the evaluation of vaccine-induced protection to suggest more practical ways to develop advanced TB vaccines. Vaccines are the most effective tools for reducing mortality and morbidity from infectious diseases, and more advanced technologies and a greater understanding of host-pathogen interactions will provide feasibility and rationale for novel vaccine design and development.
1 Introduction
Tuberculosis (TB), one of the deadliest infectious diseases, is caused by Mycobacterium tuberculosis (Mtb) and was responsible for approximately 1.6 million deaths in 2021 (World Health Organization. Global TB Report 2022). A single licensed TB vaccine called bacille Calmette-Guérin (BCG) has been employed for human use since 1921, and the degree of protection afforded by BCG vaccination varies in different regions of the world (1). Although the protective efficacy of BCG against severe TB forms such as TB meningitis and disseminated extrapulmonary TB before adolescence is well documented, worse protection with highly variable efficacies in individuals of all ages against pulmonary TB continues to be a serious concern (2, 3). Despite the global use of BCG for over 100 years, approximately a quarter of the world’s population is considered to have latent Mtb infection. Thus, the development of new TB vaccines that provide greater protection than the BCG vaccine, with the aim of preventing pulmonary TB, is critical for all age groups.
More than 20 TB vaccine candidates with various purposes have entered clinical trials, and 14 candidates are being actively evaluated. However, the unsatisfactory outcomes (for example, the MVA85A and AERAS-422 trials) (4–6) prompt us to try to further understand the complexity of the key protective immune response to Mtb infection and the way to develop vaccines that afford lifelong protection. These trials highlight our current knowledge gaps about protective correlates and controlling factors that can affect vaccine efficacies and outcomes. In this review, we discuss five points that should be considered in the individual stages of vaccine development, from the proposal of novel concepts for next-generation TB vaccines to considerations for practical development.
2 The first hurdle: purpose of vaccines
2.1 Prevention of infection
A vaccine developed for the prevention of infection (POI), given prior to Mtb exposure, should control the incipient infection stage. With much higher rates of infection than evident TB disease in endemic settings, POI trials are shorter and less costly than prevention of disease (POD) trials (7, 8). Therefore, the POI trial can be used as a viable opportunity to understand the mechanisms of vaccine efficacy in humans, providing a platform to select lead candidates for further testing. A major challenge is that there is no available standardized test to measure directly the acquisition, persistence, and clearance of asymptomatic Mtb infection. Currently, assessment of Mtb infection mainly relies on alterations in specific T-cell responses induced after Mtb infection. One of the commercial interferon (IFN)-γ release assay (IGRA), QuantiFERON-TB Gold In-Tube (QFT), measures immunological sensitization to Mtb as a biomarker for Mtb infection. Compared to persistent QFT negatives, recent negative-to-positive QFT tests are associated with higher rates of Mtb infection. Therefore, it may be ideal for conducting clinical trials of prevention of Mtb infection (POI) by novel vaccines using QFT transformation as an efficacy endpoint. A positivity cutoff IFN-γ value (0.35 IU/ml) for QFT conversion is recommended by manufacturers and CDC (9), but the immunological and analytical variability of QFT tests potentially confounds the interpretation of QFT conversion as a clinical trial endpoint (10, 11). Although the tuberculin skin test (TST) can be used as an alternative method for detecting Mtb infection, since specificity is reduced by BCG vaccination or nontuberculous mycobacteria (NTM) infection, novel diagnostic methods for successful clinical results must be developed.
2.2 Prevention of disease
A POD vaccine can be administered either pre- or post-exposure to protect against disease progression after actual Mtb infection. Knight et al. reported epidemiological modeling suggesting that adolescents or young adults are the most effective targets for POD vaccination (12). According to this model, due to children having lower rates of TB notifications, lower proportions of smear-positive pulmonary TB, and making a smaller contribution to TB transmission, a novel TB vaccine targeted at infants shows a reduced immediate impact compared to one targeted at adolescents/adults. Vaccines targeting infants prevent a relatively small number of active cases, resulting in fewer secondary cases being prevented. In contrast, vaccines targeting adolescents/adults directly affect the population with the greatest burden of active TB, such as 10-year-olds vaccinated in schools and those individuals reached in mass campaigns, which leads to a reduction in transmission. Although most vaccine candidates in clinical phases aim to prevent TB disease, POD trials require more time and higher costs than POI trials because of the much lower rate of TB disease than Mtb infection (8). Nevertheless, POD trials can directly reveal Mtb infection because the evaluation is performed by measuring clinical symptoms, chest X-ray, and direct Mtb culture from clinical samples. A recent POD trial with the candidate M72:AS01E TB vaccine (phase 2b) was conducted in Kenya, South Africa, and Zambia. Efficacy analysis was conducted on a total of 3,283 subjects, and after a period of approximately 2.3 years, the incidence of pulmonary TB was significantly lower in the M72:AS01E group than in the placebo group (13). In this trial, M72:AS01E group showed 54% vaccine efficacy among persons already infected with Mtb, but due to the inclusion of predominantly BCG-vaccinated Mtb-infected adults, it was not possible to determine the extent to which infection-generated or childhood BCG vaccination-elicited responses influenced vaccine efficacy. Similarly, a 3-year extended follow-up study demonstrated 49.7% protection by M72:AS01E among people already infected with Mtb (14), indicating that vaccine-induced protective immune responses were maintained for at least 3 years. With these promising findings, broader applications to diverse ethnic populations in different geographic settings will be required to conduct reliable clinical trials for POD.
2.3 Prevention of recurrence
Vaccines aimed at the prevention of recurrence (POR) are administered during antibiotic therapy to prevent the recurrence of TB. TB recurrence generally occurs in approximately 2 to 8% of TB patients even after treatment completion, and the recurrence rate depends on the absence or presence of cavities, bacterial burden, treatment frequency per week, type of antibiotics used and transmission rate. As most cases of recurrent TB disease develop within one year after treatment completion, the targeted populations of POR trials can usually be designated (8), but trial design is complicated due to the long-term treatment period and intervention timing. Multiple promising candidates currently under evaluation for POR include the H56:IC31 and ID93:GLA-SE subunit vaccine candidates, which were noted to prevent reactivation or restrict progression to severe disease in nonhuman primates (NHPs) (15, 16), and the recombinant BCG vaccine candidate VPM1002.
Due to the characteristics of TB, a large number of subjects for trials are needed because approximately 10% of infected individuals are at the onset of the disease. In addition, long-term monitoring is required because the timing of onset is different for each individual. These characteristics make the rapidly increasing economic problem more difficult as the number of clinical trial subjects and the test period increases. Therefore, to overcome these problems, it is important to recruit a reasonably sized experimental group and set endpoints according to the purpose of the experiment, and it is important to discover a correlate of protection (COP) that can predict vaccine efficacy, which will be addressed later in this review.
3 The second hurdle: a gap between experiments and the natural history of TB
Current concepts for the development of TB vaccines depend on experiments emphasizing T-helper type 1 (Th1)-biased immunity, based on early observations (17). For successful vaccine development, an appropriate vaccine model and translation to evaluate vaccine candidates is essential. Therefore, factors such as which animal model to select, which strain of Mtb to use for infection, and which dose to use for challenge are important.
3.1 Mtb infection dose
According to reports, the infection dose that causes TB disease is 1-200 colony-forming units (CFU). TB is transmitted via droplets containing Mtb generated through coughing or sneezing, and the number of droplets generated through a single cough is approximately 1-400 CFU (18, 19). According to another study of TB patients, the average number of aerosolized CFU generated by coughing for 5 minutes was 16 (20). When an individual is infected with Mtb, the actual infection dose may be much lower than the number of bacteria released by coughing because not all aerosols generated by the infected person are inhaled. In addition, it has been reported that symptomatic TB patients release Mtb aerosols not only by cough but also by exhalation, with an aerosol size of 0.5-5 μm, showing that actual infection can be achieved in the context of a sustained low-dose of bacteria (21, 22). However, animal models for vaccine research in the preclinical stage can be established through a single, sufficient infection dose and used to evaluate vaccine efficacy. It is unclear whether the reduction in the bacterial burden by vaccination in the context of single-dose infection is a good predictor of actual clinical vaccine performance. This single-dose challenge could overwhelm or bypass the relevant immunological cascade and mask the full potential of candidate vaccines (23). In a mouse model, ultra-low-dose aerosol infection with 1-3 CFU resulted in characteristics more similar characteristics to human TB, such as highly heterogeneous bacterial burdens and well-circumscribed granulomas, than conventional-dose infection with 50-100 CFU (24). Recently, Dijkman et al. tested the efficacy of pulmonary BCG vaccination in a rhesus macaque model with a 1 CFU Mtb infection every day for 11 days and noted the importance of Th17 cells and IL-10 (25). This study does not represent all situations in which infection occurs, but it does provide a model that accounts for specific persistent and practical infection situations, such as household contacts. These studies suggest that it is necessary to reconsider the infection dose used in preclinical vaccine studies.
3.2 Experimental models
Most individuals who become infected can remain asymptomatic for a long time. Although environmental, cultural, geographical, and contextual characteristics can affect whether infection occurs, TB susceptibility due to host genetic differences has been reported as one of the determinants of TB disease (26). In the case of TB studies, more than 60% of preclinical studies have used mouse models, susceptibility to Mtb differs depending on the mouse strain. The most widely used C57BL/6 mice or BALB/c mice have relatively low susceptibility, whereas DBA/2, CBA/J, I/St, C3H, and A/J strains have relatively high susceptibility (27). In addition, it has been reported that necrotic granulomas found in patients with active TB are not formed in BALB/c or C57BL/6 mice, whereas they are formed in the TB-susceptible mouse strains I/St and C3H (28, 29). Recently, it was confirmed that human-like necrotic granulomas were formed by Mtb infection in a humanized mouse model, and caseous necrotic granulomas showed an immune phenotype and spatial organization similar to those observed in TB patients (30). Arrey et al. presented the utility of this model for the evaluation of a preclinical model of anti-TB drugs in an in vivo environment. Zelmer et al. immunized different strains of inbred mice, such as A/J, DBA/2, C57BL/6, and 129S2, displaying different susceptibilities to Mtb with BCG (31). Smith et al. used a “collaborative cross” model system created by crossing inbred and outbred mice to understand the broader host genetic susceptibility spectrum and for use in vaccine efficacy testing (32). These models can confirm the genetic immunological correlation associated with TB vaccine efficacy and can simultaneously be used to identify potential improvements and key defense factors for the development of a robust TB vaccine.
To date, most studies with NHPs have been used to model only acute TB, which is much less prevalent than latent TB in humans (33). Rhesus macaques develop active TB in approximately 90% of the infected population, whereas cynomolgus macaques develop active TB in only 60% of the infected population. It has also been reported that BCG showed a higher protective efficacy in cynomolgus macaques than in rhesus macaques (34). Rhesus macaques and cynomolgus macaques can develop acute, chronic, or latent TB depending on the route of infection, dose, and Mtb strain used for inoculation.
Vaccine trials employing the NHP model are expensive, but they can serve as a checkpoint for clinical trials, resulting in significant cost savings. Areas-402 induced a strong T-cell response but did not protect rhesus macaques against infection with 200 CFU of Mtb Erdman (35). Conversely, a clinical study of MVA85A without efficacy evaluation was performed with the NHP model, and although it was expensive, the efficacy was not proven in the clinical stage (36, 37). These results suggest that validation of efficacy for TB vaccines via the NHP model to enter the clinical stage may accelerate TB vaccine development.
In an evaluation of vaccine efficacy in animal models, the bacterial burden between vaccinated and non-vaccinated groups is one of the key factors. Previous studies have demonstrated the presence of non-replicating bacterial populations in sputum samples obtained from TB patients (38, 39). These non-replicating subpopulations have been attributed to the existence of persister-like bacilli in a non-replicating state (39). Even in preclinical TB vaccine models, nonculturable or persistent mycobacterial subpopulations may arise due to immunological pressures resulting from the characteristics of the vaccine candidate or vaccine model, which can hinder the accurate evaluation of vaccine efficacy. Resuscitation-promoting factors (Rpfs) are bacterial proteins which are primarily identified by their ability to resuscitate nonreplicating cells in vitro and in vivo (40, 41). It has been reported that non-replicating bacteria in a patient’s sputum can be revived by Rpfs and the culture time can be shortened (42, 43). The application of Rpfs to bacterial culture in conventional media has the potential to reduce errors in vaccine efficacy evaluation that can be caused by nonculturable or persistent subpopulations.
3.3 Translation and interpretation: differential analysis of samples between humans and animals
NHPs show anatomical and physiological similarities with humans as well as a wide range of clinical symptoms of TB, including pulmonary and extrapulmonary signs and symptoms. The NHP model enables the analysis of infected tissue, which is difficult in clinical stages, and at the same time, the disease course can be monitored by measuring parameters on radiographic images and examining body fluid samples, which can also be performed in humans. In addition, the NHP model allows the use of computed tomography and positron emission tomography to observe the progression of Mtb infection to disease in an individual (44). In the clinical phase, blood samples are used to measure immunogenicity. Currently, the Ag-specific T-cell response, multifunctionality of the Ag-specific T-cell response, and Ag-specific IgG antibody titers are commonly evaluated to demonstrate immunogenicity after vaccination (Table 1). However, in the case of vaccine candidates, when the efficacy is evaluated through animal experiments, the analysis is not based on blood but rather on tissues, such as lung, spleen, and lymph nodes. Therefore, it is difficult to apply COPs from tissue-based analysis in preclinical studies to clinical studies. The NHP model enables analysis of indicators applicable to human clinical studies such as blood, urine, and PET-CT results, and analysis of indicators that can be measured only after sacrificing animals, which is possible only in preclinical models. Exploration and verification of significant indicators through this model can lead to an acceleration of vaccine development. Therefore, studies employing the NHP model before the clinical stage can provide meaning beyond simply being the gateway to the clinical stage.
4 The third hurdle: antigen selection
4.1 Universal antigens
Mtb contains approximately 4,000 individual proteins, and most Ags included in current subunit vaccines have been adopted mainly based on their immunodominant properties for T-cell responses in preclinical and clinical settings. Currently, approximately 100 Ags in the preclinical stage (approximately 3% of all Mtb Ags) have been studied (Table 2). Most of the Ags for TB vaccine candidates are abundantly secreted and cell wall-associated proteins, including ESAT6, Ag85B, Ag85A, HSPX, and MPT64. In addition, cell wall-associated or virulence-associated Pro-Glu/Pro-Pro-Glu (PE/PPE) family proteins, a component of M72 subunit and ID93 subunit vaccine candidates, and heparin-binding hemagglutinin also produced promising vaccine-induced protection in mouse models (103, 104). Ags related to latency (DosR, resuscitation-promoting factor) and hypoxia-related proteins are being used for vaccine testing. Furthermore, hypothetical proteins are also used, for example, Rv1767, which is produced by the pathogen during the first week of infection of human cells. Aagaard et al. reported that the dimers EsxD-EsxC, EsxG-EsxH and EsxW-EsxV produced by the ESAT6 secretion system (ESX) were highly immunogenic. Integrating these in a fusion protein form called H65 resulted in a formulation that demonstrated protection efficacy equivalent to that of BCG without interfering with current ESAT6- and CFP10-based diagnostics (105). Liu et al. also purified 1,250 Mtb proteins with an E. coli expression system and evaluated cellular and humoral immune responses in human PBMCs and serum, respectively. They eventually identified four Ag candidates, Rv0232, Rv1031, Rv1198, and Rv2016, displaying high immunogenicity (106). Currently, only 11 Ags have been selected as a component, in the form of fusion proteins, in formulations eventually entered into clinical trials (Table 3).
4.2 Rational antigen selection
The challenge of Ag screening is complicated by the roles of Ags in multiple stages of Mtb infection, particularly chronic and latent infection stages. During Mtb infection in a mouse model, ESAT6 is consistently expressed, but Ag85B is mainly expressed at an early stage when Mtb is actively replicating (116). Mtb infection induced the accumulation of ESAT6-specific CD4+ T cells in the mouse lung parenchyma, but the T cells became functionally exhausted due to chronic stimulation of Ag. Whereas, Ag85B-specific CD4+ T cells maintain memory cell features during infection but contract in numbers by reduced Ag expression during persistent infection (116). These results have important implications for the rational design of TB vaccines tailored to optimize the protection conferred by specific CD4+ T cells that recognize Ag expressed at distinct stages of Mtb infection.
Although immunodominant Ags are generally accepted to induce superior vaccine efficacy, some studies suggest that Mtb can modulate host immunity through immunodominant Ags. T-cell epitopes among well-known immunodominant Mtb Ags are highly conserved, suggesting the possibility that being recognized by the host through immunodominant Ags may be beneficial for Mtb (117, 118). In addition, T-cell responses to some immunodominant Mtb Ags have been found to be notably greater in active TB patients than in individuals latently infected with Mtb (119, 120), indicating that enhanced T-cell responses may be associated with deteriorated lung inflammation, resulting in subsequent transmission. Therefore, to confirm this possibility, Orr et al. confirmed the efficacy of immune subdominant Ags as a TB vaccine candidate in a mouse model, but little correlation has been found between vaccine efficacy and the immunodominance of Ags during Mtb infection (121).
Furthermore, it is also important to characterize the vaccine potential of Ags likely to be associated with reactivation from latent Mtb infection. A well-characterized bacterial regulon can induce the dormant state of Mtb that is controlled by DosR–DosS, which is induced by immunological pressure of the host such as local hypoxia, nitric oxide, and carbon monoxide (122). These host immune responses can be induced by the vaccine with immunodominant Ag in an active state of Mtb, indicating the potential for immune evasion of Mtb against immune responses produced by vaccines targeting immunodominant Ags in an activated state. Therefore, it is also important to characterize the vaccine potential of Ags likely to be associated with reactivation of latent Mtb infection. Hence, developing novel vaccines that encode genes expressed during the reactivation of a dormant state, such as rpf, would be a strategic approach. In vitro and in vivo transcriptional profiling studies have shown that five rpf of Mtb are expressed at varying levels in a growth stage-dependent manner (123, 124). RpfB, one of the five Rpfs produced by Mtb, plays an important role in the resuscitation and growth in a dormant state. In addition, delayed reactivation induced by aminoguanidine in chronic TB was observed in mice infected with a strain lacking rpfB (125), and significantly higher T cell responses to recombinant RpfB and RpfE were detected in LTBI than in active TB patients (126), indicating that Rpfs are involved in the reactivation process in vivo. Moreover, RpfB has been studied as a promising candidate for DNA vaccines, shown to induce a modest but significant cellular immune response against TB with higher levels of IL-2 and IFN-γ (74). In addition, the RpfB domain can induce a humoral response, and monoclonal antibodies against Rpfs could inhibit TB relapse (127).
4.3 Strategy for fusion proteins
Vaccine strategies using fusion proteins can be designed to include multiple Ags, so they can induce a broader immune response than single Ag vaccines. In addition, this strategy has the advantage of inducing an effective immune response by fusing a protein with low immunogenicity with a protein or peptide with high immunogenicity. The M72 vaccine candidate was formed through a fusion of the Mtb32A and Mtb39A proteins, selected for their ability to provoke T-cell responses in TST-positive healthy adults. On the other hand, multistage subunit vaccines, such as H56 (which contains the latency-associated Ag Rv2660c fused with Ag85B and ESAT6), as well as LT70 and ID93, also incorporate multiple Mtb Ags differentially involved in bacterial growth, virulence, and metabolism (111, 128, 129). A new TB vaccine candidate, H107, that integrates eight individually protective Ags (PPE68, ESAT6, EspI, EspC, EspA, MPT64, MPT70, and MPT83) is highly immunogenic in both mice and humans (102). This fusion protein is composed of 4 ESAT6 molecules in the middle, which led to a significant increase in ESAT-6-specific immunogenicity. H107 with the BCG vaccine could increase Ag coverage to induce robust protective immune responses in a diverse human population by including as many protective/recognizable Ags as possible. While traditional vaccines containing BCG-shared Ags show in vivo cross-reactivity to BCG, H107 demonstrates no cross-reactivity and does not impede BCG colonization. Instead, co-administering H107 with BCG results in enhanced adaptive responses against both H107 and BCG (102).
5 The fourth hurdle: immune correlates and protection biomarkers
Unveiling reliable predictive correlates to the immunogenicity and efficacy of TB vaccines allows estimation of vaccine efficacy well in advance of the time required to confirm vaccine efficacy against Mtb infection in the clinical stage. In addition, after the commercialization of a vaccine, successful vaccination can be tracked through reliable COP measurement of vaccinated individuals, and as a result, herd immunity through vaccination can be effectively achieved. Therefore, attempts have been made to identify reliable COPs of TB vaccines, but it is still unclear. Currently, in most vaccine studies in clinical phases, immunogenicity or vaccine-induced protection-related biomarkers are limited to immunological markers, especially IFN-γ-producing T cell responses, polyfunctional T-cell responses, or antibody titers in response to Mtb Ag. Recently, the possibility of developing protective immunity and vaccines for donor unrestricted T cells (DURTs), Th17 cells, antibodies, B cells, and innate immunity beyond Th1 immunity has been reconsidered for TB vaccines (130). Moreover, the association between TB progression and type I IFNs in active TB disease has been reported, but clinical studies on the efficacy and markers of TB vaccines are still lacking. These results raise questions about the sufficiency of T-cell responses induced by vaccination for protection and force us to explore additional biomarkers of vaccine efficacy.
5.1 Correlates of protection in innate immune response to the TB vaccine
Continuous research on innate immune factors related to the efficacy of TB vaccines has been conducted. Strategies that target these innate immune factors have been shown to improve vaccine efficacy. In addition, the characteristics of innate immune factors that correlate with vaccine efficacy show potential as biomarkers of vaccine efficacy (Figure 1).
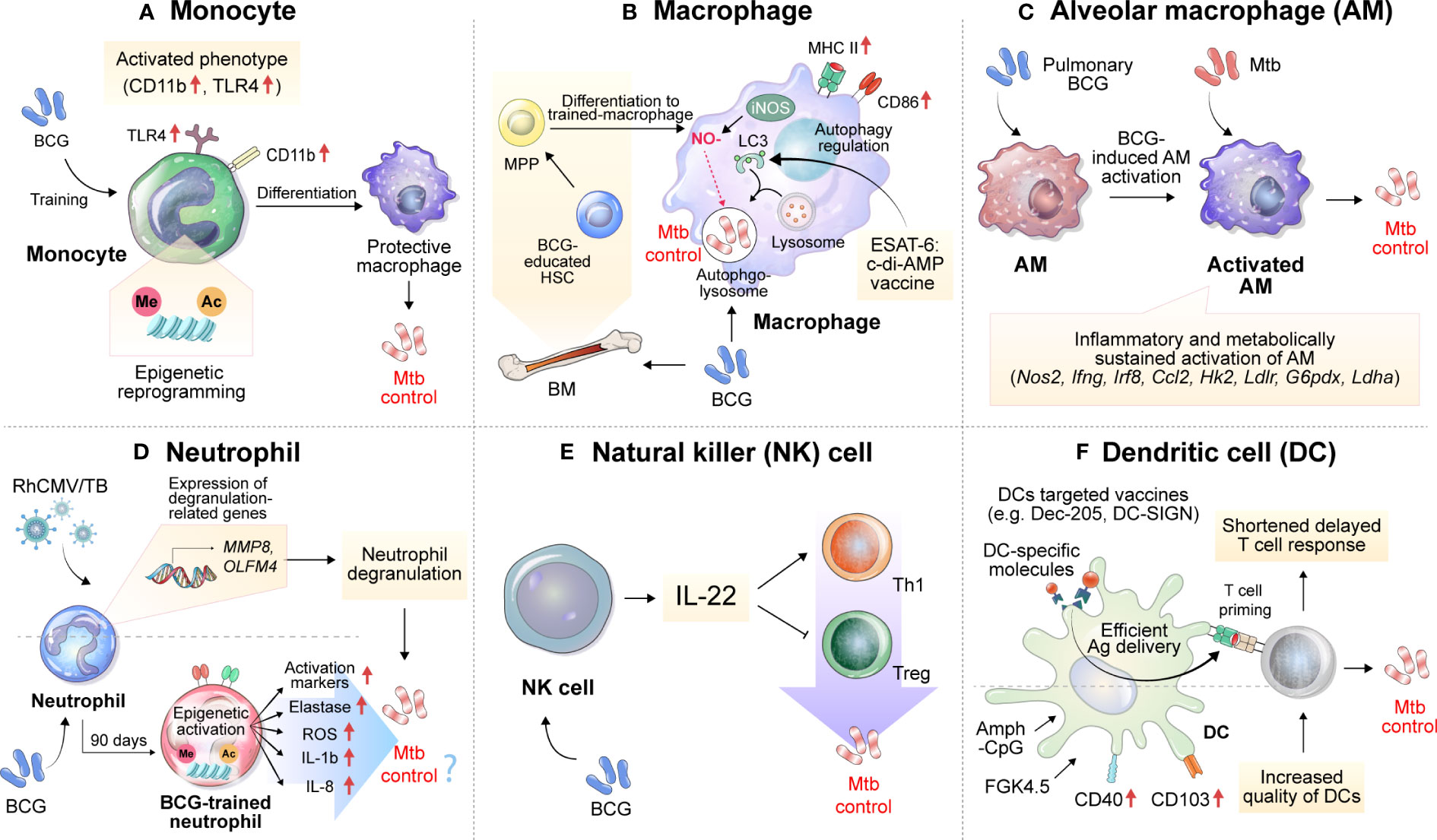
Figure 1 Implications of innate immune cells and relevant immune responses for the development of TB vaccines. Immune responses related to TB vaccines are mainly focused on adaptive immunity, especially T cells, but many studies imply the importance of innate immune responses. (A) BCG vaccination induces the histone epigenetic reprogramming of monocytes, resulting in an activated phenotype with increased CD11b and TLR4 expression. (B) BCG can activate macrophages and educate hematopoietic stem cells (HSCs) to differentiate into more protective macrophages against Mtb infection. Vaccination with ESAT6:c-di-AMP can control Mtb growth by regulating autophagy. (C) Alveolar macrophages, which act as first-line defenders against pathogens entering the lungs, are inflammatory and sustainably metabolically activated by BCG mucosal vaccination, which controls the dissemination and growth of Mtb. (D) The expression of genes related to neutrophil degranulation such as MMP8 and OLFM4 was suggested as a correlate of protection in the RhCMV/TB-vaccinated rhesus macaque model. BCG vaccination in healthy humans induces long-lasting changes in the neutrophil phenotype, characterized by increased expression of activation markers and antimicrobial function, which is associated with genome-wide epigenetic modifications in trimethylation at lysine 4 on histone 3. The enhanced function of human neutrophils persists for at least 3 months after vaccination. (E) Depletion of NK cells during BCG vaccination reduces protection against Mtb infection, concomitant with decreased Th1 response and increased Treg levels. The complementation of IL-22 restores the vaccine efficacy of BCG against Mtb infection, which was reduced by NK cell depletion. (F) Vaccines targeting DCs by using DC-specific molecules, such as Dec-205 and DC-SIGN can effectively deliver Ags to DCs. An increase in the quality of DCs through treatment with Amph-CpG and FGK4.5 can increase vaccine efficacy via effective T-cell priming. MPP, multipotent progenitor; HSCs, hematopoietic stem cells; NO, nitric oxide; iNOS, inducible nitric oxide synthase; c-di-AMP, c-di-adenosine monophosphate; BM, bone marrow; LC3, microtubule-associated protein 1A/1B-light chain 3; Amph-CpG, amphiphilic form of CpG.
5.1.1 Monocytes
The monocytes to lymphocytes (ML) ratio in peripheral blood has been reported to be correlated with TB disease risk among HIV-infected patients (131, 132). In addition, it was reported that the ML ratio increased in severe TB patients and more so in males than females even within the TB patient group, showing a correlation with TB progression (133). Interestingly, Zelmer et al. reported that upon inoculating inbred mouse strains of different genetic backgrounds with BCG, the ML ratio correlated with BCG-induced vaccine efficacy against Mtb infection, suggesting that monocytes are deeply involved in the vaccine-induced immune response (31). BCG vaccination induces an increase in inflammatory mediator production by monocytes through histone modifications and specific gene activation (134). After BCG immunization, circulating monocytes in healthy volunteers released two times more cytokines, such as IL-1β and tumor necrosis factor (TNF)-α, upon stimulation with TB nonspecific pathogens. These BCG-trained monocytes had increased expression of CD11b and Toll-like receptor 4 (TLR4), and these immune effects were related to histone epigenetic reprogramming induced by activation of the NOD2 receptor to increase trimethylation of lysine 4 on histone 3 (H3K4m3) (Figure 1A). Interestingly, the effectiveness of trained immunity was maintained for up to one year, and heterogeneous protection by BCG vaccination in terms of neonatal death from other infectious diseases was significantly increased in the infant group aged 1 to 5 years (135). Recently, protection by BCG revaccination has been reported at the clinical stage (50), but the specific protective mechanism has not yet been fully elucidated.
5.1.2 Macrophages
Recently, vaccination with ESAT6:cyclic diadenosine monophosphate (c-di-AMP) was shown to cause significant reductions in the bacterial burdens of the lungs and spleens in a mouse model by regulating autophagy in Mtb-infected macrophages (136). In addition, mouse bone marrow-derived macrophages infected with BCG become epigenetically modified to provide better protection against Mtb infection (137). This macrophage activation phenotype was also reported by Mate et al., and increases in MHC II, CD86, and inducible nitric oxide synthase levels were observed after intranasal (IN)-BCG vaccination but not after subcutaneous (SC) vaccination (Figure 1B).
Alveolar macrophages (AMs) may serve as the first line of defense against respiratory pathogens. However, a mouse model study showed that AM depletion has a protective effect on lung Mtb infection (138). Mtb becomes an exclusive niche for up to 10 days after Mtb infection (139). In addition, Mtb induces a Th1 response by inducing rapid dissemination of bacilli to the lymph nodes in an IL-1 receptor-dependent manner after AM infection, but poorly transmissible Mtb delays this process, residing inside AMs and developing and promoting the Th17 response (140). These reports suggest that AMs induce a delay in the early immune response during Mtb infection, leading to a delay in protective T-cell immunity.
On the other hand, it has been reported that mucosal vaccination with BCG is effective in inhibiting early dissemination of Mtb by inducing activation while BCG is present in AMs (141). In addition, the formation of trained immunity in mouse AMs through vaccination or infection has been reported (142, 143), and in this context, pulmonary BCG vaccination increases Mtb growth control in AMs early in infection and, through IL-1 signal-dependent Mtb transmission (140), may lead to shortening of the T-cell response delay (Figure 1C). Recently, aerosol vaccination with a human serotype-5 adenovirus (Ad)-vectored TB vaccine (AdHu5Ag85A) was reported in a clinical phase 1b trial. Transcriptomic analysis of AMs isolated from the aerosol AdHu5Ag85A-immunized group in this study showed that aerosol vaccination with AdHu5Ag85A induced persistent transcriptional changes in AMs related to the response to anoxia, inflammatory response to Ag stimulation, tyrosine phosphorylation of signal transducer and activator of transcription proteins, regulation of IL-10 production, response to IL-1 and histone demethylation (144).
5.1.3 Neutrophils
The importance of neutrophil in TB is evidenced by the identification of prominent neutrophil transcription signatures in the blood of TB patients (145). The formation of neutrophil extracellular traps (NETs) induced by type I IFN promotes bacterial growth and disease severity in Mtb-infected mice (146). Given the critical function of neutrophils in TB pathogenesis, it is important to understand their properties in vaccine immune responses.
Monalisa et al. reported that the depletion of neutrophils during vaccination with Mycobacterium smegmatis expressing CMX induced a decrease in protection against Mtb infection in a mouse model, with a decrease in Th1 and Th17 responses in lung tissue and spleen, suggesting the function of neutrophils in the formation of T-cell responses (147). Thomas et al. reported the role of neutrophils in the formation of protective immunity by BCG vaccination (148). Seven days after BCG inoculation via the SC route, a slight increase in the frequency of neutrophils was observed in the lung tissue. In addition, the protective immunity induced by BCG was independent of T cells, and it was reported that this effect was maintained until 30 days after vaccination in T cell- or TNF-α-deficient mice. After BCG inoculation, depletion of neutrophils using an anti-Ly6G antibody resulted in protection provided by BCG being reduced by half, and this phenomenon was confirmed regardless of the presence of neutrophils at the time of Mtb infection (148). These results suggest that neutrophils contribute to the generation of protective innate immunity in the early stage of infection rather than direct killing of Mtb. In addition, BCG vaccination of healthy individuals generated phenotypic alterations in neutrophils, with enhanced antimicrobial function as well as upregulation of activation marker expression. The change in human neutrophils lasts for at least three months, along with genome-wide epigenetic remodeling via H3K4m3 modifications (149) (Figure 1D).
Recently, Hansen et al. administered the rhesus cytomegalovirus vectors (RhCMV) encoding Mtb Ag inserts (RhCMV/TB) vaccine to BCG-vaccinated or unvaccinated rhesus macaques (150). Before Mtb-challenge, the transcriptomic analysis of whole blood revealed that the gene expression levels predictive of the RhCMV/TB vaccine effect were predominantly from neutrophils. These genes were linked to innate immunity and pathways related to neutrophil degranulation, which encompassed genes encoding neutrophil granule effector molecules such as MMP8 and CTSG (Figure 1D). However, in the group vaccinated with BCG + RhCMV/TB, a specific set of genes associated with protection, such as MMP8, CTSG, and CD52, showed reduced expression compared to the group vaccinated with RhCMV/TB alone at the early phase of Mtb challenge. These transcriptional profiles correlated with a lower protective ability of BCG + RhCMV/TB than RhCMV/TB vaccine.
5.1.4 Natural killer cells
NK cells accumulate in the lungs during Mtb infection and produce IFN-γ and perforin, but studies on the function of NK cells in vaccine responses are still lacking. BCG-vaccinated mice had an increased number of NK cells in the spleen and peripheral lymph nodes. To determine the function of BCG-induced NK cells, anti-NK1.1 antibodies were administered to BCG-vaccinated mice to deplete NK cells, resulting in decreased protective efficacy of BCG and an increased number of regulatory T cells (Tregs) and a diminished T-cell response (151). The depletion of NK cells resulted in the induction of Tregs and a reduction in T-cell activity after Mtb infection, but supplementation with recombinant IL-22 rescued BCG-induced protection, suggesting the importance of IL-22 in NK cell-mediated protection against BCG vaccination (151) (Figure 1E). On the other hand, Thomas et al. infected mice with H37Rv after depleting NK cells by treatment with an anti-asialo-GM1 antibody during BCG vaccination but found no difference in efficacy after 30 days (148).
5.1.5 Dendritic cells
Delayed T-cell responses are one of the typical characteristics of TB, and to control them, the formation of a protective T-cell response and the accumulation of a considerable number of T cells at the site of inflammation are important. In this process, proper dendritic cell (DC) activation, rapid DC migration, and interaction with T cells are important. According to previous studies with a mouse model, vaccination relieved the delayed T-cell response of the host to some extent, but a delayed CD4+ T-cell response still occurred in the vaccinated host (152, 153), which may be the reason why vaccine-induced TB control is not effective. There have been studies that have focused on the role of DC frequency or activation in the delay of T cell response in vaccination. In a mouse model, vaccination with recombinant BCG-producing FMS-like tyrosine kinase 3 ligand or granulocyte-macrophage colony-stimulating factor (GM-CSF) increased the frequency of DCs. This increase in DC frequency demonstrated enhanced protection against Mtb infection (154, 155). In addition, in the analysis of the RNA expression profile related to vaccine immunogenicity and efficacy in the PBMCs of recipients of the TB vaccine candidate M72/AS01E, it was confirmed that the increase in the number of activated DCs was induced by vaccination (156). Griffiths et al. reported that after BCG vaccination, an increase in CD103 and CD40 expression on DCs induced through CpG and anti-CD40 antibody (FGK4.5) stimulation increased the number of DCs and strengthened the interaction ability with T cells in the lung, resulting in increased vaccine efficacy against Mtb infection (157) (Figure 1F). These findings indicate that increasing the frequency or quality of DCs can directly affect vaccine efficacy. Moreover, efficient Ag delivery is also directly related to the efficacy of the TB vaccine. Griffiths et al. confirmed that the transfer of DCs loaded with Mtb Ag85B accelerated the delayed T-cell response of mice immunized with BCG or Mtb Ag and increased the vaccine efficacy, showing the importance of DCs in vaccination (157). DC-targeted vaccines through DC-specific molecules such as DEC-205 or DC-SIGN (158, 159) show increased T cell response and vaccine efficacy in mouse model, which emphasis the importance of DCs in TB vaccination (Figure 1F). However, there are still few data on the response of DCs induced by vaccines.
5.2 Correlates of protection in adaptive immune response to the TB vaccine
Protection against Mtb afforded by a TB vaccine in a mouse model appears to correlate with the TCM phenotype, but data are limited. Tissue-resident memory T (TRM) cells, parenchymal-resident noncirculating memory cells that have been studied only relatively recently, reside in tissues for early recognition of infected cells (160). Vaccines that elicit a rapidly accessible T-cell response to the pathogen early in Mtb infection are thought to enable more efficient protection via TRM or TEM cells. Furthermore, the protective role of antibodies in the pathogenesis of TB highlights the need for continuous exploration of the adaptive immune response as a biomarker for vaccine efficacy.
5.2.1 Tissue-resident memory T cells
Since Mtb is transmitted via the aerosol route, generating a pulmonary memory immune response is important for protective immunity, which enables an immediate immune cell response to an infection site. The generation of TRM cells has been shown to correlate with protection against Mtb and is characteristically induced, particularly upon mucosal vaccination (161, 162) (Figure 2A). Mucosal transfer of TRM cells from BCG-vaccinated mice to naïve mice showed that both CD4+ and CD8+ TRM subpopulations can provide partial protection against Mtb infection (163) and can restrict intracellular Mtb survival in vitro (164). A recent study using human tissue from surgically resected lungs also demonstrated that the number of IL-17-producing Mtb-specific TRM-like cells in the lungs was inversely correlated with IL-1β levels in the blood, indicating that Mtb-specific TRM cells producing IL-17 may play an important role in controlling Mtb in the human lung (165). These reports suggest that TRM cells are correlated with protection in TB vaccination models, and this cell population could be a target for vaccine strategies for protection against TB. In several disease models, including TB, a strategy called “prime and pull” to recruit memory T cells through booster vaccination to target tissues after prime vaccination has been carried out (166–168). Roces et al. reported a vaccine strategy to boost immunization with H56 protein in the lung mucosa through inhalation after immunization with CAF01:H56, a clinical TB vaccine candidate, by the SC route (169). For the booster vaccination, the poly lactic-co-glycolic acid delivery system, which has been recognized for its safety, was used, and the experiment was designed based on the manufacturing of a powder containing H56. Haddadi et al. parenterally immunized mice using a recombinant replication-deficient chimpanzee Ad-based TB vaccine expressing Ag85A (AdCh68Ag85A) and then immunized the mice with Ag85 complex via the IN route (170). In this study, immunization with Ag85 via the IN route was able to induce an almost 2-log reduction in the bacterial burden in the lung tissue upon Mtb H37Rv infection compared to that in the group that received only parenteral immunization. Importantly, these results demonstrate that the prime and pull strategy for the respiratory mucosa can promote the development of TRM cells as well as the recruitment of Ag-specific T cells into lung tissue. In addition, to effectively pull memory T cells into the respiratory mucosa, it was confirmed that booster vaccination should be given at a time when T cells mainly form a memory type rather than after a short period of time when effector T cells are mainly present after prime vaccination (170).
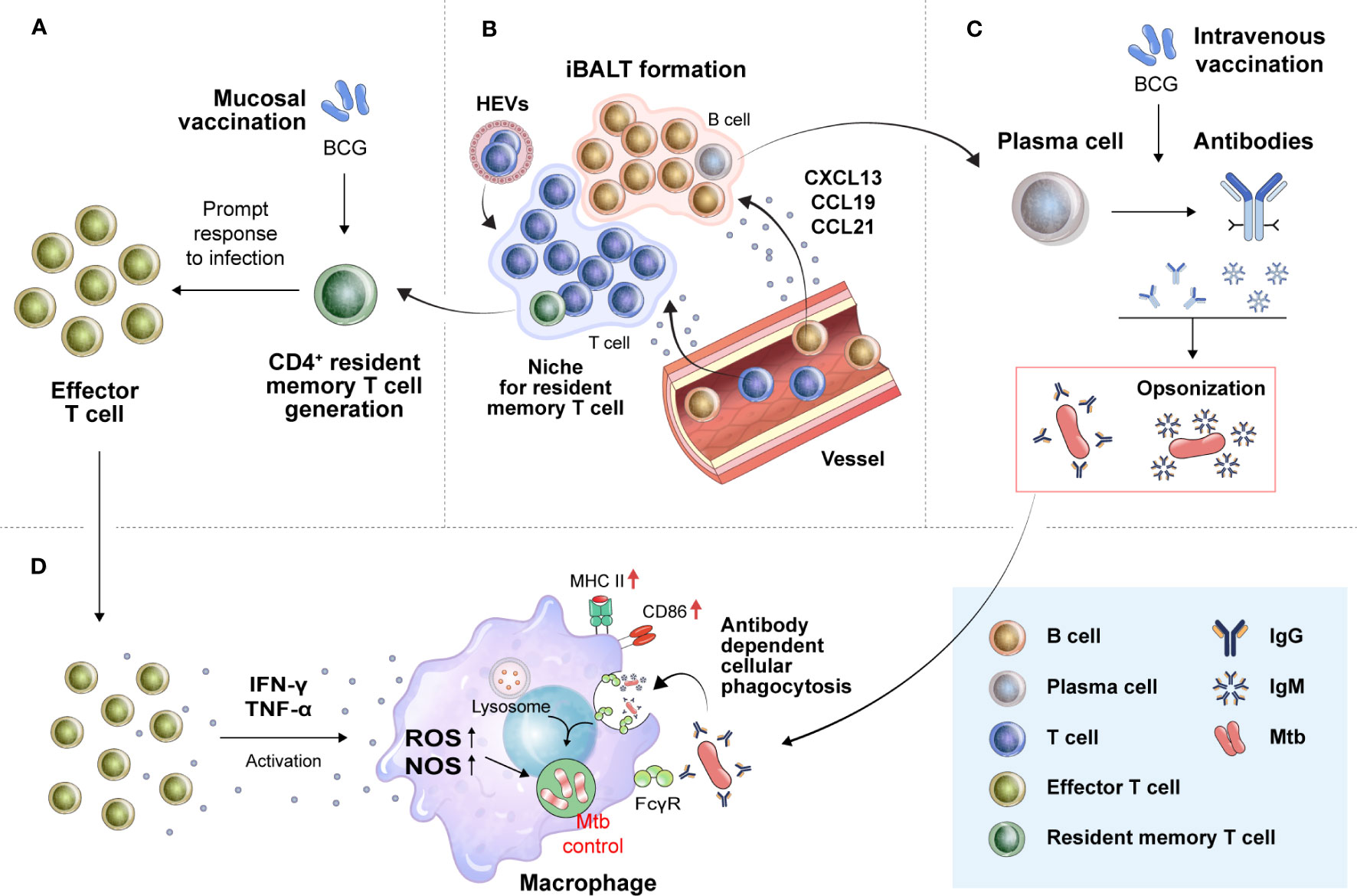
Figure 2 Translation of novel immune correlates found in preclinical animal models into humans for the development of more effective TB vaccines. (A) Mucosal vaccination can strongly induce TRM cell development. In the early stage of Mtb infection, TRM cells proliferate promptly to effector T cells to combat the bacilli by secreting proinflammatory cytokines. (B) TRM cells residing in the lung parenchyma, especially CD4+TRM cells, are known to be located in tertiary lymphoid structures, such as iBALTs. The formation of iBALTs is regulated by cytokines (IL-17, IL-22, and IL-23) and chemokines (CCL19, CCL21, CXCL12, and CXCL13). Structured iBALT consists of the T-cell zone and B-cell zone. These ectopic lymphoid-like structures provide a place where follicular helper T cells mediate the selection and survival of B cells, resulting in the differentiation of long-lived plasma cells. These processes make it possible to induce in situ protective humoral immunity by secreting protective immunoglobulins, such as IgM and IgG. (C) These humoral and cellular in situ immune responses can create an environment favorable for the host to control Mtb. Immunoglobulins from B cells aggregate Mtb, resulting in the formation of pathogen-antibody complexes. (D) The formation of these complexes enhances phagocytic killing activity of macrophages. Proinflammatory cytokines from T cells (IFN-γ and TNF-α) activate macrophages to kill Mtb. TRM cells, tissue-resident memory T cells; iBALTs, inducible bronchus-associated lymphoid tissues; ROS, reactive oxygen species; NOS, nitric oxide synthase; FcgR, Fc gamma receptor.
Direct immunization to the lung, the site of infection, has been reported to be beneficial for the formation of TRM cells. However, Darrah et al. confirmed the level of TRM cells in lung tissue 4 weeks after BCG immunization in an NHP model, BCG delivered by the intravenous (IV) route was able to induce higher levels of TRM cells than BCG injected by the aerosol route (171). Of note, six months after Mtb challenge, nine out of ten macaques with BCG immunization via the IV route produced a significant Ag-specific T-cell response accompanied by highly protective vaccine efficacy compared with those with the intradermal (ID) or aerosol route vaccination; and six of ten macaques administered BCG via the IV route had no detectable levels of infection (171).
The formation of TRM cells was thought to proceed via differentiation from effector T cells in situ via transforming growth factor beta (TGF-β) and IL-15 signals when inflammation resolves (172). In addition, at the priming level, mouse Batf3-dependent DCs and human CD1c+CD163+ DCs producing TGF-β can prime T cells for TRM cell generation in lymphoid tissues (173). These reports may reveal the reason for the finding that systemic immunization via the IV route induces higher TRM cell levels in lung tissue than the aerosol route or direct BCG delivery to the lungs. However, it is a challenge to ensure the safety of the administration method, to analyze the TRM cells and to verify the efficacy in clinical trials.
The generation of inducible bronchus-associated lymphoid tissues (iBALTs), a type of tertiary lymphoid structure (TLS), could be pivotal because increased CXCR5+ CD4+ T cell levels were correlated with a better outcome of TB disease (174, 175) (Figure 2B). TLSs are formed at sites of infection or chronic inflammation and have also been found in autoimmune disease, allograft rejection, and cancer. Importantly, B cells that respond to tumor-associated TLSs appear to participate in antitumor immunity, as B cells cultured from TLS-containing biopsy samples produced tumor Ag-specific antibodies (176). This ectopic lymphoid structure can also act as a site for B-cell selection and maturation (177) and can provide a niche for memory B cells and T cells (178). In an influenza virus-infected mouse model, CD8+ TRM cells were mainly located in the interstitium extending into the lung parenchyma, whereas CD4+ TRM cells were found in the iBALT niche (179, 180). These reports suggest that iBALT might enable a rapid and effective response to Mtb infection.
5.2.2 Effector memory T cells
Hansen et al. recently tested RhCMV/TB vaccine capable of expressing six or nine Mtb Ags in rhesus macaques (150). Upon infection of the macaques with the Erdman strain almost 1 year after vaccination, it was confirmed that sterile immunity was induced in approximately 40% of the experimental group animals (150). In contrast to previous vaccine strategies aimed at eliciting primarily TCM cell responses, this CMV-based vaccine elicited primarily TEM cell responses. TEM cell population appears to be maintained by continuous restimulation of Mtb-specific T cells by periodic reactivation of the cytomegalovirus, and the authors suggest that protective immunity induced by the RhCMV/TB vaccine can be induced by Mtb-specific T cells from the vaccination. This phenomenon is thought to be due to the high frequency of TEM cells generated by the restimulation of cells (150). However, CMV infection can be fatal in immunocompromised humans (181), and according to a recent study conducted in South Africa, CMV seems to be related to the increase in the incidence of TB in children (182). It is believed that replicating CMV-based vaccines will be needed, but this type of vaccine needs to be proven effective.
5.2.3 IgG/IgM
While the role of humoral immunity in TB has been controversial, several reports have led to a reassessment of the significance of antibody-mediated immunity in providing protection against Mtb (183–185). Antibodies can play an important role in preventing or eliminating the initial Mtb infection (Figure 2C). Antibodies can bind Mtb and increase macrophage phagocytosis by binding Fc receptors and play an effective role in clearing other intracellular pathogens. Recent studies have shown that antibodies that prevent Mtb infection are present in humans (183, 185). The first suggestion that antibodies may be protective was reported by Teitelbaum et al. (186). Mtb was pretreated with two monoclonal antibodies specific to cell surface Ags and injected through the trachea. Pretreated bacterium-infected mice lived substantially longer than control mice (186). Moreover, Ag85A-specific IgG responses have been associated with reduced TB development (187). Alternative vaccination routes, such as mucosal and IV, result in the production of pulmonary IgA and iBALTs, reducing the bacterial burden (161, 162, 171). Recently, Edward et al. reported that IgM has a negative correlation with Mtb load upon IV-BCG vaccination in macaques (188). They examined antibody responses across several BCG vaccine regimens in NHP models to determine if particular antibody profiles were linked with better Mtb control. Correlation analysis revealed a particularly strong association between plasma and bronchoalveolar lavage IgM responses and reduced Mtb burden upon BCG vaccination. Importantly, elevated Ag-specific IgM titers were observed not only in the lungs but also in the plasma of the IV-vaccinated animals. Furthermore, IgM antibodies enhance the Mtb restriction activity in vitro. These reports show the potential of IgG or IgM as a new COP in clinical practice.
These humoral and cellular in situ immune responses can be targeted for induction by a TB vaccine. Such a strategy can create a favorable environment for the host to control Mtb. Pathogen-antibody complexes formed by IgM or IgG secreted by B cells can promote the phagocytosis of Mtb by macrophages. A rapid and appropriate T-cell response to infection can induce Mtb control by inducing the activation of macrophages in the early stages of infection (Figure 2D).
5.3 Key compensatory markers
5.3.1 Biomarkers in urine samples
Finding a biomarker for COPs of a TB vaccine in urine offers several advantages over blood with respect to collection and safety. In particular, the development of biomarkers for COPs through urine sampling can be helpful in controlling TB by using vaccines in the least-developed countries, especially in the least-developed countries with a high incidence of TB, because vaccination subjects can continually collect samples themselves after simple education.
The possibility of identifying COPs for TB vaccination via biomarkers in urine is suggested by urine analysis studies on indicators of TB development and treatment. For example, in active TB patients, inflammatory mediators such as IL-8, IL-2, TNF-α, IFN-γ, chemokine ligand (CCL) 5, macrophage inflammatory protein-1 alpha and beta were not detected in the urine, but chemokine (C-X-C motif) ligand (CXCL) 10 was persistently detected (189). Moreover, the level of CXCL10 in urine was decreased in patients treated with TB drugs compared to that in active TB patients (189, 190). However, the CXCL10 level in urine is not a specific biomarker that is increased only by pulmonary TB infection, but it can be used as a limited biomarker because it shows a similar increase in patients with other lung diseases. Lipoarabinomannan (LAM), an Mtb cell wall component detected in urine, was used to establish a urinalysis for diagnosing disseminated TB patients among human immunodeficiency virus (HIV)-infected patients (191, 192). In addition, changes in the levels of 12 metabolites in urine were reported in patients with active TB after anti-TB treatment (193).
Biomarker analysis using urine samples is also being applied in vaccine research. To evaluate the toxicity of two influenza vaccines with different toxicities in a mouse model, hydrogen-1 nuclear magnetic resonance spectroscopy was used to observe changes in urine metabolite levels, and findings were compared with existing toxicity indicators such as weight loss and leukopenia (194). In addition, analysis of changes in urinary cytokine levels, as a predictor of immunogenicity and reactogenicity, induced by the AS01E-adjuvanted hepatitis B vaccine in healthy adults was used to evaluate the effectiveness of the vaccine (NCT01777295). Upon measuring the concentrations of 24 cytokines in the urine of the saline-administered control group and the vaccine group, a transient increase in CCL2 and CXCL10 levels was observed after vaccination (195). These results show the possibility of discovering biomarkers as COPs for TB vaccination through urine analysis.
5.3.2 Type I IFNs
Detrimental roles of type I IFNs in TB pathogenesis have been extensively investigated. However, there have also been reports on the protective role of type I IFNs in relation to TB vaccines. For example, it has been reported that type I IFNs can increase the immunogenicity of the BCG vaccine in mouse models. These reports showed that vaccination with ESX-1-expressing BCG could increase vaccine efficacy against Mtb infection in murine models by increasing ESX-1-dependent type I IFN production (196, 197). In the case of MTBVAC, the double deletion of phoP and fadD26 resulted in a 25- to 45-fold increase in c-di-AMP levels compared to those with Mtb or BCG, which resulted in attenuation of toxicity and high vaccine efficacy in a mouse model through an ESX-1 system-dependent type I IFN response (198, 199). Additionally, vaccination with BCGΔBCG1419c in mouse models had a higher vaccination efficacy than normal BCG vaccination (200). The BCG1419c gene encodes a cyclic diguanosine monophosphate (c-di-GMP) phosphodiesterase that normally functions to hydrolyze c-di-GMP. Vaccination with BCGΔBCG1419c is expected to result in the production of more c-di-GMP, which is thought to have a protective effect by inducing an increase in type I IFN signaling through the TANK-binding kinase 1 and interferon regulatory factor 3 cascade (200, 201). In addition, the administration of IFN-α in combination with BCG vaccination has been shown to increase the efficacy of the TB vaccine in a mouse model (202). These results indicate that type I IFNs may have different functions in TB pathogenesis and vaccine-induced immunity and the positive role of type I IFNs shows their potential as biomarkers for the efficacy of vaccine candidates.
6 The fifth hurdle: further considerations
6.1 Factors affecting vaccine efficacy: preexposure to related and unrelated pathogens
6.1.1 Helminth
Chronic infection with helminths has been well documented in cells secreting IL-10 or TGF-β and induces the induction of Tregs, which downregulate both Th1 and Th2 immune responses and mainly interfere with the function of effector Th1 cells (203). These immunological properties of helminths can affect the efficacy of TB vaccines. BCG immunogenicity was found to be lower in individuals with helminth infection than in those treated with anti-helminthic drugs (204). The reduced responses were associated with decreased purified protein derivative (PPD)-specific IFN-γ and IL-12 production and with an enhanced PPD-specific TGF-β response rather than an increase in the PPD-specific Th2 response itself. Likewise, helminth-infected college students aged 18 to 24 years in Ethiopia who received deworming therapy prior to BCG vaccination displayed relatively more PPD-specific immune responses than untreated control individuals (205). Similarly, maternal infection with helminths during pregnancy negatively influenced the frequency of IFN-γ-producing T cells in the cord blood of neonates (206) as well as the development of Th1 immunity in offspring vaccinated with BCG (207). Recently, Schick et al. reported that Nippostrongylus brasiliensis infection-induced production of IL-4 or IL-13 suppressed the H1/CAF01 vaccination-induced Th1/Th17 response in a mouse model (208). These reports show that immunization by vaccination or infection with helminth after vaccination can affect vaccine efficacy. This problem is prominent in most of the world’s tropical and subtropical developing countries that have populations that are susceptible to helminth infection.
6.1.2 Nontuberculous mycobacteria
(NTM): NTM have been reported to have cross-reactivity with BCG in humans (209), which is thought to be a factor that may affect the vaccine efficacy of BCG. BCG vaccination has been reported to show some protective effects against NTM infection in humans (210). In a mouse model, exposure to NTM after BCG vaccination also enhances BCG efficacy against Mtb infection (211), suggesting that the impact of NTM infection on BCG efficacy varies depending on factors such as the timing of exposure, route of infection, and viability of NTM. However, prior sensitization to NTM has the potential to stop BCG proliferation, prevent the induction of an effective BCG-directed immune response, and ultimately inhibit the protective effect against Mtb infection (212). Another study reported that oral exposure to Mycobacterium avium after BCG vaccination reduced the efficacy of BCG vaccination against Mtb infection in a mouse model (213). Humans are inevitably exposed to NTM via multiple infection sources such as shower water, soil, and pool water. Thus, the effect of NTM exposure and its precise mechanism of action on immunological responses are worth further investigation.
6.2 Vaccine application in the context of underlying diseases
TB is the leading cause of death among people living with HIV, affecting the immune system and eventually waning defense systems against infections, leading to an increase in the risk of TB. It is well-reported that people living with HIV are more than 20 times more susceptible to developing active TB. Therefore, protection against these two diseases is of complementary importance. Because of HIV-related immunosuppression, the TB vaccine may be less immunogenic and less effective in people with HIV infection than in people without HIV infection (214). Because HIV-infected people are a large subpopulation at a high risk of TB infection and disease, it is important to include them in TB vaccine trials. A vaccine that is expected to have a protective effect against HIV is being developed based on a promising vaccine candidate in the TB vaccine clinical stage or BCG vaccine (215).
Diabetes prevalence affects TB incidence and TB mortality, resulting in two to three times the probability of developing TB, two times the risk of death during TB treatment, four times the risk of TB recurrence after completion of treatment, and two times the risk of infection with multidrug-resistant TB (MDR-TB). A cohort study reported that the longer the period of diabetes was, the more associated with TB disease, and TB was more commonly identified in patients with a fasting plasma glucose level over 202 mg/dL (216). In addition, it was confirmed that the higher the glucose concentration in the blood of diabetic patients was, the weaker the adaptive immune response to Mtb (217). Verma et al. established a latent TB infection mouse model and induced diabetes in Mtb-infected mice by administering streptozotocin to investigate the relationship between latent TB and diabetes. These hyperglycemic conditions led to a decrease in MCP-1 and MMP9 levels and increased MMP1 levels in latent TB infection, which may lead to reactivation of latent TB infection by disrupting granulomas (218). Clement et al. reported that metabolic stress caused by hyperglycemia decreases Ag presentation ability and inhibits the proliferation of CD4+ T cells (219). These reports suggest the possibility that diabetes can affect the formation of TB vaccine-induced protective immunity.
6.3 Vaccination for elderly people
In old age, lung structural degeneration as well as changes in immune cell functions make people vulnerable to respiratory diseases, and these age-related immunological changes may also affect vaccine efficacy. The incidence of TB is common in elderly individuals and increases progressively with age, and mortality from TB is also higher in older patients (220). This phenomenon is related to the reactivation of lesions from a dormant state, which is affected by changes in the immune system with aging. In addition, chronic inflammation in aging individuals disrupts T-cell responses, followed by decreased vaccine efficacy. For example, the application of a delayed-type hypersensitivity model of BCG vaccination and TST of aged NHPs showed that the immune response to antigenic challenges between the tissue site and the periphery is compromised, restricting the optimal immune memory response (221). A follow-up study showed reduced or delayed T-cell recall responses to lung infection sites in aged BCG-vaccinated rhesus macaques (222).
Recently, nonspecific protective efficacy of the BCG vaccine was confirmed against respiratory diseases such as COVID-19 through immunological changes favorable to respiratory infections in elderly people (223, 224). Many findings reveal that this nonspecific protection is generated from innate immune memory via metabolomic and epigenetic reprogramming, also known as trained immunity. Blood samples before and 1 month after BCG vaccination were compared in 82 subjects between the ages of 60 and 80 years (225). It was confirmed that BCG vaccination induced reductions in the levels of pro-inflammatory cytokines (TNF-α, IL-6, IL-1β) and chemokines (CCL2 and CXCL10), acute phase proteins such as C-reactive protein, and matrix metalloproteinases (225, 226). Considering the immune activation by BCG vaccination in elderly people and the positive results of BCG revaccination, BCG revaccination in elderly people may be a beneficial strategy to reduce elderly mortality due to TB.
6.4 Oral vaccination: an alternative route for TB vaccine
TB vaccine candidates currently in the clinical stage are vaccinated through the intramuscular (IM) route or ID route. In addition, studies with noteworthy results in preclinical stages through the aerosol route or the intravenous route have recently been reported. However, studies on oral route vaccination are still limited. In the case of BCG, since the safety of the ID route of BCG for mass vaccination was confirmed by Scandinavian researchers in the 1930s, it has been used until now (227). However, the BCG vaccine was initially developed as an oral vaccine and was used in that form until an incident in Germany in 1930, when the oral BCG vaccine was contaminated with Mtb. In Brazil, oral BCG vaccination was administered to newborns until 1976. Recently, Hoft et al. demonstrated the safety of oral BCG vaccination through a comparative analysis of 68 healthy adults who received BCG via the intradermal (ID) route and the oral route (228). ID-BCG vaccination induced a higher systemic Th1 response than the oral route. In contrast, oral route BCG vaccination produced more elevated Mtb-specific secretory IgA and Mtb-specific bronchoalveolar lavage T cell responses than ID-BCG vaccination (228). A lipid-based formulation has been developed for oral BCG vaccination, and the results of vaccination in BALB/c mice showed increased vaccine efficacy compared to conventional BCG vaccination (229). In addition, to increase the efficacy of oral vaccination of lipid-formulated BCG vaccination, improved vaccine efficacy was confirmed through aerosol infection after oral vaccination with recombinant BCG expressing Ag85B-ESAT6 fusion protein in a guinea pig model (230). The effectiveness of oral vaccination was also confirmed with a subunit vaccine model. Although oral immunization was less effective as a priming vaccination of fusion protein ESAT-6-Ag85B with detoxified monophosphoryl lipid A (MPL), heterogeneous priming and boosting vaccination strategies combined with oral boost induced significant systemic Th1 response, providing protection similar to or exceeding vaccination via the SC route against Mtb infection (231).
Oral vaccination is an appealing route due to the absence of needles, which eliminates the risk of cross-infection, and the ability to administer vaccines without the need for specialized healthcare professionals. Exploring the properties of this unconventional vaccine route presents an additional potential strategy for TB vaccination.
7 Conclusions
Despite recent progress in clinical trials of several vaccine candidates and anti-TB drugs, the World Health Organization (WHO)’s “End TB strategy” milestone of the year 2025 has become challenging due to the coronavirus disease 2019 (COVID-19) pandemic. With the continued high prevalence and the death rate returning to the levels observed 10 years ago, researchers’ endeavors to find novel strategies to combat TB have been crippled. Nevertheless, advanced knowledge on new immune factors and consistent efforts to develop vaccine candidates will reveal promising ways to combat TB.
Most vaccine studies have focused on Th1 cells and the effector cytokine IFN-γ as potential indicators of vaccination success and vaccine efficacy. However, as the protective functions of IL-10, which have been considered negative, or novel protective functions of Th17 cells have been revealed through numerous studies, the narrow view of vaccine immunity has been expanded. From this point of view, the understanding of the functions of currently known factors is unlikely to be complete, as the factors can perform different functions in a temporally and spatially diverse immune environment. Novel analyses, such as those based on novel immune indicators, metabolomics and transcriptome analysis, may provide further insight into the complex immune environment and control of TB with vaccines. Furthermore, to progress beyond the existing ‘one-size-fits-all’ treatment approach, the prescription of a treatment strategy classified according to the patient’s condition is being considered (232, 233). These considerations should account for individual characteristics such as underlying disease and epidemiological status. For example, live attenuated vaccines, including the BCG vaccine, can be lethal in HIV-positive patients. In particular, elderly people over the age of 65 years who are very vulnerable to infection can be an important target.
The seriousness of the recent COVID-19 crisis and the quick response of humans to overcome it provide a positive message for overcoming existing diseases such as TB. However, reports of side effects such as myocarditis and severe allergic reactions (234, 235) indicate that immune balance is an important consideration for vaccine development. More than 100 years have passed since Koch first identified Mtb, and many researchers have made efforts, with many advances, to control these vicious bacilli that have killed a tremendous number of people. With numerous vaccine candidates being evaluated in clinical trials, the direction of TB vaccine development seems much more sophisticated than in the past, but achieving the intended goal remains challenging. Although several candidates showing protective efficacy in animal models eventually failed to exhibit vaccine efficacy in clinical trials, the collection and analysis of data for each candidate, whether successful or not, are obviously valuable to reduce the probability of failure. In addition, the use of BCG or BCG revaccination should be maximized and optimized in combination with other types of vaccine candidates (236). Finally, heterogeneous vaccine strategies with candidates in different phases of clinical trials, such as adjuvanted subunit priming with a vector-based candidate boost, can be another strategy for better inducing pleiotropic protective immunity.
Author contributions
HK and SS elaborated on the subject of the review. HK, H-GC, and SS wrote the manuscript. SS helped write the manuscript, provided helpful ideas and corrected the manuscript. All authors contributed to the article and approved the submitted version.
Funding
This work was supported by a grant (22202MFDS173) from the Ministry of Food and Drug Safety in 2023 and the Korean Health Technology R&D Project through the Korea Health Industry Development Institute (KHIDI), funded by the Ministry of Health and Welfare, Republic of Korea (HV22C0079). The funders had no role in the decision to publish or preparation of the manuscript.
Acknowledgments
The authors thank Medical Illustration & Design (MID), a part of the Medical Research Support Services of Yonsei University College of Medicine, for all artistic support related to this work.
Conflict of interest
The authors declare that the research was conducted in the absence of any commercial or financial relationships that could be construed as a potential conflict of interest.
Publisher’s note
All claims expressed in this article are solely those of the authors and do not necessarily represent those of their affiliated organizations, or those of the publisher, the editors and the reviewers. Any product that may be evaluated in this article, or claim that may be made by its manufacturer, is not guaranteed or endorsed by the publisher.
References
1. Brazier B, McShane H. Towards new TB vaccines. Semin Immunopathol (2020) 42(3):315–31. doi: 10.1007/s00281-020-00794-0
2. Colditz GA, Berkey CS, Mosteller F, Brewer TF, Wilson ME, Burdick E, et al. The efficacy of bacillus Calmette-Guérin vaccination of newborns and infants in the prevention of tuberculosis: meta-analyses of the published literature. Pediatrics (1995) 96(1 Pt 1):29–35.
3. Mangtani P, Abubakar I, Ariti C, Beynon R, Pimpin L, Fine PE, et al. Protection by BCG vaccine against tuberculosis: a systematic review of randomized controlled trials. Clin Infect Dis (2014) 58(4):470–80. doi: 10.1093/cid/cit790
4. Tameris MD, Hatherill M, Landry BS, Scriba TJ, Snowden MA, Lockhart S, et al. Safety and efficacy of MVA85A, a new tuberculosis vaccine, in infants previously vaccinated with BCG: a randomised, placebo-controlled phase 2b trial. Lancet (9871) 2013:1021–8:381. doi: 10.1016/s0140-6736(13)60177-4
5. Ndiaye BP, Thienemann F, Ota M, Landry BS, Camara M, Dièye S, et al. Safety, immunogenicity, and efficacy of the candidate tuberculosis vaccine MVA85A in healthy adults infected with HIV-1: a randomised, placebo-controlled, phase 2 trial. Lancet Respir Med (2015) 3(3):190–200. doi: 10.1016/S2213-2600(15)00037-5
6. Hoft DF, Blazevic A, Selimovic A, Turan A, Tennant J, Abate G, et al. Safety and immunogenicity of the recombinant BCG vaccine AERAS-422 in healthy BCG-naïve adults: A randomized, active-controlled, first-in-human phase 1 trial. EBioMedicine (2016) 7:278–86. doi: 10.1016/j.ebiom.2016.04.010
7. Garcia-Basteiro AL, White RG, Tait D, Schmidt AC, Rangaka MX, Quaife M, et al. End-point definition and trial design to advance tuberculosis vaccine development. Eur Respir Rev (2022) 31(164). doi: 10.1183/16000617.0044-2022
8. Hatherill M, Tait D, McShane H. Clinical testing of tuberculosis vaccine candidates. Microbiol Spectr (2016) 4(5). doi: 10.1128/microbiolspec.TBTB2-0015-2016
9. Mazurek GH, Jereb J, Vernon A, LoBue P, Goldberg S, Castro K. Updated guidelines for using Interferon Gamma Release Assays to detect Mycobacterium tuberculosis infection - United States, 2010. MMWR Recomm Rep (2010) 59(Rr-5):1–25.
10. Nemes E, Rozot V, Geldenhuys H, Bilek N, Mabwe S, Abrahams D, et al. Optimization and interpretation of serial quantiFERON testing to measure acquisition of mycobacterium tuberculosis infection. Am J Respir Crit Care Med (2017) 196(5):638–48. doi: 10.1164/rccm.201704-0817OC
11. Tagmouti S, Slater M, Benedetti A, Kik SV, Banaei N, Cattamanchi A, et al. Reproducibility of interferon gamma (IFN-γ) release Assays. A systematic review. Ann Am Thorac Soc (2014) 11(8):1267–76. doi: 10.1513/AnnalsATS.201405-188OC
12. Knight GM, Griffiths UK, Sumner T, Laurence YV, Gheorghe A, Vassall A, et al. Impact and cost-effectiveness of new tuberculosis vaccines in low- and middle-income countries. Proc Natl Acad Sci U S A. (2014) 111(43):15520–5. doi: 10.1073/pnas.1404386111
13. Van Der Meeren O, Hatherill M, Nduba V, Wilkinson RJ, Muyoyeta M, Van Brakel E, et al. Phase 2b controlled trial of M72/AS01(E) vaccine to prevent tuberculosis. N Engl J Med (2018) 379(17):1621–34. doi: 10.1056/NEJMoa1803484
14. Tait DR, Hatherill M, van der Meeren O, Ginsberg AM, Van Brakel E, Salaun B, et al. Final analysis of a trial of M72/AS01(E) vaccine to prevent tuberculosis. N Engl J Med (2019) 381(25):2429–39. doi: 10.1056/NEJMoa1909953
15. Coler RN, Bertholet S, Pine SO, Orr MT, Reese V, Windish HP, et al. Therapeutic immunization against Mycobacterium tuberculosis is an effective adjunct to antibiotic treatment. J Infect Dis (2013) 207(8):1242–52. doi: 10.1093/infdis/jis425
16. Lin PL, Dietrich J, Tan E, Abalos RM, Burgos J, Bigbee C, et al. The multistage vaccine H56 boosts the effects of BCG to protect cynomolgus macaques against active tuberculosis and reactivation of latent Mycobacterium tuberculosis infection. J Clin Invest (2012) 122(1):303–14. doi: 10.1172/JCI46252
17. Nunes-Alves C, Booty MG, Carpenter SM, Jayaraman P, Rothchild AC, Behar SM. In search of a new paradigm for protective immunity to TB. Nat Rev Microbiol (2014) 12(4):289–99. doi: 10.1038/nrmicro3230
18. Balasubramanian V, Wiegeshaus EH, Taylor BT, Smith DW. Pathogenesis of tuberculosis: pathway to apical localization. Tuber Lung Dis (1994) 75(3):168–78. doi: 10.1016/0962-8479(94)90002-7
19. Sakamoto K. The pathology of Mycobacterium tuberculosis infection. Vet Pathol (2012) 49(3):423–39. doi: 10.1177/0300985811429313
20. Fennelly KP, Jones-López EC, Ayakaka I, Kim S, Menyha H, Kirenga B, et al. Variability of infectious aerosols produced during coughing by patients with pulmonary tuberculosis. Am J Respir Crit Care Med (2012) 186(5):450–7. doi: 10.1164/rccm.201203-0444OC
21. Dinkele R, Gessner S, McKerry A, Leonard B, Leukes J, Seldon R, et al. Aerosolization of mycobacterium tuberculosis by tidal breathing. Am J Respir Crit Care Med (2022) 206(2):206–16. doi: 10.1164/rccm.202110-2378OC
22. Scriba TJ, Dinkele R, Warner DF, Mizrahi V. Challenges in TB research. J Exp Med (2022) 219(12). doi: 10.1084/jem.20221334
23. Roederer M. Parsimonious determination of the optimal infectious dose of a pathogen for nonhuman primate models. PloS Pathog (2015) 11(8):e1005100. doi: 10.1371/journal.ppat.1005100
24. Plumlee CR, Duffy FJ, Gern BH, Delahaye JL, Cohen SB, Stoltzfus CR, et al. Ultra-low dose aerosol infection of mice with mycobacterium tuberculosis more closely models human tuberculosis. Cell Host Microbe (2021) 29(1):68–82.e5. doi: 10.1016/j.chom.2020.10.003
25. Dijkman K, Sombroek CC, Vervenne RAW, Hofman SO, Boot C, Remarque EJ, et al. Prevention of tuberculosis infection and disease by local BCG in repeatedly exposed rhesus macaques. Nat Med (2019) 25(2):255–62. doi: 10.1038/s41591-018-0319-9
26. Cadena AM, Fortune SM, Flynn JL. Heterogeneity in tuberculosis. Nat Rev Immunol (2017) 17(11):691–702. doi: 10.1038/nri.2017.69
27. Chackerian AA, Behar SM. Susceptibility to Mycobacterium tuberculosis: lessons from inbred strains of mice. Tuberculosis (Edinb) (2003) 83(5):279–85. doi: 10.1016/S1472-9792(03)00017-9
28. Kramnik I, Beamer G. Mouse models of human TB pathology: roles in the analysis of necrosis and the development of host-directed therapies. Semin Immunopathol (2016) 38(2):221–37. doi: 10.1007/s00281-015-0538-9
29. Kondratieva E, Logunova N, Majorov K, Averbakh M, Apt A. Host genetics in granuloma formation: human-like lung pathology in mice with reciprocal genetic susceptibility to M. tuberculosis and M. avium. PloS One (2010) 5(5):e10515. doi: 10.1371/journal.pone.0010515
30. Arrey F, Löwe D, Kuhlmann S, Kaiser P, Moura-Alves P, Krishnamoorthy G, et al. Humanized mouse model mimicking pathology of human tuberculosis for in vivo evaluation of drug regimens. Front Immunol (2019) 10:89. doi: 10.3389/fimmu.2019.00089
31. Zelmer A, Stockdale L, Prabowo SA, Cia F, Spink N, Gibb M, et al. High monocyte to lymphocyte ratio is associated with impaired protection after subcutaneous administration of BCG in a mouse model of tuberculosis. F1000Res (2018) 7:296. doi: 10.12688/f1000research.14239.2
32. Smith CM, Proulx MK, Olive AJ, Laddy D, Mishra BB, Moss C, et al. Tuberculosis susceptibility and vaccine protection are independently controlled by host genotype. mBio (2016) 7(5). doi: 10.1128/mBio.01516-16
33. Peña JC, Ho WZ. Monkey models of tuberculosis: lessons learned. Infect Immun (2015) 83(3):852–62. doi: 10.1128/IAI.02850-14
34. Langermans JA, Andersen P, van Soolingen D, Vervenne RA, Frost PA, van der Laan T, et al. Divergent effect of bacillus Calmette-Guérin (BCG) vaccination on Mycobacterium tuberculosis infection in highly related macaque species: implications for primate models in tuberculosis vaccine research. Proc Natl Acad Sci U S A. (2001) 98(20):11497–502. doi: 10.1073/pnas.201404898
35. Darrah PA, Bolton DL, Lackner AA, Kaushal D, Aye PP, Mehra S, et al. Aerosol vaccination with AERAS-402 elicits robust cellular immune responses in the lungs of rhesus macaques but fails to protect against high-dose Mycobacterium tuberculosis challenge. J Immunol (2014) 193(4):1799–811. doi: 10.4049/jimmunol.1400676
36. Sharpe SA, McShane H, Dennis MJ, Basaraba RJ, Gleeson F, Hall G, et al. Establishment of an aerosol challenge model of tuberculosis in rhesus macaques and an evaluation of endpoints for vaccine testing. Clin Vaccine Immunol (2010) 17(8):1170–82. doi: 10.1128/CVI.00079-10
37. Verreck FA, Vervenne RA, Kondova I, van Kralingen KW, Remarque EJ, Braskamp G, et al. MVA.85A boosting of BCG and an attenuated, phoP deficient M. tuberculosis vaccine both show protective efficacy against tuberculosis in rhesus macaques. PloS One (2009) 4(4):e5264. doi: 10.1371/journal.pone.0005264
38. Daniel J, Deb C, Dubey VS, Sirakova TD, Abomoelak B, Morbidoni HR, et al. Induction of a novel class of diacylglycerol acyltransferases and triacylglycerol accumulation in Mycobacterium tuberculosis as it goes into a dormancy-like state in culture. J Bacteriol (2004) 186(15):5017–30. doi: 10.1128/JB.186.15.5017-5030.2004
39. Garton NJ, Waddell SJ, Sherratt AL, Lee SM, Smith RJ, Senner C, et al. Cytological and transcript analyses reveal fat and lazy persister-like bacilli in tuberculous sputum. PloS Med (2008) 5(4):e75. doi: 10.1371/journal.pmed.0050075
40. Rosser A, Stover C, Pareek M, Mukamolova GV. Resuscitation-promoting factors are important determinants of the pathophysiology in Mycobacterium tuberculosis infection. Crit Rev Microbiol (2017) 43(5):621–30. doi: 10.1080/1040841X.2017.1283485
41. Downing KJ, Mischenko VV, Shleeva MO, Young DI, Young M, Kaprelyants AS, et al. Mutants of Mycobacterium tuberculosis lacking three of the five rpf-like genes are defective for growth in vivo and for resuscitation in vitro. Infect Immun (2005) 73(5):3038–43. doi: 10.1128/IAI.73.5.3038-3043.2005
42. Mukamolova GV, Turapov O, Malkin J, Woltmann G, Barer MR. Resuscitation-promoting factors reveal an occult population of tubercle Bacilli in Sputum. Am J Respir Crit Care Med (2010) 181(2):174–80. doi: 10.1164/rccm.200905-0661OC
43. Huang W, Qi Y, Diao Y, Yang F, Zha X, Ren C, et al. Use of resuscitation-promoting factor proteins improves the sensitivity of culture-based tuberculosis testing in special samples. Am J Respir Crit Care Med (2014) 189(5):612–4. doi: 10.1164/rccm.201310-1899LE
44. White AG, Maiello P, Coleman MT, Tomko JA, Frye LJ, Scanga CA, et al. Analysis of 18FDG PET/CT imaging as a tool for studying mycobacterium tuberculosis infection and treatment in non-human primates. J Vis Exp (2017) 127. doi: 10.3791/56375
45. Tameris M, Mearns H, Penn-Nicholson A, Gregg Y, Bilek N, Mabwe S, et al. Live-attenuated Mycobacterium tuberculosis vaccine MTBVAC versus BCG in adults and neonates: a randomised controlled, double-blind dose-escalation trial. Lancet Respir Med (2019) 7(9):757–70. doi: 10.1016/S2213-2600(19)30251-6
46. Spertini F, Audran R, Chakour R, Karoui O, Steiner-Monard V, Thierry AC, et al. Safety of human immunisation with a live-attenuated Mycobacterium tuberculosis vaccine: a randomised, double-blind, controlled phase I trial. Lancet Respir Med (2015) 3(12):953–62. doi: 10.1016/S2213-2600(15)00435-X
47. Grode L, Ganoza CA, Brohm C, Weiner J 3rd, Eisele B, Kaufmann SH. Safety and immunogenicity of the recombinant BCG vaccine VPM1002 in a phase 1 open-label randomized clinical trial. Vaccine (2013) 31(9):1340–8. doi: 10.1016/j.vaccine.2012.12.053
48. Loxton AG, Knaul JK, Grode L, Gutschmidt A, Meller C, Eisele B, et al. Safety and immunogenicity of the recombinant mycobacterium bovis BCG vaccine VPM1002 in HIV-unexposed newborn infants in South Africa. Clin Vaccine Immunol (2017) 24(2). doi: 10.1128/CVI.00439-16
49. Cotton MF, Madhi SA, Luabeya AK, Tameris M, Hesseling AC, Shenje J, et al. Safety and immunogenicity of VPM1002 versus BCG in South African newborn babies: a randomised, phase 2 non-inferiority double-blind controlled trial. Lancet Infect Dis (2022) 22(10):1472–83. doi: 10.1016/S1473-3099(22)00222-5
50. Nemes E, Geldenhuys H, Rozot V, Rutkowski KT, Ratangee F, Bilek N, et al. Prevention of M. tuberculosis infection with H4:IC31 vaccine or BCG revaccination. N Engl J Med (2018) 379(2):138–49. doi: 10.1056/NEJMoa1714021
51. Rakshit S, Ahmed A, Adiga V, Sundararaj BK, Sahoo PN, Kenneth J, et al. BCG revaccination boosts adaptive polyfunctional Th1/Th17 and innate effectors in IGRA+ and IGRA- Indian adults. JCI Insight (2019) 4(24). doi: 10.1172/jci.insight.130540
52. Bekker LG, Dintwe O, Fiore-Gartland A, Middelkoop K, Hutter J, Williams A, et al. A phase 1b randomized study of the safety and immunological responses to vaccination with H4:IC31, H56:IC31, and BCG revaccination in Mycobacterium tuberculosis-uninfected adolescents in Cape Town, South Africa. EClinicalMedicine (2020) 21:100313. doi: 10.1016/j.eclinm.2020.100313
53. Montoya J, Solon JA, Cunanan SR, Acosta L, Bollaerts A, Moris P, et al. A randomized, controlled dose-finding Phase II study of the M72/AS01 candidate tuberculosis vaccine in healthy PPD-positive adults. J Clin Immunol (2013) 33(8):1360–75. doi: 10.1007/s10875-013-9949-3
54. Penn-Nicholson A, Geldenhuys H, Burny W, van der Most R, Day CL, Jongert E, et al. Safety and immunogenicity of candidate vaccine M72/AS01E in adolescents in a TB endemic setting. Vaccine (2015) 33(32):4025–34. doi: 10.1016/j.vaccine.2015.05.088
55. Gillard P, Yang PC, Danilovits M, Su WJ, Cheng SL, Pehme L, et al. Safety and immunogenicity of the M72/AS01E candidate tuberculosis vaccine in adults with tuberculosis: A phase II randomised study. Tuberculosis (Edinb) (2016) 100:118–27. doi: 10.1016/j.tube.2016.07.005
56. Kumarasamy N, Poongulali S, Beulah FE, Akite EJ, Ayuk LN, Bollaerts A, et al. Long-term safety and immunogenicity of the M72/AS01E candidate tuberculosis vaccine in HIV-positive and -negative Indian adults: Results from a phase II randomized controlled trial. Med (Baltimore) (2018) 97(45):e13120. doi: 10.1097/MD.0000000000013120
57. Tkachuk AP, Bykonia EN, Popova LI, Kleymenov DA, Semashko MA, Chulanov VP, et al. Safety and immunogenicity of the gamTBvac, the recombinant subunit tuberculosis vaccine candidate: A phase II, multi-center, double-blind, randomized, placebo-controlled study. Vaccines (Basel) (2020) 8(4). doi: 10.3390/vaccines8040652
58. Vasina DV, Kleymenov DA, Manuylov VA, Mazunina EP, Koptev EY, Tukhovskaya EA, et al. First-in-human trials of gamTBvac, a recombinant subunit tuberculosis vaccine candidate: safety and immunogenicity assessment. Vaccines (Basel) (2019) 7(4). doi: 10.3390/vaccines7040166
59. Penn-Nicholson A, Tameris M, Smit E, Day TA, Musvosvi M, Jayashankar L, et al. Safety and immunogenicity of the novel tuberculosis vaccine ID93 + GLA-SE in BCG-vaccinated healthy adults in South Africa: a randomised, double-blind, placebo-controlled phase 1 trial. Lancet Respir Med (2018) 6(4):287–98. doi: 10.1016/S2213-2600(18)30077-8
60. Day TA, Penn-Nicholson A, Luabeya AKK, Fiore-Gartland A, Du Plessis N, Loxton AG, et al. Safety and immunogenicity of the adjunct therapeutic vaccine ID93 + GLA-SE in adults who have completed treatment for tuberculosis: a randomised, double-blind, placebo-controlled, phase 2a trial. Lancet Respir Med (2021) 9(4):373–86. doi: 10.1016/S2213-2600(20)30319-2
61. Luabeya AK, Kagina BM, Tameris MD, Geldenhuys H, Hoff ST, Shi Z, et al. First-in-human trial of the post-exposure tuberculosis vaccine H56:IC31 in Mycobacterium tuberculosis infected and non-infected healthy adults. Vaccine (2015) 33(33):4130–40. doi: 10.1016/j.vaccine.2015.06.051
62. Lu JB, Chen BW, Wang GZ, Fu LL, Shen XB, Su C, et al. Recombinant tuberculosis vaccine AEC/BC02 induces antigen-specific cellular responses in mice and protects Guinea pigs in a model of latent infection. J Microbiol Immunol Infect (2015) 48(6):597–603. doi: 10.1016/j.jmii.2014.03.005
63. Méndez-Samperio P. Development of tuberculosis vaccines in clinical trials: Current status. Scand J Immunol (2018) 88(4):e12710. doi: 10.1111/sji.12710
64. Hu Z, Lu SH, Lowrie DB, Fan XY. Research advances for virus-vectored tuberculosis vaccines and latest findings on tuberculosis vaccine development. Front Immunol (2022) 13:895020. doi: 10.3389/fimmu.2022.895020
65. Jiang MJ, Liu SJ, Su L, Zhang X, Li YY, Tang T, et al. Intranasal vaccination with Listeria ivanovii as vector of Mycobacterium tuberculosis antigens promotes specific lung-localized cellular and humoral immune responses. Sci Rep (2020) 10(1):302. doi: 10.1038/s41598-019-57245-6
66. Singh SK, Kumari R, Singh DK, Tiwari S, Singh PK, Sharma S, et al. Putative roles of a proline-glutamic acid-rich protein (PE3) in intracellular survival and as a candidate for subunit vaccine against Mycobacterium tuberculosis. Med Microbiol Immunol (2013) 202(5):365–77. doi: 10.1007/s00430-013-0299-9
67. Singh SK, Tripathi DK, Singh PK, Sharma S, Srivastava KK. Protective and survival efficacies of Rv0160c protein in murine model of Mycobacterium tuberculosis. Appl Microbiol Biotechnol (2013) 97(13):5825–37. doi: 10.1007/s00253-012-4493-2
68. Niu H, Hu L, Li Q, Da Z, Wang B, Tang K, et al. Construction and evaluation of a multistage Mycobacterium tuberculosis subunit vaccine candidate Mtb10.4-HspX. Vaccine (2011) 29(51):9451–8. doi: 10.1016/j.vaccine.2011.10.032
69. Xin Q, Niu H, Li Z, Zhang G, Hu L, Wang B, et al. Subunit vaccine consisting of multi-stage antigens has high protective efficacy against Mycobacterium tuberculosis infection in mice. PloS One (2013) 8(8):e72745. doi: 10.1371/journal.pone.0072745
70. Mao L, Xu L, Wang X, Xing Y, Wang J, Zhang Y, et al. Enhanced immunogenicity of the tuberculosis subunit Rv0572c vaccine delivered in DMT liposome adjuvant as a BCG-booster. Tuberculosis (Edinb) (2022) 134:102186. doi: 10.1016/j.tube.2022.102186
71. Tian M, Zhou Z, Tan S, Fan X, Li L, Ullah N. Formulation in DDA-MPLA-TDB Liposome Enhances the Immunogenicity and Protective Efficacy of a DNA Vaccine against Mycobacterium tuberculosis Infection. Front Immunol (2018) 9:310. doi: 10.3389/fimmu.2018.00310
72. Xiao Y, Sha W, Tian Z, Chen Y, Ji P, Sun Q, et al. Adenylate kinase: a novel antigen for immunodiagnosis and subunit vaccine against tuberculosis. J Mol Med (Berl) (2016) 94(7):823–34. doi: 10.1007/s00109-016-1392-5
73. Skeiky YA, Ovendale PJ, Jen S, Alderson MR, Dillon DC, Smith S, et al. T cell expression cloning of a Mycobacterium tuberculosis gene encoding a protective antigen associated with the early control of infection. J Immunol (2000) 165(12):7140–9. doi: 10.4049/jimmunol.165.12.7140
74. ROmano M, Aryan E, Korf H, Bruffaerts N, Franken CL, Ottenhoff TH, et al. Potential of Mycobacterium tuberculosis resuscitation-promoting factors as antigens in novel tuberculosis sub-unit vaccines. Microbes Infect (2012) 14(1):86–95. doi: 10.1016/j.micinf.2011.08.011
75. Yu Q, Wang X, Fan X. A new adjuvant MTOM mediates mycobacterium tuberculosis subunit vaccine to enhance th1-type T cell immune responses and IL-2(+) T cells. Front Immunol (2017) 8:585. doi: 10.3389/fimmu.2017.00585
76. Ma J, Tian M, Fan X, Yu Q, Jing Y, Wang W, et al. Mycobacterium tuberculosis multistage antigens confer comprehensive protection against pre- and post-exposure infections by driving Th1-type T cell immunity. Oncotarget (2016) 7(39):63804–15. doi: 10.18632/oncotarget.11542
77. Lee J, Kim J, Lee J, Shin SJ, Shin EC. DNA immunization of Mycobacterium tuberculosis resuscitation-promoting factor B elicits polyfunctional CD8(+) T cell responses. Clin Exp Vaccine Res (2014) 3(2):235–43. doi: 10.7774/cevr.2014.3.2.235
78. Stylianou E, Harrington-Kandt R, Beglov J, Bull N, Pinpathomrat N, Swarbrick GM, et al. Identification and evaluation of novel protective antigens for the development of a candidate tuberculosis subunit vaccine. Infect Immun (2018) 86(7). doi: 10.1128/IAI.00014-18
79. Li Q, Yu H, Zhang Y, Wang B, Jiang W, Da Z, et al. Immunogenicity and protective efficacy of a fusion protein vaccine consisting of antigen Ag85B and HspX against Mycobacterium tuberculosis infection in mice. Scand J Immunol (2011) 73(6):568–76. doi: 10.1111/j.1365-3083.2011.02531.x
80. Counoupas C, Pinto R, Nagalingam G, Britton WJ, Petrovsky N, Triccas JA. Delta inulin-based adjuvants promote the generation of polyfunctional CD4(+) T cell responses and protection against Mycobacterium tuberculosis infection. Sci Rep (2017) 7(1):8582. doi: 10.1038/s41598-017-09119-y
81. Liang Y, Zhang X, Xiao L, Bai X, Wang X, Yang Y, et al. Immunogenicity and therapeutic effects of pVAX1- rv1419 DNA from Mycobacterium tuberculosis. Curr Gene Ther (2016) 16(4):249–255. doi: 10.2174/1566523216666161102170123
82. Wang Y, Li Z, Wu S, Fleming J, Li C, Zhu G, et al. Systematic evaluation of mycobacterium tuberculosis proteins for antigenic properties identifies rv1485 and rv1705c as potential protective subunit vaccine candidates. Infect Immun (2021) 89(3). doi: 10.1128/IAI.00585-20
83. Levillain F, Kim H, Woong Kwon K, Clark S, Cia F, Malaga W, et al. Preclinical assessment of a new live attenuated Mycobacterium tuberculosis Beijing-based vaccine for tuberculosis. Vaccine (2020) 38(6):1416–23. doi: 10.1016/j.vaccine.2019.11.085
84. Coppola M, van den Eeden SJ, Wilson L, Franken KL, Ottenhoff TH, Geluk A. Synthetic Long Peptide Derived from Mycobacterium tuberculosis Latency Antigen Rv1733c Protects against Tuberculosis. Clin Vaccine Immunol (2015) 22(9):1060–9. doi: 10.1128/CVI.00271-15
85. King TH, Shanley CA, Guo Z, Bellgrau D, Rodell T, Furney S, et al. GI-19007, a Novel Saccharomyces cerevisiae-Based Therapeutic Vaccine against Tuberculosis. Clin Vaccine Immunol (2017) 24(12). doi: 10.1128/CVI.00245-17
86. Speranza V, Colone A, Cicconi R, Palmieri G, Giovannini D, Grassi M, et al. Recombinant BCG-Rv1767 amount determines, in vivo, antigen-specific T cells location, frequency, and protective outcome. Microb Pathog (2010) 48(5):150–9. doi: 10.1016/j.micpath.2010.02.003
87. Sulman S, Savidge BO, Alqaseer K, Das MK, Nezam Abadi N, Pearl JE, et al. Balance between Protection and Pathogenic Response to Aerosol Challenge with Mycobacterium tuberculosis (Mtb) in Mice Vaccinated with TriFu64, a Fusion Consisting of Three Mtb Antigens. Vaccines (Basel) (2021) 9(5). doi: 10.3390/vaccines9050519
88. Choi S, Choi HG, Back YW, Park HS, Lee KI, Gurmessa SK, et al. A dendritic cell-activating rv1876 protein elicits mycobacterium bovis BCG-prime effect via th1-immune response. Biomolecules (2021) 11(9). doi: 10.3390/biom11091306
89. Lv W, He P, Ma Y, Tan D, Li F, Xie T, et al. Optimizing the boosting schedule of subunit vaccines consisting of BCG and "Non-BCG" Antigens to induce long-term immune memory. Front Immunol (2022) 13:862726. doi: 10.3389/fimmu.2022.862726
90. Vasilyev K, Shurygina AP, Zabolotnykh N, Sergeeva M, ROmanovskaya-ROmanko E, Pulkina A, et al. Enhancement of the Local CD8(+) T-Cellular Immune Response to Mycobacterium tuberculosis in BCG-Primed Mice after Intranasal Administration of Influenza Vector Vaccine Carrying TB10.4 and HspX Antigens. Vaccines (Basel) (2021) 9(11). doi: 10.3390/vaccines9111273
91. Sergeeva M, ROmanovskaya-ROmanko E, Zabolotnyh N, Pulkina A, Vasilyev K, Shurigina AP, et al. Mucosal Influenza Vector Vaccine Carrying TB10.4 and HspX Antigens Provides Protection against Mycobacterium tuberculosis in Mice and Guinea Pigs. Vaccines (Basel) (2021) 9(4). doi: 10.3390/vaccines9040394
92. Back YW, Shin KW, Choi S, Park HS, Lee KI, Choi HG, et al. Mycobacterium tuberculosis rv2005c induces dendritic cell maturation and th1 responses and exhibits immunotherapeutic activity by fusion with the rv2882c protein. Vaccines (Basel) (2020) 8(3). doi: 10.3390/vaccines8030370
93. Liu W, Li J, Niu H, Lin X, Li R, Wang Y, et al. Immunogenicity and protective efficacy of multistage vaccine candidates (Mtb8.4-HspX and HspX-Mtb8.4) against Mycobacterium tuberculosis infection in mice. Int Immunopharmacol (2017) 53:83–9. doi: 10.1016/j.intimp.2017.10.015
94. Niu H, Peng J, Bai C, Liu X, Hu L, Luo Y, et al. Multi-Stage Tuberculosis Subunit Vaccine Candidate LT69 Provides High Protection against Mycobacterium tuberculosis Infection in Mice. PloS One (2015) 10(6):e0130641. doi: 10.1371/journal.pone.0130641
95. Liang J, Teng X, Yuan X, Zhang Y, Shi C, Yue T, et al. Enhanced and durable protective immune responses induced by a cocktail of recombinant BCG strains expressing antigens of multistage of Mycobacterium tuberculosis. Mol Immunol (2015) 66(2):392–401. doi: 10.1016/j.molimm.2015.04.017
96. da Costa AC, Costa-Júnior Ade O, de Oliveira FM, Nogueira SV, Rosa JD, Resende DP, et al. A new recombinant BCG vaccine induces specific Th17 and Th1 effector cells with higher protective efficacy against tuberculosis. PloS One (2014) 9(11):e112848. doi: 10.1371/journal.pone.0112848
97. Commandeur S, van den Eeden SJ, Dijkman K, Clark SO, van Meijgaarden KE, Wilson L, et al. The in vivo expressed Mycobacterium tuberculosis (IVE-TB) antigen Rv2034 induces CD4+ T-cells that protect against pulmonary infection in HLA-DR transgenic mice and Guinea pigs. Vaccine (2014) 32(29):3580–8. doi: 10.1016/j.vaccine.2014.05.005
98. Choi HG, Choi S, Back YW, Paik S, Park HS, Kim WS, et al. Rv2299c, a novel dendritic cell-activating antigen of Mycobacterium tuberculosis, fused-ESAT-6 subunit vaccine confers improved and durable protection against the hypervirulent strain HN878 in mice. Oncotarget (2017) 8(12):19947–67. doi: 10.18632/oncotarget.15256
99. Clemmensen HS, Knudsen NPH, Billeskov R, Rosenkrands I, Jungersen G, Aagaard C, et al. Rescuing ESAT-6 specific CD4 T cells from terminal differentiation is critical for long-term control of murine mtb infection. Front Immunol (2020) 11:585359. doi: 10.3389/fimmu.2020.585359
100. Kwon KW, Kim WS, Kim H, Han SJ, Hahn MY, Lee JS, et al. Novel vaccine potential of Rv3131, a DosR regulon-encoded putative nitroreductase, against hyper-virulent Mycobacterium tuberculosis strain K. Sci Rep (2017) 7:44151. doi: 10.1038/srep44151
101. Jones GJ, Khatri BL, Garcia-Pelayo MC, Kaveh DA, Bachy VS, Hogarth PJ, et al. Development of an unbiased antigen-mining approach to identify novel vaccine antigens and diagnostic reagents for bovine tuberculosis. Clin Vaccine Immunol (2013) 20(11):1675–82. doi: 10.1128/CVI.00416-13
102. Woodworth JS, Clemmensen HS, Battey H, Dijkman K, Lindenstrøm T, Laureano RS, et al. A Mycobacterium tuberculosis-specific subunit vaccine that provides synergistic immunity upon co-administration with Bacillus Calmette-Guérin. Nat Commun (2021) 12(1):6658. doi: 10.1038/s41467-021-26934-0
103. Leroux-Roels I, Forgus S, De Boever F, Clement F, Demoitié MA, Mettens P, et al. Improved CD4+ T cell responses to Mycobacterium tuberculosis in PPD-negative adults by M72/AS01 as compared to the M72/AS02 and Mtb72F/AS02 tuberculosis candidate vaccine formulations: a randomized trial. Vaccine (2013) 31(17):2196–206. doi: 10.1016/j.vaccine.2012.05.035
104. Pethe K, Alonso S, Biet F, Delogu G, Brennan MJ, Locht C, et al. The heparin-binding haemagglutinin of M. tuberculosis is required for extrapulmonary dissemination. Nature (2001) 412(6843):190–4. doi: 10.1038/35084083
105. Knudsen NP, Nørskov-Lauritsen S, Dolganov GM, Schoolnik GK, Lindenstrøm T, Andersen P, et al. Tuberculosis vaccine with high predicted population coverage and compatibility with modern diagnostics. Proc Natl Acad Sci U S A. (2014) 111(3):1096–101. doi: 10.1073/pnas.1314973111
106. Liu L, Zhang WJ, Zheng J, Fu H, Chen Q, Zhang Z, et al. Exploration of novel cellular and serological antigen biomarkers in the ORFeome of Mycobacterium tuberculosis. Mol Cell Proteomics (2014) 13(3):897–906. doi: 10.1074/mcp.M113.032623
107. Brennan MJ, Clagett B, Fitzgerald H, Chen V, Williams A, Izzo AA, et al. Preclinical evidence for implementing a prime-boost vaccine strategy for tuberculosis. Vaccine (2012) 30(18):2811–23. doi: 10.1016/j.vaccine.2012.02.036
108. Brennan MJ, Thole J. Tuberculosis vaccines: a strategic blueprint for the next decade. Tuberculosis (Edinb) (2012) 92 Suppl 1:S6–13. doi: 10.1016/S1472-9792(12)70005-7
109. Coler RN, Skeiky YA, Vedvick T, Bement T, Ovendale P, Campos-Neto A, et al. Molecular cloning and immunologic reactivity of a novel low molecular mass antigen of Mycobacterium tuberculosis. J Immunol (1998) 161(5):2356–64. doi: 10.4049/jimmunol.161.5.2356
110. Skeiky YA, Lodes MJ, Guderian JA, Mohamath R, Bement T, Alderson MR, et al. Cloning, expression, and immunological evaluation of two putative secreted serine protease antigens of Mycobacterium tuberculosis. Infect Immun (1999) 67(8):3998–4007. doi: 10.1128/IAI.67.8.3998-4007.1999
111. Aagaard C, Hoang T, Dietrich J, Cardona PJ, Izzo A, Dolganov G, et al. A multistage tuberculosis vaccine that confers efficient protection before and after exposure. Nat Med (2011) 17(2):189–94. doi: 10.1038/nm.2285
112. Jenum S, Tonby K, Rueegg CS, Rühwald M, Kristiansen MP, Bang P, et al. A Phase I/II randomized trial of H56:IC31 vaccination and adjunctive cyclooxygenase-2-inhibitor treatment in tuberculosis patients. Nat Commun (2021) 12(1):6774. doi: 10.1038/s41467-021-27029-6
113. Coler RN, Day TA, Ellis R, Piazza FM, Beckmann AM, Vergara J, et al. The TLR-4 agonist adjuvant, GLA-SE, improves magnitude and quality of immune responses elicited by the ID93 tuberculosis vaccine: first-in-human trial. NPJ Vaccines (2018) 3:34. doi: 10.1038/s41541-018-0057-5
114. Liu W, Xu Y, Yan J, Shen H, Yang E, Wang H. Ag85B synergizes with ESAT-6 to induce efficient and long-term immunity of C57BL/6 mice primed with recombinant Bacille Calmette-Guerin. Exp Ther Med (2017) 13(1):208–14. doi: 10.3892/etm.2016.3944
115. Farsiani H, Mosavat A, Soleimanpour S, Sadeghian H, Akbari Eydgahi MR, Ghazvini K, et al. Fc-based delivery system enhances immunogenicity of a tuberculosis subunit vaccine candidate consisting of the ESAT-6:CFP-10 complex. Mol Biosyst (2016) 12(7):2189–201. doi: 10.1039/C6MB00174B
116. Moguche AO, Musvosvi M, Penn-Nicholson A, Plumlee CR, Mearns H, Geldenhuys H, et al. Antigen availability shapes T cell differentiation and function during tuberculosis. Cell Host Microbe (2017) 21(6):695–706.e5. doi: 10.1016/j.chom.2017.05.012
117. Comas I, Chakravartti J, Small PM, Galagan J, Niemann S, Kremer K, et al. Human T cell epitopes of Mycobacterium tuberculosis are evolutionarily hyperconserved. Nat Genet (2010) 42(6):498–503. doi: 10.1038/ng.590
118. Copin R, Coscollá M, Seiffert SN, Bothamley G, Sutherland J, Mbayo G, et al. Sequence diversity in the pe_pgrs genes of Mycobacterium tuberculosis is independent of human T cell recognition. mBio (2014) 5(1):e00960–13. doi: 10.1128/mBio.00960-13
119. Sutherland JS, Adetifa IM, Hill PC, Adegbola RA, Ota MO. Pattern and diversity of cytokine production differentiates between Mycobacterium tuberculosis infection and disease. Eur J Immunol (2009) 39(3):723–9. doi: 10.1002/eji.200838693
120. Caccamo N, Guggino G, Joosten SA, Gelsomino G, Di Carlo P, Titone L, et al. Multifunctional CD4(+) T cells correlate with active Mycobacterium tuberculosis infection. Eur J Immunol (2010) 40(8):2211–20. doi: 10.1002/eji.201040455
121. Orr MT, Ireton GC, Beebe EA, Huang PW, Reese VA, Argilla D, et al. Immune subdominant antigens as vaccine candidates against Mycobacterium tuberculosis. J Immunol (2014) 193(6):2911–8. doi: 10.4049/jimmunol.1401103
122. Kundu M, Basu J. Applications of transcriptomics and proteomics for understanding dormancy and resuscitation in mycobacterium tuberculosis. Front Microbiol (2021) 12:642487. doi: 10.3389/fmicb.2021.642487
123. Tufariello JM, Jacobs WR Jr., Chan J. Individual Mycobacterium tuberculosis resuscitation-promoting factor homologues are dispensable for growth in vitro and in vivo. Infect Immun (2004) 72(1):515–26. doi: 10.1128/IAI.72.1.515-526.2004
124. Gupta RK, Srivastava BS, Srivastava R. Comparative expression analysis of rpf-like genes of Mycobacterium tuberculosis H37Rv under different physiological stress and growth conditions. Microbiol (Reading) (2010) 156(Pt 9):2714–22. doi: 10.1099/mic.0.037622-0
125. Tufariello JM, Mi K, Xu J, Manabe YC, Kesavan AK, Drumm J, et al. Deletion of the Mycobacterium tuberculosis resuscitation-promoting factor Rv1009 gene results in delayed reactivation from chronic tuberculosis. Infect Immun (2006) 74(5):2985–95. doi: 10.1128/IAI.74.5.2985-2995.2006
126. Schuck SD, Mueller H, Kunitz F, Neher A, Hoffmann H, Franken KL, et al. Identification of T-cell antigens specific for latent mycobacterium tuberculosis infection. PloS One (2009) 4(5):e5590. doi: 10.1371/journal.pone.0005590
127. Fan A, Jian W, Shi C, Ma Y, Wang L, Peng D, et al. Production and characterization of monoclonal antibody against Mycobacterium tuberculosis RpfB domain. Hybridoma (Larchmt) (2010) 29(4):327–32. doi: 10.1089/hyb.2010.0007
128. Bertholet S, Ireton GC, Ordway DJ, Windish HP, Pine SO, Kahn M, et al. A defined tuberculosis vaccine candidate boosts BCG and protects against multidrug-resistant Mycobacterium tuberculosis. Sci Transl Med (2010) 2(53):53ra74. doi: 10.1126/scitranslmed.3001094
129. Liu X, Peng J, Hu L, Luo Y, Niu H, Bai C, et al. A multistage mycobacterium tuberculosis subunit vaccine LT70 including latency antigen Rv2626c induces long-term protection against tuberculosis. Hum Vaccin Immunother (2016) 12(7):1670–7. doi: 10.1080/21645515.2016.1141159
130. Dijkman K, Coler RN, Joosten SA. Editorial: Beyond Th1: Novel concepts in tuberculosis vaccine immunology. Front Immunol (2022) 13:1059011. doi: 10.3389/fimmu.2022.1059011
131. Naranbhai V, Hill AV, Abdool Karim SS, Naidoo K, Abdool Karim Q, Warimwe GM, et al. Ratio of monocytes to lymphocytes in peripheral blood identifies adults at risk of incident tuberculosis among HIV-infected adults initiating antiretroviral therapy. J Infect Dis (2014) 209(4):500–9. doi: 10.1093/infdis/jit494
132. Naranbhai V, Moodley D, Chipato T, Stranix-Chibanda L, Nakabaiito C, Kamateeka M, et al. The association between the ratio of monocytes: lymphocytes and risk of tuberculosis among HIV-infected postpartum women. J Acquir Immune Defic Syndr (2014) 67(5):573–5. doi: 10.1097/QAI.0000000000000353
133. Buttle TS, Hummerstone CY, Billahalli T, Ward RJB, Barnes KE, Marshall NJ, et al. The monocyte-to-lymphocyte ratio: Sex-specific differences in the tuberculosis disease spectrum, diagnostic indices and defining normal ranges. PloS One (2021) 16(8):e0247745. doi: 10.1371/journal.pone.0247745
134. Kleinnijenhuis J, Quintin J, Preijers F, Joosten LA, Ifrim DC, Saeed S, et al. Bacille Calmette-Guerin induces NOD2-dependent nonspecific protection from reinfection via epigenetic reprogramming of monocytes. Proc Natl Acad Sci U S A. (2012) 109(43):17537–42. doi: 10.1073/pnas.1202870109
135. Nankabirwa V, Tumwine JK, Mugaba PM, Tylleskär T, Sommerfelt H. Child survival and BCG vaccination: a community based prospective cohort study in Uganda. BMC Public Health (2015) 15:175. doi: 10.1186/s12889-015-1497-8
136. Ning H, Zhang W, Kang J, Ding T, Liang X, Lu Y, et al. Subunit vaccine ESAT-6:c-di-AMP delivered by intranasal route elicits immune responses and protects against mycobacterium tuberculosis infection. Front Cell Infect Microbiol (2021) 11:647220. doi: 10.3389/fcimb.2021.647220
137. Kaufmann E, Sanz J, Dunn JL, Khan N, Mendonça LE, Pacis A, et al. BCG educates hematopoietic stem cells to generate protective innate immunity against tuberculosis. Cell (2018) 172(1-2):176–90.e19. doi: 10.1016/j.cell.2017.12.031
138. Leemans JC, Juffermans NP, Florquin S, van Rooijen N, Vervoordeldonk MJ, Verbon A, et al. Depletion of alveolar macrophages exerts protective effects in pulmonary tuberculosis in mice. J Immunol (2001) 166(7):4604–11. doi: 10.4049/jimmunol.166.7.4604
139. Rothchild AC, Olson GS, Nemeth J, Amon LM, Mai D, Gold ES, et al. Alveolar macrophages generate a noncanonical NRF2-driven transcriptional response to Mycobacterium tuberculosis in vivo. Sci Immunol (2019) 4(37). doi: 10.1126/sciimmunol.aaw6693
140. Lovey A, Verma S, Kaipilyawar V, Ribeiro-Rodrigues R, Husain S, Palaci M, et al. Early alveolar macrophage response and IL-1R-dependent T cell priming determine transmissibility of Mycobacterium tuberculosis strains. Nat Commun (2022) 13(1):884. doi: 10.1038/s41467-022-28506-2
141. Mata E, Tarancon R, Guerrero C, Moreo E, Moreau F, Uranga S, et al. Pulmonary BCG induces lung-resident macrophage activation and confers long-term protection against tuberculosis. Sci Immunol (2021) 6(63):eabc2934. doi: 10.1126/sciimmunol.abc2934
142. Gu H, Zeng X, Peng L, Xiang C, Zhou Y, Zhang X, et al. Vaccination induces rapid protection against bacterial pneumonia via training alveolar macrophage in mice. Elife (2021) 10. doi: 10.7554/eLife.69951
143. Yao Y, Jeyanathan M, Haddadi S, Barra NG, Vaseghi-Shanjani M, Damjanovic D, et al. Induction of autonomous memory alveolar macrophages requires T cell help and is critical to trained immunity. Cell (2018) 175(6):1634–50.e17. doi: 10.1016/j.cell.2018.09.042
144. Jeyanathan M, Fritz DK, Afkhami S, Aguirre E, Howie KJ, Zganiacz A, et al. Aerosol delivery, but not intramuscular injection, of adenovirus-vectored tuberculosis vaccine induces respiratory-mucosal immunity in humans. JCI Insight (2022) 7(3). doi: 10.1172/jci.insight.155655
145. Berry MP, Graham CM, McNab FW, Xu Z, Bloch SA, Oni T, et al. An interferon-inducible neutrophil-driven blood transcriptional signature in human tuberculosis. Nature (2010) 466(7309):973–7. doi: 10.1038/nature09247
146. Moreira-Teixeira L, Stimpson PJ, Stavropoulos E, Hadebe S, Chakravarty P, Ioannou M, et al. Type I IFN exacerbates disease in tuberculosis-susceptible mice by inducing neutrophil-mediated lung inflammation and NETosis. Nat Commun (2020) 11(1):5566. doi: 10.1038/s41467-020-19412-6
147. Trentini MM, de Oliveira FM, Kipnis A, Junqueira-Kipnis AP. The Role of Neutrophils in the Induction of Specific Th1 and Th17 during Vaccination against Tuberculosis. Front Microbiol (2016) 7:898. doi: 10.3389/fmicb.2016.00898
148. Bickett TE, McLean J, Creissen E, Izzo L, Hagan C, Izzo AJ, et al. Characterizing the BCG induced macrophage and neutrophil mechanisms for defense against mycobacterium tuberculosis. Front Immunol (2020) 11:1202. doi: 10.3389/fimmu.2020.01202
149. Moorlag S, Rodriguez-Rosales YA, Gillard J, Fanucchi S, Theunissen K, Novakovic B, et al. BCG vaccination induces long-term functional reprogramming of human neutrophils. Cell Rep (2020) 33(7):108387. doi: 10.1016/j.celrep.2020.108387
150. Hansen SG, Zak DE, Xu G, Ford JC, Marshall EE, Malouli D, et al. Prevention of tuberculosis in rhesus macaques by a cytomegalovirus-based vaccine. Nat Med (2018) 24(2):130–43. doi: 10.1038/nm.4473
151. Dhiman R, Periasamy S, Barnes PF, Jaiswal AG, Paidipally P, Barnes AB, et al. NK1.1+ cells and IL-22 regulate vaccine-induced protective immunity against challenge with Mycobacterium tuberculosis. J Immunol (2012) 189(2):897–905. doi: 10.4049/jimmunol.1102833
152. Khader SA, Bell GK, Pearl JE, Fountain JJ, Rangel-Moreno J, Cilley GE, et al. IL-23 and IL-17 in the establishment of protective pulmonary CD4+ T cell responses after vaccination and during Mycobacterium tuberculosis challenge. Nat Immunol (2007) 8(4):369–77. doi: 10.1038/ni1449
153. Monin L, Griffiths KL, Slight S, Lin Y, Rangel-Moreno J, Khader SA. Immune requirements for protective Th17 recall responses to Mycobacterium tuberculosis challenge. Mucosal Immunol (2015) 8(5):1099–109. doi: 10.1038/mi.2014.136
154. Nambiar JK, Ryan AA, Kong CU, Britton WJ, Triccas JA. Modulation of pulmonary DC function by vaccine-encoded GM-CSF enhances protective immunity against Mycobacterium tuberculosis infection. Eur J Immunol (2010) 40(1):153–61. doi: 10.1002/eji.200939665
155. Triccas JA, Shklovskaya E, Spratt J, Ryan AA, Palendira U, Fazekas de St Groth B, et al. Effects of DNA- and Mycobacterium bovis BCG-based delivery of the Flt3 ligand on protective immunity to Mycobacterium tuberculosis. Infect Immun (2007) 75(11):5368–75. doi: 10.1128/IAI.00322-07
156. van den Berg RA, De Mot L, Leroux-Roels G, Bechtold V, Clement F, Coccia M, et al. Adjuvant-associated peripheral blood mRNA profiles and kinetics induced by the adjuvanted recombinant protein candidate tuberculosis vaccine M72/AS01 in bacillus calmette-guérin-vaccinated adults. Front Immunol (2018) 9:564. doi: 10.3389/fimmu.2018.00564
157. Griffiths KL, Ahmed M, Das S, Gopal R, Horne W, Connell TD, et al. Targeting dendritic cells to accelerate T-cell activation overcomes a bottleneck in tuberculosis vaccine efficacy. Nat Commun (2016) 7:13894. doi: 10.1038/ncomms13894
158. Silva-Sánchez A, Meza-Pérez S, Flores-Langarica A, Donis-Maturano L, Estrada-García I, Calderón-Amador J, et al. ESAT-6 Targeting to DEC205+ Antigen Presenting Cells Induces Specific-T Cell Responses against ESAT-6 and Reduces Pulmonary Infection with Virulent Mycobacterium tuberculosis. PloS One (2015) 10(4):e0124828. doi: 10.1371/journal.pone.0124828
159. Velasquez LN, Stüve P, Gentilini MV, Swallow M, Bartel J, Lycke NY, et al. Targeting Mycobacterium tuberculosis Antigens to Dendritic Cells via the DC-Specific-ICAM3-Grabbing-Nonintegrin Receptor Induces Strong T-Helper 1 Immune Responses. Front Immunol (2018) 9:471. doi: 10.3389/fimmu.2018.00471
160. Schenkel JM, Fraser KA, Vezys V, Masopust D. Sensing and alarm function of resident memory CD8+ T cells. Nat Immunol (2013) 14(5):509–13. doi: 10.1038/ni.2568
161. Christensen D, Mortensen R, Rosenkrands I, Dietrich J, Andersen P. Vaccine-induced Th17 cells are established as resident memory cells in the lung and promote local IgA responses. Mucosal Immunol (2017) 10(1):260–70. doi: 10.1038/mi.2016.28
162. Counoupas C, Ferrell KC, Ashhurst A, Bhattacharyya ND, Nagalingam G, Stewart EL, et al. Mucosal delivery of a multistage subunit vaccine promotes development of lung-resident memory T cells and affords interleukin-17-dependent protection against pulmonary tuberculosis. NPJ Vaccines (2020) 5(1):105. doi: 10.1038/s41541-020-00255-7
163. Perdomo C, Zedler U, Kühl AA, Lozza L, Saikali P, Sander LE, et al. Mucosal BCG vaccination induces protective lung-resident memory T cell populations against tuberculosis. mBio (2016) 7(6). doi: 10.1128/mBio.01686-16
164. Yang Q, Zhang M, Chen Q, Chen W, Wei C, Qiao K, et al. Cutting edge: characterization of human tissue-resident memory T cells at different infection sites in patients with tuberculosis. J Immunol (2020) 204(9):2331–6. doi: 10.4049/jimmunol.1901326
165. Ogongo P, Tezera LB, Ardain A, Nhamoyebonde S, Ramsuran D, Singh A, et al. Tissue-resident-like CD4+ T cells secreting IL-17 control Mycobacterium tuberculosis in the human lung. J Clin Invest (2021) 131(10). doi: 10.1172/JCI142014
166. He Q, Jiang L, Cao K, Zhang L, Xie X, Zhang S, et al. A systemic prime-intrarectal pull strategy raises rectum-resident CD8+ T cells for effective protection in a murine model of LM-OVA infection. Front Immunol (2020) 11:571248. doi: 10.3389/fimmu.2020.571248
167. Çuburu N, Kim R, Guittard GC, Thompson CD, Day PM, Hamm DE, et al. A prime-pull-amplify vaccination strategy to maximize induction of circulating and genital-resident intraepithelial CD8(+) memory T cells. J Immunol (2019) 202(4):1250–64. doi: 10.4049/jimmunol.1800219
168. Bernstein DI, Cardin RD, Bravo FJ, Awasthi S, Lu P, Pullum DA, et al. Successful application of prime and pull strategy for a therapeutic HSV vaccine. NPJ Vaccines (2019) 4:33. doi: 10.1038/s41541-019-0129-1
169. Roces CB, Hussain MT, Schmidt ST, Christensen D, Perrie Y. Investigating prime-pull vaccination through a combination of parenteral vaccination and intranasal boosting. Vaccines (Basel) (2019) 8(1). doi: 10.3390/vaccines8010010
170. Haddadi S, Vaseghi-Shanjani M, Yao Y, Afkhami S, D'Agostino MR, Zganiacz A, et al. Mucosal-pull induction of lung-resident memory CD8 T cells in parenteral TB vaccine-primed hosts requires cognate antigens and CD4 T cells. Front Immunol (2019) 10:2075. doi: 10.3389/fimmu.2019.02075
171. Darrah PA, Zeppa JJ, Maiello P, Hackney JA, Wadsworth MH 2nd, Hughes TK, et al. Prevention of tuberculosis in macaques after intravenous BCG immunization. Nature (2020) 577(7788):95–102. doi: 10.1038/s41586-019-1817-8
172. Hirai T, Yang Y, Zenke Y, Li H, Chaudhri VK, de la Cruz Diaz JS, et al. Competition for active TGFβ Cytokine allows for selective retention of antigen-specific tissue- resident memory T cells in the epidermal niche. Immunity (2021) 54(1):84–98.e5. doi: 10.1016/j.immuni.2020.10.022
173. Bourdely P, Anselmi G, Vaivode K, Ramos RN, Missolo-Koussou Y, Hidalgo S, et al. Transcriptional and functional analysis of CD1c(+) human dendritic cells identifies a CD163(+) subset priming CD8(+)CD103(+) T cells. Immunity (2020) 53(2):335–52.e8. doi: 10.1016/j.immuni.2020.06.002
174. Khader SA, Guglani L, Rangel-Moreno J, Gopal R, Junecko BA, Fountain JJ, et al. IL-23 is required for long-term control of Mycobacterium tuberculosis and B cell follicle formation in the infected lung. J Immunol (2011) 187(10):5402–7. doi: 10.4049/jimmunol.1101377
175. Slight SR, Rangel-Moreno J, Gopal R, Lin Y, Fallert Junecko BA, Mehra S, et al. CXCR5+ T helper cells mediate protective immunity against tuberculosis. J Clin Invest (2013) 123(2):712–26. doi: 10.1172/JCI65728
176. Germain C, Gnjatic S, Tamzalit F, Knockaert S, Remark R, Goc J, et al. Presence of B cells in tertiary lymphoid structures is associated with a protective immunity in patients with lung cancer. Am J Respir Crit Care Med (2014) 189(7):832–44. doi: 10.1164/rccm.201309-1611OC
177. Tan HX, Esterbauer R, Vanderven HA, Juno JA, Kent SJ, Wheatley AK. Inducible bronchus-associated lymphoid tissues (iBALT) serve as sites of B cell selection and maturation following influenza infection in mice. Front Immunol (2019) 10:611. doi: 10.3389/fimmu.2019.00611
178. Moyron-Quiroz JE, Rangel-Moreno J, Hartson L, Kusser K, Tighe MP, Klonowski KD, et al. Persistence and responsiveness of immunologic memory in the absence of secondary lymphoid organs. Immunity (2006) 25(4):643–54. doi: 10.1016/j.immuni.2006.08.022
179. Takamura S, Yagi H, Hakata Y, Motozono C, McMaster SR, Masumoto T, et al. Specific niches for lung-resident memory CD8+ T cells at the site of tissue regeneration enable CD69-independent maintenance. J Exp Med (2016) 213(13):3057–73. doi: 10.1084/jem.20160938
180. Takamura S. Niches for the long-term maintenance of tissue-resident memory T cells. Front Immunol (2018) 9:1214. doi: 10.3389/fimmu.2018.01214
181. Gompels UA, Larke N, Sanz-Ramos M, Bates M, Musonda K, Manno D, et al. Human cytomegalovirus infant infection adversely affects growth and development in maternally HIV-exposed and unexposed infants in Zambia. Clin Infect Dis (2012) 54(3):434–42. doi: 10.1093/cid/cir837
182. Martinez L, Nicol MP, Wedderburn CJ, Stadler A, Botha M, Workman L, et al. Cytomegalovirus acquisition in infancy and the risk of tuberculosis disease in childhood: a longitudinal birth cohort study in Cape Town, South Africa. Lancet Glob Health (2021) 9(12):e1740–e9. doi: 10.1016/S2214-109X(21)00407-1
183. Li H, Wang XX, Wang B, Fu L, Liu G, Lu Y, et al. Latently and uninfected healthcare workers exposed to TB make protective antibodies against Mycobacterium tuberculosis. Proc Natl Acad Sci U.S.A. (2017) 114(19):5023–8. doi: 10.1073/pnas.1611776114
184. Zimmermann N, Thormann V, Hu B, Köhler AB, Imai-Matsushima A, Locht C, et al. Human isotype-dependent inhibitory antibody responses against Mycobacterium tuberculosis. EMBO Mol Med (2016) 8(11):1325–39. doi: 10.15252/emmm.201606330
185. Lu LL, Chung AW, Rosebrock TR, Ghebremichael M, Yu WH, Grace PS, et al. A functional role for antibodies in tuberculosis. Cell (2016) 167(2):433–43.e14. doi: 10.1016/j.cell.2016.08.072
186. Teitelbaum R, Glatman-Freedman A, Chen B, Robbins JB, Unanue E, Casadevall A, et al. A mAb recognizing a surface antigen of Mycobacterium tuberculosis enhances host survival. Proc Natl Acad Sci U S A. (1998) 95(26):15688–93. doi: 10.1073/pnas.95.26.15688
187. Fletcher HA, Snowden MA, Landry B, Rida W, Satti I, Harris SA, et al. T-cell activation is an immune correlate of risk in BCG vaccinated infants. Nat Commun (2016) 7:11290. doi: 10.1038/ncomms11290
188. Irvine EB, O'Neil A, Darrah PA, Shin S, Choudhary A, Li W, et al. Robust IgM responses following intravenous vaccination with Bacille Calmette-Guérin associate with prevention of Mycobacterium tuberculosis infection in macaques. Nat Immunol (2021) 22(12):1515–23. doi: 10.1038/s41590-021-01066-1
189. Cannas A, Calvo L, Chiacchio T, Cuzzi G, Vanini V, Lauria FN, et al. IP-10 detection in urine is associated with lung diseases. BMC Infect Dis (2010) 10:333. doi: 10.1186/1471-2334-10-333
190. Kim SY, Kim J, Kim DR, Kang YA, Bong S, Lee J, et al. Urine IP-10 as a biomarker of therapeutic response in patients with active pulmonary tuberculosis. BMC Infect Dis (2018) 18(1):240. doi: 10.1186/s12879-018-3144-3
191. Kerkhoff AD, Barr DA, Schutz C, Burton R, Nicol MP, Lawn SD, et al. Disseminated tuberculosis among hospitalised HIV patients in South Africa: a common condition that can be rapidly diagnosed using urine-based assays. Sci Rep (2017) 7(1):10931. doi: 10.1038/s41598-017-09895-7
192. Lawn SD, Kerkhoff AD, Burton R, Schutz C, Boulle A, Vogt M, et al. Diagnostic accuracy, incremental yield and prognostic value of Determine TB-LAM for routine diagnostic testing for tuberculosis in HIV-infected patients requiring acute hospital admission in South Africa: a prospective cohort. BMC Med (2017) 15(1):67. doi: 10.1186/s12916-017-0822-8
193. Fitzgerald BL, Islam MN, Graham B, Mahapatra S, Webb K, Boom WH, et al. Elucidation of a human urine metabolite as a seryl-leucine glycopeptide and as a biomarker of effective anti-tuberculosis therapy. ACS Infect Dis (2019) 5(3):353–64. doi: 10.1021/acsinfecdis.8b00241
194. Sasaki E, Kusunoki H, Momose H, Furuhata K, Hosoda K, Wakamatsu K, et al. Changes of urine metabolite profiles are induced by inactivated influenza vaccine inoculations in mice. Sci Rep (2019) 9(1):16249. doi: 10.1038/s41598-019-52686-5
195. Burny W, Hervé C, Caubet M, Yarzabal JP, Didierlaurent AM. Utility of urinary cytokine levels as predictors of the immunogenicity and reactogenicity of AS01-adjuvanted hepatitis B vaccine in healthy adults. Vaccine (2022) 40(19):2714–22. doi: 10.1016/j.vaccine.2022.03.050
196. Gröschel MI, Sayes F, Shin SJ, Frigui W, Pawlik A, Orgeur M, et al. Recombinant BCG expressing ESX-1 of mycobacterium marinum combines low virulence with cytosolic immune signaling and improved TB protection. Cell Rep (2017) 18(11):2752–65. doi: 10.1016/j.celrep.2017.02.057
197. Bottai D, Frigui W, Clark S, Rayner E, Zelmer A, Andreu N, et al. Increased protective efficacy of recombinant BCG strains expressing virulence-neutral proteins of the ESX-1 secretion system. Vaccine (2015) 33(23):2710–8. doi: 10.1016/j.vaccine.2015.03.083
198. Pérez I, Campos-Pardos E, Díaz C, Uranga S, Sayes F, Vicente F, et al. The Mycobacterium tuberculosis PhoPR virulence system regulates expression of the universal second messenger c-di-AMP and impacts vaccine safety and efficacy. Mol Ther Nucleic Acids (2022) 27:1235–48. doi: 10.1016/j.omtn.2022.02.011
199. El Zowalaty ME, Ashour HM. Paving the way to a new class of efficient and safe tuberculosis vaccines: The role of c-di-AMP in Mycobacterium tuberculosis immunity and virulence. Mol Ther Nucleic Acids (2022) 30:13–4. doi: 10.1016/j.omtn.2022.08.034
200. Aceves-Sánchez MJ, Flores-Valdez MA, Pedroza-Roldán C, Creissen E, Izzo L, Silva-Angulo F, et al. Vaccination with BCGΔBCG1419c protects against pulmonary and extrapulmonary TB and is safer than BCG. Sci Rep (2021) 11(1):12417. doi: 10.1038/s41598-021-91993-8
201. Velázquez-Fernández JB, Ferreira-Souza GHM, Rodríguez-Campos J, Aceves-Sánchez MJ, Bravo-Madrigal J, Vallejo-Cardona AA, et al. Proteomic characterization of a second-generation version of the BCGΔBCG1419c vaccine candidate by means of electrospray-ionization quadrupole time-of-flight mass spectrometry. Pathog Dis (2021) 79(1). doi: 10.1093/femspd/ftaa070
202. Rivas-Santiago CE, Guerrero GG. IFN-α Boosting of Mycobacterium bovis Bacillus Calmette Güerin-Vaccine Promoted Th1 Type Cellular Response and Protection against M. tuberculosis Infection. BioMed Res Int (2017) 2017:8796760. doi: 10.1155/2017/8796760
203. Babu S, Blauvelt CP, Kumaraswami V, Nutman TB. Regulatory networks induced by live parasites impair both Th1 and Th2 pathways in patent lymphatic filariasis: implications for parasite persistence. J Immunol (2006) 176(5):3248–56. doi: 10.4049/jimmunol.176.5.3248
204. Elias D, Britton S, Aseffa A, Engers H, Akuffo H. Poor immunogenicity of BCG in helminth infected population is associated with increased in vitro TGF-beta production. Vaccine (2008) 26(31):3897–902. doi: 10.1016/j.vaccine.2008.04.083
205. Elias D, Wolday D, Akuffo H, Petros B, Bronner U, Britton S. Effect of deworming on human T cell responses to mycobacterial antigens in helminth-exposed individuals before and after bacille Calmette-Guérin (BCG) vaccination. Clin Exp Immunol (2001) 123(2):219–25. doi: 10.1046/j.1365-2249.2001.01446.x
206. Gebreegziabiher D, Desta K, Desalegn G, Howe R, Abebe M. The effect of maternal helminth infection on maternal and neonatal immune function and immunity to tuberculosis. PloS One (2014) 9(4):e93429. doi: 10.1371/journal.pone.0093429
207. Malhotra I, Mungai P, Wamachi A, Kioko J, Ouma JH, Kazura JW, et al. Helminth- and Bacillus Calmette-Guérin-induced immunity in children sensitized in utero to filariasis and schistosomiasis. J Immunol (1999) 162(11):6843–8. doi: 10.4049/jimmunol.162.11.6843
208. Schick J, Altunay M, Lacorcia M, Marschner N, Westermann S, Schluckebier J, et al. IL-4 and helminth infection downregulate MINCLE-dependent macrophage response to mycobacteria and Th17 adjuvanticity. Elife (2023) 12. doi: 10.7554/eLife.72923
209. Abate G, Hamzabegovic F, Eickhoff CS, Hoft DF. BCG Vaccination Induces M. avium and M. abscessus Cross-Protective Immunity. Front Immunol (2019) 10:234. doi: 10.3389/fimmu.2019.00234
210. Zimmermann P, Finn A, Curtis N. Does BCG vaccination protect against nontuberculous mycobacterial infection? A systematic review and meta-analysis. J Infect Dis (2018) 218(5):679–87. doi: 10.1093/infdis/jiy207
211. Poyntz HC, Stylianou E, Griffiths KL, Marsay L, Checkley AM, McShane H. Non-tuberculous mycobacteria have diverse effects on BCG efficacy against Mycobacterium tuberculosis. Tuberculosis (Edinb) (2014) 94(3):226–37. doi: 10.1016/j.tube.2013.12.006
212. Brandt L, Feino Cunha J, Weinreich Olsen A, Chilima B, Hirsch P, Appelberg R, et al. Failure of the Mycobacterium bovis BCG vaccine: some species of environmental mycobacteria block multiplication of BCG and induction of protective immunity to tuberculosis. Infect Immun (2002) 70(2):672–8. doi: 10.1128/IAI.70.2.672-678.2002
213. Flaherty DK, Vesosky B, Beamer GL, Stromberg P, Turner J. Exposure to Mycobacterium avium can modulate established immunity against Mycobacterium tuberculosis infection generated by Mycobacterium bovis BCG vaccination. J Leukoc Biol (2006) 80(6):1262–71. doi: 10.1189/jlb.0606407
214. Scriba TJ, Tameris M, Smit E, van der Merwe L, Hughes EJ, Kadira B, et al. A phase IIa trial of the new tuberculosis vaccine, MVA85A, in HIV- and/or Mycobacterium tuberculosis-infected adults. Am J Respir Crit Care Med (2012) 185(7):769–78. doi: 10.1164/rccm.201108-1548OC
215. Sharan R, Kaushal D. Vaccine strategies for the Mtb/HIV copandemic. NPJ Vaccines (2020) 5:95. doi: 10.1038/s41541-020-00245-9
216. Yoo JE, Kim D, Han K, Rhee SY, Shin DW, Lee H. Diabetes status and association with risk of tuberculosis among Korean adults. JAMA Netw Open (2021) 4(9):e2126099. doi: 10.1001/jamanetworkopen.2021.26099
217. Ajie M, van Heck JIP, Janssen AWM, Meijer RI, Tack CJ, Stienstra R. Disease duration and chronic complications associate with immune activation in individuals with longstanding type 1 diabetes. J Clin Endocrinol Metab (2023) 108(8):1909–1920. doi: 10.1210/clinem/dgad087
218. Verma A, Kaur M, Luthra P, Singh L, Aggarwal D, Verma I, et al. Immunological aspects of host-pathogen crosstalk in the co-pathogenesis of diabetes and latent tuberculosis. Front Cell Infect Microbiol (2022) 12:957512. doi: 10.3389/fcimb.2022.957512
219. Clement CC, Nanaware PP, Yamazaki T, Negroni MP, Ramesh K, Morozova K, et al. Pleiotropic consequences of metabolic stress for the major histocompatibility complex class II molecule antigen processing and presentation machinery. Immunity (2021) 54(4):721–36.e10. doi: 10.1016/j.immuni.2021.02.019
220. Chambers ES, Akbar AN. Can blocking inflammation enhance immunity during aging? J Allergy Clin Immunol (2020) 145(5):1323–31. doi: 10.1016/j.jaci.2020.03.016
221. Scordo JM, Piergallini TJ, Reuter N, Headley CA, Hodara VL, Gonzalez O, et al. Local immune responses to tuberculin skin challenge in Mycobacterium bovis BCG-vaccinated baboons: a pilot study of younger and older animals. Immun Ageing (2021) 18(1):16. doi: 10.1186/s12979-021-00229-w
222. Scordo JM, Piergallini TJ, Olmo-Fontánez AM, Thomas A, Raué HP, Slifka M, et al. Recall responses in the lung environment are impacted by age in a pilot study of Mycobacterium bovis-BCG vaccinated rhesus macaques. Exp Gerontol (2022) 167:111904. doi: 10.1016/j.exger.2022.111904
223. Giamarellos-Bourboulis EJ, Tsilika M, Moorlag S, Antonakos N, Kotsaki A, Domínguez-Andrés J, et al. Activate: randomized clinical trial of BCG vaccination against infection in the elderly. Cell (2020) 183(2):315–23.e9. doi: 10.1016/j.cell.2020.08.051
224. Tsilika M, Taks E, Dolianitis K, Kotsaki A, Leventogiannis K, Damoulari C, et al. ACTIVATE-2: A double-blind randomized trial of BCG vaccination against COVID-19 in individuals at risk. Front Immunol (2022) 13:873067. doi: 10.3389/fimmu.2022.873067
225. Schultze JL, Aschenbrenner AC. COVID-19 and the human innate immune system. Cell (2021) 184(7):1671–92. doi: 10.1016/j.cell.2021.02.029
226. Pavan Kumar N, Padmapriyadarsini C, Rajamanickam A, Marinaik SB, Nancy A, Padmanaban S, et al. Effect of BCG vaccination on proinflammatory responses in elderly individuals. Sci Adv (2021) 7(32). doi: 10.1126/sciadv.abg7181
227. Monteiro-Maia R, Pinho RT. Oral bacillus Calmette-Guérin vaccine against tuberculosis: why not? Mem Inst Oswaldo Cruz (2014) 109(6):838–45. doi: 10.1590/0074-0276140091
228. Hoft DF, Xia M, Zhang GL, Blazevic A, Tennant J, Kaplan C, et al. PO and ID BCG vaccination in humans induce distinct mucosal and systemic immune responses and CD4(+) T cell transcriptomal molecular signatures. Mucosal Immunol (2018) 11(2):486–95. doi: 10.1038/mi.2017.67
229. Aldwell FE, Tucker IG, de Lisle GW, Buddle BM. Oral delivery of Mycobacterium bovis BCG in a lipid formulation induces resistance to pulmonary tuberculosis in mice. Infect Immun (2003) 71(1):101–8. doi: 10.1128/IAI.71.1.101-108.2003
230. Clark SO, Kelly DL, Badell E, Castello-Branco LR, Aldwell F, Winter N, et al. Oral delivery of BCG Moreau Rio de Janeiro gives equivalent protection against tuberculosis but with reduced pathology compared to parenteral BCG Danish vaccination. Vaccine (2010) 28(43):7109–16. doi: 10.1016/j.vaccine.2010.07.087
231. Doherty TM, Olsen AW, van Pinxteren L, Andersen P. Oral vaccination with subunit vaccines protects animals against aerosol infection with Mycobacterium tuberculosis. Infect Immun (2002) 70(6):3111–21. doi: 10.1128/IAI.70.6.3111-3121.2002
232. Imperial MZ, Nahid P, Phillips PPJ, Davies GR, Fielding K, Hanna D, et al. A patient-level pooled analysis of treatment-shortening regimens for drug-susceptible pulmonary tuberculosis. Nat Med (2018) 24(11):1708–15. doi: 10.1038/s41591-018-0224-2
233. Dartois VA, Rubin EJ. Anti-tuberculosis treatment strategies and drug development: challenges and priorities. Nat Rev Microbiol (2022) 20(11):685–701. doi: 10.1038/s41579-022-00731-y
234. Pillay J, Gaudet L, Wingert A, Bialy L, Mackie AS, Paterson DI, et al. Incidence, risk factors, natural history, and hypothesised mechanisms of myocarditis and pericarditis following covid-19 vaccination: living evidence syntheses and review. Bmj (2022) 378:e069445. doi: 10.1136/bmj-2021-069445
235. Chu DK, Abrams EM, Golden DBK, Blumenthal KG, Wolfson AR, Stone CA Jr., et al. Risk of second allergic reaction to SARS-coV-2 vaccines: A systematic review and meta-analysis. JAMA Intern Med (2022) 182(4):376–85. doi: 10.1001/jamainternmed.2021.8515
Keywords: Mycobacterium tuberculosis, next-generation TB vaccines, immune correlates, immunogenicity, biomarkers
Citation: Kim H, Choi H-G and Shin SJ (2023) Bridging the gaps to overcome major hurdles in the development of next-generation tuberculosis vaccines. Front. Immunol. 14:1193058. doi: 10.3389/fimmu.2023.1193058
Received: 24 March 2023; Accepted: 27 July 2023;
Published: 11 August 2023.
Edited by:
Lanbo Shi, Rutgers University, Newark, United StatesReviewed by:
Joanna Kirman, University of Otago, New ZealandMangalakumari Jeyanathan, McMaster University, Canada
Copyright © 2023 Kim, Choi and Shin. This is an open-access article distributed under the terms of the Creative Commons Attribution License (CC BY). The use, distribution or reproduction in other forums is permitted, provided the original author(s) and the copyright owner(s) are credited and that the original publication in this journal is cited, in accordance with accepted academic practice. No use, distribution or reproduction is permitted which does not comply with these terms.
*Correspondence: Sung Jae Shin, c2pzaGluQHl1aHMuYWM=