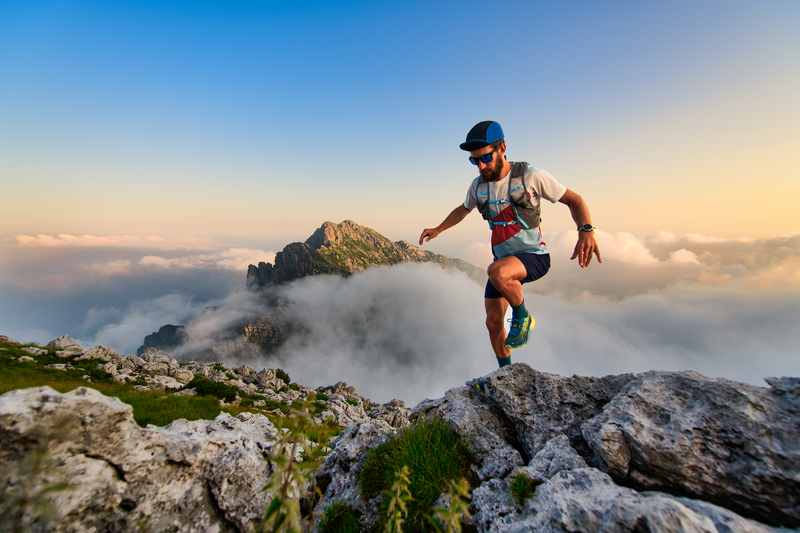
94% of researchers rate our articles as excellent or good
Learn more about the work of our research integrity team to safeguard the quality of each article we publish.
Find out more
REVIEW article
Front. Immunol. , 18 July 2023
Sec. Cancer Immunity and Immunotherapy
Volume 14 - 2023 | https://doi.org/10.3389/fimmu.2023.1192907
This article is part of the Research Topic Community Series in NK Cell Modifications to Advance their Anti-tumor Activities: Volume II View all 4 articles
Gene-engineered immune cell therapies have partially transformed cancer treatment, as exemplified by the use of chimeric antigen receptor (CAR)-T cells in certain hematologic malignancies. However, there are several limitations that need to be addressed to target more cancer types. Natural killer (NK) cells are a type of innate immune cells that represent a unique biology in cancer immune surveillance. In particular, NK cells obtained from heathy donors can serve as a source for genetically engineered immune cell therapies. Therefore, NK-based therapies, including NK cells, CAR-NK cells, and antibodies that induce antibody-dependent cellular cytotoxicity of NK cells, have emerged. With recent advances in genetic engineering and cell biology techniques, NK cell-based therapies have become promising approaches for a wide range of cancers, viral infections, and senescence. This review provides a brief overview of NK cell characteristics and summarizes diseases that could benefit from NK-based therapies. In addition, we discuss recent preclinical and clinical investigations on the use of adoptive NK cell transfer and agents that can modulate NK cell activity.
Natural killer (NK) cells are innate immune cells that account for 5–10% of peripheral blood (PB) lymphocytes (1). NK cells are classified as cytotoxic lymphocytes that play a crucial role in the recognition and elimination of malignant or infected cells. The expression of major histocompatibility complex class I (MHC-I) molecules in cells is often suppressed by neoplastic transformation and viral infection, making these cells “missing self” targets for NK cells. This mechanism allows NK cells to overcome suppressed immunosurveillance by CD8+ T cells, which require antigen presentation on MHC-I molecules (2). Unlike T cells, NK cells can be primed with interleukin-2 (IL-2) or IL-15 without licensing. Mature NK cells express various receptors that modulate their activity against target cells. These unique characteristics make NK cells an attractive option for adoptive immune cell therapy. Allogeneic transfer of NK cells derived from healthy donors or the use of chimeric antigen receptor (CAR)-NK cells has shown promising results for the treatment of various types of cancers. In addition, there is growing interest in exploring the use of NK cell therapies for other indications, such as viral infections or aging. Several therapeutic strategies have been developed to enhance NK cell function. This review provides an overview of the NK cell mechanisms and recent advancements in NK cell therapy. The first part describes these pathways during the developmental process and the intracellular signaling pathways. The second section discusses the application of NK cell therapies to various diseases, followed by an introduction to the genetic engineering of NK cells in the third section. Finally, the fourth section covers approaches that can stimulate NK cells, which may be combined with genetic engineering for NK cell therapies in the future.
NK cells originate from common lymphoid progenitors (CLPs) that are derived from hematopoietic stem cells (HSCs) in the bone marrow (BM) (3). Unlike T and B cells, which generate antigen receptors via gene rearrangements, NK cells express a broad range of germline-encoding receptors (4). The development of NK cells is facilitated by cytokine IL-15, which binds to the IL-15 receptor subunit alpha, CD122 (IL-2/IL-15 receptor subunit beta), and the common gamma chain on NK progenitors (NKPs), resulting in the commitment of these cells to the NK cell lineage (5). Although IL-2 promotes NK cell proliferation during ex vivo culture, it is not essential for the development of NK cells in mice (6). NKPs differentiate into immature NK cells (iNKs), which are identified by the expression of NK1.1 marker in mice and the NKp46 activation receptor in both mice and humans (7). In humans, CD56bright NK cells are abundant in secondary lymphoid tissues and mature into CD56dim NK cells in PB (8). Usually, CD56dim NK cells are more cytotoxic and express the CD16 (Fc gamma RIIIA) receptor, while CD56bright NK cells produce more cytokines and have lower levels of CD16 expression (9). CD56dim NK cells are generally considered as a more mature form of NK cells (mNK); however, the precise mechanism governing the differentiation of these two subsets is not yet well understood. NK cells can be classified into group 1 innate lymphoid cells (ILCs), as they produce type 1 cytokines, interferon gamma (IFN-γ) and tumor necrosis factor (TNF) (10–13). NK cells also have tissue-specific subsets with distinct functions that potentially modulate immune responses in different contexts (14–16).
NK cell development is regulated by several transcription factors including E4BP4, TBX21, EOMES, GATA3, and ID2 (17). For instance, mice lacking E4bp4 exhibit decreased levels of NK1.1+ NKPs and impaired NK cell-mediated cytotoxicity (18). In mice, CD27+ NK cells mature into CD27-CD11bhigh NK cells. A deficiency of Aiolos (Ikzf3), a member of the Ikaros family of zinc-finger transcription factors, was found to prevent the maturation of CD27+ to CD27- NK cells, while proliferation of NK1.1+CD122+ iNKs was enhanced in Aiolos-deficient cells following IL-15 stimulation (19). Likewise, researchers have investigated the gene network involved in NK cell development and the regulation of NK cell function. Detailed markers and the distribution of NK cells during development are shown in Figure 1.
Figure 1 Developmental process of NK cells. NK cells originates from HSCs and CLPs in the bone marrow. The immature NK cells express CD122 and NCRs, such as NKp46, NKp30, and NKp44. Chemokine receptors, including CXCR3, CX3CR1, and S1P5R, are involved in the egression of NK cells. In the blood, two types of NK cells are majorly found, CD56bright and CD56dim, with CD56dim NK cells expressing CD16. Long-lived NK cells can be distinguished by increased expression of CD57. Tissue-resident NK cells express CD49a, CD103, CD69, and CD56. The blocked arrows with dotted lines suggest that further research is required to fully understand these processes. HSC, hematopoietic stem cell; LMPP, lympho-myeloid primed progenitor; CLP, common lymphoid progenitor; NKP, NK progenitor; iNK, immature NK; mNK, mature NK; NCR, NK cell receptor; KIR, killer cell immunoglobulin-like receptor; VLA-4, very late antigen-4.
NK cells eliminate target cells that appear to be missing self or stressed, such as those infected with viruses, cancer, or those undergoing cellular senescence. NK cell killing can be achieved through the activation of receptors such as NKG2D and natural cytotoxic receptors (NCRs) NKp30, NKp44, and NKp46. Ligands for these receptors include major histocompatibility complex class I (MHC-I) chain-related polypeptide A (MICA) and MICB proteins, a family of six cytomegaloviral unique long 16 (UL16)-binding proteins (ULBP 1-6) (20–22), or murine retinoic acid early transcript 1 (RAET1), histocompatibility H60 (H60), and murine UL16-binding protein-like transcript 1 (MULT1), and antigens from pathogens (23–25). DNAX accessary molecule 1 (DNAM1) and signaling lymphocytic activation molecule (SLAM) family receptors, including 2B4, SLAMF7, and NKp80, act as co-receptors to enhance NK cell activity (26). Upon activation, NK cells use an immunoreceptor tyrosine-based activation motif (ITAM) or tyrosine-based signaling motif (YINM) to activate receptors to stimulate protein kinases such as SYK and ZAP70, resulting in the secretion of perforins and granzymes (27). This process leads to apoptosis of the target cells. Furthermore, CD56dim NK cells in PB can mediate antibody-dependent cellular cytotoxicity (ADCC) via the Fc gamma receptor CD16.
The killer cell immunoglobulin-like receptor (KIR) family and CD94/NKG2A heterodimer are inhibitory receptors on NK cells that recognize MHC Class I molecules (28). Humans have 14 KIR genes specific to each individual (29). KIRs are composed of two or three immunoglobulin-like (Ig-like) domains: KIR2D and KIR3D. KIRs have either activating or inhibitory functions. Generally, KIRs with a short cytoplasmic tail are activated, while those with long cytoplasmic tails are inhibitory. Activating KIRs, such as KIR2DS1, KIR2DS2, KIR2DS3, KIR2DS4, KIR2DS5, and KIR3DS1 associate with the transmembrane adaptor DAP12 to transduce activation signals in NK cells. Inhibitory KIRs, such as KIR2DL1, KIR2DL2, KIR2DL3, KIR2DL5, KIR3DL1, KIR3DL2, and KIR3DL3 have an immunoreceptor tyrosine-based inhibitory motif (ITIM) (30). PB NK cells have a diverse repertoire owing to the random expression of KIR genes in each NK cell, resulting in various NK cell clones with distinct receptor expression patterns. Consequently, a subset of NK cell clones may recognize a unique ligand expressed by a particular tumor cell (31, 32). Inhibitory receptors on NK cells include KLRG1, SIGLEC7, SIGLEC9, leukocyte immunoglobulin-like receptor subfamily B member 1 (LILRB1), CD161, TIGIT, LAG3, TIM3, and PD-1, which recruit protein tyrosine phosphatases, such as SHIP1 (also known as PTPN6) and SHP2 (also known as PTPN11), to eliminate tyrosine phosphorylation (27).
NK cells produce chemokines and express various receptors. Resting CD56bright NK cells preferentially express chemokine receptors related to BM or lymph node residency, such as CCR2, CCR7, CXCR3, and CXCR4. In contrast, CD56dim NK cells primarily express CX3CR1, ChemR23 or CXCR1 (33, 34). CXCR1 is a cognate receptor of CXCL8, a senescence-associated secretory phenotype (SASP) factor that can also be released from NK cells (35, 36).
Upon recognition of NK cell activation receptors, the phosphorylation of the adaptor proteins DAP10 and DAP12 initiates downstream signal transduction (37, 38). The activation of phosphoinositide 3-kinase (PI3K) plays a crucial role in NK cells by recruiting AKT (also known as protein kinase B), which promotes cell survival and metabolism (39, 40). The MAPK pathway is also activated, leading to sequential phosphorylation events involving extracellular signal-regulated kinase (ERK), c-Jun N-terminal kinase (JNK), and p38, ultimately resulting in gene transcription and cytokine secretion (41–43). Phospholipase Cγ2 (PLCγ2) generates inositol 1,4,5-triphosphate (IP3) and diacylglycerol (DAG), which trigger calcium ion release from the endoplasmic reticulum and activate protein kinase C (PKC). Increased intracellular calcium levels stimulate the Ca2+/calmodulin (CaM)-dependent phosphatase calcineurin, which dephosphorylates nuclear factor of activated T cells (NFAT). Dephosphorylated NFAT translocates into the nucleus, promoting the transcription of genes involved in NK cell cytotoxicity and cytokine production, as well as inducing NK cell development by inducing EOMES and TBX21 upregulation (44).
The mechanistic/mammalian target of rapamycin (mTOR) signaling pathway is upregulated by cytokines or growth factors, and inhibition of mTOR suppresses NK cell activity (45, 46). In the immune suppressive tumor microenvironment, TGF-β1 has been found to inhibit IL-15-promoted NK cell activation via the mTOR pathway (47). Upon activation, NK cells release cytotoxic granules containing perforin and granzymes. Granzymes induce target cell apoptosis and perforin forms pores in the target cell membrane. Downstream signaling pathways of NK cell receptors activate transcription factors, such as nuclear factor-kappa B (NF-κB) (48) and STAT4, promoting IFN-γ gene transcription. IFN-γ is secreted by activated NK cells in response to viral infections or cell transformation. The signaling pathways involved in NK cell activation are summarized in Figure 2.
Figure 2 A schematic overview of signal transduction pathway for NK cell activation. Upon recognition of ligands, activation receptors on NK cell surface initiate intracellular signaling via adaptor proteins DAP10 and DAP12. These signaling pathways stimulate the transcription of genes involved in cytokines and cytotoxicity, which are key functions of NK cell surveillance. DAP12, DNAX-activating protein of 12 kDa; DAP10, DNAX-activating protein 10; SAP, slam-associated protein; PI3K, phosphoinositide 3-kinase; Grb2, growth factor receptor-bound protein 2; PAK, p21-activated kinase; JNK, c-Jun N-terminal kinase; SLP-76, Src homology 2 domain-containing leukocyte protein of 76 kDa; PIP2, phosphatidylinositol 4,5-bisphosphate; PIP3, phosphatidylinositol 3,4,5-triphosphate; PLCγ, phospholipase C γ; IP3, inositol 1,4,5-triphosphate; CaM, calmodulin; CaN, calcineurin; NFAT, nuclear factor of activated T-cells; DAG, diacylglycerol; PKC, protein kinase C; MSK1, mitogen and stress activated protein kinase-1; IKK, IκB kinase; IκB, inhibitor of NFκB; NFκB, nuclear factor kappa B; PDK, phosphoinositide dependent kinase; Akt, protein kinase B; TSC, tuberous sclerosis complex; RHEB, ras homologue enriched in brain; mTORC1, mammalian target of rapamycin complex 1; 4E-BP1, eIF4E-binding protein 1; eIF-4E, eukaryotic initiation factor 4E; S6K1, ribosomal protein S6 kinase beta-1.
Immunosenescence, which typically involves a decrease in the frequency of naïve T and B cells, and an increase in the number of effector/terminally differentiated memory cells, has been examined and discussed in many studies. Although the number of NK cells tends to remain stable or increase with age, their function seems to be impaired (49). Although IL-2 stimulates NK cell responsiveness, cells from older donors are less activated than those from younger donors (50). Notably, NK cell dysfunction has been linked to the development of diseases, particularly an elevated risk of cancer with age (51–53).
The age-related decline in NK cell activity can be attributed to several intrinsic and extrinsic factors. Although changes in perforin levels and KIR diversity have been investigated, other mechanisms may be involved (54, 55). Human NK cells exhibit telomere shortening with age in both the CD56bright and CD56dim populations (56). Extrinsically, research has focused on changes in endocrine factors with age, as some appear to be associated with NK cell function (57–59). For example, glucocorticoids, which inhibit NK cell function by reducing perforin and granzyme B levels (60, 61), are upregulated in humans (62). Additionally, the plasma level of TGF-β1 increases as senescent fibroblasts secrete more of it (63).
Recently, the coronavirus disease 2019 (COVID-19) pandemic has highlighted advanced age as a major risk factor for mortality, with 95% of deaths occurring individuals over 50 years of age (64). This suggests that immunosenescence in the elderly population may partially play a role in determining the severity of viral infections (65, 66). Several studies have used clinical samples to investigate these mechanisms. For instance, it has been shown that type I IFN signaling is increased in mild cases of COVID-19, while impaired in severe cases (67). Single-cell RNA sequencing (scRNA seq) revealed a decrease in the expression of the human leukocyte antigen-DR isotype (HLA-DR) on monocytes in patients with moderate or mild COVID-19 compared to healthy controls (68). Notably, reduced numbers and cytolytic activity of NK cells have been associated with severe COVID-19 (69). In a study comparing severely young and old immune cells, it was found that the functional exhaustion of NK cells was more pronounced in older individuals (70).
The level of CD57 on NK cells is a marker commonly used to measure the number of long-lived NK cells in the blood, with higher levels typically observed in older individuals (Figure 1). These populations were also considered less cytotoxic. However, CD57 expression levels can vary depending on infection, chronic diseases, and age. Furthermore, NKG2C+CD57+ NK cells have been suggested to have strong and rapid antiviral and antitumor effects, indicating an adaptive immune response (71–73). Killer cell lectin-like receptor subfamily G member 1 (KLRG1) is an inhibitory receptor found in terminally differentiated effector lymphocytes, including memory CD8+ T and NK cells. It has been shown that an increase of KLRG1+CD57+ NK cells was induced by COVID-19 infection, particularly in older individuals, indicating NK cell exhaustion (70). Therefore, the significance of CD57+ NK cells should be interpreted in the context of disease and their function as long-lived and/or memory NK cell populations.
Researchers have found that HLA haplotype-mismatched grafts with KIR ligand incompatibility can greatly reduce relapse in post-transplant acute myeloid leukemia (AML) (74). Moreover, T cell-depleted allogeneic hematopoietic stem cell transplantation (alloHSCT) is a beneficial therapeutic approach for cancer (75). Adoptive transfer of NK cells is a safer option than CAR-T cell therapy for cytokine release syndrome (CRS) or neurotoxicity (76, 77). This can also reduce costs. This section focuses on diseases that have been targeted by adoptive NK cell therapies, including CAR-NK cells, and potential target diseases. The list of CAR-NK cells that are under development for the treatment of diseases, such as solid tumors and hematologic malignancies, is displayed in Table 1.
Hematologic malignancies, including AML, chronic lymphocytic leukemia (CLL), lymphoma, and multiple myeloma (MM), have become targetable diseases by adoptive immune cell therapies (86, 87). These therapies are effective because the administered immune cells can efficiently travel to tumor site in hematopoietic organs (88). In addition, hematopoietic tumor cells often express relatively homogeneous antigens such as CD19 in diffuse large B-cell lymphoma (DLBCL) and B-cell maturation antigen (BCMA) in MM, making it easier to apply CAR or monoclonal antibody (mAb) therapies. Since its approval by the US Food and Drug Administration (FDA) in 2017, six CAR-T cell therapies have been developed. Four of these target CD19, including the first CAR-T cell therapy and the remaining target, BCMA (89, 90). They are used to treat refractory or relapsed B cell ALL, B cell non-Hodgkin’s lymphoma, follicular lymphoma, mantle cell lymphoma, and MM (91). Notably, a phase I/II clinical trial of anti-CD19 CAR-transduced cord blood-derived NK cells showed promising results, with a 73% response rate and long-term survival of infused CAR-NK cells without CRS toxicity (92). CAR-NK cells have the potential to benefit from the intrinsic characteristics of NK cell-activating receptors, which may maintain therapeutic efficacy even after the CAR is lost (93). As a result, CAR-NK cells are being developed for hematological malignancies such as T-cell ALL, and preclinical studies have shown the effectiveness of CAR-NK cells specific for antigens such as CD5 and CD7 (94, 95).
Various studies have shown that a higher number of NK cells in the PB is associated with better outcomes in solid tumors such as non-small cell lung cancer (NSCLC) (96), melanoma (97), and colorectal cancers (98). Similarly, increased tumor-infiltrating NK cells are related to better prognosis in solid tumors, such as hepatocellular carcinoma (HCC) (99), prostate cancer (100), and renal cell carcinoma (101). However, the function of NK cells is often impaired by the tumor microenvironment, which leads to a significant decrease in both the frequency and function of NK cells in solid tumors (102–105). It was demonstrated that NK cells exhibit lower levels of cytokines like IFN-γ and TNFα in gastric cancer (106). Studies have also found that NK cells had higher expression of inhibitory receptors while showing decreased levels of activating receptors in breast cancer or pancreatic cancer samples (107). Furthermore, the presence of the soluble form of B7-H6, a ligand of NKp30, has been associated with reduced NKp30 expression in NK cells, which may contribute to their suppression (108, 109). Markers of exhaustion such as PD-1 (110, 111), TIM3 (112), and TIGIT (113), which have been studied in T cells, are also expressed in NK cells from cancer patients, indicating an exhaustive phenotype in NK cells similar to that in T cells (113).
Among peripheral tissues, the lungs are rich in NK cells, comprising 10–20% of lung lymphocytes (114, 115). Lung cancer is a significant form of cancer, as it is one of the most frequently diagnosed cancers and remains a leading cause of cancer-related deaths (116, 117). Therefore, the anti-tumor effects of NK cells against lung cancer have been suggested (118, 119). As a therapeutic approach for treating NSCLC, allogeneic NK cells obtained from healthy donors were combined with pembrolizumab, a PD-1 inhibitor. The results of this study suggest that the addition of allogeneic NK cells enhances the anti-tumor immune function of pembrolizumab. The study showed a reduction in PD-1 levels in PB NK cells, an increase in IFN-γ, an increased proportion of NK cells, and a significant decrease in circulating tumor cells (CTCs) (120).
The phenotype of NK cells in the lungs is mostly CD56dimCD16+, which is similar to that in PB but distinct from those in other organs, such as the liver and secondary lymphoid organs (121). Although PB-derived CD56dimCD16+ NK cells demonstrate greater differentiation and target cell-killing effects, lung NK cells exhibit weaker responses to target cells (122). In contrast, CD56bright NK cells in the lung co-express CD49a, CD103, and CD69, indicating they are tissue-resident NK cells (123, 124). Furthermore, NKG2C+ adaptive-like NK cells have been detected in both the blood and lungs, with a subset of CD49a+KIR+NKG2C+CD56brightCD16- lung NK cells demonstrating heightened responsiveness to target cells (125). Therefore, these NK cell subsets may be potential candidates for novel anti-tumor NK cell therapies.
The liver receives a substantial amount of blood flow and encounters numerous foreign antigens. It harbors a diverse range of immune cells, with NK cells comprising approximately half of all hepatic lymphocytes (126). HCC is a highly prevalent malignancy worldwide and the second leading cause of cancer-related mortality (127). Studies have indicated a notable correlation between a decrease in the proportion of NK cells producing IFN-γ and the severity of HCC, as well as an increased likelihood of HCC recurrence following treatment (128). Thus, strategies aimed at activating NK cells have been investigated, including the transfusion of CAR-NK cells or compounds that promote NK cell function. Among these approaches, CAR-NK-92 cells specifically targeting glypican-3 (GPC3) are effective in combating tumors (129). Cytokines such as IL-12, IL-15, and IL-18 enhance the anti-tumor capabilities of NK cells in vivo, leading to the suppression of liver tumorigenesis (126). Furthermore, the antifungal agent lomofungin was found to decrease the activity of soluble MICA, potentially augmenting the cytotoxicity of NK cells against HCC (130). Recent evidence has shown that combining NK cell immunotherapy with irreversible electroporation (IRE), a non-thermal method of tissue ablation, results in enhanced clinical outcomes, including improved progression-free and overall survival, compared with IRE ablation alone in patients with liver cancer (131). These results suggest that adoptive NK cell transfer in combination with diverse strategies holds promise as a beneficial approach for treating advanced HCC.
Pancreatic cancer is a highly aggressive disease with a predicted future increase in its incidence. Similar to CAR-T cells, CAR-NK cells have been tested for their ability to target this disease. In a mouse model of metastatic humanized pancreatic cancer, cord blood-derived NK cells transduced with an anti-prostate stem cell antigen (PSCA) CAR construct containing a soluble form of IL-15 showed efficacy (132). Additionally, in an individual with pancreatic cancer and liver metastasis, anti-ROBO1 CAR NK-92 cells showed minor adverse events and stable disease status for a certain period (78). Clinical trials testing the feasibility of CAR-NK cells or allogeneic NK cell infusion for the treatment of solid tumors are possible strategies (133).
Metastasis increases with higher tumor stage and is a significant contributor to cancer-related deaths (134). Metastasis is a complex process and its occurrence is influenced by various factors, including the composition of the tumor microenvironment and the plasticity of cancer cells. Cancer cells undergo several steps during metastasis, such as invasion and dissemination, during which they break away from the primary tumor site. These cells then enter the bloodstream or lymphatic vessels, spread to other parts of the body, and extravasate from the circulation. These processes are known as circulation and colonization, respectively. Afterwards, the cells may enter a dormant state before forming new tumors (135). Studies have shown that polyclonal clusters of cancer cells have better survival and colonization capabilities than monoclonal or single clusters (136–138). Polyclonal clusters are heterogeneous, making them difficult to control. Furthermore, CTCs that are likely to metastasize or respond to treatment can be detected in the blood and protected by platelet adhesion, preventing detection and elimination by NK cells (139).
Accordingly, multiple studies have reported that NK cell-based therapy can potentially target metastatic cancers by eliminating disseminated cancer cells in the circulation or those deposited in other organs (140–142). To understand the mechanism of immune surveillance during the metastatic progression of cancer, it is essential to analyze the characteristics of cancer cells during this process. Initially, invasive cells express keratin-14 (K14) and p63, leading to collective invasion (143). K14 is a basal epithelial marker critical for metastasis. K14-positive cells evade immune surveillance (144, 145). Metastatic cells possess genes related to epithelial and mesenchymal cells (146) as well as cell survival (147). Interestingly, monoclonal and polyclonal clusters of cancer cells exert distinct effects on NK cell resistance. Lo et al. (148) engrafted fluorescently labeled mouse mammary cells, either in a mixed or single population, into the mammary fat pad of recipient mice. The recipient mice included wild-type mice, nude mice lacking T cells, and NOD-Rag1nullIL2rgnull (NRG) mice lacking B, T, and NK cells. The results showed that NRG mice had an increased number of monoclonal metastatic lesions, whereas polyclonal clusters were more dominant in other mice. These findings suggest that these effects may be dependent on immune cells. When NK cells are present, monoclonal clusters can be removed; however, polyclonal clusters are resistant to NK cell surveillance (148). These cells may lose K14, while E-cadherin plays a role in cluster metastasis (149). Similarly, non-cluster-forming cancer cells that are sensitive to NK cell killing express lower levels of genes related to cell-cell adhesion but higher levels of genes encoding ligands for NK cell receptor activation (148). Therefore, altering the epithelial features of metastatic cancer cells, in addition to adoptive NK cell transfer, may provide a strategy for targeting metastasis.
Cancer cells that migrate to different parts of the body enter dormancy. This indicates that these cells undergo cell cycle arrest and remain hidden from the immune system (134, 150). When dormant cancer cells become active again and start dividing, they become sensitive to NK cell killing by decreasing the expression of genes related to MHC class I molecules and increasing the expression of genes related to ligands against NK cell-activating receptors (151). In preclinical models, it was found that in mice lacking B, T, and NK cells, dormant cancer cells increased metastasis, whereas in mice lacking T cells, they decreased (152). Although these findings suggest that NK cells can target metastatic cells under certain conditions, the exact mechanisms by which this occurs, particularly the intrinsic pathways of cancer cells, are not yet fully understood.
Despite the evidence that NK cells can target metastatic cancer, several studies have demonstrated that cancer cells reprogram NK cells to support metastasis. Chan et al. (153) showed that tumor-exposed NK (teNK) cells promoted tumor cell colony growth in a 3D co-culture platform. teNK cells exhibit increased TIGIT and KLRG1 expression and upregulated DNA methyltransferases, such as DNMT1, DNMT3a, and DNMT3b, compared to healthy NK cells, indicating that epigenetic control by cancer cells occurs in teNK cells. Similarly, it is well known that TGF-β signaling induces NK cell-derived ILC1 that show higher expression of immune cell exhaustion markers, including CTLA4 and LAG3 (11). Therefore, the tumor microenvironment and cancer cells can alter NK cells to promote metastasis, which could explain why clinical observations do not always show a correlation between increased NK cell numbers and overall survival in patients with cancer (154).
Many countries are becoming increasingly aging societies. All tissues, including those of the immune system, become senescent. Systemic immunosenescence can lead to a decrease in the efficient clearance of harmful cells, resulting in an increase in inflammatory or infectious diseases (155). In this section, we discuss the relationship between age-related diseases and the potential use of NK cell therapies.
Cancer incidence in humans increases with age (156). Many biological processes that contribute to cancer and aging share characteristics such as telomere attrition, cellular senescence, genomic instability, and inflammation (157). The development of cancer cells can occur through the evasion of apoptosis or senescence or through the accumulation of senescent cells (158). However, the cellular processes involved in aging and cancer are not yet fully understood, and their relationships are complex. Long-lived species have evolved tumor-suppressing mechanisms and cancer-resistant features (159, 160). Cellular senescence can lead to irreversible cell growth arrest (161), typically in response to damage. This plays a critical role in suppressing abnormal cell proliferation and preventing tumor growth. Senescent cells have been shown to trigger an immune response that aids in tumor clearance. In contrast, studies have demonstrated that senescent cells can promote tumor growth, angiogenesis, and invasion by secreting factors such as extracellular matrix components and cytokines, similar to an immunosuppressive tumor microenvironment. In addition, research has shown that aged fibroblasts can drive lung metastasis and therapy resistance in a mouse melanoma model (162). Understanding the complex interplay between aging and cancer may lead to the development of novel strategies for cancer treatment in aging populations.
Senescent cells contribute to inflammation primarily by releasing a senescent-associated secretory phenotype (SASP) (163), and their accumulation can lead to various side effects in tissues, such as fibrosis. For instance, sarcopenia, the loss of regenerative capacity in skeletal muscle with age, can lead to the replacement of muscle tissue with fat and fibrotic tissues (164). Interestingly, a recent study conducted on mice revealed that senescent cells in muscle tissue can induce age-related changes such as inflammation and increased fibrosis, as well as alterations in gene expression (165). Removing senescent cells from tissues promotes tissue regeneration and suppresses muscle inflammation, suggesting potential beneficial effects.
Recently, it has been questioned whether aging can be cured, particularly by removing senescent cells using senolytic agents (166–168). Studies in mice have shown that the removal of senescent cells can reverse age-related pathologies (169, 170). Therefore, it has been suggested that methods used for anti-tumor immune cell therapies can be applied to target senescent cells. Immune cells such as NK cells, macrophages, and T cells can recognize senescent cells (171). Furthermore, senescent cells have increased ligands for NK cell-activating receptors such as NKG2D (172). However, senescent cells can also be resistant to immune surveillance owing to immunosenescence, immune cell dysfunction, or alterations in MHC molecules. A previous study indicated that senescent fibroblasts, which are induced either by the natural aging of human skin fibroblasts or exposure to ionizing radiation, exhibit upregulation of HLA-E, a non-classical MHC molecule, and the pro-inflammatory cytokine IL-6. This upregulation inhibits NK cell surveillance (173). The authors proposed that mAbs targeting the inhibitory receptor NKG2A, similar to existing cancer treatments, could be employed as a senolytic strategy to modulate NK cell activity (174).
Senolytics such as dasatinib in combination with quercetin have been widely used to remove senescent cells, and new senolytic treatments are being developed (175, 176). A recent study showed that infusing NK cells with acein, a non-apeptide that secretes dopamine (177), reversed senescent markers in a mouse model (178). Furthermore, researchers have proposed the use of senolytic CAR-T cells that target urokinase-type plasminogen activator receptor (uPAR) in senescent cells. uPAR was initially identified in senescent models, and the effectiveness of uPAR CAR-T cells in animal models of liver fibrosis has been demonstrated (179). These findings suggest that adoptive immune cell therapies that target senescent cells hold promise as potential approaches for the treatment of aging and aging-related diseases.
NK cells play a crucial role in directly eliminating virus-infected cells and stimulating antiviral immune responses through IFN-γ production. The number of NK cells is inversely associated with the severity and recurrence of viral infections in humans (180). Chronic viral infections can contribute to tumor progression and autoimmune disorders, and NK cell dysfunction has been implicated in the pathogenesis of these virus-mediated diseases. Notably, the immunomodulatory roles of NK cells have been investigated in various autoimmune diseases such as multiple sclerosis (MS), rheumatoid arthritis, and systemic lupus erythematosus. NK cells can promote dendritic cell (DC) differentiation during adaptive immunity while also exhibiting the ability to eliminate immature DCs, activated macrophages, and T cells (181). Impaired NK cell cytotoxicity has been observed in patients with autoimmune diseases, suggesting its involvement in disease onset. However, the precise mechanism of action must be contextually considered. For instance, in MS patients, an accumulation of CD56bright NK cells was found in the cerebrospinal fluid, and treatment with daclizumab (a humanized anti-IL-2Rα antibody) led to a significant expansion of CD56bright NK cells accompanied by a reduction in CD4 T cells (182). These observations highlight the critical role of functional NK cells in antiviral immunity and the regulation of autoimmune diseases, although investigations into NK cell therapies for these conditions are still in the early stages of development. Notably, several clinical trials exploring NK cell therapy for the treatment of adults with COVID-19 have recently begun (183). These studies indicate the potential use of NK and CAR-NK cells as therapeutic approaches for managing viral infections.
In NK cell clinical trials, a large quantity of cells is used for infusion, ranging from 5×106 to 1×108 CD3-CD56+ NK cells per kilogram body weight (184). With the development of techniques and success of CAR-T cell therapies, NK cells have become the next generation of genetically engineered immune cell therapies. This involves the transduction of CARs or other genes that stimulate NK cell function. Gene transfer is accomplished using viruses such as lentiviruses, retroviruses, and adeno-associated viruses (185). The CRISPR-Cas9 system can also be used to delete genes associated with NK cell suppression. A recent report demonstrated efficient knockout of genes using a single guided RNAs and Cas9 protein (RNP) nucleofection method, and the edited NK cells were successfully expanded (186). The authors suggested a clinically relevant protocol using cryopreserved PB NK cells, while ensuring the purity and safety of gene-edited cell therapies as the ultimate goals. The following sections present current strategies aimed at genetically engineering NK cells to enhance their functions, including target cell recognition, NK cell persistence, and tumor infiltration.
Different sources of NK cells have been used to develop NK cell therapies, including peripheral blood mononuclear cells (PBMC) (187), umbilical cord blood (UCB) (92), NK-92 cells (188), and induced pluripotent stem cells (iPSCs) (189). Although NK-92 cells derived from NK lymphoma offer a valuable research tool for exploring the function of CARs and genetically engineered cells, their effectiveness may be limited due to the absence of CD16 and the need for irradiation before being administered to patients. In contrast, primary NK cells derived from UCB or PB contain not only cytotoxic NK cells but also CD34+ hematopoietic stem cells, which can be expanded and differentiated into mature NK cells. Both UCB- and PB-derived NK cells exhibit therapeutic efficacy against leukemia following CAR gene transduction. Notably, UCB-derived NK cells demonstrate higher proliferative capacity than PB-derived NK cells (190). Additionally, cytokine-induced memory-like NK cells sustained by IL-12, IL-15, and IL-18 in primary NK cells have shown promising clinical outcomes in patients with myeloid neoplasms (187). However, the isolation of large numbers of primary NK cells and hematopoietic stem cells is challenging, and purifying NK cells to deplete CD3 and CD19 positive cells is difficult during allogeneic NK cell transfer. By contrast, iPSC-derived NK cells are an unlimited source of homogeneous human NK cells. These iPSCs can be genetically modified to express or deplete specific genes, followed by therapeutic NK cell differentiation. However, safety concerns remain owing to the presence of undifferentiated iPSCs and the complexity of the differentiation protocol (185).
CARs enhance immune cell function by combining an intracellular signaling domain with an antigen-specific single-chain variable fragment (scFv) that recognizes a specific antigen. There are four to five generations of CARs with increasing complexity of the intracellular domains, whereas the recognition of antigens is dependent on the ectodomains present in each CAR (191). The second generation, which contains either CD28 or 41BB as a costimulatory domain, is effective and widely used. Second-generation CAR-T cells have been utilized in all FDA-approved CAR-T cell therapies to date. CAR-NK cells are also being developed, with promising results reported in 2020 for anti-CD19 CAR-NK cells for the treatment of certain blood cancers, as previously described (92). At least 30 clinical trials of CAR-NK cells using different sources of NK cells are currently underway worldwide (192). CAR-NK cells are being developed to target different diseases, including hematological malignancies (82–85), solid tumors (78–81), and COVID-19 (Table 1).
Despite the ability of second-generation CARs to stimulate NK cells such as T cells, researchers have been investigating novel intracellular CAR domains designed for NK cell activation. Adaptor proteins such as DAP10 and DAP12, which recruit PI3K in association with activating NK cell receptors (Figure 2), have been investigated as potential replacements for CD3z in CAR-NK cells (193, 194). Furthermore, NKG2D-engineered CAR (NKG2D-DAP10-CD3z)-NK cells, which targets NKG2D ligands in tumor cells, have been tested (195). Notably, iPSC-derived NK cells were used to test various CAR constructs, and the results showed that NKG2D is a transmembrane domain, followed by 2B4 and CD3z in iPSC-NK cells, producing optimized activity (189). Similarly, DNAM1 expression was found to be more effective than that of CD28 as an intracellular domain in anti-GPC3 CAR-NK-92 cells (196). A better understanding of the mechanisms underlying NK cell biology may lead to the development of novel CAR-NK cell therapies.
In addition to CARs, various genes have been investigated to enhance the persistence and function of NK cells in vivo. Expression of the membrane-bound form of IL-15 in human PB-derived NK cells has been shown to improve NK cell survival and in vivo anti-tumor activity (197). Anti-CD19 CAR, IL-15, and inducible caspase-9-based suicide genes were introduced into CB-derived NK cells, resulting in the extended survival of modified NK cells in a mouse model (198). Scalable and uniformly edited NK cells can be generated from iPSCs. Quadruple-gene-modified iPSC-derived NK cells, called FT576, were created by Cichocki et al. (199) for the treatment of MM by incorporating anti-BCMA CAR (200), a non-cleavable version of the CD16a Fc receptor (201, 202), and a membrane-bound IL-15/IL-15R fusion molecule. These modified NK cells can target BCMA-expressing tumor cells and display improved ADCC and in vivo persistence. Additionally, CD38 has been knocked out in NK cells (203), enabling a combination approach with an anti-CD38 mAb for the treatment of relapsed or refractory MM (204).
CRISPR-Cas9 gene editing has been used in many studies to delete negative regulators in NK cells. For example, iPSC-derived NK cells have been edited to remove the cytokine-inducible SH2-containing proteins (CIS), encoded by CISH, resulting in potential IL-15-dependent NK activation (205). Tumor necrosis factor-α (TNF-α)-induced protein-8 like-2 (TIPE2) negatively regulates the immune response of NK cells against tumors. This discovery led to the development of a novel approach involving the use of TIPE2-deficient NK cells, which could potentially enhance the effectiveness of cancer treatment in combination with CISH knockout (206). In animal models, when TIPE2-defecient NK cells are transferred to the host, they activate the IL-15-mTORC1 pathway, leading to improved antitumor activity and providing support for T cell-based immunotherapy (207).
Genetic disruption of NKG2A has been used to modulate inhibitory signaling in NK cells, resulting in superior tumor control in a mouse model (208). In the tumor microenvironment, adenosine A2A receptor is knocked out in CAR-NK cells to counteract the immunosuppressive effects of adenosine (209). Deletion of TGF-β receptor 2 in CB-derived NK cells (210) or downregulation of TGF-β-induced miR-27a-5p has been used to block TGF-β signaling and improve NK cell function (211). In MM, the therapeutic mAb daratumumab targets CD38, which is expressed by both malignant and NK cells, leading to fratricide. To overcome this issue, CRISPR-modified CD38 knockout NK cells have recently shown improved efficacy (212, 213).
Adoptive NK cell therapy has limited efficacy against solid tumors because of the immunosuppressive tumor microenvironment. To improve the targeting of solid tumors, NK cells have been engineered to overexpress chemokine receptors such as CXCR1, CXCR2, CXCR3, CCR7, and CXCR4 in various animal models of cancer (214–218). In addition, the overexpression of a high-affinity dominant-negative TGF-β receptor (TGF-β DNRII) has been used to inhibit TGF-β signaling (219, 220). Clinical trials on patients with prostate cancer have shown the feasibility of expressing TGF-β DNRII and T cells in combination with the antiprostate-specific membrane antigen CAR (221). Another approach involves targeting the inhibitory receptors on NK cells via viral transduction. For instance, human PB NK cells were transduced with a retrovirus to express NKG2A protein expression blocker (PEBL), which abrogates NKG2A expression (208). The PEBL construct contained an anti-NKG2A scFv linked to four different endoplasmic reticulum retention domains, which resulted in enhanced NK cell cytotoxicity and in vivo anti-tumor activity.
Immunotherapeutic approaches to enhance NK cell function, such as monotherapy and combination with other strategies, have rapidly developed. This section covers various types of NK cell activators, including those specifically designed based on NK cell biology and those that have been found to activate NK cells. These agents can be combined with NK cell or CAR-NK cell therapies. Various adoptive NK cell therapies in different combinations are presented in Table 2. An overview of current NK cell therapies and targetable diseases is summarized in Figure 3.
Table 2 A list of current strategies of adoptive NK cell therapy in combination with other treatments (chemotherapy, radiation therapy and immunotherapy).
Figure 3 An overview of current and emerging approaches for harnessing NK cell activity. Adoptive transfer of NK cells has demonstrated efficacy in treating tumors, and various strategies have been employed to further improve their function. These include introducing CARs, chemokine receptors, and other modifications via genome editing using CRISPR-Cas system. Additionally, ADCC has been shown to efficiently induce NK cell killing activity. Clinical trials are underway for various therapies for inducing ADCC, including mAbs, BiKEs, and TriKEs. Researches on developing TME inhibitors and cytokines to enhance cell activity, and combinations of these agents with NK cells or other treatments are also being explored. NK cell therapy is also being investigated for novel indications such as viral infections and aging, using therapeutic NK cells to eliminate damaged cells. CAR, chimeric antigen receptor; mAb, monoclonal antibody; BiKE, bi-specific killer engager; TriKE, tri-specific killer engager; sMIC, soluble MHC I chain-related molecules A and B; DPP4, dipeptidyl peptidase-4; IDO, indoleamine-pyrrole 2,3-dioxygenase; TME, tumor microenvironment; DNRII, dominant-negative TGF-β receptor 2.
NK cells exert their effector function through the ADCC-mediated killing of IgG1- or IgG3-opsonized target cells, which are recognized by CD16 in NK cells. Therapeutic antibody development often excludes IgG3 because of its long hinge region and polymorphic nature (224). Several ADCC-inducing antibodies such as rituximab (anti-CD20), cetuximab (anti-EGFR), and trastuzumab (anti-HER2) for CLL, colorectal cancer, and breast cancer, respectively, have been developed and approved by the FDA for tumor treatment (222). Notably, patients with the CD16 polymorphism at position 158F (phenylalanine) have a limited response rate owing to their low affinity for the Fc region of antibodies, in contrast to those with the 158V (valine) variant, which exhibits a high affinity (225, 226). To overcome this problem, the development of mAbs that induce ADCC has focused on improving affinity of low-affinity variant. Elotuzumab and daratumumab target SLAMF7 and CD38, respectively, and induced robust ADCC in myeloma cells (204, 227). Margetuximab, an anti-HER2 antibody, also shows improved binding to low-affinity CD16 and has received approval from the US FDA (228). Avelumab, an IgG1 antibody against PD-L1, provides the additional benefit of inducing checkpoint inhibition in addition to ADCC (229, 230). Furthermore, anti-MICA antibodies have been suggested to enhance NK cell cytotoxicity via NKG2D and ADCC engagement (231).
The use of bi- and tri-specific killer engagers (BiKEs and TriKEs, respectively) in therapeutic approaches is similar to that of ADCC-inducing antibodies. These molecules facilitate interactions between effector and target cells, leading to the activation of NK cells through CD16 engagement. Unlike Fc-targeted antibodies, BiKEs and TriKEs only target CD16 via Fv fragments, thereby reducing the off-target effects. AFM13, a BiKE targeting CD30 for B- and T-cell lymphomas (232), has been granted orphan drug designation for the treatment of peripheral T-cell lymphoma (NCT4101331, NCT04074746) (233). Another BiKE, AFM24, that targets EGFR in solid cancers, has also been studied (NCT04259450). During the development of TriKEs, moieties such as IL-15, which enhance NK cell function, can be incorporated in addition to BiKEs. Studies have investigated the effectiveness of TriKEs containing IL-15 against several tumor antigens, such as CD133, CD19, CLEC12, and CD33 (234–236). TriKEs also target additional NK cell receptors, such as NKp46 (237).
Currently, immune checkpoint inhibitors (ICIs) such as anti-PD1, PD-L1, and CTLA4 antibodies have shown success in stimulating the immune system to eradicate tumors (238). The main mechanism of action is thought to be prevention of T cell exhaustion. Nevertheless, it has been suggested that NK cells could also benefit from ICIs (239, 240). By activating NK cells through the ADCC-mediated induction of PD-L1 on the tumor cell surface, combining ICIs with ADCC immunotherapy may have a synergistic anti-tumor effect (238). Targeting other immune checkpoints such as LAG3, TIM3, and TIGIT is currently being investigated because these receptors are also expressed on tumor-infiltrating NK cells (241, 242). Previous studies have demonstrated that TIGIT antibody can increase NK-mediated antitumor response (113).
Efforts have been made to develop ICIs that are specific to NK cells, owing to their possession of various inhibitory receptors that could potentially act as immune checkpoints. Lirilumab, a KIR antibody, was developed to target the HLA-C-specific family of KIR2D receptors, which can be either inhibitory or activating (243). However, the clinical use of lirilumab alone has not resulted in significant responses. Therefore, combination strategies are being explored (NCT02813135). Another potential target for NK-specific immune checkpoint inhibition is NKG2A, as its ligand, HLA-E, is often upregulated in tumors and senescent fibroblasts (173, 244). Monalizumab (IPH2201), an NKG2A antibody, is currently being investigated in clinical trials in combination with other agents for the treatment of solid tumors, such as in head and neck cancer and breast cancer (NCT04590963, NCT02643550, NCT04307329, and NCT02671435). Notably, inhibitory KIR plays a crucial role in NK cell education and licensing, and NKG2A is important for maintaining NK cell immune tolerance; thus, caution should be exercised when targeting these receptors (245).
There are several ways to enhance the activity of NK cells against tumor cells, including pharmacological interventions or the use of cytokines. Immunomodulatory imide drugs (IMiDs) such as lenalidomide and pomalidomide, which interact with cereblon (CRBN), can activate NK cells via CRBN-dependent or independent mechanisms (246, 247). IMiDs activate ZAP70 and increase granzyme B expression in NK cells (248). In addition, IMiDs make tumor cells more susceptible to NK cell killing by upregulating ligands for NKG2D, DNAM1, MICA, and PVR in tumor cells (249). Bortezomib, a proteasome inhibitor, has been shown to have similar effects on tumor cells, including downregulation of MHC-I expression (250). Other pharmacological interventions that increase the vulnerability of cancer cells to NK cell killing have also been reported. BH3 mimetics enhance NK cell-mediated killing by inducing mitochondrial apoptosis in target tumor cells (251). ONC021, an orally administered antitumor agent, activates TNF-related apoptosis-inducing ligand (TRAIL) and stimulates NK cells at the tumor site, leading to reduced metastasis. Clinical trials for ONC021 are currently underway (NCT02525692) (252).
The direct stimulation of NK cells has also been extensively studied, particularly in the context of the tumor microenvironment, where immune cells are often suppressed. For example, HIF1α expression induced by a hypoxic tumor microenvironment can inhibit NK cell activity, but inhibition of HIF1α or using Hif1α-/- NK cells has been shown to potentiate NK cell activity against tumors in in vitro and in vivo experiments (253). Various pharmacological inhibitors against the TGF-β signaling pathway have been tested in preclinical and clinical studies. It has been shown that the TGFβRI kinase inhibitor galunisertib (LY2157299) enhances NK cell-mediated anti-tumor activity (254). On the other hand, ADAM17, a metalloproteinase that cleaves CD16 on the surface of NK cells, can suppress ADCC responses (255). Although ADAM17 inhibitors have been proposed to enhance ADCC in NK cells, the potential advantage of using ADAM17 inhibitors remains a matter of ongoing debate (256).
The cGMP-AMP synthase (cGAS)-stimulator of interferon gene protein (STING) pathway is crucial for immune cell activation via the production of type I IFNs (IFNα and IFNβ) (257). Interestingly, cyclic dinucleotides (CDNs), which are STING agonists, have been shown to activate NK cells (258). NK cell activation appears to be driven by increased IL-15 production in dendritic cells treated with CDNs, in response to increased type I IFNs. Notably, several STING agonists, including CDNs and small molecules, are currently undergoing clinical testing for tumor treatment (259).
Numerous cytokines have been identified as stimulators of NK cell proliferation and activation and cytokine mixtures are being studied to develop adoptive NK cell maturation strategies for cancer treatment. Furthermore, efforts are being made to develop cytokines that can be used as direct cancer treatments, including IL-2 (260), IL-15 (261), IL-12 (262), IL-18, and IL-21 (263). However, most of these cytokines have toxic adverse effects and limited efficacy when used as natural proteins. Consequently, novel approaches are being explored such as combining cytokines with other therapeutics or improving their delivery to target tissues. Among these cytokines, IL-15 potentiates NK and CD8+ T cell activity. Clinical trials are currently underway for heterodimeric fusion (hetIL-15) packaged in extracellular vesicles (264) and the superagonists IL-15 and N-803 (223, 265). Delivery methods for IL-12 such as direct intratumoral gene delivery or plasmids in combination with PD-1 inhibitors have also been tested (266). Furthermore, a modified form of the proinflammatory member of the IL-1 cytokine family, IL-18, has been suggested (267).
There are multiple subsets of NK cells and their activities can vary within each organ because of their dynamic nature. Exploiting the cytotoxic efficacy of NK cells through therapeutic strategies, such as adoptive NK cell therapy and BiKEs, shows great promise. Although safety is advantageous in adoptive NK cell therapy, optimizing combination approaches by understanding NK cell-specific mechanisms may enhance their efficacy. For example, combining cytokine-induced memory-like NK cells (187) with CAR (268) or BiKE (269) expression results in improved responses. Novel indications for efficient NK cell therapies, such as for virus infection, fibrosis, and aging, are emerging. With the advent of immune surveillance against abnormal cells, some researchers have shifted their focus to targeting senescent cells. Further investigations on NK cell immunity and cell-cell interactions can greatly support these new phenomena. Advances in genome editing, immune cell expansion, stem cell biology, and antibody design technologies are crucial for the progress in this field. Thus, NK cells have great potential as novel therapeutic agents against various diseases.
J-YN wrote the manuscript; ES, SB, JK, and TP produced figures and tables; ES, S-RY and HJ edited the manuscript. J-YN supervised the overall project and edited the manuscript. All authors contributed to the article and approved the submitted version.
This research was supported by the National Research Foundation of Korea (NRF) grant funded by the Korea Government (MSIT) (No. 2016R1C1B3009116; 2021R1C1C1011899), National Research Council of Science & Technology (NST) Aging Convergence Research Center (CRC22011-300), and the Korea Research Institute of Bioscience and Biotechnology (KRIBB) Research Initiative Program (KGS1142322; KGM5502322).
We would like to thank Editage (www.editage.co.kr) for English language editing.
The authors declare that the research was conducted in the absence of any commercial or financial relationships that could be construed as a potential conflict of interest.
All claims expressed in this article are solely those of the authors and do not necessarily represent those of their affiliated organizations, or those of the publisher, the editors and the reviewers. Any product that may be evaluated in this article, or claim that may be made by its manufacturer, is not guaranteed or endorsed by the publisher.
1. Blum KS, Pabst R. Lymphocyte numbers and subsets in the human blood: do they mirror the situation in all organs? Immunol Lett (2007) 108:45–51. doi: 10.1016/j.imlet.2006.10.009
2. Garrido F, Garrido F. MHC/HLA class I loss in cancer cells. Adv Exp Med Biol (2019) 1151:15–78. doi: 10.1007/978-3-030-17864-2_2
3. Freud AG, Caligiuri MA. Human natural killer cell development. Immunol Rev (2006) 214:56–72. doi: 10.1111/j.1600-065X.2006.00451.x
4. Campbell KS, Hasegawa J. Natural killer cell biology: an update and future directions. J Allergy Clin Immunol (2013) 132:536–44. doi: 10.1016/j.jaci.2013.07.006
5. Luetke-Eversloh M, Killig M, Romagnani C. Signatures of human NK cell development and terminal differentiation. Front Immunol (2013) 4:499. doi: 10.3389/fimmu.2013.00499
6. Vosshenrich CA, Ranson T, Samson SI, Corcuff E, Colucci F, Rosmaraki EE, et al. Roles for common cytokine receptor γ-chain-dependent cytokines in the generation, differentiation, and maturation of NK cell precursors and peripheral NK cells in vivo. J Immunol (2005) 174:1213–21. doi: 10.4049/jimmunol.174.3.1213
7. Ma S, Caligiuri MA, Yu J. A four-stage model for murine natural killer cell development in vivo. J Hematol Oncol (2022) 15:31. doi: 10.1186/s13045-022-01243-1
8. Orange JS. Unraveling human natural killer cell deficiency. J Clin Invest (2012) 122:798–801. doi: 10.1172/JCI62620
9. Jacobs R, Hintzen G, Kemper A, Beul K, Kempf S, Behrens G, et al. CD56bright cells differ in their KIR repertoire and cytotoxic features from CD56dim NK cells. Eur J Immunol (2001) 31:3121–6. doi: 10.1002/1521-4141(2001010)31:10<3121::aid-immu3121>3.0.co;2-4
10. Spits H, Artis D, Colonna M, Diefenbach A, Di Santo JP, Eberl G, et al. Innate lymphoid cells–a proposal for uniform nomenclature. Nat Rev Immunol (2013) 13:145–9. doi: 10.1038/nri3365
11. Gao Y, Souza-Fonseca-Guimaraes F, Bald T, Ng SS, Young A, Ngiow SF, et al. Tumor immunoevasion by the conversion of effector NK cells into type 1 innate lymphoid cells. Nat Immunol (2017) 18:1004–15. doi: 10.1038/ni.3800
12. Chiossone L, Dumas P-Y, Vienne M, Vivier E. Natural killer cells and other innate lymphoid cells in cancer. Nat Rev Immunol (2018) 18:671–88. doi: 10.1038/s41577-018-0061-z
13. Kim CH, Hashimoto-Hill S, Kim M. Migration and tissue tropism of innate lymphoid cells. Trends Immunol (2016) 37:68–79. doi: 10.1016/j.it.2015.11.003
14. Sun H, Liu L, Huang Q, Liu H, Huang M, Wang J, et al. Accumulation of tumor-infiltrating CD49a+ NK cells correlates with poor prognosis for human hepatocellular CarcinomaAccumulation of CD49a+ NK cells in HCC. Cancer Immunol Res (2019) 7:1535–46. doi: 10.1158/2326-6066.CIR-18-0757
15. Dogra P, Rancan C, Ma W, Toth M, Senda T, Carpenter DJ, et al. Tissue determinants of human NK cell development, function, and residence. Cell (2020) 180:749–63. doi: 10.1016/j.cell.2020.01.022
16. Crinier A, Milpied P, Escalière B, Piperoglou C, Galluso J, Balsamo A, et al. High-dimensional single-cell analysis identifies organ-specific signatures and conserved NK cell subsets in humans and mice. Immunity (2018) 49:971–86. doi: 10.1016/j.immuni.2018.09.009
17. Renoux VM, Zriwil A, Peitzsch C, Michaëlsson J, Friberg D, Soneji S, et al. Identification of a human natural killer cell lineage-restricted progenitor in fetal and adult tissues. Immunity (2015) 43:394–407. doi: 10.1016/j.immuni.2015.07.011
18. Gascoyne DM, Long E, Veiga-Fernandes H, De Boer J, Williams O, Seddon B, et al. The basic leucine zipper transcription factor E4BP4 is essential for natural killer cell development. Nat Immunol (2009) 10:1118–24. doi: 10.1038/ni.1787
19. Holmes ML, Huntington ND, Thong RP, Brady J, Hayakawa Y, Andoniou CE, et al. Peripheral natural killer cell maturation depends on the transcription factor aiolos. EMBO J (2014) 33:2721–34. doi: 10.15252/embj.201487900
20. Bauer S, Groh V, Wu J, Steinle A, Phillips JH, Lanier LL, et al. Activation of NK cells and T cells by NKG2D, a receptor for stress-inducible MICA. Science (1999) 285:727–9. doi: 10.1126/science.285.5428.727
21. Holmes MA, Li P, Petersdorf EW, Strong RK. Structural studies of allelic diversity of the MHC class I homolog MIC-b, a stress-inducible ligand for the activating immunoreceptor NKG2D. J Immunol (2002) 169:1395–400. doi: 10.4049/jimmunol.169.3.1395
22. Cosman D, Müllberg J, Sutherland CL, Chin W, Armitage R, Fanslow W, et al. ULBPs, novel MHC class I–related molecules, bind to CMV glycoprotein UL16 and stimulate NK cytotoxicity through the NKG2D receptor. Immunity (2001) 14:123–33. doi: 10.1016/s1074-7613(01)00095-4
23. Dhar P, Wu JD. NKG2D and its ligands in cancer. Curr Opin Immunol (2018) 51:55–61. doi: 10.1016/j.coi.2018.02.004
24. Diefenbach A, Jamieson AM, Liu SD, Shastri N, Raulet DH. Ligands for the murine NKG2D receptor: expression by tumor cells and activation of NK cells and macrophages. Nat Immunol (2000) 1:119–26. doi: 10.1038/77793
25. Cerwenka A, Bakker AB, McClanahan T, Wagner J, Wu J, Phillips JH, et al. Retinoic acid early inducible genes define a ligand family for the activating NKG2D receptor in mice. Immunity (2000) 12:721–7. doi: 10.1016/s1074-7613(00)80222-8
26. Bartel Y, Bauer B, Steinle A. Modulation of NK cell function by genetically coupled c-type lectin-like receptor/ligand pairs encoded in the human natural killer gene complex. Front Immunol (2013) 4:362. doi: 10.3389/fimmu.2013.00362
27. MacFarlane IVA, Campbell K. Signal transduction in natural killer cells. Curr Top Microbiol Immunol (2006) 298:23–57. doi: 10.1007/3-540-27743-9_2
28. Harel-Bellan A, Quillet A, Marchiol C, DeMars R, Tursz T, Fradelizi D. Natural killer susceptibility of human cells may be regulated by genes in the HLA region on chromosome 6. Proc Natl Acad Sci USA (1986) 83:5688–92. doi: 10.1073/pnas.83.15.5688
29. Marsh SG, Parham P, Dupont B, Geraghty DE, Trowsdale J, Middleton D, et al. Killer-cell immunoglobulin-like receptor (KIR) nomenclature report, 2002. Hum Immunol (2003) 55:220–6. doi: 10.1016/s0198-8859(03)00067-3
30. Campbell KS, Purdy AK. Structure/function of human killer cell immunoglobulin-like receptors: lessons from polymorphisms, evolution, crystal structures and mutations. Immunology (2011) 132:315–25. doi: 10.1111/j.1365-2567.2010.03398.x
31. Horowitz A, Strauss-Albee DM, Leipold M, Kubo J, Nemat-Gorgani N, Dogan OC, et al. Genetic and environmental determinants of human NK cell diversity revealed by mass cytometry. Sci Transl Med (2013) 5:208ra145. doi: 10.1126/scitranslmed.3006702
32. Strauss-Albee DM, Fukuyama J, Liang EC, Yao Y, Jarrell JA, Drake AL, et al. Human NK cell repertoire diversity reflects immune experience and correlates with viral susceptibility. Sci Transl Med (2015) 7:297ra115. doi: 10.1126/scitranslmed.aac5722
33. Campbell JJ, Qin S, Unutmaz D, Soler D, Murphy KE, Hodge MR, et al. Unique subpopulations of CD56+ NK and NK-T peripheral blood lymphocytes identified by chemokine receptor expression repertoire. J Immunol (2001) 166:6477–82. doi: 10.4049/jimmunol.166.11.6477
34. Berahovich RD, Lai NL, Wei Z, Lanier LL, Schall TJ. Evidence for NK cell subsets based on chemokine receptor expression. J Immunol (2006) 177:7833–40. doi: 10.4049/jimmunol.177.11.7833
35. Smyth MJ, Zachariae C, Norihisa Y, Ortaldo JR, Hishinuma A, Matsushima K. IL-8 gene expression and production in human peripheral blood lymphocyte subsets. J Immunol (1991) 146:3815–23. doi: 10.4049/jimmunol.146.11.3815
36. Walle T, Kraske JA, Liao B, Lenoir B, Timke C, von Bohlen und Halbach E, et al. Radiotherapy orchestrates natural killer cell dependent antitumor immune responses through CXCL8. Sci Adv (2022) 8:eabh4050. doi: 10.1126/sciadv.abh4050
37. Trinchieri G. The choices of a natural killer. Nat Immunol (2003) 4:509–10. doi: 10.1038/ni0603-509
38. Molfetta R, Quatrini L, Zitti B, Capuano C, Galandrini R, Santoni A, et al. Regulation of NKG2D expression and signaling by endocytosis. Trends Immunol (2016) 37:790–802. doi: 10.1016/j.it.2016.08.015
39. Jiang K, Zhong B, Gilvary DL, Corliss BC, Hong-Geller E, Wei S, et al. Pivotal role of phosphoinositide-3 kinase in regulation of cytotoxicity in natural killer cells. Nat Immunol (2000) 1:419–25. doi: 10.1038/80859
40. Schymeinsky J, Then C, Sindrilaru A, Gerstl R, Jakus Z, Tybulewicz VL, et al. Syk-mediated translocation of PI3Kδ to the leading edge controls lamellipodium formation and migration of leukocytes. PloS One (2007) 2:e1132. doi: 10.1371/journal.pone.0001132
41. Wagner EF, Nebreda AR. Signal integration by JNK and p38 MAPK pathways in cancer development. Nat Rev Cancer (2009) 9:537–49. doi: 10.1038/nrc2694
42. Pua LJW, Mai C-W, Chung FF-L, Khoo AS-B, Leong C-O, Lim W-M, et al. Functional roles of JNK and p38 MAPK signaling in nasopharyngeal carcinoma. Int J Mol Sci (2022) 23:1108. doi: 10.3390/ijms23031108
43. Lau CM, Wiedemann GM, Sun JC. Epigenetic regulation of natural killer cell memory. Immunol Rev (2022) 305:90–110. doi: 10.1111/imr.13031
44. Chen Y, Lu D, Churov A, Fu R. Research progress on NK cell receptors and their signaling pathways. Mediators Inflammation (2020) 2020:6437057. doi: 10.1155/2020/6437057
45. Marçais A, Marotel M, Degouve S, Koenig A, Fauteux-Daniel S, Drouillard A, et al. High mTOR activity is a hallmark of reactive natural killer cells and amplifies early signaling through activating receptors. Elife (2017) 6:e26423. doi: 10.7554/eLife.26423
46. Wang F, Meng M, Mo B, Yang Y, Ji Y, Huang P, et al. Crosstalks between mTORC1 and mTORC2 variagate cytokine signaling to control NK maturation and effector function. Nat Commun (2018) 9:4874. doi: 10.1038/s41467-018-07277-9
47. Viel S, Marçais A, Guimaraes FS-F, Loftus R, Rabilloud J, Grau M, et al. TGF-β inhibits the activation and functions of NK cells by repressing the mTOR pathway. Sci Signal (2016) 9:ra19. doi: 10.1126/scisignal.aad1884
48. Reber L, Vermeulen L, Haegeman G, Frossard N. Ser276 phosphorylation of NF-kB p65 by MSK1 controls SCF expression in inflammation. PloS One (2009) 4:e4393. doi: 10.1371/journal.pone.0004393
49. Brauning A, Rae M, Zhu G, Fulton E, Admasu TD, Stolzing A, et al. Aging of the immune system: focus on natural killer cells phenotype and functions. Cells (2022) 11:1017. doi: 10.3390/cells11061017
50. Kutza J, Murasko DM. Age-associated decline in IL-2 and IL-12 induction of LAK cell activity of human PBMC samples. Mech Ageing Dev (1996) 90:209–22. doi: 10.1016/0047-6374(96)01772-1
51. Carson WE, Fehniger TA, Haldar S, Eckhert K, Lindemann MJ, Lai C-F, et al. A potential role for interleukin-15 in the regulation of human natural killer cell survival. J Clin Invest (1997) 99:937–43. doi: 10.1172/JCI119258
52. Moon WY, Powis SJ. Does natural killer cell deficiency (NKD) increase the risk of cancer? NKD may increase the risk of some virus induced cancer. Front Immunol (2019) 10:1703. doi: 10.3389/fimmu.2019.01703
53. Balducci L, Ershler WB. Cancer and ageing: a nexus at several levels. Nat Rev Cancer (2005) 5:655–62. doi: 10.1038/nrc1675
54. Hazeldine J, Hampson P, Lord JM. Reduced release and binding of perforin at the immunological synapse underlies the age-related decline in natural killer cell cytotoxicity. Aging Cell (2012) 11:751–9. doi: 10.1111/j.1474-9726.2012.00839.x
55. Listi F, Caruso C, Colonna-Romano G, Lio D, Nuzzo D, Candore G. HLA and KIR frequencies in Sicilian centenarians. Rejuvenation Res (2010) 13:314–8. doi: 10.1089/rej.2009.0984
56. Fali T, Papagno L, Bayard C, Mouloud Y, Boddaert J, Sauce D, et al. New insights into lymphocyte differentiation and aging from telomere length and telomerase activity measurements. J Immunol (2019) 202:1962–9. doi: 10.4049/jimmunol.1801475
57. Luz C, Dornelles F, Preissler T, Collaziol D, da Cruz IM, Bauer ME. Impact of psychological and endocrine factors on cytokine production of healthy elderly people. Mech Ageing Dev (2003) 124:887–95. doi: 10.1016/s0047-6374(03)00148-9
58. Capellino S, Claus M, Watzl C. Regulation of natural killer cell activity by glucocorticoids, serotonin, dopamine, and epinephrine. Cell Mol Immunol (2020) 17:705–11. doi: 10.1038/s41423-020-0477-9
59. Matt S, Gaskill P. Where is dopamine and how do immune cells see it?: dopamine-mediated immune cell function in health and disease. J Neuroimmune Pharmacol (2020) 15:114–64. doi: 10.1007/s11481-019-09851-4
60. Rukavina D, Laskarin G, Rubesa G, Strbo N, Bedenicki I, Manestar D, et al. Age-related decline of perforin expression in human cytotoxic T lymphocytes and natural killer cells. Blood (1998) 92:2410–20. doi: 10.1182/blood.V92.7.2410
61. Eddy JL, Krukowski K, Janusek L, Mathews HL. Glucocorticoids regulate natural killer cell function epigenetically. Cell Immunol (2014) 290:120–30. doi: 10.1016/j.cellimm.2014.05.013
62. Yiallouris A, Tsioutis C, Agapidaki E, Zafeiri M, Agouridis AP, Ntourakis D, et al. Adrenal aging and its implications on stress responsiveness in humans. Front Endocrinol (2019) 10:54. doi: 10.3389/fendo.2019.00054
63. Frippiat C, Chen QM, Zdanov S, Magalhaes J-P, Remacle J, Toussaint O. Subcytotoxic H2O2 stress triggers a release of transforming growth factor-β1, which induces biomarkers of cellular senescence of human diploid fibroblasts. J Biol Chem (2001) 276:2531–7. doi: 10.1074/jbc.M006809200
64. Chen Y, Klein SL, Garibaldi BT, Li H, Wu C, Osevala NM, et al. Aging in COVID-19: vulnerability, immunity and intervention. Ageing Res Rev (2021) 65:101205. doi: 10.1016/j.arr.2020.101205
65. Hazeldine J, Lord JM. Immunesenescence: a predisposing risk factor for the development of COVID-19? Front Immunol (2020) 11:573662. doi: 10.3389/fimmu.2020.573662
66. Perrotta F, Corbi G, Mazzeo G, Boccia M, Aronne L, D’Agnano V, et al. COVID-19 and the elderly: insights into pathogenesis and clinical decision-making. Aging Clin Exp Res (2020) 32:1599–608. doi: 10.1007/s40520-020-01631-y
67. Hadjadj J, Yatim N, Barnabei L, Corneau A, Boussier J, Smith N, et al. Impaired type I interferon activity and inflammatory responses in severe COVID-19 patients. Science (2020) 369:718–24. doi: 10.1126/science.abc6027
68. Kuri-Cervantes L, Pampena MB, Meng W, Rosenfeld AM, Ittner CA, Weisman AR, et al. Comprehensive mapping of immune perturbations associated with severe COVID-19. Sci Immunol (2020) 5:eabd7114. doi: 10.1126/sciimmunol.abd7114
69. Maucourant C, Filipovic I, Ponzetta A, Aleman S, Cornillet M, Hertwig L, et al. Natural killer cell immunotypes related to COVID-19 disease severity. Sci Immunol (2020) 5:eabd6832. doi: 10.1126/sciimmunol.abd6832
70. Lewis SA, Sureshchandra S, Zulu MZ, Doratt B, Jankeel A, Ibraim IC, et al. Differential dynamics of peripheral immune responses to acute SARS-CoV-2 infection in older adults. Nat Aging (2021) 1:1038–52. doi: 10.1038/s43587-021-00127-2
71. Cerwenka A, Lanier LL. Natural killer cell memory in infection, inflammation and cancer. Nat Rev Immunol (2016) 16:112–23. doi: 10.1038/nri.2015.9
72. Foley B, Cooley S, Verneris MR, Pitt M, Curtsinger J, Luo X, et al. Cytomegalovirus reactivation after allogeneic transplantation promotes a lasting increase in educated NKG2C+ natural killer cells with potent function. Blood (2012) 119:2665–74. doi: 10.1182/blood-2011-10-386995
73. Lopez-Vergès S, Milush JM, Schwartz BS, Pando MJ, Jarjoura J, York VA, et al. Expansion of a unique CD57+ NKG2Chi natural killer cell subset during acute human cytomegalovirus infection. Proc Natl Acad Sci USA (2011) 108:14725–32. doi: 10.1073/pnas.1110900108
74. Ruggeri L, Capanni M, Urbani E, Perruccio K, Shlomchik WD, Tosti A, et al. Effectiveness of donor natural killer cell alloreactivity in mismatched hematopoietic transplants. Science (2002) 295:2097–100. doi: 10.1126/science.1068440
75. Yoon SR, Lee Y, Yang S, Ahn K, Lee J-H, Lee J-H, et al. Generation of donor natural killer cells from CD34+ progenitor cells and subsequent infusion after HLA-mismatched allogeneic hematopoietic cell transplantation: a feasibility study. Bone Marrow Transplant (2010) 45:1038–46. doi: 10.1038/bmt.2009.304
76. Silla L, Valim V, Pezzi A, da Silva M, Wilke I, Nobrega J, et al. Adoptive immunotherapy with double-bright (CD56bright/CD16bright) expanded natural killer cells in patients with relapsed or refractory acute myeloid leukaemia: a proof-of-concept study. Br J Haematol (2021) 195:710–21. doi: 10.1111/bjh.17751
77. Rubio-Azpeitia E, Pérez-Corral AM, Dorado-Herrero N, Monsalvo S, Pérez-Balsera G, Fernández-Santos ME, et al. Clinical grade production of IL-15 stimulated NK cells for early infusion in adult AML patients undergoing haploidentical stem cell transplantation with post-transplant cyclophosphamide. Transfusion (2022) 62:374–85. doi: 10.1111/trf.16790
78. Li C, Yang N, Li H, Wang Z. Robo1-specific chimeric antigen receptor natural killer cell therapy for pancreatic ductal adenocarcinoma with liver metastasis. J Cancer Res Ther (2020) 16:393–6. doi: 10.4103/jcrt.JCRT_190_20
79. Strecker M, Wlotzka K, Strassheimer F, Roller B, Ludmirski G, König S, et al. AAV-mediated gene transfer of a checkpoint inhibitor in combination with HER2-targeted CAR-NK cells as experimental therapy for glioblastoma. OncoImmunology (2022) 11:2127508. doi: 10.1080/2162402X.2022.2127508
80. Zhang X, Guo Y, Ji Y, Gao Y, Zhang M, Liu Y, et al. Cytokine release syndrome after modified CAR-NK therapy in an advanced non-small cell lung cancer patient: a case report. Cell Transplant (2022) 31:09636897221094244. doi: 10.1177/09636897221094244
81. Xiao L, Cen D, Gan H, Sun Y, Huang N, Xiong H, et al. Adoptive transfer of NKG2D CAR mRNA-engineered natural killer cells in colorectal cancer patients. Mol Ther (2019) 27:1114–25. doi: 10.1016/j.ymthe.2019.03.011
82. Tang X, Yang L, Li Z, Nalin AP, Dai H, Xu T, et al. First-in-man clinical trial of CAR NK-92 cells: safety test of CD33-CAR NK-92 cells in patients with relapsed and refractory acute myeloid leukemia. Am J Cancer Res (2018) 8:1083–9.
83. Ng YY, Du Z, Zhang X, Chng WJ, Wang S. CXCR4 and anti-BCMA CAR co-modified natural killer cells suppress multiple myeloma progression in a xenograft mouse model. Cancer Gene Ther (2022) 29:475–83. doi: 10.1038/s41417-021-00365-x
84. Juckett M, Mailankody S, Eghtedar A, Bickers C, Zong X, Wong L, et al. A phase I study of FT538, an off-the-Shelf, multiplexed-engineered, iPSC-derived NK cell therapy in combination with daratumumab in Relapsed/Refractory multiple myeloma. Blood (2022) 140:10327–8. doi: 10.1182/blood-2022-166728
85. Bachanova V, Ghobadi A, Patel K, Park JH, Flinn IW, Shah P, et al. Safety and efficacy of FT596, a first-in-class, multi-antigen targeted, off-the-shelf, iPSC-derived CD19 CAR NK cell therapy in relapsed/refractory b-cell lymphoma. Blood (2021) 138:823. doi: 10.1182/blood-2021-151185
86. Noh J-Y, Seo H, Lee J, Jung H. Immunotherapy in hematologic malignancies: emerging therapies and novel approaches. Int J Mol Sci (2020) 21:8000. doi: 10.3390/ijms21218000
87. Leivas A, Perez-Martinez A, Blanchard MJ, Martín-Clavero E, Fernández L, Lahuerta JJ, et al. Novel treatment strategy with autologous activated and expanded natural killer cells plus anti-myeloma drugs for multiple myeloma. Oncoimmunology (2016) 5:e1250051. doi: 10.1080/2162402X.2016.1250051
88. Ran GH, Lin YQ, Tian L, Zhang T, Yan DM, Yu JH, et al. Natural killer cell homing and trafficking in tissues and tumors: from biology to application. Signal Transduct Target Ther (2022) 7:205. doi: 10.1038/s41392-022-01058-z
89. Schuster SJ, Bishop MR, Tam CS, Waller EK, Borchmann P, McGuirk JP, et al. Tisagenlecleucel in adult relapsed or refractory diffuse large b-cell lymphoma. N Engl J Med (2019) 380:45–56. doi: 10.1056/NEJMoa1804980
90. Raje N, Berdeja J, Lin Y, Siegel D, Jagannath S, Madduri D, et al. Anti-BCMA CAR T-cell therapy bb2121 in relapsed or refractory multiple myeloma. N Engl J Med (2019) 380:1726–37. doi: 10.1056/NEJMoa1817226
91. Wang M, Munoz J, Goy A, Locke FL, Jacobson CA, Hill BT, et al. KTE-X19 CAR T-cell therapy in relapsed or refractory mantle-cell lymphoma. N Engl J Med (2020) 382:1331–42. doi: 10.1056/NEJMoa1914347
92. Liu E, Marin D, Banerjee P, Macapinlac HA, Thompson P, Basar R, et al. Use of CAR-transduced natural killer cells in CD19-positive lymphoid tumors. N Engl J Med (2020) 382:545–53. doi: 10.1056/NEJMoa1910607
93. Oei VYS, Siernicka M, Graczyk-Jarzynka A, Hoel HJ, Yang W, Palacios D, et al. Intrinsic functional potential of NK-cell subsets constrains retargeting driven by chimeric antigen ReceptorsIntrinsic functionality of NK cells affects CAR retargeting. Cancer Immunol Res (2018) 6:467–80. doi: 10.1158/2326-6066.CIR-17-0207
94. Xu Y, Liu Q, Zhong M, Wang Z, Chen Z, Zhang Y, et al. 2B4 costimulatory domain enhancing cytotoxic ability of anti-CD5 chimeric antigen receptor engineered natural killer cells against T cell malignancies. J Hematol Oncol (2019) 12:1–13. doi: 10.1186/s13045-019-0732-7
95. You F, Wang Y, Jiang L, Zhu X, Chen D, Yuan L, et al. A novel CD7 chimeric antigen receptor-modified NK-92MI cell line targeting T-cell acute lymphoblastic leukemia. Am J Cancer Res (2019) 9:64.
96. Soo RA, Chen Z, Teng RSY, Tan H-L, Iacopetta B, Tai BC, et al. Prognostic significance of immune cells in non-small cell lung cancer: meta-analysis. Oncotarget (2018) 9:24801. doi: 10.18632/oncotarget.24835
97. Cursons J, Souza-Fonseca-Guimaraes F, Foroutan M, Anderson A, Hollande F, Hediyeh-Zadeh S, et al. A gene signature predicting natural killer cell infiltration and improved survival in melanoma PatientsA gene signature for NK infiltration and melanoma survival. Cancer Immunol Res (2019) 7:1162–74. doi: 10.1158/2326-6066.CIR-18-0500
98. Tang Y-P, Xie M-Z, Li K-Z, Li J-L, Cai Z-M, Hu B-L. Prognostic value of peripheral blood natural killer cells in colorectal cancer. BMC Gastroenterol (2020) 20:1–8. doi: 10.1186/s12876-020-1177-8
99. Wu M, Mei F, Liu W, Jiang J. Comprehensive characterization of tumor infiltrating natural killer cells and clinical significance in hepatocellular carcinoma based on gene expression profiles. BioMed Pharmacother (2020) 121:109637. doi: 10.1016/j.biopha.2019.109637
100. Gannon PO, Poisson AO, Delvoye N, Lapointe R, Mes-Masson A-M, Saad F. Characterization of the intra-prostatic immune cell infiltration in androgen-deprived prostate cancer patients. J Immunol Methods (2009) 348:9–17. doi: 10.1016/j.jim.2009.06.004
101. Donskov F, von der Maase H. Impact of immune parameters on long-term survival in metastatic renal cell carcinoma. J Clin Oncol (2006) 24:1997–2005. doi: 10.1200/JCO.2005.03.9594
102. Maskalenko NA, Zhigarev D, Campbell KS. Harnessing natural killer cells for cancer immunotherapy: dispatching the first responders. Nat Rev Drug Discovery (2022) 21:559–77. doi: 10.1038/s41573-022-00413-7
103. Cózar B, Greppi M, Carpentier S, Narni-Mancinelli E, Chiossone L, Vivier E. Tumor-infiltrating natural killer CellsTumor-infiltrating natural killer cells. Cancer Discovery (2021) 11:34–44. doi: 10.1158/2159-8290.CD-20-0655
104. Concha-Benavente F, Kansy B, Moskovitz J, Moy J, Chandran U, Ferris RL. PD-L1 mediates dysfunction in activated PD-1+ NK cells in head and neck cancer PatientsPD-1/PD-L1 axis prevents NK cell cytotoxicity in HNC. Cancer Immunol Res (2018) 6:1548–60. doi: 10.1158/2326-6066.CIR-18-0062
105. Stankovic B, Bjørhovde HAK, Skarshaug R, Aamodt H, Frafjord A, Müller E, et al. Immune cell composition in human non-small cell lung cancer. Front Immunol (2019) 9:3101. doi: 10.3389/fimmu.2018.03101
106. Peng L-S, Zhang J-Y, Teng Y-S, Zhao Y-L, Wang T-T, Mao F-Y, et al. Tumor-associated Monocytes/Macrophages impair NK-cell function via TGFβ1 in human gastric CancerNK-cell functional impairment in gastric cancer. Cancer Immunol Res (2017) 5:248–56. doi: 10.1158/2326-6066.CIR-16-0152
107. Mamessier E, Sylvain A, Thibult M-L, Houvenaeghel G, Jacquemier J, Castellano R, et al. Human breast cancer cells enhance self tolerance by promoting evasion from NK cell antitumor immunity. J Clin Invest (2011) 121:3609–22. doi: 10.1172/JCI45816
108. Semeraro M, Rusakiewicz S, Minard-Colin V, Delahaye NF, Enot D, Vély F, et al. Clinical impact of the NKp30/B7-H6 axis in high-risk neuroblastoma patients. Sci Transl Med (2015) 7:283ra55–ra55. doi: 10.1126/scitranslmed.aaa2327
109. Mantovani S, Oliviero B, Lombardi A, Varchetta S, Mele D, Sangiovanni A, et al. Deficient natural killer cell NKp30-mediated function and altered NCR3 splice variants in hepatocellular carcinoma. Hepatology (2019) 69:1165–79. doi: 10.1002/hep.30235
110. Pesce S, Greppi M, Tabellini G, Rampinelli F, Parolini S, Olive D, et al. Identification of a subset of human natural killer cells expressing high levels of programmed death 1: a phenotypic and functional characterization. J Allergy Clin Immunol (2017) 139:335–46 e3. doi: 10.1016/j.jaci.2016.04.025
111. Liu Y, Cheng Y, Xu Y, Wang Z, Du X, Li C, et al. Increased expression of programmed cell death protein 1 on NK cells inhibits NK-cell-mediated anti-tumor function and indicates poor prognosis in digestive cancers. Oncogene (2017) 36:6143–53e3. doi: 10.1038/onc.2017.209
112. Seo H, Jeon I, Kim B-S, Park M, Bae E-A, Song B, et al. IL-21-mediated reversal of NK cell exhaustion facilitates anti-tumour immunity in MHC class I-deficient tumours. Nat Commun (2017) 8:15776. doi: 10.1038/ncomms15776
113. Zhang Q, Bi J, Zheng X, Chen Y, Wang H, Wu W, et al. Blockade of the checkpoint receptor TIGIT prevents NK cell exhaustion and elicits potent anti-tumor immunity. Nat Immunol (2018) 19:723–32. doi: 10.1038/s41590-018-0132-0
114. Hervier B, Russick J, Cremer I, Vieillard V. NK cells in the human lungs. Front Immunol (2019) 10:1263. doi: 10.3389/fimmu.2019.01263
115. Grégoire C, Chasson L, Luci C, Tomasello E, Geissmann F, Vivier E, et al. The trafficking of natural killer cells. Immunol Rev (2007) 220:169–82. doi: 10.1111/j.1600-065X.2007.00563.x
116. Jemal A, Bray F, Center MM, Ferlay J, Ward E, Forman D. Global cancer statistics. CA Cancer J Clin (2011) 61:69–90. doi: 10.3322/caac.20107
117. Zhou Y, Chen C, Zhang X, Fu S, Xue C, Ma Y, et al. Immune-checkpoint inhibitor plus chemotherapy versus conventional chemotherapy for first-line treatment in advanced non-small cell lung carcinoma: a systematic review and meta-analysis. J Immunother Cancer (2018) 6:1–11. doi: 10.1186/s40425-018-0477-9
118. Jia L, Chen N, Chen X, Niu C, Liu Z-L, Ma K, et al. Sintilimab plus autologous NK cells as second-line treatment for advanced non-small-cell lung cancer previous treated with platinum-containing chemotherapy. Front Immunol (2022) 13:1074906. doi: 10.3389/fimmu.2022.1074906
119. Hong G, Chen X, Sun X, Zhou M, Liu B, Li Z, et al. Effect of autologous NK cell immunotherapy on advanced lung adenocarcinoma with EGFR mutations. Precis Clin Med (2019) 2:235–45. doi: 10.1093/pcmedi/pbz023
120. Lin M, Luo H, Liang S, Chen J, Liu A, Niu L, et al. Pembrolizumab plus allogeneic NK cells in advanced non–small cell lung cancer patients. J Clin Invest (2020) 130:2560–9. doi: 10.1172/JCI132712
121. Tomasello E, Yessaad N, Gregoire E, Hudspeth K, Luci C, Mavilio D, et al. Mapping of NKp46+ cells in healthy human lymphoid and non-lymphoid tissues. Front Immunol (2012) 3:344. doi: 10.3389/fimmu.2012.00344
122. Robinson B, Pinkston P, Crystal RG. Natural killer cells are present in the normal human lung but are functionally impotent. J Clin Invest (1984) 74:942–50. doi: 10.1172/JCI111513
123. Marquardt N, Kekäläinen E, Chen P, Lourda M, Wilson JN, Scharenberg M, et al. Unique transcriptional and protein-expression signature in human lung tissue-resident NK cells. Nat Commun (2019) 10:3841. doi: 10.1038/s41467-019-11632-9
124. Cooper GE, Ostridge K, Khakoo SI, Wilkinson TM, Staples KJ. Human CD49a+ lung natural killer cell cytotoxicity in response to influenza a virus. Front Immunol (2018) 9:1671. doi: 10.3389/fimmu.2018.01671
125. Brownlie D, Scharenberg M, Mold JE, Hård J, Kekäläinen E, Buggert M, et al. Expansions of adaptive-like NK cells with a tissue-resident phenotype in human lung and blood. Proc Natl Acad Sci USA (2021) 118:e2016580118. doi: 10.1073/pnas.2016580118
126. Mikulak J, Bruni E, Oriolo F, Di Vito C, Mavilio D. Hepatic natural killer cells: organ-specific sentinels of liver immune homeostasis and physiopathology. Front Immunol (2019) 10:946. doi: 10.3389/fimmu.2019.00946
127. Akinyemiju T, Abera S, Ahmed M, Alam N, Alemayohu MA, Allen C, et al. The burden of primary liver cancer and underlying etiologies from 1990 to 2015 at the global, regional, and national level: results from the global burden of disease study 2015. JAMA Oncol (2017) 3:1683–91. doi: 10.1001/jamaoncol.2017.3055
128. Lee HA, Goh HG, Lee Y-S, Jung YK, Kim JH, Yim HJ, et al. Natural killer cell activity is a risk factor for the recurrence risk after curative treatment of hepatocellular carcinoma. BMC Gastroenterol (2021) 21:1–10. doi: 10.1186/s12876-021-01833-2
129. Yu M, Luo H, Fan M, Wu X, Shi B, Di S, et al. Development of GPC3-specific chimeric antigen receptor-engineered natural killer cells for the treatment of hepatocellular carcinoma. Mol Ther (2018) 26:366–78. doi: 10.1016/j.ymthe.2017.12.012
130. Arai J, Goto K, Tanoue Y, Ito S, Muroyama R, Matsubara Y, et al. Enzymatic inhibition of MICA sheddase ADAM17 by lomofungin in hepatocellular carcinoma cells. Int J Cancer (2018) 143:2575–83. doi: 10.1002/ijc.31615
131. Yang Y, Qin Z, Du D, Wu Y, Qiu S, Mu F, et al. Safety and short-term efficacy of irreversible electroporation and allogenic natural killer cell immunotherapy combination in the treatment of patients with unresectable primary liver cancer. Cardiovasc Intervent Radiol (2019) 42:48–59. doi: 10.1007/s00270-018-2069-y
132. Teng K-Y, Mansour AG, Zhu Z, Li Z, Tian L, Ma S, et al. Off-the-Shelf prostate stem cell antigen–directed chimeric antigen receptor natural killer cell therapy to treat pancreatic cancer. Gastroenterology (2022) 162:1319–33. doi: 10.1053/j.gastro.2021.12.281
133. Yeo D, Giardina C, Saxena P, Rasko JE. The next wave of cellular immunotherapies in pancreatic cancer. Mol Ther Oncolytics (2022) 24:561–76. doi: 10.1016/j.omto.2022.01.010
134. Phan TG, Croucher PI. The dormant cancer cell life cycle. Nat Rev Cancer (2020) 20:398–411. doi: 10.1038/s41568-020-0263-0
135. Chan IS, Ewald AJ. The changing role of natural killer cells in cancer metastasis. J Clin Invest (2022) 132(6):e143762. doi: 10.1172/JCI143762
136. Aceto N, Bardia A, Miyamoto DT, Donaldson MC, Wittner BS, Spencer JA, et al. Circulating tumor cell clusters are oligoclonal precursors of breast cancer metastasis. Cell (2014) 158:1110–22. doi: 10.1016/j.cell.2014.07.013
137. Maddipati R, Stanger BZ. Pancreatic cancer metastases harbor evidence of PolyclonalityClonality of metastases. Cancer Discovery (2015) 5:1086–97. doi: 10.1158/2159-8290.CD-15-0120
138. Liu X, Taftaf R, Kawaguchi M, Chang Y-F, Chen W, Entenberg D, et al. Homophilic CD44 interactions mediate tumor cell aggregation and polyclonal metastasis in patient-derived breast cancer ModelsHomophilic CD44 interactions mediate tumor cell aggregation. Cancer Discovery (2019) 9:96–113. doi: 10.1158/2159-8290.CD-18-0065
139. Placke T, Salih HR, Kopp H-G. GITR ligand provided by thrombopoietic cells inhibits NK cell antitumor activity. J Immunol (2012) 189:154–60. doi: 10.4049/jimmunol.1103194
140. Myers JA, Miller JS. Exploring the NK cell platform for cancer immunotherapy. Nat Rev Clin Oncol (2021) 18:85–100. doi: 10.1038/s41571-020-0426-7
141. Demaria O, Cornen S, Daëron M, Morel Y, Medzhitov R, Vivier E. Harnessing innate immunity in cancer therapy. Nature (2019) 574:45–56. doi: 10.1038/s41586-019-1593-5
142. Beffinger M, de Lara PT, Tugues S, Vermeer M, Montagnolo Y, Ohs I, et al. CSF1R-dependent myeloid cells are required for NK−mediated control of metastasis. JCI Insight (2018) 3(10):e97792. doi: 10.1172/jci.insight.97792
143. Cheung KJ, Gabrielson E, Werb Z, Ewald AJ. Collective invasion in breast cancer requires a conserved basal epithelial program. Cell (2013) 155:1639–51. doi: 10.1016/j.cell.2013.11.029
144. Kärre K, Ljunggren HG, Piontek G, Kiessling R. Selective rejection of h–2-deficient lymphoma variants suggests alternative immune defence strategy. Nature (1986) 319:675–8. doi: 10.1038/319675a0
145. Cheung KJ, Padmanaban V, Silvestri V, Schipper K, Cohen JD, Fairchild AN, et al. Polyclonal breast cancer metastases arise from collective dissemination of keratin 14-expressing tumor cell clusters. Proc Natl Acad Sci USA (2016) 113:E854–E63. doi: 10.1073/pnas.1508541113
146. Campbell K, Rossi F, Adams J, Pitsidianaki I, Barriga FM, Garcia-Gerique L, et al. Collective cell migration and metastases induced by an epithelial-to-mesenchymal transition in drosophila intestinal tumors. Nat Commun (2019) 10:2311. doi: 10.1038/s41467-019-10269-y
147. Donato C, Kunz L, Castro-Giner F, Paasinen-Sohns A, Strittmatter K, Szczerba BM, et al. Hypoxia triggers the intravasation of clustered circulating tumor cells. Cell Rep (2020) 32:108105. doi: 10.1016/j.celrep.2020.108105
148. Lo HC, Xu Z, Kim IS, Pingel B, Aguirre S, Kodali S, et al. Resistance to natural killer cell immunosurveillance confers a selective advantage to polyclonal metastasis. Nat Cancer (2020) 1:709–22. doi: 10.1038/s43018-020-0068-9
149. Padmanaban V, Krol I, Suhail Y, Szczerba BM, Aceto N, Bader JS, et al. E-cadherin is required for metastasis in multiple models of breast cancer. Nature (2019) 573:439–44. doi: 10.1038/s41586-019-1526-3
150. Kim K, Marquez-Palencia M, Malladi S. Metastatic latency, a veiled threat. Front Immunol (2019) 10:1836. doi: 10.3389/fimmu.2019.01836
151. Laughney AM, Hu J, Campbell NR, Bakhoum SF, Setty M, Lavallee V-P, et al. Regenerative lineages and immune-mediated pruning in lung cancer metastasis. Nat Med (2020) 26:259–69. doi: 10.1038/s41591-019-0750-6
152. Malladi S, Macalinao DG, Jin X, He L, Basnet H, Zou Y, et al. Metastatic latency and immune evasion through autocrine inhibition of WNT. Cell (2016) 165:45–60. doi: 10.1016/j.cell.2016.02.025
153. Chan IS, Knútsdóttir H, Ramakrishnan G, Padmanaban V, Warrier M, Ramirez JC, et al. Cancer cells educate natural killer cells to a metastasis-promoting cell state. J Cell Biol (2020) 219(9):e202001134. doi: 10.1083/jcb.202001134
154. de Jonge K, Ebering A, Nassiri S, Maby-El Hajjami H, Ouertatani-Sakouhi H, Baumgaertner P, et al. Circulating CD56bright NK cells inversely correlate with survival of melanoma patients. Sci Rep (2019) 9:4487. doi: 10.1038/s41598-019-40933-8
155. Palmer S, Albergante L, Blackburn CC, Newman T. Thymic involution and rising disease incidence with age. Proc Natl Acad Sci USA (2018) 115:1883–8. doi: 10.1073/pnas.1714478115
156. Laconi E, Marongiu F, DeGregori J. Cancer as a disease of old age: changing mutational and microenvironmental landscapes. Br J Cancer (2020) 122:943–52. doi: 10.1038/s41416-019-0721-1
157. López-Otín C, Blasco MA, Partridge L, Serrano M, Kroemer G. Hallmarks of aging: an expanding universe. Cell (2023) 186(2):243–78. doi: 10.1016/j.cell.2022.11.001
158. Mooi W, Peeper D. Oncogene-induced cell senescence–halting on the road to cancer. N Engl J Med (2006) 355:1037–46. doi: 10.1056/NEJMra062285
159. Seluanov A, Gladyshev VN, Vijg J, Gorbunova V. Mechanisms of cancer resistance in long-lived mammals. Nat Rev Cancer (2018) 18:433–41. doi: 10.1038/s41568-018-0004-9
160. Vincze O, Colchero F, Lemaître J-F, Conde DA, Pavard S, Bieuville M, et al. Cancer risk across mammals. Nature (2022) 601:263–7. doi: 10.1038/s41586-021-04224-5
161. Peacocke M, Campisi J. Cellular senescence: a reflection of normal growth control, differentiation, or aging? J Cell Biochem (1991) 45:147–55. doi: 10.1002/jcb.240450205
162. Ecker BL, Kaur A, Douglass SM, Webster MR, Almeida FV, Marino GE, et al. Age-related changes in HAPLN1 increase lymphatic permeability and affect routes of melanoma MetastasisAge-induced lymphatic permeability increases metastasis. Cancer Discovery (2019) 9:82–95. doi: 10.1158/2159-8290.CD-18-0168
163. Wiley CD, Campisi J. The metabolic roots of senescence: mechanisms and opportunities for intervention. Nat Metab (2021) 3:1290–301. doi: 10.1038/s42255-021-00483-8
164. Roubenoff R, Hughes VA. Sarcopenia: current concepts. J Gerontol A BIol Sci Med Sci (2000) 55:M716–M24. doi: 10.1093/gerona/55.12.M716
165. Moiseeva V, Cisneros A, Sica V, Deryagin O, Lai Y, Jung S, et al. Senescence atlas reveals an aged-like inflamed niche that blunts muscle regeneration. Nature (2022):1–10. doi: 10.1038/s41586-023-05765-7
166. Calcinotto A, Kohli J, Zagato E, Pellegrini L, Demaria M, Alimonti A. Cellular senescence: aging, cancer, and injury. Physiol Rev (2019) 99:1047–78. doi: 10.1152/physrev.00020.2018
167. Bektas A, Schurman SH, Sen R, Ferrucci L. Aging, inflammation and the environment. Exp Gerontol (2018) 105:10–8. doi: 10.1016/j.exger.2017.12.015
168. Childs BG, Gluscevic M, Baker DJ, Laberge R-M, Marquess D, Dananberg J, et al. Senescent cells: an emerging target for diseases of ageing. Nat Rev Drug Discovery (2017) 16:718–35. doi: 10.1038/nrd.2017.116
169. Baker DJ, Childs BG, Durik M, Wijers ME, Sieben CJ, Zhong J, et al. Naturally occurring p16Ink4a-positive cells shorten healthy lifespan. Nature (2016) 530:184–9. doi: 10.1038/nature16932
170. Baker DJ, Wijshake T, Tchkonia T, LeBrasseur NK, Childs BG, Van De Sluis B, et al. Clearance of p16Ink4a-positive senescent cells delays ageing-associated disorders. Nature (2011) 479:232–6. doi: 10.1038/nature10600
171. Eggert T, Wolter K, Ji J, Ma C, Yevsa T, Klotz S, et al. Distinct functions of senescence-associated immune responses in liver tumor surveillance and tumor progression. Cancer Cell (2016) 30:533–47. doi: 10.1016/j.ccell.2016.09.003
172. Sagiv A, Burton DG, Moshayev Z, Vadai E, Wensveen F, Ben-Dor S, et al. NKG2D ligands mediate immunosurveillance of senescent cells. Aging (Albany NY) (2016) 8:328–44. doi: 10.18632/aging.100897
173. Pereira BI, Devine OP, Vukmanovic-Stejic M, Chambers ES, Subramanian P, Patel N, et al. Senescent cells evade immune clearance via HLA-e-mediated NK and CD8+ T cell inhibition. Nat Commun (2019) 10:2387. doi: 10.1038/s41467-019-10335-5
174. Ruggeri L, Urbani E, André P, Mancusi A, Tosti A, Topini F, et al. Effects of anti-NKG2A antibody administration on leukemia and normal hematopoietic cells. Haematologica (2016) 101:626. doi: 10.3324/haematol.2015.135301
175. van Deursen JM. Senolytic therapies for healthy longevity. Science (2019) 364:636–7. doi: 10.1126/science.aaw1299
176. Chang J, Wang Y, Shao L, Laberge R-M, Demaria M, Campisi J, et al. Clearance of senescent cells by ABT263 rejuvenates aged hematopoietic stem cells in mice. Nat Med (2016) 22:78–83. doi: 10.1038/nm.4010
177. Neasta J, Valmalle C, Coyne AC, Carnazzi E, Subra G, Galleyrand JC, et al. The novel nonapeptide acein targets angiotensin converting enzyme in the brain and induces dopamine release. Br J Pharmacol (2016) 173:1314–28. doi: 10.1111/bph.13424
178. Bai Z, Yang P, Yu F, Li Z, Yao Z, Martinez J, et al. Combining adoptive NK cell infusion with a dopamine-releasing peptide reduces senescent cells in aged mice. Cell Death Dis (2022) 13:305. doi: 10.1038/s41419-022-04562-w
179. Amor C, Feucht J, Leibold J, Ho Y-J, Zhu C, Alonso-Curbelo D, et al. Senolytic CAR T cells reverse senescence-associated pathologies. Nature (2020) 583:127–32. doi: 10.1038/s41586-020-2403-9
180. Deng X, Terunuma H, Nieda M. Immunosurveillance of cancer and viral infections with regard to alterations of human NK cells originating from lifestyle and aging. Biomedicines (2021) 9:557. doi: 10.3390/biomedicines9050557
181. Fogel LA, Yokoyama WM, French AR. Natural killer cells in human autoimmune disorders. Arthritis Res Ther (2013) 15:1–9. doi: 10.1186/ar4232
182. Bielekova B, Catalfamo M, Reichert-Scrivner S, Packer A, Cerna M, Waldmann TA, et al. Regulatory CD56bright natural killer cells mediate immunomodulatory effects of IL-2Rα-targeted therapy (daclizumab) in multiple sclerosis. Proc Natl Acad Sci USA (2006) 103:5941–6. doi: 10.1073/pnas.0601335103
183. Zafarani A, Razizadeh MH, Pashangzadeh S, Amirzargar MR, Taghavi-Farahabadi M, Mahmoudi M. Natural killer cells in COVID-19: from infection, to vaccination and therapy. Future Virol (2023) 18:177–91. doi: 10.2217/fvl-2022-0040
184. Wu S-Y, Fu T, Jiang Y-Z, Shao Z-M. Natural killer cells in cancer biology and therapy. Mol Cancer (2020) 19:1–26. doi: 10.1186/s12943-020-01238-x
185. Heipertz EL, Zynda ER, Stav-Noraas TE, Hungler AD, Boucher SE, Kaur N, et al. Current perspectives on “off-the-shelf” allogeneic NK and CAR-NK cell therapies. Front Immunol (2021) 12:732135. doi: 10.3389/fimmu.2021.732135
186. Huang R-S, Lai M-C, Shih H-A, Lin S. A robust platform for expansion and genome editing of primary human natural killer cells. J Exp Med (2021) 218(3):e20201529. doi: 10.1084/jem.20201529
187. Romee R, Rosario M, Berrien-Elliott MM, Wagner JA, Jewell BA, Schappe T, et al. Cytokine-induced memory-like natural killer cells exhibit enhanced responses against myeloid leukemia. Sci Transl Med (2016) 8:357ra123. doi: 10.1126/scitranslmed.aaf2341
188. Gong J-H, Maki G, Klingemann HG. Characterization of a human cell line (NK-92) with phenotypical and functional characteristics of activated natural killer cells. Leukemia (1994) 8:652–8.
189. Li Y, Hermanson DL, Moriarity BS, Kaufman DS. Human iPSC-derived natural killer cells engineered with chimeric antigen receptors enhance anti-tumor activity. Cell Stem Cell (2018) 23:181–92. doi: 10.1016/j.stem.2018.06.002
190. Herrera L, Santos S, Vesga M, Anguita J, Martin-Ruiz I, Carrascosa T, et al. Adult peripheral blood and umbilical cord blood NK cells are good sources for effective CAR therapy against CD19 positive leukemic cells. Sci Rep (2019) 9:18729. doi: 10.1038/s41598-019-55239-y
191. Rafiq S, Hackett CS, Brentjens RJ. Engineering strategies to overcome the current roadblocks in CAR T cell therapy. Nat Rev Clin Oncol (2020) 17:147–67. doi: 10.1038/s41571-019-0297-y
192. Li H, Song W, Li Z, Zhang M. Preclinical and clinical studies of CAR-NK-cell therapies for malignancies. Front Immunol (2022) 13:992232. doi: 10.3389/fimmu.2022.992232
193. Wang Q-M, Tang PM-K, Lian G-Y, Li C, Li J, Huang X-R, et al. Enhanced cancer immunotherapy with Smad3-silenced NK-92 cells. Cancer Immunol Res (2018) 6:965–77. doi: 10.1158/2326-6066.CIR-17-0491
194. Töpfer K, Cartellieri M, Michen S, Wiedemuth R, Müller N, Lindemann D, et al. DAP12-based activating chimeric antigen receptor for NK cell tumor immunotherapy. J Immunol (2015) 194:3201–12. doi: 10.4049/jimmunol.1400330
195. Wang J, Lupo KB, Chambers AM, Matosevic S. Purinergic targeting enhances immunotherapy of CD73+ solid tumors with piggyBac-engineered chimeric antigen receptor natural killer cells. J Immunother Cancer (2018) 6:1–14. doi: 10.1186/s40425-018-0441-8
196. Huang Y, Zeng J, Liu T, Xu Q, Song X, Zeng J. DNAM1 and 2B4 costimulatory domains enhance the cytotoxicity of anti-GPC3 chimeric antigen receptor-modified natural killer cells against hepatocellular cancer cells in vitro. Cancer Manag Res (2020) 12:3247–55. doi: 10.2147/CMAR.S253565
197. Imamura M, Shook D, Kamiya T, Shimasaki N, Chai SM, Coustan-Smith E, et al. Autonomous growth and increased cytotoxicity of natural killer cells expressing membrane-bound interleukin-15. Blood (2014) 124:1081–8. doi: 10.1182/blood-2014-02-556837
198. Liu E, Tong Y, Dotti G, Shaim H, Savoldo B, Mukherjee M, et al. Cord blood NK cells engineered to express IL-15 and a CD19-targeted CAR show long-term persistence and potent antitumor activity. Leukemia (2018) 32:520–31. doi: 10.1038/leu.2017.226
199. Cichocki F, Bjordahl R, Goodridge JP, Mahmood S, Gaidarova S, Abujarour R, et al. Quadruple gene-engineered natural killer cells enable multi-antigen targeting for durable antitumor activity against multiple myeloma. Nat Commun (2022) 13:7341. doi: 10.1038/s41467-022-35127-2
200. Bluhm J, Kieback E, Marino SF, Oden F, Westermann J, Chmielewski M, et al. CAR T cells with enhanced sensitivity to b cell maturation antigen for the targeting of b cell non-hodgkin’s lymphoma and multiple myeloma. Mol Ther (2018) 26:1906–20. doi: 10.1016/j.ymthe.2018.06.012
201. Wu J, Edberg JC, Redecha PB, Bansal V, Guyre PM, Coleman K, et al. A novel polymorphism of FcgammaRIIIa (CD16) alters receptor function and predisposes to autoimmune disease. J Clin Invest (1997) 100:1059–70. doi: 10.1172/JCI119616
202. Zhu H, Blum RH, Bjordahl R, Gaidarova S, Rogers P, Lee TT, et al. Pluripotent stem cell–derived NK cells with high-affinity noncleavable CD16a mediate improved antitumor activity. Blood (2020) 135:399–410. doi: 10.1182/blood.2019000621
203. Woan KV, Kim H, Bjordahl R, Davis ZB, Gaidarova S, Goulding J, et al. Harnessing features of adaptive NK cells to generate iPSC-derived NK cells for enhanced immunotherapy. Cell Stem Cell (2021) 28:2062–75. doi: 10.1016/j.stem.2021.08.013
204. Dimopoulos MA, Oriol A, Nahi H, San-Miguel J, Bahlis NJ, Usmani SZ, et al. Daratumumab, lenalidomide, and dexamethasone for multiple myeloma. N Engl J Med (2016) 375:1319–31. doi: 10.1056/NEJMoa1607751
205. Zhu H, Blum RH, Bernareggi D, Ask EH, Wu Z, Hoel HJ, et al. Metabolic reprograming via deletion of CISH in human iPSC-derived NK cells promotes in vivo persistence and enhances anti-tumor activity. Cell Stem Cell (2020) 27:224–37. doi: 10.1016/j.stem.2020.05.008
206. Bi J, Huang C, Jin X, Zheng C, Huang Y, Zheng X, et al. TIPE2 deletion improves the therapeutic potential of adoptively transferred NK cells. J Immunother Cancer (2023) 11:e006002. doi: 10.1136/jitc-2022-006002
207. Bi J, Jin X, Zheng C, Huang C, Zhong C, Zheng X, et al. Checkpoint TIPE2 limits the helper functions of NK cells in supporting antitumor CD8+ T cells. Adv Sci (2023) 10:2207499. doi: 10.1002/advs.202207499
208. Kamiya T, Seow SV, Wong D, Robinson M, Campana D. Blocking expression of inhibitory receptor NKG2A overcomes tumor resistance to NK cells. J Clin Invest (2019) 129:2094–106. doi: 10.1172/JCI123955
209. Young A, Ngiow SF, Gao Y, Patch A-M, Barkauskas DS, Messaoudene M, et al. A2AR adenosine signaling suppresses natural killer cell maturation in the tumor MicroenvironmentAdenosine impairs proliferation of terminal NK cells. Cancer Res (2018) 78:1003–16. doi: 10.1158/0008-5472.CAN-17-2826
210. Shaim H, Shanley M, Basar R, Daher M, Gumin J, Zamler DB, et al. Targeting the αv integrin/TGF-β axis improves natural killer cell function against glioblastoma stem cells. J Clin Invest (2021) 131:e142116. doi: 10.1172/JCI142116
211. Kim T-D, Lee SU, Yun S, Sun H-N, Lee SH, Kim JW, et al. Human microRNA-27a* targets Prf1 and GzmB expression to regulate NK-cell cytotoxicity. Blood (2011) 118:5476–86. doi: 10.1182/blood-2011-04-347526
212. Naeimi Kararoudi M, Nagai Y, Elmas E, de Souza Fernandes Pereira M, Ali SA, Imus PH, et al. CD38 deletion of human primary NK cells eliminates daratumumab-induced fratricide and boosts their effector activity. Blood (2020) 136:2416–27. doi: 10.1182/blood.2020006200
213. Gurney M, Stikvoort A, Nolan E, Kirkham-McCarthy L, Khoruzhenko S, Shivakumar R, et al. CD38 knockout natural killer cells expressing an affinity optimized CD38 chimeric antigen receptor successfully target acute myeloid leukemia with reduced effector cell fratricide. Haematologica (2022) 107:437–45. doi: 10.3324/haematol.2020.271908
214. Ng YY, Tay JC, Wang S. CXCR1 expression to improve anti-cancer efficacy of intravenously injected CAR-NK cells in mice with peritoneal xenografts. Mol Ther Oncolytics (2020) 16:75–85. doi: 10.1016/j.omto.2019.12.006
215. Kremer V, Ligtenberg MA, Zendehdel R, Seitz C, Duivenvoorden A, Wennerberg E, et al. Genetic engineering of human NK cells to express CXCR2 improves migration to renal cell carcinoma. J Immunother Cancer (2017) 5:73. doi: 10.1186/s40425-017-0275-9
216. Bonanni V, Antonangeli F, Santoni A, Bernardini G. Targeting of CXCR3 improves anti-myeloma efficacy of adoptively transferred activated natural killer cells. J Immunother Cancer (2019) 7:290. doi: 10.1186/s40425-019-0751-5
217. Carlsten M, Levy E, Karambelkar A, Li L, Reger R, Berg M, et al. Efficient mRNA-based genetic engineering of human NK cells with high-affinity CD16 and CCR7 augments rituximab-induced ADCC against lymphoma and targets NK cell migration toward the lymph node-associated chemokine CCL19. Front Immunol (2016) 7:105. doi: 10.3389/fimmu.2016.00105
218. Müller N, Michen S, Tietze S, Töpfer K, Schulte A, Lamszus K, et al. Engineering NK cells modified with an EGFRvIII-specific chimeric antigen receptor to overexpress CXCR4 improves immunotherapy of CXCL12/SDF-1α-secreting glioblastoma. J Immunother (2015) 38:197–210. doi: 10.1097/CJI.0000000000000082
219. Yvon ES, Burga R, Powell A, Cruz CR, Fernandes R, Barese C, et al. Cord blood natural killer cells expressing a dominant negative TGF-β receptor: implications for adoptive immunotherapy for glioblastoma. Cytotherapy (2017) 19:408–18. doi: 10.1016/j.jcyt.2016.12.005
220. Burga R, Williams E, Yvon ES, Fernandes R, Cruz CRY, Bollard CM. Engineering the TGFβ receptor to enhance the therapeutic potential of natural killer cells as an immunotherapy for neuroblastoma. Clin Cancer Res (2018) 25:4400–12. doi: 10.1158/1078-0432.CCR-18-3183
221. Narayan V, Barber-Rotenberg JS, Jung I-Y, Lacey SF, Rech AJ, Davis MM, et al. PSMA-targeting TGFβ-insensitive armored CAR T cells in metastatic castration-resistant prostate cancer: a phase 1 trial. Nat Med (2022) 28:724–34. doi: 10.1038/s41591-022-01726-1
222. Lim CM, Liou A, Poon M, Koh LP, Tan LK, Loh KS, et al. Phase I study of expanded natural killer cells in combination with cetuximab for recurrent/metastatic nasopharyngeal carcinoma. Cancer Immunol Immunother (2022) 71:2277–86. doi: 10.1007/s00262-022-03158-9
223. Berrien-Elliott MM, Becker-Hapak M, Cashen AF, Jacobs M, Wong P, Foster M, et al. Systemic IL-15 promotes allogeneic cell rejection in patients treated with natural killer cell adoptive therapy. Blood (2022) 139:1177–83. doi: 10.1182/blood.2021011532
224. Stewart R, Hammond SA, Oberst M, Wilkinson RW. The role of fc gamma receptors in the activity of immunomodulatory antibodies for cancer. J Immunother Cancer (2014) 2:29. doi: 10.1186/s40425-014-0029-x
225. Treon S, Hansen M, Branagan A, Emmanouilides C, Kimby E, Frankel S, et al. Polymorphisms in FcγRIIIA (CD16) receptor expression are associated with clinical response to rituximab in waldenstrom's macroglobulinemia. J Clin Oncol (2004) 22:6556–. doi: 10.1200/JCO.2005.06.059
226. Temming AR, de Taeye SW, de Graaf EL, de Neef LA, Dekkers G, Bruggeman CW, et al. Functional attributes of antibodies, effector cells, and target cells affecting NK cell–mediated antibody-dependent cellular cytotoxicity. J Immunol (2019) 203:3126–35. doi: 10.4049/jimmunol.1900985
227. Jakubowiak A, Offidani M, Pégourie B, de la Rubia J, Garderet L, Laribi K, et al. Randomized phase 2 study: elotuzumab plus bortezomib/dexamethasone vs bortezomib/dexamethasone for relapsed/refractory MM. Blood (2016) 127:2833–40. doi: 10.1182/blood-2016-01-694604
228. Bang Y, Giaccone G, Im S, Oh D, Bauer T, Nordstrom J, et al. First-in-human phase 1 study of margetuximab (MGAH22), an fc-modified chimeric monoclonal antibody, in patients with HER2-positive advanced solid tumors. Ann Oncol (2017) 28:855–61. doi: 10.1093/annonc/mdx002
229. Boyerinas B, Jochems C, Fantini M, Heery CR, Gulley JL, Tsang KY, et al. Antibody-dependent cellular cytotoxicity activity of a novel anti–PD-L1 antibody avelumab (MSB0010718C) on human tumor CellsAn anti–PD-L1 mAb that mediates ADCC of human tumor cells. Cancer Immunol Res (2015) 3:1148–57. doi: 10.1158/2326-6066.CIR-15-0059
230. Juliá EP, Amante A, Pampena MB, Mordoh J, Levy EM. Avelumab, an IgG1 anti-PD-L1 immune checkpoint inhibitor, triggers NK cell-mediated cytotoxicity and cytokine production against triple negative breast cancer cells. Front Immunol (2018) 9:2140. doi: 10.3389/fimmu.2018.02140
231. Du C, Bevers J, Cook R, Lombana TN, Rajasekaran K, Matsumoto M, et al. MICA immune complex formed with alpha 3 domain-specific antibody activates human NK cells in a fc-dependent manner. J Immunother Cancer (2019) 7:207. doi: 10.1186/s40425-019-0687-9
232. Ellwanger K, Reusch U, Fucek I, Wingert S, Ross T, Müller T, et al. Redirected optimized cell killing (ROCK®): a highly versatile multispecific fit-for-purpose antibody platform for engaging innate immunity. In: MAbs. Taylor & Francis (2019).
233. Dahlén E, Veitonmäki N, Norlén P. Bispecific antibodies in cancer immunotherapy. Ther Adv Vaccines Immunother (2018) 6:3–17. doi: 10.1177/2515135518763280
234. Schmohl JU, Felices M, Oh F, Lenvik AJ, Lebeau AM, Panyam J, et al. Engineering of anti-CD133 trispecific molecule capable of inducing NK expansion and driving antibody-dependent cell-mediated cytotoxicity. Cancer Res Treat (2017) 49:1140–52. doi: 10.4143/crt.2016.491
235. Felices M, Kodal B, Hinderlie P, Kaminski MF, Cooley S, Weisdorf DJ, et al. Novel CD19-targeted TriKE restores NK cell function and proliferative capacity in CLL. Blood Adv (2019) 3:897–907. doi: 10.1182/bloodadvances.2018029371
236. Arvindam US, van Hauten PM, Schirm D, Schaap N, Hobo W, Blazar BR, et al. A trispecific killer engager molecule against CLEC12A effectively induces NK-cell mediated killing of AML cells. Leukemia (2021) 35:1586–96. doi: 10.1038/s41375-020-01065-5
237. Gauthier L, Morel A, Anceriz N, Rossi B, Blanchard-Alvarez A, Grondin G, et al. Multifunctional natural killer cell engagers targeting NKp46 trigger protective tumor immunity. Cell (2019) 177:1701–13. doi: 10.1016/j.cell.2019.04.041
238. Yamashita K, Iwatsuki M, Yasuda-Yoshihara N, Morinaga T, Nakao Y, Harada K, et al. Trastuzumab upregulates programmed death ligand-1 expression through interaction with NK cells in gastric cancer. Br J Cancer (2021) 124:595–603. doi: 10.1038/s41416-020-01138-3
239. Barry KC, Hsu J, Broz ML, Cueto FJ, Binnewies M, Combes AJ, et al. A natural killer–dendritic cell axis defines checkpoint therapy–responsive tumor microenvironments. Nat Med (2018) 24:1178–91. doi: 10.1038/s41591-018-0085-8
240. Bezman NA, Jhatakia A, Kearney AY, Brender T, Maurer M, Henning K, et al. PD-1 blockade enhances elotuzumab efficacy in mouse tumor models. Blood Adv (2017) 1:753–65. doi: 10.1182/bloodadvances.2017004382
241. Chauvin J-M, Ka M, Pagliano O, Menna C, Ding Q, DeBlasio R, et al. IL15 stimulation with TIGIT blockade reverses CD155-mediated NK-cell dysfunction in MelanomaIL15 and TIGIT blockade reverse NK dysfunction in melanoma. Clin Cancer Res (2020) 26:5520–33. doi: 10.1158/1078-0432.CCR-20-0575
242. Ohs I, Ducimetière L, Marinho J, Kulig P, Becher B, Tugues S. Restoration of natural killer cell antimetastatic activity by IL12 and checkpoint BlockadeHarnessing NK cell–mediated immunity against metastasis. Cancer Res (2017) 77:7059–71. doi: 10.1158/0008-5472.CAN-17-1032
243. Romagné F, André P, Spee P, Zahn S, Anfossi N, Gauthier L, et al. Preclinical characterization of 1-7F9, a novel human anti–KIR receptor therapeutic antibody that augments natural killer–mediated killing of tumor cells. Blood (2009) 114:2667–77. doi: 10.1182/blood-2009-02-206532
244. Borst L, van der Burg SH, van Hall T. The NKG2A–HLA-E axis as a novel checkpoint in the tumor MicroenvironmentNKG2A as a novel checkpoint in the tumor microenvironment. Clin Cancer Res (2020) 26:5549–56. doi: 10.1158/1078-0432.CCR-19-2095
245. Carlsten M, Korde N, Kotecha R, Reger R, Bor S, Kazandjian D, et al. Checkpoint inhibition of KIR2D with the monoclonal antibody IPH2101 induces contraction and hyporesponsiveness of NK cells in patients with myeloma. Clin Cancer Res (2016) 22:5211–22. doi: 10.1158/1078-0432.CCR-16-1108
246. Pan B, Lentzsch S. The application and biology of immunomodulatory drugs (IMiDs) in cancer. Pharmacol Ther (2012) 136:56–68. doi: 10.1016/j.pharmthera.2012.07.004
247. Ito T, Ando H, Suzuki T, Ogura T, Hotta K, Imamura Y, et al. Identification of a primary target of thalidomide teratogenicity. science (2010) 327:1345–50. doi: 10.1126/science.1177319
248. Hideshima T, Ogiya D, Liu J, Harada T, Kurata K, Bae J, et al. Immunomodulatory drugs activate NK cells via both zap-70 and cereblon-dependent pathways. Leukemia (2021) 35:177–88. doi: 10.1038/s41375-020-0809-x
249. Fionda C, Abruzzese MP, Zingoni A, Cecere F, Vulpis E, Peruzzi G, et al. The IMiDs targets IKZF-1/3 and IRF4 as novel negative regulators of NK cell-activating ligands expression in multiple myeloma. Oncotarget (2015) 6:23609–30. doi: 10.18632/oncotarget.4603
250. Shi J, Tricot GJ, Garg TK, Malaviarachchi PA, Szmania SM, Kellum RE, et al. Bortezomib down-regulates the cell-surface expression of HLA class I and enhances natural killer cell–mediated lysis of myeloma. Blood (2008) 111:1309–17. doi: 10.1182/blood-2007-03-078535
251. Pan R, Ryan J, Pan D, Wucherpfennig KW, Letai A. Augmenting NK cell-based immunotherapy by targeting mitochondrial apoptosis. Cell (2022) 185:1521–38. doi: 10.1016/j.cell.2022.03.030
252. Wagner J, Kline CL, Zhou L, Campbell KS, MacFarlane AW, Olszanski AJ, et al. Dose intensification of TRAIL-inducing ONC201 inhibits metastasis and promotes intratumoral NK cell recruitment. J Clin Invest (2018) 128:2325–38. doi: 10.1172/JCI96711
253. Ni J, Wang X, Stojanovic A, Zhang Q, Wincher M, Bühler L, et al. Single-cell RNA sequencing of tumor-infiltrating NK cells reveals that inhibition of transcription factor HIF-1α unleashes NK cell activity. Immunity (2020) 52:1075–87. doi: 10.1016/j.immuni.2020.05.001
254. Tran HC, Wan Z, Sheard MA, Sun J, Jackson JR, Malvar J, et al. TGFβR1 blockade with galunisertib (LY2157299) enhances anti-neuroblastoma activity of the anti-GD2 antibody dinutuximab (ch14. 18) with natural killer CellsGalunisertib with anti-GD2 plus NK therapy for neuroblastoma. Clin Cancer Res (2017) 23:804–13. doi: 10.1158/1078-0432.CCR-16-1743
255. Romee R, Foley B, Lenvik T, Wang Y, Zhang B, Ankarlo D, et al. NK cell CD16 surface expression and function is regulated by a disintegrin and metalloprotease-17 (ADAM17). Blood (2013) 121:3599–608. doi: 10.1182/blood-2012-04-425397
256. Srpan K, Ambrose A, Karampatzakis A, Saeed M, Cartwright AN, Guldevall K, et al. Shedding of CD16 disassembles the NK cell immune synapse and boosts serial engagement of target cells. J Cell Biol (2018) 217:3267–83. doi: 10.1083/jcb.201712085
257. Chen Q, Sun L, Chen ZJ. Regulation and function of the cGAS–STING pathway of cytosolic DNA sensing. Nat Immunol (2016) 17:1142–9. doi: 10.1038/ni.3558
258. Nicolai CJ, Wolf N, Chang I-C, Kirn G, Marcus A, Ndubaku CO, et al. NK cells mediate clearance of CD8+ T cell–resistant tumors in response to STING agonists. Sci Immunol (2020) 5:eaaz2738. doi: 10.1126/sciimmunol.aaz2738
259. Kong X, Zuo H, Huang H-D, Zhang Q, Chen J, He C, et al. STING as an emerging therapeutic target for drug discovery: perspectives from the global patent landscape. J Adv Res (2022) 44:119–33. doi: 10.1016/j.jare.2022.05.006
260. Lopes JE, Fisher JL, Flick HL, Wang C, Sun L, Ernstoff MS, et al. ALKS 4230: a novel engineered IL-2 fusion protein with an improved cellular selectivity profile for cancer immunotherapy. J Immunother Cancer (2020) 8:e000673. doi: 10.1136/jitc-2020-000673
261. Waldmann TA. The biology of interleukin-2 and interleukin-15: implications for cancer therapy and vaccine design. Nat Rev Immunol (2006) 6:595–601. doi: 10.1038/nri1901
262. Lewis ND, Sia CL, Kirwin K, Haupt S, Mahimkar G, Zi T, et al. Exosome surface display of IL12 results in tumor-retained pharmacology with superior potency and limited systemic exposure compared with recombinant IL12Exosomes expressing IL12 promote antitumor immunity. Mol Cancer Ther (2021) 20:523–34. doi: 10.1158/1535-7163.MCT-20-0484
263. Konjević GM, Vuletić AM, Martinović KMM, Larsen AK, Jurišić VB. The role of cytokines in the regulation of NK cells in the tumor environment. Cytokine (2019) 117:30–40. doi: 10.1016/j.cyto.2019.02.001
264. Bergamaschi C, Pandit H, Nagy BA, Stellas D, Jensen SM, Bear J, et al. Heterodimeric IL-15 delays tumor growth and promotes intratumoral CTL and dendritic cell accumulation by a cytokine network involving XCL1, IFN-γ, CXCL9 and CXCL10. J Immunother Cancer (2020) 8:e000599. doi: 10.1136/jitc-2020-000599
265. Han K-P, Zhu X, Liu B, Jeng E, Kong L, Yovandich JL, et al. IL-15: IL-15 receptor alpha superagonist complex: high-level co-expression in recombinant mammalian cells, purification and characterization. Cytokine (2011) 56:804–10. doi: 10.1016/j.cyto.2011.09.028
266. Algazi AP, Twitty CG, Tsai KK, Le M, Pierce R, Browning E, et al. Phase II trial of IL-12 plasmid transfection and PD-1 blockade in immunologically quiescent melanoma. Clin Cancer Res (2020) 26:2827–37. doi: 10.1158/1078-0432.CCR-19-2217
267. Zhou T, Damsky W, Weizman O-E, McGeary MK, Hartmann KP, Rosen CE, et al. IL-18BP is a secreted immune checkpoint and barrier to IL-18 immunotherapy. Nature (2020) 583:609–14. doi: 10.1038/s41586-020-2422-6
268. Gang M, Marin ND, Wong P, Neal CC, Marsala L, Foster M, et al. CAR-modified memory-like NK cells exhibit potent responses to NK-resistant lymphomas. Blood (2020) 136:2308–18. doi: 10.1182/blood.2020006619
269. Kerbauy LN, Marin ND, Kaplan M, Banerjee PP, Berrien-Elliott MM, Becker-Hapak M, et al. Combining AFM13, a bispecific CD30/CD16 antibody, with cytokine-activated blood and cord blood–derived NK cells facilitates CAR-like responses against CD30+ MalignanciesAFM13-loaded NK cells as therapy for CD30+ tumors. Clin Cancer Res (2021) 27:3744–56. doi: 10.1158/1078-0432.CCR-21-0164
Keywords: natural killer cell, chimeric antigen receptor, immunotherapy, cancer, aging, immune surveillance
Citation: Shin E, Bak SH, Park T, Kim JW, Yoon S-R, Jung H and Noh J-Y (2023) Understanding NK cell biology for harnessing NK cell therapies: targeting cancer and beyond. Front. Immunol. 14:1192907. doi: 10.3389/fimmu.2023.1192907
Received: 24 March 2023; Accepted: 30 June 2023;
Published: 18 July 2023.
Edited by:
Hind Rafei, University of Texas MD Anderson Cancer CenterReviewed by:
Frank M. Cichocki, University of Minnesota Twin Cities, United StatesCopyright © 2023 Shin, Bak, Park, Kim, Yoon, Jung and Noh. This is an open-access article distributed under the terms of the Creative Commons Attribution License (CC BY). The use, distribution or reproduction in other forums is permitted, provided the original author(s) and the copyright owner(s) are credited and that the original publication in this journal is cited, in accordance with accepted academic practice. No use, distribution or reproduction is permitted which does not comply with these terms.
*Correspondence: Ji-Yoon Noh, bm9oajE2QGtyaWJiLnJlLmty
Disclaimer: All claims expressed in this article are solely those of the authors and do not necessarily represent those of their affiliated organizations, or those of the publisher, the editors and the reviewers. Any product that may be evaluated in this article or claim that may be made by its manufacturer is not guaranteed or endorsed by the publisher.
Research integrity at Frontiers
Learn more about the work of our research integrity team to safeguard the quality of each article we publish.