- 1Guangdong Key Laboratory of Animal Breeding and Nutrition, Collaborative Innovation Center of Aquatic Sciences, Institute of Animal Science, Guangdong Academy of Agricultural Sciences, Guangzhou, China
- 2College of Fisheries, Guangdong Ocean University, Zhanjiang, China
The effects of dietary supplementation of Clostridium butyricum (CB) on growth performance, serum biochemistry, antioxidant activity, mRNA levels of immune-related genes and resistance to hypoxia stress were studied in largemouth bass. Feed with CB0 (control, 0 CFU/kg), CB1 (4.3×108 CFU/kg), CB2 (7.5×108 CFU/kg), CB3 (1.5×109 CFU/kg) and CB4 (3.2×109 CFU/kg) CB for 56 days, and then a 3 h hypoxic stress experiment was performed. The results showed that dietary CB significantly increased the WGR (weight gain rate), SGR (specific growth rate), PDR (protein deposition rate) and ISI (Intestosomatic index) of largemouth bass (P<0.05). Hepatic GH (growth hormone)/IGF-1 (insulin-like growth factor-1) gene expression was significantly upregulated in the CB3 and CB4 groups compared with the CB0 group (P<0.05), while the FC (feed conversion) was significantly decreased (P<0.05). Serum TP (total protein) and GLU (glucose) levels were significantly higher in the CB4 group than in the CB0 group (P<0.05), while the contents of serum AST (aspartate transaminase), ALT (alanine transaminase), AKP (alkline phosphatase) and UN (urea nitrogen) in CB4 were significantly lower than those in CB0 (P<0.05). T-AOC (total antioxidant capacity), SOD (superoxide dismutase), CAT (catalase), POD (peroxidase) and GSH-Px (glutathione peroxidase) activities were significantly higher in CB3 and CB4 groups than in CB0 group (P<0. 05). The liver MDA (malondialdehyde) content of CB1, CB2, CB3 and CB4 groups was significantly higher than that of CB0 group (P<0. 05). The relative expressions of IL-1β (interleukin 1β), TNF-α (tumor necrosis factor α) and TLR22 (toll-like receptor-22) genes in CB2, CB3 and CB4 groups were significantly lower than those in CB0 group (P<0.05). The relative expression of IL-8 (malondialdehyde) and MyD88 (Myeloid differentiation factor 88) genes in the CB4 group was significantly lower than that in the CB0 group (P<0.05). The liver LZM (lysozyme) content of CB2, CB3 and CB4 groups was significantly higher than that of CB0 group (P<0. 05). The relative expression of IL-10 (interleukin 10) and TGF-β (transforming growth factor β) genes in the CB4 group was significantly higher than that in the CB0 group (P<0.05). Under hypoxic stress for 3 h, the CMR of CB0 group was significantly higher than that of CB1, CB2, CB3 and CB4 groups (P<0.05). Dietary CB can improve the growth performance and resistance to hypoxic stress of largemouth bass by regulating the expression of GH/IGF-1 gene and inflammatory factors and inhibiting TLR22/MyD88 signaling pathway.
1 Introduction
The extensive use of antibiotics in intensive farming to control bacterial diseases exacerbates the development and spread of antibiotic resistance (1). The incidence of infectious diseases caused by antibiotic-resistant bacteria is currently increasing, resulting in a higher mortality rate than cancer (2). The misuse of antibiotics results in microbial dysbiosis and the emergence of antimicrobial resistance, thereby posing significant public health challenges (3, 4). Consequently, the utilization of antibiotics as feed additives was prohibited in the European Union, United States, and China in 2006, 2014, and 2020 correspondingly (5). Therefore, it is imperative to explore alternatives that can substitute antibiotics. Probiotics are defined as live microorganisms that optimize the microbial balance and promote health (6). Probiotics have been successfully used in farmed freshwater or marine fish, including Nile tilapia (Oreochromis niloticus), Javanese carp (Puntius gonionotus), and rainbow trout (Oncorhynchus mykiss), among others (7–9). Probiotics are the preferred therapeutic agents for managing inflammatory disorders, such as diarrheal disease and inflammatory bowel disease. A plethora of studies have demonstrated that dietary supplementation with probiotics can significantly augment fish health (5, 10–12).
Clostridium butyricum (CB) is a Gram-positive bacterium that exerts beneficial effects on growth promotion, inflammation suppression, and pathogenic bacteria reduction (13, 14). In recent years, CB has gained widespread application in the livestock and aquatic animal industries, primarily for enhancing growth performance and bolstering disease resistance (15, 16). Additionally, due to its ability to withstand low pH and high temperatures, CB is frequently utilized in fish as a preventative measure against fish pathogens or antibiotic resistance (17). Studies have shown that the addition of CB in the range of 107 - 1011 CFU/kg significantly improved the growth performance of silver pomfret (Pampus argenteus) (18), large yellow croaker (Larimichthys crocea) (19), and Nile tilapia (20), enhanced the immune response of giant freshwater prawn (Macrobrachium rosenbergii) (21), and increased the antioxidant activity of kuruma shrimp (Marsupenaeus japonicas) (22). However, the impact of dietary supplementation with CB on growth, antioxidant activity, immune response and hypoxic stress resistance in largemouth bass remains uncertain.
Largemouth bass (Micropterus salmoides), a freshwater farmed fish, commands high market value owing to its rapid growth and delectable flesh (23). Meanwhile, due to its immense popularity among Chinese consumers, it constitutes a significant portion of China’s breeding industry (24, 25). However, nutritional deficiencies, high densities, and environmental changes often lead to metabolic disorders in fish, which can result in reduced disease resistance and increased susceptibility to disease outbreaks (26). Dietary nutrients and supplementation significantly boost growth of Largemouth bass (27–29). Studies have demonstrated that probiotics have been efficaciously employed in numerous aquaculture species to stimulate growth, optimize feed utilization, and augment organismal resistance against diseases (30). Revised sentence: However, there is a lack of research on the utilization of CB as a feed additive for largemouth bass, and the mechanisms underlying CB’s promotion of growth and enhancement of disease resistance remain unclear. The objective of this investigation was to examine the impact of CB on growth performance, antioxidant capacity, immune response, and hypoxic stress in juvenile largemouth bass.
2 Materials and methods
2.1 Experimental diets
The CB with a count of 8×108 colony-forming units (colony-forming units, CFU)/ml was obtained from China Organic Biotechnology Co., Ltd., China. Juvenile largemouth bass were fed with five isonitrogenous (50%) and isolipidic (9%) diets, which were supplemented with CB at 0 (CB0), 2.5 (CB1), 5 (CB2), 10 (CB3), and 20 (CB4) ml/kg of diet, respectively. The final CB concentrations in the five diets were 0, 4.3×108, 7.5×108, 1.5×109 and 3.2×109 CFU/kg, which were determined by the plate count method (31). The raw materials were weighed in accordance with the feed formulation, crushed, and sieved. Prior to mixing into the raw materials, the CB solution was mixed with water. All diets were prepared and pelleted into 1.5 mm diameter by twin screw extruder (SLX-80, South China University of Technology, China). Dry at 55°C for 6 h and store the feed at -20°C after packaging in groups. The ingredients and proximate composition of experimental diets are presented in Table 1.
2.2 Feeding and management
This feeding experiment was carried out in an indoor recirculating aquaculture system in the aquatic laboratory of the Institute of Animal Science, Guangdong Academy of Agricultural Sciences (Guangzhou, China) and lasted for 56 d. The fish were purchased from Guangzhou, China, and were acclimated with the control diet for 1 week in an indoor recirculating aquaculture system. Six hundred fish (5.02 ± 0.01 g) were stocked into fifteen cylindrical fiberglass tanks (water volume 300 L) at 40 fish per tank to conduct the experiment. Five experimental diets were randomly allocated to triplicate groups of fish. During the 56-day feeding trial, the water temperature ranged from 25 to 32°C, pH 7.8-8.0, and dissolved oxygen > 8.0 mg/L. Fish were reared under 12 h light: 12 h dark dialcycle photoperiod. All the fish were manually fed to satiation with the experimental diets two times daily (8:30 and 18:30) (initially 5% to 6% of body weight per day and then gradually increased).
2.3 Sample collection and analysis
After the end of the 8-week feeding experiment, the fish were fasted for 24 h, and the final body weight of each cage was weighed, and the survival rate (SR) was calculated. Eighteen fish were randomly selected from each replicate and anesthetized in 120 mg/L tricaine methanesulfonate (MS-222) solution, and 3 fish were stored at -20 °C for the determination of routine nutrient composition of whole fish. The body weight, body length, intestines weight and viscera weight of six fish were measured to calculate weight gain rate (WGR), specific growth rate (SGR), protein deposition rate (PDR), feed conversion (FC), intestosomatic index (ISI) and condition factor (CF). Blood samples were collected from the tail vein of 9 fish, left at room temperature for 4 h, centrifuged at 3500 r/min for 10 min, and the supernatant was taken to prepare serum and stored at -80° C until use. The blood of 9 fish was collected and placed on ice for rapid dissection, and the liver of 3 fish was determined for immune antioxidant indexes. Livers from three fish were fixed in 10% formalin solution and stored at room temperature to produce liver paraffin sections. The routine nutrient composition of experimental diets and whole fish was determined as follows: Water content was determined by oven drying to constant weight at 105 °C (GB/T 6435-2014), crude protein content (N×6.25) was determined by semi-automatic Kjeldner nitrogen determination method (GB/T 6432-2018), crude fat content was determined by ether extraction method (GB/T 6433-2006), crude ash content was determined by the method of burning to constant weight at 550 °C (GB/T 6433-2006).
2.4 Serum biochemical analysis
After the fish were anesthetized, blood was collected from the tail vein, and the blood samples were centrifuged (3500 × g, 10 min, 4°C) to separate the serum. Serum total protein (TP) content was determined by biuret method. alanine transaminase (ALT) activity was measured by spectrophotometry. glucose (GLU) content was measured by glucose oxidase method. UN), triglyceride (TG) and aspartate transaminase (AST) activity were measured by enzyme coupling rate method, enzymatic method and spectrophotometric method, respectively. High-density lipoprotein cholesterol (HDL), low-density lipoprotein cholesterol (LDL), The contents of total cholesterol (TCHO) and alkaline phosphatase (AKP) were determined by enzymatic method. 1. The Hitachi 7600 fully automated analyzer was utilized to measure all parameters at Guangzhou Jinyu Medical Testing Center (Guangzhou, China).
2.5 Enzyme activity analysis
The liver samples were added with normal saline according to the ratio of weight liver: volume of normal saline (1:9), and the liver samples were fully broken under the condition of ice water bath. After the broken liver samples were centrifuged for 10 min (2500 r/min, 4 °C), the supernatant was taken after centrifugation for enzyme activity detection. The activity of peroxidase (POD) was determined by A084-2 colorimetric method, the activity of Lysozyme (LZM) was determined by A050 turbidimetric method, and the activity of superoxide dismutase was determined by superoxide dismutase. SOD activity was determined by A001-1 hydroxylamine method, catalase (CAT) activity was determined by A007-1 visible light method, total antioxidant capacity, T-AOC capacity was determined by A015 colorimetric method. Content of malondialdehyde (MDA) and activity of glutathione peroxidase (GSH-Px) were determined by A003-1 TBA method. The enzyme activity kit was purchased from Nanjing Jiancheng Bioengineering Institute (Nanjing, China), and the measurement procedure, principle and calculation formula were referred to the kit manual.
2.6 qRT-PCR analysis
Total RNA was extracted from the whole liver of largemouth bass using RNA Isolation Kit (Vazyme, Nanjing, China), following the protocol of the manufacturer, and electrophoresed on a 1.2% denaturing agarose gel to test the integrity. The RNA reverse was then transcribed to cDNA using HiScript®III RT SuperMix for qPCR kit (Vazyme, Nanjing, China). The qPCR assay was carried out using ChamQ Universal SYBQ qPCR Master Mix kit (Vazyme, Nanjing, China). The amplification was carried out in a 20 μL reaction volume containing 10 μL SYBQ Green Master Mix, 0.4 μL of each respective primer (10 μmol/L), 2 μL cDNA product, and 7.2 μL RNA-free water. The specific primers and primer sequences of the housekeeping gene (β-actin) are shown in Table 2, and all primers were synthesized by Shanghai Sangon Biotechnology Co., LTD. (Shanghai, China). β-actin was used as a nonregulated reference gene to normalize target gene transcript levels in largemouth bass studies, furthermore, β-actin gene expression of the intestine was also stable and was not significantly affected by dietary CB in our present research. All reactions were performed in duplicate, and each assay was repeated three times. The gene expression levels were analyzed using the 2−ΔΔCT method (32).
2.7 Hypoxia stress test
At the end of the culture experiment, 16 fish were collected from each experimental group and subjected to hypoxic stress experiments according to the method described by Zeng et al. (33). Drain the water in the tank to the water surface to the bottom 10 cm of the tank (approximately 50 L), cover the tank with transparent plastic film and stop oxygen supply, causing an oxygen deficient environment. Dissolved oxygen (DO) was measured at 30-minute intervals during the experiment (Seven2Go, Mettler Toledo, USA). The DO level decreased from 7.6 mg L-1 to 0 mg L-1 after 1.5 h of continuous testing and remained at this level. When the cumulative mortality rate (CMR) of the control group (CB0) reached 50%, the experiment was stopped. Observation of the experimental fish was confirmed as a dead state when it did not respond to external stimuli. Fasting was done during the trial, and the number of fish stocks was counted to calculate the CMR after the trial.
2.8 Statistical analysis
SPSS 25.0 software (Chicago, USA) was used for statistical analysis. One-way analysis of variance (ANOVA) and multipole difference test (Tukey’s test) were used to determine significant differences (P< 0.05). Data are expressed as mean ± standard error (SEM).
2.9 Calculations
The parameters are calculated according to the following formula:
In the formula: F initial is the initial mantissa; F final is the final mantissa; W is the body weight; L is the body length; W initial is the initial fish weight; W final is the final fish body weight; W dead is the dead fish weight; CP initial is the protein content of the initial fish body; CP final is the protein content of the final fish; CP feed is the protein content of the feed; D total is the total weight of the feed; D is the feed intake; T is the breeding time; W intestinal is the intestinal weight.
3 Results
3.1 Growth performance
The growth performance is shown in Figure 1. WGR and PDR were significantly higher in CB2, CB3 and CB4 groups than in CB0 group (P< 0.05). The SGR of CB3 and CB4 groups was significantly higher than that of CB0 group (P< 0.05). ISI was significantly higher in CB1, CB2, CB3, and CB4 groups than in CB0 group (P< 0.05). FC was significantly lower in CB3 and CB4 groups than in CB0 group (P< 0.05). SR was 100% in all groups, and the difference between groups was not statistically significant (P >0.05). No significant change in CF between groups (P >0.05).
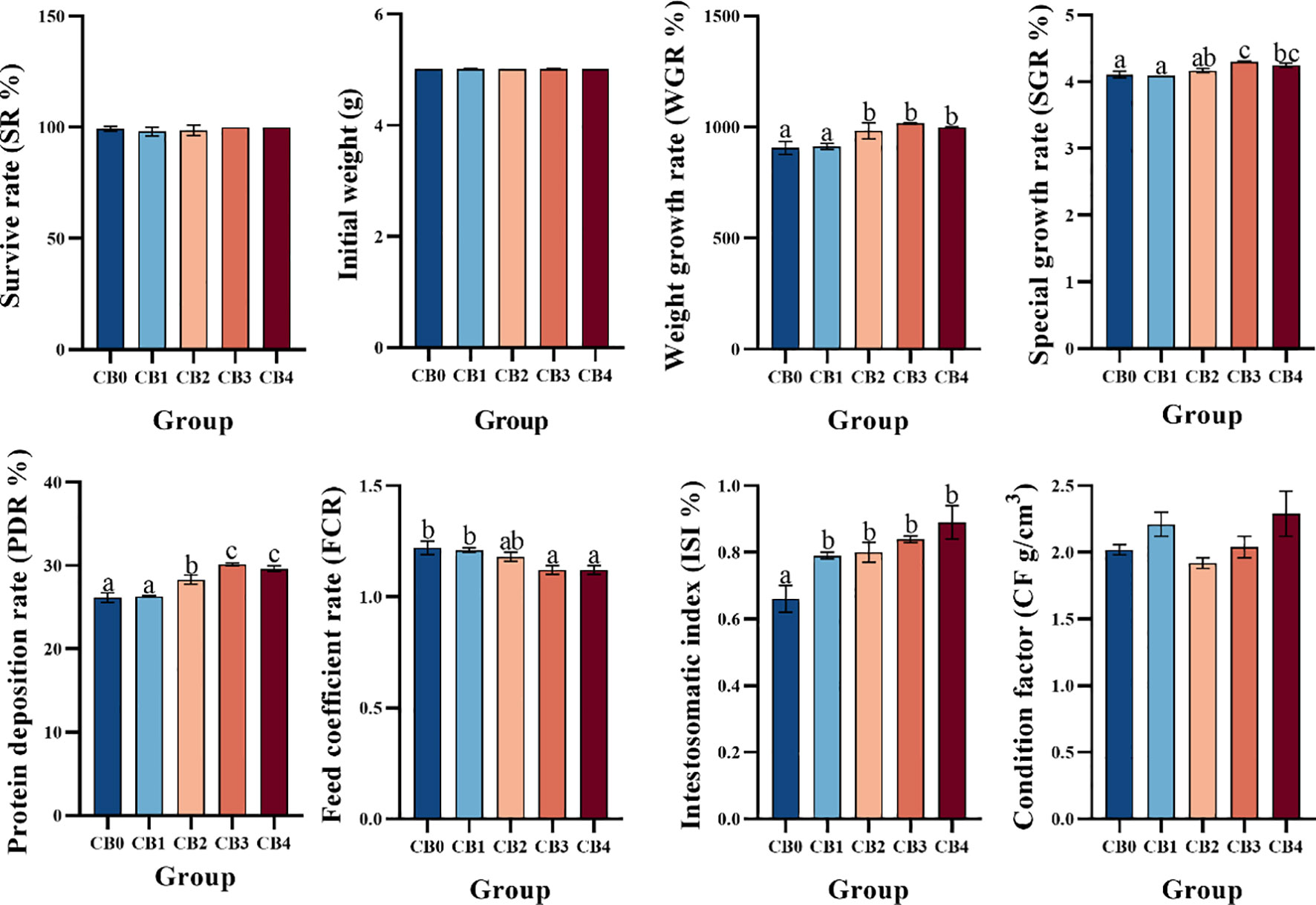
Figure 1 Effects of dietary CB growth performance, feed efficiency, and intestinal growth of juvenile largemouth bass Micropterus salmoides. a,b,c Bars with different superscripts represent significant difference (P< 0.05). Data presented are means ± SEM of 3 replicates. The same picture below.
3.2 Liver GH-IGF system
GH-IGF signaling pathway mRNA expression is shown in Figure 2. The IGF-I mRNA expression levels in CB3 and CB4 groups were significantly higher than those in CB0 group (P< 0.05). The expression of GH mRNA in CB2, CB3, and CB4 was significantly higher than that in CB0 (P< 0.05).
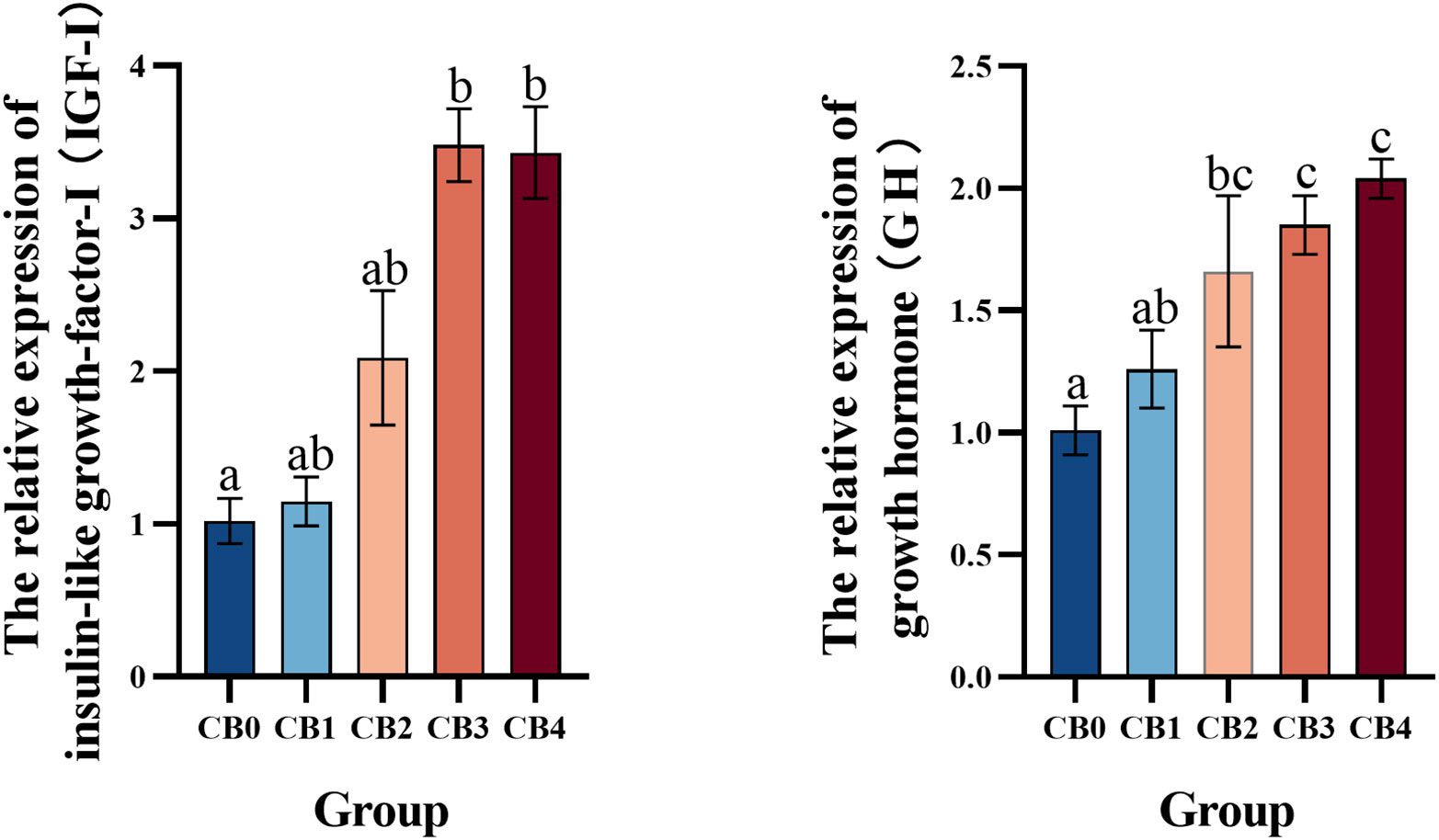
Figure 2 Effect of dietary CB levels on the relative expression of GH and IGF-I in the liver of largemouth bass.
3.3 Serum biochemistry
The serum biochemical indices are shown in Figure 3. AST and AKP were significantly lower in CB3 and CB4 groups than in CB0 group (P< 0.05). ALT, UN and TG were significantly lower in the CB4 group than in the CB0 group (P< 0.05). CB2, CB3 and CB4 serum TP levels were significantly higher than CB0 (P< 0.05). No significant changes between groups in TC, HDL, LDL (P >0.05).
3.4 Antioxidative parameters
Antioxidant activity is shown in Figure 4. TP and T-AOC were significantly higher in CB1, CB2, CB3 and CB4 groups than in CB0 group (P< 0.05). CAT and SOD were significantly higher in CB2, CB3 and CB4 groups than in CB0 group (P< 0.05). POD and GSH-Px were significantly higher in CB3 and CB4 than in CB0 (P< 0.05). The MDA of CB3 and CB4 groups was significantly lower than that of CB0 group (P< 0.05).
3.5 Immune parameters
Immune-related gene expression is shown in Figure 5. TLR22, TNF-α and IL-1β were significantly lower in CB2, CB3 and CB4 groups than in CB0 group (P< 0.05). IL-8 was significantly lower in CB4 than in CB0 (P< 0.05). MyD88 was significantly lower in CB3 and CB4 groups than in CB0 group (P< 0.05). IL-10 and TGF-β were significantly higher in the CB4 group than in the CB0 group (P< 0.05). LZM was significantly higher in CB2, CB3 and CB4 groups than in CB0 group (P< 0.05).
3.6 Hypoxic stress test
Hypoxic stress mortality is shown in Figure 6. CMR of largemouth bass significantly increased 3 hours into the experiment. The CMRs of fish were 68.75% (CB0 DO 0), 54.17% (CB1 DO 0), 52.08% (CB2 DO 0), 31.25% (CB3 DO 0) and 39.58% (CB4 DO 0) after 3 h.
4 Discussion
Dietary supplementation with CB significantly enhanced the growth performance of largemouth bass, as evidenced by increased WGR, SGR, ISI, and PDR and decreased FC. Consistent with our findings, CB has been observed to have beneficial effects on the growth of various livestock and fish species, including broilers (16), weaned piglets (34), Pacific white shrimp (Penaeus vannamei) (35), Yellow Catfish(Pelteobagrus fulvidraco) (36), large yellow croaker (19), tilapia (Oreochromis niloticus) (4), and silver pomfret (silver pomfret) (18). The GH-IGF-I signaling pathway is an endocrine signaling pathway that regulates growth and responds to the body’s nutrient metabolism (37–39). The liver is also highly responsive to the nutritional status of the host and exerts significant regulatory effects on host nutrient metabolism and immune function (40–42). The findings of this investigation indicate that the inclusion of CB in the diet significantly upregulated GH and IGF-I mRNA expression, with GH and IGF-I being particularly responsive to varying levels of CB. This promotion of GH and IGF-I mRNA expression by dietary CB may account for its growth-promoting effects on largemouth bass.
For largemouth bass, it is equally important to ensure their health and optimize growth performance. Blood biochemical indicators can provide valuable insights into the fish’s overall health status, nutritional condition, and adaptability to its environment (43–46). Therefore, the effect of dietary additives on blood biochemical indicators must be verified before application. The content of TP in blood plays a crucial role in maintaining normal osmotic pressure and pH of blood vessels, serving as the basis for protein metabolism intake by the body (47). The findings of this study demonstrate that dietary CB can significantly elevate the serum total protein (TP) and glucose (GLU) levels in largemouth bass, which is consistent with the growth trend of protein deposition efficiency in fish. This suggests that CB supplementation can effectively enhance nutrient metabolism and promote protein deposition in largemouth bass. This indirectly indicates an enhancement in the growth performance of largemouth bass, which may be correlated. Serum ALT, AST and AKP serve as crucial serum markers for identifying animal nutrition and health status (48, 49). The changes in the activities of enzymes, including ALT, AST, and AKP, are directly correlated with the extent of organ-specific cellular damage. As an extracellular enzyme, AKP plays a crucial role in various biological processes including growth, cell differentiation, protein synthesis and immune metabolism (50–52). Elevated serum AKP activity indicates compromised cell membrane permeability and integrity, resulting in cellular damage and reflecting the occurrence of host hepatobiliary inflammation (53, 54). The findings of this investigation demonstrate that dietary supplementation with CB can significantly modulate the serum AKP activity in largemouth bass, both increasing and decreasing it. Moreover, when liver inflammation occurs, CB has the potential to enhance phagocytic activity of immune cells against foreign bodies and thus protect the liver from toxic invasion. The results also indicates that dietary CB significantly enhances nutrient digestion, a finding corroborated in yellow catfish. ALT and AST are important transaminases in the human body, and their content can reflect the health status of the liver. Normally, the activity of serum aminotransferases is low. However, elevated levels of ALT and AST indicate liver damage (44). UN, or blood urea nitrogen, is not a protein; however, it serves as an indicator of the body’s protein metabolism status (54–57). Under the experimental conditions, it was observed that the control group exhibited elevated levels of serum AST, ALT, and UN, indicating potential hepatic inflammation or other pathological states. Notably, dietary supplementation of CB resulted in a significant reduction in serum AST, ALT and UN levels, indicating the hepatoprotective effect of CB on largemouth bass. However, further investigation is required to elucidate the underlying mechanism. Therefore, our objective was to identify immune and antioxidant genes in the liver of largemouth bass and comprehensively analyze the protective mechanism of CB against liver inflammation and disease states in this species.
The liver plays a crucial role in the response to oxidative stress and immune challenges (58). Oxygen free radical and lipid peroxidation reactions are integral components of the body’s metabolic processes (50, 52, 59, 60). Under normal circumstances, the two systems work in concert to maintain a multitude of physiological, biochemical, and immunological responses within the body (48, 51). SOD, POD, GSH-Px and CAT are crucial enzymes in the biological antioxidant defense system that play a vital role in scavenging reactive oxygen species within the body to safeguard cell membranes and nucleic acids (49, 61). T-AOC reflects the body’s compensatory ability to external stimuli and the status of free radical metabolism (62, 63). The MDA content serves as an indicator of the extent of cellular damage (63–66). The findings of this experiment indicate that dietary CB significantly enhances the hepatic activities of T-AOC, SOD, CAT, POD and GSH-Px in largemouth bass, which is consistent with the results obtained from a previous study on hybrid grouper (♀Epinephelus fuscoguttatus × ♂ E. lanceolatu) (60). It was also observed that dietary CB significantly reduced the MDA content in the liver of freshwater shrimp, which is consistent with the results of the study on yellow catfish (36), freshwater prawn (21), and Pacific white shrimp (67). The findings indicated that dietary supplementation of CB could significantly enhance the antioxidant enzyme activity in largemouth bass, decrease the level of MDA, suppress hepatic lipid peroxidation and safeguard against oxidative stress-induced damage. The incorporation of CB significantly augmented the antioxidant potential of largemouth bass, potentially ascribed to its pivotal role in scavenging highly reactive oxygen free radicals and producing antioxidant enzymes, thereby mitigating lipid peroxidation and effectively quenching free radicals, ultimately conferring cellular protection against oxidative damage (21).
Oxidative stress and inflammatory response are interrelated processes that significantly contribute to the body’s response to various stressors (68). The inflammatory response is initiated and modulated by pro-inflammatory cytokines (69, 70). Interleukin-8 (IL-8), a pro-inflammatory cytokine, stimulates the activation of macrophages and neutrophils, thereby promoting tissue regeneration in response to damage (71–73). Interleukin-1β (IL-1β) and tumor necrosis factor-α (TNF-α) primarily elicit inflammatory responses by modulating the expression of other cytokines (74). Interleukin-10 (IL-10) and transforming growth factor β (TGF-β) exert inhibitory effects on the proliferation, activation, and migration of T and B lymphocytes, thereby limiting the inflammatory response (75–78). Lysozyme (LZM) is a crucial antibacterial agent that can disrupt bacterial cell walls, stimulate the alternative complement pathway and phagocytic activity, and contribute to the body’s non-specific immune defense (74, 79–81). Toll-like receptors (TLRs) recognize invading microbial pathogens and initiate cellular signaling pathways. Toll-like receptor 22 (TLR22) and myeloid differentiation factor 88 (MyD88) are implicated in the regulation of inflammatory processes (82–84). Upregulation of IL-6 and TNF-α expression may be attributed to tissue damage caused by infection or oxidative stress (85, 86). Under the current experimental conditions, dietary CB significantly suppressed the expression of hepatic IL-8, IL-1β, TNF-α, MyD88 and TLR22 genes in largemouth bass. Notably, the expression of IL-10 and TGF-β genes was significantly upregulated in the liver of largemouth bass, while the activity of LZM was also significantly elevated. The findings indicated that dietary supplementation of CB could activate Toll receptors in largemouth bass, mediate MyD88-dependent signaling pathway, ultimately inhibit the release of proinflammatory cytokines IL-8, IL-1β and TNF-α, activate the acquired immune response, ultimately regulate immune function, enhance body resistance and mitigate liver inflammatory response. Similar studies have demonstrated that dietary CB significantly reduces serum levels of tumor necrosis factor-alpha (TNF-α) and increases interleukin-10 (IL-10) expression in children (87), markedly inhibits the expression of IL-6 and TNF-α in chicken intestines (88), substantially elevates serum IL-10 content and decreases the content of IL-1β in weanling piglets (89), and significantly reduces the expression of IL-1β and IL-8 in yellow catfish (36). In summary, dietary CB can protect the liver from oxidative damage by regulating antioxidant enzyme activity and alleviate the hepatic inflammatory response by upregulating the expression of anti-inflammatory factors and inhibiting the expression of pro-inflammatory factors. Interestingly, the inclusion of CB in the diet resulted in a significant upregulation of TLR22 expression in piglet ileum and promoted MyD88 gene expression, which contrasts with the findings of this experiment. The expression of MyD88 is modulated by its associated signaling cascade. In general, Toll-like receptors are the only ones stimulated by pathogens. MyD88 is transcriptionally activated by anti-inflammatory factors through NF-kB family genes. Under the experimental conditions, when largemouth bass was exposed to oxidative stress, the liver underwent pathological changes and the body was stimulated by bacteria or toxins. Toll-like receptors (TLRs) mediated immune responses through the MyD88-dependent pathway. The inclusion of CB in the diet resulted in a significant increase in hepatic LZM activity and stimulated phagocytic function. The expression of pro-inflammatory cytokines IL-8, IL-1β and TNF-α was significantly reduced, while the genes for anti-inflammatory cytokines IL-10 and TGF-β were up-regulated, leading to suppression of the inflammatory response. The comprehensive analysis revealed that CB exerted significant effects on the expression of TLR22 pathway-associated genes in largemouth bass. CB has the potential to modulate TLR22 pathway-related factors, thereby mitigating inflammatory response and enhancing immune function.
Hypoxia is a common stressor experienced by aquatic animals in aquaculture, which can result in inhibited behavior and physiological metabolism of fish, ultimately leading to oxidative damage across various organs. Fish can enhance their antioxidant activities by increasing the activity of antioxidant enzymes. The dietary addition of CB significantly boosts the antioxidant capacity of tilapia, Macrobrachium robertsoni, Penaeus vannamei, and Penaeus prefixus. Reaffirming the findings of previous studies, the results of our current experiment demonstrate that dietary CB significantly enhances the activities of antioxidant enzymes in a similar manner. Interestingly, the results of the 3-hour hypoxic stress experiment on largemouth bass revealed that CB1, CB2, CB3 and CB4 had significantly lower CMRs than CB0 by 21.22%, 24.25%, 54.55% and 42.43% respectively, indicating a significant improvement in hypoxia stress survival rate due to dietary CB. The enhanced anti-hypoxic activity of CB in promoting survival may be attributed to the upregulated hepatic antioxidant enzyme activities (SOD, GSH-Px, CAT, POD) and decreased hepatic MDA concentration observed in this study, which ultimately improved the antioxidative status of largemouth bass by elevating hepatic T-AOC levels.
5 Conclusions
Dietary CB (1.5×109 CFU/kg, CB3) can enhance the growth, antioxidant activity and hypoxic stress resistance of largemouth bass. By regulating GH/IGF-1 gene expression and inflammatory factors, as well as inhibiting TLR22/MyD88 signaling pathway, dietary CB can improve the growth performance and hypoxic stress resistance of largemouth bass.
Data availability statement
The datasets presented in this study can be found in online repositories. The names of the repository/repositories and accession number(s) can be found in the article/supplementary material.
Ethics statement
The animal study was reviewed and approved by Collaborative Innovation Center of Aquatic Sciences, Guangdong Key Laboratory of Animal Breeding and Nutrition, Institute of Animal Science, Guangdong Academy of Agricultural Sciences.
Author contributions
PL wrote the paper; XC and BC performed the experiments; DH performed the experiments; KP performed the experiments; WH analyzed the data; JC designed the experiments; HZ conceived the experiments.
Funding
This study was supported by the National Natural Science Foundation of China (31402307), the Guangdong Provincial Science and Technology Program (KTP20210322), and the Science and Technology Program of Guangzhou (202002020008).
Conflict of interest
The authors declare that the research was conducted in the absence of any commercial or financial relationships that could be construed as a potential conflict of interest.
Publisher’s note
All claims expressed in this article are solely those of the authors and do not necessarily represent those of their affiliated organizations, or those of the publisher, the editors and the reviewers. Any product that may be evaluated in this article, or claim that may be made by its manufacturer, is not guaranteed or endorsed by the publisher.
References
1. Defoirdt T, Sorgeloos P, Bossier P. Alternatives to antibiotics for the control of bacterial disease in aquaculture. Curr Opin Microbiol (2011) 14:251–8. doi: 10.1016/j.mib.2011.03.004
2. McAllister TA, Wang Y, Diarra MS, Alexander T, Stanford K. Challenges of a one-health approach to the development of alternatives to antibiotics. Anim Front (2018) 8:10–20. doi: 10.1093/af/vfy002
3. Wang Y, Zhou J, Wang G, Cai S, Zeng X, Qiao S. Advances in low-protein diets for swine. J Anim Sci Biotechnol (2018) 9:1–14. doi: 10.1186/s40104-018-0276-7
4. Li H, Zhou Y, Ling H, Luo L, Qi D, Feng L. The effect of dietary supplementation with Clostridium butyricum on the growth performance, immunity, intestinal microbiota and disease resistance of tilapia (Oreochromis niloticus). PloS One (2019) 14:e0223428. doi: 10.1371/journal.pone.0223428
5. Fu J, Wang T, Xiao X, Cheng Y, Wang F, Jin M, et al. Clostridium butyricum ZJU-F1 benefits the intestinal barrier function and immune response associated with its modulation of gut microbiota in weaned piglets. Cells (2021) 10:527. doi: 10.3390/cells10030527
6. Hasan KN, Banerjee G. Recent studies on probiotics as beneficial mediator in aquaculture: a review. J Basic Appl Zool (2020) 81:1–16. doi: 10.1186/s41936-020-00190-y
7. Vendrell D, Balcázar JL, de Blas I, Ruiz-Zarzuela I, Gironés O, Muzquiz JL. Protection of rainbow trout (Oncorhynchus mykiss) from lactococcosis by probiotic bacteria. Comp Immunol Microbiol Infect Dis (2008) 31:337–45. doi: 10.1016/j.cimid.2007.04.002
8. Allameh SK, Yusoff FM, Ringø E, Daud HM, Saad CR, Ideris A. Effects of dietary mono-and multiprobiotic strains on growth performance, gut bacteria and body composition of J avanese carp (Puntius gonionotus, B leeker 1850). Aquac Nutr (2016) 22:367–73. doi: 10.1111/anu.12265
9. Van Doan H, Lumsangkul C, Jaturasitha S, Meidong R, Hoseinifar SH, Dawood MAO. Modulation of growth, skin mucus and serum immunities, and disease resistance of Nile tilapia fed host-associated probiotic (Lactobacillus paracasei l61-27b). Aquac Nutr (2021) 27:3–12. doi: 10.1111/anu.13314
10. Nayak SK. Probiotics and immunity: a fish perspective. Fish Shellfish Immunol (2010) 29:2–14. doi: 10.1016/j.fsi.2010.02.017
11. Miao R, Zhu X, Wan C, Wang Z, Wen Y, Li Y. Effect of Clostridium butyricum supplementation on the development of intestinal flora and the immune system of neonatal mice. Exp Ther Med (2018) 15:1081–6. doi: 10.3892/etm.2017.5461
12. Mohammadian T, Nasirpour M, Tabandeh MR, Heidary AA, Ghanei-Motlagh R, Hosseini SS. Administrations of autochthonous probiotics altered juvenile rainbow trout Oncorhynchus mykiss health status, growth performance and resistance to Lactococcus garvieae, an experimental infection. Fish Shellfish Immunol (2019) 86:269–79. doi: 10.1016/j.fsi.2018.11.052
13. Liu L, Zeng D, Yang M, Wen B, Lai J, Zhou Y, et al. Probiotic Clostridium butyricum improves the growth performance, immune function, and gut microbiota of weaning rex rabbits. Probiot Antimicrob Proteins (2019) 11:1278–92. doi: 10.1007/s12602-018-9476-x
14. Tran NT, Li Z, Ma H, Zhang Y, Zheng H, Gong Y, et al. Clostridium butyricum: a promising probiotic confers positive health benefits in aquatic animals. Rev Aquac (2020) 12:2573–89. doi: 10.1111/raq.12459
15. Hsiao Y-P, Chen H-L, Tsai J-N, Lin M-Y, Liao J-W, Wei M-S, et al. Administration of Lactobacillus reuteri combined with Clostridium butyricum attenuates cisplatin-induced renal damage by gut microbiota reconstitution, increasing butyric acid production, and suppressing renal inflammation. Nutrients (2021) 13:2792. doi: 10.3390/nu13082792
16. Xu L, Sun X, Wan X, Li K, Jian F, Li W, et al. Dietary supplementation with Clostridium butyricum improves growth performance of broilers by regulating intestinal microbiota and mucosal epithelial cells. Anim Nutr (2021) 7:1105–14. doi: 10.1016/j.aninu.2021.01.009
17. Zhang L, Cao GT, Zeng XF, Zhou L, Ferket PR, Xiao YP, et al. Effects of Clostridium butyricum on growth performance, immune function, and cecal microflora in broiler chickens challenged with Escherichia coli K88. Poult Sci (2014) 93:46–53. doi: 10.3382/ps.2013-03412
18. Gao Q, Xiao C, Min M, Zhang C, Peng S, Shi Z. Effects of probiotics dietary supplementation on growth performance, innate immunity and digestive enzymes of silver pomfret, Pampus argenteus. Indian J Anim Res (2016) 50:936–41. doi: 10.18805/ijar.9640
19. Yin Z, Liu Q, Liu Y, Gao S, He Y, Yao C, et al. Early life intervention using probiotic clostridium butyricum improves intestinal development, immune response, and gut microbiota in large yellow croaker (Larimichthys crocea) larvae. Front Immunol (2021) 12:640767. doi: 10.3389/fimmu.2021.640767
20. Poolsawat L, Li X, He M, Ji D, Leng X. Clostridium butyricum as probiotic for promoting growth performance, feed utilization, gut health and microbiota community of tilapia (Oreochromis niloticus× O. aureus). Aquac Nutr (2020) 26:657–70. doi: 10.1111/anu.13025
21. Wangari MR, Gao Q, Sun C, Liu B, Song C, Tadese DA, et al. Effect of dietary Clostridium butyricum and different feeding patterns on growth performance, antioxidant and immune capacity in freshwater prawn (Macrobrachium rosenbergii). Aquac Res (2021) 52:12–22. doi: 10.1111/are.14865
22. Duan Y, Dong H, Wang Y, Zhang Y, Zhang J. Effects of the dietary probiotic Clostridium butyricum on intestine digestive and metabolic capacities, SCFA content and body composition in Marsupenaeus japonicus. J Ocean Univ China (2018) 17:690–6. doi: 10.1007/s11802-018-3464-3
23. Li S, Sang C, Turchini GM, Wang A, Zhang J, Chen N. Starch in aquafeeds: the benefits of a high amylose to amylopectin ratio and resistant starch content in diets for the carnivorous fish, largemouth bass (Micropterus salmoides). Br J Nutr (2020) 124:1145–55. doi: 10.1017/S0007114520002214
24. Gong Y, Yang F, Hu J, Liu C, Liu H, Han D, et al. Effects of dietary yeast hydrolysate on the growth, antioxidant response, immune response and disease resistance of largemouth bass (Micropterus salmoides). Fish Shellfish Immunol (2019) 94:548–57. doi: 10.1016/j.fsi.2019.09.044
25. Yusuf A, Huang X, Chen N, Apraku A, Wang W, Cornel A, et al. Impact of dietary vitamin c on plasma metabolites, antioxidant capacity and innate immunocompetence in juvenile largemouth bass, Micropterus salmoides. Aquac Rep (2020) 17:100383. doi: 10.1016/j.aqrep.2020.100383
26. Adeoye AA, Yomla R, Jaramillo-Torres A, Rodiles A, Merrifield DL, Davies SJ. Combined effects of exogenous enzymes and probiotic on Nile tilapia (Oreochromis niloticus) growth, intestinal morphology and microbiome. Aquaculture (2016) 463:61–70. doi: 10.1016/j.aquaculture.2016.05.028
27. Dawood MAO, Koshio S, Esteban MÁ. Beneficial roles of feed additives as immunostimulants in aquaculture: a review. Rev Aquac (2018) 10:950–74. doi: 10.1111/raq.12209
28. Bharathi S, Antony C, Cbt R, Arumugam U, Ahilan B, Aanand S. Functional feed additives used in fish feeds. Int J Fish Aquat Stud (2019) 7:44–52.
29. Ogunkalu O. Effects of feed additives in fish feed for improvement of aquaculture. Eurasian J Food Sci Technol (2019) 3:49–57.
30. Elsabagh M, Mohamed R, Moustafa EM, Hamza A, Farrag F, Decamp O, et al. Assessing the impact of Bacillus strains mixture probiotic on water quality, growth performance, blood profile and intestinal morphology of Nile tilapia, Oreochromis niloticus. Aquac Nutr (2018) 24:1613–22. doi: 10.1111/anu.12797
31. Wei CL, Chao SH, Tsai WB, et al. Analysis of bacterial diversity during the fermentation of inyu, a high- temperature fermented soy sauce, using nested PCR-denaturing gradient gel electrophoresis and the plate count method. Food Microbiol (2013) 33(2):252–61.
32. Livak KJ, Schmittgen TD. Analysis of relative gene expression data using real-time quantitative PCR and the 2–ΔΔCT method. methods (2001) 25:402–8. doi: 10.1006/meth.2001.1262
33. Zeng L, Wang Y-H, Ai C-X, Zheng J-L, Wu C-W, Cai R. Effects of β-glucan on ROS production and energy metabolism in yellow croaker (Pseudosciaena crocea) under acute hypoxic stress. Fish Physiol Biochem (2016) 42:1395–405. doi: 10.1007/s10695-016-0227-1
34. Liang J, Kou S, Chen C, Raza SHA, Wang S, Ma X, et al. Effects of Clostridium butyricum on growth performance, metabonomics and intestinal microbial differences of weaned piglets. BMC Microbiol (2021) 21:1–16. doi: 10.1186/s12866-021-02143-z
35. Luo K, Tian X, Wang B, Wei C, Wang L, Zhang S, et al. Evaluation of paraprobiotic applicability of Clostridium butyricum CBG01 in improving the growth performance, immune responses and disease resistance in Pacific white shrimp, Penaeus vannamei. Aquaculture (2021) 544:737041. doi: 10.1016/j.aquaculture.2021.737041
36. Li P, Hou D, Zhao H, Wang H, Peng K, Cao J. Dietary Clostridium butyricum Improves Growth Performance and Resistance to Ammonia Stress in Yellow Catfish (Pelteobagrus fulvidraco). Aquac Nutr (2022) 2022:6965174. doi: 10.1155/2022/6965174
37. Yi C-C, Liu C-H, Chuang K-P, Chang Y-T, Hu S-Y. A potential probiotic Chromobacterium aquaticum with bacteriocin-like activity enhances the expression of indicator genes associated with nutrient metabolism, growth performance and innate immunity against pathogen infections in zebrafish (Danio rerio). Fish Shellfish Immunol (2019) 93:124–34. doi: 10.1016/j.fsi.2019.07.042
38. Yang P, Wang W, Chi S, Mai K, Song F, Wang L. Effects of dietary lysine on regulating GH-IGF system, intermediate metabolism and immune response in largemouth bass (Micropterus salmoides). Aquac Rep (2020) 17:100323. doi: 10.1016/j.aqrep.2020.100323
39. Al-Samerria S, Radovick S. The role of insulin-like growth factor-1 (IGF-1) in the control of neuroendocrine regulation of growth. Cells (2021) 10:2664. doi: 10.3390/cells10102664
40. Sheridan MA, Hagemeister AL. Somatostatin and somatostatin receptors in fish growth. Gen Comp Endocrinol (2010) 167:360–5. doi: 10.1016/j.ygcen.2009.09.002
41. Norbeck LA, Sheridan MA. An in vitro model for evaluating peripheral regulation of growth in fish: effects of 17β-estradiol and testosterone on the expression of growth hormone receptors, insulin-like growth factors, and insulin-like growth factor type 1 receptors in rainbow trout (Oncorhynchus mykiss). Gen Comp Endocrinol (2011) 173:270–80. doi: 10.1016/j.ygcen.2011.06.009
42. Mohammed-Geba K, Martos-Sitcha JA, Galal-Khallaf A, Mancera JM, Martínez-Rodríguez G. Insulin-like growth factor 1 (IGF-1) regulates prolactin, growth hormone, and IGF-1 receptor expression in the pituitary gland of the gilthead sea bream Sparus aurata. Fish Physiol Biochem (2016) 42:365–77. doi: 10.1007/s10695-015-0144-8
43. Bonvini E, Bonaldo A, Parma L, Mandrioli L, Sirri R, Grandi M, et al. Feeding European sea bass with increasing dietary fibre levels: Impact on growth, blood biochemistry, gut histology, gut evacuation. Aquaculture (2018) 494:1–9. doi: 10.1016/j.aquaculture.2018.05.017
44. Guardiola FA, Saraiva-Fraga M, Cuesta A, Esteban MA. Changes in natural haemolytic complement activity induced by stress in gilthead seabream (Sparus aurata L.). Fish Shellfish Immunol (2018) 78:317–21. doi: 10.1016/j.fsi.2018.04.056
45. Parma L, Pelusio NF, Gisbert E, Esteban MA, D’Amico F, Soverini M, et al. Effects of rearing density on growth, digestive conditions, welfare indicators and gut bacterial community of gilthead sea bream (Sparus aurata, L. 1758) fed different fishmeal and fish oil dietary levels. Aquaculture (2020) 518:734854. doi: 10.1016/j.aquaculture.2019.734854
46. Pelusio NF, Bonaldo A, Gisbert E, Andree KB, Esteban MA, Dondi F, et al. Different fish meal and fish oil dietary levels in European sea bass: welfare implications after acute confinement stress. Front Mar Sci (2022) 8:2003. doi: 10.3389/fmars.2021.779053
47. Saurav K, Raman RP, Kundan K, Pandey PK, Neeraj K, Mallesh B, et al. Effect of azadirachtin on haematological and biochemical parameters of Argulus-infested goldfish Carassius auratus (Linn. 1758). Fish Physiol Biochem (2013) 39:733–47. doi: 10.1007/s10695-012-9736-8
48. Akbary P, Sartipi Yarahmadi S, Jahanbakhshi A. Hematological, hepatic enzymes’ activity and oxidative stress responses of gray mullet (Mugil cephalus) after sub-acute exposure to copper oxide. Environ Sci Pollut Res (2018) 25:1800–8. doi: 10.1007/s11356-017-0582-1
49. Ao H, Jiang J, Liu L, Liu Y, Hu B, Chen Y. Effects of dietary fermentation products of kitchen waste on growth, apparent digestibility, digestive enzyme activities and serum biochemistry in juvenile allogynogenetic gibel carp (Carassius auratus gibelio) var. CAS III. J World Aquac Soc (2021) 52:895–912. doi: 10.1111/jwas.12826
50. Dufour DR, Lott JA, Nolte FS, Gretch DR, Koff RS, Seeff LB. Diagnosis and monitoring of hepatic injury. I. Performance characteristics of laboratory tests. Clin Chem (2000) 46:2027–49. doi: 10.1093/clinchem/46.12.2027
51. Zhang C, Zhang Q, Pang Y, Song X, Zhou N, Wang J, et al. The protective effects of melatonin on oxidative damage and the immune system of the Chinese mitten crab (Eriocheir sinensis) exposed to deltamethrin. Sci Total Environ (2019) 653:1426–34. doi: 10.1016/j.scitotenv.2018.11.063
52. Wu YC, Li RM, Shen G, Huang F, Yang Q, Tan B, et al. Effects of dietary small peptides on growth, antioxidant capacity, nonspecific immunity and ingut microflora structure of Litopenaeus vannamei. J Guangdong Ocean Univ (2021) 41:1–9.
53. Lin H, Tan X, Zhou C, Niu J, Xia D, Huang Z, et al. Effect of dietary arginine levels on the growth performance, feed utilization, non-specific immune response and disease resistance of juvenile golden pompano Trachinotus ovatus. Aquaculture (2015) 437:382–9. doi: 10.1016/j.aquaculture.2014.12.025
54. Tan X, Sun Z, Chen S, Chen S, Huang Z, Zhou C, et al. Effects of dietary dandelion extracts on growth performance, body composition, plasma biochemical parameters, immune responses and disease resistance of juvenile golden pompano Trachinotus ovatus. Fish Shellfish Immunol (2017) 66:198–206. doi: 10.1016/j.fsi.2017.05.028
55. Lin S-M, Shi C-M, Mu M-M, Chen Y-J, Luo L. Effect of high dietary starch levels on growth, hepatic glucose metabolism, oxidative status and immune response of juvenile largemouth bass, Micropterus salmoides. Fish Shellfish Immunol (2018) 78:121–6. doi: 10.1016/j.fsi.2018.04.046
56. Yi Y, Zhang Z, Zhao F, Liu H, Yu L, Zha J, et al. Probiotic potential of Bacillus velezensis JW: antimicrobial activity against fish pathogenic bacteria and immune enhancement effects on Carassius auratus. Fish Shellfish Immunol (2018) 78:322–30. doi: 10.1016/j.fsi.2018.04.055
57. Chen X-Q, Zhao W, Xie S-W, Xie J-J, Zhang Z-H, Tian L-X, et al. Effects of dietary hydrolyzed yeast (Rhodotorula mucilaginosa) on growth performance, immune response, antioxidant capacity and histomorphology of juvenile Nile tilapia (Oreochromis niloticus). Fish Shellfish Immunol (2019) 90:30–9. doi: 10.1016/j.fsi.2019.03.068
58. Jin H, Yan C, Xiao T, Yan N, Xu J, Zhou L, et al. High fish oil diet promotes liver inflammation and activates the complement system. Mol Med Rep (2018) 17:6852–8. doi: 10.3892/mmr.2018.8687
59. Zhao J, Liu Y, Jiang J, Wu P, Jiang W, Li S, et al. Effects of dietary isoleucine on the immune response, antioxidant status and gene expression in the head kidney of juvenile Jian carp (Cyprinus carpio var. Jian). Fish Shellfish Immunol (2013) 35:572–80. doi: 10.1016/j.fsi.2013.05.027
60. Ni P-J, Jiang W-D, Wu P, Liu Y, Kuang S-Y, Tang L, et al. Dietary low or excess levels of lipids reduced growth performance, and impaired immune function and structure of head kidney, spleen and skin in young grass carp (Ctenopharyngodon idella) under the infection of Aeromonas hydrophila. Fish Shellfish Immunol (2016) 55:28–47. doi: 10.1016/j.fsi.2016.03.163
61. Li Y, Wu J, Chen X, Chu Z, Jin J, Tang D. Dietary myo-inositol requirements of juvenile hybrid sturgeon (Acipenser baerii♀× A. schrenckii♂). Aquac Res (2020) 51:1143–51. doi: 10.1111/are.14461
62. Tan X, Lin H, Huang Z, Zhou C, Wang A, Qi C, et al. Effects of dietary leucine on growth performance, feed utilization, non-specific immune responses and gut morphology of juvenile golden pompano Trachinotus ovatus. Aquaculture (2016) 465:100–7. doi: 10.1016/j.aquaculture.2016.08.034
63. Kong Y, Li M, Chu G, Liu H, Shan X, Wang G, et al. The positive effects of single or conjoint administration of lactic acid bacteria on Channa argus: Digestive enzyme activity, antioxidant capacity, intestinal microbiota and morphology. Aquaculture (2021) 531:735852. doi: 10.1016/j.aquaculture.2020.735852
64. Cheng C-H, Yang F-F, Ling R-Z, Liao S-A, Miao Y-T, Ye C-X, et al. Effects of ammonia exposure on apoptosis, oxidative stress and immune response in pufferfish (Takifugu obscurus). Aquat Toxicol (2015) 164:61–71. doi: 10.1016/j.aquatox.2015.04.004
65. Lan Y, Ye T, Xue Y, Liu H, Zhang H, Cheng D, et al. Physiological and immunological responses to mass mortality in noble scallop Chlamys nobilis cultured in Nan’ao waters of Shantou, China. Fish Shellfish Immunol (2018) 82:453–9. doi: 10.1016/j.fsi.2018.08.049
66. Yu Z, Wu X-Q, Zheng L-J, Dai Z-Y, Wu L-F. Effect of acute exposure to ammonia and BFT alterations on Rhynchocypris lagowski: Digestive enzyme, inflammation response, oxidative stress and immunological parameters. Environ Toxicol Pharmacol (2020) 78:103380. doi: 10.1016/j.etap.2020.103380
67. Duan Y, Zhang Y, Dong H, Wang Y, Zheng X, Zhang J. Effect of dietary Clostridium butyricum on growth, intestine health status and resistance to ammonia stress in Pacific white shrimp Litopenaeus vannamei. Fish Shellfish Immunol (2017) 65:25–33. doi: 10.1016/j.fsi.2017.03.048
68. Zhang J-X, Guo L-Y, Feng L, Jiang W-D, Kuang S-Y, Liu Y, et al. Soybean β-conglycinin induces inflammation and oxidation and causes dysfunction of intestinal digestion and absorption in fish. PloS One (2013) 8:e58115. doi: 10.1371/journal.pone.0058115
69. Zhao J, Feng L, Liu Y, Jiang W, Wu P, Jiang J, et al. Effect of dietary isoleucine on the immunity, antioxidant status, tight junctions and microflora in the intestine of juvenile Jian carp (Cyprinus carpio var. Jian). Fish Shellfish Immunol (2014) 41:663–73. doi: 10.1016/j.fsi.2014.10.002
70. Chen L, Feng L, Jiang W-D, Jiang J, Wu P, Zhao J, et al. Intestinal immune function, antioxidant status and tight junction proteins mRNA expression in young grass carp (Ctenopharyngodon idella) fed riboflavin deficient diet. Fish Shellfish Immunol (2015) 47:470–84. doi: 10.1016/j.fsi.2015.09.037
71. Stalnikowitz DK, Weissbrod AB. Liver fibrosis and inflammation. A review. Ann Hepatol (2003) 2:159–63. doi: 10.1016/S1665-2681(19)32127-1
72. Yin G, Li W, Lin Q, Lin XI, Lin J, Zhu Q, et al. Dietary administration of laminarin improves the growth performance and immune responses in Epinephelus coioides. Fish Shellfish Immunol (2014) 41:402–6. doi: 10.1016/j.fsi.2014.09.027
73. El-Leithy AAA, Hemeda SA, El Naby WSHA, El Nahas AF, Hassan SAH, Awad ST, et al. Optimum salinity for Nile tilapia (Oreochromis niloticus) growth and mRNA transcripts of ion-regulation, inflammatory, stress-and immune-related genes. Fish Physiol Biochem (2019) 45:1217–32. doi: 10.1007/s10695-019-00640-7
74. Zhang C, Zhang J, Ren H, Zhou B, Wu Q, Sun P. Effect of tributyltin on antioxidant ability and immune responses of zebrafish (Danio rerio). Ecotoxicol Environ Saf (2017) 138:1–8. doi: 10.1016/j.ecoenv.2016.12.016
75. Li MO, Flavell RA. Contextual regulation of inflammation: a duet by transforming growth factor-β and interleukin-10. Immunity (2008) 28:468–76. doi: 10.1016/j.immuni.2008.03.003
76. Giri SS, Sen SS, Chi C, Kim HJ, Yun S, Park SC, et al. Effect of guava leaves on the growth performance and cytokine gene expression of Labeo rohita and its susceptibility to Aeromonas hydrophila infection. Fish Shellfish Immunol (2015) 46:217–24. doi: 10.1016/j.fsi.2015.05.051
77. Fabregat I, Moreno-Càceres J, Sánchez A, Dooley S, Dewidar B, Giannelli G, et al. TGF-β signalling and liver disease. FEBS J (2016) 283:2219–32. doi: 10.1111/febs.13665
78. Zhang D, Tang J, Zhang J, Hu CX. Responses of pro-and anti-inflammatory cytokines in zebrafish liver exposed to sublethal doses of Aphanizomenon flosaquae DC-1 aphantoxins. Aquat Toxicol (2019) 215:105269. doi: 10.1016/j.aquatox.2019.105269
79. Chiu C-H, Cheng C-H, Gua W-R, Guu Y-K, Cheng W. Dietary administration of the probiotic, Saccharomyces cerevisiae P13, enhanced the growth, innate immune responses, and disease resistance of the grouper, Epinephelus coioides. Fish Shellfish Immunol (2010) 29:1053–9. doi: 10.1016/j.fsi.2010.08.019
80. Li Z-H, Zlabek V, Grabic R, Li P, Machova J, Velisek J, et al. Effects of exposure to sublethal propiconazole on the antioxidant defense system and Na+–K+-ATPase activity in brain of rainbow trout, Oncorhynchus mykiss. Aquat Toxicol (2010) 98:297–303. doi: 10.1016/j.aquatox.2010.02.017
81. Liu H, Wang S, Cai Y, Guo X, Cao Z, Zhang Y, et al. Dietary administration of Bacillus subtilis HAINUP40 enhances growth, digestive enzyme activities, innate immune responses and disease resistance of tilapia, Oreochromis niloticus. Fish Shellfish Immunol (2017) 60:326–33. doi: 10.1016/j.fsi.2016.12.003
82. Ding X, Lu D, Hou Q, Li S, Liu X, Zhang Y, et al. Orange-spotted grouper (Epinephelus coioides) toll-like receptor 22: molecular characterization, expression pattern and pertinent signaling pathways. Fish Shellfish Immunol (2012) 33:494–503. doi: 10.1016/j.fsi.2012.05.034
83. Zhang J, Kong X, Zhou C, Li L, Nie G, Li X. Toll-like receptor recognition of bacteria in fish: ligand specificity and signal pathways. Fish Shellfish Immunol (2014) 41:380–8. doi: 10.1016/j.fsi.2014.09.022
84. Zhao S, Chen Z, Zheng J, Dai J, Ou W, Xu W, et al. Citric acid mitigates soybean meal induced inflammatory response and tight junction disruption by altering TLR signal transduction in the intestine of turbot, Scophthalmus maximus L. Fish Shellfish Immunol (2019) 92:181–7. doi: 10.1016/j.fsi.2019.06.004
85. Takano T, Kondo H, Hirono I, Saito-Taki T, Endo M, Aoki T. Identification and characterization of a myeloid differentiation factor 88 (MyD88) cDNA and gene in Japanese flounder, Paralichthys olivaceus. Dev Comp Immunol (2006) 30:807–16. doi: 10.1016/j.dci.2005.11.003
86. Heng J, Su J, Huang T, Dong J, Chen L. The polymorphism and haplotype of TLR3 gene in grass carp (Ctenopharyngodon idella) and their associations with susceptibility/resistance to grass carp reovirus. Fish Shellfish Immunol (2011) 30:45–50. doi: 10.1016/j.fsi.2010.09.004
87. Chen C-C, Kong M-S, Lai M-W, Chao H-C, Chang K-W, Chen S-Y, et al. Probiotics have clinical, microbiologic, and immunologic efficacy in acute infectious diarrhea. Pediatr Infect Dis J (2010) 29:135–8. doi: 10.1097/INF.0b013e3181b530bf
88. Yang CM, Cao GT, Ferket PR, Liu TT, Zhou L, Zhang L, et al. Effects of probiotic, Clostridium butyricum, on growth performance, immune function, and cecal microflora in broiler chickens. Poult Sci (2012) 91:2121–9. doi: 10.3382/ps.2011-02131
89. Chen L, Li S, Zheng J, Li W, Jiang X, Zhao X, et al. Effects of dietary Clostridium butyricum supplementation on growth performance, intestinal development, and immune response of weaned piglets challenged with lipopolysaccharide. J Anim Sci Biotechnol (2018) 9:1–14. doi: 10.1186/s40104-018-0275-8
Keywords: Clostridium butyricum, Largemouth bass, growth performance, antioxidant status, immune response
Citation: Li P, Chen X, Hou D, Chen B, Peng K, Huang W, Cao J and Zhao H (2023) Positive effects of dietary Clostridium butyricum supplementation on growth performance, antioxidant capacity, immunity and viability against hypoxic stress in largemouth bass. Front. Immunol. 14:1190592. doi: 10.3389/fimmu.2023.1190592
Received: 21 March 2023; Accepted: 16 August 2023;
Published: 30 August 2023.
Edited by:
Willem Van Eden, Utrecht University, NetherlandsReviewed by:
Chaobin Qin, Henan Normal University, ChinaZheng-Yong Wen, Neijiang Normal University, China
Copyright © 2023 Li, Chen, Hou, Chen, Peng, Huang, Cao and Zhao. This is an open-access article distributed under the terms of the Creative Commons Attribution License (CC BY). The use, distribution or reproduction in other forums is permitted, provided the original author(s) and the copyright owner(s) are credited and that the original publication in this journal is cited, in accordance with accepted academic practice. No use, distribution or reproduction is permitted which does not comply with these terms.
*Correspondence: Hongxia Zhao, emhhb2hvbmd4aWE4ODY2QDE2My5jb20=
†These authors have also contributed to this work