- 1Department of Microbiology and Immunology, Peter Doherty Institute for Infection and Immunity, University of Melbourne, Melbourne, VIC, Australia
- 2Department of Biomedical Engineering, University of Michigan, Ann Arbor, MI, United States
Vaccine efficacy determined within the controlled environment of a clinical trial is usually substantially greater than real-world vaccine effectiveness. Typically, this results from reduced protection of immunologically vulnerable populations, such as children, elderly individuals and people with chronic comorbidities. Consequently, these high-risk groups are frequently recommended tailored immunisation schedules to boost responses. In addition, diverse groups of healthy adults may also be variably protected by the same vaccine regimen. Current population-based vaccination strategies that consider basic clinical parameters offer a glimpse into what may be achievable if more nuanced aspects of the immune response are considered in vaccine design. To date, vaccine development has been largely empirical. However, next-generation approaches require more rational strategies. We foresee a generation of precision vaccines that consider the mechanistic basis of vaccine response variations associated with both immunogenetic and baseline health differences. Recent efforts have highlighted the importance of balanced and diverse extra-neutralising antibody functions for vaccine-induced protection. However, in immunologically vulnerable populations, significant modulation of polyfunctional antibody responses that mediate both neutralisation and effector functions has been observed. Here, we review the current understanding of key genetic and inflammatory modulators of antibody polyfunctionality that affect vaccination outcomes and consider how this knowledge may be harnessed to tailor vaccine design for improved public health.
Introduction
Vaccines provide variable protection to different demographics as a result of complex interactions between host and environmental factors (1). This host diversity, if appropriately defined and characterised, may inform an era of precision vaccinology that accounts for inherent immunological differences between both individuals and populations (2–7). As vaccine clinical trials typically only recruit healthy adults and, unintentionally, often only from dominant ethnicities in developed countries, the data is typically not representative of vaccine efficacy in vulnerable populations (8–10). In an attempt to counter these known biases, vaccination recommendations frequently suggest prioritising early and additional doses for elderly and other immunocompromised individuals who experience reduced vaccine immunogenicity, as well as increased susceptibility to disease (11–15). Consequently, present vaccination regimens targeting specific populations are largely guided by rudimentary demographic and clinical parameters such as age and baseline health status (16–20).
However, rapid advances in molecular and systems biology along with materials science may facilitate a new frontier in population-based vaccination strategies informed by molecular mechanisms (6, 21–28). Technological and conceptual developments in vaccinology have led to numerous vaccination strategy modifications that can enhance immunogenicity and protection (1, 20, 29, 30). Concurrently, systems biology analyses of these vaccine regimens are beginning to elucidate the spectrum of protective immune interactomes (24, 27, 31, 32). These computational approaches facilitate investigation of complex biological interactions. As such, in-depth immune profiling of antibody features beyond the typically examined measures of titre and neutralisation has revealed nuanced qualitative features of antibodies that promote protection and distinguish individuals with impaired immunity (21, 33–37). Notably, a common signature associated with protection is the presence of antibody features that promote polyfunctional antibody effector functions (21, 33–37). These data may be key to informing the design of vaccines tailored to vulnerable populations.
Importance of antibodies for vaccine-induced protection
Antibodies have been identified as a correlate of protection or control of numerous infectious diseases (38). Neutralising antibodies provide sterilising immunity by binding target epitopes leading to steric hindrance that prevents pathogen entry into host cells or inhibits toxin activity. As such, elevated neutralising titres are the principal goal of most vaccination strategies and are highly predictive of protection against many viral and bacterial diseases (38, 39). However, while neutralisation is ideal as a primary humoral defence, eliciting broadly neutralising antibodies (bnAbs) via vaccination against complex, rapidly evolving, or diverse pathogens such as malaria (40), influenza (41), human immunodeficiency virus type 1 (HIV-1) (42), and severe acute respiratory syndrome coronavirus 2 (SARS-CoV-2) (43) remains an elusive goal.
Antibodies comprise of two functional components: the fragment antigen binding (Fab) region which determines target specificity and is essential for neutralisation, and the fragment crystallisable (Fc) region which engages the innate immune system via numerous mechanisms (Figure 1). As such, Fc functions bridge the innate and adaptive immune systems by enhancing viral, bacterial, and parasite degradation and clearance as well as lysis of infected host cells in an antigen-specific manner (44). Importantly, unlike neutralising antibodies, the specificities of Fc functional antibodies are not restricted by proximity to amino acids involved in pathogen binding and fusion with host cells. Instead, Fc functional antibodies can target any conformationally accessible epitope, making these antibodies less sensitive to pathogen mutation (45–53). Nevertheless, studies of HIV-1, influenza A virus, Ebola, and SARS-CoV-2 have demonstrated that antibody specificity can substantially alter Fc potency (49, 54–57). For this reason, vaccination strategies eliciting robust extra-neutralising functions against carefully selected epitopes may be an effective approach to counter the challenges associated with bnAb generation.
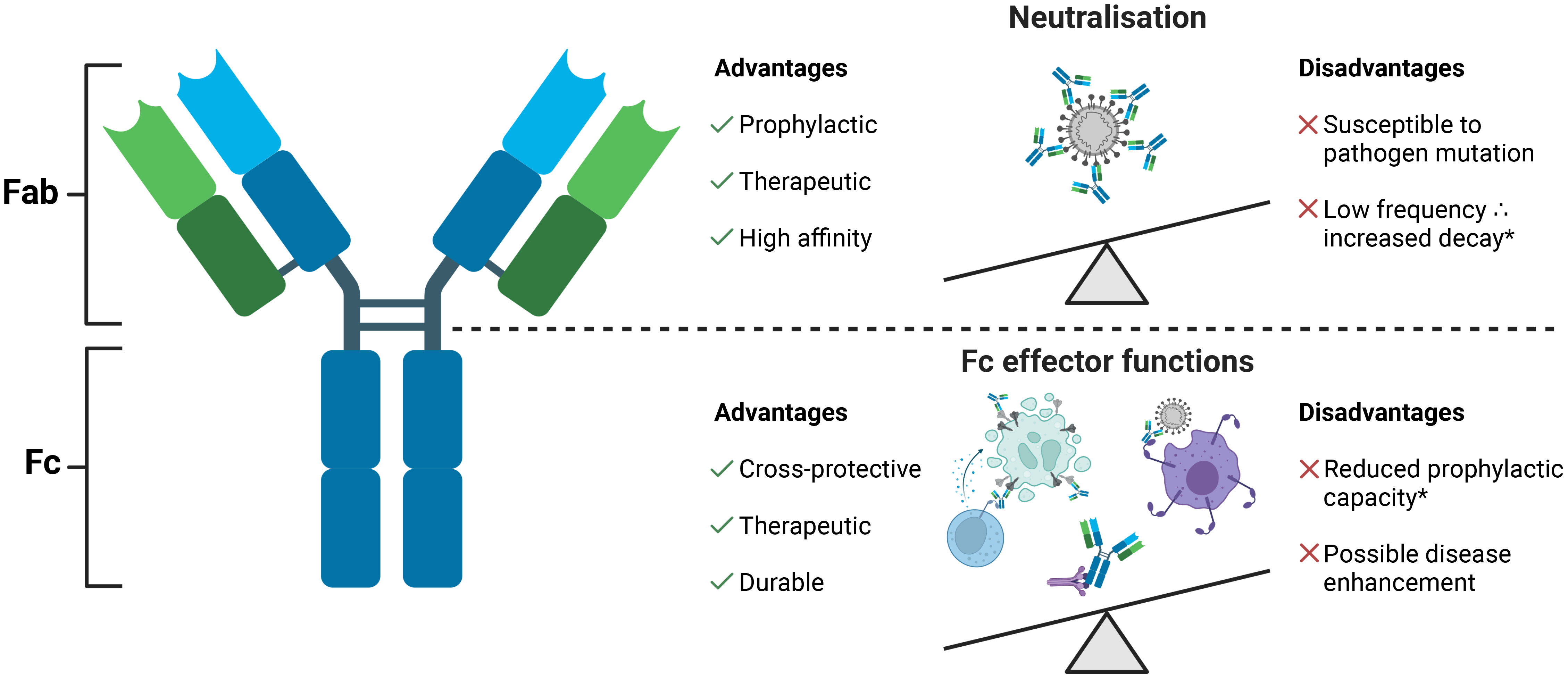
Figure 1 Antibodies comprise two fragment antigen binding (Fab) regions and one fragment crystallisable (Fc) region connected by a ladder-like hinge region. The Fab is responsible for antigen recognition and mediates pathogen and toxin neutralisation. The Fc engages effector cells and molecules of the innate immune system to mediate Fc effector functions. Neutralisation and Fc effector functions each have various advantages and disadvantages but largely counterbalance the shortcomings of the other. *Durable neutralisation capacity and prophylactic Fc functions observed for antibodies against some bacterial pathogens.
Fc effector functions enhance antibody-mediated protection
Beyond neutralisation, target-bound antibodies can initiate a range of potent effector functions via simultaneous Fc region engagement with host activating Fc receptors (FcR) on various phagocytic and cytotoxic effector cells. In addition, engagement with the neonatal Fc receptor (FcRn) increases antibody half-life (58, 59). Table 1 and Figure 2 detail the multifaceted Fc functions that antibodies mediate along with the key effector cells and molecules involved in each process. Figure 2 also defines abbreviations of key antibody mediated functions that are referenced subsequently throughout this review.
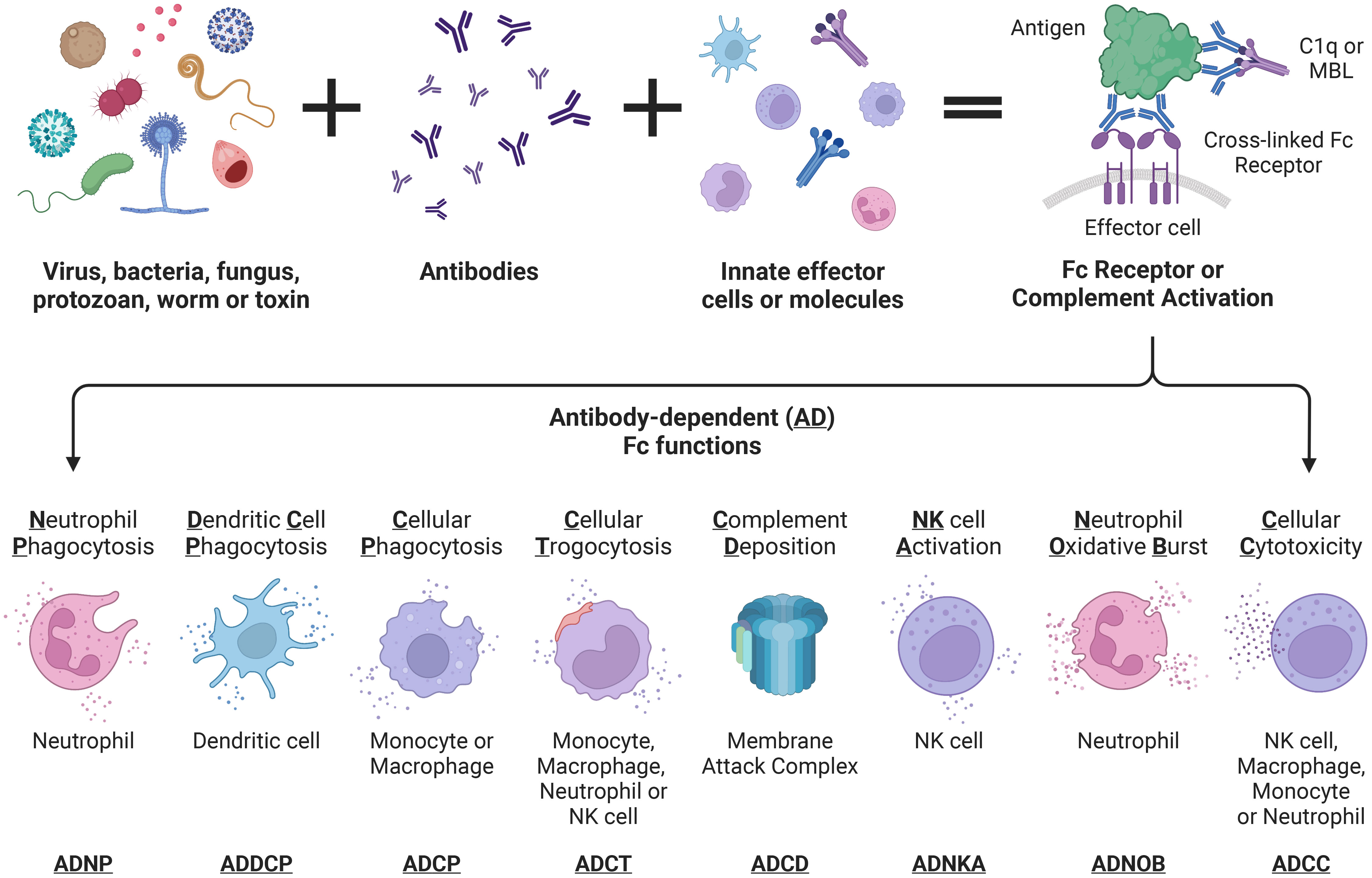
Figure 2 Antibody-dependent Fc effector functions referenced throughout this review. Fc effector functions are initiated upon simultaneous antibody engagement with a pathogen antigen and an innate effector molecule (complement component 1q (C1q) or mannose-binding lectin (MBL)) or Fc receptor (FcR) expressed by innate immune cells. Activation of C1q or MBL following antigen binding triggers the complement cascade leading to pathogen or infected cell death via antibody-dependent complement deposition. FcR cross-linking via antibody-antigen complexes triggers downstream signalling cascades within innate effector cells leading to pathogen killing and clearance via a range of antibody-dependent cellular effector functions, listed in the figure. Finally, these effector functions trigger downstream cytokine release which may enable further recruitment of effector cells.
Fc effector functions are appreciated as correlates of protection for multiple bacterial pathogens (38). Bactericidal antibodies underpin protection following meningococcal vaccination (67), and vaccine-induced antibody-dependent neutrophil phagocytosis (ADNP) is recognised as a correlate of protection against Streptococcus pneumoniae (68). Fc effector functions have also been highlighted as a key correlate of malaria protection in studies of the RTS,S/AS01 vaccine (69–72). Antibody titres alone were not associated with protection, however, protection following parasite challenge was predicted by capacity for antibody-dependent cellular phagocytosis (ADCP) and FcγRIIIa engagement (69, 71) as well as an immunoglobulin G (IgG) subclass distribution skewed towards IgG3 and away from IgG2 which would favour enhanced FcR engagement and effector functions (70, 72). Furthermore, in the case of HIV-1 (73), human papillomavirus (HPV) (74, 75), influenza (76, 77), and SARS-CoV-2 (78), neutralising antibodies do not fully explain vaccination-induced humoral protection, suggesting a pertinent role for Fc effector functions in antibody-mediated immunity (51, 79, 80). This phenomenon has been well-described for the only moderately protective HIV-1 vaccine trial, RV144, which demonstrated partial efficacy in the absence of bnAbs (73, 81); further antibody profiling indicated this phenomenon to be a consequence of robust Fc effector functions (73, 82, 83). Similarly, protection from respiratory syncytial virus (RSV) is poorly predicted by serum IgG levels or neutralising titres. Instead, Fc effector functions may be a better correlate of vaccine-induced protection (84, 85).
The importance of Fc functions in protection against pathogens has been demonstrated in animal models of HIV-1 (86), SARS-CoV-2 (87–89) and influenza challenge (49) in which neutralising monoclonal antibodies (mAbs) required Fc-functional capacity for optimal prophylaxis and treatment. The value of Fc functions was demonstrated in macaque models of HIV-1 infection whereby administration of neutralising mAbs with an Fc LALA mutation (two consecutive leucine to alanine substitutions which abolish antibody binding to FcγRs) impaired protection compared to intact mAbs (86). In the case of SARS-CoV-2, humanised mice and Syrian hamsters administered Fc-functional mAbs exhibited reduced viral load and immunopathology compared to those administered mAbs with an Fc LALA mutation (88). These protective effects were only observed in the presence of monocytes, but the absence of neutrophils or NK cells had no effect on weight loss, indicating a dominant role for ADCP (88). In addition, mAbs containing the GASDALIE mutation that promotes enhanced FcγRIIIa binding showed improved protection against lethal SARS-CoV-2 challenge compared to wild-type mAbs (87). In the case of influenza, while bnAbs against the variable head region of the hemagglutinin (HA) protein did not require Fc functional capacity for protection, bnAbs directed against the conserved stalk region required FcγR-driven antibody-dependent cellular cytotoxicity (ADCC) to confer protection against lethal H1N1 challenge (49). Given the importance of cross-reactive anti-HA stalk antibodies to counter the high mutation rates of influenza, Fc functions have great value in influenza protection (90).
Immune responses associated with reduced infection risk and severity can guide vaccine development. Indeed, the parallel identification of ADCP and antibody-dependent natural killer cell activation (ADNKA) as correlates of protection against malaria in both vaccinated and unvaccinated individuals (69, 71, 91–93) suggests a level of homology between the protective mechanisms induced by vaccination and those required for disease resolution. Similarly, ADNKA has been associated with protection against RSV following vaccination or infection (84, 94, 95). In addition, enhanced ADCC is associated with HIV-1 viremic control (96–99) and was also identified as a correlate of protection following RV144 HIV-1 vaccination (34, 82). Given the wide-ranging benefits of a coordinated Fc response, it follows that robust Fc functions are implicated in protection against most diseases for which vaccines are licenced or in clinical trial (Table 2). Furthermore, for highly fatal infections, such as Ebola virus disease (57, 193) and Marburg virus disease (159), Fc effector functions promote protection and survival, as well as reduction of long-term sequelae. Therefore, targeting generation of broad and highly potent Fc effector functions is likely a valuable goal of many vaccines currently under development.
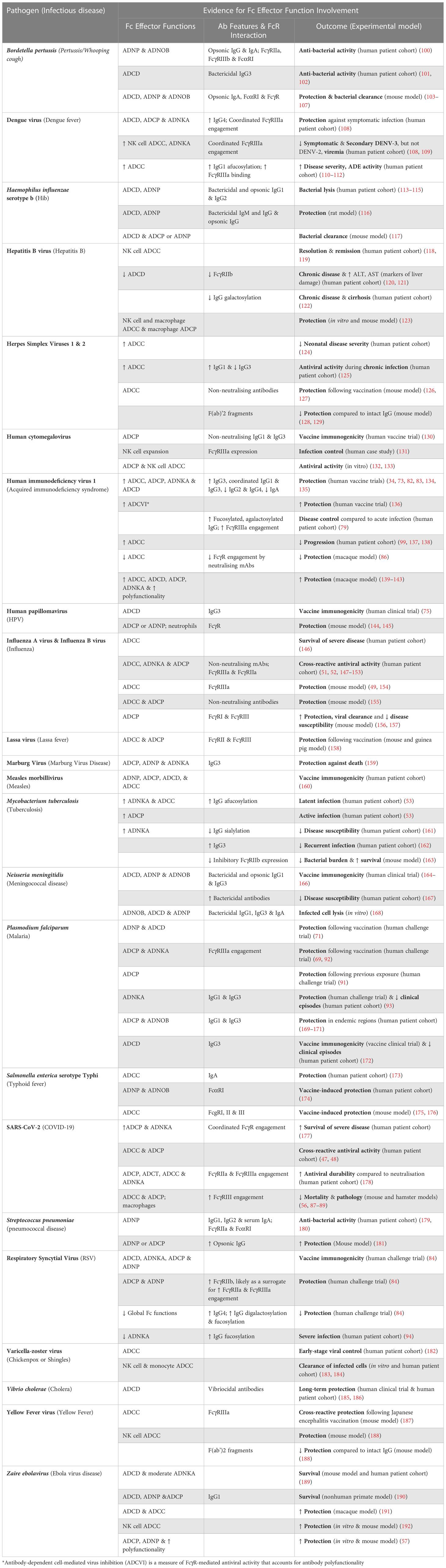
Table 2 Infectious diseases for which Fc effector functions are involved in protection or antimicrobial activity, and for which vaccines are licenced or in clinical trial.
Advantages of Fc mediated functions
Even when sterilising immunity is achievable via vaccination, neutralisation escape is frequent as a result of viral evolution. The effect of even a few amino acid mutations upon neutralisation has been extensively studied in the face of SARS-CoV-2 variants, where significantly weaker neutralising titres are observed against Omicron subvariants in comparison to the ancestral strain, and this remains true despite repeated vaccine boosts (194–196). Although boosting with Omicron BA.5 or BA.4/BA.5 adapted bivalent booster vaccination recovers neutralisation of the BA.4/BA.5 variant, neutralisation capacity is again lost against more recently emerged variants such as BQ.1.1 and XBB.1 (195). Given that perpetually updating vaccines to protect against continuously emerging viral variants is highly challenging, design of vaccines eliciting broadly protective functions, such as Fc-effector functions, is warranted. Indeed, the extent of this Fc functional antibody cross-reactivity is demonstrated by the ability of a chimeric Japanese encephalitis virus (JEV) vaccine (consisting of JEV structural genes upon the yellow fever virus vaccine YFV-17D scaffold) to protect mice against lethal yellow fever virus challenge via FcγRIIIa-mediated ADCC (187). Of note, although ADCC-mediating antibodies may exert selective pressure on HIV-1 evolution (197), the likelihood of Fc functions to drive evolution of viral escape mutations is reduced compared to that of neutralising antibodies (198, 199). This constraint of neutralisation escape mechanisms further supports prioritisation of Fc functions in vaccine development.
Fc-functional antibodies are also more durable than neutralising antibodies (178) given the increased abundance of non-neutralising antibodies, which, for example, may constitute up to 95% of antibodies against the SARS-CoV-2 spike protein (46). In human cohorts, a study characterising various antibody features of convalescent plasma from 36 mild-moderate coronavirus disease 2019 (COVID-19) recovered patients up to five months post-infection, 100% and 94% of participants maintained ADCP and ADCC functions, respectively, while neutralisation was only detectable in 70% of participants (178). Independent studies have also detected persistence of neutralising antibodies against SARS-CoV-2 five months following infection (200), however, the longevity of the response is dependent upon disease severity (201). Similar to the kinetics of post-infection responses, neutralising antibodies induced by SARS-CoV-2 vaccination have been found to decay within four to six months, particularly against SARS-CoV-2 variants of concern (202) and among immunocompromised populations (43). As such, this data reinforces the value of Fc functions in protecting vulnerable populations against evolving pandemics.
A further benefit of Fc functional antibodies is their dual capacity for both protection against infection as well as control of disease through collaboration with neutralising antibodies and T cells, respectively, as demonstrated by both mechanistic (203) and systems serology (78, 204) studies of SARS-CoV-2. Furthermore, enhanced Fc engagement with FcγRIIa supports increased dendritic cell maturation and CD8+ T cell responses, facilitating improved protection against influenza (205). In the case of SARS-CoV-2, although neutralising titres remain predictive of protection against symptomatic disease in the face of variants (206), with up to log-fold reductions in neutralisation (195), cross-reactive Fc functions likely mitigate, at least in part, the severe disease outcomes that might be expected with such drastic losses in neutralisation. As such, it is likely that, along with T cell responses (207), highly conserved Fc effector functions directed against novel variants (47, 48) may protect against severe outcomes.
Fc modifications predict effector functions
Despite the Fc portion belonging to the antibody constant region, numerous Fc modifications contribute to antibody diversity (208, 209). Antibody quality can be enhanced by heritable and inducible genetic variation of the Fc region in the form of antibody isotypes, subclasses, and allotypes, as well as post-translational Fc modifications such as glycosylation. This variation can greatly impact FcR interactions, and therefore, alter potency of Fc functions, with antibody isotype and subclass modulation typically having the greatest effects (208). Importantly, changes to the abundance of various antibody isotypes, subclasses, and glycosylation patterns can be induced via both vaccination and disease (110, 210–213). Critically, regulation of these Fc modifications is a complex, multilayered process influenced by a range of innate and adaptive immune cells and cytokines (214–218).
Isotypes and subclasses
Upon B cell activation, early IgD+ and IgM+ lymphocytes undergo affinity maturation and DNA recombination in the form of class switch recombination (CSR). CSR enables selective usage of a single immunoglobulin heavy constant (IGH) gene (Cμ through to Cα2) per transcript, with a bias towards downstream genes with increasing antigen exposure. As such, this process converts antibody constant regions to more mature isotypes (Figure 3), generating higher affinity, Fc-functional IgA, IgG, or IgE antibodies, depending on the antigen encountered. IgA production is largely driven by mucosal antigen exposure, with IgA1:IgA2 subclass ratios partially dependent on the anatomical site of exposure (219) and host age (220). IgG1-4 subclasses may be selectively induced depending on antigen characteristics, exposure frequency, or host age (213, 220, 221). CSR is further influenced by signalling molecules as well as numerous immune cell subsets, including antigen presenting cells, conventional T cells, and unconventional T cells, as discussed in detail in the following reviews (214–218). Importantly, cytokines secreted by CD4+ helper T (Th) cells, including interleukin (IL)-4, IL-10, IL-13, and IL-21, have dominant roles in class switching to IgG, with IgG subclass distributions influenced by Th cell subset ratios and innate immune cells (222–227).
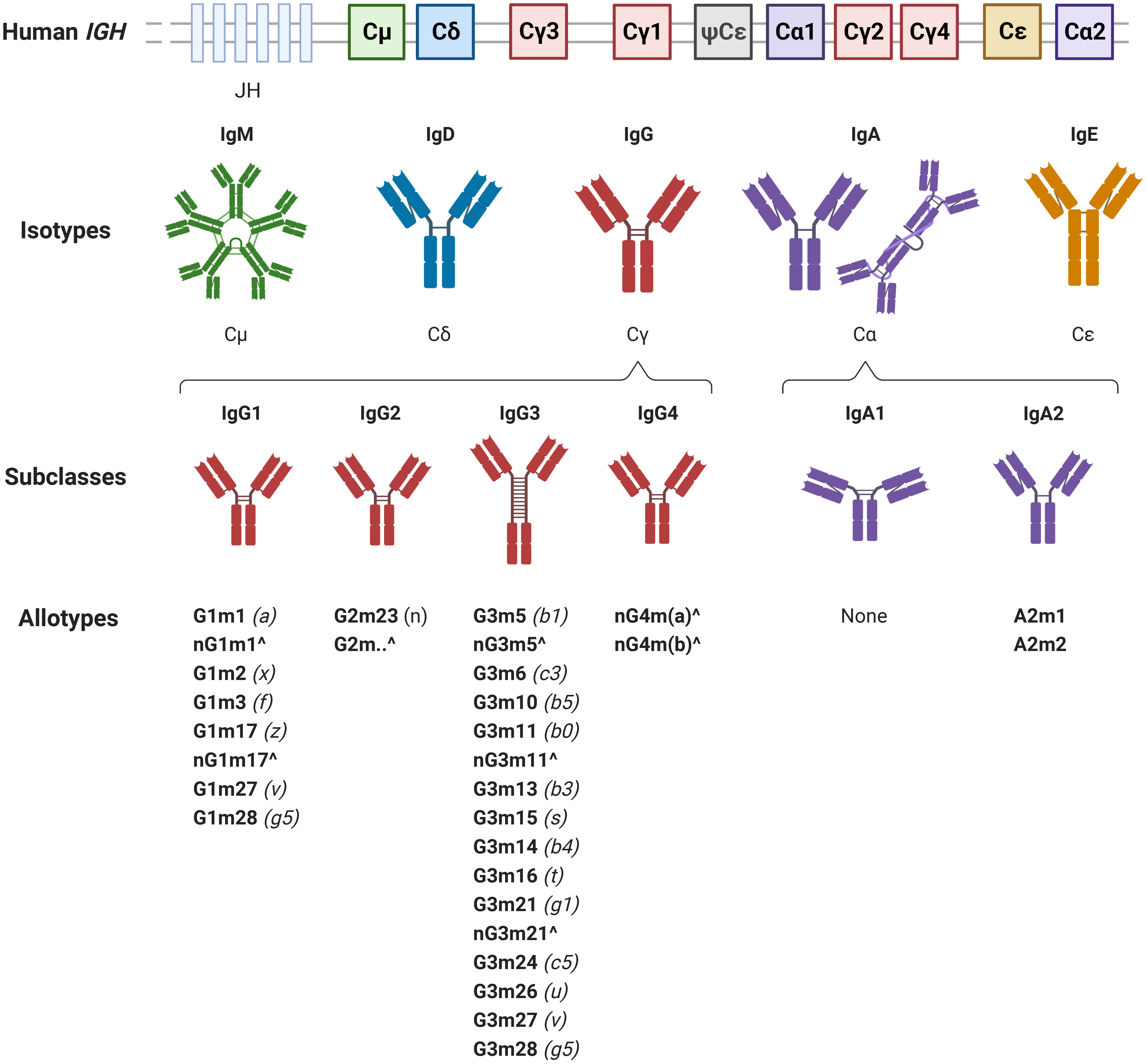
Figure 3 The immunoglobulin heavy (IGH) locus encodes the constant regions of immunoglobulin (Ig) M, IgD, IgG, IgA, and IgE. The constant heavy genes are located downstream of the joining region heavy (JH) genes. One pseudogene (ψCϵ) is also located within the IGH locus. IgG and IgA comprise four and two subclasses, respectively. Additional antibody variation is introduced by the single nucleotide polymorphisms which, alone or in combination, define a range of IgG1, IgG2, IgG3, IgG4, and IgA2 allotypes. Allotypes are listed according to the WHO/IUIS nomenclature in bold, followed by the previous alphabetical notation italicised in brackets. ‘Gm’ or ‘Am’ designates a marker of IgG1-4 or IgA, respectively, followed by a number corresponding to the named allele. ^The prefix ‘n’ or suffix '..' indicates the absence of the allotypic marker at the named allele; these are referred to as isoallotypes and contain an amino acid distinct to the subclass but common across the isotype. (Note that ‘nG1m1’ may be written as ‘G1m-1’ to indicate the absence of the G1m1 allotype). Each named allele is located at a distinct amino position except G1m17 and G1m3 which represent allotypes at the same position.
IgG3, followed by IgG1, has the highest affinity for FcγRs and, consequently, the greatest Fc-functional capacity, granting this subclass its so-called ‘cytophilic’ nature (228, 229) (Table 1). As such, elevated levels of IgG1 and IgG3 are correlated with superior protection against a range of diseases following infection or vaccination (229, 230). The robust polyfunctionality of IgG3 can be further complemented by the increased neutralisation potency observed for certain IgG3 variants (231–233). On the other hand, IgG2- or IgG4-skewed responses with reduced Fc functionality have been associated with non-protective HIV-1 trials (34, 82, 234). However, in diseases such dengue fever in which a hyperinflammatory response can be pathological, increased induction of IgG4 is more protective (108).
CSR is coordinated by multiple enzymes with dual functionality in somatic hypermutation (SHM)—the process enabling antibody Fab region diversification. Most notably, activation-induced cytidine deaminase (AID) initiates CSR and SHM and is indispensable for these mechanisms (235). The importance of AID to polyfunctional antibody responses is demonstrated by the positive association of AID expression with increased neutralisation breadth, IgG subclass diversity, and Fc responses following HIV-1 infection (236), as well as the diminished production of mature isotypes and reduced affinity maturation in individuals with impaired AID expression, such as the elderly or those with chronic inflammatory conditions (237–239).
Allotypes
Evolutionary pressures imposed by pathogens, particularly malaria, upon human populations for millennia have made immunoglobulin genes are a key target for genetic diversification mechanisms (240–242). As such, single nucleotide polymorphisms (SNPs), and combinations thereof, within the antibody constant region introduce a further layer of variability to the variable Fc-functional capacity of IgG subclasses. Initially defined via serological detection methods and termed ‘allotypes’, these antibody variants now form part of a continuously growing collection of IGH gamma (IGHG) chain alleles (243–245). IgG1 possess four classical allotypic markers present only in the IgG1 subclass, as well as two supernumerary markers occurring in IgG3 in some populations; one allotype is present in IgG2, and 13 IgG3 allotypes exist, including the two IgG1 supernumerary markers. In addition, two IgG4 isoallotypes which possess amino acids unique within the subclass but occurring in other antibodies across the isotype have been identified (245, 246) (Figure 3). Notably, IGHG genes are inherited in a Mendelian fashion and are in linkage disequilibrium such that specific allotypes are typically inherited within haplotype blocks (247–249). This is particularly evident in IgG3 which exhibits exceptional allelic diversity, and as such, IgG3 nomenclature is simplified to indicate commonly inherited combinations of alleles, annotated as G3m5* or G3m21*, for example (229). Notably, the G1m1 allotype is commonly inherited with G1m17 and, to a slightly lesser extent, G3m21* (250). As such the antithetical high prevalence allotype is Gm-1,3,5*.
Notably, advances in molecular biology and inclusion of Indigenous populations in biomedical research has enabled recent identification of additional polymorphisms (209, 243, 251). This extensive antibody diversity likely reflects the variable evolutionary selective pressures of different disease burdens imposed upon distinct populations, resulting in the selection of numerous low frequency polymorphisms in genetically isolated populations (252). However, a subset of dominant allotypes underpin variable responses to infection and vaccination. Across a diverse array of viral, bacterial, and protozoan infections, these IgG variants are associated with altered disease susceptibility possibly driven by IgG subclass distribution and titres of antigen-specific antibodies (Supplementary Table). In addition, IgG allotypes are reported to influence subclass titres and distribution of total IgG (253, 254). These variations to subclass distribution are suggested to impact Fc effector functions if antibody subclasses with reduced Fc functional capacity, such as IgG2 and IgG4, are expressed at the expense of more functional subclasses such as IgG3.
Allotype-associated regulation of Fc-functional capacity remains under-studied (244). However, recent monoclonal antibody studies revealed that IgG3 allotypes bind FcγRIIIa with different affinities and, therefore, have varied capacity to trigger ADCC, ADCP, and antibody-dependent cellular trogocytosis (ADCT) (233, 255). In addition, substitution of arginine to histidine at position 435 in some IgG3 allotypes can triple the half-life of this typically short-lived subclass via enhanced binding to FcRn (256–258). This polymorphism has been associated with increased transplacental transfer of malaria-specific IgG and improved protection against malaria during infancy (257). However, the mechanisms by which other IgG polymorphisms confer altered protection against infectious diseases or why allotypes are associated with drastic changes in IgG subclass expression remains poorly understood and warrants further investigation.
N-linked glycosylation
Beyond genetic polymorphisms and gene rearrangements which impact protein sequence and structure, post-translational glycosylation of IgG is an additional key regulator of Fc functions. Enzymatic addition of polysaccharide chains to the antibody Fab, hinge, and Fc regions can modify both antigen specificity and Fc receptor engagement, with Fc glycosylation at asparagine 297 (N297) within the constant heavy chain two (CH2) region influencing antibody polyfunctionality most substantially via modulation of Fc effector functions (259–263). Typical IgG Fc glycan chains are biantennary in nature, consisting of N-acetylglucosamine (GlcNAc) subunits from which mannose subunits form two branching structures allowing for the orderly addition of further GlcNAc, followed by galactose and then sialic acid. In addition, monomeric fucose can be linked to the N297 proximal GlcNAc (Figure 4). Variations to this N-linked glycosylation are associated with modulation of the inflammatory capacity of IgG, given the associated changes in affinity during IgG-FcγR interactions (264). It follows, therefore, that Fc glycosylation patterns predict antibody effector functions (265).
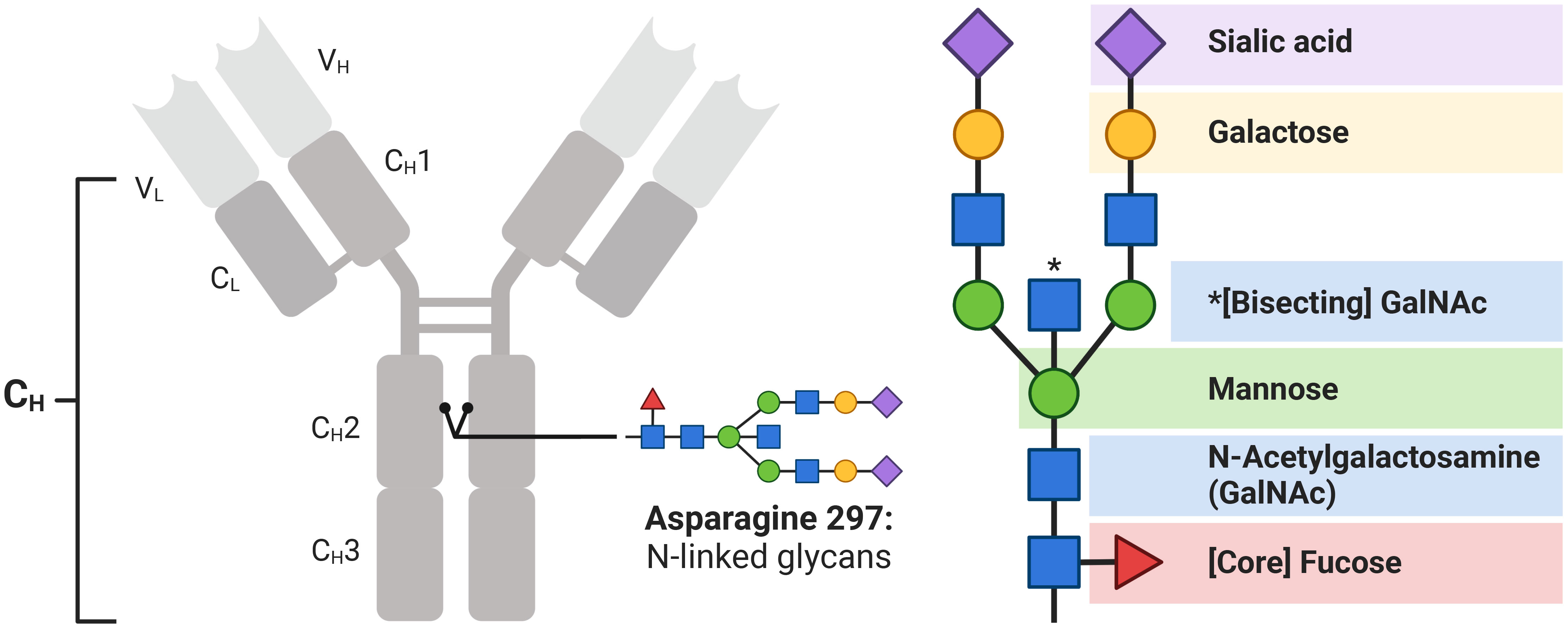
Figure 4 IgG is post-translationally glycosylated. Biantennary N-linked glycan chains are added at asparagine 297 within the Fc portion of the constant heavy (CH) regions of IgG. Two N-acetylglucosamine (GlcNAc) subunits and three mannose subunits form two branching structures upon which additional GlcNAc, followed by galactose and then sialic acid are added. Fucose can be linked to the N297 proximal GlcNAc and is present on the majority of human IgG.
Fucose has the best characterised role in modulating IgG-FcγR interactions and downstream Fc effector functions. A unique carbohydrate-carbohydrate interface exists between the glycans of afuscosylated IgG and FcγRIIIa that greatly enhances affinity compared to when core fucose is present and consequently interferes with formation of this interface (266). As such, afuscosylation is associated with upregulated FcγRIIIa signalling and enhanced ADCC and possibly ADCP (266–271).
Galactose is reported to modulate Fc effector functions, with increased galactosylation associated with increased IgG1 and IgG3 binding to complement component 1q (C1q) and, therefore, enhanced antibody-dependent complement deposition (ADCD) (271, 272). Increased galactosylation is also correlated with enhanced FcγRIIIa engagement and ADCC (271, 273, 274). However, galactose only subtly improves affinity for FcγRIIIa and does not further promote ADCC in an environment of highly afucosylated IgG (273, 275). Most critically, as galactose is the building block required for addition of sialic acid, it is essential for the anti-inflammatory properties associated with sialyation (276).
Sialic acid may inhibit FcγRIIIa binding and activation by IgG, thereby downregulating ADCC (263). However, the mechanism by which this occurs remains disputed owing to conflicting structural data (277–279). Alternatively, sialic acid may dampen inflammation by upregulating expression of inhibitory FcγRIIb (280, 281) or shifting IgG Fc receptor specificity towards C-type lectins that mediate anti-inflammatory functions (277, 282, 283). Nevertheless, these explanations which purportedly underpin the anti-inflammatory properties of intravenous immunoglobulin (280–283), are also contested (284, 285). Importantly, given the dominant role of afucosylation in modulating ADCC via enhanced FcγRIIIa binding, Fc sialyation has been suggested to only adversely impact the ADCC capacity of fucosylated, but not afucosylated IgG (286).
Critically, Fc glycosylation is under the control of a combination of genetic, hormonal, and cytokine regulatory mechanisms (287) which remain to be fully elucidated. However, IL-6 and IL-23 play relatively well-described roles in modulating Fc sialyation in mice (288, 289). IL-6 and IL-23 promote IL-17 secretion by T follicular helper 17 (Tfh17) cells which downregulates β-galactoside α-2,6-sialyltransferase I (St6gal1) expression in germinal center B cells and consequently inhibits IgG Fc sialyation (288). Furthermore, IL-23-activation of Th17 cells drives decreased Fc sialyation via IL-21 and IL-22-dependent downregulation of St6gal1 expression in plasmablasts and plasma cells (289).
Given the direct role of IgG Fc glycosylation in Fc effector functions which are both influenced and regulated by inflammation (264), glycosylation has been identified as a useful biomarker of chronic and acute inflammation as well as disease progression and severity in the context of both infectious and noncommunicable diseases (110, 111, 290–297). IgG afucosylation is a pro-inflammatory hallmark, owing largely to ADCC upregulation (296). Afucosylation is associated with heightened COVID-19 and dengue fever severity owing to the excessive inflammation to which afucosylated IgG contributes (110, 111, 290, 292, 296). However, in the setting of chronic infection, upregulated effector functions may be a protective adaptation enabling relatively slower disease progression. As such, reduced fucose abundance is associated with favourable disease outcomes, contributing to HIV-1 control and tuberculosis (TB) latency (53, 79). Whether increased abundance of fucosylated IgG is ultimately pathogenic or protective is highly disease specific and is underpinned by whether enhanced ADCC can promote pathogen clearance without inducing detrimental hyperinflammatory responses.
Reduced IgG galactosylation during chronic infection may be beneficial or detrimental to disease control depending upon the protective capacity of the upregulated Fc effector functions in the specific disease context (271–274). Indeed, agalactosylation of both bulk and antigen-specific IgG is associated with spontaneous HIV-1 control (79) as well as longer time to viral rebound following cessation of antiretroviral therapy (298), while increased IgG galactosylation is associated with tuberculosis latency (53). On the other hand, galactose is a key biomarker for the progression of non-communicable inflammatory diseases (297). Increased galactosylation of total IgG is generally associated with improved metabolic health (299, 300), while increased total IgG agalactosylation is associated with progression of inflammatory and autoimmune diseases such as rheumatoid arthritis (297, 301) and systemic lupus erythematous (302). Although this observation appears somewhat counterintuitive given the role of galactose in enhancing inflammatory processes such as ADCD and ADCC, it has been hypothesised that discrepancies in total compared to antigen-specific glycosylation may mediate this effect (303). When global IgG agalactosylation is high, thereby impairing general FcγR engagement, this environment would favour enhanced C1q engagement and FcγR activation by more highly galactosylated antigen-specific autoimmune antibodies with a consequently increased affinity for FcγRs. When global IgG agalactosylation is low, total IgG outcompetes autoantigen-specific autoimmune antibodies for FcγR binding, thereby increasing the threshold required for immune activation by pathologic antibodies (303). In addition, via a separate FcγR-mediated mechanism, terminal galactosylation of IgG1 immune complexes mediates anti-inflammatory activity by promoting FcγRIIb driven inhibition of complement-dependent inflammatory pathways (304).
IgG glycosylation is central to maintaining the fine balance between induction of protective and pathogenic Fc functions, highlighting a critical immunomodulatory role for Fc glycosylation in control of infectious disease, but also the regulatory influence of inflammation upon Fc glycosylation. Indeed, post-translational glycosylation is dynamic and highly sensitive to changes within the B cell microenvironment (288, 305, 306), and as such, may undergo relatively rapid modification dependent upon hormonal (307), vaccine or pathogen-derived stimuli (287, 308), as well as more gradual changes associated with ageing and disease (299, 309).
Dysregulated Fc effector functions characterise vulnerable populations
Priority populations can be defined by key host factors that influence the vaccine response, including age, sex, immunogenetics, pregnancy, chronic comorbidities, and malignancies (1, 4, 310–313). These clinical and demographic features are further associated with changes to well-characterised and emerging molecular predictors of vaccine-induced protection (22, 314). Some of these predictive biomarkers are highly linked to lifestyle and health status, such as baseline host inflammation and the gut microbiota (315, 316). Other features are more closely tied to age and genetics, such as pre-existing immunity as a result of prior antigen exposure, immune cell frequencies and activation, antibody titre and function, and capacity for antigen processing (37, 317–326). Notably, characteristic modulation of these host variables results in distinct vaccine responses within specific populations (22). Consequently, tailoring vaccine design to elicit the precise immune features lacking in target populations may prove essential for enhancing vaccine effectiveness.
The underlying mechanisms of immune dysregulation observed in immunocompromised populations is an active area of investigation. However, a perturbed cytokine milieu appears to be central to impaired vaccine responses (327, 328). Notably, many of these immunologically vulnerable groups, including the elderly, individuals with chronic inflammatory conditions and autoimmune disorders, as well as cancer patients, share characteristic patterns of cytokine dysregulation related to imbalances in CD4+ T cell subsets (329–334), immunoglobulin class switching (323), and IgG glycosylation (299, 309, 335), both between and within groups (Figure 5 illustrates IgG glycosylation-specific population trends). As cytokines secreted by CD4+ T cells are important B cell stimuli for the regulation of both class switching (336–338) and IgG glycosylation (305)—features which heavily influence Fc functions—a perturbed baseline cytokine milieu may drive Fc effector function dysregulation.
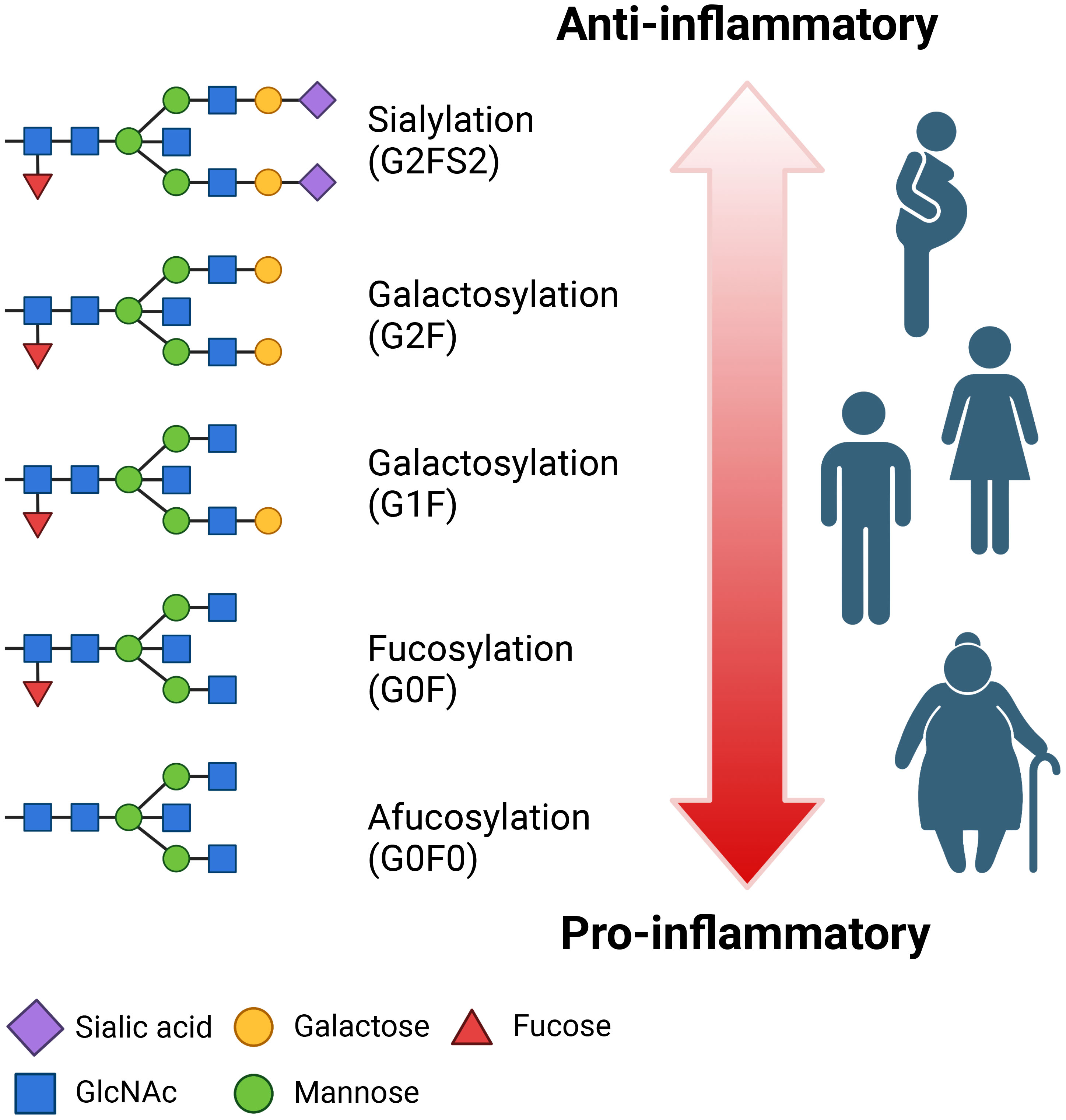
Figure 5 IgG Fc glycan structures have variable inflammatory properties. IgG Fc glycans differentially modulate Fc effector functions and, therefore, inflammation, depending on the interactions of the sugars with various Fc receptors and complement proteins. In general, lack of fucose is highly inflammatory while the presence of galactose and sialic acid is anti-inflammatory. Total IgG Fc glycosylation varies considerably with age, sex, and health status. In general, there is a greater abundance of pro-inflammatory Fc glycans in elderly individuals with chronic comorbidities, such as obesity, and this is particularly elevated in post-menopausal women. On the other end of the spectrum, pregnancy is associated with increased abundance of anti-inflammatory Fc glycans. Among healthy young adults, women typically have a slightly more anti-inflammatory Fc glycan profile.
Although poor vaccine immunogenicity in vulnerable populations may, in some instances, be restored by additional vaccine doses (15), boosting is not a universally effective strategy for all vaccines and across all immunocompromised groups (339). Furthermore, modelling suggests that the benefits of boosting may be transient for some immunosuppressed individuals (340). Therefore, in order to design more effective vaccines for immunocompromised groups, a deep understanding of the dysregulated immune networks characteristic of these populations, as well as how these altered immune responses are influenced by different vaccination strategies, is required. Recent systems serology studies have highlighted differences in Fc functions between young, healthy, non-pregnant adults and various vulnerable populations including, children, pregnant women, elderly individuals and patients with various co-morbidities (37, 310, 324, 341–343). Importantly, identification of shared characteristic immunomodulatory mechanisms underlying impaired protection across multiple immunocompromised groups (328, 330, 341) may enable design of more broadly generalised first-generation population-specific vaccine modifications.
Pregnant women
During pregnancy, to ensure the developing foetus is not rejected, the body maintains a precisely modulated immunosuppressive, anti-inflammatory state that is reflected in generally diminished Fc functions (310, 344) underpinned by a global decrease in inflammatory glycan structures (344, 345). Distinct Fc effector functions have been observed in pregnant and lactating women compared to healthy controls following prime-boost SARS-CoV-2 vaccination, despite equivalent vaccine-specific antibody titres post-boost (310). Pregnant and lactating women displayed delayed Fc kinetics, requiring two doses to generate responses that were comparable, though still reduced, to nonpregnant controls (310). In contrast, post-boost, ADNKA and ADNP trended higher in lactating women than in both pregnant and nonpregnant women (310). Varied functional antibody responses have also been described during pregnancy following influenza vaccination. Compared to their non-pregnant counterparts, pregnant women demonstrated impaired overall Fc function driven by reduced capacity for ADCP and ADCD, which was linked to an increase in anti-inflammatory Fc fucosylation and sialyation (344). Nevertheless, increased galactosylation of both bulk and vaccine-specific Fc antibodies was correlated with improved ADNKA in pregnant compared to non-pregnant influenza vaccinated women (344). Finally, the timing of maternal vaccination may impact Fc-mediated protection, with trends of higher functional antibody responses induced by third trimester SARS-CoV-2 vaccination, followed by first then second trimester vaccination (346).
Chronic infection may further influence pregnancy-induced differences in Fc capacity. For example, pregnancy during HIV-1 infection creates a complex environment of opposing immunomodulatory mechanisms (347). Pregnancy-driven immunosuppression competes with HIV-1 associated chronic inflammation thereby driving a unique IgG Fc glycan profile of decreased galactosylation in pregnant women living with HIV-1 (WLWH) (348). Influenza vaccine-induced Fc effector functions are variably regulated in pregnant WLWH compared to HIV-1-uninfected women (349). Following vaccination, ADCP boosting was evident in otherwise healthy pregnant women but not in pregnant WLWH; ADCD was boosted in both groups but was significantly higher in uninfected women (349). Altogether, these differences in Fc effector capacities may point to baseline IgG glycosylation impacting post-vaccination antigen-specific Fc glycoforms, and therefore, effector functions. These studies suggest there may be value in further tailoring vaccination strategies for vulnerable populations who fall into more than one risk group given the marked effect of highly nuanced baseline inflammation on Fc effector functions.
Neonates and infants
The health of neonates and infants is inextricably linked to that of the mother (350–353). As such, pregnancy is a unique window during which maternal and infant health can simultaneously be benefitted by a single course of vaccination (354–357). Placental transfer of maternal antibodies is a key mechanism of neonate protection against numerous infectious diseases, including RSV, influenza, pertussis, measles, and tetanus (358, 359). However, studies of HIV-1, malaria, and SARS-CoV-2 infected pregnant women have revealed that placental transfer of related and unrelated antibodies can be compromised by maternal infection (360–364). This outcome may partially explain the increased childhood disease susceptibility of HIV-1 exposed but uninfected infants as well as infants affected by placental malaria (365, 366). Critically, altered IgG subclass distribution and Fc glycosylation has been implicated in the mechanism of impaired placental transfer of antibodies generated both during and prior to infection (361, 367).
In healthy pregnant women, digalactosylated Fc functional antibodies are preferentially transferred during the gestational period in contrast to antibodies lacking the capacity to bind FcRn, FcγRIIa, and FcγRIIIa (358, 359). Most notably, there is preferential transfer of ADNKA capacity to neonates correlating with enhanced binding of digalactosylated IgG1 to FcRn and FcγRIIIa (358). In contrast, ADCP functionality is retained by the mother (358). Furthermore, equivalent antibody Fc functional capacity has been demonstrated in preterm and full term neonates with robust early transfer of ADNKA capacity (359). This early selective sieving of Fc functional capacity, ADNKA, likely points to an evolutionary advantage of increased Fc capacity in early life (358, 359). Indeed, placentally transferred NK cell activating antibodies drive elevated cytokine release by umbilical cord NK cells compared to adult NK cells (358).
The nature of this placental sieve has implications for the rational design and timing of vaccines administered to pregnant women. For example, vaccine regimens that elicit highly galactosylated antibodies with enhanced affinity for FcRn may be more efficiently transferred and, therefore, afford elevated neonate protection. Indeed, increased placental transfer efficacy of Fc functional SARS-CoV-2 specific antibodies has been observed following mRNA-1273 or BN162b2 lipid nanoparticle mRNA vaccination compared to Ad26.COV2.S adenoviral vector vaccination, with further subtle increases elicited by mRNA-1273 compared to BN162b2 vaccination (346). This suggests that vaccine formulation may substantially alter the functional capacity of antibodies transferred to neonates. On the other hand, maternal antibodies may limit humoral responses in infants following vaccination (368). Although the mechanism remains contested, epitope masking and inhibitory FcγRIIb engagement by maternal antibodies may contribute to this outcome (369, 370). Given that different epitopes drive differential Fc functions (371), immunogen selection for maternal vaccines should also consider the possible impacts on early childhood vaccine responses.
Children
Children under five are highly susceptible to infectious diseases. Numerous cellular and humoral deficiencies define the immature immune system, however, altered antibody class switching (220, 221) and IgG glycosylation (372, 373) confer young children unique Fc effector profiles. Rational vaccine design which exploits the elevated Fc capacity (37, 324, 374, 375) of childhood humoral immunity may promote optimised protection.
While age-related variation in IgG glycosylation is well-recognised (309, 335, 376), detailed data from paediatric cohorts is limited. Nevertheless, evidence exists for variation across childhood and adolescence, with an overall trend of decreased inflammatory agalactosylation with increasing age (372, 373, 376–378). However, further dissection of IgG glycosylation patterns in the first two years of life has revealed increased anti-inflammatory IgG glycoforms with increased digalactosylation, sialyation, and core fucosylation in children aged 9 months to 2 years compared to older children up to 5 years. Between ages 2 to 5 years, IgG glycosylation shifts to a more pro-inflammatory profile of increased agalactosylation and reduced sialyation, before the production of increasingly galactosylated IgG commences (372, 373). Notably, IgG glycosylation patterns have been identified as a potential biomarker of recurrent respiratory infections (RRI) in childhood (372). Interestingly, increased anti-inflammatory digalactosylated and sialyated IgG were enriched in the RRI group—suggesting that decreased effector potency of these antibodies could leave children more vulnerable to repeated infections.
Increased class switching to more mature IgG2 and IgG4 isotypes gradually occurs from infancy to adolescence (220). As such, the baseline production of increased levels of IgG1 and IgG3 in young children under 3 years may be advantageous for the generation of highly Fc functional antibodies by early childhood vaccines. Indeed, children develop elevated IgG1 titres and enhanced Fc functional responses, including ADNP and ADCD, as well as FcγRIIIa binding upon SARS-CoV-2 vaccination in comparison to adults (324). This increase in Fc functional capacity was especially evident when children were administered the full adult dose of Moderna mRNA-1273 vaccine, rather than the reduced paediatric dose (324), underscoring the impact of vaccine dosage on Fc functions.
Robust Fc effector functions in antiretroviral therapy-naïve HIV-1 infected children have also been observed (379, 380), and are especially elevated in paediatric HIV-1 non-progressors (PNP; i.e., children who maintain normal CD4+ T cell counts despite ongoing high viral replication in the absence of antiretroviral therapy) compared to in progressors (380). ADNKA, likely driven by robust IgG1 responses, is consistently observed across cohorts (379, 380), and, along with decreased Fc fucosylation, may contribute to disease control in PNP (380). Notably, coordination of Fc effector responses (379) and increased antigen-specific IgG Fc sialylation (380) were positively associated with neutralisation breadth, suggesting dual benefit to vaccines targeting the generation of Fc functional antibodies.
Elderly individuals
The ageing humoral immune system is characterised by immunosenescence induced by both chronic low-grade inflammation and prior antigen exposure leading to reduced antibody titres and largely diminished vaccine responses (327, 334, 381). The resultant upregulation of inflammatory cytokines such as IL-6, IL-1β, tumour necrosis factor α, as well as decreased anti-inflammatory cytokines such as IL-10, may contribute to impairments across a broad range of humoral immune system features, including B cell activation, antibody class switching, affinity maturation, and Fc glycosylation (300, 382–384). Reduced expression of AID, associated with transcription factor E47 downregulation, is suggested to dampen capacity for CSR, as reflected by the diminished pool of switched memory B cells in elderly individuals (385). Consequently, class switching to cytophilic IgG1 and IgG3, may be diminished in elderly individuals (237, 238, 386, 387). Increased age is also associated with increased baseline abundance of pro-inflammatory agalactosylated and asialylated IgG (300, 309, 335, 376) which may contribute to generation of dampened or uncoordinated Fc effector functions upon vaccination. Overall, these antibody impairments likely underpin the decreased FcγR binding and Fc effector functions observed in elderly individuals (37, 388).
Beyond the current approach of early and additional vaccine doses for elderly individuals, a combination of more targeted strategies may benefit this population (13, 15, 389). In the case of influenza, poor vaccine immunogenicity in the elderly may be partially overcome by high-dose vaccination (390, 391) and inclusion of adjuvants such as MF59 (391, 392) and AS03 (393). However, this population may further benefit from vaccines specifically formulated to elicit potent Fc effector functions upon a background of dysregulated IgG class switching and Fc glycosylation. Although MF59 selectively boosts IgG3 titres and may bolster generation of Fc functional antibodies (394) when class switching is impaired, eliciting Fc glycosylation patterns that support enhanced FcγR engagement may further improve vaccine effectiveness. Notably, the influence of age upon FcγR engagement and effector functions following SARS-CoV-2 vaccination is conflicting, with studies reporting positive (395), negative (396), and no association (50). However, these trends were determined via small to moderately sized patient cohorts, underscoring the need for larger clinical trials to adequately address this critical question.
Patients with chronic comorbidities
Many non-communicable diseases associated with chronic low-grade inflammation have increased in prevalence in recent decades, particularly in high- and middle-income countries (397–399). This phenomenon may reduce effectiveness of vaccines which are typically less immunogenic in patients experiencing chronic inflammation as a result of malignancies, autoimmune diseases, and obesity. Furthermore, both immunosuppressant drugs—used to mitigate symptoms of inflammatory autoimmune diseases and manage solid organ transplants (400)—as well as the chemotherapy and radiation regimens—used to treat malignancies—may render vaccines less immunogenic.
Rheumatoid arthritis, systemic lupus erythematous, renal disease, and inflammatory bowel disease as well as other chronic conditions associated with dysregulated inflammation, such as obesity and type 2 diabetes, contribute substantially to the global burden of comorbidities that reduce vaccine effectiveness (401). Most notably, research investigating vaccine responses in obese patients has revealed a proinflammatory cytokine milieu associated with a dysregulated humoral response, similar to that observed in elderly individuals (330, 332, 402, 403). Impaired humoral immunity upon vaccination is most readily evidenced by reduced antibody titres and neutralisation capacity (311, 404, 405). However, Fc effector capacity in these populations may also be highly dysregulated, largely driven by aberrant IgG Fc glycosylation underpinned by increased IgG Fc agalactosylation, asialyation, and afucosylation (299, 302).
Large networks of genes which regulate Fc glycosylation are pleiotropic with inflammatory diseases such as rheumatoid arthritis and inflammatory bowel disease (406). However, it has long been appreciated that increased pro-inflammatory agalactosylation is a biomarker of disease onset and severity for many of these conditions (301, 302, 377). Increased proinflammatory IgG glycosylation has also been defined for a variety of malignancies, including multiple myeloma (407), colorectal cancer (CRC) (408, 409), thyroid cancer (335), and ovarian cancer (410). Notably, in a study of patients receiving allogeneic hematopoietic stem cell transplantation, post-procedural recipient IgG glycosylation more closely resembled their pre-transplantation profiles than that of donor IgG glycosylation (411). This reinforces the predominant role for the B cell microenvironment in driving IgG glycosylation patterns and suggests that the persistence of patient-specific immunomodulation such as hormone dysregulation, CD4+ T cell perturbances, and inflammatory cytokines may have long-term consequences for the vaccination of patients with haematological malignancies.
Although likely dysregulated, Fc functions appear to be better preserved than neutralisation capacity in immunosuppressed populations. In a study of SARS-CoV-2 vaccination of cancer patients, anti-spike antibody titres were generally concordant with neutralising titres against the wild-type virus (405). However, this trend was not observed against variants of concern where over half of individuals generating anti-spike antibody responses were unable to neutralise SARS-CoV-2 variants (405). Although alterations to IgG Fc glycosylation and effector functions are heavily studied in the context of tumour clearance and cancer progression and survival (269, 412, 413), the effect of these malignancy-induced modifications upon immune responses to vaccination and infection remains understudied.
As IgG Fc glycosylation contributes substantially to Fc effector function potency, designing vaccines that counter perturbed IgG Fc glycosylation patterns and elicit coordinated Fc functions may enhance protective responses in populations experiencing dysregulated inflammation. Indeed, pro-inflammatory IgG glycan abundance has been associated with impaired influenza (414) and SARS-CoV-2 (395) vaccination. Increased baseline level of agalactosylated total IgG was a signature of influenza vaccine non-responders, while elevated IgG galactose abundance predicted robust vaccine response (414). Similarly, elevated baseline abundance of anti-inflammatory galactosylated, sialylated, and fucosylated IgG1 correlated with higher anti-SARS-CoV-2 IgG titres following vaccination (395).
Finally, dysbiosis of the gut microbiome is frequent in obese individuals, as well as patients with malignancies and chronic inflammatory conditions (401, 415–421). There is an established role for the gut microbiome in regulating antibody titres following vaccination (315, 316, 422). Hence, it is plausible that gut dysbiosis may also impair Fc effector functions by modulating inflammatory cytokine levels and subsequently influencing IgG Fc glycosylation and downstream effector functions.
Modulation of Fc effector functions in healthy adult populations
Distinct groups of healthy individuals may also benefit from population-based vaccination strategies. Biological sex-specific differences can impact both antibody quantity and quality, with age-dependent variation in glycosylation patterns (423) likely influencing Fc functional responses (37). Immunogenetics further impact functional antibody responses via allotype associated variations in IgG subclass distribution and FcγR polymorphisms that alter affinity for IgG. Finally, the gut microbiome within healthy individuals may also influence Fc functions by promoting inflammatory processes that modulate IgG glycosylation.
Sex-based differences in vaccine responses
Across age groups, females typically generate more robust humoral responses to many vaccines than do males, with higher antibody titres observed following vaccination against influenza, HBV, yellow fever virus, dengue virus, and measles, mumps and rubella (424–426). However, females may generate a more functional antibody response with increased class switching to IgG3 directing more robust Fc effector functions against some pathogens (427) while males may generate increased titres of poorly functional IgG4 (428). Furthermore, young to middle-aged women typically have increased abundance of anti-inflammatory galactosylated IgG than their male counterparts. However, elderly women have increased abundance of agalactosylated IgG—a phenomenon associated with onset of menopause, likely owing to reduced estrogen levels (307, 309, 429). In addition, females typically have increased phagocytic effector cell frequencies while males have higher NK cell counts but with decreased effector capacity compared to females (430, 431). Differences in IgG Fc glycosylation and innate cell frequencies result in nuanced differences in effector functions between the sexes. For example, males typically generate more robust ADCC in the context of measles (432) or HIV-1 infection (433). As such, men and women may benefit differently from vaccination regimens that aim to either elevate antibody titre or enhance FcγR engagement. Males may benefit from inclusion of adjuvants that enhance class switching to IgG3 (e.g. MF59). On the other hand, given heightened vaccine immunogenicity and reactogenicity, females may benefit from reduced dose regimens that elicit more coordinated Fc functions and fewer adverse effects.
Immunogenetics
Polymorphisms within IGHG and FCGR genes, as well as FCGR copy number variations, are associated with differential responses to infection and vaccination for a range of pathogens (Supplementary Table). The potential for IgG allotypes to modulate Fc functions is largely driven by the altered subclass distributions associated with different haplotypes, and to a much lesser extent, the altered FcγRIIIa affinity of specific allotypes, as previously discussed (255). On the other hand, FcγR polymorphisms influence Fc functions via the increased affinity of FcγRIIa-131H and FcγRIIIa-158V for IgG subclasses (60).
Epistatic interaction of FcγRIIIa polymorphisms and IgG1 allotypes has been observed in HSV-1 infection such that, as a result of enhanced ADCC, the high affinity FcγRIIIa-158V/V genotype was only associated with asymptomatic infection in individuals homozygous for the G1m3 IgG1 allotype (434), typically linked to reduced IgG1 responses against viral infections. This protective effect may not have been observed in G1m17 homozygotes given the increased affinity of G1m17 IgG1 for the HSV-1 decoy FcγR compared to the antithetical G1m3 allotype (435), possibly resulting in increased clearance of G1m17 IgG1. Notably, whether high or low affinity FcγR alleles confer a protective or deleterious effect is likely a disease specific phenomenon, which is presented in detail within the Supplementary Table.
In addition, the influence of human leukocyte antigen (HLA) alleles has long been understood to impact vaccine outcomes. Given the ethnic clustering of HLA allomorphs, different populations demonstrate varying levels of vaccine-induced protection and disease susceptibility (312, 436–440). Although not directly responsible for shaping the functional antibody response, certain HLA allomorphs have been associated with increased antibody titres against SARS-CoV-2 (438), and the potential interaction of HLA, immunoglobulin kappa chain, IgG constant region and FcγR polymorphisms cannot be ignored in the design of population-based vaccines informed by immunogenetic features (434, 441–443). The interplay of these genetic polymorphisms is of particular importance in Indigenous populations who are frequently underrepresented in vaccine studies (8, 9) and whose unique immunogenetic backgrounds may underlie differential vaccine responses and infection susceptibility (250, 437, 439).
The potential value of considering immunogenetic influences upon vaccine responses has recently emerged through comparisons of analogous HIV-1 vaccine trial efficacies derived from different study populations. The RV144 trial, conducted in Thailand with participants of predominantly South-East Asian ethnicity, demonstrated 31.2% efficacy (81). When the RV144-inspired HVTN702 follow up trial was conducted in South Africa, modified to reflect the dominant circulating HIV-1 subtype, the vaccine showed no efficacy (444). Subsequent computational analyses indicated immunogenetics may have contributed to variable protective outcomes between the trials (445, 446). Host immunogenetic diversity, particularly within the IGHG locus varies substantially between the Thai and South African populations (447). Importantly, given IGHG, FCGR, and HLA genotypes show distinct geographic clustering (248, 447, 448), the possibility exists for their influence to be modelled into future population-based vaccines. Figure 6 illustrates the geographic clustering of dominant IgG haplotypes.
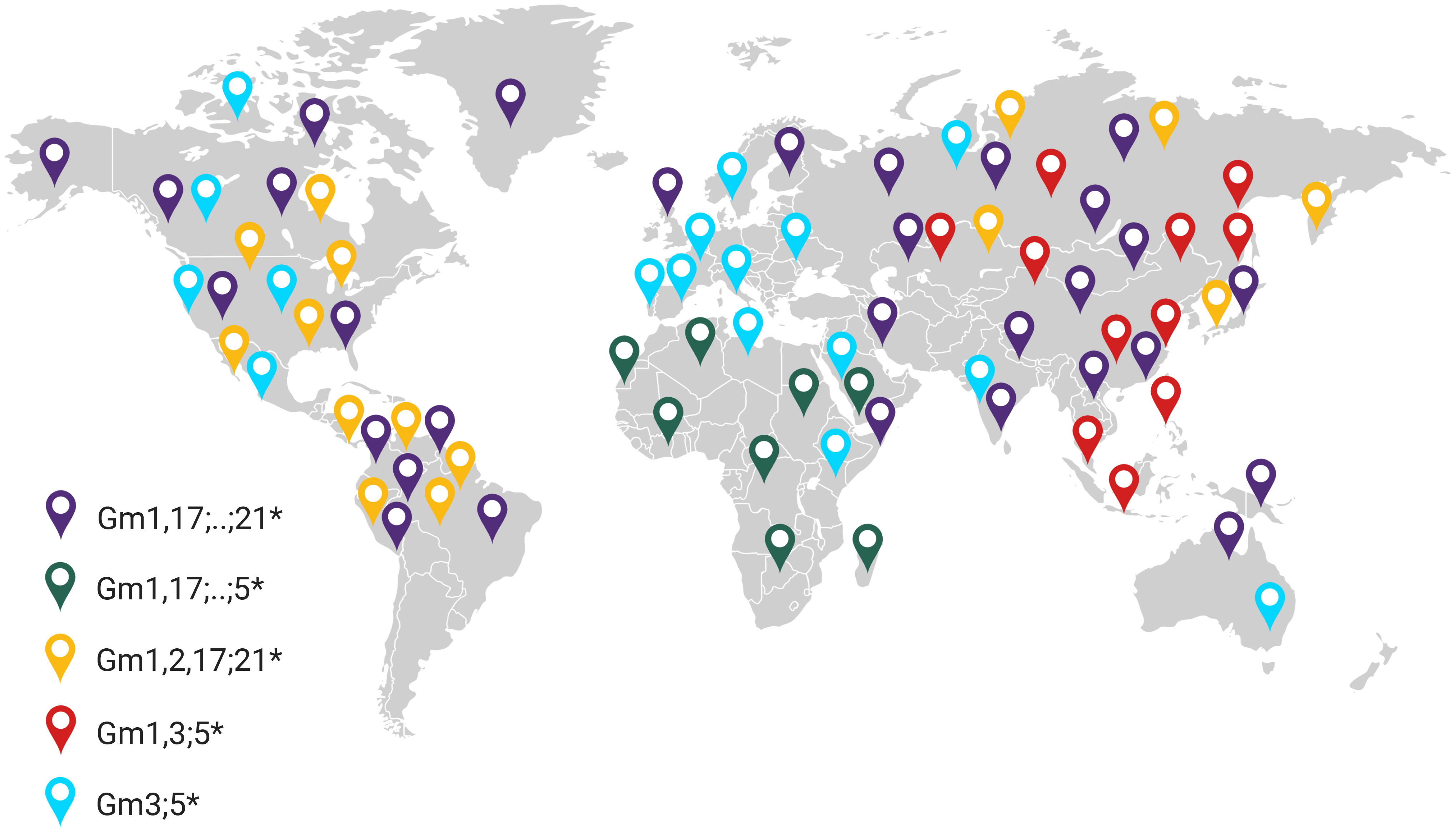
Figure 6 Geographic distribution of dominant IgG haplotypes. IgG allotypes are inherited as haplotype blocks and thus show geographic clustering within ethnicities. Data compiled from (449).
Vaccination strategies modulate Fc effector functions
There is growing consensus that precise modulation of Fc effector functions is a valuable goal of future vaccines and may be key to optimising vaccine responses in certain populations (445, 450). Dysregulated total IgG subclass ratios and global IgG glycosylation are not altered following vaccination (210, 308, 414). However, antigen-specific IgG subclass distribution and antigen-specific IgG glycosylation—key modulators of Fc effector functions—are tuneable via vaccination (210, 211). Furthermore, vaccination can override differences in baseline IgG glycosylation observed between healthy populations from distinct geographic locations (210, 308). As such, these antibody features are rational targets of vaccines designed to boost Fc effector functions in vulnerable populations.
However, given the challenge of eliciting precisely selected Fc functions, most data indicating vaccination-induced differences in functional antibody responses are derived from serendipitous observations following variations to immunogen, vaccine platform, adjuvant, dosage, and administration route. As such, defining the mechanisms of modulation along with strategies that enable fine-tuning of Fc effector functions via vaccination is of high priority for the precision vaccination field.
Immunogen selection
Although Fc effector functions can theoretically be initiated upon binding to any epitope, certain epitopes can drive more potent effector functions than others. The influence of Fab specificity upon Fc-FcR interactions and effector functions has recently been demonstrated in studies of influenza, HIV-1, and Ebola (49, 54, 55, 371). Emerging data has also implicated Fab-FcγR interactions in ADCC potency (451). In addition, antigen valency may impact Fc functions in a Fab-specific manner given that FcγR activation requires dimerisation facilitated by cooperation between at least two antibodies. Furthermore, IgG is glycosylated in an antigen-specific manner (70, 213). Different antigens from the same pathogen (210) or even the same protein (452), may induce differential IgG glycosylation which may impact Fc effector functions. As such, carefully informed choice of immunogen is critical to rational vaccine design.
However, immunogen-specific modulation of Fc functions is largely underpinned by the conformational accessibility of different epitopes as both Fab-antigen and Fc-FcR interactions must be simultaneously accommodated. Indeed, angle of approach of certain Fab-antigen interactions may result in steric hinderance of Fc-FcR engagement (56) or allosteric changes to antibody conformation upon binding which promote or impair FcR interactions (453, 454). Epitope proximity to the viral envelope or target cell membrane has been suggested to influence Fc functions (57, 455, 456). However, studies of ADCP in this context are conflicting, with reports of both enhanced (455) and impaired (456) potency with increasing distance from target cells. Nevertheless, studies of mAbs against the Ebola virus surface glycoprotein indicated that antibodies against epitopes farthest from viral envelope were the most polyfunctional (57, 457). These findings resonate with observations across of a variety of antigens that IgG1 and IgG3 hinge length polymorphisms contribute to ADCC and ADCP potency, with increasing hinge length promoting enhanced ADCP (228, 232, 233, 458) but decreased ADCC (255).
In a study of influenza A mAbs, anti-HA stalk-specific antibodies induced ADCC while those targeting the head region antibodies did not (49). However, the authors later demonstrated that this observation was not a broadly generalisable rule and that other anti-HA head antibodies mediate protective Fc functions (154). This suggests that the precise antibody footprint may have a greater influence over ADCC induction than the general epitope location. Nevertheless, a separate study found that across mouse and human mAbs, as well as polyclonal human IgG, anti-HA head antibodies did not induce ADCC and further inhibited anti-stalk mAb directed ADCC (54). Consequently, suggestions have been posited to increase Fc effector function via immunogen design. For example, shielding the immunodominant HA head via glycosylation may bias responses towards the stalk and enhance Fc functional responses (459).
Vaccine platform
Recent innovations in materials science have expanded licenced vaccine platform options beyond traditional live attenuated, inactivated, recombinant, and viral vector vaccines to include a range of nanoparticle vaccines (28). Of note, the value of lipid nanoparticle mRNA vaccines was demonstrated by their increased effectiveness in comparison to traditional platforms during the COVID-19 pandemic (39, 460). Unique features of each vaccine platform enable varied interactions with the immune system. As such, delivery of the same antigen via different modalities can elicit markedly different responses, including distinct changes to IgG glycosylation and downstream antibody effector functions, which impact vaccine efficacy.
Previous studies have identified an increased abundance of vaccine-specific galactosylated and sialylated IgG following tetanus toxoid and inactivated influenza and vaccination (308, 452). However, comparison of different SARS-CoV-2 vaccines regimens has allowed for more granular dissection of the impacts of vaccine formulation upon IgG glycosylation (212). Pfizer BioNTech BNT162b2 SARS-CoV-2 mRNA vaccination induces an initial transient pattern of increased spike-specific IgG1 Fc galactosylation and sialylation but decreased fucosylation (212, 461). Over time, antigen-specific IgG1 fucosylation levels gradually increased to above that of total IgG1, while galactosylation and sialylation levels gradually decreased, with levels of galactosylated vaccine-specific IgG1 falling below that of total IgG1 by day 190 post-vaccination (212). On the other hand, vaccination with AstraZeneca SARS-CoV-2 AZD1222 adenoviral vector produced a less pronounced decrease in fucosylation immediately post-vaccination, falling to only 95% as compared to the 80% fucosylation induced by BNT162b2 vaccination (212). Similarly, the increase in IgG1 Fc galactosylation was less pronounced after one dose of AZD1222 than BNT162b2 vaccination (212). These kinetics of IgG glycosylation following SARS-CoV-2 mRNA vaccination are in line with previous studies reporting increased antigen-specific IgG fuscoylation in the weeks following vaccination (395, 462), which may promote coordinated Fc effector functions by limiting inflammation (462).
The mechanisms by which varied vaccination platforms induce distinct Fc functions is poorly understood. However, nanomaterial vaccine platforms appear to offer a more defined strategy for enhancing antibody polyfunctionality. Nanomaterials facilitate highly ordered, repetitive antigen array that mimics the immunogenicity of many live pathogens (28). Compared to soluble antigen, the multivalent antigen presentation afforded by nanomaterials drives swift trafficking and concentration within germinal centres (463) as well as rapid B cell activation and differentiation (464). This increased antigen deposition and lymph node expansion facilitating improved B cell and Tfh cell responses is associated with generation of higher antibody titres and improved protection against influenza challenge (465, 466). Nevertheless, although B cell stimuli are known to impact IgG glycosylation (305), knowledge gaps remain in our understanding of the mechanism by which nanomaterials alter IgG Fc glycosylation and effector functions.
Recently, a nanomaterial-based HIV-1 vaccine was demonstrated to induce potent Fc effector functions correlating with unique antigen-specific antibody glycosylation (467). Q11—a frequently utilised vaccine nanomaterial—was conjugated to gp120, and co-administered with the Fc effector function enhancing adjuvant STR8S-C. This combination stimulated increased neutralisation and antibody breadth, as well as enhanced ADCC and, to a lesser degree, ADCP in rabbits (467). These enhancements to ADCC were correlated with changes in IgG Fc glycosylation patterns, including increased fucosylation and monogalactosylation of gp120-specific antibodies. Furthermore, similar glycan profiles were observed for both mice and rabbits vaccinated with or without the STR8S-C adjuvant. Altogether, these findings suggest that while the impact of STR8S-C adjuvant on glycosylation could not be excluded, the Q11 nanofiber itself was responsible for Fc glycosylation modifications. Afucsoyation of IgG Fc glycans is one of the best-defined divers of increased engagement with ADCC-mediating FcγRIIIa (266–268). That the Q11-gp120 vaccine elicited robust ADCC regardless of overall fucose abundance (467) raises important questions regarding the effects of specific combinations of Fc glycans upon antibody-dependent effector functions and warrants further investigation.
There is emerging appreciation of the role of Fc glycosylation in enhancing neutralisation breadth and affinity maturation (380, 468). The Q11-gp120 vaccine induced both increased IgG Fc sialyation and increased Fab binding breadth (467). Increased sialyation has previously been shown to enhance HIV-1 antigen-antibody complex deposition which in turn was associated with increased neutralising antibody breadth, suggesting that specific Fc glycosylation patterns may impact affinity maturation (380, 468). Fc sialylated immune complexes have similarly been shown to enhance affinity maturation and breadth of anti-influenza antibodies (452, 469). Mechanistically, this is explained by increased binding of sialylated immune complexes to inhibitory FcγRIIb, thereby elevating the B cell receptor threshold of activation (452, 469). As such, it is possible that the improved binding breadth generated by Q11-gp120 vaccination was a consequence of increased sialylation. This would suggest dual benefit to precise modulation of IgG glycosylation for the generation of robust polyfunctional antibody responses: enhanced Fc effector functions and increased Fab binding breadth.
The HIV-1 vaccine trials VAX003 and B003/IPCAVD-004/HVTN 091 comprising vaccines based upon recombinant protein subunit and adenoviral vector systems, respectively, induced differently glycosylated IgG against gp120—a key HIV-1 viral entry envelope glycoprotein (210). The VAX003 regimen induced a more inflammatory response of decreased IgG sialylation and galactosylation compared to that raised by B003/IPCAVD-004/HVTN 091 participants (210). VAX003 consisted of 7 doses of recombinant gp120 protein which resulted in reduced IgG3 titres but enhanced IgG4 titres that inhibited both ADCC and ADCP, potentially contributing to the inefficacy of VAX003 (82, 83). In comparison, the moderately protective RV144 vaccine regimen consisted of a canarypox vector prime followed by only two doses of the same gp120 recombinant protein boost used in VAX003 and was associated with increased antigen-specific IgG3, which was identified as a correlated of protection (82, 83). Intriguingly, low IgG4 levels, similar to that observed in RV144 vaccinees, were observed after only two doses of VAX003, suggesting that repeated protein boosting may have contributed to skewed IgG4 subclass profile (82). On the other hand, the zoster vaccine, consisting of two doses of adjuvanted recombinant glycoprotein E elicited improved ADCC against herpes zoster compared to the live virus vaccine (388). This suggests that muted functional responses are not necessarily inherent to recombinant protein vaccine platforms and are likely also influenced by number of doses.
Interestingly, repeated mRNA SARS-CoV-2 vaccination with either Pfizer BioNTech BNT162b2 or Moderna mRNA-1273 has been shown to induce elevated titres of noninflammatory IgG2 and IgG4 against the viral spike protein (211, 212). Six months following second dose vaccination, in a cohort of 29 individuals, IgG4 increased from 0.04% to 4.82%, which further increased to 19.27% six months following third dose vaccination. Increased IgG4 correlated with increased avidity. However, in line with the non-inflammatory properties of IgG4, this shift in subclass distribution hindered Fc effector functions, with significantly decreased ADCP and ADCD observed following the third compared to the second mRNA vaccine dose (211). In contrast, this phenomenon of elevated IgG4 induction was not observed for adenoviral based SARS-CoV-2 vaccines (212). However, primary two-dose mRNA-1273 vaccination followed by Novavax NVX-CoV2373 recombinant protein nanoparticle booster also promoted elevated IgG4 titres in rhesus macaques (470). Whether the increase in IgG4 and associated decrease in Fc effector functions reduces protection or is beneficial to mitigating potential ADCC-driven immunopathology following SARS-CoV-2 infection remains to be determined (211).
Dosing quantity and schedule
The magnitude and timing of vaccine doses can also vastly impact the quality and quantity of antibody responses. For example, two highly similar vaccination platforms delivered in an altered format and dose (Moderna mRNA-1273 and Pfizer BioNTech BNT162b2 SARS-CoV-2 mRNA lipid nanoparticle vaccines) have recently been shown to yield differential functional responses (471). Compared to BNT162b2, mRNA-1273 vaccination induced higher levels of ADNP and ADNKA (471). It has been suggested that the increased dosing interval of the mRNA-1273 vaccine regimen allowed for a more coordinated functional response to develop (471). Likewise, a subsequent study observed that an increased BNT162b2 dosing interval was also associated with enhanced vaccine immunogenicity (340). However, it is possible that the greater mRNA-1273 antigen dose or lipid nanoparticle formulation and mRNA modifications specific to each vaccine may additionally contribute to generation of superior Fc effector capacities via mRNA-1273 vaccination. Nevertheless, this study highlights the potential for fine-tuning Fc effector functions via precision vaccination strategies.
Characterisation of the impact of SARS-CoV-2 adenoviral and protein subunit vaccine dosage has provided more detailed evidence that the quality and durability of antibody Fc effector functions is regulated by antigen quantity per exposure. However, these trends do not appear consistent across different vaccine platforms (78, 204). A nonhuman primate study of the dose-dependent effects of Johnson & Johnson Ad26.CoV2.S adenoviral vector vaccination observed that increased FcγR receptor binding and Fc functional antibody levels, trended strongly with increased dosage, whereas neutralising antibody titres and T cell responses were minimally affected (204). In contrast, a second nonhuman primate study using the Novavax NVX-CoV2373 recombinant SARS-CoV-2 spike protein nanoparticle vaccine with Matrix M adjuvant demonstrated that increased ADNP and ADNKA were associated with the administration of a lower antigen dose (78). Importantly, lower ADCP, ADNP, and ADNKA were observed within single dose groups as compared to their two dose counterparts (78). As such, a single NVX-CoV2373 dose provided only partial protection, in contrast to the near-complete protection of two doses associated with marked maturation of Fc effector functions. In addition, although long priming of germinal centres with sustained antigen delivery in escalating dose vaccination strategies has been shown to improve antibody titres and affinity (472, 473), investigation into the impact of escalating dosage upon Fc effector functions is warranted.
A systems serology analysis identified that delayed fractional dosing of the RTS,S/AS01 vaccine in controlled human malaria infection models increased Fc polyfunctionality (69). Qualitative enhancement of both the Fab and Fc in vaccinees was underpinned by increased ADCP, ADNP, and antibody-dependent dendritic cell phagocytosis (ADDCP), particularly against subdominant epitopes, and correlated with increased Fab region avidity (69). Importantly, this malaria vaccine regimen maintained the immunodominant NANP6 region-specific ADCP and ADNKA (69) which were previously defined as correlates of protection for the standard vaccination schedule (92). However, a separate study associated delayed fractional dosing of the RTS,S/AS01 regimen with increased IgG4 titres that inhibited phagocytosis (474). Although delayed fractional dosing increased vaccine efficacy in malaria naïve adults (475), the efficacy of this regimen in malaria exposed populations for whom the vaccine is most relevant remains controversial (476). Nevertheless, the substantially different Fc functions induced by different dosage and timing of the RTS,S/AS01 vaccine (69) reiterate the importance of both antigen quantity and dosing interval in driving an optimised Fc response.
In addition to the effects of antigen dosage within a single vaccine regimen, prior antigen exposure as a result of infection or vaccination may further influence the magnitude, breadth, and function of post-vaccination antibody responses via several mechanisms (477). Prior antigen exposure can induce immune imprinting which can restrict de novo immune responses to antigens related to those previously encountered (478). Furthermore, increased pre-vaccination antibody titres may drive accelerated clearance of immune complexes and a decreased window of vaccine antigen presentation (478). However, the presence of pre-existing antibodies can also be beneficial. Influenza vaccination studies have observed that elevated baseline FcγRIIb binding, along with elevated pre-existing IgG2 and decreased pre-existing IgM levels, are associated with increased neutralisation breadth (479). It has been suggested that this may result from decreased immune complex clearance owing to poor FcγR engagement by IgG2, as well as enhanced antigen presentation on follicular dendritic cells via FcγRIIb-binding antibodies. In addition, engagement with inhibitory FcγRIIb on B cells may increase the threshold of activation, thereby driving selection of higher affinity antibodies (480).
Finally, in early childhood vaccines, the timing of initial vaccination may play a role in functional antibody durability. The long-term functional capacity of measles-specific antibodies appeared to be more durable if children were vaccinated at 14 months compared to those vaccination between 6-8 months (160). Despite similar functional antibody responses in both groups one-year post-vaccination, children vaccinated later in life had more robust anti-measles functional responses at three years post-vaccination (160). No variation in isotypes or IgG subclasses were observed between age groups, suggesting that other mechanisms of Fc modulation may have contributed to functional differences. For example, variable B cell programming during different stages of early childhood may have led to enduring differences in IgG glycosylation patterns (160), and should be considered when designing childhood immunisation schedules. Alternatively, variable epitope selection driven by waning interference from maternal antibodies with increasing age (369, 370) may have contributed to differential Fc functional responses.
Adjuvants
A wide range of adjuvants that enhance vaccine immunogenicity—via diverse mechanisms leading to distinct immunological profiles—are approved for human use or in trial (481). This may be highly advantageous for the design of precision vaccines tailored to the unique requirements of distinct immunologically vulnerable populations. Most notably, emulsion adjuvants, such as MF59 and AS03, as well as toll-like receptor (TLR) agonist adjuvants, particularly when used in combination, have been advantageous for generating polyfunctional antibodies and further boosting Fc functional capacity of nanoparticle-based vaccines (467, 482, 483). Nevertheless, comprehensive studies that systematically compare the impact of a spectrum of adjuvants upon Fc functions are lacking. However, systems serology has reiterated the value of TLR agonist-based and emulsion adjuvants for enhancing Fc effector functions (484, 485).
Various TLR agonists that drive differential IgG glycosylation are employed as adjuvants. Macaque studies of simian immunodeficiency virus (SIV) vaccination have shown that distinct TLR agonist adjuvant combinations induce unique antibody Fc functions (486). Further studies have defined a role for differential Fc glycosylation in the modulation of Fc functions by TLR agonists (487). Although different adjuvants induced equivalent protective antibody responses of similar magnitudes, quantitative antibody differences were evident between the groups. A TLR4 plus TLR7 agonist (glucopyranosyl lipid plus imiquimod) adjuvant system stimulated increased ADNP and ADCC, while the TLR4 agonist plus the saponin-derivative adjuvant QS21 was associated with ADCD and anti-inflammatory digalactosylated monosialylated IgG. Although the healthy macaques were equivalently protected, differentially induced Fc effector functions may be of value to vulnerable human vaccinees experiencing dysregulated baseline inflammation. Dissecting which qualitative antibody features may have the greatest protective capacity in this context remains to be determined.
MF59—a squalene oil and surfactant adjuvant—boosts CD4+ T cell and Tfh cell activity to induce robust germinal centre responses (488). This leads to long-lived plasma and memory B cells, as well B cell repertoire expansion, resulting in elevated antibody titres. Importantly, however, MF59 may also support class switching to IgG in a CD4+ T cell-independent manner (489). Consequently, MF59 is a useful adjuvant for boosting responses in individuals experiencing reduced vaccine immunogenicity and has been successfully trialled in influenza vaccines for the elderly (490, 491), with especially pronounced benefits for elderly individuals with chronic comorbidities (492). Importantly, MF59 also induces a highly functional antibody response with selective induction of IgG3, resulting in pronounced boosting of Fc effector functions (394). An H5N1 avian influenza human vaccine immunogenicity trial revealed that participants adjuvanted with MF59 had elevated IgG1 and IgG3 titres, as well as ADNP and ADCD, in comparison to those boosted with alum (394). As such, MF59 may override the unfavourable subclass biases or impaired class switching experienced by certain vulnerable populations.
Non-human primate studies of MF59 compared to alum adjuvanted HIV-1 vaccines further point to the ability of adjuvant selection to regulate IgG glycosylation and Fc effector functions (493). A comparison of SIV vaccination with gp120, adjuvanted with either MF59 or alum indicated that although MF59 induced the expected higher titre antibody responses, the alum adjuvanted vaccine was associated with increased protection (493). The alum adjuvant induced decreased galactosylated IgG which was suggested to drive a more coordinated polyfunctional antibody response compared to MF59. On the other hand, MF59 induced elevated titres of anti-inflammatory sialylated gp120 antibodies. Indeed, observations in human trials of H5N1 vaccines suggested MF59 associated improvements to ADCP were driven by titre rather than Fc glycosylation modulation (394). In addition, the lack of enhanced FcγRIIIa engagement and ADCC despite increased IgG1 and IgG3, suggest an inability of MF59 to induce coordinated Fc functional responses, possibly associated with inhibitory Fc glycosylation (394).
In a second rhesus macaque study of a HIV-1 poxvirus vector vaccine administered with either alum alone or a liposomal monophosphoryl lipid A formulation plus alum (Army Liposome Formulation (ALFA)), the ALFA adjuvanted regimen was associated with enhanced ADNP and ADCP and 90% protection against mucosal challenge compared to the 100% infection risk observed for the alum adjuvanted regimen (494). The discrepancy between these studies regarding the effects of alum upon Fc functions may be related to use of different combinations of antigen choice, vaccine platform, or dosage. As such, these data underscore the need for detailed understanding of the interactions between various vaccine modifications upon polyfunctional antibodies.
Fortunately, a more detailed mechanistic understanding regarding how adjuvants alter IgG subclass ratios and IgG glycosylation is beginning to develop (288). Experimental water-in-oil emulsion and Mycobacterium tuberculosis-derived adjuvants appear to selectively program germinal centres to produce differentially Fc-glycosylated antibodies. Mouse model studies of the mechanism by which these adjuvants influence Fc functional antibodies identified unique transcriptome alterations distinguished by St6gal1 mRNA levels which control sialyltransferase expression and, therefore, IgG Fc sialylation (288). The use of water-in-oil emulsion adjuvants and mycobacterium cord factor created germinal centre environments enriched in IL-6 which programmed Tfh cells to stimulate germinal centre B cells into producing IgG with reduced IgG Fc sialylation (288). Given the inflammatory properties of reduced Fc sialylation, inclusion of these adjuvants may support rational vaccine design targeting antibodies with enhanced effector functions. In addition, priming viral immunisation schedules with the unrelated TB Bacillus Calmette–Guérin (BCG) vaccine modulates production of IL-6, as well as other pro-inflammatory cytokines, upon target antigen stimulation (495–498). This suggests a possible role for BCG vaccination in the modulation of IgG Fc glycosylation, and consequently, regulation of Fc effector functions.
Route of administration
Comparison of systemic versus mucosal vaccination is important for the many pathogens entering via the mucosa, such as HIV-1 and respiratory viruses. For example, for respiratory viruses, vaccines administered at the anatomical site of entry (i.e., intranasally) may elicit more biologically relevant, and therefore, protective, responses. However, traditionally, most vaccines are delivered intramuscularly, and historically have not facilitated optimal humoral immune responses at mucosal surfaces for influenza, SARS-CoV-2, and HIV-1 vaccination (499). Insights into the regulation of Fc effector functions can be gleaned from the comparison of intramuscular and aerosol delivery of a SIV vaccine in nonhuman primates (500). These two delivery modes of an otherwise identical vaccine mediated equivalent protection, but distinct effector functions. Intramuscular delivery facilitated enhanced IgG-driven ADCP, while aerosol delivery facilitated enhanced ADNP bolstered by IgA activity. Although a mechanism for this observation was not fully explored, shared patterns of IgG galactosylation were associated with the different modes of phagocytosis induced by each immunisation route, highlighting key glycoforms of potential clinical relevance (500).
In addition, combining different routes of administration within prime-boost vaccination regimens against infections where both mucosal and systemic protection is required may prove beneficial. Systems serology studies have shown COVID-19 convalescent vaccinated individuals induce markedly altered Fc functions compared to those exposed only to vaccination (501). Individuals with hybrid immunity (SARS-CoV-2 infected individuals who then received a single SARS-CoV-2 intramuscular vaccination) had increased Fc functional capacity in comparison to otherwise healthy individuals given two doses of intramuscular vaccination despite antibody titres being comparable between the two groups (501). This suggests combined aerosol and intramuscular vaccine delivery may be beneficial for the generation of enhanced functional antibody responses, potentially leading to improved protection. Alternatively, FcRn-targeting vaccines with the capacity to generate antibodies that are selectively transferred from systemic circulation to mucosal sites may overcome the issues associated with conflicting anatomical sites of vaccination and infection.
Computational strategies to inform population-based vaccine design
Computational approaches can serve as safe, rapid, and cost-effective hypothesis testing tools to screen through multiple complex antibody scenarios, integrating and assessing for the influence of different geographic, genetic and clinical parameters that are rarely accounted for in traditional vaccine efficacy trials. The primary strength of these approaches is that they are able to integrate large amounts of complex data to gain insight into mechanisms that underpin variability in vaccine-induced protection (34, 502). Computational methods in systems serology can be divided into two groups, depending on the questions being asked and the data that is available.
Data-driven modelling involves the application of statistical and machine learning methods to high-throughput serology data to uncover ‘signatures’ of antibody features associated with a vaccine outcome (21). These methods can also be used to classify subpopulations of vaccinees based on responses within a given cohort (21). The advantage of data-driven approaches is that they require little prior knowledge of mechanism, making them broadly applicable to any data set of interest (21, 503). Data-driven approaches applied to plasma samples from the RV144, VAX003, HVTN204, and IPCAVD001 HIV vaccine trials identified antibody signatures that defined each vaccine response uniquely (34). Further, these approaches could also select for the humoral features (i.e., IgG titres, IgG-FcγR engagement) and functional responses (i.e., ADCP) most closely associated with protection against HIV infection (34). Results highlighted the key antibody features and functions that may be unique to each vaccine platform that was evaluated. Similarly, computational approaches applied to convalescent plasma data from COVID-19 patients revealed a signature of antibody features, primarily driven by SARS-CoV-2 Spike-specific IgG3 titres, that was associated with disease severity (504). Separate analysis of SARS-CoV-2 convalescent plasma samples has noted key differences in humoral profiles between children and elderly patients, with mature IgG and IgA responses to SARS-CoV-2 Spike 2 and Nuclear Protein antigens being associated more with elderly patients (37). These analysis reveals both the differences in immune signatures between populations and a possible reason for the vastly different clinical outcomes between them. In all, these findings illustrate how data-driven computational approaches can classify responses and identify subpopulations based on a distinct humoral signature rather than any single antibody feature.
In contrast, mechanistic (“theory-driven”) modelling requires knowledge of the underlying system and uses mathematical relationships to link system components. This trade-off sacrifices broad applicability for added depth of analysis. As these models describe the underlying system in detail, they allow for the investigation of the relationships between the components (e.g., antibodies and FcγR engagement) even at the individual level, thus allowing for the incorporation of personalised parameters (e.g., clinical history or immunogenetics) to evaluate how one individual may respond to a vaccine differently from another individual with different personalised parameters (446). Further, it can be used to evaluate not just how individual changes affect the system output (e.g., vaccine-induced antibody responses), but how combinations of changes to multiple system parameters (e.g., combinations of different immunogenetics and/or clinical history) can result in synergistic changes that are greater than the sum of individual perturbations.
Understanding these mechanistic details will be of high value for future efforts to optimise precision vaccines. For example, IgG1 allotypes and FcγR polymorphisms have the potential to influence protective responses via associated changes in antibody concentration and binding to Fc receptors as previously discussed in the sections above (60, 254). Mechanistic-modelling approaches have been applied to unravel mechanisms by which IgG1 allotypes and Fc receptor polymorphisms influence protective Fc effector functions following HIV-1 vaccination (445). An ordinary differential equation model illustrated how individuals with the G1m-1,3 IgG1 allotype would be predicted to be more responsive to changes in IgG1 concentration (titres) that arise from traditional boosting regimens, whereas G1m1 and G1m1,3 individuals may require a modification to IgG1-FcγR affinity (via glycosylation) to improve Fc effector functions. Furthermore, results suggested that Fc receptor engagement may be unaffected by FcγR polymorphisms until IgG titres reached a very high level, such as those that would be acquired with vaccine boosting. The model was also able to test vaccine design hypotheses in simulated populations of individuals with heterogeneous genetic compositions and suggest specific interventions that would be most effective. Combined, these insights provide specific target design criteria for vaccines tailored to different populations.
Improvements to these computational methods combined with broader application of the techniques will continue to increase the utility of computational approaches for population-based vaccine design. These approaches may directly inform current strategies, such as vaccine boosting, by identifying which populations may benefit most from a given intervention based on infection history, host genetics, or other clinical parameters that influence antibody levels. For vaccine parameters that are not yet modifiable, computational approaches will help prioritize targets for future modifications, overcoming challenges related to time and cost.
Potential pitfalls of Fc functional antibody targeting vaccines
Eliciting potent Fc-functional responses via vaccination in immunologically vulnerable populations has clear benefits for the generation of durable, cross-reactive humoral protection. However, the potential to induce adverse antibody responses should also be acknowledged. Antibody-dependent enhancement (ADE) of disease has been observed following administration of the formalin inactivated RSV (505) and measles vaccines (506) as well as tetravalent dengue vaccine (507, 508). Although these vaccines did not specifically aim to elicit Fc functions, aberrant FcγR engagement appears to have facilitated viral replication within FcγR-expressing cells, and consequently, more severe disease outcomes when vaccinees encountered the virus (61). In the case of vaccines specifically designed to elicit potent Fc functions, careful delineation of protective versus pathogenic Fc responses is required to ensure vaccine safety (509).
Induction of beneficial Fc functional responses is a careful balancing act between protective and pathogenic inflammation. Downstream, Fc functions may trigger inflammatory cytokine release which in turn regulates further recruitment and programming of innate and adaptive immune cells (510). Notably, ADCC must balance viral clearance with immune activation and, therefore, if poorly regulated, can lead to increased morbidity and mortality during some diseases such as dengue fever (507, 508). Although ADCC may contribute to SARS-CoV-2 control (511, 512), uncoordinated Fc functions are a feature of COVID-19, contributing to excess FcγR mediated activation of the innate immune system and consequent induction of cytokine storms (513, 514). The requirement of precise induction of select Fc effector functions has also been demonstrated in protection against Salmonella Typhi (174). Following vaccination, ADNP and antibody-dependent neutrophil oxidative burst (ADNOB) were associated with vaccine-induced protection, whereas breakthrough infection was associated with elevated ADCD, ADCP, and ADNKA (174).
In addition, given the importance of Fc functions in protection against infectious diseases, several pathogens have evolved Fc evasion mechanisms (515–517). Notably, the decoy Fc receptors expressed by members of the herpes virus family, such as glycoproteins gp34 and gp68 in human cytomegalovirus (HCMV) and gE and gI in HSV (518–520), may present unique challenges for the development of vaccines aimed at eliciting protective Fc functional antibodies. Strategies to promote preferential engagement with host FcγRs rather than viral decoy receptors will need to be devised. Several HSV-1 and HSV-2 vaccine candidates have been trialled, but without sufficient efficacy for licensure (125, 521). Interestingly, individuals bearing different IgG1 allotypes have been shown to differently engage HSV decoy Fc receptors (435), suggesting possible differences in susceptibility to HSV infection. Efficacy of HCMV candidate vaccines has similarly been low, particularly in vulnerable target populations such as pregnant women and transplant recipients (522). However, it is suggested that generation of robust Fc effector functions may provide protective responses against infection. As such, it is important to consider that HCMV expresses similar decoy Fc receptors with preferential binding by some human IgG1 allotypes (523, 524) which may add further complexity to the design of vaccines targeting robust FcγR engagement.
Conclusion and future perspectives
The success of current population-based vaccination strategies which prioritise immunocompromised and vulnerable individuals for early, additional, and/or high-dose vaccines has demonstrated the value of selectively tailoring immunisation programmes (15, 390, 391). Furthermore, this precedent lays the groundwork for what can be achieved, if more subtle immunological differences between distinct populations are considered in vaccine implementation and design.
Immunogenetic regulation, age- and disease-induced differences in host inflammatory status have emerged as potent modulators of vaccine-induced antibody responses (1) (Figure 7). Importantly, given that antibody features are similarly dysregulated across multiple vulnerable groups, there may be potential for broad implementation of population-based vaccination strategies aimed at bolstering protective Fc functions. For example, the elderly and individuals with chronic inflammatory comorbidities share similar dysregulated inflammatory Fc glycosylation states and impaired Fab affinity maturation (328, 330, 341). Therefore, future studies should consider focusing on the identification of appropriate adjuvants or vaccine platforms that modulate antibody glycosylation (288). In addition, strategies that increase antigen-specific antibody binding to FcγRIIb may be beneficial, given that FcγRIIb binding has been associated with enhanced neutralisation breadth as a result of increased affinity maturation (479). However, eliciting an optimally functional antibody response will require systematic assessment of the ideal combination of vaccine platform, adjuvant, dosage, and administrative route (Figure 7). Unfortunately, few clinical trials, or even licenced vaccine platforms, have assessed vulnerable groups for such nuanced variation. This highlights the need for more extensive population-based vaccine immunogenicity studies as well as standardisation of assays to assess functional antibody responses.
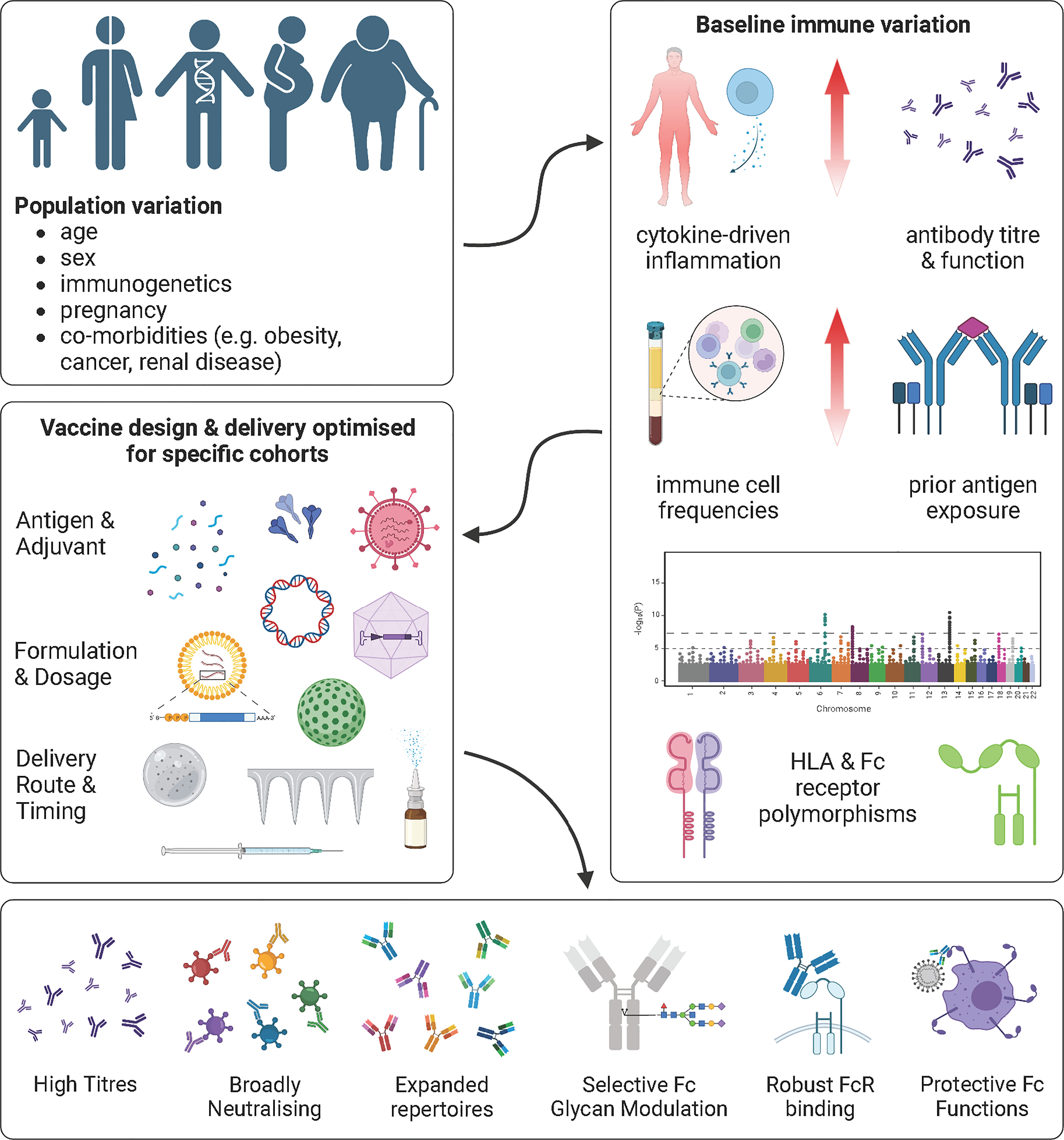
Figure 7 Considerations for the design of precision vaccines for vulnerable populations. Age, sex, immunogenetic, and baseline health variations within a vaccinated population can impact vaccine effectiveness. This population variation influences immune features known to modulate vaccine immunogenicity. However, precision vaccines designed to selectively boost the immune features that are impaired or dysregulated in vulnerable populations may enhance vaccine-induced protection. Design of such population-based precision vaccine strategies will require elucidation of the best combinations of antigen and adjuvant, vaccine formulation, and delivery mode in order to elicit an optimised polyfunctional antibody response and promote increased protection response.
It is also important to note that the precise antibody features and effector functions constituting a coordinated Fc response are highly pathogen-specific. For example, while afucosylated IgG and excessive ADCC are detrimental for dengue fever (110, 111, 290, 292, 296), they are beneficial for HIV-1 and Tuberculosis (53, 79). Therefore, elucidation of the antibody features that should be targeted via vaccination will require detailed characterisation of the protective mechanisms employed against each disease, before being tailored to specific vulnerable populations. Despite the undeniable complexity of eliciting protective, polyfunctional antibodies, advances in vaccine formulation and administration may enable more precise modulation of IgG subclass ratios and Fc glycosylation which mitigate dysregulated Fc functions in vulnerable populations. However other target strategies should not be overlooked, such as the optimisation of FcRn engagement for vaccines which can be administered during pregnancy to simultaneously protect mother and infant, as well as systemic vaccines against mucosal pathogens.
Although personalised vaccination against infectious disease is likely not imminently practical at the individual level, population-based precision vaccination approaches appear feasible within the current global health infrastructure (5, 22, 525). Ideally, next-generation vaccination strategies will promote maximal responses not only in at-risk populations, but also in healthy individuals bearing genetic variations that necessitate differential boosting of specific immune features. Such population-based vaccination accounting for immunogenetic variation may be enabled by the ethnic and, therefore, geographic clustering of key heritable genetic features (313, 445, 446).
Finally, translating the observed differences between vaccine regimens into actionable vaccine design improvements for vulnerable populations remains a key public health priority. Indeed, a generation of population-based vaccination strategies informed by molecular mechanisms may enhance vaccine effectiveness against a broad range of diseases. Critically, such strategies may enable not only maximised protection of diverse, healthy individuals, but also markedly improved protection of the globally increasing population of immunologically vulnerable individuals.
Author contributions
RP conceived and wrote the manuscript. RT contributed to writing. KA, AC, and KS edited the manuscript and supervised the project. All authors contributed to the article and approved the submitted version.
Funding
This study was supported by a Medical Research Future Fund (MRFF) GNT #2016062 and a National Health and Medical Research Council (NHMRC) Investigator grant #2008092 to AC.
Acknowledgments
We thank Ebene R. Haycroft for assistance in reviewing this manuscript. All figures were created with BioRender.com.
Conflict of interest
The authors declare that the research was conducted in the absence of any commercial or financial relationships that could be construed as a potential conflict of interest.
Publisher’s note
All claims expressed in this article are solely those of the authors and do not necessarily represent those of their affiliated organizations, or those of the publisher, the editors and the reviewers. Any product that may be evaluated in this article, or claim that may be made by its manufacturer, is not guaranteed or endorsed by the publisher.
Supplementary material
The Supplementary Material for this article can be found online at: https://www.frontiersin.org/articles/10.3389/fimmu.2023.1183727/full#supplementary-material
References
1. Zimmermann P, Curtis N. Factors that influence the immune response to vaccination. Clin Microbiol Rev (2019) 32(2):e00084–18. doi: 10.1128/CMR.00084-18
2. Duffy D. Milieu intérieur: defining the boundaries of a healthy immune response for improved vaccination strategies. Hum Vaccin Immunother (2018) 14(9):2217–21. doi: 10.1080/21645515.2018.1466764
3. Kennedy RB, Ovsyannikova IG, Lambert ND, Haralambieva IH, Poland GA. The personal touch: strategies toward personalized vaccines and predicting immune responses to them. Expert Rev Vaccines (2014) 13(5):657–69. doi: 10.1586/14760584.2014.905744
4. Poland GA, Ovsyannikova IG, Jacobson RM, Smith DI. Heterogeneity in vaccine immune response: the role of immunogenetics and the emerging field of vaccinomics. Clin Pharmacol Ther (2007) 82(6):653–64. doi: 10.1038/sj.clpt.6100415
5. Poland GA, Ovsyannikova IG, Jacobson RM. Personalized vaccines: the emerging field of vaccinomics. Expert Opin Biol Ther (2008) 8(11):1659–67. doi: 10.1517/14712598.8.11.1659
6. Poland GA, Kennedy RB, Ovsyannikova IG. Vaccinomics and personalized vaccinology: is science leading us toward a new path of directed vaccine development and discovery? PloS Pathog (2011) 7(12):e1002344. doi: 10.1371/journal.ppat.1002344
7. Poland GA, Ovsyannikova IG, Kennedy RB. Personalized vaccinology: a review. Vaccine (2018) 36(36):5350–7. doi: 10.1016/j.vaccine.2017.07.062
8. Andrasik MP, Broder GB, Wallace SE, Chaturvedi R, Michael NL, Bock S, et al. Increasing black, indigenous and people of color participation in clinical trials through community engagement and recruitment goal establishment. PloS One (2021) 16(10):e0258858. doi: 10.1371/journal.pone.0258858
9. Flores LE, Frontera WR, Andrasik MP, Del Rio C, Mondríguez-González A, Price SA, et al. Assessment of the inclusion of Racial/Ethnic minority, female, and older individuals in vaccine clinical trials. JAMA Netw Open (2021) 4(2):e2037640. doi: 10.1001/jamanetworkopen.2020.37640
10. Sethi S, Kumar A, Mandal A, Shaikh M, Hall CA, Kirk JMW, et al. The UPTAKE study: implications for the future of COVID-19 vaccination trial recruitment in UK and beyond. Trials (2021) 22(1):296. doi: 10.1186/s13063-021-05250-4
11. Garland SM, Brotherton JML, Moscicki AB, Kaufmann AM, Stanley M, Bhatla N, et al. HPV vaccination of immunocompromised hosts. Papillomavirus Res (2017) 4:35–8. doi: 10.1016/j.pvr.2017.06.002
12. Maltezou HC, Theodoridou K, Poland G. Influenza immunization and COVID-19. Vaccine (2020) 38(39):6078–9. doi: 10.1016/j.vaccine.2020.07.058
13. Müller L, Andrée M, Moskorz W, Drexler I, Walotka L, Grothmann R, et al. Age-dependent immune response to the Biontech/Pfizer BNT162b2 coronavirus disease 2019 vaccination. Clin Infect Dis (2021) 73(11):2065–72. doi: 10.1093/cid/ciab381
14. Rosdahl A, Herzog C, Frösner G, Norén T, Rombo L, Askling HH. An extra priming dose of hepatitis a vaccine to adult patients with rheumatoid arthritis and drug induced immunosuppression - a prospective, open-label, multi-center study. Travel Med Infect Dis (2018) 21:43–50. doi: 10.1016/j.tmaid.2017.12.004
15. Renia L, Goh YS, Rouers A, Le Bert N, Chia WN, Chavatte JM, et al. Lower vaccine-acquired immunity in the elderly population following two-dose BNT162b2 vaccination is alleviated by a third vaccine dose. Nat Commun (2022) 13(1):4615. doi: 10.1038/s41467-022-32312-1
16. Baguelin M, Flasche S, Camacho A, Demiris N, Miller E, Edmunds WJ. Assessing optimal target populations for influenza vaccination programmes: an evidence synthesis and modelling study. PloS Med (2013) 10(10):e1001527. doi: 10.1371/journal.pmed.1001527
17. Hogan AB, Winskill P, Watson OJ, Walker PGT, Whittaker C, Baguelin M, et al. Within-country age-based prioritisation, global allocation, and public health impact of a vaccine against SARS-CoV-2: a mathematical modelling analysis. Vaccine (2021) 39(22):2995–3006. doi: 10.1016/j.vaccine.2021.04.002
18. Moore S, Hill EM, Dyson L, Tildesley MJ, Keeling MJ. Modelling optimal vaccination strategy for SARS-CoV-2 in the UK. PloS Comput Biol (2021) 17(5):e1008849. doi: 10.1371/journal.pcbi.1008849
19. Parker EPK, Desai S, Marti M, Nohynek H, Kaslow DC, Kochhar S, et al. Response to additional COVID-19 vaccine doses in people who are immunocompromised: a rapid review. Lancet Glob Health (2022) 10(3):e326–e8. doi: 10.1016/S2214-109X(21)00593-3
20. Pollard AJ, Bijker EM. A guide to vaccinology: from basic principles to new developments. Nat Rev Immunol (2021) 21(2):83–100. doi: 10.1038/s41577-020-00479-7
21. Arnold KB, Chung AW. Prospects from systems serology research. Immunology (2018) 153(3):279–89. doi: 10.1111/imm.12861
22. Cotugno N, Ruggiero A, Santilli V, Manno EC, Rocca S, Zicari S, et al. OMIC technologies and vaccine development: from the identification of vulnerable individuals to the formulation of invulnerable vaccines. J Immunol Res (2019) 2019:8732191. doi: 10.1155/2019/8732191
23. Dowling DJ, Levy O. A precision adjuvant approach to enhance severe acute respiratory syndrome coronavirus 2 (SARS-CoV-2) vaccines optimized for immunologically distinct vulnerable populations. Clin Infect Dis (2022) 75(Supplement_1):S30–s6. doi: 10.1093/cid/ciac342
24. Nakaya HI, Wrammert J, Lee EK, Racioppi L, Marie-Kunze S, Haining WN, et al. Systems biology of vaccination for seasonal influenza in humans. Nat Immunol (2011) 12(8):786–95. doi: 10.1038/ni.2067
25. Nanishi E, Dowling DJ, Levy O. Toward precision adjuvants: optimizing science and safety. Curr Opin Pediatr (2020) 32(1):125–38. doi: 10.1097/MOP.0000000000000868
26. Poland GA, Ovsyannikova IG, Kennedy RB, Haralambieva IH, Jacobson RM. Vaccinomics and a new paradigm for the development of preventive vaccines against viral infections. Omics (2011) 15(9):625–36. doi: 10.1089/omi.2011.0032
27. Querec TD, Akondy RS, Lee EK, Cao W, Nakaya HI, Teuwen D, et al. Systems biology approach predicts immunogenicity of the yellow fever vaccine in humans. Nat Immunol (2009) 10(1):116–25. doi: 10.1038/ni.1688
28. Shin MD, Shukla S, Chung YH, Beiss V, Chan SK, Ortega-Rivera OA, et al. COVID-19 vaccine development and a potential nanomaterial path forward. Nat Nanotechnol (2020) 15(8):646–55. doi: 10.1038/s41565-020-0737-y
29. Brisse M, Vrba SM, Kirk N, Liang Y, Ly H. Emerging concepts and technologies in vaccine development. Front Immunol (2020) 11:583077. doi: 10.3389/fimmu.2020.583077
30. Excler JL, Saville M, Berkley S, Kim JH. Vaccine development for emerging infectious diseases. Nat Med (2021) 27(4):591–600. doi: 10.1038/s41591-021-01301-0
31. Fourati S, Tomalin LE, Mulè MP, Chawla DG, Gerritsen B, Rychkov D, et al. Pan-vaccine analysis reveals innate immune endotypes predictive of antibody responses to vaccination. Nat Immunol (2022) 23(12):1777–87. doi: 10.1038/s41590-022-01329-5
32. Hagan T, Gerritsen B, Tomalin LE, Fourati S, Mulè MP, Chawla DG, et al. Transcriptional atlas of the human immune response to 13 vaccines reveals a common predictor of vaccine-induced antibody responses. Nat Immunol (2022) 23(12):1788–98. doi: 10.1038/s41590-022-01328-6
33. Ackerman ME, Barouch DH, Alter G. Systems serology for evaluation of HIV vaccine trials. Immunol Rev (2017) 275(1):262–70. doi: 10.1111/imr.12503
34. Chung AW, Kumar MP, Arnold KB, Yu WH, Schoen MK, Dunphy LJ, et al. Dissecting polyclonal vaccine-induced humoral immunity against HIV using systems serology. Cell (2015) 163(4):988–98. doi: 10.1016/j.cell.2015.10.027
35. Chung AW, Alter G. Systems serology: profiling vaccine induced humoral immunity against HIV. Retrovirology (2017) 14(1):57. doi: 10.1186/s12977-017-0380-3
36. Francica JR, Flynn BJ, Foulds KE, Noe AT, Werner AP, Moore IN, et al. Protective antibodies elicited by SARS-CoV-2 spike protein vaccination are boosted in the lung after challenge in nonhuman primates. Sci Transl Med (2021) 13(607):eabi4547. doi: 10.1126/scitranslmed.abi4547
37. Selva KJ, van de Sandt CE, Lemke MM, Lee CY, Shoffner SK, Chua BY, et al. Systems serology detects functionally distinct coronavirus antibody features in children and elderly. Nat Commun (2021) 12(1):2037. doi: 10.1038/s41467-021-22236-7
38. Plotkin SA. Correlates of protection induced by vaccination. Clin Vaccine Immunol (2010) 17(7):1055–65. doi: 10.1128/CVI.00131-10
39. Khoury DS, Cromer D, Reynaldi A, Schlub TE, Wheatley AK, Juno JA, et al. Neutralizing antibody levels are highly predictive of immune protection from symptomatic SARS-CoV-2 infection. Nat Med (2021) 27(7):1205–11. doi: 10.1038/s41591-021-01377-8
40. Douglas AD, Williams AR, Illingworth JJ, Kamuyu G, Biswas S, Goodman AL, et al. The blood-stage malaria antigen PfRH5 is susceptible to vaccine-inducible cross-strain neutralizing antibody. Nat Commun (2011) 2:601. doi: 10.1038/ncomms1615
41. Miller MS, Gardner TJ, Krammer F, Aguado LC, Tortorella D, Basler CF, et al. Neutralizing antibodies against previously encountered influenza virus strains increase over time: a longitudinal analysis. Sci Transl Med (2013) 5(198):198ra07. doi: 10.1126/scitranslmed.3006637
42. Stephenson KE, Wagh K, Korber B, Barouch DH. Vaccines and broadly neutralizing antibodies for HIV-1 prevention. Annu Rev Immunol (2020) 38:673–703. doi: 10.1146/annurev-immunol-080219-023629
43. Levin EG, Lustig Y, Cohen C, Fluss R, Indenbaum V, Amit S, et al. Waning immune humoral response to BNT162b2 covid-19 vaccine over 6 months. N Engl J Med (2021) 385(24):e84. doi: 10.1056/NEJMoa2114583
44. Hogarth PM, Pietersz GA. Fc receptor-targeted therapies for the treatment of inflammation, cancer and beyond. Nat Rev Drug Discovery (2012) 11(4):311–31. doi: 10.1038/nrd2909
45. Bartsch YC, Tong X, Kang J, Avendano MJ, Serrano EF, Garcia-Salum T, et al. Omicron variant spike-specific antibody binding and fc activity are preserved in recipients of mRNA or inactivated COVID-19 vaccines. Sci Transl Med (2022) 14(642):eabn9243. doi: 10.1126/scitranslmed.abn9243
46. Amanat F, Thapa M, Lei T, Ahmed SMS, Adelsberg DC, Carreño JM, et al. SARS-CoV-2 mRNA vaccination induces functionally diverse antibodies to NTD, RBD, and S2. Cell (2021) 184(15):3936–48.e10. doi: 10.1016/j.cell.2021.06.005
47. Richardson SI, Manamela NP, Motsoeneng BM, Kaldine H, Ayres F, Makhado Z, et al. SARS-CoV-2 beta and delta variants trigger fc effector function with increased cross-reactivity. Cell Rep Med (2022) 3(2):100510. doi: 10.1016/j.xcrm.2022.100510
48. Richardson SI, Madzorera VS, Spencer H, Manamela NP, van der Mescht MA, Lambson BE, et al. SARS-CoV-2 omicron triggers cross-reactive neutralization and fc effector functions in previously vaccinated, but not unvaccinated, individuals. Cell Host Microbe (2022) 30(6):880–6.e4. doi: 10.1016/j.chom.2022.03.029
49. DiLillo DJ, Tan GS, Palese P, Ravetch JV. Broadly neutralizing hemagglutinin stalk-specific antibodies require FcγR interactions for protection against influenza virus in vivo. Nat Med (2014) 20(2):143–51. doi: 10.1038/nm.3443
50. Kaplonek P, Fischinger S, Cizmeci D, Bartsch YC, Kang J, Burke JS, et al. mRNA-1273 vaccine-induced antibodies maintain fc effector functions across SARS-CoV-2 variants of concern. Immunity (2022) 55(2):355–65.e4. doi: 10.1016/j.immuni.2022.01.001
51. Jegaskanda S, Laurie KL, Amarasena TH, Winnall WR, Kramski M, De Rose R, et al. Age-associated cross-reactive antibody-dependent cellular cytotoxicity toward 2009 pandemic influenza a virus subtype H1N1. J Infect Dis (2013) 208(7):1051–61. doi: 10.1093/infdis/jit294
52. Jegaskanda S, Weinfurter JT, Friedrich TC, Kent SJ. Antibody-dependent cellular cytotoxicity is associated with control of pandemic H1N1 influenza virus infection of macaques. J Virol (2013) 87(10):5512–22. doi: 10.1128/JVI.03030-12
53. Lu LL, Chung AW, Rosebrock TR, Ghebremichael M, Yu WH, Grace PS, et al. A functional role for antibodies in tuberculosis. Cell (2016) 167(2):433–43.e14. doi: 10.1016/j.cell.2016.08.072
54. He W, Tan GS, Mullarkey CE, Lee AJ, Lam MM, Krammer F, et al. Epitope specificity plays a critical role in regulating antibody-dependent cell-mediated cytotoxicity against influenza a virus. Proc Natl Acad Sci USA (2016) 113(42):11931–6. doi: 10.1073/pnas.1609316113
55. Alsahafi N, Bakouche N, Kazemi M, Richard J, Ding S, Bhattacharyya S, et al. An asymmetric opening of HIV-1 envelope mediates antibody-dependent cellular cytotoxicity. Cell Host Microbe (2019) 25(4):578–87.e5. doi: 10.1016/j.chom.2019.03.002
56. Schäfer A, Muecksch F, Lorenzi JCC, Leist SR, Cipolla M, Bournazos S, et al. Antibody potency, effector function, and combinations in protection and therapy for SARS-CoV-2 infection in vivo. J Exp Med (2021) 218(3):e20201993. doi: 10.1084/jem.20201993
57. Saphire EO, Schendel SL, Fusco ML, Gangavarapu K, Gunn BM, Wec AZ, et al. Systematic analysis of monoclonal antibodies against Ebola virus GP defines features that contribute to protection. Cell (2018) 174(4):938–52.e13. doi: 10.1016/j.cell.2018.07.033
58. Roopenian DC, Akilesh S. FcRn: the neonatal fc receptor comes of age. Nat Rev Immunol (2007) 7(9):715–25. doi: 10.1038/nri2155
59. Pyzik M, Sand KMK, Hubbard JJ, Andersen JT, Sandlie I, Blumberg RS. The neonatal fc receptor (FcRn): a misnomer? Front Immunol (2019) 10:1540. doi: 10.3389/fimmu.2019.01540
60. Bruhns P, Iannascoli B, England P, Mancardi DA, Fernandez N, Jorieux S, et al. Specificity and affinity of human fcγ receptors and their polymorphic variants for human IgG subclasses. Blood (2009) 113(16):3716–25. doi: 10.1182/blood-2008-09-179754
61. Bournazos S, Gupta A, Ravetch JV. The role of IgG fc receptors in antibody-dependent enhancement. Nat Rev Immunol (2020) 20(10):633–43. doi: 10.1038/s41577-020-00410-0
62. Anania JC, Chenoweth AM, Wines BD, Hogarth PM. The human FcγRII (CD32) family of leukocyte FcR in health and disease. Front Immunol (2019) 10:464. doi: 10.3389/fimmu.2019.00464
63. Golay J, Valgardsdottir R, Musaraj G, Giupponi D, Spinelli O, Introna M. Human neutrophils express low levels of FcγRIIIA, which plays a role in PMN activation. Blood (2019) 133(13):1395–405. doi: 10.1182/blood-2018-07-864538
64. Bruhns P, Jönsson F. Mouse and human FcR effector functions. Immunol Rev (2015) 268(1):25–51. doi: 10.1111/imr.12350
65. Zhao W, Kepley CL, Morel PA, Okumoto LM, Fukuoka Y, Schwartz LB. Fc gamma RIIa, not fc gamma RIIb, is constitutively and functionally expressed on skin-derived human mast cells. J Immunol (2006) 177(1):694–701. doi: 10.4049/jimmunol.177.1.694
66. Davis SK, Selva KJ, Kent SJ, Chung AW. Serum IgA fc effector functions in infectious disease and cancer. Immunol Cell Biol (2020) 98(4):276–86. doi: 10.1111/imcb.12306
67. Maslanka SE, Tappero JW, Plikaytis BD, Brumberg RS, Dykes JK, Gheesling LL, et al. Age-dependent neisseria meningitidis serogroup c class-specific antibody concentrations and bactericidal titers in sera from young children from Montana immunized with a licensed polysaccharide vaccine. Infect Immun (1998) 66(6):2453–9. doi: 10.1128/IAI.66.6.2453-2459.1998
68. Romero-Steiner S, Musher DM, Cetron MS, Pais LB, Groover JE, Fiore AE, et al. Reduction in functional antibody activity against streptococcus pneumoniae in vaccinated elderly individuals highly correlates with decreased IgG antibody avidity. Clin Infect Dis (1999) 29(2):281–8. doi: 10.1086/520200
69. Das J, Fallon JK, Yu TC, Michell A, Suscovich TJ, Linde C, et al. Delayed fractional dosing with RTS,S/AS01 improves humoral immunity to malaria via a balance of polyfunctional NANP6- and Pf16-specific antibodies. Med (N Y) (2021) 2(11):1269–86.e9. doi: 10.1016/j.medj.2021.10.003
70. Dobaño C, Santano R, Vidal M, Jiménez A, Jairoce C, Ubillos I, et al. Differential patterns of IgG subclass responses to plasmodium falciparum antigens in relation to malaria protection and RTS,S vaccination. Front Immunol (2019) 10:439. doi: 10.3389/fimmu.2019.00439
71. Kurtovic L, Atre T, Feng G, Wines BD, Chan JA, Boyle MJ, et al. Multifunctional antibodies are induced by the RTS,S malaria vaccine and associated with protection in a phase 1/2a trial. J Infect Dis (2021) 224(7):1128–38. doi: 10.1093/infdis/jiaa144
72. Seaton KE, Spreng RL, Abraha M, Reichartz M, Rojas M, Feely F 2nd, et al. Subclass and avidity of circumsporozoite protein specific antibodies associate with protection status against malaria infection. NPJ Vaccines (2021) 6(1):110. doi: 10.1038/s41541-021-00372-x
73. Haynes BF, Gilbert PB, McElrath MJ, Zolla-Pazner S, Tomaras GD, Alam SM, et al. Immune-correlates analysis of an HIV-1 vaccine efficacy trial. N Engl J Med (2012) 366(14):1275–86. doi: 10.1056/NEJMoa1113425
74. Quang C, Chung AW, Frazer IH, Toh ZQ, Licciardi PV. Single-dose HPV vaccine immunity: is there a role for non-neutralizing antibodies? Trends Immunol (2022) 43(10):815–25. doi: 10.1016/j.it.2022.07.011
75. Roy V, Jung W, Linde C, Coates E, Ledgerwood J, Costner P, et al. Differences in HPV-specific antibody fc-effector functions following gardasil® and cervarix® vaccination. NPJ Vaccines (2023) 8(1):39. doi: 10.1038/s41541-023-00628-8
76. Yassine HM, Boyington JC, McTamney PM, Wei CJ, Kanekiyo M, Kong WP, et al. Hemagglutinin-stem nanoparticles generate heterosubtypic influenza protection. Nat Med (2015) 21(9):1065–70. doi: 10.1038/nm.3927
77. Impagliazzo A, Milder F, Kuipers H, Wagner MV, Zhu X, Hoffman RM, et al. A stable trimeric influenza hemagglutinin stem as a broadly protective immunogen. Science (2015) 349(6254):1301–6. doi: 10.1126/science.aac7263
78. Gorman MJ, Patel N, Guebre-Xabier M, Zhu AL, Atyeo C, Pullen KM, et al. Fab and fc contribute to maximal protection against SARS-CoV-2 following NVX-CoV2373 subunit vaccine with matrix-m vaccination. Cell Rep Med (2021) 2(9):100405. doi: 10.1016/j.xcrm.2021.100405
79. Ackerman ME, Crispin M, Yu X, Baruah K, Boesch AW, Harvey DJ, et al. Natural variation in fc glycosylation of HIV-specific antibodies impacts antiviral activity. J Clin Invest (2013) 123(5):2183–92. doi: 10.1172/JCI65708
80. Overbaugh J, Morris L. The antibody response against HIV-1. Cold Spring Harb Perspect Med (2012) 2(1):a007039. doi: 10.1101/cshperspect.a007039
81. Rerks-Ngarm S, Pitisuttithum P, Nitayaphan S, Kaewkungwal J, Chiu J, Paris R, et al. Vaccination with ALVAC and AIDSVAX to prevent HIV-1 infection in Thailand. N Engl J Med (2009) 361(23):2209–20. doi: 10.1056/NEJMoa0908492
82. Chung AW, Ghebremichael M, Robinson H, Brown E, Choi I, Lane S, et al. Polyfunctional fc-effector profiles mediated by IgG subclass selection distinguish RV144 and VAX003 vaccines. Sci Transl Med (2014) 6(228):228ra38. doi: 10.1126/scitranslmed.3007736
83. Yates NL, Liao HX, Fong Y, deCamp A, Vandergrift NA, Williams WT, et al. Vaccine-induced env V1-V2 IgG3 correlates with lower HIV-1 infection risk and declines soon after vaccination. Sci Transl Med (2014) 6(228):228ra39. doi: 10.1126/scitranslmed.3007730
84. Bartsch YC, Cizmeci D, Kang J, Zohar T, Periasamy S, Mehta N, et al. Antibody effector functions are associated with protection from respiratory syncytial virus. Cell (2022) 185(26):4873–86.e10. doi: 10.1016/j.cell.2022.11.012
85. van Erp EA, Luytjes W, Ferwerda G, van Kasteren PB. Fc-mediated antibody effector functions during respiratory syncytial virus infection and disease. Front Immunol (2019) 10:548. doi: 10.3389/fimmu.2019.00548
86. Hessell AJ, Hangartner L, Hunter M, Havenith CE, Beurskens FJ, Bakker JM, et al. Fc receptor but not complement binding is important in antibody protection against HIV. Nature (2007) 449(7158):101–4. doi: 10.1038/nature06106
87. Ullah I, Prévost J, Ladinsky MS, Stone H, Lu M, Anand SP, et al. Live imaging of SARS-CoV-2 infection in mice reveals that neutralizing antibodies require fc function for optimal efficacy. Immunity (2021) 54(9):2143–58.e15. doi: 10.1016/j.immuni.2021.08.015
88. Winkler ES, Gilchuk P, Yu J, Bailey AL, Chen RE, Chong Z, et al. Human neutralizing antibodies against SARS-CoV-2 require intact fc effector functions for optimal therapeutic protection. Cell (2021) 184(7):1804–20.e16. doi: 10.1016/j.cell.2021.02.026
89. Mackin SR, Desai P, Whitener BM, Karl CE, Liu M, Baric RS, et al. Fc-γR-dependent antibody effector functions are required for vaccine-mediated protection against antigen-shifted variants of SARS-CoV-2. Nat Microbiol (2023) 8(4):569–80. doi: 10.1038/s41564-023-01359-1
90. Vanderven HA, Kent SJ. The protective potential of fc-mediated antibody functions against influenza virus and other viral pathogens. Immunol Cell Biol (2020) 98(4):253–63. doi: 10.1111/imcb.12312
91. Musasia FK, Nkumama IN, Frank R, Kipkemboi V, Schneider M, Mwai K, et al. Phagocytosis of plasmodium falciparum ring-stage parasites predicts protection against malaria. Nat Commun (2022) 13(1):4098. doi: 10.1038/s41467-022-31640-6
92. Suscovich TJ, Fallon JK, Das J, Demas AR, Crain J, Linde CH, et al. Mapping functional humoral correlates of protection against malaria challenge following RTS,S/AS01 vaccination. Sci Transl Med (2020) 12(553):eabb4757. doi: 10.1126/scitranslmed.abb4757
93. Odera DO, Tuju J, Mwai K, Nkumama IN, Fürle K, Chege T, et al. Anti-merozoite antibodies induce natural killer cell effector function and are associated with immunity against malaria. Sci Transl Med (2023) 15(682):eabn5993. doi: 10.1126/scitranslmed.abn5993
94. van Erp EA, Lakerveld AJ, de Graaf E, Larsen MD, Schepp RM, Hipgrave Ederveen AL, et al. Natural killer cell activation by respiratory syncytial virus-specific antibodies is decreased in infants with severe respiratory infections and correlates with fc-glycosylation. Clin Transl Immunol (2020) 9(2):e1112. doi: 10.1002/cti2.1112
95. Zohar T, Hsiao JC, Mehta N, Das J, Devadhasan A, Karpinski W, et al. Upper and lower respiratory tract correlates of protection against respiratory syncytial virus following vaccination of nonhuman primates. Cell Host Microbe (2022) 30(1):41–52.e5. doi: 10.1016/j.chom.2021.11.006
96. Forthal DN, Finzi A. Antibody-dependent cellular cytotoxicity in HIV infection. Aids (2018) 32(17):2439–51. doi: 10.1097/QAD.0000000000002011
97. Lee WS, Richard J, Lichtfuss M, Smith AB 3rd, Park J, Courter JR, et al. Antibody-dependent cellular cytotoxicity against reactivated HIV-1-Infected cells. J Virol (2016) 90(4):2021–30. doi: 10.1128/JVI.02717-15
98. Stratov I, Chung A, Kent SJ. Robust NK cell-mediated human immunodeficiency virus (HIV)-specific antibody-dependent responses in HIV-infected subjects. J Virol (2008) 82(11):5450–9. doi: 10.1128/JVI.01952-07
99. Chung AW, Mabuka JM, Ndlovu B, Licht A, Robinson H, Ramlakhan Y, et al. Viral control in chronic HIV-1 subtype c infection is associated with enrichment of p24 IgG1 with fc effector activity. Aids (2018) 32(10):1207–17. doi: 10.1097/QAD.0000000000001812
100. Rodriguez ME, Hellwig SM, Hozbor DF, Leusen J, van der Pol WL, van de Winkel JG. Fc receptor-mediated immunity against bordetella pertussis. J Immunol (2001) 167(11):6545–51. doi: 10.4049/jimmunol.167.11.6545
101. Weiss AA, Mobberley PS, Fernandez RC, Mink CM. Characterization of human bactericidal antibodies to bordetella pertussis. Infect Immun (1999) 67(3):1424–31. doi: 10.1128/IAI.67.3.1424-1431.1999
102. Barnes MG, Weiss AA. Activation of the complement cascade by bordetella pertussis. FEMS Microbiol Lett (2003) 220(2):271–5. doi: 10.1016/S0378-1097(03)00132-0
103. Hellwig SM, van Spriel AB, Schellekens JF, Mooi FR, van de Winkel JG. Immunoglobulin a-mediated protection against bordetella pertussis infection. Infect Immun (2001) 69(8):4846–50. doi: 10.1128/IAI.69.8.4846-4850.2001
104. Raeven RH, Brummelman J, Pennings JL, Nijst OE, Kuipers B, Blok LE, et al. Molecular signatures of the evolving immune response in mice following a bordetella pertussis infection. PloS One (2014) 9(8):e104548. doi: 10.1371/journal.pone.0104548
105. Kirimanjeswara GS, Agosto LM, Kennett MJ, Bjornstad ON, Harvill ET. Pertussis toxin inhibits neutrophil recruitment to delay antibody-mediated clearance of bordetella pertussis. J Clin Invest (2005) 115(12):3594–601. doi: 10.1172/JCI24609
106. Moreno G, Errea A, Van Maele L, Roberts R, Léger H, Sirard JC, et al. Toll-like receptor 4 orchestrates neutrophil recruitment into airways during the first hours of bordetella pertussis infection. Microbes Infect (2013) 15(10-11):708–18. doi: 10.1016/j.micinf.2013.06.010
107. Pishko EJ, Kirimanjeswara GS, Pilione MR, Gopinathan L, Kennett MJ, Harvill ET. Antibody-mediated bacterial clearance from the lower respiratory tract of mice requires complement component C3. Eur J Immunol (2004) 34(1):184–93. doi: 10.1002/eji.200324234
108. Dias AG Jr., Atyeo C, Loos C, Montoya M, Roy V, Bos S, et al. Antibody fc characteristics and effector functions correlate with protection from symptomatic dengue virus type 3 infection. Sci Transl Med (2022) 14(651):eabm3151. doi: 10.1126/scitranslmed.abm3151
109. Laoprasopwattana K, Libraty DH, Endy TP, Nisalak A, Chunsuttiwat S, Ennis FA, et al. Antibody-dependent cellular cytotoxicity mediated by plasma obtained before secondary dengue virus infections: potential involvement in early control of viral replication. J Infect Dis (2007) 195(8):1108–16. doi: 10.1086/512860
110. Bournazos S, Vo HTM, Duong V, Auerswald H, Ly S, Sakuntabhai A, et al. Antibody fucosylation predicts disease severity in secondary dengue infection. Science (2021) 372(6546):1102–5. doi: 10.1126/science.abc7303
111. Wang TT, Sewatanon J, Memoli MJ, Wrammert J, Bournazos S, Bhaumik SK, et al. IgG antibodies to dengue enhanced for FcγRIIIA binding determine disease severity. Science (2017) 355(6323):395–8. doi: 10.1126/science.aai8128
112. García G, Arango M, Pérez AB, Fonte L, Sierra B, Rodríguez-Roche R, et al. Antibodies from patients with dengue viral infection mediate cellular cytotoxicity. J Clin Virol (2006) 37(1):53–7. doi: 10.1016/j.jcv.2006.04.010
113. Fothergill LD, Wright J. Influenzal meningitis: the relation of age incidence to the bactericidal power of blood against the causal Organism1. J Immunol (1933) 24(4):273–84. doi: 10.4049/jimmunol.24.4.273
114. Townsend K, Ladhani SN, Findlow H, Borrow R. Evaluation and validation of a serum bactericidal antibody assay for haemophilus influenzae type b and the threshold of protection. Vaccine (2014) 32(43):5650–6. doi: 10.1016/j.vaccine.2014.08.010
115. Amir J, Scott MG, Nahm MH, Granoff DM. Bactericidal and opsonic activity of IgG1 and IgG2 anticapsular antibodies to haemophilus influenzae type b. J Infect Dis (1990) 162(1):163–71. doi: 10.1093/infdis/162.1.163
116. Schreiber JR, Barrus V, Cates KL, Siber GR. Functional characterization of human IgG, IgM, and IgA antibody directed to the capsule of haemophilus influenzae type b. J Infect Dis (1986) 153(1):8–16. doi: 10.1093/infdis/153.1.8
117. Toews GB, Vial WC, Hansen EJ. Role of C5 and recruited neutrophils in early clearance of nontypable haemophilus influenzae from murine lungs. Infect Immun (1985) 50(1):207–12. doi: 10.1128/iai.50.1.207-212.1985
118. Yu WH, Cosgrove C, Berger CT, Cheney PC, Krykbaeva M, Kim AY, et al. ADCC-mediated CD56(DIM) NK cell responses are associated with early HBsAg clearance in acute HBV infection. Pathog Immun (2018) 3(1):2–18. doi: 10.20411/pai.v3i1.228
119. Bortolotti F, Realdi G, Diodati G, Fattovich G. Antibody dependent cellular cytotoxicity (ADCC) in acute hepatitis b and in chronic active hepatitis. Clin Exp Immunol (1978) 33(2):211–6.
120. Jin J, Liu Y, Xu X, Wang Z, Niu J. The association between fc gamma RIIb expression levels and chronic hepatitis b virus infection progression. BMC Infect Dis (2021) 21(1):1235. doi: 10.1186/s12879-021-06918-7
121. Ning G, Zhen LM, Xu WX, Li XJ, Wu LN, Liu Y, et al. Suppression of complement component 2 expression by hepatitis b virus contributes to the viral persistence in chronic hepatitis b patients. J Viral Hepat (2020) 27(10):1071–81. doi: 10.1111/jvh.13319
122. Ho CH, Chien RN, Cheng PN, Liu JH, Liu CK, Su CS, et al. Aberrant serum immunoglobulin G glycosylation in chronic hepatitis b is associated with histological liver damage and reversible by antiviral therapy. J Infect Dis (2015) 211(1):115–24. doi: 10.1093/infdis/jiu388
123. Li D, He W, Liu X, Zheng S, Qi Y, Li H, et al. A Potent human neutralizing antibody fc-dependently reduces established HBV infections. Elife (2017) 6:e26738. doi: 10.7554/eLife.26738
124. Kohl S, West MS, Prober CG, Sullender WM, Loo LS, Arvin AM. Neonatal antibody-dependent cellular cytotoxic antibody levels are associated with the clinical presentation of neonatal herpes simplex virus infection. J Infect Dis (1989) 160(5):770–6. doi: 10.1093/infdis/160.5.770
125. Mahant AM, Guerguis S, Blevins TP, Cheshenko N, Gao W, Anastos K, et al. Failure of herpes simplex virus glycoprotein d antibodies to elicit antibody-dependent cell-mediated cytotoxicity: implications for future vaccines. J Infect Dis (2022) 226(9):1489–98. doi: 10.1093/infdis/jiac284
126. Petro CD, Weinrick B, Khajoueinejad N, Burn C, Sellers R, Jacobs WR Jr., et al. HSV-2 ΔgD elicits FcγR-effector antibodies that protect against clinical isolates. JCI Insight (2016) 1(12):e88529. doi: 10.1172/jci.insight.88529
127. Burn C, Ramsey N, Garforth SJ, Almo S, Jacobs WR Jr., Herold BC. A herpes simplex virus (HSV)-2 single-cycle candidate vaccine deleted in glycoprotein d protects Male mice from lethal skin challenge with clinical isolates of HSV-1 and HSV-2. J Infect Dis (2018) 217(5):754–8. doi: 10.1093/infdis/jix628
128. McKendall RR. IgG-mediated viral clearance in experimental infection with herpes simplex virus type 1: role for neutralization and fc-dependent functions but not c' cytolysis and C5 chemotaxis. J Infect Dis (1985) 151(3):464–70. doi: 10.1093/infdis/151.3.464
129. Hayashida I, Nagafuchi S, Hayashi Y, Kino Y, Mori R, Oda H, et al. Mechanism of antibody-mediated protection against herpes simplex virus infection in athymic nude mice: requirement of fc portion of antibody. Microbiol Immunol (1982) 26(6):497–509. doi: 10.1111/j.1348-0421.1982.tb00203.x
130. Nelson CS, Huffman T, Jenks JA, Cisneros de la Rosa E, Xie G, Vandergrift N, et al. HCMV glycoprotein b subunit vaccine efficacy mediated by nonneutralizing antibody effector functions. Proc Natl Acad Sci USA (2018) 115(24):6267–72. doi: 10.1073/pnas.1800177115
131. Kuijpers TW, Baars PA, Dantin C, van den Burg M, van Lier RA, Roosnek E. Human NK cells can control CMV infection in the absence of T cells. Blood (2008) 112(3):914–5. doi: 10.1182/blood-2008-05-157354
132. Vlahava VM, Murrell I, Zhuang L, Aicheler RJ, Lim E, Miners KL, et al. Monoclonal antibodies targeting nonstructural viral antigens can activate ADCC against human cytomegalovirus. J Clin Invest (2021) 131(4):e139296. doi: 10.1172/JCI139296
133. Forthal DN, Phan T, Landucci G. Antibody inhibition of cytomegalovirus: the role of natural killer and macrophage effector cells. Transpl Infect Dis (2001) 3 Suppl 2:31–4. doi: 10.1034/j.1399-3062.2001.00006.x
134. Perez LG, Martinez DR, deCamp AC, Pinter A, Berman PW, Francis D, et al. V1V2-specific complement activating serum IgG as a correlate of reduced HIV-1 infection risk in RV144. PloS One (2017) 12(7):e0180720. doi: 10.1371/journal.pone.0180720
135. Neidich SD, Fong Y, Li SS, Geraghty DE, Williamson BD, Young WC, et al. Antibody fc effector functions and IgG3 associate with decreased HIV-1 risk. J Clin Invest (2019) 129(11):4838–49. doi: 10.1172/JCI126391
136. Forthal DN, Gilbert PB, Landucci G, Phan T. Recombinant gp120 vaccine-induced antibodies inhibit clinical strains of HIV-1 in the presence of fc receptor-bearing effector cells and correlate inversely with HIV infection rate. J Immunol (2007) 178(10):6596–603. doi: 10.4049/jimmunol.178.10.6596
137. Baum LL, Cassutt KJ, Knigge K, Khattri R, Margolick J, Rinaldo C, et al. HIV-1 gp120-specific antibody-dependent cell-mediated cytotoxicity correlates with rate of disease progression. J Immunol (1996) 157(5):2168–73. doi: 10.4049/jimmunol.157.5.2168
138. Chung AW, Navis M, Isitman G, Wren L, Silvers J, Amin J, et al. Activation of NK cells by ADCC antibodies and HIV disease progression. J Acquir Immune Defic Syndr (2011) 58(2):127–31. doi: 10.1097/QAI.0b013e31822c62b9
139. Barouch DH, Stephenson KE, Borducchi EN, Smith K, Stanley K, McNally AG, et al. Protective efficacy of a global HIV-1 mosaic vaccine against heterologous SHIV challenges in rhesus monkeys. Cell (2013) 155(3):531–9. doi: 10.1016/j.cell.2013.09.061
140. Barouch DH, Alter G, Broge T, Linde C, Ackerman ME, Brown EP, et al. Protective efficacy of adenovirus/protein vaccines against SIV challenges in rhesus monkeys. Science (2015) 349(6245):320–4. doi: 10.1126/science.aab3886
141. Alter G, Yu WH, Chandrashekar A, Borducchi EN, Ghneim K, Sharma A, et al. Passive transfer of vaccine-elicited antibodies protects against SIV in rhesus macaques. Cell (2020) 183(1):185–96.e14. doi: 10.1016/j.cell.2020.08.033
142. Bradley T, Pollara J, Santra S, Vandergrift N, Pittala S, Bailey-Kellogg C, et al. Pentavalent HIV-1 vaccine protects against simian-human immunodeficiency virus challenge. Nat Commun (2017) 8:15711. doi: 10.1038/ncomms15711
143. Sahoo A, Jones AT, Cheedarla N, Gangadhara S, Roy V, Styles TM, et al. A clade c HIV-1 vaccine protects against heterologous SHIV infection by modulating IgG glycosylation and T helper response in macaques. Sci Immunol (2022) 7(73):eabl4102. doi: 10.1126/sciimmunol.abl4102
144. Wang JW, Wu WH, Huang TC, Wong M, Kwak K, Ozato K, et al. Roles of fc domain and exudation in L2 antibody-mediated protection against human papillomavirus. J Virol (2018) 92(15):e00572–18. doi: 10.1128/JVI.00572-18
145. Day PM, Kines RC, Thompson CD, Jagu S, Roden RB, Lowy DR, et al. In vivo mechanisms of vaccine-induced protection against HPV infection. Cell Host Microbe (2010) 8(3):260–70. doi: 10.1016/j.chom.2010.08.003
146. Vanderven HA, Liu L, Ana-Sosa-Batiz F, Nguyen TH, Wan Y, Wines B, et al. Fc functional antibodies in humans with severe H7N9 and seasonal influenza. JCI Insight (2017) 2(13):e92750. doi: 10.1172/jci.insight.92750
147. Jegaskanda S, Job ER, Kramski M, Laurie K, Isitman G, de Rose R, et al. Cross-reactive influenza-specific antibody-dependent cellular cytotoxicity antibodies in the absence of neutralizing antibodies. J Immunol (2013) 190(4):1837–48. doi: 10.4049/jimmunol.1201574
148. Ana-Sosa-Batiz F, Johnston APR, Hogarth PM, Wines BD, Barr I, Wheatley AK, et al. Antibody-dependent phagocytosis (ADP) responses following trivalent inactivated influenza vaccination of younger and older adults. Vaccine (2017) 35(47):6451–8. doi: 10.1016/j.vaccine.2017.09.062
149. Ana-Sosa-Batiz F, Vanderven H, Jegaskanda S, Johnston A, Rockman S, Laurie K, et al. Influenza-specific antibody-dependent phagocytosis. PloS One (2016) 11(4):e0154461. doi: 10.1371/journal.pone.0154461
150. Liu Y, Tan HX, Koutsakos M, Jegaskanda S, Esterbauer R, Tilmanis D, et al. Cross-lineage protection by human antibodies binding the influenza b hemagglutinin. Nat Commun (2019) 10(1):324. doi: 10.1038/s41467-018-07970-9
151. Valkenburg SA, Fang VJ, Leung NH, Chu DK, Ip DK, Perera RA, et al. Cross-reactive antibody-dependent cellular cytotoxicity antibodies are increased by recent infection in a household study of influenza transmission. Clin Transl Immunol (2019) 8(11):e1092. doi: 10.1002/cti2.1092
152. Wines BD, Vanderven HA, Esparon SE, Kristensen AB, Kent SJ, Hogarth PM. Dimeric FcγR ectodomains as probes of the fc receptor function of anti-influenza virus IgG. J Immunol (2016) 197(4):1507–16. doi: 10.4049/jimmunol.1502551
153. Vanderven HA, Ana-Sosa-Batiz F, Jegaskanda S, Rockman S, Laurie K, Barr I, et al. What lies beneath: antibody dependent natural killer cell activation by antibodies to internal influenza virus proteins. EBioMedicine (2016) 8:277–90. doi: 10.1016/j.ebiom.2016.04.029
154. DiLillo DJ, Palese P, Wilson PC, Ravetch JV. Broadly neutralizing anti-influenza antibodies require fc receptor engagement for in vivo protection. J Clin Invest (2016) 126(2):605–10. doi: 10.1172/JCI84428
155. Ko YA, Yu YH, Wu YF, Tseng YC, Chen CL, Goh KS, et al. A non-neutralizing antibody broadly protects against influenza virus infection by engaging effector cells. PloS Pathog (2021) 17(8):e1009724. doi: 10.1371/journal.ppat.1009724
156. Huber VC, Lynch JM, Bucher DJ, Le J, Metzger DW. Fc receptor-mediated phagocytosis makes a significant contribution to clearance of influenza virus infections. J Immunol (2001) 166(12):7381–8. doi: 10.4049/jimmunol.166.12.7381
157. He W, Chen CJ, Mullarkey CE, Hamilton JR, Wong CK, Leon PE, et al. Alveolar macrophages are critical for broadly-reactive antibody-mediated protection against influenza a virus in mice. Nat Commun (2017) 8(1):846. doi: 10.1038/s41467-017-00928-3
158. Abreu-Mota T, Hagen KR, Cooper K, Jahrling PB, Tan G, Wirblich C, et al. Non-neutralizing antibodies elicited by recombinant lassa-rabies vaccine are critical for protection against lassa fever. Nat Commun (2018) 9(1):4223. doi: 10.1038/s41467-018-06741-w
159. Ilinykh PA, Huang K, Santos RI, Gilchuk P, Gunn BM, Karim MM, et al. Non-neutralizing antibodies from a marburg infection survivor mediate protection by fc-effector functions and by enhancing efficacy of other antibodies. Cell Host Microbe (2020) 27(6):976–91.e11. doi: 10.1016/j.chom.2020.03.025
160. Brinkman ID, Butler AL, de Wit J, van Binnendijk RS, Alter G, van Baarle D. Measles vaccination elicits a polyfunctional antibody response, which decays more rapidly in early vaccinated children. J Infect Dis (2022) 225(10):1755–64. doi: 10.1093/infdis/jiab318
161. Lu LL, Smith MT, Yu KKQ, Luedemann C, Suscovich TJ, Grace PS, et al. IFN-γ-independent immune markers of mycobacterium tuberculosis exposure. Nat Med (2019) 25(6):977–87. doi: 10.1038/s41591-019-0441-3
162. Fischinger S, Cizmeci D, Shin S, Davies L, Grace PS, Sivro A, et al. A mycobacterium tuberculosis specific IgG3 signature of recurrent tuberculosis. Front Immunol (2021) 12:729186. doi: 10.3389/fimmu.2021.729186
163. Maglione PJ, Xu J, Casadevall A, Chan J. Fc gamma receptors regulate immune activation and susceptibility during mycobacterium tuberculosis infection. J Immunol (2008) 180(5):3329–38. doi: 10.4049/jimmunol.180.5.3329
164. Aase A, Bjune G, Høiby EA, Rosenqvist E, Pedersen AK, Michaelsen TE. Comparison among opsonic activity, antimeningococcal immunoglobulin G response, and serum bactericidal activity against meningococci in sera from vaccinees after immunization with a serogroup b outer membrane vesicle vaccine. Infect Immun (1995) 63(9):3531–6. doi: 10.1128/iai.63.9.3531-3536.1995
165. Andrews N, Borrow R, Miller E. Validation of serological correlate of protection for meningococcal c conjugate vaccine by using efficacy estimates from postlicensure surveillance in England. Clin Diagn Lab Immunol (2003) 10(5):780–6. doi: 10.1128/CDLI.10.5.780-786.2003
166. Holst J, Feiring B, Fuglesang JE, Høiby EA, Nøkleby H, Aaberge IS, et al. Serum bactericidal activity correlates with the vaccine efficacy of outer membrane vesicle vaccines against neisseria meningitidis serogroup b disease. Vaccine (2003) 21(7-8):734–7. doi: 10.1016/S0264-410X(02)00591-1
167. Goldschneider I, Gotschlich EC, Artenstein MS. Human immunity to the meningococcus. I role humoral antibodies J Exp Med (1969) 129(6):1307–26. doi: 10.1084/jem.129.6.1307
168. Vidarsson G, van der Pol WL, van Den Elsen JM, Vilé H, Jansen M, Duijs J, et al. Activity of human IgG and IgA subclasses in immune defense against neisseria meningitidis serogroup b. J Immunol (2001) 166(10):6250–6. doi: 10.4049/jimmunol.166.10.6250
169. Kana IH, Garcia-Senosiain A, Singh SK, Tiendrebeogo RW, Chourasia BK, Malhotra P, et al. Cytophilic antibodies against key plasmodium falciparum blood stage antigens contribute to protection against clinical malaria in a high transmission region of Eastern India. J Infect Diseases (2018) 218(6):956–65. doi: 10.1093/infdis/jiy258
170. Osier FH, Feng G, Boyle MJ, Langer C, Zhou J, Richards JS, et al. Opsonic phagocytosis of plasmodium falciparum merozoites: mechanism in human immunity and a correlate of protection against malaria. BMC Med (2014) 12:108. doi: 10.1186/1741-7015-12-108
171. Joos C, Marrama L, Polson HE, Corre S, Diatta AM, Diouf B, et al. Clinical protection from falciparum malaria correlates with neutrophil respiratory bursts induced by merozoites opsonized with human serum antibodies. PloS One (2010) 5(3):e9871. doi: 10.1371/journal.pone.0009871
172. Boyle MJ, Reiling L, Feng G, Langer C, Osier FH, Aspeling-Jones H, et al. Human antibodies fix complement to inhibit plasmodium falciparum invasion of erythrocytes and are associated with protection against malaria. Immunity (2015) 42(3):580–90. doi: 10.1016/j.immuni.2015.02.012
173. Tagliabue A, Villa L, De Magistris MT, Romano M, Silvestri S, Boraschi D, et al. IgA-driven T cell-mediated anti-bacterial immunity in man after live oral Ty 21a vaccine. J Immunol (1986) 137(5):1504–10. doi: 10.4049/jimmunol.137.5.1504
174. Jin C, Hill J, Gunn BM, Yu WH, Dahora LC, Jones E, et al. Vi-specific serological correlates of protection for typhoid fever. J Exp Med (2021) 218(2):e20201116. doi: 10.1084/jem.20201116
175. Menager N, Foster G, Ugrinovic S, Uppington H, Verbeek S, Mastroeni P. Fcgamma receptors are crucial for the expression of acquired resistance to virulent salmonella enterica serovar typhimurium in vivo but are not required for the induction of humoral or T-cell-mediated immunity. Immunology (2007) 120(3):424–32. doi: 10.1111/j.1365-2567.2006.02527.x
176. Das S, Chowdhury R, Ghosh S, Das S. A recombinant protein of salmonella typhi induces humoral and cell-mediated immune responses including memory responses. Vaccine (2017) 35(35 Pt B):4523–31. doi: 10.1016/j.vaccine.2017.07.035
177. Zohar T, Loos C, Fischinger S, Atyeo C, Wang C, Slein MD, et al. Compromised humoral functional evolution tracks with SARS-CoV-2 mortality. Cell (2020) 183(6):1508–19.e12. doi: 10.1016/j.cell.2020.10.052
178. Lee WS, Selva KJ, Davis SK, Wines BD, Reynaldi A, Esterbauer R, et al. Decay of fc-dependent antibody functions after mild to moderate COVID-19. Cell Rep Med (2021) 2(6):100296. doi: 10.1016/j.xcrm.2021.100296
179. Rodriguez ME, van der Pol W-L, Sanders LAM, van de Winkel JGJ. Crucial role of FcγRIIa (CD32) in assessment of functional anti–streptococcus pneumoniae antibody activity in human sera. J Infect Diseases (1999) 179(2):423–33. doi: 10.1086/314603
180. van der Pol W-L, Vidarsson G, Vilé HA, van de Winkel JGJ, Rodriguez ME. Pneumococcal capsular polysaccharide–specific IgA triggers efficient neutrophil effector functions via FcαRI (CD89). J Infect Diseases (2000) 182(4):1139–45. doi: 10.1086/315825
181. Johnson SE, Rubin L, Romero-Steiner S, Dykes JK, Pais LB, Rizvi A, et al. Correlation of opsonophagocytosis and passive protection assays using human anticapsular antibodies in an infant mouse model of bacteremia for streptococcus pneumoniae. J Infect Dis (1999) 180(1):133–40. doi: 10.1086/314845
182. Ihara T, Kato T, Torigoe S, Oitani K, Isaji M, Ito M, et al. Antibody response determined with antibody-dependent cell-mediated cytotoxicity (ADCC), neutralizing antibody, and varicella skin test in children with natural varicella and after varicella immunization. Acta Paediatr Jpn (1991) 33(1):43–9. doi: 10.1111/j.1442-200X.1991.tb01518.x
183. Ito M, Ihara T, Grose C, Starr S. Human leukocytes kill varicella-zoster virus-infected fibroblasts in the presence of murine monoclonal antibodies to virus-specific glycoproteins. J Virol (1985) 54(1):98–103. doi: 10.1128/jvi.54.1.98-103.1985
184. Ihara T, Ito M, Starr SE. Human lymphocyte, monocyte and polymorphonuclear leucocyte mediated antibody-dependent cellular cytotoxicity against varicella-zoster virus-infected targets. Clin Exp Immunol (1986) 63(1):179–87.
185. Mosley WH, Benenson AS, Barui R. A serological survey for cholear antibodies in rural east pakistan. 1. the distribution of antibody in the control population of a cholera-vaccine field-trial area and the relation of antibody titre to the pattern of endemic cholera. Bull World Health Organ (1968) 38(3):327–34.
186. Mosley WH, Ahmad S, Benenson AS, Ahmed A. The relationship of vibriocidal antibody titre to susceptibility to cholera in family contacts of cholera patients. Bull World Health Organ (1968) 38(5):777–85.
187. Mishra N, Boudewijns R, Schmid MA, Marques RE, Sharma S, Neyts J, et al. A chimeric Japanese encephalitis vaccine protects against lethal yellow fever virus infection without inducing neutralizing antibodies. mBio (2020) 11(2):e02494–19. doi: 10.1128/mBio.02494-19
188. Schlesinger JJ, Foltzer M, Chapman S. The fc portion of antibody to yellow fever virus NS1 is a determinant of protection against YF encephalitis in mice. Virology (1993) 192(1):132–41. doi: 10.1006/viro.1993.1015
189. Gunn BM, Lu R, Slein MD, Ilinykh PA, Huang K, Atyeo C, et al. A fc engineering approach to define functional humoral correlates of immunity against Ebola virus. Immunity (2021) 54(4):815–28.e5. doi: 10.1016/j.immuni.2021.03.009
190. Meyer M, Gunn BM, Malherbe DC, Gangavarapu K, Yoshida A, Pietzsch C, et al. Ebola Vaccine-induced protection in nonhuman primates correlates with antibody specificity and fc-mediated effects. Sci Transl Med (2021) 13(602):eabg6128. doi: 10.1126/scitranslmed.abg6128
191. Warfield KL, Swenson DL, Olinger GG, Kalina WV, Aman MJ, Bavari S. Ebola Virus-like particle-based vaccine protects nonhuman primates against lethal Ebola virus challenge. J Infect Dis (2007) 196 Suppl 2:S430–7. doi: 10.1086/520583
192. Liu Q, Fan C, Li Q, Zhou S, Huang W, Wang L, et al. Antibody-dependent-cellular-cytotoxicity-inducing antibodies significantly affect the post-exposure treatment of Ebola virus infection. Sci Rep (2017) 7:45552. doi: 10.1038/srep45552
193. Paquin-Proulx D, Gunn BM, Alrubayyi A, Clark DV, Creegan M, Kim D, et al. Associations between antibody fc-mediated effector functions and long-term sequelae in Ebola virus survivors. Front Immunol (2021) 12:682120. doi: 10.3389/fimmu.2021.682120
194. Lyke KE, Atmar RL, Islas CD, Posavad CM, Szydlo D, Paul Chourdhury R, et al. Rapid decline in vaccine-boosted neutralizing antibodies against SARS-CoV-2 omicron variant. Cell Rep Med (2022) 3(7):100679. doi: 10.1016/j.xcrm.2022.100679
195. Kurhade C, Zou J, Xia H, Liu M, Chang HC, Ren P, et al. Low neutralization of SARS-CoV-2 omicron BA.2.75.2, BQ.1.1 and XBB.1 by parental mRNA vaccine or a BA.5 bivalent booster. Nat Med (2022) 29(2):344–7. doi: 10.1038/s41591-022-02162-x
196. Lassaunière R, Polacek C, Frische A, Boding L, Sækmose SG, Rasmussen M, et al. Neutralizing antibodies against the SARS-CoV-2 omicron variant (BA.1) 1 to 18 weeks after the second and third doses of the BNT162b2 mRNA vaccine. JAMA Netw Open (2022) 5(5):e2212073. doi: 10.1001/jamanetworkopen.2022.12073
197. Chung AW, Isitman G, Navis M, Kramski M, Center RJ, Kent SJ, et al. Immune escape from HIV-specific antibody-dependent cellular cytotoxicity (ADCC) pressure. Proc Natl Acad Sci USA (2011) 108(18):7505–10. doi: 10.1073/pnas.1016048108
198. Mielke D, Bandawe G, Pollara J, Abrahams MR, Nyanhete T, Moore PL, et al. Antibody-dependent cellular cytotoxicity (ADCC)-mediating antibodies constrain neutralizing antibody escape pathway. Front Immunol (2019) 10:2875. doi: 10.3389/fimmu.2019.02875
199. Mielke D, Bandawe G, Zheng J, Jones J, Abrahams MR, Bekker V, et al. ADCC-mediating non-neutralizing antibodies can exert immune pressure in early HIV-1 infection. PloS Pathog (2021) 17(11):e1010046. doi: 10.1371/journal.ppat.1010046
200. Wajnberg A, Amanat F, Firpo A, Altman DR, Bailey MJ, Mansour M, et al. Robust neutralizing antibodies to SARS-CoV-2 infection persist for months. Science (2020) 370(6521):1227–30. doi: 10.1126/science.abd7728
201. Seow J, Graham C, Merrick B, Acors S, Pickering S, Steel KJA, et al. Longitudinal observation and decline of neutralizing antibody responses in the three months following SARS-CoV-2 infection in humans. Nat Microbiol (2020) 5(12):1598–607. doi: 10.1038/s41564-020-00813-8
202. Pegu A, O'Connell SE, Schmidt SD, O'Dell S, Talana CA, Lai L, et al. Durability of mRNA-1273 vaccine-induced antibodies against SARS-CoV-2 variants. Science (2021) 373(6561):1372–7. doi: 10.1126/science.abj4176
203. Beaudoin-Bussières G, Chen Y, Ullah I, Prévost J, Tolbert WD, Symmes K, et al. A fc-enhanced NTD-binding non-neutralizing antibody delays virus spread and synergizes with a nAb to protect mice from lethal SARS-CoV-2 infection. Cell Rep (2022) 38(7):110368. doi: 10.1016/j.celrep.2022.110368
204. Zhu DY, Gorman MJ, Yuan D, Yu J, Mercado NB, McMahan K, et al. Defining the determinants of protection against SARS-CoV-2 infection and viral control in a dose-down Ad26.CoV2.S vaccine study in nonhuman primates. PloS Biol (2022) 20(5):e3001609. doi: 10.1371/journal.pbio.3001609
205. Bournazos S, Corti D, Virgin HW, Ravetch JV. Fc-optimized antibodies elicit CD8 immunity to viral respiratory infection. Nature (2020) 588(7838):485–90. doi: 10.1038/s41586-020-2838-z
206. Cromer D, Steain M, Reynaldi A, Schlub TE, Wheatley AK, Juno JA, et al. Neutralising antibody titres as predictors of protection against SARS-CoV-2 variants and the impact of boosting: a meta-analysis. Lancet Microbe (2022) 3(1):e52–61. doi: 10.1016/S2666-5247(21)00267-6
207. Keeton R, Tincho MB, Ngomti A, Baguma R, Benede N, Suzuki A, et al. T Cell responses to SARS-CoV-2 spike cross-recognize omicron. Nature (2022) 603(7901):488–92. doi: 10.1038/s41586-022-04460-3
208. Saunders KO. Conceptual approaches to modulating antibody effector functions and circulation half-life. Front Immunol (2019) 10:1296. doi: 10.3389/fimmu.2019.01296
209. Warrender AK, Kelton W. Beyond allotypes: the influence of allelic diversity in antibody constant domains. Front Immunol (2020) 11:2016. doi: 10.3389/fimmu.2020.02016
210. Mahan AE, Jennewein MF, Suscovich T, Dionne K, Tedesco J, Chung AW, et al. Antigen-specific antibody glycosylation is regulated via vaccination. PloS Pathog (2016) 12(3):e1005456. doi: 10.1371/journal.ppat.1005456
211. Irrgang P, Gerling J, Kocher K, Lapuente D, Steininger P, Habenicht K, et al. Class switch toward noninflammatory, spike-specific IgG4 antibodies after repeated SARS-CoV-2 mRNA vaccination. Sci Immunol (2023) 8(79):eade2798. doi: 10.1126/sciimmunol.ade2798
212. Buhre JS, Pongracz T, Künsting I, Lixenfeld AS, Wang W, Nouta J, et al. mRNA vaccines against SARS-CoV-2 induce comparably low long-term IgG fc galactosylation and sialylation levels but increasing long-term IgG4 responses compared to an adenovirus-based vaccine. Front Immunol (2022) 13:1020844. doi: 10.3389/fimmu.2022.1020844
213. Tongren JE, Drakeley CJ, McDonald SL, Reyburn HG, Manjurano A, Nkya WM, et al. Target antigen, age, and duration of antigen exposure independently regulate immunoglobulin G subclass switching in malaria. Infect Immun (2006) 74(1):257–64. doi: 10.1128/IAI.74.1.257-264.2006
214. Swain SL, McKinstry KK, Strutt TM. Expanding roles for CD4+ T cells in immunity to viruses. Nat Rev Immunol (2012) 12(2):136–48. doi: 10.1038/nri3152
215. Snapper CM, Mond JJ. Towards a comprehensive view of immunoglobulin class switching. Immunol Today (1993) 14(1):15–7. doi: 10.1016/0167-5699(93)90318-F
216. Vinuesa CG, Linterman MA, Yu D, MacLennan IC. Follicular helper T cells. Annu Rev Immunol (2016) 34:335–68. doi: 10.1146/annurev-immunol-041015-055605
217. Qiu L, Zhang Y, Zeng X. The function of γδ T cells in humoral immune responses. Inflammation Res (2023) 72(4):747–55. doi: 10.1007/s00011-023-01704-4
218. Vinuesa CG, Chang PP. Innate b cell helpers reveal novel types of antibody responses. Nat Immunol (2013) 14(2):119–26. doi: 10.1038/ni.2511
219. Lin M, Du L, Brandtzaeg P, Pan-Hammarström Q. IgA subclass switch recombination in human mucosal and systemic immune compartments. Mucosal Immunol (2014) 7(3):511–20. doi: 10.1038/mi.2013.68
220. Ghraichy M, Galson JD, Kovaltsuk A, von Niederhäusern V, Pachlopnik Schmid J, Recher M, et al. Maturation of the human immunoglobulin heavy chain repertoire with age. Front Immunol (2020) 11:1734. doi: 10.3389/fimmu.2020.01734
221. Blanco E, Pérez-Andrés M, Arriba-Méndez S, Contreras-Sanfeliciano T, Criado I, Pelak O, et al. Age-associated distribution of normal b-cell and plasma cell subsets in peripheral blood. J Allergy Clin Immunol (2018) 141(6):2208–19.e16. doi: 10.1016/j.jaci.2018.02.017
222. Jeannin P, Lecoanet S, Delneste Y, Gauchat JF, Bonnefoy JY. IgE versus IgG4 production can be differentially regulated by IL-10. J Immunol (1998) 160(7):3555–61. doi: 10.4049/jimmunol.160.7.3555
223. Avery DT, Bryant VL, Ma CS, de Waal Malefyt R, Tangye SG. IL-21-induced isotype switching to IgG and IgA by human naive b cells is differentially regulated by IL-4. J Immunol (2008) 181(3):1767–79. doi: 10.4049/jimmunol.181.3.1767
224. Punnonen J, de Vries JE. IL-13 induces proliferation, ig isotype switching, and ig synthesis by immature human fetal b cells. J Immunol (1994) 152(3):1094–102. doi: 10.4049/jimmunol.152.3.1094
225. Fujieda S, Zhang K, Saxon A. IL-4 plus CD40 monoclonal antibody induces human b cells gamma subclass-specific isotype switch: switching to gamma 1, gamma 3, and gamma 4, but not gamma 2. J Immunol (1995) 155(5):2318–28. doi: 10.4049/jimmunol.155.5.2318
226. Pène J, Gauchat JF, Lécart S, Drouet E, Guglielmi P, Boulay V, et al. Cutting edge: IL-21 is a switch factor for the production of IgG1 and IgG3 by human b cells. J Immunol (2004) 172(9):5154–7. doi: 10.4049/jimmunol.172.9.5154
227. Dienz O, Eaton SM, Bond JP, Neveu W, Moquin D, Noubade R, et al. The induction of antibody production by IL-6 is indirectly mediated by IL-21 produced by CD4+ T cells. J Exp Med (2009) 206(1):69–78. doi: 10.1084/jem.20081571
228. Richardson SI, Ayres F, Manamela NP, Oosthuysen B, Makhado Z, Lambson BE, et al. HIV Broadly neutralizing antibodies expressed as IgG3 preserve neutralization potency and show improved fc effector function. Front Immunol (2021) 12:733958. doi: 10.3389/fimmu.2021.733958
229. Damelang T, Rogerson SJ, Kent SJ, Chung AW. Role of IgG3 in infectious diseases. Trends Immunol (2019) 40(3):197–211. doi: 10.1016/j.it.2019.01.005
230. Ackerman ME, Mikhailova A, Brown EP, Dowell KG, Walker BD, Bailey-Kellogg C, et al. Polyfunctional HIV-specific antibody responses are associated with spontaneous HIV control. PloS Pathog (2016) 12(1):e1005315. doi: 10.1371/journal.ppat.1005315
231. Kallolimath S, Sun L, Palt R, Stiasny K, Mayrhofer P, Gruber C, et al. Highly active engineered IgG3 antibodies against SARS-CoV-2. Proc Natl Acad Sci USA (2021) 118(42):e2107249118. doi: 10.1073/pnas.2107249118
232. Moyo-Gwete T, Scheepers C, Makhado Z, Kgagudi P, Mzindle NB, Ziki R, et al. Enhanced neutralization potency of an identical HIV neutralizing antibody expressed as different isotypes is achieved through genetically distinct mechanisms. Sci Rep (2022) 12(1):16473. doi: 10.1038/s41598-022-20141-7
233. Richardson SI, Lambson BE, Crowley AR, Bashirova A, Scheepers C, Garrett N, et al. IgG3 enhances neutralization potency and fc effector function of an HIV V2-specific broadly neutralizing antibody. PloS Pathog (2019) 15(12):e1008064. doi: 10.1371/journal.ppat.1008064
234. Alter G, Dowell KG, Brown EP, Suscovich TJ, Mikhailova A, Mahan AE, et al. High-resolution definition of humoral immune response correlates of effective immunity against HIV. Mol Syst Biol (2018) 14(3):e7881. doi: 10.15252/msb.20177881
235. Shinkura R, Ito S, Begum NA, Nagaoka H, Muramatsu M, Kinoshita K, et al. Separate domains of AID are required for somatic hypermutation and class-switch recombination. Nat Immunol (2004) 5(7):707–12. doi: 10.1038/ni1086
236. Richardson SI, Chung AW, Natarajan H, Mabvakure B, Mkhize NN, Garrett N, et al. HIV-Specific fc effector function early in infection predicts the development of broadly neutralizing antibodies. PloS Pathog (2018) 14(4):e1006987. doi: 10.1371/journal.ppat.1006987
237. Frasca D, van der Put E, Riley RL, Blomberg BB. Reduced ig class switch in aged mice correlates with decreased E47 and activation-induced cytidine deaminase. J Immunol (2004) 172(4):2155–62. doi: 10.4049/jimmunol.172.4.2155
238. Powers DC. Effect of age on serum immunoglobulin G subclass antibody responses to inactivated influenza virus vaccine. J Med Virol (1994) 43(1):57–61. doi: 10.1002/jmv.1890430111
239. Frasca D, Ferracci F, Diaz A, Romero M, Lechner S, Blomberg BB. Obesity decreases b cell responses in young and elderly individuals. Obes (Silver Spring) (2016) 24(3):615–25. doi: 10.1002/oby.21383
240. Yan SM, Sherman RM, Taylor DJ, Nair DR, Bortvin AN, Schatz MC, et al. Local adaptation and archaic introgression shape global diversity at human structural variant loci. Elife (2021) 10:e67615. doi: 10.7554/eLife.67615
241. Browning SR, Browning BL, Zhou Y, Tucci S, Akey JM. Analysis of human sequence data reveals two pulses of archaic denisovan admixture. Cell (2018) 173(1):53–61.e9. doi: 10.1016/j.cell.2018.02.031
242. Kwiatkowski DP. How malaria has affected the human genome and what human genetics can teach us about malaria. Am J Hum Genet (2005) 77(2):171–92. doi: 10.1086/432519
243. Calonga-Solís V, Malheiros D, Beltrame MH, Vargas LB, Dourado RM, Issler HC, et al. Unveiling the diversity of immunoglobulin heavy constant gamma (IGHG) gene segments in Brazilian populations reveals 28 novel alleles and evidence of gene conversion and natural selection. Front Immunol (2019) 10:1161. doi: 10.3389/fimmu.2019.01161
244. Vidarsson G, Dekkers G, Rispens T. IgG subclasses and allotypes: from structure to effector functions. Front Immunol (2014) 5:520. doi: 10.3389/fimmu.2014.00520
245. Jefferis R, Lefranc MP. Human immunoglobulin allotypes: possible implications for immunogenicity. MAbs (2009) 1(4):332–8. doi: 10.4161/mabs.1.4.9122
246. van Loghem E, de Lange G, van Leeuwen AM, van Eede PH, Nijenhuis LE, Lefranc MP, et al. Human IgG allotypes co-occurring in more than one IgG subclass. Vox Sang (1982) 43(6):301–9. doi: 10.1111/j.1423-0410.1982.tb00028.x
247. Dard P, Sanchez-Mazas A, Dugoujon JM, De Lange G, Langaney A, Lefranc MP, et al. DNA Analysis of the immunoglobulin IGHG loci in a mandenka population from eastern Senegal: correlation with gm haplotypes and hypotheses for the evolution of the ig CH region. Hum Genet (1996) 98(1):36–47. doi: 10.1007/s004390050156
248. Dard P, Lefranc MP, Osipova L, Sanchez-Mazas A. DNA Sequence variability of IGHG3 alleles associated to the main G3m haplotypes in human populations. Eur J Hum Genet (2001) 9(10):765–72. doi: 10.1038/sj.ejhg.5200700
249. Oxelius VA, Pandey JP. Human immunoglobulin constant heavy G chain (IGHG) (Fcγ) (GM) genes, defining innate variants of IgG molecules and b cells, have impact on disease and therapy. Clin Immunol (2013) 149(3):475–86. doi: 10.1016/j.clim.2013.10.003
250. Hensen L, Nguyen THO, Rowntree LC, Damelang T, Koutsakos M, Aban M, et al. Robust and prototypical immune responses toward influenza vaccines in the high-risk group of indigenous australians. Proc Natl Acad Sci U.S.A (2021) 118(41):e2109388118. doi: 10.1073/pnas.2109388118
251. Rodriguez OL, Gibson WS, Parks T, Emery M, Powell J, Strahl M, et al. A novel framework for characterizing genomic haplotype diversity in the human immunoglobulin heavy chain locus. Front Immunol (2020) 11:2136. doi: 10.3389/fimmu.2020.02136
252. Pandey JP, Nasr A, Rocca KM, Troy-Blomberg M, Elghazali G. Significant differences in GM allotype frequencies between two sympatric tribes with markedly differential susceptibility to malaria. Parasite Immunol (2007) 29(5):267–9. doi: 10.1111/j.1365-3024.2007.00938.x
253. Sarvas H, Vesterinen P, Mäkelä O. Serum IgG2 concentration is associated with gm-allotypes of IgG2 but not with the R131H polymorphism of human fc-gamma receptor type IIa. J Clin Immunol (2002) 22(2):92–7. doi: 10.1023/A:1014431819413
254. Pandey JP, French MA. GM phenotypes influence the concentrations of the four subclasses of immunoglobulin G in normal human serum. Hum Immunol (1996) 51(2):99–102. doi: 10.1016/S0198-8859(96)00205-4
255. de Taeye SW, Bentlage AEH, Mebius MM, Meesters JI, Lissenberg-Thunnissen S, Falck D, et al. FcγR binding and ADCC activity of human IgG allotypes. Front Immunol (2020) 11:740. doi: 10.3389/fimmu.2020.00740
256. Stapleton NM, Andersen JT, Stemerding AM, Bjarnarson SP, Verheul RC, Gerritsen J, et al. Competition for FcRn-mediated transport gives rise to short half-life of human IgG3 and offers therapeutic potential. Nat Commun (2011) 2:599. doi: 10.1038/ncomms1608
257. Dechavanne C, Dechavanne S, Sadissou I, Lokossou AG, Alvarado F, Dambrun M, et al. Associations between an IgG3 polymorphism in the binding domain for FcRn, transplacental transfer of malaria-specific IgG3, and protection against plasmodium falciparum malaria during infancy: a birth cohort study in Benin. PloS Med (2017) 14(10):e1002403. doi: 10.1371/journal.pmed.1002403
258. Recke A, Konitzer S, Lemcke S, Freitag M, Sommer NM, Abdelhady M, et al. The p.Arg435His variation of IgG3 with high affinity to FcRn is associated with susceptibility for pemphigus vulgaris-analysis of four different ethnic cohorts. Front Immunol (2018) 9:1788. doi: 10.3389/fimmu.2018.01788
259. Kiyoshi M, Tsumoto K, Ishii-Watabe A, Caaveiro JMM. Glycosylation of IgG-fc: a molecular perspective. Int Immunol (2017) 29(7):311–7. doi: 10.1093/intimm/dxx038
260. Borrok MJ, Jung ST, Kang TH, Monzingo AF, Georgiou G. Revisiting the role of glycosylation in the structure of human IgG fc. ACS Chem Biol (2012) 7(9):1596–602. doi: 10.1021/cb300130k
261. Xue J, Zhu LP, Wei Q. IgG-fc n-glycosylation at Asn297 and IgA O-glycosylation in the hinge region in health and disease. Glycoconj J (2013) 30(8):735–45. doi: 10.1007/s10719-013-9481-y
262. van de Bovenkamp FS, Hafkenscheid L, Rispens T, Rombouts Y. The emerging importance of IgG fab glycosylation in immunity. J Immunol (2016) 196(4):1435–41. doi: 10.4049/jimmunol.1502136
263. Scallon BJ, Tam SH, McCarthy SG, Cai AN, Raju TS. Higher levels of sialylated fc glycans in immunoglobulin G molecules can adversely impact functionality. Mol Immunol (2007) 44(7):1524–34. doi: 10.1016/j.molimm.2006.09.005
264. Wang TT. IgG fc glycosylation in human immunity. Curr Top Microbiol Immunol (2019) 423:63–75. doi: 10.1007/82_2019_152
265. Chung AW, Crispin M, Pritchard L, Robinson H, Gorny MK, Yu X, et al. Identification of antibody glycosylation structures that predict monoclonal antibody fc-effector function. Aids (2014) 28(17):2523–30. doi: 10.1097/QAD.0000000000000444
266. Ferrara C, Grau S, Jäger C, Sondermann P, Brünker P, Waldhauer I, et al. Unique carbohydrate-carbohydrate interactions are required for high affinity binding between FcgammaRIII and antibodies lacking core fucose. Proc Natl Acad Sci USA (2011) 108(31):12669–74. doi: 10.1073/pnas.1108455108
267. Cohen Saban N, Yalin A, Landsberger T, Salomon R, Alva A, Feferman T, et al. Fc glycoengineering of a PD-L1 antibody harnesses fcγ receptors for increased antitumor efficacy. Sci Immunol (2023) 8(81):eadd8005. doi: 10.1126/sciimmunol.add8005
268. Liu SD, Chalouni C, Young JC, Junttila TT, Sliwkowski MX, Lowe JB. Afucosylated antibodies increase activation of FcγRIIIa-dependent signaling components to intensify processes promoting ADCC. Cancer Immunol Res (2015) 3(2):173–83. doi: 10.1158/2326-6066.CIR-14-0125
269. Nimmerjahn F, Ravetch JV. Divergent immunoglobulin g subclass activity through selective fc receptor binding. Science (2005) 310(5753):1510–2. doi: 10.1126/science.1118948
270. Shields RL, Lai J, Keck R, O'Connell LY, Hong K, Meng YG, et al. Lack of fucose on human IgG1 n-linked oligosaccharide improves binding to human fcgamma RIII and antibody-dependent cellular toxicity. J Biol Chem (2002) 277(30):26733–40. doi: 10.1074/jbc.M202069200
271. Dekkers G, Treffers L, Plomp R, Bentlage AEH, de Boer M, Koeleman CAM, et al. Decoding the human immunoglobulin G-glycan repertoire reveals a spectrum of fc-receptor- and complement-Mediated-Effector activities. Front Immunol (2017) 8:877. doi: 10.3389/fimmu.2017.00877
272. Peschke B, Keller CW, Weber P, Quast I, Lünemann JD. Fc-galactosylation of human immunoglobulin gamma isotypes improves C1q binding and enhances complement-dependent cytotoxicity. Front Immunol (2017) 8:646. doi: 10.3389/fimmu.2017.00646
273. Thomann M, Reckermann K, Reusch D, Prasser J, Tejada ML. Fc-galactosylation modulates antibody-dependent cellular cytotoxicity of therapeutic antibodies. Mol Immunol (2016) 73:69–75. doi: 10.1016/j.molimm.2016.03.002
274. Kumpel BM, Rademacher TW, Rook GA, Williams PJ, Wilson IB. Galactosylation of human IgG monoclonal anti-d produced by EBV-transformed b-lymphoblastoid cell lines is dependent on culture method and affects fc receptor-mediated functional activity. Hum Antibodies Hybridomas (1994) 5(3-4):143–51.
275. Shinkawa T, Nakamura K, Yamane N, Shoji-Hosaka E, Kanda Y, Sakurada M, et al. The absence of fucose but not the presence of galactose or bisecting n-acetylglucosamine of human IgG1 complex-type oligosaccharides shows the critical role of enhancing antibody-dependent cellular cytotoxicity. J Biol Chem (2003) 278(5):3466–73. doi: 10.1074/jbc.M210665200
276. Wang TT, Ravetch JV. Functional diversification of IgGs through fc glycosylation. J Clin Invest (2019) 129(9):3492–8. doi: 10.1172/JCI130029
277. Sondermann P, Pincetic A, Maamary J, Lammens K, Ravetch JV. General mechanism for modulating immunoglobulin effector function. Proc Natl Acad Sci USA (2013) 110(24):9868–72. doi: 10.1073/pnas.1307864110
278. Crispin M, Yu X, Bowden TA. Crystal structure of sialylated IgG fc: implications for the mechanism of intravenous immunoglobulin therapy. Proc Natl Acad Sci USA (2013) 110(38):E3544–6. doi: 10.1073/pnas.1310657110
279. Ahmed AA, Giddens J, Pincetic A, Lomino JV, Ravetch JV, Wang LX, et al. Structural characterization of anti-inflammatory immunoglobulin G fc proteins. J Mol Biol (2014) 426(18):3166–79. doi: 10.1016/j.jmb.2014.07.006
280. Samuelsson A, Towers TL, Ravetch JV. Anti-inflammatory activity of IVIG mediated through the inhibitory fc receptor. Science (2001) 291(5503):484–6. doi: 10.1126/science.291.5503.484
281. Kaneko Y, Nimmerjahn F, Ravetch JV. Anti-inflammatory activity of immunoglobulin G resulting from fc sialylation. Science (2006) 313(5787):670–3. doi: 10.1126/science.1129594
282. Anthony RM, Wermeling F, Karlsson MC, Ravetch JV. Identification of a receptor required for the anti-inflammatory activity of IVIG. Proc Natl Acad Sci USA (2008) 105(50):19571–8. doi: 10.1073/pnas.0810163105
283. Anthony RM, Kobayashi T, Wermeling F, Ravetch JV. Intravenous gammaglobulin suppresses inflammation through a novel T(H)2 pathway. Nature (2011) 475(7354):110–3. doi: 10.1038/nature10134
284. Temming AR, Dekkers G, van de Bovenkamp FS, Plomp HR, Bentlage AEH, Szittner Z, et al. Human DC-SIGN and CD23 do not interact with human IgG. Sci Rep (2019) 9(1):9995. doi: 10.1038/s41598-019-46484-2
285. Siragam V, Crow AR, Brinc D, Song S, Freedman J, Lazarus AH. Intravenous immunoglobulin ameliorates ITP via activating fc gamma receptors on dendritic cells. Nat Med (2006) 12(6):688–92. doi: 10.1038/nm1416
286. Li T, DiLillo DJ, Bournazos S, Giddens JP, Ravetch JV, Wang LX. Modulating IgG effector function by fc glycan engineering. Proc Natl Acad Sci USA (2017) 114(13):3485–90. doi: 10.1073/pnas.1702173114
287. Jennewein MF, Alter G. The immunoregulatory roles of antibody glycosylation. Trends Immunol (2017) 38(5):358–72. doi: 10.1016/j.it.2017.02.004
288. Bartsch YC, Eschweiler S, Leliavski A, Lunding HB, Wagt S, Petry J, et al. IgG fc sialylation is regulated during the germinal center reaction following immunization with different adjuvants. J Allergy Clin Immunol (2020) 146(3):652–66.e11. doi: 10.1016/j.jaci.2020.04.059
289. Pfeifle R, Rothe T, Ipseiz N, Scherer HU, Culemann S, Harre U, et al. Regulation of autoantibody activity by the IL-23-T(H)17 axis determines the onset of autoimmune disease. Nat Immunol (2017) 18(1):104–13. doi: 10.1038/ni.3579
290. Chakraborty S, Gonzalez J, Edwards K, Mallajosyula V, Buzzanco AS, Sherwood R, et al. Proinflammatory IgG fc structures in patients with severe COVID-19. Nat Immunol (2021) 22(1):67–73. doi: 10.1038/s41590-020-00828-7
291. Petrović T, Vijay A, Vučković F, Trbojević-Akmačić I, Ollivere BJ, Marjanović D, et al. IgG n-glycome changes during the course of severe COVID-19: an observational study. EBioMedicine (2022) 81:104101. doi: 10.1016/j.ebiom.2022.104101
292. Hoepel W, Chen HJ, Geyer CE, Allahverdiyeva S, Manz XD, de Taeye SW, et al. High titers and low fucosylation of early human anti-SARS-CoV-2 IgG promote inflammation by alveolar macrophages. Sci Transl Med (2021) 13(596):eabf8654. doi: 10.1126/scitranslmed.abf8654
293. Alter G, Ottenhoff THM, Joosten SA. Antibody glycosylation in inflammation, disease and vaccination. Semin Immunol (2018) 39:102–10. doi: 10.1016/j.smim.2018.05.003
294. Irvine EB, Alter G. Understanding the role of antibody glycosylation through the lens of severe viral and bacterial diseases. Glycobiology (2020) 30(4):241–53. doi: 10.1093/glycob/cwaa018
295. Pongracz T, Nouta J, Wang W, van Meijgaarden KE, Linty F, Vidarsson G, et al. Immunoglobulin G1 fc glycosylation as an early hallmark of severe COVID-19. EBioMedicine (2022) 78:103957. doi: 10.1016/j.ebiom.2022.103957
296. Larsen MD, de Graaf EL, Sonneveld ME, Plomp HR, Nouta J, Hoepel W, et al. Afucosylated IgG characterizes enveloped viral responses and correlates with COVID-19 severity. Science (2021) 371(6532):eabc8378. doi: 10.1126/science.abc8378
297. van de Geijn FE, Wuhrer M, Selman MH, Willemsen SP, de Man YA, Deelder AM, et al. Immunoglobulin G galactosylation and sialylation are associated with pregnancy-induced improvement of rheumatoid arthritis and the postpartum flare: results from a large prospective cohort study. Arthritis Res Ther (2009) 11(6):R193. doi: 10.1186/ar2892
298. Offersen R, Yu WH, Scully EP, Julg B, Euler Z, Sadanand S, et al. HIV Antibody fc n-linked glycosylation is associated with viral rebound. Cell Rep (2020) 33(11):108502. doi: 10.1016/j.celrep.2020.108502
299. Gudelj I, Lauc G, Pezer M. Immunoglobulin G glycosylation in aging and diseases. Cell Immunol (2018) 333:65–79. doi: 10.1016/j.cellimm.2018.07.009
300. Plomp R, Ruhaak LR, Uh HW, Reiding KR, Selman M, Houwing-Duistermaat JJ, et al. Subclass-specific IgG glycosylation is associated with markers of inflammation and metabolic health. Sci Rep (2017) 7(1):12325. doi: 10.1038/s41598-017-12495-0
301. Parekh RB, Dwek RA, Sutton BJ, Fernandes DL, Leung A, Stanworth D, et al. Association of rheumatoid arthritis and primary osteoarthritis with changes in the glycosylation pattern of total serum IgG. Nature (1985) 316(6027):452–7. doi: 10.1038/316452a0
302. Vučković F, Krištić J, Gudelj I, Teruel M, Keser T, Pezer M, et al. Association of systemic lupus erythematosus with decreased immunosuppressive potential of the IgG glycome. Arthritis Rheumatol (2015) 67(11):2978–89. doi: 10.1002/art.39273
303. Dekkers G, Rispens T, Vidarsson G. Novel concepts of altered immunoglobulin G galactosylation in autoimmune diseases. Front Immunol (2018) 9:553. doi: 10.3389/fimmu.2018.00553
304. Karsten CM, Pandey MK, Figge J, Kilchenstein R, Taylor PR, Rosas M, et al. Anti-inflammatory activity of IgG1 mediated by fc galactosylation and association of FcγRIIB and dectin-1. Nat Med (2012) 18(9):1401–6. doi: 10.1038/nm.2862
305. Wang J, Balog CI, Stavenhagen K, Koeleman CA, Scherer HU, Selman MH, et al. Fc-glycosylation of IgG1 is modulated by b-cell stimuli. Mol Cell Proteomics (2011) 10(5):M110.004655. doi: 10.1074/mcp.M110.004655
306. Cao Y, Song Z, Guo Z, Zhao X, Gong Y, Zhao K, et al. Cytokines in the immune microenvironment change the glycosylation of IgG by regulating intracellular glycosyltransferases. Front Immunol (2021) 12:724379. doi: 10.3389/fimmu.2021.724379
307. Deriš H, Kifer D, Cindrić A, Petrović T, Cvetko A, Trbojević-Akmačić I, et al. Immunoglobulin G glycome composition in transition from premenopause to postmenopause. iScience (2022) 25(3):103897. doi: 10.1016/j.isci.2022.103897
308. Selman MH, de Jong SE, Soonawala D, Kroon FP, Adegnika AA, Deelder AM, et al. Changes in antigen-specific IgG1 fc n-glycosylation upon influenza and tetanus vaccination. Mol Cell Proteomics (2012) 11(4):M111.014563. doi: 10.1074/mcp.M111.014563
309. Štambuk J, Nakić N, Vučković F, Pučić-Baković M, Razdorov G, Trbojević-Akmačić I, et al. Global variability of the human IgG glycome. Aging (Albany NY) (2020) 12(15):15222–59. doi: 10.18632/aging.103884
310. Atyeo C, DeRiso EA, Davis C, Bordt EA, De Guzman RM, Shook LL, et al. COVID-19 mRNA vaccines drive differential antibody fc-functional profiles in pregnant, lactating, and nonpregnant women. Sci Transl Med (2021) 13(617):eabi8631. doi: 10.1126/scitranslmed.abi8631
311. Fendler A, de Vries EGE, GeurtsvanKessel CH, Haanen JB, Wörmann B, Turajlic S, et al. COVID-19 vaccines in patients with cancer: immunogenicity, efficacy and safety. Nat Rev Clin Oncol (2022) 19(6):385–401. doi: 10.1038/s41571-022-00610-8
312. Poland GA, Ovsyannikova IG, Jacobson RM. Immunogenetics of seasonal influenza vaccine response. Vaccine (2008) 26 Suppl 4(Suppl 4):D35–40. doi: 10.1016/j.vaccine.2008.07.065
313. Poland GA, Ovsyannikova IG, Jacobson RM. Vaccine immunogenetics: bedside to bench to population. Vaccine (2008) 26(49):6183–8. doi: 10.1016/j.vaccine.2008.06.057
314. Fourati S, Cristescu R, Loboda A, Talla A, Filali A, Railkar R, et al. Pre-vaccination inflammation and b-cell signalling predict age-related hyporesponse to hepatitis b vaccination. Nat Commun (2016) 7:10369. doi: 10.1038/ncomms10369
315. Lynn DJ, Benson SC, Lynn MA, Pulendran B. Modulation of immune responses to vaccination by the microbiota: implications and potential mechanisms. Nat Rev Immunol (2022) 22(1):33–46. doi: 10.1038/s41577-021-00554-7
316. Lynn DJ, Pulendran B. The potential of the microbiota to influence vaccine responses. J Leukoc Biol (2018) 103(2):225–31. doi: 10.1189/jlb.5MR0617-216R
317. Scepanovic P, Alanio C, Hammer C, Hodel F, Bergstedt J, Patin E, et al. Human genetic variants and age are the strongest predictors of humoral immune responses to common pathogens and vaccines. Genome Med (2018) 10(1):59. doi: 10.1186/s13073-018-0568-8
318. Cheung F, Apps R, Dropulic L, Kotliarov Y, Chen J, Jordan T, et al. Sex and prior exposure jointly shape innate immune responses to a live herpesvirus vaccine. Elife (2023) 12:e80652. doi: 10.7554/eLife.80652
319. Yang B, Lessler J, Zhu H, Jiang CQ, Read JM, Hay JA, et al. Life course exposures continually shape antibody profiles and risk of seroconversion to influenza. PloS Pathog (2020) 16(7):e1008635. doi: 10.1371/journal.ppat.1008635
320. Cuapio A, Boulouis C, Filipovic I, Wullimann D, Kammann T, Parrot T, et al. NK cell frequencies, function and correlates to vaccine outcome in BNT162b2 mRNA anti-SARS-CoV-2 vaccinated healthy and immunocompromised individuals. Mol Med (2022) 28(1):20. doi: 10.1186/s10020-022-00443-2
321. Gustafson CE, Kim C, Weyand CM, Goronzy JJ. Influence of immune aging on vaccine responses. J Allergy Clin Immunol (2020) 145(5):1309–21. doi: 10.1016/j.jaci.2020.03.017
322. Kratochvil S, McKay PF, Chung AW, Kent SJ, Gilmour J, Shattock RJ. Immunoglobulin G1 allotype influences antibody subclass distribution in response to HIV gp140 vaccination. Front Immunol (2017) 8:1883. doi: 10.3389/fimmu.2017.01883
323. Frasca D, Blomberg BB. Aging induces b cell defects and decreased antibody responses to influenza infection and vaccination. Immun Ageing (2020) 17(1):37. doi: 10.1186/s12979-020-00210-z
324. Bartsch YC, St Denis KJ, Kaplonek P, Kang J, Lam EC, Burns MD, et al. SARS-CoV-2 mRNA vaccination elicits robust antibody responses in children. Sci Transl Med (2022) 14(672):eabn9237. doi: 10.1126/scitranslmed.abn9237
325. Rahmatpanah F, Agrawal S, Scarfone VM, Kapadia S, Mercola D, Agrawal A. Transcriptional profiling of age-associated gene expression changes in human circulatory CD1c+ myeloid dendritic cell subset. J Gerontol A Biol Sci Med Sci (2019) 74(1):9–15. doi: 10.1093/gerona/gly106
326. Mogilenko DA, Shchukina I, Artyomov MN. Immune ageing at single-cell resolution. Nat Rev Immunol (2022) 22(8):484–98. doi: 10.1038/s41577-021-00646-4
327. Crooke SN, Ovsyannikova IG, Poland GA, Kennedy RB. Immunosenescence and human vaccine immune responses. Immun Ageing (2019) 16:25. doi: 10.1186/s12979-019-0164-9
328. Thomas AL, Alarcon PC, Divanovic S, Chougnet CA, Hildeman DA, Moreno-Fernandez ME. Implications of inflammatory states on dysfunctional immune responses in aging and obesity. Front Aging (2021) 2:732414. doi: 10.3389/fragi.2021.732414
329. Lambert K, Moo KG, Arnett A, Goel G, Hu A, Flynn KJ, et al. Deep immune phenotyping reveals similarities between aging, down syndrome, and autoimmunity. Sci Transl Med (2022) 14(627):eabi4888. doi: 10.1126/scitranslmed.abi4888
330. Tam BT, Morais JA, Santosa S. Obesity and ageing: two sides of the same coin. Obes Rev (2020) 21(4):e12991. doi: 10.1111/obr.12991
331. Kumar N, Chugh H, Tomar R, Tomar V, Singh VK, Chandra R. Exploring the interplay between autoimmunity and cancer to find the target therapeutic hotspots. Artif Cells Nanomed Biotechnol (2018) 46(4):658–68. doi: 10.1080/21691401.2017.1350188
332. Frasca D, Diaz A, Romero M, Blomberg BB. Ageing and obesity similarly impair antibody responses. Clin Exp Immunol (2017) 187(1):64–70. doi: 10.1111/cei.12824
333. Schmitt V, Rink L, Uciechowski P. The Th17/Treg balance is disturbed during aging. Exp Gerontol (2013) 48(12):1379–86. doi: 10.1016/j.exger.2013.09.003
334. Jo N, Hidaka Y, Kikuchi O, Fukahori M, Sawada T, Aoki M, et al. Impaired CD4(+) T cell response in older adults is associated with reduced immunogenicity and reactogenicity of mRNA COVID-19 vaccination. Nat Aging (2023) 3(1):82–92. doi: 10.1038/s43587-022-00343-4
335. Chen G, Wang Y, Qiu L, Qin X, Liu H, Wang X, et al. Human IgG fc-glycosylation profiling reveals associations with age, sex, female sex hormones and thyroid cancer. J Proteomics (2012) 75(10):2824–34. doi: 10.1016/j.jprot.2012.02.001
336. Stavnezer J. Immunoglobulin class switching. Curr Opin Immunol (1996) 8(2):199–205. doi: 10.1016/S0952-7915(96)80058-6
337. Xu Z, Zan H, Pone EJ, Mai T, Casali P. Immunoglobulin class-switch DNA recombination: induction, targeting and beyond. Nat Rev Immunol (2012) 12(7):517–31. doi: 10.1038/nri3216
338. Malisan F, Brière F, Bridon JM, Harindranath N, Mills FC, Max EE, et al. Interleukin-10 induces immunoglobulin G isotype switch recombination in human CD40-activated naive b lymphocytes. J Exp Med (1996) 183(3):937–47. doi: 10.1084/jem.183.3.937
339. Anderson GP, Irving LB, Jarnicki A, Kedzierska K, Koutsakos M, Kent S, et al. Prime-boost, double-dose influenza vaccine immunity in COPD: a pilot observational study. ERJ Open Res (2023) 9(2):00641–2021. doi: 10.1183/23120541.00641-2021
340. Voutouri C, Hardin CC, Naranbhai V, Nikmaneshi MR, Khandekar MJ, Gainor JF, et al. Mechanistic model for booster doses effectiveness in healthy, cancer, and immunosuppressed patients infected with SARS-CoV-2. Proc Natl Acad Sci USA (2023) 120(3):e2211132120. doi: 10.1073/pnas.2211132120
341. Yu KK, Fischinger S, Smith MT, Atyeo C, Cizmeci D, Wolf CR, et al. Comorbid illnesses are associated with altered adaptive immune responses to SARS-CoV-2. JCI Insight (2021) 6(6):e146242. doi: 10.1172/jci.insight.146242
342. McLean MR, Wragg KM, Lopez E, Kiazyk SA, Ball TB, Bueti J, et al. Serological and cellular inflammatory signatures in end-stage kidney disease and latent tuberculosis. Clin Transl Immunol (2021) 10(11):e1355. doi: 10.1002/cti2.1355
343. Tosif S, Neeland MR, Sutton P, Licciardi PV, Sarkar S, Selva KJ, et al. Immune responses to SARS-CoV-2 in three children of parents with symptomatic COVID-19. Nat Commun (2020) 11(1):5703. doi: 10.1038/s41467-020-19545-8
344. Jennewein MF, Kosikova M, Noelette FJ, Radvak P, Boudreau CM, Campbell JD, et al. Functional and structural modifications of influenza antibodies during pregnancy. iScience (2022) 25(4):104088. doi: 10.1016/j.isci.2022.104088
345. Damelang T, Aitken EH, Hasang W, Lopez E, Killian M, Unger HW, et al. Antibody mediated activation of natural killer cells in malaria exposed pregnant women. Sci Rep (2021) 11(1):4130. doi: 10.1038/s41598-021-83093-4
346. Atyeo CG, Shook LL, Brigida S, De Guzman RM, Demidkin S, Muir C, et al. Maternal immune response and placental antibody transfer after COVID-19 vaccination across trimester and platforms. Nat Commun (2022) 13(1):3571. doi: 10.1038/s41467-022-31169-8
347. Schnittman SR, Byakwaga H, Boum Y, Kabakyenga J, Matthews LT, Burdo TH, et al. Changes in immune activation during pregnancy and the postpartum period in treated HIV infection. Open Forum Infect Dis (2021) 8(6):ofab245. doi: 10.1093/ofid/ofab245
348. Taylor SA, Sharma S, Remmel CAL, Holder B, Jones CE, Marchant A, et al. HIV-Associated alterations of the biophysical features of maternal antibodies correlate with their reduced transfer across the placenta. J Infect Dis (2022) 226(8):1441–50. doi: 10.1093/infdis/jiac222
349. Motsoeneng BM, Dhar N, Nunes MC, Krammer F, Madhi SA, Moore PL, et al. Influenza vaccination results in differential hemagglutinin stalk-specific fc-mediated functions in individuals living with or without HIV. Front Immunol (2022) 13:873191. doi: 10.3389/fimmu.2022.873191
350. Lassi ZS, Majeed A, Rashid S, Yakoob MY, Bhutta ZA. The interconnections between maternal and newborn health–evidence and implications for policy. J Matern Fetal Neonatal Med (2013) 26 Suppl 1:3–53. doi: 10.3109/14767058.2013.784737
351. Donald K, Petersen C, Turvey SE, Finlay BB, Azad MB. Secretory IgA: linking microbes, maternal health, and infant health through human milk. Cell Host Microbe (2022) 30(5):650–9. doi: 10.1016/j.chom.2022.02.005
352. Arabin B, Baschat AA. Pregnancy: an underutilized window of opportunity to improve long-term maternal and infant health-an appeal for continuous family care and interdisciplinary communication. Front Pediatr (2017) 5:69. doi: 10.3389/fped.2017.00069
353. Lebold KM, Jacoby DB, Drake MG. Inflammatory mechanisms linking maternal and childhood asthma. J Leukoc Biol (2020) 108(1):113–21. doi: 10.1002/JLB.3MR1219-338R
354. Marshall H, McMillan M, Andrews RM, Macartney K, Edwards K. Vaccines in pregnancy: the dual benefit for pregnant women and infants. Hum Vaccin Immunother (2016) 12(4):848–56. doi: 10.1080/21645515.2015.1127485
355. Jarvis JR, Dorey RB, Warricker FDM, Alwan NA, Jones CE. The effectiveness of influenza vaccination in pregnancy in relation to child health outcomes: systematic review and meta-analysis. Vaccine (2020) 38(7):1601–13. doi: 10.1016/j.vaccine.2019.12.056
356. Sakala IG, Honda-Okubo Y, Fung J, Petrovsky N. Influenza immunization during pregnancy: benefits for mother and infant. Hum Vaccin Immunother (2016) 12(12):3065–71. doi: 10.1080/21645515.2016.1215392
357. Beharier O, Plitman Mayo R, Raz T, Nahum Sacks K, Schreiber L, Suissa-Cohen Y, et al. Efficient maternal to neonatal transfer of antibodies against SARS-CoV-2 and BNT162b2 mRNA COVID-19 vaccine. J Clin Invest (2021) 131(13):e150319. doi: 10.1172/JCI150319
358. Jennewein MF, Goldfarb I, Dolatshahi S, Cosgrove C, Noelette FJ, Krykbaeva M, et al. Fc glycan-mediated regulation of placental antibody transfer. Cell (2019) 178(1):202–15.e14. doi: 10.1016/j.cell.2019.05.044
359. Dolatshahi S, Butler AL, Pou C, Henckel E, Bernhardsson AK, Gustafsson A, et al. Selective transfer of maternal antibodies in preterm and fullterm children. Sci Rep (2022) 12(1):14937. doi: 10.1038/s41598-022-18973-4
360. Alonso S, Vidal M, Ruiz-Olalla G, González R, Manaca MN, Jairoce C, et al. Reduced placental transfer of antibodies against a wide range of microbial and vaccine antigens in HIV-infected women in Mozambique. Front Immunol (2021) 12:614246. doi: 10.3389/fimmu.2021.614246
361. Martinez DR, Fong Y, Li SH, Yang F, Jennewein MF, Weiner JA, et al. Fc characteristics mediate selective placental transfer of IgG in HIV-infected women. Cell (2019) 178(1):190–201.e11. doi: 10.1016/j.cell.2019.05.046
362. Cumberland P, Shulman CE, Maple PA, Bulmer JN, Dorman EK, Kawuondo K, et al. Maternal HIV infection and placental malaria reduce transplacental antibody transfer and tetanus antibody levels in newborns in Kenya. J Infect Dis (2007) 196(4):550–7. doi: 10.1086/519845
363. Brair ME, Brabin BJ, Milligan P, Maxwell S, Hart CA. Reduced transfer of tetanus antibodies with placental malaria. Lancet (1994) 343(8891):208–9. doi: 10.1016/S0140-6736(94)90991-1
364. Dolatshahi S, Butler AL, Siedner MJ, Ngonzi J, Edlow AG, Adong J, et al. Altered maternal antibody profiles in women with human immunodeficiency virus drive changes in transplacental antibody transfer. Clin Infect Dis (2022) 75(8):1359–69. doi: 10.1093/cid/ciac156
365. Dauby N, Goetghebuer T, Kollmann TR, Levy J, Marchant A. Uninfected but not unaffected: chronic maternal infections during pregnancy, fetal immunity, and susceptibility to postnatal infections. Lancet Infect Dis (2012) 12(4):330–40. doi: 10.1016/S1473-3099(11)70341-3
366. Afran L, Garcia Knight M, Nduati E, Urban BC, Heyderman RS, Rowland-Jones SL. HIV-Exposed uninfected children: a growing population with a vulnerable immune system? Clin Exp Immunol (2014) 176(1):11–22. doi: 10.1111/cei.12251
367. Atyeo C, Pullen KM, Bordt EA, Fischinger S, Burke J, Michell A, et al. Compromised SARS-CoV-2-specific placental antibody transfer. Cell (2021) 184(3):628–42.e10. doi: 10.1016/j.cell.2020.12.027
368. Vono M, Eberhardt CS, Auderset F, Mastelic-Gavillet B, Lemeille S, Christensen D, et al. Maternal antibodies inhibit neonatal and infant responses to vaccination by shaping the early-life b cell repertoire within germinal centers. Cell Rep (2019) 28(7):1773–84.e5. doi: 10.1016/j.celrep.2019.07.047
369. Semmes EC, Chen JL, Goswami R, Burt TD, Permar SR, Fouda GG. Understanding early-life adaptive immunity to guide interventions for pediatric health. Front Immunol (2020) 11:595297. doi: 10.3389/fimmu.2020.595297
370. Niewiesk S. Maternal antibodies: clinical significance, mechanism of interference with immune responses, and possible vaccination strategies. Front Immunol (2014) 5:446. doi: 10.3389/fimmu.2014.00446
371. Gohain N, Tolbert WD, Acharya P, Yu L, Liu T, Zhao P, et al. Cocrystal structures of antibody N60-i3 and antibody JR4 in complex with gp120 define more cluster a epitopes involved in effective antibody-dependent effector function against HIV-1. J Virol (2015) 89(17):8840–54. doi: 10.1128/JVI.01232-15
372. Cheng HD, Tirosh I, de Haan N, Stöckmann H, Adamczyk B, McManus CA, et al. IgG fc glycosylation as an axis of humoral immunity in childhood. J Allergy Clin Immunol (2020) 145(2):710–3.e9. doi: 10.1016/j.jaci.2019.10.012
373. de Haan N, Reiding KR, Driessen G, van der Burg M, Wuhrer M. Changes in healthy human IgG fc-glycosylation after birth and during early childhood. J Proteome Res (2016) 15(6):1853–61. doi: 10.1021/acs.jproteome.6b00038
374. Bartsch YC, Chen JW, Kang J, Burns MD, St Denis KJ, Sheehan ML, et al. BNT162b2 induces robust cross-variant SARS-CoV-2 immunity in children. NPJ Vaccines (2022) 7(1):158. doi: 10.1038/s41541-022-00575-w
375. Tomasi L, Thiriard A, Heyndrickx L, Georges D, Van den Wijngaert S, Olislagers V, et al. Younger children develop higher effector antibody responses to SARS-CoV-2 infection. Open Forum Infect Dis (2022) 9(11):ofac554. doi: 10.1093/ofid/ofac554
376. Parekh R, Roitt I, Isenberg D, Dwek R, Rademacher T. Age-related galactosylation of the n-linked oligosaccharides of human serum IgG. J Exp Med (1988) 167(5):1731–6. doi: 10.1084/jem.167.5.1731
377. Ercan A, Barnes MG, Hazen M, Tory H, Henderson L, Dedeoglu F, et al. Multiple juvenile idiopathic arthritis subtypes demonstrate proinflammatory IgG glycosylation. Arthritis Rheumatol (2012) 64(9):3025–33. doi: 10.1002/art.34507
378. Pucic M, Muzinic A, Novokmet M, Skledar M, Pivac N, Lauc G, et al. Changes in plasma and IgG n-glycome during childhood and adolescence. Glycobiology (2012) 22(7):975–82. doi: 10.1093/glycob/cws062
379. Nduati EW, Gorman MJ, Sein Y, Hermanus T, Yuan D, Oyaro I, et al. Coordinated fc-effector and neutralization functions in HIV-infected children define a window of opportunity for HIV vaccination. Aids (2021) 35(12):1895–905. doi: 10.1097/QAD.0000000000002976
380. Muenchhoff M, Chung AW, Roider J, Dugast AS, Richardson S, Kløverpris H, et al. Distinct immunoglobulin fc glycosylation patterns are associated with disease nonprogression and broadly neutralizing antibody responses in children with HIV infection. mSphere (2020) 5(6):e00880–20. doi: 10.1128/mSphere.00880-20
381. Fulop T, Larbi A, Dupuis G, Le Page A, Frost EH, Cohen AA, et al. Immunosenescence and inflamm-aging as two sides of the same coin: friends or foes? Front Immunol (2017) 8:1960. doi: 10.3389/fimmu.2017.01960
382. Santoro A, Bientinesi E, Monti D. Immunosenescence and inflammaging in the aging process: age-related diseases or longevity? Ageing Res Rev (2021) 71:101422. doi: 10.1016/j.arr.2021.101422
383. Krištić J, Lauc G, Pezer M. Immunoglobulin G glycans - biomarkers and molecular effectors of aging. Clin Chim Acta (2022) 535:30–45. doi: 10.1016/j.cca.2022.08.006
384. Collier DA, Ferreira I, Kotagiri P, Datir RP, Lim EY, Touizer E, et al. Age-related immune response heterogeneity to SARS-CoV-2 vaccine BNT162b2. Nature (2021) 596(7872):417–22. doi: 10.1038/s41586-021-03739-1
385. Frasca D, Diaz A, Romero M, Landin AM, Blomberg BB. Age effects on b cells and humoral immunity in humans. Ageing Res Rev (2011) 10(3):330–5. doi: 10.1016/j.arr.2010.08.004
386. Frasca D, Diaz A, Romero M, Mendez NV, Landin AM, Blomberg BB. Effects of age on H1N1-specific serum IgG1 and IgG3 levels evaluated during the 2011-2012 influenza vaccine season. Immun Ageing (2013) 10(1):14. doi: 10.1186/1742-4933-10-14
387. Stepanova L, Naykhin A, Kolmskog C, Jonson G, Barantceva I, Bichurina M, et al. The humoral response to live and inactivated influenza vaccines administered alone and in combination to young adults and elderly. J Clin Virol (2002) 24(3):193–201. doi: 10.1016/S1386-6532(01)00246-3
388. Park SY, Levin MJ, Canniff J, Johnson M, Schmid DS, Weinberg A. Development of antibody-dependent cellular cytotoxicity in response to recombinant and live-attenuated herpes zoster vaccines. NPJ Vaccines (2022) 7(1):123. doi: 10.1038/s41541-022-00545-2
389. Nanishi E, Angelidou A, Rotman C, Dowling DJ, Levy O, Ozonoff A. Precision vaccine adjuvants for older adults: a scoping review. Clin Infect Dis (2022) 75(Suppl 1):S72–s80. doi: 10.1093/cid/ciac302
390. DiazGranados CA, Dunning AJ, Kimmel M, Kirby D, Treanor J, Collins A, et al. Efficacy of high-dose versus standard-dose influenza vaccine in older adults. N Engl J Med (2014) 371(7):635–45. doi: 10.1056/NEJMoa1315727
391. Ng TWY, Cowling BJ, Gao HZ, Thompson MG. Comparative immunogenicity of enhanced seasonal influenza vaccines in older adults: a systematic review and meta-analysis. J Infect Dis (2019) 219(10):1525–35. doi: 10.1093/infdis/jiy720
392. Domnich A, Arata L, Amicizia D, Puig-Barberà J, Gasparini R, Panatto D. Effectiveness of MF59-adjuvanted seasonal influenza vaccine in the elderly: a systematic review and meta-analysis. Vaccine (2017) 35(4):513–20. doi: 10.1016/j.vaccine.2016.12.011
393. McElhaney JE, Beran J, Devaster JM, Esen M, Launay O, Leroux-Roels G, et al. AS03-adjuvanted versus non-adjuvanted inactivated trivalent influenza vaccine against seasonal influenza in elderly people: a phase 3 randomised trial. Lancet Infect Dis (2013) 13(6):485–96. doi: 10.1016/S1473-3099(13)70046-X
394. Boudreau CM, Yu WH, Suscovich TJ, Talbot HK, Edwards KM, Alter G. Selective induction of antibody effector functional responses using MF59-adjuvanted vaccination. J Clin Invest (2020) 130(2):662–72. doi: 10.1172/JCI129520
395. Farkash I, Feferman T, Cohen-Saban N, Avraham Y, Morgenstern D, Mayuni G, et al. Anti-SARS-CoV-2 antibodies elicited by COVID-19 mRNA vaccine exhibit a unique glycosylation pattern. Cell Rep (2021) 37(11):110114. doi: 10.1016/j.celrep.2021.110114
396. Bates TA, Lu P, Kang YJ, Schoen D, Thornton M, McBride SK, et al. BNT162b2-induced neutralizing and non-neutralizing antibody functions against SARS-CoV-2 diminish with age. Cell Rep (2022) 41(4):111544. doi: 10.1016/j.celrep.2022.111544
397. Kaplan GG, Ng SC. Understanding and preventing the global increase of inflammatory bowel disease. Gastroenterology (2017) 152(2):313–21.e2. doi: 10.1053/j.gastro.2016.10.020
398. Popkin BM, Slining MM. New dynamics in global obesity facing low- and middle-income countries. Obes Rev (2013) 14 Suppl 2(0 2):11–20. doi: 10.1111/obr.12102
399. Malik VS, Willet WC, Hu FB. Nearly a decade on - trends, risk factors and policy implications in global obesity. Nat Rev Endocrinol (2020) 16(11):615–6. doi: 10.1038/s41574-020-00411-y
400. Lederer K, Bettini E, Parvathaneni K, Painter MM, Agarwal D, Lundgreen KA, et al. Germinal center responses to SARS-CoV-2 mRNA vaccines in healthy and immunocompromised individuals. Cell (2022) 185(6):1008–24.e15. doi: 10.1016/j.cell.2022.01.027
401. Furman D, Campisi J, Verdin E, Carrera-Bastos P, Targ S, Franceschi C, et al. Chronic inflammation in the etiology of disease across the life span. Nat Med (2019) 25(12):1822–32. doi: 10.1038/s41591-019-0675-0
402. Hulme KD, Noye EC, Short KR, Labzin LI. Dysregulated inflammation during obesity: driving disease severity in influenza virus and SARS-CoV-2 infections. Front Immunol (2021) 12:770066. doi: 10.3389/fimmu.2021.770066
403. Burdin N, Van Kooten C, Galibert L, Abrams JS, Wijdenes J, Banchereau J, et al. Endogenous IL-6 and IL-10 contribute to the differentiation of CD40-activated human b lymphocytes. J Immunol (1995) 154(6):2533–44. doi: 10.4049/jimmunol.154.6.2533
404. Thomson T, Prendecki M, Gleeson S, Martin P, Spensley K, De Aguiar RC, et al. Immune responses following 3rd and 4th doses of heterologous and homologous COVID-19 vaccines in kidney transplant recipients. EClinicalMedicine (2022) 53:101642. doi: 10.1016/j.eclinm.2022.101642
405. Fendler A, Shepherd STC, Au L, Wilkinson KA, Wu M, Byrne F, et al. Adaptive immunity and neutralizing antibodies against SARS-CoV-2 variants of concern following vaccination in patients with cancer: the CAPTURE study. Nat Canc (2021) 2(12):1305–20. doi: 10.1038/s43018-021-00274-w
406. Klarić L, Tsepilov YA, Stanton CM, Mangino M, Sikka TT, Esko T, et al. Glycosylation of immunoglobulin G is regulated by a large network of genes pleiotropic with inflammatory diseases. Sci Adv (2020) 6(8):eaax0301. doi: 10.1126/sciadv.aax0301
407. Aurer I, Lauc G, Dumić J, Rendić D, Matisić D, Milos M, et al. Aberrant glycosylation of igg heavy chain in multiple myeloma. Coll Antropol (2007) 31(1):247–51.
408. Theodoratou E, Thaçi K, Agakov F, Timofeeva MN, Štambuk J, Pučić-Baković M, et al. Glycosylation of plasma IgG in colorectal cancer prognosis. Sci Rep (2016) 6:28098. doi: 10.1038/srep28098
409. Vučković F, Theodoratou E, Thaçi K, Timofeeva M, Vojta A, Štambuk J, et al. IgG glycome in colorectal cancer. Clin Cancer Res (2016) 22(12):3078–86. doi: 10.1158/1078-0432.CCR-15-1867
410. Ruhaak LR, Kim K, Stroble C, Taylor SL, Hong Q, Miyamoto S, et al. Protein-specific differential glycosylation of immunoglobulins in serum of ovarian cancer patients. J Proteome Res (2016) 15(3):1002–10. doi: 10.1021/acs.jproteome.5b01071
411. de Haan N, van Tol MJD, Driessen GJ, Wuhrer M, Lankester AC. Immunoglobulin G fragment crystallizable glycosylation after hematopoietic stem cell transplantation is dissimilar to donor profiles. Front Immunol (2018) 9:1238. doi: 10.3389/fimmu.2018.01238
412. Wang M, Zhu J, Lubman DM, Gao C. Aberrant glycosylation and cancer biomarker discovery: a promising and thorny journey. Clin Chem Lab Med (2019) 57(4):407–16. doi: 10.1515/cclm-2018-0379
413. Thomas D, Rathinavel AK, Radhakrishnan P. Altered glycosylation in cancer: a promising target for biomarkers and therapeutics. Biochim Biophys Acta Rev Canc (2021) 1875(1):188464. doi: 10.1016/j.bbcan.2020.188464
414. Wang JR, Guan WD, Yau LF, Gao WN, Zhan YQ, Liu L, et al. Glycomic signatures on serum IgGs for prediction of postvaccination response. Sci Rep (2015) 5:7648. doi: 10.1038/srep07648
415. Virtue AT, McCright SJ, Wright JM, Jimenez MT, Mowel WK, Kotzin JJ, et al. The gut microbiota regulates white adipose tissue inflammation and obesity via a family of microRNAs. Sci Transl Med (2019) 11(496):eaav1892. doi: 10.1126/scitranslmed.aav1892
416. Wei M, Huang F, Zhao L, Zhang Y, Yang W, Wang S, et al. A dysregulated bile acid-gut microbiota axis contributes to obesity susceptibility. EBioMedicine (2020) 55:102766. doi: 10.1016/j.ebiom.2020.102766
417. Aggeletopoulou I, Konstantakis C, Assimakopoulos SF, Triantos C. The role of the gut microbiota in the treatment of inflammatory bowel diseases. Microb Pathog (2019) 137:103774. doi: 10.1016/j.micpath.2019.103774
418. Wilkins LJ, Monga M, Miller AW. Defining dysbiosis for a cluster of chronic diseases. Sci Rep (2019) 9(1):12918. doi: 10.1038/s41598-019-49452-y
419. Wang J, Chen WD, Wang YD. The relationship between gut microbiota and inflammatory diseases: the role of macrophages. Front Microbiol (2020) 11:1065. doi: 10.3389/fmicb.2020.01065
420. Allen J, Sears CL. Impact of the gut microbiome on the genome and epigenome of colon epithelial cells: contributions to colorectal cancer development. Genome Med (2019) 11(1):11. doi: 10.1186/s13073-019-0621-2
421. Zhuang H, Cheng L, Wang Y, Zhang YK, Zhao MF, Liang GD, et al. Dysbiosis of the gut microbiome in lung cancer. Front Cell Infect Microbiol (2019) 9:112. doi: 10.3389/fcimb.2019.00112
422. Hagan T, Cortese M, Rouphael N, Boudreau C, Linde C, Maddur MS, et al. Antibiotics-driven gut microbiome perturbation alters immunity to vaccines in humans. Cell (2019) 178(6):1313–28.e13. doi: 10.1016/j.cell.2019.08.010
423. Baković MP, Selman MH, Hoffmann M, Rudan I, Campbell H, Deelder AM, et al. High-throughput IgG fc n-glycosylation profiling by mass spectrometry of glycopeptides. J Proteome Res (2013) 12(2):821–31. doi: 10.1021/pr300887z
424. Bouman A, Heineman MJ, Faas MM. Sex hormones and the immune response in humans. Hum Reprod Update (2005) 11(4):411–23. doi: 10.1093/humupd/dmi008
425. Furman D, Hejblum BP, Simon N, Jojic V, Dekker CL, Thiébaut R, et al. Systems analysis of sex differences reveals an immunosuppressive role for testosterone in the response to influenza vaccination. Proc Natl Acad Sci USA (2014) 111(2):869–74. doi: 10.1073/pnas.1321060111
426. Klein SL, Jedlicka A, Pekosz A. The xs and y of immune responses to viral vaccines. Lancet Infect Dis (2010) 10(5):338–49. doi: 10.1016/S1473-3099(10)70049-9
427. Simon B, Kundi M, Puchhammer-Stöckl E. Association of HCMV specific IgG subclass antibody levels with gender and age. Exp Gerontol (2013) 48(5):472–5. doi: 10.1016/j.exger.2013.02.011
428. Rastawicki W, Smietańska K, Rokosz-Chudziak N, Jagielski M. [Serum immunoglobulin IgG subclass distribution of antibody responses to pertussis toxin and filamentous hemagglutinin of bordetella pertussis in patients with whooping cough]. Med Dosw Mikrobiol (2013) 65(4):269–74.
429. Ercan A, Kohrt WM, Cui J, Deane KD, Pezer M, Yu EW, et al. Estrogens regulate glycosylation of IgG in women and men. JCI Insight (2017) 2(4):e89703. doi: 10.1172/jci.insight.89703
430. Cheng MI, Li JH, Riggan L, Chen B, Tafti RY, Chin S, et al. The X-linked epigenetic regulator UTX controls NK cell-intrinsic sex differences. Nat Immunol (2023) 24(5):780–91. doi: 10.1038/s41590-023-01463-8
431. Klein SL, Flanagan KL. Sex differences in immune responses. Nat Rev Immunol (2016) 16(10):626–38. doi: 10.1038/nri.2016.90
432. Atabani S, Landucci G, Steward MW, Whittle H, Tilles JG, Forthal DN. Sex-associated differences in the antibody-dependent cellular cytotoxicity antibody response to measles vaccines. Clin Diagn Lab Immunol (2000) 7(1):111–3. doi: 10.1128/CDLI.7.1.111-113.2000
433. Baum LL, Cassutt KJ, Knigge K, Khattri R, Margolick J, Rinaldo C, et al. HIV-1 gp120-specific antibody-dependent cell-mediated cytotoxicity correlates with rate of disease progression. J Immunol (1996) 157(5):2168–73.
434. Moraru M, Black LE, Muntasell A, Portero F, López-Botet M, Reyburn HT, et al. NK cell and ig interplay in defense against herpes simplex virus type 1: epistatic interaction of CD16A and IgG1 allotypes of variable affinities modulates antibody-dependent cellular cytotoxicity and susceptibility to clinical reactivation. J Immunol (2015) 195(4):1676–84. doi: 10.4049/jimmunol.1500872
435. Atherton A, Armour KL, Bell S, Minson AC, Clark MR. The herpes simplex virus type 1 fc receptor discriminates between IgG1 allotypes. Eur J Immunol (2000) 30(9):2540–7. doi: 10.1002/1521-4141(200009)30:9<2540::AID-IMMU2540>3.0.CO;2-S
436. Gartland AJ, Li S, McNevin J, Tomaras GD, Gottardo R, Janes H, et al. Analysis of HLA A*02 association with vaccine efficacy in the RV144 HIV-1 vaccine trial. J Virol (2014) 88(15):8242–55. doi: 10.1128/JVI.01164-14
437. Hensen L, Illing PT, Bridie Clemens E, Nguyen THO, Koutsakos M, van de Sandt CE, et al. CD8(+) T cell landscape in indigenous and non-indigenous people restricted by influenza mortality-associated HLA-A*24:02 allomorph. Nat Commun (2021) 12(1):2931. doi: 10.1038/s41467-021-23212-x
438. Mentzer AJ, O'Connor D, Bibi S, Chelysheva I, Clutterbuck EA, Demissie T, et al. Human leukocyte antigen alleles associate with COVID-19 vaccine immunogenicity and risk of breakthrough infection. Nat Med (2022) 29(1):147–57. doi: 10.1038/s41591-022-02078-6
439. Quiñones-Parra S, Grant E, Loh L, Nguyen TH, Campbell KA, Tong SY, et al. Preexisting CD8+ T-cell immunity to the H7N9 influenza a virus varies across ethnicities. Proc Natl Acad Sci USA (2014) 111(3):1049–54. doi: 10.1073/pnas.1322229111
440. Lambert ND, Haralambieva IH, Kennedy RB, Ovsyannikova IG, Pankratz VS, Poland GA. Polymorphisms in HLA-DPB1 are associated with differences in rubella virus-specific humoral immunity after vaccination. J Infect Dis (2015) 211(6):898–905. doi: 10.1093/infdis/jiu553
441. Deepe RN, Kistner-Griffin E, Martin JN, Deeks SG, Pandey JP. Epistatic interactions between fc (GM) and FcγR genes and the host control of human immunodeficiency virus replication. Hum Immunol (2012) 73(3):263–6. doi: 10.1016/j.humimm.2011.12.008
442. Fall A, Dechavanne C, Sabbagh A, Guitard E, Milet J, Garcia A, et al. Susceptibility to plasmodium falciparum malaria: influence of combined polymorphisms of IgG3 gm allotypes and fc gamma receptors IIA, IIIA, and IIIB. Front Immunol (2020) 11:608016. doi: 10.3389/fimmu.2020.608016
443. Pandey JP, Luo Y, Elston RC, Wu Y, Philp FH, Astemborski J, et al. Immunoglobulin allotypes influence IgG antibody responses to hepatitis c virus envelope proteins E1 and E2. Hum Immunol (2008) 69(3):158–64. doi: 10.1016/j.humimm.2008.01.019
444. Gray GE, Bekker LG, Laher F, Malahleha M, Allen M, Moodie Z, et al. Vaccine efficacy of ALVAC-HIV and bivalent subtype c gp120-MF59 in adults. N Engl J Med (2021) 384(12):1089–100. doi: 10.1056/NEJMoa2031499
445. Lemke MM, Theisen RM, Bozich ER, McLean MR, Lee CY, Lopez E, et al. A quantitative approach to unravel the role of host genetics in IgG-FcγR complex formation after vaccination. Front Immunol (2022) 13:820148. doi: 10.3389/fimmu.2022.820148
446. Lemke MM, McLean MR, Lee CY, Lopez E, Bozich ER, Rerks-Ngarm S, et al. A systems approach to elucidate personalized mechanistic complexities of antibody-fc receptor activation post-vaccination. Cell Rep Med (2021) 2(9):100386. doi: 10.1016/j.xcrm.2021.100386
447. Bashirova AA, Zheng W, Akdag M, Augusto DG, Vince N, Dong KL, et al. Population-specific diversity of the immunoglobulin constant heavy G chain (IGHG) genes. Genes Immun (2021) 22(7-8):327–34. doi: 10.1038/s41435-021-00156-2
448. Torkildsen O, Utsi E, Mellgren SI, Harbo HF, Vedeler CA, Myhr KM. Ethnic variation of fc gamma receptor polymorphism in sami and Norwegian populations. Immunology (2005) 115(3):416–21. doi: 10.1111/j.1365-2567.2005.02158.x
449. Dugoujon JM, Hazout S, Loirat F, Mourrieras B, Crouau-Roy B, Sanchez-Mazas A. GM haplotype diversity of 82 populations over the world suggests a centrifugal model of human migrations. Am J Phys Anthropol (2004) 125(2):175–92. doi: 10.1002/ajpa.10405
450. Richardson SI, Moore PL. Targeting fc effector function in vaccine design. Expert Opin Ther Targets (2021) 25(6):467–77. doi: 10.1080/14728222.2021.1907343
451. Sun Y, Izadi S, Callahan M, Deperalta G, Wecksler AT. Antibody-receptor interactions mediate antibody-dependent cellular cytotoxicity. J Biol Chem (2021) 297(1):100826. doi: 10.1016/j.jbc.2021.100826
452. Wang TT, Maamary J, Tan GS, Bournazos S, Davis CW, Krammer F, et al. Anti-HA glycoforms drive b cell affinity selection and determine influenza vaccine efficacy. Cell (2015) 162(1):160–9. doi: 10.1016/j.cell.2015.06.026
453. Zhao J, Nussinov R, Ma B. Antigen binding allosterically promotes fc receptor recognition. MAbs (2019) 11(1):58–74. doi: 10.1080/19420862.2018.1522178
454. Al Qaraghuli MM, Kubiak-Ossowska K, Ferro VA, Mulheran PA. Antibody-protein binding and conformational changes: identifying allosteric signalling pathways to engineer a better effector response. Sci Rep (2020) 10(1):13696. doi: 10.1038/s41598-020-70680-0
455. Bakalar MH, Joffe AM, Schmid EM, Son S, Podolski M, Fletcher DA. Size-dependent segregation controls macrophage phagocytosis of antibody-opsonized targets. Cell (2018) 174(1):131–42.e13. doi: 10.1016/j.cell.2018.05.059
456. Cleary KLS, Chan HTC, James S, Glennie MJ, Cragg MS. Antibody distance from the cell membrane regulates antibody effector mechanisms. J Immunol (2017) 198(10):3999–4011. doi: 10.4049/jimmunol.1601473
457. Bournazos S, DiLillo DJ, Goff AJ, Glass PJ, Ravetch JV. Differential requirements for FcγR engagement by protective antibodies against Ebola virus. Proc Natl Acad Sci USA (2019) 116(40):20054–62. doi: 10.1073/pnas.1911842116
458. Chu TH, Crowley AR, Backes I, Chang C, Tay M, Broge T, et al. Hinge length contributes to the phagocytic activity of HIV-specific IgG1 and IgG3 antibodies. PloS Pathog (2020) 16(2):e1008083. doi: 10.1371/journal.ppat.1008083
459. Bajic G, Maron MJ, Adachi Y, Onodera T, McCarthy KR, McGee CE, et al. Influenza antigen engineering focuses immune responses to a subdominant but broadly protective viral epitope. Cell Host Microbe (2019) 25(6):827–35.e6. doi: 10.1016/j.chom.2019.04.003
460. Pilkington EH, Suys EJA, Trevaskis NL, Wheatley AK, Zukancic D, Algarni A, et al. From influenza to COVID-19: lipid nanoparticle mRNA vaccines at the frontiers of infectious diseases. Acta Biomater (2021) 131:16–40. doi: 10.1016/j.actbio.2021.06.023
461. Van Coillie J, Pongracz T, Rahmöller J, Chen HJ, Geyer CE, van Vught LA, et al. The BNT162b2 mRNA SARS-CoV-2 vaccine induces transient afucosylated IgG1 in naive but not in antigen-experienced vaccinees. EBioMedicine (2023) 87:104408. doi: 10.1016/j.ebiom.2022.104408
462. Chakraborty S, Gonzalez JC, Sievers BL, Mallajosyula V, Chakraborty S, Dubey M, et al. Early non-neutralizing, afucosylated antibody responses are associated with COVID-19 severity. Sci Transl Med (2022) 14(635):eabm7853. doi: 10.1126/scitranslmed.abm7853
463. Tokatlian T, Read BJ, Jones CA, Kulp DW, Menis S, Chang JYH, et al. Innate immune recognition of glycans targets HIV nanoparticle immunogens to germinal centers. Science (2019) 363(6427):649–54. doi: 10.1126/science.aat9120
464. Kato Y, Abbott RK, Freeman BL, Haupt S, Groschel B, Silva M, et al. Multifaceted effects of antigen valency on b cell response composition and differentiation in vivo. Immunity (2020) 53(3):548–63.e8. doi: 10.1016/j.immuni.2020.08.001
465. Kelly HG, Tan HX, Juno JA, Esterbauer R, Ju Y, Jiang W, et al. Self-assembling influenza nanoparticle vaccines drive extended germinal center activity and memory b cell maturation. JCI Insight (2020) 5(10):e136653. doi: 10.1172/jci.insight.136653
466. Vu MN, Kelly HG, Tan HX, Juno JA, Esterbauer R, Davis TP, et al. Hemagglutinin functionalized liposomal vaccines enhance germinal center and follicular helper T cell immunity. Adv Healthc Mater (2021) 10(10):e2002142. doi: 10.1002/adhm.202002142
467. Chen JL, Fries CN, Berendam SJ, Rodgers NS, Roe EF, Wu Y, et al. Self-assembling peptide nanofiber HIV vaccine elicits robust vaccine-induced antibody functions and modulates fc glycosylation. Sci Adv (2022) 8(38):eabq0273. doi: 10.1126/sciadv.abq0273
468. Lofano G, Gorman MJ, Yousif AS, Yu WH, Fox JM, Dugast AS, et al. Antigen-specific antibody fc glycosylation enhances humoral immunity via the recruitment of complement. Sci Immunol (2018) 3(26):eaat7796. doi: 10.1126/sciimmunol.aat7796
469. Maamary J, Wang TT, Tan GS, Palese P, Ravetch JV. Increasing the breadth and potency of response to the seasonal influenza virus vaccine by immune complex immunization. Proc Natl Acad Sci USA (2017) 114(38):10172–7. doi: 10.1073/pnas.1707950114
470. Routhu NK, Stampfer SD, Lai L, Akhtar A, Tong X, Yuan D, et al. Efficacy of mRNA-1273 and novavax ancestral or BA.1 spike booster vaccines against SARS-CoV-2 BA.5 infection in non-human primates. Sci Immunol (2023) 0:eadg7015. doi: 10.1126/sciimmunol.adg7015
471. Kaplonek P, Cizmeci D, Fischinger S, Collier AR, Suscovich T, Linde C, et al. mRNA-1273 and BNT162b2 COVID-19 vaccines elicit antibodies with differences in fc-mediated effector functions. Sci Transl Med (2022) 14(645):eabm2311. doi: 10.1126/scitranslmed.abm2311
472. Tam HH, Melo MB, Kang M, Pelet JM, Ruda VM, Foley MH, et al. Sustained antigen availability during germinal center initiation enhances antibody responses to vaccination. Proc Natl Acad Sci USA (2016) 113(43):E6639–e48. doi: 10.1073/pnas.1606050113
473. Lee JH, Sutton HJ, Cottrell CA, Phung I, Ozorowski G, Sewall LM, et al. Long-primed germinal centres with enduring affinity maturation and clonal migration. Nature (2022) 609(7929):998–1004. doi: 10.1038/s41586-022-05216-9
474. Chaudhury S, Regules JA, Darko CA, Dutta S, Wallqvist A, Waters NC, et al. Delayed fractional dose regimen of the RTS,S/AS01 malaria vaccine candidate enhances an IgG4 response that inhibits serum opsonophagocytosis. Sci Rep (2017) 7(1):7998. doi: 10.1038/s41598-017-08526-5
475. Regules JA, Cicatelli SB, Bennett JW, Paolino KM, Twomey PS, Moon JE, et al. Fractional third and fourth dose of RTS,S/AS01 malaria candidate vaccine: a phase 2a controlled human malaria parasite infection and immunogenicity study. J Infect Dis (2016) 214(5):762–71. doi: 10.1093/infdis/jiw237
476. Ntoumi F, Kremsner PG. Vaccination with fractional doses: promise or illusion? Lancet Infect Dis (2022) 22(9):1258–9. doi: 10.1016/S1473-3099(22)00310-3
477. White MT, Verity R, Griffin JT, Asante KP, Owusu-Agyei S, Greenwood B, et al. Immunogenicity of the RTS,S/AS01 malaria vaccine and implications for duration of vaccine efficacy: secondary analysis of data from a phase 3 randomised controlled trial. Lancet Infect Dis (2015) 15(12):1450–8. doi: 10.1016/S1473-3099(15)00239-X
478. Dangi T, Sanchez S, Lew MH, Awakoaiye B, Visvabharathy L, Richner JM, et al. Pre-existing immunity modulates responses to mRNA boosters. Cell Rep (2023) 42(3):112167. doi: 10.1016/j.celrep.2023.112167
479. Boudreau CM, JSt B, AL R, MJ G, Mundle S, Lingwood D, et al. Pre-existing fc profiles shape the evolution of neutralizing antibody breadth following influenza vaccination. Cell Rep Med (2023) 4(3):100975. doi: 10.1016/j.xcrm.2023.100975
480. Nimmerjahn F, Ravetch JV. Antibody-mediated modulation of immune responses. Immunol Rev (2010) 236:265–75. doi: 10.1111/j.1600-065X.2010.00910.x
481. Knudsen NP, Olsen A, Buonsanti C, Follmann F, Zhang Y, Coler RN, et al. Different human vaccine adjuvants promote distinct antigen-independent immunological signatures tailored to different pathogens. Sci Rep (2016) 6:19570. doi: 10.1038/srep19570
482. Zilker C, Kozlova D, Sokolova V, Yan H, Epple M, Überla K, et al. Nanoparticle-based b-cell targeting vaccines: tailoring of humoral immune responses by functionalization with different TLR-ligands. Nanomedicine (2017) 13(1):173–82. doi: 10.1016/j.nano.2016.08.028
483. Kaplonek P, Cizmeci D, Lee JS, Shin SA, Fischinger S, Gobeil P, et al. Robust induction of functional humoral response by a plant-derived coronavirus-like particle vaccine candidate for COVID-19. NPJ Vaccines (2023) 8(1):13. doi: 10.1038/s41541-023-00612-2
484. Loos C, Coccia M, Didierlaurent AM, Essaghir A, Fallon JK, Lauffenburger D, et al. Systems serology-based comparison of antibody effector functions induced by adjuvanted vaccines to guide vaccine design. NPJ Vaccines (2023) 8(1):34. doi: 10.1038/s41541-023-00613-1
485. Arunachalam PS, Walls AC, Golden N, Atyeo C, Fischinger S, Li C, et al. Adjuvanting a subunit COVID-19 vaccine to induce protective immunity. Nature (2021) 594(7862):253–8. doi: 10.1038/s41586-021-03530-2
486. Moody MA, Santra S, Vandergrift NA, Sutherland LL, Gurley TC, Drinker MS, et al. Toll-like receptor 7/8 (TLR7/8) and TLR9 agonists cooperate to enhance HIV-1 envelope antibody responses in rhesus macaques. J Virol (2014) 88(6):3329–39. doi: 10.1128/JVI.03309-13
487. Singh S, Ramírez-Salazar EG, Doueiri R, Valentin A, Rosati M, Hu X, et al. Control of heterologous simian immunodeficiency virus SIV(smE660) infection by DNA and protein coimmunization regimens combined with different toll-Like-Receptor-4-Based adjuvants in macaques. J Virol (2018) 92(15):e00281–18. doi: 10.1128/JVI.00281-18
488. O'Hagan DT, van der Most R, Lodaya RN, Coccia M, Lofano G. "World in motion" - emulsion adjuvants rising to meet the pandemic challenges. NPJ Vaccines (2021) 6(1):158. doi: 10.1038/s41541-021-00418-0
489. Ko EJ, Lee YT, Kim KH, Jung YJ, Lee Y, Denning TL, et al. Effects of MF59 adjuvant on induction of isotype-switched IgG antibodies and protection after immunization with T-dependent influenza virus vaccine in the absence of CD4+ T cells. J Virol (2016) 90(15):6976–88. doi: 10.1128/JVI.00339-16
490. De Donato S, Granoff D, Minutello M, Lecchi G, Faccini M, Agnello M, et al. Safety and immunogenicity of MF59-adjuvanted influenza vaccine in the elderly. Vaccine (1999) 17(23-24):3094–101. doi: 10.1016/S0264-410X(99)00138-3
491. Coleman BL, Sanderson R, Haag MDM, McGovern I. Effectiveness of the MF59-adjuvanted trivalent or quadrivalent seasonal influenza vaccine among adults 65 years of age or older, a systematic review and meta-analysis. Influenza Other Respir Viruses (2021) 15(6):813–23. doi: 10.1111/irv.12871
492. Banzhoff A, Nacci P, Podda A. A new MF59-adjuvanted influenza vaccine enhances the immune response in the elderly with chronic diseases: results from an immunogenicity meta-analysis. Gerontology (2003) 49(3):177–84. doi: 10.1159/000069172
493. Vaccari M, Gordon SN, Fourati S, Schifanella L, Liyanage NP, Cameron M, et al. Adjuvant-dependent innate and adaptive immune signatures of risk of SIVmac251 acquisition. Nat Med (2016) 22(7):762–70. doi: 10.1038/nm.4105
494. Om K, Paquin-Proulx D, Montero M, Peachman K, Shen X, Wieczorek L, et al. Adjuvanted HIV-1 vaccine promotes antibody-dependent phagocytic responses and protects against heterologous SHIV challenge. PloS Pathog (2020) 16(9):e1008764. doi: 10.1371/journal.ppat.1008764
495. Messina NL, Germano S, McElroy R, Rudraraju R, Bonnici R, Pittet LF, et al. Off-target effects of bacillus calmette-guérin vaccination on immune responses to SARS-CoV-2: implications for protection against severe COVID-19. Clin Transl Immunol (2022) 11(4):e1387. doi: 10.1002/cti2.1387
496. Leentjens J, Kox M, Stokman R, Gerretsen J, Diavatopoulos DA, van Crevel R, et al. BCG Vaccination enhances the immunogenicity of subsequent influenza vaccination in healthy volunteers: a randomized, placebo-controlled pilot study. J Infect Dis (2015) 212(12):1930–8. doi: 10.1093/infdis/jiv332
497. Arts RJW, Moorlag S, Novakovic B, Li Y, Wang SY, Oosting M, et al. BCG Vaccination protects against experimental viral infection in humans through the induction of cytokines associated with trained immunity. Cell Host Microbe (2018) 23(1):89–100.e5. doi: 10.1016/j.chom.2017.12.010
498. Moorlag S, Arts RJW, van Crevel R, Netea MG. Non-specific effects of BCG vaccine on viral infections. Clin Microbiol Infect (2019) 25(12):1473–8. doi: 10.1016/j.cmi.2019.04.020
499. Lavelle EC, Ward RW. Mucosal vaccines - fortifying the frontiers. Nat Rev Immunol (2022) 22(4):236–50. doi: 10.1038/s41577-021-00583-2
500. Ackerman ME, Das J, Pittala S, Broge T, Linde C, Suscovich TJ, et al. Route of immunization defines multiple mechanisms of vaccine-mediated protection against SIV. Nat Med (2018) 24(10):1590–8. doi: 10.1038/s41591-018-0161-0
501. Bowman KA, Stein D, Shin S, Ferbas KG, Tobin NH, Mann C, et al. Hybrid immunity shifts the fc-effector quality of SARS-CoV-2 mRNA vaccine-induced immunity. mBio (2022) 13(5):e0164722. doi: 10.1128/mbio.01647-22
502. Pittala S, Morrison KS, Ackerman ME. Systems serology for decoding infection and vaccine-induced antibody responses to HIV-1. Curr Opin HIV AIDS (2019) 14(4):253–64. doi: 10.1097/COH.0000000000000558
503. Benedict KF, Lauffenburger DA. Insights into proteomic immune cell signaling and communication via data-driven modeling. Curr Top Microbiol Immunol (2013) 363:201–33. doi: 10.1007/82_2012_249
504. Zhang W, Chua BY, Selva KJ, Kedzierski L, Ashhurst TM, Haycroft ER, et al. SARS-CoV-2 infection results in immune responses in the respiratory tract and peripheral blood that suggest mechanisms of disease severity. Nat Commun (2022) 13(1):2774. doi: 10.1038/s41467-022-30088-y
505. Kim HW, Canchola JG, Brandt CD, Pyles G, Chanock RM, Jensen K, et al. Respiratory syncytial virus disease in infants despite prior administration of antigenic inactivated vaccine. Am J Epidemiol (1969) 89(4):422–34. doi: 10.1093/oxfordjournals.aje.a120955
506. Nader PR, Horwitz MS, Rousseau J. Atypical exanthem following exposure to natural measles: eleven cases in children previously inoculated with killed vaccine. J Pediatrics (1968) 72(1):22–8. doi: 10.1016/S0022-3476(68)80396-8
507. Dejnirattisai W, Jumnainsong A, Onsirisakul N, Fitton P, Vasanawathana S, Limpitikul W, et al. Cross-reacting antibodies enhance dengue virus infection in humans. Science (2010) 328(5979):745–8. doi: 10.1126/science.1185181
508. Sridhar S, Luedtke A, Langevin E, Zhu M, Bonaparte M, Machabert T, et al. Effect of dengue serostatus on dengue vaccine safety and efficacy. N Engl J Med (2018) 379(4):327–40. doi: 10.1056/NEJMoa1800820
509. Lee WS, Wheatley AK, Kent SJ, DeKosky BJ. Antibody-dependent enhancement and SARS-CoV-2 vaccines and therapies. Nat Microbiol (2020) 5(10):1185–91. doi: 10.1038/s41564-020-00789-5
510. Vogelpoel LT, Baeten DL, de Jong EC, den Dunnen J. Control of cytokine production by human fc gamma receptors: implications for pathogen defense and autoimmunity. Front Immunol (2015) 6:79. doi: 10.3389/fimmu.2015.00079
511. Yu Y, Wang M, Zhang X, Li S, Lu Q, Zeng H, et al. Antibody-dependent cellular cytotoxicity response to SARS-CoV-2 in COVID-19 patients. Signal Transduct Target Ther (2021) 6(1):346. doi: 10.1038/s41392-021-00759-1
512. Tso FY, Lidenge SJ, Poppe LK, Peña PB, Privatt SR, Bennett SJ, et al. Presence of antibody-dependent cellular cytotoxicity (ADCC) against SARS-CoV-2 in COVID-19 plasma. PloS One (2021) 16(3):e0247640. doi: 10.1371/journal.pone.0247640
513. Junqueira C, Crespo Â, Ranjbar S, de Lacerda LB, Lewandrowski M, Ingber J, et al. FcγR-mediated SARS-CoV-2 infection of monocytes activates inflammation. Nature (2022) 606(7914):576–84. doi: 10.1038/s41586-022-04702-4
514. Selva KJ, Chung AW. Insights into how SARS-CoV2 infection induces cytokine storms. Trends Immunol (2022) 43(6):417–9. doi: 10.1016/j.it.2022.04.007
515. Lambris JD, Ricklin D, Geisbrecht BV. Complement evasion by human pathogens. Nat Rev Microbiol (2008) 6(2):132–42. doi: 10.1038/nrmicro1824
516. Vossen MT, Westerhout EM, Söderberg-Nauclér C, Wiertz EJ. Viral immune evasion: a masterpiece of evolution. Immunogenetics (2002) 54(8):527–42. doi: 10.1007/s00251-002-0493-1
517. Foster TJ, Geoghegan JA, Ganesh VK, Höök M. Adhesion, invasion and evasion: the many functions of the surface proteins of staphylococcus aureus. Nat Rev Microbiol (2014) 12(1):49–62. doi: 10.1038/nrmicro3161
518. Jenks JA, Goodwin ML, Permar SR. The roles of host and viral antibody fc receptors in herpes simplex virus (HSV) and human cytomegalovirus (HCMV) infections and immunity. Front Immunol (2019) 10:2110. doi: 10.3389/fimmu.2019.02110
519. Corrales-Aguilar E, Hoffmann K, Hengel H. CMV-encoded fcγ receptors: modulators at the interface of innate and adaptive immunity. Semin Immunopathol (2014) 36(6):627–40. doi: 10.1007/s00281-014-0448-2
520. Corrales-Aguilar E, Trilling M, Hunold K, Fiedler M, Le VT, Reinhard H, et al. Human cytomegalovirus fcγ binding proteins gp34 and gp68 antagonize fcγ receptors I, II and III. PloS Pathog (2014) 10(5):e1004131. doi: 10.1371/journal.ppat.1004131
521. Awasthi S, Friedman HM. An mRNA vaccine to prevent genital herpes. Transl Res (2022) 242:56–65. doi: 10.1016/j.trsl.2021.12.006
522. Plotkin SA, Wang D, Oualim A, Diamond DJ, Kotton CN, Mossman S, et al. The status of vaccine development against the human cytomegalovirus. J Infect Dis (2020) 221(Suppl 1):S113–s22. doi: 10.1093/infdis/jiz447
523. Namboodiri AM, Pandey JP. The human cytomegalovirus TRL11/IRL11-encoded FcγR binds differentially to allelic variants of immunoglobulin G1. Arch Virol (2011) 156(5):907–10. doi: 10.1007/s00705-011-0937-8
524. Pandey JP, Namboodiri AM, Radwan FF, Nietert PJ. The decoy fcγ receptor encoded by the cytomegalovirus UL119-UL118 gene has differential affinity to IgG proteins expressing different GM allotypes. Hum Immunol (2015) 76(8):591–4. doi: 10.1016/j.humimm.2015.09.005
Keywords: antibody, allotype, polymorphism, Fc receptor, Fc function, computational modelling, vaccine design, IgG glycosylation
Citation: Purcell RA, Theisen RM, Arnold KB, Chung AW and Selva KJ (2023) Polyfunctional antibodies: a path towards precision vaccines for vulnerable populations. Front. Immunol. 14:1183727. doi: 10.3389/fimmu.2023.1183727
Received: 10 March 2023; Accepted: 30 May 2023;
Published: 27 June 2023.
Edited by:
Ofer Levy, Boston Children’s Hospital and Harvard Medical School, United StatesReviewed by:
Dapeng Zhou, Tongji University, ChinaHans Verkerke, Emory University, United States
Kelly Seaton, Duke University, United States
Copyright © 2023 Purcell, Theisen, Arnold, Chung and Selva. This is an open-access article distributed under the terms of the Creative Commons Attribution License (CC BY). The use, distribution or reproduction in other forums is permitted, provided the original author(s) and the copyright owner(s) are credited and that the original publication in this journal is cited, in accordance with accepted academic practice. No use, distribution or reproduction is permitted which does not comply with these terms.
*Correspondence: Amy W. Chung, YXdjaHVuZ0B1bmltZWxiLmVkdS5hdQ==; Kevin J. Selva, a2V2aW4uc2VsdmFAdW5pbWVsYi5lZHUuYXU=