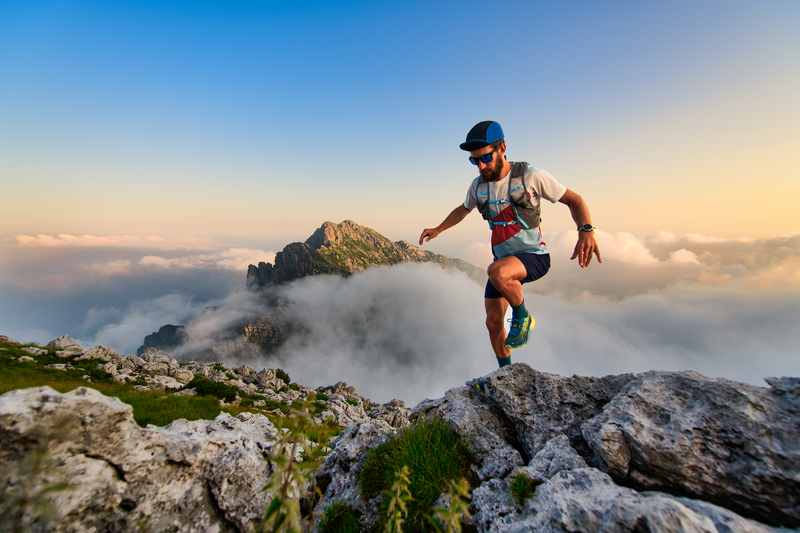
94% of researchers rate our articles as excellent or good
Learn more about the work of our research integrity team to safeguard the quality of each article we publish.
Find out more
HYPOTHESIS AND THEORY article
Front. Immunol. , 26 April 2023
Sec. Vaccines and Molecular Therapeutics
Volume 14 - 2023 | https://doi.org/10.3389/fimmu.2023.1183258
This article is part of the Research Topic Overcoming Challenges in Vaccines and Molecular Therapeutics: 2022 View all 12 articles
COVID-19 vaccination is a life-saving intervention. However, it does not come up without a risk of rare adverse events, which frequency varies between vaccines developed using different technological platforms. The increased risk of Guillain-Barré syndrome (GBS) has been reported for selected adenoviral vector vaccines but not for other vaccine types, including more widely used mRNA preparations. Therefore, it is unlikely that GBS results from the cross-reactivity of antibodies against the SARS-CoV-2 spike protein generated after the COVID-19 vaccination. This paper outlines two hypotheses according to which increased risk of GBS following adenoviral vaccination is due to (1) generation of anti-vector antibodies that may cross-react with proteins involved in biological processes related to myelin and axons, or (2) neuroinvasion of selected adenovirus vectors to the peripheral nervous system, infection of neurons and subsequent inflammation and neuropathies. The rationale behind these hypotheses is outlined, advocating further epidemiological and experimental research to verify them. This is particularly important given the ongoing interest in using adenoviruses in developing vaccines against various infectious diseases and cancer immunotherapeutics.
The COVID-19 pandemic has been met with unprecedented scientific efforts, including developing and clinical testing numerous vaccine candidates at an unseen pace (1, 2). China reported the first cluster of cases in late December 2019, the first genomic data of its etiological agent, later named severe acute respiratory syndrome coronavirus 2 (SARS-CoV-2), became publicly available in the first half of January 2020 (3), while the first COVID-19 vaccines were authorized by the end of 2020. By the end of 2021, over twenty different COVID-19 vaccines were already in use in various world regions (4). These included preparations based on the inactivated virus (e.g., BIBP-CorV by Sinopharm and CoronaVac by Sinovac Biotech), mRNA technology (e.g., BNT162b2 by BioNTech/Pfizer, and mRNA-1273 by Moderna), non-replicating adenoviral vector (e.g., AZD1222 by AstraZeneca, Ad26.COV2.S by Janssen/Johnson&Johnson, Ad5-nCoV by Cansino Biologics, and Sputnik V by Gamaleya Research Institute of Epidemiology and Microbiology), plasmid DNA (ZyCoV-D by Cadila Healthcare), and subunit protein (e.g., NVX-CoV2373 by Novavax) (4).
The COVID-19 vaccination has been proven to be a life-saving intervention. As estimated, it reduced COVID-19 mortality by two-thirds in 2021, averting globally 19.8 million deaths (5). It was also associated with lower admissions rates to intensive care units and hospitalizations (6–8), and evidence shows that it also reduced long-term consequences of SARS-CoV-2 infections, known as post-COVID syndrome (6, 7). With time it became evident that booster doses are required to restore gradually decreasing levels of neutralizing antibodies and address the novel SARS-CoV-2 variants that revealed varying levels of immune evasion (8, 9). Moreover, in response to the emergence of the Omicron variant and its further evolution, the bivalent mRNA vaccines, containing the second component optimized for Omicron BA.1 or BA.4/BA.5 subvariants, were authorized in the second half of 2022. All in all, this resulted in repeated administration of COVID-19 vaccines, with over 13 billion doses administrated by the end of 2022 and 69% of the world population receiving at least one dose (10).
Although the clinical trials have demonstrated a good safety profile of COVID-19 vaccines, with the reactogenicity represented mainly by short-term local (e.g., injection-site pain, redness, swelling) or systemic responses (e.g., fatigue, fever, headache), one should note that these studies, despite thousands of participants, were not designed to detect very rare adverse events that may occur during the massive vaccination campaign. For example, after authorization, mRNA vaccination has been associated with an increased risk of myocarditis and pericarditis (11), while unusual thrombotic events associated with thrombocytopenia have been recorded following the administration of adenoviral vector vaccines (12, 13). Moreover, some adenoviral vaccines were also associated with the risk of Guillain-Barré syndrome (GBS), a rare, immune-mediated neurologic disorder characterized by varying degrees of weakness, sensory abnormalities, and autonomic dysfunction due to damage to peripheral nerves and nerve roots (14). GBS is usually reversible, but in severe cases, it may lead to breathing difficulties, require mechanical ventilation, and leave permanent neurological alterations (15). Its worldwide annual incidence is estimated at 0.8-1.9 cases per 100,000 persons, with frequency increasing with age and being more common in men (16).
In 2021, the population-based study employing data from the National Health Service in England identified that administration of the first dose of AZD1222, based on a modified chimpanzee adenovirus (ChAdOx1), was associated with an excess of GBS risk of 0.58 cases per 100,000 doses (17). In India, the reported frequency of GBS following AZD1222 was 1.4- to 10-fold higher than expected for a population and included severe cases progressing to areflexic quadriplegia (18), while in Australia, it exceeded the background level by 4.7-fold (19). In 2021, a preliminary safety concern for GBS following a single dose of Ad26.COV2.S vaccination, based on modified human adenovirus serotype 26 (HAdV26), was detected in the United States, with the observed-to-expected ratio of 4.2 over the 42-day observation (20). More recently, this concern was confirmed by the analysis of the U.S. Vaccine Adverse Event Reporting System (21). As observed, the administration of Ad26.COV2.S vaccine was associated with an increased risk of GBS, with the observed-to-expected ratio of 3.8 and 2.3 within 21 and 42 days of vaccination, respectively (21). Analysis of VigiBase, which contains spontaneous reports of adverse drug reactions from 149 countries, again indicated that adenoviral vector COVID-19 vaccines AZD1222 and Ad26.COV2.S are associated with the increased observed-to-expected ratio of GBS consistently exceeding 2.0 for all analyzed countries (22). Cases of GBS were also reported following the administration of the first dose of the Sputnik V adenoviral vaccine (containing HAdV26), and its modification Sputnik Light (that is also based on the same adenoviral vector), was also reported in the literature (23, 24). However, no comprehensive epidemiological surveillance data for these vaccines encompassing GBS was published. Interestingly though, no increased risk of GBS was seen in the case of Ad5-nCoV (although GBS cases after its administration were reported) (25, 26), which is the only adenoviral COVID-19 vaccine based solely on human adenovirus serotype 5 (HAdV5) given as a single dose (27).
Although the GBS risk is increased following the administration of certain adenoviral COVID-19 vaccines, cases of this condition remain rare. This indicates the potential existence of a susceptible group of individuals among vaccinees. Such a susceptibility may be due to genetic predisposition linked, albeit not without controversies, to genes encoding human leukocyte antigens, a cluster of differentiation 1A, FAS, Fc gamma receptors, intercellular adhesion molecule-1, nucleotide oligomerization domain, toll-like receptor 4, tumor necrosis factor-α, and various interleukins (28). However, the association between COVID-19 vaccination and polygenic GBS risk was not subject to any study that the author of this paper is aware of. Nevertheless, such genetic predisposition cannot solely explain why GBS cases following COVID-19 vaccination are biased toward some of the adenoviral vector vaccines. Examining the mechanisms behind this phenomenon is pivotal in taking precautionary measures, adjusting recommendations, and managing vaccine hesitancy. Similarly to the need to understand whether an increased risk of peri- and myocarditis is associated solely with COVID-19 mRNA vaccines or mRNA technology in general (29, 30), it is essential to elucidate the exact nature of the relationship between GBS and adenoviral vaccines. Such knowledge can be potentially beneficial in improving the technological platforms employing adenoviruses as gene delivery systems. It is also imperative given that non-replicating and single-cycle adenoviruses have been recognized as suitable vaccine vectors as they can be easily modified and manufactured, revealing a broad spectrum of host cell tropism and high gene expression, and, consequently, inducing a robust innate and adaptive response (31). Currently, adenoviruses are utilized in authorized vaccines against COVID-19 (Table 1) and filoviruses (Ad26.ZEBOV by Janssen/Johnson&Johnson) (32). No safety signals regarding GBS were observed during clinical trials of Ad26.ZEBOV vaccine (based on adenovirus type 26, similarly to Ad26.COV2.S) (33–36), although one should note that such studies are not designed to detect very rare adverse events, while at the same time, contrary to Ad26.COV2.S, there is no broad post-authorization experience with this vaccine.
The clinical trials of various adenoviral vector vaccine candidates are ongoing, including preparations against other coronaviruses (MERS-CoV), influenza, malaria, RSV, tuberculosis, and Chikungunya virus, and even more candidates are in earlier stages of testing (37–40). In addition to classical intramuscular injections, adenoviral vaccine candidates for oral, intradermal, and intranasal administration have also been developed. Such routes are substantially less invasive and painless, hence more attractive for individuals with needle phobia, while potentially offering additional immunological benefits (e.g., sterilizing immunity in the case of intranasal preparations) (41–43). There is also an interest in exploring adenoviral-based cancer gene therapies (44, 45). In other words, a portfolio of medical use of adenoviruses is likely to increase in the future; thus, understanding all potential safety issues of their administration is highly important.
This paper hypothesizes that the administration of selected adenoviruses employed in the vector vaccines is triggering the adaptive response to the viral vector that can eventually lead to autoimmunity against proteins of the peripheral nervous system and induce GBS. An alternative hypothesis is also presented and implies the neuroinvasion of selected adenoviral vectors, their interaction with peripheral neurons, and the induction of subsequent inflammation and neuropathies associated with GBS.
In general, the etiology of GBS remains not fully known. It is suggested to be predominantly an autoimmune condition resulting from molecular mimicry. Under this scenario, autoantibodies, induced by an external factor, target epitopes on peripheral nerves and induce axonal damage and neuronal demyelination (46, 47). Therefore, some authors suggested that GBS following the administration of the COVID-19 adenoviral vector vaccine is due to cross-reaction between generated antibodies against SARS-CoV-2 spike protein and neuronal proteins (48–50). Moreover, using a bioinformatic approach the molecular mimicry was predicted between spike protein epitopes and sequences of eight proteins involved in biological processes related to myelin and axons, i.e., neural cell adhesion molecule, receptor-type tyrosine-protein phosphatase zeta, teneurin-4, receptor tyrosine-protein kinase erbB-2-, integrin alpha-X, integrin beta-1, attractin, and myelin-associated glycoprotein (51). Therefore, the cross-reactivity of anti-spike antibodies with these proteins could potentially lead to severe neurological conditions, such as GBS.
However, if this assumption holds, GBS should be frequently observed in COVID-19 patients and individuals vaccinated with any COVID-19 vaccine since they all use spike protein as an antigen. A systematic review of 79 papers published until February 2021 identified 109 GBS cases related to COVID-19, including 16 cases in the United States (52). However, by the time this review was performed, over 103 million cases of SARS-CoV-2 had been confirmed worldwide, of which nearly 26.5 million were reported in the United States (10). It indicates that the frequency of GBS in COVID-19 is much lower than expected when assuming the involvement of anti-spike antibodies, especially considering that most infected patients produce their detectable levels (53–55). Moreover, some COVID-19 patients who presented with GBS tested positive for antibodies against antigangliosides (52), which can be implicated in selected variants of this condition (56). Furthermore, the reported GBS cases were more common in men, which aligns with the general tendency observed for this condition (16). Therefore, it can be suggested that GBS is a rare complication in COVID-19, which pathogenesis is unlikely due to cross-reactive anti-spike antibodies, but possibly result from other causes, e.g., neuroinvasion of SARS-CoV-2 (as there are reports on its presence in cerebrospinal fluid and brain tissue) (57). It may also be due to particular genetic predispositions postulated to influence the GBS risk (28).
Moreover, although GBS cases were observed in individuals vaccinated with different COVID-19 vaccines, its increased risk was only found for adenoviral vector vaccines (19, 21, 22, 58). Therefore, European Medicine Agency included a warning of GBS in the updated versions of package information of AZD1222 and Ad26.COV2.S vaccines (59, 60), but not for other COVID-19 vaccines. In many developed regions, e.g., the United States and European Union, the vaccination campaigns were disproportionally based on mRNA vaccines (e.g., nearly 1 billion doses of mRNA vaccines vs. approximately 185 million doses of adenoviral vector vaccines were given in the European Economic Area by early February 2023) (61). Therefore, if the post-vaccination GBS was related to cross-reactive anti-spike antibodies generated after immunization, it should be predominantly observed after mRNA, not adenoviral vaccination, but the evidence points to the contrary (19, 21, 58). Moreover, the varying risk of GBS observed for COVID-19 vaccines cannot be explained by the difference in the prefusion stability of full-length spike protein encoded by adenoviral, and mRNA vaccines, and present in subunit protein vaccines. Although ChAdOx1 adenovirus in AZD1222 encodes wild-type viral spike, which can lead to the sporadic presentation of post-fusion spike conformation on the cell surface (62), Ad26.COV2.S vaccine utilizes modified human adenovirus encoding stabilized prefusion spike protein (by substituting two residues with proline), similar to the spike encoded by both mRNA vaccines and present in NVX-CoV2373 subunit vaccine (63–66).
In summary, the evidence indicates that the increased risk of GBS following adenoviral vector vaccines against COVID-19 is more likely related to the response to administrated adenoviruses than the effect of cross-reactivity of anti-spike antibodies induced following immunization. This tentatively supports the hypothesis presented in this paper and suggests focusing further on selected adenoviral vectors as potential etiological agents of GBS.
Pathogen infections, including the gram-negative bacteria Campylobacter jejuni (32% of cases) and Mycoplasma pneumoniae (5%), and DNA viruses human cytomegalovirus (10-15%) and Epstein-Barr virus (8-10%), were implicated as one of the precipitants of GBS (67, 68). Such association with adenoviruses, potentially providing additional clues in understanding the increased risk of GBS after adenoviral vector vaccines, was substantially less explored. This is likely because adenoviral infections in immunocompetent individuals are mostly asymptomatic or self-limiting and do not require any specific treatment apart from supportive care (69). The first study addressing the potential association between adenoviral infections and GBS that included 92 GBS cases was published in 1977 and did not find such a relationship; instead, it highlighted that cytomegalovirus might be a common agent involved in the pathogenesis of GBS (70). The second study was published 25 years ago, analyzed 154 GBS cases, and reported the anti-adenovirus antibodies in 1% of samples while highlighting the association between antecedent C. jejuni infection and GBS and antiganglioside antibodies (68). However, the employed determinations in these studies were based on complement fixation, an old method, later suppressed by more accurate enzyme-linked immunosorbent assays (71, 72). Nevertheless, the findings of these studies likely caused no interest in further exploration of links between adenovirus infections and GBS using a more sensitive approach. The necessity to conduct such research reemergences now, after increased GBS risk was evidenced for selected adenoviral vector vaccines against COVID-19. Moreover, to evaluate the hypothesis put forward in the present paper, such investigations should distinguish between infections caused by different adenoviruses, with a primary focus on HAdV26, a component of Ad26.COV2.S vaccine, which administration was associated with an excess of GBS cases. In this context, the research should primarily focus on selected African and Asian populations since they demonstrate the highest seroprevalence of HAdV26 (73, 74). Although these regions are characterized by the lower reported GBS morbidity, it is acknowledged that this might be due to resource-limited settings resulting in a significant underestimation of cases (75). Including HAdV5 infections for comparison in such research would also be informative in understanding why no association between Ad5-nCoV vaccination and GBS was observed.
Most human adenoviruses are not associated with severe disease, and their infections are often asymptomatic (69). Therefore, there was no urgent need to develop, test and authorize the broadly available vaccine against adenoviruses. However, human adenovirus serotype 4 (HAdV4) and serotype 7 (HAdV7) have been reported to induce febrile acute respiratory disease and became a leading cause of hospitalization of U.S. Army personnel. To mitigate it, live oral vaccines have been used since the 1970s to protect U.S. soldiers from severe HAdV4 and HAdV7 infections (76, 77). The latest vaccine against these viruses, developed by Barr Labs, Inc., was licensed by the U.S. Food and Drug Administration in 2011 for military personnel aged 17-50. If exposure to adenoviral vectors was potentially a precipitant of GBS, one could expect to see cases of this condition following the administration of the adenovirus vaccine. In line with this, the analysis of the U.S. Vaccine Adverse Event Reporting System for reports among individuals who were immunized with the adenovirus vaccine between 2011 and 2018 found that GBS was the most frequently reported severe adverse events with the median onset time of 24 days from vaccination (78). However, one should note that the frequency of GBS was approximately 1.0 per 100,000 vaccinated individuals, which is within the background level (16). The authors speculate that these cases may also be due to the common co-administration of other vaccines and prophylactic intramuscular antibiotics in military personnel, complicating an understanding of the direct link with vaccination against adenoviruses (78). Nevertheless, such a link cannot be excluded and advocates further research, particularly in the light of GBS cases reported following adenoviral vector COVID-19 vaccines and the hypothesis outlined in the present paper.
Administration of adenoviruses as vaccine vectors is associated with the induction of anti-vector antibodies, also in the case of modified non-replicating adenoviruses, which are classically obtained by deletion of E1/E3 region and lead to transient infection. As shown in phase 1 and 2/3 clinical trials of the AZD1222 vaccine, the administration of the first dose elicited anti-vector (anti-ChAdOx1) antibodies across different age groups, which remained detectable at high levels at least 84 days (last time point assessed) since vaccination (79, 80). ChAdOx1 is based on chimpanzee adenovirus Y25 with a very low baseline seroprevalence in the human population, which predisposed it as a good vector candidate (since pre-existing anti-vector immunity may decrease the efficacy of the vaccination). However, it was known that its broad use during the COVID-19 vaccination campaigns would increase the seroprevalence of anti-ChAdOx1 antibodies, while the potential cross-reactivity of these antibodies was not investigated.
Other authorized vector COVID-19 vaccines were based on human adenoviruses, HAdV5 and HAdV26 (Table 2). Administration of both of them should generate adaptive responses against them. For example, immunization with Ad26.COV2.S was associated with increased titers of antibodies neutralizing a HAdV26 that persisted for at least 71 days post-vaccination (last time point assessed) (88). According to the comprehensive international seroepidemiological study, the pre-pandemic seroprevalence of HAdV26 was significantly lower in all studied regions than that of HAdV5, for which it was widespread (73). This predisposed HAdV26 as a better candidate for the vaccine vector due to concern that pre-existing immunity to it could decrease the efficacy of immunization (89). However, further studies have shown that pre-existing immunity to a vector does not necessarily always prevent them from inducing a robust adaptive response to the target antigen, or if this is the case, such an effect can be overcome by increasing the dose of viral particles (90, 91). Pre-existing anti-HAdV26 antibodies did not compromise SARS-COV-2 neutralizing antibody responses to a booster (third) dose of the Sputnik V vaccine (based on the HAdV26 vector) (92). Moreover, as shown experimentally, anti-HAdV5 antibodies did not prevent HAdV5 from infecting muscle cells but contributed to the more rapid elimination of the vector, likely via effector mechanisms (93). Considering that the large epidemiological studies did not detect an increased risk of GBS following the administration of the Ad5-nCoV vaccine (25, 26) suggests that the pre-existing immunity to HAdV5 may play a protective role or that GBS risk is increased only during the first exposure to the virus, i.e., through natural infection. Contrary to HAdV5-based vaccines, a substantial number of individuals vaccinated with Ad26.CoV2.S did not have pre-existing immunity to a vector. Whether anti-HAdV26 antibodies may reveal cross-reaction with neuronal proteins associated with axonal and myelin function remains unclear.
Therefore, to address this knowledge gap and test the hypothesis outlined in the present paper, research based on a bioinformatic approach (e.g., through the construction of a protein-protein interaction network) and experimental studies (e.g., using purified anti-ChAdOx1, anti-HAdV26, and anti-HAdV5 antibodies and neuronal cell lines) are encouraged.
In addition to the above-discussed hypothesis on the role of anti-vector antibodies in GBS induction after administering adenoviral vector vaccines, this paper also offers an alternative hypothesis. According to it, the adenoviruses used in such vaccines can invade the peripheral nervous system, interact with a receptor on the surface of neurons, infect them, and trigger the immunological response that, sporadically, leads to GBS. Experimental in vivo studies have demonstrated that intramuscular injection of adenoviral vectors, including replication-deficient ones, can be transferred to the peripheral nervous system and deliver the genes of interest (94–96). As evidenced in animal studies, the administrated adenoviral vectors, including those which are replication-defective, can persist for weeks, not only locally but also in distant sites, e.g., the liver (97, 98). This makes their transfer to the peripheral nervous system more possible. In turn, as shown by the studies focusing on the central nervous system, the presence of adenovirus can stimulate T-cell responses leading to the elimination of the vector, but accompanied by inflammation (99, 100). What is important in light of the outlined hypothesis, this process can lead to local demyelination in the central nervous system (100–102). If this phenomenon is also plausible in the peripheral nervous system following intramuscular delivery of adenoviral vectors, it would explain the increased risk of GBS associated with administering selected adenoviral vector vaccines against COVID-19. As shown, the ChAdOx1 vector reveals a high affinity to the coxsackie and adenovirus receptor (CAR) (86), which is also expressed on the surface of neurons (103). Therefore, it is plausible that ChAdOx1 trafficking to the peripheral nervous system could, in some cases, induce the cascade leading to GBS. Whether the risk of such events is modified by the genetic predisposition postulated in GBS (28) remains to be studied. However, it has to be noted that HAdV5 also utilizes CAR as a primary cell receptor (104). Thus, the question arises as to why, contrary to AZD1222, the HAdV5-based Ad5-nCoV vaccination was not associated with excess GBS. One of the potential explanations that would require confirmatory studies is that pre-existing immunity to HAdV5 in vaccinated individuals, which had to be widespread, was efficient enough to suppress the translocation of vector to the peripheral nerves. The experimental observations support this scenario that increased baseline levels of anti-HadV5 antibodies contributed to more rapid elimination of the vector (93).
HAdV26 can also interact with CAR but reveals only a weak affinity. It has been previously suggested that this adenovirus utilizes the CD46 (105), which is also expressed on the surface of human neurons. More recent investigations have shown that CD46 is also unlikely a primary cell entry receptor for HAdV26 and established that this role is played by sialic acid–bearing glycans (87). Importantly, gangliosides, sialylated glycosphingolipids, are the most prevalent sialoglycans of nerve cells that reside primarily in the outer leaflet of the plasma membrane (106). Immune responses against gangliosides have been recognized to play a role in demyelinating immune-mediated neuropathies, including GBS (107). These observations tentatively favor the hypothesis outlined in this paper’s subsection. Whether HAdV26 can interact with gangliosides and induce such events remains to be studied in vitro and in vivo.
The risk of GBS following COVID-19 vaccination appears to be increased exclusively in the case of selected adenoviral vaccines, indicating that it is not the encoded antigen (spike protein) but a vector that is likely responsible for this neuropathy. This paper hypothesizes that some adenoviruses employed in vector vaccines can trigger an adaptive humoral immune response against themselves that, in some cases, can lead to the interaction of antibodies with neurological factors and induce GBS. Indeed, immunization with such vaccines is known to induce the production of anti-vector antibodies, which before the COVID-19 pandemic, were nearly non-existence in the case of anti-ChAdOx1 antibodies or had a relatively low prevalence for anti-HAdV26. In turn, the cross-reactivity of these antibodies was not studied.
According to the second hypothesis outlined in the present paper, the adenoviral particles in vector vaccines can, in some cases, invade the peripheral nervous system, interact with surface receptors (e.g., CAR), infect neurons, and induce an immune response that leads to GBS, the severity of which depends on the spectrum of neuronal inflammation. Such penetration and further consequences are plausible because the adenoviral vectors, including those devoid of replication potency, will persist at low levels for weeks after administration. Interestingly, GBS risk was not increased when using the COVID-19 vaccine based on the HAdV5 vector, for which the pre-existing immunity was widespread prior to the COVID-19 vaccination campaign. This suggests that using HAdV5 as a vaccine vector may potentially be beneficial in decreasing the vaccination-associated GBS risk due to pre-existing immunity to the vector and increased pace of vector elimination. However, this assumption would require further research confirmation.
Research testing the hypotheses mentioned above is urgently needed due to the ongoing interest in using adenoviruses in preventive vaccines against various infectious diseases and as immunotherapeutic agents in cancer treatment. Elucidating the underlying mechanism behind the GBS following adenoviral administration should be perceived as a pathway to increase the acceptance of vector vaccines because such knowledge will bring a better understanding of the risks and enable its elimination or precautionary actions.
The original contributions presented in the study are included in the article/supplementary material. Further inquiries can be directed to the corresponding author.
PR conceptualized the study, outlined the hypotheses and wrote the manuscript.
The author reports consultation and lecture fees from Pfizer and Moderna.
All claims expressed in this article are solely those of the authors and do not necessarily represent those of their affiliated organizations, or those of the publisher, the editors and the reviewers. Any product that may be evaluated in this article, or claim that may be made by its manufacturer, is not guaranteed or endorsed by the publisher.
1. Nowakowska J, Sobocińska J, Lewicki M, Lemańska Ż, Rzymski P. When science goes viral: the research response during three months of the COVID-19 outbreak. BioMed Pharmacother (2020) 129:110451. doi: 10.1016/j.biopha.2020.110451
2. Le TT, Cramer JP, Chen R, Mayhew S. Evolution of the COVID-19 vaccine development landscape. NRDD (2020) 19:667–8. doi: 10.1038/d41573-020-00151-8
3. Novel 2019 coronavirus genome, in: Virological (2020). Available at: https://virological.org/t/novel-2019-coronavirus-genome/319 (Accessed February 12, 2023).
4. Mohamed K, Rzymski P, Islam MS, Makuku R, Mushtaq A, Khan A, et al. COVID-19 vaccinations: the unknowns, challenges, and hopes. J Med Virol (2021) 94:1336–49. doi: 10.1002/jmv.27487
5. Watson OJ, Barnsley G, Toor J, Hogan AB, Winskill P, Ghani AC. Global impact of the first year of COVID-19 vaccination: a mathematical modelling study. Lancet Infect Dis (2022) 22:1293–302. doi: 10.1016/S1473-3099(22)00320-6
6. Notarte KI, Catahay JA, Velasco JV, Pastrana A, Ver AT, Pangilinan FC, et al. Impact of COVID-19 vaccination on the risk of developing long-COVID and on existing long-COVID symptoms: a systematic review. EClinicalMedicine (2022) 53:101624. doi: 10.1016/j.eclinm.2022.101624
7. Gao P, Liu J, Liu M. Effect of COVID-19 vaccines on reducing the risk of long COVID in the real world: a systematic review and meta-analysis. Int J Environ Res Public Health (2022) 19:12422. doi: 10.3390/ijerph191912422
8. Zarębska-Michaluk D, Hu C, Brzdęk M, Flisiak R, Rzymski P. COVID-19 vaccine booster strategies for omicron SARS-CoV-2 variant: effectiveness and future prospects. Vaccines (Basel) (2022) 10:1223. doi: 10.3390/vaccines10081223
9. Rzymski P, Camargo CA, Fal A, Flisiak R, Gwenzi W, Kelishadi R, et al. COVID-19 vaccine boosters: the good, the bad, and the ugly. Vaccines (2021) 9:1299. doi: 10.3390/vaccines9111299
10. Mathieu E, Ritchie H, Rodés-Guirao L, Appel C, Giattino C, Hasell J, et al. Coronavirus pandemic (COVID-19), in: Our world in data (2020). Available at: https://ourworldindata.org/covid-hospitalizations (Accessed December 14, 2022).
11. Gao J, Feng L, Li Y, Lowe S, Guo Z, Bentley R, et al. A systematic review and meta-analysis of the association between SARS-CoV-2 vaccination and myocarditis or pericarditis. Am J Prev Med (2022) 64:275–84. doi: 10.1016/j.amepre.2022.09.002
12. Rzymski P, Perek B, Flisiak R. Thrombotic thrombocytopenia after COVID-19 vaccination: in search of the underlying mechanism. Vaccines (Basel) (2021) 9:559. doi: 10.3390/vaccines9060559
13. Li X, Burn E, Duarte-Salles T, Yin C, Reich C, Delmestri A, et al. Comparative risk of thrombosis with thrombocytopenia syndrome or thromboembolic events associated with different covid-19 vaccines: international network cohort study from five European countries and the US. BMJ (2022) 379:e071594. doi: 10.1136/bmj-2022-071594
14. Hughes RAC, Cornblath DR. Guillain-Barré Syndrome. Lancet (2005) 366:1653–66. doi: 10.1016/S0140-6736(05)67665-9
15. Shang P, Feng J, Wu W, Zhang H-L. Intensive care and treatment of severe guillain-Barré syndrome. Front Pharmacol (2021) 12:608130. doi: 10.3389/fphar.2021.608130
16. Sejvar JJ, Baughman AL, Wise M, Morgan OW. Population incidence of Guillain-Barré syndrome: a systematic review and meta-analysis. Neuroepidemiology (2011) 36:123–33. doi: 10.1159/000324710
17. Keh RYS, Scanlon S, Datta-Nemdharry P, Donegan K, Cavanagh S, Foster M, et al. COVID-19 vaccination and Guillain-Barré syndrome: analyses using the national immunoglobulin database. Brain (2022) 146:739–48. doi: 10.1093/brain/awac067
18. Maramattom BV, Krishnan P, Paul R, Padmanabhan S, Cherukudal Vishnu Nampoothiri S, Syed AA, et al. Guillain-Barré Syndrome following ChAdOx1-S/nCoV-19 vaccine. Ann Neurol (2021) 90:312–4. doi: 10.1002/ana.26143
19. Osowicki J, Morgan HJ, Harris A, Clothier HJ, Buttery JP, Kiers L, et al. Guillain-Barré Syndrome temporally associated with COVID-19 vaccines in Victoria, Australia. Vaccine (2022) 40:7579–85. doi: 10.1016/j.vaccine.2022.10.084
20. Woo EJ, Mba-Jonas A, Dimova RB, Alimchandani M, Zinderman CE, Nair N. Association of receipt of the Ad26.COV2.S COVID-19 vaccine with presumptive Guillain-Barré syndrome, February-July 2021. JAMA (2021) 326:1606–13. doi: 10.1001/jama.2021.16496
21. Abara WE, Gee J, Marquez P, Woo J, Myers TR, DeSantis A, et al. Reports of Guillain-Barré syndrome after COVID-19 vaccination in the united states. JAMA Netw Open (2023) 6:e2253845. doi: 10.1001/jamanetworkopen.2022.53845
22. Atzenhoffer M, Auffret M, Pegat A, Masmoudi K, Khouri C, Bertin B, et al. Guillain-Barré Syndrome associated with COVID-19 vaccines: a perspective from spontaneous report data. Clin Drug Investig (2022) 42:581–92. doi: 10.1007/s40261-022-01164-4
23. Karimi N, Boostani R, Fatehi F, Panahi A, Asghar Okhovat A, Ziaadini B, et al. Guillain-Barre syndrome and COVID-19 vaccine: a report of nine patients. Basic Clin Neurosci (2021) 12:703–10. doi: 10.32598/bcn.2021.3565.1
24. Abu-Arish H, Abuturki A. Guillain-Barré Syndrome after receiving sputnik light COVID-19 vaccine: a case report form Palestine. J Clin Stud Rev Rep (2022) 4:1–3. Al-Quds University, Faculty of Medicine, Jerusalem, State of PalestineAl-Ahli Hospital, Hebron, State of Palestine. doi: 10.47363/JCCSR/2021(4)199
25. World health organization. interim recommendations for use of the CanSinoBIO Ad5-nCoV-S [recombinant] vaccine (ConvideciaTM) against COVID-19: interim guidance, first issued 19 may 2022 (2022). World Health Organization. Available at: https://apps.who.int/iris/handle/10665/354409 (Accessed February 13, 2023).
26. García-Grimshaw M, Galnares-Olalde JA, Bello-Chavolla OY, Michel-Chávez A, Cadena-Fernández A, Briseño-Godínez ME, et al. Incidence of Guillain–Barré syndrome following SARS-CoV -2 immunization: analysis of a nationwide registry of recipients of 81 million doses of seven vaccines. Eur J Neurol (2022) 29:3368–79. doi: 10.1111/ene.15504
27. Halperin SA, Ye L, MacKinnon-Cameron D, Smith B, Cahn PE, Ruiz-Palacios GM, et al. Final efficacy analysis, interim safety analysis, and immunogenicity of a single dose of recombinant novel coronavirus vaccine (adenovirus type 5 vector) in adults 18 years and older: an international, multicentre, randomised, double-blinded, placebo-controlled phase 3 trial. Lancet (2022) 399:237–48. doi: 10.1016/S0140-6736(21)02753-7
28. Khanmohammadi S, Malekpour M, Jabbari P, Rezaei N. Genetic basis of Guillain-barre syndrome. J Neuroimmunol (2021) 358:577651. doi: 10.1016/j.jneuroim.2021.577651
29. Rzymski P, Szuster-Ciesielska A, Dzieciątkowski T, Gwenzi W, Fal A. mRNA vaccines: the future of prevention of viral infections? J Med Virol (2023) 95:e28572. doi: 10.1002/jmv.28572
30. Rzymski P, Fal A. To aspirate or not to aspirate? considerations for the COVID-19 vaccines. Pharmacol Rep (2022). doi: 10.1007/s43440-022-00361-4
31. Chang J. Adenovirus vectors: excellent tools for vaccine development. Immune Netw (2021) 21:e6. doi: 10.4110/in.2021.21.e6
32. Sakurai F, Tachibana M, Mizuguchi H. Adenovirus vector-based vaccine for infectious diseases. Drug Metab Pharmacokinet (2022) 42:100432. doi: 10.1016/j.dmpk.2021.100432
33. Manno D, Bangura A, Baiden F, Kamara AB, Ayieko P, Kallon J, et al. Safety and immunogenicity of an Ad26.ZEBOV booster dose in children previously vaccinated with the two-dose heterologous Ad26.ZEBOV and MVA-BN-Filo Ebola vaccine regimen: an open-label, non-randomised, phase 2 trial. Lancet Infect Dis (2023) 23:352–60. doi: 10.1016/S1473-3099(22)00594-1
34. Barry H, Mutua G, Kibuuka H, Anywaine Z, Sirima SB, Meda N, et al. Safety and immunogenicity of 2-dose heterologous Ad26.ZEBOV, MVA-BN-Filo Ebola vaccination in healthy and HIV-infected adults: a randomised, placebo-controlled phase II clinical trial in Africa. PLoS Med (2021) 18:e1003813. doi: 10.1371/journal.pmed.1003813
35. Ishola D, Manno D, Afolabi MO, Keshinro B, Bockstal V, Rogers B, et al. Safety and long-term immunogenicity of the two-dose heterologous Ad26.ZEBOV and MVA-BN-Filo Ebola vaccine regimen in adults in Sierra Leone: a combined open-label, non-randomised stage 1, and a randomised, double-blind, controlled stage 2 trial. Lancet Infect Dis (2022) 22:97–109. doi: 10.1016/S1473-3099(21)00125-0
36. Afolabi MO, Ishola D, Manno D, Keshinro B, Bockstal V, Rogers B, et al. Safety and immunogenicity of the two-dose heterologous Ad26.ZEBOV and MVA-BN-Filo Ebola vaccine regimen in children in Sierra Leone: a randomised, double-blind, controlled trial. Lancet Infect Dis (2022) 22:110–22. doi: 10.1016/S1473-3099(21)00128-6
37. Elkashif A, Alhashimi M, Sayedahmed EE, Sambhara S, Mittal SK. Adenoviral vector-based platforms for developing effective vaccines to combat respiratory viral infections. Clin Transl Immunol (2021) 10:e1345. doi: 10.1002/cti2.1345
38. Saramago S, Magalhães J, Pinheiro M. Tuberculosis vaccines: an update of recent and ongoing clinical trials. Appl Sci (Basel) (2021) 11:9250. doi: 10.3390/app11199250
39. Tiono AB, Nébié I, Anagnostou N, Coulibaly AS, Bowyer G, Lam E, et al. First field efficacy trial of the ChAd63 MVA ME-TRAP vectored malaria vaccine candidate in 5-17 months old infants and children. PLoS One (2018) 13:e0208328. doi: 10.1371/journal.pone.0208328
40. Folegatti PM, Harrison K, Preciado-Llanes L, Lopez FR, Bittaye M, Kim YC, et al. A single dose of ChAdOx1 chik vaccine induces neutralizing antibodies against four chikungunya virus lineages in a phase 1 clinical trial. Nat Commun (2021) 12:4636. doi: 10.1038/s41467-021-24906-y
41. Zhang Z, Zhao Z, Wang Y, Wu S, Wang B, Zhang J, et al. Comparative immunogenicity analysis of intradermal versus intramuscular immunization with a recombinant human adenovirus type 5 vaccine against Ebola virus. Front Immunol (2022) 13:963049. doi: 10.3389/fimmu.2022.963049
42. Mendonça SA, Lorincz R, Boucher P, Curiel DT. Adenoviral vector vaccine platforms in the SARS-CoV-2 pandemic. NPJ Vaccines (2021) 6:97. doi: 10.1038/s41541-021-00356-x
43. Dhama K, Dhawan M, Tiwari R, Emran TB, Mitra S, Rabaan AA, et al. COVID-19 intranasal vaccines: current progress, advantages, prospects, and challenges. Hum Vaccin Immunother (2022) 18:2045853. doi: 10.1080/21645515.2022.2045853
44. Shaw AR, Suzuki M. Immunology of adenoviral vectors in cancer therapy. Mol Ther Methods Clin Dev (2019) 15:418–29. doi: 10.1016/j.omtm.2019.11.001
45. Zhao Y, Liu Z, Li L, Wu J, Zhang H, Zhang H, et al. Oncolytic adenovirus: prospects for cancer immunotherapy. Front Microbiol (2021) 12:707290. doi: 10.3389/fmicb.2021.707290
46. van den Berg B, Walgaard C, Drenthen J, Fokke C, Jacobs BC, van Doorn PA. Guillain–Barré Syndrome: pathogenesis, diagnosis, treatment and prognosis. Nat Rev Neurol (2014) 10:469–82. doi: 10.1038/nrneurol.2014.121
47. van Doorn PA, Ruts L, Jacobs BC. Clinical features, pathogenesis, and treatment of Guillain-Barré syndrome. Lancet Neurol (2008) 7:939–50. doi: 10.1016/S1474-4422(08)70215-1
48. Hai PD, Phuong LL, Tot NH, Anh NLP, Tung ND, Quan NH. Guillain-Barré Syndrome after COVID-19 vaccination: report of two cases from Vietnam. J Infect Dev Ctries (2022) 16:1703–5. doi: 10.3855/jidc.16998
49. Thant HL, Morgan R, Paese MM, Persaud T, Diaz J, Hurtado L. Guillain-Barré Syndrome after Ad26.COV2.S vaccination. Am J Case Rep (2022) 23:e935275. doi: 10.12659/AJCR.935275
50. Kadkhoda K. Post-adenoviral-based vaccines Guillain-barre syndrome: a proposed mechanism. Med Hypotheses (2022) 160:110792. doi: 10.1016/j.mehy.2022.110792
51. Felipe Cuspoca A, Isaac Estrada P, Velez-van-Meerbeke A. Molecular mimicry of SARS-CoV-2 spike protein in the nervous system: a bioinformatics approach. Comput Struct Biotechnol J (2022) 20:6041–54. doi: 10.1016/j.csbj.2022.10.022
52. Aladawi M, Elfil M, Abu-Esheh B, Abu Jazar D, Armouti A, Bayoumi A, et al. Guillain Barre syndrome as a complication of COVID-19: a systematic review. Can J Neurol Sci (2022) 49:38–48. doi: 10.1017/cjn.2021.102
53. Poniedziałek B, Hallmann E, Sikora D, Szymański K, Kondratiuk K, Żurawski J, et al. Relationship between humoral response in COVID-19 and seasonal influenza vaccination. Vaccines (Basel) (2022) 10:1621. doi: 10.3390/vaccines10101621
54. Alfego D, Sullivan A, Poirier B, Williams J, Adcock D, Letovsky S. A population-based analysis of the longevity of SARS-CoV-2 antibody seropositivity in the united states. EClinicalMedicine (2021) 36:100902. doi: 10.1016/j.eclinm.2021.100902
55. Lumley SF, Wei J, O’Donnell D, Stoesser NE, Matthews PC, Howarth A, et al. The duration, dynamics, and determinants of severe acute respiratory syndrome coronavirus 2 (SARS-CoV-2) antibody responses in individual healthcare workers. Clin Infect Dis (2021) 73:e699–709. doi: 10.1093/cid/ciab004
56. Nagashima T, Koga M, Odaka M, Hirata K, Yuki N. Continuous spectrum of pharyngeal-cervical-brachial variant of Guillain-Barré syndrome. Arch Neurol (2007) 64:1519–23. doi: 10.1001/archneur.64.10.1519
57. Pacheco-Herrero M, Soto-Rojas LO, Harrington CR, Flores-Martinez YM, Villegas-Rojas MM, León-Aguilar AM, et al. Elucidating the neuropathologic mechanisms of SARS-CoV-2 infection. Front Neurol (2021) 12:660087. doi: 10.3389/fneur.2021.660087
58. Tamborska AA, Singh B, Leonhard SE, Hodel EM, Stowe J, Watson-Fargie T, et al. Guillain-Barré Syndrome following SARS-CoV-2 vaccination in the UK: a prospective surveillance study. BMJ Neurol Open (2022) 4:e000309.
59. European Medicines agency. vaxzevria product information. Available at: https://www.ema.europa.eu/en/documents/product-information/vaxzevria-previously-covid-19-vaccine-astrazeneca-epar-product-information_en.pdf (Accessed February 13, 2023).
60. European Medicines agency. jcovden product information. Available at: https://www.ema.europa.eu/en/documents/product-information/jcovden-previously-covid-19-vaccine-janssen-epar-product-information_en.pdf (Accessed February 13, 2023).
61. European Centre for disease prevention and control. COVID-19 vaccine tracker. Available at: https://vaccinetracker.ecdc.europa.eu (Accessed February 13, 2023).
62. Watanabe Y, Mendonça L, Allen ER, Howe A, Lee M, Allen JD, et al. Native-like SARS-CoV-2 spike glycoprotein expressed by ChAdOx1 nCoV-19/AZD1222 vaccine. ACS Cent Sci (2021) 7:594–602. doi: 10.1021/acscentsci.1c00080
63. Sadoff J, Gray G, Vandebosch A, Cárdenas V, Shukarev G, Grinsztejn B, et al. Safety and efficacy of single-dose Ad26.COV2.S vaccine against covid-19. N Engl J Med (2021) 384:2187–201. doi: 10.1056/NEJMoa2101544
64. Jackson LA, Anderson EJ, Rouphael NG, Roberts PC, Makhene M, Coler RN, et al. An mRNA vaccine against SARS-CoV-2 — preliminary report. N Engl J Med (2020) 383:1920–31. doi: 10.1056/NEJMoa2022483
65. Vogel AB, Kanevsky I, Che Y, Swanson KA, Muik A, Vormehr M, et al. BNT162b vaccines protect rhesus macaques from SARS-CoV-2. Nature (2021) 592:283–9. doi: 10.1038/s41586-021-03275-y
66. Tian J-H, Patel N, Haupt R, Zhou H, Weston S, Hammond H, et al. SARS-CoV-2 spike glycoprotein vaccine candidate NVX-CoV2373 immunogenicity in baboons and protection in mice. Nat Commun (2021) 12:372. doi: 10.1038/s41467-020-20653-8
67. Hartung HP. Infections and the guillain-Barré syndrome. J Neurol Neurosurg Psychiatry (1999) 66:277. doi: 10.1136/jnnp.66.3.277
68. Jacobs BC, Rothbarth PH, van der Meché FG, Herbrink P, Schmitz PI, de Klerk MA, et al. The spectrum of antecedent infections in Guillain-Barré syndrome. Neurology (1998) 51:1110–5. doi: 10.1212/WNL.51.4.1110
70. Dowling P, Menonna J, Cook S. Cytomegalovirus cornplement fixation antibody in Guillain-Barré syndrome. Neurology (1977) 27:1153–3. doi: 10.1212/WNL.27.12.1153
71. Dussaix E, Slim A, Tournier P. Comparison of enzyme-linked immunosorbent assay (ELISA) and complement fixation test for detection of mycoplasma pneumoniae antibodies. J Clin Pathol (1983) 36:228–32. doi: 10.1136/jcp.36.2.228
72. Hinchliffe P. The detection of complement fixing antibodies by ELISA (COMPELISA) in brucella serology. Dev Biol Stand (1984) 56:465–9.
73. Barouch DH, Kik SV, Weverling GJ, Dilan R, King SL, Maxfield LF, et al. International seroepidemiology of adenovirus serotypes 5, 26, 35, and 48 in pediatric and adult populations. Vaccine (2011) 29:5203–9. doi: 10.1016/j.vaccine.2011.05.025
74. Mennechet FJD, Paris O, Ouoba AR, Salazar Arenas S, Sirima SB, Takoudjou Dzomo GR, et al. A review of 65 years of human adenovirus seroprevalence. Expert Rev Vaccines (2019) 18:597–613. doi: 10.1080/14760584.2019.1588113
75. Bragazzi NL, Kolahi A-A, Nejadghaderi SA, Lochner P, Brigo F, Naldi A, et al. Global, regional, and national burden of Guillain-Barré syndrome and its underlying causes from 1990 to 2019. J Neuroinflamm (2021) 18:264. doi: 10.1186/s12974-021-02319-4
76. Top FH Jr. Control of adenovirus acute respiratory disease in U.S. army trainees. Yale J Biol Med (1975) 48:185–95.
77. Goffin E, Javaux J, Destexhe E, Pretto CD, Spindler KR, Machiels B, et al. Oral vaccination with replication-competent adenovirus in mice reveals dissemination of the viral vaccine beyond the gastrointestinal tract. J Virol (2019) 93:e00237–19. doi: 10.1128/JVI.00237-19
78. McNeil MM, Paradowska-Stankiewicz I, Miller ER, Marquez PL, Seshadri S, Collins LC Jr, et al. Adverse events following adenovirus type 4 and type 7 vaccine, live, oral in the vaccine adverse event reporting system (VAERS), united states, October 2011-July 2018. Vaccine (2019) 37:6760–7. doi: 10.1016/j.vaccine.2019.08.087
79. Ramasamy MN, Minassian AM, Ewer KJ, Flaxman AL, Folegatti PM, Owens DR, et al. Safety and immunogenicity of ChAdOx1 nCoV-19 vaccine administered in a prime-boost regimen in young and old adults (COV002): a single-blind, randomised, controlled, phase 2/3 trial. Lancet (2021) 396:1979–93. doi: 10.1016/S0140-6736(20)32466-1
80. Barrett JR, Belij-Rammerstorfer S, Dold C, Ewer KJ, Folegatti PM, Gilbride C, et al. Phase 1/2 trial of SARS-CoV-2 vaccine ChAdOx1 nCoV-19 with a booster dose induces multifunctional antibody responses. Nat Med (2021) 27:279–88. doi: 10.1038/s41591-020-01179-4
81. Dicks MDJ, Spencer AJ, Edwards NJ, Wadell G, Bojang K, Gilbert SC, et al. A novel chimpanzee adenovirus vector with low human seroprevalence: improved systems for vector derivation and comparative immunogenicity. PLoS One (2012) 7:e40385. doi: 10.1371/journal.pone.0040385
82. Yi H, Wang Q, Deng J, Li H, Zhang Y, Chen Z, et al. Seroprevalence of neutralizing antibodies against adenovirus type 26 and 35 in healthy populations from guangdong and Shandong provinces, China. Virol Sin (2022) 37:716–23. doi: 10.1016/j.virs.2022.06.006
83. Mast TC, Kierstead L, Gupta SB, Nikas AA, Kallas EG, Novitsky V, et al. International epidemiology of human pre-existing adenovirus (Ad) type-5, type-6, type-26 and type-36 neutralizing antibodies: correlates of high Ad5 titers and implications for potential HIV vaccine trials. Vaccine (2010) 28:950–7. doi: 10.1016/j.vaccine.2009.10.145
84. Abbink P, Lemckert AAC, Ewald BA, Lynch DM, Denholtz M, Smits S, et al. Comparative seroprevalence and immunogenicity of six rare serotype recombinant adenovirus vaccine vectors from subgroups b and d. J Virol (2007) 81:4654–63. doi: 10.1128/JVI.02696-06
85. Vogels R, Zuijdgeest D, van Rijnsoever R, Hartkoorn E, Damen I, de Beíthune M-P, et al. Replication-deficient human adenovirus type 35 vectors for gene transfer and vaccination: efficient human cell infection and bypass of preexisting adenovirus immunity. J Virol (2003) 77:8263–71. doi: 10.1128/JVI.77.15.8263-8271.2003
86. Baker AT, Boyd RJ, Sarkar D, Teijeira-Crespo A, Chan CK, Bates E, et al. ChAdOx1 interacts with CAR and PF4 with implications for thrombosis with thrombocytopenia syndrome. Sci Adv (2021) 7:eabl8213. doi: 10.1126/sciadv.abl8213
87. Baker AT, Mundy RM, Davies JA, Rizkallah PJ, Parker AL. Human adenovirus type 26 uses sialic acid-bearing glycans as a primary cell entry receptor. Sci Adv (2019) 5:eaax3567. doi: 10.1126/sciadv.aax3567
88. Stephenson KE, Le Gars M, Sadoff J, de Groot AM, Heerwegh D, Truyers C, et al. Immunogenicity of the Ad26.COV2.S vaccine for COVID-19. JAMA (2021) 325:1535–44. doi: 10.1001/jama.2021.3645
89. Fausther-Bovendo H, Kobinger GP. Pre-existing immunity against ad vectors: humoral, cellular, and innate response, what’s important? Hum Vaccin Immunother (2014) 10:2875–84. doi: 10.4161/hv.29594
90. Steffensen MA, Jensen BAH, Holst PJ, Bassi MR, Christensen JP, Thomsen AR. Pre-existing vector immunity does not prevent replication deficient adenovirus from inducing efficient CD8 T-cell memory and recall responses. PLoS One (2012) 7:e34884. doi: 10.1371/journal.pone.0034884
91. Pandey A, Singh N, Vemula SV, Couëtil L, Katz JM, Donis R, et al. Impact of preexisting adenovirus vector immunity on immunogenicity and protection conferred with an adenovirus-based H5N1 influenza vaccine. PLoS One (2012) 7:e33428. doi: 10.1371/journal.pone.0033428
92. Byazrova MG, Astakhova EA, Minnegalieva AR, Sukhova MM, Mikhailov AA, Prilipov AG, et al. Anti-Ad26 humoral immunity does not compromise SARS-COV-2 neutralizing antibody responses following gam-COVID-Vac booster vaccination. NPJ Vaccines (2022) 7:145. doi: 10.1038/s41541-022-00566-x
93. Sanchez S, Palacio N, Dangi T, Ciucci T, Penaloza-MacMaster P. Fractionating a COVID-19 Ad5-vectored vaccine improves virus-specific immunity. Sci Immunol (2021) 6:eabi8635. doi: 10.1126/sciimmunol.abi8635
94. Haase G, Pettmann B, Vigne E, Castelnau-Ptakhine L, Schmalbruch H, Kahn A. Adenovirus-mediated transfer of the neurotrophin-3 gene into skeletal muscle of pmn mice: therapeutic effects and mechanisms of action. J Neurol Sci (1998) 160 Suppl 1:S97–105. doi: 10.1016/S0022-510X(98)00207-X
95. Glatzel M, Flechsig E, Navarro B, Klein MA, Paterna JC, Büeler H, et al. Adenoviral and adeno-associated viral transfer of genes to the peripheral nervous system. Proc Natl Acad Sci U S A (2000) 97:442–7. doi: 10.1073/pnas.97.1.442
96. Watanabe TS, Ohtori S, Koda M, Aoki Y, Doya H, Shirasawa H, et al. Adenoviral gene transfer in the peripheral nervous system. J Orthop Sci (2006) 11:64–9. doi: 10.1007/s00776-005-0971-z
97. Tatsis N, Fitzgerald JC, Reyes-Sandoval A, Harris-McCoy KC, Hensley SE, Zhou D, et al. Adenoviral vectors persist in vivo and maintain activated CD8+ T cells: implications for their use as vaccines. Blood (2007) 110:1916–23. doi: 10.1182/blood-2007-02-062117
98. Jager L, Ehrhardt A. Persistence of high-capacity adenoviral vectors as replication-defective monomeric genomes in vitro and in murine liver. Hum Gene Ther (2009) 20:883–96. doi: 10.1089/hum.2009.020
99. Byrnes AP, Rusby JE, Wood MJ, Charlton HM. Adenovirus gene transfer causes inflammation in the brain. Neuroscience (1995) 66:1015–24. doi: 10.1016/0306-4522(95)00068-T
100. Byrnes AP, MacLaren RE, Charlton HM. Immunological instability of persistent adenovirus vectors in the brain: peripheral exposure to vector leads to renewed inflammation, reduced gene expression, and demyelination. J Neurosci (1996) 16:3045–55. doi: 10.1523/JNEUROSCI.16-09-03045.1996
101. Wood MJ, Charlton HM, Wood KJ, Kajiwara K, Byrnes AP. Immune responses to adenovirus vectors in the nervous system. Trends Neurosci (1996) 19:497–501. doi: 10.1016/S0166-2236(96)10060-6
102. Fathallah-Shaykh HM, Kafrouni AI, Zhao LJ, Diaz-Arrastia R, Garcia JA, Frawley WH, et al. Demyelination but no cognitive, motor or behavioral deficits after adenovirus-mediated gene transfer into the brain. Gene Ther (2000) 7:2094–8. doi: 10.1038/sj.gt.3301346
103. Zussy C, Salinas S. Study of adenovirus and CAR axonal transport in primary neurons. Methods Mol Biol (2014) 1089:71–8. doi: 10.1007/978-1-62703-679-5_5
104. Zhang Y, Bergelson JM. Adenovirus receptors. J Virol (2005) 79:12125–31. doi: 10.1128/JVI.79.19.12125-12131.2005
105. Li H, Rhee EG, Masek-Hammerman K, Teigler JE, Abbink P, Barouch DH. Adenovirus serotype 26 utilizes CD46 as a primary cellular receptor and only transiently activates T lymphocytes following vaccination of rhesus monkeys. J Virol (2012) 86:10862–5. doi: 10.1128/JVI.00928-12
106. Schnaar RL, Gerardy-Schahn R, Hildebrandt H. Sialic acids in the brain: gangliosides and polysialic acid in nervous system development, stability, disease, and regeneration. Physiol Rev (2014) 94:461–518. doi: 10.1152/physrev.00033.2013
Keywords: Guillain - Barré, SARS-CoV-2, pandemic, Adenoviral (Ad) vector, COVID-19 vaccination
Citation: Rzymski P (2023) Guillain-Barré syndrome and COVID-19 vaccines: focus on adenoviral vectors. Front. Immunol. 14:1183258. doi: 10.3389/fimmu.2023.1183258
Received: 09 March 2023; Accepted: 12 April 2023;
Published: 26 April 2023.
Edited by:
Salman Sadullah Usmani, Albert Einstein College of Medicine, United StatesCopyright © 2023 Rzymski. This is an open-access article distributed under the terms of the Creative Commons Attribution License (CC BY). The use, distribution or reproduction in other forums is permitted, provided the original author(s) and the copyright owner(s) are credited and that the original publication in this journal is cited, in accordance with accepted academic practice. No use, distribution or reproduction is permitted which does not comply with these terms.
*Correspondence: Piotr Rzymski, cnp5bXNraXBpb3RyQHVtcC5lZHUucGw=
Disclaimer: All claims expressed in this article are solely those of the authors and do not necessarily represent those of their affiliated organizations, or those of the publisher, the editors and the reviewers. Any product that may be evaluated in this article or claim that may be made by its manufacturer is not guaranteed or endorsed by the publisher.
Research integrity at Frontiers
Learn more about the work of our research integrity team to safeguard the quality of each article we publish.