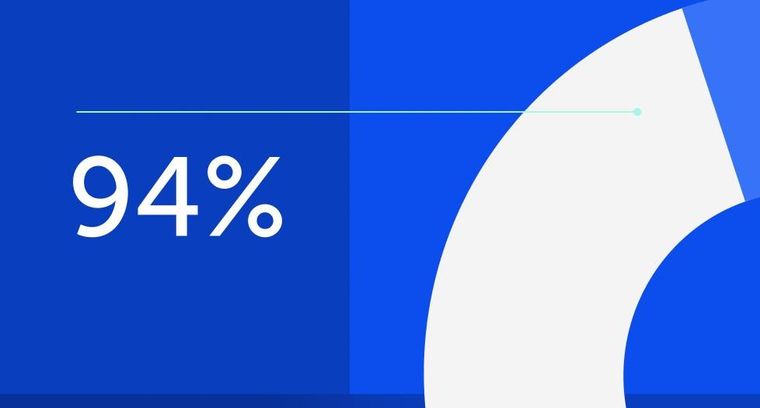
94% of researchers rate our articles as excellent or good
Learn more about the work of our research integrity team to safeguard the quality of each article we publish.
Find out more
REVIEW article
Front. Immunol., 17 May 2023
Sec. Alloimmunity and Transplantation
Volume 14 - 2023 | https://doi.org/10.3389/fimmu.2023.1179355
Vascularized composite allotransplantation (VCA) is an evolving field of reconstructive surgery that has revolutionized the treatment of patients with devastating injuries, including those with limb losses or facial disfigurement. The transplanted units are typically comprised of different tissue types, including skin, mucosa, blood and lymphatic vasculature, muscle, and bone. It is widely accepted that the antigenicity of some VCA components, such as skin, is particularly potent in eliciting a strong recipient rejection response following transplantation. The fine line between tolerance and rejection of the graft is orchestrated by different cell types, including both donor and recipient-derived lymphocytes, macrophages, and other immune and donor-derived tissue cells (e.g., endothelium). Here, we delineate the role of different cell and tissue types during VCA rejection. Rejection of VCA grafts and the necessity of life-long multidrug immunosuppression remains one of the major challenges in this field. This review sheds light on recent developments in decoding the cellular signature of graft rejection in VCA and how these may, ultimately, influence the clinical management of VCA patients by way of novel therapies that target specific cellular processes.
Devastating injuries such as severe facial disfigurement (e.g., loss of nose and lips) that cannot be adequately addressed by conventional reconstructive surgical techniques, including local tissue rearrangement and free tissue transfer. Although flaps, grafts, or local tissue rearrangement are the gold standard for reconstruction, they merely achieve wound coverage but result in substantial amount of donor-site morbidity. Vascularized composite allotransplants (e.g., face or limb transplants) have revolutionized functional and aesthetic restoration with promising short and long-term outcomes (1–3). Yet, this groundbreaking reconstructive biotechnology is also associated with challenges, mainly owing to the strong rejection response that invariably occurs following transplantation. VCAs are composed of a variety of tissue types, including, but not limited to, skin, mucosa, blood vessels, lymphatics, nerves, muscle, and bone, with suspected varying degrees of antigenicity between the tissues (4). To ensure graft survival, patients are usually maintained on high doses of systemic immunosuppression with potentially severe long-term side effects (5).
Different cell types such as endothelial cells (EC), B cells, T cell variants, natural killer (NK) cells, and antigen-presenting cells (APC) have been implicated in the pathogenesis of rejection. Based on the current evidence, there is a clear hierarchy of effector-target cell interactions. For acute cellular rejection, CD8+ T cells of both donor and recipient origin are the primary effector cells targeting epithelial and follicular stem cells and microvascular endothelium. During chronic rejection, the most relevant effector-target cell combinations are donor and recipient CD8+ effector T cells that target endothelium (partially chimeric), leading to arteritis with chronic remodeling. Of note, CD4+ T cells and antibodies seem to play an additional pivotal role in this process (6, 7). Secondary effector and modulator cells of the immune response (NK-cells, endothelial cells, granulocytes, Tregs, macrophages and APC, immunosuppressive dermal mesenchymal cells, keratinocytes, mast cells) have also been recognized to play a role in modulating the primary effector-target cell interactions (8–11). Additionally, it has been demonstrated that both donor and recipient-derived immune cells may contribute to the development of allograft rejection (12). Given the recognition of CD8+ effector T cells as the main (but not only) protagonists of VCA allograft rejection, this cell type is predominantly targeted by current clinical immunosuppression regimens (Tacrolimus, Steroids, mycophenolate mofetil (MMF)) (13). To further improve our understanding of VCA pathoimmunology, there is a critical need to review the current understanding of how different cell types contribute to VCA rejection (Supplementary Figure 1).
VCA grafts are comprised of different tissue types (e.g., muscle, skin, mucosa, lymphatics, vasculature, adipose tissue), each of which may feature specific immunological properties and thus be differentially targeted by rejection (5, 14). Skin is largely viewed as the most immunogenic tissue and most studies focus on the rejection mechanisms in skin itself. The phenomenon of split tolerance (rejection of one tissue with tolerance towards another) has been observed in several studies, characterized by the rejection of skin components without evidence of rejection in the remaining tissue types (13, 15–17). The preferential targeting of the donor skin by the recipient immune system might be due to the transfer of a rich skin-resident donor-derived immune system, including T cells and APC (such as dendritic cells). It has been shown that the skin is home to more resident T cells when compared to the peripheral circulation, and given the constant exposure of skin to foreign antigens, a large number of T effector cells are present in the skin (particularly CD8+ memory T cells) (17). These cells potentially fuel detrimental interactions between the two immune (i.e., innate and adaptive) systems (12). Recent work by our group identified the mucosal tissue of the oral and nasal cavities as another main target of rejection in facial VCAs (14, 15, 18, 19). Interestingly, the mucosa consistently shows more distinct microscopic changes indicative of acute rejection events when compared to skin biopsies (14, 15, 20). Since most studies on comparative antigenicity were performed in limb allografts, the dogma that skin is the most immunogenic may need to be re-evaluated.
Few studies have detailed the precise role of different cell types involved in allograft rejection. However, since most therapeutics target a specific cell type (predominantly T and/or B cells), it is important to examine the relevance of specific cell types for allograft rejection and how these cell types interact. To decipher the hierarchy of effector-target cell interactions in allograft rejection, we will first delineate the functions of the different cell types in acute rejection. Of note, the transition from acute rejection episodes into chronic stages represents most likely a gradual process rather than two clearly differentiable phases (21, 22). Thus, the cell types discussed in this review are likely to be involved in both acute and chronic rejection. Yet, further research, especially on chronic VCA rejection, is needed to match cell type and fate with either acute and/or chronic rejection.
Mounting body of evidence points toward CD8+ effector T cells as the hallmark effectors of VCA rejection targeting epidermal and follicular stem cells (and to a lesser extent the microvascular endothelium), thus driving acute cellular rejection reactions (16, 23, 24). Besides these key effector-target cell interactions, multiple secondary effectors, in addition to other cellular targets, are involved in VCA rejection (17).
In acute VCA rejection, the primary effector-target cell pair are CD8+-T-cells and epithelial/follicular stem cells (25–28). This interaction will be discussed first followed by an outline of the role of endothelial cells which represent both targets and effectors in VCA. Lastly, secondary effectors (e.g., NK cells, APC, granulocytes with APC-like cellular characteristics) will be discussed (11, 29–31).
Acute rejection (AR) is usually defined as a typically reversible immune-mediated attack on the allograft, orchestrated at the cellular level by cytotoxic cells (predominantly T cells) or through donor-specific antibodies (DSA) produced by plasma cells (antibody mediated rejection, AMR). The primary effector/target cell combination are T cells that target epidermal and follicular stem cells. Nearly 85% of VCA patients experience at least one episode of AR within the first postoperative year; in fact, more than 50% of cases report multiple AR episodes (32). The reported incidence of AR is, thus, significantly higher than in the field of solid organ transplantation (SOT) (33). It is hypothesized that this is, at least partly, due to easy visual monitoring of the graft (8). Indeed, any suspicious skin changes or cutaneous abnormality should trigger a prompt biopsy and histologic examination. In cases of histologically confirmed AR, immediate therapy is often initiated, usually in the form of steroid bolus administration and immunotherapeutic regimen optimization (34). Of note, Win et al. found distinctive genetic features in AMR (on postoperative day 5) compared with T cell mediated rejection episodes (by 12, 21, and 24 months postoperative) in one VCA facial allograft. Genes that were particularly upregulated during the suspected AMR episode included ICAM1, VCAM1, and SELE, which are all linked with endothelial activation and leukocyte-endothelial cell interactions. In contrast, granzyme B was specifically upregulated during the T cell mediated rejection episodes. Granzyme B is commonly expressed by CD8+ cytotoxic T cells and natural killer cells upon activation (35).
Histologically, AR of the skin in VCA shows different levels of perivascular and/or interstitial mononuclear cell infiltration with epidermal and/or adnexal involvement (36) (Figure 1). Bhan et al. provided initial evidence in 1980 that both CD4+ and CD8+ T-cells infiltrate human skin allografts (37). In a study of 113 biopsies from full-facial transplants, Lian et al. showed that T cells also play a crucial role during acute rejection of facial VCAs (12). Notably, Lian et al. found that some of the involved immune cells were of donor origin, with an immunophenotype typical of the resident memory T cell subset. While cell-mediated rejection primarily affects the skin in the sense of epidermal targeting, the endothelium of graft vessels is the main site of damage in antibody-mediated rejection (AMR) (36). During AMR, DSA trigger rejection by complement activation. In the classical pathway, along with C4a and C4b, C4d is subsequently cleaved and can covalently bind to the endothelium, serving as a diagnostic tool of AMR in solid organ transplantation (38, 39). Yet, AMR remains rarely reported in VCA recipients (40). In the few cases of AMR in VCA, no clear correlation between C4d deposition and DSA formation was found (41, 42).
Figure 1 Target cells in acute rejection of facial VCA skin. (A) Spatial associations of lymphoid cells with target cell injury in facial allografts. Lymphocytes at tips of epidermal rete ridges surrounded target cells (arrows, top row H&E staining) to form ‘satellitosis’. Donor (Bw4) and CD8+ T cells precisely corresponded to these patterns of satellitosis (bottom row). Sites of follicular targeting in the bulge region, as well as microvascular targeting, also correlated with the presence of donor T cells that often were located within vessel lumens (intraluminal donor T cell in apposition to degenerating endothelial cells within square; intrafollicular apoptotic target cell encircled). Dotted line=dermal–epidermal and dermal–follicular junctions). (B) Dual labeling for donor/recipient histocompatibility antigens and T-cell phenotype (color of font=fluorochrome used; patient 5). The majority of cells in epidermal infiltrates (top and middle rows, far left) and follicular infiltrates (top and middle rows, middle) were CD3-positive (green) T cells of donor origin (Bw4, red; co-expression=yellow–orange). Cells expressing resident memory T-cell markers (CD69, green) co-expressed donor (Bw4, red) but not recipient (B7) antigen markers (top and middle rows, far right) consistent with their origin in the facial allograft. Donor resident memory T cells (CD69- or CD103-positive cells, red) were predominantly CD8 positive (green) in the epidermis and CD4 positive (green) in hair follicles (bottom row). Color mixing of red and green epitopes=yellow or yellow–orange; blue=DAPI nuclear stain. Please note: Figure 1 and the respective caption are adapted and modified from Lian et al. (12). Permission to reuse was granted by Springer Nature.
T cells are the protagonists of adaptive cell-mediated immunity and the major cell type involved in VCA rejection (43). Two main populations of T-effector cells exist, which are divided based on their CD4 or CD8 glycoprotein that is associated with a respective TCR on the cell surface: CD4+ T-helper cells (TH-Cells) and CD8+ cytotoxic (cytolytic) T lymphocytes (CTL). T-helper cells produce cytokines and modulate immunological processes such as CTL, macrophage, and B-cell activation, whereas CTL bind to target cells and induce apoptosis in target cells via secretion of perforins and granzyme. These cell populations were noted to be involved in skin allograft rejection as early as in 1982 by Bhan et al. At the time, two pathways of T cell-mediated rejection mediated by T cells of recipient origin were proposed: a direct pathway of epithelial injury mediated by CD8+ CTLs, and an indirect pathway via T cell-mediated endothelial microvascular injury (37). With the clinical advent of VCA, we were able to better characterize functions and cell populations involved in skin rejection.
T cells are specifically trained to recognize donor-derived antigens. The indirect pathway of antigen presentation is the conventional mechanism of antigen presentation of bacterial/viral antigens, whereas dendritic cells acquire an antigen via endocytosis, process it into peptide fragments, and present it on their surface via MHC molecules (= antigen presentation) (8, 16, 43). A processed antigen fragment (peptide) is presented on the surface of APC via self MHC I (for CD8+) or II (for CD4+ T cells), and the T cell binds via TCR. During transplant rejection, processed antigen is mostly a donor-derived MHC molecule. In the direct pathway of antigen recognition, T cells recognize intact (unprocessed) foreign MHC molecules on the surface of donor-derived cells (44). Naïve T cell activation requires professional APC (dendritic cell) activation to become an effector T cell. In addition to the antigen-specific signal, a costimulatory signal is required for T cell activation (43, 44). The B7:CD28 and CD80/CD86:CD40 pathways are the most widely known costimulatory pathways, and the costimulatory molecules are expressed in high density on APC.
Cellular infiltrates of human skin rejection samples in hand transplantation consist predominantly of CD4+ and CD8+ lymphocytes, with some B-cells and CD68+ cells (histiocytes, macrophages) (45). In a detailed analysis of facial VCA skin samples, Lian et al. demonstrated that stem cell-rich epidermal rete ridges, follicles, and dermal microvessels were primarily targeted during the acute rejection process (12). Initially, it was assumed that most of the target cell damage is caused by recipient-derived lymphocytes that infiltrate the graft and react towards alloantigens. However, Lian et al. identified that both donor- and recipient-derived T-lymphocytes are involved in the rejection process. In the epidermis and hair follicles, most infiltrating lymphocytes were of donor origin (>90%) and stained positive mainly for CD8 (8). The authors demonstrated that lymphocytes surround epithelial target cells (“satellitosis”). Near vasculature, both donor and recipient lymphocytes of comparable quantity were identified, with donor-derived T cells often located intraluminally next to the injured endothelium (lymphocytic vasculitis). Multiplex gene expression profiling of both facial and limb VCA samples has recently been performed (13, 46). In face transplant samples, findings demonstrated that in higher grade rejection, mostly T cell activation pathways are activated with upregulation of genes associated with T cell infiltration (CD3D, CD3G, CD4, CD8A), T cell co-stimulation (TNFRSF4, CD28, ICOS, TNFRSF9), Th1 chemokines (e.g. CXCL9,10,11) and effector molecules such as granzyme A, granzyme B, granzyme K that are responsible for cytotoxicity (13). In biopsy samples from three limb transplant recipients, chemokines including CCL18 were shown to be significantly upregulated during allograft rejection. CCL18, which commonly binds to the CCR8 receptor, has been linked to an increased recruitment of allo-T cells (CD4+ and CD8+ T cells) to skin xenografts, leading to signs of accelerated graft rejection. Using a humanized skin transplantation model, the authors could show that blockade of CCR8 remarkedly decreased CCL18-induced allo-T cell infiltration (46).
Stem cells represent unspecialized cells of the human body (47). Following several steps of specialization, developmental potency is reduced with each step (48). Of note, both epithelial and follicular stem cells are characterized by high developmental capacity, which classifies them as multipotent stem cells. Epithelial stem cells, for example, can differentiate into keratinocytes (49). Follicular stem cells, in turn, have shown even stronger multipotency and can differentiate into various cell types such as keratinocytes, melanocytes, mesenchymal cells, neurons, and glial cells. In addition, they have also been shown to contribute to angiogenesis (50, 51). Stem cells are also involved in various immune-related diseases, such as cancer, cardiovascular, and autoimmune diseases, where they can both promote disease and contribute to healing (52–54). Cancer stem cells, for example, have been implicated with tumorigenesis and therapy resistance while other stem cell types can promote tissue regeneration and healing in cardiovascular and autoimmune diseases (55, 56). In acute VCA rejection, epithelial and follicular stem cells have been proposed as the primary target cells (12).
Cutaneous stem cells, such as epithelial and follicular stem cells, play an important role in skin regeneration, and complex interactions with skin resident and infiltrating immune cells have been observed. These interactions not only make the skin particularly susceptible for immune-related diseases like autoimmune disorders (e.g., systemic lupus erythematosus) but also may play a pivotal role in allograft rejection. Epithelial stem cells, for example, can be affected by various immune cell types including Tregs and macrophages. Tregs in close spatial proximity to hair follicles have been demonstrated to regulate the activity of follicular stem cells, thus impacting hair growth (57). Macrophages, in turn, have been shown to exert suppressive effects on follicular stem cells (58). Since hair follicles have the capacity to recruit a variety of immune cells in response to damage, follicular stem cells may be at particular risk of immune cell targeting and subsequent inflammation (59). The above-mentioned interactions may be based on various specific properties of cutaneous stem cells. For instance, several signaling pathways including JAK-STAT, β-catenin/Wnt, and Jag1-Notch exist, mediating the interaction of cutaneous stem cells with immune cells (60). Of additional interest, transcriptional profiling of human epithelial stem cells has shown increased expression of encoding surface receptors and cell adhesion molecules such as leukocyte differentiation antigens and activated leukocyte cell adhesion molecule (ALCAM), potentially further enhancing interactions with immune cells (61). Most interestingly, it has been shown that MHC class I and II expression can be increased in follicular stem cells under pathological conditions such as autoimmune disease, thus enhancing the potential for immune cell interactions. Intriguingly, this mechanism may also play a role in alloimmunity and explain why the skin is seen as a predominant target of the immune response in VCA (62, 63). In line with this observation, biopsies from facial transplant recipients, for example, have shown lymphocyte accumulation in epithelial stem cell-rich regions. Of note, intra-epidermal and intra-follicular cells were mainly targeted by cells exhibiting a donor-derived T cell phenotype (12). This was observed in a manner that mirrors other cytotoxic immune reactions in the skin such as acute graft-versus-host disease. Additional studies in face transplant recipients have reported that hair follicles are also involved in chronic rejection with hair loss as an early feature presenting prior to sclerosis or vasculopathy (64). More importantly, our own group has described epidermal thinning with a loss of rete ridges in chronic face transplant rejection (65). As epidermal stem cells are located within rete ridges, this indicates that skin stem cells do not only represent a target of alloimmunity in acute, but also in chronic rejection (66). In addition, studies of cutaneous graft versus host disease after bone marrow transplant have shown that epithelial stem cells are particularly targeted by effector T cells. Mechanistically, they have been shown to be especially prone to the pro-apoptotic effects of cytotoxic cytokines produced by allostimulated T cells (67). Of note, this susceptibility may be due to increased expression of cytokine-inducible adhesion molecules such as CD106 (68). Moreover, it has been shown that proinflammatory cytokines such as TNF-α may prime epithelial stem cells towards apoptosis by interacting with apoptosis-regulators such as p73, a member of the p53 family. This may ultimately result in increased vulnerability to the effects of allostimulated T cells (69). Taken together, these mechanisms may also play an important role in VCA rejection, thus potentially explaining the predominant targeting of skin stem cells.
EC are the first line of defense against blood-borne pathogens and represent the interface between graft tissue and recipient-derived immune cells following VCA (70). In general, EC can act as “semiprofessional APC”, meaning that they can express the genes involved in antigen processing and presentation while lacking the surface receptors CD80 and CD86 (71). Furthermore, EC drive regeneration of the vasculature (72) and they can promote a local antithrombotic environment by activation of antithrombin-3 (73). Platt et al. have demonstrated that the activated complement system (i.e., the membrane attack complex (MAC)) and preformed DSAs trigger intravascular coagulation and EC vulnerability to oxygen radicals (74). In response to these processes, EC acquire pro-coagulant functions followed by activation of the tissue factor (TF) and thrombus formation (75–77).
EC hold a key position in acute VCA rejection as they activate and recruit lymphocytes through the upregulated expression of HLA-II and adhesion molecules (e.g., E-selectin) on their cell surface (12). Ligation to the HLA-II molecule drives phosphorylation of protein kinase B (PKB), which increases IL-6 secretion of EC (78). Rising IL-6 levels contribute to the expansion of the Th17 subset of T helper cells while reducing the immunosuppressive regulatory T cells (Tregs). The Th17 subset has been shown to navigate neutrophil granulocytes to the inflammation site via IL-8 and produce proinflammatory IL-17A and IL-22 (79). Furthermore, E-selectin orchestrates overall leukocyte trafficking and allows transendothelial migration of lymphocytes to the skin. This migration process is due to T cells harboring the cutaneous lymphocyte antigen (CLA; a ligand for E-selectin) which is commonly expressed on <5% of T cells. However, in skin lesion biopsies, >80% of T cells were found to be CLA+ (80). Further, EC can lead to increased levels of vasoactive messenger substances such as bradykinin, prostacyclin, and nitric oxide (NO) during acute VCA rejection (12, 81). This can be due to increased levels of IL-6 resulting in elevated NO levels (82). Mechanistically, high-level NO leads to phosphorylation of vascular endothelial (VE)-cadherin Y685 which promotes the dismantling of adherens junctions between the EC (83). This cascade entails the disruption of the endothelial barrier that is crucial for the fluid, gas, and metabolic homeostasis of the VCA transplant (84). Because of the limited vessel diameters, especially at the capillary level (≤5 μm versus activated T cells measuring 8- 12 μm), the EC inevitably interact with T cells (85). This phenomenon may explain how EC can activate resting memory T cells in acute VCA rejection, unlike for example fibroblasts, despite both cell types expressing HLA-II molecules (85).
During acute VCA rejection, EC can be involved in cellular- (CMR) or antibody-mediated rejection (AMR) processes. In terms of CMR, EC of the allograft act via the presentation of donor HLA molecules which are recognized by T cells and trigger inflammatory pathways. Further, VCA grafts host an array of expanded populations of donor T cells expressing CD69, CD103, and CLA (i.e., markers of resident memory T cells (Trm)). The increased prevalence of this cell subset can be due to the majority of VCA patients having undergone previous blood transfusions and/or surgical procedures, thereby inducing donor antigen sensitization and generating long-lived (i.e., occurring up to 23 months following facial transplants), donor-specific memory T cells (12, 86). This subset has been implicated with early-onset VCA rejection on postoperative day five. Vice versa, it remains to be determined whether donor-derived T cells target recipient EC that are derived from blood vessels that grow into the VCA graft (12). Remarkably, AMR has thus far occurred less frequently in VCA than in SOT. This might be due to the ingrowth of recipient blood vessels and/or migration of EC into the VCA graft, which prevents binding of DSA to the EC (25). Further, tissue damage in the aftermath of VCA surgery may trigger the release of donor antigens which can lead to clonal suppression (i.e., they interact with the respective B cell antigen receptors and suppress the production of DSA) as well as directly bind to DSA, hindering their interaction with donor EC (87). The deposition of C4d on EC – a hallmark of AMR in SOT – has also been demonstrated in bilateral hand transplant recipients, as well as forearm and full-face transplants, but its diagnostic value in VCA rejection remains controversial (10, 41).
EC lining the endothelial wall in deep donor arteries, as well as synovial and sentinel skin graft vessels, have been associated with chronic VCA graft rejection (88). The first face transplant patient re-presented with fibrosing EC, which impaired the functionality of the endothelial barrier and (presumably) caused EC-driven thrombosis of VCA vessels (88). The Lyon group outlined the key role of capillary thrombosis in chronic VCA rejection after studying 10 VCA recipients (21). Interestingly, the involvement of fibrosing EC in chronic VCA rejection has also been proposed by Mundinger et al. utilizing a nonhuman primate model of face transplantation (89). Lymphoid follicle formation, which has been reported in chronic VCA rejection by Mundinger et al. but also separately in hand transplants, seems to be intertwined with EC: follicles were found to be surrounded by endothelial venule-like vessels (89, 90). In addition, accelerated arteriosclerosis has been found in SOT, which may be mediated by various processes including ischemic injury of vessels during transplant, viral infections of the graft, and side effects of immunosuppressive drugs such as corticosteroid-induced hyperlipoproteinemia (91). Of note, arteriosclerosis-like changes such as intimal thickening have also been observed in acute VCA rejection. Moreover, rejecting VCA patients are often treated with high-dose corticosteroids. Thus, accelerated arteriosclerosis may play a key role in chronic VCA rejection and contribute to graft damage (92).
In terms of medication regimens targeting EC in VCA rejection, ustekinumab and secukinumab have demonstrated beneficial effects by inhibiting Th17 cell proliferation and blocking IL-17A in SOT and were therefore tested in an osteomyocutaneous radial forearm flap model of non-human primates by Atia et al. (93, 94). Of note, the authors combined ustekinumab and secukinumab with belatacept, which blocks CD86-CD28 interactions, but they did not find improved outcomes when compared to the standard triple immunosuppressive regimen (i.e., tacrolimus, mycophenolate mofetil (MMF), and methylprednisolone). They hypothesized that those results were based on CD28-independent co-signaling pathways in Th17 cells and high-level CTLA-4 expression inducing resistance to belatacept (95). Complement system activation leading to C4d disposition on endothelia as the hallmark of AMR represents another drug target in VCA medication therapy. To this end, eculizumab that blocks the cleavage of C5 into C5a and C5b fragments has been applied in a highly presensitized full-face transplant patient in combination with total plasma exchange therapy and intravenous immunoglobulin administration. This drug protocol antagonized symptoms of VCA rejection in a full-face allotransplant recipient within 1-month post-transplantation and allowed reduced medication therapy compared to the standard triple immunosuppression regiment (41). Furthermore, when investigating the molecular biology of skin rejection in VCA, Hautz et al. observed a close correlation between the severity of rejection with ICAM-1 and E-selectin (45). Both E- and P-selection mediate the binding and rolling of immune cells in the vasculature (96, 97). The therapeutic potential of these adhesion molecules was then investigated in a rat hind limb allotransplant model by locally administering efomycine E (a specific E- and P-selectin inhibitor) (45). When combined with antithymocyte globulin and low-dose tacrolimus, a weekly subcutaneous injection of efomycine E led to a significantly prolonged skin and allograft survival. Thus, targeting adhesion molecules may be a promising adjunctive treatment to reduce the burdensome immunosuppressive regimen. Nevertheless, the administration of efomycine E alone remained ineffective, indicating the need for combined therapies.
NK cells are effector lymphocytes of the innate immune system. While NK cells lack the clonotypic TCR and CD3ϵ (i.e., the associated signal-transducing adaptor), they express the low-affinity Fc receptor CD16 and are therefore capable of detecting antibody-coated target cells, exerting antibody-dependent cell cytotoxicity. NK cell activation is initiated by the interplay of different activating and inhibitory signals involving ITAM (immunoreceptor tyrosine-based activation motif)-bearing molecules (98). NK cells can secrete the cytotoxic reagents perforin and granzyme B (99). Further, NK cells interact with EC for example via binding of α4β1 integrin to vascular cell adhesion protein-1 (VCAM-1) (100). NK cells can also mediate DC homeostasis via IFN-γ and TNF-α. NK cells can prime Th1 cells through IFN-γ secretion (101). Regarding B cells, NK cells have been demonstrated to increase IgG and IgM antibody production and facilitate immunoglobulin class switching (102, 103).
Friedman et al. identified multiple cytokines (e.g., IL-10 and IL-18) and chemokines (e.g., CXCL-9 and CX3CL-1) that were upregulated in VCA rejection (104). CX3CL-1 is expressed by EC and directs C-X3-C motif receptor-1 (CX3CR-1) expressing cells like macrophages and NK cells into inflammatory sites. In response to this ligation, NK cells produce IFN-γ which drives CX3CL-1 expression on EC, pointing towards a paracrine feedback loop between CX3CL-1 and CX3CR-1 (105). IFN-γ induces Th1 responses and increases ROS levels, leading to endothelial damage in VCA grafts (104). Moris et al. further proposed an activating effect of Trm on NK cells in VCA rejection (24). In NK cells, this interaction coordinates increased granzyme B secretion (106). Granzyme B, a serine protease found in the lytic granules of NK cells, orchestrates cell apoptosis, especially through Bid (i.e., a Bcl-2 family member) (107). Interestingly, elevated levels of granzyme B have been shown to increase mesenchymal stem cell (MSC) populations (108). Conversely, MSCs suppress the activity of NK and other immune cells for example by increasing tryptophan metabolites (109, 110). Kuo et al. translated the basic biological links between NK cells and MSCs into the field of VCA and corroborated the modulatory effects of MSCs on NK cells (109). Of note, Win et al. directly compared the potency of NK cells versus CD3+ T cells during rejection by collecting skin biopsies from 7 face transplant patients. The authors revealed that T cells accounted for the majority of secreted granzyme B and outnumbered NK cells 4-fold. Yet, their work demonstrated that 56% of NK cells were activated (i.e., CD56+CD107+) in comparison to 21% of CD3+ T cells expressing the activation marker CD40L. To evaluate the relative contributions of T cells and NK cells to cytotoxic injury, the authors immunostained for caspase-8 (a marker of cytotoxic cell death) and found that T cells mediated significantly more cytotoxicity than NK cells and were responsible for a mean 71% of cytotoxic events in VCA grafts (versus 29% attributed to NK cell cytotoxicity) (13). While the relative NK cell count following VCA surgery remains to be ascertained, preliminary data from Belike et al. demonstrated that anti-NK treatment (HB-191) administered one day prior to transplantation of islet allografts did not delay acute rejection in a diabetic murine model (111). Their findings may point towards a subordinate role of NK cells in the acute rejection phase which remains to be investigated in further studies.
NK cells in VCA rejection can be targeted by repetitive injections of MSCs. Their potent effects have been demonstrated in a rodent hindlimb model by Kuo et al. They found that the combination of adipose-derived MSCs (administered on day 7, 14, and 21 post-transplantation), anti-lymphocyte serum, and cyclosporin A yielded prolonged allograft survival when compared to the untreated control and the subgroup receiving only anti-lymphocyte serum and cyclosporin A (112). The same group confirmed the beneficial use of MSC injections for VCA graft survival in both a swine hindlimb model as well as a swine hemifacial model (113, 114). Expanding on the modulatory effects of MSCs on NK cells, there is a complex interplay between these cell types. MSCs can shield against NK cell-mediated lysis by expressing serine protease inhibitor 9 or TLR-3 (115). Vice versa, IDO and prostaglandin E2 (PGE2) are pivotal mediators in MSC-induced suppression of NK cells as they interfere with IL-2 which is crucial for NK cell proliferation (116). Friedman et al. introduced CX3CR-1 on the NK cell surface as a potential point of leverage in VCA rejection therapy (104). In murine models, CX3CR-1-/- mice have shown prolonged cardiac allograft survival, though this remains to be translated to VCA settings (117).
The bone marrow hosts the initial phases of B cell development and facilitates the deletion of B cells which bind self-antigens (i.e., negative selection) and the assembly of the B cell receptor. In response to antigen contact, naïve B cells differentiate into plasma or memory B cells (29, 118, 119). Memory B cell populations can shift into long-lived plasma cells or GC B cells following antigen rechallenge (29). The interplay with T follicular helper (Tfh) cells further impacts B cell fate as Tfh cells act on GC B cells for example by IL-4 and IL-21 secretion and CD40L-CD40-interaction (120). B cell memory shields human health via long-lived plasma cells in the bone marrow that secrete highly specific antibodies recognizing recurrent homologous pathogens (121). Variant pathogens that may have evaded this first line of defense are targeted by memory B cells accumulating a broader set of antigen affinities and specificities (122). Mucosal B cells populate the gut, respiratory, and urogenital mucosae as well as the skin, salivary, mammary, and lacrimal glands. Their main purpose is to engage with secretory epithelia to provide a first-line defense through the secretion of immunoglobulin A (IgA) (123). B cells are also classified as APC as they form immunological synapses in an actin-dependent manner to capture, process, and present antigens on HLA-II molecules to CD4+ T cells upon B cell receptor signaling (124).
The B cell-based production of DSAs holds a pivotal role in the overlap of B cell biology and VCA and can be subdivided into preexisting versus de-novo DSAs, with the latter typically occurring within 3 months post-transplantation (25, 40, 125, 126). In theory, the immunogenic components of VCA grafts (especially skin and mucosal tissue) and the frequently encountered presensitization (i.e., the formation of preformed potentially donor-specific antibodies) of VCA recipients through previous blood transfusions and/or surgical procedures may predispose this patient population to AMR. However, AMR is inconsistently observed in VCA patients. This might be due to (i) blood vessels in VCA grafts partially sprouting from the recipient’s vasculature and thus not expressing antigens recognized by DSA (this effect is potentiated by the fact that >80% of immunoglobulins circulate with the bloodstream, exposing the intravascular surface as the main location of arising alloreactivity), (ii) antigen shed from the graft resulting in clonal suppression (i.e., suppression of the DSA production) and enhancement (i.e., blockade of antigen recognition by DSAs), (iii) the interplay of DSAs with the graft tissue inducing resistance to AMR, and (iv) the prolonged normothermic ischemia time and higher frequency of reperfusions potentially reducing ischemic reperfusion injury (IRI) and development of DSAs (25, 42, 127). Further, the incidence of de-novo DSAs does not seem to be higher in VCA than SOT, as recent work by the Oxford VCA program found that 6/16 (37%) abdominal wall transplant patients developed de-novo DSAs versus eight of 13 (61%) intestinal or multi-visceral transplant recipients. Interestingly, they reported no case of AMR in their patient cohort, no difference in 1- and 3-year graft survival when stratified for the formation of de-novo DSAs, and they did not find any correlation between the incidence of de-novo DSAs and previous blood transfusions. The authors proposed that rejection in VCA patients was treated before triggering significant tissue damage and formation of de-novo DSAs (128). Following-up on six face transplant recipients, Borges et al. reported on one patient with AMR who – in contrast to the other recipients – displayed a persistent dominance of Th17 (129). This T cell subset has been implicated in the induction of B cell proliferation, increased antibody production cells, and elevated levels of the inducible T cell costimulator (ICOS) molecule further promoting B cell differentiation (130). Biopsies taken from the first face-allograft recipient nine years post-transplantation due to skin rejection symptoms showed dermal infiltration of CD20+ B cells in the facial allograft, as well as the sentinel skin graft. Here, CD20+ B cells formed tertiary lymphoid organs (TLOs), which are considered pathological hallmarks of chronic VCA rejection (88). Interestingly, TLOs TLS can mimic functions of GCs and drive the proliferation and/or differentiation of autoreactive B cells for example via the activation-induced cytidine deaminase (AID) (131).
Current immunosuppressive protocols are often focused on antagonizing alloreactive T cell responses with, for example, tacrolimus and cyclosporin A showing limited effects on B cells (40, 125). Sutter et al. utilized the Brown Norway-to-Lewis hind limb model to evaluate the effects of in situ forming rapamycin implants on VCA acceptance. While the authors observed increased levels of multilineage mixed chimerism and frequency of Tregs, they found no significantly different B cell frequencies in peripheral blood and bone marrow when compared to the untreated control group (132). Belatacept is a fusion protein (CTLA4-IgG1) that targets the CD28/B7 co-stimulation between T and B cells resulting in inhibition of DSA formation, as shown by Grahammer et al. in four hand-transplanted patients (133). De-novo belatacept-based treatment has led to sufficient rejection prophylaxis and reduced side effects in a hand transplant recipient when compared to calcineurin inhibitor-based protocols (134). The Innsbruck group reported the first case of a primarily B cell-driven rejection episode in a forearm recipient at nine years post-transplant. While the patient did not respond to steroid treatment, administration of rituximab (i.e., an anti-CD20 monoclonal antibody) resulted in complete remission of clinical symptoms (10). While the therapeutic benefits of Bregs in controlling VCA rejection remain to be ascertained, the NK cell-mediated effects of Bregs in inducing allograft tolerance have been demonstrated by Schuetz et al. in a murine islet model. Of note, the authors did not find quantitative changes in Bregs population when NK cells had previously been depleted and therefore hypothesized that NK cells mainly influence the donor-specific regulatory function of Bregs (135).
APC are involved in the rejection process via the direct and indirect pathways of allorecognition. In the direct pathway, donor-derived APC are recognized by recipient T-cells due to their foreign MHC molecules, whereas in the indirect pathway, recipient-derived APC have processed donor-derived antigens and present them via self-MHC to recipient T-cells (136). Dendritic cells (DCs), macrophages, and B cells represent professional APC and express pattern recognition receptors (PRRs) such as Toll-like receptors to recognize pathogen-associated molecular patterns (PAMPs), as well as damage-associated molecular patterns (DAMPs) (137). APC internalize the target molecule via endocytosis, pinocytosis, or phagocytosis (138). The internalized molecules are processed and presented by MHC-I to CD8+ T cells and MHC-II to CD4+ T helper cells, respectively. In a reciprocal manner, APC and T cells promote their interactions for example via co-stimulatory signal involving B7 molecules (i.e., CD80 and CD86) and CD28 or CD40 and CD40L (139–141).
In facial VCAs, dendritic-appearing CD8+ T cells have been identified, representing a subclass of dendritic Trm (12). DCs and CD8+ Trm both express for example CD103L to bind E-cadherin, which is a hallmark in mucosal and epithelial barrier function (142). While they constitute a dendritic-like, not dendritic-identical phenotype, a potential cross-talk between DCs and Trm in VCA seems plausible and has already been demonstrated in cancer research (143). Biopsy staining from four face transplants further revealed a novel role of tissue-resident macrophages in the surveillance of VCA graft integrity (144). Given their dependence on colony-stimulating factor 1 receptor (CSF1R), tissue-resident macrophages are defined as “M2-like” macrophages with a CD169+ subset displaying distinctive immunoregulatory properties (145). In keeping with results from rodent studies, Ualiyeva proposed the loss of this subset to be indicative of VCA rejection and identified T cell immunoglobin mucin-4 (TIM-4) as a potential driver of CD169+ tissue-resident macrophages migration to inflammatory/rejection sites via its phosphatidylserine binding pocket (144). Further, VCA graft-infiltrating macrophages produce IL-18 and reactive oxygen species (ROS) (104). IL-18 has been shown to trigger the secretion of proinflammatory interferon-γ (IFN-γ), and ROS can trigger pro-apoptotic B cell lymphoma-2 (Bcl-2) family proteins as well as impair mitochondrial function (146, 147) (for in-depth analysis of B cells in VCA please refer to “B cells”).
In VCA rejection, DCs can be therapeutically targeted by administration of the mammalian target of rapamycin (mTOR) inhibitor sirolimus (rapamycin) which conditions DC to stimulate immunosuppressive Tregs while reducing alloreactive T cell populations (148, 149). Interestingly, donor alloantigen-pulsed immature recipient DCs in combination with low-dose cyclosporine plus antilymphocyte serum regimen prolonged graft survival (32 versus 18 days in the cyclosporine-only control group) in a rat hindlimb model (150). This effect might be due to the induction of T cell hyporesponsiveness and reduced IFN-γ secretion. APC produce IL-12 leading to increased IL-4 levels, which have been demonstrated to promote M2 macrophage differentiation and function, as well as represent a marker for acute VCA rejection (151). This macrophage subset has been implicated with graft rejection (152, 153). Thus, targeting IL-4 via IL-4Rα blocker dupilumab seems an intriguing strategy for VCA rejection therapy and has already been successfully applied in the treatment of atopic dermatitis (154). Ustekinumab, an anti-IL-12/23 antibody, has shown potent effects in VCA rejection (please see “Endothelial Cells”) (93). Yet, its interplay with APC in the context of VCA remains to be determined.
Granulocytes are the most abundant subpopulation of leukocytes and can be subdivided into neutrophils, eosinophils, and basophils (155). Neutrophils express HLA-II and costimulatory molecules (e.g., CD80 and CD86) needed for antigen presentation and acquire DC-like functionality after exposure to cytokines (156, 157). Eosinophils have been involved shuttling of antigens to lymphoid tissues, while MHC-II molecules can be expressed in response to cytokine contact (158). Murine data points towards basophils acting as APC, while Eckl-Dorna et al. showed that basophils from allergic patients did not exert antigen-presenting functions (155, 159, 160).
Utilizing a groin free-flap rat model, Friedman et al. provided evidence of high overall granulocyte contents amongst infiltrating leukocytes within inflamed graft regions (104). The Innsbruck group reported that granulocytes were located interstitially and toward the epidermis in biopsies from a VCA patient eleven years after hand transplantation (161). Etra et al. found neutrophilic inflammation to be significantly correlated with severe VCA rejection in swine hindlimb transplants but not specific to the pathogenesis of rejection. They further identified granulocytes (neutrophils and eosinophils) in rejecting animal skin samples, whilst the vast majority of infiltrating inflammatory cells were lymphocytes (162). Overall, further studies are needed to determine the specific role of the different granulocytic subsets in VCA rejection and translate previous findings on the pharmacological targeting of granulocytes into the field of VCA.
Neutrophils express HLA-II and costimulatory molecules (e.g., CD80 and CD86) needed for antigen presentation with both immature and mature neutrophils being able to acquire DC-like functionality after exposure to cytokines such as IFN-γ, TNF-α, or IL-4. Alternatively, depletion of anti-granulocyte receptor-1 (Gr-1) or combined CXCR2–formyl peptide receptor 1 antagonism have been demonstrated to reduce neutrophil infiltration in a murine hepatocyte injury model (163). In allergy therapy, blocking antibodies (Benralizumab) targeting the IL-5 receptor, as well as anti-IL-5 neutralizing antibodies (Reslizumab) have been demonstrated to reduce eosinophils count (164, 165). Blocking key eosinophil molecules such as IL-33 and CD48 represents another therapeutic option. Addressing inhibitory receptors (e.g., Siglec-8 and CD300a) may allow eosinophils to be directly targeted, regardless of the underlying activating stimulus (166). CD48 and CD300a have also been researched in the context of basophil-centered allergy therapies (167). Mylotarg, a CD33-targeted drug, has been implicated with a reduced frequency of basophils without driving basophil-derived histamine secretion (168).
Regulatory T cells (i.e., CD4+CD25+CD127- T cells; Tregs) exert their immunosuppressive effects through direct cell-cell interaction with target immune cells or through the expression of immunosuppressive cytokines and anti-inflammatory molecules (169–171). Sagoo et al. demonstrated the beneficial effect of Tregs cells in preventing skin allograft rejection utilizing humanized mouse models (172). Tregs can be equipped with a chimeric antigen receptor (CAR) representing another, yet bioengineered cell type in VCA grafts (173). Such CAR-Tregs are not MHC-dependent in their activation process and instead specifically migrate to the rejection site, where they unleash more potent immunosuppression than conventional polyclonal Tregs (3).
Different types of stem cells have been shown to play important immunoregulatory roles in allograft rejection, including MSC and adipose-derived stem cells (ASC). Experimental administration of MSC, for example, has been shown to mitigate acute allograft rejection in a hindlimb VCA model, thereby significantly prolonging graft survival. Mechanistically, these findings were associated with elevated levels of regulatory T cells (Tregs) in the peripheral blood and skin of recipients. These observations are supported by in vitro evidence showing increased Treg proliferation in co-cultures with MSC and T cells when compared to T cells alone. Of histological interest, MSC accumulated in the subcutaneous layer of both donor and recipient skin, whereas no significant accumulation in muscle or bone marrow tissue was detected (109, 174). Of note, another study investigating the effects of MSC in a tracheal transplant model also observed beneficial effects on allograft survival with augmented microvascular blood flow and oxygenation of the graft. Mechanistically, these results have been associated with upregulation of Tregs and increased levels of cytokines IL-5, IL-10, and IL-15 (175). ASC have also been reported to prolong allograft survival in experimental VCA models. Consistently, these observations have been associated with elevated Treg levels in the peripheral blood and skin compartment of the allograft, along with increased TGF-β1 and IL-10 expression. Moreover, in vitro experiments demonstrated attenuating effects of ASC on T cell proliferation (112). Further studies have confirmed the regulatory effects of ASC on T cells with decreased T cell proliferation and increased numbers of Tregs (176). Of additional interest, ASC also appear to play an important role in regulating B cell responses in the setting of transplantation. More specifically, ASC administration was associated with increased amounts of CD45Ra+/Foxp3+ regulatory B cell subsets. Investigating complement activation in the same study, C4d, a marker of cellular- and antibody-mediated rejection, has been found to be decreased in transplanted alloskin tissue when administering ASC (177). Moreover, studies investigating the effects of ASC on graft vasculopathy have observed reduced intima/media ratios in arterioles of allograft skin and muscle, indicating beneficial effects of ASC treatment (178).
Langerhans cells (LC) are a subset of immature dendritic cells and as such are a type of APC that can be found in the epidermis (179). Upon phagocytosis of antigens, they migrate to regional lymph nodes where they initiate T cell responses by antigen presentation (180). Most interestingly, LC have also been shown to exert immunosuppressive effects by selectively promoting the expansion and activation of skin-resident Tregs (181, 182). Hence, it can be assumed that LC not only possess stimulatory, but also regulatory capabilities, emphasizing the complexity of the skin immune microenvironment (181). In tolerated VCA, recipient-derived LC have been shown to rapidly infiltrate allografts after transplantation, thereby establishing LC chimerism. However, in rejecting VCA, initial dilution of graft-resident LC has been observed, followed by extensive infiltration by recipient-derived LC, ultimately leading to a near-complete loss of donor cell contribution. This infiltration of rejecting VCA most likely occurs via transvascular migration, as previous studies have observed perivascular infiltrates in early VCA rejection phases (16, 183, 184). In summary, these findings suggest that durable chimerism of LC is an important contributor to immune homeostasis and long-term graft tolerance in VCA (185). Moreover, previous studies have shown that large amounts of donor lymphocytes are released into the recipient circulation upon graft reperfusion, which may further stimulate recipient dendritic cells and thereby drive infiltration (186).
Mast cells (tissue-resident, innate immune cells orchestrating inflammatory response and tissue homeostasis; MC) have also been shown to be implicated in allograft rejection. In experimental skin graft models, MC have been observed to promote allograft rejection by inducing proinflammatory responses through degranulation, with elevated levels of cytokines such as keratinocyte chemoattractant (KC), macrophage inflammatory protein 2 (MIP-2), and TNF-α. Consequently, increased infiltration of neutrophils has been described, further accelerating graft rejection. Accordingly, administration of MC-stabilizing drugs such as cromolyn significantly delayed allograft rejection (187). Of additional interest, studies investigating the role of mast cells in skeletal muscle ischemia reperfusion injury (IRI) observed increased muscle viability in mast cell-deficient mice following ischemic injury when compared to wild-type mice. Histologically, this was accompanied by increased muscle necrosis in wild-type animals, suggesting that MC are a major source of necrosis mediators in muscle IRI (188). Additional studies on VCA have shown that several inflammatory mediators, including IL-4, IL-12p70 and TNF-α, can serve as predictors for alloskin rejection. For muscle rejection, in turn, IL-12p70 and TNF-α have been identified as particularly accurate classifiers. Of note, MC appear to be the main source of TNF-α in the skin, entailing the initiation of T cell responses with subsequent tissue injury and allograft dysfunction. Thus, MC may be a key initiator of TNF-α-mediated rejection processes in VCA (189–191).
Recently, several chronic rejection (CR) cases have been reported in VCA (17, 22, 92, 192, 193). Chronic rejection is typically defined as an irreversible later-onset change of the allograft that leads to loss of function and accelerated aging. The mechanisms leading to CR in fVCA are poorly understood but both cellular responses (against MHC bearing endothelium as proposed by Libby and Tanaka) and antibody mediated injury to graft vasculature may play a role (91). As sparse as the understanding is, the consequences of CR in VCA are drastic: CR in VCA irreversibly leads to gradual destruction, with graft dysfunction and loss of architecture as ultimate endpoints (88). In human VCA recipients, the skin and vasculature were identified as the main targets of CR (21, 22, 65, 192–196): Clinically documented manifestations of CR ranged from cutaneous lesions to changes in skin adnexa (loss of hair and nails). Krezdorn et al. collected biopsies of seven fVCA patients for up to an 8-year interval postoperative. The authors found that sclerotic zones in the allografts demonstrated upregulation of AP-1 pathway components such as JunB and c-Fos. These genes have been implicated with overproduction of type I dermal collagen in the setting of autoimmune cutaneous disorders (e.g., systemic sclerosis) (65). Further, Lee et al. used digital spatial proteomic profiling to show the expression of pathway components involved in atherogenic responses, including IDO1 and STING, as well as proteins expressed by activated cytotoxic T cells and macrophages (197).
The main pathological correlates of CR were chronic endothelial changes leading to graft vasculopathy with capillary thrombosis and accelerated arteriosclerosis (3, 7, 198) (Figure 2). In addition to the changes in the vessels, fibrosis, thinning, and atrophy of the skin layers were described as pathological findings. Another frequently documented characteristic of CR in VCA is the increased appearance of tertiary lymphoid organs (TLOs) (i.e., highly ordered structures resembling the cellular composition of lymphoid follicles) (22). These TLOs are believed to be local sites of alloantibody production and T cell activation (90). Further, cases of simultaneous targeting of skin plus vessels, and isolated damage to cutaneous or vascular structures, respectively, have been reported (198). Accordingly, skin and vasculature appear to be independent CR targets. It is hypothesized that the skin may be more prone to cellular rejection mechanisms, while vascular structures may be more likely to be the target of humoral immune responses. Although it is widely agreed upon that vasculature and skin are main targets of chronic rejection in VCA, there is little evidence that vascular changes are necessary for chronic changes of the skin component. In a case published by our workgroup, we thoroughly studied the explant of a facial VCA that was removed after ten years following the patient’s death due to unrelated reasons (194). There was no graft vasculopathy despite obvious chronic skin changes (pale appearance of the graft, telangiectasis, epidermal thinning, loss of rete ridges, papillary dermal sclerosis) and numerous acute rejection episodes over the course of follow-up. Repeated (sub-)clinical acute T-cell mediated rejection of the skin part was hypothesized to have induced a profibrotic change over time. Given steady motor function scoring, it seems like underlying musculature was spared of such chronic changes. Thus, we assumed that two phenotypes of chronic rejection may exist, one that involved chronic immune-mediated arteriosclerotic change of the vasculature and one that spares the vasculature and predominantly affects the skin component. While acute VCA rejection is mainly based on the interaction of CD8+ effector T cells targeting follicular and epithelial stem cells, T and B cells are likely to represent the primary effectors in chronic rejection (40). In contrast, EC may be considered a primary target during chronic rejection with changes of vasculature. However, there are also chronic pathologic changes (e.g., loss of rete ridges) independent of the vasculature (194). Thus, the cellular landscape of chronic VCA rejection represents an ongoing area of research and remains to be further elucidated.
Figure 2 Chronic allograft changes in Fvca. Chronic rejection is associated with different histopathological changes such as allograft vasculopathy with capillary thrombosis, accelerated arteriosclerosis, as well as the destruction of the epidermal architecture. (A) Histopathological findings of T-cell mediated rejection involving lip mucosa of face transplant show interface change with scattered apoptotic target epithelial cells with associated dense subepithelial inflammatory infiltrate. (B) Chronic face transplant rejection of skin as shown in this biopsy specimen demonstrates epidermal thinning with intraepidermal colloid body formation and occasional lymphocyte-associated apoptotic keratinocytes. Evidence of chronicity in allograft transplant rejection also includes fibrous thickening of subepidermal basement membrane zone and deformation of hair follicles. (C) Representative image of lymphocytic vasculitis seen in acute and chronic rejections of VCA. Histopathology of deep dermal vascular injury shows lymphocytes surrounding and infiltrating vessel walls associated with endothelial cell damage.
Detailed knowledge of cellular crosstalk after VCA surgery is critical to further improve allograft survival, functional long-term results, and patient-reported outcomes (199–201). The current standard immunosuppressive drug regimen based on SOT protocols consists of anti-thymoglobulin/alemtuzumab/rituximab combinations as induction therapy, followed by a high-dose maintenance protocol, most commonly including tacrolimus, MMF, and prednisone (5, 15). Commonly, is recommended to maintain high tacrolimus levels (10–15 ng/ml) during the first three months posttransplant and then tapering it down to 5–10 ng/ml. Prednisone doses are also tapered to be maintained at lower doses (5–15 mg/day) for six to twelve months in the majority of VCA recipients. Based on these general considerations, individual therapy protocols are administered to minimize renal side effects, stabilize glycemic control, and prevent neurotoxicity as well as myointimal hyperproliferation of the vasculature (202–204). Despite extensive immunosuppression, about 85% of VCA recipients experience at least one episode of acute allograft rejection in the first year. This might be due to certain cell types being less sensitive to, or even triggered by, current immunosuppressive protocols. For example, tacrolimus mainly targets T cell subsets by inhibiting the IL-2 activation pathway, whereas Wai et al. found tacrolimus has no effect on NK cell cytotoxicity (205, 206). While corticosteroids have been shown to efficiently hinder Th cell immunity at various stages of the activation cascade and impair cytokine production, as well as effector function, their effects on B cells seem to be more complex (207). Cupps et al. found corticosteroids to have only minor effects on B cell proliferation and even drive immunoglobulin secretion when stimulated with B cell growth factor in vitro (208). Interestingly, Cooper et al. have demonstrated that prednisone can indeed decrease NK cell concentration but does so only in a minority of patients (209). In contrast, MMF has been shown to enhance cytotoxicity and chemotaxis in NK cells (210). Thus, more profound knowledge of cell types involved in VCA tolerance and rejection may help to target the respective cellular subsets and reduce medication side effects more specifically. Of note, there has been little change regarding the immunosuppressive drug regimen administered following VCA surgery for the past two decades. For more detailed information, please refer to some elegant work by Kauke-Navarro et al. (5).
The role of each cell types within the context of the respective tissue is important. For example, Lian et al. identified that Trm in skin tissue is a pivotal cell subset in facial VCA rejection (12). Yet, the same cell subset might have different or even opposite functional and phenotypic properties in the mucosa, which has been recently identified as another promising target to modulate the VCA rejection reaction (14, 15, 18, 19). Further, our knowledge on the relevance of passenger immune cells (PICs) in acute and chronic rejection remains limited. PICs refer broadly to all the graft-derived immune cells that are transferred to the host secondary lymphoid tissue and trigger allograft rejection by direct recognition of the alloantigen (211, 212). However, PICs seem to contribute to allograft rejection and tolerance induction at the same time. In rat liver allograft models, irradiation of the allograft prior to transplantation triggered transplant rejection in otherwise tolerant recipients, underscoring the potential tolerance induction role of donor-derived graft-resident PICs (213, 214). Yet, direct recognition of the alloantigen by CD4+ T cells was considered to persist at early time points after SOT and was highly correlated with the lifespan of graft DCs (215). As an alternative pathway to the insult of donor endothelium by recipient immune cells, PICs were hypothesized to attack the recipient endothelium that has chimerically populated donor-derived vasculature during chronic transplant rejection (12). In facial allograft rejection, Lian et al. discovered that immune cells spatially associated with vascular, pilosebaceous, and epidermal sites of injury were mainly Trm (please see “Endothelial Cells” for further details) of donor origin (12). This finding represents a novel research perspective as the current dogma assumes that skin allograft rejection is mediated by recipient T cells. The local association of donor T cells with sites of direct cell injury reinforces the hypothesis of more complex cross-talks between immune cell during the rejection process. Overall, a more advanced and biomarker-based approach to the assessment of VCA rejection is still needed.
The cell types relevant to VCA are multifunctional and interact through complex pathways (Table 1 and Figure 3). It is important to note that each cell line represents a mere piece of the puzzle, however a clear hierarchy of effector-target cell interactions can be formulated based on current understanding of the pathomechanisms of acute and chronic cellular rejection in VCA. Some cells are more important than others with CD8+-T-cells posing as the protagonists, mainly targeting epithelial and follicular stem cells. Researchers and clinicians should be aware of this complex, closely interlinked network. Despite the predominance of T-cells and effective suppression by current immunosuppressive regiments, it is important to note that other cell types fuel the rejection process and further studies are needed to define the exact impact of such secondary effector (and target cells). Rejection of VCAs frequently occurs and several longer-term studies demonstrate chronic allograft changes. This demonstrates that current treatment regimens are not perfect at mitigating the immune response, indicating that other cell types not currently (sufficiently) targeted by the standard triple maintenance regimen, may be more relevant than previously thought. Future studies are needed to better define the cellular network leading to acute and chronic VCA rejections.
Table 1 Summary of cell types involved in acute and chronic rejection of vascularized composite allotransplantation (VCA).
Figure 3 Cellular cross-talks in the field of VCA. The various cell types in VCA interact via multiple pathways and their balance determines surgical VCA outcomes. Therefore, an isolated approach impacting single cell types is a challenging, yet potentially high-yield leverage point. Different cell types are involved both in acute and chronic rejection and can trigger various pathological processes, e.g., epidermal thinning and loss of rate ridges, follicular plugging, hyperkeratosis, vascular ectasia subepidermal sclerosis with collagen type I deposition and collagen type I shift into the papillary dermis as published by Krezdorn et al., 2019 (65).
The authors state that this manuscript represents their own work. Figure 1 has already been published by Lian et al., while all the other figures and supplements have been created by the author’s team (12).
LK: writing and manuscript preparation. SK: writing and manuscript preparation. AP: reviewing and figures. CaL: reviewing and figures. SS: histopathological background. LH: cellular background. VS: reviewing and immunity background. AS: reviewing immunity data. BK: reviewing immunity data. VM: reviewing rejection paragraphs. ChL: reviewing rejection paragraphs. ST: reviewing. GM: reviewing and figures. BP: reviewing and manuscript. MK-N: reviewing and manuscript. All authors contributed to the article and approved the submitted version.
The authors declare that the research was conducted in the absence of any commercial or financial relationships that could be construed as a potential conflict of interest.
All claims expressed in this article are solely those of the authors and do not necessarily represent those of their affiliated organizations, or those of the publisher, the editors and the reviewers. Any product that may be evaluated in this article, or claim that may be made by its manufacturer, is not guaranteed or endorsed by the publisher.
The Supplementary Material for this article can be found online at: https://www.frontiersin.org/articles/10.3389/fimmu.2023.1179355/full#supplementary-material
Supplementary Figure 1 | Flow chart. The key points of this work include the definition of types of rejection in VCA surgery followed by an in-depth description of target and effector cells for acute and chronic rejection reactions.
1. Park SH, Eun SC, Kwon ST. Hand transplantation: current status and immunologic obstacles. Exp Clin Transplant (2019) 17(1):97–104. doi: 10.6002/ect.2018.0163
2. Siemionow M. The decade of face transplant outcomes. J Mater Sci Mater Med (2017) 28(5):64. doi: 10.1007/s10856-017-5873-z
3. Tchiloemba B, Kauke M, Haug V, Abdulrazzak O, Safi AF, Kollar B, et al. Long-term outcomes after facial allotransplantation: systematic review of the literature. Transplantation (2021) 105(8):1869–80. doi: 10.1097/TP.0000000000003513
4. Gallay B, DeMattos A. Immunology of transplant protocols. Curr Otorhinolaryngol Rep (2014) 2(3):184–91. doi: 10.1007/s40136-014-0057-6
5. Kauke M, Safi AF, Panayi AC, Palmer WJ, Haug V, Kollar B, et al. A systematic review of immunomodulatory strategies used in skin-containing preclinical vascularized composite allotransplant models. J Plast Reconstr Aesthet Surg (2022) 75(2):586–604. doi: 10.1016/j.bjps.2021.11.003
6. Justiz Vaillant AA, Mohseni M. Chronic transplantation rejection. In: StatPearls. Treasure Island (FL: StatPearls Publishing LLC (2022).
7. Puscz F, Dadras M, Dermietzel A, Jacobsen F, Lehnhardt M, Behr B, et al. A chronic rejection model and potential biomarkers for vascularized composite allotransplantation. PloS One (2020) 15(6):e0235266. doi: 10.1371/journal.pone.0235266
8. Fischer S, Lian CG, Kueckelhaus M, Strom TB, Edelman ER, Clark RA, et al. Acute rejection in vascularized composite allotransplantation. Curr Opin Organ Transplant (2014) 19(6):531–44. doi: 10.1097/MOT.0000000000000140
9. Cetrulo CL Jr., Ng ZY, Winograd JM, Eberlin KR. The advent of vascularized composite allotransplantation. Clin Plast Surg (2017) 44(2):425–9. doi: 10.1016/j.cps.2016.12.007
10. Weissenbacher A, Hautz T, Zelger B, Zelger BG, Mayr V, Brandacher G, et al. Antibody-mediated rejection in hand transplantation. Transpl Int (2014) 27(2):e13–7. doi: 10.1111/tri.12233
11. Karahan GE, Claas FHJ, Heidt S. B cell immunity in solid organ transplantation. Front Immunol (2017) 7. doi: 10.3389/fimmu.2016.00686
12. Lian CG, Bueno EM, Granter SR, Laga AC, Saavedra AP, Lin WM, et al. Biomarker evaluation of face transplant rejection: association of donor T cells with target cell injury. Modern Pathol (2014) 27(6):788–99. doi: 10.1038/modpathol.2013.249
13. Win TS, Crisler WJ, Dyring-Andersen B, Lopdrup R, Teague JE, Zhan Q, et al. Immunoregulatory and lipid presentation pathways are upregulated in human face transplant rejection. J Clin Invest (2021) 131(8). doi: 10.1172/JCI135166
14. Kauke-Navarro M, Tchiloemba B, Haug V, Kollar B, Diehm Y, Safi AF, et al. Pathologies of oral and sinonasal mucosa following facial vascularized composite allotransplantation. J Plast Reconstr Aesthet Surg (2021) 74(7):1562–71. doi: 10.1016/j.bjps.2020.11.028
15. Kauke M, Safi AF, Zhegibe A, Haug V, Kollar B, Nelms L, et al. Mucosa and rejection in facial vascularized composite allotransplantation: a systematic review. Transplantation (2020) 104(12):2616–24. doi: 10.1097/TP.0000000000003171
16. Leonard DA, Amin KR, Giele H, Fildes JE, Wong JKF. Skin immunology and rejection in VCA and organ transplantation. Curr Transplant Rep (2020) 7(4):251–9. doi: 10.1007/s40472-020-00310-1
17. Iske J, Nian Y, Maenosono R, Maurer M, Sauer IM, Tullius SG. Composite tissue allotransplantation: opportunities and challenges. Cell Mol Immunol (2019) 16(4):343–9. doi: 10.1038/s41423-019-0215-3
18. Kauke M, Safi AF, Palmer WJ, Kollar B, Nelms L, Tchiloemba B, et al. Dynamic maxillary sinus changes of facial vascularized composite allotransplants. Plast Reconstr Surg (2021) 147(3):722–7. doi: 10.1097/PRS.0000000000007673
19. Kauke M, Tchiloemba B, Haug V, Kollar B, Safi AF, Pomahac B. Partial loss of nasal tissue in a facial vascularized composite allograft patient. Plast Reconstr Surg Glob Open (2020) 8(8):e3038. doi: 10.1097/GOX.0000000000003038
20. Kauke M, Panayi AC, Tchiloemba B, Diehm YF, Haug V, Kollar B, et al. Face transplantation in a black patient - racial considerations and early outcomes. N Engl J Med (2021) 384(11):1075–6. doi: 10.1056/NEJMc2033961
21. Kanitakis J, Petruzzo P, Gazarian A, Karayannopoulou G, Buron F, Dubois V, et al. Capillary thrombosis in the skin: a pathologic hallmark of Severe/Chronic rejection of human vascularized composite tissue allografts? Transplantation (2016) 100(4):954–7. doi: 10.1097/TP.0000000000000882
22. Kanitakis J, Petruzzo P, Badet L, Gazarian A, Thaunat O, Testelin S, et al. Chronic rejection in human vascularized composite allotransplantation (Hand and face recipients): an update. Transplantation (2016) 100(10):2053–61. doi: 10.1097/TP.0000000000001248
23. Milek D, Reed LT, Echternacht SR, Shanmugarajah K, Cetrulo CL Jr, Lellouch AG, et al. A systematic review of the reported complications related to facial and upper extremity vascularized composite allotransplantation. J Surg Res (2023) 281:164–75. doi: 10.1016/j.jss.2022.08.023
24. Moris D, Wang J, Selim MA, Song M, Stempora L, Parker W, et al. Vascularized composite allotransplants as a mechanistic model for allograft rejection - an experimental study. Transpl Int (2021) 34(3):572–84. doi: 10.1111/tri.13820
25. Platt JL, Kaufman CL, Garcia de Mattos Barbosa M, Cascalho M. Accommodation and related conditions in vascularized composite allografts. Curr Opin Organ Transplant (2017) 22(5):470–6. doi: 10.1097/MOT.0000000000000446
26. Wang S, Zhang C, Wang J, Yang C, Xu M, Rong R, et al. Corrigendum to "Endothelial cells in antibody-mediated rejection of kidney transplantation: pathogenesis mechanisms and therapeutic implications". J Immunol Res 2019 (2019) p:9691679. doi: 10.1155/2019/9691679
27. Orosz CG. Endothelial activation and chronic allograft rejection. Clin Transplant (1994) 8(3 Pt 2):299–303.
28. Al-Lamki RS, Bradley JR, Pober JS. Endothelial cells in allograft rejection. Transplantation (2008) 86(10):1340–8. doi: 10.1097/TP.0b013e3181891d8b
29. Akkaya M, Kwak K, Pierce SK. B cell memory: building two walls of protection against pathogens. Nat Rev Immunol (2020) 20(4):229–38. doi: 10.1038/s41577-019-0244-2
30. Schmitz R, Fitch ZW, Schroder PM, Choi AY, Jackson AM, Knechtle SJ, et al. B cells in transplant tolerance and rejection: friends or foes? Transpl Int (2020) 33(1):30–40. doi: 10.1111/tri.13549
31. Clark MR, Massenburg D, Siemasko K, Hou P, Zhang M. B-cell antigen receptor signaling requirements for targeting antigen to the MHC class II presentation pathway. Curr Opin Immunol (2004) 16(3):382–7. doi: 10.1016/j.coi.2004.03.007
32. Sarhane KA, Tuffaha SH, Broyles JM, Ibrahim AE, Khalifian S, Baltodano P, et al. A critical analysis of rejection in vascularized composite allotransplantation: clinical, cellular and molecular aspects, current challenges, and novel concepts. Front Immunol (2013) 4:406. doi: 10.3389/fimmu.2013.00406
33. Kueckelhaus M, Fischer S, Seyda M, Bueno EM, Aycart MA, Alhefzi M, et al. Vascularized composite allotransplantation: current standards and novel approaches to prevent acute rejection and chronic allograft deterioration. Transpl Int (2016) 29(6):655–62. doi: 10.1111/tri.12652
34. Alhefzi M, Aycart MA, Bueno EM, Kiwanuka H, Krezdorn N, Pomahac B, et al. Treatment of rejection in vascularized composite allotransplantation. Curr Transplant Rep (2016) 3(4):404–9. doi: 10.1007/s40472-016-0128-3
35. Win TS, Murakami N, Borges TJ, Chandraker A, Murphy G, Lian C, et al. Longitudinal immunological characterization of the first presensitized recipient of a face transplant. JCI Insight (2017) 2(13). doi: 10.1172/jci.insight.93894
36. Etra JW, Raimondi G, Brandacher G. Mechanisms of rejection in vascular composite allotransplantation. Curr Opin Organ Transplant (2018) 23(1):28–33. doi: 10.1097/MOT.0000000000000490
37. Bhan AK, Mihm MC Jr., Dvorak HF. T Cell subsets in allograft rejection. in situ characterization of T cell subsets in human skin allografts by the use of monoclonal antibodies. J Immunol (1982) 129(4):1578–83.
38. Murata K, Baldwin WM 3rd. Mechanisms of complement activation, C4d deposition, and their contribution to the pathogenesis of antibody-mediated rejection. Transplant Rev (Orlando) (2009) 23(3):139–50. doi: 10.1016/j.trre.2009.02.005
39. Haas M, Sis B, Racusen LC, Solez K, Glotz D, Colvin RB, et al. Banff 2013 meeting report: inclusion of c4d-negative antibody-mediated rejection and antibody-associated arterial lesions. Am J Transplant (2014) 14(2):272–83. doi: 10.1111/ajt.12590
40. Kaufman CL, Cascalho M, Ozyurekoglu T, Jones CM, Ramirez A, Roberts T, et al. The role of b cell immunity in VCA graft rejection and acceptance. Hum Immunol (2019) 80(6):385–92. doi: 10.1016/j.humimm.2019.03.002
41. Chandraker A, Arscott R, Murphy GF, Lian CG, Bueno EM, Marty FM, et al. The management of antibody-mediated rejection in the first presensitized recipient of a full-face allotransplant. Am J Transplant (2014) 14(6):1446–52. doi: 10.1111/ajt.12715
42. Kaufman CL, Ouseph R, Blair B, Kutz JE, Tsai TM, Scheker LR, et al. Graft vasculopathy in clinical hand transplantation. Am J Transplant (2012) 12(4):1004–16. doi: 10.1111/j.1600-6143.2011.03915.x
43. Sayegh MH, Turka LA. The role of T-cell costimulatory activation pathways in transplant rejection. N Engl J Med (1998) 338(25):1813–21. doi: 10.1056/NEJM199806183382506
44. Siu JHY, Surendrakumar V, Richards JA, Pettigrew GJ. T Cell allorecognition pathways in solid organ transplantation. Front Immunol (2018) 9:2548. doi: 10.3389/fimmu.2018.02548
45. Hautz T, Zelger B, Grahammer J, Krapf C, Amberger A, Brandacher G, et al. Molecular markers and targeted therapy of skin rejection in composite tissue allotransplantation. Am J Transplant (2010) 10(5):1200–9. doi: 10.1111/j.1600-6143.2010.03075.x
46. Lian CG, Bueno EM, Granter SR, Laga AC, Saavedra AP, Lin WM, et al. T Cell-attracting CCL18 chemokine is a dominant rejection signal during limb transplantation. Cell Rep Med (2022) 3(3):100559. doi: 10.1016/j.xcrm.2022.100559
47. Borges TJ, Abarzua P, Gassen RB, Kollar B, Lima-Filho M, Aoyama BT, et al. Stem cells: past, present, and future. Stem Cell Res Ther (2019) 10(1):68. doi: 10.1186/s13287-019-1165-5
48. Martello G, Smith A. The nature of embryonic stem cells. Annu Rev Cell Dev Biol (2014) 30:647–75. doi: 10.1146/annurev-cellbio-100913-013116
49. Yang R, Liu F, Wang J, Chen X, Xie J, Xiong K. Epidermal stem cells in wound healing and their clinical applications. Stem Cell Res Ther (2019) 10(1):229. doi: 10.1186/s13287-019-1312-z
50. Babakhani A, Hashemi P, Mohajer Ansari J, Ramhormozi P, Nobakht M. In vitro differentiation of hair follicle stem cell into keratinocyte by simvastatin. Iran BioMed J (2019) 23(6):404–11. doi: 10.29252/ibj.23.6.404
51. Yari A, Teimourian S, Amidi F, Bakhtiyari M, Heidari F, Sajedi N, et al. The role of biodegradable engineered random polycaprolactone nanofiber scaffolds seeded with nestin-positive hair follicle stem cells for tissue engineering. Adv BioMed Res (2016) 5:22. doi: 10.4103/2277-9175.175911
52. Hayat H, Hayat H, Dwan BF, Gudi M, Bishop JO, Wang P. A concise review: the role of stem cells in cancer progression and therapy. Onco Targets Ther (2021) 14:2761–72. doi: 10.2147/OTT.S260391
53. Nasser MI, Qi X, Zhu S, He Y, Zhao M, Guo H, et al. Current situation and future of stem cells in cardiovascular medicine. BioMed Pharmacother (2020) 132:110813. doi: 10.1016/j.biopha.2020.110813
54. Rosa SB, Voltarelli JC, Chies JA, Pranke P. The use of stem cells for the treatment of autoimmune diseases. Braz J Med Biol Res (2007) 40(12):1579–97. doi: 10.1590/S0100-879X2006005000166
55. Yu Z, Pestell TG, Lisanti MP, Pestell RG. Cancer stem cells. Int J Biochem Cell Biol (2012) 44(12):2144–51. doi: 10.1016/j.biocel.2012.08.022
56. Gattinoni L, Speiser DE, Lichterfeld M, Bonini C. T Memory stem cells in health and disease. Nat Med (2017) 23(1):18–27. doi: 10.1038/nm.4241
57. Ali N, Zirak B, Rodriguez RS, Pauli ML, Truong HA, Lai K, et al. Regulatory T cells in skin facilitate epithelial stem cell differentiation. Cell (2017) 169(6):1119–1129.e11. doi: 10.1016/j.cell.2017.05.002
58. Wang ECE, Dai Z, Ferrante AW, Drake CG, Christiano AM. A subset of TREM2(+) dermal macrophages secretes oncostatin m to maintain hair follicle stem cell quiescence and inhibit hair growth. Cell Stem Cell (2019) 24(4):654–669.e6. doi: 10.1016/j.stem.2019.01.011
59. Agudo J. Immune privilege of skin stem cells: what do we know and what can we learn? Exp Dermatol (2021) 30(4):522–8. doi: 10.1111/exd.14221
60. Rahmani W, Sinha S, Biernaskie J. Immune modulation of hair follicle regeneration. NPJ Regener Med (2020) 5:9. doi: 10.1038/s41536-020-0095-2
61. Kocer SS, Djuric PM, Bugallo MF, Simon SR, Matic M. Transcriptional profiling of putative human epithelial stem cells. BMC Genomics (2008) 9:359. doi: 10.1186/1471-2164-9-359
62. Li KN, Tumbar T. Hair follicle stem cells as a skin-organizing signaling center during adult homeostasis. EMBO J (2021) 40(11):e107135. doi: 10.15252/embj.2020107135
63. Hautz T, Zelger BG, Weissenbacher A, Zelger B, Brandacher G, Landin L, et al. Standardizing skin biopsy sampling to assess rejection in vascularized composite allotransplantation. Clin Transplant (2013) 27(2):E81–90. doi: 10.1111/ctr.12086
64. Akdogan N, Ersoy-Evans S, Gokoz O, Erdem Y, Nasir S. Early recognition of chronic rejection in a face allotransplant patient with alopecia. J Cutan Pathol (2021) 48(10):1286–97. doi: 10.1111/cup.14069
65. Krezdorn N, Lian CG, Wells M, Wo L, Tasigiorgos S, Xu S, et al. Chronic rejection of human face allografts. Am J Transplant (2019) 19(4):1168–77. doi: 10.1111/ajt.15143
66. Aleemardani M, Trikić MZ, Green NH, Claeyssens F. The importance of mimicking dermal-epidermal junction for skin tissue engineering: a review. Bioengineering (Basel) (2021) 8(11). doi: 10.3390/bioengineering8110148
67. Zhan Q, Korngold R, Lezcano C, McKeon F, Murphy GF. Graft-versus-host disease-related cytokine-driven apoptosis depends on p73 in cytokeratin 15-positive target cells. Biol Blood Marrow Transplant (2012) 18(6):841–51. doi: 10.1016/j.bbmt.2012.02.004
68. Kim JC, Whitaker-Menezes D, Deguchi M, Adair BS, Korngold R, Murphy GF. Novel expression of vascular cell adhesion molecule-1 (CD106) by squamous epithelium in experimental acute graft-versus-host disease. Am J Pathol (2002) 161(3):763–70. doi: 10.1016/S0002-9440(10)64235-6
69. Whitaker-Menezes D, Jones SC, Friedman TM, Korngold R, Murphy GF. An epithelial target site in experimental graft-versus-host disease and cytokine-mediated cytotoxicity is defined by cytokeratin 15 expression. Biol Blood Marrow Transplant (2003) 9(9):559–70. doi: 10.1016/S1083-8791(03)00288-X
70. Kummer L, Zaradzki M, Vijayan V, Arif R, Weigand MA, Immenschuh S, et al. Vascular signaling in allogenic solid organ transplantation - the role of endothelial cells. Front Physiol (2020) 11:443. doi: 10.3389/fphys.2020.00443
71. Sheikh S, Rainger GE, Gale Z, Rahman M, Nash GB. Exposure to fluid shear stress modulates the ability of endothelial cells to recruit neutrophils in response to tumor necrosis factor-alpha: a basis for local variations in vascular sensitivity to inflammation. Blood (2003) 102(8):2828–34. doi: 10.1182/blood-2003-01-0080
72. Tombor LS, John D, Glaser SF, Luxán G, Forte E, Furtado M, et al. Single cell sequencing reveals endothelial plasticity with transient mesenchymal activation after myocardial infarction. Nat Commun (2021) 12(1):681. doi: 10.1038/s41467-021-20905-1
73. Madonna R, De Caterina R. Potential roles of vessel wall heparan sulfate proteoglycans in atherosclerosis. Vascul Pharmacol (2014) 60(2):49–51. doi: 10.1016/j.vph.2013.12.002
74. Platt JL, Vercellotti GM, Lindman BJ, Oegema TR, Bach FH, Dalmasso AP. Release of heparan sulfate from endothelial cells. implications for pathogenesis of hyperacute rejection. J Exp Med (1990) 171(4):1363–8.
75. Pober JS, Sessa WC. Evolving functions of endothelial cells in inflammation. Nat Rev Immunol (2007) 7(10):803–15. doi: 10.1038/nri2171
76. Wang M, Hao H, Leeper NJ, Zhu L. Thrombotic regulation from the endothelial cell perspectives. Arteriosclerosis Thrombosis Vasc Biol (2018) 38(6):e90–5. doi: 10.1161/ATVBAHA.118.310367
77. Witkowski M, Weithauser A, Tabaraie T, Steffens D, Kränkel N, Witkowski M, et al. Micro-RNA-126 reduces the blood thrombogenicity in diabetes mellitus via targeting of tissue factor. Arterioscler Thromb Vasc Biol (2016) 36(6):1263–71. doi: 10.1161/ATVBAHA.115.306094
78. Lion J, Taflin C, Cross AR, Robledo-Sarmiento M, Mariotto E, Savenay A, et al. HLA class II antibody activation of endothelial cells promotes Th17 and disrupts regulatory T lymphocyte expansion. Am J Transplant (2016) 16(5):1408–20. doi: 10.1111/ajt.13644
79. Dong C. Defining the TH17 cell lineage. Nat Rev Immunol (2021) 21(10):618–8. doi: 10.1038/s41577-021-00596-x
80. Picker LJ, Michie SA, Rott LS, Butcher EC. A unique phenotype of skin-associated lymphocytes in humans. preferential expression of the HECA-452 epitope by benign and malignant T cells at cutaneous sites. Am J Pathol (1990) 136(5):1053–68.
81. Di Lorenzo A, Lin MI, Murata T, Landskroner-Eiger S, Schleicher M, Kothiya M, et al. eNOS-derived nitric oxide regulates endothelial barrier function through VE-cadherin and rho GTPases. J Cell Sci (2013) 126(Pt 24):5541–52.
82. Adams V, Nehrhoff B, Späte U, Linke A, Schulze PC, Baur A, et al. Induction of iNOS expression in skeletal muscle by IL-1beta and NFkappaB activation: an in vitro and in vivo study. Cardiovasc Res (2002) 54(1):95–104. doi: 10.1016/S0008-6363(02)00228-6
83. Ninchoji T, Love DT, Smith RO, Hedlund M, Vestweber D, Sessa WC, et al. eNOS-induced vascular barrier disruption in retinopathy by c-src activation and tyrosine phosphorylation of VE-cadherin. eLife (2021) 10:e64944. doi: 10.7554/eLife.64944.sa2
84. Adil A, Xu M, Haykal S. Recellularization of bioengineered scaffolds for vascular composite allotransplantation. Front Surg (2022) 9. doi: 10.3389/fsurg.2022.843677
85. Du M, Kalia N, Frumento G, Chen F, Zhang Z. Biomechanical properties of human T cells in the process of activation based on diametric compression by micromanipulation. Med Eng Phys (2017) 40:20–7. doi: 10.1016/j.medengphy.2016.11.011
86. Li XC, Kloc M, Ghobrial RM. Memory T cells in transplantation - progress and challenges. Curr Opin Organ Transplant (2013) 18(4):387–92. doi: 10.1097/MOT.0b013e3283626130
87. Edtinger K, Yang X, Uehara H, Tullius SG. Current status of vascularized composite tissue allotransplantation. Burns Trauma (2014) 2(2):53–60.
88. Morelon E, Petruzzo P, Kanitakis J. Chronic rejection in vascularized composite allotransplantation. Curr Opin Organ Transplant (2018) 23(5):582–91. doi: 10.1097/MOT.0000000000000571
89. Mundinger GS, Munivenkatappa R, Drachenberg CB, Ha JS, Vaca EE, Shipley ST, et al. Histopathology of chronic rejection in a nonhuman primate model of vascularized composite allotransplantation. Transplantation (2013) 95(10):1204–10. doi: 10.1097/TP.0b013e31828d1528
90. Hautz T, Zelger BG, Nasr IW, Mundinger GS, Barth RN, Rodriguez ED, et al. Lymphoid neogenesis in skin of human hand, nonhuman primate, and rat vascularized composite allografts. Transpl Int (2014) 27(9):966–76. doi: 10.1111/tri.12358
91. Libby P, Tanaka H. The pathogenesis of coronary arteriosclerosis ("chronic rejection") in transplanted hearts. Clin Transplant (1994) 8(3 Pt 2):313–8.
92. Morelon E, Petruzzo P, Kanitakis J, Dakpé S, Thaunat O, Dubois V, et al. Face transplantation: partial graft loss of the first case 10 years later. Am J Transplant (2017) 17(7):1935–40. doi: 10.1111/ajt.14218
93. Atia A, Moris D, McRae M, Song M, Stempora L, Leopardi F, et al. Th17 cell inhibition in a costimulation blockade-based regimen for vascularized composite allotransplantation using a nonhuman primate model. Transpl Int (2020) 33(10):1294–301. doi: 10.1111/tri.13612
94. Kwan T, Chadban SJ, Ma J, Bao S, Alexander SI, Wu H. IL-17 deficiency attenuates allograft injury and prolongs survival in a murine model of fully MHC-mismatched renal allograft transplantation. Am J Transplant (2015) 15(6):1555–67. doi: 10.1111/ajt.13140
95. Krummey SM, Cheeseman JA, Conger JA, Jang PS, Mehta AK, Kirk AD, et al. High CTLA-4 expression on Th17 cells results in increased sensitivity to CTLA-4 coinhibition and resistance to belatacept. Am J Transplant (2014) 14(3):607–14. doi: 10.1111/ajt.12600
96. Hirata T, Furie BC, Furie B. P-, e-, and l-selectin mediate migration of activated CD8+ T lymphocytes into inflamed skin. J Immunol (2002) 169(8):4307–13. doi: 10.4049/jimmunol.169.8.4307
97. Staite ND, Justen JM, Sly LM, Beaudet AL, Bullard DC. Inhibition of delayed-type contact hypersensitivity in mice deficient in both e-selectin and p-selectin. Blood (1996) 88(8):2973–9. doi: 10.1182/blood.V88.8.2973.bloodjournal8882973
98. Abel AM, Yang C, Thakar MS, Malarkannan S. Natural killer cells: development, maturation, and clinical utilization. Front Immunol (2018) 9. doi: 10.3389/fimmu.2018.01869
99. Sanchez D, Azaceta G, Muntasell A, Aguilo N, Núñez D, Galvez E, et al. Human NK cells activated by EBV + lymphoblastoid cells overcome anti-apoptotic mechanisms of drug resistance in haematological cancer cells. OncoImmunology (2015) 4:00–0.
100. Yoneda O, Imai T, Goda S, Inoue H, Yamauchi A, Okazaki T, et al. Fractalkine-mediated endothelial cell injury by NK cells. J Immunol (2000) 164(8):4055–62. doi: 10.4049/jimmunol.164.8.4055
101. Lu L, Ikizawa K, Hu D, Werneck MB, Wucherpfennig KW, Cantor H. Regulation of activated CD4+ T cells by NK cells via the qa-1-NKG2A inhibitory pathway. Immunity (2007) 26(5):593–604. doi: 10.1016/j.immuni.2007.03.017
102. Pierce S, Geanes ES, Bradley T. Targeting natural killer cells for improved immunity and control of the adaptive immune response. Front Cell Infection Microbiol (2020) 10. doi: 10.3389/fcimb.2020.00231
103. Snapper CM, Yamaguchi H, Moorman MA, Mond JJ. An in vitro model for T cell-independent induction of humoral immunity. a requirement for NK cells. J Immunol (1994) 152(10):4884–92.
104. Friedman O, Carmel N, Sela M, Abu Jabal A, Inbal A, Ben Hamou M, et al. Immunological and inflammatory mapping of vascularized composite allograft rejection processes in a rat model. PloS One (2017) 12(7):e0181507. doi: 10.1371/journal.pone.0181507
105. Umehara H, Bloom ET, Okazaki T, Nagano Y, Yoshie O, Imai T. Fractalkine in vascular biology. Arteriosclerosis Thrombosis Vasc Biol (2004) 24(1):34–40. doi: 10.1161/01.ATV.0000095360.62479.1F
106. Beura LK, Rosato PC, Masopust D. Implications of resident memory T cells for transplantation. Am J Transplant (2017) 17(5):1167–75. doi: 10.1111/ajt.14101
107. Sutton VR, Davis JE, Cancilla M, Johnstone RW, Ruefli AA, Sedelies K, et al. Initiation of apoptosis by granzyme b requires direct cleavage of bid, but not direct granzyme b-mediated caspase activation. J Exp Med (2000) 192(10):1403–14. doi: 10.1084/jem.192.10.1403
108. Abbasi B, Shamsasenjan K, Ahmadi M, Beheshti SA, Saleh M. Mesenchymal stem cells and natural killer cells interaction mechanisms and potential clinical applications. Stem Cell Res Ther (2022) 13(1):97. doi: 10.1186/s13287-022-02777-4
109. Kuo Y-R, Chen C-C, Goto S, Lin P-Y, Wei F-C, Chen C-L. Mesenchymal stem cells as immunomodulators in a vascularized composite allotransplantation. Clin Dev Immunology 2012 (2012) p:854846. doi: 10.1155/2012/854846
110. Meisel R, Zibert A, Laryea M, Göbel U, Däubener W, Dilloo D. Human bone marrow stromal cells inhibit allogeneic T-cell responses by indoleamine 2, 3-dioxygenase–mediated tryptophan degradation. Blood (2004) 103(12):4619–21. doi: 10.1182/blood-2003-11-3909
111. Beilke JN, Edwards L, Gill RG. NK CELLS RESTRAIN CD8 T CELLS DURING TOLERANCE INDUCTION TO ISLET ALLOGRAFTS. Transplantation (2006) 82(1):367.
112. Kuo Y-R, Chen C-C, Goto S, Lee I-T, Huang C-W, Tsai C-C, et al. Modulation of immune response and T-cell regulation by donor adipose-derived stem cells in a rodent hind-limb allotransplant model. Plast Reconstructive Surg (2011) 128(6):661e–72e. doi: 10.1097/PRS.0b013e318230c60b
113. Kuo Y-R, Chen C-C, Shih H-S, Goto S, Huang C-W, Wang C-T, et al. Prolongation of composite tissue allotransplant survival by treatment with bone marrow mesenchymal stem cells is correlated with T-cell regulation in a swine hind-limb model. Plast Reconstructive Surg (2011) 127(2):569–79. doi: 10.1097/PRS.0b013e318200a92c
114. Kuo Y-R, Chen C-C, Goto S, Huang Y-T, Wang C-T, Tsai C-C, et al. Immunomodulatory effects of bone marrow-derived mesenchymal stem cells in a swine hemi-facial allotransplantation model. PloS One (2012) 7(4):e35459. doi: 10.1371/journal.pone.0035459
115. Reinders ME, Hoogduijn MJ. NK cells and MSCs: possible implications for MSC therapy in renal transplantation. J Stem Cell Res Ther (2014) 4(2):1000166. doi: 10.4172/2157-7633.1000166
116. Spaggiari GM, Capobianco A, Abdelrazik H, Becchetti F, Mingari MC, Moretta L. Mesenchymal stem cells inhibit natural killer–cell proliferation, cytotoxicity, and cytokine production: role of indoleamine 2,3-dioxygenase and prostaglandin E2. Blood (2008) 111(3):1327–33. doi: 10.1182/blood-2007-02-074997
117. Haskell CA, Hancock WW, Salant DJ, Gao W, Csizmadia V, Peters W, et al. Targeted deletion of CX(3)CR1 reveals a role for fractalkine in cardiac allograft rejection. J Clin Invest (2001) 108(5):679–88. doi: 10.1172/JCI12976
118. Sandel PC, Monroe JG. Negative selection of immature b cells by receptor editing or deletion is determined by site of antigen encounter. Immunity (1999) 10(3):289–99. doi: 10.1016/S1074-7613(00)80029-1
119. Hamilton JA, Hsu HC, Mountz JD. Autoreactive b cells in SLE, villains or innocent bystanders? Immunol Rev (2019) 292(1):120–38. doi: 10.1111/imr.12815
120. Papa I, Vinuesa CG. Synaptic interactions in germinal centers. Front Immunol (2018) 9:1858. doi: 10.3389/fimmu.2018.01858
121. Lightman SM, Utley A, Lee KP. Survival of long-lived plasma cells (LLPC): piecing together the puzzle. Front Immunol (2019) 10. doi: 10.3389/fimmu.2019.00965
122. Parra D, Takizawa F, Sunyer JO. Evolution of b cell immunity. Annu Rev Anim Biosci (2013) 1:65–97. doi: 10.1146/annurev-animal-031412-103651
123. Spencer J, Sollid LM. The human intestinal b-cell response. Mucosal Immunol (2016) 9(5):1113–24. doi: 10.1038/mi.2016.59
124. Yuseff M-I, Pierobon P, Reversat A, Lennon-Duménil A-M. How b cells capture, process and present antigens: a crucial role for cell polarity. Nat Rev Immunol (2013) 13(7):475–86. doi: 10.1038/nri3469
125. Kaufman CL, Marvin MR, Chilton PM, Hoying JB, Williams SK, Tien H, et al. Immunobiology in VCA. Transpl Int (2016) 29(6):644–54. doi: 10.1111/tri.12764
126. Moris D, Cendales LC. Sensitization and desensitization in vascularized composite allotransplantation. Front Immunol (2021) 12. doi: 10.3389/fimmu.2021.682180
127. Koch CA, Kanazawa A, Nishitai R, Knudsen BE, Ogata K, Plummer TB, et al. Intrinsic resistance of hepatocytes to complement-mediated injury. J Immunol (2005) 174(11):7302–9. doi: 10.4049/jimmunol.174.11.7302
128. Weissenbacher A, Vrakas G, Chen M, Reddy S, Allan P, Giele H, et al. De novo donor-specific HLA antibodies after combined intestinal and vascularized composite allotransplantation - a retrospective study. Transpl Int (2018) 31(4):398–407. doi: 10.1111/tri.13096
129. Borges TJ, O'Malley JT, Wo L, Murakami N, Smith B, Azzi J, et al. Codominant role of interferon-γ- and interleukin-17-Producing T cells during rejection in full facial transplant recipients. Am J Transplant (2016) 16(7):2158–71. doi: 10.1111/ajt.13705
130. Patakas A, Benson RA, Withers DR, Conigliaro P, McInnes IB, Brewer JM, et al. Th17 effector cells support b cell responses outside of germinal centres. PloS One (2012) 7(11):e49715. doi: 10.1371/journal.pone.0049715
131. Corsiero E, Delvecchio FR, Bombardieri M, Pitzalis C. B cells in the formation of tertiary lymphoid organs in autoimmunity, transplantation and tumorigenesis. Curr Opin Immunol (2019) 57:46–52. doi: 10.1016/j.coi.2019.01.004
132. Sutter D, Dzhonova DV, Prost JC, Bovet C, Banz Y, Rahnfeld L, et al. Delivery of rapamycin using in situ forming implants promotes immunoregulation and vascularized composite allograft survival. Sci Rep (2019) 9(1):9269. doi: 10.1038/s41598-019-45759-y
133. Grahammer J, Weissenbacher A, Zelger B, Zelger B, Boesmueller C, Ninkovic M, et al. Benefits and limitations of belatacept in 4 hand-transplanted patients. Am J Transplant (2017) 17(12):3228–35. doi: 10.1111/ajt.14440
134. Cendales LC, Ruch DS, Cardones AR, Potter G, Dooley J, Dore D, et al. De novo belatacept in clinical vascularized composite allotransplantation. Am J Transplant (2018) 18(7):1804–9. doi: 10.1111/ajt.14910
135. Schuetz C, Lee KM, Scott R, Kojima L, Washburn L, Liu L, et al. Regulatory b cell-dependent islet transplant tolerance is also natural killer cell dependent. Am J Transplant (2017) 17(6):1656–62. doi: 10.1111/ajt.14265
136. Roche PA, Furuta K. The ins and outs of MHC class II-mediated antigen processing and presentation. Nat Rev Immunol (2015) 15(4):203–16. doi: 10.1038/nri3818
137. Pishesha N, Harmand TJ, Ploegh HL. A guide to antigen processing and presentation. Nat Rev Immunol (2022). doi: 10.1038/s41577-022-00707-2
138. Kaksonen M, Roux A. Mechanisms of clathrin-mediated endocytosis. Nat Rev Mol Cell Biol (2018) 19(5):313–26. doi: 10.1038/nrm.2017.132
139. Zhang YQ, Joost van Neerven RJ, Van Gool SW, Coorevits L, de Boer M, Ceuppens JL. B7-CD28 interaction is a late acting co-stimulatory signal for human T cell responses. Int Immunol (1997) 9(8):1095–102. doi: 10.1093/intimm/9.8.1095
140. Suresh M, Whitmire JK, Harrington LE, Larsen CP, Pearson TC, Altman JD, et al. Role of CD28-B7 interactions in generation and maintenance of CD8 T cell memory. J Immunol (2001) 167(10):5565. doi: 10.4049/jimmunol.167.10.5565
141. Watanabe M, Lu Y, Breen M, Hodes RJ. B7-CD28 co-stimulation modulates central tolerance via thymic clonal deletion and treg generation through distinct mechanisms. Nat Commun (2020) 11(1):6264. doi: 10.1038/s41467-020-20070-x
142. Topham DJ, Reilly EC, Emo KL, Sportiello M. Formation and maintenance of tissue resident memory CD8+ T cells after viral infection. Pathogens (2019) 8(4). doi: 10.3390/pathogens8040196
143. Menares E, Gálvez-Cancino F, Cáceres-Morgado P, Ghorani E, López E, Díaz X, et al. Tissue-resident memory CD8+ T cells amplify anti-tumor immunity by triggering antigen spreading through dendritic cells. Nat Commun (2019) 10(1):4401. doi: 10.1038/s41467-019-12319-x
144. Ualiyeva S. The role of tissue-resident macrophages in transplant immunity (2017). Available at: http://nrs.harvard.edu/urn-3:HUL.InstRepos:33820489.
145. Davies LC, Jenkins SJ, Allen JE, Taylor PR. Tissue-resident macrophages. Nat Immunol (2013) 14(10):986–95. doi: 10.1038/ni.2705
146. Okamura H, Kashiwamura S, Tsutsui H, Yoshimoto T, Nakanishi K. Regulation of interferon-gamma production by IL-12 and IL-18. Curr Opin Immunol (1998) 10(3):259–64. doi: 10.1016/S0952-7915(98)80163-5
147. Amin KR, Wong JKF, Fildes JE. Strategies to reduce ischemia reperfusion injury in vascularized composite allotransplantation of the limb. J Handb Surg Am (2017) 42(12):1019–24. doi: 10.1016/j.jhsa.2017.09.013
148. Safi AF, Kauke M, Nelms L, Palmer WJ, Tchiloemba B, Kollar B, et al. Local immunosuppression in vascularized composite allotransplantation (VCA): a systematic review. J Plast Reconstr Aesthet Surg (2021) 74(2):327–35. doi: 10.1016/j.bjps.2020.10.003
149. Leonard DA, McGrouther DA, Kurtz JM, Cetrulo CL. Tolerance induction strategies in vascularized composite allotransplantation: mixed chimerism and novel developments. Clin Dev Immunology 2012 (2012) p:863264. doi: 10.1155/2012/863264
150. Ikeguchi R, Sacks JM, Unadkat JV, Solari M, Horibe EK, Thomson AW, et al. Long-term survival of limb allografts induced by pharmacologically conditioned, donor alloantigen-pulsed dendritic cells without maintenance immunosuppression. Transplantation (2008) 85(2):237–46. doi: 10.1097/TP.0b013e31815e870e
151. Aral AM, Zamora R, Barclay D, Yin J, El-Dehaibi F, Erbas VE, et al. The effects of tacrolimus on tissue-specific, protein-level inflammatory networks in vascularized composite allotransplantation. Front Immunol (2021) 12. doi: 10.3389/fimmu.2021.591154
152. Salehi S, Reed EF. The divergent roles of macrophages in solid organ transplantation. Curr Opin Organ Transplant (2015) 20(4):446–53. doi: 10.1097/MOT.0000000000000209
153. Horne PH, Zimmerer JM, Fisher MG, Lunsford KE, Nadasdy G, Nadasdy T, et al. Critical role of effector macrophages in mediating CD4-dependent alloimmune injury of transplanted liver parenchymal cells. J Immunol (2008) 181(2):1224–31. doi: 10.4049/jimmunol.181.2.1224
154. Chiricozzi A, Maurelli M, Peris K, Girolomoni G. Targeting IL-4 for the treatment of atopic dermatitis. Immunotargets Ther (2020) 9:151–6. doi: 10.2147/ITT.S260370
155. Lin A, Loré K. Granulocytes: new members of the antigen-presenting cell family. Front Immunol (2017) 8:1781. doi: 10.3389/fimmu.2017.01781
156. Oehler L, Majdic O, Pickl WF, Stöckl J, Riedl E, Drach J, et al. Neutrophil granulocyte–committed cells can be driven to acquire dendritic cell characteristics. J Exp Med (1998) 187(7):1019–28. doi: 10.1084/jem.187.7.1019
157. Gosselin E, Wardwell K, Rigby W, Guyre P. Induction of MHC class II on human polymorphonuclear neutrophils by granulocyte/macrophage colony-stimulating factor, IFN-gamma, and IL-3. J Immunol (1993) 151(3):1482–90. doi: 10.4049/jimmunol.151.3.1482
158. Wang H-B, Ghiran I, Matthaei K, Weller PF. Airway eosinophils: allergic inflammation recruited professional antigen-presenting cells. J Immunol (2007) 179(11):7585–92. doi: 10.4049/jimmunol.179.11.7585
159. Voskamp AL, Prickett SR, Mackay F, Rolland JM, O'Hehir RE. MHC class II expression in human basophils: induction and lack of functional significance. PloS One (2013) 8(12):e81777. doi: 10.1371/journal.pone.0081777
160. Eckl‐Dorna J, Ellinger A, Blatt K, Ghanim V, Steiner I, Pavelka M, et al. Basophils are not the key antigen-presenting cells in allergic patients. Allergy (2012) 67(5):601–8. doi: 10.1111/j.1398-9995.2012.02792.x
161. Weissenbacher A, Hautz T, Pierer G, Ninkovic M, Zelger BG, Zelger B, et al. Hand transplantation in its fourteenth year: the Innsbruck experience. Vascularized Composite Allotransplantation (2014) 1(1-2):11–21. doi: 10.4161/23723505.2014.973798
162. Etra JW, Grzelak MJ, Fidder SAJ, Kolegraff K, Bonawitz S, Shores J, et al. A skin rejection grading system for vascularized composite allotransplantation in a preclinical Large animal model. Transplantation (2019) 103(7):1385–91. doi: 10.1097/TP.0000000000002695
163. Marques PE, Amaral SS, Pires DA, Nogueira LL, Soriani FM, Lima BH, et al. Chemokines and mitochondrial products activate neutrophils to amplify organ injury during mouse acute liver failure. Hepatology (2012) 56(5):1971–82. doi: 10.1002/hep.25801
164. Walsh GM. Profile of reslizumab in eosinophilic disease and its potential in the treatment of poorly controlled eosinophilic asthma. Biologics (2013) 7:7–11.
165. Agache I, Beltran J, Akdis C, Akdis M, Canelo-Aybar C, Canonica GW, et al. Efficacy and safety of treatment with biologicals (benralizumab, dupilumab, mepolizumab, omalizumab and reslizumab) for severe eosinophilic asthma. a systematic review for the EAACI guidelines - recommendations on the use of biologicals in severe asthma. Allergy (2020) 75(5):1023–42.
166. Landolina NAC, Levi-Schaffer F. Eosinophils as a pharmacological target for the treatment of allergic diseases. Curr Opin Pharmacol (2014) 17:71–80. doi: 10.1016/j.coph.2014.07.014
167. Harvima IT, Levi-Schaffer F, Draber P, Friedman S, Polakovicova I, Gibbs BF, et al. Molecular targets on mast cells and basophils for novel therapies. J Allergy Clin Immunol (2014) 134(3):530–44. doi: 10.1016/j.jaci.2014.03.007
168. Krauth M-T, Böhm A, Agis H, Sonneck K, Samorapoompichit P, Florian S, et al. Effects of the CD33-targeted drug gemtuzumab ozogamicin (Mylotarg) on growth and mediator secretion in human mast cells and blood basophils. Exp Hematol (2007) 35(1):108–16. doi: 10.1016/j.exphem.2006.09.008
169. Burt TD. Fetal regulatory T cells and peripheral immune tolerance in utero: implications for development and disease. Am J Reprod Immunol (2013) 69(4):346–58. doi: 10.1111/aji.12083
170. Sojka DK, Huang YH, Fowell DJ. Mechanisms of regulatory T-cell suppression - a diverse arsenal for a moving target. Immunology (2008) 124(1):13–22. doi: 10.1111/j.1365-2567.2008.02813.x
171. Vignali DA, Collison LW, Workman CJ. How regulatory T cells work. Nat Rev Immunol (2008) 8(7):523–32. doi: 10.1038/nri2343
172. Sagoo P, Ali N, Garg G, Nestle FO, Lechler RI, Lombardi G. Human regulatory T cells with alloantigen specificity are more potent inhibitors of alloimmune skin graft damage than polyclonal regulatory T cells. Sci Transl Med (2011) 3(83):83ra42. doi: 10.1126/scitranslmed.3002076
173. Kauke-Navarro M, Knoedler S, Panayi AC, Knoedler L, Noel OF, Pomahac B. Regulatory T cells: liquid and living precision medicine for the future of VCA. Transplantation (2022). doi: 10.1097/TP.0000000000004342
174. Kuo YR, Huang CW, Goto S, Wang CT, Hsu LW, Lin YC, et al. Alloantigen-pulsed host dendritic cells induce T-cell regulation and prolong allograft survival in a rat model of hindlimb allotransplantation. J Surg Res (2009) 153(2):317–25. doi: 10.1016/j.jss.2008.05.034
175. Khan MA, Alanazi F, Ahmed HA, Shamma T, Kelly K, Hammad MA, et al. iPSC-derived MSC therapy induces immune tolerance and supports long-term graft survival in mouse orthotopic tracheal transplants. Stem Cell Res Ther (2019) 10(1):290. doi: 10.1186/s13287-019-1397-4
176. Jeong SH, Ji YH, Yoon ES. Immunosuppressive activity of adipose tissue-derived mesenchymal stem cells in a rat model of hind limb allotransplantation. Transplant Proc (2014) 46(5):1606–14. doi: 10.1016/j.transproceed.2013.12.069
177. Chen CC, Chen RF, Shao JS, Li YT, Wang YC, Brandacher G, et al. Adipose-derived stromal cells modulating composite allotransplant survival is correlated with b cell regulation in a rodent hind-limb allotransplantation model. Stem Cell Res Ther (2020) 11(1):478. doi: 10.1186/s13287-020-01961-8
178. Schweizer R, Klein H, Fuchs N, Waldner M, Kollar B, Kamat P, et al. Adipose-derived stromal cells attenuate acute rejection and graft vasculopathy in rodent vascularized composite allotransplantation. Transplantation (2018) 102:S50. doi: 10.1097/01.tp.0000542613.71454.22
179. Clayton K, Vallejo AF, Davies J, Sirvent S, Polak ME. Langerhans cells-programmed by the epidermis. Front Immunol (2017) 8:1676. doi: 10.3389/fimmu.2017.01676
180. Deckers J, Hammad H, Hoste E. Langerhans cells: sensing the environment in health and disease. Front Immunol (2018) 9:93. doi: 10.3389/fimmu.2018.00093
181. Seneschal J, Clark RA, Gehad A, Baecher-Allan CM, Kupper TS. Human epidermal langerhans cells maintain immune homeostasis in skin by activating skin resident regulatory T cells. Immunity (2012) 36(5):873–84. doi: 10.1016/j.immuni.2012.03.018
182. van der Aar AM, Picavet DI, Muller FJ, de Boer L, van Capel TM, Zaat SA, et al. Langerhans cells favor skin flora tolerance through limited presentation of bacterial antigens and induction of regulatory T cells. J Invest Dermatol (2013) 133(5):1240–9. doi: 10.1038/jid.2012.500
183. Zamfirescu DG, Owen E, Lascar I, Molitor M, Zegrea I, Popescu M, et al. Sentinel skin allograft-a reliable marker for monitoring of composite tissue transplant rejection. Transplant Proc (2009) 41(2):503–8. doi: 10.1016/j.transproceed.2009.01.023
184. Kanitakis J, Jullien D, Petruzzo P, Hakim N, Claudy A, Revillard JP, et al. Clinicopathologic features of graft rejection of the first human hand allograft. Transplantation (2003) 76(4):688–93. doi: 10.1097/01.TP.0000079458.81970.9A
185. Leonard DA, Powell HR, Defazio MW, Shanmugarajah K, Mastroianni M, Rosales IA, et al. Cutaneous leukocyte lineages in tolerant large animal and immunosuppressed clinical vascularized composite allograft recipients. Am J Transplant (2021) 21(2):582–92. doi: 10.1111/ajt.16230
186. Amin KR, Ball AL, Chhina C, Edge RJ, Stone JP, Critchley WR, et al. Ex-vivo flush of the limb allograft reduces inflammatory burden prior to transplantation. J Plast Reconstr Aesthet Surg (2018) 71(2):140–6. doi: 10.1016/j.bjps.2017.11.002
187. Ngo Nyekel F, Pacreau E, Benadda S, Msallam R, Abrink M, Pejler G, et al. Mast cell degranulation exacerbates skin rejection by enhancing neutrophil recruitment. Front Immunol (2018) 9:2690. doi: 10.3389/fimmu.2018.02690
188. Lazarus B, Messina A, Barker JE, Hurley JV, Romeo R, Morrison WA, et al. The role of mast cells in ischaemia-reperfusion injury in murine skeletal muscle. J Pathol (2000) 191(4):443–8. doi: 10.1002/1096-9896(2000)9999:9999<::AID-PATH666>3.0.CO;2-L
189. Grenz A, Schenk M, Zipfel A, Viebahn R. TNF-alpha and its receptors mediate graft rejection and loss after liver transplantation. Clin Chem Lab Med (2000) 38(11):1183–5.
190. Walsh LJ, Trinchieri G, Waldorf HA, Whitaker D, Murphy GF. Human dermal mast cells contain and release tumor necrosis factor alpha, which induces endothelial leukocyte adhesion molecule 1. Proc Natl Acad Sci U.S.A. (1991) 88(10):4220–4. doi: 10.1073/pnas.88.10.4220
191. Wolfram D, Starzl R, Hackl H, Barclay D, Hautz T, Zelger B, et al. Insights from computational modeling in inflammation and acute rejection in limb transplantation. PloS One (2014) 9(6):e99926. doi: 10.1371/journal.pone.0099926
192. Petruzzo P, Kanitakis J, Testelin S, Pialat JB, Buron F, Badet L, et al. Clinicopathological findings of chronic rejection in a face grafted patient. Transplantation (2015) 99(12):2644–50. doi: 10.1097/TP.0000000000000765
193. Kauke M, Panayi AC, Safi AF, Haug V, Perry B, Kollar B, et al. Full facial retransplantation in a female patient-technical, immunologic, and clinical considerations. Am J Transplant (2021) 21(10):3472–80. doi: 10.1111/ajt.16696
194. Kollar B, Rizzo NM, Borges TJ, Haug V, Abdulrazzak O, Kauke M, et al. Accelerated chronic skin changes without allograft vasculopathy: a 10-year outcome report after face transplantation. Surgery (2020) 167(6):991–8. doi: 10.1016/j.surg.2020.01.010
195. Kanitakis J, Karayannopoulou G, Lanzetta M, Petruzzo P. Graft vasculopathy in the skin of a human hand allograft: implications for diagnosis of rejection of vascularized composite allografts. Transpl Int (2014) 27(11):e118–23. doi: 10.1111/tri.12399
196. Kanitakis J, McGregor B, Badet L, Petruzzo P, Morelon E, Devauchelle B, et al. Absence of c4d deposition in human composite tissue (hands and face) allograft biopsies: an immunoperoxidase study. Transplantation (2007) 84(2):265–7. doi: 10.1097/01.tp.0000266899.93315.52
197. Lee CAA, Wang D, Kauke-Navarro M, Russell-Goldman E, Xu S, Mucciarone KN, et al. Insights from immunoproteomic profiling of a rejected full face transplant. Am J Transplant (2023). doi: 10.1016/j.ajt.2023.04.008
198. Kaufman CL, Kanitakis J, Weissenbacher A, Brandacher G, Mehra MR, Amer H, et al. Defining chronic rejection in vascularized composite allotransplantation-the American society of reconstructive transplantation and international society of vascularized composite allotransplantation chronic rejection working group: 2018 American society of reconstructive transplantation meeting report and white paper research goals in defining chronic rejection in vascularized composite allotransplantation. SAGE Open Med (2020) 8:2050312120940421. doi: 10.1177/2050312120940421
199. Alberti FB, Hoyle V. Face transplants: an international history. J History Med Allied Sci (2021) 76(3):319–45. doi: 10.1093/jhmas/jrab019
200. Shores JT, Brandacher G, Lee WPA. Hand and upper extremity transplantation: an update of outcomes in the worldwide experience. Plast Reconstr Surg (2015) 135(2):351e–60e. doi: 10.1097/PRS.0000000000000892
201. Lúcio MJ, Horta R. Hand transplantation-risks and benefits. J Handb Microsurg (2021) 13(4):207–15. doi: 10.1055/s-0040-1715427
202. Ravindra KV, Ildstad ST. Immunosuppressive protocols and immunological challenges related to hand transplantation. Handb Clin (2011) 27(4):467–79, ix. doi: 10.1016/j.hcl.2011.07.001
203. Steinbrink JM, Wolfe CR. Infectious complications of vascularized composite allograft transplantation. Curr Opin Organ Transplant (2020) 25(4):377–82. doi: 10.1097/MOT.0000000000000780
204. Schena FP, Pascoe MD, Alberu J, del Carmen Rial M, Oberbauer R, Brennan DC, et al. Conversion from calcineurin inhibitors to sirolimus maintenance therapy in renal allograft recipients: 24-month efficacy and safety results from the CONVERT trial. Transplantation (2009) 87(2):233–42. doi: 10.1097/TP.0b013e3181927a41
205. Wallin EF, Hill DL, Linterman MA, Wood KJ. The calcineurin inhibitor tacrolimus specifically suppresses human T follicular helper cells. Front Immunol (2018) 9:1184. doi: 10.3389/fimmu.2018.01184
206. Meehan AC, Mifsud NA, Nguyen THO, Levvey BJ, Snell GI, Kotsimbos TC, et al. Impact of commonly used transplant immunosuppressive drugs on human NK cell function is dependent upon stimulation condition. PloS One (2013) 8(3):e60144. doi: 10.1371/journal.pone.0060144
207. Taves MD, Ashwell JD. Glucocorticoids in T cell development, differentiation and function. Nat Rev Immunol (2021) 21(4):233–43. doi: 10.1038/s41577-020-00464-0
208. Cupps TR, Gerrard TL, Falkoff RJ, Whalen G, Fauci AS. Effects of in vitro corticosteroids on b cell activation, proliferation, and differentiation. J Clin Invest (1985) 75(2):754–61. doi: 10.1172/JCI111757
209. Cooper S, Laird SM, Mariee N, Li TC, Metwally M. The effect of prednisolone on endometrial uterine NK cell concentrations and pregnancy outcome in women with reproductive failure. a retrospective cohort study. J Reprod Immunol (2019) 131:1–6. doi: 10.1016/j.jri.2018.10.001
210. Maghazachi A, Sand K, Salim Z. Glatiramer acetate, dimethyl fumarate, and monomethyl fumarate upregulate the expression of CCR10 on the surface of natural killer cells and enhance their chemotaxis and cytotoxicity. Front Immunol (2016) 7. doi: 10.3389/fimmu.2016.00437
211. Elkins WL, Guttmann RD. Pathogenesis of a local graft versus host reaction: immunogenicity of circulating host leukocytes. Science (1968) 159(3820):1250–1. doi: 10.1126/science.159.3820.1250
212. Talmage DW, Dart G, Radovich J, Lafferty KJ. Activation of transplant immunity: effect of donor leukocytes on thyroid allograft rejection. Science (1976) 191(4225):385–8. doi: 10.1126/science.1082167
213. Sun J, McCAUGHAN GW, Gallagher ND, Sheil A, Bishop GA. Deletion of spontaneous rat liver allograft acceptance by donor irradiation. Transplantation (1995) 60(3):233–6. doi: 10.1097/00007890-199508000-00004
214. Zhang Y, Zhao H, Bo L, Yang Y, Lu X, Sun J, et al. Total body irradiation of donors can alter the course of tolerance and induce acute rejection in a spontaneous tolerance rat liver transplantation model. Sci China Life Sci (2012) 55(9):774–81. doi: 10.1007/s11427-012-4370-3
Keywords: transplant, reconstructive surgery, vascularized composite allotransplantation, VCA, alloimmune response, acute rejection, chronic rejection
Citation: Knoedler L, Knoedler S, Panayi AC, Lee CAA, Sadigh S, Huelsboemer L, Stoegner VA, Schroeter A, Kern B, Mookerjee V, Lian CG, Tullius SG, Murphy GF, Pomahac B and Kauke-Navarro M (2023) Cellular activation pathways and interaction networks in vascularized composite allotransplantation. Front. Immunol. 14:1179355. doi: 10.3389/fimmu.2023.1179355
Received: 04 March 2023; Accepted: 28 April 2023;
Published: 17 May 2023.
Edited by:
Malgorzata Kloc, Houston Methodist Research Institute, United StatesReviewed by:
Philippe Lemaitre, Columbia University, United StatesCopyright © 2023 Knoedler, Knoedler, Panayi, Lee, Sadigh, Huelsboemer, Stoegner, Schroeter, Kern, Mookerjee, Lian, Tullius, Murphy, Pomahac and Kauke-Navarro. This is an open-access article distributed under the terms of the Creative Commons Attribution License (CC BY). The use, distribution or reproduction in other forums is permitted, provided the original author(s) and the copyright owner(s) are credited and that the original publication in this journal is cited, in accordance with accepted academic practice. No use, distribution or reproduction is permitted which does not comply with these terms.
*Correspondence: Martin Kauke-Navarro, a2F1a2UtbmF2YXJyby5tYXJ0aW5AeWFsZS5lZHU=; Leonard Knoedler, TGVvbmFyZC5Lbm9lZGxlckB1ci5kZQ==
†These authors have contributed equally to this work
Disclaimer: All claims expressed in this article are solely those of the authors and do not necessarily represent those of their affiliated organizations, or those of the publisher, the editors and the reviewers. Any product that may be evaluated in this article or claim that may be made by its manufacturer is not guaranteed or endorsed by the publisher.
Research integrity at Frontiers
Learn more about the work of our research integrity team to safeguard the quality of each article we publish.