- 1Cell and Molecular Biology Graduate Program, Colorado State University, Fort Collins, CO, United States
- 2Flint Animal Cancer Center, Colorado State University, Fort Collins, CO, United States
Macrophages are ancient, phagocytic immune cells thought to have their origins 500 million years ago in metazoan phylogeny. The understanding of macrophages has evolved to encompass their foundational roles in development, homeostasis, tissue repair, inflammation, and immunity. Notably, macrophages display high plasticity in response to environmental cues, capable of a strikingly wide variety of dynamic gene signatures and phenotypes. Macrophages are also involved in many pathological states including neural disease, asthma, liver disease, heart disease, cancer, and others. In cancer, most tumor-associated immune cells are macrophages, coined tumor-associated macrophages (TAMs). While some TAMs can display anti-tumor properties such as phagocytizing tumor cells and orchestrating an immune response, most macrophages in the tumor microenvironment are immunosuppressive and pro-tumorigenic. Macrophages have been implicated in all stages of cancer. Therefore, interest in manipulating macrophages as a therapeutic strategy against cancer developed as early as the 1970s. Companion dogs are a strong comparative immuno-oncology model for people due to documented similarities in the immune system and spontaneous cancers between the species. Data from clinical trials in humans and dogs can be leveraged to further scientific advancements that benefit both species. This review aims to provide a summary of the current state of knowledge on macrophages in general, and an in-depth review of macrophages as a therapeutic strategy against cancer in humans and companion dogs.
Introduction
Macrophages are ancient, phagocytic immune cells thought to have their origins 500 million years ago in metazoan phylogeny (1). Ilya Metchnikoff, a Russian zoologist, biologist, and Nobel laureate, first described macrophages in 1882 when he observed large, mobile cells that moved to areas of injury in starfish larvae and phagocytized foreign debris (2). The understanding of macrophages has evolved since then to encompass their foundational roles in development, homeostasis, tissue repair, inflammation, and immunity. Notably, macrophages display high plasticity in response to environmental cues, capable of a strikingly wide variety of dynamic gene signatures and phenotypes. In addition to macrophages derived from blood monocytes, most tissues in the body have resident macrophages uniquely adapted to their local environment.
Regardless of their remarkable diversity, a few core genetic programs establish macrophages’ key role as phagocytes (3, 4). Like other myeloid cells of the innate immune system, macrophages display trained immunity, in which responsiveness to secondary stimulation is enhanced over their first response, and immunological tolerance, in which responsiveness to repeated exposures is diminished (5). Macrophages are also involved in a variety of pathological states including neural disease, asthma, liver disease, heart disease, cancer, and others (6).
In cancer, most tumor-associated immune cells are macrophages, coined tumor-associated macrophages (TAMs) (7). While some TAMs can display anti-tumor properties such as phagocytizing tumor cells and orchestrating an immune response, most macrophages in the tumor microenvironment are pro-tumorigenic (8). Macrophages have been implicated in all stages of cancer. They contribute to the initiation of cancer through smoldering inflammation, leading to a mutagenic environment (9, 10). They support the progression of cancer by inducing angiogenesis, supporting the migration and invasion of cancer cells, and enhancing anti-tumor immunity. They also drive metastasis by preparing the metastatic niche and enhancing tumor cell extravasation and survival during the metastatic cascade (7, 9). Therefore, interest in manipulating macrophages as a therapeutic strategy against cancer developed as early as the 1970s (11–13). Companion dogs are a strong comparative immuno-oncology model for people due to documented similarities of the immune system and spontaneous cancers between the species (14). Data from clinical trials in humans and dogs can be leveraged to further scientific advancements that benefit both species. This review therefore aims to provide a summary of the current state of knowledge on macrophages in general, and an in-depth review of macrophages as a therapeutic strategy against cancer in people and companion dogs.
Macrophage ontogeny
The understanding of the ontogeny of macrophages has undergone a somewhat dramatic evolution since their initial discovery. Although Metchnikoff popularized macrophages and the theory of phagocytosis, there were earlier observations of phagocytic cells (2, 15). As scientists fought to understand the complexities of the immune system, macrophages were included in an evolving number of classification schemes. The reticuloendothelial system was proposed in 1924, representing a group of cells found in blood vessels capable of forming networks in tissues. Macrophages were observed to differentiate from monocytes in 1925 and documented to respond to sites of inflammation in several experiments in the following decades (16–18). The mononuclear phagocyte system was therefore coined in 1969 as an updated classification scheme, comprised of monocytes and monocyte-derived macrophages (15, 19, 20). Dendritic cells (DCs) would shortly be recognized as distinct cells in the mononuclear phagocyte system as well (21). A bone marrow-derived progenitor cell of monocytes, macrophages and DCs was isolated in 2006 in the mouse, although this finding has since been debated in favor of the theory that there is not a common macrophage-dendritic cell precursor (22, 23). The current understanding indicates that hematopoietic stem cells (HSCs) differentiate into myeloid progenitors and granulocyte-macrophage precursors in adults. A series of intermediates including a common monocyte-restricted progenitor result in mature blood monocytes, which can differentiate into macrophages or monocyte-derived DCs. Other DC subsets likely differentiate from a very early branch point off early myeloid progenitors (24, 25). There is ongoing debate about the exact branching pattern from early progenitors that lead to all known subsets of monocyte, macrophages and DCs (24, 26). It should be noted that monocytes themselves have been recognized as increasingly heterogeneous in origin and function, with multiple defined subsets (26, 27). To help with standardization in the face of this complexity, ontogeny-based classifications schemes of myeloid cells should take priority over schemes that rely primarily on function, location, or cell-surface markers (28). A more comprehensive outline of the history of macrophage research is presented in several good reviews (15, 29).
Monocyte-derived macrophages (MDMs) are continually renewed via the bone marrow and spleen during periods of high demand such as inflammation or tissue reparation (28, 30). While MDMs remain an important subset of macrophages in vertebrates, in the past two decades a finer understanding of macrophage ontogeny and heterogeneity has evolved. It is now known that the MDMs recruited to inflammation are distinct from tissue-resident macrophages (TRMs). There are numerous populations of TRMs that are singularly adapted to their anatomical location and unique in their ability to self-renew through proliferation (31, 32). TRMs were hypothesized as early as 1971 when Ken Hashimoto identified a resident population of Langerhans cells in the dermis and noted they were self-perpetuating phagocytes (33). Examples of TRMs include the Kupffer cells of the liver, alveolar macrophages of the lung, and microglia of the brain in the adult. Their origins can be traced back to embryogenesis, during which mammalian embryos produce waves of erythro-myeloid progenitor cells prior to the definitive establishment of HSCs in the bone marrow. The yolk sac of the embryo first produces primitive macrophages that develop into microglia in the adult. Subsequent waves of cells from the yolk sac and fetal liver seed resident macrophages in most tissues (34). Fate-mapping studies have shown that these TRMs are replaced to varying degrees by MDMs in the adult (35). Some tissues, like the intestines and skin, appear to rely primarily on MDMs. Other tissues, like the lung, brain, liver, spleen, and peritoneum, rely primarily on their respective TRMs with minimal contributions from MDMs. These balances can be upset after pathological insults (36–38). The exact origin and function of fetal macrophage progenitors is the topic of ongoing debate and is nuanced beyond the scope of this review; several excellent reviews of macrophage ontogeny and nomenclature are recommended (34, 39). A simplified outline of the ontogeny of macrophages is shown in Figure 1. The interplay and contribution of MDMs and TRMs to states of health and disease is not yet fully elucidated. Ontogeny, and not just external stimuli, has been established as an important contributing factor to how macrophages respond to physiologic and pathologic stimuli, underlying the importance of further understanding in this area (43, 44).
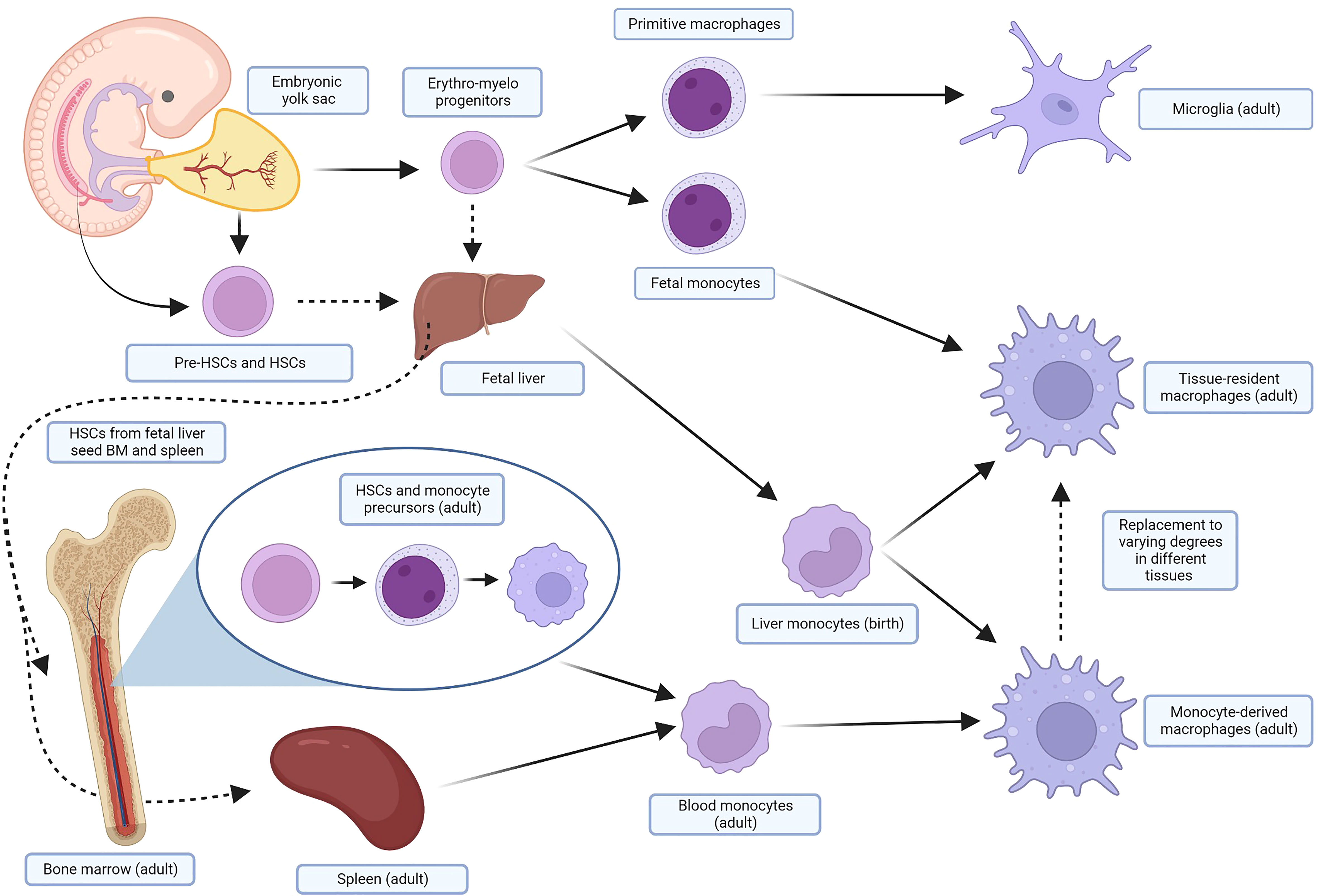
Figure 1 Simplified ontogeny of macrophages. In several waves of primitive hematopoiesis stemming initially from the embryonic yolk sac, erythro-myeloid progenitors differentiate into primitive macrophages and fetal monocytes that eventually become adult microglia and TRMs, respectively. These progenitors also seed the fetal liver, along with pre-HSCs and HSCs from the embryo, yolk sac, and placenta. The fetal liver, the major site of hematopoiesis, subsequently produces liver monocytes, which likely contribute perinatally to macrophage pools in the adult. HSCs, after maturing in the fetal liver, also seed the fetal bone marrow and spleen. In the adult, HSCs from the bone marrow differentiate through several monocyte precursors before being released into the blood as monocytes. These cells are recruited by inflammation and injury in the tissue, and terminally differentiate into MDMS. The spleen also holds a pool of monocytes that can be mobilized when needed. TRMs will be replaced to varying degrees in different tissues by MDMs in the adult. Many of these steps are areas of ongoing research and debate in murine and human models; similar fate-mapping studies have not been undertaken in dogs. For more detail, focused reviews are recommended (34, 40–42). Created with BioRender.com. TRMs, tissue-resident macrophages; HSCs, hematopoietic stem cells; MDMs, monocyte-derived macrophages.
Macrophage polarization
Macrophage activation or polarization is a complex topic, in part because of the lack of standardized nomenclature. Both terms are used frequently in macrophage research, without clear definitions for either. Additionally, these terms do not encompass a single switch in a macrophage. Rather, polarization towards a certain phenotype involves multiple steps that trigger changes in concert with an evolving microenvironment (45). In general, activation or polarization refers to the effect of cytokines or growth factors on macrophages, producing changes to their phenotype, metabolism, gene expression, cell surface markers, and function. Macrophage phenotype is determined by a complex network of extrinsic factors, the tissue microenvironment, and genetic and epigenetic regulation (29, 43, 46). Examples of stimuli that impact polarization in addition to cytokines and growth factors include hypoxia, toll-like receptor (TLR) binding, microbes and microbial products, glucocorticoids, tissue damage and nucleotides (29). It is worthwhile to note that many polarization experiments have been performed only in mice and have not been fully replicated in humans (47). A thorough review of the important differences between murine and human macrophages is recommended (48).
The term “activation” was in use by the early 1970s. Peter Alexander and Robert Evans did significant early work with macrophages at the Institute of Cancer Research in London, demonstrating that macrophages could be “armed” and made cytotoxic by exposure to specially sensitized lymphoid cells (13, 49). Veterinarian Isaiah Fidler injected activated macrophages intravenously to inhibit pulmonary metastasis in a mouse model of melanoma around the same time (11). The discovery of specific factors that could activate macrophages was made in the 1980s when it was demonstrated that cytokines such as interferon-gamma (IFN-γ) could increase the antimicrobial activity and oxidative metabolism of macrophages (50). It was thus generally believed that type 1 T helper (Th1) lymphocytes produced IFN-γ to activate macrophages and type 2 T helper (Th2) lymphocyte responses characterized by interleukin (IL)-4 and IL-10 inhibited macrophages. Then, in 1992, Stein et al. described the activation of macrophages by IL-4. As this contrasted with the classical IFN-γ activation, the term “alternatively activated” was used to denote a distinct function and phenotype (51). In 2000, the M1/M2 terminology was formalized in an experiment with Th1 mouse strains (C57BL/6) and Th2 mouse strains (BALB/c) that presented the idea that persists to this day that M2 macrophages have increased arginine metabolism. It was noted that IFN-γ and lipopolysaccharide (LPS) could activate macrophages in the Th1 strains to produce nitrous oxide more easily, while LPS activated arginine macrophage metabolism in the Th2 strains (52). However, a discovery since then has shown that C57BL/6 mice have a mutation that impacts their arginine handling, and therefore the differences seen in arginine metabolism is likely multifactorial (53). Regardless, metabolic reprogramming contributes significantly to macrophage polarization, with differences documented in multiple pathways including glycolysis, arginine metabolism, oxidative phosphorylation, the Krebs cycle, and others. Several good reviews are recommended for more detail (54–56). In 2002 Montovani et al. formalized the knowledge that M1/M2 phenotypes represented “extremes of a continuum of functional states” (57). Presently, the work of transcriptome and single-cell analysis has significantly increased the granularity and depth of the understanding of macrophage phenotype (discussed further below). Table 1 provides an overview of generally accepted characteristics of the two extremes of macrophage polarization, although as already noted this framework should be applied with caution to in vivo systems.
It is easy to see that these dichotomous labels fall short of capturing the complexity of macrophage reality, which is best described as a multidimensional spectrum (61). One barrier in macrophage research is how to apply results from in vitro conditions to in vivo reality, as in vitro macrophages that are perturbed in a variety of ways merely represent one snapshot in time of these dynamic systems. Experimentally stimulated macrophages in vitro have significantly different gene signatures than in vivo activated macrophages (62). For example, while granulocyte macrophage colony-stimulating factor (GM-CSF) and macrophage colony-stimulating factor (M-CSF) are often associated with the M1 and M2 phenotype respectively, these growth factors would not be found alone in vivo (60). One solution to better capture the complexities of macrophages in vivo is to describe additional categories of alternative activation including M2a through 2d (54, 63–66). Other groups have investigated mesenchymal stem cell-activated macrophages as a distinct phenotype as well (67, 68). While the general behavior of these subtypes is largely consistent across experiments, it is easy to find discrepancies in the literature regarding stimuli, markers and secretory products attributed to each (69, 70). As these categories grow, so do the problems of standardization and replication across experiments. In 2014 a group of macrophage researchers informally met to propose unofficial guidelines to attempt to improve clarity and reproducibility within the field (71). They recommend several steps worth summarizing. For example, M1/M2 should only refer to in vitro experimental conditions, specifically using IFN-γ and IL-4, respectively, as these activators have been extensively studied (6). They provide comprehensive guidelines on minimum reporting standards for in vitro macrophage experiments, including stringent descriptors of the population under study, the isolation and culture method used, and the details of external stimuli used. They also recommend nomenclature that specifies activators, such as M(LPS) rather than M1, to avoid confusion across experiments (71).
Macrophage phenotype has been investigated in dogs. Heinrich et al. used canine MDMs, defining their populations as M0 (unstimulated), M1 (GM-CSF, LPS, IFN γ-stimulated) and M2 (M-CSF, IL-4-stimulated). Using immunofluorescence (IF), only cluster of differentiation (CD)206 was able to distinguish M2-polarized macrophages from the other phenotypes. Routinely used murine and human M1-markers (CD16, CD32, major histocompatibility complex [MHC] class II and inducible nitric oxide synthase [iNOS]) and additional M2-markers (CD163 and arginase-1) were not useful in discriminating the subtypes. Global microarray analysis showed significant differences in the transcriptomes of the polarized macrophage subsets. Interestingly, the identification of gene sets from prototypical literature-based human and murine macrophage gene sets only had minor overlap with gene sets of the polarized macrophages (72).
Herrmann et al. used canine and human MDMs activated with combinations of M-CSF, IL-4, and IL-13 to activate an M2a allergen subtype and GM-CSF, LPS, and IFN-γ for an M1 subtype. On flow cytometric surface marker analysis, only the high-affinity immunoglobulin (Ig) E receptor FcϵR1 was significantly upregulated in canine M2a macrophages, whereas CD86 was upregulated in human M1 macrophages. They did not find significant differences in the expression of another well-established M1-marker CD80 or the M2-marker CD206 in canine MDMs (although CD206 had a statistically insignificant increase in both human and canine M2a macrophages). Reverse transcription-quantitative polymerase chain reaction did show expected changes such as the upregulation of pro-inflammatory genes in the M1 subtype (73).
Chow et al. also investigated canine macrophage phenotype. They cultured canine MDMs in M-CSF and polarized them with IFN-γ (M1) or IL-13 and IL-4 (M2). Flow cytometric analysis of cell surface markers CD86, MHC class II and CD40 were not able to discriminate between M1 and M2 macrophages. Activated macrophages (both M1 and M2) upregulated MHC class II, a finding supported by Heinrich et al. as well (72, 74). Using IF, however, M1 macrophages were shown to significantly upregulate intracellular iNOS, while M2 macrophages upregulated intracellular CD206, transglutaminase 2 and suppressor of cytokine signaling 1 (SOCS1) as compared to M1 macrophages. This group also found significant differences in function as measured by cytokine secretion and phagocytosis, with M2 macrophages displaying an impaired ability to phagocytose and kill intracellular pathogens. (It is worthwhile to note that although it is widely accepted that M2 macrophages have decreased ability to respond to infectious insults, some conflicting data in the human literature describe M2 macrophages as highly phagocytic (75, 76)). RNA-sequencing (RNA-seq) was used to identify unique canine gene signatures of polarized macrophages, resulting in 6 distinct clusters of macrophage genes (74). Macrophage phenotype has also been studied in a variety of canine pathologies, including (non-exhaustively) intestinal disease, spinal cord disease and Leishmania infections (77–79).
Tumor-associated macrophages
Tumors maintain a tumor microenvironment (TME) via secretion of cellular factors such as chemokines and cytokines, other environmental factors such as hypoxia and lactic acid, and by the recruitment of local and distant immune and stromal cells. Although most intuitive with solid tumors, blood-borne cancers also maintain a TME composed of a diversity of cells (80). Macrophages are the most abundant immune cell in the TME and comprise a strikingly heterogenous group with multiple ontogenies, phenotypes and roles represented (81). There are differing accounts of the ontogeny of TAMs; both MDMs and TRMs are likely recruited by tumors to varying degrees (82). Some evidence from mice also suggests that a subset of myeloid-derived suppressor cells (MDSCs), a distinct, immature myeloid cell that contributes to the immunosuppression, also differentiate into mature TAMs once in the TME (83–85).There is evidence that subsets of TAMs with differing ontogenies take up different functions in the TME. For example, in a mouse model of lung carcinoma, TRMs were associated with tumor cell growth, while recruited MDMs were more associated with metastasis (86). Even in protected spaces such as the central nervous system, both MDMs and tissue-resident microglia with distinct transcriptional profiles and functions are represented in the population of TAMs in primary brain tumors (43, 87).
TAMs are generally described with the permissive language of “M2-like”, sometimes specifically associated with the M2d subset. The M2d phenotype is associated with angiogenesis, matrix remodeling and immunosuppression (88). However, TAMs can simultaneously express gene signatures from multiple subsets including canonical M1 signatures, and some TAM subsets are more closely aligned solely with M1 phenotypes (89–91). Additionally, TAM maturation has been shown as distinct from typical alternative activation of macrophages (92). Although initially aligned most strongly with tumor-associated inflammation, it is now known that TAMs interact with cancer cells, other immune cells, and stromal cells to facilitate several other hallmarks of cancer (10, 93). TAMs help induce or access vasculature, promote invasion and metastasis, help avoid immune destruction and contribute to sustained proliferative signaling (93, 94).
TAMs have complex interactions in the TME with cancer cells. TAMs and cancer cells engage in various constitutive feedback loops, in which cancer cells recruit TAMs via factors such as colony stimulating factor-1 (CSF-1, a major driver of macrophage maturation). In turn, TAMs then secrete cellular factors such as protein kinase C, which upregulates production of CSF-1 from colon cancer cells (95). Similar feedback loops have been established in gastric cancer (hypoxia induced C-X-C motif chemokine ligand [CXCL]8 production from TAMs stimulates IL-10 secretion from cancer cells resulting in M2 polarization), breast cancer (reciprocal secretion of GM-CSF and C-C motif chemokine ligand [CCL]18 recruits TAMs and induces epithelial-mesenchymal transition), and others (96–98). Many of these same pathways reveal how TAMs promote the progression and metastasis of cancer cells. Once recruited, TAMs produce factors that remodel the TME to better support cancer cells, increase invasion via epithelial-mesenchymal transition, improve tumor vascularization, increase intravasation and extravasation, improve survival of circulating tumor cells, and help prepare the pre-metastatic niche. For example, TAMs produce matrix metalloproteinases and cathepsins, which disrupt cell junctions and basement membranes to aid in tumor invasion into surrounding tissues and blood vessels (99, 100). Another interesting example demonstrates that primary tumor secretion of factors such as vascular endothelial growth factor-A and transforming growth factor-beta induce distant lung endothelium to produce a variety of monocyte chemoattractants, thus preparing the metastatic niche (101). Each of these processes are complex, and detailed reviews have been written (96, 102, 103). The unique metabolism of TAMs also supports cancer cell growth. Their handling of glucose, amino acids, and lipids increases the availability of critical nutrients such as iron in the TME, contributes to immunosuppression, and provides strong growth signals to cancer cells (55, 104). Their universal metabolism of L-arginine, for example, results in extracellular depletion, rendering it unavailable to auxotrophic T cells that require it for proliferation (105).
The crosstalk between TAMs and cancer cells does not exist in a vacuum. Stromal cells and other immune cells are intimately involved in these processes as well. The biomechanical properties of the extracellular matrix (such as stiffness, topography, etc.) in the TME contributes to TAM polarization and function (106). Stromal cells themselves can recruit and polarize TAMs. Cancer-associated fibroblasts recruit TAMs, support M2 polarization, and inhibit cancer fighting immune cells from entering the TME (107, 108). Mesenchymal stromal cells, in addition to directly promoting tumor progression, secrete various angiogenic factors that polarize TAMs to an M2-like phenotype (109). TAMs are also involved in constant, reciprocal feedback with other immune cells. In hepatocellular carcinoma, for example, tumor-associated neutrophils recruit TAMs and regulatory T cells (Tregs) to the TME, promoting the immunosuppressive environment (110). In the TME, TAMs inhibit CD4+ and CD8+ T cells and natural killer (NK) cells, induce Treg differentiation, and recruit natural Tregs (111). Exhausted T cells and TAMs have been shown to maintain a positive feedback loop which supports the persistence of both populations in the TME (112).
There is some evidence from the veterinary literature supporting how macrophages may contribute to the immunosuppressive TME. Hartley et al. evaluated programmed death-ligand 1 (PD-L1) expression in primary canine MDMs, showing that expression was significantly induced by exposure to IFN-γ (113). Although IFN-γ is classically considered a pro-inflammatory cytokine, it is better understood now to have both immunostimulatory and immunosuppressive functions as well (114). Upregulation of PD-L1 on MDMs after exposure to IFN-γ may therefore be one mechanism of immunosuppression in the TME (113). Eto et al. demonstrated that damage-associated molecules released from necrotic canine cancer cells exerted immunosuppressive effects on macrophages via prostaglandin E2. This was hypothesized to be one mechanism by which dysregulation of the response to inflammation may contribute to tumorigenesis (115).
TAMs serve as prognostic biomarkers in many cases, as well as predictive biomarkers for response to treatment. TAMs are also associated with resistance to chemotherapy, immunotherapy, endocrine therapy, radiation therapy and targeted therapy (116–118). One meta-review of the prognostic significance of TAMs in human solid tumors identified a negative effect of TAM density on overall survival (OS) in gastric, breast, bladder, ovarian, oral, and thyroid cancer. In contrast, a positive effect was noted for OS in colorectal cancer, and no effect of TAM phenotype was found for any tumor type (119). However, the literature on this topic is vast, and as expected there are many conflicting conclusions. Table 2 outlines key prognostic indicators of TAMs in select human and canine cancers. It can be difficult to comprehensively apply conclusions regarding TAMs and prognosis to large groups of patients, as the immune composition of the TME varies widely not only between tumor types, but also within tumor type dependent on patient factors such as age, sex, and body weight (146–148).
Single-cell RNA-seq (scRNA-seq) techniques, first reported in 2009, have made incredible progress in elucidating the complexity of TAMs a priori (91, 149, 150). In brief, these techniques resolve the transcriptomes of single cells otherwise masked in bulk sequencing techniques. Several recent publications have explored the complexity and heterogeneity of TAM subsets across many cancer types using scRNA-seq (91, 151). A consistent finding across studies is that individual TAMs can express both canonical M1 and M2 gene profiles at the single-cell level (43, 91). A review article that attempted to integrate the findings of multiple scRNA-seq studies on TAMs proposed names for seven TAM subsets with distinct transcriptional profiles and metabolic pathways across many cancer types. For example, angio-TAMs had signatures enriched for angiogenesis, while IFN-TAMs were enriched for interferon-regulated genes and M1 markers. These seven subsets were not exhaustive, with many other subsets found in fewer numbers of cancers (152).
scRNA-seq of TAMs has not only been used to characterize new subsets of macrophages but also to identify macrophage-based prognostic markers or personalized treatment strategies (153, 154). For example, malignant gliomas in people are characterized by robust angiogenesis, a process aided significantly by TAMs. It has been demonstrated that the contribution of MDMs and TRMs to this process differs between primary and recurrent tumors, and therefore strategies targeting blood-derived TAMs versus resident microglia may have varying degrees of success at different time points in the course of disease (43, 155). Single-cell sequencing has also progressed beyond RNA, with new multi-omics techniques providing information at the single-cell level on epigenetic modifications, including histone modifications, DNA methylation, and chromatin openness (156). For example, combining epigenetic information with scRNA-seq data has produced data on TAM chromatin accessibility and cis-regulatory sequences (152). Single-cell sequencing data in companion animal species is in its infancy, although there are increasing numbers of publications in the past few years (157–162). ScRNA-seq data on canine TAMs has not yet been published to the authors’ knowledge.
TAMs in companion dogs
In companion dogs, a large body of work exists regarding TAMs and mammary carcinoma due to its relative frequency and relevance as a translational model for breast cancer. Several groups have investigated the molecular crosstalk and changes in gene expression that occurs between canine mammary carcinoma cells co-cultured with macrophages (163–165). One notable conclusion was that carcinoma cells co-cultured with canine macrophages had increased migration and invasion compared to carcinoma cells in monoculture (163). Another group showed that carcinoma cells inhibited LPS activation of co-cultured macrophages, suggesting cancer-mediated immune suppression (164). Another demonstrated that TAMs increased the secretion of pro-angiogenic factors from canine mammary cancer stem-like cells (165). Multiple groups have published on associations between higher levels of TAMs in canine mammary tumors and shorter OS, higher metastatic rate, higher histologic grade, higher levels of intra-tumoral vascular endothelial growth factor and hormone receptor negativity (137–140, 166). One study found that, in contrast, higher numbers of macrophages in canine mammary carcinoma samples correlated with longer survival time. However, when taking the M2 TAMs alone a correlation with lymph node metastasis was noted (141). It should be noted that M1 and M2/TAM markers vary by study, and so results should be interpreted considering this fact. Some of the discrepancies arise from differing use of the term TAM, to either signify all macrophages in the TME versus only those with a pro-tumorigenic/M2-like phenotype. Table 3 summarizes markers investigated for canine macrophages in the veterinary literature (most of which have been chosen based on human and murine data and have not been validated as able to discern between macrophage phenotypes in the dog).
TAMs and their significance have also been investigated in other canine tumor types. Correlations between increased TAM infiltration and higher-grade disease has been identified in canine gliomas, lymphoma, soft tissue sarcomas and mast cell tumors (123, 124, 131, 134, 171, 173). In an analysis of canine hemangiosarcoma tumors, 67% contained macrophages that co-expressed an M2 marker and PD-L1. These tumors with PD-L1-expressing M2 macrophages had lower numbers of T cells in the TME. Additionally, M2 polarization and PD-L1 expression could be induced by tumor-conditioned media (167). In samples of canine tumors, pulmonary metastases from hemangiosarcoma were shown to have greater numbers of monocytes compared to metastases from other tumor types, as well as significantly higher CCL2 production, a monocyte chemoattractant protein (also called MCP-1) (174). In canine oral melanomas, M2 macrophages were significantly higher in malignant disease and associated with nuclear atypia and mitotic count. M2-marker CD163 positivity by itself was associated with metastatic disease and tumor-related death (168). In contrast to the above studies, in canine osteosarcoma, a higher percentage of M2 macrophages was correlated with a longer disease-free interval in one study. This same group analyzed paired primary tumor and metastasis samples of canine osteosarcoma and found the metastases to have a significantly higher degree of T cells, B cells and M2 macrophages, suggesting a role of these cells in the metastatic immune environment (128, 169). A preprint study from the National Cancer Institute confirmed these findings, showing a potential benefit from abundant M2 macrophages in canine and human osteosarcoma by transcriptomic analysis. These authors suggest this may be due in part to the unique properties of bone, namely that cytokines responsible for M2 differentiation can also inhibit osteoclast formation (170). In canine colorectal cancer, one study found no significant difference in macrophage counts between control tissue, adenomas, and carcinomas, while another found lower levels of macrophages in adenomas and carcinomas as compared to controls (144, 145).
There is also a body of literature that investigates the prognostic value of peripheral blood monocytes in dogs with cancer. Peripheral blood may provide insight into the TME’s systemic immunosuppressive impacts and can provide useful prognostic information in many tumor types. On analysis of peripheral blood, MCP-1 was increased in dogs with histiocytic sarcoma and lymphoma compared to healthy controls, while dogs with osteosarcoma were shown to have decreased chemotactic function of peripheral blood monocytes compared to controls (176–178). Increased monocyte counts or decreased lymphocyte-to-monocyte ratios have also been shown to be poor prognostic factors in canine lymphoma and osteosarcoma, similar to findings in many human cancers (178–182). A retrospective study of adjuvant carboplatin in dogs with hemangiosarcoma found an increased median survival time (MST) in dogs whose monocyte counts decreased post-operatively compared to those whose counts increased (265 days versus 66 days, respectively) (183). Interestingly, recent attention has been paid to circulating macrophage-like cells in peripheral blood. One veterinary study found that of 39 complete blood counts from dogs with circulating macrophage-like cells, 46% had a diagnosis of cancer (including both histiocytic and non-histiocytic origin) (184). These cells have the potential to be detectable in higher numbers than circulating tumor cells as sources of phagocytized tumor DNA (185).
Therapy (preclinical/human)
When targeting macrophages as an anti-cancer therapy, there are a few general approaches. Broadly, one may attempt to deplete the TME of TAMs, inhibit the recruitment of monocytes/macrophages to the TME, or re-educate TAMs from tumorigenic to anti-tumor. (Although these categories are discussed separately below, it is likely that many of these therapies impact different macrophage subsets in multiple ways). Since the early work of Evans, Alexander and Fidler (discussed above), significant progress has been made in macrophage-based therapies, with many ongoing clinical trials. Table 4 outlines some noteworthy macrophage-based cancer therapies in people and dogs. As the understanding of myeloid cells in the TME progresses, several anti-cancer therapies initially understood to have other targets have subsequently been found to have significant impacts on TAMs, very likely contributing to the anti-cancer effect. Examples include paclitaxel (via TLR-4 activation similar to LPS), imatinib (via the M-CSF receptor), and ibrutinib (via Bruton’s tyrosine kinase inhibition) (200–202).
TAM depletion
Macrophage depletion has long been performed in vitro with bisphosphonates (BPs), initially agents of interest for anti-resorptive properties for skeletal metastasis (203–205). BPs are phagocytized by macrophages resulting in apoptosis and have been demonstrated in vivo to deplete TAMs and inhibit tumor growth (206, 207). They are still actively studied clinically for their macrophage-specific anti-cancer effects (208). There are other ways to selectively induce apoptosis in TAMs. Trabectedin, a marine-based DNA-binding small molecule, and its analogue lurbinectedin are approved for use in soft tissue sarcoma (trabectedin) and ovarian cancer and non-small cell lung cancer (lurbinectedin). While they likely have multiple mechanisms of action, they have preferential cytotoxicity for monocytes and macrophages via tumor necrosis factor-related apoptosis ligand (TRAIL) mediated apoptosis (209–211).
Another approach to TAM depletion is accomplished via blocking ligand-receptor interactions with small molecules or monoclonal antibodies. One popular strategy is the targeting of the M-CSF receptor, also called colony-stimulating factor 1 receptor (CSF-1R), or one of its ligands, M-CSF/CSF-1 (212). This pathway is responsible for the survival and differentiation of macrophages. Selective inhibitor of CSF-1R pexidartinib has shown significant clinical benefit for a rare tumor that overexpresses CSF-1 (tenosynovial giant cell tumor) (213). In other trials of CSF-1/R inhibitors for advanced solid tumors, stable disease or partial responses are observed with combinatorial therapies, and there are many other CSF-1/R inhibitors under investigation (212, 214, 215).
There are other interesting, experimental approaches to TAM depletion. One group engineered a hybrid peptide, consisting of melittin (a polypeptide that binds preferentially to TAMs) and a pro-apoptotic peptide. Injection of this hybrid peptide into a mouse model of lung carcinoma resulted in selective apoptosis of M2-like macrophages while sparing other immune cells (216). Chimeric antigen receptor (CAR)-T cells have been engineered to deplete TAMs via folate receptor beta binding, resulting in improved antitumor immunity and survival in a mouse model (217). Bi- and tri-valent T-cell engagers have been made that recognize CD3 on T-cells and a specific M2 marker on TAMs such as CD206 or folate receptor beta, resulting in selective T-cell mediated TAM depletion (218).
Blocking TAM recruitment
Blocking the recruitment of MDMs to the tumor site is another therapeutic strategy. The chemokine CCL2 is crucial for the recruitment of c-c chemokine receptor type 2 (CCR2)-expressing monocytes to the TME. Of several compounds targeting CCL2/CCR2 to enter phase I clinical trials, CCX872, a CCR2-specific antagonist, showed the best anti-cancer activity, although the benefit was still modest. When given in combination with standard-of-care chemotherapy to advanced pancreatic cancer patients, it modestly improved OS in comparison to a historical cohort of patients receiving chemotherapy alone (219). Monoclonal antibodies against CCL2 have not been as effective (220). Another important pathway for monocyte recruitment is CCL5/CCR5. Several CCR5 antagonists in phase I clinical trials are repurposed drugs, initially developed for human immunodeficiency virus therapy (186, 221, 222). Therapeutic hurdles to blocking TAM recruitment include a rebound of recruited monocytes to the TME that can be seen after discontinuation of treatment, as well as redundancy in monocyte recruitment mechanisms (223, 224). However, decreasing recruitment or depleting TAMs can still enhance other immunotherapies, such as checkpoint inhibitors and cancer vaccines (225–227).
TAM repolarization
TAMs may also be re-educated (or “repolarized”) from their generally immunosuppressive, pro-tumorigenic roles to have anti-cancer functions. Re-programming TAMs not only encourages macrophage-specific tumor cell killing but may also activate NK cells and T cells in the TME to kill tumor cells as well (228–231). In 2011, while testing CD40 activation (a co-stimulatory molecule expressed on antigen-presenting cells) on antitumor T cell responses, Beatty et al. found that CD40 agonism robustly activated macrophages in the TME, resulting in infiltration and depletion of tumor cells and tumor stroma (228). Since then, multiple agonists of CD40 have progressed to phase I/II clinical trials (186). A CD40 monoclonal antibody showed promising clinical in combination with chemotherapy for metastatic pancreatic adenocarcinoma (232). Checkpoint inhibitors are another avenue for TAM repolarization. TAMs, in addition to T cells, express PD-1, resulting in tumor immunity via PD-L1 expression from tumor cells (233). PD-L1 inhibition was shown to successfully rescue phagocytosis and re-educate TAMs in a mouse model (233, 234). Myeloid-specific checkpoint inhibitors have also been developed. Many tumor cells overexpress CD47, the prototypical “don’t eat me” signal that inhibits phagocytosis on normal cells from signal regulatory protein alpha (SIRPα)-expressing myeloid cells. This pathway can be blocked to increase phagocytosis of cancer cells and antigen presentation by macrophages, and promising clinical activity has been demonstrated in hematological malignancies and solid tumors (231, 235, 236). Increased Fc-mediated phagocytosis appears especially beneficial when combined with antibody-mediated opsonization (186, 237). As might be expected from a ubiquitously expressed self-marker, significant toxicity can be seen, and so different strategies have been employed to circumvent this limitation. Strategies include manipulation of the antibody structure or increasing specificity with a bispecific molecule target CD47 and a tumor antigen (186, 231). Other myeloid checkpoint inhibitor targets are under investigation such as the inhibitory leukocyte immunoglobulin-like receptor subfamily B member 1 receptor (LILRB1), an MHC class I-binding protein that suppresses phagocytosis, and CD24, another anti-phagocytic signal that binds through sialic-acid-binding Ig-like lectin (Siglec)-10 (238, 239). There are various other targets that repolarize TAMs including agonism or inhibition of TLRs, scavenger receptors (CD206, Clever-1, macrophage receptor with collagenous structure (MARCO), stimulator of interferon genes (STING), phosphoinositide 3-kinases gamma (PI3Kγ), or histone deacetylase (HDAC). Some of these therapies have broad impacts on anti-tumor immunity, although TAM re-polarization is recognized as an important contributing factor (186, 240, 241). A phase I/II clinical trial with a Clever-1 inhibiting antibody resulted in reversed immunosuppression in the TME and some clinical responses in patients (242). Conventional radiation therapy, proton irradiation, cryo-thermal therapy and cryosurgery have also been used to repolarize TAMs in addition to directly killing cancer cells. These therapies have multiple anti-cancer mechanisms, but in general cause activation of previously immunotolerant macrophages (243–246).
Toll-like receptors, a type of pattern recognition receptor, sense and respond to exogenous and endogenous danger signals. Their stimulation largely results in inflammatory, anti-cancer responses mediated by various immune cells including macrophages, and so make attractive targets for TAM repolarization. Historically, systemic administration of TLR agonists was inefficient and resulted in significant toxicity. However, with the advancements of nanomedicine, TLR agonists are able to be administered with fewer adverse effects, significantly improved tumor trafficking, and prolonged persistence in the TME (247, 248). Repolarization of TAMs has been investigated pre-clinically using nanoparticles (NPs) loaded or linked with various TLR agonists including CpG oligodeoxynucleotides, type I IFNs, imidazoquinolinone, and many others (247–252). Interestingly, empty NPs themselves have been shown to stimulate TLRs (247). As with many immunotherapies, combination therapy is of high interest. Imiquimod, a TLR-7 agonist, and anti-CD47 antibodies were delivered together on a nanoscale metal framework, leading to tumor eradication when combined with checkpoint inhibitors in a colorectal tumor model (253).
As mentioned above, the metabolism of TAMs and other myeloid cells in the TME is unique and contributes to immunosuppression and treatment resistance. Altering their metabolic pathways has therefore become an interesting therapeutic target. CB-1158, a small molecule developed to inhibit arginine metabolism and reverse immunosuppression in the TME, has entered phase I/II trials alone and in combination with checkpoint inhibitors (105, 254). Other interventions are under study that reprogram TAM metabolism towards an M1 phenotype, including suppressing glycolysis (resulting in decreased lactate in the TME), inhibiting hypoxia, and regulating iron handling. As might be expected, metabolic manipulation can have complex downstream effects, and the metabolism of TAMs even within a solitary tumor is significantly heterogenous (81, 104).
Cell-based therapies with macrophages deserve special mention. Combining cell therapy with nanotechnology has been used in of interesting ways to target TAMs. The first approach is to load M1-like macrophages with a variety of drug-laden NPs, inducing tumor cell killing via both the NP load and their natural M1 functions (255, 256). The second approach involves using NPs to deliver messages to TAMs to induce repolarization. One group used mannose-coated nanoparticles to introduce mRNA encoding M1-polarizing transcription factors into TAMs, subsequently inducing an M1 phenotype with anti-tumor properties (257). These approaches can be combined, for example, by using doxorubicin-laden NPs anchored to macrophages via LPS. When these reached their target, tumor cells were killed via M1 macrophages and doxorubicin, and TAMs were re-educated via the LPS (258). Macrophages have been modified in a variety of other ways as well for cell-based therapy, including engineered IFN-γ-laden “backpacks” and lentivirus-driven genetic engineering to express therapeutic IL-12, a pro-inflammatory cytokine (259, 260). Most interestingly, macrophages have been identified as candidates for CAR therapy. Large numbers of macrophages can be obtained after monocyte-apheresis or from HSCs (261, 262). Despite initial technological challenges, macrophages can now be engineered to express CARs against a cancer-specific antigen, called CAR-M cells (263, 264). It has been suggested that CAR-M cells possess an advantage over CAR-T cells in their ability to infiltrate into the TME and bypass the immunosuppressive environment, as well as re-educate “bystander” M2 macrophages (263, 265). Recently, the first-in-human CAR-M trial was launched, targeting human epidermal growth factor receptor 2 (HER2)-positive solid tumors (clinical trial NCT04660929). Several other targets are under preclinical investigation for CAR-M therapy (186, 264).
TAM-specific imaging
Dynamic TAM-specific imaging is an important arm in treatment strategies. This has been investigated with both magnetic resonance imaging (MRI) and positron emission tomography (PET). TAM-targeting contrast agents used with MRI are phagocytosed by monocytes and then accumulate in tumors or leak directly into tumors due to the increased permeability of tumor vessels and are subsequently phagocytosed by macrophages. Alternatively, some agents are labeled with antibodies that bind macrophage receptors. Gadolinium, iron oxide nanoparticles and fluorine-19 have all been studied preclinically. Generally, these agents are unable to distinguish M1-like versus M2-like TAMs (266). Therapeutic uses of contrast agents have also been studied. One group used magnetic resonance targeting to direct iron oxide-labeled macrophages carrying an oncolytic virus to the sites of primary and metastatic tumors in mice. This resulted in increased macrophage infiltration and decreased tumor burden (267). With PET, radiotracers can be used to distinguish generally pro-inflammatory and anti-inflammatory macrophages. Tracers are in development for several prototypical M2 markers. Another approach is to target radiotracers to macrophage function, such as phagocytosis or antigen presentation (268). Imaging probes can be modified to include immunomodulatory therapeutics, resulting in imaging techniques that are both diagnostic and therapeutic. As discussed above, CSF-1/R blockade is a popular investigational approach for TAM depletion. Ligands of CSF-1R have been radiolabeled for PET tracers and investigated in mouse models (269). Several other targets of macrophage-based therapy are under similar investigation as potential imaging tracers. In theory, these tracers could be used to stratify patients pre-treatment who may benefit from macrophage-based therapy, deliver macrophage-targeted therapies and monitor response to therapy (269).
Therapy (companion dogs)
In companion dogs, there are historically very few macrophage-specific therapies in cancer, although this is starting to change. As mentioned above, several therapeutics in use likely significantly impact TAMs, whether intentionally or not. As in humans, depletion of TAMs via BPs has been attempted. Liposomal clodronate (LC) was evaluated for the treatment of 13 dogs with soft tissue sarcoma (STS), and serial biopsies from 5 of them demonstrated significantly decreased numbers of infiltrating macrophages after the administration of LC. However, a decrease in TAMs was not correlated with tumor regression in this small sample (270). This group also used canine histiocytic sarcoma (HS) cells to evaluate sensitivity to LC in vitro, demonstrating apoptotic cell death in HS cell lines, but not other cell lines. They also showed tumor regression in 2 of 5 dogs with spontaneous HS treated with LC. As histiocytic neoplasms arise from DC or macrophage origin, the mechanism leading to apoptosis is likely similar to primary macrophages (271). They also demonstrated an increased sensitivity to chemotherapy in canine HS cells co-treated with clodronate (272). Regarding the CSF-1/R axis for TAM depletion, a blocking antibody against canine CSF-1R has been developed, although follow-up work has not yet been published (188).
Attempts to block the recruitment of TAMs have also been evaluated in dogs. Losartan, a type I angiotensin II receptor blocker, has recently gained interest as a newly recognized specific inhibitor of the CCL2-CCR2 axis mentioned above. Its ability to block monocyte/macrophage recruitment was initially evaluated in inflammatory and atherosclerotic diseases (273). Regan et al. were the first to evaluate its anti-cancer role as a TAM-targeting therapy in dogs. They initially demonstrated a reduction in pulmonary metastasis in a mouse model associated with a significant decrease in monocytes recruited to the lung through the inhibition of CCL2 (189). A follow-up study demonstrated that losartan inhibited monocyte migration to human and canine osteosarcoma cells in vitro. A prospective clinical trial evaluating safety and efficacy in combination with toceranib was performed on dogs with metastatic osteosarcoma. In the high-dose cohort, 4 of 16 dogs experienced a partial response of their lung metastasis for a median duration of 163 days, and another 4 dogs experienced stable disease for a median of 139 days (190). These findings were significant as toceranib alone had been shown to have minimal activity in metastatic osteosarcoma in dogs (274). This same group used losartan in combination with propranolol (shown to have MDSC depletion activity) and a cancer stem cell vaccine in canine glioma. Of 10 dogs (6 with high-grade tumors and 4 with low-grade tumors), 2 experienced a partial response and 8 had stable disease, with an overall MST of 351 days (191). This MST is comparable to other studies for high-grade disease (275, 276).
The final approach of re-polarizing or activating TAMs has also been investigated (largely unintentionally). In all examples noted below, other than when explicitly stated, macrophage-specific data were not reported, even when other immune cells were profiled. Several bacterial-based immunotherapies which cause broad immunomodulation in the TME undoubtedly impact TAMs in some way. Starting in the 1890s, a New York City surgeon Dr. William Coley was treating cancer patients with systemic or intra-tumoral injections of bacteria and bacterial products referred to as Coley’s toxins. Although he went through several iterations, the most successful concoction was heat-killed streptococcal organisms and Serratia marcescens, importantly anaerobic organisms that would flourish in a hypoxic tumor (277). Although his results eventually came under fire for inconsistencies, the kernel of his ideas has lived on in other experiments (278, 279). Importantly for macrophages, Coley’s toxins and other bacterial products are known to activate TLRs and other pathogen-associated molecular pattern receptors (280, 281). This bacterial activation of macrophages is the precursor to more specific TLR agonists used in macrophage-based therapies presently (240, 280).
Multiple bacterial-based anti-cancer therapies have been evaluated in dogs. These include a phase I trial of Salmonella typhimurium in dogs with spontaneous tumors, intra-tumoral injection of Clostridium novyi spores in dogs with spontaneous tumors (largely STSs), and Listeria- and Salmonella-based vaccine strategies in dogs with osteosarcoma (278, 279, 282–284). Two vaccine-based treatments for canine OS showed promise (one with immunogenic peptides from Salmonella-infected canine osteosarcoma cells and one with a HER2-targeting Listeria). Improvements in time to metastasis and survival were seen compared to historical cohorts receiving the standard of care in these preliminary studies (282, 284). Bacillus Calmette-Guerin (BCG), a live-attenuated strain of Mycobacterium bovis, is thought to activate macrophages predominantly through TLR-2, although it introduces a massive cytokine response and likely works through other mechanisms as well (285, 286). In the 1970s and 1980s it was used in a wide variety of canine cancers with varying degrees of success, although the treatment of transmissible venereal tumors seemed most promising (287–289). A more recent study used it in combination with vincristine in dogs with transmissible venereal tumors, resulting in shorter times to regression and increased macrophages in post-treatment biopsy samples (290).
Like the theory that the intentional administration of bacterial products may be beneficial in cancer, the survival benefits of post-limb salvage surgical site infections in dogs and people with osteosarcoma are well-established. This is likely attributable in part to the upregulation of NK cells, monocytes, and macrophages (291–293). Laboratory studies have demonstrated that chronic bacterial osteomyelitis-mediated suppression of tumor growth can be abrogated by the depletion of NK cells and monocytes (294).
Another example is liposome-encapsulated muramyl tripeptide phosphatidylethanolamine (L-MTP-PE), a synthetic derivative of a bacterial cell wall component. The base compounds, muramyl dipeptide or tripeptide, were studied in the 1980s by Fidler’s group, who demonstrated that these compounds activate monocytes and macrophages to kill tumor cells (295, 296). L-MTP-PE is a ligand of the Nod-like receptor and is taken up via phagocytosis, increasing inflammatory cytokines such as tumor necrosis factor alpha (TNF-α), IL-6, and C-reactive protein (193, 297). It appeared to improve OS and disease-free interval compared to the standard of care in dogs with osteosarcoma, hemangiosarcoma, and stage I oral melanoma in several studies from the 1990s (193, 194, 297). A similar study in dogs with mammary carcinoma did not show any benefit to L-MTP-PE treatment but did report the mild toxicity observed (similar in people), namely fever and shivering for 10-24 hours after dosing (195). Interestingly, this early work in companion dogs assisted the advancement of this compound through phase II/III clinical trials in people, and while it is approved for use in dozens of countries outside the USA, it was denied approval by the FDA in 2007 (192).
Other ways to re-polarize TAMs have also been investigated in dogs. A canine monoclonal agonist antibody against CD40, the macrophage-activating receptor, has been developed. In a phase I dose escalation trial, 27 dogs with STS were treated with intralesional agonist anti-CD40 canine antibody and IL-2. A clinical benefit was observed in 13 of 19 evaluable dogs at one month, including 2 partial responses. Seven of the 11 dogs with stable disease at one month continued to have stable disease for at least 50 days (197). As mentioned above, paclitaxel, imatinib, and BTK inhibitors can repolarize TAMs, and have been used in companion dogs with cancer (298–301). Chloroquine, an anti-malarial drug, also repolarizes TAMs via calcium-mediated nuclear factor kappa B (NF-kB) activation and was evaluated in combination with doxorubicin for dogs with lymphoma (302, 303). Of a total of 30 dogs, the response rate for this combination therapy was 93.3% and the median progression-free interval (PFI) was 5 months (302). Although head-to-head trials are needed, a retrospective evaluation of doxorubicin alone showed a similar PFI and a response rate of 84% (304). Another group treated canine MDMs with all-trans retinoic acid (ATRA) to reduce immunosuppressive secretions from the macrophages, with possible applications to reversing immunosuppression in the TME (305). There is human data, however, that shows ATRA may support M2 polarization (306).
Cancer vaccines rely in part on the presentation of tumor antigens to innate immune cells, including macrophages (307). In addition to the bacterial-based vaccines mentioned above, several other cancer vaccines have been looked at in veterinary medicine. An oncolytic vaccinia virus was used in xenograft models of canine STS and prostatic cancer, significantly increasing tumor macrophage infiltration and causing tumor regression (308). Similarly, an oncolytic herpes virus was used for canine gliomas. Pre-treatment biopsy samples showed significant infiltration with macrophages, and transcriptome analysis indicated myeloid cell activation after treatment (172). A whole-cell autologous cancer vaccine for metastatic canine hemangiosarcoma utilized a protein immune adjuvant known to activate macrophages and other immune cells (309, 310). Dogs with hemangiosarcoma received surgery followed by either chemotherapy or the vaccine; MSTs were the same (142 days) and either therapy conferred a benefit over surgery alone (309). Monophosphoryl lipid A, a TLR-4 agonist, has been evaluated as an adjuvant for anti-cancer vaccines in people, and an in vitro study showed it can activate canine macrophages as well (175, 311). Other examples abound (312–316).
Cytokine-based therapies have obvious applications to macrophage strategies as they are major drivers of macrophage polarization and function. Kurzman et al. exposed canine pulmonary alveolar macrophages to recombinant canine TNF-α and IFN-γ and found increased cytotoxicity against osteosarcoma cells (317). A phase I trial evaluating PEGylated TNF-α in dogs with spontaneous tumors showed increased tumor blood flow after administration and was well-tolerated. Minor or transient responses in melanoma, squamous cell carcinoma and mammary carcinoma were noted (318). Inhaled IL-2 and IL-15 have also been used to treat dogs with pulmonary metastatic disease, and a statistically insignificant increase in macrophage numbers in bronchoalveolar lavage samples was noted after IL-2 therapy (319, 320). These latter cytokines are largely studied for their anti-tumor activation of T cells and NK cells, although there is evidence that they activate macrophages as well (321–323).
Checkpoint inhibitors are areas of ongoing research interest in veterinary medicine. Although PD-1/PD-L1 blockade is most associated with T cells, as mentioned above TAMs may also express PD-1 and/or PD-L1. The blockade of this checkpoint has been shown to increase tumor cell phagocytosis by PD-1-positive TAMs (113, 324). Canine monoclonal antibodies blocking this axis have been developed and used in a pilot clinical study (325–329). Similarly, the CD47/SIRPα axis is conserved in dogs. A xenograft model of canine diffuse large B-cell lymphoma was sensitive to combinatorial anti-CD20 and CD47 blockade therapy (198).
Conclusions and future directions
Tumor-associated macrophages have undeniable impacts on cancer progression and response to therapy. Veterinary therapeutics targeting macrophages and other myeloid cells lag behind human therapies, although progress is being made in recent years. As with other cell-based therapies, the cost of macrophage adoptive cell therapy will likely be a major obstacle in veterinary species. Regardless, there is a strong potential to use companion dogs as a streamlined pipeline for macrophage-based therapy discovery due to similarities in spontaneous cancers and immune systems between both species. When other immune parameters are being studied, monocyte/macrophage-specific data should also be collected whenever possible. In polarization experiments, precise and complete reporting of experimental conditions should be provided to improve reproducibility. Many topics tangentially related to TAMs are routinely studied in veterinary medicine, including radiotherapy, nanomedicine, and advanced imaging such as PET. Future studies should explore these topics in relation to TAMs in companion species, including the impact of radiotherapy on TAM polarization, the use of NPs to reverse immunosuppression in the TME and improve outcomes in veterinary oncology patients, and the use of novel PET tracers to explore macrophage-based imaging. Additionally, scRNA-seq techniques should be employed to deepen the understanding of TAMs in dogs and other companion species.
Author contributions
RB drafted this review article, revised it critically, provided approval for publication, and agrees to be responsible for all aspects of this work. DT conceptualized this review article, revised it critically, provided approval for publication, and agrees to be responsible for all aspects of this work. All authors contributed to the article and approved the submitted version.
Funding
The authors wish to thank the AKC Canine Health Foundation Clinician-Scientist Fellowship Program (award number 03033-E), the Morris Animal Foundation (award number D22CA-500), the National Institutes of Health Ruth Kirschstein Institutional National Research Service Award Training Grant (award number T32OD010437), and the Flint Animal Cancer Center for support and funding.
Conflict of interest
The authors declare that the research was conducted in the absence of any commercial or financial relationships that could be construed as a potential conflict of interest.
Publisher’s note
All claims expressed in this article are solely those of the authors and do not necessarily represent those of their affiliated organizations, or those of the publisher, the editors and the reviewers. Any product that may be evaluated in this article, or claim that may be made by its manufacturer, is not guaranteed or endorsed by the publisher.
References
1. Gaudet RG, Bradfield CJ, MacMicking JD. Evolution of cell-autonomous effector mechanisms in macrophages versus non-immune cells. Microbiol Spectr (2016) 4(6). doi: 10.1128/MICROBIOLSPEC.MCHD-0050-2016
2. Tauber AI. Metchnikoff and the phagocytosis theory. Nat Rev Mol Cell Biol (2003) 4:897–901. doi: 10.1038/nrm1244
3. Gautiar EL, Shay T, Miller J, Greter M, Jakubzick C, Ivanov S, et al. Gene-expression profiles and transcriptional regulatory pathways that underlie the identity and diversity of mouse tissue macrophages. Nat Immunol (2012) 13:1118–28. doi: 10.1038/ni.2419
4. Martinez FO, Helming L, Milde R, Varin A, Melgert BN, Draijer C, et al. Genetic programs expressed in resting and IL-4 alternatively activated mouse and human macrophages: similarities and differences. Blood (2013) 121:e57–69. doi: 10.1182/BLOOD-2012-06-436212
5. Zubair K, You C, Kwon G, Kang K. Two faces of macrophages: Training and tolerance. Biomedicines (2021) 9(11):1596. doi: 10.3390/BIOMEDICINES9111596
6. Wynn TA, Chawla A, Pollard JW. Macrophage biology in development, homeostasis and disease. Nature (2013) 496:445–55. doi: 10.1038/nature12034
7. Dallavalasa S, Beeraka NM, Basavaraju CG, Tulimilli SV, Sadhu SP, Rajesh K, et al. The role of tumor associated macrophages (TAMs) in cancer progression, chemoresistance, angiogenesis and metastasis - current status. Curr Med Chem (2021) 28:8203–36. doi: 10.2174/0929867328666210720143721
8. Kerneur C, Cano CE, Olive D. Major pathways involved in macrophage polarization in cancer. Front Immunol (2022) 13:1026954/BIBTEX. doi: 10.3389/FIMMU.2022.1026954/BIBTEX
9. Qian BZ, Pollard JW. Macrophage diversity enhances tumor progression and metastasis. Cell (2010) 141:39. doi: 10.1016/J.CELL.2010.03.014
10. Balkwill F, Charles KA, Mantovani A. Smoldering and polarized inflammation in the initiation and promotion of malignant disease. Cancer Cell (2005) 7:211–7. doi: 10.1016/j.ccr.2005.02.013
11. Fidler IJ. Inhibition of pulmonary metastasis by intravenous injection of specifically activated macrophages. Cancer Res (1974) 34:1074–8.
12. Alexander P, Evans R. Endotoxin and double stranded RNA render macrophages cytotoxic. Nat New Biol (1971) 232:76–8. doi: 10.1038/NEWBIO232076A0
13. Evans R, Alexander P. Rendering macrophages specifically cytotoxic by a factor released from immune lymphoid cells. Transplantation (1971) 12:227–9. doi: 10.1097/00007890-197109000-00015
14. Leblanc AK, Mazcko CN, Khanna C. Defining the value of a comparative approach to cancer drug development. Clin Cancer Res (2016) 22:2133–8. doi: 10.1158/1078-0432.CCR-15-2347
15. Yona S, Gordon S. From the reticuloendothelial to mononuclear phagocyte system – the unaccounted years. Front Immunol (2015) 6:328. doi: 10.3389/FIMMU.2015.00328
16. Takahashi K. Development and differentiation of macrophages and related cells historical review and current concepts. J Clin Exp hematop (2001) 41:1–1. doi: 10.3960/JSLRT.41.1
17. Ebert RH, Florey HW. The extravascular development of the monocyte observed In vivo. Br J Exp Pathol (1939) 20(4):342–356.
18. Lewis MR, Lewis WH. The transformation of white blood cells: Into clasmatocytes (Macrophages), epithelioid cells, and giant cells. J Am Med Assoc (1925) 84:798–9. doi: 10.1001/JAMA.1925.02660370008002
19. van Furth R, Cohn ZA, Hirsch JG, Humphrey JH, Spector WG, Langevoort HL. The mononuclear phagocyte system: a new classification of macrophages, monocytes, and their precursor cells. Bull World Health Organ (1972) 46(6):845–52.
20. Aschoff L. Das reticulo-endotheliale system. Ergeb Inn Med Kinderheilkd (1924) 26:1–118. doi: 10.1007/978-3-642-90639-8_1
21. Steinman RM, Cohn ZA. Identification of a novel cell type in peripheral lymphoid organs of mice i. morphology, quantitation, tissue distribution. J Exp Med (1973) 137:1142–62. doi: 10.1084/JEM.137.5.1142
22. Sathe P, Metcalf D, Vremec D, Naik SH, Langdon WY, Huntington ND, et al. Lymphoid tissue and plasmacytoid dendritic cells and macrophages do not share a common macrophage-dendritic cell-restricted progenitor. Immunity (2014) 41:104–15. doi: 10.1016/J.IMMUNI.2014.05.020
23. Fogg DK, Sibon C, Miled C, Jung S, Aucouturier P, Littman DR, et al. A clonogenic bone marrow progenitor specific for macrophages and dendritic cells. Science (2006) 311:83–7. doi: 10.1126/SCIENCE.1117729
24. Kawamura S, Onai N, Miya F, Sato T, Tsunoda T, Kurabayashi K, et al. Identification of a human clonogenic progenitor with strict monocyte differentiation potential: A counterpart of mouse cMoPs. Immunity (2017) 46:835–848.e4. doi: 10.1016/j.immuni.2017.04.019
25. Poulin LF, Lasseaux C, Chamaillard M. Understanding the cellular origin of the mononuclear phagocyte system sheds light on the myeloid postulate of immune paralysis in sepsis. Front Immunol (2018) 9:823. doi: 10.3389/FIMMU.2018.00823
26. Wolf AA, Yáñez A, Barman PK, Goodridge HS. The ontogeny of monocyte subsets. Front Immunol (2019) 10:1642. doi: 10.3389/fimmu.2019.01642
27. Guilliams M, Mildner A, Yona S. Developmental and functional heterogeneity of monocytes. Immunity (2018) 49:595–613. doi: 10.1016/J.IMMUNI.2018.10.005
28. Teh YC, Ding JL, Ng LG, Chong SZ. Capturing the fantastic voyage of monocytes through time and space. Front Immunol (2019) 10:834/BIBTEX. doi: 10.3389/FIMMU.2019.00834/BIBTEX
29. Murray PJ. Macrophage polarization. Annu Rev Physiol (2017) 79:541–66. doi: 10.1146/ANNUREV-PHYSIOL-022516-034339
30. Kapellos TS, Bonaguro L, Gemünd I, Reusch N, Saglam A, Hinkley ER, et al. Human monocyte subsets and phenotypes in major chronic inflammatory diseases. Front Immunol (2019) 10:2035/BIBTEX. doi: 10.3389/FIMMU.2019.02035/BIBTEX
31. Davies LC, Taylor PR. Tissue-resident macrophages: then and now. Immunology (2015) 144:541. doi: 10.1111/IMM.12451
32. Gordon S, Taylor PR. Monocyte and macrophage heterogeneity. Nat Rev Immunol (2005) 5:953–64. doi: 10.1038/NRI1733
33. Hashimoto K. Langerhans’ cell granule: An endocytotic organelle. Arch Dermatol (1971) 104:148–60. doi: 10.1001/ARCHDERM.1971.04000200036007
34. Hoeffel G, Ginhoux F. Ontogeny of tissue-resident macrophages. Front Immunol (2015) 6:486/BIBTEX. doi: 10.3389/FIMMU.2015.00486/BIBTEX
35. Liu Z, Gu Y, Chakarov S, Bleriot C, Kwok I, Chen X, et al. Fate mapping via Ms4a3-expression history traces monocyte-derived cells. Cell (2019) 178:1509–1525.e19. doi: 10.1016/J.CELL.2019.08.009
36. Guilliams M, van de Laar L. A hitchhiker’s guide to myeloid cell subsets: Practical implementation of a novel mononuclear phagocyte classification system. Front Immunol (2015) 6:406. doi: 10.3389/FIMMU.2015.00406
37. Hashimoto D, Chow A, Noizat C, Teo P, Beasley MB, Leboeuf M, et al. Tissue-resident macrophages self-maintain locally throughout adult life with minimal contribution from circulating monocytes. Immunity (2013) 38:792–804. doi: 10.1016/J.IMMUNI.2013.04.004
38. Natoli G, Ostuni R. Adaptation and memory in immune responses. Nat Immunol (2019) 20:783–92. doi: 10.1038/s41590-019-0399-9
39. Wu Y, Hirschi KK. Tissue-resident macrophage development and function. Front Cell Dev Biol (2021) 8:617879/BIBTEX. doi: 10.3389/FCELL.2020.617879/BIBTEX
40. Gao X, Xu C, Asada N, Frenette PS. The hematopoietic stem cell niche: from embryo to adult. Development (2018) 145(2):dev139691. doi: 10.1242/dev.139691
41. Hoeffel G, Ginhoux F. Fetal monocytes and the origins of tissue-resident macrophages. Cell Immunol (2018) 330:5–15. doi: 10.1016/J.CELLIMM.2018.01.001
42. Li F, Okreglicka KM, Pohlmeier LM, Schneider C, Kopf M. Fetal monocytes possess increased metabolic capacity and replace primitive macrophages in tissue macrophage development. EMBO J (2020) 39(3):e103205. doi: 10.15252/EMBJ.2019103205
43. Müller S, Kohanbash G, Liu SJ, Alvarado B, Carrera D, Bhaduri A, et al. Single-cell profiling of human gliomas reveals macrophage ontogeny as a basis for regional differences in macrophage activation in the tumor microenvironment. Genome Biol (2017) 18:1–14. doi: 10.1186/S13059-017-1362-4
44. Lokhonina A, Elchaninov A, Fatkhudinov T, Makarov A, Arutyunyan I, Grinberg M, et al. Activated macrophages of monocytic origin predominantly express proinflammatory cytokine genes, whereas kupffer cells predominantly express anti-inflammatory cytokine genes. BioMed Res Int (2019) 2019:3912142. doi: 10.1155/2019/3912142
45. Stout RD, Suttles J. Functional plasticity of macrophages: reversible adaptation to changing microenvironments. J Leukoc Biol (2004) 76:509–13. doi: 10.1189/JLB.0504272
46. Ivashkiv LB. Epigenetic regulation of macrophage polarization and function. Trends Immunol (2013) 34:216–23. doi: 10.1016/J.IT.2012.11.001
47. Murray PJ, Wynn TA. Obstacles and opportunities for understanding macrophage polarization. J Leukoc Biol (2011) 89:557–63. doi: 10.1189/JLB.0710409
48. Monnier M, Paolini L, Vinatier E, Mantovani A, Delneste Y, Jeannin P. Antitumor strategies targeting macrophages: the importance of considering the differences in differentiation/polarization processes between human and mouse macrophages. J Immunother Cancer (2022) 10(10):e005560. doi: 10.1136/JITC-2022-005560
49. Evans R, Alexander P. Cooperation of immune lymphoid cells with macrophages in tumour immunity. Nature (1970) 228:620–2. doi: 10.1038/228620A0
50. Nathan CF, Murray HW, Wlebe IE, Rubin BY. Identification of interferon-gamma as the lymphokine that activates human macrophage oxidative metabolism and antimicrobial activity. J Exp Med (1983) 158:670–89. doi: 10.1084/JEM.158.3.670
51. Stein M, Keshav S, Harris N, Gordon S. Interleukin 4 potently enhances murine macrophage mannose receptor activity: a marker of alternative immunologic macrophage activation. J Exp Med (1992) 176:287–92. doi: 10.1084/JEM.176.1.287
52. Mills CD, Kincaid K, Alt JM, Heilman MJ, Hill AM. M-1/M-2 macrophages and the Th1/Th2 paradigm. J Immunol (2000) 164:6166–73. doi: 10.4049/JIMMUNOL.164.12.6166
53. Sans-Fons MG, Yeramian A, Pereira-Lopes S, Santamaría-Babi LF, Modolell M, Lloberas J, et al. Arginine transport is impaired in C57Bl/6 mouse macrophages as a result of a deletion in the promoter of Slc7a2 (CAT2), and susceptibility to leishmania infection is reduced. J Infect Dis (2013) 207:1684–93. doi: 10.1093/INFDIS/JIT084
54. Viola A, Munari F, Sánchez-Rodríguez R, Scolaro T, Castegna A. The metabolic signature of macrophage responses. Front Immunol (2019) 10:1462. doi: 10.3389/FIMMU.2019.01462
55. Wculek SK, Dunphy G, Heras-Murillo I, Mastrangelo A, Sancho D. Metabolism of tissue macrophages in homeostasis and pathology. Cell Mol Immunol (2021) 19:384–408. doi: 10.1038/s41423-021-00791-9
56. Liu Y, Xu R, Gu H, Zhang E, Qu J, Cao W, et al. Metabolic reprogramming in macrophage responses. biomark Res (2021) 9:1–17. doi: 10.1186/S40364-020-00251-Y
57. Mantovani A, Sozzani S, Locati M, Allavena P, Sica A. Macrophage polarization: tumor-associated macrophages as a paradigm for polarized M2 mononuclear phagocytes. Trends Immunol (2002) 23:549–55. doi: 10.1016/S1471-4906(02)02302-5
58. Ambarus CA, Krausz S, Van Eijk M, Hamann J, Radstake TRDJ, Reedquist KA, et al. Systematic validation of specific phenotypic markers for in vitro polarized human macrophages. J Immunol Methods (2011) 375(1–2):196–206. doi: 10.1016/j.jim.2011.10.013
59. Yao Y, Xu XH, Jin L. Macrophage polarization in physiological and pathological pregnancy. Front Immunol (2019) 10:792. doi: 10.3389/FIMMU.2019.00792
60. Martinez FO, Gordon S. The M1 and M2 paradigm of macrophage activation: time for reassessment. F1000Prime Rep (2014) 6:13. doi: 10.12703/P6-13
61. Ginhoux F, Schultze JL, Murray PJ, Ochando J, Biswas SK. New insights into the multidimensional concept of macrophage ontogeny, activation and function. Nat Immunol (2016) 17:34–40. doi: 10.1038/NI.3324
62. Orecchioni M, Ghosheh Y, Pramod AB, Ley K. Macrophage polarization: Different gene signatures in M1(LPS+) vs. classically and M2(LPS-) vs. alternatively activated macrophages. Front Immunol (2019) 10:1084. doi: 10.3389/FIMMU.2019.01084
63. Ferrante CJ, Pinhal-Enfield G, Elson G, Cronstein BN, Hasko G, Outram S, et al. The adenosine-dependent angiogenic switch of macrophages to an M2-like phenotype is independent of interleukin-4 receptor alpha (IL4Rα) signaling. Inflammation (2013) 36(4):921–31. doi: 10.1007/s10753-013-9621-3
64. Edwards JP, Zhang X, Frauwirth KA, Mosser DM. Biochemical and functional characterization of three activated macrophage populations. J Leukoc Biol (2006) 80:1298–307. doi: 10.1189/JLB.0406249
65. Mantovani A, Sica A, Sozzani S, Allavena P, Vecchi A, Locati M. The chemokine system in diverse forms of macrophage activation and polarization. Trends Immunol (2004) 25:677–86. doi: 10.1016/J.IT.2004.09.015
66. Roszer T. Understanding the mysterious M2 macrophage through activation markers and effector mechanisms. Mediators Inflammation (2015) 2015:816460. doi: 10.1155/2015/816460
67. Kim J, Hematti P. Mesenchymal stem cell-educated macrophages: A novel type of alternatively activated macrophages. Exp Hematol (2009) 37:1445–53. doi: 10.1016/J.EXPHEM.2009.09.004
68. Vasandan AB, Jahnavi S, Shashank C, Prasad P, Kumar A, Jyothi Prasanna S. Human mesenchymal stem cells program macrophage plasticity by altering their metabolic status via a PGE2-dependent mechanism. Sci Rep (2016) 6:38308. doi: 10.1038/SREP38308
69. Abdelaziz MH, Abdelwahab SF, Wan J, Cai W, Huixuan W, Jianjun C, et al. Alternatively activated macrophages; a double-edged sword in allergic asthma. J Transl Med (2020) 18:1–12. doi: 10.1186/S12967-020-02251-W
70. Guo Y, Tsai HI, Zhang L, Zhu H. Mitochondrial DNA on tumor-associated macrophages polarization and immunity. Cancers (Basel) (2022) 14(6):1452. doi: 10.3390/CANCERS14061452
71. Murray PJ, Allen JE, Biswas SK, Fisher EA, Gilroy DW, Goerdt S, et al. Macrophage activation and polarization: Nomenclature and experimental guidelines. Immunity (2014) 41:14–20. doi: 10.1016/J.IMMUNI.2014.06.008
72. Heinrich F, Lehmbecker A, Raddatz BB, Kegler K, Tipold A, Stein VM, et al. Morphologic, phenotypic, and transcriptomic characterization of classically and alternatively activated canine blood-derived macrophages in vitro. PloS One (2017) 12(8):e0183572. doi: 10.1371/JOURNAL.PONE.0183572
73. Herrmann I, Gotovina J, Fazekas-Singer J, Fischer MB, Hufnagl K, Bianchini R, et al. Canine macrophages can like human macrophages be in vitro activated toward the M2a subtype relevant in allergy. Dev Comp Immunol (2018) 82:118–27. doi: 10.1016/J.DCI.2018.01.005
74. Chow L, Soontararak S, Wheat W, Ammons D, Dow S. Canine polarized macrophages express distinct functional and transcriptomic profiles. Front Vet Sci (2022) 9:988981/BIBTEX. doi: 10.3389/FVETS.2022.988981/BIBTEX
75. Jaggi U, Yang M, Matundan HH, Hirose S, Shah PK, Sharifi BG, et al. Increased phagocytosis in the presence of enhanced M2-like macrophage responses correlates with increased primary and latent HSV-1 infection. PloS Pathog (2020) 16:e1008971. doi: 10.1371/JOURNAL.PPAT.1008971
76. Schulz D, Severin Y, Zanotelli VRT, Bodenmiller B. In-depth characterization of monocyte-derived macrophages using a mass cytometry-based phagocytosis assay. Sci Rep (2019) 9:1–12. doi: 10.1038/s41598-018-38127-9
77. Venturin GL, Chiku VM, Silva KLO, de Almeida BFM, de Lima VMF. M1 polarization and the effect of PGE2 on TNF-α production by lymph node cells from dogs with visceral leishmaniasis. Parasite Immunol (2016) 38:698–704. doi: 10.1111/PIM.12353
78. Wagner A, Junginger J, Lemensieck F, Hewicker-Trautwein M. Immunohistochemical characterization of gastrointestinal macrophages/phagocytes in dogs with inflammatory bowel disease (IBD) and non-IBD dogs. Vet Immunol Immunopathol (2018) 197:49–57. doi: 10.1016/J.VETIMM.2018.01.011
79. Spitzbarth I, Moore SA, Stein VM, Levine JM, Olby NJ, Gjessing KM, et al. Current insights into the pathology of canine intervertebral disc extrusion-induced spinal cord injury. Front Vet Sci (2020) 7:595796/BIBTEX. doi: 10.3389/FVETS.2020.595796/BIBTEX
80. Li Y, You MJ, Yang Y, Hu D, Tian C. The role of tumor-associated macrophages in leukemia. Acta Haematol (2020) 143:112–7. doi: 10.1159/000500315
81. Li S, Yu J, Huber A, Kryczek I, Wang Z, Jiang L, et al. Metabolism drives macrophage heterogeneity in the tumor microenvironment. Cell Rep (2022) 39(1):110609. doi: 10.1016/J.CELREP.2022.110609
82. Laviron M, Boissonnas A. Ontogeny of tumor-associated macrophages. Front Immunol (2019) 10:1799/BIBTEX. doi: 10.3389/FIMMU.2019.01799/BIBTEX
83. Kusmartsev S, Gabrilovich DI. Inhibition of myeloid cell differentiation in cancer: the role of reactive oxygen species. J Leukoc Biol (2003) 74:186–96. doi: 10.1189/JLB.0103010
84. Kusmartsev S, Gabrilovich DI. STAT1 signaling regulates tumor-associated macrophage-mediated T cell deletion. J Immunol (2005) 174:4880–91. doi: 10.4049/JIMMUNOL.174.8.4880
85. Veglia F, Sanseviero E, Gabrilovich DI. Myeloid-derived suppressor cells in the era of increasing myeloid cell diversity. Nat Rev Immunol (2021) 21:485–98. doi: 10.1038/s41577-020-00490-y
86. Loyher PL, Hamon P, Laviron M, Meghraoui-Kheddar A, Goncalves E, Deng Z, et al. Macrophages of distinct origins contribute to tumor development in the lung. J Exp Med (2018) 215:1–18. doi: 10.1084/JEM.20180534
87. Bowman RL, Klemm F, Akkari L, Pyonteck SM, Sevenich L, Quail DF, et al. Macrophage ontogeny underlies differences in tumor-specific education in brain malignancies. Cell Rep (2016) 17:2445. doi: 10.1016/J.CELREP.2016.10.052
88. Sica A, Schioppa T, Mantovani A, Allavena P. Tumour-associated macrophages are a distinct M2 polarised population promoting tumour progression: potential targets of anti-cancer therapy. Eur J Cancer (2006) 42:717–27. doi: 10.1016/J.EJCA.2006.01.003
89. Cassetta L, Fragkogianni S, Sims AH, Swierczak A, Forrester LM, Zhang H, et al. Human tumor-associated macrophage and monocyte transcriptional landscapes reveal cancer-specific reprogramming, biomarkers, and therapeutic targets. Cancer Cell (2019) 35:588–602.e10. doi: 10.1016/J.CCELL.2019.02.009
90. Bi K, He MX, Bakouny Z, Kanodia A, Napolitano S, Wu J, et al. Tumor and immune reprogramming during immunotherapy in advanced renal cell carcinoma. Cancer Cell (2021) 39:649–661.e5. doi: 10.1016/J.CCELL.2021.02.015
91. Cheng S, Li Z, Gao R, Xing B, Gao Y, Yang Y, et al. A pan-cancer single-cell transcriptional atlas of tumor infiltrating myeloid cells. Cell (2021) 184:792–809.e23. doi: 10.1016/J.CELL.2021.01.010
92. Franklin RA, Liao W, Sarkar A, Kim MV, Bivona MR, Liu K, et al. The cellular and molecular origin of tumor-associated macrophages. Sci (1979) (2014) 344:921–5. doi: 10.1126/SCIENCE.1252510
93. Pan Y, Yu Y, Wang X, Zhang T. Tumor-associated macrophages in tumor immunity. Front Immunol (2020) 11:583084/BIBTEX. doi: 10.3389/FIMMU.2020.583084/BIBTEX
94. Hanahan D. Hallmarks of cancer: New dimensions. Cancer Discovery (2022) 12:31–46. doi: 10.1158/2159-8290.CD-21-1059
95. Wang H, Shao Q, Sun J, Ma C, Gao W, Wang Q, et al. Interactions between colon cancer cells and tumor-infiltrated macrophages depending on cancer cell-derived colony stimulating factor 1. Oncoimmunology (2016) 5(4):e1122157. doi: 10.1080/2162402X.2015.1122157
96. Ge Z, Ding S. The crosstalk between tumor-associated macrophages (TAMs) and tumor cells and the corresponding targeted therapy. Front Oncol (2020) 10:590941/BIBTEX. doi: 10.3389/FONC.2020.590941/BIBTEX
97. Su S, Liu Q, Chen J, Chen J, Chen F, He C, et al. A positive feedback loop between mesenchymal-like cancer cells and macrophages is essential to breast cancer metastasis. Cancer Cell (2014) 25:605–20. doi: 10.1016/J.CCR.2014.03.021
98. Piao H, Fu L, Wang Y, Liu Y, Wang Y, Meng X, et al. A positive feedback loop between gastric cancer cells and tumor-associated macrophage induces malignancy progression. J Exp Clin Cancer Res (2022) 41:174. doi: 10.1186/S13046-022-02366-6
99. Quintero-Fabián S, Arreola R, Becerril-Villanueva E, Torres-Romero JC, Arana-Argáez V, Lara-Riegos J, et al. Role of matrix metalloproteinases in angiogenesis and cancer. Front Oncol (2019) 9:1370/BIBTEX. doi: 10.3389/FONC.2019.01370/BIBTEX
100. Yan D, Wang HW, Bowman RL, Joyce JA. STAT3 and STAT6 signaling pathways synergize to promote cathepsin secretion from macrophages via IRE1α activation. Cell Rep (2016) 16:2914–27. doi: 10.1016/J.CELREP.2016.08.035
101. Hiratsuka S, Watanabe A, Aburatani H, Maru Y. Tumour-mediated upregulation of chemoattractants and recruitment of myeloid cells predetermines lung metastasis. Nat Cell Biol (2006) 8(12):1369–75. doi: 10.1038/ncb1507
102. Doak GR, Schwertfeger KL, Wood DK. Distant relations: Macrophage functions in the metastatic niche HHS public access. Trends Cancer (2018) 4:445–59. doi: 10.1016/j.trecan.2018.03.011
103. Lin Y, Xu J, Lan H. Tumor-associated macrophages in tumor metastasis: biological roles and clinical therapeutic applications. J Hemat Oncol (2019) 12:1–16. doi: 10.1186/S13045-019-0760-3
104. Vitale I, Manic G, Coussens LM, Kroemer G, Galluzzi L. Macrophages and metabolism in the tumor microenvironment. Cell Metab (2019) 30:36–50. doi: 10.1016/J.CMET.2019.06.001
105. Steggerda SM, Bennett MK, Chen J, Emberley E, Huang T, Janes JR, et al. Inhibition of arginase by CB-1158 blocks myeloid cell-mediated immune suppression in the tumor microenvironment. J Immunother Cancer (2017) 5(1):101. doi: 10.1186/S40425-017-0308-4
106. Hoffmann EJ, Ponik SM. Biomechanical contributions to macrophage activation in the tumor microenvironment. Front Oncol (2020) 10:787. doi: 10.3389/FONC.2020.00787
107. Zhang R, Qi F, Zhao F, Li G, Shao S, Zhang X, et al. Cancer-associated fibroblasts enhance tumor-associated macrophages enrichment and suppress NK cells function in colorectal cancer. Cell Death Dis (2019) 10(4):273. doi: 10.1038/S41419-019-1435-2
108. Mao X, Xu J, Wang W, Liang C, Hua J, Liu J, et al. Crosstalk between cancer-associated fibroblasts and immune cells in the tumor microenvironment: new findings and future perspectives. Mol Cancer (2021) 20:1–30. doi: 10.1186/S12943-021-01428-1
109. Yamada K, Uchiyama A, Uehara A, Perera B, Ogino S, Yokoyama Y, et al. MFG-E8 drives melanoma growth by stimulating mesenchymal stromal cell-induced angiogenesis and M2 polarization of tumor-associated macrophages. Cancer Res (2016) 76:4283–92. doi: 10.1158/0008-5472.CAN-15-2812
110. Zhou SL, Zhou ZJ, Hu ZQ, Huang XW, Wang Z, Chen EB, et al. Tumor-associated neutrophils recruit macrophages and T-regulatory cells to promote progression of hepatocellular carcinoma and resistance to sorafenib. Gastroenterology (2016) 150:1646–1658.e17. doi: 10.1053/J.GASTRO.2016.02.040
111. Mun JY, Leem SH, Lee JH, Kim HS. Dual relationship between stromal cells and immune cells in the tumor microenvironment. Front Immunol (2022) 13:864739. doi: 10.3389/FIMMU.2022.864739
112. Kersten K, Hu KH, Combes AJ, Samad B, Harwin T, Ray A, et al. Spatiotemporal co-dependency between macrophages and exhausted CD8+ T cells in cancer. Cancer Cell (2022) 40:624–638.e9. doi: 10.1016/J.CCELL.2022.05.004
113. Hartley G, Faulhaber E, Caldwell A, Coy J, Kurihara J, Guth A, et al. Immune regulation of canine tumour and macrophage PD-L1 expression. Vet Comp Oncol (2017) 15:534–49. doi: 10.1111/vco.12197
114. Mojic M, Takeda K, Hayakawa Y. Molecular sciences the dark side of IFN-γ: Its role in promoting cancer immunoevasion. J Mol Sci (2018) 19:89. doi: 10.3390/ijms19010089
115. Eto S, Yanai H, Hangai S, Kato D, Nishimura R, Nakagawa T. The impact of damage-associated molecules released from canine tumor cells on gene expression in macrophages. Sci Rep (2021) 11:1–13. doi: 10.1038/s41598-021-87979-1
116. Xiao M, He J, Yin L, Chen X, Zu X, Shen Y. Tumor-associated macrophages: Critical players in drug resistance of breast cancer. Front Immunol (2021) 12:799428/BIBTEX. doi: 10.3389/FIMMU.2021.799428/BIBTEX
117. Chen Y, Song Y, Du W, Gong L, Chang H, Zou Z. Tumor-associated macrophages: an accomplice in solid tumor progression. J BioMed Sci (2019) 26(1):78. doi: 10.1186/S12929-019-0568-Z
118. Ruffell B, Coussens LM. Macrophages and therapeutic resistance in cancer. Cancer Cell (2015) 27:462–72. doi: 10.1016/J.CCELL.2015.02.015
119. Zhang Qw, Liu L, Gong Cy, Shi Hs, Zeng Yh, Wang X, et al. Prognostic significance of tumor-associated macrophages in solid tumor: A meta-analysis of the literature. PloS One (2012) 7:e50946. doi: 10.1371/JOURNAL.PONE.0050946
120. Wang LJ, Xue Y, Lou Y. Tumor-associated macrophages related signature in glioma. Aging (Albany NY) (2022) 14:2720. doi: 10.18632/AGING.203968
121. Zeiner PS, Preusse C, Golebiewska A, Zinke J, Iriondo A, Muller A, et al. Distribution and prognostic impact of microglia/macrophage subpopulations in gliomas. Brain Pathol (2019) 29:513–29. doi: 10.1111/BPA.12690
122. Andersen JK, Miletic H, Hossain JA. Tumor-associated macrophages in gliomas–basic insights and treatment opportunities. Cancers (Basel) (2022) 14(5):1319. doi: 10.3390/CANCERS14051319
123. Krane GA, O’Dea CA, Malarkey DE, Miller AD, Miller CR, Tokarz DA, et al. Immunohistochemical evaluation of immune cell infiltration in canine gliomas. Vet Pathol (2021) 58:952–63. doi: 10.1177/03009858211023946
124. Toedebusch R, Grodzki AC, Dickinson PJ, Woolard K, Vinson N, Sturges B, et al. Glioma-associated microglia/macrophages augment tumorigenicity in canine astrocytoma, a naturally occurring model of human glioma. Neurooncol Adv (2021) 3(1):vdab062. doi: 10.1093/NOAJNL/VDAB062
125. Luo ZW, Liu PP, Wang ZX, Chen CY, Xie H. Macrophages in osteosarcoma immune microenvironment: Implications for immunotherapy. Front Oncol (2020) 10:586580. doi: 10.3389/FONC.2020.586580
126. Buddingh EP, Kuijjer ML, Duim RAJ, Bürger H, Agelopoulos K, Myklebost O, et al. Tumor-infiltrating macrophages are associated with metastasis suppression in high-grade osteosarcoma: a rationale for treatment with macrophage activating agents. Clin Cancer Res (2011) 17:2110–9. doi: 10.1158/1078-0432.CCR-10-2047
127. Gomez-Brouchet A, Illac C, Gilhodes J, Bouvier C, Aubert S, Guinebretiere JM, et al. CD163-positive tumor-associated macrophages and CD8-positive cytotoxic lymphocytes are powerful diagnostic markers for the therapeutic stratification of osteosarcoma patients: An immunohistochemical analysis of the biopsies from the French OS2006 phase 3 trial. Oncoimmunology (2017) 6(9):e1331193. doi: 10.1080/2162402X.2017.1331193
128. Withers SS, Skorupski KA, York D, Choi JW, Woolard KD, Laufer-Amorim R, et al. Association of macrophage and lymphocyte infiltration with outcome in canine osteosarcoma. Vet Comp Oncol (2019) 17:49–60. doi: 10.1111/VCO.12444
129. Li YL, Shi ZH, Wang X, Gu KS, Zhai ZM. Tumor-associated macrophages predict prognosis in diffuse large b-cell lymphoma and correlation with peripheral absolute monocyte count. BMC Cancer (2019) 19:1–10. doi: 10.1186/S12885-019-6208-X
130. Lin M, Ma S, Sun L, Qin Z. The prognostic value of tumor-associated macrophages detected by immunostaining in diffuse large b cell lymphoma: A meta-analysis. Front Oncol (2023) 12:1094400/FULL. doi: 10.3389/FONC.2022.1094400/FULL
131. Vázquez S, Vallejo R, Espinosa J, Arteche N, Vega JA, Pérez V. Immunohistochemical characterization of tumor-associated macrophages in canine lymphomas. Animals (2021) 11(8):2301. doi: 10.3390/ani11082301
132. Umakoshi M, Nakamura A, Tsuchie H, Li Z, Kudo-Asabe Y, Miyabe K, et al. Macrophage numbers in the marginal area of sarcomas predict clinical prognosis. Sci Rep (2023) 13:1–15. doi: 10.1038/s41598-023-28024-1
133. Fujiwara T, Healey J, Ogura K, Yoshida A, Kondo H, Hata T, et al. Role of tumor-associated macrophages in sarcomas. Cancers (Basel) (2021) 13:1–17. doi: 10.3390/CANCERS13051086
134. Finotello R, Whybrow K, Scarin G, Ressel L. Correlation between tumour associated macrophage (TAM) infiltration and mitotic activity in canine soft tissue sarcomas. Anim (Basel) (2021) 11:1–8. doi: 10.3390/ani11030684
135. Wang C, Lin Y, Zhu H, Zhou Y, Mao F, Huang X, et al. The prognostic and clinical value of tumor-associated macrophages in patients with breast cancer: A systematic review and meta-analysis. Front Oncol (2022) 12:905846/FULL. doi: 10.3389/FONC.2022.905846/FULL
136. Zhao X, Qu J, Sun Y, Wang J, Liu X, Wang F, et al. Prognostic significance of tumor-associated macrophages in breast cancer: a meta-analysis of the literature. Oncotarget (2017) 8:30576. doi: 10.18632/ONCOTARGET.15736
137. Monteiro LN, Rodrigues MA, Gomes DA, Salgado BS, Cassali GD. Tumour-associated macrophages: Relation with progression and invasiveness, and assessment of M1/M2 macrophages in canine mammary tumours. Vet J (2018) 234:119–25. doi: 10.1016/j.tvjl.2018.02.016
138. Parisi F, Tesi M, Millanta F, Gnocchi M, Poli A. M1 and M2 tumour-associated macrophages subsets in canine malignant mammary tumours: An immunohistochemical study. Res Vet Sci (2021) 136:32–8. doi: 10.1016/J.RVSC.2021.02.007
139. Raposo T, Gregório H, Pires I, Prada J, Queiroga FL. Prognostic value of tumour-associated macrophages in canine mammary tumours. Vet Comp Oncol (2014) 12:10–9. doi: 10.1111/j.1476-5829.2012.00326.x
140. Seung BJ, Lim HY, Il SJ, HW K, SH C, SH K, et al. CD204-expressing tumor-associated macrophages are associated with malignant, high-grade, and hormone receptor–negative canine mammary gland tumors. Vet Pathol (2018) 55:417–24. doi: 10.1177/0300985817750457
141. Vieira-Filho CHC, Barrouin-Melo SM, Damasceno KA, Araújo MSS, Borges NF, Silva FL, et al. Tumor-associated macrophage is correlated with survival and SOCS protein expression in canine mammary carcinoma. Braz J Vet Res Anim Sci (2018) 38(10). doi: 10.1590/1678-5150-PVB-5638
142. Wang H, Tian T, Zhang J. Tumor-associated macrophages (TAMs) in colorectal cancer (CRC): From mechanism to therapy and prognosis. Int J Mol Sci (2021) 22(16):8470. doi: 10.3390/IJMS22168470
143. Yahaya MAF, Lila MAM, Ismail S, Zainol M, Afizan NARNM. Tumour-associated macrophages (TAMs) in colon cancer and how to reeducate them. J Immunol Res (2019) 2019:2368249. doi: 10.1155/2019/2368249
144. Woldemeskel M, Hawkins I, Whittington L. Ki-67 protein expression and tumor associated inflammatory cells (macrophages and mast cells) in canine colorectal carcinoma. BMC Vet Res (2017) 13(1):111. doi: 10.1186/S12917-017-1030-7
145. Herstad KMV, Gunnes G, Rørtveit R, Kolbjørnsen Ø, Tran L, Skancke E. Immunohistochemical expression of β-catenin, Ki67, CD3 and CD18 in canine colorectal adenomas and adenocarcinomas. BMC Vet Res (2021) 17(1):119. doi: 10.1186/S12917-021-02829-6
146. Ringel AE, Drijvers JM, Baker GJ, Catozzi A, García-Cañaveras JC, Gassaway BM, et al. Obesity shapes metabolism in the tumor microenvironment to suppress anti-tumor immunity. Cell (2020) 183:1848–1866.e26. doi: 10.1016/J.CELL.2020.11.009
147. Fan T, Li C, He J. Prognostic value of immune-related genes and comparative analysis of immune cell infiltration in lung adenocarcinoma: sex differences. Biol Sex Differ (2021) 12:1–17. doi: 10.1186/S13293-021-00406-Y
148. Duong L, Pixley FJ, Nelson DJ, Jackaman C. Aging leads to increased monocytes and macrophages with altered CSF-1 receptor expression and earlier tumor-associated macrophage expansion in murine mesothelioma. Front Aging Neurosci (2022) 3:848925. doi: 10.3389/FRAGI.2022.848925
149. Van Ginderachter JA, Xu D, Wu K, Lin K, Li X, Yuan X, et al. Redefining tumor-associated macrophage subpopulations and functions in the tumor microenvironment. Front Immunol (2020) 11:1731. doi: 10.3389/fimmu.2020.01731
150. Tang F, Barbacioru C, Wang Y, Nordman E, Lee C, Xu N, et al. mRNA-seq whole-transcriptome analysis of a single cell. Nat Methods (2009) 6:377–82. doi: 10.1038/NMETH.1315
151. Mulder K, Patel AA, Kong WT, Piot C, Halitzki E, Dunsmore G, et al. Cross-tissue single-cell landscape of human monocytes and macrophages in health and disease. Immunity (2021) 54:1883–1900.e5. doi: 10.1016/J.IMMUNI.2021.07.007
152. Ma RY, Black A, Qian BZ. Macrophage diversity in cancer revisited in the era of single-cell omics. Trends Immunol (2022) 43:546–63. doi: 10.1016/J.IT.2022.04.008
153. Wang X, Xu Y, Sun Q, Zhou X, Ma W, Wu J, et al. New insights from the single-cell level: Tumor associated macrophages heterogeneity and personalized therapy. BioMed Pharmacother (2022) 153:113343. doi: 10.1016/J.BIOPHA.2022.113343
154. Ardighieri L, Missale F, Bugatti M, Gatta LB, Pezzali I, Monti M, et al. Infiltration by CXCL10 secreting macrophages is associated with antitumor immunity and response to therapy in ovarian cancer subtypes. Front Immunol (2021) 12:690201/BIBTEX. doi: 10.3389/FIMMU.2021.690201/BIBTEX
155. Pombo Antunes AR, Scheyltjens I, Lodi F, Messiaen J, Antoranz A, Duerinck J, et al. Single-cell profiling of myeloid cells in glioblastoma across species and disease stage reveals macrophage competition and specialization. Nat Neurosci (2021) 24:595–610. doi: 10.1038/s41593-020-00789-y
156. Zhang X, Qiu H, Zhang F, Ding S. Advances in single-cell multi-omics and application in cardiovascular research. Front Cell Dev Biol (2022) 10:883861. doi: 10.3389/FCELL.2022.883861
157. Ayers J, Milner RJ, Cortés-Hinojosa G, Riva A, Bechtel S, Sahay B, et al. Novel application of single-cell next-generation sequencing for determination of intratumoral heterogeneity of canine osteosarcoma cell lines. J Vet Diagn Invest (2021) 33:261–78. doi: 10.1177/1040638720985242
158. Fastrès A, Pirottin D, Fievez L, Tutunaru AC, Bolen G, Merveille AC, et al. Identification of pro-fibrotic macrophage populations by single-cell transcriptomic analysis in West highland white terriers affected with canine idiopathic pulmonary fibrosis. Front Immunol (2020) 11:611749. doi: 10.3389/FIMMU.2020.611749
159. Jackson K, Milner RJ, Doty A, Hutchison S, Cortes-Hinojosa G, Riva A, et al. Analysis of canine myeloid-derived suppressor cells (MDSCs) utilizing fluorescence-activated cell sorting, RNA protection mediums to yield quality RNA for single-cell RNA sequencing. Vet Immunol Immunopathol (2021) 231:110144. doi: 10.1016/J.VETIMM.2020.110144
160. Fastrès A, Pirottin D, Fievez L, Marichal T, Desmet CJ, Bureau F, et al. Characterization of the bronchoalveolar lavage fluid by single cell gene expression analysis in healthy dogs: A promising technique. Front Immunol (2020) 11:1707/BIBTEX. doi: 10.3389/FIMMU.2020.01707/BIBTEX
161. Skidmore ZL, Rindt H, Chu S, Fisk B, Fronick C, Fulton R, et al. Single cell T cell receptor repertoire profiling for dogs. BioRxiv (2021) 2021:450365. doi: 10.1101/2021.06.29.450365
162. Gingrich AA, Reiter TE, Judge SJ, York D, Yanagisawa M, Razmara A, et al. Comparative immunogenomics of canine natural killer cells as immunotherapy target. Front Immunol (2021) 12:670309/BIBTEX. doi: 10.3389/FIMMU.2021.670309/BIBTEX
163. Król M, Pawłowski KM, Majchrzak K, Gajewska M, Majewska A, Motyl T. Global gene expression profiles of canine macrophages and canine mammary cancer cells grown as a co-culture in vitro. BMC Vet Res (2012) 8:1–20. doi: 10.1186/1746-6148-8-16
164. Beirão BCB, Raposo T, Pang LY, Argyle DJ. Canine mammary cancer cells direct macrophages toward an intermediate activation state between M1/M2. BMC Vet Res (2015) 11:1–14. doi: 10.1186/S12917-015-0473-Y
165. Rybicka A, Król M. Tumour-associated macrophages influence canine mammary cancer stem-like cells enhancing their pro-angiogenic properties. J Physiol Pharmacol (2016) 67(4):491–500.
166. Raposo TP, Pires I, Carvalho MI, Prada J, Argyle DJ, Queiroga FL. Tumour-associated macrophages are associated with vascular endothelial growth factor expression in canine mammary tumours. Vet Comp Oncol (2015) 13:464–74. doi: 10.1111/VCO.12067
167. Gulay KCM, Aoshima K, Maekawa N, Suzuki T, Konnai S, Kobayashi A, et al. Hemangiosarcoma cells induce M2 polarization and PD-L1 expression in macrophages. Sci Rep (2022) 12:2124. doi: 10.1038/s41598-022-06203-w
168. Porcellato I, Sforna M, Lo Giudice A, Bossi I, Musi A, Tognoloni A, et al. Tumor-associated macrophages in canine oral and cutaneous melanomas and melanocytomas: Phenotypic and prognostic assessment. Front Vet Sci (2022) 0:878949. doi: 10.3389/FVETS.2022.878949
169. Withers SS, York D, Choi JW, Woolard KD, Laufer-Amorim R, Sparger EE, et al. Metastatic immune infiltrates correlate with those of the primary tumour in canine osteosarcoma. Vet Comp Oncol (2019) 17:242–52. doi: 10.1111/vco.12459
170. Mannheimer J, Tawa G, Gerhold D, Braisted J, Sayers C, McEachron T, et al. Transcriptional profiling of canine osteosarcoma identifies prognostic gene expression signatures with translational value for humans. Res Sq (2022). doi: 10.21203/RS.3.RS-2331111/V1
171. Costa VR, Soileau AM, Liu CC, Moeller CE, Carossino M, Langohr IM, et al. Exploring the association of intratumoral immune cell infiltrates with histopathologic grade in canine mast cell tumors. Res Vet Sci (2022) 147:83–91. doi: 10.1016/J.RVSC.2022.04.005
172. Chambers MR, Foote JB, Bentley RT, Botta D, Crossman DK, Della MDL, et al. Evaluation of immunologic parameters in canine glioma patients treated with an oncolytic herpes virus. J Transl Genet Genom (2021) 5:423. doi: 10.20517/JTGG.2021.31
173. Boozer LB, Davis TW, Borst LB, Zseltvay KM, Olby NJ, Mariani CL. Characterization of immune cell infiltration into canine intracranial meningiomas. Vet Pathol (2012) 49:784–95. doi: 10.1177/0300985811417249
174. Regan DP, Escaffi A, Coy J, Kurihara J, Dow SW. Role of monocyte recruitment in hemangiosarcoma metastasis in dogs. Vet Comp Oncol (2017) 15:1309. doi: 10.1111/VCO.12272
175. Guldner D, Hwang JK, Cardieri MCD, Eren M, Ziaei P, Norton MG, et al. In vitro evaluation of the biological responses of canine macrophages challenged with PLGA nanoparticles containing monophosphoryl lipid a. PloS One (2016) 11:e0165477. doi: 10.1371/JOURNAL.PONE.0165477
176. Nikolic Nielsen L, Kjelgaard-Hansen M, Kristensen AT. Monocyte chemotactic protein-1 and other inflammatory parameters in bernese mountain dogs with disseminated histiocytic sarcoma. Vet J (2013) 198:424–8. doi: 10.1016/J.TVJL.2013.07.030
177. Tuohy JL, Lascelles BDX, Griffith EH, Fogle JE. Association of canine osteosarcoma and monocyte phenotype and chemotactic function. J Vet Intern Med (2016) 30:1167. doi: 10.1111/JVIM.13983
178. Perry JA, Thamm DH, Eickhoff J, Avery AC, Dow SW. Increased monocyte chemotactic protein-1 concentration and monocyte count independently associate with a poor prognosis in dogs with lymphoma. Vet Comp Oncol (2011) 9:55–64. doi: 10.1111/J.1476-5829.2010.00235.X
179. Marconato L, Martini V, Stefanello D, Moretti P, Ferrari R, Comazzi S, et al. Peripheral blood lymphocyte/monocyte ratio as a useful prognostic factor in dogs with diffuse large b-cell lymphoma receiving chemoimmunotherapy. Vet J (2015) 206:226–30. doi: 10.1016/J.TVJL.2015.07.009
180. Sottnik JL, Rao S, Lafferty MH, Thamm DH, Morley PS, Withrow SJ, et al. Association of blood monocyte and lymphocyte count and disease-free interval in dogs with osteosarcoma. J Vet Intern Med (2010) 24:1439–44. doi: 10.1111/J.1939-1676.2010.0591.X
181. Nishijima TF, Muss HB, Shachar SS, Tamura K, Takamatsu Y. Prognostic value of lymphocyte-to-monocyte ratio in patients with solid tumors: A systematic review and meta-analysis. Cancer Treat Rev (2015) 41:971–8. doi: 10.1016/J.CTRV.2015.10.003
182. Gu L, Li H, Chen L, Ma X, Li X, Gao Y, et al. Prognostic role of lymphocyte to monocyte ratio for patients with cancer: evidence from a systematic review and meta-analysis. Oncotarget (2016) 7:31926. doi: 10.18632/ONCOTARGET.7876
183. Faulhaber EA, Janik E, Thamm DH. Adjuvant carboplatin for treatment of splenic hemangiosarcoma in dogs: Retrospective evaluation of 18 cases (2011-2016) and comparison with doxorubicin-based chemotherapy. J Vet Intern Med (2021) 35:1929–34. doi: 10.1111/JVIM.16212
184. Mochizuki H, Stowe DM. Hematologic and clinical characteristics of dogs with circulating macrophage-like cells. Vet Clin Pathol (2021) 50:37–44. doi: 10.1111/VCP.12962
185. Sutton TL, Patel RK, Anderson AN, Bowden SG, Whalen R, Giske NR, et al. Circulating cells with macrophage-like characteristics in cancer: The importance of circulating neoplastic-immune hybrid cells in cancer. Cancers (Basel) (2022) 14(16):3871. doi: 10.3390/CANCERS14163871
186. Mantovani A, Allavena P, Marchesi F, Garlanda C. Macrophages as tools and targets in cancer therapy. Nat Rev Drug Discovery (2022) 21:799–820. doi: 10.1038/s41573-022-00520-5
187. Benner B, Good L, Quiroga D, Schultz TE, Kassem M, Carson WE, et al. Pexidartinib, a novel small molecule CSF-1R inhibitor in use for tenosynovial giant cell tumor: A systematic review of pre-clinical and clinical development. Drug Des Devel Ther (2020) 14:1693–704. doi: 10.2147/DDDT.S253232
188. Beirão BCB, Raposo TP, Imamura LM, Ingberman M, Hupp T, Vojtěšek B, et al. A blocking antibody against canine CSF-1R maturated by limited CDR mutagenesis. Antib Ther (2020) 3:193–204. doi: 10.1093/abt/tbaa018
189. Regan DP, Coy JW, Chahal KK, Chow L, Kurihara JN, Guth AM, et al. The angiotensin receptor blocker losartan suppresses growth of pulmonary metastases via AT1R-independent inhibition of CCR2 signaling and monocyte recruitment. J Immunol (2019) 202:3087–102. doi: 10.4049/JIMMUNOL.1800619
190. Regan DP, Chow L, Das S, Haines L, Palmer E, Kurihara JN, et al. Losartan blocks osteosarcoma-elicited monocyte recruitment, and combined with the kinase inhibitor toceranib, exerts significant clinical benefit in canine metastatic osteosarcoma. Clin Cancer Res (2022) 28:662–76. doi: 10.1158/1078-0432.CCR-21-2105
191. Ammons DT, Guth A, Rozental AJ, Kurihara J, Marolf AJ, Chow L, et al. Reprogramming the canine glioma microenvironment with tumor vaccination plus oral losartan and propranolol induces objective responses. Cancer Res Commun (2022) 2:1657–67. doi: 10.1158/2767-9764.CRC-22-0388
192. Meyers PA. Muramyl tripeptide-phosphatidyl ethanolamine encapsulated in liposomes (L-MTP-PE) in the treatment of osteosarcoma. Adv Exp Med Biol (2020) 1257:133–9. doi: 10.1007/978-3-030-43032-0_11/COVER
193. Vail DM, MacEwen EG, Kurzman ID, Dubielzig RR, Helfand SC, Kisseberth WC, et al. Liposome-encapsulated muramyl tripeptide phosphatidylethanolamine adjuvant immunotherapy for splenic hemangiosarcoma in the dog: a randomized multi-institutional clinical trial. Clin Cancer Res (1995) 1(10):1165–70.
194. MacEwen EG, Kurzman ID, Vail DM, Dubielzig RR, Everlith K, Madewell BR, et al. Adjuvant therapy for melanoma in dogs: Results of randomized clinical trials using surgery, liposome-encapsulated muramyl tripeptide, and granulocyte macrophage colony-stimulating factor. Clin Cancer Res (1999) 5(12):4249–58.
195. Teske E, Rutteman GR, Ted TSGAM, Van Noort R, Misdorp W. Liposome-encapsulated muramyl tripeptide phosphatidylethanolamine (L-MTP-PE): A randomized clinical trial in dogs with mammary carcinoma. Anticancer Res (1998) 18(2A):1015–9.
196. Macewen EG, Kurzman ID, Helfand S, Vail D, London C, Kisseberth W, et al. Current studies of liposome muramyl tripeptide (CGP 19835a lipid) therapy for metastasis in spontaneous tumors: A progress review. J Drug Target (1994) 2:391–6. doi: 10.3109/10611869408996814
197. Proksch SF, Matthysen CP, Jardine JE, Wyatt KM, Finlay JR, Nelson DJ. Developing a translational murine-to-canine pathway for an IL-2/agonist anti-CD40 antibody cancer immunotherapy. Vet Comp Oncol (2022) 20:602–12. doi: 10.1111/VCO.12813
198. Weiskopf K, Anderson KL, Ito D, Schnorr PJ, Tomiyasu H, Ring AM, et al. Eradication of canine diffuse Large b-cell lymphoma in a murine xenograft model with CD47 blockade and anti-CD20. Cancer Immunol Res (2016) 4:1072. doi: 10.1158/2326-6066.CIR-16-0105
199. ClinicalTrials.gov. CAR-macrophages for the treatment of HER2 overexpressing solid tumors (2022). Available at: https://clinicaltrials.gov/ct2/show/NCT04660929 (Accessed February 5, 2023).
200. Dewar AL, Cambareri AC, Zannettino ACW, Miller BL, Doherty KV, Hughes TP, et al. Macrophage colony-stimulating factor receptor c-fms is a novel target of imatinib. Blood (2005) 105:3127–32. doi: 10.1182/BLOOD-2004-10-3967
201. Wanderley CW, Colón DF, Luiz JPM, Oliveira FF, Viacava PR, Leite CA, et al. Paclitaxel reduces tumor growth by reprogramming tumor-associated macrophages to an M1 profile in a TLR4-dependent manner. Cancer Res (2018) 78:5891–900. doi: 10.1158/0008-5472.CAN-17-3480
202. Ping L, Ding N, Shi Y, Feng L, Li J, Liu Y, et al. The bruton’s tyrosine kinase inhibitor ibrutinib exerts immunomodulatory effects through regulation of tumor-infiltrating macrophages. Oncotarget (2017) 8:39218. doi: 10.18632/ONCOTARGET.16836
203. van Rooijen N, van Nieuwmegen R. Elimination of phagocytic cells in the spleen after intravenous injection of liposome-encapsulated dichloromethylene diphosphonate. an enzyme-histochemical study. Cell Tissue Res (1984) 238:355–8. doi: 10.1007/BF00217308
204. Claassen I, Van Rooijen N, Claassen E. A new method for removal of mononuclear phagocytes from heterogeneous cell populations in vitro, using the liposome-mediated macrophage “suicide” technique. J Immunol Methods (1990) 134:153–61. doi: 10.1016/0022-1759(90)90376-7
205. Rogers TL, Holen I. Tumour macrophages as potential targets of bisphosphonates. J Transl Med (2011) 9:177. doi: 10.1186/1479-5876-9-177
206. Griesmann H, Drexel C, Milosevic N, Sipos B, Rosendahl J, Gress TM, et al. Pharmacological macrophage inhibition decreases metastasis formation in a genetic model of pancreatic cancer. Gut (2017) 66:1278–85. doi: 10.1136/gutjnl-2015-310049
207. Opperman KS, Vandyke K, Clark KC, Coulter EA, Hewett DR, Mrozik KM, et al. Clodronate-liposome mediated macrophage depletion abrogates multiple myeloma tumor establishment. In Vivo Neoplasia (2019) 21:777–87. doi: 10.1016/J.NEO.2019.05.006
208. Van Acker HH, Anguille S, Willemen Y, Smits EL, Van Tendeloo VF. Bisphosphonates for cancer treatment: Mechanisms of action and lessons from clinical trials. Pharmacol Ther (2016) 158:24–40. doi: 10.1016/J.PHARMTHERA.2015.11.008
209. Germano G, Frapolli R, Belgiovine C, Anselmo A, Pesce S, Liguori M, et al. Role of macrophage targeting in the antitumor activity of trabectedin. Cancer Cell (2013) 23:249–62. doi: 10.1016/J.CCR.2013.01.008
210. Liguori M, Buracchi C, Pasqualini F, Bergomas F, Pesce S, Sironi M, et al. Functional TRAIL receptors in monocytes and tumor-associated macrophages: A possible targeting pathway in the tumor microenvironment. Oncotarget (2016) 7:41662–76. doi: 10.18632/ONCOTARGET.9340
211. Belgiovine C, Bello E, Liguori M, Craparotta I, Mannarino L, Paracchini L, et al. Lurbinectedin reduces tumour-associated macrophages and the inflammatory tumour microenvironment in preclinical models. Br J Cancer (2017) 117:628. doi: 10.1038/BJC.2017.205
212. Cannarile MA, Weisser M, Jacob W, Jegg AM, Ries CH, Rüttinger D. Colony-stimulating factor 1 receptor (CSF1R) inhibitors in cancer therapy. J Immunother Cancer (2017) 5(1):53. doi: 10.1186/S40425-017-0257-Y
214. Denny WA, Flanagan JU. Small-molecule CSF1R kinase inhibitors; review of patents 2015-present. Expert Opin Ther Pat (2021) 31:107–17. doi: 10.1080/13543776.2021.1839414
215. Manji GA, van Tine BA, Lee SM, Raufi AG, Pellicciotta I, Hirbe AC, et al. A phase I study of the combination of pexidartinib and sirolimus to target tumor-associated macrophages in unresectable sarcoma and malignant peripheral nerve sheath tumors. Clin Cancer Res (2021) 27:5519–27. doi: 10.1158/1078-0432.CCR-21-1779
216. Lee C, Jeong H, Bae Y, Shin K, Kang S, Kim H, et al. Targeting of M2-like tumor-associated macrophages with a melittin-based pro-apoptotic peptide. J Immunother Cancer (2019) 7(1):147. doi: 10.1186/S40425-019-0610-4
217. Rodriguez-Garcia A, Lynn RC, Poussin M, Eiva MA, Shaw LC, O’Connor RS, et al. CAR-T cell-mediated depletion of immunosuppressive tumor-associated macrophages promotes endogenous antitumor immunity and augments adoptive immunotherapy. Nat Commun (2021) 12(1):877. doi: 10.1038/S41467-021-20893-2
218. Scott EM, Jacobus EJ, Lyons B, Frost S, Freedman JD, Dyer A, et al. Bi- and tri-valent T cell engagers deplete tumour-associated macrophages in cancer patient samples. J Immunother Cancer (2019) 7(1):320. doi: 10.1186/S40425-019-0807-6
219. Linehan D, Noel MS, Hezel AF, Wang-Gillam A, Eskens F, Sleijfer S, et al. Overall survival in a trial of orally administered CCR2 inhibitor CCX872 in locally advanced/metastatic pancreatic cancer: Correlation with blood monocyte counts. J Clin Oncol (2018) 36:92. doi: 10.1200/JCO.2018.36.5_suppl.92
220. Pienta KJ, Machiels JP, Schrijvers D, Alekseev B, Shkolnik M, Crabb SJ, et al. Phase 2 study of carlumab (CNTO 888), a human monoclonal antibody against CC-chemokine ligand 2 (CCL2), in metastatic castration-resistant prostate cancer. Invest New Drugs (2013) 31:760–8. doi: 10.1007/S10637-012-9869-8
221. Cendrowicz E, Sas Z, Bremer E, Rygiel TP. The role of macrophages in cancer development and therapy. Cancers (Basel) (2021) 13:1946. doi: 10.3390/cancers13081946
222. Haag GM, Springfeld C, Grün B, Apostolidis L, Zschäbitz S, Dietrich M, et al. Pembrolizumab and maraviroc in refractory mismatch repair proficient/microsatellite-stable metastatic colorectal cancer - the PICCASSO phase I trial. Eur J Cancer (2022) 167:112–22. doi: 10.1016/J.EJCA.2022.03.017
223. Roy RM, Wüthrich M, Klein BS. Chitin elicits CCL2 from airway epithelial cells and induces CCR2-dependent innate allergic inflammation in the lung. J Immunol (2012) 189:2545–52. doi: 10.4049/JIMMUNOL.1200689
224. Bonapace L, Coissieux MM, Wyckoff J, Mertz KD, Varga Z, Junt T, et al. Cessation of CCL2 inhibition accelerates breast cancer metastasis by promoting angiogenesis. Nature (2014) 515:130–3. doi: 10.1038/nature13862
225. Zhu Y, Knolhoff BL, Meyer MA, Nywening TM, West BL, Luo J, et al. CSF1/CSF1R blockade reprograms tumor-infiltrating macrophages and improves response to T-cell checkpoint immunotherapy in pancreatic cancer models. Cancer Res (2014) 74:5057–69. doi: 10.1158/0008-5472.CAN-13-3723
226. Neubert NJ, Schmittnaegel M, Bordry N, Nassiri S, Wald N, Martignier C, et al. T Cell-induced CSF1 promotes melanoma resistance to PD1 blockade. Sci Transl Med (2018) 10(436):eaan3311. doi: 10.1126/SCITRANSLMED.AAN3311
227. Fridlender ZG, Buchlis G, Kapoor V, Cheng G, Sun J, Singhal S, et al. CCL2 blockade augments cancer immunotherapy. Cancer Res (2010) 70:109–18. doi: 10.1158/0008-5472.CAN-09-2326
228. Beatty GL, Chiorean EG, Fishman MP, Saboury B, Teitelbaum UR, Sun W, et al. CD40 agonists alter tumor stroma and show efficacy against pancreatic carcinoma in mice and humans. Sci (1979) (2011) 331:1612–6. doi: 10.1126/SCIENCE.1198443/SUPPL_FILE/BEATTY.SOM.PDF
229. Elgueta R, Benson MJ, De Vries VC, Wasiuk A, Guo Y, Noelle RJ. Molecular mechanism and function of CD40/CD40L engagement in the immune system. Immunol Rev (2009) 229:152–72. doi: 10.1111/J.1600-065X.2009.00782.X
230. Eisinger S, Sarhan D, Boura VF, Ibarlucea-Benitez I, Tyystjärvi S, Oliynyk G, et al. Targeting a scavenger receptor on tumor-associated macrophages activates tumor cell killing by natural killer cells. Proc Natl Acad Sci USA (2020) 117:32005–16. doi: 10.1073/pnas.2015343117
231. Jalil AAR, Andrechak JC, Discher DE. Macrophage checkpoint blockade: results from initial clinical trials, binding analyses, and CD47-SIRPα structure–function. Antib Ther (2020) 3:80–94. doi: 10.1093/ABT/TBAA006
232. O’Hara MH, O’Reilly EM, Varadhachary G, Wolff RA, Wainberg ZA, Ko AH, et al. CD40 agonistic monoclonal antibody APX005M (sotigalimab) and chemotherapy, with or without nivolumab, for the treatment of metastatic pancreatic adenocarcinoma: an open-label, multicentre, phase 1b study. Lancet Oncol (2021) 22:118–31. doi: 10.1016/S1470-2045(20)30532-5
233. Gordon SR, Maute RL, Dulken BW, Hutter G, George BM, McCracken MN, et al. PD-1 expression by tumour-associated macrophages inhibits phagocytosis and tumour immunity. Nature (2017) 545:495–9. doi: 10.1038/NATURE22396
234. Sun N-Y, Chen Y-L, Wu W-Y, Lin H-W, Chiang Y-C, Chang C-F, et al. Blockade of PD-L1 enhances cancer immunotherapy by regulating dendritic cell maturation and macrophage polarization. Cancers (Basel) (2019) 11:1400. doi: 10.3390/cancers11091400
235. Advani R, Flinn I, Popplewell L, Forero A, Bartlett NL, Ghosh N, et al. CD47 blockade by Hu5F9-G4 and rituximab in non-hodgkin’s lymphoma. N Engl J Med (2018) 379:1711–21. doi: 10.1056/NEJMOA1807315
236. Fisher GA, Lakhani NJ, Eng C, Hecht JR, Bendell JC, Philip PA, et al. A phase Ib/II study of the anti-CD47 antibody magrolimab with cetuximab in solid tumor and colorectal cancer patients. J Clin Oncol (2020) 38:114–4. doi: 10.1200/JCO.2020.38.4_SUPPL.114
237. Ring NG, Herndler-Brandstetter D, Weiskopf K, Shan L, Volkmer JP, George BM, et al. Anti-SIRPα antibody immunotherapy enhances neutrophil and macrophage antitumor activity. Proc Natl Acad Sci U.S.A. (2017) 114:E10578–85. doi: 10.1073/PNAS.1710877114
238. Barkal AA, Brewer RE, Markovic M, Kowarsky M, Barkal SA, Zaro BW, et al. CD24 signalling through macrophage siglec-10 is a target for cancer immunotherapy. Nature (2019) 572:392–6. doi: 10.1038/S41586-019-1456-0
239. Chen HM, van der Touw W, Wang YS, Kang K, Mai S, Zhang J, et al. Blocking immunoinhibitory receptor LILRB2 reprograms tumor-associated myeloid cells and promotes antitumor immunity. J Clin Invest (2018) 128:5647–62. doi: 10.1172/JCI97570
240. Van Dalen FJ, Van Stevendaal MHME, Fennemann FL, Verdoes M, Ilina O. Molecular repolarisation of tumour-associated macrophages. Molecules (2018) 24(1):9. doi: 10.3390/MOLECULES24010009
241. Tan Y, Wang M, Zhang Y, Ge S, Zhong F, Xia G, et al. Tumor-associated macrophages: A potential target for cancer therapy. Front Oncol (2021) 11:693517. doi: 10.3389/FONC.2021.693517
242. Virtakoivu R, Rannikko JH, Viitala M, Vaura F, Takeda A, Lonnberg T, et al. Systemic blockade of clever-1 elicits lymphocyte activation alongside checkpoint molecule downregulation in patients with solid tumors: Results from a phase I/II clinical trial. Clin Cancer Res (2021) 27:4205–20. doi: 10.1158/1078-0432.CCR-20-4862
243. Genard G, Wera AC, Huart C, Le Calve B, Penninckx S, Fattaccioli A, et al. Proton irradiation orchestrates macrophage reprogramming through NFκB signaling. Cell Death Dis (2018) 9(7):728. doi: 10.1038/S41419-018-0757-9
244. Genard G, Lucas S, Michiels C. Reprogramming of tumor-associated macrophages with anticancer therapies: Radiotherapy versus chemo- and immunotherapies. Front Immunol (2017) 8:828. doi: 10.3389/FIMMU.2017.00828
245. He K, Jia S, Lou Y, Liu P, Xu LX. Cryo-thermal therapy induces macrophage polarization for durable anti-tumor immunity. Cell Death Dis (2019) 10(3):216. doi: 10.1038/S41419-019-1459-7
246. Ou W, Stewart S, White A, Kwizera EA, Xu J, Fang Y, et al. In-situ cryo-immune engineering of tumor microenvironment with cold-responsive nanotechnology for cancer immunotherapy. Nat Commun (2023) 14:1–20. doi: 10.1038/s41467-023-36045-7
247. Huang L, Ge X, Liu Y, Li H, Zhang Z. The role of toll-like receptor agonists and their nanomedicines for tumor immunotherapy. Pharmaceutics (2022) 14(6):1228. doi: 10.3390/PHARMACEUTICS14061228
248. Hu G, Guo M, Xu J, Wu F, Fan J, Huang Q, et al. Nanoparticles targeting macrophages as potential clinical therapeutic agents against cancer and inflammation. Front Immunol (2019) 10:1998. doi: 10.3389/FIMMU.2019.01998
249. Bolli E, Scherger M, Arnouk SM, Pombo Antunes AR, Straßburger D, Urschbach M, et al. Targeted repolarization of tumor-associated macrophages via imidazoquinoline-linked nanobodies. Adv Sci (Weinh) (2021) 8:2004574. doi: 10.1002/ADVS.202004574
250. Shan H, Dou W, Zhang Y, Qi M. Targeted ferritin nanoparticle encapsulating CpG oligodeoxynucleotides induces tumor-associated macrophage M2 phenotype polarization into M1 phenotype and inhibits tumor growth. Nanoscale (2020) 12:22268–80. doi: 10.1039/D0NR04520A
251. Müller E, Speth M, Christopoulos PF, Lunde A, Avdagic A, Øynebråten I, et al. Both type I and type II interferons can activate antitumor M1 macrophages when combined with TLR stimulation. Front Immunol (2018) 9:2520. doi: 10.3389/FIMMU.2018.02520
252. Rodell CB, Arlauckas SP, Cuccarese MF, Garris CS, Li R, Ahmed MS, et al. TLR7/8-agonist-loaded nanoparticles promote the polarization of tumour-associated macrophages to enhance cancer immunotherapy. Nat BioMed Eng (2018) 2:578. doi: 10.1038/S41551-018-0236-8
253. Ni K, Luo T, Culbert A, Kaufmann M, Jiang X, Lin W. Nanoscale metal-organic framework Co-delivers TLR-7 agonists and anti-CD47 antibodies to modulate macrophages and orchestrate cancer immunotherapy. J Am Chem Soc (2020) 142:12579–84. doi: 10.1021/JACS.0C05039
254. ClinicalTrials.gov. Arginase inhibitor INCB001158 as a single agent and in combination with immune checkpoint therapy in patients with Advanced/Metastatic solid tumors . Available at: https://clinicaltrials.gov/ct2/show/NCT02903914 (Accessed March 21, 2023).
255. Ding X, Sun X, Cai H, Wu L, Liu Y, Zhao Y, et al. Engineering macrophages via nanotechnology and genetic manipulation for cancer therapy. Front Oncol (2022) 11:786913/XML/NLM. doi: 10.3389/FONC.2021.786913/XML/NLM
256. Pang L, Zhu Y, Qin J, Zhao W, Wang J. Primary M1 macrophages as multifunctional carrier combined with PLGA nanoparticle delivering anticancer drug for efficient glioma therapy. Drug Delivery (2018) 25:1922–31. doi: 10.1080/10717544.2018.1502839
257. Zhang F, Parayath NN, Ene CI, Stephan SB, Koehne AL, Coon ME, et al. Genetic programming of macrophages to perform anti-tumor functions using targeted mRNA nanocarriers. Nature (2019) 10(1):3974. doi: 10.1038/s41467-019-11911-5
258. Guo L, Zhang Y, Wei R, Wang C, Feng M. Lipopolysaccharide-anchored macrophages hijack tumor microtube networks for selective drug transport and augmentation of antitumor effects in orthotopic lung cancer. Theranostics (2019) 9:6936–48. doi: 10.7150/THNO.37380
259. Shields CW, Evans MA, Li L, -Wen W, Baugh N, Iyer S, et al. Cellular backpacks for macrophage immunotherapy. Sci Adv (2020) 6(18):eaaz6579. doi: 10.1126/sciadv.aaz6579
260. Brempelis KJ, Cowan CM, Kreuser SA, Labadie KP, Prieskorn BM, Lieberman NAP, et al. Genetically engineered macrophages persist in solid tumors and locally deliver therapeutic proteins to activate immune responses. J Immunother Cancer (2020) 8:1356. doi: 10.1136/jitc-2020-001356
261. Faradji A, Bohbot A, Schmitt-Goguel M, Siffert JC, Dumont S, Wiesel ML, et al. Large Scale isolation of human blood monocytes by continuous flow centrifugation leukapheresis and counterflow centrifugation elutriation for adoptive cellular immunotherapy in cancer patients. J Immunol Methods (1994) 174:297–309. doi: 10.1016/0022-1759(94)90033-7
262. Paasch D, Meyer J, Stamopoulou A, Lenz D, Kuehle J, Kloos D, et al. Ex vivo generation of CAR macrophages from hematopoietic stem and progenitor cells for use in cancer therapy. Cells (2022) 11(6):994. doi: 10.3390/CELLS11060994
263. Klichinsky M, Ruella M, Shestova O, Lu XM, Best A, Zeeman M, et al. Human chimeric antigen receptor macrophages for cancer immunotherapy. Nat Biotechnol (2020) 38:947–53. doi: 10.1038/S41587-020-0462-Y
264. Sloas C, Gill S, Klichinsky M. Engineered CAR-macrophages as adoptive immunotherapies for solid tumors. Front Immunol (2021) 12:783305. doi: 10.3389/FIMMU.2021.783305
265. Pan K, Farrukh H, Chittepu VCSR, Xu H, xian PC, Zhu Z. CAR race to cancer immunotherapy: from CAR T, CAR NK to CAR macrophage therapy. J Exp Clin Cancer Res (2022) 41(1):119. doi: 10.1186/s13046-022-02327-z
266. Yang R, Sarkar S, Yong VW, Dunn JF. In vivo MR imaging of tumor-associated macrophages: The next frontier in cancer imaging. Magn Reson Insights (2018) 11:1178623X1877197. doi: 10.1177/1178623X18771974
267. Muthana M, Kennerley AJ, Hughes R, Fagnano E, Richardson J, Paul M, et al. Directing cell therapy to anatomic target sites in vivo with magnetic resonance targeting. Nat Commun (2015) 6:8009. doi: 10.1038/NCOMMS9009
268. Mukherjee S, Sonanini D, Maurer A, Daldrup-Link HE. The yin and yang of imaging tumor associated macrophages with PET and MRI. Theranostics (2019) 9:7730–48. doi: 10.7150/THNO.37306
269. Fernandes B, Feltes PK, Luft C, Nazario LR, Jeckel CMM, Antunes IF, et al. Potential PET tracers for imaging of tumor-associated macrophages. EJNMMI Radiopharm Chem (2022) 7:1–19. doi: 10.1186/S41181-022-00163-2
270. Guth AM, Hafeman SD, Elmslie RE, Dow SW. Liposomal clodronate treatment for tumour macrophage depletion in dogs with soft-tissue sarcoma. Vet Comp Oncol (2013) 11:296–305. doi: 10.1111/J.1476-5829.2012.00319.X
271. Hafeman S, London C, Elmslie R, Dow S. Evaluation of liposomal clodronate for treatment of malignant histiocytosis in dogs. Cancer Immunol Immunother (2010) 59:441–52. doi: 10.1007/S00262-009-0763-Y
272. Hafeman SD, Varland D, Dow SW. Bisphosphonates significantly increase the activity of doxorubicin or vincristine against canine malignant histiocytosis cells. Vet Comp Oncol (2012) 10:44–56. doi: 10.1111/j.1476-5829.2011.00274.x
273. Dai Q, Xu M, Yao M, Sun B. Angiotensin AT1 receptor antagonists exert anti-inflammatory effects in spontaneously hypertensive rats. Br J Pharmacol (2007) 152:1042. doi: 10.1038/SJ.BJP.0707454
274. Laver T, London C, Vail D, Biller B, Coy J, Thamm D. Prospective evaluation of toceranib phosphate in metastatic canine osteosarcoma. Vet Comp Oncol (2018) 16:E23–9. doi: 10.1111/vco.12328
275. Merickel JL, Pluhar GE, Rendahl A, O’Sullivan MG. Prognostic histopathologic features of canine glial tumors. Vet Pathol (2021) 58:945–51. doi: 10.1177/03009858211025795
276. Hubbard ME, Arnold S, Bin Zahid A, McPheeters M, Gerard O’Sullivan M, Tabaran AF, et al. Naturally occurring canine glioma as a model for novel therapeutics. Cancer Invest (2018) 36:415–23. doi: 10.1080/07357907.2018.1514622
277. Tsung K, Norton JA. Lessons from coley’s toxin. Surg Oncol (2006) 15:25–8. doi: 10.1016/J.SURONC.2006.05.002
278. Thamm DH, Kurzman ID, King I, Li Z, Sznol M, Dubielzig RR, et al. Systemic administration of an attenuated, tumor-targeting salmonella typhimurium to dogs with spontaneous neoplasia: phase I evaluation. Clin Cancer Res (2005) 11:4827–34. doi: 10.1158/1078-0432.CCR-04-2510
279. Roberts NJ, Zhang L, Janku F, Collins A, Bai RY, Staedtke V, et al. Intratumoral injection of clostridium novyi-NT spores induces antitumor responses. Sci Transl Med (2014) 6(249):249ra111. doi: 10.1126/SCITRANSLMED.3008982
280. Feng Y, Mu R, Wang Z, Xing P, Zhang J, Dong L, et al. A toll-like receptor agonist mimicking microbial signal to generate tumor-suppressive macrophages. Nat Commun (2019) 10:1–14. doi: 10.1038/s41467-019-10354-2
281. Orange M, Reuter U, Hobohm U. Coley’s lessons remembered: Augmenting mistletoe therapy. Integr Cancer Ther (2016) 15:502–11. doi: 10.1177/1534735416649916
282. Mason NJ, Gnanandarajah JS, Engiles JB, Gray F, Laughlin D, Gaurnier-Hausser A, et al. Immunotherapy with a HER2-targeting listeria induces HER2-specific immunity and demonstrates potential therapeutic effects in a phase I trial in canine osteosarcoma. Clin Cancer Res (2016) 22:4380–90. doi: 10.1158/1078-0432.CCR-16-0088
283. Musser ML, Berger EP, Tripp CD, Clifford CA, Bergman PJ, Johannes CM. Safety evaluation of the canine osteosarcoma vaccine, live listeria vector. Vet Comp Oncol (2021) 19:92–8. doi: 10.1111/VCO.12642
284. Marconato L, Melacarne A, Aralla M, Sabattini S, Tiraboschi L, Ferrari V, et al. A target animal effectiveness study on adjuvant peptide-based vaccination in dogs with non-metastatic appendicular osteosarcoma undergoing amputation and chemotherapy. Cancers (Basel) (2022) 14(5):1347. doi: 10.3390/cancers14051347
285. Kremenovic M, Schenk M, Lee DJ. Clinical and molecular insights into BCG immunotherapy for melanoma. J Intern Med (2020) 288:625–40. doi: 10.1111/JOIM.13037
286. Kresowik TP, Griffith TS. Bacillus calmette–guerin immunotherapy for urothelial carcinoma of the bladder. Immunotherapy (2009) 1:281. doi: 10.2217/1750743X.1.2.281
287. Owen LN, Bostock DE. Effects of intravenous BCG in normal dogs and in dogs with spontaneous osteosarcoma. Eur J Cancer (1974) 10:775–80. doi: 10.1016/0014-2964(74)90133-9
288. Weiden PL, Deeg HJ, Graham TC, Storb R. Canine osteosarcoma failure of intravenous or intralesional BCG as adjuvant immunotherapy. Cancer Immunol Immunother (1981) 11:69–72. doi: 10.1007/BF00205777
289. Hess AD, Catchatourian R, Zander AR, Epstein RN. Intralesional bacillus calmette-guerin immunotherapy of canine venereal tumors(1977) (Accessed January 18, 2023).
290. Mukaratirwa S, Chitanga S, Chimatira T, Makuleke C, Sayi ST, Bhebhe E. Combination therapy using intratumoral bacillus calmette-guerin (BCG) and vincristine in dogs with transmissible venereal tumours: therapeutic efficacy and histological changes. J S Afr Vet Assoc (2009) 80:92–6. doi: 10.4102/JSAVA.V80I2.178
291. Hans EC, Pinard C, van Nimwegen SA, Kirpensteijn J, Singh A, MacEachern S, et al. Effect of surgical site infection on survival after limb amputation in the curative-intent treatment of canine appendicular osteosarcoma: a veterinary society of surgical oncology retrospective study. Vet Surg (2018) 47:E88–96. doi: 10.1111/VSU.13105
292. Lascelles BDX, Dernell WS, Correa MT, Lafferty M, Devitt CM, Kuntz CA, et al. Improved survival associated with postoperative wound infection in dogs treated with limb-salvage surgery for osteosarcoma. Ann Surg Oncol (2005) 12:1073–83. doi: 10.1245/ASO.2005.01.011
293. Chen Y, Xu S-F, Xu M, Yu X-C. Postoperative infection and survival in osteosarcoma patients: Reconsideration of immunotherapy for osteosarcoma. Mol Clin Oncol (2015) 3:495. doi: 10.3892/MCO.2015.528
294. Sottnik JL, U’Ren LW, Thamm DH, Withrow SJ, Dow SW. Chronic bacterial osteomyelitis suppression of tumor growth requires innate immune responses. Cancer Immunol Immunother (2010) 59:367–78. doi: 10.1007/S00262-009-0755-Y
295. Fidler IJ, Fogler WE, Schroit AJ, Schumann G, Braun DG, Schroit AJ. Systemic activation of macrophages and treatment of cancer metastases by liposomes containing hydrophilic or lipophilic muramyl dipeptide. Adv Immunopharmacol (1983), 235–41. doi: 10.1016/B978-0-08-029775-0.50035-3
296. Killion JJ, Fidler IJ. Systemic targeting of liposome-encapsulated immunomodulators to macrophages for treatment of cancer metastasis. Immunomethods (1994) 4(3):273–9. doi: 10.1006/IMMU.1994.1029
297. Macewen EG, Kurzman ID, Helfand S, Vail D, London C, Kisseberth W, et al. Current studies of liposome muramyl tripeptide (CGP 19835A lipid) therapy for metastasis in spontaneous tumors: A progress review*. J Drug Target (1994) 2:391–6. doi: 10.3109/10611869408996814
298. Khanna C, Rosenberg M, Vail DM. A review of paclitaxel and novel formulations including those suitable for use in dogs. J Vet Intern Med (2015) 29:1006–12. doi: 10.1111/JVIM.12596
299. Harrington BK, Gardner HL, Izumi R, Hamdy A, Rothbaum W, Coombes KR, et al. Preclinical evaluation of the novel BTK inhibitor acalabrutinib in canine models of b-cell non-Hodgkin lymphoma. PloS One (2016) 11(7):e0159607. doi: 10.1371/JOURNAL.PONE.0159607
300. London CA. Tyrosine kinase inhibitors in veterinary medicine. Top Companion Anim Med (2009) 24:106–12. doi: 10.1053/J.TCAM.2009.02.002
301. Honigberg LA, Smith AM, Sirisawad M, Verner E, Loury D, Chang B, et al. The bruton tyrosine kinase inhibitor PCI-32765 blocks b-cell activation and is efficacious in models of autoimmune disease and b-cell malignancy. Proc Natl Acad Sci U.S.A. (2010) 107:13075–80. doi: 10.1073/pnas.1004594107
302. Barnard RA, Wittenburg LA, Amaravadi RK, Gustafson DL, Thorburn A, Thamm DH. Phase I clinical trial and pharmacodynamic evaluation of combination hydroxychloroquine and doxorubicin treatment in pet dogs treated for spontaneously occurring lymphoma. Autophagy (2014) 10:1415. doi: 10.4161/AUTO.29165
303. Chen D, Xie J, Fiskesund R, Dong W, Liang X, Lv J, et al. Chloroquine modulates antitumor immune response by resetting tumor-associated macrophages toward M1 phenotype. Nat Commun (2018) 9(1):873. doi: 10.1038/S41467-018-03225-9
304. Al-Nadaf S, Rebhun RB, Curran KM, Venable RO, Skorupski KA, Willcox JL, et al. Retrospective analysis of doxorubicin and prednisone as first-line therapy for canine b-cell lymphoma. BMC Vet Res (2018) 14:1–8. doi: 10.1186/S12917-018-1688-5/TABLES/3
305. Soileau AM, Quick CN, Moeller CE, Schaumburg JC, Withers SS. The effect of arginase on canine T-lymphocyte functions and its modulation by all-trans retinoid acid (ATRA) in canine monocyte-derived macrophages. Vet Sci (2022) 9(7):374. doi: 10.3390/VETSCI9070374
306. Meunier E, Silvestre R, Lopes MF, Vellozo NS, Pereira-Marques ST, Cabral-Piccin MP, et al. All-trans retinoic acid promotes an M1- to M2-phenotype shift and inhibits macrophage-mediated immunity to leishmania major. Immunol (2017) 8:1560. doi: 10.3389/fimmu.2017.01560
307. Sayour EJ, Mitchell DA. Manipulation of innate and adaptive immunity through cancer vaccines. J Immunol Res (2017) 2017:3145742. doi: 10.1155/2017/3145742
308. Patil SS, Gentschev I, Adelfinger M, Donat U, Hess M, Weibel S, et al. Virotherapy of canine tumors with oncolytic vaccinia virus GLV-1h109 expressing an anti-VEGF single-chain antibody. PloS One (2012) 7:e47472. doi: 10.1371/JOURNAL.PONE.0047472
309. Lucroy MD, Clauson RM, Suckow MA, El-Tayyeb F, Kalinauskas A. Evaluation of an autologous cancer vaccine for the treatment of metastatic canine hemangiosarcoma: a preliminary study. BMC Vet Res (2020) 16(1):447. doi: 10.1186/s12917-020-02675-y
310. Aachoui Y, Ghosh SK. Extracellular matrix from porcine small intestinal submucosa (SIS) as immune adjuvants. PloS One (2011) 6:e27083. doi: 10.1371/JOURNAL.PONE.0027083
311. Cluff CW, Monophosphoryl Lipid A. (MPL) as an adjuvant for anti-cancer vaccines: Clinical results. Adv Exp Med Biol (2013) 667:111–23. doi: 10.1007/978-1-4419-1603-7_10
312. Konduri V, Halpert MM, Baig YC, Coronado R, Rodgers JR, Levitt JM, et al. Dendritic cell vaccination plus low-dose doxorubicin for the treatment of spontaneous canine hemangiosarcoma. Cancer Gene Ther (2019) 26:282. doi: 10.1038/S41417-019-0080-3
313. U’Ren LW, Biller BJ, Elmslie RE, Thamm DH, Dow SW. Evaluation of a novel tumor vaccine in dogs with hemangiosarcoma. J Vet Intern Med (2007) 21:113–20. doi: 10.1111/J.1939-1676.2007.TB02936.X
314. Bergman PJ, Camps-Palau MA, McKnight JA, Leibman NF, Craft DM, Leung C, et al. Development of a xenogeneic DNA vaccine program for canine malignant melanoma at the animal medical center. Vaccine (2006) 24:4582–5. doi: 10.1016/J.VACCINE.2005.08.027
315. Yannelli JR, Wouda R, Masterson TJ, Avdiushko MG, Cohen DA. Development of an autologous canine cancer vaccine system for resectable malignant tumors in dogs. Vet Immunol Immunopathol (2016) 182:95–100. doi: 10.1016/J.VETIMM.2016.10.011
316. Sorenmo KU, Krick E, Coughlin CM, Overley B, Gregor TP, Vonderheide RH, et al. CD40-activated b cell cancer vaccine improves second clinical remission and survival in privately owned dogs with non-hodgkin’s lymphoma. PloS One (2011) 6:e24167. doi: 10.1371/JOURNAL.PONE.0024167
317. Kurzman ID, Shi F, Vail DM, MacEwen EG. In vitro and In vivo enhancement of canine pulmonary alveolar macrophage cytotoxic activity against canine osteosarcoma cells. Cancer Biother Radiopharm (2009) 14:121–8. doi: 10.1089/CBR.1999.14.121
318. Thamm DH, Kurzman ID, Clark MA, Ehrhart EJ, Kraft SL, Gustafson DL, et al. Preclinical investigation of PEGylated tumor necrosis factor alpha in dogs with spontaneous tumors: phase I evaluation. Clin Cancer Res (2010) 16:1498–508. doi: 10.1158/1078-0432.CCR-09-2804
319. Khanna C. Interleukin-2 liposome inhalation therapy is safe and effective for dogs with spontaneous pulmonary metastases. Cancer (2000) 79:1409–21. doi: 10.1002/(SICI)1097-0142(19970401)79:7<1409::AID-CNCR19>3.0.CO;2-3
320. Rebhun RB, York D, Cruz SM, Judge SJ, Razmara AM, Farley LE, et al. Inhaled recombinant human IL-15 in dogs with naturally occurring pulmonary metastases from osteosarcoma or melanoma: a phase 1 study of clinical activity and correlates of response. J Immunother Cancer (2022) 10:e004493. doi: 10.1136/JITC-2022-004493
321. Bendickova K, Fric J. Roles of IL-2 in bridging adaptive and innate immunity, and as a tool for cellular immunotherapy. J Leukoc Biol (2020) 108:427–37. doi: 10.1002/JLB.5MIR0420-055R
322. Jackaman C, Dye DE, Nelson DJ. IL-2/CD40-activated macrophages rescue age and tumor-induced T cell dysfunction in elderly mice. Age (Omaha) (2014) 36:1315–28. doi: 10.1007/S11357-014-9655-Y/FIGURES/6
323. Rückert R, Brandt K, Ernst M, Marienfeld K, Csernok E, Metzler C, et al. Interleukin-15 stimulates macrophages to activate CD4+ T cells: A role in the pathogenesis of rheumatoid arthritis? Immunology (2009) 126:63–73. doi: 10.1111/J.1365-2567.2008.02878.X
324. Gordon SR, Maute RL, Dulken BW, Hutter G, George BM, McCracken MN, et al. PD-1 expression by tumor-associated macrophages inhibits phagocytosis and tumor immunity. Nature (2017) 545:495. doi: 10.1038/NATURE22396
325. Maekawa N, Konnai S, Ikebuchi R, Okagawa T, Adachi M, Takagi S, et al. Expression of PD-L1 on canine tumor cells and enhancement of IFN-γ production from tumor-infiltrating cells by PD-L1 blockade. PloS One (2014) 9:e98415. doi: 10.1371/JOURNAL.PONE.0098415
326. Coy J, Caldwell A, Chow L, Guth A, Dow S. PD-1 expression by canine T cells and functional effects of PD-1 blockade. Vet Comp Oncol (2017) 15:1487–502. doi: 10.1111/VCO.12294
327. Nemoto Y, Shosu K, Okuda M, Noguchi S, Mizuno T. Development and characterization of monoclonal antibodies against canine PD-1 and PD-L1. Vet Immunol Immunopathol (2018) 198:19–25. doi: 10.1016/J.VETIMM.2018.02.007
328. Maekawa N, Konnai S, Takagi S, Kagawa Y, Okagawa T, Nishimori A, et al. A canine chimeric monoclonal antibody targeting PD-L1 and its clinical efficacy in canine oral malignant melanoma or undifferentiated sarcoma. Sci Rep (2017) 7:1–12. doi: 10.1038/s41598-017-09444-2
Keywords: dog (canine), oncology, cancer, immunotherapy, tumor-associated macrophage, tumor microenvironment
Citation: Brady RV and Thamm DH (2023) Tumor-associated macrophages: Prognostic and therapeutic targets for cancer in humans and dogs. Front. Immunol. 14:1176807. doi: 10.3389/fimmu.2023.1176807
Received: 28 February 2023; Accepted: 24 March 2023;
Published: 05 April 2023.
Edited by:
Elena Chaves-Pozo, Spanish Institute of Oceanography, SpainReviewed by:
Fernando Torres Andón, University of Santiago de Compostela, SpainShadab Kazmi, University of Missouri, United States
Copyright © 2023 Brady and Thamm. This is an open-access article distributed under the terms of the Creative Commons Attribution License (CC BY). The use, distribution or reproduction in other forums is permitted, provided the original author(s) and the copyright owner(s) are credited and that the original publication in this journal is cited, in accordance with accepted academic practice. No use, distribution or reproduction is permitted which does not comply with these terms.
*Correspondence: Douglas H. Thamm, ZG91Zy50aGFtbUBjb2xvc3RhdGUuZWR1