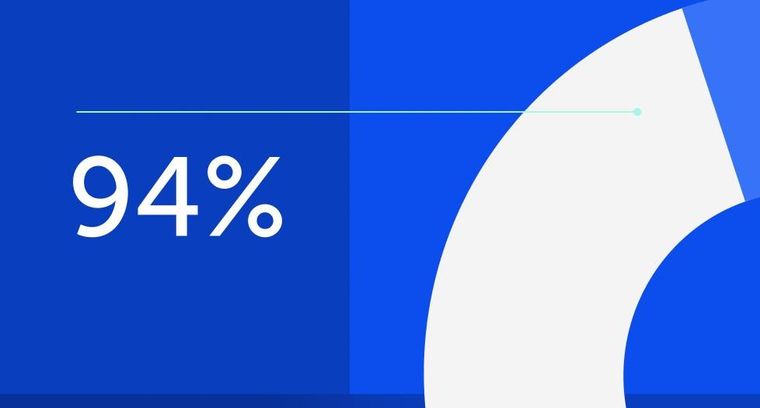
94% of researchers rate our articles as excellent or good
Learn more about the work of our research integrity team to safeguard the quality of each article we publish.
Find out more
ORIGINAL RESEARCH article
Front. Immunol., 15 May 2023
Sec. Molecular Innate Immunity
Volume 14 - 2023 | https://doi.org/10.3389/fimmu.2023.1175786
This article is part of the Research TopicBroadening Our View on Nucleic Acid Sensing: Novel Sensors, Signaling Pathways, and involvement in Non-Infectious DiseasesView all 9 articles
Background: The plant immune response to DNA is highly self/nonself-specific. Self-DNA triggered stronger responses by early immune signals such as H2O2 formation than nonself-DNA from closely related plant species. Plants lack known DNA receptors. Therefore, we aimed to investigate whether a differential sensing of self-versus nonself DNA fragments as damage- versus pathogen-associated molecular patterns (DAMPs/PAMPs) or an activation of the DNA-damage response (DDR) represents the more promising framework to understand this phenomenon.
Results: We treated Arabidopsis thaliana Col-0 plants with sonicated self-DNA from other individuals of the same ecotype, nonself-DNA from another A. thaliana ecotype, or nonself-DNA from broccoli. We observed a highly self/nonself-DNA-specific induction of H2O2 formation and of jasmonic acid (JA, the hormone controlling the wound response to chewing herbivores) and salicylic acid (SA, the hormone controlling systemic acquired resistance, SAR, to biotrophic pathogens). Mutant lines lacking Ataxia Telangiectasia Mutated (ATM) or ATM AND RAD3-RELATED (ATR) – the two DDR master kinases – retained the differential induction of JA in response to DNA treatments but completely failed to induce H2O2 or SA. Moreover, we observed H2O2 formation in response to in situ-damaged self-DNA from plants that had been treated with bleomycin or SA or infected with virulent bacteria Pseudomonas syringae pv. tomato DC3000 or pv. glycinea carrying effector avrRpt2, but not to DNA from H2O2-treated plants or challenged with non-virulent P. syringae pv. glycinea lacking avrRpt2.
Conclusion: We conclude that both ATM and ATR are required for the complete activation of the plant immune response to extracellular DNA whereas an as-yet unknown mechanism allows for the self/nonself-differential activation of the JA-dependent wound response.
The immunogenic effects of DNA are known since Alick Isaacs’ group reported in 1963 on nonself-nucleic acids as a stimulus to produce interferon (1, 2) although it is worth to note that already Mechnikov in his Nobel Speech in 1908 had mentioned “surgeons who introduce … nucleic acid or other substance, with the object of bringing to the scene a protective army of phagocytes to ward the microbes off” (3). Since then, tremendous progress has been achieved in our understanding of how the mammalian immune system detects bacterial or viral nucleic acids as pathogen-associated molecular patterns (PAMPs) in order to mount an adequate immune response (4–9). “The dark side of DNA” (10) - immune responses to self-DNA – has only recently been considered feasible, violating the immunological paradigm of self-tolerance and thereby sparking controversy. Inappropriate activation of DNA sensors such as cyclic GMP-AMP synthase (cGAS) or Toll-like receptor (TLR 9) by self-DNA has been related to aberrant type I interferon signalling and ongoing inflammation in autoimmune and autoinflammatory disorders as well as in infectious (usually viral) diseases (11–15). Nevertheless, the preference of TLR9 for unmethylated CpG motifs and the expression of cGAS and other DNA sensors in the cytoplasm usually enables the mammalian immune system to limit activation by self-DNA to situations in which delocalized fragments of self-DNA are sensed as a ‘damage-associated molecular patterns’ (DAMPs): endogenous molecules that have an ‘all-day job’ in the intact cell but serve as danger signals when they appear in aberrant compartments (16). A search in Clarivate Web of Science™ (17) for ‘DNA sensing’ currently yields over 25,000 results (search term ‘DNA sensing’ in ‘topic’ performed on 29th of January 2023). By contrast, only a handful of studies over the last 15 years have investigated DNA-activated immunity in plants (Table 1).
Treatments with plant DNA, genomic or plasmid bacterial DNA, or synthetic single stranded oligodeoxynucleotides (ssODNs), were shown to trigger early immune responses that are highly conserved between plants and mammals, including Ca2+ fluxes, membrane depolarization, the formation of reactive oxygen species (ROS) such as hydrogen peroxide (H2O2), or the activation of mitogen-activated protein kinases (MAPKs) (9, 20–22, 25, 27, 34, 35). The responses include massive transcriptomic reprogramming (24, 25, 35), and studies at the phenotypic level reported not only increased immunity (‘resistance’) to microbial pathogens, but also increased ‘defence’ to plant-specific pests such as leaf and sap-sucking herbivores (21, 23, 26, 27, 29, 33–36). Strikingly, stronger responses to self- in comparison to nonself-DNA were reported in most, although not all, of the studies that compared DNA from different sources. Even the ‘Mazzoleni-effect’ – a dosage-dependent inhibition of growth by self-DNA, but not nonself-DNA that has been described originally by the group of Stefano Mazzoleni – could subsequently be confirmed in different models, including a tree (Alnus glutinosa), freshwater and marine algae and the nematode, Caenorhabditis elegans (18, 21, 22, 30, 31, 35, 37, 38). Differential responses to self- versus nonself-DNA are significant even when nonself-DNA from closely related genotypes (species of the same genus or ecotypes of the same species) is used (21, 26, 31, 35, 36, 38).
Plants coordinate their responses to attack by biological enemies via two major signalling pathways: the jasmonic acid (JA)-dependent wound response (WR) and salicylic acid (SA)-dependent systemic acquired resistance (SAR). The WR is activated upon mechanical damage, feeding by herbivores or infection by necrotrophic pathogens that cause intensive tissue damage. Detection of these events depends on membrane-bound pattern recognition receptors (PRRs) that sense molecular nonself patterns from the herbivore (i.e., herbivore-associated molecular patterns, HAMPs) or DAMPs that are released upon cell disruption, including sucrose, glutamate, cell wall components such as oligogalacturonides, signalling peptides, extracellular ATP, high mobility group box proteins (HMGB) and – most likely - self-DNA (21, 39–44). Upon sensing of HAMPs or DAMPs, metabolic reprogramming allows for the induction of numerous JA-responsive mechanical, biochemical and chemical mechanisms that exert repellent, toxic or otherwise detrimental effects on enemies whose feeding habit exposes them to the intracellular contents of their host. In contrast, SAR is activated in response to biotrophic pathogens and sucking herbivores that generate only minor physical damage. Perception of these events also relies on PRR sensing of PAMPs and the subsequent sensing of effectors which lead to accumulation of ROS, phytoalexins, programmed cell death and the systemic induction of multiple SA-dependent pathogenesis-related (PR) proteins including PR1, chitinases, β-1,3-glucanases and thaumatin-like proteins. Both the WR and SAR usually lead to a systemic so-called ‘pattern-triggered immunity’ (PTI) even after local attack. Considering the different roles of DAMPs and PAMPs in the activation of PTI, it seems reasonable to assume that self- and nonself DNA is sensed as a DAMP or a PAMP respectively, similar to the roles that place DNA sensors such as cGAS and TLR9 inflammasome-forming DNA receptors at the centre of mammalian innate immunity to pathogens, cancer and damage by abiotic factors (6, 15, 45, 46).
Alternatively, exogenously applied DNA could trigger the DNA damage response (DDR): A highly conserved system enabling eukaryotes to detect and repair damage to their genomic, plastid and mitochondrial DNA resulting from the effects of external abiotic and biotic factors but also during vital processes including photosynthesis, oxidative phosphorylation and DNA transcription and synthesis (47–51). Pharmacological studies that link DNA damage to the resistance of pea plants to fungal pathogens date back to the early 1970s (52–54), reviewed in (55, 56). Since then, it has become increasingly evident that activation of the DDR and of innate immunity are closely related (57–62). Current knowledge does not provide sufficient evidence to favour one of the proposed models (DAMP/PAMP-triggered PTI versus DDR activation) over the other. In principle, PTI, being a mechanism which evolved for the perception of exogenous threats, could be considered more likely to respond in a self/nonself-specific way to exogenously applied DNA fragments than the DDR, which evolved to detect damaged self-DNA. However, on the one hand, an induction by DNA has been reported for traits that are typically controlled via WR as well as SAR. Further, more recent support for the PTI model comes from a study that linked resistance-induction by self-DNA in peach (Prunus persica) fruits to the brassinosteroid insensitive 1-associated receptor kinase 1 (BAK1) – a cytoplasmatic co-receptor that interacts with various PRRs of DAMPs and PAMPs (34) - and from two studies that reported self-DNA-induced JA-synthesis in A. thaliana and common bean (Phaseolus vulgaris) (35, 36). On the other hand, self-/nonself specific effects are mainly reported for early, not enemy-specific immune signals which have also been reported for the DDR, and as far as we know, no PRRs that could act as dsDNA receptors have been reported for plants or could be predicted by in silico approaches (4, 63–65). Therefore, the main goal of our present study was to investigate which of the before mentioned models provides the more promising framework to guide future research and to compare the effects of sonicated DNA to DNA fragments produced under more realistic scenarios. We hypothesized that the DDR, as the more conserved mechanism of sensing damaged DNA, should play a role in the innate immunity induction by fragmented DNA but perhaps not be sufficient to control the differential responses to DNA from different sources.
To characterise the self/nonself-specific immune response to DNA in the model plant, Arabidopsis thaliana Col-0, we treated Col-0 plants with sonicated self-DNA from other plants of the same ecotype, nonself-DNA from A. thaliana ecotype Cape Verde islands (Cvi-0) or nonself-DNA from broccoli (Brassica oleracea var. italica, ‘Br’) and quantified H2O2 as an early, nonspecific signal and the hormones, JA and SA, as later signals specifically involved in the WR or SAR, respectively. ROS including H2O2 are known as endogenous DNA-damaging factors that are generated during all basal metabolic processes that include oxidative phosphorylation, but they also serve as local defence against invading pathogens and play a central role as signals that trigger the local and in the form of the ROS-wave, systemic activation of innate immune responses (66, 67). Although ROS had originally been considered mainly in the context of SAR, more recent evidence demonstrates their role in WR. The cognate receptor of eATP, a well-established DAMP in plants, is a lectin-type receptor like kinase that upon sensing eATP activates the ROS-wave by inducing NADPH oxidase-dependent superoxide activation (68). The role of JA as the mobile signal in the WR and the essential role of SA in the systemic tissue response (although not as the mobile signal) are generally accepted. SA is important for SAR activation since the SA receptor, “NO pathogenesis-related protein 1 (NPR1)” serves as a transcription factor for PR1 expression (69, 70). In homeostasis, NPR1 is a cytoplasmic oligomer, but upon binding SA, NPR1 monomers are formed and translocated to the nucleus, where they bind to the PR1 promoter facilitating its expression (69). NPR1 also seems to be involved in coordinating the WR-SAR trade-off. However, doubts remain concerning to which precursors liberated from different substrates and two major biosynthetic pathways contribute to the accumulation of JA and SA in response to wounding and infection, respectively (71–78). Therefore, we opted for a quantification of the hormones themselves.
As a first step to elucidate a potential role for the DDR, we used T- DNA insertion lines of the two master kinases: Ataxia Telangiectasia Mutated (ATM) and ATM AND RAD3-RELATED (ATR) (47, 51, 62, 79, 80). Although neither of these kinases are directly involved in damage recognition, they associate with DNA binding proteins, recruit and/or activate additional proteins and thus, organise large protein complexes that are required for a rapid and efficient repair. While ATR is mainly activated by single-strand breaks (SSBs), ATM is activated by double-strand breaks (DSBs) (47, 51, 62, 79, 80).
In WT, JA increased in response to DNA from all three sources, although with self/nonself-specific quantitative differences, whereas SA and H2O2 increased only in response to DNA from the two A. thaliana ecotypes. Surprisingly, the atm and atr mutants were unaffected in the self/nonself-specific induction of JA, whereas both failed to respond to DNA with a detectable increase in H2O2 or SA. Interestingly, all three sources of DNA induced phenotypic resistance to Pst DC3000 in atr but not atm mutant lines. We interpret these results as a preliminary demonstration of a role for the DDR in the induction of SA by exogenous DNA, whereas a DDR-independent mechanism enables plants to perceive self-DNA as a DAMP to activate a JA-dependent wound response.
Wild type Arabidopsis thaliana Col-0 plants were donated by Stefano Mazzoleni (Naples, Italy), T-DNA insertion lines atm-1 (SALK_040423C) and atr-2 (SALK_032841C) were acquired from European Arabidopsis Stock Centre, Nottingham, UK (NASC), A. thaliana ecotype Cape Verde islands (Cvi-0) were donated by Jean-Philippe Vielle-Calzada (Irapuato, Mexico) and Brassica oleracea seeds were purchased from Rancho Los Molinos (Tepoztlán, Morelos, Mexico). We used A. thaliana Col-0 as sources of self -DNA and A. thaliana Cvi-0 and B. oleraceaas sources of non-self-DNA. A. thaliana plants were grown in a growth chamber with a photoperiod of 16 h/8 h light/dark at 23°C with 70% relative humidity for 3 week in a substrate composed of 3:1:1 (v:v:v) of Sunshine Mix #3 (Sungro horticulture Agawam, MA, USA), vermiculite (Sungro) and perlite (Termolita, Santa Catarina, Mx). B. oleracea plants were cultivated in the same substrate in a greenhouse under natural light and photoperiod conditions at an average temperature of 28°C (day) and 20°C (night). T-DNA insertion lines were genotyped using the protocol recommended by NASC (81) and the primers listed in Supplementary Figure S5. PCR conditions using a C1000 Thermal cycler (Biorad, Hercules, CA, USA) were as follows: initial denaturalization at 94°C for 3 min, followed by 30 cycles as follows: 94°C for 30 s, 55°C (for atr samples) or 58°C (for atm samples) for 30 s, 72°C for 2 min and a final extension at 72°C for 10 min.
Pseudomonas syringae pv. tomato DC3000 (Pst DC3000) were donated by John Délano-Frier (Irapuato, Mexico) and Pseudomonas syringae pv. glycinea carrying vector pVSP61 [Psg (–)] or the vector pV288 containing the effector avrRpt2 [Psg (+)] were donated by Andrew F. Bent (Madison, WI, USA) (82). These strains were cultured at 28°C with peptone-yeast-glycerol agar (NYGA) (83) containing 25 µg ml-1 kanamycin (Sigma-Aldrich, St. Louis, MO, USA).
The extraction of DNA from leaves of A. thaliana Col-0, A. thaliana Cvi-0 and B. oleracea was based on a method reported by Healey & col (84). A total of 20 ml of cetyltrimethylammonium bromide (CTAB) buffer (1.5% CTAB, 100 mM Tris-HCl pH 8, 20 mM EDTA pH 8, 1.4 M NaCl and 2% β-mercaptoethanol in water, all from Sigma-Aldrich) were added per 5 g of ground tissue in 50 ml tubes, shaken on a vortex and heated to 65°C for 30 min in a water bath. Then, 20 ml of 24:1 chloroform:isoamyl alcohol (Karal, Leon, Gto, Mexico) was added and shaken on a vortex. The tubes were centrifuged at 3000 g for 5 min at 4°C and the supernatant was transferred to a new 50 ml tube. Next, 20 ml of precooled isopropanol and 2 ml of ammonium acetate 7.5 M (Karal, Leon, Gto, Mexico) were added to the supernatant, which was kept at –20°C. After 1 h, the tubes were centrifuged at 3000 g for 20 min at 4°C, the supernatant was discarded, and the pellet was dried for 5 min at room temperature before washing by adding 5 ml of 70% ethanol (Karal, Leon, Gto, Mexico) and shaking. After further centrifugation at 3000 g for 10 min at 4°C, the supernatant was discarded, the pellet was dried for 5 min and re-suspended in 1 ml of sterile distilled water for subsequent purification using a Maxi DNA purification Kit (Qiagen, Hilden, Germany). The DNA was quantified using a NanoDrop 2000 spectrometer (Thermo Fisher Scientific, Waltham, MA, USA). To obtain fragments shorter than 1000 bp, a solution of 500 μg ml–1 of DNA in sterile distilled water was sonicated using a CP505 ultrasonic processor (Cole Parmer, Vernon Hills, IL, USA) for 3:30 min at 55% of amplitude and using pulse mode (1 s pulse ‘On’ and a 1 s pulse ‘Off’). The successful fragmentation of DNA was verified by gel electrophoresis on a 2.2% agarose gel using ethidium bromide staining (Thermo Fisher) (Supplementary Figure S1).
To characterise the ROS response to exogenous DNA fragments, two initial experiments were carried out. In the first one, we treated plants with fragmented self-DNA at 5 µg ml−1 or 50 µg ml− 1 in 0.05% v v−1 Tween 20 and sampled at 0, 5, 10, 15, 30, 45 and 60 min after treatment, in the second one, we treated plants with 0, 0.005, 0.05, 0.5, 5 or 50 µg ml−1 self-DNA and sampled at 15 min after treatment. The rosettes of 15 plants were cut off at the base, pooled in groups of three rosettes to give 5 biologically independent replicates per time point and ground in liquid nitrogen. Subsequently, we added 0.1% trichloroacetic acid (250 µl) (Sigma-Aldrich), 250 µl of potassium phosphate buffer 10mM, pH 5.8 and 500 µl of sodium iodide 1M (both from JT Baker, Phillipsburg, NJ, USA) to 150 mg of ground tissue, samples were shaken and incubated at 4°C for 10 min in the dark. After centrifugation at 12,000 g for 15 min at 4°C, 200 µl of supernatant were placed in microplate wells and incubated in the dark for 20 min at room temperature. Hydrogen peroxide quantification (85) was carried out using NaI (JT Baker) instead of KI and absorbance was measured at 350 nm in a µQuant microplate reader (BioTek Instruments, Winooski, VT, USA). Samples and blanks were compared to a calibration curve obtained using H2O2 at concentrations of 0–250 nmol ml-1. To visually detect the accumulation of ROS, after 15 min, leaves were stained with 3,3-diaminobenzidine (Sigma-Aldrich) as described previously (86). For subsequent experiments DNA was applied at 5 µg ml−1 self-DNA and samples were taken at 15 min after treatment.
To quantify the levels of JA and SA, fragmented self and nonself-DNA (5 µg ml−1 in 0.05% v v−1 Tween 20, Sigma-Aldrich) was sprayed on both sides of the rosette leaves of each plant until the leaf surface was soaked. Samples for JA analysis were collected at 0, 5, 10, 15, 30, 45 or 60 min after treatment and those for SA analysis at 0, 12, 24 or 48 h as described above to yield 5 biologically independent replicates per time point.
The extraction of JA was carried out as described in (87). 0.5 ml of ethyl acetate and 20 µl of 0.1 mg ml-1 (9,10- H2)-dihydrojasmonic acid as an internal standard (both from Sigma-Aldrich) were added to 250 mg of tissue, shaken and kept at 4°C overnight. After centrifugation at 14000 g for 15 min at 4°C, the supernatant was collected, and the pellet was re-extracted with 0.5 ml of ethyl acetate and centrifuged. The supernatants were combined and evaporated in a miVac concentrator (GeneVac, Warminster, PA, USA) with gaseous nitrogen. The residue was derivatized by adding 100 µL of N´N´-disopropylethylamine, 100 µl of chloroform and 10 µl of pentafluorobenzyl bromide (Sigma-Aldrich) at 60°C under an extraction hood (88). After 30 min, the resultant liquid was cooled on ice and evaporated with gaseous nitrogen. The residue was re-suspended with 100 µl of HPLC grade methanol (Sigma-Aldrich) and used for JA quantification.
The extraction of SA was based on published methods (89, 90). In brief, 750 µl of 90% methanol and 250 ng ml-1 of ortho-anisic acid (as internal standard, Thermo Fisher) were added to 250 mg of ground tissue and extracted at 4°C overnight. After centrifugation at 13000 g for 15 min at 4°C, the supernatant was collected, the pellet was re-extracted with 750 μl of 90% methanol, and both supernatants were combined and evaporated in a miVac Concentrator plus (GeneVac) for 4 h. The residue was re-suspended with 500 μl of trichloroacetic acid 5% and centrifuged at 4000 g for 10 min at 4°C. The supernatant was mixed with two volumes of ethyl acetate-hexane (1: 1 v v-1, Sigma-Aldrich) and incubated at room temperature for 10 min. The organic phase was recovered and dried with gaseous nitrogen. The residue was derivatized by mixing with 20 µl of pyridine (JT Baker) and 80 μl of N,O-bis(trimethylsilyl)trifluoroacetamide (BSTFA; Sigma-Aldrich) and incubated at 80°C for 1 h, using an extraction hood. The resulting mixture was used for SA quantification using gas chromatography.
Both hormones were quantified using gas chromatography - electronic impact ionization mass spectrometry (GC-EIMS) in a 7890A Gas Chromatograph equipped with a DB-1MS UI column (60 m × 0.25 mm × 0.25 µm) coupled to a MSD 5973 mass spectrometer (Agilent Technologies, Santa Clara, CA, USA) in SIM mode, using ions 141, 181, 390 and 392 m z–1 for JA and 73, 135, 267 and 282 m z–1 for SA. An injection volume of 1 µl of sample was used in the splitless mode. For JA, the operating conditions (91) were an injector temperature of 200°C and an initial oven temperature of 150°C for 3 min, which was then ramped at 4°C min–1 to 300°C, with the final temperature maintained for 20 min. For SA we used an injector temperature of 200°C and an initial oven temperature of 150°C for 3 min, which was then ramped at 4°C min–1 to 260°C, with the final temperature maintained for 25 min. Helium was used as a carrier gas with a constant flow of 1 ml min–1 and standard curves were prepared using pure compounds (JA, Sigma-Aldrich; SA, JT Baker) to quantify the respective amounts of JA and SA based on peak areas with reference to the internal standard.
To elucidate whether the effects of sonicated DNA are similar to those of naturally damaged DNA, we inoculated Col-0 plants with one of three bacterial strains: virulent Pst DC3000 and Psg (+) or the non-virulent Psg (–) (82, 92). The two virulent bacteria, Pst DC3000 and Psg avrRpt2 + have been reported to inflict damage to the DNA of their host plant (93). For inoculation, bacteria were resuspended in 10 mM MgCl2 (Sigma-Aldrich). After adjusting the bacterial suspension following an established protocol (A.F. Bent, personal communication) by quantifying optical density at 600nm (where od 0.1 = bacterial density of 108 cells) in a µQuant microplate reader (BioTek Instruments, Winooski, VT, USA) A. thaliana Col-0 plants were inoculated by spraying the rosette with a suspension of 108 colony forming units (CFUs) ml-1 in 10 mM MgCl2. Alternatively, the plants were syringe infiltrated with 100 µl of an aqueous solution of a DNA-damaging agent (56, 59, 93–95): 0.6 µg ml-1 bleomycin (Sigma-Aldrich), 100 µM SA (Sigma-Aldrich), or 80 mM H2O2 (Jaloma, Guadalajara, Jal, Mexico).
Damaged DNA was retrieved from bacteria-inoculated plants after 7 days and from plants infiltrated with SA, bleomycin and H2O2 after 24 h, 12 h or 3 h, respectively, adapting a method developed to quantify the leakage of NAD(P) from leaf disks floating on water (96). Thirty leaf discs of 6 mm diameter were harvested from 10 individual plants per treatment and pools of 10 leaf disks were placed in test tubes with 600 µl CTAB buffer. After shaking for 10 minutes, the buffer was recollected in a microcentrifuge tube and the leaked DNA was precipitated with 400 µl of isopropanol and 60 µl of ammonium acetate 7.5 M (Karal Leon, Gto, Mexico) and quantified using a NanoDrop 2000 spectrometer (Thermo Fisher Scientific, Waltham, MA, USA). To control for any artefacts resulting from DNA fragmentation during this process, we applied a mechanical stress control. For this we collected leaf discs from untreated plants, placed them in the buffer and gently pressed them repeatedly between a plastic stick and the tube, avoiding visible tissue disruption.
To evaluate the in situ fragmentation caused by the beforementioned treatments in comparison with the pattern observed in sonicated DNA, the DNA was visualized on a 2.2% agarose gel using ethidium bromide staining and the signal intensity of the fragments that fell within a size range of < 1000 bp in each lane was estimated using Image Lab (Biorad). To test these fragments for their ROS-inducing activities, we treated Col-0 plants with the eluted DNA and sampled these plants 15 min later to quantify H2O2-levels as described in section 2.3.1.
The expected total amount of DNA was calculated based on assuming a total number of 130,000 cells in a leaf with an area of 200 mm2 (97) and a 2C-value of 0.32pg (98). Based on these assumptions, we calculated an area of 0.0015 mm2 and a radius of 0.022 mm for the average cell, which translates to a total number of 18,400 cells in the entire leaf disc and of 850 cells in the circumference, with an expected total amount of 5.88 ng DNA in the entire disc and of 0.27 ng in the circumference (Table 2).
Table 2 Parameters and calculated values to estimate the expected total amount of DNA in leaf discs of 6mm.
To determine importance of CpG motifs (23), the ODNs IMT504 (5’-CATCATTTTGTCATTTTGTCATT-3’) and 2006 (5’- TCGTCGTTTTGTCGTTTTGT-3’) and their complementary sequences (IMT504c: 5’- AATGACAAAATGACAAAATGATG-3’; 2006c: 5’-ACAAAACGACAAAACGACGA-3’) were purchased from Sigma Aldrich and used as single stranded DNA (ssDNA). To generate double stranded DNA (dsDNA), each ODN was annealed with its complementary sequence in a C1000 Thermal cycler (Biorad) by heating to 95°C for 3 minutes and cooling to room temperature.
In order to obtain DNA with completely methylated CpG motifs, sonicated DNA of Col-0, Cvi-0 or Br was used as substrate for the CpG DNA methyltransferase from Spiroplasma sp. strain MW1 (M.SssI, Thermo Scientific #EM0821) (99). Methylation was performed according to the product manual. To evaluate the degree of methylation, non-fragmented DNA was treated with M.SssI and aliquots of 1 µg µl–1 of the product were digested with each of two restriction enzymes, MspI (Thermo-Scientific #ER0541) and HpaII (Invitrogen INVN093-6), according to the product manuals (Supplementary Figure S2).
To generate unmethylated DNA, genome amplification was performed using 15-mer random primers and non-fragmented DNA of Col-0, Cvi-0 or Br as a template for amplification via PCR in a C1000 Thermal cycler (Biorad), using the following conditions: initial denaturalization at 94°C for 2 min followed by 50 cycles as follows: 94°C for 1 min, alignment ramping from 28 to 55°C (0.1 °C s-1), 55°C for 4 min, extension at 68°C for 30 s and a final extension at 68°C for 8 min. PCR products were separated by gel electrophoresis on a 2.2% agarose gel.
All statistical analyses and plots were performed using R version 4.2.0 (100) with Rstudio version 2022.12.0.353 (101) as integrated development environment. Global data from each experimental set was tested by either one- or two-way analysis of variance (ANOVA), when significant differences were found (p < 0.05) a Tukey test was performed. For the dose response curve analyses, we used the drc package (102), while the tidyverse (103), multcompView (104), ggbeeswarm (105), ggtext (106), ggrepel (107) and rstatix (108) packages were used for creating the plots and general data wrangling. R scripts used for the statistical analyses and for creating the figures are available at GitHub as IsaacVegaM/PAPER_Vega_2023_exDNA_DDR.
Earlier studies observed dosage-dependent effects of DNA on immune signals and seedling growth and revealed certain species-specific differences in the timing of the responses. Therefore, we first characterised the time course of H2O2 levels after treatment with different concentrations of self-DNA. We observed that H2O2 levels started to increase at 10 min, reached peak values at 15 min and returned to a base level at 60 min after treatment with 5 µg·ml-1 DNA but not 50 µg·ml-1 DNA (Figure 1A). Both DNA concentration and time after treatment had highly significant effects on H2O2 levels that were subject to a significant interaction (Figure 1A, see Supplementary Datasheet 1 for details). Based on these results, 15 min after treatment was selected as a standard sampling time for H2O2 quantification in all subsequent experiments.
Figure 1 The Arabidopsis thaliana ROS response to exogenous DNA. The level of ROS in [nmol H2O2 g-1 leaf fresh weight] was determined at different time points (A) or 15 min (B) after treating A. thaliana Col-0 plants with a solution of self-DNA in 0.05% v v−1 Tween 20. Controls (black symbols) were treated with 0.05% v v−1 Tween 20 in water. Different DNA concentrations are indicated as different saturations of red, symbols represent individual data points. In (A) dotted lines represent means and different letters indicate statistically significant differences at each time point among plants treated with different concentrations (p < 0.05, post hoc Tukey tests; p < 0.001 for time and for concentration, and p = 0.002 for the interaction according to two-way ANOVA, n = 3 biologically independent replicates per concentration and time point). In (B) the line shows the result of a log-logistic dose-response model adjusted to estimate the effective dose to trigger effects in 50% (ED50) of the individuals (n = 5 biologically independent replicates per concentration). See Supplementary Datasheet 1 for detailed results of statistical analyses.
In a second experiment, the threshold concentration of DNA required to trigger a significant ROS response was determined. Self-DNA was applied at the beforementioned concentrations and additionally at 0.5, 0.05 and 0.005 µg·ml-1 and H2O2 levels were quantified 15 min later (n = 5 independent biological replicates for each concentration, Figure 1B). Using a log-logistic dose-response model we determined 0.74 and 5.15 µg·ml-1 as the effective doses that cause 50% and 99% responses (ED50 and ED99) respectively (Supplementary Datasheet 1). Based on these data, we selected 5 µg·ml-1 of DNA as a standard concentration for all subsequent experiments.
To confirm the self/nonself specific effects of DNA on H2O2 formation previously reported in other models and investigate whether this specificity also applied to the induction of the two major defence hormones, we first characterised the induction of JA and SA by self-DNA over time (Supplementary Figure S3) and selected 30 min as the sampling time for JA and 24 h for SA. Subsequently, we treated Col-0 plants with self-and nonself-DNA. DNA treatment had a significant and species-specific effect on the levels of H2O2, JA and SA at the respective sampling time (Figure 2). Post hoc Tukey analysis revealed that JA was induced by DNA from all three species, although with species-specific differences, while H2O2 and SA were induced by self-DNA and by nonself-DNA from A. thaliana Cvi-0, but not by broccoli DNA (Figure 2).
Figure 2 Self-nonself-specific induction of H2O2 and defence hormones by exogenous DNA. The levels of ROS [in nmol H2O2 g-1 leaf fresh weight], jasmonic acid [in ng JA g-1 leaf fresh weight] and salicylic acid [in µg SA g-1 leaf fresh weight] were determined at 15 min (H2O2, A), 30 min (JA, B) and 24 h (SA, C) after treating Arabidopsis thaliana Col-0 plants with 5 µg·DNA ml-1 in 0.05% v v−1 Tween 20 of self-DNA (red symbols), nonself-DNA from A. thaliana ecotype Cape Verde islands (Cvi-0, dark grey symbols), or nonself-DNA from broccoli (Br, Brassica oleracea, light grey symbols). Controls (black symbols) were treated with 0.05% v v−1 Tween 20 in water. In all three panels, circles represent individual data points, horizontal lines indicate means, and different letters indicate statistically significant differences among plants treated with DNA from different origins (p < 0.05, post hoc Tukey tests). The p-values shown in the figure indicate the treatment effect on H2O2, JA and SA, according to separate one-way ANOVAs, n = 5 biologically independent replicates). See Supplementary Datasheet 1 for detailed results of statistical analyses.
To investigate whether the effects of sonicated DNA on H2O2 levels are comparable to those elicited by naturally damaged DNA, we treated Col-0 plants with each of three bacterial pathogens or with DNA-damaging agents and collected the complete (fragmented and non-fragmented) DNA from subsequently excised leaf discs. This method yielded on average 0.8 ng DNA per leaf disc, with no statistically significant differences among treatments (Figure 3A). Based on our estimation, the total amount of DNA would have been ca 5.8 ng in the entire disc and 0.03 ng in the circumference (dotted lines in Figure 3A). Gel-electrophoresis of the recovered DNA revealed a strong fragmentation of DNA in leaves that had been inoculated with either of the two virulent bacterial strains, Pst DC3000 and Psg (+) or infiltrated with Bleomycin or SA. An analysis with Image Lab (Biorad) confirmed that most of the fragments fell within a size range of < 1000 bp, in the same range as in sonicated DNA. In contrast, we retrieved significantly lower levels of fragmented DNA from leaves inoculated with the nonvirulent Psg (–) strain or infiltrated with H2O2 and from non-treated leaves subjected to the mechanical stress control (Inset in Figure 3A; Supplementary Figure S4).
Figure 3 In situ damage by virulent bacteria or DNA-damaging molecules generates fragments with ROS-inducing activity. Arabidopsis thaliana Col-0 plants were pre-treated with pathogenic bacteria or DNA-damaging molecules and the DNA retrieved from leaf discs was analysed by gel electrophoresis for fragmentation (A) and for ROS-inducing activity (B). Pre-treatments were inoculation with a suspension of 108 bacterial cells of: Psg (–), avirulent Pseudomonas syringae pv. glycinea carrying vector pVSP61; Psg (+), virulent Pseudomonas syringae pv. glycinea carrying vector pV288 with effector avrRpt2; Pst DC3000, Pseudomonas syringae pv. tomato DC3000; or infiltration with 100µl of: Bleomycin, 0.6 µg ml-1 of bleomycin; SA, 100 µM salicylic acid; H2O2, 80 mM hydrogen peroxide. Control, leaf disks from plants with no prior treatment, MSC, mechanical stress control. (A) The mean amount of DNA [ng DNA per leaf disc] retrieved from 10 discs pooled from at least three individual plants is shown as circles that represent individual data points. We detected no significant effect of the pre-treatment on the amount of retrieved DNA (p = 0.908 according to one-way ANOVA, n = 3 biologically independent replicates). Dotted lines represent the estimated quantity of DNA in the cells of the circumference and the entire leaf disc, respectively. The photo shows a 2.2% agarose gel stained with ethidium bromide that was used to visually analyse the DNA for fragmentation. (B) The levels of ROS [in nmol H2O2 g-1 leaf fresh weight] were determined 15 min after treating Col-0 plants with a solution of 5 µg·DNA ml-1 in 0.05% v v−1 Tween 20 of the retrieved DNA. No additional treatment for fragmentation was used except for the sonicated DNA that served as a positive control. In both panels, circles represent individual data points, horizontal lines indicate means, and different letters in (B) indicate statistically significant differences among treatments (p < 0.05, post hoc Tukey tests). The p-value shown in (B) indicates the treatment effect on H2O2 according to one-way ANOVA, n = 5 biologically independent replicates. See Supplementary Datasheet 1 for detailed results of statistical analyses.
In a subsequent experiment, we used this DNA to treat Col-0 plants. We sampled leaves 15 min later and observed a highly significant effect of the previous treatment on the H2O2-levels in the DNA-treated leaves (p < 0.001, One-Way ANOVA, n = 5 biologically independent replicates, see Supplementary Datasheet 1 for details). We detected a significant induction of H2O2 by DNA from Pst DC3000 infected or Psg (+) infected plants and by DNA from plants previously infiltrated with Bleomycin or SA (p < 0.05, post hoc Tukey tests, n = 5 biologically independent replicates, Figure 3B, see Supplementary Datasheet 1 for details). In fact, we found no statistically significant difference (p =0.99) between the H2O2-inducing effect of DNA from Pst DC3000- infected leaves and of sonicated DNA. In contrast, DNA from plants inoculated with the avirulent Psg avrRpt2+, infiltrated with H2O2 or from mechanically damaged leaves had no statistically significant effect on H2O2 levels (p =0.99, p = 0.71, p = 0.99, respectively).
To investigate whether central steps of the DNA-damage response are involved in induction of H2O2, JA or SA by exogenously applied DNA, T-DNA insertion lines atm-1 (SALK_040423C) and atr-2 (SALK_032841C) confirmed via genotyping to be homozygous (Supplementary Figure S5). We treated these plants with self and nonself-DNA from the before mentioned three sources, quantified H2O2 levels 15 min later and observed significant differences between the responses of the mutants and the wild type plants (p < 0.001 for DNA treatment, genotype and the treatment genotype interaction, Two-Way ANOVA, n = 5 biologically independent replicates, see Supplementary Datasheet 1 for details). We could detect no statistically significant effects of DNA treatment on H2O2 levels in the atm or the atr plants (p = 0.087 and 0.736, respectively, One-Way ANOVA, n = 5 biologically independent replicates, see Supplementary Datasheet 1 for details). Intriguingly however atm plants but not atr plants, were characterized by higher baseline H2O2 levels than WT plants (Figure 4A). In fact, Tukey post hoc tests revealed that the H2O2 levels in the atm plants, including untreated controls, were significantly higher than in WT control plants and not statistically different from self-DNA treated WT plants. In contrast, we could not detect any statistically significant differences when comparing the H2O2 levels in untreated WT control plants with those in atr plants in any of the treatment groups (Figure 4A; Supplementary Datasheet 1).
Figure 4 Arabidopsis DDR mutant lines atm and atr show self/nonself-specific JA induction by DNA but no induction of H2O2 and SA. The levels of ROS [in nmol H2O2 g-1 leaf fresh weight], jasmonic acid [in ng JA g-1 leaf fresh weight] and salicylic acid [in µg SA g-1 leaf fresh weight] were determined at 15 min (H2O2, A), 30 min (JA, B) and 24 h (SA, C) after treating Arabidopsis thaliana Col-0 wildtype (WT) plants or T-DNA insertion lines of Ataxia Telangiectasia Mutated (ATM) and ATM AND RAD3-RELATED (ATR) with a solution of 5 µg·DNA ml-1 in 0.05% v v−1 Tween 20 of self-DNA (red symbols), nonself-DNA from A. thaliana ecotype Cape Verde islands (Cvi-0, dark grey symbols), or nonself-DNA from broccoli (Br, Brassica oleracea, light grey symbols). Controls (black symbols) were treated with 0.05% v v−1 Tween 20 in water. Circles represent individual data points, horizontal lines indicate means, and different letters indicate statistically significant differences among plants treated with DNA from different origins (p < 0.05, post hoc Tukey tests, n = 5, see Supplementary data sheet 2 for detailed results of statistical analyses). The p-values shown in the figure indicate the treatment effect on H2O2, JA and SA, according to separate two-way ANOVAs, and according to separate one-way ANOVAS for each genotype (n = 5 biologically independent replicates in all cases, see Supplementary Datasheet 1 for details).
Whereas the ROS response to DNA in atr and atm mutants clearly differed from the response in WT, we observed a seemingly normal induction of JA by exogenously applied DNA in the mutants (Figure 4B). In fact, we observed a highly significant treatment effect on JA in the entire dataset and also when analysing each genotype with individual ANOVAs, while we detected only marginally significant differences among the mutants and the WT (Figure 4B, p = 0.012 for genotype and p = 0.453 for the interaction). By contrast, none of the mutants showed a detectable induction of SA (Figure 4C, p = 0.945 for atm and P = 0.832 for atr). In fact, even with post hoc tests comparing the SA levels in atm or atr plants of any of the treatment groups with those in untreated controls of the WT, we detected no significant differences (Figure 4C: p > 0.05 in all cases, see Supplementary Datasheet 1 for details).
To study the effects of DNA treatment on the resistance to bacterial pathogens we treated WT, atm and atr plants with each of the three types of DNA and challenged them 48 h later with Pst DC3000. Common disease symptoms like chlorosis (yellowing due to the loss of chlorophyll) and necrosis (browning due to premature cell death) were recorded and determined visually (Figure 5A) and bacterial densities were quantified as CFUs (Figure 5B) seven days post infection. In WT plants previously treated with different sources of DNA we could observe mild severity of both chlorosis and necrosis in comparison to the control treatment. The atm and atr mutants suffered from slightly stronger symptoms (a mix of chlorosis and necrosis symptoms) than the wildtype. However, while atm mutants previously treated with DNA still showed clear disease symptoms independently of the type of DNA, DNA treatment of atr mutants strongly reduced the severity of the symptoms, similar to levels as seen in treated WT. In summary, the evaluation of disease symptoms revealed that DNA-treatment triggered a significant resistance induction that did not depend on the source of the DNA in WT and atr plants, but not in atm plants. The overall analysis of CFU numbers confirmed a highly significant treatment effect (p < 0.001) but marginally significant effects of the genotype (p = 0.021) with a significant (p = 0.002) interaction. Moreover, individual ANOVAs confirmed a significant effect for the WT and for atr, while no significant effect of DNA treatment on CFU numbers could be detected for atm (p = 0.327, Figure 5B). Intriguingly, post hoc tests did not allow to detect significant differences among WT or atr plants treated with the different types of DNA and thereby confirmed that DNA induces resistance to Pst DC300 in a self/nonself-independent way (Figure 5B).
Figure 5 Arabidopsis DDR mutant line atm but not atr is affected in the immunity to a bacterial pathogen. Disease symptoms like chlorosis (yellowing due to the loss of chlorophyll) and necrosis (browning due to premature cell death) (A) and bacterial density [in colony forming units, CFUs, per leaf disc] (B) are shown seven days after inoculating Arabidopsis thaliana Col-0 wildtype (WT) plants or T-DNA insertion lines of Ataxia Telangiectasia Mutated (ATM) and ATM AND RAD3-RELATED (ATR) with a suspension of 108 bacterial cells of Pseudomonas syringae pv. tomato DC3000. Inoculation was performed two days after treating the plants with a solution of 5 µg·DNA ml-1 in 0.05% v v−1 Tween 20 of self-DNA (red symbols), nonself-DNA from A. thaliana ecotype Cape Verde islands (Cvi-0, dark grey symbols), or nonself-DNA from broccoli (Br, Brassica oleracea, light grey symbols). Controls (black symbols in B) were treated with in 0.05% v v−1 Tween 20 in water 2 days before inoculation. Circles in (B) indicate individual data points, horizontal lines indicate means, and different letters indicate statistically significant differences among plants treated with DNA from different origins (p < 0.05, post hoc Tukey tests). The p-values in (B) indicate the treatment effect on CFU numbers according to two-way ANOVA and according to separate one-way ANOVAS for each genotype (n = 5 biologically independent replicates in all cases, see Supplementary Datasheet 1 for details).
In the mammalian immune system, TLR9 binds preferentially to DNA that is rich in unmethylated CpG motifs while all other dsDNA sensors known so far bind DNA in a sequence-independent way (6, 7, 64, 109). Since the few studies that tested for a role of the unmethylated CpG motif in the immune response of plants show inconclusive results, we aimed at providing further evidence, by taking two independent approaches. First, we compared the H2O2-inducing properties of DNA with completely methylated CpG motifs (generated from natural self-or nonself-DNA that was treated with M.SssI, Supplementary Figure S2) versus completely unmethylated DNA (produced by a PCR with 15-mer random primers from natural DNA of all three species as templates). In both cases, the manipulated DNA had a significant effect on H2O2 levels, but with no detectable differences between DNA produced from the three different sources (Figure 6A). In fact, the treatment effect was significant in the complete experimental design and also when analysing the effects of natural DNA, methylated DNA and PCR-generated DNA separately, but post hoc tests identified significant differences only between controls and DNA-treated plants, while we could detect no significant effects for comparisons among methylated DNAs from different sources (p > 0.99, Figure 6A, see Supplementary Datasheet 1 for details).
Figure 6 Presence and methylation of CpG motifs have minor effects on ROS-inducing properties of DNA. The level of ROS in [nmol H2O2 g-1 leaf fresh weight] was determined at 15 min after treating Arabidopsis thaliana Col-0 plants with 5 µg·ml-1 DNA with different degrees of CpG methylation (A) or with synthetic oligodeoxynucleotides (ODNs), the CpG-containing 2006 and the CpG-free IMT504 (B). In (A), self-DNA from Col-0 plants (red symbols), nonself-DNA from A. thaliana ecotype Cape Verde islands (Cvi-0, dark grey symbols) or nonself-DNA from broccoli (Br, Brassica oleracea, light grey symbols) was used in its ‘natural’ state (only sonicated), after complete cytosine methylation in the CpG motif using the methyltransferase M.SssI, or served as template for genome amplification using 15-mer random primers. In (B) the CpG-containing ODN 2006 (5’- TCGTCGTTTTGTCGTTTTGT-3’) and the CpG-free ODN IMT504 (5’-CATCATTTTGTCATTTTGTCATT-3’) as well as their complementary sequence (2006c and IMT504c) were applied either as single-stranded (ss)ODNs or as annealed double stranded (ds)ODNs. Different letters indicate statistically significant differences (p < 0.05, post hoc Tukey tests, n = 5 biologically independent replicates in all cases). The p-values in (A) indicate the treatment effect on according to two-way ANOVA and according to separate one-way ANOVAS for DNA of each methylation status, and in (B) the treatment effect according to one-way ANOVA (n = 5 biologically independent replicates in all cases, see Supplementary Datasheet 1 for details).
In the second approach we used the CpG- containing ODN 2006 and the CpG-free ODN IMT504 and observed a significant effect on H2O2 levels (Figure 6B). However, the H2O2-inducing effect was independent of whether we applied the ODNs or their complementary sequence (IMT504c and 2006c) either as single-stranded (ss)ODNs or as annealed double stranded (ds)ODNs. In fact, post hoc tests identified only the differences between the control condition and the treated plants to be significant, but they did not show any significant differences between the different treatments (p > 0.85 for all pairwise comparisons, Figure 6B).
The accumulation of fragmented DNA in the cytoplasm or the extracellular space is a signal of danger. Mammals and plants respond to this danger with an activation of innate immunity. Our present study confirms earlier reports that plants exhibit a self/nonself-specific response to DNA from related species at a particularly fine taxonomic resolution: A. thaliana plants of ecotype Col-0 responded to treatments with self-DNA from other Col-0 plants with significantly stronger increases in the levels of H2O2 and JA than after treatments with nonself-DNA from another ecotype of the same species (Figure 2). In general terms, an immune response to ‘self’ contradicts the classical immunological paradigm as expressed in the title of Charles Janeway’s seminal publication “The immune system evolved to discriminate infectious nonself from noninfectious self” (110) but rather, provides strong empirical support for Polly Matzinger’s statement “The immune system is more concerned with entities that do damage than with those that are foreign” (111). Both perspectives provided the framework for important immunological breakthroughs, from the discovery of PRRs guided by the Janeway paradigm to providing the explanation of pro-inflammatory self-molecules as DAMPs, which were predicted by Matzinger and discovered empirically by Walter Land in 1994 (112, 113) and since then are increasingly being identified as drivers of important beneficial as well as detrimental functions of the immune system (44, 114, 115).
Our knowledge on the mechanisms that control DAMPs-triggered plant immunity is still very limited and few receptors have been identified so far (41, 116–118). Considering the opportunities provided by the mutants and multiple other genetic and molecular tools existing for Arabidopsis thaliana for future research into response to DNA we characterised the dose-response relations and the time course the ROS-response to self-DNA, and identified 5µg DNA ml-1 as a concentration that in 99% of cases should induce a significant H2O2 accumulation that reaches peak – and likely maximum – levels at 15 min (Figure 1). The levels of JA peaked at 30 min and those of SA at 24 h (Figure 2; Supplementary Figure S3). Subsequent treatments revealed self/nonself-specific effects of DNA on H2O2 and both hormones and in all cases, strongest effects were triggered by self-DNA (Figure 2), similar to earlier observations, although with minor differences between species (20–22, 26, 35, 36). The response times and levels reached by the three signals fall clearly within the standard kinetics of the three signals (119–121).
In spite of numerous reports that associate infection or herbivore feeding with damage to the host’s DNA, it remains unknown whether the release of self-DNA fragments is a common outcome of an attack by herbivores or pathogens. Moreover, doubts remained as to which degree the exogenous application of sonicated plant DNA causes effects that are comparable to those when DNA is damaged in situ in more realistic scenarios. Therefore, we aimed to compare the ROS-inducing properties in situ-damaged DNA to the effects of sonicated DNA.
To this end, we challenged Col-0 plants with various pathogenic bacteria or treated them with H2O2, SA or bleomycin (a radiomimetic drug that triggers DSBs (94, 122, 123)). Aiming to collect only DNA that had leaked into the apoplast we followed a method developed to study extracellular NAD+ a SAR-triggering DAMP (96). The results revealed the presence of massive amounts of DNA fragments in a size range between ca 100 bp and 1000 bp obtained from leaves previously infected with Pst DC3000 or Psg (+) or treated with Bleomycin or SA. In contrast, infection with Psg (–) and treatment with H2O2 did not cause major fragmentation of plant DNA, at least in terms of generating visible amounts of fragments in our assay. For the bacteria, this pattern confirms earlier reports that characterised the first two strains as virulent bacteria that inflict intensive damage to their host´s genomic DNA, whereas Psg (–) exhibits a very low level of virulence although it can reproduce in Arabidopsis (92, 93, 124, 125). Similarly, our results confirm for bleomycin and SA, but not H2O2, that the in situ effects of widely used DNA-damaging agents comprise the generation of fragments within the same size range as sonication. At the first glance, the lack of massive DNA fragmentation upon H2O2, treatment seems counterintuitive. While the role of SA as a DNA-damaging agent that had been proposed by the group of Xinnian Dong (59) has later been questioned by a study that reported damage-induced SA to be involved in subsequent repair (93), the role of oxidative stress as a DNA-damaging factor can be considered as generally accepted (126–128). However, studies using a wide variety of experimental models from the plant and animal kingdom identify SA and H2O2 as ‘double-edged swords’ that act as DNA damaging agents but also serve as signals to induce adequate countermeasures (129–132). Considering that most of the involved processes are dosage-dependent, follow different kinetics and are likely to interact via diverse direct and indirect mechanisms, it should not come as a surprise that the net outcome quantified at any single time point yields seemingly contrasting results.
Originally, the motivation for this experiment was to demonstrate the leakage of DNA fragments from intact cells into the apoplast. We reasoned that our method would allow the recovery of leaked DNA from most cells in the leaf disc, whereas in a scenario in which DNA release requires mechanical disruption of cells, only DNA from cells in the circumference would be retrieved. To this end, we estimated the total amount of DNA in the leaf disc versus the circumference. Comparison of the average amount of DNA retrieved from each leaf disc to these hypothetical values indicates that the retrieved DNA had most likely had been released only from the cells in the circumference that were mechanically damaged when cutting out the leaf discs. Thus, further work will be required to provide unambiguous evidence for the release of DNA fragments from stressed but still intact cells. More importantly, a mechanical stress control based on application of gentle pressure on leaf discs from untreated plants during the DNA elution process clearly demonstrates that mechanical stress during the collection procedure did not generate detectable amounts of DNA fragments (last lane, Figure 3A; Supplementary Figure S4). Treatments of Col-0 plants with the collected DNA confirmed that infection with virulent bacteria or DNA-damaging agents generates fragments of DNA with similar H2O2-inducing properties as those of sonicated DNA (Figure 3B).
Based on this validation of sonicated DNA as an experimental model, it seems safe to conclude that plants exhibit stronger immune responses to self-DNA than to nonself-DNA from closely related sources. This specificity resembles the effects of leaf homogenates, which arguably contain a cocktail of DAMPs (86) and thereby, supports a role of self-DNA as DAMP (133). Unlike mammals, which express several dsDNA receptors in different subcellular compartments, no dsDNA receptors are known from plants. Evidently, a lack of published evidence does not exclude the possibility that plant dsDNA sensors simply remain to be discovered. Nevertheless, it appears difficult to envision receptors able to differentiate between the fragmented genomes of two closely related plant species. For mammals, the differential activation of murine versus human plasmacytoid Dendritic Cells (pdCs) by single-stranded RNA of Human Immunodeficiency Virus Type 1 (HIV-1) could be attributed to TLR 7 and 8 (134) and most recently, species-specific differences have been discovered among the mechanism used for DNA binding by cGAS from different mammalian species (46). Still, the only mammalian dsDNA sensor known to distinguish DNA from organisms belonging to different phylogenetic groups seems to be TLR9, which binds preferably DNA that is rich in unmethylated CpG motifs and thus allows for differential immune responses to bacterial and viral DNA versus genomic DNA of eukaryotes (135–137), i.e., a differentiation at the level of domains.
This particular feature of TLR9 motivated earlier studies to test for a role of CpG motifs and/or cytosin methylation in the plant immune response to DNA. Yakushiji and colleagues (9) observed that the accumulation of ROS in Arabidopsis in response to genomic or plasmid bacterial DNA decreased after methylation of the cytosine residues in the 5’-CG-3’ sequence using the DNA methyltransferase M.SssI (99). Moreover, the authors used the same methyltransferase in combination with restriction enzymes to corroborate the methylation of natural herring DNA and thereby could attribute the lower ROS-inducing activity of herring DNA to a TLR9-like mechanism that requires unmethylated CpG motifs (9). Similarly, a recent study using the same three enzymes to generate DNA free of unmethylated CpG motifs from naturally methylated DNA of common bean and observed that any enzymatic manipulation decreased the H2O2-inducing properties as compared to the natural self-DNA, with no detectable differences among fragments with completely methylated CpG motifs and fragments in which all unmethylated CpG motifs had been cleaved enzymatically (36). These results would be consistent with a mechanism in which DNA triggers a partial induction of H2O2 that is independent of CpG-methylation whereas the full response requires additional activation by unmethylated CpG motifs (36). However, another recent study (23) discovered that treatments with the CpG containing ssODN 2006 and the CpG-free ssODN 504 triggered various immune responses in Arabidopsis, including H2O2 formation and phenotypic resistance to a fungus and the bacterial pathogen Pst DC3000 (Table 1), again with no detectable differences between the two ODNs (23).
In the present study we observed a reduction in the H2O2-inducing effect after M.SssI-mediated methylation that eliminated the self/nonself-specific differences among DNA from both Arabidopsis ecotypes and from broccoli (Figure 6A).We also observed an induction of H2O2 when using synthetic – and thus, completely unmethylated - DNA that was statistically not different from the levels observed after treatments with completely methylated DNA, but quantitatively lower than the level triggered by natural self-DNA (Figure 6A). Furthermore, we repeated the crucial experiment reported by Toum and colleague (23) and observed that synthetic ODNs induced H2O2 to levels that were similar to those triggered by completely methylated or complete unmethylated DNA, and with no detectable differences between CpG-containing and CpG-free or single-stranded versus double-stranded ODNs (Figure 6B).
We consider it difficult to envision a straightforward explanation of these results within a framework that is exclusively based on a TLR9-like recognition of unmethylated CpG motifs and conclude that other properties of natural plant DNA – perhaps the plant-specific methylation in CHG and CHH motifs – could contribute to the full immunogenic effects of self-DNA. The proposed role of methylation in CHG and CHH would be consistent with the induction of SA to similar levels by Col-0 and Cvi-0 DNA (Figure 2C; p = 0.985 for the difference between the sources, see Supplementary Datasheet 1 for details). The Arabidopsis ecotype Cvi-0 is well known for having the lowest degree of CpG methylation in genes bodies among more than 1000 A. thaliana ecotypes, but Cvi-0 and Col-0 have very similar global methylation patterns in CHG and CHH motifs (138). Evidently, future work will be required to confirm the suggested role of methylation in non-CpG motifs and even if confirmed, it would remain to be verified if the minor differences between Col-0 and Cvi-0 shown suffice to explain the significantly different effects on H2O2 and JA.
Taken together, the beforementioned observations open promising perspectives, but they do not present a basis to exclude that a process connected to the DDR is contributing to the activation of immune signalling by extracellular DNA fragments. The DDR evolved for the repair of an organism´s own genome and thus, it seems plausible to assume that some of the involved elements work best for the own DNA but only partially when dealing with nonself-DNA; similar to the recently discovered differences among the mechanisms that control the self-DNA reactivity of cGAS molecules from different primates (46). We used mutant lines of atr and atm because both kinases are conserved among plants and mammals and well-known for their central role in the coordination of the DDR (139). While ATR is mainly activated by single-strand breaks, ATM is activated by double-strand breaks (DSB) and necessary for their repair, thus playing a crucial role for DNA repair via homologous recombination (47, 51, 62, 79, 80, 140). Moreover, a transcriptomic analysis of atm and atr mutants of Arabidopsis after exposure to γ-radiation revealed hundreds of upregulated genes, and the induction of ‘virtually all’ of these genes depended on ATM, but not ATR (79). Thus, it seems safe to assume that ATM should play a crucial role in DDR-related mechanisms that are activated during infection and thus, potentially linked to SAR induction.
Both mutants responded to DNA with a self/nonself-specific induction of JA that could not be distinguished from the response of the wild type (Figure 4B). The induction of JA via a DDR-independent mechanism should not come as a surprise: while links between SA and DNA damage have been reported in numerous studies for plants and mammals (59, 130, 132, 141–146), we are not aware of a study that liked the DDR to JA-signalling. In contrast, both mutants failed to respond to self- or nonself-DNA with a detectable induction of H2O2 or SA; at least, we could not detect any statistically significant effect of DNA the levels of H2O2 or of SA in any of the mutants (Figures 4A, C). Post hoc tests indicate that in the case of H2O2, this lack of inducibility was likely due to increased baseline levels in atm, but not in atr. Correspondingly, atm plants exhibited a slightly increased basal level of resistance to Pst DC3000 (CFU numbers not statistically different from DNA-treated WT plants) but failed to induce resistance upon DNA treatment (no detectable treatment effect, Figure 5B). This observation is consistent with the assumption that an increased accumulation of unrepaired DNA damage in atm mutants triggers ongoing downstream signalling which ultimately exacerbates an efficient response to infection. Similarly, this scenario would be consistent with the observation that the atm and atr plants exhibited basal SA levels between basal levels and self-DNA-induced levels in WT. In fact, post hoc tests confirmed for the WT a significant induction of SA by self-DNA, but did not allow to detect a significant difference between the SA-levels in any of the atm or atr treatment groups and the SA levels in WT controls, but also when compared with the self-DNA induced SA levels (with three exceptions, p > 0.5 in all comparisons): thus, post hoc tests definitively place the basal SA level in the mutants quantitatively between the basal and the self-DNA inducible level in WT (See Supplementary Datasheet 1).
Surprisingly, though, atr plants exhibited a DNA-inducible resistance to Pst DC3000 that did not depend on the source of DNA and thus, resembled the pattern observed in wildtype. We lack a convincing explanation of this phenomenon. Resistance in Arabidopsis to Pst DC3000 is generally assumed to be under the control of SA. An SA-independent but JA-dependent signalling controls induced systemic resistance (ISR) triggered by Rhizobacteria and is mainly based on priming, rather than direct induction of resistance trait expression (147). Therefore, a similar, priming-based phenomenon might explain our observation.
Pathogen attack, mechanical damage and even sucrose treatment (a DAMP mimic triggering JA accumulation and JA-dependent defence activation in lima bean (148)) have been reported to induce nuclease activities and DNA cleavage in the host plant (149): a scenario that would link damage to DNA inflicted by DNases to the WR, rather than SA-mediated signalling. Likewise, the induction of ROS by ssODNs (23) and the lack of any differences among the responses to ssODNs and dsODNs (Figure 6 of the present study) hardly fit into a classical DDR scenario.
Nevertheless, numerous observations link DNA damage and the DDR to the induction of SA-dependent SAR. For general reviews on these topics, we refer to recent reviews for different aspects of the DDR, of SA-mediated SAR and of the DAMPS-mediated SWR (4, 40, 41, 47, 51, 55, 69, 129, 133, 150, 151). Since the late 1960s, pharmacological studies reported on the resistance-inducing effects of DNA-damaging compounds (53, 54, 95, 152). More recently, a direct genetic connection among both processes has been demonstrated in studies that identified the transcription repressor SUPPRESSOR OF NPR1-1, INDUCIBLE 1 (SNI1) as part of the STRUCTURAL MAINTENANCE OF CHROMOSOME (SMC) complex (70) or reported that RAD51 induces SAR by replacing SNI1 from the promotor regions of PR1 (58, 59, 144). The SMC complex controls homologous recombination (59, 144), an error-free ATM-dependent (79) mechanism for the repair of DSB in which the RAD51 filament facilitates homology search and subsequent DNA strand invasion followed by DNA synthesis using the homologous strand as template (144). Homologous repair is essential for crossing over during meiosis (153), but it has also been reported repeatedly to occur at increased frequencies in pathogen-infected plants (57, 58, 154). The recruitment of ATM to DSB depends on the Meiotic Recombination 11(MRE11) RADIATION SENSITIVE 50 (RAD50) Nijmegen breakage syndrome 1(NBS1) (MRN) complex (47, 155). The processing of DSB by the MRN-complex depends on the nuclease activity of its elements, in particular MRE11, which leads to the formation of 3’ ssDNA overhangs (156, 157). These ssDNA overhangs explain why the activation of ATR upon DSBs requires ATM and the MRN complex (155, 158). Intriguingly, in the absence of NBS1, MRE11 and RAD50 localize to the cytoplasm. Therefore, MRE11 has been brought forward as a cytosolic sensor for dsDNA, at least in vertebrates. The role of NBS1 for the nuclear localization of the MRN complex and the subsequent recruitment and activation of ATM could be confirmed for Arabidopsis (140).
Nevertheless, doubts remain concerning the role of SA and concerning the role of SA-induced NPR1 in PR gene expression. SA has been brought forward as a DNA-damaging agent in a study published by the group of Xinnian Dong (59) while others found damage-induced SA to reduce damage (93). Therefore, Nisa and colleagues (51) consider the causal reasons of DNA damage during infection as ‘unknown’. Moreover, the beforementioned se observations would place RAD51 within the ATM-controlled mechanisms that are activated upon DSBs. However, Xinnian Dong´s group considers ATR/RAD17 as inducers of RAD51 (59, 144). RAD51 can either move to the site of DNA damage and facilitate homologous recombination or move to the PR-1 gene promotor to facilitate expression, either by replacing SNI1 or by binding to the promotor and thus trigger expression in an NPR1-independent pathway (144, 145). In honour of Xinnian Dong, we hereinafter use the term ‘Dong-pathway’ for the SA-independent induction of PR-1 gene expression by RAD51 which upon activation by ATR/RAD17 downstream to SA-inflicted SSB directly moves to the PR-1 promotor (58, 59), while in the presence of SA-activated NPR1, RAD51 can favour PR-1-expression by removing SNI1 from the PR-1 promotor (144).
In some cases, seemingly incongruent findings could reflect species-specific differences (159), mutants that generate changes in the phenotypic resistance levels via changes in the sensitivity to a certain signal rather than signal intensity (160), the context-dependent action of alternative mechanisms, e.g., during different parts of the cell cycle (79), different times of the day or different contributions of partly redundant elements. For example, plants possess two pathways for the synthesis of SA, the isochorismate-pathway in which ISOCHORISMATE SYNTHASE (ICS) catalyses the synthesis of the central precursor molecule and the PHENYLALANINE AMMONIA LYASE (PAL) pathway in which PAL, the first enzyme in phenylpropanoid synthesis, converts phenylalanine to trans-cinnamic acid (77). Although pathogen-induced SA synthesis has been considered to be mostly dependent on the ICS pathway (74, 78), this pattern has been questioned by others (72). Indeed, a study transforming Arabidopsis lines that overexpressed RAD51A from maize (ZmRAD51A) reported increased SA levels and observed RAD51 to be induced by SA and vice-versa. Moreover, the same plants exhibited a strong expression of PAL upon pathogen challenge – but not of ICS1 (161).
Similarly, an existence of both NPR1- dependent and NPR1-independent mechanisms of PR1 induction as proposed by the Dong group (58, 59) could result in scenarios attributing from ‘fine-tuning’ to ‘essential’ roles to SNI1 (69).
Even the NPR1-independent SAR induction via the ‘Dong-pathway’ requires SA as the DNA-damaging agent. Moreover, most studies assign partly overlapping, additive or even redundant functions to ATM and ATR (162, 163), mainly because both kinases signal through the shared SUPPRESSOR OF GAMMA RESPONSE 1 (SOG1) (80, 139, 164, 165). With exception of the sterility of double mutants of atm and atr (163), it seems difficult to envision a strictly ATM – and ATR-dependent process as it is indicated by our data for the DNA-induced increased in SA (Figure 4C).
Based on the results of the present study and published information on resistance induction and the DDR (mainly – but not exclusively - in plants) we propose the following working model aimed at guiding the next steps in research (See Figure 7 for a graphical representation, numbers of specific steps as cited in the following text in round brackets refer to the number used to identify the respective arrow in this Figure).
Figure 7 Graphical presentation of a working model fitting SAR induction by DNA fragments into the DDR. Exogenously applied DNA fragments could activate ATM via the MRN-complex to induce RAD51 and subsequently the ISOCHORISMATE SYNTHASE (ICS) and/or the PHENYLALANINE AMMONIA LYASE (PAL) biosynthetic pathway of salicylic acid (SA), which triggers DNA damage and subsequently, ATR, thereby connecting the ATM-based and NPR1-dependent pathway to the ATR-dependent and NPR1-independent Dong-pathway to PR1 expression. Functions corresponding to the arrows and supporting references are listed in Table 3 according to the number used to identify the respective arrow. Black arrows indicate published evidence, red arrows highlight evidence from DNA-treated plants and grey arrows numbered H1-H5 indicate the different hypotheses underlying this model. See main text section ‘4.5. Fitting SAR induction by DNA fragments into the DDR: a working model’ for further references and explanations.
Table 3 Functions and supporting references corresponding to the arrows in the working model (Figure 7).
Processing of DSBs by the MRN complex generates – besides 3’ ssDNA overhangs – ssODNs, which can activate ATM independently of DSB, at least in vertebrates, as demonstrated by treatments with annealed oligonucleotides consisting of 70 bases of random complementary sequences or poly-dA70/poly-dT70 (pA70/pT70) (173). Assuming that DNases and other factors during infection or herbivore attack generate fragments of DNA that accumulate in the extracellular space (arrow H1) and assuming further, that these fragments can enter the cell and activate ATM via the MRN complex (H2), exogenously applied fragments of self- and nonself-DNA could activate ATM and thereby induce the synthesis of SA, which triggers DNA damage (H3) and thereby activates ATR (arrow 7) to close the circle by connecting ATM-based and NPR1-dependent PR1 expression to the ATR-dependent and NPR1-independent Dong-pathway (4–6).
In case that this mechanism existed in plants, it could be the pathway via which exogenously applied DNA fragments or DNA-damaging agents induce RAD51 expression and subsequent SA accumulation via a DDR-mediated pathway that depends on ATM and ATR. This assumption remains to be empirically supported, although the observation that atmre11 mutant lines failed to respond to bleomycin treatment with increased expression of RAD51 provides some indirect support (123). More importantly, the proposed mechanism would be consistent with the complete failure of both mutant lines to exhibit a DNA-induced SA accumulation. Taking into account that the DDR is considered as independent of cytosine methylation (172), this scenario would also explain the responses to ssODNs and dsODNs as well to completely methylated versus unmethylated DNA.
As alternative scenario, ROS have been reported to activate ATM via an SA-independent pathway (17) (168–171). Moreover, following an initial oxidative burst, plant cells attain a more reducing environment due to the accumulation of antioxidants, such as reduced glutathione (GSH), which is considered to be one of the most important ROS scavengers (166, 174). Although glutathione-S-transferases perform a variety of pivotal functions, their most important effect is to catalyze peroxidase reactions to control oxidative stress (175). Increases in the ratio of reduced with oxidised glutathione were found to be associated with the increased appearance the reduced monomeric form of NPR1 monomers which then moves into the nucleus to control SAR-related gene expression (69, 166). Therefore, exogenously applied fragments of self- and nonself-DNA could contribute to the activation of ATM and NPR1 via their ROS-inducing effect Figures (20) (H4/H5). In fact, significant changes in the expression of numerous glutathione-S-transferases have been observed upon DNA treatment of Arabidopsis (Supplementary Table S1). Therefore, it seems possible that DNA fragments activate NPR1 via the induction of ROS and downstream redox changes.
However, self- and nonself-DNA trigger increases in SA in wild type Arabidopsis plants and common bean (this study and (35, 36)) and they induced ICS1 and ISC2, PAL, transcription factors controlling ICS1 and other central steps of SA synthesis (22, 25) (Supplementary Table S1). In addition, even fungal DNA induced PAL expression in Chili pepper plants (27). Considering this evidence for an induction of both pathways of SA biosynthesis (21, 22, 27) by exogenously applied DNA fragments and several earlier reports that identified the induction of PAL activity as crucial for resistance induction by DNA-damaging agents like actinomycin D or even by histone fragments (54, 95, 152, 176), we consider an activation of ATM via the MRN complex (H2) and subsequent SA synthesis as the more likely scenario.
If the MRN-dependent activation of ATM by extracellular DNA fragments was to be used as a model for studying the plant immune response to DNA, fragments of DNA that are generated during enemy attack should enter via the same pathway (H1). Pathogen-derived DNases have been brought forward repeatedly to link DNA damage to PR gene expression. Indeed, several pathogens and even herbivores of plants secrete DNases as effectors or as part of their nutrient acquisition strategy (177–182). The putative relevance of extracellular self-DNA fragments in the immunity of plants to biological enemies has gained empirical support by the recent discovery of extracellular DNases in the salivary secretion of the brown plant hopper (Laodelphax striatellus) and the fungal pathogen Cochliobolus heterostrophus (181, 182). However, the benefits of these DNases were attributed to a function of extracellular DNA as a DAMP in one study and as a direct defence mechanism in the other study, and we are not aware of direct evidence for a release of DNA fragments into the extracellular space in this context. Our first attempt to provide this evidence has, seemingly, failed (Figure 3). Therefore, the suggested role of H2 in the DDR-dependent plant immune response to DNA during natural enemy attack remains a hypothesis (H5) that requires further investigation.
Our present study validates the exogenous application of sonicated as a suitable model to study immune responses in plants to fragmented DNA and shows that Arabidopsis exhibits a highly self/nonself-DNA-specific induction of ROS and the defence hormones, JA and SA. We also consider our observations as a clear support of an involvement of ATM and ATR in the induction of SA by DNA. The recruitment of ATM and ATR to damaged DNA sites depends on the MRN complex and – although in DNA repair must take place in the nucleus – two elements of this complex are also expressed in the cytoplasm. In this scenario, a component of the MRN complex – most likely the nuclease MRE11 – would play the role of a cytoplasmatic DNA sensor in plants, as it has already been suggested for mammals (157).
To guide future investigation, we propose a model that – although as yet merely hypothetical – would be consistent with diverse results published by others and that offers a possibility how two seemingly contrasting roles of SA could be merged into a single mechanism. In this context, it seems worth to highlight that the induction of elements of both SA biosynthetic pathways by exogenously applied DNA validates our decision to quantify the defence hormones themselves as a more integrative outread, rather than focusing on the expression of pre-selected marker genes which in our case likely would have missed the induction of the PAL pathway by DNA. More importantly, our model is based on two assumptions that remain to be empirically supported and it does not explain the most interesting feature of the response. Considering the induction of numerous nucleases by DNA and the continuing discovery of new classes of nucleases (183), it seems plausible that DNA damage by pathogens or herbivores can generate DNA fragments that trigger plant immunity via the same pathway that is activated by exogenously applied sonicated DNA. Similarly, the overall grade of conservation that characterises the DDR can serve as an argument that the assumed MRN-dependent activation of ATM (that is known from vertebrates but not yet form plants) is not too speculative. Nevertheless, the self/nonself-specific induction remains without an explanation. Although two transcriptomic studies revealed that exogenously applied fragments of self- and nonself-DNA induced expression of JA-biosynthetic and responsive genes in A. thaliana (24, 35), we observed that both mutants exhibited the self/nonself-DNA specific induction of JA that showed no detectable differences from the WT. We conclude that intact DDR machinery is required to activate the SA-dependent plant immunity response to extracellular DNA whereas an as-yet unknown mechanism controls the species-specific perception of DNA fragments as DAMPs that activate the JA-dependent wound response. Future studies will have to investigate whether the recently reported different mechanisms by which cGAS molecules from different mammalian species recognise their respective self-DNA (46) provide a starting point to identify the mechanisms that allow for a differential recognition of DNA in a single plant species.
The original contributions presented in the study are included in the article/Supplementary Materials, further inquiries can be directed to the corresponding author.
IV-M, AH-E, and MH conceived and designed the experiments, IV-M performed the experiments and evaluated the data, IV-M and OM performed the statistical analysis, MH and IV-M wrote the first draft of the manuscript. All authors contributed to the article and approved the submitted version.
This research was funded by the Consejo Nacional de Ciencia y Tecnología (CONACYT) de México, grants number 661846 to IV-M and 278283 to MH.
The authors thank Rosa María Adame-Álvarez and Silvia Sánchez-García for help with lab routine work, Stefano Mazzoleni for donating the Arabidopsis thaliana ecotype Col-0 seeds, Jean-Philippe Vielle-Calzada for donating the Arabidopsis thaliana ecotype Cvi-0 seeds, John Délano-Frier for donating the Pseudomonas syringae pv Tomato DC3000 strain and Andrew Bent for donating the Pseudomonas syringae pv Glycinea AvrRpt2 (-) and AvrRpt2 (+) strains. We are indebted Jurriaan Ton for his insightful comments on our observations and help with interpretation of the results, to both referees for their positive and helpful comments and to June Kilpatrick Simpson and Graciela García Guzmán for correcting and reviewing an earlier version of this manuscript.
The authors declare that the research was conducted in the absence of any commercial or financial relationships that could be construed as a potential conflict of interest.
All claims expressed in this article are solely those of the authors and do not necessarily represent those of their affiliated organizations, or those of the publisher, the editors and the reviewers. Any product that may be evaluated in this article, or claim that may be made by its manufacturer, is not guaranteed or endorsed by the publisher.
The Supplementary Material for this article can be found online at: https://www.frontiersin.org/articles/10.3389/fimmu.2023.1175786/full#supplementary-material
1. Isaacs A, Rotem Z, Cox RA. Foreign nucleic acids as stimulus to make interferon. Lancet (1963) 2:113–6. doi: 10.1016/S0140-6736(63)92585-6
2. Rotem Z, Cox RA, Isaacs A. Inhibition of virus multiplication by foreign nucleic acid. Nature (1963) 197:564–6. doi: 10.1038/197564a0
3. Mechnikov I. On the present state of the question of immunity in infectious diseases (1908). Available at: https://www.nobelprize.org/prizes/medicine/1908/mechnikov/lecture/ (Accessed 28 Feb 2019).
4. Gallucci S, Maffei M. DNA Sensing across the tree of life. Trends Immunol (2017) 38:719–32. doi: 10.1016/j.it.2017.07.012
6. Ablasser A, Chen ZJJ. cGAS in action: expanding roles in immunity and inflammation. Science (2019) 363:eaat8657. doi: 10.1126/science.aat8657
7. Schlee M, Hartmann G. Discriminating self from non-self in nucleic acid sensing. Nat Rev Immunol (2016) 16:566–80. doi: 10.1038/nri.2016.78
8. Dempsey A, Bowie AG. Innate immune recognition of DNA: a recent history. Virology (2015) 479–480:146–52. doi: 10.1016/j.virol.2015.03.013
9. Yakushiji S, Ishiga Y, Inagaki Y, Toyoda K, Shiraishi T, Ichinose Y. Bacterial DNA activates immunity in Arabidopsis thaliana. J Gen Plant Pathol (2009) 75:227–34. doi: 10.1007/s10327-009-0162-4
10. O’Neill LAJ. Sensing the dark side of DNA. Science (2013) 339:763–4. doi: 10.1126/science.1234724
11. Naqvi I, Giroux N, Olson L, Morrison SA, Llanga T, Akinade TO, et al. DAMPs/PAMPs induce monocytic TLR activation and tolerance in COVID-19 patients; nucleic acid binding scavengers can counteract such TLR agonists. Biomaterials (2022) 283:121393. doi: 10.1016/j.biomaterials.2022.121393
12. Di Domizio J, Gulen MF, Saidoune F, Thacker VV, Yatim A, Sharma K, et al. The cGAS-STING pathway drives type I IFN immunopathology in COVID-19. Nature (2022) 603:145–51. doi: 10.1038/s41586-022-04421-w
13. Lind NA, Rael VE, Pestal K, Liu B, Barton GM. Regulation of the nucleic acid-sensing toll-like receptors. Nat Rev Immunol (2022) 22:224–35. doi: 10.1038/s41577-021-00577-0
14. Vora SM, Lieberman J, Wu H. Inflammasome activation at the crux of severe COVID-19. Nat Rev Immunol (2021) 21:694–703. doi: 10.1038/s41577-021-00588-x
15. Paludan SR, Reinert LS, Hornung V. DNA-Stimulated cell death: implications for host defence, inflammatory diseases and cancer. Nat Rev Immunol (2019) 19:141–53. doi: 10.1038/s41577-018-0117-0
16. Vénéreau E, Ceriotti C, Bianchi ME. DAMPs from death to new life. Front Immunol (2015) 6:422. doi: 10.3389/fimmu.2015.00422
17. Clarivate Web of Science™. DNA Sensing (2023). Available at: https://www.webofscience.com/wos/woscc/summary/2861046b-2626-44ae-a4ae-3e26ba4659f0-80bd4f46/relevance/1 (Accessed January 29, 2023).
18. Mazzoleni S, Bonanomi G, Incerti G, Chiusano ML, Termolino P, Mingo A, et al. Inhibitory and toxic effects of extracellular self-DNA in litter: a mechanism for negative plant–soil feedbacks? New Phytol (2015) 205:1195–210. doi: 10.1111/nph.13121
19. Lee B, Park YS, Lee S, Song GC, Ryu CM. Bacterial RNAs activate innate immunity in arabidopsis. New Phytol (2016) 209:785–97. doi: 10.1111/nph.13717
20. Barbero F, Guglielmotto M, Capuzzo A, Maffei ME. Extracellular self-DNA (esDNA), but not heterologous plant or insect DNA (etDNA), induces plasma membrane depolarization and calcium signaling in Lima bean (Phaseolus lunatus) and maize (Zea mays). Internat J Mol Sci (2016) 17:1659. doi: 10.3390/ijms17101659
21. Duran-Flores D, Heil M. Extracellular self-DNA as a damage-associated molecular pattern (DAMP) that triggers self-specific immunity induction in plants. Brain Behav Immun (2018) 72:78–88. doi: 10.1016/j.bbi.2017.10.010
22. Vega-Muñoz I, Feregrino-Pérez AA, Torres-Pacheco I, Guevara-González RG. Exogenous fragmented DNA acts as a damage-associated molecular pattern (DAMP) inducing changes in CpG DNA methylation and defence-related responses in Lactuca sativa. Funct Plant Biol (2018) 45:1065–72. doi: 10.1071/FP18011
23. Toum L, Conti G, Guerriero FC, Conforte VP, Garolla FA, Asurmendi S, et al. Single-stranded oligodeoxynucleotides induce plant defence in Arabidopsis thaliana. Ann Bot (2020) 126:413–22. doi: 10.1093/aob/mcaa061
24. Chiusano ML, Incerti G, Colantuono C, Termolino P, Palomba E, Monticolo F, et al. Arabidopsis thaliana response to extracellular DNA: self versus nonself exposure. Plants (2021) 10:1744. doi: 10.3390/plants10081744
25. Barbero F, Guglielmotto M, Islam M, Maffei ME. Extracellular fragmented self-DNA is involved in plant responses to biotic stress. Front Plant Sci (2021) 12:686121. doi: 10.3389/fpls.2021.686121
26. Rassizadeh L, Cervero R, Flors V, Gamir J. Extracellular DNA as an elicitor of broad-spectrum resistance in Arabidopsis thaliana. Plant Sci (2021) 312:111036. doi: 10.1016/j.plantsci.2021.111036
27. Serrano-Jamaica LM, Villordo-Pineda E, González-Chavira MM, Guevara-González RG, Medina-Ramos G. Effect of fragmented DNA from plant pathogens on the protection against wilt and root rot of Capsicum annuum l. plants. Front Plant Sci (2021) 11:581891. doi: 10.3389/fpls.2020.581891
28. Meitha K, Esyanti RR, Iriawati RH. Hanisia, and rohyani, green pesticide: tapping to the promising roles of plant secreted small RNAs and responses towards extracellular DNA. Non-coding RNA Res (2021) 6:42–50. doi: 10.1016/j.ncrna.2021.02.001
29. Ferrusquía-Jiménez NI, Serrano-Jamaica LM, Martínez-Camacho JE, Sáenz de la O D, Villagomez-Aranda AL, González-Chavira MM, et al. Extracellular self-DNA plays a role as a damage-associated molecular pattern (DAMP) delaying zoospore germination rate and inducing stress-related responses in Phytophthora capsici. Plant Pathol (2022) 71:1066–75. doi: 10.1111/ppa.13545
30. Bonanomi G, Zotti M, Idbella M, Termolino P, De Micco V, Mazzoleni S. Field evidence for litter and self-DNA inhibitory effects on Alnus glutinosa roots. New Phytol (2022) 236:399–412. doi: 10.1111/nph.18391
31. Palomba E, Chiaiese P, Termolino P, Paparo R, Filippone E, Mazzoleni S, et al. Effects of extracellular self- and nonself-DNA on the freshwater microalga Chlamydomonas reinhardtii and on the marine microalga Nannochloropsis gaditana. Plants (2022) 11:1436. doi: 10.3390/plants11111436
32. Lanzotti V, Grauso L, Mangoni A, Termolino P, Palomba E, Anzano A, et al. Metabolomics and molecular networking analyses in arabidopsis thaliana show that extracellular self-DNA affects nucleoside/nucleotide cycles with accumulation of cAMP, cGMP and N6-methyl-AMP. Phytochemistry (2022) 204:113453. doi: 10.1016/j.phytochem.2022.113453
33. Carbajal-Valenzuela IA, Guzmán-Cruz R, González-Chavira MM, Medina-Ramos G, Serrano-Jamaica LM, Torres-Pacheco I, et al. Response of plant immunity markers to early and late application of extracellular DNA from different sources in tomato (Solanum lycopersicum). Agriculture (2022) 12:1587. doi: 10.3390/agriculture12101587
34. Li C, Wang K, Zou Y, Lei C, Chen Z, Zheng Y. Extracellular self-DNA induced a PTI-related local defence against Rhizopus rot in postharvest peach fruit. Postharvest Biol Technol (2023) 200:112306. doi: 10.1016/j.postharvbio.2023.112306
35. Zhou X, Gao H, Zhang X, Khashi u Rahman M, Mazzoleni S, Du M, et al. Plant extracellular self-DNA inhibits growth and induces immunity via the jasmonate signaling pathway. Plant Physiol (2023). doi: 10.1093/plphys/kiad195
36. Duran-Flores D, Heil M. The CpG-dependent plant immune response to self-DNA triggers defence hormone signalling and improves fitness, 03 march 2023, PREPRINT (Version 1). Res Square preprint (2023). doi: 10.21203/rs.3.rs-2649049/v1
37. Mazzoleni S, Cartenì F, Bonanomi G, Senatore M, Termolino P, Giannino F, et al. Inhibitory effects of extracellular self-DNA: a general biological process? New Phytol (2015) 206:127–32. doi: 10.1111/nph.13306
38. Germoglio M, Adamo A, Incerti G, Cartenì F, Gigliotti S, Storlazzi A, et al. Self-DNA exposure induces developmental defects and germline DNA damage response in Caenorhabditis elegans. Biology (2022) 11:262. doi: 10.3390/biology11020262
39. Klosterman SJ, Hadwiger LA. Plant HMG proteins bearing the AT-hook motif. Plant Sci (2002) 162:855–66. doi: 10.1016/s0168-9452(02)00056-0
40. Jewell JB, Sowders JM, He RF, Willis MA, Gang DR, Tanaka K. Extracellular ATP shapes a defense-related transcriptome both independently and along with other defense signaling pathways. Plant Physiol (2019) 179:1144–58. doi: 10.1104/pp.18.01301
41. Tanaka K, Heil M. Damage-associated molecular patterns (DAMPs) in plant innate immunity: applying the danger model and evolutionary perspectives. Annu Rev Phytopathol (2021) 59:53–75. doi: 10.1146/annurev-phyto-082718-100146
42. Duran-Flores D, Heil M. Sources of specificity in plant damaged-self recognition. Curr Opin Plant Biol (2016) 32:77–87. doi: 10.1016/j.pbi.2016.06.019
43. Vega-Muñoz I, Duran-Flores D, Fernández-Fernández Á.D., Heyman J, Ritter A, Stael S. Breaking bad news: dynamic molecular mechanisms of wound response in plants. Front Plant Sci (2020) 11:610445. doi: 10.3389/fpls.2020.610445
44. Heil M, Land WG. Danger signals - damaged-self recognition across the tree of life. Front Plant Sci (2014) 5:578. doi: 10.3389/fpls.2014.00578
45. Decout A, Katz JD, Venkatraman S, Ablasser A. The cGAS–STING pathway as a therapeutic target in inflammatory diseases. Nat Rev Immunol (2021) 21:548–69. doi: 10.1038/s41577-021-00524-z
46. Mosallanejad K, Kennedy SN, Bahleda KM, Slavik KM, Zhou W, Govande AA, et al. Species-specific self-DNA detection mechanisms by mammalian cyclic GMP-AMP synthases. Sci Immunol (2023) 8:eabp9765. doi: 10.1126/sciimmunol.abp9765
47. Maréchal A, Zou L. DNA Damage sensing by the ATM and ATR kinases. Cold Spring Harb Perspect Biol (2013) 5:a012716. doi: 10.1101/cshperspect.a012716
48. Rong Z, Tu P, Xu P, Sun Y, Yu F, Tu N, et al. The mitochondrial response to DNA damage. Front Cell Dev Biol (2021) 9:669379. doi: 10.3389/fcell.2021.669379
49. Duan S, Hu L, Dong B, Jin H-L, Wang H-B. Signaling from plastid genome stability modulates endoreplication and cell cycle during plant development. Cell Rep (2020) 32:108019. doi: 10.1016/j.celrep.2020.108019
50. Boesch P, Weber-Lotfi F, Ibrahim N, Tarasenko V, Cosset A, Paulus F, et al. DNA Repair in organelles: pathways, organization, regulation, relevance in disease and aging. Biochim Biophys Acta Mol Cell Res (2011) 1813:186–200. doi: 10.1016/j.bbamcr.2010.10.002
51. Nisa MU, Huang Y, Benhamed M, Raynaud C. The plant DNA damage response: signaling pathways leading to growth inhibition and putative role in response to stress conditions. Front Plant Sci (2019) 10:653. doi: 10.3389/fpls.2019.00653
52. Schwochau ME, Hadwiger LA. Regulation of gene expression by actinomycin d and other compounds which change the conformation of DNA. Arch Biochem Biophys (1969) 134:34–41. doi: 10.1016/0003-9861(69)90247-1
53. Hadwiger LA, Schwochau ME. Role of dyes and other DNA intercalating compounds in activating genes for phytoalexin production. Phytopathology (1970) 60:1294. doi: 10.1094/Phyto-60-332
54. Hadwiger LA, Schwochau ME. Specificity of deoxyribonucleic acid intercalating compounds in the control of phenylalanine ammonia lyase and pisatin levels. Plant Physiol (1971) 47:346–51. doi: 10.1104/pp.47.3.346
55. Hadwiger LA, Tanaka K. DNA Damage and chromatin conformation changes confer nonhost resistance: a hypothesis based on effects of anti-cancer agents on plant defense responses. Front Plant Sci (2018) 9:1056. doi: 10.3389/fpls.2018.01056
56. Hadwiger LA, Tanaka K. Non-host resistance: DNA damage is associated with SA signaling for induction of PR genes and contributes to the growth suppression of a pea pathogen on pea endocarp tissue. Front Plant Sci (2017) 8:446. doi: 10.3389/fpls.2017.00446
57. Lucht JM, Mauch-Mani B, Steiner H-Y, Metraux J-P, Ryals J, Hohn B. Pathogen stress increases somatic recombination frequency in arabidopsis. Net Genet (2002) 30:311–4. doi: 10.1038/ng846
58. Durrant WE, Wang S, Dong X. Arabidopsis SNI1 and RAD51D regulate both gene transcription and DNA recombination during the defense response. Proc Natl Acad Sci USA (2007) 104:4223–7. doi: 10.1073/pnas.0609357104
59. Yan S, Wang W, Marqués J, Mohan R, Saleh A, Wendy E, et al. Salicylic acid activates DNA damage responses to potentiate plant immunity. Mol Cell (2013) 52:602–10. doi: 10.1016/j.molcel.2013.09.019
60. Yoshiyama KO, Aoshima N, Takahashi N, Sakamoto T, Hiruma K, Saijo Y, et al. SUPPRESSOR OF GAMMA RESPONSE 1 acts as a regulator coordinating crosstalk between DNA damage response and immune response in Arabidopsis thaliana. Plant Mol Biol (2020) 103:321–40. doi: 10.1007/s11103-020-00994-0
61. Rodriguez E, Chevalier J, El Ghoul H, Voldum-Clausen K, Mundy J, Petersen M. DNA Damage as a consequence of NLR activation. PloS Genet (2018) 14:e1007235. doi: 10.1371/journal.pgen.1007235
62. Shiloh Y, Ziv Y. The ATM protein kinase: regulating the cellular response to genotoxic stress, and more. Nat Rev Mol Cell Biol (2013) 14:197–210. doi: 10.1038/nrm3546
63. Bhat A, Ryu C-M. Plant perceptions of extracellular DNA and RNA. Mol Plant (2016) 9:956–8. doi: 10.1016/j.molp.2016.05.014
64. Heil M, Vega-Muñoz I. Nucleic acid sensing in mammals and plants - facts and caveats. Int Rev Cell Mol Biol (2019) 345:225–85. doi: 10.1016/bs.ircmb.2018.10.003
65. Pavlopoulou A, Karaca E, Balestrazzi A, Georgakilas AG. In silico phylogenetic and structural analyses of plant endogenous danger signaling molecules upon stress. Oxid Med Cell Longev (2019) 2019):8683054. doi: 10.1155/2019/8683054
66. Fichman Y, Mittler R. Rapid systemic signaling during abiotic and biotic stresses: is the ROS wave master of all trades? Plant J (2020) 102:887–96. doi: 10.1111/tpj.14685
67. Mittler R, Vanderauwera S, Suzuki N, Miller G, Tognetti VB, Vandepoele K, et al. ROS signaling: the new wave? Trends Plant Sci (2011) 16:300–9. doi: 10.1016/j.tplants.2011.03.007
68. Myers RJ Jr, Fichman Y, Stacey G, Mittler R. Extracellular ATP plays an important role in systemic wound response activation. Plant Physiol (2022) 189:1314–25. doi: 10.1093/plphys/kiac148
69. Backer R, Naidoo S, van den Berg N. The NONEXPRESSOR OF PATHOGENESIS-RELATED GENES 1 (NPR1) and related family: mechanistic insights in plant disease resistance. Front Plant Sci (2019) 10:102. doi: 10.3389/fpls.2019.00102
70. Cao H, Glazebrook J, Clarke JD, Volko S, Dong XN. The Arabidopsis NPR1 gene that controls systemic acquired resistance encodes a novel protein containing ankyrin repeats. Cell (1997) 88:57–63. doi: 10.1016/S0092-8674(00)81858-9
71. Wasternack C, Feussner I. The oxylipin pathways: biochemistry and function. In: Merchant SS, editor. Annual review of plant biology, vol. 69. (Palo Alto, CA: Annual Reviews) (2018). p. 363–86.
72. Lefevere H, Bauters L, Gheysen G. Salicylic acid biosynthesis in plants. Front Plant Sci (2020) 11:338. doi: 10.3389/fpls.2020.00338
73. Wang C, Zien CA, Afitlhile M, Welti R, Hildebrand DF, Wang X. Involvement of phospholipase d in wound-induced accumulation of jasmonic acid in arabidopsis. Plant Cell (2000) 12:2237–46. doi: 10.1105/tpc.12.11.2237
74. Ding P, Ding Y. Stories of salicylic acid: a plant defense hormone. Trends Plant Sci (2020) 25:549–65. doi: 10.1016/j.tplants.2020.01.004
75. Zhao J. Phospholipase d and phosphatidic acid in plant defence response: from protein–protein and lipid–protein interactions to hormone signalling. J Exp Bot (2015) 66:1721–36. doi: 10.1093/jxb/eru540
76. Ellinger D, Stingl N, Kubigsteltig II, Bals T, Juenger M, Pollmann S, et al. DONGLE and DEFECTIVE IN ANTHER DEHISCENCE1 lipases are not essential for wound- and pathogen-induced jasmonate biosynthesis: redundant lipases contribute to jasmonate formation. Plant Physiol (2010) 153:114–27. doi: 10.1104/pp.110.155093
77. Klessig DF, Choi HW, Dempsey DA. Systemic acquired resistance and salicylic acid: past, present, and future. Mol Plant-Microbe Interact (2018) 31:871–88. doi: 10.1094/mpmi-03-18-0067-cr
78. Durrant WE, Dong X. Systemic acquired resistance. Annu Rev Phytopathol (2004) 42:185–209. doi: 10.1146/annurev.phyto.42.040803.140421
79. Culligan KM, Robertson CE, Foreman J, Doerner P, Britt AB. ATR and ATM play both distinct and additive roles in response to ionizing radiation. Plant J (2006) 48:947–61. doi: 10.1111/j.1365-313X.2006.02931.x
80. Yoshiyama KO, Kobayashi J, Ogita N, Ueda M, Kimura S, Maki H, et al. ATM-Mediated phosphorylation of SOG1 is essential for the DNA damage response in arabidopsis. EMBO Rep (2013) 14:817–22. doi: 10.1038/embor.2013.1122
81. SIGnAL. T-DNA Primer Design. (2010). Available at: http://signal.salk.edu/tdnaprimers.2.html (Accessed 15 January, 2021).
82. Yu IC, Parker J, Bent AF. Bent, gene-for-gene disease resistance without the hypersensitive response in Arabidopsis dnd1 mutant. Proc Natl Acad Sci USA (1998) 95:7819–24. doi: 10.1073/pnas.95.13.7819
83. Daniels MJ, Barber CE, Turner PC, Cleary WG, Sawczyc MK. Isolation of mutants of Xanthomonas campestris pv. campestris showing altered pathogenicity. Microbiology (1984) 130:2447–55. doi: 10.1099/00221287-130-9-2447
84. Healey A, Furtado A, Cooper T, Henry RJ. Protocol: a simple method for extracting next-generation sequencing quality genomic DNA from recalcitrant plant species. Plant Methods (2014) 10:21. doi: 10.1186/1746-4811-10-21
85. Junglee S, Urban L, Sallanon H, Lopez-Lauri F. Optimized assay for hydrogen peroxide determination in plant tissue using potassium iodide. Am J Analytical Chem (2014) 5:730–6. doi: 10.4236/ajac.2014.511081
86. Duran-Flores D, Heil M. Damaged-self recognition in common bean (Phaseolus vulgaris) shows taxonomic specificity and depends on reactive oxygen species (ROS) signalling. Front Plant Sci (2014) 5:585. doi: 10.3389/fpls.2014.00585
87. Pluskota WE, Qu N, Maitrejean M, Boland W, Baldwin IT. Jasmonates and its mimics differentially elicit systemic defence responses in Nicotiana attenuata. J Exp Bot (2007) 58:4071–82. doi: 10.1093/jxb/erm263
88. Mueller MJ, Brodschelm W. Quantification of jasmonic acid by capillary gas chromatography-negative chemical ionization-mass spectrometry. Analyt Biochem (1994) 218:425–35. doi: 10.1006/abio.1994.1202
89. Malamy J, Hennig J, Klessig DF. Temperature-dependent induction of salicylic acid and its conjugates during the resistance response to Tobacco mosaic virus infection. Plant Cell (1992) 4:359–36. doi: 10.2307/3869546
90. Meuwly P, Metraux JP. Ortho-anisic acid as internal standard for the simultaneous quantitation of salicylic acid and its putative biosynthetic precursors in cucumber leaves. Analyt Biochem (1993) 214:500–5. doi: 10.1006/abio.1993.1529
91. Ramírez-Chavez E, López-Bucio J, Herrera-Estrella L, Molina-Torres J. Alkamides isolated from plants promote growth and alter root development in arabidopsis. Plant Physiol (2004) 134:1058–68. doi: 10.1104/pp.103.034553
92. Whalen MC, Innes RW, Bent AF, Staskawicz BJ. Identification of pseudomonas syringae pathogens of arabidopsis and a bacterial locus determining avirulence on both arabidopsis and soybean. Plant Cell (1991) 3:49–59. doi: 10.1105/tpc.3.1.49
93. Song JQ, Bent AF. Microbial pathogens trigger host DNA double-strand breaks whose abundance is reduced by plant defense responses. PloS Pathog (2014) 10:e1004030. doi: 10.1371/journal.ppat.1004030
94. Chen IP, Haehnel U, Altschmied L, Schubert I, Puchta H. The transcriptional response of arabidopsis to genotoxic stress - a high-density colony array study (HDCA). Plant J (2003) 35:771–86. doi: 10.1046/j.1365-313X.2003.01847.x
95. Choi JJ, Klosterman SJ, Hadwiger LA. A comparison of the effects of DNA-damaging agents and biotic elicitors on the induction of plant defense genes, nuclear distortion, and cell death. Plant Physiol (2001) 125:752–62. doi: 10.1104/pp.125.2.752
96. Wang CG, Huang XE, Li Q, Zhang YP, Li JL, Mou ZL. Extracellular pyridine nucleotides trigger plant systemic immunity through a lectin receptor kinase/BAK1 complex. Nat Comm (2019) 10:4810. doi: 10.1038/s41467-019-12781-7
97. Gonzalez N, De Bodt S, Sulpice R, Jikumaru Y, Chae E, Dhondt S, et al. Increased leaf size: different means to an end. Plant Physiol (2010) 153:1261–79. doi: 10.1104/pp.110.156018
98. Leitch IJ, Johnston E, Pellicer J, Hidalgo O, Bennett MD. Plant DNA c-values. Release 7.1. Kew Botanical Gardens (2019). Available at: https://cvalues.science.kew.org/ (Accessed 15 Dec 2022).
99. Renbaum P, Abrahamove D, Fainsod A, Wilson GG, Rottem S, Razin A. Cloning, characterization, and expression in Escherichia coli of the gene coding for the GpG DNA methylase from Spiroplasma sp. strain MW1 (M.SssI). Nucleic Acids Res (1990) 18:1145–52. doi: 10.1093/nar/18.5.1145
100. R Core Team. : a language and environment for statistical computing. version (2022). Available at: https://www.R-project.org/.
101. Posit Team. RStudio: integrated development environment for r. version (2022). Available at: http://www.posit.co/.
102. Ritz C, Baty F, Streibig JC, Gerhard D. Dose-response analysis using r. PloS One (2016) 10:e0146021. doi: 10.1371/journal.pone.0146021
103. Wickham H, Averick M, Jennifer B, Chang W, D’Agostino McGowan LF, Romain, et al. Welcome to the tidyverse. J Open Source Software (2019) 4:1686. doi: 10.21105/joss.01686
104. Graves S, Piepho H-P, Selzer L, Dorai-Raj S. multcompView: visualizations of paired comparisons. version 0.1-8 (2019). Available at: https://CRAN.R-project.org/package=multcompView.
105. Clarke E, Sherril-Mix S, Dawson C. Ggbeeswarm: categorical scatter (Violin point) plots. version 0.6.0 (2016). Available at: https://CRAN.R-project.org/package=ggbeeswarm.
106. Wilke CO, Wiernik ggtext BM. Improved text rendering support for ‘ggplot2’. version 0.1.2 (2022). Available at: https://CRAN.R-project.org/package=ggt.
107. Slowikowski K, Schep A, Hughes S, Dang TK, Lukauskas S, Irisson J-O, et al. Ggrepel: automatically position non-overlapping text labels with ‘ggplot2’. version 0.9.1 (2021). Available at: https://CRAN.R-project.org/package=ggrepel.
108. Kassambara A. Rstatix: pipe-friendly framework for basic statistical tests. version 0.7.0 (2021). Available at: https://CRAN.R-project.org/package=rstatix.
109. Okude H, Ori D, Kawai T. Signaling through nucleic acid sensors and their roles in inflammatory diseases. Front Immunol (2021) 11:625833. doi: 10.3389/fimmu.2020.625833
110. Janeway CA. The immune system evolved to discriminate infectious nonself from noninfectious self. Immunol Today (1992) 13:11–6. doi: 10.1016/0167-5699(92)90198-G
111. Matzinger P. The danger model: a renewed sense of self. Science (2002) 296:301–5. doi: 10.1126/science.1071059
112. Matzinger P. Tolerance, danger, and the extended family. Annu Rev Immunol (1994) 12:991–1045. doi: 10.1146/annurev.iy.12.040194.005015
113. Land W, Schneeberger H, Schleibner S, Illner WD, Abendroth D, Rutili G, et al. The beneficial effect of human recombinant superoxide dismutase on acute and chronic rejection events in recipients of cadaveric renal transplants. Transplantation (1994) 57:211–7. doi: 10.1097/00007890-199401001-00010
114. Land WG. How evolution tells us to induce allotolerance. Exp Clin Transplant (2015) 13:46–54. doi: 10.6002/ect.mesot2014.L69
115. Land WG. The role of damage-associated molecular patterns in human diseases: part I - promoting inflammation and immunity. SQU Med J (2015) 15:e9–e21.
116. Zipfel C. Pattern-recognition receptors in plant innate immunity. Curr Opin Immunol (2008) 20:10–6. doi: 10.1016/j.coi.2007.11.003
117. Li Q, Wang CG, Mou ZL. Perception of damaged self in plants. Plant Physiol (2020) 182:1545–65. doi: 10.1104/pp.19.01242
118. Mermigka G, Amprazi M, Mentzelopoulou A, Amartolou A, Sarris PF. Plant and animal innate immunity complexes: fighting different enemies with similar weapons. Trends Plant Sci (2020) 25:80–91. doi: 10.1016/j.tplants.2019.09.008
119. McConn M, Creelman RA, Bell E, Mullet JE, Browse J. Jasmonate is essential for insect defense in Arabidopsis. Proc Natl Acad Sci USA (1997) 94:5473–7. doi: 10.1073/pnas.94.10.5473
120. Glauser G, Grata E, Dubugnon L, Rudaz S, Farmer EE, Wolfender J-L. Spatial and temporal dynamics of jjasmonate synthesis and accumulation in arabidopsis in response to wounding. J Biol Chem (2008) 283:16400–7. doi: 10.1074/jbc.M801760200
121. Ogawa T, Ara T, Aoki K, Suzuki H, Shibata D. Transient increase in salicylic acid and its glucose conjugates after wounding in arabidopsis leaves. Plant Biotechnol (2010) 27:205–9. doi: 10.5511/plantbiotechnology.27.205
122. Smetana O, Široký J, Houlné G, Opatrný Z, Chabouté M-E. Non-apoptotic programmed cell death with paraptotic-like features in bleomycin-treated plant cells is suppressed by inhibition of ATM/ATR pathways or NtE2F overexpression. J Exp Bot (2012) 63:2631–44. doi: 10.1093/jxb/err439
123. Šamanić I, Cvitanić R, Simunić J, Puizina J. Arabidopsis thaliana MRE11 is essential for activation of cell cycle arrest, transcriptional regulation and DNA repair upon the induction of double-stranded DNA breaks. Plant Biol (2016) 18:681–94. doi: 10.1111/plb.12453
124. Century KS, Holub EC, Staskawicz BJ. NDRJ, a locus of Arabidopsis thaliana that is required for disease resistance to both a bacterial and a fungal pathogen. Proc Natl Acad Sci USA (1995) 92:6597–601. doi: 10.1073/pnas.92.14.6597
125. Kunkel BN, Bent AF, Dahlbeck D, Innes RW, Staskawicz BJ. RPS2, an arabidopsis disease resistance locus specifying recognition of Pseudomonas syringae strains expressing the avirulence gene avrRpt2. Plant Cell (1993) 5:865–75. doi: 10.2307/3869655
126. Nakad R, Schumacher B. DNA Damage response and immune defense: links and mechanisms. Front Genet (2016) 7:147. doi: 10.3389/fgene.2016.00147
127. Tripathi D, Nam A, Oldenburg DJ, Bendich AJ. Reactive oxygen species, antioxidant agents, and DNA damage in developing maize mitochondria and plastids. Front Plant Sci (2020) 11:596. doi: 10.3389/fpls.2020.00596
128. Azzouz D, Palaniyar N. ROS and DNA repair in spontaneous versus agonist-induced NETosis: context matters. Front Immunol (2022) 13:1033815. doi: 10.3389/fimmu.2022.1033815
129. Cimini S, Gualtieri C, Macovei A, Balestrazzi A, De Gara L, Locato V. Redox balance-DDR-miRNA triangle: relevance in genome stability and stress responses in plants. Front Plant Sci (2019) 10:989. doi: 10.3389/fpls.2019.00989
130. Gómez-Oliván LM, Galar-Martínez M, Islas-Flores H, García-Medina S, SanJuan-Reyes N. DNA Damage and oxidative stress induced by acetylsalicylic acid in Daphnia magna. Com Biochem Physiol Part - C Toxicol Pharmacol (2014) 164:21–6. doi: 10.1016/j.cbpc.2014.04.004
131. Chernov MV, Stark GR. The p53 activation and apoptosis induced by DNA damage are reversibly inhibited by salicylate. Oncogene (1997) 14:2503–10. doi: 10.1038/sj.onc.1201104
132. Woloschak GE, Panozzo J, Schreck S, Libertin CR. Salicylic acid inhibits ultraviolet-induced and cis-platinum-induced human immunodeficiency-virus expression. Cancer Res (1995) 55:1696–700.
133. Heil M. Damaged-self recognition in plant herbivore defence. Trends Plant Sci (2009) 14:356–63. doi: 10.1016/j.tplants.2009.04.002
134. Heil F, Hemmi H, Hochrein H, Ampenberger F, Kirschning C, Akira S, et al. Species-specific recognition of single-stranded RNA via toll-like receptor 7 and 8. Science (2004) 303:1526–9. doi: 10.1126/science.1093620
135. Krieg AM, Yi A-K, Matson S, Waldschmidt TJ, Bishop GA, Teasdale R, et al. CpG motifs in bacterial DNA trigger direct b-cell activation. Nature (1995) 374:546–9. doi: 10.1038/374546a0
136. Hemmi H, Takeuchi O, Kawai T, Kaisho T, Sato S, Sanjo H, et al. A toll-like receptor recognizes bacterial DNA. Nature (2000) 408:740–5. doi: 10.1038/35047123
137. Bauer S, Kirschning CJ, Häcker H, Redecke V, Hausmann S, Akira S, et al. Human TLR9 confers responsiveness to bacterial DNA via species-specific CpG motif recognition. Proc Natl Acad Sci USA (2001) 98:9237–42. doi: 10.1073/pnas.161293498
138. Lee J, Shin S-Y, Lee S-K, Park K, Gill H, Hyun Y, et al. Contribution of RdDM to the ecotype-specific differential methylation on conserved as well as highly variable regions between arabidopsis ecotypes. BMC Genom (2023) 24:36. doi: 10.1186/s12864-023-09128-4
139. Mahapatra K, Roy S. SOG1 transcription factor promotes the onset of endoreduplication under salinity stress in arabidopsis. Sci Rep (2021) 11:11659. doi: 10.1038/s41598-021-91293-1
140. Waterworth WM, Altun C, Armstrong SJ, Roberts N, Dean PJ, Young K, et al. NBS1 is involved in DNA repair and plays a synergistic role with ATM in mediating meiotic homologous recombination in plants. Plant J (2007) 52:41–52. doi: 10.1111/j.1365-313X.2007.03220.x
141. Choi HW, Wang L, Powell AF, Strickler SR, Wang D, Dempsey DMA, et al. A genome-wide screen for human salicylic acid (SA)-binding proteins reveals targets through which SA may influence development of various diseases. Sci Rep (2019) 9:13084. doi: 10.1038/s41598-019-49234-6
142. Fingrut O, Flescher E. Plant stress hormones suppress the proliferation and induce apoptosis in human cancer cells. Leukemia (2002) 16:608–16. doi: 10.1038/sj.leu.2402419
143. Zebell SG, Dong X. Cell-cycle regulators and cell death in immunity. Cell Host Microbe (2015) 18:402–7. doi: 10.1016/j.chom.2015.10.001
144. Song JQ, Durrant WE, Wang S, Yan SP, Tan EH, Dong XN. DNA Repair proteins are directly involved in regulation of gene expression during plant immune response. Cell Host Microbe (2011) 9:115–24. doi: 10.1016/j.chom.2011.01.011
145. Wang S, Durrant WE, Song J, Spivey NW, Dong X. Arabidopsis BRCA2 and RAD51 proteins are specifically involved in defense gene transcription during plant immune responses. Proc Natl Acad Sci USA (2010) 107:22716–21. doi: 10.1073/pnas.1005978107
146. Hartney S, Carson J, Hadwiger LA. The use of chemical genomics to detect functional systems affecting the non-host disease resistance of pea to Fusarium solani f. sp phaseoli. Plant Sci (2007) 172:45–56. doi: 10.1016/j.plantsci.2006.07.014
147. Verhagen BWM, Glazebrook J, Zhu T, Chang HS, van Loon LC, Pieterse CMJ. The transcriptome of rhizobacteria-induced systemic resistance in Arabidopsis. Mol Plant-Microbe Interact (2004) 17:895–908. doi: 10.1094/mpmi.2004.17.8.895
148. Heil M, Ibarra-Laclette E, Adame-Álvarez RM, Martínez O, Ramirez-Chávez E, Molina-Torres J, et al. How plants sense wounds: damaged-self recognition is based on plant-derived elicitors and induces octadecanoid signaling. PloS One (2012) 7:e30537. doi: 10.1371/journal.pone.003053
149. Mittler R, Lam E. Characterization of nuclease activities and DNA fragmentation induced upon hypersensitive response cell death and mechanical stress. Plant Mol Biol (1997) 34:209–21. doi: 10.1023/A:1005868402827
150. Kollist H, Zandalinas SI, Sengupta S, Nuhkat M, Kangasjarvi J, Mittler R. Rapid responses to abiotic stress: priming the landscape for the signal transduction network. Trends Plant Sci (2019) 24:25–37. doi: 10.1016/j.tplants.2018.10.003
151. Vlot AC, Sales JH, Lenk M, Bauer K, Brambilla A, Sommer A, et al. Systemic propagation of immunity in plants. New Phytol (2021) 229:1234–50. doi: 10.1111/nph.16953
152. Schwochau ME, Hadwiger LA. Stimulation of pisatin production in Pisum sativum by actinomycin d and other compounds. Arch Biochem Biophys (1968) 126:731–3. doi: 10.1016/0003-9861(68)90463-3
153. Yao Y, Li X, Chen W, Liu H, Mi L, Ren D, et al. ATM Promotes RAD51-mediated meiotic DSB repair by inter-sister-chromatid recombination in Arabidopsis. Front Plant Sci (2020) 11:839. doi: 10.3389/fpls.2020.00839
154. Schuermann D, Molinier J, Fritsch O, Hohn B. The dual nature of homologous recombination in plants. Trends Genet (2005) 21:172–81. doi: 10.1016/j.tig.2005.01.002
155. Adams KE, Medhurst AL, Dart DA, Lakin ND. Recruitment of ATR to sites of ionising radiation-induced DNA damage requires ATM and components of the MRN protein complex. Oncogene (2006) 25:3894–904. doi: 10.1038/sj.onc.1209426
156. Shen MM, Nie YS, Chen YY, Zhang XF, Zhao J. OsMre11 is required for mitosis during rice growth and development. Internat J Mol Sci (2021) 22:169. doi: 10.3390/ijms22010169
157. Kondo T, Kobayashi J, Saitoh T, Maruyama K, Ishii KJ, Barber GN, et al. DNA Damage sensor MRE11 recognizes cytosolic double-stranded DNA and induces type I interferon by regulating STING trafficking. Proc Natl Acad Sci USA (2013) 110:2969–74. doi: 10.1073/pnas.1222694110
158. Ricaud L, Proux C, Renou J-P, Pichon O, Fochesato S, Ortet P, et al. ATM-Mediated transcriptional and developmental responses to γ-rays in arabidopsis. PloS One (2007) 2:e430. doi: 10.1371/journal.pone.0000430
159. Pedroza-Garcia JA, Eekhout T, Achon I, Nisa MU, Coussens G, Vercauteren I, et al. Maize ATR safeguards genome stability during kernel development to prevent early endosperm endocycle onset and cell death. Plant Cell (2021) 33:2662–84. doi: 10.1093/plcell/koab158
160. Kumimoto RW, Ellison CT, Toruño TY, Bak A, Zhang H, Casteel CL, et al. XAP5 CIRCADIAN TIMEKEEPER affects both DNA damage responses and immune signaling in arabidopsis. Front Plant Sci (2021) 12:707923. doi: 10.3389/fpls.2021.707923
161. Liu F, Xu YJ, Zhou LY, Ali A, Jiang HY, Zhu SW, et al. DNA Repair gene ZmRAD51A improves rice and arabidopsis resistance to disease. Internat J Mol Sci (2019) 20:ijms20040807. doi: 10.3390/ijms20040807
162. Liu C-H, Finke A, Díaz M, Rozhon W, Poppenberger B, Baubec T, et al. Repair of DNA damage induced by the cytidine analog zebularine requires ATR and ATM in arabidopsis. Plant Cell (2015) 27:1788–800. doi: 10.1105/tpc.114.135467
163. Culligan K, Tissier A, Britt A. ATR regulates a G2-phase cell-cycle checkpoint in Arabidopsis thaliana. Plant Cell (2004) 16:1091–104. doi: 10.1105/tpc.018903
164. Yoshiyama KO. SOG1: a master regulator of the DNA damage response in plants. Genes Genet Syst (2015) 90:209–16. doi: 10.1266/ggs.15-00011
165. Waterworth WM, Latham R, Wang D, Alsharif M, West CE. Seed DNA damage responses promote germination and growth in Arabidopsis thaliana. Proc Natl Acad Sci USA (2022) 119:e2202172119. doi: 10.1073/pnas.2202172119
166. Mou Z, Fan W, Dong X. Inducers of plant systemic acquired resistance regulate NPR1 function through redox changes. Cell (2003) 113:935–44. doi: 10.1016/S0092-8674(03)00429-X
167. Zhang Y, Xu S, Ding P, Wang D, Cheng YT, He J, et al. Control of salicylic acid synthesis and systemic acquired resistance by two members of a plant-specific family of transcription factors. Proc Natl Acad Sci USA (2010) 107:18220–5. doi: 10.1073/pnas.1005225107
168. Wuerzberger-Davis SM, Nakamura Y, Seufzer BJ, Miyamoto S. NF-κB activation by combinations of NEMO SUMOylation and ATM activation stresses in the absence of DNA damage. Oncogene (2007) 26:641–51. doi: 10.1038/sj.onc.1209815
169. Guo Z, Kozlov S, Lavin MF, Person MD, Paull TT. ATM Activation by oxidative stress. Science (2010) 330:517–21. doi: 10.1126/science.1192912
170. Berger ND, Stanley FKT, Moore S, Goodarzi AA. ATM-Dependent pathways of chromatin remodelling and oxidative DNA damage responses. Phil Transact Roy Soc B (2017) 372:20160283. doi: 10.1098/rstb.2016.0283
171. Paull TT. Mechanisms of ATM activation. Annu Rev Biochem (2015) 84:711–38. doi: 10.1146/annurev-biochem-060614-034335
172. Xiang Y, Laurent B, Hsu C-H, Nachtergaele S, Lu Z, Sheng W, et al. RNA m6A methylation regulates the ultraviolet-induced DNA damage response. Nature (2017) 543:573–6. doi: 10.1038/nature21671
173. Jazayeri A, Balestrini A, Garner E, Haber JE, Costanzo V. Mre11–Rad50–Nbs1-dependent processing of DNA breaks generates oligonucleotides that stimulate ATM activity. EMBO J (2008) 27:1953–62. doi: 10.1038/emboj.2008.128
174. Zitka O, Skalickova S, Gumulec J, Masarik M, Adam V, Hubalek J, et al. Redox status expressed as GSH : GSSG ratio as a marker for oxidative stress in paediatric tumour patients. Oncol Lett (2012) 4:1247–53. doi: 10.3892/ol.2012.931
175. Moons A. Regulatory and functional interactions of plant growth regulators and plant glutathione s-transferases (GSTs). In: Litwack G, editor. Vitamins & hormones. (San Diego, CA: Academic Press) (2005). p. 155–202.
176. Hadwiger LA, Schwochau WE. Induction of phenylalanine ammonia lyase and pisatin in pea pods by poly-lysine, spermidine or histone fractions. Biochem Biophys Res Comm (1970) 38:683–91. doi: 10.1016/0006-291X(70)90635-2
177. Milsted C, Dai B, Garcia N, Yin L, He Y, Kianian S, et al. Genome-wide investigation of maize RAD51 binding affinity through phage display. BMC Genom (2022) 23:199. doi: 10.1186/s12864-022-08419-6
178. Hadwiger LA, Druffel K, Humann JL, Schroeder BK. Nuclease released by Verticillium dahliae is a signal for non-host resistance. Plant Sci (2013) 201:98–107. doi: 10.1016/j.plantsci.2012.11.011
179. Hadwiger LA, Polashock J. Fungal mitochondrial DNases: effectors with the potential to activate plant defenses in nonhost resistance. Phytopathology (2013) 103:81–90. doi: 10.1094/phyto-04-12-0085-r
180. Klosterman SJ, Chen JP, Choi JJ, Chinn EE, Hadwiger LA. Characterization of a 20 kDa DNase elicitor from Fusarium solani f. sp phaseoli and its expression at the onset of induced resistance in Pisum sativum. Mol Plant Pathol (2001) 2:147–58. doi: 10.1046/j.1364-3703.2001.00062.x
181. Park HJ, Wang WW, Curlango-Rivera G, Xiong ZG, Lin ZR, Huskey DA, et al. A DNase from a fungal phytopathogen is a virulence factor likely deployed as counter defense against host-secreted extracellular DNA. mBIO (2019) 10:e02805–18. doi: 10.1128/mBio.02805-18
182. Huang H-J, Cui J-R, Xia X, Chen J, Ye Y-X, Zhang C-X, et al. Salivary DNase II from Laodelphax striatellus acts as an effector that suppresses plant defense. New Phytol (2019) 224:860–74. doi: 10.1111/nph.15792
Keywords: damaged-self recognition, defence signalling, DNA-triggered immunity, eDNA, Mazzoleni effect, plant immune response to DNA, innate immunity
Citation: Vega-Muñoz I, Herrera-Estrella A, Martínez-de la Vega O and Heil M (2023) ATM and ATR, two central players of the DNA damage response, are involved in the induction of systemic acquired resistance by extracellular DNA, but not the plant wound response. Front. Immunol. 14:1175786. doi: 10.3389/fimmu.2023.1175786
Received: 28 February 2023; Accepted: 27 April 2023;
Published: 15 May 2023.
Edited by:
Nadine Laguette, Center for the National Scientific Research (CNRS), FranceReviewed by:
Edmund Kozieł, Warsaw University of Life Sciences, PolandCopyright © 2023 Vega-Muñoz, Herrera-Estrella, Martínez-de la Vega and Heil. This is an open-access article distributed under the terms of the Creative Commons Attribution License (CC BY). The use, distribution or reproduction in other forums is permitted, provided the original author(s) and the copyright owner(s) are credited and that the original publication in this journal is cited, in accordance with accepted academic practice. No use, distribution or reproduction is permitted which does not comply with these terms.
*Correspondence: Martin Heil, bWFydGluLmhlaWxAY2ludmVzdGF2Lm14
Disclaimer: All claims expressed in this article are solely those of the authors and do not necessarily represent those of their affiliated organizations, or those of the publisher, the editors and the reviewers. Any product that may be evaluated in this article or claim that may be made by its manufacturer is not guaranteed or endorsed by the publisher.
Research integrity at Frontiers
Learn more about the work of our research integrity team to safeguard the quality of each article we publish.