- 1Department of Infectious Diseases, Chongqing Public Health Medical Center, Chongqing, China
- 2Clinical Research Center, Chongqing Public Health Medical Center, Chongqing, China
- 3Cancer Center, Medical Research Institute, Southwest University, Chongqing, China
- 4Infectious Diseases and Immunity in Global Health Program, Research Institute, McGill University Health Centre, Montréal, QC, Canada
- 5Chronic Viral Illness Service, McGill University Health Centre, Montréal, QC, Canada
- 6Canadian HIV Trials Network, Canadian Institutes for Health Research, Vancouver, BC, Canada
- 7Division of Hematology, McGill University Health Centre, Montréal, QC, Canada
The intestinal barrier has the daunting task of allowing nutrient absorption while limiting the entry of microbial products into the systemic circulation. HIV infection disrupts the intestinal barrier and increases intestinal permeability, leading to microbial product translocation. Convergent evidence has shown that gut damage and an enhanced level of microbial translocation contribute to the enhanced immune activation, the risk of non-AIDS comorbidity, and mortality in people living with HIV (PLWH). Gut biopsy procedures are invasive, and are not appropriate or feasible in large populations, even though they are the gold standard for intestinal barrier investigation. Thus, validated biomarkers that measure the degree of intestinal barrier damage and microbial translocation are needed in PLWH. Hematological biomarkers represent an objective indication of specific medical conditions and/or their severity, and should be able to be measured accurately and reproducibly via easily available and standardized blood tests. Several plasma biomarkers of intestinal damage, i.e., intestinal fatty acid-binding protein (I-FABP), zonulin, and regenerating islet-derived protein-3α (REG3α), and biomarkers of microbial translocation, such as lipopolysaccharide (LPS) and (1,3)-β-D-Glucan (BDG) have been used as markers of risk for developing non-AIDS comorbidities in cross sectional analyses and clinical trials, including those aiming at repair of gut damage. In this review, we critically discuss the value of different biomarkers for the estimation of gut permeability levels, paving the way towards developing validated diagnostic and therapeutic strategies to repair gut epithelial damage and to improve overall disease outcomes in PLWH.
Introduction
The introduction and extensive usage of antiretroviral therapy (ART) for HIV infection has resulted in persistent inhibition of viral replication, and a dramatic decline in morbidity and mortality in people living with HIV (PLWH). However, ART does not comprehensively restore the compromised immune system, and a chronic state of inflammation persists in PLWH on ART, even after long-term viral suppression. This chronic inflammation is positively associated with non-AIDS comorbidities and premature aging (1–5).
The gut epithelial barrier acts as an essential player in maintaining intestinal homeostasis, and in restricting the entry of microbes and their pro-inflammatory products through the mucosa and into the systemic circulation (6–12). The human gut is inhabited by a microbiota population comprising nearly 100 trillion individual organisms (bacteria, archaea, fungi, and viruses) (13, 14). Host-microbial mutualism in the intestine contributes to intestinal homeostasis (11, 13, 15). However, the gut is one of the earliest targets of HIV, as the virus is known to induce dramatic alterations to the gut microbiota and the gut mucosa (16–19). Epithelial damage allows microbial products to translocate from the gut lumen into the systemic circulation, to subsequently participate in and contribute to the chronic inflammatory state present in PLWH (2, 20–22).
A direct method to determine gut integrity is via intestinal biopsy during endoscopy; however, this is relatively invasive and is not suitable or appropriate in large populations (23). Another method to measure gut integrity is to determine the urinary excretion of a sugar probe or other labeled molecule that is not usually absorbed by the intestine [such as the lactulose-mannitol test (24) and the 51Cr-EDTA test (25)], which indirectly reflects intestinal permeability. However, these tests are time-consuming, lack standardization, and have relatively limited validity (24, 26).
Plasma or serum biomarkers can easily be identified from blood samples and are considered to be non-invasive tests for the accurate diagnosis and prognosis of disease. Several gut damage biomarkers, including intestinal fatty acid-binding protein (I-FABP), zonulin, and regenerating islet-derived protein-3α (REG3α) have been validated as biomarkers of gut damage, as well as markers of microbial translocation such as lipopolysaccharide (LPS), LPS-binding protein (LBP), sCD14, (1, 3) β-D-Glucan (BDG). These markers are mainly used in studies related to colonic inflammation (and only more recently in HIV studies), where each marker may have differing values for diagnosis and prognosis (27–32). Herein, we summarize published information regarding these gut damage and microbial translocation biomarkers in PLWH, and also discuss potential therapeutic strategies to potentially improve the integrity of the intestinal barrier assessed using these biomarkers.
Key factors associated with intestinal damage in PLWH
Intestinal structural and immunological damage is common in PLWH, leading to increased gut permeability, microbial translocation, and subsequent immune activation (33–36). An overview of this process is summarized in Figure 1, and the mechanisms whereby immunological activation is induced by some microbial products are illustrated in Figure 2. Cumulative evidence has shown that during HIV infection, the processes of intestinal crypt hyperproliferation and villous shortening results in partial villous atrophy during all stages of HIV infection (37–40). Li et al., reported that in rhesus macaques, simian immunodeficiency virus (SIV) infection induces massive apoptosis of intestinal epithelial cells, and this apoptosis is directly related to the depletion of gut lamina propria CD4+ T-cells (41). A study by Epple et al., using immunofluorescence visualized increased apoptosis of duodenal epithelial cells in patients with acute and chronic HIV infection (42). Another intestinal permeability study showed higher lactulose-mannitol ratios in HIV-infected patients compared to uninfected control individuals, indicating that gut permeability is increased in PLWH (43). Moreover, various other gut microbial products, such as LPS, 16S rDNA, and BDG, have now been identified in the circulation of PLWH (2, 21, 29, 44).
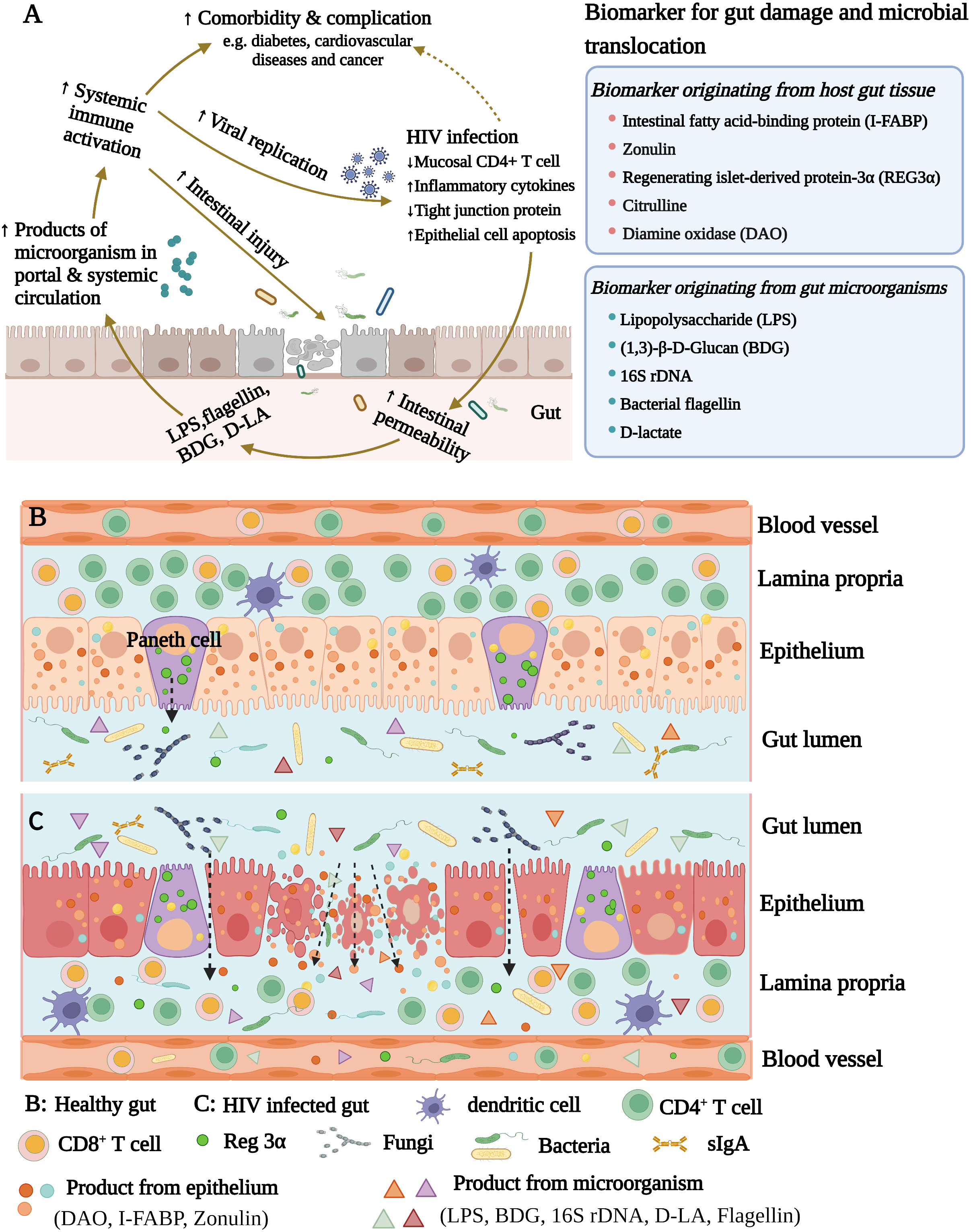
Figure 1 (A) The microbial translocation caused by HIV infection increases systemic inflammation. HIV infection damages the intestinal barrier and increases intestinal permeability. The intestinal microorganisms and their products subsequently enter into blood circulation, inducing immune activation and increasing the systemic inflammatory response. (B) Healthy gut. (C) HIV infected gut. HIV infection damages the intestinal barrier and promotes intestinal permeability.
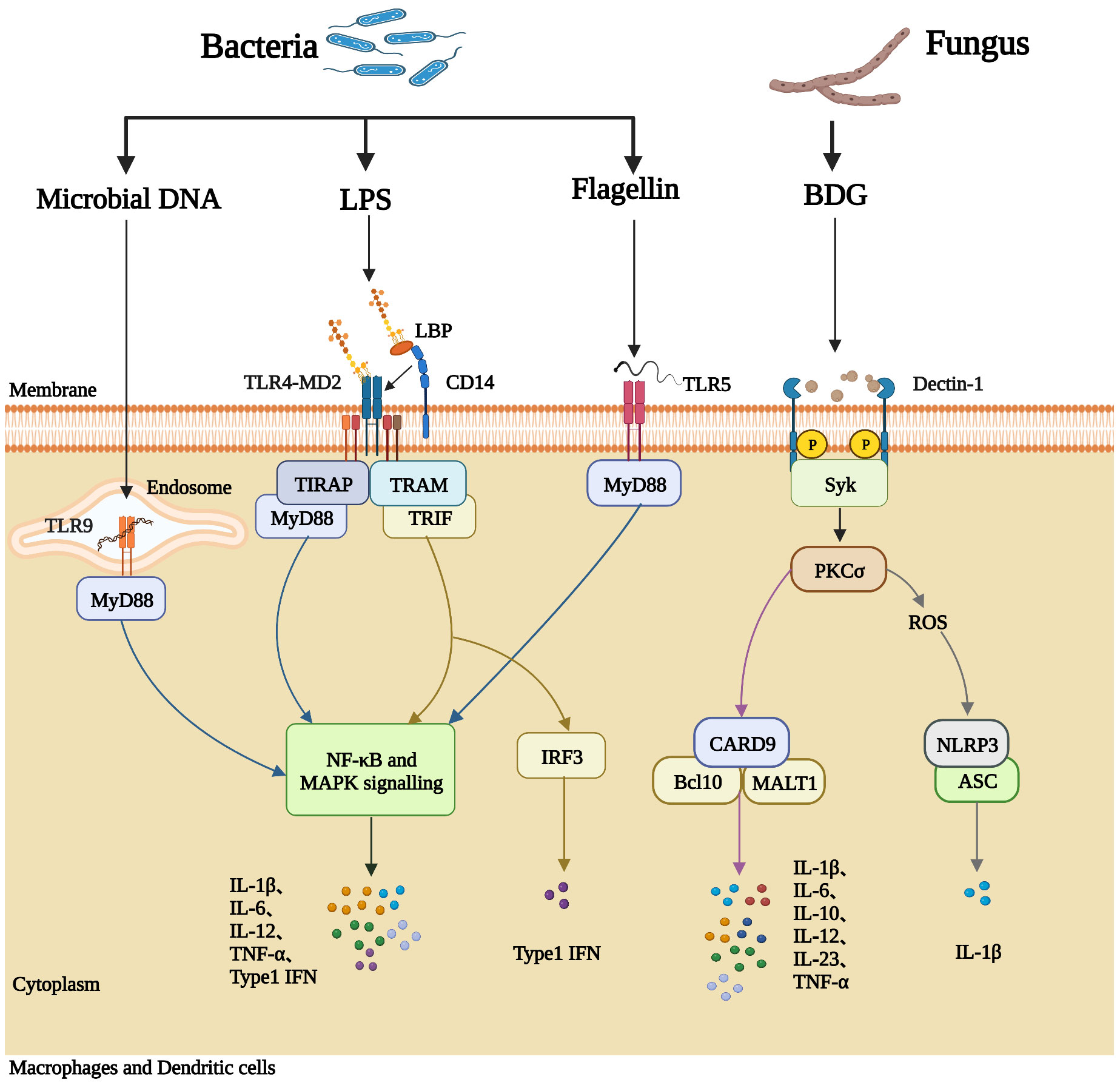
Figure 2 Microbial products induce immune activation in PLWH. Macrophages and dendritic cells express pattern recognition receptors (PRRs) on the cell membrane, which can recognize pathogen-associated molecular patterns (PAMPs) on the surface of microorganisms, including bacterial DNA, LPS, flagellin, and fungal BDG, and subsequently generate inflammatory responses. LPS-binding protein (LBP) and CD14 deliver LPS to the signaling receptor complex TLR4/MD2 on the outer member of the cell. The TLR4/MD2/LPS complex activates the NF-κB signaling pathway and the mitogen-activated protein kinase (MAPK) signaling pathway, causing the synthesis and release of pro-inflammatory cytokines into the blood (such as IL-6, IL-12, TNF-α, and IL- 1β). Membrane TLR5 recognizes bacterial flagellin and activates NF-κB through MyD88. Bacterial DNA can be recognized by TLR9 located in the endosome, activating the MyD88-dependent pathway. BDG from fungi is recognized by the C-type lectin receptor Dectin-1 and mediates inflammatory cytokine production by activating Syk-dependent pathways. The downstream signal PKCσ from Syk (complex phosphatase SHP2) leads to the production of reactive oxygen species (ROS) and activates CARD9/Bcl-10/Malt-1 complex, causing the synthesis and release of pro-inflammatory cytokines into the blood (such as IL-6, IL-10, IL-12).
Several factors contribute to the gut damage seen in PLWH. Firstly, HIV may directly induce epithelial apoptosis and thus directly damage the gut epithelial barrier (45–47). In vitro, exposure to HIV-1 gp120 (a surface envelope glycoprotein) impairs mucosal epithelial barrier integrity by reduction of tight junction (TJ) proteins, resulting in increased epithelial permeability and microbial translocation (46). Other reports have observed that the HIV-1 Tat protein (an HIV regulatory protein) may inhibit proliferation of, and promote apoptosis in intestinal epithelial cells, in studies using the Caco-2 cell line (45, 47).
Additionally, HIV infection dramatically depletes intestinal CD4+ T-cells, which plays a prominent role in the mucosal immunity of the gut barrier (35, 36, 48). The gut-associated lymphoid tissue (GALT) is deemed to be the largest lymphoid organ in the human body (49), within which about 70% of CD4+ T-cells express the C-C chemokine receptor type 5 (CCR5), a vital co-receptor for HIV entry into cells. In contrast, only 20% of peripheral blood CD4+ T-cells express CCR5 (16, 17, 50). Therefore, GALT can be seen to be the primary target and replication site for HIV, and CD4+ T-cells present in gut tissue are largely depleted after HIV infection. One subset of CD4+ T-cells, the T helper 17 (Th17) cell, promotes neutrophil recruitment and secretes antimicrobial peptides, interleukin 17 (IL-17), and interleukin 22 (IL-22) (36, 51). The depletion of Th17 cells may thus result in microbial overgrowth as well as the inhibition of epithelial regeneration (35, 51, 52). One study in rhesus macaques observed that depletion of mucosal Th17 cells by SIV infection promotes dissemination of gut Salmonella typhimurium into the peripheral blood (53).
Numerous studies have now shown that HIV infection is associated with intestinal microbiota dysbiosis, which has been considered to be the underlying factor in a diverse range of pathological processes (54–59). Specifically, in PLWH potential pathogens such as Enterobacteriaceae and Erysipelotrichaceae are enriched, while “protective” intestinal bacteria such as Bacteroidaceae, Ruminococcaceae, and Akkermansia muciniphila (A. muciniphila) are depleted (54, 60). A. muciniphila is a symbiont in the intestine and has been observed to thicken the mucus layer and fortify the integrity of epithelium (60–62). Bifidobacteria and Lactobacillus are considered to be “beneficial” microorganisms and, are reduced in PLWH (63), and past clinical trials have shown that supplementation with Bifidobacteria- and/or Lactobacillus-rich drugs may reduce levels of C-reactive protein (CRP), IL-6, and CD4+ T-cell activation in HIV infection (64–67). Butyrate is a major short-chain fatty acid (SCFA) in the gut, which serves as an energy source for epithelial cells, and promotes epithelial barrier integrity (68, 69). HIV infection reduces the frequency of butyrate-producing bacterial genera (e.g., Roseburia, Coprococcus, Faecalibacterium prausnitzii, and Eubacterium rectale) (53, 70, 71). Moreover, multiple past studies have shown the Proteobacteria phylum to be more abundant in HIV-infected individuals (55, 72–75), and includes several pathogens, such as Pseudomonas (56), Desulfovibrio (75), and Shigella (76). Pseudomonas is known to be capable of impairing host mucus production (77, 78), and Shigella has been shown to induce disruption of tight junctions (76, 79). Additionally, fungal communities are also significantly altered in PLWH (80). The abundance of Candida albicans (C. albicans), an opportunistic pathogen, is increased in the intestines of PLWH (80). Invasion by C. albicans actively contributes to enterocyte damage, with consequent cell death (81–83). Clostridium difficile (C. difficile) -related diarrhea is also common in PLWH (84, 85), and toxins from C. difficile (TcdA and TcdB) may disrupt intestinal tight junction integrity, thus promoting intestinal permeability (86–88).
Once the gut barrier is impaired by the collective and cumulative contributions of the preceding factors, products originating from both gut epithelial cells and the various gut microorganisms translocate into the blood. It is thus practicable and convenient to directly measure these circulating products (Table 1) in blood as the surrogates of gut damage and microbial translocation.
Plasma markers of gut damage and microbial translocation
Biomarkers originating from host gut tissue
I-FABP
I-FABP is a member of the FABP family, has a molecular weight of approximately 15 kilodaltons (kDa), and plays a key role in the transportation and metabolism of long-chain fatty acids. It is specific to and abundant in the epithelial cells of the small intestine (102). Upon intestinal mucosal injury, I-FABP is released from epithelial cells into the circulating blood, and levels of plasma I-FABP have been reported to correlate with the severity of intestinal disease [e.g., ulcerative colitis, Crohn’s disease (CD), mesenteric ischemia, coeliac disease], and is considered a marker of intestinal epithelial cell tight junction disruption and cell death (103–107).
I-FABP has been shown to be an indicator of intestinal damage in PLWH. One prospective trial of ART-naïve HIV-infected individuals observed a remarkable elevation in I-FABP from baseline to week 96, and this elevation correlates with viral replication (108). Sustained effective ART significantly reduces immune activation and tends to ameliorate peripheral T-cell immunophenotypic imbalances; however, this positive and beneficial immunological response to targeted drug therapy is observed to be insufficient to adequately and comprehensively restore intestinal permeability and integrity, and plasma I-FABP levels remain markedly elevated subsequent to ART treatment (109). In chronic HIV-infected patients, I-FABP is increased to a much greater degree than in elite controllers and correlates with nutritional intake and body composition (body mass index, visceral and subcutaneous adipose tissue distribution) in HIV progressors (90). As an intestinal epithelial barrier marker, I-FABP may also act as a predictor of mortality in treated HIV-infected patients. Hunt et al., reported that, similar to other markers of intestinal barrier integrity (Zonulin-1) and some inflammatory factors, I-FABP strongly predicts mortality in treated HIV-infected individuals, with higher plasma I-FABP levels being associated with higher soluble CD14 (sCD14) levels, kynurenine-to-tryptophan (K/T) ratios, IL-6, and D-dimer levels, and lower proximal CD4+ T-cell counts (110). It is also worth noting that levels of plasma I-FABP have also been reported to correlate with other non-inflammatory factors (111–113). Our previous study observed that levels of I-FABP were affected by circadian rhythm, and plasma levels of I-FABP at 16h00 were significantly lower, compared to levels at 08h00 and 04h00 (111). Auinger et al., have demonstrated that I-FABP expression significantly correlates with fatty acid intake in food as well as I-FABP gene polymorphisms (112).
Zonulin
Zonulin is a protein with a molecular mass of 47 kDa, is specifically expressed at the cell surface and secreted by the intestinal epithelium, and is the only physiological enzyme discovered thus far that is known to regulate intestinal permeability via reversibly disassembling the intercellular tight junctions between intestinal epithelial cells (114–116). Serum zonulin has been reported to be elevated in inflammatory and autoimmune disorders [e.g., type 1 diabetes, inflammatory bowel disease, ankylosing spondylitis (AS), and CD], and its levels correlate with the opening of tight junctions and increased intestinal permeability when zonulin production is dysregulated (117–119). It has also been observed that the synthetic TJ regulator larazotide (which acts in an inhibitory capacity) blocks zonulin enzyme activity with excellent efficacy in type 1 diabetes and celiac disease (118, 120, 121).
The zonulin-driven intestinal cellular immune response has been validated during in vitro experiments (122). When the small intestine is exposed to microbes, there is an increase in the secretion of zonulin from the intestinal lumen. Zonulin disassembles TJs by separating the zonula occludens-1 protein from the TJ complexes, decreasing transepithelial electrical resistance (TEER), and increasing permeability (122). Likewise, plasma zonulin levels are elevated in PLWH and are associated with monocyte and T-cell activation (92, 123). Perinatally HIV-infected infants show increased circulating levels of zonulin despite viral suppression by treatment with early ART, and these levels are significantly higher than in HIV-exposed but uninfected infants at 5 months of age (124). Compared to chronically HIV-infected patients, plasma zonulin levels in primary HIV infection (PHI) are significantly elevated and correlate with high viral replication. Plasma zonulin also demonstrates the best accuracy to identify PHI among HIV-infected individuals {AUC=0.85 [95% CI 0.75-0.94]}. Using a cutoff value of plasma zonulin >8.75 ng/mL, the model identified PHI with 87.7% sensitivity and 69.2% specificity (93).
Additionally, elevated zonulin levels have also been associated with disease progression in PLWH. Hunt et al., reported that zonulin has the strong capacity to predict mortality in treated PLWH who had an AIDS history (110). In perinatally HIV-infected children with a history of breastfeeding, zonulin is associated with multiple markers of systemic inflammation, including CRP, IL-6, and D-dimer (92). Improvement and amelioration of the intestinal barrier via serum bovine immunoglobulin significantly reduces circulating levels of I-FABP and zonulin, and systemic inflammation is also ameliorated (125).
REG3α
REG3α, is a C-type lectin antimicrobial peptide secreted by Paneth cells in the gut lumen, and helps contain bacterial infection by binding to peptidoglycans in the cell wall of certain bacteria, and has the capacity to kill some gram-positive bacteria (126, 127). REG3α also helps maintain intestinal barrier integrity by reducing apoptosis of intestinal epithelial cells (128). When the integrity of the intestinal epithelial barrier is disrupted, REG3α crosses through the epithelium, translocates to the lamina propria, and subsequently enters into the systemic circulation (28, 129). Thus, circulating REG3α levels are considered to be a marker of intestinal permeability.
REG3α has long been proposed as a biomarker of intestinal epithelial damage in multiple inflammatory diseases in which plasma REG3α levels are significantly elevated, such as CD, celiac disease, ulcerative colitis, nonalcoholic steatohepatitis, and gastrointestinal graft-versus-host disease (129–133). Heavy alcohol consumption is known to disrupt the integrity of the gut epithelium. Yang et al., used REG3α and Trefoil factor 3 as biomarkers to assess intestinal damage and microbial translocation in patients with alcoholic hepatitis, and observed that circulating levels of these markers are highly elevated, and differentially correlates with disease severity, sCD14 levels, and IL-6 levels (134). Darnaud et al., studied the mechanism by which REG3α maintains intestinal homeostasis and affects inflammatory responses in genetically engineered C57BL/6 mice. The preceding authors observed that REG3α is a potent reactive oxygen species (ROS) scavenger that reduces oxidative stress and inflammatory responses in intestinal epithelial cells, reduces host susceptibility to colitis, and alters the murine gut microflora composition (inducing an increase in Clostridium and a decrease in Bacteroides and Proteus) by reducing ROS levels (135).
Given that REG3α is a reliable marker of intestinal barrier damage, REG3α has been also used in HIV patients to assess the degree of gut damage and systemic immune activation. As reported by Isnard et al., plasma REG3α levels are elevated in untreated and ART-treated PLWH (including elite controllers), when compared to HIV-negative individuals. In contrast, plasma REG3α levels are decreased in PLWH who initiate ART, compared with untreated patients. REG3α levels also negatively correlate with CD4+ T-cell counts and CD4+/CD8+ ratios, and positively correlate with HIV viral load, fungal translocation products, and inflammatory markers in all PLWH (28). Immune non-responders (INRs) are PLWH who fail to adequately restore CD4+ T-cell numbers after effective ART and are known to have irreversibly impaired intestinal mucosal barrier function. It has been observed that INRs have higher levels of plasma I-FABP and REG3α, compared to immune responders (136), and that mucosal CD4+ T-cells positively correlate with I-FABP and REG3α (91).
Citrulline
Citrulline is a non-essential amino acid synthesized from glutamine, and plays a role associated with inflammatory disease (137–142). In several organ exclusion experiments, it has been observed that most citrulline is synthesized in the intestinal epithelium, and subsequently enters into the blood circulation; thus, the intestinal epithelium is the main source of circulating citrulline under physiological conditions (138, 143–145). When intestinal epithelial injury occurs, the production of citrulline decreases, and consequently, in contrast to the trend seen for other biomarkers, the plasma concentration of citrulline decreases.
Circulating citrulline has been used to estimate the degree of intestinal injury, and circulating levels negatively correlate with disease severity in intestinal enteropathies (94, 137, 146, 147). Crenn et al., analyzed the relationship between plasma citrulline concentrations and villous atrophy in celiac disease (148). Their results showed that plasma citrulline concentration is lower in patients with villous atrophy than in healthy subjects, and negatively correlates with the severity of villous atrophy. The results of an investigation in pediatric and adolescent cases of CD by Diamanti et al., showed that CD patients have a reduced concentration of plasma citrulline compared to controls, and that plasma citrulline levels are significantly lower in patients with small bowel localization of CD than in patients with ileo-colon disease (149). Nuzzo et al., analyzed plasma citrulline concentrations in acute mesenteric ischemia (AMI), and plasma citrulline concentrations were observed to be significantly lower in AMI patients compared to controls; however, its practicality for the diagnosis of AMI was found to not be satisfactory, with an area under the receiver operating curve (AUROC) sensitivity and specificity of 0.68, 56%, and 84%, respectively (137). Kulu et al., also observed similar results, with plasma citrulline concentrations in AMI patients being lower, and the AUROC sensitivity and specificity for the diagnosis of AMI were 0.72, 39%, and 100%, respectively, with the best cut-off value being 15.82 µmol/L (150). Furthermore, Fragkos et al., used a meta-analytical method to assess citrulline as a biomarker of gut damage (147), and their results observed that plasma citrulline negatively correlates with disease severity in intestinal enteropathies. The preceding authors have advocated for the use of citrulline as a marker for acute and chronic intestinal insufficiency. In PLWH, Papadia et al., reported that median citrulline levels in HIV positive individuals is significantly lower than that in HIV-negative individuals, and that there are statistically significant correlations between citrulline and villous atrophy in HIV positive individuals (94). Thus, citrulline has been identified as a biomarker for intestinal injury; however, further studies are warranted in order to validate its accuracy among different subgroups of PLWH.
Diamine oxidase
Human diamine oxidase (hDAO) is encoded by the amine oxidase copper containing 1 (AOC1) gene, located on chromosome 7q35 (151). DAO is mainly expressed in the human intestinal mucosal epithelium, the placenta, and the kidney, and catalyzes the oxidation of diamines such as histamine, putrescine, and cadaverine. High activity of this enzyme is found in the mature upper villus cells of the intestinal mucosa, and the intestine is the sole source of plasma diamine oxidase (152–156). DAO is normally present in exceedingly small quantities in the systemic circulation, is stable in the circulation, and high plasma levels are associated with poor integrity of the intestinal mucosa (156, 157).
DAO has been used as a serum marker of intestinal injury (156, 157). It has been reported that plasma DAO activity is associated with the histological and biochemical changes indicative of injury and recovery in a rat model of intestinal injury (155). DAO activity has also been observed in some diseases causing intestinal impairment in humans, including irritable bowel syndrome (IBS) and inflammatory bowel disease (IBD) (158–161). Meng et al., studied the intestinal injury induced by high dose methotrexate in children with acute lymphoblastic leukemia (158), and they observed that levels of plasma LPS and DAO at 1h, 24h, and 44h gradually increased after treatment (158). Zhang et al., reported a similar tendency in DAO levels in patients with heatstroke (160). Additionally, Ji and colleagues observed higher DAO activity levels in 60 patients with IBS compared to 20 healthy controls, and DAO activity levels correlated with disease severity (162). It is worth noting, however, that some investigations observed decreased plasma DAO levels in some severe instances of intestinal disease, such as IBD (161, 163), and anticancer drug treatment (95, 164). A probable reason that may explain these contrasting observations could be related to the fact that DAO-producing intestinal epithelial cells were significantly reduced in the latter specific instances (163, 165, 166). Moreover, several factors may affect DAO activity, such as genetic variability, the pH-value and temperature of the surrounding milieu, and medication use (167–169). Ayuso et al., identified three variants (Thr16Met, Ser332Phe, and His645Asp) of the AOC1 gene, and reported that individuals carrying the His645Asp variant displayed lower serum DAO activity as compared with noncarriers, with a significant gene-dose effect (168). In vitro experimental results have observed a potent DAO inhibitory effect (of greater than 90%) associated with chloroquine and clavulanic acid (167).
It is, therefore, suggested that DAO may be used as a candidate biomarker to evaluate the extent of intestinal injury when few other factors may be discernable, especially in the initial stages of intestinal injury.
Biomarkers originating from gut microorganisms
LPS, sCD14, and 16S rDNA
Lipopolysaccharide (LPS), a critical component of the cell wall of gram-negative bacteria, consists of hydrophobic lipids and hydrophilic sugars, and is soluble in both water and lipids, thus facilitating diffusion (170, 171). The intestinal barrier prevents microorganisms and their products, such as LPS, from entering into the systemic circulation (36, 48). In PLWH, however, the disrupted intestinal barrier together with an increased permeability allows LPS to translocate into the blood circulation (36). In addition, when the monocyte/macrophage receptor for LPS, i.e., CD14, binds with circulating LPS, this induces activation and systemic inflammation (172, 173). CD14 is cleaved and released upon cell activation. Plasma LPS and sCD14 levels are increased in PLWH (36), and plasma levels of sCD14 have been generally shown to positively correlate with circulating LPS levels (174). Thus, circulating LPS and sCD14 have been frequently utilized as biomarkers of bacterial translocation, and have been observed to be associated with systemic immune activation and HIV disease progression (34, 171, 173, 175, 176). Nevertheless, some studies have also reported that LPS either negatively correlates with or does not correlate with sCD14 at all (177–179). This ambiguity may relate either to the CD14 genomic polymorphism (180), multifarious LPSs from different bacteria (181), a different extent of influence from ART, or opportunistic coinfections in these patients (177).
Immunohistochemistry results in SIV-infected rhesus macaques illustrate multifocal compromises and epithelial breaches in the normally intact epithelial barrier in gut tissues. In concert with this, quantitative image analysis of microbial translocation has revealed the emergence of LPS in the gut as well as parenteral tissue of rhesus macaques (1). Numerous studies have shown that levels of LPS and sCD14 are raised in PLWH (2, 34, 96, 175). Brenchley et al., reported that LPS levels are mildly increased in acute/early HIV-infected individuals, compared to HIV-uninfected individuals (2). However, significantly higher LPS levels are observed in chronic HIV-infected individuals, and sCD14 levels are raised in all cohorts of PLWH (2). LPS levels have also been associated with increased activation of activated CD4+ and CD8+ T-cells and plasma Interferon-alpha (IFN-α) levels (2). Even elite controllers have higher LPS levels than HIV-negative individuals, and the higher plasma LPS levels are associated with higher activated CD8+ T-cell counts (97). Furthermore, research by Marchetti et al., observed that compared with immunological responders, immunological non-responders, additionally, had higher LPS levels, which correlates significantly with frequencies of activated CD4+ and CD8+ T-cells (96).
A robust association has also been observed between clinical outcomes and levels of sCD14 and LPS (182–184). A study by Marchetti et al., observed that in a cohort of 379 HIV-infected individuals, circulating LPS level is a strong predictor of disease progression, independently of CD4+ T-cell counts and plasma viral load counts (175). PLWH with higher LPS levels showed a substantially accelerated disease progression rate, with a median time to clinical event of 1.5 years, compared with 4 years for patients with lower LPS levels (175). Jumare and colleagues observed that compared with uninfected controls, plasma levels of sCD14 were significantly higher in PLWH, and among PLWH, those with neurocognitive impairment had significantly higher sCD14 levels compared with neurocognitively unimpaired individuals (182).
Presence of circulating fragments of microbial DNA is generally accepted to be valid evidence for bacterial translocation (185, 186). DNA sequences encoding bacterial ribosomal 16S RNA (16S rDNA) corresponds to rRNA on bacterial chromosomes, with a length of about 1542 base pairs (bp) (187). 16S rDNA exists in all bacterial chromosomal genes, with homologous functions, and the oldest of these genes (known as “bacterial fossils”) are highly conserved in structure and function (188). As an important bacterial product, 16S rDNA of gut origin is highly likely to translocate into the systemic circulation in the presence of compromised gut integrity (189). Thus, measurement of 16S rDNA levels in plasma has the capacity to effectively reflect levels of microbial translocation (186, 189, 190).
By quantitative PCR (qPCR) determination of 16S rDNA fragments, Jiang et al., observed that plasma 16S rDNA levels in HIV-infected individuals are significantly higher than in uninfected individuals, and correlates with LPS levels (21, 178, 190). Plasma 16S rDNA level increases with duration of HIV infection (98), and treatment with ART may reduce, but does not fully eliminate plasma levels of bacterial 16S rDNA (109). Higher levels of 16S rDNA during treatment are strongly associated with higher T-cell activation and lower CD4+ T-cell recovery, regardless of plasma HIV RNA viral load (21). In the context of non-human primate lentiviral infection, 16S rDNA levels and CD8+ T-cell activation inversely correlate with the Th17/regulatory T-cell (Th17/Treg) ratio, suggesting that the extent of microbial translocation and T-cell activation in progressive HIV disease is closely related to skewed maturation along the TH17/Treg axis (191). These findings highlight the importance of microbial translocation markers such as LPS and sCD14 in the context of immunodeficiency and T-cell homeostasis in chronic HIV infection (2, 21, 192).
Plasma 16S rDNA is strongly associated with persistent immune activation in HIV disease. Among HIV-Hepatitis C virus (HCV) co-infected patients, bacterial 16S rDNA levels are notably higher than in the ART-controlled HIV-positive group, and 16S rDNA levels have been seen to increase with duration of HIV infection (98, 193). In HIV-positive patients with neuro-inflammation, Jaime et al., observed a significant correlation between plasma concentrations of 16S rDNA and increased expression of translocated proteins in brain regions with markedly active microglia, such as the basal ganglia and the globus pallidus (194). Plasma 16S rDNA concentrations are also associated with increased white matter tract density (194). One study by Bossola et al. (195), observed that plasma levels of bacteria-derived 16S rDNA in whole blood are statistically significantly associated with higher levels of CRP and IL-6 in patients undergoing chronic hemodialysis. Furthermore, 16S rDNA may bind to Toll-like receptors (TLR) and stimulate immune cells (196). These induce Natural Killer (NK) cell activity and the release of IFN-γ, tumor necrosis factor alpha (TNF-α), and IL-6 from mononuclear cells (197–200). Similarly, in treated HIV-infected patients, higher levels of inflammatory markers (IL-6 and TNF-α) are associated with microbial translocation (16S rDNA, sCD14) and previous cardiovascular events (201).
BDG
Fungi are also an integral component of the gut microbiome and is second only to bacterial frequency in terms of numbers of organisms (80, 202, 203). Similar to translocation of bacterial products, higher circulating levels of fungal products have also been found in PLWH (44, 123, 204). As a major component of most fungal cell walls, (1, 3) β-D-Glucan (BDG) has now been validated as a fungal translocation biomarker (27, 29), and is contemporarily used for the clinical diagnosis of invasive fungal infections (IFI) (205, 206).
Leelahavanichkul et al., tested serum BDG levels in several murine models of gastrointestinal leakage (including dextran sulfate solution administration, LPS injection, and cecal ligation and puncture sepsis), and observed increased levels of the fungal product, BDG, in the systemic circulation (207). Morris et al., first reported that the fungal product, BDG, is present in the blood of PLWH, and that those individuals with higher plasma BDG are associated with lower CD4+ T-cell counts, a higher viral load, and cardiopulmonary comorbidity (208). Subsequently, other investigators also reported higher BDG levels in PLWH (92, 209–211). An investigation by Mehraj et al., showed that, in a similar manner to LPS levels indicating translocation of bacterial products, plasma BDG levels are elevated in all HIV-positive patients without IFI, compared with HIV-negative controls (44). The preceding group prospectively assessed the levels of BDG in early and chronic HIV infection and observed increasing levels over time, in absence of treatment (44). Similarly, a cross-sectional study conducted in Ugandan children showed that compared with HIV-exposed uninfected children, those who acquired HIV perinatally had higher plasma BDG levels that correlated with IL-6 and D-dimer levels (92). A longitudinal investigation in 451 participants who were ART-naïve at baseline showed that BDG levels had decreased after 48 weeks of ART treatment, and higher BDG levels are associated with increased risk of non-AIDS events (209). Hoenigl et al., reported that plasma BDG concentrations are not affected by plant BDG-rich food, further indicating that translocated BDG may be deemed as a reliable marker of intestinal fungal translocation (212). These studies indicate BDG may function as a potential biomarker to indicate whether to initiate antifungal prophylaxis in the early stages of HIV infection, or not.
Higher blood BDG levels have also been reported to correlate with immune activation and risk of developing non-AIDS comorbidities (27, 29, 44, 213). Among PLWH on ART, higher plasma BDG levels are associated with higher levels of activated CD4+ and CD8+ T-cells, and positively correlates with the K/T ratio (44), which is linked to gut damage and bacterial translocation during HIV infection (214–216). Weiner et al., reported that higher BDG levels are associated with the inflammatory cytokines sCD14, IP-10, D-dimer, and sCD163 (213). A study by Hoenigl et al., reported that higher plasma BDG levels were related significantly to worse neurocognitive performance among HIV-infected individuals with suppressed viral loads (217). An investigation by Isnard et al., observed the association between elevated plasma BDG levels and subclinical coronary atherosclerotic plaques in PLWH. Interestingly, BDG levels (and not LPS levels) were elevated significantly in ART-treated PLWH with subclinical coronary atherosclerosis and correlates with total plaque volume (89).
Bacterial flagellin
Flagellin is a structural protein present on the flagella of most motile bacteria in the intestine. Flagellin is recognized and bound by TLR5 on the cell membranes of immune cells, resulting in activation of these cells (218). Bacterial flagellin acts as a pathogen-associated molecular pattern (PAMP) with strong antigenicity, and plays a significant role under conditions of gut damage, including in inflammatory bowel disease (218–220), necrotizing enterocolitis (221), and diarrheal diseases (222, 223). Flagellin is considered to be the major immune antigen in Crohn’s disease, with bacterial flagellin antibody detected in approximately half of Crohn’s disease patients (224, 225). Anti-flagellin antibodies recognize the TLR5 and PATJ (PALS-1-associated tight junction protein) and induce monocyte activation and increased intestinal permeability in Crohn’s disease (226). Svärd et al., reported that circulating flagellin correlates with anti-flagellin levels, and is associated with activation of monocytes in chronic HIV-1-infected individuals (227).
Flagellin promotes virus entry into epithelial cells (228), and enhances HIV-1 induced mucosal immunity in the intestine (229). In vitro, the flagellin/TLR5 complex has been observed to directly trigger viral replication in HIV-infected cells (100). Thibault and colleagues have reported that flagellin, by itself, is able to activate latent HIV-1 provirus in T-lymphoid cells and provoke virus gene expression in central memory CD4+ T-cells (230). A study by Brichacek et al., showed that flagellin enhances HIV-1 replication, and activation of CD4+ T-cells in tonsillar tissue ex vivo (231). In PLWH, elevated levels of anti-flagellin immunoglobulin G (IgG) are present in ART-naïve HIV infected individuals, as compared to HIV-negative controls, and after ART treatment, the level of anti-flagellin IgG decreases (100, 232, 233). The outcomes of these studies imply that in PLWH, flagellin may accelerate the depletion of immune cells in the intestinal mucosa, which in turn worsens the integrity of the intestinal barrier and further encourages the entry of flagellin into the circulation.
D-lactate
D-lactate (D-LA) is a product released by bacteria residing in the human gut, and is not known to be produced in other tissues; thus, the D-LA present in plasma can be assumed to originate from the gastrointestinal tract (234–237). In other words, plasma D-LA levels may also be used as a surrogate for the degree of gut permeability and epithelial damage.
Increased plasma D-LA and LPS levels have been observed in some disease models that lead to intestinal mucosal damage. Increased D-LA levels are associated with diseases which induce severe intestinal injury (238–240). Cai et al., observed and reported on the correlation between D-LA and Crohn’s disease activity. Their study found that serum D-LA levels in patients with active Crohn’s disease and that of those in disease remission were 16.08 ± 4.8 mg/L and 11.16 ± 3.17 mg/L, respectively. Serum D-lactate levels were significantly higher in the active phase compared to the remission phase of Crohn’s disease (241). Teng et al., observed that serum D-LA is associated with acute gastrointestinal injury (AGI) and failure in critically ill patients. D-LA and LPS levels were observed to be higher in patients in the gastrointestinal dysfunction (GID) group or the gastrointestinal failure (GIF) group, than in the healthy control group (101).
Similarly, plasma D-LA can also be used as a marker to predict the degree of damage to the intestinal barrier in HIV patients, and D-LA is associated with recovery of CD4+ T-cell counts. HIV-positive individuals who are immunological non-responders despite ART are prone to malnutrition and compromised gut barriers, which further exacerbates chronic immune activation and inflammation (242). Geng et al., conducted enteral nutritional intervention in these populations and found that serum D-LA and LPS levels were significantly lower in PLWH, with good immune reconstitution after intervention, compared with levels of these markers pre-intervention. Also, D-LA levels negatively correlate with the recovery of CD4+ T-cells, and positively correlate with levels of the inflammatory factor, IL-1β (243).
Plasma biomarkers are widely used to assess the adequacy of therapeutic strategies in PLWH
In view of the significant structural and functional changes caused by HIV infection to the intestinal epithelial barrier, exploration of methods to potentially restore intestinal barrier function is emerging as a research priority, in addition to improvements to ART for PLWH (Table 2).
Therapeutic strategies that target gut microbial composition to potentially restore the physiological gut microbiome and gut barrier integrity have been evaluated in the past. At present, a number of methods capable of altering and modifying gut microbial composition have been reported, including supplementation with probiotics, prebiotics, and synbiotics, fecal microbiota transplantation (FMT), and antibiotic use; however, it remains a confounding exercise to coherently interpret the effects and consequences of these methods on gut microbial composition because of the heterogeneity of recent studies with respect to study design, participant ethnicity, HIV status, ART regimen used, etc. Probiotics and prebiotics are deemed as effective adjuvant therapeutic strategies for PLWH. D’Ettorre et al., conducted a clinical trial of oral probiotics (with an abundance of Streptococcus salivarius and Bifidobacteria) in ART-treated PLWH over 48 weeks, and microbial translocation and immune activation were evaluated by blood sCD14, LBP, and CRP levels. Results indicated that the activation of CD4+ T-cells and levels of sCD14, LBP, and CRP were all decreased after probiotic supplementation (64). A study with ART-naïve HIV-infected individuals showed that prebiotic supplementation (with an oligosaccharide mixture) improved bifidobacterial levels, and decreased C. lituseburense/C. histolyticum levels and sCD14 concentrations in plasma (66). Moreover, it has been reported that HIV infection induces a depletion of A. muciniphila in the intestine (60, 249). Supplementation with A. muciniphila can relieve the inflammation of chronic colitis (250, 251). Liu et al., assessed intestinal permeability in A. muciniphila treated mice with bone fractures by measuring plasma LPS and Fluorescein Isothiocyanate (FITC)-dextran, and reported that A. muciniphila treatment decreases LPS and FITC-dextran levels, and increases mRNA expression of tight junction proteins, including occludin, jam3, claudin-2, -3, and -15 (252). Hence, elevating the level of A. muciniphila in the gut appears to be a potentially effective treatment strategy to foster the integrity of the gut barrier to some extent. In addition, a recent study by Wang et al., has shown that an extract of the Chinese traditional medicine, Painong-San, alleviates colitis by upregulating the expression of tight junction proteins [claudin-1, occludin, and zonula occludens-1 (ZO-1)], and increases the abundance of probiotic organisms, including Lactobacillus, Bifidobacterium, and A. muciniphila in a murine model (253).
FMT is known to be an effective therapy for recurrent C. difficile infection through transplantation of fecal microbiota from a healthy donor into the gastrointestinal tract of a recipient (254–257). A study by Konturek et al., has shown that the serum level of pro-inflammatory cytokines (TNF-α, IL-1β, IL-6, IL-8, and IL-12) decreases significantly post FMT, and the abundance of beneficial bacterial species such as Lactobacillaceae, Ruminococcaceae, Desulfovibrionaceae, Sutterellaceae, and Porphyromonodacea increase after FMT in patients with C. difficile infection (254). Cheng et al., used serum DAO activity and D-lactate to assess the gut barrier in a gut-injured piglet model, and observed that serum DAO activity and D-lactate levels reduced significantly in FMT-treated piglets. FMT has also been observed to increase the protein expression of ZO-1 and occludin in the colonic mucosa, and to increase the abundance of beneficial bacteria, such as Lactobacillus and Succinivibrio, and decrease the abundance of Enterobacteriaceae and Proteobacteria (258). In PLWH, the safety of FMT was investigated by Vujkovic-Cvijin et al., and they observed that no serious adverse effects occurred during 24 weeks of follow-up after one-time FMT, and during the 8 weeks post-FMT, recipients demonstrate partial engraftment of the donor microbiota, and no differences in sCD14 levels were observed (259). In addition, in a pilot FMT study in HIV-infected individuals, a significant 0.5-fold decrease of I-FABP was observed in the FMT group, while no statistically significant decrease in sCD14 and LBP levels was detected. They also observed that there was a significant increase in the alpha diversity of the constituent microbiota of the gut microbiome, and an increase in several members of the Lachnospiraceae family, including Anaerostipes spp., Blautia spp., Dorea spp., and Fusicatenibacter spp. after FMT (245).
The use of antibiotics is a direct method to alter microbial composition in the gut. Administration of Rifaximin (a nonabsorbable antibiotic) combined with sulfasalazine (an anti-inflammatory drug), has been shown to decrease LPS and sCD14 in SIV-infected pigtailed macaques (260). However, a study by Tenorio et al., observed that there were no significant changes in LPS and sCD14 levels in Rifaximin-treated PLWH (261). Moreover, cotrimoxazole (trimethoprim + sulfamethoxazole) is commonly used to prevent Pneumocystis jirovecii infection in HIV-infected patients, and an investigation by Vesterbacka et al., has observed that levels of LPS and sCD14 show no additional reduction in PLWH who initiated ART together with cotrimoxazole in over two years of use (244).
Improving intestinal epithelial function and reducing the entry of microbial products into the bloodstream may also be useful. Sevelamer, a phosphate-lowering drug, has been reported to decrease circulating LPS levels in subjects with chronic kidney disease (262, 263), and also decreases LPS levels by 80% and reduces CRP levels by 78% in subjects on hemodialysis (264). In a SIV-infected pigtailed macaque model, Kristoff et al., assessed intestinal damage via plasma LPS and sCD14 levels, and revealed that sevelamer treatment (2400mg, 3 times per day) reduces LPS, sCD14, and SIV viral loads, and decreases the frequency of HLA-DR+CD38+CD8+ T-cells (265). However, Sandler et al., designed a clinical trial of sevelamer (1600mg, 3 times per day) administration over 8 weeks, and their results observed an absence of a statistically significant decrease in LPS and sCD14 levels in ART-naïve HIV-infected patients (246). Moreover, larazotide acetate (also called AT-1001), an inhibitor of zonulin, has been shown to inhibit TJ disassembly and dysfunction caused by endogenous and exogenous stimuli in intestinal epithelial cells (121, 266–268). In vivo, larazotide acetate significantly reduces the frequency of gastrointestinal symptoms, particularly diarrhea in coeliac disease subjects, and generates a 70% decrease of intestinal permeability (121). Glutamine is a major amino acid in the human body, and is a common substrate used by intestinal cells. Multiple lines of evidence indicate that glutamine regulates the expression of tight junction proteins (269, 270). The depletion of glutamine results in enterocyte atrophy and a subsequent increase in permeability of the intestinal barrier, and supplementation with glutamine has the potential capacity to promote enterocyte proliferation, fortify intestinal membrane integrity, and reduce microbial translocation (269, 271–273).
Immune activation induced by microbial translocation plays a vital role in gut damage. Microbial products may provoke pro-inflammatory responses by binding to numerous receptors i.e., the nucleotide-binding oligomerization domain, as well as multiple TLRs. These receptors are expressed by a number of immune cells, including monocytes, macrophages, and dendritic cells. Once the microbial substrates bind to the receptors, a signaling cascade is activated, subsequently inducing the secretion of many inflammatory cytokines, e.g., IL-1β, IL-6, TNF, and type I IFNs (36, 272, 274). Novel approaches targeting microbial products or their downstream effects, thus attenuating immune activation, may also be a potentially effective therapeutic strategy. The 5-ASA preparations (e.g. mesalazine, sulfasalazine) are clinically effective drugs for the treatment of IBD (which causes intestinal damage), and act by modulating several gut inflammatory pathways [including those associated with Peroxisome proliferator-activated receptor gamma (PPARγ), arachidonic acid and leukotriene biosynthesis, NF-kappaB (NF-κB), and mechanistic target of rapamycin (mTOR)] (275–279). In an SIV-infected animal model, Pandrea et al., used LPS and sCD14 to assess the levels of microbial translocation in SIV-infected pigtailed macaques, and their results indicate that LPS and sCD14 levels are significantly reduced in Rifaximin- and sulfasalazine-treated acutely SIV-infected pigtailed macaques; however, their use induces no statistically significant reductions in LPS and sCD14 levels in chronic SIV-infected pigtailed macaques (260). Furthermore, rifaximin and sulfasalazine treatment significantly reduces the levels of CD4+ and CD8+ T-cell activation and improves hypercoagulation in acute SIV-infected pigtailed macaques (260). In PLWH, a randomized crossover trial (280) of oral mesalazine for 12 weeks showed that, compared to placebo-treated subjects, plasma sCD14 levels did not significantly decrease, and there is no evidence of an effect of mesalazine on CD8+ and CD4+ T-cell activation, IL-6 levels, D-dimer levels, or the K/T ratio at any time point. However, an investigation by Michelini et al. (247), observed that, compared to HIV-infected patients, the plasma levels of sCD14 were significantly lower in mesalazine treated HIV-infected patients with ulcerative colitis; however, the levels of I-FABP were found to not be statistically different.
Furthermore, glucocorticoids (GCs, e.g. dexamethasone, betamethasone, prednisolone) are therapeutically used for their anti-inflammatory and immunosuppressive effects in clinical medicine (281). In vitro experiments indicate that GCs regulate the intestinal tight junction barrier in the intestinal epithelial Caco-2 cell line model (282, 283). Fische and colleagues (282) reported that dexamethasone induces a time- and dose-dependent increase in transepithelial electrical resistance on Caco-2 cell monolayers, which is an in vitro model of the intestinal epithelial barrier, and the expression of claudin 2 (which is involved in pore formation) was downregulated, while expression of claudin 4 (which contributes in the sealing of TJs) was elevated in the dexamethasone treated Caco-2 cell line. Similarly, prednisone treatment of patients with active Crohn’s disease demonstrates a significant reduction in intestinal permeability in the majority of treated individuals, as assessed by the lactulose-mannitol ratio (284). Nockher et al., reported that prednisolone suppressed expression and release of sCD14 in vitro and in vivo (285). An investigation by Kasang et al., in HIV+ patients observed that low-dose prednisolone significantly decreases levels of sCD14, LBP, and soluble urokinase plasminogen activated receptor (suPAR) in untreated patients; however, there were no significant changes in ART-treated patients (248). GCs have also been reported to decrease LPS-induced inflammatory responses (286–288), reduce HIV viral loads, and to postpone CD4+ T-cell loss (289, 290), and the progression to AIDS (291). However, these therapies remain experimental, and the utilization of GCs must be reserved for specific circumstances only and should be strictly controlled, as their use may raise multiple issues, including adverse effects (292, 293) and the interactions of GCs with antiretroviral-boosting agents (e.g., ritonavir and cobicistat) that are currently included in ART regimens (294, 295). The inflammatory cytokine TNF-α has been reported to increase epithelial cell shedding and increases intestinal epithelial tight junction permeability, along with enhancing gut permeability (283, 296, 297). The TNF-α inhibitors infliximab and adalimumab have been used in the treatment of IBD, and exert their effects by inhibiting the TNF pathway, thus decreasing inflammation and restoring mucosal integrity (298–301). A trial conducted in 23 patients with active CD showed that gut permeability, CRP, and the Crohn’s Disease Activity Index (CDAI) significantly decreased after a single infusion of 5 mg/kg infliximab (301). High levels of TNF-α have been reported at all stages of HIV infection, and correlate with high viral load, depletion of CD4+ T-cells and poor disease progression (302–304). The activation of the TNF pathway by TNF-α may facilitate HIV infection and immune activation through multiple pathways (305), and therefore a TNF-α inhibitor may be an effective therapeutic strategy. Nevertheless, only a limited number of clinical studies have reported on the safety and effectiveness of TNF-α inhibitors in PLWH. One review by Gallitano et al., summarized the use of TNF-α inhibitors in 27 published cases of patients with HIV/AIDS, and advises that TNF-α inhibitors may induce improvement in PLWH (306). However, anti-TNF-α therapy has a risk of inducing opportunistic infections and comorbid complications, such as infections by Pneumocystis jirovecii, invasive mycoses, and listeriosis (307), and its use in HIV infection must be carefully monitored. Further clinical studies are required to provide definitive data regarding the safety and effectiveness of TNF-α inhibitors in PLWH.
Conclusion
In PLWH, HIV-related gut damage enhances gut permeability and promotes microbial translocation, which plays a critical role in the chronic immune activation seen in these patients. We have summarized information regarding multiple plasma biomarkers of gut damage and microbial translocation (Table 1), some of which have been widely used, to assess potential therapeutic strategies in PLWH. However, we cannot confidently conclude that the attenuation of microbial translocation would definitely lead to a decrease in immune activation. Other than microbial translocation, chronic immune activation in PLWH may also be driven by other factors, including persistence of the HIV reservoir, depletion of regulatory T-cells, and coinfection with other viruses or organisms (308–310). Furthermore, discordant results exist among different studies due to their heterogenous study design, and more robust and concordant evidence is required to validate the role that these biomarkers may potentially play in the management of PLWH in clinical settings or during interventions that target repair of the gut lining. Moreover, the validity of some biomarkers have not been confidently supported by histological evidence, which is deemed the gold standard to observe the intestinal barrier. Some factors may also interfere with the capability and the value of these biomarkers, such as food intake, genetic differences, medications. Numerous adjunctive therapeutic strategies, such as probiotic use, FMT, and antibiotic use have been investigated to optimize the condition of the gut; however, there remains a dearth of satisfactorily targeted therapeutic options to accurately and adequately repair the structural integrity of the leaky gut in a manner that would effectively prevent microbial translocation and the consequent systemic inflammation seen in PLWH. In the future, further targeted investigations are warranted to develop biomarkers and possible therapeutic strategies for the leaky gut present in PLWH, and should include collaborative efforts encompassing microbiology, clinical care, and pharmacology.
Author contributions
JO, JY, and XZ contributed to revision of the literature and preparation of the initial draft of the manuscript. SI, VH, and HC critically reviewed and copy-edited the manuscript. J-PR and YC contributed to conception of the manuscript and provided critical revision. All authors have read and endorsed the submitted version of the manuscript. All authors contributed to the article.
Funding
This work was supported by the Research Project of China Public Health Clinical Alliance (GWLM202024), the Chongqing Talent Cultivation Program (cstc2021ycjh-bgzxm0275), Chongqing Science and Technology Bureau (cstc2020jscx-cylhX0001), the Chongqing First Batch Key Public Health Disciplines Improvement Project and Chongqing Research Center for Disease Control and Prevention and Public Health Project.
Conflict of interest
The authors declare that the research was conducted in the absence of any commercial or financial relationships that could be construed as a potential conflict of interest.
Publisher’s note
All claims expressed in this article are solely those of the authors and do not necessarily represent those of their affiliated organizations, or those of the publisher, the editors and the reviewers. Any product that may be evaluated in this article, or claim that may be made by its manufacturer, is not guaranteed or endorsed by the publisher.
References
1. Estes JD, Harris LD, Klatt NR, Tabb B, Pittaluga S, Paiardini M, et al. Damaged intestinal epithelial integrity linked to microbial translocation in pathogenic simian immunodeficiency virus infections. PloS Pathog (2010) 6(8):e1001052. doi: 10.1371/journal.ppat.1001052
2. Brenchley JM, Price DA, Schacker TW, Asher TE, Silvestri G, Rao S, et al. Microbial translocation is a cause of systemic immune activation in chronic HIV infection. Nat Med (2006) 12(12):1365–71. doi: 10.1038/nm1511
3. Ishizaka A, Koga M, Mizutani T, Parbie PK, Prawisuda D, Yusa N, et al. Unique gut microbiome in HIV patients on antiretroviral therapy (ART) suggests association with chronic inflammation. Microbiol Spectr (2021) 9(1):e0070821. doi: 10.1128/Spectrum.00708-21
4. Schmiedel Y, Zimmerli S. Common invasive fungal diseases: an overview of invasive candidiasis, aspergillosis, cryptococcosis, and pneumocystis pneumonia. Swiss Med Wkly (2016) 146:w14281. doi: 10.4414/smw.2016.14281
5. Sim JH, Mukerji SS, Russo SC, Lo J. Gastrointestinal dysfunction and HIV comorbidities. Curr HIV/AIDS Rep (2021) 18(1):57–62. doi: 10.1007/s11904-020-00537-8
6. Villoslada-Blanco P, Perez-Matute P, Oteo JA. Lights and shadows of microbiota modulation and cardiovascular risk in HIV patients. Int J Environ Res Public Health (2021) 18(13):6837. doi: 10.3390/ijerph18136837
7. Das B, Nair GB. Homeostasis and dysbiosis of the gut microbiome in health and disease. J Biosci (2019) 44(5):117. doi: 10.1007/s12038-019-9926-y
8. Takiishi T, Fenero CIM, Camara NOS. Intestinal barrier and gut microbiota: shaping our immune responses throughout life. Tissue Barriers (2017) 5(4):e1373208. doi: 10.1080/21688370.2017.1373208
9. Wells JM, Brummer RJ, Derrien M, MacDonald TT, Troost F, Cani PD, et al. Homeostasis of the gut barrier and potential biomarkers. Am J Physiol Gastrointest Liver Physiol (2017) 312(3):G171–G93. doi: 10.1152/ajpgi.00048.2015
10. Thaiss CA, Zmora N, Levy M, Elinav E. The microbiome and innate immunity. Nature (2016) 535(7610):65–74. doi: 10.1038/nature18847
11. Ghaisas S, Maher J, Kanthasamy A. Gut microbiome in health and disease: linking the microbiome-gut-brain axis and environmental factors in the pathogenesis of systemic and neurodegenerative diseases. Pharmacol Ther (2016) 158:52–62. doi: 10.1016/j.pharmthera.2015.11.012
12. Walker AW, Lawley TD. Therapeutic modulation of intestinal dysbiosis. Pharmacol Res (2013) 69(1):75–86. doi: 10.1016/j.phrs.2012.09.008
13. Jandhyala SM, Talukdar R, Subramanyam C, Vuyyuru H, Sasikala M, Nageshwar Reddy D. Role of the normal gut microbiota. World J Gastroenterol (2015) 21(29):8787–803. doi: 10.3748/wjg.v21.i29.8787
14. Backhed F, Ley RE, Sonnenburg JL, Peterson DA, Gordon JI. Host-bacterial mutualism in the human intestine. Science (2005) 307(5717):1915–20. doi: 10.1126/science.1104816
15. Lynch SV, Pedersen O. The human intestinal microbiome in health and disease. N Engl J Med (2016) 375(24):2369–79. doi: 10.1056/NEJMra1600266
16. Anton PA, Elliott J, Poles MA, McGowan IM, Matud J, Hultin LE, et al. Enhanced levels of functional HIV-1 co-receptors on human mucosal T cells demonstrated using intestinal biopsy tissue. AIDS (2000) 14(12):1761–5. doi: 10.1097/00002030-200008180-00011
17. Lapenta C, Boirivant M, Marini M, Santini SM, Logozzi M, Viora M, et al. Human intestinal lamina propria lymphocytes are naturally permissive to HIV-1 infection. Eur J Immunol (1999) 29(4):1202–8. doi: 10.1002/(SICI)1521-4141(199904)29:04<1202::AID-IMMU1202>3.0.CO;2-O
18. Mowat AM, Viney JL. The anatomical basis of intestinal immunity. Immunol Rev (1997) 156:145–66. doi: 10.1111/j.1600-065x.1997.tb00966.x
19. Cerf-Bensussan N, Guy-Grand D. Intestinal intraepithelial lymphocytes. Gastroenterol Clinics North America (1991) 20(3):549–76. doi: 10.1016/S0889-8553(21)00570-7
20. Noguera-Julian M, Rocafort M, Guillen Y, Rivera J, Casadella M, Nowak P, et al. Gut microbiota linked to sexual preference and HIV infection. EBioMedicine (2016) 5:135–46. doi: 10.1016/j.ebiom.2016.01.032
21. Jiang W, Lederman MM, Hunt P, Sieg SF, Haley K, Rodriguez B, et al. Plasma levels of bacterial DNA correlate with immune activation and the magnitude of immune restoration in persons with antiretroviral-treated HIV infection. J Infect Dis (2009) 199(8):1177–85. doi: 10.1086/597476
22. Clayton F, Kapetanovic S, Kotler DP. Enteric microtubule depolymerization in HIV infection: a possible cause of HIV-associated enteropathy. Aids (2001) 15(1):123–4. doi: 10.1097/00002030-200101050-00019
23. Ishigami H, Matsumura T, Kasamatsu S, Hamanaka S, Taida T, Okimoto K, et al. Endoscopy-guided evaluation of duodenal mucosal permeability in functional dyspepsia. Clin Transl Gastroenterol (2017) 8(4):e83. doi: 10.1038/ctg.2017.12
24. Seethaler B, Basrai M, Neyrinck AM, Nazare JA, Walter J, Delzenne NM, et al. Biomarkers for assessment of intestinal permeability in clinical practice. Am J Physiol Gastrointest Liver Physiol (2021) 321(1):G11–G7. doi: 10.1152/ajpgi.00113.2021
25. Leclercq S, Cani PD, Neyrinck AM, Starkel P, Jamar F, Mikolajczak M, et al. Role of intestinal permeability and inflammation in the biological and behavioral control of alcohol-dependent subjects. Brain behavior Immun (2012) 26(6):911–8. doi: 10.1016/j.bbi.2012.04.001
26. Camilleri M. Leaky gut: mechanisms, measurement and clinical implications in humans. Gut (2019) 68(8):1516–26. doi: 10.1136/gutjnl-2019-318427
27. Isnard S, Lin J, Bu S, Fombuena B, Royston L, Routy JP. Gut leakage of fungal-related products: turning up the heat for HIV infection. Front Immunol (2021) 12:656414. doi: 10.3389/fimmu.2021.656414
28. Isnard S, Ramendra R, Dupuy FP, Lin J, Fombuena B, Kokinov N, et al. Plasma levels of c-type lectin REG3alpha and gut damage in people with human immunodeficiency virus. J Infect Dis (2020) 221(1):110–21. doi: 10.1093/infdis/jiz423
29. Ramendra R, Isnard S, Mehraj V, Chen J, Zhang Y, Finkelman M, et al. Circulating LPS and (1–>3)-beta-D-Glucan: a folie a deux contributing to HIV-associated immune activation. Front Immunol (2019) 10:465. doi: 10.3389/fimmu.2019.00465
30. Troseid M, Manner IW, Pedersen KK, Haissman JM, Kvale D, Nielsen SD. Microbial translocation and cardiometabolic risk factors in HIV infection. AIDS Res Hum Retroviruses (2014) 30(6):514–22. doi: 10.1089/AID.2013.0280
31. Larabi A, Barnich N, Nguyen HTT. New insights into the interplay between autophagy, gut microbiota and inflammatory responses in IBD. Autophagy (2020) 16(1):38–51. doi: 10.1080/15548627.2019.1635384
32. Luchetti MM, Ciccia F, Avellini C, Benfaremo D, Rizzo A, Spadoni T, et al. Gut epithelial impairment, microbial translocation and immune system activation in inflammatory bowel disease-associated spondyloarthritis. Rheumatol (Oxford) (2021) 60(1):92–102. doi: 10.1093/rheumatology/keaa164
33. Zevin AS, McKinnon L, Burgener A, Klatt NR. Microbial translocation and microbiome dysbiosis in HIV-associated immune activation. Curr Opin HIV AIDS (2016) 11(2):182–90. doi: 10.1097/COH.0000000000000234
34. Marchetti G, Tincati C, Silvestri G. Microbial translocation in the pathogenesis of HIV infection and AIDS. Clin Microbiol Rev (2013) 26(1):2–18. doi: 10.1128/CMR.00050-12
35. Klatt NR, Funderburg NT, Brenchley JM. Microbial translocation, immune activation, and HIV disease. Trends Microbiol (2013) 21(1):6–13. doi: 10.1016/j.tim.2012.09.001
36. Sandler NG, Douek DC. Microbial translocation in HIV infection: causes, consequences and treatment opportunities. Nat Rev Microbiol (2012) 10(9):655–66. doi: 10.1038/nrmicro2848
37. Sakai E, Higurashi T, Ohkubo H, Hosono K, Ueda A, Matsuhashi N, et al. Investigation of small bowel abnormalities in HIV-infected patients using capsule endoscopy. Gastroenterol Res Pract (2017) 2017:1932647. doi: 10.1155/2017/1932647
38. Ullrich R, Riecken EO, Zeitz M. Human immunodeficiency virus-induced enteropathy. Immunol Res (1991) 10(3-4):456–64. doi: 10.1007/BF02919742
39. Ullrich R, Zeitz M, Heise W, L’Age M, Hoffken G, Riecken EO. Small intestinal structure and function in patients infected with human immunodeficiency virus (HIV): evidence for HIV-induced enteropathy. Ann Intern Med (1989) 111(1):15–21. doi: 10.7326/0003-4819-111-1-15
40. Batman PA, Miller AR, Forster SM, Harris JR, Pinching AJ, Griffin GE. Jejunal enteropathy associated with human immunodeficiency virus infection: quantitative histology. J Clin Pathol (1989) 42(3):275–81. doi: 10.1136/jcp.42.3.275
41. Li Q, Estes JD, Duan L, Jessurun J, Pambuccian S, Forster C, et al. Simian immunodeficiency virus-induced intestinal cell apoptosis is the underlying mechanism of the regenerative enteropathy of early infection. J Infect Dis (2008) 197(3):420–9. doi: 10.1086/525046
42. Epple HJ, Allers K, Troger H, Kuhl A, Erben U, Fromm M, et al. Acute HIV infection induces mucosal infiltration with CD4+ and CD8+ T cells, epithelial apoptosis, and a mucosal barrier defect. Gastroenterology (2010) 139(4):1289–300. doi: 10.1053/j.gastro.2010.06.065
43. Obinna FC, Cook G, Beale T, Dave S, Cunningham D, Fleming SC, et al. Comparative assessment of small intestinal and colonic permeability in HIV-infected homosexual men. AIDS (1995) 9(9):1009–16. doi: 10.1097/00002030-199509000-00005
44. Mehraj V, Ramendra R, Isnard S, Dupuy FP, Ponte R, Chen J, et al. Circulating (1–>3)-beta-D-glucan is associated with immune activation during human immunodeficiency virus infection. Clin Infect Dis (2020) 70(2):232–41. doi: 10.1093/cid/ciz212
45. Buccigrossi V, Laudiero G, Nicastro E, Miele E, Esposito F, Guarino A. The HIV-1 transactivator factor (Tat) induces enterocyte apoptosis through a redox-mediated mechanism. PloS One (2011) 6(12):e29436. doi: 10.1371/journal.pone.0029436
46. Nazli A, Chan O, Dobson-Belaire WN, Ouellet M, Tremblay MJ, Gray-Owen SD, et al. Exposure to HIV-1 directly impairs mucosal epithelial barrier integrity allowing microbial translocation. PloS Pathog (2010) 6(4):e1000852. doi: 10.1371/journal.ppat.1000852
47. Canani RB, Cirillo P, Mallardo G, Buccigrossi V, Secondo A, Annunziato L, et al. Effects of HIV-1 tat protein on ion secretion and on cell proliferation in human intestinal epithelial cells. Gastroenterology (2003) 124(2):368–76. doi: 10.1053/gast.2003.50056
48. Tincati C, Douek DC, Marchetti G. Gut barrier structure, mucosal immunity and intestinal microbiota in the pathogenesis and treatment of HIV infection. AIDS Res Ther (2016) 13:19. doi: 10.1186/s12981-016-0103-1
49. Galperin C, Gershwin ME. Immunopathogenesis of gastrointestinal and hepatobiliary diseases. JAMA (1997) 278(22):1946–55. doi: 10.1001/jama.1997.03550220152020
50. Mehandru S, Tenner-Racz K, Racz P, Markowitz M. The gastrointestinal tract is critical to the pathogenesis of acute HIV-1 infection. J Allergy Clin Immunol (2005) 116(2):419–22. doi: 10.1016/j.jaci.2005.05.040
51. Stadhouders R, Lubberts E, Hendriks RW. A cellular and molecular view of T helper 17 cell plasticity in autoimmunity. J Autoimmun (2018) 87:1–15. doi: 10.1016/j.jaut.2017.12.007
52. Liu JZ, Pezeshki M, Raffatellu M. Th17 cytokines and host-pathogen interactions at the mucosa: dichotomies of help and harm. Cytokine (2009) 48(1-2):156–60. doi: 10.1016/j.cyto.2009.07.005
53. Raffatellu M, Santos RL, Verhoeven DE, George MD, Wilson RP, Winter SE, et al. Simian immunodeficiency virus-induced mucosal interleukin-17 deficiency promotes salmonella dissemination from the gut. Nat Med (2008) 14(4):421–8. doi: 10.1038/nm1743
54. Gootenberg DB, Paer JM, Luevano JM, Kwon DS. HIV-Associated changes in the enteric microbial community: potential role in loss of homeostasis and development of systemic inflammation. Curr Opin Infect Dis (2017) 30(1):31–43. doi: 10.1097/QCO.0000000000000341
55. Mutlu EA, Keshavarzian A, Losurdo J, Swanson G, Siewe B, Forsyth C, et al. A compositional look at the human gastrointestinal microbiome and immune activation parameters in HIV infected subjects. PloS Pathog (2014) 10(2):e1003829. doi: 10.1371/journal.ppat.1003829
56. Vujkovic-Cvijin I, Dunham RM, Iwai S, Maher MC, Albright RG, Broadhurst MJ, et al. Dysbiosis of the gut microbiota is associated with HIV disease progression and tryptophan catabolism. Sci Transl Med (2013) 5(193):193ra91. doi: 10.1126/scitranslmed.3006438
57. Cho I, Blaser MJ. The human microbiome: at the interface of health and disease. Nat Rev Genet (2012) 13(4):260–70. doi: 10.1038/nrg3182
58. Littman DR, Pamer EG. Role of the commensal microbiota in normal and pathogenic host immune responses. Cell Host Microbe (2011) 10(4):311–23. doi: 10.1016/j.chom.2011.10.004
59. Ellis RJ, Iudicello JE, Heaton RK, Isnard S, Lin J, Routy JP, et al. Markers of gut barrier function and microbial translocation associate with lower gut microbial diversity in people with HIV. Viruses (2021) 13(10):1891. doi: 10.3390/v13101891
60. Rocafort M, Noguera-Julian M, Rivera J, Pastor L, Guillen Y, Langhorst J, et al. Evolution of the gut microbiome following acute HIV-1 infection. Microbiome (2019) 7(1):73. doi: 10.1186/s40168-019-0687-5
61. Derrien M, Vaughan EE, Plugge CM, de Vos WM. Akkermansia muciniphila gen. nov., sp. nov., a human intestinal mucin-degrading bacterium. Int J Syst Evol Microbiol (2004) 54(Pt 5):1469–76. doi: 10.1099/ijs.0.02873-0
63. Cunningham-Rundles S, Ahrne S, Johann-Liang R, Abuav R, Dunn-Navarra AM, Grassey C, et al. Effect of probiotic bacteria on microbial host defense, growth, and immune function in human immunodeficiency virus type-1 infection. Nutrients (2011) 3(12):1042–70. doi: 10.3390/nu3121042
64. d’Ettorre G, Ceccarelli G, Giustini N, Serafino S, Calantone N, De Girolamo G, et al. Probiotics reduce inflammation in antiretroviral treated, HIV-infected individuals: results of the “Probio-HIV” clinical trial. PloS One (2015) 10(9):e0137200. doi: 10.1371/journal.pone.0137200
65. Perez-Santiago J, Gianella S, Massanella M, Spina CA, Karris MY, Var SR, et al. Gut lactobacillales are associated with higher CD4 and less microbial translocation during HIV infection. AIDS (2013) 27(12):1921–31. doi: 10.1097/qad.0b013e3283611816
66. Gori A, Rizzardini G, Van’t Land B, Amor KB, van Schaik J, Torti C, et al. Specific prebiotics modulate gut microbiota and immune activation in HAART-naive HIV-infected adults: results of the “COPA” pilot randomized trial. Mucosal Immunol (2011) 4(5):554–63. doi: 10.1038/mi.2011.15
67. Kirpich IA, Solovieva NV, Leikhter SN, Shidakova NA, Lebedeva OV, Sidorov PI, et al. Probiotics restore bowel flora and improve liver enzymes in human alcohol-induced liver injury: a pilot study. Alcohol (2008) 42(8):675–82. doi: 10.1016/j.alcohol.2008.08.006
68. Kelly CJ, Zheng L, Campbell EL, Saeedi B, Scholz CC, Bayless AJ, et al. Crosstalk between microbiota-derived short-chain fatty acids and intestinal epithelial HIF augments tissue barrier function. Cell Host Microbe (2015) 17(5):662–71. doi: 10.1016/j.chom.2015.03.005
69. Donohoe DR, Garge N, Zhang X, Sun W, O’Connell TM, Bunger MK, et al. The microbiome and butyrate regulate energy metabolism and autophagy in the mammalian colon. Cell Metab (2011) 13(5):517–26. doi: 10.1016/j.cmet.2011.02.018
70. Wang Z, Qi Q. Gut microbial metabolites associated with HIV infection. Future Virol (2019) 14(5):335–47. doi: 10.2217/fvl-2019-0002
71. Louis P, Flint HJ. Diversity, metabolism and microbial ecology of butyrate-producing bacteria from the human large intestine. FEMS Microbiol Lett (2009) 294(1):1–8. doi: 10.1111/j.1574-6968.2009.01514.x
72. Ling Z, Jin C, Xie T, Cheng Y, Li L, Wu N. Alterations in the fecal microbiota of patients with HIV-1 infection: an observational study in a Chinese population. Sci Rep (2016) 6:30673. doi: 10.1038/srep30673
73. Lozupone CA, Rhodes ME, Neff CP, Fontenot AP, Campbell TB, Palmer BE. HIV-Induced alteration in gut microbiota: driving factors, consequences, and effects of antiretroviral therapy. Gut Microbes (2014) 5(4):562–70. doi: 10.4161/gmic.32132
74. McHardy IH, Li X, Tong M, Ruegger P, Jacobs J, Borneman J, et al. HIV Infection is associated with compositional and functional shifts in the rectal mucosal microbiota. Microbiome (2013) 1(1):26. doi: 10.1186/2049-2618-1-26
75. Lozupone CA, Li M, Campbell TB, Flores SC, Linderman D, Gebert MJ, et al. Alterations in the gut microbiota associated with HIV-1 infection. Cell Host Microbe (2013) 14(3):329–39. doi: 10.1016/j.chom.2013.08.006
76. Sakaguchi T, Kohler H, Gu X, McCormick BA, Reinecker HC. Shigella flexneri regulates tight junction-associated proteins in human intestinal epithelial cells. Cell Microbiol (2002) 4(6):367–81. doi: 10.1046/j.1462-5822.2002.00197.x
77. de Bentzmann S, Plesiat P. The pseudomonas aeruginosa opportunistic pathogen and human infections. Environ Microbiol (2011) 13(7):1655–65. doi: 10.1111/j.1462-2920.2011.02469.x
78. McGuckin MA, Linden SK, Sutton P, Florin TH. Mucin dynamics and enteric pathogens. Nat Rev Microbiol (2011) 9(4):265–78. doi: 10.1038/nrmicro2538
79. Sarkar P, Saha T, Sheikh IA, Chakraborty S, Aoun J, Chakrabarti MK, et al. Zinc ameliorates intestinal barrier dysfunctions in shigellosis by reinstating claudin-2 and -4 on the membranes. Am J Physiol Gastrointest Liver Physiol (2019) 316(2):G229–G46. doi: 10.1152/ajpgi.00092.2018
80. Hager CL, Ghannoum MA. The mycobiome in HIV. Curr Opin HIV AIDS (2018) 13(1):69–72. doi: 10.1097/COH.0000000000000432
81. Fusco A, Savio V, Donniacuo M, Perfetto B, Donnarumma G. Antimicrobial peptides human beta-Defensin-2 and -3 protect the gut during candida albicans infections enhancing the intestinal barrier integrity: In Vitro study. Front Cell Infect Microbiol (2021) 11:666900. doi: 10.3389/fcimb.2021.666900
82. Allert S, Förster TM, Svensson CM, Richardson JP, Pawlik T, Hebecker B, et al. Candida albicans-induced epithelial damage mediates translocation through intestinal barriers. mBio (2018) 9(3):e00915–18. doi: 10.1128/mBio.00915-18
83. Goyer M, Loiselet A, Bon F, L’Ollivier C, Laue M, Holland G, et al. Intestinal cell tight junctions limit invasion of candida albicans through active penetration and endocytosis in the early stages of the interaction of the fungus with the intestinal barrier. PloS One (2016) 11(3):e0149159. doi: 10.1371/journal.pone.0149159
84. Revolinski SL, Munoz-Price LS. Clostridium difficile in immunocompromised hosts: a review of epidemiology, risk factors, treatment, and prevention. Clin Infect Dis (2019) 68(12):2144–53. doi: 10.1093/cid/ciy845
85. Collini PJ, Kuijper E, Dockrell DH. Clostridium difficile infection in patients with HIV/AIDS. Curr HIV/AIDS Rep (2013) 10(3):273–82. doi: 10.1007/s11904-013-0162-z
86. Schenck LP, Hirota SA, Hirota CL, Boasquevisque P, Tulk SE, Li Y, et al. Attenuation of clostridium difficile toxin-induced damage to epithelial barrier by ecto-5’-nucleotidase (CD73) and adenosine receptor signaling. Neurogastroenterol Motil (2013) 25(6):e441–53. doi: 10.1111/nmo.12139
87. Chen ML, Pothoulakis C, LaMont JT. Protein kinase c signaling regulates ZO-1 translocation and increased paracellular flux of T84 colonocytes exposed to clostridium difficile toxin a. J Biol Chem (2002) 277(6):4247–54. doi: 10.1074/jbc.M109254200
88. Nusrat A, von Eichel-Streiber C, Turner JR, Verkade P, Madara JL, Parkos CA. Clostridium difficile toxins disrupt epithelial barrier function by altering membrane microdomain localization of tight junction proteins. Infect Immun (2001) 69(3):1329–36. doi: 10.1128/IAI.69.3.1329-1336.2001
89. Isnard S, Fombuena B, Sadouni M, Lin J, Richard C, Routy B, et al. Circulating beta-d-Glucan as a marker of subclinical coronary plaque in antiretroviral therapy-treated people with human immunodeficiency virus. Open Forum Infect Dis (2021) 8(6):ofab109. doi: 10.1093/ofid/ofab109
90. Cheru LT, Park EA, Saylor CF, Burdo TH, Fitch KV, Looby S, et al. I-FABP is higher in people with chronic HIV than elite controllers, related to sugar and fatty acid intake and inversely related to body fat in people with HIV. Open Forum Infect Dis (2018) 5(11):ofy288. doi: 10.1093/ofid/ofy288
91. Lorvik KB, Meyer-Myklestad MH, Kushekar K, Handeland C, Medhus AW, Lund-Iversen M, et al. Enhanced gut-homing dynamics and pronounced exhaustion of mucosal and blood CD4(+) T cells in HIV-infected immunological non-responders. Front Immunol (2021) 12:744155. doi: 10.3389/fimmu.2021.744155
92. Dirajlal-Fargo S, El-Kamari V, Weiner L, Shan L, Sattar A, Kulkarni M, et al. Altered intestinal permeability and fungal translocation in Ugandan children with human immunodeficiency virus. Clin Infect Dis (2020) 70(11):2413–22. doi: 10.1093/cid/ciz561
93. Pastor L, Langhorst J, Schroder D, Casellas A, Ruffer A, Carrillo J, et al. Different pattern of stool and plasma gastrointestinal damage biomarkers during primary and chronic HIV infection. PloS One (2019) 14(6):e0218000. doi: 10.1371/journal.pone.0218000
94. Papadia C, Kelly P, Caini S, Corazza GR, Shawa T, Franze A, et al. Plasma citrulline as a quantitative biomarker of HIV-associated villous atrophy in a tropical enteropathy population. Clin Nutr (2010) 29(6):795–800. doi: 10.1016/j.clnu.2010.04.008
95. Namikawa T, Fukudome I, Kitagawa H, Okabayashi T, Kobayashi M, Hanazaki K. Plasma diamine oxidase activity is a useful biomarker for evaluating gastrointestinal tract toxicities during chemotherapy with oral fluorouracil anti-cancer drugs in patients with gastric cancer. Oncology (2012) 82(3):147–52. doi: 10.1159/000336799
96. Marchetti G, Bellistri GM, Borghi E, Tincati C, Ferramosca S, La Francesca M, et al. Microbial translocation is associated with sustained failure in CD4+ T-cell reconstitution in HIV-infected patients on long-term highly active antiretroviral therapy. AIDS (2008) 22(15):2035–8. doi: 10.1097/QAD.0b013e3283112d29
97. Hunt PW, Brenchley J, Sinclair E, McCune JM, Roland M, Page-Shafer K, et al. Relationship between T cell activation and CD4+ T cell count in HIV-seropositive individuals with undetectable plasma HIV RNA levels in the absence of therapy. J Infect Dis (2008) 197(1):126–33. doi: 10.1086/524143
98. Tudesq JJ, Dunyach-Remy C, Combescure C, Doncesco R, Laureillard D, Lavigne JP, et al. Microbial translocation is correlated with HIV evolution in HIV-HCV co-infected patients. PloS One (2017) 12(9):e0183372. doi: 10.1371/journal.pone.0183372
99. George V, Harrison L, Roach M, Li XD, Tierney C, Fischl MA, et al. Associations of plasma cytokine and microbial translocation biomarkers with immune reconstitution inflammatory syndrome. J Infect Dis (2017) 216(9):1159–63. doi: 10.1093/infdis/jix460
100. Nowak P, Abdurahman S, Lindkvist A, Troseid M, Sonnerborg A. Impact of HMGB1/TLR ligand complexes on HIV-1 replication: possible role for flagellin during HIV-1 infection. Int J Microbiol (2012) 2012:263836. doi: 10.1155/2012/263836
101. Teng J, Xiang L, Long H, Gao C, Lei L, Zhang Y. The serum citrulline and d-lactate are associated with gastrointestinal dysfunction and failure in critically ill patients. Int J Gen Med (2021) 14:4125–34. doi: 10.2147/IJGM.S305209
102. Funaoka H, Kanda T, Fujii H. [Intestinal fatty acid-binding protein (I-FABP) as a new biomarker for intestinal diseases]. Rinsho Byori (2010) 58(2):162–8.
103. Wang L, Llorente C, Hartmann P, Yang AM, Chen P, Schnabl B. Methods to determine intestinal permeability and bacterial translocation during liver disease. J Immunol Methods (2015) 421:44–53. doi: 10.1016/j.jim.2014.12.015
104. Adriaanse MP, Tack GJ, Passos VL, Damoiseaux JG, Schreurs MW, van Wijck K, et al. Serum I-FABP as marker for enterocyte damage in coeliac disease and its relation to villous atrophy and circulating autoantibodies. Aliment Pharmacol Ther (2013) 37(4):482–90. doi: 10.1111/apt.12194
105. Wiercinska-Drapalo A, Jaroszewicz J, Siwak E, Pogorzelska J, Prokopowicz D. Intestinal fatty acid binding protein (I-FABP) as a possible biomarker of ileitis in patients with ulcerative colitis. Regul Pept (2008) 147(1-3):25–8. doi: 10.1016/j.regpep.2007.12.002
106. Pelsers MM, Namiot Z, Kisielewski W, Namiot A, Januszkiewicz M, Hermens WT, et al. Intestinal-type and liver-type fatty acid-binding protein in the intestine. tissue distribution and clinical utility. Clin Biochem (2003) 36(7):529–35. doi: 10.1016/s0009-9120(03)00096-1
107. Lieberman JM, Sacchettini J, Marks C, Marks WH. Human intestinal fatty acid binding protein: report of an assay with studies in normal volunteers and intestinal ischemia. Surgery (1997) 121(3):335–42. doi: 10.1016/s0039-6060(97)90363-9
108. El Kamari V, Sattar A, McComsey GA. Brief report: gut structural damage: an ongoing process in chronically untreated HIV infection. J acquired Immune deficiency syndromes (2019) 80(2):242–5. doi: 10.1097/QAI.0000000000001910
109. Ancona G, Merlini E, Tincati C, Barassi A, Calcagno A, Augello M, et al. Long-term suppressive cART is not sufficient to restore intestinal permeability and gut microbiota compositional changes. Front Immunol (2021) 12:639291. doi: 10.3389/fimmu.2021.639291
110. Hunt PW, Sinclair E, Rodriguez B, Shive C, Clagett B, Funderburg N, et al. Gut epithelial barrier dysfunction and innate immune activation predict mortality in treated HIV infection. J Infect Dis (2014) 210(8):1228–38. doi: 10.1093/infdis/jiu238
111. Ouyang J, Isnard S, Lin J, Fombuena B, Chatterjee D, Wiche Salinas TR, et al. Daily variations of gut microbial translocation markers in ART-treated HIV-infected people. AIDS Res Ther (2020) 17(1):15. doi: 10.1186/s12981-020-00273-4
112. Auinger A, Helwig U, Rubin D, Herrmann J, Jahreis G, Pfeuffer M, et al. Human intestinal fatty acid binding protein 2 expression is associated with fat intake and polymorphisms. J Nutr (2010) 140(8):1411–7. doi: 10.3945/jn.109.118034
113. Levy E, Menard D, Delvin E, Montoudis A, Beaulieu JF, Mailhot G, et al. Localization, function and regulation of the two intestinal fatty acid-binding protein types. Histochem Cell Biol (2009) 132(3):351–67. doi: 10.1007/s00418-009-0608-y
114. Yu J, Shen Y, Zhou N. Advances in the role and mechanism of zonulin pathway in kidney diseases. Int Urol Nephrol (2021) 53(10):2081–8. doi: 10.1007/s11255-020-02756-9
115. Tripathi A, Lammers KM, Goldblum S, Shea-Donohue T, Netzel-Arnett S, Buzza MS, et al. Identification of human zonulin, a physiological modulator of tight junctions, as prehaptoglobin-2. Proc Natl Acad Sci U.S.A. (2009) 106(39):16799–804. doi: 10.1073/pnas.0906773106
116. Wang W, Uzzau S, Goldblum SE, Fasano A. Human zonulin, a potential modulator of intestinal tight junctions. J Cell Sci (2000) 113 Pt 24:4435–40. doi: 10.1242/jcs.113.24.4435
117. Fasano A. All disease begins in the (leaky) gut: role of zonulin-mediated gut permeability in the pathogenesis of some chronic inflammatory diseases. F1000Res (2020) 9:F1000. doi: 10.12688/f1000research.20510.1
118. Fasano A. Zonulin and its regulation of intestinal barrier function: the biological door to inflammation, autoimmunity, and cancer. Physiol Rev (2011) 91(1):151–75. doi: 10.1152/physrev.00003.2008
119. Fasano A, Not T, Wang W, Uzzau S, Berti I, Tommasini A, et al. Zonulin, a newly discovered modulator of intestinal permeability, and its expression in coeliac disease. Lancet (2000) 355(9214):1518–9. doi: 10.1016/S0140-6736(00)02169-3
120. Leffler DA, Kelly CP, Abdallah HZ, Colatrella AM, Harris LA, Leon F, et al. A randomized, double-blind study of larazotide acetate to prevent the activation of celiac disease during gluten challenge. Am J Gastroenterol (2012) 107(10):1554–62. doi: 10.1038/ajg.2012.211
121. Paterson BM, Lammers KM, Arrieta MC, Fasano A, Meddings JB. The safety, tolerance, pharmacokinetic and pharmacodynamic effects of single doses of AT-1001 in coeliac disease subjects: a proof of concept study. Aliment Pharmacol Ther (2007) 26(5):757–66. doi: 10.1111/j.1365-2036.2007.03413.x
122. El Asmar R, Panigrahi P, Bamford P, Berti I, Not T, Coppa GV, et al. Host-dependent zonulin secretion causes the impairment of the small intestine barrier function after bacterial exposure. Gastroenterology (2002) 123(5):1607–15. doi: 10.1053/gast.2002.36578
123. Dirajlal-Fargo S, Albar Z, Bowman E, Labbato D, Sattar A, Karungi C, et al. Increased monocyte and T-cell activation in treated HIV+ Ugandan children: associations with gut alteration and HIV factors. AIDS (2020) 34(7):1009–18. doi: 10.1097/QAD.0000000000002505
124. Koay WLA, Lindsey JC, Uprety P, Bwakura-Dangarembizi M, Weinberg A, Levin MJ, et al. Intestinal integrity biomarkers in early antiretroviral-treated perinatally HIV-1-Infected infants. J Infect Dis (2018) 218(7):1085–9. doi: 10.1093/infdis/jiy271
125. Utay NS, Somasunderam A, Hinkle JE, Petschow BW, Detzel CJ, Somsouk M, et al. Serum bovine immunoglobulins improve inflammation and gut barrier function in persons with HIV and enteropathy on suppressive ART. Pathog Immun (2019) 4(1):124–46. doi: 10.20411/pai.v4i1.276
126. Shin JH, Seeley RJ. Reg3 proteins as gut hormones? Endocrinology (2019) 160(6):1506–14. doi: 10.1210/en.2019-00073
127. Bevins CL, Salzman NH. Paneth cells, antimicrobial peptides and maintenance of intestinal homeostasis. Nat Rev Microbiol (2011) 9(5):356–68. doi: 10.1038/nrmicro2546
128. Chen Z, Downing S, Tzanakakis ES. Four decades after the discovery of regenerating islet-derived (Reg) proteins: current understanding and challenges. Front Cell Dev Biol (2019) 7:235. doi: 10.3389/fcell.2019.00235
129. Marafini I, Di Sabatino A, Zorzi F, Monteleone I, Sedda S, Cupi ML, et al. Serum regenerating islet-derived 3-alpha is a biomarker of mucosal enteropathies. Aliment Pharmacol Ther (2014) 40(8):974–81. doi: 10.1111/apt.12920
130. Zhao D, Kim YH, Jeong S, Greenson JK, Chaudhry MS, Hoepting M, et al. Survival signal REG3alpha prevents crypt apoptosis to control acute gastrointestinal graft-versus-host disease. J Clin Invest (2018) 128(11):4970–9. doi: 10.1172/JCI99261
131. Bluemel S, Wang L, Martino C, Lee S, Wang Y, Williams B, et al. The role of intestinal c-type regenerating islet derived-3 lectins for nonalcoholic steatohepatitis. Hepatol Commun (2018) 2(4):393–406. doi: 10.1002/hep4.1165
132. Wang L, Fouts DE, Starkel P, Hartmann P, Chen P, Llorente C, et al. Intestinal REG3 lectins protect against alcoholic steatohepatitis by reducing mucosa-associated microbiota and preventing bacterial translocation. Cell Host Microbe (2016) 19(2):227–39. doi: 10.1016/j.chom.2016.01.003
133. Ferrara JL, Harris AC, Greenson JK, Braun TM, Holler E, Teshima T, et al. Regenerating islet-derived 3-alpha is a biomarker of gastrointestinal graft-versus-host disease. Blood (2011) 118(25):6702–8. doi: 10.1182/blood-2011-08-375006
134. Yang J, Syed F, Xia Y, Sanyal AJ, Shah VH, Chalasani N, et al. Blood biomarkers of intestinal epithelium damage regenerating islet-derived protein 3alpha and trefoil factor 3 are persistently elevated in patients with alcoholic hepatitis. Alcohol Clin Exp Res (2021) 45(4):720–31. doi: 10.1111/acer.14579
135. Darnaud M, Dos Santos A, Gonzalez P, Augui S, Lacoste C, Desterke C, et al. Enteric delivery of regenerating family member 3 alpha alters the intestinal microbiota and controls inflammation in mice with colitis. Gastroenterology (2018) 154(4):1009–23 e14. doi: 10.1053/j.gastro.2017.11.003
136. Meyer-Myklestad MH, Medhus AW, Lorvik KB, Seljeflot I, Hansen SH, Holm K, et al. Human immunodeficiency virus-infected immunological nonresponders have colon-restricted gut mucosal immune dysfunction. J Infect Dis (2022) 225(4):661–74. doi: 10.1093/infdis/jiaa714
137. Nuzzo A, Guedj K, Curac S, Hercend C, Bendavid C, Gault N, et al. Accuracy of citrulline, I-FABP and d-lactate in the diagnosis of acute mesenteric ischemia. Sci Rep (2021) 11(1):18929. doi: 10.1038/s41598-021-98012-w
138. Maric S, Restin T, Muff JL, Camargo SM, Guglielmetti LC, Holland-Cunz SG, et al. Citrulline, biomarker of enterocyte functional mass and dietary supplement. metabolism, transport, and current evidence for clinical use. Nutrients (2021) 13(8):2794. doi: 10.3390/nu13082794
139. Ye F, Ning J, Fardous Z, Katsube T, Li Q, Wang B. Citrulline, a potential biomarker of radiation-induced small intestine damage. Dose Response (2020) 18(3):1559325820962341. doi: 10.1177/1559325820962341
140. Curis E, Crenn P, Cynober L. Citrulline and the gut. Curr Opin Clin Nutr Metab Care (2007) 10(5):620–6. doi: 10.1097/MCO.0b013e32829fb38d
141. Vossenaar ER, Zendman AJ, van Venrooij WJ, Pruijn GJ. PAD, a growing family of citrullinating enzymes: genes, features and involvement in disease. Bioessays (2003) 25(11):1106–18. doi: 10.1002/bies.10357
142. Rogers GE, Harding HW, Llewellyn-Smith IJ. The origin of citrulline-containing proteins in the hair follicle and the chemical nature of trichohyalin, an intracellular precursor. Biochim Biophys Acta (1977) 495(1):159–75. doi: 10.1016/0005-2795(77)90250-1
143. Flynn NE, Wu G. An important role for endogenous synthesis of arginine in maintaining arginine homeostasis in neonatal pigs. Am J Physiol (1996) 271(5 Pt 2):R1149–55. doi: 10.1152/ajpregu.1996.271.5.R1149
144. Hoogenraad N, Totino N, Elmer H, Wraight C, Alewood P, Johns RB. Inhibition of intestinal citrulline synthesis causes severe growth retardation in rats. Am J Physiol (1985) 249(6 Pt 1):G792–9. doi: 10.1152/ajpgi.1985.249.6.G792
145. Windmueller HG, Spaeth AE. Source and fate of circulating citrulline. Am J Physiol (1981) 241(6):E473–80. doi: 10.1152/ajpendo.1981.241.6.E473
146. Blaser A, Padar M, Tang J, Dutton J, Forbes A. Citrulline and intestinal fatty acid-binding protein as biomarkers for gastrointestinal dysfunction in the critically ill. Anaesthesiol Intensive Ther (2019) 51(3):230–9. doi: 10.5114/ait.2019.86049
147. Fragkos KC, Forbes A. Citrulline as a marker of intestinal function and absorption in clinical settings: a systematic review and meta-analysis. United Eur Gastroenterol J (2018) 6(2):181–91. doi: 10.1177/2050640617737632
148. Crenn P, Vahedi K, Lavergne-Slove A, Cynober L, Matuchansky C, Messing B. Plasma citrulline: a marker of enterocyte mass in villous atrophy-associated small bowel disease. Gastroenterology (2003) 124(5):1210–9. doi: 10.1016/s0016-5085(03)00170-7
149. Diamanti A, Knafelz D, Panetta F, Bracci F, Gambarara M, Papadatou B, et al. Plasma citrulline as surrogate marker of intestinal inflammation in pediatric and adolescent with crohn’s disease: preliminary report. Int J Colorectal Dis (2011) 26(11):1445–51. doi: 10.1007/s00384-011-1255-z
150. Kulu R, Akyildiz H, Akcan A, Ozturk A, Sozuer E. Plasma citrulline measurement in the diagnosis of acute mesenteric ischaemia. ANZ J Surg (2017) 87(9):E57–60. doi: 10.1111/ans.13524
151. Maintz L, Novak N. Histamine and histamine intolerance. Am J Clin Nutr (2007) 85(5):1185–96. doi: 10.1093/ajcn/85.5.1185
152. Elmore BO, Bollinger JA, Dooley DM. Human kidney diamine oxidase: heterologous expression, purification, and characterization. J Biol Inorg Chem (2002) 7(6):565–79. doi: 10.1007/s00775-001-0331-1
153. Rokkas T, Vaja S, Taylor P, Murphy GM, Dowling RH. Is the intestine the sole source of heparin-stimulated plasma diamine oxidase? acute effects of jejunectomy, ileectomy and total enterectomy. Digestion (1990) 46 Suppl 2:439–46. doi: 10.1159/000200419
154. Bieganski T, Kusche J, Lorenz W, Hesterberg R, Stahlknecht CD, Feussner KD. Distribution and properties of human intestinal diamine oxidase and its relevance for the histamine catabolism. Biochim Biophys Acta (1983) 756(2):196–203. doi: 10.1016/0304-4165(83)90092-2
155. Luk GD, Vaughan WP, Burke PJ, Baylin SB. Diamine oxidase as a plasma marker of rat intestinal mucosal injury and regeneration after administration of 1-beta-D-arabinofuranosylcytosine. Cancer Res (1981) 41(6):2334–7.
156. Luk GD, Bayless TM, Baylin SB. Diamine oxidase (histaminase). a circulating marker for rat intestinal mucosal maturation and integrity. J Clin Invest (1980) 66(1):66–70. doi: 10.1172/JCI109836
157. Wolvekamp MC, de Bruin RW. Diamine oxidase: an overview of historical, biochemical and functional aspects. Dig Dis (1994) 12(1):2–14. doi: 10.1159/000171432
158. Meng Y, Zhang Y, Liu M, Huang YK, Zhang J, Yao Q, et al. Evaluating intestinal permeability by measuring plasma endotoxin and diamine oxidase in children with acute lymphoblastic leukemia treated with high-dose methotrexate. Anticancer Agents Med Chem (2016) 16(3):387–92. doi: 10.2174/1871520615666150812125955
159. Miyoshi J, Miyamoto H, Goji T, Taniguchi T, Tomonari T, Sogabe M, et al. Serum diamine oxidase activity as a predictor of gastrointestinal toxicity and malnutrition due to anticancer drugs. J Gastroenterol Hepatol (2015) 30(11):1582–90. doi: 10.1111/jgh.13004
160. Zhang L, Fan X, Zhong Z, Xu G, Shen J. Association of plasma diamine oxidase and intestinal fatty acid-binding protein with severity of disease in patient with heat stroke. Am J Emerg Med (2015) 33(7):867–71. doi: 10.1016/j.ajem.2015.01.047
161. Honzawa Y, Nakase H, Matsuura M, Chiba T. Clinical significance of serum diamine oxidase activity in inflammatory bowel disease: importance of evaluation of small intestinal permeability. Inflammation Bowel Dis (2011) 17(2):E23–5. doi: 10.1002/ibd.21588
162. Ji M, Huang H, Lan X. Correlation between intestinal microflora in irritable bowel syndrome and severity. Dis Markers (2022) 2022:1031844. doi: 10.1155/2022/1031844
163. Mennigen R, Kusche J, Streffer C, Krakamp B. Diamine oxidase activities in the large bowel mucosa of ulcerative colitis patients. Agents Actions (1990) 30(1-2):264–6. doi: 10.1007/BF01969056
164. Fukudome I, Kobayashi M, Dabanaka K, Maeda H, Okamoto K, Okabayashi T, et al. Diamine oxidase as a marker of intestinal mucosal injury and the effect of soluble dietary fiber on gastrointestinal tract toxicity after intravenous 5-fluorouracil treatment in rats. Med Mol Morphol (2014) 47(2):100–7. doi: 10.1007/s00795-013-0055-7
165. Thompson JS, Burnett DA, Markin RS, Vaughan WP. Intestinal mucosa diamine oxidase activity reflects intestinal involvement in crohn’s disease. Am J Gastroenterol (1988) 83(7):756–60.
166. Kusche J, Mennigen R, Erpenbach K. The intestinal diamine oxidase activity under the influence of adaptive proliferation of the intestinal mucosa–a proliferation terminating principle? Agents Actions (1988) 23(3-4):354–6. doi: 10.1007/BF02142586
167. Comas-Baste O, Sanchez-Perez S, Veciana-Nogues MT, Latorre-Moratalla M, Vidal-Carou MDC. Histamine intolerance: the current state of the art. Biomolecules (2020) 10(8):1181. doi: 10.3390/biom10081181
168. Ayuso P, Garcia-Martin E, Martinez C, Agundez JA. Genetic variability of human diamine oxidase: occurrence of three nonsynonymous polymorphisms and study of their effect on serum enzyme activity. Pharmacogenet Genomics (2007) 17(9):687–93. doi: 10.1097/FPC.0b013e328012b8e4
169. Kettner L, Seitl I, Fischer L. Recent advances in the application of microbial diamine oxidases and other histamine-oxidizing enzymes. World J Microbiol Biotechnol (2022) 38(12):232. doi: 10.1007/s11274-022-03421-2
170. Konovalova A, Kahne DE, Silhavy TJ. Outer membrane biogenesis. Annu Rev Microbiol (2017) 71:539–56. doi: 10.1146/annurev-micro-090816-093754
171. Beutler B, Rietschel ET. Innate immune sensing and its roots: the story of endotoxin. Nat Rev Immunol (2003) 3(2):169–76. doi: 10.1038/nri1004
172. Ikegame A, Kondo A, Kitaguchi K, Sasa K, Miyoshi M. Presepsin production in monocyte/macrophage-mediated phagocytosis of neutrophil extracellular traps. Sci Rep (2022) 12(1):5978. doi: 10.1038/s41598-022-09926-y
173. Ciesielska A, Matyjek M, Kwiatkowska K. TLR4 and CD14 trafficking and its influence on LPS-induced pro-inflammatory signaling. Cell Mol Life Sci (2021) 78(4):1233–61. doi: 10.1007/s00018-020-03656-y
174. Romero-Sanchez M, Gonzalez-Serna A, Pacheco YM, Ferrando-Martinez S, Machmach K, Garcia-Garcia M, et al. Different biological significance of sCD14 and LPS in HIV-infection: importance of the immunovirology stage and association with HIV-disease progression markers. J Infect (2012) 65(5):431–8. doi: 10.1016/j.jinf.2012.06.008
175. Marchetti G, Cozzi-Lepri A, Merlini E, Bellistri GM, Castagna A, Galli M, et al. Microbial translocation predicts disease progression of HIV-infected antiretroviral-naive patients with high CD4+ cell count. AIDS (2011) 25(11):1385–94. doi: 10.1097/QAD.0b013e3283471d10
176. da Silva B, Singer W, Fong IW, Ottaway CA. In vivo Cytokine and neuroendocrine responses to endotoxin in human immunodeficiency virus-infected subjects. J Infect Dis (1999) 180(1):106–15. doi: 10.1086/314819
177. Cassol E, Malfeld S, Mahasha P, van der Merwe S, Cassol S, Seebregts C, et al. Persistent microbial translocation and immune activation in HIV-1-infected south africans receiving combination antiretroviral therapy. J Infect Dis (2010) 202(5):723–33. doi: 10.1086/655229
178. Byakwaga H, Kelly M, Purcell DF, French MA, Amin J, Lewin SR, et al. Intensification of antiretroviral therapy with raltegravir or addition of hyperimmune bovine colostrum in HIV-infected patients with suboptimal CD4+ T-cell response: a randomized controlled trial. J Infect Dis (2011) 204(10):1532–40. doi: 10.1093/infdis/jir559
179. Sandler NG, Wand H, Roque A, Law M, Nason MC, Nixon DE, et al. Plasma levels of soluble CD14 independently predict mortality in HIV infection. J Infect Dis (2011) 203(6):780–90. doi: 10.1093/infdis/jiq118
180. Meiler C, Muhlbauer M, Johann M, Hartmann A, Schnabl B, Wodarz N, et al. Different effects of a CD14 gene polymorphism on disease outcome in patients with alcoholic liver disease and chronic hepatitis c infection. World J Gastroenterol (2005) 11(38):6031–7. doi: 10.3748/wjg.v11.i38.6031
181. Alhawi M, Stewart J, Erridge C, Patrick S, Poxton IR. Bacteroides fragilis signals through toll-like receptor (TLR) 2 and not through TLR4. J Med Microbiol (2009) 58(Pt 8):1015–22. doi: 10.1099/jmm.0.009936-0
182. Jumare J, Akolo C, Ndembi N, Bwala S, Alabi P, Okwuasaba K, et al. Elevated plasma levels of sCD14 and MCP-1 are associated with HIV associated neurocognitive disorders among antiretroviral-naive individuals in Nigeria. J Acquir Immune Defic Syndr (2020) 84(2):196–202. doi: 10.1097/QAI.0000000000002320
183. Manner IW, Baekken M, Kvale D, Oektedalen O, Pedersen M, Nielsen SD, et al. Markers of microbial translocation predict hypertension in HIV-infected individuals. HIV Med (2013) 14(6):354–61. doi: 10.1111/hiv.12015
184. Lyons JL, Uno H, Ancuta P, Kamat A, Moore DJ, Singer EJ, et al. Plasma sCD14 is a biomarker associated with impaired neurocognitive test performance in attention and learning domains in HIV infection. J Acquir Immune Defic Syndr (2011) 57(5):371–9. doi: 10.1097/QAI.0b013e3182237e54
185. Bruns T, Reuken PA, Stengel S, Gerber L, Appenrodt B, Schade JH, et al. The prognostic significance of bacterial DNA in patients with decompensated cirrhosis and suspected infection. Liver Int (2016) 36(8):1133–42. doi: 10.1111/liv.13095
186. Merlini E, Bellistri GM, Tincati C, d’Arminio Monforte A, Marchetti G. Sequencing of bacterial microflora in peripheral blood: our experience with HIV-infected patients. J Vis Exp (2011) 52:2830. doi: 10.3791/2830
187. Garcia-Mazcorro JF, Barcenas-Walls JR. Thinking beside the box: should we care about the non-coding strand of the 16S rRNA gene? FEMS Microbiol Lett (2016) 363(16):fnw171. doi: 10.1093/femsle/fnw171
188. Drancourt M, Bollet C, Carlioz A, Martelin R, Gayral JP, Raoult D. 16S ribosomal DNA sequence analysis of a large collection of environmental and clinical unidentifiable bacterial isolates. J Clin Microbiol (2000) 38(10):3623–30. doi: 10.1128/JCM.38.10.3623-3630.2000
189. Lichtfuss GF, Hoy J, Rajasuriar R, Kramski M, Crowe SM, Lewin SR. Biomarkers of immune dysfunction following combination antiretroviral therapy for HIV infection. biomark Med (2011) 5(2):171–86. doi: 10.2217/bmm.11.15
190. Kramski M, Gaeguta AJ, Lichtfuss GF, Rajasuriar R, Crowe SM, French MA, et al. Novel sensitive real-time PCR for quantification of bacterial 16S rRNA genes in plasma of HIV-infected patients as a marker for microbial translocation. J Clin Microbiol (2011) 49(10):3691–3. doi: 10.1128/JCM.01018-11
191. Favre D, Mold J, Hunt PW, Kanwar B, Loke P, Seu L, et al. Tryptophan catabolism by indoleamine 2,3-dioxygenase 1 alters the balance of TH17 to regulatory T cells in HIV disease. Sci Transl Med (2010) 2(32):32ra6. doi: 10.1126/scitranslmed.3000632
192. Brenchley JM, Price DA, Douek DC. HIV Disease: fallout from a mucosal catastrophe? Nat Immunol (2006) 7(3):235–9. doi: 10.1038/ni1316
193. Sacchi P, Cima S, Corbella M, Comolli G, Chiesa A, Baldanti F, et al. Liver fibrosis, microbial translocation and immune activation markers in HIV and HCV infections and in HIV/HCV co-infection. Dig Liver Dis (2015) 47(3):218–25. doi: 10.1016/j.dld.2014.11.012
194. Vera JH, Guo Q, Cole JH, Boasso A, Greathead L, Kelleher P, et al. Neuroinflammation in treated HIV-positive individuals: a TSPO PET study. Neurology (2016) 86(15):1425–32. doi: 10.1212/WNL.0000000000002485
195. Bossola M, Sanguinetti M, Scribano D, Zuppi C, Giungi S, Luciani G, et al. Circulating bacterial-derived DNA fragments and markers of inflammation in chronic hemodialysis patients. Clin J Am Soc Nephrol (2009) 4(2):379–85. doi: 10.2215/CJN.03490708
196. Schindler R, Beck W, Deppisch R, Aussieker M, Wilde A, Gohl H, et al. Short bacterial DNA fragments: detection in dialysate and induction of cytokines. J Am Soc Nephrol (2004) 15(12):3207–14. doi: 10.1097/01.ASN.0000145049.94888.26
197. Gomila M, Gasco J, Busquets A, Gil J, Bernabeu R, Buades JM, et al. Identification of culturable bacteria present in haemodialysis water and fluid. FEMS Microbiol Ecol (2005) 52(1):101–14. doi: 10.1016/j.femsec.2004.10.015
198. Vollmer J, Janosch A, Laucht M, Ballas ZK, Schetter C, Krieg AM. Highly immunostimulatory CpG-free oligodeoxynucleotides for activation of human leukocytes. Antisense Nucleic Acid Drug Dev (2002) 12(3):165–75. doi: 10.1089/108729002760220761
199. Verthelyi D, Ishii KJ, Gursel M, Takeshita F, Klinman DM. Human peripheral blood cells differentially recognize and respond to two distinct CPG motifs. J Immunol (2001) 166(4):2372–7. doi: 10.4049/jimmunol.166.4.2372
200. Ballas ZK, Rasmussen WL, Krieg AM. Induction of NK activity in murine and human cells by CpG motifs in oligodeoxynucleotides and bacterial DNA. J Immunol (1996) 157(5):1840–5. doi: 10.4049/jimmunol.157.5.1840
201. Reus Banuls S, Portilla Sogorb J, Sanchez-Paya J, Boix Martinez V, Giner Oncina L, Frances R, et al. [Association between inflammatory markers and microbial translocation in patients with human immunodeficiency virus infection taking antiretroviral treatment]. Med Clin (Barc) (2014) 142(2):47–52. doi: 10.1016/j.medcli.2013.05.044
202. Nash AK, Auchtung TA, Wong MC, Smith DP, Gesell JR, Ross MC, et al. The gut mycobiome of the human microbiome project healthy cohort. Microbiome (2017) 5(1):153. doi: 10.1186/s40168-017-0373-4
203. Hallen-Adams HE, Suhr MJ. Fungi in the healthy human gastrointestinal tract. Virulence (2017) 8(3):352–8. doi: 10.1080/21505594.2016.1247140
204. Dirajlal-Fargo S, Moser C, Rodriguez K, El-Kamari V, Funderburg NT, Bowman E, et al. Changes in the fungal marker beta-D-Glucan after antiretroviral therapy and association with adiposity. Open Forum Infect Dis (2019) 6(11):ofz434. doi: 10.1093/ofid/ofz434
205. Farhour Z, Mehraj V, Chen J, Ramendra R, Lu H, Routy JP. Use of (1–>3)-beta-d-glucan for diagnosis and management of invasive mycoses in HIV-infected patients. Mycoses (2018) 61(10):718–22. doi: 10.1111/myc.12797
206. He S, Hang JP, Zhang L, Wang F, Zhang DC, Gong FH. A systematic review and meta-analysis of diagnostic accuracy of serum 1,3-beta-D-glucan for invasive fungal infection: focus on cutoff levels. J Microbiol Immunol Infect (2015) 48(4):351–61. doi: 10.1016/j.jmii.2014.06.009
207. Leelahavanichkul A, Worasilchai N, Wannalerdsakun S, Jutivorakool K, Somparn P, Issara-Amphorn J, et al. Gastrointestinal leakage detected by serum (1–>3)-beta-D-Glucan in mouse models and a pilot study in patients with sepsis. Shock (2016) 46(5):506–18. doi: 10.1097/SHK.0000000000000645
208. Morris A, Hillenbrand M, Finkelman M, George MP, Singh V, Kessinger C, et al. Serum (1–>3)-beta-D-glucan levels in HIV-infected individuals are associated with immunosuppression, inflammation, and cardiopulmonary function. J Acquir Immune Defic Syndr (2012) 61(4):462–8. doi: 10.1097/QAI.0b013e318271799b
209. Hoenigl M, Moser CB, Funderburg N, Bosch R, Kantor A, Zhang Y, et al. Soluble urokinase plasminogen activator receptor is predictive of non-AIDS events during antiretroviral therapy-mediated viral suppression. Clin Infect Dis (2019) 69(4):676–86. doi: 10.1093/cid/ciy966
210. Hoenigl M, Perez-Santiago J, Nakazawa M, de Oliveira MF, Zhang Y, Finkelman MA, et al. (1–>3)-beta-d-Glucan: a biomarker for microbial translocation in individuals with acute or early HIV infection? Front Immunol (2016) 7:404. doi: 10.3389/fimmu.2016.00404
211. Hoenigl M, de Oliveira MF, Perez-Santiago J, Zhang Y, Woods SP, Finkelman M, et al. Correlation of (1–>3)-beta-D-glucan with other inflammation markers in chronically HIV infected persons on suppressive antiretroviral therapy. GMS Infect Dis (2015) 3:Doc3. doi: 10.3205/id000018
212. Hoenigl M, Lin J, Finkelman M, Zhang Y, Karris MY, Letendre SL, et al. Glucan rich nutrition does not increase gut translocation of beta-glucan. Mycoses (2021) 64(1):24–9. doi: 10.1111/myc.13161
213. Weiner LD, Retuerto M, Hager CL, El Kamari V, Shan L, Sattar A, et al. Fungal translocation is associated with immune activation and systemic inflammation in treated HIV. AIDS Res Hum Retroviruses (2019) 35(5):461–72. doi: 10.1089/AID.2018.0252
214. Mehraj V, Jenabian MA, Ponte R, Lebouche B, Costiniuk C, Thomas R, et al. The plasma levels of soluble ST2 as a marker of gut mucosal damage in early HIV infection. AIDS (2016) 30(10):1617–27. doi: 10.1097/QAD.0000000000001105
215. Jenabian MA, El-Far M, Vyboh K, Kema I, Costiniuk CT, Thomas R, et al. Immunosuppressive tryptophan catabolism and gut mucosal dysfunction following early HIV infection. J Infect Dis (2015) 212(3):355–66. doi: 10.1093/infdis/jiv037
216. Ciorba MA. Indoleamine 2,3 dioxygenase in intestinal disease. Curr Opin Gastroenterol (2013) 29(2):146–52. doi: 10.1097/MOG.0b013e32835c9cb3
217. Hoenigl M, de Oliveira MF, Perez-Santiago J, Zhang Y, Morris S, McCutchan AJ, et al. (1–>3)-beta-D-Glucan levels correlate with neurocognitive functioning in HIV-infected persons on suppressive antiretroviral therapy: a cohort study. Med (Baltimore) (2016) 95(11):e3162. doi: 10.1097/MD.0000000000003162
218. Hajam IA, Dar PA, Shahnawaz I, Jaume JC, Lee JH. Bacterial flagellin-a potent immunomodulatory agent. Exp Mol Med (2017) 49(9):e373. doi: 10.1038/emm.2017.172
219. Luo S, Deng X, Liu Q, Pan Z, Zhao Z, Zhou L, et al. Emodin ameliorates ulcerative colitis by the flagellin-TLR5 dependent pathway in mice. Int Immunopharmacol (2018) 59:269–75. doi: 10.1016/j.intimp.2018.04.010
220. Winstanley C, Morgan JAW. The bacterial flagellin gene as a biomarker for detection, population genetics and epidemiological analysis. Microbiol (Reading) (1997) 143(Pt 10):3071–84. doi: 10.1099/00221287-143-10-3071
221. Claud EC, Lu L, Anton PM, Savidge T, Walker WA, Cherayil BJ. Developmentally regulated IkappaB expression in intestinal epithelium and susceptibility to flagellin-induced inflammation. Proc Natl Acad Sci U.S.A. (2004) 101(19):7404–8. doi: 10.1073/pnas.0401710101
222. Gosselin KB, Aboud S, McDonald CM, Moyo S, Khavari N, Manji K, et al. Etiology of diarrhea, nutritional outcomes, and novel intestinal biomarkers in Tanzanian infants. J Pediatr Gastroenterol Nutr (2017) 64(1):104–8. doi: 10.1097/MPG.0000000000001323
223. Dlugosz A, Nowak P, D’Amato M, Mohammadian Kermani G, Nystrom J, Abdurahman S, et al. Increased serum levels of lipopolysaccharide and antiflagellin antibodies in patients with diarrhea-predominant irritable bowel syndrome. Neurogastroenterol Motil (2015) 27(12):1747–54. doi: 10.1111/nmo.12670
224. Targan SR, Landers CJ, Yang H, Lodes MJ, Cong Y, Papadakis KA, et al. Antibodies to CBir1 flagellin define a unique response that is associated independently with complicated crohn’s disease. Gastroenterology (2005) 128(7):2020–8. doi: 10.1053/j.gastro.2005.03.046
225. Lodes MJ, Cong Y, Elson CO, Mohamath R, Landers CJ, Targan SR, et al. Bacterial flagellin is a dominant antigen in crohn disease. J Clin Invest (2004) 113(9):1296–306. doi: 10.1172/JCI20295
226. Lunardi C, Bason C, Dolcino M, Navone R, Simone R, Saverino D, et al. Antiflagellin antibodies recognize the autoantigens toll-like receptor 5 and pals 1-associated tight junction protein and induce monocytes activation and increased intestinal permeability in crohn’s disease. J Intern Med (2009) 265(2):250–65. doi: 10.1111/j.1365-2796.2008.02013.x
227. Svard J, Paquin-Proulx D, Buggert M, Noyan K, Barqasho B, Sonnerborg A, et al. Role of translocated bacterial flagellin in monocyte activation among individuals with chronic HIV-1 infection. Clin Immunol (2015) 161(2):180–9. doi: 10.1016/j.clim.2015.08.018
228. Benedikz EK, Bailey D, Cook CNL, Goncalves-Carneiro D, Buckner MMC, Blair JMA, et al. Bacterial flagellin promotes viral entry via an NF-kB and toll like receptor 5 dependent pathway. Sci Rep (2019) 9(1):7903. doi: 10.1038/s41598-019-44263-7
229. Vassilieva EV, Wang BZ, Vzorov AN, Wang L, Wang YC, Bozja J, et al. Enhanced mucosal immune responses to HIV virus-like particles containing a membrane-anchored adjuvant. mBio (2011) 2(1):e00328–10. doi: 10.1128/mBio.00328-10
230. Thibault S, Imbeault M, Tardif MR, Tremblay MJ. TLR5 stimulation is sufficient to trigger reactivation of latent HIV-1 provirus in T lymphoid cells and activate virus gene expression in central memory CD4+ T cells. Virology (2009) 389(1-2):20–5. doi: 10.1016/j.virol.2009.04.019
231. Brichacek B, Vanpouille C, Kiselyeva Y, Biancotto A, Merbah M, Hirsch I, et al. Contrasting roles for TLR ligands in HIV-1 pathogenesis. PloS One (2010) 5(9):e12831. doi: 10.1371/journal.pone.0012831
232. Abdurahman S, Barqasho B, Nowak P, Cuong do D, Amogne W, Larsson M, et al. Pattern of microbial translocation in patients living with HIV-1 from Vietnam, Ethiopia and Sweden. J Int AIDS Soc (2014) 17:18841. doi: 10.7448/IAS.17.1.18841
233. Vesterbacka J, Nowak P, Barqasho B, Abdurahman S, Nystrom J, Nilsson S, et al. Kinetics of microbial translocation markers in patients on efavirenz or lopinavir/r based antiretroviral therapy. PloS One (2013) 8(1):e55038. doi: 10.1371/journal.pone.0055038
234. Levitt MD, Levitt DG. Quantitative evaluation of d-lactate pathophysiology: new insights into the mechanisms involved and the many areas in need of further investigation. Clin Exp Gastroenterol (2020) 13:321–37. doi: 10.2147/CEG.S260600
235. Smith SM, Eng RH, Buccini F. Use of d-lactic acid measurements in the diagnosis of bacterial infections. J Infect Dis (1986) 154(4):658–64. doi: 10.1093/infdis/154.4.658
236. Perlmutter DH, Boyle JT, Campos JM, Egler JM, Watkins JB. D-lactic acidosis in children: an unusual metabolic complication of small bowel resection. J Pediatr (1983) 102(2):234–8. doi: 10.1016/s0022-3476(83)80527-7
237. Stolberg L, Rolfe R, Gitlin N, Merritt J, Mann L Jr., Linder J, et al. D-lactic acidosis due to abnormal gut flora: diagnosis and treatment of two cases. N Engl J Med (1982) 306(22):1344–8. doi: 10.1056/NEJM198206033062207
238. Assadian A, Assadian O, Senekowitsch C, Rotter R, Bahrami S, Furst W, et al. Plasma d-lactate as a potential early marker for colon ischaemia after open aortic reconstruction. Eur J Vasc Endovasc Surg (2006) 31(5):470–4. doi: 10.1016/j.ejvs.2005.10.031
239. Szalay L, Umar F, Khadem A, Jafarmadar M, Furst W, Ohlinger W, et al. Increased plasma d-lactate is associated with the severity of hemorrhagic/traumatic shock in rats. Shock (2003) 20(3):245–50. doi: 10.1097/00024382-200309000-00008
240. Sun XQ, Fu XB, Zhang R, Lu Y, Deng Q, Jiang XG, et al. Relationship between plasma d(-)-lactate and intestinal damage after severe injuries in rats. World J Gastroenterol (2001) 7(4):555–8. doi: 10.3748/wjg.v7.i4.555
241. Cai J, Chen H, Weng M, Jiang S, Gao J. Diagnostic and clinical significance of serum levels of d-lactate and diamine oxidase in patients with crohn’s disease. Gastroenterol Res Pract (2019) 2019:8536952. doi: 10.1155/2019/8536952
242. Massanella M, Negredo E, Clotet B, Blanco J. Immunodiscordant responses to HAART–mechanisms and consequences. Expert Rev Clin Immunol (2013) 9(11):1135–49. doi: 10.1586/1744666X.2013.842897
243. Geng ST, Zhang JB, Wang YX, Xu Y, Lu D, Zhang Z, et al. Pre-digested protein enteral nutritional supplementation enhances recovery of CD4(+) T cells and repair of intestinal barrier in HIV-infected immunological non-responders. Front Immunol (2021) 12:757935. doi: 10.3389/fimmu.2021.757935
244. Vesterbacka J, Barqasho B, Haggblom A, Nowak P. Effects of Co-trimoxazole on microbial translocation in HIV-1-Infected patients initiating antiretroviral therapy. AIDS Res Hum Retroviruses (2015) 31(8):830–6. doi: 10.1089/AID.2014.0366
245. Serrano-Villar S, Talavera-Rodriguez A, Gosalbes MJ, Madrid N, Perez-Molina JA, Elliott RJ, et al. Fecal microbiota transplantation in HIV: a pilot placebo-controlled study. Nat Commun (2021) 12(1):1139. doi: 10.1038/s41467-021-21472-1
246. Sandler NG, Zhang X, Bosch RJ, Funderburg NT, Choi AI, Robinson JK, et al. Sevelamer does not decrease lipopolysaccharide or soluble CD14 levels but decreases soluble tissue factor, low-density lipoprotein (LDL) cholesterol, and oxidized LDL cholesterol levels in individuals with untreated HIV infection. J Infect Dis (2014) 210(10):1549–54. doi: 10.1093/infdis/jiu305
247. Michelini Z, Baroncelli S, Fantauzzi A, Pasquale C, Galluzzo CM, Sanchez M, et al. Reduced plasma levels of sCD14 and I-FABP in HIV-infected patients with mesalazine-treated ulcerative colitis. HIV Clin trials (2016) 17(2):49–54. doi: 10.1080/15284336.2015.1125077
248. Kasang C, Ulmer A, Donhauser N, Schmidt B, Stich A, Klinker H, et al. HIV Patients treated with low-dose prednisolone exhibit lower immune activation than untreated patients. BMC Infect Dis (2012) 12:14. doi: 10.1186/1471-2334-12-14
249. Grander C, Adolph TE, Wieser V, Lowe P, Wrzosek L, Gyongyosi B, et al. Recovery of ethanol-induced akkermansia muciniphila depletion ameliorates alcoholic liver disease. Gut (2018) 67(5):891–901. doi: 10.1136/gutjnl-2016-313432
250. Wang L, Tang L, Feng Y, Zhao S, Han M, Zhang C, et al. A purified membrane protein from akkermansia muciniphila or the pasteurised bacterium blunts colitis associated tumourigenesis by modulation of CD8(+) T cells in mice. Gut (2020) 69(11):1988–97. doi: 10.1136/gutjnl-2019-320105
251. Zhai R, Xue X, Zhang L, Yang X, Zhao L, Zhang C. Strain-specific anti-inflammatory properties of two akkermansia muciniphila strains on chronic colitis in mice. Front Cell Infect Microbiol (2019) 9:239. doi: 10.3389/fcimb.2019.00239
252. Liu JH, Yue T, Luo ZW, Cao J, Yan ZQ, Jin L, et al. Akkermansia muciniphila promotes type h vessel formation and bone fracture healing by reducing gut permeability and inflammation. Dis Model Mech (2020) 13(11):dmm043620. doi: 10.1242/dmm.043620
253. Wang K, Guo J, Chang X, Gui S. Painong-San extract alleviates dextran sulfate sodium-induced colitis in mice by modulating gut microbiota, restoring intestinal barrier function and attenuating TLR4/NF-kappaB signaling cascades. J Pharm BioMed Anal (2022) 209:114529. doi: 10.1016/j.jpba.2021.114529
254. Konturek PC, Koziel J, Dieterich W, Haziri D, Wirtz S, Glowczyk I, et al. Successful therapy of clostridium difficile infection with fecal microbiota transplantation. J Physiol Pharmacol (2016) 67(6):859–66.
255. Cammarota G, Ianiro G, Tilg H, Rajilic-Stojanovic M, Kump P, Satokari R, et al. European Consensus conference on faecal microbiota transplantation in clinical practice. Gut (2017) 66(4):569–80. doi: 10.1136/gutjnl-2016-313017
256. Song Y, Garg S, Girotra M, Maddox C, von Rosenvinge EC, Dutta A, et al. Microbiota dynamics in patients treated with fecal microbiota transplantation for recurrent clostridium difficile infection. PloS One (2013) 8(11):e81330. doi: 10.1371/journal.pone.0081330
257. Gough E, Shaikh H, Manges AR. Systematic review of intestinal microbiota transplantation (fecal bacteriotherapy) for recurrent clostridium difficile infection. Clin Infect Dis (2011) 53(10):994–1002. doi: 10.1093/cid/cir632
258. Cheng S, Ma X, Geng S, Jiang X, Li Y, Hu L, et al. Fecal microbiota transplantation beneficially regulates intestinal mucosal autophagy and alleviates gut barrier injury. mSystems (2018) 3(5):e00137–18. doi: 10.1128/mSystems.00137-18
259. Vujkovic-Cvijin I, Rutishauser RL, Pao M, Hunt PW, Lynch SV, McCune JM, et al. Limited engraftment of donor microbiome via one-time fecal microbial transplantation in treated HIV-infected individuals. Gut Microbes (2017) 8(5):440–50. doi: 10.1080/19490976.2017.1334034
260. Pandrea I, Xu C, Stock JL, Frank DN, Ma D, Policicchio BB, et al. Antibiotic and antiinflammatory therapy transiently reduces inflammation and hypercoagulation in acutely SIV-infected pigtailed macaques. PloS Pathog (2016) 12(1):e1005384. doi: 10.1371/journal.ppat.1005384
261. Tenorio AR, Chan ES, Bosch RJ, Macatangay BJ, Read SW, Yesmin S, et al. Rifaximin has a marginal impact on microbial translocation, T-cell activation and inflammation in HIV-positive immune non-responders to antiretroviral therapy - ACTG A5286. J Infect Dis (2015) 211(5):780–90. doi: 10.1093/infdis/jiu515
262. Sun PP, Perianayagam MC, Jaber BL. Sevelamer hydrochloride use and circulating endotoxin in hemodialysis patients: a pilot cross-sectional study. J Ren Nutr (2009) 19(5):432–8. doi: 10.1053/j.jrn.2009.01.022
263. Perianayagam MC, Jaber BL. Endotoxin-binding affinity of sevelamer hydrochloride. Am J Nephrol (2008) 28(5):802–7. doi: 10.1159/000135691
264. Stinghen AE, Goncalves SM, Bucharles S, Branco FS, Gruber B, Hauser AB, et al. Sevelamer decreases systemic inflammation in parallel to a reduction in endotoxemia. Blood Purif (2010) 29(4):352–6. doi: 10.1159/000302723
265. Kristoff J, Haret-Richter G, Ma D, Ribeiro RM, Xu C, Cornell E, et al. Early microbial translocation blockade reduces SIV-mediated inflammation and viral replication. J Clin Invest (2014) 124(6):2802–6. doi: 10.1172/JCI75090
266. Tajik N, Frech M, Schulz O, Schalter F, Lucas S, Azizov V, et al. Targeting zonulin and intestinal epithelial barrier function to prevent onset of arthritis. Nat Commun (2020) 11(1):1995. doi: 10.1038/s41467-020-15831-7
267. Gopalakrishnan S, Tripathi A, Tamiz AP, Alkan SS, Pandey NB. Larazotide acetate promotes tight junction assembly in epithelial cells. Peptides (2012) 35(1):95–101. doi: 10.1016/j.peptides.2012.02.016
268. Gopalakrishnan S, Durai M, Kitchens K, Tamiz AP, Somerville R, Ginski M, et al. Larazotide acetate regulates epithelial tight junctions. Vitro vivo. Peptides (2012) 35(1):86–94. doi: 10.1016/j.peptides.2012.02.015
269. Kim MH, Kim H. The roles of glutamine in the intestine and its implication in intestinal diseases. Int J Mol Sci (2017) 18(5):1051. doi: 10.3390/ijms18051051
270. Li N, Lewis P, Samuelson D, Liboni K, Neu J. Glutamine regulates caco-2 cell tight junction proteins. Am J Physiol Gastrointest Liver Physiol (2004) 287(3):G726–33. doi: 10.1152/ajpgi.00012.2004
271. Wang B, Wu G, Zhou Z, Dai Z, Sun Y, Ji Y, et al. Glutamine and intestinal barrier function. Amino Acids (2015) 47(10):2143–54. doi: 10.1007/s00726-014-1773-4
272. Camilleri M, Madsen K, Spiller R, Greenwood-Van Meerveld B, Verne GN. Intestinal barrier function in health and gastrointestinal disease. Neurogastroenterol Motil (2012) 24(6):503–12. doi: 10.1111/j.1365-2982.2012.01921.x
273. Souba WW, Klimberg VS, Plumley DA, Salloum RM, Flynn TC, Bland KI, et al. The role of glutamine in maintaining a healthy gut and supporting the metabolic response to injury and infection. J Surg Res (1990) 48(4):383–91. doi: 10.1016/0022-4804(90)90080-l
274. Cario E, Gerken G, Podolsky DK. Toll-like receptor 2 controls mucosal inflammation by regulating epithelial barrier function. Gastroenterology (2007) 132(4):1359–74. doi: 10.1053/j.gastro.2007.02.056
275. Baan B, Dihal AA, Hoff E, Bos CL, Voorneveld PW, Koelink PJ, et al. 5-aminosalicylic acid inhibits cell cycle progression in a phospholipase d dependent manner in colorectal cancer. Gut (2012) 61(12):1708–15. doi: 10.1136/gutjnl-2011-301626
276. Joo K, Lee Y, Choi D, Han J, Hong S, Kim YM, et al. An anti-inflammatory mechanism of taurine conjugated 5-aminosalicylic acid against experimental colitis: taurine chloramine potentiates inhibitory effect of 5-aminosalicylic acid on IL-1beta-mediated NFkappaB activation. Eur J Pharmacol (2009) 618(1-3):91–7. doi: 10.1016/j.ejphar.2009.07.009
277. Rousseaux C, Lefebvre B, Dubuquoy L, Lefebvre P, Romano O, Auwerx J, et al. Intestinal antiinflammatory effect of 5-aminosalicylic acid is dependent on peroxisome proliferator-activated receptor-gamma. J Exp Med (2005) 201(8):1205–15. doi: 10.1084/jem.20041948
278. Weber CK, Liptay S, Wirth T, Adler G, Schmid RM. Suppression of NF-kappaB activity by sulfasalazine is mediated by direct inhibition of IkappaB kinases alpha and beta. Gastroenterology (2000) 119(5):1209–18. doi: 10.1053/gast.2000.19458
279. Nielsen OH, Bukhave K, Elmgreen J, Ahnfelt-Ronne I. Inhibition of 5-lipoxygenase pathway of arachidonic acid metabolism in human neutrophils by sulfasalazine and 5-aminosalicylic acid. Dig Dis Sci (1987) 32(6):577–82. doi: 10.1007/BF01296156
280. Somsouk M, Dunham RM, Cohen M, Albright R, Abdel-Mohsen M, Liegler T, et al. The immunologic effects of mesalamine in treated HIV-infected individuals with incomplete CD4+ T cell recovery: a randomized crossover trial. PloS One (2014) 9(12):e116306. doi: 10.1371/journal.pone.0116306
281. Timmermans S, Souffriau J, Libert C. A general introduction to glucocorticoid biology. Front Immunol (2019) 10:1545. doi: 10.3389/fimmu.2019.01545
282. Fischer A, Gluth M, Weege F, Pape UF, Wiedenmann B, Baumgart DC, et al. Glucocorticoids regulate barrier function and claudin expression in intestinal epithelial cells via MKP-1. Am J Physiol Gastrointest Liver Physiol (2014) 306(3):G218–28. doi: 10.1152/ajpgi.00095.2013
283. Boivin MA, Ye D, Kennedy JC, Al-Sadi R, Shepela C, Ma TY. Mechanism of glucocorticoid regulation of the intestinal tight junction barrier. Am J Physiol Gastrointest Liver Physiol (2007) 292(2):G590–8. doi: 10.1152/ajpgi.00252.2006
284. Wild GE, Waschke KA, Bitton A, Thomson AB. The mechanisms of prednisone inhibition of inflammation in crohn’s disease involve changes in intestinal permeability, mucosal TNFalpha production and nuclear factor kappa b expression. Aliment Pharmacol Ther (2003) 18(3):309–17. doi: 10.1046/j.1365-2036.2003.01611.x
285. Nockher WA, Scherberich JE. Expression and release of the monocyte lipopolysaccharide receptor antigen CD14 are suppressed by glucocorticoids. Vivo vitro. J Immunol (1997) 158(3):1345–52. doi: 10.4049/jimmunol.158.3.1345
286. Li Z, Trakooljul N, Hadlich F, Ponsuksili S, Wimmers K, Murani E. Transcriptome analysis of porcine PBMCs reveals lipopolysaccharide-induced immunomodulatory responses and crosstalk of immune and glucocorticoid receptor signaling. Virulence (2021) 12(1):1808–24. doi: 10.1080/21505594.2021.1948276
287. Van Looveren K, Timmermans S, Vanderhaeghen T, Wallaeys C, Ballegeer M, Souffriau J, et al. Glucocorticoids limit lipopolysaccharide-induced lethal inflammation by a double control system. EMBO Rep (2020) 21(7):e49762. doi: 10.15252/embr.201949762
288. Wang J, Wang R, Yang J, Yang X, Hu S, Wang H, et al. Glucocorticoids differentially regulate the innate immune responses of TLR4 and the cytosolic DNA sensing pathway. Int Immunopharmacol (2017) 47:190–8. doi: 10.1016/j.intimp.2017.03.022
289. Ulmer A, Muller M, Bertisch-Mollenhoff B, Frietsch B. Low-dose prednisolone has a CD4-stabilizing effect in pre-treated HIV-patients during structured therapy interruptions (STI). Eur J Med Res (2005) 10(6):227–32.
290. Ulmer A, Muller M, Bertisch-Mollenhoff B, Frietsch B. Low dose prednisolone reduces CD4+ T cell loss in therapy-naive HIV-patients without antiretroviral therapy. Eur J Med Res (2005) 10(3):105–9.
291. Andrieu JM, Lu W. Long-term clinical, immunologic and virologic impact of glucocorticoids on the chronic phase of HIV infection. BMC Med (2004) 2:17. doi: 10.1186/1741-7015-2-17
292. Oray M, Abu Samra K, Ebrahimiadib N, Meese H, Foster CS. Long-term side effects of glucocorticoids. Expert Opin Drug Saf (2016) 15(4):457–65. doi: 10.1517/14740338.2016.1140743
293. Damba JJ, Laskine M, Peet MM, Jin Y, Sinyavskaya L, Durand M. Corticosteroids use and incidence of severe infections in people living with HIV compared to a matched population. J Int Assoc Provid AIDS Care (2022) 21:23259582221107196. doi: 10.1177/23259582221107196
294. Peyro-Saint-Paul L, Besnier P, Demessine L, Biour M, Hillaire-Buys D, de Canecaude C, et al. Cushing’s syndrome due to interaction between ritonavir or cobicistat and corticosteroids: a case-control study in the French pharmacovigilance database. J Antimicrob Chemother (2019) 74(11):3291–4. doi: 10.1093/jac/dkz324
295. Renjifo B, van Wyk J, Salem AH, Bow D, Ng J, Norton M. Pharmacokinetic enhancement in HIV antiretroviral therapy: a comparison of ritonavir and cobicistat. AIDS Rev (2015) 17(1):37–46.
296. Kiesslich R, Goetz M, Angus EM, Hu Q, Guan Y, Potten C, et al. Identification of epithelial gaps in human small and large intestine by confocal endomicroscopy. Gastroenterology (2007) 133(6):1769–78. doi: 10.1053/j.gastro.2007.09.011
297. Ma TY, Iwamoto GK, Hoa NT, Akotia V, Pedram A, Boivin MA, et al. TNF-alpha-induced increase in intestinal epithelial tight junction permeability requires NF-kappa b activation. Am J Physiol Gastrointest Liver Physiol (2004) 286(3):G367–76. doi: 10.1152/ajpgi.00173.2003
298. Xu P, Elamin E, Elizalde M, Bours P, Pierik MJ, Masclee AAM, et al. Modulation of intestinal epithelial permeability by plasma from patients with crohn’s disease in a three-dimensional cell culture model. Sci Rep (2019) 9(1):2030. doi: 10.1038/s41598-018-38322-8
299. Fischer A, Gluth M, Pape UF, Wiedenmann B, Theuring F, Baumgart DC. Adalimumab prevents barrier dysfunction and antagonizes distinct effects of TNF-alpha on tight junction proteins and signaling pathways in intestinal epithelial cells. Am J Physiol Gastrointest Liver Physiol (2013) 304(11):G970–9. doi: 10.1152/ajpgi.00183.2012
300. Danese S. New therapies for inflammatory bowel disease: from the bench to the bedside. Gut (2012) 61(6):918–32. doi: 10.1136/gutjnl-2011-300904
301. Suenaert P, Bulteel V, Lemmens L, Noman M, Geypens B, Van Assche G, et al. Anti-tumor necrosis factor treatment restores the gut barrier in crohn’s disease. Am J Gastroenterol (2002) 97(8):2000–4. doi: 10.1111/j.1572-0241.2002.05914.x
302. Wada NI, Jacobson LP, Margolick JB, Breen EC, Macatangay B, Penugonda S, et al. The effect of HAART-induced HIV suppression on circulating markers of inflammation and immune activation. AIDS (2015) 29(4):463–71. doi: 10.1097/QAD.0000000000000545
303. Vaidya SA, Korner C, Sirignano MN, Amero M, Bazner S, Rychert J, et al. Tumor necrosis factor alpha is associated with viral control and early disease progression in patients with HIV type 1 infection. J Infect Dis (2014) 210(7):1042–6. doi: 10.1093/infdis/jiu206
304. Aukrust P, Liabakk NB, Muller F, Lien E, Espevik T, Froland SS. Serum levels of tumor necrosis factor-alpha (TNF alpha) and soluble TNF receptors in human immunodeficiency virus type 1 infection–correlations to clinical, immunologic, and virologic parameters. J Infect Dis (1994) 169(2):420–4. doi: 10.1093/infdis/169.2.420
305. Pasquereau S, Kumar A, Herbein G. Targeting TNF and TNF receptor pathway in HIV-1 infection: from immune activation to viral reservoirs. Viruses (2017) 9(4):64. doi: 10.3390/v9040064
306. Gallitano SM, McDermott L, Brar K, Lowenstein E. Use of tumor necrosis factor (TNF) inhibitors in patients with HIV/AIDS. J Am Acad Dermatol (2016) 74(5):974–80. doi: 10.1016/j.jaad.2015.11.043
307. Shale MJ, Seow CH, Coffin CS, Kaplan GG, Panaccione R, Ghosh S. Review article: chronic viral infection in the anti-tumour necrosis factor therapy era in inflammatory bowel disease. Aliment Pharmacol Ther (2010) 31(1):20–34. doi: 10.1111/j.1365-2036.2009.04112.x
308. Crespo-Bermejo C, de Arellano ER, Lara-Aguilar V, Valle-Millares D, Gomez-Lus ML, Madrid R, et al. Persistent low-level viremia in persons living with HIV undertreatment: an unresolved status. Virulence (2021) 12(1):2919–31. doi: 10.1080/21505594.2021.2004743
309. Isnard S, Ramendra R, Lin J, Kant S, Fombuena B, Ouyang J, et al. Anti-cytomegalovirus immunoglobulin G is linked to CD4 T-cell count decay in human immunodeficiency virus (HIV) elite controllers. Clin Infect Dis (2021) 73(1):144–7. doi: 10.1093/cid/ciaa1129
Keywords: HIV infection, biomarker, intestine, microbial translocation, inflammation
Citation: Ouyang J, Yan J, Zhou X, Isnard S, Harypursat V, Cui H, Routy J-P and Chen Y (2023) Relevance of biomarkers indicating gut damage and microbial translocation in people living with HIV. Front. Immunol. 14:1173956. doi: 10.3389/fimmu.2023.1173956
Received: 25 February 2023; Accepted: 10 April 2023;
Published: 21 April 2023.
Edited by:
Javier Leceta, Complutense University of Madrid, SpainReviewed by:
Simona Alexandra Iacob, Carol Davila University of Medicine and Pharmacy, RomaniaJingjing Meng, University of Miami, United States
Copyright © 2023 Ouyang, Yan, Zhou, Isnard, Harypursat, Cui, Routy and Chen. This is an open-access article distributed under the terms of the Creative Commons Attribution License (CC BY). The use, distribution or reproduction in other forums is permitted, provided the original author(s) and the copyright owner(s) are credited and that the original publication in this journal is cited, in accordance with accepted academic practice. No use, distribution or reproduction is permitted which does not comply with these terms.
*Correspondence: Jean-Pierre Routy, amVhbi1waWVycmUucm91dHlAbWNnaWxsLmNh; Yaokai Chen, eWFva2FpY2hlbkBob3RtYWlsLmNvbQ==
†These authors have contributed equally to this work