- 1Cancer Therapeutics Program, Ottawa Hospital Research Institute, Ottawa, Canada
- 2Department of Medicine, University of Ottawa, Ottawa, Canada
- 3Department of Biochemistry, Microbiology and Immunology, University of Ottawa, Ottawa, Canada
- 4Center for Infection, Immunity and Inflammation, University of Ottawa, Ottawa, Canada
- 5Human Health Therapeutics Research Centre, National Research Council Canada, Ottawa, Canada
- 6Department of Medicine, University of Ottawa, Ottawa Hospital Research Institute, Ottawa, Canada
Advancements in chimeric antigen receptor engineered T-cell (CAR-T) therapy have revolutionized treatment for several cancer types over the past decade. Despite this success, obstacles including the high price tag, manufacturing complexity, and treatment-associated toxicities have limited the broad application of this therapy. Chimeric antigen receptor engineered natural killer cell (CAR-NK) therapy offers a potential opportunity for a simpler and more affordable “off-the-shelf” treatment, likely with fewer toxicities. Unlike CAR-T, CAR-NK therapies are still in early development, with few clinical trials yet reported. Given the challenges experienced through the development of CAR-T therapies, this review explores what lessons we can apply to build better CAR-NK therapies. In particular, we explore the importance of optimizing the immunochemical properties of the CAR construct, understanding factors leading to cell product persistence, enhancing trafficking of transferred cells to the tumor, ensuring the metabolic fitness of the transferred product, and strategies to avoid tumor escape through antigen loss. We also review trogocytosis, an important emerging challenge that likely equally applies to CAR-T and CAR-NK cells. Finally, we discuss how these limitations are already being addressed in CAR-NK therapies, and what future directions may be possible.
Introduction
Although chimeric antigen receptor engineered T-cells (CAR-T) have produced astounding remission rates in patients with hematological cancers, response rates have been much lower in patients with solid tumors (1). Furthermore, long-term follow up has shown that approximately half of the patients achieving an initial complete response with CAR-T will ultimately relapse (2, 3). Key barriers leading to suboptimal CAR therapy responses include limited persistence of transferred cells, poor metabolic fitness of cellular products, issues with trafficking of CAR-T cells to sites of disease, and loss of target antigen on malignant cells (Figure 1) (4). Another emerging challenge for CAR therapies is trogocytosis, wherein cell membranes are transferred from target cells to immune cells, resulting in antigen loss on target cells and fratricidal destruction of CAR cells (Figure 2) (5). Importantly, as clinical experience with CAR-NK is very limited compared to CAR-T, how much each of these factors contribute to CAR-NK clinical efficacy remains unclear. This offers researchers and clinicians a unique opportunity to proactively apply lessons learned while developing CAR-T products to the CAR-NK field. For the remainder of this mini review, we will provide updates on the well-identified barriers and then discuss at length the concept of trogocytosis and the work that has been done to address this increasingly recognized phenomenon.
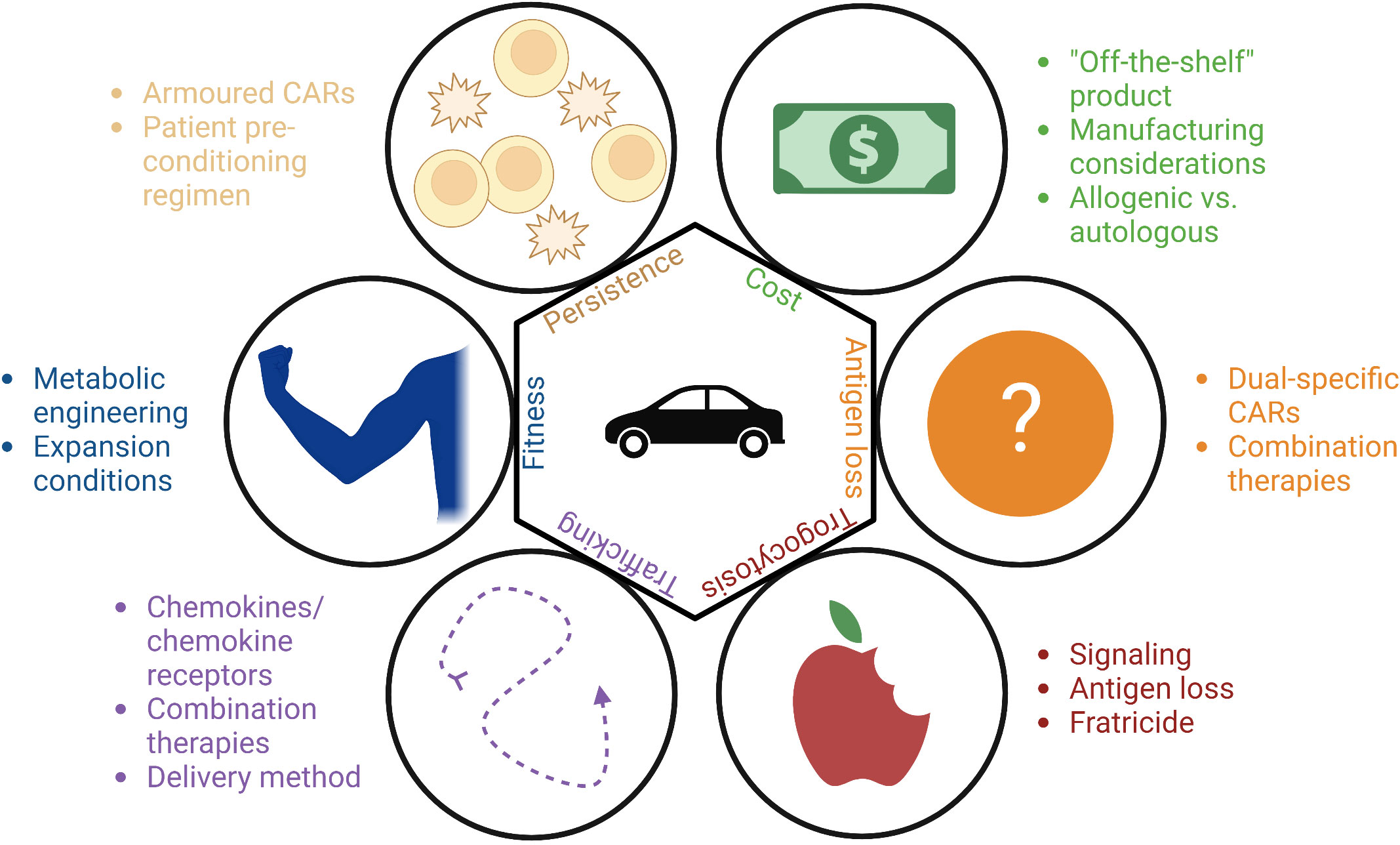
Figure 1 Methods to overcome the barriers to the CAR-T and CAR-NK fields: cost, antigen loss, trogocytosis, trafficking, fitness, and persistence.
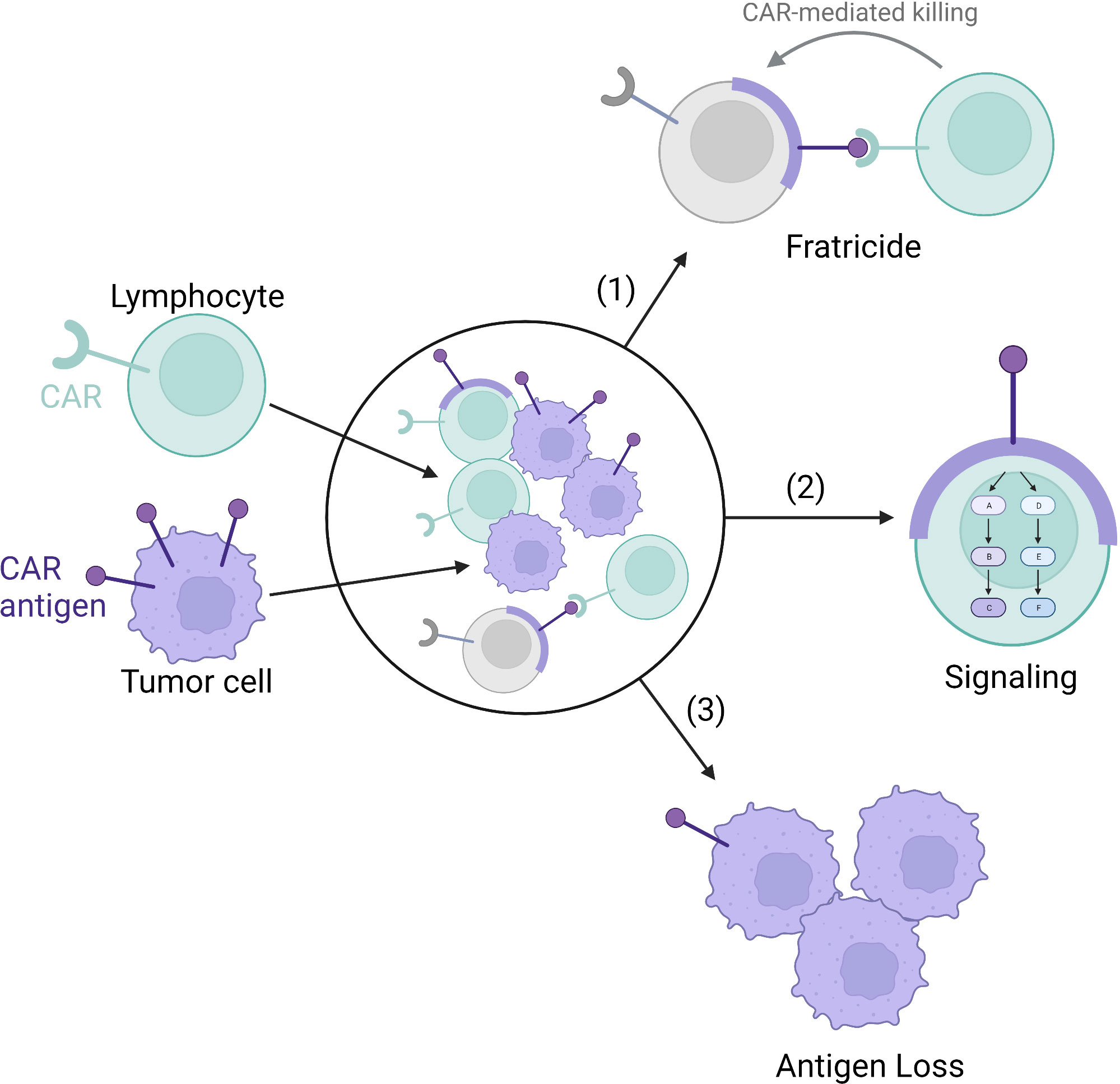
Figure 2 Trogocytosis-mediated antigen procurement leads to trogocytosis-mediated signaling, antigen loss leading to tumor escape, and fratricide. The tumor cell membrane (purple) and CAR antigen is transferred to the CAR-lymphocytes (green) via trogocytosis. (1) The transferred membrane acts as a target for CAR-mediated fratricide leading to lymphocyte cell death (grey). (2) The transferred membrane can also signal within the CAR-lymphocyte. (3) Finally, the transfer of the cell membrane results in antigen loss on the tumor cells themselves.
Manufacturing and cost
CD19-targeted CAR-T has achieved unprecedented clinical response in leukemia and lymphoma, and now B-cell maturation antigen (BCMA)-targeted CAR-T is yielding remarkable results in patients with multiple myeloma (6, 7). High cost and complex manufacturing for these personalized CAR-T limit access to these therapies (8). Recently, studies have reported the use of distributed and highly automated CAR-T manufacturing as a strategy to significantly reduce manufacturing costs relative to centralized processes (9–11). An alternative cost-reduction strategy would be to develop an “off the shelf” therapeutic, wherein modified cells are manufactured in large numbers at a central location and used allogeneically to treat many patients (12).
Currently, CAR-T products approved for commercial use are all produced using lentiviral or retroviral vector transduction, and are only used autologously due to the risk of graft versus host disease (GvHD). It is important to note though that it may be possible to safely apply donor derived allogenic CAR-T molecules in post-haematopoietic cell transplantion (HCT) settings without acute GvHD (13). Gene editing to remove T cell receptor expression from CAR-T cells has been employed to eliminate the risk of GvHD with TCR-edited allogeneic CAR-T products. However, this increases manufacturing complexity and cost, and the safety profile of such gene-edited CAR-T cells is not yet fully understood (14–16). Unlike CAR-T cells, CAR-NK cells do not cause GvHD and can thus be applied allogeneically without such gene editing (17).
To maximize the benefit of an off-the-shelf therapy, as with gene-edited CAR-T, it will also be vital to identify manufacturing approaches that can scale up CAR-NK products to treat as many patients as possible per product batch (18–20). Like CAR-T cells, cytokines alone can achieve expansion of NK cells to clinically relevant doses (21, 22). NK expansion can be greatly increased with the use of feeder cells, however, feeder cells can be difficult to completely remove from the culture, therefore leading to safety concerns (23–26). An alternative feeder-free method being explored involves the use of dissolveable polymer-based microspheres that slowly release growth factors and nutrients to facilitate cell expansion (27).
Vesicular-stomatitis-virus-G protein (VSV-G) lentivirus is the most commonly used pseudotyping receptor applied in manufacturing CAR-T cells. Unlike T cells though, NK cells have low expression of the low density lipoprotein receptor, a major entry receptor for VSV-G (28). Baboon envelope pseudotyped lentiviral vector (BaEV-LV) has been shown to greatly improve transduction efficiency in NK cells (29), and can also be used for the delivery of CRISPR-genome editing components through viral like particles (30). We have recently shown that CRISPR-loaded BaEV-partcles can be used for efficient simultaneous genome editing of primary NK cells and CAR-transgene delivery, potentially offering a promising opportunity to clinical scale deployment of gene edited CAR-NK therapies (31).
The relative risk of insertional mutagenesis represents another potential issue for CAR-NK therapies manufactured via viral gene transfer. There are at least two cases of T-cell lymphomas arising from CAR-modified cells, though it is important to note that both of these occurred in transposon modified cells, a process that can lead to many insertion events within a single cell (32). In contrast to this, thousands of patients have now been treated with CAR-T therapies generated via retro- or lentiviral transduction, with follow-up times as long as 20-years, and no malignant T-cell lymphomas have yet been reported (33). There are at least two case reports of insertional disruption of a specific gene being associated with clonal CAR-T hyperexpansion (34, 35). Remarkably, in both cases CAR-T expansion drove a strong therapeutic response without creating a T-cell malignancy. Whether the impressive safety record of virally modified CAR-T cells can also be extended to CAR-NK cells remains to be seen. Publication of pre-clinical and clinical reports with insertion site mapping in CAR-NK cells will be critical to address this question in future.
Persistence
Dogma dictates that NK cells are inherently less likely to demonstrate long term persistence than T cells (36). Indeed, the short lifespan of transferred cells has been proposed as an explanation for the limited clinical efficacy of NK cell therapies in clinical trials (37–39). To date, much of the work in optimizing CAR constructs for NK cells has focused on improving cytotoxicity as opposed to evaluating persistence (18, 40, 41), though it is known that CAR-NK cell persistence can be improved by engineering cells with immunostimulatory cytokines such as IL-15 (42–49). A clinical trial employing IL-15 engineered CD19-targeted CAR-NK cells in relapsed/refractory hematologic malignancies is currently underway, and has already reported to be safe and show at least short term efficacy in a cohort of patients [(50); NCT05092451].
Short-term exposure to IL-12, IL-15, and IL-18 produces memory-like NK cells with longer persistence (51), which can be combined with CAR-NK to increase efficacy (52). In CAR-T products, expression of certain costimulatory domains is known to enrich for memory phenotype CAR-T cells and is associated with improved persistence and more durable responses in preclinical models and clinical trials (53–55). Engineering of CAR-T cells with immunostimulatory cytokines (secreted or membrane bound) such as IL-7, IL-12, IL-15, IL-18, IL-21, and IL-23, to create “armoured CARs”, has also been shown to improve persistence and is being explored in several clinical trials (56, 57).
Another method to increase CAR cell functionality, safety, and specificity is the use of inducible promoters, which become active upon recognition of a tumor associated antigen, metabolite, drug, or through activation of cell signaling pathways. Ultimately, the goal is to facilitate a more directed delivery of an additional transgene without systemic toxicity (58). This has been shown to be effective in CAR-T cells using a nuclear factor of activated T-cells (NFAT) promoter (59). This type of sense-and-respond engineering of CAR-T cells is extremely flexible and could be applied to deliver a wide variety of TME-modifying payloads to improve CAR-T or CAR-NK penetration and survival within tumours (60, 61). This approach has also been tried with CAR-NK cells; the use of a nuclear factor kappa B (NFκB) inducible promoter to induce IL-12 secretion upon CAR-NK activation led to increased cytotoxicity and monocyte recruitment (62).
A major clinical determinant of NK cell persistence is the lymphodepleting pre-conditioning regimen used to prepare patients before cell infusion. Thusfar, the role of lymphodepletion with CAR-NK therapy has been extrapolated from CAR-T studies, where lymphodepletion has been shown to be vital for the efficacy of CAR-T (63). Typically, a combination of fludarabine/cyclophosphamide is used prior to receiving CAR-NK therapy to reduce NK cell rejection by the host, reprogram the immunosuppressive TME, and decrease tumor burden (18). Comparing high-dose and low-dose lymphodepletion regimens prior to adoptive transfer of unmodified NK cell therapies has demonstrated that high-dose regimens resulted in in vivo NK expansion and persistence while low-dose regimens did not (64). The use of CD52-targeting monoclonal antibodies in combination with CD52-knockout CAR-T cells is increasingly being employed as a selective lymphodepletion strategy which can be employed concurrently with cellular infusion (65), whether such an approach can work with CAR-NK cells has not yet been investigated. To our knowledge, there have been no clinical trials comparing lympdepleting regimens in CAR-NK therapies, and this is an area where further investigation will be needed. The need for further study will be even more critical when applying CAR-NK in solid tumours, where the question of the value of lymphodepletion is quite controversial due to the potential for such immune suppression to harm endogenous anti-tumor immune responses.
Ultimately, long-term persistence of CAR-NK cells may not be necessary to achieve long term remissions. While loss of functional CAR-T cells has been established in some clinical trials as the single best predictor of relapse, particularly in the setting of some acute and chronic B-cell leukemias (34, 66), persistence of CAR products may be less important in other malignancies, such as lymphomas (53, 67, 68). In diffuse large B cell lymphoma (a non-hodgkin lymphoma) for example, patients can have durable responses without prolonged CAR-T persistence (67, 69, 70). In line with these observations, optimization of CAR design to maximize short-term or long-term responses appears to be disease specific rather than a one size fits all approach (54). Given this evolution in thinking with regard to CAR-T persistence, it is likely that the need for longer term persistence of CAR-NK cells may similarly be disease specific (53). Clinical studies applying different CAR-NK approaches in different disease settinge will be vital to understand how CAR-NK persistence correlates with outcome. Furthermore, an off-the-shelf CAR-NK therapy could make it easier to compensate for lack of persistence through using multiple infusions to maintain a sufficient number of circulating cells for ongoing disease control.
Trafficking
Issues with CAR-T or CAR-NK cells in locating and penetrating into the tumor microenvironment (TME) are thought to be an important limit for the efficacy of the therapy in solid tumors (58). One method to improve CAR trafficking is to engineer cells to express chemokine receptors that can directly enhance their ability to track tumor sites. This strategy has been evaluated in pre-clinical studies (71) and is being explored in an early phase clinical trial where CXCR4 co-expression on an anti- B-cell maturation antigen (BCMA) CAR-T was added to increase trafficking to the bone marrow (NCT04727008). Similar to CAR-T cells, NK cells could also benefit from engineered expression of chemokine receptors, as NK cells are known to use chemokine signaling in natural settings (72). NK cells modified to express CXCR2, CXCR4, CCR5, or CCR7 have all been shown to have enhanced tumor control in mice (73–76). In addition, CAR-NK cells engineered to express CXCR1 or CXCR4 also experienced enhanced trafficking to the tumor (74, 77).
Strategies to improve CAR-T trafficking also include attempts to combine with other therapies to make the tumor microenvironment more amenable to lymphocyte recruitment, or modifying the delivery method (direct tumoral injection or systemic delivery) of the CAR therapy (58, 78). The tumor microenvironment can be modified using tools such as oncolytic viruses (OVs) (79), or radiotherapy (80) to increase tumor inflammation (stimulating an immune response) and increase CAR efficacy. While there are substantial studies on combination therapies for CAR-T, combination studies in CAR-NK cells with radiotherapy or OVs are lacking (81). Finally, local injection of CAR-T cells to the tumour, rather than the peripheral blood, improved responses (82–84). This method has also been shown to be safe and efficacious for CAR-NK (85).
Fitness
For CAR-T therapy to be successful after finding the tumor, CAR-expressing cells need to also survive and function in the harsh TME, where considerable barriers prevent the normal function of immune cells. Barriers include: hypoxia, lack of nutrients, low pH, and elevated levels of various metabolic waste products (86). CAR-T cell products with optimized metabolic functions, such as higher oxidative phosphorylation, have been shown to be more efficacious in the clinic as they can overcome these limitations in the TME (87, 88). Two strategies to metabolically improve T cell function in the TME are: i) by direct manipulation of cell metabolism during ex vivo expansion, or ii) genetically engineering T cells to better cope with the TME (89). As in the case of CAR-T cells, NK cells can also be metabolically optimized during expansion either through genetic alterations or by manipulating expansion conditions (90–93).
Looking at examples of ex vivo metabolic engineering of T cells, CD8+ T cells cultured in glutamine restricted conditions had enhanced expression of pro-survival transcription factors and were able to expand better in mice upon reinfusion (94). T cells expanded with mitogen-activated protein kinase kinase (MEK) inhibitors led to decreased tumor burden in mice (95). Finally, T cells were expanded in the presence of phosphoinositide 3-kinase inhibitor (PI3K) to enrich for memory T cells in an ongoing BCMA CAR-T clinical trial which is reported to show promising signs of improved efficacy (96). Even the choice of media can have a major impact on the function and metabolism of the T cells (97). Work in NK cells has been carried out to examine the impact of changing feeder cells used for expansion, and how this can affect metabolic fitness and cytotoxicity (98, 99).
Changes to the CAR itself are also known to alter metabolism, as different signaling domains can significantly change glucose or oxidative metabolism in CAR-T cells (100). Additionally, alteration of the cell by knock-out or overexpression of metabolic genes can increase CAR-T cell efficacy. For example, the TME contains low levels of arginine, so overexpressing enzymes for arginine synthesis in T cells has been shown to improve their efficacy in mouse models of leukemia and solid tumors (101). Similarly, overexpression of D-2-hydroxyglutarate dehydrogenase in CAR-T cells overcomes the immunosuppressive effects of D-2-hydroxyglutarate in tumors with isocitrate dehydrogenase mutation (102). Genetically engineering NK cells to overcome the immune suppressive effects of metabolites, such as hydrogen peroxide through the expression of peroxiredoxin-1 has been shown to improve NK cell function (103). Much opportunity remains to metabolically optimize CAR-NK therapeutics to thrive in the TME.
Antigen loss
Tumor intrinsic factors also contribute to suboptimal CAR-T responses. This can include immune suppression in the TME through the presence of immunosuppressive cells (e.g. due to cells such as regulatory T cells, myloid derived suppressor cells, tumor associated macrophages, and stromal cells) (104). It can also include resistance due to loss of adhesion molecules on the tumor cells, such as CD58 or ICAM-1 (105, 106), as well as increased expression of apoptotic moleucles (107). The tumor intrinsic factor we will focus on for this section is antigen loss.
Loss of target antigen on tumor cells is an obvious mechanism by which CAR-T therapies fail (108, 109). This may be exacerbated in solid tumors where there is a greater degree of inconsistency in antigen expression (110, 111). Several strategies are under development to circumvent this limitation. Dual-specificity CAR-T cells capable of targeting two separate antigens have achieved strong complete response rates in clinical trials (112–115). Even then, relapses following the loss of at least one antigen have been observed (113–115). Longer follow up and larger trials are needed to determine if these strategies are truly beneficial in reducing relapse and what effect multi-antigen targeting has on CAR-T persistence. Sequential administration of different CAR products has also been explored as a strategy to mitigate tumor antigen loss (116, 117). Furthermore, in preclinical models, combinations with oncolytic viruses, multi-antigen targeting using ankyrin repeat motif CARs, and engineering CAR-T cells to modulate the endogenous immune system are all strategies that have shown promise in limiting relapse by overcoming antigen loss (118–120).
Given the broader antigen independent killing capacity of NK cells, it is possible that CAR-NK cells would have a built-in mechanism for resisting tumor escape due to the loss of CAR target antigen (121, 122). That is, NK cells can rely on their innate killing capabilities rather than on antigen recognition through the CAR alone. However, it is also well established that tumors have the capacity to adapt and evade NK cells both in the context of immunoediting and NK-cell based immunotherapies (123, 124). The implementation of dual target approaches in CAR-NK cells is currently being explored in pre-clinical development (46, 125–128). Clinical experience will show whether loss of multiple antigens is a concern in the context of CAR-NK therapies. However, with either CAR-T or CAR-NKs, identifying better strategies to select the optimal antigen combinations will be critical to creating safe and effective therapies.
Trogocytosis
The term trogocytosis was used in the 1970s to describe the process in which the amoeba, Naegleria fowleri, destroys other cells (129). In 2002, it was adapted to describe the transfer of plasma membrane fragments and associated surface molecules from one cell to another (130). Since then, trogocytosis has been well studied in T and NK cells (131). It has been proposed that trogocytosis provides a mechanism for tissue adaptation of immune cells but can also act as a potent mechanism of immune cell deactivation (132). Trogocytosis has also been documented more recently with CAR-T and CAR-NK cells, causing fratricidal killing and potentially limiting therapeutic efficacy. We will review the consequences of trogocytosis-mediated antigen procurement. Specifically, how this leads to trogocytosis-mediated signaling, target cell antigen loss, and fratricide (Figure 2).
Trogocytosis-mediated signaling
It has been shown that molecules can be transferred between cells via trogocytosis within minutes of contact, and these molecules retain their ability to signal (131), remaining on the cell surface for days (133). Thus, it is important to consider how these acquired proteins change the function of the receiving cell. On one hand, trogocytosis can have positive consequences on NK cell function. For example, NK cells can trogocytose chemokine receptors such as CCR5, CXCR4, or CCR7 (134–136). The acquisition of CCR7 leads to increased NK cell homing to the lymph nodes (135). The acquisition of some molecules, such as TYR03, also increases effector function and proliferation (137). In contrast, trogocytosis can also inhibit NK cell cytotoxicity through the acquisition of immunosuppressive proteins (138–142). In the context of cancer, we found that NK cells acquire PD-1 from leukemia cells (138). In ovarian cancer, NK cells acquire CD9 from ovarian cancer tumor cells, supressing cytotoxicity (139). Despite many studies investigating trogocytosis-mediated signaling, there are few studies proposing methods to counteract these effects. One method to overcome trogocytosis-mediated signaling is to use blocking antibodies against the target antigen. In the case of CD9, NK cell cytotoxicity was restored using a CD9 blocking antibody in vitro (139).
To the best of our knowledge, signaling from receptors transferred via trogocytosis to CAR-T and CAR-NK cells has not yet been explored. Given that antigens targeted by the CAR can be important signaling molecules in tumor cells (such as BCMA in plasma cells, or mesothelin in solid tumors) (143, 144). Acquisition of target molecules by T or NK cells through trogocytosis may also alter cell function in the CAR cells. Future studies could explore the signaling of trogocytosis-acquired molecules in CAR cells and determine if this is a necessary consideration in designing CAR therapies.
Antigen loss
Another consequence of trogocytosis is antigen loss on the target cells. While this has been shown in CAR-NK (145), it is more highly studied in the context of CAR-T (146–148). Since antigen density impacts CAR functionality, downregulation or internalization of the target antigen can lead to tumor escape. Therefore, methods to overcome trogocytosis-induced antigen loss could improve tumor clearance. Trogocytosis may be overcome by tweaking the affinity of the CAR for the target antigen, as low affinity constructs are thought to lead to less trogocytosis while maintaining efficacy (149). Several reports have shown that lower affinity CARs present increased efficacy by preventing tumor escape (147, 149–151); it is tempting to speculate that lower trogocytosis might contribute to this improved function.
Another option to overcome trogocytosis-mediated antigen loss is to adjust the signaling domain of the CAR, as trogocytosis has been shown to affect CARs with CD28 or 4-1BB signaling domain differentially (147). Finally, one interesting study showed that overexpression of cholesterol 25-hydroxylase (CH25H), can lower trogocytosis in CAR-T cells through altered cholesterol metabolism, leading to better outcomes in xenograft models (152). For both T and NK cells, it is not clear at this time whether CAR-expression increases trogocytic transfer by increasing the strength and length of CAR-target cell interaction, or if the antigen-specific binding of the CAR is directly implicated in the process of antigen transfer. More mechanistic studies of trogocytosis in the context of CAR signaling will be needed to better understand the underlying biological processes and how this applies to antigen loss.
Fratricide
In some cases, the ultimate consequence of trogocytosis-mediated antigen transfer to NK cells is NK-mediated killing of other NK cells, a process known as fratricide. In non-genetically modified NK cells trogocytosis of MHC class I-related chain A (MICA) in humans or retinoic acid early-inducible protein 1 (Rae-1) in mice has been shown to lead to fratricidal NK-mediated killing of NK cells (153, 154). This is also a common problem in CAR therapy, when the CAR target antigen is transferred from the tumor to the CAR-T or CAR-NK cell, leading to fratricide of CAR cells (145, 146). In the context of endogenous T or NK antigens like CD7 or CD38 respectively, knockout of the target antigen in the effector cell can overcome fratricidal killing (155, 156), but such an approach would not work for antigens which are transferred to effector cells via trogocytosis. As described above, design of the CAR to limit trogocytosis in CAR-T cells could not only improve antigen loss, but it can also prevent fratricide (147, 149, 152). In the case of NK-CARs, a recent elegant study used an inhibitory receptor targeting an NK-restricted antigen along with the tumour-targeted CAR in order to prevent trogocytosis and improve therapeutic activity (145). Future studies are needed to assess whether specific changes in the design of the various domains of the CAR construct can reduce trogocytosis mediated antigen loss and/or fratricide, and thereby improve the long-term efficacy of both CAR-T and CAR-NK therapies.
Conclusion
While not yet as well understood as CAR-T, CAR-NK have shown promise in early studies treating both hematologic malignancies and solid tumors. However, despite the clear conceptual advantages to NK cell-based CAR therapies, and a robust clinical safety record for NK therapies in general, CAR-NK treatments have not had the strong efficacy and/or long-term persistence that are needed. The multiple reasons underlying the struggles for CAR-NK therapy have yet to be fully elucidated but certainly include many of the same challenges which limit CAR-T therapies. Hopefully by applying lessons learned over many years of experience in the CAR-T field, those working with CAR-NK cells will be able to capitalize on the unique assets offered by NK cell biology and create the next generation of revolutionary accessible and affordable cellular therapies.
Author contributions
MK, DB, SL, MA, SM, and AV wrote, edited, and conceptualized the manuscript. All authors contributed to the article and approved the submitted version.
Funding
SM was funded by the National Research Council Canada Disruptive Technology Solutions Cell and Gene Therapy challenge program. AV was funded by the Myeloma Canada Aldo Del Research Grant. MA was funded by CIHR and a Faculty of Medicine Translational Research Grant.
Conflict of interest
MA received monetary compensation from Alloy Therapeutics for consulting. MA is under a contract agreement to perform sponsored research with Actym Therapeutics and Dragonfly Therapeutics. Neither consulting nor sponsored research is related to the present article.
The remaining authors declare that the research was conducted in the absence of any commercial or financial relationships that could be constructed as a potential conflict of interest.
Publisher’s note
All claims expressed in this article are solely those of the authors and do not necessarily represent those of their affiliated organizations, or those of the publisher, the editors and the reviewers. Any product that may be evaluated in this article, or claim that may be made by its manufacturer, is not guaranteed or endorsed by the publisher.
References
1. Grigor EJM, Fergusson D, Kekre N, Montroy J, Atkins H, Seftel MD, et al. Risks and benefits of chimeric antigen receptor T-cell (CAR-T) therapy in cancer: a systematic review and meta-analysis. Transfus Med Rev (2019) 33:98–110. doi: 10.1016/j.tmrv.2019.01.005
2. Aamir S, Anwar MY, Khalid F, Khan SI, Ali MA, Khattak ZE. Systematic review and meta-analysis of CD19-specific CAR-T cell therapy in Relapsed/Refractory acute lymphoblastic leukemia in the pediatric and young adult population: safety and efficacy outcomes. Clin Lymphoma Myeloma Leuk (2021) 21:e334–47. doi: 10.1016/j.clml.2020.12.010
3. Grover P, Veilleux O, Tian L, Sun R, Previtera M, Curran E, et al. Chimeric antigen receptor T-cell therapy in adults with b-cell acute lymphoblastic leukemia. Blood Adv (2022) 6:1608–18. doi: 10.1182/bloodadvances.2020003482
4. Sterner RC, Sterner RM. CAR-T cell therapy: current limitations and potential strategies. Blood Cancer J (2021) 11:1–11. doi: 10.1038/s41408-021-00459-7
5. Miao L, Zhang Z, Ren Z, Tang F, Li Y. Obstacles and coping strategies of CAR-T cell immunotherapy in solid tumors. Front Immunol (2021) 12:687822. doi: 10.3389/fimmu.2021.687822
6. June CH, O’Connor RS, Kawalekar OU, Ghassemi S, Milone MC. CAR T cell immunotherapy for human cancer. Science (2018) 359:1361–5. doi: 10.1126/science.aar6711
7. Roex G, Timmers M, Wouters K, Campillo-Davo D, Flumens D, Schroyens W, et al. Safety and clinical efficacy of BCMA CAR-t-cell therapy in multiple myeloma. J Hematol Oncol J Hematol Oncol (2020) 13:1–14. doi: 10.1186/s13045-020-01001-1
8. Rebecca Borgert P. Improving outcomes and mitigating costs associated with CAR T-cell therapy (2021). Available at: https://www.ajmc.com/view/improving-outcomes-and-mitigating-costs-associated-with-car-t-cell-therapy.
9. Palani HK, Arunachalam AK, Yasar M, Venkatraman A, Kulkarni U, Lionel SA, et al. Decentralized manufacturing of anti CD19 CAR-T cells using CliniMACS prodigy®: real-world experience and cost analysis in India. Bone Marrow Transpl (2022) 58, 160–167. doi: 10.1038/s41409-022-01866-5
10. Kekre N, Hay KA, Webb JR, Mallick R, Balasundaram M, Sigrist MK, et al. CLIC-01: manufacture and distribution of non-cryopreserved CAR-T cells for patients with CD19 positive hematologic malignancies. Front Immunol (2022) 13:1074740. doi: 10.3389/fimmu.2022.1074740
11. Ran T, Eichmüller SB, Schmidt P, Schlander M. Cost of decentralized CAR T-cell production in an academic nonprofit setting. Int J Cancer (2020) 147:3438–45. doi: 10.1002/ijc.33156
12. Cutmore LC, Marshall JF. Current perspectives on the use of off the shelf CAR-T/NK cells for the treatment of cancer. Cancers (2021) 13:1926. doi: 10.3390/cancers13081926
13. Sanber K, Savani B, Jain T. Graft-versus-host disease risk after chimeric antigen receptor T-cell therapy: the diametric opposition of T cells. Br J Haematol (2021) 195:660–8. doi: 10.1111/bjh.17544
14. Mailankody S, Matous JV, Chhabra S, Liedtke M, Sidana S, Oluwole OO, et al. Allogeneic BCMA-targeting CAR T cells in relapsed/refractory multiple myeloma: phase 1 UNIVERSAL trial interim results. Nat Med (2023) 29:422–429. doi: 10.1038/s41591-022-02182-7
15. Depil S, Duchateau P, Grupp SA, Mufti G, Poirot L. ‘Off-the-shelf’ allogeneic CAR T cells: development and challenges. Nat Rev Drug Discovery (2020) 19:185–99. doi: 10.1038/s41573-019-0051-2
16. Sidaway P. Allogeneic CAR T cells show promise. Nat Rev Clin Oncol (2022) 19:748–8. doi: 10.1038/s41571-022-00703-4
17. Daher M, Rezvani K. Next generation natural killer cells for cancer immunotherapy: the promise of genetic engineering. Curr Opin Immunol (2018) 51:146–53. doi: 10.1016/j.coi.2018.03.013
18. Xie G, Dong H, Liang Y, Ham JD, Rizwan R, Chen J. CAR-NK cells: a promising cellular immunotherapy for cancer. EBioMedicine (2020) 59:102975. doi: 10.1016/j.ebiom.2020.102975
19. Mueller S, Sohmen M, Kostyra J, Mekes A, Nitsche M, Luevano ME, et al. GMP-compliant, automated process for generation of CAR NK cells in a closed system for clinical use. Cytotherapy (2020) 22:S205–6. doi: 10.1016/j.jcyt.2020.04.085
20. Oberschmidt O, Morgan M, Huppert V, Kessler J, Gardlowski T, Matthies N, et al. Development of automated separation, expansion, and quality control protocols for clinical-scale manufacturing of primary human NK cells and alpharetroviral chimeric antigen receptor engineering. Hum Gene Ther Methods (2019) 30:102–20. doi: 10.1089/hgtb.2019.039
21. Sutlu T, Stellan B, Gilljam M, Quezada HC, Nahi H, Gahrton G, et al. Clinical-grade, large-scale, feeder-free expansion of highly active human natural killer cells for adoptive immunotherapy using an automated bioreactor. Cytotherapy (2010) 12:1044–55. doi: 10.3109/14653249.2010.504770
22. Wagner J, Pfannenstiel V, Waldmann A, Bergs JWJ, Brill B, Huenecke S, et al. A two-phase expansion protocol combining interleukin (IL)-15 and IL-21 improves natural killer cell proliferation and cytotoxicity against rhabdomyosarcoma. Front Immunol (2017) 8:676. doi: 10.3389/fimmu.2017.00676
23. Liu S, Galat V, Galat4 Y, Lee YKA, Wainwright D, Wu J. NK cell-based cancer immunotherapy: from basic biology to clinical development. J Hematol Oncol J Hematol Oncol (2021) 14:7. doi: 10.1186/s13045-020-01014-w
24. Gurney M, Kundu S, Pandey S, O’Dwyer M. Feeder cells at the interface of natural killer cell activation, expansion and gene editing. Front Immunol (2022) 13:802906. doi: 10.3389/fimmu.2022.802906
25. Lapteva N, Durett AG, Sun J, Rollins LA, Huye LL, Fang J, et al. Large-Scale ex vivo expansion and characterization of natural killer cells for clinical applications. Cytotherapy (2012) 14:1131–43. doi: 10.3109/14653249.2012.700767
26. Fujisaki H, Kakuda H, Shimasaki N, Imai C, Ma J, Lockey T, et al. Expansion of highly cytotoxic human natural killer cells for cancer cell therapy. Cancer Res (2009) 69:4010–7. doi: 10.1158/0008-5472.CAN-08-3712
27. Johnson CDL, Zale NE, Frary ED, Lomakin JA. Feeder-Cell-Free and serum-free expansion of natural killer cells using cloudz microspheres, G-Rex6M, and human platelet lysate. Front Immunol (2022) 13:803380. doi: 10.3389/fimmu.2022.803380
28. Gong Y, Klein Wolterink RGJ, Wang J, Bos GMJ, Germeraad WTV. Chimeric antigen receptor natural killer (CAR-NK) cell design and engineering for cancer therapy. J Hematol Oncol J Hematol Oncol (2021) 14:1–35. doi: 10.1186/s13045-021-01083-5
29. Colamartino ABL, Lemieux W, Bifsha P, Nicoletti S, Chakravarti N, Sanz J, et al. Efficient and robust NK-cell transduction with baboon envelope pseudotyped lentivector. Front Immunol (2019) 10:2873. doi: 10.3389/fimmu.2019.02873
30. Gutierrez-Guerrero A, Abrey Recalde MJ, Mangeot PE, Costa C, Bernadin O, Périan S, et al. Baboon envelope pseudotyped “Nanoblades” carrying Cas9/gRNA complexes allow efficient genome editing in human T, b, and CD34+ cells and knock-in of AAV6-encoded donor DNA in CD34+ cells. Front Genome Ed (2021) 3:604371. doi: 10.3389/fgeed.2021.604371
31. Jo D-H, Kaczmarek S, Shin O, Wang L, Cowan J, McComb S, et al. Simultaneous engineering of natural killer cells for CAR transgenesis and CRISPR-Cas9 knockout using retroviral particles. Mol Ther - Methods Clin Dev (2023) 29:173–184. doi: 10.1016/j.omtm.2023.03.006
32. Micklethwaite KP, Gowrishankar K, Gloss BS, Li Z, Street JA, Moezzi L, et al. Investigation of product-derived lymphoma following infusion of piggyBac-modified CD19 chimeric antigen receptor T cells. Blood (2021) 138:1391–405. doi: 10.1182/blood.2021010858
33. Moretti A, Ponzo M, Nicolette CA, Tcherepanova IY, Biondi A, Magnani CF, et al. Present, and future of non-viral CAR T cells. Front Immunol (2022) 13:867013. doi: 10.3389/fimmu.2022.867013
34. Fraietta JA, Lacey SF, Orlando EJ, Pruteanu-Malinici I, Gohil M, Lundh S, et al. Determinants of response and resistance to CD19 chimeric antigen receptor (CAR) T cell therapy of chronic lymphocytic leukemia. Nat Med (2018) 24:563–71. doi: 10.1038/s41591-018-0010-1
35. Shah NN, Qin H, Yates B, Su L, Shalabi H, Raffeld M, et al. Clonal expansion of CAR T cells harboring lentivector integration in the CBL gene following anti-CD22 CAR T-cell therapy. Blood Adv (2019) 3:2317–22. doi: 10.1182/bloodadvances.2019000219
36. Lowry LE, Zehring WA. Potentiation of natural killer cells for cancer immunotherapy: a review of literature. Front Immunol (2017) 8:1061. doi: 10.3389/fimmu.2017.01061
37. Shaffer BC, Le Luduec J-B, Forlenza C, Jakubowski AA, Perales M-A, Young JW, et al. Phase II study of haploidentical natural killer cell infusion for treatment of relapsed or persistent myeloid malignancies following allogeneic hematopoietic cell transplantation. Biol Blood Marrow Transpl J Am Soc Blood Marrow Transpl (2016) 22:705–9. doi: 10.1016/j.bbmt.2015.12.028
38. Bachanova V, Burns LJ, McKenna DH, Curtsinger J, Panoskaltsis-Mortari A, Lindgren BR, et al. Allogeneic natural killer cells for refractory lymphoma. Cancer Immunol Immunother CII (2010) 59:1739–44. doi: 10.1007/s00262-010-0896-z
39. Nguyen R, Wu H, Pounds S, Inaba H, Ribeiro RC, Cullins D, et al. A phase II clinical trial of adoptive transfer of haploidentical natural killer cells for consolidation therapy of pediatric acute myeloid leukemia. J Immunother Cancer (2019) 7:81. doi: 10.1186/s40425-019-0564-6
40. Xiao L, Cen D, Gan H, Sun Y, Huang N, Xiong H, et al. Adoptive transfer of NKG2D CAR mRNA-engineered natural killer cells in colorectal cancer patients. Mol Ther J Am Soc Gene Ther (2019) 27:1114–25. doi: 10.1016/j.ymthe.2019.03.011
41. Xu Y, Liu Q, Zhong M, Wang Z, Chen Z, Zhang Y, et al. 2B4 costimulatory domain enhancing cytotoxic ability of anti-CD5 chimeric antigen receptor engineered natural killer cells against T cell malignancies. J Hematol Oncol J Hematol Oncol (2019) 12:49. doi: 10.1186/s13045-019-0732-7
42. Christodoulou I, Ho WJ, Marple A, Ravich JW, Tam A, Rahnama R, et al. Engineering CAR-NK cells to secrete IL-15 sustains their anti-AML functionality but is associated with systemic toxicities. J Immunother Cancer (2021) 9:e003894. doi: 10.1136/jitc-2021-003894
43. Du Z, Ng YY, Zha S, Wang S. piggyBac system to co-express NKG2D CAR and IL-15 to augment the in vivo persistence and anti-AML activity of human peripheral blood NK cells. Mol Ther Methods Clin Dev (2021) 23:582–96. doi: 10.1016/j.omtm.2021.10.014
44. Morgan MA, Kloos A, Lenz D, Kattre N, Nowak J, Bentele M, et al. Improved activity against acute myeloid leukemia with chimeric antigen receptor (CAR)-NK-92 cells designed to target CD123. Viruses (2021) 13:1365. doi: 10.3390/v13071365
45. Teng K-Y, Mansour AG, Zhu Z, Li Z, Tian L, Ma S, et al. Off-the-Shelf prostate stem cell antigen–directed chimeric antigen receptor natural killer cell therapy to treat pancreatic cancer. Gastroenterology (2022) 162:1319–33. doi: 10.1053/j.gastro.2021.12.281
46. Cichocki F, Goodridge JP, Bjordahl R, Mahmood S, Davis ZB, Gaidarova S, et al. Dual antigen–targeted off-the-shelf NK cells show durable response and prevent antigen escape in lymphoma and leukemia. Blood (2022) 140:2451–62. doi: 10.1182/blood.2021015184
47. Cichocki F, Bjordahl R, Goodridge JP, Mahmood S, Gaidarova S, Abujarour R, et al. Quadruple gene-engineered natural killer cells enable multi-antigen targeting for durable antitumor activity against multiple myeloma. Nat Commun (2022) 13:7341. doi: 10.1038/s41467-022-35127-2
48. Xu D-L, He Y-Q, Xiao B, Si Y, Shi J, Liu X-A, et al. A novel sushi-IL15-PD1 CAR-NK92 cell line with enhanced and PD-L1 targeted cytotoxicity against pancreatic cancer cells. Front Oncol (2022) 12:726985. doi: 10.3389/fonc.2022.726985
49. Liu E, Tong Y, Dotti G, Shaim H, Savoldo B, Mukherjee M, et al. Cord blood NK cells engineered to express IL-15 and a CD19-targeted CAR show long-term persistence and potent antitumor activity. Leukemia (2018) 32:520–31. doi: 10.1038/leu.2017.226
50. Liu E, Marin D, Banerjee P, Macapinlac HA, Thompson P, Basar R, et al. Use of CAR-transduced natural killer cells in CD19-positive lymphoid tumors. N Engl J Med (2020) 382:545–53. doi: 10.1056/NEJMoa1910607
51. Foltz JA, Berrien-Elliott MM, Neal C, Foster M, McClain E, Schappe T, et al. Cytokine-induced memory-like (ML) NK cells persist for > 2 months following adoptive transfer into leukemia patients with a MHC-compatible hematopoietic cell transplant (HCT). Blood (2019) 134:1954. doi: 10.1182/blood-2019-126004
52. Gang M, Marin ND, Wong P, Neal CC, Marsala L, Foster M, et al. CAR-modified memory-like NK cells exhibit potent responses to NK-resistant lymphomas. Blood (2020) 136:2308–18. doi: 10.1182/blood.2020006619
53. Gupta A, Gill S. CAR-T cell persistence in the treatment of leukemia and lymphoma. Leuk Lymphoma (2021) 62:2587–99. doi: 10.1080/10428194.2021.1913146
54. Weinkove R, George P, Dasyam N, McLellan AD. Selecting costimulatory domains for chimeric antigen receptors: functional and clinical considerations. Clin Transl Immunol (2019) 8:e1049. doi: 10.1002/cti2.1049
55. Deng Q, Han G, Puebla-Osorio N, Ma MCJ, Strati P, Chasen B, et al. Characteristics of anti-CD19 CAR T cell infusion products associated with efficacy and toxicity in patients with large b cell lymphomas. Nat Med (2020) 26:1878–87. doi: 10.1038/s41591-020-1061-7
56. Zhang Z, Miao L, Ren Z, Tang F, Li Y. Gene-edited interleukin CAR-T cells therapy in the treatment of malignancies: present and future. Front Immunol (2021) 12:718686. doi: 10.3389/fimmu.2021.718686
57. Yeku OO, Brentjens RJ. Armored CAR T-cells: utilizing cytokines and pro-inflammatory ligands to enhance CAR T-cell anti-tumour efficacy. Biochem Soc Trans (2016) 44:412–8. doi: 10.1042/BST20150291
58. White LG, Goy HE, Rose AJ, McLellan AD. Controlling cell trafficking: addressing failures in CAR T and NK cell therapy of solid tumours. Cancers (2022) 14:978. doi: 10.3390/cancers14040978
59. Chmielewski M, Abken H, Talleur AC TRUCKs: the fourth generation of CARs. Expert Opin Biol Ther (2015) 15(8):1145–54. doi: 10.1517/14712598.2015.1046430
60. Guo T, Ma D, Lu TK. Sense-and-Respond payload delivery using a novel antigen-inducible promoter improves suboptimal CAR-T activation. ACS Synth Biol (2022) 11:1440–53. doi: 10.1021/acssynbio.1c00236
61. Choi BD, Yu X, Castano AP, Bouffard AA, Schmidts A, Larson RC, et al. CAR-T cells secreting BiTEs circumvent antigen escape without detectable toxicity. Nat Biotechnol (2019) 37:1049–58. doi: 10.1038/s41587-019-0192-1
62. Rudek LS, Zimmermann K, Galla M, Meyer J, Kuehle J, Stamopoulou A, et al. Generation of an NFκB-driven alpharetroviral “All-in-One” vector construct as a potent tool for CAR NK cell therapy. Front Immunol (2021) 12:751138. doi: 10.3389/fimmu.2021.751138
63. Amini L, Silbert SK, Maude SL, Nastoupil LJ, Ramos CA, Brentjens RJ, et al. Preparing for CAR T cell therapy: patient selection, bridging therapies and lymphodepletion. Nat Rev Clin Oncol (2022) 19:342–55. doi: 10.1038/s41571-022-00607-3
64. Miller JS, Soignier Y, Panoskaltsis-Mortari A, McNearney SA, Yun GH, Fautsch SK, et al. Successful adoptive transfer and in vivo expansion of human haploidentical NK cells in patients with cancer. Blood (2005) 105:3051–7. doi: 10.1182/blood-2004-07-2974
65. Caldwell KJ, Gottschalk S, Talleur AC. Allogeneic CAR cell therapy–more than a pipe dream. Front Immunol (2021) 11:618427. doi: 10.3389/fimmu.2020.618427
66. Gardner RA, Finney O, Annesley C, Brakke H, Summers C, Leger K, et al. Intent-to-treat leukemia remission by CD19 CAR T cells of defined formulation and dose in children and young adults. Blood (2017) 129:3322–31. doi: 10.1182/blood-2017-02-769208
67. Locke FL, Ghobadi A, Jacobson CA, Miklos DB, Lekakis LJ, Oluwole OO, et al. Long-term safety and activity of axicabtagene ciloleucel in refractory large b-cell lymphoma (ZUMA-1): a single-arm, multicentre, phase 1-2 trial. Lancet Oncol (2019) 20:31–42. doi: 10.1016/S1470-2045(18)30864-7
68. Majzner RG, Mackall CL. Clinical lessons learned from the first leg of the CAR T cell journey. Nat Med (2019) 25:1341–55. doi: 10.1038/s41591-019-0564-6
69. Kochenderfer JN, Somerville RPT, Lu T, Yang JC, Sherry RM, Feldman SA, et al. Long-duration complete remissions of diffuse Large b cell lymphoma after anti-CD19 chimeric antigen receptor T cell therapy. Mol Ther (2017) 25:2245–53. doi: 10.1016/j.ymthe.2017.07.004
70. Cappell KM, Sherry RM, Yang JC, Goff SL, Vanasse DA, McIntyre L, et al. Long-term follow-up of anti-CD19 chimeric antigen receptor T-cell therapy. J Clin Oncol (2020) 38:3805–15. doi: 10.1200/JCO.20.01467
71. Foeng J, Comerford I, McColl SR. Harnessing the chemokine system to home CAR-T cells into solid tumors. Cell Rep Med (2022) 3:100543. doi: 10.1016/j.xcrm.2022.100543
72. He Ran G, Qing Lin Y, Tian L, Zhang T, Mei Yan D, Hua Yu J, et al. Natural killer cell homing and trafficking in tissues and tumors: from biology to application. Signal Transduction Targeting Ther (2022) 7:1–21. doi: 10.1038/s41392-022-01058-z
73. Kremer V, Ligtenberg MA, Zendehdel R, Seitz C, Duivenvoorden A, Wennerberg E, et al. Genetic engineering of human NK cells to express CXCR2 improves migration to renal cell carcinoma. J Immunother Cancer (2017) 5:73. doi: 10.1186/s40425-017-0275-9
74. Ng YY, Du Z, Zhang X, Chng WJ, Wang S. CXCR4 and anti-BCMA CAR co-modified natural killer cells suppress multiple myeloma progression in a xenograft mouse model. Cancer Gene Ther (2022) 29:475–83. doi: 10.1038/s41417-021-00365-x
75. Schomer NT, Jiang ZK, Lloyd MI, Klingemann H, Boissel L. CCR7 expression in CD19 chimeric antigen receptor-engineered natural killer cells improves migration toward CCL19-expressing lymphoma cells and increases tumor control in mice with human lymphoma. Cytotherapy (2022) 24:827–34. doi: 10.1016/j.jcyt.2022.02.006
76. Li F, Sheng Y, Hou W, Sampath P, Byrd D, Thorne S, et al. CCL5-armed oncolytic virus augments CCR5-engineered NK cell infiltration and antitumor efficiency. J Immunother Cancer (2020) 8:e000131. doi: 10.1136/jitc-2019-000131
77. Ng YY, Tay JCK, Wang S. CXCR1 expression to improve anti-cancer efficacy of intravenously injected CAR-NK cells in mice with peritoneal xenografts. Mol Ther - Oncolytics (2020) 16:75–85. doi: 10.1016/j.omto.2019.12.006
78. Lin Y-J, Mashouf LA, Lim M. CAR T cell therapy in primary brain tumors: current investigations and the future. Front Immunol (2022) 13:817296. doi: 10.3389/fimmu.2022.817296
79. Ma R, Li Z, Chiocca EA, Caligiuri MA, Yu J. The emerging field of oncolytic virus-based cancer immunotherapy. Trends Cancer (2023) 9:122–39. doi: 10.1016/j.trecan.2022.10.003
80. Qin VM, Haynes NM, D’Souza C, Neeson PJ, Zhu JJ. CAR-T plus radiotherapy: a promising combination for immunosuppressive tumors. Front Immunol (2022) 12:813832. doi: 10.3389/fimmu.2021.813832
81. Lamers-Kok N, Panella D, Georgoudaki A-M, Liu H, Özkazanc D, Kučerová L, et al. Natural killer cells in clinical development as non-engineered, engineered, and combination therapies. J Hematol Oncol J Hematol Oncol (2022) 15:164. doi: 10.1186/s13045-022-01382-5
82. Sagnella SM, White AL, Yeo D, Saxena P, van Zandwijk N, Rasko JEJ. Locoregional delivery of CAR-T cells in the clinic. Pharmacol Res (2022) 182:106329. doi: 10.1016/j.phrs.2022.106329
83. Li H, Wang Z, Ogunnaike EA, Wu Q, Chen G, Hu Q, et al. Scattered seeding of CAR T cells in solid tumors augments anticancer efficacy. Natl Sci Rev (2022) 9:nwab172. doi: 10.1093/nsr/nwab172
84. Agliardi G, Liuzzi AR, Hotblack A, De Feo D, Núñez N, Stowe CL, et al. Intratumoral IL-12 delivery empowers CAR-T cell immunotherapy in a pre-clinical model of glioblastoma. Nat Commun (2021) 12:444. doi: 10.1038/s41467-020-20599-x
85. Bae WK, Lee BC, Kim H-J, Lee J-J, Chung I-J, Cho SB, et al. A phase I study of locoregional high-dose autologous natural killer cell therapy with hepatic arterial infusion chemotherapy in patients with locally advanced hepatocellular carcinoma. Front Immunol (2022) 13:879452. doi: 10.3389/fimmu.2022.879452
86. Jenkins Y, Zabkiewicz J, Ottmann O, Jones N. Tinkering under the hood: metabolic optimisation of CAR-T cell therapy. Antibodies (2021) 10:17. doi: 10.3390/antib10020017
87. van Bruggen JAC, Martens AWJ, Fraietta JA, Hofland T, Tonino SH, Eldering E, et al. Chronic lymphocytic leukemia cells impair mitochondrial fitness in CD8+ T cells and impede CAR T-cell efficacy. Blood (2019) 134:44–58. doi: 10.1182/blood.2018885863
88. Bai Z, Lundh S, Kim D, Woodhouse S, Barrett DM, Myers RM, et al. Single-cell multiomics dissection of basal and antigen-specific activation states of CD19-targeted CAR T cells. J Immunother Cancer (2021) 9:e002328. doi: 10.1136/jitc-2020-002328
89. O’Sullivan D, Sanin DE, Pearce EJ, Pearce EL. Metabolic interventions in the immune response to cancer. Nat Rev Immunol (2019) 19:324–35. doi: 10.1038/s41577-019-0140-9
90. Choi C, Finlay DK. Optimising NK cell metabolism to increase the efficacy of cancer immunotherapy. Stem Cell Res Ther (2021) 12:320. doi: 10.1186/s13287-021-02377-8
91. Krug A, Martinez-Turtos A, Verhoeyen E. Importance of T, NK, CAR T and CAR NK cell metabolic fitness for effective anti-cancer therapy: a continuous learning process allowing the optimization of T, NK and CAR-based anti-cancer therapies. Cancers (2021) 14:183. doi: 10.3390/cancers14010183
92. Mangal JL, Handlos JL, Esrafili A, Inamdar S, Mcmillian S, Wankhede M, et al. Engineering metabolism of chimeric antigen receptor (CAR) cells for developing efficient immunotherapies. Cancers (2021) 13:1123. doi: 10.3390/cancers13051123
93. Gardiner CM. NK cell metabolism and the potential offered for cancer immunotherapy. Immunometabolism (2019) 1(1):e190005. doi: 10.20900/immunometab20190005
94. Nabe S, Yamada T, Suzuki J, Toriyama K, Yasuoka T, Kuwahara M, et al. Reinforce the antitumor activity of CD8+ T cells via glutamine restriction. Cancer Sci (2018) 109:3737–50. doi: 10.1111/cas.13827
95. Verma V, Jafarzadeh N, Boi S, Kundu S, Jiang Z, Fan Y, et al. MEK inhibition reprograms CD8 + T lymphocytes into memory stem cells with potent antitumor effects. Nat Immunol (2021) 22:53–66. doi: 10.1038/s41590-020-00818-9
96. Raje NS, Shah N, Jagannath S, Kaufman JL, Siegel DS, Munshi NC, et al. Updated clinical and correlative results from the phase I CRB-402 study of the BCMA-targeted CAR T cell therapy bb21217 in patients with relapsed and refractory multiple myeloma. Blood (2021) 138:548. doi: 10.1182/blood-2021-146518
97. MacPherson S, Keyes S, Kilgour M, Smazynski J, Sudderth J, Turcotte T, et al. “Clinically-relevant T cell expansion protocols activate distinct cellular metabolic programs and phenotypes”. Molecular Therapy - Methods & Clinical Development (2021), 24, 380–93. doi: 10.1101/2021.08.24.457536
98. Yang Y, Badeti S, Tseng H, Ma MT, Liu T, Jiang J-G, et al. Superior expansion and cytotoxicity of human primary NK and CAR-NK cells from various sources via enriched metabolic pathways. Mol Ther Methods Clin Dev (2020) 18:428–45. doi: 10.1016/j.omtm.2020.06.014
99. Liu E, Ang SOT, Kerbauy L, Basar R, Kaur I, Kaplan M, et al. GMP-compliant universal antigen presenting cells (uAPC) promote the metabolic fitness and antitumor activity of armored cord blood CAR-NK cells. Front Immunol (2021) 12:626098. doi: 10.3389/fimmu.2021.626098
100. Kawalekar OU, O’Connor RS, Fraietta JA, Guo L, McGettigan SE, Posey AD, et al. Distinct signaling of coreceptors regulates specific metabolism pathways and impacts memory development in CAR T cells. Immunity (2016) 44:380–90. doi: 10.1016/j.immuni.2016.01.021
101. Fultang L, Booth S, Yogev O, Martins da Costa B, Tubb V, Panetti S, et al. Metabolic engineering against the arginine microenvironment enhances CAR-T cell proliferation and therapeutic activity. Blood (2020) 136:1155–60. doi: 10.1182/blood.2019004500
102. Yang Q, Hao J, Chi M, Wang Y, Li J, Huang J, et al. D2HGDH-mediated D2HG catabolism enhances the anti-tumor activities of CAR-T cells in an immunosuppressive microenvironment. Mol Ther (2022) 30:1188–200. doi: 10.1016/j.ymthe.2022.01.007
103. Klopotowska M, Bajor M, Graczyk-Jarzynka A, Kraft A, Pilch Z, Zhylko A, et al. PRDX-1 supports the survival and antitumor activity of primary and CAR-modified NK cells under oxidative stress. Cancer Immunol Res (2022) 10:228–44. doi: 10.1158/2326-6066.CIR-20-1023
104. Liu Z, Zhou Z, Dang Q, Xu H, Lv J, Li H, et al. Immunosuppression in tumor immune microenvironment and its optimization from CAR-T cell therapy. Theranostics (2022) 12:6273–90. doi: 10.7150/thno.76854
105. Yan X, Chen D, Ma X, Wang Y, Guo Y, Wei J, et al. CD58 loss in tumor cells confers functional impairment of CAR T cells. Blood Adv (2022) 6:5844–56. doi: 10.1182/bloodadvances.2022007891
106. Ramkumar P, Abarientos AB, Tian R, Seyler M, Leong JT, Chen M, et al. CRISPR-based screens uncover determinants of immunotherapy response in multiple myeloma. Blood Adv (2020) 4:2899–911. doi: 10.1182/bloodadvances.2019001346
107. Rasche L, Vago L, Mutis T. Tumour escape from CAR-T cells. In: Kröger N, Gribben J, Chabannon C, Yakoub-Agha I, Einsele H, editors. The EBMT/EHA CAR-T cell handbook. Cham: Springer International Publishing (2022). p. 15–22. doi: 10.1007/978-3-030-94353-0_4
108. Ruella M, Maus MV. Catch me if you can: leukemia escape after CD19-directed T cell immunotherapies. Comput Struct Biotechnol J (2016) 14:357–62. doi: 10.1016/j.csbj.2016.09.003
109. Maude SL, Laetsch TW, Buechner J, Rives S, Boyer M, Bittencourt H, et al. Tisagenlecleucel in children and young adults with b-cell lymphoblastic leukemia. N Engl J Med (2018) 378:439–48. doi: 10.1056/NEJMoa1709866
110. Majzner RG, Mackall CL. Tumor antigen escape from CAR T-cell therapy. Cancer Discovery (2018) 8:1219–26. doi: 10.1158/2159-8290.CD-18-0442
111. Martinez M, Moon EK. CAR T cells for solid tumors: new strategies for finding, infiltrating, and surviving in the tumor microenvironment. Front Immunol (2019) 10:128. doi: 10.3389/fimmu.2019.00128
112. Mei H, Li C, Jiang H, Zhao X, Huang Z, Jin D, et al. A bispecific CAR-T cell therapy targeting BCMA and CD38 in relapsed or refractory multiple myeloma. J Hematol Oncol J Hematol Oncol (2021) 14:161. doi: 10.1186/s13045-021-01170-7
113. Spiegel JY, Patel S, Muffly L, Hossain NM, Oak J, Baird JH, et al. CAR T cells with dual targeting of CD19 and CD22 in adult patients with recurrent or refractory b cell malignancies: a phase 1 trial. Nat Med (2021) 27:1419–31. doi: 10.1038/s41591-021-01436-0
114. Dai H, Wu Z, Jia H, Tong C, Guo Y, Ti D, et al. Bispecific CAR-T cells targeting both CD19 and CD22 for therapy of adults with relapsed or refractory b cell acute lymphoblastic leukemia. J Hematol Oncol J Hematol Oncol (2020) 13:30. doi: 10.1186/s13045-020-00856-8
115. Furqan F, Shah NN. Bispecific CAR T-cells for b-cell malignancies. Expert Opin Biol Ther (2022) 22:1005–15. doi: 10.1080/14712598.2022.2086043
116. Yan L, Qu S, Shang J, Shi X, Kang L, Xu N, et al. Sequential CD19 and BCMA-specific CAR T-cell treatment elicits sustained remission of relapsed and/or refractory myeloma. Cancer Med (2021) 10:563–74. doi: 10.1002/cam4.3624
117. Meng Y, Deng B, Rong L, Li C, Song W, Ling Z, et al. Short-interval sequential CAR-T cell infusion may enhance prior CAR-T cell expansion to augment anti-lymphoma response in b-NHL. Front Oncol (2021) 11:640166. doi: 10.3389/fonc.2021.640166
118. Wing A, Fajardo CA, Posey AD, Shaw C, Da T, Young RM, et al. Improving CART-cell therapy of solid tumors with oncolytic virus-driven production of a bispecific T-cell engager. Cancer Immunol Res (2018) 6:605–16. doi: 10.1158/2326-6066.CIR-17-0314
119. Balakrishnan A, Rajan A, Salter AI, Kosasih PL, Wu Q, Voutsinas J, et al. Multispecific targeting with synthetic ankyrin repeat motif chimeric antigen receptors. Clin Cancer Res Off J Am Assoc Cancer Res (2019) 25:7506–16. doi: 10.1158/1078-0432.CCR-19-1479
120. Kuhn NF, Purdon TJ, van Leeuwen DG, Lopez AV, Curran KJ, Daniyan AF, et al. CD40 ligand-modified chimeric antigen receptor T cells enhance antitumor function by eliciting an endogenous antitumor response. Cancer Cell (2019) 35:473–488.e6. doi: 10.1016/j.ccell.2019.02.006
121. Valeri A, García-Ortiz A, Castellano E, Córdoba L, Maroto-Martín E, Encinas J, et al. Overcoming tumor resistance mechanisms in CAR-NK cell therapy. Front Immunol (2022) 13:953849. doi: 10.3389/fimmu.2022.953849
122. Elahi R, Heidary AH, Hadiloo K, Esmaeilzadeh A. Chimeric antigen receptor-engineered natural killer (CAR NK) cells in cancer treatment; recent advances and future prospects. Stem Cell Rev Rep (2021) 17:2081–106. doi: 10.1007/s12015-021-10246-3
123. Sordo-Bahamonde C, Vitale M, Lorenzo-Herrero S, López-Soto A, Gonzalez S. Mechanisms of resistance to NK cell immunotherapy. Cancers (2020) 12:893. doi: 10.3390/cancers12040893
124. Groth A, Klöss S, von Strandmann EP, Koehl U, Koch J. Mechanisms of tumor and viral immune escape from natural killer cell-mediated surveillance. J Innate Immun (2011) 3:344–54. doi: 10.1159/000327014
125. Davis Z, Cichocki F, Felices M, Wang H, Hinderlie P, Juckett M, et al. A novel dual-antigen targeting approach enables off-the-Shelf CAR NK cells to effectively recognize and eliminate the heterogenous population associated with AML. Blood (2022) 140:10288–9. doi: 10.1182/blood-2022-168981
126. Roex G, Campillo-Davo D, Flumens D, Shaw PAG, Krekelbergh L, De Reu H, et al. Two for one: targeting BCMA and CD19 in b-cell malignancies with off-the-shelf dual-CAR NK-92 cells. J Transl Med (2022) 20:124. doi: 10.1186/s12967-022-03326-6
127. Luanpitpong S, Poohadsuan J, Klaihmon P, Issaragrisil S. Selective cytotoxicity of single and dual anti-CD19 and anti-CD138 chimeric antigen receptor-natural killer cells against hematologic malignancies. J Immunol Res (2021) 2021:5562630. doi: 10.1155/2021/5562630
128. Wang J, Toregrosa-Allen S, Elzey BD, Utturkar S, Lanman NA, Bernal-Crespo V, et al. Multispecific targeting of glioblastoma with tumor microenvironment-responsive multifunctional engineered NK cells. Proc Natl Acad Sci U S A (2021) 118:e2107507118. doi: 10.1073/pnas.2107507118
129. Brown T. Observations by immunofluorescence microscopy and electron microscopy on the cytopathogenicity of naegleria fowleri in mouse embryo-cell cultures. J Med Microbiol (1979) 12:363–71. doi: 10.1099/00222615-12-3-363
130. Joly E, Hudrisier D. What is trogocytosis and what is its purpose? Nat Immunol (2003) 4:815. doi: 10.1038/ni0903-815
131. Reed J, Reichelt M, Wetzel SA. Lymphocytes and trogocytosis-mediated signaling. Cells (2021) 10:1478. doi: 10.3390/cells10061478
132. Miyake K, Karasuyama H. The role of trogocytosis in the modulation of immune cell functions. Cells (2021) 10:1255. doi: 10.3390/cells10051255
133. Vanherberghen B, Andersson K, Carlin LM, Nolte-`t Hoen ENM, Williams GS, Höglund P, et al. Human and murine inhibitory natural killer cell receptors transfer from natural killer cells to target cells. Proc Natl Acad Sci U S A (2004) 101:16873–8. doi: 10.1073/pnas.0406240101
134. Vo D-N, Leventoux N, Campos-Mora M, Gimenez S, Corbeau P, Villalba M. NK cells acquire CCR5 and CXCR4 by trogocytosis in people living with HIV-1. Vaccines (2022) 10:688. doi: 10.3390/vaccines10050688
135. Somanchi SS, Somanchi A, Cooper LJN, Lee DA. Engineering lymph node homing of ex vivo–expanded human natural killer cells via trogocytosis of the chemokine receptor CCR7. Blood (2012) 119:5164–72. doi: 10.1182/blood-2011-11-389924
136. Marcenaro E, Cantoni C, Pesce S, Prato C, Pende D, Agaugué S, et al. Uptake of CCR7 and acquisition of migratory properties by human KIR+ NK cells interacting with monocyte-derived DC or EBV cell lines: regulation by KIR/HLA-class I interaction. Blood (2009) 114:4108–16. doi: 10.1182/blood-2009-05-222265
137. Lu T, Ma R, Li Z, Mansour AG, Teng K-Y, Chen L, et al. Hijacking TYRO3 from tumor cells via trogocytosis enhances NK-cell effector functions and proliferation. Cancer Immunol Res (2021) 9:1229–41. doi: 10.1158/2326-6066.CIR-20-1014
138. Hasim MS, Marotel M, Hodgins JJ, Vulpis E, Makinson OJ, Asif S, et al. When killers become thieves: trogocytosed PD-1 inhibits NK cells in cancer. Sci Adv (2022) 8:eabj3286. doi: 10.1126/sciadv.abj3286
139. Gonzalez VD, Huang Y-W, Delgado-Gonzalez A, Chen S-Y, Donoso K, Sachs K, et al. High-grade serous ovarian tumor cells modulate NK cell function to create an immune-tolerant microenvironment. Cell Rep (2021) 36(9):109632. doi: 10.1016/j.celrep.2021.109632
140. Caumartin J, Favier B, Daouya M, Guillard C, Moreau P, Carosella ED, et al. Trogocytosis-based generation of suppressive NK cells. EMBO J (2007) 26:1423–33. doi: 10.1038/sj.emboj.7601570
141. López-Cobo S, Romera-Cárdenas G, García-Cuesta EM, Reyburn HT, Valés-Gómez M. Transfer of the human NKG2D ligands UL16 binding proteins (ULBP) 1–3 is related to lytic granule release and leads to ligand retransfer and killing of ULBP-recipient natural killer cells. Immunology (2015) 146:70–80. doi: 10.1111/imm.12482
142. Roda-Navarro P, Vales-Gomez M, Chisholm SE, Reyburn HT. Transfer of NKG2D and MICB at the cytotoxic NK cell immune synapse correlates with a reduction in NK cell cytotoxic function. Proc Natl Acad Sci U S A (2006) 103:11258–63. doi: 10.1073/pnas.0600721103
143. Avula LR, Rudloff M, El-Behaedi S, Arons D, Albalawy R, Chen X, et al. Mesothelin enhances tumor vascularity in newly forming pancreatic peritoneal metastases. Mol Cancer Res MCR (2020) 18:229–39. doi: 10.1158/1541-7786.MCR-19-0688
144. Coquery CM, Erickson LD. Regulatory roles of the tumor necrosis factor receptor BCMA. Crit Rev Immunol (2012) 32:287–305. doi: 10.1615/CritRevImmunol.v32.i4.10
145. Li Y, Basar R, Wang G, Liu E, Moyes JS, Li L, et al. KIR-based inhibitory CARs overcome CAR-NK cell trogocytosis-mediated fratricide and tumor escape. Nat Med (2022) 28:2133–44. doi: 10.1038/s41591-022-02003-x
146. Schoutrop E, Renken S, Micallef Nilsson I, Hahn P, Poiret T, Kiessling R, et al. Trogocytosis and fratricide killing impede MSLN-directed CAR T cell functionality. OncoImmunology (2022) 11:2093426. doi: 10.1080/2162402X.2022.2093426
147. Hamieh M, Dobrin A, Cabriolu A, van der Stegen SJC, Giavridis T, Mansilla-Soto J, et al. CAR T cell trogocytosis and cooperative killing regulate tumour antigen escape. Nature (2019) 568:112–6. doi: 10.1038/s41586-019-1054-1
148. Camviel N, Wolf B, Croce G, Gfeller D, Zoete V, Arber C, et al. And antibody-fragment-based CAR T cells for myeloma induce BCMA downmodulation by trogocytosis and internalization. J Immunother Cancer (2022) 10:e005091. doi: 10.1136/jitc-2022-005091
149. Olson ML, Mause ERV, Radhakrishnan SV, Brody JD, Rapoport AP, Welm AL, et al. Low-affinity CAR T cells exhibit reduced trogocytosis, preventing rapid antigen loss, and increasing CAR T cell expansion. Leukemia (2022) 36:1943–6. doi: 10.1038/s41375-022-01585-2
150. Ghorashian S, Kramer AM, Onuoha S, Wright G, Bartram J, Richardson R, et al. Enhanced CAR T cell expansion and prolonged persistence in pediatric patients with ALL treated with a low-affinity CD19 CAR. Nat Med (2019) 25:1408–14. doi: 10.1038/s41591-019-0549-5
151. Mao R, Kong W, He Y. The affinity of antigen-binding domain on the antitumor efficacy of CAR T cells: moderate is better. Front Immunol (2022) 13:1032403. doi: 10.3389/fimmu.2022.1032403
152. Lu Z, McBrearty N, Chen J, Tomar VS, Zhang H, Rosa GD, et al. ATF3 and CH25H regulate effector trogocytosis and anti-tumor activities of endogenous and immunotherapeutic cytotoxic T lymphocytes. Cell Metab (2022) 34:1342–1358.e7. doi: 10.1016/j.cmet.2022.08.007
153. McCann FE, Eissmann P, Onfelt B, Leung R, Davis DM. The activating NKG2D ligand MHC class I-related chain a transfers from target cells to NK cells in a manner that allows functional consequences. J Immunol Baltim Md 1950 (2007) 178:3418–26. doi: 10.4049/jimmunol.178.6.3418
154. Nakamura K, Nakayama M, Kawano M, Amagai R, Ishii T, Harigae H, et al. Fratricide of natural killer cells dressed with tumor-derived NKG2D ligand. Proc Natl Acad Sci U S A (2013) 110:9421–6. doi: 10.1073/pnas.1300140110
155. Cooper ML, Choi J, Staser K, Ritchey JK, Devenport JM, Eckardt K, et al. An ‘off-the-shelf’ fratricide-resistant CAR-T for the treatment of T cell hematologic malignancies. Leukemia (2018) 32:1970–83. doi: 10.1038/s41375-018-0065-5
156. Gurney M, Stikvoort A, Nolan E, Kirkham-McCarthy L, Khoruzhenko S, Shivakumar R, et al. CD38 knockout natural killer cells expressing an affinity optimized CD38 chimeric antigen receptor successfully target acute myeloid leukemia with reduced effector cell fratricide. Haematologica (2020) 107:437–45. doi: 10.3324/haematol.2020.271908
Keywords: natural kill cell, T cell, chimeric antigen receptor, trogocytosis, immunotherapy, cancer
Citation: Kilgour MK, Bastin DJ, Lee S-H, Ardolino M, McComb S and Visram A (2023) Advancements in CAR-NK therapy: lessons to be learned from CAR-T therapy. Front. Immunol. 14:1166038. doi: 10.3389/fimmu.2023.1166038
Received: 14 February 2023; Accepted: 12 April 2023;
Published: 02 May 2023.
Edited by:
Nicolas Jacquelot, University of Calgary, CanadaReviewed by:
Jonathan Fisher, University College London, United KingdomMichael ODwyer, University of Galway, Ireland
Copyright © 2023 Kilgour, Bastin, Lee, Ardolino, McComb and Visram. This is an open-access article distributed under the terms of the Creative Commons Attribution License (CC BY). The use, distribution or reproduction in other forums is permitted, provided the original author(s) and the copyright owner(s) are credited and that the original publication in this journal is cited, in accordance with accepted academic practice. No use, distribution or reproduction is permitted which does not comply with these terms.
*Correspondence: Alissa Visram, alisvisram@toh.ca
†These authors have contributed equally to this work