- 14+4 Medical Doctor Program, Chinese Academy of Medical Sciences and Peking Union Medical College, Dongcheng, Beijing, China
- 2Department of General Surgery, Peking Union Medical College Hospital (CAMS), Beijing, China
- 3Center for National Cancer, Cancer Hospital, Chinese Academy of Medical Sciences and Peking Union Medical College, Beijing, China
Immunotherapy has brought a paradigm shift in the treatment of tumors in recent decades. However, a significant proportion of patients remain unresponsive, largely due to the immunosuppressive tumor microenvironment (TME). Tumor-associated macrophages (TAMs) play crucial roles in shaping the TME by exhibiting dual identities as both mediators and responders of inflammation. TAMs closely interact with intratumoral T cells, regulating their infiltration, activation, expansion, effector function, and exhaustion through multiple secretory and surface factors. Nevertheless, the heterogeneous and plastic nature of TAMs renders the targeting of any of these factors alone inadequate and poses significant challenges for mechanistic studies and clinical translation of corresponding therapies. In this review, we present a comprehensive summary of the mechanisms by which TAMs dynamically polarize to influence intratumoral T cells, with a focus on their interaction with other TME cells and metabolic competition. For each mechanism, we also discuss relevant therapeutic opportunities, including non-specific and targeted approaches in combination with checkpoint inhibitors and cellular therapies. Our ultimate goal is to develop macrophage-centered therapies that can fine-tune tumor inflammation and empower immunotherapy.
1 Introduction
Tumor-associated macrophages (TAMs) represent the most abundant and heterogeneous cell population in the tumor microenvironment (TME). The M0/M1/M2 model has been widely adopted to describe their broad spectrum of phenotypes and functions (Figure 1). Briefly, Toll-like receptors (TLRs) and type 1 cytokines stimulate the pro-inflammatory M1 phenotype. In contrast, alternatively activated M2 macrophages are further classified into subtypes including M2a, M2b, M2c, and M2d. Anti-inflammatory M2a macrophages with characteristic CD206 and TGF-β expression are induced by interleukin (IL)-4 and IL-13. M2b is closely associated with type 2 immunity and T helper 2 differentiation in response to parasitic and fungal infections. Glucocorticoids promote the deactivation of M2c macrophages (1). In the TME, tumor-associated factors such as adenosine favor the differentiation of M2d macrophages with high expression of IL-10 and VEGF (2). In vitro stimulation of cell lines under controlled experimental conditions often leads to highly reproducible results in terms of the aforementioned markers. However, these results are frequently disjointed with real-world situations.
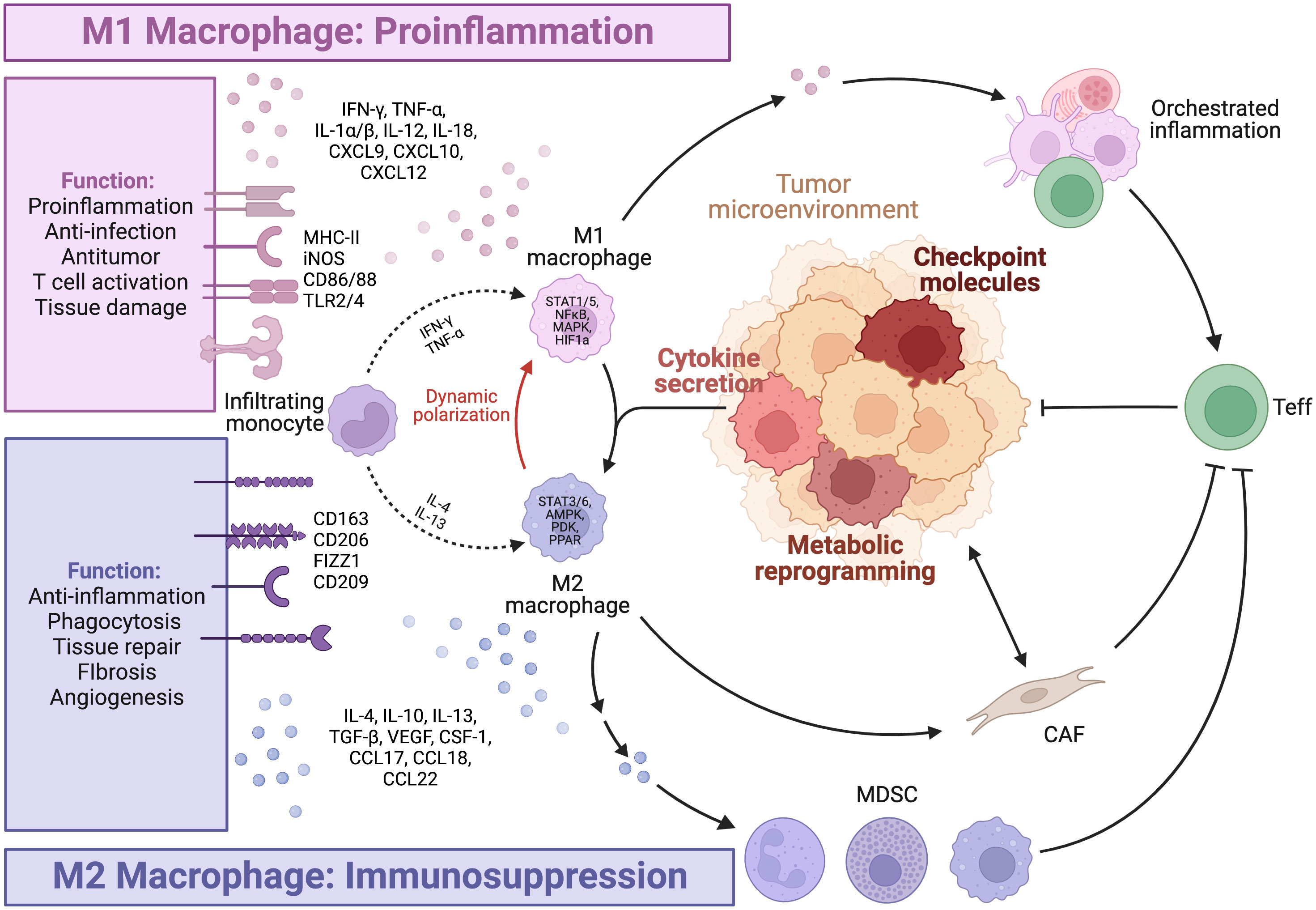
Figure 1 Macrophage polarization. The figure is created with BioRender.com.
The emergence of high-throughput and multi-omic technologies has led to advancements in the understanding of TAMs in patient samples, revealing the heterogeneity and plasticity of TAMs, particularly in the TME. TAM subtypes are dynamic spectrums, rather than fixed states of terminal differentiation, influenced by both ontogeny and environmental factors. This complexity exhibits patterns, as large-scale pan-cancer studies at the transcriptomic level have identified seven subtypes of TAMs that are conserved across multiple tumor types. These subtypes include the interferon-primed, immune regulatory, inflammatory cytokine-enriched, lipid-associated, pro-angiogenic, resident-tissue-macrophage-like, and proliferating subtypes (3). While such phenotype-based annotation is informative, it may not provide a complete understanding of the functional significance of these TAMs subtypes in tumor progression and immune activation.
TAMs should be redefined in functional classes that exhibit either tumor-promoting or tumor-suppressive effects. Multiple factors, particularly those with similar functional effects, are under orchestrated regulation and exhibit patterns of co-expression. Antigen presentation, costimulatory molecules and activating cytokines, including IFN-γ, TNF-α, and IL-2, are needed for synergistic amplification of the immune response cascade (4). On the other hand, M2 cytokines (5) such as IL-4, IL-13, TGF-β, and IL-10, and inhibitory ligands collectively establish feedback loops where regulatory T cell (Treg)-secreted IL-13 stimulates M2 to secrete IL-10, further promoting Treg differentiation (6). Notably, phenotypic markers and function can be disjointed. CD206+ M2 TAMs conventionally considered to be tumor-promoting have been shown to be capable of antigen cross-presentation, stimulation of antitumor immune responses, and tumor regression in mouse models of melanoma and colorectal cancer, due to concurrent CD80 expression (7). In contrast, M1 alveolar macrophages without CD80/86 expression failed to present antigens effectively (8). Considering the importance of evaluating the functional identity of TAMs, we will further highlight several key pro-inflammatory and immunosuppressive factors (Figure 2).
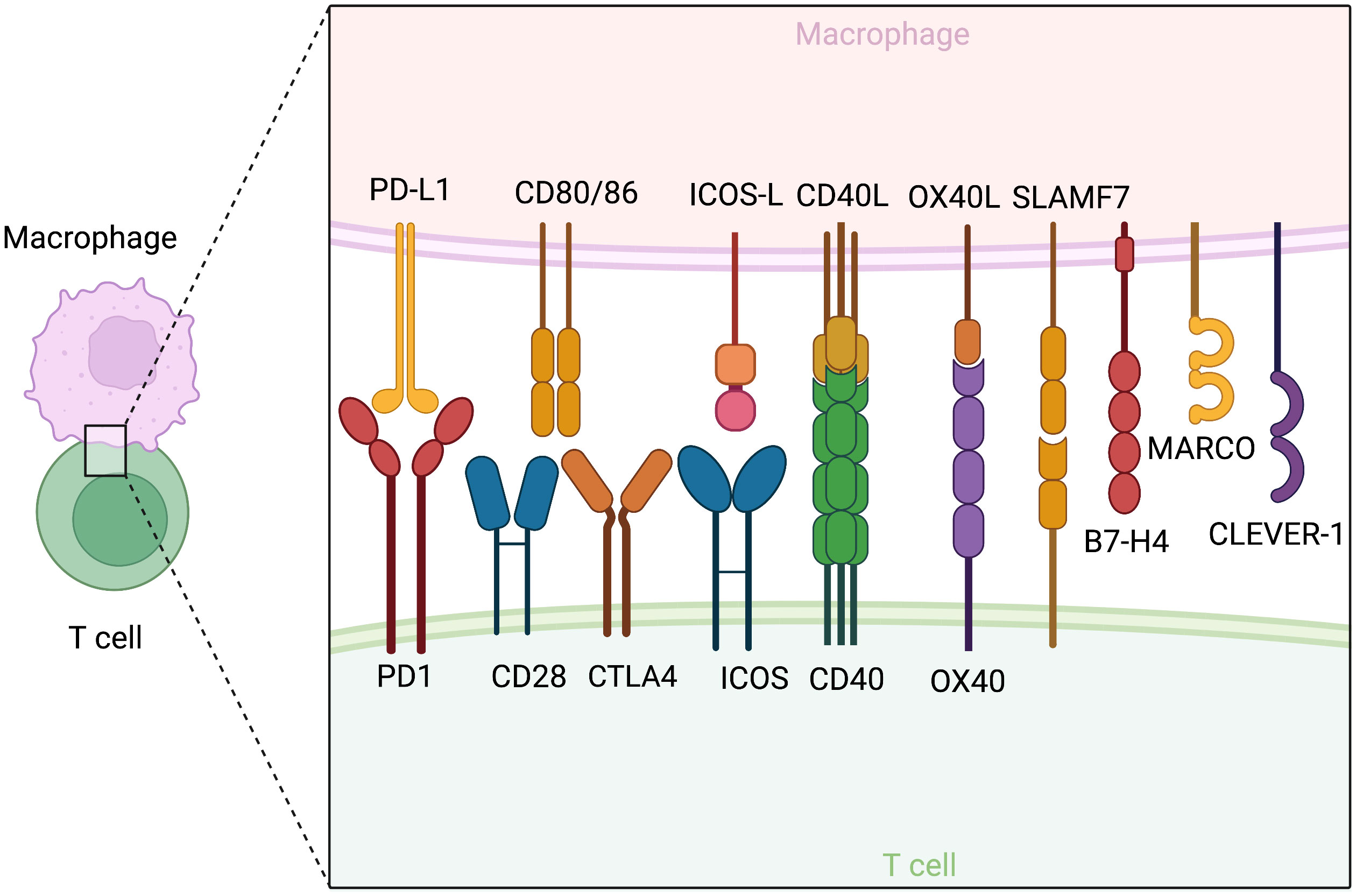
Figure 2 Co-stimulatory and co-inhibitory molecules on macrophages and their corresponding receptors on T cells. The figure is created with BioRender.com.
2 Pro-inflammatory
2.1 TNF-α
Tumor necrosis factor-alpha (TNF-α) is an inflammatory cytokine produced in large amounts by macrophages upon pattern recognition receptor (PRR) activation, and is considered a key antitumor agent responsible for the suppression of tumor growth by activated macrophages (9–12). However, chronic exposure to TNF-α in a detrimental inflammatory context has pro-tumor functions, including angiogenesis, metastasis, T cell apoptosis, and exhaustion (13–15). Therefore, both activators and inhibitors of TNF-α have been attempted in clinical use and will be discussed later in the Therapeutics section.
2.2 IFN-γ
Interferon-gamma (IFN-γ), plays a crucial role in innate stimulation, antigen presentation, T cell activation, and effector function. While activated T and natural killer (NK) cells are the major sources of IFN-γ, TAMs also produce this cytokine (16–21). IFN-γ production is under intricate regulation, as it is enhanced by NFκB activation downstream of IL-12 and IL-18 stimuli (22–25) and STAT4 phosphorylation (25), but inhibited by IL-27 treatment (22). Studies in CAR-T and anti-PDL1 antibody therapies, as well as a mouse model of bladder cancer, have demonstrated that antitumor TAMs rely on both antigen presentation and IFN-γ secretion to activate CD4+ T cells. These CD4+ T cells then secrete IFN-γ to stimulate M1 polarization, establishing reciprocal amplification that sets the antitumor immune response in motion (26, 27).
2.3 MHC-II
Macrophages are capable of processing and loading antigens onto MHC-II, whose variable regions bind to specific antigenic peptides recognizable by their corresponding T cell receptor (TCR). Formation of this complex leads to TCR activation as the first signal of T cell activation. T cells are often localized in TAM-rich regions due to the ability of TAMs to present tumor-associated antigens and mediate T cell chemotaxis. However, impaired immunological synapses between TAMs and T cells can lead to T cell anergy and notably forming M2-Treg interactions, even in tumor-draining lymph nodes (28–30). Furthermore, prolonged interaction between TAMs and CD8+ T cells is a significant factor of T cell exhaustion (31) and can impede T cell infiltration (32).
2.4 Co-stimulatory molecules
CD80 (B7-1) and CD86 (B7-2) are classical M1 markers and serve as ligands for both the co-stimulatory CD28 and inhibitory CTLA-4 of the B7 family expressed by T cells. The expression level and density of CD80/86 determine their effects. Low-level expression favors CTLA4 binding and immunosuppression, while only high-density expression can effectively stimulate T activation in models of colorectal cancer (33). A study in dendritic cells revealed a transcription factor, PU.1, with the ability to bind to the CD80/86 promoter and induce transcription (34). The expression of CD80/86 is temporally dynamic, peaking after 24-48 hours of culture and decreasing at 60 hours, in line with the timeframe of macrophage exhaustion and deactivation (35). In addition to CD80/86, other costimulatory molecules, such as the ICOS, OX40, 41BB, and CD40 signaling axis, are induced by primary activating signals (36–42). Many therapeutic attempts have been developed based on these checkpoints, which will be discussed in the Therapeutics section.
3 Immunosuppressive
3.1 VEGF
Vascular endothelial growth factors (VEGFs) have well-characterized functions in promoting angiogenesis and TME remodeling. However, in addition to these functions, VEGF secreted by TAMs has been shown to exhibit strong immunosuppressive effects through autocrine signaling that favors M2 polarization and upregulates PD-L1 expression (43). Interestingly, VEGF-A and VEGF-C are considered crucial for angiogenesis and lymphogenesis, respectively (44, 45), while the expression of latter by perivascular TAMs helps contain lung metastasis (46). By selectively modulating these processes, it may be possible to promote good angiogenesis and lymphogenesis over the undesirable angiogenesis, thereby converting immunologically “cold,” poorly perfused tumors to “hot” tumors with increased immune infiltration and better responses to immunotherapies, all without increasing tumor metastasis.
3.2 TGF-β
Transforming growth factor-beta (TGF-β) is a M2 cytokine with crucial roles in normal physiology, mediating inflammation resolution and apoptotic clearance (47–50). TGF-β secreted by TAMs shapes the TME by monitoring immune cell statuses and exerting immunosuppressive functions (51). Both TAMs and tumor cells share the downstream pathway of TGF-β/SNAIL (52–55). In tumor cells, TGF-β signaling induces thrombospondin-1 (TSP1) secretion and Treg differentiation (56). In TAMs, TGF-β exhibits potent pro-M2 functions and promotes its own expression in an immunosuppressive feedback loop (52, 57–59). However, studies in injury and inflammatory bowel disease models suggest that the transient expression and activation of SNAIL is essential for macrophage recruitment to their site of function by affecting chemotaxis and motility (60).
3.3 Co-inhibitory molecules
TAMs, rather than tumor cells, are the primary source of PD-L1 with CD8 suppressive functions and a key driver of response to anti-PD1/PDL1 therapy (61–70). However, TAM PD-L1 expression may result from a reactive response to various inflammatory stimuli in an immunologically “hot” tumor (65), such as GM-CSF (71), S100A8/TLR4/MyD88 (69, 72), IL10 and IL-27 (73), IL32/PFKFB3 (74), TGF-β/PKM2 (75), and classical M1 TNF-α/NFkB and MAPK (73, 76–78) through the activation of STAT1 and, in particular, STAT3 (79, 80). TAMs are also known to take up tumor cell PD-L1 for expression (81). In addition to inhibiting T cell function, TAM PD-1 suppresses phagocytosis and impairs antigen presentation (82). However, it also mediates pro-inflammatory macrophage differentiation and secretion (83). Apart from PD-L1, several other molecules have been identified as potential targets for fine-tuning the immunomodulatory functions of TAMs. SLAMF7 and VISTA/PSGL1 are novel checkpoint molecules expressed by TAMs to drive T cell exhaustion (84–86). CLEVER-1 and B7-H4 have been identified as specific to suppressive TAMs correlated with dysfunctional cytokine production and T cell dysfunction (87, 88).
4 TME remodeling
4.1 Fibroblast
Cancer-associated fibroblasts (CAFs) are mesenchymal cells that undergo reshaping by the TME to achieve activation, differentiation, metabolic and epigenetic programming. CAFs display a high level of heterogeneity and dynamism and can be classified into three major subtypes with different capacities in stromal remodeling and inflammatory modulation, including inflammatory CAFs (iCAFs), myofibroblastic CAFs (myCAFs), and antigen-presenting CAFs (apCAFs) (89, 90). Despite their heterogeneity, CAFs are widely regarded as pro-tumorigenic and immunosuppressive cells that hinder immune cell infiltration, impair T cell activation and effector function, and promote T cell exhaustion. As the two primary components of the tumor stroma, TAMs and CAFs are extensively co-localized, collaborating to promote immunosuppressive desmoplasia (91) (Figure 3).
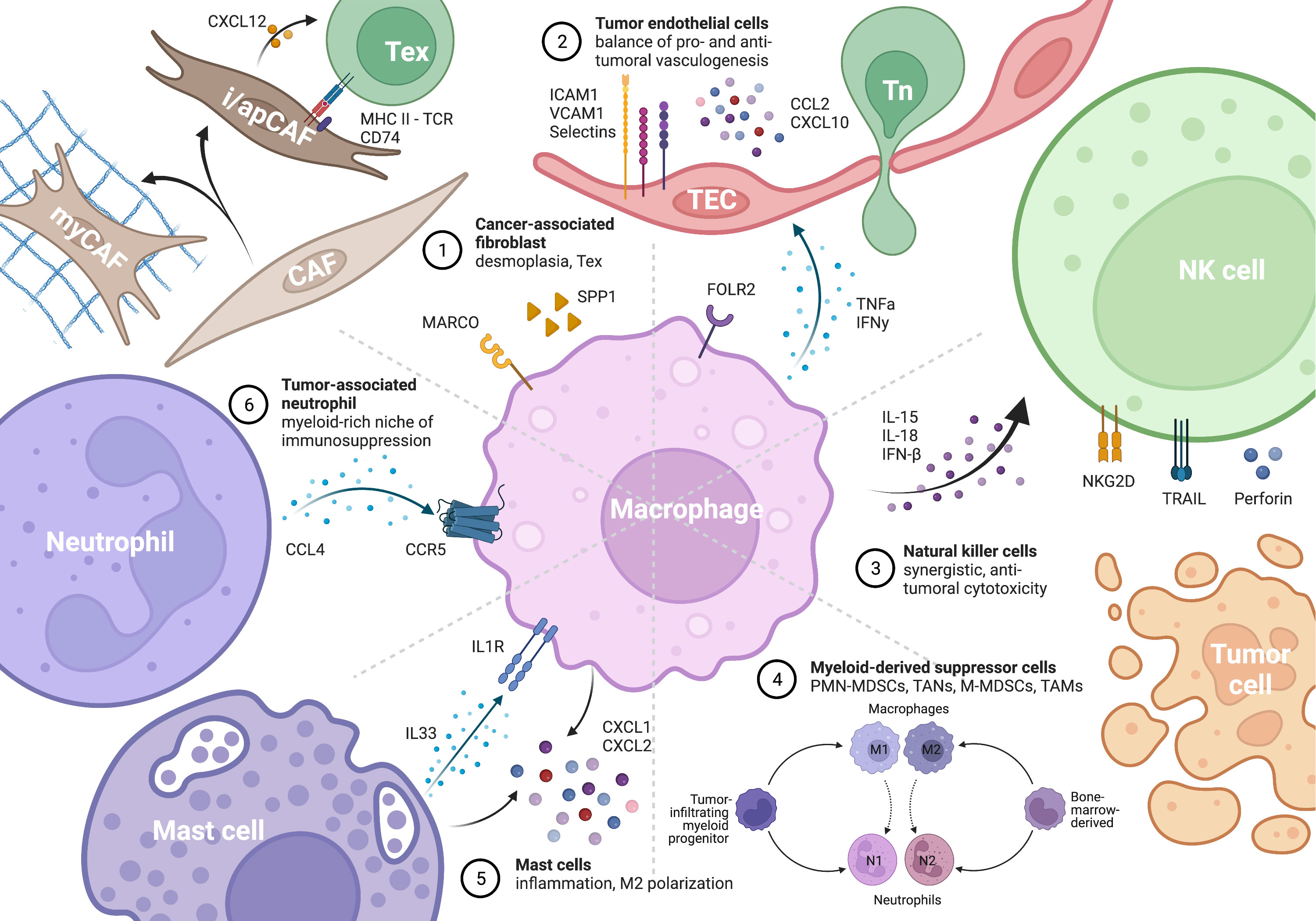
Figure 3 Macrophage as the key remodeler of TME inflammation by interacting with different cells. The figure is created with BioRender.com.
4.1.1 T cell suppression through ECM remodeling
TAMs and myCAFs work together in extracellular matrix (ECM) deposition and remodeling, creating a desmoplastic microenvironment that acts as a barrier to T cell infiltration. A subset of TAMs expressing SPP1, also known as osteopontin (OPN), has been identified as markers of poor prognosis and immune checkpoint blockade (ICB) resistance in several types of tumors, including lung adenocarcinoma, hepatocellular carcinoma, and colorectal cancer (92–100). These SPP1+ TAMs are also characterized by high expression of the S100 family proteins, ECM-associated genes, and lipid metabolism features (101) and are often regarded as pro-inflammatory M1 macrophages. However, due to their low MHC-II expression and lack of co-stimulatory molecules, their antigen presentation capability is impaired, resulting in futile inflammation that fails to stimulate T cells effectively. Instead, SPP1+ TAMs interact with FAP+ CAFs to activate ECM deposition and inflammatory desmoplasia through SDC2 and MMP-2 interaction (94), IL-1, TGF-β (93), and CSF-induced granulin (102). Similar pro-fibrotic functions have also been reported in lung (103) and liver fibrosis (101, 104). Further studies have shown that the expression of SPP1 is induced by IL-17 or type 3 inflammation (104), metabolic reprogramming such as HIF-1 (93) and PGC-1α (105), and contact with tumor cells (106) or chemerin+ TGFβ+ CAFs (93). In vitro experiments in a mouse lung adenocarcinoma model have suggested that SPP1 mediates M2 polarization and the expression of IL-10, Arg1, and PD-L1, ultimately inhibiting CD4 T cells (106).
Much effort aimed at alleviating the immunosuppressive effects of the ECM (107, 108). The ECM provides not only integrin cross-talk (109) but also structural-biological coupling of mechanical sensing and signal transduction (110). Stiffness has extensive effects on T cell activation and antigen reactivity, cellular processes that are highly dependent on the spatial arrangement and proximity of receptors (111). High-density matrix hinders T cell proliferation and effector functions, favoring an immunosuppressive high ratio of CD4+/CD8+T cells (112), potentially through metabolic reprogramming (113). TAMs are a major source of matrix-remodeling enzymes: TAM-derived lysyl hydroxylase and lysyl oxidase mediate collagen cross-linking, matrix stiffening, and worse prognosis in breast cancer (114). The boundary between matrix-remodeling stromal cells has been further blurred by the discovery of SMAD3-dependent macrophage-myCAF transition (115).
4.1.2 Direct suppression of T cells
TAMs can activate CAFs, resulting in the direct suppression of T cells. In pancreatic cancer, FAPα+ IL-6+ iCAF, TAMs and T cells form “reactive areas”, which inhibit T cell proliferation via both contact-dependent PD-L1 and PD-L2 mediated T cell exhaustion, as well as contact-independent PGE2 secretion (116–118). This ultimately leads to poor patient survival. In triple-negative breast cancer, CXCL12+ iCAF-induced T cell dysfunction is evidenced by a decoupling between survival benefit and T cell infiltration (119). In esophageal cancer, FGF2+ iCAF upregulates SPRY1 expression in T cells, a potent transcription factor for T cell exhaustion (120).
Recent studies have reported the presence of a rare subset of MHC-II+ CD74+ apCAFs in pancreatic (121–123), lung (118), breast, and colorectal (124) cancer, but not in prostate cancer. MSLN+ apCAFs from pancreatic cancer lack co-stimulatory molecules and can activate CD4+ T cells in an antigen-specific fashion to promote Treg differentiation. Targeted depletion of these cells is a major mechanism behind the therapeutic effect of anti-MSLN antibodies (122). In lung cancer, apCAFs express co-inhibitory molecules (CD73, IL-6, and IL-27) under the stimulation of CD39+ exhausted T cells, thereby creating a negative feedback loop of T cell exhaustion (125). Additionally, conditioned-medium from colorectal cancer cells up-regulates CTSS and immunosuppressive antigen cross-presentation in apCAFs (124).
Targeting these reciprocal interactions between TAMs, CAFs, and T cells to break the detrimental feedback loops may produce leveraged effects in alleviating immunosuppression (126). Overall, a deeper understanding of the complex interplay between TAMs, CAFs, and T cells in the TME could lead to novel therapeutic approaches for cancer treatment.
4.2 TEC function and angiogenesis
Endothelial cells play a crucial role in initiating immune responses and facilitating T cell trafficking. The three-step process of T-cell adhesion, extravasation, and infiltration relies on the expression of cell-cell interaction molecules such as ICAM-1, VCAM-1, and E/P-selectin, as well as secreted factors including CCL2 and CXCL10, by activated endothelial cells (127–129). However, tumor-associated endothelial cells (TECs) are reprogrammed by the TME into the first line of defense against incoming T cells (Figure 3). Secretions from TAMs, particularly the well-studied TNF-α and VEGF, play a critical role in determining the success or failure of angiogenesis (130).
4.2.1 T cell recruitment by endothelial cells
TAMs can promote “good angiogenesis,” as TAM-dependent pro-inflammatory angiogenesis forms reactive areas of TAMs, T cells, and tumor endothelial cells (TECs) in pancreatic adenocarcinoma. TAMs are the major source of TNF-α in the TME, which activates TECs to allow for immunosurveillance (15, 131, 132). Inadequate TNF-α stimulation has been reported to reduce the expression of ICAM-1 on TECs, leading to impaired survival in patients with gut microbiota dysbiosis (133, 134). IFN-γ stimulates antigen presentation by tumor-associated lymphoid endothelial cells (135). Moreover, high endothelial venules (HEVs), which are essential for immune cell entry and ICB response, are supported by a specialized perivascular niche of enriched TAMs and sialomucin+ E/P-selectin+ TECs (136, 137). Such arrangements have been shown to potently attract CD8 T cell chemotaxis in cerebral malaria (138) and tumors, leading to markedly perivascular primed CD8 T cells and better survival in breast cancer (139).
4.2.2 Pro-metastatic angiogenesis
On the other hand, TAMs are also the major source of VEGF in the TME. The combined use of anti-VEGFR and ICB has achieved impressive success in multiple clinical settings (140). VEGF stimulation leads to the development of immunosuppressive tumor endothelial cells (TECs) expressing GPNMB (141) and PD-L1 (142) in hepatocellular carcinoma and melanoma, respectively, resulting in T cell exhaustion. The combination of a VEGF inhibitor and ICB led to high endothelial venule (HEV) formation and T cell infiltration (143). Specific delivery of LIGHT to tumor vessels through vascular targeting peptide (VTP), known as the LIGHT-VTP therapy, potently induced tertiary lymphoid structures (144). Macrophages, which naturally express LIGHT in adipose inflammatory responses (145) and atherogenesis (146), are promising targets for the induction and amplification of LIGHT expression.
TAMs are the key to promoting good angiogenesis over immunosuppressive and pro-metastatic angiogenesis (147, 148). Specific targeting of VEGF+ TAM subsets responsible for immunosuppressive angiogenesis, while sparing the T cell attractant FOLR2+ and peri-HEV TAMs, may improve the TME and sensitize the tumor to ICB treatment (149).
4.3 Crosstalks with other immune cells
TAMs actively interact with other immune cells through surface molecules and cytokine secretions. These powerful engines are central to the formation, amplification and maintenance of inflammation in the TME. Such activities warrant antitumor immune response, but also end up in inflammation-mediated immunosuppression and exhaustion. The concept of tertiary lymphoid structures (TLS) refers to organized clusters of immune cells, including TAMs, dendritic cells, B cells, and T cells, which play a crucial role in refreshing adaptive immunity (150, 151). In this context, TAMs act as amplifiers and sustainers of inflammation by engaging in reciprocal interactions with other immune cells, including the recruitment of B cells, which produce IgG to further activate TAMs (152). Interestingly, TAMs and TLS exhibit different prognostic values across different types of cancer: while they are favorable in pancreatic and hepatocellular cancer, they are considered hazardous in breast and colorectal cancer (153). This suggests that the effects of inflammation are context-dependent: TAMs have the potential to promote immune cell infiltration into immunologically “cold” tumors, but for tumors challenged by chronic inflammation, inhibiting sustained TAM activation may alleviate inflammatory fibrosis and T cell exhaustion (154).
4.3.1 NK cells
As part of the innate immune system, the activation and effector function of NK cells depend on a balance of inhibitory and stimulatory signals, allowing them to recognize and kill MHC-I deficient tumor cells, and at the same time rendering them susceptible to modulation by macrophages (155) (Figure 3). The cooperation between M1 macrophages and NK cells is crucial for effective immune response in both infection and tumor settings (156–158). Mechanistically, cytokines produced by M1 macrophages, such as IL-15, IL-18, and IFN-β, can upregulate NKG2D expression on NK cells and enhance their cytotoxic activity (159). However, in the TME, tumor cells and VCAM1+ CAFs can polarize macrophages towards an M2 phenotype, indirectly suppressing NK cell activity and promoting immune evasion (160, 161).
4.3.2 Neutrophils
Myeloid-derived suppressor cells (MDSCs) are a heterogeneous population of cells of myeloid origin that are capable of potently suppressing T and NK cells. This population is often immature and exists in various states of differentiation, making consensus classification and therapeutic development challenging. MDSCs can be broadly classified into two major groups: polymorphonuclear (PMN-MDSC) and monocytic (M-MDSC) (Figure 3). Unlike TAMs or tumor-associated neutrophils (TANs), which refer to macrophages and neutrophils infiltrating into the TME, MDSCs are derived from the bone marrow under the remote influence of tumors and can be found outside the TME in peripheral blood and spleen. Despite differences in origin and cellular markers, MDSCs share many similarities in effector functions and extensivel cross-talks (162, 163). M-MDSCs and TAMs have been reported to be more potent in immunosuppression than PMN-MDSCs and TANs, although neutrophils usually outnumber macrophages in the TME (164). The myeloid-rich immunosuppressive landscape in liver, stomach, and breast cancer is constituted by reciprocal induction and synergistic action between CCR5+ TAMs and CCL4+ TANs (164–168). However, mutual exclusion of the two species has also been reported in breast cancer, with TANs being the more resistant group to ICB (169).
4.3.3 Mast cells
Mast cells, a type of myeloid cell with specialized granules containing histamine and heparin, have been relatively overlooked in tumor immunology despite their importance in immune surveillance of normal tissue. In lung cancer, the formation of TAM-mast cell islets allows for mutually enhancing, synergized CXCL1/2 secretion, which has potent immune attracting and antitumor effects that are beneficial for survival (170–172). However, mast cells have been shown to exert pro-tumor functions in prostate, stomach, and pancreatic cancers, mechanistically through IL33-mediated M2 polarization (173–176). A deeper understanding of the dual roles of TAM-mast cell interaction in the TME may reveal novel therapeutic opportunities (Figure 3).
5 Metabolism
5.1 Glucose metabolism
Tumor cells primarily rely on aerobic glycolysis to produce energy, leading to the accumulation of lactate, acidic pH, and limited glucose availability in a metabolically hostile microenvironment (177, 178). Altered pathway activities, such as the key pathways AMPK/PGC1, HIF-1α, AKT/mTOR, and MAPK, lead to profound changes in metabolic preferences between glycolysis and oxidative phosphorylation. Subsequent metabolic adaptations give rise to phenotypic and functional outcomes in terms of pro- and anti-inflammatory activities, as summarized in the introduction section. These intricately regulated processes are not specific to any particular cell type but often share common molecular mechanisms across diverse cellular contexts, resulting in context-specific consequences. These phenotype-function correlations highlight the importance of master metabolic regulators and the crosstalk between pathways (Figure 4).
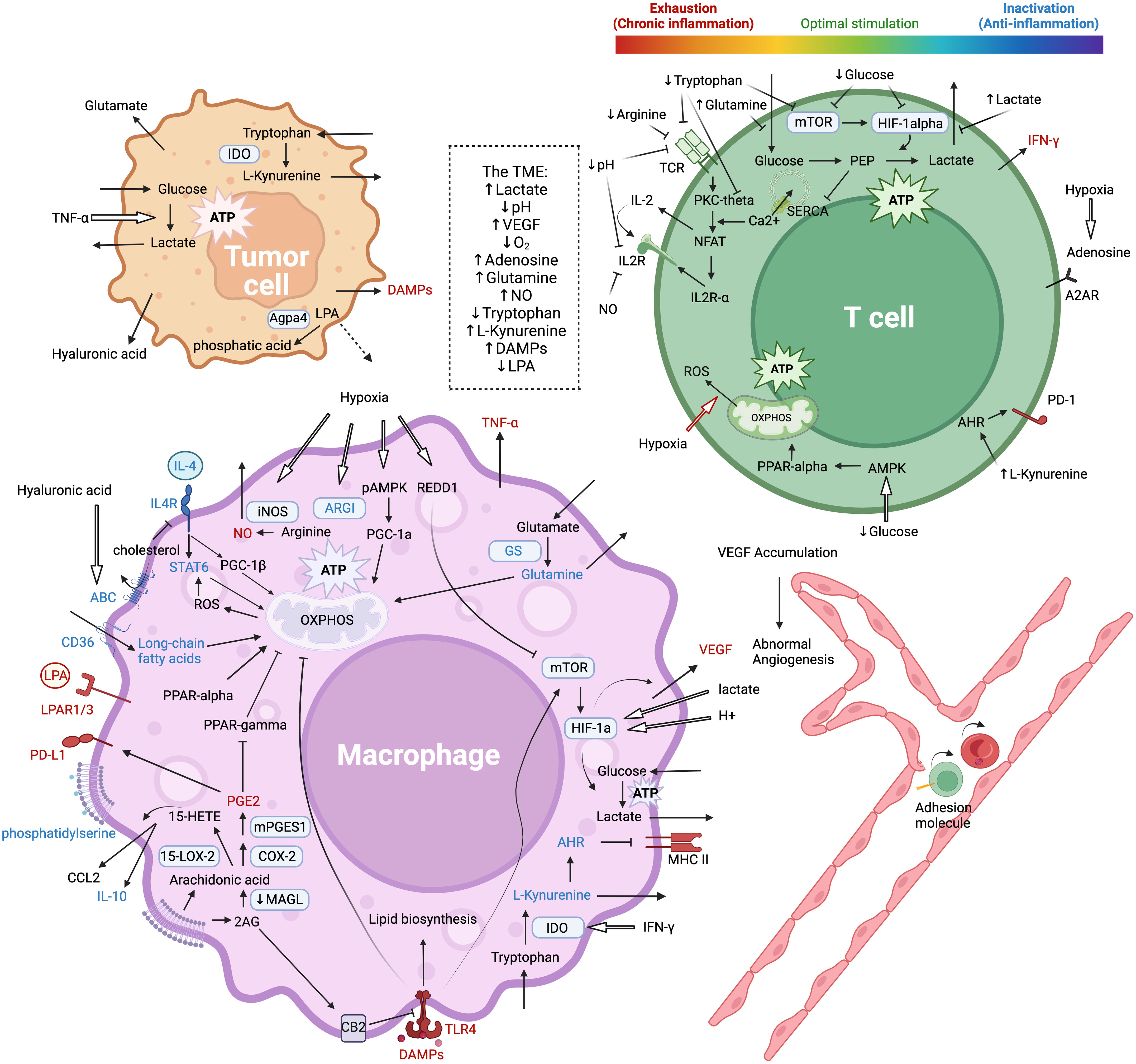
Figure 4 Metabolic interplay between macrophages, tumor cells, and T cells, and their effects on the inflammatory state of the TME and on T cell functions. Molecules in red and blue are clear pro-inflammatory and anti-inflammatory mediators respectively. The figure is created with BioRender.com.
5.1.1 TAMs
Different signaling pathways and metabolic programs can independently upregulate either M1 or M2 specific characteristics without affecting each other, indicating a mutually-independent regulation mechanism (179, 180). Signaling through HIF-1α, AKT/mTOR, and MAPK pathways promotes aerobic glycolysis and converge on the downstream effector JAK/STAT, leading to subsequent M1 features, while the stress-responsive AMPK/PGC1 pathway and PDK signaling promotes mitochondrial function and oxidative phosphorylation, leading to M2 polarization (52, 65, 179, 181–196).
However, single cell sequencing studies have identified anti-inflammatory TAMs that perform both glycolysis and oxidative phosphorylation (197). Also, hypoxia also lead to increased production of M2 secretions by TAMs, especially VEGF and TGF-β, as yin and yang to keep immune reactions in check (47, 198, 199). However, such mechanisms in the TME exacerbate glucose and oxygen deficiency and further metabolic challenges (183, 187, 200–204). Interestingly, HIF-2α has the opposite role and stimulates the production of secreted VEGF receptor (sVEGFR-1) to neutralize the biological activity of VEGF-A, reducing angiogenesis and tumor growth (205–207). However, the effects of some stimuli are dependent on the baseline activation status of TAMs, leading to differing outcomes between M1 and M2 (47, 208). The response to external stimuli is coupled to finely regulated signaling pathways, with multiple targetable points and well-developed activators and inhibitors. Therefore, understanding the links between these pathway activities, metabolic states, and functional phenotypes in TAMs would guide the development of TAM-centered metabolic regulators to modulate the inflammatory state of the TME (181, 191, 209, 210).
5.1.2 T cells
High oxygen consumption in the oxidative phosphorylation process of TAMs in the TME can lead to hypoxia, which inhibits the function of T cells in several ways. The hypoxic TME leads to abnormal angiogenesis and dysfunctional endothelial cells that express lower levels of cell adhesion molecules, making it difficult for T cells to attach and infiltrate (134). Adenosine accumulation activates the inhibitory A2A receptor on CD8+ T cells (211, 212). Hypoxia also leads to long-lasting interactions between TAMs and CD8+ T cells, eventually causing T cell exhaustion (31). The acidic pH of a hypoxic TME decreases the expression of TCR and IL-2Ra and leads to T cell inhibition, reversible with proton pump inhibitors (213). Mechanistically, lactate accumulation in the extracellular compartment directly prevents the secretion of lactate from T cells, driving the glycolysis equilibrium to the left and hindering T cell functions (214).
Upon activation, T cells switch from oxidative phosphorylation to aerobic glycolysis to meet the high energy demands while minimizing reactive oxygen species (ROS) production (215). However, in response to the metabolic challenges of TME, activated T cells preferentially utilize the AMPK/PGC1 pathway instead of the HIF-1α and PAM pathways, suppressing glycolysis (216). Subsequent ROS overproduction and mitochondrial stress lead to T-cell exhaustion (217), while decreased availability of a key metabolite phosphoenolpyruvate alleviates SERCA inhibition to disrupt Ca2+-dependent NFAT signaling and impair effector function (218). Despite these detrimental effects of oxidative phosphorylation, it is also essential for the viability and activity of T cells. PPAR-α improves the metabolic competitiveness of T cells, indicating the importance of a balanced metabolic state for T cell function (216, 219)
5.2 Amino acid metabolism
5.2.1 Glutamine
TAMs express glutamine synthetase (GS), an important enzyme in the conversion of glutamate secreted by tumors to glutamine, which is then released back into the TME as a building block for tumor cells (189, 220–222). This process not only supports tumor anabolism but also affects TAM polarization. Inhibiting GS and allowing succinate to accumulate promotes HIF-1α signaling, resulting in increased glucose flux through glycolysis and the pro-inflammatory M1 phenotype, restoring the proliferation of co-cultured T cells and promoting good angiogenesis in vivo (189, 223). Additionally, high levels of glutamine in the TME directly suppress T cells, possibly by competing with glucose transporters and suppressing glycolysis (224).
5.2.2 Arginine
Under metabolic challenges of the TME, TAMs can be polarized to preferentially express arginase-1 (ARG1) or inducible nitric oxide synthase (iNOS), resulting in different functional phenotypes (200, 225). iNOS-produced nitric oxide (NO) is a pro-inflammatory product of M1 phenotype, whereas ARG1 converts arginine into ornithine and urea, making it an immunosuppressive M2 marker due to competition with iNOS for the limited arginine pool (226–228). High expression of the arginine transporter and ARG1 in TAMs can deplete arginine from the TME, leading to the loss of the zeta-chain of CD3 in activated T cells and impaired antitumor immune activity (228). Surprisingly, arginine-depletion therapy paradoxically increases CD8+ T cells and pro-inflammatory TAMs in the TME, possibly due to the fact that T cells expressing argininosuccinate synthase 1 (ASS1) can synthesize arginine from citrulline and succinate, whereas ARG1+ TAMs and tumor cells are more dependent on extrinsic arginine (229–231). Moreover, recent studies have shown that citrulline depletion by ASS1 activity in the urea cycle is important for the pro-inflammatory functions of macrophages, which could partly explain how arginine depletion may potentially help reprogram TAMs (232, 233). Thus, the competition for arginine between TAMs and T cells in the TME is a critical metabolic checkpoint that can influence the balance between immunosuppressive and inflammatory responses.
5.2.3 Tryptophan
Tryptophan is an essential amino acid required by T cells. Its metabolism by indoleamine 2,3-dioxygenase (IDO) through the kynurenine pathway generates immunosuppressive catabolites, particularly kynurenine. The expression of IDO is induced by inflammatory stimuli to prevent excessive immune responses. However, this process is often exploited by tumors and immunosuppressive cells in the TME. TAMs interaction with specific CD69+CD8+ T cells enhances IFN-γ secretion to upregulate IDO in TAMs, creating a negative feedback loop to keep T cell activities in check (234–236).
Tryptophan starvation and catabolites decrease mTOR and PKC-θ signaling, resulting in reduced CD3ζ expression and cell cycle arrest in T cells (237–239). L-Kynurenine, the major kynurenine pathway metabolite, enters cells through transporters SLC7A8 and PAT4 to activate the aryl hydrocarbon receptor (AHR), a cytoplasmic transcription factor expressed in T cells and TAMs (240–242). In T cells, AHR activity inhibits cytokine secretion and promotes PD-1 expression (240). In TAMs, AHR lowers MHC-II expression but enhances interaction with regulatory T cells (241). Notably, L-Kynurenine is produced not only by IDO+ TAMs or tumor cells but also by microorganisms such as Lactobacillus from pancreatic cancer (243). In this case, a low-tryptophan diet is beneficial by increasing the number of cytotoxic CD8+ T cells in the TME (243).
5.3 Lipid metabolism
5.3.1 Fatty acids
Pro-inflammatory TAMs are characterized by active lipid biosynthesis, which is coupled to the PAM pathway and glycolysis, as previously described in the section on glucose metabolism (191, 202). In contrast, fatty acid oxidation (FAO) is associated with other immunosuppressive and pro-M2 factors including oxidative phosphorylation, IL4/STAT6 signaling, AMPK/PGC1 pathways, and ROS activity (190, 192, 244, 245). The accumulation of long-chain unsaturated fatty acids, oxLDL, and lipid droplets in the lipid-enriched TME allows preferences of FAO over other methods of energy production (49, 246, 247). Active FAO depends on several important factors involved in lipid transport, metabolic enzymes, and regulatory molecules. The transcription factor PPAR-α drives the expression of FAO and OXPHOS genes (248). ABHD5 is a coactivator for HSL, the rate-limiting enzyme in triacylglycerol hydrolysis, and a stimulator of PPAR-α (249–252). APOE lipid transporter (253) and CD36 fatty acid translocase (49) both mediate the accumulation of intracellular fatty acids. The activity of NFκB and RIPK3 promotes the degradation of the aforementioned molecules and counters FAO (209). These molecules are often highly expressed in tumor cells and some TAM subsets, while downregulated in specific inflammatory TAMs, mediating differential reprogramming of lipid metabolism in a context-dependent fashion. Therefore, further research on these key molecules may enable the differentiation of TAMs from tumor cells and the cell-type-specific metabolic rewiring. Understanding the regulation and role of FAO in TAMs is critical as it can shape the tumor microenvironment and the immune response.
5.3.2 Phospholipids and derivatives
Phospholipids and their derivatives are not only major components of the plasma membrane with structural functions, but also important bioactive molecules with potent signaling functions. Different species of phospholipids have distinct effects, whose production and metabolism are often harnessed by tumor cells and TAMs in the TME. For example, phosphatidylserine (PS) exposure on the outer plasma membrane is a key feature of apoptotic cells. Together with other “eat me” signals, PS promotes phagocytosis and TGF-β production, making it a targetable point to stimulate inflammation (254, 255). Lysophosphatidic acid (LPA) has dual effects depending on the available receptors. In models of colorectal cancer, secreted LPA by cancer cells is recognized by LPAR1-3 on TAMs, driving the expression of inflammatory genes. However, in the ascites of patients with ovarian cancer, LPA binding to LPAR5/6 is associated with M2 polarization, immunosuppression, tumor metastasis, and poor outcomes (256). AGPA4 expressed by cancer cells converts TME LPA to phosphatidic acid, undermining its pro-inflammatory effects (257). 15-LOX-2+ TAMs from renal cell carcinoma catalyze the degradation of arachidonic acid into 15-HETE, which stimulates CCL2 and IL-10 production to recruit immunosuppressive TAMs (258, 259). In murine bladder and prostate cancer, specific TAMs express mPGES1 and COX-2 to produce large amounts of prostaglandin E2 (PGE2), a molecule with well-investigated immunosuppressive effects through NFκB-mediated PD-1/PD-L1 expression in both TAMs and T cells, unleashing the inhibitory effect of PPAR-γ on fatty acid oxidation and oxidative phosphorylation, and increasing secretion of immunosuppressive factors especially VEGF (260–265). In colorectal cancer, tumor-induced downregulation of monoacylglycerol lipase (MAGL) in TAMs is associated with the accumulation of tri-, di-, and mono-glycerides along with arachidonoylglycerol, enhancing CB2 receptor activity to antagonize TLR4 signaling and mediate immunosuppression (266).
5.3.3 Cholesterol
Cholesterol and its derivatives have been shown to have immunomodulatory effects. In the context of metastatic ovarian cancer, cholesterol is considered beneficial. Tumor-derived hyaluronic acid has been shown to stimulate TAMs to express ABC transporters, which facilitate cholesterol efflux and lipid raft depletion, leading to IL-4 signaling and immunosuppressive reprogramming (267, 268). The liver X receptor (LXR), which is activated by cholesterol derivatives, has been shown to favor the expression of pro-inflammatory genes in TAMs. Pharmacological manipulation of the cholesterol represents a promising strategy for reprogramming TAMs towards an anti-tumor phenotype (269, 270). However, in certain situations, the cholesterol family may also have immunosuppressive and pro-tumor effects.
6 Therapeutics
Therapeutic targeting of TAMs by antibody/cytokine administration or depletion is rather unspecific. However, recent drug developments attempted more precise approaches to achieve better efficacy and reduce off-target effects.
6.1 Polarization
Despite the phenotypical diversity of TAMs, the classical model of M1-inflammatory and M2-immunosuppressive TAMs remains a useful reference framework for the overall polarization direction, which is closely linked to the functional and phenotypic status of the TAMs.
6.1.1 Chemotherapy, radiation therapy and TKI
Conventional therapies are generally considered immunostimulatory (271–276) by inducing cancer cell death and increasing the release of damage-associated molecular patterns (DAMPs) into the TME (277, 278). TAMs express several PRRs such as TLR4, which can recognize DAMPs and activate downstream signaling pathways including NF-kB and inflammasome, leading to phenotypical, metabolic and functional changes, and the expression of pro-inflammatory genes (191, 202, 279). The synergistic effect between conventional therapies and immunotherapies, with the former priming for the latter, leads to improved efficacy of combined therapy compared to monotherapy. However, the suboptimal effect and non-specificity, along with the exhausting effect of chronic futile inflammation, highlight the need for further development of regulated immune stimulation (280, 281).
6.1.2 Innate activation
Numerous natural and synthetic compounds have been found to activate the innate immune system, making them potential therapeutic agents that are currently under active pre-clinical development and clinical translation (282). PRR agonists have shown promising results in preliminary clinical trials (283, 284), particularly when combined with other immunostimulatory agents. For example, the TLR4 agonist monophosphoryl lipid A (MPLA) has been combined with IFN-γ (285), and the TLR7/8 agonist has been linked to anti-HER2 to form PRR-antibody-drug conjugates (286) (Table 1). These agonists are capable of promoting M1 polarization and TME inflammation. STING agonists have also been widely used in pre-clinical and clinical studies. They are administered through direct intratumoral injection or nanoparticle-based intravenous administration to increase the specificity of delivery to tumor cells or TAMs (287, 288). Recently, a STING agonist derived from microbiota has been identified to be associated with improved response to ICB in mouse models, thus implicating it as a potential mechanism underlying the benefits observed in high-fat diet or fecal transplantation of human responder (289).
Systemic administration of TNF-α has been limited by adverse reactions, as phase 1 studies have generally been disappointing due to sepsis-associated symptoms and dose-limiting toxicities with little or no favorable antitumor activity (290–295). Intratumoral administration or delivery into specific arteries (isolated limb or hepatic perfusion) has achieved some therapeutic effects in selected tumors such as Kaposi’s sarcoma (296), high-grade soft tissue sarcoma (297), and liver cancer (298, 299). More targeted delivery into the TME via engineered malignant cell homing has improved response in mouse models of breast cancer and melanoma (300). TAMs can also be engineered to produce inflammatory cytokines under specific TME conditions, with the IFN-γ gene construct controlled by a synthetic promoter inducible by hypoxia (HRE3x-Tk) (301). Interestingly, anti-TNF-α antibodies, which are extensively used in the treatment of autoimmune diseases, are also potentially applicable in the treatment of inflammatory tumors (302). The combined use of Infliximab and ICB, first to control ICB-related adverse effects such as colitis, did not impair antitumor effects but rather demonstrated synergistic effects and enhanced response in animal models, melanoma, and further clinical trials (303–306).
6.1.3 CSF-1/CSF-1R
CSF1R, a receptor tyrosine kinase, plays a crucial role in the differentiation and maintenance of M2 TAMs. Many small molecules inhibitors and antagonistic antibodies against CSF-1R have been developed, among which PLX3397 (pexidartinib) has been FDA-approved for treating tenosynovial giant cell tumor in 2019 (307, 308). These agents serve as a valuable foundation for anti-TAM therapeutic strategies, exhibiting synergistic effects with chemotherapy, radiotherapy, and ICB. Anti-CSF-1R antibody is promising as an addition to reverse resistance to anti-VEGF therapy and taxane chemotherapy (309, 310). A study in pancreatic cancer demonstrated that CSF-1/CSF-1R blockade up-regulated PD-L1 and CTLA-4, justifying that the combination of TAM reprogramming therapy with ICB may yield maximum effect (311). However, the effect of CSF-1R-targeted TAM depletion in the context of the heterogeneous TME requires further investigation, as preferential depletion of inflammatory TAMs but sparing pro-angiogenic/tumorigenic TAMs may lead to unwanted effects (94).
6.1.4 Others
CD206, an M2 macrophage marker, has been utilized as a guidepost for precise targeting of immunosuppressive TAMs (312). Various approaches such as nanoparticle-based mRNAs of IRF5, IKK-β, and miRNA-155, Fe3O4-based poly(lactic-co-glycolic) acid (PLGA) nanoparticles conjugated with anti-CD206, and RP-182 peptide, a small molecule inhibitor of CD206, have been developed (313–315). However, it is important to consider the heterogeneity and dynamic nature of TAMs, and to note that CD206 expression alone may not fully define immunosuppressive TAMs. Recent studies have shown that CD206-expressing TAMs are also capable of cross-presenting tumor-associated antigens to activate T cells (7), suggesting that CD206-directed therapies may inadvertently deplete beneficial TAMs. Advancements in high-throughput technologies have identified additional markers providing novel targets for intervention. For example, anti-MARCO-antibody and anti-Clever-1 antibody (FP-1305) were both capable of causing a phenotypic switch in TAMs from immunosuppressive to pro-inflammatory, and the combination of anti-Clever-1 antibody (FP-1305) with ICB showed synergistic benefits in aggressive tumors that were unresponsive to ICB (158, 316, 317).
6.2 Metabolism
The metabolic state of TAMs is closely linked to their phenotypical and functional polarization, as they both respond to and influence the inflammatory microenvironment. Many therapies have been developed targeting the metabolism of TAMs and their associated effects on T cells.
6.2.1 A2AR antagonism
Both pro- and anti-inflammatory TAMs have been found to exacerbate TME hypoxia, leading to the accumulation of adenosine, which in turn acts on A2AR to suppress T cell activity (211, 212). A2AR antagonists have shown promising therapeutic responses in various pre-clinical studies, particularly in the setting of chimeric antigen receptor T (CAR-T) cell therapy (211, 318). Currently, several phase I/II clinical trials investigating A2AR inhibitors, some in combination with ICB, are ongoing (see Table 1).
6.2.2 Amino acid metabolism
Targeting glutamate-glutamine metabolism (189, 319) with glutamine antagonists, GS blockade, and glutamine transporter inhibition, effectively drives M1 polarization and antitumor response, especially when used in combination with ICB (189, 224, 319, 320). A promising GS inhibitor, CB-839/Telaglenastat, is currently being evaluated in several phase I/II clinical trials. Preliminary of results have shown decreased mortality but increased incidence of serious adverse events when combined with the mTOR inhibitor Everolimus (Table 1). Moreover, glutamine antagonism has been found to reduce the expression of IDO in both tumor cells and TAMs, which suggests an intrinsic link between glutamine and tryptophan metabolism (319).
Restoring tryptophan availability and depleting kynurenine by inhibiting IDO has been an attractive therapeutic approach (239–241). However, the combination of the IDO inhibitor Epacadostat with Pembrolizumab failed to show antitumor activity in a phase II trial as compared to monotherapy (320). The lack of efficacy may be attributed to enzymes with redundant functions, such as IDO1, IDO2, and tryptophan 2,3-dioxygenase (TDO), indicating the need for dual- or pan-inhibitors.
The ARG1 inhibitor CB-1158 has demonstrated promising results in pre-clinical models, both as a monotherapy and in combination with ICB as well as adoptive T or NK cell therapy (226, 228, 321). A phase I/II clinical trial of CB-1158 monotherapy suggested good tolerability (Table 1), but paradoxically, better antitumor response was observed with CB-1158 monotherapy compared to combination with pembrolizumab. The reason behind the compromised effect of combinatorial therapy requires further investigation.
6.2.3 Modulation of OXPHOS and lipid metabolism
OXPHOS and FAO are associated with immunosuppression in the TME (188). In preclinical models, the inhibition of FAO by etomoxir, a drug that targets the rate-limiting enzyme carnitine palmitoyl-transferase 1a (CPT1a), led to a reduction in tumor growth (190). Similarly, CD36 knockout had a similar effect, indicating the potential of CD36-based therapies for TAM-specific inhibition of OXPHOS. Conversely, the CB2 cannabinoid receptor has been shown to inhibit TLR4 signaling and promote fatty acid oxidation, and pharmacological antagonism of this receptor reduced tumor growth independently of CB2 expression in tumor cells (266).
Direct inhibition of the electron transport chain can also reduce OXPHOS in TAMs (322). The respiratory complex I inhibitor metformin, an anti-diabetic drug that has been repurposed to treat cancer, has shown promising results in preclinical studies (Table 1). In a phase II trial, low-dose metformin was able to reprogram an inflammatory TME by increasing the number of anti-tumor TAMs and CD8+ T cells infiltration while decreasing infiltration of Treg cells. However, there was no significant change in the growth or apoptosis markers of tumor cells (323). Future clinical trials that combine metformin with other therapeutics, particularly ICB and cellular therapy, may reveal any potential synergistic effects.
Phospholipids and cholesterol also play a crucial role in regulating TAM phenotypes (260–262, 266–268). Inhibitors of COX2 or antagonists of PGE2 receptors in combination with ICB have been shown to reprogram the TME and increase T cell infiltration (324). Additionally, in patients with colorectal cancer, treatment with the DNA methyltransferase (DNMT) inhibitor 5-aza-2’-deoxycytidine (5Aza) decreased cholesterol efflux from the ABC transporter of TAMs, leading to pro-inflammatory effects and improved function of CD4 and CD8+ T cells (325).
It is important to remember that most metabolic pathways and corresponding drugs lack cell type selectivity. Since tumor cells, TAMs, and T cells depend on similar non-specific metabolic pathways, targeting TAM glycolysis with such drugs may hinder the proliferation and activity of T cells and promote tumor growth simultaneously. For example, activated T cells generally rely on aerobic glycolysis, but in low-glucose and hypoxic conditions like the TME, OXPHOS is crucial for their survival and function. Animal models have shown that a PPAR-α agonist can stimulate OXPHOS and FAO, leading to increased CD8+ T cell cytotoxicity and enhanced efficacy of anti-PD1 (219). Conversely, the pro-M2 effects of PPAR-α on TAMs justify the use of PPAR-α antagonists in combination with nivolumab in a phase I clinical trial (Table 1). Therefore, it is essential to keep this limitation in mind and explore nanoparticle- or antibody-based delivery systems to achieve precision therapy and maximize therapeutic efficacy.
6.3 Interactions
6.3.1 Checkpoints
Anti-PD1/PDL1 therapies have shown remarkable efficacy in multiple tumor types, but their effectiveness is largely limited to patients with high PD-L1 expression prior to treatment, leaving a significant portion of the patient population in need of alternative immune checkpoint inhibitors. Chronic inflammation can induce the expression of various immune checkpoint molecules, including PD1/PDL1, SLAMF7, CLEVER1, B7H4, and VISTA, which all have potential as therapeutic targets. For instance, the VISTA-PSGL1 axis has been extensively studied, and both anti-VISTA and anti-PSGL1 are currently being developed (85, 86, 326, 327). In a mouse model of lung cancer, an anti-Clever-1 antibody demonstrated superior performance to anti-PD-1 antibodies, reducing tumor growth in a TNBC model that is resistant to anti-PD-1 treatment (328). Phase I/II trials (NCT03733990) in patients with advanced solid tumors further demonstrated safety, tolerance, and preliminary immunostimulatory and antitumor activity (329, 330).
Phagocytosis and antigen uptake by TAMs are essential for subsequent immune stimulation. Targeting the “don’t-eat-me” signal CD47 and its receptor, SIRPa, enhances phagocytosis and antigen presentation by TAMs (331, 332 NCT02953782). Dual recognition antibodies are being investigated to improve specificity and avoid off-target effects; for example, anti-CD47&CD20 treatment is under clinical investigation in B-cell lymphoma (NCT03804996). LILRB on TAMs binds to MHC-I on cancer cells and inhibits macrophage phagocytosis. LILRB is reported to mediate resistance to various immunotherapies, and anti-LILRB1 (MK-4830) has demonstrated promising antitumor effects, along with a potent ability to reprogram TAMs and increase CD8+ T cells, as monotherapy or in combination with anti-CD47 or pembrolizumab (333, NCT03564691). Anti-SIGLEC10-mAb prevents the interaction between SIGLEC10 and another “don’t-eat-me” signal CD24, improving TAMs’ phagocytosis of tumor cells (334).
6.3.2 Stimulatory
As discussed in detail in previous sections of this review, co-stimulatory interactions between TAMs and T cells are indispensable for effective antitumor immunity. Among the co-stimulatory molecules investigated, CD40, OX40, ICOS, and 4-1BB have been extensively studied (335).
The development of CD40 agonists has experience diverse molecular modification and optimization, evolving from CD40L-like structures to agonistic antibodies. However, most of these available agents demonstrated acceptable adverse effects but limited antitumor responses in monotherapy (336). Selicrelumab is an exception with promising therapeutic effects, reported to achieved a PR of 27% in patients with advanced melanoma (337). Combination with chemotherapeutic agents, other mAbs against PD-1, PD-L1, Flt3L, and VEGF, and MEKi are under active clinical investigation (32, 338–341). Interestingly, the combination of anti-CSF-1R antibody and agonistic anti-CD40 antibody transient TAM hyperpolarization and subsequently T cell activation before final depleting effect, emphasizing the importance of time (342, 343).
OX40 agonist preferentially drives M1 polarization over M1 (344, 345). A study in the Pan02 model of mouse pancreatic cancer revealed that the combination of agnostic anti-OX40 and inhibitory anti-CTLA4 led to transient decrease in ARG1 expression in TAMs, giving a therapeutic window for gemcitabine (346). However, other voices have suggested that OX40 agonist therapy actually increase ARG1 in TAM, justifying its combination with ARG1 inhibitor to improve efficacy (347). Additionally, the pro-inflammatory effects of OX40 agonist is further enhanced by Gal-3 inhibitior (belapectin) (348).
ICOS/ICOSL has dual roles with both pro- and anti-tumor activities, leading to T cell activation but also Treg differentiation, justifying the development of both anti-ICOS agonists (GSK3359609, JTX-2011) (NCT04428333, NCT02904226) and antagonists (MEDI-570, KY1044/Alomfilimab/SAR445256) (NCT02520791, NCT03829501). INDUCE-1 trial (NCT02723955) of agonist anti-ICOS-mAb in monotherapy or in combination with Pembrolizumab in patients with advanced solid tumors reported promising tolerability and antitumor activity (349). As patients treated with anti-CTLA-4 or anti-PD-1 had expanded the ICOS+FoxP3+ T cells, which are reported to be an important biomarker for clinical response, suggesting optimal response with combined therapies (350, 351). However, ICOS-ICOSL has also been considered immunosuppressive, mediates repair processes in liver damage (352) and skin wounding (61, 353), mechanistically through induction of Th2 cytokines (IL-4, IL-6, IL-10), M2 (61) and Tregs (354), leading to fever in developing anti-ICOSL immunotherapies (354, 355). Antagonistic anti-ICOS-mAbs had limited antitumor activity (356) but anti-inflammatory wound healing effects (61).
Intratumoral administration of 4-1BB agonistic Ab led to increased T cell infiltration not only through T and NK cell activation (65, 357, 358), but also activating effects in TAMs (359).
6.4 Cellular therapy
T cell-based cellular therapies have demonstrated success in treating hematogenous tumors but not solid tumors. Macrophage-based cellular therapy is a promising approach due to their superior ability to infiltrate into the hostile TME of solid tumors and potent secretory capacity. Similar to CAR-T, macrophages can also be loaded with a CAR construct against specific antigens. However, instead of direct cytotoxicity, CAR-M relies primarily on phagocytosis and antigen presentation to modulate the TME rather than directly eliminating tumor cells (360). As drivers of inflammation, CAR-M can synchronize an amplified anti-tumor immune response, transforming an immunologically cold TME into an inflamed battlefield.
Advancements in macrophage-related technologies have largely overcome their inherent resistance to expansion and genetic manipulation (361). Induction of macrophage differentiation from iPSC allowed for efficient in vitro amplification, making large-scale production for clinical application possible (362). CAR-M has demonstrated promising results in preclinical studies with cellular and animal models. Anti-HER2-CAR-M was safe and tolerable with optimistic therapeutic effects in early clinical trials (NCT04660929).
CAR-Ms, similar to endogenous macrophages, are also dynamically polarized to display pro- or anti-inflammatory functions and phenotypes. Combination with other macrophage polarization methods help prevent immunosuppressive polarization with pro-tumor effects. Adoptive cellular transfer has extra advantages as in vitro amplification provides a chance for precise genetic manipulation. Chimeric vector with co-stimulatory domains, engineered constitutive expression of IFNa (363, 364, NCT03866109), and knockout of pro-M2 genes are potential methods to maintain the desired M1 phenotype, coming into clinical testing. Similar to fourth-generation CAR-T, or TRUNKS, CAR-M can also be loaded with cytokines such as IL-12 to amplify type 1 immune response (365), or even with drug-containing nanoparticles to take advantage of their superior efficiency in tumor homing and improve drug delivery (366).
Furthermore, macrophages not only serve as tools of cellular therapy but also as targets. Despite challenges due to the heterogeneity of TAMs, CAR-Ts have the potential to specifically deplete detrimental TAMs while sparing inflammatory TAMs needed for antitumor immunity. Anti-F4/80 CAR-T in a mouse tumor model unselectively depleted all TAMs (367), while anti-FRb CAR-T, an M2 marker, allowed for specific targeting of immunosuppressive TAMs and restrained tumor growth (368).
7 Perspective
Immunotherapy revolutionized the clinical courses of tumor treatment, prolonging survival and offering patients with previously considered unresectable tumors a chance at surgery. However, only selected tumor types and a limited population of patients are responsive to anti-PD1/PDL1 antibodies, largely due to the hostile, immunosuppressive microenvironment. Much effort has been devoted to elucidating the mechanism of resistance and subsequently developing interventions, all leading to the importance of tumor immunogenicity and local inflammation.
Inflammation has a dual role in antitumor immunity, complicated by spatial-temporal factors and immune cells under influence. This double-edged sword is indispensable for the priming and activation of anti-tumor immunity, but also responsible for exhaustion and reactive desmoplasia. TAMs not only are the major cellular population of the TME, but also have superior secretory capacities. As the master regulators of TME inflammation, TAMs are capable of initiating and fine-tuning the cascade amplification of immune response, making them valuable targets. However, TAMs are highly heterogeneous and dynamic, existing in an almost inseparable spectrum of statuses, rather than the distinct M1/2 characteristics defined by in vitro stimulation or a state of terminal differentiation. TAMs are constantly affected by environmental factors including metabolic availability, stress, and cellular crosstalk through both direct contact and secreted factors. Upon sensing these stimuli, activation of coupled signaling pathways leads to transcriptional and epigenetic reprogramming, ultimately achieving functional and phenotypical polarization.
Such complexity and plasticity offer many therapeutic opportunities but at the same time pose challenges for precise and effective targeting, especially in the translation from bioinformatic data mining to experimental validation and further therapeutic strategy development. Still in the prime of its age, the development of TAM-centered therapies must adopt novel approaches to seek convergent points, striving to produce the butterfly effect in addition to specific targeting of each individual factor. In this review, we integrated recently published bioinformatic data, experimental studies, and advancements in clinical trials to provide a comprehensive understanding on the TAM polarization-inflammation process and potential therapeutic development. TAMs are promising tools to regulate TME inflammation, optimizing antitumor immune activation while minimizing protumor exhaustion and desmoplasia.
Author contributions
JH and LD contributed equally to this work. All authors contributed to the article and approved the submitted version.
Funding
This work was supported by CAMS Innovation Fund for Medical Sciences (2021-I2M-1-014).
Conflict of interest
The authors declare that the research was conducted in the absence of any commercial or financial relationships that could be construed as a potential conflict of interest.
Publisher’s note
All claims expressed in this article are solely those of the authors and do not necessarily represent those of their affiliated organizations, or those of the publisher, the editors and the reviewers. Any product that may be evaluated in this article, or claim that may be made by its manufacturer, is not guaranteed or endorsed by the publisher.
References
1. Oates T, Moura P, Cross S, Roberts K, Baum H, Haydn-Smith K, et al. Characterizing the polarization continuum of macrophage subtypes M1, M2a and M2c. Biorxiv (2022). doi: 10.1101/2022.06.13.495868
2. Ferrante CJ, Pinhal-Enfield G, Elson G, Cronstein BN, Hasko G, Outram S, et al. The adenosine-dependent angiogenic switch of macrophages to an M2-like phenotype is independent of interleukin-4 receptor alpha (IL-4Rα) signaling. Inflammation (2013) 36(4):921–31. doi: 10.1007/s10753-013-9621-3
3. Ma R-Y, Black A, Qian B-Z. Macrophage diversity in cancer revisited in the era of single-cell omics. Trends Immunol (2022) 43(7):546–63. doi: 10.1016/j.it.2022.04.008
4. Coma G, Peña R, Blanco J, Rosell A, Borras FE, Esté JA, et al. Treatment of monocytes with interleukin (IL)-12 plus IL-18 stimulates survival, differentiation and the production of CXC chemokine ligands (CXCL)8, CXCL9 and CXCL10. Clin Exp Immunol (2006) 145(3):535–44. doi: 10.1111/j.1365-2249.2006.03145.x
5. Oishi S, Takano R, Tamura S, Tani S, Iwaizumi M, Hamaya Y, et al. M2 polarization of murine peritoneal macrophages induces regulatory cytokine production and suppresses T-cell proliferation. Immunology (2016) 149(3):320–8. doi: 10.1111/imm.12647
6. Bartlett B, Ludewick HP, Misra A, Lee S, Dwivedi G. Macrophages and T cells in atherosclerosis: a translational perspective. Am J Physiol-Heart Circulatory Physiol (2019) 317(2):H375–86. doi: 10.1152/ajpheart.00206.2019
7. Modak M, Mattes A-K, Reiss D, Skronska-Wasek W, Langlois R, Sabarth N, et al. CD206+ tumor-associated macrophages cross-present tumor antigen and drive antitumor immunity. JCI Insight (2022) 7(11). doi: 10.1172/jci.insight.155022
8. Chelen CJ, Fang Y, Freeman GJ, Secrist H, Marshall JD, Hwang PT, et al. Human alveolar macrophages present antigen ineffectively due to defective expression of B7 costimulatory cell surface molecules. J Clin Invest (1995) 95(3):1415–21. doi: 10.1172/JCI117796
9. Carswell EA, Old LJ, Kassel RL, Green S, Fiore N, Williamson B. An endotoxin-induced serum factor that causes necrosis of tumors. Proc Natl Acad Sci United States America (1975) 72(9):3666–70. doi: 10.1073/pnas.72.9.3666
10. Mace KF, Ehrke MJ, Hori K, Maccubbin DL, Mihich E. Role of tumor necrosis factor in macrophage activation and tumoricidal activity. Cancer Res (1988) 48(19):5427–32.
11. Hoepel W, Newling M, Vogelpoel LTC, Sritharan L, Hansen IS, Kapsenberg ML, et al. FcγR-TLR cross-talk enhances TNF production by human monocyte-derived DCs via IRF5-dependent gene transcription and glycolytic reprogramming. Front Immunol (2019) 10.
12. Grassin-Delyle S, Abrial C, Salvator H, Brollo M, Naline E, Devillier P. The role of toll-like receptors in the production of cytokines by human lung macrophages. J Innate Immun (2020) 12(1):63–73. doi: 10.1159/000494463
13. Wang X, Lin Y. Tumor necrosis factor and cancer, buddies or foes? Acta Pharmacologica Sin (2008) 29(11):1275–88. doi: 10.1111/j.1745-7254.2008.00889.x
14. Montfort A, Colacios C, Levade T, Andrieu-Abadie N, Meyer N, Ségui B. The TNF paradox in cancer progression and immunotherapy. Front Immunol (2019) 10. doi: 10.3389/fimmu.2019.01818
15. Laha D, Grant R, Mishra P, Nilubol N. The role of tumor necrosis factor in manipulating the immunological response of tumor microenvironment. Front Immunol (2021) 12. doi: 10.3389/fimmu.2021.656908
16. Mezouar S, Mege J-L. Changing the paradigm of IFN-γ at the interface between innate and adaptive immunity: macrophage-derived IFN-γ. J Leukoc Biol (2020) 108(1):419–26. doi: 10.1002/JLB.4MIR0420-619RR
17. Su X, Yu Y, Zhong Y, Giannopoulou EG, Hu X, Liu H, et al. Interferon-γ regulates cellular metabolism and mRNA translation to potentiate macrophage activation. Nat Immunol (2015) 16(8):838–49. doi: 10.1038/ni.3205
18. Wu C, Xue Y, Wang P, Lin L, Liu Q, Li N, et al. IFN-γ primes macrophage activation by increasing phosphatase and tensin homolog via downregulation of miR-3473b. J Immunol (Baltimore Md 1950) (2014) 193(6):3036–44. doi: 10.4049/jimmunol.1302379
19. Zhang F, Mears JR, Shakib L, Beynor JI, Shanaj S, Korsunsky I, et al. IFN-γ and TNF-α drive a CXCL10+ CCL2+ macrophage phenotype expanded in severe COVID-19 lungs and inflammatory diseases with tissue inflammation. Genome Med (2021) 13(1). doi: 10.1186/s13073-021-00881-3
20. Müller E, Christopoulos PF, Halder S, Lunde A, Beraki K, Speth M, et al. Toll-like receptor ligands and interferon-γ synergize for induction of antitumor M1 macrophages. Front Immunol (2017) 8. doi: 10.3389/fimmu.2017.01383
21. Dallagi A, Girouard J, Hamelin-Morrissette J, Dadzie R, Laurent L, Vaillancourt C, et al. The activating effect of IFN-γ on monocytes/macrophages is regulated by the LIF–trophoblast–IL-10 axis via Stat1 inhibition and Stat3 activation. Cell Mol Immunol (2014) 12(3):326–41.
22. Robinson CM, O’Dee D, Hamilton T, Nau GJ. Cytokines involved in interferon-γ production by human macrophages. J Innate Immun (2009) 2(1):56–65.
23. Darwich L, Coma G, Peña R, Bellido R, Blanco EJJ, Este JA, et al. Secretion of interferon-γ by human macrophages demonstrated at the single-cell level after costimulation with interleukin (IL)-12 plus IL-18. Immunology (2009) 126(3):386–93. doi: 10.1111/j.1365-2567.2008.02905.x
24. Munder M, Mallo M, Eichmann K, Modolell M. Murine macrophages secrete interferon γ upon combined stimulation with interleukin (IL)-12 and IL-18: a novel pathway of autocrine macrophage activation. J Exp Med (1998) 187(12):2103–8. doi: 10.1084/jem.187.12.2103
25. Frucht DM, Fukao T, Bogdan C, Schindler H, O’Shea JJ, Koyasu S. IFN-γ production by antigen-presenting cells: mechanisms emerge. Trends Immunol (2001) 22(10):556–60. doi: 10.1016/S1471-4906(01)02005-1
26. Yamaguchi Y, Gibson J, Ou K, Lopez LS, Ng RH, Leggett N, et al. PD-L1 blockade restores CAR T cell activity through IFN-γ-regulation of CD163+ M2 macrophages. J ImmunoTherapy Cancer (2022) 10(6):e004400. doi: 10.1136/jitc-2021-004400
27. Perez-Diez A, Liu X, Matzinger P. Neoantigen presentation and IFN-gamma signaling on the same tumor associated macrophage are necessary for CD4 T cell mediated anti-tumor activity in mice. Cancer Res Commun (2022). doi: 10.1158/2767-9764.c.6550731.v1
28. Davidsson S, Fiorentino M, Giunchi F, Eriksson M, Erlandsson A, Sundqvist P, et al. Infiltration of M2 macrophages and regulatory T cells plays a role in recurrence of renal cell carcinoma. Eur Urol Open Science (2020) 20:62–71. doi: 10.1016/j.euros.2020.06.003
29. Staveley-O’Carroll K, Sotomayor E, Montgomery J, Borrello I, Hwang L, Fein S, et al. Induction of antigen-specific T cell anergy: an early event in the course of tumor progression. Proc Natl Acad Sci (1998) 95(3):1178–83. doi: 10.1073/pnas.95.3.1178
30. Alonso R, Flament H, Lemoine S, Sedlik C, Bottasso E, Péguillet I, et al. Induction of anergic or regulatory tumor-specific CD4+ T cells in the tumor-draining lymph node. Nat Commun (2018) 9(1). doi: 10.1038/s41467-018-04524-x
31. Kersten K, Hu KH, Combes AJ, Samad B, Harwin T, Ray A, et al. Spatiotemporal co-dependency between macrophages and exhausted CD8+ T cells in cancer. Cancer Cell (2022) 40(6):624–638.e9. doi: 10.1016/j.ccell.2022.05.004
32. Peranzoni E, Lemoine J, Vimeux L, Feuillet V, Barrin S, Kantari-Mimoun C, et al. Macrophages impede CD8 T cells from reaching tumor cells and limit the efficacy of anti–PD-1 treatment. Proc Natl Acad Sci (2018) 115(17):E4041–50. doi: 10.1073/pnas.1720948115
33. Tirapu I, Huarte E, Guiducci C, Arina A, Zaratiegui M, Murillo O, et al. Low surface expression of B7-1 (CD80) is an immunoescape mechanism of colon carcinoma. Cancer Res (2006) 66(4):2442–50. doi: 10.1158/0008-5472.CAN-05-1681
34. Kanada S, Nishiyama C, Nakano N, Suzuki R, Maeda K, Hara M, et al. Critical role of transcription factor PU.1 in the expression of CD80 and CD86 on dendritic cells. Blood (2011) 117(7):2211–22.
35. Galdiero M, Pisciotta MG, Gorga F, Petrillo G, Marinelli A, Galdiero E. Modulation of costimulatory molecules CD80/CD86 on b cells and macrophages by stress proteins GroEL, GroES and DnaK. Int J Immunopathol Pharmacol (2005) 18(4):637–44. doi: 10.1177/039463200501800404
36. Richter G, Hayden-Ledbetter M, Irgang M, Ledbetter JA, Westermann J, Körner I, et al. Tumor necrosis factor-α regulates the expression of inducible costimulator receptor ligand on CD34+ progenitor cells during differentiation into antigen presenting cells. J Biol Chem (2001) 276(49):45686–93. doi: 10.1074/jbc.M108509200
37. Kang YJ, Kim SO, Shimada S, Otsuka M, Seit-Nebi A, Kwon BS, et al. Cell surface 4-1BBL mediates sequential signaling pathways “downstream” of TLR and is required for sustained TNF production in macrophages. Nat Immunol (2007) 8(6):601–9. doi: 10.1038/ni1471
38. Ma J, Bang B-R, Lu J, Eun S-Y, Otsuka M, Croft M, et al. The TNF family member 4-1BBL sustains inflammation by interacting with TLR signaling components during late-phase activation. Sci Signaling (2013) 6(295). doi: 10.1126/scisignal.2004431
39. Buchan SL, Rogel A, Al-Shamkhani A. The immunobiology of CD27 and OX40 and their potential as targets for cancer immunotherapy. Blood (2018) 131(1):39–48. doi: 10.1182/blood-2017-07-741025
41. Imaizumi K, Kawabe T, Ichiyama S, Kikutani H, Yagita H, Shimokata K, et al. Enhancement of tumoricidal activity of alveolar macrophages via CD40-CD40 ligand interaction. Am J Physiology-Lung Cell Mol Physiol (1999) 277(1):L49–57. doi: 10.1152/ajplung.1999.277.1.L49
42. Buhtoiarov IN, Lum H, Berke G, Paulnock DM, Sondel PM, Rakhmilevich AL. CD40 ligation activates murine macrophages via an IFN-γ-Dependent mechanism resulting in tumor cell destruction In vitro. J Immunol (2005) 174(10):6013–22. doi: 10.4049/jimmunol.174.10.6013
43. Lai Y, Wahyuningtyas R, Aui S, Chang K. AutocrineVEGFsignalling on M2 macrophages regulatesPD-L1 expression for immunomodulation of T cells. J Cell Mol Med (2018). doi: 10.1111/jcmm.14027
44. Otrock ZK, Makarem JA, Shamseddine AI. Vascular endothelial growth factor family of ligands and receptors: review. Blood Cells Mol Diseases (2007) 38(3):258–68. doi: 10.1016/j.bcmd.2006.12.003
45. Hwang I, Kim JW, Ylaya K, Chung EJ, Kitano H, Perry C, et al. Tumor-associated macrophage, angiogenesis and lymphangiogenesis markers predict prognosis of non-small cell lung cancer patients. J Trans Med (2020) 18(1). doi: 10.1186/s12967-020-02618-z
46. Ferreira S de S, Wallmann T, Kerzel T, Wallerius M, Bartish M, Landwehr L-S, et al. Macrophage-derived VEGF-c decreases hematogenous metastatic dissemination by normalizing the tumor vasculature. Biorxiv (2022).
47. Xiao YQ, Freire-de-Lima CG, Schiemann WP, Bratton DL, Vandivier RW, Henson PM. Transcriptional and translational regulation of TGF-β production in response to apoptotic cells. J Immunol (2008) 181(5):3575–85. doi: 10.4049/jimmunol.181.5.3575
48. Xiong W, Frasch SC, Thomas SM, Bratton DL, Henson PM. Induction of TGF-β1 synthesis by macrophages in response to apoptotic cells requires activation of the scavenger receptor CD36. PloS One (2013) 8(8):e72772. doi: 10.1371/journal.pone.0072772
49. Huynh M-LN, Fadok VA, Henson PM. Phosphatidylserine-dependent ingestion of apoptotic cells promotes TGF-β1 secretion and the resolution of inflammation. J Clin Invest (2002) 109(1):41–50. doi: 10.1172/JCI0211638
50. Nacu N, Luzina IG, Highsmith K, Lockatell V, Pochetuhen K, Cooper ZA, et al. Macrophages produce TGF-β-Induced (β-ig-h3) following ingestion of apoptotic cells and regulate MMP14 levels and collagen turnover in fibroblasts. J Immunol (2008) 180(7):5036–44. doi: 10.4049/jimmunol.180.7.5036
51. Batlle E, Massagué J. Transforming growth factor-β signaling in immunity and cancer. Immunity (2019) 50(4):924–40. doi: 10.1016/j.immuni.2019.03.024
52. Zhang F, Wang H, Wang X, Jiang G, Liu H, Zhang G, et al. TGF-β induces M2-like macrophage polarization via SNAIL-mediated suppression of a pro-inflammatory phenotype. Oncotarget (2016) 7(32):52294–306. doi: 10.18632/oncotarget.10561
53. Cai J, Xia L, Li J, Ni S, Song H, Wu X. Tumor-associated macrophages derived TGF-β–Induced epithelial to mesenchymal transition in colorectal cancer cells through Smad2,3-4/Snail signaling pathway. Cancer Res Treat (2019) 51(1):252–66. doi: 10.4143/crt.2017.613
55. Zhu L, Fu X, Chen X, Han X, Dong P. M2 macrophages induce EMT through the TGF-β/Smad2 signaling pathway. Cell Biol Int (2017) 41(9):960–8. doi: 10.1002/cbin.10788
56. Kudo-Saito C, Shirako H, Takeuchi T, Kawakami Y. Cancer metastasis is accelerated through immunosuppression during snail-induced EMT of cancer cells. Cancer Cell (2009) 15(3):195–206. doi: 10.1016/j.ccr.2009.01.023
57. Standiford TJ, Kuick R, Bhan U, Chen J, Newstead M, Keshamouni VG. TGF-β-induced IRAK-m expression in tumor-associated macrophages regulates lung tumor growth. Oncogene (2011) 30(21):2475–84. doi: 10.1038/onc.2010.619
58. Sun X, Bernhardt SM, Glynn DJ, Hodson LJ, Woolford L, Evdokiou A, et al. Attenuated TGFB signalling in macrophages decreases susceptibility to DMBA-induced mammary cancer in mice. Breast Cancer Res (2021) 23(1). doi: 10.1186/s13058-021-01417-8
59. Lyons JG, Patel V, Roue NC, Fok SY, Soon LL, Halliday GM, et al. Snail up-regulates proinflammatory mediators and inhibits differentiation in oral keratinocytes. Cancer Res (2008) 68(12):4525–30. doi: 10.1158/1078-0432.CCR-07-6735
60. Hotz B, Visekruna A, Buhr H-J, Hotz HG. Beyond epithelial to mesenchymal transition: a novel role for the transcription factor snail in inflammation and wound healing. J Gastrointest Surg (2009) 14(2):388–97. doi: 10.1007/978-3-642-00625-8_109
61. Stoppa I, Gigliotti CL, Clemente N, Pantham D, Dianzani C, Monge C, et al. ICOSL stimulation by ICOS-fc accelerates cutaneous wound healing In vivo. Int J Mol Sci (2022) 23(13):7363. doi: 10.3390/ijms23137363
62. Tang H, Liang Y, Anders RA, Taube JM, Qiu X, Mulgaonkar A, et al. PD-L1 on host cells is essential for PD-L1 blockade–mediated tumor regression. J Clin Invest (2018) 128(2):580–8. doi: 10.1172/JCI96061
63. Lin H, Wei S, Hurt EM, Green MD, Zhao L, Vatan L, et al. Host expression of PD-L1 determines efficacy of PD-L1 pathway blockade–mediated tumor regression. J Clin Invest (2018) 128(2):805–15. doi: 10.1172/JCI96113
64. Lau J, Cheung J, Navarro A, Lianoglou S, Haley B, Totpal K, et al. Tumour and host cell PD-L1 is required to mediate suppression of anti-tumour immunity in mice. Nat Commun (2017) 8(1). doi: 10.1038/ncomms14572
65. Liu Y, Zugazagoitia J, Ahmed FS, Henick BS, Gettinger SN, Herbst RS, et al. Immune cell PD-L1 colocalizes with macrophages and is associated with outcome in PD-1 pathway blockade therapy. Clin Cancer Res (2020) 26(4):970–7. doi: 10.1158/1078-0432.CCR-19-1040
66. Lu L-G, Zhou Z-L, Wang X-Y, Liu B-Y, Lu J-Y, Liu S, et al. PD-L1 blockade liberates intrinsic antitumourigenic properties of glycolytic macrophages in hepatocellular carcinoma. Gut (2022) 71(12):2551–60. doi: 10.1136/gutjnl-2021-326350
67. Guo S, Wang X, Zhou H, Gao Y, Wang P, Zhi H, et al. PD-L1-Mediated immunosuppression in hepatocellular carcinoma: relationship with macrophages infiltration and inflammatory response activity. Biomolecules (2022) 12(9):1226. doi: 10.3390/biom12091226
68. Petty AJ, Dai R, Lapalombella R, Baiocchi RA, Benson DM, Li Z, et al. Hedgehog-induced PD-L1 on tumor-associated macrophages is critical for suppression of tumor-infiltrating CD8+ T cell function. JCI Insight (2021) 6(6). doi: 10.1172/jci.insight.146707
69. Lee AH, Sun L, Mochizuki AY, Reynoso JG, Orpilla J, Chow F, et al. Neoadjuvant PD-1 blockade induces T cell and cDC1 activation but fails to overcome the immunosuppressive tumor associated macrophages in recurrent glioblastoma. Nat Commun (2021) 12(1). doi: 10.1038/s41467-021-26940-2
70. Ene CI, Kreuser SA, Jung M, Zhang H, Arora S, White Moyes K, et al. Anti–PD-L1 antibody direct activation of macrophages contributes to a radiation-induced abscopal response in glioblastoma. Neuro-Oncology (2019) 22(5):639–51.
71. Shinchi Y, Ishizuka S, Komohara Y, Matsubara E, Mito R, Pan C, et al. The expression of PD-1 ligand 1 on macrophages and its clinical impacts and mechanisms in lung adenocarcinoma. Cancer Immunol Immunother (2022) 71(11):2645–61. doi: 10.1007/s00262-022-03187-4
72. Wen Z-F, Liu H, Gao R, Zhou M, Ma J, Zhang Y, et al. Tumor cell-released autophagosomes (TRAPs) promote immunosuppression through induction of M2-like macrophages with increased expression of PD-L1. J ImmunoTherapy Cancer (2018) 6(1). doi: 10.1186/s40425-018-0452-5
73. Horlad H, Ma C, Yano H, Pan C, Ohnishi K, Fujiwara Y, et al. An IL-27/Stat3 axis induces expression of programmed cell death 1 ligands (PD-L1/2) on infiltrating macrophages in lymphoma. Cancer Science (2016) 107(11):1696–704. doi: 10.1111/cas.13065
74. Liu Y, Yan H, Gu H, Zhang E, He J, Cao W, et al. Myeloma-derived IL-32γ induced PD-L1 expression in macrophages facilitates immune escape via the PFKFB3-JAK1 axis. OncoImmunology (2022) 11(1). doi: 10.1080/2162402X.2022.2057837
75. Xia Q, Jia J, Hu C, Lu J, Li J, Xu H, et al. Tumor-associated macrophages promote PD-L1 expression in tumor cells by regulating PKM2 nuclear translocation in pancreatic ductal adenocarcinoma. Oncogene (2021) 41(6):865–77.
76. Bloch O, Crane CA, Kaur R, Safaee M, Rutkowski MJ, Parsa AT. Gliomas promote immunosuppression through induction of B7-H1 expression in tumor-associated macrophages. Clin Cancer Res (2013) 19(12):3165–75. doi: 10.1158/1078-0432.CCR-12-3314
77. Noman MZ, Desantis G, Janji B, Hasmim M, Karray S, Dessen P, et al. PD-L1 is a novel direct target of HIF-1α, and its blockade under hypoxia enhanced MDSC-mediated T cell activation. J Exp Med (2014) 211(5):781–90. doi: 10.1084/jem.20131916
78. Hartley G, Regan D, Guth A, Dow S. Regulation of PD-L1 expression on murine tumor-associated monocytes and macrophages by locally produced TNF-α. Cancer Immunol Immunother (2017) 66(4):523–35. doi: 10.1007/s00262-017-1955-5
79. Lu D, Ni Z, Liu X, Feng S, Dong X, Shi X, et al. Beyond T cells: understanding the role of PD-1/PD-L1 in tumor-associated macrophages. J Immunol Res (2019) 2019:1–7. doi: 10.1155/2019/1919082
80. Cai H, Zhang Y, Wang J, Gu J. Defects in macrophage reprogramming in cancer therapy: the negative impact of PD-L1/PD-1. Front Immunol (2021) 12. doi: 10.3389/fimmu.2021.690869
81. Kawashima M, Carreras J, Higuchi H, Kotaki R, Hoshina T, Okuyama K, et al. PD-L1/L2 protein levels rapidly increase on monocytes via trogocytosis from tumor cells in classical Hodgkin lymphoma. Leukemia (2020) 34(9):2405–17. doi: 10.1038/s41375-020-0737-9
82. Gordon SR, Maute RL, Dulken BW, Hutter G, George BM, McCracken MN, et al. PD-1 expression by tumour-associated macrophages inhibits phagocytosis and tumour immunity. Nature (2017) 545(7655):495–9. doi: 10.1038/nature22396
83. Cao H, Xiang Y, Zhang S, Chao Y, Guo J, Ho JWK, et al. PD-L1 regulates inflammatory macrophage development from human pluripotent stem cells by maintaining interferon-gamma signal. Biorxiv (2022). doi: 10.1101/2022.12.14.520176
84. O’Connell P, Hyslop S, Blake MK, Godbehere S, Amalfitano A, Aldhamen YA. SLAMF7 signaling reprograms T cells toward exhaustion in the tumor microenvironment. J Immunol (2020) 206(1):193–205.
85. Wang L, Rubinstein R, Lines JL, Wasiuk A, Ahonen C, Guo Y, et al. VISTA, a novel mouse ig superfamily ligand that negatively regulates T cell responses. J Exp Med (2011) 208(3):577–92. doi: 10.1084/jem.20100619
86. Johnston RJ, Su LJ, Pinckney J, Critton D, Boyer E, Krishnakumar A, et al. VISTA is an acidic pH-selective ligand for PSGL-1. Nature (2019) 574(7779):565–70. doi: 10.1038/s41586-019-1674-5
87. Kryczek I, Zou L, Rodriguez P, Zhu G, Wei S, Mottram P, et al. B7-H4 expression identifies a novel suppressive macrophage population in human ovarian carcinoma. J Exp Med (2006) 203(4):871–81. doi: 10.1084/jem.20050930
88. Podojil JR, Miller SD. Potential targeting of B7-H4 for the treatment of cancer. Immunol Rev (2017) 276(1):40–51. doi: 10.1111/imr.12530
89. Kalluri R. The biology and function of fibroblasts in cancer. Nat Rev Cancer (2016) 16(9):582–98. doi: 10.1038/nrc.2016.73
90. Chen P-Y, Wei W-F, Wu H-Z, Fan L-S, Wang W. Cancer-associated fibroblast heterogeneity: a factor that cannot be ignored in immune microenvironment remodeling. Front Immunol (2021) 12. doi: 10.3389/fimmu.2021.671595
91. Raskov H, Orhan A, Gaggar S, Gögenur I. Cancer-associated fibroblasts and tumor-associated macrophages in cancer and cancer immunotherapy. Front Oncol (2021) 11. doi: 10.3389/fonc.2021.668731
92. Rittling SR. Osteopontin in macrophage function. Expert Rev Mol Med (2011) 13. doi: 10.1017/S1462399411001839
93. Qi J, Sun H, Zhang Y, Wang Z, Xun Z, Li Z, et al. Single-cell and spatial analysis reveal interaction of FAP+ fibroblasts and SPP1+ macrophages in colorectal cancer. Nat Commun (2022) 13(1). doi: 10.1038/s41467-022-29366-6
94. Zhang L, Li Z, Skrzypczynska KM, Fang Q, Zhang W, O’Brien SA, et al. Single-cell analyses inform mechanisms of myeloid-targeted therapies in colon cancer. Cell (2020) 181(2):442–459.e29. doi: 10.1016/j.cell.2020.03.048
95. Liu Y, Ye G, Dong B, Huang L, Zhang C, Sheng Y, et al. A pan-cancer analysis of the oncogenic role of secreted phosphoprotein 1 (SPP1) in human cancers. Ann Trans Med (2022) 10(6):279–9. doi: 10.21037/atm-22-829
96. Dong B, Wu C, Huang L, Qi Y. Macrophage-related SPP1 as a potential biomarker for early lymph node metastasis in lung adenocarcinoma. Front Cell Dev Biol (2021) 27:9. doi: 10.3389/fcell.2021.739358
97. Matsubara E, Komohara Y, Esumi S, Shinchi Y, Ishizuka S, Mito R, et al. SPP1 derived from macrophages is associated with a worse clinical course and chemo-resistance in lung adenocarcinoma. Cancers (2022) 14(18):4374. doi: 10.3390/cancers14184374
98. Liu L, Zhang R, Deng J, Dai X, Zhu X, Fu Q, et al. Construction of TME and identification of crosstalk between malignant cells and macrophages by SPP1 in hepatocellular carcinoma. Cancer Immunol Immunother (2021) 71(1):121–36.
99. Raineri D, Dianzani C, Cappellano G, Maione F, Baldanzi G, Iacobucci I, et al. Osteopontin binds ICOSL promoting tumor metastasis. Commun Biol (2020) 3(1). doi: 10.1038/s42003-020-01333-1
100. Raineri D, Cappellano G, Vilardo B, Maione F, Clemente N, Canciani E, et al. Inducible T-cell costimulator ligand plays a dual role in melanoma metastasis upon binding to osteopontin or inducible T-cell costimulator. Biomedicines (2021) 10(1):51. doi: 10.3390/biomedicines10010051
101. Remmerie A, Martens L, Thoné T, Castoldi A, Seurinck R, Pavie B, et al. Osteopontin expression identifies a subset of recruited macrophages distinct from kupffer cells in the fatty liver. Immunity (2020) 53(3):641–657.e14. doi: 10.1016/j.immuni.2020.08.004
102. Quaranta V, Rainer C, Nielsen SR, Raymant ML, Ahmed MS, Engle DD, et al. Macrophage-derived granulin drives resistance to immune checkpoint inhibition in metastatic pancreatic cancer. Cancer Res (2018) 78(15):4253–69. doi: 10.1158/0008-5472.CAN-17-3876
103. Morse C, Tabib T, Sembrat J, Buschur KL, Bittar HT, Valenzi E, et al. Proliferating SPP1/MERTK-expressing macrophages in idiopathic pulmonary fibrosis. Eur Respir J (2019) 54(2):1802441. doi: 10.1183/13993003.02441-2018
104. Fabre T, Barron AMS, Christensen SM, Asano S, Wadsworth MH II, Chen X, et al. Identification of a broadly fibrogenic macrophage subset induced by type 3 inflammation in human and murine liver and lung fibrosis. Biorxiv (2022). doi: 10.1101/2022.07.01.498017
105. Rowe GC, Raghuram S, Jang C, Nagy JA, Patten IS, Goyal A, et al. PGC-1α induces SPP1 to activate macrophages and orchestrate functional angiogenesis in skeletal muscle. Circ Res (2014) 115(5):504–17. doi: 10.1161/CIRCRESAHA.115.303829
106. Zhang Y, Du W, Chen Z, Xiang C. Upregulation of PD-L1 by SPP1 mediates macrophage polarization and facilitates immune escape in lung adenocarcinoma. Exp Cell Res (2017) 359(2):449–57. doi: 10.1016/j.yexcr.2017.08.028
107. Jiang Y, Zhang H, Wang J, Liu Y, Luo T, Hua H. Targeting extracellular matrix stiffness and mechanotransducers to improve cancer therapy. J Hematol Oncol (2022) 15(1). doi: 10.1186/s13045-022-01252-0
108. Deng B, Zhao Z, Kong W, Han C, Shen X, Zhou C. Biological role of matrix stiffness in tumor growth and treatment. J Trans Med (2022) 20(1). doi: 10.1186/s12967-022-03768-y
109. Kim SHJ, Hammer DA. Integrin cross-talk modulates stiffness-independent motility of CD4+ T lymphocytes. In: Weaver VM, editor. Molecular biology of the cell, vol. 32 (2021). p. 1749–57.
110. Gkretsi V, Stylianopoulos T. Cell adhesion and matrix stiffness: coordinating cancer cell invasion and metastasis. Front Oncol (2018) 8. doi: 10.3389/fonc.2018.00145
111. Chirivì M, Maiullari F, Milan M, Presutti D, Cordiglieri C, Crosti M, et al. Tumor extracellular matrix stiffness promptly modulates the phenotype and gene expression of infiltrating T lymphocytes. Int J Mol Sci (2021) 22(11):5862. doi: 10.3390/ijms22115862
112. Kuczek DE, Larsen AMH, Thorseth M-L, Carretta M, Kalvisa A, Siersbæk MS, et al. Collagen density regulates the activity of tumor-infiltrating T cells. J ImmunoTherapy Cancer (2019) 7(1). doi: 10.1186/s40425-019-0556-6
113. Ge H, Tian M, Pei Q, Tan F, Pei H. Extracellular matrix stiffness: new areas affecting cell metabolism. Front Oncol (2021) 11. doi: 10.3389/fonc.2021.631991
114. Maller O, Drain AP, Barrett AS, Borgquist S, Ruffell B, Zakharevich I, et al. Tumour-associated macrophages drive stromal cell-dependent collagen crosslinking and stiffening to promote breast cancer aggression. Nat Mater (2020) 20(4):548–59.
115. Tang PC, Chung JY, Xue VW, Xiao J, Meng X, Huang X, et al. Smad3 promotes cancer-associated fibroblasts generation via macrophage–myofibroblast transition. Adv Science (2021) 9(1):2101235.
116. Gorchs L, Fernández Moro C, Bankhead P, Kern KP, Sadeak I, Meng Q, et al. Human pancreatic carcinoma-associated fibroblasts promote expression of Co-inhibitory markers on CD4+ and CD8+ T-cells. Front Immunol (2019) 10. doi: 10.3389/fimmu.2019.00847
117. Grünwald BT, Devisme A, Andrieux G, Vyas F, Aliar K, McCloskey CW, et al. Spatially confined sub-tumor microenvironments in pancreatic cancer. Cell (2021) 184(22):5577–5592.e18.
118. Lakins MA, Ghorani E, Munir H, Martins CP, Shields JD. Cancer-associated fibroblasts induce antigen-specific deletion of CD8 T cells to protect tumour cells. Nat Commun (2018) 9(1). doi: 10.1038/s41467-018-03347-0
119. Wu SZ, Roden DL, Wang C, Holliday H, Harvey K, Cazet AS, et al. Stromal cell diversity associated with immune evasion in human triple-negative breast cancer. EMBO J (2020) 39(19). doi: 10.15252/embj.2019104063
120. Chen Q, Li Y, Wang X, Zhang X, Hu Y, Li L, et al. Tumor fibroblast–derived FGF2 regulates expression of SPRY1 in esophageal tumor–infiltrating T cells and plays a role in T-cell exhaustion. Cancer Res (2020) 80(24):5583–96. doi: 10.1158/0008-5472.CAN-20-1542
121. Elyada E, Bolisetty M, Laise P, Flynn WF, Courtois ET, Burkhart RA, et al. Cross-species single-cell analysis of pancreatic ductal adenocarcinoma reveals antigen-presenting cancer-associated fibroblasts. Cancer Discovery (2019) 9(8):1102–23. doi: 10.1158/2159-8290.CD-19-0094
122. Huang H, Wang Z, Zhang Y, Pradhan RN, Ganguly D, Chandra R, et al. Mesothelial cell-derived antigen-presenting cancer-associated fibroblasts induce expansion of regulatory T cells in pancreatic cancer. Cancer Cell (2022). doi: 10.1101/2021.02.04.429827
123. Shinkawa T, Ohuchida K, Nakamura M. Heterogeneity of cancer-associated fibroblasts and the tumor immune microenvironment in pancreatic cancer. Cancers (2022) 14(16):3994. doi: 10.3390/cancers14163994
124. Harryvan TJ, Visser M, de Bruin L, Plug L, Griffioen L, Mulder A, et al. Enhanced antigen cross-presentation in human colorectal cancer-associated fibroblasts through upregulation of the lysosomal protease cathepsin s. J ImmunoTherapy Cancer (2022) 10(3):e003591. doi: 10.1136/jitc-2021-003591
125. O’Connor RA, Chauhan V, Mathieson L, Titmarsh H, Koppensteiner L, Young I, et al. T Cells drive negative feedback mechanisms in cancer-associated fibroblasts, promoting expression of co-inhibitory ligands, CD73 and IL-27 in non-small cell lung cancer. OncoImmunology (2021) 10(1). doi: 10.1080/2162402X.2021.1940675
126. Mayer S, Milo T, Isaacson A, Halperin C, Miyara S, Stein Y, et al. A cell circuit approach to dissect fibroblast-macrophage interactions in the tumor microenvironment. Biorxiv (2022). doi: 10.1101/2022.11.17.516850
127. Ley K, Kansas GS. Selectins in T-cell recruitment to non-lymphoid tissues and sites of inflammation. Nat Rev Immunol (2004) 4(5):325–35. doi: 10.1038/nri1351
128. Amersfoort J, Eelen G, Carmeliet P. Immunomodulation by endothelial cells {{/amp]]mdash; partnering up with the immune system? Nat Rev Immunol (2022). doi: 10.1038/s41577-022-00694-4
129. Lambrechts D, Wauters E, Boeckx B, Aibar S, Nittner D, Burton O, et al. Phenotype molding of stromal cells in the lung tumor microenvironment. Nat Med (2018) 24(8):1277–89. doi: 10.1038/s41591-018-0096-5
130. Huang H, Langenkamp E, Georganaki M, Loskog A, Fuchs PF, Dieterich LC, et al. VEGF suppresses T-lymphocyte infiltration in the tumor microenvironment through inhibition of NF-κB-induced endothelial activation. FASEB J (2014) 29(1):227–38.
131. Agius E, Lacy KE, Vukmanovic-Stejic M, Jagger AL, Papageorgiou A-P, Hall S, et al. Decreased TNF-α synthesis by macrophages restricts cutaneous immunosurveillance by memory CD4+ T cells during aging. J Exp Med (2009) 206(9):1929–40. doi: 10.1084/jem.20090896
132. Wei Q, Singh O, Ekinci C, Gill J, Li M, Mamatjan Y, et al. TNFα secreted by glioma associated macrophages promotes endothelial activation and resistance against anti-angiogenic therapy. Acta Neuropathol Commun (2021) 9(1). doi: 10.1186/s40478-021-01163-0
133. Graf E. Abstract 3529: sample quality control of cell-free DNA. Cancer Res (2019) 79(13_Supplement):3529–9. doi: 10.1158/1538-7445.AM2019-3529
134. Huang Y, Kim BYS, Chan CK, Hahn SM, Weissman IL, Jiang W. Improving immune–vascular crosstalk for cancer immunotherapy. Nat Rev Immunol (2018) 18(3):195–203. doi: 10.1038/nri.2017.145
135. Garnier L, Pick R, Montorfani J, Sun M, Brighouse D, Liaudet N, et al. IFN-γ–dependent tumor-antigen cross-presentation by lymphatic endothelial cells promotes their killing by T cells and inhibits metastasis. Sci Adv (2022) 8(23). doi: 10.1126/sciadv.abl5162
136. Asrir A, Tardiveau C, Coudert J, Laffont R, Blanchard L, Bellard E, et al. Tumor-associated high endothelial venules mediate lymphocyte entry into tumors and predict response to PD-1 plus CTLA-4 combination immunotherapy. Cancer Cell (2022). doi: 10.1016/j.ccell.2022.01.002
137. Carmona-Fontaine C, Bucci V, Akkari L, Deforet M, Joyce JA, Xavier JB. Emergence of spatial structure in the tumor microenvironment due to the warburg effect. Proc Natl Acad Sci (2013) 110(48):19402–7. doi: 10.1073/pnas.1311939110
138. Qin J, Lovelace MD, Mitchell AJ, Koning-Ward T, Grau GE, Pai S. Perivascular macrophages create an intravascular niche for CD8 T cell localisation prior to the onset of fatal experimental cerebral malaria. Clin Trans Immunol (2021) 10(4). doi: 10.1002/cti2.1273
139. Nalio Ramos R, Missolo-Koussou Y, Gerber-Ferder Y, Bromley CP, Bugatti M, Núñez NG, et al. Tissue-resident FOLR2+ macrophages associate with CD8+ T cell infiltration in human breast cancer. Cell (2022) 185(7):1189–1207.e25.
140. Georganaki M, van Hooren L, Dimberg A. Vascular targeting to increase the efficiency of immune checkpoint blockade in cancer. Front Immunol (2018) 9. doi: 10.3389/fimmu.2018.03081
141. Sakano Y, Noda T, Kobayashi S, Sasaki K, Iwagami Y, Yamada D, et al. Tumor endothelial cell-induced CD8 T-cell exhaustion via GPNMB in hepatocellular carcinoma. Cancer Science (2022) 113(5):1625–38. doi: 10.1111/cas.15331
142. Taguchi K, Onoe T, Yoshida T, Yamashita Y, Tanaka Y, Ohdan H. Tumor endothelial cell–mediated antigen-specific T-cell suppression via the PD-1/PD-L1 pathway. Mol Cancer Res (2020) 18(9):1427–40. doi: 10.1158/1541-7786.MCR-19-0897
143. Allen E, Jabouille A, Rivera LB, Lodewijckx I, Missiaen R, Steri V, et al. Combined antiangiogenic and anti–PD-L1 therapy stimulates tumor immunity through HEV formation. Sci Trans Med (2017) 9(385). doi: 10.1126/scitranslmed.aak9679
144. Johansson-Percival A, He B, Li Z-J, Kjellén A, Russell K, Li J, et al. De novo induction of intratumoral lymphoid structures and vessel normalization enhances immunotherapy in resistant tumors. Nat Immunol (2017) 18(11):1207–17. doi: 10.1038/ni.3836
145. Kim H-M, Jeong C-S, Choi H-S, Kawada T, Yu R. LIGHT/TNFSF14 enhances adipose tissue inflammatory responses through its interaction with HVEM. FEBS Lett (2011) 585(3):579–84. doi: 10.1016/j.febslet.2011.01.011
146. Lee W-H, Kim S-H, Lee Y, Lee BB, Kwon B, Song H, et al. Tumor necrosis factor receptor superfamily 14 is involved in atherogenesis by inducing proinflammatory cytokines and matrix metalloproteinases. Arteriosclerosis Thrombosis Vasc Biol (2001) 21(12):2004–10. doi: 10.1161/hq1201.098945
147. Wu J, Jin Z, Lin J, Fu Y, Wang J, Shen Y. Vessel state and immune infiltration of the angiogenesis subgroup and construction of a prediction model in osteosarcoma. Front Immunol (2022) 13. doi: 10.3389/fimmu.2022.992266
148. Johansson-Percival A, He B, Ganss R. Immunomodulation of tumor vessels: it takes two to tango. Trends Immunol (2018) 39(10):801–14. doi: 10.1016/j.it.2018.08.001
149. Johansson-Percival A, Ganss R. Therapeutic induction of tertiary lymphoid structures in cancer through stromal remodeling. Front Immunol (2021) 12. doi: 10.3389/fimmu.2021.674375
150. Bobik A, Kyaw TS, Tipping P, Toh B-H. M1 macrophages, key contributors to lymphoid neogenesis in atherosclerotic aorta. Cardiovasc Res (2014) 101(3):339–41. doi: 10.1093/cvr/cvu019
151. Koscsó B, Kurapati S, Rodrigues RR, Nedjic J, Gowda K, Shin C, et al. Gut-resident CX3CR1hi macrophages induce tertiary lymphoid structures and IgA response in situ. Sci Immunol (2020) 5(46). doi: 10.1126/sciimmunol.aax0062
152. Fridman WH, Meylan M, Petitprez F, Sun C-M, Italiano A, Sautès-Fridman C. B cells and tertiary lymphoid structures as determinants of tumour immune contexture and clinical outcome. Nat Rev Clin Oncol (2022) 19(7):441–57. doi: 10.1038/s41571-022-00619-z
153. Kang W, Feng Z, Luo J, He Z, Liu J, Wu J, et al. Tertiary lymphoid structures in cancer: the double-edged sword role in antitumor immunity and potential therapeutic induction strategies. Front Immunol (2021) 12. doi: 10.3389/fimmu.2021.689270
154. Weinstein AM, Giraldo NA, Petitprez F, Julie C, Lacroix L, Peschaud F, et al. Association of IL-36γ with tertiary lymphoid structures and inflammatory immune infiltrates in human colorectal cancer. Cancer Immunol Immunother (2018) 68(1):109–20.
155. Zhou J, Zhang S, Guo C. Crosstalk between macrophages and natural killer cells in the tumor microenvironment. Int Immunopharmacol (2021), 108374. doi: 10.1016/j.intimp.2021.108374
156. Baratin M, Roetynck S, Lépolard C, Falk C, Sawadogo S, Uematsu S, et al. Natural killer cell and macrophage cooperation in MyD88-dependent innate responses to plasmodium falciparum. Proc Natl Acad Sci (2005) 102(41):14747–52. doi: 10.1073/pnas.0507355102
157. Wang C, Cui A, Bukenya M, Aung A, Pradhan D, Whittaker CA, et al. Reprogramming NK cells and macrophages via combined antibody and cytokine therapy primes tumors for elimination by checkpoint blockade. Cell Rep (2021) 37(8):110021.
158. Eisinger S, Sarhan D, Boura VF, Ibarlucea-Benitez I, Tyystjärvi S, Oliynyk G, et al. Targeting a scavenger receptor on tumor-associated macrophages activates tumor cell killing by natural killer cells. Proc Natl Acad Sci (2020) 117(50):32005–16. doi: 10.1073/pnas.2015343117
159. Zhou Z, Zhang C, Zhang J, Tian Z. Macrophages help NK cells to attack tumor cells by stimulatory NKG2D ligand but protect themselves from NK killing by inhibitory ligand qa-1. PloS One (2012) 7(5):e36928. doi: 10.1371/journal.pone.0036928
160. Zhang R, Qi F, Zhao F, Li G, Shao S, Zhang X, et al. Cancer-associated fibroblasts enhance tumor-associated macrophages enrichment and suppress NK cells function in colorectal cancer. Cell Death Dis (2019) 10(4):1–14. doi: 10.1038/s41419-019-1435-2
161. Peng L, Zhang J, Teng Y, Zhao Y, Wang T, Mao F, et al. Tumor-associated Monocytes/Macrophages impair NK-cell function via TGFβ1 in human gastric cancer. Cancer Immunol Res (2017) 5(3):248–56. doi: 10.1158/2326-6066.CIR-16-0152
162. Youn J-I, Collazo M, Shalova IN, Biswas SK, Gabrilovich DI. Characterization of the nature of granulocytic myeloid-derived suppressor cells in tumor-bearing mice. J Leukoc Biol (2011) 91(1):167–81. doi: 10.1189/jlb.0311177
163. Youn J-I, Kumar V, Collazo M, Nefedova Y, Condamine T, Cheng P, et al. Epigenetic silencing of retinoblastoma gene regulates pathologic differentiation of myeloid cells in cancer. Nat Immunol (2013) 14(3):211–20. doi: 10.1038/ni.2526
164. Wu L, Zhang XH-F. Tumor-associated neutrophils and macrophages–heterogenous but not chaotic. Front Immunol (2020) 11. doi: 10.3389/fimmu.2020.553967
165. Xue R, Zhang Q, Cao Q, Kong R, Xiang X, Liu H, et al. Liver tumour immune microenvironment subtypes and neutrophil heterogeneity. Nature (2022) 612(7938):141–7. doi: 10.1038/s41586-022-05400-x
166. He G, Zhang H, Zhou J, Wang B, Chen Y, Kong Y, et al. Peritumoural neutrophils negatively regulate adaptive immunity via the PD-L1/PD-1 signalling pathway in hepatocellular carcinoma. J Exp Clin Cancer Res (2015) 34(1). doi: 10.1186/s13046-015-0256-0
167. Wang T, Zhao Y, Peng L, Chen N, Chen W, Lv Y, et al. Tumour-activated neutrophils in gastric cancer foster immune suppression and disease progression through GM-CSF-PD-L1 pathway. Gut (2017) 66(11):1900–11. doi: 10.1136/gutjnl-2016-313075
168. Sinha P, Clements VK, Bunt SK, Albelda SM, Ostrand-Rosenberg S. Cross-talk between myeloid-derived suppressor cells and macrophages subverts tumor immunity toward a type 2 response. J Immunol (2007) 179(2):977–83. doi: 10.4049/jimmunol.179.2.977
169. Kim IS, Gao Y, Welte T, Wang H, Liu J, Janghorban M, et al. Immuno-subtyping of breast cancer reveals distinct myeloid cell profiles and immunotherapy resistance mechanisms. Nat Cell Biol (2019) 21(9):1113–26. doi: 10.1038/s41556-019-0373-7
170. Oldford SA, Marshall JS. Mast cells as targets for immunotherapy of solid tumors. Mol Immunol (2015) 63(1):113–24. doi: 10.1016/j.molimm.2014.02.020
171. Welsh TJ, Green RH, Richardson D, Waller DA, O’Byrne KJ, Bradding P. Macrophage and mast-cell invasion of tumor cell islets confers a marked survival advantage in non–Small-Cell lung cancer. J Clin Oncol (2005) 23(35):8959–67. doi: 10.1200/JCO.2005.01.4910
172. De Filippo K, Dudeck A, Hasenberg M, Nye E, van Rooijen N, Hartmann K, et al. Mast cell and macrophage chemokines CXCL1/CXCL2 control the early stage of neutrophil recruitment during tissue inflammation. Blood (2013) 121(24):4930–7. doi: 10.1182/blood-2013-02-486217
173. Komi DEA, Redegeld FA. Role of mast cells in shaping the tumor microenvironment. Clin Rev Allergy Immunol (2019) 58(3):313–25.
174. Teng LKH, Pereira BA, Keerthikumar S, Huang C, Niranjan B, Lee SN, et al. Mast cell-derived SAMD14 is a novel regulator of the human prostate tumor microenvironment. Cancers (2021) 13(6):1237. doi: 10.3390/cancers13061237
175. Chang DZ, Ma Y, Ji B, Wang H, Deng D, Liu Y, et al. Mast cells in tumor microenvironment promotes the In vivo growth of pancreatic ductal adenocarcinoma. Clin Cancer Res (2011) 17(22):7015–23. doi: 10.1158/1078-0432.CCR-11-0607
176. Eissmann MF, Dijkstra C, Jarnicki A, Phesse T, Brunnberg J, Poh AR, et al. IL-33-mediated mast cell activation promotes gastric cancer through macrophage mobilization. Nat Commun (2019) 10(1). doi: 10.1038/s41467-019-10676-1
177. Warburg O. über den stoffwechsel der carcinomzelle. Klinische Wochenschrift (1925) 4(12):534–6. doi: 10.1007/BF01726151
178. Hirschhaeuser F, Sattler UGA, Mueller-Klieser W. Lactate: a metabolic key player in cancer. Cancer Res (2011) 71(22):6921–5. doi: 10.1158/0008-5472.CAN-11-1457
179. Bögel G, Murányi J, Szokol B, Kukor Z, Móra I, Kardon T, et al. Production of NOS2 and inflammatory cytokines is reduced by selected protein kinase inhibitors with partial repolarization of HL-60 derived and human blood macrophages. Heliyon (2022) 8(1):e08670.
180. Viola A, Munari F, Sánchez-Rodríguez R, Scolaro T, Castegna A. The metabolic signature of macrophage responses. Front Immunol (2019) 10. doi: 10.3389/fimmu.2019.01462
181. Arts RJW, Plantinga TS, Tuit S, Ulas T, Heinhuis B, Tesselaar M, et al. Transcriptional and metabolic reprogramming induce an inflammatory phenotype in non-medullary thyroid carcinoma-induced macrophages. OncoImmunology (2016) 5(12):e1229725. doi: 10.1080/2162402X.2016.1229725
182. Cheng S-C, Quintin J, Cramer RA, Shepardson KM, Saeed S, Kumar V, et al. mTOR- and HIF-1 -mediated aerobic glycolysis as metabolic basis for trained immunity. Science (2014) 345(6204):1250684–4. doi: 10.1126/science.1250684
183. Jeong H, Kim S, Hong B-J, Lee C-J, Kim Y-E, Bok S, et al. Tumor-associated macrophages enhance tumor hypoxia and aerobic glycolysis. Cancer Res (2019) 79(4):795–806. doi: 10.1158/0008-5472.CAN-18-2545
184. Liu M-F, Li J-S, Weng T-H, Lei H-Y. Differential expression and modulation of costimulatory molecules CD80 and CD86 on monocytes from patients with systemic lupus erythematosus. Scandinavian J Immunol (1999) 49(1):82–7. doi: 10.1046/j.1365-3083.1999.00452.x
185. Curiel RE, Garcia CS, Rottschafer S, Bosco MC, Espinoza-Delgado I. Enhanced B7-2 gene expression by interferon-gamma in human monocytic cells is controlled through transcriptional and posttranscriptional mechanisms. Blood (1999) 94(5):1782–9. doi: 10.1182/blood.V94.5.1782
186. Li Z, Wang J, Zhang X, Liu P, Zhang X, Wang J, et al. Proinflammatory S100A8 induces PD-L1 expression in macrophages, mediating tumor immune escape. J Immunol (2020) 204(9):2589–99. doi: 10.4049/jimmunol.1900753
187. Wenes M, Shang M, Di Matteo M, Goveia J, Martín-Pérez R, Serneels J, et al. Macrophage metabolism controls tumor blood vessel morphogenesis and metastasis. Cell Metab (2016) 24(5):701–15. doi: 10.1016/j.cmet.2016.09.008
188. Furgiuele S, Descamps G, Cascarano L, Boucq A, Dubois C, Journe F, et al. Dealing with macrophage plasticity to address therapeutic challenges in head and neck cancers. Int J Mol Sci (2022) 23(12):6385. doi: 10.3390/ijms23126385
189. Palmieri EM, Menga A, Martín-Pérez R, Quinto A, Riera-Domingo C, De Tullio G, et al. Pharmacologic or genetic targeting of glutamine synthetase skews macrophages toward an M1-like phenotype and inhibits tumor metastasis. Cell Rep (2017) 20(7):1654–66. doi: 10.1016/j.celrep.2017.07.054
190. Su P, Wang Q, Bi E, Ma X, Liu L, Yang M, et al. Enhanced lipid accumulation and metabolism are required for the differentiation and activation of tumor-associated macrophages. Cancer Res (2020) 80(7):1438–50. doi: 10.1158/0008-5472.CAN-19-2994
191. Rabold K, Aschenbrenner A, Thiele C, Boahen CK, Schiltmans A, Smit JWA, et al. Enhanced lipid biosynthesis in human tumor-induced macrophages contributes to their protumoral characteristics. J ImmunoTherapy Cancer (2020) 8(2):e000638. doi: 10.1136/jitc-2020-000638
192. Vats D, Mukundan L, Odegaard JI, Zhang L, Smith KL, Morel CR, et al. Oxidative metabolism and PGC-1β attenuate macrophage-mediated inflammation. Cell Metab (2006) 4(1):13–24. doi: 10.1016/j.cmet.2006.05.011
193. Zeng Q-H, Wei Y, Lao X-M, Chen D-P, Huang C-X, Lin Q-Y, et al. B cells polarize pathogenic inflammatory T helper subsets through ICOSL-dependent glycolysis. Sci Adv (2020) 6(37). doi: 10.1126/sciadv.abb6296
194. Teichmann Lino L, Cullen JL, Kashgarian M, Dong C, Craft J, Shlomchik Mark J. Local triggering of the ICOS coreceptor by CD11c+ myeloid cells drives organ inflammation in lupus. Immunity (2015) 42(3):552–65.
195. Fu T, He Q, Sharma P. The ICOS/ICOSL pathway is required for optimal antitumor responses mediated by anti–CTLA-4 therapy. Cancer Res (2011) 71(16):5445–54. doi: 10.1158/0008-5472.CAN-11-1138
196. Miki H, Han KH, Scott D, Croft M, Kang YJ. 4-1BBL regulates the polarization of macrophages, and inhibition of 4-1BBL signaling alleviates imiquimod-induced psoriasis. J Immunol (2020) 204(7):1892–903. doi: 10.4049/jimmunol.1900983
197. Yang Q, Zhang H, Wei T, Lin A, Sun Y, Luo P, et al. Single-cell RNA sequencing reveals the heterogeneity of tumor-associated macrophage in non-small cell lung cancer and differences between sexes. Front Immunol (2021) 12. doi: 10.3389/fimmu.2021.756722
198. Kiriakidis S. VEGF expression in human macrophages is NF-kappaB-dependent: studies using adenoviruses expressing the endogenous NF-kappaB inhibitor IkappaBalpha and a kinase-defective form of the IkappaB kinase 2. J Cell Science (2002) 116(4):665–74.
199. Lewis JS, Landers RJ, Underwood JCE, Harris AL, Lewis CE. Expression of vascular endothelial growth factor by macrophages is up-regulated in poorly vascularized areas of breast carcinomas. J Pathol (2000) 192(2):150–8. doi: 10.1002/1096-9896(2000)9999:9999<::AID-PATH687>3.0.CO;2-G
200. Colegio OR, Chu N-Q, Szabo AL, Chu T, Rhebergen AM, Jairam V, et al. Functional polarization of tumour-associated macrophages by tumour-derived lactic acid. Nature (2014) 513(7519):559–63. doi: 10.1038/nature13490
201. Carmeliet P. VEGF as a key mediator of angiogenesis in cancer. Oncology (2005) 69(3):4–10. doi: 10.1159/000088478
202. Bohn T, Rapp S, Luther N, Klein M, Bruehl T-J, Kojima N, et al. Tumor immunoevasion via acidosis-dependent induction of regulatory tumor-associated macrophages. Nat Immunol (2018) 19(12):1319–29. doi: 10.1038/s41590-018-0226-8
203. Zhang A, Fang H, Chen J, He L, Chen Y. Role of VEGF-a and LRG1 in abnormal angiogenesis associated with diabetic nephropathy. Front Physiol (2020) 11. doi: 10.3389/fphys.2020.01064
204. Liu C, Zhang W, Wang J, Si T, Xing W. Tumor-associated macrophage-derived transforming growth factor-β promotes colorectal cancer progression through HIF1-TRIB3 signaling. Cancer Science (2021) 112(10):4198–207. doi: 10.1111/cas.15101
205. Staples KJ, Sotoodehnejadnematalahi F, Pearson H, Frankenberger M, Francescut L, Ziegler-Heitbrock L, et al. Monocyte-derived macrophages matured under prolonged hypoxia transcriptionally up-regulate HIF-1α mRNA. Immunobiology (2011) 216(7):832–9. doi: 10.1016/j.imbio.2010.12.005
206. Eubank TD, Roda JM, Liu H, O’Neil T, Marsh CB. Opposing roles for HIF-1α and HIF-2α in the regulation of angiogenesis by mononuclear phagocytes. Blood (2011) 117(1):323–32. doi: 10.1182/blood-2010-01-261792
207. Roda JM, Wang Y, Sumner LA, Phillips GS, Marsh CB, Eubank TD. Stabilization of HIF-2α induces sVEGFR-1 production from tumor-associated macrophages and decreases tumor growth in a murine melanoma model. J Immunol (2012) 189(6):3168–77. doi: 10.4049/jimmunol.1103817
208. Wu W-K, Llewellyn OPC, Bates DO, Nicholson LB, Dick AD. IL-10 regulation of macrophage VEGF production is dependent on macrophage polarisation and hypoxia. Immunobiology (2010) 215(9-10):796–803. doi: 10.1016/j.imbio.2010.05.025
209. Wu L, Zhang X, Zheng L, Zhao H, Yan G, Zhang Q, et al. RIPK3 orchestrates fatty acid metabolism in tumor-associated macrophages and hepatocarcinogenesis. Cancer Immunol Res (2020) 8(5):710–21. doi: 10.1158/2326-6066.CIR-19-0261
210. Laoui D, Van Overmeire E, Di Conza G, Aldeni C, Keirsse J, Morias Y, et al. Tumor hypoxia does not drive differentiation of tumor-associated macrophages but rather fine-tunes the M2-like macrophage population. Cancer Res (2013) 74(1):24–30.
211. Sitkovsky MV, Kjaergaard J, Lukashev D, Ohta A. Hypoxia-adenosinergic immunosuppression: tumor protection by T regulatory cells and cancerous tissue hypoxia. Clin Cancer Res (2008) 14(19):5947–52. doi: 10.1158/1078-0432.CCR-08-0229
212. Briceño P, Rivas-Yañez E, Rosemblatt MV, Parra-Tello B, Farías P, Vargas L, et al. CD73 ectonucleotidase restrains CD8+ T cell metabolic fitness and anti-tumoral activity. Front Cell Dev Biol (2021) 9. doi: 10.3389/fcell.2021.638037
213. Calcinotto A, Filipazzi P, Grioni M, Iero M, De Milito A, Ricupito A, et al. Modulation of microenvironment acidity reverses anergy in human and murine tumor-infiltrating T lymphocytes. Cancer Res (2012) 72(11):2746–56. doi: 10.1158/0008-5472.CAN-11-1272
214. Fischer K, Hoffmann P, Voelkl S, Meidenbauer N, Ammer J, Edinger M, et al. Inhibitory effect of tumor cell–derived lactic acid on human T cells. Blood (2007) 109(9):3812–9. doi: 10.1182/blood-2006-07-035972
215. Brand KA, Hermfisse U. Aerobic glycolysis by proliferating cells: a protective strategy against reactive oxygen species. FASEB J (1997) 11(5):388–95. doi: 10.1096/fasebj.11.5.9141507
216. Blagih J, Coulombe F, Vincent Emma E, Dupuy F, Galicia-Vázquez G, Yurchenko E, et al. The energy sensor AMPK regulates T cell metabolic adaptation and effector responses In vivo. Immunity (2015) 42(1):41–54. doi: 10.1016/j.immuni.2014.12.030
217. Scharping NE, Rivadeneira DB, Menk AV, Vignali PDA, Ford BR, Rittenhouse NL, et al. Mitochondrial stress induced by continuous stimulation under hypoxia rapidly drives T cell exhaustion. Nat Immunol (2021) 22(2):205–15. doi: 10.1038/s41590-020-00834-9
218. Ho P-C, Bihuniak JD, Macintyre AN, Staron M, Liu X, Amezquita R, et al. Phosphoenolpyruvate is a metabolic checkpoint of anti-tumor T cell responses. Cell (2015) 162(6):1217–28. doi: 10.1016/j.cell.2015.08.012
219. Zhang Y, Kurupati R, Liu L, Zhou XY, Zhang G, Hudaihed A, et al. Enhancing CD8+ T cell fatty acid catabolism within a metabolically challenging tumor microenvironment increases the efficacy of melanoma immunotherapy. Cancer Cell (2017) 32(3):377–391.e9. doi: 10.1016/j.ccell.2017.08.004
220. Kim GW, Lee DH, Jeon YH, Yoo J, Kim SY, Lee SW, et al. Glutamine synthetase as a therapeutic target for cancer treatment. Int J Mol Sci (2021) 22(4):1701. doi: 10.3390/ijms22041701
221. Zhu L, Zhu X, Wu Y. Effects of glucose metabolism, lipid metabolism, and glutamine metabolism on tumor microenvironment and clinical implications. Biomolecules (2022) 12(4):580. doi: 10.3390/biom12040580
222. Choi J, Stradmann-Bellinghausen B, Yakubov E, Savaskan NE, Régnier-Vigouroux A. Glioblastoma cells induce differential glutamatergic gene expressions in human tumor-associated microglia/macrophages and monocyte-derived macrophages. Cancer Biol Ther (2015) 16(8):1205–13. doi: 10.1080/15384047.2015.1056406
223. Mills EL, Kelly B, Logan A, Costa ASH, Varma M, Bryant CE, et al. Succinate dehydrogenase supports metabolic repurposing of mitochondria to drive inflammatory macrophages. Cell (2016) 167(2):457–70. doi: 10.1016/j.cell.2016.08.064
224. Reinfeld BI, Madden MZ, Wolf MM, Chytil A, Bader JE, Patterson AR, et al. Cell-programmed nutrient partitioning in the tumour microenvironment. Nature (2021) 593(7858):282–8. doi: 10.1038/s41586-021-03442-1
225. Doedens AL, Stockmann C, Rubinstein MP, Liao D, Zhang N, DeNardo DG, et al. Macrophage expression of hypoxia-inducible factor-1 suppresses T-cell function and promotes tumor progression. Cancer Res (2010) 70(19):7465–75. doi: 10.1158/0008-5472.CAN-10-1439
226. Mills CD. Anatomy of a discovery: M1 and M2 macrophages. Front Immunol (2015) 6. doi: 10.3389/fimmu.2015.00212
227. Nath N, Kashfi K. Tumor associated macrophages and “NO.”. Biochem Pharmacol (2020) 176:113899. doi: 10.1016/j.bcp.2020.113899
228. Rodriguez PC, Quiceno DG, Zabaleta J, Ortiz B, Zea AH, Piazuelo MB, et al. Arginase I production in the tumor microenvironment by mature myeloid cells inhibits T-cell receptor expression and antigen-specific T-cell responses. Cancer Res (2004) 64(16):5839–49. doi: 10.1158/0008-5472.CAN-04-0465
229. Hajji N, Garcia-Revilla J, Soto MS, Perryman R, Symington J, Quarles CC, et al. Arginine deprivation alters microglial polarity and synergizes with radiation to eradicate non-arginine-auxotrophic glioblastoma tumors. J Clin Invest (2022) 132(6). doi: 10.1172/JCI142137
230. Badeaux MD, Rolig AS, Agnello G, Enzler D, Kasiewicz MJ, Priddy L, et al. Arginase therapy combines effectively with immune checkpoint blockade or agonist anti-OX40 immunotherapy to control tumor growth. Cancer Immunol Res (2021) 9(4):415–29. doi: 10.1158/2326-6066.CIR-20-0317
231. Werner A, Koschke M, Leuchtner N, Luckner-Minden C, Habermeier A, Rupp J, et al. Reconstitution of T cell proliferation under arginine limitation: activated human T cells take up citrulline via l-type amino acid transporter 1 and use it to regenerate arginine after induction of argininosuccinate synthase expression. Front Immunol (2017) 8. doi: 10.3389/fimmu.2017.00864
232. Mao Y, Shi D, Li G, Jiang P. Citrulline depletion by ASS1 is required for proinflammatory macrophage activation and immune responses. Mol Cell (2022) 82(3):527–541.e7. doi: 10.1016/j.molcel.2021.12.006
233. Van de Velde L-A, Subramanian C, Smith AM, Barron L, Qualls JE, Neale G, et al. T Cells encountering myeloid cells programmed for amino acid-dependent immunosuppression use Rictor/mTORC2 protein for proliferative checkpoint decisions. J Biol Chem (2017) 292(1):15–30. doi: 10.1074/jbc.M116.766238
234. Zhao Q, Kuang D-M, Wu Y, Xiao X, Li X-F, Li T-J, et al. Activated CD69+ T cells foster immune privilege by regulating IDO expression in tumor-associated macrophages. J Immunol (2012) 188(3):1117–24. doi: 10.4049/jimmunol.1100164
235. Cibrián D, Sánchez-Madrid F. CD69: from activation marker to metabolic gatekeeper. Eur J Immunol (2017) 47(6):946–53. doi: 10.1002/eji.201646837
236. Mulder K, Patel AA, Kong WT, Piot C, Halitzki E, Dunsmore G, et al. Cross-tissue single-cell landscape of human monocytes and macrophages in health and disease. Immunity (2021) 54(8):1883–1900.e5. doi: 10.1016/j.immuni.2021.07.007
237. Lee GK, Park HJ, Macleod M, Chandler P, Munn DH, Mellor AL. Tryptophan deprivation sensitizes activated T cells to apoptosis prior to cell division. Immunology (2002) 107(4):452–60. doi: 10.1046/j.1365-2567.2002.01526.x
238. Fallarino F, Grohmann U, You S, McGrath BC, Cavener DR, Vacca C, et al. The combined effects of tryptophan starvation and tryptophan catabolites down-regulate T cell receptor ζ-chain and induce a regulatory phenotype in naive T cells. J Immunol (2006) 176(11):6752–61. doi: 10.4049/jimmunol.176.11.6752
239. Metz R, Rust S, DuHadaway JB, Mautino MR, Munn DH, Vahanian NN, et al. IDO inhibits a tryptophan sufficiency signal that stimulates mTOR. Oncoimmunology (2012) 1(9):1460–8. doi: 10.4161/onci.21716
240. Liu Y, Liang X, Dong W, Fang Y, Lv J, Zhang T, et al. Tumor-repopulating cells induce PD-1 expression in CD8+ T cells by transferring kynurenine and AhR activation. Cancer Cell (2018) 33(3):480–494.e7. doi: 10.1016/j.ccell.2018.02.005
241. Campesato LF, Budhu S, Tchaicha J, Weng C-H, Gigoux M, Cohen IJ, et al. Blockade of the AHR restricts a treg-macrophage suppressive axis induced by l-kynurenine. Nat Commun (2020) 11(1):4011. doi: 10.1038/s41467-020-17750-z
242. Carr EL, Kelman A, Wu GS, Gopaul R, Senkevitch E, Aghvanyan A, et al. Glutamine uptake and metabolism are coordinately regulated by ERK/MAPK during T lymphocyte activation. J Immunol (2010) 185(2):1037–44. doi: 10.4049/jimmunol.0903586
243. Hezaveh K, Shinde RS, Klötgen A, Halaby MJ, Lamorte S, Ciudad MT, et al. Tryptophan-derived microbial metabolites activate the aryl hydrocarbon receptor in tumor-associated macrophages to suppress anti-tumor immunity. Immunity (2022) 55(2):324–340.e8. doi: 10.1016/j.immuni.2022.01.006
244. Lin X, Wang S, Sun M, Zhang C, Wei C, Yang C, et al. miR-195-5p/NOTCH2-mediated EMT modulates IL-4 secretion in colorectal cancer to affect M2-like TAM polarization. J Hematol Oncol (2019) 12(1). doi: 10.1186/s13045-019-0810-x
245. Yang P, Qin H, Li Y, Xiao A, Zheng E, Zeng H, et al. CD36-mediated metabolic crosstalk between tumor cells and macrophages affects liver metastasis. Nat Commun (2022) 13(1). doi: 10.1038/s41467-022-33349-y
246. Ramos MA, Kuzuya M, Esaki T, Miura S, Satake S, Asai T, et al. Induction of macrophage VEGF in response to oxidized LDL and VEGF accumulation in human atherosclerotic lesions. Arteriosclerosis Thrombosis Vasc Biol (1998) 18(7):1188–96. doi: 10.1161/01.ATV.18.7.1188
247. Wu H, Han Y, Rodriguez Sillke Y, Deng H, Siddiqui S, Treese C, et al. Lipid droplet-dependent fatty acid metabolism controls the immune suppressive phenotype of tumor-associated macrophages. EMBO Mol Med (2019) 11(11). doi: 10.15252/emmm.201910698
248. Sozio MS, Lu C, Zeng Y, Liangpunsakul S, Crabb DW. Activated AMPK inhibits PPAR-α and PPAR-γ transcriptional activity in hepatoma cells. Am J Physiology-Gastrointestinal Liver Physiol (2011) 301(4):G739–47. doi: 10.1152/ajpgi.00432.2010
249. Obrowsky S, Chandak PG, Patankar JV, Povoden S, Schlager S, Kershaw EE, et al. Adipose triglyceride lipase is a TG hydrolase of the small intestine and regulates intestinal PPARα signaling. J Lipid Res (2013) 54(2):425–35. doi: 10.1194/jlr.M031716
250. Ou J, Miao H, Ma Y, Guo F, Deng J, Wei X, et al. Loss of Abhd5 promotes colorectal tumor development and progression by inducing aerobic glycolysis and epithelial-mesenchymal transition. Cell Rep (2014) 9(5):1798–811. doi: 10.1016/j.celrep.2014.11.016
251. Miao H, Ou J, Peng Y, Zhang X, Chen Y, Hao L, et al. Macrophage ABHD5 promotes colorectal cancer growth by suppressing spermidine production by SRM. Nat Commun (2016) 7(1). doi: 10.1038/ncomms11716
252. Shang S, Ji X, Zhang L, Chen J, Li C, Shi R, et al. Macrophage ABHD5 suppresses NF-κB-dependent matrix metalloproteinase expression and cancer metastasis. Cancer Res (2019). doi: 10.1158/0008-5472.CAN-19-1059
253. Duan H, Li Z, Mazzone T. Tumor necrosis factor-alpha modulates monocyte/macrophage apoprotein e gene expression. J Clin Invest (1995) 96(2):915–22. doi: 10.1172/JCI118139
254. Wang W, Wu S, Cen Z, Zhang Y, Chen Y, Huang Y, et al. Mobilizing phospholipids on tumor plasma membrane implicates phosphatidylserine externalization blockade for cancer immunotherapy. Cell Rep (2022) 41(5):111582. doi: 10.1016/j.celrep.2022.111582
255. Budhu S, Giese R, Gupta A, Fitzgerald K, Zappasodi R, Schad S, et al. Targeting phosphatidylserine enhances the anti-tumor response to tumor-directed radiation therapy in a preclinical model of melanoma. Cell Rep (2021) 34(2):108620. doi: 10.1016/j.celrep.2020.108620
256. Reinartz S, Lieber S, Pesek J, Brandt DT, Asafova A, Finkernagel F, et al. Cell type-selective pathways and clinical associations of lysophosphatidic acid biosynthesis and signaling in the ovarian cancer microenvironment. Mol Oncol (2018) 13(2):185–201.
257. Zhang D, Shi R, Xiang W, Kang X, Tang B, Li C, et al. The Agpat4/LPA axis in colorectal cancer cells regulates antitumor responses via p38/p65 signaling in macrophages. Signal Transduction Targeted Ther (2020) 5(1). doi: 10.1038/s41392-020-0117-y
258. Daurkin I, Eruslanov E, Stoffs T, Perrin GQ, Algood C, Gilbert SM, et al. Tumor-associated macrophages mediate immunosuppression in the renal cancer microenvironment by activating the 15-Lipoxygenase-2 pathway. Cancer Res (2011) 71(20):6400–9. doi: 10.1158/0008-5472.CAN-11-1261
259. Li F, Kitajima S, Kohno S, Yoshida A, Tange S, Sasaki S, et al. Retinoblastoma inactivation induces a protumoral microenvironment via enhanced CCL2 secretion. Cancer Res (2019) 79(15):3903–15. doi: 10.1158/0008-5472.CAN-18-3604
260. Prima V, Kaliberova LN, Kaliberov S, Curiel DT, Kusmartsev S. COX2/mPGES1/PGE2pathway regulates PD-L1 expression in tumor-associated macrophages and myeloid-derived suppressor cells. Proc Natl Acad Sci (2017) 114(5):1117–22. doi: 10.1073/pnas.1612920114
261. Wei J, Zhang J, Wang D, Cen B, Lang JD, DuBois RN. The COX-2–PGE2 pathway promotes tumor evasion in colorectal adenomas. Cancer Prev Res (2022) 15(5):285–96. doi: 10.1158/1940-6207.CAPR-21-0572
262. Xu M, Wang X, Li Y, Geng X, Jia X, Zhang L, et al. Arachidonic acid metabolism controls macrophage alternative activation through regulating oxidative phosphorylation in PPARγ dependent manner. Front Immunol (2021) 12.
263. Wu W-K, Dick AD, Bates DO, Nicholson LB. VEGF production in macrophages is enhanced by anti-inflammatory stimuli. Invest Ophthalmol Visual Sci (2009) 50(13):3758–8.
264. Olesch C, Sha W, Angioni C, Sha LK, Açaf E, Patrignani P, et al. MPGES-1-derived PGE2 suppresses CD80 expression on tumor-associated phagocytes to inhibit anti-tumor immune responses in breast cancer. Oncotarget (2015) 6(12):10284–96. doi: 10.18632/oncotarget.3581
265. Lin C-W, Chen C-C, Huang W-Y, Chen Y-Y, Chen S-T, Chou H-W, et al. Restoring Prohealing/Remodeling-associated M2a/c macrophages using ON101 accelerates diabetic wound healing. JID Innov (2022) 2(5):100138. doi: 10.1016/j.xjidi.2022.100138
266. Xiang W, Shi R, Kang X, Zhang X, Chen P, Zhang L, et al. Monoacylglycerol lipase regulates cannabinoid receptor 2-dependent macrophage activation and cancer progression. Nat Commun (2018) 9(1). doi: 10.1038/s41467-018-04999-8
267. Sag D, Cekic C, Wu R, Linden J, Hedrick CC. The cholesterol transporter ABCG1 links cholesterol homeostasis and tumour immunity. Nat Commun (2015) 6(1). doi: 10.1038/ncomms7354
268. Goossens P, Rodriguez-Vita J, Etzerodt A, Masse M, Rastoin O, Gouirand V, et al. Membrane cholesterol efflux drives tumor-associated macrophage reprogramming and tumor progression. Cell Metab (2019) 29(6):1376–1389.e4. doi: 10.1016/j.cmet.2019.02.016
269. González de la Aleja A, Herrero C, Torres-Torresano M, de la Rosa JV, Alonso B, Capa-Sardón E, et al. Activation of LXR nuclear receptors impairs the anti-inflammatory gene and functional profile of m-CSF-Dependent human monocyte-derived macrophages. Front Immunol (2022) 13. doi: 10.3389/fimmu.2022.835478
270. Jin H, He Y, Zhao P, Hu Y, Tao J, Chen J, et al. Targeting lipid metabolism to overcome EMT-associated drug resistance via integrin β3/FAK pathway and tumor-associated macrophage repolarization using legumain-activatable delivery. Theranostics (2019) 9(1):265–78. doi: 10.7150/thno.27246
271. Galluzzi L, Humeau J, Buqué A, Zitvogel L, Kroemer G. Immunostimulation with chemotherapy in the era of immune checkpoint inhibitors. Nat Rev Clin Oncol (2020) 17(12):725–41. doi: 10.1038/s41571-020-0413-z
272. Di Caro G, Cortese N, Castino GF, Grizzi F, Gavazzi F, Ridolfi C, et al. Dual prognostic significance of tumour-associated macrophages in human pancreatic adenocarcinoma treated or untreated with chemotherapy. Gut (2015) 65(10):1710–20.
273. Heath O, Berlato C, Maniati E, Lakhani A, Pegrum C, Kotantaki P, et al. Chemotherapy induces tumor-associated macrophages that aid adaptive immune responses in ovarian cancer. Cancer Immunol Res (2021) 9(6):665–81. doi: 10.1158/2326-6066.CIR-20-0968
274. Malesci A, Bianchi P, Celesti G, Basso G, Marchesi F, Grizzi F, et al. Tumor-associated macrophages and response to 5-fluorouracil adjuvant therapy in stage III colorectal cancer. OncoImmunology (2017) 6(12):e1342918. doi: 10.1080/2162402X.2017.1342918
275. Madeddu C, Donisi C, Liscia N, Lai E, Scartozzi M, Macciò A. EGFR-mutated non-small cell lung cancer and resistance to immunotherapy: role of the tumor microenvironment. Int J Mol Sci (2022) 23(12):6489. doi: 10.3390/ijms23126489
276. Zhou Q, Liang J, Yang T, Liu J, Li B, Li Y, et al. Carfilzomib modulates tumor microenvironment to potentiate immune checkpoint therapy for cancer. EMBO Mol Med (2021) 14(1). doi: 10.15252/emmm.202114502
277. Hernandez C, Huebener P, Schwabe RF. Damage-associated molecular patterns in cancer: a double-edged sword. Oncogene (2016) 35(46):5931–41. doi: 10.1038/onc.2016.104
278. Furukawa R, Inoue H, Yoneshima Y, Tsutsumi H, Iwama E, Ikematsu Y, et al. Cytotoxic chemotherapeutic agents and the EGFR-TKI osimertinib induce calreticulin exposure in non–small cell lung cancer. Lung Cancer (2021) 155:144–50. doi: 10.1016/j.lungcan.2021.03.018
279. Heil M, Land WG. Danger signals — damaged-self recognition across the tree of life. Front Plant Sci (2014) 5. doi: 10.3389/fpls.2014.00578
280. Wu J, Chen J, Feng Y, Zhang S, Lin L, Guo Z, et al. An immune cocktail therapy to realize multiple boosting of the cancer-immunity cycle by combination of drug/gene delivery nanoparticles. Sci Adv (2020), 6(40). doi: 10.1126/sciadv.abc7828
281. Tenold M, Ravi P, Kumar M, Bowman A, Hammers H, Choueiri TK, et al. Current approaches to the treatment of advanced or metastatic renal cell carcinoma. Am Soc Clin Oncol Educ Book (2020) 40):187–96. doi: 10.1200/EDBK_279881
282. Gowda DC, Wu X. Parasite recognition and signaling mechanisms in innate immune responses to malaria. Front Immunol (2018) 9. doi: 10.3389/fimmu.2018.03006
283. Chen X, Zhang Y, Fu Y. The critical role of toll-like receptor-mediated signaling in cancer immunotherapy. Med Drug Discovery (2022), 100122. doi: 10.1016/j.medidd.2022.100122
284. Leeper-Woodford SK, Detmer K. Acute hypoxia increases alveolar macrophage tumor necrosis factor activity and alters NF-κB expression. Am J Physiology-Lung Cell Mol Physiol (1999) 276(6):L909–16. doi: 10.1152/ajplung.1999.276.6.L909
285. Sun L, Kees T, Almeida AS, Liu B, He X-Y, Ng D, et al. Activating a collaborative innate-adaptive immune response to control metastasis. Cancer Cell (2021) 39(10):1361–1374.e9. doi: 10.1016/j.ccell.2021.08.005
286. Xu J, Li X, Du Y. Antibody–pattern recognition receptor agonist conjugates: a promising therapeutic strategy for cancer. Adv Biol (2022) 6(3):2101065. doi: 10.1002/adbi.202101065
287. Amouzegar A, Chelvanambi M, Filderman J, Storkus W, Luke J. STING agonists as cancer therapeutics. Cancers (2021) 13(11):2695. doi: 10.3390/cancers13112695
288. Yang K, Han W, Jiang X, Piffko A, Bugno J, Han C, et al. Zinc cyclic di-AMP nanoparticles target and suppress tumours via endothelial STING activation and tumour-associated macrophage reinvigoration. Nat Nanotechnology (2022) 17(12):1322–31. doi: 10.1038/s41565-022-01225-x
289. Lam KC, Araya RE, Huang A, Chen Q, Di Modica M, Rodrigues RR, et al. Microbiota triggers STING-type I IFN-dependent monocyte reprogramming of the tumor microenvironment. Cell (2021) 184(21):5338–5356.e21. doi: 10.1016/j.cell.2021.09.019
290. Roberts NJ, Zhou S, Diaz LA, Holdhoff M. Systemic use of tumor necrosis factor alpha as an anticancer agent. Oncotarget (2011) 2(10):739–51. doi: 10.18632/oncotarget.344
291. Beutler B, Greenwald D, Hulmes JD, Chang M, Pan YC, Mathison J, et al. Identity of tumour necrosis factor and the macrophage-secreted factor cachectin. Nature (1985) 316(6028):552–4. doi: 10.1038/316552a0
292. Kimura K, Taguchi T, Urushizaki I, Ohno R, Abe O, Furue H, et al. Phase I study of recombinant human tumor necrosis factor. Cancer Chemotherapy Pharmacol (1987) 20(3):223–9. doi: 10.1007/BF00570490
293. Creaven Patrick J, Plager John E, Dupere S, Huben Robert P, Takita H, Mittelman A, et al. Phase I clinical trial of recombinant human tumor necrosis factor. Cancer Chemotherapy Pharmacol (1987) 20(2). doi: 10.1007/BF00253968
294. Helson L, Budd GT. A southwest oncology group phase ii trial of recombinant tumor necrosis factor in metastatic breast cancer. Cancer (1992) 69(11):2866–6. doi: 10.1002/1097-0142(19920601)69:11<2866::AID-CNCR2820691136>3.0.CO;2-Q
295. Brown TD, Goodman P, Fleming T, Macdonald JS, Hersh EM, Braun TJ. A phase II trial of recombinant tumor necrosis factor in patients with adenocarcinoma of the pancreas: a southwest oncology group study. J Immunother (1991) 10(5):376–8. doi: 10.1097/00002371-199110000-00010
296. Kahn JO, Kaplan LD, Volberding PA, Ziegler JL, Crowe S, Saks SR, et al. Intralesional recombinant tumor necrosis factor-alpha for AIDS-associated kaposi’s sarcoma: a randomized, double-blind trial. J Acquired Immune Deficiency Syndromes (1989) 2(3):217–23.
297. van Horssen R. TNF- in cancer treatment: molecular insights, antitumor effects, and clinical utility. Oncologist (2006) 11(4):397–408. doi: 10.1634/theoncologist.11-4-397
298. Alexander HR. Current status of isolated hepatic perfusion with or without tumor necrosis factor for the treatment of unresectable cancers confined to liver. Oncologist (2000) 5(5):416–24. doi: 10.1634/theoncologist.5-5-416
299. Alexander HR, Libutti SK, Bartlett DL, Puhlmann M, Fraker DL, Bachenheimer LC. A phase I-II study of isolated hepatic perfusion using melphalan with or without tumor necrosis factor for patients with ocular melanoma metastatic to liver. Clin Cancer Res (2000) 6(8):3062–70.
300. Dondossola E, Dobroff AS, Marchiò S, Cardó-Vila M, Hosoya H, Libutti SK, et al. Self-targeting of TNF-releasing cancer cells in preclinical models of primary and metastatic tumors. Proc Natl Acad Sci (2016) 113(8):2223–8. doi: 10.1073/pnas.1525697113
301. Carta L, Pastorino S, Melillo G, Bosco MC, Massazza S, Varesio L. Engineering of macrophages to produce IFN-gamma in response to hypoxia. J Immunol (2001) 166(9):5374–80. doi: 10.4049/jimmunol.166.9.5374
302. Parameswaran N, Patial S. Tumor necrosis factor-α signaling in macrophages. Crit ReviewsTM Eukaryotic Gene Expression (2010) 20(2):87–103. doi: 10.1615/CritRevEukarGeneExpr.v20.i2.10
303. Lesage C, Longvert C, Prey S, Maanaoui S, Dréno B, Machet L, et al. Incidence and clinical impact of anti-TNFα treatment of severe immune checkpoint inhibitor-induced colitis in advanced melanoma: the mecolit survey. J Immunother (2019) 42(5):175–9. doi: 10.1097/CJI.0000000000000268
304. Badran YR, Cohen JV, Brastianos PK, Parikh AR, Hong TS, Dougan M. Concurrent therapy with immune checkpoint inhibitors and TNFα blockade in patients with gastrointestinal immune-related adverse events. J ImmunoTherapy Cancer (2019) 7(1). doi: 10.1186/s40425-019-0711-0
305. Perez-Ruiz E, Minute L, Otano I, Alvarez M, Ochoa MC, Belsue V, et al. Prophylactic TNF blockade uncouples efficacy and toxicity in dual CTLA-4 and PD-1 immunotherapy. Nature (2019) 569(7756):428–32. doi: 10.1038/s41586-019-1162-y
306. Montfort A, Dufau C, Colacios C, Andrieu-Abadie N, Levade T, Filleron T, et al. Anti-TNF, a magic bullet in cancer immunotherapy? J ImmunoTherapy Cancer (2019) 7(1). doi: 10.1186/s40425-019-0802-y
307. Wen J, Wang S, Guo R, Liu D. CSF1R inhibitors are emerging immunotherapeutic drugs for cancer treatment. Eur J Med Chem (2023) 245:114884. doi: 10.1016/j.ejmech.2022.114884
308. Lamb YN. Pexidartinib: first approval. Drugs (2019) 79(16):1805–12. doi: 10.1007/s40265-019-01210-0
309. Lyons YA, Pradeep S, Wu SY, Haemmerle M, Hansen JM, Wagner MJ, et al. Macrophage depletion through colony stimulating factor 1 receptor pathway blockade overcomes adaptive resistance to anti-VEGF therapy. Oncotarget (2017) 8(57):96496–505. doi: 10.18632/oncotarget.20410
310. Pfirschke C, Zilionis R, Engblom C, Messemaker M, Zou AE, Rickelt S, et al. Macrophage-targeted therapy unlocks antitumoral cross-talk between IFNγ-secreting lymphocytes and IL12-producing dendritic cells. Cancer Immunol Res (2021) 10(1):40–55.
311. Zhu Y, Knolhoff BL, Meyer MA, Nywening TM, West BL, Luo J, et al. CSF1/CSF1R blockade reprograms tumor-infiltrating macrophages and improves response to T-cell checkpoint immunotherapy in pancreatic cancer models. Cancer Res (2014) 74(18):5057–69. doi: 10.1158/0008-5472.CAN-13-3723
312. Xu Z-J, Gu Y, Wang C-Z, Jin Y, Wen X-M, Ma J-C, et al. The M2 macrophage marker CD206: a novel prognostic indicator for acute myeloid leukemia. OncoImmunology (2019) 9(1). doi: 10.1080/2162402X.2019.1683347
313. Jaynes JM, Sable R, Ronzetti M, Bautista W, Knotts Z, Abisoye-Ogunniyan A, et al. Mannose receptor (CD206) activation in tumor-associated macrophages enhances adaptive and innate antitumor immune responses. Sci Trans Med (2020) 12(530). doi: 10.1126/scitranslmed.aax6337
314. Zang X, Zhang X, Zhao X, Hu H, Qiao M, Deng Y, et al. Targeted delivery of miRNA 155 to tumor associated macrophages for tumor immunotherapy. Mol Pharmaceutics (2019) 16(4):1714–22. doi: 10.1021/acs.molpharmaceut.9b00065
315. Zhang F, Parayath NN, Ene CI, Stephan SB, Koehne AL, Coon ME, et al. Genetic programming of macrophages to perform anti-tumor functions using targeted mRNA nanocarriers. Nat Commun (2019) 10(1). doi: 10.1038/s41467-019-11911-5
316. Viitala M, Virtakoivu R, Tadayon S, Rannikko J, Jalkanen S, Hollmén M. Immunotherapeutic blockade of macrophage clever-1 reactivates the CD8+ T-cell response against immunosuppressive tumors. Clin Cancer Res (2019) 25(11):3289–303. doi: 10.1158/1078-0432.CCR-18-3016
317. Virtakoivu R, Rannikko JH, Viitala M, Vaura F, Takeda A, Lönnberg T, et al. Systemic blockade of clever-1 elicits lymphocyte activation alongside checkpoint molecule downregulation in patients with solid tumors: results from a phase I/II clinical trial. Clin Cancer Res (2021) 27(15):4205–20. doi: 10.1158/1078-0432.CCR-20-4862
318. Sun C, Wang B, Hao S. Adenosine-A2A receptor pathway in cancer immunotherapy. Front Immunol (2022) 13. doi: 10.3389/fimmu.2022.837230
319. Oh M-H, Sun I-H, Zhao L, Leone RD, Sun I-M, Xu W, et al. Targeting glutamine metabolism enhances tumor-specific immunity by modulating suppressive myeloid cells. J Clin Invest (2020) 130(7):3865–84. doi: 10.1172/JCI131859
320. Menga A, Serra M, Todisco S, Riera-Domingo C, Ammarah U, Ehling M, et al. Glufosinate constrains synchronous and metachronous metastasis by promoting anti-tumor macrophages. EMBO Mol Med (2020) 12(10). doi: 10.15252/emmm.201911210
321. Steggerda SM, Bennett MK, Chen J, Emberley E, Huang T, Janes JR, et al. Inhibition of arginase by CB-1158 blocks myeloid cell-mediated immune suppression in the tumor microenvironment. J ImmunoTherapy Cancer (2017) 5(1). doi: 10.1186/s40425-017-0308-4
322. Zhang Q, Wang J, Yadav DK, Bai X, Liang T. Glucose metabolism: the metabolic signature of tumor associated macrophage. Front Immunol (2021) 12. doi: 10.3389/fimmu.2021.702580
323. Wang S, Lin Y, Xiong X, Wang L, Guo Y, Chen Y, et al. Low-dose metformin reprograms the tumor immune microenvironment in human esophageal cancer: results of a phase II clinical trial. Clin Cancer Res (2020) 26(18):4921–32. doi: 10.1158/1078-0432.CCR-20-0113
324. Pelly VS, Moeini A, Roelofsen LM, Bonavita E, Bell CR, Hutton C, et al. Anti-inflammatory drugs remodel the tumor immune environment to enhance immune checkpoint blockade efficacy. Cancer Discovery (2021) 11(10):2602–19. doi: 10.1158/2159-8290.CD-20-1815
325. Shi R, Zhao K, Wang T, Yuan J, Zhang D, Xiang W, et al. 5-aza-2′-deoxycytidine potentiates anti-tumor immunity in colorectal peritoneal metastasis by modulating ABC A9-mediated cholesterol accumulation in macrophages. Theranostics (2022) 12(2):875–90. doi: 10.7150/thno.66420
326. Rogers BM, Smith L, Dezso Z, Shi X, DiGiammarino E, Nguyen D, et al. VISTA is an activating receptor in human monocytes. J Exp Med (2021) 218(8). doi: 10.1084/jem.20201601
327. Nguyen P, Phennicie R, Kauffman K, Nowakowska D, Zafari M, Komoroski V, et al. 862 targeting PSGL-1, a novel macrophage checkpoint, repolarizes suppressive macrophages, induces an inflammatory tumor microenvironment, and suppresses tumor growth. J ImmunoTherapy Cancer (2020) 8(Suppl 3). doi: 10.1136/jitc-2020-SITC2020.0862
328. Outsmarting cancer immunosuppression. Available at: https://www.nature.com/articles/d43747-020-00662-8.
329. Bono P, Hollmen M, Jaakkola P, Shetty S, Thibault A, de JMJA, et al. Immune activation with a novel immune switch anti-macrophage antibody (anti-Clever-1 mAb; FP-1305) in phase I/II first-in-human MATINS trial in patients with advanced solid tumours. Ann Oncol (2019) 30:v865–6. doi: 10.1093/annonc/mdz394.018
330. Bono P, Virtakoivu R, Vaura F, Jaakkola P, Shetty S, Thibault A, et al. Immune activation in first-in-human anti-macrophage antibody (anti-Clever-1 mAb; FP-1305) phase I/II MATINS trial: part I dose-escalation, safety, and efficacy results. J Clin Oncol (2020) 38(15_suppl):3097–7. doi: 10.1200/JCO.2020.38.15_suppl.3097
331. Tseng D, Volkmer J-P, Willingham SB, Contreras-Trujillo H, Fathman JW, Fernhoff NB, et al. Anti-CD47 antibody-mediated phagocytosis of cancer by macrophages primes an effective antitumor T-cell response. Proc Natl Acad Sci (2013) 110(27):11103–8. doi: 10.1073/pnas.1305569110
332. Zhang M, Hutter G, Kahn SA, Azad TD, Gholamin S, Xu CY, et al. Anti-CD47 treatment stimulates phagocytosis of glioblastoma by M1 and M2 polarized macrophages and promotes M1 polarized macrophages In vivo. PloS One (2016) 11(4):e0153550. doi: 10.1371/journal.pone.0153550
333. Chen H-M, van der Touw W, Wang YS, Kang K, Mai S, Zhang J, et al. Blocking immunoinhibitory receptor LILRB2 reprograms tumor-associated myeloid cells and promotes antitumor immunity. J Clin Invest (2018) 128(12):5647–62. doi: 10.1172/JCI97570
334. Barkal AA, Brewer RE, Markovic M, Kowarsky M, Barkal SA, Zaro BW, et al. CD24 signalling through macrophage siglec-10 is a target for cancer immunotherapy. Nature (2019) 572(7769):392–6. doi: 10.1038/s41586-019-1456-0
335. Beatty GL, Chiorean EG, Fishman MP, Saboury B, Teitelbaum UR, Sun W, et al. CD40 agonists alter tumor stroma and show efficacy against pancreatic carcinoma in mice and humans. Science (2011) 331(6024):1612–6. doi: 10.1126/science.1198443
336. Vonderheide RH. CD40 agonist antibodies in cancer immunotherapy. Annu Rev Med (2020) 71(1):47–58. doi: 10.1146/annurev-med-062518-045435
337. Vonderheide RH, Flaherty KT, Khalil M, Stumacher MS, Bajor DL, Hutnick NA, et al. Clinical activity and immune modulation in cancer patients treated with CP-870,893, a novel CD40 agonist monoclonal antibody. J Clin Oncol (2007) 25(7):876–83. doi: 10.1200/JCO.2006.08.3311
338. Baumann D, Hägele T, Mochayedi J, Drebant J, Vent C, Blobner S, et al. Proimmunogenic impact of MEK inhibition synergizes with agonist anti-CD40 immunostimulatory antibodies in tumor therapy. Nat Commun (2020) 11(1):1–18. doi: 10.1038/s41467-020-15979-2
339. Beatty GL, Torigian DA, Chiorean EG, Saboury B, Brothers A, Alavi A, et al. A phase I study of an agonist CD40 monoclonal antibody (CP-870,893) in combination with gemcitabine in patients with advanced pancreatic ductal adenocarcinoma. Clin Cancer Res (2013) 19(22):6286–95. doi: 10.1158/1078-0432.CCR-13-1320
340. Huffman AP, Lin JH, Kim SI, Byrne KT, Vonderheide RH. CCL5 mediates CD40-driven CD4+ T cell tumor infiltration and immunity. JCI Insight (2020) 5(10). doi: 10.1172/jci.insight.137263
341. O’Hara MH, O’Reilly EM, Varadhachary G, Wolff RA, Wainberg ZA, Ko AH, et al. CD40 agonistic monoclonal antibody APX005M (sotigalimab) and chemotherapy, with or without nivolumab, for the treatment of metastatic pancreatic adenocarcinoma: an open-label, multicentre, phase 1b study. Lancet Oncol (2021) 22(1):118–31. doi: 10.1016/S1470-2045(20)30532-5
342. Wiehagen KR, Girgis NM, Yamada DH, Smith AA, Chan SR, Grewal IS, et al. Combination of CD40 agonism and CSF-1R blockade reconditions tumor-associated macrophages and drives potent antitumor immunity. Cancer Immunol Res (2017) 5(12):1109–21. doi: 10.1158/2326-6066.CIR-17-0258
343. Hoves S, Ooi C-H, Wolter C, Sade H, Bissinger S, Schmittnaegel M, et al. Rapid activation of tumor-associated macrophages boosts preexisting tumor immunity. J Exp Med (2018) 215(3):859–76. doi: 10.1084/jem.20171440
344. Peng W, Williams LJ, Xu C, Melendez B, McKenzie JA, Chen Y, et al. Anti-OX40 antibody directly enhances the function of tumor-reactive CD8+ T cells and synergizes with PI3Kβ inhibition in PTEN loss melanoma. Clin Cancer Res (2019) 25(21):6406–16. doi: 10.1158/1078-0432.CCR-19-1259
345. Gough MJ, Ruby CE, Redmond WL, Dhungel B, Brown A, Weinberg AD. OX40 agonist therapy enhances CD8 infiltration and decreases immune suppression in the tumor. Cancer Res (2008) 68(13):5206–15. doi: 10.1158/0008-5472.CAN-07-6484
346. Friedman DJ, Young K, Baird JR, Cottam B, Savage T, Newell P, et al. Preparative immunotherapy with anti-OX40 and anti-CTLA4 improves the response to chemotherapy. J ImmunoTherapy Cancer (2014) 2(S3). doi: 10.1186/2051-1426-2-S3-P207
347. Gough MJ, Killeen N, Weinberg AD. Targeting macrophages in the tumour environment to enhance the efficacy of αOX40 therapy. Immunology (2012) 136(4):437–47. doi: 10.1111/j.1365-2567.2012.03600.x
348. Sturgill ER, Rolig AS, Linch SN, Mick C, Kasiewicz MJ, Sun Z, et al. Galectin-3 inhibition with belapectin combined with anti-OX40 therapy reprograms the tumor microenvironment to favor anti-tumor immunity. OncoImmunology (2021) 10(1). doi: 10.1080/2162402X.2021.1892265
349. Burris HA, Callahan MK, Tolcher AW, Kummar S, Falchook GS, Pachynski RK, et al. Phase 1 safety of ICOS agonist antibody JTX-2011 alone and with nivolumab (nivo) in advanced solid tumors; predicted vs observed pharmacokinetics (PK) in ICONIC. J Clin Oncol (2017) 35(15_suppl):3033–3. doi: 10.1200/JCO.2017.35.15_suppl.3033
350. Heeren AM, Koster BD, Samuels S, Ferns DM, Chondronasiou D, Kenter GG, et al. High and interrelated rates of PD-L1+CD14+ antigen-presenting cells and regulatory T cells mark the microenvironment of metastatic lymph nodes from patients with cervical cancer. Cancer Immunol Res (2015) 3(1):48–58. doi: 10.1158/2326-6066
351. Di Giacomo AM, Calabrò L, Danielli R, Fonsatti E, Bertocci E, Pesce I, et al. Long-term survival and immunological parameters in metastatic melanoma patients who responded to ipilimumab 10 mg/kg within an expanded access programme. Cancer Immunol Immunother (2013) 62(6):1021–8. doi: 10.1007/s00262-013-1418-6
352. Ramavath NN, Gadipudi LL, Provera A, Gigliotti LC, Boggio E, Bozzola C, et al. Inducible T-cell costimulator mediates Lymphocyte/Macrophage interactions during liver repair. Front Immunol (2021) 12. doi: 10.3389/fimmu.2021.786680
353. Maeda S, Fujimoto M, Matsushita T, Hamaguchi Y, Takehara K, Hasegawa M. Inducible costimulator (ICOS) and ICOS ligand signaling has pivotal roles in skin wound healing via cytokine production. Am J Pathol (2011) 179(5):2360–9. doi: 10.1016/j.ajpath.2011.07.048
354. Lee H-J, Kim S-N, Jeon M-S, Yi T, Song SU. ICOSL expression in human bone marrow-derived mesenchymal stem cells promotes induction of regulatory T cells. Sci Rep (2017) 7(1). doi: 10.1038/srep44486
355. Marinelli O, Nabissi M, Morelli MB, Torquati L, Amantini C, Santoni G. ICOS-l as a potential therapeutic target for cancer immunotherapy. Curr Protein Pept Science (2018) 19(11):1107–13. doi: 10.2174/1389203719666180608093913
356. Sainson R, Thotakura A, Parveen N, Kosmac M, Borhis G, Carvalho J, et al. Cancer res. nat biotechnol. Available at: https://www.kymab.com/media/uploads/files/11-Nov-2017-Kymab-KY1044-SITC-Poster.pdf.
357. Cendrowicz E, Jacob L, Greenwald S, Tamir A, Pecker I, Tabakman R, et al. DSP107 combines inhibition of CD47/SIRPα axis with activation of 4-1BB to trigger anticancer immunity. J Exp Clin Cancer Res (2022) 41(1). doi: 10.1186/s13046-022-02256-x
358. Innamarato P, Asby S, Morse J, Mackay A, Hall M, Kidd S, et al. Intratumoral activation of 41BB costimulatory signals enhances CD8 T cell expansion and modulates tumor-infiltrating myeloid cells. J Immunol (2020) 205(10):2893–904. doi: 10.4049/jimmunol.2000759
359. Stoll A, Bruns H, Fuchs M, Völkl S, Nimmerjahn F, Kunz M, et al. CD137 (4-1BB) stimulation leads to metabolic and functional reprogramming of human monocytes/macrophages enhancing their tumoricidal activity. Leukemia (2021) 35(12):3482–96. doi: 10.1038/s41375-021-01287-1
360. Wang S, Yang Y, Ma P, Zha Y, Zhang J, Lei A, et al. CAR-macrophage: an extensive immune enhancer to fight cancer. eBioMedicine (2022) 76:103873. doi: 10.1016/j.ebiom.2022.103873
361. Klichinsky M, Ruella M, Shestova O, Lu XM, Best A, Zeeman M, et al. Human chimeric antigen receptor macrophages for cancer immunotherapy. Nat Biotechnol (2020). doi: 10.1038/s41587-020-0462-y
362. Zhang L, Tian L, Dai X, Yu H, Wang J, Lei A, et al. Pluripotent stem cell-derived CAR-macrophage cells with antigen-dependent anti-cancer cell functions. J Hematol Oncol (2020) 13(1). doi: 10.1186/s13045-020-00983-2
363. De Palma M, Mazzieri R, Politi LS, Pucci F, Zonari E, Sitia G, et al. Tumor-targeted interferon-α delivery by Tie2-expressing monocytes inhibits tumor growth and metastasis. Cancer Cell (2008) 14(4):299–311. doi: 10.1016/j.ccr.2008.09.004
364. Shields CW, Evans MA, Wang LL-W, Baugh N, Iyer S, Wu D, et al. Cellular backpacks for macrophage immunotherapy. Sci Adv (2020) 6(18). doi: 10.1126/sciadv.aaz6579
365. Kaczanowska S, Beury DW, Gopalan V, Tycko AK, Qin H, Clements ME, et al. Genetically engineered myeloid cells rebalance the core immune suppression program in metastasis. Cell (2021) 184(8):2033–2052.e21. doi: 10.1016/j.cell.2021.02.048
366. Allavena P, Palmioli A, Avigni R, Sironi M, La Ferla B, Maeda A. PLGA based nanoparticles for the monocyte-mediated anti-tumor drug delivery system. J Biomed Nanotechnol (2020) 16(2):212–23. doi: 10.1166/jbn.2020.2881
367. Sánchez-Paulete AR, Mateus-Tique J, Mollaoglu G, Nielsen SR, Marks A, Lakshmi A, et al. Targeting macrophages with CAR T cells delays solid tumor progression and enhances antitumor immunity. Cancer Immunol Res (2022) 10(11):1354–69. doi: 10.1158/2326-6066.CIR-21-1075
Keywords: immunotherapy, tumor-associated macrophages, tumor microenvironment, inflammation, immunometabolic dysregulation, macrophage polarization
Citation: Han J, Dong L, Wu M and Ma F (2023) Dynamic polarization of tumor-associated macrophages and their interaction with intratumoral T cells in an inflamed tumor microenvironment: from mechanistic insights to therapeutic opportunities. Front. Immunol. 14:1160340. doi: 10.3389/fimmu.2023.1160340
Received: 07 February 2023; Accepted: 20 April 2023;
Published: 12 May 2023.
Edited by:
Lei Zhu, Emory University, United StatesReviewed by:
H. Atakan Ekiz, Izmir Institute of Technology, TürkiyeHao Wu, University of Würzburg, Germany
Guohao Wang, First Affiliated Hospital of Xiamen University, China
Copyright © 2023 Han, Dong, Wu and Ma. This is an open-access article distributed under the terms of the Creative Commons Attribution License (CC BY). The use, distribution or reproduction in other forums is permitted, provided the original author(s) and the copyright owner(s) are credited and that the original publication in this journal is cited, in accordance with accepted academic practice. No use, distribution or reproduction is permitted which does not comply with these terms.
*Correspondence: Fei Ma, ZHJtYWZlaUAxMjYuY29t
†These authors have contributed equally to this work