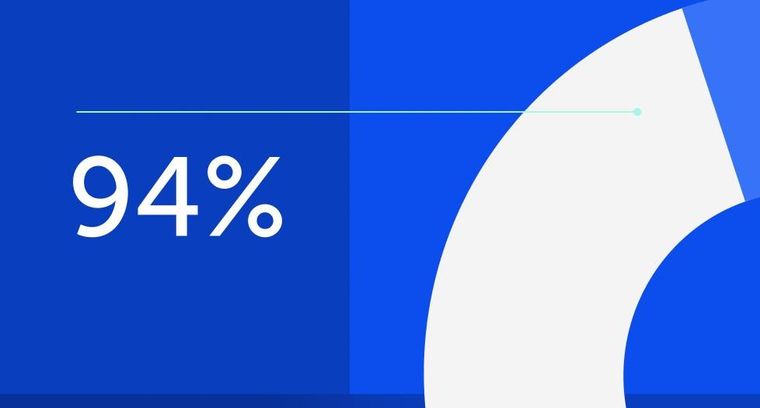
94% of researchers rate our articles as excellent or good
Learn more about the work of our research integrity team to safeguard the quality of each article we publish.
Find out more
REVIEW article
Front. Immunol., 14 March 2023
Sec. Nutritional Immunology
Volume 14 - 2023 | https://doi.org/10.3389/fimmu.2023.1154211
This article is part of the Research TopicDiet, Food Allergy, and Gut ImmunityView all 6 articles
Breast milk is an essential source of nutrition and hydration for the infant. In addition, this highly complex biological fluid contains numerous immunologically active factors such as microorganisms, immunoglobulins, cytokines and microRNAs (miRNAs). Here, we set out to predict the function of the top 10 expressed miRNAs in human breast milk, focusing on their relevance in oral tolerance development and allergy prevention in the infant. The top expressed miRNAs in human breast milk were identified on basis of previous peer-reviewed studies gathered from a recent systematic review and an updated literature search. The miRNAs with the highest expression levels in each study were used to identify the 10 most common miRNAs or miRNA families across studies and these were selected for subsequent target prediction. The predictions were performed using TargetScan in combination with the Database for Annotation, Visualization and Integrated Discovery. The ten top expressed miRNAs were: let-7-5p family, miR-148a-3p, miR-30-5p family, miR-200a-3p + miR-141-3p, miR-22-3p, miR-181-5p family, miR-146b-5p, miR-378a-3p, miR-29-3p family, miR-200b/c-3p and miR-429-3p. The target prediction identified 3,588 potential target genes and 127 Kyoto Encyclopedia of Genes and Genomes pathways; several connected to the immune system, including TGF-b and T cell receptor signaling and T-helper cell differentiation. This review highlights the role of breast milk miRNAs and their potential contribution to infant immune maturation. Indeed, breast milk miRNAs seem to be involved in several pathways that influence oral tolerance development.
Breast milk is an important source of nutrition and hydration for infant mammals, including humans (1). However, this highly complex biological fluid has functions that exceed nutrition (2, 3). Breast milk contains a number of immunological factors such as microorganisms, immunoglobulins and cytokines that represent the first postnatal immunological stimuli and protection for the infant (3, 4). The composition of breast milk changes over time to the different needs of the infant. For example, the first milk produced, colostrum, contains a higher concentration of immunoglobulin A compared to mature milk (5). Not only does the milk offer protective effects against infections in early life, but it may also have more long-lasting immune modulatory effects (6–9).
Amongst the immune regulatory components in breast milk, microRNA (miRNA) represents an abundant form. The miRNAs are small, non-coding fragments of RNA, with the potential to regulate gene expression by post-transcriptional modifications of mRNA strands (10). During the last 15 years, miRNAs have gained increasing interest as research has moved forward through new technologies and discoveries; indeed, it has been suggested that miRNAs are able to regulate up to 60% of all transcribed mRNAs (11, 12). Although present in all biological fluids, miRNAs are most abundant in breast milk and, interestingly, many of the milk-born miRNAs seem to be evolutionarily conserved between different mammal species (13–15). Although the biological relevance remains to be clarified, this conservation of sequence suggest that miRNAs serve fundamental functions. Like other breast milk components, the miRNA profile seems to vary over time, displaying daily as well as more long-term fluctuations (14, 16, 17), although empirical data is still scarce. An astonishing >1,900 different types of miRNAs have been detected in human breast milk, with potential biological implications on cell communication, fatty acid biosynthesis, regulation of actin skeleton and a vast number of immunological pathways (12).
Human milk miRNAs hypothetically regulate infant gene expression through epigenetic modifications and thereby affect various biological processes, including immune maturation. These epigenetic effects could potentially occur both up-stream and down-stream of gene transcription. Down-stream of transcription, a miRNA could target one or more mRNA and inhibit their translation into proteins. For example, miRNA-155, expressed in human milk (14), targets the signal transducer and activator of transcription (STAT) 1 mRNA and thereby, via concurrent activation of IL-2/STAT5 signaling, promote regulatory T cell (Treg) differentiation (18, 19). Up-stream of gene transcription, miRNAs could for example influence gene expression via DNA methylation, interfering with the transcriptional process. For example, DNA de/methylation regulates FOXP3 expression, a key transcription factor in the differentiation of CD4+ T cells into Tregs (20, 21). DNA methyltransferase (DNMT) 1 and DNMT3b seem to be in control of this transcriptional “switch” (22). Interestingly, one of the most highly expressed miRNAs in mammalian milk, miRNA-148a, can downregulate the expression of DNA methyltransferase 1 in vitro (23). Also, Admyre et al. (24) observed how human milk EVs could dose-dependently increase the numbers of FOXP3+CD4+CD25+ Treg cells in peripheral blood mononuclear cells. However, it remains to be further explored if such effects can be exclusively attributed to the EV born miRNAs. On this note, it should also be pointed out that although interactions between different bioactive components in the milk likely occurs – for example miR-155 and miR-181 interacts with TGF-β and IL-10 (all abundantly found in breast milk) to regulate proliferation and function of Tregs (18) - functional studies designed to uncover details in these interplays are missing. Yet, early discoveries, as highlighted here, prompts enthralling questions about the biological relevance of human breast milk miRNAs and their role in infant development and immune maturation.
Breast milk, along with all biological fluids, contain RNase (a catalyst for RNA degradation) suggesting that viable miRNAs are somehow protected from RNase activity. Breast milk-derived miRNAs are believed to primarily originate from maternal mammary epithelial cells and immune cells but could potentially also originate from cells in other parts of the body, reaching the breast milk through the blood circulation (12, 25). However, it is assumed that the majority of human breast milk miRNAs are not found freely in breast milk but rather encapsuled in “vehicle” structures; primarily extracellular vesicles (EVs) (26). The EVs are bilayer membraned vesicles involved in intercellular signaling, and transport of proteins, nucleic acids and lipids from the originating cell to the target cell. Packing of EVs involves sorting mechanisms that favor some cargos over others and is hence not random (27, 28); emphasizing the biological relevance of their content.
In vitro studies have shown that milk-derived EVs survive when exposed to the harsh conditions of gastric digestion (29–31) and are even able to subsequently enter intestinal crypt-like cells (29–31). Furthermore, in vivo animal experiments show that exogenous miRNAs can be absorbed through the digestive tract only to be further distributed throughout the body of a suckling pup or piglet (32–34), or after oral gavage in adult mice (35). Cells seem to absorb EVs via different endocytic pathways, including clathrin-dependent endocytosis, phagocytosis, macropinocytosis and caveolin-mediated uptake, but the mechanisms in how the miRNA loaded EVs are absorbed by the epithelial cells in the infants’ intestine remain to be discovered in detail (13). Nevertheless, the fact that milk-derived miRNAs to a large extent are evolutionary conserved and can survive the harsh conditions of the digestive tract to be taken up in the intestine of the offspring, points to the important influence they may have on the epigenetic development of the child.
One of the earliest biological challenges in life is the ability of the infant’s immune system to distinguish between harmful and harmless proteins, also known as antigens. An adverse immune response to food antigens is known as food allergy. The prevalence of food allergy is believed to be at an all-time high, although describing the increasing rates in exact numbers proves a challenge (36–39). Nevertheless, allergies pose a great challenge to the afflicted individual and their next of kin (40), and in the absence of a cure (41, 42) further mechanistic insight is warranted.
The immune system avoids adverse reactions to foods through the induction of oral tolerance (43); see the key factors, as described below, depicted in Figure 1. Tolerance, i.e. systemic and mucosal unresponsiveness, is maintained through interaction between intestinal cells and immune cells at mucosal surfaces. In the gastrointestinal tract (GIT), where the infant is exposed to breast milk miRNAs, the gut-associated lymphoid tissues including the mesenteric lymph nodes (44) (MLN), play a major role in these interactions. The gut-associated lymphoid tissues include both innate and adaptive immune cells and tolerance is primarily developed through interaction between regulatory dendritic cells (CD103+ DCs) and naïve T helper (Th) cells in the MLN, where the CD103+ DCs promote Th cell differentiation into Tregs. The Tregs, will favor a tolerogenic immune response by producing the anti-inflammatory cytokines IL-10 and TGF-β, inhibiting the proliferation of effector T cells and thereby mitigating adverse inflammatory responses (45). Tregs are hence at the hub of oral tolerance development. The TGF-β produced by Tregs also promotes B cell antibody class switching towards immunoglobulin (Ig) A, facilitating IgA-mediated antigen exclusion in the intestinal lumen (46). Moreover, the CD103+DCs of the MLN also imprint GIT homing, so the Tregs may leave the lymph node and migrate back to the lamina propria. In fact, induction of Treg homing back to the gut has been suggested as a vital step to install oral tolerance (46, 47). In the gut, Tregs are exposed to IL-10 producing macrophages that cause their clonal expansion and thereby facilitate their suppressive function (43, 47). As pointed out above, more than one breast milk miRNA has been previously implicated in these interactions, hence, we hypothesize that breast milk miRNAs are important for installing immunological tolerance in the infant.
Figure 1 Key players in oral tolerance. Oral tolerance is an active suppression of cellular and humoral responses to antigens. The mesenteric lymph node is the major site for Treg cell differentiation, as mediated by CD103+ DCs. Production of the anti-inflammatory cytokines IL-10 and TGF-β supports the positive feedback loop of stimulating CD103+ DCs, Tregs and tolerogenic macrophages. The TGF-β also promotes B cell antibody class switching towards IgA, thus facilitating antigen exclusion in the intestinal lumen. Interestingly, there seems to exist several breastmilk miRNAs with potential involvement in these interactions.
In this review we set out to predict the mRNA targets of the top 10 expressed miRNAs in human breast milk, focusing on their relevance in oral tolerance development and allergy prevention in the infant.
For a visual overview of the methods, please refer to Figure 2.
Figure 2 Methodological overview. Overview of the methodological process. The topmost light grey, unfilled text boxes depict the paper selection following the updated literature search. Below, depicted by the grey text boxes, follows the steps taken for the result generation: 1) and 2) illustrates how the three papers included after the new search was combined with the 16 papers analyzing miRNA in breast milk from our previous ncRNA review (14), 3) on basis of these 19 papers we identified the highest expressed breast milk miRNAs in each study (Supplementary B, Table S1), 4) thereafter the miRNAs were scored according to paragraph 2.2 (also refer to Supplementary B, Tables S2-S5) and for the last two steps denoted 5) please refer to method paragraph 2.2 and Supplementary B, Tables S6-S8.
The top 10 expressed miRNAs in human breast milk were identified based on a previous systematic review produced by us in 2021 (14) (PROSPERO Identifier: CRD42020138989), where we summarized the scientific studies on non-coding RNAs (ncRNA) in breast milk up until September 2020. To also capture relevant literature published after this date, a new structured search was conducted in the bibliographic databases MEDLINE, Cochrane Library, Embase and Web of Science. The search strategy included thesaurus- and free-text terms for the two main concepts miRNA and mothers’ milk (see Supplementary A for complete details). The searches were last updated on the 9th of August 2022. The identified records were collected in EndNote and screened for duplicates (by author S.A.P). Records previously screened in the systematic review (14) were also removed. Following duplicate removal, the new records were screened for inclusion based on titles and abstract by author E.A. and subsequently cross-checked by author M.R.S.
Please observe that in updating our previous search, we included new papers within the area of miRNA and mothers’ milk research, as opposed to the previous systematic search where we considered all ncRNAs. Hence, although this paper in some respects draws from our previous systematic review, it should be considered as a stand-alone paper built on a separate rational and aim.
Functional analysis was performed on the top 10 expressed miRNAs in breast milk based on the previous summary in Tingö et al. (14), including 16 papers (15, 23, 25, 29–31, 48–57) and adding information from 3 additional papers found in the updated literature search described above (17, 58, 59). The most frequently expressed miRNAs, as described either by the authors or calculated based on the data from their corresponding supplementary information, were based on RNA sequencing or open Array qPCR analysis and isolated from all fractions, i.e. whole, cell, skim milk, lipid or EVs (refer to Supplementary B, Table 1). From each included study, the top 10 expressed miRNAs were assigned a score between 1 and 10 that corresponded to their expression ranking (i.e., the highest expressed miRNA was assigned a score of 10; see complete scoring in Supplementary B, Table S1), to get an average of the top 10 most abundant miRNAs from the 19 included papers. The miRNAs belonging to the same family were scored together (Supplementary B, Table S2) due to their shared seed region and thus common mRNA targets. The assigned scores for each miRNA were then summarized across studies, producing a total score for each miRNA on basis of their ranking (Supplementary B, Table S3); miR-6073 was removed due to the likelihood that the high relative abundance of this miRNA in two studies may be an artifact of the adopted sequencing protocol as discussed in our previous review (14). Furthermore, the miRNA-200 family consists of miR-200a/b/c, miR-141 and miR-429, however, they are divided into two groups based on their seed region similarities; miR-200a and miR-141-3p in one group, and miR-200b/c and miR-429 in the other (60). The groups were scored separately, and subsequently included among the top 10 expressed (Supplementary B, Table S3).
Target prediction was run using TargetScan version 8.0 (61, 62), with the default settings, and an upper threshold for the cumulative weighted context++ score was set at -0.2 (62). The prediction of targets are primarily based on the mRNA matching at the “canonical” site, i.e. a base sequence matching perfectly to the miRNA seed region (62, 63), and a set of additional variables (e.g., sequence conservation, target site accessibility, flanking sequence determinants and compensatory paring outside the seed region) that contribute to reducing the number of false positive predictions (61, 64), refer to Figure 3 for visual illustration. However, contrary to many other prediction algorithms, TargetScan also base the predictions on an additional set of variables, such as sequence conservation, target site accessibility, flanking sequence determinants, and compensatory pairing outside the seed region, which reduce the number of false positive predictions (61, 64).
Figure 3 Seed region matching. The figure illustrates the different types of canonical site paring between the miRNA and its mRNA target, i.e. 8mer, 7mer-1A, 7mer-m8 and 6mer paring. The 8mer paring has a complete matched seed region of 7 bases, including an additional match at the 8th position, and an adenine opposite of the first position; the 7mer-1A has a match between position 2-7 and an adenine opposite of the first position; the 7mer-m8 has a match between position 2-8, and the 6mer has a match only in position 2-7. Notably, most miRNAs also have additional pairing beyond the seed region, called 3’-supplementary site.
The lists of predicted target genes were subsequently uploaded to the Database for Annotation, Visualization and Integrated Discovery (DAVID) 2021 (65, 66), and potential biological functions of the top 10 miRNAs were analyzed using the Kyoto Encyclopedia of Genes and Genomes (KEGG) pathway enrichment analysis. An FDR corrected p-value < 0.05 was considered of interest.
A total of 1671 records were identified by the updated literature search. After removal of duplicates, and records previously screened in our systematic review on ncRNAs in human breast milk (14), 418 unique and new records were identified and subjected to further screening. Out of these 418 records, 5 studies were read in full text and 3 were subsequently judged as relevant for this review. The results of these 3 studies were subsequently combined with the results of the other 16 studies originating from the systematic review, producing a grand total of 19 scientific studies (based on 25 unique data sets) serving as the basis of this review.
The top expressed miRNAs, from the 19 included studies, were scored and ranked; for ranking see Table 1, and for further details in the scoring system refer to the method section and Supplementary B, Tables S1-S5). Accordingly, the list of the most highly expressed breastmilk miRNAs in falling order is as follows: let-7-5p family, miR-30-5p family, miR-148a-3p, miR-200a-3p + miR-141-3p, miR-22-3p, miR-181-5p family, miR-146b-5p, miR-378a-3p, miR-29-3p family and lastly miR-200b/c-3p.
Note that we consider the familywise ranking of the miRNAs to be our main result (see Table 1, left column). The rationale behind this is that miRNAs sharing seed region will interact with the same mRNA targets and are thus likely to produce a more pronounced physiological effect collectively, as compared to a single miRNA. Table 1, however, also shows the top expressed miRNAs ranked individually as this may be interesting complementary information.
In the target prediction search we identified 3,588 potential target genes using TargetScan 8 (see complete list in Supplementary B, Table S6). The predicted genes resulted in 127 KEGG pathways of interest based on FDR corrected p-values < 0.05. Several of the pathways were directly connected to the immune system, including TGF-beta signaling, T cell receptor signaling, Toll-like receptor signaling, Jak-STAT signaling, and Th1 and Th2 cell differentiation (see complete list in Supplementary B, Table S7). In addition, some major signaling pathways also connected to immune regulation, such as the PI3K-Akt signaling pathway, MAPK signaling pathway and, FoxO signaling pathway were also indicated.
This review set out to predict the mRNA targets of the top 10 expressed miRNAs in human breast milk, focusing on their possible role in infant immunological maturation and more specifically on oral tolerance development and allergy prevention. In combining the findings from a previous systematic review from our research group (14) with an updated database search, we found the following miRNAs to be the most highly expressed in human milk: let-7-5p family, miR-148a-3p, miR-30-5p family, miR-200a-3p+ miR-141-3p, miR-22-3p, miR-181-5p family, miR-146b-5p, miR-378a-3p, miR-29-3p family, miR-200b/c-3p and miR-429-3p. Together these miRNAs were predicated to interfere with 3,588 gene products and when weighed together for pathway prediction these targets were implicated in 127 KEGG pathway. Several of these pathways were directly connected to the immune system, including TGF-beta signaling, T cell receptor signaling, Toll-like receptor signaling, Jak-STAT signaling, and Th1 and Th2 cell differentiation. In addition, some major signaling pathways frequently indicated in normal physiology, but also highly relevant for immune regulation, such as the PI3K-Akt signaling, MAPK signaling, TNF signaling and FoxO signaling, were also indicated.
Here, we have used a systematic strategy to find original studies analyzing breast milk miRNA and subsequently developed a system to identify out the most commonly expressed ones. To narrow down the number of miRNAs to be addressed in this review, we chose to focus on the top 10 miRNAs from the included studies. Of course, this potentially means disregarding miRNAs that have important immunological effects albeit expressed in low amounts. For example, miR-155 did not make it onto our list. This miRNA has received rather much attention due to its presumed involvement in Treg differentiation; it has been previously demonstrated that miR‐155 promotes the differentiation of Treg in allergic rhinitis (18). Similarly, Hicks et al. (16) recently showed that breast milk miR-375 seem to decrease the risk of atopy in breastfed infants; a miRNA that did not make it on to our top 10 list. Nevertheless, while acknowledging this as a potential shortcoming, we believe that adhering to a systematic approach in selecting which miRNAs to focus on adds rigor to this review. Furthermore, for additional information on methodological differences between the studies, such as sample source, milk fraction and maternal characteristics, please refer to our previous review (14), where we systematically deal with these topics. Moreover, we choose to focus on the pathways and corresponding targets of the top 10 expressed miRNAs from an oral tolerance perspective, as we hypothesize that breast milk miRNAs are important in immunological maturation and childhood allergy prevention. In this context, it is important to note that this review, albeit based on a systematic approach, is speculative in nature. It is also important to note that we do not attempt to predict the direction of action, i.e. suppressive or stimulatory effects. In general, miRNA act inhibitory as their primary function, but in instances where a miRNA ends up suppressing a suppressor the effect will turn out stimulatory. As the databases utilized here predict direct seed matching and enrichment of targets in the indicated pathways, making predictions about such indirect suppressive actions are difficult. The inhibitory action of a miRNA is likely also a delicate balance between the potency of the miRNA and the expression of the target mRNA at different levels. Additionally, each miRNA has several targets that are also shared with other miRNAs. Due to the level of complexity of these interactions, we deemed direction of action to be outside the scope of the current review; settling on the mere fact that human milk is enriched with miRNAs of significance to these pathways/interactions gives reason to speculate about their importance in oral tolerance development. Furthermore, we make the underlying assumption that breast milk miRNAs are protected from degradation, most likely by EV encapsulation, and taken up in sufficient amounts to exert biological effects in the infant recipient cells. As outlined in the introduction, this notion has some previous support in the scientific literature as milk miRNAs are taken up by suckling animals. However, the route of uptake is not yet completely clarified. Endocytosis is most likely one prominent way of recipient cells to incorporate exosomal miRNAs (67, 68), or less supervised uptake may potentially occur in immune cells such as macrophages (69, 70). In infants, the immature state of the intestine may further facilitate uptake of EV and their miRNA cargo, as the neonatal GIT show increased permeability (as reviewed in (71)).
As further laid out in the introduction of this paper, the primary site for oral tolerance induction is the GIT, where the infant is also exposed to breast milk. In the MLN, CD103+ DCs interacts with naïve Th cells to induce Treg differentiation; this process is highly TGF-β dependent. Among the top expressed breast milk miRNAs, we find direct mRNA targets upstream of TGF-β (miR-148a-3p) and the TGF-β receptors 1/2/3 (miR-181-5p and let-7-5p). In addition, miR-148a-3p have targets within the SMAD-family; activation of TGF-β receptor leads to the activation of the Smad downstream signaling cascade, promoting FoxP3 expression (72). The chemokine receptor CCR7 is involved in the egress of Tregs from tissues to lymph nodes (73–76) and seem to be crucial for Treg induction by antigen presenting cells primed by commensal microbes (77); CCR7 is a direct target of the breast milk expressed let-7-5p. The clonal expansion of the Tregs is further promoted by IL-10 producing macrophages; the IL-10 mRNA is a direct target of let-7-5p, while miR-29-3p targets the IL-10 receptor beta. A recent study, conducted in humans and mice, demonstrated that miR‐181a regulates the expression of IL‐10 and TGF‐β in allergic rhinitis (18). In addition, IL-10 and TGF-β are also important factors in facilitating Treg inhibition of effector T cell proliferation. Importantly, previous research has shown that deficiency in TGF-β or its receptors is proven to be fatal in the first few weeks of life, due to fulminant inflammation (78). Interestingly, TGF-β deficient mouse pups remains healthy as long as they are fed milk from dams that are not TGF-β deficient, but develop severe inflammation when weaned (79). As such TGF-β is crucial for stimulation of naïve CD4+ T cells to differentiate into Foxp3+ Treg and their subsequent suppression of effector T cell activation and proliferation, as this will halt the life-threatening inflammatory response. It is noteworthy that DICER (an enzyme crucial for miRNA biogenesis) knock out mice have low number of Tregs and develops fatal autoimmune conditions (80–82).
The combination of TGF-β with other cytokines could push naïve T cells to differentiate into non-regulatory T cell subtypes, for example Th17 differentiation is promoted in the presence of IL-6 (72, 83). Th17 cells are pro-inflammatory in their action and in many instances are regarded as problematic due to their involvement in autoimmunity and related tissue damage (84, 85). Failure of oral immune therapy, aimed to treat peanut allergy, was associated with the expression of inflammatory gene signatures present in Th17 cell populations (86), also indicating a role for Th17 cells in oral immunotherapy efficacy. The mRNA of the Th17 key transcription factor RORC is a let-7-5p target, as is the IL17 receptor IL17RD. The RORC transcription factor (known as RORγt in mice), however, also seems important for a suppressive subset of Foxp3 expressing RORγt+ T cells with regulatory function against exacerbated Th2 responses and linked to oral tolerance development in mice (87). These RORγt+ Tregs can be generated under the influence of IL-2 and TGF-β, and despite their Th-17 shared characteristics they seem to have suppressive activity against antigen-specific effector T cells in vitro (88). In addition to let-7 targeting RORC and IL-17, miR-30-5p have a target in the IL-2-receptor a mRNA. The IL-17 signaling pathway was also indicated in the KEGG pathways analysis. Interestingly, these double positive Foxp3+RORγt+ T cells have been found in the small intestinal and colonic lamina propria of mice, linked to a specific, but diverse set of bacterial species (89–91). In addition, colonization of germ-free mice with Clostridia species upregulates IL-22 production by RORγt+ innate lymphoid cells and T cells in the lamina propria of the intestine, contributing to a reduced permeability to oral antigens (92). This is interesting as tight junctions between enterocytes in the GIT prevent the paracellular passing of antigens. An increased transport of intact antigens through the epithelial cells has been related to allergenic activity (93, 94). On this note, we also found several targets involved in tight junction regulation, e.g. miR-200b-c-3p targets the occludin mRNA.
Inoculation of the infant gut with the maternal microbiota during and after birth is thought to promote an accelerated transition from Th2 to a Th1/Th17-dominant immunity, as well as facilitating Treg induction in the gut and lungs (95). A Th2 skewed immune system is the prevailing antenatal programming, meant to accommodate fetal development and promoting maternal tolerance towards the fetus (96). Th2-mediated immune responses are, however, also associated with allergic reactions mediated by B-cell produced IgE antibodies. A Th1-mediated response on the other hand antagonizes IgE responses and hence, hypothetically, prevents the development of allergic disease. Therefore, a lingering post-natal Th2 programming may increase the risk of allergy development in the infant. Indeed, previous research from our group has shown that placental gene expression, including cells of fetal origin, is dominated by a Th2- and anti-inflammatory transcription profile and that enhanced Th2 deviation at birth is related to increased risk of allergy development in the child (97, 98). Tregs are key players in the calibration of Th2 as well as Th1/Th17 driven inflammatory responses and are thus crucial to avoid adverse immune reactions (99–101). The gut microbiota also seems to play a key role in oral tolerance induction, for example by regulating the phenotype of GIT DCs (mediated by macrophages and innate lymphoid cells) (102) and Tregs (103, 104). Babies delivered by Cesarean section have a different microbiota composition early in life as compared to vaginally delivered infants (105–107) and the former are also at greater risk of developing allergies later in life (106, 108). Interestingly, the disturbance of gut microbiota in cesarean delivered infants may to some extent be restored by breastfeeding (109, 110).
The toll-like receptor (TLR) signaling pathway was indicated among the KEGG pathways. The TLRs are pattern recognition receptors that respond to microbial signals, and in particular TLR2 has been implicated in oral tolerance by promoting Treg induction as mediated by DCs (111). Soluble TLR2, capable of modulating TLR2 signaling, has also been found in human milk (112). A previous study by our group suggests that probiotic supplementation in children may decrease responses to TLR2, potentially dependent on factors downstream of TLR mRNA expression (113); which hence hypothetically could be miRNA mediated. The probiotic intervention in our study also reduced allergen responsiveness (114), and IgE-associated eczema at two years of age (115). As such, TLRs may be regarded as an important “bridge” between gut microbiota and tolerance development. In addition, the C-type lectin receptor signaling pathway was also indicated among the breast milk miRNA predicted KEGG pathways; the C-type lectin domain family 2 is a target of breast milk miRNA miR-29-3p. As the TLRs, the C-type lectin receptors (CLRs) represent a family of PPRs, recognizing both pathogens associated molecular patterns and damage-associated molecular patterns. Upon activation, CLR signaling leads to cytokine secretion and immune cell recruitment, which portraits their role in inducing innate and adaptive immune responses (116). The dendritic cell-associated C-type lectin-1 (Dectin-1) is one of the most well studied CRLs, which has been implicated in bridging the innate and adaptive immune responses. For example, dectin-1 activates the transcription factor NF-κB, through both canonical and noncanonical pathways, initiating its signaling pathway to induce Th1 and Th17 cytokine production (117), and dectin-1-Syk-CARD9 signaling seems to promote DC maturation and Th17 differentiation, both in vitro and in vivo (117).
IgE-mediated allergy reactions are caused by B-cell produced IgE antibodies triggering mast cell activation through cross-linking with the high affinity Fc epsilon Receptor I (FcϵRI) on the mast-cell surface (118, 119). Notably, miR-22 together with miRNAs from the let-7-5p family have direct targets in the IL-13 mRNA and its alpha 1 receptor; IL-13 is a central regulator in IgE synthesis and has been described as an important mediator of allergic inflammation (120). In addition, we found predicted targets in FcϵRI signaling and the B-cell receptor signaling pathway. The FcϵRI exists on mast cells and basophils. Upon cross-linking with IgE, the FcϵRI induces the release of pre-made histamines through mast cell degranulation and triggers the synthesis of a wide range of pro-inflammatory cytokines and chemokines, alongside leukotrienes and prostaglandins (118, 119, 121). Similarly, IgE-mediated activation of basophils will lead to the release of various proinflammatory mediators, but especially IL-4 and IL-6 (122). Cytokine signaling is mediated through the Janus kinase-signal transducer and activator of transcription (JAK-STAT) pathway, which was also indicated among our predicted KEGG pathways. The JAK-STAT signaling cascade is responsible for transferring signals from cytokine receptors to the nucleus, making the JAK-STAT pathway heavily involved in regulation of the immune system (123). Other than the cytokines, receptors and chemokines already mentioned in this review we found that both IL-1A and IL-31, as well as IL-1RAP, IL-1RAPL2, IL-2RA, and CCL3/7/8 are targets among the top expressed miRNAs found in breastmilk.
Furthermore, concerning the connection between allergy development and balance in Th1 versus Th2 responses, miR-29 directly targets the TBX21 mRNA (also known as T-bet), the key transcription factor of Th1 cells (124), and miR-181-5p targets CD4, a co-receptor of the T cell receptor expressed on Th cells. Indeed, previous research from our group have shown that enhanced Th2 deviation at birth is related to allergy development in the child (97, 98). Furthermore, we have also shown that breast milk has anti-inflammatory effects on peripheral blood mononuclear cells from cord blood (125); the high levels of TGF-β in breast milk and inhibitory effect of colostrum on IL-4 production, suggests a possible mechanism whereby breast milk of certain composition may protect against the development of allergy. We emphasize here that “certain compositions” may be of particular interest since previous studies on the preventative effects of breast milk on allergy are inconclusive (126–130). However, there remains a possibility that varying levels of breast milk mediators may partially explain the previous conflicting findings (131). We have for example shown that the concentrations of the Th2 associated cytokines IL-4, IL-5 and IL-13 seem to be higher in breast milk from allergic compared to non-allergic mothers (132) and that breast milk cytokine levels may have geographical variations, perhaps depending on differences in microbial load (133). Indeed, members from our group recently showed that allergy development is associated with consumption of breastmilk with a reduced microbial richness in the first month of life (134). There are also indications that levels of certain breast milk miRNA differ between allergic and non-allergic women, for example, downregulation of let-7f-5p in mature milk tends to be associated with development of atopic dermatitis in breastfed infants (50). Hence, the composition likely matters; not all breastmilk is created equal. Whilst composition likely matters, the scientific literature investigating these relations is still fairly scarce, especially in terms of mechanisms and causality, and future research will have to bring further clarity to these interactions.
In this review we make a case for breastmilk miRNAs as important regulators of infant immune maturation, focusing on their potential role in oral tolerance development and allergy prevention. This review is speculative in nature and future studies are needed to confirm the ideas put forward here, and particularly to bring further clarity to the direction of action in the interactions suggested. This will require well-designed trials in an in vivo setting, but also functional experiments uncovering the underlying mechanisms of action in vitro. We would also like to point out that the 19 scientific studies serving as the basis of this review were all performed in women from high-income countries; no studies have yet been performed outside of this population segment.
Infant immune regulation is a complex process impacted by multiple factors and relies on the finetuning of multiple factors related to Th differentiation. For example, DCs and Th interactions in the MLN, and Treg GIT homing play a central role in tolerance development as the primary site of interactions between food antigens, immune cells, gut microbiota, and the immunologically active components in breast milk. Here, we highlight the role of breast milk miRNAs and their potential contribution to infant immune maturation. Indeed, breast milk miRNAs seem to be involved in several pathways that may have implications for oral tolerance development. However, clarification of the direction of action in these complex interactions will require knowledge production from cleverly designed functional experimental and further studies of the co-variation of these factors in in vivo systems.
LT, EA, AA-K and RT designed research. SP performed the updated database search. EA and MS reviewed results from the literature search. EA and MS compiled the list of the top 10 expressed miRNAs. EA performed target and pathways predictions. LT, EA, AA-K and RT wrote the paper. LT did all figures. MJ, EC, SP, MS edited the paper. LT had primary responsibility for final content. All authors contributed to the article and approved the submitted version.
The authors declare that the research was conducted in the absence of any commercial or financial relationships that could be construed as a potential conflict of interest.
All claims expressed in this article are solely those of the authors and do not necessarily represent those of their affiliated organizations, or those of the publisher, the editors and the reviewers. Any product that may be evaluated in this article, or claim that may be made by its manufacturer, is not guaranteed or endorsed by the publisher.
The Supplementary Material for this article can be found online at: https://www.frontiersin.org/articles/10.3389/fimmu.2023.1154211/full#supplementary-material
1. Le Doare K, Holder B, Bassett A, Pannaraj PS. Mother's milk: A purposeful contribution to the development of the infant microbiota and immunity. Front Immunol (2018) 9:361. doi: 10.3389/fimmu.2018.00361
2. Chutipongtanate S, Morrow AL, Newburg DS. Human milk extracellular vesicles: A biological system with clinical implications. Cells (2022) 11(15). doi: 10.3390/cells11152345
3. Newburg DS, Walker WA. Protection of the neonate by the innate immune system of developing gut and of human milk. Pediatr Res (2007) 61(1):2–8. doi: 10.1203/01.pdr.0000250274.68571.18
4. Moossavi S, Miliku K, Sepehri S, Khafipour E, Azad MB. The prebiotic and probiotic properties of human milk: Implications for infant immune development and pediatric asthma. Front Pediatr (2018) 6:197. doi: 10.3389/fped.2018.00197
5. Akhter H, Aziz F, Ullah FR, Ahsan M, Islam SN. Immunoglobulins content in colostrum, transitional and mature milk of Bangladeshi mothers: Influence of parity and sociodemographic characteristics. J Mother Child (2020) 24(3):8–15. doi: 10.34763/jmotherandchild.20202403.2032.d-20-00001
6. Yi DY, Kim SY. Human breast milk composition and function in human health: From nutritional components to microbiome and MicroRNAs. Nutrients (2021) 13(9):3094. doi: 10.3390/nu13093094
7. Lodge CJ, Tan DJ, Lau MX, Dai X, Tham R, Lowe AJ, et al. Breastfeeding and asthma and allergies: A systematic review and meta-analysis. Acta Paediatr (2015) 104(467):38–53. doi: 10.1111/apa.13132
8. Gungor D, Nadaud P, LaPergola CC, Dreibelbis C, Wong YP, Terry N, et al. Infant milk-feeding practices and food allergies, allergic rhinitis, atopic dermatitis, and asthma throughout the life span: A systematic review. Am J Clin Nutr (2019) 109(Suppl 7):772S–99S. doi: 10.1093/ajcn/nqy283
9. Harvey SM, Murphy VE, Whalen OM, Gibson PG, Jensen ME. Breastfeeding and wheeze-related outcomes in high-risk infants: A systematic review and meta-analysis. Am J Clin Nutr (2021) 113(6):1609–18. doi: 10.1093/ajcn/nqaa442
10. Gebert LFR, MacRae IJ. Regulation of microRNA function in animals. Nat Rev Mol Cell Biol (2019) 20(1):21–37. doi: 10.1038/s41580-018-0045-7
11. van Esch B, Porbahaie M, Abbring S, Garssen J, Potaczek DP, Savelkoul HFJ, et al. The impact of milk and its components on epigenetic programming of immune function in early life and beyond: Implications for allergy and asthma. Front Immunol (2020) 11:2141. doi: 10.3389/fimmu.2020.02141
12. Jiang X, You L, Zhang Z, Cui X, Zhong H, Sun X, et al. Biological properties of milk-derived extracellular vesicles and their physiological functions in infant. Front Cell Dev Biol (2021) 9:693534. doi: 10.3389/fcell.2021.693534
13. Melnik BC, Stremmel W, Weiskirchen R, John SM, Schmitz G. Exosome-derived MicroRNAs of human milk and their effects on infant health and development. Biomolecules (2021) 11(6):851. doi: 10.3390/biom11060851
14. Tingo L, Ahlberg E, Johansson L, Pedersen SA, Chawla K, Saetrom P, et al. Non-coding RNAs in human breast milk: A systematic review. Front Immunol (2021) 12:725323. doi: 10.3389/fimmu.2021.725323
15. van Herwijnen MJC, Driedonks TAP, Snoek BL, Kroon AMT, Kleinjan M, Jorritsma R, et al. Abundantly present miRNAs in milk-derived extracellular vesicles are conserved between mammals. Front Nutr (2018) 5:81. doi: 10.3389/fnut.2018.00081
16. Hicks SD, Beheshti R, Chandran D, Warren K, Confair A. Infant consumption of microRNA miR-375 in human milk lipids is associated with protection from atopy. Am J Clin Nutr (2022) 116(6):1654–62. doi: 10.1093/ajcn/nqac266
17. Raymond F, Lefebvre G, Texari L, Pruvost S, Metairon S, Cottenet G, et al. Longitudinal human milk miRNA composition over the first 3 mo of lactation in a cohort of healthy mothers delivering term infants. J Nutr (2022) 152(1):94–106. doi: 10.1093/jn/nxab282
18. Zeng Q, Liu W, Luo R, Lu G. MicroRNA-181a and microRNA-155 are involved in the regulation of the differentiation and function of regulatory T cells in allergic rhinitis children. Pediatr Allergy Immunol (2019) 30(4):434–42. doi: 10.1111/pai.13038
19. Lu LF, Thai TH, Calado DP, Chaudhry A, Kubo M, Tanaka K, et al. Foxp3-dependent microRNA155 confers competitive fitness to regulatory T cells by targeting SOCS1 protein. Immunity (2009) 30(1):80–91. doi: 10.1016/j.immuni.2008.11.010
20. Huehn J, Beyer M. Epigenetic and transcriptional control of Foxp3+ regulatory T cells. Semin Immunol (2015) 27(1):10–8. doi: 10.1016/j.smim.2015.02.002
21. Lal G, Zhang N, van der Touw W, Ding Y, Ju W, Bottinger EP, et al. Epigenetic regulation of Foxp3 expression in regulatory T cells by DNA methylation. J Immunol (2009) 182(1):259–73. doi: 10.4049/jimmunol.182.1.259
22. Lal G, Bromberg JS. Epigenetic mechanisms of regulation of Foxp3 expression. Blood (2009) 114(18):3727–35. doi: 10.1182/blood-2009-05-219584
23. Golan-Gerstl R, Elbaum Shiff Y, Moshayoff V, Schecter D, Leshkowitz D, Reif S. Characterization and biological function of milk-derived miRNAs. Mol Nutr Food Res (2017) 61(10). doi: 10.1002/mnfr.201700009
24. Admyre C, Johansson SM, Qazi KR, Filen JJ, Lahesmaa R, Norman M, et al. Exosomes with immune modulatory features are present in human breast milk. J Immunol (2007) 179(3):1969–78. doi: 10.4049/jimmunol.179.3.1969
25. Alsaweed M, Lai CT, Hartmann PE, Geddes DT, Kakulas F. Human milk miRNAs primarily originate from the mammary gland resulting in unique miRNA profiles of fractionated milk. Sci Rep (2016) 6:20680. doi: 10.1038/srep20680
26. Alsaweed M, Hartmann PE, Geddes DT, Kakulas F. MicroRNAs in breastmilk and the lactating breast: Potential immunoprotectors and developmental regulators for the infant and the mother. Int J Environ Res Public Health (2015) 12(11):13981–4020. doi: 10.3390/ijerph121113981
27. Stevanato L, Thanabalasundaram L, Vysokov N, Sinden JD. Investigation of content, stoichiometry and transfer of miRNA from human neural stem cell line derived exosomes. PloS One (2016) 11(1):e0146353. doi: 10.1371/journal.pone.0146353
28. Squadrito ML, Baer C, Burdet F, Maderna C, Gilfillan GD, Lyle R, et al. Endogenous RNAs modulate microRNA sorting to exosomes and transfer to acceptor cells. Cell Rep (2014) 8(5):1432–46. doi: 10.1016/j.celrep.2014.07.035
29. Liao Y, Du X, Li J, Lonnerdal B. Human milk exosomes and their microRNAs survive digestion in vitro and are taken up by human intestinal cells. Mol Nutr Food Res (2017) 61(11). doi: 10.1002/mnfr.201700082
30. Zhou Q, Li M, Wang X, Li Q, Wang T, Zhu Q, et al. Immune-related microRNAs are abundant in breast milk exosomes. Int J Biol Sci (2012) 8(1):118–23. doi: 10.7150/ijbs.8.118
31. Kahn S, Liao Y, Du X, Xu W, Li J, Lonnerdal B. Exosomal MicroRNAs in milk from mothers delivering preterm infants survive in vitro digestion and are taken up by human intestinal cells. Mol Nutr Food Res (2018) 62(11):e1701050. doi: 10.1002/mnfr.201701050
32. Zhou F, Ebea P, Mutai E, Wang H, Sukreet S, Navazesh S, et al. Small extracellular vesicles in milk cross the blood-brain barrier in murine cerebral cortex endothelial cells and promote dendritic complexity in the hippocampus and brain function in C57BL/6J mice. Front Nutr (2022) 9:838543. doi: 10.3389/fnut.2022.838543
33. Manca S, Upadhyaya B, Mutai E, Desaulniers AT, Cederberg RA, White BR, et al. Milk exosomes are bioavailable and distinct microRNA cargos have unique tissue distribution patterns. Sci Rep (2018) 8(1):11321. doi: 10.1038/s41598-018-29780-1
34. Carrillo-Lozano E, Sebastian-Valles F, Knott-Torcal C. Circulating microRNAs in breast milk and their potential impact on the infant. Nutrients (2020) 12(10):3066. doi: 10.3390/nu12103066
35. Lopez de Las Hazas MC, Del Pozo-Acebo L, Hansen MS, Gil-Zamorano J, Mantilla-Escalante DC, Gomez-Coronado D, et al. Dietary bovine milk miRNAs transported in extracellular vesicles are partially stable during GI digestion, are bioavailable and reach target tissues but need a minimum dose to impact on gene expression. Eur J Nutr (2022) 61(2):1043–56. doi: 10.1007/s00394-021-02720-y
36. Tang ML, Mullins RJ. Food allergy: is prevalence increasing? Intern Med J (2017) 47(3):256–61. doi: 10.1111/imj.13362
37. Koplin JJ, Mills EN, Allen KJ. Epidemiology of food allergy and food-induced anaphylaxis: is there really a Western world epidemic? Curr Opin Allergy Clin Immunol (2015) 15(5):409–16. doi: 10.1097/ACI.0000000000000196
38. Tedner SG, Asarnoj A, Thulin H, Westman M, Konradsen JR, Nilsson C. Food allergy and hypersensitivity reactions in children and adults-a review. J Intern Med (2022) 291(3):283–302. doi: 10.1111/joim.13422
39. Spolidoro GCI, Amera YT, Ali MM, Nyassi S, Lisik D, Ioannidou A, et al. Frequency of food allergy in Europe: An updated systematic review and meta-analysis. Allergy (2022) 78(2):351–368. doi: 10.1111/all.15560
40. Golding MA, Batac ALR, Gunnarsson NV, Ahlstedt S, Middelveld R, Protudjer JLP. The burden of food allergy on children and teens: A systematic review. Pediatr Allergy Immunol (2022) 33(3):e13743. doi: 10.1111/pai.13743
41. Sicherer SH, Sampson HA. Food allergy: A review and update on epidemiology, pathogenesis, diagnosis, prevention, and management. J Allergy Clin Immunol (2018) 141(1):41–58. doi: 10.1016/j.jaci.2017.11.003
42. Burks AW, Sampson HA, Plaut M, Lack G, Akdis CA. Treatment for food allergy. J Allergy Clin Immunol (2018) 141(1):1–9. doi: 10.1016/j.jaci.2017.11.004
43. Tordesillas L, Berin MC. Mechanisms of oral tolerance. Clin Rev Allergy Immunol (2018) 55(2):107–17. doi: 10.1007/s12016-018-8680-5
44. Chinthrajah RS, Hernandez JD, Boyd SD, Galli SJ, Nadeau KC. Molecular and cellular mechanisms of food allergy and food tolerance. J Allergy Clin Immunol (2016) 137(4):984–97. doi: 10.1016/j.jaci.2016.02.004
45. Akdis CA, Akdis M. Mechanisms of immune tolerance to allergens: role of IL-10 and tregs. J Clin Invest (2014) 124(11):4678–80. doi: 10.1172/JCI78891
46. Iweala OI, Nagler CR. The microbiome and food allergy. Annu Rev Immunol (2019) 37:377–403. doi: 10.1146/annurev-immunol-042718-041621
47. Hadis U, Wahl B, Schulz O, Hardtke-Wolenski M, Schippers A, Wagner N, et al. Intestinal tolerance requires gut homing and expansion of FoxP3+ regulatory T cells in the lamina propria. Immunity (2011) 34(2):237–46. doi: 10.1016/j.immuni.2011.01.016
48. Weber JA, Baxter DH, Zhang S, Huang DY, Huang KH, Lee MJ, et al. The microRNA spectrum in 12 body fluids. Clin Chem (2010) 56(11):1733–41. doi: 10.1373/clinchem.2010.147405
49. Smyczynska U, Bartlomiejczyk MA, Stanczak MM, Sztromwasser P, Wesolowska A, Barbarska O, et al. Impact of processing method on donated human breast milk microRNA content. PloS One (2020) 15(7):e0236126. doi: 10.1371/journal.pone.0236126
50. Simpson MR, Brede G, Johansen J, Johnsen R, Storro O, Saetrom P, et al. Human breast milk miRNA, maternal probiotic supplementation and atopic dermatitis in offspring. PloS One (2015) 10(12):e0143496. doi: 10.1371/journal.pone.0143496
51. Rubio M, Bustamante M, Hernandez-Ferrer C, Fernandez-Orth D, Pantano L, Sarria Y, et al. Circulating miRNAs, isomiRs and small RNA clusters in human plasma and breast milk. PloS One (2018) 13(3):e0193527. doi: 10.1371/journal.pone.0193527
52. Munch EM, Harris RA, Mohammad M, Benham AL, Pejerrey SM, Showalter L, et al. Transcriptome profiling of microRNA by next-gen deep sequencing reveals known and novel miRNA species in the lipid fraction of human breast milk. PloS One (2013) 8(2):e50564. doi: 10.1371/journal.pone.0050564
53. Leiferman A, Shu J, Upadhyaya B, Cui J, Zempleni J. Storage of extracellular vesicles in human milk, and MicroRNA profiles in human milk exosomes and infant formulas. J Pediatr Gastroenterol Nutr (2019) 69(2):235–8. doi: 10.1097/MPG.0000000000002363
54. Kupsco A, Prada D, Valvi D, Hu L, Petersen MS, Coull B, et al. Human milk extracellular vesicle miRNA expression and associations with maternal characteristics in a population-based cohort from the faroe islands. Sci Rep (2021) 11(1):5840. doi: 10.1038/s41598-021-84809-2
55. Carney MC, Tarasiuk A, DiAngelo SL, Silveyra P, Podany A, Birch LL, et al. Metabolism-related microRNAs in maternal breast milk are influenced by premature delivery. Pediatr Res (2017) 82(2):226–36. doi: 10.1038/pr.2017.54
56. Alsaweed M, Lai CT, Hartmann PE, Geddes DT, Kakulas F. Human milk cells contain numerous miRNAs that may change with milk removal and regulate multiple physiological processes. Int J Mol Sci (2016) 17(6):956. doi: 10.3390/ijms17060956
57. Alsaweed M, Hepworth AR, Lefevre C, Hartmann PE, Geddes DT, Hassiotou F. Human milk MicroRNA and total RNA differ depending on milk fractionation. J Cell Biochem (2015) 116(10):2397–407. doi: 10.1002/jcb.25207
58. Yun B, Kim Y, Park DJ, Oh S. Comparative analysis of dietary exosome-derived microRNAs from human, bovine and caprine colostrum and mature milk. J Anim Sci Technol (2021) 63(3):593–602. doi: 10.5187/jast.2021.e39
59. Hicks SD, Confair A, Warren K, Chandran D. Levels of breast milk MicroRNAs and other non-coding RNAs are impacted by milk maturity and maternal diet. Front Immunol (2021) 12:785217. doi: 10.3389/fimmu.2021.785217
60. Humphries B, Yang C. The microRNA-200 family: small molecules with novel roles in cancer development, progression and therapy. Oncotarget (2015) 6(9):6472–98. doi: 10.18632/oncotarget.3052
61. Agarwal V, Bell GW, Nam JW, Bartel DP. Predicting effective microRNA target sites in mammalian mRNAs. Elife (2015) 4. doi: 10.7554/eLife.05005
62. Lewis BP, Burge CB, Bartel DP. Conserved seed pairing, often flanked by adenosines, indicates that thousands of human genes are microRNA targets. Cell (2005) 120(1):15–20. doi: 10.1016/j.cell.2004.12.035
63. Witkos TM, Koscianska E, Krzyzosiak WJ. Practical aspects of microRNA target prediction. Curr Mol Med (2011) 11(2):93–109. doi: 10.2174/156652411794859250
64. Hammond SM. An overview of microRNAs. Adv Drug Deliv Rev (2015) 87:3–14. doi: 10.1016/j.addr.2015.05.001
65. Huang da W, Sherman BT, Lempicki RA. Systematic and integrative analysis of large gene lists using DAVID bioinformatics resources. Nat Protoc (2009) 4(1):44–57. doi: 10.1038/nprot.2008.211
66. Sherman BT, Hao M, Qiu J, Jiao X, Baseler MW, Lane HC, et al. DAVID: a web server for functional enrichment analysis and functional annotation of gene lists (2021 update). Nucleic Acids Res (2022) 50(W1):W216–21. doi: 10.1093/nar/gkac194
67. Wolf T, Baier SR, Zempleni J. The intestinal transport of bovine milk exosomes is mediated by endocytosis in human colon carcinoma caco-2 cells and rat small intestinal IEC-6 cells. J Nutr (2015) 145(10):2201–6. doi: 10.3945/jn.115.218586
68. Kusuma RJ, Manca S, Friemel T, Sukreet S, Nguyen C, Zempleni J. Human vascular endothelial cells transport foreign exosomes from cow's milk by endocytosis. Am J Physiol Cell Physiol (2016) 310(10):C800–7. doi: 10.1152/ajpcell.00169.2015
69. Lasser C, Alikhani VS, Ekstrom K, Eldh M, Paredes PT, Bossios A, et al. Human saliva, plasma and breast milk exosomes contain RNA: uptake by macrophages. J Transl Med (2011) 9:9. doi: 10.1186/1479-5876-9-9
70. Izumi H, Tsuda M, Sato Y, Kosaka N, Ochiya T, Iwamoto H, et al. Bovine milk exosomes contain microRNA and mRNA and are taken up by human macrophages. J Dairy Sci (2015) 98(5):2920–33. doi: 10.3168/jds.2014-9076
71. Westrom B, Arevalo Sureda E, Pierzynowska K, Pierzynowski SG, Perez-Cano FJ. The immature gut barrier and its importance in establishing immunity in newborn mammals. Front Immunol (2020) 11:1153. doi: 10.3389/fimmu.2020.01153
72. Yang D, Dai F, Yuan M, Zheng Y, Liu S, Deng Z, et al. Role of transforming growth factor-beta1 in regulating fetal-maternal immune tolerance in normal and pathological pregnancy. Front Immunol (2021) 12:689181. doi: 10.3389/fimmu.2021.689181
74. Bromley SK, Thomas SY, Luster AD. Chemokine receptor CCR7 guides T cell exit from peripheral tissues and entry into afferent lymphatics. Nat Immunol (2005) 6(9):895–901. doi: 10.1038/ni1240
75. Geem D, Ngo V, Harusato A, Chassaing B, Gewirtz AT, Newberry RD, et al. Contribution of mesenteric lymph nodes and GALT to the intestinal Foxp3+ regulatory T-cell compartment. Cell Mol Gastroenterol Hepatol (2016) 2(3):274–80. doi: 10.1016/j.jcmgh.2015.12.009
76. Menning A, Hopken UE, Siegmund K, Lipp M, Hamann A, Huehn J. Distinctive role of CCR7 in migration and functional activity of naive- and effector/memory-like treg subsets. Eur J Immunol (2007) 37(6):1575–83. doi: 10.1002/eji.200737201
77. Kedmi R, Najar TA, Mesa KR, Grayson A, Kroehling L, Hao Y, et al. A RORgammat(+) cell instructs gut microbiota-specific treg cell differentiation. Nature (2022) 610(7933):737–43. doi: 10.1038/s41586-022-05089-y
78. Kulkarni AB, Huh CG, Becker D, Geiser A, Lyght M, Flanders KC, et al. Transforming growth factor beta 1 null mutation in mice causes excessive inflammatory response and early death. Proc Natl Acad Sci USA (1993) 90(2):770–4. doi: 10.1073/pnas.90.2.770
79. Letterio JJ, Geiser AG, Kulkarni AB, Roche NS, Sporn MB, Roberts AB. Maternal rescue of transforming growth factor-beta 1 null mice. Science (1994) 264(5167):1936–8. doi: 10.1126/science.8009224
80. Hippen KL, Loschi M, Nicholls J, MacDonald KPA, Blazar BR. Effects of MicroRNA on regulatory T cells and implications for adoptive cellular therapy to ameliorate graft-versus-Host disease. Front Immunol (2018) 9:57. doi: 10.3389/fimmu.2018.00057
81. Liston A, Lu LF, O'Carroll D, Tarakhovsky A, Rudensky AY. Dicer-dependent microRNA pathway safeguards regulatory T cell function. J Exp Med (2008) 205(9):1993–2004. doi: 10.1084/jem.20081062
82. Zhou X, Jeker LT, Fife BT, Zhu S, Anderson MS, McManus MT, et al. Selective miRNA disruption in T reg cells leads to uncontrolled autoimmunity. J Exp Med (2008) 205(9):1983–91. doi: 10.1084/jem.20080707
83. Johnston CJ, Smyth DJ, Dresser DW, Maizels RM. TGF-beta in tolerance, development and regulation of immunity. Cell Immunol (2016) 299:14–22. doi: 10.1016/j.cellimm.2015.10.006
84. Yasuda K, Takeuchi Y, Hirota K. The pathogenicity of Th17 cells in autoimmune diseases. Semin Immunopathol (2019) 41(3):283–97. doi: 10.1007/s00281-019-00733-8
85. McGeachy MJ, Cua DJ, Gaffen SL. The IL-17 family of cytokines in health and disease. Immunity (2019) 50(4):892–906. doi: 10.1016/j.immuni.2019.03.021
86. Monian B, Tu AA, Ruiter B, Morgan DM, Petrossian PM, Smith NP, et al. Peanut oral immunotherapy differentially suppresses clonally distinct subsets of T helper cells. J Clin Invest (2022) 132(2):e150634. doi: 10.1172/JCI150634
87. Martinez-Blanco M, Lozano-Ojalvo D, Perez-Rodriguez L, Benede S, Molina E, Lopez-Fandino R. Retinoic acid induces functionally suppressive Foxp3(+)RORgammat(+) T cells in vitro. Front Immunol (2021) 12:675733. doi: 10.3389/fimmu.2021.675733
88. Kim BS, Lu H, Ichiyama K, Chen X, Zhang YB, Mistry NA, et al. Generation of RORgammat(+) antigen-specific T regulatory 17 cells from Foxp3(+) precursors in autoimmunity. Cell Rep (2017) 21(1):195–207. doi: 10.1016/j.celrep.2017.09.021
89. Lochner M, Peduto L, Cherrier M, Sawa S, Langa F, Varona R, et al. In vivo equilibrium of proinflammatory IL-17+ and regulatory IL-10+ Foxp3+ RORgamma t+ T cells. J Exp Med (2008) 205(6):1381–93. doi: 10.1084/jem.20080034
90. Ohnmacht C, Park JH, Cording S, Wing JB, Atarashi K, Obata Y, et al. MUCOSAL IMMUNOLOGY. the microbiota regulates type 2 immunity through RORgammat(+) T cells. Science (2015) 349(6251):989–93. doi: 10.1126/science.aac4263
91. Sefik E, Geva-Zatorsky N, Oh S, Konnikova L, Zemmour D, McGuire AM, et al. MUCOSAL IMMUNOLOGY. individual intestinal symbionts induce a distinct population of RORgamma(+) regulatory T cells. Science (2015) 349(6251):993–7. doi: 10.1126/science.aaa9420
92. Stefka AT, Feehley T, Tripathi P, Qiu J, McCoy K, Mazmanian SK, et al. Commensal bacteria protect against food allergen sensitization. Proc Natl Acad Sci USA (2014) 111(36):13145–50. doi: 10.1073/pnas.1412008111
93. Perrier C, Corthesy B. Gut permeability and food allergies. Clin Exp Allergy (2011) 41(1):20–8. doi: 10.1111/j.1365-2222.2010.03639.x
94. Tordesillas L, Gomez-Casado C, Garrido-Arandia M, Murua-Garcia A, Palacin A, Varela J, et al. Transport of pru p 3 across gastrointestinal epithelium - an essential step towards the induction of food allergy? Clin Exp Allergy (2013) 43(12):1374–83. doi: 10.1111/cea.12202
95. Gao Y, Nanan R, Macia L, Tan J, Sominsky L, Quinn TP, et al. The maternal gut microbiome during pregnancy and offspring allergy and asthma. J Allergy Clin Immunol (2021) 148(3):669–78. doi: 10.1016/j.jaci.2021.07.011
96. Wang W, Sung N, Gilman-Sachs A, Kwak-Kim J. T Helper (Th) cell profiles in pregnancy and recurrent pregnancy losses: Th1/Th2/Th9/Th17/Th22/Tfh cells. Front Immunol (2020) 11:2025. doi: 10.3389/fimmu.2020.02025
97. Abelius MS, Ernerudh J, Berg G, Matthiesen L, Nilsson LJ, Jenmalm MC. High cord blood levels of the T-helper 2-associated chemokines CCL17 and CCL22 precede allergy development during the first 6 years of life. Pediatr Res (2011) 70(5):495–500. doi: 10.1203/PDR.0b013e31822f2411
98. Abelius MS, Janefjord C, Ernerudh J, Berg G, Matthiesen L, Duchen K, et al. The placental immune milieu is characterized by a Th2- and anti-inflammatory transcription profile, regardless of maternal allergy, and associates with neonatal immunity. Am J Reprod Immunol (2015) 73(5):445–59. doi: 10.1111/aji.12350
99. Ozdemir C, Akdis M, Akdis CA. T Regulatory cells and their counterparts: masters of immune regulation. Clin Exp Allergy (2009) 39(5):626–39. doi: 10.1111/j.1365-2222.2009.03242.x
100. Li SL, Yu Y, Yang P, Wang H, Zhang C, Liu M, et al. Trichloroethylene alters Th1/Th2/Th17/Treg paradigm in mice: A novel mechanism for chemically induced autoimmunity. Int J Toxicol (2018) 37(2):155–63. doi: 10.1177/1091581818757036
101. Cottrez F, Hurst SD, Coffman RL, Groux H. T Regulatory cells 1 inhibit a Th2-specific response in vivo. J Immunol (2000) 165(9):4848–53. doi: 10.4049/jimmunol.165.9.4848
102. Mortha A, Chudnovskiy A, Hashimoto D, Bogunovic M, Spencer SP, Belkaid Y, et al. Microbiota-dependent crosstalk between macrophages and ILC3 promotes intestinal homeostasis. Science (2014) 343(6178):1249288. doi: 10.1126/science.1249288
103. Arpaia N, Campbell C, Fan X, Dikiy S, van der Veeken J, deRoos P, et al. Metabolites produced by commensal bacteria promote peripheral regulatory T-cell generation. Nature (2013) 504(7480):451–5. doi: 10.1038/nature12726
104. Tan J, McKenzie C, Vuillermin PJ, Goverse G, Vinuesa CG, Mebius RE, et al. Dietary fiber and bacterial SCFA enhance oral tolerance and protect against food allergy through diverse cellular pathways. Cell Rep (2016) 15(12):2809–24. doi: 10.1016/j.celrep.2016.05.047
105. Rodriguez JM, Murphy K, Stanton C, Ross RP, Kober OI, Juge N, et al. The composition of the gut microbiota throughout life, with an emphasis on early life. Microb Ecol Health Dis (2015) 26:26050. doi: 10.3402/mehd.v26.26050
106. Jakobsson HE, Abrahamsson TR, Jenmalm MC, Harris K, Quince C, Jernberg C, et al. Decreased gut microbiota diversity, delayed bacteroidetes colonisation and reduced Th1 responses in infants delivered by caesarean section. Gut (2014) 63(4):559–66. doi: 10.1136/gutjnl-2012-303249
107. Shao Y, Forster SC, Tsaliki E, Vervier K, Strang A, Simpson N, et al. Stunted microbiota and opportunistic pathogen colonization in caesarean-section birth. Nature (2019) 574(7776):117–21. doi: 10.1038/s41586-019-1560-1
108. Sandall J, Tribe RM, Avery L, Mola G, Visser GH, Homer CS, et al. Short-term and long-term effects of caesarean section on the health of women and children. Lancet (2018) 392(10155):1349–57. doi: 10.1016/S0140-6736(18)31930-5
109. Liu Y, Qin S, Song Y, Feng Y, Lv N, Xue Y, et al. The perturbation of infant gut microbiota caused by cesarean delivery is partially restored by exclusive breastfeeding. Front Microbiol (2019) 10:598. doi: 10.3389/fmicb.2019.00598
110. Coker MO, Laue HE, Hoen AG, Hilliard M, Dade E, Li Z, et al. Infant feeding alters the longitudinal impact of birth mode on the development of the gut microbiota in the first year of life. Front Microbiol (2021) 12:642197. doi: 10.3389/fmicb.2021.642197
111. Tunis MC, Marshall JS. Toll-like receptor 2 as a regulator of oral tolerance in the gastrointestinal tract. Mediators Inflamm (2014) 2014:606383. doi: 10.1155/2014/606383
112. LeBouder E, Rey-Nores JE, Rushmere NK, Grigorov M, Lawn SD, Affolter M, et al. Soluble forms of toll-like receptor (TLR)2 capable of modulating TLR2 signaling are present in human plasma and breast milk. J Immunol (2003) 171(12):6680–9. doi: 10.4049/jimmunol.171.12.6680
113. Forsberg A, Abrahamsson TR, Bjorksten B, Jenmalm MC. Pre- and postnatal administration of lactobacillus reuteri decreases TLR2 responses in infants. Clin Transl Allergy (2014) 4:21. doi: 10.1186/2045-7022-4-21
114. Forsberg A, Abrahamsson TR, Bjorksten B, Jenmalm MC. Pre- and post-natal lactobacillus reuteri supplementation decreases allergen responsiveness in infancy. Clin Exp Allergy (2013) 43(4):434–42. doi: 10.1111/cea.12082
115. Abrahamsson TR, Jakobsson T, Bottcher MF, Fredrikson M, Jenmalm MC, Bjorksten B, et al. Probiotics in prevention of IgE-associated eczema: a double-blind, randomized, placebo-controlled trial. J Allergy Clin Immunol (2007) 119(5):1174–80. doi: 10.1016/j.jaci.2007.01.007
116. Li M, Zhang R, Li J, Li J. The role of c-type lectin receptor signaling in the intestinal microbiota-Inflammation-Cancer axis. Front Immunol (2022) 13:894445. doi: 10.3389/fimmu.2022.894445
117. Gringhuis SI, den Dunnen J, Litjens M, van der Vlist M, Wevers B, Bruijns SC, et al. Dectin-1 directs T helper cell differentiation by controlling noncanonical NF-kappaB activation through raf-1 and syk. Nat Immunol (2009) 10(2):203–13. doi: 10.1038/ni.1692
118. Gri G, Frossi B, D'Inca F, Danelli L, Betto E, Mion F, et al. Mast cell: an emerging partner in immune interaction. Front Immunol (2012) 3:120. doi: 10.3389/fimmu.2012.00120
119. Sibilano R, Frossi B, Pucillo CE. Mast cell activation: a complex interplay of positive and negative signaling pathways. Eur J Immunol (2014) 44(9):2558–66. doi: 10.1002/eji.201444546
120. Rael EL, Lockey RF. Interleukin-13 signaling and its role in asthma. World Allergy Organ J (2011) 4(3):54–64. doi: 10.1097/WOX.0b013e31821188e0
121. Li Y, Leung PSC, Gershwin ME, Song J. New mechanistic advances in FcepsilonRI-mast cell-mediated allergic signaling. Clin Rev Allergy Immunol (2022) 63(3):431-446. doi: 10.1007/s12016-022-08955-9
122. Miyake K, Karasuyama H. Emerging roles of basophils in allergic inflammation. Allergol Int (2017) 66(3):382–91. doi: 10.1016/j.alit.2017.04.007
123. Villarino AV, Kanno Y, O'Shea JJ. Mechanisms and consequences of jak-STAT signaling in the immune system. Nat Immunol (2017) 18(4):374–84. doi: 10.1038/ni.3691
124. Zhu J, Yamane H, Paul WE. Differentiation of effector CD4 T cell populations (*). Annu Rev Immunol (2010) 28:445–89. doi: 10.1146/annurev-immunol-030409-101212
125. Bottcher MF, Fredriksson J, Hellquist A, Jenmalm MC. Effects of breast milk from allergic and non-allergic mothers on mitogen- and allergen-induced cytokine production. Pediatr Allergy Immunol (2003) 14(1):27–34. doi: 10.1034/j.1399-3038.2003.02119.x
126. Mennini M, Arasi S, Fiocchi AG. Allergy prevention through breastfeeding. Curr Opin Allergy Clin Immunol (2021) 21(2):216–21. doi: 10.1097/ACI.0000000000000718
127. Lin B, Dai R, Lu L, Fan X, Yu Y. Breastfeeding and atopic dermatitis risk: A systematic review and meta-analysis of prospective cohort studies. Dermatology (2020) 236(4):345–60. doi: 10.1159/000503781
128. Garcia-Larsen V, Ierodiakonou D, Jarrold K, Cunha S, Chivinge J, Robinson Z, et al. Diet during pregnancy and infancy and risk of allergic or autoimmune disease: A systematic review and meta-analysis. PloS Med (2018) 15(2):e1002507. doi: 10.1371/journal.pmed.1002507
129. Halken S, Muraro A, de Silva D, Khaleva E, Angier E, Arasi S, et al. EAACI guideline: Preventing the development of food allergy in infants and young children (2020 update). Pediatr Allergy Immunol (2021) 32(5):843–58. doi: 10.1111/pai.13496
130. Fleischer DM, Chan ES, Venter C, Spergel JM, Abrams EM, Stukus D, et al. A consensus approach to the primary prevention of food allergy through nutrition: Guidance from the American academy of allergy, asthma, and immunology; American college of allergy, asthma, and immunology; and the Canadian society for allergy and clinical immunology. J Allergy Clin Immunol Pract (2021) 9(1):22–43 e4. doi: 10.1016/j.jaip.2020.11.002
131. Danielewicz H. Breastfeeding and allergy effect modified by genetic, environmental, dietary, and immunological factors. Nutrients (2022) 14(15):3011. doi: 10.3390/nu14153011
132. Bottcher MF, Jenmalm MC, Garofalo RP, Bjorksten B. Cytokines in breast milk from allergic and nonallergic mothers. Pediatr Res (2000) 47(1):157–62. doi: 10.1203/00006450-200001000-00026
133. Tomicic S, Johansson G, Voor T, Bjorksten B, Bottcher MF, Jenmalm MC. Breast milk cytokine and IgA composition differ in Estonian and Swedish mothers-relationship to microbial pressure and infant allergy. Pediatr Res (2010) 68(4):330–4. doi: 10.1203/PDR.0b013e3181ee049d
Keywords: micro-RNA, non-coding RNA, dendritic cells, oral tolerance, regulatory T cell, allergy, infant - age, breast milk
Citation: Ahlberg E, Al-Kaabawi A, Thune R, Simpson MR, Pedersen SA, Cione E, Jenmalm MC and Tingö L (2023) Breast milk microRNAs: Potential players in oral tolerance development. Front. Immunol. 14:1154211. doi: 10.3389/fimmu.2023.1154211
Received: 30 January 2023; Accepted: 28 February 2023;
Published: 14 March 2023.
Edited by:
Qiaozhi Zhang, Zhejiang Gongshang University, ChinaReviewed by:
Hirohisa Izumi, Morinaga Milk Industry Co Ltd., JapanCopyright © 2023 Ahlberg, Al-Kaabawi, Thune, Simpson, Pedersen, Cione, Jenmalm and Tingö. This is an open-access article distributed under the terms of the Creative Commons Attribution License (CC BY). The use, distribution or reproduction in other forums is permitted, provided the original author(s) and the copyright owner(s) are credited and that the original publication in this journal is cited, in accordance with accepted academic practice. No use, distribution or reproduction is permitted which does not comply with these terms.
*Correspondence: Lina Tingö, bGluYS50aW5nb0BsaXUuc2U=
†These authors share first authorship
Disclaimer: All claims expressed in this article are solely those of the authors and do not necessarily represent those of their affiliated organizations, or those of the publisher, the editors and the reviewers. Any product that may be evaluated in this article or claim that may be made by its manufacturer is not guaranteed or endorsed by the publisher.
Research integrity at Frontiers
Learn more about the work of our research integrity team to safeguard the quality of each article we publish.