- 1CINBIO, Universidade de Vigo, Inmunology Group, Vigo, Spain
- 2Instituto de Investigación Sanitaria Galicia Sur (IIS Galicia Sur), SERGAS-UVIGO, Vigo, Spain
- 3I3S-Instituto de Investigação e Inovação em Saúde, Universidade do Porto, Porto, Portugal
- 4IBMC-Instituto de Biologia Molecular e Celular, Universidade do Porto, Porto, Portugal
- 5ICBAS-Instituto de Ciências Biomédicas de Abel Salazar, Universidade do Porto, Porto, Portugal
Purpose: The growing incidence and lethality of pancreatic cancer urges the development of new therapeutic approaches. Anti-tumoral vaccines can potentiate the immune response against the tumor, targeting specific antigens expressed only on tumor cells. In this work, we designed new vaccines for pancreatic cancer, composed by chitosan nanocapsules (CS NCs) containing imiquimod (IMQ) as adjuvant, and targeting the K-Ras mutation G12V.
Experimental design: We tested the immunogenicity of our vaccines in mice, carrying different combinations of K-Ras mutated peptides. Then, we analyzed their prophylactic and therapeutic efficacy in mice bearing heterotopic pancreatic cancer.
Results: Unexpectedly, although good results were observed at short time points, the different combinations of our CS NCs vaccines seemed to potentiate tumor growth and reduce survival rate. We propose that this effect could be due to an inadequate immune response, partially because of the induction of a regulatory tolerogenic response.
Conclusion: Our results call for caution in the use of some NCs containing IMQ in the immunotherapy against pancreatic cancer.
1 Introduction
Pancreatic ductal adenocarcinoma (PDAC) is one of the deadliest types of cancer, with poor prognosis due to the difficulty of an early diagnosis (1, 2). Several types of cancer, including PDAC, often accumulate mutations in the KRAS oncogene. The mutations commonly lead to a permanently activation of the protein, which ultimately promotes tumor transformation and proliferation. KRAS mutations are found in around 90% of PDAC cancers, often involving codon 12 (3, 4).
For a long time, mutations in the Ras family have been prioritized as therapeutic targets (5). These approaches include the development of anti-tumoral vaccines that could help in the recognition and further attack against the malignant cells by the immune system. Although vaccines targeting K-Ras mutations had reached clinical trials, they have shown modest benefits (reviewed in (6)). Some strategies have combined K-Ras vaccination with classic chemotherapy or used it after after tumor resection surgery.
The development of new vaccines and adjuvants offers novel possibilities for either therapeutic or preventive cancer vaccines. Nanoparticles have been successfully proven as good vaccine delivery systems due to their properties: 1) they can encapsulate antigens and adjuvants, protecting them from premature degradation; 2) their characteristics at the nanoscale (size, shape, charge) and similarity to pathogens. They can facilitate the uptake by antigen-presenting cells (APCs), allowing good immunogenic properties (7); and 3) they can easily drain to the closest lymph nodes from the administration site, including the tumor-draining lymph nodes (8).
Moreover, the incorporation of additional immunostimulants could improve the vaccine immunogenicity. Imiquimod (IMQ) is used in creams as a drug to treat precancerous growths (like actinic keratosis) and genital warts on adults. IMQ interacts with Toll-like receptor 7 on innate immune cells, inducing the expression of cytokines favoring a Th1-type immune response. IMQ can act as adjuvant in vaccines for infectious diseases (9, 10), but also in anti-tumoral vaccines, increasing the immunogenicity of a tumor-lysate vaccine against T cell lymphoma (11), or melanoma (12), among others. However, some publications reported opposite effects (13), presumably due to the specific induction of immunosuppressive reactions.
In our study, we designed a chitosan-based nanovaccine carrying K-Ras G12V mutated peptides, and IMQ as adjuvant, included in the oily core of the nanocapsule. We assessed its immunogenicity and tested its efficacy either as a preventive or therapeutic vaccine against PDAC in mice. Furthermore, we compared our vaccine with another K-Ras-mutated vaccine in clinical trials, TG02 (from the Targovax company), that incorporates granulocyte macrophage colony-stimulating factor (GM-CSF) as adjuvant, either used alone or in combination with our nanovaccine.
2 Materials and methods
2.1 K-Ras mutated peptide design
Peptides from the K-Ras mutated protein were designed using Immune Epitope Database (IEDB) major histocompatibility complex (MHC) binding prediction tool (http://tools.iedb.org/mhci/), taking into account the class I and class II MHC molecules from the mouse strain to be used (C57BL/6) and the K-Ras mutated sequence (G12V). Peptides with the lowest percentile rank to C57BL/6 were selected for MHC-II (YKLVVVGAVGVGKSA peptide p1, KLVVVGAVGVGKSAL peptide p2) and MHC-I (AVGVGKSAL peptide p3). Mutated K-Ras peptides will be hereafter referred to as Krasm. More information about peptides can be found in Table S1.
2.2 Nanovaccines’ synthesis
Polymeric chitosan nanocapsules (CS NCs) or inulin/polyarginine nanocapsules (INU/pArg NCs) with IMQ (14) as adjuvant were synthetized as described previously (10). Mixture of the NCs with the peptides were prepared just before each usage in a 1:1.5 ratio (polymer:antigen). The mixture was incubated with constant orbital shaking (300 rpm) at room temperature (RT) for one hour to allow antigen adsorption to the polymer surface. In this way, 10 µg of IMQ and 200 µg of peptides were added per dose. Estimated IMQ encapsulation efficiency was 60–70% (10). Peptide absorption on the nanocapsule surface was 50-60%, determined by liquid chromatography–mass spectrometry (LC-MS), and similar to the antigen adsorption previously described for an intranasal vaccine (10). Following the same procedure, antigen not bound to the NCs was not removed from the suspension. Complete CS and INU/pArg nanovaccines containing the peptides will be referred to as CS-Krasm and INU/pArg-Krasm, respectively.
In addition to our CS-Krasm, we also worked with a commercial vaccine, TG02, belonging to the company TARGOVAX ASA (Olso, Norway). It is a cocktail of 8 peptides of 17 amino acids with the K-Ras G12V mutation of interest, apart from other classic mutations of this protein at that point. The peptide sequences are KLVVVGAGCVGKSALTI and KLVVVGAVGVGKSALTI (amino acid indicated in bold can be a C or a D in the first one and A, C, D, R, S or V in the second one (https://patents.google.com/patent/EP3140320B1/en).
2.3 Cell culture
Pancreatic tumor cells ATQ303G were gently provided by Dr. Carmen Guerra and Prof. Mariano Barbacid from Centro Nacional de Investigaciones Oncológicas (CNIO, Madrid, Spain). Cells were generated from PDAC tumors developed by Elastase-tTA; Tet-O-Cre; K-Ras+/LSLG12Vgeo; p53lox/lox mice (C57BL6/129 background) described in (15). Pancreatic cells were cultured in presence of DMEM High Glucose + Glutamax (Gibco; Waltham, MA, USA).
THP-1 and Jurkat cell lines were purchased from the American Type Culture Collection (ATCC) (VA, USA), while human peripheral blood mononuclear cells (hPBMCs) were obtained from three healthy donors by isopycnic or density gradient centrifugation (Ficoll, GE Healthcare; Boston, MA, USA) from whole blood. For these cells, RPMI (Roswell Park Memorial Institute) 1640 culture medium (Corning; Corning, NY, USA) was used.
In all cases, culture medium was supplemented with 10% Fetal Bovine Serum (Sigma-Aldrich; Burlington, MA, USA) and 1% Penicillin-Streptomycin (Gibco). Cells were maintained at 37°C and 5% CO2.
2.4 Apoptotic assay
Cytotoxicity of NCs was determined by flow cytometry using the apoptosis detection kit (Immunostep S.L; Salamanca, Spain) by double labeling Annexin V-FITC and Propidium Iodide (PI). The following cell populations were determined: Annexin V– IP– (live cells), Annexin V+ IP– (early apoptotic cells), Annexin V+ IP+ (late apoptotic cells) and Annexin V– IP+ (necrotic cells).
For this, 100,000 Jurkat or hPBMCs cells/well were seeded in flat-bottom 96-well plates (Costar, Corning). 24 hours later, NCs were added at 50 or 100 µg/mL. After 24 hours of incubation, cells were collected and labeled with Annexin V-FITC and IP according to the protocol described by the manufacturer. Cells were read in the FC500 cytometer (Beckman Coulter, IN, USA) and data analysis was performed using FlowLogic™ software (version 7.1, Miltenyi Biotec; Bergisch Gladbach, Germany).
2.5 xCELLigence assay
ATQ303G cells were seeded at a density of 7,500 cells/well in special 16-well plates with gold electrodes from the xCELLigence® RTCA DP Instrument system (Roche Diagnostics, version 1.2.1). The equipment measures impedance to monitor cell growth and cell death in real time. It transforms the electrical signal into numerical values represented as a Cell Index. Cells were kept in culture until reaching the exponential phase of growth. Once achieved, 100 µg/mL of CS NCs were added. Untreated cells and culture medium alone are used as controls. The Cell Index was normalized to the time of NC addition, and the percentage of cell capacity was normalized with respect to the negative control.
2.6 Hemolysis
Blood from healthy mice or humans was obtained by cardiac or intravenous puncture, respectively, and treated with heparin. Blood was diluted to 3% in PBS (w/v) and 80 µL of this erythrocyte suspension was incubated with 80 µL of the NCs (50 and 100 µg/mL). PBS was used as negative control and 1% Triton X-100 in PBS was used as positive control. After 4 hours of incubation at 37°C, cells were centrifugated at 1000 x gg for 10 min at 4°C. 80 µL of the supernatant were transferred to a new plate to read the optical density (OD) at 558 in the multidetector EnVision (Perkin-Elmer Inc.; Norwalk, CT, EEUU).
The hemolytic percentage was calculated assuming that PBS is 0% and PBS-Triton X-100 is 100% from the following formula “hemolysis (%) = [(OD sample – OD PBS)/(OD Triton – OD PBS)] × 100”. Per the protocol of the American Society for Testing and Materials (ASTM) International E2524-08 hemolysis percentages above 5% were considered positive. Percentages between 5 and 2% are considered as partially hemolytic, while percentages lower than 2% were considered non-hemolytic (16).
2.7 Coagulation assay
It was carried out according to the Method ITA-12 V1.1 protocol (Laboratory NC. Assay Cascade Protocols. Available: http://ncl.cancer.gov/working_assay-cascade.asp) by determining the prothrombin time (PT), the activated partial thromboplastin time (APTT) and thrombin time (TT).
Blood from several healthy donors was obtained by intravenous puncture and in a tube with sodium citrate as an anticoagulant. The plasma was recovered after centrifugation of the blood for 10 min at 2500 x gg and 21°C. 900 µL of pooled plasma were incubated for 30 min at 37°C with 100 µL of the NCs diluted in PBS at three concentrations (25, 50 and 100 µg/mL). PBS was used as a negative control. After the incubation time, the reading was carried out in the Start 4 coagulometer (Diagnostica Stago; Parsippany, NJ, USA) by adding the corresponding coagulation-inducing reagents. Table S2 shows the conditions used.
2.8 Macrophage polarization
The THP-1 monocytic cell line was used for this experiment. 400,000 cells/well were plated in a 48-well plate (Costar) with a final phorbol 12-myristate 13-acetate (PMA, Abcam) concentration of 10 ng/mL for their differentiation into macrophages. After 72 hours, the treatments for specific phenotype differentiation (20 ng/mL of IFN-γ (Miltenyi Biotec) + 5 ng/mL of lipopolysaccharide (LPS) (InvivoGen; San Diego, CA, USA) for the M1 phenotype and 20 ng/mL of IL-4 (Sigma-Aldrich) + 10 ng/mL de M-CSF (Miltenyi Biotec) for the M2 phenotype) were added for 24 hours. Then, 100 µg/mL of CS NCs were added. The culture medium was used as negative control. After 24 hours of the addition of the different treatments, cells were harvested and centrifugated at 1200 rpm for 5 min. Then, cells were resuspended in 10 µL of FcR blocking reagent (Immunostep S.L) and incubated for 15 min at RT. Next, 5 µL of the following antibodies were added: anti- CD206, CD163, HLA-DR, CD86 and CD14 (Supplementary Table S3). Cells were incubated with the antibodies for 30 min at 4°C. Subsequently, they were washed and resuspended in PBS for analysis by flow cytometry (Cytoflex S, Beckman Coulter). Data analysis was performed with the CytExpert analysis program (Beckman Coulter, version 2.3.1.22).
2.9 Mice
Seven-week-old C57BL/6JRj female mice were purchased from Janvier Labs (Le Genest-Saint-Isle, France) (or C57BL/6J imported from Charles River to i3s Animal Facility of Instituto de investigação e inovação em saúde, University of Porto, specifically for one experiment). Mice were maintained under specific pathogen-free conditions at the animal facility of Servizo de Bioexperimentación Universidade de Vigo, Spain (or i3s Animal Facility in one experiment), with constant temperature (20 ± 1°C) and humidity (55 ± 10%). The animals were fed with sterile water and diet water ad libitum under standardized light-controlled conditions (12 h light and dark periods). Mice were acclimatized for at least 1 week before use. Animal experiments were performed with ethical approval from the hosting institutions and according to the national regulations and legislation of that country.
2.10 Vaccination
Mice were vaccinated three times separated 21 days. 100 µL of prepared vaccines were subcutaneously administered per injection in the left flank. Mice vaccinated with our vaccines received a total of 200 µg of the peptide mixture (three peptides), subcutaneously in the left flank. Mice vaccinated with TG02 vaccine received 800 µg of the peptide mixture subcutaneously in the left flank and 2.5×105 U of GM-CSF 15-30 min later.
2.11 Tumor induction and monitoring
Mice were anesthetized with isoflurane and 3×106 cells in PBS: Matrigel (Corning) (1:1 proportion) were injected subcutaneously in the right flank (100 µL). Mice were supervised and weighed every day, and tumor diameter was measured with a caliper. Tumor volume was calculated using the formula “volume = (wide2 × length)/2”.
2.12 IgG antibody specific ELISA
Peptides were coupled to Bovine serum albumin (BSA) protein (VWR; Radnor, PA, USA) to improve their attached to plastic plates and favor the detection of peptide-specific antibodies in ELISA. 2 mM N-(3-Dimethylaminopropyl)-N′-ethylcarbodiimide hydrochloride (Sigma-Aldrich) and 5 mM N-Hydroxysulfosuccinimide sodium salt (Sigma-Aldrich) were added to BSA 1 mg/mL and reacted for 15 minutes at RT. Then, p1, p2 and p3 peptides were added in a 15:1 peptides:BSA ratio (5:1 for each peptide) and reacted for 2 hours at RT.
ELISA plates (Thermo Fisher Scientific; Waltham, MA, USA) were coated with BSA-peptides conjugated for 3 hours at RT. Then, plates were blocked with PBS 1% BSA at 4°C overnight. Diluted serum was incubated for 1 hour at RT. Wells were washed three times with PBS-Tween 0.05% and incubated with secondary antibodies: goat anti-mouse Igs linked to horseradish peroxidase (HRP) (1:5000) (BioRad; Hercules, CA, USA). Wells were washed three times and TMB substrate (3,3’,5,5’-Tetramethylbenzidine) was added for 15 min at RT. Reaction was stopped by adding H2SO4. Optical density (OD) was measured at 450 nm using an Envision Multilabel Plate Reader (Perkin Elmer; Waltham, MA, USA).
To eliminate background noise, the OD value from blank wells (incubated with PBS) was subtracted. OD values from wells coated with BSA but without peptides, were also subtracted from the corresponding OD of peptide-coated wells.
2.13 Tumor infiltrating immune fraction analysis
Mice were sacrificed with CO2 and subcutaneous tumors were extracted. Half tumor was processed to study immune infiltrating fraction. Tumors were cut with a scalpel into pieces of 2-3 mm in diameter and incubated with 1 mg/mL Collagenase (Gibco) at 37°C for 30 min. Then, tumors were homogenized and filtered through a 100 mm nylon mesh cell. Immune fraction was isolated using density gradient Ficoll Premium 1084 (GE Healthcare; Chicago, IL, USA) and centrifuged at 1800 rpm for 30 min at 22°C. Immune cells were washed and stained for flow cytometry.
Tumor infiltrating cells were incubated with Fixable Viability Stain 510 and antibodies anti-CD45, CD3, CD4, CD8, NK1.1 and MHC-II for 30 min at 4°C. Then, cells were fixed and permeabilized using mouse Foxp3 fixation buffer (BD Pharmigen; San Diego, CA, USA) for 30 min 4°C in the dark and permeabilized using mouse Foxp3 permeabilization buffer (BD Pharmigen) 30 min 37°C in the dark. Next, cells were stained with anti-Foxp3 antibody and fixed with paraformaldehyde (PFA) 2% for 30 min at 4°C. Samples were run on an CytoFLEX S flow cytometer (Beckman Coulter; Brea, CA, USA), and data were analyzed using CytExpert (version 2.3.1.22, Beckman Coulter) (or FlowLogic (version 7.1, FlowLogic; UK) for one experiment) software. A list of antibodies used, and references can be found in Table S2.
2.14 Splenocytes analysis
Spleens were homogenized and filtered through a 100 mm nylon mesh cell. Red blood cells (RBC) were lysed with 3 mL of RBC lysis buffer for mouse (Alfa Aesar, Thermo Fisher Scientific) for 4 min. Then, cells were washed with RPMI 1640 (Corning) media supplemented with 10% Fetal Bovine Serum (Sigma-Aldrich) 1% Penicillin-Streptomycin (Gibco) 50 nM β-mercaptoethanol (Sigma-Aldrich) and 10 mM Hepes (VWR).
Splenocytes from tumor-bearing mice were processed and kept in liquid nitrogen to be plated and analyzed simultaneously. Cells were stored in a cryopreserving solution (Fetal Bovine Serum with 10% Dimethyl sulfoxide (Sigma-Aldrich) and kept at –196°C in liquid nitrogen until further use. Live cells were counted and 5×105 splenocytes per well were plated in a 96-well plate. Cells were stimulated with a final concentration of 100 µg/mL of either 3-peptide-mixture or 100 µg/mL of TG02-peptide-mixture at 37°C with 5% CO2 for 2 days.
During the last 4 hours of stimulus, 10 µg/mL of Brefeldin A (Sigma-Aldrich) were added to block Golgi apparatus protein processing. Cells were then centrifuged, and supernatants were kept for cytokine analysis. Splenocytes were incubated with Fixable Viability Stain 510 and antibodies anti-CD3, CD4, CD8, CD25, CD62L and CD44 for 30 min at 4°C. Then, cells were fixed using mouse Foxp3 fixation buffer (BD Pharmigen) for 30 min 4°C in the dark and permeabilized using mouse Foxp3 permeabilization buffer (BD Pharmigen) 30 min 37°C in the dark. Next, cells were stained with anti-Foxp3, -IFN-γ and -IL-4 antibodies in Brilliant Stain Buffer (BD Pharmigen) for 30 min at 4°C. Cells were then washed and fixed with paraformaldehyde (PFA) 2% for 30 min at 4°C. Samples were run on an CytoFLEX S flow cytometer (Beckman Coulter) (or BD FACSCanto™ II for one experiment), and data were analyzed using CytExpert (version 2.3.1.22, Beckman Coulter) (or Flowlogic (version 7.1, FlowLogic; UK) for one experiment) software. A list of antibodies used and references can be found in Table S3.
2.15 Multiplex cytokine assay
Supernatants from splenocyte cultures were used for cytokine detection using the customized MILLIPLEX MAP kit Mouse Th17 Magnetic Bead Panel (GM-CSF, IFN-γ, IL-1β, IL-2, IL-4, IL-6, IL-10, IL-12p70, IL-17A, TNF-α) (Merk; Kenilworth, NJ, USA). Immunoassay was performed following manufacturer’s instructions. Samples were analyzed in triplicates with a MAGPIX reader (Luminex; Austin, TX, USA) and the results were analyzed using the xPonent 4.2 software.
2.16 TNF-α assay for assessing trained immunity.
Peritoneal macrophages were extracted from C57BL/6 mice. Mice were sacrificed with CO2 and 5 mL of RPMI 1640 media (Corning), supplemented with 10% Fetal Bovine Serum (Sigma-Aldrich) and 1% Penicillin-Streptomycin (Gibco), was injected into the peritoneal cavity. The abdomen was gently massaged for 5 minutes to dislodge attached macrophages. Then, peritoneal fluid was recovered using a Pasteur pipette.
5×105 macrophages per well were seeded on 96-well plates and let rest for 2 hours at 37°C 5% CO2. CS NCs were added at 20 µg/mL (or free media) and incubated overnight. Conditions were tested in biological duplicates. Media was replaced every two days. At day 7, macrophages were incubated with 0.1 µg/mL LPS (Sigma-Aldrich) (or free media) for 3 hours. Then, cells were incubated with new media overnight. At day 8, cells were centrifuged and supernatants recovered.
Tumor necrosis factor α (TNF-α) levels were measured using Mouse TNF alpha Uncoated ELISA kit (Invitrogen), following manufacturer’s instructions. TNF-α standard control was used to determine cytokine levels. OD was measured at 450 nm using an Envision Multilabel Plate Reader (Perkin Elmer).
2.17 Statistical analysis
Statistical analyses were performed using the GraphPad Prism 8 software. A Shapiro-Wilk test was carried out to determine the normal distribution of the samples. Statistical tests used are specified in each experiment description.
3 Results
3.1 Characterization of CS-nanocapsules
3.1.1 Physicochemical characterization
CS NCs were designed following the procedure previously described (10), containing IMQ at the oily core. The antigen selected corresponds to a combination of 2 or 3 peptides containing the K-Ras G12V mutation (Supplementary Table S1, Figure S1A). CS NCs, both alone and with incorporated peptides, were shown to be homogeneous with a polydispersity index (PDI) ≤ 0.1, a mean size of 178 nm and positive charge (Table 1).
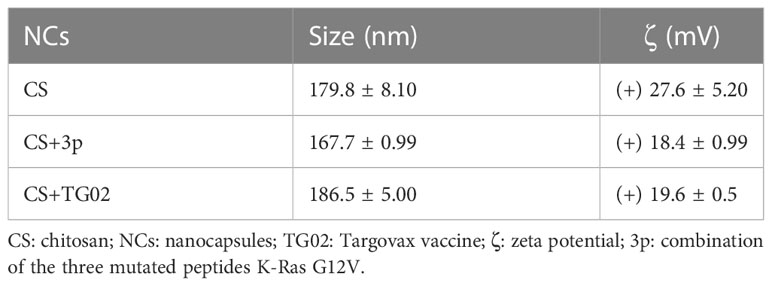
Table 1 Physical-chemical characterization of nanocapsules of CS in the absence and presence of mutated antigenic peptides.
3.1.2 Biological characterization
Previous data indicated that CS NCs were cytocompatible on macrophages and lung epithelial cells and induced the production of reactive oxygen species (ROS) at long incubation times, the release of cytokines (mainly TNF-α and IL-6), and were able to activate the complement system, which could favour the vaccine efficacy (10). Here, the assessment of the cytotoxicity was expanded by studying their potential harmful effect on different immune cells. The Jurkat cell line (immortalized human T lymphocyte cells) and human peripheral blood mononuclear cell (hPBMCs) were incubated with CS NCs at two different concentrations (100 and 50 µg/mL) for 24 hours. As expected, CS NCs showed to be cytocompatible in both cell types at the concentrations tested (Supplementary Figure S1B).
The interaction with blood cells-components was assessed by hemolytic assay and coagulation time. For hemolysis assay, unwashed whole blood was used to mimic the most physiological environment possible (17). In mouse erythrocytes CS NCs did not induce hemolysis (< 2%), except at the highest concentration tested (100 µg/mL), with a hemolytic percentage of 4.75 ± 0.8, indicative of partial hemolytic activity (<5%). These results were similar to the data obtained with other CS NPs (18). The toxic effect of CS NCs was also tested on human blood from three healthy donors. In this case, CS NCs did not reach hemolytic levels at none of the concentrations tested (100 and 50 µg/mL) (Supplementary Figure S1C). As it can be observed in Supplementary Figure S1D, the CS NCs did not induce effects on any of the coagulation times analysed: prothrombin time (PT), activated partial thromboplastin time (APTT) and the thrombin time (TT). This indicates that CS NCs do not interfere with the coagulation cascade.
3.2 Immunization with CS-Krasm induces immunogenic response
To test the immunogenic effect of the vaccines, C57BL/6 mice were vaccinated three times at 21-day intervals. Mouse groups received PBS (control), CS (empty CS NCs), CS+2p (CS NCs containing Krasm peptides p1 and p2) or CS+3p (CS NCs containing the combination of the three Krasm peptides p1, p2 and p3). The vaccines were proven to be safe and not to induce weight loss on mice along the experiment.
Blood was collected 10 days after 2nd and 3rd immunization (Figure 1A) to analyze the existence of specific IgGs that recognize Krasm peptides. No significant differences among groups were found after the two first immunizations (Figure 1B). Nonetheless, specific antibodies were found after the third boost in both CS+2p and CS+3p-vaccinated mice, with significantly higher levels in the group receiving CS+3p (Figure 1C).
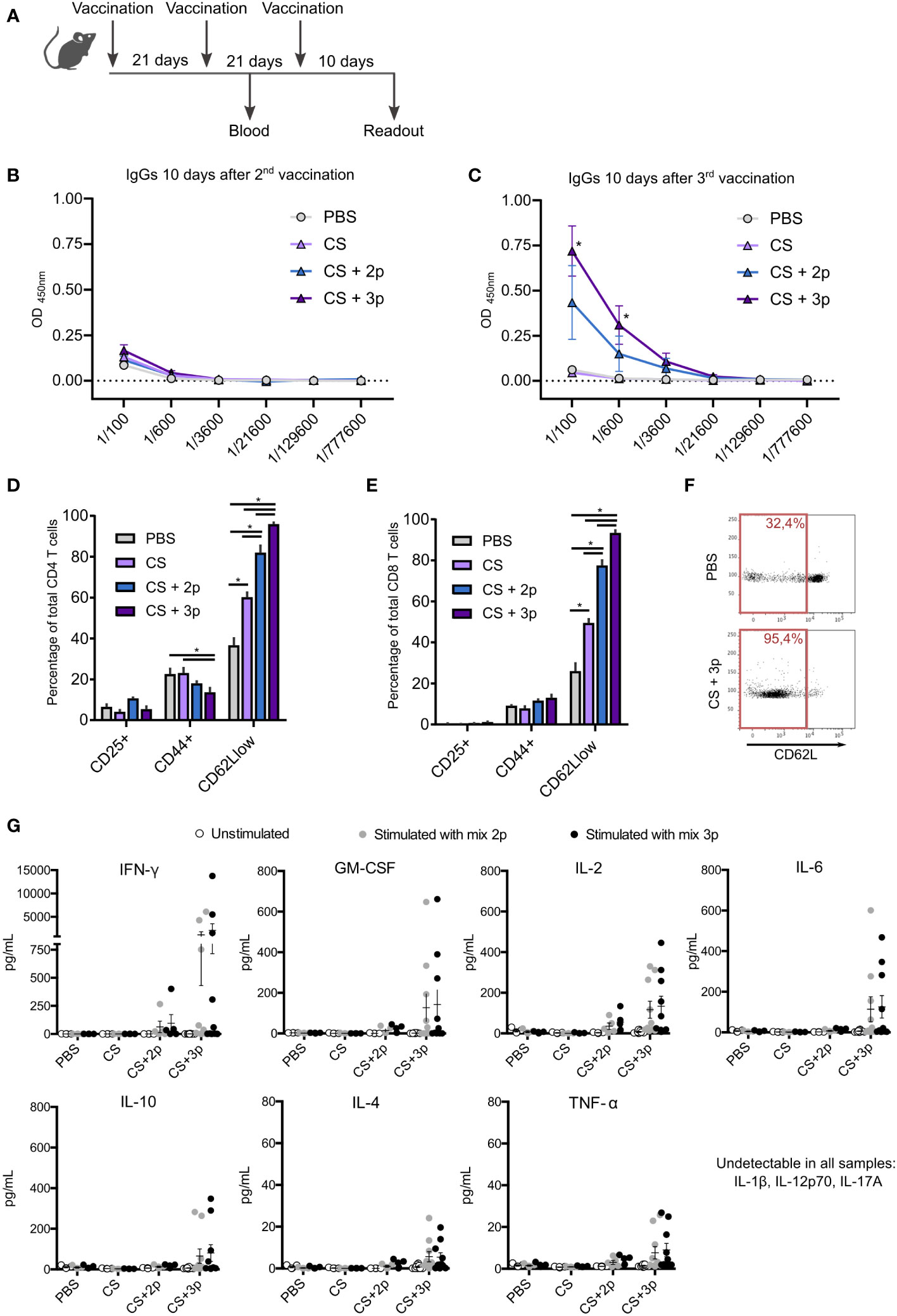
Figure 1 Characterization of the immune response generated by CS-Krasm vaccines. (A) Vaccination regimen is depicted. C57BL/6 mice 8-weeks old were vaccinated three times at 21-day intervals (n=5-10 per group). Blood was collected 10 days after 2nd and 3rd immunization. Ten days after 3rd immunization, mice were sacrificed, and spleens were collected. The levels of specific IgGs that recognize a mixture of Krasm peptides (peptide 1, peptide 2 and peptide 3) were determined by ELISA immunoassay using serum collected ten days after the 2nd (B) and 3rd (C) immunization. Splenocytes were cultivated for 72 hours with the 3-peptides mixture. Then, lymphocyte biomarkers were evaluated by flow cytometry in CD4 T (D) and CD8 T cells (E). Kruskal–Wallis and Dunn’s multiple comparison test were used for statistical analysis. *p < 0.05. (F) Representative example of the flow cytometry dot plot showing CD62L expression in PBS-vaccinated mice and CS+3p-vaccinated mice, with their respective percentages of CD62L low-expressing cells from ancestor gate. (G) Splenocytes were left unstimulated or incubated with either the 2-peptides mixture (peptide 1+ peptide 2) or the 3-peptides mixture (peptide 1 + peptide 2 + peptide 3) for 72 hours. Supernatants were collected and levels of cytokines GM-CSF, IFN-γ, IL-2, IL-4, IL-6, IL-10, TNF-α, IL-1β, IL-12p70 and IL-17A were measured using a multiplex cytokine assay.
After their incubation with the 3-peptides mixture, T cells obtained from mice vaccinated with CS+2p or CS+3p showed a higher percentage of CD62Llow lymphocytes, indicative of effector T cells, in both CD4+ and CD8+ subtypes (Figures 1D–F). Intriguingly, CD4+ cells had a lower proportion of activated CD44+ cells elicited by groups CS+2p and CS+3p (Figure 1D). No differences among vaccination groups were found in the percentage of CD25+ T lymphocytes. Cells were also incubated with the individual peptides or left unstimulated, but no remarkable differences were found among culture conditions (Supplementary Figure S2).
Cytokine levels were measured in supernatants of cultured splenocytes stimulated with either the mixture of peptides p1+p2, or the mixture of the three peptides. Mice vaccinated with CS+3p produced the highest levels of cytokines, including GM-CSF, interferon-γ (IFN-γ), interleukin (IL)-2, IL-4, IL-6, IL-10 and tumor necrosis factor-α (TNF-α) (Figure 1G). Markedly lower levels of these cytokines were detected in the supernatants of splenocytes from mice vaccinated with the prototype CS+2p. The cytokines IL-1β, IL-12p70 or IL-17A were not detected in any case. Moreover, unstimulated cells generated no detectable levels of cytokines in any analyzed group.
Overall, our results demonstrate that both prototypes CS+2p and CS+3p induced in C57BL/6 mice the production of antibodies recognizing the Kras-mutated peptides and an immunogenic cellular response. However, prototype CS+3p seemed to induce the best immunogenic response.
3.3 Prophylactic effect of CS-Krasm vaccines
Based on the immunogenic evaluation, we selected the vaccine candidate CS+3p to test its possible preventive anti-tumoral effect and TG02 (from Targovax company) as control vaccine. As mentioned above, the TG02 vaccine contains a mixture of mutated peptides in combination with GM-CSF. We aimed to compare the anti-tumoral effect of our vaccine with that one induced by TG02, but also to test its efficacy combined with our CS nanoparticle formulation. Thus, several mouse groups followed different vaccination regimens, receiving either PBS, CS, CS+3p, TG02, CS+TG02 or CS+3p+TG02 (Supplementary Figure S3A). Eight days after vaccination, the pancreatic tumor cells were injected in one flank, and tumor growth was daily monitored. Mice were sacrificed following ethical endpoints.
The first 30 days after tumor injection, all vaccination groups showed the same survival rate (Supplementary Figure S3B). Between days 40 and 50, the percentage decreased in all groups, except for mice vaccinated with the combination CS+3p+TG02. This group remained with a 100% of viability for over 60 days.
We continued monitoring tumor growth until day 110 after tumor injection. At the end of the experiment, we observed that the highest survival ratios were obtained in the TG02-vaccinated group, whereas the remaining groups reached less survival than the control group. We analyzed for the presence of specific IgGs directed against Krasm peptides in blood at the moment of sacrifice. Nonetheless, no significant differences were found among vaccination groups (Supplementary Figure S3C).
The systemic immune response was also assessed through the culture of splenocytes, collected at the endpoint-sacrifice. Cells were cultured with antigens for 48 hours and further evaluated by flow cytometry. In this case, no phenotypic changes in the spleen immune populations were found among the different groups (Supplementary Figure S3D).
3.4 Therapeutic CS-Krasm vaccines do not prevent tumor growth, but rather favor it
We assessed whether the vaccines would have any beneficial effects when administered after tumor induction. This setting simulates better the most plausible scenario in the clinic: the administration of a therapeutic vaccine after tumor diagnosis.
Pancreatic tumor cells were subcutaneously injected and, when tumors reached a volume of 100-150 mm3, the first vaccine dose was administered. Mice were vaccinated following the same regimen with either PBS, CS, CS+3p, TG02 or CS+3p+TG02 (Figure 2A; Supplementary Figure S4).
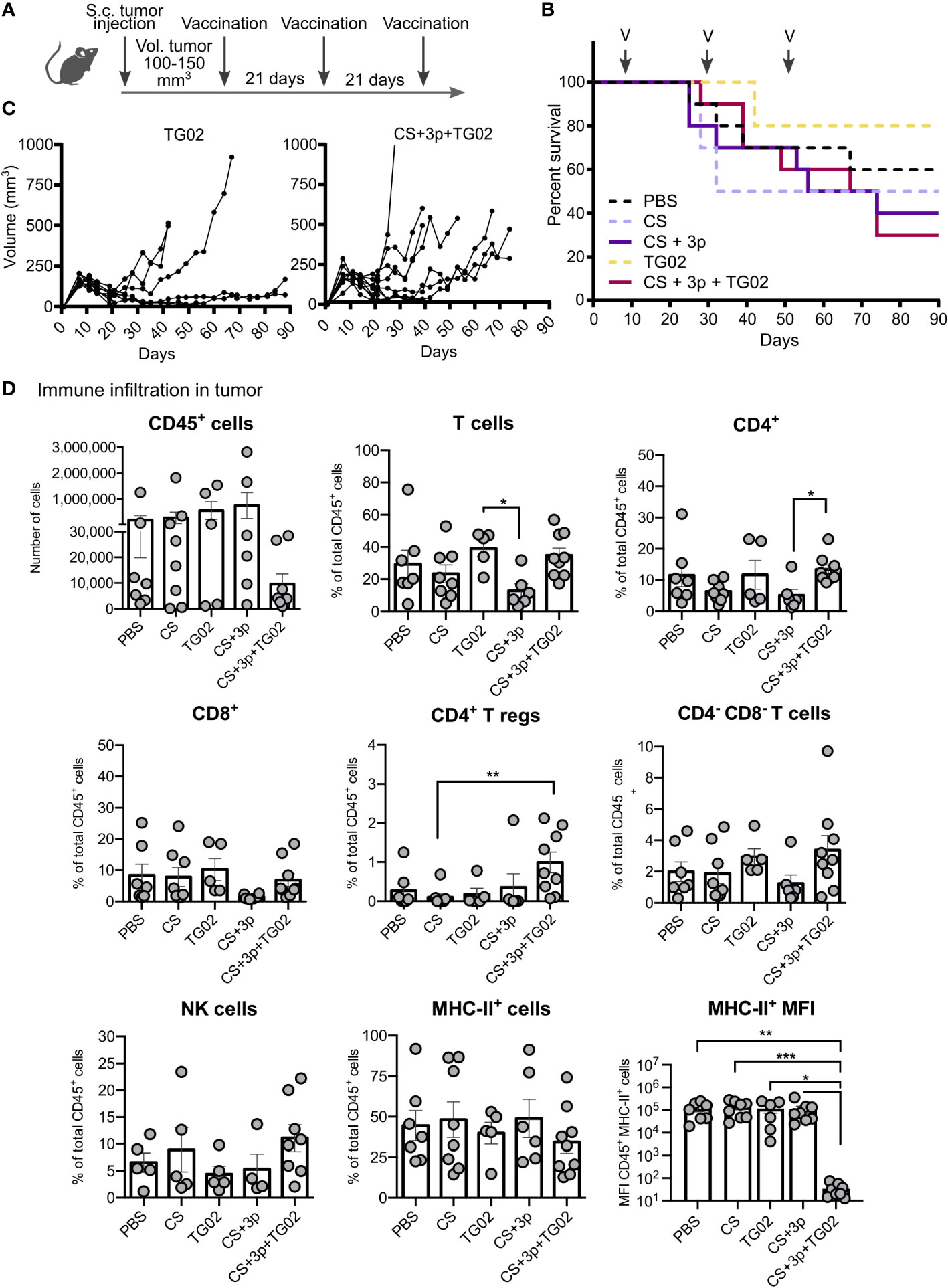
Figure 2 Effect of CS-Krasm used as therapeutic-vaccination in tumor-bearing mice. (A) Experimental protocol is depicted. C57BL/6 mice 8-weeks old were subcutaneously injected ATQ303G pancreatic cells in the left flank. When tumors reached a volume of 100-150 mm3, mice were vaccinated three times at 21-days intervals (n=10 per group). Blood was collected ten days after 2nd and 3rd immunization. Whenever mice reached their ethical time-point, mice were sacrificed, with blood and spleens collected. (B) Mice percentage of survival from day 0 (tumor injection) to 90. Vaccination days are indicated with an arrow and a V. (C) Tumor volume of TG02 and CS+3p+TG02 -vaccinated groups during the experiment. (D) Analysis of the immune infiltrate in tumors by flow cytometry. Data is represented in terms of total number of cells (CD45 biomarker), in percentage of total CD45+ leukocyte fraction or representing median fluorescence intensity (MFI) values for marker MHC-II in CD45+MHCII+ population (MHC-II MFI graphic). Kruskal–Wallis test and Dunn’s multiple comparison test were used for statistical analysis. *p < 0.05; **p < 0.01, ***p < 0.001.
The first 20 days after tumor injection, all vaccination groups shared the same survival rate (Figure 2B). Around day 40, TG02 and CS+3p+TG02 groups displayed again the best survival percentages. Nonetheless, by day 90, animals receiving CS, CS+3p and CS+3p+TG02, accumulated less survival than the control PBS-vaccinated group. We also observed an enhancement of the tumor-growth in these groups, especially from day 40 and later (Figure 2C).
We analyzed the immune cell infiltrates in those tumors by flow cytometry. Tumors displayed heterogeneous levels of immune cells (CD45+), regardless their vaccination group (Figure 2D). Attending to the diverse immune subtypes, no major differences were found, excepting for an increment on T regulatory cells (Tregs) (CD4+ Foxp3+) and on the total percentage of CD4+ T cells in the group receiving the combined vaccine CS+3p+TG02. Although no differences among groups were observed in the percentage of immune cells expressing MHC-II, we also analyzed the expression levels of that marker. By comparing the median fluorescence intensity of MHC-II in the CD45+ MHC-II positive population, we discovered that group CS+TG02+3p had statistically significant lower MHC-II expression compared to the other vaccination groups.
The analysis of the systemic immune response generated by those vaccines in mice-bearing tumors showed that specific anti-Krasm IgGs in the serum were only found 10 days after the 3rd boost, mostly in the CS+3p+TG02 group (Supplementary Figure S5B), although their levels were not statistically significant compared with those obtained in the other groups. After the 2nd boost or at sacrifice time (Supplementary Figures S5A–C, respectively), no increment in the level of anti-Krasm specific IgGs were detected. Moreover, no differences in the spleen immune T cell populations were detected after incubation with antigens for 48 hours (Supplementary Figure S5D).
3.5 In vitro effect of CS NCs on pancreatic tumoral cells and innate immune cells
Our CS-Krasm vaccines containing IMQ as adjuvant, not only failed to prevent or reduce tumor growth in vivo, but they seemed to promote it. This effect was associated with a detected increase in the percentage of tumor infiltrating Tregs in the mouse group vaccinated with CS+3p+TG02.
We attempted to decipher the mechanisms that could be acting to promote this in vivo tumor growth. We studied the effect of CS NCs on the mouse tumoral pancreatic ATQ303G cell line (K-Ras G12V and knock out for p53), using the xCELLigence system. We found an increase in the Cell Index during 50-72 hours of incubation, in both untreated and incubated with CS NCs cells, being maintained for approximately another 24 hours (Figure 3A). After 5 days, tumoral cells treated with CS NCs had less viability than untreated cells, pointing towards a cytotoxic effect of the CS NCs on the tumoral cells.
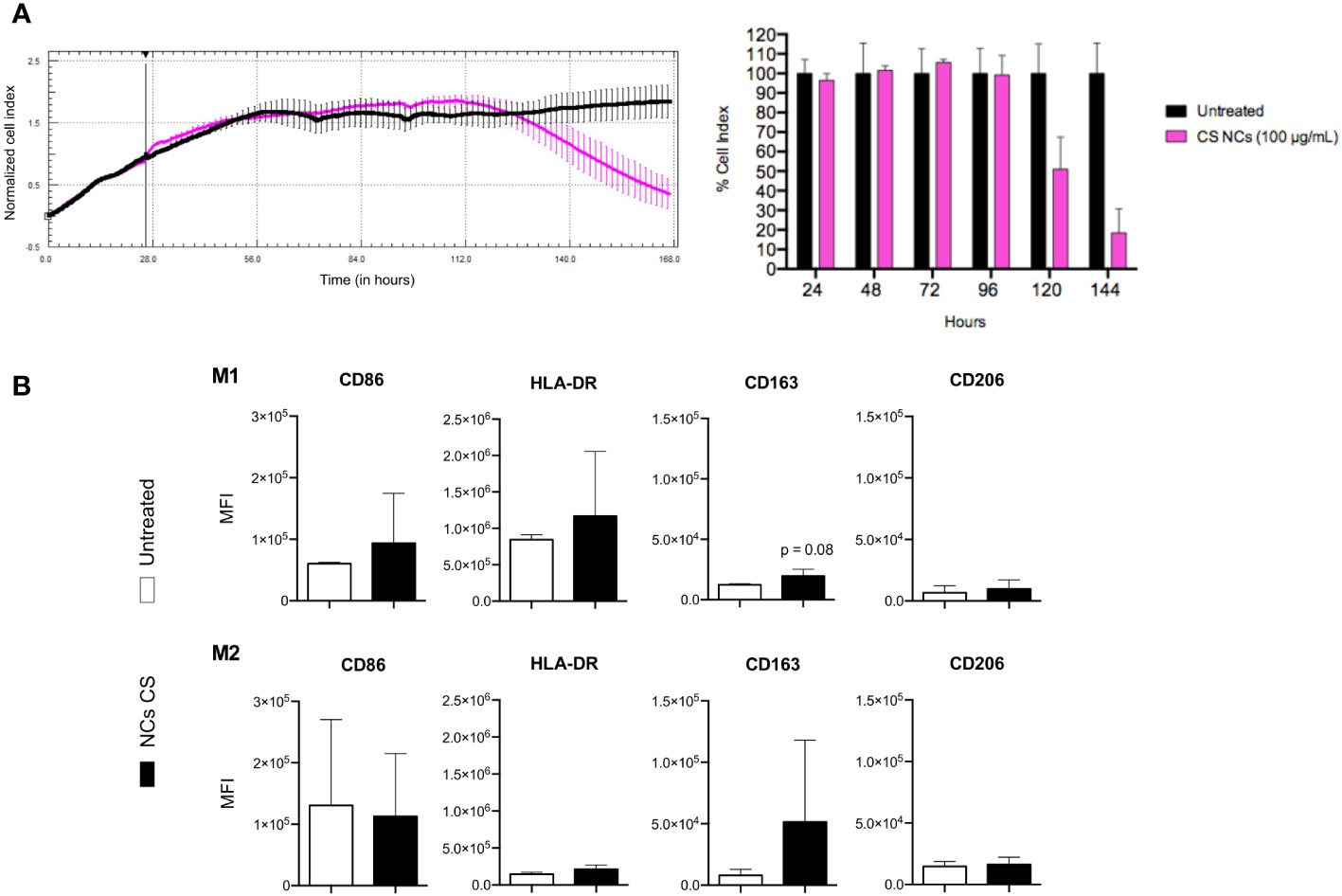
Figure 3 Effect of CS NCs in tumor cells in vitro. (A) Kinetics of the normalized cell index of ATQ303G in the presence of the CS NCs (left) and the cell index quantification (right). Untreated cells (black color) were used as control. (B) Modulation of marker expression on THP-1 cells differentiated to M1 and M2 after incubation with RMPI culture medium (white color) or CS NCs (black color) at 100 µg/mL for 24 h. The values are normalized to those obtained for the differentiated M1 and M2. For differentiation to M1, THP-1 cells were incubated for 48 h with 20 ng/mL IFN-γ + 5 ng/mL LPS + 10 ng/mL of GM-CSF, while for M2 they were incubated with 20 ng/mL IL-4 + 10 ng/mL M-CSF.
Then, we decided to study the potential involvement of the innate cell population. Tumor immune microenvironment is known to be extremely important in a tumor’s fate. Diverse signals and materials can modulate, for instance, the macrophage phenotype, polarizing them into M1 (pro-inflammatory - anti-tumoral phenotype) or M2 type (anti-inflammatory - repair - pro-tumoral phenotype).
We studied the effect of CS NCs on previously differentiated and polarized M1 or M2 macrophages, analyzing the expression of some markers mainly associated to M1 (Major histocompatibility complex), or M2 profiles [CD163 is a scavenger receptor used as a typical marker for M2 macrophages (19)]. We observed a tendency of a higher expression of the CD163 marker in both types of polarized macrophages (Figure 3B). No prominent differences in other markers were found.
We asked then whether CS NCs could be inducing mouse innate trained immunity, but in this case by modulating tolerance (favoring therefore tumor growth). We performed an in vitro assay using peritoneal macrophages collected from C57BL/6 mice. We incubated the macrophages overnight with CS nanocapsules, and after 7 days, the cells were exposed to LPS to assess their pro-inflammatory response through TNF-α quantitation (Supplementary Figure S6A). Macrophages previously incubated with CS NCs produced slightly lower levels of TNF-α (363.23 pg/mL mean) than naïve macrophages (434.24 pg/mL mean), but differences were not statistically significant (Supplementary Figure S6B). We verified that all samples shared similar levels of cell viability at the final time-point (Supplementary Figure S6C). Thus, the hypothesis that CS nanocapsules could induce tolerance through innate trained immunity was not confirmed by the in vitro approach.
The pro-tumoral effect induced by the CS NCs nanovaccines seemed to appear mostly in vivo, either used before and after tumor induction. No clear evidence was found in this study about the mechanisms underlying this effect, except that CS NCs are promoting an immunosuppressive response, including an augment of T regulatory cells within the tumor environment in the therapeutic vaccination with CS+TG02+3p or that CS-NCs are inducing a slight promotion of the macrophage polarization towards M2 phenotype.
3.6 Pro-tumorigenic effect of prophylactic inulin/polyarginine-Krasm vaccine
To assess whether the observed effects were due to the polymeric composition of the CS-NCs, we replaced the polymeric composition of the nanocapsules by designing another nanovaccine based on inulin and polyarginine shell (INU/pArg NCs). Both polymers have been proved to be biocompatible, safe and with adjuvant properties (20, 21). The complete INU/pArg nanovaccine also carried IMQ adjuvant in the lipidic core and antigens (our Krasm peptides or the TG02 peptides) adsorbed on the surface (Supplementary Figure S7A).
The INU/pArg NCs were characterised (Supplementary Table S5), but they proved to be less cytocompatible and hemocompatible than CS NCs (Supplementary Figures S7B–D). However, they demonstrated some immunogenic properties, based on their induction of ROS and secretion of cytokines (mainly INF-γ) (10).
Then, we tested the prophylactic effect of the INU/pArg-Krasm vaccines. Mice received three doses of either PBS, INU/pArg alone, INU/pArg+3p, INU/pArg+TG02 or INU/pArg+3p+TG02. After eight days, tumors were induced following the same scheme depicted in Supplementary Figure S3A. In this case, mice vaccinated with the INU/pArg nanocapsules (without peptides) displayed the lowest survival rates, with all individuals deceased by day 66 (Supplementary Figure S6E). Again, all the remaining vaccinated groups had less survival percentage than the control group (receiving PBS) by the end of the experiment.
These results indicate a detrimental effect of IMQ adjuvant in PDAC cancer vaccines. The pro-tumoral effect must be induced systemically, probably involving the immune response, as the in vitro incubation of the tumor cell line with INU/pArg NCs displayed a clear anti-tumoral effect (Supplementary Figure S6F).
4 Discussion
PDAC is a highly aggressive and lethal cancer with poor prognosis, with a growing incidence in many countries. The poor outcome is due to several factors like a delay in diagnosis (impending surgery), but also to some special PDAC characteristics including low vascularization and dense stroma and immune-suppressor microenvironment. These altogether lead to multidrug resistance capacity. There is a clear urgency for the development of new and effective therapies, as it is estimated that pancreatic cancer will double its associated mortality in the next 40 years (22).
In this work, we designed a new cancer vaccine that targets the K-Ras protein mutated at the G12V position, composed by CS nanocapsules with IMQ as adjuvant. The underlying rationale was that CS nanoparticles had previously showed good results as vaccines in several anti-tumoral therapies, especially as drug-delivery systems (23–25). Moreover, IMQ is widely used as an immunostimulant in skin cancer treatments.
We first assessed the effect of our vaccines in healthy mice. Mutations in the 12 codon of K-Ras have been described to induce specific CD8+ and CD4+ T-cell immune responses (26, 27). We designed three peptides of K-Ras containing G12V mutation to be presented in either MHC class I or class II molecules. We tested two peptide combinations: CS+2p, containing peptides p1 and p2, that should be presented via class II MHC, and CS+3p, containing three peptides (p1, p2 and p3) designed to be presented by both MHC class I and II. Both vaccines incremented the percentage of effector CD4+ and CD8+ T lymphocytes cells, losing the expression of the CD62L marker. Importantly, the vaccines also induced specific antibodies targeting the K-Ras G12V mutation. In both cases, better results were achieved with the combination of the three peptides (CS+3p group).
Furthermore, splenocytes of mice vaccinated with CS+3p secreted higher levels of some Th1 pro-inflammatory cytokines such as IFN-γ, GM-CSF, IL-2 or TNF-α, following antigen stimulation. However, we also reported the secretion of some immunomodulatory Th2 cytokines, including IL-4 or IL-10, and IL-6.
After the promising in vitro results, especially with the CS+3p combination, we assessed the tumor efficacy in vivo. We first tested its potential as a prophylactic vaccine. Currently, in the oncology field, there are only two preventive vaccines authorized for clinical use: one against papillomavirus-associated cervical cancer (28) and another against hepatitis B virus-associated hepatocellular carcinoma (29).
At short term, our preventive vaccination with the CS+3p prototype was successful, achieving a good survival rate (>60%) up to 45 days after tumor induction. However, when the study was prolonged beyond this time, there was an increase in mortality of all groups immunized with CS NCs. In these mice, we did not find K-Ras peptide-specific IgG antibodies in the blood, probably because their disappearance with time. We could not find neither any differences in the immune splenocyte populations after antigen stimulation in vitro.
No therapeutic efficacy associated with vaccination was observed neither when the tumors pre-existed at the beginning of the immunization regimen. Again, all groups vaccinated with CS NCs showed less survival rates than the PBS control group, having the CS+3p+TG02 combination the worst long-term outcome. It is worthy to indicate that we found an increase on the percentage of tumor-infiltrating Tregs in this group of mice receiving CS+3p+TG02, although no relevant differences in the immune populations were observed in the spleen.
Despite our encouraging first results, our vaccines seemed to induce a pro-tumoral effect in PDAC tumor-bearing mice, especially in the long-term, similarly to what it has been described for another K-Ras vaccine that favored tumor growth in skin cancer (in this case with a G12R mutation) (30). In this work, tumors grew faster and became much larger in the vaccinated mouse group. The authors could not clarify the mechanisms underlying this proliferation, but they proposed that an insufficient immune response could be stimulating the tumor growth, rather than inhibiting it (31, 32).
In our case, the increase of Tregs infiltrating the tumors of CS+3p+TG02-vaccinated mice could cause an immunosuppressive and tolerogenic effect helping tumor progression. The increase of Tregs after immunization with peptide vaccines together with cytokines (GM-CSF or IL-2), was also described in patients with different types of cancer carrying a mutated Ras (33). It is described that Tregs play a significant role in self-tolerance as well as in so-called immunosurveillance (34, 35). In addition, they are a fundamental part of the immunosuppressive microenvironment which is a distinctive trait of many cancers including PDAC (36). Moreover, this agrees with the observed high IL-10 production, the main cytokine produced by Tregs.
The second significant finding was the decrease of MHC-II expression, but not a decrease in the number of MHC-II-positive cells. An important mechanism of tumor scape is to impede maturation of dendritic cells (DCs), being immature DCs characterized by a lower expression of MHC class II molecule (37). Our observations suggest that vaccination with CS+3p+TG02 could be favoring the accumulation of immature DCs in the tumor environment, which might be contributing to a tolerogenic immune response. However, further analysis should be performed to characterise the antigen-presenting cells within the tumor and their functions.
Chitosan nanoparticles have been previously proven by our group and others to be good candidates in anti-pathogen vaccine designs (10, 38) or as a drug delivery system in cancer therapy (reviewed in (39)). However, their direct effects on tumor cells have been controversial, with several works reporting either anti (25, 40, 41) or pro-tumorigenic properties (42). Some of these pro-tumoral effects could be through their capacity to activate complement, promoting tumorigenesis and cancer progression (43). Despite the variability found by the cell differentiation model itself, it could be indicated that CS NCs favour an anti-inflammatory environment. Various studies corroborate our results, showing how CS in different forms is capable of inducing a polarization of macrophages towards an anti-inflammatory M2 phenotype (44, 45, 46).
In our hands, the in vitro incubation of tumoral cells with the CS NCs or their individual components did not induce significant tumoral growth at long-term. Although a certain increase in tumor growth and viability was observed within the first 48 hours, it is unlikely to affect the in vivo assay, since the nanovaccine was injected at the opposite flank of the tumor. Furthermore, in both in vivo experiments (prophylactic or therapeutic), we observed differences depending on the type of peptides (+3p, +TG02 or +3p+TG02) included on the CS NCs, pointing towards the specific action of the immune response. As far as we know, this is the first time that CS NCs have been tested in a pancreatic cancer vaccine.
An important factor to reckon in this prototype is the use of mutated self-antigens, instead of foreign antigens from infectious pathogens (10). This, altogether with a small peptide size and an insufficient immunostimulation, could deviate the immune response towards a tolerogenic state, as proposed by Siegel et al. (30). We also do not know whether the length of the peptides (9-15 mers) and their quantity, could affect antigen presentation (intracellular loading or direct binding) and how that affects to the subsequent immune activation.
We have previously described that CS NCs loaded with IMQ, but not empty CS NCs, induce the production of IL-10, IL-6 and TNF-α in mouse peritoneal macrophages in a dose dependent manner (9). These three cytokines were also found in the supernatant of splenocytes from those mice vaccinated with the CS+3p combination, along with other cytokines. It has been described that under specific circumstances, IL-6 and IL-10 could favor cancer development (47). We wondered then whether the pro-tumorigenic effect might be shaped by IMQ.
When we replaced the polymeric CS shell of the nanovaccine for INU/pArg, maintaining IMQ inside the core, immunization with our preventive vaccines also enhanced the subcutaneous tumor growth. These results support the hypothesis that the pro-tumoral effect might be influenced by IMQ. However, IMQ has been described to induce anti-tumoral effects when directly used (48–50) or loaded inside some nanocapsules (51), and it shows good immunogenic properties as vaccine adjuvant (11, 12). Nevertheless, it has also been observed that it could produce the opposite effect by favouring the production of IL-10 and Treg cell stimulation (13). This, altogether with our results, exposes a warning for the use of IMQ for cancer therapies, although further studies are needed to understand completely its mechanism of action. It is also important to perform experiments at short and long term (>60 days), because, as shown in this work, an anti-tumoral effect induced by a vaccine at short time, can be modified to generate negative effects months later.
Overall, the presence of Tregs infiltrating the tumor, the reduction of MHC-II expression, as well as the slight polarization of the macrophages in vitro towards an anti-inflammatory phenotype, support that our vaccines may exert a tolerogenic effect. However, Tregs were only found in one of the CS NCs-vaccinated group.
In the immunogenicity study using healthy mice, our vaccines seemed to induce a good immune response in both, lymphocyte cell activation and generation of specific antibodies recognizing mutated K-Ras, shortly after immunization. Thus, immunization with our vaccines did not directly induce T cell tolerance, but it may lead to a long-term insufficient response that promotes tumor growth. Because of that, we propose that the vaccine could be producing a dual effect, very much affected by the tumor environment. This idea is supported by the facts that in tumor-bearing mice vaccinated with CS+3p, less specific antibodies, decreased MHC-II expression and more Tregs infiltrating the tumor were found.
The mechanisms underlying the immunosuppression induced by our NCs in the pancreatic tumor remain to be solved. We propose that the effect might be driven by a combination of factors, being IMQ an important participant.
Our results open the way to further explore many factors that could contribute to this pro-tumorogenic results. We mainly focused on the lymphocyte response (activation, surface markers and specific antibody production), but there are other cell populations with the ability to induce tolerance in vivo (e.g., immature dendritic cells, myeloid derived suppressor cells) that remain to be characterised in further detail. If other concentrations of antigen or adjuvants will affect the outcome, also remain as appealing and unsolved questions. New prototypes combining CS or INU/pArg nanocapsules with different adjuvants (such as Poly I:C, MPLA, AS04, CpG oligonucleotides or GM-CSF) could be tested to clarify the adjuvant contribution to the tolerogenic situation. The effect of our prototypes in other types of cancers carrying G12V KRAS mutations also remains to be tested, maybe leading to a better prognosis in less immunosuppressive environments. This could help to understand why PDAC is so special regarding its absence of response to many different drugs or to the monoclonal antibodies against the immune check point inhibitors. Moreover, the ability of CS NCs to polarize macrophages into M2 phenotype must be an issue of concern in the design of future anti-tumoral vaccines.
5 Conclusions
Our prophylactic vaccine against pancreatic cancer (based on CS NCs, IMQ and containing specific mutated K-Ras peptides at G12V position), showed certain efficacy at short time. However, at long-term it showed detrimental pro-tumorigenic effects, used either as a preventive or therapeutic treatment. Another anti-K-Ras vaccine, TG02, using GM-CSF as adjuvant, showed the best survival data in both cases. We observed that our NCs exerted a tolerogenic effect by inducing a slight re-polarization of pro-inflammatory macrophages M1 towards an anti-inflammatory M2 phenotype and an increment on the tumor infiltrating Tregs in some mice. Tumor growth also increased after vaccination with similar NCs based on INU/pArg, which included IMQ as adjuvant too.
Although we could not completely decipher yet the mechanisms that promoted the tumor growth, the observations call for caution in the use of IMQ and CS or INU/pArg nanocapsules in PDAC cancer therapy.
Data availability statement
The raw data supporting the conclusions of this article will be made available by the authors, without undue reservation.
Ethics statement
The studies involving human participants were reviewed and approved by CEEA Xunta de Galicia, code 2018/269. The patients/participants provided their written informed consent to participate in this study. The animal study was reviewed and approved by CEEA Xunta de Galicia, code ES360570215601/18/INV MED 02/CANC.[3]/C. Experiment done at i3S was approved by the institutional board responsible for animal welfare (ORBEA) and authorization was issued by the competent Portuguese authority (DGAV), code 014036/2019-07-24.
Author contributions
AM-P, LD-G and AC performed the experiments and analyzed the data. MV and AC provided counselling. RS-V and AG-F conceived the study and contributed with funding acquisition. AM-P wrote the paper with input from all other authors. All the authors have read and approved the manuscript.
Funding
This work was supported by the government of Spain (BIO2017-84974-R), Xunta de Galicia (Grupo de Referencia Competitiva [ED431C 2020/02]), and Centro singular de investigación de Galicia accreditation funded by European Regional Development Fund (ERDF)-[ED431G2019/06]. AM-P and LD-G acknowledge fellowships from Xunta de Galicia Programa de axudas á etapa predoutoral (Consellería de Cultura, Educación e Ordenación Universitaria) ED481A-2018/230 and ED481A-2018/294.
Acknowledgments
The authors thank Targovax company for kindly providing the TG02 peptide mixture, and Carmen Guerra and Mariano Barbacid (CNIO, Spain) for the donation of the ATQ303G pancreatic cell line. We also thank Mercedes Peleteiro (Flow Cytometry Core, CINBIO) for her constant support and guidance in flow cytometry.
Conflict of interest
The authors declare that the research was conducted in the absence of any commercial or financial relationships that could be construed as a potential conflict of interest.
Publisher’s note
All claims expressed in this article are solely those of the authors and do not necessarily represent those of their affiliated organizations, or those of the publisher, the editors and the reviewers. Any product that may be evaluated in this article, or claim that may be made by its manufacturer, is not guaranteed or endorsed by the publisher.
Supplementary material
The Supplementary Material for this article can be found online at: https://www.frontiersin.org/articles/10.3389/fimmu.2023.1153724/full#supplementary-material
Supplementary Figure 1 | Physicochemical and biological characterization of CS-nanoparticles. (A) Polymeric nanovaccine prototype against the mutated protein K-Ras G12V. (B) Cellular apoptosis assay after 24 hours of incubation with CS NCs at 50 and 100 µg/mL in Jurkat and hPMBCs. One-way ANOVA and Dunnett’s multiple comparison tests were used for statistical analysis. (C) Percentage of hemolysis induced by CS NCs in mouse and human blood. The NCs were tested at different concentrations (25, 50 and 100 µg/mL). PBS and 1% Triton X-100 were used as negative and positive control, respectively. (D) Determination of Prothrombin time (PT), Activated partial thromboplastin time (APTT) and Thrombin time (TT) after incubation of plasma with CS NCs at three different concentrations (25, 50 and 100 µg/mL). Mann-Whitney test was used for statistical analysis comparing to PBS negative control. *p < 0.05.
Supplementary Figure 2 | Lymphocyte biomarkers in splenocytes from vaccinated mice, left unstimulated or after stimulation with individual Krasm peptides. Vaccination regimen is depicted in Figure 1A. Ten days after 3rd immunization spleens were collected and cultured. Splenocytes were left unstimulated or incubated with the individual Krasm peptides (peptide 1, peptide 2 or peptide 3) for 72 hours. Then, lymphocyte biomarkers were evaluated by flow cytometry in CD4+ T (A) and CD8+ T cell (B) populations. The Kruskal–Wallis test and Dunn’s multiple comparison test were used for statistical analysis. *p < 0.05.
Supplementary Figure 3 | Effect of CS-Krasm used as preventive-vaccination in tumor-bearing mice. (A) Experimental protocol is depicted. C57BL/6 mice 8-weeks old were vaccinated three times at 21-days intervals (n=5 mice per group). Eight days after last boost, mice were subcutaneously injected ATQ303G pancreatic cells in the left flank. Whenever mice reached their ethical time-point, mice were sacrificed, with blood and spleens collected. (B) Mice percentage of survival from day 0 (tumor injection) to day 110. (C) The levels of specific IgGs in serum that recognize a mixture of Krasm peptides (peptide 1, peptide 2 and peptide 3) at their readout time-point were determined by ELISA immunoassay. (D) Splenocytes were stimulated for 48 hours. CS+3p group was incubated with the 3-peptide-mixture; TG02 and CS+TG02 groups were incubated with the TG02-peptide-mixture; and the remaining groups with the combination 3-peptide and TG02-peptide-mixtures. After that, lymphocyte biomarkers were evaluated by flow cytometry.
Supplementary Figure 4 | Tumor volumes of vaccinated mice. C57BL/6 mice 8-weeks old were subcutaneously injected with ATQ303G pancreatic cells in the left flank. Changes in tumor volumes for each vaccination group are displayed throughout the duration of the experiment. When tumors reached a volume of 100-150 mm3, mice were vaccinated three times at 21-days intervals (n=10 per group). Whenever mice reached their ethical time-point, mice were sacrificed.
Supplementary Figure 5 | Characterization of the immune systemic response generated by CS-Krasm therapeutic-vaccination in tumor-bearing mice. Experimental protocol is depicted in The levels of specific IgGs in serum that recognize a mixture of Krasm peptides (peptide 1, peptide 2 and peptide 3) were determined by ELISA immunoassay from serum collected ten days after 2nd (A) and 3rd (B) immunizations or at the readout (C) time-point. (D) Splenocytes were stimulated for 48 hours. CS+3p group was incubated with the 3-peptide-mixture; TG02 group was incubated with the TG02-peptide-mixture; and the remaining groups with both, 3-peptides and TG02-peptide-mixtures. After that, lymphocyte biomarkers were evaluated by flow cytometry.
Supplementary Figure 6 | Trained-immunity effect of CS NCs in vitro. (A) Experimental protocol is depicted. Briefly, peritoneal macrophages from mice were incubated overnight with CS nanoparticles (day 0), washed and rest for 6 days. At day 7, LPS was added during 3 hours, cells were washed and supernatants were collected after overnight resting. (B) TNF-α levels at day 8 measured using ELISA assay. (C) Percentages of macrophage viability at day 8 measured using the MTS assay, which was performed as described in (10). Percentages are normalized to untreated control.
Supplementary Figure 7 | Physicochemical and biological characterization of INU/pArg-nanoparticles and its effect as a preventive vaccination in tumor-bearing mice. (A) Polymeric INU/pArg nanovaccine prototype against the mutated protein K-Ras G12V. (B) Cellular apoptosis assay after 24 hours of incubation with INU/pArg NCs at 50 and 100 µg/mL in Jurkat and hPMBCs. One-way ANOVA and Dunnett’s multiple comparison tests were used for statistical analysis. *p < 0.05 compared to untreated cells. (C) Percentage of hemolysis induced by INU/pArg NCs in mouse and human blood. The NCs were tested at different concentrations (25, 50 and 100 µg/mL). PBS and 1% Triton X-100 were used as negative and positive control, respectively. (D) Determination of Prothrombin time (PT), Activated partial thromboplastin time (APTT) and Thrombin time (TT) after incubation of plasma with INU/pArg NCs at three different concentrations (25, 50 and 100 µg/mL). Mann-Whitney test was used for statistical analysis comparing to PBS negative control. *p < 0.05. (E) 8-weeks old C57BL/6 mice were vaccinated three times at 21-days intervals (n=5 mice per group), and eight days after the last boost they were subcutaneously injected ATQ303G pancreatic cells in the left flank. The graph depicts the percentage of survival from day 0 (tumor injection) to day 110.
Supplementary Table 1 | Selected peptides using IEDB MHC binding prediction tool.
Supplementary Table 2 | Summary of the times and volumes used for each reagent for the different coagulation tests.
Supplementary Table 3 | List of antibodies used in flow cytometry - macrophage polarization.
Supplementary Table 4 | List of antibodies and reagents used in flow cytometry – splenocytes and tumor analysis.
Supplementary Table 5 | Physico-chemical characterization of nanocapsules of INU/pArg in the absence and presence of mutated antigenic peptides.
References
1. Singhi AD, Koay EJ, Chari ST, Maitra A. Early detection of pancreatic cancer: Opportunities and challenges. Gastroenterology (2019) 156(7):P2024–2040. doi: 10.1053/j.gastro.2019.01.259
2. Siegel RL, Miller KD, Fuchs HE, Jemal A. Cancer statistics 2021. CA: A Cancer J Clin (2021) 71(1):7–33. doi: 10.3322/caac.21654
3. Prior IA, Lewis PD, Mattos C. A comprehensive survey of ras mutations in cancer. Cancer Res (2012) 72(10):2457–67. doi: 10.1158/0008-5472.CAN-11-2612
4. Singhi AD, George B, Greenbowe JR, Chung J, Suh J, Maitra A, et al. Real-time targeted genome profile analysis of pancreatic ductal adenocarcinomas identifies genetic alterations that might be targeted with existing drugs or used as biomarkers. Gastroenterology (2019) 156(7):P2024–2040. doi: 10.1053/j.gastro.2019.02.037
5. Malumbres M, Barbacid M. RAS oncogenes: The first 30 years. Nat Rev Cancer (2003) 3(6):459–655. doi: 10.1038/nrc1097
6. Zhang Y, Ma JA, Zhang HX, Jiang YN, Luo WH. Cancer vaccines: Targeting KRAS-driven cancers. Expert Rev Vaccines (2020) 19(2):163–735. doi: 10.1080/14760584.2020.1733420
7. Pati R, Shevtsov M, Sonawane A. Nanoparticle vaccines against infectious diseases. Front Immunol (2018) 9. doi: 10.3389/fimmu.2018.02224
8. Ding Y, Li Z, Jaklenec A, Hu Q. Vaccine delivery systems toward lymph nodes. Adv Drug Deliv Rev (2021) 179:113914. doi: 10.1016/j.addr.2021.113914
9. Vicente S, Peleteiro M, Díaz-Freitas B, Sanchez A, González-Fernández Á, Alonso M. Co-Delivery of viral proteins and a TLR7 agonist from polysaccharide nanocapsules: A needle-free vaccination strategy. J Controlled Release (2013) 172(3):773–81. doi: 10.1016/j.jconrel.2013.09.012
10. Diego-González L, Crecente-Campo J, Paul MJ, Singh M, Reljic R, Alonso MJ, et al. Design of polymeric nanocapsules for intranasal vaccination against mycobacterium tuberculosis: Influence of the polymeric shell and antigen positioning. Pharmaceutics (2020) 12(6):489. doi: 10.3390/pharmaceutics12060489
11. Sciullo PD, Menay F, Cocozza F, Gravisaco MJ, Waldner CI, Mongini C. Systemic administration of imiquimod as an adjuvant improves immunogenicity of a tumor-lysate vaccine inducing the rejection of a highly aggressive T-cell lymphoma. Clin Immunol (2019) 203:154–61. doi: 10.1016/j.clim.2019.04.013
12. Adams S, O’Neill DW, Nonaka D, Hardin E, Chiriboga L, Siu K, et al. Immunization of malignant melanoma patients with full-length NY-ESO-1 protein using TLR7 agonist imiquimod as vaccine adjuvant. J Immunol (2008) 181(1):776–784. doi: 10.4049/jimmunol.181.1.776
13. Dang Y, Wagner WM, Gad E, Rastetter L, Berger CM, Holt GE, et al. Dendritic cell-activating vaccine adjuvants differ in the ability to elicit antitumor immunity due to an adjuvant- specific induction of immunosuppressive cells. Clin Cancer Res (2012) 18(11):3122–31. doi: 10.1158/1078-0432.CCR-12-0113
14. Stanley MA. Imiquimod and the imidazoquinolones: Mechanism of action and therapeutic potential. Clin Exp Dermatol (2002) 27(7):571–7. doi: 10.1046/j.1365-2230.2002.01151.x
15. Guerra C, Collado M, Navas C, Schuhmacher AJ, Hernández-Porras I, Cañamero M, et al. Pancreatitis-induced inflammation contributes to pancreatic cancer by inhibiting oncogene-induced senescence. Cancer Cell (2011) 19(6):728–39. doi: 10.1016/j.ccr.2011.05.011
16. Dobrovolskaia MA, McNeil SE. Understanding the correlation between in vitro and in vivo immunotoxicity tests for nanomedicines. J Controlled Release (2013) 172(2):456–66. doi: 10.1016/j.jconrel.2013.05.025
17. Aggarwal P, Hall JB, McLeland CB, Dobrovolskaia MA, McNeil SE. Nanoparticle interaction with plasma proteins as it relates to particle biodistribution, biocompatibility and therapeutic efficacy. Adv Drug Deliv Rev (2009) 61(6):428–37. doi: 10.1016/j.addr.2009.03.009
18. Jesus S, Marques AP, Duarte A, Soares E, Costa JP, Colaço M, et al. Chitosan nanoparticles: Shedding light on immunotoxicity and hemocompatibility. Front Bioengineering Biotechnol (2020) 8. doi: 10.3389/fbioe.2020.00100
19. Takiguchi H, Yang CX, Yang CWT, Sahin B, Whalen BA, Milne S, et al. Macrophages with reduced expressions of classical M1 and M2 surface markers in human bronchoalveolar lavage fluid exhibit pro-inflammatory gene signatures. Sci Rep (2021) 11:8282. doi: 10.1038/s41598-021-87720-y
20. Wedemeyer H, Schuller E, Schlaphoff V, Stauber RE, Wiegand J, Schiefke I, et al. Therapeutic vaccine IC41 as late add-on to standard treatment in patients with chronic hepatitis c. Vaccine (2009) 27(37):5142–51. doi: 10.1016/j.vaccine.2009.06.027
21. Crecente-Campo J, Virgilio T, Morone D, Calviño-Sampedro C, Fernández-Mariño I, Olivera A, et al. Design of polymeric nanocapsules to improve their lympho-targeting capacity. Nanomedicine (2019) 13:23. doi: 10.2217/nnm-2019-0206
22. Lippi G, Mattiuzzi C. The global burden of pancreatic cancer. Arch Med Sci (2020) 16(4):820–4. doi: 10.5114/aoms.2020.94845
23. Kim JH, Kim YS, Park K, Lee S, Nam HY, Min KH, et al. Antitumor efficacy of cisplatin-loaded glycol chitosan nanoparticles in tumor-bearing mice. J Controlled Release (2008) 127(1):41–9. doi: 10.1016/j.jconrel.2007.12.014
24. Khan MdA, Zafaryab Md, Mehdi SH, Ahmad I, Rizvi MMA. Characterization and anti-proliferative activity of curcumin loaded chitosan nanoparticles in cervical cancer. Int J Biol Macromolecules (2016) 93(Part A):242–53. doi: 10.1016/j.ijbiomac.2016.08.050
25. Jiang Y, Yu X, Su C, Zhao L, Shi Y. Chitosan nanoparticles induced the antitumor effect in hepatocellular carcinoma cells by regulating ROS-mediated mitochondrial damage and endoplasmic reticulum stress. Artif Cells Nanom Biotechnol (2019) 47(1):747–56. doi: 10.1080/21691401.2019.1577876
26. Abrams SI, Khleif SN, Bergmann-Leitner ES, Kantor JA, Chung Y, Michael Hamilton J, et al. Generation of stable CD4+ and CD8+ T cell lines from patients immunized with ras oncogene-derived peptides reflecting codon 12 mutations. Cell Immunol (1997) 182(2):137–51. doi: 10.1006/cimm.1997.1224
27. Gjertsen MK, Gaudernack G. Mutated ras peptides as vaccines in immunotherapy of cancer. Vox Sanguinis (1998) 74(S2):489–95. doi: 10.1111/j.1423-0410.1998.tb05462.x
28. Markowitz LE, Tsu V, Deeks SL, Cubie H, Wang SA, Vicari AS, et al. Human papillomavirus vaccine introduction - the first five years. Vaccine (2012) 30(Supplement 5):F139–48. doi: 10.1016/j.vaccine.2012.05.039
29. Hu Y, Yu H. Prevention strategies of mother-to-Child transmission of hepatitis b virus (HBV) infection. Pediatr Invest (2020) 4(2):133–7. doi: 10.1002/ped4.12205
30. Siegel CT, Schreiber K, Meredith SC, Beck-Engeser GB, Lancki DW, Lazarski CA, et al. Enhanced growth of primary tumors in cancer-prone mice after immunization against the mutant region of an inherited oncoprotein. J Exp Med (2000) 191(11):1945–56. doi: 10.1084/jem.191.11.1945
31. Prehn RT. The immune reaction as a stimulator of tumor growth. Science (1972) 176(4031):170–1. doi: 10.1126/science.176.4031.170
32. Prehn RT. Stimulatory effects of immune reactions upon the growths of untransplanted tumors. Cancer Res (1994) 54(4):908–14.
33. Rahma OE, Hamilton JM, Wojtowicz M, Dakheel O, Bernstein S, Liewehr DJ, et al. The immunological and clinical effects of mutated ras peptide vaccine in combination with IL-2, GM-CSF, or both in patients with solid tumors. J Trans Med (2014) 12:55. doi: 10.1186/1479-5876-12-55
34. Ohue Y, Nishikawa H. Regulatory T (Treg) cells in cancer: Can treg cells be a new therapeutic target? Cancer Sci (2019) 110(7):2080–895. doi: 10.1111/cas.14069
35. Togashi Y, Shitara K, Nishikawa H. Regulatory T cells in cancer immunosuppression — implications for anticancer therapy. Nat Rev Clin Oncol (2019) 16:356–71. doi: 10.1038/s41571-019-0175-7
36. Hatzioannou A, Boumpas A, Papadopoulou M, Papafragkos I, Varveri A, Alissafi T, et al. Regulatory T cells in autoimmunity and cancer: A duplicitous lifestyle. Front Immunol (2021) 12. doi: 10.3389/fimmu.2021.731947
37. Almand B, Clark JI, Nikitina E, Beynen Jv, English NR, Knight SC, et al. “Increased production of immature myeloid cells in cancer patients: A mechanism of immunosuppression in cancer. J Immunol (2001) 166(1):678–895. doi: 10.4049/jimmunol.166.1.678
38. Lebre F, Bento D, Jesus S, Borges O. Chitosan-based nanoparticles as a hepatitis b antigen delivery system. Methods Enzymol (2012) 509:127–42. doi: 10.1016/B978-0-12-391858-1.00007-1
39. Fu S, Xia J, Wu J. Functional chitosan nanoparticles in cancer treatment. J Biomed Nanotechnol (2016) 12(8):1585–603. doi: 10.1166/jbn.2016.2228
40. David KI, Jaidev LR, Sethuraman S, Krishnan UM. Dual drug loaded chitosan nanoparticles-Sugar-Coated arsenal against pancreatic cancer. Colloids Surfaces B: Biointerfaces (2015) 135:689–98. doi: 10.1016/j.colsurfb.2015.08.038
41. Potdar PD, Shetti AU. Evaluation of anti-metastatic effect of chitosan nanoparticles on esophageal cancer-associated fibroblasts. J Cancer Metastasis Treat (2016) 2:259–67. doi: 10.20517/2394-4722.2016.25
42. Chang PoH, Sekine K, Chao HM, Hsu SH, Chern E. Chitosan promotes cancer progression and stem cell properties in association with wnt signaling in colon and hepatocellular carcinoma cells. Sci Rep (2017) 7:45751. doi: 10.1038/srep45751
43. Zhang R, Liu Q, Li T, Liao Q, Zhao Y. Role of the complement system in the tumor microenvironment. Cancer Cell Int (2019) 19:300. doi: 10.1186/s12935-019-1027-3
44. Kazimierczak P, Koziol M, Przekora A. The Chitosan/Agarose/Nanoha bone scaffold-induced M2 macrophage polarization and its effect on osteogenic differentiation in vitro. Int J Mol Sci (2021) 22(3):1109. doi: 10.3390/ijms22031109
45. Vasconcelos DP, Fonseca AC, Costa M, Amaral IF, Barbosa MA, Águas AP, et al. Macrophage polarization following chitosan implantation. Biomaterials (2013) 34(38):9952–59. doi: 10.1016/j.biomaterials.2013.09.012
46. Boxberg Yv, Soares S, Giraudon C, David L, Viallon M, Montembault A, et al. Macrophage polarization in vitro and in vivo modified by contact with fragmented chitosan hydrogel. J Biomed Materials Res - Part A (2022) 110(4):773–87. doi: 10.1002/jbm.a.37326
47. Briukhovetska D, Dörr J, Endres S, Libby P, Dinarello CA, Kobold S. Interleukins in cancer: From biology to therapy. Nat Rev Cancer (2021) 21:481–99. doi: 10.1038/s41568-021-00363-z
48. Beutner KR, Geisse JK, Helman D, Fox TL, Ginkel A, Owens ML. Therapeutic response of basal cell carcinoma to the immune response modifier imiquimod 5% cream. J Am Acad Dermatol (1999) 41(6):1002–7. doi: 10.1016/S0190-9622(99)70261-6
49. Salasche SJ, Levine N, Morrison L. Cycle therapy of actinic keratoses of the face and scalp with 5% topical imiquimod cream: An open-label trial. J Am Acad Dermatol (2002) 47(4):571–7. doi: 10.1067/mjd.2002.126257
50. Smyth EC, Flavin M, Pulitzer MP, Gardner GJ, Costantino PD, Chi DS, et al. Treatment of locally recurrent mucosal melanoma with topical imiquimod. J Clin Oncol (2011) 29(33):e809–11. doi: 10.1200/JCO.2011.36.8829
Keywords: KRAS, nanovaccine, pancreatic cancer, imiquimod (IMQ), chitosan, polyarginine
Citation: Martínez-Pérez A, Diego-González L, Vilanova M, Correia A, Simón-Vázquez R and González-Fernández Á (2023) Immunization with nanovaccines containing mutated K-Ras peptides and imiquimod aggravates heterotopic pancreatic cancer induced in mice. Front. Immunol. 14:1153724. doi: 10.3389/fimmu.2023.1153724
Received: 30 January 2023; Accepted: 27 March 2023;
Published: 12 April 2023.
Edited by:
Wenxue Ma, University of California, San Diego, United StatesReviewed by:
Rajeev K Tyagi, Institute of Microbial Technology (CSIR), IndiaErkko Ylösmäki, Rokote Laboratories Finland Oy, Finland
Copyright © 2023 Martínez-Pérez, Diego-González, Vilanova, Correia, Simón-Vázquez and González-Fernández. This is an open-access article distributed under the terms of the Creative Commons Attribution License (CC BY). The use, distribution or reproduction in other forums is permitted, provided the original author(s) and the copyright owner(s) are credited and that the original publication in this journal is cited, in accordance with accepted academic practice. No use, distribution or reproduction is permitted which does not comply with these terms.
*Correspondence: Amparo Martínez-Pérez, YW1tYXJ0aW5lekB1dmlnby5lcw==