- 1State Key Laboratory of Biotherapy and Cancer Center, West China Hospital, Sichuan University, Chengdu, China
- 2Division of Arboviral Vaccines, National Institutes for Food and Drug Control, Beijing, China
- 3Department of Preclinical Vaccine Research, Suzhou Abogen Biosciences Co., Ltd., Suzhou, China
- 4Division of HIV/AIDS and Sex-Transmitted Virus Vaccines, National Institutes for Food and Drug Control, Beijing, China
Chikungunya fever (CHIKF) has spread to more than 100 countries worldwide, with frequent outbreaks in Europe and the Americas in recent years. Despite the relatively low lethality of infection, patients can suffer from long-term sequelae. Until now, no available vaccines have been approved for use; however, increasing attention is being paid to the development of vaccines against chikungunya virus (CHIKV), and the World Health Organization has included vaccine development in the initial blueprint deliverables. Here, we developed an mRNA vaccine using the nucleotide sequence encoding structural proteins of CHIKV. And immunogenicity was evaluated by neutralization assay, Enzyme-linked immunospot assay and Intracellular cytokine staining. The results showed that the encoded proteins elicited high levels of neutralizing antibody titers and T cell-mediated cellular immune responses in mice. Moreover, compared with the wild-type vaccine, the codon-optimized vaccine elicited robust CD8+ T-cell responses and mild neutralizing antibody titers. In addition, higher levels of neutralizing antibody titers and T-cell immune responses were obtained using a homologous booster mRNA vaccine regimen of three different homologous or heterologous booster immunization strategies. Thus, this study provides assessment data to develop vaccine candidates and explore the effectiveness of the prime-boost approach.
1 Introduction
The chikungunya virus (CHIKV) is a pathogen that causes chikungunya fever (CHIKF) in humans; Aedes aegypti and A. albopictus are its hosts. Symptoms, such as high fever, rash, and headache, appear in the acute phase after infection, and there is a high incidence of joint pain in the chronic phase (1). In recent decades, CHIKF has spread to over 100 countries worldwide, thereby leading to a number of epidemics (2). Some infected patients suffer from arthralgia for years or even decades after infection, even though other symptoms have disappeared (3). This causes great suffering to patients and serious social and economic development problems. CHIKV has four genotypes, but is generally believed to have only one serotype; therefore, a vaccine developed using one genotype should achieve cross-protection against all genotypes (4). Although this simplifies the development of a CHIKV vaccine to some extent, no vaccine has yet been approved. As Arboviral diseases continue to receive international attention, several CHIKV vaccines have been developed and are in clinical trials, including inactivated vaccines (5, 6), live-attenuated vaccines (LAV) (7), recombinant vector vaccines (8, 9), virus-like particle (VLP) vaccines (10), and mRNA vaccines (11).
In just three decades since their large-scale deployment, mRNA vaccines have become one of the most important vaccine strategies to address infectious disease epidemics owing to their simplicity, short process cycle, and less restrictive manufacturing environment. Several mRNA vaccines have been approved for the market or are in clinical trials (12). For instance, mRNA-1273 from Moderna and BNT162b2 from BioNTech were the first coronavirus disease 2019 (COVID-19) vaccines approved for use in several countries worldwide and have been shown to have broad immunogenicity (13–16). In addition, clinical trials for various prime-boost strategies have been initiated. Homologous prime-boost immunization strategies are used with most vaccines to achieve good immunity. Some vaccines, including vaccines against human immunodeficiency viruses (HIVs), Ebola virus disease (EVD), malaria, tuberculosis, influenza, and hepatitis B, have undergone heterologous prime-boost investigation with the expectation of improving vaccine-induced immunity. A mix-and-match immunity approach combined an Ebola adenovirus vector and a modified vaccinia virus Ankara (MVA) vector to produce a vaccine, which has exhibited good safety and immunogenicity in clinical trials (17). However, this technique has not been successful for HIV or malaria vaccines (18, 19). A heterologous prime-boost clinical program for COVID-19 is underway and includes different combinations of the Moderna, Pfizer, AstraZeneca, Johnson & Johnson, Sinopharm, and Sinovac vaccines (20).
In this study, we constructed a CHIKV vaccine using an mRNA vaccine platform. We used a structural protein gene of CHIKV as the target antigen gene, which is similar to recombinant vectored vaccine and mRNA vaccine candidates under preclinical development (2). The expression of the entire structural cassette polyprotein promotes folding of the correct antigen and resembles the proper formation that mimic the protein structure of CHIKV (21). mRNA is obtained by in vitro transcription and self-assembly formulation with lipid nanoparticles (LNPs), which are then purified to prepare the vaccine. The immunogenicity of the vaccine was evaluated in mice. In a previous study, we constructed a recombinant CHIKV vaccine that expressed the same antigen as the mRNA vaccine using a replication-defective human type 5 adenovirus (Ad5) as the vector, which also exhibited a good immune response. We used the recombinant adenovirus vector vaccine and mRNA vaccine in a prime-boost strategy of sequential immunization, with the aim of further improving efficacy.
2 Materials and methods
2.1 Cells and virus
Both 293T and DC 2.4 cells were maintained in DMEM (Gibco) supplemented with 10% heat-inactivated fetal bovine serum (FBS, Gibco) and penicillin–streptomycin (100 U/ml, Gibco). Pseudovirus pSG-cmv-flu-chikv (22) was stored at −80°C and titrated using the 50% tissue culture infectious dose (TCID50) assay in 293T cells.
2.2 mRNA preparation and formulation
The cDNAs encoding wild-type (WT) or codon-optimized (OP) structural protein C-E3-E2-6K-E1 genes from CHIKV strain LR2006 OPY1 (23) were cloned into plasmid ABOP-028 (GENEWIZ), which contained 5′ and 3′ untranslated regions (UTRs) and a poly A tail. The mRNA was synthesized in vitro using T7 polymerase-mediated transcription from the linearized plasmid DNA template.
Formulation was performed as described previously for the COVID-19 vaccine (24). Briefly, lipids were dissolved in ethanol containing an ionizable lipid, 1,2-distearoyl-sn-glycero-3-phosphocholine (DSPC), cholesterol, and PEG-lipid (molar ratios of 50:10:38.5:1.5). The lipid mixture was run through a T-mixer with mRNA dissolved in 20 mM citrate buffer (pH = 4.0) at a ratio of 1:2. The generated mRNA-LNPs were diafiltrated against PBS (pH = 7.4) in a dialysis cassette with 20 kD MWCO overnight, passed through a 0.22-μm filter, and stored at 2°C–8°C until use. The product was characterized for particle size and distribution using a particle size analyzer (Malvern Panalytical), RNA concentration using HPLC (Thermo Fisher Scientific), and encapsulation using RiboGreen reagent (Thermo Fisher Scientific).
2.3 mRNA transfection
DC 2.4 cells were seeded in 24-well plates at 500,000 cells/well and then transfected with 1 μg of mRNA to form cell monolayers using Lipofectamine 2000 Transfection Reagent (Thermo Fisher Scientific). After 6 h, the medium was replaced with DMEM supplemented with 3% FBS. Following 24 h post-transfection, cells were fixed with ice-cold acetone for 10 min and incubated with a polyclonal mouse anti-CHIKV antibody (prepared in our laboratory) and then FITC-labeled goat anti-mouse IgG (Abcam). Nuclei were counterstained with DAPI (Thermo Fisher Scientific). Fluorescence was observed using a fluorescent microscope (Olympus).
After 16–26 h post-transfection, cells were lysed with RIPA buffer plus proteinase inhibitor (Sigma), clarified by centrifugation at 12,000 × g, and then 5 × SDS loading buffer was added, and cells were kept at 95°C for 10 min. The lysates were run on a 10% NuPAGE Bis-Tris gels (Invitrogen) followed by transferring proteins to a nitrocellulose membrane. The membrane was incubated with a polyclonal mouse anti-CHIKV antibody (prepared in our laboratory) and HRP-labeled goat anti-mouse IgG (Abcam). DC 2.4 cells with lysing buffer and E2 protein were used as negative and positive controls (ProSpec), respectively. Blots were developed using ECL reagents (GE).
2.4 Animal experiments
Female C57BL/6 mice were obtained from the Institute for Laboratory Animal Resources at the National Institutes for Food and Drug Control (NIFDC). For neutralizing antibody responses, mice aged 6–8 weeks were injected via an intramuscular (im) route with 100 μl of the indicated vaccine or negative control. Serum was collected from the submandibular vein before and/or post-prime immunization. For cellular immune responses, mice aged 6–8 weeks were immunized via the im route with 100 μl of the indicated vaccine or negative control. Spleen tissues were collected after immunization. Housing and experimentation of mice were performed strictly in accordance with the guidelines set by the Association for the Assessment and Accreditation of Laboratory Animal Care (AAALAC). The study protocol was approved by the Animal Care and Use Committee of the NIFDC.
2.5 Homologous and heterogeneous prime-boost
The vaccines used in this experiment were the mRNA vaccine and Ad5 vaccine (developed in our laboratory). Both vaccines contain the prototype gene of structural protein. Mice were immunized using different vaccination regimens: same vaccination routes (2×Ad5, 2×mRNA), different vaccination routes (Ad5 + mRNA), and negative control groups. The prime vaccination day was set as day 0, and the boost was performed on day 14. Serum and spleen tissues were collected before and after prime immunization.
2.6 Neutralization assays
The neutralization assay using pseudovirus was similar to that used for severe acute respiratory syndrome coronavirus 2 (SARS-CoV-2) as described previously (25). Serial threefold diluted heat-inactivated serum obtained from C57BL/6 mice was incubated with 400 TCID50 of the pseudovirus for 1 h at 37°C. The 293T cells were added to serum–virus complexes in 96-well plates at 50,000 cells/well and incubated for approximately 48 h, together with the virus control and cell control in wells. The supernatant was then removed and luciferase substrate (Perkin Elmer) was added to each well, followed by incubation for 2 min. Luminescence was measured for pseudovirus titration. The 50% inhibitory dilution (EC50) was defined as the serum dilution at which the relative light units (RLUs) were reduced by 50% compared with the virus control after subtraction of the background RLUs in control cells.
2.7 Enzyme-linked immunospot assay
Spleens were isolated from mice and dispersed in a 40-μm cell strainer with mouse lymphocyte separation medium (Dakewe). The tissues were centrifuged at 800 × g for 20 min and covered with 1 ml of RPMI-1640 medium (Hyclone). After centrifugation, splenocytes were resuspended in serum-free medium (Dakewe). Interferon gamma (IFN-γ)- or interleukin-2 (IL-2)-positive cells were assessed using precoated enzyme-linked immunospot (ELISpot) kits (MabTech) according to the manufacturer’s protocol. Briefly, 96-well plates were blocked with RPMI-1640 medium containing 10% FBS for at least 2 h at 24°C. The splenocytes were transferred to the wells at 500,000 cells/well and stimulated at 37°C for 18 h with Ad5 containing entire structural proteins C-E3-E2-6K-E1. Phorbol ester (PMA)/Ionomycin (Dakewe) and RPMI-1640 media were used as the positive and blank control, respectively. The plates were incubated with anti-mouse IFN-γ or IL-2 antibody for 2 h and streptavidin-horseradish peroxidase for 1 h at ~25°C. The visualized immunoprecipitate revealed that the following treatment with TMB substrate solution was subsequently imaged and quantified using an ImmunoSpot S6 Universal instrument (Cellular Technology Limited).
2.8 Intracellular cytokine staining
Splenocytes were transferred into wells at 500,000 cells/well and stimulated at 37°C for 8 h with Ad5 containing entire structural proteins C-E3-E2-6K-E1. Brefeldin A (BioLegend) was then added to block cytokine secretion and incubation continued for 4 h. Following two washes with PBS, splenocytes were incubated with the following antibodies against lineage markers: PE anti-mouse CD3e, FITC anti-mouse CD4, and PerCP/Cy 5.5 anti-mouse CD8a (all from BioLegend). After two washes with PBS, cells were fixed, permeabilized with Cytofix/Cytoperm (BD Biosciences), and incubated with the following antibodies against intracellular markers: APC anti-mouse IFN-γ and PE/Cy7 anti-mouse IL-2 (all from BioLegend). Cells were analyzed using a FACS Lyric analyzer (BD Biosciences).
2.9 Statistical analysis
Data were plotted and analyzed using GraphPad Prism 8.0 and presented as geometric means ± geometric standard deviation. Neutralization antibody levels were compared by two-way analysis of variance (ANOVA), and an unpaired t-test was used for comparison of cellular immune levels. A p-value of <0.05 was considered statistically significant (*p < 0.05; ** p < 0.01; *** p < 0.001; **** p < 0.0001; ns, no significance).
3 Results
3.1 Construction of mRNA vaccine encoding a CHIKV structural protein
The structural proteins C-E3-E2-6K-E1 of WT CHIKV virus strain LR2006 OPY1 were selected as the target antigens for the mRNA coding sequence. Moreover, 5′ and 3′ UTRs were added to increase mRNA stability and regulation of translation. A poly(A) tail of ~100–130 bp was synthesized to follow the 3′ UTR. Transcription of the linearized DNA template to mRNA was mediated by the T7 promoter, and the complete mRNA was obtained by adding the Cap-1 structure (N7mGpppGm) to the 5′ end of the RNA using a vaccinia capping enzyme. We also constructed an mRNA-OP using OP sequences (26) (Figure 1A) and a sequence optimization strategy for mRNA vaccines as previously described (24). The resulting mRNA was transfected into DC 2.4 cells. Immunofluorescence assays using anti-CHIKV structural protein polyclonal antibodies indicated that the mRNA-WT and -OP translated targeted proteins were expressed intracellularly and on the cell membrane (Figure 1B). Cell lysates were collected, immunoblotting assays were performed using anti-CHIKV structural protein polyclonal antibodies, and specific protein expression was detected in both mRNA-WT and -OP transfected cell samples (Figure 1C). mRNA was encapsulated in LNPs for diafiltration and purification; formulation with an average particle size of ~79 nm and an encapsulation rate of over 90% were obtained (Table S1, Figure S1).
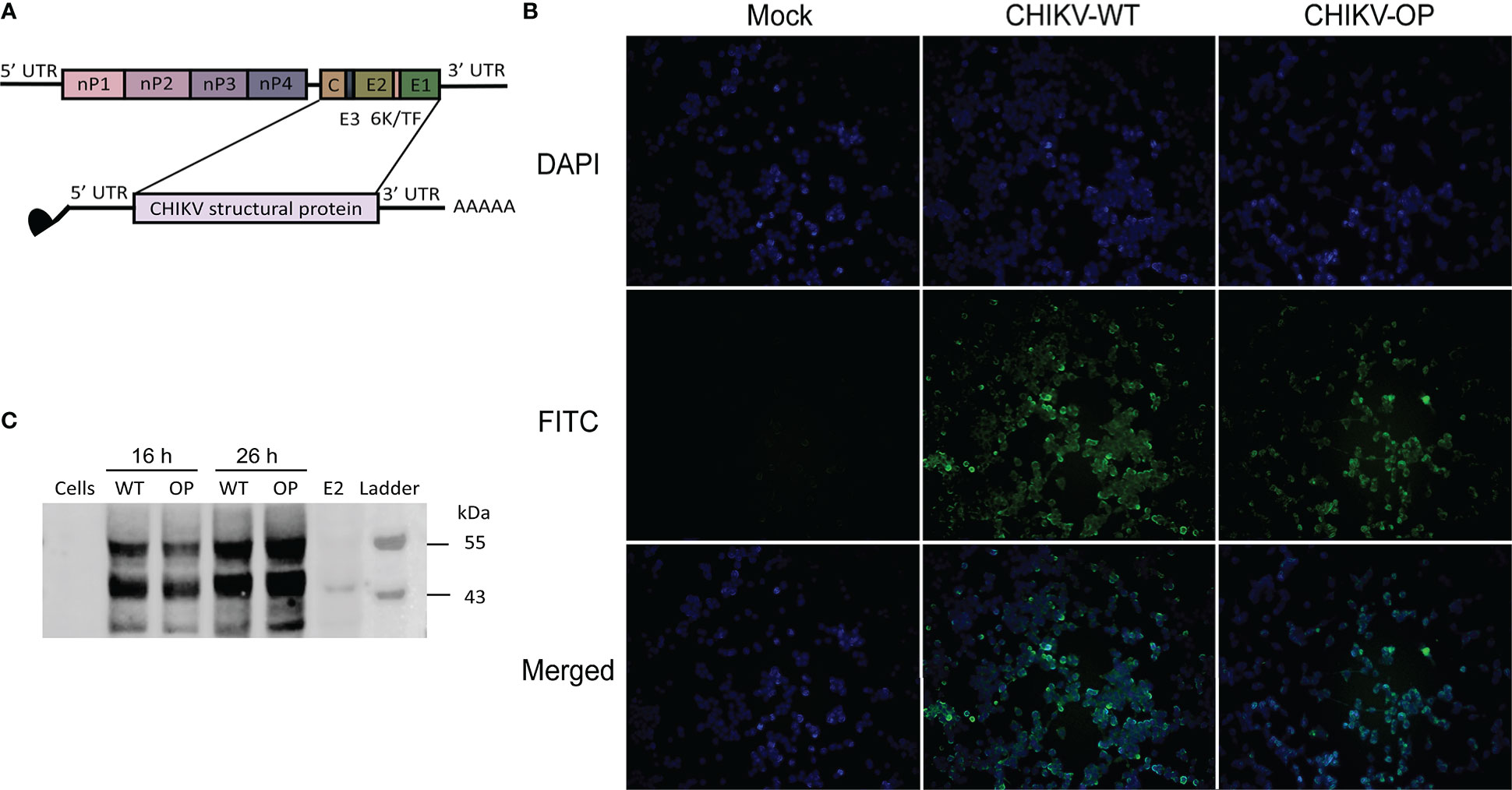
Figure 1 Design and protein expression of the CHIKV mRNA vaccine. (A) Schematic of the CHIKV genome structure and mRNA construct. (B) Structural protein expression following mRNA transfection with wild-type (WT) or codon-optimized (OP) mRNA as determined using FITC immunofluorescence staining (scale bar, 400 μm). (C) Western blot analysis of structural protein expression from CHIKV mRNA-WT or -OP in DC 2.4 cells and lysate at 16 or 26 h post-transfection. E2, control structural protein.
3.2 Neutralizing antibody analysis
In the single-dose immunization regimen, C57BL/6 mice received an im injection of 1, 5, or 10 μg WT or OP vaccine, with PBS solution as the negative control. Serum was collected before immunization and on days 7, 14, 28, 42, 56, and 70 post-immunization for neutralizing antibody testing using a pseudovirus assay (Figures 2A–C). For the 1-μg WT dose, the EC50 antibody titer was maintained at a low level approaching negative control; the EC50 antibody titer exhibited a high agreement between that of the 5- and 10-μg doses (p = 0.9958), which reached ~20,000 on day 42 post-immunization and remained steady. At the 1-µg OP dose, antibody was not induced; at the 10 μg dose, the EC50 antibody titer reached a peak of 26,833 on day 42 post-immunization, which was approximately twice that of the 5-μg dose, although the EC50 antibody titer showed no significant difference with the 10-μg dose of OP (p = 0.0827). The neutralizing antibody titers of the WT vaccine were better than those of the OP vaccine at the 5-μg dose, which suggests that base changes in the target genes may have affected humoral immunity.
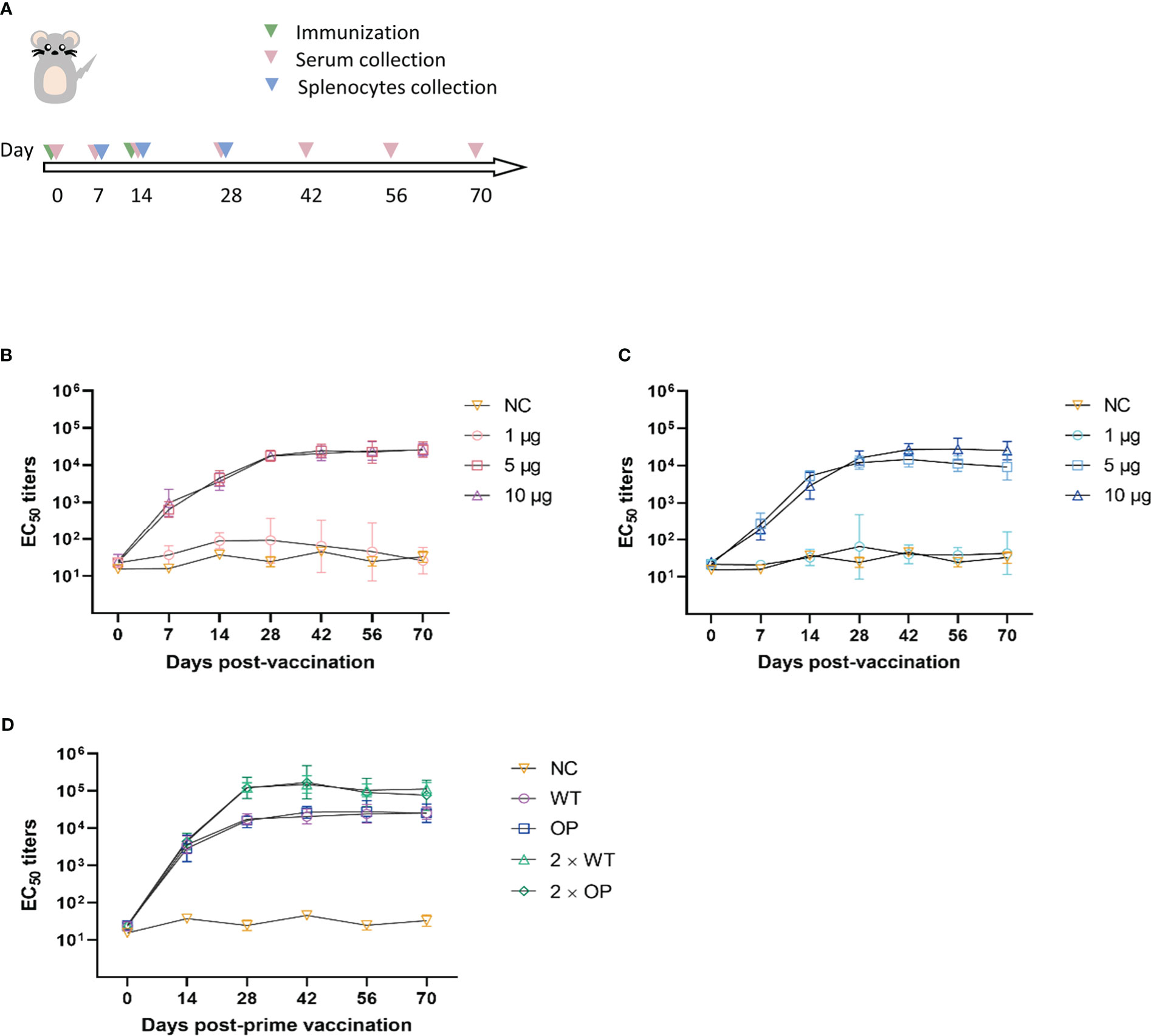
Figure 2 Neutralizing antibody responses to the CHIKV mRNA vaccine in mice. (A) Mice were immunized with one or two doses, and serum and splenocytes were collected at the indicated time points. EC50 values were assessed using CHIKV pseudovirus. (B–D) Post-vaccination neutralization curves of EC50 following (B) a single dose of wild-type (WT) vaccine, (C) a single dose of codon-optimized (OP) vaccine, and (D) prime-boost vaccines. n = 6 mice per group; one data point represents the geometric mean titer per group at each time point. Bars represent geometric means ± geometric standard deviation (SD). NC, negative control.
In the booster immunization regimen, WT and OP vaccines were given at a dose of 10 μg and boosted with an equal dose of vaccine on day 14 post-initial immunization. Serum was collected before immunization and on days 7, 14, 28, 42, 56, and 70 post-prime immunization for neutralizing antibody testing (Figure 2D). Neutralizing antibody levels for both vaccines increased substantially on day 28 post-immunization, with a greater than sixfold increase compared with that of single-dose immunization. Furthermore, EC50 antibody titers reached over 100,000, peaking on day 42 post-immunization for the WT and OP vaccines. Neutralizing antibody titers were significantly higher following booster immunization than single-dose immunization (WT: p < 0.0001; OP: p = 0.0028). The results indicate that booster immunization with both WT and OP vaccines induced relatively high levels of neutralizing antibodies in mice.
3.3 Cellular immunity response
C57BL/6 mice were immunized with a single dose of 10 μg of WT or OP vaccine, with PBS solution as the negative control. Splenocytes were harvested from the mice on days 7, 14, and 28 after immunization (Figure 2A) and stimulated with Ad5 containing CHIKV structural proteins; ELISpot and intracellular cytokine staining (ICS) assays were performed.
The levels of IFN-γ and IL-2 secretion by splenocytes were analyzed by the ELISpot assay (Figure 3). The WT and OP vaccines induced strong IFN-γ (WT: p = 0.0025; OP: p = 0.0002) and IL-2 (WT: p < 0.0001; OP: p = 0.0009) levels on day 7 post-immunization compared with the negative control group. There was no statistically significant difference in the levels of cytokine-secreting cell number between the WT and OP vaccine groups (IFN-γ: p = 0.1484; IL-2: p = 0.8606). Furthermore, both vaccines displayed stable cellular responses within 28 days post-immunization.
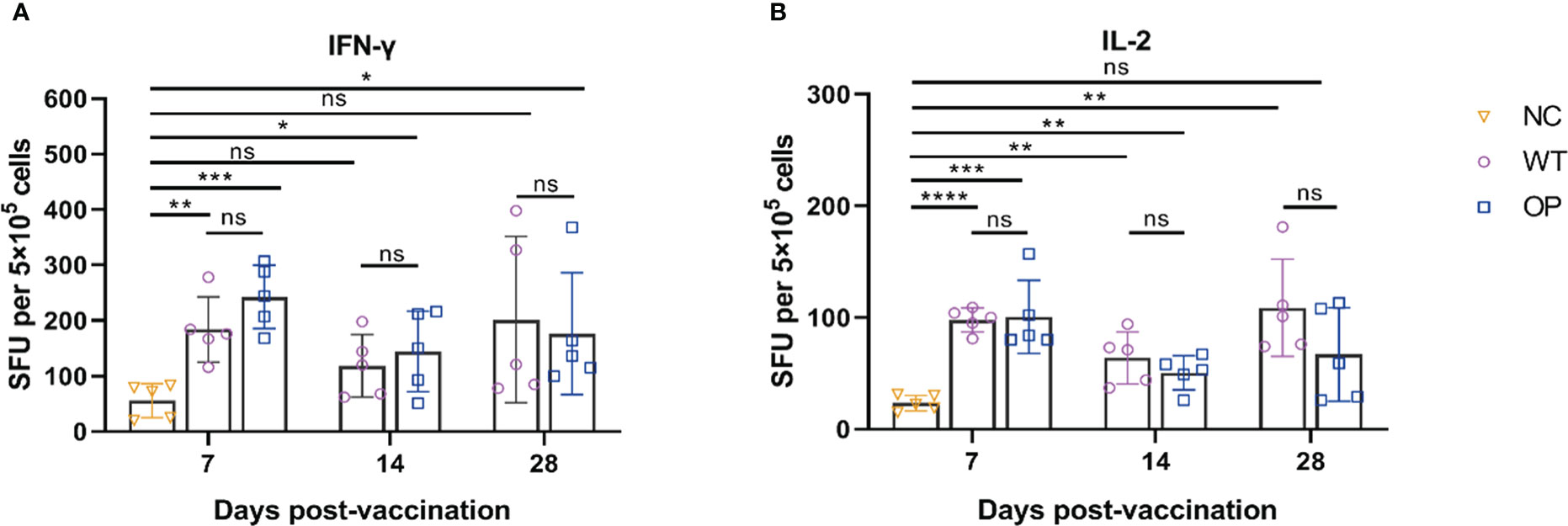
Figure 3 CHIKV-specific T-cell responses induced by CHIKV mRNA vaccine. mRNA vaccine was administered to mice and the splenocytes were harvested and analyzed. (A) IFN-γ-secreting cells and (B) IL-2-secreting cells were quantified by ELISpot assay. n = 5 mice per group; each data point represents the mean number of spots per well. * p < 0.05; ** p < 0.01; *** p < 0.001; **** p < 0.0001; ns: p > 0.05. NC, negative control; SFU, spot forming units; WT, wild-type vaccine; OP, codon-optimized vaccine.
Both mRNA vaccines induced CD4+ and CD8+ T-cell responses (Figure 4). However, the WT vaccine induced IFN-γ CD4+ T-cell response on day 7, but not on day 14 and day 28. Interestingly, IFN-γ CD4+ T cells were not clearly detected or were detected at very low levels in the OP vaccine group. In contrast, both vaccines induced IL-2 CD4+ T-cell responses with the OP vaccine group having a lower T-cell number on day 28 (p = 0.0010) than the WT vaccine. The OP vaccine induced a higher IFN-γ CD8+ T-cell response than the WT group. The latter showed a very low level of IFN-γ CD8+ T-cell responses. The IL-2 T-cell response showed a similar trend with the OP group having a higher IL-2 CD8+ T-cell response although the differences between the two groups were not significant on day 14 (p = 0.3622) and day 28 (p = 0.2874).
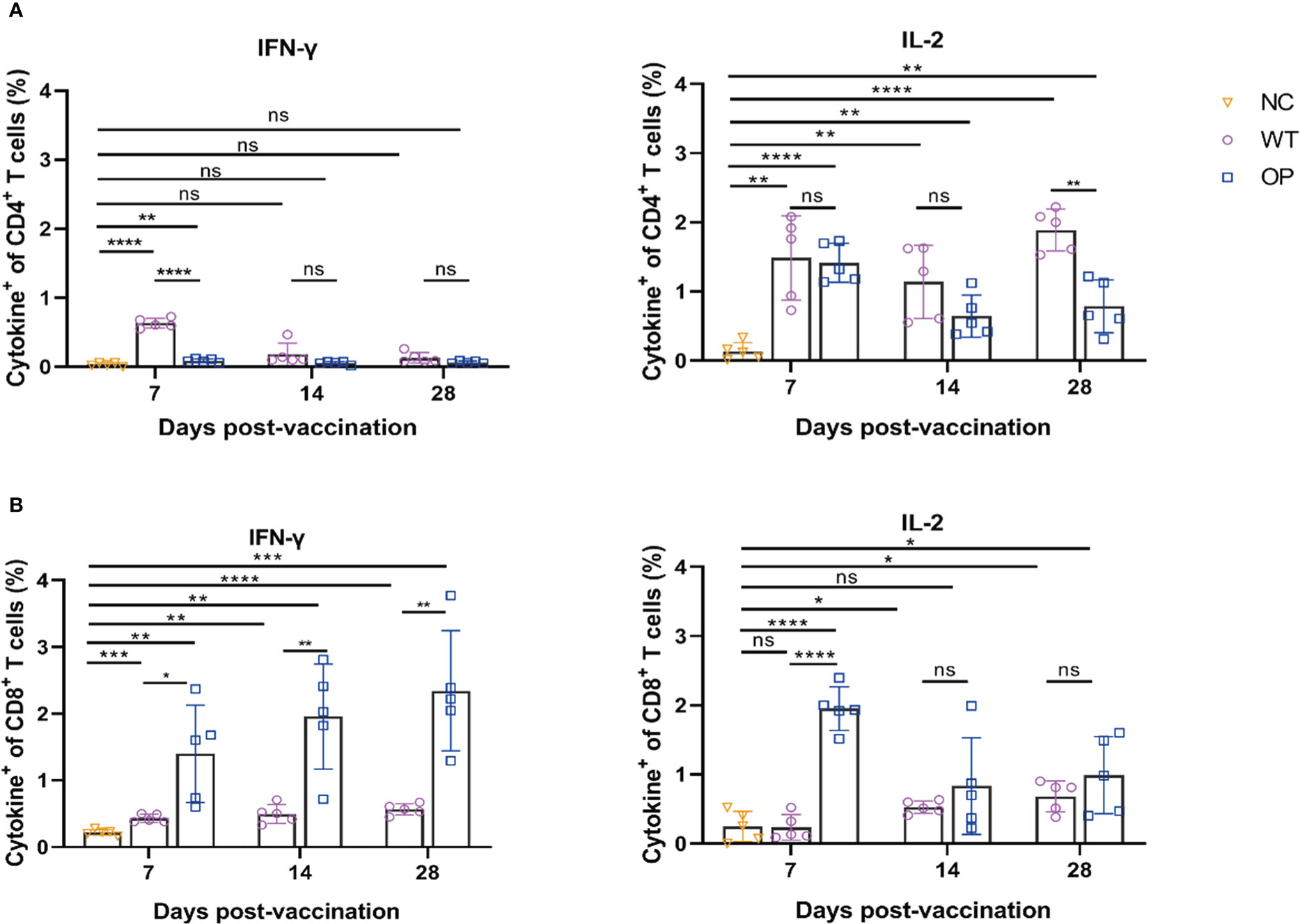
Figure 4 CD4+ and CD8+ T-cell responses induced by CHIKV mRNA vaccine. mRNA vaccine was administered to mice and the splenocytes were harvested and analyzed. Percentage of intracellular (A) IFN-γ and IL-2 CD4+ T cells, and (B) CD8+ T cells as determined by ICS. n = 5 mice per group; each data point represents the mean number of spots per well. * p < 0.05; ** p < 0.01; *** p < 0.001; **** p < 0.0001; ns: p > 0.05. NC, negative control; WT, wild-type vaccine; OP, codon-optimized vaccine.
3.4 Homologous and heterologous prime-boost
To compare the immune responses of the different prime-boost regimens, we used the Ad5 CHIKV vaccine constructed in our previous study, which was obtained by cloning the complete CHIKV structural protein genes (C-E3-E2-6K-E1) into a shuttle plasmid. We employed homologous recombination with the plasmid using the AdEasy adenovirus packaging system, transfecting Ad293 cells and assembling them into a mature recombinant adenovirus, which was amplified and purified to obtain the Ad5 vaccine expressing the CHIKV antigen.
As illustrated for the immunization regimen in Figure 5, mice were immunized using different vaccination regimens: prime-boosted homologously with mRNA vaccine, or with Ad5 vaccine, or primed with Ad5 vaccine followed by heterologous boosting with mRNA vaccine at a 14-day interval. The overall scheme for group design and immunization is shown in Table 1. A total of 107 infectious units (IFU) of Ad5 vaccine or 10 μg of mRNA vaccine was used for vaccination.
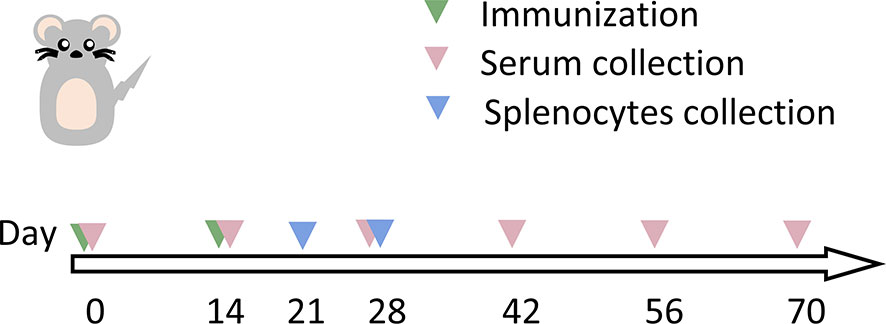
Figure 5 Schematic representation of the schedule for immunization and immunological characterization of the different immunization regimens. Mice were immunized with two doses of vaccine (as indicated in Table 1), and serum and splenocytes were collected at the indicated time points. NC, negative control; PBS, phosphate-buffered saline; Ad5, replication-defective human type 5 adenovirus-vectored vaccine.
Serum was collected on day 0 before immunization and on days 14, 28, 42, 56, and 70 post-prime immunization (Figure 6). The EC50 antibody titers of all immunization groups peaked on day 42 post-prime immunization. The EC50 antibody titer of the 2×mRNA immunization group was 149,428, which was significantly higher than that of the Ad5 + mRNA group (p = 0.0004) and the 2×Ad5 group (p < 0.0001), and it was maintained above 100,000 thereafter. There was a statistically significant difference in the titers between the Ad5+mRNA and 2×Ad5 groups (p = 0.0014), with peak antibody titers of 43,679 and 13,335, respectively. No significant increase in neutralizing antibody titers was observed with heterologous immunization compared to the homologous regimen.
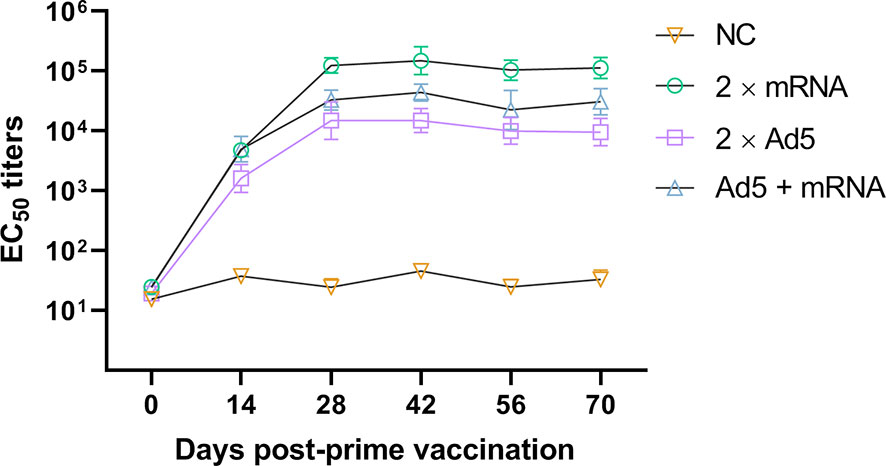
Figure 6 Neutralizing antibody responses to different immunization regimens. Mice in three groups were immunized with different vaccine combinations including homologous immunization with the developed mRNA vaccine, replication-defective human type 5 adenovirus-vectored vaccine (Ad5) vaccine, and priming immunization with Ad5 vaccine followed by mRNA vaccine. Neutralizing EC50 values were assessed using the CHIKV pseudovirus. n = 6 mice per group, one data point represents the geometric mean titer per group at each time point. Bars represent geometric means ± geometric standard deviation (SD). NC, negative control.
Splenocytes were harvested on days 21 and 28 post-prime immunization, and ELISpot and ICS assays were performed. The ELISpot assay (Figure 7) showed that the 2×Ad5 group failed to induce IFN-γ (day 21: p = 0.0519; day 28: p = 0.0561), but inducing IL-2 response (day 21: p = 0.0117; day 28: p = 0.0003), while IFN-γ and IL-2 were induced in the other two groups (2×mRNA and Ad5+mRNA). On day 21 post-prime immunization, the cytokine levels in the 2×mRNA group were higher than those in the other two groups with a statistically significant difference compared with the Ad5 + mRNA group (IFN-γ: p = 0.0041; IL-2: p = 0.0002). On day 28 post-initial immunization, the cytokine levels in the Ad5+mRNA group were significantly higher, while they decreased in the other two groups. Moreover, cytokine levels in the Ad5+mRNA group showed a statistically significant difference from those in the 2×Ad5 group (IFN-γ: p = 0.0003; IL-2: p < 0.0001); IL-2 levels were higher than those in the 2×mRNA group (p = 0.0004), while the Ad5+mRNA group showed similar levels of IFN-γ induction to those of the 2×mRNA group (p = 0.0564).
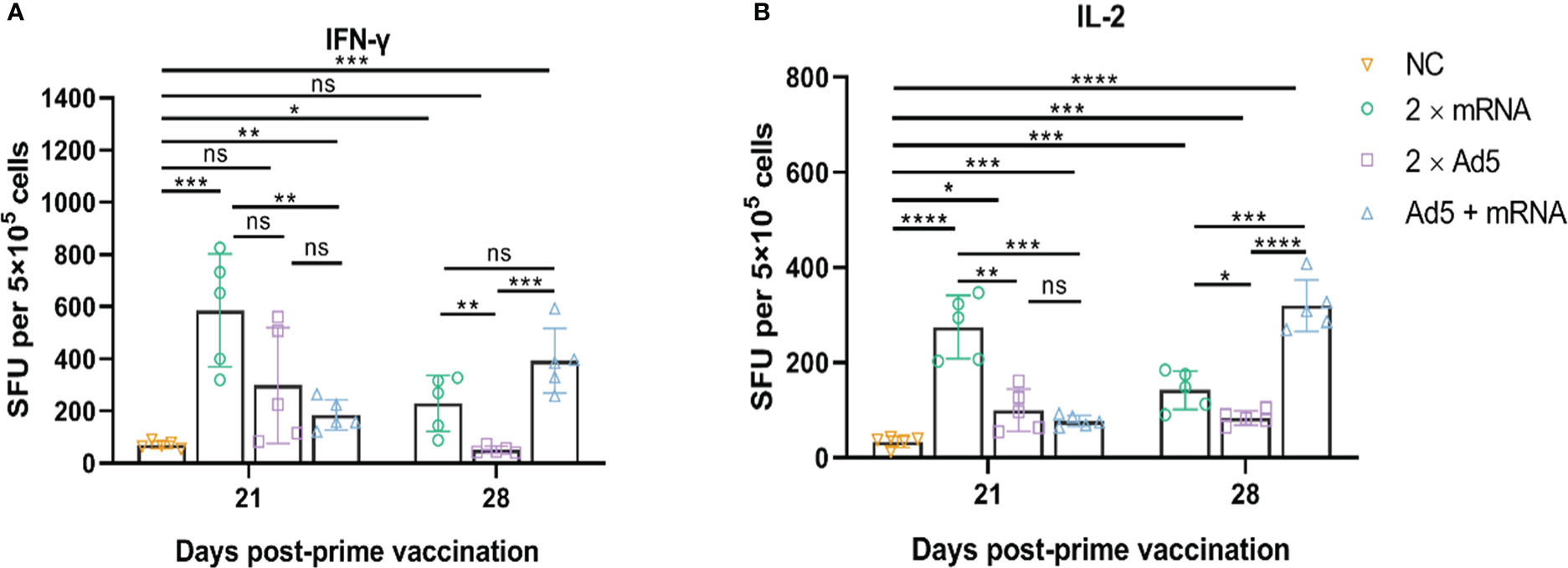
Figure 7 CHIKV-specific T-cell responses induced by different immunization regimens. Mice in three groups were immunized with different prime-boost strategies and splenocytes were harvested and analyzed. (A) IFN-γ-secreting cells and (B) IL-2-secreting cells were quantified by ELISpot assay. n = 5 per group; each data point represents the mean number of spots from wells. * p < 0.05; ** p < 0.01; *** p < 0.001; **** p < 0.0001; ns: p > 0.05. NC, negative control; SFU, spot forming units; Ad5, replication-defective human type 5 adenovirus-vectored vaccine.
ICS results (Figure 8) showed that CD4+ and CD8+ T cells in the Ad5+mRNA group exhibited higher levels of induced IFN-γ on both day 21 and day 28 post-prime immunization, while IFN-γ in the other two groups was induced primarily by CD8+ T cells. CD4+ and CD8+ T cells in all three immunization groups induced comparable levels of IL-2 on day 28 post-prime immunization.
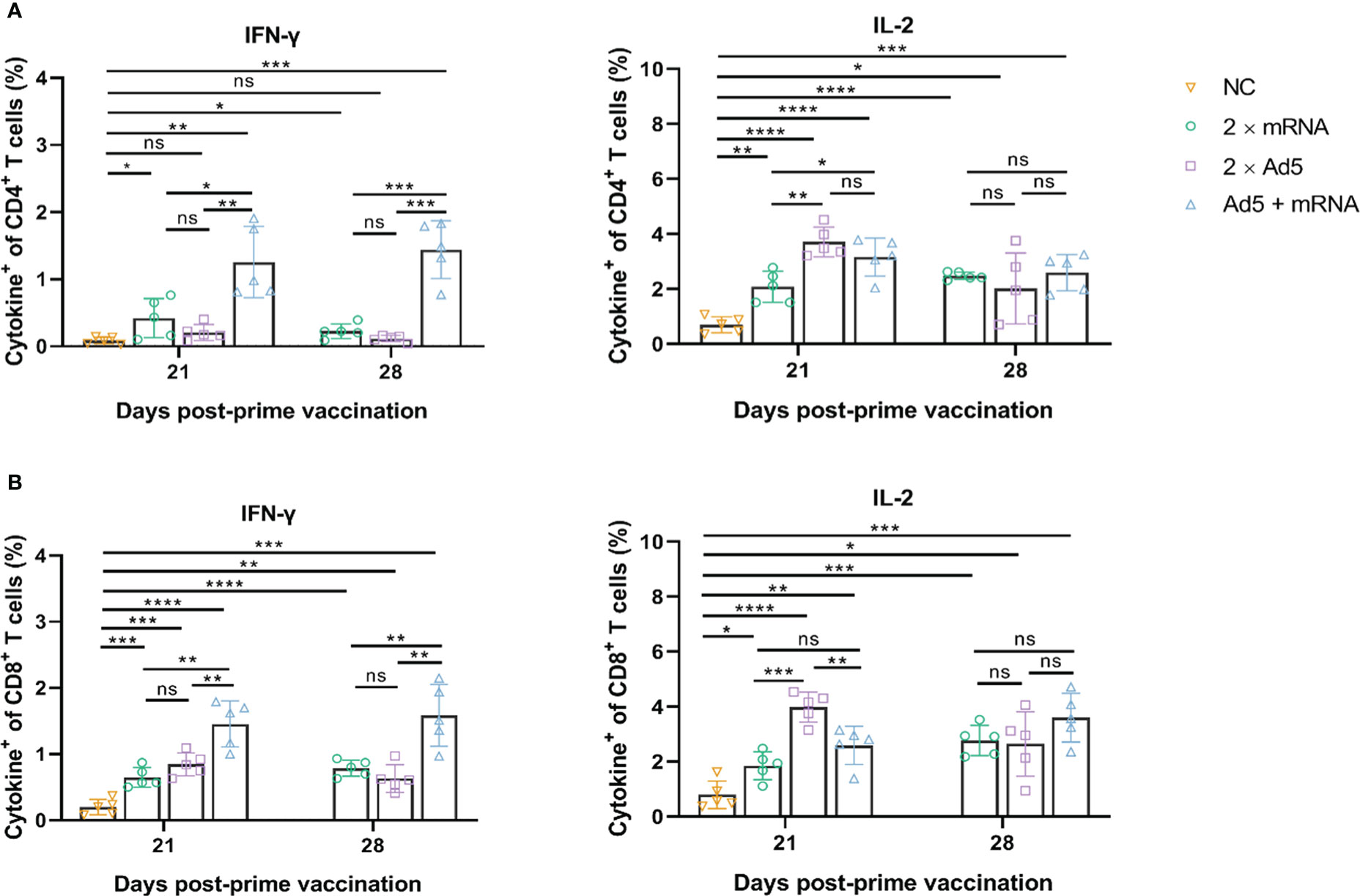
Figure 8 CD4+ and CD8+ T-cell responses induced by different immunization regimens. Mice in four groups were immunized with different prime-boost strategies and splenocytes were harvested and analyzed. Percentage of intracellular (A) IFN-γ and IL-2 positive CD4+ T cells, and (B) CD8+ T cells determined by ICS. n = 5 per group; each data point represents the mean number of spots per well. * p < 0.05; ** p < 0.01; *** p < 0.001; **** p < 0.0001; ns: p > 0.05. NC, negative control; Ad5, replication-defective human type 5 adenovirus-vectored vaccine.
4 Discussion
Here, we developed an mRNA vaccine against CHIKV encoding structural proteins (C-E3-E2-6K-E1) of the highly infectious LR2006 OPY1 strain from the IOL lineage (23). The vaccine was based on the COVID-19 mRNA-LNP vaccine platform (24), which has been shown to have satisfactory safety and efficacy in clinical trials (27).
The genes encoding CHIKV structural proteins can be successfully expressed in cells and the mRNA vaccine induced high-level titers of neutralizing antibodies and strong cellular immune responses in mice. Humoral immunity plays a crucial role in protection against CHIKV infection (28, 29). Neutralizing antibody titers reached up to 169,564 when mice were immunized with two doses of WT vaccine (10 μg) and remained at high levels. In a previous publication by Campos, two-dose immunization with the ChAdOx1 Chik vaccine candidate resulted in a neutralizing antibody titer of 15,300, and a single-dose immunization with the vaccine protected mice from a lethal viral attack (21, 30). The neutralizing antibody titers induced by the mRNA vaccine in the current study were much higher than those reported by Campos, indicating that the mRNA vaccine may have the potential to confer sufficient protection efficacy.
In respect to cellular immunity, single-dose immunization (WT, 10 μg) induced IFN-γ and IL-2 on day 7 and maintained high cytokine levels. IFN-γ was induced primarily by CD8+ T cells, and IL-2 was induced by CD4+ and CD8+ T cells. Although a strong T-cell immune response was induced, the role of cellular immunity in regulating CHIKV virus replication and clearance requires further investigation. Studies have shown that CD4+ and CD8+ T cells can induce a long-lasting immune response after CHIKV infection, reducing viral replication capacity, but they do not appear to protect against acute CHIKV infection (31). Moreover, virus clearance does not solely depend on CD4+ and CD8+ T cells in a mouse model (32).
The OP mRNA vaccine in the study induced less persistent neutralizing antibodies than the WT vaccine, while cellular immunity, especially IFN-γ secreted by CD8+ T cells, was significantly higher than that observed from the WT vaccine group. mRNA can directly interact with some pattern recognition receptor to stimulate the innate immune response. It was reported that mRNA-induced upregulation of type-I IFN tends to upregulate CD8+ T-cell cytotoxicity, attenuating the helper T cells’ effect in humoral immune responses. As a consequence, it may lead to reduced antigen expression and diminished vaccine efficacy (33, 34). Codon optimization is thought to influence mRNA translation and stability (35). However, rapid translation could prevent adequate folding of the target protein, as shown in a codon-optimized firefly luciferase mRNA that reduced the expression capacity of fluorescent proteins (36). In addition, highly stable secondary structures may hinder ribosome binding, and may be recognized and eliminated by the innate immune response (37). We speculated that the OP vaccine may enhance a stronger innate immune response, allowing activated CD8+ T cells to clear the mRNA, thereby limiting the translation of effective antigens and leading to a decrease in neutralizing antibodies. Only the larger dosage (10 μg) could achieve the same results as that of the WT vaccine. Thus, how to reach a balance between innate and adaptive immunity that allows mRNA to serve as an adjuvant, while also allowing effective translation, is a major goal in mRNA vaccine development.
We conducted a study on the immunogenicity of homologous and heterologous booster immunization to evaluate whether we could effectively improve the immune response to mRNA vaccines. Heterologous booster immunization did not result in higher neutralizing antibody titers; instead, homologous booster immunization with mRNA vaccine yielded better neutralizing antibody titers than the other groups. With respect to the cellular immune response, heterologous booster immunization showed delayed kinetics in the induction of high levels of cytokines, while CD4+ and CD8+ T cells induced more balanced levels of cytokines. Moreover, homologous booster immunization with the mRNA vaccine still exhibited a stronger T-cell immune response, and homologous immunization with the Ad5 vaccine induced more stable levels of cytokines over 28 days. This result differs from that of the COVID-19 vaccine in that the first dose of ChAdOx1-S, followed by an mRNA vaccine, elicited higher anti-COVID-19 neutralizing antibody titers and T-cell immune responses in mouse models (38). The sequence of administering ChAdOx1-S in the first dose followed by the mRNA vaccine in the second dose yielded higher immunogenicity than the reverse order of immunization (39, 40). The results of ELISpot and ICS appear to be inconsistent, as previously reported (41, 42). This may be related to the characteristics of the two methods. ELISpot is better able to measure low-level responses owing to its level of sensitivity. However, ICS allows for discrimination and phenotypic analysis of individual cytokine-producing cells across a broad dynamic range (41). A previous finding showed that peptides were more effective in stimulating responses than whole-protein antigens (43). To ensure a cellular response, a combination of several methods should be used. For most vaccines, booster immunization results in more effective protection. Activation of innate immunity leads to specific antibody production and memory T cells after primary immunization; re-activation of innate and adaptive immunity results in enhanced antigen presentation, cytokine secretion, and other functions that further promote differentiation of secondary memory cells after booster immunization (44). Several aspects may influence the immune response in heterologous booster immunity. For example, different properties of vectors may induce different immune responses, different immunization regimens may lead to attenuation of anti-vector immunity, and different antigens may produce different effects in induced T-cell immune responses (45). More data are needed to support the safety and efficacy of heterologous booster immunization.
Our study has several limitations. First, we did not measure the vaccine protection against a lethal challenge and viremia because of the limited resources because live CHIKV experiments should be performed in a biosafety level 3 laboratory or biosafety animal level 3 laboratory, and protection against CHIKV challenge needs to be confirmed in further studies. Neutralization of the antibody titer induced by the mRNA vaccine was superior to that of the ChAdOx1 Chik vaccine, which could protect mice from a lethal viral attack. Second, we used Ad5 containing entire structural proteins C-E3-E2-6K-E1 as a stimulant rather than the peptide pool, which may have weakened the cellular response.
In summary, we developed an mRNA vaccine encoding the structural proteins of CHIKV, and showed that it induced a robust immune response in a mouse model. Furthermore, the immunogenicity of homologous booster vaccination was superior to that of Ad5 vaccine and the heterologous immunization regimen evaluated. Thus, the mRNA vaccine against CHIKV constructed in this study has promising potential for development as a CHIKV vaccine candidate.
Data availability statement
The raw data supporting the conclusions of this article will be made available by the authors, without undue reservation.
Ethics statement
The animal study was reviewed and approved by The Animal Care and Use Committee in National Institutes for Food and Drug Control.
Author contributions
YL and BY conceived and supervised the project. JJL and XSL designed the experiments. JJL, XSL, XXL, EF, WL, XHL, and ZZ performed the experiments. JJL, XXL, and JL analyzed the data. WH provided material and technical supports. JJL drafted the first version of the manuscript. HS and YL revised the manuscript. All authors approved the submitted version.
Acknowledgments
We sincerely thank Huayuan Yan and Dandan Ling (Suzhou Abogen Biosciences Co., Ltd.) for preparation of vaccine samples. We also appreciate the support of Professor Yongxin Yu (National Institutes for Food and Drug Control).
Conflict of interest
XSL, SH, and BY are employed by Suzhou Abogen Biosciences Co., Ltd.
The remaining authors declare that the research was conducted in the absence of any commercial or financial relationships that could be construed as a potential conflict of interest.
Publisher’s note
All claims expressed in this article are solely those of the authors and do not necessarily represent those of their affiliated organizations, or those of the publisher, the editors and the reviewers. Any product that may be evaluated in this article, or claim that may be made by its manufacturer, is not guaranteed or endorsed by the publisher.
Supplementary material
The Supplementary Material for this article can be found online at: https://www.frontiersin.org/articles/10.3389/fimmu.2023.1129118/full#supplementary-material
Supplementary Table 1 | Characterization of mRNA formulation between wild-type (WT) and codon-optimized (OP) vaccines. Quality control parameters were examined after mRNA production.
Supplementary Figure 1 | Representative data examined for the wild-type (WT) and codon-optimized (OP) vaccines. Intensity size graphs for the (A) WT and (B) OP vaccines measured using the dynamic light-scattering method. mRNA content for (C) WT and (D) OP vaccines tested by Chromatographic graphs.
References
1. Burt FJ, Rolph MS, Rulli NE, Mahalingam S, Heise MT. Chikungunya: a re-emerging virus. Lancet (2012) 379:662–71. doi: 10.1016/S0140-6736(11)60281-X
2. de Lima Cavalcanti TYV, Pereira MR, de Paula SO, de O Franca RF. A review on chikungunya virus epidemiology, pathogenesis and current vaccine development. Viruses (2022) 14:969. doi: 10.3390/v14050969
3. Kumar R, Ahmed S, Parray HA, Das S. Chikungunya and arthritis: An overview. Travel Med Infect Dis (2021) 44:102168. doi: 10.1016/j.tmaid.2021.102168
4. Langsjoen RM, Haller SL, Roy CJ, Vinet-Oliphant H, Bergren NA, Erasmus JH, et al. Chikungunya virus strains show lineage-specific variations in virulence and cross-protective ability in murine and nonhuman primate models. mBio (2018) 9:e02449–17. doi: 10.1128/mBio.02449-17
5. Harrison VR, Eckels KH, Bartelloni PJ, Hampton C. Production and evaluation of a formalin-killed chikungunya vaccine. J Immunol (1971) 107:643–7.
6. Tiwari M, Parida M, Santhosh SR, Khan M, Dash PK, Rao PVL. Assessment of immunogenic potential of vero adapted formalin inactivated vaccine derived from novel ECSA genotype of chikungunya virus. Vaccine (2009) 27:2513–22. doi: 10.1016/j.vaccine.2009.02.062
7. Wressnigg N, Hochreiter R, Zoihsl O, Fritzer A, Bézay N, Klingler A, et al. Single-shot live-attenuated chikungunya vaccine in healthy adults: A phase 1, randomised controlled trial. Lancet Infect Dis (2020) 20:1193–203. doi: 10.1016/S1473-3099(20)30238-3
8. Folegatti PM, Harrison K, Preciado-Llanes L, Lopez FR, Bittaye M, Kim YC, et al. A single dose of ChAdOx1 chik vaccine induces neutralizing antibodies against four chikungunya virus lineages in a phase 1 clinical trial. Nat Commun (2021) 12:4636. doi: 10.1038/s41467-021-24906-y
9. Reisinger EC, Tschismarov R, Beubler E, Wiedermann U, Firbas C, Loebermann M, et al. Immunogenicity, safety, and tolerability of the measles-vectored chikungunya virus vaccine MV-CHIK: a double-blind, randomised, placebo-controlled and active-controlled phase 2 trial. Lancet (2019) 392:2718–27. doi: 10.1016/S0140-6736(18)32488-7
10. Bennett SR, McCarty JM, Ramanathan R, Mendy J, Richardson JS, Smith J, et al. Safety and immunogenicity of PXVX0317, an aluminium hydroxide-adjuvanted chikungunya virus-like particle vaccine: A randomised, double-blind, parallel-group, phase 2 trial. Lancet Infect Dis (2022) 22:1343–55. doi: 10.1016/S1473-3099(22)00226-2
11. Ge N, Sun J, Liu Z, Shu J, Yan H, Kou Z, et al. An mRNA vaccine encoding chikungunya virus E2-E1 protein elicits robust neutralizing antibody responses and CTL immune responses. Virol Sin (2022) 37:266–76. doi: 10.1016/j.virs.2022.01.032
12. Aldrich C, Leroux-Roels I, Huang KB, Bica MA, Loeliger E, Schoenborn-Kellenberger O, et al. Proof-of-concept of a low-dose unmodified mRNA-based rabies vaccine formulated with lipid nanoparticles in human volunteers: A phase 1 trial. Vaccine (2021) 39:1310–8. doi: 10.1016/j.vaccine.2020.12.070
13. Baden LR, El Sahly HM, Essink B, Kotloff K, Frey S, Novak R, et al. Efficacy and safety of the mRNA-1273 SARS-CoV-2 vaccine. N Engl J Med (2021) 384:403–16. doi: 10.1056/NEJMoa2035389
14. Creech CB, Anderson E, Berthaud V, Yildirim I, Atz AM, Melendez Baez I, et al. Evaluation of mRNA-1273 covid-19 vaccine in children 6 to 11 years of age. N Engl J Med (2022) 386:2011–23. doi: 10.1056/NEJMoa2203315
15. Moreira ED, Kitchin N, Xu X, Dychter SS, Lockhart S, Gurtman A, et al. Safety and efficacy of a third dose of BNT162b2 covid-19 vaccine. N Engl J Med (2022) 386:1910–21. doi: 10.1056/NEJMoa2200674
16. Polack FP, Thomas SJ, Kitchin N, Absalon J, Gurtman A, Lockhart S, et al. Safety and efficacy of the BNT162b2 mRNA covid-19 vaccine. N Engl J Med (2020) 383:2603–15. doi: 10.1056/NEJMoa2034577
17. Venkatraman N, Ndiaye BP, Bowyer G, Wade D, Sridhar S, Wright D, et al. Safety and immunogenicity of a heterologous prime-boost Ebola virus vaccine regimen in healthy adults in the united kingdom and Senegal. J Infect Dis (2019) 219:1187–97. doi: 10.1093/infdis/jiy639
18. Brown SA, Surman SL, Sealy R, Jones BG, Slobod KS, Branum K, et al. Heterologous prime-boost HIV-1 vaccination regimens in pre-clinical and clinical trials. Viruses (2010) 2:435–67. doi: 10.3390/v2020435
19. Ockenhouse CF, Regules J, Tosh D, Cowden J, Kathcart A, Cummings J, et al. Ad35.CS.01-RTS,S/AS01 heterologous prime boost vaccine efficacy against sporozoite challenge in healthy malaria-naïve adults. PLoS One (2015) 10:e0131571. doi: 10.1371/journal.pone.0131571
20. Siddiqui A, Adnan A, Abbas M, Taseen S, Ochani S, Essar MY. Revival of the heterologous prime-boost technique in COVID-19: An outlook from the history of outbreaks. Health Sci Rep (2022) 5:e531. doi: 10.1002/hsr2.531
21. López-Camacho C, Kim YC, Blight J, Lazaro Moreli M, Montoya-Diaz E, Huiskonen JT, et al. Assessment of immunogenicity and neutralisation efficacy of viral-vectored vaccines against chikungunya virus. Viruses (2019) 11:E322. doi: 10.3390/v11040322
22. Wu J, Zhao C, Liu Q, Huang W, Wang Y. Development and application of a bioluminescent imaging mouse model for chikungunya virus based on pseudovirus system. Vaccine (2017) 35:6387–94. doi: 10.1016/j.vaccine.2017.10.007
23. Parola P, de Lamballerie X, Jourdan J, Rovery C, Vaillant V, Minodier P, et al. Novel chikungunya virus variant in travelers returning from Indian ocean islands. Emerg Infect Dis (2006) 12:1493–9. doi: 10.3201/eid1210.060610
24. Zhang N-N, Li X-F, Deng Y-Q, Zhao H, Huang Y-J, Yang G, et al. A thermostable mRNA vaccine against COVID-19. Cell (2020) 182:1271–83.e16. doi: 10.1016/j.cell.2020.07.024
25. Nie J, Li Q, Wu J, Zhao C, Hao H, Liu H, et al. Establishment and validation of a pseudovirus neutralization assay for SARS-CoV-2. Emerg Microbes Infect (2020) 9:680–6. doi: 10.1080/22221751.2020.1743767
26. Kudla G, Lipinski L, Caffin F, Helwak A, Zylicz M. High guanine and cytosine content increases mRNA levels in mammalian cells. PLoS Biol (2006) 4:e180. doi: 10.1371/journal.pbio.0040180
27. Chen G-L, Li X-F, Dai X-H, Li N, Cheng M-L, Huang Z, et al. Safety and immunogenicity of the SARS-CoV-2 ARCoV mRNA vaccine in Chinese adults: A randomised, double-blind, placebo-controlled, phase 1 trial. Lancet Microbe (2022) 3:e193–202. doi: 10.1016/S2666-5247(21)00280-9
28. Hallengärd D, Kakoulidou M, Lulla A, Kümmerer BM, Johansson DX, Mutso M, et al. Novel attenuated chikungunya vaccine candidates elicit protective immunity in C57BL/6 mice. J Virol (2014) 88:2858–66. doi: 10.1128/JVI.03453-13
29. Lum F-M, Teo T-H, Lee WWL, Kam Y-W, Rénia L, Ng LFP. An essential role of antibodies in the control of chikungunya virus infection. J Immunol (2013) 190:6295–302. doi: 10.4049/jimmunol.1300304
30. Campos RK, Preciado-Llanes L, Azar SR, Lopez-Camacho C, Reyes-Sandoval A, Rossi SL. A single and un-adjuvanted dose of a chimpanzee adenovirus-vectored vaccine against chikungunya virus fully protects mice from lethal disease. Pathogens (2019) 8:E231. doi: 10.3390/pathogens8040231
31. Wauquier N, Becquart P, Nkoghe D, Padilla C, Ndjoyi-Mbiguino A, Leroy EM. The acute phase of chikungunya virus infection in humans is associated with strong innate immunity and T CD8 cell activation. J Infect Dis (2011) 204:115–23. doi: 10.1093/infdis/jiq006
32. Teo T-H, Lum F-M, Claser C, Lulla V, Lulla A, Merits A, et al. A pathogenic role for CD4+ T cells during chikungunya virus infection in mice. J Immunol (2013) 190:259–69. doi: 10.4049/jimmunol.1202177
33. Iavarone C, O’hagan DT, Yu D, Delahaye NF, Ulmer JB. Mechanism of action of mRNA-based vaccines. Expert Rev Vaccines (2017) 16:871–81. doi: 10.1080/14760584.2017.1355245
34. Karikó K, Muramatsu H, Ludwig J, Weissman D. Generating the optimal mRNA for therapy: HPLC purification eliminates immune activation and improves translation of nucleoside-modified, protein-encoding mRNA. Nucleic Acids Res (2011) 39:e142. doi: 10.1093/nar/gkr695
35. Hanson G, Coller J. Codon optimality, bias and usage in translation and mRNA decay. Nat Rev Mol Cell Biol (2018) 19:20–30. doi: 10.1038/nrm.2017.91
36. Spencer PS, Siller E, Anderson JF, Barral JM. Silent substitutions predictably alter translation elongation rates and protein folding efficiencies. J Mol Biol (2012) 422:328–35. doi: 10.1016/j.jmb.2012.06.010
37. Linares-Fernández S, Lacroix C, Exposito J-Y, Verrier B. Tailoring mRNA vaccine to balance Innate/Adaptive immune response. Trends Mol Med (2020) 26:311–23. doi: 10.1016/j.molmed.2019.10.002
38. Li W, Li X, Zhao D, Liu J, Wang L, Li M, et al. Heterologous prime-boost with AdC68- and mRNA-based COVID-19 vaccines elicit potent immune responses in mice. Signal Transduct Target Ther (2021) 6:419. doi: 10.1038/s41392-021-00843-6
39. He Q, Mao Q, An C, Zhang J, Gao F, Bian L, et al. Heterologous prime-boost: breaking the protective immune response bottleneck of COVID-19 vaccine candidates. Emerging Microbes Infections (2021) 10:629–37. doi: 10.1080/22221751.2021.1902245
40. Liu X, Shaw RH, Stuart ASV, Greenland M, Aley PK, Andrews NJ, et al. Safety and immunogenicity of heterologous versus homologous prime-boost schedules with an adenoviral vectored and mRNA COVID-19 vaccine (Com-COV): a single-blind, randomised, non-inferiority trial. Lancet (2021) 398:856–69. doi: 10.1016/S0140-6736(21)01694-9
41. Karlsson AC, Martin JN, Younger SR, Bredt BM, Epling L, Ronquillo R, et al. Comparison of the ELISPOT and cytokine flow cytometry assays for the enumeration of antigen-specific T cells. J Immunol Methods (2003) 283:141–53. doi: 10.1016/j.jim.2003.09.001
42. Tassignon J, Burny W, Dahmani S, Zhou L, Stordeur P, Byl B, et al. Monitoring of cellular responses after vaccination against tetanus toxoid: Comparison of the measurement of IFN-γ production by ELISA, ELISPOT, flow cytometry and real-time PCR. J Immunol Methods (2005) 305:188–98. doi: 10.1016/j.jim.2005.07.014
43. Maecker HT, Dunn HS, Suni MA, Khatamzas E, Pitcher CJ, Bunde T, et al. Use of overlapping peptide mixtures as antigens for cytokine flow cytometry. J Immunol Methods (2001) 255:27–40. doi: 10.1016/S0022-1759(01)00416-1
44. Palgen J-L, Feraoun Y, Dzangué-Tchoupou G, Joly C, Martinon F, Le Grand R, et al. Optimize Prime/Boost vaccine strategies: Trained immunity as a new player in the game. Front Immunol (2021) 12:612747. doi: 10.3389/fimmu.2021.612747
Keywords: chikungunya virus, mRNA vaccine, immunogenicity, sequential immunization, prime-boost
Citation: Liu J, Lu X, Li X, Huang W, Fang E, Li W, Liu X, Liu M, Li J, Li M, Zhang Z, Song H, Ying B and Li Y (2023) Construction and immunogenicity of an mRNA vaccine against chikungunya virus. Front. Immunol. 14:1129118. doi: 10.3389/fimmu.2023.1129118
Received: 21 December 2022; Accepted: 28 February 2023;
Published: 15 March 2023.
Edited by:
Julia Kzhyshkowska, Heidelberg University, GermanyReviewed by:
Yan-Peng Xu, Capital Institute of Pediatrics, ChinaArun Iyer, Boehringer Ingelheim, United States
Copyright © 2023 Liu, Lu, Li, Huang, Fang, Li, Liu, Liu, Li, Li, Zhang, Song, Ying and Li. This is an open-access article distributed under the terms of the Creative Commons Attribution License (CC BY). The use, distribution or reproduction in other forums is permitted, provided the original author(s) and the copyright owner(s) are credited and that the original publication in this journal is cited, in accordance with accepted academic practice. No use, distribution or reproduction is permitted which does not comply with these terms.
*Correspondence: Yuhua Li, bGl5dWh1YUBuaWZkYy5vcmcuY24=; Bo Ying, Ym8ueWluZ0BhYm9nZW5iaW8uY29t
†These authors have contributed equally to this work