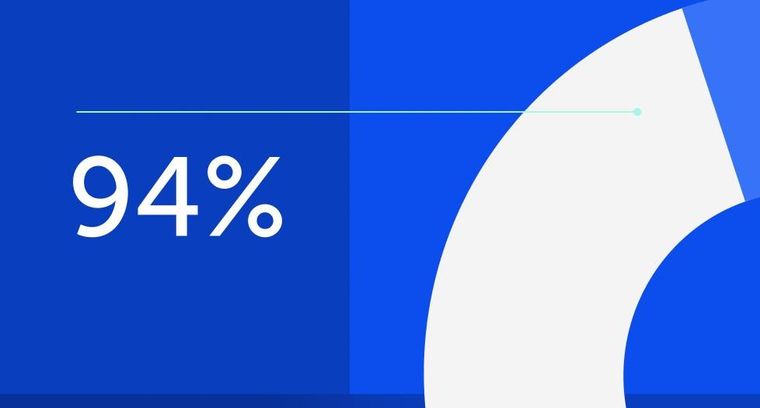
94% of researchers rate our articles as excellent or good
Learn more about the work of our research integrity team to safeguard the quality of each article we publish.
Find out more
REVIEW article
Front. Immunol., 28 February 2023
Sec. Molecular Innate Immunity
Volume 14 - 2023 | https://doi.org/10.3389/fimmu.2023.1128840
This article is part of the Research TopicCutting-edge approaches to investigate the immune response to nanomaterials: advances in nanodiagnostic, nanomedicine and nanotoxicologyView all 5 articles
Manganese (Mn), a nutrient inorganic trace element, is necessary for a variety of physiological processes of animal body due to their important roles in oxidative regulation effects and other aspects of activities. Moreover, manganese ion (Mn2+) has widely reported to be crucial for the regulations of different immunological responses, thus showing promising application as potential adjuvants and immunotherapeutics. Taking the advantages of Mn-based biological and immunological activities, Manganese dioxide nanoparticles (MnO2 NPs) are a new type of inorganic nanomaterials with numerous advantages, including simple preparation, low cost, environmental friendliness, low toxicity, biodegradable metabolism and high bioavailability. MnO2 NPs, as a kind of drug carrier, have also shown the ability to catalyze hydrogen peroxide (H2O2) to produce oxygen (O2) under acidic conditions, which can enhance the efficacy of radiotherapy, chemotherapy and other therapeutics for tumor treatment by remodeling the tumor microenvironment. More importantly, MnO2 NPs also play important roles in immune regulations both in innate and adaptive immunity. In this review, we summarize the biological activities of Manganese, followed by the introduction for the biological and medical functions and mechanisms of MnO2 NPs. What’s more, we emphatically discussed the immunological regulation effects and mechanisms of MnO2 NPs, as well as their potentials to serve as adjuvants and immunomodulators, which might benefit the development of novel vaccines and immunotherapies for more effective disease control.
As an essential trace element for human body, manganese (Mn) plays an important role in promoting the normal growth and development of bones, maintaining normal glucose, lipid metabolism and the function of central nervous system. Manganese is also an important component and activator of some critical enzymes in the body that regulate oxidative stress (1), antioxidant status (2), mitochondrial function (3) and neurotransmitter synthesis (4). Moreover, manganese ions (Mn2+) has also shown promises as a functional intracellular MR imaging contrast agent through its ability to evaluate cellular integrity, activity, and neural connectivity (5, 6). Taking the advantages of these important roles in human health, manganese-based compounds and materials have been considered as a kind of potential candidates for novel diagnosis strategy, vaccine and drug development.
Interestingly, increasing evidences are indicating that manganese can regulate the complicated immunological responses in different conditions (7, 8). The immune function of manganese has been introduced in terms of nutritional immunity (9) due to its roles as a nutrient inorganic micronutrient necessary for a variety of physiological processes, including metabolism, antioxidant defense and antibody production (10, 11). Although the precise mechanisms are still needed to be further investigated, manganese homeostasis has been proved to be critical for different immune responses, especially for its role as an alarm protein of innate immunity for host anti-cancer and anti-infection defense (12). And based on the ability to control innate immunity, manganese has also been proved to possess strong function for the regulation of adaptive immunity.
Nanotechnology is defined as the science and engineering involved in the design, synthesis, characterization and application of materials and devices with molecular precision (13), which is based on a variety of traditional science, including chemistry, physics, materials science, biology and medicine (13, 14). Nanotechnology has demonstrated plenty of applications in biology and medicine, including in situ and multimodal imaging, gene/protein/drug delivery and immunological regulations, which therefore shows promising potentials for diagnostic, vaccine and therapeutic strategy development (15, 16).
Manganese can also be designed into functional nanomaterials with different manganese valence with different associated functions. And among these manganese- based nanomaterials, manganese dioxide nanoparticles (MnO2 NPs) are one of the most stable and functional nanomaterials with some important biological activities and immunological response regulatory activities (5). Under acidic conditions, MnO2 NPs can efficiently catalyze the production of O2 by interact with H2O2 to release Mn2+ due to its peroxidase-like activity (17). Additionally, MnO2 NPs can also interact with the intracellular glutathione (GSH) to release Mn2+ (18, 19). These abilities not only allow MnO2 NPs to regulate the cellular oxidative stress, but also endow MnO2 NPs the potentials to regulate multiple immunological responses by the oxidative associated mechanisms and Mn2+ associated mechanisms (7, 20).
In this review, we summarized the basic biological functions of manganese and MnO2 NPs, as well as their relevant biological and medical application. And due to the increasing attentions paid onto the immune therapy and vaccine development, we emphatically discussed the immunological regulation effects of MnO2 NPs, followed by their promising application in different diseased conditions, which is expected to benefit the future development of novel vaccines and therapies based on MnO2 NPs.
As a kind of typical nanomaterial MnO2 NPs can be used as carriers to design targeted and controlled delivery systems. MnO2 NPs with mesoporous shell have been widely proved to show the ability to load chemotherapy drugs for efficient targeted drug delivery with controlled drug release behaviors (21, 22). MnO2 NPs or MnO2 nanosheets can also be used as the carrier of the photosensitizer drugs to achieve the controlled intracellular release for enhanced phototherapy efficiency (23). The main role of MnO2 nanocarriers for drug delivery is as a “gatekeeper” for the encapsulated drugs, which decompositions at the acidic pH after reaching the targeted site to release the encapsulated drugs, thereby improving the bioavailability, efficacy selectivity of drugs for more effective therapy (19).
Except for the drug carrier roles, MnO2 NPs can also be used as nucleic acid vector for nucleic acid delivery-based therapies. For example, MnO2 nanomaterials can be used also as siRNA loading system for targeted delivery and controlled release siRNA for more effective gene expression regulation, which therefore allowed tumor-related gene therapy (24, 25). In addition to the ability to load siRNA, MnO2 nanomaterials can also be used as DNA nanocarrier, which allows the conjugation of DNA aptamers onto the system to achieve the binding of DNA aptamers to their targets for more effective therapy and diagnostic strategy developments (26, 27). Additionally, DNA enzyme can also be adsorbed onto MnO2 nanomaterials to effectively deliver DNAzyme into cells (23, 28), which enables DNAzyme to catalyze multiple reactions (e.g., RNA or DNA cutting and linking or DNA phosphorylation) for gene therapy (29). Moreover, MnO2 NPs can also be used to wrap proteins, which can combine the advantages of engineered proteins and nanocarriers to provide an intelligent strategy to efficiently deliver functional proteins in vivo (30). The advanced property of MnO2 NPs to serve as drug/nucleic acid/protein carriers provide novel possibilities for the development of more effective therapeutics and diagnostics.
MnO2 NPs can also be used as a T1-weighted magnetic resonance imaging (MRI) probe to significantly enhance MRI signals. The Mn2+, which can be generated by MnO2 NPs decomposition, has been approved by FDA as a clinical T1-weighted magnetic resonance (MR) imaging contrast agent. Mn2+ improves the signal intensity and specificity of MRI by shortening T1 relaxation, resulting in efficient positive contrast enhancement and brighter images. MnO2 NPs have been widely proved to release Mn2+ after their entry into cells by reacting with H2O2 or GSM. Thus, MnO2 NPs are also expected to be used as T1-weighted MRI probes due to their ability to release Mn2+, which provides new possibility to develop MnO2 NPs into novel diagnostic and therapeutic agents (5, 31).
Taking the advantages of the outstanding biological activities of Mn, MnO2 NPs have also been proved to show plenty of biological functions that directly regulate tumor growth. Tumor microenvironment (TME) is typically characterized by its hypoxia and acidic pH, where cancer cells can produce large amounts of H2O2 and GSH for their metabolism and resistance to immunological killings. Under the acidic conditions of TME, MnO2 NPs can effectively catalyze the in situ production of O2 by reacting with endogenous H2O2 to release Mn2+ due to its peroxidase-like activity, thus significantly relieving tumor hypoxia (Figure 1).
Similarly, MnO2 NPs can react with GSH in tumor cells to generate GSSH and Mn2+, which would result in the in uncontrolled reactive oxygen species (ROS) levels in tumor cells due to the decreased GSH level (Figure 2). The ability of MnO2 NPs to regulate TME by catalyzing REDOX activity therefore allows MnO2 NPs to enhance the sensitivity of anti-tumor therapy (17, 32, 33).
In addition, viral or bacterial infections are often accompanied by inflammation.ROS production increases in tissues under inflammation, and the concentration of ROS such as H2O2 can be up to more than 100 times that of normal tissues (34, 35). Besides, hypoxia is a common microenvironmental feature in the inflammatory process related to bacterial infection (36), especially in the site of abscess caused by infection and the bacterial biofilm of chronic wounds, which is characterized by hypoxia, acidic pH and high H2O2 content (37, 38). MnO2 NPs can catalyze H2O2 to produce O2 and release Mn2+ under acidic conditions and even physiological conditions (pH=7.4) (39, 40), so MnO2 NPs can continue to produce oxygen and improve oxidative stress, thus reducing inflammation associated with infection.Therefore, it is believed that MnO2 NPs can also reshape the environment of the infected site to a certain extent.
Phototherapy, including photodynamic therapy (PDT) and photothermal therapy (PTT), relies on photosensitizers (PS) or photothermal agents (PTA) to convert external light energy into monomorphic oxygen (O2) and heat for direct tumor cell killings. Phototherapy is heavily dependent on the production of O2, and the hypoxia of TME will greatly limit the efficacy of phototherapy. The REDOX function of MnO2 NPs can overcome the hypoxia of TME, thus improving the efficacy of phototherapy against tumor (17, 41). Moreover, phototherapy can also activate acute inflammation or induce immunogenic cell death (ICD) to enhance tumor immunogenicity, which could turn the non-immunogenic tumor into the more sensitive immunogenic tumor for immunotherapy (42, 43). Sonodynamic therapy (SDT), similar to PDT, can activate sound sensitizers to produce ROS and overcome the limitation of light penetration depth in phototherapy. However, SDT is also limited by the hypoxic TME, which can also mediate the resistance of tumor to radiotherapy (RT). Based on the ability to catalyze REDOX activity, MnO2 NPs can also be applied to enhance the efficacy of SDT and RT for tumor treatment (44–46).
Interestingly, by regulating REDOX, MnO2 NPs can decompose and release Mn2+, which makes MnO2 NPs can be used as a CDT agent. L/D-MnO2@Pt NPs could be specifically internalized by tumor cells and efficiently deplete the glutathione (GSH) through redox reaction to release Mn2+, while the released Mn2+ could exhibit strong chemodynamic effects through Fenton-like reactions for enhanced tumor therapy efficiency (32). Similarly, stimuli-responsive manganese carbonate-indocyanine green complexes (MnCO3-ICG) can also release “ion reactors” of Mn2+ and ICG in acidic tumor environment, which accelerated the generation of hydroxyl radicals for the oxidative stress damage of tumors cells by catalyzing Mn-mediated Fenton-like reaction to suppress tumor growth more effectively (47). These results strongly suggested that MnO2 NPs based drug delivery system not only introduce the enhanced targeting effects of drugs, but also allowed MnO2 NPs to be used as a CDT agent to improve the catalytic efficiency of Fenton-like reaction to improve CDT performance (48).
As mentioned above, MnO2 NPs have many biological properties, which might be very beneficial for the treatment of diseases. The toxicity and biocompatibility of MnO2 NPs are the most critical issues if MnO2 NPs are to be effectively and practically used in clinical treatment. Interestingly, the whole-body biocompatibility of MnO2 NPs has been demonstrated in tumor models (49). Moreover, some literatures have shown that MnO2 has a crucial advantage -it can be decomposed to release water-soluble Mn2+ in the presence of H2O2 under acidic conditions, and more importantly, Mn2+ can be easily and quickly filtered out of the body by the kidney under physiological conditions (39, 50). Some related works also showed that even when high doses of MnO2 NPs were injected into healthy mice, their liver function markers, kidney function markers and blood routine indexes were no different from normal values, suggesting that MnO2 NPs did not cause significant liver and kidney dysfunction in vivo (40). Thus, unlike many other non-biodegradable inorganic nanomaterials, MnO2 NPs are a kind of biodegradable nanomaterials that can be used in vivo without long-term toxicity concerns. In addition, MnO2 NPs can also be modified with some good biocompatible and biodegradable materials, such as glycol chitosan (GC) polymer and polyethylene glycol (PEG) (18). Loading MnO2 NPs with GC or PEG can further enhance the biocompatibility of nanoparticles and improve their colloidal stability (30, 49). In addition, albumin, such as human albumin (HSA) and bovine albumin (BSA), also have excellent biocompatibility and low immunogenicity, which make them very suitable for wrapping MnO2 NPs (41, 51). Moreover, modification of nanoparticles with hyaluronic acid (HA) or folic acid (FA) can also make MnO2 NPs more biocompatible in treatment (30, 31).
In addition, MnO2 NPs also have great potential for immunotherapy or vaccine development due to the key role of Mn2+ in triggering innate immunity and enhancing adaptive immunity. In conclusion, MnO2 NPs can be used as a kind of novel agents to deliver drugs, regulate REDOX, regulate TME, increase MRI signals, enhance the efficiency of photodynamic therapy, photothermal therapy, sonodynamic therapy, radiotherapy and as a CDT agent to improve chemodynamic therapy performance, activate innate and adaptive immunity with high biocompatibility, which therefore allow MnO2 NPs to be used for novel vaccine, diagnostic, therapeutic, therapeutic vaccine or diagnosis-therapy combined strategy development.
MnO2 NPs have the ability to regulate ROS levels due to the peroxidase activity of MnO2 (17), and the released Mn2+ from MnO2 NPs by catalyzing REDOX reactions also has the ability to regulate ROS levels (32). Additionally, MnO2 can also act as a kind of novel oxidants to consume excess GSH production in tumors to reduce ·OH depletion and release Mn2+ (18, 32). The released Mn2+ can in turn mediate Fenton-like reactions by catalyzing the generation of highly active ·OH from H2O2 (32) to induce high oxidative stress in tumor cells and the production of proinflammatory factors, such as TNF-α (52–55). The high oxidative stress burdens could induce the damage of tumors cells, which is helpful for the tumor antigens release to stimulate anti-tumor immune responses (56–58). And the increased production of proinflammatory factors could induce acute inflammation in tumor, which can stimulate the maturation of DC (7), and thus further enhance the body’s innate anti-tumor immune responses. These effects allow MnO2 NPs to remodel TME and enhance the immunogenicity of tumor cells for enhanced anti-tumor immunity, which suggested MnO2 NPs as immunomodulators and immune activators to regulate innate anti-tumor immune response. Moreover, the inflammatory microenvironment caused by infection is also characterized by hypoxia, acidic pH, accumulation of lactic acid and overexpression of ROS. The functions of MnO2 NPs are also helpful to reverse these microenvironment associated inflammatory responses (59–62). Therefore, MnO2 NPs are also expected to play important roles in innate immune defense against infections.
It’s well known that tumor cells produce a large amount of lactic acid due to high metabolism and insufficient blood supply in solid tumors, and they also produce large amounts of GSH as antioxidants to cause hypoxia and acidic pH, which are recognized as the characteristics of TME (18, 41, 63). Hypoxia not only causes the accumulation of immunosuppressive metabolites (such as adenosine and lactic acid) in solid tumors, but also restricts the infiltration of immune cells, thus inhibiting the body’s anti-tumor immune responses. For example, the accumulated lactic acid induced by hypoxia could reduce antigen presentation and inhibit NK cell activity by inhibiting DC differentiation, which would further promote the infiltration of M2-TAMs (tumor-associated macrophages) and other immunosuppressive cells into the solid tumors (7).
Hypoxia helps tumor cells recruit macrophages, and the recruitment of tumor-associated macrophages (TAMs) into avascular areas sustains tumor progression (64). Classic M1 phenotype macrophages is a type of proinflammatory cell with anti-proliferative and cytotoxic activity due to its ability to secrete reactive nitrogen and reactive oxygen species (e.g., hydrogen peroxide and NO) (31). Moreover, M1 can directly engulf tumor cells (65) or target tumor cells by releasing pro-inflammatory cytokines (66), and can also act as APC to activate T cells, thus enhancing adaptive anti-tumor immunity (67, 68). However, the continued action of TME can polarize M1 into M2-phenotypic macrophages. In contrast to M1 macrophages, M2 macrophages promote immunosuppression by producing various cytokines and growth factors (69), and can promote tumor cell survival in fragile environments such as TME or infection by limiting alternating activation of interleukin-13 and 4 (70, 71). Interestingly, the alleviating effect of MnO2 NPs on TME hypoxia can repolarize the M2 phenotype of TAM to the classic M1 phenotype (72, 73), while the released Mn2+ from MnO2 NPs also promotes M2 macrophages to M1 macrophages by activating interferon signaling (67).
DC is the main supervisor of the immune system (74). DC can recognize, capture and treat the antigen of pathogens at the invasion site, convert the antigen into polypeptides and present the antigenic peptide (75) to cytotoxic CD8+T(CTL) cells and pro-inflammatory CD4+T cells (Th1). Meanwhile, DC also secretes IL-12 (76), which plays an important role in the growth and activity of NK cells and T cells. However, the acidic pH of TME and the accumulation of immunosuppressors (e.g., lactic acid) inhibited the immunostimulatory function of DC (77, 78) and limited the differentiation of monocytes into DC (63). MnO2 NPs can also block the production of lactic acid while increasing the production of oxygen (79), which not only enhances the mature activation of DC (80) but also reverses the inhibitory effect of lactic acid on DC (65). By targeting hypoxia of TME immunosuppression, MnO2 NPs can exert their peroxide-like activity to react with tumor endogenous H2O2, which could generate in situ O2 to relieve TME hypoxia to remit the immune suppression in solid tumors (33, 81). The remission of hypoxia can remodel TME (82), which can also promote the differentiation of monocytes into DC (67), promote the transformation of M2 into anti-tumor immune type M1 (22, 67, 73), and enhance the anti-tumor activity of NK cells (65, 83).By enhancing the anti-tumor activity of immune cells and promoting the infiltration of immune cells in solid tumors, MnO2 NPs can significantly enhance the innate immunological responses against tumor (Figure 3).
Tissue hypoxia and lactic acid buildup due to hypoxia are common microenvironmental features of inflammatory processes associated with bacterial infections (84–86). Meanwhile, H2O2 is also overexpressed in the inflammatory microenvironment. The peroxidase-like activity of MnO2 NPs also catalyzes H2O2 to relieve tissue hypoxia in inflammatory microenvironments and inhibits lactic acid production by producing in situ oxygen (60–62). MnO2 NPs can enhance the effects of DC and macrophages by remodeling inflammatory microenvironment, and enhance the innate immunity against bacteria infections (Figure 3). More importantly, some H2O2-producing bacteria, such as Streptococcus pneumoniae and Streptococcus oral, release large amounts of H2O2 to oxidatively inactivate inflammatosomes in immune cells (87). Moreover, excess ROS production by immune cells is also associated with the decreased activation of inflammasome (88–91). However, inflammasome is a substance that plays a crucial role in the body’s innate immunity against bacterial pathogens (88, 92, 93). Therefore, it is reasonable to speculate that MnO2 NPs might also inhibit the decreased activation of inflammasome by clearing excessive H2O2, and indirectly promote the body’s anti-bacterial innate immune responses. Other studies have also shown (94) that MnO2 nanosheets can directly contact with the bacterial cell membrane and produce a large amount of ROS(mainly ·O2-) at the contact site, which would cause damage to the cell membrane, resulting in leakage of electrolytes/intracellular contents and reduction ATPase activity for bacterial death.
The relief of MnO2 NPs in hypoxic environment enhanced the activity of macrophages, DC and NK cells. Macrophages and DC can secrete IFN-I, and Mn2+ released by MnO2 could promote the production of IFN-I through the cGAS-STING pathway in the presence of viral infection (95). IFN, a group of signaling proteins produced and secreted by host cells in response to pathogen infection (96, 97), has been used in the treatment of hepatitis B virus infection (98–100). IFN-I could induce multiple innate immune responses, such as limiting virus invasion, replication, assembly, and transmission (67). More importantly, the hypoxic state facilitates the transition of Epstein-Barr virus (EBV,HHV-4) and Kaposi Sarcoma-associated herpes virus (KSHV,HHV-8) from latent to dissolved mode (101). And hypoxia could increase the expression of Zta, a protein that mediates the transition between latent and lytic EBV infection, which could in turn increase the amount of viral DNA replication in infected cells (102). Therefore, the ability of MnO2 NPs to increase IFN-I production and relief the hypoxic environment by oxygen production also provides novel possibilities to enhance the innate immune response against viruses.
The released Mn2+ from MnO2 NPs has also been proved to show critical roles in immune regulations in different diseased conditions. After DNA virus infection, host cells release Mn2+ from membrane organelles to cytosol, which is accumulated to activate cGAS-STING signaling pathway to induce IRF3 phosphorylation, activate NF-κB pathway and promote IFN-I production for antiviral effects. The cGAS-STING pathway can also be activated by tumor-derived dsDNA to induce IFN-I, which can promote the maturation/activation of tumor-infiltrating dendritic cell (DCs) or macrophages and enhance the presentation of tumor-specific antigens to activate CD8+T cells and NK cells for tumor immune responses (41, 42). Therefore, Mn2+ released by MnO2 NPs after catalytic REDOX can also trigger innate immunity by activating cGAS-STING signaling pathway. cGAS can be activated by any dsDNA in a sequence independent manner to activate cGAS-STING signaling pathway, which has been reported to be a critical pathway for anti-tumor, antiviral and anti-bacterial defense (53, 103). dsDNA can be detected by the membrane localization protein cGAS (cyclic GMP-AMP(cGAMP) synthetase) (104), leading to the synthesis of the second messenger cGAMP and its binding to STING(stimulator of interferon gene), followed by activation of interferon regulator IRF3 and transcription factor NF-kB. These effects would result in the expression of proinflammatory factors and IFN-I (53, 105), which, in addition to its effects, can promote the maturation and activation of DC and macrophages and enhance NK cell activity (106), thereby further enhancing the innate immune responses (Figure 4). However, Mn2+ itself has been reported to be an effective innate immune stimulator by activating cGAS-STING pathway. Even if there is only a low level of dsDNA (less than 104mg/ml) or even no dsDNA in the cytoplasm, Mn2+ can activate the cGAS-STING signaling pathway to play the similar anti-tumor, antiviral and anti-bacterial roles (107). Mn2+ may promote cGAS-STING pathway activation through two mechanisms. Firstly, Mn2+ can directly bind to cGAS and induce the activation of cGAS protein to form a compact conformation that is more easily to be further activated. The direct interactions between Mn2+ and cGAS proteins may enhance the sensitivity and enzymatic activity of cGAS to dsDNA, which makes very low concentration of dsDNA can also activate cGAS and initiate noncanonical 2’3’ -cGAMP synthesis as a second messenger (108).The second potential mechanism is the ability of Mn2+ to enhance the affinity of cGAMP and STING on the surface of the ER, which could finally enhance the activity of STING (9) and promote the activation of cGAS at the same time (9, 41).
In addition, activation of cGAS by Mn2+ can also alarm the immune system to inhibit cancer development by promoting tumor cell senescence and cytokine production (103). And based on these results, cGAS-STING agonists are therefore being developed as a novel cancer therapeutic, and a greater understanding of cGAS-STING pathway regulation is leading to a broadened list of candidate immune regulatory targets (109). Therefore, MnO2 NPs, with the ability to produce Mn2+, can act as novel cGAS-STING agonists to ameliorate innate immune responses.
Some studies have also identified the intrinsic immune regulatory properties of MnO2 NPs as a unique inducer of nutritionally responsive immunogenic cell death (ICD). While ICDs can directly kill tumor cells, they also highly expose damage-related molecular patterns (DAMPs) on tumor cells (110–112). Lots of evidences support the notion that DAMPs contribute to adaptive immunity in vertebrates (113, 114), and the exposure or release of DAMPs is a key event in the initiation of adaptive immunity (115, 116). DAMPs can not only bind to pattern recognition receptors (PRR) to subsequently promote adaptive immunity by inducing DC activation, but can also enhance adaptive immune responses by affecting the presentation of antigens associated with dying cells, promoting phagocytic action (“ eat-me signaling, “e.g., calreticulin), and facilitating antigen extraction and processing (“ present-me signaling,” e.g., F-actin or HMGB1) (111, 115, 117).
MnO2 nanoparticles have been widely employed in cancer immunotherapy, playing a subsidiary role in assisting immunostimulatory drugs by improving their pharmacokinetics and/or creating a favorable microenvironment. Moreover, Yang et al. introduced an intrinsic immunomodulatory property of MnO2 NPs as a unique nutrient-responsive immunogenic cell death (ICD) inducer, capable of directly modulating immunosurveillance toward tumor cells (110). The underlying mechanism of MnO2 NPs-mediated selective ICD induction might be associated with the concurrently upregulated oxidative stress and autophagy. This starvation- immunotherapy method based on MnO2 NPs is realized by the cut off intratumoral nutrient supply, eliciting potent efficacy for suppressing local and distant tumors. Their findings open up a new avenue toward biomedical applications of MnO2 NPs by manipulating ICD, enabling an innovative therapeutics paradigm with great clinical significance.
By activating innate immune responses, MnO2 NPs can further promote innate immune response and adaptive immunity for disease treatment. It’s widely known that hypoxia helps tumor cells to evade the body’s adaptive immune responses. Under hypoxia, accumulation of hypoxia-inducable factor-1 (HIF-1) activates hypoxia- adapted genes, which induces the expression of the immunosuppressive molecule PD-L1 in tumor cells, thereby increasing the resistance of tumor cells to cytotoxic lymphocyte (CTL)-mediated lysis and inducing CTL apoptosis (118–123). MnO2 NPs can catalyze tumor-derived H2O2 to produce O2 in situ under the acidic pH environment in TME to alleviate hypoxia (22, 23, 45, 124, 125), which could inhibit immune evasion promoted by hypoxia, and indirectly promote adaptive immunity against tumor cells (67, 72, 126, 127) (Figure 3). By controlling Mn2+, MnO2 NPs can also activate cGAS-STING signaling pathway to trigger adaptive immune responses through the antigen presentation by APCs. It is well known that APCs are the bridge between innate immunity and adaptive immunity, among which DC plays a critical role (74, 80). Mn2+ can induce the production of IFN-I by activating cGAS-STING pathway to promote the maturation and activation of tumor-infiltrating macrophages and DCs, and enhance the presentation of tumor-specific antigens (126), which is a key step in activating CD8+T cells and increasing the proportion of memory CD8+T cells (128, 129). IFN-I can also up-regulate costimulatory molecules on the surface of APCs, such as CD80 and CD86 (76, 105). Costimulatory molecules bind to the receptor CD28 protein on the surface of T cells to stimulate the activation, proliferation and differentiation of tumor-specific T cells into memory T cells. This also has significant implications for the treatment of abscesses caused by bacterial infections, given that the immune mimicking effects of Mn2+, which can be released by MnO2 NPs, to induce robust immune memory (38). Similarly, the adaptive immunity triggered by APCs is of great significance to the body’s antibacterial activity. When the host needs to resist pathogen invasion, DC could present antigenic peptides to CD8+T cells and Th1 cells to activate T lymphocytes through direct cell-cell contact (75) (Figure 3). Studies have shown that Mn2+ promotes HBs–AG antibody, ALT, and IFN-β production after HBs–AG immunization to regulate antigen presentation and CD4+T cell activation (95). Moreover, IFN-I induced by Mn2+ is also involved in the regulation of cellular and humoral immunity mediated by CD8+T cells (130), further suggesting that MnO2 NPs might be beneficial for the adaptive immunity against viruses by regulating cellular and humoral immunity. In conclusion, MnO2 NPs can be used as a kind of novel immunological modulators to enhance innate immune responses, increase the efficacy of ICD inducers and improve adaptive immune responses by overcoming hypoxia, regulating ROS levels and controlling cGAS-STING signaling pathway. The ability of MnO2 NPs to release Mn2+ has been regarded as one of the key reasons to activate innate immune responses and trigger adaptive immunity through APCs, which is therefore expected to show promising applications in future vaccine and therapeutic strategy development.
In the past few decades, the research on the biological and medical application of MnO2 NPs has been rapidly developed to show many profound advances. Here, we have reviewed the biological activities of Mn2+ and MnO2 NPs, especially their immunological regulation effects, as well as their potential application. Due to the important physiological roles of Mn, MnO2 NPs are expected to play promising roles in the development of novel nanomedicines.
Taking the advantages of the advancing biological activities of Mn, MnO2 NPs also exert promising biological and immunological activity, and can also be used as a drug carrier for targeted drug delivery. The MnO2 nano preparations can enhance the drug permeability and retention effect, and also enhance the stability of the drugs in the complicated physiological environment and allow the accumulation of drugs at the targeted site, which always lead to enhanced efficacy by combining the drug effects and biological activity of MnO2 NPs (131).
More importantly, MnO2 NPs also possess strong immunological regulation effects to regulate both innate and adaptive immunity. In tumor, hypoxia not only limits the efficacy of RT, PT, etc., but also causes the accumulation of immunosuppressors in tumor and limits the infiltration of immune cells. MnO2 NPs exert the activity of peroxidase to produce O2 in situ in the tumor to improve the immunosuppression while reshaping tumor microenvironment, and further enhance the anti-tumor immunity by promoting the infiltration of innate immune cells (7). MnO2 can relieve hypoxia at the tumor site by REDOX with excess H2O2 and GSH in TME, and enhance the antitumor efficacy of RT (44), PT (17, 41), SDT (45), CDT (23, 32) and their synergistic treatment (5, 17). After the entry into cells, MnO2 NPs can interact with endogenous H2O2 to increase OH· levels, by depleting GSH and mediating Fenton-like reactions, thereby enhancing the antitumor efficacy of chemodynamic therapy and chemotherapy (32).
During the interactions between MnO2 NPs and GSH or H2O2, Mn2+ can be released to show multiple biological functions. The obtained Mn2+ can directly bind with cGAS to enhance their enzyme activity and their sensitivity to dsDNA. The binding of Mn2+ with cGAS could also activate STING by enhancing the affinity between cGAMP and STING to promote the CGAS-STING signaling pathway, which would induce the production of IFN-I to promote innate immunity. IFN-I can further promote the mature activation of infiltrating APCs to trigger adaptive immunity, which therefore introduces the potential of Mn2+ to be used as an immune adjuvant (9, 38, 107, 128).
Mn2+ released by MnO2 NPs after catalyzing H2O2 can mediate Fenton-like reaction to produce OH·, which induces oxidative stress in tumor cells and acute inflammatory response, thus enhancing DC maturation and TNF-α production to further promote innate immune responses (32, 55). MnO2 NPs can also indirectly improve adaptive immunity by producing in situ O2 to overcome immune escape caused by HIF-1α-dependent PD-L1 expression in tumor cells under hypoxia conditions (121). MnO2 NPs can also be used as a unique nutrition-responsive ICD inducer, selectively inducing ICD in nutrition-deficient tumor cells to regulate adaptive immunity (110).
Based on these properties, MnO2 NPs have shown great potentials as novel immune adjuvants and agonists due to its various biological activities and immune regulatory functions. Due to the development of Mn2+ -based adjuvants, the use of MnO2 NPs for potential adjuvant uses might also attract increasing attentions. Additionally, more and more researchers are working on how to integrate MnO2 NPs into multiple mode agents for diagnostic and therapeutic uses simultaneously. The development of such multiple mode agents based on MnO2 NPs might introduce more important advances, including its immunotherapy potentials, multi-mode synergistic therapy, and MRI guided chemotherapy or immunotherapy.
However, in order to apply MnO2 NPs for effective clinical uses, its biocompatibility and toxicity, and their associated metabolism and degradation are the most important issues that need to be further investigated. And among these issues, biocompatibility and toxicity are the most urgent issues for the further uses of MnO2 NPs. Some studies have proposed (41, 132) that the wrap of MnO2 NPs with HSA could introduce MnO2 NPs better biocompatibility and appropriate size, which is conducive to enhancing the permeability and retention effect of nanoparticles. In addition, studies (18, 38) have shown that loading MnO2 NPs with GC or PEG can also enhance their biocompatibility and improve their colloid stability More studies have pointed out that polydopamine (PDA) is a “connector” of functional materials due to its strong adhesion and chemical reactivity, and MnO2 NPs can also be connected to PDA with good biocompatibility (133). The encapsulation of MnO2 NPs in a carrier constructed with HA could also make MnO2 NPs more biocompatible for cancer treatment (63). Although lots of attempts have been made to improve the biocompatibility and reduce the toxicity of MnO2 NPs, more attentions are still needed to promote the final clinic uses of MnO2 NPs. Moreover, the systemic metabolism and degradation behaviors and mechanisms are also need to be further evaluated. On the premise of maintaining the therapeutic effect of biological system, attention should be paid to reducing or reducing the toxicity in vivo and speeding up its biodegradability.In addition to the primary issue of biocompatibility, the absence of a perfect preparation method is also the key hindering the clinical application of MnO2 NPs.Currently, there are many methods to prepare nanomaterials, which have their own advantages but also their own obvious disadvantages, such as difficult preparation methods and high cost, difficult to control the consistency of nanomaterials, potential dangers and environmental pollution (134, 135). Therefore, it remains a major challenge to explore a simple, low-cost, safe and environmentally friendly method for the controlled synthesis of MnO2 NPs.
Thus, although MnO2 NPs have shown promising application in plenty of biological and medical uses, such as their excellent immunoregulation effects, there are still many obstacles that affect their clinical use, which requires more researches and investigations. However, we believe that as one of the most functional nanomaterials with strong immunoregulation activities, MnO2 NPs would play more and more important roles for the development of novel vaccines or immunotherapies, which would finally benefit the more effective disease precaution and treatments.
YuH and YR drafted this manuscript, YM, DC, TZ, SF, WL, YiH, HL helped to revise the manuscript, J-FX, JP and BZ helped to revise the manuscript and were responsible for leading this work. All authors contributed to the article and approved the submitted version.
This work was supported by National Natural Science Foundation of China (82272348, 82270013 and 81870016), High Talent Project of Guangdong Province (2021QN02Y720), Natural Science Foundation of Guangdong Province (2023A1515030195, 2022A1515010525 and 2022A1515011223), Characteristic Innovation Project of Universities in Guangdong Province (2021KTSCX038), Key Project of Universities in Guangdong Province (2022ZDZX2021), Innovation Team Project of Universities in Guangdong Province (2022KCXTD010), Key Project of Science and Technology of Dongguan (20211800905072), Funds for Ph.D. Researchers of Guangdong Medical University in 2021 (4SG22209G), Discipline Construction Project of Guangdong Medical University (4SG21229GDGFY01), Open Research Fund of Songshan Lake Materials Laboratory (2021SLABFN10) and Open Research Fund for Key Laboratory of Tropical Disease Control Sun Yat-sen University, Ministry of Education (2022kfkt01).
We extend our apologies to those authors whose deserving research was not cited in this manuscript. We also thank Figdraw (www.figdraw.com ) for the assistance in creating all figures.
The authors declare that the research was conducted in the absence of any commercial or financial relationships that could be construed as a potential conflict of interest.
All claims expressed in this article are solely those of the authors and do not necessarily represent those of their affiliated organizations, or those of the publisher, the editors and the reviewers. Any product that may be evaluated in this article, or claim that may be made by its manufacturer, is not guaranteed or endorsed by the publisher.
1. Michalke B, Fernsebner K. New insights into manganese toxicity and speciation. J Trace Elements Med Biol (2014) 28:106–16. doi: 10.1016/j.jtemb.2013.08.005
2. Buettner GR, Ng CF, Wang M, Rodgers VGJ, Schafer FQ. A new paradigm: Manganese superoxide dismutase influences the production of H2O2 in cells and thereby their biological state. Free Radical Biol Med (2006) 41:1338–50. doi: 10.1016/j.freeradbiomed.2006.07.015
3. Gunter TE, Gerstner B, Lester T, Wojtovich AP, Malecki J, Swarts SG, et al. An analysis of the effects of Mn2+ on oxidative phosphorylation in liver, brain, and heart mitochondria using state 3 oxidation rate assays. Toxicol Appl Pharmacol (2010) 249:65–75. doi: 10.1016/j.taap.2010.08.018
4. Wedler FC, Denman RB. Glutamine synthetase: The major Mn(II) enzyme in mammalian brain. In: Current topics in cellular regulation. Elsevier, United States (1984). p. 153–4. doi: 10.1016/B978-0-12-152824-9.50021-6
5. Lopes SV, Walczak P, Janowski M, Reis RL, Silva-Correia J, Oliveira JM. Cytocompatible manganese dioxide-based hydrogel nanoreactors for MRI imaging. Biomaterials Adv (2022) 134:112575. doi: 10.1016/j.msec.2021.112575
6. Boretius S, Frahm J. Manganese-enhanced magnetic resonance imaging. In: Schröder L, Faber C, editors. In vivo NMR imaging. methods in molecular biology. Totowa, NJ: Humana Press (2011). p. 531–68. doi: 10.1007/978-1-61779-219-9_28
7. Zhang K, Qi C, Cai K. Advanced materials. United States, Wiley-Blackwell (2022). p. 2205409. doi: 10.1002/adma.202205409
8. Wu Q, Mu Q, Xia Z, Min J, Wang F. Manganese homeostasis at the host-pathogen interface and in the host immune system. Semin Cell Dev Biol (2021) 115:45–53. doi: 10.1016/j.semcdb.2020.12.006
9. Haase H. Innate immune cells speak manganese. Immunity (2018) 48:616–8. doi: 10.1016/j.immuni.2018.03.031
10. Horning KJ, Caito SW, Tipps KG, Bowman AB, Aschner M. Manganese is essential for neuronal health. Annu Rev Nutr (2015) 35:71–108. doi: 10.1146/annurev-nutr-071714-034419
11. Kwakye G, Paoliello M, Mukhopadhyay S, Bowman A, Aschner M. Manganese-induced parkinsonism and parkinson’s disease: Shared and distinguishable features. IJERPH (2015) 12:7519–40. doi: 10.3390/ijerph120707519
12. Chen X, Liu Z, Ge X, Luo X, Huang S, Zhou Y, et al. Associations between manganese exposure and multiple immunological parameters in manganese-exposed workers healthy cohort. J Trace Elements Med Biol (2020) 59:126454. doi: 10.1016/j.jtemb.2020.126454
13. Saini R, Saini S, Sharma S. Nanotechnology: The future medicine. J Cutan Aesthet Surg (2010) 3:32. doi: 10.4103/0974-2077.63301
14. Gökçay B, Arda B. Nanotechnology, nanomedicine. Ethical Aspects (2017) 12:1–12. doi: 10.1186/1477-3155-2-3
15. Salata O. Applications of nanoparticles in biology and medicine. J Nanobiotechnology (2004) 6:1–6.
16. Jones E, He J, VanBrocklin H, Franc B, Seo Y. Nanoprobes for medical diagnosis: Current status of nanotechnology in molecular imaging. CNANO (2008) 4:17–29. doi: 10.2174/157341308783591843
17. Li M, Xiao M, Pan Q, Xiong J. Multifunctional nanoplatform based on g-C3N4, loaded with MnO2 and CuS nanoparticals for oxygen self-generation photodynamic/photothermal synergistic therapy. Photodiagnosis Photodyn Ther (2022) 37:102684. doi: 10.1016/j.pdpdt.2021.102684
18. Zhu J, Xiao T, Zhang J, Che H, Shi Y, Shi X, et al. Surface-Charge-Switchable nanoclusters for magnetic resonance imaging-guided and glutathione depletion-enhanced photodynamic therapy. ACS Nano (2020) 14:11225–37. doi: 10.1021/acsnano.0c03080
19. Zhang Z, Ji Y, Lin C, Tao L. Thermosensitive hydrogel-functionalized gold nanorod/mesoporous MnO2 nanoparticles for tumor cell-triggered drug delivery. Materials Sci Engineering: C (2021) 131:112504. doi: 10.1016/j.msec.2021.112504
20. Li Y, Du L, Li F, Deng Z, Zeng S. Intelligent nanotransducer for deep-tumor hypoxia modulation and enhanced dual-photosensitizer photodynamic therapy. ACS Appl Mater Interfaces (2022) 14:14944–52. doi: 10.1021/acsami.1c24172
21. Cheng M, Yu Y, Huang W, Fang M, Chen Y, Wang C, et al. Monodisperse hollow MnO 2 with biodegradability for efficient targeted drug delivery. ACS Biomater Sci Eng (2020) 6:4985–92. doi: 10.1021/acsbiomaterials.0c00507
22. Dong C, Huang Q, Cheng H, Zheng D, Hong S, Yan Y, et al. Neisseria meningitidis opca Protein/MnO 2 hybrid nanoparticles for overcoming the blood–brain barrier to treat glioblastoma. Advanced Materials (2022) 34:2109213. doi: 10.1002/adma.202109213
23. Fan H, Yan G, Zhao Z, Hu X, Zhang W, Liu H, et al. A smart photosensitizer–manganese dioxide nanosystem for enhanced photodynamic therapy by reducing glutathione levels in cancer cells. Angew Chem Int Ed (2016) 55:5477–82. doi: 10.1002/anie.201510748
24. Shi M, Wang S, Zheng S, Hou P, Dong L, He M, et al. Activatable MRI-monitoring gene delivery for the theranostic of renal carcinoma. Colloids Surfaces B: Biointerfaces (2020) 185:110625. doi: 10.1016/j.colsurfb.2019.110625
25. Ming L, Song L, Xu J, Wang R, Shi J, Chen M, et al. Smart manganese dioxide-based lanthanide nanoprobes for triple-negative breast cancer precise gene synergistic chemodynamic therapy. ACS Appl Mater Interfaces (2021) 13:35444–55. doi: 10.1021/acsami.1c08927
26. Zhao Z, Fan H, Zhou G, Bai H, Liang H, Wang R, et al. Activatable Fluorescence/MRI bimodal platform for tumor cell imaging via MnO 2 nanosheet–aptamer nanoprobe. J Am Chem Soc (2014) 136:11220–3. doi: 10.1021/ja5029364
27. Zhao M, Song X, Lu J, Liu S, Sha X, Wang Q, et al. DNA Aptamer-based dual-responsive nanoplatform for targeted MRI and combination therapy for cancer. RSC Adv (2022) 12:3871–82. doi: 10.1039/D1RA08373B
28. Gong X, Li R, Wang J, Wei J, Ma K, Liu X, et al. A smart theranostic nanocapsule for spatiotemporally programmable photo-gene therapy. Angew Chem Int Ed (2020) 59:21648–55. doi: 10.1002/anie.202008413
29. Liu J, Cao Z, Lu Y. Functional nucleic acid sensors. Chem Rev (2009) 109:1948–98. doi: 10.1021/cr030183i
30. Zhu X, Tang R, Wang S, Chen X, Hu J, Lei C, et al. A protein at inorganic nano-dumpling system for high-loading protein delivery with activatable fluorescence and magnetic resonance bimodal imaging capabilities. ACS Nano (2020) 43:2172–2182. doi: 10.1021/acsnano.9b09024
31. Song M, Liu T, Shi C, Zhang X, Chen X. Bioconjugated manganese dioxide nanoparticles enhance chemotherapy response by priming tumor-associated macrophages toward M1-like phenotype and attenuating tumor hypoxia. ACS Nano (2016) 10:633–47. doi: 10.1021/acsnano.5b06779
32. Gao F, Sun M, Zhang J, Chang Y, Gao W, Ma G, et al. Fenton-like reaction and glutathione depletion by chiral manganese dioxide nanoparticles for enhanced chemodynamic therapy and chemotherapy. J Colloid Interface Sci (2022) 616:369–78. doi: 10.1016/j.jcis.2022.02.060
33. Xiao J, Cheng L, Fang T, Zhang Y, Zhou J, Cheng R, et al. Nanoparticle-embedded electrospun fiber–covered stent to assist intraluminal photodynamic treatment of oesophageal cancer. Small (2019) 15:1904979. doi: 10.1002/smll.201904979
34. Mittal M, Siddiqui MR, Tran K, Reddy SP, Malik AB. Reactive oxygen species in inflammation and tissue injury. Antioxidants Redox Signaling (2014) 20:1126–67. doi: 10.1089/ars.2012.5149
35. Wang P, Gong Q, Hu J, Li X, Zhang X. Reactive oxygen species (ROS)-responsive prodrugs, probes, and theranostic prodrugs: Applications in the ROS-related diseases. J Med Chem (2021) 64:298–325. doi: 10.1021/acs.jmedchem.0c01704
36. Schaffer K, Taylor CT. The impact of hypoxia on bacterial infection. FEBS J (2015) 282:2260–6. doi: 10.1111/febs.13270
37. Xiu W, Gan S, Wen Q, Qiu Q, Dai S, Dong H, et al. Biofilm microenvironment-responsive nanotheranostics for dual-mode imaging and hypoxia-Relief-Enhanced photodynamic therapy of bacterial infections. Research (2020) 2020):9426453. doi: 10.34133/2020/9426453
38. Wang C, Xiao Y, Zhu W, Chu J, Xu J, Zhao H, et al. Photosensitizer-modified MnO 2 nanoparticles to enhance photodynamic treatment of abscesses and boost immune protection for treated mice. Small (2020) 16:2000589. doi: 10.1002/smll.202000589
39. Yang G, Xu L, Chao Y, Xu J, Sun X, Wu Y, et al. Hollow MnO2 as a tumor-microenvironment-responsive biodegradable nano-platform for combination therapy favoring antitumor immune responses. Nat Commun (2017) 8:902. doi: 10.1038/s41467-017-01050-0
40. Hu D, Chen L, Qu Y, Peng J, Chu B, Shi K, et al. Oxygen-generating hybrid polymeric nanoparticles with encapsulated doxorubicin and chlorin e6 for trimodal imaging-guided combined chemo-photodynamic therapy. Theranostics (2018) 8:1558–74. doi: 10.7150/thno.22989
41. Chen Q, Feng L, Liu J, Zhu W, Dong Z, Wu Y, et al. Intelligent albumin-MnO 2 nanoparticles as pH-/H 2 O 2 -responsive dissociable nanocarriers to modulate tumor hypoxia for effective combination therapy. Adv Mater (2016) 28:7129–36. doi: 10.1002/adma.201601902
42. Li M, Guo R, Wei J, Deng M, Li J, Tao Y, et al. Polydopamine-based nanoplatform for photothermal ablation with long-term immune activation against melanoma and its recurrence. Acta Biomaterialia (2021) 136:546–57. doi: 10.1016/j.actbio.2021.09.014
43. Li X, Gao Y, Liu X, Hu X, Li Y, Sun J, et al. Ultrasound and laser-promoted dual-gas nano-generator for combined photothermal and immune tumor therapy. Front Bioeng Biotechnol (2022) 10:1005520. doi: 10.3389/fbioe.2022.1005520
44. Abbasi AZ, Gordijo CR, Amini MA, Maeda A, Rauth AM, DaCosta RS, et al. Hybrid manganese dioxide nanoparticles potentiate radiation therapy by modulating tumor hypoxia. Cancer Res (2016) 76:6643–56. doi: 10.1158/0008-5472.CAN-15-3475
45. Pan X, Wang H, Wang S, Sun X, Wang L, Wang W, et al. Sonodynamic therapy (SDT): a novel strategy for cancer nanotheranostics. Sci China Life Sci (2018) 61:415–26. doi: 10.1007/s11427-017-9262-x
46. Qian X, Zheng Y, Chen Y. Micro/Nanoparticle-augmented sonodynamic therapy (SDT): Breaking the depth shallow of photoactivation. Adv Mater (2016) 28:8097–129. doi: 10.1002/adma.201602012
47. Feng Y. Intracellular marriage of bicarbonate and Mn ions as “immune ion reactors” to regulate redox homeostasis and enhanced antitumor immune responses. Journal of nanobiotechnology (2022) 17:1–17. doi: 10.1186/s12951-022-01404-x
48. Wang X, Zhong X, Liu Z, Cheng L. Recent progress of chemodynamic therapy-induced combination cancer therapy. Nano Today (2020) 35:100946. doi: 10.1016/j.nantod.2020.100946
49. Kumar S, Adjei IM, Brown SB, Liseth O, Sharma B. Manganese dioxide nanoparticles protect cartilage from inflammation-induced oxidative stress. Biomaterials (2019) 224:119467. doi: 10.1016/j.biomaterials.2019.119467
50. Zhu W, Dong Z, Fu T, Liu J, Chen Q, Li Y, et al. Modulation of hypoxia in solid tumor microenvironment with MnO 2 nanoparticles to enhance photodynamic therapy. Adv Funct Mater (2016) 26:5490–8. doi: 10.1002/adfm.201600676
51. Zhang M, Xing L, Ke H, He Y-J, Cui P-F, Zhu Y, et al. MnO 2 -based nanoplatform serves as drug vehicle and MRI contrast agent for cancer theranostics. ACS Appl Mater Interfaces (2017) 9:11337–44. doi: 10.1021/acsami.6b15247
52. Marin E, Tapeinos C, Lauciello S, Ciofani G, Sarasua JR, Larrañaga A. Encapsulation of manganese dioxide nanoparticles into layer-by-layer polymer capsules for the fabrication of antioxidant microreactors. Materials Sci Engineering: C (2020) 117:111349. doi: 10.1016/j.msec.2020.111349
53. Ablasser A, Chen ZJ. cGAS in action: Expanding roles in immunity and inflammation. Science (2019) 363:eaat8657. doi: 10.1126/science.aat8657
54. Chandra J, Teoh SM, Kuo P, Tolley L, Bashaw AA, Tuong ZK, et al. Manganese-doped silica-based nanoparticles promote the efficacy of antigen-specific immunotherapy. JI (2021) 206:987–98. doi: 10.4049/jimmunol.2000355
55. Zhao H, Wu L, Yan G, Chen Y, Zhou M, Wu Y, et al. Inflammation and tumor progression: Signaling pathways and targeted intervention. Sig Transduct Target Ther (2021) 6:263. doi: 10.1038/s41392-021-00658-5
56. Weinberg F, Ramnath N, Nagrath D. Reactive oxygen species in the tumor microenvironment: An overview. Cancers (2019) 11:1191. doi: 10.3390/cancers11081191
57. Wang J, Sun D, Huang L, Wang S, Jin Y. Targeting reactive oxygen species capacity of tumor cells with repurposed drug as an anticancer therapy. Oxid Med Cell Longevity (2021) 2021:1–17. doi: 10.1155/2021/8532940
58. Chen X, Stewart E, Shelat AA, Qu C, Bahrami A, Hatley M, et al. Targeting oxidative stress in embryonal rhabdomyosarcoma. Cancer Cell (2013) 24:710–24. doi: 10.1016/j.ccr.2013.11.002
59. Lu X, Chen R, Lv J, Xu W, Chen H, Ma Z, et al. High-resolution bimodal imaging and potent antibiotic/photodynamic synergistic therapy for osteomyelitis with a bacterial inflammation-specific versatile agent. Acta Biomaterialia (2019) 99:363–72. doi: 10.1016/j.actbio.2019.08.043
60. Li C, Zhao Z, Luo Y, Ning T, Liu P, Chen Q, et al. Macrophage-disguised manganese dioxide nanoparticles for neuroprotection by reducing oxidative stress and modulating inflammatory microenvironment in acute ischemic stroke. Advanced Sci (2021) 8:2101526. doi: 10.1002/advs.202101526
61. Shen J, Chen A, Cai Z, Chen Z, Cao R, Liu Z, et al. Exhausted local lactate accumulation via injectable nanozyme-functionalized hydrogel microsphere for inflammation relief and tissue regeneration. Bioactive Materials (2022) 12:153–68. doi: 10.1016/j.bioactmat.2021.10.013
62. Tu C, Lu H, Zhou T, Zhang W, Deng L, Cao W, et al. Promoting the healing of infected diabetic wound by an anti-bacterial and nano-enzyme-containing hydrogel with inflammation-suppressing, ROS-scavenging, oxygen and nitric oxide-generating properties. Biomaterials (2022) 286:121597. doi: 10.1016/j.biomaterials.2022.121597
63. Gao S, Wang G, Qin Z, Wang X, Zhao G, Ma Q, et al. Oxygen-generating hybrid nanoparticles to enhance fluorescent/photoacoustic/ultrasound imaging guided tumor photodynamic therapy. Biomaterials (2017) 112:324–35. doi: 10.1016/j.biomaterials.2016.10.030
64. Casazza A, Laoui D, Wenes M, Rizzolio S, Bassani N, Mambretti M, et al. Impeding macrophage entry into hypoxic tumor areas by Sema3A/Nrp1 signaling blockade inhibits angiogenesis and restores antitumor immunity. Cancer Cell (2013) 24:695–709. doi: 10.1016/j.ccr.2013.11.007
65. Wang JX, Choi SYC, Niu X, Kang N, Xue H, Killam J, et al. Lactic acid and an acidic tumor microenvironment suppress anticancer immunity. IJMS (2020) 21:8363. doi: 10.3390/ijms21218363
66. Hao N-B, Lü M-H, Fan Y-H, Cao Y-L, Zhang Z-R, Yang S-M. Macrophages in tumor microenvironments and the progression of tumors. Clin Dev Immunol (2012) 2012:1–11. doi: 10.1155/2012/948098
67. Sun Y, Yin Y, Gong L, Liang Z, Zhu C, Ren C, et al. Manganese nanodepot augments host immune response against coronavirus. Nano Res (2021) 14:1260–72. doi: 10.1007/s12274-020-3243-5
68. Poltavets AS, Vishnyakova PA, Elchaninov AV, Sukhikh GT, TKh F. Macrophage modification strategies for efficient cell therapy. Cells (2020) 9:1535. doi: 10.3390/cells9061535
69. Hughes R, Qian B-Z, Rowan C, Muthana M, Keklikoglou I, Olson OC, et al. Perivascular M2 macrophages stimulate tumor relapse after chemotherapy. Cancer Res (2015) 75:3479–91. doi: 10.1158/0008-5472.CAN-14-3587
70. Gordon S, Martinez FO. Alternative activation of macrophages: Mechanism and functions. Immunity (2010) 32:593–604. doi: 10.1016/j.immuni.2010.05.007
71. Chanmee T, Ontong P, Konno K, Itano N. Tumor-associated macrophages as major players in the tumor microenvironment. Cancers (2014) 6:1670–90. doi: 10.3390/cancers6031670
72. Chang C-C, Dinh TK, Lee Y-A, Wang F-N, Sung Y-C, Yu P-L, et al. Nanoparticle delivery of MnO 2 and antiangiogenic therapy to overcome hypoxia-driven tumor escape and suppress hepatocellular carcinoma. ACS Appl Mater Interfaces (2020) 12:44407–19. doi: 10.1021/acsami.0c08473
73. Nath A, Pal R, Singh LM, Saikia H, Rahaman H, Ghosh SK, et al. Gold−manganese oxide nanocomposite suppresses hypoxia and augments pro-inflammatory cytokines in tumor associated macrophages. Int Immunopharmacol (2018) 57:157–64. doi: 10.1016/j.intimp.2018.02.021
74. Steinman RM. Decisions about dendritic cells: Past, present, and future. Annu Rev Immunol (2012) 30:1–22. doi: 10.1146/annurev-immunol-100311-102839
75. Winning S, Fandrey J. Dendritic cells under hypoxia: How oxygen shortage affects the linkage between innate and adaptive immunity. J Immunol Res (2016) 2016:1–8. doi: 10.1155/2016/5134329
76. Banchereau J, Steinman RM. Dendritic cells and the control of immunity. Nature (1998) 392:245–52. doi: 10.1038/32588
77. Hinshaw DC, Shevde LA. The tumor microenvironment innately modulates cancer progression. Cancer Res (2019) 79:4557–66. doi: 10.1158/0008-5472.CAN-18-3962
78. Monti M, Vescovi R, Consoli F, Farina D, Moratto D, Berruti A, et al. Plasmacytoid dendritic cell impairment in metastatic melanoma by lactic acidosis. Cancers (2020) 12:2085. doi: 10.3390/cancers12082085
79. Cassavaugh J, Lounsbury KM. Hypoxia-mediated biological control. J Cell Biochem (2011) 112:735–44. doi: 10.1002/jcb.22956
80. Yang M, Li J, Gu P, Fan X. The application of nanoparticles in cancer immunotherapy: Targeting tumor microenvironment. Bioactive Materials (2021) 6:1973–87. doi: 10.1016/j.bioactmat.2020.12.010
81. Wang W, Liu X, Ding L, Jin HJ, Li X. RNA Hydrogel combined with MnO2 nanoparticles as a nano-vaccine to treat triple negative breast cancer. Front Chem (2021) 9:797094. doi: 10.3389/fchem.2021.797094
82. Fan W, Bu W, Shen B, He Q, Cui Z, Liu Y, et al. Intelligent MnO 2 nanosheets anchored with upconversion nanoprobes for concurrent pH-/H 2 O 2 -responsive UCL imaging and oxygen-elevated synergetic therapy. Adv Mater (2015) 27:4155–61. doi: 10.1002/adma.201405141
83. Murphy DA, Cheng H, Yang T, Yan X, Adjei IM. Reversing hypoxia with PLGA-encapsulated manganese dioxide nanoparticles improves natural killer cell response to tumor spheroids. Mol Pharmaceutics (2021) 18:2935–46. doi: 10.1021/acs.molpharmaceut.1c00085
84. Eltzschig HK. Hypoxia and inflammation. New Engl J Med (2011) 364:656–665. doi: 10.1056/NEJMra0910283
85. Palazon A, Goldrath AW, Nizet V, Johnson RS. HIF transcription factors, inflammation, and immunity. Immunity (2014) 41:518–28. doi: 10.1016/j.immuni.2014.09.008
86. Bhandari T, Nizet V. Hypoxia-inducible factor (HIF) as a pharmacological target for prevention and treatment of infectious diseases. Infect Dis Ther (2014) 3:159–74. doi: 10.1007/s40121-014-0030-1
87. Erttmann SF, Gekara NO. Hydrogen peroxide release by bacteria suppresses inflammasome-dependent innate immunity. Nat Commun (2019) 10:3493. doi: 10.1038/s41467-019-11169-x
88. Erttmann SF, Härtlova A, Sloniecka M, Raffi FAM, Hosseinzadeh A, Edgren T, et al. Loss of the DNA damage repair kinase ATM impairs inflammasome-dependent anti-bacterial innate immunity. Immunity (2016) 45:106–18. doi: 10.1016/j.immuni.2016.06.018
89. Meissner F, Molawi K, Zychlinsky A. Superoxide dismutase 1 regulates caspase-1 and endotoxic shock. Nat Immunol (2008) 9:866–72. doi: 10.1038/ni.1633
90. Mao K, Chen S, Chen M, Ma Y, Wang Y, Huang B, et al. Nitric oxide suppresses NLRP3 inflammasome activation and protects against LPS-induced septic shock. Cell Res (2013) 23:201–12. doi: 10.1038/cr.2013.6
91. Hernandez-Cuellar E, Tsuchiya K, Hara H, Fang R, Sakai S, Kawamura I, et al. Cutting edge: Nitric oxide inhibits the NLRP3 inflammasome. JI (2012) 189:5113–7. doi: 10.4049/jimmunol.1202479
92. von Moltke J, Ayres JS, Kofoed EM, Chavarría-Smith J, Vance RE. Recognition of bacteria by inflammasomes. Annu Rev Immunol (2013) 31:73–106. doi: 10.1146/annurev-immunol-032712-095944
93. Fang R, Tsuchiya K, Kawamura I, Shen Y, Hara H, Sakai S, et al. Critical roles of ASC inflammasomes in caspase-1 activation and host innate resistance to Streptococcus pneumoniae infection. JI (2011) 187:4890–9. doi: 10.4049/jimmunol.1100381
94. Du T, Chen S, Zhang J, Li T, Li P, Liu J, et al. Antibacterial activity of manganese dioxide nanosheets by ROS-mediated pathways and destroying membrane integrity. Nanomaterials (2020) 10:1545. doi: 10.3390/nano10081545
95. Lin M, Guo R, Ma C, Zeng D, Su Z. Manganese breaks the immune tolerance of HBs-Ag. Open Forum Infect Dis (2021) 8:ofab028. doi: 10.1093/ofid/ofab028
96. De Andrea M, Ravera R, Gioia D, Gariglio M, Landolfo S. The interferon system: An overview. Eur J Paediatric Neurol (2002) 6:A41–6. doi: 10.1053/ejpn.2002.0573
97. Kalvakolanu DV, Borden EC. An overview of the interferon system: Signal transduction and mechanisms of action. Cancer Invest (1996) 14:25–53. doi: 10.3109/07357909609018435
98. Sundaram V, Kowdley K. Management of chronic hepatitis b infection. BMJ (2015) 351:h4263–3. doi: 10.1136/bmj.h4263
99. Lee Y-S, Suh DJ, Lim Y-S, Jung SW, Kim KM, Lee HC, et al. Increased risk of adefovir resistance in patients with lamivudine-resistant chronic hepatitis b after 48 weeks of adefovir dipivoxil monotherapy. Hepatology (2006) 43:1385–91. doi: 10.1002/hep.21189
100. Belloni L, Allweiss L, Guerrieri F, Pediconi N, Volz T, Pollicino T, et al. IFN-α inhibits HBV transcription and replication in cell culture and in humanized mice by targeting the epigenetic regulation of the nuclear cccDNA minichromosome. J Clin Invest (2012) 122:529–37. doi: 10.1172/JCI58847
101. Reyes A, Duarte LF, Farías MA, Tognarelli E, Kalergis AM, Bueno SM, et al. Impact of hypoxia over human viral infections and key cellular processes. IJMS (2021) 22:7954. doi: 10.3390/ijms22157954
102. Jiang J-H, Wang N, Li A, Liao W-T, Pan Z-G, Mai S-J, et al. Hypoxia can contribute to the induction of the Epstein-Barr virus (EBV) lytic cycle. J Clin Virol (2006) 37:98–103. doi: 10.1016/j.jcv.2006.06.013
103. Zhang X, Bai X, Chen ZJ. Structures and mechanisms in the cGAS-STING innate immunity pathway. Immunity (2020) 53:43–53. doi: 10.1016/j.immuni.2020.05.013
104. Barnett KC, Coronas-Serna JM, Zhou W, Ernandes MJ, Cao A, Kranzusch PJ, et al. Phosphoinositide interactions position cGAS at the plasma membrane to ensure efficient distinction between self- and viral DNA. Cell (2019) 176:1432–1446.e11. doi: 10.1016/j.cell.2019.01.049
105. Cai X, Chiu Y-H, Chen ZJ. The cGAS-cGAMP-STING pathway of cytosolic DNA sensing and signaling. Mol Cell (2014) 54:289–96. doi: 10.1016/j.molcel.2014.03.040
106. Capobianchi MR, Uleri E, Caglioti C, Dolei A. Type I IFN family members: Similarity, differences and interaction. Cytokine Growth Factor Rev (2015) 26:103–11. doi: 10.1016/j.cytogfr.2014.10.011
107. Wang C, Guan Y, Lv M, Zhang R, Guo Z, Wei X, et al. Manganese increases the sensitivity of the cGAS-STING pathway for double-stranded DNA and is required for the host defense against DNA viruses. Immunity (2018) 48:675–687.e7. doi: 10.1016/j.immuni.2018.03.017
108. Zhao Z, Ma Z, Wang B, Guan Y, Su X-D, Jiang Z. Mn2+ directly activates cGAS and structural analysis suggests Mn2+ induces a noncanonical catalytic synthesis of 2′3′-cGAMP. Cell Rep (2020) 32:108053. doi: 10.1016/j.celrep.2020.108053
109. Flood BA, Higgs EF, Li S, Luke JJ, Gajewski TF. STING pathway agonism as a cancer therapeutic. Immunol Rev (2019) 290:24–38. doi: 10.1111/imr.12765
110. Yang Y, Gu Z, Tang J, Zhang M, Yang Y, Song H, et al. MnO 2 nanoflowers induce immunogenic cell death under nutrient deprivation: Enabling an orchestrated cancer starvation-immunotherapy. Adv Sci (2021) 8:2002667. doi: 10.1002/advs.202002667
111. Zhang S, Wang J, Kong Z, Sun X, He Z, Sun B, et al. Emerging photodynamic nanotherapeutics for inducing immunogenic cell death and potentiating cancer immunotherapy. Biomaterials (2022) 282:121433. doi: 10.1016/j.biomaterials.2022.121433
112. Yan W, Lang T, Qi X, Li Y. Engineering immunogenic cell death with nanosized drug delivery systems improving cancer immunotherapy. Curr Opin Biotechnol (2020) 66:36–43. doi: 10.1016/j.copbio.2020.06.007
113. Marichal T, Ohata K, Bedoret D, Mesnil C, Sabatel C, Kobiyama K, et al. DNA Released from dying host cells mediates aluminum adjuvant activity. Nat Med (2011) 17:996–1002. doi: 10.1038/nm.2403
114. Casares N, Pequignot MO, Tesniere A, Ghiringhelli F, Roux S, Chaput N, et al. Caspase-dependent immunogenicity of doxorubicin-induced tumor cell death. J Exp Med (2005) 202:1691–701. doi: 10.1084/jem.20050915
115. Zelenay S, Reis e Sousa C. Adaptive immunity after cell death. Trends Immunol (2013) 34:329–35. doi: 10.1016/j.it.2013.03.005
116. Seong S-Y, Matzinger P. Hydrophobicity: An ancient damage-associated molecular pattern that initiates innate immune responses. Nat Rev Immunol (2004) 4:469–78. doi: 10.1038/nri1372
117. Theruvath J, Menard M, Smith BAH, Linde MH, Coles GL, Dalton GN, et al. Anti-GD2 synergizes with CD47 blockade to mediate tumor eradication. Nat Med (2022) 28:333–44. doi: 10.1038/s41591-021-01625-x
118. Barsoum IB, Smallwood CA, Siemens DR, Graham CH. A mechanism of hypoxia-mediated escape from adaptive immunity in cancer cells. Cancer Res (2014) 74:665–74. doi: 10.1158/0008-5472.CAN-13-0992
119. Noman MZ, Hasmim M, Messai Y, Terry S, Kieda C, Janji B, et al. Hypoxia: a key player in antitumor immune response. A review in the theme: Cellular responses to hypoxia. Am J Physiology-Cell Physiol (2015) 309:C569–79. doi: 10.1152/ajpcell.00207.2015
120. Shimizu K, Iyoda T, Okada M, Yamasaki S, Fujii S. Immune suppression and reversal of the suppressive tumor microenvironment. Int Immunol (2018) 30:445–55. doi: 10.1093/intimm/dxy042
121. Graham C, Barsoum I, Kim J, Black M, Siemens RD. Mechanisms of hypoxia-induced immune escape in cancer and their regulation by nitric oxide. Redox Biol (2015) 5:417. doi: 10.1016/j.redox.2015.09.022
122. Noman MZ, Buart S, Van Pelt J, Richon C, Hasmim M, Leleu N, et al. The cooperative induction of hypoxia-inducible factor-1α and STAT3 during hypoxia induced an impairment of tumor susceptibility to CTL-mediated cell lysis. J Immunol (2009) 182:3510–21. doi: 10.4049/jimmunol.0800854
123. Juneja VR, McGuire KA, Manguso RT, LaFleur MW, Collins N, Haining WN, et al. PD-L1 on tumor cells is sufficient for immune evasion in immunogenic tumors and inhibits CD8 T cell cytotoxicity. J Exp Med (2017) 214:895–904. doi: 10.1084/jem.20160801
124. Wu M, Chen T, Wang L, Akakuru OU, Ma X, Xu J, et al. The strategy of precise targeting and in situ oxygenating for enhanced triple-negative breast cancer chemophototherapy. Nanoscale (2022) 14:8349–61. doi: 10.1039/D2NR00985D
125. Prasad P, Gordijo CR, Abbasi AZ, Maeda A, Ip A, Rauth AM, et al. Multifunctional albumin–MnO 2 nanoparticles modulate solid tumor microenvironment by attenuating hypoxia, acidosis, vascular endothelial growth factor and enhance radiation response. ACS Nano (2014) 8:3202–12. doi: 10.1021/nn405773r
126. Tang S, Zhou L, He H, Cui L, Ren Z, Tai Y, et al. MnO2-melittin nanoparticles serve as an effective anti-tumor immunotherapy by enhancing systemic immune response. Biomaterials (2022) 288:121706. doi: 10.1016/j.biomaterials.2022.121706
127. Yu M, Duan X, Cai Y, Zhang F, Jiang S, Han S, et al. Multifunctional nanoregulator reshapes immune microenvironment and enhances immune memory for tumor immunotherapy. Adv Sci (2019) 6:1900037. doi: 10.1002/advs.201900037
128. Lv M, Chen M, Zhang R, Zhang W, Wang C, Zhang Y, et al. Manganese is critical for antitumor immune responses via cGAS-STING and improves the efficacy of clinical immunotherapy. Cell Res (2020) 30:966–79. doi: 10.1038/s41422-020-00395-4
129. Zitvogel L, Galluzzi L, Kepp O, Smyth MJ, Kroemer G. Type I interferons in anticancer immunity. Nat Rev Immunol (2015) 15:405–14. doi: 10.1038/nri3845
130. Kumar H, Kawai T, Akira S. Pathogen recognition by the innate immune system. Int Rev Immunol (2011) 30:16–34. doi: 10.3109/08830185.2010.529976
131. Xin Y, Huang Q, Tang J-Q, Hou X-Y, Zhang P, Zhang LZ, et al. Nanoscale drug delivery for targeted chemotherapy. Cancer Lett (2016) 379:24–31. doi: 10.1016/j.canlet.2016.05.023
132. Tian L, Chen Q, Yi X, Chen J, Liang C, Chao Y, et al. Albumin-templated manganese dioxide nanoparticles for enhanced radioisotope therapy. Small (2017) 13:1700640. doi: 10.1002/smll.201700640
133. Mo Z, Qiu M, Zhao K, Hu H, Xu Q, Cao J, et al. Multifunctional phototheranostic nanoplatform based on polydopamine-manganese dioxide-IR780 iodide for effective magnetic resonance imaging-guided synergistic photodynamic/photothermal therapy. J Colloid Interface Sci (2022) 611:193–204. doi: 10.1016/j.jcis.2021.12.071
134. Chen J, Meng H, Tian Y, Yang R, Du D, Li Z, et al. Recent advances in functionalized MnO 2 nanosheets for biosensing and biomedicine applications. Nanoscale Horiz (2019) 4:321–38. doi: 10.1039/C8NH00274F
Keywords: manganese dioxide nanoparticles, manganese ion, immune regulation, vaccines, immunotherapies
Citation: Huang Y, Ruan Y, Ma Y, Chen D, Zhang T, Fan S, Lin W, Huang Y, Lu H, Xu J-F, Pi J and Zheng B (2023) Immunomodulatory activity of manganese dioxide nanoparticles: Promising for novel vaccines and immunotherapeutics. Front. Immunol. 14:1128840. doi: 10.3389/fimmu.2023.1128840
Received: 21 December 2022; Accepted: 09 February 2023;
Published: 28 February 2023.
Edited by:
Lorella Pascolo, Institute for Maternal and Child Health Burlo Garofolo (IRCCS), ItalyReviewed by:
Xinzeyu Yi, Wuhan University, ChinaCopyright © 2023 Huang, Ruan, Ma, Chen, Zhang, Fan, Lin, Huang, Lu, Xu, Pi and Zheng. This is an open-access article distributed under the terms of the Creative Commons Attribution License (CC BY). The use, distribution or reproduction in other forums is permitted, provided the original author(s) and the copyright owner(s) are credited and that the original publication in this journal is cited, in accordance with accepted academic practice. No use, distribution or reproduction is permitted which does not comply with these terms.
*Correspondence: Jun-Fa Xu, eHVqdW5mYUBnZG11LmVkdS5jbg==; Jiang Pi, amlhbmdwaUBnZG11LmVkdS5jbg==; Biying Zheng, emJ5ODhrckBnZG11LmVkdS5jbg==
†These authors have contributed equally to this work and share first authorship
Disclaimer: All claims expressed in this article are solely those of the authors and do not necessarily represent those of their affiliated organizations, or those of the publisher, the editors and the reviewers. Any product that may be evaluated in this article or claim that may be made by its manufacturer is not guaranteed or endorsed by the publisher.
Research integrity at Frontiers
Learn more about the work of our research integrity team to safeguard the quality of each article we publish.