- 1IrsiCaixa AIDS Research Institute, Badalona, Spain
- 2Autonomous University of Barcelona, Cerdanyola del Vallès, Catalonia, Spain
- 3Skin Neglected Tropical Diseases and Sexually Transmitted Infections Department, Germans Trias i Pujol Hospital, Badalona, Spain
- 4Fight Infections Foundation, Germans Trias i Pujol Hospital, Badalona, Catalonia, Spain
- 5Centre for Health and Social Care Research (CESS), Faculty of Medicine, University of Vic – Central University of Catalonia (UVic – UCC), Vic, Catalonia, Spain
- 6Germans Trias i Pujol Research Institute (IGTP), Badalona, Spain
- 7CIBERINFEC, Instituto de Salut Carlos III (ISCIII), Madrid, Spain
Syphilis is a sexually or vertically (mother to fetus) transmitted disease caused by the infection of Treponema pallidum subspecie pallidum (TPA). The incidence of syphilis has increased over the past years despite the fact that this bacterium is an obligate human pathogen, the infection route is well known, and the disease can be successfully treated with penicillin. As complementary measures to preventive campaigns and early treatment of infected individuals, development of a syphilis vaccine may be crucial for controlling disease spread and/or severity, particularly in countries where the effectiveness of the aforementioned measures is limited. In the last century, several vaccine prototypes have been tested in preclinical studies, mainly in rabbits. While none of them provided protection against infection, some prototypes prevented bacteria from disseminating to distal organs, attenuated lesion development, and accelerated their healing. In spite of these promising results, there is still some controversy regarding the identification of vaccine candidates and the characteristics of a syphilis-protective immune response. In this review, we describe what is known about TPA immune response, and the main mechanisms used by this pathogen to evade it. Moreover, we emphasize the importance of integrating this knowledge, in conjunction with the characterization of outer membrane proteins (OMPs), to expedite the development of a syphilis vaccine that can protect against TPA infection.
1 Introduction
Syphilis is a sexual transmitted infection caused by the spirochete Treponema pallidum subspecie pallidum (TPA). Although the origin of the syphilis epidemic is unclear, the first cases of the disease were reported in Europe in the late 15th century (1, 2). In 1905, Schaudinn and Hoffmann discovered the bacteria causing this venereal disease, and in 1943, the first cases of syphilis were successfully treated with penicillin (3). Over half a century later, penicillin remains one of the most efficacious treatments (4).
Syphilis and the three nonvenereal treponematoses (yaws, bejel and pinta) were at first believed to be caused by the same agent, despite the fact that their clinical manifestations are different. Since pathogenic treponemes are morphologically and antigenically very similar (> 95% DNA homology), genomic sequencing was needed to identify distinct subspecies of Treponema pallidum that cause nonvenereal treponematoses: Treponema pallidum endemicum (causative agent of bejel), and Treponema pallidum pertenue (causative agent yaws). Pinta is caused by a different species of spirochete bacteria, namely Treponema carateum (4, 5). All of them are obligate human pathogens characterized by its invasiveness and immune evasiveness (6–9).
A syphilis infection consists of three main stages (5). Primary syphilis usually begins approximately 2-3 weeks after contact with the pathogen, and it is characterized by the presence of an ulcerated lesion, called chancre. Typically, this lesion appears on the genital area, or other body parts related to sexual contact, usually accompanied by regional lymphadenopathy (5, 10). In the absence of treatment, primary lesion resolves spontaneously in 3-6 weeks. Secondary syphilis develops as a result of bacteria dissemination (10). Clinical manifestations include malaise, headache, fever, diffuse lymphadenopathy, and maculopapular rashes with either discrete or widespread body involvement (5, 10). Unless treated, secondary lesions can take up to several months to resolve. The disease then enters in a latent stage without exhibiting clinical manifestations (5, 6, 10). It remains unclear how TPA establishes latency, and which tissues or organs act as reservoirs (6). A relapse of infection from these reservoirs can occur in 25% of untreated patients within two years after secondary syphilis resolution, and present new secondary-like clinical manifestations (6, 10). After years or even decades, 15-40% of untreated and latently infected individuals will develop tertiary syphilis. This stage involves serious cardiovascular, neurological, bony and visceral affections that may eventually result on death of infected individuals (5, 10). Remarkably, penicillin administration prevents syphilis progression to its secondary and tertiary stages, and cures the infection. However, treated patients remain susceptible to reinfection, because the previous one fails to produce a protective immune response (11–13).
According to WHO data, in 2016, 19.9 million people had syphilis, and there were 6.3 million of new cases per year (14). The prevalence of congenital syphilis was 0.69% in 2016, with a rate of 473 cases per 100.000 live births (15). Syphilis is considered the second cause of stillbirths after malaria (16). A high prevalence of syphilis is observed in low-income countries, and it has been rising in high-middle income states during the last decade (5, 14, 17, 18). Particularly, this increase has been observed in men who have sex with men (MSM) with multiple sexual partners, as well as sexual networks of heterosexual individuals. In this setting, syphilis is also associated with higher risk of HIV infection (5, 17–19).
Importantly, although syphilis can be easily diagnosed, treated with an inexpensive antibiotic, and no animal reservoir has been identified to date, syphilis continues to be a significant global health problem. Therefore, the development of a syphilis vaccine is urgently needed to complement disease control and prevention measures. In this review, we focus on: syphilis vaccine development, use of outer membrane proteins (OMPs) as putative immunogens, anti-TPA immune responses, as well as TPA evasion mechanisms, to provide information about potential antigen selection in future vaccine and immunization strategies.
2 Treponema pallidum subsp. pallidum
TPA is a flat-wave spirochete of 5-15 μm and 0.2 μm of diameter (20) that belongs to Spirochaetaceae family, particularly Treponema genus. Treponemes are usually classified as Gram-negative bacteria due to their double membrane structure (21). However, the composition of their outer membrane (OM) is noticeably different (22, 23). The TPA OM is characterized by the paucity of surface-exposed proteins (24–26), the presence of phosphatidylcholine, phosphatidylglycerol, phosphatidylserine, and the lack of cardiolipins (23) and lipopolysaccharide (LPS) (27, 28). Interestingly, cardiolipins are present in the cytoplasmic membrane (23), and represent the main lipid antigen targeted by anti-TPA antibodies of infected individuals (23). However, other constituents of the OM (e.g. glycolipids) do not present immune reactivity (23, 29). The lipid composition and poor protein content of OM are key features of TPA, and may contribute to the poor immunogenicity of this pathogen (22–24, 26).
TPA is actively motile, although its motile system differs from other flagellar bacteria (30). Axis filaments (known as endoflagella) are found in the periplasmatic space and extend from cell poles through the entire cell body length (31). These filaments comprise three core protein (FlaB1, FlaB2, and FlaB3) and an external protein, which surround the filament core (FlaA) (28, 31). When endoflagella rotate in one direction, the cell body moves in the opposite one. This torsion results in a corkscrew-like motion that, together with the action of metalloprotease and adhesin proteins, allows spirochetes to cross tissues and disseminate through the body (32, 33).
Until recently, TPA had not been grown in vitro (34), and bacteria propagation in rabbits was the only strategy to obtain sufficient live and infective organisms for experimental investigation (35). The lack of the tricarboxylic acid cycle and microaerophilic requirements make this bacterium totally dependent on host cells for the acquisition of purines, pyrimidines, and most amino acids (6, 8, 28). Therefore, TPA cannot survive outside the host, and loses its infectious capability within few hours (8, 36).
Besides humans, rabbits are one of the few mammalians that are susceptible to TPA infection, and has become the reference animal model to study syphilis immune protection. After infection, rabbits develop primary and secondary stage-like clinical signs, and a humoral response similar to the one observed in humans (37–39). Intradermal TPA inoculation induces dermal lesions that resemble human chancres, and bacteria can disseminate to distal organs, mainly secondary lymphoid organs (i.e. spleen) (32, 40). However, invasion of the central nervous system is not frequently observed and it depends on the utilized TPA strain (41). In addition to rabbits, there are other species that are also susceptible to TPA (i.e. non-human primates, hamsters, guinea pigs, and mice). While only non-human primates and rabbits develop clinical signs similar to humans (40, 42), mice and other rodents may be useful to study bacteria dissemination (43).
The TPA genome is a circular chromosome of approximately 1138 kilobase pairs that contains 1041 predicted ORFs (28). Its genome is small compared to other pathogenic bacteria (28). The genome sequence confirms that TPA cannot synthetize de novo enzyme cofactors, fatty acids, tricarboxylic pathway enzymes, or nucleotides, even though it contains 57 ORFs encoding transport proteins and is able to use the glycolytic pathway (28). TPA lacks genes encoding superoxide dismutase, catalase, or peroxidase, which could explain its susceptibility to oxygen. Additionally, several genes have also been identified that are involved in the synthesis of motile proteins and lipoproteins (4, 8, 28). Genome comparison among pathogenic Treponema pallidum subspecies has shown that they are similar in size and structure, and differ less than 0.2%-0.4% in their genome sequence (44, 45).
3 Infection course and immune response
Syphilis is generally transmitted through sexual contact or from mother to child. Spirochetes gain access to the host through epidermal micro-abrasions or directly penetrating mucosal membranes (46, 47). Once TPA has entered the body, it adheres to epithelial cells and extracellular matrix and locally multiplies with an estimate rate of once every 30-33 hours (48, 49). In vitro binding studies have shown that laminin and fibronectin are among the anchor molecules involved in these interactions (50–53). Since TPA lacks cytotoxic toxins or other virulence factors (28), tissue destruction and chancre lesions are probably caused by the inflammatory response at the entry site (47). The initial immune response clears bacteria locally, and the primary stage lesions resolve spontaneously within 3-6 weeks. Meanwhile spirochetes spread throughout the body and the infection rapidly becomes systemic, triggering secondary syphilis. Afterward, spirochetes penetrate deeper tissues and induce the expression of inflammatory signals, promoting the migration of immune cells to the infected tissues (47). In vitro studies have shown that TPA can induce the expression of the adhesion molecules ICAM-1, VCAM-1 and E-selectin in endothelial cells (54–56). In addition, metalloprotease activity plays a major role in the penetration and dissemination of TPA through extracellular matrix and intercellular junctions (57, 58). In the final stage, infection and the associated immune response damage various organs (e.g. heart and brain), resulting in debilitating health problems (47).
TPA infection triggers a complex immune response that fails to control the spread of the bacteria and the progression of the disease. In primary syphilis, polymorphonuclear leukocytes (PMNs) are the first immune cells to infiltrate the infection site (59) (Figure 1A). These cells may contribute to the initial control of the infection by secreting anti-microbial peptides (60) and clearing spirochetes by phagocytosis (59, 61). However, the initial control of the infection is limited as bacteria dissemination occurs in virtually all cases. Besides PMN, dendritic cells (DCs) (Figure 1A) can also phagocyte whole or bacteria-derived fragments, thereby stimulating their maturation and antigen-presentation capacity. Thus, DCs increase the production of pro-inflammatory cytokines, such as IL-12, IL-6 and IL-1B, and upregulate the expression of CD54, CD83, CD80, CD86, and HLA-DR (62, 63). Mature DCs migrate to lymph nodes where they present treponemal antigens to T cells, inducing antigen-specific T cells responses. These T cells can be detected in secondary lymphoid organs of infected animals three days post-infection and progressively accumulate at the infection site (64–66), coinciding with maximal TPA burden (67, 68). Rabbits infected with TPA showed a rapid hyperplasia of T cell zones in secondary lymphoid organs (lymph node and spleen) (38, 66). Although Th1 CD4+ T cells dominate the T cell infiltrate (38, 61, 69)(Figure 1A), cytotoxic T cells (CD8+) can also be found in primary lesions, where their role remains unclear. CD8+ T cell may be required for the elimination of TPA reservoir inside non-phagocytic cells in early syphilis lesions (69–71). In this sense, perforin and granzyme are detected in early lesions, suggesting cytolytic activity (72). Furthermore, CD8+ T cells, as well as NK cells, could contribute to the secretion of interferon-γ (IFN-γ) (9, 73). Th1 cytokines can promote the migration and activation of macrophages (Figure 1A), whose number increase rapidly by day 10 after infection at the entry site (68). Activated macrophages phagocytose and destroy spirochetes (64, 74) until they are completely cleared from the infection site. Interestingly, this process can be enhanced by TPA opsonizing antibodies and IFN-γ, particularly through the Fc-Fcγ receptor interaction (73–77). However, other less efficient mechanisms, such as non-opsonic phagocytosis and active direct invasion, may also participate in TPA-macrophage interplay (78). In response to TPA infection, macrophages polarize to M1 phenotype and secrete proinflammatory cytokines (i.e. IL-1β, TNF-α, IL-12, and IL-15) (77, 79), which can ultimately promote necrosis and ulcer formation typical of primary chancre lesions.
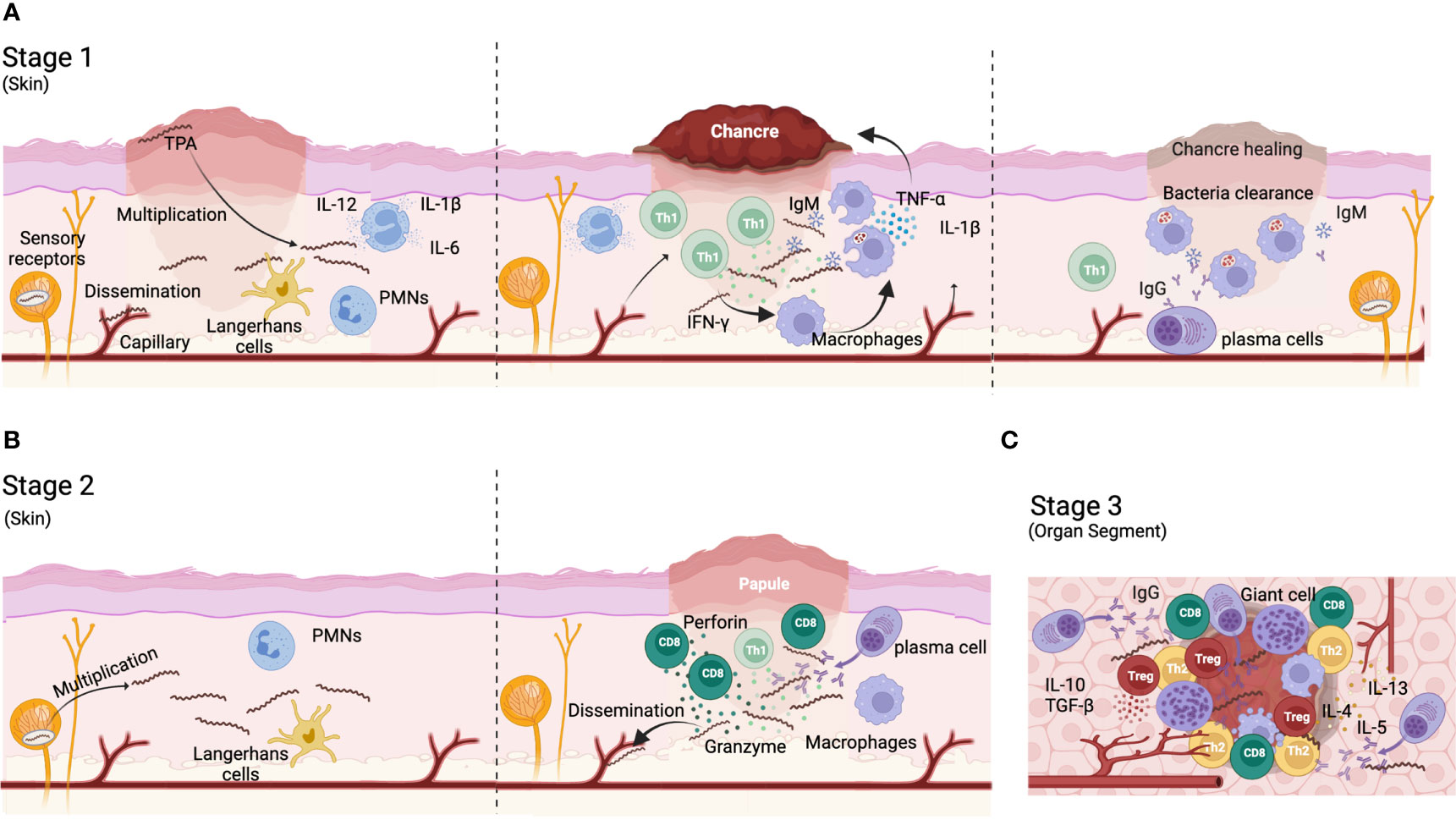
Figure 1 Immune response to TPA infection. (A) During primary syphilis, a local lesion called chancre appear in the site of infection. After bacteria entry, the microorganisms proliferate and disseminate to distal organs. Bacteria are phagocyted by polymorphonuclear cells (PMN) and dendritic cells that migrate to draining lymph nodes to activate CD4+ Th cells. Th1 T cells migrate to the bacteria proliferation foci and produce Th1 cytokines (IFN-γ) for recruiting and activate macrophages. M1 macrophages phagocyte bacteria and produce TNF-α and IL-1β that contribute to chancre development and eventually necrosis. IgM antibodies and complement contribute to bacteria opsonization favoring their removal by macrophages. (B) During secondary syphilis, TPA expands from reservoirs with systematic affectation. A typical rash appears in most cases. Anti-TPA humoral responses are mainly IgGs and plasma cells are visible in the infiltrate of secondary cutaneous lesions. CD8+ T-cells are more represented than CD4+ T-cells in lesion infiltrates. (C) Tertiary syphilis affects deep organs like brain and heart. A Th1/Th2 switch progressively occur and the immune response is down regulated by the presence of regulatory T cells. Plasma cells are well represented. Gummas can be developed in different locations (i.e., liver, skin, brain). Gummas are a granuloma-like structure characterized by a necrotic hyaline nucleus surrounded by immune cells (i.e. plasma cells, macrophages and giant cells) and fibroblasts.
Regarding the humoral response, anti-TPA antibodies can be detected as early as 6 days post-infection (80). However, the antibody response kinetics vary based on the target protein and TPA strain. For example, antibodies targeting Tpr Subfamily I and II rise between days 10 and 45 after infection (81). During primary infection, IgM dominates the anti-TPA humoral response, while IgG levels gradually increase (80, 82) (Figure 1A). Antibodies are not only involved in opsonization processes, they can also block bacteria dissemination and dermal lesions. Furthermore, the complement system is also involved in limiting treponemal activity, specially working in conjunction with antibodies (83–85). TPA accumulates sialic acid on its surface, making it resistant to complement lysis by the alternative pathway (86–89). Thus, classical complement activation pathway is key to bacterial clearance. This data suggest that the immune response generated against TPA during primo-infection is a delayed type hypersensitivity (DTH) immune response, where sensitized T cells play a major role. In fact, it has been hypothesized that syphilis prognosis depends on the balance between DTH and humoral responses. Thus, it has been proposed that a strong DTH immune response may be required to clear the infection, whereas latency may result from intermediate DTH responses. Tertiary disease would be related to a weak DTH and a strong humoral response (68, 90). Despite this, antibodies may be crucial for preventing infection (68, 91). Accordingly, rabbits receiving immune serum showed a delay in the appearance of lesions that were also less severe (92). Further, patients who have been previously treated for syphilis remain susceptible to reinfection because they do not develop an effective anti-TPA humoral response (68).
Both cellular and humoral responses are maintained for months after clearance of primary syphilis lesions (39, 93, 94), and their magnitude correlate with the persistence of TPA as latent infection (95–98). From this state, relapse of active infection can occur leading to secondary and tertiary syphilis. Secondary syphilis manifests as inflammatory cutaneous affection that differs in histological appearance from primary syphilis. The histology of secondary syphilis is variable but, in general, lymphocytes, macrophages, and plasma cells are commonly present in secondary lesions whereas polymorphonuclear and eosinophilic infiltrates are found in a smaller proportion (99–101) (Figure 1B). Furthermore, CD8+ T cells are highly represented (Figure 1B) (99). Contrary to primary syphilis, IgGs dominate the anti-TPA humoral immune response during secondary syphilis (Figure 1B) (82, 102, 103). Moreover, whereas IgG1 is the main IgG subclass in primary syphilis, both IgG1 and IgG3 subclass can be equally found in secondary syphilis (104).
Finally, tertiary syphilis is characterized by a multiorgan affection and the development of gummas in different tissues (such as liver and skin). Gummas are granuloma-like structures characterized by a necrotic nucleus surrounded by macrophages, giant multinucleate cells, lymphocytes, and plasma cells (68) (Figure 1C). Neurosyphilis is characterized by an increase in the number of CD8+ T cells in blood (105). Moreover, a switch in the production of Th1 to Th2 cytokines is observed during the evolution of the disease. While Th1 cytokines are mainly detected during primary syphilis, Th2 cytokines increase in late states of the disease (106) (Figure 1C). This correlates with cell depletion in the diffuse cortex, and a follicular hyperplasia in lymph nodes with plasma cells accumulation within the interfollicular areas during tertiary syphilis (68). These changes in the immune response during syphilis are closely related with disease progression.
4 Immunization studies and vaccine strategy
Despite public health campaigns and the availability of efficacious treatment, syphilis prevalence has increased worldwide in the last decade (17), suggesting the need for additional measures to control the transmission of the infection. The development of a syphilis vaccine could be a valuable tool. However, after several decades of research, an effective vaccine for syphilis remains elusive. A variety of strategies have been tested, including inactivated bacteria and subunit recombinant proteins, even though with limited success. Interestingly, Miller et al. demonstrated protection of rabbits against TPA infection following 60 immunizations with γ-irradiated bacteria for 37 weeks (107). Although this experimental strategy is far from being applicable to humans, it serves as a proof of concept that a syphilis vaccine is feasible. In addition, human challenge studies performed in the 50’s showed that latently infected patients were resistant to reinfection with a heterologous TPA strain (108). Accordingly, Marra and colleagues reported that previous syphilis infection may attenuate the manifestation of subsequent TPA infection (109). Thus, those individuals that experimented three or more episodes of syphilis were more likely to develop latent early syphilis after subsequent infections. These studies indicate that it will take a long time to establish a protective immunity against TPA, further emphasizing the low immunogenicity of this pathogen.
Until 2018, one of the main handicaps in the development of a syphilis vaccine was the inability to grow the bacteria in vitro (34). This technical limitation shifted the focus of most studies towards recombinant OMPs (110). Although none of these proteins provided complete protection against in vivo TPA challenge, promising results were observed; namely, the induction of a strong humoral response, less ulcerative lesions, faster recovery of lesions, or inhibition of bacterial dissemination to distal organs. These studies provided meaningful knowledge about OMPs, immunization regimens, and potential vaccine targets. The advancement of bioinformatic tools allowed the identification of additional putative OMPs, as well as the prediction of structure models and B cell epitopes (111, 112). The employment of newly-developed approaches (e.g. genetic engineering), and refined bioinformatic tools enable to delve further into OMPs knowledge, benefiting future vaccine studies (113, 114).
5 The outer membrane proteins
One of the major features that differentiate TPA from other bacteria is the paucity of surface-exposed OMPs (24–26). It has been calculated that the density of OMP in TPA is approximately 100-fold less than that in E. coli (22). The identification and characterization of TPA OMPs has been challenging due to the inability to genetically manipulate and cultivate TPA in vitro (until recently) (34), and the fragility of its OM, which generated a strong controversy in some studies. The intrinsic properties of the OM (i.e lack of LPS and low density of proteins) makes it can be easily damaged by common experimental manipulations (e.g. centrifugation, resuspension, or using low concentration of non-ionic detergent) (22, 26). OM disruption leads to potential exposure of lipoproteins that are normally present in the periplasmic space and cytoplasmic membrane, and could erroneously pinpoint proteins as OMPs. Due to these technical limitations, the identification of OMP was often based on prediction of their sequence and structure or by comparison with functional orthologs from other species (111). Thus, OMPs can be classified in three main groups according to their putative functions:
5.1 Transport function
Since nutrient import and toxics efflux are crucial for bacteria surveillance, several TPA OMPs have been involved in transport function.
5.1.1 Tpr protein family
The Treponema pallidum repeat (Tpr) family of proteins include 12 members that are divided into three subfamilies according to their amino acid composition: subfamily I (TprC, D, F, I), subfamily II (TprE, G, J) and subfamily III (TprA, B, H, K, L). Among them, subfamily I and TprK (subfamily III) are the most widely studied. Regarding Tpr protein location, sequence analysis predicted that TprB, TprC, TprD, TprE, TprG, TprH, TprI, TprJ, TprK and TprL could be located in the OM (22). Supporting this prediction, a putative cleavable signal peptide was identified in most of the Tprs superfamily members, including TprC, D, F, I, E, G, J, A, B and TprK proteins (111, 115).
The Tpr family is related to the major outer sheath protein (MOSP) of Treponema denticola (115), a surface-exposed protein with adhesive and porin function (116–118). Most Tprs include three main domains: 1) a N-terminal domain related to the N-terminal domain of Treponema denticola MOSP, 2) a central region, and 3) a C-terminal domain related to the C-terminal domain of Treponema dentiola MOSP, that is lacking in TprF and TprA proteins. Recently, the presence of short molecular recognition features (MoRFs) was predicted in most Tprs. These motifs may interact with specific proteins and undergo disorder-to-order transition upon binding (111). Sequence analysis has shown that the N- and C-terminal domains of the Tpr proteins from subfamily I and II are relatively conserved, whereas central domains are variable in length and sequence (6). Terminal domains present amphipathic regions and flank hydrophilic regions of the central domains. According to Hawley et al., the N- and C-terminal domains from all Tprs adopt a β-stranded structure, while the central regions may be mostly α-helices (111).
Structural analysis of the subfamily I has shown that these proteins form a β-barrel trimeric channel. However, unlike classical porins, which the entire polypeptide forms a β-barrel, Tpr subfamily I possesses bipartite topology. The C-terminal domain forms a β-barrel structure and is surface-exposed, while the N-terminal and central domains anchor the β-barrel into the peptidoglycan sacculus of the periplasmatic region (119, 120). Accordingly, while the expression of TprC/D in E. coli is surface-exposed, TprF lacks the C-terminal domain, and therefore, it is entirely periplasmatic (22). The immunodetection of Tpr proteins by electron microscopy using purified rabbit anti-Tpr I immunoglobulins, confirmed the presence of Tpr proteins in OM and periplasmic spaces, which could be due to the bipartite structure previously described or because the anti-Tpr I antibody recognized proteins with distinct location (121). More recently, Hawley et al., confirmed that the bipartite membrane topology identified in subfamily I is common to all Tprs with three full domains (111). Based on sequence and structure analysis, it has been shown that the Tpr family may be involved in the import of small soluble molecules. In this sense, the integration of some Tpr proteins in liposomes increased their permeability (119, 120). Therefore, changes in protein expression and/or variation of their channel-forming β-barrel region could be used to adapt TPA nutritional requirements to the environment. Accordingly, the expression of Tpr proteins can vary among strains, and in the same strain overtime (122), which may explain why the anti-Tpr humoral response differ among TPA isolates (81). This observation suggests the existence of mechanisms that can regulate the expression of these genes. Thus, a hypervariable homopolymeric guanosine (poly-G) tract and a cAMP receptor protein (CRP) binding motif have been identified upstream of the transcription start site of the Tpr subfamily II and III (123–125). In fact, Tp0262 (a TPA CRP homologue) can bind to these promoters and regulate the surface expression of these proteins during infection (123). As a result, the specificity of the humoral response elicited against Tpr proteins differ among TPA isolates (81).
Of the Tpr superfamily, TprK is one of the best characterized proteins. It has been previously showed that recombinant TprK is a monomeric porin by negative-staining electron microscopy (126). Anti-TprK antibodies can be detected early during infection (81). However, TprK exhibits sequence variation that contributes to immune escape. Thus, several alleles have been identified among TPA strains (127, 128). Moreover, this protein shows seven variable domains whose sequence changes by non-reciprocal recombination, mainly with regions downstream of the TprD gene (127, 128), a mechanism that is enhanced under immune pressure (129, 130). The existence of this mechanism could explain why TprK variability was higher in TPA isolates from patients affected with secondary syphilis than those with primary syphilis (128). However, TprK genetic variation can also occur in the absence of immune pressure (131). Interestingly, it has been reported that humoral response in vivo targets variable regions, while cellular response recognizes conserved TprK epitopes (132, 133). Moreover, animal challenges using TPA with TprK homologous to the one used in immunization regimen show better protection than those animals challenged with a strain expressing heterologous TprK (132). Despite that, there are controversies on the role of anti-TprK antibodies, and TprK protective efficacy as immunogen and its location. Centurion-Lara and colleagues described that TprK is located on the OM, and TprK-specific antibodies had opsonization capacity (115). Interestingly, rabbits immunized with this protein did not develop ulcerative lesions. These lesions healed faster than those observed in unvaccinated control animals and contained fewer spirochetes, when analyzed by darkfield microscopy (115). Additionally, Morgan et al. identified the N-terminal region of TprK (37-273 amino acids) and, to a lesser extent, the C-terminal portion (349-478 amino acids), as the parts of the protein that induced the previously-described protective immune response (134). Conversely, Hazlett and colleagues described that TprK was located in the periplasmic space, anti-TprK antibodies lacked opsonization activity, and immunization with recombinant TprK did not induce a protective immune response nor TprK sequence variation (135). Further investigation would be needed to clarified these discrepancies.
Regarding the immunity elicited against other Tprs members, anti-TprI antibodies were detected in 98% of syphilis-affected individuals. These antibodies mainly targeted the conserved N-terminal region. The lack of reactivity was associated with early infection (121). Remarkably, experiments performed in rabbits showed that TprI immunization did not protect rabbits from infection (136). However, cutaneous lesions did not ulcerate and healed faster than those generated in unvaccinated animals. This protein elicited strong humoral and cellular responses mainly targeting the conserved N-terminal region of the protein during TPA infection (81, 136). Not surprisingly, immunization with the conserved N-terminal region of TrpF, which is also common to all subfamily I members, did not protect rabbits from TPA infection after challenge. However, TprF vaccination attenuated lesion development, prevented ulceration, and reduced bacterial burden in skin lesions (136). In regards to TprC and TprD, epitope mapping has shown that the humoral response detected in rabbit sera is directed against exposed loops (112). Moreover, the N- and C- terminal regions contain the most reactive epitopes. Surprisingly, Anand et al. showed that sera from infected individuals do not recognize TprC, while TPA-infected rabbits develop antibodies with opsonization activity (119). One explanation could be the low expression of TprC and the different magnitude of the humoral response found among various syphilis strains and subspecies.
5.1.2 Tp0515
Tp0515 is a structural ortholog of LptD protein, which is part of a multiprotein complex involved in LPS trafficking to the OM in Gram-negative bacteria (22). Thus, since LptD is embedded in the OM, Tp0515 is inferred to be an OMP. However, sequence analysis of TPA genome failed to identify LPS biosynthetic pathway (28). Notably, orthologous proteins for several components of the LPS transport pathway have been found in TPA (111), suggesting that even without LPS, these proteins could be involved in translocation of other cellular products to the OM, as glycolipids (22). Tp0515 structural modelling found that this protein is a 26-stranded β-barrel embedded in OM with exposed extracellular loops (111). Moreover, Hawley et al. identified B cells epitopes inside these extracellular loops using bioinformatic analysis (111). However, the immunogenicity and capacity of this protein to induce protective immune response still need to be empirically demonstrated.
5.1.3 Tp0126 and other OmpW/AlkL orthologs
Four proteins that are orthologs of OmpW (Tp0126 and Tp0733) and OprG (Tp0479 and Tp0698) have been identified in TPA (111). Both E. coli OmpW and Pseudomonas aeruginosa OprG proteins are members of the OmpW/AlkL family of proteins, which have been suggested to be involved in bacterial adaption to environment stress and nutrient acquisition (137, 138). OmpW and OprG have an eight-stranded β-barrel architecture and form a peculiar hydrophobic channel, which allows the transport of hydrophobic molecules directly to the membrane, bypassing the hydrophilic periplasmatic space (111, 139). Thus, Tp0126 could be involved in fatty acid transport (unable to be synthesized by TPA alone) (140). Interestingly, this protein is highly conserved among TPA strains. Its transcription is regulated by the presence of guanidine homopolymers of various length located upstream of its promoter, which is consistent with phase variation (141).
Remarkably, Tp0126 may show low immunogenicity. Anti-Tp0126 antibody levels in serum samples from patients with latent syphilis were lower than those targeting Tp0574, a highly expressed and immunogenic TPA protein (141). In addition, anti-Tp0126 antibodies were detected in Tp0126-immunized rabbits only after the third boost, highlighting the potentially low immunogenicity of this protein (141). Interestingly, these antibodies targeted Tp0126-external loops and were able of opsonize TPA. However, immunization with Tp0126 showed poor protective capacity, if any, in rabbits. In fact, treponemes isolates from lesions of immunized and challenged rabbits showed lower transcription levels of Tp0126, which was associated with a longer polyG region (≥ 9 G’s), when compared to treponemes obtained from the challenge inoculum (141). These results suggest that the expression of Tp0126 may be reduced during infection, which could explain the absence of protection observed in immunization and challenge experiments.
Like Tp0126, other members of the TPA OmpW/AlkL ortholog group (Tp0733, Tp0479, and Tp0698) (111) are predicted to form a membrane-channel and being involved in transport of small molecules. Hawley et al. predicted multiple surface-exposed B cell epitopes in their extracellular loops, although the density of these epitopes differs from one to another protein (111). Future experimental studies with these OMPs are envisaged to confirm their in silico predictions, and to inform about potential immunogenicity and capacity of inducing protective immune responses.
5.1.4 FadL-like proteins
Due to the low OM permeability of Gram-negative bacteria, these bacteria have an OM protein machinery involved in the uptake of long-chain fatty acids, such as the FadL (Long-chain fatty acid transport protein). TPA is unable to synthesize long-chain fatty acids (28). However, since TPA OM is more permeable to fatty acids than other Gram-negative bacteria (142), membrane diffusion may be one of the mechanisms that this microorganism uses to uptake these molecules. Nevertheless, five FadL orthologs (Tp0548, Tp0856, Tp0858, Tp0859, and Tp0865) have been identified in TPA, suggesting that passive diffusion of fatty acids is not the only mechanism (111). Structural modelling of these orthologs predicted that all TPA FadL-like proteins form a 14-stranded β-barrel with N-terminal in the barrel lumen (111). Moreover, all five proteins contain one or more predicted B cell epitopes (111). Interestingly, Delgado et al. recently described the presence of IgG antibodies and IgG+ B cells in TPA-infected rabbits that recognized the extracellular loops 2 and 4 from Tp0856 and Tp0858 (143), indicating that these proteins could be useful in vaccine design.
5.1.5 TolC-like proteins
TolC is an OM protein from E. coli, which is part of an efflux pump complex involved in the removal of toxic substances (144). To date, four TolC orthologs (Tp0966, Tp0967, Tp0968, and Tp0969) have been described in TPA (145). Structural modelling of these proteins identified that they have a TolC-like topology based on four β-strands with two large extracellular loops and six α-helices (111). Furthermore, Hawley et al. predicted that all four TolC-like TPA proteins, particularly Tp0969, enclose surface-exposed B cells epitopes (111). Thus, these predicted OMPs could be targeted by the immune system. However, further in vivo analysis of their immunogenicity is required to determine their potential in vaccine development.
5.1.6 Treponema rare outer membrane proteins (TROMPs)
TROMP family includes three proteins: TROMP-1 (31 kDa), TROMP-2 (28 kDa) and TROMP-3 (65 kDa) (146). TROMP-1 (also called TroA or Tp0163) was firstly described as a surface-exposed protein with porin-like properties (147). However, it was later identified to be a periplasmatic metalloprotein anchored to the cytoplasmatic membrane (148–150). TROMP-2 (also called Tp0663) was localized on the OM when it was expressed as recombinant protein in E. coli. However, the isolation of TROMP-2 from E. coli OM showed low porin insertional events, casting doubts on its porin function (29). Interestingly, both TROMP-1 and TROMP-2 were targeted by antibodies present in sera from infected rabbits and humans (146). In fact, TROMP-2 could be a potential candidate for serodiagnosis of all syphilis stages (151). TPA challenge experiments performed in Tp0663-immunized rabbits showed partial protection, with high titers of anti-Tp0663 antibodies. The authors observed attenuated lesions with an increase cellular infiltration. Importantly, no bacteria dissemination to distal organs was detected in immunized animals (152). Of note, TROMP-3 is expressed at a lower concentration than the other two members of this family, and its function and structure remain unknown (29).
5.2 Adhesion function
Adhesion to cells and extracellular matrix is crucial to the establishment of infection and the dissemination of TPA.
5.2.1 Tp0136
Tp0136 has been identified as a fibronectin-binding TPA OMP that binds more efficiently to cellular than plasma fibronectin (50, 51). This selective binding involves different domains of this protein. Thus, the conserved N-terminal region is mainly responsible for binding to plasma fibronectin. Residues in the C-terminal end, and also in the central portion of the protein, participate in binding to the cellular form of fibronectin (51). Interestingly, Tp0136 shows sequence variability among TPA strains, and its transcription is regulated during infection (50, 51). The interaction of Tp0136 with fibronectin expressed on cell surface can promote bacteria attachment to tissues, facilitating body dissemination and favoring the colonization of distal endothelial tissues, central nervous system, or placenta (153).
In addition to its adhesion function, Tp0136 may also play an important role in chancre healing by promoting fibroblast and microvascular endothelial cell migration, and the activation and aggregation of platelets (154).
Immunization and challenge experiments performed in rabbits using recombinant Tp0136 produced in E. coli showed a delay in lesion ulceration but not in their development, indicating no protection against infection (50). Remarkably, sera obtained from immunized animals reduced the attachment of the bacteria to fibronectin, although at lower levels than sera obtained from unvaccinated and infected rabbits (50). Interestingly, Xu et al. showed that rabbits immunized with Tp0136 elicited high levels of antigen-specific antibodies, attenuated lesion development with increased cellular infiltration, and limited treponemal dissemination to distant organs (152). Although there are discrepancies between both studies that could be explained by differences in adjuvant, immunization schedule, and the amount of live inoculated bacteria, these results suggest that antibodies developed against Tp0136 might be protective. However, the fact that antibodies elicited during experimental infection blocked more efficiently TPA binding to fibronectin than vaccine-induced antibodies elicited against Tp0136, suggest that, during vaccine design, other specificities should be also targeted to more efficiently block bacteria from binding to the extracellular matrix (51).
5.2.2 Tp0155
It has been described that Tp0155 preferably binds to fibronectin matrix and, therefore, it might be involved in the dissemination of TPA (155). Tp0155 shows two lysin motifs (LysM) domains in its N-terminal portion that can play a major role in binding to fibronectin and peptidoglycans, and a M23 peptidase domain in the C-terminal region, which might show peptidoglycan lytic activities (156).
There is some controversy regarding Tp0155 surface location. Inhibition assays showed that recombinant Tp0155 reduced live TPA binding to fibronectin-coated slides, suggesting that Tp0155 is expressed on the OM (155). This result was in line with the fact that TDE2318, a fibronectin-binding protein with high homology to Tp0155, was found in the surface of T. denticola according to immunofluorescence microscopy data (156). However, immunofluorescence studies using agarose microencapsulated treponemes showed that Tp0155 is not exposed on the OM of TPA (157). According to this observation, Tp0155-immunized rabbits, which elicited high antigen-specific antibody titers, were not protected against an intradermal challenge with live treponemes (157). Thus, further investigation will be needed to determine whether Tp0155 would be a bona fide OMP or not.
Remarkably, studies performed with human sera showed that approximately 70% of syphilis patients did not show antibodies against Tp0155, indicating that the expression of this protein or its immunogenicity may be low in natural infection (157, 158).
5.2.3 Tp0435
Tp0435 is a highly immunogenic 14 kDa lipoprotein with adhesin function, also known as Tp17. To identify the location of Tp0435 in TPA, Chan and colleagues performed a study that analyzed the Tp0435 surface expression in TPA and Tp0435-transformed B. burgdorferi cells (159). The authors concluded that Tp0435 may be post-translationally modified, generating several variants that can be differentially located between the surface and the periplasmic space. Contrarily, Cox et al., did not find Tp0435 surface-exposure evidences (160). Structurally, Tp0435 encompasses eight-stranded anti-parallel β-barrel with a basin-like domain located at one end (161). According to Chan et al., Tp0435 favors the attachment of the spirochetes to mammalian cell lines (159). Interestingly, human sera from infected patients are reactive against Tp0435 (158), and immunization of rabbits with a Tp0435-expressing B. burgdorferi strain induced a strong immune response against Tp0435. However, this immune response failed to protect from infection or lesion development after experimental challenge (162). Thus, further studies may be needed to determine the location of Tp0435 and its potential as vaccine candidate.
5.2.4 Tp0483
Tp0483 can bind both extracellular matrix and soluble fibronectins (155). The C-terminal portion of Tp0483 (179-374 amino acids) also showed reactivity against laminin (163). Little is known about the structure of Tp0483. However, a peptide encompassing 316-333 amino acids inhibited binding of Tp0483 to fibronectin, suggesting that this peptide, or a near region of Tp0483, should be responsible for binding to adhesive glycoprotein (164). Similar to Tp0155, Cameron et al. indirectly suggested that Tp0483 was an OMP (155). Contrarily, Tomson and colleagues did not find evidences of the Tp0483 OM location. Even though rabbits immunized with this protein elicited high antibody titers, the authors did not detect protection after challenge (157).
5.2.5 Tp0750
Tp0750 binds to fibrinogen and the fibrinolytic receptor complex protein Annexin A2. Tp0750 shows serine metalloprotease activity, and it is able to degrade fibrinogen and fibronectin, inhibiting the coagulation cascade, but cannot degrade fibrin clots (57). Structurally, Tp0750 shows metal ion-dependent adhesion site (MIDAS)-containing von Willebrand Factor type A domains (region V29-T147). The MIDAS domains bind to primary calcium. This protein, as well as Tp0751 (see section 5.2.6), are co-transcribed and might coexist as heterodimeric complex. However, unlike Tp0751, Tp0750 shows a limited laminin binding capacity (57). Sequence analyses of both proteins have shown that Tp0750 is highly conserved among pathogenic treponemes species, whereas Tp0751 is less conserved, except for the most invasive treponemes species. Interestingly, Tp0750 and Tp0751 orthologs from the less invasive treponemes species do not bind or degrade host proteins, which strengthen the idea that both adhesion function as well as degradative capabilities are important to syphilis progression (165).
5.2.6 Tp0751
While there is a degree of agreement in regards to the structure of Tp0751, there are discrepancies regarding its function, location, and the relevance of the immune response elicited against this protein. From the structural point of view, Tp0751 presents a bipartite topology with a disorganized N-terminal region that is joined through an α-helix domain to a C-terminal portion arranged in eight anti-parallel β-sheets (52, 166).
It has been previously described that Tp0751 binds to different forms of laminin in a dose-dependent manner, and can work as vascular adhesin, promoting bacterial dissemination (53, 167). Additionally, Tp0751 showed metalloprotease activity, and can degrade fibrinogen and laminin, promoting clot dissolution, and favoring bacterial dissemination (58). However, Luthra and colleagues showed that Tp0751 is located in the periplasmic space and its main role is binding to small molecules along the barrel rim, such as hemes (166).
While Cameron and colleagues described that Tp0751 is recognized by antibodies in patient and infected rabbit sera (163), and these antibodies can opsonize the bacterium, Luthra et al. observed opposite results. In their study, Tp0751 induced a weak antibody response in infected human and challenged rabbits, and anti-Tp0751 antibodies lack opsonization capacity, probably due to the low levels of Tp0751 expression on the OM (166). Immunization and challenge experiment in rabbits also provided contradictory results. Lithgow and colleagues showed that Tp0751-immunized animals showed attenuated lesions with low bacteria burden. Although immunization did not prevent infection, it successfully inhibited bacteria dissemination (168). Conversely, Luthra et al. showed no protection against local or disseminated infection following intradermal TPA challenge in Tp0751-immunized animals (166). Therefore, further research will be needed to clarify these discrepancies.
5.2.7 Tp0954
Tp0954 has been recently described as a surface lipoprotein, and may have a major role in congenital syphilis (169). According to its structure, this protein is predicted to have a tetratricopeptide repeat (TPR) structural motif with tandem α-helices. Moreover, Tp0954 sequence is conserved among several TPA strains (e.g. Nichols, Chicago, Mexico A and Amoy) (169). Primus et al. also described that Tp0954-transformed B. burgdorferi B314 bacteria, which is known to be a poorly adherent strain, gained binding to mammalian epithelial cell lines, including a human placental cell line [i.e. BeWo (CCL-98)] (169). Unlike other TPA adhesins, Tp0954 can mediate adhesion and bacteria dissemination through binding to glycosaminoglycans present in human placenta, such as dermatan sulfate, heparin, and heparan sulfate, which are components of the extracellular matrix (169). Dermatan sulfate is associated with fetal blood vessels and syncytial surface, whereas heparan sulfate is located in trophoblast layers (170–173). Thus, it is possible that Tp0954 might facilitate congenital infection through binding to placental glycosaminoglycans.
5.3 Other functions
A number of proteins in the OM of TPA are not related with neither transport or adhesion function.
5.3.1 Tp0326
Tp0326 (or Tp92) shows homology with BamA orthologs from other Gram-negative bacteria. BamA-like proteins are characterized by a bipartite structure with a β-barrel domain located in the C-terminal portion, and at least one polypeptide-transport-associated (POTRA) domain in the N-terminal end. Particularly, Tp0326 was predicted to contain five N-terminal POTRA and one C-terminal β-barrel domains (174, 175). According to Desrosiers et al., the N-terminal POTRA domains are periplasmic, whereas the C-terminal β-barrel is embedded in the OM forming a pore (175).The N-terminal end can bind to multiple periplasmatic proteins through POTRA domains, establishing a protein complex that is crucial for membrane synthesis. As other BamA-like proteins, Tp0326 is suggested to be part of a protein machinery involved in the translocation and insertion of proteins from the periplasmatic space to the TPA OM (175). Interestingly, human antibodies are mainly directed against the Tp0326 periplasmic segment, while rabbit antibodies target both N-terminal and C-terminal regions (175). Indeed, Luthra et al. found that POTRA domains are immunodominant over the β-barrel domains in both rabbits and humans (174). In addition, these authors described that the Tp0326 central pore exposes eight loops to the extracellular space. Among them, loop 4 is specially targeted by antibodies with opsonizing capabilities from infected rabbits and humans with secondary syphilis (174). By contrast, Tomson and colleges failed to detect the expression of Tp0326 on the OM of treponemes encapsulated within agarose gel microdroplets using indirect immunofluorescence (157). In addition, they did not observe protection in Tp0326-immunized animals after TPA challenge. Conversely, Cameron et al. showed that, although all Tp0326-immunized rabbits were infected after challenge, there was some degree of protection in the vaccinated animals that had high antigen-specific antibody titers (176).
5.3.2 Tp0257
Tp0257 (or GpD) was identified using a treponema genetic expression library and opsonized rabbit immune sera. This protein presents homology with H. influenzae GplQ protein, a glycerolphosphodiester phosphodiesterase protein, which is partially expressed on the surface (177). Thus, it is possible that Tp0257 may be an OM protein. Accordingly, Cameron et al. reported indirect evidences of its surface location by immunoblot analysis of TPA lysates. The authors observed positive staining in the preparation that included the OM, but not in the OM-removed TPA lysate fraction (178). Moreover, GpD was isolated from OM preparations (179). Interestingly, rabbits immunized with recombinant Tp0257 exhibited a reduction in lesions development and bacterial proliferation after TPA challenge (178). Contrarily, Shevchenko et al., showed that Tp0257 could be a periplasmic protein, since they did not identify surface-exposed evidences by multiple assays, and Tp0257-immunization failed to protect rabbits from TPA challenge (180). More recently, a DNA vaccine encoding a Tp0257-IL-2 fusion protein showed a decrease in ulcerative lesions, as well as, in the number of lesions containing TPA (181). The inclusion of IL-2 in the vaccine enhanced anti-GpD humoral responses and the levels of IFN-γ. Remarkably, rabbits immunized with a Tp0257-IL2 DNA prime and intranasal boost with recombinant protein adjuvanted with CpG-ODN induced mucosal and systemic immunity, and showed a faster lesion recover after TPA inoculation (182).
Interestingly, Tp0257 is conserved among TPA strains, as well as in Treponema pallidum endemicum and Treponema pallidum pertenue (183). These results make this protein an attractive immunogen candidate for vaccine design.
5.3.3 Tp1038
Tp1038, also known as TpF1 or antigen 4D, is a bacterioferritin with ferroxidase activity playing a major role in iron uptake (184). Radolf et al. showed that Tp1038 is surface-exposed since sera from Tp1038-immunized rabbits was reactive to immobilized TPA in the presence of complement (185).
Tp1038 is able to induce diverse immune responses. For example, this protein might be involved in the proinflammatory response during primary syphilis, since it can activate the inflammasome complex in monocytes, and thereby, induces the release of IL-1β, IL-6, and TNF-α (186, 187), which could result in tissue damage associated with this stage. However, Babolin et al. described that Tp1038 may also drive a T regulatory response (186). CD4+ CD25+ Foxp3+ T cells from patients with secondary syphilis produce TGF-β under Tp1038 stimulation, and Tp1038-stimulated monocytes produce IL-10 and TGF-β, two cytokines linked to Treg cell differentiation (186). Therefore, TpF1 might be a virulence factor involves in the persistence of TPA infection through the downregulation of the immune response (186). Regarding the humoral response, anti-Tp1038 antibodies are detectable in all syphilis stages (186, 188). Finally, Pozzobon and colleagues identified that patients with tertiary syphilis have Tp1038-specific T cells, which stimulate monocyte and human umbilical vein endothelial cells to produce tissue factor, IL-8, and CCL-20 (189). These cytokines are involved in angiogenesis, thus Tp1038 may also be implicated in tertiary syphilis, in which vascular inflammation and angiogenesis characterize the lesions of this stage (189). Thus, Tp1038 is an interesting target for vaccine development since it is engaged in all syphilis stages.
6 Immune evasion strategies
While an immune response is triggered against TPA, it does not protect against bacterial dissemination and disease progression to the second or third stage. In addition, reinfections are quite common, with an incidence around 5-22% (190–192), indicating that TPA developed some mechanisms to evade the immune system (Figure 2).
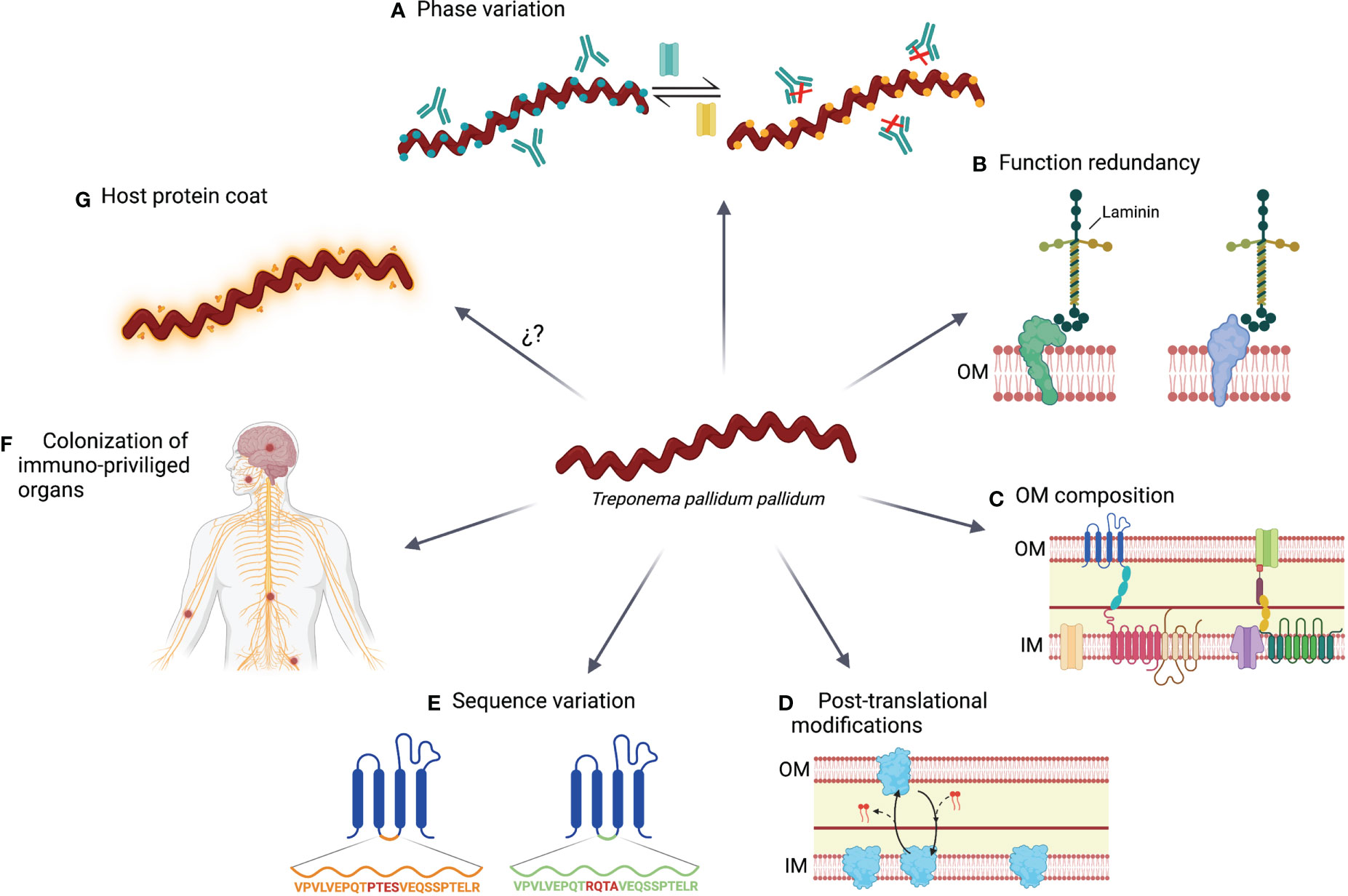
Figure 2 Immune evasions mechanisms use by TPA. (A) During its adaptation to environment changes, including the immune system pressure, TPA modulates the expression of certain genes by a switch on/off mechanism. (B) Linked with the prior mechanism; function redundancy ensure that bacterial homeostasis is not compromised by changing the expression of proteins with vital functions. (C) OM of TPA is characterized by a paucity of protein compare with the inner membrane (IM), the lack of LPS and the presence of anti-inflammatory phospholipids (i.e., phosphatidylserine and phosphatidylcholine). (D) Some proteins experience post-translational modifications that result in variants with different location preference. (E) Proteins can accumulate sequence variations by point mutation, gene conversion or recombination. (F) TPA can persist in immune privileged organs, such as central nervous system or nerve fibers, and prolong their survival by remaining hidden to the immune system. (G) TPA may be coated by host serum proteins, reducing its immunogenicity.
6.1 Phase variation or switch on/off
TPA can modulate the expression of several OMPs during its life cycle (122). However, the mechanisms controlling the expression of those proteins are not well characterized. In the case of subfamilies I and II of Tpr proteins, guanosine homopolymer (polyG) repeats have been identified upstream of the transcriptional start site. The length of the polyG sequences differ among treponemal isolates and modulate the expression of Tpr proteins at the transcriptional level (124). PolyG signals have also been found in other OMPs, such as the Tp0126 protein (140). Phase variation based on hypervariable homopolymeric repeats have been previously reported in several other bacteria such as Neisseria meningitidis, Helicobacter pylori, or Haemophilus influenzae, and it is crucial for the survival of pathogens facing host immunity (193–195). Moreover, other transcriptional regulatory mechanisms have been identified, such as the G4FS cis-acting DNA elements, which form guanine-quadruplexes and induce recombination and gene conversion of Tp0136 gene (196). Using these mechanisms, TPA can modify its OMP repertoire to adapt to the environment conditions and evade the immune response. Consequently, the specificity of the humoral response against Tpr proteins may vary between animals or humans that are infected with the same or different TPA strain, thereby supporting the existence of a variable repertoire of OMPs (81).
6.2 Functional redundancy
Several OMPs present similar functions in transport or cell adhesion. Functional redundancy may be advantageous since the replacement of one protein by a functional homologous can guarantee that phase variation and post-translational modifications will not adversely affect cell homeostasis (22). As an example, Salmonella has been reported to have two distinct flagellin genes, fliC and fliB, with the same function but different structure. By switching their expression from one flagellin protein to the other, the immune system becomes ineffective against assembled flagella (197).
6.3 Outer membrane composition
TPA is characterized by a low number of OMPs, which may encompass an advantage by limiting the number of exposed antigens to the immune system. Moreover, the spacing distribution of these proteins may promote that antibodies bind to them just with one arm, reducing the avidity of the interaction. This mechanism has been previously described in Human Immunodeficiency Virus (HIV) infection (198), which also presents low density of Envelope glycoprotein on the virion surface. In HIV, polyreactivity of neutralizing antibodies may increase binding avidity by heteroligation with membrane components (198). However, the development of these antibodies could be limited by tolerance mechanisms (199). Whether this phenomenon is also related to TPA immunity requires further investigation, but it could partially explain the low antibody efficacy at controlling infection. Since the OM lacks LPS (27, 28), and it is enriched in anti-inflammatory phospholipids (e.g. phosphatidylserine and phosphatidylcholine), these characteristics may also hamper the development of an efficient immune response (200, 201).
6.4 Post-translational modifications
Post-translational protein modifications play a crucial role in different processes of prokaryote cells, including persistence and virulence (202). These modifications have been found in the TPA proteome. For example, there are several variants of Tp0435 that may coexist and were generated by palmitoylation (159). Palmitoylation consists in the addition of lipid chains to terminal cysteine residues. This process has been previously reported in other bacteria and contributes to their infectivity and recognition of the immune system (203). Tp0435 variants may be located on the surface or into periplasmatic spaces, being the latter one the most abundant (159), which could explain why there is no consensus about the Tp0435 location. In fact, this protein has been the first description of post-translationally modified protein variants in TPA. However, other OMPs could be also susceptible to post-translational modifications.
6.5 Sequence variation
Genetic variation of several OMPs has been described in TPA, including laboratory-adapted or primary isolated strains (127, 129, 204). Some of these variations have been reported in the Tpr family, including TprC, TprD, TprG and TprK proteins (127, 204, 205). Interestingly, TprK accumulates sequence variation in variable regions (particularly in V6) by non-reciprocal recombination with silent cassettes, which is enhanced under immune pressure (129, 130). Additionally, several tprk alleles have been identified in different TPA strains (127), which contributes to increase the variability of this protein. Besides Tpr proteins, others OMPs, such as Tp0326 (BamA), also show genetic variants (204, 205). This protein has accumulated mutations on the extracellular hydrophilic loops predicted to contain B cell epitopes (204). Sequence variation was also confirmed in FadL orthologs proteins ranging from fully conserved Tp0856 to deeply variable Tp0548, as well as in Tp0136, Tp0868, Tp0966 and Tp0967 (206–208).
6.6 Colonization of immune privileged organs
TPA can colonize distal immune privileged organs, such as the central nervous system, placenta, or eyes. In these tissues, the action of the immune system may be limited, contributing to the persistence of this pathogen (209–211). In fact, studies performed using the rabbit model showed that TPA can be detected in nerves early after infection (68).
6.7 Host proteins coat
Similarly to other bacteria; for example, some streptococcal species (212), TPA immune evasion may be associated with host proteins forming a surface coat, also known as antigenic disguise. Alderete and Baseman described that both rabbit and human albumins, as well as other host proteins, could be adsorbed on the surface of TPA (213). Egesten et al. showed that Group G streptococci express protein G on their surface, and it is able to bind human albumin, and therefore, inhibit anti-microbial action of CXCL9 (214). Accordingly, it has been proposed that the capacity of Tp0483 to bind soluble fibronectin could be related to the evasion of the humoral response (164). However, Cox and colleagues did not detect serum proteins on the surface of TPA by immunoelectron microscopy (26), stating that the low density of OMP might be the main immune evasion mechanism of TPA.
7 Discussion
Syphilis is a multistage disease caused by TPA, for which an effective vaccine remains elusive. Despite several decades of research, the impossibility to genetically manipulate and grow TPA in vitro, the paucity of OMPs, the OM fragility, and the lack of an appropriate animal model that recapitulates all stages of human syphilis have hampered the field progress. In addition, many controversies remain unresolved with respect to OMPs identification, location, function, the potential of these proteins to induce protective immune responses, or the characteristics of these responses. Researchers have hypothesized that a DTH immune response is essential to control and clear TPA infection, but it is also responsible for the development of tissue damage, including an ulcerative lesion that forms chancres. As DTH is primarily mediated by Th1 CD4+ T cells, the presentation of antigens by DCs, macrophages, or other professional antigen-presenting cells is crucial. Of note, this process is independent of antigen location, and both intracellular and surface-exposed antigens can be indistinctly processed and presented to T cells. Therefore, both antigens might generate protective Th1 CD4+ T cell responses. Thus, rabbits immunized with intracellular endoflagellar antigens that have been directly isolated from TPA showed faster development of lesions (215). However, bacteria were not detected in these lesions, which may be explained by an accelerated memory DTH response. In contrast, rabbits immunized with a plasmid coding for FlaB3 showed attenuated lesion development that was associated with an enhanced cellular infiltration, and an inhibition of bacteria dissemination to distal organs (216). In line with this observation, rabbits immunized with TprF, a periplasmic protein, displayed attenuated skin lesions with reduced bacteria burden (136). The reasons behind these different outcomes are not completely understood, but differences in vaccine formulation and delivery routes, the magnitude of vaccine-induced T cell responses, and the relative representation of antigens (flagellin and TprF) within the whole TPA proteome could be involved. Similar results to TprF immunization were also observed when OMPs were used as antigens. Therefore, DTH could be a protective immune response that modifies lesion development and clears bacteria from infection sites. However, none of TPA proteins tested as immunogens to date protected against infection, indicating that additional immune responses are likely to be required.
Despite some controversy (68), strong evidence supports a protective role of antibodies, facilitating bacteria opsonization and killing by innate immune cells, as well as blocking their interaction with components of the extracellular matrix, such as laminin and fibronectin. These functions strictly depend on the exposure of antigens on the OM and, in some cases (e.g. bacteria phagocytosis), the interaction with Fcγ receptors expressed on the surface of innate immune cells, such as macrophages. IgG-FcγR interaction may also improve antigen presentation by antigen-presenting cells and the generation of Th1 cellular responses (217). Therefore, to develop a protective TPA vaccine, it is crucial to define which types of antigens can induce synergic anti-TPA immune responses. It is also essential to considerer immune evasion strategies developed by TPA, such as functional redundancy and phase variation. In this sense, targeting several proteins with two or more functions, such as transport and adhesion, could increase vaccine efficacy, as it was previously observed (218, 219).
The ideal syphilis vaccine should provide protection against TPA infection. However, even if the vaccine is unable to completely prevent infection, it could still worth considering as a means of limiting bacteria dissemination and tissue invasiveness, blocking the establishment of latency, or preventing the disease from progressing to secondary and tertiary stages (113). Additionally, a vaccine that reduces bacterial persistence or prevents TPA transplacental invasion might also reduce the number of congenital syphilis in endemic areas, where the number of non-diagnosed infected individuals might be high, and the access to health care may be limited. Moreover, a syphilis vaccine has to be effective against possible reinfections with similar or different TPA strains or isolates, indicating the importance of using conserved antigens. Recently, there has been evidence that TPA can be cultured in vitro and that genetic manipulation of this pathogen can be accomplished (114, 220, 221). These scientific advances are likely to open the gateway to new research lines that can shed light on the current controversies.
Author contributions
CÁ-N, OM, BC, JB and JC designed the scientific content. CÁ-N, NP-L and JC drafted the manuscript and figures. OM, BC and JB edited the manuscript and participate in content discussion. All authors have read and approved the content of the manuscript.
Funding
This work was supported by the CERCA Program (2017 SGR 252; Generalitat de Catalunya), Direcció General de Recerca i Innovació en Salut (Generalitat de Catalunya) (projects SLD0015 and SLD0016), the Carlos III Health Institute (PI17/01518 and PI18/01332). JB is supported by the Health Department of the Catalan Government (Generalitat de Catalunya). CÁ-N was supported by a predoctoral grant from Generalitat de Catalunya and Fons Social Europeu (2020 FI_B_0742). This study was also supported by CIBER - Consorcio Centro de Investigación Biomédica en Red (CB 2021), Carlos III Health Institute, Ministerio de Ciencia e Innovación and Unión Europea – NextGenerationEU.Funders had no role in study design, data analysis, decision to publish, or manuscript preparation.
Acknowledgments
Figure 1 and Figure 2 were created with BioRender.com.
Conflict of interest
The authors declare that the research was conducted in the absence of any commercial or financial relationships that could be construed as a potential conflict of interest.
Publisher’s note
All claims expressed in this article are solely those of the authors and do not necessarily represent those of their affiliated organizations, or those of the publisher, the editors and the reviewers. Any product that may be evaluated in this article, or claim that may be made by its manufacturer, is not guaranteed or endorsed by the publisher.
References
1. Harper KN, Zuckerman MK, Harper ML, Kingston JD, Armelagos GJ. The origin and antiquity of syphilis revisited: An appraisal of old world pre-Columbian evidence for treponemal infection. Am J Phys Anthropol (2011) 146:99–133. doi: 10.1002/ajpa.21613
2. De Melo FL, De Mello JCM, Fraga AM, Nunes K, Eggers S. Syphilis at the crossroad of phylogenetics and paleopathology. PloS Negl Trop Dis (2010) 4:e575. doi: 10.1371/journal.pntd.0000575
3. Mahoney JF, Arnold RC, Harris A. Penicillin treatment of early syphilis-a preliminary report. Am J Public Health Nations Health (1943) 33:1387–91. doi: 10.2105/ajph.33.12.1387
4. Singh AE, Romanowski B. Syphilis: Review with emphasis on clinical, epidemiologic, and some biologic features. Clinical Microbiology Reviews (1999) 12:187–209. doi: 10.1128/cmr.12.2.187
5. Peeling RW, Mabey D, Kamb ML, Chen X-S, Radolf JD, Benzaken AS. Syphilis. Nature Reviews Disease Primers (2017) 3:17073. doi: 10.1038/nrdp.2017.73
6. Radolf JD, Deka RK, Anand A, Šmajs D, Norgard MV, Yang XF. Treponema pallidum, the syphilis spirochete: Making a living as a stealth pathogen. Nat Rev Microbiol (2016) 14:744–59. doi: 10.1038/nrmicro.2016.141
7. Denee Thomas D, Navabo M, Haaket DA, Fogelmant AM, Miller JN, Lovett MA. Treponema pallidum invades intercellular junctions of endothelial cell monolayers. Proc Natl Acad Sci USA (1988) 85(10):3608–12. doi: 10.1073/pnas.85.10.3608
8. Lafond RE, Lukehart SA. Biological basis for syphilis. Clin Microbiol Rev (2006) 19:29–49. doi: 10.1128/cmr.19.1.29-49.2006
9. Cruz AR, Ramirez LG, Zuluaga AV, Pillay A, Abreu C, Valencia CA, et al. Immune evasion and recognition of the syphilis spirochete in blood and skin of secondary syphilis patients: Two immunologically distinct compartments. PloS Negl Trop Dis (2012) 6(7):e1717. doi: 10.1371/journal.pntd.0001717
11. Luo Z, Zhu L, Ding Y, Yuan J, Li W, Wu Q, et al. Factors associated with syphilis treatment failure and reinfection: A longitudinal cohort study in shenzhen, China. BMC Infect Dis (2017) 17:1–5. doi: 10.1186/s12879-017-2715-z
12. Brewer TH, Peterman TA, Newman DR, Schmitt K. Reinfections during the Florida syphilis epidemic, 2000-2008. Sex Transm Dis (2011) 38:12–7. doi: 10.1097/OLQ.0b013e3181e9afc7
13. STD Facts - syphilis (Detailed). Available at: https://www.cdc.gov/std/syphilis/stdfact-syphilis-detailed.htm (Accessed March 18, 2020).
14. Rowley J, Vander Hoorn S, Korenromp E, Low N, Unemo M, Abu-Raddad LJ, et al. Chlamydia, gonorrhoea, trichomoniasis and syphilis: Global prevalence and incidence estimates, 2016. Bull World Health Organ (2019) 97(8):548–62. doi: 10.2471/blt.18.228486
15. Korenromp Id EL, Rowley J, Alonso M, Mello MB, Saman N, Id W, et al. Global burden of maternal and congenital syphilis and associated adverse birth outcomes-estimates for 2016 and progress since 2012. PloS One (2019) 14(2):e0211720. doi: 10.1371/journal.pone.0211720
16. Lawn JE, Blencowe H, Waiswa P, Amouzou A, Mathers C, Hogan D, et al. Stillbirths: Rates, risk factors, and acceleration towards 2030. Lancet (2016) 387:587–603. doi: 10.1016/S0140-6736(15)00837-5
17. Kojima N, Klausner JD. An update on the global epidemiology of syphilis. Curr Epidemiol Rep (2018) 5(1):24–38. doi: 10.1007/s40471-018-0138-z
18. Ghanem KG, Ram S, Rice PA. The modern epidemic of syphilis. New Engl J Med (2020) 382:845–54. doi: 10.1056/nejmra1901593
19. European Centre for Disease Prevention and Control. Syphilis and congenital syphilis in Europe - A review of epidemiological trends (2007 2018) and options for response. Stockholm: ECDC. (2019). doi: 10.2900/578824
20. Izard J, Renken C, Hsieh CE, Desrosiers DC, Dunham-Ems S, La Vake C, et al. Cryo-electron tomography elucidates the molecular architecture of treponema pallidum, the syphilis spirochete. J Bacteriol (2009) 191:7566–80. doi: 10.1128/jb.01031-09
21. Madigan MT, Martinko JM, Parker J. Brock Biology of microorganisms. 8th Edition. New York: Prentice Hall International, Inc. (1997).
22. Radolf JD, Kumar S. The treponema pallidum outer membrane. Curr Top Microbiol Immunol (2018) 415:1–38. doi: 10.1007/82_2017_44
23. Radolf JD, Robinson EJ, Bourell KW, Akins DR, Porcella SF, Weigel LM, et al. Characterization of outer membranes isolated from treponema pallidum, the syphilis spirochete. Infect Immun (1995) 63:4244–52. doi: 10.1128/iai.63.11.4244-4252.1995
24. Bailey DMJ. The outer membrane of treponema pallidum: Biological significance and biochemical properties. J Gen Microbiol (1985) 131(9):2349–57. doi: 10.1099/00221287-131-9-2349
25. Radolf JD, Norgardt MV, Schulz WW. Outer membrane ultrastructure explains the limited antigenicity of virulent treponema pallidum. Proc Natl Acad Sci USA (1989) 86(6):2051–5. doi: 10.1073/pnas.86.6.2051
26. Cox DL, Chang P, McDowall AW, Radolf JD. The outer membrane, not a coat of host proteins, limits antigenicity of virulent treponema pallidum. Infect Immun (1992) 60:1076–83. doi: 10.1128/iai.60.3.1076-1083.1992
27. Hardy PH, Levin J. Lack of endotoxin in borrelia hispanica and treponema pallidum1. Proc Soc Exp Biol Med (1983) 174(1):47–52. doi: 10.3181/00379727-174-41702
28. Fraser CM, Norris SJ, Weinstock GM, White O, Sutton GG, Dodson R, et al. Complete genome sequence of treponema pallidum, the syphilis spirochete. Science (1998) 281:375–88. doi: 10.1126/science.281.5375.375
29. Blanco DR, Miller JN, Lovett MA. Surface antigens of the syphilis spirochete and their potential as virulence determinants. Emerg Infect Dis (1997) 3:11–20. doi: 10.3201/eid0301.970102
30. Charon NW, Cockburn A, Li C, Liu J, Miller KA, Miller MR, et al. The unique paradigm of spirochete motility and chemotaxis. Annu Rev Microbiol (2012) 66:349–70. doi: 10.1146/ANNUREV-MICRO-092611-150145
31. Liu J, Howell JK, Bradley SD, Zheng Y, Hong Zhou Z, Norris SJ. Cellular architecture of treponema pallidum: Novel flagellum, periplasmic cone, and cell envelope as revealed by cryo-electron tomography. J Mol Biol (2010) 403(4):546–61. doi: 10.1016/j.jmb.2010.09.020
32. Salazar JC, Rathi A, Michael NL, Radolf JD, Jagodzinski LL. Assessment of the kinetics of treponema pallidum dissemination into blood and tissues in experimental syphilis by real-time quantitative PCR. Infect Immun (2007) 75:2954–8. doi: 10.1128/iai.00090-07
33. Harman M, Vig DK, Radolf JD, Wolgemuth CW. Viscous dynamics of Lyme disease and syphilis spirochetes reveal flagellar torque and drag. Biophys J (2013) 105:2273–80. doi: 10.1016/J.bpj.2013.10.004
34. Edmondson DG, Hu B, Norris SJ. Long-term In vitro culture of the syphilis spirochete treponema pallidum subsp. pallidum. mBio (2018) 9(3):e01153–18. doi: 10.1128/mbio.01153-18
35. Lukehart SA, Marra CM. Isolation and laboratory maintenance of treponema pallidum. Curr Protoc Microbiol (2007) 7:12A.1.1–12A.1.18. doi: 10.1002/9780471729259.mc12a01s7
36. Willcox RR, Guthe T. Treponema pallidum. a bibliographical review of the morphology, culture and survival of t. pallidum and associated organisms. Bull World Health Organ (1966) 35(1):1–16.
37. Izzat NN, Knox JM, Werth JA, Dacres WG. Evolution of syphilitic chancres with virulent treponema pallidum in the rabbit. Brit J vener Dis (1970) 47:67. doi: 10.1136/sti.47.2.67
38. Baker-Zander S, Sell S. A histopathologic and immunologic study of the course of syphilis in the experimentally infected rabbit. demonstration of long-lasting cellular immunity. Am J Pathol (1980) 101:387–413.
39. Hanff PA, Fehniger TE, Miller JN, Lovett MA. Humoral immune response in human syphilis to polypeptides of treponema pallidum. J Immunol (1982) 129(3):1287–91.
40. Esteves PJ, Abrantes J, Baldauf HM, BenMohamed L, Chen Y, Christensen N, et al. The wide utility of rabbits as models of human diseases. Exp Mol Med (2018) 50(5):1–10. doi: 10.1038/s12276-018-0094-1
41. Tantalo LC, Lukehart SA, Marra CM. Treponema pallidum strain-specific differences in neuroinvasion and clinical phenotype in a rabbit model. J Infect Dis (2005) 191(1):75–80. doi: 10.1086/426510
42. Tansey C, Zhao C, Hopkins A, Ritter JM, Fakile YF, Pillay A, et al. A nonhuman primate model for rectally transmitted syphilis. J Infect Dis (2018) 217(7):1139–44. doi: 10.1093/infdis/jix669
43. Lu S, Zheng K, Wang J, Xu M, Xie Y, Yuan S, et al. Characterization of treponema pallidum dissemination in C57BL/6 mice. Front Immunol (2021) 11:577129/bibtex. doi: 10.3389/fimmu.2020.577129/bibtex
44. Čejková DC, Zobaníková M, Chen L, Pospíšilová P, Strouhal M, Qin X, et al. Whole genome sequences of three treponema pallidum ssp. pertenue strains: Yaws and syphilis treponemes differ in less than 0.2% of the genome sequence. PloS Negl Trop Dis (2012) 6(1):e1471. doi: 10.1371/journal.pntd.0001471
45. Mikalová L, Strouhal M, Čejková DC, Zobaníková M, Pospíšilová P, Norris SJ, et al. Genome analysis of treponema pallidum subsp. pallidum and subsp. pertenue strains: Most of the genetic differences are localized in six regions. PloS One (2010) 5:15713. doi: 10.1371/journal.pone.0015713
47. Ho EL, Lukehart SA. Syphilis: using modern approaches to understand an old disease. J Clin Invest (2011) 121:4584. doi: 10.1172/jci57173
48. Magnuson HJ, Eagle H, Fleischman R. The minimal infectious inoculum of spirochaeta pallida (Nichols strain) and a consideration of its rate of multiplication in vivo. Am J Syph Gonorrhea Vener Dis (1948) 32(1):1–18.
49. Cumberland MC, Turner TB. The rate of multiplication of treponema pallidum in normal and immune rabbits. Am J Syph Gonorrhea Vener Dis (1949) 33(3):201–12.
50. Brinkman MB, McGill MA, Pettersson J, Rogers A, Matějková P, Šmajs D, et al. A novel treponema pallidum antigen, TP0136, is an outer membrane protein that binds human fibronectin. Infect Immun (2008) 76(5):1848–57. doi: 10.1128/iai.01424-07
51. Ke W, Molini BJ, Lukehart SA, Giacani L. Treponema pallidum subsp. pallidum TP0136 protein is heterogeneous among isolates and binds cellular and plasma fibronectin via its NH 2-terminal end. PLOS Neglected Tropical Diseases (2015) 9(3):. doi: 10.1371/journal.pntd.0003662
52. Parker ML, Houston S, Pětrošová H, Lithgow KV, Hof R, Wetherell C, et al. The structure of treponema pallidum Tp0751 (Pallilysin) reveals a non-canonical lipocalin fold that mediates adhesion to extracellular matrix components and interactions with host cells. PloS Pathog (2016) 12(9):e1005919. doi: 10.1371/journal.ppat.1005919
53. Cameron CE, Brouwer NL, Tisch LM, Kuroiwa JMY. Defining the interaction of the treponema pallidum adhesin Tp0751 with laminin. Infect Immun (2005) 73(11):7485–94. doi: 10.1128/iai.73.11.7485-7494.2005
54. Lee KH, Choi HJ, Lee MG, Lee JB. Virulent treponema pallidum 47 kDa antigen regulates the expression of cell adhesion molecules and binding of T-lymphocytes to cultured human dermal microvascular endothelial cells. Yonsei Med J (2000) 41(5):623–33. doi: 10.3349/ymj.2000.41.5.623
55. Riley BS, Oppenheimer-Marks N, Hansen EJ, Radolf JD, Norgard MV. Virulent treponema pallidum activates human vascular endothelial cells. J Infect Dis (1992) 165(3):484–93. doi: 10.1093/infdis/165.3.484
56. Zhang RL, Zhang JP, Wang QQ. Recombinant treponema pallidum protein Tp0965 activates endothelial cells and increases the permeability of endothelial cell monolayer. PloS One (2014) 9(12):e115134. doi: 10.1371/journal.pone.0115134
57. Houston S, Russell S, Hof R, Roberts AK, Cullen P, Irvine K, et al. The multifunctional role of the pallilysin-associated T reponema pallidum protein, Tp0750, in promoting fibrinolysis and extracellular matrix component degradation. Mol Microbiol (2013) 91(3):618–34. doi: 10.1111/mmi.12482
58. Houston S, Hof R, Francescutti T, Hawkes A, Boulanger MJ, Cameron CE. Bifunctional role of the treponema pallidum extracellular matrix binding adhesin Tp0751. Infect Immun (2011) 79(3):1386–98. doi: 10.1128/iai.01083-10
59. Sell S, Salman J, Norris SJ. Reinfection of chancre-immune rabbits with treponema pallidum i. light and immunofluorescence studies. Am J Pathol (1985) 118(2):248–55.
60. Borenstein LA, Ganz T, Sell S, Lehrer RI, Miller’ AJN. Contribution of rabbit leukocyte defensins to the host response in experimental syphilis. Infect Immun (1991) 59(4):1368–77. doi: 10.1128/iai.59.4.1368-1377.1991
61. Musher DM, Hague-Park M, Gyorkey F, Anderson DC, Baughn RE. The interaction between treponema pallidum and human polymorphonuclear leukocytes. J Infect Dis (1983) 147(1):77–86. doi: 10.1093/infdis/147.1.77
62. Shin JL, Chung KY, Kang JM, Lee TH, Lee MG. The effects of treponema pallidum on human dendritic cells. Yonsei Med J (2004) 45(3):515–22. doi: 10.3349/ymj.2004.45.3.515
63. Bouis DA, Popova TG, Takashima A, Norgard MV. Dendritic cells phagocytose and are activated by treponema pallidum. Infect Immun (2001) 69(1):518–28. doi: 10.1128/iai.69.1.518-528.2001
64. Sell S, Baker-Zander S, Powell HC. Experimental syphilitic orchitis in rabbits: Ultrastructural appearance of treponema pallidum during phagocytosis and dissolution by macrophages in vivo. Lab Invest (1982) 46(4):355–64.
65. Lukehart SA, Baker-Zander SA, Lloyd RMC, Sell S. Characterization of lymphocyte responsiveness in early experimental syphilis. II. nature of cellular infiltration and treponema pallidum distribution in testicular lesions. J Immunol (1980) 124(1):461–7.
66. Sell S, Baker-Zander SA, Lloyd RM. T-Cell hyperplasia of lymphoid tissues of rabbits infected with treponema pallidum: Evidence for a vigorous immune response. Sex Transm Dis (1980) 7(2):74–84. doi: 10.1097/00007435-198004000-00009
67. Leader BT, Godornes C, VanVoorhis WC, Lukehart SA. CD4+ lymphocytes and gamma interferon predominate in local immune responses in early experimental syphilis. Infect Immun (2007) 75(6):3021–6. doi: 10.1128/iai.01973-06
68. Carlson JA, Dabiri G, Cribier B, Sell S, Physician R. The immunopathobiology of syphilis: the manifestations and course of syphilis are determined by the level of delayed-type hypersensitivity. Am J Dermatopathol (2011) 33(5):433–60. doi: 10.1097/dad.0b013e3181e8b587
69. Sykes JA, Kalan J. Intracellular treponema pallidum in cells of a syphilitic lesion of the uterine cervix. Am J Obstet Gynecol (1975) 122(3):361–7. doi: 10.1016/0002-9378(75)90185-4
70. Sykes JA, Miller JN. Intracellular location of treponema pallidum (Nichols strain) in the rabbit testis. Infect Immun (1971) 4(3):307–14. doi: 10.1128/iai.4.3.307-314.1971
71. Sykes JA, Miller JN, Kalan AJ. Treponema pallidum within cells of a primary chancre from a human female. Br J Venereal Dis (1974) 50(1):40–4. doi: 10.1136/sti.50.1.40
72. Van Voorhis WC, Barrett LK, Nasio JM, Plummer FA, Lukehart SA. Lesions of primary and secondary syphilis contain activated cytolytic T cells. Infect Immun (1996) 64(3):1048–50. doi: 10.1128/iai.64.3.1048-1050.1996
73. Moore MW, Cruz AR, LaVake CJ, Marzo AL, Eggers CH, Salazar JC, et al. Phagocytosis of borrelia burgdorferi and treponema pallidum potentiates innate immune activation and induces gamma interferon production. Infect Immun (2007) 75(4):2046–62. doi: 10.1128/iai.01666-06
74. Lukehart SA, Miller JN. Demonstration of the in vitro phagocytosis of treponema pallidum by rabbit peritoneal macrophages. J Immunol (1978) 121(5):2014–24. doi: 10.4049/jimmunol.121.5.2014
75. Baker-Zander SA, Lukehart SA. Macrophage-mediated killing of opsonized treponema pallidum. J Infect Dis (1992) 165(1):69–74. doi: 10.1093/infdis/165.1.69
76. Baker-Zander SA, Shaffer JM, Lukehart SA. Characterization of the serum requirement for macrophage-mediated killing of treponema pallidum ssp. pallidum: Relationship to the development of opsonizing antibodies. FEMS Immunol Med Microbiol (1993) 6(4):273–9. doi: 10.1111/j.1574-695x.1993.tb00339.x
77. Hawley KL, Cruz AR, Benjamin SJ, La Vake CJ, Cervantes JL, LeDoyt M, et al. IFNγ enhances CD64-potentiated phagocytosis of treponema pallidum opsonized with human syphilitic serum by human macrophages. Immunol (2017) 8:1227. doi: 10.3389/fimmu.2017.01227
78. Chen H, Tong ML, Liu LL, Lin LR, Yang TC. The whole process of macrophage-treponema pallidum interactions: Opsonic phagocytosis, nonopsonic phagocytosis and active invasion. Int Immunopharmacol (2022) 107:108657. doi: 10.1016/j.intimp.2022.108657
79. Lin L-R, Liu W, Zhu X-Z, Chen Y-Y, Gao Z-X, Gao K, et al. Treponema pallidum promotes macrophage polarization and activates the NLRP3 inflammasome pathway to induce interleukin-1β production. BMC Immunol (2018) 19(1):28. doi: 10.1186/s12865-018-0265-9
80. Lukehart SA, Baker-Zander SA, Sell S. Characterization of the humoral immune response of the rabbit to antigens of treponema pallidum after experimental infection and therapy. Sex Transm Dis (1986) 13(1):9–15. doi: 10.1097/00007435-198601000-00003
81. Leader BT, Hevner K, Molini BJ, Barrett LK, Van Voorhis WC, Lukehart SA. Antibody responses elicited against the treponema pallidum repeat proteins differ during infection with different isolates of treponema pallidum subsp. pallidum. Infect Immun (2003) 71(10):6054–7. doi: 10.1128/iai.71.10.6054-6057.2003
82. Moskophidis M. Analysis of the humoral immune response to treponema pallidum in the different stages of untreated human syphilis. Zentralblatt fur Bakteriologie (1989) 271(2):171–9. doi: 10.1016/S0934-8840(89)80070-2
83. Fitzgerald TJ. Activation of the classical and alternative pathways of complement by treponema pallidum subsp. pallidum and treponema vincentii. Infection and Immunity (1987) . 55(9):2066–73. doi: 10.1128/iai.55.9.2066-2073.1987
84. Blanco DR, Walker EM, Haake DA, Champion CI, Miller JN, Lovett MA. Complement activation limits the rate of in vitro treponemicidal activity and correlates with antibody-mediated aggregation of treponema pallidum rare outer membrane protein. J Immunol (1990) 144(5):1914–21. doi: 10.4049/jimmunol.144.5.1914
85. Rice M, Fitzgerald TJ. Immune immobilization of treponema pallidum: Antibody and complement interactions revisited. Can J Microbiol (1985) 31(12):1147–51. doi: 10.1139/m85-216
86. Fearon DT. Regulation by membrane sialic acid of β1H-dependent decay-dissociation of amplification C3 convertase of the alternative complement pathway. Proc Natl Acad Sci USA (1978) 75(4):1971–5. doi: 10.1073/pnas.75.4.1971
87. Meri S, Pangburnt MK. Discrimination between activators and nonactivators of the alternative pathway of complement: Regulation via a sialic acid/polyanion binding site on factor h. Proc Natl Acad Sci USA (1990) 87(10):3982–6. doi: 10.1073/pnas.87.10.3982
88. Ram S, Sharma AK, Simpson SD, Gulati S, Mcquillen DP, Pangburn MK, et al. A novel sialic acid binding site on factor h mediates serum resistance of sialylated neisseria gonorrhoeae. J Exp Med (1998) 187(5):743–52:Rice. doi: 10.1084/jem.187.5.743
89. Jarvis GA, Vedros NA. Sialic acid of group b neisseria meningitidis regulates alternative complement pathway activation. Infect Immun (1987) 55(1):174–80. doi: 10.1128/iai.55.1.174-180.1987
90. Sell S, Hsu PL. Delayed hypersensitivity, immune deviation, antigen processing and T-cell subset selection in syphilis pathogenesis and vaccine design. Immunol Today (1993) 14(12):576–82. doi: 10.1016/0167-5699(93)90196-r
91. Bishop NH, Miller JN. Humoral immunity in experimental syphilis. II. the relationship of neutralizing factors in immune serum to acquired resistance. J Immunol (1976) 117(1):197–207.
93. Hanff PA, Bishop NH, Miller JN, Lovett MA. Humoral immune response in experimental syphilis to polypeptides of treponema pallidum. J Immunol (1983) 131(4):1973–7. doi: 10.4049/jimmunol.131.4.1973
94. Arroll TW, Centurion-Lara A, Lukehart SA, Van Voorhis WC. T-Cell responses to treponema pallidum subsp. pallidum antigens during the course of experimental syphilis infection. Infect Immun (1999) 67(9):4757–63. doi: 10.1128/IAI.67.9.4757-4763.1999
95. Sell S, Gamboa D, Baker-Zander SA, Lukehart SA, Miller JN. Host response to treponema pallidum in intradermally-infected rabbits: Evidence for persistence of infection at local and distant sites. J Invest Dermatol (1980) 75(6):470–5. doi: 10.1111/1523-1747.ep12524230
96. Yogeswari L, Chacko CW. Persistence of t. pallidum and its significance in penicillin-treated seropositive late syphilis. Br J Vener Dis (1971) 47(5):339–47. doi: 10.1136/sti.47.5.339
97. Wicher K, Abbruscato F, Wicher V, Collins DN, Auger I, Horowitz HW. Identification of persistent infection in experimental syphilis by PCR. Infect Immun (1998) 66(6):2509–13. doi: 10.1128/IAI.66.6.2509-2513.1998
98. Castro R, Prieto E, Águas MJ, Manata MJ, Botas J, Santo I, et al. Detection of treponema pallidum sp pallidum DNA in latent syphilis. Int J STD AIDS (2007) 18(12):842–5. doi: 10.1258/095646207782716901
99. Engelkens HJ, Ten Kate FJ, Judanarso J, Vuzevski VD, Van Lier JB, Godschalk JC, et al. The localisation of treponemes and characterisation of the inflammatory infiltrate in skin biopsies from patients with primary or secondary syphilis, or early infectious yaws. Genitourin Med (1993) 69(2):102–7. doi: 10.1136/sti.69.2.102
100. Singh R, Teranno D. Unusual presentation of a skin rash. J Clin Pathol (2017) 70(12):1088. doi: 10.1136/jclinpath-2016-204007
101. Alessi E, Innocenti M, Ragusa G. Secondary syphilis. clinical morphology and histopathology. Am J Dermatopathol (1983) 5(1):11–7. doi: 10.1097/00000372-198302000-00004
102. Baker-Zander SA, Roddy RE, Handsfield HH, Lukehart SA. IgG and IgM antibody reactivity to antigens of treponema pallidum after treatment of syphilis. Sex Transm Dis (1986) 13(4):214–20. doi: 10.1097/00007435-198610000-00002
103. Breeze AC. Infectious diseases of the fetus and newborn infant, 6th edn. Arch Dis Child Fetal Neonatal Ed (2007) 92:F156. doi: 10.1136/adc.2006.102566
104. Baughn RE, Jorizzo JL, Adams CB, Musher DM. Ig class and IgG subclass responses to treponema pallidum in patients with syphilis. J Clin Immunol (1988) 8(2):128–39. doi: 10.1007/bf00917901
105. Liu LL, Chao PL, Zhang HL, Tong ML, Liu GL, Lin LR, et al. Analysis of lymphocyte subsets in HIV-negative neurosyphilis patients. Diagn Microbiol Infect Dis (2013) 75(2):165–8. doi: 10.1016/j.diagmicrobio.2012.10.007
106. Podwinska J, Lusiak M, Zaba R, Bowszyc J. The pattern and level of cytokines secreted by Th1 and Th2 lymphocytes of syphilitic patients correlate to the progression of the disease. FEMS Immunol Med Microbiol (2000) 28(1):1–14. doi: 10.1111/j.1574-695x.2000.tb01451.x
107. Miller JN. Immunity in experimental syphilis. VI. successful vaccination of rabbits with treponema pallidum, nichols strain, attenuated by -irradiation. J Immunol (1973) 110(5):1206–15. doi: 10.4049/jimmunol.110.6.np
108. Magnuson HJ, Thomas EW, Olansky S, Kaplan BI, de Mello L, Cutler JC. Inoculation syphilis in human volunteers. Medicine (1956) 35(1):33–82. doi: 10.1097/00005792-195602000-00002
109. Marra CM, Maxwell CL, Sahi SK, Tantalo LC, Dunaway SB, Lukehart SA. Previous syphilis alters the course of subsequent episodes of syphilis. Clin Infect Dis (2022) 74:e1–5. doi: 10.1093/cid/ciab287
110. Cullen PA, Haake DA, Adler B. Outer membrane proteins of pathogenic spirochetes. FEMS Microbiol Rev (2004) 28:291–318. doi: 10.1016/j.femsre.2003.10.004
111. Hawley KL, Montezuma-Rusca JM, Delgado KN, Singh N, Uversky VN, Caimano MJ, et al. Structural modeling of the treponema pallidum outer membrane protein repertoire: A road map for deconvolution of syphilis pathogenesis and development of a syphilis vaccine. J Bacteriol (2021) 203:82–103. doi: 10.1128/JB
112. Molini B, Fernandez MC, Godornes C, Vorobieva A, Lukehart SA, Giacani L. B-cell epitope mapping of TprC and TprD variants of treponema pallidum subspecies informs vaccine development for human treponematoses. Front Immunol (2022) 13:862491/bibtex. doi: 10.3389/fimmu.2022.862491/bibtex
113. Kojima N, Konda KA, Klausner JD. Notes on syphilis vaccine development. Front Immunol (2022) 13:952284. doi: 10.3389/fimmu.2022.952284
114. Romeis E, Tantalo L, Lieberman N, Phung Q, Greninger A, Giacani L. Genetic engineering of treponema pallidum subsp. pallidum, the syphilis spirochete. PloS Pathog (2021) 17(7):e1009612. doi: 10.1371/journal.ppat.1009612
115. Centurion-Lara A, Castro C, Barrett L, Cameron C, Mostowfi M, Van Voorhis WC, et al. Treponema pallidum major sheath protein homologue tpr K is a target of opsonic antibody and the protective immune response. J Exp Med (1999) 189(4):647–56. doi: 10.1084/jem.189.4.647
116. Edwards AM, Jenkinson HF, Woodward MJ, Dymock D. Binding properties and adhesion-mediating regions of the major sheath protein of treponema denticola ATCC 35405. Infect Immun (2005) 73(5):2891–8. doi: 10.1128/iai.73.5.2891-2898.2005
117. Fenno JC, Müller KH, McBride BC. Sequence analysis, expression, and binding activity of recombinant major outer sheath protein (Msp) of treponema denticola. J Bacteriol (1996) 178(9):2489–97. doi: 10.1128/jb.178.9.2489-2497.1996
118. Mathers DA, Leung WK, Fenno JC, Hong Y, McBride BC. The major surface protein complex of treponema denticola depolarizes and induces ion channels in HeLa cell membranes. Infect Immun (1996) 64(8):2904–10. doi: 10.1128/iai.64.8.2904-2910.1996
119. Anand A, Luthra A, Dunham-Ems S, Caimano MJ, Karanian C, LeDoyt M, et al. TprC/D (Tp0117/131), a trimeric, pore-forming rare outer membrane protein of treponema pallidum, has a bipartite domain structure. J Bacteriol (2012) 194(2):2321–33. doi: 10.1128/jb.00101-12
120. Anand A, Ledoyt M, Karanian C, Luthra A, Koszelak-Rosenblum M, Malkowski MG, et al. Bipartite topology of treponema pallidum repeat proteins C/D and I: Outer membrane insertion, trimerization, and porin function require a c-Terminal-Barrel domain. J Biol Chem (2015) 290(19):12313–31. doi: 10.1074/jbc.m114.629188
121. Giacani L, Sambri V, Marangoni A, Cavrini F, Storni E, Donati M, et al. Immunological evaluation and cellular location analysis of the TprI antigen of treponema pallidum subsp. pallidum. Infect Immun (2005) 73(6):3817–22. doi: 10.1128/iai.73.6.3817-3822.2005
122. Giacani L, Molini B, Godornes C, Barrett L, Van Voorhis W, Centurion-Lara A, et al. Quantitative analysis of tpr gene expression in treponema pallidum isolates: Differences among isolates and correlation with T-cell responsiveness in experimental syphilis. Infect Immun (2007) 75(1):104–12. doi: 10.1128/iai.01124-06
123. Giacani L, Godornes C, Puray-Chavez M, Guerra-Giraldez C, Tompa M, Lukehart SA, et al. TP0262 is a modulator of promoter activity of tpr subfamily II genes of treponema pallidum ssp. pallidum. Mol Microbiol (2009) 72(5):1087–99. doi: 10.1111/j.1365-2958.2009.06712.x
124. Giacani L, Lukehart S, Centurion-Lara A. Length of guanosine homopolymeric repeats modulates promoter activity of subfamily II tpr genes of treponema pallidum ssp. pallidum. FEMS Immunol Med Microbiol (2007) 51(2):289–301. doi: 10.1111/j.1574-695X.2007.00303.x
125. Haynes AM, Fernandez M, Romeis E, Mitjà O, Konda KA, Vargas SK, et al. Transcriptional and immunological analysis of the putative outer membrane protein and vaccine candidate TprL of treponema pallidum. PloS Negl Trop Dis (2021) 15(1):e0008812. doi: 10.1371/journal.pntd.0008812
126. Lian T, Zhang B, Giacani L, Kou C, Yang X, Zhang R, et al. Full-length TprK of treponema pallidum subsp. pallidum in lipid nanodiscs is a monomeric porin. Enzyme Microb Technol (2022) 153:109897. doi: 10.1016/j.enzmictec.2021.109897
127. Centurion-Lara A, Godornes C, Castro C, Van Voorhis WC, Lukehart SA. The tprK gene is heterogeneous among treponema pallidum strains and has multiple alleles. Infect Immun (2000) 68(2):824–31. doi: 10.1128/IAI.68.2.824-831.2000
128. Addetia A, Lin MJ, Phung Q, Xie H, Huang ML, Ciccarese G, et al. Estimation of full-length tprk diversity in treponema pallidum subsp. pallidum. mBio (2020) 11(5):1–19. doi: 10.1128/mbio.02726-20
129. Lafond RE, Centurion-lara A, Godornes C, Van Voorhis WC, Lukehart SA. TprK sequence diversity accumulates during infection of rabbits with treponema pallidum subsp. pallidum nichols strain. Infection and Immunity (2006) 74(3):1896–906. doi: 10.1128/iai.74.3.1896
130. Giacani L, Molini BJ, Kim EY, Godornes BC, Leader BT, Tantalo LC, et al. Antigenic variation in treponema pallidum: TprK sequence diversity accumulates in response to immune pressure during experimental syphilis. J Immunol (2010) 184(7):3822–9. doi: 10.4049/jimmunol.0902788
131. Lin MJ, Haynes AM, Addetia A, Lieberman NAP, Phung Q, Xie H, et al. Longitudinal TprK profiling of in vivo and in vitro-propagated treponema pallidum subsp. pallidum reveals accumulation of antigenic variants in absence of immune pressure. PloS Negl Trop Dis (2021) 15(9):e0009753. doi: 10.1371/JOURNAL.PNTD.0009753
132. Morgan CA, Lukehart SA, Van Voorhis WC. Protection against syphilis correlates with specificity of antibodies to the variable regions of treponema pallidum repeat protein K. Infect Immun (2003) 71(10):5605–12. doi: 10.1128/iai.71.10.5605-5612.2003
133. Morgan CA, Molini BJ, Lukehart SA, Van Voorhis WC. Segregation of b and T cell epitopes of treponema pallidum repeat protein K to variable and conserved regions during experimental syphilis infection. J Immunol (2002) 169(2):952–7. doi: 10.4049/jimmunol.169.2.952
134. Morgan CA, Lukehart SA, Van Voorhis WC. Immunization with the n-terminal portion of treponema pallidum repeat protein K attenuates syphilitic lesion development in the rabbit model. Infect Immun (2002) 70(12):6811. doi: 10.1128/IAI.70.12.6811-6816.2002
135. Hazlett KR, Sellati TJ, Nguyen TT, Cox DL, Clawson ML, Caimano MJ, et al. The TprK protein of treponema pallidum is periplasmic and is not a target of opsonic antibody or protective immunity. J Exp Med (2001) 193(9):1015–26. doi: 10.1084/jem.193.9.1015
136. Sun ES, Molini BJ, Barrett LK, Centurion-Lara A, Lukehart SA, Van Voorhis WC. Subfamily I treponema pallidum repeat protein family: sequence variation and immunity. Microbes Infect (2004) 6:725–37. doi: 10.1016/j.micinf.2004.04.001
137. Nandi B, Nandy RK, Sarkar A, Ghose AC. Structural features, properties and regulation of the outer-membrane protein W (OmpW) of vibrio cholerae. Microbiology (2005) 151:2975–86. doi: 10.1099/mic.0.27995-0
138. Hu WS, Li PC, Cheng CY. Correlation between ceftriaxone resistance of salmonella enterica serovar typhimurium and expression of outer membrane proteins OmpW and Ail/OmpX-like protein, which are regulated by BaeR of a two-component system. Antimicrob Agents Chemother (2005) 49(9):3955–8. doi: 10.1128/aac.49.9.3955-3958.2005
139. Hong H, Patel DR, Tamm LK, Van Den Berg B. The outer membrane protein OmpW forms an eight-stranded beta-barrel with a hydrophobic channel. J Biol Chem (2006) 281(11):7568–77. doi: 10.1074/jbc.m512365200
140. Giacani L, Brandt SL, Ke W, Reid TB, Molini BJ, Iverson-Cabral S, et al. Transcription of TP0126, treponema pallidum putative OmpW homolog, is regulated by the length of a homopolymeric guanosine repeat. Infect Immun (2015) 83(6):2275–89. doi: 10.1128/iai.00360-15
141. Haynes AM, Godornes C, Ke W, Giacani L. Evaluation of the protective ability of the treponema pallidum subsp. pallidum Tp0126 OmpW homolog in the rabbit model of syphilis. Infect Immun (2019) 87(8):e00323–19. doi: 10.1128/IAI.00323-19
142. Cox DL, Radolf JD. Insertion of fluorescent fatty acid probes into the outer membranes of the pathogenic spirochaetes treponema pallidum and borrelia burgdorferi. Microbiology (2001) 147:1161–9. doi: 10.1099/00221287-147-5-1161
143. Delgado KN, Montezuma-Rusca JM, Orbe IC, Caimano MJ, La Vake CJ, Luthra A, et al. Extracellular loops of the treponema pallidum FadL orthologs TP0856 and TP0858 elicit IgG antibodies and IgG+-specific b-cells in the rabbit model of experimental syphilis. mBio (2022) 13(4):e0163922. doi: 10.1128/mbio.01639-22
144. Bleuel C, Große C, Taudte N, Scherer J, Wesenberg D, Krauß GJ, et al. TolC is involved in enterobactin efflux across the outer membrane of escherichia coli. J Bacteriol (2005) 187(19):6701–7. doi: 10.1128/jb.187.19.6701-6707.2005
145. Brautigam CA, Deka RK, Ouyang Z, Machius M, Knutsen G, Tomchick DR, et al. Biophysical and bioinformatic analyses implicate the treponema pallidum tp34 lipoprotein (tp0971) in transition metal homeostasis. J Bacteriol (2012) 194(24):6771–81. doi: 10.1128/JB.01494-12
146. Kubanov A, Runina A, Deryabin D. Novel treponema pallidum recombinant antigens for syphilis diagnostics: Current status and future prospects. BioMed Res Int (2017) 2017:1436080. doi: 10.1155/2017/1436080
147. Blanco DR, Champion CI, Exner MM, Shang ES, Skare JT, Hancock REW, et al. Recombinant treponema pallidum rare outer membrane protein 1 (Tromp1) expressed in escherichia coli has porin activity and surface antigenic exposure. J Bacteriol (1996) 178(23):6685–92. doi: 10.1128/jb.178.23.6685-6692.1996
148. Deka RK, Lee YH, Hagman KE, Shevchenko D, Lingwood CA, Hasemann CA, et al. Physicochemical evidence that treponema pallidum TroA is a zinc-containing metalloprotein that lacks porin-like structure. J Bacteriol (1999) 181(14):4420–3. doi: 10.1128/jb.181.14.4420-4423.1999
149. Akins DR, Robinson E, Shevchenko D, Elkins C, Cox DL, Radolf JD. Tromp1, a putative rare outer membrane protein, is anchored by an uncleaved signal sequence to the treponema pallidum cytoplasmic membrane. J Bacteriol (1997) 179(16):5076–86. doi: 10.1128/jb.179.16.5076-5086.1997
150. Hardham JM, Stamm LV, Porcella SF, Frye JG, Barnes NY, Howell JK, et al. Identification and transcriptional analysis of a treponema pallidum operon encoding a putative ABC transport system, an iron-activated repressor protein homolog, and a glycolytic pathway enzyme homolog. Gene (1997) 197(1):47–64. doi: 10.1016/S0378-1119(97)00234-5
151. Xu M, Xie Y, Jiang C, Xiao Y, Kuang X, Zhao F, et al. A novel ELISA using a recombinant outer membrane protein, rTp0663, as the antigen for serological diagnosis of syphilis. Int J Infect Dis (2016) 43:51–7. doi: 10.1016/j.ijid.2015.12.013
152. Xu M, Xie Y, Zheng K, Luo H, Tan M, Zhao F, et al. Two potential syphilis vaccine candidates inhibit dissemination of treponema pallidum. Front Immunol (2021) 12:759474. doi: 10.3389/fimmu.2021.759474
153. Djokic V, Giacani L, Parveen N. Analysis of host cell binding specificity mediated by the Tp0136 adhesin of the syphilis agent treponema pallidum subsp. pallidum. PloS Negl Trop Dis (2019) 13(5):e0007401. doi: 10.1371/journal.pntd.0007401
154. Xu QY, Wang YJ, Lin LR, Liu LL, Yang TC. The outer membrane lipoprotein Tp0136 stimulates human platelet activation and aggregation through PAR1 to enhance Gq/Gi signaling. Front Immunol (2022) 13:818151. doi: 10.3389/fimmu.2022.818151
155. Cameron CE, Brown EL, Kuroiwa JMY, Schnapp LM, Brouwer NL. Treponema pallidum fibronectin-binding proteins. Journal of Bacteriology (2004) 186(20):7019–22. doi: 10.1128/jb.186.20.7019
156. Bamford CV, Francescutti T, Cameron CE, Jenkinson HF, Dymock D. Characterization of a novel family of fibronectin-binding proteins with M23 peptidase domains from treponema denticola. Mol Oral Microbiol (2010) 25(6):369–83. doi: 10.1111/j.2041-1014.2010.00584.x
157. Tomson FL, Conley PG, Norgard MV, Hagman KE. Assessment of cell-surface exposure and vaccinogenic potentials of treponema pallidum candidate outer membrane proteins. Microbes Infect (2007) 9(11):1267–75. doi: 10.1016/j.micinf.2007.05.018
158. Van Voorhis WC, Barrett LK, Lukehart SA, Schmidt B, Schriefer M, Cameron CE. Serodiagnosis of syphilis: Antibodies to recombinant Tp0453, Tp92, and gpd proteins are sensitive and specific indicators of infection by treponema pallidum. J Clin Microbiol (2003) 41(8):3668. doi: 10.1128/jcm.41.8.3668-3674.2003
159. Chan K, Nasereddin T, Alter L, Centurion-Lara A, Giacani L, Parveen N. Treponema pallidum lipoprotein TP0435 expressed in borrelia burgdorferi produces multiple Surface/Periplasmic isoforms and mediates adherence. Sci Rep (2016) 6:1–13. doi: 10.1038/srep25593
160. Cox DL, Akins DR, Porcella SF, Norgard MV, Radolf JD. Treponema pallidum in gel microdroplets: a novel strategy for investigation of treponemal molecular architecture. Mol Microbiol (1995) 15(6):1151–64. doi: 10.1111/j.1365-2958.1995.tb02288.x
161. Brautigam CA, Deka RK, Liu WZ, Norgard MV. Insights into the potential function and membrane organization of the TP0435 (Tp17) lipoprotein from treponema pallidum derived from structural and biophysical analyses. Protein Sci (2015) 24(1):11–9. doi: 10.1002/pro.2576
162. Parveen N, Fernandez MC, Haynes AM, Zhang RL, Godornes BC, Centurion-Lara A, et al. Non-pathogenic borrelia burgdorferi expressing treponema pallidum TprK and Tp0435 antigens as a novel approach to evaluate syphilis vaccine candidates. Vaccine (2019) . 37(13):1807–18. doi: 10.1016/j.vaccine.2019.02.022
163. Cameron CE. Identification of a treponema pallidum laminin-binding protein. Infect Immun (2003) 71(5):2525–33. doi: 10.1128/iai.71.5.2525-2533.2003
164. Dickerson MT, Abney MB, Cameron CE, Knecht M, Bachas LG, Anderson KW. Fibronectin binding to the treponema pallidum adhesin protein fragment rTp0483 on functionalized self-assembled monolayers. Bioconjug Chem (2012) 23(2):184–95. doi: 10.1021/bc200436x
165. Houston S, Taylor JS, Denchev Y, Hof R, Zuerner RL, Cameron CE. Conservation of the host-interacting proteins Tp0750 and pallilysin among treponemes and restriction of proteolytic capacity to treponema pallidum. Infect Immun (2015) 83(11):4204–16. doi: 10.1128/iai.00643-15
166. Luthra A, Montezuma-Rusca JM, la Vake CJ, LeDoyt M, Delgado KN, Davenport TC, et al. Evidence that immunization with TP0751, a bipartite treponema pallidum lipoprotein with an intrinsically disordered region and lipocalin fold, fails to protect in the rabbit model of experimental syphilis. PloS Pathog (2020) 16(9):e1008871. doi: 10.1371/journal.ppat.1008871
167. Kao WCA, Pětrošová H, Ebady R, Lithgow KV, Rojas P, Zhang Y, et al. Identification of Tp0751 (Pallilysin) as a treponema pallidum vascular adhesin by heterologous expression in the Lyme disease spirochete. Sci Rep (2017) 7(1):1–13. doi: 10.1038/s41598-017-01589-4
168. Lithgow KV, Hof R, Wetherell C, Phillips D, Houston S, Cameron CE. A defined syphilis vaccine candidate inhibits dissemination of treponema pallidum subspecies pallidum. Nat Commun (2017) 8(1):1–10. doi: 10.1038/ncomms14273
169. Primus S, Rocha SC, Giacani L, Parveen N. Identification and functional assessment of the first placental adhesin of treponema pallidum that may play critical role in congenital syphilis. Front Microbiol (2020) 11:621654. doi: 10.3389/FMICB.2020.621654
170. Giri TK, Tollefsen DM. Placental dermatan sulfate: isolation, anticoagulant activity, and association with heparin cofactor II. Blood (2006) 107(7):2753. doi: 10.1182/blood-2005-09-3755
171. Suga N, Sugimura M, Koshiishi T, Yorifuji T, Makino S, Takeda S. Heparin/heparan sulfate/CD44-v3 enhances cell migration in term placenta-derived immortalized human trophoblast cells. Biol Reprod (2012) 86(5):134, 1–8. doi: 10.1095/biolreprod.111.093690
172. Lala PK, Nandi P. Mechanisms of trophoblast migration, endometrial angiogenesis in preeclampsia: The role of decorin. Cell Adh Migr (2016) 10(1-2):111–25. doi: 10.1080/19336918.2015.1106669
173. de Oliveira GB, do Vale AM, dos Santos AC, de Moura CEB, Rocha HA de O, de Oliveira MF. Composition and significance of glycosaminoglycans in the uterus and placenta of mammals. Braz Arch Biol Technol (2015) 58(4):512–20. doi: 10.1590/S1516-8913201500281
174. Luthra A, Anand A, Hawley KL, LeDoyt M, La Vake CJ, Caimano MJ, et al. A homology model reveals novel structural features and an immunodominant surface Loop/Opsonic target in the treponema pallidum BamA ortholog TP_0326. J Bacteriol (2015) 197(11):1906–20. doi: 10.1128/jb.00086-15
175. Desrosiers DC, Anand A, Luthra A, Dunham-Ems SM, Ledoyt M, Cummings MAD, et al. TP0326, a treponema pallidum β-barrel assembly machinery a (BamA) orthologue and rare outer membrane protein. Mol Microbiol (2011) 80(6):1496–515. doi: 10.1111/j.1365-2958.2011.07662.x
176. Cameron CE, Lukehart SA, Castro C, Molini B, Godornes C, Van Voorhis WC. Opsonic potential, protective capacity, and sequence conservation of the treponema pallidum subspecies pallidum Tp92. J Infect Dis (2000) 181(4):1401–13. doi: 10.1086/315399
177. Stebeck CE, Shaffer JM, Arroll TW, Lukehart SA, Van Voorhis WC. Identification of the treponema pallidum subsp. pallidum glycerophosphodiester phosphodiesterase homologue. FEMS Microbiol Lett (1997) 154(2):303–10. doi: 10.1111/J.1574-6968.1997.TB12660.X
178. Cameron CE, Castro C, Lukehart SA, Van Voorhis WC. Function and protective capacity of treponema pallidum subsp. pallidum glycerophosphodiester phosphodiesterase. Infection and Immunity (1998) 66(12):5763–70. doi: 10.1128/IAI.66.12.5763-5770.1998
179. Shevchenko DV, Akins DR, Robinson EJ, Li M, Shevchenko OV, Radolf JD. Identification of homologs for thioredoxin, peptidyl prolyl cis-trans isomerase, and glycerophosphodiester phosphodiesterase in outer membrane fractions from treponema pallidum, the syphilis spirochete. Infect Immun (1997) 65(10):4179–89. doi: 10.1128/iai.65.10.4179-4189.1997
180. Shevchenko DV, Sellati TJ, Cox DL, Shevchenko OV, Robinson EJ, Radolf JD. Membrane topology and cellular location of the treponema pallidum glycerophosphodiester phosphodiesterase (GlpQ) ortholog. Infect Immun (1999) 67(5):2266. doi: 10.1128/iai.67.5.2266-2276.1999
181. Zhao F, Wang S, Zhang X, Gu W, Yu J, Liu S, et al. Protective efficacy of a treponema pallidum gpd DNA vaccine vectored by chitosan nanoparticles and fused with interleukin-2. Can J Microbiol (2012) 123:117–23. doi: 10.1139/w11-115
182. Zhang X, Zhao T, Zeng T, Wu N, Xiao Y, Liu S, et al. Intramuscular primary immunization by nucleic acid vaccine pcDNA/Gpd-IL-2 and enhanced immunization with mucosal adjuvant CpG-ODN and gpd-IL-2 recombinant protein effectively induced strong mucosal immune responses and immune protective effects against treponema pallidum skin infection. Exp Ther Med (2018) 15(3):2533. doi: 10.3892/etm.2018.5689
183. Cameron CE, Castro C, Lukehart SA, Van Voorhis WC. Sequence conservation of glycerophosphodiester phosphodiesterase among treponema pallidum strains. Infect Immun (1999) 67(6):3168. doi: 10.1128/iai.67.6.3168-3170.1999
184. Thumiger A, Polenghi A, Papinutto E, Battistutta R, Montecucco C, Zanotti G. Crystal structure of antigen TpF1 from treponema pallidum. Proteins: Structure Funct Genet (2006) 62(3):827–30. doi: 10.1002/prot.20828
185. Radolf JD, Fehniger TE, Silverblatt FJ, Miller JN, Lovett MA. The surface of virulent treponema pallidum: resistance to antibody binding in the absence of complement and surface association of recombinant antigen 4D. Infect Immun (1986) 52(2):579–85. doi: 10.1128/iai.52.2.579-585.1986
186. Babolin C, Amedei A, Ozoliņš D, Žileviča A, D’Elios MM, de Bernard M. TpF1 from treponema pallidum activates inflammasome and promotes the development of regulatory T cells. J Immunol (2011) 187(3):1377–84. doi: 10.4049/jimmunol.1100615
187. Lu D-P, Jia J, Wei S-F, Zhang W-L, Liang R, Liu T, et al. Treponema pallidum (Syphilis) antigen TpF1 induces activation of macrophages and accelerates P2X7R-induced NLRP3-dependent release of IL-1β. Endocr Metab Immune Disord Drug Targets (2022) 22(4):425–32. doi: 10.2174/1871530321666211015091109
188. McGill MA, Edmondson DG, Carroll JA, Cook RG, Orkiszewski RS, Norris SJ. Characterization and serologic analysis of the treponema pallidum proteome. Infect Immun (2010) 78(6):2631–43. doi: 10.1128/iai.00173-10
189. Pozzobon T, Facchinello N, Bossi F, Capitani N, Benagiano M, Di Benedetto G, et al. Treponema pallidum (syphilis) antigen TpF1 induces angiogenesis through the activation of the IL-8 pathway. Sci Rep (2016) 6:1–14. doi: 10.1038/srep18785
190. Cohen SE, Chew Ng RA, Katz KA, Bernstein KT, Samuel MC, Kerndt PR, et al. Repeat syphilis among men who have sex with men in California 2002-2006: Implications for syphilis elimination efforts. Am J Public Health (2012) 102(1):2002–6. doi: 10.2105/ajph.2011.300383
191. de Almeida VC, Donalisio MR, Cordeiro R. Factors associated with reinfection of syphilis in reference centers for sexually transmitted infections. Rev Saude Publica (2017) 51:64. doi: 10.1590/S1518-8787.2017051006432
192. Jain J, Santos GM, Scheer S, Gibson S, Crouch PC, Kohn R, et al. Rates and correlates of syphilis reinfection in men who have sex with men. LGBT Health (2017) 4(3):232–6. doi: 10.1089/lgbt.2016.0095
193. Van der Ende A, Hopman CTP, Zaat S, Essink BBO, Berkhout B, Dankert J. Variable expression of class 1 outer membrane protein in neisseria meningitidis is caused by variation in the spacing between the -10 and -35 regions of the promoter. J Bacteriol (1995) 177(9):2475–80. doi: 10.1128/jb.177.9.2475-2480.1995
194. Saunders NJ, Peden JF, Hood DW, Moxon ER. Simple sequence repeats in the helicobacter pylori genome. Mol Microbiol (1998) 27(6):1091–8. doi: 10.1046/j.1365-2958.1998.00768.x
195. Van Der Woude MW, Bäumler AJ. Phase and antigenic variation in bacteria. Clin Microbiol Rev (2004) 17(3):581–611. doi: 10.1128/cmr.17.3.581-611.2004
196. Osbak KK, Houston S, Lithgow KV, Meehan CJ, Strouhal M, Šmajs D, et al. Characterizing the syphilis-causing treponema pallidum ssp. pallidum proteome using complementary mass spectrometry. PloS Negl Trop Dis (2016) 10(9):e0004988. doi: 10.1371/journal.pntd.0004988
197. Bonifield HR, Hughes KT. Flagellar phase variation in salmonella enterica is mediated by a posttranscriptional control mechanism. J Bacteriol (2003) 185(12):3567–74. doi: 10.1128/jb.185.12.3567-3574.2003
198. Mouquet H, Scheid JF, Zoller MJ, Krogsgaard M, Ott RG, Shukair S, et al. Polyreactivity increases the apparent affinity of anti-HIV antibodies by heteroligation. Nature (2010) 467(7315):591–5. doi: 10.1038/nature09385
199. Wardemann H, Yurasov S, Schaefer A, Young JW, Meffre E, Nussenzweig MC. Predominant autoantibody production by early human b cell precursors. Science (2003) 301(5638):1374–7. doi: 10.1126/science.1086907
200. Birge RB, Boeltz S, Kumar S, Carlson J, Wanderley J, Calianese D, et al. Phosphatidylserine is a global immunosuppressive signal in efferocytosis, infectious disease, and cancer. Cell Death Differentiation (2016) 23(6):962–78. doi: 10.1038/cdd.2016.11
201. Treede I, Braun A, Sparla R, Kühnel M, Giese T, Turner JR, et al. Anti-inflammatory effects of phosphatidylcholine. J Biol Chem (2007) 282(37):27155–64. doi: 10.1074/jbc.m704408200
202. Macek B, Forchhammer K, Hardouin J, Weber-Ban E, Grangeasse C, Mijakovic I. Protein post-translational modifications in bacteria. Nat Rev Microbiol (2019) 17(11):651–64. doi: 10.1038/s41579-019-0243-0
203. Sobocińska J, Roszczenko-Jasińska P, Ciesielska A, Kwiatkowska K. Protein palmitoylation and its role in bacterial and viral infections. Front Immunol (2018) 8:2003. doi: 10.3389/fimmu.2017.02003
204. Kumar S, Caimano MJ, Anand A, Dey A, Hawley KL, LeDoyt ME, et al. Sequence variation of rare outer membrane protein β-barrel domains in clinical strains provides insights into the evolution of treponema pallidum subsp. pallidum , the syphilis spirochete. mBio (2018) 9(3):e01006–18. doi: 10.1128/mBio.01006-18
205. Centurion-Lara A, Giacani L, Godornes C, Molini BJ, Brinck Reid T, Lukehart SA. Fine analysis of genetic diversity of the tpr gene family among treponemal species, subspecies and strains. PloS Negl Trop Dis (2013) 7(5):e2222. doi: 10.1371/journal.pntd.0002222
206. Hawley K, Luthra A, La VC, Ledoyt M, Chen W, Heping Z, et al. O01.2 genetic, structural, and surface antigenic variation of treponema pallidum’s OMPeome: steps towards a global syphilis vaccine. Sex Transm Infect (2019) 95:A37–7. doi: 10.1136/sextrans-2019-sti.105
207. Grillová L, Oppelt J, Mikalová L, Nováková M, Giacani L, Niesnerová A, et al. Directly sequenced genomes of contemporary strains of syphilis reveal recombination-driven diversity in genes encoding predicted surface-exposed antigens. Front Microbiol (2019) 10:1691. doi: 10.3389/fmicb.2019.01691
208. Lieberman NAP, Lin MJ, Xie HI, Shrestha L, Nguyen T, Huang M-L, et al. Treponema pallidum genome sequencing from six continents reveals variability in vaccine candidate genes and dominance of nichols clade strains in Madagascar. PloS Negl Trop Dis (2021) 15(12):e0010063. doi: 10.1371/journal.pntd.0010063
209. Ovcinnikov NM, Delektorskij VV. Treponema pallidum in nerve fibres. Br J Venereal Dis (1975) 51(1):10. doi: 10.1136/sti.51.1.10
210. Sell S, Salman J. Demonstration of treponema pallidum in axons of cutaneous nerves in experimental chancres of rabbits. Sex Transm Dis (1992) 19(1):1–6. doi: 10.1097/00007435-199201000-00001
211. Medici MA. The immunoprotective niche–a new pathogenic mechanism for syphilis, the systemic mycoses and other infectious diseases. J Theor Biol (1972) 36(3):617–25. doi: 10.1016/0022-5193(72)90012-4
212. Kronvall G, Simmons A, Myhre EB, Jonsson S. Specific absorption of human serum albumin, immunoglobulin a, and immunoglobulin G with selected strains of group a and G streptococci. Infect Immun (1979) 25(1):1–10. doi: 10.1128/iai.25.1.1-10.1979
213. Alderete JF, Baseman JB. Surface-associated host proteins on virulent treponema pallidum. Infect Immun (1979) 26(3):1048–56. doi: 10.1128/iai.26.3.1048-1056.1979
214. Egesten A, Frick IM, Mörgelin M, Olin AI, Björck L. Binding of albumin promotes bacterial survival at the epithelial surface. J Biol Chem (2011) 286(4):2469. doi: 10.1074/jbc.m110.148171
215. Champion CI, Miller JN, Borenstein LA, Lovett MA, Blanco DR. Immunization with treponema pallidum endoflagella alters the course of experimental rabbit syphilis. Infect Immun (1990) 58(9):3158–61. doi: 10.1128/iai.58.9.3158-3161.1990
216. Zheng K, Xu M, Xiao Y, Luo H, Xie Y, Yu J, et al. Immunogenicity and protective efficacy against treponema pallidum in new Zealand rabbits immunized with plasmid DNA encoding flagellin. Emerg Microbes Infect (2018) 7(1):177. doi: 10.1038/s41426-018-0176-0
217. Nimmerjahn F, Ravetch JV. Fcγ receptors as regulators of immune responses. Nat Rev Immunol (2008) 8(1):34–47. doi: 10.1038/nri2206
218. Lukehart SA, Molini B, Gomez A, Godornes C, Hof R, Fernandez MC, et al. Immunization with a tri-antigen syphilis vaccine significantly attenuates chancre development, reduces bacterial load, and inhibits dissemination of treponema pallidum. Vaccine (2022) 40(52):7676–92. doi: 10.1016/J.vaccine.2022.11.002
219. Haake DA, Mazel MK, Mccoy AM, Milward F, Chao G, Matsunaga J, et al. Leptospiral outer membrane proteins OmpL1 and LipL41 exhibit synergistic immunoprotection. Infect Immun (1999) 67(12):6572–82. doi: 10.1128/iai.67.12.6572-6582.1999
220. Edmondson DG, Norris SJ. In vitro cultivation of the syphilis spirochete treponema pallidum. Curr Protoc (2021) 1(2):e44. doi: 10.1002/cpz1.44
Keywords: syphilis, treponema pallidum, vaccine, immune response, outer membrane proteins
Citation: Ávila-Nieto C, Pedreño-López N, Mitjà O, Clotet B, Blanco J and Carrillo J (2023) Syphilis vaccine: challenges, controversies and opportunities. Front. Immunol. 14:1126170. doi: 10.3389/fimmu.2023.1126170
Received: 17 December 2022; Accepted: 27 March 2023;
Published: 06 April 2023.
Edited by:
Catherine Mary O’Connell, University of North Carolina at Chapel Hill, United StatesReviewed by:
Elizabeth De Gaspari, Adolfo Lutz Institute, BrazilKelly Lynn Hawley, University of Connecticut Health Center, United States
Copyright © 2023 Ávila-Nieto, Pedreño-López, Mitjà, Clotet, Blanco and Carrillo. This is an open-access article distributed under the terms of the Creative Commons Attribution License (CC BY). The use, distribution or reproduction in other forums is permitted, provided the original author(s) and the copyright owner(s) are credited and that the original publication in this journal is cited, in accordance with accepted academic practice. No use, distribution or reproduction is permitted which does not comply with these terms.
*Correspondence: Jorge Carrillo, amNhcnJpbGxvQGlyc2ljYWl4YS5lcw==; Carlos Ávila-Nieto, Y2F2aWxhQGlyc2ljYWl4YS5lcw==