- 1Department of Plastic and Reconstructive Surgery, Shanghai Ninth People’s Hospital, Shanghai Jiao Tong University School of Medicine, Shanghai, China
- 2Key Laboratory of Tissue Microenvironment and Tumor, Shanghai Institute of Nutrition and Health, University of Chinese Academy of Sciences, Chinese Academy of Sciences, Shanghai, China
Adipose tissue is a widely distributed organ that plays a critical role in age-related physiological dysfunctions as an important source of chronic sterile low-grade inflammation. Adipose tissue undergoes diverse changes during aging, including fat depot redistribution, brown and beige fat decrease, functional decline of adipose progenitor and stem cells, senescent cell accumulation, and immune cell dysregulation. Specifically, inflammaging is common in aged adipose tissue. Adipose tissue inflammaging reduces adipose plasticity and pathologically contributes to adipocyte hypertrophy, fibrosis, and ultimately, adipose tissue dysfunction. Adipose tissue inflammaging also contributes to age-related diseases, such as diabetes, cardiovascular disease and cancer. There is an increased infiltration of immune cells into adipose tissue, and these infiltrating immune cells secrete proinflammatory cytokines and chemokines. Several important molecular and signaling pathways mediate the process, including JAK/STAT, NFκB and JNK, etc. The roles of immune cells in aging adipose tissue are complex, and the underlying mechanisms remain largely unclear. In this review, we summarize the consequences and causes of inflammaging in adipose tissue. We further outline the cellular/molecular mechanisms of adipose tissue inflammaging and propose potential therapeutic targets to alleviate age-related problems.
1 Background
Adipose tissue is a widespread organ roughly divided into white adipose tissue (WAT) and brown adipose tissue (BAT) (1). In addition, beige adipocytes are known to differentiate from progenitors resident in WAT while exhibiting BAT-like morphology and function (1). According to the depots, WAT can also be categorized into subcutaneous adipose tissue (SAT) and visceral adipose tissue (VAT). SAT is located beneath the skin, while VAT surrounds internal organs and is usually found in the mesentery and omentum (2). The function of adipose tissue, including both SAT or VAT, is mainly to store energy, regulate temperature, modulate immune responses, facilitate wound healing and promote tissue regeneration (2).
Aging is considered to be associated with an increasing prevalence of obesity, type 2 diabetes, and other comorbidities (3). These age-related diseases are usually related to adipose tissue, which not only mediates an organism’s adaptation and response to aging but also plays a pivotal role in age-related metabolic dysfunction and longevity (4).
Aging adipose tissue has several characteristics. First, the ratio of VAT to SAT is increased in aged individuals (5). Second, brown and beige fat are reduced during the aging process (3). Third, the function of adipose stem cells and progenitor cells is decreased (6). Fourth, there is an accumulation of senescent cells in aging adipose tissue (7). Finally, but most importantly, aging adipose tissue is linked to a chronic, low-grade inflammation termed inflammaging, which is a central characteristic as it may promote other aging characteristics and influence the overall health status. The thermogenic capability of brown adipose tissue is compromised by proinflammatory cytokines, which may suppress the uncoupled activity of protein-1 (UCP-1) (8). Proinflammatory cytokines also compromise the adipogenic capacity of adipose stem cells (9). Senescent cells promote an inflammatory environment, while proinflammatory cytokines also promote senescent cells (10). Adipose tissue inflammaging is related to an increased body mass, elevated adipocyte size, emerging fragile states and chronic degenerative disorders (11). Therefore, inflammation in adipose tissue may be a potential therapeutic target in antiaging therapy.
The progenitor cell decline phenomenon is observed mainly in aging WAT (3). Similarly, adipokine changes are only observed in aging WAT rather than BAT (3). Briefly, WAT has a more dramatic response to aging than BAT. Thus, we emphasize WAT inflammaging.
In this review, we first discuss the impact of inflammaging on aging adipose tissue and the overall health status and then reveal the factors contributing to adipose tissue inflammation. Furthermore, we frame the alteration in immune cells in aging adipose tissue and the underlying molecular mechanism of inflammaging. Finally, we summarize a potential strategy for antiaging therapy through adipose tissue.
2 The impact of inflammaging on adipose tissue
2.1 Adipose plasticity
During the process of inflammaging, the plasticity and function of ADSCs are regulated by specific factors. Taha et al. cultured ADSCs treated with TNFα to trigger a strong inflammatory response, and then, deep next-generation mRNA sequencing was performed to evaluate the inflammatory responses of the ADSCs (12). The results showed that the ADSCs exhibited a strong response when exposed to an inflammatory environment. Adipogenesis is also reduced by inflammaging. Liu et al. showed that after the deletion of proinflammatory macrophages in SAT, the differentiation of preadipocytes was upregulated, and the expression of differentiation genes was increased (9). Inflammaging is strongly related to hypoxia (13). Chol et al. found that ADSCs cultured under low-oxygen conditions exhibited a higher proliferative ability, significantly higher basal migration, and reduced lipid production. ADSCs maintain an undifferentiated status in a hypoxic environment, and their potential to differentiate into adipocytes is decreased (14).
2.2 Adipocyte remodeling
Adipose tissue inflammaging, as a consequence of proinflammatory immune cell infiltration, may disrupt the recruitment of new adipocytes, leading to adipocyte hypertrophy (15). Adipocyte hypertrophy is closely related to metabolic diseases. Initially, adipocyte hypertrophy is an adaptive reaction to excessive nutrition, which is beneficial in lean objects as it can protect tissues other than adipose tissue from lipotoxicity. However, in some obese or aging patients, the adipocyte buffering ability may be exceeded, reaching the hypertrophic threshold, leading to ectopic lipid deposition in other tissues (16). Adipocyte hypertrophy further exacerbates adipose tissue hypoxia and inflammation, leading to adipose tissue fibrosis and adipocyte apoptosis.
2.3 Adipose tissue fibrosis
In aging adipose tissue, the insufficient angiogenic potential, inappropriate ECM remodeling and unresolved inflammation result in adipose tissue fibrosis. An insufficient angiogenic potential leads to hypoxia, which stimulates the transcription of HIF1α (5, 17). On the one hand, the activation of HIF1α inhibits preadipocyte differentiation and initiates adipose tissue fibrosis. On the other hand, HIF1α may induce a change in the cellular redox status, which, in turn, affects enzymes involved in collagen crosslinking and stabilization (18). Fibrosis is characterized by an imbalance in ECM homeostasis, including the balance between ECM production and ECM degradation (19). Studies in aged mice (~30 months of age) demonstrated an increase in WAT collagen staining, indicating more fibrosis in this tissue (20). Two cells play important roles in ECM production, M1-type macrophages and mast cells. Macrophage-inducible C-type lectin (Mincle) production is induced in macrophages through the saturated fatty acid/TLR4/NF-κB pathway and contributes to ECM production. Mast cells can promote fibroblast growth and collagen production by releasing cytokines, chemokines, proteases, etc., eventually leading to ECM production (21, 22). Fibroblasts and macrophages are the primary cell types that mediate collagen internalization and degradation. Studies have shown that an increase in proinflammatory cytokines is linked to the downregulation of metalloproteinase (MMP) expression. MMPs have the ability to cleave ECM components; thus, the increase in proinflammatory cytokines inhibits ECM degradation (21, 22). The size of both VAT and SAT is reduced in aged animals, suggesting that senescence affects lipid processing in adipose tissue, promoting ectopic lipid accumulation.
2.4 Ectopic lipid accumulation
Inflammaging caused by proinflammatory immune cells largely damages the function of adipose tissue and eventually leads to adipose tissue fibrosis. As the function and composition of VAT and SAT are affected, ectopic lipid storage is promoted (23). When the dietary buffer cannot be addressed by senescent adipose tissue, lipotoxicity mediated by the ectopic deposition of lipids occurs in the liver and skeletal muscle. Lipotoxicity in these tissues increases the ROS levels and activates serine threonine kinases, such as c-jun N-terminal kinase (JNK), IκB kinase (IKK), and protein kinase C (PKC). These events not only disrupt insulin receptor signaling cascades and promote insulin resistance but also are associated with the development of hepatic steatosis and muscle dysfunction and may trigger the development of sarcopenia (24).
3 The impact of adipose tissue inflammaging on the overall health status
3.1 Metabolic diseases
Aging-induced proinflammatory cytokines can directly interfere with the insulin signaling pathway in adipocytes (25). In addition, NLRP3 activated by DAMPs mediates chronic inflammation and insulin resistance. The activation of NLRP3 contributes to a higher expression of IL-1β, and IL-1β is a key cytokine in the etiology of type 2 diabetes. Briefly, IL-1β can affect insulin signaling, reduce glucose transporter type 4 (GLUT4) expression, and have proapoptotic effects on β-cells (mediated by the MAPK and NF-κB signaling pathways) (26).
3.2 Cardiovascular disease
Adipose tissue can act as an important source of inflammatory mediators, thus promoting systemic inflammaging (27). Chronic inflammation significantly increases the risk of CVD. The proinflammatory cytokines released by inflamed adipose tissue may force perivascular adipose tissue to modify its composition and accelerate atherosclerosis (28). Adipose tissue-derived proinflammatory cytokines, such as IL-1β and TNF, induce the expression of endothelial cell adhesion molecules, which further promote vascular inflammation (29). Exosomes derived from inflamed VAT have been shown to promote the M1 proinflammatory polarization of macrophages and promote atherosclerosis (30).
3.3 Cancer
Cancer can arise at a site of inflammation, and a proinflammatory microenvironment is an essential component of cancer. Chronic inflammation can initiate cancer, promote its progression and support its metastatic diffusion (31). Adipose tissue inflammation may also be the driver of cancer (32).
4 Factors contributing to adipose tissue inflammaging
4.1 Senescent cell accumulation and cell death
A central reason for adipose tissue dysfunction and inflammation during aging is the accumulation of senescent cells. Cellular senescence is a basic aging mechanism that results in organ dysfunction and chronic inflammation (33). Upregulated ROS are the main drivers of adipose tissue senescence. Following ROS upregulation, the DNA damage response (DDR) triggers the p53/p21 signaling pathway, followed by the consequent promotion of the senescence phenotype along with exacerbated TNF-α/IL-6 secretion and β-galactosidase activities. Such DNA injury can eventually pave the path for ATM/p53/p21 upregulation (34). Senescent cells can release several proinflammatory cytokines, which are currently considered hallmark components of the SASP (35). These proinflammatory factors continue to accumulate in tissues as the clearance of senescent cells is compromised during aging (4).
Key essential cells within adipose tissue develop the senescence phenotype. Adipose-derived stem cells (ADSCs) gradually lose the ability to replicate before entering cellular senescence, a state characterized by the upregulation of senescence markers, such as p16INK4a, p21Waf1 and caveolin-1 (36). p16INK4a is upregulated through p38 MAPK influence, possibly contributing to cellular senescence. In addition, p53 MAPK is involved in age-related ADSC functional transformation. Its activation impairs mitochondrial function. Mitochondrial activities are essential players in maintaining stem cell pluripotency (37). Additionally, ADSCs expand in dimensions, morphology and structural complexity along with the decreased expression of CD105 during the natural aging process (38). Cellular senescence of preadipocytes can progress to widespread shifts in preadipocyte function, such as reduced proliferation, adipogenesis, and exacerbated production of proinflammatory cytokines and extracellular matrix–modifying proteases (39). Senescent preadipocytes also negatively influence adipogenicity within surrounding progenitors and induce them to senesce (34). Cellular senescence is also linked to downregulated PPARγ and the triggering of downstream targets in endothelial cells, suggesting that capabilities, such as responding to fatty acids and promoting lipid transport, are significantly diminished. Moreover, p53 protein upregulation typically occurs in endothelial cells. Senescent endothelial cells lose the capability to discharge endogenous lipid PPARγ ligands, consequently leading to detrimental influences on the differentiation and function of human adipocytes (34). Adipocyte senescence is also induced by elevated DNA damage (40). In addition to adipocyte senescence, adipocyte death occurs upon obesity or during aging (41, 42). Dying adipocytes can attract macrophages, and these macrophages produce various types of cytokines according to the type of cell death. Apoptotic cells establish the production of anti-inflammatory cytokines, whereas necrotic cells establish proinflammatory cytokine production characterized by the secretion of IL-1 by the macrophage population (43). The NOD-like receptor (NLR) family of pattern recognition receptors (PRRs) can sense obesity- or aging-induced signals, such as damage-associated molecular patterns (DAMPs), originating from stressed adipocytes. In macrophages, the activation of NLR activates the NLRP3 inflammasome (44). The inflammasome consists of a multiprotein intracellular complex that develops as a stress-triggering response, leading to the secretion of the proinflammatory cytokines IL-1β and IL-18 (45). IL-1β upregulates IL-2 and TNFα, generating tissue inflammatory activities by triggering cyclooxygenase-2, consequently generating prostaglandin E2, inducible intercellular adhesion molecules and NO (45).
4.2 Hypoxia, mechanical stress and obesity caused by adipocyte hypertrophy
Aging leads to adipocyte hypertrophy, even within a single white adipose tissue (WAT) depot, and the cell diameters of different adipocytes can dramatically vary, ranging from less than 20 µm to 300 μm (46). An increased adipocyte size results in decreased oxygen diffusion. In addition, the blood supply to adipocytes is reduced during aging (5). These two factors generate a hypoxic environment in aged adipose tissue, which has been shown to occur with aging as revealed by immunohistochemistry and direct measurements of the interstitial partial pressure of oxygen (47). Hypoxia may induce a reaction mediated by hypoxia-inducible factors (HIFs) to promote angiogenesis and complement oxygen levels (17). However, as the angiogenic potential of ADSCs declines and the expression of VEGF and the density of blood vessels decrease with aging, compensation from the vasculature becomes inadequate. As a result, the reduced oxygen diffusion is aggravated due to insufficient compensation by the vasculature (48).
The expression of HIFs is usually induced by hypoxia, and HIF isoforms have defined, nonredundant functions concerning adipocyte function. Even though HIF-2α expression within adipocytes is beneficial since it exerts key senescence prophylaxis-related metabolic effects, including enhanced vascularization possibilities (49), HIF-1α could perform opposing functions. HIF-1α triggering did not activate typical VEGFα-vascularization responses; rather, HIF-1α induced a collagen-driven profibrotic response that paved the path for maladaptive adipose tissue remodeling and insulin resistance (49). HIF-1α may also reduce the expression of genes in mitochondrial complex IV such that the reduced mitochondrial activity contributes to adipocyte hypertrophy. A previous study showed that the knockout of HIF-1α improved mitochondrial function and reduced adipocyte hypertrophy in middle-aged mice (5). Studies have shown that cultivating adipose tissue in a hypoxic environment induces alterations in gene expression, including the upregulation of inflammation-related genes (50). Furthermore, evidence suggests that the NF-κB signaling pathway is enhanced in hypoxic adipose tissue (13).
Mechanical stress also induces inflammation in adipose tissue. As adipocyte hypertrophy is facilitated by aging, the pathological expansion of the extracellular matrix (ECM) has also been observed, leading to altered mechanical stress (51). Furthermore, mechanical stress is exerted by enlarged lipid droplets within the cell (52). Although the pathways controlled by mechanical stress in adipocytes have not been elucidated, the NFκB signaling pathway may be influenced by mechanical stress via the RhoA-Rock signaling pathway (53).
As a metabolic syndrome, obesity frequently develops during old age and is a critical factor associated with adipose tissue inflammaging. Typically, obesity is mediated by adipocyte hypertrophy or hyperplasia. Adipocyte hyperplasia is more metabolically friendly than adipocyte hypertrophy. Adipocyte hypertrophy caused by obesity can result in a hypoxic environment, leading to adipose tissue inflammation. Multiple studies have shown that PDGFα+CD9low proadipogenic adipose progenitor cells (APCs) switch to PDGFα+CD9high profibrotic progenitor cells when influenced by inflammation, ultimately promoting adipose tissue fibrosis and reducing adipocyte hyperplasia (54). The increased adipocyte hypertrophy with reduced adipocyte hyperplasia contributes to adipose tissue inflammation through aging (55). However, obesity may further worsen immunosenescence by enabling the activation and differentiation of immune cells passing through the adipose tissue microvasculature. Previous research has shown that adipose tissue dysfunction in obesity enables immunological aging along with excessive inflammatory responses (56).
4.3 Exogenous and endogenous fatty acids and exogenous lipopolysaccharide
Exogenous and endogenous fatty acids and exogenous lipopolysaccharide (LPS) are capable of inducing inflammation in adipose tissue through the activation of toll-like receptors (TLRs) expressed in both adipocytes and macrophages (57). Gut-derived LPS binds TLR4, while free fatty acids (FFAs) can activate inflammatory signaling through either TLR4 or TLR2 (58).
4.4 Dysregulation of immune cells in aging adipose tissue
Immunosenescence, which results in a defective immune response to pathogens and is often coupled with excessive inflammatory activities, is also found in aging adipose tissue. In particular, VAT shows immune cell activation and inflammation compared to other tissues (59). The abnormal activation of immune cells was first detected in WAT depots in middle age and is considered a hallmark of aging (32). In old age, the adipose immune system shifts toward being more unregulated. At this age, various resident regulatory cell populations are dwindling and substituted by inflammatory cells due to phenotypic switching in resident adipose immune cells or the infiltration of inflammatory immune cells from the periphery (60). Prior studies have mainly focused on macrophages, ILCs and eosinophils in adipose tissue. Among these cell types, M2-like macrophages tend to be lost in aged adipose tissue (61). Moreover, there are differential age-related changes between SAT and VAT. For example, the number of eosinophils is substantially reduced in elderly VAT but remains unaffected in SAT (62). Other changes in immune cells are also discussed in this review (Figure 1).
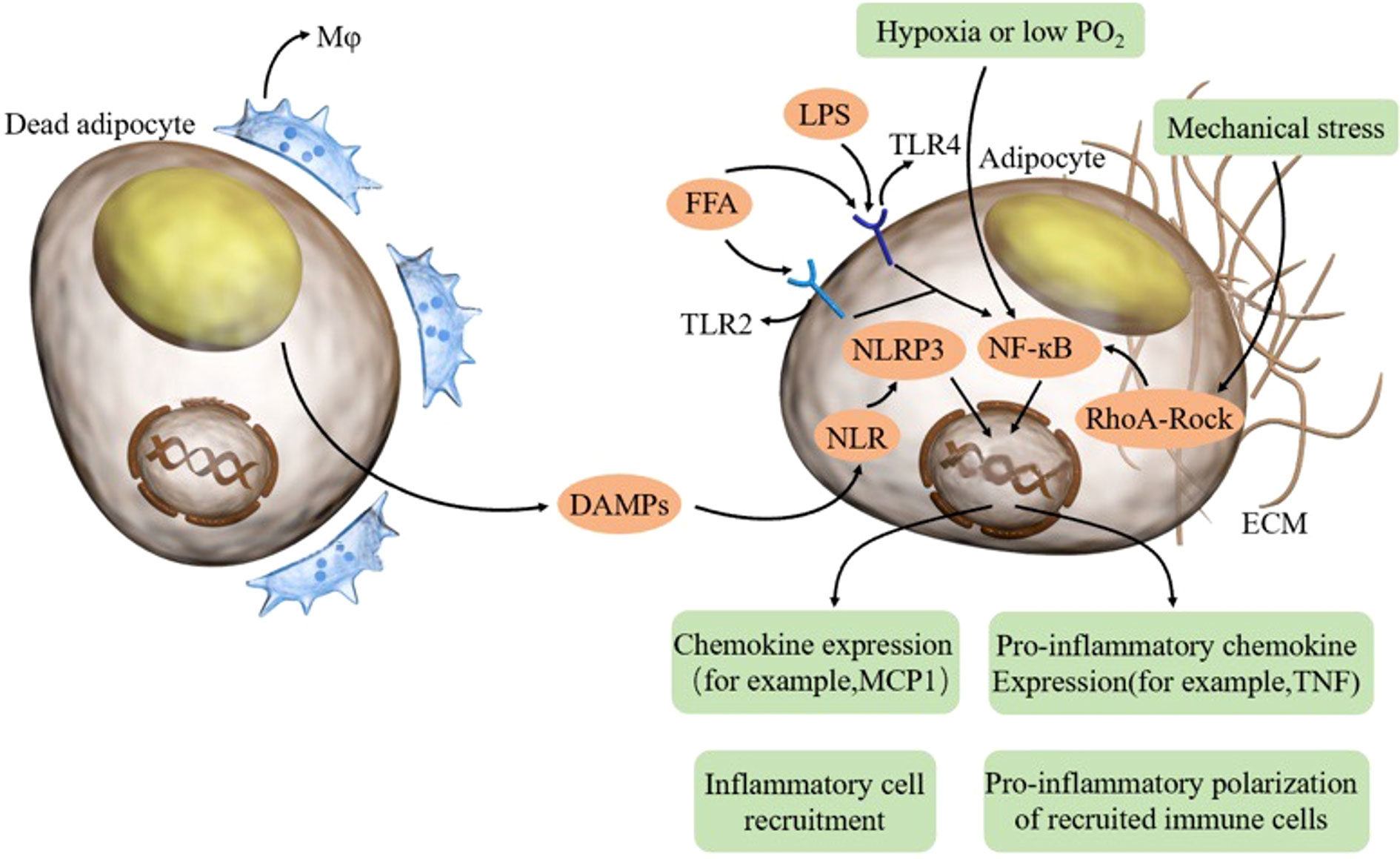
Figure 1 In aging adipose tissue, dead adipocytes induce macrophages and DAMPs, thereby activating the NLRP3 inflammasome in adipocytes. FFA and LPS combine TLR on adipocytes and activate NFκB. Hypoxia and mechanical stress can also activate NFκB. Adipocytes can produce proinflammatory cytokines and chemokines, thus recruiting inflammatory cells and promoting the proinflammatory polarization of recruited immune cells. FFA free fatty acid, LPS lipopolysaccharide, TLR toll-like receptor, DAMP damage-associated molecular patterns, NLR NOD-like receptor.
5 The cellular mechanisms mediating adipose tissue inflammaging
5.1 Proinflammatory adipose tissue resident immune cells
5.1.1 Innate immune cells
M1-type macrophages release proinflammatory cytokines, such as TNF-α and IL-1β, to promote adipose tissue inflammation (63, 64). Monocyte chemotactic protein-1 (MCP-1) recruits circulating monocytes to adipose tissue, where they become adipose tissue macrophages (ATMs). LTB4 also promotes macrophage activation and chemotaxis into adipose tissue (65).
An increase in M1-type macrophages and a decrease in M2-type macrophages are observed in aged adipose tissue (66). Inositol-requiring enzyme 1α (IRE1α) induces M1-type macrophage polarization while reducing polarization in M2-type macrophages (67). Similarly, the IRE1α signaling pathway impairs white adipose tissue browning, leading to the development of obesity during aging (68). Further studies have shown that glucose may activate M1 macrophages via the ROCK/JNK and ROCK/ERK pathways (69). The Notch 1 signaling pathway is important for M1 polarization and is negatively regulated by the microRNA miR-30 (70). In addition, endoplasmic reticulum (ER) stress signaling helps promote M1 polarization. CHOP, one of its downstream components, is induced by a high-fat diet (71).
Many important signaling pathways are involved in macrophage-induced adipose tissue inflammation. Long-chain saturated fatty acids induce macrophages to produce an inflammatory response through the activation of the JNK signaling pathway (61). TLR signaling is important for macrophage-induced inflammation, acting in conjunction with the Wnt signaling pathway to amplify the release of proinflammatory cytokines (72). Moreover, TLR4 induces the NLRP3 inflammasome in macrophages (73).
Senescent macrophages display increased JNK phosphorylation. The JNK signaling pathway contributes to inflammation and plays a key role in reshaping the metabolic status. Moreover, senescent macrophages show a reduction in SIRT1 expression (74). P38MAPK signaling is increased in senescent macrophages, which is promoted by Arginase-II (Arg-II). In turn, Arg-II reduces Arg-I expression and activity, induces interleukin (IL)-6 expression and secretion, and increases active P38MAPK in aging senescent adipose tissue macrophages (75).
Approximately 80-90% of dendritic cells in adipose tissue are CD11c+ conventional DCs (cDCs), and the other dendritic cells are CD123+ plasmacytoid DCs (pDCs) (76). cDCs are further subdivided into cDC1s and cDC2s (77). cDC1s are characterized by an activated Wnt/β-catenin pathway, whereas cDC2s show activation of the PPARγ pathway, which suppresses NFκB target genes and leads to the reduced expression of inflammatory genes (61, 78, 79). In a homeostatic state in young and lean subjects, adipocytes can activate the Wnt/β-catenin pathway in cDC1s, leading to the production of the anti-inflammatory cytokine IL-10 (80). In addition, dietary lipids secreted by adipocytes can induce PPARγ signaling in cDC2s, suppressing the activation of inflammatory DCs (81). Both signaling pathways are able to suppress toll-like receptor-4 (TLR4)-induced inflammation in VAT (77). However, when the homeostasis of adipose tissue is compromised, antigen or lipid uptake by ATDCs induces the activation of MAPK signaling, resulting in increased MHC-II expression and cellular maturation (81).
Neutrophils are recruited by the LTB4-BCT1 axis and cytoplasmic phospholipase A2α (cPLA2α). Neutrophils have recently been shown to promote inflammation through the activation of NFκB signaling, and neutrophil activation is closely related to its interaction with adipocytes. Elgazar-Carmon et al. reported that CD11b on neutrophils and ICAM1 on adipocytes mediate their interaction (82). The interaction with adipocytes is critical for the expression of IL-1β via NFκB activation in adipose tissue neutrophils (82).
Innate lymphoid cells1/3 are proinflammatory. The activation and proliferation of ILC1 proinflammatory immune cells is promoted by JAK3/STAT5 signaling, while Lnk/Sh2b3 (Lnk) induced by high-fat diet (HFD) signaling suppresses JAK3 (83). IL-15 has also been proven to be important for ILC1 proliferation (83). Similarly, IL-12 could activate adipose ILC1s, leading to the production of IFN-γ and the polarization of M1 macrophages in adipose tissue at the early stages of HFD consumption (84). Studies have shown that ILC1s contribute to not only adipose tissue inflammation but also fibrosis, and this process depends on IFN-γ (85). Furthermore, ILC1s promote fibrogenesis through the activation of CD11c+ macrophages and TGF-β1/Smad3 signaling, with TGF-β1/Smad3 activation contributing to persistent and aberrant ECM remodeling in VAT (86). Studies investigating ILC3s are limited, but their proinflammatory and pro-obesity characteristics are well defined. On a mechanistic level, some studies have shown that ILC3s induce obesity through the lymphotoxin/IL-23/IL-22 pathway (87).
Human adipose tissue-resident NK cells are predominantly CD56brightCD16- NK cells (88, 89). NK cells and other ILC1s in adipose tissue are often stimulated by proinflammatory factors, such as IL-12, IL-15, and NKp46 ligands produced by macrophages and stressed adipocytes, to promote the production of IFN-γ, TNF-α and IL-6, thereby aggravating the inflammatory response in adipose tissue (Figure 2).
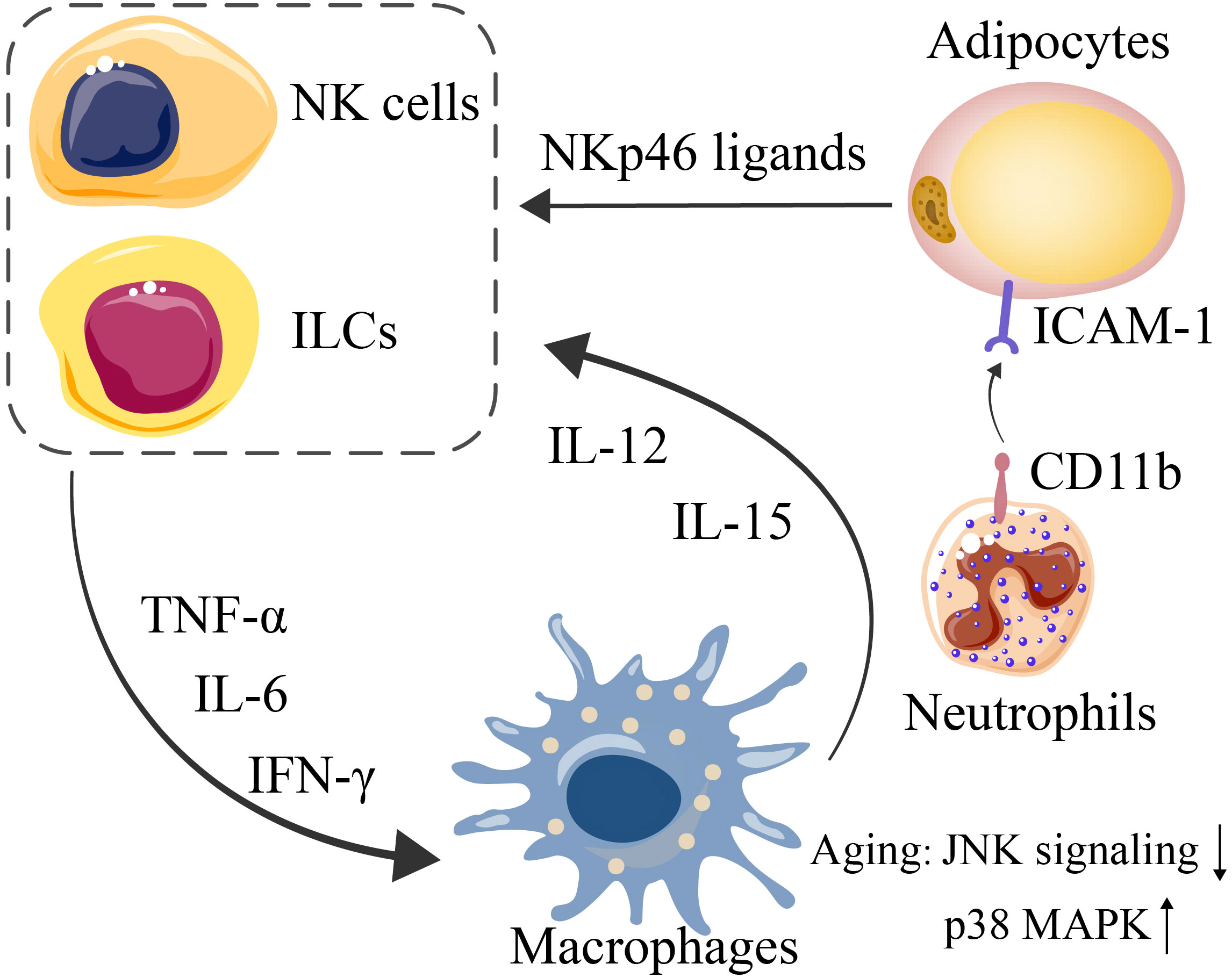
Figure 2 Interactions among adipocytes, NK cells and ILCs (mediated by NKp46 ligands, expressed by stressed adipocytes) lead to further exacerbated expression of IFN-γ, TNF-α, and IL-6. Since macrophages are triggered by such proinflammatory cytokines, IL-12 and IL-15 are consequently secreted by macrophages to induce NK-cell and ILC proliferation. CD11b on neutrophils and ICAM1 on adipocytes mediate their interaction.
5.1.2 Adaptive immune cells
T cells are easily affected by aging and tend to polarize into a proinflammatory phenotype. T cells from both SAT or VAT exhibit senescent features, including the loss of CD28 and expansion of CD44+CD62- memory T cells. With aging, there are increases in the numbers of CD4+ and CD8+ T cells (90–92).
CD4+ T cells are activated by MHC-II, and the expression of MHC-II on adipocytes is induced by free fatty acids possibly via the activation of JAK and STAT1, which may further activate CIITA, a prime regulator of MHC-II (93). MHC-II is also expressed on other APCs, and in macrophages, CD40 signaling in adipose tissue macrophages regulates MHC II and CD86 expression to control the expansion of CD4+ T cells (94). Subtype changes among CD4+ T cells represent an important modulator mechanism in different metabolic diseases. Adipose tissue contains a large amount of TGFβ and IL-6. The TGFβ/Smad pathway limits Th1 and Th2 differentiation through the downregulation of T-bet/GATA-3 expression, leading to increased Th17 differentiation (95). IL-6-induced STAT3 can promote the differentiation of Th17 cells (96). In addition, MAP4K4 in T cells can phosphorylate TRAF2, thus downregulating the expression of IL-6, leading to the inhibition of Th17 differentiation and preventing insulin resistance (95). Adiponectin has been shown to directly enhance Th1 differentiation by activating the p38-STAT4-T-bet axis (97). Nevertheless, studies have shown that the PD-L1:PD-1 axis can inhibit Th1 proliferation and promote Th2 polarization, thus limiting the inflammation induced by T cells (98). Regarding CD8+ T cells, it has been shown that CD40-TRAF2/3/5/6 signaling is important for CD8+ T cells to promote adipose tissue inflammation (99).
Recent studies have shown that the numbers of γδT cells are increased in adipose tissue during aging and contribute to adipose tissue inflammation (100). However, γδT cells also play a role in maintaining adipose tissue homeostasis through adipose tissue browning. Studies have shown that γδT cells and IL-17F upregulate the expression of TGFβ1 in adipocytes by signaling through IL-17RC. In turn, adipocyte-derived TGFβ1 promotes sympathetic innervation, promoting adipose tissue browning (101).
B2 cells accumulate in adipose tissue prior to T cells. LTB4R1 expression on B2 cells is important for B2 cell chemotaxis. B-cell recruitment to fat-associated lymphoid clusters (FALC) and VAT is also mediated by the CXCR5 signaling axis (102). IgG derived from B2 cells can contribute to adipose tissue inflammation. Aging induces the accumulation of B2 cells in VAT, and B2 cells become more inflammatory once they infiltrate VAT. The following two B-cell types emerge in aging mice: aged adipose B cells (AAB) and aging-related B cells (ABC). The percentage of follicular B cells is reduced, while ABC is increased in VAT in aging mice. Further studies have shown that ABC originates from follicular B cells. AAB primarily reside in FALC, and the expansion of AAB along with their secretion of proinflammatory cytokines and monocyte-recruiting chemokines further aggravate adipose tissue inflammation (103). The activation of NLRP3 is essential for increasing the AAB numbers, FALCS number, and lipolysis by upregulating IL-18 and the IL-1β/IL-1βR axis (104). IL-1R signaling is critical for AAB proliferation (103). Furthermore, activated monocytes can convert innate B1a cells into 4BL cells (4–1BBL+ B1a cells), inducing cytolytic CD8+ T cells and insulin resistance in elderly individuals (105) (Figure 3).
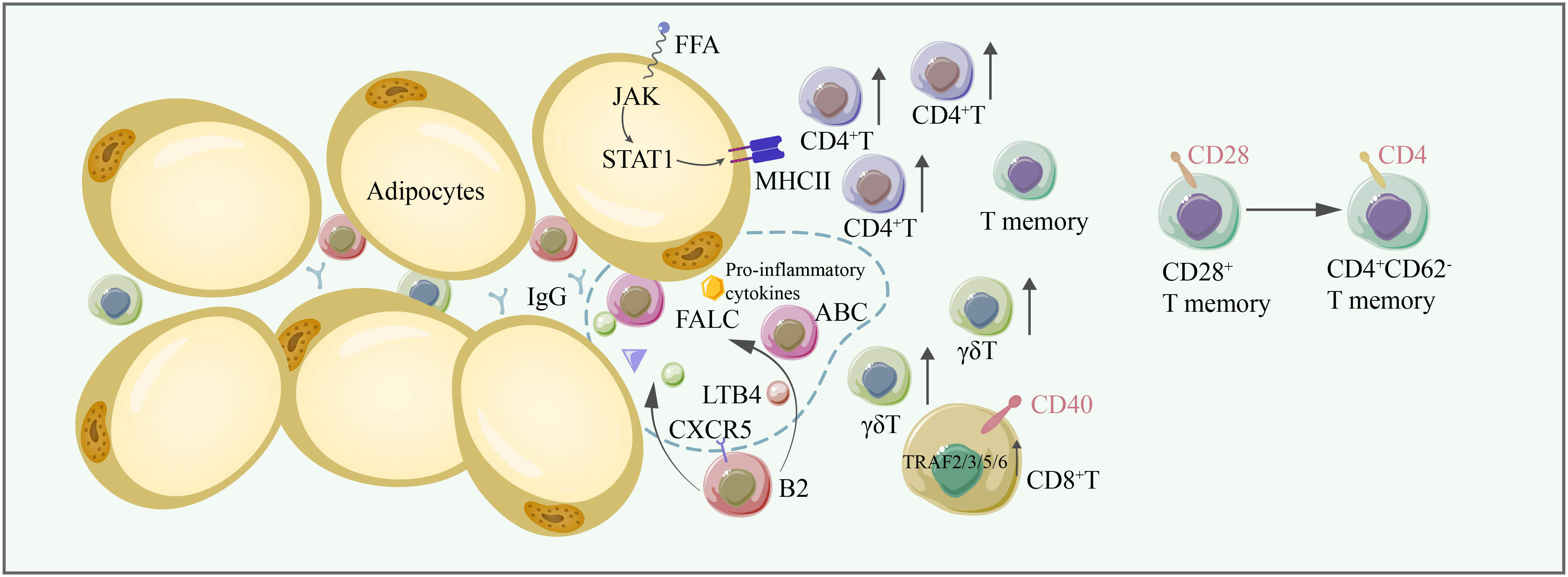
Figure 3 In aging adipose tissues, the number of conventional T cells, γδT cells, increases, thus contributing to adipose tissue inflammation. CD28+ T memory cells are converted to CD4+ CD62- memory T cells. FFA induces the expression of MHC-II through the JAK/STAT1 signaling pathway, facilitating antigen presentation to CD4+ T cells. CD40/TRAF2/3/5/6 signaling is critical for CD8+ T-cell-induced inflammation. B2 cells are recruited to FALC by LTB4, and CXCR5 on B2 cells is important for their recruitment to FALC. Some B2 cells become ABCs. B2 cells and ABC are proinflammatory, producing IgG and exacerbating inflammation in adipose tissue. FFA free fatty acid, FALC fat-associated lymphoid cluster, LTB4 leukotriene B4, CXCR5 C-X-C chemokine receptor type 5.
5.2 Anti-inflammatory adipose tissue resident immune cells
5.2.1 Innate immune cells
M2-type macrophages and type 2 cytokine signaling are crucial for adipose tissue homeostasis (63). The JAK/STAT pathway is known to control macrophage biology. STAT1 induces M1 macrophage polarization, while STAT6 mediates IL-4a signaling and regulates many M2 signature genes (106). PPAR-γ promotes primary human monocytes to differentiate toward an M2 phenotype (107). Macrophage PPARγ inhibits IFNβ production by interfering with the IRF3-mediated transcription of IFNβ. Interactions between PPARγ and STAT6 facilitate the induction of PPARγ-regulated genes (108). PPARγ activation in murine macrophages induces miR223 expression by binding upstream of miR223, which, in turn, inhibits the expression of NFAT-5 and RAS p21 protein activator 1, promoting the development of an anti-inflammatory M2-like phenotype (109, 110). Several members of the CCAAT-enhancer-binding protein (C/EBP) family play important roles in macrophage activation. While C/EBPσ was shown to induce M1-like proinflammatory responses, cAMP response element-binding protein (CREB) inhibits the expression of M1-associated genes through p38-mediated induction of IL-10 (111). Interferon regulatory factors (IRFs) also play important roles in macrophage polarization. IRF5 was reported to promote M1 macrophage polarization, while IRF3, 4, 6, and 9 promote M2 macrophage polarization (110). The metabolic regulators SIRT and AMPK perform important functions in macrophage polarization. The adipocyte-specific knockout of SIRT accelerates the recruitment of macrophages and polarizes cells into the M1 type. Moreover, the levels of SIRT1 are inversely correlated with BMI (112). AMPK can inhibit proinflammatory responses in macrophages and promote macrophage polarization into an anti-inflammatory phenotype. AMPK can interfere with inflammation by inhibiting NF-κB signaling through the regulation of downstream mediators of NF-κB signaling, including SIRT1, PGC-1α, p53, and Forkhead box O (FoxO) factors (61). AMPK can enhance SIRT1 expression by increasing the NAD/NADH ratio (113).
Although cDC2s are proinflammatory, cDC1s play pivotal roles in circumventing obesity development during aging through a reduction of inflammatory activities, affecting the iNKT and NK-cell abundance. The presence of cDC1s is not typically affected by aging, while there are increasing numbers of cDC2s in VAT in aged mice (114). Regulatory DCs in adipose tissue have been shown to play an important role in the prevention of adipose tissue inflammation as they inhibit specialized autoreactive T cells through perforin (115). However, recent studies have shown that regulatory DCs are reduced with aging, resulting in greatly reduced immune tolerance in adipose tissue (115).
Eosinophils, identified by the CD45+CD14-CD16-CD117-Siglec-8+CD66b+ phenotype, play a key protective role in the innate immune response (116). One of their functions includes the induction of M2-type macrophages through the production of IL-4 (117). Eosinophils are also critical immune cells involved in adipose tissue browning that may be capable of driving the activation of adipocyte beiging through paracrine signaling mechanisms (118). The FGF21-CCL11 axis is critical for type 2 immune cell activation and the beiging of SAT, which is critical for adipose tissue homeostasis (119). FGF21 is usually induced in adipocytes by cold exposure through cyclic AMP-mediated activation of protein kinase A and p38 MAPK, which, in turn, phosphorylates the transcription factor ATF2 for the transactivation of the FGF21 gene promoter (120). Then, FGF21 stimulates CCL11 production through the KLB-ERK1/2 signaling cascade. CCL11 further recruits eosinophils to SAT and contributes to the recruitment of M2-type macrophages (121). Furthermore, iNKT cells may also induce FGF21 (122). IL-4 is expressed and secreted by eosinophils and binds its receptor IL-4R on adipocytes, where it activates the PI3K-AKT and MAPK/ERK signaling pathways, promoting adipocyte beiging. IL-13 produced by ILC2s has the same properties as IL-4 (118). The IL4/13-IL4Rα-STAT6 pathway is required for the biogenesis of functional beige fat (118). Limited studies have shown that the distribution and function of ATEs change throughout aging, leading to defects in adipose tissue homeostasis and low-grade chronic inflammation. First, the migration and regulatory functions of ATEs significantly decrease (123). Second, the level of CCL11 (eotaxin-1), a potent ATE chemoattractant, systemically increases with aging (124). However, CCL11 is negatively correlated with the distribution of ATEs. Third, the decreased ATE/ATM ratio in elderly individuals leads to significant increases in IL-6, IL-1β, and adipocyte hypertrophy, which are closely related to adipose tissue inflammation (125).
ILC2s (CD45+Lin–CD127+CD161+CRTH2+) play essential roles in adipose tissue homeostasis by maintaining eosinophils and M2-type macrophages (126). However, a significant loss of ILC2s is observed during aging. Moreover, IL-33 normally stimulates the expansion of ILC2s and promotes an anti-inflammatory profile. The binding of IL-33 to the IL-1 receptor–related protein ST2 coupled to an IL-1RAcP (IL-1 receptor accessory protein) unit leads to the release of NFκB (127). Activated NFκB promotes the transcription of GATA binding protein 3 (GATA3), ST2, and consequently, IL-5 and IL-13, leading to the activation of ILC2s and a type 2 immune response (128). IL-33, however, can also stimulate the accumulation of pathogenic ILC2s, which leads to adipose tissue inflammation.
5.2.2 Adaptive immune cells
Aging leads to an increase in Tregs in VAT that continues as mice age (129). IL-33 efficiently induces the process (130). Middle-aged to old mice exhibit a 7- to 11-fold increase in adipose tissue Tregs compared to young mice, with these cells accounting for more than 50% of all CD4+ T cells in adipose tissue (131). Interestingly, Treg depletion in young mice may increase the levels of several inflammatory markers in adipose tissue (132). However, the depletion of adipose tissue Tregs in aging mice does not significantly enhance systemic or tissue inflammation (131). Tregs play a protective role in adipose tissue homeostasis. Studies have shown that inducible T-cell costimulator (ICOS) signaling negatively regulates the recruitment of Tregs via PI3k-dependent mechanisms. In contrast, the absence of ICOS signaling enhances the recruitment of Tregs and increases the expression of CCR3 (133). The maintenance of Tregs in lean adipose tissue depends on PPARγ, IRF4, BATF, Blimp1, and IL-33 signaling, with PPARγ being a prime activator of Treg accumulation (98). The combination of PPARγ and Foxp3 can further enhance and promote the expression of a Treg-specific transcriptome (134). PPARγ+ Tregs can be affected by IFN-γ released by pDCs and eventually become apoptotic (135). The IL-33 receptor ST2 plays important roles in Tregs as follows: insulin signaling can drive the transition of CD73hiST2lo into CD73loST2hi adipose Treg cell subsets through the HIF-1α–Med23–PPARγ axis (136). Furthermore, PLZF+ γδT cells were shown to induce the abundance of IL-33 and ST2 through enhanced IL-17A expression (129). The IL-33/ST2 axis is also important for the differentiation of Tregs, and the downstream signaling of IL-33 is mediated by MyD88 (137). In addition to IL-33, a recent study showed that IL-2 can upregulate hydroxy-prostaglandin dehydrogenase (HPGD) in Tregs through JAK3/STAT5 signaling, and HPGD can further inhibit conventional T cells through PPARγ, thus maintaining the homeostasis of adipose tissue (98). Furthermore, KLF10 is an important protein regulating the differentiation and chemotaxis of Tregs. A decrease in KLF10 in obese subjects impairs the PI3K-Akt-mTOR signaling pathway in Tregs, leading to the impaired migration and decreased accumulation of Tregs in adipose tissue (138). AKT signaling, however, is reported to reverse the ability of Treg cells to inhibit TNF-α production by macrophages (98). TCR signaling is another important signaling pathway for the induction of Treg precursors in VAT (139). Similarly, Stat6/Pten signaling plays an important role in the induction of Tregs into adipose tissue during cold stimulation (134). Tregs, however, can also play a negative role in adipose tissue homeostasis by producing IL-10, which is mediated by Blimp-1. IL-10 can directly suppress thermogenesis in adipocytes through a STAT3-dependent signaling pathway (140).
Invariant natural killer T cells (iNKT) play a protective role in adipose tissue homeostasis. iNKT produce anti-inflammatory cytokines, such as IL-4 and IL-10, and regulate the function of M2 macrophages. iNKT can also upregulate the expression of FGF21 in both BAT and SAT, which drives the activation of BAT and browning of WAT (141). In addition, in obese subjects, iNKT cells can help eliminate hypertrophic adipocytes while promoting adipogenesis through the FAS/FASL pathway, contributing to adipose tissue homeostasis (142). However, recent studies have identified heterogeneity in iNKT subtypes; NK1.1- iNKT cells have upregulated IRE1α/XBP1 signaling, exerting an anti-inflammatory profile, while NK1.1+ iNKT cells can release IFNγ, promoting adipose tissue inflammation (143). iNKT cells decrease in aging adipose tissue (90–92).
IgM derived from B1 cells blocks inflammation. IgM antibodies can clear self-antigens and play a regulatory role by promoting B-cell tolerance. They can also induce M2 macrophage polarization in adipose tissue (102) (Figure 4 and Tables 1, 2).
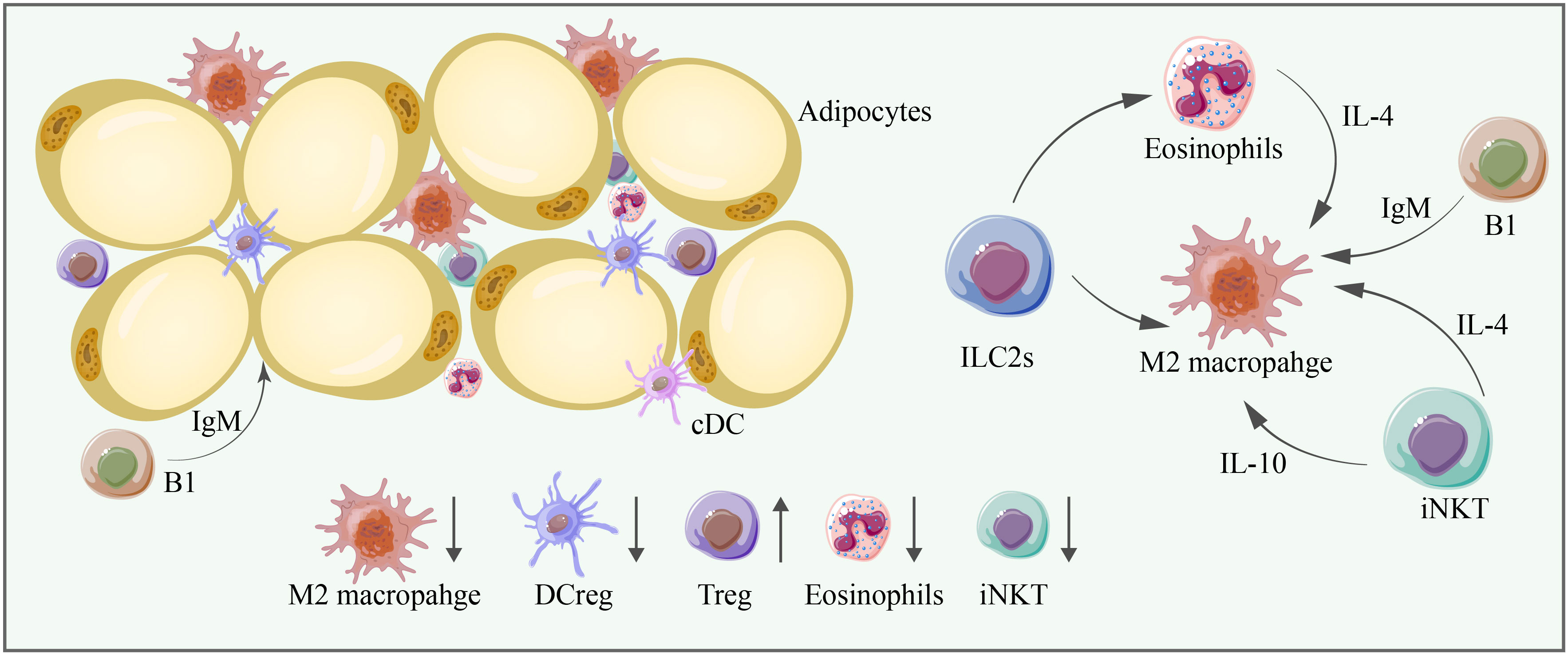
Figure 4 M2 macrophages, DCregs, Tregs, eosinophils, and iNKT cells are significant anti-inflammatory immune cells in adipose tissue, and their abundance is mostly decreased in aging adipose tissue. Cell–cell interactions are essential for adipose tissue homeostasis. ILC2s can promote the proliferation of eosinophils and M2 macrophages, and eosinophils can promote the proliferation of M2 macrophages through IL-4. iNKT cells can promote the proliferation of M2 macrophages through IL-4 and IL-10. B1 cells produce IgM, which exerts anti-inflammatory effects and promotes the proliferation of M2 macrophages.
6 The molecular mechanisms mediating adipose tissue inflammaging
6.1 JAK/STAT signaling pathway
Over the past two decades, the JAK/STAT signaling pathway has been shown to play vital roles in the regulation of lipid metabolism, glucose metabolism, and adipokine secretion in adipose tissue (158). While early research mainly focused on the role of JAK/STAT signaling in adipogenesis, subsequent studies further demonstrated a role in mediating inflammation in adipose tissue (159). The JAK/STAT signaling pathway mediates the activation of peripheral blood B cells and promotes the activation and proliferation of ILC1s in adipose tissue (83). Adipocytes also express several receptors for JAK/STAT-activating cytokines and hormones, including immune cytokines acting in a paracrine manner to induce JAK/STAT signaling in adipocytes (160). For example, IFN-γ can induce JAK1/STAT1 signaling in human adipocytes and promote inflammation and insulin resistance (161). STAT proteins heterodimerize with phosphorylated IRFs to activate the expression of inflammatory gene signatures (162). Ligands, including IL-6 and platelet-derived growth factor receptors (PDGFRs), also signal through the JAK/STAT signaling pathway (163). Oncostatin M (OSM) is a cytokine expressed in immune cells in adipose tissue, while its receptor OSMR is expressed on adipocytes (164). The loss of OSMR expression is accompanied by decreased STAT5 phosphorylation in adipocytes and a reduction in the expression of proinflammatory cytokines and chemokines (165). Recent studies have reported crosstalk between the JAK/STAT and TGFβ signaling pathways. Transient inflammation caused by lipolysis leads to the upregulation of IL-6 and activation of JAK/STAT3 signaling in adipose progenitors, alleviating the inhibitory effect of TGFβ on adipogenic lineage commitment and thermogenic beige adipocyte differentiation (166).
6.2 Wnt/β-catenin and PI3K/AKT signaling pathways
Wnt signaling plays pivotal roles in modulating the adipose tissue microenvironment. Wnt signals can alter important steps of insulin utilization within cells, leading to the progression of insulin resistance (167). The binding of Wnt ligands to their receptors activates the Wnt pathway and causes adipose tissue inflammation, obesity, and glucose homeostasis. Wnt signaling can be further divided into canonical and noncanonical Wnt signaling. Noncanonical Wnt ligands, such as Wnt5a, are expressed in adipocytes at high levels when the diet consists of fatty substances, which contributes to obesity-associated inflammation (168). Wnt5a can also activate JNK and promote insulin resistance (169). The Wnt signaling pathway is activated in cDC1s, leading to the production of the anti-inflammatory cytokine IL-10 (80). However, TLR signaling acts in conjunction with the Wnt signaling pathway to amplify the release of proinflammatory cytokines in macrophage-induced inflammation (72). PI3K signaling is also closely related to chronic inflammation and insulin resistance. Inflammation or other stress stimuli block the PI3K signaling pathway downstream of the insulin receptor through the activation of several serine/threonine kinases, contributing to insulin resistance in adipocytes (170). PI3K signaling and its downstream effectors, including protein kinase B (AKT), help preserve beneficial macrophage subpopulations (75). However, the PI3K/AKT-mediated anti-inflammatory effects are inhibited in aging adipose tissue DCs (171). PI3Kγ can promote neutrophil infiltration.
6.3 NF-κB signaling pathway
The NF-κB and JNK signaling pathways are two central mediators of the inflammatory response in adipose inflammation. Specific dietary saturated fatty acids induce the expression of MCP-1 and SAA in adipocytes through the activation of the NF-κB and ROS pathways (172). The activation of NF-κB signaling increases the expression of TNF-α, IL-6 and MCP-1, leading to serine phosphorylation of IRS-1, thus blocking insulin signaling downstream of insulin receptors (26). The transcription of NF-κB is mainly controlled by the phosphorylation of inhibitor of NF-κB (IκB) by the upstream IκB kinase (IKK). Canonical IKKs, including IKKα and IKKβ, phosphorylate IκB and other subunits of NF-κB to induce the expression of NF-κB target genes. IKKα phosphorylates IRS-1 and, thus, induces insulin resistance (153). Panahi et al. found that IKKβ deficiency in adipocytes prevents the expression of proinflammatory cytokines, such as IL-6 and TNF-α, induced by free fatty acids. In contrast, the activation of IKKβ inhibits the expression of anti-inflammatory cytokines, such as adiponectin and leptin (173). In addition to canonical IKKα and IKKβ, two noncanonical IKKs, IKKϵ and tank-binding kinase 1 (TBK1), play roles in adipocyte biology (174). Proinflammatory cytokines, such as TNF-α, activate TBK1, which attenuates adipose tissue inflammation by repressing the atypical NF-κB pathway. In this pathway, NF-κB-inducing kinase (NIF) phosphorylates Ser176 to activate IKKα, which, in turn, activates the RelB (NF-κB2) precursor p100, inducing its maturation. This pathway induces the expression of target genes, such as CCL2, and promotes infiltration by macrophages. TBK1 can further inhibit the metabolic regulator AMPK, thus promoting inflammation (175). Moreover, NFκB activation in adipose tissue neutrophils is important for its expression of IL-1β (82). Activated NFκB also promotes the activation of ILC2s and a type 2 immune response (128). DR3, a recently defined receptor expressed on ILC2s, can also induce and activate ILC2s through NFκB pathways (176).
6.4 MAPK signaling pathway
The following two distinct MAPK signaling pathways play important roles in adipocyte inflammation: the p38 pathway and the JNK pathway. JNK signaling is activated in adipocytes in obese humans and mice and promotes insulin resistance through the phosphorylation of IRS, thereby decreasing PI3K/PKB signaling downstream of insulin receptors (153). The activation of JNK can further activate the transcriptional regulator AP-1 and induce the expression of inflammatory genes, such as IL-6 and TNF, inhibiting insulin signaling and causing insulin resistance (25). In contrast to JNK, p38α activity is reduced in obese mice, with concurrent activation of other p38 isoforms, including p38g and p38d (177). The p38 pathway is activated in adipose tissue immune cells, such as macrophages, to promote the production of inflammatory cytokines and the recruitment of monocytes, leading to adipose tissue inflammation (178). Activated p38MAPK signaling in mast cells can also contribute to metabolic dysfunction in SAT (179). The p38 pathway is also implicated in adipocytes as the inhibition of p38 in adipocytes results in a decreased secretion of TNF-α-induced IL-6 (180). JNK plays a role in lipolysis induced by TNF-α, while the role of p38 in this process needs further elucidation (181). When MAPK signaling is activated in DCs, the expression of MHC-II and cellular maturation are increased (81).
6.5 AMPK signaling pathway
The final signaling pathway we aim to discuss here is the AMPK pathway, an important anti-inflammatory signaling pathway in adipose tissue. AMPK signaling can inhibit the synthesis of proinflammatory cytokines, including IL-6 and IL-8, in adipocytes, while a deficiency in AMPK leads to an increased production of proinflammatory cytokines. The knockdown of AMPKα1 in 3T3-L1 adipocytes leads to an increase in the mRNA levels of the proinflammatory cytokines TNF-α, IL-1β and MCP-1 in response to FA treatment (182). In a recent study, the following more detailed mechanism was elucidated: AMPK might block a proinflammatory IL-6 trans-signaling mechanism involving IL-6/soluble IL-6R that can stimulate JAK, thus influencing the JAK/STAT3 signaling pathway in adipocytes. Furthermore, AMPK can block IL-1β-stimulated IRAK-4, thus influencing the JNK signaling pathway in adipocytes. Moreover, AMPK can block TNF-α-stimulated IKKβ, thus influencing the NF-κB signaling pathway in adipocytes (183). Thus, AMPK plays a critical role as a master regulator of inflammation by regulating several inflammatory pathways (82). In addition, AMPK can promote M2-type macrophage polarization (61) and compromise the function of ILC2s by interacting with the NFκB pathway (184) (Figure 5).
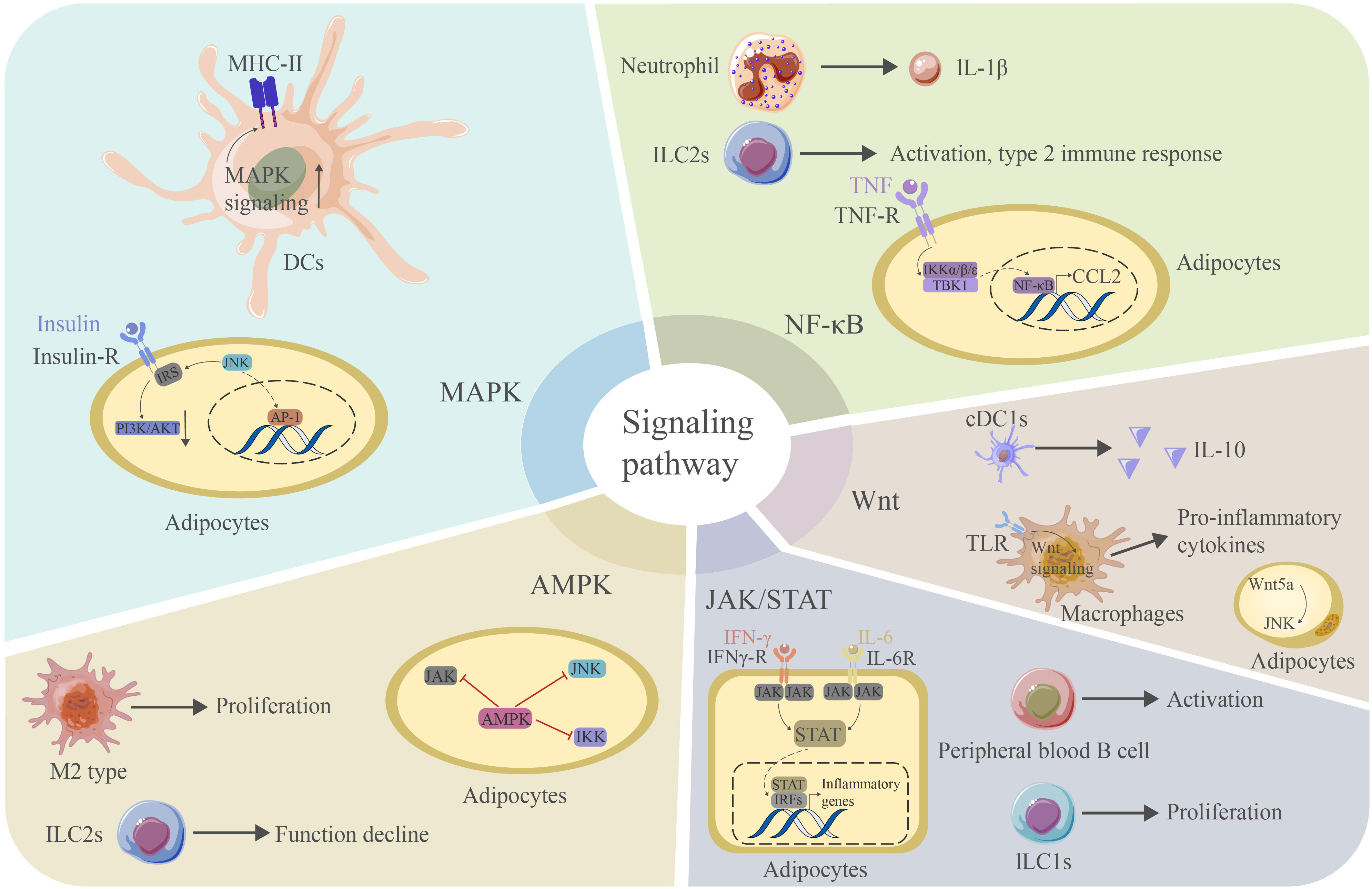
Figure 5 The MAPK signaling pathway is critical for the expression of MHC-II in DCs. JNK signaling can interact with IRS to reduce PI3K/AKT signaling and contribute. JNK can further promote AP-1 production and promote inflammation in adipose tissue. The NF-κB signaling pathway mediates the production of IL-1β in neutrophils and the activation of ILC2s. This pathway is also essential for the adipocyte response to TNF and the production of CCL2. Wnt signaling mediates IL-10 production in CDC1s and binds TLR to produce proinflammatory cytokines in macrophages. Wnt5a can induce JNK in adipocytes. The JAK/STAT signaling pathway is essential for activating peripheral blood B cells and the proliferation of ILC1s. This pathway is also critical for the adipocyte response to IFN-γ. STATs can further bind IRFs to induce the production of proinflammatory cytokines. The AMPK signaling pathway mediates the proliferation of M2-type macrophages and blocks the proinflammatory signaling pathway in adipocytes. However, it may cause a decrease in the function of ILC2s. AP-1 activator protein 1, CCL2 chemokine ligand 2, TLR toll-like receptor, IRF interferon regulation factor.
7 Adipose tissue as a therapeutic target in aging
In a recent targeted pathway proteomics study, the researchers revealed that aging has tissue-specific effects on WAT in mice, with alterations in metabolic and inflammatory pathways, suggesting that WAT could be critical for an organism’s adaptation and response to aging (185). It has also been shown using bulk RNA-sequencing of 17 organs and plasma proteomics at 10 ages across the mouse lifespan that immune cell activation was first detected in white adipose depots during middle age (186). Many classic mechanisms of aging, such as cellular senescence, chronic inflammation, and metabolic disorders, occur in adipose tissue. Therefore, adipose tissue should be considered a significant therapeutic target in antiaging treatments. Emerging interventions against aging targeting adipose tissue have recently been developed. Many studies have demonstrated that reducing the WAT mass and ameliorating WAT dysfunction through many methods, such as exercise, caloric restriction, senolytics and other signaling pathways, can extend health and the lifespan in various organisms (187–195).
7.1 Caloric restriction as the foundation of anti-aging therapies
Caloric restriction (CR) without malnutrition has been the foundation of aging for decades (196). A reduction in food intake prolongs the lifespan and delays the onset of age-related diseases in diverse species. The life-prolonging effect of CR is due to changes in many physiological processes, and the biology of AT is closely related (197). As we previously mentioned, hypoxia, mechanical stress and obesity caused by adipocyte hypertrophy are factors contributing to adipose tissue inflammaging. Research has found that the surgical removal of VAT in rats offered approximately 20% of the effect of CR on longevity, preventing insulin resistance and glucose intolerance of aging (189). A reduction in the fat mass, specifically visceral fat, may be a possible underlying mechanism of the antiaging effect of CR (190). However, a recent study has shown that 30% CR alone without fasting or circadian alignment accounts for a 10% extension of the lifespan; however, a daily fasting interval and circadian alignment of feeding act together to extend the lifespan by 35% in male C57BL/6J mice, with improvements in inflammation and immune and metabolic function (198), which are consistent with the results of other recent studies in C57BL/6J male mice (199). Moreover, researchers have found that CR and fasting have overlapping effects on gene expression by performing transcriptomic profiling of inguinal white adipose tissue (iWAT), where CR and fasting altered many Kyoto Encyclopedia of Genes and Genomes (KEGG) pathways, including PPAR, insulin, TGF-β and AMPK signaling and various metabolic pathways (198–201). Many molecules have been discovered to function in regulating the lifespan by dietary restriction; among these, SIRT1, an NAD-dependent deacetylase that participates in cell cycle regulation, is the best established longevity determinant. Studies have shown that SIRT1 mediates the effect of CR on longevity by suppressing lipid accumulation and enhancing adipocyte lipolysis (202, 203). SIRT1 also participates in the regulation of other signaling pathways related to aging. The transactivation of PPARγ, which is essential for proper adipose tissue development and function, is repressed in WAT by SIRT1 (204). Previous studies suggested that experimental Pparg2-deficient mouse models with a lower expression of PPARγ in WAT exhibited a reduction in the lifespan (205). In addition, a new study identified reduced expression of platelet-activating factor acetylhydrolase (PLA2G7) in adipose tissue from people undergoing CR for 2 years by using gene expression profiles, and PLA2G7-deficient mice showed decreased age-related inflammation, lower NLRP3 inflammasome activation, and improved adipose tissue metabolism. These findings demonstrate that PLA2G7 may become an immunometabolic regulator of CR and could potentially be used to lower inflammation and extend the lifespan (206). Moreover, the pathologic expansion of adipose tissue leads to the excessive production of FFA, thereby stimulating TLR4 signaling through the TLR4-NFkB pathway, resulting in the release of proinflammatory cytokines. A study suggested that the expression of three major proinflammatory cytokines (IL-6, MCP1 and TNF-α) in adipose tissue is significantly reduced in old TLR4-KO mice compared to old wild-type mice, showing that TLR4-deficient mice are protected from adipose tissue inflammation during aging (207). Thus, the manipulation of the TLR4 pathway might have great therapeutic potential in aging. The possible molecular pathways described above link caloric restriction to life extension in mammals, providing new insight into the targets of anti-aging treatments.
7.2 Senothrerapeutics: senolytics and senomorphics
Over the past decade, the search for strategies that can achieve the beneficial effects of CR without reducing calorie intake has undergone considerable expansion (208). The accumulation of senescent cells (SnCs) is one of the hallmarks of aging, which leads to tissue and organismal aging, and the selective elimination of SnCs in animal models extends the health span (209). Therefore, pharmacological interventions targeting SnCs, also known as senotherapeutics, might be a potential strategy for longevity and the prevention of age-related diseases. Senolytics, drugs that specifically kill SnCs, have shown efficacy against atherosclerosis (210), osteoarthritis (211) and other age-related diseases (212–214). The first senolytics reported by Zhu et al. in 2015 were a drug combination of dasatinib (D), a protein tyrosine kinase inhibitor, and quercetin (Q), a plant flavonoid. By using a transcriptome analysis of senescent and nonsenescent human preadipocytes, the authors revealed that SnCs protect themselves from apoptosis through senescent cell antiapoptotic pathways (SCAPs), including ephrin receptors, BCL-2/BCL-XL family members, P13K/AKT, HIF-1α, etc. (213). In vivo, the D + Q combination reduced the senescent cell burden in fat tissue by targeting SCAPs with the benefit of reduced frailty and an extended healthspan (194, 213). To date, D + Q treatment has been tested in several human clinical trials; for example, a clinical trial of D + Q in individuals with diabetic kidney disease found that the D + Q treatment alleviated adipose tissue and the skin senescent cell burden, decreased the resulting adipose tissue macrophage accumulation, enhanced the adipocyte progenitor replicative potential, and reduced key circulating SASP factors (215).
In addition, SnCs can lead to extensive microenvironment dysfunction and cause damage to surrounding cells and tissues due to their proinflammatory SASP (216). Senomorphics, another class of senotherapeutics, is known for modulating the phenotypes of SnCs by interfering with inflammaging, senescence-related signaling pathways, and SASP without inducing apoptosis (217). Resveratrol, a plant-derived polyphenol, is the most potent of the natural SIRT1 activators, and several studies have reported that it can extend the lifespan of various organisms (218–221). In addition to the features of SIRT1 mentioned above, SIRT1 exerts anti-inflammatory activity by inhibiting NF-κB, a key regulator of the immune response and inflammaging (222, 223). Research involving rhesus monkeys fed a high-fat, high-sugar diet suggested that resveratrol improves adipose insulin signaling and reduces the inflammatory response in WAT with increased SIRT 1 expression and decreased NF-κB activation (224). Moreover, SASP in SnCs is regulated by the JAK/STAT pathway, and using the JAK inhibitor roxolitinib in aged mice for 10 weeks reduced both adipose tissue and systemic inflammation and enhanced physical function (225). Metformin, originally approved for the treatment of type 2 diabetes, has been found to have therapeutic effects on age-related diseases, such as insulin resistance, obesity and cardiovascular diseases (226). Numerous studies have proven that metformin is effective in inhibiting cellular senescence and SASPs and preventing age-associated dysfunctions in many model organisms. Metformin modulates aging-related protein synthesis by regulating AMPK/mTOR signaling and enhancing autophagy to increase aging-related protein degradation (227). A recent study demonstrated that metformin reduced cell cycle progression and mTOR signaling and decreased the secretion of most proinflammatory SASP cytokines in mature human adipocytes, exerting anti-inflammatory effects on adipose tissue function (228). Rapamycin, a specific mTOR inhibitor, has been regarded as one of the most well-established senomorphics that reduce cell senescence, suppress SASPs and extend the lifespan. A study focusing on the effects of rapamycin on inflammation in gonadal white adipose tissue (gWAT) of HET3 mice revealed that rapamycin led to a 56% increase in CD45+ leukocytes in gWAT, where the majority of these are ATMs. Interestingly, rapamycin led to an increase in M1 type ATMs, suggesting that rapamycin may achieve life-span extension partially through adipose tissue inflammation (229). L-carnitine, an inhibitor of the JNK/p53 pathway that can prevent apoptosis, has been found to attenuate aging adipose tissue dysfunction by reducing the expression of SASP factors in the WAT of aged (> 18 months old) rats (230).
7.3 Immune therapy as an antiaging strategy
Since immune cells play a key role as sources and integrators of inflammatory signals, the regulation of immune cell phenotypes could be a target for intervention to limit ‘inflammaging’ and restore repair capacity in older organisms. Heterochronic parabiosis, a model system in which two animals of different ages are joined to share a common circulatory system, represents an important milestone in aging biology (231). Various circulatory factors have been identified as mediators of the prorejuvenation and proaging systemic effects of heterochronic parabiosis. A recent study demonstrated that transferring eosinophils from young mice reduces WAT and systemic low-grade inflammation, with lower levels of inflammatory factors (such as IL-6, CCL2 and IL-1β), resulting in the restoration of adipose immune homeostasis and widespread rejuvenating consequences for the aging host (125). Another study suggested that adoptive NK-cell infusion reduces senescent markers (p16 and p21) and decreases the SASP phenotype in human adipose tissue (232). Therefore, immune therapy could be a promising strategy for intervention in aging in the future.
7.4 Antiaging therapy targeting potential signaling pathways
The JAK/STAT pathway is of great importance in regulating cytokine production and has been investigated as a therapeutic target for many diseases (233–236). Studies have found that the JAK pathway is more highly activated in fat tissue from old than young animals and senescent than nonsenescent cells, and 2 months of administration of ruxolitinib, a specific JAK1/2 inhibitor, reduced systemic inflammation, enhanced physical capacity, preserved fat tissue homeostasis, and improved metabolic function in 22– to 24-month-old mice (237, 238).
As we previously discussed, the p38MAPK pathway also plays a vital role in adipose tissue inflammaging. Studies have proven that the l-arginine-metabolizing enzyme arginase-II (Arg-II) promotes IL-6 production in aging adipose tissues through the p38MAPK pathway. There is more macrophage accumulation in visceral adipose tissues in old WT mice than Arg-II knockout mice. The treatment of aging adipose tissues in WT mice with the specific p38mapk inhibitor SB203580 reduces IL-6 secretion, suggesting that targeting Arg-II or inhibiting p38mapk could be beneficial in reducing age-associated adipose tissue inflammation (239).
In addition, studies have shown that Rolipram is a selective phosphodiesterase 4 (PDE4) inhibitor that activates the AMPK-SIRT6 pathway to reduce adipose deposition and inflammation in aged mice, suggesting that targeting the AMPK-SIRT6 pathway and selective PDE4 inhibitors may be useful agents for the treatment of age-related metabolic dysfunction and diseases (240).
In summary, adipose tissue aging is of great value for studying the basic mechanisms of aging and is an effective therapeutic target for developing new strategies to combat aging and age-related disease (Table 3).
8 Conclusions and future prospective
Adipose tissue is essential for age-related dysfunction such as metabolic diseases, while aging can also generate multiple effects on adipose tissue, including redistribution of deposits and composition, adipose tissue plasticity reduction, senescent cell accumulation and inflammaging. Among them, adipose tissue inflammation is the most important. This chronic inflammation is usually promoted by senescent/dead cell accumulation, adipocyte hypertrophy, FFA and LPS, and immune cell dysregulation. Various cellular and molecular mechanisms regulate adipose tissue inflammaging. Immune cells are recruited to adipose tissue by different chemokines, and undergo tremendous changes in both their numbers and characteristics during aging. Proinflammatory signaling pathways, including the JAK/STAT, Wnt/β-catenin, NF-κB, and MAPK signaling pathways, control the process of adipose tissue inflammaging in different way. Indeed, Increased inflammaging in aging impacts adipose tissue, leading to adipose tissue dysfunction and ectopic lipid accumulation, further impacting the overall health status. Systemic diseases, such as type II diabetes, CVD and cancer, are somewhat caused by adipose tissue inflammation. Since adipose tissue inflammaging plays pivotal roles, emerging anti-aging interventions have recently been developed targeting adipose tissue. In this review, we summarize the latest approaches that can extend healthy lifespan and delay the onset of age-related diseases including caloric restriction, senothrerapeutics, immune therapies and other strategies targeting adipose tissue inflammaging related signaling pathways. Further research may need to focus on whether suppressing the inflammatory response in adipose tissue can reverse the senescent phenotype, an approach that may identify new targets to relieve aging-associated complications.
Author contributions
Y-XZ and Z-HY wrote the manuscript and drew the figures. M-YO supervised the manuscript and modified the figures. YS provided a critical review and helped edit the manuscript. S-BZ and Q-FL conceived the idea and supervised the manuscript. All authors contributed to the article and approved the submitted version.
Funding
The authors acknowledge the financial support from the NSFC (81971848, 81620108019), the Clinical Research Plan of SHDC (SHDC2020CR1019B, SHC2020CR402), Shanghai Municipal Key Clinical Specialty (shslczdzk00901), the Innovative Research Team of High-level Local University in Shanghai (SSMU-ZDCX20180700).
Conflict of interest
The authors declare that the research was conducted in the absence of any commercial or financial relationships that could be construed as a potential conflict of interest.
The reviewer JL declared a shared parent affiliation with the authors Y-XZ, M-YO, Z-HY, S-BZ and Q-FL to the handling editor at the time of review.
Publisher’s note
All claims expressed in this article are solely those of the authors and do not necessarily represent those of their affiliated organizations, or those of the publisher, the editors and the reviewers. Any product that may be evaluated in this article, or claim that may be made by its manufacturer, is not guaranteed or endorsed by the publisher.
References
1. Park A, Kim WK, Bae K-H. Distinction of white, beige and brown adipocytes derived from mesenchymal stem cells. World J Stem Cells (2014) 6:33–42. doi: 10.4252/wjsc.v6.i1.33
2. Mittal B. Subcutaneous adipose tissue & visceral adipose tissue. Indian J Med Res (2019) 149:571–3. doi: 10.4103/ijmr.IJMR_1910_18
3. Zoico E, Rubele S, De Caro A, Nori N, Mazzali G, Fantin F, et al. Brown and beige adipose tissue and aging. Front Endocrinol (2019) 10:368–8. doi: 10.3389/fendo.2019.00368
4. Mau T, Yung R. Adipose tissue inflammation in aging. Exp Gerontol (2018) 105:27–31. doi: 10.1016/j.exger.2017.10.014
5. Von Bank H, Kirsh C, Simcox J. Aging adipose: Depot location dictates age-associated expansion and dysfunction. Ageing Res Rev (2021) 67:101259. doi: 10.1016/j.arr.2021.101259
6. Sadie-Van Gijsen H. Is adipose tissue the fountain of youth? The impact of adipose stem cell aging on metabolic homeostasis, longevity, and cell-based therapies. Adv Exp Med Biol (2021) 1286:225–50. doi: 10.1007/978-3-030-55035-6_16
7. Frasca D, Blomberg BB. Adipose tissue, immune aging, and cellular senescence. Semin Immunopathol (2020) 42:573–87. doi: 10.1007/s00281-020-00812-1
8. Goto T, Naknukool S, Yoshitake R, Hanafusa Y, Tokiwa S, Li Y, et al. Proinflammatory cytokine interleukin-1β suppresses cold-induced thermogenesis in adipocytes. Cytokine (2016) 77:107–14. doi: 10.1016/j.cyto.2015.11.001
9. Liu LF, Craig CM, Tolentino LL, Choi O, Morton J, Rivas H, et al. Adipose tissue macrophages impair preadipocyte differentiation in humans. PloS One (2017) 12:e0170728. doi: 10.1371/journal.pone.0170728
10. Ghosh AK, O'Brien M, Mau T, Qi N, Yung R. Adipose tissue senescence and inflammation in aging is reversed by the young milieu. journals gerontol Ser A Biol Sci Med Sci (2019) 74:1709–15. doi: 10.1093/gerona/gly290
11. Zamboni M, Nori N, Brunelli A, Zoico E. How does adipose tissue contribute to inflammageing? Exp Gerontol (2021) 143:111162. doi: 10.1016/j.exger.2020.111162
12. Taha S, Volkmer E, Haas E, Alberton P, Straub T, David-Rus D, et al. Differences in the inflammatory response of white adipose tissue and adipose-derived stem cells. Int J Mol Sci (2020) 21. doi: 10.3390/ijms21031086
13. Rius J, Guma M, Schachtrup C, Akassoglou K, Zinkernagel AS, Nizet V, et al. NF-kappaB links innate immunity to the hypoxic response through transcriptional regulation of HIF-1alpha. Nature (2008) 453:807–11. doi: 10.1038/nature06905
14. Shin S, El-Sabbagh AS, Lukas BE, Tanneberger SJ, Jiang Y. Adipose stem cells in obesity: challenges and opportunities. Biosci Rep (2020) 40. doi: 10.1042/BSR20194076
15. Koenen TB, Stienstra R, van Tits LJ, de Graaf J, Stalenhoef AF, Joosten LA, et al. Hyperglycemia activates caspase-1 and TXNIP-mediated IL-1beta transcription in human adipose tissue. Diabetes (2011) 60:517–24. doi: 10.2337/db10-0266
16. Muir LA, Neeley CK, Meyer KA, Baker NA, Brosius AM, Washabaugh AR, et al. Adipose tissue fibrosis, hypertrophy, and hyperplasia: Correlations with diabetes in human obesity. Obes (Silver Spring Md.) (2016) 24:597–605. doi: 10.1002/oby.21377
17. Hashimoto T, Shibasaki F. Hypoxia-inducible factor as an angiogenic master switch. Front Pediatr (2015) 3. doi: 10.3389/fped.2015.00033
18. Kawai T, Autieri MV, Scalia R. Adipose tissue inflammation and metabolic dysfunction in obesity. Am J Physiol Cell Physiol (2021) 320:C375–c391. doi: 10.1152/ajpcell.00379.2020
19. Sun K, Tordjman J, Clément K, Scherer PE. Fibrosis and adipose tissue dysfunction. Cell Metab (2013) 18:470–7. doi: 10.1016/j.cmet.2013.06.016
20. Donato AJ, Henson GD, Hart CR, Layec G, Trinity JD, Bramwell RC, et al. The impact of ageing on adipose structure, function and vasculature in the B6D2F1 mouse: evidence of significant multisystem dysfunction. J Physiol (2014) 592:4083–96. doi: 10.1113/jphysiol.2014.274175
21. Tanaka M, Ikeda K, Suganami T, Komiya C, Ochi K, Shirakawa I, et al. Macrophage-inducible c-type lectin underlies obesity-induced adipose tissue fibrosis. Nat Commun (2014) 5:4982. doi: 10.1038/ncomms5982
22. DeBari MK, Abbott RD. Adipose tissue fibrosis: Mechanisms, models, and importance. Int J Mol Sci (2020) 21:6030. doi: 10.3390/ijms21176030
23. Johnson AA, Stolzing A. The role of lipid metabolism in aging, lifespan regulation, and age-related disease. Aging Cell (2019) 18:e13048. doi: 10.1111/acel.13048
24. Mancuso P, Bouchard B. The impact of aging on adipose function and adipokine synthesis. Front Endocrinol (2019) 10. doi: 10.3389/fendo.2019.00137
25. Fernø J, Strand K, Mellgren G, Stiglund N, Björkström NK. Natural killer cells as sensors of adipose tissue stress. Trends Endocrinol Metab (2020) 31:3–12. doi: 10.1016/j.tem.2019.08.011
26. Zatterale F, Longo M, Naderi J, Raciti GA, Desiderio A, Miele C, et al. Chronic adipose tissue inflammation linking obesity to insulin resistance and type 2 diabetes. Front Physiol (2020) 10. doi: 10.3389/fphys.2019.01607
27. Oikonomou EK, Antoniades C. The role of adipose tissue in cardiovascular health and disease. Nat Rev Cardiol (2019) 16:83–99. doi: 10.1038/s41569-018-0097-6
28. Tanaka K, Sata M. Roles of perivascular adipose tissue in the pathogenesis of atherosclerosis. Front Physiol (2018) 9. doi: 10.3389/fphys.2018.00003
29. Oikonomou EK, Antoniades C. Immunometabolic regulation of vascular redox state: The role of adipose tissue. Antioxid Redox Signal (2018) 29:313–36. doi: 10.1089/ars.2017.7017
30. Xie Z, Wang X, Liu X, Du H, Sun C, Shao X, et al. Adipose-derived exosomes exert proatherogenic effects by regulating macrophage foam cell formation and polarization. J Am Heart Assoc (2018) 7. doi: 10.1161/JAHA.117.007442
31. Leonardi GC, Accardi G, Monastero R, Nicoletti F, Libra M. Ageing: From inflammation to cancer. Immun Ageing I A (2018) 15:1–1. doi: 10.1186/s12979-017-0112-5
32. Ou MY, Zhang H, Tan PC, Zhou SB, Li QF. Adipose tissue aging: Mechanisms and therapeutic implications. Cell Death Dis (2022) 13:300. doi: 10.1038/s41419-022-04752-6
33. Ghosh AK, O’Brien M, Mau T, Qi N, Yung R. Adipose tissue senescence and inflammation in aging is reversed by the young milieu. The journals of gerontology. is J Gerontol A Biol Sci Med Sci (2018) 74:1709–15. doi: 10.1093/gerona/gly290
34. Smith U, Li Q, Rydén M, Spalding KL. Cellular senescence and its role in white adipose tissue. Int J Obes (2021) 45:934–43. doi: 10.1038/s41366-021-00757-x
35. Kumari R, Jat P. Mechanisms of cellular senescence: Cell cycle arrest and senescence associated secretory phenotype. Front Cell Dev Biol (2021) 9. doi: 10.3389/fcell.2021.645593
36. Schosserer M, Grillari J, Wolfrum C, Scheideler M. Age-induced changes in white, brite, and brown adipose depots: A mini-review. Gerontology (2018) 64:229–36. doi: 10.1159/000485183
37. Mantovani C, Terenghi G, Magnaghi V. Senescence in adipose-derived stem cells and its implications in nerve regeneration. Neural regen Res (2014) 9:10–5. doi: 10.4103/1673-5374.125324
38. Truong NC, Bui KH, Van Pham P. Characterization of senescence of human adipose-derived stem cells after long-term expansion. Adv Exp Med Biol (2019) 1084:109–28. doi: 10.1007/5584_2018_235
39. Yu P, Yuan R, Yang X, Qi Z. Adipose tissue, aging, and metabolism. Curr Opin Endocr Metab Res (2019) 5:11–20. doi: 10.1016/j.coemr.2019.02.003
40. Toussaint O, Royer V, Salmon M, Remacle J. Stress-induced premature senescence and tissue ageing. Biochem Pharmacol (2002) 64:1007–9. doi: 10.1016/S0006-2952(02)01170-X
41. Kuroda M, Sakaue H. Adipocyte death and chronic inflammation in obesity. J Med Invest (2017) 64:193–6. doi: 10.2152/jmi.64.193
42. De Carvalho FG, Justice JN, Freitas E, Kershaw EE, Sparks LM. Adipose tissue quality in aging: How structural and functional aspects of adipose tissue impact skeletal muscle quality. Nutrients (2019) 11:2553. doi: 10.3390/nu11112553
43. Lindhorst A, Raulien N, Wieghofer P, Eilers J, Rossi FMV, Bechmann I, et al. Adipocyte death triggers a pro-inflammatory response and induces metabolic activation of resident macrophages. Cell Death Dis (2021) 12:579. doi: 10.1038/s41419-021-03872-9
44. Rock KL, Lai JJ, Kono H. Innate and adaptive immune responses to cell death. Immunol Rev (2011) 243:191–205. doi: 10.1111/j.1600-065X.2011.01040.x
45. Guo H, Callaway JB, Ting JP. Inflammasomes: Mechanism of action, role in disease, and therapeutics. Nat Med (2015) 21:677–87. doi: 10.1038/nm.3893
46. Liu F, He J, Wang H, Zhu D, Bi Y. Adipose morphology: A critical factor in regulation of human metabolic diseases and adipose tissue dysfunction. Obes Surg (2020) 30:5086–100. doi: 10.1007/s11695-020-04983-6
47. Rausch ME, Weisberg S, Vardhana P, Tortoriello DV. Obesity in C57BL/6J mice is characterized by adipose tissue hypoxia and cytotoxic T-cell infiltration. Int J Obes (2008) 32:451–63. doi: 10.1038/sj.ijo.0803744
48. Krock BL, Skuli N, Simon MC. Hypoxia-induced angiogenesis: good and evil. Genes Cancer (2011) 2:1117–33. doi: 10.1177/1947601911423654
49. Michailidou Z. Fundamental roles for hypoxia signalling in adipose tissue metabolism and inflammation in obesity. Curr Opin Physiol (2019) 12:39–43. doi: 10.1016/j.cophys.2019.09.005
50. Trayhurn P. Hypoxia and adipose tissue function and dysfunction in obesity. Physiol Rev (2013) 93:1–21. doi: 10.1152/physrev.00017.2012
51. Kang L, Ayala JE, Lee-Young RS, Zhang Z, James FD, Neufer PD, et al. Diet-induced muscle insulin resistance is associated with extracellular matrix remodeling and interaction with integrin alpha2beta1 in mice. Diabetes (2011) 60:416–26. doi: 10.2337/db10-1116
52. Datta R, Podolsky MJ, Atabai K. Fat fibrosis: Friend or foe? JCI Insight (2018) 3. doi: 10.1172/jci.insight.122289
53. Ruiz-Ojeda FJ, Méndez-Gutiérrez A, Aguilera CM, Plaza-Díaz J. Extracellular matrix remodeling of adipose tissue in obesity and metabolic diseases. Int J Mol Sci (2019) 20:4888. doi: 10.3390/ijms20194888
54. Pyrina I, Chung K-J, Michailidou Z, Koutsilieris M, Chavakis T, Chatzigeorgiou A. Fate of adipose progenitor cells in obesity-related chronic inflammation. Front Cell Dev Biol (2020) 8. doi: 10.3389/fcell.2020.00644
55. Marcelin G, Silveira ALM, Martins LB, Ferreira AV, Clément K. Deciphering the cellular interplays underlying obesity-induced adipose tissue fibrosis. J Clin Invest (2019) 129:4032–40. doi: 10.1172/JCI129192
56. Trim W, Turner JE, Thompson D. Parallels in immunometabolic adipose tissue dysfunction with ageing and obesity. Front Immunol (2018) 9:169. doi: 10.3389/fimmu.2018.00169
57. Rogero MM, Calder PC. Obesity, inflammation, toll-like receptor 4 and fatty acids. Nutrients (2018) 10:432. doi: 10.3390/nu10040432
58. Hoareau L, Bencharif K, Rondeau P, Murumalla R, Ravanan P, Tallet F, et al. Signaling pathways involved in LPS induced TNFalpha production in human adipocytes. J Inflammation (Lond) (2010) 7:1. doi: 10.1186/1476-9255-7-1
59. Dahlquist KJV, Camell CD. Aging leukocytes and the inflammatory microenvironment of the adipose tissue. Diabetes (2021) 71:23–30. doi: 10.2337/dbi21-0013
60. Kane H, Lynch L. Innate immune control of adipose tissue homeostasis. Trends Immunol (2019) 40:857–72. doi: 10.1016/j.it.2019.07.006
61. Lu B, Huang L, Cao J, Li L, Wu W, Chen X, et al. Adipose tissue macrophages in aging-associated adipose tissue function. J Physiol Sci (2021) 71:38. doi: 10.1186/s12576-021-00820-2
62. Khan S, Chan YT, Revelo XS, Winer DA. The immune landscape of visceral adipose tissue during obesity and aging. Front Endocrinol (2020) 11. doi: 10.3389/fendo.2020.00267
63. Chung KJ, Nati M, Chavakis T, Chatzigeorgiou A. Innate immune cells in the adipose tissue. Rev Endocr Metab Disord (2018) 19:283–92. doi: 10.1007/s11154-018-9451-6
64. Hildreth AD, Ma F, Wong YY, Sun R, Pellegrini M, O’Sullivan TE. Single-cell sequencing of human white adipose tissue identifies new cell states in health and obesity. Nat Immunol (2021) 22:639–53. doi: 10.1038/s41590-021-00922-4
65. Thomas D, Apovian C. Macrophage functions in lean and obese adipose tissue. Metabolism (2017) 72:120–43. doi: 10.1016/j.metabol.2017.04.005
66. Garg SK, Delaney C, Shi H, Yung R. Changes in adipose tissue macrophages and T cells during aging. Crit Rev Immunol (2014) 34:1–14. doi: 10.1615/CritRevImmunol.2013006833
67. Batista A, Rodvold JJ, Xian S, Searles SC, Lew A, Iwawaki T, et al. IRE1α regulates macrophage polarization, PD-L1 expression, and tumor survival. PloS Biol (2020) 18:e3000687–e3000687. doi: 10.1371/journal.pbio.3000687
68. Shan B, Wang X, Wu Y, Xu C, Xia Z, Dai J, et al. The metabolic ER stress sensor IRE1α suppresses alternative activation of macrophages and impairs energy expenditure in obesity. Nat Immunol (2017) 18:519–29. doi: 10.1038/ni.3709
69. Torres-Castro I, Arroyo-Camarena ÚD, Martínez-Reyes CP, Gómez-Arauz AY, Dueñas-Andrade Y, Hernández-Ruiz J, et al. Human monocytes and macrophages undergo M1-type inflammatory polarization in response to high levels of glucose. Immunol Lett (2016) 176:81–9. doi: 10.1016/j.imlet.2016.06.001
70. Miranda K, Yang X, Bam M, Murphy EA, Nagarkatti PS, Nagarkatti M. MicroRNA-30 modulates metabolic inflammation by regulating notch signaling in adipose tissue macrophages. Int J Obes (2018) 42:1140–50. doi: 10.1038/s41366-018-0114-1
71. Li Y, Yun K, Mu R. A review on the biology and properties of adipose tissue macrophages involved in adipose tissue physiological and pathophysiological processes. Lipids Health Dis (2020) 19:164. doi: 10.1186/s12944-020-01342-3
72. Aamir K, Khan HU, Sethi G, Hossain MA, Arya A. Wnt signaling mediates TLR pathway and promote unrestrained adipogenesis and metaflammation: Therapeutic targets for obesity and type 2 diabetes. Pharmacol Res (2020) 152:104602. doi: 10.1016/j.phrs.2019.104602
73. Moraes-Vieira PM, Yore MM, Sontheimer-Phelps A, Castoldi A, Norseen J, Aryal P, et al. Retinol binding protein 4 primes the NLRP3 inflammasome by signaling through toll-like receptors 2 and 4. Proc Natl Acad Sci United States America (2020) 117:31309–18. doi: 10.1073/pnas.2013877117
74. Matacchione G, Perugini J, Di Mercurio E, Sabbatinelli J, Prattichizzo F, Senzacqua M, et al. Senescent macrophages in the human adipose tissue as a source of inflammaging. GeroScience (2022). doi: 10.1007/s11357-022-00536-0
75. Brunner JS, Vogel A, Lercher A, Caldera M, Korosec A, Pühringer M, et al. The PI3K pathway preserves metabolic health through MARCO-dependent lipid uptake by adipose tissue macrophages. Nat Metab (2020) 2:1427–42. doi: 10.1038/s42255-020-00311-5
76. Mráz M, Cinkajzlová A, Kloučková J, Lacinová Z, Kratochvílová H, Lipš M, et al. Dendritic cells in subcutaneous and epicardial adipose tissue of subjects with type 2 diabetes, obesity, and coronary artery disease. Mediators Inflammation (2019) 2019:5481725. doi: 10.1155/2019/5481725
77. Soedono S, Cho KW. Adipose tissue dendritic cells: Critical regulators of obesity-induced inflammation and insulin resistance. Int J Mol Sci (2021) 22:8666. doi: 10.3390/ijms22168666
78. Swafford D, Manicassamy S. Wnt signaling in dendritic cells: Its role in regulation of immunity and tolerance. Discovery Med (2015) 19:303–10.
79. LaMarche NM, Lynch L. Adipose dendritic cells come out of hiding. Cell Metab (2018) 27:485–6. doi: 10.1016/j.cmet.2018.02.014
80. Macdougall CE, Wood EG, Loschko J, Scagliotti V, Cassidy FC, Robinson ME, et al. Visceral adipose tissue immune homeostasis is regulated by the crosstalk between adipocytes and dendritic cell subsets. Cell Metab (2018) 27:588–601.e584. doi: 10.1016/j.cmet.2018.02.007
81. Wculek SK, Khouili SC, Priego E, Heras-Murillo I, Sancho D. Metabolic control of dendritic cell functions: Digesting information. Front Immunol (2019) 10. doi: 10.3389/fimmu.2019.00775
82. Jeon S-M. Regulation and function of AMPK in physiology and diseases. Exp Mol Med (2016) 48:e245–5. doi: 10.1038/emm.2016.81
83. Mori T, Suzuki-Yamazaki N, Takaki S. Lnk/Sh2b3 regulates adipose inflammation and glucose tolerance through group 1 ILCs. Cell Rep (2018) 24:1830–41. doi: 10.1016/j.celrep.2018.07.036
84. Saetang J, Sangkhathat S. Role of innate lymphoid cells in obesity and metabolic disease (Review). Mol Med Rep (2018) 17:1403–12. doi: 10.3892/mmr.2017.8038
85. Murphy JM, Ngai L, Mortha A, Crome SQ. Tissue-dependent adaptations and functions of innate lymphoid cells. Front Immunol (2022) 13. doi: 10.3389/fimmu.2022.836999
86. Wang H, Shen L, Sun X, Liu F, Feng W, Jiang C, et al. Adipose group 1 innate lymphoid cells promote adipose tissue fibrosis and diabetes in obesity. Nat Commun (2019) 10:3254. doi: 10.1038/s41467-019-11270-1
87. Sasaki T, Moro K, Kubota T, Kubota N, Kato T, Ohno H, et al. Innate lymphoid cells in the induction of obesity. Cell Rep (2019) 28:202–217.e207. doi: 10.1016/j.celrep.2019.06.016
88. Björkström NK, Ljunggren HG, Michaëlsson J. Emerging insights into natural killer cells in human peripheral tissues. Nat Rev Immunol (2016) 16:310–20. doi: 10.1038/nri.2016.34
89. Stiglund N, Strand K, Cornillet M, Stål P, Thorell A, Zimmer CL, et al. Retained NK cell phenotype and functionality in non-alcoholic fatty liver disease. Front Immunol (2019) 10:1255. doi: 10.3389/fimmu.2019.01255
90. Lumeng CN, Liu J, Geletka L, Delaney C, Delproposto J, Desai A, et al. Aging is associated with an increase in T cells and inflammatory macrophages in visceral adipose tissue. J Immunol (2011) 187:6208–16. doi: 10.4049/jimmunol.1102188
91. Nishimura S, Manabe I, Nagasaki M, Eto K, Yamashita H, Ohsugi M, et al. CD8+ effector T cells contribute to macrophage recruitment and adipose tissue inflammation in obesity. Nat Med (2009) 15:914–20. doi: 10.1038/nm.1964
92. Jiang E, Perrard XD, Yang D, Khan IM, Perrard JL, Smith CW, et al. Essential role of CD11a in CD8+ T-cell accumulation and activation in adipose tissue. Arterioscler Thromb Vasc Biol (2014) 34:34. doi: 10.1161/ATVBAHA.113.302077
93. Kalathookunnel Antony A, Lian Z, Wu H. T Cells in adipose tissue in aging. Front Immunol (2018) 9. doi: 10.3389/fimmu.2018.02945
94. Morris DL, Oatmen KE, Mergian TA, Cho KW, DelProposto JL, Singer K, et al. CD40 promotes MHC class II expression on adipose tissue macrophages and regulates adipose tissue CD4+ T cells with obesity. J Leukoc Biol (2016) 99:1107–19. doi: 10.1189/jlb.3A0115-009R
95. Chuang H-C, Sheu WHH, Lin Y-T, Tsai C-Y, Yang C-Y, Cheng Y-J, et al. HGK/MAP4K4 deficiency induces TRAF2 stabilization and Th17 differentiation leading to insulin resistance. Nat Commun (2014) 5:4602. doi: 10.1038/ncomms5602
96. Zhou L, Ivanov II, Spolski R, Min R, Shenderov K, Egawa T, et al. IL-6 programs T(H)-17 cell differentiation by promoting sequential engagement of the IL-21 and IL-23 pathways. Nat Immunol (2007) 8:967–74. doi: 10.1038/ni1488
97. Zhou H, Liu F. Regulation, communication, and functional roles of adipose tissue-resident CD4(+) T cells in the control of metabolic homeostasis. Front Immunol (2018) 9:1961. doi: 10.3389/fimmu.2018.01961
98. Schwartz C, Schmidt V, Deinzer A, Hawerkamp HC, Hams E, Bayerlein J, et al. Innate PD-L1 limits T cell-mediated adipose tissue inflammation and ameliorates diet-induced obesity. Sci Transl Med (2022) 14:eabj6879. doi: 10.1126/scitranslmed.abj6879
99. Wang L, Sun P, Wu Y, Wang L. Metabolic tissue-resident CD8(+) T cells: A key player in obesity-related diseases. Obes Rev (2021) 22:e13133. doi: 10.1111/obr.13133
100. Bruno MEC, Mukherjee S, Powell WL, Mori SF, Wallace FK, Balasuriya BK, et al. Accumulation of γδ T cells in visceral fat with aging promotes chronic inflammation. Geroscience (2022). doi: 10.1007/s11357-022-00572-w
101. Hu B, Jin C, Zeng X, Resch JM, Jedrychowski MP, Yang Z, et al. γδ T cells and adipocyte IL-17RC control fat innervation and thermogenesis. Nature (2020) 578:610–4. doi: 10.1038/s41586-020-2028-z
102. Srikakulapu P, McNamara CA. B lymphocytes and adipose tissue inflammation. Arterioscler Thromb Vasc Biol (2020) 40:1110–22. doi: 10.1161/ATVBAHA.119.312467
103. Khan S, Tsai S, Winer DA. Adipose tissue b cells come of age: The AABs of fat inflammation. Cell Metab (2019) 30:997–9. doi: 10.1016/j.cmet.2019.11.007
104. Camell CD, Günther P, Lee A, Goldberg EL, Spadaro O, Youm YH, et al. Aging induces an Nlrp3 inflammasome-dependent expansion of adipose b cells that impairs metabolic homeostasis. Cell Metab (2019) 30:1024–1039.e1026. doi: 10.1016/j.cmet.2019.10.006
105. Reyes-Farias M, Fos-Domenech J, Serra D, Herrero L, Sánchez-Infantes D. White adipose tissue dysfunction in obesity and aging. Biochem Pharmacol (2021) 192:114723. doi: 10.1016/j.bcp.2021.114723
106. Richard AJ, Stephens JM. The role of JAK-STAT signaling in adipose tissue function. Biochim Biophys Acta (2014) 1842:431–9. doi: 10.1016/j.bbadis.2013.05.030
107. Bouhlel MA, Derudas B, Rigamonti E, Dièvart R, Brozek J, Haulon S, et al. PPARgamma activation primes human monocytes into alternative M2 macrophages with anti-inflammatory properties. Cell Metab (2007) 6:137–43. doi: 10.1016/j.cmet.2007.06.010
108. Lee Y-J, Kim B-M, Ahn Y-H, Choi JH, Choi Y-H, Kang JL. STAT6 signaling mediates PPARγ activation and resolution of acute sterile inflammation in mice. Cells (2021) 10:501. doi: 10.3390/cells10030501
109. Tellechea M, Buxadé M, Tejedor S, Aramburu J, López-Rodríguez C. NFAT5-regulated macrophage polarization supports the proinflammatory function of macrophages and T lymphocytes. J Immunol (2018) 200:305–15. doi: 10.4049/jimmunol.1601942
110. Li C, Xu MM, Wang K, Adler AJ, Vella AT, Zhou B. Macrophage polarization and meta-inflammation. Trans Res (2018) 191:29–44. doi: 10.1016/j.trsl.2017.10.004
111. Li C, Qu L, Farragher C, Vella A, Zhou B. MicroRNA regulated macrophage activation in obesity. J Trans Internal Med (2019) 7:46–52. doi: 10.2478/jtim-2019-0011
112. Hui X, Zhang M, Gu P, Li K, Gao Y, Wu D, et al. Adipocyte SIRT1 controls systemic insulin sensitivity by modulating macrophages in adipose tissue. EMBO Rep (2017) 18:645–57. doi: 10.15252/embr.201643184
113. Cantó C, Gerhart-Hines Z, Feige JN, Lagouge M, Noriega L, Milne JC, et al. AMPK regulates energy expenditure by modulating NAD+ metabolism and SIRT1 activity. Nature (2009) 458:1056–60. doi: 10.1038/nature07813
114. Hernández-García E, Cueto FJ, Cook ECL, Redondo-Urzainqui A, Charro-Zanca S, Robles-Vera I, et al. Conventional type 1 dendritic cells protect against age-related adipose tissue dysfunction and obesity. Cell Mol Immunol (2022) 19:260–75. doi: 10.1038/s41423-021-00812-7
115. Zlotnikov-Klionsky Y, Nathansohn-Levi B, Shezen E, Rosen C, Kagan S, Bar-On L, et al. Perforin-positive dendritic cells exhibit an immuno-regulatory role in metabolic syndrome and autoimmunity. Immunity (2015) 43:776–87. doi: 10.1016/j.immuni.2015.08.015
116. Larrick JW, Mendelsohn AR. Eosinophils and white fat: Protection from worms and inflammaging. Rejuvenation Res (2020) 23:349–52. doi: 10.1089/rej.2020.2375
117. Wu D, Molofsky AB, Liang HE, Ricardo-Gonzalez RR, Jouihan HA, Bando JK, et al. Eosinophils sustain adipose alternatively activated macrophages associated with glucose homeostasis. Science (2011) 332:243–7. doi: 10.1126/science.1201475
118. Qiu Y, Nguyen Khoa D, Odegaard Justin I, Cui X, Tian X, Locksley Richard M, et al. Eosinophils and type 2 cytokine signaling in macrophages orchestrate development of functional beige fat. Cell (2014) 157:1292–308. doi: 10.1016/j.cell.2014.03.066
119. Geng L, Lam KSL, Xu A. The therapeutic potential of FGF21 in metabolic diseases: from bench to clinic. Nat Rev Endocrinol (2020) 16:654–67. doi: 10.1038/s41574-020-0386-0
120. Jeanson Y, Ribas F, Galinier A, Arnaud E, Ducos M, André M, et al. Lactate induces FGF21 expression in adipocytes through a p38-MAPK pathway. Biochem J (2016) 473:685–92. doi: 10.1042/BJ20150808
121. Huang Z, Zhong L, Lee JTH, Zhang J, Wu D, Geng L, et al. The FGF21-CCL11 axis mediates beiging of white adipose tissues by coupling sympathetic nervous system to type 2 immunity. Cell Metab (2017) 26:493–508.e494. doi: 10.1016/j.cmet.2017.08.003
122. Vohralik EJ, Psaila AM, Knights AJ, Quinlan KGR. EoTHINophils: Eosinophils as key players in adipose tissue homeostasis. Clin Exp Pharmacol Physiol (2020) 47:1495–505. doi: 10.1111/1440-1681.13304
123. Mathur SK, Schwantes EA, Jarjour NN, Busse WW. Age-related changes in eosinophil function in human subjects. Chest (2008) 133:412–9. doi: 10.1378/chest.07-2114
124. Teixeira AL, Gama CS, Rocha NP, Teixeira MM. Revisiting the role of eotaxin-1/CCL11 in psychiatric disorders. Front Psychiatry (2018) 9. doi: 10.3389/fpsyt.2018.00241
125. Brigger D, Riether C, van Brummelen R, Mosher KI, Shiu A, Ding Z, et al. Eosinophils regulate adipose tissue inflammation and sustain physical and immunological fitness in old age. Nat Metab (2020) 2:688–702. doi: 10.1038/s42255-020-0228-3
126. Brüggen MC, Strobl J, Koszik F, Naito R, Vierhapper M, Li N, et al. Subcutaneous white adipose tissue of healthy young individuals harbors a leukocyte compartment distinct from skin and blood. J Invest Dermatol (2019) 139:2052–2055.e2057. doi: 10.1016/j.jid.2019.02.034
127. Wang C, Deng L, Hong M, Akkaraju GR, Inoue J, Chen ZJ. TAK1 is a ubiquitin-dependent kinase of MKK and IKK. Nature (2001) 412:346–51. doi: 10.1038/35085597
128. Mjösberg J, Bernink J, Golebski K, Karrich Julien J, Peters Charlotte P, Blom B, et al. The transcription factor GATA3 is essential for the function of human type 2 innate lymphoid cells. Immunity (2012) 37:649–59. doi: 10.1016/j.immuni.2012.08.015
129. Kohlgruber AC, Gal-Oz ST, LaMarche NM, Shimazaki M, Duquette D, Koay HF, et al. γδ T cells producing interleukin-17A regulate adipose regulatory T cell homeostasis and thermogenesis. Nat Immunol (2018) 19:464–74. doi: 10.1038/s41590-018-0094-2
130. Zhang H, Xue R, Zhu S, Fu S, Chen Z, Zhou R, et al. M2-specific reduction of CD1d switches NKT cell-mediated immune responses and triggers metaflammation in adipose tissue. Cell Mol Immunol (2018) 15:506–17. doi: 10.1038/cmi.2017.11
131. Bapat SP, Suh JM, Fang S, Liu S, Ye Z. Depletion of fat-resident treg cells prevents age-associated insulin resistance. Nature (2015) 528:137. doi: 10.1038/nature16151
132. Feuerer M, Herrero L, Cipolletta D, Naaz A, Wong J, Nayer A, et al. Lean, but not obese, fat is enriched for a unique population of regulatory T cells that affect metabolic parameters. Nat Med (2009) 15:930–9. doi: 10.1038/nm.2002
133. Mittelsteadt KL, Hayes ET, Campbell DJ. ICOS signaling limits regulatory T cell accumulation and function in visceral adipose tissue. J Exp Med (2021) 218. doi: 10.1084/jem.20201142
134. Kälin S, Becker M, Ott VB, Serr I, Hosp F, Mollah MMH, et al. A Stat6/Pten axis links regulatory T cells with adipose tissue function. Cell Metab (2017) 26:475–492.e477. doi: 10.1016/j.cmet.2017.08.008
135. Li C, Wang G, Sivasami P, Ramirez RN, Zhang Y, Benoist C, et al. Interferon-α-producing plasmacytoid dendritic cells drive the loss of adipose tissue regulatory T cells during obesity. Cell Metab (2021) 33:1610–1623.e1615. doi: 10.1016/j.cmet.2021.06.007
136. Li Y, Lu Y, Lin SH, Li N, Han Y, Huang Q, et al. Insulin signaling establishes a developmental trajectory of adipose regulatory T cells. Nat Immunol (2021) 22:1175–85. doi: 10.1038/s41590-021-01010-3
137. Hu ZQ, Zhao WH. The IL-33/ST2 axis is specifically required for development of adipose tissue-resident regulatory T cells. Cell Mol Immunol (2015) 12:521–4. doi: 10.1038/cmi.2015.49
138. Wara AK, Wang S, Wu C, Fang F, Haemmig S, Weber BN, et al. KLF10 deficiency in CD4(+) T cells triggers obesity, insulin resistance, and fatty liver. Cell Rep (2020) 33:108550. doi: 10.1016/j.celrep.2020.108550
139. Sivasami P, Li C. Derivation and differentiation of adipose-tissue regulatory T cells: A stepwise, multi-site process. Front Immunol (2020) 11:599277. doi: 10.3389/fimmu.2020.599277
140. Beppu LY, Mooli RGR, Qu X, Marrero GJ, Finley CA, Fooks AN, et al. Tregs facilitate obesity and insulin resistance via a blimp-1/IL-10 axis. JCI Insight (2021) 6. doi: 10.1172/jci.insight.140644
141. Wang Q, Wu H. T Cells in adipose tissue: Critical players in immunometabolism. Front Immunol (2018) 9. doi: 10.3389/fimmu.2018.02509
142. Park J, Huh JY, Oh J, Kim JI, Han SM, Shin KC, et al. Activation of invariant natural killer T cells stimulates adipose tissue remodeling via adipocyte death and birth in obesity. Genes Dev (2019) 33:1657–72. doi: 10.1101/gad.329557.119
143. LaMarche NM, Kane H, Kohlgruber AC, Dong H, Lynch L, Brenner MB. Distinct iNKT cell populations use IFNγ or ER stress-induced IL-10 to control adipose tissue homeostasis. Cell Metab (2020) 32:243–258.e246. doi: 10.1016/j.cmet.2020.05.017
144. Varghese M, Griffin C, McKernan K, Eter L, Abrishami S, Singer K. Female adipose tissue has improved adaptability and metabolic health compared to males in aged obesity. Aging (Albany NY) (2020) 12:1725–46. doi: 10.18632/aging.102709
145. Albright JM, Dunn RC, Shults JA, Boe DM, Afshar M, Kovacs EJ. Advanced age alters monocyte and macrophage responses. Antioxid Redox Signaling (2016) 25:805–15. doi: 10.1089/ars.2016.6691
146. Sundara Rajan S, Longhi MP. Dendritic cells and adipose tissue. Immunology (2016) 149:353–61. doi: 10.1111/imm.12653
147. Hadad N, Burgazliev O, Elgazar-Carmon V, Solomonov Y, Wueest S, Item F, et al. Induction of cytosolic phospholipase a2α is required for adipose neutrophil infiltration and hepatic insulin resistance early in the course of high-fat feeding. Diabetes (2013) 62:3053–63. doi: 10.2337/db12-1300
148. Tam TH, Chan KL, Boroumand P, Liu Z, Brozinick JT, Bui HH, et al. Nucleotides released from palmitate-activated murine macrophages attract neutrophils. J Biol Chem (2020) 295:4902–11. doi: 10.1074/jbc.RA119.010868
149. Suffiotti M, Carmona SJ, Jandus C, Gfeller D. Identification of innate lymphoid cells in single-cell RNA-seq data. Immunogenetics (2017) 69:439–50. doi: 10.1007/s00251-017-1002-x
150. Pan X-X, Yao K-L, Yang Y-F, Ge Q, Zhang R, Gao P-J, et al. Senescent T cell induces brown adipose tissue “Whitening” Via secreting IFN-γ. Front Cell Dev Biol (2021) 9. doi: 10.3389/fcell.2021.637424
151. Carter S, Miard S, Caron A, Sallé-Lefort S, St-Pierre P, Anhê FF, et al. Loss of OcaB prevents age-induced fat accretion and insulin resistance by altering b-lymphocyte transition and promoting energy expenditure. Diabetes (2018) 67:1285–96. doi: 10.2337/db17-0558
152. Muir LA, Kiridena S, Griffin C, DelProposto JB, Geletka L, Martinez-Santibañez G, et al. Frontline science: Rapid adipose tissue expansion triggers unique proliferation and lipid accumulation profiles in adipose tissue macrophages. J Leukoc Biol (2018) 103:615–28. doi: 10.1002/JLB.3HI1017-422R
153. Michailidou Z, Gomez-Salazar M, Alexaki VI. Innate immune cells in the adipose tissue in health and metabolic disease. J Innate Immun (2022) 14:4–30. doi: 10.1159/000515117
154. Wu H, Ballantyne CM. Metabolic inflammation and insulin resistance in obesity. Circ Res (2020) 126:1549–64. doi: 10.1161/CIRCRESAHA.119.315896
155. Żelechowska P, Agier J, Kozłowska E, Brzezińska-Błaszczyk E. Mast cells participate in chronic low-grade inflammation within adipose tissue. Obes Rev (2018) 19:686–97. doi: 10.1111/obr.12670
156. Altintas MM, Nayer B, Walford EC, Johnson KB, Gaidosh G, Reiser J, et al. Leptin deficiency-induced obesity affects the density of mast cells in abdominal fat depots and lymph nodes in mice. Lipids Health Dis (2012) 11:21. doi: 10.1186/1476-511X-11-21
157. Bonamichi B, Lee J. Unusual suspects in the development of obesity-induced inflammation and insulin resistance: NK cells, iNKT cells, and ILCs. Diabetes Metab J (2017) 41:229–50. doi: 10.4093/dmj.2017.41.4.229
158. Shi SY, Luk CT, Brunt JJ, Sivasubramaniyam T, Lu S-Y, Schroer SA, et al. Adipocyte-specific deficiency of janus kinase (JAK) 2 in mice impairs lipolysis and increases body weight, and leads to insulin resistance with ageing. Diabetologia (2014) 57:1016–26. doi: 10.1007/s00125-014-3185-0
159. Richard AJ, Stephens JM. The role of JAK–STAT signaling in adipose tissue function. Biochim Biophys Acta (BBA) - Mol Basis Dis (2014) 1842:431–9. doi: 10.1016/j.bbadis.2013.05.030
160. Burrell JA, Boudreau A, Stephens JM. Latest advances in STAT signaling and function in adipocytes. Clin Sci (Lond) (2020) 134:629–39. doi: 10.1042/CS20190522
161. McGillicuddy FC, Chiquoine EH, Hinkle CC, Kim RJ, Shah R, Roche HM, et al. Interferon gamma attenuates insulin signaling, lipid storage, and differentiation in human adipocytes via activation of the JAK/STAT pathway. J Biol Chem (2009) 284:31936–44. doi: 10.1074/jbc.M109.061655
162. Toubal A, Treuter E, Clément K, Venteclef N. Genomic and epigenomic regulation of adipose tissue inflammation in obesity. Trends Endocrinol Metab (2013) 24:625–34. doi: 10.1016/j.tem.2013.09.006
163. Bijland S, Mancini Sarah J, Salt Ian P. Role of AMP-activated protein kinase in adipose tissue metabolism and inflammation. Clin Sci (2013) 124:491–507. doi: 10.1042/CS20120536
164. Sanchez-Infantes D, White UA, Elks CM, Morrison RF, Gimble JM, Considine RV, et al. Oncostatin m is produced in adipose tissue and is regulated in conditions of obesity and type 2 diabetes. J Clin Endocrinol Metab (2014) 99:E217–25. doi: 10.1210/jc.2013-3555
165. Elks CM, Zhao P, Grant RW, Hang H, Bailey JL, Burk DH, et al. Loss of oncostatin m signaling in adipocytes induces insulin resistance and adipose tissue inflammation in vivo. J Biol Chem (2016) 291:17066–76. doi: 10.1074/jbc.M116.739110
166. Babaei R, Schuster M, Meln I, Lerch S, Ghandour RA, Pisani DF, et al. Jak-TGFβ cross-talk links transient adipose tissue inflammation to beige adipogenesis. Sci Signal (2018) 11. doi: 10.2337/db14-1164
167. Fuster JJ, Zuriaga MA, Ngo DT-M, Farb MG, Aprahamian T, Yamaguchi TP, et al. Noncanonical wnt signaling promotes obesity-induced adipose tissue inflammation and metabolic dysfunction independent of adipose tissue expansion. Diabetes (2014) 64:1235–48. doi: 10.2337/db14-1164
168. Das B, Das M, Kalita A, Baro MR. The role of wnt pathway in obesity induced inflammation and diabetes: A review. J Diabetes Metab Disord (2021) 20:1871–82. doi: 10.1007/s40200-021-00862-8
169. Ouchi N, Higuchi A, Ohashi K, Oshima Y, Gokce N, Shibata R, et al. Sfrp5 is an anti-inflammatory adipokine that modulates metabolic dysfunction in obesity. Science (2010) 329:454–7. doi: 10.1126/science.1188280
170. Beretta M, Bauer M, Hirsch E. PI3K signaling in the pathogenesis of obesity: The cause and the cure. Adv Biol Regul (2015) 58:1–15. doi: 10.1016/j.jbior.2014.11.004
171. Agrawal A, Agrawal S, Cao JN, Su H, Osann K, Gupta S. Altered innate immune functioning of dendritic cells in elderly humans: A role of phosphoinositide 3-kinase-signaling pathway. J Immunol (2007) 178:6912–22. doi: 10.4049/jimmunol.178.11.6912
172. Watanabe Y, Nagai Y, Honda H, Okamoto N, Yanagibashi T, Ogasawara M, et al. Bidirectional crosstalk between neutrophils and adipocytes promotes adipose tissue inflammation. FASEB J (2019) 33:11821–35. doi: 10.1096/fj.201900477RR
173. Jiao P, Ma J, Feng B, Zhang H, Diehl JA, Chin YE, et al. FFA-induced adipocyte inflammation and insulin resistance: Involvement of ER stress and IKKβ pathways. Obes (Silver Spring) (2011) 19:483–91. doi: 10.1038/oby.2010.200
174. Zhao P, Saltiel AR. Interaction of adipocyte metabolic and immune functions through TBK1. Front Immunol (2020) 11:592949. doi: 10.3389/fimmu.2020.592949
175. Zhao P, Wong KI, Sun X, Reilly SM, Uhm M, Liao Z, et al. TBK1 at the crossroads of inflammation and energy homeostasis in adipose tissue. Cell (2018) 172:731–743.e712. doi: 10.1016/j.cell.2018.01.007
176. Shafiei-Jahani P, Hurrell BP, Galle-Treger L, Helou DG, Howard E, Painter J, et al. DR3 stimulation of adipose resident ILC2s ameliorates type 2 diabetes mellitus. Nat Commun (2020) 11:4718. doi: 10.1038/s41467-020-18601-7
177. Aouadi M, Laurent K, Prot M, Le Marchand-Brustel Y, Binétruy B, Bost F. Inhibition of p38MAPK increases adipogenesis from embryonic to adult stages. Diabetes (2006) 55:281–9. doi: 10.2337/diabetes.55.02.06.db05-0963
178. Cereijo R, Gavaldà-Navarro A, Cairó M, Quesada-López T, Villarroya J, Morón-Ros S, et al. CXCL14, a brown adipokine that mediates brown-Fat-to-Macrophage communication in thermogenic adaptation. Cell Metab (2018) 28:750–763.e756. doi: 10.1016/j.cmet.2018.07.015
179. Gurung P, Moussa K, Adams-Huet B, Devaraj S, Jialal I. Increased mast cell abundance in adipose tissue of metabolic syndrome: Relevance to the proinflammatory state and increased adipose tissue fibrosis. Am J Physiol Endocrinol Metab (2019) 316:E504–e509. doi: 10.1152/ajpendo.00462.2018
180. Trujillo ME, Lee MJ, Sullivan S, Feng J, Schneider SH, Greenberg AS, et al. Tumor necrosis factor alpha and glucocorticoid synergistically increase leptin production in human adipose tissue: Role for p38 mitogen-activated protein kinase. J Clin Endocrinol Metab (2006) 91:1484–90. doi: 10.1210/jc.2005-1901
181. Leiva M, Matesanz N, Pulgarín-Alfaro M, Nikolic I, Sabio G. Uncovering the role of p38 family members in adipose tissue physiology. Front Endocrinol (2020) 11. doi: 10.3389/fendo.2020.572089
182. Zhang W, Zhang X, Wang H, Guo X, Li H, Wang Y, et al. AMP-activated protein kinase α1 protects against diet-induced insulin resistance and obesity. Diabetes (2012) 61:3114–25. doi: 10.2337/db11-1373
183. Mancini SJ, White AD, Bijland S, Rutherford C, Graham D, Richter EA, et al. Activation of AMP-activated protein kinase rapidly suppresses multiple pro-inflammatory pathways in adipocytes including IL-1 receptor-associated kinase-4 phosphorylation. Mol Cell Endocrinol (2017) 440:44–56. doi: 10.1016/j.mce.2016.11.010
184. Wang L, Luo Y, Luo L, Wu D, Ding X, Zheng H, et al. Adiponectin restrains ILC2 activation by AMPK-mediated feedback inhibition of IL-33 signaling. J Exp Med (2021) 218. doi: 10.1084/jem.20191054
185. Yu Q, Xiao H, Jedrychowski MP, Schweppe DK, Navarrete-Perea J, Knott J, et al. Sample multiplexing for targeted pathway proteomics in aging mice. Proc Natl Acad Sci United States America (2020) 117:9723–32. doi: 10.1073/pnas.1919410117
186. Schaum N, Lehallier B, Hahn O, Pálovics R, Hosseinzadeh S, Lee SE, et al. Ageing hallmarks exhibit organ-specific temporal signatures. Nature (2020) 583:596–602. doi: 10.1038/s41586-020-2499-y
187. Barzilai N, Banerjee S, Hawkins M, Chen W, Rossetti L. Caloric restriction reverses hepatic insulin resistance in aging rats by decreasing visceral fat. J Clin Invest (1998) 101:1353–61. doi: 10.1172/JCI485
188. López-Otín C, Galluzzi L, Freije JMP, Madeo F, Kroemer G. Metabolic control of longevity. Cell (2016) 166:802–21. doi: 10.1016/j.cell.2016.07.031
189. Gabriely I, Ma XH, Yang XM, Atzmon G, Rajala MW, Berg AH, et al. Removal of visceral fat prevents insulin resistance and glucose intolerance of aging: An adipokine-mediated process? Diabetes (2002) 51:2951–8. doi: 10.2337/diabetes.51.10.2951
190. Muzumdar R, Allison DB, Huffman DM, Ma X, Atzmon G, Einstein FH, et al. Visceral adipose tissue modulates mammalian longevity. Aging Cell (2008) 7:438–40. doi: 10.1111/j.1474-9726.2008.00391.x
191. Fontana L, Partridge L, Longo VD. Extending healthy life span–from yeast to humans. Sci (New York N.Y.) (2010) 328:321–6. doi: 10.1126/science.1172539
192. Colman RJ, Anderson RM, Johnson SC, Kastman EK, Kosmatka KJ, Beasley TM, et al. Caloric restriction delays disease onset and mortality in rhesus monkeys. Sci (New York N.Y.) (2009) 325:201–4. doi: 10.1126/science.1173635
193. Mattison JA, Colman RJ, Beasley TM, Allison DB, Kemnitz JW, Roth GS, et al. Caloric restriction improves health and survival of rhesus monkeys. Nat Commun (2017) 8:14063. doi: 10.1038/ncomms14063
194. Xu M, Pirtskhalava T, Farr JN, Weigand BM, Palmer AK, Weivoda MM, et al. Senolytics improve physical function and increase lifespan in old age. Nat Med (2018) 24:1246–56. doi: 10.1038/s41591-018-0092-9
195. Hahn O, Drews LF, Nguyen A, Tatsuta T, Gkioni L, Hendrich O, et al. A nutritional memory effect counteracts benefits of dietary restriction in old mice. Nat Metab (2019) 1:1059–73. doi: 10.1038/s42255-019-0121-0
196. Rhoads Timothy W, Anderson Rozalyn M. Caloric restriction has a new player. Science (2022) 375:620–1. doi: 10.1126/science.abn6576
197. Picard F, Guarente L. Molecular links between aging and adipose tissue. Int J Obes (2005) 29:S36–9. doi: 10.1038/sj.ijo.0802912
198. Acosta-Rodríguez V, Rijo-Ferreira F, Izumo M, Xu P, Wight-Carter M, Green CB, et al. Circadian alignment of early onset caloric restriction promotes longevity in male C57BL/6J mice. Sci (New York N.Y.) (2022). doi: 10.1126/science.abk0297
199. Pak HH, Haws SA, Green CL, Koller M, Lavarias MT, Richardson NE, et al. Fasting drives the metabolic, molecular and geroprotective effects of a calorie-restricted diet in mice. Nat Metab (2021) 3:1327–41. doi: 10.1038/s42255-021-00466-9
200. Green CL, Mitchell SE, Derous D, Wang Y, Chen L, Han J-DJ, et al. The effects of graded levels of calorie restriction: IX. Global metabolomic screen reveals modulation of carnitines, sphingolipids and bile acids in the liver of C57BL/6 mice. Aging Cell (2017) 16:529–40. doi: 10.1111/acel.12570
201. Derous D, Mitchell SE, Green CL, Wang Y, Han JDJ, Chen L, et al. The effects of graded levels of calorie restriction: X. Transcriptomic responses of epididymal adipose tissue. Journals Gerontol Ser A Biol Sci Med Sci (2018) 73:279–88. doi: 10.1093/gerona/glx101
202. Chen C, Zhou M, Ge Y, Wang X. SIRT1 and aging related signaling pathways. Mech Ageing Dev (2020) 187:111215. doi: 10.1016/j.mad.2020.111215
203. Cantó C, Auwerx J. Caloric restriction, SIRT1 and longevity. Trends In Endocrinol Metab: TEM (2009) 20:325–31. doi: 10.1016/j.tem.2009.03.008
204. Picard F, Kurtev M, Chung N, Topark-Ngarm A, Senawong T, Machado De Oliveira R, et al. Sirt1 promotes fat mobilization in white adipocytes by repressing PPAR-gamma. Nature (2004) 429:771–6. doi: 10.1038/nature02583
205. Argmann C, Dobrin R, Heikkinen S, Auburtin A, Pouilly L, Cock T-A, et al. Ppargamma2 is a key driver of longevity in the mouse. PloS Genet (2009) 5:e1000752. doi: 10.1371/journal.pgen.1000752
206. Spadaro O, Youm Y, Shchukina I, Ryu S, Sidorov S, Ravussin A, et al. Caloric restriction in humans reveals immunometabolic regulators of health span. Sci (New York N.Y.) (2022) 375:671–7. doi: 10.1126/science.abg7292
207. Ghosh AK, O'Brien M, Mau T, Yung R. Toll-like receptor 4 (TLR4) deficient mice are protected from adipose tissue inflammation in aging. Aging (2017) 9:1971–82. doi: 10.18632/aging.101288
208. Roth GS, Ingram DK. Manipulation of health span and function by dietary caloric restriction mimetics. Ann N Y Acad Sci (2016) 1363:5–10. doi: 10.1111/nyas.12834
209. Baker DJ, Childs BG, Durik M, Wijers ME, Sieben CJ, Zhong J, et al. Naturally occurring p16(Ink4a)-positive cells shorten healthy lifespan. Nature (2016) 530:184–9. doi: 10.1038/nature16932
210. Childs BG, Baker DJ, Wijshake T, Conover CA, Campisi J, van Deursen JM. Senescent intimal foam cells are deleterious at all stages of atherosclerosis. Sci (New York N.Y.) (2016) 354:472–7. doi: 10.1126/science.aaf6659
211. Jeon OH, Kim C, Laberge R-M, Demaria M, Rathod S, Vasserot AP, et al. Local clearance of senescent cells attenuates the development of post-traumatic osteoarthritis and creates a pro-regenerative environment. Nat Med (2017) 23:775–81. doi: 10.1038/nm.4324
212. Chang J, Wang Y, Shao L, Laberge R-M, Demaria M, Campisi J, et al. Clearance of senescent cells by ABT263 rejuvenates aged hematopoietic stem cells in mice. Nat Med (2016) 22:78–83. doi: 10.1038/nm.4010
213. Zhu Y, Tchkonia T, Pirtskhalava T, Gower AC, Ding H, Giorgadze N, et al. The achilles' heel of senescent cells: From transcriptome to senolytic drugs. Aging Cell (2015) 14:644–58. doi: 10.1111/acel.12344
214. Yosef R, Pilpel N, Tokarsky-Amiel R, Biran A, Ovadya Y, Cohen S, et al. Directed elimination of senescent cells by inhibition of BCL-W and BCL-XL. Nat Commun (2016) 7:11190. doi: 10.1038/ncomms11190
215. Hickson LJ, Langhi Prata LGP, Bobart SA, Evans TK, Giorgadze N, Hashmi SK, et al. Senolytics decrease senescent cells in humans: Preliminary report from a clinical trial of dasatinib plus quercetin in individuals with diabetic kidney disease. EBioMedicine (2019) 47:446–56. doi: 10.1016/j.ebiom.2019.08.069
216. Kim E-C, Kim J-R. Senotherapeutics: Emerging strategy for healthy aging and age-related disease. BMB Rep (2019) 52:47–55. doi: 10.5483/BMBRep.2019.52.1.293
217. Childs BG, Gluscevic M, Baker DJ, Laberge R-M, Marquess D, Dananberg J, et al. Senescent cells: An emerging target for diseases of ageing. Nat Rev Drug Discovery (2017) 16:718–35. doi: 10.1038/nrd.2017.116
218. Howitz KT, Bitterman KJ, Cohen HY, Lamming DW, Lavu S, Wood JG, et al. Small molecule activators of sirtuins extend saccharomyces cerevisiae lifespan. Nature (2003) 425:191–6. doi: 10.1038/nature01960
219. Wood JG, Rogina B, Lavu S, Howitz K, Helfand SL, Tatar M, et al. Sirtuin activators mimic caloric restriction and delay ageing in metazoans. Nature (2004) 430:686–9. doi: 10.1038/nature02789
220. Rascón B, Hubbard BP, Sinclair DA, Amdam GV. The lifespan extension effects of resveratrol are conserved in the honey bee and may be driven by a mechanism related to caloric restriction. Aging (2012) 4:499–508. doi: 10.18632/aging.100474
221. Baur JA, Pearson KJ, Price NL, Jamieson HA, Lerin C, Kalra A, et al. Resveratrol improves health and survival of mice on a high-calorie diet. Nature (2006) 444:337–42. doi: 10.1038/nature05354
222. Morris BJ. Seven sirtuins for seven deadly diseases of aging. Free Radical Biol Med (2013) 56:133–71. doi: 10.1016/j.freeradbiomed.2012.10.525
223. Tilstra JS, Clauson CL, Niedernhofer LJ, Robbins PD. NF-κB in aging and disease. Aging Dis (2011) 2:449–65.
224. Jimenez-Gomez Y, Mattison JA, Pearson KJ, Martin-Montalvo A, Palacios HH, Sossong AM, et al. Resveratrol improves adipose insulin signaling and reduces the inflammatory response in adipose tissue of rhesus monkeys on high-fat, high-sugar diet. Cell Metab (2013) 18:533–45. doi: 10.1016/j.cmet.2013.09.004
225. Xu M, Tchkonia T, Ding H, Ogrodnik M, Lubbers ER, Pirtskhalava T, et al. JAK inhibition alleviates the cellular senescence-associated secretory phenotype and frailty in old age. Proc Natl Acad Sci United States America (2015) 112:E6301–10. doi: 10.1073/pnas.1515386112
226. Foretz M, Guigas B, Bertrand L, Pollak M, Viollet B. Metformin: From mechanisms of action to therapies. Cell Metab (2014) 20:953–66. doi: 10.1016/j.cmet.2014.09.018
227. Hu D, Xie F, Xiao Y, Lu C, Zhong J, Huang D, et al. Metformin: A potential candidate for targeting aging mechanisms. Aging Dis (2021) 12:480–93. doi: 10.14336/AD.2020.0702
228. Li Q, Hagberg CE, Silva Cascales H, Lang S, Hyvönen MT, Salehzadeh F, et al. Obesity and hyperinsulinemia drive adipocytes to activate a cell cycle program and senesce. Nat Med (2021) 27:1941–53. doi: 10.1038/s41591-021-01501-8
229. Mau T, O'Brien M, Ghosh AK, Miller RA, Yung R. Life-span extension drug interventions affect adipose tissue inflammation in aging. Journals Gerontol Ser A Biol Sci Med Sci (2020) 75:89–98. doi: 10.1093/gerona/glz177
230. Yang L-W, Song M, Li Y-L, Liu Y-P, Liu C, Han L, et al. L-carnitine inhibits the senescence-associated secretory phenotype of aging adipose tissue by JNK/p53 pathway. Biogerontology (2019) 20:203–11. doi: 10.1007/s10522-018-9787-z
231. Eggel A, Wyss-Coray T. A revival of parabiosis in biomedical research. Swiss Med Week (2014) 144:w13914. doi: 10.4414/smw.2014.13914
232. Bai Z, Yang P, Yu F, Li Z, Yao Z, Martinez J, et al. Combining adoptive NK cell infusion with a dopamine-releasing peptide reduces senescent cells in aged mice. Cell Death Dis (2022) 13:305. doi: 10.1038/s41419-022-04562-w
233. Meyer SC, Levine RL. Molecular pathways: Molecular basis for sensitivity and resistance to JAK kinase inhibitors. Clin Cancer Res an Off J Am Assoc For Cancer Res (2014) 20:2051–9. doi: 10.1158/1078-0432.CCR-13-0279
234. Kralovics R, Passamonti F, Buser AS, Teo S-S, Tiedt R, Passweg JR, et al. A gain-of-function mutation of JAK2 in myeloproliferative disorders. New Engl J Med (2005) 352:1779–90. doi: 10.1056/NEJMoa051113
235. Shabbir M, Stuart R. Lestaurtinib, a multitargeted tyrosine kinase inhibitor: from bench to bedside. Expert Opin On Investig Drugs (2010) 19:427–36. doi: 10.1517/13543781003598862
236. Fridman JS, Scherle PA, Collins R, Burn TC, Li Y, Li J, et al. Selective inhibition of JAK1 and JAK2 is efficacious in rodent models of arthritis: Preclinical characterization of INCB028050. J Immunol (Baltimore Md. 1950) (2010) 184:5298–307. doi: 10.4049/jimmunol.0902819
237. Xu M, Palmer AK, Ding H, Weivoda MM, Pirtskhalava T, White TA, et al. Targeting senescent cells enhances adipogenesis and metabolic function in old age. ELife (2015) 4:e12997. doi: 10.7554/eLife.12997
238. Xu M, Tchkonia T, Kirkland JL. Perspective: Targeting the JAK/STAT pathway to fight age-related dysfunction. Pharmacol Res (2016) 111:152–4. doi: 10.1016/j.phrs.2016.05.015
239. Huang J, Liu C, Ming X-F, Yang Z. Inhibition of p38mapk reduces adipose tissue inflammation in aging mediated by arginase-II. Pharmacology (2020) 105:491–504. doi: 10.1159/000507635
Keywords: aging, adipose tissue, inflammaging, metabolic disease, immune aging
Citation: Zhang Y-X, Ou M-Y, Yang Z-H, Sun Y, Li Q-F and Zhou S-B (2023) Adipose tissue aging is regulated by an altered immune system. Front. Immunol. 14:1125395. doi: 10.3389/fimmu.2023.1125395
Received: 21 December 2022; Accepted: 30 January 2023;
Published: 17 February 2023.
Edited by:
Jinghua Pan, Jinan University, ChinaCopyright © 2023 Zhang, Ou, Yang, Sun, Li and Zhou. This is an open-access article distributed under the terms of the Creative Commons Attribution License (CC BY). The use, distribution or reproduction in other forums is permitted, provided the original author(s) and the copyright owner(s) are credited and that the original publication in this journal is cited, in accordance with accepted academic practice. No use, distribution or reproduction is permitted which does not comply with these terms.
*Correspondence: Yu Sun, c3VueXVAc2licy5hYy5jbg==; Qing-Feng Li, ZHIubGlxaW5nZmVuZ0BzaHNtdS5lZHUuY24=; Shuang-Bai Zhou, c2h1YW5nYmFpemhvdUB5YWhvby5jb20=
†These authors have contributed equally to this work