- 1Department of Rheumatology and Immunology, China-Japan Union Hospital, Jilin University, Changchun, China
- 2Scientific Research Center, China-Japan Union Hospital, Jilin University, Changchun, China
- 3Department of Stomatology, China-Japan Union Hospital, Jilin University, Changchun, China
Systemic sclerosis (SSc) is an intricate systemic autoimmune disease with pathological features such as vascular injury, immune dysregulation, and extensive fibrosis of the skin and multiple organs. Treatment options are limited; however, recently, mesenchymal stem cell-derived extracellular vesicles (MSC-EVs) have been acknowledged in preclinical and clinical trials as being useful in treating autoimmune diseases and are likely superior to MSCs alone. Recent research has also shown that MSC-EVs can ameliorate SSc and the pathological changes in vasculopathy, immune dysfunction, and fibrosis. This review summarizes the therapeutic effects of MSC-EVs on SSc and the mechanisms that have been discovered to provide a theoretical basis for future studies on the role of MSC-EVs in treating SSc.
1 Introduction
Systemic sclerosis (SSc) is a complex and chronic connective tissue disorder with an incidence of 17.6 per 10,000 (1). The pathogenesis of SSc is dominated by disorders in three major areas of pathophysiology: vasculopathy, immune dysfunction, and fibrosis. The disease progression is cumulative with amplified effects that primarily involve endothelial cell (EC) activation and the recruitment of inflammatory cells, followed by the release of various factors. This results in fibroblast activation and the deposition of extracellular matrix (ECM) proteins (2).
However, the etiology of SSc is complex and unclear since epigenetics, environmental factors, and a history of infection and drugs may all contribute to SSc. Genetics is the primary factor, and a survey has shown the percentage of relatives of patients with SSc suffering from the disease is higher than that of the general population (3). In addition, genetic factor studies at different levels of genetic information have advanced considerably with the development of technology and lower costs. There is a close relationship between human leukocyte antigen (HLA) locus genes and SSc, and 32 non-HLA loci have been identified (4). This opens up promising possibilities for the use of precision medicine for SSc patients. It has been shown that viruses could trigger SSc, in particular parvovirus B19 (5), cytomegalovirus, Epstein–Barr virus, and retroviruses (6). Intriguingly, recent findings that SARS-CoV-2 may be involved in the occurrence of SSc have been disputed. Although COVID-19 in patients only affects the incidence of SSc minimally (7), both diseases have manifestations of endothelial damage, making it possible for them to correlate (8).
Clinically, SSc is primarily characterized by skin fibrosis and the accelerated progression of organ-associated complications, which include the early appearance of arthropathy, gastrointestinal dysmotility, myositis, and Raynaud’s phenomenon (9), and late stages of severe pulmonary or cardiac complications with significant morbidity and mortality rates (10). Further characteristics of SSc include a prevalence rate that is approximately four times higher in women than that in men, an age of onset between 30 and 60 years (1), an incidence rate higher in North America than that in northern Europe (11), and a more rapid and severe disease progression in African-American patients (12).
Faced with cumulative and persistent multi-organ symptoms, no single approach to the treatment of SSc has proven uniformly effective. Current clinical treatments are mainly palliative, and current management strategies focus on treating the symptoms, accompanied by systemic immunotherapy (13). Early intervention with drugs for vascular modulation, especially during the early symptoms of vascular injury, substantially mitigates sclerosis associated with pulmonary hypertension and reduces the risk of mortality associated with scleroderma renal crisis (14, 15). Immunosuppressive drugs are typically used during the active and diffuse phases of the disease and may result in a poor prognosis for patients with SSc (13). In addition, hematopoietic stem cell transplantation and several agents targeting potential drivers of disease pathogenesis, such as T cells, B cells, transforming germinal factor (TGF) β, and interleukin (IL)-6, are under evaluation as possible therapeutic agents in clinical trials (16–18).
Mesenchymal stem cells (MSCs) have the capacity to not only modulate immune cell activity but also stimulate tissue regeneration, mainly by secreting extracellular vesicles (EVs), which in turn play a role in the functional treatment of diseases (19). EVs secreted by MSCs contain a variety of bioactive substances such as DNA, mRNA, long non-coding RNA (lncRNA), proteins, and lipids and may be classified into three subtypes based on their size: exosomes (Exos; 30–100 nm), microvesicles (50–2,000 nm), and apoptotic bodies (50–5,000 nm) (20). Exos have the highest degree of homogeneity and are the most complex and versatile of the three types. Therefore, due to the highest value of theory and application, Exos receive the most attention in academic papers on EV. MSC-EVs can be classified as adipose tissue-derived MSCs (ASCs), bone marrow MSCs (BM-MSCs), umbilical cord MSCs (UC-MSCs), menstrual fluid MSCs (MenSCs), human-induced pluripotent stem cell MSCs, and human amniotic fluid MSCs (AF-MSCs) (21). EVs from ASCs, UC-MSCs, and BM-MSCs exhibit a capacity for wound healing; however, BM-MSC-EVs have stronger induction effects on fibroblasts, and the greatest induction of keratinocytes belongs to UC-MSC-EVs (22). Thus, when considering their use, it should be noted that EVs from different sources of MSCs can differ in efficacy.
There are still some challenges in the clinical application of MSC-EVs, for instance, high and sustained production, prolonged in vivo action, and avoidance of macrophage clearance (23, 24). However, compared to MSC transplantation, MSC-EVs possess a number of advantageous characteristics, including smaller size, singularity, long circulatory half-life, low immunogenicity, easy coating of therapeutic substances, easy crossing of the blood–brain barrier, easy production and storage, and no tumorigenicity (25). Therefore, various clinical studies are currently underway regarding the therapeutic applications of MSC-derived EVs (MSC-EVs) in autoimmune diseases (ADs) (26), including SSc. This review summarizes the possible pathogenesis of SSc and explores the potential use of MSC-EVs in SSc treatment. MSC-EVs may contribute to the treatment of SSc by improving vascular lesions, regulating immune dysfunction, and inhibiting fibrosis.
2 Pathophysiology of systemic sclerosis
Despite the fact that the exact causes of SSc are not well understood, numerous studies have shown that endogenous and exogenous environmental factors or risk factors trigger gene activation. The subsequent onset of SSc is associated with endothelial damage, microvascular injury, inflammation, and autoimmune activation (27, 28). These factors inevitably cause abnormal differentiation of fibroblasts and the accumulation of collagen and ECM proteins in tissues. SSc progression is discussed below in three parts: vascular injury, the immune response, and fibrosis.
2.1 Vascular injury and microangiopathy
EC activation is the main event at the beginning of SSc (29), wherein the enhanced expression of adhesion molecules, such as vascular cell adhesion protein 1, intercellular adhesion molecule, and E-selectin, lead to activation of the abnormal secretion of vasoactive factors (30). The adhesion molecules with adhesion mainly recruit inflammatory cells, while the disturbed vasoactive factors lead to frequent and constant fluctuations in microvascular tone (31). Platelet activation, which is caused by vascular changes, increases microvessel permeability; therefore, microvascular leaks can develop (32). Moreover, platelet activation enhances the proliferation of vascular smooth muscle cells (VSMCs) and pericytes, leading to a thickening of the vessel wall and luminal narrowing (31). These events then cause microvascular damage, tissue hypoxia, and oxidative stress (33).
2.2 Inflammation and the immune response
In the early inflammatory phase of SSc, Toll-like receptor (TLR) signaling, which acts as a significant indicator of inflammation, can be provoked by non-specific or pathogenic injury, which may result in inflammation induction and the activation of innate immune cells (monocytes/macrophages, plasmacytoid dendritic cells (pDCs), and others). The upregulated production of CXCL4 in plasmacytoid DCs can lead to the differentiation of monocytes into pro-inflammatory DCs that enhance TLR-mediated cytokine expression and impact T cells (34, 35). In addition, monocytes participate in fibrosis through the inflammatory response and differentiate into macrophages or fibroblast-like cells. Macrophages are also engaged in the inflammatory and fibrotic aspects of SSc. Macrophages can generate classically activated (M1) and/or alternatively activated (M2) macrophages that are distinguishable based on their different surface markers. M1 macrophages are effector phagocytes that increase significantly in the early stage of SSc inflammation and generate pro-inflammatory cytokines, such as tumor necrosis factor-alpha (TNF-α), IL-6, and IL-1 (36). M2 macrophages restrain M1 responses by releasing anti-inflammatory cytokines, including IL-4, IL-13, and IL-10 when the repair mechanism is initiated after sustained damage. In addition, they facilitate the production of ECM proteins and pro-fibrotic cytokines and reinforce the anti-inflammatory response by triggering Th2 effector activity (36). Thus, M2 macrophages are considered to be important pathogenic factors in SSc.
Among the various adaptive immune responses, T cells participate significantly in the pathophysiology of SSC and markedly affect the synthesis of autoantibodies (37). CD4+ and CD8+ T cells have been confirmed in the skin (38, 39) and lungs (40, 41) of patients with SSc. Initial CD4+ T cells (Th0) can differentiate into Th1, Th2, and Th17, as well as Treg (T-regulatory) and Tfh (T-follicular helper) cells (42–44). Th2 cells, characterized by the secretion of anti-inflammatory cytokines IL-4 and IL-13, predominate over Th1 cells (45). Moreover, both Th17 cells and IL-17 production have been recognized as being elevated in patients with SSc, and they aggravate early inflammatory responses (46, 47). Treg cells could result in the advancement of SSc by transforming into pathogenic effector T cells, and their number can be reduced (48, 49) to inhibit immune activation (50). Therefore, an imbalance of Th1/Th2/Th17/Treg cytokines is a crucial causal factor for SSc. B cells also play a role in SSc, and their efficacy is linked to antigen presentation, DC maturation, and autoantibody production, varying across phenotypes (51). Different phenotypes exhibit different CD antigens on the cell surface, such as memory B cells with increased expression of CD95, CD80, and CD86, and peripheral blood B cells with a higher expression of CD19 (52–55).
2.3 Fibrosis
Due to persistent tissue damage, inflammation, and immune cell activation, SSc comprises a gradual fibrotic condition affecting tissues and organs (56). Clinical and pathological SSc is characterized by fibrosis accompanied by massive α-SMA-positive myofibroblasts, accumulation of ECM proteins (collagens, elastin, glycosaminoglycans, tenascin, and fibronectin) in tissue, and regulation of growth factor (TGF)-β and other profibrotic mediators (57). Circulating CD14 monocyte precursor pericytes and ECs have been suggested as potential sources of myofibroblast overproduction through epithelial–mesenchymal transition and endothelial–mesenchymal transition (58). The subsequent steady accumulation of ECM proteins stiffens the skin and organs while decreasing elasticity, thereby leading to mechanical stress. Mechanical stress further maintains fibroblast activation and intensifies the progression of the fibrotic process in tissues (59). This may be due to fibroblasts in patients with SSc exhibiting structural focal adhesion kinase activation, which integrates TGF-β signaling and integrin-mediated mechanical transduction so as to promote continuous myofibroblast differentiation and reactive oxygen species production (59).
The soluble mediators related to fibrosis in SSc are TGF-β, connective tissue growth factor (CTGF), and platelet-derived growth factor (PDGF). TGF-β, a pleiotropic factor secreted by macrophages and other cells or stored in the ECM, is believed to be the master regulator of fibrosis. As an inactive precursor, TGF-β-associated signaling cascades are consistently activated in fibrotic tissues (60). CTGF, a cysteine-rich matricellular protein, has a synergistic effect together with TGF-β, endothelin-1, and angiotensin II in inducing fibrosis (61). CTGF levels are significantly higher in the sera of SSc patients than those in the sera of healthy individuals and are positively correlated with the degree of fibrosis (62). PDGFs, which are heterodimeric peptides produced by platelets, macrophages, ECs, and fibroblasts, play an important role in fibrosis as well. PDGFs act as powerful mitogens and chemoattractants and convert mesenchymal cells into profibrotic cell types (63).
3 Potential therapeutic effects of MSC-EVs in SSc
MSC-EV transplantation, which is an emerging and novel therapy, has been confirmed to be beneficial for SSc in bleomycin or hypochlorous acid (HOCl)-induced, or chronic graft-versus-host disease (cGVHD) mouse models, and TGF-β1-induced model of human myofibroblast (Table 1). These preclinical studies are mainly focused on the antifibrotic effect of MSC-EVs in SSc and the mechanisms involved (64, 65). The discovery of effective improvements of MSC-EVs in SSc in vitro and in vivo is a breakthrough in the field of SSc therapeutic approaches. Recent advances have been made in the exploration of the biogenesis, cargo, and biological potential of MSC-EVs and in understanding their molecular mechanisms in angiogenesis and immunomodulation, which is the key to SSc treatment. These advances have led to promising improvements being observed in other diseases through MSC-EV treatment (71, 72). In view of the heterogeneity of pathways involved in SSc pathogenesis and progression, as well as the existence of crosstalk among vascular injury, the immune response, and fibrosis in SSc, long-term single-target treatment could lead to adverse reactions (73, 74). Thus, for SSc, MSC-EVs enriched with multiple efficacious biokines perhaps improve through various pathways, which may include angiogenesis and the modulation of inflammation and fibrosis (Figure 1). This evidence points out that MSC-EVs may become a potential tool for SSc. The effects of MSC-EVs on vascular injury, immune imbalance, and fibrosis in different disease models are described below.
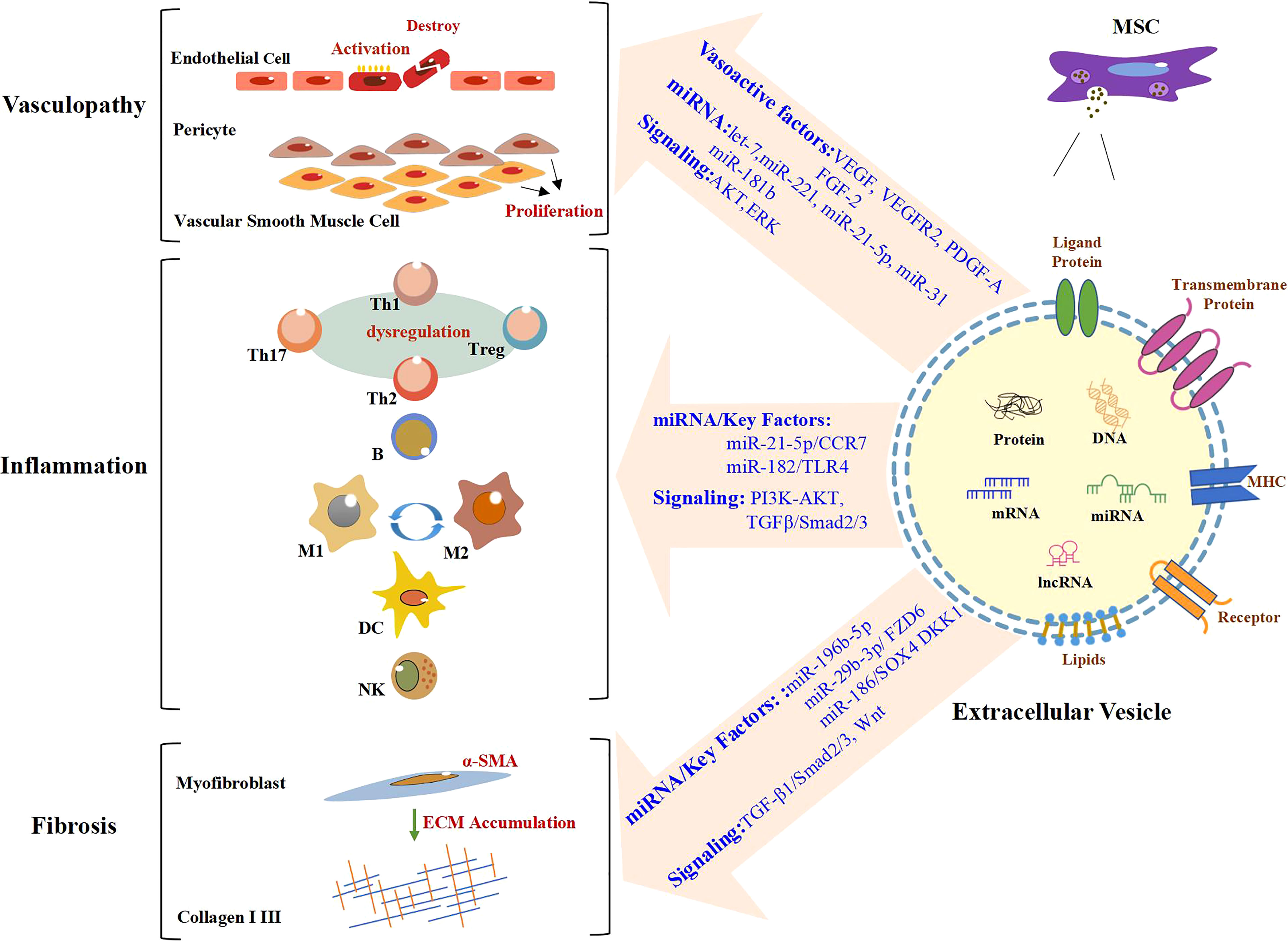
Figure 1 Role of MSC-EVs in the modulation of SSc. In the stage of vasculopathy in SSc, endothelial cells are activated and even destroyed. Vascular smooth muscle cells and pericytes proliferate abnormally. A large number of immune cells also participate in the process of SSc. Likewise, there are large numbers of α-SMA-positive myofibroblasts, which produce excessive amounts of extracellular matrix (ECM), in particular Collagen I and III, resulting in fibrosis. MSC-EVs promote angiogenesis by regulating vasoactive factors and signaling pathways and carrying miRNA. MiRNA/key factors and signaling pathways are involved in the modulation of inflammation and fibrosis by MSC-EVs. Text in blue indicates mechanisms involved in SSc. MSC-EVs, mesenchymal stem cell-derived extracellular vesicles; SSc, systemic sclerosis.
3.1 Pro-angiogenic effects
Vascular injury and the thickening of the vessel wall are key components of SSc. In the early stage of SSc, if the extent of the vascular injury can be reduced, subsequent fibrosis can be considerably inhibited (75). Recent studies have demonstrated the MSC-EV-mediated delivery of cytokines, proteins, microRNA (miRNA), mRNA, and lncRNA as a significant component of the angiogenic process (72, 76). MSC-EVs have been shown to have pro-angiogenic properties in wound-healing models and ischemic conditions such as diabetic foot ulcers (DFUs), full-thickness wounds, myocardial infarction (MI), and acute kidney injury.
3.1.1 Angiogenic function of MSC-EVs
ECs boost angiogenesis (the formation of fresh blood vessels from existing ones) and the secretion of several factors, including nitric oxide, endothelin, and prostacyclin (77). Therefore, they are valuable targets under ischemic conditions and require vascular regeneration. Studies have indicated that MSC-EVs enhance the migration of human umbilical vein endothelial cells (HUVECs) and promote proliferation and vessel-like structure formation in a dose-dependent manner in human microvascular endothelial cells (HMECs) in vivo and in vitro (78, 79). Another research has shown that MSC-Exos are internalized by HUVECs and accumulate around the nucleus (80). The characteristics of MSC-EVs can influence pathological changes in vascular-rich tissue, similar to Raynaud’s phenomenon, which affects over 95% of SSc patients and comprises occasional color changes in the extremities in cold environments (81). SSc-associated interstitial lung disease (SSc-ILD), one of the causes of death, is chiefly caused by damage to the alveolar epithelium and blood vessels in the lung region (82). Similarly, MSC-EVs are effective against other structural cells that are associated with blood vessels. Studies on abnormal proliferation and the migration of smooth muscle cells have demonstrated that MSC-EVs can alleviate asthma or pulmonary arterial hypertension in vivo and/or in vitro (83, 84). On this basis, MSC-EVs are promising as prospective therapeutic targets for SSc-related vasculopathy.
3.1.2 Angiogenic molecular mechanisms of MSC-EVs
MSC-EVs most likely promote angiogenesis by upregulating vasoactive factors such as vascular endothelial-derived growth factor (VEGF), VEGF receptor (R)2, PDGF-A, and fibroblast growth factor (FGF)-2 in HUVECs in vitro and in vivo skin injury models (85). Studies conducted on primary MSCs originating from BM, ASCs, and UCs have revealed that all of the aforementioned cell-derived Exos promote angiogenesis by secreting angiogenesis-mediated factors such as VEGF-A, FGF-2, hepatocyte growth factor, and PDGF-BB during wound healing (22). In addition, exposure to vasoactive factors can strengthen the therapeutic effect of MSC-EVs and encourage better angiogenesis. Lopatina et al. previously showed that PDGF pretreatment of human ASC-EVs reinforces pro-angiogenic capacity by elevating the levels of secreted pro-angiogenic proteins. Conditioned media derived from AF-MSCs incorporating VEGF and TGF-β1 have been found to improve proliferation, the migration of human skin fibroblasts in vitro, and wound healing in vivo (86). As for the angiogenic effects of MSC-EVs, vasoactive factors may be targeted by MSC-EVs, which would influence the properties of MSC-EVs.
Other angiogenic mechanisms that warrant further study are the signaling pathways that involve MSC-derived proteins. A comprehensive analysis previously showed that ASC-EVs contain proteins that are linked to signal transduction pathways. A total of 277 proteins associated with the ECM, glycoproteins, angiogenesis, TGF-β signaling, the inflammatory response, and blood coagulation have been found to be enriched in EVs. It has been found that ASC-EVs facilitate angiogenesis through increased expression of Ang-1 and Flk-1 in HUVECs, involving the let-7/argonaut 1/VEGF signaling pathway in a fat grafting nude mouse model (87). Additionally, ASC-MVs have been found to be readily internalized by HUVECs. ASC-MV proliferation, migration, and angiogenesis are promoted through the AKT and ERK signaling pathways in vitro and in an in vivo skin injury model (85). The activation of these signaling pathways often involves not only proteins but also genetic materials such as miRNA, mRNA, and lncRNA.
It has been found that although MSC-EVs carry different genes than their parent cells, they can still regulate angiogenesis. Eirin et al. found that 386 miRNAs were enriched in ASC-EVs compared to parental cells. MicroRNA-148, one of these detected miRNAs, regulates angiogenesis aimed at transcription factors, inhibits tumor angiogenesis, and suppresses the sprouting of ECs from vessels in vivo and/or in vitro (88–90). Other miRNAs and even genes in their families also act independently from the key factors related to regulatory angiogenesis (91–94). Additionally, miR-221 was found to impact vasoactivity by exerting effects on VSMCs and ECs (95). Meanwhile, EVs obtained from MSCs have been known to exhibit a high level of pro-angiogenic miRNA-21-5p. The pro-angiogenic function of miRNA-21-5p in MSC-EVs was verified in DFU models by knockdown and overexpression of the gene, and it was also further determined that miRNA-21-5p may promote angiogenesis and improve ischemic tissue by stimulating VEGFR and by activating serine/threonine kinase AKT and mitogen-activated protein kinase in receptor cells such as ECs (96). Furthermore, for ischemic diseases, studies revealed that miR-31 was rich in ASC-MVs, especially in endothelial differentiation medium-pretreated ASC-MVs, and that miR-31 has been strongly implicated in angiogenesis in HUVECs and endothelial progenitor cells (97) in promoting migration and tube formation in vitro and in vivo. Moreover, both the miR-31-miR-720 and the VEGF-miR-31 pathways may be involved (98, 99). In addition, factor-inhibiting HIF-1, an anti-angiogenic gene, has been identified as a target of miR-31 in HUVECs, and together they may mediate angiogenesis (79). Additional studies have shown that MSC-Exos and its Exo-transmitted miR-125a can promote endothelial tip cell generation for EC, resulting in vascular sprouting by inhibiting the expression of DLL4, an angiogenesis inhibitor in vivo and in vitro (100). ASC-Exos promote the mobility and angiogenesis of brain microvascular endothelial cells after oxygen-glucose deprivation via the microRNA-181b/TRPM7 axis in vitro (101). Significant progress has been made in the study of miRNA in MSC-EVs. These miRNAs are not only involved in MSC-EVs promoting angiogenesis but also have immunomodulatory and antifibrosis effects (Table 2). MSC-EV delivery can protect miRNAs from degradation by ribonucleases, thereby ensuring that the miRNAs are able to perform their crucial roles in recipient cells. Interestingly, another study showed that proteins in EVs do not originate from the transcription of miRNAs.
3.2 Immunomodulatory effects
Abnormal immune cells were exhibited in SSc focusing on the dysregulation of the Th1/Th2/Th17/Treg and M1/M2 cytokine (2). MSC-EVs have an auto-immunosuppressive property that attenuates inflammation and immune responses in inflammatory and autoimmune diseases (119). MSCs act as immunomodulators by delivering EVs that modulate the generation, differentiation, efficacy, and interactions of adaptive and innate immune cells, such as T cells, B cells, macrophages, natural killer (NK) cells, and DCs in inflammatory diseases (120). Likewise, the anti-inflammatory and immunomodulatory properties of MSC-EVs are probably of great significance in the treatment of SSc.
3.2.1 T cells
In the early stages of SSc, the inhibition of inflammation is an important goal of therapy. At this stage, M1 macrophages and Th1 cells are the main cells involved in the upregulation of type I interferon (IFN) (121). In tissues, M1 macrophages with IFNs are transformed into M2 macrophages to promote fibrosis, after synergistic involvement with the responses of Th2 to exposure to IL-6 and IL-13 (122). Increased numbers of Treg cells facilitate the inhibition of immune activation and prevent themselves from transferring to Th2- and Th17-like cells for antifibrotic progress in skin tissues (123). As MSC-EVs activate different effector substances depending on different biological environments, the disorder in SSc could be regulated by them.
3.2.1.1 Th cells
MSC-EVs modulate the functions and activities of Th cells. It has been demonstrated that MSC-EVs regulate CD4 T cells in the conversion between Th1 and Th2 and decrease the Th17 differentiation of peripheral blood mononuclear cells in asthmatic mice (124). In dextran sulfate sodium-induced colitis models, olfactory ecto-derived MSC-EVs have been found to remarkably reduce Th1/Th17 subpopulations, accompanied by reduced serum levels of IL-17, IL-6, and IFN-γ and elevated levels of TGF-β and IL-10 secreted by T cells (125). Interestingly, pro-inflammatory cytokines, such as IL-10 (126) and IFN preconditioned MSC, could enhance CD4 T-cell inhibition while inducing Treg cells and Th17. In addition, the molecular mechanisms of the MSC-EV-mediated regulation of Th cells focus on cargoes in EV, for example, by transferring miR-23a-3p to regulate the Treg/Th17 balance in aplastic anemia (102), miR-146a-5p/IRAK1 axis to regulate the Th17/Treg imbalance in immune thrombocytopenia (103), miR-125a and miR-125b to inhibit Th17 cell differentiation in colitis (104), and miR-10a loading to promote Th17 and Treg responses while decreasing Th1 responses (105).
3.2.1.2 Treg cells
MSC-EVs substantially promote the conversion of monocytes to Treg cells and the immune-suppression capacity of Treg cells (127, 128), which is possibly triggered by TGF-β from MSC-EVs exposed to IFN-γ (129). Meanwhile, MSC-EVs could lead to the differentiation of Treg cells to reverse the imbalance between T-effector and Treg cells, which has been confirmed regarding the imbalance of Th1/Th4/Th17/Treg cells (105). It has been noted that Treg regulation occurs mainly through antigen-presenting cells (APCs) and is not dependent on CD4+ T cells in asthmatic mice (124). Subsequent studies have also confirmed that the differentiation of Treg cells is mediated by activated APCs, which are induced by MSC-EVs in a myeloid differentiation primary response-dependent manner (128). Treg differentiation can also suppress the proliferation of T cells indirectly, which is a property of MSC-Exos, but not of MSCs (130). The ability of MSC-EVs to regulate the function, homing, and phenotype of immune cells may result in the development of novel therapies for inflammatory diseases and ADs (26, 120). Nevertheless, further research is needed to elucidate the molecular mechanisms of the MSC-EV-mediated regulation of immune cell activities.
3.2.2 B lymphocytes
B lymphocytes play a major role in the adaptive immune response and MSC-EVs have been shown to regulate the proliferation and function of B cells. Khare et al. (131) sequenced MSC-EV co-cultured with B cells and identified upregulated genes related to B-cell proliferation and Ca2+ mobilization via B-cell receptors in vitro. Guo et al. earlier revealed that MSC-EVs suppressed fibrosis in a mouse model of sclerodermatous chronic graft-versus-host disease by inhibiting Tfh/germinal center B-cell interactions and reducing the frequency of B cell-activating factor (BAFF)-expressing B cells (70). Furthermore, MSC-EVs have been found to regulate Breg cells through the PI3K-AKT signaling pathway in vitro, but the role of MSCs in regulating Breg cells still needs to be explored (132).
3.2.3 Macrophages
Macrophages orchestrate both the initiation and resolution of inflammation. An imbalance in the phenotypes and activation of macrophages has been considered critical in the development of inflammatory, autoimmune, and fibrotic diseases. Accumulating evidence has suggested that MSC-EVs promote macrophage polarization and phagocytic capacity (133). The regulation of EVs in the conversion between M1 and M2 macrophages has also been found in some cases of SSc (134). Meanwhile, MSC-EVs reduce macrophage infiltration in tissues by carrying miRNAs in SSc (68). Interestingly, in renal interstitial fibrosis, Hu et al. (107) showed that MSC-Exos may inhibit macrophage activation by delivering miR-34c-5p in Exos to macrophages via CD81-epidermal growth factor receptor (EGFR) complex aids. In another context, it was noted that MSC-EVs could reverse the polarization of M1 to M2 macrophages; further, it was found that miR-182 from MSC-EVs can suppress TLR4, as part of its underlying mechanism of action in myocardial ischemia–reperfusion injury (106).
3.2.4 DCs and NK cells
DCs and NK cells are essential for the innate immune response, and the effect of MSC-EVs on both cells has been explored in some studies in vitro. Several reports have shown that MSC-EVs could suppress the proliferation and maturation of DCs, thus regulating the immune response. The coincubation of DCs derived from BM with MSC-EVs has been found to reduce IL-6 release, increase IL-10 and TGF-β levels, and downregulate lymphocyte proliferation (135). MSC-EVs loaded with miR-21-5p are able to depress the target gene C–C chemokine receptor type 7 (CCR7) and reduce the migratory capacity of the CCR7-ligand CCL21, thereby suppressing the secretion of inflammatory cytokines (136). Previous studies have demonstrated that MSC-EVs could suppress the proliferation of NK cells in a manner similar to DCs (130). In recent research, an analogous study showed that human fetal liver-derived MSC-Exos not only inhibited NK-cell proliferation but also suppressed NK-cell activation and cytotoxicity via latency-associated peptides and TGF-β and thrombospondin 1 and induced downstream TGF-β/Smad2/3 signaling. Nevertheless, research on the topic remains limited. Despite the growing research on MSC-EVs and immune cells, the precise molecular mechanisms underlying their interactions needs additional study (137). Innate immune responses also occur in the course of SSc. Regulation of DCs and NK cells by MSC-EVs should play a role in SSc.
3.3 Antifibrotic effects
MSC-EV treatment can ameliorate fibrosis of the skin and lungs, which is a clear sign of SSc. Furthermore, the clinical fibrotic manifestations of SSc involve alterations in the heart, kidney, and colon, and several experimental studies have examined the antifibrotic effect of MSC-EVs in other diseases (Table 3). Presently, investigations of fibrosis are restricted to the preclinical phase. Many preclinical trials have been conducted to set up various SSc models. There are many methods to induce SSc given the various underlying causes of fibrosis (Supplementary Table 1); however, no experimental models have been able to perfectly reproduce the pathophysiological spectrum of SSc.
3.3.1 Antifibrotic function of MSC-EVs
MSC-EVs have been confirmed to be beneficial for SSc due to their antifibrotic effects. Rozier et al. evaluated the antifibrotic function of ASCs and their EVs by co-culturing them with TGF-β1-induced myofibroblasts (65). However, the underlying therapeutic mechanisms remain unclear. Several studies have investigated the potential antifibrotic properties of MSC-EVs in bleomycin-induced mouse models of scleroderma (64, 134). These effects were exhibited in the amelioration of the following aspects. MSC-EVs can suppress myofibroblast differentiation and inhibit the expression of collagen types I and III in a skin-defect mouse model (111). Studies on the molecular markers of fibrosis and remodeling have shown that MSC-EVs might diminish scar formation by decreasing the ratios between collagen types I and III in a diabetic mouse model (138). In addition, ASC-EVs decrease scar formation via modulating the ratios of type I and III collagen, TGF-β1 and TGF-β3, matrix metalloproteinase-3 and tissue inhibitor of metalloproteinases 1, and abnormal activation of fibroblasts in vivo (139). These effects have been noted in many fibrotic diseases (140).
3.3.2 Antifibrotic molecular mechanisms of MSC-EVs
Many studies have confirmed that MSC-EVs, typified by Exos, can act as antifibrotic agents through various mechanisms of action in the treatment of fibrotic diseases. Among the signaling pathways involved, TGF-β and Wnt signaling are regarded as the core pathways involved in fibrosis in SSc (141, 142). In addition, the effects of Exos on reducing fibrotic markers through the TGF pathway have also been confirmed in SSc (64). The phosphorylation levels of α-SMA, Smad2/3, and collagen I and III in fibroblasts treated with Exos have been found to be markedly decreased. Furthermore, MSC-derived Exos have been found to suppress the transition of dermal fibroblasts to myofibroblasts by inhibiting the NF-κB, Hedgehog, Wnt/β-catenin, PI3K/Akt, Erk1/2, and TGF-β1/Smad2/3 signaling pathways (140). Moreover, it has been shown that EV alleviates fibrosis by carrying cargo, such as RNA, through activation of the aforementioned signaling pathways. Results of high-throughput RNA sequencing and functional analysis of MSC-EVs suggest that a group of specific microRNAs can prevent excessive myofibroblast revitalization by blocking the TGF-β/Smad2 signaling pathways in vivo (111). MiR-29b-3p, carried by BM-MSC-EVs in bilayered thiolated alginate/PEG diacrylate hydrogels, inhibits the proliferation and migration of ECs and fibroblasts by curbing the PI3K/Akt, Erk1/2, and TGF-β1/Smad3 signaling pathways in a full-thickness skin defect model of rats and rabbit ears, thereby allowing for scar-free wound healing (110). In a study on BMSC-EV-based treatment, the gene activity in the Wnt signaling pathway that was hyperactivated by bleomycin stimulation was significantly lowered or related to ECM–receptor interactions and the cell cycle (68).
Several investigations have explored the role of MSC-EV-carried miRNAs in fibrosis. In SSc, miR-29a-3p from MSCs and ASCs has been confirmed to be beneficial for fibrosis in HOCl-induced mice (69). In other fibrotic diseases, a group of uMSC-EVs composed of miR-21, miR-23a, miR-imno125b, and miR-145, whose expression is changed, was thought to be related to the modulation of pro-fibrotic genes (110, 111). Among the various features of fibrosis, MSC-EVs also display efficacy in the inhibition of fibroblast proliferation. MiR-29b-3p and miR-186, both secreted by BMMSC-EVs, have been reported to downregulate FZD6, SOX4, and DKK1 expression to inhibit fibroblast activation and proliferation in idiopathic pulmonary fibrosis, respectively (108, 109). Likewise, elevated collagen levels are one of the main factors contributing to ECM deposition in fibrosis. MiR-212-5p, miR-133b (143), miR-192-5p (144), miR-196b-5p (67), and let-7a (145) have been found to reduce the levels of type I and/or type III collagens, and miR-212-5p downstream may inhibit the NLRC5/VEGF/TGF-β1/SMAD axis, while miR-192-5p targets IL-17RA. Similarly, fibrosis and remodeling factors are targets of miRNAs from MSC-EVs. EVs from MSCs and ASCs exhibit a mechanism of action that comprises the secretion of miR-29a-3p, which could diminish the levels of several profibrotic, remodeling, and anti-apoptotic factors and methylases in HOCl-induced mice (69). In the aforementioned studies, MSC-EVs further exerted a positive effect on fibrosis. Despite the progress that has been made in recent studies, ameliorating fibrosis with MSC-EVs remains in the preclinical stage.
4 Conclusions and prospects
It is possible that MSC-EVs could ameliorate SSc and the pathological changes in vasculopathy, immune dysfunction, and fibrosis by regulating key factors and signaling pathways. Despite the positive effects of MSC-EVs in SSc, further extensive clinical studies are required to establish the applicability of EVs in treating SSc. A focus area is modification and engineering, which could enhance the therapeutic effects of MSCs-EVs. Future exploration of strategies, including chemical stimulation from MSCs, MSC genetic modification, and physical variables of MSCs, could generate more effective MSCs-EVs for supporting the desired outcomes. Undoubtedly, enhancing the effectiveness of MSC-EV treatment will be an important trend in future clinical applications.
Author contributions
NS participated in the investigation. JJ and PL reviewed and supervised the manuscript. All authors contributed to the paper and approved the submitted draft. All authors contributed to the article and approved the submitted version.
Funding
Jilin Provincial Development and Reform Commission (2022C044-8), Jilin Provincial Department of Education (JJKH20211146), Natural Science Foundation of Jilin Provincial Department of Science and Technology (YDZJ202201ZYTS104).
Conflict of interest
The authors declare that the research was conducted in the absence of any commercial or financial relationships that could be construed as a potential conflict of interest.
Publisher’s note
All claims expressed in this article are solely those of the authors and do not necessarily represent those of their affiliated organizations, or those of the publisher, the editors and the reviewers. Any product that may be evaluated in this article, or claim that may be made by its manufacturer, is not guaranteed or endorsed by the publisher.
Supplementary material
The Supplementary Material for this article can be found online at: https://www.frontiersin.org/articles/10.3389/fimmu.2023.1125257/full#supplementary-material
References
1. Bairkdar M, Rossides M, Westerlind H, Hesselstrand R, Arkema EV, Holmqvist M. Incidence and prevalence of systemic sclerosis globally: a comprehensive systematic review and meta-analysis. Rheumatol (Oxford) (2021) 60(7):3121–33. doi: 10.1093/rheumatology/keab190
2. Cutolo M, Soldano S, Smith V. Pathophysiology of systemic sclerosis: current understanding and new insights. Expert Rev Clin Immunol (2019) 15(7):753–64. doi: 10.1080/1744666X.2019.1614915
3. Kuo CF, Luo SF, Yu KH, See LC, Zhang W, Doherty M. Familial risk of systemic sclerosis and Co-aggregation of autoimmune diseases in affected families. Arthritis Res Ther (2016) 18(1):231. doi: 10.1186/s13075-016-1127-6
4. Villanueva-Martin G, Martin J, Bossini-Castillo L. Recent advances in elucidating the genetic basis of systemic sclerosis. Curr Opin Rheumatol (2022) 34(6):295–301. doi: 10.1097/BOR.0000000000000897
5. Arvia R, Zakrzewska K, Giovannelli L, Ristori S, Frediani E, Del Rosso M, et al. Parvovirus B19 induces cellular senescence in human dermal fibroblasts: putative role in systemic sclerosis-associated fibrosis. Rheumatol (Oxford) (2022) 61(9):3864–74. doi: 10.1093/rheumatology/keab904
6. Randone SB, Guiducci S, Cerinic MM. Systemic sclerosis and infections. Autoimmun Rev (2008) 8(1):36–40. doi: 10.1016/j.autrev.2008.07.022
7. Chang R, Yen-Ting Chen T, Wang SI, Hung YM, Chen HY, Wei CJ. Risk of autoimmune diseases in patients with covid-19: a retrospective cohort study. EClinicalMedicine (2023) 56:101783. doi: 10.1016/j.eclinm.2022.101783
8. Matucci-Cerinic M, Hughes M, Taliani G, Kahaleh B. Similarities between covid-19 and systemic sclerosis early vasculopathy: a "Viral" challenge for future research in scleroderma. Autoimmun Rev (2021) 20(10):102899. doi: 10.1016/j.autrev.2021.102899
9. Varga J, Abraham D. Systemic sclerosis: a prototypic multisystem fibrotic disorder. J Clin Invest (2007) 117(3):557–67. doi: 10.1172/JCI31139
10. Condliffe R, Kiely DG, Peacock AJ, Corris PA, Gibbs JS, Vrapi F, et al. Connective tissue disease-associated pulmonary arterial hypertension in the modern treatment era. Am J Respir Crit Care Med (2009) 179(2):151–7. doi: 10.1164/rccm.200806-953OC
11. Bergamasco A, Hartmann N, Wallace L, Verpillat P. Epidemiology of systemic sclerosis and systemic sclerosis-associated interstitial lung disease. Clin Epidemiol (2019) 11:257–73. doi: 10.2147/CLEP.S191418
12. Gelber AC, Manno RL, Shah AA, Woods A, Le EN, Boin F, et al. Race and association with disease manifestations and mortality in scleroderma: a 20-year experience at the johns Hopkins scleroderma center and review of the literature. Med (Baltimore) (2013) 92(4):191–205. doi: 10.1097/MD.0b013e31829be125
13. Volkmann ER, Andreasson K, Smith V. Systemic sclerosis. Lancet (2023) 401(10373):304–18. doi: 10.1016/S0140-6736(22)01692-0
14. Zanatta E, Polito P, Favaro M, Larosa M, Marson P, Cozzi F, et al. Therapy of scleroderma renal crisis: state of the art. Autoimmun Rev (2018) 17(9):882–9. doi: 10.1016/j.autrev.2018.03.012
15. Humbert M, Coghlan JG, Ghofrani HA, Grimminger F, He JG, Riemekasten G, et al. Riociguat for the treatment of pulmonary arterial hypertension associated with connective tissue disease: results from patent-1 and patent-2. Ann Rheum Dis (2017) 76(2):422–6. doi: 10.1136/annrheumdis-2015-209087
16. Ebata S, Oba K, Kashiwabara K, Ueda K, Uemura Y, Watadani T, et al. Predictors of rituximab effect on modified rodnan skin score in systemic sclerosis: a machine-learning analysis of the desires trial. Rheumatol (Oxford) (2022) 61(11):4364–73. doi: 10.1093/rheumatology/keac023
17. van Laar JM, Farge D, Sont JK, Naraghi K, Marjanovic Z, Larghero J, et al. Autologous hematopoietic stem cell transplantation vs intravenous pulse cyclophosphamide in diffuse cutaneous systemic sclerosis: a randomized clinical trial. JAMA (2014) 311(24):2490–8. doi: 10.1001/jama.2014.6368
18. Khanna D, Lin CJF, Furst DE, Goldin J, Kim G, Kuwana M, et al. Tocilizumab in systemic sclerosis: a randomised, double-blind, placebo-controlled, phase 3 trial. Lancet Respir Med (2020) 8(10):963–74. doi: 10.1016/S2213-2600(20)30318-0
19. Jeppesen DK, Fenix AM, Franklin JL, Higginbotham JN, Zhang Q, Zimmerman LJ, et al. Reassessment of exosome composition. Cell (2019) 177(2):428–45.e18. doi: 10.1016/j.cell.2019.02.029
20. van Niel G, D'Angelo G, Raposo G. Shedding light on the cell biology of extracellular vesicles. Nat Rev Mol Cell Biol (2018) 19(4):213–28. doi: 10.1038/nrm.2017.125
21. Mushahary D, Spittler A, Kasper C, Weber V, Charwat V. Isolation, cultivation, and characterization of human mesenchymal stem cells. Cytometry A (2018) 93(1):19–31. doi: 10.1002/cyto.a.23242
22. Hoang DH, Nguyen TD, Nguyen HP, Nguyen XH, Do PTX, Dang VD, et al. Differential wound healing capacity of mesenchymal stem cell-derived exosomes originated from bone marrow, adipose tissue and umbilical cord under serum- and xeno-free condition. Front Mol Biosci (2020) 7:119. doi: 10.3389/fmolb.2020.00119
23. Imai T, Takahashi Y, Nishikawa M, Kato K, Morishita M, Yamashita T, et al. Macrophage-dependent clearance of systemically administered B16bl6-derived exosomes from the blood circulation in mice. J Extracell Vesicles (2015) 4:26238. doi: 10.3402/jev.v4.26238
24. Wen S, Dooner M, Papa E, Del Tatto M, Pereira M, Borgovan T, et al. Biodistribution of mesenchymal stem cell-derived extracellular vesicles in a radiation injury bone marrow murine model. Int J Mol Sci (2019) 20(21):5468. doi: 10.3390/ijms20215468
25. Barreca MM, Cancemi P, Geraci F. Mesenchymal and induced pluripotent stem cells-derived extracellular vesicles: the new frontier for regenerative medicine? Cells (2020) 9(5):1163. doi: 10.3390/cells9051163
26. Liu H, Chen Y, Yin G, Xie Q. Therapeutic prospects of micrornas carried by mesenchymal stem cells-derived extracellular vesicles in autoimmune diseases. Life Sci (2021) 277:119458. doi: 10.1016/j.lfs.2021.119458
27. Gabrielli A, Avvedimento EV, Krieg T. Scleroderma. N Engl J Med (2009) 360(19):1989–2003. doi: 10.1056/NEJMra0806188
28. Fuschiotti P. Current perspectives on the immunopathogenesis of systemic sclerosis. Immunotargets Ther (2016) 5:21–35. doi: 10.2147/ITT.S82037
29. Geyer M, Muller-Ladner U. The pathogenesis of systemic sclerosis revisited. Clin Rev Allergy Immunol (2011) 40(2):92–103. doi: 10.1007/s12016-009-8193-3
30. Mostmans Y, Cutolo M, Giddelo C, Decuman S, Melsens K, Declercq H, et al. The role of endothelial cells in the vasculopathy of systemic sclerosis: a systematic review. Autoimmun Rev (2017) 16(8):774–86. doi: 10.1016/j.autrev.2017.05.024
31. Kahaleh B. The microvascular endothelium in scleroderma. Rheumatol (Oxford) (2008) 47 Suppl 5:v14–5. doi: 10.1093/rheumatology/ken279
32. Bruni C, Frech T, Manetti M, Rossi FW, Furst DE, De Paulis A, et al. Vascular leaking, a pivotal and early pathogenetic event in systemic sclerosis: should the door be closed? Front Immunol (2018) 9:2045. doi: 10.3389/fimmu.2018.02045
33. Trojanowska M. Cellular and molecular aspects of vascular dysfunction in systemic sclerosis. Nat Rev Rheumatol (2010) 6(8):453–60. doi: 10.1038/nrrheum.2010.102
34. Carvalheiro T, Zimmermann M, Radstake T, Marut W. Novel insights into dendritic cells in the pathogenesis of systemic sclerosis. Clin Exp Immunol (2020) 201(1):25–33. doi: 10.1111/cei.13417
35. Lu TT. Dendritic cells: novel players in fibrosis and scleroderma. Curr Rheumatol Rep (2012) 14(1):30–8. doi: 10.1007/s11926-011-0215-5
36. Funes SC, Rios M, Escobar-Vera J, Kalergis AM. Implications of macrophage polarization in autoimmunity. Immunology (2018) 154(2):186–95. doi: 10.1111/imm.12910
37. Fuschiotti P. T Cells and cytokines in systemic sclerosis. Curr Opin Rheumatol (2018) 30(6):594–9. doi: 10.1097/BOR.0000000000000553
38. Sakkas LI, Xu B, Artlett CM, Lu S, Jimenez SA, Platsoucas CD. Oligoclonal T cell expansion in the skin of patients with systemic sclerosis. J Immunol (2002) 168(7):3649–59. doi: 10.4049/jimmunol.168.7.3649
39. Kalogerou A, Gelou E, Mountantonakis S, Settas L, Zafiriou E, Sakkas L. Early T cell activation in the skin from patients with systemic sclerosis. Ann Rheum Dis (2005) 64(8):1233–5. doi: 10.1136/ard.2004.027094
40. Luzina IG, Atamas SP, Wise R, Wigley FM, Choi J, Xiao HQ, et al. Occurrence of an activated, profibrotic pattern of gene expression in lung Cd8+ T cells from scleroderma patients. Arthritis Rheum (2003) 48(8):2262–74. doi: 10.1002/art.11080
41. Kowal-Bielecka O, Kowal K, Highland KB, Silver RM. Bronchoalveolar lavage fluid in scleroderma interstitial lung disease: technical aspects and clinical correlations: review of the literature. Semin Arthritis Rheum (2010) 40(1):73–88. doi: 10.1016/j.semarthrit.2008.10.009
42. Zhang M, Zhang S. T Cells in fibrosis and fibrotic diseases. Front Immunol (2020) 11:1142. doi: 10.3389/fimmu.2020.01142
43. Ricard L, Jachiet V, Malard F, Ye Y, Stocker N, Riviere S, et al. Circulating follicular helper T cells are increased in systemic sclerosis and promote plasmablast differentiation through the il-21 pathway which can be inhibited by ruxolitinib. Ann Rheum Dis (2019) 78(4):539–50. doi: 10.1136/annrheumdis-2018-214382
44. Baraut J, Michel L, Verrecchia F, Farge D. Relationship between cytokine profiles and clinical outcomes in patients with systemic sclerosis. Autoimmun Rev (2010) 10(2):65–73. doi: 10.1016/j.autrev.2010.08.003
45. Chizzolini C, Parel Y, Scheja A, Dayer JM. Polarized subsets of human T-helper cells induce distinct patterns of chemokine production by normal and systemic sclerosis dermal fibroblasts. Arthritis Res Ther (2006) 8(1):R10. doi: 10.1186/ar1860
46. Dufour AM, Borowczyk-Michalowska J, Alvarez M, Truchetet ME, Modarressi A, Brembilla NC, et al. Il-17a dissociates inflammation from fibrogenesis in systemic sclerosis. J Invest Dermatol (2020) 140(1):103–12.e8. doi: 10.1016/j.jid.2019.05.026
47. Chizzolini C, Dufour AM, Brembilla NC. Is there a role for il-17 in the pathogenesis of systemic sclerosis? Immunol Lett (2018) 195:61–7. doi: 10.1016/j.imlet.2017.09.007
48. Mathian A, Parizot C, Dorgham K, Trad S, Arnaud L, Larsen M, et al. Activated and resting regulatory T cell exhaustion concurs with high levels of interleukin-22 expression in systemic sclerosis lesions. Ann Rheum Dis (2012) 71(7):1227–34. doi: 10.1136/annrheumdis-2011-200709
49. Klein S, Kretz CC, Ruland V, Stumpf C, Haust M, Hartschuh W, et al. Reduction of regulatory T cells in skin lesions but not in peripheral blood of patients with systemic scleroderma. Ann Rheum Dis (2011) 70(8):1475–81. doi: 10.1136/ard.2009.116525
50. Slobodin G, Rimar D. Regulatory T cells in systemic sclerosis: a comprehensive review. Clin Rev Allergy Immunol (2017) 52(2):194–201. doi: 10.1007/s12016-016-8563-6
51. Thoreau B, Chaigne B, Mouthon L. Role of b-cell in the pathogenesis of systemic sclerosis. Front Immunol (2022) 13:933468. doi: 10.3389/fimmu.2022.933468
52. Sato S, Fujimoto M, Hasegawa M, Takehara K. Altered blood b lymphocyte homeostasis in systemic sclerosis: expanded naive b cells and diminished but activated memory b cells. Arthritis Rheum (2004) 50(6):1918–27. doi: 10.1002/art.20274
53. Whitfield ML, Finlay DR, Murray JI, Troyanskaya OG, Chi JT, Pergamenschikov A, et al. Systemic and cell type-specific gene expression patterns in scleroderma skin. Proc Natl Acad Sci U.S.A. (2003) 100(21):12319–24. doi: 10.1073/pnas.1635114100
54. Lafyatis R, O'Hara C, Feghali-Bostwick CA, Matteson E. B cell infiltration in systemic sclerosis-associated interstitial lung disease. Arthritis Rheum (2007) 56(9):3167–8. doi: 10.1002/art.22847
55. Mavropoulos A, Simopoulou T, Varna A, Liaskos C, Katsiari CG, Bogdanos DP, et al. Breg cells are numerically decreased and functionally impaired in patients with systemic sclerosis. Arthritis Rheumatol (2016) 68(2):494–504. doi: 10.1002/art.39437
56. Khan K, Xu S, Nihtyanova S, Derrett-Smith E, Abraham D, Denton CP, et al. Clinical and pathological significance of interleukin 6 overexpression in systemic sclerosis. Ann Rheum Dis (2012) 71(7):1235–42. doi: 10.1136/annrheumdis-2011-200955
57. van Caam A, Vonk M, van den Hoogen F, van Lent P, van der Kraan P. Unraveling ssc pathophysiology; the myofibroblast. Front Immunol (2018) 9:2452. doi: 10.3389/fimmu.2018.02452
58. Piera-Velazquez S, Li Z, Jimenez SA. Role of endothelial-mesenchymal transition (Endomt) in the pathogenesis of fibrotic disorders. Am J Pathol (2011) 179(3):1074–80. doi: 10.1016/j.ajpath.2011.06.001
59. Ho YY, Lagares D, Tager AM, Kapoor M. Fibrosis–a lethal component of systemic sclerosis. Nat Rev Rheumatol (2014) 10(7):390–402. doi: 10.1038/nrrheum.2014.53
60. Varga J, Pasche B. Transforming growth factor beta as a therapeutic target in systemic sclerosis. Nat Rev Rheumatol (2009) 5(4):200–6. doi: 10.1038/nrrheum.2009.26
61. Leask A, Denton CP, Abraham DJ. Insights into the molecular mechanism of chronic fibrosis: the role of connective tissue growth factor in scleroderma. J Invest Dermatol (2004) 122(1):1–6. doi: 10.1046/j.0022-202X.2003.22133.x
62. Abraham D. Connective tissue growth factor: growth factor, matricellular organizer, fibrotic biomarker or molecular target for anti-fibrotic therapy in ssc? Rheumatol (Oxford) (2008) 47 Suppl 5:v8–9. doi: 10.1093/rheumatology/ken278
63. Trojanowska M. Role of pdgf in fibrotic diseases and systemic sclerosis. Rheumatol (Oxford) (2008) 47 Suppl 5:v2–4. doi: 10.1093/rheumatology/ken265
64. Li M, Zhang HP, Wang XY, Chen ZG, Lin XF, Zhu W. Mesenchymal stem cell-derived exosomes ameliorate dermal fibrosis in a murine model of bleomycin-induced scleroderma. Stem Cells Dev (2021) 30(19):981–90. doi: 10.1089/scd.2021.0112
65. Rozier P, Maumus M, Bony C, Maria ATJ, Sabatier F, Jorgensen C, et al. Extracellular vesicles are more potent than adipose mesenchymal stromal cells to exert an anti-fibrotic effect in an in vitro model of systemic sclerosis. Int J Mol Sci (2021) 22(13):6837. doi: 10.3390/ijms22136837
66. Rozier P, Maumus M, Maria ATJ, Toupet K, Jorgensen C, Guilpain P, et al. Lung fibrosis is improved by extracellular vesicles from ifngamma-primed mesenchymal stromal cells in murine systemic sclerosis. Cells (2021) 10(10):2727. doi: 10.3390/cells10102727
67. Baral H, Uchiyama A, Yokoyama Y, Sekiguchi A, Yamazaki S, Amalia SN, et al. Antifibrotic effects and mechanisms of mesenchymal stem cell-derived exosomes in a systemic sclerosis mouse model: possible contribution of mir-196b-5p. J Dermatol Sci (2021) 104(1):39–47. doi: 10.1016/j.jdermsci.2021.08.006
68. Jin J, Ou Q, Wang Z, Tian H, Xu JY, Gao F, et al. Bmsc-derived extracellular vesicles intervened the pathogenic changes of scleroderma in mice through mirnas. Stem Cell Res Ther (2021) 12(1):327. doi: 10.1186/s13287-021-02400-y
69. Rozier P, Maumus M, Maria ATJ, Toupet K, Lai-Kee-Him J, Jorgensen C, et al. Mesenchymal stromal cells-derived extracellular vesicles alleviate systemic sclerosis Via mir-29a-3p. J Autoimmun (2021) 121:102660. doi: 10.1016/j.jaut.2021.102660
70. Guo L, Lai P, Wang Y, Huang T, Chen X, Geng S, et al. Extracellular vesicles derived from mesenchymal stem cells prevent skin fibrosis in the cgvhd mouse model by suppressing the activation of macrophages and b cells immune response. Int Immunopharmacol (2020) 84:106541. doi: 10.1016/j.intimp.2020.106541
71. Ha DH, Kim HK, Lee J, Kwon HH, Park GH, Yang SH, et al. Mesenchymal stem/stromal cell-derived exosomes for immunomodulatory therapeutics and skin regeneration. Cells (2020) 9(5). doi: 10.3390/cells9051157
72. Rautiainen S, Laaksonen T, Koivuniemi R. Angiogenic effects and crosstalk of adipose-derived mesenchymal Stem/Stromal cells and their extracellular vesicles with endothelial cells. Int J Mol Sci (2021) 22(19):10890. doi: 10.3390/ijms221910890
73. Gyorfi AH, Matei AE, Distler JHW. Targeting tgf-beta signaling for the treatment of fibrosis. Matrix Biol (2018) 68-69:8–27. doi: 10.1016/j.matbio.2017.12.016
74. Bergmann C, Distler JH. Canonical wnt signaling in systemic sclerosis. Lab Invest (2016) 96(2):151–5. doi: 10.1038/labinvest.2015.154
75. Guiducci S, Giacomelli R, Cerinic MM. Vascular complications of scleroderma. Autoimmun Rev (2007) 6(8):520–3. doi: 10.1016/j.autrev.2006.12.006
76. Bian D, Wu Y, Song G, Azizi R, Zamani A. The application of mesenchymal stromal cells (Mscs) and their derivative exosome in skin wound healing: a comprehensive review. Stem Cell Res Ther (2022) 13(1):24. doi: 10.1186/s13287-021-02697-9
77. Kruger-Genge A, Blocki A, Franke RP, Jung F. Vascular endothelial cell biology: an update. Int J Mol Sci (2019) 20(18):4411. doi: 10.3390/ijms20184411
78. Mou S, Zhou M, Li Y, Wang J, Yuan Q, Xiao P, et al. Extracellular vesicles from human adipose-derived stem cells for the improvement of angiogenesis and fat-grafting application. Plast Reconstr Surg (2019) 144(4):869–80. doi: 10.1097/PRS.0000000000006046
79. Kang T, Jones TM, Naddell C, Bacanamwo M, Calvert JW, Thompson WE, et al. Adipose-derived stem cells induce angiogenesis Via microvesicle transport of mirna-31. Stem Cells Transl Med (2016) 5(4):440–50. doi: 10.5966/sctm.2015-0177
80. Lopatina T, Bruno S, Tetta C, Kalinina N, Porta M, Camussi G. Platelet-derived growth factor regulates the secretion of extracellular vesicles by adipose mesenchymal stem cells and enhances their angiogenic potential. Cell Commun Signal (2014) 12:26. doi: 10.1186/1478-811X-12-26
81. Wigley FM, Flavahan NA. Raynaud's phenomenon. N Engl J Med (2016) 375(6):556–65. doi: 10.1056/NEJMra1507638
82. Bruni C, Occhipinti M, Pienn M, Camiciottoli G, Bartolucci M, Bosello SL, et al. Lung vascular changes as biomarkers of severity in systemic sclerosis-associated interstitial lung disease. Rheumatol (Oxford) (2023) 62(2):696–706. doi: 10.1093/rheumatology/keac311
83. Shan L, Liu S, Zhang Q, Zhou Q, Shang Y. Human bone marrow-mesenchymal stem cell-derived exosomal microrna-188 reduces bronchial smooth muscle cell proliferation in asthma through suppressing the Jarid2/Wnt/Beta-catenin axis. Cell Cycle (2022) 21(4):352–67. doi: 10.1080/15384101.2021.2020432
84. Zhang Z, Ge L, Zhang S, Wang J, Jiang W, Xin Q, et al. The protective effects of msc-exo against pulmonary hypertension through regulating Wnt5a/Bmp signalling pathway. J Cell Mol Med (2020) 24(23):13938–48. doi: 10.1111/jcmm.16002
85. Ren S, Chen J, Duscher D, Liu Y, Guo G, Kang Y, et al. Microvesicles from human adipose stem cells promote wound healing by optimizing cellular functions Via akt and erk signaling pathways. Stem Cell Res Ther (2019) 10(1):47. doi: 10.1186/s13287-019-1152-x
86. Jun EK, Zhang Q, Yoon BS, Moon JH, Lee G, Park G, et al. Hypoxic conditioned medium from human amniotic fluid-derived mesenchymal stem cells accelerates skin wound healing through tgf-Beta/Smad2 and Pi3k/Akt pathways. Int J Mol Sci (2014) 15(1):605–28. doi: 10.3390/ijms15010605
87. Li X, Xie X, Lian W, Shi R, Han S, Zhang H, et al. Exosomes from adipose-derived stem cells overexpressing Nrf2 accelerate cutaneous wound healing by promoting vascularization in a diabetic foot ulcer rat model. Exp Mol Med (2018) 50(4):1–14. doi: 10.1038/s12276-018-0058-5
88. Eirin A, Riester SM, Zhu XY, Tang H, Evans JM, O'Brien D, et al. Microrna and mrna cargo of extracellular vesicles from porcine adipose tissue-derived mesenchymal stem cells. Gene (2014) 551(1):55–64. doi: 10.1016/j.gene.2014.08.041
89. Yu J, Li Q, Xu Q, Liu L, Jiang B. Mir-148a inhibits angiogenesis by targeting Erbb3. J BioMed Res (2011) 25(3):170–7. doi: 10.1016/S1674-8301(11)60022-5
90. Kim H, Ko Y, Park H, Zhang H, Jeong Y, Kim Y, et al. Microrna-148a/B-3p regulates angiogenesis by targeting neuropilin-1 in endothelial cells. Exp Mol Med (2019) 51(11):1–11. doi: 10.1038/s12276-019-0344-x
91. Lee DY, Deng Z, Wang CH, Yang BB. Microrna-378 promotes cell survival, tumor growth, and angiogenesis by targeting sufu and fus-1 expression. Proc Natl Acad Sci U S A (2007) 104(51):20350–5. doi: 10.1073/pnas.0706901104
92. Kuehbacher A, Urbich C, Zeiher AM, Dimmeler S. Role of dicer and drosha for endothelial microrna expression and angiogenesis. Circ Res (2007) 101(1):59–68. doi: 10.1161/CIRCRESAHA.107.153916
93. Ma J, Zhang J, Wang Y, Long K, Wang X, Jin L, et al. Mir-532-5p alleviates hypoxia-induced cardiomyocyte apoptosis by targeting Pdcd4. Gene (2018) 675:36–43. doi: 10.1016/j.gene.2018.06.087
94. Huang B, Huang LF, Zhao L, Zeng Z, Wang X, Cao D, et al. Microvesicles (Mivs) secreted from adipose-derived stem cells (Adscs) contain multiple micrornas and promote the migration and invasion of endothelial cells. Genes Dis (2020) 7(2):225–34. doi: 10.1016/j.gendis.2019.04.005
95. Chistiakov DA, Sobenin IA, Orekhov AN, Bobryshev YV. Human mir-221/222 in physiological and atherosclerotic vascular remodeling. BioMed Res Int (2015) 2015:354517. doi: 10.1155/2015/354517
96. Huang C, Luo W, Wang Q, Ye Y, Fan J, Lin L, et al. Human mesenchymal stem cells promote ischemic repairment and angiogenesis of diabetic foot through exosome mirna-21-5p. Stem Cell Res (2021) 52:102235. doi: 10.1016/j.scr.2021.102235
97. Wang HW, Huang TS, Lo HH, Huang PH, Lin CC, Chang SJ, et al. Deficiency of the microrna-31-Microrna-720 pathway in the plasma and endothelial progenitor cells from patients with coronary artery disease. Arterioscler Thromb Vasc Biol (2014) 34(4):857–69. doi: 10.1161/ATVBAHA.113.303001
98. Suarez Y, Fernandez-Hernando C, Yu J, Gerber SA, Harrison KD, Pober JS, et al. Dicer-dependent endothelial micrornas are necessary for postnatal angiogenesis. Proc Natl Acad Sci U S A (2008) 105(37):14082–7. doi: 10.1073/pnas.0804597105
99. Greco S, De Simone M, Colussi C, Zaccagnini G, Fasanaro P, Pescatori M, et al. Common micro-rna signature in skeletal muscle damage and regeneration induced by duchenne muscular dystrophy and acute ischemia. FASEB J (2009) 23(10):3335–46. doi: 10.1096/fj.08-128579
100. Liang X, Zhang L, Wang S, Han Q, Zhao RC. Exosomes secreted by mesenchymal stem cells promote endothelial cell angiogenesis by transferring mir-125a. J Cell Sci (2016) 129(11):2182–9. doi: 10.1242/jcs.170373
101. Yang Y, Cai Y, Zhang Y, Liu J, Xu Z. Exosomes secreted by adipose-derived stem cells contribute to angiogenesis of brain microvascular endothelial cells following oxygen-glucose deprivation in vitro through microrna-181b/Trpm7 axis. J Mol Neurosci (2018) 65(1):74–83. doi: 10.1007/s12031-018-1071-9
102. Shi QZ, Yu HM, Chen HM, Liu M, Cheng X. Exosomes derived from mesenchymal stem cells regulate Treg/Th17 balance in aplastic anemia by transferring mir-23a-3p. Clin Exp Med (2021) 21(3):429–37. doi: 10.1007/s10238-021-00701-3
103. He Y, Ji D, Lu W, Li F, Huang X, Huang R, et al. Bone marrow mesenchymal stem cell-derived exosomes induce the Th17/Treg imbalance in immune thrombocytopenia through mir-146a-5p/Irak1 axis. Hum Cell (2021) 34(5):1360–74. doi: 10.1007/s13577-021-00547-7
104. Yang R, Huang H, Cui S, Zhou Y, Zhang T, Zhou Y. Ifn-gamma promoted exosomes from mesenchymal stem cells to attenuate colitis Via mir-125a and mir-125b. Cell Death Dis (2020) 11(7):603. doi: 10.1038/s41419-020-02788-0
105. Bolandi Z, Mokhberian N, Eftekhary M, Sharifi K, Soudi S, Ghanbarian H, et al. Adipose derived mesenchymal stem cell exosomes loaded with mir-10a promote the differentiation of Th17 and treg from naive Cd4(+) T cell. Life Sci (2020) 259:118218. doi: 10.1016/j.lfs.2020.118218
106. Zhao J, Li X, Hu J, Chen F, Qiao S, Sun X, et al. Mesenchymal stromal cell-derived exosomes attenuate myocardial ischaemia-reperfusion injury through mir-182-Regulated macrophage polarization. Cardiovasc Res (2019) 115(7):1205–16. doi: 10.1093/cvr/cvz040
107. Hu X, Shen N, Liu A, Wang W, Zhang L, Sui Z, et al. Bone marrow mesenchymal stem cell-derived exosomal mir-34c-5p ameliorates rif by inhibiting the core fucosylation of multiple proteins. Mol Ther (2022) 30(2):763–81. doi: 10.1016/j.ymthe.2021.10.012
108. Wan X, Chen S, Fang Y, Zuo W, Cui J, Xie S. Mesenchymal stem cell-derived extracellular vesicles suppress the fibroblast proliferation by downregulating Fzd6 expression in fibroblasts Via micrrna-29b-3p in idiopathic pulmonary fibrosis. J Cell Physiol (2020) 235(11):8613–25. doi: 10.1002/jcp.29706
109. Zhou J, Lin Y, Kang X, Liu Z, Zhang W, Xu F. Microrna-186 in extracellular vesicles from bone marrow mesenchymal stem cells alleviates idiopathic pulmonary fibrosis Via interaction with Sox4 and Dkk1. Stem Cell Res Ther (2021) 12(1):96. doi: 10.1186/s13287-020-02083-x
110. Shen Y, Xu G, Huang H, Wang K, Wang H, Lang M, et al. Sequential release of small extracellular vesicles from bilayered thiolated Alginate/Polyethylene glycol diacrylate hydrogels for scarless wound healing. ACS Nano (2021) 15(4):6352–68. doi: 10.1021/acsnano.0c07714
111. Fang S, Xu C, Zhang Y, Xue C, Yang C, Bi H, et al. Umbilical cord-derived mesenchymal stem cell-derived exosomal micrornas suppress myofibroblast differentiation by inhibiting the transforming growth factor-Beta/Smad2 pathway during wound healing. Stem Cells Transl Med (2016) 5(10):1425–39. doi: 10.5966/sctm.2015-0367
112. Kim YS, Kim JY, Cho R, Shin DM, Lee SW, Oh YM. Adipose stem cell-derived nanovesicles inhibit emphysema primarily Via an Fgf2-dependent pathway. Exp Mol Med (2017) 49(1):e284. doi: 10.1038/emm.2016.127
113. Chaubey S, Thueson S, Ponnalagu D, Alam MA, Gheorghe CP, Aghai Z, et al. Early gestational mesenchymal stem cell secretome attenuates experimental bronchopulmonary dysplasia in part Via exosome-associated factor tsg-6. Stem Cell Res Ther (2018) 9(1):173. doi: 10.1186/s13287-018-0903-4
114. Mansouri N, Willis GR, Fernandez-Gonzalez A, Reis M, Nassiri S, Mitsialis SA, et al. Mesenchymal stromal cell exosomes prevent and revert experimental pulmonary fibrosis through modulation of monocyte phenotypes. JCI Insight (2019) 4(21):e128060. doi: 10.1172/jci.insight.128060
115. Feng Y, Huang W, Wani M, Yu X, Ashraf M. Ischemic preconditioning potentiates the protective effect of stem cells through secretion of exosomes by targeting Mecp2 Via mir-22. PLoS One (2014) 9(2):e88685. doi: 10.1371/journal.pone.0088685
116. Qiu Z, Zhong Z, Zhang Y, Tan H, Deng B, Meng G. Human umbilical cord mesenchymal stem cell-derived exosomal mir-335-5p attenuates the inflammation and tubular epithelial-myofibroblast transdifferentiation of renal tubular epithelial cells by reducing Adam19 protein levels. Stem Cell Res Ther (2022) 13(1):373. doi: 10.1186/s13287-022-03071-z
117. Yang J, Zhou CZ, Zhu R, Fan H, Liu XX, Duan XY, et al. Mir-200b-Containing microvesicles attenuate experimental colitis associated intestinal fibrosis by inhibiting epithelial-mesenchymal transition. J Gastroenterol Hepatol (2017) 32(12):1966–74. doi: 10.1111/jgh.13797
118. Wu H, Fan H, Shou Z, Xu M, Chen Q, Ai C, et al. Extracellular vesicles containing mir-146a attenuate experimental colitis by targeting Traf6 and Irak1. Int Immunopharmacol (2019) 68:204–12. doi: 10.1016/j.intimp.2018.12.043
119. Shen Z, Huang W, Liu J, Tian J, Wang S, Rui K, et al. Effects of Mesenchymal Stem Cell-Derived Exosomes on Autoimmune Diseases. Am Front Immunol (2021) 12: 749192. doi: 10.3389/fimmu.2021.749192
120. Harrell CR, Jovicic N, Djonov V, Arsenijevic N, Volarevic V. Mesenchymal stem cell-derived exosomes and other extracellular vesicles as new remedies in the therapy of inflammatory diseases. Cells (2019) 8(12):1605. doi: 10.3390/cells8121605
121. Jaguin M, Houlbert N, Fardel O, Lecureur V. Polarization profiles of human m-Csf-Generated macrophages and comparison of M1-markers in classically activated macrophages from gm-csf and m-csf origin. Cell Immunol (2013) 281(1):51–61. doi: 10.1016/j.cellimm.2013.01.010
122. Lescoat A, Lecureur V, Varga J. Contribution of monocytes and macrophages to the pathogenesis of systemic sclerosis: recent insights and therapeutic implications. Curr Opin Rheumatol (2021) 33(6):463–70. doi: 10.1097/BOR.0000000000000835
123. MacDonald KG, Dawson NAJ, Huang Q, Dunne JV, Levings MK, Broady R. Regulatory T cells produce profibrotic cytokines in the skin of patients with systemic sclerosis. J Allergy Clin Immunol (2015) 135(4):946–55.e9. doi: 10.1016/j.jaci.2014.12.1932
124. Du YM, Zhuansun YX, Chen R, Lin L, Lin Y, Li JG. Mesenchymal stem cell exosomes promote immunosuppression of regulatory T cells in asthma. Exp Cell Res (2018) 363(1):114–20. doi: 10.1016/j.yexcr.2017.12.021
125. Tian J, Zhu Q, Zhang Y, Bian Q, Hong Y, Shen Z, et al. Olfactory ecto-mesenchymal stem cell-derived exosomes ameliorate experimental colitis Via modulating Th1/Th17 and treg cell responses. Front Immunol (2020) 11:598322. doi: 10.3389/fimmu.2020.598322
126. Li Y, Ren X, Zhang Z, Duan Y, Li H, Chen S, et al. Effect of small extracellular vesicles derived from il-10-Overexpressing mesenchymal stem cells on experimental autoimmune uveitis. Stem Cell Res Ther (2022) 13(1):100. doi: 10.1186/s13287-022-02780-9
127. Riazifar M, Mohammadi MR, Pone EJ, Yeri A, Lasser C, Segaliny AI, et al. Stem cell-derived exosomes as nanotherapeutics for autoimmune and neurodegenerative disorders. ACS Nano (2019) 13(6):6670–88. doi: 10.1021/acsnano.9b01004
128. Nojehdehi S, Soudi S, Hesampour A, Rasouli S, Soleimani M, Hashemi SM. Immunomodulatory effects of mesenchymal stem cell-derived exosomes on experimental type-1 autoimmune diabetes. J Cell Biochem (2018) 119(11):9433–43. doi: 10.1002/jcb.27260
129. Zhang Q, Fu L, Liang Y, Guo Z, Wang L, Ma C, et al. Exosomes originating from mscs stimulated with tgf-beta and ifn-gamma promote treg differentiation. J Cell Physiol (2018) 233(9):6832–40. doi: 10.1002/jcp.26436
130. Di Trapani M, Bassi G, Midolo M, Gatti A, Kamga PT, Cassaro A, et al. Differential and transferable modulatory effects of mesenchymal stromal cell-derived extracellular vesicles on T, b and nk cell functions. Sci Rep (2016) 6:24120. doi: 10.1038/srep24120
131. Khare D, Or R, Resnick I, Barkatz C, Almogi-Hazan O, Avni B. Mesenchymal stromal cell-derived exosomes affect mrna expression and function of b-lymphocytes. Front Immunol (2018) 9:3053. doi: 10.3389/fimmu.2018.03053
132. Adamo A, Brandi J, Caligola S, Delfino P, Bazzoni R, Carusone R, et al. Extracellular vesicles mediate mesenchymal stromal cell-dependent regulation of b cell Pi3k-akt signaling pathway and actin cytoskeleton. Front Immunol (2019) 10:446. doi: 10.3389/fimmu.2019.00446
133. Phinney DG, Di Giuseppe M, Njah J, Sala E, Shiva S, St Croix CM, et al. Mesenchymal stem cells use extracellular vesicles to outsource mitophagy and shuttle micrornas. Nat Commun (2015) 6:8472. doi: 10.1038/ncomms9472
134. Yu Y, Shen L, Xie X, Zhao J, Jiang M. The therapeutic effects of exosomes derived from human umbilical cord mesenchymal stem cells on scleroderma. Tissue Eng Regener Med (2022) 19(1):141–50. doi: 10.1007/s13770-021-00405-5
135. Shahir M, Mahmoud Hashemi S, Asadirad A, Varahram M, Kazempour-Dizaji M, Folkerts G, et al. Effect of mesenchymal stem cell-derived exosomes on the induction of mouse tolerogenic dendritic cells. J Cell Physiol (2020) 235(10):7043–55. doi: 10.1002/jcp.29601
136. Reis M, Mavin E, Nicholson L, Green K, Dickinson AM, Wang XN. Mesenchymal stromal cell-derived extracellular vesicles attenuate dendritic cell maturation and function. Front Immunol (2018) 9:2538. doi: 10.3389/fimmu.2018.02538
137. Fan Y, Herr F, Vernochet A, Mennesson B, Oberlin E, Durrbach A. Human fetal liver mesenchymal stem cell-derived exosomes impair natural killer cell function. Stem Cells Dev (2019) 28(1):44–55. doi: 10.1089/scd.2018.0015
138. Dalirfardouei R, Jamialahmadi K, Jafarian AH, Mahdipour E. Promising effects of exosomes isolated from menstrual blood-derived mesenchymal stem cell on wound-healing process in diabetic mouse model. J Tissue Eng Regener Med (2019) 13(4):555–68. doi: 10.1002/term.2799
139. Wang L, Hu L, Zhou X, Xiong Z, Zhang C, Shehada HMA, et al. Exosomes secreted by human adipose mesenchymal stem cells promote scarless cutaneous repair by regulating extracellular matrix remodelling. Sci Rep (2017) 7(1):13321. doi: 10.1038/s41598-017-12919-x
140. Huang Y, Yang L. Mesenchymal stem cell-derived extracellular vesicles in therapy against fibrotic diseases. Stem Cell Res Ther (2021) 12(1):435. doi: 10.1186/s13287-021-02524-1
141. Wei J, Fang F, Lam AP, Sargent JL, Hamburg E, Hinchcliff ME, et al. Wnt/Beta-catenin signaling is hyperactivated in systemic sclerosis and induces smad-dependent fibrotic responses in mesenchymal cells. Arthritis Rheum (2012) 64(8):2734–45. doi: 10.1002/art.34424
142. Gillespie J, Ross RL, Corinaldesi C, Esteves F, Derrett-Smith E, McDermott MF, et al. Transforming growth factor beta activation primes canonical wnt signaling through down-regulation of axin-2. Arthritis Rheumatol (2018) 70(6):932–42. doi: 10.1002/art.40437
143. Cao D, Wang Y, Zhang Y, Zhang Y, Huang Q, Yin Z, et al. Regulation of connective tissue growth factor expression by mir-133b for the treatment of renal interstitial fibrosis in aged mice with unilateral ureteral obstruction. Stem Cell Res Ther (2021) 12(1):171. doi: 10.1186/s13287-021-02210-2
144. Li Y, Wang F, Guo R, Zhang Y, Chen D, Li X, et al. Exosomal sphingosine 1-phosphate secreted by mesenchymal stem cells regulated Treg/Th17 balance in aplastic anemia. IUBMB Life (2019) 71(9):1284–92. doi: 10.1002/iub.2035
145. Wang B, Yao K, Huuskes BM, Shen HH, Zhuang J, Godson C, et al. Mesenchymal stem cells deliver exogenous microrna-Let7c Via exosomes to attenuate renal fibrosis. Mol Ther (2016) 24(7):1290–301. doi: 10.1038/mt.2016.90
Glossary
Keywords: systemic sclerosis, fibrosis, mesenchymal stem cell, extracellular vesicle, vascular injury
Citation: Zhao K, Kong C, Shi N, Jiang J and Li P (2023) Potential angiogenic, immunomodulatory, and antifibrotic effects of mesenchymal stem cell-derived extracellular vesicles in systemic sclerosis. Front. Immunol. 14:1125257. doi: 10.3389/fimmu.2023.1125257
Received: 16 December 2022; Accepted: 24 April 2023;
Published: 12 May 2023.
Edited by:
Oded Shamriz, Hadassah Medical Center, IsraelReviewed by:
Yaoxiang Sun, The Affiliated Yixing Hospital of Jiangsu University, ChinaEdoardo Rosato, Sapienza University of Rome, Italy
Philippe Guilpain, Université de Montpellier, France
Nicolò Costantino Brembilla, University of Geneva, Switzerland
Copyright © 2023 Zhao, Kong, Shi, Jiang and Li. This is an open-access article distributed under the terms of the Creative Commons Attribution License (CC BY). The use, distribution or reproduction in other forums is permitted, provided the original author(s) and the copyright owner(s) are credited and that the original publication in this journal is cited, in accordance with accepted academic practice. No use, distribution or reproduction is permitted which does not comply with these terms.
*Correspondence: Jinlan Jiang, jiangjinlan@jlu.edu.cn; Ping Li, l i_ping@jlu.edu.cn
†These authors have contributed equally to this work and share first authorship