- 1Department of Pharmacy, The First Affiliated Hospital of Zhengzhou University, Zhengzhou, Henan, China
- 2Division of Nephrology, Department of Medicine, School of Medicine, University of Connecticut Health Center, Farmington, CT, United States
- 3Department of Immunology, School of Medicine, University of Connecticut Health Center, Farmington, CT, United States
The emergence of severe acute respiratory syndrome coronavirus 2 (SARS-CoV-2) caused a pandemic named coronavirus disease 2019 (COVID-19) that has become the greatest worldwide public health threat of this century. Recent studies have unraveled numerous mysteries of SARS-CoV-2 pathogenesis and thus largely improved the studies of COVID-19 vaccines and therapeutic strategies. However, important questions remain regarding its therapy. In this review, the recent research advances on COVID-19 mechanism are quickly summarized. We mainly discuss current therapy strategies for COVID-19, with an emphasis on antiviral agents, neutralizing antibody therapies, Janus kinase inhibitors, and steroids. When necessary, specific mechanisms and the history of therapy are present, and representative strategies are described in detail. Finally, we discuss key outstanding questions regarding future directions of the development of COVID-19 treatment.
Introduction
At the end of 2019, a new coronavirus that quickly causes severe respiratory syndrome and lethal pneumonia emerged in Wuhan, China, and, 3 months later, the World Health Organization characterized the outbreak as a severe acute respiratory syndrome coronavirus 2 (SARS-CoV-2)–induced pandemic that is coronavirus disease 2019 (COVID-19) (1). The pandemic has led to a profound strike on medical care systems, economic progress, and social cohesion around the world. The magnificent research work on developing an effective COVID-19 vaccine has resulted in several safe and effective options (2–4). However, there is still a need to focus on developing potential drug candidates for treating patients with severe clinical symptoms. During the COVID-19 public health emergency, the Food and Drug Administration (FDA) issued Emergency Use Authorization (EUA) for various new drugs and medical products without full FDA approval. Currently, the primary treatments for the disease are antiviral drugs, immunomodulators, neutralizing antibody, and cell and gene therapies (5, 6). Our understanding about the effect of different categories of potential treatments due to their diversity has significantly improved. This study summarized the current therapeutic approaches (Figure 1) for COVID-19 with a simple review of SARS-CoV-2 infection pathogenesis, aiming to help researchers and doctors involved in the epidemic to improve their further work.
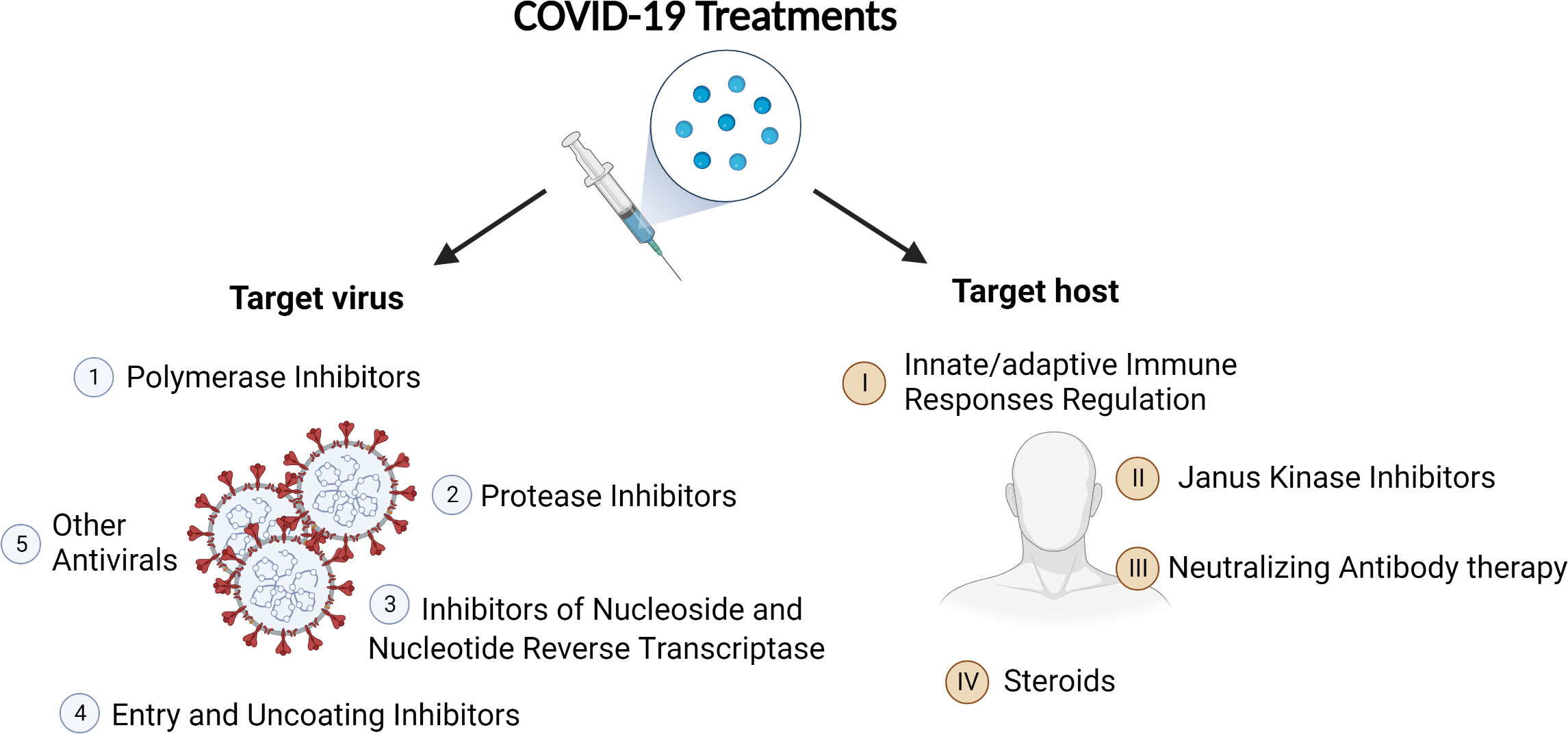
Figure 1 Category of COVID-19 treatments. On the basis of their targets, the treatments can be divided into two big categories: antiviral agents and therapies targeting host.
Mechanism of COVID-19
Coronaviruses have ignited big-scale pandemics for three times over the past 20 years: SARS from 2002 to 2003, Middle Eastern Respiratory Syndrome in 2012, and COVID-19 emerged at the end of 2019. The COVID-19, caused by SARS-CoV-2, led to an outbreak of unusual viral pneumonia that has spread worldwide and becomes the greatest global public health crisis of this century (7, 8). As of August 2022, there have been over 59 million confirmed COVID-19 cases, and more than 6 million COVID-19–related deaths worldwide. Although a total of 1.2 billion vaccine doses have been administered, COVID-19 is still a huge threat to life due to the lack of effective medical treatment (1).
SARS-CoV-2 characteristics
CoVs, including SARS-CoV-2, are enveloped viruses with positive-sense single-stranded RNA that possess the largest genomes (~30 kb) among the known RNA viruses, which belong to the Betacoronavirus genus of the family Coroaviridae (9). The genome of SARS-CoV-2 comprises 14 open reading frames encoding nine accessory proteins; four structural proteins of spike (S), envelope (E), membrane (M), and nucleocapsid (N); and 16 nonstructural proteins (nsp1–16). In all these viral proteins, protein S mediates host cell entry of SARS-CoV-2 by direct contact with its cellular receptor–angiotensin-converting enzyme 2 (ACE2). Moreover, the transmembrane protease serine 2 and cathepsin L can also facilitate SARS-CoV-2 cellular entry using a different manner at the plasma membrane surface and endosomal compartments (7, 10, 11). Once enter the host cell, cytosol release of RNA genome of SARS-CoV-2 is activated and starts to replicate. New virions are then assembled and secreted to the intercellular space for infection of neighbor cells (12). SARS-CoV-2 infections often lead to “flu-like” symptoms such as headache, fever, sore throat, backache, cough, and loss of taste or smell. Although plenty of infection cases are asymptomatic or mild, there are certain cases that show severe outcomes and are associated with systemic inflammation, acute respiratory distress syndrome, tissue damage, and cardiac injury. The severe COVID-19 disease with multiorgan damage can be fatal, and its risk largely depends on comorbidities including diabetes, obesity, hypertension, and others (8, 13, 14).
Host innate immune responses and cytokine storm
Once SARS-CoV-2 invasion happens, the host innate immune response will be rapidly activated followed by the involvement of adaptive immune system (15). As the frontline defense of host, innate immune system employs different strategies for virus detection and elimination to fight against SARS-CoV-2. During the virus infection, the innate immune cells, including natural killer cells, macrophages, monocytes, dendritic cells, and neutrophils, are rapidly recruited and activated first to produce inflammatory cytokines efficiently, such as type I interferons (IFNs) for antiviral activities. Later, B and T lymphocytes are activated for the engagement of immunological memory (16). Host innate immune system primarily relies on pattern recognition receptors (PRRs) to sense virus, bacteria, and other pathogen-associated molecular patterns and/or damage-associated molecular patterns to trigger inflammatory responses that limit viral lifecycle, promote viral clearance, and accelerate the establishment of adaptive immunity (17). The PRRs, expressed by epithelial cells and innate immune cells, are classified into several families: retinoic acid–inducible gene I (RIG-I)–like receptors (RLRs) [including RIG-I and melanoma differentiation-associated protein 5 (MDA5)], Toll-like receptors (TLRs), nucleotide-binding oligomerization domain (NOD)–like receptors (NLRs), the cyclic GMP-AMP synthase (cGAS), absent in melanoma 2–like receptors, and C-type lectin receptors (18). In all the PRRs, intracellular RLR pathways are essential for sensing RNA virus invasion to trigger innate antiviral immune response. Following activation by viral RNA, RIG-I and MDA5 translocate and bind to mitochondria to activate the adaptor protein mitochondrial antiviral signaling (MAVS) that subsequently induces TANK-binding kinase activation and IFN regulatory factor 3 (IRF3) phosphorylation, which, in turn, promotes production of IFNs to prevent virus infection (19, 20). SARS-CoV-2, belonging to single-stranded RNA viruses, can also be detected by MDA5 and RIG-I. However, their roles in regulating SARS-CoV-2 infection seem different (21–24). By screening RNA sensors for SARS-CoV-2 infection in a model of human lung epithelial cells (Calu-3), Yin and colleagues identified MDA5 and LGP2 as the dominant sensors to trigger IFN production in response to SARS-CoV-2 infection (21). Another two independent research groups found that knocking out genes encoding MDA5 or MAVS in human lung epithelial cells leads to impairment of SARS-CoV-2–induced type I IFN production (22, 23). These studies demonstrate the indispensable role of MDA5 in mediating type I IFN expression in response to SARS-CoV-2 infection. However, RIG-I seems to function in a MAVS-IFN–independent way. RIG-I deletion either by small interfering RNA or CRISPR-Cas9 in Calu-3 failed to reduce IFN-β production but still enhanced viral replication (21–23). RIG-I likely restrains full-length ACE2 expression or binds the 3′ untranslated region of the viral RNA genome via its helicase domains and consequently restricts cellular entry or replication of virus independently of IFNs (22, 25).TLRs and NLRs also are reported to play roles in anti–SARS-CoV-2 responses. As a classic innate immune signaling, TLRs express widely from tissue cells to innate immune cells, which generally trigger MyD88 or TRIF to transduce signals via nuclear factor–κB, mitogen-activated protein kinases, and IRFs to mediate transcriptional activation of pro-inflammatory cytokines (26). Upon SARS-CoV-2 infection, TLR2 senses E protein to enhance inflammatory responses and TLR4 can be activated by S protein to contribute to the release of cytokines (27, 28). In patients with COVID-19, NLRP3 inflammasome activation and its dependent caspase-1 and GSDMD cleavage as well as subsequently IL-1β secretion have been demonstrated, suggesting that NLRs and inflammasome sensors are involved in SARS-CoV-2 infection as well (29–31). In addition, the cGAS–STING signaling pathway, triggered by cytosolic DNA, is also involved in the campaign of fighting against SARS-CoV-2 invasion (32–34). In the case of severe COVID-19, PRRs and cGAS–STING signaling engaged by SARS-CoV-2 induce the expression of both IFNs and numerous pro-inflammatory cytokines, including TNF, IL-6, IL-1β, IL-12, and IL-17 (35, 36). These cytokines act as a two-edged sword, not only aiding in clearing virus infections but also contributing to life-threatening condition caused by cytokine storm. A recent study shows the combination of TNF-α and IFN-γ promotes inflammatory programmed cell death-PANoptosis by activating the Janus kinase (JAK)/signal transducer and activator of transcription (STAT1)/IRF1 axis and caspase-8/FADD signaling (36). The lethal shock phenotype observed in mice administrated with TNF-α and IFN-γ combination mirrors the cytokine storm syndrome in patients with severe COVID-19, emphasizing the link between the dysregulated release of cytokines and the multiorgan damage in patients with SARS-CoV-2 infection (37–39). In addition, the identification of elevated IL-6 levels in serum as a strong predictor of respiratory failure in patients with COVID-19 makes IL-6 one of the critical cytokines in the COVID-19–related hyperinflammatory syndrome (40, 41).
Adaptive immunity and vaccines
Adaptative immune response, established by activation of T and B cells, sent us a powerful weapon to fight against SARS-CoV-2 pandemic: the vaccine. The activation of innate immune cells during SARS-CoV-2 infection drives T and B cells to respond efficiently to secrete specific antibodies and to kill infected cells, which accelerates the development of acquired immunological memory. The adaptive immunity produced by B and T cells in response to SARS-CoV-2 infection and the vaccines have been discussed in other reviews (42, 43).
Treatments
All the treatments can be subcategorized into two big groups on the basis of their targets: antiviral agents and therapies targeting host.
Antiviral agents
Antiviral agents against COVID-19 reported mainly include polymerase inhibitors, protease inhibitors, inhibitors of nucleoside and nucleotide reverse transcriptase, entry and uncoating inhibitors, and other antivirals.
Polymerase inhibitors
Remdesivir is a nucleotide prodrug, and its active metabolite can inhibit the activity of RNA polymerases, which is a key enzyme for the replication of many viruses, including coronaviridae. Remdesivir showed antiviral effect on SARS-CoV-2 (44, 45), and it was approved by FDA for treating COVID-19. However, the clinical antiviral effect of remdesivir against SARS-CoV-2 remains controversial. One study reported a clinical trial of non-hospitalized patients with COVID-19. Among the enrolled patients, the safety was acceptable following 3 days of treatment with remdesivir, and the risk of hospitalization or death was reduced by 87% compared with the placebo (46). Another clinical trial demonstrated that remdesivir outperforms placebo. The recovery time of adults hospitalized with COVID-19 and lower respiratory tract infection is shortened after receiving remdesivir treatment (47). Whereas, other studies including a multicenter trial conducted in 10 hospitals in Hubei, China, showed that there was no statistically significant difference in the clinical status of patients with COVID-19 receiving remdesivir compared with standard care (48–52). In addition, the researchers also evaluated the effect of baricitinib combined with remdesivir in hospitalized adults with COVID-19. In terms of shortening the recovery time of patients with COVID-19 and speeding up the improvement of their clinical symptoms, remdesivir combined with baricitinib was more effective than remdesivir alone (53).
Favipiravir, an antiviral drug, selectively inhibits the RNA polymerase of viral and has antiviral effects on a variety of RNA viruses (54, 55). A clinical study demonstrated that standard supportive care plus early oral favipiravir monotherapy significantly decreased the recovery time of patients with mild-to-moderate COVID-19 compared with the standard supportive care alone (56).
Protease inhibitors
Protease is one of the key enzymes in the processing of coronavirus polyproteins. Many studies have been carried out on protease inhibitors for treating COVID-19 in recent years. Lopinavir is a viral protease inhibitor and is primarily used to treat human immunodeficiency virus (HIV). Ritonavir can increase the serum concentration of lopinavir in vivo by inhibiting CYP3A-mediated metabolism of lopinavir (57). Therefore, lopinavir/ritonavir is marketed as a combination product. Cao et al. conducted a clinical trial involving 199 hospitalized adult patients with COVID-19. The results showed that lopinavir-ritonavir treatment had no effect on adult patients with severe COVID-19 (58). Moreover, another clinical trial with more participants enrolled at 176 hospitals in the UK was reported subsequently. In this study, 1,616 patients were assigned to lopinavir/ritonavir group and 3,424 patients to the usual care group. Similarly, no efficacy was observed in hospitalized patients with COVID-19 treated with lopinavir/ritonavir (59).
Lopinavir/ritonavir might be more effective if combined with other antiviral regimens. In one study, four patients with COVID-19 were given antiviral treatment, including lopinavir/ritonavir, arbidol, and Shufeng Jiedu Capsule (a traditional Chinese medicine). The pneumonia-related symptoms of three patients were significantly improved; however, the efficacy of the combinational treatment still needs further studies to confirm (60). Moreover, the results of a phase 2 trial showed that early triple antiviral therapy (combined IFN-β1b, lopinavir/ritonavir, and ribavirin) was safe and had a better effect in patients with mild-to-moderate COVID-19 for alleviating symptoms, shortening the time of viral shedding and hospital stay than lopinavir/ritonavir alone (61). Nirmatrelvir is an inhibitor of the SARS-CoV-2 main protease (Mpro) enzyme (62). A phase 2–3 clinical trial was performed in symptomatic, unvaccinated, nonhospitalized adults at high risk for progression to severe COVID-19. In this study, 1,120 patients received nirmatrelvir plus ritonavir therapy and 1,126 patients received placebo. Symptomatic patients with COVID-19 treated with nirmatrelvir plus ritonavir had an 89% lower risk of developing severe COVID-19 than placebo (63).
Inhibitors of nucleoside and nucleotide reverse transcriptase
Azvudine (FNC), a nucleoside reverse transcriptase inhibitor, has broad-spectrum antivirus activity including HIV-1. FNC had been approved by the national medical products administration (NMPA, China) for AIDS treatment on 21 July 2021. One clinical trial of FNC confirmed that oral FNC (5 mg, qd) could cure patients with COVID-19, and the viral RNA turned negative in about 3.29 ± 2.22 days. The results demonstrated that FNC could be used against SARS-CoV-2 (64). Another clinical trial was performed in China to investigate the anti–COVID-19 effect of FNC. The results of this study showed that FNC could shorten the time of nucleic acid turning negative compared with the standard antiviral drugs for patients with mild and common COVID-19. This work also suggests that FNC is effective for COVID-19, and larger–sample size clinical trials of FNC for treating COVID-19 are needed (65, 66). Recently, NMPA conditionally approved FNC to treat common COVID-19 in adults on 25 July 2022.
Molnupiravir is a small-molecule ribonucleoside prodrug of N-hydroxycytidine and has activity against coronaviruses including SARS-CoV-2 (67). Molnupiravir reduced the risk of hospital admission or death by approximately 50% in nonhospitalized adults with mild-to-moderate COVID-19 who were at risk for poor outcomes (68). To evaluate the efficacy and safety of treatment with molnupiravir in nonhospitalized, unvaccinated adults with mild-to-moderate COVID-19, a phase 3 clinical trial was conducted. Study results suggested that the risk of hospitalization or death of unvaccinated adults with COVID-19 could be reduced by early treatment with molnupiravir (69). Another study showed that molnupiravir was active against the three predominant circulating variants (delta, gamma, and mu) of SARS-CoV-2 and showed a modest antiviral effect (70, 71). Moreover, the UK’s Medicines regulator and the US FDA have authorized the emergency use of molnupiravir for treating mild-to-moderate COVID-19 in adults.
Entry and uncoating inhibitors
Amantadine can block the early stage of viral replication, which has been used to treat influenza A (72). Amantadine, because of its lipophilic and alkaline physicochemical properties, could cross the lysosome membrane and prevents the release of viral RNA into the cells (73). One study has shown that adamantane may have protective effects against COVID-19. This study has limitations such as a small sample size, and further research is needed to confirm it (74). Enfuvirtide, an HIV-1 fusion inhibitor peptide, could be used as a potent SARS-CoV-2 fusion inhibitor (75). Clinical trials need to be performed to confirm the effect of enfuvirtide against COVID-19.
Other antivirals
Azithromycin is a synthetic macrolide antibiotic with a broad range of antibacterial, anti-inflammatory, and antiviral properties (76). A prospective, randomized superiority trial done at 19 hospitals in the UK reported that adding azithromycin to standard care treatment did not reduce the risk of subsequent hospital admission or death in patients with mild-to-moderate COVID-19 (77). Moreover, another study also showed that the routine use of azithromycin did not reduce the recovery time or risk of hospitalization for people who were suspected with COVID-19 (78).
Hydroxychloroquine and chloroquine, used to treat malaria and rheumatologic conditions, have been suggested as potential treatments for COVID-19. Currently, at least 80 trials of chloroquine, hydroxychloroquine, or both, sometimes in combination with other drugs, are registered worldwide (79). In one study of 1,561 patients with COVID-19 treated with hydroxychloroquine and 3,155 in usual care, hydroxychloroquine did not lower patient mortality compared with usual care (80). Moreover, hydroxychloroquine did not provide significant improvement in symptom severity for early, mild COVID-19 outpatients (81), and could not prevent symptomatic infection after SARS-CoV-2 exposure (82, 83). In addition, studies had shown that comparing standard care, hydroxychloroquine, alone or with azithromycin did not improve clinical outcomes for patients with COVID-19 (84, 85). Therefore, there is no need to combine azithromycin with hydroxychloroquine in patients with COVID-19.
IFNs, as one of many inflammatory mediators induced by SARS-CoV-2 infection, have been noticed since the beginning of the pandemic, but the effect of IFNs of type I (IFN-I) or type III (IFN-III) families remains controversial (86). Research reported that, unlike other infectious or noninfectious lung pathologies, the expression of INFs was increased in the lower respiratory tract of patients with severe COVID-19. The results indicated that IFNs played opposing roles at distinct anatomical sites of patients with SARS-CoV-2 (86). The hyperinflammation makes the patients succumb rapidly to COVID-19 without the help of IFN. Therefore, IFN improves the prognosis of patients with COVID-19 (87). Kalil et al. found that IFN-β1a plus remdesivir was not superior to remdesivir alone in hospitalized patients with COVID-19 pneumonia (88). Another study showed that IFN-α decreased the mean days of virus clearance and the average days of hospitalization. This study suggests that early administration of IFN-α could be a promising treatment for COVID-19 (89).
Targeting host
The treatments targeting host include neutralizing antibody therapy, Janus kinase inhibitors, and steroids.
Neutralizing antibody therapy
The history of antibody therapy can date back to the early 1890s, and, at that time, Dr. Behring and Dr. Kitasato found that the serum from an animal recovered from diphtheria infection could protect diphtheria- and tetanus-infected patients and developed the first serum therapy. Forty years later, serum therapy was widely applied in the treatment of various infectious diseases. However, it had been abandoned with the development of the first antibiotics by the late 1940s. In 1959, a big discovery about the molecular formula of antibodies was made by Gerald Edelman and Rodney Porter (90, 91). Thirty years later, the first antibody “muromonab” was approved in clinics around the world (92). However, the application of the therapeutic antibody was still limited because of the restricted resource at that time. In the 1990s, with the development of antibody engineering technologies (93), the restriction of the antibody resource was broken, which made it possible to check the effectiveness of antibody treatment on a large scale. Since then, antibody treatment has been quickly developed. So far, over 80 therapeutic monoclonal or polyclonal antibodies have been endorsed in the world, which have been mainly adopted in passive immunotherapy. However, with the outburst of COVID-19 and following severe and emergent world health situations, the interest in plasma therapy has been renewed.
Convalescent plasma
Convalescent plasma from patients who recovered from infection was adopted to treat severe patients. In 2019, the first peer-reviewed study about the effect of convalescent plasma was carried out in China (94). Compared with patients who received standard treatment, 103 patients with severe COVID-19 did not show a statistical difference after transfusion of convalescent plasma. Unfortunately, the trial was halted because of slow and limited enrollment. However, similar studies were carried out in other countries on a smaller scale in 2020. A phase 1 clinical trial study was carried out to inspect the potential of convalescent plasma in Switzerland. Thirty inpatients with COVID-19 were transfused 3 units of 200 ml plasma in 3 consecutive days, followed by comprehensive longitudinal monitoring for over 70 days. The safety of convalescent plasma therapy was confirmed with the absence of transfusion-related adverse events throughout the whole process as a consequence of smaller plasma volumes being adopted in the treatment. Furthermore, faster virus clearance and fewer comorbidities were confirmed in the following monitoring (95). However, this trial was less persuasive by virtue of the small sample size and shortage of control. In later 2020, Spanish scientists did another a higher-scale trial that is multicenter, double-blind, and randomized placebo-controlled. A total of 376 patients with mild-to-moderate COVID-19 with were recruited to receive 250–300 ml of convalescent plasma with high anti–SARS-CoV-2 IgG titers or 250-ml sterile 0.9% saline solution as the control group. The authors did not see a significant viral load decrease and the prevention of progression of the illness at the end of the trial. In addition, one patient showed a serious adverse event after infusion for 7 days (96). At the same time, another trial with 1,181 patients across 23 sites in the US was carried out with the opposite conclusion. This study found that 37 COVID-19–related hospitalization occurred in 589 patients who received control plasma, whereas only 17 of the 592 patients who were infused with convalescent plasma showed disease progression, leading to hospitalization, which means convalescent plasma greatly reduced the hospitalization risk. However, 149 participants among these 1,181 patients were fully vaccinated, and participants older than 65 years only account for 6.8%, all of which made the effectiveness of the treatment controversial (97). Another large convalescent plasma conducted in the UK involving 11,558 patients confirmed that high-titer convalescent plasma treatment failed to improve the survival of hospitalized patients with COVID-19 (98). Estcourt et al. discussed that the reason for the discrepancy between different trials was the standard of patients’ enrollment (99). Moreover, they mentioned another two ongoing trials: the COVID-19 trial and the REMAP-CAP trial, which may bring additional clarity to the effectiveness of convalescent plasma.
Neutralizing monoclonal and polyclonal antibody therapies
Although convalescent plasma showed partial effectiveness in selected patients, its potential is still controversial. In addition, only part of plasma antibodies will be neutralizing, and those non-neutralizing antibodies will bind to non-spike protein viral antigens, which will sabotage antibody reactions to further cause tissue damage. Indeed, in some convalescent plasma trials, allergic responses and lung damage occurred. Furthermore, the antibody titer in convalescent plasma is low and the resource of the blood is constrained. All these disadvantages restricted the application of convalescent plasma therapy in clinics. In contrast, monoclonal/polyclonal antibodies therapy, as another type of passive immunotherapy, can precisely target the neutralizing sites, and they can be massively produced and easily scalable, which conquers all the disadvantages of convalescent plasma. All these advantages attract medical scientists to put more effort into monoclonal or polyclonal antibodies to develop more potent therapies. As above mentioned, the S protein can bind to receptors for ACE2 to enter host cells. For the early stage, many monoclonal antibody trials targeting S protein were conducted since the routes of the virus entering host cells have been uncovered.
The first monoclonal antibody that was found effective for COVID-19 infection was LY-CoV555. The effect of LY-CoV555 in anti–SARS-CoV-2 infection in nonhuman primates was first reported by Jones et al. (100, 101). In this study, LY-CoV555 (Bamlanivimab) showed strong binding to ACE2 and neutralizing activity. It could reduce viral load in respiratory tract samples even at a low dose. Later, the effectiveness of LY-CoV555 was tested on outpatients and hospitalized patients (102, 103). For the outpatients’ trials, 309 patients were injected with 700, 2,800, or 7,000 mg of LY-CoV555. The viral load of the patients was checked on day 11. Compared with placebo patients, viral RNA showed a significant decrease in the 2,800-mg group patients. In addition, LY-CoV555 decreased the ratio of hospitalization or visiting emergency (102, 104). Two months later, another trial was conducted for the efficacy of LY-CoV555 on hospitalized patients. A total of 163 hospitalized patients were infused with LY-CoV555 and Remdesivir. However, the status of patients was not improved by LY-CoV555 (103, 105). Monoclonal antibodies are restricted only to the same or single epitope due to their monovalent affinity, which may be ineffective against the virus variance. To solve this problem, researchers combined two monoclonal antibodies. Etesevimab, as another monoclonal antibody, was always used jointly with bamlanivimab. A randomized phase 2/3 trial with 613 participants enrolled was carried out at 49 US centers to compare the efficacy of Bamlanivimab as monotherapy or together with etesevimab (106, 107). Bamlanivimab/Etesevimab showed stronger efficacy than bamlanivimab monotherapy in decreasing viral amount in outpatients with mild-to-moderate symptoms. The same conclusion was drawn by another larger trial that bamlanivimab plus etesevimab could decrease the potential of COVID-19–related hospitalization and death (108, 109). Because of their strong efficacy in patients with mild-to-moderate COVID-19, they were issued together for emergency use on 9 February 2021. However, because of the high frequency of the Omicron variant, FDA already retracted this monoclonal antibody for the post-exposure prevention or treatment under the EUA. Later, the study published by VanBlargan et al. also confirmed the futility of LY-CoV555 to Omicron variance (109, 110). So far, the only neutralizing monoclonal antibody issued by FDA for emergency use is bebtelovimab. Iketani et al. confirmed three sublineages of Omicron showed resistance to 17 neutralizing antibodies except for bebtelovimab (111, 112). Westendorf et al. were able to isolate bebtelovimab through a high-throughput B cell screening pipeline. The authors uncovered the LY-CoV1404 epitope is highly conserved in contact residues, which is why they still show neutralizing activity against omicron variance (113, 114). The efficacy of bebtelovimab was also confirmed by another study conducted by Wang et al. and published 1 month ago (115, 116). In this study, they also identified that the Omicron variance showed more transmissible and more evasive to antibodies.
Janus kinase inhibitors
Serum concentrations of proinflammatory cytokines and chemokines—including IFN-γ, TNF-α, IP-10, G-CSF, IL-2, IL-6, IL-8, IL-9, IL-10, and IL-17—were increased in patients with severe COVID-19 and strongly correlated with disease outcome (117). The JAK/STAT pathway regulates a number of inflammatory cytokines and growth factors by transferring signals from receptors in the cell membrane to the nucleus, leading to hematopoiesis, lactation, and the immune system and mammary gland development (118, 119). JAKs are tyrosine kinases that bind to the cytoplasmic domains of type I and type II cytokine receptors. When ligands bind to their receptors, the intracellular portion of JAKs will be activated, which recruits and phosphorylates STATs. Activated STATs translocate into the nucleus and bind to the promoters of related genes, inducing the expression of specific genes (120–123). These cytokines are highly important in initiating and orchestrating innate and adaptive immune responses but may also be a source of excessive or uncontrolled inflammation and tissue damage in patients with COVID-19. The importance of JAK/STAT pathway in malignancies and autoimmune diseases has been reported (124–131); therefore, inhibition of the JAK/STAT pathway is a promising approach for the treatment of various diseases.
JAK inhibitors can competitively bind to the adenosine triphosphate-binding site of JAKs and interfere with the phosphorylation of STATs proteins, thereby inhibiting the expression of downstream inflammatory genes and growth factors (132, 133). Currently, JAK inhibitors have achieved efficacy in a wide range of immune-mediated inflammatory diseases, such as rheumatoid arthritis (RA) (134), myelofibrosis, and polycythemia vera (135–137). The severity of COVID-19 is strongly associated with SARS-CoV-2–induced hypercytokinemia and inflammation (138, 139). Up to now, some JAKi (such as Baricitinib, Ruxolitinib, Tofacitinib, and Nerizutinib) have had significant clinical impacts on improving clinical outcomes of hospitalized patients with COVID-19. These kinase inhibitors are used as treatments for COVID-19 because they inhibit virus-induced immune activation and signaling of inflammation (140).
Baricitinib
Baricitinib is a JAK1/JAK2 inhibitor that blocks cytokine and growth factor receptor stimulation, thereby reducing downstream immune cell function (117). Baricitinib is used for the treatment of RA and has shown success in clinical studies in RA. Baricitinib is reliably absorbed when administered orally and is therefore highly bioavailable and well tolerated in patients with RA (141, 142). COVID-19 infection–induced cytokine signaling pathways—including IL-6, IL-2, IL-10, IFN-γ, and GM-CSF—are elevated in hyperinflammatory conditions but are disrupted by baricitinib (53, 117). Furthermore, baricitinib may also have direct anti–SARS-CoV-2 activity by interfering with viral endocytosis, thus hindering SARS-CoV-2 entry and infection of susceptible cells (143).
Numerous studies have established the potency of baricitinib in hospitalized participants with COVID-19. Improved oxygenation and reduced levels of systemic inflammatory cytokines have been reported in patients with COVID-19 treated with baricitinib (144–146). A meta-analysis of randomized controlled trials shows that treatment with JAK inhibitors (including baricitinib) in hospitalized patients with COVID-19 can significantly reduce the risk for COVID-19 death by 43%, whereas it led to a significant decrease in the risk for mechanical ventilation or ECMO (extracorporeal membrane oxygenation) initiation by 36% (147). A study demonstrated that baricitinib plus standard of care including corticosteroids predominantly reduced mortality at 28 days and 60 days in patients who were receiving National Institute of Allergy and Infectious Disease (NIAID) ordinal scale score 7 population (NIAID-OS 7; hospitalized and on IMV or ECMO). Overall, 28-day all-cause mortality among patients on IMV or ECMO at baseline was 58% among those receiving placebo and 39% among participants receiving baricitinib. Sixty-day mortality was significantly lower in the baricitinib group compared with that in the placebo (45% vs. 62%, respectively) (148). In addition, another two studies showed that baricitinib absolutely reduced mortality in patients with moderate-to-severe or severe COVID-19 (149, 150). Another meta-analysis of randomized controlled trials evaluated the safety and efficacy of baricitinib in hospitalized patients with COVID-19 and showed that baricitinib could lead to better clinical outcomes for hospitalized patients with COVID-19 (151).
Since baricitinib’s continued success in COVID-19 clinical studies, FDA issues the first EUA for baricitinib in combination with remdesivir for the treatment of hospitalized adults with COVID-19 and pediatric patients 2 years or older requiring assisted invasive mechanical ventilation or ECMO. The EUA, revised on 28 July 2021, to address safety concerns and protect public health, authorizes baricitinib as a stand-alone therapy. In May 2022, baricitinib was approved by the US FDA for the treating hospitalized adults with COVID-19 requiring supplemental oxygen, mechanical ventilation, or ECMO.
Tofacitinib
Tofacitinib, an orally available JAK inhibitor, can inhibit inflammatory cytokines such as IL-2, IL-4, IL-6, and IL-7 through inhibiting JAK3 and JAK1, which is approved for treating autoimmune diseases and RA (152–155). Many studies suggest that tofacitinib therapy can be continued in patients with COVID-19.
A double-blind, interventional phase 3 trial (NCT04469114) including 289 patients evaluated the safety and efficacy of tofacitinib in hospitalized patients with COVID-19 with pneumonia (156). The cumulative incidence of death or respiratory failure through day 28 was 18.1% in the tofacitinib group and 29.0% in the placebo group. Specifically, 28-day all-cause mortality was significantly lower in the tofacitinib group than in the placebo group (2.8% vs. 5.5%, respectively) (156). In another study by Roman and colleagues on 62 patients with severe COVID-19, it has shown that tofacitinib group showed lower mortality and the incidence of admission to intensive care than the control group (16.6% vs. 40.0% and 15.6% vs. 50.0%) (157). Furthermore, an ongoing randomized, double-blinded, placebo-controlled phase 2 study developed by Yale University evaluated the safety and efficacy of tofacitinib in 60 hospitalized patients with COVID-19 (18–99 years old) who require supplemental oxygen and have serologic markers of inflammation but do not need mechanical ventilation (NCT04415151). Analysis of these RCT studies demonstrated the promising efficacy of tofacitinib in mortality and the incidence of invasive mechanical ventilation.
Ruxolitinib
Ruxolitinib, a JAK1 and JAK2 protein kinase inhibitor, can suppress cytokine production associated with myelofibrosis, polycythemia vera, and acute graft-versus-host disease (158–163), which is similar to SARS-CO-V2 infection. The antiviral potency of ruxolitinib has also been shown to be effective against HIV and Epstein–Barr virus infection (164, 165). Since ruxolitinib has been successfully used to treat the diseases associated with hyperimmune syndrome, it has been employed in patients with COVID-19.
Several studies are being conducted with ruxolitinib in several clinical settings. In a global, double-blind, placebo-controlled, 29-day, multicenter phase 3 trial, 432 patients were randomly assigned to receive ruxolitinib (n = 287) or placebo (n = 145) plus the standard of care to assess the efficacy and safety of ruxolitinib in hospitalized patients with COVID-19. In this study, the primary objective was missing: the composite endpoint was 12% in both ruxolitinib-treated patients and placebo-treated patients; the mortality rate by day 29 was 3% in patients receiving ruxolitinib, compared with 2% in the placebo group. In addition, 8% ruxolitinib-treated patients had received invasive ventilation compared with 7% of 145 placebo-treated patients, and 11% ruxolitinib-treated patients had received ICU care compared with 12% placebo-treated patients (166). Another phase 3 study was conducted to assess the efficacy and safety of ruxolitinib with 5 mg bidaily or 15 mg bidaily in patients with COVID-19–associated ARDS who require mechanical ventilation. There was no statistically significant improvement in mortality in ruxolitinib group at day 29 compared with that in the placebo. However, it is significant when US study participants were analyzed separately, and when data from both treatment arms were pooled, it could also be detected for the overall population (167). In addition, a beneficial effect of ruxolitinib was reported in COVID-19 pneumonia in a multicenter study with 43 participants. In this study, 90% of ruxolitinib-treated patients showed computed tomography improvement at day 14 compared with 61.9% of placebo-treated patients. Three patients in the control group died of respiratory failure, with an overall mortality rate of 14.3% on day 28; no patients in the ruxolitinib group died. This study demonstrates that ruxolitinib tends to improve the clinical status of patients with COVID-19 in a shorter time (168).
Nezulcitinib
Nezulcitinib, an inhaled, lung-selective, pan-JAK inhibitor, has shown strong, broad inhibition of JAK/STAT signaling in the respiratory tract in experimental mouse models, which is a promising therapy for broad intervention in excessive immune response in the airways. Therefore, nezutinib, administered by nebulization, may provide a new therapeutic modality to inhibit cytokine release and decrease morbidity and mortality in patients with COVID-19 with acute lung injury. A phase 2 clinical trial with inhaled nezulcitinib 3 doses (1, 3, and 10 mg once daily for 7 days) explored the safety and efficacy in 25 patients with severe COVID-19 and initially reported trends toward improving the clinical status and decreasing mortality in patients requiring supplemental oxygen (169). The lower mortality rate and earlier clinical recovery in patients receiving nezulcitinib compared with that in patients receiving a placebo suggest that it is a promising therapy for cytokine-driven lung inflammation.
Steroids
The latest research shows that SARS-CoV-2 infection can motivate the immune system and ignite inflammation, which occasionally causes lethal cytokine storm. Corticosteroids have been used to treat inflammation related diseases in the last decade, such as RA and asthma. However, the trials of corticosteroids in patients with COVID were not encouraged in the beginning considering their function in suppressing the immune system. After several randomized clinical trials, corticosteroids have been proved to be able to improve survival in severe COVID-19 (170), which will be discussed in the following part.
Dexamethasone
In 2020, a controlled and open-label trial, including around 6,425 hospitalized patients, that lasted for 28 days was started in the UK (171). A total of 4,321 patients receiving usual care were taken as control, whereas another 2,104 patients received dexamethasone treatment. After 28 days, dexamethasone decreased the death rate of patients rely on invasive mechanical ventilation or oxygen. Another clinical trial stated that intravenous dexamethasone was able to increase ventilator-free days in patients with COVID-19 with moderate or severe ARDS in comparison with standard care alone (172). However, long-term treatment with 12 or 6 mg of dexamethasone for 10 days in patients with COVID-19 with severe hypoxemia did not show improvement in mortality or health-related quality of life (173). Similarly, another clinical trial study that lasted for 10 days showed that dexamethasone at 12 mg/day compared with that at 6 mg/day did not decrease the death rate of patients with COVID-19 and severe hypoxemia in the absence of life support (174), whereas a 90-day follow-up of which revealed the benefits of the high dose compared with that of the low dose (175). Furthermore, an open-label and randomized clinical trial demonstrated that high-dose dexamethasone, compared with low-dose, improved clinical symptoms within 11 days in hospitalized patients with COVID-19 who rely on oxygen therapy (176).
Budesonide
The study carried out in Spain and Argentina stated that inhaled budesonide was safe and could reduce the incidence of severe syndrome in inpatients with COVID-19 (177). Another similar study was accomplished in the UK community, which showed that inhaled budesonide could promote the recovery rate and reduces hospitalization or death in patients with COVID-19 (178). In addition, a phase 2 randomized controlled trial indicated that administration of inhaled budesonide in early COVID-19 could reduce the chance of worsening, which may be due to its inflammatory modulating effect through improving T-cell response (179, 180).
Ciclesonide
CONTAIN phase 2 randomized controlled trial exhibited that patients with inhalation and intranasal ciclesonide showed less severe symptoms than the patients in placebo groups, which did not show in young patients. However, further research is required due to insufficient evidence (181). Another randomized clinical trial of the efficacy of inhaled ciclesonide in outpatients showed that ciclesonide did not reduce the recovery time in adolescents and adult patients (182). Nevertheless, it is still early to get a conclusion about the effect of ciclesonide.
Glucocorticoids
Hydrocortisone and methylprednisolone all showed positive effects in patients with COVID-19 with severe symptoms revealed by two clinical trials (183, 184). Prednisolone showed a protective effect in patients with post–COVID-19 diffuse parenchymal abnormalities, which did not show dose dependency (185).
Conclusions
COVID-19, as a worldwide spreading virus with high transfection and lethal, caused millions of people to die, exposing the weakness of the human healthcare system in coping with emergent and serious health risks. All the above-illustrated treatments with different advantages or disadvantages (Figure 2) have been developed, targeting the virus or the host, which indeed saved lots of lives before effective vaccines were produced. Although some trials failed, they still played positive roles in the human healthcare development. The effect of many treatments is compromised or controversial, such as the antiviral agents and neutral antibody therapies, which may be caused by viral variants, and combinational treatment can be considered based on the results of some clinical trials. Many studies reported combinational therapies showing better effects than single treatment, such as molnupiravir/fluvoxamine/Paxlovid, IFN-β1b/lopinavir/ritonavir/ribavirin, and lopinavir/ritonavir/arbidol/Shufeng Jiedu Capsule. In general, combinational therapy showed more advantages than single treatment. In addition, compared with antiviral agents and neutral antibody treatments, JAK inhibitors show more promising effects, which may be worthy to continue in future studies. For the effect of steroids, it is still early to get a conclusion. Although current vaccines restrain the development of the invisible “war” ignited by COVID-19, humans should be getting ready anytime for future pandemic threats that may jeopardize the whole world. It is impossible for humans to predict the next pandemic, which makes it impractical to prepare effective vaccines in advance. However, effective treatments targeting symptoms caused by infection are still significant, with respect to being prepared for another potential pandemic threat. Hence, more efforts are still needed to be put into developing effective treatments for COVID-19.
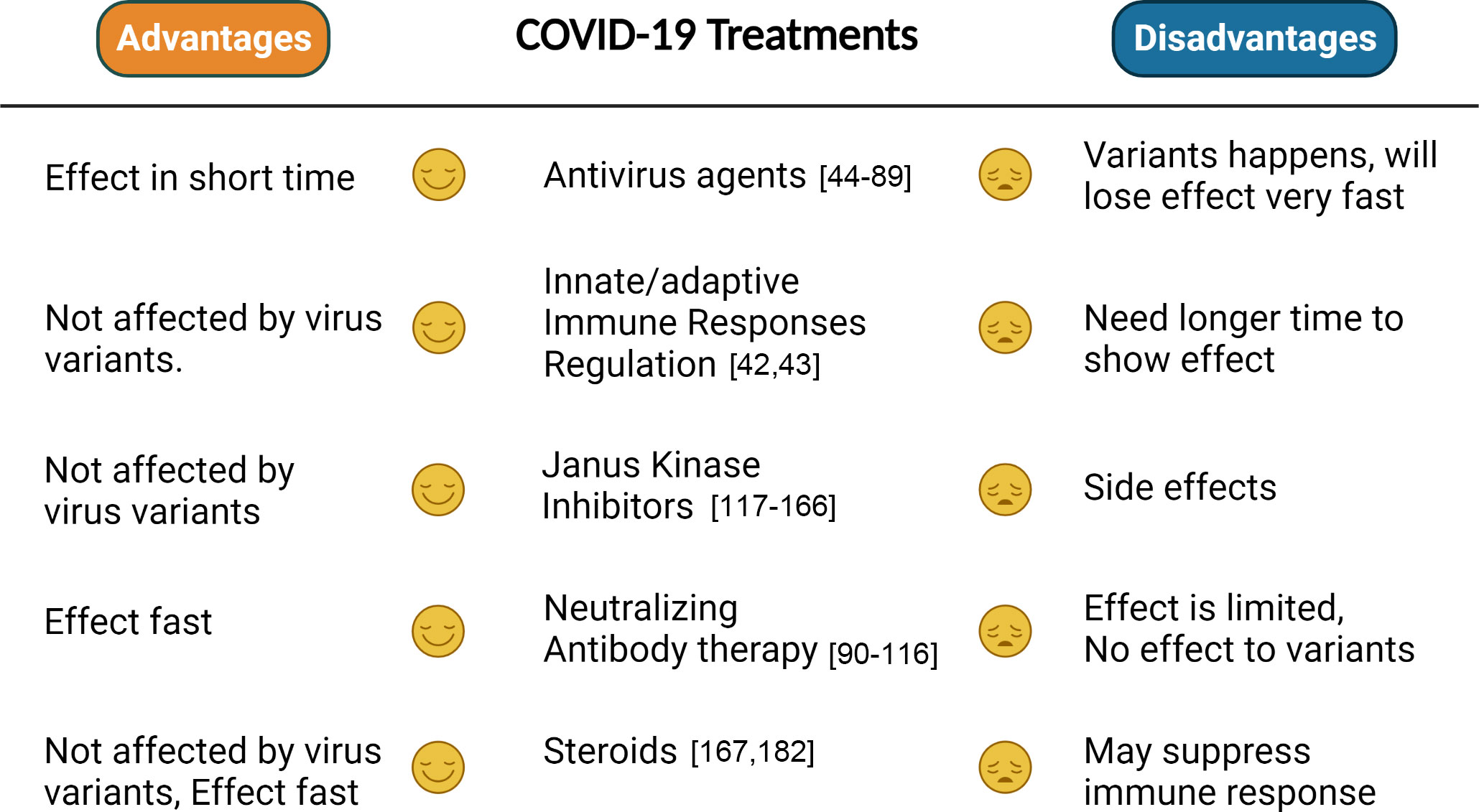
Figure 2 Comparison between different treatments. The advantages and disadvantages of each treatment are summarized on the basis of whether they will be efficient to variants, have side effects, or effect fast.
Author contributions
YY and DY arranged an overview of the content. All authors contributed to the article and approved the submitted version.
Funding
This study was supported by the program of the National natural science Foundation of China.(Grant number 81903874 and 81903720).
Conflict of interest
The authors declare that the research was conducted in the absence of any commercial or financial relationships that could be construed as a potential conflict of interest.
Publisher’s note
All claims expressed in this article are solely those of the authors and do not necessarily represent those of their affiliated organizations, or those of the publisher, the editors and the reviewers. Any product that may be evaluated in this article, or claim that may be made by its manufacturer, is not guaranteed or endorsed by the publisher.
References
1. Cucinotta D, Vanelli M. WHO declares COVID-19 a pandemic. Acta BioMed (2020) 91(1):157–60. doi: 10.23750/abm.v91i1.9397
2. Abbasi J. Fourth COVID-19 vaccine dose increases low antibodies. JAMA (2022) 327(6):517. doi: 10.1001/jama.2022.0727
3. Altmann DM, Boyton RJ. COVID-19 vaccination: The road ahead. Science (2022) 375(6585):1127–32. doi: 10.1126/science.abn1755
4. Dube E, MacDonald NE. COVID-19 vaccine hesitancy. Nat Rev Nephrol (2022) 18(7):409–10. doi: 10.1038/s41581-022-00571-2
5. Niknam Z, Golchin A, Danesh Pouya F, Nemati M, Rezaei-Tavirani M. Potential therapeutic options for COVID-19: an update on current evidence. Eur J Med Res (2022) 27(1):6. doi: 10.1186/s40001-021-00626-3
6. Drozdzal S, Rosik J, Lechowicz K, Machaj F, Szostak B, Przybycinski J, et al. An update on drugs with therapeutic potential for SARS-CoV-2 (COVID-19) treatment. Drug Resist Update (2021) 59:100794. doi: 10.1016/j.drup.2021.100794
7. Zhou P, Yang XL, Wang XG, Hu B, Zhang L, Zhang W, et al. A pneumonia outbreak associated with a new coronavirus of probable bat origin. Nature (2020) 579(7798):270–3. doi: 10.1038/s41586-020-2012-7
8. Huang C, Wang Y, Li X, Ren L, Zhao J, Hu Y, et al. Clinical features of patients infected with 2019 novel coronavirus in wuhan, China. Lancet (2020) 395(10223):497–506. doi: 10.1016/S0140-6736(20)30183-5
9. Coronaviridae Study Group of the International Committee on Taxonomy of, V. The species severe acute respiratory syndrome-related coronavirus: classifying 2019-nCoV and naming it SARS-CoV-2. Nat Microbiol (2020) 5(4):536–44. doi: 10.1038/s41564-020-0695-z
10. Lu R, Zhao X, Li J, Niu P, Yang B, Wu H, et al. Genomic characterisation and epidemiology of 2019 novel coronavirus: implications for virus origins and receptor binding. Lancet (2020) 395(10224):565–74. doi: 10.1016/S0140-6736(20)30251-8
11. Hoffmann M, Kleine-Weber H, Schroeder S, Kruger N, Herrler T, Erichsen S, et al. SARS-CoV-2 cell entry depends on ACE2 and TMPRSS2 and is blocked by a clinically proven protease inhibitor. Cell (2020) 181(2):271–280 e8. doi: 10.1016/j.cell.2020.02.052
12. Harrison AG, Lin T, Wang P. Mechanisms of SARS-CoV-2 transmission and pathogenesis. Trends Immunol (2020) 41(12):1100–15. doi: 10.1016/j.it.2020.10.004
13. Mizrahi B, Shilo S, Rossman H, Kalkstein N, Marcus K, Barer Y, et al. Longitudinal symptom dynamics of COVID-19 infection. Nat Commun (2020) 11(1):6208. doi: 10.1038/s41467-020-20053-y
14. Subramanian A, Nirantharakumar K, Hughes S, Myles P, Williams T, Gokhale KM, et al. Symptoms and risk factors for long COVID in non-hospitalized adults. Nat Med (2022) 28(8):1706–14. doi: 10.1038/s41591-022-01909-w
15. Zhang W, Chua BY, Selva KJ, Kedzierski L, Ashhurst TM, Haycroft ER, et al. SARS-CoV-2 infection results in immune responses in the respiratory tract and peripheral blood that suggest mechanisms of disease severity. Nat Commun (2022) 13(1):2774. doi: 10.1038/s41467-022-30088-y
16. Brodin P. Immune determinants of COVID-19 disease presentation and severity. Nat Med (2021) 27(1):28–33. doi: 10.1038/s41591-020-01202-8
17. Li D, Wu M. Pattern recognition receptors in health and diseases. Signal Transduct Target Ther (2021) 6(1):291. doi: 10.1038/s41392-021-00687-0
18. Kanneganti TD. Intracellular innate immune receptors: Life inside the cell. Immunol Rev (2020) 297(1):5–12. doi: 10.1111/imr.12912
19. Loo YM, Gale M Jr. Immune signaling by RIG-i-like receptors. Immunity (2011) 34(5):680–92. doi: 10.1016/j.immuni.2011.05.003
20. Horner SM, Liu HM, Park HS, Briley J, Gale M Jr. Mitochondrial-associated endoplasmic reticulum membranes (MAM) form innate immune synapses and are targeted by hepatitis c virus. Proc Natl Acad Sci U.S.A. (2011) 108(35):14590–5. doi: 10.1073/pnas.1110133108
21. Yin X, Riva L, Pu Y, Martin-Sancho L, Kanamune J, Yamamoto Y, et al. MDA5 governs the innate immune response to SARS-CoV-2 in lung epithelial cells. Cell Rep (2021) 34(2):108628. doi: 10.1016/j.celrep.2020.108628
22. Yang DM, Geng TT, Harrison AG, Wang PH. Differential roles of RIG-I like receptors in SARS-CoV-2 infection. Mil Med Res (2021) 8(1):49. doi: 10.1186/s40779-021-00340-5
23. Rebendenne A, Valadao ALC, Tauziet M, Maarifi G, Bonaventure B, McKellar J, et al. SARS-CoV-2 triggers an MDA-5-dependent interferon response which is unable to control replication in lung epithelial cells. J Virol (2021) 95(8):e02415–20. doi: 10.1128/JVI.02415-20
24. Thorne LG, Reuschl AK, Zuliani-Alvarez L, Whelan MVX, Turner J, Noursadeghi M. SARS-CoV-2 sensing by RIG-I and MDA5 links epithelial infection to macrophage inflammation. EMBO J (2021) 40(15):e107826. doi: 10.15252/embj.2021107826
25. Yamada T, Sato S, Sotoyama Y, Orba Y, Sawa H, Yamauchi H, et al. RIG-I triggers a signaling-abortive anti-SARS-CoV-2 defense in human lung cells. Nat Immunol (2021) 22(7):820–8. doi: 10.1038/s41590-021-00942-0
26. Akira S, Takeda K. Toll-like receptor signalling. Nat Rev Immunol (2004) 4(7):499–511. doi: 10.1038/nri1391
27. Zheng M, Karki R, Williams EP, Yang D, Fitzpatrick E, Vogel P, et al. TLR2 senses the SARS-CoV-2 envelope protein to produce inflammatory cytokines. Nat Immunol (2021) 22(7):829–38. doi: 10.1038/s41590-021-00937-x
28. Zhao Y, Kuang M, Li J, Zhu L, Jia Z, Guo X, et al. SARS-CoV-2 spike protein interacts with and activates TLR41. Cell Res (2021) 31(7):818–20. doi: 10.1038/s41422-021-00495-9
29. Pan P, Shen M, Yu Z, Ge W, Chen K, Tian M, et al. SARS-CoV-2 n protein promotes NLRP3 inflammasome activation to induce hyperinflammation. Nat Commun (2021) 12(1):4664. doi: 10.1038/s41467-021-25015-6
30. Rodrigues TS, de Sa KSG, Ishimoto AY, Becerra A, Oliveira S, Almeida L, et al. Inflammasomes are activated in response to SARS-CoV-2 infection and are associated with COVID-19 severity in patients. J Exp Med (2021) 218(3):e20201707. doi: 10.1084/jem.20201707
31. Ferreira AC, Soares VC, de Azevedo-Quintanilha IG, Dias S, Fintelman-Rodrigues N, Sacramento CQ, et al. SARS-CoV-2 engages inflammasome and pyroptosis in human primary monocytes. Cell Death Discovery (2021) 7(1):43. doi: 10.1038/s41420-021-00428-w
32. Rui Y, Su J, Shen S, Hu Y, Huang D, Zheng W, et al. Unique and complementary suppression of cGAS-STING and RNA sensing- triggered innate immune responses by SARS-CoV-2 proteins. Signal Transduct Target Ther (2021) 6(1):123. doi: 10.1038/s41392-021-00515-5
33. Li M, Ferretti M, Ying B, Descamps H, Lee E, Dittmar M, et al. Pharmacological activation of STING blocks SARS-CoV-2 infection. Sci Immunol (2021) 6(59):eabi9007. doi: 10.1126/sciimmunol.abi9007
34. Humphries F, Shmuel-Galia L, Jiang Z, Wilson R, Landis P, Ng SL, et al. A diamidobenzimidazole STING agonist protects against SARS-CoV-2 infection. Sci Immunol (2021) 6(59):eabi9002. doi: 10.1126/sciimmunol.abi9002
35. Hadjadj J, et al. Impaired type I interferon activity and inflammatory responses in severe COVID-19 patients. Science (2020) 369(6504):718–24. doi: 10.1126/science.abc6027
36. Karki R, Sharma BR, Tuladhar S, Williams EP, Zalduondo L, Samir P, et al. Synergism of TNF-alpha and IFN-gamma triggers inflammatory cell death, tissue damage, and mortality in SARS-CoV-2 infection and cytokine shock syndromes. Cell (2021) 184(1):149–168 e17. doi: 10.1016/j.cell.2020.11.025.
37. Zhang F, Mears JR, Shakib L, Beynor JI, Shanaj S, Korsunsky I, et al. IFN-gamma and TNF-alpha drive a CXCL10+ CCL2+ macrophage phenotype expanded in severe COVID-19 lungs and inflammatory diseases with tissue inflammation. Genome Med (2021) 13(1):64. doi: 10.1186/s13073-021-00881-3
38. Belhadjer Z, Meot M, Bajolle F, Khraiche D, Legendre A, Abakka S, et al. Acute heart failure in multisystem inflammatory syndrome in children in the context of global SARS-CoV-2 pandemic. Circulation (2020) 142(5):429–36. doi: 10.1161/CIRCULATIONAHA.120.048360
39. Mehandru S, Merad M. Pathological sequelae of long-haul COVID. Nat Immunol (2022) 23(2):194–202. doi: 10.1038/s41590-021-01104-y
40. Herold T, Jurinovic V, Arnreich C, Lipworth BJ, Hellmuth JC, von Bergwelt-Baildon M, et al. Elevated levels of IL-6 and CRP predict the need for mechanical ventilation in COVID-19. J Allergy Clin Immunol (2020) 146(1):128–136 e4. doi: 10.1016/j.jaci.2020.05.008
41. Chen X, Zhao B, Qu Y, Chen Y, Xiong J, Feng Y, et al. Detectable serum severe acute respiratory syndrome coronavirus 2 viral load (RNAemia) is closely correlated with drastically elevated interleukin 6 level in critically ill patients with coronavirus disease 2019. Clin Infect Dis (2020) 71(8):1937–42. doi: 10.1093/cid/ciaa449
42. Roltgen K, Boyd SD. Antibody and b cell responses to SARS-CoV-2 infection and vaccination. Cell Host Microbe (2021) 29(7):1063–75. doi: 10.1016/j.chom.2021.06.009
43. Moss P. The T cell immune response against SARS-CoV-2. Nat Immunol (2022) 23(2):186–93. doi: 10.1038/s41590-021-01122-w
44. Wang M, Cao R, Zhang L, Yang X, Liu J, Xu M, et al. Remdesivir and chloroquine effectively inhibit the recently emerged novel coronavirus (2019-nCoV) in vitro. Cell Res (2020) 30(3):269–71. doi: 10.1038/s41422-020-0282-0
45. Williamson BN, Feldmann F, Schwarz B, Meade-White K, Porter DP, Schulz J, et al. Clinical benefit of remdesivir in rhesus macaques infected with SARS-CoV-2. Nature (2020) 585(7824):273–6. doi: 10.1038/s41586-020-2423-5
46. Gottlieb RL, Vaca CE, Paredes R, Mera J, Webb BJ, Perez G, et al. Early remdesivir to prevent progression to severe covid-19 in outpatients. N Engl J Med (2022) 386(4):305–15. doi: 10.1056/NEJMoa2116846
47. Beigel JH, Tomashek KM, Dodd LE, Mehta AK, Zingman BS, Kalil AC, et al. Remdesivir for the treatment of covid-19 - final report. N Engl J Med (2020) 383(19):1813–26. doi: 10.1056/NEJMoa2007764
48. Spinner CD, Gottlieb RL, Criner GJ, Arribas Lopez JR, Cattelan AM, Soriano Viladomiu A, et al. Effect of remdesivir vs standard care on clinical status at 11 days in patients with moderate COVID-19: A randomized clinical trial. JAMA (2020) 324(11):1048–57. doi: 10.1001/jama.2020.16349
49. Goldman JD, Lye DCB, Hui DS, Marks KM, Bruno R, Montejano R, et al. Remdesivir for 5 or 10 days in patients with severe covid-19. N Engl J Med (2020) 383(19):1827–37. doi: 10.1056/NEJMoa2015301
50. Singh KP, Tong SYC. In adults hospitalized with COVID-19, adding remdesivir to standard care did not reduce in-hospital mortality. Ann Intern Med (2022) 175(5):JC51. doi: 10.7326/J22-0025
51. Ader F, Bouscambert-Duchamp M, Hites M, Peiffer-Smadja N, Poissy J, Belhadi D, et al. Remdesivir plus standard of care versus standard of care alone for the treatment of patients admitted to hospital with COVID-19 (DisCoVeRy): a phase 3, randomised, controlled, open-label trial. Lancet Infect Dis (2022) 22(2):209–21. doi: 10.1016/S1473-3099(21)00485-0
52. Wang Y, Zhang D, Du G, Du R, Zhao J, Jin Y, et al. Remdesivir in adults with severe COVID-19: a randomised, double-blind, placebo-controlled, multicentre trial. Lancet (2020) 395(10236):1569–78. doi: 10.1016/S0140-6736(20)31022-9
53. Kalil AC, Patterson TF, Mehta AK, Tomashek KM, Wolfe CR, Ghazaryan V, et al. Baricitinib plus remdesivir for hospitalized adults with covid-19. N Engl J Med (2021) 384(9):795–807. doi: 10.1056/NEJMoa2031994
54. Delang L, Abdelnabi R, Neyts J. Favipiravir as a potential countermeasure against neglected and emerging RNA viruses. Antiviral Res (2018) 153:85–94. doi: 10.1016/j.antiviral.2018.03.003
55. Furuta Y, Gowen BB, Takahashi K, Shiraki K, Smee DF, Barnard DL. Favipiravir (T-705), a novel viral RNA polymerase inhibitor. Antiviral Res (2013) 100(2):446–54. doi: 10.1016/j.antiviral.2013.09.015
56. Udwadia ZF, Singh P, Barkate H, Patil S, Rangwala S, Pendse A, et al. Efficacy and safety of favipiravir, an oral RNA-dependent RNA polymerase inhibitor, in mild-to-moderate COVID-19: A randomized, comparative, open-label, multicenter, phase 3 clinical trial. Int J Infect Dis (2021) 103:62–71. doi: 10.1016/j.ijid.2020.11.142
57. Yao TT, Qian JD, Zhu WY, Wang Y, Wang GQ. A systematic review of lopinavir therapy for SARS coronavirus and MERS coronavirus-a possible reference for coronavirus disease-19 treatment option. J Med Virol (2020) 92(6):556–63. doi: 10.1002/jmv.25729
58. Cao B, Wang Y, Wen D, Liu W, Wang J, Fan G, et al. A trial of lopinavir-ritonavir in adults hospitalized with severe covid-19. N Engl J Med (2020) 382(19):1787–99. doi: 10.1056/NEJMoa2001282
59. Group RC. Lopinavir-ritonavir in patients admitted to hospital with COVID-19 (RECOVERY): a randomised, controlled, open-label, platform trial. Lancet (2020) 396(10259):1345–52. doi: 10.1016/S0140-6736(20)32013-4
60. Wang Z, Chen X, Lu Y, Chen F, Zhang W. Clinical characteristics and therapeutic procedure for four cases with 2019 novel coronavirus pneumonia receiving combined Chinese and Western medicine treatment. Biosci Trends (2020) 14(1):64–8. doi: 10.5582/bst.2020.01030
61. Hung IF, Lung KC, Tso EY, Liu R, Chung TW, Chu MY, et al. Triple combination of interferon beta-1b, lopinavir-ritonavir, and ribavirin in the treatment of patients admitted to hospital with COVID-19: an open-label, randomised, phase 2 trial. Lancet (2020) 395(10238):1695–704. doi: 10.1016/S0140-6736(20)31042-4
62. Owen DR, Allerton CMN, Anderson AS, Aschenbrenner L, Avery M, Berritt S, et al. An oral SARS-CoV-2 m(pro) inhibitor clinical candidate for the treatment of COVID-19. Science (2021) 374(6575):1586–93. doi: 10.1126/science.abl4784
63. Hammond J, Leister-Tebbe H, Gardner A, Abreu P, Bao W, Wisemandle W, et al. Oral nirmatrelvir for high-risk, nonhospitalized adults with covid-19. N Engl J Med (2022) 386(15):1397–408. doi: 10.1056/NEJMoa2118542
64. Zhang JL, Li YH, Wang LL, Liu HQ, Lu SY, Liu Y, et al. Azvudine is a thymus-homing anti-SARS-CoV-2 drug effective in treating COVID-19 patients. Signal Transduct Target Ther (2021) 6(1):414. doi: 10.1038/s41392-021-00835-6
65. Ren Z, Luo H, Yu Z, Song J, Liang L, Wang L, et al. A randomized, open-label, controlled clinical trial of azvudine tablets in the treatment of mild and common COVID-19, a pilot study. Adv Sci (Weinh) (2020) 7(19):e2001435. doi: 10.1002/advs.202001435
66. Yu B, Chang J. Azvudine (FNC): a promising clinical candidate for COVID-19 treatment. Signal Transduct Target Ther (2020) 5(1):236. doi: 10.1038/s41392-020-00351-z
67. Wahl A, Gralinski LE, Johnson CE, Yao W, Kovarova M, Dinnon KH 3rd, et al. SARS-CoV-2 infection is effectively treated and prevented by EIDD-2801. Nature (2021) 591(7850):451–7. doi: 10.1038/s41586-021-03312-w
68. Mahase E. Covid-19: Molnupiravir reduces risk of hospital admission or death by 50% in patients at risk, MSD reports. BMJ (2021) 375:n2422. doi: 10.1136/bmj.n2422
69. Jayk Bernal A, Gomes da Silva MM, Musungaie DB, Kovalchuk E, Gonzalez A, Delos Reyes V, et al. Molnupiravir for oral treatment of covid-19 in nonhospitalized patients. N Engl J Med (2022) 386(6):509–20. doi: 10.1056/NEJMoa2116044
70. Mahase E. Covid-19: UK becomes first country to authorise antiviral molnupiravir. BMJ (2021) 375:n2697. doi: 10.1136/bmj.n2697
71. Whitley R. Molnupiravir - a step toward orally bioavailable therapies for covid-19. N Engl J Med (2022) 386(6):592–3. doi: 10.1056/NEJMe2117814
72. Araujo R, Aranda-Martinez JD, Aranda-Abreu GE. Amantadine treatment for people with COVID-19. Arch Med Res (2020) 51(7):739–40. doi: 10.1016/j.arcmed.2020.06.009
73. Smieszek SP, Przychodzen BP, Polymeropoulos MH. Amantadine disrupts lysosomal gene expression: A hypothesis for COVID19 treatment. Int J Antimicrob Agents (2020) 55(6):106004. doi: 10.1016/j.ijantimicag.2020.106004
74. Rejdak K, Grieb P. Adamantanes might be protective from COVID-19 in patients with neurological diseases: multiple sclerosis, parkinsonism and cognitive impairment. Mult Scler Relat Disord (2020) 42:102163. doi: 10.1016/j.msard.2020.102163
75. Ahmadi K, Farasat A, Rostamian M, Johari B, Madanchi H. Enfuvirtide, an HIV-1 fusion inhibitor peptide, can act as a potent SARS-CoV-2 fusion inhibitor: an in silico drug repurposing study. J Biomol Struct Dyn (2022) 40(12):5566–76. doi: 10.1080/07391102.2021.1871958
76. Oliver ME, Hinks TSC. Azithromycin in viral infections. Rev Med Virol (2021) 31(2):e2163. doi: 10.1002/rmv.2163
77. Dontcheff L. [Discontinuous evolution of oxygen consumption in relation to weight, during fasting in rats]. C R Seances Soc Biol Fil (1970) 164(6):1370–1.
78. Group PTC. Azithromycin for community treatment of suspected COVID-19 in people at increased risk of an adverse clinical course in the UK (PRINCIPLE): a randomised, controlled, open-label, adaptive platform trial. Lancet (2021) 397(10279):1063–74. doi: 10.1016/S0140-6736(21)00461-X
79. Ferner RE, Aronson JK. Chloroquine and hydroxychloroquine in covid-19. BMJ (2020) 369:m1432. doi: 10.1136/bmj.m1432
80. Group RC, Horby P, Mafham M, Linsell L, Bell JL, Staplin N, et al. Effect of hydroxychloroquine in hospitalized patients with covid-19. N Engl J Med (2020) 383(21):2030–40. doi: 10.1056/NEJMoa2022926
81. Correction: Hydroxychloroquine in nonhospitalized adults with early COVID-19. Ann Intern Med (2021) 174(3):435. doi: 10.7326/L20-1220
82. Boulware DR, Pullen MF, Bangdiwala AS, Pastick KA, Lofgren SM, Okafor EC, et al. A randomized trial of hydroxychloroquine as postexposure prophylaxis for covid-19. N Engl J Med (2020) 383(6):517–25. doi: 10.1056/NEJMoa2016638
83. Mitja O, Pullen MF, Bangdiwala AS, Pastick KA, Lofgren SM, Okafor EC, et al. A cluster-randomized trial of hydroxychloroquine for prevention of covid-19. N Engl J Med (2021) 384(5):417–27. doi: 10.1056/NEJMoa2021801
84. Furtado RHM, Berwanger O, Fonseca HA, Correa TD, Ferraz LR, Lapa MG, et al. Azithromycin in addition to standard of care versus standard of care alone in the treatment of patients admitted to the hospital with severe COVID-19 in Brazil (COALITION II): a randomised clinical trial. Lancet (2020) 396(10256):959–67. doi: 10.1016/S0140-6736(20)31862-6
85. Cavalcanti AB, Zampieri FG, Rosa RG, Azevedo LCP, Veiga VC, Avezum A, et al. Hydroxychloroquine with or without azithromycin in mild-to-Moderate covid-19. N Engl J Med (2020) 383(21):2041–52. doi: 10.1056/NEJMoa2019014
86. Sposito B, Broggi A, Pandolfi L, Crotta S, Clementi N, Ferrarese R, et al. The interferon landscape along the respiratory tract impacts the severity of COVID-19. Cell (2021) 184(19):4953–4968 e16. doi: 10.1016/j.cell.2021.08.016
87. Ramasamy S, Subbian S. Erratum for ramasamy and subbian, “Critical determinants of cytokine storm and type I interferon response in COVID-19 pathogenesis”. Clin Microbiol Rev (2021) 34(4):e0016321. doi: 10.1128/CMR.00163-21
88. Kalil AC, Mehta AK, Patterson TF, Erdmann N, Gomez CA, Jain MK, et al. Efficacy of interferon beta-1a plus remdesivir compared with remdesivir alone in hospitalised adults with COVID-19: a double-bind, randomised, placebo-controlled, phase 3 trial. Lancet Respir Med (2021) 9(12):1365–76. doi: 10.1016/S2213-2600(21)00384-2
89. Nakhlband A, Fakhari A, Azizi H. Interferon-alpha position in combating with COVID-19: A systematic review. J Med Virol (2021) 93(9):5277–84. doi: 10.1002/jmv.27072
90. Porter RR. The hydrolysis of rabbit y-globulin and antibodies with crystalline papain. Biochem J (1959) 73(1):119–26. doi: 10.1042/bj0730119
91. Edelman GM, Benacerraf B, Ovary Z, Poulik MD. Structural differences among antibodies of different specificities. Proc Natl Acad Sci U.S.A. (1961) 47(11):1751–8. doi: 10.1073/pnas.47.11.1751
92. Todd PA, Brogden RN. Muromonab CD3. a review of its pharmacology and therapeutic potential. Drugs (1989) 37(6):871–99. doi: 10.2165/00003495-198937060-00004
93. Rajewsky K. The advent and rise of monoclonal antibodies. Nature (2019) 575(7781):47–9. doi: 10.1038/d41586-019-02840-w
94. Li L, Zhang W, Hu Y, Tong X, Zheng S, Yang J, et al. Effect of convalescent plasma therapy on time to clinical improvement in patients with severe and life-threatening COVID-19: A randomized clinical trial. JAMA (2020) 324(5):460–70. doi: 10.1001/jama.2020.10044
95. Marconato M, Abela IA, Hauser A, Schwarzmüller M, Katzensteiner R, Braun DL, et al. Antibodies from convalescent plasma promote SARS-CoV-2 clearance in individuals with and without endogenous antibody response. J Clin Invest (2022) 132(12):e158190. doi: 10.1172/JCI158190
96. Alemany A, Millat-Martinez P, Corbacho-Monne M, Malchair P, Ouchi D, Ruiz-Comellas A, et al. High-titre methylene blue-treated convalescent plasma as an early treatment for outpatients with COVID-19: a randomised, placebo-controlled trial. Lancet Respir Med (2022) 10(3):278–88. doi: 10.1016/S2213-2600(21)00545-2
97. Sullivan DJ, Gebo KA, Shoham S, Bloch EM, Lau B, Shenoy AG, et al. Early outpatient treatment for covid-19 with convalescent plasma. N Engl J Med (2022) 386(18):1700–11. doi: 10.1056/NEJMoa2119657
98. RECOVERY Collaborative Group. Convalescent plasma in patients admitted to hospital with COVID-19 (RECOVERY): a randomised controlled, open-label, platform trial. Lancet (2021) 397(10289):2049–59. doi: 10.1016/S0140-6736(21)00897-7
99. Estcourt L, Callum J. Convalescent plasma for covid-19 - making sense of the inconsistencies. N Engl J Med (2022) 386(18):1753–4. doi: 10.1056/NEJMe2204332
100. Jones BE, Brown-Augsburger PL, Corbett KS, Westendorf K, Davies J, Cujec TP, et al. The neutralizing antibody, LY-CoV555, protects against SARS-CoV-2 infection in nonhuman primates. Sci Transl Med (2021) 13(593):eabf1906. doi: 10.1126/scitranslmed.abf1906
101. Matz AJ, Qu L, Karlinsey K, Zhou B. MicroRNA-regulated b cells in obesity. Immunometabolism (Cobham) (2022) 4(3):e00005. doi: 10.1097/IN9.0000000000000005
102. Chen P, Nirula A, Heller B, Gottlieb RL, Boscia J, Morris J, et al. SARS-CoV-2 neutralizing antibody LY-CoV555 in outpatients with covid-19. N Engl J Med (2021) 384(3):229–37. doi: 10.1056/NEJMoa2029849
103. ACTIV-3/TICO LY-CoV555 Study Group, Lundgren JD, Grund B, Barkauskas CE, Holland TL, Gottlieb RL, et al. A neutralizing monoclonal antibody for hospitalized patients with covid-19. N Engl J Med (2021) 384(10):905–14. doi: 10.1056/NEJMoa2033130
104. Matz A, Qu L, Karlinsey K, Zhou B. Impact of microRNA regulated macrophage actions on adipose tissue function in obesity. Cells (2022) 11(8):1336. doi: 10.3390/cells11081336
105. Qu L, Matz AJ, Karlinsey K, Cao Z, Vella AT, Zhou B, et al. Macrophages at the crossroad of meta-inflammation and inflammaging. Genes (Basel) (2022) 13(11):2074. doi: 10.3390/genes13112074
106. Gottlieb RL, Nirula A, Chen P, Boscia J, Heller B, Morris J, et al. Effect of bamlanivimab as monotherapy or in combination with etesevimab on viral load in patients with mild to moderate COVID-19: A randomized clinical trial. JAMA (2021) 325(7):632–44. doi: 10.1001/jama.2021.0202
107. Karlinsey K, Qu L, Matz AJ, Zhou B. A novel strategy to dissect multifaceted macrophage function in human diseases. J Leukoc Biol (2022) 112(6):1535–42. doi: 10.1002/JLB.6MR0522-685R
108. Dougan M, Nirula A, Azizad M, Mocherla B, Gottlieb RL, Chen P, et al. Bamlanivimab plus etesevimab in mild or moderate covid-19. N Engl J Med (2021) 385(15):1382–92. doi: 10.1056/NEJMoa2102685
109. Li C, Phoon YP, Karlinsey K, Tian YF, Thapaliya S, Thongkum A, et al. A high OXPHOS CD8 T cell subset is predictive of immunotherapy resistance in melanoma patients. J Exp Med (2022) 219(1):e20202084. doi: 10.1084/jem.20202084
110. VanBlargan LA, Errico JM, Halfmann PJ, Zost SJ, Crowe JE Jr, Purcell LA, et al. An infectious SARS-CoV-2 B.1.1.529 omicron virus escapes neutralization by therapeutic monoclonal antibodies. Nat Med (2022) 28(3):490–5. doi: 10.1038/s41591-021-01678-y
111. Iketani S, Liu L, Guo Y, Liu L, Chan JF, Huang Y, et al. Antibody evasion properties of SARS-CoV-2 omicron sublineages. Nature (2022) 604(7906):553–6. doi: 10.1038/s41586-022-04594-4
112. Li C, Qu L, Matz AJ, Murphy PA, Liu Y, Manichaikul AW, et al. AtheroSpectrum reveals novel macrophage foam cell gene signatures associated with atherosclerotic cardiovascular disease risk. Circulation (2022) 145(3):206–18. doi: 10.1161/CIRCULATIONAHA.121.054285
113. Westendorf K, Zentelis S, Wang L, Foster D, Vaillancourt P, Wiggin M, et al. LY-CoV1404 (bebtelovimab) potently neutralizes SARS-CoV-2 variants. Cell Rep (2022) 39(7):110812. doi: 10.1016/j.celrep.2022.110812
114. Li C, Qu L, Farragher C, Vella A, Zhou B. MicroRNA regulated macrophage activation in obesity. J Transl Int Med (2019) 7(2):46–52. doi: 10.2478/jtim-2019-0011
115. Wang Q, Guo Y, Iketani S, Nair MS, Li Z, Mohri H, et al. Antibody evasion by SARS-CoV-2 omicron subvariants BA.2.12.1, BA.4 and BA.5. Nature (2022) 608(7923):603–8. doi: 10.1038/s41586-022-05053-w
116. Qu LL, Yu B, Li Z, Jiang WX, Jiang JD, Kong WJ. Gastrodin ameliorates oxidative stress and proinflammatory response in nonalcoholic fatty liver disease through the AMPK/Nrf2 pathway. Phytother Res (2016) 30(3):402–11. doi: 10.1002/ptr.5541
117. Hoang TN, Pino M, Boddapati AK, Viox EG, Starke CE, Upadhyay AA, et al. Baricitinib treatment resolves lower-airway macrophage inflammation and neutrophil recruitment in SARS-CoV-2-infected rhesus macaques. Cell (2021) 184(2):460–475 e21. doi: 10.1016/j.cell.2020.11.007
118. Duan X, Li S, Holmes JA, Tu Z, Li Y, Cai D, et al. MicroRNA 130a regulates both hepatitis c virus and hepatitis b virus replication through a central metabolic pathway. J Virol (2018) 92(7):e02009-17. doi: 10.1128/JVI.02009-17
119. Chen X, Ye H, Li S, Jiao B, Wu J, Zeng P, et al. Severe fever with thrombocytopenia syndrome virus inhibits exogenous type I IFN signaling pathway through its NSs invitro. PloS One (2017) 12(2):e0172744. doi: 10.1371/journal.pone.0172744
120. Hu X, Li J, Fu M, Zhao X, Wang W. The JAK/STAT signaling pathway: from bench to clinic. Signal Transduct Target Ther (2021) 6(1):402. doi: 10.1038/s41392-021-00791-1
121. Jiao B, Shi X, Chen Y, Ye H, Yao M, Hong W, et al. Insulin receptor substrate-4 interacts with ubiquitin-specific protease 18 to activate the Jak/STAT signaling pathway. Oncotarget (2017) 8(62):105923–35. doi: 10.18632/oncotarget.22510
122. Shi X, Jiao B, Chen Y, Li S, Chen L. MxA is a positive regulator of type I IFN signaling in HCV infection. J Med Virol (2017) 89(12):2173–80. doi: 10.1002/jmv.24867
123. Villarino AV, Gadina M, O'Shea JJ, Kanno Y. SnapShot: Jak-STAT signaling II. Cell (2020) 181(7):1696–1696 e1. doi: 10.1016/j.cell.2020.04.052
124. O’Shea JJ, Schwartz DM, Villarino AV, Gadina M, McInnes IB, Laurence A. The JAK-STAT pathway: impact on human disease and therapeutic intervention. Annu Rev Med (2015) 66:311–28. doi: 10.1146/annurev-med-051113-024537
125. Li Y, Li S, Duan X, Chen Y, Jiao B, Ye H, et al. Interferon-stimulated gene 15 conjugation stimulates hepatitis b virus production independent of type I interferon signaling pathway in vitro. Mediators Inflammation (2016) 2016:7417648. doi: 10.1155/2016/7417648
126. Chen Y, Jiao B, Yao M, Shi X, Zheng Z, Li S, et al. ISG12a inhibits HCV replication and potentiates the anti-HCV activity of IFN-alpha through activation of the Jak/STAT signaling pathway independent of autophagy and apoptosis. Virus Res (2017) 227:231–9. doi: 10.1016/j.virusres.2016.10.013
127. Jiao B, An C, Du H, Tran M, Wang P, Zhou D, et al. STAT6 deficiency attenuates myeloid fibroblast activation and macrophage polarization in experimental folic acid nephropathy. Cells (2021) 10(11):3057. doi: 10.3390/cells10113057
128. Banerjee S, Biehl A, Gadina M, Hasni S, Schwartz DM. JAK-STAT signaling as a target for inflammatory and autoimmune diseases: Current and future prospects. Drugs (2017) 77(5):521–46. doi: 10.1007/s40265-017-0701-9
129. Villarino AV, Kanno Y, O’Shea JJ. Mechanisms and consequences of jak-STAT signaling in the immune system. Nat Immunol (2017) 18(4):374–84. doi: 10.1038/ni.3691
130. O’Shea JJ, Plenge R. JAK and STAT signaling molecules in immunoregulation and immune-mediated disease. Immunity (2012) 36(4):542–50. doi: 10.1016/j.immuni.2012.03.014
131. Jiao B, An C, Tran M, Du H, Wang P, Zhou D, et al. Pharmacological inhibition of STAT6 ameliorates myeloid fibroblast activation and alternative macrophage polarization in renal fibrosis. Front Immunol (2021) 12:735014. doi: 10.3389/fimmu.2021.735014
132. Lin CM, Cooles FA, Isaacs JD. Basic mechanisms of JAK inhibition. Mediterr J Rheumatol (2020) 31(Suppl 1):100–4. doi: 10.31138/mjr.31.1.100
133. Zarrin AA, Bao K, Lupardus P, Vucic D. Kinase inhibition in autoimmunity and inflammation. Nat Rev Drug Discovery (2021) 20(1):39–63. doi: 10.1038/s41573-020-0082-8
134. Baldini C, Moriconi FR, Galimberti S, Libby P, De Caterina R. The JAK-STAT pathway: an emerging target for cardiovascular disease in rheumatoid arthritis and myeloproliferative neoplasms. Eur Heart J (2021) 42(42):4389–400. doi: 10.1093/eurheartj/ehab447
135. Wolfe L. Ruxolitinib in myelofibrosis and polycythemia Vera. J Adv Pract Oncol (2016) 7(4):436–44.
136. Sorensen AL, Mikkelsen SU, Knudsen TA, Bjorn ME, Andersen CL, Bjerrum OW, et al. Ruxolitinib and interferon-alpha2 combination therapy for patients with polycythemia vera or myelofibrosis: a phase II study. Haematologica (2020) 105(9):2262–72. doi: 10.3324/haematol.2019.235648
137. Alvarez-Larran A, Garrote M, Ferrer-Marin F, Perez-Encinas M, Mata-Vazquez MI, Bellosillo B, et al. Real-world analysis of main clinical outcomes in patients with polycythemia vera treated with ruxolitinib or best available therapy after developing resistance/intolerance to hydroxyurea. Cancer (2022) 128(13):2441–8. doi: 10.1002/cncr.34195
138. Shrock E, Fujimura E, Kula T, Timms RT, Lee IH, Leng Y, et al. Viral epitope profiling of COVID-19 patients reveals cross-reactivity and correlates of severity. Science (2020) 370(6520):eabd4250. doi: 10.1126/science.abd4250
139. Carvalho T, Krammer F, Iwasaki A. The first 12 months of COVID-19: a timeline of immunological insights. Nat Rev Immunol (2021) 21(4):245–56. doi: 10.1038/s41577-021-00522-1
140. Zhang W, Zhao Y, Zhang F, Wang Q, Li T, Liu Z, et al. The use of anti-inflammatory drugs in the treatment of people with severe coronavirus disease 2019 (COVID-19): The perspectives of clinical immunologists from China. Clin Immunol (2020) 214:108393. doi: 10.1016/j.clim.2020.108393
141. Smolen JS, Landewe RBM, Bijlsma JWJ, Burmester GR, Dougados M, Kerschbaumer A, et al. EULAR recommendations for the management of rheumatoid arthritis with synthetic and biological disease-modifying antirheumatic drugs: 2019 update. Ann Rheum Dis (2020) 79(6):685–99. doi: 10.1136/annrheumdis-2019-216655
142. Kerschbaumer A, Sepriano A, Smolen JS, van der Heijde D, Dougados M, van Vollenhoven R, et al. Efficacy of pharmacological treatment in rheumatoid arthritis: a systematic literature research informing the 2019 update of the EULAR recommendations for management of rheumatoid arthritis. Ann Rheum Dis (2020) 79(6):744–59. doi: 10.1136/annrheumdis-2019-216656
143. Stebbing J, Phelan A, Griffin I, Tucker C, Oechsle O, Smith D, et al. COVID-19: combining antiviral and anti-inflammatory treatments. Lancet Infect Dis (2020) 20(4):400–2. doi: 10.1016/S1473-3099(20)30132-8
144. Kulkarni S, Fisk M, Kostapanos M, Banham-Hall E, Bond S, Hernan-Sancho E, et al. Repurposed immunomodulatory drugs for covid-19 in pre-ICu patients - mulTi-arm therapeutic study in pre-ICu patients admitted with covid-19 - repurposed drugs (TACTIC-r): A structured summary of a study protocol for a randomised controlled trial. Trials (2020) 21(1):626. doi: 10.1186/s13063-020-04535-4
145. Moreno-Gonzalez G, Mussetti A, Albasanz-Puig A, Salvador I, Sureda A, Gudiol C, et al. A phase I/II clinical trial to evaluate the efficacy of baricitinib to prevent respiratory insufficiency progression in onco-hematological patients affected with COVID19: A structured summary of a study protocol for a randomised controlled trial. Trials (2021) 22(1):116. doi: 10.1186/s13063-021-05072-4
146. Bronte V, Ugel S, Tinazzi E, Vella A, De Sanctis F, Cane S, et al. Baricitinib restrains the immune dysregulation in patients with severe COVID-19. J Clin Invest (2020) 130(12):6409–16. doi: 10.1172/JCI141772
147. Patoulias D, Doumas M, Papadopoulos C, Karagiannis A. Janus kinase inhibitors and major COVID-19 outcomes: time to forget the two faces of janus! a meta-analysis of randomized controlled trials. Clin Rheumatol (2021) 40(11):4671–4. doi: 10.1007/s10067-021-05884-4
148. Ely EW, Ramanan AV, Kartman CE, de Bono S, Liao R, Piruzeli MLB, et al. Efficacy and safety of baricitinib plus standard of care for the treatment of critically ill hospitalised adults with COVID-19 on invasive mechanical ventilation or extracorporeal membrane oxygenation: an exploratory, randomised, placebo-controlled trial. Lancet Respir Med (2022) 10(4):327–36. doi: 10.1016/S2213-2600(22)00006-6
149. Stebbing J, Sánchez Nievas G, Falcone M, Youhanna S, Richardson P, Ottaviani S, et al. JAK inhibition reduces SARS-CoV-2 liver infectivity and modulates inflammatory responses to reduce morbidity and mortality. Sci Adv (2021) 7(1):eabe4724. doi: 10.1126/sciadv.abe4724
150. Abizanda P, Calbo Mayo JM, Mas Romero M, Cortes Zamora EB, Tabernero Sahuquillo MT, Romero Rizos L, et al. Baricitinib reduces 30-day mortality in older adults with moderate-to-severe COVID-19 pneumonia. J Am Geriatr Soc (2021) 69(10):2752–8. doi: 10.1111/jgs.17357
151. Chen CY, Chen WC, Hsu CK, Chao CM, Lai CC. Clinical efficacy and safety of janus kinase inhibitors for COVID-19: A systematic review and meta-analysis of randomized controlled trials. Int Immunopharmacol (2021) 99:108027. doi: 10.1016/j.intimp.2021.108027
152. Pillaiyar T, Laufer S. Kinases as potential therapeutic targets for anti-coronaviral therapy. J Med Chem (2022) 65(2):955–82. doi: 10.1021/acs.jmedchem.1c00335
153. Mease P, Hall S, FitzGerald O, van der Heijde D, Merola JF, Avila-Zapata F, et al. Tofacitinib or adalimumab versus placebo for psoriatic arthritis. N Engl J Med (2017) 377(16):1537–50. doi: 10.1056/NEJMoa1615975
154. Dhillon S. Tofacitinib: A review in rheumatoid arthritis. Drugs (2017) 77(18):1987–2001. doi: 10.1007/s40265-017-0835-9
155. Wollenhaupt J, Lee EB, Curtis JR, Silverfield J, Terry K, Soma K, et al. Safety and efficacy of tofacitinib for up to 9.5 years in the treatment of rheumatoid arthritis: final results of a global, open-label, long-term extension study. Arthritis Res Ther (2019) 21(1):89. doi: 10.1186/s13075-019-1866-2
156. Guimaraes PO, Quirk D, Furtado RH, Maia LN, Saraiva JF, Antunes MO, et al. Tofacitinib in patients hospitalized with covid-19 pneumonia. N Engl J Med (2021) 385(5):406–15. doi: 10.1056/NEJMoa2101643
157. Maslennikov R, Ivashkin V, Vasilieva E, Chipurik M, Semikova P, Semenets V, et al. Tofacitinib reduces mortality in coronavirus disease 2019 tofacitinib in COVID-19. Pulm Pharmacol Ther (2021) 69:102039. doi: 10.1016/j.pupt.2021.102039
158. Yan B, Freiwald T, Chauss D, Wang L, West E, Mirabelli C, et al. SARS-CoV-2 drives JAK1/2-dependent local complement hyperactivation. Sci Immunol (2021) 6(58):eabg0833. doi: 10.1126/sciimmunol.abg0833
159. Verstovsek S, Mesa RA, Gotlib J, Levy RS, Gupta V, DiPersio JF, et al. A double-blind, placebo-controlled trial of ruxolitinib for myelofibrosis. N Engl J Med (2012) 366(9):799–807. doi: 10.1056/NEJMoa1110557
160. Mascarenhas J, Hoffman R, Talpaz M, Gerds AT, Stein B, Gupta V, et al. Pacritinib vs best available therapy, including ruxolitinib, in patients with myelofibrosis: A randomized clinical trial. JAMA Oncol (2018) 4(5):652–9. doi: 10.1001/jamaoncol.2017.5818
161. Vannucchi AM, Kiladjian JJ, Griesshammer M, Masszi T, Durrant S, Passamonti F, et al. Ruxolitinib versus standard therapy for the treatment of polycythemia vera. N Engl J Med (2015) 372(5):426–35. doi: 10.1056/NEJMoa1409002
162. Zeiser R, von Bubnoff N, Butler J, Mohty M, Niederwieser D, Or R, et al. Ruxolitinib for glucocorticoid-refractory acute graft-versus-Host disease. N Engl J Med (2020) 382(19):1800–10. doi: 10.1056/NEJMoa1917635
163. Gratwohl A. Ruxolitinib for acute graft-versus-Host disease. N Engl J Med (2020) 383(5):501–2. doi: 10.1056/NEJMc2020763
164. Zhang Q, Zhao YZ, Ma HH, Wang D, Cui L, Li WJ, et al. A study of ruxolitinib response-based stratified treatment for pediatric hemophagocytic lymphohistiocytosis. Blood (2022) 139(24):3493–504. doi: 10.1182/blood.2021014860
165. Marconi VC, Moser C, Gavegnano C, Deeks SG, Lederman MM, Overton ET, et al. Randomized trial of ruxolitinib in antiretroviral-treated adults with human immunodeficiency virus. Clin Infect Dis (2022) 74(1):95–104. doi: 10.1093/cid/ciab212
166. Han MK, Antila M, Ficker JH, Gordeev I, Guerreros A, Bernus AL, et al. Ruxolitinib in addition to standard of care for the treatment of patients admitted to hospital with COVID-19 (RUXCOVID): a randomised, double-blind, placebo-controlled, phase 3 trial. Lancet Rheumatol (2022) 4(5):e351–61. doi: 10.1016/S2665-9913(22)00044-3
167. Levy G, Guglielmelli P, Langmuir P, Constantinescu SN. JAK inhibitors and COVID-19. J Immunother Cancer (2022) 10(4):e002838. doi: 10.1136/jitc-2021-002838
168. Cao Y, Wei J, Zou L, Jiang T, Wang G, Chen L, et al. Ruxolitinib in treatment of severe coronavirus disease 2019 (COVID-19): A multicenter, single-blind, randomized controlled trial. J Allergy Clin Immunol (2020) 146(1):137–146 e3. doi: 10.1016/j.jaci.2020.05.019
169. Singh D, Bogus M, Moskalenko V, Lord R, Moran EJ, Crater GD, et al. A phase 2 multiple ascending dose study of the inhaled pan-JAK inhibitor nezulcitinib (TD-0903) in severe COVID-19. Eur Respir J (2021) 58(4):2100673. doi: 10.1183/13993003.00673-2021
170. WHO Rapid Evidence Appraisal for COVID-19 Therapies (REACT) Working Group, Sterne JAC, Murthy S, Diaz JV, Slutsky AS, Villar J, et al. Association between administration of systemic corticosteroids and mortality among critically ill patients with COVID-19: A meta-analysis. JAMA (2020) 324(13):1330–41. doi: 10.1001/jama.2020.17023
171. RECOVERY Collaborative Group, Horby P, Lim WS, Emberson JR, Mafham M, Bell JL, et al. Dexamethasone in hospitalized patients with covid-19. N Engl J Med (2021) 384(8):693–704. doi: 10.1056/NEJMoa2021436
172. Tomazini BM, Maia IS, Cavalcanti AB, Berwanger O, Rosa RG, Veiga VC, et al. Effect of dexamethasone on days alive and ventilator-free in patients with moderate or severe acute respiratory distress syndrome and COVID-19: The CoDEX randomized clinical trial. JAMA (2020) 324(13):1307–16. doi: 10.1001/jama.2020.17021
173. Granholm A, Kjaer MN, Munch MW, Myatra SN, Vijayaraghavan BKT, Cronhjort M, et al. Long-term outcomes of dexamethasone 12 mg versus 6 mg in patients with COVID-19 and severe hypoxaemia. Intensive Care Med (2022) 48(5):580–9. doi: 10.1007/s00134-022-06677-2
174. Incorrect equivalent dose and p values in figure 3. JAMA (2022) 327(3):286. doi: 10.1001/jama.2021.24153
175. Granholm A, Munch MW, Myatra SN, Vijayaraghavan BKT, Cronhjort M, Wahlin RR, et al. Dexamethasone 12 mg versus 6 mg for patients with COVID-19 and severe hypoxaemia: a pre-planned, secondary Bayesian analysis of the COVID STEROID 2 trial. Intensive Care Med (2022) 48(1):45–55. doi: 10.1007/s00134-021-06573-1
176. Taboada M, Rodríguez N, Varela PM, Rodríguez MT, Abelleira R, González A, et al. Effect of high versus low dose of dexamethasone on clinical worsening in patients hospitalised with moderate or severe COVID-19 pneumonia: an open-label, randomised clinical trial. Eur Respir J (2022) 60(2):2102518. doi: 10.1183/13993003.02518-2021
177. Agusti A, De Stefano G, Levi A, Muñoz X, Romero-Mesones C, Sibila O, et al. Add-on inhaled budesonide in the treatment of hospitalised patients with COVID-19: a randomised clinical trial. Eur Respir J (2022) 59(3):2103036. doi: 10.1183/13993003.03036-2021
178. Yu LM, Bafadhel M, Dorward J, Hayward G, Saville BR, Gbinigie O, et al. Inhaled budesonide for COVID-19 in people at high risk of complications in the community in the UK (PRINCIPLE): a randomised, controlled, open-label, adaptive platform trial. Lancet (2021) 398(10303):843–55. doi: 10.1016/S0140-6736(21)01744-X
179. Ramakrishnan S, Nicolau DV, Jr, Langford B, Mahdi M, Jeffers H, Mwasuku C, et al, et al. Inhaled budesonide in the treatment of early COVID-19 (STOIC): a phase 2, open-label, randomised controlled trial. Lancet Respir Med (2021) 9(7):763–72. doi: 10.1016/S2213-2600(21)00160-0
180. Baker JR, Mahdi M, Nicolau DV Jr, Ramakrishnan S, Barnes PJ, Simpson JL, et al. Early Th2 inflammation in the upper respiratory mucosa as a predictor of severe COVID-19 and modulation by early treatment with inhaled corticosteroids: a mechanistic analysis. Lancet Respir Med (2022) 10(6):545–56. doi: 10.1016/S2213-2600(22)00002-9
181. Ezer N, Belga S, Daneman N, Chan A, Smith BM, Daniels SA, et al. Inhaled and intranasal ciclesonide for the treatment of covid-19 in adult outpatients: CONTAIN phase II randomised controlled trial. BMJ (2021) 375:e068060. doi: 10.1136/bmj-2021-068060
182. Clemency BM, Varughese R, Gonzalez-Rojas Y, Morse CG, Phipatanakul W, Koster DJ, et al. Efficacy of inhaled ciclesonide for outpatient treatment of adolescents and adults with symptomatic COVID-19: A randomized clinical trial. JAMA Intern Med (2022) 182(1):42–9. doi: 10.1001/jamainternmed.2021.6759
183. Angus DC, Derde L, Al-Beidh F, Annane D, Arabi Y, Beane A, et al. Effect of hydrocortisone on mortality and organ support in patients with severe COVID-19: The REMAP-CAP COVID-19 corticosteroid domain randomized clinical trial. JAMA (2020) 324(13):1317–29. doi: 10.1001/jama.2020.17022
184. Edalatifard M, Akhtari M, Salehi M, Naderi Z, Jamshidi A, Mostafaei S, et al. Intravenous methylprednisolone pulse as a treatment for hospitalised severe COVID-19 patients: results from a randomised controlled clinical trial. Eur Respir J (2020) 56(6):2002808. doi: 10.1183/13993003.02808-2020
185. Dhooria S, Chaudhary S, Sehgal IS, Agarwal R, Arora S, Garg M, et al. High-dose versus low-dose prednisolone in symptomatic patients with post-COVID-19 diffuse parenchymal lung abnormalities: an open-label, randomised trial (the COLDSTER trial). Eur Respir J (2022) 59(2):2102930. doi: 10.1183/13993003.02930-2021
Keywords: COVID-19, treatments, antiviral agents, neutralizing antibody therapy, Janus kinase inhibitors
Citation: Yuan Y, Jiao B, Qu L, Yang D and Liu R (2023) The development of COVID-19 treatment. Front. Immunol. 14:1125246. doi: 10.3389/fimmu.2023.1125246
Received: 16 December 2022; Accepted: 03 January 2023;
Published: 26 January 2023.
Edited by:
Chang Li, Chinese Academy of Agricultural Sciences (CAAS), ChinaReviewed by:
Xiaozhou Feng, New Jersey Medical School, Rutgers, The State University of New Jersey, United StatesXiaoyang Ye, Institute for Systems Biology (ISB), United States
Copyright © 2023 Yuan, Jiao, Qu, Yang and Liu. This is an open-access article distributed under the terms of the Creative Commons Attribution License (CC BY). The use, distribution or reproduction in other forums is permitted, provided the original author(s) and the copyright owner(s) are credited and that the original publication in this journal is cited, in accordance with accepted academic practice. No use, distribution or reproduction is permitted which does not comply with these terms.
*Correspondence: Ruijuan Liu, rj182@163.com; Duomeng Yang, dyang@uchc.edu
†These authors have contributed equally to this work