- 1Laboratory of Malaria Research, Oswaldo Cruz Institute, Oswaldo Cruz Foundation, Rio de Janeiro, RJ, Brazil
- 2Malaria Research, Diagnosis and Training Center (CPD-Mal) of Oswaldo Cruz Foundation, Rio de Janeiro, RJ, Brazil
- 3Laboratory on Leishmaniasis Research, Oswaldo Cruz Institute, Oswaldo Cruz Foundation, Rio de Janeiro, RJ, Brazil
- 4Rio de Janeiro Research Network on Neuroinflammation, Oswaldo Cruz Institute, Oswaldo Cruz Foundation, Rio de Janeiro, RJ, Brazil
Malaria and leishmaniasis are endemic parasitic diseases in tropical and subtropical countries. Although the overlap of these diseases in the same host is frequently described, co-infection remains a neglected issue in the medical and scientific community. The complex relationship of concomitant infections with Plasmodium spp. and Leishmania spp. is highlighted in studies of natural and experimental co-infections, showing how this “dual” infection can exacerbate or suppress an effective immune response to these protozoa. Thus, a Plasmodium infection preceding or following Leishmania infection can impact the clinical course, accurate diagnosis, and management of leishmaniasis, and vice versa. The concept that in nature we are affected by concomitant infections reinforces the need to address the theme and ensure its due importance. In this review we explore and describe the studies available in the literature on Plasmodium spp. and Leishmania spp. co-infection, the scenarios, and the factors that may influence the course of these diseases.
1 Introduction
The risk of contracting an infection is inherent in the life of any living being. The same individual can encounter a variety of viruses, bacteria, helminths, fungi, or protozoa throughout a lifetime and often in the same period of time, increasing the chances of a co-infection (1, 2). Co-infection is defined as infection of the same host by two or more microorganisms belonging to different species or strains (1, 3).
The effect of co-infection on disease pathogenesis is related to the type of interaction that the parasites establish with each other and with the host, including the immune response triggered against these pathogens (3–5). In a co-infection, the parasites may cause a similar immune response profile (which may exacerbate this response) or a different one, bringing limitations to the immune response, with increased susceptibility and lack of control of the infection (6). Therefore, interactions between parasites that inhabit the same host simultaneously can be neutral, synergistic or antagonistic (1–3).
The order of arrival of pathogens in the host is another crucial factor to be considered. When we talk about co-infection, it is immediately assumed that at least two parasites infect the host simultaneously, but this only happens in specific cases, when a particular vector carries different pathogens and transmits them to the host at the same time (5). However, what is more feasible in nature is sequential co-infection, where the first parasite infects the host and then the second parasite, within a certain period (which can be from days to years), finds the same host and infects it (5). Therefore, at the time of co-infection, the host environment is metabolically and immunologically different compared to an uninfected host. Thus, the manifestation of a given disease is not only dependent on host susceptibility, pathogen virulence and environmental factors, but can also be directly influenced by co-infections (Figure 1).
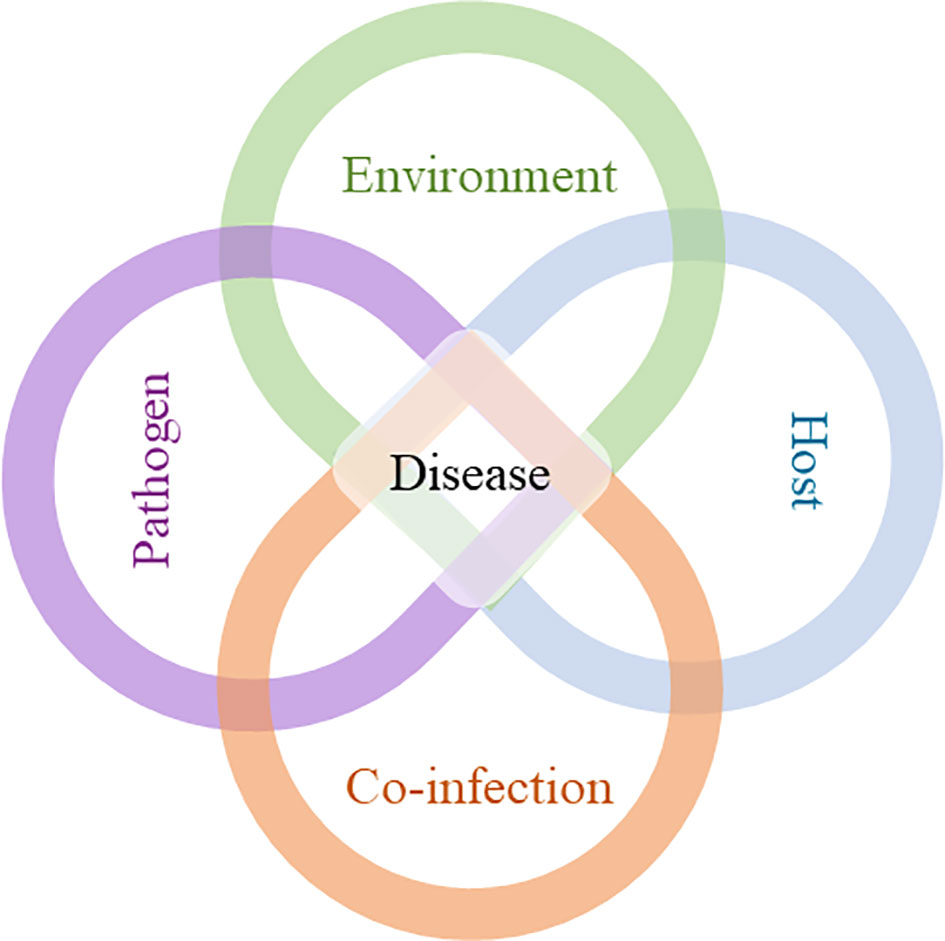
Figure 1 Development of a disease: interplay between environment, host, pathogen, and co-infection. The course of disease development cannot be sufficiently predicted by the interaction of host, pathogen and environment alone. Co-infection (s), along with the other factors, can interfere with the duration of infection, level of infectivity, clinical manifestations, and transmission, which can compromise disease control.
The geographical overlap of malaria and leishmaniasis has been reported through the presence of the parasites and specific vectors circulating in the same endemic regions (7, 8), showing that there is a great potential for interaction between these pathogens in the same host. Indeed, human co-infection with Plasmodium and Leishmania parasites has been described in nature (9–27). Despite that, and the impact that the coexistence of the two parasites in the same host can have on the course of the diseases, there are few studies in the literature that address this interaction. The purpose of this review is to provide an overview of the knowledge available in the literature on co-infection by the parasites that cause malaria and leishmaniasis. Articles detailing this interaction permeate natural co-infection in humans and experimental models of co-infection (Table 1).
2 Malaria and leishmaniasis: An overview
Malaria is an infectious parasitic disease caused by protozoa of the genus Plasmodium and transmitted by the bite of the Anopheles mosquito (7). Despite being an ancient disease, it remains a major public health problem worldwide. According to the World Malaria Report (37), an estimated 247 million cases of malaria occurred in 2021, with 619,000 deaths. In 2020, there was a significant increase in the numbers of malaria deaths (620,000) compared to the previous year (568,000). Overall, between 2019 and 2021, an estimated 13.4 million additional cases of malaria and 63,000 deaths can largely be attributed to the disruption of malaria prevention, diagnosis, and treatment program during the COVID-19 pandemic (37–39). Human malaria is mainly caused by five species of Plasmodium: P. falciparum, P. vivax, P. malariae and P. ovale curtisi and P. ovale wallikeri (7, 40, 41). However, there are three other zoonotically transmitted species that are also capable of infecting humans: P. knowlesi, P. cynomolgi and P. simium (40–45).
Plasmodium species show differences in their geographic distribution, pathological severity, and biology. P. falciparum is the predominant species on the African continent and is found in other tropical and subtropical regions. It is considered the species with the greatest impact on public health due to the high morbidity and mortality rates associated with severe forms of the disease, in addition to drug resistance (46). P. vivax is the most widely distributed species in the world. This parasite is the leading cause of malaria outside Africa and is found in regions of Asia and the Americas (46, 47). In the early stages, malaria has nonspecific symptoms, such as fever, chills, sweating, headache, and muscle pain. This set of symptoms is observed in most patients, but some cases, the disease can progress to more severe manifestations, such as pulmonary malaria, severe anemia, and cerebral malaria.
According to the WHO guidelines, the recommended treatment for adults (excluding pregnant women in their first trimester) and children with uncomplicated malaria caused by P. falciparum, P. vivax, P. ovale, P. malariae or P. knowlesi is with artemisinin-based combination therapies (ACT). This can include artemether + lumefantrine, artesunate + amodiaquine, artesunate + sulfadoxine–pyrimethamine (SP), and others (48). Chloroquine can also be used to treat uncomplicated non-P. falciparum malaria in areas with chloroquine-susceptible infections. In specific cases, to prevent P. vivax or P. ovale relapse, treatment should be carried out with primaquine, paying attention to the risk of G6PD deficiency. For severe malaria, the treatment of choice is intravenous or intramuscular administration of artesunate, followed by combination ACT therapy (48).
Leishmaniasis is a group of neglected infectious diseases caused by protozoan parasites of the genus Leishmania and transmitted by the bite of infected sand flies. It is estimated that 700,000 to 1 million new cases occur annually, and 1 billion people live in endemic areas and are at risk of infection (8) Endemic in 98 countries and 3 territories (49), in tropical and subtropical regions and in the Mediterranean basin, leishmaniasis is associated with lack of financial resources, precarious housing, climate change, population displacement, malnutrition and compromised immune system (8, 50, 51). More than 50 species of Leishmania have been identified, distributed in regions of the Old World (Europe, Asia, and Africa) and the New World (Americas). Of these, 20 species are known to infect humans (50, 52).
The disease, leishmaniasis, comprise a wide spectrum of clinical manifestations grouped in three main forms: Visceral Leishmaniasis (VL), Cutaneous Leishmaniasis (CL) and Mucocutaneous Leishmaniasis (ML) (8, 50). The VL form, also called kala-azar, is the most severe form of leishmaniasis. In the Old World, it is associated with infection by L. donovani and L. infantum, and in the New World by L. infantum. It is characterized as a systemic infection, with symptoms that include irregular fever, fatigue, abdominal pain, weight loss, splenomegaly and hepatomegaly (51, 52). Without treatment, VL is fatal in 95% of cases (8, 52). The CL form is the most common form of the disease. In the Old World, CL was mainly caused by L. major and L. tropica, and in the New World by more than eight different species of the L. (Leishmania) and L. (Viannia) subgenera, including L. (L.) amazonensis, L. (L.) mexicana, L. (V.) braziliensis, L. (V.) panamensis, L. (V.) peruviana and L. (V.) guyanensis (51, 53). The clinical presentation of CL varies according to the infecting species, the genetic background of the host, and immune response triggered. Typically, the lesions occur in the region of the sand fly bite and present as a single non-ulcerative and painless papule. However, multiple lesions can also occur as well as ulcerating dry or wet lesions with raised edges. Cutaneous lesions can heal spontaneously or after therapeutic interventions, but others can persist and be refractory to the treatment (51, 52, 54, 55). Between 2-14% of patients with CL caused by parasites of the L. (Viannia) subgenus develop ML (52, 56). In ML, the parasite disseminates from the skin to the oral and nasal mucosa through the lymphatic and hematogenous system, with slow and progressive destruction of these tissues (51, 52, 54, 57).
During the last six decades, the different forms of have been treated with a reduced option of drugs, including pentavalent antimonials, paromomycin, miltefosine, pentamidine isethionate and amphotericin B (52, 58, 59). Monotherapy with pentavalent antimonial was the first-line treatment for CL and VL worldwide, but due to the emergence of resistance or failure during treatment with this drug, its use is no longer recommended in some regions of the world (59, 60). Oral miltefosine monotherapy is being implemented by more countries in the last decade, as well as combination regimens of antimonial, paromomycin, liposomal amphotericin B, amphotericin B deoxycholate, pentoxifylline and miltefosine, according to the disease form and particular conditions of pediatric and adult patients (52, 58, 59).
It is well known that genetic factors influence resistance or susceptibility to infectious diseases, including malaria and leishmaniasis. However, few genome-wide association studies (GWAS) have been conducted to identify human gene polymorphisms associated with these diseases. Some genetic variations, such as those found in the hemoglobin subunit beta (HBB), ABO blood group (ABO), ATPase plasma membrane Ca2+ transporting 4 (ATP2B4), glucose-6-phosphate dehydrogenase (G6PD) and CD40 ligand (CD40LG) loci, have been associated with attenuation of severe malaria (61). In addition, polymorphism in the erythrocyte Duffy antigen/receptor for chemokines (DARC) gene is associated with resistance to P. vivax malaria (62, 63). Specific TNF and DDX39B haplotypes have also been linked to increased susceptibility to P. vivax infections in Brazil (64, 65). DDX39B encodes an RNA helicase that has been described as a negative regulator for the expression of TNF and IL-6.
Regarding leishmaniasis, recently, a robust GWAS identified lysosomal associated membrane protein 3 (LAMP3), Syntaxin 7 (STX7), Keratin 80 (KRT80), IFNG antisense RNA 1 (IFNG-AS1), Cytokine receptor like factor 3 (CRLF3), and Serpin family B member 10 (SERPINB10) as plausible genetic risk factors for CL caused by L. braziliensis (66). This study suggested that lower levels of IFN-γ and TNF, regulated by IFNG-AS1, increase the risk of CL. For VL, GWAS analysis identified a polymorphic HLA-DR-DQ region within the major histocompatibility complex of immune-related genes as the single major genetic determinant of VL (67). Interestingly, this association was found in three independent cohorts from India and Brazil, and represented infections caused by different parasite species, including L. donovani and L. infantum. Sakthianandeswaren A, et al. (68) and Bharati, K (69), have also described in their revisions several gene variations and their relationships with the course of different forms of leishmaniasis (68, 69). Despite the mentioned studies on genetic polymorphisms and parasitic diseases, to date, there has been no description of the association of certain polymorphisms with resistance or susceptibility to Plasmodium and Leishmania co-infection. This remains a challenging field for future studies.
The geographical distribution of countries with reported malaria cases (39) or VL and CL cases in the world (70) reveals an overlapping of malaria, VL and/or CL cases in the Americas (Central and South), Africa and Asia, and suggests that at least 38 countries are at risk of co-infection (Figure 2). In brief, overlapping cases of malaria and CL are reported in Central America in countries such as Mexico, Costa Rica, Panama, Honduras, and Nicaragua, and in South America in Ecuador, Peru, Guyana, and Suriname. In addition, cases of malaria, CL and VL are observed in Guatemala, Colombia, Venezuela, Bolivia and Brazil (39, 56, 70, 71). On the African continent, cases of malaria and CL overlap mainly in the north of the continent, in countries with a predominantly tropical climate, such as Senegal, Burkina Faso and Nigeria. Also, countries in northeastern Africa, such as Somalia, Uganda, Djibouti, Tanzania, Eritrea, and South Sudan, have reported cases of malaria and VL (39, 70). And yet, cases of malaria, CL, and VL have been reported in Cameroon, Chad, Sudan, Ethiopia, and Kenya. In West Asia, malaria and CL are endemic in Saudi Arabia, while all three diseases are present in Yemen. In South Asia, cases of malaria and CL are found in Pakistan, and cases of malaria, CL and VL are found in Iran and Afghanistan (39, 71, 72). Malaria and VL cases have been reported only in India, Nepal, Bangladesh and Thailand (39, 70), while malaria and CL cases have been reported in Bhutan, a country located between China and India. Isolated cases of malaria or leishmaniasis are observed in several other countries, but without reports of co-endemicity.
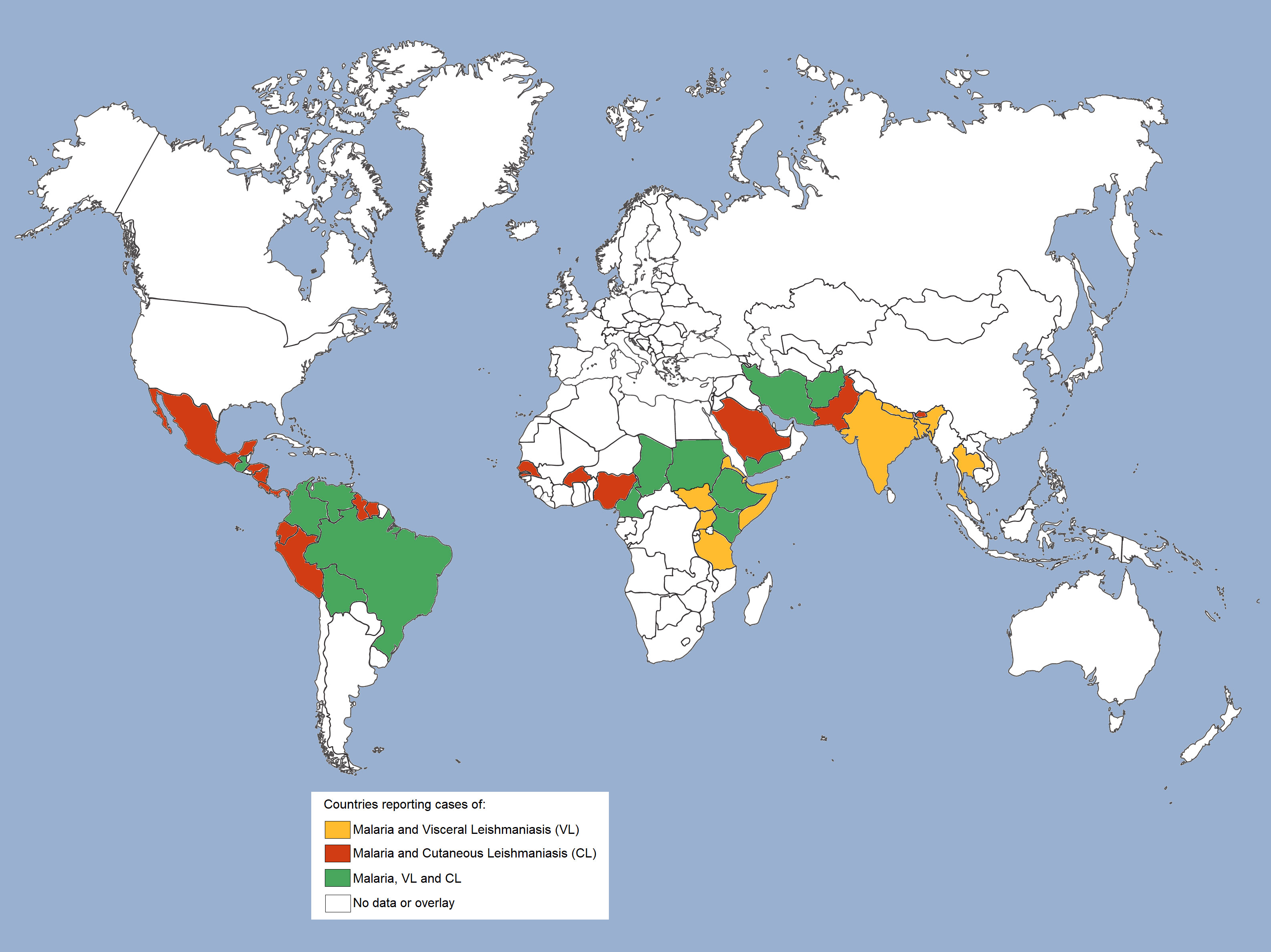
Figure 2 Geographical overlap of malaria and leishmaniasis cases worldwide. Geographical distribution of countries co-endemic for malaria and leishmaniasis. Cases of malaria and visceral leishmaniasis (VL) are reported in countries highlighted in mustard; cases of malaria and cutaneous leishmaniasis (CL) are reported in countries highlighted in red, and cases of malaria and both leishmaniasis (VL and CL) are reported in countries highlighted in green.
3 Plasmodium spp. and Leishmania spp. co-infections
3.1 Natural co-infections
Seminal reports addressing natural co-infection by the parasites that cause malaria and leishmaniasis are from the 1930 and 1940 decades. To our knowledge, the first report of natural co-infection by Plasmodium spp. and Leishmania spp. in areas endemic for both parasites was made by Henderson et al. (9). Conducted on 300 patients with VL from a province in Sudan between 1933 and 1936, this study found the occurrence of concomitant infection with Plasmodium spp. in 30% of patients with VL (9). A few years later, Yoeli (10) observed the presence of non-pigmented malaria parasites, with characteristics similar to P. vivax in the bone marrow of a patient hospitalized with VL in Athens (10).
Since then, natural co-infection has been described in different regions of the world involving different Plasmodium and Leishmania species (Table 1). When assessing the concomitance of other infections in patients with leishmaniasis, Plasmodium was responsible for 10.7% of co-infection cases during a VL epidemic in southern Sudan in 1988 (20). Plasmodium co-infection was also described in 9,3% of VL cases in eastern Sudan in a retrospective study conducted from January 2013 to June 2014 (12) and, in 6.4% of VL cases in Pokot territory, located in western Kenya and eastern Uganda, a few years earlier, in 2006 (23). However, two other studies also conducted in Pokot territory, between 2000-2006, with a larger number of study subjects, describe a higher co-infection rate of 19-20.8% among patients with VL (24, 26). In Ethiopia, a study conducted between 2013 and 2018 showed that out of a total of 434 VL cases, Plasmodium co-infection is seen in 6.4% of cases in the Oromia region (17). Another study conducted in 2014 in northwest Ethiopia, on the border with Sudan, showed that 4.2% of VL patients were co-infected with Plasmodium (13). Overall, a meta-analysis study with data from 1991 to 2020 suggests that the prevalence of Plasmodium co-infection among individuals with VL ranges from 7 to 18% in Africa and Asia, according to the endemicity of VL and malaria in the evaluated area (18).
To assess the prevalence and risk factors of co-infection by Leishmania and Plasmodium parasites, van den Bogaart et al. (26) followed patients admitted to a hospital in Uganda between 2000 and 2006. As mentioned above, of the 2,414 VL patients, 19% were diagnosed with malaria (26). Most of the co-infected patients were male, and age was considered a risk factor of co-infection, since the highest incidence of concurrent VL and malaria was observed in children of 0 to 9 years old. Children in this age group are twice as likely to be co-infected as adults over the age of 30 (26). The same group of investigators also conducted a study in three hospitals in Sudan between the years 2005-2010 evaluating malaria parasite co-infection in VL-hospitalized patients. Of the 1,295 VL patients, 31.2% were co-diagnosed with malaria (mainly caused by P. falciparum) at the time of hospital admission or during hospitalization (27), corroborating the prevalence of Leishmania and Plasmodium co-infection reported previously in the same region (22). Gender and age were considered risk factors for co-infection (27). The findings by Ferede et al. (13) also indicated that age was associated with co-infection by the parasites that cause malaria and leishmaniasis. They noted that the diagnosis of both diseases was significantly higher (33%) in children under 5 years old, who would possibly be more prone to co-infection because they have a less robust immune system (13). The second age group with the highest cases of co-infection was patients aged 15-29 years, where a 4% prevalence of dual diagnosis of VL and malaria was observed (13). Focusing on migrant workers, mostly male and aged between 15 and 29 years, Aschale et al. (16) found that the prevalence of co-infection among workers was 2.8% (16).
The concomitant occurrence of both infections in the same individual has implications for the clinical course of malaria and leishmaniasis. In a study conducted in East Africa, malaria is cited as a risk factor for the development of VL (23). The opposite, however, has not been thoroughly investigated. Although no differences in the prognosis of VL have been observed (as long as the Plasmodium infection is identified and treated effectively), co-infected patients have greater morbidity evidenced by loss of body mass, jaundice, and malaise (26, 27). Interestingly, an increased Leishmania parasite load was observed in lymph nodes or bone marrow aspirates in co-infected patients, suggesting a potential suppressive effect exerted by malaria parasites on Leishmania infection (27). On the other hand, reduced levels of P. falciparum parasitemia were detected in the co-infected patients compared to malaria-only patients, suggesting a protective effect of co-infection on Plasmodium infection (11). This effect may be related to the increased systemic levels of pro-inflammatory cytokines (TNF-α and IFN-γ) observed in the co-infected group when compared to patients infected with Plasmodium alone (11). The data indicate that the co-occurrence of the two diseases in the same individual interferes with the host’s immune response.
Co-infection cases have also been reported in India, accounting for 5.9% of patients with splenomegaly and fever (21). Interestingly, all patients diagnosed with VL, and malaria had symptoms that could be related to both diseases, such as fever, splenomegaly and hepatomegaly (15, 21). In a region of Nepal bordering India, another case of co-infection was reported, this time in a 5-year-old child, infected with P. vivax and L. donovani, with a history of fever, abdominal pain, constipation, and hepatomegaly (14). The data suggest that the clinical symptoms of co-infected patients hinder accurate diagnosis and, consequently, the initiation of appropriate treatment . Thus, delay in the recommended treatment may compromise treatment success and the course of the diseases (13, 15, 18, 25). However, co-infection is not reported to affect the efficacy of the recommended treatment for malaria or leishmaniasis if it is done in a timely manner.
Traditionally, we look for cases of co-infections with pathogens endemic to the same geographic area, but with the intense migratory activities and war refugees, this condition no longer applies. There may be imported cases of a particular infection, which is not endemic to that region. In an interesting case report, a patient from Nepal, a VL endemic area, while going to work in Malaysia (a malaria endemic area) tested positive for P. vivax two weeks after his arrival in the country. But even after complete treatment for malaria, the patient still had high fever, lack of appetite, splenomegaly, hepatomegaly, and pancytopenia. When the bone marrow aspirate was evaluated, amastigotes forms of Leishmania were found inside macrophages, showing that this was a case of co-infection by Leishmania and Plasmodium. This situation is considered challenging, since the patient probably contracted the L. donovani infection first at a different location and the Plasmodium infection, contracted later, interfered with the VL diagnosis, which could have been fatal (25).
3.2 Experimental co-infection: In vivo and in vitro models
Experimental animal models are valuable tools to investigate the pathological mechanisms of co-infections. The first study carried out to understand the dynamics of co-infection used Syrian golden hamsters (Mesocricetus auratus) inoculated with L. infantum and P. berghei. In this study, the authors observed that previous infection with L. infantum inhibited the multiplication of malaria parasites. On the other hand, an infection already initiated by P. berghei did not inhibit the multiplication of L. infantum (28). Using the same rodent model, the effect of P. yoelii infection on the course of infection by L. enriettii, a natural parasite of guinea pigs, was also evaluated. In contrast to what was observed by Adler et al. (28), when both infections were performed simultaneously or when P. yoelii was inoculated few days after L. enriettii infection, there was an increase in skin lesions in these two groups of co-infected animals when compared to the L. enriettii mono-infected group (29).
Like hamsters, murine models are excellent experimental models, being susceptible or resistant to different strains of Plasmodium spp. and Leishmania spp. according to their genetic background. Coleman et al. in their first studies on multiple infections, evaluated the co-infection by P. yoelii 17x (a non-lethal strain) and L. amazonensis in BALB/c and C57BL/6 mice, murine models considered susceptible and resistant to L. amazonensis infection, respectively (30–33). BALB/c mice infected first with L. amazonensis and a few days later (2 days, 3 days or 3 weeks) infected with P. yoelii 17x showed worsening of the skin lesion and Plasmodium infection with high parasitemia, marked hypothermia and elevated mortality rate (30, 33, 36). Similarly, C57BL/6 mice, when initially inoculated with L. amazonensis and subsequently (2 days or 3 weeks later) infected with P. yoelii, also showed increased footpad lesion and greater P. yoelli parasitemia compared to mono-infected animals (31, 33). Interestingly, in some co-infected animals, dissemination of the L. amazonensis was observed, with the appearance of a lesion in the contralateral uninfected paw, suggesting that concomitant infection with Plasmodium would have a potential to suppress the immune response, increasing the severity of cutaneous leishmaniasis in this model (32).
The impact of P. yoelii 17XNL infection on BALB/c mice parasitized by L. braziliensis was different from that observed in co-infection with L. amazonensis and P. yoelli (36). Animals co-infected with L. braziliensis and P. yoelii had lower parasitemia and smaller and less ulcerative lesions compared to mono-infected groups (36). Finally, a preliminary study in C57BL/6 mice infected first with P. chabaudi and then with L. infantum describes a higher susceptibility to leishmaniasis in co-infected animals. Co-infection, on the other hand, did not interfere with the course of malaria (34).
The effect of co-occurrence of the two infections in the same host on the elicited immune response was evaluated by Pinna et al. (36). Co-infection of BALB/c mice with L. amazonensis and P. yoelii or L. braziliensis and P. yoelii reduced serum levels of the cytokines IFN-γ, TNF-α, IL-6 and IL-10 induced by the malaria parasite. The data suggest a modulating effect of Leishmania infection on the immune response triggered by the Plasmodium parasite (36). Note that in malaria, the optimal immune response capable of controlling the parasite and causing no harm to the host involves an initial pro-inflammatory type 1 response, which later switches to a more regulatory response (73). Reduced serum levels of IL-10, an important regulatory cytokine, may have triggered an imbalance in the immune response and the worsening of malaria (74–77), contributing to the higher morbidity observed in animals infected with L. amazonensis and P. yoelii. From the standpoint of Leishmania infection, co-infection with P. yoelii increased serum levels of pro-inflammatory cytokines compared to animals infected with Leishmania alone. This immune response profile may have contributed to the reduced severity of Leishmania lesions observed in co-infected animals (36).
In summary, although the data suggest that co-infection shapes the susceptibility of the host to one or other infection, there is a considerable difference between the results obtained by the experimental co-infection studies. These differences are possibly related to the different species and/or strains of parasites involved in the studies, the genetic background of the host, and the time and order in which the infections occurred. All of these factors can dictate the clinical course and severity of the diseases (28–30, 33, 34, 36).
Similar to the in vivo experimental models, the in vitro co-culture system also provides important clues to understand how the immune response to a pathogen is triggered. Thus, it is quite common to use the in vitro co-culture system to assess how a specific parasite interacts with a particular cell type and modulates its function. In this regard, several studies have shown that exposure to Plasmodium-infected erythrocytes or hemozoin, a product of hemoglobin metabolism, negatively interferes with the antigen-presenting function of dendritic cells (DCs) (78–84). This negative impact on DC activation and maturation is also described in studies evaluating the interaction of the promastigote form of Leishmania with DCs (85–92). On the other hand, uptake of amastigote forms of Leishmania by DC induces its maturation and activation of T cells to a Th1 profile (92, 93). However, only one study so far has evaluated what happens to DC when interacting with both parasites, Plasmodium and Leishmania, simultaneously. In this study, monocyte-derived DCs (obtained from healthy donors) and co-stimulated with different concentrations of L. donovani promastigotes forms and P. falciparum-parasitized erythrocytes developed a semi-mature to immature profile, as the parasite/cell ratio increased, similar to DCs cultured with promastigotes forms of L. donovani alone (35). Suggesting a dominant effect of the Leishmania parasite-induced stimulus, over Plasmodium-induced stimulus, on the activation profile of DCs.
4 Conclusion
The geographical overlapping of the Plasmodium and Leishmania parasites, their vectors, and cases of natural co-infection are more than proven. Studies on natural and experimental co-infection, discussed in this review, suggest that the co-occurrence of the two parasites in the same host interferes with the duration of infection, level of infectivity and/or clinical manifestations. Some of these factors can influence the transmission and compromise the control of one or both diseases. This review also evidences the dangers of co-infection for populations residing in areas where these diseases are co-endemic. As the search for co-infections is not usually performed in these regions, the diagnosis of one infection may compromise the accurate and timely diagnosis of the other, implying a delay in treatment and possibly leading to patient death. Thus, more efforts should be made in an attempt to reduce the lack of knowledge about concomitant infections, and encouragement for the creation of programs that seek to diagnose co-infections by Plasmodium spp. and Leishmania spp. in areas co-endemic for both diseases.
Author contributions
UO-G drafted the manuscript. UO-G, PC, and FL reviewed, edited, and prepared its final version. All authors contributed to the article and approved the submitted version.
Funding
This work was supported by Fundação de Amparo à Pesquisa do Estado do Rio de Janeiro - FAPERJ (FL grant # E26/211.323/2019; PC Emergentes grant # E-26/010.002168/2019, and PC JCNE E-26/203.253/2017), and FIOCRUZ -PAEF (PC grant No. IOC-023-FIO-18-2-63). FL and PC are PQ-fellows of the Conselho Nacional de Desenvolvimento Científico e Tecnológico – CNPq (FL Process No. 308809/2019-0; PC Process No. 312573/2020-0). This work was developed in the framework of the Rio de Janeiro Research Network on Neuroinflammation (FAPERJ - grant # E-26/211.571/2019).
Acknowledgments
UO-G is grateful to the Postgraduate Program in Cellular and and Molecular Biology of the Instituto Oswaldo Cruz (IOC), Fiocruz, for the academic support and to Fundação de Amparo à Pesquisa do Estado do Rio de Janeiro (FAPERJ) and Coordenação de Aperfeiçoamento de Pessoal de Nível Superior (CAPES) for MSc and doctoral fellowship.
Conflict of interest
The authors declare that the research was conducted in the absence of any commercial or financial relationships that could be construed as a potential conflict of interest.
Publisher’s note
All claims expressed in this article are solely those of the authors and do not necessarily represent those of their affiliated organizations, or those of the publisher, the editors and the reviewers. Any product that may be evaluated in this article, or claim that may be made by its manufacturer, is not guaranteed or endorsed by the publisher.
References
1. Cox FEG. Concomitant infections, parasites and immune responses. Parasitology (2001) 122:23–38. doi: 10.1017/s003118200001698x
2. Griffiths EC, Pedersen AB, Fenton A, Petchey OL. Analysis of a summary network of co-infection in humans reveals that parasites interact most via shared resources. Proc R Soc B Biol Sci (2014) 281. doi: 10.1098/rspb.2013.2286
3. Birger RB, Kouyos RD, Cohen T, Griffiths EC, Huijben S, Mina MJ, et al. The potential impact of coinfection on antimicrobial chemotherapy and drug resistance. Trends Microbiol (2015) 23:537–44. doi: 10.1016/j.tim.2015.05.002
4. Susi H, Barrès B, Vale PF, Laine A-L. Co-Infection alters population dynamics of infectious disease. Nat Commun (2015) 6:5975. doi: 10.1038/ncomms6975
5. Karvonen A, Jokela J, Laine A-L. Importance of sequence and timing in parasite coinfections. Trends Parasitol (2019) 35:109–18. doi: 10.1016/j.pt.2018.11.007
6. Supali T, Verweij JJ, Wiria AE, Djuardi Y, Hamid F, Kaisar MMM, et al. Polyparasitism and its impact on the immune system. Int J Parasitol (2010) 40:1171–6. doi: 10.1016/j.ijpara.2010.05.003
9. Henderson L. Clinical observations on kala-azar in the province of the Sudan. Trans R Soc Trop Med Hyg (1937) 31:179–90. doi: 10.1016/S0035-9203(37)90036-1
10. Yoeli M, July I, Cross TR. Non-pigmented malaria parasites in the bone marrow from a mixed infection of Leishmania and Plasmodium vivax. Trans R Soc Trop Med Hyg. (1948) 42:99–101. doi: 10.1016/0035-9203(48)90355-1
11. van den Bogaart E, Talha AA, Straetemans M, Mens PF, Adams ER, Grobusch MP, et al. Cytokine profiles amongst Sudanese patients with visceral leishmaniasis and malaria co-infections. BMC Immunol (2014) 15:16. doi: 10.1186/1471-2172-15-16
12. Hashim BM, AbdelAziem AA, Mubarak II, Khalid MG, Mona MY, Abdalazeem AI, et al. Prevalence of hepatitis b, hepatitis c, HIV and malaria Co infection among patients infected with visceral leishmaniasis in gedarif, Eastern Sudan. Glob J Infect Dis Clin Res (2016) 2:021–4. doi: 10.17352/2455-5363.000010
13. Ferede G, Diro E, Getie S, Getnet G, Takele Y, Amsalu A, et al. Visceral leishmaniasis-malaria coinfection and their associated factors in patients attending metema hospital, Northwest Ethiopia : Suggestion for integrated vector management. Malar Res Treat (2017) 2017:6816913. doi: 10.1155/2017/6816913
14. Ghimire PG, Ghimire P, Adhikari J, Chapagain A. A case report of visceral leishmaniasis and malaria co-infection with pancytopenia and splenomegaly- a diagnostic challenge. BMC Infect Dis (2019) 19:4–6. doi: 10.1186/s12879-019-4478-1
15. Topno RK, Kumar R, Pandey K, Verma N, Das VR, Sahoo GC, et al. A co-infection with malaria and visceral leishmaniasis in Eastern state of India. Presse Med (2019) 48:328–31. doi: 10.1016/j.lpm.2018.04.017
16. Aschale Y, Ayehu A, Worku L, Tesfa H, Birhanie M, Lemma W. Malaria-visceral leishmaniasis co-infection and associated factors among migrant laborers in West armachiho district, north West Ethiopia: community based cross-sectional study. BMC Infect Dis (2019) 19:239. doi: 10.1186/s12879-019-3865-y
17. Tekalign S, Adera C, den Boer M, Miecha H, Zewde A, Mulugeta D, et al. Clinical features and treatment outcomes of visceral leishmaniasis patients admitted to three centers in oromia, Ethiopia. J Infect Dev Ctries (2020) 14:42S–7S. doi: 10.3855/jidc.11731
18. Wilairatana P, Chanmol W, Rattaprasert P, Masangkay FR, Milanez GDJ, Kotepui KU, et al. Prevalence and characteristics of malaria co-infection among individuals with visceral leishmaniasis in Africa and Asia: a systematic review and meta-analysis. Parasites Vectors (2021) 14:1–18. doi: 10.1186/s13071-021-05045-1
19. Saini I, Joshi J, Kaur S. Unwelcome prevalence of leishmaniasis with several other infectious diseases. Int Immunopharmacol (2022) 110:109059. doi: 10.1016/j.intimp.2022.109059
20. Beer P, Harith A, Deng LL, Semiao-Santos SJ, Chantal B, Grootheest MV. A killing disease epidemic among displaced sudanese population identified as visceral leishmaniasis. Am J Trop Med Hyg (1991) 44:283–9. doi: 10.4269/ajtmh.1991.44.283
21. Nandy A, Addyl M, Guhaz SK, Majil AK. Co-Existent kala-azar and malaria in India. Trans R Soc Trop Med Hyg (1995) 89(5):516. doi: 10.1016/0035-9203(95)90091-8
22. Mueller M, Ritmeijer K, Balasegaram M, Koummuki Y, Santana MR, Davidson R. Unresponsiveness to AmBisome in some Sudanese patients with kala-azar. Trans R Soc Trop Med Hyg (2007) 101:19–24. doi: 10.1016/j.trstmh.2006.02.005
23. Kolaczinski JH, Reithinger R, Worku DT, Ocheng A, Kasimiro J, Kabatereine N, et al. Risk factors of visceral leishmaniasis in East Africa: A case-control study in pokot territory of Kenya and Uganda. Int J Epidemiol (2008) 37:344–52. doi: 10.1093/ije/dym275
24. Mueller Y, Mbulamberi DB, Odermatt P, Hoffmann A, Loutan L, Chappuis F. Risk factors for in-hospital mortality of visceral leishmaniasis patients in eastern Uganda. Trop Med Int Heal (2009) 14:910–7. doi: 10.1111/j.1365-3156.2009.02305.x
25. Ab Rahman AK, Abdullah FH. Visceral leishmaniasis (kala-azar) and malaria coinfection in an immigrant in the state of terengganu, Malaysia: A case report. J Microbiol Immunol Infect (2011) 44:72–6. doi: 10.1016/j.jmii.2011.01.014
26. van den Bogaart E, Berkhout MMZ, Adams ER, Mens PF, Sentongo E, Mbulamberi DB, et al. Prevalence, features and risk factors for malaria Co-infections amongst visceral leishmaniasis patients from amudat hospital, Uganda. PloS Negl Trop Dis (2012) 6:e1617. doi: 10.1371/journal.pntd.0001617
27. van den Bogaart E, Berkhout MM, Nour AB, Mens PF, Talha A-BA, Adams ER, et al. Concomitant malaria among visceral leishmaniasis in-patients from gedarif and sennar states, Sudan: a retrospective case-control study. BMC Public Health (2013) 13:332. doi: 10.1186/1471-2458-13-332
28. Adler S OBE, M.B. MRCP. The behaviour of Plasmodium berghei in the golden hamster mesocricetus auratus infected with visceral leishmaniasis. Trans R Soc Trop Med Hyg (1954) 48:431–40. doi: 10.1016/0035-9203(54)90145-5
29. Belehu A, Poulter LW, Turk JL. Influence of rodent malaria on the course of Leishmania enriettii infection of hamsters. Infect Immun (1976) 14:457–62. doi: 10.1128/iai.14.2.457-462.1976
30. Coleman RE, Edman JD, Semprevivo LH. Leishmania mexicana: Effect of concomitant malaria on cutaneous leishmaniasis. development of lesions in a leishmania-susceptible (BALB/c) strain of mouse. Exp Parasitol (1988) 65:269–76. doi: 10.1016/0014-4894(88)90131-2
31. Coleman RE, Edman JD, Semprevivo LH. Interactions between Plasmodium yoelii and Leishmania mexicana amazonensis in leishmania resistant C57B1/6 mice. Am J Trop Med Hyg (1988) 39:540–4. doi: 10.4269/ajtmh.1988.39.540
32. Coleman RE, Edman JD, Semprevivo LH. Metastasis of Leishmania mexicana in a leishmania-resistant mouse strain (C/57) following concomitant malarial infection. Ann Trop Med Parasitol (1988) 82:399–401. doi: 10.1080/00034983.1988.11812265
33. Coleman RE, Edman JD, Semprevivo LH. Interactions between malaria (Plasmodium yoelii) and leishmaniasis (Leishmania mexicana amazonensis): effect of concomitant infection on host activity, host body temperature, and vector engorgement success. J Med Entomol (1988) 25:467–71. doi: 10.1093/jmedent/25.6.467
34. Marques CS, Rolão N, Centeno-Lima S, Lousada H, Maia C, Campino L, et al. Studies in a co-infection murine model of plasmodium chabaudi chabaudi and Leishmania infantum: interferon-gamma and interleukin-4 mRNA expression. Mem Inst Oswaldo Cruz (2005) 100:889–92. doi: 10.1590/s0074-02762005000800011
35. van den Bogaart E, de Bes HM, Balraadjsing PPS, Mens PF, Adams ER, Grobusch MP, et al. Leishmania donovani infection drives the priming of human monocyte-derived dendritic cells during Plasmodium falciparum co-infections. Parasite Immunol (2015) 37:453–69. doi: 10.1111/pim.12214
36. Pinna RA, Silva-dos-Santos D, Perce-da-Silva DS, Oliveira-Ferreira J, Villa-Verde DMS, De Luca PM, et al. Malaria-cutaneous leishmaniasis co-infection: Influence on disease outcomes and immune response. Front Microbiol (2016) 7:982. doi: 10.3389/fmicb.2016.00982
37. World Health Organization. World malaria report. (2022). Geneva: World Health Organization. Licence: CC BY-NC-SA 3.0 IGO. Avaiable at: https://www.who.int/publications/i/item/9789240064898 (Accessed January 5, 2023).
38. World Health Organization. World malaria report: 20 years of global progress and challenges. (2020). Geneva: World Health Organization. Licence: CC BY-NC-SA 3.0IGO. Avaiable at: https://apps.who.int/iris/handle/10665/337660 (Accessed November 11, 2022)
39. WHO. Word malaria report 2021. (2021). Geneva: World Health Organization Licence: CC BY-NC-SA 3.0 IGO. Available at: https://www.who.int/teams/global-malaria-programme/reports/world-malaria-report-2021 (Accessed November 11, 2022).
40. Sutherland CJ, Tanomsing N, Nolder D, Oguike M, Jennison C, Pukrittayakamee S, et al. Two nonrecombining sympatric forms of the human malaria parasite plasmodium ovale occur globally. J Infect Dis (2010) 201:1544–50. doi: 10.1086/652240
41. Mourier T, de Alvarenga DAM, Kaushik A, de Pina-Costa A, Douvropoulou O, Guan Q, et al. The genome of the zoonotic malaria parasite plasmodium simium reveals adaptations to host switching. BMC Biol (2021) 19:219. doi: 10.1186/s12915-021-01139-5
42. Cox-Singh J, Davis TME, Lee K-S, Shamsul SSG, Matusop A, Ratnam S, et al. Plasmodium knowlesi malaria in humans is widely distributed and potentially life threatening. Clin Infect Dis (2008) 46:165–71. doi: 10.1086/524888
43. Ta TH, Hisam S, Lanza M, Jiram AI, Ismail N, Rubio JM. First case of a naturally acquired human infection with plasmodium cynomolgi. Malar J (2014) 13:68. doi: 10.1186/1475-2875-13-68
44. Brasil P, Zalis MG, de Pina-Costa A, Siqueira AM, Júnior CB, Silva S, et al. Outbreak of human malaria caused by plasmodium simium in the Atlantic forest in Rio de Janeiro: a molecular epidemiological investigation. Lancet Glob Heal (2017) 5:e1038–46. doi: 10.1016/S2214-109X(17)30333-9
45. Grignard L, Shah S, Chua TH, William T, Drakeley CJ, Fornace KM. Natural human infections with plasmodium cynomolgi and other malaria species in an elimination setting in sabah, Malaysia. J Infect Dis (2019) 220:1946–9. doi: 10.1093/infdis/jiz397
47. Carlton JM, Sina BJ, Adams JH. Why is Plasmodium vivax a neglected tropical disease? PloS Negl Trop Dis (2011) 5:3–6. doi: 10.1371/journal.pntd.0001160
48. World Health Organization. WHO guidelines for malaria. (2022). Geneva: World Health Organization. License: CC BY-NC-SA 3.0 IGO. Avaiable at: https://www.who.int/publications/i/item/guidelines-for-malaria (Accessed January 11, 2023).
49. Alvar J, Vélez ID, Bern C, Herrero M, Desjeux P, Cano J, et al. Leishmaniasis worldwide and global estimates of its incidence. PloS One (2012) 7. doi: 10.1371/journal.pone.0035671
50. Akhoundi M, Kuhls K, Cannet A, Votýpka J, Marty P, Delaunay P, et al. A historical overview of the classification, evolution, and dispersion of leishmania parasites and sandflies. PloS Negl Trop Dis (2016) 10:1–40. doi: 10.1371/journal.pntd.0004349
51. Mann S, Frasca K, Scherrer S, Henao-Martínez AF, Newman S, Ramanan P, et al. A review of leishmaniasis: Current knowledge and future directions. Curr Trop Med Rep (2021) 8:121–32. doi: 10.1007/s40475-021-00232-7
52. Burza S, Croft SL, Boelaert M. Leishmaniasis. Lancet (2018) 392:951–70. doi: 10.1016/S0140-6736(18)31204-2
53. Weigle K, Saravia NG. Natural history, clinical evolution, and the host-parasite interaction in new world cutaneous leishmaniasis. Clin Dermatol (1996) 14:433–50. doi: 10.1016/0738-081X(96)00036-3
54. David CV, Craft N. Cutaneous and mucocutaneous leishmaniasis. Dermatol Ther (2009) 22:491–502. doi: 10.1111/j.1529-8019.2009.01272.x
55. Abadías-Granado I, Diago A, Cerro PA, Palma-Ruiz AM, Gilaberte Y. Cutaneous and mucocutaneous leishmaniasis. Actas Dermosifiliogr (2021) 112:601–18. doi: 10.1016/j.ad.2021.02.008
56. Pan American Health Organization. Leishmaniasis: Epidemiological report of the americas, no. 10. December 2021. (2021). Washington, DC: PAHO. Licence: CCBY-NC-SA3.0IGO. Avaiable at: https://iris.paho.org/handle/10665.2/51742 (Accessed November 19, 2022).
57. Machado-Coelho GLL, Caiaffa WT, Genaro O, Magalhães PA, Mayrink W. Risk factors for mucosal manifestation of American cutaneous leishmaniasis. Trans R Soc Trop Med Hyg (2005) 99:55–61. doi: 10.1016/j.trstmh.2003.08.001
58. Alves F, Bilbe G, Blesson S, Goyal V, Monnerat S, Mowbray C, et al. Recent development of visceral leishmaniasis treatments: Successes, pitfalls, and perspectives. Clin Microbiol Rev (2018) 31:1–30. doi: 10.1128/CMR.00048-18
59. Pan American Health Organization. Guideline for the treatment of leishmaniasis in the americas. (2022). Washington, DC: PAHO. Licence: CC BY-NC-SA 3.0 IGO. Available at: https://iris.paho.org/handle/10665.2/56120 (Accessed January 11, 2023).
60. Sundar S, More DK, Singh MK, Singh VP, Sharma S, Makharia A, et al. Failure of pentavalent antimony in visceral leishmaniasis in India: Report from the center of the Indian epidemic. Clin Infect Dis (2000) 31:1104–7. doi: 10.1086/318121
61. Rockett KA, Clarke GM, Fitzpatrick K, Hubbart C, Jeffreys AE, Rowlands K, et al. Reappraisal of known malaria resistance loci in a large multicenter study. Nat Genet (2014) 46:1197–204. doi: 10.1038/ng.3107
62. Tournamille C, Colin Y, Cartron JP, Le Van Kim C. Disruption of a GATA motif in the Duffy gene promoter abolishes erythroid gene expression in Duffy–negative individuals. Nat Genet (1995) 10:224–8. doi: 10.1038/ng0695-224
63. King CL, Adams JH, Xianli J, Grimberg BT, McHenry AM, Greenberg LJ, et al. Fy a/Fy b antigen polymorphism in human erythrocyte Duffy antigen affects susceptibility to Plasmodium vivax malaria. Proc Natl Acad Sci (2011) 108:20113–8. doi: 10.1073/pnas.1109621108
64. Sortica VA, Cunha MG, Ohnishi MDO, Souza JM, Ribeiro-dos-Santos ÂK, Santos NP, et al. IL1B, IL4R, IL12RB1 and TNF gene polymorphisms are associated with Plasmodium vivax malaria in Brazil. Malar J (2012) 11:409. doi: 10.1186/1475-2875-11-409
65. Mendonça VR, Souza LC, Garcia GC, Magalhães BM, Lacerda MV, Andrade BB, et al. DDX39B (BAT1), TNF and IL6 gene polymorphisms and association with clinical outcomes of patients with Plasmodium vivax malaria. Malar J (2014) 13:278. doi: 10.1186/1475-2875-13-278
66. Castellucci LC, Almeida L, Cherlin S, Fakiola M, Francis RW, Carvalho EM, et al. A genome-wide association study identifies SERPINB10, CRLF3, STX7, LAMP3, IFNG-AS1, and KRT80 as risk loci contributing to cutaneous leishmaniasis in Brazil. Clin Infect Dis (2021) 72:e515–25. doi: 10.1093/cid/ciaa1230
67. Fakiola M, Strange A, Cordell HJ, Miller EN, Pirinen M, Su Z, et al. Common variants in the HLA-DRB1–HLA-DQA1 HLA class II region are associated with susceptibility to visceral leishmaniasis. Nat Genet (2013) 45:208–13. doi: 10.1038/ng.2518
68. Sakthianandeswaren A, Foote SJ, Handman E. The role of host genetics in leishmaniasis. Trends Parasitol (2009) 25:383–91. doi: 10.1016/j.pt.2009.05.004
69. Bharati K. Human genetic polymorphism and leishmaniasis. Infect Genet Evol (2022) 98:105203. doi: 10.1016/j.meegid.2021.105203
71. Ministério da Saúde. Secretaria de vigilância em saúde. boletim epidemiológico - malária. (2021). Brasil: Ministério da Saúde. Avaiable at: https://www.gov.br/saude/pt-br/centrais-de-conteudo/publicacoes/boletins/epidemiologicos/especiais/2021/boletim_epidemiologico_especial_malaria_2021.pdf (Accessed November 11, 2022).
72. Al-Awadhi M, Ahmad S, Iqbal J. Current status and the epidemiology of malaria in the middle East region and beyond. Microorganisms (2021) 9:338. doi: 10.3390/microorganisms9020338
73. Li C, Seixas E, Langhorne J. Rodent malarias: The mouse as a model for understanding immune responses and pathology induced by the erythrocytic stages of the parasite. Med Microbiol Immunol (2001) 189:115–26. doi: 10.1007/s430-001-8017-8
74. Nussenblatt V, Mukasa G, Metzger A, Ndeezi G, Garrett E, Semba RD. Anemia and interleukin-10, tumor necrosis factor alpha, and erythropoietin levels among children with acute, uncomplicated Plasmodium falciparum malaria. Clin Diagn Lab Immunol (2001) 8:1164–70. doi: 10.1128/CDLI.8.6.1164-1170.2001
75. Couper KN, Blount DG, Wilson MS, Hafalla JC, Belkaid Y, Kamanaka M, et al. IL-10 from CD4+CD25-Foxp3-CD127 - adaptive regulatory T cells modulates parasite clearance and pathology during malaria infection. PloS Pathog (2008) 4. doi: 10.1371/journal.ppat.1000004
76. Niikura M, Kamiya S, Kita K, Kobayashi F. Coinfection with nonlethal murine malaria parasites suppresses pathogenesis caused by Plasmodium berghei NK65. J Immunol (2008) 180:6877–84. doi: 10.4049/jimmunol.180.10.6877
77. Niikura M, Inoue SI, Kobayashi F. Role of interleukin-10 in malaria: Focusing on coinfection with lethal and nonlethal murine malaria parasites. J BioMed Biotechnol (2011) 2011. doi: 10.1155/2011/383962
78. Urban BC, Ferguson DJP, Pain A, Willcox N, Plebanski M, Austyn JM, et al. Plasmodium falciparum infected erythrocytes modulate the maturation of dendritic cells. Nature (1999) 400:73–7. doi: 10.1038/21900
79. Urban BC, Mwangi T, Ross A, Kinyanjui S, Mosobo M, Kai O, et al. Peripheral blood dendritic cells in children with acute Plasmodium falciparum malaria. Blood (2001) 98:2859–61. doi: 10.1182/blood.V98.9.2859
80. Urban BC, Cordery D, Shafi MJ, Bull PC, Newbold CI, Williams TN, et al. The frequency of BDCA3-positive dendritic cells is increased in the peripheral circulation of Kenyan children with severe malaria. Infect Immun (2006) 74:6700–6. doi: 10.1128/IAI.00861-06
81. Elliott SR, Spurck TP, Dodin JM, Maier AG, Voss TS, Yosaatmadja F, et al. Inhibition of dendritic cell maturation by malaria is dose dependent and does not require Plasmodium falciparum erythrocyte membrane protein 1. Infect Immun (2007) 75:3621–32. doi: 10.1128/IAI.00095-07
82. Skorokhod OA, Alessio M, Mordmüller B, Arese P, Schwarzer E. Hemozoin (Malarial pigment) inhibits differentiation and maturation of human monocyte-derived dendritic cells: A peroxisome proliferator-activated receptor-γ-Mediated effect. J Immunol (2004) 173:4066–74. doi: 10.4049/jimmunol.173.6.4066
83. Götz A, Tang MS, Ty MC, Arama C, Ongoiba A, Doumtabe D, et al. Atypical activation of dendritic cells by Plasmodium falciparum. Proc Natl Acad Sci U.S.A. (2017) 114:E10568–77. doi: 10.1073/pnas.1708383114
84. Osii RS, Otto TD, Garside P, Ndungu FM, Brewer JM. The impact of malaria parasites on dendritic cell–T cell interaction. Front Immunol (2020) 11:1597. doi: 10.3389/fimmu.2020.01597
85. Revest M, Donaghy L, Cabillic F, Guiguen C, Gangneux JP. Comparison of the immunomodulatory effects of l. donovani and L. major excreted-secreted antigens, particulate and soluble extracts and viable parasites on human dendritic cells. Vaccine (2008) 26:6119–23. doi: 10.1016/j.vaccine.2008.09.005
86. Favali C, Tavares N, Clarencio J, Barral A, Barral-Netto M, Brodskyn C. Leishmania amazonensis infection impairs differentiation and function of human dendritic cells. J Leukoc Biol (2007) 82:1401–6. doi: 10.1189/jlb.0307187
87. Tejle K, Lindroth M, Magnusson KE, Rasmusson B. Wild-type Leishmania donovani promastigotes block maturation, increase integrin expression and inhibit detachment of human monocyte-derived dendritic cells - the influence of phosphoglycans. FEMS Microbiol Lett (2008) 279:92–102. doi: 10.1111/j.1574-6968.2007.01013.x
88. Xin L, Li K, Soong L. Down-regulation of dendritic cell signaling pathways by Leishmania amazonensis amastigotes. Mol Immunol (2008) 45:3371–82. doi: 10.1016/j.molimm.2008.04.018
89. Bennett CL, Misslitz A, Colledge L, Aebischer T, Clare Blackburn C. Silent infection of bone marrow-derived dendritic cells by Leishmania mexicana amastigotes. Eur J Immunol (2001) 31:876–83. doi: 10.1002/1521-4141(200103)31:3<876::AID-IMMU876>3.0.CO;2-I
90. Contreras I, Estrada JA, Guak H, Martel C, Borjian A, Ralph B, et al. Impact of Leishmania mexicana infection on dendritic cell signaling and functions. PloS Negl Trop Dis (2014) 8. doi: 10.1371/journal.pntd.0003202
91. Akhtar MN, Kumar S, Sen P. Leishmania donovani impedes antileishmanial immunity by suppressing dendritic cells via the TIM-3 receptor. MBio (2022) 13:1–25. doi: 10.1128/mbio.03309-21
92. De Trez C, Brait M, Leo O, Aebischer T, Torrentera FA, Carlier Y, et al. Myd88-dependent In vivo maturation of splenic dendritic cells induced by Leishmania donovani and other leishmania species. Infect Immun (2004) 72:824–32. doi: 10.1128/IAI.72.2.824-832.2004
Keywords: co-infection, tropical diseases, Plasmodium, Leishmania, immune response
Citation: Ornellas-Garcia U, Cuervo P and Ribeiro-Gomes FL (2023) Malaria and leishmaniasis: Updates on co-infection. Front. Immunol. 14:1122411. doi: 10.3389/fimmu.2023.1122411
Received: 12 December 2022; Accepted: 06 February 2023;
Published: 21 February 2023.
Edited by:
Dario S. Zamboni, University of São Paulo, BrazilReviewed by:
Fariborz Bahrami, Pasteur Institute of Iran, IranSonali Das, National Heart, Lung, and Blood Institute (NIH), United States
Copyright © 2023 Ornellas-Garcia, Cuervo and Ribeiro-Gomes. This is an open-access article distributed under the terms of the Creative Commons Attribution License (CC BY). The use, distribution or reproduction in other forums is permitted, provided the original author(s) and the copyright owner(s) are credited and that the original publication in this journal is cited, in accordance with accepted academic practice. No use, distribution or reproduction is permitted which does not comply with these terms.
*Correspondence: Flávia Lima Ribeiro-Gomes, ZmxhdmlhLmdvbWVzQGlvYy5maW9jcnV6LmJy