Corrigendum: The mast cell: A Janus in kidney transplants
- 1Department of Pathology and Clinical Bioinformatics, Erasmus University Center Rotterdam, Rotterdam, Netherlands
- 2Department of Internal Medicine, Division of Allergy and Clinical Immunology, Erasmus University Medical Center Rotterdam, Rotterdam, Netherlands
- 3Department of Internal Medicine, Division of Nephrology and Transplantation, Erasmus University Medical Center Rotterdam, Rotterdam, Netherlands
- 4Department of Pathology, Necker Hospital, APHP, Paris, France
- 5Institute of Experimental Medicine and Systems Biology, RWTH Aachen University, Aachen, Germany
- 6Division of Nephrology and Clinical Immunology, RWTH Aachen University Hospital, Aachen, Germany
Mast cells (MCs) are innate immune cells with a versatile set of functionalities, enabling them to orchestrate immune responses in various ways. Aside from their known role in allergy, they also partake in both allograft tolerance and rejection through interaction with regulatory T cells, effector T cells, B cells and degranulation of cytokines and other mediators. MC mediators have both pro- and anti-inflammatory actions, but overall lean towards pro-fibrotic pathways. Paradoxically, they are also seen as having potential protective effects in tissue remodeling post-injury. This manuscript elaborates on current knowledge of the functional diversity of mast cells in kidney transplants, combining theory and practice into a MC model stipulating both protective and harmful capabilities in the kidney transplant setting.
1 Introduction
Kidney transplant (KTx) recipients often experience progressive transplant injury and loss of function. Within 10 years, approximately 50% of KTx from deceased donors and 30% of KTx from living donors suffer complete graft loss (1). Although improved donor-recipient matching and better immunosuppressive drug combination therapy has resulted in a decrease of early rejection and graft loss over the past decades, late rejection and graft loss still remain a significant problem for KTx patients (2–5). While modern immunosuppression can halt an episode of acute rejection, in approximately half of all patients their graft function will not return to baseline and they remain at high risk for subsequent graft loss (6). Using the Banff classification of Renal Allograft Pathology, renal allograft rejection can be broadly categorized into T cell-mediated rejection (TCMR) and antibody-mediated rejection (AMR) (7). Both innate and adaptive immune systems are involved in graft-injury. General tissue injury initially triggers the innate immune system, potentially leading to activation of the adaptive immune system by donor or recipient innate cell antigen presentation and mediator release through interaction with T cells (8). Interstitial fibrosis (IF) results from an abundant deposition of extracellular matrix (ECM) in the tubulointerstitial compartment, eventually leading to scar formation (9). IF is a marker for graft dysfunction (10), and fibrosis with inflammation is a strong predictor of subsequent graft dysfunction and graft loss (10–12). Inflammation within areas of IF and tubular atrophy (i-IFTA) is a transitional phase between initial inflammation and tubulitis and either resolved fibrosis or chronic i-IFTA with progressive fibrosis. i-IFTA is a strong predictor of graft failure in TMCR, but a diverse gene expression pattern is witnessed, including B cell, plasma cell and mast cell transcripts (13).
Mast cells (MCs) are a critical component of both innate and adaptive immune responses, for example in allergy and anaphylaxis (14, 15), and host defense against parasites and animal toxins (16–18). MCs are also associated with various fibrotic diseases, although their exact role in fibrosis remains controversial (19, 20). To what extent MCs contribute to the formation of graft fibrosis and its relation to transplant outcome remains unclear (2). This manuscript will first focus on the current knowledge of MCs within the KTx setting, elaborating on the functional diversity of MCs in KTx. Thereafter, an integrative model of MCs in kidney tolerance and rejection will be proposed.
2 Current knowledge on functionality of mast cell in kidney allografts
21 Mast cell development and function
The exact origin of MCs remains unclear, with both a bi-potent basophil/mast cell progenitor (21) and a unique progenitor line besides the known myeloid cell line having been proposed (22, 23). MCs have a long lifespan, sometimes outlasting an entire immune response, aiding the process of clearing pathogens, including helminths (nematodes), reptile and arthropod venoms and certain tick species (16–18). MCs also partake in and help regulate host defence against viral and bacterial pathogens (24). As first responders, MCs possess sensory and regulatory functions in inflammatory processes, such as pathogen detection, mediator release, cellular and vascular tissue activation (25), antigen presentation (26) and pathogen removal (27–31). MCs mature and reside within peripheral tissues and can be found in almost all vascularized tissue, being most abundant in and around skin and mucosal surfaces (17). Furthermore, they have a different molecular expression profile depending on the tissue they reside and mature in, but share a common transcriptional MC signature of 128 genes (32). Additionally, they contain granules filled with premade mediators, including vasoactive amines (serotonin and histamine), proteoglycans, proteases (tryptase and chymase) and cytokines (33).
As innate immune cells, MCs possess toll-like receptors (TLRs), which can be activated by pathogen- or damage-associated molecular pattern molecules (25) to effect certain MC functions like mediator release, their antigen-presenting cell (APC) capabilities and interaction with dendritic cells (DCs) (34, 35) or interaction with other immune cells (18, 19). They respond to cell injury independently of TLRs through IL-33 activation and many other mediators (36). As an ‘unprofessional’ APC they can, in conjunction with DCs, fine-tune a type 2 immune response through promoting DC migration to draining lymph nodes, thereby priming an adequate T helper 2 (Th2) cell response. MCs and DCs secrete IL-10, interferons and tissue growth factor beta (TGF-β), thereby assisting regulatory T cells (Tregs) in their immune-protective actions against alloreactive T cells (37); thus, they can both activate and inhibit T cell-mediated responses (18, 34, 38).
There are two types of mast cells described in mice based on their phenotypical characteristics and their location, namely connective tissue-type and mucosal type. The first is found more often in serosal cavities, around venules and near nerves and the latter more often in the mucosa of the gut and respiratory tract (39). In the human setting, there are two main types of MCs: those that contain tryptase granules (MCT), and those containing both tryptase and chymase granules (MCTC) (40). MCTC also contain cathepsin G, a serine protease similar to chymase (41). In lung tissue, MC subtype occurrence depends on its surrounding tissue; MCs around smooth muscle tissue are mostly MCTCs while MCs in alveoli are more often MCTs (42). Interestingly, in the mucosa of small intestine most mast cells are MCTs and in the submucosa the MCTs are only scarcely represented (43). Differences in the type of mast cell therefor represent their function within the different microenvironments. Distribution patterns have not been studied in kidneys, but the MCT is presumed to be the most prevalent in the normal tubular interstitium (44), although an MCTCs count of 54% has been observed (40).
2.2 Mast cells and organ transplant rejection
Chronic rejection is associated with an increase in MCs within the solid organ transplant, including kidney (40, 44–47), intestine (48), lung (49), heart (50, 51) and liver (52, 53). An increase in MCs was also observed during acute rejection (50, 53–56), although not consistently (51, 52, 57). The increase in MCs could, however, be secondary to the inflammatory response of rejection, as it is related to both IF and time post-transplantation, suggesting that MCs are a marker for cumulative burden of tissue injury (58). Due to the minimal amount of data investigating mast cells numbers in transplantation in relation to time post transplantation, it is not known whether it is time dependent.
In KTx rejection, the number of MCTCs is increased in comparison to native kidneys, constituting approximately 57-60% of MCs (40, 44), although a subset of patients with rejection had a low MCCT to MCT ratio (40). A higher total MC count as well as a higher MCTC : MCT ratio is related to fibrosis and rejection (40, 44). Interestingly, both the absolute and relative amount of MCTCs was drastically increased in patients with poorer transplant outcome, suggestion a more potent role of chymase in rejection and IF and a phenotype switch of MC subtype in transplant disease, a phenomenon also observed in lung Tx (59).
2.2.1 Mast cell recruitment and activation
Stem cell factor (SCF) is important in MC development, maturation, activation, recruitment and chemotaxis of (im)mature MCs (60–62). SCF is secreted by endothelial cells and fibroblasts (63, 64) and binds to the c-KIT receptor. It is found in soluble form (sSCF) and membrane bound form (mSCF), the latter being cleaved into sSCF by chymase (65) and matrix metalloproteinase-9 (MMP-9) (66), both of which are released by MCs. This suggests a positive feedback loop of degranulation, with chymase release resulting in more sSCF and thus increased MC recruitment (65). SCF is linked to increased MC infiltration, fibrosis and interstitial alpha smooth muscle actin (α-SMA) (63, 67), as well as tissue remodeling (68). SCF stimulation has a protective role on (c-KIT positive) tubular epithelium and kidney function after ischemia-reperfusion injury (66, 69) and can be a predictive factor of eGFR in healthy, aging kidneys (70).
IL-9 has the ability to recruit MCs and is secreted by different cell types, including Th cells, Tregs and MCs (71). Naive Th cells express IL-9 after TGF-β and IL-4 exposure, while Th2 cells expresses IL-9 after IL-1 stimulation. IL-10 and SCF exposure enhance IL-9 synthesis by MCs, resulting in a positive feedback loop (72). Finally, IgE bound antigens can induce MC chemotaxis (61, 73). Donor specific anti-HLA I and II IgE has been found in transplant studies in both mice and humans, linking it to rejection (74). Although IgE presence in the kidney transplant is much higher in AMR, non-anti-HLA IgE antibodies are also found in areas with interstitial fibrosis and tubular atrophy (IF/TA) (47).
FcϵRI is a high affinity IgE receptor, giving MCs their infamous reputation in anaphylaxis. This receptor can be highly fine-tuned depending on the type of stimulation (75, 76); stimulation is at its strongest when bound to high concentrations of IgE with high antigen affinity and proximate IgE epitopes within the antigen (33). IgE-independent activation of MCs has also been described, for example by compound 48/80 (a synthetic ‘histamine liberator’ used to study MC degranulation (77, 78)), substance P and the Mas-related G protein-coupled receptor-X2 (MRGPRX2 or MRGX2), although MRGPRX2 is presumably absent in renal mast cells (78). Finally, MC expression of high-affinity IgG receptor FCγRI has also been reported (79). Interestingly, low dose antigen exposure of MCs can result in desensitization of the FcϵRI and MC tolerance to the antigen (75). Unique to the immune system, MCs can recover and resynthesize new granules after IgE (80, 81) or compound 48/80 mediated degranulation, after which they can be reactivated again by either mechanism (82). MCs can release granules with mixed mediator contents, or specific mediators, depending on the type of activation (33). MC stimulation and exocytosis can be highly fine-tuned, with focused or ‘piecemeal exocytosis’, multi-vesicular exocytosis and compound exocytosis (mass degranulation), depending on the amount of intracellular Ca2+ and type of activation (83, 84).
Combinations of IgE and substance P stimulation can result in either very localized (piecemeal) or systemic (compound) degranulation of MCs. Piecemeal exocytosis is related to complement factors C3a and C5a, endothelin and, most importantly, substance P (83). C3a and C5a are chemo-attractants for MCs in allergy and result in a rapid release of intracellular Ca2+ when activating MCs (85), which also has been observed in rejection (74).
After mast cell degranulation, proinflammatory cytokines as TNF-α, IL-6 and IL-8 are rapidly released. These cytokines also contribute to the inflammatory process as described in the following sections. In contrast, inhibition of IgE-dependent mast cell activation can be achieved by the cytokine TGF-β can inhibit mast cell degranulation and TNF-α production (86).
2.2.2 Mast cells and pro-inflammatory pathways
MCs can produce and release pro-inflammatory cytokines upon various different stimuli. IgE stimulates MCs to release TNF-α, a pro-inflammatory cytokine, resulting in the recruitment of innate immune cells like neutrophils (25, 87), DCs and T cells. Mouse models have shown that after MC degranulation, histamine and serotonin increase vascular permeability (88, 89). In human models, MCs have been shown to also selectively release vascular endothelial growth factor (VEGF) together with IL-6 and IL-8 (90, 91). This combination can increase local vascular permeability and stimulate leukocyte and lymphocyte infiltration, which can result in a transplant can result in transplant dysfunction, due to rejection. Indirect communication with other innate cells occurs when MC granules are ingested by DCs and macrophages (25, 34).
2.2.3 Crosstalk with T cells
Activated MCs primed with IgE can interact with various T cells, mainly through MHC-TCR interaction with co-stimulation of OX40L-OX40. TNF-α upregulates OX40L expression by MCs, and it is a potent factor in MC-T cell interaction (92). When linked with CD4+ Th cells, (co-)stimulation of TNF-α, IL-6 and MHC II antigen presentation will result in activation and proliferation of Th cells and release of pro-inflammatory cytokines (35, 93). In MC cross-talk with CD8+ T cells, (co-)stimulation with CCL5, 4-1BBL, TNF-α and MHC I antigen presentation will result in CD8+ recruitment, activation, proliferation and cytokine release (93, 94). While the OX40L-OX40 interaction activates T cells, it inhibits MC degranulation (94). Treg cells react differently to IgE activated MCs compared to CD4+ and CD8+ T cells; MCs suppress Treg activity through OX40L-OX40 cross-linking, in combination with histamine and IL-6 release (93). The crosstalk with T-cells, and in particular with CD8+ T cells can result in the development of an acute t cell-mediated rejection in the KTx as it is known that CD8+ T cells are a main player in transplant rejection (95).
2.2.4 Crosstalk with B cells
MC interaction with B cells has been described in mice after migration of MCs from the skin to a draining lymph node (96). It is there where proliferation of B cells is achieved by OX40-OX40L as well as CD40-CD40L interaction in combination with MC derived IL-6 stimulation after IgE sensitization (97, 98). While most of these pathways lead to B cell activation and IgA, IgE or IgG producing plasma cells, pathways leading to IL-10 producing regulatory B cells have also been suggested (96, 98). It is in antibody mediated rejection that B-cells have a prominent function and the role of MC crosstalk with B-cells should also be further studied in this context (99).
2.3 Mast cells and allograft tolerance
MC tolerance to a specific antigen can be accomplished in several ways. Treg-MC interaction through OX40L-OX40 and IL-9 induces a tolerogenic state in MCs and perhaps the entire allograft (71, 94, 100, 101), provided the MC is not activated by IgE. OX40L activation in MCs will inhibit IgE-mediated degranulation. Tregs increase intracellular cAMP in MCs, resulting in lower levels of Ca2+, further inhibiting degranulation (102). IL-9, secreted by Tregs, Th9 and Th17 cells, regulates MCs, promoting their immune-suppressive functions and decreasing pro-inflammatory release (103). MCs produce IL-10 and TGF-β, which enhances Treg differentiation and recruitment, subsequently promoting Foxp3 expression (37, 94, 100). MCs secrete GM-SCF and TNF-α, resulting in a tolerogenic state of DCs (37, 104). In turn, tolerogenic DCs (tDCs) also increase tolerance through Treg proliferation, again through IL-10 and TGF-β (105). Although TNF-α is considered pro-inflammatory, it also enhances tolerance trough tDC stimulation (37). MC mediators that inhibit effector T cell proliferation and function include Mast Cell Protease 6 (MCP6), a tryptase inhibiting the pro-inflammatory IL-6 cytokine and Th7 cells (106), TGF-β, IL-10 (94, 107), and histamine (37). IL-10 and TGF-β induce anergy of naïve CD4+ and CD8+ T cells, or T cells cross-linked to APCs (108). Together with DCs, MCs can induce type 1 regulatory T cells (Tr1), which are immunosuppressive cells similar to Tregs (108, 109). Tr1s show suppressed alloreactivity to specific antigens and inhibit other naive alloreactive CD4+ T cells (110) by producing IL-10 and TGF-β themselves (108). TGF-β and IL-10 also inhibit FcϵRI function, implying MC self-regulation and DC inhibition of MC degranulation (86, 111). MC-derived IL-10, in co-stimulation with IL-4, results in suppression of progenitor MC recruitment and survival, thereby countering positive feedback loops of MC recruitment (112). IL-10 has anti-fibrotic capabilities (64), and together with tDCs, Tregs and Tr1s, MCs thus potentially modulate inflammation and fibrogenesis in KTx (113, 114).
IgE-mediated MC degranulation inhibits peripheral tolerance in multiple ways: the balance between effector T cells and Tregs is distorted, alloreactivity within T cells is restored and an efflux of Tregs out of the Tx is observed (115). Thus, MC degranulation in tolerant transplants can theoretically promote T cell-mediated rejection. It is important to note that even local degranulation can lead to systemic breakdown of peripheral tolerance.
2.3 Mast cells and fibrosis
Stressed or injured epithelial cells (e.g. due to hypoxia) can acquire a mesenchymal phenotype, a process known as epithelial-to-mesenchymal transition (EMT). In the kidney this process has been controversial, and most recently has been defined as partial EMT. The latter indicates mesenchymal transition of epithelial cells that do not become myofibroblasts but are important drivers of inflammation and fibrosis through cross-talk with immune cells and mesenchyme (116).
EMT is linked to myofibroblast activation and proliferation, Smad pathway activation and IF in the kidney, both dependently and independently of TGF-β (117, 118). Myofibroblasts originate from both fibroblasts and pericytes, and express α-SMA and high amounts of extracellular matrix upon activation of various pathways including but not limited to TGF-β, inflammatory, and extracellular matrix pathways (116).
An important factor in tissue TGF-β synthesis is the renin-angiotensin system (RAS) and its end-product angiotensin II (ANG II) (9). Angiotensin converting enzyme (ACE), mostly derived from lung capillaries, is required for conversion of ANG I to ANG II. MC-derived chymase is capable of cleaving ANG I, leading to an ACE-independent ANG II and subsequent TGF-β formation. Thus, kidney resident MCTCs can contribute to intra-renal ANG II, TGF-β synthesis and fibrosis (119). MCs are also capable of releasing TGF-β as well as fibroblast growth factor-2 (FGF-2) (35, 120, 121). MMP-9, an ECM degrading enzyme secreted by MCs (also known as gelatinase B) (122), is another source of matrix-bound TGF-β activation and fibroblast contraction, further increasing MC potential to activate (myo)fibroblasts independently of RAS. Chymase can activate the plasmin system (123) and degrade fibrin/fibrinogen (124), thus countering the pro-fibrotic actions of the coagulation system. MCs have also been shown to crosstalk and form adhesion with tissue fibroblasts through c-KIT and CADM1 receptors. Crosstalk in combination with tryptase secretion leads to mostly pro-fibrotic activation and enhanced MC survival in co-culture studies, although select MC cultures exhibit anti-fibrotic activities (19).
Studies investigating MCs and fibrosis in human KTx patients are rare, but one study found a pro-fibrotic role of MCs, especially chymase positive MCs (44). Mouse models investigating MC influence on fibrosis shows the relationship to be more complex. Investigations using MC deficient mice show increased amount of fibrosis in aminonucleoside-nephrosis (125) and the unilateral ureteral obstruction model (126).
While MCs are regarded as inflictors of tissue fibrosis, MCs are also capable of modulating tissue remodeling. Local IL-10 release reduces collagen I deposition and decreased α-SMA and other fibroblast gene expression (127). Besides promoting fibroblasts, chymase also activates MMP-1 and MMP-3 function, both remodeling factors that degrade collagen fibers. Additionally, chymase cleaves and inactivates a latent factor called tissue inhibitor of metalloproteinase (TIMP-2), which inhibits MMP-2 within the ECM (128). MCs can express MMP-2 and MMP-9 themselves (122, 128, 129) after T cell mediated TNF-a stimulation (122). MCs are also capable of secreting, activating and removing inhibition of MMPs within the tubulo-interstitial compartment, a function unique to MCs. The contributory role of MMPs in fibrosis is complex, as e.g. TGF-ß increases both MMP-2 expression and release of its antagonist TIMP-2 (130).
3 Discussion
Mast cells are a pluripotent cell type that can either enhance or resolve injury, depending on their real-time environment. In this manuscript we propose a model depicting the multifaceted contribution of mast cells in the setting of kidney transplantation, namely in tolerance, rejection and chronic damage/fibrosis.
As discussed in this mini review, MCs contain a vast set of mediators and can act independently or in interaction with locoregional (immune) cells. There are distinct pro-inflammatory and tolerogenic patterns of interaction, with modulation of fibrogenesis. In kidney transplantation, IgE-mediated activation leads to the most profound degranulation, resulting in activation of pro-inflammatory and pro-fibrotic pathways, but IgE-independent activation also occurs. A hypothetical model, split between MC actions in rejection and transplant tolerance, is shown in Figures 1, 2. This model portrays the most important pathways of all MC actions within the transplant.
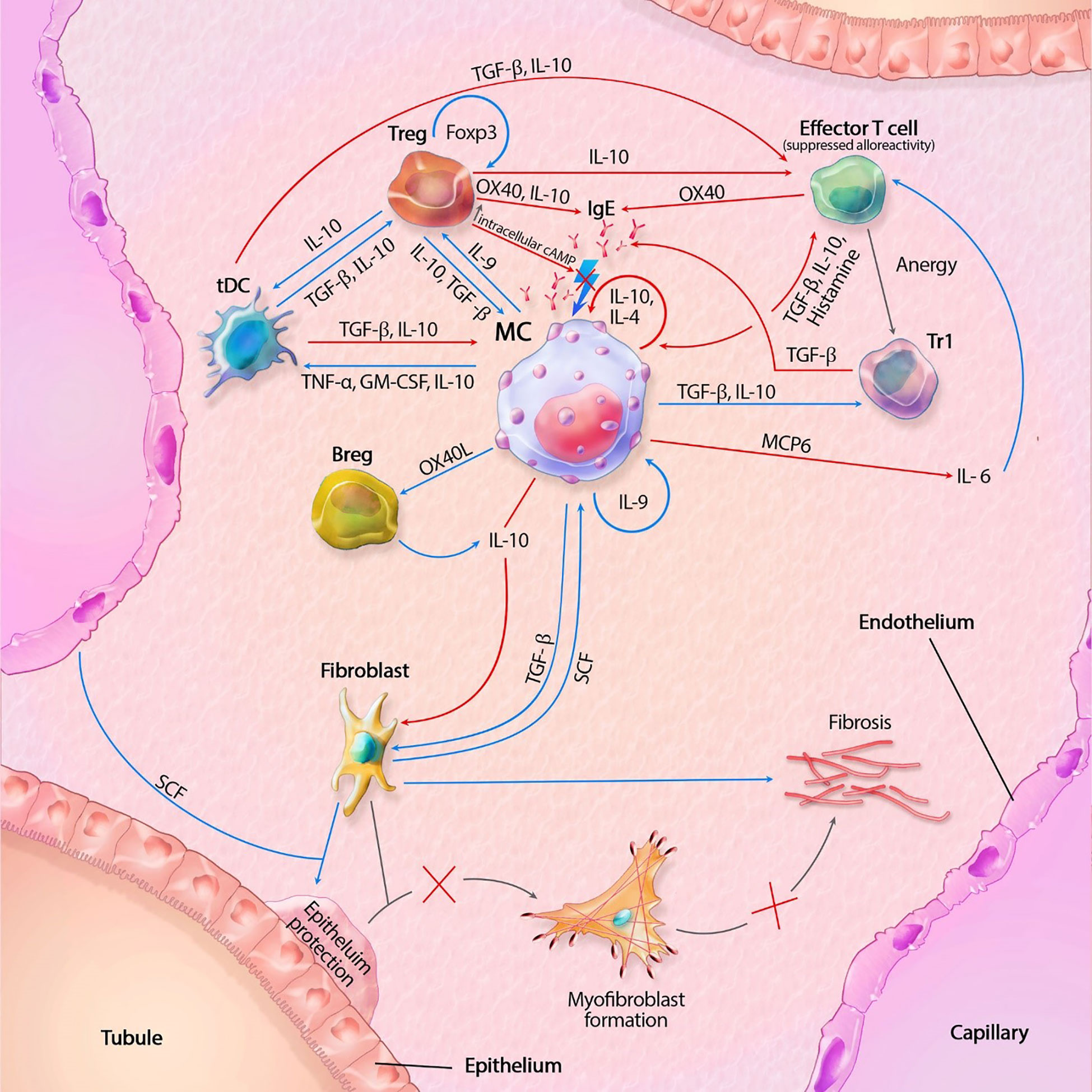
Figure 1 Mast cell (MC) interactions within the transplant during tolerance. FcϵRI activity is inhibited by TGF-β, IL-10 and OX40 ligation. Tregs also inhibit degranulation by lowering intracellular Ca2+ levels through increased cAMP. IL-10 suppresses alloreactivity within CD4+ and CD8+ T cells and promote anergy and regulatory functions of CD4+ T cells. IL-10 mediated inhibition of fibroblasts also inhibit subsequent formation of myofibroblasts. IL-10 with co-stimulation of IL-4 decrease MC proliferation, while IL-9 increases proliferation. GM-CSF, granulocyte-macrophage colony-stimulating factor; IL, interleukin; MCP6, mat cell protease 6; SCF, stem cell factor; tDC, tolerogenic dendritic cell; TGF-β, tissue growth factor beta; TNF-α, tissue necrotic factor alpha; Tr1, regulatory T cell type 1 (induced); Treg, regulatory T cell (natural); Blue lines symbolize activating pathways, red lines inhibitory pathways, gray lines symbolize subsequent events. Lighting icons are used in the most profound activation patterns, which are inhibited in tolerogenic environments.
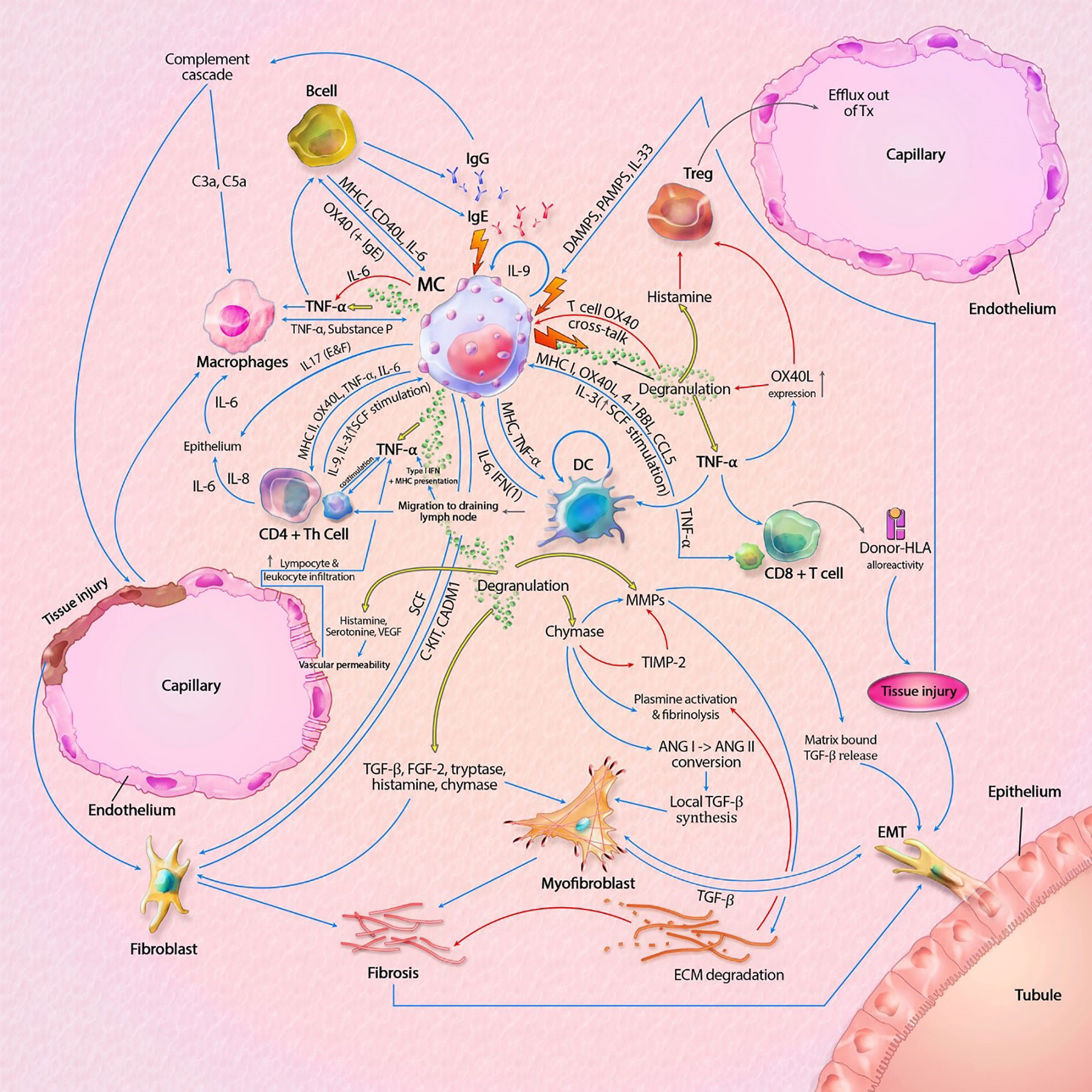
Figure 2 Mast cell (MC) interactions within the graft during rejection. Pathways can include both cytokines (like TNF-α) and membrane bound interaction (like MHC I-TLR interaction). MC-T cell interaction through OX40L-OX40 cross-linking inhibits MC degranulation, represented by the inhibitory pathway towards degranulation. Innate immune cells can also result in tissue injury, which is not shown in this model. Interaction between APCs, T cells and B cells, resulting in antigen production is also not shown in this model. The model shows almost no inhibitory pathways, explaining the progressive state of fibrosis within KTx even when immunosuppressive drugs are taken. Detailed description of the model can be found within the text. ANG, angiotensin; C3a/C5a, complement component; ECM, extracellular matrix; EMT, epithelial-mesenchymal transition; FGF-2; fibroblast growth factor-2; Ig, immunoglobulin; IL, interleukin; MHC, major histocompatibility complex; MMPs, matrix metalloproteinase; SCF, stem cell factor; tDC, tolerogenic dendritic cell; TGF-β, tissue growth factor beta; Th cell, T helper cell; TIMP-2, tissue inhibitor of metalloproteinase-2; TNF-α, tissue necrotic factor alpha; Treg, regulatory T cell (natural); VEGF, Vascular Endothelial Growth Factor. Blue lines symbolize activating pathways, red lines inhibitory pathways, yellow lines represent pre-formed mediators within MCs. Grey lines represent subsequent events. Lighting icons are used in the most profound activation patterns.
Ultimately, the current model may constitute a paradigm shift: stimulating donor-tolerance should be considered, rather than focusing on immunosuppressive drugs, undermining the patient’s immune system (131). This would lessen the (therapeutic) burden of transplant recipients, could potentially prevent transplant rejection and would result in a more natural state of self-induced tolerance. Treg-based therapies are already being investigated, although long-term stability of said tolerance is unknown (3, 132). As our model shows, MCs could play an important role in inducing and upholding this state of tolerance towards the KTx. So, rather than eradicating or fully inhibiting MCs, MC modulation toward tolerogenic action should be investigated.
Author contributions
MC-VG and TB conceptualized the review topic. GE gathered and summarized the relevant literature. GE and HV wrote the initial manuscript and TB, CB, JD-VH, MH, DH, RK, MR, MR, JT and MC-VG critically revised the manuscript. All authors contributed to the editing and finalization of the manuscript and approved the submitted version.
Funding
This article is funded by the dept. of Pathology, Erasmus Medical Center, Rotterdam, The Netherlands.
Acknowledgments
We would like to kindly thank Mr. Bernard Elsman, illustrator, for the excellent work he provided with the beautiful illustrations of the models depicted in Figures 1, 2.
Conflict of interest
The authors declare that the research was conducted in the absence of any commercial or financial relationships that could be construed as a potential conflict of interest.
Publisher’s note
All claims expressed in this article are solely those of the authors and do not necessarily represent those of their affiliated organizations, or those of the publisher, the editors and the reviewers. Any product that may be evaluated in this article, or claim that may be made by its manufacturer, is not guaranteed or endorsed by the publisher.
References
1. Hart A, Lentine KL, Smith JM, Miller JM, Skeans MA, Prentice M, et al. OPTN/SRTR 2019 annual data report: Kidney. Am J Transplant (2021) 21 Suppl 2:21–137. doi: 10.1111/ajt.16502
2. Nankivell BJ, Agrawal N, Sharma A, Taverniti A, P'Ng CH, Shingde M, et al. The clinical and pathological significance of borderline T cell-mediated rejection. Am J Transplant (2019) 19(5):1452–63. doi: 10.1111/ajt.15197
3. Wekerle T, Segev D, Lechler R, Oberbauer R. Strategies for long-term preservation of kidney graft function. Lancet (2017) 389(10084):2152–62.doi: 10.1016/S0140-6736(17)31283-7
4. Coemans M, Callemeyn J, Naesens M. Long-term survival after kidney transplantation. N Engl J Med (2022) 386(5):497–8. doi: 10.1056/NEJMc2115207sa1
5. Hariharan S, Israni AK, Danovitch G. Long-term survival after kidney transplantation. N Engl J Med (2021) 385(8):729–43. doi: 10.1056/NEJMra2014530
6. Srinivas TR, Oppenheimer F. Identifying endpoints to predict the influence of immunosuppression on long-term kidney graft survival. Clin Transplant (2015) 29(7):644–53. doi: 10.1111/ctr.12554
7. Roufosse C, Simmonds N, Clahsen-van Groningen M, Haas M, Henriksen KJ, Horsfield C, et al. A 2018 reference guide to the banff classification of renal allograft pathology. Transplantation (2018) 102(11):1795–814. doi: 10.1097/TP.0000000000002366
8. Wood KJ, Goto R. Mechanisms of rejection: current perspectives. Transplantation (2012) 93(1):1–10. doi: 10.1097/TP.0b013e31823cab44
9. Zhou D, Liu Y. Renal fibrosis in 2015: Understanding the mechanisms of kidney fibrosis. Nat Rev Nephrol (2016) 12(2):68–70. doi: 10.1038/nrneph.2015.215
10. Park WD, Griffin MD, Cornell LD, Cosio FG, Stegall MD. Fibrosis with inflammation at one year predicts transplant functional decline. J Am Soc Nephrol (2010) 21(11):1987–97. doi: 10.1681/ASN.2010010049
11. Cosio FG, Grande JP, Wadei H, Larson TS, Griffin MD, Stegall MD. Predicting subsequent decline in kidney allograft function from early surveillance biopsies. Am J Transplant (2005) 5(10):2464–72. doi: 10.1111/j.1600-6143.2005.01050.x
12. Mannon RB, Matas AJ, Grande J, Leduc R, Connett J, Kasiske B, et al. Inflammation in areas of tubular atrophy in kidney allograft biopsies: A potent predictor of allograft failure. Am J Transplant (2010) 10(9):2066–73. doi: 10.1111/j.1600-6143.2010.03240.x
13. Nankivell BJ, Shingde M, Keung KL, Fung CL, Borrows RJ, O'Connell PJ, et al. The Causes, significance and consequences of inflammatory fibrosis in kidney transplantation: The banff i-IFTA lesion. Am J Transplant (2018) 18(2):364–76. doi: 10.1111/ajt.14609
14. He SH, Zhang HY, Zeng XN, Chen D, Yang PC. Mast cells and basophils are essential for allergies: mechanisms of allergic inflammation and a proposed procedure for diagnosis. Acta Pharmacol Sin (2013) 34(10):1270–83. doi: 10.1038/aps.2013.88
15. Gould HJ, Sutton BJ, Beavil AJ, Beavil RL, McCloskey N, Coker HA, et al. The biology of IGE and the basis of allergic disease. Annu Rev Immunol (2003) 21:579–628. doi: 10.1146/annurev.immunol.21.120601.141103
16. Galli SJ. The mast cell-IgE paradox: From homeostasis to anaphylaxis. Am J Pathol (2016) 186(2):212–24. doi: 10.1016/j.ajpath.2015.07.025
17. Mukai K, Tsai M, Starkl P, Marichal T, Galli SJ. IgE and mast cells in host defense against parasites and venoms. Semin Immunopathol (2016) 38(5):581–603. doi: 10.1007/s00281-016-0565-1
18. Dudeck A, Koberle M, Goldmann O, Meyer N, Dudeck J, Lemmens S, et al. Mast cells as protectors of health. J Allergy Clin Immun (2019) 144(4):S4–S18. doi: 10.1016/j.jaci.2018.10.054
19. Bradding P, Pejler G. The controversial role of mast cells in fibrosis. Immunol Rev (2018) 282(1):198–231. doi: 10.1111/imr.12626
20. Wilgus TA, Ud-Din S, Bayat A. A review of the evidence for and against a role for mast cells in cutaneous scarring and fibrosis. Int J Mol Sci (2020) 21(24):9673. doi: 10.3390/ijms21249673
21. Dahlin JS, Hallgren J. Mast cell progenitors: origin, development and migration to tissues. Mol Immunol (2015) 63(1):9–17. doi: 10.1016/j.molimm.2014.01.018
22. Fodinger M, Fritsch G, Winkler K, Emminger W, Mitterbauer G, Gadner H, et al. Origin of human mast-cells - development from transplanted hematopoietic stem-cells after allogeneic bone-marrow transplantation. Blood (1994) 84(9):2954–9. doi: 10.1182/blood.V84.9.2954.2954
23. Valent P, Akin C, Hartmann K, Nilsson G, Reiter A, Hermine O, et al. Mast cells as a unique hematopoietic lineage and cell system: From Paul ehrlich's visions to precision medicine concepts. Theranostics (2020) 10(23):10743–68. doi: 10.7150/thno.46719thnov10p10743
24. Jimenez M, Cervantes-Garcia D, Cordova-Davalos LE, Perez-Rodriguez MJ, Gonzalez-Espinosa C, Salinas E. Responses of mast cells to pathogens: Beneficial and detrimental roles. Front Immunol (2021) 12:685865. doi: 10.3389/fimmu.2021.685865
25. St John AL, Abraham SN. Innate immunity and its regulation by mast cells. J Immunol (2013) 190(9):4458–63. doi: 10.4049/jimmunol.1203420
26. Galli SJ, Gaudenzio N. Human mast cells as antigen-presenting cells: When is this role important. vivo? J Allergy Clin Immun (2018) 141(1):92–3. doi: 10.1016/j.jaci.2017.05.029
27. Della Rovere F, Granata A, Monaco M, Basile G. Phagocytosis of cancer cells by mast cells in breast cancer. Anticancer Res (2009) 29(8):3157–61
28. Lima HG, Pinke KH, Gardizani TP, Souza-Junior DA, Carlos D, Avila-Campos MJ, et al. Mast cells act as phagocytes against the periodontopathogen aggregatibacter actinomycetemcomitans. J Periodontol (2013) 84(2):265–72. doi: 10.1902/jop.2012.120087
29. Malaviya R, Ross EA, MacGregor JI, Ikeda T, Little JR, Jakschik BA, et al. Mast cell phagocytosis of FimH-expressing enterobacteria. J Immunol (1994) 152(4):1907–14. doi: 10.4049/jimmunol.152.4.1907
30. von Kockritz-Blickwede M, Goldmann O, Thulin P, Heinemann K, Norrby-Teglund A, Rohde M, et al. Phagocytosis-independent antimicrobial activity of mast cells by means of extracellular trap formation. Blood (2008) 111(6):3070–80. doi: 10.1182/blood-2007-07-104018
31. Mezouar S, Vitte J, Gorvel L, Ben Amara A, Desnues B, Mege JL. Mast cell cytonemes as a defense mechanism against coxiella burnetii. mBio (2019) 10(2):e02669–18. doi: 10.1128/mBio.02669-18
32. Dwyer DF, Barrett NA, Austen KF, Conso IGP. Expression profiling of constitutive mast cells reveals a unique identity within the immune system. Nat Immunol (2016) 17(7):878–+. doi: 10.1038/ni.3445
33. Bulfone-Paus S, Nilsson G, Draber P, Blank U, Levi-Schaffer F. Positive and negative signals in mast cell activation. Trends Immunol (2017) 38(9):657–67. doi: 10.1016/j.it.2017.01.008
34. Dudeck J, Froebel J, Kotrba J, Lehmann CHK, Dudziak D, Speier S, et al. Engulfment of mast cell secretory granules on skin inflammation boosts dendritic cell migration and priming efficiency. J Allergy Clin Immunol (2019) 143(5):1849–64 e4. doi: 10.1016/j.jaci.2018.08.052
35. Elieh Ali Komi D, Ribatti D. Mast cell-mediated mechanistic pathways in organ transplantation. Eur J Pharmacol (2019) 857:172458. doi: 10.1016/j.ejphar.2019.172458
36. Lunderius-Andersson C, Enoksson M, Nilsson G. Mast cells respond to cell injury through the recognition of IL-33. Front Immunol (2012) 3:82. doi: 10.3389/fimmu.2012.00082
37. de Vries VC, Noelle RJ. Mast cell mediators in tolerance. Curr Opin Immunol (2010) 22(5):643–8. doi: 10.1016/j.coi.2010.08.015
38. Schuijs MJ, Hammad H, Lambrecht BN. Professional and 'Amateur' antigen-presenting cells in type 2 immunity. Trends Immunol (2019) 40(1):22–34. doi: 10.1016/j.it.2018.11.001
39. Reber LL, Sibilano R, Mukai K, Galli SJ. Potential effector and immunoregulatory functions of mast cells in mucosal immunity. Mucosal Immunol (2015) 8(3):444–63. doi: 10.1038/mi.2014.131
40. Yamada M, Ueda M, Naruko T, Tanabe S, Han YS, Ikura Y, et al. Mast cell chymase expression and mast cell phenotypes in human rejected kidneys. Kidney Int (2001) 59(4):1374–81. doi: 10.1046/j.1523-1755.2001.0590041374.x
41. Schechter NM, Irani AM, Sprows JL, Abernethy J, Wintroub B, Schwartz LB. Identification of a cathepsin G-like proteinase in the MCTC type of human mast cell. J Immunol (1990) 145(8):2652–61. doi: 10.4049/jimmunol.145.8.2652
42. Erjefalt JS. Mast cells in human airways: The culprit? Eur Respir Rev (2014) 23(133):299–307. doi: 10.1183/09059180.00005014
43. Irani AA, Schechter NM, Craig SS, DeBlois G, Schwartz LB. Two types of human mast cells that have distinct neutral protease compositions. Proc Natl Acad Sci USA (1986) 83(12):4464–8. doi: 10.1073/pnas.83.12.4464
44. Ishida T, Hyodo Y, Ishimura T, Takeda M, Hara I, Fujisawa M. Mast cell numbers and protease expression patterns in biopsy specimens following renal transplantation from living-related donors predict long-term graft function. Clin Transplant (2005) 19(6):817–24. doi: 10.1111/j.1399-0012.2005.00427.x
45. Pardo J, Diaz L, Errasti P, Idoate M, de Alava E, Sola I, et al. Mast cells in chronic rejection of human renal allografts. Virchows Arch (2000) 437(2):167–72. doi: 10.1007/s004280000211
46. Roberts IS, Brenchley PE. Mast cells: the forgotten cells of renal fibrosis. J Clin Pathol (2000) 53(11):858–62. doi: 10.1136/jcp.53.11.858
47. Rascio F, Pontrelli P, Netti GS, Manno E, Infante B, Simone S, et al. IgE-mediated immune response and antibody-mediated rejection. Clin J Am Soc Nephrol (2020) 15(10):1474–83. doi: 10.2215/CJN.02870320
48. Walgenbach KJ, Heeckt PF, Stanson JD, Whiteside TL, Bauer AJ. Increased presence of mast cells and interleukin-4 during chronic rejection of rat intestinal allografts. Transplant Proc (1996) 28(5):2454.
49. Yousem SA. The potential role of mast cells in lung allograft rejection. Hum Pathol (1997) 28(2):179–82. doi: 10.1016/s0046-8177(97)90103-9
50. Li QY, Raza-Ahmad A, MacAulay MA, Lalonde LD, Rowden G, Trethewey E, et al. The relationship of mast cells and their secreted products to the volume of fibrosis in posttransplant hearts. Transplantation (1992) 53(5):1047–51. doi: 10.1097/00007890-199205000-00015
51. Koskinen PK, Kovanen PT, Lindstedt KA, Lemstrom KB. Mast cells in acute and chronic rejection of rat cardiac allografts–a major source of basic fibroblast growth factor. Transplantation (2001) 71(12):1741–7. doi: 10.1097/00007890-200106270-00007
52. O'Keeffe C, Baird AW, Nolan N, McCormick PA. Mast cell hyperplasia in chronic rejection after liver transplantation. Liver Transpl (2002) 8(1):50–7. doi: 10.1053/jlts.2002.30343
53. El-Refaie AM, Burt AD. Mast cells and c-kit expression in liver allograft rejection. Histopathology (2005) 47(4):375–81. doi: 10.1111/j.1365-2559.2005.02239.x
54. Zweifel M, Hirsiger H, Matozan K, Welle M, Schaffner T, Mohacsi P. Mast cells in ongoing acute rejection: Increase in number and expression of a different phenotype in rat heart transplants. Transplantation (2002) 73(11):1707–16. doi: 10.1097/00007890-200206150-00004
55. Greenstein SM, Katz JP, Hakki A, Simonian S, Sowinski J, Katz SM. Mast cells in ureters from rejected allografts. Transplant Proc (1989) 21(1 Pt 1):286–8.
56. Lajoie G, Nadasdy T, Laszik Z, Blick KE, Silva FG. Mast cells in acute cellular rejection of human renal allografts. Mod Pathol (1996) 9(12):1118–25.
57. Minami M, Nakahara K, Matsumura A, Mizuta T, Yoon HE, Sakaguchi M, et al. Histamine release from pulmonary mast cells after lung transplantation in rats. J Heart Lung Transplant (1995) 14(3):505–11.
58. Papadimitriou JC, Drachenberg CB, Ramos E, Ugarte R, Haririan A. Mast cell quantitation in renal transplant biopsy specimens as a potential marker for the cumulative burden of tissue injury. Transplant Proc (2013) 45(4):1469–71. doi: 10.1016/j.transproceed.2013.01.078
59. Banga A, Han YC, Wang XF, Hsieh FH. Mast cell phenotypes in the allograft after lung transplantation. Clin Transplant (2016) 30(7):845–51. doi: 10.1111/ctr.12758
60. Levi-Schaffer F, Piliponsky AM. Tryptase, a novel link between allergic inflammation and fibrosis. Trends Immunol (2003) 24(4):158–61. doi: 10.1016/s1471-4906(03)00058-9
61. Draber P, Halova I, Polakovicova I, Kawakami T. Signal transduction and chemotaxis in mast cells. Eur J Pharmacol (2016) 778:11–23. doi: 10.1016/j.ejphar.2015.02.057
62. Galli SJ, Zsebo KM, Geissler EN. The kit ligand, stem cell factor. Adv Immunol (1994) 55:1–96. doi: 10.1016/s0065-2776(08)60508-8
63. El-Koraie AF, Baddour NM, Adam AG, El Kashef EH, El Nahas AM. Role of stem cell factor and mast cells in the progression of chronic glomerulonephritides. Kidney Int (2001) 60(1):167–72. doi: 10.1046/j.1523-1755.2001.00783.x
64. Heinrich MC, Dooley DC, Freed AC, Band L, Hoatlin ME, Keeble WW, et al. Constitutive expression of steel factor gene by human stromal cells. Blood (1993) 82(3):771–83 doi: 10.1182/blood.V82.3.771.771
65. Longley BJ, Tyrrell L, Ma Y, Williams DA, Halaban R, Langley K, et al. Chymase cleavage of stem cell factor yields a bioactive, soluble product. Proc Natl Acad Sci USA (1997) 94(17):9017–21. doi: 10.1073/pnas.94.17.9017
66. Bengatta S, Arnould C, Letavernier E, Monge M, de Preneuf HM, Werb Z, et al. MMP9 and SCF protect from apoptosis in acute kidney injury. J Am Soc Nephrol (2009) 20(4):787–97. doi: 10.1681/ASN.2008050515
67. Liu H, Liu F, Peng Y, Liu Y, Li L, Tu X, et al. Role of mast cells, stem cell factor and protease-activated receptor-2 in tubulointerstitial lesions in IgA nephropathy. Inflammation Res (2010) 59(7):551–9. doi: 10.1007/s00011-010-0159-7
68. El Kossi MM, Haylor JL, Johnson TS, El Nahas AM. Stem cell factor in a rat model of serum nephrotoxic nephritis. Nephron Exp Nephrol (2008) 108(1):e1–e10. doi: 10.1159/000112518
69. Stokman G, Stroo I, Claessen N, Teske GJ, Weening JJ, Leemans JC, et al. Stem cell factor expression after renal ischemia promotes tubular epithelial survival. PloS One (2010) 5(12):e14386. doi: 10.1371/journal.pone.0014386
70. Zhang W, Jia L, Liu DLX, Chen L, Wang Q, Song K, et al. Serum stem cell factor level predicts decline in kidney function in healthy aging adults. J Nutr Health Aging (2019) 23(9):813–20. doi: 10.1007/s12603-019-1253-3
71. Lu LF, Lind EF, Gondek DC, Bennett KA, Gleeson MW, Pino-Lagos K, et al. Mast cells are essential intermediaries in regulatory T-cell tolerance. Nature (2006) 442(7106):997–1002. doi: 10.1038/nature05010
72. Stassen M, Arnold M, Hultner L, Muller C, Neudorfl C, Reineke T, et al. Murine bone marrow-derived mast cells as potent producers of IL-9: Costimulatory function of IL-10 and kit ligand in the presence of IL-1. J Immunol (2000) 164(11):5549–55. doi: 10.4049/jimmunol.164.11.5549
73. Halova I, Draberova L, Draber P. Mast cell chemotaxis - chemoattractants and signaling pathways. Front Immunol (2012) 3:119. doi: 10.3389/fimmu.2012.00119
74. Farkas AM, Baranyi U, Bohmig GA, Unger L, Hopf S, Wahrmann M, et al. Allograft rejection is associated with development of functional IgE specific for donor MHC antigens. J Allergy Clin Immunol (2019) 143(1):335–45 e12. doi: 10.1016/j.jaci.2018.06.034
75. Ra C, Nunomura S, Okayama Y. Fine-tuning of mast cell activation by FcepsilonRIbeta chain. Front Immunol (2012) 3:112. doi: 10.3389/fimmu.2012.00112
76. Espinosa E, Valitutti S. New roles and controls of mast cells. Curr Opin Immunol (2018) 50:39–47. doi: 10.1016/j.coi.2017.10.012
77. Byrne RD, Rosivatz E, Parsons M, Larijani B, Parker PJ, Ng T, et al. Differential activation of the PI 3-kinase effectors AKT/PKB and p70 S6 kinase by compound 48/80 is mediated by PKCalpha. Cell Signal (2007) 19(2):321–9. doi: 10.1016/j.cellsig.2006.07.004
78. Tatemoto K, Nozaki Y, Tsuda R, Konno S, Tomura K, Furuno M, et al. Immunoglobulin e-independent activation of mast cell is mediated by mrg receptors. Biochem Biophys Res Commun (2006) 349(4):1322–8. doi: 10.1016/j.bbrc.2006.08.177
79. Okayama Y, Kirshenbaum AS, Metcalfe DD. Expression of a functional high-affinity IgG receptor On human mast cells: Up-regulation by IFN-gamma. J Immunol (2000) 164(8):4332–9. doi: 10.4049/jimmunol.164.8.4332
80. Xiang Z, Block M, Lofman C, Nilsson G. IgE-mediated mast cell degranulation and recovery monitored by time-lapse photography. J Allergy Clin Immunol (2001) 108(1):116–21. doi: 10.1067/mai.2001.116124
81. Dvorak AM, Schleimer RP, Lichtenstein LM. Human mast cells synthesize new granules during recovery from degranulation. In Vitro Stud mast Cells purified Hum lungs. Blood (1988) 71(1):76–85.
82. Liao H, Peng X, Ge Y, Liang Y, Yin Y, Li J, et al. Novel reactivation and degranulation of mast cells. BioMed Pharmacother (2020) 127:110157. doi: 10.1016/j.biopha.2020.110157
83. Gaudenzio N, Sibilano R, Marichal T, Starkl P, Reber LL, Cenac N, et al. Different activation signals induce distinct mast cell degranulation strategies. J Clin Invest (2016) 126(10):3981–98. doi: 10.1172/Jci85538
84. Blank U. The mechanisms of exocytosis in mast cells. Adv Exp Med Biol (2011) 716:107–22. doi: 10.1007/978-1-4419-9533-9_7
85. Hartmann K, Henz BM, Kruger-Krasagakes S, Kohl J, Burger R, Guhl S, et al. C3a and C5a stimulate chemotaxis of human mast cells. Blood (1997) 89(8):2863–70. doi: 10.1182/blood.V89.8.2863
86. Gomez G, Ramirez CD, Rivera J, Patel M, Norozian F, Wright HV, et al. TGF-beta 1 inhibits mast cell fc epsilon RI expression. J Immunol (2005) 174(10):5987–93. doi: 10.4049/jimmunol.174.10.5987
87. Young JDE, Liu CC, Butler G, Cohn ZA, Galli SJ. Identification, purification, and characterization of a mast cell-associated cytolytic factor related to tumor necrosis factor. P Natl Acad Sci USA (1987) 84(24):9175–9. doi: 10.1073/pnas.84.24.9175
88. Michel CC, Kendall S. Differing effects of histamine and serotonin on microvascular permeability in anaesthetized rats. J Physiol (1997) 501(Pt 3):657–62. doi: 10.1111/j.1469-7793.1997.657bm.x
89. Goromaru T, Sendo T, Itoh Y, Sakai N, Teshima D, Oishi R. Evidence for involvement of mast cell degranulation and subsequent stimulation of histamine H1 and H2 receptors in radiographic contrast media-increased vascular permeability in rats. Naunyn Schmiedebergs Arch Pharmacol (2002) 366(6):605–12. doi: 10.1007/s00210-002-0618-y
90. Theoharides TC, Kempuraj D, Tagen M, Conti P, Kalogeromitros D. Differential release of mast cell mediators and the pathogenesis of inflammation. Immunol Rev (2007) 217:65–78. doi: 10.1111/j.1600-065X.2007.00519.x
91. Grutzkau A, Kruger-Krasagakes S, Baumeister H, Schwarz C, Kogel H, Welker P, et al. Synthesis, storage, and release of vascular endothelial growth factor/vascular permeability factor (VEGF/VPF) by human mast cells: Implications for the biological significance of VEGF206. Mol Biol Cell (1998) 9(4):875–84. doi: 10.1091/mbc.9.4.875
92. Nakae S, Suto H, Iikura M, Kakurai M, Sedgwick JD, Tsai M, et al. Mast cells enhance T cell activation: Importance of mast cell costimulatory molecules and secreted TNF. J Immunol (2006) 176(4):2238–48. doi: 10.4049/jimmunol.176.4.2238
93. Bulfone-Paus S, Bahri R. Mast cells as regulators of T cell responses. Front Immunol (2015) 6:394. doi: 10.3389/fimmu.2015.00394
94. Elieh Ali Komi D, Grauwet K. Role of mast cells in regulation of T cell responses in experimental and clinical settings. Clin Rev Allergy Immunol (2018) 54(3):432–45. doi: 10.1007/s12016-017-8646-z
95. Rocha PN, Plumb TJ, Crowley SD, Coffman TM. Effector mechanisms in transplant rejection. Immunol Rev (2003) 196:51–64. doi: 10.1046/j.1600-065x.2003.00090.x
96. Byrne SN, Limon-Flores AY, Ullrich SE. Mast cell migration from the skin to the draining lymph nodes upon ultraviolet irradiation represents a key step in the induction of immune suppression. J Immunol (2008) 180(7):4648–55. doi: 10.4049/jimmunol.180.7.4648
97. Merluzzi S, Frossi B, Gri G, Parusso S, Tripodo C, Pucillo C. Mast cells enhance proliferation of b lymphocytes and drive their differentiation toward IgA-secreting plasma cells. Blood (2010) 115(14):2810–7. doi: 10.1182/blood-2009-10-250126
98. Palma AM, Hanes MR, Marshall JS. Mast cell modulation of b cell responses: An under-appreciated partnership in host defence. Front Immunol (2021) 12:718499. doi: 10.3389/fimmu.2021.718499
99. Chong AS. Mechanisms of organ transplant injury mediated by b cells and antibodies: Implications for antibody-mediated rejection. Am J Transplant (2020) 20 Suppl 4(Suppl 4):23–32. doi: 10.1111/ajt.15844
100. Zhao YB, Yang SH, Shen J, Deng K, Li Q, Wang Y, et al. Interaction between regulatory T cells and mast cells via IL-9 and TGF-beta production. Oncol Lett (2020) 20(6):360. doi: 10.3892/ol.2020.12224
101. Eller K, Wolf D, Huber JM, Metz M, Mayer G, McKenzie AN, et al. IL-9 production by regulatory T cells recruits mast cells that are essential for regulatory T cell-induced immune suppression. J Immunol (2011) 186(1):83–91. doi: 10.4049/jimmunol.1001183
102. Frossi B, Gri G, Tripodo C, Pucillo C. Exploring a regulatory role for mast cells: 'MCregs'? Trends Immunol (2010) 31(3):97–102. doi: 10.1016/j.it.2009.12.007
104. de Vries VC, Pino-Lagos K, Nowak EC, Bennett KA, Oliva C, Noelle RJ. Mast cells condition dendritic cells to mediate allograft tolerance. Immunity (2011) 35(4):550–61. doi: 10.1016/j.immuni.2011.09.012
105. Maldonado RA, von Andrian UH. How tolerogenic dendritic cells induce regulatory T cells. Adv Immunol (2010) 108:111–65. doi: 10.1016/B978-0-12-380995-7.00004-5
106. de Vries VC, Elgueta R, Lee DM, Noelle RJ. Mast cell protease 6 is required for allograft tolerance. Transplant Proc (2010) 42(7):2759–62. doi: 10.1016/j.transproceed.2010.05.168
107. Jungraithmayr W. The putative role of mast cells in lung transplantation. Am J Transplant (2015) 15(3):594–600. doi: 10.1111/ajt.13126
108. Levings MK, Bacchetta R, Schulz U, Roncarolo MG. The role of IL-10 and TGF-beta in the differentiation and effector function of T regulatory cells. Int Arch Allergy Immunol (2002) 129(4):263–76. doi: 10.1159/000067596
109. Hara M, Kingsley CI, Niimi M, Read S, Turvey SE, Bushell AR, et al. IL-10 is required for regulatory T cells to mediate tolerance to alloantigens. vivo. J Immunol (2001) 166(6):3789–96. doi: 10.4049/jimmunol.166.6.3789
110. Chen ZM, O'Shaughnessy MJ, Gramaglia I, Panoskaltsis-Mortari A, Murphy WJ, Narula S, et al. IL-10 and TGF-beta induce alloreactive CD4+CD25- T cells to acquire regulatory cell function. Blood (2003) 101(12):5076–83. doi: 10.1182/blood-2002-09-2798S0006-4971(20)50682-4
111. Kennedy Norton S, Barnstein B, Brenzovich J, Bailey DP, Kashyap M, Speiran K, et al. IL-10 suppresses mast cell IgE receptor expression and signaling. Vitro vivo. J Immunol (2008) 180(5):2848–54. doi: 10.4049/jimmunol.180.5.2848
112. Speiran K, Bailey DP, Fernando J, Macey M, Barnstein B, Kolawole M, et al. Endogenous suppression of mast cell development and survival by IL-4 and IL-10. J Leukoc Biol (2009) 85(5):826–36. doi: 10.1189/jlb.0708448
113. Jin Y, Liu R, Xie J, Xiong H, He JC, Chen N. Interleukin-10 deficiency aggravates kidney inflammation and fibrosis in the unilateral ureteral obstruction mouse model. Lab Invest (2013) 93(7):801–11. doi: 10.1038/labinvest.2013.64
114. Steen EH, Wang X, Balaji S, Butte MJ, Bollyky PL, Keswani SG. The role of the anti-inflammatory cytokine interleukin-10 in tissue fibrosis. Adv Wound Care (New Rochelle) (2020) 9(4):184–98. doi: 10.1089/wound.2019.1032
115. de Vries VC, Wasiuk A, Bennett KA, Benson MJ, Elgueta R, Waldschmidt TJ, et al. Mast cell degranulation breaks peripheral tolerance. Am J Transplant (2009) 9(10):2270–80. doi: 10.1111/j.1600-6143.2009.02755.x
116. Kuppe C, Ibrahim MM, Kranz J, Zhang X, Ziegler S, Perales-Paton J, et al. Decoding myofibroblast origins in human kidney fibrosis. Nature (2021) 589(7841):281–6. doi: 10.1038/s41586-020-2941-1
117. Sun YB, Qu X, Caruana G, Li J. The origin of renal fibroblasts/myofibroblasts and the signals that trigger fibrosis. Differentiation (2016) 92(3):102–7. doi: 10.1016/j.diff.2016.05.008
118. Farris AB, Colvin RB. Renal interstitial fibrosis: Mechanisms and evaluation. Curr Opin Nephrol Hypertens (2012) 21(3):289–300. doi: 10.1097/MNH.0b013e3283521cfa
119. Wasse H, Naqvi N, Husain A. Impact of mast cell chymase on renal disease progression. Curr Hypertens Rev (2012) 8(1):15–23. doi: 10.2174/157340212800505007
120. Qu Z, Huang X, Ahmadi P, Stenberg P, Liebler JM, Le AC, et al. Synthesis of basic fibroblast growth factor by murine mast cells. regulation by transforming growth factor beta, tumor necrosis factor alpha, and stem cell factor. Int Arch Allergy Immunol (1998) 115(1):47–54. doi: 10.1159/000023829
121. Qu Z, Kayton RJ, Ahmadi P, Liebler JM, Powers MR, Planck SR, et al. Ultrastructural immunolocalization of basic fibroblast growth factor in mast cell secretory granules. morphological evidence for bfgf release through degranulation. J Histochem Cytochem (1998) 46(10):1119–28. doi: 10.1177/002215549804601004
122. Baram D, Vaday GG, Salamon P, Drucker I, Hershkoviz R, Mekori YA. Human mast cells release metalloproteinase-9 on contact with activated T cells: Juxtacrine regulation by TNF-alpha. J Immunol (2001) 167(7):4008–16. doi: 10.4049/jimmunol.167.7.4008
123. Sillaber C, Baghestanian M, Bevec D, Willheim M, Agis H, Kapiotis S, et al. The mast cell as site of tissue-type plasminogen activator expression and fibrinolysis. J Immunol (1999) 162(2):1032–41. doi: 10.4049/jimmunol.162.2.1032
124. Lipitsa T, Siiskonen H, Naukkarinen A, Harvima IT. Mast cell chymase degrades fibrinogen and fibrin. Br J Dermatol (2019) 181(2):296–303. doi: 10.1111/bjd.17534
125. Miyazawa S, Hotta O, Doi N, Natori Y, Nishikawa K, Natori Y. Role of mast cells in the development of renal fibrosis: use of mast cell-deficient rats. Kidney Int (2004) 65(6):2228–37. doi: 10.1111/j.1523-1755.2004.00629.xS0085-2538(15)49968-5
126. Kim DH, Moon SO, Jung YJ, Lee AS, Kang KP, Lee TH, et al. Mast cells decrease renal fibrosis in unilateral ureteral obstruction. Kidney Int (2009) 75(10):1031–8. doi: 10.1038/ki.2009.1
127. Sziksz E, Pap D, Lippai R, Beres NJ, Fekete A, Szabo AJ, et al. Fibrosis related inflammatory mediators: Role of the IL-10 cytokine family. Mediators Inflammation (2015) 2015:764641. doi: 10.1155/2015/764641
128. Frank BT, Rossall JC, Caughey GH, Fang KC. Mast cell tissue inhibitor of metalloproteinase-1 is cleaved and inactivated extracellularly by alpha-chymase. J Immunol (2001) 166(4):2783–92. doi: 10.4049/jimmunol.166.4.2783
129. Kobayashi T, Kim H, Liu X, Sugiura H, Kohyama T, Fang Q, et al. Matrix metalloproteinase-9 activates TGF-beta and stimulates fibroblast contraction of collagen gels. Am J Physiol Lung Cell Mol Physiol (2014) 306(11):L1006–15. doi: 10.1152/ajplung.00015.2014
130. Schnaper HW, Hayashida T, Hubchak SC, Poncelet AC. TGF-beta signal transduction and mesangial cell fibrogenesis. Am J Physiol Renal Physiol (2003) 284(2):F243–52. doi: 10.1152/ajprenal.00300.2002
131. Adams DH, Sanchez-Fueyo A, Samuel D. From immunosuppression to tolerance. J Hepatol (2015) 62(1 Suppl):S170–85. doi: 10.1016/j.jhep.2015.02.042
Keywords: mast cell (MC), kidney transplant, rejection, fibrosis, tolerance
Citation: van der Elst G, Varol H, Hermans M, Baan CC, Duong-van Huyen JP, Hesselink DA, Kramann R, Rabant M, Reinders MEJ, von der Thüsen JH, van den Bosch TPP and Clahsen-van Groningen MC (2023) The mast cell: A Janus in kidney transplants. Front. Immunol. 14:1122409. doi: 10.3389/fimmu.2023.1122409
Received: 12 December 2022; Accepted: 30 January 2023;
Published: 20 February 2023.
Edited by:
Christian Morath, Heidelberg University, GermanyReviewed by:
Luc Colas, INSERM U1064 Centre de Recherche en Transplantation et Immunologie, FranceCopyright © 2023 van der Elst, Varol, Hermans, Baan, Duong-van Huyen, Hesselink, Kramann, Rabant, Reinders, von der Thüsen, van den Bosch and Clahsen-van Groningen. This is an open-access article distributed under the terms of the Creative Commons Attribution License (CC BY). The use, distribution or reproduction in other forums is permitted, provided the original author(s) and the copyright owner(s) are credited and that the original publication in this journal is cited, in accordance with accepted academic practice. No use, distribution or reproduction is permitted which does not comply with these terms.
*Correspondence: M. C. Clahsen-van Groningen, bS5jbGFoc2VuLXZhbmdyb25pbmdlbkBlcmFzbXVzbWMubmw=
†These authors have contributed equally to this work and share senior authorship