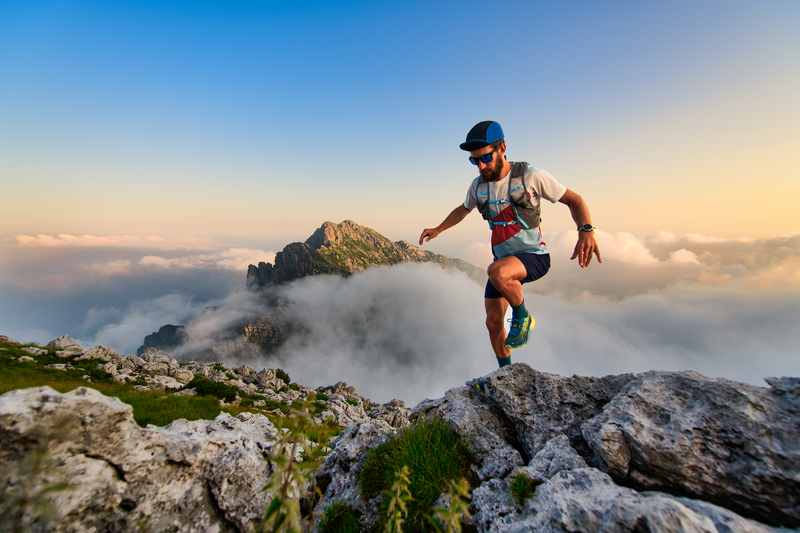
95% of researchers rate our articles as excellent or good
Learn more about the work of our research integrity team to safeguard the quality of each article we publish.
Find out more
REVIEW article
Front. Immunol. , 21 April 2023
Sec. Cancer Immunity and Immunotherapy
Volume 14 - 2023 | https://doi.org/10.3389/fimmu.2023.1121603
Cyclic guanosine monophosphate–adenosine monophosphate (cGAMP) synthase (cGAS) detects infections or tissue damage by binding to microbial or self-DNA in the cytoplasm. Upon binding DNA, cGAS produces cGAMP that binds to and activates the adaptor protein stimulator of interferon genes (STING), which then activates the kinases IKK and TBK1 to induce the secretion of interferons and other cytokines. Recently, a series of studies demonstrated that the cGAS-STING pathway, a vital component of host innate immunity, might play an important role in anticancer immunity, though its mechanism remains to be elucidated. In this review, we highlight the latest understanding of the cGAS-STING pathway in tumor development and the advances in combination therapy of STING agonists and immunotherapy.
Innate immunity, as the first line of defense against pathogenic infections, depends on pattern recognition receptors that identify pathogen-associated molecular patterns (PAMPs) and damage-associated molecular patterns (DAMPs). PAMPs and DAMPs, which include aberrant RNA or DNA, RNA-DNA hybrids, or cyclic dinucleotides from either pathogens or the microbiome, elicit a series of immune responses (1, 2). The immune system serves as an essential defense mechanism against cancer, as it recognizes and eliminates neoplastic cells during immunosurveillance. Therefore, strong innate immunity is critical for adaptive antitumor immunity (3).
Recent evidence suggests that tumor-derived DNA is the primary DAMP driving the host antitumor immune response (4). However, although immune checkpoint inhibitor-based immunotherapy has become the standard therapy for many cancers, its efficacy depends on the antitumor immune effect triggered. The critical cytoplasmic DNA pattern recognition receptor, cyclic guanosine monophosphate–adenosine monophosphate synthase (cGAS), binds to double-stranded DNA (dsDNA) and activates stimulator of interferon (IFN) genes (STING), which in turn induces the release of type I IFNs and other inflammatory cytokines that trigger an adaptive immune response (5–7). Therefore, this pathway has great potential as a target for improving immunotherapeutic efficacy. In this review, we summarize the latest mechanism and roles of the cGAS-STING pathway in tumor development and highlight associated treatments in combination with the latest research.
The catalytic activity of cGAS is activated when it binds to dsDNA, which triggers a conformational change. The C-terminal nucleotidyltransferase of cGAS, which is crucial to its function, consists of a catalytic structural domain, dsDNA recognition domains, and a conserved zinc ion binding site. When the two DNA binding sites are in close proximity, a stable “ladder-like” dimer complex is formed, which activates cGAS (8). The complex then alters the catalytic structural domain to convert GTP and ATP into cyclized 2’3’-cyclic GMP–AMP (cGAMP), which has a higher affinity for STING. While cGAS activation is primarily dependent on dsDNA length, single-stranded DNA, single-stranded RNA, and dsRNA can also bind to cGAS, but they cannot activate the domain (9, 10). The DNA-induced liquid–liquid phase separation mechanism of cGAS is also crucial for its activation. Polyvalent interactions between cGAS and DNA cause the formation of droplets that function as microreactors through which cGAS can be more efficiently activated by DNA. Together with zinc ions, the complex forms liquid droplets, leading to dimerization and activation (11–13). Since liquid–liquid phase separation relies on polyvalent interactions, long DNA activates cGAS more efficiently than short DNA. This also prevents the pseudo-activation of cGAS by limited and short dsDNA, thereby providing an important intrinsic protection mechanism for living cells (14).
In addition, intracellular stresses such as oxidative stress or DNA damage can lead to the release of manganese ions, which are preferred by cGAS for the efficient synthesis of cGAMP (15). Previous studies by Tao Li’s team (16, 17) have shown that the stress granule protein G3BP1 pre-assembles cGAS through liquid–liquid phase separation, enabling a rapid response to DNA stimulation. They also discovered that the G3BP1 inhibitor, epigallocatechin gallate, can prevent cGAS activation. The combination of cGAS and dsDNA triggers the production of cGAMP, a second messenger and agonist of STING, which is considered a critical first step (11).
Cancer cells are typically characterized by an abundance of cytoplasmic dsDNA derived from various sources, such as extracellular DNA, mitochondrial DNA, and genomic DNA, which is not commonly observed in normal cells.
The induction of non-apoptotic tumor cell death by exogenous stimuli can release unprogrammed extracellular DNA, apoptosis-derived DNA, and exosomes (18). This DNA can be degraded by 3-prime repair exonuclease 1 (TREX1), which prevents aberrant nucleic acid recognition. However, tumor-derived DNA that remains undegraded can activate the cGAS-STING pathway, induce type I IFNs, and drive immune cell recruitment (19). Moreover, mitochondrial endonuclease G deficiency, mitochondrial protease YME1L deficiency, or chemotherapy drug induction can cause mitochondrial DNA stress, leading to resistance to TREX1 clearance through the reactive oxygen species pathway and mitochondrial DNA accumulation (20–22). The accumulated DNA can enter the cytoplasmic matrix through macropores in the outer mitochondrial membrane via BAX/BAK and voltage-dependent anion channel proteins VDAC1 and VDAC3, activating the cGAS-STING pathway (18, 23–25). Furthermore, tumor cells with genomic instability are prone to chromosome loss or division during mitosis due to oncogenic mutations, oxidative stress, and hypermetabolism. This can cause DNA leakage in the form of micronuclei, chromatin fragmentation, and free telomeric DNA, which can also be sensed by cGAS and affect antitumor immunity (26, 27).
cGAMP is a second messenger that binds to STING, which is anchored on the endoplasmic reticulum (ER). cGAMP binding induces a conformational change in STING, which subsequently translocates from the ER to the Golgi apparatus. This process is thought to liberate the STING carboxyl terminus to subsequently recruit and activate TANK-binding kinase 1 (TBK1) and IFN regulatory factor 3 (IRF3) via a phosphorylation-dependent mechanism. STING also activates NF-κB, which functions together with IRF3 to activate the transcription of type I IFNs and other cytokines. TBK1, a kinase that binds to the C-terminal tail of STING via a conserved PLPLRT/SD motif, tightly controls the activation of IRF3. Upon binding, TBK1 phosphorylates Ser366 within the C-terminal tail, resulting in the upregulation of IRF3. IRF3 is then phosphorylated and dimerized by TBK1, before entering the nucleus to activate the transcription of type I IFNs and IFN-stimulated genes (ISGs) (28). IFNs have been shown to have multiple effects on different immune cells, and recent evidence suggests that the effectiveness of antitumor immunotherapy largely depends on IFN signaling (29).
The STING pathway also activates NF-κB-dependent transcription. The IκB kinase phosphorylates IκBα, an inhibitor of NF-κB, which leads to its degradation via the ubiquitin–proteasome pathway, thereby freeing NF-κB to enter the nucleus and trigger canonical NF-κB signaling. NF-κB collaborates with IRF3 and other transcription factors to release IFNs (e.g., IFNα and IFNβ) and inflammatory cytokines (e.g., tumor necrosis factor [TNF], interleukin [IL]-1β, and IL-6). Moreover, mitogen-activated protein kinase 14 phosphorylates the NFκB2/p100 subunit that complexes with RelB, a proto-oncogene that is part of the NF-κB subunits (12). Phosphorylated p100 undergoes proteasomal degradation to form p52, which forms a heterodimer with RelB, triggering non-canonical NF-κB signaling (12, 30, 31). Notably, cGAS-STING also promotes non-canonical NF-κB by inducing p52-RelB nuclear translocation. Thus, the cGAS-STING pathway acts as a key negative regulator of the STING effector mechanism by suppressing STING-driven signaling of type I IFNs and canonical NF-κB signaling. As a result, the cGAS-STING signaling pathway may have important implications in limiting cancer immune escape and metastasis (32). Figure 1 illustrates the cGAS-STING signaling cascade.
Figure 1 Schematic representation of the cGAS-STING cascade. DNA derived from various sources including viruses, dying tumor cells or nuclei and mitochondria binds to and activates the cytosolic DNA sensor cGAS. cGAS catalyzes the synthesis of 2′3′-cGAMP in the presence of ATP and GTP, and then 2′3′-cGAMP binds to the ER adaptor STING, which also can be activated by CDNs derived from bacteria. Upon activation, STING translocate from the ER to the Golgi compartments, where it activates TBK1 and IKK, which phosphorylate IRF3 and IκBα respectively.
Research suggests that when STING is activated on the ER–Golgi intermediate compartment, it binds to microtubule-associated protein 1-light chain 3 on the autophagic membrane, inducing lipidation and STING degradation. This ultimately terminates signal transduction and triggers autophagy, which removes DNA and viruses from the cytoplasmic matrix via a mechanism independent of TBK1 or IFN (33). This implies that autophagy induction by STING may be a primordial function of the cGAS pathway. Importantly, autophagy may prevent sustained STING phosphorylation, but not NF-κB activation (34). This process also contributes to the destruction and removal of microbial DNA present in the cytoplasm via enzymatic degradation within lysosomes (35, 36). Additionally, cGAS-STING activation mediated by the cGAS-STING-TBK1-IRF3 assembly complex can occur directly in the autophagosome (34). Clinical trials that have tested autophagy inhibitors in combination with other chemotherapeutic agents (37) indicate that autophagy inhibitors combined with pathway agonists have great potential in antitumor immunotherapy.
The state in which defective cells enter permanent cell cycle arrest, known as cellular senescence, has been historically regarded as a tumor-suppressive mechanism. This process is largely attributed to the accumulation of damaged DNA and activation of the p53 or p16 pathway (38). Senescent cells secrete inflammatory cytokines, growth factors, and proteases; this is known as the senescence-associated secretory phenotype (SASP) and can inhibit tumorigenesis (39).
There is evidence that suggests the cGAS-STING pathway is initiated by self-derived cytoplasmic DNA substrates produced by genotoxic stress-induced cellular senescence, leading to STING-mediated production of SASP in an NF-κB-dependent manner (40, 41). This indicates the potential of the STING pathway in regulating senescence (42). Short-term exposure to SASP factors drives the recruitment of immune cells to clear pre-malignant cells and senescent cells, thereby preventing tumorigenesis (42, 43). Therefore, modulating the activity of the cGAS-STING pathway could benefit cancer immunotherapy and inhibit cancer progression by promoting antitumor immunity and inducing SASP.
The cGAS-STING pathway plays a significant role in regulating tumorigenesis. Its activation in tumor cells increases their immunogenicity by inducing IFNα and IFNβ, which could bind to IFN receptors on immune cells in an autocrine or paracrine manner. IFNα can activate the JAK-STAT pathway, promoting the expression of ISGs such as IFIT1, ISG15, and CXCL10. Among these, CXCL10 is crucial in recruiting CD8+ T cells and enhancing cytotoxicity. On the other hand, IFNβ can stimulate the mobilization of immune cells such as dendritic cells (DCs) and T cells, and induce the presentation of tumor-associated antigens or neoantigens by MHC molecules on the surface of DCs to CD8+ T cells, thus eliciting an antitumor immune response (27, 44).
Pattern recognition receptors are present on immune cells such as DCs, macrophages, T cells, and natural killer (NK) cells in the tumor microenvironment. Damaged nucleic acids released from dying tumor cells, regarded as DAMPs, can activate the cGAS-STING pathway and induce the release of type I IFNs, enhancing antitumor immunity (45, 46). DCs are one of the most important antigen-presenting cells that produce type I IFNs via the activated cGAS-STING pathway in their cytoplasm. These IFNs bind to IFN receptors on the surfaces of their own or neighboring DCs, as well as on other immune cells, in a paracrine or autocrine manner. This induces the expression of MHC-I and co-stimulatory molecules, promotes the maturation of DCs, cross-presentation of tumor antigens, and enhances the response of antitumor cytotoxic T lymphocytes (CTLs). Thus, type I IFNs produced by DCs exhibit a combination of innate and adaptive immunity (47, 48). Additionally, these IFNs promote the production of chemokines such as CXCL9, CXCL10, and CXCL11, which enhance the homing in of antigen-presenting cells, movement, and migration of CD8+ T cells and NK cells (47). The STING-dependent expression of type I IFNs induces an established antitumor immune response, with STING-IRF3 signaling required for the initiation of tumor-specific CD8+ T cell responses. Tumors with reduced DNA repair capacity and high mutational burden often present as immunologically “hot” tumors and exhibit better immunotherapeutic outcomes. Recent studies have shown that STING activation leads to antitumor effects independent of the type of immune cells but dependent on activation of the NF-κB pathway. CD8+ T cell depletion in melanoma B16-bearing mice did not affect intra-tumor injection of cGAMP’s ability to reduce tumor load, suggesting that STING activation promotes antitumor activities independent of CD8+ T cells. STING-dependent type I IFN signaling has also been reported to induce antitumor NK cell activation (45, 49). While normal somatic cells undergo senescence as their telomeres shorten with each cell division, 90% of tumor cells are immortalized due to continuously extending telomeres. 6-thio-dG, a nucleoside analogue, is detected by telomerase and incorporated into nascent telomeres, which become imbalanced and cause massive DNA damage in tumor cells. These DNA fragments are captured by DCs, which activate type I IFN to further promote the proliferation and activation of antigen-specific T cells and enhance antitumor immunity (50). Epigenetic modulation is also vital in tumor immune escape; Wu et al. demonstrated that inhibition of the histone demethylase KDM5B activates type I IFN through the cGAS-STING pathway to suppress the anti-immune response (51). Activation of DNA immune recognition is also accompanied by AKT pathway upregulation. The HER2-AKT oncogenic pathway inhibits cGAS-STING pathway-mediated DNA immune recognition by suppressing STING-TBK1 interaction, as well as TBK1 K63-type ubiquitination, thereby inhibiting type I IFN production, cellular senescence, and apoptosis in tumor cells (52).
The expression of cGAS and STING has been observed to be significantly reduced in CD8+ T cells derived from cancer patients. Furthermore, in mouse models, T cell therapy was less effective when CD8+ T cells lacked cGAS or STING. Endogenous activation of cGAS-STING was found to enhance the antitumor immune response by promoting the differentiation of stem cell-like CD8+ T cells, which offers novel approaches to improving the efficacy of chimeric antigen receptor T-cell therapy (53).
Tumor cells have developed intrinsic inhibitory mechanisms to prevent activation of the cGAS-STING axis, which enables them to evade immune surveillance.
The expression of cGAS and STING is often epigenetically altered in many tumors. For instance, in lung cancer, the expression of cGAS and STING is repressed by the epigenetic regulator DNMT1. Knockdown of the long non-coding RNA NEAT1 reduces the enrichment of DNMT1 at the promoters of cGAS and STING, which in turn promotes the production of IFNβ, CXCL10, and CCL5 (54). Hence, it is essential to unravel epigenetic mechanisms that impede the innate immune response and identify potential targets to enhance the antitumor immune response.
Zhang et al. reported that the deubiquitinase USP35 directly interacts with STING (55). Knockdown of USP35 led to enhanced phosphorylation of STING, TBK1, and IRF3, improved endogenous interactions between STING and TBK1, and promoted the expression of type I IFNs. High levels of USP35 were associated with reduced CD8+ T cell infiltration and poor prognosis in patients with ovarian cancer. In addition, the β-galactoside-binding protein Gal-9 was found to be associated with shorter survival in patients with nasopharyngeal carcinoma. The carbohydrate recognition domain 1 of Gal-9 directly interacts with the STING C-terminus and recruits TRIM29, which mediates the K48 chain of STING ubiquitination, leading to STING degradation (56). Therefore, it is crucial to develop cGAS-STING agonists with individualized dosing regimens to enhance antitumor immunity.
The potential for cGAS-STING to trigger innate immunity within the tumor microenvironment makes it a promising target for tumor therapy (57, 58). Figure 2 illustrates the relationship between cGAS-STING agonists and antitumor immunity. Ongoing preclinical and clinical studies have demonstrated that various therapeutic modalities can synergize with cGAS-STING agonists to remodel the tumor immune microenvironment and enhance antitumor effects (47).
Figure 2 Schematic representation of the agonists of cGAS-STING and antitumor immunity. Treatment such as radiotherapy and chemotherapy can lead to DNA damage, resulting in increased free intracellular DNA and damaged chromatin, and lead to the formation of micronuclei which can activate the cGAS-STING pathway. Released inflammatory mediators act on T cells to promote the proliferation, activation, and stem-like changes of T cells. However, some STING agonists can promote the death of CTLs and the secretion of NK inhibitory factors by B cells, which is not conducive to antitumor immunity. Combining ICIs or targeting adverse effects will improve the efficacy of STING agonists.
Over the past decade, numerous human STING agonists have been developed, some of which, including cyclic dinucleotides (CDNs) and their derivatives, as well as non-CDN-based STING agonists and their analogues, have entered clinical trials (listed in Table 1) (100). Notably, several experiments have demonstrated that intra-tumor administration of cGAMP and other CDN stimuli results in significant antitumor responses in animal models such as 4T1 breast cancer, squamous cell carcinoma, CT26 colon cancer, and B16F10 melanoma. These responses were accompanied by increased secretion of TNFα and various chemokines, providing strong evidence for the involvement of the cGAS-STING pathway in antitumor immunity (101). However, the hydrophilicity of STING agonists may result in reduced antitumor efficacy, making effective drug delivery systems such as nanocarriers, particles, hydrogels, and others essential (12). Recently, Li et al. reported that nanoparticles of the novel polymer agonist PC7A, coupled with cGAMP, specifically target STING within immune cells and show great therapeutic potential in a tumor-bearing mouse model (102).
There is increasing evidence of the involvement of non-CDN drugs in cGAS-STING activation. For instance, DMXAA, a flavonoid STING agonist with anti-angiogenic properties, has been shown to induce the expression of IFNβ and enhance the proliferation and infiltration of CD8+ T cells (58). However, despite its promising preclinical data, DMXAA failed to demonstrate efficacy in a phase III clinical trial for non-small cell lung cancer (103). Subsequently, it was discovered that although DMXAA activates the STING pathway in mice, it does not have the same effect in humans or rats (104).
In response to this phenomenon, a study explained that the protective immune effect provided by S100, a STING agonist, was mainly driven by CD8+ T cells. However, the response to the tumor showed a bell-shaped curve with the increase of S100 concentration, indicating the importance of dose control. High concentrations of S100 led to T cell death, which may be one of the reasons for the lack of clinical therapeutic effect of S100 (105). Similarly, Wu et al. showed that STING agonists could induce T cell death in an IFN-independent way and that inhibitors targeting STING palmitoylation effectively blocked STING-mediated T cell death in vitro. However, these inhibitors also suppressed other IFN-dependent STING pathway activations, such as macrophages, thus resulting in a new requirement for STING inhibitors for precise targeting (106) (Figure 2).
Despite the unsatisfactory clinical results of DMXAA, these valuable works have prompted efforts to design analogues with a higher affinity for hSTING (11). Quan et al. have shown that α-mangostin, a derivative of DMXAA, can activate hSTING more effectively than mSTING (107). These findings suggest that the rational design of DMXAA analogues will stimulate the emergence of novel antitumor therapies.
The aforementioned findings suggest that activation of the STING axis alone may not be sufficient for immunotherapeutic tumor clearance. Several studies have demonstrated that the STING agonist cGAMP induces the expression of IL-35 in B cells through an IFN-independent and IRF3-dependent mechanism. This results in the inhibition of NK cell proliferation and attenuation of their antitumor response. To enhance the efficacy of STING agonists, the combination of these agents with IL-35 antagonists may be a viable approach (106) (Figure 2).
The field of tumor immunotherapy has made significant progress through the introduction of immune checkpoint inhibitors (ICIs). A study by Sivick et al. demonstrated that the combination of PD-1 and CTLA4 antibodies increased the sensitivity of mouse tumors to ICI treatment, leading to significant tumor control (105). However, the lack of T-cell infiltration makes most tumors insensitive to ICI treatment. STING agonists can induce the expression of ISGs (such as CXCL9 and CXCL10) and the infiltration of CTLs, which can convert “cold tumors” into “hot tumors” and overcome resistance to ICIs (46, 108). Additionally, the STING axis can enhance the sensitivity of tumor cells to NK cells and CTLs (109). STING agonists also inhibit the depletion of MHC molecules on tumor cells, which is vital for tumors to evade immune surveillance (47). Therefore, the combination of STING agonists and ICIs has the potential to enhance the sensitivity of tumor cells to the latter (Figure 2). Solid tumors with DNA mismatch repair deletion or microsatellite high instability (dMMR/MSI-H) produce a substantial amount of tumor antigens that enable PD-1 antibodies to enhance the antitumor response (110). It has been found that dMMR/MSI-H tumors can activate the cGAS-STING pathway by regulating the activity of the nucleic acid Exo1, and that immunotherapy is ineffective in similar tumors with abnormal cGAS-STING activity. Thus, the expression levels of cGAS-STING-encoding genes can also be used to predict dMMR tumor response to immunotherapy (111, 112).
STING agonists are important in cancer vaccine adjuvants, as they are used to enhance tumor-specific immunity and overcome tolerance (47, 108). The innate immune response activates antigen-presenting cells, promoting the immunogenicity of tumor-associated antigens (47). STINGVAX, the first STING-based cancer vaccine designed using granulocyte–macrophage colony-stimulating factor (GM-CSF)-secreting cancer cells and CDNs, has been found to induce a superior antitumor response in vivo compared to unformulated GM-CSF-secreting tumor cell vaccines in models of CT26 colon cancer, upper gastrointestinal squamous cell carcinoma (SCCFVII), and pancreatic cancer (PANC02) (113).
Radiotherapy, chemotherapy, and other targeted therapies are frequently utilized in clinical cancer treatments due to their ability to activate the cGAS-STING pathway by inducing DNA damage, ultimately promoting an antitumor immune response.
In addition to directly destroying DNA strands and causing tumor cell death, radiation therapy can activate the cGAS-STING pathway through various mechanisms. These mechanisms include DNA damage-induced micronucleus formation, cytoplasmic chromatin fragmentation induced by aging, and the ZBP1-MLKL pathway-dependent release of mitochondrial DNA (11, 114, 115). It is worth noting that activation of the cGAS-STING pathway through radiation therapy is dependent on the radiation dose. Low doses of radiation can prevent TREX1 activation, enabling cGAS-STING induction. In contrast, high doses of radiation (20-30 Gy) often inhibit pathway activation by increasing TREX1 (116) (see Figure 2 for a summary of the mechanisms of cGAS-STING activation by radiation therapy).
In clinical practice, drugs targeting DNA damage repair are also utilized. The DNA damage response (DDR) kinases, ATM and ATR, work together during DNA replication and repair to initiate DNA damage sensing and activate the DDR pathway. The ATM inhibitor, KU-60019, induces microglial cytosolic DNA accumulation and promotes the secretion of proinflammatory cytokines in a STING-dependent manner (117). Combining ATM inhibitors with PD-L1 antibodies has been shown to increase therapeutic efficacy in mice bearing ARID1A-deficient tumors (118). Poly ADP-ribose polymerase 1 (PARP1), an enzyme that interacts with damaged DNA, is crucial for mediating the DDR pathway, regulating chromatin structure, and maintaining genomic stability. PARP inhibitors are approved for the standard treatment of breast or ovarian cancers with BRCA mutations. Research has demonstrated that PARP inhibitors effectively activate the STING pathway, inducing CD8+ T cell aggregation in patients with BRCA1/2-mutated triple-negative breast cancer (119). Furthermore, in combination with the SHP2 agonist lovastatin and ATR inhibitors, PARP1 can activate the cGAS-STING pathway and play an antitumor role in colon cancer and PBRM1-deficient renal clear cell carcinoma (120, 121).
Checkpoint kinase 1 (CHK1) functions as a DNA damage monitor during replication, detecting any abnormalities and regulating replication-driven activation while also acting on S-phase progression. Prexasertib, a CHK1 inhibitor, has been found to activate the STING pathway in small cell lung cancer cells, including in immunocompetent models. This activation results in increased chemokine levels, promoting the activation of CTLs (122, 123).
Some drugs that target DNA replication damage can activate the cGAS-STING pathway and induce antitumor immunity. Topotecan, Teniposide, and etoposide, which are topoisomerase inhibitors, can cause replication fork collision and abnormal DNA activation, leading to the activation of STING signaling and its downstream NF-κB and type I IFN pathways in a cGAS-dependent or independent manner, thereby promoting the infiltration of DCs and CD8+ T lymphocytes and inhibiting tumor growth (35, 123–125). Antimetabolites such as hydroxyurea upregulate chemokine expression in a cGAS-STING-dependent way by inducing DNA damage, inhibiting ribonucleoside diphosphate reductase, and blocking replication forks (126). In addition, cross-linking agents such as cisplatin can form covalent adducts on cellular DNA, which alter DNA structure and hinder replication forks, inducing DNA damage and activating the cGAS-STING pathway (127).
The microtubule-targeting agent paclitaxel has recently been found to interfere with chromosome segregation and induce type I IFNs and TNFα, promoting the production of pro-apoptotic vesicles via the cGAS-STING pathway in primary human breast tumors (128). This mechanism enhances antitumor immunity.
Immunotherapies for cancer, represented by PD-1/PD-L1 antibodies and CAR-T cell therapy, have been widely used in clinical practice, but many patients still do not benefit from these treatments. PD-1/PD-L1 antibodies and CAR-T cell therapy mainly intervene in the adaptive immune process of cancer patients; however, therapies targeting innate immunity are still lacking. As a vital component of host innate immunity, cGAS-STING and its downstream cytokines, especially type I IFNs, link innate and adaptive immunity, making STING an attractive target for cancer immunotherapy. A growing preclinical study has demonstrated that the cGAS-STING pathway plays pivotal roles in DC-mediated antigen cross-presentation and subsequent priming of tumor-specific CD8+ T cells. Although numerous human STING agonists have been developed and entered clinical trials, the efficacy of STING agonist monotherapy is poor, highlighting the need to conduct more in-depth research on the mechanism of the cGAS-STING pathway in the tumor environment. Recent studies have found that the cGAS-STING pathway and its agonists may suppress the function of antitumor immune cells, including CD8+ T cells and NK cells (105, 106). Therefore, further research is required to explore the role of the cGAS-STING pathway in different tumor-infiltrating immune cells in specific tumors. In terms of drug design, designing STING agonists with higher affinity and tumor-specific carriers can also help improve their clinical effectiveness. With the advancement of basic and clinical research, targeting the cGAS-STING pathway has the potential to provide new strategies and drug combinations for cancer immunotherapy.
RC and MXL wrote the manuscript, QHJ collected and analyzed data, XBM and JMW conceived and designed this review. All authors contributed to the article and approved the submitted version.
This work was supported by Shandong Provincial Natural Science Foundation (ZR2019MH122 and ZR2021LSW007) and the Wu Jieping Medical Foundation (320.6750.2020-01-31).
The authors declare that the research was conducted in the absence of any commercial or financial relationships that could be construed as a potential conflict of interest.
All claims expressed in this article are solely those of the authors and do not necessarily represent those of their affiliated organizations, or those of the publisher, the editors and the reviewers. Any product that may be evaluated in this article, or claim that may be made by its manufacturer, is not guaranteed or endorsed by the publisher.
1. Tan X, Sun L, Chen J, Chen ZJ. Detection of microbial infections through innate immune sensing of nucleic acids. Annu Rev Microbiol (2018) 72:447–78. doi: 10.1146/annurev-micro-102215-095605
2. Mankan AK, Schmidt T, Chauhan D, Goldeck M, Höning K, Gaidt M, et al. Cytosolic RNA:DNA hybrids activate the cGAS-STING axis. EMBO J (2014) 33(24):2937–46. doi: 10.15252/embj.201488726
3. Sayour EJ, Mitchell DA. Manipulation of innate and adaptive immunity through cancer vaccines. J Immunol Res (2017) 2017:3145742. doi: 10.1155/2017/3145742
4. Woo SR, Corrales L, Gajewski TF. Innate immune recognition of cancer. Annu Rev Immunol (2015) 33:445–74. doi: 10.1146/annurev-immunol-032414-112043
5. Khoo LT, Chen LY. Role of the cGAS-STING pathway in cancer development and oncotherapeutic approaches. EMBO Rep (2018) 19(12). doi: 10.15252/embr.201846935
6. Chen Q, Sun L, Chen ZJ. Regulation and function of the cGAS-STING pathway of cytosolic DNA sensing. Nat Immunol (2016) 17(10):1142–9. doi: 10.1038/ni.3558
7. Margolis SR, Wilson SC, Vance RE. Evolutionary origins of cGAS-STING signaling. Trends Immunol (2017) 38(10):733–43. doi: 10.1016/j.it.2017.03.004
8. Boyer JA, Spangler CJ, Strauss JD, Cesmat AP, Liu P, McGinty RK, et al. Structural basis of nucleosome-dependent cGAS inhibition. Science (2020) 370(6515):450–4. doi: 10.1126/science.abd0609
9. Andreeva L, Hiller B, Kostrewa D, Lässig C, de Oliveira Mann CC, Jan Drexler D, et al. cGAS senses long and HMGB/TFAM-bound U-turn DNA by forming protein-DNA ladders. Nature (2017) 549(7672):394–8. doi: 10.1038/nature23890
10. Luecke S, Holleufer A, Christensen MH, Jønsson KL, Boni GA, Sørensen LK, et al. cGAS is activated by DNA in a length-dependent manner. EMBO Rep (2017) 18(10):1707–15. doi: 10.15252/embr.201744017
11. Zheng J, Mo J, Zhu T, Zhuo W, Yi Y, Hu S, et al. Comprehensive elaboration of the cGAS-STING signaling axis in cancer development and immunotherapy. Mol Cancer (2020) 19(1):133. doi: 10.1186/s12943-020-01250-1
12. Wang Y, Luo J, Alu A, Han X, Wei Y, Wei X. cGAS-STING pathway in cancer biotherapy. Mol Cancer (2020) 19(1):136. doi: 10.1186/s12943-020-01247-w
13. Zhang X, Bai XC, Chen ZJ. Structures and mechanisms in the cGAS-STING innate immunity pathway. Immunity (2020) 53(1):43–53. doi: 10.1016/j.immuni.2020.05.013
14. Du M, Chen ZJ. DNA-Induced liquid phase condensation of cGAS activates innate immune signaling. Science (2018) 361(6403):704–9. doi: 10.1126/science.aat1022
15. Zhao Z, Ma Z, Wang B, Guan Y, Su XD, Jiang Z. Mn 2+ directly activates cGAS and structural analysis suggests Mn 2+ induces a noncanonical catalytic synthesis of 2'3'-cGAMP. Cell Rep (2020) 32(7):108053. doi: 10.1016/j.celrep.2020.108053
16. Liu Z, Cai H, Xue W, Wang M, Xia T, Li W, et al. G3BP1 promotes DNA binding and activation of cGAS. Nat Immunol (2019) 20(1):18–28. doi: 10.1038/s41590-018-0262-4
17. Zhao M, Xia T, Xing J, Yin L, Li X, Pan J, et al. The stress granule protein G3BP1 promotes pre-condensation of cGAS to allow rapid responses to DNA. EMBO Rep (2021):e53166. doi: 10.15252/embr.202153166
18. Kwon J, Bakhoum SF. The cytosolic DNA-sensing cGAS-STING pathway in cancer. Cancer Discovery (2020) 10(1):26–39. doi: 10.1158/2159-8290.CD-19-0761
19. Hemphill WO, Simpson SR, Liu M, Salsbury FR Jr., Hollis T, Grayson JM, et al. TREX1 as a novel immunotherapeutic target. Front Immunol (2021) 12:660184. doi: 10.3389/fimmu.2021.660184
20. Oduro PK, Zheng X, Wei J, Yang Y, Wang Y, Zhang H, et al. The cGAS-STING signaling in cardiovascular and metabolic diseases: future novel target option for pharmacotherapy. Acta Pharm Sin B (2022) 12(1):50–75. doi: 10.1016/j.apsb.2021.05.011
21. Wang W, Li J, Tan J, Wang M, Yang J, Zhang ZM, et al. Endonuclease G promotes autophagy by suppressing mTOR signaling and activating the DNA damage response. Nat Commun (2021) 12(1):476. doi: 10.1038/s41467-020-20780-2
22. Sprenger HG, MacVicar T, Bahat A, Fiedler KU, Hermans S, Ehrentraut D, et al. Cellular pyrimidine imbalance triggers mitochondrial DNA-dependent innate immunity. Nat Metab (2021) 3(5):636–50. doi: 10.1038/s42255-021-00385-9
23. Wolf P, Schoeniger A, Edlich F. Pro-apoptotic complexes of BAX and BAK on the outer mitochondrial membrane. Biochim Biophys Acta Mol Cell Res (2022) 1869(10):119317. doi: 10.1016/j.bbamcr.2022.119317
24. Decout A, Katz JD, Venkatraman S, Ablasser A. The cGAS-STING pathway as a therapeutic target in inflammatory diseases. Nat Rev Immunol (2021) 21(9):548–69. doi: 10.1038/s41577-021-00524-z
25. Ablasser A, Hur S. Regulation of cGAS- and RLR-mediated immunity to nucleic acids. Nat Immunol (2020) 21(1):17–29. doi: 10.1038/s41590-019-0556-1
26. Mackenzie KJ, Carroll P, Martin CA, Murina O, Fluteau A, Simpson DJ, et al. cGAS surveillance of micronuclei links genome instability to innate immunity. Nature (2017) 548(7668):461–5. doi: 10.1038/nature23449
27. Hong C, Tijhuis AE, Foijer F. The cGAS paradox: contrasting roles for cGAS-STING pathway in chromosomal instability. Cells (2019) 8(10):1228. doi: 10.3390/cells8101228
28. Tao J, Zhou X, Jiang Z. cGAS-cGAMP-STING: the three musketeers of cytosolic DNA sensing and signaling. IUBMB Life (2016) 68(11):858–70. doi: 10.1002/iub.1566
29. Zhou L, Zhang Y, Wang Y, Zhang M, Sun W, Dai T, et al. A dual role of type I interferons in antitumor immunity. Adv Biosyst (2020) 4(11):e1900237. doi: 10.1002/adbi.201900237
30. Galluzzi L, Vanpouille-Box C, Bakhoum SF, Demaria S. SnapShot: CGAS-STING signaling. Cell (2018) 173(1):276–e1. doi: 10.1016/j.cell.2018.03.015
31. Hou Y, Liang H, Rao E, Zheng W, Huang X, Deng L, et al. Non-canonical NF-κB antagonizes STING sensor-mediated DNA sensing in radiotherapy. Immunity (2018) 49(3):490–503.e4. doi: 10.1016/j.immuni.2018.07.008
32. Bakhoum SF, Ngo B, Laughney AM, Cavallo J-A, Murphy CJ, Ly P, et al. Chromosomal instability drives metastasis through a cytosolic DNA response. Nature (2018) 553(7689):467–72. doi: 10.1038/nature25432
33. Gui X, Yang H, Li T, Tan X, Shi P, Li M, et al. Autophagy induction via STING trafficking is a primordial function of the cGAS pathway. Nature (2019) 567(7747):262–6. doi: 10.1038/s41586-019-1006-9
34. Konno H, Konno K, Barber GN. Cyclic dinucleotides trigger ULK1 (ATG1) phosphorylation of STING to prevent sustained innate immune signaling. Cell (2013) 155(3):688–98. doi: 10.1016/j.cell.2013.09.049
35. Skopelja-Gardner S, An J, Elkon KB. Role of the cGAS-STING pathway in systemic and organ-specific diseases. Nat Rev Nephrol (2022) 18(9):558–72. doi: 10.1038/s41581-022-00589-6
36. Cheng Z, Dai T, He X, Zhang Z, Xie F, Wang S, et al. The interactions between cGAS-STING pathway and pathogens. Signal Transduct Target Ther (2020) 5(1):91. doi: 10.1038/s41392-020-0198-7
37. Li Y, Chen H, Yang Q, Wan L, Zhao J, Wu Y, et al. Increased Drp1 promotes autophagy and ESCC progression by mtDNA stress mediated cGAS-STING pathway. J Exp Clin Cancer Res (2022) 41(1):76. doi: 10.1186/s13046-022-02262-z
38. Milanovic M, Fan DNY, Belenki D, Däbritz JHM, Zhao Z, Yu Y, et al. Senescence-associated reprogramming promotes cancer stemness. Nature (2018) 553(7686):96–100. doi: 10.1038/nature25167
39. Birch J, Gil J. Senescence and the SASP: many therapeutic avenues. Genes Dev (2020) 34(23-24):1565–76. doi: 10.1101/gad.343129.120
40. Glück S, Guey B, Gulen MF, Wolter K, Kang TW, Schmacke NA, et al. Innate immune sensing of cytosolic chromatin fragments through cGAS promotes senescence. Nat Cell Biol (2017) 19(9):1061–70. doi: 10.1038/ncb3586
41. Li T, Chen ZJ. The cGAS-cGAMP-STING pathway connects DNA damage to inflammation, senescence, and cancer. J Exp Med (2018) 215(5):1287–99. doi: 10.1084/jem.20180139
42. Dou Z, Ghosh K, Vizioli MG, Zhu J, Sen P, Wangensteen KJ, et al. Cytoplasmic chromatin triggers inflammation in senescence and cancer. Nature (2017) 550(7676):402–6. doi: 10.1038/nature24050
43. Takahashi A, Loo TM, Okada R, Kamachi F, Watanabe Y, Wakita M, et al. Downregulation of cytoplasmic DNases is implicated in cytoplasmic DNA accumulation and SASP in senescent cells. Nat Commun (2018) 9(1):1249. doi: 10.1038/s41467-018-03555-8
44. Reisländer T, Groelly FJ, Tarsounas M. DNA Damage and cancer immunotherapy: a STING in the tale. Mol Cell (2020) 80(1):21–8. doi: 10.1016/j.molcel.2020.07.026
45. Marcus A, Mao AJ, Lensink-Vasan M, Wang L, Vance RE, Raulet DH. Tumor-derived cGAMP triggers a STING-mediated interferon response in non-tumor cells to activate the NK cell response. Immunity (2018) 49(4):754–63.e4. doi: 10.1016/j.immuni.2018.09.016
46. Schadt L, Sparano C, Schweiger NA, Silina K, Cecconi V, Lucchiari G, et al. Cancer-Cell-Intrinsic cGAS expression mediates tumor immunogenicity. Cell Rep (2019) 29(5):1236–48.e7. doi: 10.1016/j.celrep.2019.09.065
47. Li A, Yi M, Qin S, Song Y, Chu Q, Wu K. Activating cGAS-STING pathway for the optimal effect of cancer immunotherapy. J Hematol Oncol (2019) 12(1):35. doi: 10.1186/s13045-019-0721-x
48. Bose D. cGAS/STING pathway in cancer: Jekyll and Hyde story of cancer immune response. Int J Mol Sci (2017) 18(11):2456. doi: 10.3390/ijms18112456
49. Demaria O, De Gassart A, Coso S, Gestermann N, Di Domizio J, Flatz L, et al. STING activation of tumor endothelial cells initiates spontaneous and therapeutic antitumor immunity. Proc Natl Acad Sci USA (2015) 112(50):15408–13. doi: 10.1073/pnas.1512832112
50. Mender I, Zhang A, Ren Z, Han C, Deng Y, Siteni S, et al. Telomere stress potentiates STING-dependent anti-tumor immunity. Cancer Cell (2020) 38(3):400–11.e6. doi: 10.1016/j.ccell.2020.05.020
51. Wu L, Cao J, Cai WL, Lang SM, Horton JR, Jansen DJ, et al. KDM5 histone demethylases repress immune response via suppression of STING. PloS Biol (2018) 16(8):e2006134. doi: 10.1371/journal.pbio.2006134
52. Wu S, Zhang Q, Zhang F, Meng F, Liu S, Zhou R, et al. HER2 recruits AKT1 to disrupt STING signalling and suppress antiviral defence and antitumour immunity. Nat Cell Biol (2019) 21(8):1027–40. doi: 10.1038/s41556-019-0352-z
53. Li W, Lu L, Lu J, Wang X, Yang C, Jin J, et al. cGAS-STING-mediated DNA sensing maintains CD8+ T cell stemness and promotes antitumor T cell therapy. Sci Transl Med (2020) 12(549):eaay9013. doi: 10.1126/scitranslmed.aay9013
54. Zou SS, Qiao Y, Zhu S, Gao B, Yang N, Liu YJ, et al. Intrinsic strategies for the evasion of cGAS-STING signaling-mediated immune surveillance in human cancer: how therapy can overcome them. Pharmacol Res (2021) 166:105514. doi: 10.1016/j.phrs.2021.105514
55. Zhang J, Chen Y, Chen X, Zhang W, Zhao L, Weng L, et al. Deubiquitinase USP35 restrains STING-mediated interferon signaling in ovarian cancer. Cell Death Differ (2021) 28(1):139–55. doi: 10.1038/s41418-020-0588-y
56. Zhang C-x, Huang D-j, Baloche V, Zhang L, Xu J-x, Li B-w, et al. Galectin-9 promotes a suppressive microenvironment in human cancer by enhancing STING degradation. Oncogenesis (2020) 9(7):65. doi: 10.1038/s41389-020-00248-0
57. Pepin G, Gantier MP. cGAS-STING activation in the tumor microenvironment and its role in cancer immunity. Adv Exp Med Biol (2017) 1024:175–94. doi: 10.1007/978-981-10-5987-2_8
58. Feng X, Liu D, Li Z, Bian J. Bioactive modulators targeting STING adaptor in cGAS-STING pathway. Drug Discovery Today (2020) 25(1):230–7. doi: 10.1016/j.drudis.2019.11.007
59. Marill J, Mohamed Anesary N, Paris S. DNA Damage enhancement by radiotherapy-activated hafnium oxide nanoparticles improves cGAS-STING pathway activation in human colorectal cancer cells. Radiother Oncol (2019) 141:262–6. doi: 10.1016/j.radonc.2019.07.029
60. Benkovics T, Peng F, Phillips EM, An C, Bade RS, Chung CK, et al. Diverse catalytic reactions for the stereoselective synthesis of cyclic dinucleotide MK-1454. J Am Chem Soc (2022) 144(13):5855–63. doi: 10.1021/jacs.1c12106
61. Jang SC, Moniz RJ, Sia CL, Harrison RA, Houde D, Ross N, et al. Abstract 944: exoSTING: an engineered exosome therapeutic that selectively delivers STING agonist to the tumor resident antigen-presenting cells resulting in improved tumor antigen-specific adaptive immune response. Cancer Res (2019) 79(13_Supplement):944. doi: 10.1158/1538-7445.AM2019-944
62. Meric-Bernstam F, Sweis RF, Hodi FS, Messersmith WA, Andtbacka RHI, Ingham M, et al. Phase I dose-escalation trial of MIW815 (ADU-S100), an intratumoral STING agonist, in patients with Advanced/Metastatic solid tumors or lymphomas. Clin Cancer Res (2022) 28(4):677–88. doi: 10.1158/1078-0432.CCR-21-1963
63. Harrington K, Parkes E, Weiss J, Ingham M, Cervantes A, Calvo E, et al. Abstract CT217: phase I, first-in-human trial evaluating BI 1387446 (STING agonist) alone and in combination with ezabenlimab (BI 754091; anti-PD-1) in solid tumors. Cancer Res (2021) 81(13_Supplement):CT217–CT. doi: 10.1158/1538-7445.AM2021-CT217
64. Huang KC, Chanda D, McGrath S, Dixit V, Zhang C, Wu J, et al. Pharmacologic activation of STING in the bladder induces potent antitumor immunity in non-muscle invasive murine bladder cancer. Mol Cancer Ther (2022) 21(6):914–24. doi: 10.1158/1535-7163.MCT-21-0780
65. Leventhal DS, Sokolovska A, Li N, Plescia C, Kolodziej SA, Gallant CW, et al. Immunotherapy with engineered bacteria by targeting the STING pathway for anti-tumor immunity. Nat Commun (2020) 11(1):2739. doi: 10.1038/s41467-020-16602-0
66. Diamond JR, Henry JT, Falchook GS, Olszanski AJ, Singh H, Leonard EJ, et al. Abstract CT249: first-in-human study of TAK-500, a novel STING agonist immune stimulating antibody conjugate (ISAC), alone and in combination with pembrolizumab in patients with select advanced solid tumors. Cancer Res (2022) 82(12_Supplement):CT249–CT. doi: 10.1158/1538-7445.AM2022-CT249
67. Appleman VA, Berger AJ, Roberts ER, Maldonado-Lopez AE, Ganno ML, Christensen CI, et al. Abstract 3448: the IV STING agonist, TAK-676, enhances immune-mediated anti-tumor activity of radiation in syngeneic mouse models. Cancer Res (2022) 82(12_Supplement):3448. doi: 10.1158/1538-7445.AM2022-3448
68. Hatton BA, Grenley M, Ditzler S, Klinghoffer RA, Berger AJ, Malek KS, et al. Abstract 620: intratumoral microdosing via the CIVO® platform reveals anti-tumor immune responses induced by the STING agonist TAK-676 alone and in combination with chemotherapies. Cancer Res (2022) 82(12_Supplement):620. doi: 10.1158/1538-7445.AM2022-620
69. Cooper B, Chmura SJ, Luke JJ, Shiao SL, Basho RK, Iams WT, et al. Abstract CT243: phase 1 study of TAK-676 + pembrolizumab following radiation therapy in patients with advanced non-small-cell lung cancer (NSCLC), triple-negative breast cancer (TNBC), or squamous-cell carcinoma of the head and neck (SCCHN). Cancer Res (2022) 82(12_Supplement):CT243–CT. doi: 10.1158/1538-7445.AM2022-CT243
70. Duvall JR, Bukhalid RA, Cetinbas NM, Catcott KC, Lancaster K, Bentley KW, et al. Abstract 3503: XMT-2056, a HER2-targeted immunosynthen STING-agonist antibody-drug conjugate, binds a novel epitope of HER2 and shows increased anti-tumor activity in combination with trastuzumab and pertuzumab. Cancer Res (2022) 82(12_Supplement):3503. doi: 10.1158/1538-7445.AM2022-3503
71. Duvall JR, Bukhalid RA, Cetinbas NM, Catcott KC, Slocum K, Avocetien K, et al. Abstract 1738: XMT-2056, a well-tolerated, immunosynthen-based STING-agonist antibody-drug conjugate which induces anti-tumor immune activity. Cancer Res (2021) 81(13_Supplement):1738. doi: 10.1158/1538-7445.AM2021-1738
72. Amalfitano A, Alyaqoub FS, Aldhamen YA, Abbas A, Pereira-Hicks C, Godbehere S, et al. Abstract 2994: engineered STING-targeting immunotherapy delays B16 melanoma tumor growth and significantly improves animal survival as compared to an immune-checkpoint inhibitor. Cancer Res (2017) 77(13_Supplement):2994. doi: 10.1158/1538-7445.AM2017-2994
73. Jekle A, Thatikonda S, Stevens S, Williams C, Kinkade A, Ren S, et al. Abstract 4520: preclinical characterization of ALG-031048, a novel STING agonist with potent anti-tumor activity in mice. Cancer Res (2020) 80(16_Supplement):4520. doi: 10.1158/1538-7445.AM2020-4520
74. Cetinbas NM, Catcott KC, Monnell T, Soomer-James J, Bentley K, Clardy S, et al. Abstract 2114: tumor cell-targeted STING-agonist antibody-drug conjugates achieve potent anti-tumor activity by delivering STING agonist specifically to tumor cells andFcγRI-expressing subset of myeloid cells. Cancer Res (2022) 82(12_Supplement):2114. doi: 10.1158/1538-7445.AM2022-2114
75. Miyagi S, Watanabe T, Hara Y, Arata M, Uddin MK, Mantoku K, et al. A STING inhibitor suppresses EBV-induced b cell transformation and lymphomagenesis. Cancer Sci (2021) 112(12):5088–99. doi: 10.1111/cas.15152
76. Binder GA, Gambino CS, Kharitonova A, Metcalf RS, Daniel KG, Guida WC. Abstract 6: computationally assisted target screening of STING agonist for immunologic therapy. Cancer Res (2019) 79(13_Supplement):6. doi: 10.1158/1538-7445.AM2019-6
77. Liu Z, Zhou J, Xu W, Deng W, Wang Y, Wang M, et al. A novel STING agonist-adjuvanted pan-sarbecovirus vaccine elicits potent and durable neutralizing antibody and T cell responses in mice, rabbits and NHPs. Cell Res (2022) 32(3):269–87. doi: 10.1038/s41422-022-00612-2
78. Li A, Song Y, Dong C, Chen X, Yang J. Abstract 3317: discovery of novel STING agonists with robust anti-tumor activity. Cancer Res (2020) 80(16_Supplement):3317. doi: 10.1158/1538-7445.AM2020-3317
79. Ramanjulu JM, Pesiridis GS, Yang J, Concha N, Singhaus R, Zhang SY, et al. Design of amidobenzimidazole STING receptor agonists with systemic activity. Nature (2018) 564(7736):439–43. doi: 10.1038/s41586-018-0705-y
80. Yang J, Adam M, Clemens J, Creech K, Schneck J, Pasikanti K, et al. Abstract 5554: preclinical characterization of GSK532, a novel STING agonist with potent anti-tumor activity. Cancer Res (2018) 78(13_Supplement):5554. doi: 10.1158/1538-7445.AM2018-5554
81. Kogan AA, Topper MJ, Stojanovic L, McLaughlin LJ, Kingsbury TJ, Baer MR, et al. Abstract 6301: DNA methyltransferase inhibitors increase ERV reactivation and STING-dependent interferon/inflammasome signaling in TP53 mutant AML. Cancer Res (2022) 82(12_Supplement):6301. doi: 10.1158/1538-7445.AM2022-6301
82. Ager CR, Zhang H, Wei Z, Jones P, Curran MA, Di Francesco ME. Discovery of IACS-8803 and IACS-8779, potent agonists of stimulator of interferon genes (STING) with robust systemic antitumor efficacy. Bioorg Med Chem Lett (2019) 29(20):126640. doi: 10.1016/j.bmcl.2019.126640
83. Boudreau CE, Najem H, Ott M, Horbinski C, Fang D, DeRay CM, et al. Intratumoral delivery of STING agonist results in clinical responses in canine glioblastoma. Clin Cancer Res (2021) 27(20):5528–35. doi: 10.1158/1078-0432.CCR-21-1914
84. Jung HR, Jo S, Jeon MJ, Lee H, Chu Y, Lee J, et al. Development of small-molecule STING activators for cancer immunotherapy. Biomedicines (2022) 10(1):33. doi: 10.3390/biomedicines10010033
85. Saulters E, Woolley JF, Varadarajan S, Jones TM, Dahal LN. STINGing viral tumors: what we know from head and neck cancers. Cancer Res (2021) 81(15):3945–52. doi: 10.1158/0008-5472.CAN-21-0785
86. Miller J, Luo M, Wang H, Wang Z, Ding X, Campbell A, et al. Abstract 4577: ONM-500: a STING-activating therapeutic nanovaccine platform for cancer immunotherapy. Cancer Res (2020) 80(16_Supplement):4577. doi: 10.1158/1538-7445.AM2020-4577
87. Li S, Wang J, Wilhelm J, Su Q, Bharadwaj G, Miller J, et al. Abstract 4234: ONM-501: a polyvalent STING agonist for oncology immunotherapy. Cancer Res (2022) 82(12_Supplement):4234. doi: 10.1158/1538-7445.AM2022-4234
88. Yu Y, Noman MZ, Parpal S, Kleinendorst SC, Saether KB, Alexeyenko A, et al. Abstract 2116: VPS34 inhibitor SB02024 activates cGAS-STING signaling and sensitizes tumors to STING agonist. Cancer Res (2022) 82(12_Supplement):2116. doi: 10.1158/1538-7445.AM2022-2116
89. Song C, Liu D, Liu S, Li D, Horecny I, Zhang X, et al. SHR1032, a novel STING agonist, stimulates anti-tumor immunity and directly induces AML apoptosis. Sci Rep (2022) 12(1):8579. doi: 10.1038/s41598-022-12449-1
90. Hong Z, Mei J, Li C, Bai G, Maimaiti M, Hu H, et al. STING inhibitors target the cyclic dinucleotide binding pocket. Proc Natl Acad Sci USA (2021) 118(24):e2105465118. doi: 10.1073/pnas.2105465118
91. Lemos H, Mohamed E, Ou R, McCardle C, Zheng X, McGuire K, et al. Co-Treatments to boost IDO activity and inhibit production of downstream catabolites induce durable suppression of experimental autoimmune encephalomyelitis. Front Immunol (2020) 11:1256. doi: 10.3389/fimmu.2020.01256
92. Stazzoni S, Böhmer DFR, Hernichel F, Özdemir D, Pappa A, Drexler D, et al. Novel poxin stable cGAMP-derivatives are remarkable STING agonists. Angew Chem Int Ed Engl (2022) 61(40):e202207175. doi: 10.1002/anie.202207175
93. Liu J, Yuan L, Ruan Y, Deng B, Yang Z, Ren Y, et al. Novel CRBN-recruiting proteolysis-targeting chimeras as degraders of stimulator of interferon genes with In Vivo anti-inflammatory efficacy. J Med Chem (2022) 65(9):6593–611. doi: 10.1021/acs.jmedchem.1c01948
94. Huang R, Shi Q, Zhang S, Lin H, Han C, Qian X, et al. Inhibition of the cGAS-STING pathway attenuates lung Ischemia/Reperfusion injury via regulating endoplasmic reticulum stress in alveolar epithelial type II cells of rats. J Inflammation Res (2022) 15:5103–19. doi: 10.2147/JIR.S365970
95. Weston A, Thode T, Munoz R, Daniel S, Soldi R, Kaadige M, et al. Abstract 3077: preclinical studies of SR-8314, a highly selective ENPP1 inhibitor and an activator of STING pathway. Cancer Res (2019) 79(13_Supplement):3077. doi: 10.1158/1538-7445.AM2019-3077
96. Weston AS, Thode TG, Rodriguez del Villar R, Dana S, Kasibhatla S, Kaadige MR, et al. Abstract LB-118: SR8541A is a potent inhibitor of ENPP1 and exhibits dendritic cell mediated antitumor activity. Cancer Res (2020) 80(16_Supplement):LB–118-LB. doi: 10.1158/1538-7445.AM2020-LB-118
97. Bukhalid RA, Duvall JR, Cetinbas NM, Catcott KC, Avocetien K, Bentley KW, et al. Abstract 6706: systemic administration of STING agonist antibody-drug conjugates elicit potent anti-tumor immune responses with minimal induction of circulating cytokines. Cancer Res (2020) 80(16_Supplement):6706. doi: 10.1158/1538-7445.AM2020-6706
98. Song Y, Li A, Li H, Li X, Huang Z, Yang J, et al. Abstract 2094: conjugates of TLR9 and STING agonists achieved profound synergistic effects in vitro and in vivo. Cancer Res (2022) 82(12_Supplement):2094. doi: 10.1158/1538-7445.AM2022-2094
99. Ager CR, Boda A, Rajapakshe K, Lea ST, Di Francesco ME, Jayaprakash P, et al. High potency STING agonists engage unique myeloid pathways to reverse pancreatic cancer immune privilege. J Immunother Cancer (2021) 9(8):e003246. doi: 10.1136/jitc-2021-003246
100. Ablasser A, Chen ZJ. cGAS in action: expanding roles in immunity and inflammation. Science (2019) 363(6431).
101. Ohkuri T, Kosaka A, Ishibashi K, Kumai T, Hirata Y, Ohara K, et al. Intratumoral administration of cGAMP transiently accumulates potent macrophages for anti-tumor immunity at a mouse tumor site. Cancer Immunol Immunother (2017) 66(6):705–16. doi: 10.1007/s00262-017-1975-1
102. Li S, Luo M, Wang Z, Feng Q, Wilhelm J, Wang X, et al. Prolonged activation of innate immune pathways by a polyvalent STING agonist. Nat BioMed Eng (2021) 5(5):455–66. doi: 10.1007/s00262-017-1975-1
103. Lara PN Jr, Douillard JY, Nakagawa K, von Pawel J, McKeage MJ, Albert I, et al. Randomized phase III placebo-controlled trial of carboplatin and paclitaxel with or without the vascular disrupting agent vadimezan (ASA404) in advanced non-small-cell lung cancer. J Clin Oncol (2011) 29(22):2965–71. doi: 10.1200/JCO.2011.35.0660
104. Conlon J, Burdette DL, Sharma S, Bhat N, Thompson M, Jiang Z, et al. Mouse, but not human STING, binds and signals in response to the vascular disrupting agent 5,6-dimethylxanthenone-4-acetic acid. J Immunol (2013) 190(10):5216–25.
105. Sivick KE, Desbien AL, Glickman LH, Reiner GL, Corrales L, Surh NH, et al. Magnitude of therapeutic STING activation determines CD8+ T cell-mediated anti-tumor immunity. Cell Rep (2018) 25(11):3074–85.e5. doi: 10.1016/j.celrep.2018.11.047
106. Li S, Mirlekar B, Johnson BM, Brickey WJ, Wrobel JA, Yang N, et al. STING-induced regulatory b cells compromise NK function in cancer immunity. Nature (2022) 610(7931):373–80. doi: 10.1038/s41586-022-05254-3
107. Vanpouille-Box C, Alard A, Aryankalayil MJ, Sarfraz Y, Diamond JM, Schneider RJ, et al. DNA Exonuclease Trex1 regulates radiotherapy-induced tumour immunogenicity. Nat Commun (2017) 8:15618. doi: 10.1038/ncomms15618
108. Jiang M, Chen P, Wang L, Li W, Chen B, Liu Y, et al. cGAS-STING, an important pathway in cancer immunotherapy. J Hematol Oncol (2020) 13(1):81. doi: 10.1186/s13045-020-00916-z
109. Tan YS, Sansanaphongpricha K, Xie Y, Donnelly CR, Luo X, Heath BR, et al. Mitigating SOX2-potentiated immune escape of head and neck squamous cell carcinoma with a STING-inducing nanosatellite vaccine. Clin Cancer Res (2018) 24(17):4242–55. doi: 10.1158/1078-0432.CCR-17-2807
110. Germano G, Lamba S, Rospo G, Barault L, Magri A, Maione F, et al. Inactivation of DNA repair triggers neoantigen generation and impairs tumour growth. Nature (2017) 552(7683):116–20. doi: 10.1038/nature24673
111. Lu C, Guan J, Lu S, Jin Q, Rousseau B, Lu T, et al. DNA Sensing in mismatch repair-deficient tumor cells is essential for anti-tumor immunity. Cancer Cell (2021) 39(1):96–108.e6. doi: 10.1016/j.ccell.2020.11.006
112. Guan J, Lu C, Jin Q, Lu H, Chen X, Tian L, et al. MLH1 deficiency-triggered DNA hyperexcision by exonuclease 1 activates the cGAS-STING pathway. Cancer Cell (2021) 39(1):109–21.e5. doi: 10.1016/j.ccell.2020.11.004
113. Fu J, Kanne DB, Leong M, Glickman LH, McWhirter SM, Lemmens E, et al. STING agonist formulated cancer vaccines can cure established tumors resistant to PD-1 blockade. Sci Transl Med (2015) 7(283):283ra52. doi: 10.1126/scitranslmed.aaa4306
114. Storozynsky Q, Hitt MM. The impact of radiation-induced DNA damage on cGAS-STING-Mediated immune responses to cancer. Int J Mol Sci (2020) 21(22):8877. doi: 10.3390/ijms21228877
115. Ng KW, Marshall EA, Bell JC, Lam WL. cGAS-STING and cancer: dichotomous roles in tumor immunity and development. Trends Immunol (2018) 39(1):44–54.
116. Yang Y, Wu M, Cao D, Yang C, Jin J, Wu L, et al. ZBP1-MLKL necroptotic signaling potentiates radiation-induced antitumor immunity via intratumoral STING pathway activation. Sci Adv (2021) 7(41):eabf6290. doi: 10.1126/sciadv.abf6290
117. Helt CE, Cliby WA, Keng PC, Bambara RA, O'Reilly MA. Ataxia telangiectasia mutated (ATM) and ATM and Rad3-related protein exhibit selective target specificities in response to different forms of DNA damage. J Biol Chem (2005) 280(2):1186–92. doi: 10.1074/jbc.M410873200
118. Song X, Ma F, Herrup K. Accumulation of cytoplasmic DNA due to ATM deficiency activates the microglial viral response system with neurotoxic consequences. J Neurosci (2019) 39(32):6378–94. doi: 10.1523/JNEUROSCI.0774-19.2019
119. Pantelidou C, Sonzogni O, De Oliveria Taveira M, Mehta AK, Kothari A, Wang D, et al. PARP inhibitor efficacy depends on CD8(+) T-cell recruitment via intratumoral STING pathway activation in BRCA-deficient models of triple-negative breast cancer. Cancer Discovery (2019) 9(6):722–37. doi: 10.1158/2159-8290.CD-18-1218
120. Wei B, Xu L, Guo W, Wang Y, Wu J, Li X, et al. SHP2-mediated inhibition of DNA repair contributes to cGAS-STING activation and chemotherapeutic sensitivity in colon cancer. Cancer Res (2021) 81(12):3215–28. doi: 10.1158/0008-5472.CAN-20-3738
121. Chabanon RM, Morel D, Eychenne T, Colmet-Daage L, Bajrami I, Dorvault N, et al. PBRM1 deficiency confers synthetic lethality to DNA repair inhibitors in cancer. Cancer Res (2021) 81(11):2888–902. doi: 10.1158/0008-5472.CAN-21-0628
122. Maya-Mendoza A, Petermann E, Gillespie DAF, Caldecott KW, Jackson DA. Chk1 regulates the density of active replication origins during the vertebrate s phase. EMBO J (2007) 26(11):2719–31. doi: 10.1038/sj.emboj.7601714
123. Kitai Y, Kawasaki T, Sueyoshi T, Kobiyama K, Ishii KJ, Zou J, et al. DNA-Containing exosomes derived from cancer cells treated with topotecan activate a STING-dependent pathway and reinforce antitumor immunity. J Immunol (2017) 198(4):1649–59. doi: 10.4049/jimmunol.1601694
124. You F, Gao C. Topoisomerase inhibitors and targeted delivery in cancer therapy. Curr Top Med Chem (2019) 19(9):713–29. doi: 10.2174/1568026619666190401112948
125. Wang Z, Chen J, Hu J, Zhang H, Xu F, He W, et al. cGAS/STING axis mediates a topoisomerase II inhibitor-induced tumor immunogenicity. J Clin Invest (2019) 129(11):4850–62. doi: 10.1172/JCI127471
126. Flanagan JM, Howard TA, Mortier N, Avlasevich SL, Smeltzer MP, Wu S, et al. Assessment of genotoxicity associated with hydroxyurea therapy in children with sickle cell anemia. Mutat Res (2010) 698(1-2):38–42. doi: 10.1016/j.mrgentox.2010.03.001
127. Lewis CW, Golsteyn RM. Cancer cells that survive checkpoint adaptation contain micronuclei that harbor damaged DNA. Cell Cycle (2016) 15(22):3131–45. doi: 10.1080/15384101.2016.1231287
Keywords: cGAS-STING, tumor, immune regulation, immunotherapy, clinical application
Citation: Chen R, Liu M, Jiang Q, Meng X and Wei J (2023) The cyclic guanosine monophosphate synthase-stimulator of interferon genes pathway as a potential target for tumor immunotherapy. Front. Immunol. 14:1121603. doi: 10.3389/fimmu.2023.1121603
Received: 12 December 2022; Accepted: 07 April 2023;
Published: 21 April 2023.
Edited by:
Yan Gong, Wuhan University, ChinaReviewed by:
Xueping Jiang, Zhongnan Hospital of Wuhan University, ChinaCopyright © 2023 Chen, Liu, Jiang, Meng and Wei. This is an open-access article distributed under the terms of the Creative Commons Attribution License (CC BY). The use, distribution or reproduction in other forums is permitted, provided the original author(s) and the copyright owner(s) are credited and that the original publication in this journal is cited, in accordance with accepted academic practice. No use, distribution or reproduction is permitted which does not comply with these terms.
*Correspondence: Junmin Wei, d2VpanVubWluQHNkdS5lZHUuY24=; Xiangbo Meng, eGJtZW5nQHNkdS5lZHUuY24=
†These authors have contributed equally to this work
Disclaimer: All claims expressed in this article are solely those of the authors and do not necessarily represent those of their affiliated organizations, or those of the publisher, the editors and the reviewers. Any product that may be evaluated in this article or claim that may be made by its manufacturer is not guaranteed or endorsed by the publisher.
Research integrity at Frontiers
Learn more about the work of our research integrity team to safeguard the quality of each article we publish.