- 1Department of Paediatrics and Adolescent Medicine, The University of Hong Kong, Hong Kong, Hong Kong SAR, China
- 2School of Public Health, The University of Hong Kong, Hong Kong, Hong Kong SAR, China
- 3HKU-Pasteur Research Pole, School of Public Health, The University of Hong Kong, Hong Kong, Hong Kong SAR, China
- 4Research Institute for Bioresources and Biotechnology, Ishikawa Prefectural University, Nonoichi, Japan
- 5Department of Microbiology and Immunology, Peter Doherty Institute for Infection and Immunity, University of Melbourne, Melbourne, VIC, Australia
- 6Centre for Immunology & Infection C2i, Hong Kong, Hong Kong SAR, China
Introduction: Two doses of inactivated SARS-CoV-2 vaccine CoronaVac cannot elicit high efficacy against symptomatic COVID-19, especially against the Omicron variant, but that can be improved by a third dose in adults. The use of a third dose of CoronaVac in adolescents may be supported by immunobridging studies in the absence of efficacy data.
Methods: With an immunobridging design, our study (NCT04800133) tested the non-inferiority of the binding and neutralizing antibodies and T cell responses induced by a third dose of CoronaVac in healthy adolescents (N=94, median age 14.2 years, 56% male) compared to adults (N=153, median age 48.1 years, 44% male). Responses against wild-type (WT) and BA.1 SARS-CoV-2 were compared in adolescents. Safety and reactogenicity were also monitored.
Results: A homologous third dose of CoronaVac further enhanced antibody response in adolescents compared to just 2 doses. Adolescents mounted non-inferior antibody and T cell responses compared to adults. Although S IgG and neutralizing antibody responses to BA.1 were lower than to WT, they remained detectable in 96% and 86% of adolescents. T cell responses to peptide pools spanning only the mutations of BA.1 S, N and M in adolescents were preserved, increased, and halved compared to WT respectively. No safety concerns were identified.
Discussion: The primary vaccination series of inactivated SARS-CoV-2 vaccines for adolescents should include 3 doses for improved humoral immunogenicity.
Highlights
1. A third dose of CoronaVac is needed for improved immunogenicity in healthy adolescents
2. Non-inferiority of antibody and T cell responses in adolescents versus adults
3. BA.1 S IgG and neutralizing antibodies were detectable in 96% and 86% adolescents after dose 3
4. T cell responses against BA.1 mutations in S, N and M were preserved, increased, and halved, respectively
Introduction
Inactivated vaccines against COVID-19 such as CoronaVac are widely used with more than 4 billion doses distributed worldwide because of simpler manufacturing requirements and greater vaccine stability during transport (1). Real-world vaccine effectiveness studies have also shown that 2 doses of inactivated vaccines could strongly protect against severe COVID-19 but less so against mild disease (2, 3). In comparison to the mRNA COVID-19 vaccines widely in use, 2 doses of inactivated COVID-19 vaccines elicit weaker neutralization responses yet higher T cell responses in adults (4), as well as in adolescents as we have shown (5). There is a growing consensus that the primary series of inactivated COVID-19 vaccines should include 3 doses, similar to other routinely used inactivated vaccines such as the inactivated polio vaccine. Homologous third dose of CoronaVac has been shown to improve vaccine effectiveness against mild and severe COVID-19 in adults (3, 6). However, as of September 2022, there is currently no published data on the paediatric use of 3 doses of CoronaVac.
Vaccine effectiveness, especially against mild disease, is susceptible to waning over time as well as to antigenically divergent variants of concern (3, 7). Neutralizing antibody escape by the newly emergent Omicron variant may account for high transmission in populations with high vaccine coverage (8, 9). On the other hand, T cell responses in adults are mostly (~80%) preserved against the Omicron variant as most immunodominant T cell epitopes are unaffected (10–12), which may explain the preservation of vaccine effectiveness against severe outcomes with Omicron variant (3). Data from the United Kingdom showed that in contrast to adults, adolescents are not at significantly lower risk of hospitalization due to Omicron relative to Delta (13), and paediatric COVID-19-associated hospitalizations increased rapidly during the Omicron outbreak in South Africa (14). In adults, a third dose of COVID-19 vaccine boosted neutralizing antibody and T cell response against the Omicron variant (11, 15), yet this remains unknown in adolescents.
To inform the paediatric use of CoronaVac, an inactivated COVID-19 vaccine, amid the spread of Omicron, we sought to determine the safety and immunogenicity of a third dose of CoronaVac in healthy adolescents. We adopted an immunobridging design, where adolescents were tested for whether various immunogenicity outcomes, including antibody binding and avidity, neutralizing and non-neutralizing antibody functions, and T cell responses against the wild-type (WT) virus (5), were non-inferior to those in adults. The goal is to support the extension of age group indication for the third dose of CoronaVac in the absence of efficacy data in adolescents, based on the established effectiveness of a homologous third dose of CoronaVac in adults (3, 6). In addition, immunogenicity against Omicron BA.1 was also assessed.
Methods
Study design
COVID-19 Vaccination in Adolescents and Children (COVAC; NCT04800133) is a non-randomized immunobridging study of BNT162b2 and CoronaVac in adolescents and children, as previously described (5, 16). The University of Hong Kong (HKU)/Hong Kong West Cluster Hospital Authority Institutional Review Board (UW21-157) authorized this study. ClinicalTrials.gov
Participants
The current analysis included adolescents aged 11-17 years and adults ≥18 years at the time of dose 1 who received 3 intramuscular doses of CoronaVac. The exclusion were history of COVID-19, severe allergy, major neuropsychiatric issues, immune compromise conditions, blood transfusion within 60 days, significant bleeding tendency, and pregnancy or breastfeeding.
Procedures
Participants were recruited in Hong Kong from schools, media, or referral. Written informed consent was obtained from participants aged ≥18 years or above. Informed assent was obtained from underage participants and written consent was obtained from their parents or legally acceptable representatives. Vaccination consisted of three doses of 0.5 mL inactivated virus vaccine that contains 600SU of SARS-CoV-2 CZ02 strain whole virus antigen. Doses 1 and 2 were administered 28-35 days apart, while dose 3 was given ≥84 days after dose 1. The vaccination interval was chosen after the finding of limited durability of the 2-dose antibody response during an evolving pandemic and likely benefits of more persistent prime-boost interval (17). Blood was sampled on the day of dose 3 and 13-42 days following dose 3 to detect the expected peak antibody response and short-term cellular response after dose 3 (18).
Safety data collection
Participants were observed for 15 minutes after each vaccine injection. Prespecified adverse reactions (ARs) were recorded in an online or paper-based diary for 7 days after vaccine administration. Unsolicited adverse events were captured for 28 days after each vaccine dose. There will be ongoing surveillance for severe adverse events include hospitalizations, life-threatening complications, disabilities, deaths, birth defects in offspring, and breakthrough COVID-19 for 3 years. The study investigators determined whether there was causal relationship of the study vaccine with the reported adverse effects.
S-RBD IgG, N IgG and N-CTD IgG, surrogate virus neutralization test (sVNT) and plaque reduction neutralization test (PRNT)
Clotted blood and the serum from the participants was maintained at -80° C. Sera were inactivated at 56° C for 30 minutes before performance of the SARS-CoV-2 S receptor-binding domain (S-RBD) IgG, N and N-CTD IgG enzyme-linked immunosorbent assay (ELISA), sVNT (GenScript Inc, Piscataway, USA) and PRNT as previously described and validated according to the manufacturer’s instructions (19–21). The cut-offs for ELISA-based tests were derived from mean of OD + 3SD of pre-pandemic samples. For sVNT, the cut-off was provided by the manufacturer. The cut-off for the PRNT was set at 1:10, which was the lowest dilution demonstrating inhibition to the virus.
In summary, S-RBD IgG ELISA plates were coated with 100 ng/well of purified recombinant S-RBD in PBS buffer overnight and 100 μL Chonblock Blocking/Sample Dilution (CBSD) ELISA buffer (Chondrex Inc, Redmond, USA) was added. This mixture remained at room temperature (RT) for 2 hours. Sera at 1:100 dilution in CBSD ELISA buffer were added to the wells at 37 for 2 hours. The wells were washed with PBS containing 0.1% Tween 20, followed by the addition of horseradish peroxidase (HRP)-conjugated goat anti-human IgG (1:5,000) (GE Healthcare, Chicago, USA) for 1 hour at 37°C. These were washed with PBS containing 0.1% Tween 20 for five times, and then 100 μL HRP substrate (Ncm TMB One, New Cell & Molecular Biotech Co. Ltd, China) was added and kept for 15 minutes. This reaction was ceased with 50 μL 2 M H2SO4. The OD of the mixture was analyzed in a Sunrise absorbance microplate reader (Tecan, Männedorf, Switzerland) at 450 nm wavelength. The background OD in the PBS-coated control wells with the sera was subtracted from each final OD reading. OD450 values below the cut-off of 0.5 were imputed as 0.25.
For N IgG and N-CTD IgG, the 96-well ELISA plates (Nunc MaxiSorp, Thermo Fisher Scientific) were coated with 125 ng (N) or 40.3 ng (N-CTD) purified recombinant protein in PBS buffer overnight. 100 μL Chonblock blocking/sample dilution ELISA buffer (Chondrex Inc, Redmon, US) was added to the plates, which were incubated for 1 hour at room temperature. Afterwards, the sera were diluted to 1:100 in Chonblock blocking/sample dilution ELISA buffer. Sera were added to the ELISA plates, which were incubated at 37 for 2 hours. Each well was washed with PBS containing 0.1% Tween 20 and incubated at 37 for 1 hour with anti-human IgG secondary antibody (1:2500, Thermo Fisher Scientific). The plates were washed five times with PBS containing 0.1% Tween 20, and 100 μL of HRP substrate (Ncm TMB One; New Cell and Molecular Biotech Co. Ltd, Suzhou, China) was added into each well. After a 15-minute incubation period, the reaction was stopped with 50 μL of 2M H2SO4 solution. OD450 was analyzed using an absorbance microplate reader.
10 μL of each sera was used for sVNT, with positive and negative controls prepared by dilution of 1:10 mixed with same volume of HRP-conjugated WT SARS-CoV-2 S-RBD (6 ng). The mixtures were incubated at 37 for 30 minutes, followed by the addition of 100 μL of sample to the microtitre plate wells coated with the recombinant angiotensin-converting enzyme-2 (ACE-2) receptor. The plates were sealed for 15 minutes at 37 and then washed with wash-solution and tapped dry. 100 μL of 3,3’,5,5’-tetramethylbenzidine (TMB) was then added, followed by incubation for 15 minutes at RT in the dark. 50 μL of Stop Solution was added. The absorbance was recorded at 450 nm. The % inhibition was calculated using the formula: (1-sample OD value/negative control OD value) x100%. Inhibition % below 30%, which was the limit of quantification (LOQ), was imputed as 15%.
PRNT duplicates were performed in a biosafety level 3 facility. Serial serum dilutions at 1:10 to 1:320 were incubated with ~30 plaque-forming units of SARS-CoV-2 BetaCoV/Hong Kong/VM20001061/2020 virus (WT) or hCoV-19/Hong Kong/VM21044713_WHP5047-S5/2021 (Omicron BA.1) for 1 hour at 37 in culture plates (Techno Plastic Products AG, Trasadingen, Switzerland) (8). We added the virus-sera mixtures onto Vero-E6 TMPRSS2 cell monolayers, which were then placed in a 5% CO2 incubator for 1 hour at 37°C. After overlaying with 1% agarose in cell culture medium, these plates were incubated for 3 days while fixed and stained. The antibody titres were defined as the reciprocal of the highest dilution of serum resulting in a >=90% (PRNT90) or >50% (PRNT50) reduction in the plaque numbers. Values above 1:320 were imputed as 1:640 and those below 10 were imputed as 5.
S IgG, avidity and FcγRIIIa-binding
We diluted the antigens for antibody detection in PBS and coated the plates (Nunc MaxiSorp, Thermofisher Scientific) with 250 ng/mL WT (AcroBiosystems) or Omicron BA.1 (AcroBiosystems) SARS-CoV-2 S protein for IgG and IgG avidity assessment and 500 ng/mL ancestral (Sinobiological) or Omicron BA.1 (AcroBiosystems) S for FcγRIIIa-binding detection. ORF8 protein of 300 ng/mL was coated at 37 for 2 hours. The plates were blocked with 1% FBS in PBS for 1 hour, followed by incubation with 1:100 HI sera diluted in 0.05% Tween-20/0.1% FBS in PBS for 2 hours for IgG detection, and 1:50 for 1 hour at 37 for FcγRIIIa-binding detection, prior to rinsing. For avidity, plates with 8M were washed with urea 3 times. IgG was measured after 2 hours of incubation period with anti-IgG-HRP (1:5000; G18-145, BD), HRP revealed with addition of stabilized hydrogen peroxide and tetramethylbenzidine (R&D systems) for 20 minutes. The reaction was terminated with 2N H2SO4, which was then analyzed at 450 nm wavelength with an absorbance microplate reader (Tecan Life Sciences). Similarly, FcγRIIIa-binding antibodies were assessed after incubation with biotinylated FcγRIIIa-V158 at 100 ng/mL for 1 hour at 37 after streptavidin-HRP (1:10000, Pierce).
T cell responses
Peripheral blood mononuclear cells (PBMCs) were extracted and maintained at -80° C. Thawed PBMCs were placed in 10% human AB serum supplemented RPMI medium for 2 hours. The PBMCs were stimulated with sterile ddH2O or 1 µg/mL overlapping peptide pools representing the WT SARS-CoV-2 S, N and M proteins (Miltenyi Biotec, Bergisch Gladbach, Germany), or BA.1 S mutation pool and WT S reference pool (Miltenyi Biotec, Bergisch Gladbach, Germany), Omicron BA.1 N mutation pool, WT N reference pool, BA.1 M mutation pool and WT M reference pool (peptide sequences in Supplementary Table 6; synthesized by ChinaPeptides Co., Ltd) in 1 µg/mL anti-CD28 and anti-CD49d costimulatory antibodies (clones CD28.2 and 9F10, Biolegend, San Diego, USA) for 16 hours, followed by the addition of 10 µg/mL brefeldin A (Sigma, Kawasaki, Japan) (22). The PBMCs were then washed and stained for CD3 (HIT3a, 1:60), CD4 (OKT4, 1:60), CD8 (HIT8a, 1:60), IFN-γ (B27, 1:15), IL-2 (MQ1-17H12, 1:15) (Biolegend, San Diego, USA) and fixable viability dye (eBioscience, Santa Clara, USA, 1:60). Flow cytometry was performed by the LSR II (BD Biosciences, Franklin Lakes, USA). Flowjo v10 software (BD, Ashland, USA) was used to analyze the data. Calculation of measured IFN-γ+ or IL-2+ T cells were performed by deducting the background (sterile ddH2O) data, which are presented as the percentages of CD4+ or CD8+ T cells (23). T cell responses against the peptide pool was considered positive if the cytokine-expressing cell frequency was ≥0.005% and the stimulation index was >2. Negative values were imputed as 0.0025%. The total T cell responses against S, N and M peptide pools were summed and the cut-off of 0.01% was used.
Outcomes
For the current analysis, the primary immunogenicity outcomes were S-specific antibody markers, which included the S IgG and S-RBD IgG levels, sVNT %inhibition, 90% and 50% PRNT titres, S IgG avidity and FcγRIIIa-binding, and the total and separate S, N and M-specific IFN-γ+ and IL-2+ CD4+ and CD8+ T cell responses measured by flow cytometry 13-42 days after the third dose of CoronaVac. The primary reactogenicity outcomes were ARs and anti-pyretic use within 7 days after vaccine injection.
The secondary immunogenicity outcomes were N and N-CTD IgG levels, and antibody and T cell responses against Omicron BA.1. For safety, the secondary outcomes were AEs within 28 days post-vaccination and SAEs during the study period.
Statistical analyses
Sample size and power estimation
G*Power (Heinrich-Heine-Universität Düsseldorf, Düsseldorf, Germany) and Sampsize (sampsize.sourceforge.net) were used for the power calcuation. For primary immunogenicity objectives, when comparing the peak geometric mean (GM) immunogenicity outcomes between adolescents and adults, 61 participants in each group would allow two-sided tests with α=0.05 and 99% power to detect a difference of 0.51 after natural logarithm transformation and the standard deviation (SD) of 0.65 within group on the natural logarithmic scale, with the Cohen’s d value=0.78. Sample sizes were reduced when the feasibility for assays with higher technical requirements were limited, such as PRNT and assays which required greater volumes of blood, such as Omicron-specific tests, as participants with samples tested in earlier timepoints and earlier collection dates or higher blood volume collected chosen. For the proportion of participants with a positive result in immunogenicity outcomes or ARs, with the assumption of a prevalence of 80%, 62 participants would yield a 95% chance to detect the true value within 10% precision.
Analysis sets
The primary immunogenicity analysis was performed in healthy participants in the evaluable analysis population. This consisted of participants who were uninfected before and during the study period, which was based on clinical history, baseline S-RBD IgG negativity, and ORF8 IgG negativity, generally healthy status with no major protocol deviations, receipt of dose 3 ≥84 days after dose 1, had blood sampled days 13-42 post-dose 3, and had valid results for the relevant test (Protocol in Supplementary Materials). The expanded analysis population were more relaxed, which permitted inclusion of those who received dose 3 ≥56 days after dose 1 and had blood sampled days 6-56 post-dose 3 (Protocol in Supplementary Materials). Geometric mean ratios (GMRs) included two-sided 95% CI, corresponding to a one-sided 97.5% CI, which was used for testing non-inferiority at the 0.60 margin. This threshold promotes rapid delivery of study results that requires a smaller sample size amid the evolving pandemic, a practice deemed allowable by the World Health Organization Expert Committee on Biological Standardization and adopted in another recent landmark COVID-19 vaccine study (24, 25). The inferiority analyses were confirmed in the expanded analysis population. Superiority was reached if the lower bound of the 95% CI for GMR was >1, or inferiority was declared if the upper bound of the 95% CI was <1. When both non-inferiority and inferiority were not met, the results were considered as inconclusive. Geometric mean fold rises (GMFR) were calculated for those who had valid results at both timepoints. When there were negative immunogenicity outcome data, values that were half the cut-off were imputed. Unpaired t test after natural logarithmic transformation was performed for comparisons of immunogenicity outcomes between groups. Proportions of positive or negative results were given in percentages with 95% Clopper-Pearson CI. The Fisher exact test was used for comparisons of proportions between groups.
Reactogenicity and safety outcomes were assessed in healthy, uninfected participants who had reported any safety or ARs post-dose 3 and before the study database was locked for this interim analysis in the adolescent group (the healthy safety population). In this primary reactogenicity analysis, the proportions of participants that had reported each of the ARs according to maximum severity and anti-pyretic use were shown as percentages with the 95% Clopper-Pearson CI. The incidences of AEs by severity and SAEs that were reported by the post-dose 3 study visit (28 days after dose 3) were presented as counts and events-per-participant.
Vaccine efficacy estimation
Vaccine efficacies (VEs) were estimated as a secondary objective by extrapolation according to the neutralizing titres, as previously established (5, 26). The mean neutralizing level (fold of convalescent) was based on the GMTs of PRNT50 for SARS-CoV-2 WT or BA.1 in evaluable adolescents divided by that of 102 convalescent sera from patients aged ≥18 years on days 28-59 after the onset of illness (21, 27). The point estimates of VE were extracted from the best fit of the logistic model using the plot digitizer tool (https://automeris.io/WebPlotDigitizer/, version 4.5).
Results
Enrolment and study completion
Among 327 participants in the COVID-19 Vaccination in Adolescents and Children study (COVAC; NCT04800133) who received 2 doses of CoronaVac, 259 participants received a third dose of CoronaVac by January 31, 2022 (Supplementary Figure 1). Excluding participants who were infected during the study as determined by ORF8 serology assay or contributed no safety data and did not attend follow-up clinic, 94 adolescents aged 11-17 years and 153 adults aged 18 years or above were included in healthy safety analysis, with comparable demographic characteristics (Supplementary Table 1). Doses 1 and 2 were given 28-35 days apart while dose 3 was given at least 84 days after dose 1. Blood sampling was performed on the day of dose 3 and 13-42 days after dose 3. Primary immunogenicity analyses were performed in the evaluable analysis population which included participants with valid and timely immunogenicity results and no protocol deviations (adolescents N=60, adults N=119). Immunogenicity analyses were repeated in the expanded analysis population with relaxed vaccination and blood sampling intervals to further confirm the findings (adolescents N=82, adults N=149; see Methods). Protocol and Statistical Analysis Plan are available in Supplementary Materials.
Immunogenicity outcomes before and after the third dose in adolescents
We first assessed the durability of antibody responses against the WT virus after 2 doses of CoronaVac, including SARS-CoV-2 Spike receptor-binding domain (S-RBD) IgG by enzyme-linked immunosorbent assay (ELISA) and ACE2-blocking antibody by surrogate virus neutralization test (sVNT), as well as interferon-γ (IFN-γ)+ and interleukin-2 (IL-2)+ CD4+ and CD8+ T cells responses specific to WT SARS-CoV-2 S, Nucleocapsid (N), and Membrane (M) peptide pools by flow cytometry (see Methods). In evaluable adolescents with paired sera across all timepoints, S-RBD IgG and ACE2-blocking antibody declined significantly with geometric mean (GM) fold reduction of 1.60 and 2.12 fold respectively from post-dose 2 (mean 28 days after dose 2) to pre-dose 3 (Figure 1A). Total SNM-specific IFN-γ+ and IL-2+ CD4+ and IL-2+ CD8+ T cells showed a reducing trend after 2 doses in evaluable adolescents, yet none of the paired analyses between the post-dose 2 and pre-dose 3 timepoints were significant, suggesting T cell responses were preserved (Figure 1B). Results for T cell responses to separate S, N and M peptide pools were presented in Supplementary Figure 2A-C.
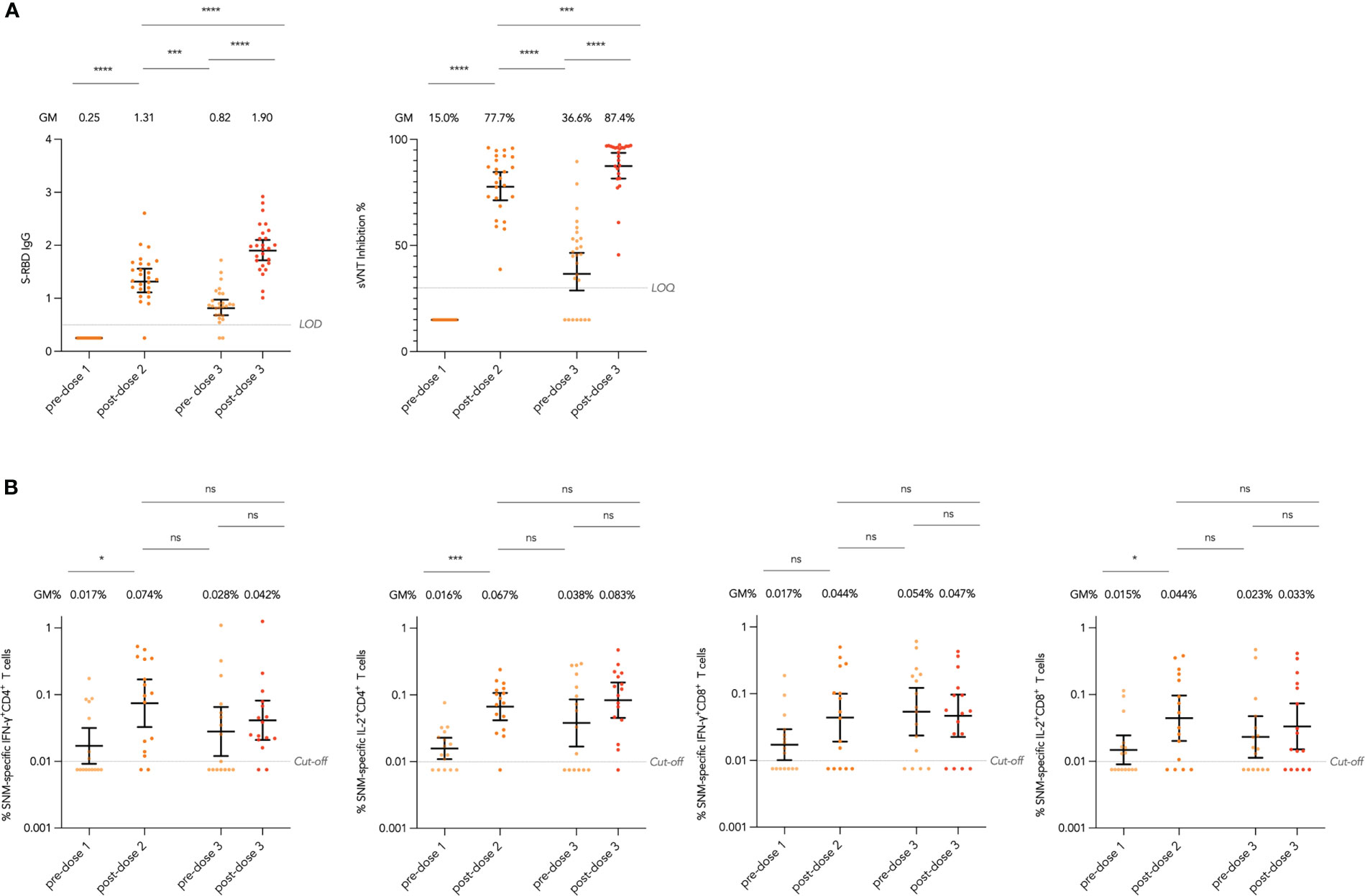
Figure 1 Longitudinal humoral and cellular immunogenicity in healthy evaluable adolescents receiving 3 doses of CoronaVac. (A) Longitudinal analysis of Spike receptor-binding domain (S-RBD) IgG OD450 values and surrogate virus neutralisation test (sVNT) inhibition % in evaluable adolescents. (B) Longitudinal analysis of total Spike (S), Nucleocapsid (N) and Membrane (M) protein-specific interferon-γ (IFN-γ)+ and interleukin-2 (IL-2)+ CD4+ and CD8+ T cells responses in evaluable adolescents. Geometric means (GM) are shown with centre lines and stated above each column, with corresponding 95% confidence intervals shown by error bars. Samples from the same participant were paired across timepoints and compared with paired t test after natural logarithmic transformation with p-values denoted (*, P<0.05; ***, P<0.001; ****, P<0.0001; ns, not significant). Limits of detection and quantification (LOD and LOQ) and cut-offs were drawn as grey lines.
At the post-dose 3 timepoint (mean 19 days after dose 3), evaluable adolescents were assessed for all primary humoral and cellular immunogenicity outcomes against the WT virus (see Methods). All adolescents had positive S-RBD IgG and S-RBD ACE2-blocking antibody post-dose 3 (Table 1). On plaque reduction neutralization test (PRNT), 100% and 78.3% adolescents were positive for 50% and 90% PRNT at a limit of detection of 1 in 10, and with GM 50% and 90% PRNT of 55.3 and 17.8 respectively. As CoronaVac is a whole-virion inactivated vaccine, N IgG and N-C terminal domain (N-CTD) IgG were also assessed with 98.3% seropositivity for both. SARS-CoV-2 S IgG, S IgG avidity and S IgG Fcγ receptor IIIa (FcγRIIIa)-binding testing were available in 56 evaluable adolescents, with S IgG and S IgG FcγRIIIa-binding detected in 98.2% tested evaluable adolescents, and GM S IgG avidity of 38.5%. Results in the expanded analysis population were similar (Supplementary Table 2). When compared to pre-dose 3 timepoint, evaluable adolescents showed significant GM fold rises in S-RBD IgG of 2.32 fold and sVNT inhibition of 2.39 fold (Figure 1A).
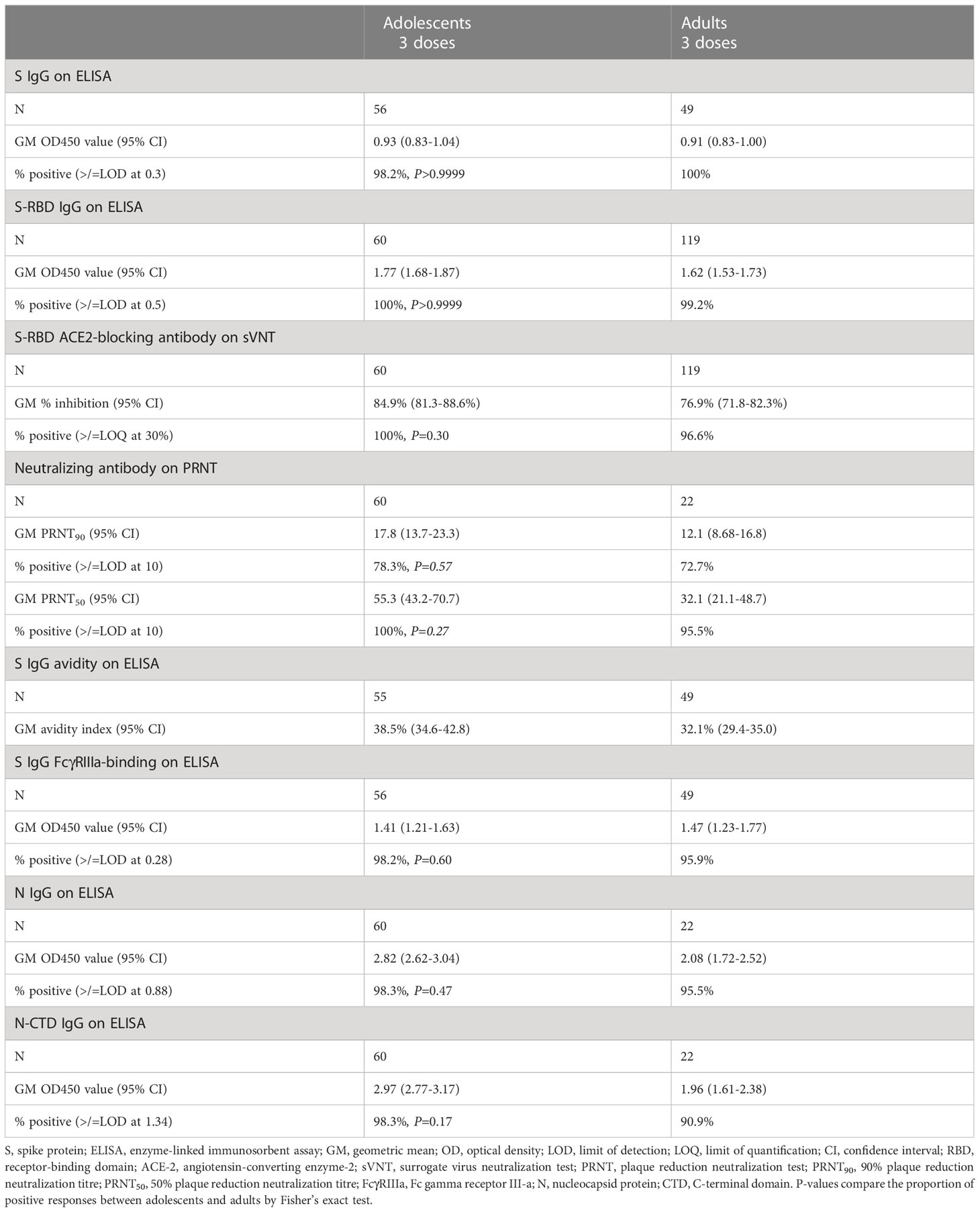
Table 1 Humoral immunogenicity outcomes against wild-type SARS-CoV-2 after the third dose of CoronaVac in evaluable analysis population.
For cellular immunogenicity outcomes, among 58 evaluable adolescents tested, most participants tested positive for total WT SNM-specific IFN-γ+ and IL-2+ CD4+ T cell responses (74.1% and 79.3% respectively) on flow cytometry at a cut-off of 0.01% (Table 2; see Methods). Yet, for IFN-γ+ and IL-2+ CD8+ T cell responses, a lower but still high proportion of participants (62.1% and 65.5%) tested positive. When broken down into T cell responses against separate peptide pools, T cell responses appeared to be lowest for the M peptide pool, which elicited IFN-γ+ and IL-2+ CD4+ T cells in 23.7% and 25.4% tested evaluable adolescents, and IFN-γ+ and IL-2+ CD8+ T cells in 13.6% and 18.6% (Supplementary Table 3). Similar results were yielded in the expanded analysis population (Supplementary Table 4). When compared to pre-dose 3 timepoint, evaluable adolescents showed statistically insignificant increases in total SNM-specific IFN-γ+ and IL-2+ CD4+ and IL-2+ CD8+ T cell responses after dose 3 (Figure 1B).
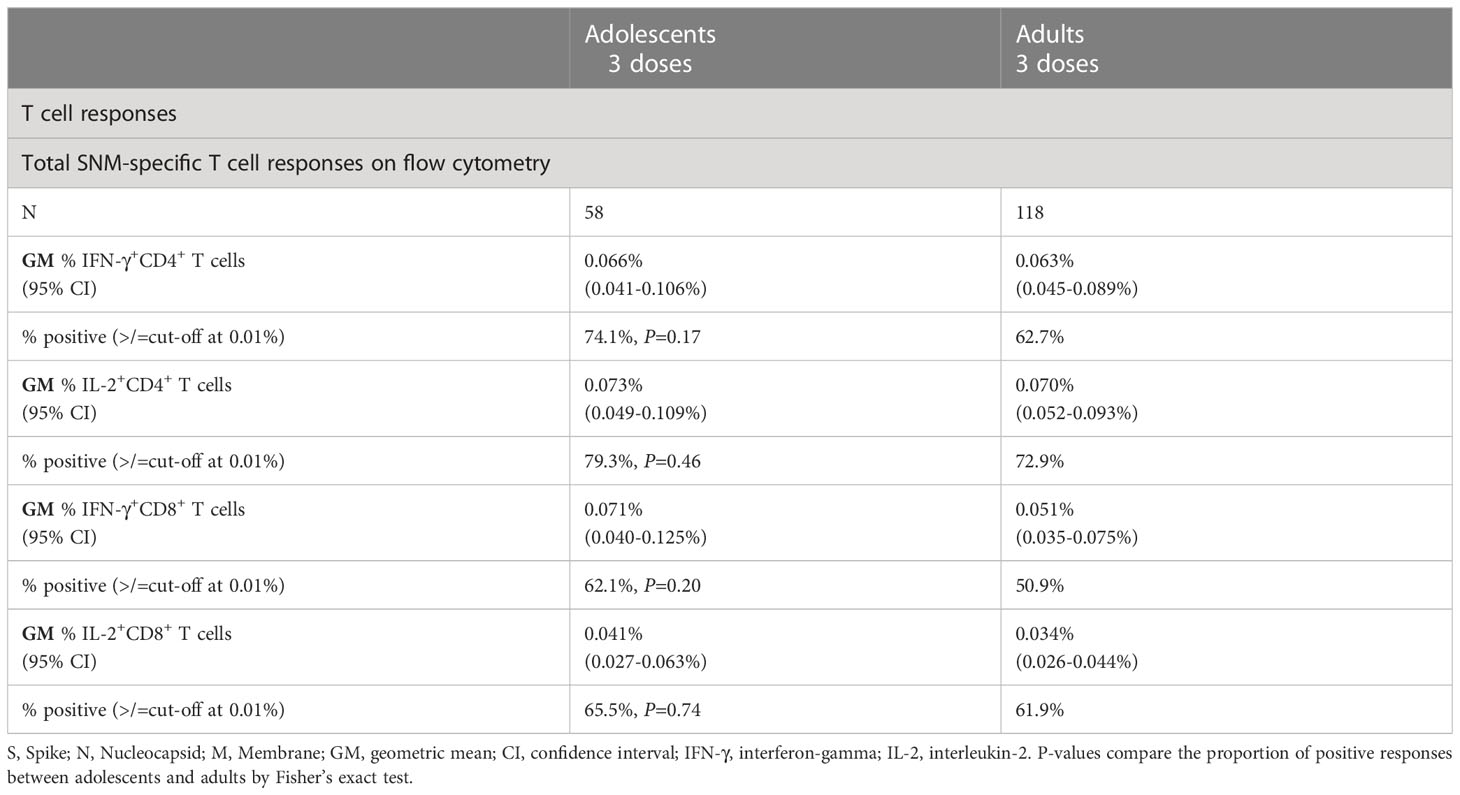
Table 2 Cellular immunogenicity outcomes against wild-type SARS-CoV-2 after the third dose of CoronaVac in evaluable analysis population.
Non-inferiority hypothesis testing of immunogenicity outcomes between adolescents and adults
To support the use of CoronaVac in adolescents without the availability of efficacy data, we calculated the geometric mean ratios (GMRs) of various immunogenicity outcomes as a primary analysis (see Methods). Nine humoral immunogenicity outcomes assessed were all non-inferior in adolescents as the lower bounds of their two-sided 95% confidence intervals (CI) were at least 0.60 (Figure 2A), with 50% PRNT, S IgG avidity, N IgG and N-CTD IgG responses satisfying the criterion for superiority as well. These findings were confirmed by secondary analyses in the expanded analysis population (Supplementary Figure 3). On the other hand, total SNM-specific IL-2+ CD4+ and IFN-γ+ and IL-2+ CD8+ T cells were non-inferior (Figure 2B), while it was inconclusive for total SNM-specific IFN-γ+ CD4+ T cells. When we considered separate S, N and M peptide pools-specific T cell responses, M-specific IL-2+ CD4+ T cell responses were inferior in evaluable adolescents (Supplementary Figure 4). Cellular immunogenicity outcomes were also confirmed in the expanded analysis population, yet total WT SNM-specific IFN-γ+ CD4+ T cells also tested non-inferior (Supplementray Figure 5).
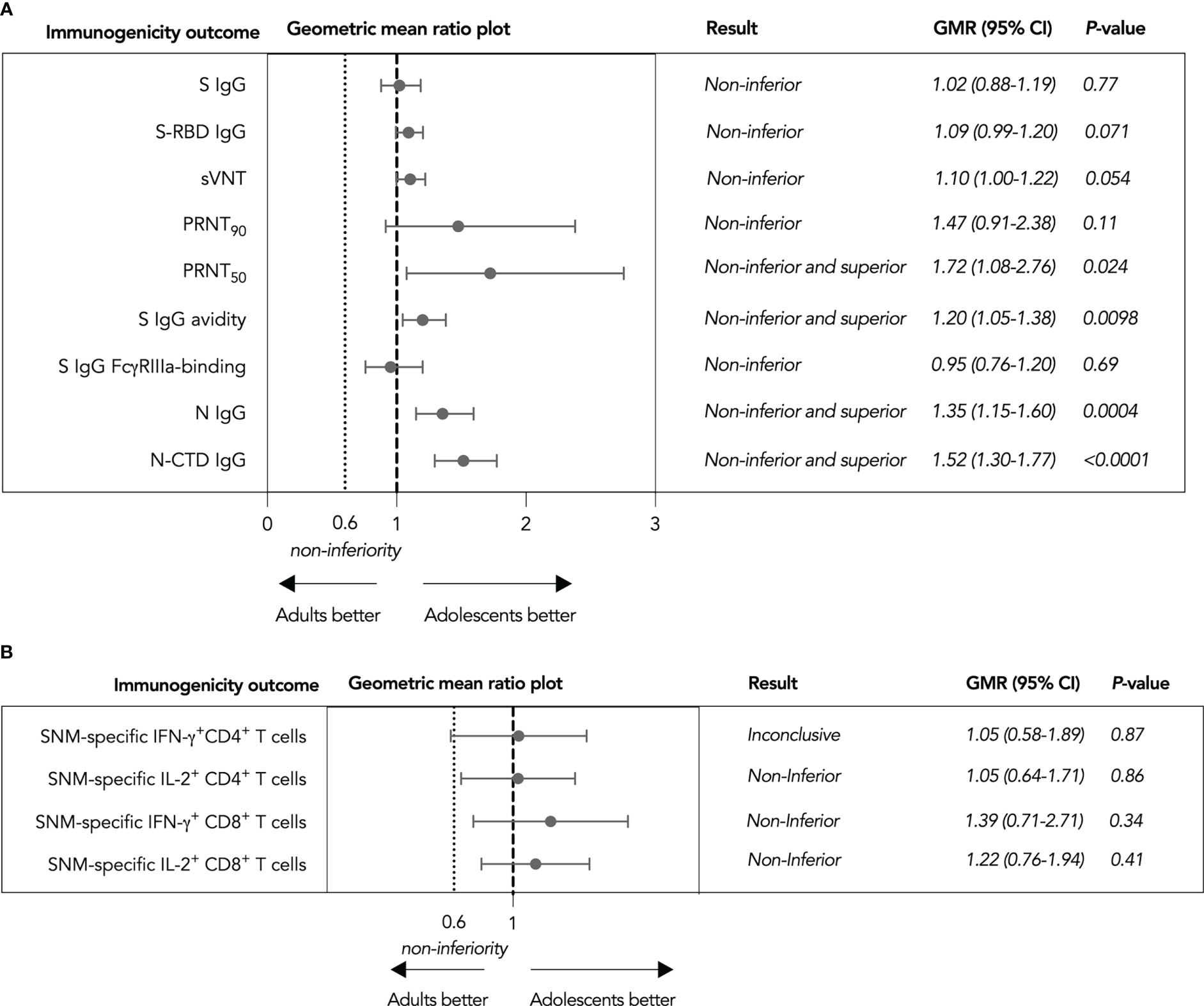
Figure 2 Non-inferiority hypothesis testing of humoral and cellular immunogenicity outcomes against wild-type SARS-CoV-2 after the third dose of CoronaVac in evaluable analysis population. (A) Non-inferiority testing of SARS-CoV-2 Spike (S) IgG, S-receptor binding domain (S-RBD) IgG, surrogate virus neutralization test (sVNT), plaque reduction neutralization test (PRNT), S IgG avidity, S IgG Fcγ receptor IIIa (FcγRIIIa)-binding, Nucleocapsid (N) IgG, and N-C-terminal domain (N-CTD) IgG (B Non-inferiority testing of total S, N and Membrane (M) protein-specific interferon-γ (IFN-γ)+ and interleukin-2 (IL-2)+ CD4+ and CD8+ T cells Geometric mean ratios (GMR) and two-tailed 95% confidence intervals (CI) were plotted.
Humoral and cellular immunogenicity against Omicron in adolescents
As vaccine efficacy (VE) against SARS-CoV-2 infection may be susceptible to immune escape by novel variants, we included immunogenicity against variants of concern as a secondary objective. At the time of analysis, Omicron has emerged as the dominant variant worldwide and has amino acid substitutions predominantly in the S protein, although also some across the rest of the proteome. We investigated whether Omicron BA.1 could escape S IgG, neutralizing antibodies and T cells elicited by CoronaVac. For Omicron-specific binding antibody responses, we interrogated Omicron BA.1 S IgG binding, avidity, and FcγRIIIa-binding in subsets of adolescents and adults and compared these to the WT assay. As expected, S IgG was significantly reduced in BA.1 compared to WT in adolescents and adults (Figure 3A). S IgG avidity was reduced against BA.1 in adolescents as well, yet interestingly, S IgG FcγRIIIa-binding was not significantly reduced. In terms of neutralizing antibodies, GM 50% PRNT was reduced by 5.19 fold against BA.1, but neutralizing antibodies remained detectable in 86.2%.
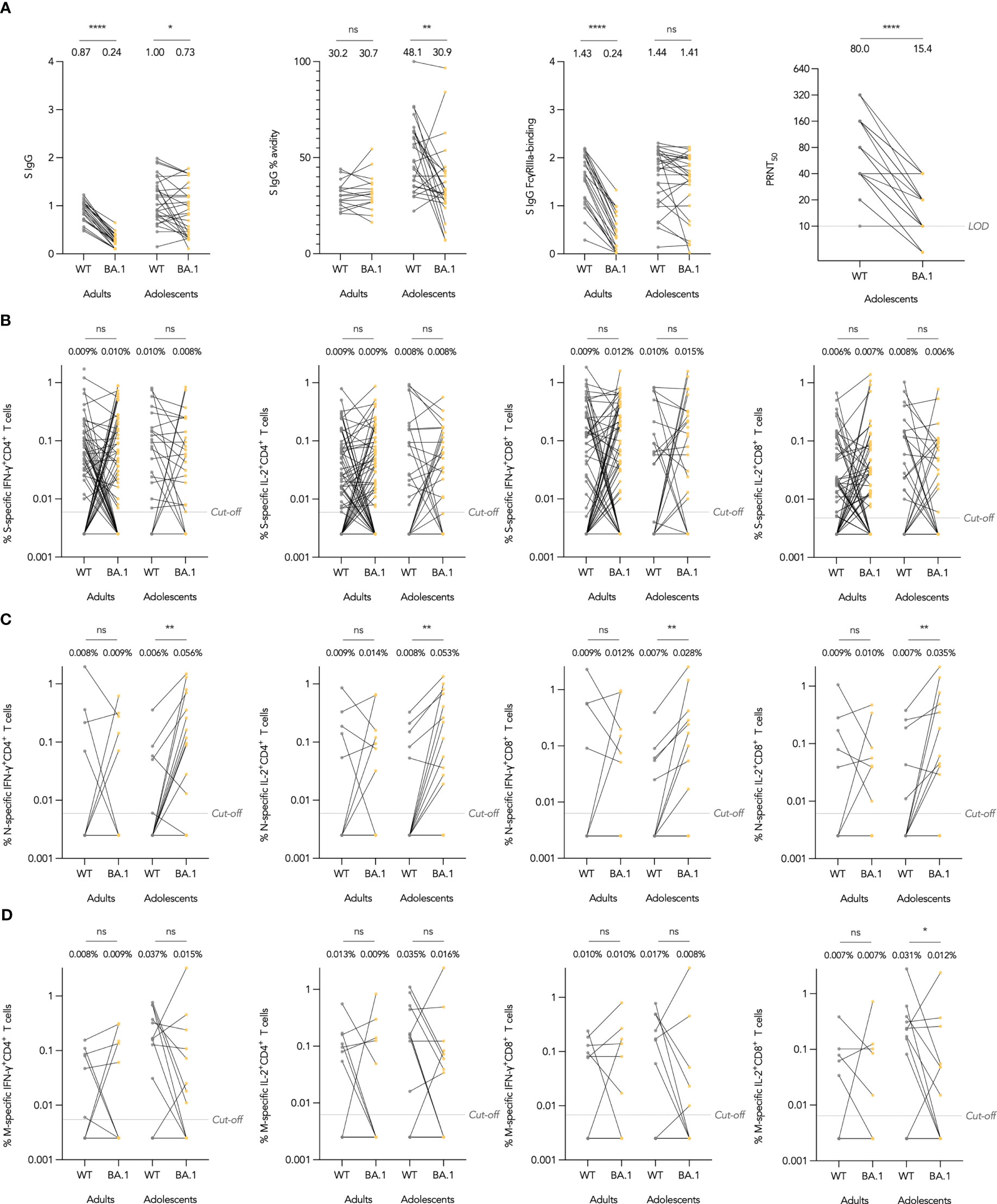
Figure 3 Omicron BA.1-specific humoral and cellular immunogenicity after the third dose of CoronaVac in healthy evaluable adolescents and adults. (A) Wild-type (WT) and BA.1 SARS-CoV-2 Spike (S) IgG OD450 values, S IgG avidity index, and S IgG Fcγ receptor IIIa (FcγRIIIa)-binding OD450 values, and 50% plaque reduction neutralization titers (PRNT). (B-D) Separate S, N and Membrane (M) protein WT reference pool and BA.1 mutation pool-specific interferon-γ (IFN-γ)+ and interleukin-2 (IL-2)+ CD4+ and CD8+ T cell frequencies. Samples from the same participant were paired between WT and BA.1 and compared with paired t test after natural logarithmic transformation with p-values denoted (*, P<0.05; **, P<0.01; ****, P<0.0001; ns, not significant).
To assess whether Omicron BA.1 mutations could lead to escape from T cell responses, we focused on BA.1-associated mutations and utilized S, N and M mutation pools which only contained peptides covering BA.1-associated mutations (37, 3 and 3 mutations in S, N and M respectively), and compared their T cell responses against those from WT reference peptide pools containing only the homologous WT peptides (Methods). As expected, no differences between WT and BA.1-S-specific T cells were found in both adolescents and adults (Figure 3B). Interestingly, BA.1-associated mutations in N increased IFN-γ+ and IL-2+ CD4+ and CD8+ T cell responses, differences which were significant in adolescents (Figure 3C). Meanwhile, T cell responses against BA.1 M mutation pool were reduced in comparison to WT reference pool, with the difference significant only for IL-2+ CD8+ T cells in adolescents, which had a 2.58-fold reduction (Figure 3D).
Reactogenicity and safety of the third dose of CoronaVac in adolescents
Among 94 adolescents in the healthy safety population, very common adverse reactions (ARs) included pain at the injection site (35.1% grade 1 and 8.5% grade 2) and fatigue (22.3% grade 1 and 4.3% grade 2) (Figure 4). Almost all ARs reported were of grades 1 and 2 severity; one grade 3 AR (diarrhoea) was reported. Only a single grade 1 adverse event (peripheral swelling) was reported within 28 days after vaccination in adolescents (Supplementary Table 5), and it was not considered to have been likely caused by vaccination. There were no serious adverse events reported in the follow-up period.
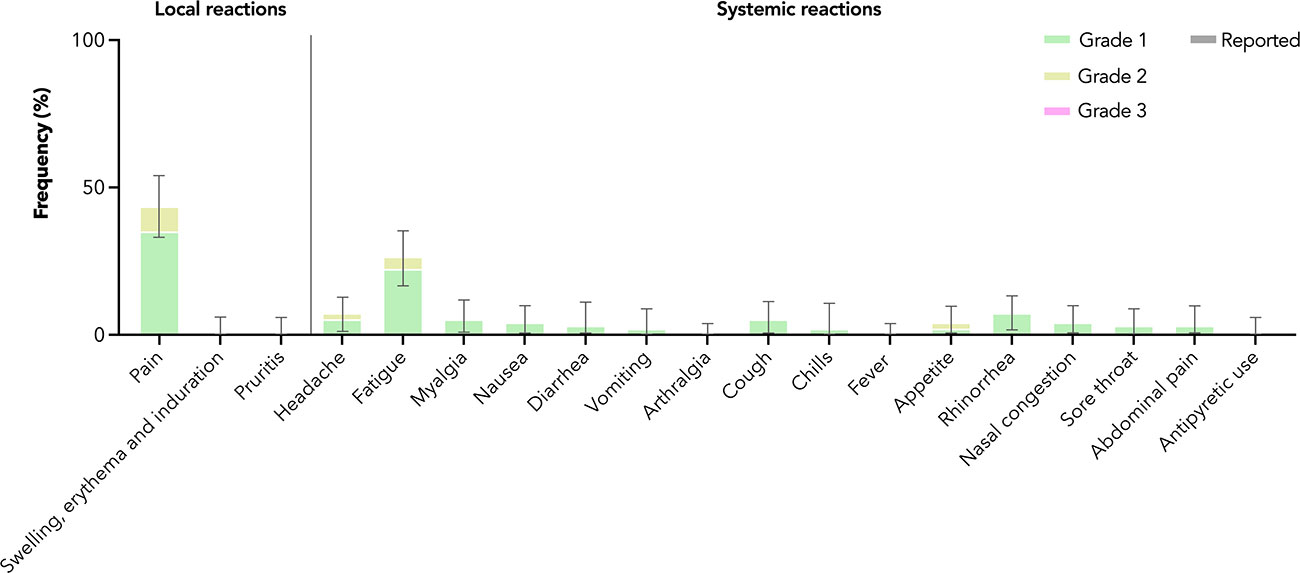
Figure 4 Adverse reactions in adolescents after the third dose of CoronaVac. Adverse reactions were reported by maximal severity (grade 1 – green, grade 2 – yellow, grade 3 – pink) within 7 days after vaccination. Antipyretic use was also captured (reported – grey). 95% confidence intervals are derived from the Clopper-Pearson method and marked by error bars.
Estimation of VE based on neutralization titres against WT and BA.1 SARS-CoV-2 in adolescents
We extrapolated VE estimates against symptomatic COVID-19 from WT and BA.1 PRNT50 results in evaluable adolescents as established by Khoury et al. (Methods) (21, 26, 27). The PRNT results were normalized to 102 in-house convalescent sera collected on days 28-59 post-onset of illness in patients aged ≥18 years, and yielded mean neutralization levels against WT and BA.1 of 0.40 and 0.11, which extrapolated to 66% and 36% VE, respectively (Figure 5). These estimates will need to be validated in real-world effectiveness studies.
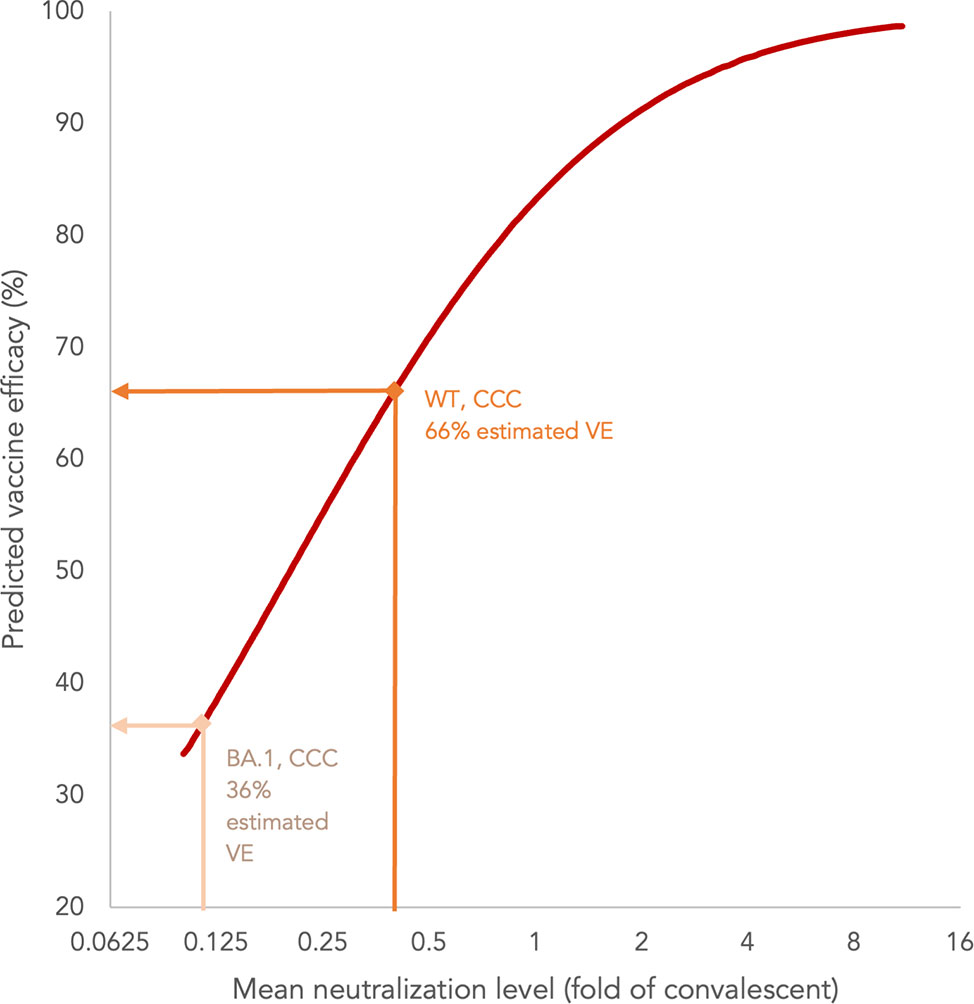
Figure 5 Estimation of vaccine efficacy (VE) of three doses of CoronaVac (CCC) based on neutralization titres against wild-type (WT) and BA.1 SARS-CoV-2 in adolescents.
Discussion
This study is the first to assess the reactogenicity and immunogenicity of third dose of CoronaVac in healthy adolescents. We found a third dose of CoronaVac further boosted antibody responses after 2 doses in adolescents. Immunobridging analyses showed non-inferior and superior binding and neutralizing antibody responses as well as T cell responses in adolescents when benchmarked against adults. Mutations associated with Omicron BA.1 attenuated binding and neutralizing antibody responses in adolescents who received a third dose, yet binding and neutralizing antibodies remained detectable in most. Adolescents had divergent responses toward mutation pools of Omicron BA.1 S, N and M proteins. ARs were mild, and there were no safety issues observed.
Our finding in adolescents is comparable to that observed in healthy adults who had a further increase in antibody responses after a third dose of CoronaVac (15, 17, 28). As there is evidence of waning protection against symptomatic disease after 2 doses, a booster after the 2-dose primary series of mRNA and adenoviral vector vaccines has been authorized in many countries. For inactivated vaccines, Hong Kong and Singapore have both opined that 3 doses, rather than 2, should form the primary series due to more rapid waning of antibody responses and failure to seroconvert in a minority of healthy vaccinees (29–31). Yet, there have been no published immunogenicity and safety data to inform the use of a third dose in adolescents to date. Our findings of inadequate and rapidly waning antibody responses after 2 doses, and non-inferior antibody responses after a third dose in adolescents compared to adults, support the authorization of a homologous third dose in this age group.
In addition to antibody response, we also surveyed T cell responses, with no waning detected after 2 doses in adolescents, and they were unaltered by a third dose in adolescents. There is limited evidence in literature on waning of T cell responses after 2 doses of CoronaVac in adults. One study in Chile showed an age-dependent preservation of T cell responses with no decline in adults aged 18-59 years and a more significant decline in adults aged 60 years or above (15). In studies of natural infection, half-life of convalescent T cell responses was variably estimated to be 3-7 months (32–35). As our study includes a 3-year follow-up, we will investigate the longevity of T cell responses after a third dose in adolescents. On the other hand, when adolescents were compared against adults after the third dose in our study, T cell responses against SNM in total were non-inferior. Yet, T cell responses against M protein trended lower in adolescents, including IL-2+ CD4+ T cells which were statistically inferior. Our data hint at differences in targets of T cell reactivity in adolescents versus adults. Previously, our group also found IFN-γ+ CD4+ and CD8+ T cell responses in children aged 1-13 years infected with SARS-CoV-2 appeared to favour non-structural proteins by flow cytometry, although individual proteins were not studied (36). In another study in the United Kingdom where an IFN-γ ELISpot assay was used, T cell responses in seropositive children aged 3-11 years were stronger to the S peptide pool than the combined NM peptide pool, while the responses appeared to be balanced in seropositive adults (37). These observations are possibly due to differential history of antigenic experience with common cold coronaviruses in different age groups, affecting cross-reactive T cell responses (38).
Omicron emerged in most parts of the world during the second year of COVID-19 vaccine rollout, and many studies in adults have pointed to dramatic escape of neutralizing antibodies (8, 9). Sixty-three percent adult vaccinees who received three doses of CoronaVac had detectable neutralizing antibodies against BA.1 in another study by our group (8). Using the same experimental platform, we found sera from a higher proportion (86%) of adolescents who received three doses of CoronaVac neutralized BA.1, suggesting adolescent vaccinees can make more cross-neutralizing antibodies. The neutralization data are in alignment with superior WT S IgG avidity observed in our study, and may lead to preserved VE against symptomatic disease with Omicron. As for T cells, we detected no difference in S-specific T cell response against WT and BA.1 mutated sequences, in agreement with previous studies (10, 11). Interestingly, we found a significant increase in both CD4+ and CD8+ T cell response against BA.1 mutations in N. It may be because 2 out of 3 mutations in N (31_33delERS, 203_204delRGinsKR) were at the fringes of the immunodominant antigenic regions of WT N protein (39, 40), and the mutations could have enhanced T cell reactivity (41). In contrast, our study revealed CD4+ and CD8+ T cell responses were both approximately halved against BA.1-associated mutations in M. Divergent changes in T cell responses towards BA.1-associated mutations in different SARS-CoV-2 proteins support that T cells exert very limited or absent selection pressure against SARS-CoV-2 (42). It is also noteworthy while our experimental design allowed us to zoom in on BA.1-associated mutations in each of S, N and M proteins. These changes in T cell response towards mutated sequences, albeit dramatic, should be considered in the context of the entire protein antigen, especially for N and M which contain only three small-scale mutations along the entire protein sequence. Overall, we do not expect a reduction of T cell response or any reduction in vaccine effectiveness against severe disease in vaccinees who received CoronaVac with Omicron BA.1. This conclusion is likely applicable towards other Omicron subvariants, which contain mostly point mutations only, supported by effectiveness data from Hong Kong’s experience with BA.2 (3, 43).
Our study had several strengths and limitations. In addition to neutralizing antibodies, which is a well-established correlate of protection against symptomatic COVID-19 and the basis for other immunobridging studies (26, 44–47), we also studied binding antibodies and T cell responses which also play important roles in protection (48, 49). We were able to track both antibody and T cell responses in healthy adolescent vaccinees from pre-vaccine to post-dose 3, and excluded infection in our participants before or during the study with ORF8 serology at the last timepoint. This was possible also because Hong Kong maintained extremely low levels of local transmission of SARS-CoV-2 during the study period. Non-randomized study design may lead to bias. Sample sizes varied between immunogenicity outcomes as various humoral and cellular assays had different technical and blood volume requirements, and samples were prioritised based on whether the participant had the same test performed at an earlier timepoint, earlier date of sample collection, and sample volume available. We assayed T cell responses by peptide pool-stimulated intracellular IFN-γ and IL-2 cytokine staining, as IFN-γ is an important Th/c1 effector cytokine and IL-2+ T cell populations are associated with long-term memory (36, 50, 51). We did not study other antiviral cytokines for polyfunctionality, nor memory and exhaustion markers. We estimated a VE against WT and BA.1 based on PRNT, though that will need to be validated in large-scale effectiveness studies. We only included uninfected adolescents aged 11-17 with good past health in the present analysis, so these findings may not be applicable to infected or younger children as well as paediatric patients with comorbidities. We did not investigate heterologous vaccination or responses against other Omicron subvariants.
In conclusion, our findings support the authorization of a homologous third dose of CoronaVac in healthy adolescents for optimized antibody response. To determine whether a fourth dose of CoronaVac will be needed as a booster in this age group, we will further track the durability of immunogenicity after this third dose and hybrid immunity in this population.
Data availability statement
The original contributions presented in the study are included in the article/Supplementary Material. Further inquiries can be directed to the corresponding authors.
Ethics statement
The studies involving human participants were reviewed and approved by The University of Hong Kong Institutional Review Board. Written informed consent to participate in this study was provided by the participants, their parents, or legally acceptable representatives.
Author contributions
YL conceptualized the study. YL, MP, WT, WL, DL, JR, and XW designed the study. YL led the acquisition of funding. YL, WT, and MP supervised the project. SMC, DL, XM, XW, SMSC, IYST, and JHL led the study administrative procedures. WW provided software support. SMC and WW contributed to recruitment of participants. YL and JR provided clinical assessments and follow-up. DL, SMC, JHL, JR, and YL collected safety data. SMSC, SC, KK, KC, JKL, LL, LT, NC, and MP developed and performed S-RBD IgG, N IgG, N-CTD IgG sVNT and neutralization antibody assays. CC and SV developed and performed the S IgG, IgG avidity, S IgG Fcγ receptor IIIa-binding and ORF8 antibody assays. MM provided and developed the specialised ORF8 protein. XW, XM, YZ, MW, WZ, and WT developed and performed the T cell assays. DL and JHL curated and analysed the data. DL, SMSC, YL, and MP performed the vaccine efficacy extrapolation. DL and JHL visualized the data. DL, XM, XW, SMSC, JR, CC, WW, JHL, and SMC validated the data. DL wrote the first draft supervised by YL, with input from JR, XM, XW, SMSC, and CC. All authors contributed to the article and approved the submitted version.
Funding
This study was supported by the research grant, COVID19F02, COVID19F10, T11-705/21-N and T11-712/19-N, from the Hong Kong SAR Government Health Bureau, which was not involved in the study design, data collection, laboratory assays, statistical computation, interpretation, or final conclusions of this project.
Acknowledgments
We thank the HKUMed Community Vaccination Centre at Ap Lei Chau Sports Centre and Gleneagles Hospital Hong Kong, led by Dr Victoria WY Wong of HKU, Ms Cindy HS Man and Dr Hon-Kuan Tong. The investigators are grateful to all clinical research team members and laboratory staff of Department of Paediatrics and Adolescent Medicine, including Mr KW Chan and Dr Davy CW Lee, for their research support. We are most thankful to the study participants.
Conflict of interest
The authors declare that the research was conducted in the absence of any commercial or financial relationships that could be construed as a potential conflict of interest.
Publisher’s note
All claims expressed in this article are solely those of the authors and do not necessarily represent those of their affiliated organizations, or those of the publisher, the editors and the reviewers. Any product that may be evaluated in this article, or claim that may be made by its manufacturer, is not guaranteed or endorsed by the publisher.
Supplementary material
The Supplementary Material for this article can be found online at: https://www.frontiersin.org/articles/10.3389/fimmu.2023.1106837/full#supplementary-material
References
1. Mallapaty S, Callaway E, Kozlov M, Ledford H, Pickrell J, Van Noorden R. How covid vaccines shaped 2021 - in eight powerful charts. Nature (2021) 600:580–3. doi: 10.1038/d41586-021-03686-x
2. Jara A, Undurraga EA, Gonzalez C, Paredes F, Fontecilla T, Jara G, et al. Effectiveness of an inactivated SARS-CoV-2 vaccine in Chile. N Engl J Med (2021) 385:875–84. doi: 10.1056/NEJMoa2107715
3. McMenamin ME, Nealon J, Lin Y, Wong JY, Cheung JK, Lau EHY, et al. Vaccine effectiveness of two and three doses of BNT162b2 and CoronaVac against COVID-19 in Hong Kong. medRxiv (2022). doi: 10.1101/2022.03.22.22272769
4. Mok CKP, Cohen CA, Cheng SMS, Chen C, Kwok KO, Yiu K, et al. Comparison of the immunogenicity of BNT162b2 and CoronaVac COVID-19 vaccines in Hong Kong. Respirology (2021) 4:301–10. doi: 10.1111/resp.14191
5. Rosa Duque J, Wang X, Leung D, Cheng S, Cohen C, Mu X, et al. Immunogenicity and reactogenicity of SARS-CoV-2 mRNA and inactivated vaccines in healthy adolescents (Accepted). Nat Commun (2022). doi: 10.21203/rs.3.rs-1327020/v1
6. Jara A, Undurraga EA, Zubizarreta JR, González C, Pizarro A, Acevedo J, et al. Effectiveness of homologous and heterologous booster doses for an inactivated SARS-CoV-2 vaccine: a large-scale prospective cohort study. Lancet Global Health (2022) 10:e798–806. doi: 10.1016/s2214-109x(22)00112-7
7. Feikin DR, Higdon MM, Abu-Raddad LJ, Andrews N, Araos R, Goldberg Y, et al. Duration of effectiveness of vaccines against SARS-CoV-2 infection and COVID-19 disease: results of a systematic review and meta-regression. Lancet (2022) 399:924–44. doi: 10.1016/s0140-6736(22)00152-0
8. Cheng SMS, Mok CKP, Leung YWY, Ng SS, Chan KCK, Ko FW, et al. Neutralizing antibodies against the SARS-CoV-2 omicron variant following homologous and heterologous CoronaVac or BNT162b2 vaccination. Nat Med (2022) 28(3):486–9. doi: 10.1038/s41591-022-01704-7
9. Cele S, Jackson L, Khoury DS, Khan K, Moyo-Gwete T, Tegally H, et al. Omicron extensively but incompletely escapes pfizer BNT162b2 neutralization. Nature (2022) 602:654–6. doi: 10.1038/s41586-021-04387-1
10. Gao Y, Cai C, Grifoni A, Muller TR, Niessl J, Olofsson A, et al. Ancestral SARS-CoV-2-specific T cells cross-recognize the omicron variant. Nat Med (2022) 28:472–6. doi: 10.1038/s41591-022-01700-x
11. Naranbhai V, Nathan A, Kaseke C, Berrios C, Khatri A, Choi S, et al. T Cell reactivity to the SARS-CoV-2 omicron variant is preserved in most but not all individuals. Cell (2022) 185:1041–1051 e1046. doi: 10.1016/j.cell.2022.01.029
12. Choi SJ, Kim DU, Noh JY, Kim S, Park SH, Jeong HW, et al. T Cell epitopes in SARS-CoV-2 proteins are substantially conserved in the omicron variant. Cell Mol Immunol (2022) 19:447–8. doi: 10.1038/s41423-022-00838-5
13. Nyberg T, Ferguson NM, Nash SG, Webster HH, Flaxman S, Andrews N, et al. Comparative analysis of the risks of hospitalisation and death associated with SARS-CoV-2 omicron (B.1.1.529) and delta (B.1.617.2) variants in England: A cohort study. Lancet (2022) 399(10332):1303–12. doi: 10.1016/s0140-6736(22)00462-7
14. Cloete J, Kruger A, Masha M, du Plessis NM, Mawela D, Tshukudu M, et al. Paediatric hospitalisations due to COVID-19 during the first SARS-CoV-2 omicron (B.1.1.529) variant wave in south Africa: A multicentre observational study. Lancet Child Adolesc Health (2022) 6(5):294–302. doi: 10.1016/S2352-4642(22)00027-X
15. Schultz BM, Melo-Gonzalez F, Duarte LF, Galvez NM, Pacheco GA, Soto JA, et al. A booster dose of an inactivated SARS-CoV-2 vaccine increases neutralizing antibodies and T cells that recognize delta and omicron variants of concern. medRxiv (2022). doi: 10.1101/2021.11.16.21266350
16. Ma AL-T, Leung D, Chan EY-H, Chim S, Cheng S, Ho FT-W, et al. Antibody responses to 2 doses of mRNA COVID-19 vaccine in pediatric patients with kidney diseases. Kidney Int (2022) 101:1069–72. doi: 10.1016/j.kint.2022.01.035
17. Zeng G, Wu Q, Pan H, Li M, Yang J, Wang L, et al. Immunogenicity and safety of a third dose of CoronaVac, and immune persistence of a two-dose schedule, in healthy adults: interim results from two single-centre, double-blind, randomised, placebo-controlled phase 2 clinical trials. Lancet Infect Dis (2022) 22:483–95. doi: 10.1016/s1473-3099(21)00681-2
18. Orange JS, Ballow M, Stiehm ER, Ballas ZK, Chinen J, de la Morena M, et al. Use and interpretation of diagnostic vaccination in primary immunodeficiency: A working group report of the basic and clinical immunology interest section of the American academy of allergy, asthma & immunology. J Allergy Clin Immunol (2012) 130:S1–24. doi: 10.1016/j.jaci.2012.07.002
19. Perera RA, Mok CK, Tsang OT, Lv H, Ko RL, Wu NC, et al. Serological assays for severe acute respiratory syndrome coronavirus 2 (SARS-CoV-2), march 2020. Euro Surveill (2020) 25(16):2000421. doi: 10.2807/1560-7917.ES.2020.25.16.2000421
20. Wu C, Qavi AJ, Hachim A, Kavian N, Cole AR, Moyle AB, et al. Characterization of SARS-CoV-2 nucleocapsid protein reveals multiple functional consequences of the c-terminal domain. iScience (2021) 24:102681. doi: 10.1016/j.isci.2021.102681
21. Lau EHY, Tsang OTY, Hui DSC, Kwan MYW, Chan WH, Chiu SS, et al. Neutralizing antibody titres in SARS-CoV-2 infections. Nat Commun (2021) 12:63. doi: 10.1038/s41467-020-20247-4
22. Sattler A, Schrezenmeier E, Weber UA, Potekhin A, Bachmann F, Straub-Hohenbleicher H, et al. Impaired humoral and cellular immunity after SARS-CoV-2 BNT162b2 (tozinameran) prime-boost vaccination in kidney transplant recipients. J Clin Invest (2021) 131(14):e150175. doi: 10.1172/JCI150175
23. Mateus J, Dan JM, Zhang Z, Rydyznski Moderbacher C, Lammers M, Goodwin B, et al. Low-dose mRNA-1273 COVID-19 vaccine generates durable memory enhanced by cross-reactive T cells. Science (2021) 374(6566). doi: 10.1126/science.abj9853
24. Liu X, Shaw RH, Stuart ASV, Greenland M, Aley PK, Andrews NJ, et al. Safety and immunogenicity of heterologous versus homologous prime-boost schedules with an adenoviral vectored and mRNA COVID-19 vaccine (Com-COV): a single-blind, randomised, non-inferiority trial. Lancet (2021) 398:856–69. doi: 10.1016/s0140-6736(21)01694-9
25. WHO Expert Committee on Biological Standardization Sixty-seventh report. Guidelines on clinical evaluation of vaccines: regulatory expectations. World Health Organization (2017).
26. Khoury DS, Cromer D, Reynaldi A, Schlub TE, Wheatley AK, Juno JA, et al. Neutralizing antibody levels are highly predictive of immune protection from symptomatic SARS-CoV-2 infection. Nat Med (2021) 27:1205–11. doi: 10.1038/s41591-021-01377-8
27. Lau EH, Hui DS, Tsang OT, Chan WH, Kwan MY, Chiu SS, et al. Long-term persistence of SARS-CoV-2 neutralizing antibody responses after infection and estimates of the duration of protection. EClinicalMedicine (2021) 41:101174. doi: 10.1016/j.eclinm.2021.101174
28. Mok CKP, Chen C, Yiu K, Chan TO, Lai KC, Ling KC, et al. A randomized clinical trial using CoronaVac or BNT162b2 vaccine as a third dose in adults vaccinated with two doses of CoronaVac. Am J Respir Crit Care Med (2022) 205:844–7. doi: 10.1164/rccm.202111-2655LE
29. Sauré D, O’Ryan M, Torres JP, Zuniga M, Santelices E, Basso LJ. Dynamic IgG seropositivity after rollout of CoronaVac and BNT162b2 COVID-19 vaccines in Chile: a sentinel surveillance study. Lancet Infect Dis (2022) 22:56–63. doi: 10.1016/s1473-3099(21)00479-5
30. Centre for health Protection, the Government of HKSAR. Centre for health protection consensus interim recommendations on the use of COVID-19 vaccines in persons with previous COVID-19 infection and children in Hong Kong (As of 12 march 2022). (2022).
31. Ministry of health Singapore. Ministry of health Singapore vaccination information sheet SinoVac COVID-19 vaccine (CoronaVac). Ministry of health (2022).
32. Dan JM, Mateus J, Kato Y, Hastie KM, Yu ED, Faliti CE, et al. Immunological memory to SARS-CoV-2 assessed for up to 8 months after infection. Science (2021) 371(6529):eabf4063. doi: 10.1126/science.abf4063
33. Jung JH, Rha MS, Sa M, Choi HK, Jeon JH, Seok H, et al. SARS-CoV-2-specific T cell memory is sustained in COVID-19 convalescent patients for 10 months with successful development of stem cell-like memory T cells. Nat Commun (2021) 12:4043. doi: 10.1038/s41467-021-24377-1
34. Cohen KW, Linderman SL, Moodie Z, Czartoski J, Lai L, Mantus G, et al. Longitudinal analysis shows durable and broad immune memory after SARS-CoV-2 infection with persisting antibody responses and memory b and T cells. Cell Rep Med (2021) 2:100354. doi: 10.1016/j.xcrm.2021.100354
35. Wragg KM, Lee WS, Koutsakos M, Tan HX, Amarasena T, Reynaldi A, et al. Establishment and recall of SARS-CoV-2 spike epitope-specific CD4(+) T cell memory. Nat Immunol (2022) 23(5):768–80. doi: 10.1038/s41590-022-01175-5
36. Cohen CA, Li APY, Hachim A, Hui DSC, Kwan MYW, Tsang OTY, et al. SARS-CoV-2 specific T cell responses are lower in children and increase with age and time after infection. Nat Commun (2021) 12:4678. doi: 10.1038/s41467-021-24938-4
37. Dowell AC, Butler MS, Jinks E, Tut G, Lancaster T, Sylla P, et al. Children develop robust and sustained cross-reactive spike-specific immune responses to SARS-CoV-2 infection. Nat Immunol (2022) 23:40–9. doi: 10.1038/s41590-021-01089-8
38. Chou J, Thomas PG, Randolph AG. Immunology of SARS-CoV-2 infection in children. Nat Immunol (2022) 23:177–85. doi: 10.1038/s41590-021-01123-9
39. Grifoni A, Sidney J, Vita R, Peters B, Crotty S, Weiskopf D, et al. SARS-CoV-2 human T cell epitopes: Adaptive immune response against COVID-19. Cell Host Microbe (2021) 29:1076–92. doi: 10.1016/j.chom.2021.05.010
40. Keller MD, Harris KM, Jensen-Wachspress MA, Kankate VV, Lang H, Lazarski CA, et al. SARS-CoV-2–specific T cells are rapidly expanded for therapeutic use and target conserved regions of the membrane protein. Blood (2020) 136:2905–17. doi: 10.1182/blood.2020008488
41. Heide J, Schulte S, Kohsar M, Brehm TT, Herrmann M, Karsten H, et al. Broadly directed SARS-CoV-2-specific CD4+ T cell response includes frequently detected peptide specificities within the membrane and nucleoprotein in patients with acute and resolved COVID-19. PloS Pathog (2021) 17:e1009842. doi: 10.1371/journal.ppat.1009842
42. Tarke A, Coelho CH, Zhang Z, Dan JM, Yu ED, Methot N, et al. SARS-CoV-2 vaccination induces immunological T cell memory able to cross-recognize variants from alpha to omicron. Cell (2022) 185:847–859 e811. doi: 10.1016/j.cell.2022.01.015
43. Tegally H, Moir M, Everatt J, Giovanetti M, Scheepers C, Wilkinson E, et al. Continued emergence and evolution of omicron in south Africa: New BA.4 and BA.5 lineages. medRxiv (2022). doi: 10.1101/2022.05.01.22274406
44. Earle KA, Ambrosino DM, Fiore-Gartland A, Goldblatt D, Gilbert PB, Siber GR, et al. Evidence for antibody as a protective correlate for COVID-19 vaccines. Vaccine (2021) 39:4423–8. doi: 10.1016/j.vaccine.2021.05.063
45. Gilbert PB, Montefiori DC, McDermott AB, Fong Y, Benkeser D, Deng W, et al. Immune correlates analysis of the mRNA-1273 COVID-19 vaccine efficacy clinical trial. Science (2022) 375:43–50. doi: 10.1126/science.abm3425
46. Frenck RW Jr., Klein NP, Kitchin N, Gurtman A, Absalon J, Lockhart S, et al. Safety, immunogenicity, and efficacy of the BNT162b2 covid-19 vaccine in adolescents. N Engl J Med (2021) 385:239–50. doi: 10.1056/NEJMoa2107456
47. Walter EB, Talaat KR, Sabharwal C, Gurtman A, Lockhart S, Paulsen GC, et al. Evaluation of the BNT162b2 covid-19 vaccine in children 5 to 11 years of age. N Engl J Med (2022) 386:35–46. doi: 10.1056/NEJMoa2116298
48. Kalimuddin S, Tham CYL, Qui M, de Alwis R, Sim JXY, Lim JME, et al. Early T cell and binding antibody responses are associated with COVID-19 RNA vaccine efficacy onset. Med (N Y) (2021) 2:682–688 e684. doi: 10.1016/j.medj.2021.04.003
49. Openshaw PJM. Using correlates to accelerate vaccinology. Science (2022) 375:22–3. doi: 10.1126/science.abn0007
50. McKinstry KK, Strutt TM, Bautista B, Zhang W, Kuang Y, Cooper AM, et al. Effector CD4 T-cell transition to memory requires late cognate interactions that induce autocrine IL-2. Nat Commun (2014) 5:5377. doi: 10.1038/ncomms6377
Keywords: COVID-19, vaccine, CoronaVac, Omicron, adolescent
Citation: Leung D, Cohen CA, Mu X, Rosa Duque JS, Cheng SMS, Wang X, Wang M, Zhang W, Zhang Y, Tam IYS, Lam JHY, Chan SM, Chaothai S, Kwan KKH, Chan KCK, Li JKC, Luk LLH, Tsang LCH, Chu NC, Wong WHS, Mori M, Leung WH, Valkenburg S, Peiris M, Tu W and Lau YL (2023) Immunogenicity against wild-type and Omicron SARS-CoV-2 after a third dose of inactivated COVID-19 vaccine in healthy adolescents. Front. Immunol. 14:1106837. doi: 10.3389/fimmu.2023.1106837
Received: 24 November 2022; Accepted: 16 February 2023;
Published: 06 March 2023.
Edited by:
Ritthideach Yorsaeng, Chulalongkorn University, ThailandReviewed by:
Wai Shing Leung, Kwong Wah Hospital, Hong Kong SAR, ChinaTania Regina Tozetto-Mendoza, Faculty of Medicine, University of São Paulo, Brazil
Copyright © 2023 Leung, Cohen, Mu, Rosa Duque, Cheng, Wang, Wang, Zhang, Zhang, Tam, Lam, Chan, Chaothai, Kwan, Chan, Li, Luk, Tsang, Chu, Wong, Mori, Leung, Valkenburg, Peiris, Tu and Lau. This is an open-access article distributed under the terms of the Creative Commons Attribution License (CC BY). The use, distribution or reproduction in other forums is permitted, provided the original author(s) and the copyright owner(s) are credited and that the original publication in this journal is cited, in accordance with accepted academic practice. No use, distribution or reproduction is permitted which does not comply with these terms.
*Correspondence: Sophie Valkenburg, c29waGllLnZAdW5pbWVsYi5lZHUuYXU=; Malik Peiris, bWFsaWtAaGt1Lmhr; Wenwei Tu, d3d0dUBoa3UuaGs=; Yu Lung Lau, bGF1eWx1bmdAaGt1Lmhr
†These authors have contributed equally to this work and share first authorship
‡These authors have contributed equally to this work