- 1Critical Care Medicine, The Second Xiangya Hospital, Central South University, Changsha, China
- 2Department of Critical Care Medicine and Hematology, The 3rd Xiangya Hospital, Central South University, Changsha, China
- 3Department of Nephrology, The First Affiliated Hospital of Gannan Medical University, Ganzhou, China
Heatstroke, which is associated with circulatory failure and multiple organ dysfunction, is a heat stress-induced life-threatening condition characterized by a raised core body temperature and central nervous system dysfunction. As global warming continues to worsen, heatstroke is expected to become the leading cause of death globally. Despite the severity of this condition, the detailed mechanisms that underlie the pathogenesis of heatstroke still remain largely unknown. Z-DNA-binding protein 1 (ZBP1), also referred to as DNA-dependent activator of IFN-regulatory factors (DAI) and DLM-1, was initially identified as a tumor-associated and interferon (IFN)-inducible protein, but has recently been reported to be a Z-nucleic acid sensor that regulates cell death and inflammation; however, its biological function is not yet fully understood. In the present study, a brief review of the main regulators is presented, in which the Z-nucleic acid sensor ZBP1 was identified to be a significant factor in regulating the pathological characteristics of heatstroke through ZBP1-dependent signaling. Thus, the lethal mechanism of heatstroke is revealed, in addition to a second function of ZBP1 other than as a nucleic acid sensor.
1 Introduction
Heatstroke is generally regarded as one of the most dangerous illnesses, with rapid progression after onset. The primary feature of heatstroke is a high core body temperature, which is caused by strenuous exercise or exposure to hot environments, and the direct cause is the imbalance of heat metabolism, manifested as the heat generation being greater than the heat dissipation (1). Clinically, heatstroke is defined as extreme hyperthermia (usually over 40.0°C), central nervous system (CNS) dysfunction, and multiple organ dysfunction multiple organ dysfunction syndrome (MODS), which are caused by the complex interaction between thermal-related cytotoxicity, inflammatory response, and coagulation abnormalities (2–4). Depending on the trigger, heatstroke is clinically classified as either classic heatstroke (CHS) or exertional heatstroke (EHS). The direct cause of both types is high temperature; however, despite their clinical manifestations being similar, the mechanisms of the two slightly differ. CHS is mainly caused by passive exposure to high temperatures, while EHS is mainly caused by strenuous exercise. The former involves the absorption of too much heat from the environment, while the latter involves the production of too much endogenous heat due to an accelerated metabolism (3). As for the survival rate of heatstroke, the reported mortality rate was as high as 23.3%, and the death rate from heatstroke has been predicted to increase as a result of climate change and the estimated whole world increase in the frequency and intensity of heat waves (5–8). Although adequate lowering of the body temperature and timely treatment can be administered, heatstroke remains hazardous, and individuals who do survive from heatstroke may suffer long-lasting neurological sequelae (7, 9).
Z-DNA-binding protein 1 (ZBP1) was originally identified as an interferon (IFN)-inducible tumor-associated protein involved in the host response to tumors (10). The amino terminus of ZBP1 contains two nucleic acid-binding domains and two receptor-interacting protein homotypic interaction motif (RHIM) domains (11, 12). The nucleic acid-binding domains sense and bind viral nucleic acids and are involved in the innate immune response. One the other hand, the RHIM domains bind to homologous proteins and are involved in the regulation of cell death and inflammation. The carboxy-terminal of ZBP1 has been reported to be involved in signal transduction (Figure 1) (12, 15, 16).
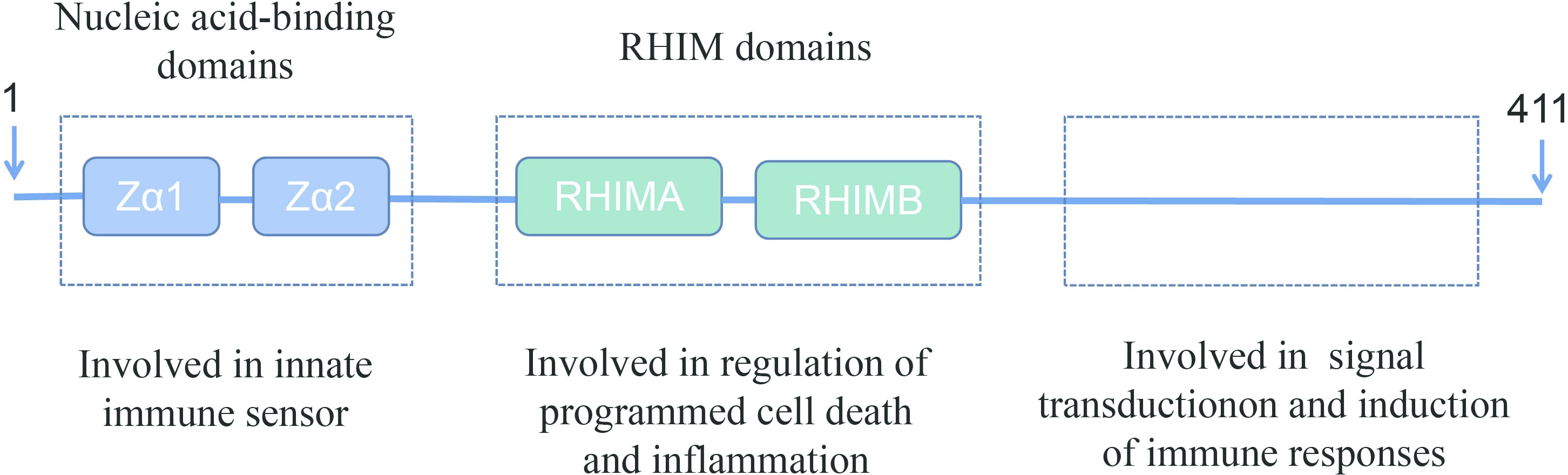
Figure 1 Schematic representation of full-length ZBP1. ZBP1 encodes two N-terminal Z-DNA-binding domains, which are reported to bind to Z-DNA, B-DNA, and RNA. ZBP1 also has two receptor-interacting protein homotypic interaction motif (RHIM) domains in the central part that facilitate the interaction with other RHIM domain-containing proteins. These RHIM domains are important in mediating ZBP1-dependent cell death and inflammatory responses (13, 14).
Numerous studies on heatstroke and ZBP1 have been published, including several review articles. Despite such research, at present, there are no reports on the relationship between heatstroke and ZBP1 (1–3, 7, 17–19). Studies on heatstroke over the past several decades have suggested that heatstroke results from the complex interaction of thermoregulatory failure with the amplification of acute-phase response (3, 4, 7, 9, 20). In a recent study by us, a novel function of ZBP1 in mediating heatstroke has been identified, which not only supplemented the understanding of the pathogenesis of heatstroke but also revealed a second function of ZBP1 other than as a nucleic acid sensor (Figure 2) (21). In the present review, the latest research advances on ZBP1 are summarized, as well as the pathogenesis and pathophysiology of heatstroke.
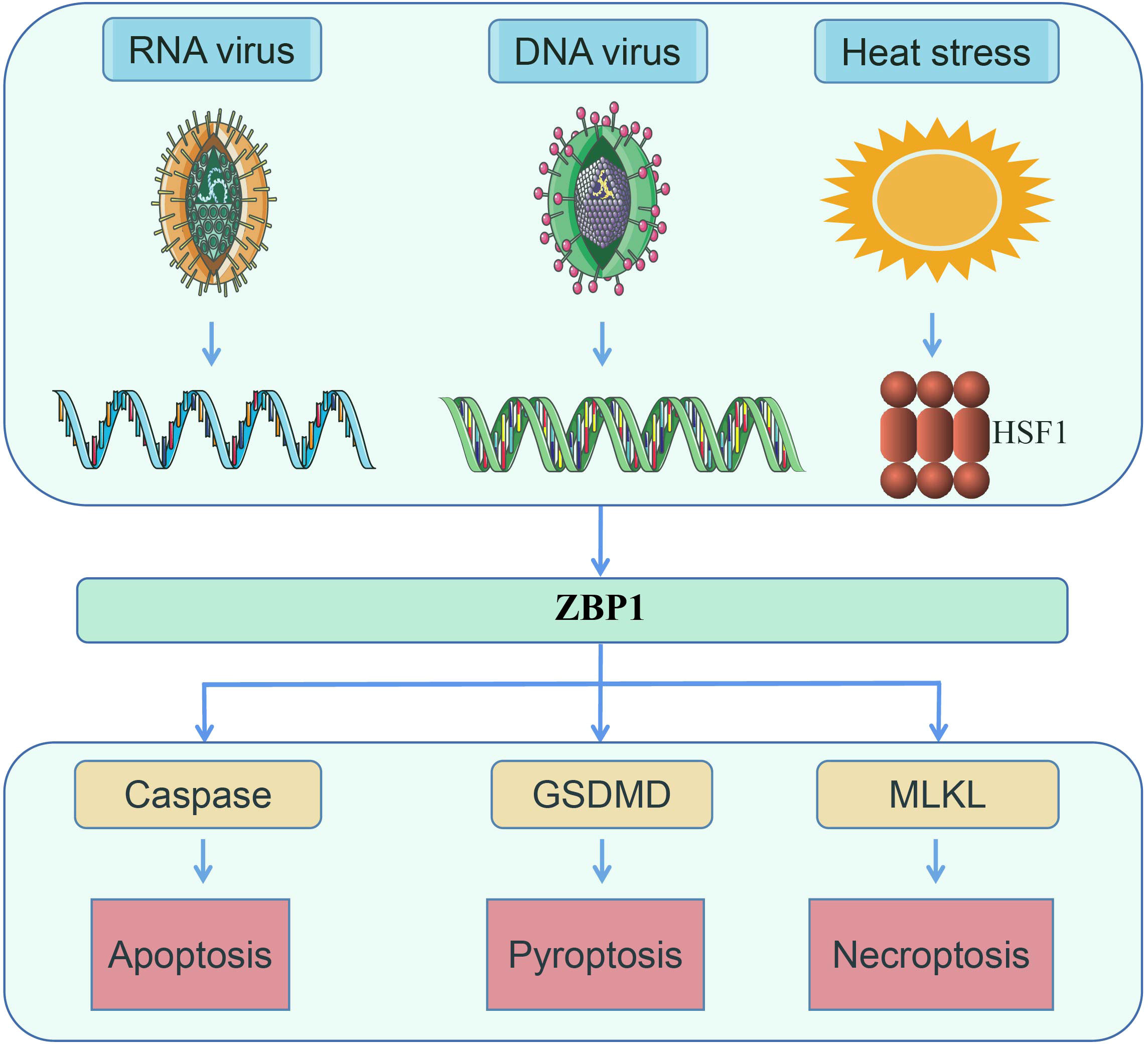
Figure 2 ZBP1 senses RNA virus infection, DNA virus infection, and heat stress, following which the necrotic complex will be assembled to induce apoptosis, pyroptosis, and necroptosis (14, 16).
2 The Role of ZBP1
2.1 ZBP1 in viruses
2.1.1 ZBP1 in influenza A virus
Influenza A virus (IAV) is a negative-sense RNA virus that is cytotoxic to most cell types in which it replicates. The primary IAV host is aquatic birds, with virus replications occurring in the gastrointestinal tract that commonly are asymptomatic. However, mammalian IAV strains replicate in respiratory tissues and produce symptoms that range from mild cases to severe and sometimes fatal (13). Owing to the high mutation rates and the genetic recombination thereof, IAV is commonly associated with epidemics (22). Several pattern recognition receptors (PRRs), including Toll-like receptors (TLRs), RIG-I-like receptors (RLRs), and nucleotide-binding domain leucine-rich repeat-containing receptors (NLRs), have been found to be involved in IAV infection (22). Virus recognition by these receptors triggers an innate immune response to restrict virus replication and clear the infection. The sensing of IAV initiates a variety of intracellular signaling pathways.
In a previous study, ZBP1 was found to be able to sense nucleoprotein (NP) and polymerase subunit PB1, which are components of IAV, and to promote activation of the nucleotide-binding domain leucine-rich repeat and pyrin domain-containing receptor 3 (NLRP3) inflammasome and pro-inflammatory cytokine production via receptor-interacting protein kinase 3 (RIPK3) signaling. At the same time, ZBP1 is regulated by IFN regulatory factor (IRF1) (23, 24). Aside from caspase-8, caspase-6 has also been reported to promote ZBP1-mediated programmed cell death (PCD) during IAV infection. The authors also found that pyroptosis, which is another PCD, could mediate cell death during IAV infection (25). In addition, regarding selectively deficient apoptosis in an IAV infection mouse model, necroptosis could completely compensate for the role of apoptosis (26). In further research, ZBP1 was also discovered to be able to sense IAV genomic RNA, bind to RIPK3, and trigger both the necroptosis and apoptosis of PCD to clear away infected cells and accordingly protect the host (27). In addition, a new pathogen-associated molecular pattern, Z-RNA, generated from replicating IAV, could activate ZBP1 via the Zα2 domain thereof in infected cells (28, 29). Despite these findings on the mechanisms promoting ZBP1 activation to induce PCD during IAV infection, further studies have confirmed the involvement of viral sensing of the IAV viral ribonucleoprotein (vrnP) complexes and sensing of the IAV RNA by retinoic acid-inducible gene I (RIG-I), which then triggered ZBP1-related cell death (30). Moreover, IAV can also induce the ubiquitination of ZBP1, and tripartite motif 34 (TRIM34) has been notably reported to be involved in regulating IAV-induced PCD by mediating the K63-linked polyubiquitination of ZBP1 (24, 30, 31). Thus, to a certain extent, these findings together explain the activation of ZBP1 during infection with IAV.
2.1.2 ZBP1 in cytomegalovirus
Cytomegaloviruses (CMVs), which belong to the herpesvirus family, are enveloped double-stranded DNA (dsDNA) viruses with a genome size of approximately 235 kbp. CMVs are slowly replicated in several specific cell types (32). The CMV genomes include over 200 independent genes with the potential to encode antigenic proteins. They also contain a common core of genes conserved in all herpesviruses, which is in the central 100 kbp. These gene families encode most of the glycoproteins that are required for virus replication (32).
As early as 2009, protein 45 of the mouse CMV (M45) was identified as a RHIM-containing protein that could bind to ZBP1 via the functional RHIMs thereof when overexpressed. At the same time, M45 can inhibit the phosphorylation of RIP3 and has the potential to disrupt ZBP1–RIP interactions, suggesting that it is significant in the suppression of cell death during mouse CMV (MCMV) infection (33). Notably, M45 was found to promote self-assembly into amyloid fibrils and interact with the RHIM-containing domain so as to form heteromeric amyloid fibrils and mediate necroptosis (34). Further studies verified that the MCMV-encoded viral inhibitor of RIP activation (vIRA) serves a similar function to M45, i.e., the targeting of ZBP1–RIP3 complexes, as well as the suppression of PCD induced by these complexes (35, 36). Recently, viral immediate-early protein 3 (IE3)-dependent transcription has been found to be indispensable for ZBP1-mediated cell death, and both the Zα2 and RHIM domains are required for MCMV-mediated necroptosis (37). Human cytomegalovirus (HCMV), much like MCMV, can also interact with ZBP1, which triggers strong antiviral immune responses (14, 38, 39).
2.1.3 ZBP1 in herpes simplex virus 1
Herpes simplex virus 1 (HSV-1) belongs to the alphaherpesvirinae subfamily. It is a dsDNA virus with a genome size of approximately 152 kbp. To date, over 80 genes encoded by HSV-1 have been confirmed (40). HSV-1 primarily infects epithelial cells, manifests vesicular eruptions (mainly in the oral or genital mucosa), and has lifelong latency in neurons (41, 42). Primary infection of HSV-1 generally occurs at a young age, with the great majority of infection causing mild clinical symptoms in immunocompetent people, in addition to the fairly high mortality rate in neonates (43). Approximately 3.7 billion individuals aged under 50 years are infected with HSV-1 worldwide (44).
In an earlier study in 2012, ZBP1 was found not necessary for HSV-1 DNA sensing in HepG2 hepatocellular carcinoma cells. However, ectopic ZBP1 expression repressing the HSV-1 viral replication depends on the Zβ and D3 domains rather than the Zα domain (45). In further research, HSV-1 ICP6 was identified as a RHIM-containing viral inhibitor analogous to vIRA/M45, which can target RIPK3 and function as a ZBP1–RIPK3 signaling inhibitor, thereby inducing death in murine cells and survival in human cells (46). ICP6 was also confirmed to promote tumor necrosis factor (TNF) receptor 1-induced necrosome assembly (47). Notably, in astrocytes, HSV-1 can also induce necroptosis through ZBP1 signaling (48). In a study on HSV-2-infected primary vaginal epithelial cells, it was found that ZBP1 was also required to recognize HSV-2 (49). In a recent study, it has been revealed that ZBP1 can interact with AIM2 and pyrin inflammasome components and mediate multiple pathways of PCD, including apoptosis, necroptosis, and pyroptosis (50).
2.1.4 ZBP1 in other viruses
In addition to IAV, CMV, and HSV, a variety of diverse viruses, including vaccinia virus (VV) (51–55), Zika virus (ZIKV) (56–58), West Nile virus (WNV) (57), severe acute respiratory syndrome coronavirus 2 (SARS-CoV-2) (59–61), Japanese encephalitis virus (JEV) (62), and human immunodeficiency virus type 1 (HIV-1) (63), have been demonstrated to induce or repress ZBP1-mediated PCD during infection. Together, these studies clearly illustrate that ZBP1-mediated PCD acts as a significant defense mechanism against viral infection.
2.1.5 ZBP1 in other pathogenic microorganisms
ZBP1 is not only involved in virus-mediated PCD, but it has also been reported in other pathogenic microorganisms such as bacteria (64–67), fungi (50, 68), and parasites (69, 70). Similar to its function in viruses, ZBP1 promotes the induced inflammasome activation and PCD of these microorganisms, but the mechanisms of the natural ligands or signals that activate the inflammasome and PCD remain unclear.
2.2 ZBP1 in tumors
ZBP1 was initially identified in the tumor stroma and tumor cells as an IFN-inducible protein that senses Z-form nucleic acids, but was later confirmed in the cytosolic sensing of both DNA and RNA (10, 11, 28). Recently, ZBP1 has been found to be involved in the occurrence and development of various tumors, including breast cancer (71–74), melanoma (75–77), colorectal cancer (76, 77), non-small cell lung cancer (76), multiple myeloma (78), ovarian cancer (79), urothelial carcinoma (80), fibrosarcoma (71), and kidney renal clear cell carcinoma (81). A common feature of these studies is the finding that ZBP1 mediates PCD in the aforementioned tumors. However, several of these aforementioned studies were performed in cell lines and there was a lack of animal experiments. Moreover, the specific mechanism of ZBP1-mediated PCD in tumor cells remains unclear. As such, further studies including animal experiments are still required.
2.3 ZBP1 in adenosine deaminase acting on RNA 1
More recently, studies have revealed that RNA-specific adenosine deaminase 1 (ADAR1) is involved in ZBP1-mediated signaling. ADAR1 belongs to the adenosine deaminase acting on RNA (ADAR) family. ADARs were initially found to have dsRNA unfolding activities (82), but were later confirmed to be adenosine-to-inosine RNA editing enzymes (83). ADAR1 has a catalytic deaminase domain and three dsRNA-binding domains, with two different functional isoforms: ADAR1p110 and ADAR1p150. The p110 isoform contains one Z-DNA-binding domain, Zβ, while the p150 isoform not only has the Zβ but also the Zα domain (84). ADAR1 also has editing-dependent functions, can regulate innate immunity, and alters miRNA maturation (85).
A breakthrough was recently made with regard to the research on ADAR1 and ZBP1. In a previous study using murine colorectal cancer and melanoma models, ADAR1 was found to suppress ZBP1-mediated inflammasome activation and PCD, including apoptosis, necroptosis, and pyroptosis (77). Most recently, several Nature articles have reported that ADAR1 could inhibit Z-RNA accumulation and ZBP1-dependent cell death by preventing the accumulation of mRNA transcripts. In parallel, further studies have identified ADAR1 as a negative regulator of sterile ZBP1 activation by means of ADAR1 mutations (75, 86). Through ADAR1 mutations, ZBP1 could also contribute to type I interferonopathies (87). With further in-depth research, the Zα domain of ADAR1 was confirmed to interact with ZBP1 and inhibit its signaling (88).
2.4 ZBP1 in heatstroke
The molecular mechanism of heatstroke pathogenesis remains unclear. The focus of previous research has been on the nucleic acid receptor function of ZBP1 and its mediation of PCD. In our recent studies, ZBP1 has been found to cause heatstroke pathology by activating the RIPK3-induced activation of mixed lineage kinase domain-like pseudokinase (MLKL)-dependent necroptosis and caspase-8-dependent cell death both in vivo in genetic mouse models and in vitro in cultured cells (21). A further study found that heat stress increased the expression of ZBP1 through HSF1 and promoted the aggregation of ZBP1 fusion proteins, which was followed by the recruitment of RIPK3, phosphorylation of MLKL, and the cleavage of caspase-8 (21).
3 Pathogenesis and pathophysiology of heatstroke
In the early phase of heatstroke, the organism is in a status of compensation, in which a balance is maintained between heat dissipation and heat generation. Upon entering the late stage of heatstroke, the organism produces more heat than it dissipates, with the cardiac output being reduced and is becoming inadequate to deal with the high thermoregulatory demands, ultimately leading to a status of decompensation. The heatstroke injury to the organism mainly occurs in the decompensation stage, which results in thermal-related cytotoxicity, inflammatory responses, and coagulation responses, eventually causing MODS, disseminated intravascular coagulation (DIC), and even death (Figure 3) (1–3, 89, 90). Such pathological features of heatstroke were found to be mediated by ZBP1 through the triggering of the RIPK3-induced activation of MLKL-dependent necroptosis and caspase-8-dependent cell death, which was identified in vivo in genetic mouse models and in vitro in cultured cells (21).
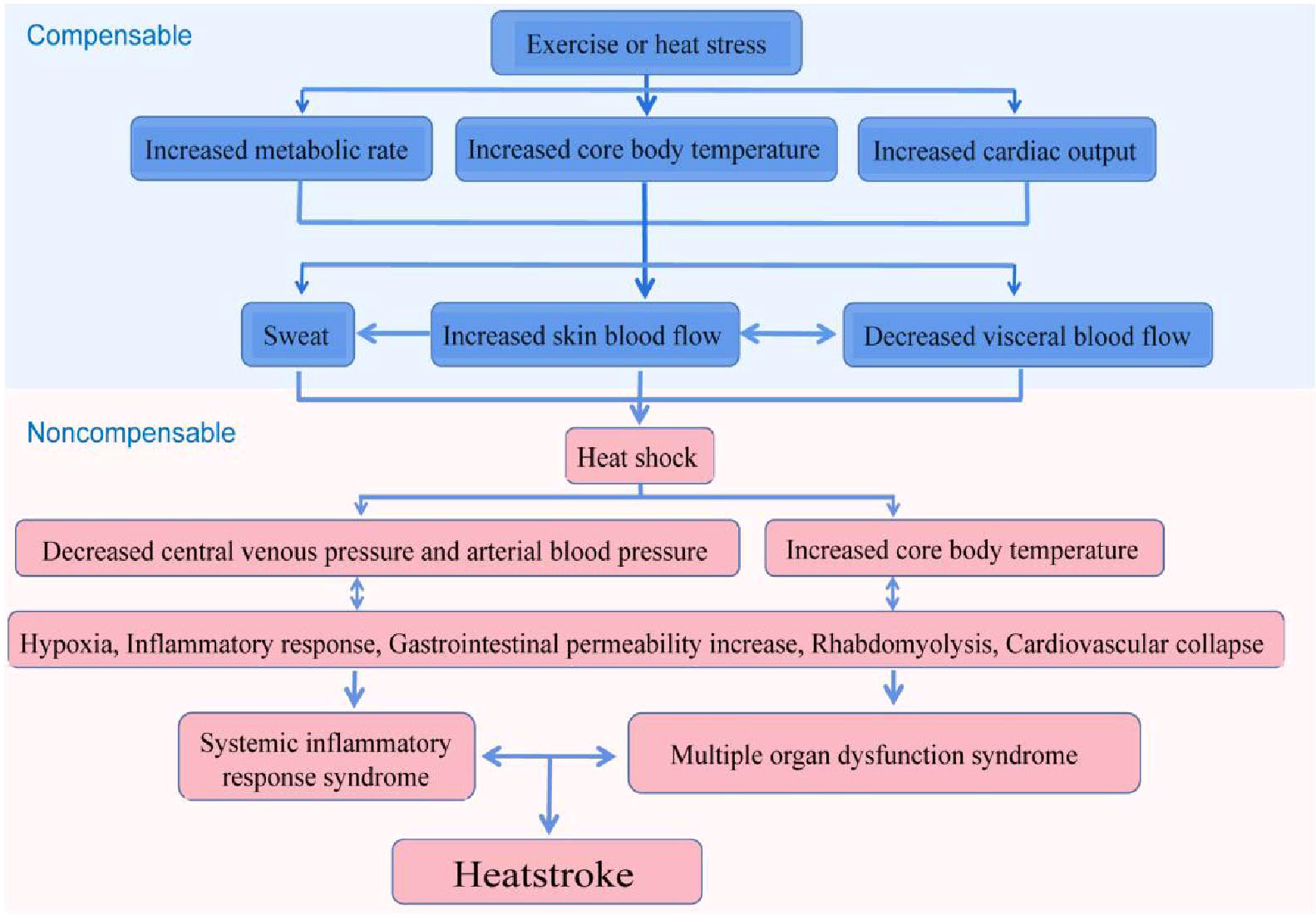
Figure 3 Possible pathophysiological pathway leading to heatstroke. The sequence of events leading to heatstroke involves a transition from a compensable thermoregulatory state to the non-compensable condition. Heat stress initiates a thermoregulatory response to maintain the balance of heat production and heat dissipation. When the arterial blood pressure begins to decrease substantially, the core temperature begins to increase rapidly and becomes non-compensable. This thermoregulatory failure aggravates pathophysiological processes, including the inflammatory response and multi-organ dysfunction, and is ultimately expressed as heatstroke (3).
3.1 Hypoxia and oxidative stress
Upon entering the non-compensable phase, the cardiac output is reduced and becomes inadequate to deal with the high thermoregulatory demands; subsequently, hypoxia occurs. Hypoxia further induces the generation of reactive oxygen species (ROS) (91–101). Several possible mechanisms cause the production of ROS during heatstroke. Li et al. (91) found that heat stress can promote the activation of the p38–MK2 signaling pathway, which promotes apoptosis by regulating the accumulation of ROS. Heat stress can also stimulate the production of ROS by inducing mitochondrial fission or inhibiting the activity of mitochondrial complex I (92, 93). Kikusato and Toyomizu (94) showed that the change in the mitochondrial membrane potential may be a significant factor in the overproduction of ROS under heat stress stimulation. Wang et al. (101) reported that heat stress can induce the production of ROS via the upregulation of Ang II and the receptor AT1 thereof.
The generated ROS can oxidatively damage lipids, proteins, and DNA, among others. The mitochondria represent the main production site of ROS and also its main active site, with damage to the mitochondria being able to induce apoptosis (91–95, 97, 102, 103). Under heat stress, the generated ROS can induce necroptosis and pyroptosis (103–105). Studies have revealed that ROS can induce apoptosis by promoting the release of apoptotic factors (105). Several studies have also demonstrated that ROS are pivotal upstream factors in the progress of apoptosis induced by heat stress (103, 106, 107). They can promote heat stress-induced apoptosis via ERK and Bcl-2 signaling (103). The accumulation of ROS, in particular O2−, promotes the elevation of Ca2+ in the cytoplasm via upregulating the inositol triphosphate receptor (IP3R), and the increased Ca2+ then induces apoptosis (108). Notably, similar results were found in another study, wherein heat stress induced apoptosis through the ROS-, Ca2+-, and p53-dependent translocation of Bax (106). Apoptosis can also be prevented by scavenging ROS (107, 109). In a recent study by us, heat stress has been found to be able to induce necroptosis, apoptosis, and pyroptosis via the ZBP1–RIPK3 signaling pathway, genetic deletion, or the targeted inhibition of ZBP1, RIPK3, or both caspase-8 and MLKL, which can prevent the multiple forms of cell death and improve survival. Although ROS was not directly detected, heat stress induced the generation of intravascular thrombin, and the occlusion of the microcirculation was attenuated by ZBP1 and RIPK3 deficiency (21). Therefore, a reasonable assumption could be made that blocking this pathway could reduce the production of ROS, then mitigating cell death. However, these findings require further validation.
3.2 Inflammatory response
Hyperthermia syndrome is an ongoing exacerbation process that consequently leads not only to a direct heat-related cytotoxicity effect but also to an inflammatory response. The heat stress-induced inflammatory response is considerably similar to that in systemic inflammatory response syndrome (SIRS) (2, 3). As is well known, SIRS can lead to rapid deterioration of the clinical status, resulting in multi-organ dysfunction, DIC, and death (110). Hence, akin to SIRS, heatstroke is considered a heat-related SIRS (2, 3). In a clinical study including patients with heatstroke, approximately 84% at the same time met the diagnostic criteria for SIRS (111). Existing studies have suggested that cytokines are involved in the heatstroke responses from patients with heatstroke to experimental animal models. Both clinical and experimental pieces of evidence have revealed multiple cytokines elevated in plasma and tissues, including both pro-inflammatory [such as interleukin-1 beta (IL-1β) and tumor necrosis factor alpha (TNF-α)] and anti-inflammatory [such as interleukin-6 (IL-6) and interleukin-10 (IL-10)] cytokines (3, 112). As such, multi-organ dysfunction is considered to be caused by heat-related cytotoxicity combined with the subsequent SIRS (2).
Cytokines are immune modulators that are released by a variety of cells, including immune and non-immune cells, e.g., macrophages, endothelial cells, T cells, B cells, and microglia, which act as intercellular chemical messengers (113). Elevated levels of cytokines can be detected in the plasma of patients and experimental animal models. These elevated cytokines include both pro-inflammatory [e.g., IL-1β, TNF-α, and interferon gamma (IFN-γ)] and anti-inflammatory (e.g., IL-6 and IL-10) cytokines (2, 3, 7, 112, 114). The plasma levels of cytokines differ during the progress of heatstroke, and loss of the balance between pro- and anti-inflammatory cytokines may result in inflammation-associated injury and immunosuppression. Previous studies in humans, rats, and mice have confirmed that heatstroke promotes systemic and local generation of pro-inflammatory cytokines (i.e., IL-1β, IL-6, IFN-γ, and TNF-α) (115–118). The levels of TNF receptors and IL-6 have been reported to be correlated with the severity of heatstroke (119, 120).
Studies conducted on the regulation of the inflammatory response of heatstroke in rat models revealed that neutralizing the inflammatory mediators with recombinant activated protein C, cytokine receptor antagonist, or corticosteroids reduced tissue injury, resulting to an improved survival (121, 122). However, increased mortality was observed in studies involving baboons that received corticosteroids and involving mice, in which the expression levels of the TNF-α receptor and IL-6 were deficient (114, 123). Consistent with prior research, a previous study by us that used a mouse model also showed elevated serum levels of pro-inflammatory cytokines (i.e., IL-1β, IL-6, TNF-α, and IL-1α) and the genetic deletion of RIPK3 or ZBP1 in mice, which significantly reduced the serum levels of the aforementioned pro-inflammatory cytokines (21). The described evidence supports the inflammatory response possibly being either beneficial or detrimental, depending on the phase and magnitude of the induced response.
3.3 Coagulopathy
Coagulation disorders are prominent features in patients with heatstroke and in animal models. Heatstroke has been identified to be associated with the activation of coagulation, anti-coagulation, and fibrinolytic systems (124–132). There are several different components of the coagulation, anti-coagulation, and fibrinolytic pathways that have been identified to be altered during heatstroke both in human and animal models, such as increased plasminogen activator inhibitor 1 (PAI-1), increased thrombin/anti-thrombin (TAT) complex, thrombocytopenia, decreased fibrinogen (Fib) levels, increased D-dimer levels, delayed prothrombin time (PT), and delayed activated partial thromboplastin time (APTT) (21, 133, 134). The most serious coagulopathy complicated by heatstroke is DIC, and the mortality rate of patients with DIC is significantly increased. Pathological manifestations such as congestion, hemorrhage, endothelial cell injury, extensive microthrombosis, and necrosis were observed in multiple organs in heatstroke rats (135). In our study recently published in Science, thrombin activation, fibrin deposition, and platelet adhesion were directly observed in a mouse model of heat stress using spinning disk confocal intravital microscopy (SD-IVM) (21). Furthermore, genetic deletion of ZBP1 or RIPK3 obviously decreased the thrombin activation, fibrin deposition, and platelet adhesion in the liver microvasculature of heat-stressed mice, while the plasma levels of the anticoagulant components PAI-1 and TAT complex were significantly decreased (21).
During the pathogenesis of heatstroke, various factors such as high temperature, ischemia, hypoxia, and inflammatory responses can lead to disorders of the coagulation system and even induce DIC. Clinically, DIC is characterized by extensive microthrombosis and bleeding due to damage to the microvascular system, activation of coagulation, excessive consumption of coagulation factors, and secondary hyperfibrinolysis (2). It is a fatal complication of heatstroke that can initiate during the recovery period and is thought to be a significant mechanism of fatality (3, 7, 129). The endothelium regulates vascular permeability and leukocyte movement, and it maintains the balance between procoagulants, anticoagulants, and fibrinolysis. Previous in vitro studies have revealed that heat stress induces a hypercoagulable state, increases vascular permeability, and promotes the expression of adhesion molecules (3, 7, 129). In vitro studies have also suggested that heat directly activates platelets, promotes platelet aggregation, and causes hyper-aggregation (129, 136, 137). Hyperthermia also results in the increased concentrations of platelet-derived microparticles (PMPs) and endothelial-derived microparticles (EMPs) in the circulation (138). EMPs have been reported to be involved in promoting endothelial oxidative stress, diminishing nitric oxide bioavailability, enhancing cell adhesion molecule expression, and impairing the endothelial vasomotor function (138, 139). PMPs, which are released as a result of platelet activation, cause endothelial injury and contribute to a hypercoagulable state (138–140). Circulating levels of several platelet aggregation-promoting components [such as von Willebrand factor (vWF) and thrombomodulin (TM)] and intercellular adhesion molecules are elevated, which indicates an interaction between endothelial cells and leukocytes in vivo (128, 141).
Tissue factor (TF) is the initiator of the coagulation process and is widely expressed in various tissues and cells. It initiates the coagulation cascade via the extrinsic coagulation pathway (142). Bouchama et al. reported that, in a baboon model of heatstroke, inhibiting TF with recombinant anticoagulant protein significantly attenuated the coagulation activation. However, neutralizing TF had no apparent effect on fibrinolysis and inflammation. These findings suggest that TF is the main trigger of coagulopathy under such conditions, but it is dispensable for tissue damage and organ dysfunction (143). At present, the specific role of TF on coagulation during heat stress remains unclear, and further research is still needed.
3.4 Tissue injury responses
Heat stress induces extensive tissue damage, including damage to the liver, lung, kidney, spleen, gastrointestinal tract, brain, and skeletal muscles. Although these injuries are commonly seen in human and animal studies (3, 4, 7, 21, 115), most of the tissue damage and organ dysfunction are attenuated after the genetic deletion of ZBP1 or Ripk3, as shown in our previous study (21). A typical symptom of heatstroke is CNS dysfunction, which manifests as varying degrees of mental status changes (8, 112, 144, 145). An elevated brain temperature is caused by the combination of an increased cerebral metabolic rate and a decreased cerebral blood flow (112). A decrease in cerebral blood flow causes cerebral ischemia, hypoxia, cerebral edema, the generation of ROS, activation of the microglia, disruption of the blood–brain barrier, an increase in the permeability of the blood–brain barrier, and further promotion of the leakage of vascular contents into the brain. The aforementioned series of responses promote the occurrence of neuroinflammation and neuronal damage. Neuroinflammation and neuronal damage may directly cause cognitive dysfunction (74, 115, 116, 118, 119, 146, 147). As reported in previous studies, 100% of patients with heatstroke presented acute neurological symptoms, with a total of 71.4% of the impaired patients developing long-term cerebellar dysfunction and approximately 34.4% of the survivors being left with long-lasting neurological damage, which may be associated with irreversible brain damage (8). However, the role of the brain in the progression of heatstroke remains unknown. Further research on the pathogenesis of encephalopathy is still needed.
Extensive peripheral tissue damage has been found in both patients and animals with heatstroke, and the commonly used index to assess the damage to peripheral tissues and organs is the alteration in the serum enzyme levels. For instance, elevation of the levels of creatine kinase, creatine kinase-MB, and alanine aminotransferase can indicate damage to different organs (3, 4). Histopathology has also fully confirmed the morphological and other pathological changes in patients and animals with heatstroke (4, 148–150). When heat stress persists, the blood flow is redistributed, which is manifested as a decreased visceral blood flow and an increased blood flow to the skin and vital organs, so as to meet the needs of heat dissipation. These factors lead to intestinal ischemia, which induces the generation of intestinal free radicals and intestinal mucosal edema and increases intestinal permeability, all of which promote intestinal disruption and lead to the release of a large number of intestinal toxins into the blood circulation (13, 109, 151–158). Renal failure is a common complication of heatstroke that is mainly manifested as tubular necrotizing acute renal failure and can be caused by massive protein deposition in the renal tubular epithelial cells after hemolysis and rhabdomyolysis (13, 21, 158–166). Liver dysfunction in patients with heatstroke and in animal models is considerably common, with the liver injury mainly occurring in the recovery period of heatstroke (167–174). Under heat stress, the liver can actively release various inflammatory mediators such as high-mobility group box-1 (HMGB1), which aggravates liver damage, suggesting that this factor may be a consequence of the inflammatory response that ensues during recovery rather than an acute response to heat stress (21, 97, 104, 112, 175). Liver injury under heat stress has also been considered to be associated with a large proportion of hepatocyte cell death, functional disorders of Kupffer cells, and abnormal expression of heat-related proteins, among other factors (21, 174). During the heatstroke process, the lungs are the most vulnerable and injured organs owing to their unique structure. Patients mainly present with acute lung injury (ALI), accompanied by pulmonary edema, pulmonary hemorrhage, pleural effusion, and exudation of inflammatory cells and cytokines (150, 176, 177). ALI was also found in mouse and rat models of heatstroke (21, 99, 158, 177–183). Rhabdomyolysis is more common in patients with EHS, and several possible mechanisms cause such phenomenon (3, 166, 184–193). One potential mechanism is the elevated muscle tension caused by strenuous activity, which increases the chance of tissue injury (184). Direct thermal cytotoxicity of hyperthermia may be another cause. Alterations of the calcium channels in muscle cell membranes have also been reported to be involved in rhabdomyolysis (7, 194–196). In parallel, more detailed studies revealed that heat stress-induced rhabdomyolysis could be partly attributed to the Ca2+-dependent increases in the levels of reactive oxygen and nitrogen, which exacerbated muscle membrane injury (7, 197). Another potential mechanism of rhabdomyolysis is dehydration, which increases blood redistribution and reduces the skeletal muscle blood flow. Such changes make the skeletal muscles ischemic, cause hypoxia, and increase Ca2+, which in turn increases the activation of the proteolytic enzymes in intramuscular Ca2+ that contribute to skeletal muscle breakdown, eventually causing rhabdomyolysis (198, 199). Ferroptosis has also been reported to mediate rhabdomyolysis (193).
3.5 Gastrointestinal integrity and flora imbalance
During heat stress, the body’s blood flow is redistributed in order to maintain the balance between heat generation and heat dissipation. This redistribution results in a decrease in splanchnic blood flow, causing gastrointestinal ischemia and hypoxia, and the generation of ROS and nitrogen species. Furthermore, the damage to the intestinal mucosa and cells is in turn aggravated and the permeability of the intestinal mucosal barrier increased, thereby allowing toxins and pathogens to leak into circulation, which further aggravates multiple organ injury (200). These factors also result in the gastrointestinal flora imbalance, including the decreased diversity and abundance of flora, which is mainly manifested as a decrease in beneficial bacteria and an increase in pathogenic bacteria (201, 202). In turn, the increase in pathogenic bacteria promotes intestinal inflammation and disrupts the intestinal barrier, resulting in a vicious cycle (203). The results of our previous study using a heatstroke mouse model also revealed the loss of intestinal integrity and inflammatory cell infiltration (21).
4 Conclusions
In the present review, the most recent advances with regard to ZBP1 and the pathogenesis of heatstroke were summarized. Recent research has indicated that the clinical characteristics of heatstroke result from the complex interaction between thermal-related cytotoxicity, inflammatory response, coagulopathy, rhabdomyolysis, and gastrointestinal disruption. In a most recent study by our group, in addition to demonstrating the features of heatstroke in a mouse model, it was also confirmed that genetic deletion or targeted inhibition of the ZBP1 pathway, this pathway being a significant factor in heatstroke, was able to significantly improve organ function, reduce multiple organ injury, inhibit DIC, and, most notably, improve survival (Figure 4). Another function of ZBP1, in addition to being a nucleic acid sensor, was also discovered. These findings are of considerable significance in revealing the pathogenic mechanism of heatstroke. However, the present understanding of the pathogenesis and pathophysiology of heatstroke is still incomplete. Additional research is still needed to fully elucidate the pathogenic mechanism of heatstroke so as to provide critical direction and basis for its clinical diagnosis and treatment, thereby reducing morbidity and improving patient survival.
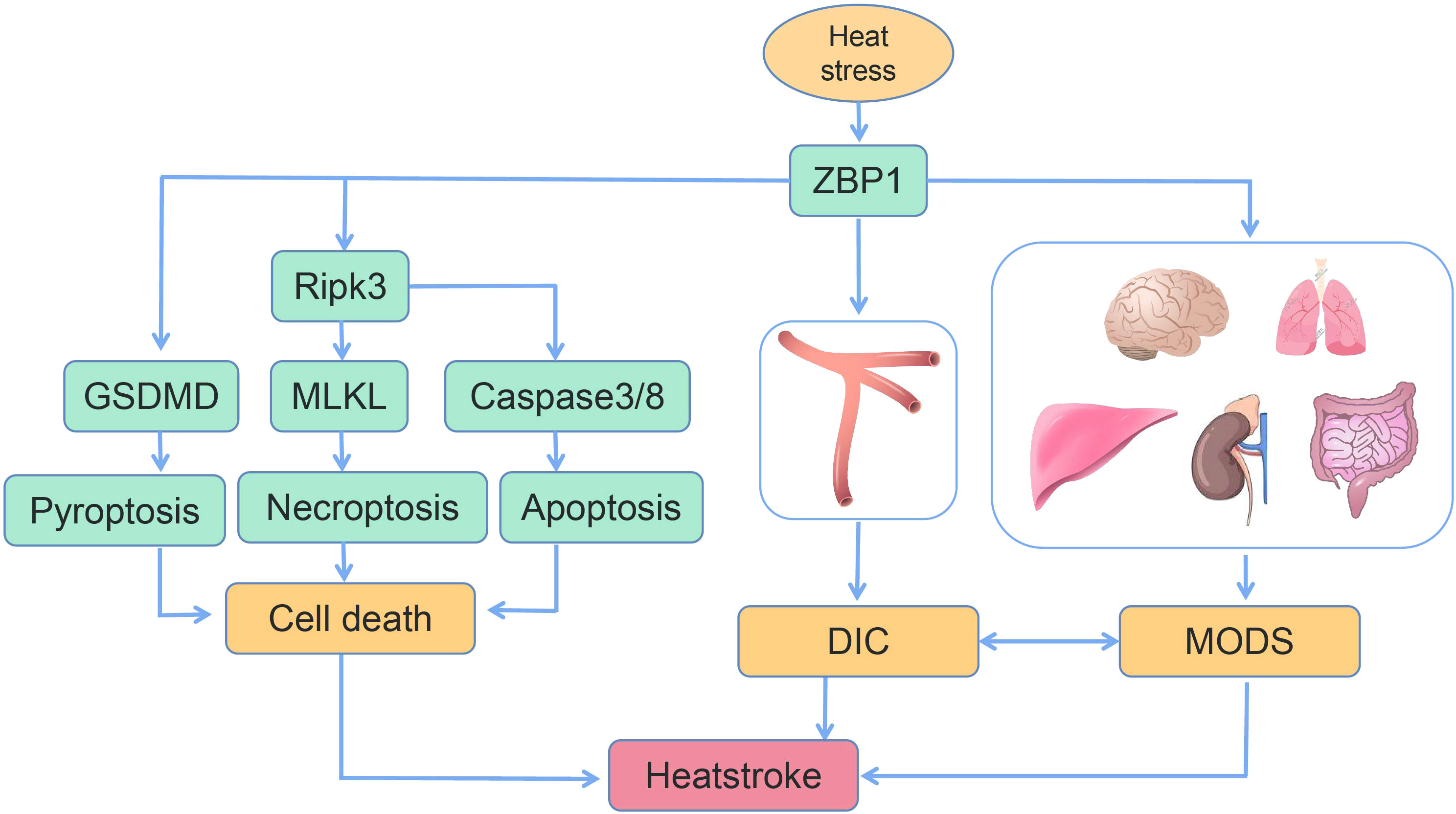
Figure 4 Schematic of the mechanism of heat stress-induced programmed cell death, disseminated intravascular coagulation (DIC), and multiple organ dysfunction syndrome (MODS) (14, 16).
Author contributions
FL, QH, and YZ wrote the main manuscript text. JD prepared Figures 1–4. All authors reviewed the manuscript. All authors contributed to the article and approved the submitted version.
Conflict of interest
The authors declare that the research was conducted in the absence of any commercial or financial relationships that could be construed as a potential conflict of interest.
Publisher’s note
All claims expressed in this article are solely those of the authors and do not necessarily represent those of their affiliated organizations, or those of the publisher, the editors and the reviewers. Any product that may be evaluated in this article, or claim that may be made by its manufacturer, is not guaranteed or endorsed by the publisher.
Glossary
Abbreviations
ZBP1, Z-DNA-binding protein 1; DAI, DNA-dependent activator of IFN-regulatory factors; CNS, central nervous system; MODS, multiple organ dysfunction syndrome; CHS, classic heatstroke; EHS, exertional heatstroke; RHIM, receptor-interacting protein homotypic interaction motif; IAV, influenza A virus; PRRs, pattern recognition receptors; TLRs, Toll-like receptors; RLRs, RIG-I-like receptors; NLRs, nucleotide-binding domain leucine-rich repeat-containing receptors; NP, nucleoprotein; NLRP3, nucleotide-binding domain leucine-rich repeat and pyrin domain-containing receptor 3; RIPK3, receptor-interacting protein kinase 3; IRF1, IFN regulatory factor; PCD, programmed cell death; RIG-I, retinoic acid-inducible gene I; TRIM34, tripartite motif 34; CMV, cytomegalovirus; M45, protein 45 of the mouse CMV; MCMV, mouse CMV; vIRA, viral inhibitor of RIP activation; IE3, immediate-early protein 3; HCMV, human cytomegalovirus; dsDNA, double-stranded DNA; VV, vaccinia virus; ZIKV, Zika virus; WNV, West Nile virus; SARS-CoV-2, severe acute respiratory syndrome coronavirus 2; JEV, Japanese encephalitis virus; HIV-1, human immunodeficiency virus type 1; HSV1, herpes simplex virus 1; AIM2, absent in melanoma 2; ADAR1, adenosine deaminase acting on RNA; DIC, disseminated intravascular coagulation; ROS, reactive oxygen species; IP3R, inositol triphosphate receptor; SIRS, systemic inflammatory response syndrome; IL-1β, interleukin-1 beta; TNF-α, tumor necrosis factor alpha; IL-6, interleukin-6; IL-10, interleukin-10; IFN-γ, interferon gamma; PAI-1, plasminogen activator inhibitor 1; TAT, thrombin–anti-thrombin; Fib, fibrinogen; PT, prothrombin time; APTT, activated partial thromboplast; SD-IVM, spinning disk confocal intravital microscopy; PMPs, platelet-derived microparticles; EMPs, endothelial-derived microparticles; vWF, von Willebrand factor; TM, thrombomodulin; TF, tissue factor; ALI, acute lung injury; GI, gastrointestinal; HMGB1, high-mobility group box-1
References
2. Bouchama A, Knochel JP. Heat stroke. N Engl J Med (2002) 346(25):1978–88. doi: 10.1056/NEJMra011089
3. Epstein Y, Yanovich R. Heatstroke. N Engl J Med (2019) 380(25):2449–59. doi: 10.1056/NEJMra1810762
4. Liu SY, Song JC, Mao HD, Zhao JB, Song Q. Expert consensus on the diagnosis and treatment of heat stroke in China. Mil Med Res (2020) 7(1):1. doi: 10.1186/s40779-019-0229-2
5. Gaudio FG, Grissom CK. Cooling methods in heat stroke. J Emerg Med (2016) 50(4):607–16. doi: 10.1016/j.jemermed.2015.09.014
6. Easterling DR, Meehl GA, Parmesan C, Changnon SA, Karl TR, Mearns LO. Climate extremes: Observations, modeling, and impacts. Science. (2000) 289(5487):2068–74. doi: 10.1126/science.289.5487.2068
7. Bouchama A, Abuyassin B, Lehe C, Laitano O, Jay O, O'Connor FG, et al. Classic and exertional heatstroke. Nat Rev Dis Primers. (2022) 8(1):8. doi: 10.1038/s41572-021-00334-6
8. Lawton EM, Pearce H, Gabb GM. Review article: Environmental heatstroke and long-term clinical neurological outcomes: A literature review of case reports and case series 2000-2016. Emerg Med Australas. (2019) 31(2):163–73. doi: 10.1111/1742-6723.12990
9. Ebi KL, Capon A, Berry P, Broderick C, Dear R, Havenith G, et al. Hot weather and heat extremes: Health risks. Lancet. (2021) 398(10301):698–708. doi: 10.1016/S0140-6736(21)01208-3
10. Fu Y, Comella N, Tognazzi K, Brown LF, Dvorak HF, Kocher O. Cloning of DLM-1, a novel gene that is up-regulated in activated macrophages, using RNA differential display. Gene. (1999) 240(1):157–63. doi: 10.1016/S0378-1119(99)00419-9
11. Takaoka A, Wang Z, Choi MK, Yanai H, Negishi H, Ban T, et al. DAI (DLM-1/ZBP1) is a cytosolic DNA sensor and an activator of innate immune response. Nature. (2007) 448(7152):501–5. doi: 10.1038/nature06013
12. Zheng M, Kanneganti TD. The regulation of the ZBP1-NLRP3 inflammasome and its implications in pyroptosis, apoptosis, and necroptosis (PANoptosis). Immunol Rev (2020) 297(1):26–38. doi: 10.1111/imr.12909
13. Wang L, Deng Z, Yuan R, Zhao Y, Yang M, Hu J, et al. Protective effect and mechanism of mesenchymal stem cells on heat stroke induced intestinal injury. Exp Ther Med (2020) 20(4):3041–50. doi: 10.3892/etm.2020.9051
14. Yoneda S, Imagawa A, Fukui K, Uno S, Kozawa J, Sakai M, et al. A histological study of fulminant type 1 diabetes mellitus related to human cytomegalovirus reactivation. J Clin Endocrinol Metab (2017) 102(7):2394–400. doi: 10.1210/jc.2016-4029
15. Ishii KJ, Kawagoe T, Koyama S, Matsui K, Kumar H, Kawai T, et al. TANK-binding kinase-1 delineates innate and adaptive immune responses to DNA vaccines. Nature. (2008) 451(7179):725–9. doi: 10.1038/nature06537
16. Kesavardhana S, Kanneganti TD. ZBP1: A STARGTE to decode the biology of z-nucleic acids in disease. J Exp Med (2020) 217(7):e20200885. doi: 10.1084/jem.20200885
17. Hifumi T, Kondo Y, Shimizu K, Miyake Y. Heat stroke. J Intensive Care (2018) 6:30. doi: 10.1186/s40560-018-0298-4
18. Kuriakose T, Kanneganti TD. ZBP1: Innate sensor regulating cell death and inflammation. Trends Immunol (2018) 39(2):123–34. doi: 10.1016/j.it.2017.11.002
19. Malireddi RKS, Kesavardhana S, Kanneganti TD. ZBP1 and TAK1: Master regulators of NLRP3 Inflammasome/Pyroptosis, apoptosis, and necroptosis (PAN-optosis). Front Cell Infect Microbiol (2019) 9:406. doi: 10.3389/fcimb.2019.00406
20. Roberts WO, Armstrong LE, Sawka MN, Yeargin SW, Heled Y, O'Connor FG. ACSM expert consensus statement on exertional heat illness: Recognition, management, and return to activity. Curr Sports Med Rep (2021) 20(9):470–84. doi: 10.1249/JSR.0000000000000878
21. Yuan F, Cai J, Wu J, Tang Y, Zhao K, Liang F, et al. Z-DNA binding protein 1 promotes heatstroke-induced cell death. Science. (2022) 376(6593):609–15. doi: 10.1126/science.abg5251
22. Iwasaki A, Pillai PS. Innate immunity to influenza virus infection. Nat Rev Immunol (2014) 14(5):315–28. doi: 10.1038/nri3665
23. Kuriakose T, Man SM, Malireddi RK, Karki R, Kesavardhana S, Place DE, et al. ZBP1/DAI is an innate sensor of influenza virus triggering the NLRP3 inflammasome and programmed cell death pathways. Sci Immunol (2016) 1(2):aag2045. doi: 10.1126/sciimmunol.aag2045
24. Kuriakose T, Zheng M, Neale G, Kanneganti TD. IRF1 is a transcriptional regulator of ZBP1 promoting NLRP3 inflammasome activation and cell death during influenza virus infection. J Immunol (2018) 200(4):1489–95. doi: 10.4049/jimmunol.1701538
25. Zheng M, Karki R, Vogel P, Kanneganti TD. Caspase-6 is a key regulator of innate immunity, inflammasome activation, and host defense. Cell. (2020) 181(3):674–687.e613. doi: 10.1016/j.cell.2020.03.040
26. Shubina M, Tummers B, Boyd DF, Zhang T, Yin C, Gautam A, et al. Necroptosis restricts influenza a virus as a stand-alone cell death mechanism. J Exp Med (2020) 217(11):e20191259. doi: 10.1084/jem.20191259
27. Thapa RJ, Ingram JP, Ragan KB, Nogusa S, Boyd DF, Benitez AA, et al. DAI senses influenza a virus genomic RNA and activates RIPK3-dependent cell death. Cell Host Microbe (2016) 20(5):674–81. doi: 10.1016/j.chom.2016.09.014
28. Zhang T, Yin C, Boyd DF, Quarato G, Ingram JP, Shubina M, et al. Influenza virus z-RNAs induce ZBP1-mediated necroptosis. Cell. (2020) 180(6):1115–1129 e1113. doi: 10.1016/j.cell.2020.02.050
29. Kesavardhana S, Malireddi RKS, Burton AR, Porter SN, Vogel P, Pruett-Miller SM, et al. The Zalpha2 domain of ZBP1 is a molecular switch regulating influenza-induced PANoptosis and perinatal lethality during development. J Biol Chem (2020) 295(24):8325–30. doi: 10.1074/jbc.RA120.013752
30. Kesavardhana S, Kuriakose T, Guy CS, Samir P, Malireddi RKS, Mishra A, et al. ZBP1/DAI ubiquitination and sensing of influenza vRNPs activate programmed cell death. J Exp Med (2017) 214(8):2217–29. doi: 10.1084/jem.20170550
31. Wang X, Xiong J, Zhou D, Zhang S, Wang L, Tian Q, et al. TRIM34 modulates influenza virus-activated programmed cell death by targeting z-DNA-binding protein 1 for K63-linked polyubiquitination. J Biol Chem (2022) 298(3):101611. doi: 10.1016/j.jbc.2022.101611
32. Hengel H, Reusch U, Gutermann A, et al. Cytomegaloviral control of MHC class I function in the mouse. Immunol Rev (1999) 168:167–76. doi: 10.1111/j.1600-065X.1999.tb01291.x
33. Rebsamen M, Heinz LX, Meylan E, Michallet MC, Schroder K, Hofmann K, et al. DAI/ZBP1 recruits RIP1 and RIP3 through RIP homotypic interaction motifs to activate NF-kappaB. EMBO Rep (2009) 10(8):916–22. doi: 10.1038/embor.2009.109
34. Pham CL, Shanmugam N, Strange M, O'Carroll A, Brown JW, Sierecki E, et al. Viral M45 and necroptosis-associated proteins form heteromeric amyloid assemblies. EMBO Rep (2019) 20(2):e46518. doi: 10.15252/embr.201846518
35. Upton JW, Kaiser WJ, Mocarski ES. DAI/ZBP1/DLM-1 complexes with RIP3 to mediate virus-induced programmed necrosis that is targeted by murine cytomegalovirus vIRA. Cell Host Microbe (2012) 11(3):290–7. doi: 10.1016/j.chom.2012.01.016
36. Upton JW, Kaiser WJ, Mocarski ES. DAI/ZBP1/DLM-1 complexes with RIP3 to mediate virus-induced programmed necrosis that is targeted by murine cytomegalovirus vIRA. Cell Host Microbe (2019) 26(4):564. doi: 10.1016/j.chom.2019.09.004
37. Sridharan H, Ragan KB, Guo H, Gilley P, Landsteiner VJ, Kaiser WJ, et al. Murine cytomegalovirus IE3-dependent transcription is required for DAI/ZBP1-mediated necroptosis. EMBO Rep (2017) 18(8):1429–41. doi: 10.15252/embr.201743947
38. DeFilippis VR, Sali T, Alvarado D, White L, Bresnahan W, Fruh KJ. Activation of the interferon response by human cytomegalovirus occurs via cytoplasmic double-stranded DNA but not glycoprotein b. J Virol (2010) 84(17):8913–25. doi: 10.1128/JVI.00169-10
39. DeFilippis VR, Alvarado D, Sali T, Rothenburg S, Fruh K. Human cytomegalovirus induces the interferon response via the DNA sensor ZBP1. J Virol (2010) 84(1):585–98. doi: 10.1128/JVI.01748-09
40. Davison AJ. Herpesvirus systematics. Vet Microbiol (2010) 143(1):52–69. doi: 10.1016/j.vetmic.2010.02.014
41. Denes CE, Everett RD, Diefenbach RJ. Tour de herpes: Cycling through the life and biology of HSV-1. Methods Mol Biol (2020) 2060:1–30. doi: 10.1007/978-1-4939-9814-2_1
42. Looker KJ, Magaret AS, May MT, Turner KME, Vickerman P, Newman LM, et al. First estimates of the global and regional incidence of neonatal herpes infection. Lancet Glob Health (2017) 5(3):e300–9. doi: 10.1016/S2214-109X(16)30362-X
43. Looker KJ, Magaret AS, May MT, Turner KM, Vickerman P, Gottlieb SL, et al. Global and regional estimates of prevalent and incident herpes simplex virus type 1 infections in 2012. PloS One (2015) 10(10):e0140765. doi: 10.1371/journal.pone.0140765
44. Saleh D, Yarrarapu SNS, Sharma S. Herpes simplex type 1. In: StatPearls. Treasure Island (FL: StatPearls Publishing (2022).
45. Pham TH, Kwon KM, Kim YE, Kim KK, Ahn JH. DNA Sensing-independent inhibition of herpes simplex virus 1 replication by DAI/ZBP1. J Virol (2013) 87(6):3076–86. doi: 10.1128/JVI.02860-12
46. Guo H, Gilley RP, Fisher A, Lane R, Landsteiner VJ, Ragan KB, et al. Species-independent contribution of ZBP1/DAI/DLM-1-triggered necroptosis in host defense against HSV1. Cell Death Dis (2018) 9(8):816. doi: 10.1038/s41419-018-0868-3
47. Ali M, Roback L, Mocarski ES. Herpes simplex virus 1 ICP6 impedes TNF receptor 1-induced necrosome assembly during compartmentalization to detergent-resistant membrane vesicles. J Biol Chem (2019) 294(3):991–1004. doi: 10.1074/jbc.RA118.004651
48. Jeffries AM, Suptela AJ, Marriott I. Z-DNA binding protein 1 mediates necroptotic and apoptotic cell death pathways in murine astrocytes following herpes simplex virus-1 infection. J Neuroinflammation. (2022) 19(1):109. doi: 10.1186/s12974-022-02469-z
49. Triantafilou K, Eryilmazlar D, Triantafilou M. Herpes simplex virus 2-induced activation in vaginal cells involves toll-like receptors 2 and 9 and DNA sensors DAI and IFI16. Am J Obstet Gynecol. (2014) 210(2):122.e121–122.e110. doi: 10.1016/j.ajog.2013.09.034
50. Lee S, Karki R, Wang Y, Nguyen LN, Kalathur RC, Kanneganti TD. AIM2 forms a complex with pyrin and ZBP1 to drive PANoptosis and host defence. Nature. (2021) 597(7876):415–9. doi: 10.1038/s41586-021-03875-8
51. Szczerba M, Subramanian S, Trainor K, McCaughan M, Kibler KV, Jacobs BL. Small hero with great powers: Vaccinia virus E3 protein and evasion of the type I IFN response. Biomedicines. (2022) 10(2):235. doi: 10.3390/biomedicines10020235
52. Koehler H, Cotsmire S, Zhang T, Balachandran S, Upton JW, Langland J, et al. Vaccinia virus E3 prevents sensing of z-RNA to block ZBP1-dependent necroptosis. Cell Host Microbe (2021) 29(8):1266–1276.e1265. doi: 10.1016/j.chom.2021.05.009
53. Koehler H, Cotsmire S, Langland J, Kibler KV, Kalman D, Upton JW, et al. Inhibition of DAI-dependent necroptosis by the z-DNA binding domain of the vaccinia virus innate immune evasion protein, E3. Proc Natl Acad Sci U S A. (2017) 114(43):11506–11. doi: 10.1073/pnas.1700999114
54. Klaas L, Vier J, Gentle IE, Hacker G, Kirschnek S. Diversity of cell death signaling pathways in macrophages upon infection with modified vaccinia virus Ankara (MVA). Cell Death Dis (2021) 12(11):1011. doi: 10.1038/s41419-021-04286-3
55. Kim YG, Muralinath M, Brandt T, Pearcy M, Hauns K, Lowenhaupt K, et al. A role for z-DNA binding in vaccinia virus pathogenesis. Proc Natl Acad Sci U S A. (2003) 100(12):6974–9. doi: 10.1073/pnas.0431131100
56. Wen C, Yu Y, Gao C, Qi X, Cardona CJ, Xing Z. RIPK3-dependent necroptosis is induced and restricts viral replication in human astrocytes infected with zika virus. Front Cell Infect Microbiol (2021) 11:637710. doi: 10.3389/fcimb.2021.637710
57. Rothan HA, Arora K, Natekar JP, Strate PG, Brinton MA, Kumar M. Z-DNA-Binding protein 1 is critical for controlling virus replication and survival in West Nile virus encephalitis. Front Microbiol (2019) 10:2089. doi: 10.3389/fmicb.2019.02089
58. Daniels BP, Kofman SB, Smith JR, Norris GT, Snyder AG, Kolb JP, et al. The nucleotide sensor ZBP1 and kinase RIPK3 induce the enzyme IRG1 to promote an antiviral metabolic state in neurons. Immunity. (2019) 50(1):64–76 e64. doi: 10.1016/j.immuni.2018.11.017
59. Rothan HA, Kumari P, Stone S, Natekar JP, Arora K, Auroni TT, et al. SARS-CoV-2 infects primary neurons from human ACE2 expressing mice and upregulates genes involved in the inflammatory and necroptotic pathways. Pathogens. (2022) 11(2). doi: 10.3390/pathogens11020257
60. Karki R, Lee S, Mall R, Pandian N, Wang Y, Sharma BR, et al. ZBP1-dependent inflammatory cell death, PANoptosis, and cytokine storm disrupt IFN therapeutic efficacy during coronavirus infection. Sci Immunol (2022) 7(74):eabo6294. doi: 10.1126/sciimmunol.abo6294
61. Geng H, Subramanian S, Wu L, Bu HF, Wang X, Du C, et al. SARS-CoV-2 ORF8 forms intracellular aggregates and inhibits IFNgamma-induced antiviral gene expression in human lung epithelial cells. Front Immunol (2021) 12:679482. doi: 10.3389/fimmu.2021.679482
62. Zhang N, Gao X, Zhang W, Xiong J, Cao X, Fu ZF, et al. JEV infection induces m-MDSC differentiation into CD3(+) macrophages in the brain. Front Immunol (2022) 13:838990. doi: 10.3389/fimmu.2022.838990
63. Hayashi T, Nishitsuji H, Takamori A, Hasegawa A, Masuda T, Kannagi M. DNA-Dependent activator of IFN-regulatory factors enhances the transcription of HIV-1 through NF-kappaB. Microbes Infect (2010) 12(12-13):937–47. doi: 10.1016/j.micinf.2010.06.003
64. Stutz MD, Ojaimi S, Allison C, Preston S, Arandjelovic P, Hildebrand JM, et al. Necroptotic signaling is primed in mycobacterium tuberculosis-infected macrophages, but its pathophysiological consequence in disease is restricted. Cell Death Differ (2018) 25(5):951–65. doi: 10.1038/s41418-017-0031-1
65. Pearson JS, Giogha C, Muhlen S, Nachbur U, Pham CL, Zhang Y, et al. EspL is a bacterial cysteine protease effector that cleaves RHIM proteins to block necroptosis and inflammation. Nat Microbiol (2017) 2:16258. doi: 10.1038/nmicrobiol.2016.258
66. Muendlein HI, Connolly WM, Magri Z, Smirnova I, Ilyukha V, Gautam A, et al. ZBP1 promotes LPS-induced cell death and IL-1beta release via RHIM-mediated interactions with RIPK1. Nat Commun (2021) 12(1):86. doi: 10.1038/s41467-020-20357-z
67. Li Y, Guo X, Hu C, Du Y, Guo C, Di W, et al. Type I IFN operates pyroptosis and necroptosis during multidrug-resistant a. baumannii infection. Cell Death Differ (2018) 25(7):1304–18. doi: 10.1038/s41418-017-0041-z
68. Banoth B, Tuladhar S, Karki R, Sharma BR, Briard B, Kesavardhana S, et al. ZBP1 promotes fungi-induced inflammasome activation and pyroptosis, apoptosis, and necroptosis (PANoptosis). J Biol Chem (2020) 295(52):18276–83. doi: 10.1074/jbc.RA120.015924
69. Pittman KJ, Cervantes PW, Knoll LJ. Z-DNA binding protein mediates host control of toxoplasma gondii infection. Infect Immun (2016) 84(10):3063–70. doi: 10.1128/IAI.00511-16
70. Cervantes PW, Martorelli Di Genova B, Erazo Flores BJ, Knoll LJ. RIPK3 facilitates host resistance to oral toxoplasma gondii infection. Infect Immun (2021) 89(5):e00021-21. doi: 10.1128/IAI.00021-21
71. Znidar K, Bosnjak M, Semenova N, Pakhomova O, Heller L, Cemazar M. Tumor cell death after electrotransfer of plasmid DNA is associated with cytosolic DNA sensor upregulation. Oncotarget. (2018) 9(27):18665–81. doi: 10.18632/oncotarget.24816
72. Saez-Freire MDM, Blanco-Gomez A, Castillo-Lluva S, Gomez-Vecino A, Galvis-Jimenez JM, Martin-Seisdedos C, et al. The biological age linked to oxidative stress modifies breast cancer aggressiveness. Free Radic Biol Med (2018) 120:133–46. doi: 10.1016/j.freeradbiomed.2018.03.012
73. Huang QF, Fang DL, Nong BB, Zeng J. Focal pyroptosis-related genes AIM2 and ZBP1 are prognostic markers for triple-negative breast cancer with brain metastases. Transl Cancer Res (2021) 10(11):4845–58. doi: 10.21037/tcr-21-2182
74. Baik JY, Liu Z, Jiao D, Kwon HJ, Yan J, Kadigamuwa C, et al. ZBP1 not RIPK1 mediates tumor necroptosis in breast cancer. Nat Commun (2021) 12(1):2666. doi: 10.1038/s41467-021-23004-3
75. Zhang T, Yin C, Fedorov A, Qiao L, Bao H, Beknazarov N, et al. ADAR1 masks the cancer immunotherapeutic promise of ZBP1-driven necroptosis. Nature. (2022) 606(7914):594–602. doi: 10.1038/s41586-022-04753-7
76. Yang Y, Wu M, Cao D, Yang C, Jin J, Wu L, et al. ZBP1-MLKL necroptotic signaling potentiates radiation-induced antitumor immunity. via intratumoral STING pathway activation. Sci Adv (2021) 7(41):eabf6290. doi: 10.1126/sciadvabf6290
77. Karki R, Sundaram B, Sharma BR, Lee S, Malireddi RKS, Nguyen LN, et al. ADAR1 restricts ZBP1-mediated immune response and PANoptosis to promote tumorigenesis. Cell Rep (2021) 37(3):109858. doi: 10.1016/j.celrep.2021.109858
78. Ponnusamy K, Tzioni MM, Begum M, Robinson ME, Caputo VS, Katsarou A, et al. The innate sensor ZBP1-IRF3 axis regulates cell proliferation in multiple myeloma. Haematologica. (2022) 107(3):721–32. doi: 10.3324/haematol2020274480
79. Liu Y, Cao H, Zhao Y, Shan L, Lan S. Fisetin-induced cell death in human ovarian cancer cell lines via zbp1-mediated necroptosis. J Ovarian Res (2022) 15(1):57. doi: 10.1186/s13048-022-00984-4
80. Cheng R, Liu X, Wang Z, Tang K. ABT737, a Bcl2 family inhibitor, has a synergistic effect with apoptosis by inducing urothelial carcinoma cell necroptosis. Mol Med Rep (2021) 23(6):412. doi: 10.3892/mmr.2021.12051
81. Chen W, Lin W, Wu L, Xu A, Liu C, Huang P. A novel prognostic predictor of immune microenvironment and therapeutic response in kidney renal clear cell carcinoma based on necroptosis-related gene signature. Int J Med Sci (2022) 19(2):377–92. doi: 10.7150/ijms.69060
82. Bass BL, Weintraub H. A developmentally regulated activity that unwinds RNA duplexes. Cell. (1987) 48(4):607–13. doi: 10.1016/0092-8674(87)90239-X
83. Bass BL, Weintraub H. An unwinding activity that covalently modifies its double-stranded RNA substrate. Cell. (1988) 55(6):1089–98. doi: 10.1016/0092-8674(88)90253-X
84. Herbert A, Alfken J, Kim YG, Mian IS, Nishikura K, Rich A. A z-DNA binding domain present in the human editing enzyme, double-stranded RNA adenosine deaminase. Proc Natl Acad Sci U S A. (1997) 94(16):8421–6. doi: 10.1073/pnas.94.16.8421
85. Song B, Shiromoto Y, Minakuchi M, Nishikura K. The role of RNA editing enzyme ADAR1 in human disease. Wiley Interdiscip Rev RNA. (2022) 13(1):e1665. doi: 10.1002/wrna.1665
86. Hubbard NW, Ames JM, Maurano M, Chu LH, Somfleth KY, Gokhale NS, et al. ADAR1 mutation causes ZBP1-dependent immunopathology. Nature. (2022) 607(7920):769–75. doi: 10.1038/s41586-022-04896-7
87. Jiao H, Wachsmuth L, Wolf S, Lohmann J, Nagata M, Kaya GG, et al. ADAR1 averts fatal type I interferon induction by ZBP1. Nature. (2022) 607(7920):776–83. doi: 10.1038/s41586-022-04878-9
88. de Reuver R, Verdonck S, Dierick E, Nemegeer J, Hessmann E, Ahmad S, et al. ADAR1 prevents autoinflammation by suppressing spontaneous ZBP1 activation. Nature. (2022) 607(7920):784–9. doi: 10.1038/s41586-022-04974-w
89. Epstein Y, Roberts WO. The pathopysiology of heat stroke: An integrative view of the final common pathway. Scand J Med Sci Sports. (2011) 21(6):742–8. doi: 10.1111/j.1600-0838.2011.01333.x
90. Lim CL. Heat sepsis precedes heat toxicity in the pathophysiology of heat stroke-a new paradigm on an ancient disease. Antioxidants (Basel). (2018) 7(11):149. doi: 10.3390/antiox7110149
91. Li H, Liu Y, Gu Z, Li L, Liu Y, Wang L, et al. p38 MAPK-MK2 pathway regulates the heat-stress-induced accumulation of reactive oxygen species that mediates apoptotic cell death in glial cells. Oncol Lett (2018) 15(1):775–82. doi: 10.3892/ol20177360
92. Yu T, Ferdjallah I, Elenberg F, Chen SK, Deuster P, Chen Y. Mitochondrial fission contributes to heat-induced oxidative stress in skeletal muscle but not hyperthermia in mice. Life Sci (2018) 200:6–14. doi: 10.1016/j.lfs.2018.02.031
93. Huang C, Jiao H, Song Z, Zhao J, Wang X, Lin H. Heat stress impairs mitochondria functions and induces oxidative injury in broiler chickens. J Anim Sci (2015) 93(5):2144–53. doi: 10.2527/jas.2014-8739
94. Kikusato M, Toyomizu M. Crucial role of membrane potential in heat stress-induced overproduction of reactive oxygen species in avian skeletal muscle mitochondria. PloS One (2013) 8(5):e64412. doi: 10.1371/journalpone0064412
95. Yi G, Li L, Luo M, He X, Zou Z, Gu Z, et al. Heat stress induces intestinal injury through lysosome- and mitochondria-dependent pathway. Vivo vitro. Oncotarget. (2017) 8(25):40741–55. doi: 10.18632/oncotarget.16580
96. Xie W, Huang W, Cai S, Chen H, Fu W, Chen Z, et al. NFkappaB/IkappaBalpha signaling pathways are essential for resistance to heat stressinduced ROS production in pulmonary microvascular endothelial cells. Mol Med Rep (2021) 24(5):149. doi: 10.3892/mmr.2021.12454
97. Zhang M, Zhu X, Tong H, Lou A, Li Y, Li Y, et al. AVE 0991 attenuates pyroptosis and liver damage after heatstroke by inhibiting the ROS-NLRP3 inflammatory signalling pathway. BioMed Res Int (2019) 2019:1806234. doi: 10.1155/2019/1806234
98. Huang W, Mao L, Xie W, Cai S, Huang Q, Liu Y, et al. Impact of UCP2 depletion on heat stroke-induced mitochondrial function in human umbilical vein endothelial cells. Int J Hyperthermia. (2022) 39(1):287–96. doi: 10.1080/02656736.2022.2032846
99. Tsai HY, Hsu YJ, Lu CY, Tsai MC, Hung WC, Chen PC, et al. Pharmacological activation of aldehyde dehydrogenase 2 protects against heatstroke-induced acute lung injury by modulating oxidative stress and endothelial dysfunction. Front Immunol (2021) 12:740562. doi: 10.3389/fimmu.2021.740562
100. Ni XX, Nie J, Xie QY, Yu RH, Su L, Liu ZF. Protective effects of hyperbaric oxygen therapy on brain injury by regulating the phosphorylation of Drp1 through ROS/PKC pathway in heatstroke rats. Cell Mol Neurobiol (2020) 40(8):1253–69. doi: 10.1007/s10571-020-00811-8
101. Wang X, Yuan B, Dong W, Yang B, Yang Y, Lin X, et al. Humid heat exposure induced oxidative stress and apoptosis in cardiomyocytes through the angiotensin II signaling pathway. Heart Vessels. (2015) 30(3):396–405. doi: 10.1007/s00380-014-0523-6
102. Pisoschi AM, Pop A. The role of antioxidants in the chemistry of oxidative stress: A review. Eur J Med Chem (2015) 97:55–74. doi: 10.1016/j.ejmech.2015.04.040
103. Li L, Tan H, Yang H, Li F, He X, Gu Z, et al. Reactive oxygen species mediate heat stress-induced apoptosis via ERK dephosphorylation and bcl-2 ubiquitination in human umbilical vein endothelial cells. Oncotarget. (2017) 8(8):12902–16. doi: 10.18632/oncotarget.14186
104. Geng Y, Ma Q, Liu YN, Peng N, Yuan FF, Li XG, et al. Heatstroke induces liver injury via IL-1beta and HMGB1-induced pyroptosis. J Hepatol (2015) 63(3):622–33. doi: 10.1016/j.jhep.2015.04.010
105. Tajeddine N. How do reactive oxygen species and calcium trigger mitochondrial membrane permeabilisation? Biochim Biophys Acta (2016) 1860(6):1079–88. doi: 10.1016/j.bbagen.2016.02.013
106. Gu ZT, Li L, Wu F, Zhao P, Yang H, Liu YS, et al. Heat stress induced apoptosis is triggered by transcription-independent p53, Ca(2+) dyshomeostasis and the subsequent bax mitochondrial translocation. Sci Rep (2015) 5:11497. doi: 10.1038/srep11497
107. Gong J, Gu Z, Su L. [Possible protective role of melatonin on heat stress induced apoptosis]. Zhonghua Wei Zhong Bing Ji Jiu Yi Xue. (2019) 31(5):658–61. doi: 10.3760/cmajissn2095-4352201905028
108. Li L, Tan H, Gu Z, Liu Z, Geng Y, Liu Y, et al. Heat stress induces apoptosis through a Ca(2)(+)-mediated mitochondrial apoptotic pathway in human umbilical vein endothelial cells. PloS One (2014) 9(12):e111083. doi: 10.3892/ol20177360
109. Li L, Tan H, Zou Z, Gong J, Zhou J, Peng N, et al. Preventing necroptosis by scavenging ROS production alleviates heat stress-induced intestinal injury. Int J Hyperthermia. (2020) 37(1):517–30. doi: 10.1080/02656736.2020.1763483
110. Font MD, Thyagarajan B, Khanna AK. Sepsis and septic shock - basics of diagnosis, pathophysiology and clinical decision making. Med Clin North Am (2020) 104(4):573–85. doi: 10.1016/j.mcna.2020.02.011
111. Zeller L, Novack V, Barski L, Jotkowitz A, Almog Y. Exertional heatstroke: Clinical characteristics, diagnostic and therapeutic considerations. Eur J Intern Med (2011) 22(3):296–9. doi: 10.1016/j.ejim.2010.12.013
112. Leon LR. Heat stroke and cytokines. Prog Brain Res (2007) 162:481–524. doi: 10.1016/S0079-6123(06)62024-4
113. Fajgenbaum DC, June CH. Cytokine storm. N Engl J Med (2020) 383(23):2255–73. doi: 10.1056/NEJMra2026131
114. Leon LR, Dineen S, Blaha MD, Rodriguez-Fernandez M, Clarke DC. Attenuated thermoregulatory, metabolic, and liver acute phase protein response to heat stroke in TNF receptor knockout mice. Am J Physiol Regul Integr Comp Physiol (2013) 305(12):R1421–1432. doi: 10.1152/ajpregu.00127.2013
115. Chauhan NR, Kapoor M, Prabha Singh L, Gupta RK, Meena Chand R, Tulsawani R, et al. Heat stress-induced neuroinflammation and aberration in monoamine levels in hypothalamus are associated with temperature dysregulation. Neuroscience. (2017) 358:79–92. doi: 10.1016/j.neuroscience.2017.06.023
116. Chang CY, Chen JY, Chen SH, Cheng TJ, Lin MT, Hu ML. Therapeutic treatment with ascorbate rescues mice from heat stroke-induced death by attenuating systemic inflammatory response and hypothalamic neuronal damage. Free Radic Biol Med (2016) 93:84–93. doi: 10.1016/j.freeradbiomed.2015.12.017
117. Biedenkapp JC, Leon LR. Increased cytokine and chemokine gene expression in the CNS of mice during heat stroke recovery. Am J Physiol Regul Integr Comp Physiol (2013) 305(9):R978–986. doi: 10.1152/ajpregu.00011.2013
118. Lee W, Moon M, Kim HG, Lee TH, Oh MS. Heat stress-induced memory impairment is associated with neuroinflammation in mice. J Neuroinflammation. (2015) 12:102. doi: 10.1186/s12974-015-0324-6
119. Li P, Shen T, Luo X, Yang J, Luo Z, Tan Y, et al. Modulation of microglial phenotypes by dexmedetomidine through TREM2 reduces neuroinflammation in heatstroke. Sci Rep (2021) 11(1):13345. doi: 10.1038/s41598-021-92906-5
120. Ikeda T, Tani N, Watanabe M, Hirokawa T, Ikeda K, Morioka F, et al. Evaluation of cytokines and structural proteins to analyze the pathology of febrile central nervous system disease. Leg Med (Tokyo). (2021) 51:101864. doi: 10.1016/j.legalmed.2021.101864
121. Chen CM, Hou CC, Cheng KC, Tian RL, Chang CP, Lin MT. Activated protein c therapy in a rat heat stroke model. Crit Care Med (2006) 34(7):1960–6. doi: 10.1097/01.CCM.0000224231.01533.B1
122. Liu CC, Chien CH, Lin MT. Glucocorticoids reduce interleukin-1 concentration and result in neuroprotective effects in rat heatstroke. J Physiol (2000) 527 Pt 2:333–43. doi: 10.1111/j.1469-7793.2000.t01-1-00333.x
123. Bouchama A, Kunzelmann C, Dehbi M, Kwaasi A, Eldali A, Zobairi F, et al. Recombinant activated protein c attenuates endothelial injury and inhibits procoagulant microparticles release in baboon heatstroke. Arterioscler Thromb Vasc Biol (2008) 28(7):1318–25. doi: 10.1161/ATVBAHA.107.161737
124. Zhong L, Wu M, Wang C, Yu B, Liu Z, Liu Z. Clinical characteristics and outcomes of patients with severe heatstroke complicated with disseminated intravascular coagulation: A case-control study. Thromb Res (2021) 197:120–3. doi: 10.1016/j.thromres.2020.11.009
125. Proctor EA, Dineen SM, Van Nostrand SC, Kuhn MK, Barrett CD, Brubaker DK, et al. Coagulopathy signature precedes and predicts severity of end-organ heat stroke pathology in a mouse model. J Thromb Haemost. (2020) 18(8):1900–10. doi: 10.1111/jth.14875
126. Ohbe H, Isogai S, Jo T, Matsui H, Fushimi K, Yasunaga H. Treatment with antithrombin or thrombomodulin and mortality from heatstroke-induced disseminated intravascular coagulation: A nationwide observational study. Semin Thromb Hemost. (2019) 45(8):760–6. doi: 10.1055/s-0039-1700520
127. Matsumoto H, Takeba J, Umakoshi K, Nakabayashi Y, Moriyama N, Annen S, et al. Successful treatment for disseminated intravascular coagulation (DIC) corresponding to phenotype changes in a heat stroke patient. J Intensive Care (2019) 7:2. doi: 10.1186/s40560-019-0359-3
128. Iba T, Helms J, Levi M, Levy JH. The role of platelets in heat-related illness and heat-induced coagulopathy. Thromb Res (2022) 17:S0049-3848(22)00342-5 . doi: 10.1016/j.thromres.2022.08.009
129. Iba T, Connors JM, Levi M, Levy JH. Heatstroke-induced coagulopathy: Biomarkers, mechanistic insights, and patient management. EClinicalMedicine. (2022) 44:101276. doi: 10.1016/j.eclinm.2022.101276
130. Hifumi T, Kondo Y, Shimazaki J, Oda Y, Shiraishi S, Wakasugi M, et al. Prognostic significance of disseminated intravascular coagulation in patients with heat stroke in a nationwide registry. J Crit Care (2018) 44:306–11. doi: 10.1016/j.jcrc.2017.12.003
131. He L, Lin Q, Zhong L, Zeng Q, Song J. Thromboelastography maximum amplitude as an early predictor of disseminated intravascular coagulation in patients with heatstroke. Int J Hyperthermia. (2022) 39(1):605–10. doi: 10.1080/02656736.2022.2066206
132. Goto K, Masuda T, Ohashi J, Nakatsu Y, Nakamura F. Biventricular thrombi associated with cardiac systolic dysfunction and disseminated intravascular coagulation from heat stroke. Int Heart J (2021) 62(3):687–94. doi: 10.1536/ihj.20-558
133. Bruchim Y, Kelmer E, Cohen A, Codner C, Segev G, Aroch I. Hemostatic abnormalities in dogs with naturally occurring heatstroke. J Vet Emerg Crit Care (San Antonio). (2017) 27(3):315–24. doi: 10.1111/vec.12590
134. Chen F, Li H, Zhu G, Chen X, Tang Z. Sodium tanshinone IIA sulfonate improves inflammation, aortic endothelial cell apoptosis, disseminated intravascular coagulation and multiple organ damage in a rat heat stroke model. Mol Med Rep (2017) 16(1):87–94. doi: 10.3892/mmr.2017.6573
135. Chen J, Song Q, Li R, Zhang T, Miao L, Wu Z. Role of heparin in early treatment of exertional heatstroke in rats. Acad J Chin PLA Med School. (2014) 16:87–94.
136. Al Ghumlas AK, Abdel Gader AG, Hussein MF, Al Haidary A, White JG. Effects of heat on camel platelet structure and function-a comparative study with humans. Platelets. (2008) 19(3):163–71. doi: 10.1080/09537100701882061
137. Thulesius O. Thermal reactions of blood vessels in vascular stroke and heatstroke. Med Princ Pract (2006) 15(4):316–21. doi: 10.1159/000092999
138. Bain AR, Ainslie PN, Bammert TD, Hijmans JG, Sekhon M, Hoiland RL, et al. Passive heat stress reduces circulating endothelial and platelet microparticles. Exp Physiol (2017) 102(6):663–9. doi: 10.1113/EP086336
139. Burger D, Montezano AC, Nishigaki N, He Y, Carter A, Touyz RM. Endothelial microparticle formation by angiotensin II is mediated via ang II receptor type I/NADPH oxidase/ rho kinase pathways targeted to lipid rafts. Arterioscler Thromb Vasc Biol (2011) 31(8):1898–907. doi: 10.1161/ATVBAHA.110.222703
140. Melki I, Tessandier N, Zufferey A, Boilard E. Platelet microvesicles in health and disease. Platelets. (2017) 28(3):214–21. doi: 10.1080/09537104.2016.1265924
141. McDonald B, Davis RP, Kim SJ, Tse M, Esmon CT, Kolaczkowska E, et al. Platelets and neutrophil extracellular traps collaborate to promote intravascular coagulation during sepsis in mice. Blood. (2017) 129(10):1357–67. doi: 10.1182/blood-2016-09-741298
142. Hisada Y, Sachetto ATA, Mackman N. Circulating tissue factor-positive extracellular vesicles and their association with thrombosis in different diseases. Immunol Rev (2022) 312(1):61–75. doi: 10.1111/imr.13106
143. Bouchama A, Al-Mohanna F, Assad L, Baturcam E, Eldali A, Owaidah T, et al. Tissue factor/factor VIIa pathway mediates coagulation activation in induced-heat stroke in the baboon. Crit Care Med (2012) 40(4):1229–36. doi: 10.1097/CCM.0b013e3182387bef
144. Khan AM, Finlay JM, Clarke P, Sol K, Melendez R, Judd S, et al. Association between temperature exposure and cognition: A cross-sectional analysis of 20,687 aging adults in the united states. BMC Public Health (2021) 21(1):1484. doi: 10.1186/s12889-021-11533-x
145. Kamidani R, Okada H, Kitagawa Y, Kusuzawa K, Ichihashi M, Kakino Y, et al. Severe heat stroke complicated by multiple cerebral infarctions: A case report. J Med Case Rep (2021) 15(1):24. doi: 10.1186/s13256-020-02596-2
146. Audet GN, Dineen SM, Quinn CM, Leon LR. Altered hypothalamic inflammatory gene expression correlates with heat stroke severity in a conscious rodent model. Brain Res (2016) 1637:81–90. doi: 10.1016/j.brainres.2016.01.048
147. Zhang ZT, Gu XL, Zhao X, He X, Shi HW, Zhang K, et al. NLRP3 ablation enhances tolerance in heat stroke pathology by inhibiting IL-1beta-mediated neuroinflammation. J Neuroinflammation. (2021) 18(1):128. doi: 10.1186/s12974-021-02179-y
148. Cabral BMI, Edding SN, Portocarrero JP, Lerma EV. Rhabdomyolysis. Dis Mon. (2020) 66(8):101015. doi: 10.1016/j.disamonth.2020.101015
149. Chen GD, Fan H, Zhu JH. Salidroside pretreatment protects against myocardial injury induced by heat stroke in mice. J Int Med Res (2019) 47(10):5229–38. doi: 10.1177/0300060519868645
150. Lin CH, Tsai CC, Chen TH, Chang CP, Yang HH. Oxytocin maintains lung histological and functional integrity to confer protection in heat stroke. Sci Rep (2019) 9(1):18390. doi: 10.1038/s41598-019-54739-1
151. Cao Y, Liu Z, Xiao W, Gu Z, Xiao G, Yuan F, et al. 4-phenylbutyrate prevents endoplasmic reticulum stress-mediated apoptosis induced by heatstroke in the intestines of mice. Shock. (2020) 54(1):102–9. doi: 10.1097/SHK.0000000000001419
152. Lian P, Braber S, Garssen J, Wichers HJ, Folkerts G, Fink-Gremmels J, et al. Beyond heat stress: Intestinal integrity disruption and mechanism-based intervention strategies. Nutrients. (2020) 12(3):734. doi: 10.3390/nu12030734
153. King MA, Rollo I, Baker LB. Nutritional considerations to counteract gastrointestinal permeability during exertional heat stress. J Appl Physiol (1985). (2021) 130(6):1754–65. doi: 10.1152/japplphysiol.00072.2021
154. Cao Y, Fan M, Pei Y, Su L, Xiao W, Chen F, et al. CCAAT/Enhancer-binding protein homologous protein (CHOP) deficiency attenuates heatstroke-induced intestinal injury. Inflammation. (2022) 45(2):695–711. doi: 10.1007/s10753-021-01577-x
155. Hii HP, Lo WZ, Fu YH, Chen MH, Shih CC, Tsao CM, et al. Improvement in heat stress-induced multiple organ dysfunction and intestinal damage through protection of intestinal goblet cells from prostaglandin E1 analogue misoprostol. Life Sci (2022) 310:121039. doi: 10.1016/j.lfs.2022.121039
156. Li YN, Tao H, Hong JH, Xiong YL, Pan XC, Liu Y, et al. The Chinese herbal formula huoxiang zhengqi dropping pills prevents acute intestinal injury induced by heatstroke by increasing the expression of claudin-3 in rats. Evid Based Complement Alternat Med (2022) 2022:9230341. doi: 10.1155/2022/9230341
157. Pan Z, He X, Shao Y, Chen W, Fang B. ROS/JNK-mediated lysosomal injury in rat intestinal epithelial-6 cells during heat stress. J Therm Biol (2022) 109:103326. doi: 10.1016/j.jtherbio.2022.103326
158. Schlader ZJ, Davis MS, Bouchama A. Biomarkers of heatstroke-induced organ injury and repair. Exp Physiol (2022) 107(10):1159–71. doi: 10.1113/EP090142
159. Sato Y, Roncal-Jimenez CA, Andres-Hernando A, et al. Increase of core temperature affected the progression of kidney injury by repeated heat stress exposure. Am J Physiol Renal Physiol (2019) 317(5):F1111–21. doi: 10.1152/ajprenal.00259.2019
160. Thongprayoon C, Qureshi F, Petnak T, Cheungpasitporn W, Chewcharat A, Cato LD, et al. Impact of acute kidney injury on outcomes of hospitalizations for heat stroke in the united states. Diseases. (2020) 8(3):28. doi: 10.3390/diseases8030028
161. Wang C, Yu B, Chen R, Su L, Wu M, Liu Z. Association of d-dimer and acute kidney injury associated with rhabdomyolysis in patients with exertional heatstroke: An over 10-year intensive care survey. Ren Fail (2021) 43(1):1561–8. doi: 10.1080/0886022X.2021.2008975
162. Wu M, Wang C, Liu Z, Zhong L, Yu B, Chengc B, et al. Clinical characteristics and risk factors associated with acute kidney injury inpatient with exertional heatstroke: An over 10-year intensive care survey. Front Med (Lausanne). (2021) 8:678434. doi: 10.3389/fmed.2021.678434
163. Xue L, Guo W, Li L, Ou S, Zhu T, Cai L, et al. Metabolomic profiling identifies a novel mechanism for heat stroke−related acute kidney injury. Mol Med Rep (2021) 23(4):241. doi: 10.3892/mmr.2021.11880
164. Zhao YH, Shen CF, Kang Y, Qi A, Xu WJ, Shi WH, et al. Curcumin prevents renal cell apoptosis in acute kidney injury in a rat model of dry-heat environment heatstroke via inhibition of the mitochondrial apoptotic pathway. Exp Ther Med (2021) 21(2):126. doi: 10.3892/etm20209558
165. Song R, Li Q, Hu J, Yi H, Mao Z, Zhou F. A mouse model of exertional heatstroke-related acute kidney injury. Ann Transl Med (2022) 10(6):276. doi: 10.21037/atm-22-715
166. Wu M, Wang C, Zhong L, Liu Z. Serum myoglobin as predictor of acute kidney injury and 90-day mortality in patients with rhabdomyolysis after exertional heatstroke: An over 10-year intensive care survey. Int J Hyperthermia. (2022) 39(1):446–54. doi: 10.1080/02656736.2022.2046183
167. Bi X, Deising A, Frenette C. Acute liver failure from exertional heatstroke can result in excellent long-term survival with liver transplantation. Hepatology. (2020) 71(3):1122–3. doi: 10.1002/hep.30938
168. Monzon B, Hegarty K, Rech MA. A knack for "NAC": Treatment for heat stroke induced acute liver injury. Am J Emerg Med (2020) 38(4):853.e851–853.e853. doi: 10.1016/j.ajem.2019.11.029
169. Ribeiro F, Bibi M, Pereira M, Ferreira S, Pessegueiro H, Araujo R. Severe acute liver injury related to heat stroke. Eur J Case Rep Intern Med (2020) 7(2):001382. doi: 10.12890/2020_001382
170. Huang W, Xie W, Zhong H, Cai S, Huang Q, Liu Y, et al. Cytosolic p53 inhibits parkin-mediated mitophagy and promotes acute liver injury induced by heat stroke. Front Immunol (2022) 13:859231. doi: 10.3389/fimmu.2022.859231
171. Li C, Su HB, Li H, Li X, Wang HM, Song Q, et al. Severe acute liver injury in patients with exertional heat stroke associated with poor short-term prognosis. World J Emerg Med (2022) 13(2):124–9. doi: 10.5847/wjem.j.1920-8642.2022.047
172. Lin JS, Zaffar D, Muhammad H, Ting PS, Woreta T, Kim A, et al. Exertional heat stroke-induced acute liver failure and liver transplantation. ACG Case Rep J (2022) 9(7):e00820. doi: 10.14309/crj.0000000000000820
173. Martins PN, Bruggenwirth IMA, McDaid J, Hertl M, Kawai T, Elias N, et al. Heat stroke as a cause of liver failure and evaluation of liver transplant. Exp Clin Transplant. (2022) 20(6):621–6. doi: 10.6002/ect.2018.0003
174. Wang F, Zhang Y, Li J, Xia H, Zhang D, Yao S. The pathogenesis and therapeutic strategies of heat stroke-induced liver injury. Crit Care (2022) 26(1):391. doi: 10.1186/s13054-022-04273-w
175. Tong H, Tang Y, Chen Y, Yuan F, Liu Z, Peng N, et al. HMGB1 activity inhibition alleviating liver injury in heatstroke. J Trauma Acute Care Surg (2013) 74(3):801–7. doi: 10.1097/TA.0b013e31827e9a65
176. Wu WS, Chou MT, Chao CM, Chang CK, Lin MT, Chang CP. Melatonin reduces acute lung inflammation, edema, and hemorrhage in heatstroke rats. Acta Pharmacol Sin (2012) 33(6):775–82. doi: 10.1038/aps.2012.29
177. Liu Z, Chen J, Hu L, Li M, Liang M, Chen J, et al. Expression profiles of genes associated with inflammatory responses and oxidative stress in lung after heat stroke. Biosci Rep (2020) 40(6):BSR20192048. doi: 10.1042/BSR20192048
178. Huang W, Xie W, Gong J, Wang W, Cai S, Huang Q, et al. Heat stress induces RIP1/RIP3-dependent necroptosis through the MAPK, NF-kappaB, and c-jun signaling pathways in pulmonary vascular endothelial cells. Biochem Biophys Res Commun (2020) 528(1):206–12. doi: 10.1016/j.bbrc.2020.04.150
179. Zhou G, Chen Z, Li J, Guo X, Qin K, Luo J, et al. Role of the receptor for advanced glycation end products in heat stress-induced endothelial hyperpermeability in acute lung injury. Front Physiol (2020) 11:1087. doi: 10.3389/fphys.2020.01087
180. Tseng CK, Liu TT, Lin TC, Cheng CP. Expression of heme oxygenase-1 in type II pneumocytes protects against heatstroke-induced lung damage. Cell Stress Chaperones. (2021) 26(1):67–76. doi: 10.1007/s12192-020-01152-7
181. Chen H, Lin X, Yi X, Liu X, Yu R, Fan W, et al. SIRT1-mediated p53 deacetylation inhibits ferroptosis and alleviates heat stress-induced lung epithelial cells injury. Int J Hyperthermia. (2022) 39(1):977–86. doi: 10.1080/02656736.2022.2094476
182. Dong X, Li JJ, Ma N, Liu AZ, Liu JW. Anti-inflammatory and anti-oxidative effects of isorhamnetin for protection against lung injury in a rat model of heatstroke in a dry-heat environment. Med Sci Monit (2022) 28:e935426. doi: 10.12659/MSM.935426
183. Xia X, Zhang C, Li L, Wang S, Ding X, He J, et al. Bovine lactoferrin alleviates pulmonary lipid peroxidation and inflammatory damage in heat stroke rats. Ther Hypothermia Temp Manage (2022) 12(4):223–8. doi: 10.1089/ther.2022.0002
184. Laitano O, Sheikh LH, Mattingly AJ, Murray KO, Ferreira LF, Clanton TL. Osmolality selectively offsets the impact of hyperthermia on mouse skeletal muscle. vitro. Front Physiol (2018) 9:1496. doi: 10.3389/fphys.2018.01496
185. Lee BH. Atypical brain imaging findings associated with heat stroke: A patient with rhabdomyolysis and acute kidney injury: A case report. Radiol Case Rep (2020) 15(5):560–3. doi: 10.1016/j.radcr.2020.02.007
186. Marlega J, Mickiewicz A, Fijalkowska J, Gruchala M, Fijalkowski M. Exertional heat stroke in an amateur runner - challenges in diagnostics and the role of unhealthy competition. J Sports Sci (2020) 38(22):2597–602. doi: 10.1080/02640414.2020.1794256
187. Thongprayoon C, Petnak T, Kanduri SR, Kovvuru K, Cheungpasitporn W, Boonpheng B, et al. Impact of rhabdomyolysis on outcomes of hospitalizations for heat stroke in the united states. Hosp Pract (1995). (2020) 48(5):276–81. doi: 10.1080/21548331.2020.1792214
188. Ubaldo OGV, Quiwa K, Rollan RE, Tripon E, Sebastian E. The fire from within: Multiorgan failure with bimodal rhabdomyolysis from exertional heat stroke. Case Rep Hepatol (2020) 2020:1305730. doi: 10.1155/2020/1305730
189. Dhingra J, Baum Y. Bone scanning findings in a patient with heat stroke-induced rhabdomyolysis. J Nucl Med Technol (2021) 49(4):362–3. doi: 10.2967/jnmt.121.262501
190. Kaewput W, Thongprayoon C, Petnak T, Cato LD, Chewcharat A, Boonpheng B, et al. Inpatient burden and mortality of heatstroke in the united states. Int J Clin Pract (2021) 75(4):e13837. doi: 10.1111/ijcp.13837
191. Wu M, Wang C, Liu Z, Liu Z. Sequential organ failure assessment score for prediction of mortality of patients with rhabdomyolysis following exertional heatstroke: A longitudinal cohort study in southern China. Front Med (Lausanne). (2021) 8:724319. doi: 10.3389/fmed.2021.724319
192. Xu Q, Tian M, Xia J, Zhu W, Yang L. Application of ultrasonography in the diagnosis of rhabdomyolysis. Ultrasound Med Biol (2021) 47(12):3349–55. doi: 10.1016/j.ultrasmedbio.2021.08.012
193. He S, Li R, Peng Y, et al. ACSL4 contributes to ferroptosis-mediated rhabdomyolysis in exertional heat stroke. J Cachexia Sarcopenia Muscle. (2022) 13(3):1717–30. doi: 10.1002/jcsm.12953
194. Gardner L, Miller DM, Daly C, Wang Z, Huang J, Meng H, et al. Investigating the genetic susceptibility to exertional heat illness. J Med Genet (2020) 57(8):531–41. doi: 10.1136/jmedgenet-2019-106461
195. Dlamini N, Voermans NC, Lillis S, Stewart K, Kamsteeg EJ, Drost G, et al. Mutations in RYR1 are a common cause of exertional myalgia and rhabdomyolysis. Neuromuscul Disord (2013) 23(7):540–8. doi: 10.1016/j.nmd.2013.03.008
196. Roux-Buisson N, Monnier N, Sagui E, Abriat A, Brosset C, Bendahan D, et al. Identification of variants of the ryanodine receptor type 1 in patients with exertional heat stroke and positive response to the malignant hyperthermia in vitro contracture test. Br J Anaesth. (2016) 116(4):566–8. doi: 10.1093/bja/aew047
197. Lanner JT, Georgiou DK, Dagnino-Acosta A, Ainbinder A, Cheng Q, Joshi AD, et al. AICAR prevents heat-induced sudden death in RyR1 mutant mice independent of AMPK activation. Nat Med (2012) 18(2):244–51. doi: 10.1038/nm.2598
198. Laitano O, Oki K, Leon LR. The role of skeletal muscles in exertional heat stroke pathophysiology. Int J Sports Med (2021) 42(8):673–81. doi: 10.1055/a-1400-9754
199. Hyatt HW, Powers SK. The role of calpains in skeletal muscle remodeling with exercise and inactivity-induced atrophy. Int J Sports Med (2020) 41(14):994–1008. doi: 10.1055/a-1199-7662
200. Snipe RMJ, Khoo A, Kitic CM, Gibson PR, Costa RJS. The impact of mild heat stress during prolonged running on gastrointestinal integrity, gastrointestinal symptoms, systemic endotoxin and cytokine profiles. Int J Sports Med (2018). doi: 10.1055/s-0043-122742
201. Karl JP, Hatch AM, Arcidiacono SM, et al. Effects of psychological, environmental and physical stressors on the gut microbiota. Front Microbiol (2018) 9:2013. doi: 10.3389/fmicb.2018.02013
202. Ducray HAG, Globa L, Pustovyy O, Morrison E, Vodyanoy V, Sorokulova I. Yeast fermentate prebiotic improves intestinal barrier integrity during heat stress by modulation of the gut microbiota in rats. J Appl Microbiol (2019) 127(4):1192–206. doi: 10.1111/jam.14361
Keywords: ZBP1, heatstroke, RIPK3, programmed cell death, necroptosis
Citation: Li F, Deng J, He Q and Zhong Y (2023) ZBP1 and heatstroke. Front. Immunol. 14:1091766. doi: 10.3389/fimmu.2023.1091766
Received: 09 November 2022; Accepted: 19 January 2023;
Published: 10 February 2023.
Edited by:
Ana Maria Gamero, Temple University, United StatesReviewed by:
Jason Upton, Auburn University, United StatesYuanyuan Zhao, Huazhong University of Science and Technology, China
Copyright © 2023 Li, Deng, He and Zhong. This is an open-access article distributed under the terms of the Creative Commons Attribution License (CC BY). The use, distribution or reproduction in other forums is permitted, provided the original author(s) and the copyright owner(s) are credited and that the original publication in this journal is cited, in accordance with accepted academic practice. No use, distribution or reproduction is permitted which does not comply with these terms.
*Correspondence: Qiuli He, aGVxbGk1MDQxNzc3MzRAMTYzLmNvbQ==; Yanjun Zhong, emhvbmd5YW5qdW5AY3N1LmVkdS5jbg==