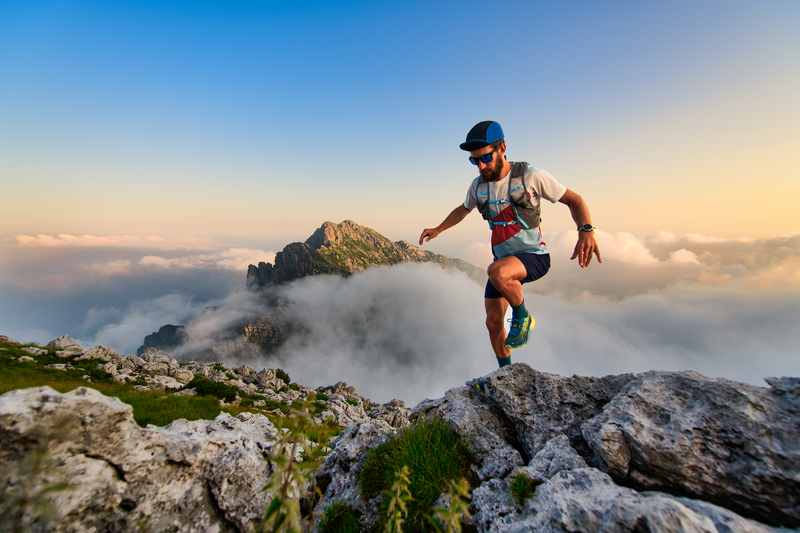
95% of researchers rate our articles as excellent or good
Learn more about the work of our research integrity team to safeguard the quality of each article we publish.
Find out more
REVIEW article
Front. Immunol. , 30 January 2023
Sec. Multiple Sclerosis and Neuroimmunology
Volume 14 - 2023 | https://doi.org/10.3389/fimmu.2023.1087815
This article is part of the Research Topic How Age and Sex Regulate Immunometabolism in Stroke Pathology View all 5 articles
Ischemic stroke is one of the world’s leading causes of death and disability. It has been established that gender differences in stroke outcomes prevail, and the immune response after stroke is an important factor affecting patient outcomes. However, gender disparities lead to different immune metabolic tendencies closely related to immune regulation after stroke. The present review provides a comprehensive overview of the role and mechanism of immune regulation based on sex differences in ischemic stroke pathology.
Stroke is well-established as the second most common cause of death worldwide (1), with significantly different outcomes between males and females (2). In this respect, current evidence suggests that females have a worse prognosis after a stroke than males, despite males having a larger incidence of stroke overall (3, 4). Generally, females are more prone to death from stroke than males (5).
An inflammatory response in the central nervous system is known as neurological inflammation. Several CNS cells, such as glial cells, endothelial cells and even immune cells in the periphery, release mediators such as cytokines, chemokines, reactive oxygen species, and secondary messengers, which play important roles in regulating CNS function. However, the immune response, a combination of innate and adaptive immune responses, is involved in normal brain growth and certain pathological conditions, including dementia and stroke (6). Inflammation caused by stroke contributes to the poor prognosis of ischemic stroke by causing neurological injury (7).
The heterogeneity in immune responses to invading and autoantigens is linked to gender differences in autoimmune illnesses, infectious disease susceptibility, vaccine efficacy and age-associated diseases, including Alzheimer’s (8, 9). It has been reported that the immune response to both foreign antigens and autoantigens is stronger in females. Current evidence suggests that females are more prone to autoimmune diseases than males (10). Compared to males, females have a higher lifetime risk of stroke, mostly during the older postmenopausal period (11). Overall, the immune systems of men and females may be fundamentally different, which may explain this discrepancy.
The immune reaction in males and females is affected by the biological differences between the sexes, which determine how immune cells react to their respective environments, as shown in Figure 1. Due to their higher sensitivity to antigens, robust immune response, ability to successfully fight off infections, propensity to produce inflammation, and higher prevalence of autoimmune diseases, females are more susceptible than males to autoimmune disorders (12). An increasing body of evidence suggests that females have more toll-like receptors and a higher abundance and function of monocytes, macrophages, and dendritic cells than males (13). The adaptive immune system and the Th1 response are also more active in females than in males (14). Furthermore, sex hormones influence the maturation and maintenance of the immune system. Gender disparities in immunological response have been associated with the three major gonadal hormones-estrogen, progesterone, and androgen. Estrogen receptors are expressed in T lymphocytes, B lymphocytes, natural killer (NK) cells, macrophages, and neutrophils (11, 15).
Figure 1 Sex difference in immunity. Estrogen, progesterone and testosterone regulate immune tendency by regulating immune cell function and cytokine release.
Current evidence indicates that estrogen plays a role in neutrophil apoptosis, chemotaxis and the development of neutrophil extracellular traps (NETs) (16). Female neutrophils suppress cellular apoptosis more effectively than their male counterparts (17). The combination of estrogen and progesterone inhibits cytochrome C-mediated spontaneous apoptosis (17). Estrogen can also increase CCR5 expression, stimulating T cell homing (18). Estrogen has been shown to decrease oxidative metabolism (19), increase the production of anti-inflammatory annexin A1, block neutrophil activation (20), and decrease the release of proinflammatory cytokines such as tumor necrosis factor-α (TNF-α), Interleukin-1β (IL-1β) and IL-6 secreted by neutrophils and macrophages (21–24), which finally suppresses nuclear factor kappa-B (NF-κB) activity (25). Estrogen can decrease the expression of pro-inflammatory cytokines and chemokines, such as IL-6, IFN-γ, IL-12, CXCL8 and CCL2, up-regulate the expression of inhibitory molecules PD-L1 and PD-L2, and regulate the expression of cytokines IL-10 and TGF-β, resulting in T helper 1 (Th1) cell activation fluctuates and bias the response towards a T helper 2(Th2) phenotype (26–28). The antiviral immune response relies on plasmacytoid dendritic cells (pDC), and estrogen plays an important role in maintaining pDC homeostasis (29).
High doses of estrogen reportedly yield an immunoregulatory effect, boosting the humoral immune response to control the inflammatory response and promoting Th2 polarization and IL-4 secretion (30–32). In other words, a small amount of estrogen can cause naive T cells to develop into effector Th1 cells and produce the proinflammatory cytokine IFN-γ (33). Antibody production is correlated with estrogen dosage (34). Estrogen can promote the activity of B cells by downregulating the expression of CD80 and increasing the total amount of IgG antibodies in B cells to improve the survival rate of B cells (35).
The activation of NK cells, macrophages and dendritic cells in mice is inhibited by progesterone. Progesterone has been shown to limit the synthesis of chemokines such as macrophage inflammatory protein-1α (MIP-1α), macrophage inflammatory protein-1β, (MIP-1β) and RANTES by CD8+ T cells in addition to reducing the production of cytokines (36). Many immune cells, such as T cells and NK cells, have progesterone receptors on their surface (37). Progesterone, like estrogen, yields an anti-inflammatory effect by modulating the activity of microglia and astrocytes and raising the secretion of Tregs while lowering the activity of CD8+ T cells, T helper cells 17(Th17) and Th1 cells (38–40). In animal stroke models, progesterone has been shown to decrease infarct volume, improve neurological damage and lengthen survival time (41, 42). Reducing oxidative damage, apoptosis, blood-brain barrier breakdown, and hemorrhagic transformation (43)are some benefits of progesterone, which also controls gamma-aminobutyric acid type A (GABAA) receptors and antagonizes excitatory toxicity (42, 44).
Combination therapy with estrogen and progesterone in a middle cerebral artery occlusion (MCAO) model has been shown to protect female rats against brain injury by decreasing the expression of cortical Iba1 and CD3, as well as by inhibiting the expression of IL-6 and chemokines (CCL2, CCL5) (45). It is widely acknowledged that the peptide hormone oxytocin promotes breastfeeding and delivery. In addition to the mammary gland and the uterus, oxytocin receptors are widely distributed throughout the brain and immune system (46). Females often have higher oxytocin secretion rates than males (47). Ischemic stroke patients may benefit from oxytocin’s neuroprotective properties (48), most likely due to the hormone’s mediation of neuroimmune and anti-inflammatory properties (46).
Testosterone and dihydrotestosterone, two types of androgens, modulate the immune system by favoring the development of a Th1 response and activating CD8+ T cells while enhancing the NK cell response, elevating TNF-α, and decreasing IL-10 (49–51). When testosterone is present in vitro, the ratio of Th1:Th2 cells in male peripheral blood shifts toward more Th1 cells (52). Testosterone’s ability to bind to androgen receptors in the brain allows it to direct genetic transcription or regulate intracellular signaling pathways, affecting apoptosis, blood-brain barrier integrity, cerebral blood flow, and neuroinflammation (53, 54). Estrogens may mediate these protective effects of androgens because many androgens are aromatized into estrogens (55). When it comes to the immunological response, androgens play a key role in controlling both the innate and adaptive arms of the immune system. It is well established that androgens have immunosuppressive effects, acting on numerous arms of the immune system to dampen their activity. Androgens inhibit the immune system by acting on several immune system components (56). Androgens have also been reported to yield immunomodulatory effects. For instance, testicular-ablated males demonstrated higher surface expression of toll-like receptor 4 (TLR4) and greater vulnerability to lipopolysaccharide (LPS)-induced shock (57).
X chromosomal variations account for most of the variance in innate immune response across sexes (58). Both innate and adaptive immunological genes (such as TLR7, IRAK1, IL2RG, FOXP3, and CD40L) (58) are located on the X chromosome. The second X chromosome’s genes are normally muted; however, as people age, some of these genes can avoid being inactivated (XCI). On the X chromosome, 15% of the genes can avoid XCI (59), and their expression is higher in females (60, 61), leading to varying immunological responses (62). TLR7, TLR8 (63, 64) and the transcription factor FoxP3 in regulatory t helper cells have been linked to the innate immune response against viral infection, and females tend to have higher expression levels of these receptors than males (65). Moreover, several microRNAs on the X chromosome affect the immune system (66).
The promoters of several innate immune genes contain hormone-responsive regions (30, 67), including TLR7, MyD88, IRF7, and TLR3. Protein interactions between hormone receptors and other transcription factors that bind to DNA, such as NF-κB, specific protein 1 (Sp1), CCAAT/enhancer binding protein β(C/EBPβ) and activator protein 1 (AP-1) (31, 68), regulate gene expression and play a role in the production of proinflammatory molecules by innate immune cells. The estrogen receptor’s association with NF-κB and C/EBP β has been reported to reduce IL-6 production, indicating that sex hormones like testosterone and estrogen are crucial for innate immune responses. The Sry gene on the Y chromosome drives the growth of the testis and the generation of testosterone during the embryonic stage, demonstrating that sex chromosomes are not fully independent (58). There are counteracting effects on the immune response from chromosome complement and sex hormones (69). A study found that the immune response to autoantigens was enhanced in ovariectomized XYSry- (mice lacking Sry expression on the Y chromosome) mice compared to ovariectomized XX mice, demonstrating the usefulness of the male sex chromosome complement. However, testosterone injection dampened the immunological response, suggesting a compensatory effect between the male chromosomal complement and testosterone (69).
A dichotomy has been proposed for macrophage activation: classic vs. alternative, also M1 and M2, respectively. Like helper T cells, immunological stimulation favors the M1 polarization of male macrophages and the M2 polarization of female macrophages (70, 71). he immunological response elicited in males and females varies in intensity and nature. Macrophages are reportedly polarized toward M1 under the influence of IFN-γ and LPS and toward M2 under the influence of IL-4 or IL-13 and engaged in symbiotic relationships with other cell types to increase diversity (72, 73). These cell types include fibroblasts, mesenchymal stem cells, endothelial cells, T cells, B cells and NK cells. In this respect, mice infected with coxsackievirus B3 exhibited sex-specific differences in macrophage polarization and myocarditis severity (74). Female macrophages are associated with M2 phenotypes, while M1 activation markers are more prevalent in infected male macrophages (74). Macrophage polarization appears gender-specific in viral myocarditis, with M1 macrophages potentially damaging the host and M2 macrophages protecting against infection. In addition, the M2 phenotype macrophage has been associated to the increased susceptibility of female mice to asthma compared to male mice (75, 76). It has been shown that macrophage abundance and TLR2, TLR3 and TLR4 expression are higher, and phagocytosis is increased in females compared to males (77).
T-cell activation in the immunological response exhibits clear sexual dimorphism. The ‘classical’ Th1 and ‘surrogate’ Th2 activation states are two of the many possible activation states of helper T cells. Th1 cell activity and secretion of related cytokines, such as IFN-γ and Interleukin-2(IL-2), are more prevalent in males, while ‘surrogate’ Th2 cell activity and the secretion of IL-4 and IL-10 are more common in females (52, 78). Mice have been found to exhibit similar sex-dependent effects, with males displaying more active Th1 responses and females displaying more active Th2 cell activity (79). Another example is the anti-inflammatory cytokine IL-10, which controls the release of T cells and Th2 cells and is associated with gender. An elevated IL-10 level is associated with a poor prognosis and immunosuppression in females, whereas this relationship is less clear in males. Stroke patients who produce high levels of IL-10 may be more susceptible to infection (80, 81). Lower levels of IL-10 production by cytotoxic T cells after stroke have been documented in males compared to females (82).
Reduced abundance of B cells throughout the body due to age and gender is associated with a loss of neurotrophic signals produced by B cells, an increase in the deleterious effects of B cell antibody production, and a decline in mental acuity (83). Besides, the protein B cell maturation antigen (BCMA) controls the division of B cells and the development of plasma cells (84). Moreover, demyelination, infiltration by inflammatory T cells and macrophages, and the severity of neuroinflammation are reportedly worse in males who lack BCMA than in females (85). Conclusions drawn from these studies suggest that the immunogenicity of the neuroinflammatory milieu is strongly influenced by gender in terms of the mechanisms governing the proliferation, survival, and differentiation of B cells. Although the overall abundance of B cells increased dramatically in women after stroke, the number of regulatory B cells decreased in the spleen compared to males (86). This discrepancy may be attributed to differences in migration to the brain. After experimental autoimmune encephalomyelitis, estrogen was found to increase the abundance of regulatory B cells in the female brain (87).
The pathogenesis of the ischemic brain is mediated by the immune response following a stroke. The neuronal cell death cascade begins with the release of inflammatory signals from immune cells triggered by brain damage. Glial cells and infiltrating leukocytes, including neutrophils, monocytes, and lymphocytes, make up the bulk of the immune system. The regulation of neuronal damage and wound healing after an ischemic stroke depends on glial cell activation and the production of proinflammatory and anti-inflammatory signals. White blood cells that have entered the damage site release inflammatory mediators, which worsen brain injury.
Microglia are macrophage-like cells in the CNS (88), making up around 5% - 12% of all brain cells (89). When the brain is injured, such as during a stroke, the first line of defense is microglia and activated macrophages, which release cytokines to entice even more immune cells to the injury site (90, 91). Microglia, when activated, can either promote inflammation by becoming M1-like or suppress it by becoming M2. Following an ischemic stroke, M1-like microglia release proinflammatory cytokines such as IL-1β, IL-6, and TNF-α, as well as nitric oxide synthase (92, 93), activating nuclear NF-κB and causing subsequent brain injury (94–96). Inhibiting immunity, releasing anti-inflammatory substances like IL-4 and IL-10, clearing away cell debris and misfolded proteins, encouraging extracellular matrix and tissue repair, and releasing neurotrophic factors account for the efficacy of M2-like substances in reducing inflammation (88, 92). These findings suggest that M1 and M2 microglia phenotypes contribute to the inflammatory response after a stroke (97).
Depending on the activation signals they receive, microglia can either promote injury or repair (98, 99). Different M2 subsets, such as M2a, M2b, M2c, and M2d, each have respective physiological features and biological roles (100). Strong anti-inflammatory and weak phagocytic capabilities, the ability to attract Th2 cells and drive tissue repair, etc., are all hallmarks of M2a induced by IL-4/IL-13 and mannose-CD206 receptors (100, 101). Those with an M2b phenotype exhibit increased expression of CD206, TGF-β, and CD163 and are involved in the immunological memory response and can both trigger inflammation and quell it. The M2c phenotype, associated with tissue remodeling, is triggered by glucocorticoids, IL-10 or apoptotic cells (100–102).
Although both M1 proinflammatory and M2 anti-inflammatory microglia undergo physical changes, their molecular signaling routes and activities evolve differently (93). Current evidence suggests that the early stages of ischemic stroke are characterized by predominant M2 polarization of microglia. The M1 type becomes more prevalent after a few days, particularly in the peri-infarct area (93), ultimately leading to blood-brain barrier breakdown and infiltration of peripheral immune cells (103). These multifaceted impacts of microglia/macrophages raise doubts about the efficacy of simply suppressing microglia/macrophages as a stroke treatment. Instead, rebalancing the ratio of good to negative responses by microglia/macrophages may be more effective.
Dendritic cells are professional antigen-presenting cells that express major histocompatibility complex(MHC) II and are essential for bridging the gap between the innate and adaptive arms of the immune system (104). By presenting antigens, dendritic cells can stimulate T cell-mediated immunological responses. Resting dendritic cells have been documented close to the blood-brain barrier (105). Dendritic cells influence the migration and maturation of neighboring dendritic cells as time passes after a stroke. Most of these cells enter the ischemic area through the bone marrow (106).
Antigen presentation and antibody synthesis are functions of B cells, which are effector cells. The cerebrospinal fluid of human stroke survivors contains immunoglobulin (107, 108). Reducing the abundance of B cells in the body causes an increase in infarct size, which is associated with a poor prognosis and a high overall death rate (109). It has been found that while the early infiltration of B lymphocytes after a stroke may have a short-term favorable effect by increasing immunosuppression and the synthesis of neurotrophic factors, the long-term effects are detrimental due to an increase in autoantibodies (110). Importantly, after an ischemic stroke, IL-10-producing regulatory B cells play a protective effect (111).
As incompletely differentiated cells, monocytes have potent phagocytosis abilities and the ability to mount an appropriate immune response based on their surroundings (112, 113). They can be classified into proinflammatory and anti-inflammatory fractions based on the expression of surface-specific markers. Chemotactic protein-1 (MCP-1, CCL2) and its receptor CCR2 are involved in the inflammatory response (114), and proinflammatory monocytes constitute the primary monocyte subgroups following brain injury (115),CCR2 is expressed high on proinflammatory monocytes while CX3CR1 is expressed low or not at all. As monocytes penetrate the damaged brain, they must express CCR2 in order to differentiate into macrophages. In stable environments, anti-inflammatory monocytes check blood arteries and engage in in situ phagocytosis (116), but they do not express CCR2. There are two main subpopulations of monocytes in rodents, distinguished by their expression of chemokine receptors and Ly-6C (Gr1). The inflammatory response is facilitated by the short half-life and active absorption of Ly6C high proinflammatory factor by the inflammatory tissue. It has been found that Ly6Clow has a long anti-inflammatory half-life and aids in vascular homeostasis (117, 118).
Blood-derived mononuclear cells undergo phenotypic and functional changes in response to the varying inflammatory conditions present during an acute ischemic stroke. The function of monocytes shifts from being proinflammatory M1 type cells to becoming anti-inflammatory M2 type cells on day 3 post-stroke (119). Through the activation of inflammasomes, M1 monocytes release reactive oxygen species (ROS), cytokines, and chemokines, and disrupt the tight connections between endothelial cells that protects the brain’s blood-brain barrier. Receptor P2X4 (P2X4R) activation enhances M1 proinflammatory phenotype polarization (120). When blood-derived mononuclear cells and other innate immune receptors collaborate, secondary inflammatory damage to the blood-brain barrier is exacerbated (121), since blood-derived mononuclear cells upregulate the triggering receptor expressed in bone marrow cells. Importantly, M2 macrophages are generated from monocytes and may shield the blood-brain barrier potentially preventing ischemia injury to the brain by vascular remodeling, physical attachment, and reduced inflammation (122, 123).
There is a favorable relationship between infarct size, stroke severity, and long-term prognosis after an ischemic stroke (124, 125). Neutrophils are white blood cells that rush to an area of illness or damage, where they consume dead cells and release inflammatory signals to draw in additional leukocytes (126). Ischemia-induced neutrophils release ROS, proteases (MMPs, protease 3, elastase), lipocalin-2(LCN-2), and NETs, all of which contribute to the breakdown of the blood-brain barrier. Connexins (primarily cadherin/β-catenin complex, occludin, ZO-1, and claudin-5) are degraded by high permeability-associated signaling pathways (e.g. MLCK, PKC, MAPK, and Rho GTPass) when there is an excess of ROS such as superoxide anion, peroxynitrite, and hydrogen peroxide (127). When it comes to the immunological response to ischemic brain injury, neutrophil adherence is a crucial first step (128). Immune cells are transported to the site of ischemic brain injury via the vascular wall, where they attach to adhesion molecules such as ICAM-1, MAC-1 (CD11b/CD18) and selectin (128). Within a few hours after a stroke, ICAM-1 expression rises in the proximal endothelial tissue of the brain damage, peaking at around 12–48 hours (129). In experimental stroke, infarct size and brain leukocyte infiltration have been found to be decreased in adhesion molecule deficient animals when ICAM-1 was blocked (130, 131). CD11b/CD18, also known as MAC-1, is expressed on the plasma membrane of neutrophils and binds to the intercellular adhesion molecule 1 (ICAM-1) on endothelial cells. Reduced infarct size, survival, and neutrophil infiltration into the ischemic brain are all observed in MAC-1-deficient transgenic mice after an ischemic stroke (132). P-selectin and E-selectin contribute to initial neutrophil recruitment (133), L-selectin induces recruitment of unstimulated neutrophils to activated endothelial regions (134), and selectin itself is a calcium-dependent transmembrane glycoprotein that is responsible for transporting neutrophils after cerebral ischemia (133). P- and E-selectin upregulation is positively linked with post-ischemic inflammatory response enhancement and injury severity in all experimental stroke models (135, 136).
T-lymphocyte activation plays a role in both innate and adaptive immunity, with the ability to promote or suppress inflammation (137). It has been established that 30% of T-lymphocytes are cytotoxic T cells (CD8+T cells), which destroy infected cells by cytotoxic processes, while 40% are helper T cells (CD4+T cells), which release cytokines to modulate adaptive and innate immune responses (138, 139). Acute cerebral ischemia causes neuroinflammation, activated and infiltrated microglia/macrophages may stimulate activated CD4+T cells to develop into Th1 or Th2 cells, generate proinflammatory or anti-inflammatory cytokines, and either harm or protect the brain (138). Proinflammatory cytokines such as IL-2, IL-12, and IFN-γ are released by Th1 cells, which may exacerbate brain injury. Anti-inflammatory cytokines such as IL-4, Interleukin-5(IL-5), IL-10, and IL-13 may be secreted by Th2 cells, which may have a neuroprotective effect on the wounded brain (140). The earliest T cells found after a stroke are CD8+T cells, which detected within hours of a stroke (141). Neuronal death and exacerbated brain injury are caused by CD8+ T lymphocytes after they come into contact with other cells and become antigen-dependently activated, releasing perforin/granzyme (142).
To mount an immune response to an inflammatory setting, certain naive T cells can independently generate ROS and inflammatory cytokines (143). Naive CD4+ T cells differentiate into specific T helper cells (Th), namely Th1, Th2, Th17 and induced T regulatory cells (iTregs) (144). Maintaining immunological homeostasis and suppressing effector T cells are the functions of naturally induced Tregs (nTregs) found in the thymus and the iTregs (145). By recognizing autoantigens and foreign antigens, iTregs can suppress an overactive immune response. The post-stroke neuroinflammatory response is mitigated by Foxp3+Tregs (146). Toll-like receptors (TLRs) and T cell receptors (TCRs) are two examples of immunological receptors that T cells express, accounting for their immune features (147). The proinflammatory cytokine IL-17, secreted by T cells, works in tandem with IL-23 to entice monocytes and neutrophils to the site of inflammation (148).
As one of the early cytokines in the inflammatory response to ischemic brain injury (149), TNF-α, is released and generated by monocytes, T cells, mast cells, macrophages, neutrophils, keratinocytes, and fibroblasts (88). Pericerebral cells are stem cells that line the surface of capillaries that TNF-α stimulates to enhance IL-6 production via activation of NF-κB (150, 151). Transmembrane (tmTNF-α) regulates local inflammation by cell-to-cell interaction, and soluble bioactive (sTNF-α) is created by tumor necrosis factor-converting enzyme (TACE) (88). sTNF-α acts systemically and locally in the central nervous system, promoting phagocytosis and cytotoxic activity of macrophages and enhancing the production of IL-6 and IL-1, mediated by binding of TNF-α to the receptors TNFR-1 and TNFR-2. Although TNFR1 mediates sTNF-α, TNFR-2 and TNFR-1 mediate tmTNF-α (149). The neurotoxic and neuroprotective actions of TNF-α in the ischemic brain highlight the central role of TNF-α in the neuroimmune genesis of stroke (152–154).
Microglia, astrocytes, leukocytes, and endothelial cells reportedly contribute to the brain injury response by releasing IL-6. There is an increasing consensus that this multi-functional proinflammatory cytokine increases leukocyte migration, regulates the production of chemokines and the expression of adhesion molecules, and activates acute phase proteins (150, 151, 155). IL-6 released after a stroke can worsen cerebral vascular damage by activating NMDI-Rs and upregulating ET-1 and JNK (156). IL-6 enhances local inflammatory responses by activating and recruiting neutrophils and monocytes and stimulating vascular endothelial cells to produce adhesion molecules and other inflammatory mediators (157). Although IL-6 is a proinflammatory cytokine, it plays a crucial role in cerebral ischemia by acting as a carrier of the inflammatory process during the early phase of stroke and as a neurotrophic factor during the late development of cerebral ischemia (158). Similar neurotrophic factors, including leukemia inhibitory factor (LIF) and ciliary neurotrophic factors, share a common receptor component, gp130 (159). Importantly, the severity of cerebral ischemia injury following an ischemic stroke may be mitigated by administering these cytokines directly into brain tissue following a stroke. Neurogenesis, angiogenesis, and neuronal differentiation are all aided by IL-6 produced by astrocytes, which also promotes Th1 polarization to Th2 and causes an immunosuppressive microenvironment (160). Additionally, IL-6 aids CNS post-traumatic recovery via endothelial cell repair, which may improve vascular reconstruction or angiogenesis following ischemic stroke (161). IL-6 protects neurons from apoptosis, enhances CNS neuron survival, and decreases N-methyl D-aspartate (NMDA) -mediated excitatory toxic neuron injury (158).
The proinflammatory cytokine IL-1 is synthesized by monocytes, macrophages, and epithelial cells (149), and the IL-1 family comprises IL-1α, IL- 1β, and IL-1RN (162). During the early stages of stroke, IL-1 mediates harmful inflammatory processes, such as the upregulation of IL-6, TNF-α, Matrix metallopeptidase 9 (MMP-9), and chemokines in astrocytes, inhibition of neurogenesis (163). IL-1 may also act on the vascular endothelium to encourage the recruitment of white blood cells (164). However, during the subacute and chronic phases of stroke, IL-1 may bring some benefits. It is widely thought that IL-1 may aid recovery from an ischemic stroke since it encourages scar formation from glial cells and boosts angiogenesis (165).
IL-1β is a cytokine that helps keep the immune system in check, and it can increase inflammation influencing nearly all the cytotypes (88). IL-1β is widely acknowledged to promote microglial activation. Indeed, microglia are pivotal in the neuroinflammatory response as effector cells. By producing potentially neurotoxic molecules like TNF-α and iNOS, they exacerbate the inflammatory response and cause secondary brain injury. The IL-1β-mediated phosphorylation and ubiquitination of inhibitor of NF-κB-a (IκB-a) by IRAK activates IκB kinase via the IRAK pathway, which promotes nuclear NF-κB expression and the transcription of target genes, including IL-8 and TNF-α (166). To further exacerbate injury caused by ischemia, IL-1β modulates the PI3K/AKT pathway, promotes IL-6 and other cytokines, and operates synergistically in the ischemic region (167). Growing evidence suggests that phosphorylation of JAK2/STAT3 is stimulated when IL-6 and other proinflammatory cytokines are upregulated (168, 169). Once within the nucleus, phosphorylated STAT3 (P-STAT3) upregulates the IL-1β, IL-6, and TNF-α genes by binding to certain DNA sequence features in the promoter region of the target gene (169). When brain cells are injured, it can be extremely challenging to repair the damage caused by the vicious cycle of inflammation that results.
IL-10 is an anti-inflammatory protein released mostly by monocytes but can also be secreted by other cell types, including Th2 lymphocytes (170). By reducing the body’s inflammatory response, IL-10 reduces the risk of stroke. By activating PI3K and STAT3, IL-10 inhibits the synthesis and activity of Th1 cells (171), reducing the expression and activity of proinflammatory cytokines such as IFN-γ, IL-1β, and TNF-α (172). Ischemic stroke is protected against thanks to IL-10 treatment’s ability to effectively down-regulate the upregulated proinflammatory signals in acute ischemic lesions (173). A study reported that transduction of the IL-10 gene prior to cerebral artery ischemia protected rat brains from ischemic and reperfused injuries by boosting heme oxygenase expression (174). Inhibiting NF-κB has been reported as another anti-inflammatory function of IL-10 (175). In a mouse model of focal cerebral ischemia (MCAO), transgenic mice overexpressing IL-32A showed decreased ischemic neuronal cell death and increased secretion of anti-neuroinflammatory factors (IL-10) by decreasing the release of neuroinflammatory factors (IL-6, IL-1β, TNF-α), thereby reducing astrocyte activation, indicating a cross-talk between IL-32 A and these other cytokines (176). Importantly, taking myelin oligodendrocyte glycoprotein (MOG) through the nose triggers Il-10 secretion from CD4+ T cells, which helps reduce stroke-related disability. The neuroprotective effect of oligodendrocyte glycoprotein treatment in MCAO mice (177, 178) may be due to IL-10 released by CD4+ T cells. Secondary infarct growth via the nitric oxide route may be facilitated by an increase in IL-10, which reduces the amount of CD11b+ cells (177).
The strong anti-inflammatory properties of IL-4 play an important role in determining the prognosis of stroke. By causing naive T cells to differentiate into Th2 cells that secrete anti-inflammatory cytokines, including IL-4, IL-10, and IL13, IL-4 inhibits the activity of Th1 inflammatory effector cells (179). T-cell differentiation and non-specific B-cell transformation are two immunological responses that IL-4 controls (180). Microglia/macrophage M2 polarization is promoted by IL-4, the most notable M2 macrophage polarization promoter. IL-4 is widely thought to be crucial during the acute stage of stroke (181), with dramatically increased serum levels hours after stroke onset (182). A lack of IL-4 causes brain damage and neurological impairment 24 hours after transient MCAO (183). Long-term healing after an ischemic stroke and microglia/macrophage M2 polarization depend heavily on IL-4. After cerebral ischemia, IL-4-deficient mice showed increased populations of M1-polarized microglia/macrophages and greater infarct sizes that caused neurological damage. Importantly, IL-4 recovery could reverse these effects (184). The neuroprotective effects of IL-4 are mediated by activating IL-4/STAT6 signal transduction and suppressing proinflammatory cytokines. Consistently, more proinflammatory cytokines, such as IL-1β and TNF-α, were produced by IL-4 knockout mice (185).
IL-23 in the brain following ischemic stroke is produced mostly by CD172a+/IRF4+ 2 dendritic cells (CDC2s), which regulate IL-17 expression in γδ T cells (186). Antigen-stimulated dendritic cells and macrophages boost Th17 cell growth during persistent inflammation by releasing interleukin-23 (186). The IL-17 produced by Th17 cells accounts for a robust inflammatory response by inducing the expression of many inflammatory cytokines. Dendritic cells migrate to the perivascular infarct area after a stroke, and cDC2s cells stimulate γδ T cells to release IL-17, which recruits neutrophils to the ischemic side of the brain (187). The synergistic effects of Vgamma4 T cell-derived IL-17A and IL-1β/IL-23 in the infarct hemisphere exacerbate the inflammatory cascade and ischemic tissue damage (188).
Stroke treatment outcomes may vary by gender due to differences in how men and women react to inflammation throughout their lives (189, 190). The cellular and molecular mechanisms of sex hormones are distinct, summarized in Figure 2. Local and systemic inflammation, including the activation of glial and myeloid cells, occurs following cerebral ischemia, mostly due to the innate immune system (191, 192). Many proinflammatory genes are upregulated in response to the damage, including TNF-α, monocyte chemoattractant protein-1 (MCP-1), and IL-6 (193, 194).
Figure 2 Sex difference involved in immune regulation after stroke. Different chromosomes host the expression of specific genes that can influence immune function. Microglia were more prone to the M1 phenotype in males and M2 phenotype in females, however their production of cytokines is also affected by age. Astrocytes in females have a more anti-inflammatory phenotype. Significant sex differences exist in the in lymphocytes and the cytokines they release.
In the infarct boundary zone of a stroke, microglia are the first to respond to neuronal injury as part of the innate immune response (195). The number of macrophages was lower in females and M2 phenotype microglia were higher in females, therefore showing reduced inflammation (54). Anti-inflammatory IL-4 and CD206 are more highly expressed in the ischemic brain of female microglia, and female microglia are more responsive to IL-4 and IL-10 (86, 196). Male microglia are more active and have a more proinflammatory phenotype (11, 54, 197) because they express higher amounts of Iba1, TLR2, and TLR4. Higher amounts of TNF-α, IL-1β, CXCL10, and KDM5C/6A are produced by microglia in older women, indicating a proinflammatory effect (54, 198). Experimental stroke in young male mice causes greater inflammation in the microglia (82, 199). In contrast to young male MCAO mice, female MCAO mice showed lower expression of microglial IL-1β and macrophage inflammatory protein-1a (200). Age-related variations in the hippocampus, amygdala, and cortex account for the gender gap in CCL4, CCL20 and CD206 (19). Mice of the male gender showed greater expression of genes encoding IL-1β, TNF-α, and CXCL10, among other inflammatory proteins. It has been established that genes involved in T-cell activity, adhesion molecules, cellular communication, MHC, co-stimulatory signals, cell death, and inflammatory cytokines are more highly expressed in the experimental post-stroke ischemic brains of young male mice than in females (199).
Astrocytes often play a more important role when ischemia lasts for several days. Sex hormone receptors have been documented in both male and female astrocytes. The gonadotropin hormone is responsible for the sex difference of astrocytes in ischemic stroke (201). Steroid hormones, including estradiol, progesterone, and testosterone, are largely produced by astrocytes in the brain and spinal cord (202). Both estrogen and progesterone prevent astrocyte activation (203, 204). Amphoteric astrocytes have a distinct reaction to gonadal hormones on top of the already observed differences in hormone levels between the sexes. Estrogen or an estrogen receptor (ER) agonist can trigger a positive feedback mechanism in female astrocytes, leading to increased ERs and progesterone expression (205, 206). Higher activity of estradiol-producing P450 and aromatase enzymes in female astrocytes may explain why they are more resistant to oxygen and glucose deprivation(OGD) and H2O2-induced oxidative stress than male astrocytes (207, 208). Stroke can cause neuroinflammation, and astrocytes play a role in this process, which is influenced by individual sexual hormone levels (209).
Inhibition of OGD and ROS release in astrocytes are directly induced by estrogen therapy (210), which polarizes astrocytes to an anti-inflammatory A2 phenotype (202). The expression of N-myc downstream regulatory gene 2 (Ndrg2) in astrocytes is increased by estrogen and suppresses astrocyte differentiation during ischemic stroke (211).
Peripheral leukocytes are activated after an ischemic stroke and move to the injury site, where they further cause neurological injury (13). An increasing body of evidence suggests that elimination of the spleen improves stroke prognosis in young male mouse models, where ischemia injury triggers the release of inflammatory immune cells into the periphery (13, 212, 213). Current evidence suggests an elevation in blood macrophages after a stroke, which is associated with spleen atrophy (214). The peripheral immune response to stroke is engendered at the level of the spleen and blood. Young male mice show a greater increase in CD4+T cells and expression of the VLA-4 adhesion molecule in the spleen than female mice do following an experimental stroke (82). A study found a significant reduction in macrophages/monocytes and activated T cells in men and a reduction in ischemic injury after splenectomy before MCAO, but these effects were not observed in women (199). Furthermore, splenectomy reduced infarct size and activated microglia in the brain in men but did not affect stroke prognosis in women (199).
There are gender disparities in the levels of cytokines in the brain. In contrast to IL-2, which exclusively harms male nerves (215), IL-13 alleviates experimental autoimmune encephalitis symptoms in females (216), showing significant functional implications associated with gender differences in the neuroimmune system. T regulatory cells and Th2 CD4+ T cells produce IL-10, the inflammatory signal after stroke; however, there are gender variations in the production of these cells. The prognosis of ischemic stroke patients can be affected by post-stroke immunosuppression due to elevated IL-10 levels (80). IL-1β and MIP-1α expression were lower in microglia grown from young female MCAO mice than in male MCAO mice (217). Stroke prognosis is significantly affected by IL-4’s potent anti-inflammatory effect. Neurons produce IL-4 after an ischemic stroke and the expression of IL-4 receptors (IL-4Rs) on microglia increases in response (218). IL-4 is reportedly crucial for the neuroprotection of young female mice following stroke. Cerebral infarction is exacerbated, and the number of inflammatory cells in the brain is elevated in female mice who lack IL-4 (183). The neuroprotective benefits of IL-4 in females are mirrored by the reduced abundance of M2 microglia in the brain of IL-4 knockout mice (183).
X-chromosome genes implicated in the signaling of natural killer cells, TNFR1 signaling and axon guidance, transforming growth factor signaling, and IL17 signaling (tissue inhibitor of matrix metalloproteinase-1) have been reported to be differentially expressed in females. Genes related to development, cell trafficking, and cellular mobility experienced male-specific changes in expression, suggestive of a more potent inflammatory response in men (126). The X chromosome’s genetic manifestations in ischemic stroke exhibit significant gender heterogeneity. In this regard, it has been found that TIMP1, DDX3X, IKBKG, PRKX, GLA, MAOA/B, and MAGE gene families’ post-translational modifications, small-molecule biochemistry, and cell-cell signal transduction functions are only controlled in females (219). Only males possess the genes EFNB1, CYSLTR1, IGBP1, and TLR-7, which are involved in cell proliferation, differentiation, transport, and apoptosis (219). The XCI escape genes kdm5c and kdm6a, which demethylate H3K4me3 and H3K27me3, respectively, and epigenetically modify the expression of interferon regulatory factor (IRF4/5) (198), are responsible for changes in the proinflammatory activity of female microglia. Some genes on Y chromosomes have been reported to be differentially expressed between male stroke victims and men in general, including VAMP7, CSF2RA, SPRY3, DHRSX, PLCXD1, EIF1AY, and DDX3Y. The immune system, RNA metabolism, vesicular fusion, and angiogenesis are all regulated by processes related to the differential expression of Y chromosomal genes (220).
Female hormones may play an important role in neuroprotection after ischemia (221, 222). In this respect, estrogen, which is generated in the brain and acts as a neuroprotective agent after stroke and an efficient anti-inflammatory agent (223), is a sex steroid hormone and a neurosteroid hormone (126). The neuroprotective effects of estrogen are mediated by its ability to dampen the immunological response that develops in the wake of brain damage caused by ischemia. Studies have demonstrated that estrogen therapy can inhibit the synthesis and secretion of proinflammatory cytokines in vitro and in vivo (25, 224). By acting as an inflammatory mediator, IL-1β is injected before estrogen is delivered to the body to precipitate the death of ischemia cells. Reduced levels of IL-1β in the rat brain’s cortex improve neurological impairment by decreasing the infarct size and blocking neutrophil infiltration into injured tissue caused by ischemia (225).
It is well-established that estrogen reduces apoptosis and oxidative stress in the brain (226), decreases NF-κB activity and the expression of IkB, iNOS, and TNF associated with ischemic neuroprotection (227–230), activates anti-apoptotic PI3K/AKT and MAPK/Erk pathways and inhibits the pro-apoptotic JNK pathway (231–233), protecting neurons from damage. Brain endothelium COX-2 induction and Il-1-mediated astrocyte activation are suppressed by estrogen (234, 235). The transcription of neurotrophic factors (IGF-1, BDNF, GDNF, and VEGF) is regulated by estrogen, reducing neuroinflammation. Importantly, estrogen also enhances the transcription of STAT3 and PPAR, inhibiting NF-κB transcriptional activity (236–240).
Low concentrations of TNF aid in promoting injury repair, while high concentrations are neurotoxic (241, 242). Estrogen regulates the production of the proinflammatory cytokine TNF, which acts on receptors of many cell types, including neurons, glial cells, and endothelial cells (243). One such inflammatory protein that estrogen regulates is nuclear NF-κB, which modulates inflammatory signaling pathways in cells, including neurons (244). After ischemia, NF-κB regulates the upregulation of inflammatory mediators, including IL-1, IL-6, IL-8, iNOS, ICAM 1, VCAM and E-selectin, and then leads to neutrophil infiltration and adhesion molecule induction. Estrogen treatment can inhibit the activation of NF-κB, reduce the activation of NF-κB-mediated delayed cell death in ischemia-reperfusion injury, alleviate inflammation and apoptosis, and protect neurons (225).
The role of sex differences in the incidence and prognosis of ischemic stroke is significant, and the immune regulation based on sex difference is one of the important reasons. However, the importance of gender factors varies at different ages, which is due to sex hormone levels, underlying disease differences and other factors. There is growing evidence that some risk factors, like hypertension and atrial fibrillation, tend to occur more frequently in older women, while others, like diabetes and smoking, disproportionately affect women. The disparity in stroke prevalence and outcome may also be influenced by sex-specific risk factors, such as the use of oral contraceptives and menopause. Evidence suggests that females are more likely than males to exhibit non-traditional acute stroke symptoms, making it more challenging for clinicians to correctly diagnose a stroke and potentially delaying the administration of thrombolytic intervention.
More regrettably, there is still no gender-specific stroke treatment, even though gender differences are a valuable risk factor in many studies. Research on immune regulation based on sex difference presents great difficulties because it is difficult to simulate human ischemic stroke state in vitro as well as in animal models, leading to a slow progress in the study of mechanisms in this area. We expect more detailed studies to support the development of gender-specific stroke treatment options, including immunotherapy agents and specific inflammatory markers.
PN and LL completed the manuscript and contributed equally to the article. YZ, ZS, BW, HL, and SZ collected and collated literature. SQ and YL designed this work. All authors contributed to the article and approved the submitted version.
The authors declare that the research was conducted in the absence of any commercial or financial relationships that could be construed as a potential conflict of interest.
All claims expressed in this article are solely those of the authors and do not necessarily represent those of their affiliated organizations, or those of the publisher, the editors and the reviewers. Any product that may be evaluated in this article, or claim that may be made by its manufacturer, is not guaranteed or endorsed by the publisher.
1. Kuriakose D, Xiao Z. Pathophysiology and treatment of stroke: Present status and future perspectives, int J mol sci, 21. Int J Mol Sci (2020) 21(20): 7609. doi: 10.3390/ijms21207609
2. Appelros P, Asberg S. Sex differences in stroke. Handb Clin Neurol (2020) 175:299–312. doi: 10.1016/B978-0-444-64123-6.00021-7
3. Branyan TE, Sohrabji F. Sex differences in stroke co-morbidities. Exp Neurol (2020) 332:113384. doi: 10.1016/j.expneurol.2020.113384
4. Vyas MV, Silver FL, Austin PC, Yu AYX, Pequeno P, Fang J, et al. Stroke incidence by sex across the lifespan. Stroke (2021) 52:447–51. doi: 10.1161/STROKEAHA.120.032898
5. Rexrode KM, Madsen TE, Yu AYX, Carcel C, Lichtman JH, Miller EC. The impact of sex and gender on stroke. Circ Res (2022) 130:512–28. doi: 10.1161/CIRCRESAHA.121.319915
6. Ransohoff RM, Schafer D, Vincent A, Blachere NE, Bar-Or A. Neuroinflammation: Ways in which the immune system affects the brain. Neurotherapeutics (2015) 12:896–909. doi: 10.1007/s13311-015-0385-3
7. Iadecola C, Buckwalter MS, Anrather J. Immune responses to stroke: mechanisms, modulation, and therapeutic potential. J Clin Invest (2020) 130:2777–88. doi: 10.1172/JCI135530
8. Fischinger S, Boudreau CM, Butler AL, Streeck H, Alter G. Sex differences in vaccine-induced humoral immunity. Semin Immunopathol (2019) 41:239–49. doi: 10.1007/s00281-018-0726-5
9. Podcasy JL, Epperson CN. Considering sex and gender in Alzheimer disease and other dementias. Dialogues Clin Neurosci (2016) 18:437–46. doi: 10.31887/DCNS.2016.18.4/cepperson
10. Bereshchenko O, Bruscoli S, Riccardi C. Glucocorticoids. Sex Hormones Immunity Front Immunol (2018) 9:1332. doi: 10.3389/fimmu.2018.01332
11. Spychala MS, Honarpisheh P, McCullough LD. Sex differences in neuroinflammation and neuroprotection in ischemic stroke. J Neurosci Res (2017) 95:462–71. doi: 10.1002/jnr.23962
12. Metcalf CJE, Roth O, Graham AL. Why leveraging sex differences in immune trade-offs may illuminate the evolution of senescence. Funct Ecol (2020) 34:129–40. doi: 10.1111/1365-2435.13458
13. Dotson AL, Offner H. Sex differences in the immune response to experimental stroke: Implications for translational research. J Neurosci Res (2017) 95:437–46. doi: 10.1002/jnr.23784
14. Villacres MC, Longmate J, Auge C, Diamond DJ. Predominant type 1 CMV-specific memory T-helper response in humans: evidence for gender differences in cytokine secretion. Hum Immunol (2004) 65:476–85. doi: 10.1016/j.humimm.2004.02.021
15. Ahnstedt H, McCullough LD. The impact of sex and age on T cell immunity and ischemic stroke outcomes. Cell Immunol (2019) 345:103960. doi: 10.1016/j.cellimm.2019.103960
16. Yasuda H, Sonoda A, Yamamoto M, Kawashima Y, Takishita Y, Morita A, et al. 17-beta-estradiol enhances neutrophil extracellular trap formation by interaction with estrogen membrane receptor. Arch Biochem Biophys (2019) 663:64–70. doi: 10.1016/j.abb.2018.12.028
17. Molloy EJ, O'Neill AJ, Grantham JJ, Sheridan-Pereira M, Fitzpatrick JM, Webb DW, et al. Sex-specific alterations in neutrophil apoptosis: the role of estradiol and progesterone. Blood (2003) 102:2653–9. doi: 10.1182/blood-2003-02-0649
18. Mo R, Chen J, Grolleau-Julius A, Murphy HS, Richardson BC, Yung RL. Estrogen regulates CCR gene expression and function in T lymphocytes. J Immunol (2005) 174:6023–9. doi: 10.4049/jimmunol.174.10.6023
19. Rettberg JR, Yao J, Brinton RD. Estrogen: a master regulator of bioenergetic systems in the brain and body. Front Neuroendocrinol (2014) 35:8–30. doi: 10.1016/j.yfrne.2013.08.001
20. Nadkarni S, Cooper D, Brancaleone V, Bena S, Perretti M. Activation of the annexin A1 pathway underlies the protective effects exerted by estrogen in polymorphonuclear leukocytes. Arterioscler Thromb Vasc Biol (2011) 31:2749–59. doi: 10.1161/ATVBAHA.111.235176
21. Toyoda Y, Miyashita T, Endo S, Tsuneyama K, Fukami T, Nakajima M, et al. Estradiol and progesterone modulate halothane-induced liver injury in mice. Toxicol Lett (2011) 204:17–24. doi: 10.1016/j.toxlet.2011.03.031
22. Murphy AJ, Guyre PM, Pioli PA. Estradiol suppresses NF-kappa b activation through coordinated regulation of let-7a and miR-125b in primary human macrophages. J Immunol (2010) 184:5029–37. doi: 10.4049/jimmunol.0903463
23. Hsu JT, Kan WH, Hsieh CH, Choudhry MA, Schwacha MG, Bland KI, et al. Mechanism of estrogen-mediated attenuation of hepatic injury following trauma-hemorrhage: Akt-dependent HO-1 up-regulation. J Leukoc Biol (2007) 82:1019–26. doi: 10.1189/jlb.0607355
24. Cuzzocrea S, Genovese T, Mazzon E, Esposito E, Di Paola R, Muia C, et al. Effect of 17beta-estradiol on signal transduction pathways and secondary damage in experimental spinal cord trauma. Shock (2008) 29:362–71. doi: 10.1097/SHK.0b013e31814545dc
25. Nadkarni S, McArthur S. Oestrogen and immunomodulation: new mechanisms that impact on peripheral and central immunity. Curr Opin Pharmacol (2013) 13:576–81. doi: 10.1016/j.coph.2013.05.007
26. Liu HY, Buenafe AC, Matejuk A, Ito A, Zamora A, Dwyer J, et al. Estrogen inhibition of EAE involves effects on dendritic cell function. J Neurosci Res (2002) 70:238–48. doi: 10.1002/jnr.10409
27. Bachy V, Williams DJ, Ibrahim MA. Altered dendritic cell function in normal pregnancy. J Reprod Immunol (2008) 78:11–21. doi: 10.1016/j.jri.2007.09.004
28. Papenfuss TL, Powell ND, McClain MA, Bedarf A, Singh A, Gienapp IE, et al. Estriol generates tolerogenic dendritic cells in vivo that protect against autoimmunity. J Immunol (2011) 186:3346–55. doi: 10.4049/jimmunol.1001322
29. Seillet C, Laffont S, Tremollieres F, Rouquie N, Ribot C, Arnal JF, et al. The TLR-mediated response of plasmacytoid dendritic cells is positively regulated by estradiol in vivo through cell-intrinsic estrogen receptor alpha signaling. Blood (2012) 119:454–64. doi: 10.1182/blood-2011-08-371831
30. Klein SL, Flanagan KL. Sex differences in immune responses. Nat Rev Immunol (2016) 16:626–38. doi: 10.1038/nri.2016.90
31. Klein SL, Jedlicka A, Pekosz A. The xs and y of immune responses to viral vaccines. Lancet Infect Dis (2010) 10:338–49. doi: 10.1016/S1473-3099(10)70049-9
32. Pfeilschifter J, Koditz R, Pfohl M, Schatz H. Changes in proinflammatory cytokine activity after menopause. Endocr Rev (2002) 23:90–119. doi: 10.1210/edrv.23.1.0456
33. Pennell LM, Galligan CL, Fish EN. Sex affects immunity. J Autoimmun (2012) 38:J282–291. doi: 10.1016/j.jaut.2011.11.013
34. Lu FX, Abel K, Ma Z, Rourke T, Lu D, Torten J, et al. The strength of b cell immunity in female rhesus macaques is controlled by CD8+ T cells under the influence of ovarian steroid hormones. Clin Exp Immunol (2002) 128:10–20. doi: 10.1046/j.1365-2249.2002.01780.x
35. Fu Y, Li L, Liu X, Ma C, Zhang J, Jiao Y, et al. Estrogen promotes b cell activation in vitro through down-regulating CD80 molecule expression. Gynecol Endocrinol (2011) 27:593–6. doi: 10.3109/09513590.2010.507281
36. Vassiliadou N, Tucker L, Anderson DJ. Progesterone-induced inhibition of chemokine receptor expression on peripheral blood mononuclear cells correlates with reduced HIV-1 infectability in vitro. J Immunol (1999) 162:7510–8. doi: 10.4049/jimmunol.162.12.7510
37. Szekeres-Bartho J. Progesterone-mediated immunomodulation in pregnancy: its relevance to leukocyte immunotherapy of recurrent miscarriage. Immunotherapy (2009) 1:873–82. doi: 10.2217/imt.09.54
38. Aryanpour R, Pasbakhsh P, Zibara K, Namjoo Z, Beigi Boroujeni F, Shahbeigi S, et al. Progesterone therapy induces an M1 to M2 switch in microglia phenotype and suppresses NLRP3 inflammasome in a cuprizone-induced demyelination mouse model. Int Immunopharmacol (2017) 51:131–9. doi: 10.1016/j.intimp.2017.08.007
39. Piccinni MP, Giudizi MG, Biagiotti R, Beloni L, Giannarini L, Sampognaro S, et al. Progesterone favors the development of human T helper cells producing Th2-type cytokines and promotes both IL-4 production and membrane CD30 expression in established Th1 cell clones. J Immunol (1995) 155:128–33. doi: 10.4049/jimmunol.155.1.128
40. Lee JH, Ulrich B, Cho J, Park J, Kim CH. Progesterone promotes differentiation of human cord blood fetal T cells into T regulatory cells but suppresses their differentiation into Th17 cells. J Immunol (2011) 187:1778–87. doi: 10.4049/jimmunol.1003919
41. Wong R, Renton C, Gibson CL, Murphy SJ, Kendall DA, Bath PM, et al. Progesterone treatment for experimental stroke: an individual animal meta-analysis. J Cereb Blood Flow Metab (2013) 33:1362–72. doi: 10.1038/jcbfm.2013.120
42. Zhu X, Frechou M, Liere P, Zhang S, Pianos A, Fernandez N, et al. A role of endogenous progesterone in stroke cerebroprotection revealed by the neural-specific deletion of its intracellular receptors. J Neurosci (2017) 37:10998–1020. doi: 10.1523/JNEUROSCI.3874-16.2017
43. Guennoun R. Progesterone in the brain: Hormone, neurosteroid and neuroprotectant. Int J Mol Sci (2020) 21(15): 5271. doi: 10.3390/ijms21155271
44. Hosie AM, Wilkins ME, da Silva HM, Smart TG. (2006). Endogenous neurosteroids regulate GABAA receptors through two discrete transmembrane sites. Nature 444(7118):486–9. doi: 10.1038/nature05324
45. Dang J, Mitkari B, Kipp M, Beyer C. Gonadal steroids prevent cell damage and stimulate behavioral recovery after transient middle cerebral artery occlusion in male and female rats. Brain Behav Immun (2011) 25:715–26. doi: 10.1016/j.bbi.2011.01.013
46. Wang P, Yang HP, Tian S, Wang L, Wang SC, Zhang F, et al. Oxytocin-secreting system: A major part of the neuroendocrine center regulating immunologic activity. J Neuroimmunol (2015) 289:152–61. doi: 10.1016/j.jneuroim.2015.11.001
47. Kunitake Y, Imamura Y, Mizoguchi Y, Matsushima J, Tateishi H, Murakawa-Hirachi T, et al. Serum oxytocin levels and logical memory in older people in rural Japan. J Geriatr Psychiatry Neurol (2021) 34:156–61. doi: 10.1177/0891988720915526
48. Karelina K, Stuller KA, Jarrett B, Zhang N, Wells J, Norman GJ, et al. Oxytocin mediates social neuroprotection after cerebral ischemia. Stroke (2011) 42:3606–11. doi: 10.1161/STROKEAHA.111.628008
49. Beagley KW, Gockel CM. Regulation of innate and adaptive immunity by the female sex hormones oestradiol and progesterone. FEMS Immunol Med Microbiol (2003) 38:13–22. doi: 10.1016/S0928-8244(03)00202-5
50. Bouman A, Schipper M, Heineman MJ, Faas MM. Gender difference in the non-specific and specific immune response in humans. Am J Reprod Immunol (2004) 52:19–26. doi: 10.1111/j.1600-0897.2004.00177.x
51. Aguilar-Castro J, Cervantes-Candelas LA, Buendia-Gonzalez FO, Fernandez-Rivera O, Nolasco-Perez TJ, Lopez-Padilla MS, et al. Testosterone induces sexual dimorphism during infection with plasmodium berghei ANKA. Front Cell Infect Microbiol (2022) 12:968325. doi: 10.3389/fcimb.2022.968325
52. Giron-Gonzalez JA, Moral FJ, Elvira J, Garcia-Gil D, Guerrero F, Gavilan I, et al. Consistent production of a higher TH1:TH2 cytokine ratio by stimulated T cells in men compared with women. Eur J Endocrinol (2000) 143:31–6. doi: 10.1530/eje.0.1430031
53. Shepherd R, Cheung AS, Pang K, Saffery R, Novakovic B. Sexual dimorphism in innate immunity: The role of sex hormones and epigenetics. Front Immunol (2020) 11:604000. doi: 10.3389/fimmu.2020.604000
54. Kerr N, Dietrich DW, Bramlett HM, Raval AP. Sexually dimorphic microglia and ischemic stroke. CNS Neurosci Ther (2019) 25:1308–17. doi: 10.1111/cns.13267
55. Li J, Siegel M, Yuan M, Zeng Z, Finnucan L, Persky R, et al. Estrogen enhances neurogenesis and behavioral recovery after stroke. J Cereb Blood Flow Metab (2011) 31:413–25. doi: 10.1038/jcbfm.2010.181
56. Trigunaite A, Dimo J, Jorgensen TN. Suppressive effects of androgens on the immune system. Cell Immunol (2015) 294:87–94. doi: 10.1016/j.cellimm.2015.02.004
57. Rettew JA, Huet-Hudson YM, Marriott I. Testosterone reduces macrophage expression in the mouse of toll-like receptor 4, a trigger for inflammation and innate immunity. Biol Reprod (2008) 78:432–7. doi: 10.1095/biolreprod.107.063545
58. Libert C, Dejager L, Pinheiro I. The X chromosome in immune functions: when a chromosome makes the difference. Nat Rev Immunol (2010) 10:594–604. doi: 10.1038/nri2815
59. Carrel L, Willard HF. X-Inactivation profile reveals extensive variability in X-linked gene expression in females. Nature (2005) 434:400–4. doi: 10.1038/nature03479
60. Gubbels Bupp MR. Sex, the aging immune system, and chronic disease. Cell Immunol (2015) 294:102–10. doi: 10.1016/j.cellimm.2015.02.002
61. Ji H, Zheng W, Wu X, Liu J, Ecelbarger CM, Watkins R, et al. Sex chromosome effects unmasked in angiotensin II-induced hypertension. Hypertension (2010) 55:1275–82. doi: 10.1161/HYPERTENSIONAHA.109.144949
62. Florijn BW, Bijkerk R, van der Veer EP, van Zonneveld AJ. Gender and cardiovascular disease: are sex-biased microRNA networks a driving force behind heart failure with preserved ejection fraction in women? Cardiovasc Res (2018) 114:210–25. doi: 10.1093/cvr/cvx223
63. Krieg AM. The toll of too much TLR7. Immunity (2007) 27:695–7. doi: 10.1016/j.immuni.2007.11.001
64. Cervantes JL, Weinerman B, Basole C, Salazar JC. TLR8: the forgotten relative revindicated. Cell Mol Immunol (2012) 9:434–8. doi: 10.1038/cmi.2012.38
65. Zheng Y, Rudensky AY. Foxp3 in control of the regulatory T cell lineage. Nat Immunol (2007) 8:457–62. doi: 10.1038/ni1455
66. Cheever A, Ceman S. Translation regulation of mRNAs by the fragile X family of proteins through the microRNA pathway. RNA Biol (2009) 6:175–8. doi: 10.4161/rna.6.2.8196
67. Hannah MF, Bajic VB, Klein SL. Sex differences in the recognition of and innate antiviral responses to Seoul virus in Norway rats. Brain Behav Immun (2008) 22:503–16. doi: 10.1016/j.bbi.2007.10.005
68. Bjornstrom L, Sjoberg M. Mechanisms of estrogen receptor signaling: convergence of genomic and nongenomic actions on target genes. Mol Endocrinol (2005) 19:833–42. doi: 10.1210/me.2004-0486
69. Palaszynski KM, Smith DL, Kamrava S, Burgoyne PS, Arnold AP, Voskuhl RR. A yin-yang effect between sex chromosome complement and sex hormones on the immune response. Endocrinology (2005) 146:3280–5. doi: 10.1210/en.2005-0284
70. Martinez FO, Gordon S. The M1 and M2 paradigm of macrophage activation: time for reassessment. F1000Prime Rep (2014) 6:13. doi: 10.12703/P6-13
71. Keselman A, Fang X, White PB, Heller NM. Estrogen signaling contributes to sex differences in macrophage polarization during asthma. J Immunol (2017) 199:1573–83. doi: 10.4049/jimmunol.1601975
72. Murray PJ, Allen JE, Biswas SK, Fisher EA, Gilroy DW, Goerdt S, et al. Macrophage activation and polarization: nomenclature and experimental guidelines. Immunity (2014) 41:14–20. doi: 10.1016/j.immuni.2014.06.008
73. Mantovani A, Allavena P. The interaction of anticancer therapies with tumor-associated macrophages. J Exp Med (2015) 212:435–45. doi: 10.1084/jem.20150295
74. Li K, Xu W, Guo Q, Jiang Z, Wang P, Yue Y, et al. Differential macrophage polarization in male and female BALB/c mice infected with coxsackievirus B3 defines susceptibility to viral myocarditis. Circ Res (2009) 105:353–64. doi: 10.1161/CIRCRESAHA.109.195230
75. Melgert BN, Oriss TB, Qi Z, Dixon-McCarthy B, Geerlings M, Hylkema MN, et al. Macrophages: regulators of sex differences in asthma? Am J Respir Cell Mol Biol (2010) 42:595–603. doi: 10.1165/rcmb.2009-0016OC
76. Galvan-Pena S, O'Neill LA. Metabolic reprograming in macrophage polarization. Front Immunol (2014) 5:420. doi: 10.3389/fimmu.2014.00420
77. Scotland RS, Stables MJ, Madalli S, Watson P, Gilroy DW. Sex differences in resident immune cell phenotype underlie more efficient acute inflammatory responses in female mice. Blood (2011) 118:5918–27. doi: 10.1182/blood-2011-03-340281
78. Engler H, Benson S, Wegner A, Spreitzer I, Schedlowski M, Elsenbruch S. Men and women differ in inflammatory and neuroendocrine responses to endotoxin but not in the severity of sickness symptoms. Brain Behav Immun (2016) 52:18–26. doi: 10.1016/j.bbi.2015.08.013
79. Huber SA, Pfaeffle B. Differential Th1 and Th2 cell responses in male and female BALB/c mice infected with coxsackievirus group b type 3. J Virol (1994) 68:5126–32. doi: 10.1128/jvi.68.8.5126-5132.1994
80. Conway SE, Roy-O'Reilly M, Friedler B, Staff I, Fortunato G, McCullough LD. Sex differences and the role of IL-10 in ischemic stroke recovery. Biol Sex Differ (2015) 6:17. doi: 10.1186/s13293-015-0035-9
81. Byrne A, Reen DJ. Lipopolysaccharide induces rapid production of IL-10 by monocytes in the presence of apoptotic neutrophils. J Immunol (2002) 168:1968–77. doi: 10.4049/jimmunol.168.4.1968
82. Banerjee A, Wang J, Bodhankar S, Vandenbark AA, Murphy SJ, Offner H. Phenotypic changes in immune cell subsets reflect increased infarct volume in male vs. female mice. Transl Stroke Res (2013) 4:554–63. doi: 10.1007/s12975-013-0268-z
83. Engler-Chiurazzi EB, Monaghan KL, Wan ECK, Ren X. Role of b cells and the aging brain in stroke recovery and treatment. Geroscience (2020) 42:1199–216. doi: 10.1007/s11357-020-00242-9
84. Shah N, Chari A, Scott E, Mezzi K, Usmani SZ. B-cell maturation antigen (BCMA) in multiple myeloma: rationale for targeting and current therapeutic approaches. Leukemia (2020) 34:985–1005. doi: 10.1038/s41375-020-0734-z
85. Banerjee A, McCullough LD. Sex-specific immune responses in stroke. Stroke (2022) 53:1449–59. doi: 10.1161/STROKEAHA.122.036945
86. Seifert HA, Benedek G, Liang J, Nguyen H, Kent G, Vandenbark AA, et al. Sex differences in regulatory cells in experimental stroke. Cell Immunol (2017) 318:49–54. doi: 10.1016/j.cellimm.2017.06.003
87. Benedek G, Zhang J, Bodhankar S, Nguyen H, Kent G, Jordan K, et al. Estrogen induces multiple regulatory b cell subtypes and promotes M2 microglia and neuroprotection during experimental autoimmune encephalomyelitis. J Neuroimmunol (2016) 293:45–53. doi: 10.1016/j.jneuroim.2016.02.009
88. Maida CD, Norrito RL, Daidone M, Tuttolomondo A, Pinto A. Neuroinflammatory mechanisms in ischemic stroke: Focus on cardioembolic stroke, background, and therapeutic approaches. Int J Mol Sci (2020) 21(18): 6454. doi: 10.3390/ijms21186454
89. Lawson LJ, Perry VH, Dri P, Gordon S. Heterogeneity in the distribution and morphology of microglia in the normal adult mouse brain. Neuroscience (1990) 39:151–70. doi: 10.1016/0306-4522(90)90229-W
90. Kissela B, Lindsell CJ, Kleindorfer D, Alwell K, Moomaw CJ, Woo D, et al. Clinical prediction of functional outcome after ischemic stroke: the surprising importance of periventricular white matter disease and race. Stroke (2009) 40:530–6. doi: 10.1161/STROKEAHA.108.521906
91. Lenzlinger PM, Hans VH, Joller-Jemelka HI, Trentz O, Morganti-Kossmann MC, Kossmann T. Markers for cell-mediated immune response are elevated in cerebrospinal fluid and serum after severe traumatic brain injury in humans. J Neurotrauma (2001) 18:479–89. doi: 10.1089/089771501300227288
92. Tang Y, Le W. Differential roles of M1 and M2 microglia in neurodegenerative diseases. Mol Neurobiol (2016) 53:1181–94. doi: 10.1007/s12035-014-9070-5
93. Hu X, Li P, Guo Y, Wang H, Leak RK, Chen S, et al. Microglia/macrophage polarization dynamics reveal novel mechanism of injury expansion after focal cerebral ischemia. Stroke (2012) 43:3063–70. doi: 10.1161/STROKEAHA.112.659656
94. Yang S, Wang H, Yang Y, Wang R, Wang Y, Wu C, et al. Baicalein administered in the subacute phase ameliorates ischemia-reperfusion-induced brain injury by reducing neuroinflammation and neuronal damage. BioMed Pharmacother (2019) 117:109102. doi: 10.1016/j.biopha.2019.109102
95. Weng L, Wu Z, Zheng W, Meng H, Han L, Wang S, et al. Malibatol a enhances alternative activation of microglia by inhibiting phosphorylation of mammalian Ste20-like kinase1 in OGD-BV-2 cells. Neurol Res (2016) 38:342–8. doi: 10.1080/01616412.2016.1174423
96. Zheng X, Huang H, Liu J, Li M, Liu M, Luo T. Propofol attenuates inflammatory response in LPS-activated microglia by regulating the miR-155/SOCS1 pathway. Inflammation (2018) 41:11–9. doi: 10.1007/s10753-017-0658-6
97. Guruswamy R, ElAli A. Complex roles of microglial cells in ischemic stroke pathobiology: New insights and future directions. Int J Mol Sci (2017) 18(3): 496. doi: 10.3390/ijms18030496
98. Ma Y, Wang J, Wang Y, Yang GY. The biphasic function of microglia in ischemic stroke. Prog Neurobiol (2017) 157:247–72. doi: 10.1016/j.pneurobio.2016.01.005
99. Al Mamun A, Chauhan A, Yu H, Xu Y, Sharmeen R, Liu F. Interferon regulatory factor 4/5 signaling impacts on microglial activation after ischemic stroke in mice. Eur J Neurosci (2018) 47:140–9. doi: 10.1111/ejn.13778
100. Franco R, Fernandez-Suarez D. Alternatively activated microglia and macrophages in the central nervous system. Prog Neurobiol (2015) 131:65–86. doi: 10.1016/j.pneurobio.2015.05.003
101. Colton CA. Heterogeneity of microglial activation in the innate immune response in the brain. J Neuroimmune Pharmacol (2009) 4:399–418. doi: 10.1007/s11481-009-9164-4
102. Chhor V, Le Charpentier T, Lebon S, Ore MV, Celador IL, Josserand J, et al. Characterization of phenotype markers and neuronotoxic potential of polarised primary microglia in vitro. Brain Behav Immun (2013) 32:70–85. doi: 10.1016/j.bbi.2013.02.005
103. Anrather J, Iadecola C. Inflammation and stroke: An overview. Neurotherapeutics (2016) 13:661–70. doi: 10.1007/s13311-016-0483-x
104. Banchereau J, Steinman RM. Dendritic cells and the control of immunity. Nature (1998) 392:245–52. doi: 10.1038/32588
105. Pashenkov M, Huang YM, Kostulas V, Haglund M, Soderstrom M, Link H. Two subsets of dendritic cells are present in human cerebrospinal fluid. Brain (2001) 124:480–92. doi: 10.1093/brain/124.3.480
106. Dai H, Thomson AW, Rogers NM. Dendritic cells as sensors, mediators, and regulators of ischemic injury. Front Immunol (2019) 10:2418. doi: 10.3389/fimmu.2019.02418
107. Rostrom B, Link B. Oligoclonal immunoglobulins in cerebrospinal fluid in acute cerebrovascular disease. Neurology (1981) 31:590–6. doi: 10.1212/WNL.31.5.590
108. Tsementzis SA, Chao SW, Hitchcock ER, Gill JS, Beevers DG. Oligoclonal immunoglobulin G in acute subarachnoid hemorrhage and stroke. Neurology (1986) 36:395–7. doi: 10.1212/WNL.36.3.395
109. Hurn PD, Subramanian S, Parker SM, Afentoulis ME, Kaler LJ, Vandenbark AA, et al. T- and b-cell-deficient mice with experimental stroke have reduced lesion size and inflammation. J Cereb Blood Flow Metab (2007) 27:1798–805. doi: 10.1038/sj.jcbfm.9600482
110. Ortega SB, Torres VO, Latchney SE, Whoolery CW, Noorbhai IZ, Poinsatte K, et al. B cells migrate into remote brain areas and support neurogenesis and functional recovery after focal stroke in mice. Proc Natl Acad Sci U.S.A. (2020) 117:4983–93. doi: 10.1073/pnas.1913292117
111. Ren X, Akiyoshi K, Dziennis S, Vandenbark AA, Herson PS, Hurn PD, et al. Regulatory b cells limit CNS inflammation and neurologic deficits in murine experimental stroke. J Neurosci (2011) 31:8556–63. doi: 10.1523/JNEUROSCI.1623-11.2011
112. Geissmann F, Manz MG, Jung S, Sieweke MH, Merad M, Ley K. Development of monocytes, macrophages, and dendritic cells. Science (2010) 327:656–61. doi: 10.1126/science.1178331
113. Ginhoux F, Jung S. Monocytes and macrophages: developmental pathways and tissue homeostasis. Nat Rev Immunol (2014) 14:392–404. doi: 10.1038/nri3671
114. Singh S, Anshita D, Ravichandiran V. MCP-1: Function, regulation, and involvement in disease. Int Immunopharmacol (2021) 101:107598. doi: 10.1016/j.intimp.2021.107598
115. Garcia-Bonilla L, Faraco G, Moore J, Murphy M, Racchumi G, Srinivasan J, et al. Spatio-temporal profile, phenotypic diversity, and fate of recruited monocytes into the post-ischemic brain. J Neuroinflamm (2016) 13:285. doi: 10.1186/s12974-016-0750-0
116. Naert G, Rivest S. A deficiency in CCR2+ monocytes: the hidden side of alzheimer's disease. J Mol Cell Biol (2013) 5:284–93. doi: 10.1093/jmcb/mjt028
117. Michaud JP, Pimentel-Coelho PM, Tremblay Y, Rivest S. The impact of Ly6Clow monocytes after cerebral hypoxia-ischemia in adult mice. J Cereb Blood Flow Metab (2014) 34:e1–9. doi: 10.1038/jcbfm.2014.80
118. Carlin LM, Stamatiades EG, Auffray C, Hanna RN, Glover L, Vizcay-Barrena G, et al. Nr4a1-dependent Ly6C(low) monocytes monitor endothelial cells and orchestrate their disposal. Cell (2013) 153:362–75. doi: 10.1016/j.cell.2013.03.010
119. Wattananit S, Tornero D, Graubardt N, Memanishvili T, Monni E, Tatarishvili J, et al. Monocyte-derived macrophages contribute to spontaneous long-term functional recovery after stroke in mice. J Neurosci (2016) 36:4182–95. doi: 10.1523/JNEUROSCI.4317-15.2016
120. Verma R, Cronin CG, Hudobenko J, Venna VR, McCullough LD, Liang BT. Deletion of the P2X4 receptor is neuroprotective acutely, but induces a depressive phenotype during recovery from ischemic stroke. Brain Behav Immun (2017) 66:302–12. doi: 10.1016/j.bbi.2017.07.155
121. Liu Q, Johnson EM, Lam RK, Wang Q, Bo Ye H, Wilson EN, et al. Peripheral TREM1 responses to brain and intestinal immunogens amplify stroke severity. Nat Immunol (2019) 20:1023–34. doi: 10.1038/s41590-019-0421-2
122. Carmona-Mora P, Ander BP, Jickling GC, Dykstra-Aiello C, Zhan X, Ferino E, et al. Distinct peripheral blood monocyte and neutrophil transcriptional programs following intracerebral hemorrhage and different etiologies of ischemic stroke. J Cereb Blood Flow Metab (2021) 41:1398–416. doi: 10.1177/0271678X20953912
123. Wang R, Liu Y, Ye Q, Hassan SH, Zhao J, Li S, et al. RNA Sequencing reveals novel macrophage transcriptome favoring neurovascular plasticity after ischemic stroke. J Cereb Blood Flow Metab (2020) 40:720–38. doi: 10.1177/0271678X19888630
124. Xue J, Huang W, Chen X, Li Q, Cai Z, Yu T, et al. Neutrophil-to-Lymphocyte ratio is a prognostic marker in acute ischemic stroke. J Stroke Cerebrovasc Dis (2017) 26:650–7. doi: 10.1016/j.jstrokecerebrovasdis.2016.11.010
125. Roy-O'Reilly MA, Ahnstedt H, Spychala MS, Munshi Y, Aronowski J, Sansing LH, et al. Aging exacerbates neutrophil pathogenicity in ischemic stroke. Aging (Albany NY) (2020) 12:436–61. doi: 10.18632/aging.102632
126. Ritzel RM, Capozzi LA, McCullough LD. Sex, stroke, and inflammation: the potential for estrogen-mediated immunoprotection in stroke. Horm Behav (2013) 63:238–53. doi: 10.1016/j.yhbeh.2012.04.007
127. Krizbai IA, Bauer H, Bresgen N, Eckl PM, Farkas A, Szatmari E, et al. Effect of oxidative stress on the junctional proteins of cultured cerebral endothelial cells. Cell Mol Neurobiol (2005) 25:129–39. doi: 10.1007/s10571-004-1378-7
128. Dimasi D, Sun WY, Bonder CS. Neutrophil interactions with the vascular endothelium. Int Immunopharmacol (2013) 17:1167–75. doi: 10.1016/j.intimp.2013.05.034
129. Danton GH, Dietrich WD. Inflammatory mechanisms after ischemia and stroke. J Neuropathol Exp Neurol (2003) 62:127–36. doi: 10.1093/jnen/62.2.127
130. Vemuganti R, Dempsey RJ, Bowen KK. Inhibition of intercellular adhesion molecule-1 protein expression by antisense oligonucleotides is neuroprotective after transient middle cerebral artery occlusion in rat. Stroke (2004) 35:179–84. doi: 10.1161/01.STR.0000106479.53235.3E
131. Liesz A, Zhou W, Mracsko E, Karcher S, Bauer H, Schwarting S, et al. Inhibition of lymphocyte trafficking shields the brain against deleterious neuroinflammation after stroke. Brain (2011) 134:704–20. doi: 10.1093/brain/awr008
132. Soriano SG, Coxon A, Wang YF, Frosch MP, Lipton SA, Hickey PR, et al. Mice deficient in mac-1 (CD11b/CD18) are less susceptible to cerebral ischemia/reperfusion injury. Stroke (1999) 30:134–9. doi: 10.1161/01.STR.30.1.134
133. Zhang R, Chopp M, Zhang Z, Jiang N, Powers C. The expression of p- and e-selectins in three models of middle cerebral artery occlusion. Brain Res (1998) 785:207–14. doi: 10.1016/S0006-8993(97)01343-7
134. Kim JS. Cytokines and adhesion molecules in stroke and related diseases. J Neurol Sci (1996) 137:69–78. doi: 10.1016/0022-510X(95)00338-3
135. Khan M, Jatana M, Elango C, Paintlia AS, Singh AK, Singh I. Cerebrovascular protection by various nitric oxide donors in rats after experimental stroke. Nitric Oxide (2006) 15:114–24. doi: 10.1016/j.niox.2006.01.008
136. Huang J, Choudhri TF, Winfree CJ, McTaggart RA, Kiss S, Mocco J, et al. Postischemic cerebrovascular e-selectin expression mediates tissue injury in murine stroke. Stroke (2000) 31:3047–53. doi: 10.1161/01.STR.31.12.3047
137. Gagliani N, Huber S. Basic aspects of T helper cell differentiation. Methods Mol Biol (2017) 1514:19–30. doi: 10.1007/978-1-4939-6548-9_2
138. Jian Z, Liu R, Zhu X, Smerin D, Zhong Y, Gu L, et al. The involvement and therapy target of immune cells after ischemic stroke. Front Immunol (2019) 10:2167. doi: 10.3389/fimmu.2019.02167
139. Bravo-Alegria J, McCullough LD, Liu F. Sex differences in stroke across the lifespan: The role of T lymphocytes. Neurochem Int (2017) 107:127–37. doi: 10.1016/j.neuint.2017.01.009
140. Filiano AJ, Gadani SP, Kipnis J. How and why do T cells and their derived cytokines affect the injured and healthy brain? Nat Rev Neurosci (2017) 18:375–84. doi: 10.1038/nrn.2017.39
141. Noh MY, Lee WM, Lee SJ, Kim HY, Kim SH, Kim YS. Regulatory T cells increase after treatment with poly (ADP-ribose) polymerase-1 inhibitor in ischemic stroke patients. Int Immunopharmacol (2018) 60:104–10. doi: 10.1016/j.intimp.2018.04.043
142. Mracsko E, Liesz A, Stojanovic A, Lou WP, Osswald M, Zhou W, et al. Antigen dependently activated cluster of differentiation 8-positive T cells cause perforin-mediated neurotoxicity in experimental stroke. J Neurosci (2014) 34:16784–95. doi: 10.1523/JNEUROSCI.1867-14.2014
143. Unutmaz D, Pileri P, Abrignani S. Antigen-independent activation of naive and memory resting T cells by a cytokine combination. J Exp Med (1994) 180:1159–64. doi: 10.1084/jem.180.3.1159
144. Zhu J, Yamane H, Paul WE. Differentiation of effector CD4 T cell populations (*). Annu Rev Immunol (2010) 28:445–89. doi: 10.1146/annurev-immunol-030409-101212
145. Liesz A, Hu X, Kleinschnitz C, Offner H. Functional role of regulatory lymphocytes in stroke: facts and controversies. Stroke (2015) 46:1422–30. doi: 10.1161/STROKEAHA.114.008608
146. Rudensky AY. Regulatory T cells and Foxp3. Immunol Rev (2011) 241:260–8. doi: 10.1111/j.1600-065X.2011.01018.x
147. Dong C. Cytokine regulation and function in T cells. Annu Rev Immunol (2021) 39:51–76. doi: 10.1146/annurev-immunol-061020-053702
148. Gelderblom M, Weymar A, Bernreuther C, Velden J, Arunachalam P, Steinbach K, et al. Neutralization of the IL-17 axis diminishes neutrophil invasion and protects from ischemic stroke. Blood (2012) 120:3793–802. doi: 10.1182/blood-2012-02-412726
149. Pawluk H, Wozniak A, Grzesk G, Kolodziejska R, Kozakiewicz M, Kopkowska E, et al. The role of selected pro-inflammatory cytokines in pathogenesis of ischemic stroke. Clin Interv Aging (2020) 15:469–84. doi: 10.2147/CIA.S233909
150. Ramiro L, Simats A, Garcia-Berrocoso T, Montaner J. Inflammatory molecules might become both biomarkers and therapeutic targets for stroke management. Ther Adv Neurol Disord (2018) 11:1756286418789340. doi: 10.1177/1756286418789340
151. Matsumoto J, Dohgu S, Takata F, Machida T, Bolukbasi Hatip FF, Hatip-Al-Khatib I, et al. TNF-alpha-sensitive brain pericytes activate microglia by releasing IL-6 through cooperation between IkappaB-NFkappaB and JAK-STAT3 pathways. Brain Res (2018) 1692:34–44. doi: 10.1016/j.brainres.2018.04.023
152. Barone FC, Arvin B, White RF, Miller A, Webb CL, Willette RN, et al. Tumor necrosis factor-alpha. a mediator of focal ischemic brain injury. Stroke (1997) 28:1233–44. doi: 10.1161/01.STR.28.6.1233
153. Yilmaz G, Arumugam TV, Stokes KY, Granger DN. Role of T lymphocytes and interferon-gamma in ischemic stroke. Circulation (2006) 113:2105–12. doi: 10.1161/CIRCULATIONAHA.105.593046
154. Lambertsen KL, Gregersen R, Meldgaard M, Clausen BH, Heibol EK, Ladeby R, et al. A role for interferon-gamma in focal cerebral ischemia in mice. J Neuropathol Exp Neurol (2004) 63:942–55. doi: 10.1093/jnen/63.9.942
155. Erta M, Quintana A, Hidalgo J. Interleukin-6, a major cytokine in the central nervous system. Int J Biol Sci (2012) 8:1254–66. doi: 10.7150/ijbs.4679
156. Esenwa CC, Elkind MS. Inflammatory risk factors, biomarkers and associated therapy in ischaemic stroke. Nat Rev Neurol (2016) 12:594–604. doi: 10.1038/nrneurol.2016.125
157. Uyama N, Tsutsui H, Wu S, Yasuda K, Hatano E, Qin XY, et al. Anti-interleukin-6 receptor antibody treatment ameliorates postoperative adhesion formation. Sci Rep (2019) 9:17558. doi: 10.1038/s41598-019-54175-1
158. Suzuki S, Tanaka K, Suzuki N. Ambivalent aspects of interleukin-6 in cerebral ischemia: inflammatory versus neurotrophic aspects. J Cereb Blood Flow Metab (2009) 29:464–79. doi: 10.1038/jcbfm.2008.141
159. Rose-John S. Interleukin-6 family cytokines. Cold Spring Harb Perspect Biol (2018) 10(2): a028415. doi: 10.1101/cshperspect.a028415
160. Yao H, Zhang Y, Shu H, Xie B, Tao Y, Yuan Y, et al. Hyperforin promotes post-stroke neuroangiogenesis via astrocytic IL-6-Mediated negative immune regulation in the ischemic brain. Front Cell Neurosci (2019) 13:201. doi: 10.3389/fncel.2019.00201
161. Swartz KR, Liu F, Sewell D, Schochet T, Campbell I, Sandor M, et al. Interleukin-6 promotes post-traumatic healing in the central nervous system. Brain Res (2001) 896:86–95. doi: 10.1016/S0006-8993(01)02013-3
162. Smith DE, Renshaw BR, Ketchem RR, Kubin M, Garka KE, Sims JE. Four new members expand the interleukin-1 superfamily. J Biol Chem (2000) 275:1169–75. doi: 10.1074/jbc.275.2.1169
163. Allan SM, Tyrrell PJ, Rothwell NJ. Interleukin-1 and neuronal injury. Nat Rev Immunol (2005) 5:629–40. doi: 10.1038/nri1664
164. Konsman JP, Drukarch B, Van Dam AM. (Peri)vascular production and action of pro-inflammatory cytokines in brain pathology. Clin Sci (Lond) (2007) 112:1–25. doi: 10.1042/CS20060043
165. Sobowale OA, Parry-Jones AR, Smith CJ, Tyrrell PJ, Rothwell NJ, Allan SM. Interleukin-1 in stroke: From bench to bedside. Stroke (2016) 47:2160–7. doi: 10.1161/STROKEAHA.115.010001
166. Liu G, Tsuruta Y, Gao Z, Park YJ, Abraham E. Variant IL-1 receptor-associated kinase-1 mediates increased NF-kappa b activity. J Immunol (2007) 179:4125–34. doi: 10.4049/jimmunol.179.6.4125
167. Cahill CM, Rogers JT. Interleukin (IL) 1beta induction of IL-6 is mediated by a novel phosphatidylinositol 3-kinase-dependent AKT/IkappaB kinase alpha pathway targeting activator protein-1. J Biol Chem (2008) 283:25900–12. doi: 10.1074/jbc.M707692200
168. Kong X, Gong Z, Zhang L, Sun X, Ou Z, Xu B, et al. JAK2/STAT3 signaling mediates IL-6-inhibited neurogenesis of neural stem cells through DNA demethylation/methylation. Brain Behav Immun (2019) 79:159–73. doi: 10.1016/j.bbi.2019.01.027
169. Zhu H, Jian Z, Zhong Y, Ye Y, Zhang Y, Hu X, et al. Janus kinase inhibition ameliorates ischemic stroke injury and neuroinflammation through reducing NLRP3 inflammasome activation via JAK2/STAT3 pathway inhibition. Front Immunol (2021) 12:714943. doi: 10.3389/fimmu.2021.714943
170. O'Garra A, Vieira PL, Vieira P, Goldfeld AE. IL-10-producing and naturally occurring CD4+ tregs: limiting collateral damage. J Clin Invest (2004) 114:1372–8. doi: 10.1172/JCI23215
171. Oleszycka E, McCluskey S, Sharp FA, Munoz-Wolf N, Hams E, Gorman AL, et al. The vaccine adjuvant alum promotes IL-10 production that suppresses Th1 responses. Eur J Immunol (2018) 48:705–15. doi: 10.1002/eji.201747150
172. Sharma S, Yang B, Xi X, Grotta JC, Aronowski J, Savitz SI. IL-10 directly protects cortical neurons by activating PI-3 kinase and STAT-3 pathways. Brain Res (2011) 1373:189–94. doi: 10.1016/j.brainres.2010.11.096
173. Bodhankar S, Chen Y, Vandenbark AA, Murphy SJ, Offner H. Treatment of experimental stroke with IL-10-producing b-cells reduces infarct size and peripheral and CNS inflammation in wild-type b-cell-sufficient mice. Metab Brain Dis (2014) 29:59–73. doi: 10.1007/s11011-013-9474-3
174. Liang QJ, Jiang M, Wang XH, Le LL, Xiang M, Sun N, et al. Pre-existing interleukin 10 in cerebral arteries attenuates subsequent brain injury caused by ischemia/reperfusion. IUBMB Life (2015) 67:710–9. doi: 10.1002/iub.1429
175. Driessler F, Venstrom K, Sabat R, Asadullah K, Schottelius AJ. Molecular mechanisms of interleukin-10-mediated inhibition of NF-kappaB activity: a role for p50. Clin Exp Immunol (2004) 135:64–73. doi: 10.1111/j.1365-2249.2004.02342.x
176. Hwang CJ, Yun HM, Jung YY, Lee DH, Yoon NY, Seo HO, et al. Reducing effect of IL-32alpha in the development of stroke through blocking of NF-kappaB, but enhancement of STAT3 pathways. Mol Neurobiol (2015) 51:648–60. doi: 10.1007/s12035-014-8739-0
177. Frenkel D, Huang Z, Maron R, Koldzic DN, Hancock WW, Moskowitz MA, et al. Nasal vaccination with myelin oligodendrocyte glycoprotein reduces stroke size by inducing IL-10-producing CD4+ T cells. J Immunol (2003) 171:6549–55. doi: 10.4049/jimmunol.171.12.6549
178. Frenkel D, Huang Z, Maron R, Koldzic DN, Moskowitz MA, Weiner HL. Neuroprotection by IL-10-producing MOG CD4+ T cells following ischemic stroke. J Neurol Sci (2005) 233:125–32. doi: 10.1016/j.jns.2005.03.022
179. Abbas AK, Murphy KM, Sher A. Functional diversity of helper T lymphocytes. Nature (1996) 383:787–93. doi: 10.1038/383787a0
180. Gadani SP, Cronk JC, Norris GT, Kipnis J. IL-4 in the brain: a cytokine to remember. J Immunol (2012) 189:4213–9. doi: 10.4049/jimmunol.1202246
181. Zhu H, Hu S, Li Y, Sun Y, Xiong X, Hu X, et al. Interleukins and ischemic stroke. Front Immunol (2022) 13:828447. doi: 10.3389/fimmu.2022.828447
182. Kim HM, Shin HY, Jeong HJ, An HJ, Kim NS, Chae HJ, et al. Reduced IL-2 but elevated IL-4, IL-6, and IgE serum levels in patients with cerebral infarction during the acute stage. J Mol Neurosci (2000) 14:191–6. doi: 10.1385/JMN:14:3:191
183. Xiong X, Xu L, Wei L, White RE, Ouyang YB, Giffard RG. IL-4 is required for sex differences in vulnerability to focal ischemia in mice. Stroke (2015) 46:2271–6. doi: 10.1161/STROKEAHA.115.008897
184. Liu X, Liu J, Zhao S, Zhang H, Cai W, Cai M, et al. Interleukin-4 is essential for Microglia/Macrophage M2 polarization and long-term recovery after cerebral ischemia. Stroke (2016) 47:498–504. doi: 10.1161/STROKEAHA.115.012079
185. Shi Q, Cai X, Shi G, Lv X, Yu J, Wang F. Interleukin-4 protects from chemotherapy-induced peripheral neuropathy in mice modal via the stimulation of IL-4/STAT6 signaling. Acta Cir Bras (2018) 33:491–8. doi: 10.1590/s0102-865020180060000003
186. Gelderblom M, Gallizioli M, Ludewig P, Thom V, Arunachalam P, Rissiek B, et al. IL-23 (Interleukin-23)-Producing conventional dendritic cells control the detrimental IL-17 (Interleukin-17) response in stroke. Stroke (2018) 49:155–64. doi: 10.1161/STROKEAHA.117.019101
187. Chien YH, Meyer C, Bonneville M. Gammadelta T cells: first line of defense and beyond. Annu Rev Immunol (2014) 32:121–55. doi: 10.1146/annurev-immunol-032713-120216
188. Lu L, Wang Y, Zhou L, Li Y, Zhang X, Hu X, et al. Vgamma4 T cell-derived IL-17A is essential for amplification of inflammatory cascades in ischemic brain tissue after stroke. Int Immunopharmacol (2021) 96:107678. doi: 10.1016/j.intimp.2021.107678
189. Leon RL, Huber JD, Rosen CL. Potential age-dependent effects of estrogen on neural injury. Am J Pathol (2011) 178:2450–60. doi: 10.1016/j.ajpath.2011.01.057
190. Liu F, Yuan R, Benashski SE, McCullough LD. Changes in experimental stroke outcome across the life span. J Cereb Blood Flow Metab (2009) 29:792–802. doi: 10.1038/jcbfm.2009.5
191. Iadecola C, Anrather J. The immunology of stroke: from mechanisms to translation. Nat Med (2011) 17:796–808. doi: 10.1038/nm.2399
192. Stoll G, Jander S, Schroeter M. Inflammation and glial responses in ischemic brain lesions. Prog Neurobiol (1998) 56:149–71. doi: 10.1016/S0301-0082(98)00034-3
193. Altinoz MA, Korkmaz R. NF-kappaB, macrophage migration inhibitory factor and cyclooxygenase-inhibitions as likely mechanisms behind the acetaminophen- and NSAID-prevention of the ovarian cancer. Neoplasma (2004) 51:239–47.
194. Wang Q, Tang XN, Yenari MA. The inflammatory response in stroke. J Neuroimmunol (2007) 184:53–68. doi: 10.1016/j.jneuroim.2006.11.014
195. Qin C, Zhou LQ, Ma XT, Hu ZW, Yang S, Chen M, et al. Dual functions of microglia in ischemic stroke. Neurosci Bull (2019) 35:921–33. doi: 10.1007/s12264-019-00388-3
196. Bodhankar S, Lapato A, Chen Y, Vandenbark AA, Saugstad JA, Offner H. Role for microglia in sex differences after ischemic stroke: importance of M2. Metab Brain Dis (2015) 30:1515–29. doi: 10.1007/s11011-015-9714-9
197. Villa A, Gelosa P, Castiglioni L, Cimino M, Rizzi N, Pepe G, et al. Sex-specific features of microglia from adult mice. Cell Rep (2018) 23:3501–11. doi: 10.1016/j.celrep.2018.05.048
198. Qi S, Al Mamun A, Ngwa C, Romana S, Ritzel R, Arnold AP, et al. X Chromosome escapee genes are involved in ischemic sexual dimorphism through epigenetic modification of inflammatory signals. J Neuroinflamm (2021) 18:70. doi: 10.1186/s12974-021-02120-3
199. Dotson AL, Wang J, Saugstad J, Murphy SJ, Offner H. Splenectomy reduces infarct volume and neuroinflammation in male but not female mice in experimental stroke. J Neuroimmunol (2015) 278:289–98. doi: 10.1016/j.jneuroim.2014.11.020
200. Bodhankar S, Chen Y, Lapato A, Vandenbark AA, Murphy SJ, Saugstad JA, et al. Regulatory CD8(+)CD122 (+) T-cells predominate in CNS after treatment of experimental stroke in male mice with IL-10-secreting b-cells. Metab Brain Dis (2015) 30:911–24. doi: 10.1007/s11011-014-9639-8
201. Morrison HW, Filosa JA. Stroke and the neurovascular unit: glial cells, sex differences, and hypertension. Am J Physiol Cell Physiol (2019) 316:C325–39. doi: 10.1152/ajpcell.00333.2018
202. Chisholm NC, Sohrabji F. Astrocytic response to cerebral ischemia is influenced by sex differences and impaired by aging. Neurobiol Dis (2016) 85:245–53. doi: 10.1016/j.nbd.2015.03.028
203. Perez-Alvarez MJ, Maza Mdel C, Anton M, Ordonez L, Wandosell F. Post-ischemic estradiol treatment reduced glial response and triggers distinct cortical and hippocampal signaling in a rat model of cerebral ischemia. J Neuroinflamm (2012) 9:157. doi: 10.1186/1742-2094-9-157
204. Djebaili M, Guo Q, Pettus EH, Hoffman SW, Stein DG. The neurosteroids progesterone and allopregnanolone reduce cell death, gliosis, and functional deficits after traumatic brain injury in rats. J Neurotrauma (2005) 22:106–18. doi: 10.1089/neu.2005.22.106
205. Micevych PE, Chaban V, Ogi J, Dewing P, Lu JK, Sinchak K. Estradiol stimulates progesterone synthesis in hypothalamic astrocyte cultures. Endocrinology (2007) 148:782–9. doi: 10.1210/en.2006-0774
206. Kuo J, Hamid N, Bondar G, Prossnitz ER, Micevych P. Membrane estrogen receptors stimulate intracellular calcium release and progesterone synthesis in hypothalamic astrocytes. J Neurosci (2010) 30:12950–7. doi: 10.1523/JNEUROSCI.1158-10.2010
207. Liu M, Hurn PD, Roselli CE, Alkayed NJ. Role of P450 aromatase in sex-specific astrocytic cell death. J Cereb Blood Flow Metab (2007) 27:135–41. doi: 10.1038/sj.jcbfm.9600331
208. Liu M, Oyarzabal EA, Yang R, Murphy SJ, Hurn PD. A novel method for assessing sex-specific and genotype-specific response to injury in astrocyte culture. J Neurosci Methods (2008) 171:214–7. doi: 10.1016/j.jneumeth.2008.03.002
209. Wang C, Jie C, Dai X. Possible roles of astrocytes in estrogen neuroprotection during cerebral ischemia. Rev Neurosci (2014) 25:255–68. doi: 10.1515/revneuro-2013-0055
210. Guo J, Duckles SP, Weiss JH, Li X, Krause DN. 17beta-estradiol prevents cell death and mitochondrial dysfunction by an estrogen receptor-dependent mechanism in astrocytes after oxygen-glucose deprivation/reperfusion. Free Radic Biol Med (2012) 52:2151–60. doi: 10.1016/j.freeradbiomed.2012.03.005
211. Wang J, Liu M, Hou W, Hou M, Zhang L, Sun M, et al. N-myc downstream-regulated gene 2 (Ndrg2): A critical mediator of estrogen-induced neuroprotection against cerebral ischemic injury. Mol Neurobiol (2022) 59:4793–804. doi: 10.1007/s12035-022-02877-5
212. Pennypacker KR, Offner H. The role of the spleen in ischemic stroke. J Cereb Blood Flow Metab (2015) 35:186–7. doi: 10.1038/jcbfm.2014.212
213. Ostrowski RP, Schulte RW, Nie Y, Ling T, Lee T, Manaenko A, et al. Acute splenic irradiation reduces brain injury in the rat focal ischemic stroke model. Transl Stroke Res (2012) 3:473–81. doi: 10.1007/s12975-012-0206-5
214. Offner H, Subramanian S, Parker SM, Wang C, Afentoulis ME, Lewis A, et al. Splenic atrophy in experimental stroke is accompanied by increased regulatory T cells and circulating macrophages. J Immunol (2006) 176:6523–31. doi: 10.4049/jimmunol.176.11.6523
215. Beck RD Jr., Wasserfall C, Ha GK, Cushman JD, Huang Z, Atkinson MA, et al. Changes in hippocampal IL-15, related cytokines, and neurogenesis in IL-2 deficient mice. Brain Res (2005) 1041:223–30. doi: 10.1016/j.brainres.2005.02.010
216. Sinha S, Kaler LJ, Proctor TM, Teuscher C, Vandenbark AA, Offner H. IL-13-mediated gender difference in susceptibility to autoimmune encephalomyelitis. J Immunol (2008) 180:2679–85. doi: 10.4049/jimmunol.180.4.2679
217. Bodhankar S, Chen Y, Lapato A, Dotson AL, Wang J, Vandenbark AA, et al. PD-L1 monoclonal antibody treats ischemic stroke by controlling central nervous system inflammation. Stroke (2015) 46:2926–34. doi: 10.1161/STROKEAHA.115.010592
218. Zhao X, Wang H, Sun G, Zhang J, Edwards NJ, Aronowski J. Neuronal interleukin-4 as a modulator of microglial pathways and ischemic brain damage. J Neurosci (2015) 35:11281–91. doi: 10.1523/JNEUROSCI.1685-15.2015
219. Stamova B, Tian Y, Jickling G, Bushnell C, Zhan X, Liu D, et al. The X-chromosome has a different pattern of gene expression in women compared with men with ischemic stroke. Stroke (2012) 43:326–34. doi: 10.1161/STROKEAHA.111.629337
220. Tian Y, Stamova B, Jickling GC, Xu H, Liu D, Ander BP, et al. Y chromosome gene expression in the blood of male patients with ischemic stroke compared with male controls. Gend Med (2012) 9:68–75.e63. doi: 10.1016/j.genm.2012.01.005
221. Alkayed NJ, Harukuni I, Kimes AS, London ED, Traystman RJ, Hurn PD. Gender-linked brain injury in experimental stroke. Stroke (1998) 29:159–65. doi: 10.1161/01.STR.29.1.159
222. Suzuki S, Gerhold LM, Bottner M, Rau SW, Dela Cruz C, Yang E, et al. Estradiol enhances neurogenesis following ischemic stroke through estrogen receptors alpha and beta. J Comp Neurol (2007) 500:1064–75. doi: 10.1002/cne.21240
223. Arevalo MA, Azcoitia I, Garcia-Segura LM. The neuroprotective actions of oestradiol and oestrogen receptors. Nat Rev Neurosci (2015) 16:17–29. doi: 10.1038/nrn3856
224. Petrone AB, Simpkins JW, Barr TL. 17beta-estradiol and inflammation: implications for ischemic stroke. Aging Dis (2014) 5:340–5. doi: 10.14336/ad.2014.0500340
225. Kim T, Chelluboina B, Chokkalla AK, Vemuganti R. Age and sex differences in the pathophysiology of acute CNS injury. Neurochem Int (2019) 127:22–8. doi: 10.1016/j.neuint.2019.01.012
226. Behl C, Skutella T, Lezoualc'h F, Post A, Widmann M, Newton CJ, et al. Neuroprotection against oxidative stress by estrogens: structure-activity relationship. Mol Pharmacol (1997) 51:535–41. doi: 10.1124/mol.51.4.535
227. Iadecola C, Ross ME. Molecular pathology of cerebral ischemia: delayed gene expression and strategies for neuroprotection. Ann N Y Acad Sci (1997) 835:203–17. doi: 10.1111/j.1749-6632.1997.tb48631.x
228. Liao SL, Chen WY, Chen CJ. Estrogen attenuates tumor necrosis factor-alpha expression to provide ischemic neuroprotection in female rats. Neurosci Lett (2002) 330:159–62. doi: 10.1016/S0304-3940(02)00754-1
229. Nagayama M, Aber T, Nagayama T, Ross ME, Iadecola C. Age-dependent increase in ischemic brain injury in wild-type mice and in mice lacking the inducible nitric oxide synthase gene. J Cereb Blood Flow Metab (1999) 19:661–6. doi: 10.1097/00004647-199906000-00009
230. Wen Y, Yang S, Liu R, Perez E, Yi KD, Koulen P, et al. Estrogen attenuates nuclear factor-kappa b activation induced by transient cerebral ischemia. Brain Res (2004) 1008:147–54. doi: 10.1016/j.brainres.2004.02.019
231. Mannella P, Brinton RD. Estrogen receptor protein interaction with phosphatidylinositol 3-kinase leads to activation of phosphorylated akt and extracellular signal-regulated kinase 1/2 in the same population of cortical neurons: a unified mechanism of estrogen action. J Neurosci (2006) 26:9439–47. doi: 10.1523/JNEUROSCI.1443-06.2006
232. Wang S, Ren P, Li X, Guan Y, Zhang YA. 17beta-estradiol protects dopaminergic neurons in organotypic slice of mesencephalon by MAPK-mediated activation of anti-apoptosis gene BCL2. J Mol Neurosci (2011) 45:236–45. doi: 10.1007/s12031-011-9500-z
233. Yao M, Nguyen TV, Pike CJ. Estrogen regulates bcl-w and bim expression: role in protection against beta-amyloid peptide-induced neuronal death. J Neurosci (2007) 27:1422–33. doi: 10.1523/JNEUROSCI.2382-06.2007
234. Friedman WJ, Thakur S, Seidman L, Rabson AB. Regulation of nerve growth factor mRNA by interleukin-1 in rat hippocampal astrocytes is mediated by NFkappaB. J Biol Chem (1996) 271:31115–20. doi: 10.1074/jbc.271.49.31115
235. Ospina JA, Brevig HN, Krause DN, Duckles SP. Estrogen suppresses IL-1beta-mediated induction of COX-2 pathway in rat cerebral blood vessels. Am J Physiol Heart Circ Physiol (2004) 286:H2010–2019. doi: 10.1152/ajpheart.00481.2003
236. Campos FL, Cristovao AC, Rocha SM, Fonseca CP, Baltazar G. GDNF contributes to oestrogen-mediated protection of midbrain dopaminergic neurones. J Neuroendocrinol (2012) 24:1386–97. doi: 10.1111/j.1365-2826.2012.02348.x
237. Pietranera L, Brocca ME, Roig P, Lima A, Garcia-Segura LM, De Nicola AF. Estrogens are neuroprotective factors for hypertensive encephalopathy. J Steroid Biochem Mol Biol (2015) 146:15–25. doi: 10.1016/j.jsbmb.2014.04.001
238. Cook S, Hung V, Duncan KA. Crosstalk between estrogen withdrawal and NFkappaB signaling following penetrating brain injury. Neuroimmunomodulation (2018) 25:193–200. doi: 10.1159/000493506
239. Sehara Y, Sawicka K, Hwang JY, Latuszek-Barrantes A, Etgen AM, Zukin RS. Survivin is a transcriptional target of STAT3 critical to estradiol neuroprotection in global ischemia. J Neurosci (2013) 33:12364–74. doi: 10.1523/JNEUROSCI.1852-13.2013
240. Kinouchi T, Kitazato KT, Shimada K, Yagi K, Tada Y, Matsushita N, et al. Activation of signal transducer and activator of transcription-3 by a peroxisome proliferator-activated receptor gamma agonist contributes to neuroprotection in the peri-infarct region after ischemia in oophorectomized rats. Stroke (2012) 43:478–83. doi: 10.1161/STROKEAHA.111.618926
241. Lambertsen KL, Biber K, Finsen B. Inflammatory cytokines in experimental and human stroke. J Cereb Blood Flow Metab (2012) 32:1677–98. doi: 10.1038/jcbfm.2012.88
242. Strom JO, Theodorsson A, Theodorsson E. Mechanisms of estrogens' dose-dependent neuroprotective and neurodamaging effects in experimental models of cerebral ischemia. Int J Mol Sci (2011) 12:1533–62. doi: 10.3390/ijms12031533
243. Koellhoffer EC, McCullough LD. The effects of estrogen in ischemic stroke. Transl Stroke Res (2013) 4:390–401. doi: 10.1007/s12975-012-0230-5
Keywords: immune regulation, ischemic stroke pathology, immune cell, cytokines, sex differences
Citation: Niu P, Li L, Zhang Y, Su Z, Wang B, Liu H, Zhang S, Qiu S and Li Y (2023) Immune regulation based on sex differences in ischemic stroke pathology. Front. Immunol. 14:1087815. doi: 10.3389/fimmu.2023.1087815
Received: 02 November 2022; Accepted: 02 January 2023;
Published: 30 January 2023.
Edited by:
Hong-Fei Zhang, Southern Medical University, ChinaReviewed by:
Zhe Gong, Hospital of Zhengzhou University, ChinaCopyright © 2023 Niu, Li, Zhang, Su, Wang, Liu, Zhang, Qiu and Li. This is an open-access article distributed under the terms of the Creative Commons Attribution License (CC BY). The use, distribution or reproduction in other forums is permitted, provided the original author(s) and the copyright owner(s) are credited and that the original publication in this journal is cited, in accordance with accepted academic practice. No use, distribution or reproduction is permitted which does not comply with these terms.
*Correspondence: Sheng Qiu, cWl1czIwMDFAMTI2LmNvbQ==; Yuntao Li, bHl0YTcyMkBsaXZlLmNvbQ==
†These authors have contributed equally to this work
Disclaimer: All claims expressed in this article are solely those of the authors and do not necessarily represent those of their affiliated organizations, or those of the publisher, the editors and the reviewers. Any product that may be evaluated in this article or claim that may be made by its manufacturer is not guaranteed or endorsed by the publisher.
Research integrity at Frontiers
Learn more about the work of our research integrity team to safeguard the quality of each article we publish.