- 1Department of Stem Cell & Regenerative Medicine, State Key Laboratory of Trauma, Burn and Combined Injury, Daping Hospital, Army Medical University, Chongqing, China
- 2Department of Research and Development, Ankerui (Shanxi) Biological Cell Co., Ltd., Shanxi, China
- 3Department of Biochemistry and Molecular Biology, College of Basic Medical Science, Army Medical University, Chongqing, China
- 4State Key Laboratory of Ophthalmology, Zhongshan Ophthalmic Center, Sun Yat-sen University, Guangzhou, China
Mesenchymal stem cells (MSCs) are pluripotent stem cells with multidirectional differentiation potential and strong immunomodulatory capacity. MSCs have been widely used in the treatment of injured, inflammatory, and immune-related diseases. Resting MSCs lack differentiation and immunomodulatory ability. Instead, they rely on microenvironmental factors to: 1) stimulate and regulate their expression of specific cell growth factors, chemokines, immunomodulatory factors, or receptors; or 2) direct their differentiation into specific tissue cells, which ultimately perform tissue regeneration and repair and immunomodulatory functions. Tumor necrosis factor (TNF)-α is central to the creation of an inflammatory microenvironment. TNF-α regulates the fate and functional reprogramming of MSCs, either alone or in combination with a variety of other inflammatory factors. TNF-α can exert opposing effects on MSCs, from inducing MSC apoptosis to enhancing their anti-tumor capacity. In addition, the immunomodulation and osteogenic differentiation capacities of MSCs, as well as their exosome or microvesicle components vary significantly with TNF-α stimulating concentration, time of administration, or its use in combination with or without other factors. Therefore, this review discusses the impact of TNF-α on the fate and functional reprogramming of MSCs in the inflammatory microenvironment, to provide new directions for improving the immunomodulatory and tissue repair functions of MSCs and enhance their therapeutic potential.
Introduction
Mesenchymal stem cells (MSCs) are important members of the stem cell family and are found in a variety of tissues of mesodermal origin, such as the bone marrow, umbilical cord, placenta, amniotic membrane, dental pulp, and adipose tissue (1). MSCs can directionally differentiate into lineages of tissue cells, including chondrocytes, adipocytes, and osteoblasts et al. On doing so, MSCs indirectly facilitate the regeneration and repair of damaged tissues by tissue replacement and cell growth factor secretion. Moreover, MSCs also directly influence the proliferation, activation, and polarization of T cells, B cells, dendritic cells (DCs), and natural killer (NK) cells by secreting immunomodulatory factors or expressing membrane receptors, resulting in their immunosuppression or immunoregulation. However, resting MSCs have no differentiation or immunomodulatory capacity, and only gain these functions after they are stimulated by microenvironmental modulators, particularly immune or inflammatory factors (2).
Targeted activated lymphocytes induce the apoptosis of MSCs by secreting tumor necrosis factor (TNF)-α, one of the main immune factors regulating the fate and function of MSCs (3). TNF-α belongs to the TNF superfamily, which accounts for approximately 70%–95% of the biological activity of TNFs, and is mostly produced by activated macrophages and immune cells such as lymphocytes (4). TNF-α plays an important role in inflammation, immune response regulation, and carcinogenesis. It is a key signaling molecule, which coordinates immune and inflammatory responses and can initiate cell apoptosis and programmed necrosis. Recently, researchers found that while a highly inflammatory environment inhibits the function of MSCs, modulation of MSCs by certain inflammatory factors might play a positive role in the treatment of many diseases (5). TNF-α is the main inflammatory factor in the microenvironment of inflammatory disease. Therefore, the question of whether TNF-α can indirectly influence the disease process or the clinical therapeutic efficacy of MSCs by regulating tissue regeneration and repair or the immunoregulatory function of MSCs needs to be addressed. Numerous studies have reported either positive or negative regulatory effects of TNF-α on the fate and function of MSCs. Dorronsoro et al. found that inhibition of the NF-κB signaling pathway by silencing IκB kinase β (IKK-β) and TNF-α receptors could significantly weaken the immunosuppressive capacity of MSCs (6). However, López-García and colleagues claimed that inflammatory factors such as TNF-α and interferon (IFN)-γ positively or negatively affect the immunomodulatory function of MSCs by modulating specific immunoregulatory molecules in their exosomes, microvesicles or micro(mi)-RNAs (7). Here, we summarize and discuss the latest research exploring the different regulatory mechanisms linking TNF-α and MSCs. We envisage that this review will offer new insights to help improve the clinical efficacy of TNF-α-modulated MSCs for the treatment of various inflammatory or immune-related diseases.
Methods
Search engines and time period
An electronic search concerning the role of TNF-α in the regulation and functional reprogramming of MSCs in an inflammatory microenvironment was conducted in NCBI (PubMed) and Web of Science. Articles with full publications that appeared in English language biomedical journals and published between 1 January 1995 and 18 December 2022 were included in the current review.
Search strategy
Public databases were searched using the combination of keywords ‘TNF-α‘ and ‘mesenchymal stem cells’ or ‘MSCs’in the Titles/Abstract of publications. This provided the initial database for our review. Initial search was performed in late June 2022, followed by two searches in September 2022, and a final update in December 2022. The PRISMA flow diagram for the literature search process was illustrated in Figure 1.
Selection criteria
Eligibility criteria for inclusion were: a) prospective clinical studies; b) animal studies; c) cell studies; d) studies reporting effect of TNF-α on MSCs in an inflammatory microenvironment; e) studies in the English language. Review articles (narrative and systematic), short communications, letters to editors, and data extraction were excluded. All publications were screened according to the PRISMA guideline. PRISMA 2020 checklist was used to assess the quality of our review.
Data synthesis
Statistics derived from each paper were summarized by pictures and tables involving the concentration of inflammatory cytokines, species, and type of MSCs and action of exchanged exosomes or microvesicles in tissue.
TNF-α inhibits MSC proliferation, while promoting their autophagy and apoptosis
The inflammatory microenvironment is a complex system, involving: 1) pro-inflammatory cells and factors such as M1 macrophages, CD4+ T cells, CD8+ T cells, interleukin (IL)-1β, IL-2, IL-6, IL-8, IL-12, IL-17, TNF-α, and IFN-γ; and 2) anti-inflammatory cells and factors such as M2 macrophages, IL-4, IL-10, IL-35, and tumor growth factor (TGF)-β (8, 9). In vitro experiments often simulate the occurrence, development, and inhibition of the inflammatory environment in vivo using combinations of pro-inflammatory factors, especially TNF-α and IFN-γ.
TNF-α inhibits MSC proliferation in an inflammatory environment
As an initiating factor of the inflammatory response, TNF-α plays a prominent role in the fate regulation and functional reprogramming of MSCs. In the inflammatory microenvironment, TNF-α acts in concert with many other inflammatory factors. Domenis et al. treated human adipose MSCs with 10–40 ng/ml TNF-α and IFN-γ for 48 h and found that the morphology and proliferative capacity of MSCs was altered and reduced, respectively, with the increase in cytokine dose (9). Similarly, Crop et al. demonstrated that after human adipose MSCs were stimulated for 7 days with a pro-inflammatory mixture of 20 ng/ml TNF-α, 50 ng/ml IFN-γ, and 10 ng/ml IL-6, their diameter increased, while their proliferative capacity declined (10). This indicates that treating hMSCs with TNF-α and IFN-γ affects their morphology and proliferation ability.
TNF-α induces MSC autophagy
Autophagy is a process that occurs under conditions of starvation and growth factor deficiency, in which cells survive by degrading their own components or organelles (11). ATG5 (12), ATG7, LC3 (13), and BECN1 are important autophagy-associated genes, which are expressed in fibroblasts, lymphocytes, and MSCs. Dang et al. found that stimulating bone marrow mice MSCs for 4 h with 20 ng/ml TNF-α significantly increase their mRNA and protein expression of BECN1. Meanwhile, stimulation of the MSCs with 50 ng/ml IFN-γ only resulted in the upregulation of Becn1 mRNA and not protein expression. However, treating the MSCs with a combination of 20 ng/ml TNF-α and 50 ng/ml IFN-γ produced the highest rise in BECN1 mRNA and protein expression, in comparison with each treatment alone (14) (Figure 2). These experiments demonstrate that TNF-α is a key factor in the induction of autophagy in bone marrow MSCs, while IFN-γ plays a synergistic role.
Similarly, 20 ng/ml TNF-α stimulation of human umbilical cord MSCs for 24 h increased the expression of autophagy-related protein microtubule-associated protein 1 light chain B-II (LC3B-II) and lowered the expression of autophagy inhibitory molecule tribbles homology protein 3 (TRIB3) (15). Therefore, in the inflammatory microenvironment, and especially during in vitro experiments, the presence of high concentrations of inflammatory factors promotes the survival of MSCs via autophagy.
TNF-α induces MSC apoptosis
TNF-α is mainly produced by macrophages and participates in various pathophysiological activities such as inflammatory cytokine production, cell survival, proliferation, and apoptosis. TNF-α performs these functions on binding to its receptors: tumor necrosis factor receptor 1 (TNFR1) and tumor necrosis factor receptor 2 (TNFR2) on the cell membrane. Li et al. treated human umbilical cord MSCs with 20 ng/ml TNF-α and 50 ng/ml IFN-γ and reported a significant upregulation in the expression of MCP and IL-6, which eventually inhibited the protein synthesis of the inhibitor of apoptosis (IAP), leading to the mass apoptosis of MSCs (16). Furthermore, Liu and colleagues found that 0–200 ng/ml IFN-γ did not induce mice MSC apoptosis, while the combination of 20 ng/ml TNF-α and 50 ng/ml IFN-γ induced MSC apoptosis more effectively than using 20 ng/ml TNF-α alone. Therefore, IFN-γ enhances the pro-apoptotic action of TNF-α. The synergistic mechanism may involve IFN-γ upregulating the expression of Fas on the cell membrane. TNF-α could then promote Fas internalization, thus enhancing Caspase-3- and Caspase-8-mediated apoptotic signaling. Alternatively, IFN-γ also decreases TNFR2 expression and the phosphorylation of NF-κB, the X-linked inhibitor of apoptosis protein (XIAP), κB kinas (IKK), and FLICE inhibitory protein (FLIP), which inhibits the anti-apoptotic pathway of MSCs and eventually results in apoptosis (17) (Figure 2).
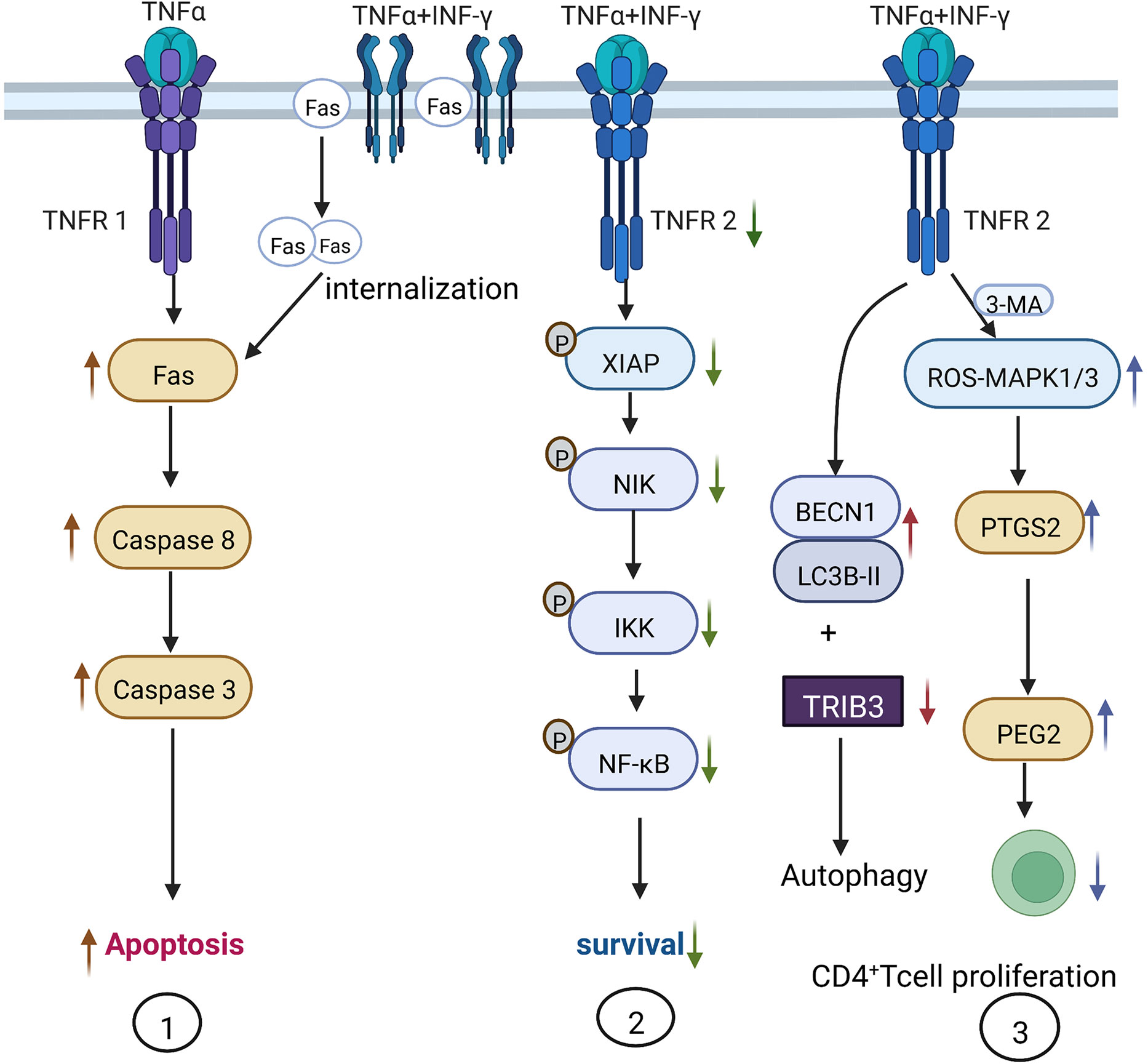
Figure 2 Mechanism of TNF-α-induced autophagy and apoptosis in MSCs. 1) IFN-γ assists TNF-α in the induction of apoptosis via TNFR1. 20–200 ng/ml of TNF-α induces MSC apoptosis in a dose-dependent manner, while 50 ng/ml IFN-γ only promotes the expression of Fas on the cell surface. Adding 20 ng/ml TNF-α promotes Fas internalization into cells to induce MSC apoptosis. 2) TNF-α and IFN-γ inhibit TNFR2 and the downstream anti-apoptotic signaling pathway. When MSCs are treated with 20 ng/ml TNF-α and 50 ng/ml IFN-γ, the phosphorylation of XIAP and NF-κB within MSCs is inhibited and the anti-apoptotic effect is suppressed. 3) Administration of 20 ng/ml TNF-α and 50 ng/ml IFN-γ increases the expression of autophagy-related genes BECN1 and LC3B-II, and decreases the expression of autophagy-inhibiting molecule TRIB3, thus increasing autophagy; 3-methyladenine (3-MA) can inhibit autophagy by activating the ROS-MAPK1/3 pathway and inhibiting CD4+ T cell proliferation by stimulating MSCs to secrete PGE-2.
Since the high concentration of TNF-α in the inflammatory environment induces MSC autophagy and apoptosis and affects MSC regeneration, immune regulation, and differentiation functions, the non-specific autophagy inhibitor 3-methyladenine (3-MA) has been used in animal experimental models to inhibit MSC autophagy. 3-MA inhibits MSC autophagy via the activation of the ROS-MAPK1/3 pathway, significantly reducing the mRNA and protein expression of TNF-α, IFN-γ, IL-6, and IL-17. At the same time, 3-MA also upregulates the production of a key immune regulatory factor prostaglandin E2 (PGE-2) (downstream from PTGS2), thereby restoring the immunomodulatory function of MSCs to suppress CD4+ T cell proliferation in nude mice (14).
Similarly, aspirin and etanercept can reduce the impact of the inflammatory environment on the apoptosis and proliferation of bone marrow MSCs by inhibiting the functions of TNF-α and IFN-γ. These drugs can restore the self-renewal and multidirectional differentiation capacity of MSCs, and have been shown to be effective in treating acute graft versus host disease and cranial defects in animal experiment (18, 19). Furthermore, Zhao et al. used a lentiviral vector (GV358) to overexpress TNFRII-Fc in human umbilical cord MSCs, neutralizing the effects of TNF-α on the apoptosis and autophagy of these cells (16).
The inflammatory environment is an important regulator of MSC function. Nevertheless, prolonged chronic inflammation or the high concentration of TNF-α inhibit the proliferation of MSCs, while promoting their autophagy or apoptosis. Therefore, in the next section we evaluate the effect of different TNF-α concentrations and administration time points on the proliferation, autophagy, and apoptosis of MSCs and provide guidelines for future clinical therapies.
TNF-α enhances the immunomodulatory capacity of MSCs
TNF-α is mainly expressed by DCs, macrophages, NK cells, and T cells, and is mostly secreted in the inflammatory environment, participating in processes such as tissue regeneration and immunomodulation (20). In the development of inflammation, TNF-α is the first factor secreted by immune cells, which can enhance or weaken the effects of other cytokines (21, 22). In the inflammatory microenvironment, the immunomodulatory capacity of MSCs is susceptible to various factors, which leads to them either promoting or reducing inflammation. MSCs exert their immunomodulatory effects mainly through direct cellular contact or the secretion of growth factors, cytokines, and chemokines.
Resting mouse MSCs constitutively express low levels of COX-2, PGE-2, TGF-β1, HGF (23) and B7H1 (24), meanwhile resting human MSCs can persistently express ICAM-1 (25), CXCL10 (26), IL-6 (26), IL-8 (27), CCL-2 (28), PD-L1 (29), MHC I (30) and IDO (31). However, in both human and mouse, either TNF-α or IFN-γ alone can increase the protein production of COX-2 and PGE-2, while PD-L1 (29, 32) and IDO (31) can only be induced by IFN-γ alone (33)in inflammatory environment.
Several articles have shown that TNF-α can enhance the immunomodulatory capacity of MSCs or to assist other cytokines to play immunomodulatory roles in the inflammatory environment. Because of the large variety of immune cells and cytokines, and the complex interplay between them, in this review, we will focus on summarizing the mechanisms by which TNF-α modulates MSC function.
TNF-α stimulation alone enhances the immunosuppressive capacity of MSCs
TNF-α stimulates MSCs to suppress the inflammatory response by promoting polarization of anti-inflammatory M2 macrophages
Macrophages are subdivided into pro-inflammatory M1 macrophages and anti-inflammatory M2 macrophages. The M1 subtype eliminates nonself-components, prevents tumor growth, and the mediates T helper (Th)1-mediated pro-inflammatory response by releasing pro-inflammatory factors such as IL-1β, TNF-α, IL-12, IL-6, and IL-23. The M2 subset is involved in Th2-mediated immune regulation by secreting anti-inflammatory factors such as IL-10 (8).
It has been reported that the coculture of human bone marrow MSCs pre-stimulated with 10 ng/ml TNF-α and macrophages promoted the release of IL-10 from M2 macrophages and reduced TNF-α levels in the culture medium by increasing the secretion of COX-2 and PGE-2 (33, 34).
TNF-α enhances the immunosuppressive capacity of MSCs by inducing TSG-6 expression
Tumor necrosis factor alpha stimulated gene-6 (TSG-6) is a key anti-inflammatory molecule synthesized by MSCs following the high expression of the TNFA1P6 gene. For example, the high expression of TSG-6 in human umbilical cord MSCs attenuates the excessive inflammatory responses induced by severe burns, by inhibiting the activation of P38 and JNK signaling pathways (35).
10 ng/ml TNF-α stimulation was shown to promote TSG-6 release from human bone marrow MSCs (36, 37). Subsequent coculture with macrophages inhibited the NF-κB signaling pathway via the expression of CD44, thus, suppressing excessive inflammatory responses (38). Similar studies have also shown that 100 ng/ml TNF-α enhanced the secretion of TSG-6 by human iPSC-MSCs and bone marrow MSCs, initiated TSG6-related inflammatory pathways, mediated hyaluronan and CD44 interactions, suppressed inflammatory responses in an Akt-dependent manner, and promoted epithelial cell proliferation, thereby accelerating mucosal healing in a mouse model of colitis (39).
The mechanism by which TNF-α regulates the immunosuppressive capacity of MSCs in concert with other factors
An immune response can develop in a pro- or anti-inflammatory direction, depending on the action of various immune cells and inflammatory factors. However, as the first molecule of the inflammatory response, TNF-α assumes a key position in the regulation of multiple immunosuppressive pathways.
IL-10 enhances the TNF-α-induced immunosuppressive pathway of MSCs
At present, only one study has reported the cooperation between TNF-α and IL-10 in the immunomodulation of hMSCs. Saldaña et al. showed that human bone marrow MSCs pre-stimulated with 10 ng/ml TNF-α and 1 ng/ml IL-10 were able to significantly reduce TNF-α production by macrophages, compared to MSCs pre-treated with 10 ng/ml TNF-α alone (34). Pre-treating MSCs with 1 ng/ml IL-10 had no effect on TNF-α concentration within the culture medium. This indicates that the role of IL-10 is to promote the TNF-α-induced immunosuppressive capacity of MSCs. Therefore, it would be interesting to investigate: 1) whether pro-inflammatory M1 macrophages secrete TNF-α to stimulate bone marrow MSCs in the early stages of inflammation in the presence of pro-inflammatory factors; and 2) if these MSCs then cause anti-inflammatory M2 macrophages to secrete IL-10 to reduce TNF-α levels by increasing COX-2 and PGE-2 expression. Thus, the inhibition of the inflammatory response is achieved by prompting TNF-α-stimulated MSCs to release PGE-2, which leads to the secretion of IL-10, which in turn synergized with TNF-α. However, how low the concentration of TNF-α needs to drop before this synergistic effect is alleviated, remains unclear.
TNF-α enhances the immunosuppressive capacity of IFN-γ-regulated MSCs via PD-L1 and IDO
Several published reports have mentioned that TNF-α and IFN-γ act synergistically in the inflammatory microenvironment to increase the expression of various cytokines (e.g., PD-L1, PD-L2, IL-8 (27), IL-6, TGF-β (40), IDO (41), and PGE-2), as well adhesion molecules in hMSCs to enable these cells to perform their immunomodulatory functions. However, the exact synergistic mechanism implicating TNF-α and IFN-γ remains elusive.
It has been reported that TNF-α alone actually reduces the expression of PD-L1 and IDO in murine MSCs. In contrast, IFN-γ, can inhibit T cell proliferation by increasing IDO and PD-L1 expression (42). However, according to a recent study, TNF-α can indirectly promote PD-L1 and IDO expression by increasing IFN-γ levels via NF-κB signaling in hMSCs. Thus, TNF-α synergistically amplifies the IFN-γ/STAT signaling pathway (29).
In another study, TNF-α and 10 ng/ml of IL-1β enhanced the expression and sensitivity of the IFN-γ receptor via NF-κB signaling in hMSCs (43). Thus, in the presence of TNF-α and IL-1β, IFN-γ could bind to its receptor more easily, activating the signal transducers and activators of transcription (STAT5) and p38-MAPK signaling pathways and leading to the increased release of IL-8 and the recruitment of polymorphonuclear granulocytes (6).
Finally, the pro-inflammatory phenotype of hMSCs induced by pre-stimulation with 0.4 mM sodium palmitate could be reversed by treating the cells with 10 ng/ml IFN-γ and 1 ng/ml TNF-α, causing the MSCs to become anti-inflammatory (44). These anti-inflammatory MSCs were able to inhibit peripheral blood mononuclear cell (PBMC) proliferation through the JAK1/JAK2 signaling pathway.
TNF-α and IFN-γ synergistically enhance ICAM-1 expression on the surface of MSCs to strengthen their immunomodulatory capacity
The intercellular adhesion molecule-1 (ICAM-1) can improve the migration ability of 6-8wk old mice MSCs and their adhesion to immune cells (45, 46). In addition, the interaction between ICAM-1 on the surface of human bone marrow MSCs and CD43 on the surface of activated T cells through cell-to-cell contact can inhibit T cell receptor (TCR)-meditated signaling (47), which is one of the most important immunoregulatory mechanisms.
50 ng/ml TNF-α stimulation of human bone marrow MSCs was shown to increase ICAM-1 expression, enhancing MSC migration and their capacity to repair damaged tissues (48). The expression of ICAM-1 also rose when human bone marrow MSCs were stimulated with either 0.5–1 ng/ml TNF-α or 5 ng/ml IFN-γ alone in an inflammatory environment; however, only IFN-γ was able to increase MHC I expression. After co-stimulation of MSCs with 1 ng/ml TNF-α and 5 ng/ml IFN-γ, their MHC I and ICAM-1 expression increased significantly, compared with MSCs subjected to each cytokine alone; however, the mechanism of this synergistic effect remains unclear (25). In brief, TNF-α synergizes with IFN-γ to increase ICAM-1 expression on MSCs, enhancing their immune cell adhesion ability and immunomodulatory capacity.
TNF-α is the first factor released by immune cells in the early stages of inflammation to promote the inflammatory response. This initial stage is followed by a rise in the levels of inflammatory factors such as IFN-γ, which stimulate multiple cells to inhibit the inflammatory process. Although the modes of TNF-α and IFN-γ action in vitro are partially understood, the complex inflammatory process in vivo is still unclear. Furthermore, investigating the effects of multiple inflammatory factors on the therapeutic efficacy of MSCs during disease progression will be important for future translation into the clinic.
TNF-α promotes homing of MSCs and enhances tissue repair
Microenvironmental disturbances are the initiating factor for stem cell homing. Various signaling molecules such as chemokines, adhesion molecules, and growth factors are released locally after tissue injury. Before MSCs can perform their functions, they must first migrate to the damaged tissue site (49) and adhere to the microvascular endothelium. On reaching the damaged tissue, MSCs secrete a variety of cytokines such as vascular endothelial growth factor (VEGF) to promote angiogenesis, increase local vascularity (50), and facilitate wound healing.
TNF-α enhances the migration and adhesion of MSCs
TNF-α enhances the migration ability of MSCs
MSCs mobilized from the bone marrow into the peripheral blood migrate to the damaged endothelium and promote endothelial repair. The SDF-1a/CXCR4 signaling pathway plays an important role in the migration of stem cells and tumor cells. Pre-stimulating rats MSCs for 24 h with 50 ng/ml TNF-α was shown to enhance their migration ability by increasing the level of phosphorylated NF-κB-p65 and the expression of CXC chemokine receptor 4 (CXCR4) on the cell surface (51).
Engulfment and cell motility protein 1 (ELMO1) is a core molecule involved in cell migration. ELMO1 binds to dedicator of cytokinesis (DOCK) proteins and participates in the cellular directional migration, neurological development, and cancer cell invasion via Rac1. It has been demonstrated that the stimulation of human bone marrow MSCs with high concentrations (100 ng/ml) of TNF-α lowered their expression of METTL14. This led to a reduction in the number of m6A modifications and slowed down the degradation of ELMO1. The binding of ELMO1 to DOCK1 then promoted the activation of Ras-related C3 botulinum toxin substrate1 (Rac1) and thus enhanced the migration capacity of MSCs, as demonstrated in the context of obligate spondylitis disease. Consistent results were obtained both in vitro and in in vivo experiments in mice (52).
TNF-α enhances the adhesion of MSCs to endothelial cells
Vascular endothelial cell adhesion molecule (VCAM-1) is an important cell surface adhesion molecule expressed by a variety of cells, including MSCs (53). It was reported that treating human bone marrow MSCs with 50 ng/ml TNF-α enhanced their adhesion to the vascular endothelium at the site of damage by activating NF-κB, ERK, and JNK signaling pathways and upregulating the expression of VCAM-1 (54, 55). Also, in an ischemic rat injury model, Wistar rats bone marrow MSCs pre-stimulated with 10 ng/ml TNF-α accumulated in muscle tissue in large numbers after tail vein injection (55).
TNF-α enhances the tissue repair ability of MSCs
MSCs have been shown to promote angiogenesis (56), together with inflammatory response control, which are essential prerequisites for wound healing. Furthermore, MSCs undergo self-renewal and multidirectional differentiation, and can secrete various reparatory factors, including VEGF, fibroblast growth factor (FGF), and matrix metalloproteinase (MMP), which play key roles in the wound healing process.
Co-stimulation of human MSCs with 20 ng/ml TNF-α and 20 ng/ml IL-1β, mimicking an inflammatory environment in vitro, transactivated the EGFR-mediated MAPK signaling pathway via the TACE/ADAM17 axis. This caused an increase in ERK1/2 phosphorylation and promoted the mRNA and protein expression of FGF2, HGF, HBEGF, and IL-6. Thus, the pre-treatment of MSCs with TNF-α and IL-1β significantly improved wound closure when the MSCs were cocultured with NCI-H292 cells (57).
In 2015, researchers reported that pre-stimulation of mice MSCs with TNF-α could: 1) increase the expression of heme oxygenase-1; 2) enhance paracrine effects; 3) upregulate growth factors (including IGF-1, bFGF, and VEGF), chemokines (including CXCL-3, CCL-2/20, TIMP-2, MMP3, and MMP-2/13), and the immunomodulatory factor TGF-β; and 4) downregulate the inflammatory response. Ultimately, these TNF-α-induced MSCs promoted intestinal stem cell regeneration and increased intestinal epithelial cell proliferation, thus improving the survival of radiation-treated mice (58, 59).
At present, it has been shown that pre-stimulation with TNF-α improves the homing and tissue repair functions of MSCs. However, whether these MSCs are also responsible for tissue repair at the damaged site is still unclear. Thus, the role of TNF-α in MSC homing to the damaged site in an inflammatory environment needs to be further explored.
The role of TNF-α in MSC differentiation
MSCs have multidirectional differentiation potential. In vitro experiments often exploit this feature to differentiate MSCs into osteogenic, lipogenic, and chondrogenic cells, which is the current criterion for the “stemness” of MSCs. However, in inflammatory environments, damaged tissue repair is often accompanied by stem cell differentiation. Thus, the effects of inflammatory factors on MSC differentiation and the underlying mechanisms need to be explored.
Different concentrations of TNF-α have opposing effects on the osteogenic differentiation of MSCs
Osterix (OSX), alkaline phosphatase (ALP), and particularly runt-related transcription factor 2 (RUNX2) are important osteogenic genes. RUNX2 is an important transcription factor in bone development, playing major regulatory roles in osteoblast differentiation, chondrocyte maturation, and extracellular matrix secretion. Currently, it is believed that TNF-α plays a dual role in the osteogenic differentiation of MSCs. This is because, in the early stages of bone injury, a low concentration of TNF-α promotes osteogenic differentiation and bone tissue repair. Conversely, prolonged exposure to high doses of TNF-α causes bone damage (60).
Low concentrations of TNF-α promote the osteogenic differentiation of MSCs
Stimulation of human bone marrow MSCs with BAY60-6583, a selective adenosine A2B receptor (A2BAR) agonist, promotes the expression of RUNX2 and ALP and accelerates the osteogenic process (61). However, the binding of G protein-coupled receptor kinase 2 (GRK2) to A2BAR increases the desensitization of A2BAR and reduces MSC mineralization. Treating bone marrow MSCs with a low concentration of TNF-α (1 ng/ml) led to the ubiquitination and degradation of GRK2 by promoting its intracellular binding to murine double minute 2 (Mdm2). This further reduced the BAY60-6583-mediated desensitization of GPCR, leading to an increase in G protein signaling. Finally, both mineralization and calcium deposition were increased, ultimately promoting the osteogenic differentiation of human bone marrow MSCs (60) (Figure 3). Moreover, TNF-α and IL-1β can stimulate human bone marrow MSCs in a dose-dependent manner to increase the expression of tissue nonspecific alkaline phosphatase (TNAP), which is required for osteoblast mineralization (62). More specifically, treating human bone marrow MSCs with 1 ng/ml TNF-α was shown to activate the NF-κB pathway, inhibit the binding of peroxisome proliferators-activated receptors (PPAR)-γ and PPRE, and upregulate the mRNA levels of TNAP, COX-2, prostaglandin E2 synthase (PGES), and prostaglandin E2 synthase (PGDS). Thus, via this mechanism, TNAP expression was increased in the osteoblast cell line MG-63, which enhanced cell mineralization (63).
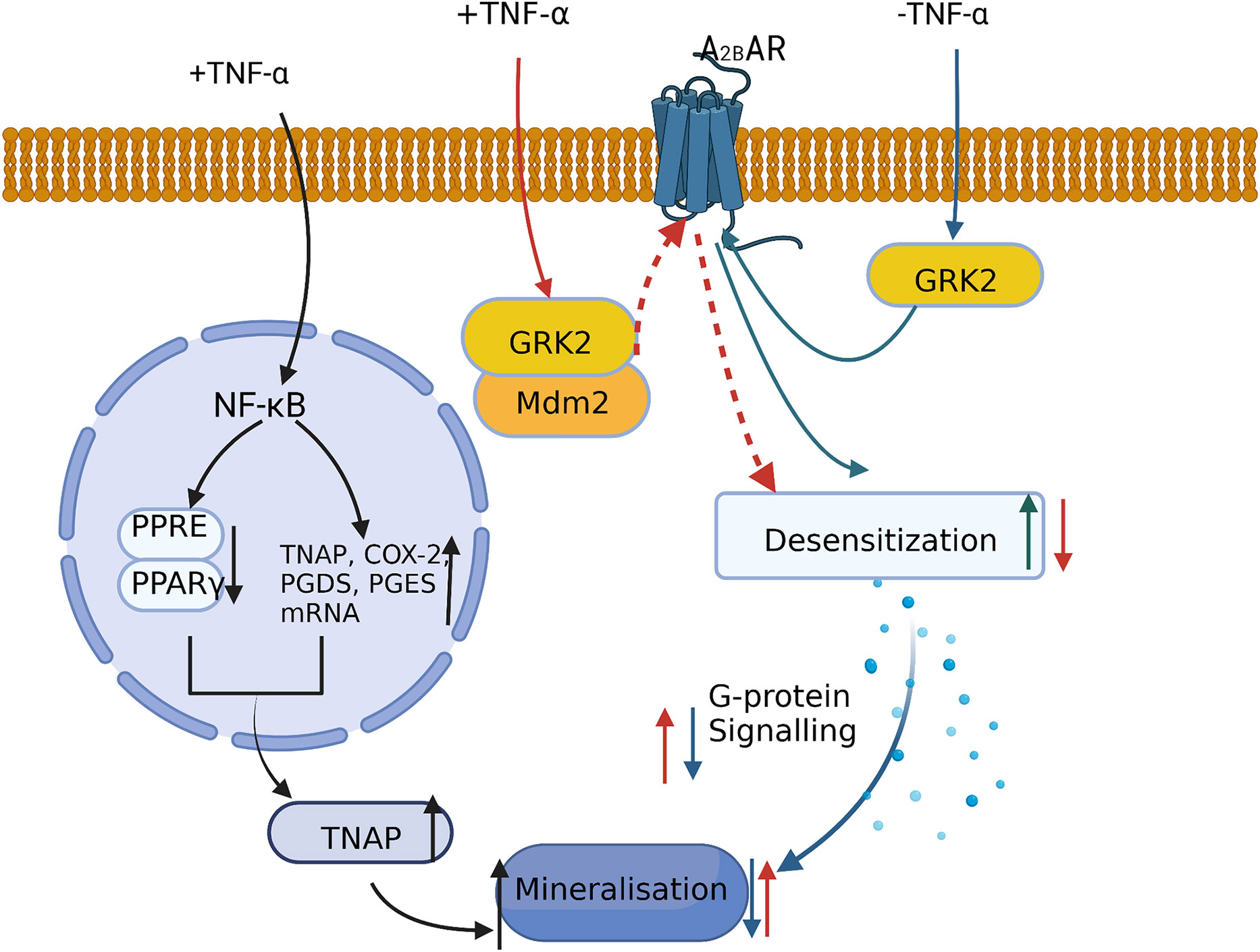
Figure 3 A low concentration of TNF-α promotes MSC mineralization and osteogenic differentiation by: inhibiting PPAR-γ activity and increasing TNAP expression and inducing the ubiquitination and degradation of GRK2 via its binding to Mdm2 and decreasing A2BAR desensitization.
High concentrations of TNF-α inhibit osteogenic differentiation of MSCs
In the inflammatory environment, TNF-α inhibits osteogenic differentiation through multiple complex signaling pathways. The degree of inhibition may be related to cell type, animal model, duration of action, and immunomodulatory factor concentration. TNF-α can directly or indirectly inhibit the conversion of osteogenic precursor cells to osteoblasts and affect the formation of mineralized nodules (64).
High concentrations of TNF-α were shown to induce the expression of miR-23b and inhibit RUNX2 expression via the NF-κB in human bone marrow MSCs (65) or the Wnt/β-catenin signaling pathways in human dental MSCs (66), thus suppressing the osteogenic differentiation of human MSCs. 10 ng/ml TNF-α also inhibited the osteogenic differentiation ability of human periodontal stem cells by decreasing miR-21 expression, leading to a rise in recombinant Sprouty Homolog 1 (SPRY1) levels, thereby suppressing ALP and RUNX2 production (67).
In cranial defect experiments in mice, TNF-α and IL-17 caused the phosphorylation of IκBα and p56, which led to the ubiquitination and degradation of β-catenin in bone marrow MSCs, the inhibition of osteogenic translation factors such as ALP, RUN2, and OSX, and the suppression of osteogenic differentiation of MSCs (68).
In addition, pre-stimulation of bone marrow MSCs from ovariectomized mice (OVE mice) with 5 ng/ml TNF-α and 50 ng/ml IFN-γ activated the NF-κB signaling pathway, increased SMAD7 expression, and lowered RUNX2 and ALP expression. This eventually led to the reduced mineralization of bone marrow MSCs and affected their osteogenic differentiation (19) (Figure 4).
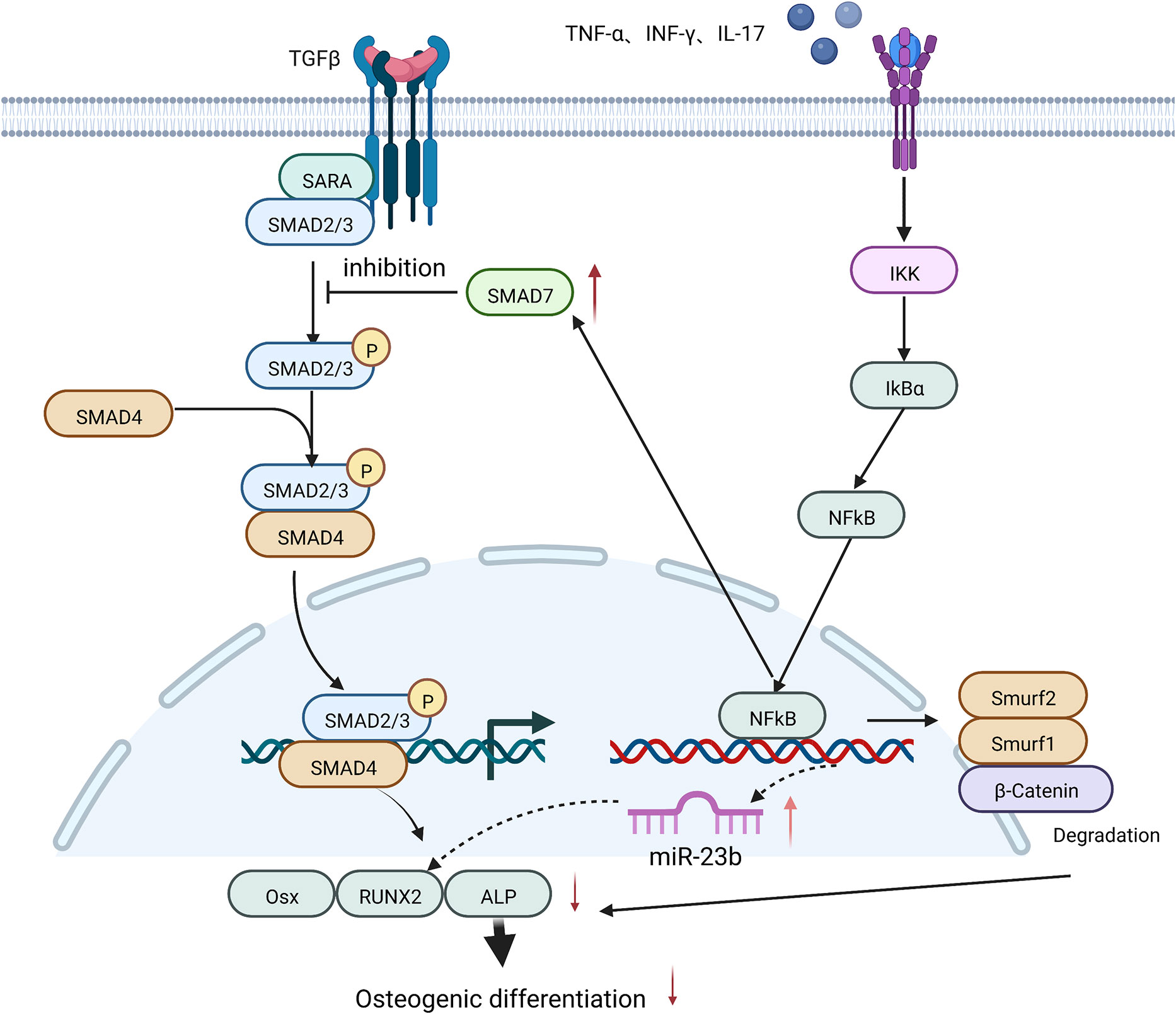
Figure 4 A high concentration of TNF-α inhibits the osteogenic differentiation of MSCs by: increasing the expression of miR-23b and SMAD7; and promoting the degradation of β-Catenin, both of which are mediated by NF-κB signaling and eventually lead to a decrease in the expression of osteogenic-related factors such as RUNX2 and ALP.
Surprisingly, the osteogenic differentiation of hMSCs could also be inhibited by 200 ng/ml IFN-γ through the activation of Fas and the upregulation of Smad6, leading to the downregulation of RUNX2, OCN, and ALP.
TNF-α inhibits lipogenic differentiation of MSCs
PPAR-γ is a major transcription factor in adipogenesis. 5 ng/ml TNF-α and 5 ng/ml IL-1β, either alone or in combination, can inhibit the lipogenic differentiation of human adipose MSCs by suppressing the expression of PPAR-γ, CAAT-enhancer binding protein α (C/EBP-α), glucose transporters (e.g., GLUT4), and lipoprotein lipase (LPL) (63, 69). Moreover, 10 ng/ml TNF-α could suppress the expression of miR-21 in human periodontal stem cells, increase SPRY1 levels, inhibit PPAR-γ and fatty-acid-binding protein 4 (FABP4) production, and attenuate the lipogenic differentiation of stem cells (67).
TNF-α inhibits chondrogenic differentiation of MSCs
SRY-Box Transcription Factor 9 (SOX9) is of great significance in the chondrogenic differentiation of MSCs. TNF-α can inhibit the synthesis of SOX9 in human MSCs via the NF-κB signaling pathway (70, 71) to inhibit chondrogenesis, while transfection with siRNA targeting TNF-α can restore this differentiation ability of MSCs.
Antitumor effects of mscs pre-stimulated with TNF-α and associated mechanisms
MSCs are double-edged swords in the process of tumor development (72). On the one hand, MSCs can promote tumor initiation and development by: 1) suppressing the immune response and enhancing the invasiveness of tumor cells (73); 2) secreting multiple trophic factors and cytokines to promote tumor vascularization (74); 3) increasing tumor cell drug resistance by paracrine mechanisms (75); 4) stimulating tumor cells to secrete Bcl-2 and Bcl-XL and inhibiting their apoptosis (76); and 5) transforming into tumor-associated fibroblasts (77). On the other hand, MSCs exhibit anti-tumor capacity by: 1) upregulating the expression of the negative regulators p21 and cyclin D2 to block tumor proliferation and induce apoptosis (78, 79); 2) enhancing the inflammatory response (80); and 3) inhibiting tumor proliferation by inducing Dickkopf-related protein 1 (DKK-1) expression via the AKT signaling pathway (81).
However, tumor initiation and progression are often accompanied by a chronic inflammatory response. Under these conditions, as the first molecule of the inflammatory response, TNF-α enhances the anti-tumor capacity of MSCs. Few studies have reported that TNF-α can stimulate MSCs to promote tumor development.
TNF-α can enhance the inflammatory response and the anti-tumor capacity of MSCs
The Toll-like receptor (TLR) family contains multiple members. TLR2/5/6 activation can induce IL-6 and IL-8 production to initiate the inflammatory response (82), while TLR3 activation can polarize hMSCs towards the anti-inflammatory phenotype via the p38 and NF-κB signaling pathways (27).
Stimulation of human adipose MSCs for 96 h with 50 ng/ml TNF-α significantly increased their TLR2 and prostaglandin-endoperoxide synthase 2 (PTGS2) expression, compared to a 24 h stimulation period (59). TLR2 and PTGS2 expression initiated downstream NF-κB signaling pathways, promoting inflammatory responses, and exerting anti-tumor effects. Similarly, the synergy between 1.5 ng/ml TNF-α and 6.5 ng/ml IFN-γ (or 15 ng/ml TNF-α and 65 ng/ml IFN-γ) led to the polarization of bone marrow MSCs towards the pro-inflammatory Th1 phenotype, thus amplifying the anti-tumor immune response. In the tumor inflammatory microenvironment, hMSC polarization can be achieved following the release of TNF-α and INF-γ from activated T cells (25, 83).
TNF-α enhances the anti-tumor capacity of MSCs by inducing TRAIL expression
It was reported that 24 h coculture of bone marrow MSCs pre-stimulated with 10 ng/ml TNF-α for 48 h with MDA tumor cells, induced tumor cell apoptosis via the intercellular interaction with TRAIL on the membrane surface of hMSCs. mRNA or DNA of apoptotic MDA cells in turn promoted the expression of TLR3 in hMSCs, activated the NF-κB signaling pathway, and caused increased TRAIL expression, inducing further apoptosis of TRAIL-sensitive tumor cells (84).
One study also described that the treatment of bone marrow MSCs with 20 ng/ml IFN-γ for 12 h induced the apoptosis of H460 cells in vitro via a TRAIL-associated mechanism. However, this was not effectively replicated in animal experiments, as the co-injection of hMSCs and tumor cells greatly enhanced tumor angiogenesis, compared with controls (85).
TNF-α inhibits the expression of cell proliferation-related factors and enhances the production of tumor suppressor molecules in MSCs after prolonged coculture, suppressing the proliferation of tumor cells
It has been previously reported that TNF-α treatment could further affect the expression of IP10 and RANTES in human bone marrow MSCs after inducing TLR3 expression in these cells (86). IP10 is an important tumor suppressor, which was shown to inhibit tumor angiogenesis by suppressing the formation of artificial blood cell clones, stimulating T cell adhesion to endothelial cells, and enhancing the killing capacity of NK cells (59, 87). Stimulation of human adipose MSCs with 50 ng/ml TNF-α for 96 h (but not 24 h) significantly decreased the expression of factors associated with cell proliferation including VEGF, RANTES, and TGF-β1. In contrast, the levels of IP10 were significantly increased after prolonged TNF-α treatment (88), which inhibited tumor cell proliferation.
MSCs pre-stimulated with TNF-α induce tumor cell apoptosis by inhibiting checkpoint molecules
Coculture of TNF-α-pre-stimulated human bone marrow MSCs with MDA tumor cells induced tumor cell apoptosis via the secretion of DKK3, which inhibited the cyclins D1 and D3, and increased P21 expression in the tumor cells. Gratifying anti-tumor effects were achieved in mice injected with TNF-α-pre-stimulated bone marrow MSCs (84) (Figure 5). Therefore, TNF-α-stimulated MSCs have some anti-tumor capacity, which could provide new directions for the treatment of tumors in the future.
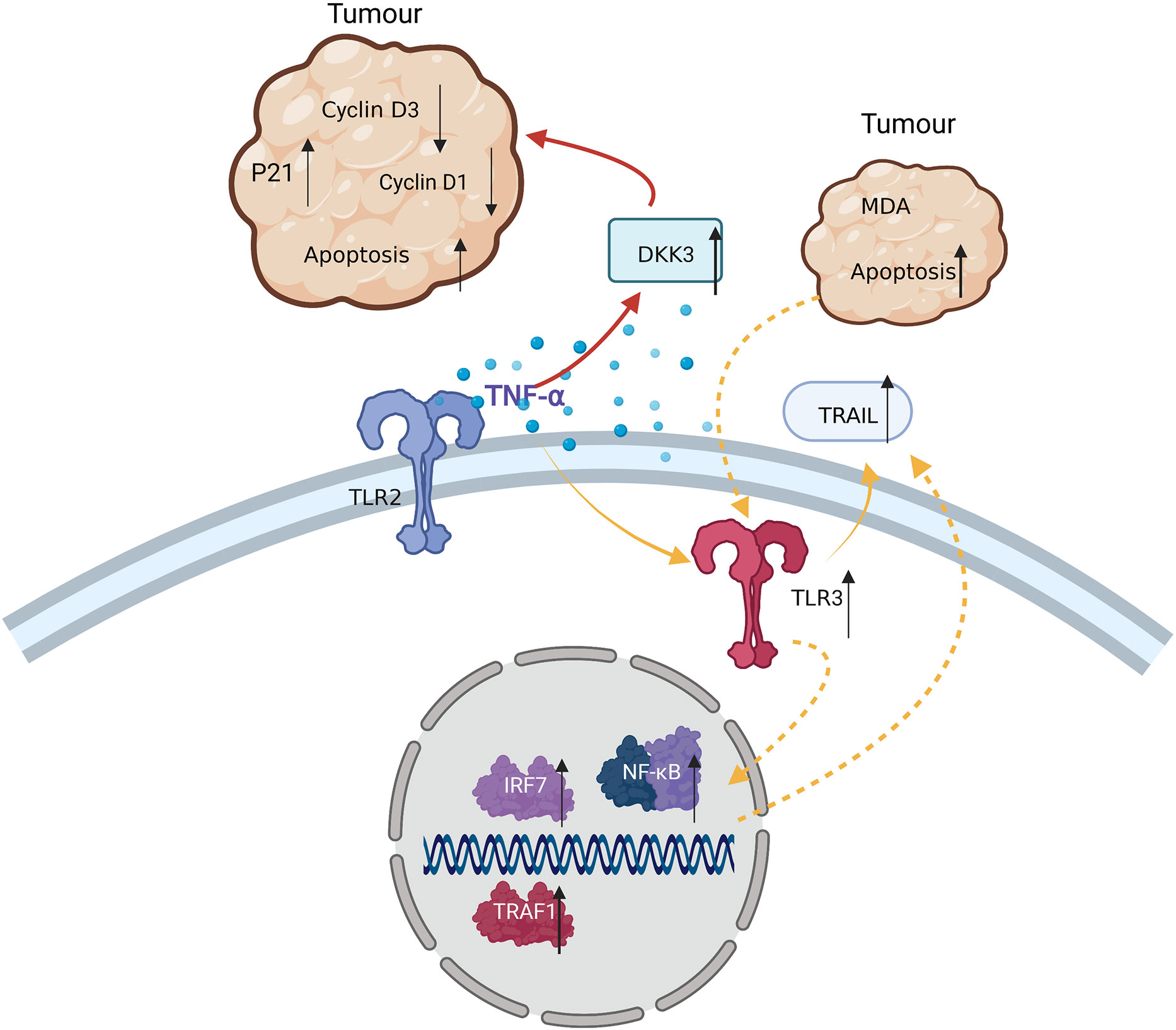
Figure 5 TNF-α exerts anti-tumor effects in TRAIL-sensitive tumor cells by: 1) inducing MDA apoptosis via TRAIL and enhancing this process through cascade effects; and 2) inducing MSCs to secrete DKK3, reducing Cyclin D3 and D1 levels in tumor cells, and leading to tumor apoptosis.
However, whether TNF-α stimulation of MSCs in the inflammatory microenvironment or during wound repair induces MSC tumorigenicity, has not been reported. Only one study mentioned that TNF-α can activate p65 to directly activate NF-κB signaling, leading to the release of the pro-inflammatory and pro-cancer factors, CCL-2 and CCL-8, in the inflammatory tumor microenvironment (89). Thus, in this context, TNF-α can recruit a subpopulation of myeloid tumor cells with significant pro-cancer effects.
In summary, TNF-α enhances the anti-tumor capacity of MSCs via a variety of mechanisms. Therefore, an in-depth exploration of the role of TNF-α in the tumor microenvironment and its mechanisms will be helpful for the development of future cancer treatment strategies.
TNF-α affects the composition of MSC exosomal microvesicles
hMSCs can repair damaged tissues by modulating the inflammatory response (90) and reconstituting the microenvironment (91). However, the presence of allogeneic genes in hMSCs (92) and the fact that only 5% of hMSCs reach damaged tissues in the body (93) greatly limits their clinical application. hMSC-derived exosomal microvesicles have MSCs-like immunomodulatory functions and are non-immunogenic, making them promising therapeutic tools (94).
Resting MSCs can autonomously secrete exosomal microvesicles containing cytokines and growth factors including ICAM-1, PGE-2, miR-145, miR-23b, miR-21, miR-146b, miR-133b, and mitochondrial (mt)DNA (95). However, the stimulation of MSCs with multiple factors such as TNF-α and IFN-γ in the inflammatory environment leads to the accumulation of specific components within the exosomal microvesicles. Table 1 summarizes the changes in the composition or abundance of MSC-derived exosomal microvesicles and how this influences the tissue microenvironment.
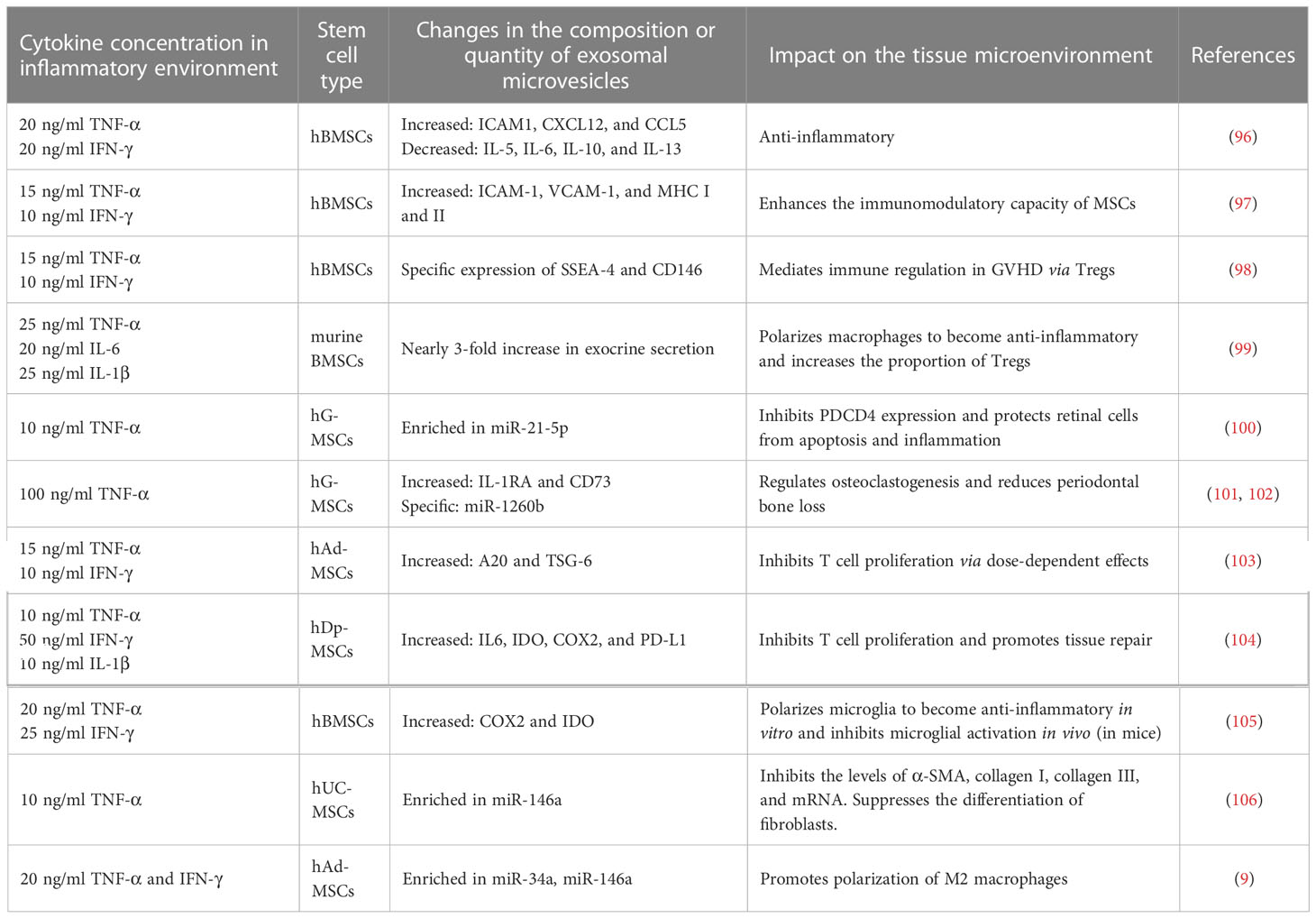
Table 1 Effect of TNF-α on the exosomal expression profile of MSCs in the inflammatory microenvironment.
Conclusion
MSCs have become a major focus of drug transport and cell therapy research because of their low immunogenicity and their ease of isolation, amplification, and differentiation in vitro (107). MSCs have now been used in preclinical and clinical studies to treat a variety of diseases such as diabetes mellitus (108), multiple sclerosis (109), cirrhosis (110), systemic lupus erythematosus (111), Crohn’s disease (112), and GVHD (113). In addition, MSCs have been used to facilitate umbilical cord blood stem cell transplantation (114). Since these MSC-based therapies are influenced by the inflammatory microenvironment, the design of in vitro studies or animal models to simulate the in vivo inflammatory microenvironment has become a major focus of MSC research (115).
In the inflammatory microenvironment, MSCs experience a gradual decline in function from autophagy to apoptosis. In the early stages of inflammation, low concentrations of TNF-α and other inflammatory factors enable MSCs to survive via autophagy. However, as inflammation develops, MSCs are driven towards apoptosis. It has also been reported that autophagy of MSCs in the inflammatory microenvironment could also affect the functions of other cells (116). Thus, the early control of inflammation is important for achieving rapid tissue repair and preserving cell function.
Live, apoptotic, and dead MSCs all have immunomodulatory capacity (117). The effect of TNF-α and other inflammatory factors on MSCs in the tissue microenvironment is a determining factor of their pro- or anti-inflammatory functions. In the non-contact state, TNF-α can both stimulate MSCs to inhibit inflammation (via PGE-2 and TSG-6) and, in the initial stage of inflammation, increase their sensitivity to IFN-γ. This promotes the release of soluble PDL1, PDL2, and IDO from MSCs and inhibits the immune response. In the contact state, PDL1 on the surface of MSCs and PD1 on the surface of T cells act as immune checkpoints (118). TNF-α cooperates with IFN-γ to increase the expression of ICAM-1 and enhance the interaction between MSCs and immune cells, thus allowing PDL1 and CTLA-4 to play a role in immune regulation. Therefore, a comprehensive understanding of the cooperation between various cytokines targeting MSCs in the inflammatory microenvironment provides a basis for the treatment of inflammatory chronic diseases and tumors.
The osteogenic, lipogenic, and chondrogenic differentiation of MSCs is an important manifestation of their “stemness”. Tissue injury is often accompanied by an inflammatory reaction and tissue repair. Under such circumstances, the effect of TNF-α on the differentiation of MSCs will be a non-negligible factor in the clinical application of MSCs. Different TNF-α concentrations, durations of stimulation, and time points of administration may affect the osteogenic differentiation of MSCs. For example, during the development of inflammation, TNF-α inhibits the activity of ALP in the early stages and inhibits the osteogenic differentiation of MSCs by suppressing their mineralization in the later stages (119). In addition, TNF-α can also affect the lipogenic and chondrogenic differentiation of MSCs via PPAR-γ and SOX9, respectively; however, the underlying mechanisms are not well understood. Therefore, studying the effects of the inflammatory environment on the differentiation of MSCs is necessary to improve the self-renewal ability of tissues and promote wound healing.
MSCs exert both anti- and pro-tumor effects. However, TNF-α-stimulated MSCs mainly exhibit anti-tumor capacity, principally by: 1) enhancing the inflammatory response to eliminate tumor cells; 2) releasing tumor apoptotic factors, such as IP10 and TRAIL; and 3) blocking the tumor cell cycle. Understanding the synergistic inhibitory effect exerted by TNF-α and MSCs on tumor cells will be useful in designing future anti-tumor cell therapies.
Exosomes or microvesicles play an important role in both the diagnosis and treatment of disease (120), and have the potential to become valuable therapeutic tools in the future (121). In this review, we focused on the differences between the expression profiles of exosomal components within MSCs in the resting and inflammatory states. MSC exosomes or microvesicles have achieved notable success in the treatment of chronic and refractory diseases (122). In addition, TNF-α may modulate the mRNA and protein expression of MSC exosome or microvesicle components for the treatment of a particular disease.
Last, species which MSCs were derived from may be a critical factor affecting the results of animal trials. There were significant differences in the expression of immunosuppressive factors in MSCs from different species. For instance, resting MSCs could produce 60 pg/ml (123) and 90 pg/ml PGE2 (23) when derived from Sprague-Dawley (SD) rats and BALB/c mice, respectively. As for human MSCs, the PGE2 level is 0.2 ng/ml from the human placenta (124), 0.75 ng/ml from the human umbilical cord (125), and 5 ng/ml from the human umbilical cord (purchased from ATCC) (126). Besides PGE2, the level of other cytokines might also be different between MSCs derived from humans and mice, and affect the result of experiments. These results suggested that when we evaluate the efficacy of MSCs, we need to select appropriate evaluation criteria according to the species or tissues of origin, and select the different tissue-derived MSCs according to the pathophysiological characteristics of the disease, which may lead to better efficacy.
The inflammatory microenvironment is complex. TNF-α initiates the inflammatory response and cooperates with multiple factors to participate in inflammation and tissues repair. A greater understanding of the processes and mechanisms underlying the differentiation, regulation, autophagy, and apoptosis of MSCs in the inflammatory microenvironment will provide guidance for future therapeutic strategies involving MSCs.
Author contributions
XX and JX conceived the article, the first draft of the manuscript was written by WL. JX, XX, critically revised the work. QL, JS, WL, performed the literature search and figure work. All authors contributed to the article and approved the submitted version.
Funding
This study was supported by National Natural Science Foundation of China (No. 82000105 and 81871568); National Defense Basic Scientific Research Project of China (2022-JCJQ-ZD-224-12); Army Medical University (No. 2019CXJSB017 and 2019XYY21); and National Natural Science Foundation of Chongqing (No. cstc2020jcyj-msxmX0227).
Conflict of interest
Author WL, QL and JS are employed by Ankerui Shanxi Biological Cell Co., Ltd.
The remaining authors declare that the research was conducted in the absence of any commercial and financial relationships that could be conducted as a potential conflict of interest.
Publisher’s note
All claims expressed in this article are solely those of the authors and do not necessarily represent those of their affiliated organizations, or those of the publisher, the editors and the reviewers. Any product that may be evaluated in this article, or claim that may be made by its manufacturer, is not guaranteed or endorsed by the publisher.
References
1. Kozlowska U, Krawczenko A, Futoma K, Jurek T, Rorat M, Patrzalek D, et al. Similarities and differences between mesenchymal stem/progenitor cells derived from various human tissues. World J Stem Cells (2019) 11:347–74. doi: 10.4252/wjsc.v11.i6.347
2. Li W, Ren G, Huang Y, Su J, Han Y, Li J, et al. Mesenchymal stem cells: a double-edged sword in regulating immune responses. Cell Death differentiation (2012) 19:1505–13. doi: 10.1038/cdd.2012.26
3. Liu Y, Wang S, Shi S. The role of recipient T cells in mesenchymal stem cell-based tissue regeneration. Int J Biochem Cell Biol (2012) 44:2044–50. doi: 10.1016/j.biocel.2012.08.003
4. Inoue M, Ando D, Kamada H, Taki S, Niiyama M, Mukai Y, et al. A trimeric structural fusion of an antagonistic tumor necrosis factor-α mutant enhances molecular stability and enables facile modification. J Biol Chem (2017) 292:6438–51. doi: 10.1074/jbc.M117.779686
5. Yang C, Chen Y, Li F, You M, Zhong L, Li W, et al. The biological changes of umbilical cord mesenchymal stem cells in inflammatory environment induced by different cytokines. Mol Cell Biochem (2018) 446:171–84. doi: 10.1007/s11010-018-3284-1
6. Dorronsoro A, Ferrin I, Salcedo JM, Jakobsson E, Fernández-Rueda J, Lang V, et al. Human mesenchymal stromal cells modulate T-cell responses through TNF-α-mediated activation of NF-κB. Eur J Immunol (2014) 44:480–8. doi: 10.1002/eji.201343668
7. López-García L, Castro-Manrreza ME. TNF-α and IFN-γ participate in improving the immunoregulatory capacity of mesenchymal Stem/Stromal cells: Importance of cell-cell contact and extracellular vesicles. Int J Mol Sci (2021) 22:9531. doi: 10.3390/ijms22179531
8. Lu D, Xu Y, Liu Q, Zhang Q. Mesenchymal stem cell-macrophage crosstalk and maintenance of inflammatory microenvironment homeostasis. Front Cell Dev Biol (2021) 9:681171. doi: 10.3389/fcell.2021.681171
9. Domenis R, Cifù A, Quaglia S, Pistis C, Moretti M, Vicario A, et al. Pro inflammatory stimuli enhance the immunosuppressive functions of adipose mesenchymal stem cells-derived exosomes. Sci Rep (2018) 8:13325. doi: 10.1038/s41598-018-31707-9
10. Crop MJ, Baan CC, Korevaar SS, Ijzermans JN, Pescatori M, Stubbs AP, et al. Inflammatory conditions affect gene expression and function of human adipose tissue-derived mesenchymal stem cells. Clin Exp Immunol (2010) 162:474–86. doi: 10.1111/j.1365-2249.2010.04256.x
11. Levine B, Deretic V. Unveiling the roles of autophagy in innate and adaptive immunity. Nat Rev Immunol (2007) 7:767–77. doi: 10.1038/nri2161
12. Cui B, Lin H, Yu J, Yu J, Hu Z. Autophagy and the immune response. Adv Exp Med Biol (2019) 1206:595–634. doi: 10.1007/978-981-15-0602-4_27
13. Levine B, Mizushima N, Virgin HW. Autophagy in immunity and inflammation. Nature (2011) 469:323–35. doi: 10.1038/nature09782
14. Dang S, Xu H, Xu C, Cai W, Li Q, Cheng Y, et al. Autophagy regulates the therapeutic potential of mesenchymal stem cells in experimental autoimmune encephalomyelitis. Autophagy (2014) 10:1301–15. doi: 10.4161/auto.28771
15. Zhao Y, Yang X, Li S, Zhang B, Li S, Wang X, et al. sTNFRII-fc modification protects human UC-MSCs against apoptosis/autophagy induced by TNF-α and enhances their efficacy in alleviating inflammatory arthritis. Stem Cell Res Ther (2021) 12:535. doi: 10.1186/s13287-021-02602-4
16. Li X, Du W, Ma FX, Feng X, Bayard F, Han ZC. High concentrations of TNF-α induce cell death during interactions between human umbilical cord mesenchymal stem cells and peripheral blood mononuclear cells. PloS One (2015) 10:e0128647. doi: 10.1371/journal.pone.0128647
17. Liu Y, Wang L, Kikuiri T, Akiyama K, Chen C, Xu X, et al. Mesenchymal stem cell-based tissue regeneration is governed by recipient T lymphocytes via IFN-γ and TNF-α. Nat Med (2011) 17:1594–601. doi: 10.1038/nm.2542
18. Ding L, Ning HM, Li PL, Yan HM, Han DM, Zheng XL, et al. Tumor necrosis factor α in aGVHD patients contributed to the impairment of recipient bone marrow MSC stemness and deficiency of their hematopoiesis-promotion capacity. Stem Cell Res Ther (2020) 11:119. doi: 10.1186/s13287-020-01615-9
19. Wang L, Zhao Y, Liu Y, Akiyama K, Chen C, Qu C, et al. IFN-γ and TNF-α synergistically induce mesenchymal stem cell impairment and tumorigenesis via NFκB signaling. Stem Cells (Dayton Ohio) (2013) 31:1383–95. doi: 10.1002/stem.1388
20. Kalliolias GD, Ivashkiv LB. TNF biology, pathogenic mechanisms and emerging therapeutic strategies. Nat Rev Rheumatol (2016) 12:49–62. doi: 10.1038/nrrheum.2015.169
21. Pierini A, Strober W, Moffett C, Baker J, Nishikii H, Alvarez M, et al. TNF-α priming enhances CD4+FoxP3+ regulatory T-cell suppressive function in murine GVHD prevention and treatment. Blood (2016) 128:866–71. doi: 10.1182/blood-2016-04-711275
22. Sohn C, Lee A, Qiao Y, Loupasakis K, Ivashkiv LB, Kalliolias GD. Prolonged tumor necrosis factor α primes fibroblast-like synoviocytes in a gene-specific manner by altering chromatin. Arthritis Rheumatol (Hoboken N.J.) (2015) 67:86–95. doi: 10.1002/art.38871
23. English K, Barry FP, Field-Corbett CP, Mahon BP. IFN-gamma and TNF-alpha differentially regulate immunomodulation by murine mesenchymal stem cells. Immunol Lett (2007) 110:91–100. doi: 10.1016/j.imlet.2007.04.001
24. Sheng H, Wang Y, Jin Y, Zhang Q, Zhang Y, Wang L, et al. A critical role of IFNgamma in priming MSC-mediated suppression of T cell proliferation through up-regulation of B7-H1. cell.Res (2008) 18:846–57. doi: 10.1038/cr.2088.80
25. Montesinos JJ, López-García L, Cortés-Morales VA, Arriaga-Pizano L, Valle-Ríos R, Fajardo-Orduña GR, et al. Human bone marrow mesenchymal Stem/Stromal cells exposed to an inflammatory environment increase the expression of ICAM-1 and release microvesicles enriched in this adhesive molecule: Analysis of the participation of TNF-α and IFN-γ. J Immunol Res (2020) 2020:8839625. doi: 10.1155/2020/8839625
26. Hoogduijn MJ, Popp F, Verbeek R, Masoodi M, Nicolaou A, Baan C, et al. The immunomodulatory properties of mesenchymal stem cells and their use for immunotherapy. Int Immunopharmacol (2010) 10:1496–500. doi: 10.1016/j.intimp.2010.06.019
27. Dumitru CA, Hemeda H, Jakob M, Lang S, Brandau S. Stimulation of mesenchymal stromal cells (MSCs) via TLR3 reveals a novel mechanism of autocrine priming. FASEB J Off Publ Fed Am Societies Exp Biol (2014) 28:3856–66. doi: 10.1096/fj.14-250159
28. Wu HJ, Yiu WH, Li RX, Wong DW, Leung JC, Chan LY, et al. Mesenchymal stem cells modulate albumin-induced renal tubular inflammation and fibrosis. PloS One (2014) 9:e90883. doi: 10.1371/journal.pone.0090883
29. Yao M, Chen Z, He X, Long J, Xia X, Li Z, et al. Cross talk between glucose metabolism and immunosuppression in IFN-γ-primed mesenchymal stem cells. Life Sci alliance (2022) 5:e202201493. doi: 10.26508/lsa.202201493
30. Chinnadurai R, Copland IB, Patel SR, Galipeau J. IDO-independent suppression of T cell effector function by IFN-gamma-licensed human mesenchymal stromal cells. J Immunol (2014) 192:1491–501. doi: 10.4049/jimmunol.1301828
31. Meisel R, Zibert A, Laryea M, Göbel U, Däubener W. Human bone marrow stromal cells inhibit allogeneic T-cell responses by indoleamine 2,3-dioxygenase mediated tryptophan degradation. Blood (2004) 103:4619–4621. doi: 10.1182/blood-2003-11-3909
32. Davies LC, Heldring N, Kadri N, Le Blanc K. Mesenchymal stromal cell secretion of programmed death-1 ligands regulates T cell mediated immunosuppression. Stem Cells (Dayton Ohio) (2017) 35:766–76. doi: 10.1002/stem.2509
33. Prasanna SJ, Gopalakrishnan D, Shankar SR, Vasandan AB. Pro-inflammatory cytokines, IFNgamma and TNFalpha, influence immune properties of human bone marrow and Wharton jelly mesenchymal stem cells differentially. PloS One (2010) 5:e9016. doi: 10.1371/journal.pone.0009016
34. Saldaña L, Bensiamar F, Vallés G, Mancebo FJ, García-Rey E, Vilaboa N. Immunoregulatory potential of mesenchymal stem cells following activation by macrophage-derived soluble factors. Stem Cell Res Ther (2019) 10:58. doi: 10.1186/s13287-019-1156-6
35. Liu L, Song H, Duan H, Chai J, Yang J, Li X, et al. TSG-6 secreted by human umbilical cord-MSCs attenuates severe burn-induced excessive inflammation via inhibiting activations of P38 and JNK signaling. Sci Rep (2016) 6:30121. doi: 10.1038/srep30121
36. Lee RH, Pulin AA, Seo MJ, Kota DJ, Ylostalo J, Larson BL, et al. Intravenous hMSCs improve myocardial infarction in mice because cells embolized in lung are activated to secrete the anti-inflammatory protein TSG-6. Cell Stem Cell (2009) 5:54–63. doi: 10.1016/j.stem.2009.05.003
37. Hamidian Jahromi S, Li Y, Davies JE. Effect of tumor necrosis factor alpha dose and exposure time on tumor necrosis factor-induced gene-6 activation by neonatal and adult mesenchymal stromal cells. Stem Cells Dev (2018) 27:44–54. doi: 10.1089/scd.2017.0179
38. Choi H, Lee RH, Bazhanov N, Oh JY, Prockop DJ. Anti-inflammatory protein TSG-6 secreted by activated MSCs attenuates zymosan-induced mouse peritonitis by decreasing TLR2/NF-κB signaling in resident macrophages. Blood (2011) 118:330–8. doi: 10.1182/blood-2010-12-327353
39. Yang H, Feng R, Fu Q, Xu S, Hao X, Qiu Y, et al. Human induced pluripotent stem cell-derived mesenchymal stem cells promote healing via TNF-α-stimulated gene-6 in inflammatory bowel disease models. Cell Death Dis (2019) 10:718. doi: 10.1038/s41419-019-1957-7
40. Petri RM, Hackel A, Hahnel K, Dumitru CA, Bruderek K, Flohe SB, et al. Activated tissue-resident mesenchymal stromal cells regulate natural killer cell immune and tissue-regenerative function. Stem Cell Rep (2017) 9:985–98. doi: 10.1016/j.stemcr.2017.06.020
41. Barrachina L, Remacha AR, Romero A, Vázquez FJ, Albareda J, Prades M, et al. Effect of inflammatory environment on equine bone marrow derived mesenchymal stem cells immunogenicity and immunomodulatory properties. Veterinary Immunol immunopathol (2016) 171:57–65. doi: 10.1016/j.vetimm.2016.02.007
42. Mohammadpour H, Pourfathollah AA, Zarif MN, Tahoori MT. TNF-α modulates the immunosuppressive effects of MSCs on dendritic cells and T cells. Int Immunopharmacol (2015) 28:1009–17. doi: 10.1016/j.intimp.2015.07.045
43. Hackel A, Aksamit A, Bruderek K, Lang S, Brandau S. TNF-α and IL-1β sensitize human MSC for IFN-γ signaling and enhance neutrophil recruitment. Eur J Immunol (2021) 51:319–30. doi: 10.1002/eji.201948336
44. Boland L, Burand AJ, Brown AJ, Boyt D, Lira VA, Ankrum JA. IFN-γ and TNF-α pre-licensing protects mesenchymal stromal cells from the pro-inflammatory effects of palmitate. Mol Ther J Am Soc Gene Ther (2018) 26:860–73. doi: 10.1016/j.ymthe.2017.12.013
45. Ren G, Zhao X, Zhang L, Zhang J, L'Huillier A, Ling W, et al. Inflammatory cytokine-induced intercellular adhesion molecule-1 and vascular cell adhesion molecule-1 in mesenchymal stem cells are critical for immunosuppression. J Immunol (2010) 184:2321–8. doi: 10.4049/jimmunol.0902023
46. Li N, Hua J. Interactions between mesenchymal stem cells and the immune system. Cell Mol Life Sci CMLS (2017) 74:2345–60. doi: 10.1007/s00018-017-2473-5
47. Zheng S, Huang K, Xia W, Shi J, Liu Q, Zhang X, et al. Mesenchymal stromal cells rapidly suppress TCR signaling-mediated cytokine transcription in activated T cells through the ICAM-1/CD43 interaction. Front Immunol (2021) 12:609544. doi: 10.3389/fimmu.2021.609544
48. Fu X, Han B, Cai S, Lei Y, Sun T, Sheng Z. Migration of bone marrow-derived mesenchymal stem cells induced by tumor necrosis factor-alpha and its possible role in wound healing. Wound Repair regeneration Off Publ Wound Healing Soc [and] Eur Tissue Repair Soc (2009) 17:185–91. doi: 10.1111/j.1524-475X.2009.00454.x
49. De Becker A, Riet IV. Homing and migration of mesenchymal stromal cells: How to improve the efficacy of cell therapy? World J Stem Cells (2016) 8:73–87. doi: 10.4252/wjsc.v8.i3.73
50. MacDonald A, Burden AD. Psoriasis: advances in pathophysiology and management. Postgraduate Med J (2007) 83:690–7. doi: 10.1136/pgmj.2007.061473
51. Bai X, Xi J, Bi Y, Zhao X, Bing W, Meng X, et al. TNF-α promotes survival and migration of MSCs under oxidative stress via NF-κB pathway to attenuate intimal hyperplasia in vein grafts. J Cell Mol Med (2017) 21:2077–91. doi: 10.1111/jcmm.13131
52. Xie Z, Yu W, Zheng G, Li J, Cen S, Ye G, et al. TNF-α-mediated m(6)A modification of ELMO1 triggers directional migration of mesenchymal stem cell in ankylosing spondylitis. Nat Commun (2021) 12:5373. doi: 10.1038/s41467-021-25710-4
53. Bühring HJ, Treml S, Cerabona F, de Zwart P, Kanz L, Sobiesiak M. Phenotypic characterization of distinct human bone marrow-derived MSC subsets. Ann New York Acad Sci (2009) 1176:124–34. doi: 10.1111/j.1749-6632.2009.04564.x
54. Lu ZY, Chen WC, Li YH, Li L, Zhang H, Pang Y, et al. TNF-α enhances vascular cell adhesion molecule-1 expression in human bone marrow mesenchymal stem cells via the NF-κB, ERK and JNK signaling pathways. Mol Med Rep (2016) 14:643–8. doi: 10.3892/mmr.2016.5314
55. Xiao Q, Wang SK, Tian H, Xin L, Zou ZG, Hu YL, et al. TNF-α increases bone marrow mesenchymal stem cell migration to ischemic tissues. Cell Biochem biophysics (2012) 62:409–14. doi: 10.1007/s12013-011-9317-y
56. Burlacu A, Grigorescu G, Rosca AM, Preda MB, Simionescu M. Factors secreted by mesenchymal stem cells and endothelial progenitor cells have complementary effects on angiogenesis in vitro. Stem Cells Dev (2013) 22:643–53. doi: 10.1089/scd.2012.0273
57. Broekman W, Amatngalim GD, de Mooij-Eijk Y, Oostendorp J, Roelofs H, Taube C, et al. TNF-α and IL-1β-activated human mesenchymal stromal cells increase airway epithelial wound healing in vitro via activation of the epidermal growth factor receptor. Respir Res (2016) 17:3. doi: 10.1186/s12931-015-0316-1
58. Chen H, Min XH, Wang QY, Leung FW, Shi L, Zhou Y, et al. Pre-activation of mesenchymal stem cells with TNF-α, IL-1β and nitric oxide enhances its paracrine effects on radiation-induced intestinal injury. Sci Rep (2015) 5:8718. doi: 10.1038/srep08718
59. Ting HK, Chen CL, Meng E, Cherng JH, Chang SJ, Kao CC, et al. Inflammatory regulation by TNF-α-Activated adipose-derived stem cells in the human bladder cancer microenvironment. Int J Mol Sci (2021) 22:3987. doi: 10.3390/ijms22083987
60. Daniele S, Natali L, Giacomelli C, Campiglia P, Novellino E, Martini C, et al. Osteogenesis is improved by low tumor necrosis factor alpha concentration through the modulation of gs-coupled receptor signals. Mol Cell Biol (2017) 37:e00442–16. doi: 10.1128/mcb.00442-16
61. Trincavelli ML, Daniele S, Giacomelli C, Taliani S, Da Settimo F, Cosimelli B, et al. Osteoblast differentiation and survival: A role for A2B adenosine receptor allosteric modulators. Biochim Biophys Acta (2014) 1843:2957–66. doi: 10.1016/j.bbamcr.2014.09.013
62. Ding J, Ghali O, Lencel P, Broux O, Chauveau C, Devedjian JC, et al. TNF-alpha and IL-1beta inhibit RUNX2 and collagen expression but increase alkaline phosphatase activity and mineralization in human mesenchymal stem cells. Life Sci (2009) 84:499–504. doi: 10.1016/j.lfs.2009.01.013
63. Lencel P, Delplace S, Hardouin P, Magne D. TNF-α stimulates alkaline phosphatase and mineralization through PPARγ inhibition in human osteoblasts. Bone (2011) 48:242–9. doi: 10.1016/j.bone.2010.09.001
64. Kotake S, Nanke Y. Effect of TNFα on osteoblastogenesis from mesenchymal stem cells. Biochim Biophys Acta (2014) 1840:1209–13. doi: 10.1016/j.bbagen.2013.12.013
65. Deng L, Hu G, Jin L, Wang C, Niu H. Involvement of microRNA-23b in TNF-α-reduced BMSC osteogenic differentiation via targeting runx2. J Bone mineral Metab (2018) 36:648–60. doi: 10.1007/s00774-017-0886-8
66. Sun X, Li M, Ban J, Li Z. miR-23b mediates TNF-α-Inhibited osteogenic differentiation of human periodontal ligament stem cells by targeting Runx2. Int J Med Sci (2021) 18:3674–83. doi: 10.7150/ijms.64312
67. Yang N, Li Y, Wang G, Ding Y, Jin Y, Xu Y. Tumor necrosis factor-α suppresses adipogenic and osteogenic differentiation of human periodontal ligament stem cell by inhibiting miR-21/Spry1 functional axis. Differentiation; Res Biol Diversity (2017) 97:33–43. doi: 10.1016/j.diff.2017.08.004
68. Chang J, Liu F, Lee M, Wu B, Ting K, Zara JN, et al. NF-κB inhibits osteogenic differentiation of mesenchymal stem cells by promoting β-catenin degradation. Proc Natl Acad Sci U.S.A. (2013) 110:9469–74. doi: 10.1073/pnas.1300532110
69. Ma H, Li YN, Song L, Liu R, Li X, Shang Q, et al. Macrophages inhibit adipogenic differentiation of adipose tissue derived mesenchymal stem/stromal cells by producing pro-inflammatory cytokines. Cell bioscience (2020) 10:88. doi: 10.1186/s13578-020-00450-y
70. Sitcheran R, Cogswell PC, Baldwin AS Jr. NF-kappaB mediates inhibition of mesenchymal cell differentiation through a posttranscriptional gene silencing mechanism. Genes Dev (2003) 17:2368–73. doi: 10.1101/gad.1114503
71. Wehling N, Palmer GD, Pilapil C, Liu F, Wells JW, Müller PE, et al. Interleukin-1beta and tumor necrosis factor alpha inhibit chondrogenesis by human mesenchymal stem cells through NF-kappaB-dependent pathways. Arthritis rheumatism (2009) 60:801–12. doi: 10.1002/art.24352
72. Liang W, Chen X, Zhang S, Fang J, Chen M, Xu Y, et al. Mesenchymal stem cells as a double-edged sword in tumor growth: focusing on MSC-derived cytokines. Cell Mol Biol Lett (2021) 26:3. doi: 10.1186/s11658-020-00246-5
73. Li HJ, Reinhardt F, Herschman HR, Weinberg RA. Cancer-stimulated mesenchymal stem cells create a carcinoma stem cell niche via prostaglandin E2 signaling. Cancer Discovery (2012) 2:840–55. doi: 10.1158/2159-8290.Cd-12-0101
74. Rajantie I, Ilmonen M, Alminaite A, Ozerdem U, Alitalo K, Salven P. Adult bone marrow-derived cells recruited during angiogenesis comprise precursors for periendothelial vascular mural cells. Blood (2004) 104:2084–6. doi: 10.1182/blood-2004-01-0336
75. Scherzed A, Hackenberg S, Froelich K, Kessler M, Koehler C, Hagen R, et al. BMSC enhance the survival of paclitaxel treated squamous cell carcinoma cells in vitro. Cancer Biol Ther (2011) 11:349–57. doi: 10.4161/cbt.11.3.14179
76. Dreuw A, Hermanns HM, Heise R, Joussen S, Rodríguez F, Marquardt Y, et al. Interleukin-6-type cytokines upregulate expression of multidrug resistance-associated proteins in NHEK and dermal fibroblasts. J Invest Dermatol (2005) 124:28–37. doi: 10.1111/j.0022-202X.2004.23499.x
77. Spaeth EL, Dembinski JL, Sasser AK, Watson K, Klopp A, Hall B, et al. Mesenchymal stem cell transition to tumor-associated fibroblasts contributes to fibrovascular network expansion and tumor progression. PloS One (2009) 4:e4992. doi: 10.1371/journal.pone.0004992
78. Lu YR, Yuan Y, Wang XJ, Wei LL, Chen YN, Cong C, et al. The growth inhibitory effect of mesenchymal stem cells on tumor cells in vitro and in vivo. Cancer Biol Ther (2008) 7:245–51. doi: 10.4161/cbt.7.2.5296
79. Glennie S, Soeiro I, Dyson PJ, Lam EW, Dazzi F. Bone marrow mesenchymal stem cells induce division arrest anergy of activated T cells. Blood (2005) 105:2821–7. doi: 10.1182/blood-2004-09-3696
80. Khakoo AY, Pati S, Anderson SA, Reid W, Elshal MF, Rovira II, et al. Human mesenchymal stem cells exert potent antitumorigenic effects in a model of kaposi's sarcoma. J Exp Med (2006) 203:1235–47. doi: 10.1084/jem.20051921
81. Qiao L, Xu ZL, Zhao TJ, Ye LH, Zhang XD. Dkk-1 secreted by mesenchymal stem cells inhibits growth of breast cancer cells via depression of wnt signalling. Cancer Lett (2008) 269:67–77. doi: 10.1016/j.canlet.2008.04.032
82. Gillaux C, Méhats C, Vaiman D, Cabrol D, Breuiller-Fouché M. Functional screening of TLRs in human amniotic epithelial cells. J Immunol (2011) 187:2766–74. doi: 10.4049/jimmunol.1100217
83. Jin P, Zhao Y, Liu H, Chen J, Ren J, Jin J, et al. Interferon-γ and tumor necrosis factor-α polarize bone marrow stromal cells uniformly to a Th1 phenotype. Sci Rep (2016) 6:26345. doi: 10.1038/srep26345
84. Lee RH, Yoon N, Reneau JC, Prockop DJ. Preactivation of human MSCs with TNF-α enhances tumor-suppressive activity. Cell Stem Cell (2012) 11:825–35. doi: 10.1016/j.stem.2012.10.001
85. Du J, Zhou L, Chen X, Yan S, Ke M, Lu X, et al. IFN-γ-primed human bone marrow mesenchymal stem cells induce tumor cell apoptosis in vitro via tumor necrosis factor-related apoptosis-inducing ligand. Int J Biochem Cell Biol (2012) 44:1305–14. doi: 10.1016/j.biocel.2012.04.015
86. Waterman RS, Tomchuck SL, Henkle SL, Betancourt AM. A new mesenchymal stem cell (MSC) paradigm: polarization into a pro-inflammatory MSC1 or an immunosuppressive MSC2 phenotype. PloS One (2010) 5:e10088. doi: 10.1371/journal.pone.0010088
87. Strieter RM, Kunkel SL, Arenberg DA, Burdick MD, Polverini PJ. Interferon gamma-inducible protein 10 (IP-10), a member of the c-X-C chemokine family, is an inhibitor of angiogenesis. Biochem Biophys Res Commun (1995) 210:51–7. doi: 10.1006/bbrc.1995.1626
88. Dai C, Wen X, He W, Liu Y. Inhibition of proinflammatory RANTES expression by TGF-beta1 is mediated by glycogen synthase kinase-3beta-dependent beta-catenin signaling. J Biol Chem (2011) 286:7052–9. doi: 10.1074/jbc.M110.174821
89. Katanov C, Lerrer S, Liubomirski Y, Leider-Trejo L, Meshel T, Bar J, et al. Regulation of the inflammatory profile of stromal cells in human breast cancer: prominent roles for TNF-α and the NF-κB pathway. Stem Cell Res Ther (2015) 6:87. doi: 10.1186/s13287-015-0080-7
90. Chen M, Su W, Lin X, Guo Z, Wang J, Zhang Q, et al. Adoptive transfer of human gingiva-derived mesenchymal stem cells ameliorates collagen-induced arthritis via suppression of Th1 and Th17 cells and enhancement of regulatory T cell differentiation. Arthritis rheumatism (2013) 65:1181–93. doi: 10.1002/art.37894
91. Shi Y, Wang Y, Li Q, Liu K, Hou J, Shao C, et al. Immunoregulatory mechanisms of mesenchymal stem and stromal cells in inflammatory diseases. Nat Rev Nephrol (2018) 14:493–507. doi: 10.1038/s41581-018-0023-5
92. Moll G, Ankrum JA, Kamhieh-Milz J, Bieback K, Ringdén O, Volk HD, et al. Intravascular mesenchymal Stromal/Stem cell therapy product diversification: Time for new clinical guidelines. Trends Mol Med (2019) 25:149–63. doi: 10.1016/j.molmed.2018.12.006
93. Uemura R, Xu M, Ahmad N, Ashraf M. Bone marrow stem cells prevent left ventricular remodeling of ischemic heart through paracrine signaling. Circ Res (2006) 98:1414–21. doi: 10.1161/01.Res.0000225952.61196.39
94. Xie M, Xiong W, She Z, Wen Z, Abdirahman AS, Wan W, et al. Immunoregulatory effects of stem cell-derived extracellular vesicles on immune cells. Front Immunol (2020) 11:13. doi: 10.3389/fimmu.2020.00013
95. Phinney DG, Pittenger MF. Concise review: MSC-derived exosomes for cell-free therapy. Stem Cells (Dayton Ohio) (2017) 35:851–8. doi: 10.1002/stem.2575
96. Harting MT, Srivastava AK, Zhaorigetu S, Bair H, Prabhakara KS, Toledano Furman NE, et al. Inflammation-stimulated mesenchymal stromal cell-derived extracellular vesicles attenuate inflammation. Stem Cells (Dayton Ohio) (2018) 36:79–90. doi: 10.1002/stem.2730
97. Di Trapani M, Bassi G, Midolo M, Gatti A, Kamga PT, Cassaro A, et al. Differential and transferable modulatory effects of mesenchymal stromal cell-derived extracellular vesicles on T, b and NK cell functions. Sci Rep (2016) 6:24120. doi: 10.1038/srep24120
98. Dal Collo G, Adamo A, Gatti A, Tamellini E, Bazzoni R, Takam Kamga P, et al. Functional dosing of mesenchymal stromal cell-derived extracellular vesicles for the prevention of acute graft-versus-host-disease. Stem Cells (Dayton Ohio) (2020) 38:698–711. doi: 10.1002/stem.3160
99. Tolomeo AM, Castagliuolo I, Piccoli M, Grassi M, Magarotto F, De Lazzari G, et al. Extracellular vesicles secreted by mesenchymal stromal cells exert opposite effects to their cells of origin in murine sodium dextran sulfate-induced colitis. Front Immunol (2021) 12:627605. doi: 10.3389/fimmu.2021.627605
100. Yu Z, Wen Y, Jiang N, Li Z, Guan J, Zhang Y, et al. TNF-α stimulation enhances the neuroprotective effects of gingival MSCs derived exosomes in retinal ischemia-reperfusion injury via the MEG3/miR-21a-5p axis. Biomaterials (2022) 284:121484. doi: 10.1016/j.biomaterials.2022.121484
101. El Moshy S, Radwan IA, Rady D, Abbass MMS, El-Rashidy AA, Sadek KM, et al. Dental stem cell-derived Secretome/Conditioned medium: The future for regenerative therapeutic applications. Stem Cells Int (2020) 2020:7593402. doi: 10.1155/2020/7593402
102. Nakao Y, Fukuda T, Zhang Q, Sanui T, Shinjo T, Kou X, et al. Exosomes from TNF-α-treated human gingiva-derived MSCs enhance M2 macrophage polarization and inhibit periodontal bone loss. Acta biomaterialia (2021) 122:306–24. doi: 10.1016/j.actbio.2020.12.046
103. Cheng A, Choi D, Lora M, Shum-Tim D, Rak J, Colmegna I. Human multipotent mesenchymal stromal cells cytokine priming promotes RAB27B-regulated secretion of small extracellular vesicles with immunomodulatory cargo. Stem Cell Res Ther (2020) 11:539. doi: 10.1186/s13287-020-02050-6
104. Gómez-Ferrer M, Villanueva-Badenas E, Sánchez-Sánchez R, Sánchez-López CM, Baquero MC, Sepúlveda P, et al. HIF-1α and pro-inflammatory signaling improves the immunomodulatory activity of MSC-derived extracellular vesicles. Int J Mol Sci (2021) 22:3416. doi: 10.3390/ijms22073416
105. Losurdo M, Pedrazzoli M, D'Agostino C, Elia CA, Massenzio F, Lonati E, et al. Intranasal delivery of mesenchymal stem cell-derived extracellular vesicles exerts immunomodulatory and neuroprotective effects in a 3xTg model of alzheimer's disease. Stem Cells Trans Med (2020) 9:1068–84. doi: 10.1002/sctm.19-0327
106. Liang YC, Wu YP, Li XD, Chen SH, Ye XJ, Xue XY, et al. TNF-α-induced exosomal miR-146a mediates mesenchymal stem cell-dependent suppression of urethral stricture. J Cell Physiol (2019) 234:23243–55. doi: 10.1002/jcp.28891
107. Wu HH, Zhou Y, Tabata Y, Gao JQ. Mesenchymal stem cell-based drug delivery strategy: from cells to biomimetic. J Controlled release Off J Controlled Release Soc (2019) 294:102–13. doi: 10.1016/j.jconrel.2018.12.019
108. Vojtassák J, Danisovic L, Kubes M, Bakos D, Jarábek L, Ulicná M, et al. Autologous biograft and mesenchymal stem cells in treatment of the diabetic foot. Neuro Endocrinol Lett (2006) 27 Suppl 2:134–7.
109. Christopeit M, Schendel M, Föll J, Müller LP, Keysser G, Behre G. Marked improvement of severe progressive systemic sclerosis after transplantation of mesenchymal stem cells from an allogeneic haploidentical-related donor mediated by ligation of CD137L. Leukemia (2008) 22:1062–4. doi: 10.1038/sj.leu.2404996
110. Kharaziha P, Hellström PM, Noorinayer B, Farzaneh F, Aghajani K, Jafari F, et al. Improvement of liver function in liver cirrhosis patients after autologous mesenchymal stem cell injection: a phase I-II clinical trial. Eur J Gastroenterol Hepatol (2009) 21:1199–205. doi: 10.1097/MEG.0b013e32832a1f6c
111. Sun L, Wang D, Liang J, Zhang H, Feng X, Wang H, et al. Umbilical cord mesenchymal stem cell transplantation in severe and refractory systemic lupus erythematosus. Arthritis rheumatism (2010) 62:2467–75. doi: 10.1002/art.27548
112. Duijvestein M, Vos AC, Roelofs H, Wildenberg ME, Wendrich BB, Verspaget HW, et al. Autologous bone marrow-derived mesenchymal stromal cell treatment for refractory luminal crohn's disease: Results of a phase I study. Gut (2010) 59:1662–9. doi: 10.1136/gut.2010.215152
113. Le Blanc K, Frassoni F, Ball L, Locatelli F, Roelofs H, Lewis I, et al. Mesenchymal stem cells for treatment of steroid-resistant, severe, acute graft-versus-host disease: A phase II study. Lancet (London England) (2008) 371:1579–86. doi: 10.1016/s0140-6736(08)60690-x
114. Macmillan ML, Blazar BR, DeFor TE, Wagner JE. Transplantation of ex-vivo culture-expanded parental haploidentical mesenchymal stem cells to promote engraftment in pediatric recipients of unrelated donor umbilical cord blood: Results of a phase I-II clinical trial. Bone marrow Transplant (2009) 43:447–54. doi: 10.1038/bmt.2008.348
115. Raffaghello L, Bianchi G, Bertolotto M, Montecucco F, Busca A, Dallegri F, et al. Human mesenchymal stem cells inhibit neutrophil apoptosis: A model for neutrophil preservation in the bone marrow niche. Stem Cells (Dayton Ohio) (2008) 26:151–62. doi: 10.1634/stemcells.2007-0416
116. Ceccariglia S, Cargnoni A, Silini AR, Parolini O. Autophagy: a potential key contributor to the therapeutic action of mesenchymal stem cells. Autophagy (2020) 16:28–37. doi: 10.1080/15548627.2019.1630223
117. Weiss ARR, Dahlke MH. Immunomodulation by mesenchymal stem cells (MSCs): Mechanisms of action of living, apoptotic, and dead MSCs. Front Immunol (2019) 10:1191. doi: 10.3389/fimmu.2019.01191
118. Augello A, Tasso R, Negrini SM, Amateis A, Indiveri F, Cancedda R, et al. Bone marrow mesenchymal progenitor cells inhibit lymphocyte proliferation by activation of the programmed death 1 pathway. Eur J Immunol (2005) 35:1482–90. doi: 10.1002/eji.200425405
119. Mountziaris PM, Dennis Lehman E, Mountziaris I, Sing DC, Kasper FK, Mikos AG. Effect of temporally patterned TNF-α delivery on in vitro osteogenic differentiation of mesenchymal stem cells cultured on biodegradable polymer scaffolds. J biomaterials sci Polymer edition (2013) 24:1794–813. doi: 10.1080/09205063.2013.803455
120. Duan P, Tan J, Miao Y, Zhang Q. Potential role of exosomes in the pathophysiology, diagnosis, and treatment of hypoxic diseases. Am J Trans Res (2019) 11:1184–201.
121. Trubiani O, Marconi GD, Pierdomenico SD, Piattelli A, Diomede F, Pizzicannella J. Human oral stem cells, biomaterials and extracellular vesicles: A promising tool in bone tissue repair. Int J Mol Sci (2019) 20:4987. doi: 10.3390/ijms20204987
122. Gholampour MA, Abroun S, Nieuwland R, Mowla SJ, Soudi S. Mesenchymal stem cell-derived extracellular vesicles conditionally ameliorate bone marrow failure symptoms in an immune-mediated aplastic anemia mouse model. J Cell Physiol (2021) 236:6055–67. doi: 10.1002/jcp.30291
123. Jin L, Zhang J, Deng Z, Liu J, Han W, Chen G, et al. Mesenchymal stem cells ameliorate myocardial fibrosis in diabetic cardiomyopathy via the secretion of prostaglandin E2. Stem Cell Res Ther (2020) 11:122. doi: 10.1186/s13287-020-01633-7
124. Cao X, Duan L, Hou H, Liu Y, Li Z. IGF-1C hydrogel improves the therapeutic effects of MSCs on colitis in mice through PGE 2 -mediated M2 macrophage polarization. theranostics (2020) 10:7697–709. doi: 10.7150/thno.45434
125. Lee BC, Kim HS, Shin TH, Kang I, Lee JY, Kim JJ, et al. PGE2 maintains self-renewal of human adult stem cells via EP2-mediated autocrine signaling and its production is regulated by cell-to-cell contact. Sci Rep (2016) 6:26298. doi: 10.1038/srep26298
Keywords: mesenchymal stem cells, TNF-α, exosomal microvesicles, immunomodulation, tissue regeneration and repair
Citation: Li W, Liu Q, Shi J, Xu X and Xu J (2023) The role of TNF-α in the fate regulation and functional reprogramming of mesenchymal stem cells in an inflammatory microenvironment. Front. Immunol. 14:1074863. doi: 10.3389/fimmu.2023.1074863
Received: 20 October 2022; Accepted: 24 January 2023;
Published: 06 February 2023.
Edited by:
Lucienne Chatenoud, Université Paris Cité, FranceReviewed by:
Elba Mónica Vermeulen, Instituto de Biología y Medicina Experimental, ArgentinaAlix Kay Berglund, North Carolina State University, United States
Monia Orciani, Marche Polytechnic University, Italy
Copyright © 2023 Li, Liu, Shi, Xu and Xu. This is an open-access article distributed under the terms of the Creative Commons Attribution License (CC BY). The use, distribution or reproduction in other forums is permitted, provided the original author(s) and the copyright owner(s) are credited and that the original publication in this journal is cited, in accordance with accepted academic practice. No use, distribution or reproduction is permitted which does not comply with these terms.
*Correspondence: Xiang Xu, WGlhbmd4dUB0bW11LmVkdS5jbg==; Jinyi Xu, MTAwMzU0MzY2OUBxcS5jb20=