- College of Life Science and Technology, Beijing University of Chemical Technology, Beijing, China
Rotavirus (RV) causes 200,000 deaths per year and imposes a serious burden to public health and livestock farming worldwide. Currently, rehydration (oral and intravenous) remains the main strategy for the treatment of rotavirus gastroenteritis (RVGE), and no specific drugs are available. This review discusses the viral replication cycle in detail and outlines possible therapeutic approaches including immunotherapy, probiotic-assisted therapy, anti-enteric secretory drugs, Chinese medicine, and natural compounds. We present the latest advances in the field of rotavirus antivirals and highlights the potential use of Chinese medicine and natural compounds as therapeutic agents. This review provides an important reference for rotavirus prevention and treatment.
1 Introduction
Rotavirus is the major cause of severe diarrhoea in infants and young animals worldwide. It causes 130 million childhood infections and over 200,000 deaths each year and contributes to significant losses to livestock production. In 2006 and 2008, two live oral attenuated rotavirus vaccines - Rotarix® (RV1) and RotaTeq ™ (RV5) - were approved for the prevention of rotavirus infection (1). In 2009, WHO made a recommendation for the global use of rotavirus vaccines, particularly in developing countries with high mortality rates of childhood diarrhoea. Prior to this recommendation, rotavirus gastroenteritis killed approximately 500,000 children under 5 years of age each year. A review of rotavirus deaths among children under 5 years of age from 2000 to 2013 showed that global rotavirus-associated deaths declined from 528,000 in 2000 to 215,000 in 2013. In 2017, 53% of rotavirus-associated deaths worldwide occurred in three countries (Nigeria, India, Democratic Republic of Congo).[https://preventrotavirus.org/rotavirus-disease/global-burden/](Figure 1) Two other live oral attenuated vaccines are used for routine vaccination in India: ROTAVAC (Bharat Biologicals) and ROTASIIL (Serum Institute of India), and several other vaccines are in development, including a non-replicating injectable vaccine (2). At present, there are two vaccines in the Chinese market: Rotavirus Vaccine for Live, Oral (Lanzhou Institute of Biological Products), which was marketed in China in 2001, and RotaTeq™ (Merck Sharp & Dohme, USA), which was introduced in 2018 (3). Although the introduction of rotavirus immunization programs has reduced mortality by 60%, vaccine efficacy in developing countries is low (4). Oral and intravenous rehydration remains the mainstay of rotavirus treatment today; however, it does not alleviate the course and severity of the diarrhoea because it is only symptomatic therapy. Therefore, there is an urgent need for the development of specific anti-rotavirus drugs.
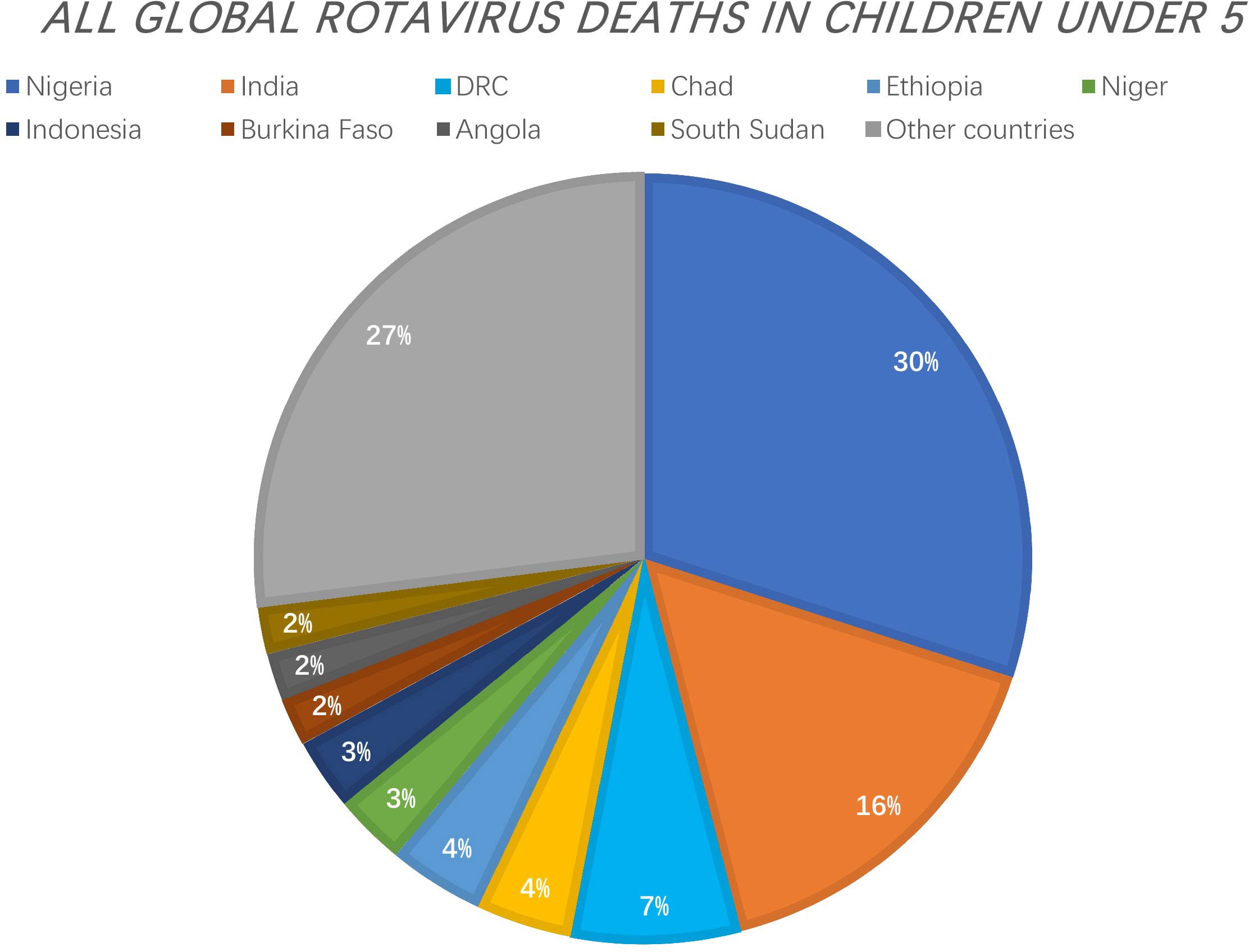
Figure 1 The Countries with the Greatest Number of Rotavirus Deaths as a Proportion of All Global Rotavirus Deaths in Children under 5. 53% of rotavirus-associated deaths worldwide occurred in three countries (Nigeria, India, Democratic Republic of Congo). [https://preventrotavirus.org/rotavirus-disease/global-burden/].
2 Overview of rotavirus
2.1 Epidemiology
Rotavirus is transmitted through faecal-oral transmission and aerosol transmission. The infected hosts are mainly young children and animals, and immunocompromised patients such as those with organ- and stem cell transplantation. The infection sites in the host are the mature villus cells and intestinal endocrine cells (intestinal chromaffin cells) in the upper middle part of the small intestine. Rotavirus infections cause diarrhoea and vomiting symptoms mainly through the destruction of enterocytes (loss of epithelial cell absorption) and activation of the enteric nervous system (ENS) by NSP4 enterotoxin (5). And the viral replication was also found in salivary gland ductal epithelial cells recently (6).
2.2 Rotavirus structure
The rotavirus genus belongs to the Reoviridae family and is an envelope-free icosahedral-shaped double-stranded RNA virus with spikes and an average diameter of 100 nm (7). It is named rotavirus because of its wheel-like appearance when viewed by electron microscopy (8). Rotavirus consists of three concentric coat layers surrounded by 11 segmented double-stranded RNA fragments with a genome length of 18,500 bp which encodes 6 structural proteins (VP1-4, VP6-7) and 5-6 non-structural proteins (NSP1-5/6). The capsid structure consists of an inner capsid layer (VP2), a middle capsid layer (VP6), and an outer capsid layer (capsid glycoprotein VP7 and hemagglutinin spike protein VP4) (9). In vitro experiments showed that trypsin cleavage of VP4 into VP5* and VP8* is necessary for virus entry into cells. Analysis of the structural domains of VP5* and VP8* reveals some information about the early interaction between the virus and the host cell (Figure 2). The viral genome and the viral proteins and functions it encodes are shown in Table 1.
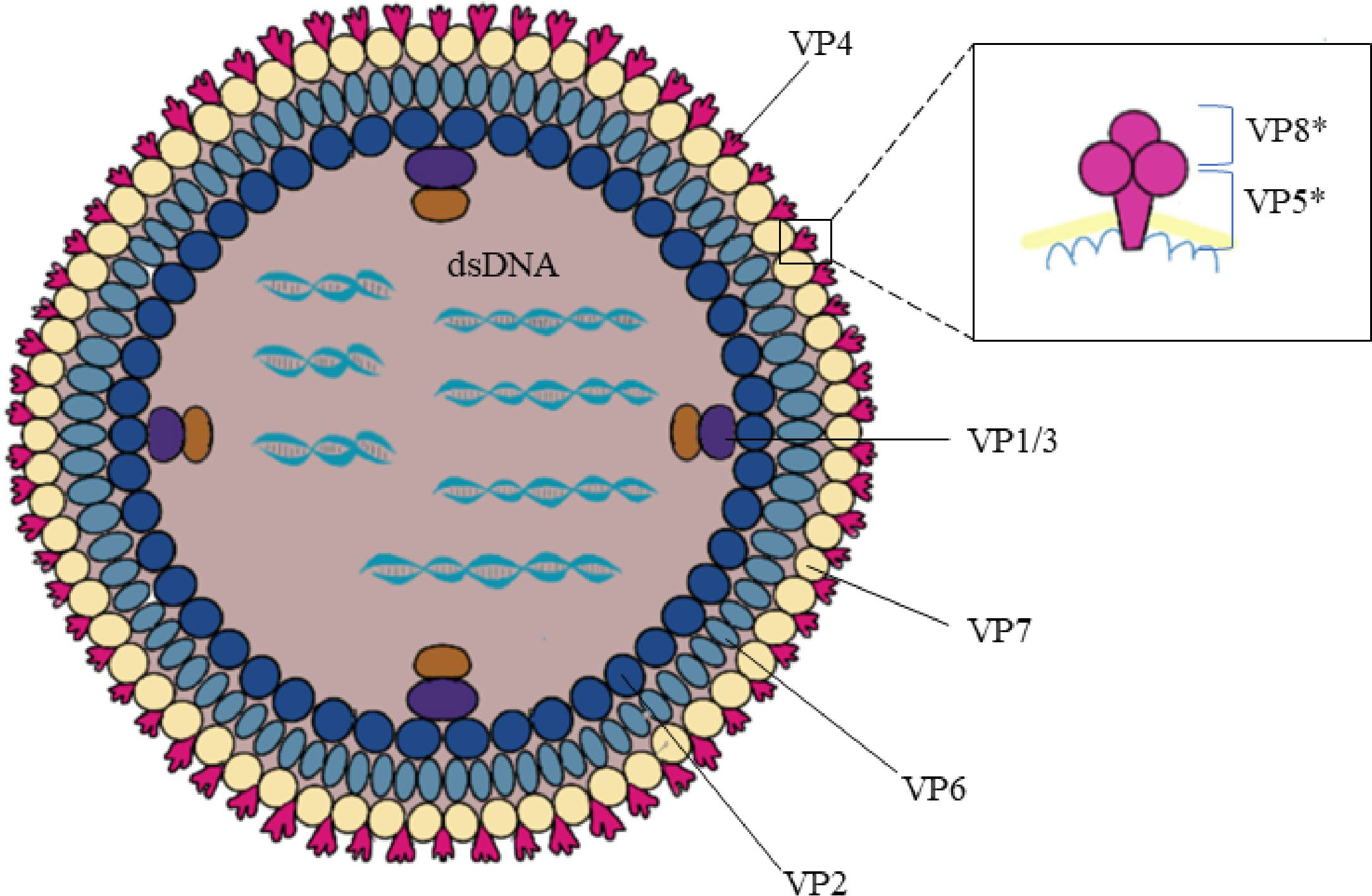
Figure 2 Rotavirus structure. Rotavirus consists of three concentric coat layers surrounded by 11 segmented double-stranded RNA fragments with a genome length of 18,500 bp which encodes 6 structural proteins (VP1-4, VP6-7) and 5-6 non-structural proteins (NSP1-5/6). The capsid structure consists of an inner capsid layer (VP2), a middle capsid layer (VP6), and an outer capsid layer (capsid glycoprotein VP7 and hemagglutinin spike protein VP4). VP4 is cleaved into VP5* and VP8* by the action of trypsin which enhances rotavirus infectivity. The bottom of the VP5* protein is half-buried inside the VP6 protein.
2.3 Rotavirus serotyping
Rotavirus is classified into 7 serotypes (A-G) according to the difference in VP6 serotypes, with A, B, and C infectious to humans. Rotavirus outer capsid proteins VP4 and VP7 play an important role in virus adsorption and entry into host cells and have neutralizing antigens; The VP7 (G) and VP4 (P) antigens can be freely combined, hence, there is a dual classification scheme: VP7 (G1-G36), VP4 P[1]-P[51], at least 51 species) (3, 10, 11).
3 Research progress of anti-rotavirus drugs
There are no FDA-approved drugs for rotavirus gastroenteritis. Most deaths are associated with excessive water and electrolyte loss due to vomiting and diarrhoea; therefore, oral intravenous rehydration is the most basic treatment strategy. Probiotics including Lactobacillus rhamnosus GG(LGG) are recommended by several guidelines around the world (12). In addition to anti-RVA secretory IgA (13, 14), breast milk is rich in oligosaccharides and proteins that inhibit rotavirus infection of the host and modulate host immune function, therefore, breastfeeding is widely recommended (15). A article published in 1982 reported that rotavirus antibodies were found in the fresh milk but not after pasteurization (16). Racecadotril is an anti-secretory drug used as an adjunct drug for acute gastroenteritis treatment yet it is not promoted; some studies support its therapeutic efficacy, while other paediatricians believe that Racecadotril with oral rehydration is ineffective (17). The inclusion of rotavirus vaccine in immunization programs has decreased reported rotavirus infections worldwide by 60%. However, deaths are still as high as 200,000 and 90% of fatal rotavirus infections occur in low-income countries where vaccine coverage is low and access to care for children is limited. This calls for the urgent development of specific anti-rotavirus drugs.
3.1 Rotavirus replication cycle
The rotavirus replication cycle is the main development direction of antiviral drugs. The related compounds targeting different stages of the life cycle are shown in Figure 3.
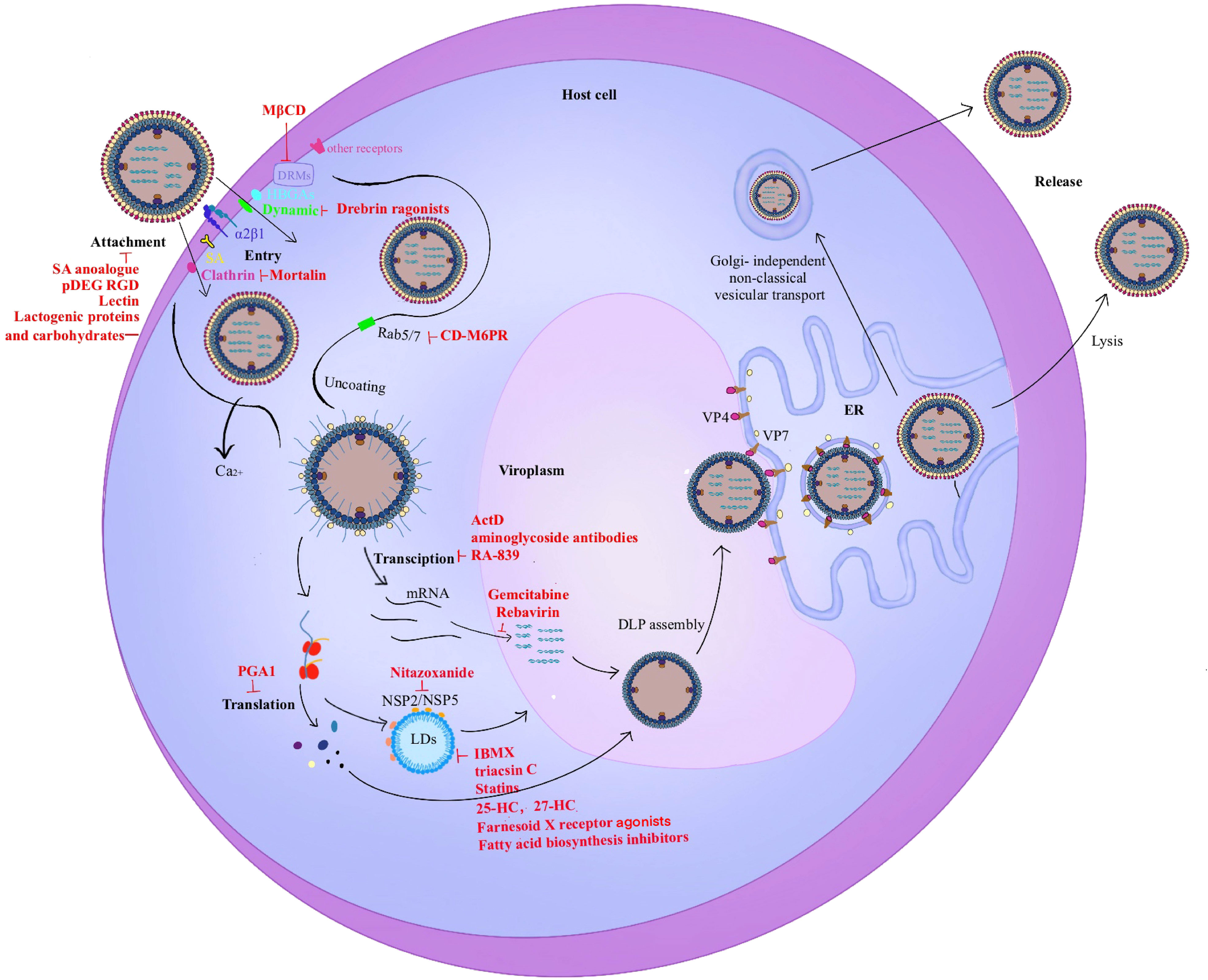
Figure 3 Rotavirus life cycle. The entry stage of rotavirus replication is complex. 1). The first step in infection is the recognition of rotavirus and host surface molecules, including sialic acid, α2β1, and histo-blood group antigens (HBGAs). Rotavirus invades cells through two different endocytosis pathways (clathrin-dependent or dynamin-dependent, depending on the strain), and a mode of direct penetration. VP5* contains a hydrophobic structural domain that promotes rotavirus penetration into cells. 2). Triple-layered particles (TLPs) entering the cytoplasm enter the same endosome and migrate from early endosomes (EEs) to mature endosomes (MEs) and late endosomes (LEs) with Rab5/7 transformation. MEs exist in Rab5 and Rab7. As the Ca2+ level decreases, the viral outer capsid disintegrates and double-layered particles (DLPs) are released into the cytoplasm which triggers viral translation and replication. 3). The viroplasm is the main site of rotavirus genome replication and packaging. The interaction of NSP2/NSP5 with lipid droplets (LDs) is the first step in the formation of viral plasmids. 4). The virus precursor DLPs completes the outer capsid assembly in the endoplasmic reticulum (ER). 5). Mature viral particles are released by cell lysis in polarized cells such as monkey embryonic kidney cells MA-104. In contrast, mature viral particles are released by budding in non-polarized cells such as human intestinal cells Caco-2 which does not cleave host cells.
3.1.1 Drugs inhibiting viral adherent cells
Rotavirus attachment to host cells is dependent on trypsin-like enzyme cleavage of viral outer capsid proteins VP4 and VP7 in the intestine to become infectious. This process cleaves VP4 into the C-terminal VP5* domain and the N-terminal VP8* domain, which contains the hemagglutination- or sialic acid binding domain necessary for the subgroup of rotaviruses that require sialic acid for infection (9, 18). Sialic acid is usually in the form of oligosaccharides, glycolipids, or glycoproteins, and binds directly to rotavirus to inhibit viral adhesion; however, this inhibition appears to be strain-dependent (19). Mucin is a negatively charged glycoprotein that is a major component of the mucus layer of the gastrointestinal tract (20). Supplementation with leucine (21) or LGG (22) promotes mucin production. The sialic acid content of mucin is proportional to its antiviral activity (23). The antiviral activity of sialic acid glycoprotein is demonstrated in vitro and in vivo (24). Many studies observed that salivary acid mimics inhibited rotavirus replication in vitro and in vivo (25–27). Liakatos A et al. (25) also showed that lactose-based salivary acid mimics were not inhibitors of rhesus rotavirus strains; however, they exhibited moderate inhibition of human rotavirus Wa strain. The neoglycolipid receptor mimic is a synthetic neoglycolipid containing sialic acid lactose. Lactose sialate is the carbohydrate fraction of GM(3) and GM(3) ganglioside receptors are required for host cell recognition by sialic acid-dependent rotavirus. However, GM(3) ganglioside production as an oral therapeutic agent in pigs is costly. The new glycolipid receptor mimic reduces rotavirus infection of host cells by over 90% using concentrations comparable to GM(3) (28). Integrin α2β1 also plays a key role in the early attachment step of infection, and an α2β1 integrin-binding motif is located in the N-terminal region of donkey lactamucin (29). A peptide containing 20 amino acids of the DGE and RGD motifs (pDGE RGD) exhibited significant antiviral activity. Thus, the authors propose developing anti-rotavirus peptides that block viral receptor binding. A similar effect was observed with ricin lectin, a galactose-specific lectin that competitively binds to monkey rotavirus SA11 and inhibits infection at concentrations as low as 10-9 M (30). A neutralizing effect of bovine lectins on rotavirus infectivity was also observed (31).
Oligosaccharides are abundant in breast milk and dairy products (32). Their structure is similar to that of viral receptors and can trap viruses and prevent viral adhesion to host cells (33, 34). Human rotavirus infectivity of strains Wa and DS1 in MA104 cells is inhibited by milk oligosaccharides. Laucirica DR et al. showed that the reduction in infectivity by oligosaccharides was mainly achieved through effects on the virus rather than that on the cells. The authors suggest that breastfeeding reduces rotavirus infection and propose adding specific oligosaccharides to infant formulas (15). Recently, breast milk oligosaccharides (2’-FL, scGOS/lcFOS) modulate the gut microbiota in neonatal rats and regulate cellular Toll-like receptor (TLR) gene expression, suggesting that breast milk oligosaccharides prevent rotavirus infection (35). Breast milk oligosaccharides (2’-FL, LNnT, 6’-SL, and 3’-SL) modulate the gut microbiota and stimulate the immune response to rotavirus in piglets (36). In addition, the anti-rotavirus activity of some major breast milk proteins (milk fat globule membrane, whey protein concentrate, and lactoferrin) appears to modulate the immune response in young rats (37, 38). Lactoferrin is an iron-binding protein enriched in mammalian milk and has several physiological functions (antibacterial, anti-inflammatory, immunomodulatory). It has antiviral activity against DNA- and RNA viruses including rotavirus, respiratory syncytial virus, herpesvirus, and human immunodeficiency virus (HIV) (39). The anti-rotavirus activity of lactoferrin occurs during the pre-attachment and entry phase of the virus (40, 41), and prevents the virus from entering the host cell by blocking cellular receptors or binding to viral particles (42). It is agreed that lactogenic proteins and carbohydrates are the main active components against pathogens (20, 43, 44). Subsequently, studies have shown that children who drink low-fat milk are 5 times more likely to visit a doctor for acute diarrheal disease compared to those who drink whole milk. Buttermilk MFGM containing polar lipid enrichment exhibits a greater rate of rotavirus inhibition than that of whey MFGM (45). Therefore, lipids such as sphingolipids and triglycerides may have antiviral- and antibacterial effects and prevent foodborne gastroenteritis.
The charged properties of polymers also seem to influence viral infectivity. Negatively charged mucins, heparin, acetyl heparin sulphate, alpha-1-acid glycoprotein, dextran sulphate, and glyoxylate (stevia extract) inhibit viral replication (46). These negatively charged polymers appear to interfere with the binding of VP7 and cellular receptors through spatial site-blocking. However, positively charged polymers (fisetin, fisetin sulphate, DEAE-dextran, and histones) enhance viral infection (47).
3.1.2 Drugs inhibiting virus entry into cells
After rotavirus attachment to the host cell surface, TLPs can enter the cell by different endocytic pathways or by directly penetrating the cell membrane. Although rotavirus is a non-enveloped, large hydrophilic viral particle, the virus appears to rely on the presence of a hydrophobic structural domain of VP5* after VP4 cleavage for direct cell entry after viral attachment to the cell surface (48–50). This cleavage process does not affect cell binding.
In general, viruses can undertake endocytic pathways including clathrin-mediated endocytosis, caveolae-mediated endocytosis, phagocytosis, and dynamin-dependent endocytosis (51). Using two rotavirus strains infecting monkey, RRV and SV40, Claudia Sa´nchez-San Martí et al. described that the important role of Dynamin in the entry of these two rotavirus strains in MA104 cells. However, later studies have shown that the endocytic pathway of rotaviruses appears to be strain dependent. VP4 appears to determine the endocytic pathway of rotavirus entry into MA104 cells, and the infectivity in MA104 cells was determined using a set of UK × RRV recombinant viruses with different combinations of viral structural proteins. Human rotavirus strain Wa, porcine strain TFR-41, and bovine strain UK appear to enter cells via clathrin-mediated endocytosis, while strain RRV is sensitive to cellular neuraminidase (NA) processing using integrins α2β1 and αvβ3, and heat shock cognate protein 70 (hsc70) as receptors, and is dependent on kinesin and a non-lattice protein-mediated pathway in the presence of cell surface cholesterol (52). The same requirement for hsc70, dynamin, and cholesterol was confirmed in independent experiments, although endocytoses in RRV is different from that of other strains (53).
Cholesterol in the plasma membrane is necessary for rotavirus infection. Lipid rafts are micro-regions within the plasma membrane bilayer that are rich in cholesterol and have higher lipoprotein ratios. The infectivity of PRV and RRV is significantly reduced in cells treated with methyl-β-cyclodextrin (MβCD) (54, 55). The lipid raft structure has no significant effect on virus attachment and mainly controls the entry phase of the virus. This suggests that reduction of the cholesterol content in the plasma membrane may help fight against rotavirus infection. Lipid raft structures are also known as detergent-resistant membrane domains (DRMs). Ganglioside GM1, integrin subunits α2 and β3, and hsc70 are rich in DRMs. The virus is excluded from the DRM if cells are treated with MβCD (55), suggesting that cholesterol-rich membrane lipid microdomains provide a platform for efficient attachment of rotaviruses and cellular receptors. And not all rotaviruses require integrins, but all tested rotaviruses require hsc70.
Drebrin is a cytoskeletal protein that inhibits dynamin mediated endocytosis, including the early entry steps of rotavirus, vesicular stomatitis virus (VSV), human adenovirus 5 (HAdV5), and SV40. It restricts the entry of multiple viral pathogens, including rotavirus into host cells (56). Since genetic deletion- or chemical inhibition of Drebrin increases rotavirus infection in vitro and increases diarrhoea incidence and viral shedding in vivo. The authors propose that Drebrin agonists should be developed for antiviral drugs. Clathrin is a trimer of heavy chain polypeptides (CLTC) and each heavy chain subunit binds a light chain subunit. Clathrin-mediated endocytosis is a well-characterized endocytic pathway and a major route of entry for rotaviruses other than RRV into cells. Mortalin is a member of the heat shock protein HSP70 family, and its carboxyl terminus interacts with the CLTC of clathrin (57). Mortalin induces CLTC degradation via the proteasome pathway, thereby inhibiting the clathrin-mediated endocytic pathway into host cells.
The receptor tyrosine kinase inhibitors (RTKIs) AG879 and tyrphostin A9 (A9) exhibit in vitro inhibitory activity against various RNA- and DNA viruses, including Sendai virus (Paramyxoviridae), herpes simplex virus (Herpesviridae), mouse hepatitis virus (Coronaviridae), and rhesus rotavirus (Reoviridae) (58). The receptor tyrosine kinase is an enzyme and a receptor capable of binding to a ligand and phosphorylating the tyrosine residues of the target protein. Rotavirus NSP5 is thought to have kinase activity and the replication process of rotavirus causes a series of phosphorylation changes. Genistein antiviral activity against monkey rotavirus strain SA11 (an bovine RF), bovine UK, Rhesus RV, nar3, porcine rotavirus strain YM, and human WA showed that rotavirus infectivity is strain-dependent (59). RTKIs reduce the affinity of integrins to bind rotavirus by blocking the “inside-out” signalling of integrins through the inhibition of intracellular receptor tyrosinase (60), which suggested that the inhibition of rotavirus replication by genistein may be achieved by upregulating AQP4 expression through the cAMP/PKA/CREB signalling pathway.
Protein disulphide isomerase (PDI) is an oxidoreductase present in mammalian cells that acts as a reducing agent in the cell membrane; it reduces the cell membrane-binding protein disulphide bond. DTNB [5,5’-dithiobis-(2-nitrobenzoic acid)] and bacteriocin inhibit the redox activity of PDI. Thiol/disulphide exchange is involved in the rotavirus entry process and redox reactions affect rotavirus infectivity. DTNB, bacitracin, and anti-PDI antibodies reduce the infectivity of rotavirus in MA104 cells (61). Comparison of anti-rotavirus drugs ibuprofen, NAC, and pioglitazone which all interfere with the NF-kB pathway showed that NAC was the most promising drug for the treatment of rotavirus infection in children, and the expression level of hsc70 and PDI in cells of the drug-treated group returned to the level of the uninfected virus group (62). Clinical data show good therapeutic efficacy of N-acetylcysteine for rotavirus infection (63). The Nrf2/ARE pathway is sensitive to cellular redox stress, and RA-839 is a recently identified agonist of the Nrf2/ARE pathway. RA-839 inhibits viral RNA and protein translation, while the anti-rotavirus activity of RA-839 is supported by two classical pharmacological activators of the Nrf2/ARE pathway (2-cyano-3, 12-dioxooleana-1, 9(11)-dien-28-oic acid methyl ester (CDDO-Me) and hemin) (64).
Rotaviruses use different endocytic pathways to enter cells. In most cases, these viruses enter EEs, while some viruses enter the cytoplasm from EEs to initiate zygotic replication. The pathways of intracellular transit are different for different strains, with hominin RRV and SA11 reaching the cytoplasm from MEs, while bovine UK, porcine TFR-41, and human Wa travel from MEs to LEs to the cytoplasm (59). Rab proteins are molecular switches in cellular vesicle transport; EEs and LEs are enriched in GTPase Rab5 and GTPase Rab7, respectively. Most rotavirus strains must be transported to LEs, and Rab5 inhibition blocks RRV infectivity, while Rab7 inhibition blocks BRV- UK infectivity. Most virulent strains need to enter the cytoplasm via LEs to acquire infectivity and require CD-M6PR (cation-dependent mannose-6-phosphate receptor) mediated histone protease activity; therefore, this receptor may be used as a drug target (65).
Prostaglandin E2 is an abundant eicosanoid produced from arachidonic acid during rotavirus infection and the site of production is the lipid droplet. Epoxygenase is necessary for prostaglandin (PG) biosynthesis. Indomethacin has an anti-rotavirus effect against human rotavirus Wa or simian rotavirus SA-11 in Caco-2 cells during the post-attachment phase of the viral infection cycle to reduce viral protein synthesis and does not affect viral RNA synthesis (66). Prostaglandin A1 (PGA1) may prevent SA-11 rotavirus maturation in MA-104 cells by inhibiting NSP4 glycosylation or VP4/VP7 synthesis (67). Prostaglandin E2 inhibitors indomethacin, CAY10502, celecoxib, and SC-560 affect SA11 viral internalization in vitro in early infection and VP6 RNA synthesis in the mid-stage in MA-104 cells (68). Furthermore, prostaglandin E2 replacement enhances rotavirus infection.
Multiple group A rotavirus strains including SA11 (genotype P[2]), Wa (genotype P[8]), DS-1 (genotype P[4]), and Azuk-1 (genotype P[29])-over-expressing type II transmembrane serine protease (TTSP) cells are not dependent on trypsin activation and TMPRSS2 and TMPRSS11D are potential targets (69). However, whether TTSP is involved in group A rotavirus infection in the presence of trypsin remains to be explored.
Gangliosides are glycosphingolipids composed of ceramide and contain one or more sialic acid residues. Downregulation of ganglioside expression reduces the infectivity of the four rotavirus strains tested: human Wa, simian RRV, porcine TFR-41, and bovine UK. Gangliosides did not affect early viral binding and post-entry replication (70) which contradicts previous findings (28). In contrast, a new glycolipid receptor mimic inhibits 90% of porcine rotavirus OSU strain and MA-104 cell binding. The reason may be that the virus relies on other sialic acid-containing cellular molecules, histo-blood group antigens (HBGAs), or other glycoproteins to bind to cells (71, 72). Further studies are required to determine whether gangliosides prevent viral recognition of cells to reduce viral infectivity.
3.1.3 Inhibition of genome replication
It is widely believed that trypsin-like proteases promote rotavirus replication in vivo and in vitro, and protease hydrolysis causes PDCoV (73), TuMV (74), influenza A and B viruses (75), reovirus, and rotavirus (76). The small intestine is the main site of rotavirus replication where there are high levels of protease activity. Protease inhibitor blocks virus replication when added at different times after rotavirus inoculation, inhibits VP4 activation, and prevents intercellular transmission of the virus (77).
A decrease in calcium ion concentration enhances viral infection. The removal of calcium ions from the endosome disintegrates the outer layer of TLPs and releases DLPs into the cytoplasm to activate VP1 and VP3 and trigger viral ssRNA replication and transcription (7, 78). Chelators such as EDTA and EGTA remove Ca2+ from viral particles (79); EDTA treatment disrupts the rotavirus outer capsid and hinders infectivity.
3.1.4 Inhibition of protein translation
Hsp90 inhibitors affect the formation of functionally active mature NSP3 (dimer) by blocking the direct interaction between Hsp90 and NSP3, which causes nuclear translocation of poly(A)-binding proteins and reduces viral protein translation (80).
Actinomycin D (Act D) is an antibiotic embedded in DNA that inhibits DNA-dependent transcription. It may bind to viral RNA and prevent newly synthesized mRNA from being translated; thereby inhibiting SA-11 replication in MA-104 cells (81). Neomycin B inhibits rotavirus proliferation by affecting the transcription- and replication of the viral genome. Other aminoglycoside antibiotics including levamycin, palomycin, and tobramycin have similar antiviral effects (82). Although numerous antibiotics have significant antiviral effects against rotavirus, their use should be limited due to side effects such as accelerated virus evolution, disruption of normal human flora, and damage to kidney function.
3.1.5 Drugs inhibiting intracellular viral replication
Rotaviruses use energy and raw materials from host cells and rely on their own VP1 (RdRp) to complete viral genome replication. Many broad-spectrum antiviral drugs target RdRp to inhibit viral replication by interfering with nucleotide synthesis, including nucleotide analogues (gemcitabine) (83) and nucleoside analogues (ribavirin) (84–88).
3.1.6 Drugs inhibiting viroplasm formation
The viroplasm is the main site of rotavirus genome replication and packaging. Its formation is associated with LDs and viral proteins, especially NSP2 and NSP5. After translation of viral proteins, LDs interact with NSP2 as the first step in viroplasm formation (89). Therefore, drugs that directly target LDs or viroplasm formation are a viable antiviral strategy.
Nitazoxanide is a nitrothiazole derivative of salicylamide (90) that was initially developed as an antiparasitic drug. It has broad-spectrum antiviral activity (91), and is clinically effective in the treatment of rotavirus-induced diarrhoea (92, 93). The combination of nitazoxanide and probiotics significantly reduces the duration of diarrhoea in children infected with rotavirus (94). Thiazolides interferes with viral NSP2/NSP5 interactions to affect viroplasm formation and size alteration, thereby reducing dsRNA formation (89).
Lipid droplets are organelles involved in lipid metabolism and are responsible for the storage of triacylglycerols, cholesterol, and cholesteryl esters. The relationship between LDs and viroplasm is prompted by the insertion of phosphoproteins in a cyclic form on their surfaces. β-adrenergic agonist isoproterenol and the phosphodiesterase inhibitor isobutylmethylxanthine (IBMX) promotes the degradation of LDs and catecholamines, with the hormones involved in this process playing an important role. Triacsin C is a specific inhibitor of long chain acyl coenzyme A synthetases that blocks LD formation. The above compounds prevent rotavirus infection by reducing the amount of viroplasm to inhibit dsRNA replication and mature viral particle production (95). In recent years, the importance of lipid metabolism for virus-host interactions was recognized for hepatitis C virus (HCV), dengue virus, GB virus B, flavivirus, bunyavirus, intracellular parasites Chlamydia, and SARS-CoV-2 (96, 97).
Bile acids and synthetic farnesoid X receptors (FXR) play important roles in lipid metabolism. Bile acids and synthetic FXR agonists reduce rotavirus infection by downregulating lipid synthesis in vitro and in vivo (98). In addition, statins are widely known as lipid-lowering agents that reduce total cholesterol levels by competitively inhibiting the endogenous cholesterol synthesis rate-limiting enzyme HMG-CoA reductase and blocking the intracellular hydroxymevalonate metabolic pathway. Statins may also have beneficial effects for the treatment of rotavirus infections. Rotavirus replication is significantly inhibited after statin treatment (99) or HMGCR knockdown (100). Notably, viral titres in the drug-treated group of cells decrease by 2 log values while viral mRNA remains relatively unchanged, suggesting that statins affect viral assembly. Similarly, oxysterols are cholesterol oxidation derivatives involved in cholesterol biosynthesis. 25-hydroxycholesterol (25-HC) and 27-hydroxycholesterol (27-HC) have significant antiviral activity against three non-enveloped viruses, including (human papillomavirus-16 (HPV-16), human rotavirus (HRoV), and human rhinovirus (HRhV) (101). Meanwhile, hydroxysterols from breast milk may have antiviral properties (102). Fatty acid biosynthesis is important for lipid homeostasis, and neutral fats stored in LDs are also synthesized by this pathway. Treatment with fatty acid biosynthesis inhibitors results in a 3.2-fold decrease in rotavirus titre and a 1.2-fold decrease in viral RNA level, suggesting a role for LDs in rotavirus assembly or release (103).
3.1.7 Inhibition of virus maturation
The endoplasmic reticulum is the site of viral maturation where viral precursor DLPs formed by virion assembly outgrow across the endoplasmic reticulum, briefly acquire an envelope, acquire VP4 (60 trimers) and VP7 (260 trimers), then remove the envelope to complete the assembly of mature infectious TLPs. Mature viral particles are released by cell lysis in polarized cells (such as MA-104). Meanwhile, mature viral particles are released by an unconventional vesicular transport mechanism in non-polarized cells (such as Caco-2): direct transport from the endoplasmic reticulum to the cytoplasmic membrane without passing through the Golgi complex, in a non-cleaved host cell budding manner (104).
3.2 Immunotherapy
Immunotherapy may complete the long-term control of the virus without specific antiviral therapy by relying on the patient’s own immunity. Therefore, drug development to activate the cellular immune response, modulate the inflammatory response, and further restore the adaptive immunity of the body is a therapeutic strategy (Figure 4).
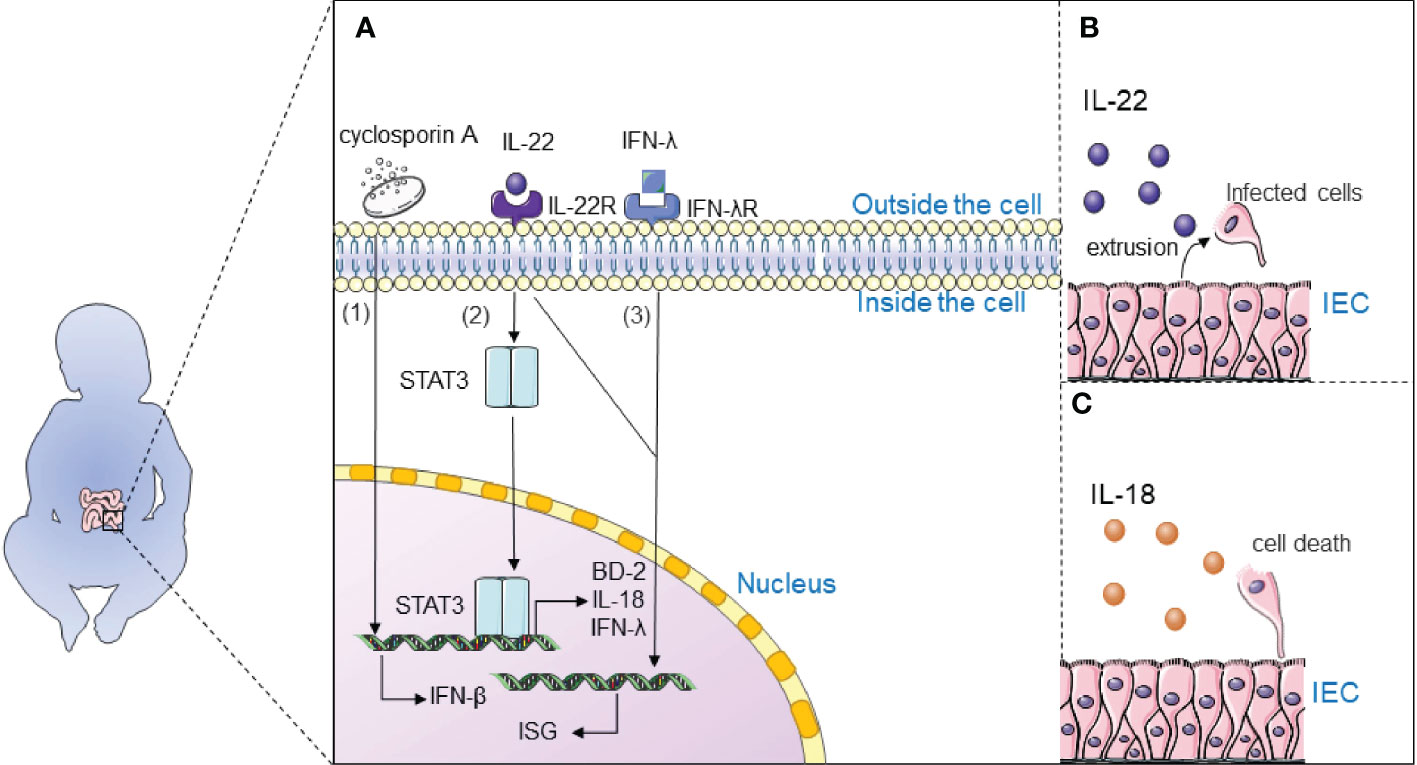
Figure 4 Immunotherapy inhibits rotavirus infection. (A) 1) Cyclosporine A may inhibit rotavirus infection by regulating the expression of IFN-β to activate the intracellular IFN1-based immune response; 2) Interleukin-22 (IL-22) inhibits rotavirus infection by activating the STAT3 signaling pathway and upregulates the expression of antimicrobial genes in the gut, including antimicrobial peptide β-defensin (BD-2), cytokine IL-18, and interferon-λ (IFN-λ); 3) Interferon-λ and IL-22 synergistically induce interferon-stimulated genes (ISG) and control rotavirus infection; (B) IL-22 extrudes cells from tissues by stimulating the proliferation of infected intestinal epithelial cells (IECs) and their migration toward the tips of the villi; (C) IL-18 promotes death of infected IECs to directly interrupt the viral replication cycle.
It is widely believed that interleukin-22 (IL-22) inhibits rotavirus infection by activating the STAT3 signaling pathway and upregulates the expression of antimicrobial genes in the gut, including antimicrobial peptide β-defensin (BD-2), cytokine IL-18, and interferon- λ (IFN-λ) (105, 106). TLR5-mediated IL-22- and NLRC4-mediated IL-18 cytokines induce the expression of each other and inhibit the replication of rotavirus. IL-22 extrudes cells from tissues by stimulating the proliferation of infected intestinal epithelial cells (IECs) and their migration toward the tips of the villi. IL-18 promotes death of infected IECs to directly interrupt the viral replication cycle. Combination therapy with IL-18 and IL-22 may be a treatment for rotavirus infection since IL-22 and IL-18 can induce expression of one another. Interestingly, IL-22 and IFN-λ have a close relationship: Il22ra1 encoding the α chain IL-22 receptor, and Ifnlr1 encoding the IFN-λR1 chain, are closely located on human and mouse chromosomes; IL-22Rα and IFN-λR1 are associated with the IL-10Rβ chain (also known as IL-10R2) to form functional heterodimeric receptor complexes. Interferon-λ and IL-22 synergistically induce interferon-stimulated genes (ISG) and control rotavirus infection (107). It is generally accepted that IFN plays an important role in antiviral defence; Type 3 III plays an important role in rotavirus infection and induces type I IFN to some extent (108). Type I IFN induces the expression of ISGs through activation of the JAK/STAT signaling pathway, which induces entry into the antiviral state. Natural human IFN-α successfully treats rotavirus infection in neonates and piglets (109).
Organ transplant patients and other immunocompromised individuals are more susceptible to rotavirus infection, and the choice of immunosuppressant is critical for their treatment. Cyclosporine is a commonly used immunosuppressive agent in clinical practice. It is proposed that cyclosporine A may inhibit rotavirus infection by regulating the expression of cytokines (especially IFN-β) to activate the intracellular IFN1-based immune response (110, 111). Mycophenolic acid (MPA) has anti-rotavirus activity as an immunosuppressant, and it inhibits IMPDH (inosine-5’-phosphate dehydrogenase) activity which can lead to guanylate depletion and inhibition of rotavirus replication (112). However, rotavirus resistance to MPA is a barrier for drug application. mTOR inhibitor rapamycin inhibits PI3K-Akt-mTOR signaling and activates autophagy which facilitates anti-rotavirus effects (113). A similar conclusion was reached in another study which identified a virus-like small RNA (RV-vsRNA1755) that inhibits PI3K-Akt-mTOR signaling and activates autophagy (114).
Evidence has shown that immunoglobulins derived from bovine colostrum, egg yolk of immunized hens, or human pooled plasma may be promising passive immunotherapy for children, but not neonates. Llama-derived heavy chain antibody fragments (VHHs), designated as ARP1 and ARP3 (ARP: anti-rotavirus protein) was shown to significantly reduce stool output in severe cases of infantile rotaviral diarrhea (115). SusanaLópez et al. found a hybridoma secreting a monoclonal antibody (MAb) (2D9) can specifically block the infectivity of wild-type RRV and its sialic acid-independent variant nar3 (116).
3.3 Intestinal function
The infection of rotavirus triggers watery diarrhea. The mechanisms for the potential drugs targeting intestine have been intensively studied (Table 2).
3.3.1 Probiotics
Probiotics are active microorganisms that exert beneficial effects by modulating the host’s intestinal flora or interacting with the host’s immune system. Growing evidence supports the idea that probiotics are effective in the prevention and treatment of rotavirus infections. The use of L. rhamnosus GG (LGG) and Saccharomyces boulardii as supplemental therapy with oral rehydration solutions is recommended by several guidelines around the world and the anti-rotavirus activity of LGG is widely studied (12, 117–119). The anti-rotavirus activity of LGG was first discovered in 1995 (120). LGG rapidly alleviates secretory diarrhoea within a few hours by inhibiting chloride secretion. Long-term LGG supplementation is thought to have a postbiotic effect through regulating intestinal flora, protecting enterocytes, and relieving osmotic diarrhoea (121). Lactobacillus acidophilus, Lactobacillus reuteri, and Bifidobacterium modulate the immune response to rotavirus infection (122–125) and appear to block rotavirus infection by directly interacting with the virus (126).
3.3.2 Suppression of intestinal secretory diarrhoea
Racecadotril inhibits intestinal secretion through selective- and reversible inhibition of enkephalinase activity. Many clinical trials have successfully used Racecadotril to treat acute gastroenteritis in children, including rotavirus gastroenteritis (127–129). It is widely believed that secretory diarrhoea is due to activation of the enteric nervous system (ENS), and rotavirus NSP4 is an enterotoxin that stimulates an increase in intracellular calcium concentration which triggers the release of amines or peptides in the gut, and further activates the enteric secretory nerves. Therefore, inhibition of the ENS may be a target for drug development. Four drugs that inhibit ENS function (tetrodotoxin, lidocaine, hexamethonium, and mecamylamine) all significantly inhibit rotavirus-induced enteric secretion (5).
Chloride (Cl-1) channel inhibitors may be an antisecretory and antimotor drug for the widespread treatment of diarrhoea. Rotavirus enterotoxin NSP4 activates transmembrane protein 16A (TMEM16A) and inhibits Na+ absorption to induce diarrhoea. TMEM16A inhibitors such as leukodendrin block TMEM16A-mediated calcium activation of Cl- currents. Plumbagin inhibits secretory diarrhoea in neonatal mice infected with rotavirus by 50% (130). Similarly, trans-δ-glucoside prevents secretory diarrhoea in mice by inhibiting TMEM16A-mediated Cl- currents (131, 132), while iOWH032 is a synthetic Cl- channel inhibitor used to treat secretory diarrhoea (133).
Serotonin secretion is Ca2+ dependent, and calcium ions, 5-hydroxytryptamine, and gastrointestinal hormone ions may interact to influence the ENS (134). 5-hydroxytryptamine (5-HT) and vasoactive intestinal peptide (VIP) are involved in rotavirus-induced gastroenteritis (135). Ondansetron is a 5-HT receptor antagonist, and the FDA states that it is safe for use in children as young as 1 month old (136). Animal studies and clinical trials have confirmed its efficacy in rotavirus gastroenteritis treatment (137, 138). In addition, clinical observations of er xie ting granules (139) and lunxieting paste (140) showed good results for rotavirus enteritis treatment in children and infants and high compliance in children.
Rotavirus infection causes intercellular signaling which manifests as intercellular calcium waves (ICW) (140). This calcium signal is caused by the release of ADP which activates the purinergic receptor P2Y1 in neighbouring cells and induces the secretion of chloride and 5-hydroxytryptamine, leading to diarrhoea and vomiting. The authors blocked intercellular calcium waves by P2Y1 antagonists or by knocking out the P2Y1 receptor gene (141). Secretory diarrhoea caused by rotavirus is induced by enterotoxin NSP4. Enterocytes are stimulated by NSP4 early in the infection to increase chloride ion and water influx into the intestinal lumen. This causes an increase in intracellular Ca2+; therefore, targeting plasma membrane Ca2+ permeability may be a target for drug development. Viral protein glycosylation and calcium channel formation is blocked using clathrin and brefeldin A to prevent the formation of infectious viral particles (142). Calmodulin (CaM) interacts with viral protein VP6 in the presence of Ca2+ to promote viral replication, and CaM inhibitor (W-7) and Ca2+ chelator (BAPTA-AM) reduces the formation of infectious viral particles.
3.3.3 Increasing intestinal barrier function
Rotavirus NSP4 causes damage to the intestinal barrier; therefore, drugs that protect intestinal cells from viral invasion should be considered. The anti-diarrheal effect of transmontane saponite is demonstrated (143). Recently, a study reported that diosmectite blocks the interaction with enterocytes by trapping SARS-CoV-2, thus preventing NF-κB activation (144). The relief of diarrhoea in rotavirus infected piglets by lentinan administration may be the result of improved intestinal microbiota and attenuation of apoptosis (145). Animal tests of Qiwei Baizhu powder confirmed that the drug reduces pathological damage such as small intestinal mucosa and villi damage and improves small intestinal absorption (146). Oral administration of transforming growth factor alpha promotes the recovery of mucosal damage induced by rotavirus enteritis in pigs (147).
3.4 Chinese medicine and natural compounds
Natural compounds are natural treasures, and many natural products with pharmaceutical activity (antiviral, anti-inflammatory and antibacterial) were developed and applied (Table 3). Shenling Baizhu powder (SBP) mainly composed of Panax ginseng C.A.Mey (Ren Shen), Wolfiporia cocos(Fu Ling), Atractylodes macrocephala Koidz(Bai Zhu), is a classical prescription medicine in Chinese medicine for the treatment of digestive tract diseases. The antiviral mechanism of SBP was investigated based on the effective curative effect of SBP on rotavirus enteritis in clinical practice (148). The protein-protein interaction (PPI) network analysis suggests that the TLR4/MyD88/NF-κB signalling pathway may be activated and SBP treatment restores the upregulation of inflammatory factors and downregulation of IFN-β due to rotavirus infection. GeGen Qinlian decoction (GGQLD) composed of Puerariae Lobatae Radix (Gegen), Scutellariae Radix (Huangqin), Coptidis Rhizoma (Huanglian), glycyrrhiza (Gancao), is the most traditional formula for diarrhoea treatment in Chinese medicine. A network pharmacology-based approach identified 130 active ingredients from GGHD and their targets were mainly related to the calcium signaling pathway, 5-HT, and gastrointestinal hormone ions which interact closely with the ENS (134). Clinical trials using GGHD in the treatment of rotavirus enteritis in children provide important insights into Chinese medicine (149). An evaluation of 34 herbs against rotavirus shows that the fruit of Citrus aurantium (golden mandarin) is the most effective, with the main active ingredients being neohesperidin (EC50 = 25 mM) and hesperidin (EC50 = 10 mM) (150).
Liquorice extracts show good results in in vivo and in vitro experiments with the main active component (18β-glycyrrhetinic acid, 18β-GA) inhibiting Fas/FasL signalling and apoptosis after rotavirus infection (CC50 = 86.92 µg/mL, EC50 = 3.14 µg/mL, SI = 27.68) (151, 152). Tissue cells have elevated chemokine expression after 18β-GA treatment which is indicative of B-cell recruitment to the intestine (153). This finding provides a rationale for oral drugs to promote intestinal lymphocyte enrichment and maintain the intestinal barrier.
Clinical data shows that tormentil root extract has good therapeutic effects on rotavirus in vivo and in vitro (154). Combination therapy of Sophora flavescens extract and stevioside produces a significant antiviral effect (155). Nordiacontanol is a major active ingredient of Sophora flavescens that inhibits viral replication by blocking the toll-like receptor 3 (TLR3)-mediated pro-inflammatory signaling pathway (156). Boraginaceae is a traditional European and American herb belonging to the poppy family that contains several alkaloids. A recent report shows that it has anti-rotavirus activity and improves intestinal inflammation by inhibiting the JAK2/STAT3 pathway (157). Resveratrol flavonoid is widely found in berries, and nuts, and possesses anti-rotavirus activity in cellular and animal experiments; 20 μM resveratrol inhibits viral replication in Caco-2 cells. Furthermore, it inhibits the expression of hsp90 mRNA and protein and blocks the early stages of the rotavirus replication cycle (158).
Vitamin D is a steroid hormone, and hydroxyvitamin D3 inhibits rotavirus replication in IPEC-J2 cells by activating the RIG-I signaling pathway, while vitamin D activates the autophagic pathway (159). Vitamin D inhibits rotavirus infection by targeting the TBK1/IRF3 signaling pathway via microRNA-155-5p, and 1-100 nM of 1α,25-dihydroxy VD3 (the hydroxylated product of vitamin D) in a dose-dependent manner (160). Garlic aqueous extract potentially inhibits PRV in MA104 cells, possibly by blocking the ERK/MAPK signaling pathway (EC50 = 0.03 mg/mL) (161, 162). The sea cucumber tegument extract (Pattalus mollis) has a significant inhibitory effect on HRV and acts before the virus enters the cells (CC50 = 27,042.10 μg/mL, IC50 = 64.29 μg/mL) (163).
Ursolic acid is a natural triterpene carboxylic acid and 10 µM of this molecule inhibits rotavirus replication at all stages in vitro; there is a significant reduction in the number and size of virplasms at 4 h of viral infection, and viral particle maturation is affected at 15 h (164). Ziyuglycoside II is a triterpene saponin extracted from the traditional Chinese medicine, Sanguisorba officinalis L.; it reverses the upregulation of inflammatory factors caused by rotavirus and inhibits the TLR4/NF-κB pathway (165).
Fruits and vegetables, medicinal plants, and herbs are the main sources of polyphenols. Gopalsamy Rajiv Gandhi et.el (166) reviewed natural compounds with potential anti-rotavirus activity, of which polyphenols were the most studied, also including phenolic acids, stilbenes, tannins, pectins, terpenoids, and flavonoid glycosides, and suggested the potential of polyphenols as future anti-rotavirus drug candidates with potential anti-rotavirus activity (166). Thirty-four out of sixty flavonoid compounds exhibit anti-rotavirus activity (SI≥ 4) (167). The antiviral activity of metformin and hesperidin is validated in vitro (EC50 = 10 mM) (168). Soy isoflavones affect all stages of viral replication, and 30 μM of genistein significantly inhibits RV infection, in the binding phase or post-binding phase (169)
Ginseng is one of the medicinal plants used to treat diarrheal diseases in traditional oriental medicine and is now used as a functional supplement which can be regularly taken to improve gastrointestinal function and prevent diarrhoea. Its main medicinal active components are saponins, polysaccharides, and flavonoids. Active ginseng polysaccharides reduce viral infection by interfering with the adhesion of viruses and host cells (170). Saponins are glycosides consisting of non-polar glycosides and varying amounts of monosaccharides, and are the main medicinal components of traditional medicinal plants such as ginseng, liquorice, and panax ginseng. The ginsenoside component of Korean red ginseng prevents diarrhoea in new-born mice (171). Roner MR (172) and Tam KI et al. (173) successively showed that Quillaja saponaria Molina extract blocks rotavirus adhesion in vitro and in vivo, and saponins appear to prevent rotavirus adhesion by coated/modified (wrapped/modified) host cell membranes. Saponins are water soluble and are approved as food additives. The authors suggest that oral administration of saponin with water is an affordable measure for rotavirus disruption. Baicalin is a flavonoid isolated from the root of Radix scutellariae. It was recently shown that the anti-rotavirus attachment and immunomodulatory effects of baicalin involve STAT3 and p-JNK-PDK1-AKT-SIK2 signalling pathways (174, 175). In addition, Alpinia katsumadai extracts strongly interact with viral hemagglutinin protein and prevent virus adsorption by hemagglutination inhibition assays (176). Tannins are macromolecular phenolic compounds that can form strong complexes with carbohydrates or proteins. The active substances in tannins acting against pathogens are divided into two main groups: proanthocyanidins and hydrolysable tannins (177). Proanthocyanidins are abundant in berries such as Vaccinium macrocarpon and Vitis labrusca; their juice has anti-rotavirus effects. Epigallocatechin gallate (EGCG) is a major component of tea polyphenols and its combination with proanthocyanidin-containing nutraceuticals shows synergy against rotavirus (178). The anti-rotavirus mechanism of these flavonoids is through binding to viral proteins (179) or mediating the aggregation of viral proteins (180).
4 Conclusion
Some progress has been made in immune protection against rotavirus. More than half of the countries in the world now introduce oral rotavirus attenuated vaccines into their national immunization programs (2); however, hundreds of thousands of rotavirus deaths continue to occur each year (181). This review focuses on recent advances in targeting different stages of the viral replication cycle, improving host immunity and gut function, and utilizing natural compounds and Chinese medicine to develop anti-rotavirus drugs. At present, oral rehydration solution, montmorillonite, probiotics, and nitazoxanide commonly used in the treatment of rotavirus enteritis in Western medicine do not achieve good results. Traditional Chinese medicine is effective in the treatment of infantile rotavirus enteritis. In recent years, there are increasing reports on natural compounds and Chinese medicine for rotavirus enteritis. Several studies (148, 151, 156, 159, 170, 171) showed that plant extracts such as ginseng and Atractylodis macrocephalae, Pueraria Mirifica, 18β-glycyrrhetinic acid, vitamin D, and Sophora flavescens extract have antiviral activity against rotavirus. Due to the anti-rotavirus effects of SBP and GHHD observed in vitro, we anticipated that SBP and GHHD would exert a protective effect against RV infection via modulation of inflammatory cytokines in the gut. In addition, SBP was first discovered in Taiping Huimin Heji Ju Fang compounded by Song Dynasty officials (1,078–1085 A.D.) and was recorded in the Pharmacopoeia of the People’s Republic of China, 2020. GHHD first recorded in the book of shanghan zabing lun (200 ~ 210 A.D.) written by Zhang Zhongjing (148, 149). These two formulas have been widely used in traditional Chinese medicine to clear away the heat and dry dampness. Clinical trials are needed to further verify their effectiveness in treating rotavirus.
Author contributions
LJ assisted with the design of the study, participated in the acquisition of data, analysis, drafted and co-wrote the manuscript. AT participated in the acquisition of data and analysis, co-wrote the manuscript. LS assisted with the design of the study, participated in the interpretation of data, drafted and co-wrote the manuscript. YT assisted with the design of the study, participated in the interpretation of data, drafted and co-wrote the manuscript. HF assisted with the design of the study, participated in the acquisition of data, analysis and the interpretation of data, drafted and co-wrote the manuscript. All authors contributed to the article and approved the submitted version.
Funding
This research was supported by National Natural Science Foundation of China (Grant No. 82202492, 82151224), National Key Research and Development Program of China (Grant No. 2020YFA0712102, 20SWAQK22 and BWS21J025), Key Project of Beijing University of Chemical Technology (Grant No. XK1803-06, XK2020-02), Fundamental Research Funds for Central Universities (Grant No. BUCTZY2022), and H&H Global Research and Technology Center (Grant No. H2021028).
Conflict of interest
The authors declare that the research was conducted in the absence of any commercial or financial relationships that could be construed as a potential conflict of interest.
Publisher’s note
All claims expressed in this article are solely those of the authors and do not necessarily represent those of their affiliated organizations, or those of the publisher, the editors and the reviewers. Any product that may be evaluated in this article, or claim that may be made by its manufacturer, is not guaranteed or endorsed by the publisher.
References
1. Hibbs BF, Miller ER, Shimabukuro T. Notes from the field: rotavirus vaccine administration errors–united states, 2006-2013. MMWR Morb Mortal Wkly Rep (2014) 63(4):81. doi: 10.15585/mmwr.mm6720a4
2. Hasso-Agopsowicz M, Ladva CN, Lopman B, Sanderson C, Cohen AL, Tate JE, et al. Global review of the age distribution of rotavirus disease in children aged <5 years before the introduction of rotavirus vaccination. Clin Infect Dis (2019) 69(6):1071–8. doi: 10.1093/cid/ciz060
3. Hong L, Song YY, Tao ZX, Xu AQ, Wang HT. Rotavirus gastroenteritis and its vaccination. Zhonghua Yu Fang Yi Xue Za Zhi. (2020) 54(7):787–92. doi: 10.3760/cma.j.cn112150-20190816-00653
4. Parashar UD, Johnson H, Steele AD, Tate JE. Health impact of rotavirus vaccination in developing countries: Progress and way forward. Clin Infect Dis (2016) 62(Suppl 2):S91–5. doi: 10.1093/cid/civ1015
5. Lundgren O, Peregrin AT, Persson K, Kordasti S, Uhnoo I, Svensson L. Role of the enteric nervous system in the fluid and electrolyte secretion of rotavirus diarrhea. Science. (2000) 287(5452):491–5. doi: 10.1126/science.287.5452.491
6. Ghosh S, Kumar M, Santiana M, Mishra A, Zhang M, Labayo H, et al. Enteric viruses replicate in salivary glands and infect through saliva. Nature. (2022) 607(7918):345–50. doi: 10.1038/s41586-022-04895-8
7. Tohmé MJ, Delgui LR. Advances in the development of antiviral compounds for rotavirus infections. mBio. (2021) 12(3):e00111-21. doi: 10.1128/mBio.00111-21
8. Flewett TH, Bryden AS, Davies H, Woode GN, Bridger JC, Derrick JM. Relation between viruses from acute gastroenteritis of children and newborn calves. Lancet. (1974) 2(7872):61–3. doi: 10.1016/S0140-6736(74)91631-6
9. Crawford SE, Mukherjee SK, Estes MK, Lawton JA, Shaw AL, Ramig RF, et al. Trypsin cleavage stabilizes the rotavirus VP4 spike. J Virol (2001) 75(13):6052–61. doi: 10.1128/JVI.75.13.6052-6061.2001
10. Trask SD, McDonald SM, Patton JT. Structural insights into the coupling of virion assembly and rotavirus replication. Nat Rev Microbiol (2012) 10(3):165–77. doi: 10.1038/nrmicro2673
11. Gouvea V, Santos N, Timenetsky Mdo C. VP4 typing of bovine and porcine group a rotaviruses by PCR. J Clin Microbiol (1994) 32(5):1333–7. doi: 10.1128/jcm.32.5.1333-1337.1994
12. Jiang Y, Ye L, Cui Y, Yang G, Yang W, Wang J, et al. Effects of lactobacillus rhamnosus GG on the maturation and differentiation of dendritic cells in rotavirus-infected mice. Benef Microbes (2017) 8(4):645–56. doi: 10.3920/BM2016.0157
13. Rogier EW, Frantz AL, Bruno ME, Wedlund L, Cohen DA, Stromberg AJ, et al. Secretory antibodies in breast milk promote long-term intestinal homeostasis by regulating the gut microbiota and host gene expression. Proc Natl Acad Sci U S A. (2014) 111(8):3074–9. doi: 10.1073/pnas.1315792111
14. Blutt SE, Miller AD, Salmon SL, Metzger DW, Conner ME. IgA is important for clearance and critical for protection from rotavirus infection. Mucosal Immunol (2012) 5(6):712–9. doi: 10.1038/mi.2012.51
15. Hester SN, Chen X, Li M, Monaco MH, Comstock SS, Kuhlenschmidt TB, et al. Human milk oligosaccharides inhibit rotavirus infectivity in vitro and in acutely infected piglets. Br J Nutr (2013) 110(7):1233–42. doi: 10.1017/S0007114513000391
17. Bui T, Li G, Kim I, Wen K, Twitchell EL, Hualei S, et al. Effects of racecadotril on weight loss and diarrhea due to human rotavirus in neonatal gnotobiotic pigs (Sus scrofa domesticus). Comp Med (2017) 67(2):157–64.
18. López S, Arias CF. Multistep entry of rotavirus into cells: a versaillesque dance. Trends Microbiol (2004) 12(6):271–8. doi: 10.1016/j.tim.2004.04.003
19. Kiefel MJ, Beisner B, Bennett S, Holmes ID, von Itzstein M. Synthesis and biological evaluation of n-acetylneuraminic acid-based rotavirus inhibitors. J Med Chem (1996) 39(6):1314–20. doi: 10.1021/jm950611f
20. Inagaki M, Muranishi H, Yamada K, Kakehi K, Uchida K, Suzuki T, et al. Bovine κ-casein inhibits human rotavirus (HRV) infection via direct binding of glycans to HRV. J Dairy Sci (2014) 97(5):2653–61. doi: 10.3168/jds.2013-7792
21. Mao X, Liu M, Tang J, Chen H, Chen D, Yu B, et al. Dietary leucine supplementation improves the mucin production in the jejunal mucosa of the weaned pigs challenged by porcine rotavirus. PloS One (2015) 10(9):e0137380. doi: 10.1371/journal.pone.0137380
22. Liu F, Li G, Wen K, Wu S, Zhang Y, Bui T, et al. Lactobacillus rhamnosus GG on rotavirus-induced injury of ileal epithelium in gnotobiotic pigs. J Pediatr Gastroenterol Nutr (2013) 57(6):750–8. doi: 10.1097/MPG.0b013e3182a356e1
23. Chen CC, Baylor M, Bass DM. Murine intestinal mucins inhibit rotavirus infection. Gastroenterology. (1993) 105(1):84–92. doi: 10.1016/0016-5085(93)90013-3
24. Yolken RH, Willoughby R, Wee SB, Miskuff R, Vonderfecht S. Sialic acid glycoproteins inhibit in vitro and in vivo replication of rotaviruses. J Clin Invest. (1987) 79(1):148–54. doi: 10.1172/JCI112775
25. Liakatos A, Kiefel MJ, Fleming F, Coulson B, von Itzstein M. The synthesis and biological evaluation of lactose-based sialylmimetics as inhibitors of rotaviral infection. Bioorg Med Chem (2006) 14(3):739–57. doi: 10.1016/j.bmc.2005.08.057
26. Takahashi K, Ohashi K, Abe Y, Mori S, Taniguchi K, Ebina T, et al. Protective efficacy of a sulfated sialyl lipid (NMSO3) against human rotavirus-induced diarrhea in a mouse model. Antimicrob Agents Chemother (2002) 46(2):420–4. doi: 10.1128/AAC.46.2.420-424.2002
27. Konishi K, Gu Y, Hatano I, Ushijima H. Effect of sulfated colominic acid on enteric virus (rotavirus, poliovirus and coxsackievirus) infections in vitro. Jpn J Infect Dis (2000) 53(2):62–6.
28. Bergner DW, Kuhlenschmidt TB, Hanafin WP, Firkins LD, Kuhlenschmidt MS. Inhibition of rotavirus infectivity by a neoglycolipid receptor mimetic. Nutrients. (2011) 3(2):228–44. doi: 10.3390/nu3020228
29. Civra A, Giuffrida MG, Donalisio M, Napolitano L, Takada Y, Coulson BS, et al. Identification of equine lactadherin-derived peptides that inhibit rotavirus infection via integrin receptor competition. J Biol Chem (2015) 290(19):12403–14. doi: 10.1074/jbc.M114.620500
30. Jolly CL, Beisner BM, Holmes IH. Rotavirus infection of MA104 cells is inhibited by ricinus lectin and separately expressed single binding domains. Virology. (2000) 275(1):89–97. doi: 10.1006/viro.2000.0470
31. Reading PC, Holmskov U, Anders EM. Antiviral activity of bovine collectins against rotaviruses. J Gen Virol (1998) 79(Pt 9):2255–63. doi: 10.1099/0022-1317-79-9-2255
32. Morozov V, Hansman G, Hanisch FG, Schroten H, Kunz C. Human milk oligosaccharides as promising antivirals. Mol Nutr Food Res (2018) 62(6):e1700679. doi: 10.1002/mnfr.201700679
33. Yolken RH, Peterson JA, Vonderfecht SL, Fouts ET, Midthun K, Newburg DS. Human milk mucin inhibits rotavirus replication and prevents experimental gastroenteritis. J Clin Invest. (1992) 90(5):1984–91. doi: 10.1172/JCI116078
34. Laucirica DR, Triantis V, Schoemaker R, Estes MK, Ramani S. Milk oligosaccharides inhibit human rotavirus infectivity in MA104 cells. J Nutr (2017) 147(9):1709–14. doi: 10.3945/jn.116.246090
35. Azagra-Boronat I, Massot-Cladera M, Knipping K, Van't Land B, Tims S, Stahl B, et al. Oligosaccharides modulate rotavirus-associated dysbiosis and TLR gene expression in neonatal rats. Cells (2019) 8(8):876. doi: 10.3390/cells8080876
36. Li M, Monaco MH, Wang M, Comstock SS, Kuhlenschmidt TB, Fahey GC, et al. Human milk oligosaccharides shorten rotavirus-induced diarrhea and modulate piglet mucosal immunity and colonic microbiota. ISME J (2014) 8(8):1609–20. doi: 10.1038/ismej.2014.10
37. Monaco MH, Gross G, Donovan SM. Whey protein lipid concentrate high in milk fat globule membrane components inhibit porcine and human rotavirus in vitro. Front Pediatr (2021) 9:731005. doi: 10.3389/fped.2021.731005
38. Pérez-Cano FJ, Marín-Gallén S, Castell M, Rodríguez-Palmero M, Rivero M, Castellote C, et al. Supplementing suckling rats with whey protein concentrate modulates the immune response and ameliorates rat rotavirus-induced diarrhea. J Nutr (2008) 138(12):2392–8. doi: 10.3945/jn.108.093856
39. van der Strate BW, Beljaars L, Molema G, Harmsen MC, Meijer DK. Antiviral activities of lactoferrin. Antiviral Res (2001) 52(3):225–39. doi: 10.1016/S0166-3542(01)00195-4
40. Superti F, Siciliano R, Rega B, Giansanti F, Valenti P, Antonini G. Involvement of bovine lactoferrin metal saturation, sialic acid and protein fragments in the inhibition of rotavirus infection. Biochim Biophys Acta (2001) 1528(2-3):107–15. doi: 10.1016/S0304-4165(01)00178-7
41. Grover M, Giouzeppos O, Schnagl RD, May JT. Effect of human milk prostaglandins and lactoferrin on respiratory syncytial virus and rotavirus. Acta Paediatr (1997) 86(3):315–6. doi: 10.1111/j.1651-2227.1997.tb08896.x
42. Superti F, Ammendolia MG, Valenti P, Seganti L. Antirotaviral activity of milk proteins: lactoferrin prevents rotavirus infection in the enterocyte-like cell line HT-29. Med Microbiol Immunol (1997) 186(2-3):83–91. doi: 10.1007/s004300050049
43. Parrón JA, Ripollés D, Pérez MD, Calvo M, Rasmussen JT, Sánchez L. Antirotaviral activity of bovine and ovine dairy byproducts. J Agric Food Chem (2017) 65(21):4280–8. doi: 10.1021/acs.jafc.7b01059
44. Bojsen A, Buesa J, Montava R, Kvistgaard AS, Kongsbak MB, Petersen TE, et al. Inhibitory activities of bovine macromolecular whey proteins on rotavirus infections in vitro and in vivo. J Dairy Sci (2007) 90(1):66–74. doi: 10.3168/jds.S0022-0302(07)72609-7
45. Fuller KL, Kuhlenschmidt TB, Kuhlenschmidt MS, Jiménez-Flores R, Donovan SM. Milk fat globule membrane isolated from buttermilk or whey cream and their lipid components inhibit infectivity of rotavirus in vitro. J Dairy Sci (2013) 96(6):3488–97. doi: 10.3168/jds.2012-6122
46. Takahashi K, Matsuda M, Ohashi K, Nakagomi O, Abe Y, Mori S, et al. Analysis of anti-rotavirus activity of extract from stevia rebaudiana. Antiviral Res (2001) 49(1):15–24. doi: 10.1016/S0166-3542(00)00134-0
47. Superti F, Marziano ML, Tinari A, Donelli G. Effect of polyions on the infectivity of SA-11 rotavirus in LCC-MK2 cells. Comp Immunol Microbiol Infect Dis (1993) 16(1):55–62. doi: 10.1016/0147-9571(93)90061-9
48. Suzuki H. Rotavirus replication: Gaps of knowledge on virus entry and morphogenesis. Tohoku J Exp Med (2019) 248(4):285–96. doi: 10.1620/tjem.248.285
49. Denisova E, Dowling W, LaMonica R, Shaw R, Scarlata S, Ruggeri F, et al. Rotavirus capsid protein VP5* permeabilizes membranes. J Virol (1999) 73(4):3147–53. doi: 10.1128/JVI.73.4.3147-3153.1999
50. Dowling W, Denisova E, LaMonica R, Mackow ER. Selective membrane permeabilization by the rotavirus VP5* protein is abrogated by mutations in an internal hydrophobic domain. J Virol (2000) 74(14):6368–76. doi: 10.1128/JVI.74.14.6368-6376.2000
51. Sánchez-San Martín C, López T, Arias CF, López S. Characterization of rotavirus cell entry. J Virol (2004) 78(5):2310–8. doi: 10.1128/JVI.78.5.2310-2318.2004
52. Díaz-Salinas MA, Romero P, Espinosa R, Hoshino Y, López S, Arias CF. The spike protein VP4 defines the endocytic pathway used by rotavirus to enter MA104 cells. J Virol (2013) 87(3):1658–63. doi: 10.1128/JVI.02086-12
53. Gutiérrez M, Isa P, Sánchez-San Martin C, Pérez-Vargas J, Espinosa R, Arias CF, et al. Different rotavirus strains enter MA104 cells through different endocytic pathways: the role of clathrin-mediated endocytosis. J Virol (2010) 84(18):9161–9. doi: 10.1128/JVI.00731-10
54. Dou X, Li Y, Han J, Zarlenga DS, Zhu W, Ren X, et al. Cholesterol of lipid rafts is a key determinant for entry and post-entry control of porcine rotavirus infection. BMC Vet Res (2018) 14(1):45. doi: 10.1186/s12917-018-1366-7
55. Isa P, Realpe M, Romero P, López S, Arias CF. Rotavirus RRV associates with lipid membrane microdomains during cell entry. Virology. (2004) 322(2):370–81. doi: 10.1016/j.virol.2004.02.018
56. Li B, Ding S, Feng N, Mooney N, Ooi YS, Ren L, et al. Drebrin restricts rotavirus entry by inhibiting dynamin-mediated endocytosis. Proc Natl Acad Sci U S A. (2017) 114(18):E3642–51. doi: 10.1073/pnas.1619266114
57. Fan B, Zhu L, Chang X, Zhou J, Guo R, Zhao Y, et al. Mortalin restricts porcine epidemic diarrhea virus entry by downregulating clathrin-mediated endocytosis. Vet Microbiol (2019) 239:108455. doi: 10.1016/j.vetmic.2019.108455
58. Kumar N, Sharma NR, Ly H, Parslow TG, Liang Y. Receptor tyrosine kinase inhibitors that block replication of influenza a and other viruses. Antimicrob Agents Chemother (2011) 55(12):5553–9. doi: 10.1128/AAC.00725-11
59. López T, López S, Arias CF. The tyrosine kinase inhibitor genistein induces the detachment of rotavirus particles from the cell surface. Virus Res (2015) 210:141–8. doi: 10.1016/j.virusres.2015.07.020
60. Huang H, Liao D, Liang L, Song L, Zhao W. Genistein inhibits rotavirus replication and upregulates AQP4 expression in rotavirus-infected caco-2 cells. Arch Virol (2015) 160(6):1421–33. doi: 10.1007/s00705-015-2404-4
61. Calderon MN, Guerrero CA, Acosta O, Lopez S, Arias CF. Inhibiting rotavirus infection by membrane-impermeant thiol/disulfide exchange blockers and antibodies against protein disulfide isomerase. Intervirology. (2012) 55(6):451–64. doi: 10.1159/000335262
62. Guerrero CA, Murillo A, Acosta O. Inhibition of rotavirus infection in cultured cells by n-acetyl-cysteine, PPARγ agonists and NSAIDs. Antiviral Res (2012) 96(1):1–12. doi: 10.1016/j.antiviral.2012.06.011
63. Guerrero CA, Torres DP, García LL, Guerrero RA, Acosta O. N-acetylcysteine treatment of rotavirus-associated diarrhea in children. Pharmacotherapy. (2014) 34(11):e333–40. doi: 10.1002/phar.1489
64. Patra U, Mukhopadhyay U, Sarkar R, Mukherjee A, Chawla-Sarkar M. RA-839, a selective agonist of Nrf2/ARE pathway, exerts potent anti-rotaviral efficacy in vitro. Antiviral Res (2019) 161:53–62. doi: 10.1016/j.antiviral.2018.11.009
65. Díaz-Salinas MA, Silva-Ayala D, López S, Arias CF. Rotaviruses reach late endosomes and require the cation-dependent mannose-6-phosphate receptor and the activity of cathepsin proteases to enter the cell. J Virol (2014) 88(8):4389–402. doi: 10.1128/JVI.03457-13
66. Rossen JW, Bouma J, Raatgeep RH, Büller HA, Einerhand AW. Inhibition of cyclooxygenase activity reduces rotavirus infection at a postbinding step. J Virol (2004) 78(18):9721–30. doi: 10.1128/JVI.78.18.9721-9730.2004
67. Superti F, Amici C, Tinari A, Donelli G, Santoro MG. Inhibition of rotavirus replication by prostaglandin a: evidence for a block of virus maturation. J Infect Dis (1998) 178(2):564–8. doi: 10.1086/517475
68. Sander WJ, Kemp G, Hugo A, Pohl CH, Neill HG. Rotavirus-mediated prostaglandin E(2) production in MA104 cells promotes virus attachment and internalisation, resulting in an increased viral load. Front Physiol (2022) 13:805565. doi: 10.3389/fphys.2022.805565
69. Sasaki M, Itakura Y, Kishimoto M, Tabata K, Uemura K, Ito N, et al. Host serine proteases TMPRSS2 and TMPRSS11D mediate proteolytic activation and trypsin-independent infection in group a rotaviruses. J Virol (2021) 95(11). doi: 10.1128/JVI.00398-21
70. Martínez MA, López S, Arias CF, Isa P. Gangliosides have a functional role during rotavirus cell entry. J Virol (2013) 87(2):1115–22. doi: 10.1128/JVI.01964-12
71. Sun X, Li D, Duan Z. Structural basis of glycan recognition of rotavirus. Front Mol Biosci (2021) 8:658029. doi: 10.3389/fmolb.2021.658029
72. Hu L, Crawford SE, Czako R, Cortes-Penfield NW, Smith DF, Le Pendu J, et al. Cell attachment protein VP8* of a human rotavirus specifically interacts with a-type histo-blood group antigen. Nature. (2012) 485(7397):256–9. doi: 10.1038/nature10996
73. Yang YL, Meng F, Qin P, Herrler G, Huang YW, Tang YD. Trypsin promotes porcine deltacoronavirus mediating cell-to-cell fusion in a cell type-dependent manner. Emerg Microbes Infect (2020) 9(1):457–68. doi: 10.1080/22221751.2020.1730245
74. Thompson S, Fraser RS, Barnden KL. A beneficial effect of trypsin on the purification of turnip mosaic virus (TuMV) and other potyviruses. J Virol Methods (1988) 20(1):57–64. doi: 10.1016/0166-0934(88)90040-7
75. Daikoku T, Okuda T, Kawai M, Morita N, Tanaka T, Takemoto M, et al. Growth activation of influenza virus by trypsin and effect of T-705 (favipiravir) on trypsin-optimized growth condition. Acta Virol (2019) 63(3):309–15. doi: 10.4149/av_2019_311
76. Clark SM, Roth JR, Clark ML, Barnett BB, Spendlove RS. Trypsin enhancement of rotavirus infectivity: mechanism of enhancement. J Virol (1981) 39(3):816–22. doi: 10.1128/jvi.39.3.816-822.1981
77. Vonderfecht SL, Miskuff RL, Wee SB, Sato S, Tidwell RR, Geratz JD, et al. Protease inhibitors suppress the in vitro and in vivo replication of rotavirus. J Clin Invest. (1988) 82(6):2011–6. doi: 10.1172/JCI113821
78. Bednarz-Prashad AJ, John EI. Effect of clioquinol, an 8-hydroxyquinoline derivative, on rotavirus infection in mice. J Infect Dis (1983) 148(3):613. doi: 10.1093/infdis/148.3.613
79. Dormitzer PR, Greenberg HB. Calcium chelation induces a conformational change in recombinant herpes simplex virus-1-expressed rotavirus VP7. Virology. (1992) 189(2):828–32. doi: 10.1016/0042-6822(92)90616-W
80. Dutta D, Chattopadhyay S, Bagchi P, Halder UC, Nandi S, Mukherjee A, et al. Active participation of cellular chaperone Hsp90 in regulating the function of rotavirus nonstructural protein 3 (NSP3). J Biol Chem (2011) 286(22):20065–77. doi: 10.1074/jbc.M111.231878
81. Stefanelli CC, Castilho JG, Botelho MV, Linhares RE, Nozawa CM. Effect of actinomycin d on simian rotavirus (SA11) replication in cell culture. Braz J Med Biol Res (2002) 35(4):445–9. doi: 10.1590/S0100-879X2002000400006
82. Manchego A, Spencer E. Effect of neomycin b on rotavirus plus- and minus-strand RNA synthesis. Arch Virol (2003) 148(6):1071–84. doi: 10.1007/s00705-003-0004-1
83. Chen S, Wang Y, Li P, Yin Y, Bijvelds MJ, de Jonge HR, et al. Drug screening identifies gemcitabine inhibiting rotavirus through alteration of pyrimidine nucleotide synthesis pathway. Antiviral Res (2020) 180:104823. doi: 10.1016/j.antiviral.2020.104823
84. Kitaoka S, Konno T, De Clercq E. Comparative efficacy of broad-spectrum antiviral agents as inhibitors of rotavirus replication in vitro. Antiviral Res (1986) 6(1):57–65. doi: 10.1016/0166-3542(86)90039-2
85. Van Dycke J, Arnoldi F, Papa G, Vandepoele J, Burrone OR, Mastrangelo E, et al. A single nucleoside viral polymerase inhibitor against norovirus, rotavirus, and sapovirus-induced diarrhea. J Infect Dis (2018) 218(11):1753–8. doi: 10.1093/infdis/jiy398
86. Tsoukala E, Agelis G, Dolinsek J, Botić T, Cencic A, Komiotis D. An efficient synthesis of 3-fluoro-5-thio-xylofuranosyl nucleosides of thymine, uracil, and 5-fluorouracil as potential antitumor or/and antiviral agents. Bioorg Med Chem (2007) 15(9):3241–7. doi: 10.1016/j.bmc.2007.02.031
87. Manta S, Agelis G, Botić T, Cencic A, Komiotis D. Fluoro-ketopyranosyl nucleosides: synthesis and biological evaluation of 3-fluoro-2-keto-beta-D-glucopyranosyl derivatives of N4-benzoyl cytosine. Bioorg Med Chem (2007) 15(2):980–7. doi: 10.1016/j.bmc.2006.10.033
88. Agelis G, Tzioumaki N, Botić T, Cencic A, Komiotis D. Exomethylene pyranonucleosides: efficient synthesis and biological evaluation of 1-(2,3,4-trideoxy-2-methylene-beta-d-glycero-hex-3-enopyranosyl)thymine. Bioorg Med Chem (2007) 15(16):5448–56. doi: 10.1016/j.bmc.2007.05.055
89. La Frazia S, Ciucci A, Arnoldi F, Coira M, Gianferretti P, Angelini M, et al. Thiazolides, a new class of antiviral agents effective against rotavirus infection, target viral morphogenesis, inhibiting viroplasm formation. J Virol (2013) 87(20):11096–106. doi: 10.1128/JVI.01213-13
90. Krátký M, Vinšová J. Antiviral activity of substituted salicylanilides–a review. Mini Rev Med Chem (2011) 11(11):956–67. doi: 10.2174/138955711797068382
91. Rossignol JF. Nitazoxanide: a first-in-class broad-spectrum antiviral agent. Antiviral Res (2014) 110:94–103. doi: 10.1016/j.antiviral.2014.07.014
92. Rossignol JF, Abu-Zekry M, Hussein A, Santoro MG. Effect of nitazoxanide for treatment of severe rotavirus diarrhoea: randomised double-blind placebo-controlled trial. Lancet. (2006) 368(9530):124–9. doi: 10.1016/S0140-6736(06)68852-1
93. Rossignol JF, El-Gohary YM. Nitazoxanide in the treatment of viral gastroenteritis: a randomized double-blind placebo-controlled clinical trial. Aliment Pharmacol Ther (2006) 24(10):1423–30. doi: 10.1111/j.1365-2036.2006.03128.x
94. Teran CG, Teran-Escalera CN, Villarroel P. Nitazoxanide vs. probiotics for the treatment of acute rotavirus diarrhea in children: a randomized, single-blind, controlled trial in Bolivian children. Int J Infect Dis (2009) 13(4):518–23. doi: 10.1016/j.ijid.2008.09.014
95. Cheung W, Gill M, Esposito A, Kaminski CF, Courousse N, Chwetzoff S, et al. Rotaviruses associate with cellular lipid droplet components to replicate in viroplasms, and compounds disrupting or blocking lipid droplets inhibit viroplasm formation and viral replication. J Virol (2010) 84(13):6782–98. doi: 10.1128/JVI.01757-09
96. Lever A, Desselberger U. Rotavirus replication and the role of cellular lipid droplets: New therapeutic targets. J Formos Med Assoc (2016) 115(6):389–94. doi: 10.1016/j.jfma.2016.02.004
97. Kim TY, Jeon S, Jang Y, Gotina L, Won J, Ju YH, et al. A natural component of platycodon grandiflorum, prevents both lysosome- and TMPRSS2-driven SARS-CoV-2 infection by hindering membrane fusion. Exp Mol Med (2021) 53(5):956–72. doi: 10.1038/s12276-021-00624-9
98. Kim Y, Chang KO. Inhibitory effects of bile acids and synthetic farnesoid X receptor agonists on rotavirus replication. J Virol (2011) 85(23):12570–7. doi: 10.1128/JVI.05839-11
99. Ding S, Yu B, van Vuuren AJ. Statins significantly repress rotavirus replication through downregulation of cholesterol synthesis. Gut Microbes (2021) 13(1):1955643. doi: 10.1080/19490976.2021.1955643
100. Mohan KV, Muller J, Atreya CD. Defective rotavirus particle assembly in lovastatin-treated MA104 cells. Arch Virol (2008) 153(12):2283–90. doi: 10.1007/s00705-008-0261-0
101. Civra A, Cagno V, Donalisio M, Biasi F, Leonarduzzi G, Poli G, et al. Inhibition of pathogenic non-enveloped viruses by 25-hydroxycholesterol and 27-hydroxycholesterol. Sci Rep (2014) 4:7487. doi: 10.1038/srep07487
102. Civra A, Leoni V, Caccia C, Sottemano S, Tonetto P, Coscia A, et al. Antiviral oxysterols are present in human milk at diverse stages of lactation. J Steroid Biochem Mol Biol (2019) 193:105424. doi: 10.1016/j.jsbmb.2019.105424
103. Gaunt ER, Cheung W, Richards JE, Lever A, Desselberger U. Inhibition of rotavirus replication by downregulation of fatty acid synthesis. J Gen Virol (2013) 94(Pt 6):1310–7. doi: 10.1099/vir.0.050146-0
104. Jourdan N, Maurice M, Delautier D, Quero AM, Servin AL, Trugnan G. Rotavirus is released from the apical surface of cultured human intestinal cells through nonconventional vesicular transport that bypasses the golgi apparatus. J Virol (1997) 71(11):8268–78. doi: 10.1128/jvi.71.11.8268-8278.1997
105. Schnepf D, Hernandez P, Mahlakõiv T, Crotta S, Sullender ME, Peterson ST, et al. Rotavirus susceptibility of antibiotic-treated mice ascribed to diminished expression of interleukin-22. PloS One (2021) 16(8):e0247738. doi: 10.1371/journal.pone.0247738
106. Xue M, Zhao J, Ying L, Fu F, Li L, Ma Y, et al. IL-22 suppresses the infection of porcine enteric coronaviruses and rotavirus by activating STAT3 signal pathway. Antiviral Res (2017) 142:68–75. doi: 10.1016/j.antiviral.2017.03.006
107. Hernández PP, Mahlakoiv T, Yang I, Schwierzeck V, Nguyen N, Guendel F, et al. Interferon-λ and interleukin 22 act synergistically for the induction of interferon-stimulated genes and control of rotavirus infection. Nat Immunol (2015) 16(7):698–707. doi: 10.1038/ni.3180
108. Feng N, Kim B, Fenaux M, Nguyen H, Vo P, Omary MB, et al. Role of interferon in homologous and heterologous rotavirus infection in the intestines and extraintestinal organs of suckling mice. J Virol (2008) 82(15):7578–90. doi: 10.1128/JVI.00391-08
109. Lecce JG, Cummins JM, Richards AB. Treatment of rotavirus infection in neonate and weanling pigs using natural human interferon alpha. Mol Biother. (1990) 2(4):211–6.
110. Shen Z, He H, Wu Y, Li J. Cyclosporin a inhibits rotavirus replication and restores interferon-beta signaling pathway in vitro and in vivo. PloS One (2013) 8(8):e71815. doi: 10.1371/journal.pone.0071815
111. Shen Z, Tian Z, He H, Zhang J, Li J, Wu Y. Antiviral effects of cyclosporine a in neonatal mice with rotavirus-induced diarrhea. J Pediatr Gastroenterol Nutr (2015) 60(1):11–7. doi: 10.1097/MPG.0000000000000493
112. Yin Y, Wang Y, Dang W, Xu L, Su J, Zhou X, et al. Mycophenolic acid potently inhibits rotavirus infection with a high barrier to resistance development. Antiviral Res (2016) 133:41–9. doi: 10.1016/j.antiviral.2016.07.017
113. Yin Y, Dang W, Zhou X, Xu L, Wang W, Cao W, et al. PI3K-Akt-mTOR axis sustains rotavirus infection via the 4E-BP1 mediated autophagy pathway and represents an antiviral target. Virulence. (2018) 9(1):83–98. doi: 10.1080/21505594.2017.1326443
114. Zhou Y, Geng P, Liu Y, Wu J, Qiao H, Xie Y, et al. Rotavirus-encoded virus-like small RNA triggers autophagy by targeting IGF1R via the PI3K/Akt/mTOR pathway. Biochim Biophys Acta Mol Basis Dis (2018) 1864(1):60–8. doi: 10.1016/j.bbadis.2017.09.028
115. Ghosh S, Malik YS, Kobayashi N. Therapeutics and immunoprophylaxis against noroviruses and rotaviruses: The past, present, and future. Curr Drug Metab (2018) 19(3):170–91. doi: 10.2174/1389200218666170912161449
116. Lanata CF, Franco M. Nitazoxanide for rotavirus diarrhoea. Lancet. (2006) 368(9530):100–1. doi: 10.1016/S0140-6736(06)68981-2
117. Santosa S, Farnworth E, Jones PJ. Probiotics and their potential health claims. Nutr Rev (2006) 64(6):265–74. doi: 10.1111/j.1753-4887.2006.tb00209.x
118. Marteau PR, de Vrese M, Cellier CJ, Schrezenmeir J. Protection from gastrointestinal diseases with the use of probiotics. Am J Clin Nutr (2001) 73(2 Suppl):430S–6S. doi: 10.1093/ajcn/73.2.430s
119. Szymański H, Chmielarczyk A, Strus M, Pejcz J, Jawień M, Kochan P, et al. Colonisation of the gastrointestinal tract by probiotic l. rhamnosus strains in acute diarrhoea in children. Dig Liver Dis (2006) 38(Suppl 2):S274–6. doi: 10.1016/S1590-8658(07)60009-7
120. Majamaa H, Isolauri E, Saxelin M, Vesikari T. Lactic acid bacteria in the treatment of acute rotavirus gastroenteritis. J Pediatr Gastroenterol Nutr (1995) 20(3):333–8. doi: 10.1097/00005176-199504000-00012
121. Buccigrossi V, Poeta M, Cioffi V, Terranova S, Nunziata F, Lo Vecchio A, et al. Lacticaseibacillus rhamnosus GG counteracts rotavirus-induced ion secretion and enterocyte damage by inhibiting oxidative stress and apoptosis through specific effects of living and postbiotic preparations. Front Cell Infect Microbiol (2022) 12:854989. doi: 10.3389/fcimb.2022.854989
122. Qiao H, Duffy LC, Griffiths E, Dryja D, Leavens A, Rossman J, et al. Immune responses in rhesus rotavirus-challenged BALB/c mice treated with bifidobacteria and prebiotic supplements. Pediatr Res (2002) 51(6):750–5. doi: 10.1203/00006450-200206000-00015
123. Gagnon M, Vimont A, Darveau A, Fliss I, Jean J. Study of the ability of bifidobacteria of human origin to prevent and treat rotavirus infection using colonic cell and mouse models. PloS One (2016) 11(10):e0164512. doi: 10.1371/journal.pone.0164512
124. Kawahara T, Makizaki Y, Oikawa Y, Tanaka Y, Maeda A, Shimakawa M, et al. Oral administration of bifidobacterium bifidum G9-1 alleviates rotavirus gastroenteritis through regulation of intestinal homeostasis by inducing mucosal protective factors. PloS One (2017) 12(3):e0173979. doi: 10.1371/journal.pone.0173979
125. Shu Q, Qu F, Gill HS. Probiotic treatment using bifidobacterium lactis HN019 reduces weanling diarrhea associated with rotavirus and escherichia coli infection in a piglet model. J Pediatr Gastroenterol Nutr (2001) 33(2):171–7. doi: 10.1097/00005176-200108000-00014
126. Fernandez-Duarte KP, Olaya-Galán NN, Salas-Cárdenas SP, Lopez-Rozo J, Gutierrez-Fernandez MF. Bifidobacterium adolescentis (DSM 20083) and lactobacillus casei (Lafti L26-DSL): Probiotics able to block the In vitro adherence of rotavirus in MA104 cells. Probiotics Antimicrob Proteins. (2018) 10(1):56–63. doi: 10.1007/s12602-017-9277-7
127. Salazar-Lindo E, Santisteban-Ponce J, Chea-Woo E, Gutierrez M. Racecadotril in the treatment of acute watery diarrhea in children. N Engl J Med (2000) 343(7):463–7. doi: 10.1056/NEJM200008173430703
128. Lehert P, Chéron G, Calatayud GA, Cézard JP, Castrellón PG, Garcia JM, et al. Racecadotril for childhood gastroenteritis: an individual patient data meta-analysis. Dig Liver Dis (2011) 43(9):707–13. doi: 10.1016/j.dld.2011.03.001
129. Kang G, Thuppal SV, Srinivasan R, Sarkar R, Subashini B, Venugopal S, et al. Racecadotril in the management of rotavirus and non-rotavirus diarrhea in under-five children: Two randomized, double-blind, placebo-controlled trials. Indian Pediatr (2016) 53(7):595–600. doi: 10.1007/s13312-016-0894-0
130. Yu B, Zhu X, Yang X, Jin L, Xu J, Ma T, et al. Plumbagin prevents secretory diarrhea by inhibiting CaCC and CFTR channel activities. Front Pharmacol (2019) 10:1181. doi: 10.3389/fphar.2019.01181
131. Yu B, Jiang Y, Zhang B, Yang H, Ma T. Resveratrol dimer trans-ϵ-viniferin prevents rotaviral diarrhea in mice by inhibition of the intestinal calcium-activated chloride channel. Pharmacol Res (2018) 129:453–61. doi: 10.1016/j.phrs.2017.11.016
132. Yu B, Xie R, Jin L, Tian X, Niu Y, Ma T, et al. Trans-δ-Viniferin inhibits Ca(2+)-activated cl(-) channels and improves diarrhea symptoms. Fitoterapia. (2019) 139:104367. doi: 10.1016/j.fitote.2019.104367
133. de Hostos EL, Choy RK, Nguyen T. Developing novel antisecretory drugs to treat infectious diarrhea. Future Med Chem (2011) 3(10):1317–25. doi: 10.4155/fmc.11.87
134. Zhong P, Song L, Gao M, Wang X, Tan W, Lu H, et al. Network pharmacology-based strategy for predicting active ingredients and potential targets of gegen qinlian decoction for rotavirus enteritis. Evid Based Complement Alternat Med (2020) 2020:2957567. doi: 10.1155/2020/2957567
135. Kordasti S, Sjövall H, Lundgren O, Svensson L. Serotonin and vasoactive intestinal peptide antagonists attenuate rotavirus diarrhoea. Gut. (2004) 53(7):952–7. doi: 10.1136/gut.2003.033563
136. Hagbom M, Hellysaz A, Istrate C, Nordgren J, Sharma S, de-Faria FM, et al. The 5-HT(3) receptor affects rotavirus-induced motility. J Virol (2021) 95(15):e0075121. doi: 10.1128/JVI.00751-21
137. Freedman SB, Hall M, Shah SS, Kharbanda AB, Aronson PL, Florin TA, et al. Impact of increasing ondansetron use on clinical outcomes in children with gastroenteritis. JAMA Pediatr (2014) 168(4):321–9. doi: 10.1001/jamapediatrics.2013.4906
138. Bialowas S, Hagbom M, Nordgren J, Karlsson T, Sharma S, Magnusson KE, et al. Rotavirus and serotonin cross-talk in diarrhoea. PloS One (2016) 11(7):e0159660. doi: 10.1371/journal.pone.0159660
139. Lou JG, Zeng YM, Xu JY, Wang XQ, You JY, Dong C, et al. A multicenter randomized parallel-controlled study on the efficacy and safety of er xie ting granules in children with acute diarrhea. Zhonghua Er Ke Za Zhi (2013) 51(9):670–5.
140. Wu Y, Chen WQ, Jin Y. Clinical observation on treatment of infantile rotavirus enteritis by umbilical application of lunxieting paste. Zhongguo Zhong Xi Yi Jie He Za Zhi. (2010) 30(12):1275–8.
141. Chang-Graham AL, Perry JL, Engevik MA, Engevik KA, Scribano FJ, Gebert JT, et al. Rotavirus induces intercellular calcium waves through ADP signaling. Science (2020) 370(6519). doi: 10.1126/science.abc3621
142. Ruiz MC, Díaz Y, Peña F, Aristimuño OC, Chemello ME, Michelangeli F. Ca2+ permeability of the plasma membrane induced by rotavirus infection in cultured cells is inhibited by tunicamycin and brefeldin a. Virology. (2005) 333(1):54–65. doi: 10.1016/j.virol.2004.12.032
143. Dupont C, Foo JL, Garnier P, Moore N, Mathiex-Fortunet H, Salazar-Lindo E. Oral diosmectite reduces stool output and diarrhea duration in children with acute watery diarrhea. Clin Gastroenterol Hepatol (2009) 7(4):456–62. doi: 10.1016/j.cgh.2008.12.007
144. Poeta M, Cioffi V, Buccigrossi V, Nanayakkara M, Baggieri M, Peltrini R, et al. Diosmectite inhibits the interaction between SARS-CoV-2 and human enterocytes by trapping viral particles, thereby preventing NF-kappaB activation and CXCL10 secretion. Sci Rep (2021) 11(1):21725. doi: 10.1038/s41598-021-01217-2
145. Mao X, Hu H, Xiao X, Chen D, Yu B, He J, et al. Lentinan administration relieves gut barrier dysfunction induced by rotavirus in a weaned piglet model. Food Funct (2019) 10(4):2094–101. doi: 10.1039/C8FO01764F
146. He ST, He FZ, Wu CR, Li SX, Liu W, Yang YF, et al. Treatment of rotaviral gastroenteritis with qiwei baizhu powder. World J Gastroenterol (2001) 7(5):735–40. doi: 10.3748/wjg.v7.i5.735
147. Rhoads JM, Ulshen MH, Keku EO, Chen W, Kandil HM, Woodard JP, et al. Oral transforming growth factor-alpha enhances jejunal mucosal recovery and electrical resistance in piglet rotavirus enteritis. Pediatr Res (1995) 38(2):173–81. doi: 10.1203/00006450-199508000-00007
148. Wang X, Yang Q, Zhou X, Chen T, Dou L, Wang F, et al. Shenling baizhu powder inhibits RV-SA11-Induced inflammation and rotavirus enteritis via TLR4/MyD88/NF-κB signaling pathway. Front Pharmacol (2021) 12:642685. doi: 10.3389/fphar.2021.642685
149. Wu Y, Tu X, Liang X, Chen J, Wan X, Zhang T, et al. Gegen huangqin huanglian decoction for children rotavirus enteritis: A protocol for systematic review and meta-analysis. Med (Baltimore). (2020) 99(49):e23376. doi: 10.1097/MD.0000000000023376
150. Kim DH, Song MJ, Bae EA, Han MJ. Inhibitory effect of herbal medicines on rotavirus infectivity. Biol Pharm Bull (2000) 23(3):356–8. doi: 10.1248/bpb.23.356
151. Alfajaro MM, Kim HJ, Park JG, Ryu EH, Kim JY, Jeong YJ, et al. Anti-rotaviral effects of glycyrrhiza uralensis extract in piglets with rotavirus diarrhea. Virol J (2012) 9:310. doi: 10.1186/1743-422X-9-310
152. Kwon HJ, Kim HH, Ryu YB, Kim JH, Jeong HJ, Lee SW, et al. In vitro anti-rotavirus activity of polyphenol compounds isolated from the roots of glycyrrhiza uralensis. Bioorg Med Chem (2010) 18(21):7668–74. doi: 10.1016/j.bmc.2010.07.073
153. Hendricks JM, Hoffman C, Pascual DW, Hardy ME. 18β-glycyrrhetinic acid delivered orally induces isolated lymphoid follicle maturation at the intestinal mucosa and attenuates rotavirus shedding. PloS One (2012) 7(11):e49491. doi: 10.1371/journal.pone.0049491
154. Subbotina MD, Timchenko VN, Vorobyov MM, Konunova YS, Aleksandrovih YS, Shushunov S. Effect of oral administration of tormentil root extract (Potentilla tormentilla) on rotavirus diarrhea in children: a randomized, double blind, controlled trial. Pediatr Infect Dis J (2003) 22(8):706–11. doi: 10.1097/01.inf.0000078355.29647.d0
155. Alfajaro MM, Rho MC, Kim HJ, Park JG, Kim DS, Hosmillo M, et al. Anti-rotavirus effects by combination therapy of stevioside and sophora flavescens extract. Res Vet Sci (2014) 96(3):567–75. doi: 10.1016/j.rvsc.2014.03.011
156. Oh HM, Lee SW, Park MH, Kim MH, Ryu YB, Kim MS, et al. Norkurarinol inhibits toll-like receptor 3 (TLR3)-mediated pro-inflammatory signaling pathway and rotavirus replication. J Pharmacol Sci (2012) 118(2):161–70. doi: 10.1254/jphs.11077FP
157. Jiang C, Yang H, Chen X, Qiu S, Wu C, Zhang B, et al. Macleaya cordata extracts exert antiviral effects in newborn mice with rotavirus-induced diarrhea via inhibiting the JAK2/STAT3 signaling pathway. Exp Ther Med (2020) 20(2):1137–44. doi: 10.3892/etm.2020.8766
158. Huang H, Liao D, Zhou G, Zhu Z, Cui Y, Pu R. Antiviral activities of resveratrol against rotavirus in vitro and in vivo. Phytomedicine (2020) 77:153230. doi: 10.1016/j.phymed.2020.153230
159. Zhao Y, Yu B, Mao X, He J, Huang Z, Zheng P, et al. Effect of 25-hydroxyvitamin D3 on rotavirus replication and gene expressions of RIG-I signalling molecule in porcine rotavirus-infected IPEC-J2 cells. Arch Anim Nutr (2015) 69(3):227–35. doi: 10.1080/1745039X.2015.1034522
160. Zhao Y, Ran Z, Jiang Q, Hu N, Yu B, Zhu L, et al. Vitamin d alleviates rotavirus infection through a microrna-155-5p mediated regulation of the TBK1/IRF3 signaling pathway in vivo and In vitro. Int J Mol Sci (2019) 20(14):3562. doi: 10.3390/ijms20143562
161. Rouf R, Uddin SJ, Sarker DK, Islam MT, Ali ES, Shilpi JA, et al. Antiviral potential of garlic (Allium sativum) and its organosulfur compounds: A systematic update of pre-clinical and clinical data. Trends Food Sci Technol (2020) 104:219–34. doi: 10.1016/j.tifs.2020.08.006
162. Jafri M, Donnelly B, McNeal M, Ward R, Tiao G. MAPK signaling contributes to rotaviral-induced cholangiocyte injury and viral replication. Surgery. (2007) 142(2):192–201. doi: 10.1016/j.surg.2007.03.008
163. Garcia-Candela E, Pariona-Velarde C, Mondragón-Martínez A, Chumpitaz-Cerrate V. Antiviral activity of the sea cucumber tegument extract (Pattalus mollis) on human rotavirus a (RVA). Nat Prod Res (2021) 35(6):1014–8. doi: 10.1080/14786419.2019.1611807
164. Tohmé MJ, Giménez MC, Peralta A, Colombo MI, Delgui LR. Ursolic acid: A novel antiviral compound inhibiting rotavirus infection in vitro. Int J Antimicrob Agents (2019) 54(5):601–9. doi: 10.1016/j.ijantimicag.2019.07.015
165. Chen X, Liu L, Chen W, Qin F, Zhou F, Yang H. Ziyuglycoside II inhibits rotavirus induced diarrhea possibly via TLR4/NF-κB pathways. Biol Pharm Bull (2020) 43(6):932–7. doi: 10.1248/bpb.b19-00771
166. Gandhi GR, Barreto PG, Lima BD, Quintans JS, Araújo AA, Narain N, et al. Medicinal plants and natural molecules with in vitro and in vivo activity against rotavirus: A systematic review. Phytomedicine. (2016) 23(14):1830–42. doi: 10.1016/j.phymed.2016.11.005
167. Savi LA, Caon T, de Oliveira AP, Sobottka AM, Werner W, Reginatto FH, et al. Evaluation of antirotavirus activity of flavonoids. Fitoterapia. (2010) 81(8):1142–6. doi: 10.1016/j.fitote.2010.07.017
168. Bae EA, Han MJ, Lee M, Kim DH. In vitro inhibitory effect of some flavonoids on rotavirus infectivity. Biol Pharm Bull (2000) 23(9):1122–4. doi: 10.1248/bpb.23.1122
169. Andres A, Donovan SM, Kuhlenschmidt TB, Kuhlenschmidt MS. Isoflavones at concentrations present in soy infant formula inhibit rotavirus infection in vitro. J Nutr (2007) 137(9):2068–73. doi: 10.1093/jn/137.9.2068
170. Baek SH, Lee JG, Park SY, Bae ON, Kim DH, Park JH. Pectic polysaccharides from panax ginseng as the antirotavirus principals in ginseng. Biomacromolecules. (2010) 11(8):2044–52. doi: 10.1021/bm100397p
171. Yang H, Oh KH, Kim HJ, Cho YH, Yoo YC. Ginsenoside-Rb2 and 20(S)-Ginsenoside-Rg3 from Korean red ginseng prevent rotavirus infection in newborn mice. J Microbiol Biotechnol (2018) 28(3):391–6. doi: 10.4014/jmb.1801.01006
172. Roner MR, Tam KI, Kiesling-Barrager M. Prevention of rotavirus infections in vitro with aqueous extracts of quillaja saponaria Molina. Future Med Chem (2010) 2(7):1083–97. doi: 10.4155/fmc.10.206
173. Tam KI, Roner MR. Characterization of in vivo anti-rotavirus activities of saponin extracts from quillaja saponaria Molina. Antiviral Res (2011) 90(3):231–41. doi: 10.1016/j.antiviral.2011.04.004
174. Song L, Zhong P, Zhu X, Zhou R, Gao M, Lan Q, et al. The anti-rotavirus effect of baicalin via the gluconeogenesis-related p-JNK-PDK1-AKT-SIK2 signaling pathway. Eur J Pharmacol (2021) 897:173927. doi: 10.1016/j.ejphar.2021.173927
175. Shen J, Chen JJ, Zhang BM, Zhao J, Chen L, Ye QY, et al. Baicalin is curative against rotavirus damp heat diarrhea by tuning colonic mucosal barrier and lung immune function. Dig Dis Sci (2020) 65(8):2234–45. doi: 10.1007/s10620-019-05977-w
176. Kim HH, Kwon HJ, Ryu YB, Chang JS, Cho KO, Hosmillo MD, et al. Antiviral activity of alpinia katsumadai extracts against rotaviruses. Res Vet Sci (2012) 92(2):320–3. doi: 10.1016/j.rvsc.2010.11.012
177. Serrano J, Puupponen-Pimiä R, Dauer A, Aura AM, Saura-Calixto F. Tannins: current knowledge of food sources, intake, bioavailability and biological effects. Mol Nutr Food Res (2009) 53(Suppl 2):S310–29. doi: 10.1002/mnfr.200900039
178. Lipson SM, Sethi L, Cohen P, Gordon RE, Tan IP, Burdowski A, et al. Antiviral effects on bacteriophages and rotavirus by cranberry juice. Phytomedicine. (2007) 14(1):23–30. doi: 10.1016/j.phymed.2006.11.009
179. Mukoyama A, Ushijima H, Nishimura S, Koike H, Toda M, Hara Y, et al. Inhibition of rotavirus and enterovirus infections by tea extracts. Jpn J Med Sci Biol (1991) 44(4):181–6. doi: 10.7883/yoken1952.44.181
180. Ueda K, Kawabata R, Irie T, Nakai Y, Tohya Y, Sakaguchi T. Inactivation of pathogenic viruses by plant-derived tannins: strong effects of extracts from persimmon (Diospyros kaki) on a broad range of viruses. PloS One (2013) 8(1):e55343. doi: 10.1371/journal.pone.0055343
Keywords: rotavirus, diarrhoea, Chinese medicine, natural compounds, viral replication cycle, immunotherapy
Citation: Jiang L, Tang A, Song L, Tong Y and Fan H (2023) Advances in the development of antivirals for rotavirus infection. Front. Immunol. 14:1041149. doi: 10.3389/fimmu.2023.1041149
Received: 10 September 2022; Accepted: 28 February 2023;
Published: 17 March 2023.
Edited by:
Yuebang Yin, Nankai University, ChinaReviewed by:
Viviana Parreño, Instituto Nacional de Tecnología Agropecuaria, ArgentinaRameez Raja, Cleveland Clinic, United States
Aravinthkumar Jayabalan, Johns Hopkins University, United States
Copyright © 2023 Jiang, Tang, Song, Tong and Fan. This is an open-access article distributed under the terms of the Creative Commons Attribution License (CC BY). The use, distribution or reproduction in other forums is permitted, provided the original author(s) and the copyright owner(s) are credited and that the original publication in this journal is cited, in accordance with accepted academic practice. No use, distribution or reproduction is permitted which does not comply with these terms.
*Correspondence: Huahao Fan, ZmFuaHVhaGFvQG1haWwuYnVjdC5lZHUuY24=; Yigang Tong, dG9uZ3lpZ2FuZ0BtYWlsLmJ1Y3QuZWR1LmNu; Lihua Song, c29uZ2xpaHVhQG1haWwuYnVjdC5lZHUuY24=