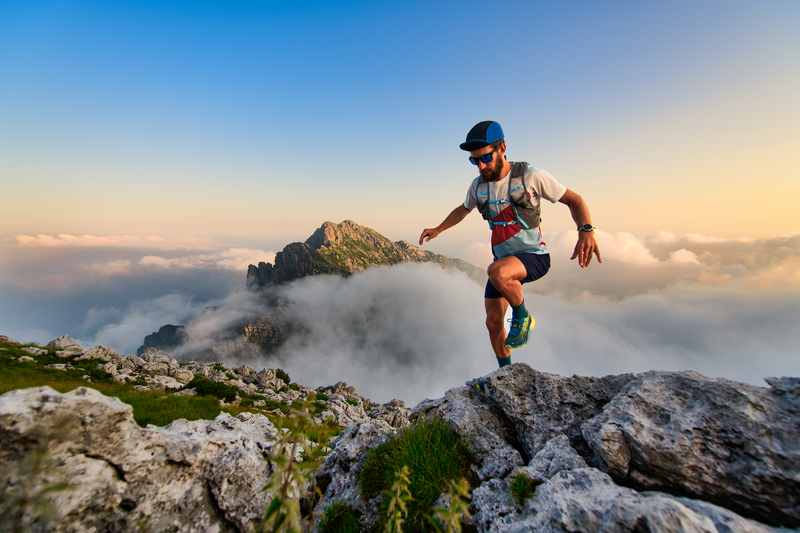
95% of researchers rate our articles as excellent or good
Learn more about the work of our research integrity team to safeguard the quality of each article we publish.
Find out more
REVIEW article
Front. Immunol. , 06 October 2022
Sec. Cancer Immunity and Immunotherapy
Volume 13 - 2022 | https://doi.org/10.3389/fimmu.2022.999549
This article is part of the Research Topic Macrophage Immunity and Metabolism in Cancer: Novel Diagnostic and Therapeutic Strategies View all 5 articles
The immunosuppressive tumor microenvironment (TME) remains one of the most prevailing barriers obstructing the implementation of effective immunotherapy against solid-state cancers. Eminently composed of immunosuppressive tumor associated macrophages (TAMs) and myeloid-derived suppressor cells (MDSCs) among others, the TME attenuates the effects of immune checkpoint blockade and adoptive cell therapies, mandating a novel therapy capable of TME remediation. In this review we explore the potential of three innate-like T cell subsets, invariant natural killer T (iNKT), mucosal-associated invariant T (MAIT) cells, and gamma delta T (γδT) cells, that display an intrinsic anti-TAM/MDSC capacity. Exhibiting both innate and adaptive properties, innate-like T cell types express a subset-specific TCR with distinct recombination, morphology, and target cell recognition, further supplemented by a variety of NK activating receptors. Both NK activating receptor and TCR activation result in effector cell cytotoxicity against targeted immunosuppressive cells for TME remediation. In addition, innate-like T cells showcase moderate levels of tumor cell killing, providing dual antitumor and anti-TAM/MDSC function. This latent antitumor capacity can be further bolstered by chimeric antigen receptor (CAR) engineering for recognition of tumor specific antigens to enhance antitumor targeting. In contrast with established CAR-T cell therapies, adoption of these innate-like cell types provides an enhanced safety profile without the risk of graft versus host disease (GvHD), due to their non-recognition of mismatched major histocompatibility complex (MHC) molecules, for use as widely accessible, allogeneic “off-the-shelf” cancer immunotherapy.
For solid state cancers in particular, the development of a localized tumor microenvironment (TME) has been associated with disease progression, facilitating resistance against targeted immunotherapies. A wide array of cells including tumor-associated macrophages (TAMs), myeloid-derived suppressor cells (MDSCs), cancer-associated fibroblasts (CAFs) and T regulatory cells aggregate within the TME and dampen effector cell response (Figure 1A) (1, 2). These immunosuppressive cells have been observed to attenuate immune cell antitumor immunity and promote tumor growth and metastasis (3). Inhibition of T cell-mediated antitumor capacity develops through upregulation of immune checkpoint ligands, such as programmed death-ligand 1 and 2 (PD-L1 and PD-L2), and secretion of immunosuppressive factors, such as transforming growth factor-β (TGF-β), tumor necrosis factor-α (TNF-α), IL-10 and CCL-22 (4). Furthermore, production of pro-angiogenic cytokines and growth factors, including ornithine, TGF-β, vascular endothelial growth factor (VEGF), basic fibroblast growth factor (bFGF) and colony stimulating factor 1 (CSF1), provides nutrient factors that promote tumor angiogenesis and vessel co-option wherein tumor cells hijack the existing patient vasculature, therefore enhancing tumor progression (4, 5).
Figure 1 Cell-based therapy to target immunosuppressive cells in solid tumor microenvironment (TME). (A) The TME is composed of a heterogeneous milieu of tumor and immune cells. Various immunosuppressive cells such as tumor-associated macrophages (TAMs), myeloid-derived suppressor cells (MDSCs), cancer-associated fibroblasts (CAFs) and T regulatory cells, suppress or reprogram the antitumor immune response. (B) Different chimeric antigen receptors (CARs) were utilized to generate CAR-T cells, including fibroblast activation protein (FAP) CAR, Folate receptor β (FRβ) CAR, CD123 CAR and C-C motif chemokine receptor 4 (CCR4) CAR. (C) Innate T cells, including invariant natural killer T (iNKT) cells, mucosal-associated invariant T (MAIT) cells, and gamma delta T (γδT) cells, efficiently target immunosuppressive cells, and these innate T cells could be further engineered with CARs and achieve both tumor cell and immunosuppressive cell elimination.
In order to restore TME-dampened antitumor capacity, therapeutics targeting associated immunosuppressive cells, especially TAMs and MDSCs, to modulate the solid TME have gained traction as an attractive avenue for cancer immunotherapy. One prominent method employs CCR2 antagonism to block recruitment and infiltration of immunosuppressive monocytes/macrophages to the tumor site (6–8). More direct approaches utilize a variety of TAM depleting agents, including clodronate-liposome, melittin-based pro-apoptotic peptide, and mannose-conjugated nanoparticles, to reduce their impact within the TME (9–11). Other approaches harness antineoplastic agents such as natural compound baicalin, paclitaxel, cyclophosphamide, gemcitabine, targeted nanocarrier delivering M1-polarizing transcription factor mRNAs, and CD163-targeted corosolic acid-containing liposomes to reprogram TAMs and restore their proinflammatory phenotype (12–17). Despite the preliminary successes of these immunotherapeutic agents, their transient retention period within the solid TME greatly restricts their interventive potential. These limitations call for a novel therapeutic that persists within the TME for sustained suppression or remediation of immunosuppressive cellular activity.
Recently studies have investigated the potential for cell-based immunotherapy, especially chimeric antigen receptor (CAR)-engineered T (CAR-T) cell therapy, to target the TME. Various CARs, such as folate receptor β (FRβ)-, fibroblast activation protein (FAP)-, CD123-, CCR4- and VEGFR-2-targeting CARs, have been applied to eliminate immunosuppressive cells in the TME with promising effect (Figure 1B) (18, 19). FRβ-targeting CAR-T cells have shown the capacity to deplete FRβ+ TAMs, delaying tumor progression and prolonging survival in mice (20); FAP is a membrane protease highly expressed on CAFs, FAP-targeting CAR-T cells have been developed to target CAFs in multiple solid tumors, such as mesothelioma, lung and pancreatic cancers (21, 22); The upregulation of CD123 on myelodysplastic syndrome (MDS) clones as well as MDSCs provides a compelling rationale for targeting CD123 antigen by monoclonal antibodies and CD123-targeting CAR-T cells in the immunosuppressive TME of MDS patients (23, 24); CCR4 is highly expressed in T cell malignancies as well as in CD4+CD25+Foxp3+ T regulatory cells, CCR4-targeting CAR-T cells displayed powerful cytotoxicity against a wide spectrum of aberrant T cells, including adult T cell leukemia/lymphoma (ATL), cutaneous T cell lymphoma (CTCL), and anaplastic large cell lymphoma (ALCL) (25, 26).
Innate T cells, including invariant natural killer T (iNKT) cells, mucosal-associated invariant T (MAIT) cells, and gamma delta T (γδT) cells, exhibit intrinsic anti-TAM capacity, and are potent models for CAR-engineering to achieve dual elimination of tumor cells and TAMs (Figure 1C and Table 1) (27, 28). In this review, we summarized the potential of innate T cell-based therapy for targeting the TME, introducing the immunosuppressive cell-targeting capacity of iNKT, MAIT and γδT cells and reviewing their genetic engineering, preclinical application and translational potential in cancer immunotherapy.
Invariant natural killer T (iNKT) cells are an uncommon subset of αβT cells that exhibit features of both innate and adaptive immune responses (29). iNKT cells present with a specific TCR complex that differs from those of conventional αβT cells; mouse iNKT TCR expresses the Vα14-Jα18 chain paired with a limited number of Vβ chains, typically Vβ2, Vβ7 or Vβ8, whereas human iNKT TCR expresses the Vα24-Jα18 chain with limited Vβ chains, predominantly Vβ11 (30–34). This semi-invariant TCR specifically recognizes lipid and glycolipid antigens presented by the class I MHC-like glycoprotein CD1d (29, 35, 36). Specialized development of iNKT cells in the thymus initially follows that of classical αβ T cells but then diverges during the CD4+CD8+ double positive (DP) stage (37). Positive selection of the iNKT TCR from antigen-loaded CD1d presentation by cortical thymic epithelial cells (TECs) induces expression of innate NK markers (e.g., CD161) and transcription factor PLZF to produce the mature iNKT cytokine profile and phenotype (38). In addition, iNKT cells can also be activated in a TCR-independent manner in response to antigen presenting cell (APC)-derived IL-12 and IL-18 (39, 40). Upon stimulation, iNKT cells acquire cytotoxicity and secrete large quantities of effector cytokines that stimulate downstream activation of other immune effector cells including NK cells, dendritic cells (DCs), and CD4 helper and CD8 cytotoxic T cells (29, 41). Since their powerful antitumor activity remains independent of antigen priming and MHC restrictions, iNKT cells have become a major focus in the development of novel cell-based immunotherapies. Additionally, implementation of iNKT cells as a strategy for cell-based immunotherapy offers several other advantages, such as eliminating the risk of graft versus host disease (GvHD) from lack of MHC engagement as well as ancillary remediation of the TME through cytotoxic killing of CD1d-expressing TAMs and MDSCs (29, 41–43).
Among macrophages, selective expression of CD1d on TAMs renders iNKT therapy an ideal method for precise disruption of TAM immunosuppression while preserving the pro-inflammatory function of classically activated macrophages (44, 45). CD1d cross-presentation of tumor-derived glycolipids from the surrounding environment enables iNKT cells to eliminate TAMs and dampen their effects (45). Furthermore, iNKT expression of NK activating receptors (e.g., NKG2D, NKp33, NKp40 and DNAM-1) provide a secondary method for these cells to recognize TAMs independently of CD1d presentation (27, 46–48). Recognition of NK receptor ligands on TAMs activates Perforin/Granzyme-mediated lysis and IFN-γ secretion, suppressing the pro-tumoral environment generated by TAMs (46, 49). Previous studies have shown that human peripheral blood mononuclear cell (PBMC)-derived iNKT cells could effectively eliminate M2-polarized macrophages when stimulated with alpha galactosylceramide (α-GalCer or αGC), a synthetic iNKT lipid antigen; iNKT engagement against macrophages was validated through addition of anti-CD1d antibody to block the CD1d/iNKT TCR pathway, which produced diminished killing of CD1d+ M2-polarized macrophages (27, 41). Interestingly, in the absence of αGC, iNKT cells could also kill M2 macrophages albeit at a reduced efficacy (27, 41). This intrinsic killing despite the absence of TCR engagement emerges due to high expression levels of NK activating receptors in iNKT cells for potent NK-mediated cytotoxicity (Figures 2A, B) (50, 51). The capacity of iNKT cells to target TAMs through two distinct pathways provides an attractive method to reengineer the tumor microenvironment and stimulate endogenous CD8 cytotoxic T cells and NK cells (52). Another study reported that neuroblastoma TAMs were capable of cross-presenting neuroblastoma-derived endogenous glycosphingolipids from the TME, which could specifically activate iNKT cells and induce iNKT cell-mediated TAM killing (45). The interaction of iNKT cells and CD1d+ TAMs within the TME may explain the association between iNKT infiltration with favorable outcome in neuroblastoma and other solid tumors (45). In cases of murine prostate cancer, mouse iNKT cells directly targeted M2-like macrophages through CD1d recognition and engagement of Fas-FasL mediated killing to reduce tumor burden; in addition, CD40L presentation to APCs motivated crosstalk with other effector cells to dampen the pro-angiogenic and immunosuppressive capabilities of tumor-infiltrating immune cells, delaying prostate tumor growth (53). Overall, through CD1d-iNKT TCR recognition, iNKT cells are poised to target TAMs within the solid TME, leading to improved outcomes for cancer patients.
Figure 2 Targeting tumor and immunosuppressive cells by innate T cells. (A) Diagram showing iNKT cells target CD1d+ tumor cells using NK/TCR double mechanisms. MIC, MHC class I chain-related protein. ULBP, UL16 binding protein. (B) Diagram showing iNKT cells target CD1d+ TAMs using NK/TCR double mechanisms. (C) Diagram showing MAIT cells target CD1d+ TAMs using NK/TCR double mechanisms. MR1, major histocompatibility complex, class I-related protein. 5-OP-RU, 5-(2-oxopropylideneamino)-6-D-ribitylaminouracil. 5-OP-RU is a microbial riboflavin-derived antigen and can specifically activate MAIT cells. (D) Diagram showing γδT cells target CD1d+ TAMs using NK/TCR double mechanisms. Zoledronate is a nitrogen-containing bisphosphonate and can activate γδT cells.
The predominant clinical platforms implementing iNKT immunotherapy utilize autologously engrafted PBMC-derived iNKT cells that are activated prior using in vitro glycolipid presentation (54–56). While iNKT-based therapy could be repurposed for TAM depletion, limitations on PBMC iNKT purity and yield demand a novel platform for the generation of iNKT cells. To overcome this hurdle, our group explored in vitro generation of allogenic hematopoietic stem cell (HSC)-engineered iNKT (AlloHSC-iNKT) cells through cord blood HSC engineering; notably the final cell product displayed near one-thousand-fold expansion, providing a scalable platform for extensive generation of AlloHSC-iNKT cells (48, 50). In agreement with the anti-TAM function of PBMC iNKT cells, significant depletion of M2 macrophages and TAMs by AlloHSC-iNKT cells was similarly observed, validating their efficacy (48, 50). Importantly, these engineered iNKT cells specifically depleted virus-infected monocytes through CD1d recognition, suggesting that virus-infected monocytes or TAMs present greater amounts of stress molecules and glycolipids, enhancing cytotoxic recognition of AlloHSC-iNKT cells using intrinsic NK pathways and iNKT TCR pathways (48). Despite their promise, current AlloHSC-iNKT cell products and other stem cell-derived cell therapies confront certain limitations that preclude implementation. During human stem cell culture, induction of mouse-derived stromal feeder cells (e.g., OP9 and MS5 cells) could potentially increase the risk of mouse cell contamination. The manufacturing process can be improved through replacement with a feeder-free culture system to improve the safety profile of cell products and accelerate the clinical development (43, 57). In addition, the highly inflammatory and cytotoxic function of AlloHSC-iNKT cells in conjunction with their rapid in vitro and in vivo cell proliferation may induce cytokine release/storm syndrome (CRS) as a side-effect during massive lysis of tumor cells and TAMs. Indeed, CRS is a salient concern for current CAR-T therapies (58). So far, the fast evolution of CAR-T cell therapy has accumulated valuable clinical experiences for CRS management (e.g., anti-IL-6 antibody treatment), that can be adapted for AlloHSC-iNKT cell therapy and other stem cell-based cell therapies. Moreover, a suicide gene “safety switch” (e.g., sr39TK, iCasp9, RQR8) could be incorporated in these cellular products to provide an additional safety control (59, 60).
To further overcome tumor development, the AlloHSC-iNKT platform could adopt CAR-engineered iNKT (CAR-iNKT) mechanisms to enhance dual targeting of TAMs and tumor cells. The AlloHSC-iNKT cell product demonstrated cytotoxicity against multiple tumor cell lines (50); the baseline level of tumor killing mediated by NKG2D and other activating NK receptors (52) could thus be further enhanced using CARs for high-fidelity recognition. Successful preliminary trials incorporating CAR-iNKT therapy have targeted a variety of cancer-specific antigens including CD19 (61), B cell maturation antigen (BCMA) (62), and GD2 (63) for treatment of B cell lymphoma, multiple myeloma, and neuroblastoma, respectively (Table 2). Precise elimination of tumor cells through CAR targeting reduces secretion of immunosuppressive cytokines (e.g. IL-10 and TGFβ) that induce TAM polarization and persistence (72), thereby minimizing TAM-related immunosuppression. CAR-iNKT cells are highly potent effector cells that remediate the TME through simultaneous depletion of TAMs and tumors using iNKT TCR/CD1d and CAR recognition, respectively, as well as generalized elimination of both mediated by NK receptors (3, 42, 57, 73). Refinement of the HSC-iNKT platform with CAR-engineering provides a powerful method to address the TME, which remains a significant barrier to the efficacy of cell-based treatments, to produce a powerful alternative for current cancer immunotherapies.
Further incorporation of immune enhancing genes (e.g., IL-15) and depleting checkpoint molecules (e.g., PD-1 and CTLA-4) in iNKT cell- and other immune cell-based therapy have been explored to expand CAR performance. Self-sustaining secretion of human IL-15 through CAR integration presages activation of essential signaling for iNKT and NK cell development and homeostasis, enhancing in vivo persistence and antitumor function (74–77). A GD2-targeting CAR-armed, IL-15-enhanced iNKT cell product was applied to clinical trials for treating children with relapsed neuroblastoma, demonstrating encouraging therapeutic outcomes, safety, and feasibility (63, 78). Blockade of checkpoints such as PD-1 and CTLA-4 significantly enhanced the antitumor immunity of human iNKT and other immune cells (79, 80). In a similar vein, CRIPSR-Cas 9 technology has been successfully used to knock out checkpoints to enhance antitumor immunity in cytotoxic T lymphocytes, and such technology could be easily applied to other cell types (81–83). Considering TAMs engineer immunosuppression through upregulation of PD-1 ligands (e.g., PD-L1 and PD-L2) (3, 84), depleting cognate checkpoint molecules in iNKT cells and other immune cells provides another promising strategy to enhance their anti-TAM capacities and persistence, therefore augmenting tumor treatment.
Mucosal-associated invariant T (MAIT) cells are another innate-like T cell subset that recognize small molecule biosynthetic derivatives produced during microbial riboflavin synthesis, which are then presented on MHC-related protein-1 (MR1) by APCs (85–88). Mouse MAIT TCR is comprised of a semi-invariant TCR α chain Vα19–Jα33 predominantly paired with TCRβ chain Vβ6/Vβ8; human MAIT TCR uses Vα7.2–Jα33/12/20 associated with Vβ2/Vβ13 (89, 90). MAIT cell development in the thymus derives from a common CD4+CD8+ DP αβ T cell progenitor as conventional T cells; MR1-mediated positive selection of MAIT TCR by TECs induces differential co-expression of CD161 and CD8αα markers, controlled by PLZF transcription factor (91, 92). Two ligands specifically, 5-(2-oxopropylideneamino)-6-D-ribitylaminouracil (5-OP-RU) and 5-(2-oxoethylideneamino)-6-D-ribitylaminouracil (5-OE-RU), are produced by several strains of bacteria and yeast during riboflavin synthesis; through specific presentation to MAIT TCR these ligands could induce MAIT cell activation, akin to αGC stimulation of iNKT cells (93). Activated MAIT cells expand rapidly and produce an innate-like immune response with potent effector function through secretion of inflammatory cytokines, chemokines, and cytotoxic molecules to eliminate target cells.
Both bone marrow-derived APCs, including monocytes, macrophages, and DCs, and non-bone marrow-derived epithelial cells express high levels of MR1 for MAIT cell activation (94–97). Specifically, elevated expression of MR1 on healthy donor PBMC-derived M2-polarized macrophages and cancer patient endogenous TAMs suggest that MAIT cells could mobilize a powerful anti-TAM response to engineer a TME with pro-inflammatory character (27). Similar to PBMC-derived iNKT cells, MAIT cells could directly target M2 macrophages through intrinsic NK activating receptors; in addition, the application of 5-OP-RU could induce TCR activation and further enhance MAIT cell anti-TAM capacity, which was blocked by the anti-MR1 antibody (Figure 2C) (27). Macrophage-killing by MAIT cells was also correlated with upregulation of activation markers, such as CD25, and secretion of pro-inflammatory cytokines, such as IFN-γ (27). Furthermore, MAIT cells could undergo CAR engineering, wherein the mesothelin CAR-armed MAIT (MCAR-MAIT) cells demonstrated dual killing of mesothelin+ ovarian tumor cells and MR1+ TAMs to enhance antitumor reactivity (27).
Gamma delta T (γδT) cells, another scarce population of unconventional T cells, express rearranged TCR γδ chains instead of conventional TCR αβ chains. Unlike αβT cells, γδT cells possess features of both innate and adaptive immune cells and can be activated in the absence of their cognate TCR ligands through APC cytokine signaling alone (98, 99). γδT cells arise from a common CD4-CD8- double negative (DN) progenitor as conventional αβ T cells, but productive rearrangement of γδ TCR rearrangement between DN2 (CD44+CD25+) and DN3 (CD44-CD25+) stages induces fate commitment towards the γδ subtype (100). Enrichment through TEC positive selection of γδ TCR induces differential expression of CD73, which is persistently expressed by peripheral γδT cells; CD73 can therefore be used as an early indicator of γδ fate commitment (101, 102). When activated, γδT cells generate a burst of inflammatory cytokines that subsequently induce an inflammatory response from adaptive effector cells (98, 99). These features poise γδT cells as a potent upstream effector T cell that mediates the immune cascade in inflamed tissues (103).
As mentioned previously, γδT cells do not require MHC antigen presentation for recognition and function, evading onset of GvHD observed in allogeneic engraftment of classical αβT cells (104, 105). Amino bisphosphonate class drugs such as zoledronate have been shown to effectively induce γδT cell expansion both in vitro and in vivo (106, 107). Profiling of in vitro expanded Vγ9Vδ2 T cells show potent antitumor functions that hold attractive promise for adoptive immunotherapy (108). Since γδT cells demonstrate intrinsic anti-tumor reactivity and can be safely applied for allogeneic therapies (27), engineering γδT cells with CAR expression provides an off-the-shelf approach to target tumors with higher antigen heterogeneity and lower antigen density, which present an obstacle for conventional CAR-T cells.
Despite their significant antitumor potential, the capacity of γδT cells to modulate the TME remain controversial. Previous studies reported that engraftment of mouse γδT cells induced an undesirable increase in the quantity of MDSCs and mobilized MDSC infiltration, exacerbating the immunosuppressive TME through MDSC-mediated CD8+ T cell exhaustion (109, 110). An additional human study related to colorectal cancer revealed that γδT17 cell secretion of IL-17, G-CSF, and GM-CSF cytokine could mobilize polymorphonuclear MDSCs into the tumor, eliciting immunosuppression (111). However, mitigation of γδT cell pro-tumoral effects is achievable through modulation of their cytokine profile to prevent MDSC recruitment; a study of γδ17 in a murine breast cancer model demonstrated the capacity to minimize accumulation and pro-tumoral polarization of neutrophils through ablation of IL-17 and G-CSF pathways (112). In conjunction, an opposing study indicated the strong anti-tumoral capacity of γδT cells through synergistic application of Zoledronate to stimulate cytotoxicity against monocytes, and therefore TAMs, although the γδT cells lacked the capacity to localize to the tumor site (112). We previously utilized an in vitro mixed macrophage/γδT cell assay to study the anti-TAM function of γδT cells and verified the killing capacity of allogeneic PBMC-derived γδT against M2 macrophages in the presence of Zoledronate (Figure 2D) (27). While elimination of IL-17, G-CSF, and GM-CSF cytokines could potentially be applied to therapeutic γδT cells to reduce their pro-tumoral effects, the impact of such treatment on their anti-tumoral capacity is still unknown (27, 112, 113).
Although the exact mechanism of recognition as well as interplay with MDSC-mediated exhaustion remains under investigation, allogeneic γδT cells could be another promising candidate to target immunosuppressive cells and modulate the TME.
Immunosuppressive cells, especially M2-like TAMs and MDSCs, have been shown to play a role in the progression, metastasis, and chemoresistance of solid tumors (114, 115). Given their role in promoting an immunosuppressive TME in cancer, the specific targeting of TAMs and MDSCs may potentially provide an effective therapeutic route to stimulate patient immune response (116). To date, there have been several proposed methods of targeting M2-like TAMs in cancer via various strategies, including the use of immunotherapies, small molecule inhibitors, and nanoparticles (116). The overall goal of these targeted therapies is either outright elimination of TAMs in the TME to prevent further recruitment of TAMs or repolarization of M2-like TAMs towards a pro-inflammatory M1-like phenotype (7, 117).
However, these current treatment strategies are still plagued with their own drawbacks and limitations. The administration of bisphosphonates remains under questionable consideration due to a lack of target specificity within the TAM population; the inability to specifically eliminate M2-like, pro-tumoral TAMs can result in wide-spread TAM depletion that may result in an overactive immune response for potential patients (118). Use of CSF-1R inhibition has also generated inconsistent results, yielding limited clinical and single-agent success (116, 119). Additionally, CSF-1R inhibition for TAM elimination within the TME may undesirably increase MDSC infiltration worsening patient symptomatology (119). For CCL2-directed inhibition therapy, termination of the drug regiment adversely enhanced metastatic progression and decreased survival in mouse breast cancer models (120). Due to the limitations of the current therapies geared towards eliminating TAMs in the TME, a safe, effective alternative is necessary.
Innate T cells, including iNKT, MAIT and γδT cells are unconventional T cell subsets that have the potential to deplete TAMs through powerful NK receptor- and TCR-mediated cytotoxicity (27, 28, 54). These innate-like T cells are activated independently of MHC antigen presentation, and therefore do not recognize mismatch or protein alloantigen to induce GvHD (42, 121–123). The GvHD-free safety profile situate these innate T cell subsets as ideal candidates for the development of an “off-the-shelf” allogeneic cell therapy. Further engineering, such as arming with CARs, incorporating immune enhanced genes (e.g., IL-15), and depleting checkpoint molecules (e.g., PD-1 and CTLA-4), could improve the antitumor immunity of these therapeutic cells and provide an approach to simultaneously target both tumor and immunosuppressive cells. Further enhancement of anti-TAM capacity has been achieved using engineered FRβ CAR-T cells for depletion of FRβ+ TAMs (20); incorporation of the aforementioned FRβ CAR on allogeneic innate T cells could achieve powerful anti-TAM killing through an NK/TCR/FRβ CAR triple targeting mechanism.
One of the major limitations for innate T cell-based therapy is their low frequency and number in human. Human blood contains low numbers of iNKT (0.001-1%), MAIT (0.1-5%) and γδT (0.1-5%) cells, making it very difficult to reliably grow large numbers of innate T cells for CAR-engineering (28, 124, 125). Therefore, the initial cell materials require optimized expansion protocols, usually involving agonist (e.g., α-GalCer, 5-OP-RU, and Zoledronate)-loaded feeder cells and cytokines, followed by enrichment, purification and subsequent cell engineering (57). In addition, low viral transduction rate on some innate T cells may limit their CAR engineering and CAR-mediated antitumor functions, and strategies to improve the efficiency of viral transduction on innate T cells can be developed (28, 126).
Although the scarcity of innate T cells in human peripheral blood hinder the application of these cells, stem cell engineering and in vitro differentiation provide another opportunity to generate these cells at high yield and purity (Figure 3A) (48, 50). Multiple stem cell sources (e.g., HSCs, ESCs, and iPSCs) and stem cell culture approaches (e.g., OP9-DL, artificial thymic organoid, and Feeder-Free culture) have been employed to generate innate T cells that bear close resemblance to healthy donor PBMC-derived immune cells and maintain their potent tumor targeting capabilities (Figure 3B) (48, 50, 127, 128). For example, the OP9-DL system, which is based on a mouse stromal cell line OP9 overexpressing the Notch ligand, Delta-like ligand 1 (DLL-1) or 4 (DLL-4), was utilized to generate iPSC-derived iNKT and MAIT cells; the artificial thymic organoid (ATO) culture system, which is based on DLL-1- or DLL-4- overexpressed mouse stromal cell line MS5, was used to develop HSC-engineered iNKT cells; feeder-free, serum-free culture system has also been developed recently to generate iNKT cells with high yield, purity, and safety profile (43, 48, 50, 127–130). Overall, in vitro generation of CAR-engineered innate iNKT, MAIT, and γδT cells have the potential to effectively target both tumor cells and immunosuppressive cells, thus highlighting the capacity of innate T cell-based therapy for treatment of solid tumors, especially in the absence of inflammatory signaling, a defect characteristic of TME afflicted “cold” tumors.
Figure 3 Two approaches to generate CAR-engineered innate T cells. (A) Innate T cells are enriched from healthy donor peripheral blood mononuclear cells (PBMCs) via cell sorting, cultured in vitro and engineered with CARs. The generated CAR-engineered innate T cells could target both tumor and immunosuppressive cells. However, these cells have limited expansion fold and yield; therefore, the cell products could be adoptively transferred to less cancer patients. (B) Stem cells such as hematopoietic stem cells (HSCs) and pluripotent stem cells (PSCs; include embryonic stem cells and induced PSCs) could be engineered with innate T cell TCR and then cultured in the in vitro differentiation systems where the stem cells develop into mature innate T cells. The platforms could be easily combined with CAR engineering and other genetic modifications such as CRISPR-Cas9. The stem cell-derived innate T cells have high yield and purity and can be transferred to more cancer patients.
This manuscript was written by Y-RL, MW, and LY. All authors contributed to the article and approved the submitted version.
This work was supported by a Partnering Opportunity for Translational Research Projects Award and a Partnering Opportunity for Discovery Stage Award from the California Institute for Regenerative Medicine (CIRM TRAN1-12250 and DISC2-13505, to LY), a UCLA BSCRC Innovation Award (to LY), and an Ablon Scholars Award (to LY).
The authors would like to acknowledge the members of the Yang Lab for contributing insights and discussion surrounding the topic. Figures were created in biorender.com.
Y-RL and LY are inventors on patents relating to this article filed by UCLA. LY is a scientific advisor to AlzChem and Amberstone Biosciences, and a co-founder, stockholder, and advisory board member of Appia Bio. None of the declared companies contributed to or directed any of the research reported in this article.
The remaining authors declare that the research was conducted in the absence of any commercial or financial relationships that could be construed as a potential conflict of interest.
All claims expressed in this article are solely those of the authors and do not necessarily represent those of their affiliated organizations, or those of the publisher, the editors and the reviewers. Any product that may be evaluated in this article, or claim that may be made by its manufacturer, is not guaranteed or endorsed by the publisher.
TME, tumor microenvironment; TAM, tumor-associated macrophage; MDSC, myeloid-derived suppressor cell; iNKT, invariant natural killer T; MAIT, mucosal-associated invariant T; γδT, gamma delta T; CAR, chimeric antigen receptor; GvHD, graft versus host disease; CAF, cancer-associated fibroblasts; PD-L1, programmed death-ligand 1; TGF-β, transforming growth factor-β; TNF-α, tumor necrosis factor-α; VEGF, vascular endothelial growth factor; bFGF, basic fibroblast growth factor; CSF1, colony stimulating factor 1; G-CSF, Granulocyte colony stimulating factor; GM-CSF, Granulocyte macrophage colony stimulating factor; CRS, cytokine release/storm syndrome; DC, dendritic cell; APC, antigen-presenting cell; MR1, MHC-related protein-1; HSC, hematopoietic stem cell; ESC, embryonic stem cell; iPSC, induced pluripotent stem cell; ATO, artificial thymic organoid.
1. Wu T, Dai Y. Tumor microenvironment and therapeutic response. Cancer Lett (2017) 387:61–8. doi: 10.1016/j.canlet.2016.01.043
2. Denton AE, Roberts EW, Fearon DT. Stromal cells in the tumor microenvironment. Adv Exp Med Biol (2018) 1060:99–114. doi: 10.1007/978-3-319-78127-3_6
3. Li Y-R, Yu Y, Kramer A, Hon R, Wilson M, Brown J, et al. An ex vivo 3D tumor microenvironment-mimicry culture to study TAM modulation of cancer immunotherapy. Cells (2022) 11:1583. doi: 10.3390/cells11091583
4. Caux C, Ramos RN, Prendergast GC, Bendriss-Vermare N, Ménétrier-Caux C. A milestone review on how macrophages affect tumor growth. Cancer Res (2016) 76:6439–42. doi: 10.1158/0008-5472.CAN-16-2631
5. Donnem T, Hu J, Ferguson M, Adighibe O, Snell C, Harris AL, et al. Vessel co-option in primary human tumors and metastases: An obstacle to effective anti-angiogenic treatment? Cancer Med (2013) 2:427–36. doi: 10.1002/cam4.105
6. Fei L, Ren X, Yu H, Zhan Y. Targeting the CCL2/CCR2 axis in cancer immunotherapy: One stone, three birds? Front Immunol (2021) 12:771210. doi: 10.3389/fimmu.2021.771210
7. Petty AJ, Owen DH, Yang Y, Huang X. Targeting tumor-associated macrophages in cancer immunotherapy. Cancers (Basel) (2021) 13:5318. doi: 10.3390/cancers13215318
8. Li M, He L, Zhu J, Zhang P, Liang S. Targeting tumor-associated macrophages for cancer treatment. Cell Biosci (2022) 12:85. doi: 10.1186/s13578-022-00823-5
9. Lee C, Jeong H, Bae Y, Shin K, Kang S, Kim H, et al. Targeting of M2-like tumor-associated macrophages with a melittin-based pro-apoptotic peptide. J Immunother Cancer (2019) 7:147. doi: 10.1186/s40425-019-0610-4
10. Opperman KS, Vandyke K, Clark KC, Coulter EA, Hewett DR, Mrozik KM, et al. Clodronate-liposome mediated macrophage depletion abrogates multiple myeloma tumor establishment In vivo. Neoplasia (2019) 21:777–87. doi: 10.1016/j.neo.2019.05.006
11. Li Y, Wu H, Ji B, Qian W, Xia S, Wang L, et al. Targeted imaging of CD206 expressing tumor-associated M2-like macrophages using mannose-conjugated antibiofouling magnetic iron oxide nanoparticles. ACS Appl Bio Mater (2020) 3:4335–47. doi: 10.1021/acsabm.0c00368
12. Zhang F, Parayath NN, Ene CI, Stephan SB, Koehne AL, Coon ME, et al. Genetic programming of macrophages to perform anti-tumor functions using targeted mRNA nanocarriers. Nat Commun (2019) 10:3974. doi: 10.1038/s41467-019-11911-5
13. Andersen MN, Etzerodt A, Graversen JH, Holthof LC, Moestrup SK, Hokland M, et al. STAT3 inhibition specifically in human monocytes and macrophages by CD163-targeted corosolic acid-containing liposomes. Cancer Immunol Immunother (2019) 68:489–502. doi: 10.1007/s00262-019-02301-3
14. Wanderley CW, Colón DF, Luiz JPM, Oliveira FF, Viacava PR, Leite CA, et al. Paclitaxel reduces tumor growth by reprogramming tumor-associated macrophages to an M1 profile in a TLR4-dependent manner. Cancer Res (2018) 78:5891–900. doi: 10.1158/0008-5472.CAN-17-3480
15. Tan H-Y, Wang N, Man K, Tsao S-W, Che C-M, Feng Y. Autophagy-induced RelB/p52 activation mediates tumour-associated macrophage repolarisation and suppression of hepatocellular carcinoma by natural compound baicalin. Cell Death Dis (2015) 6:e1942. doi: 10.1038/cddis.2015.271
16. Buhtoiarov IN, Sondel PM, Wigginton JM, Buhtoiarova TN, Yanke EM, Mahvi DA, et al. Anti-tumour synergy of cytotoxic chemotherapy and anti-CD40 plus CpG-ODN immunotherapy through repolarization of tumour-associated macrophages. Immunology (2011) 132:226–39. doi: 10.1111/j.1365-2567.2010.03357.x
17. Di Caro G, Cortese N, Castino GF, Grizzi F, Gavazzi F, Ridolfi C, et al. Dual prognostic significance of tumour-associated macrophages in human pancreatic adenocarcinoma treated or untreated with chemotherapy. Gut (2016) 65:1710–20. doi: 10.1136/gutjnl-2015-309193
18. Rodriguez-Garcia A, Palazon A, Noguera-Ortega E, Powell DJ Jr., Guedan S. CAR-T cells hit the tumor microenvironment: Strategies to overcome tumor escape. Front Immunol (2020) 11:1109. doi: 10.3389/fimmu.2020.01109
19. Ruella M, Kenderian SS. Next-generation chimeric antigen receptor T-cell therapy: Going off the shelf. BioDrugs (2017) 31:473–81. doi: 10.1007/s40259-017-0247-0
20. Rodriguez-Garcia A, Lynn RC, Poussin M, Eiva MA, Shaw LC, O’Connor RS, et al. CAR-T cell-mediated depletion of immunosuppressive tumor-associated macrophages promotes endogenous antitumor immunity and augments adoptive immunotherapy. Nat Commun (2021) 12:877. doi: 10.1038/s41467-021-20893-2
21. Bughda R, Dimou P, D’Souza RR, Klampatsa A. Fibroblast activation protein (FAP)-targeted CAR-T cells: Launching an attack on tumor stroma. ImmunoTargets Ther (2021) 10:313–23. doi: 10.2147/ITT.S291767
22. Petrausch U, Schuberth PC, Hagedorn C, Soltermann A, Tomaszek S, Stahel R, et al. Re-directed T cells for the treatment of fibroblast activation protein (FAP)-positive malignant pleural mesothelioma (FAPME-1). BMC Cancer (2012) 12:615. doi: 10.1186/1471-2407-12-615
23. Uckun FM, Watts J. CD123-directed bispecific antibodies for targeting MDS clones and immunosuppressive myeloid-derived suppressor cells (MDSC) in high-risk adult MDS patients. Front Aging (2021) 2:757276. doi: 10.3389/fragi.2021.757276
24. Gustafson M, Lin Y, Maas M, Gastineau D, Dietz A. A method for non-overlapping identification of human myeloid derived suppressor cells. J Immunother Cancer (2014) 2:P150. doi: 10.1186/2051-1426-2-S3-P150
25. Perera LP, Zhang M, Nakagawa M, Petrus MN, Maeda M, Kadin ME, et al. Chimeric antigen receptor modified T cells that target chemokine receptor CCR4 as a therapeutic modality for T-cell malignancies. Am J Hematol (2017) 92:892–901. doi: 10.1002/ajh.24794
26. Nicolay JP, Albrecht JD, Alberti-Violetti S, Berti E. CCR4 in cutaneous T-cell lymphoma: Therapeutic targeting of a pathogenic driver. Eur J Immunol (2021) 51:1660–71. doi: 10.1002/eji.202049043
27. Li Y-R, Brown J, Yu Y, Lee D, Zhou K, Dunn ZS, et al. Targeting immunosuppressive tumor-associated macrophages using innate T cells for enhanced antitumor reactivity. Cancers (2022) 14:2749. doi: 10.3390/cancers14112749
28. Cortés-Selva D, Dasgupta B, Singh S, Grewal IS. Innate and innate-like cells: The future of chimeric antigen receptor (CAR) cell therapy. Trends Pharmacol Sci (2021) 42:45–59. doi: 10.1016/j.tips.2020.11.004
29. Van Kaer L, Parekh VV, Wu L. Invariant natural killer T cells: Bridging innate and adaptive immunity. Cell Tissue Res (2011) 343:43–55. doi: 10.1007/s00441-010-1023-3
30. Zhou Y, Li Y-R, Zeng S, Yang L. Methods for studying mouse and human invariant natural killer T cells. Methods Mol Biol (2021) 2388:35–57. doi: 10.1007/978-1-0716-1775-5_4
31. Juno JA, Keynan Y, Fowke KR. Invariant NKT cells: Regulation and function during viral infection. PloS Pathog (2012) 8:e1002838. doi: 10.1371/journal.ppat.1002838
32. Nelson A, Lukacs JD, Johnston B. The current landscape of NKT cell immunotherapy and the hills ahead. Cancers (2021) 13:5174. doi: 10.3390/cancers13205174
33. Park SH, Weiss A, Benlagha K, Kyin T, Teyton L, Bendelac A. The mouse CD1d-restricted repertoire is dominated by a few autoreactive T cell receptor families. J Exp Med (2001) 193:893–904. doi: 10.1084/jem.193.8.893
34. Cardell S, Tangri S, Chan S, Kronenberg M, Benoist C, Mathis D. CD1-restricted CD4+ T cells in major histocompatibility complex class II-deficient mice. J Exp Med (1995) 182:993–1004. doi: 10.1084/jem.182.4.993
35. Kawano T, Cui J, Koezuka Y, Toura I, Kaneko Y, Motoki K, et al. CD1d-restricted and TCR-mediated activation of valpha14 NKT cells by glycosylceramides. Science (1997) 278:1626–9. doi: 10.1126/science.278.5343.1626
36. Brossay L, Chioda M, Burdin N, Koezuka Y, Casorati G, Dellabona P, et al. CD1d-mediated recognition of an alpha-galactosylceramide by natural killer T cells is highly conserved through mammalian evolution. J Exp Med (1998) 188:1521–8. doi: 10.1084/jem.188.8.1521
37. Gapin L, Matsuda JL, Surh CD, Kronenberg M. NKT cells derive from double-positive thymocytes that are positively selected by CD1d. Nat Immunol (2001) 2:971–8. doi: 10.1038/ni710
38. Baranek T, de Amat Herbozo C, Mallevaey T, Paget C. Deconstructing iNKT cell development at single-cell resolution. Trends Immunol (2022) 43:503–12. doi: 10.1016/j.it.2022.04.012
39. Nagarajan NA, Kronenberg M. Invariant NKT cells amplify the innate immune response to lipopolysaccharide. J Immunol (2007) 178:2706–13. doi: 10.4049/jimmunol.178.5.2706
40. Reilly EC, Wands JR, Brossay L. Cytokine dependent and independent iNKT cell activation. Cytokine (2010) 51:227–31. doi: 10.1016/j.cyto.2010.04.016
41. Zhu Y, Smith DJ, Zhou Y, Li YR, Yu J, Lee D, et al. Development of hematopoietic stem cell-engineered invariant natural killer T cell therapy for cancer. Cell Stem Cell (2019) 25:542–557.e9. doi: 10.1016/j.stem.2019.08.004
42. Li Y-R, Dunn ZS, Zhou Y, Lee D, Yang L. Development of stem cell-derived immune cells for off-the-Shelf cancer immunotherapies. Cells (2021) 10:3497. doi: 10.3390/cells10123497
43. Li Y-R, Zeng S, Dunn ZS, Zhou Y, Li Z, Yu J, et al. Off-the-shelf third-party HSC-engineered iNKT cells for ameliorating GvHD while preserving GvL effect in the treatment of blood cancers. iScience (2022) 25:104859. doi: 10.1016/j.isci.2022.104859
44. Metelitsa LS. Anti-tumor potential of type-I NKT cells against CD1d-positive and CD1d-negative tumors in humans. Clin Immunol (2011) 140:119–29. doi: 10.1016/j.clim.2010.10.005
45. Song L, Asgharzadeh S, Salo J, Engell K, Wu H, Sposto R, et al. Vα24-invariant NKT cells mediate antitumor activity via killing of tumor-associated macrophages. J Clin Invest (2009) 119:1524–36. doi: 10.1172/JCI37869
46. Lanier LL. NKG2D receptor and its ligands in host defense. Cancer Immunol Res (2015) 3:575–82. doi: 10.1158/2326-6066.CIR-15-0098
47. An B, Lim J-Y, Jeong S, Shin D-M, Choi EY, Min C-K, et al. CD1d is a novel cell-surface marker for human monocytic myeloid-derived suppressor cells with T cell suppression activity in peripheral blood after allogeneic hematopoietic stem cell transplantation. Biochem Biophys Res Commun (2018) 495:519–25. doi: 10.1016/j.bbrc.2017.11.010
48. Li YR, Dunn ZS, Garcia G Jr., Carmona C, Zhou Y, Lee D, et al. Development of off − the − shelf hematopoietic stem cell − engineered invariant natural killer T cells for COVID − 19 therapeutic intervention. Stem Cell Res Ther (2022) 13:1–15. doi: 10.1186/s13287-022-02787-2
49. Aoki T, Takami M, Takatani T, Motoyoshi K, Ishii A, Hara A, et al. Activated invariant natural killer T cells directly recognize leukemia cells in a CD1d-independent manner. Cancer Sci (2020) 111:2223–33. doi: 10.1111/cas.14428
50. Li Y-R, Zhou Y, Kim YJ, Zhu Y, Ma F, Yu J, et al. Development of allogeneic HSC-engineered iNKT cells for off-the-shelf cancer immunotherapy. Cell Rep Med (2021) 2:100449. doi: 10.1016/j.xcrm.2021.100449
51. Krijgsman D, Hokland M, Kuppen PJK. The role of natural killer T cells in cancer-a phenotypical and functional approach. Front Immunol (2018) 9:367. doi: 10.3389/fimmu.2018.00367
52. Fuertes MB, Domaica CI, Zwirner NW. Leveraging NKG2D ligands in immuno-oncology. Front Immunol (2021) 12:713158. doi: 10.3389/fimmu.2021.713158
53. Cortesi F, Delfanti G, Grilli A, Calcinotto A, Gorini F, Pucci F, et al. Bimodal CD40/Fas-dependent crosstalk between iNKT cells and tumor-associated macrophages impairs prostate cancer progression. Cell Rep (2018) 22:3006–20. doi: 10.1016/j.celrep.2018.02.058
54. Delfanti G, Dellabona P, Casorati G, Fedeli M. Adoptive immunotherapy with engineered iNKT cells to target cancer cells and the suppressive microenvironment. Front Med (2022) 9:897750. doi: 10.3389/fmed.2022.897750
55. Gao Y, Guo J, Bao X, Xiong F, Ma Y, Tan B, et al. Adoptive transfer of autologous invariant natural killer T cells as immunotherapy for advanced hepatocellular carcinoma: A phase I clinical trial. Oncologist (2021) 26:e1919–30. doi: 10.1002/onco.13899
56. Cheng X, Wang J, Qiu C, Jin Y, Xia B, Qin R, et al. Feasibility of iNKT cell and PD-1+CD8+ T cell-based immunotherapy in patients with lung adenocarcinoma: Preliminary results of a phase I/II clinical trial. Clin Immunol (2022) 238:108992. doi: 10.1016/j.clim.2022.108992
57. Li Y-R, Zhou Y, Kramer A, Yang L. Engineering stem cells for cancer immunotherapy. Trends Cancer (2021) 7:1059–73. doi: 10.1016/j.trecan.2021.08.004
58. Brudno JN, Kochenderfer JN. Toxicities of chimeric antigen receptor T cells: recognition and management. Blood (2016) 127:3321–30. doi: 10.1182/blood-2016-04-703751
59. Di Stasi A, Tey S-K, Dotti G, Fujita Y, Kennedy-Nasser A, Martinez C, et al. Inducible apoptosis as a safety switch for adoptive cell therapy. N Engl J Med (2011) 365:1673–83. doi: 10.1056/nejmoa1106152
60. Straathof KC, Pulè MA, Yotnda P, Dotti G, Vanin EF, Brenner MK, et al. An inducible caspase 9 safety switch for T-cell therapy. Blood (2005) 105:4247–54. doi: 10.1182/blood-2004-11-4564
61. Rotolo A, Caputo VS, Holubova M, Baxan N, Dubois O, Chaudhry MS, et al. Enhanced anti-lymphoma activity of CAR19-iNKT cells underpinned by dual CD19 and CD1d targeting. Cancer Cell (2018) 34:596–610.e11. doi: 10.1016/j.ccell.2018.08.017
62. Poels R, Drent E, Lameris R, Katsarou A, Themeli M, van der Vliet HJ, et al. Preclinical evaluation of invariant natural killer T cells modified with CD38 or BCMA chimeric antigen receptors for multiple myeloma. Int J Mol Sci (2021) 22:1096. doi: 10.3390/ijms22031096
63. Xu X, Huang W, Heczey A, Liu D, Guo L, Wood M, et al. NKT cells coexpressing a GD2-specific chimeric antigen receptor and IL15 show enhanced in vivo persistence and antitumor activity against neuroblastoma. Clin Cancer Res (2019) 25:7126–38. doi: 10.1158/1078-0432.CCR-19-0421
64. Simon B, Wiesinger M, März J, Wistuba-Hamprecht K, Weide B, Schuler-Thurner B, et al. The generation of CAR-transfected natural killer T cells for the immunotherapy of melanoma. Int J Mol Sci (2018) 19:2365. doi: 10.3390/ijms19082365
65. Tian G, Courtney AN, Jena B, Heczey A, Liu D, Marinova E, et al. CD62L+ NKT cells have prolonged persistence and antitumor activity in vivo. J Clin Invest (2016) 126:2341–55. doi: 10.1172/JCI83476
66. Simonetta F, Lohmeyer JK, Hirai T, Maas-Bauer K, Alvarez M, Wenokur AS, et al. Allogeneic CAR invariant natural killer T cells exert potent antitumor effects through host CD8 T-cell cross-priming. Clin Cancer Res an Off J Am Assoc Cancer Res (2021) 27:6054–64. doi: 10.1158/1078-0432.CCR-21-1329
67. Ngai H, Tian G, Courtney AN, Ravari SB, Guo L, Liu B, et al. IL-21 selectively protects CD62L(+) NKT cells and enhances their effector functions for adoptive immunotherapy. J Immunol (2018) 201:2141–53. doi: 10.4049/jimmunol.1800429
68. Heczey A, Liu D, Tian G, Courtney AN, Wei J, Marinova E, et al. Invariant NKT cells with chimeric antigen receptor provide a novel platform for safe and effective cancer immunotherapy. Blood (2014) 124:2824–33. doi: 10.1182/blood-2013-11-541235
69. Deniger DC, Switzer K, Mi T, Maiti S, Hurton L, Singh H, et al. Bispecific T-cells expressing polyclonal repertoire of endogenous γδ T-cell receptors and introduced CD19-specific chimeric antigen receptor. Mol Ther (2013) 21:638–47. doi: 10.1038/mt.2012.267
70. Rozenbaum M, Meir A, Aharony Y, Itzhaki O, Schachter J, Bank I, et al. Gamma-delta CAR-T cells show CAR-directed and independent activity against leukemia. Front Immunol (2020) 11:1347. doi: 10.3389/fimmu.2020.01347
71. Ang WX, Ng YY, Xiao L, Chen C, Li Z, Chi Z, et al. Electroporation of NKG2D RNA CAR improves Vγ9Vδ2 T cell responses against human solid tumor xenografts. Mol Ther Oncol (2020) 17:421–30. doi: 10.1016/j.omto.2020.04.013
72. Sica A, Larghi P, Mancino A, Rubino L, Porta C, Totaro MG, et al. Macrophage polarization in tumour progression. Semin Cancer Biol (2008) 18:349–55. doi: 10.1016/j.semcancer.2008.03.004
73. Li Y-R, Zhou Y, Wilson M, Kramer A, Hon R, Zhu Y, et al. Tumor-localized administration of α-GalCer to recruit invariant natural killer T cells and enhance their antitumor activity against solid tumors. Int J Mol Sci (2022) 23:7547. doi: 10.3390/ijms23147547
74. Liu E, Marin D, Banerjee P, Macapinlac HA, Thompson P, Basar R, et al. Use of CAR-transduced natural killer cells in CD19-positive lymphoid tumors. N Engl J Med (2020) 382:545–53. doi: 10.1056/nejmoa1910607
75. Christodoulou I, Ho WJ, Marple A, Ravich JW, Tam A, Rahnama R, et al. Engineering CAR-NK cells to secrete IL-15 sustains their anti-AML functionality but is associated with systemic toxicities. J Immunother Cancer (2021) 9:e003894. doi: 10.1136/jitc-2021-003894
76. Christodoulou I, Koldobskiy M, Ho WJ, Marple A, Ravich WJ, Rahnama R, et al. Engineered interleukin-15 autocrine signaling invigorates anti-CD123 CAR-NK cells. Blood (2021) 138:2806. doi: 10.1182/blood-2021-146609
77. Du Z, Ng YY, Zha S, Wang S. piggyBac system to co-express NKG2D CAR and IL-15 to augment the in vivo persistence and anti-AML activity of human peripheral blood NK cells. Mol Ther - Methods Clin Dev (2021) 23:582–96. doi: 10.1016/j.omtm.2021.10.014
78. Heczey A, Courtney AN, Montalbano A, Robinson S, Liu K, Li M, et al. Anti-GD2 CAR-NKT cells in patients with relapsed or refractory neuroblastoma: An interim analysis. Nat Med (2020) 26:1686–90. doi: 10.1038/s41591-020-1074-2
79. Kamata T, Suzuki A, Mise N, Ihara F, Takami M, Makita Y, et al. Blockade of programmed death-1/programmed death ligand pathway enhances the antitumor immunity of human invariant natural killer T cells. Cancer Immunol Immunother (2016) 65:1477–89. doi: 10.1007/s00262-016-1901-y
80. Catafal-Tardos E, Baglioni MV, Bekiaris V. Inhibiting the unconventionals: Importance of immune checkpoint receptors in γδ t, mait, and nkt cells. Cancers (Basel) (2021) 13:4647. doi: 10.3390/cancers13184647
81. Zhao Z, Shi L, Zhang W, Han J, Zhang S, Fu Z, et al. CRISPR knock out of programmed cell death protein 1 enhances anti-tumor activity of cytotoxic T lymphocytes. Oncotarget (2018) 9:5208–15. doi: 10.18632/oncotarget.23730
82. McGowan E, Lin Q, Ma G, Yin H, Chen S, Lin Y. PD-1 disrupted CAR-T cells in the treatment of solid tumors: Promises and challenges. BioMed Pharmacother (2020) 121:109625. doi: 10.1016/j.biopha.2019.109625
83. Shi L, Meng T, Zhao Z, Han J, Zhang W, Gao F, et al. CRISPR knock out CTLA-4 enhances the anti-tumor activity of cytotoxic T lymphocytes. Gene (2017) 636:36–41. doi: 10.1016/j.gene.2017.09.010
84. Lin Y, Xu J, Lan H. Tumor-associated macrophages in tumor metastasis: Biological roles and clinical therapeutic applications. J Hematol Oncol (2019) 12:76. doi: 10.1186/s13045-019-0760-3
85. Eckle SBG, Corbett AJ, Keller AN, Chen Z, Godfrey DI, Liu L, et al. Recognition of vitamin b precursors and byproducts by mucosal associated invariant T cells. J Biol Chem (2015) 290:30204–11. doi: 10.1074/jbc.R115.685990
86. Gao Y, Williams AP. Role of innate T cells in anti-bacterial immunity. Front Immunol (2015) 6:302. doi: 10.3389/fimmu.2015.00302
87. Godfrey DI, Uldrich AP, McCluskey J, Rossjohn J, Moody DB. The burgeoning family of unconventional T cells. Nat Immunol (2015) 16:1114–23. doi: 10.1038/ni.3298
88. Lamichhane R, Ussher JE. Expression and trafficking of MR1. Immunology (2017) 151:270–9. doi: 10.1111/imm.12744
89. Reantragoon R, Corbett AJ, Sakala IG, Gherardin NA, Furness JB, Chen Z, et al. Antigen-loaded MR1 tetramers define T cell receptor heterogeneity in mucosal-associated invariant T cells. J Exp Med (2013) 210:2305–20. doi: 10.1084/jem.20130958
90. Lepore M, Kalinichenko A, Colone A, Paleja B, Singhal A, Tschumi A, et al. Parallel T-cell cloning and deep sequencing of human MAIT cells reveal stable oligoclonal TCRβ repertoire. Nat Commun (2014) 5:3866. doi: 10.1038/ncomms4866
91. Seach N, Guerri L, Le Bourhis L, Mburu Y, Cui Y, Bessoles S, et al. Double-positive thymocytes select mucosal-associated invariant T cells. J Immunol (2013) 191:6002–9. doi: 10.4049/jimmunol.1301212
92. Koay H-F, Gherardin NA, Enders A, Loh L, Mackay LK, Almeida CF, et al. A three-stage intrathymic development pathway for the mucosal-associated invariant T cell lineage. Nat Immunol (2016) 17:1300–11. doi: 10.1038/ni.3565
93. Hinks TSC, Zhang X-W. MAIT cell activation and functions. Front Immunol (2020) 11:1014. doi: 10.3389/fimmu.2020.01014
94. van Wilgenburg B, Scherwitzl I, Hutchinson EC, Leng T, Kurioka A, Kulicke C, et al. MAIT cells are activated during human viral infections. Nat Commun (2016) 7:11653. doi: 10.1038/ncomms11653
95. Salerno-Goncalves R, Rezwan T, Sztein M. B cells modulate mucosal associated invariant T cell immune responses. Front Immunol (2014) 4:511. doi: 10.3389/fimmu.2013.00511
96. Huimeng W, Lars K-N, Mai S, Criselle D, Pediongco TJ, Hanwei C, et al. IL-23 costimulates antigen-specific MAIT cell activation and enables vaccination against bacterial infection. Sci Immunol (2019) 4:eaaw0402. doi: 10.1126/sciimmunol.aaw0402
97. Dusseaux M, Martin E, Serriari N, Péguillet I, Premel V, Louis D, et al. Human MAIT cells are xenobiotic-resistant, tissue-targeted, CD161hi IL-17–secreting T cells. Blood (2011) 117:1250–9. doi: 10.1182/blood-2010-08-303339
98. Born WK, Jin N, Aydintug MK, Wands JM, French JD, Roark CL, et al. γδ T lymphocytes–selectable cells within the innate system? J Clin Immunol (2007) 27:133–44. doi: 10.1007/s10875-007-9077-z
99. Lalor SJ, Dungan LS, Sutton CE, Basdeo SA, Fletcher JM, Mills KHG. Caspase-1–processed cytokines IL-1β and IL-18 promote IL-17 production by γδ and CD4 T cells that mediate autoimmunity. J Immunol (2011) 186:5738 LP – 5748. doi: 10.4049/jimmunol.1003597
100. Passoni L, Hoffman ES, Kim S, Crompton T, Pao W, Dong MQ, et al. Intrathymic delta selection events in gammadelta cell development. Immunity (1997) 7:83–95. doi: 10.1016/s1074-7613(00)80512-9
101. Coffey F, Lee S-Y, Buus TB, Lauritsen J-PH, Wong GW, Joachims ML, et al. The TCR ligand-inducible expression of CD73 marks γδ lineage commitment and a metastable intermediate in effector specification. J Exp Med (2014) 211:329–43. doi: 10.1084/jem.20131540
102. Fahl SP, Coffey F, Wiest DL. Origins of γδ T cell effector subsets: a riddle wrapped in an enigma. J Immunol (2014) 193:4289–94. doi: 10.4049/jimmunol.1401813
103. Malik S, Want MY, Awasthi A. The emerging roles of gamma–delta T cells in tissue inflammation in experimental autoimmune encephalomyelitis. Front Immunol (2016) 7:14. doi: 10.3389/fimmu.2016.00014
104. Legut M, Cole DK, Sewell AK. The promise of γδ T cells and the γδ T cell receptor for cancer immunotherapy. Cell Mol Immunol (2015) 12:656–68. doi: 10.1038/cmi.2015.28
105. Airoldi I, Bertaina A, Prigione I, Zorzoli A, Pagliara D, Cocco C, et al. γδ T-cell reconstitution after HLA-haploidentical hematopoietic transplantation depleted of TCR-αβ+/CD19+ lymphocytes. Blood (2015) 125:2349–58. doi: 10.1182/blood-2014-09-599423
106. Fournié J-J, Sicard H, Poupot M, Bezombes C, Blanc A, Romagné F, et al. What lessons can be learned from γδ T cell-based cancer immunotherapy trials? Cell Mol Immunol (2013) 10:35–41. doi: 10.1038/cmi.2012.39
107. Sugie T, Murata-Hirai K, Iwasaki M, Morita CT, Li W, Okamura H, et al. Zoledronic acid-induced expansion of γδ T cells from early-stage breast cancer patients: Effect of IL-18 on helper NK cells. Cancer Immunol Immunother (2013) 62:677—87. doi: 10.1007/s00262-012-1368-4
108. Deniger DC, Moyes JS, Cooper LJN. Clinical applications of gamma delta T cells with multivalent immunity. Front Immunol (2014) 5:636. doi: 10.3389/fimmu.2014.00636
109. Kong X, Sun R, Chen Y, Wei H, Tian Z. γδT cells drive myeloid-derived suppressor cell-mediated CD8+ T cell exhaustion in hepatitis b virus-induced immunotolerance. J Immunol (2014) 193:1645–53. doi: 10.4049/jimmunol.1303432
110. Qu P, Wang L-Z, Lin PC. Expansion and functions of myeloid-derived suppressor cells in the tumor microenvironment. Cancer Lett (2016) 380:253–6. doi: 10.1016/j.canlet.2015.10.022
111. Wu P, Wu D, Ni C, Ye J, Chen W, Hu G, et al. γδT17 cells promote the accumulation and expansion of myeloid-derived suppressor cells in human colorectal cancer. Immunity (2014) 40:785–800. doi: 10.1016/j.immuni.2014.03.013
112. Fowler DW, Copier J, Dalgleish AG, Bodman-Smith MD. Zoledronic acid causes γδ T cells to target monocytes and down-modulate inflammatory homing. Immunology (2014) 143:539–49. doi: 10.1111/imm.12331
113. Coffelt SB, Kersten K, Doornebal CW, Weiden J, Vrijland K, Hau C-S, et al. IL-17-producing γδ T cells and neutrophils conspire to promote breast cancer metastasis. Nature (2015) 522:345–8. doi: 10.1038/nature14282
114. Cheng H, Wang Z, Fu L, Xu T. Macrophage polarization in the development and progression of ovarian cancers: An overview. Front Oncol (2019) 9:421. doi: 10.3389/fonc.2019.00421
115. Nowak M, Klink M. The role of tumor-associated macrophages in the progression and chemoresistance of ovarian cancer. Cells (2020) 9:1299. doi: 10.3390/cells9051299
116. Guerriero JL. Macrophages: The road less traveled, changing anticancer therapy. Trends Mol Med (2018) 24:472–89. doi: 10.1016/j.molmed.2018.03.006
117. Li C, Xu X, Wei S, Jiang P, Xue L, Wang J. Tumor-associated macrophages: potential therapeutic strategies and future prospects in cancer. J Immunother Cancer (2021) 9:e001341. doi: 10.1136/jitc-2020-001341
118. Xiang X, Wang J, Lu D, Xu X. Targeting tumor-associated macrophages to synergize tumor immunotherapy. Signal Transduct Target Ther (2021) 6:75. doi: 10.1038/s41392-021-00484-9
119. Kumar V, Donthireddy L, Marvel D, Condamine T, Wang F, Lavilla-Alonso S, et al. Cancer-associated fibroblasts neutralize the anti-tumor effect of CSF1 receptor blockade by inducing PMN-MDSC infiltration of tumors. Cancer Cell (2017) 32:654–668.e5. doi: 10.1016/j.ccell.2017.10.005
120. Bonapace L, Coissieux M-M, Wyckoff J, Mertz KD, Varga Z, Junt T, et al. Cessation of CCL2 inhibition accelerates breast cancer metastasis by promoting angiogenesis. Nature (2014) 515:130–3. doi: 10.1038/nature13862
121. Haraguchi K, Takahashi T, Hiruma K, Kanda Y, Tanaka Y, Ogawa S, et al. Recovery of Valpha24+ NKT cells after hematopoietic stem cell transplantation. Bone Marrow Transplant (2004) 34:595–602. doi: 10.1038/sj.bmt.1704582
122. Bohineust A, Tourret M, Derivry L, Caillat-Zucman S. Mucosal-associated invariant T (MAIT) cells, a new source of universal immune cells for chimeric antigen receptor (CAR)-cell therapy. Bull Cancer (2021) 108:S92–5. doi: 10.1016/j.bulcan.2021.07.003
123. Morandi F, Yazdanifar M, Cocco C, Bertaina A, Airoldi I. Engineering the bridge between innate and adaptive immunity for cancer immunotherapy: Focus on γδ T and NK cells. Cells (2020) 9:1757. doi: 10.3390/cells9081757
124. Vivier E, Ugolini S, Blaise D, Chabannon C, Brossay L. Targeting natural killer cells and natural killer T cells in cancer. Nat Rev Immunol (2012) 12:239–52. doi: 10.1038/nri3174
125. Godfrey DI, Le Nours J, Andrews DM, Uldrich AP, Rossjohn J. Unconventional T cell targets for cancer immunotherapy. Immunity (2018) 48:453–73. doi: 10.1016/j.immuni.2018.03.009
126. Sutton KS, Dasgupta A, McCarty D, Doering CB, Spencer HT. Bioengineering and serum free expansion of blood-derived γδ T cells. Cytotherapy (2016) 18:881–92. doi: 10.1016/j.jcyt.2016.04.001
127. Kitayama S, Zhang R, Liu TY, Ueda N, Iriguchi S, Yasui Y, et al. Cellular adjuvant properties, direct cytotoxicity of re-differentiated Vα24 invariant NKT-like cells from human induced pluripotent stem cells. Stem Cell Rep (2016) 6:213–27. doi: 10.1016/j.stemcr.2016.01.005
128. Wakao H, Yoshikiyo K, Koshimizu U, Furukawa T, Enomoto K, Matsunaga T, et al. Expansion of functional human mucosal-associated invariant T cells via reprogramming to pluripotency and redifferentiation. Cell Stem Cell (2013) 12:546–58. doi: 10.1016/j.stem.2013.03.001
129. Seet CS, He C, Bethune MT, Li S, Chick B, Gschweng EH, et al. Generation of mature T cells from human hematopoietic stem and progenitor cells in artificial thymic organoids. Nat Methods (2017) 14:521–30. doi: 10.1038/nmeth.4237
Keywords: tumor microenvironment (TME), tumor-associated macrophage (TAM), myeloid-derived suppressor cell (MDSC), innate T cell, invariant natural killer T (iNKT) cell, mucosal-associated invariant T (MAIT) cell, gamma delta T (γδT) cell, cell-based immunotherapy
Citation: Li Y-R, Wilson M and Yang L (2022) Target tumor microenvironment by innate T cells. Front. Immunol. 13:999549. doi: 10.3389/fimmu.2022.999549
Received: 21 July 2022; Accepted: 23 September 2022;
Published: 06 October 2022.
Edited by:
Yongsheng Li, Cancer Hospital, Chongqing University, ChinaReviewed by:
Sadiya Parveen, Johns Hopkins University, United StatesCopyright © 2022 Li, Wilson and Yang. This is an open-access article distributed under the terms of the Creative Commons Attribution License (CC BY). The use, distribution or reproduction in other forums is permitted, provided the original author(s) and the copyright owner(s) are credited and that the original publication in this journal is cited, in accordance with accepted academic practice. No use, distribution or reproduction is permitted which does not comply with these terms.
*Correspondence: Lili Yang, bGlsaXlhbmdAdWNsYS5lZHU=
†These authors have contributed equally to this work
Disclaimer: All claims expressed in this article are solely those of the authors and do not necessarily represent those of their affiliated organizations, or those of the publisher, the editors and the reviewers. Any product that may be evaluated in this article or claim that may be made by its manufacturer is not guaranteed or endorsed by the publisher.
Research integrity at Frontiers
Learn more about the work of our research integrity team to safeguard the quality of each article we publish.