- 1Key Laboratory of Aquaculture Nutrition and Feed (Ministry of Agriculture and Rural Affairs) and Key Laboratory of Mariculture (Ministry of Education), Ocean University of China, Qingdao, China
- 2Tongwei Co., Ltd., Chengdu, China
- 3Healthy Aquaculture Key Laboratory of Sichuan Province, Chengdu, China
- 4Laboratory for Marine Fisheries Science and Food Production Processes, Qingdao National Laboratory for Marine Science and Technology, Qingdao, China
In the 21st century, intestinal homeostatic imbalance has emerged as a growing health challenge worldwide. Accumulating evidence reveals that excessive intake of saturated fatty acid (SFA) induces intestinal homeostatic imbalance. However, the potential molecular mechanism is still unclear. In the present study, we found that palm oil or palmitic acid (PA) treatment disturbed lipid metabolism homeostasis and triggered endoplasmic reticulum (ER) stress and inflammation in the intestine or intestinal cells of large yellow croaker (Larimichthys crocea). Interestingly, PA treatment significantly decreased phosphatidylethanolamine (PE) content in the intestinal cells. PE supplementation decreased triglyceride content in the intestinal cells induced by PA treatment by inhibiting fatty acid uptake and lipogenesis. PE supplementation suppressed ER stress. Meanwhile, PE supplementation alleviated inflammatory response through p38 MAPK-p65 pathway, reducing the damage of intestinal cells caused by PA treatment to some extent. Our work revealed that intestinal homeostatic imbalance caused by PA treatment was partly due to the decrease of PE content. PE consumption might be a nutritional strategy to regulate intestinal homeostasis in fish and even human beings.
Introduction
Effective function of intestine is important in regulating physiology, metabolism, and immunity in the whole body (1). Current studies have demonstrated that the intestinal homeostasis is closely related to multiple factors, including genetic mutations, environment, gut microbiota and dietary factors (2). With the rapid urbanization of developed and developing countries, excessive Western diet consumption which is rich in statured fatty acid (SFA) is one of the severe challenges to intestinal homeostasis. Previous study has demonstrated that intake of palmitic acid (PA) could induce inflammatory cytokine production (3) and lipid metabolism disorder (4) in the intestinal cells. However, the underlying mechanism is not well understood.
Phospholipid is not only an essential component of intestine, but also an important signal molecule, which is involved in maintaining metabolic and immune homeostasis (5). Increasing studies have demonstrated that phospholipid treatment inhibited proinflammatory gene expression via direct inhibition of NF-κB in intestinal epithelial cells (6, 7). Moreover, clinical study has revealed that phospholipid supplementation could alleviate inflammatory response in those patients with ulcerative colitis (8). Ethanolamine which is the base constituent of phosphatidylethanolamine (PE) is required for the intestinal development and promotes intestinal functions (9). Our previous study has found that PA treatment induced adverse effects that might be associated with PE content in macrophage (10), which is one of the most abundant phospholipid in cells (11). Thus, we hypothesized that PE metabolism may be involved in PA-induced intestinal homeostatic imbalance.
Fish are the largest group of vertebrates in the world (12). Although fish are less evolved than mammals, the nutrient-sensing and immunity are conservated to some extent (13). In aquaculture, different nutritional components can influence fish health by affecting the intestinal homeostasis (14–16), while the potential molecular mechanisms are still poorly understood. The method of isolating and culturing intestinal cells of fish are mature (17). Therefore, fish are good model animals to study the pathogenesis of intestinal homeostatic imbalance (12, 18). Accordingly, the present study investigated the mechanism of PA on lipid metabolism and immune homeostasis in the intestine and aimed to develop nutritional strategies to promote intestinal homeostasis of fish and human beings.
Materials and methods
Animal ethics
All animal experiments in the present were carried out in strict standard operation with the Management Rule of Laboratory Animals (Chinese Order No. 676 of the State Council, revised March 1, 2017).
Diet formulation, fish culture and sample collection
Two diets were formulated in the present study as follows: a fish oil diet (FO) and a palm oil diet (PO) (Table 1) (19). Healthy juvenile large yellow croaker (average weight 15.8 ± 0.14 g) was obtained from Ningde, China. After two weeks of domestication, total fish were randomly divided into two groups (three seawater cages in each group). Fish were fed twice a day for 10 weeks. At end of the feeding trial, fish were anaesthetized with MS-222 (Sigma, USA), then intestines of fish were collected for further study.
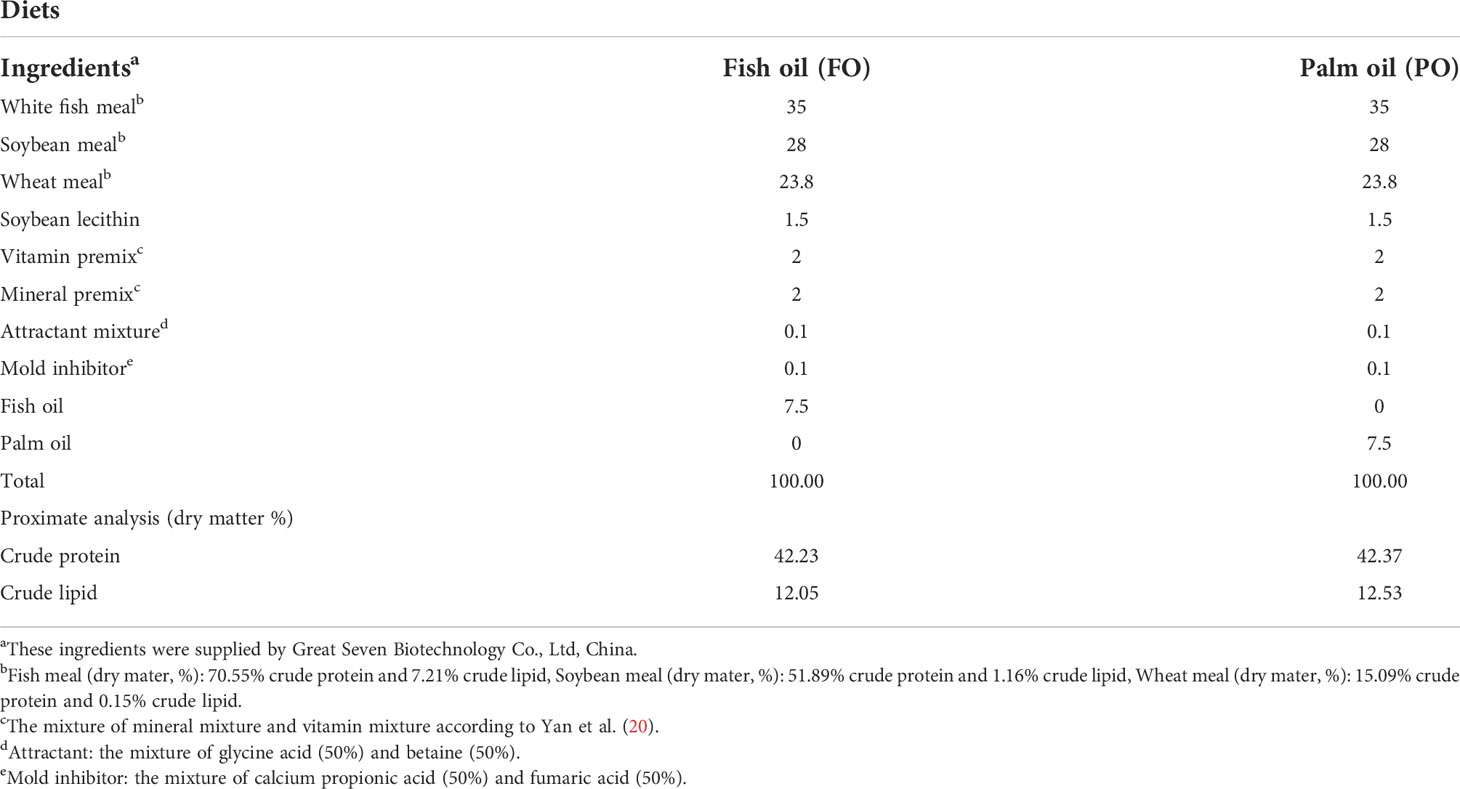
Table 1 Formulation and proximate composition of experimental diets (% dry weight) (19).
Cell culture and treatment
Intestinal cells were isolated and cultured in the six-well plates in DMEM/F12 medium with 15% fetal bovine serum (BI, Israel) at 27°C and 5% CO2 atmosphere, according to our previous study (17). To investigate the effects of PA on lipid metabolism, ER stress and inflammation in the intestinal cells, cells were treated with 100 μM PA (Sigma, USA) for 24h. To prove the function of PE in the intestinal cells, cells were co-treated with 10uM PE (Sigma) and 100uM PA for 24h.
Detection of triglyceride and PE content
The content of TG in the intestine and intestinal cells were measured by a commercial kit (Applygen, China), according to our previous study (21). Meanwhile, the content of PE was measured using an ELISA kit (Fankew, China), according to manufacturer’s instructions.
Gene expression quantification
Intestine and cell samples were processed for total RNA extraction using TRIzol reagent (Takara, Japan). Genomic DNA was removed at 42 °C for 2 min using the PrimeScript™ RT reagent kit (Takara), and then cDNA was synthesized. The program of cDNA synthesis consisted of 37°C for 15 min and 85°C for 5 s. Relative gene expression was performed with quantitative real-time polymerase chain reaction (qRT-PCR) using SYBR kit (Takara) and calculated by the method according to a previous study (22). β-actin was used as the internal reference gene. The primers for qRT-PCR were listed in the Table 2.
Western blotting analysis
Intestine and cell samples were homogenized on ice using RIPA lysis buffer (Solarbio, China). Then the homogenate was centrifuged at 4°C for 10 min to obtain supernatant. The protein supernatant was separated on 10% SDS-PAGE and transferred into PVDF membranes. Membranes were blocked with 5% skimmed milk and incubated with specific primary antibodies at 4°C overnight. The primary antibodies were listed in the Table S1. After that, the membranes were incubated with secondary antibody (Golden Bridge, China) and visualized by using an electrochemiluminescence kit (Beyotime, China).
Data analysis
All data in the present study were performed with SPSS 17.0 software (IBM, USA) by using independent sample t-test or one-way analysis of variance (ANOVA) followed by Tukey’s test. All data were expressed as mean ± standard deviation (SD). The level of significance was set at P < 0.05.
Results
Effect of PO or PA treatment on lipid metabolism in the intestine or intestinal cells
Compared to the control group, the content of TG in the intestine was significantly higher in the PO group (Figure 1A). The mRNA expression of fatty acid uptake-related genes in the intestine was significantly increased in the PO group, including cd36, fatp1, fabp2 and fabp3 (Figure 1B). Dietary PO significantly up-regulated the mRNA expression of lipid synthesis-related genes (Figure 1C). Meanwhile, the mRNA expression of chylomicron assembly and secretion-related genes, including apob48, sar1b, and sec23 was basically up-regulated in the PO group (Figure S1A). Dietary PO significantly increased the mRNA level of aco which plays a vital role in fatty acid β-oxidation (Figure S1B). Moreover, the protein levels of CD36, cleavage of SREBP1c, SAR1B, CPT1α and PPARα in the PO group were significantly higher than those in the control group (Figure 1D and Figure S1C), while the protein levels of APOB48 and SEC13 were not remarkably changed (Figure S1C). Thus, dietary PO induced intestinal abnormal lipid accumulation in large yellow croaker through disturbing intestinal lipid homeostasis.
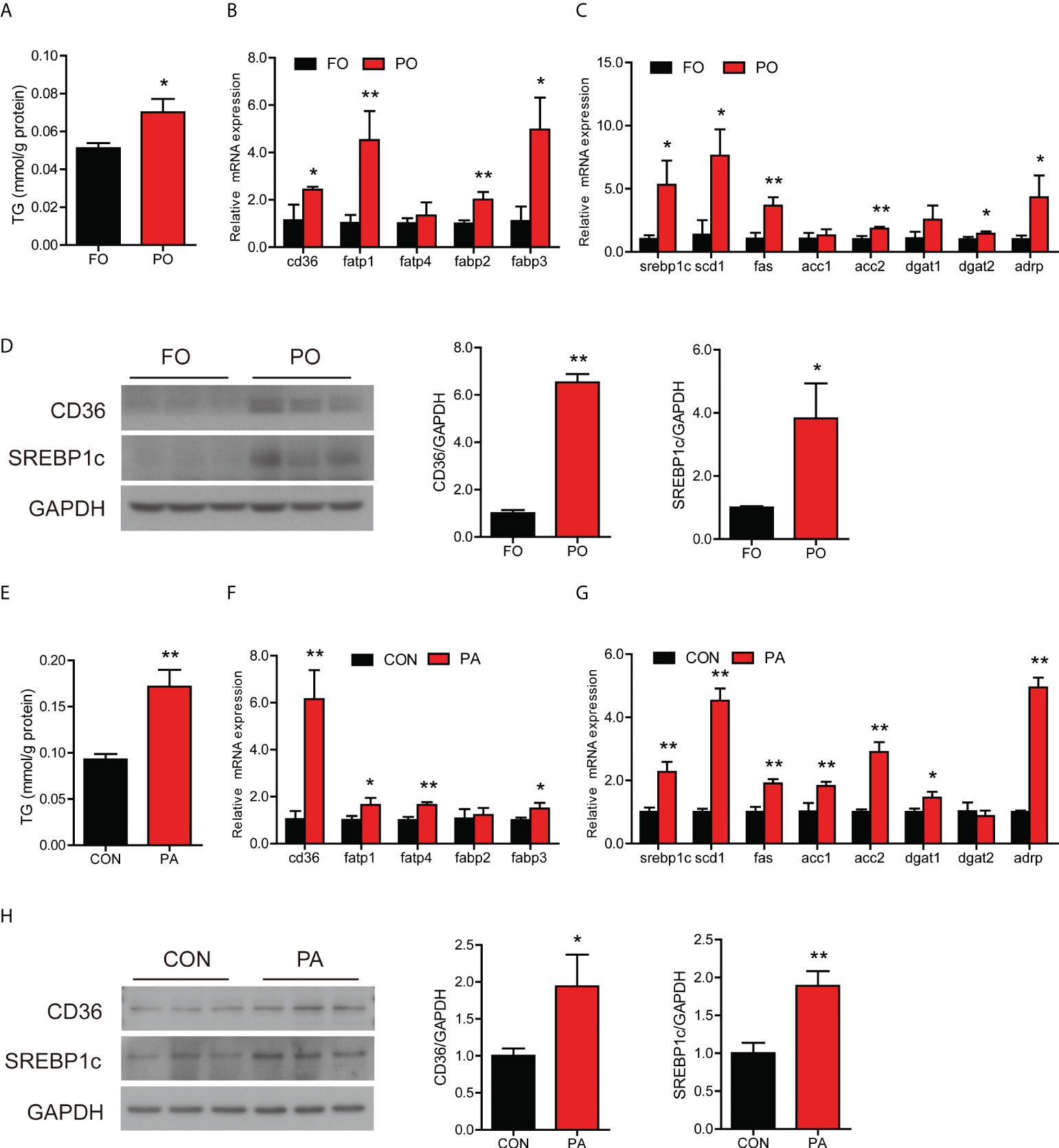
Figure 1 Effect of PO or PA treatment on lipid metabolism in the intestine or intestinal cells of large yellow croaker. (A) TG content in the intestine after different diets (n = 3). (B) Fatty acid uptake and (C) lipid synthesis-related genes expression in the intestine after different diets (n = 3). (D) Protein levels of CD36 and cleavage of SREBP1c in the intestine after different diets (n = 3). (E) TG content in the intestinal cells after BSA or PA treatment (n = 3). (F) Fatty acid uptake and (G) lipid synthesis-related genes expression in the intestinal cells after BSA or PA treatment (n = 3). (H) Protein levels of CD36 and cleavage of SREBP1c in the intestinal cells after BSA or PA treatment (n = 3). Results were presented as mean ± standard deviation (SD) and analyzed using independent t-test (*P < 0.05, **P < 0.01). FO, fish oil; PO, palm oil; CON, bovine serum albumin treatment; PA, palmitic acid treatment.
To further investigate whether PO disturbed intestinal lipid metabolism, we treated the intestinal cells with PA in vitro experiment. PA treatment significantly induced TG accumulation in cells (Figure 1E). The mRNA expression of cd36, fatp1, fatp4 and fabp3 was significantly increased in the PA group than the control group (Figure 1F), which suggested that PA treatment promoted fatty acid uptake in cells. Meanwhile, the transcription of genes related to lipid synthesis was significantly increased after PA treatment (Figure 1G). In terms of lipid secretion, the gene expression showed an upward trend after PA treatment (Figure S1D). In addition, PA treatment significantly upregulated the gene expression involved in fatty acid β-oxidation, including pparα, aco and cpt1α (Figure S1E). Moreover, the protein levels of CD36, cleavage of SREBP1c, APOB48, CPT1α and PPARα were significantly increased after PA treatment (Figure 1H, Figure S1F). These results above indicated that PO or PA treatment induced abnormal lipid accumulation in the intestine or intestinal cells of large yellow croaker through disturbing lipid homeostasis.
Effect of PO or PA treatment on ER stress in the intestine or intestinal cells
The transcription of grp78, xbp1, atf4, atf6 and chop was higher in the PO group (Figure 2A). Moreover, PO replacement significantly upregulated the protein level of GRP78 compared to the control group (Figure 2B).
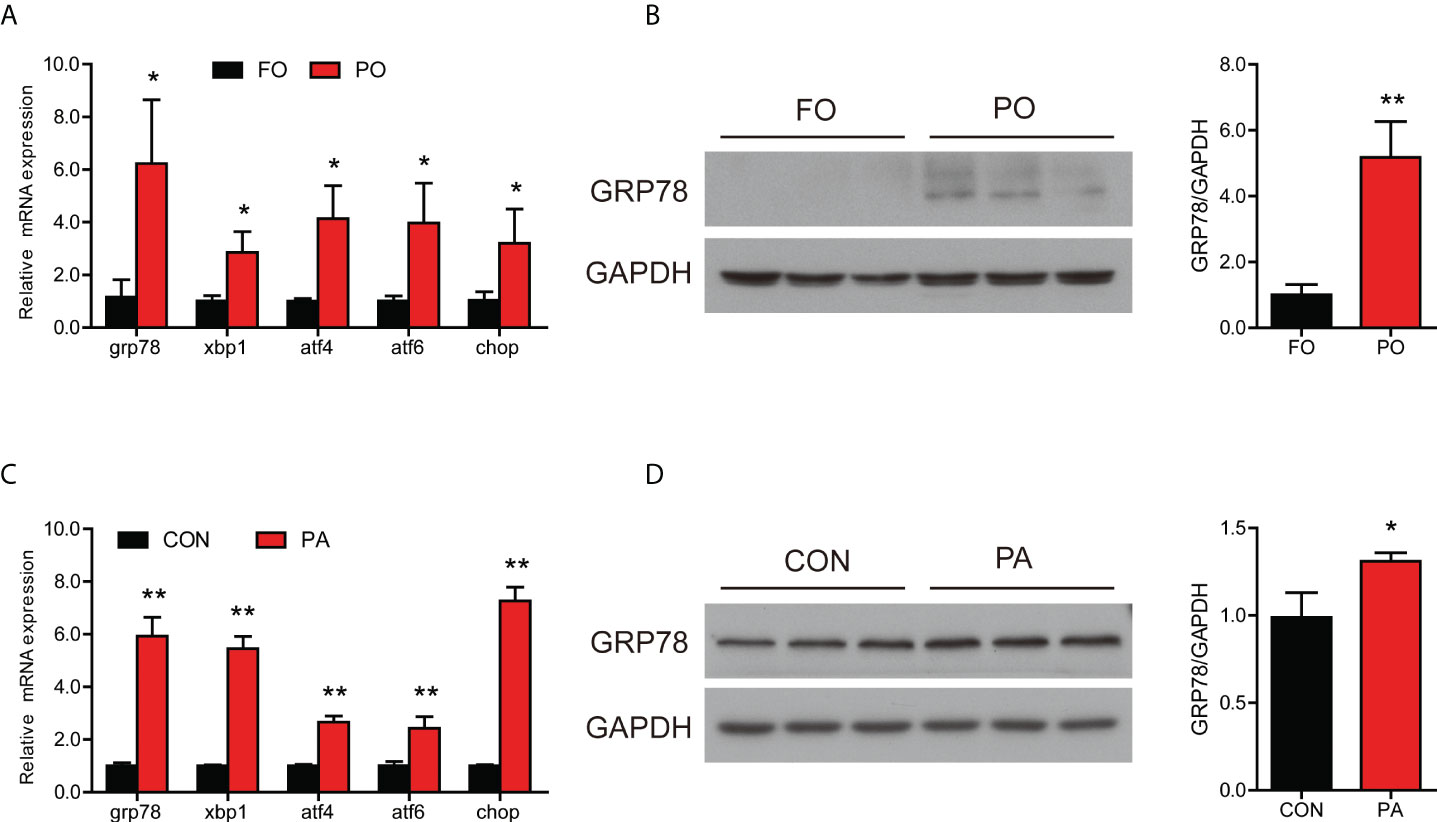
Figure 2 Effect of PO or PA treatment on ER stress and UPR pathway in the intestine or intestinal cells of large yellow croaker. (A) Relative mRNA expression of ER stress-related genes in the intestine after different diets (n = 3). (B) Protein level of GRP78 in the intestine after different diets (n = 3). (C) Relative mRNA expression of ER stress-related genes in the intestinal cells after BSA or PA treatment (n = 3). (D) Protein level of GRP78 in the intestinal cells after BSA or PA treatment (n = 3). Results were presented as mean ± standard deviation (SD) and analyzed using independent t-test (*P < 0.05, **P < 0.01). FO, fish oil; PO, palm oil; CON, bovine serum albumin treatment; PA, palmitic acid treatment.
Consistent with experiments in vivo, incubation of intestinal cells with PA significantly increased the transcription of grp78, xbp1, atf4, atf6 and chop (Figure 2C). Moreover, the protein level of GRP78 was significantly increased after PA treatment (Figure 2D). These results suggested that PO or PA treatment induced ER stress in the intestine or intestinal cells of large yellow croaker.
Effect of PO or PA treatment on inflammation in the intestine or intestinal cells
The mRNA level of il-1β was significantly higher and the gene expression of il-6, il-8 and cox2 followed an upward trend in the PO group compared to the FO group (Figure 3A). We further examined the protein levels involved in inflammation and MAPK pathway. Dietary PO significantly upregulated the ratio of p38 MAPK to MAPK and decreased the ratio of p-ERK1/2 to ERK1/2 (Figure 3B). Meanwhile, the protein level of nuclear p65 was significantly increased in the PO group (Figure 3C).
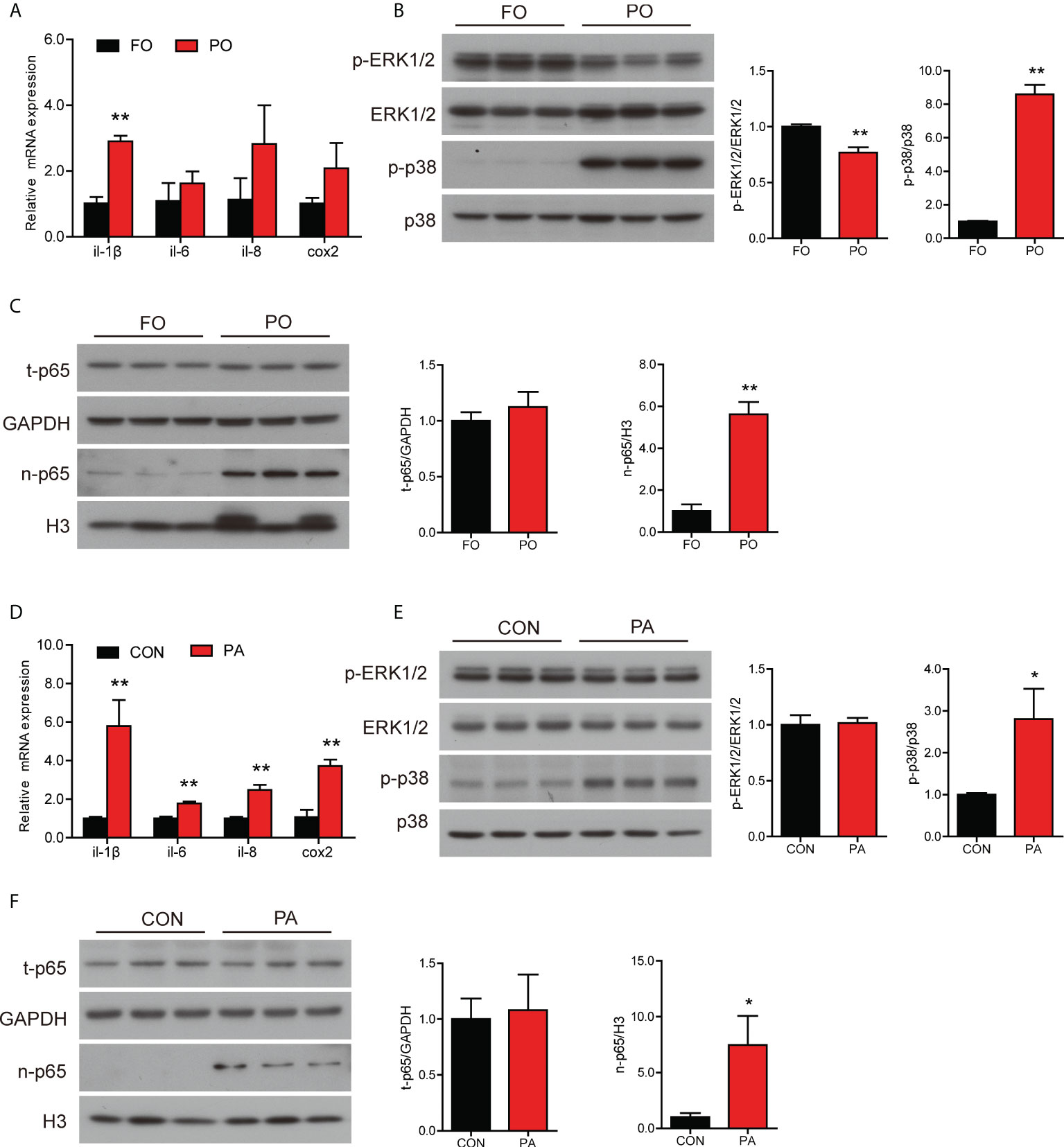
Figure 3 Effect of PO or PA treatment on inflammation in the intestine or intestinal cells of large yellow croaker. (A) Relative mRNA expression of proinflammatory genes in the intestine after different diets (n = 3). (B) Protein levels of p-ERK1/2, ERK1/2, p-p38 MAPK and p38 MAPK in the intestine after different diets (n = 3). (C) Protein levels of total p65 and nuclear p65 in the intestine after different diets. (D) Relative mRNA expression of proinflammatory genes in the intestinal cells after BSA or PA treatment (n = 3). (E) Protein levels of p-ERK1/2, ERK1/2, p-p38 MAPK and p38 MAPK in the intestinal cells after BSA or PA treatment (n = 3). (F) Protein levels of total p65 and nuclear p65 in the intestinal cells after BSA or PA treatment (n = 3). Results were presented as mean ± standard deviation (SD) and analyzed using independent t-test (*P < 0.05, **P < 0.01). FO, fish oil; PO, palm oil; CON, bovine serum albumin treatment; PA, palmitic acid treatment.
Next, we further measured the gene and protein levels related to inflammation in the intestinal cells with PA treatment. The transcription of proinflammation genes was significantly higher in the PA group, including il-1β, il-6, il-8 and cox2 (Figure 3D). Results of western blotting analysis were similar to those in vivo. The ratio of p38 MAPK to MAPK was significantly higher after PA treatment, while the ratio of p-ERK1/2 to ERK1/2 was not remarkably changed (Figure 3E). Moreover, the protein level of nuclear p65 was significantly rose in the PA group (Figure 3F). Thus, these results showed that PO or PA treatment induced inflammation in the intestine or intestinal cells of large yellow croaker.
Effect of PO or PA treatment on the content of PE in the intestine or intestinal cells
Our previous had found that PA-induced inflammation in macrophage might be related to the decrease of PE content (10). Next, we measured the content of PE in the intestinal cells in vivo and in vitro. The content of PE was significantly decreased in the PO group (Figure 4A), while the expression of genes related to PE synthesis were significantly upregulated, including etnk1, pcyt2 and pisd (Figure 4B). Consistence with in vivo results, PA treatment significantly reduced the level of PE in the intestinal cells (Figure 4C), while the gene expression of etnk1, etnk2, pcyt2, selenoi and pisd were significantly upregulated (Figure 4D). These results above indicated that PO or PA treatment decreased the content of PE in the intestine or intestinal cells of large yellow croaker.
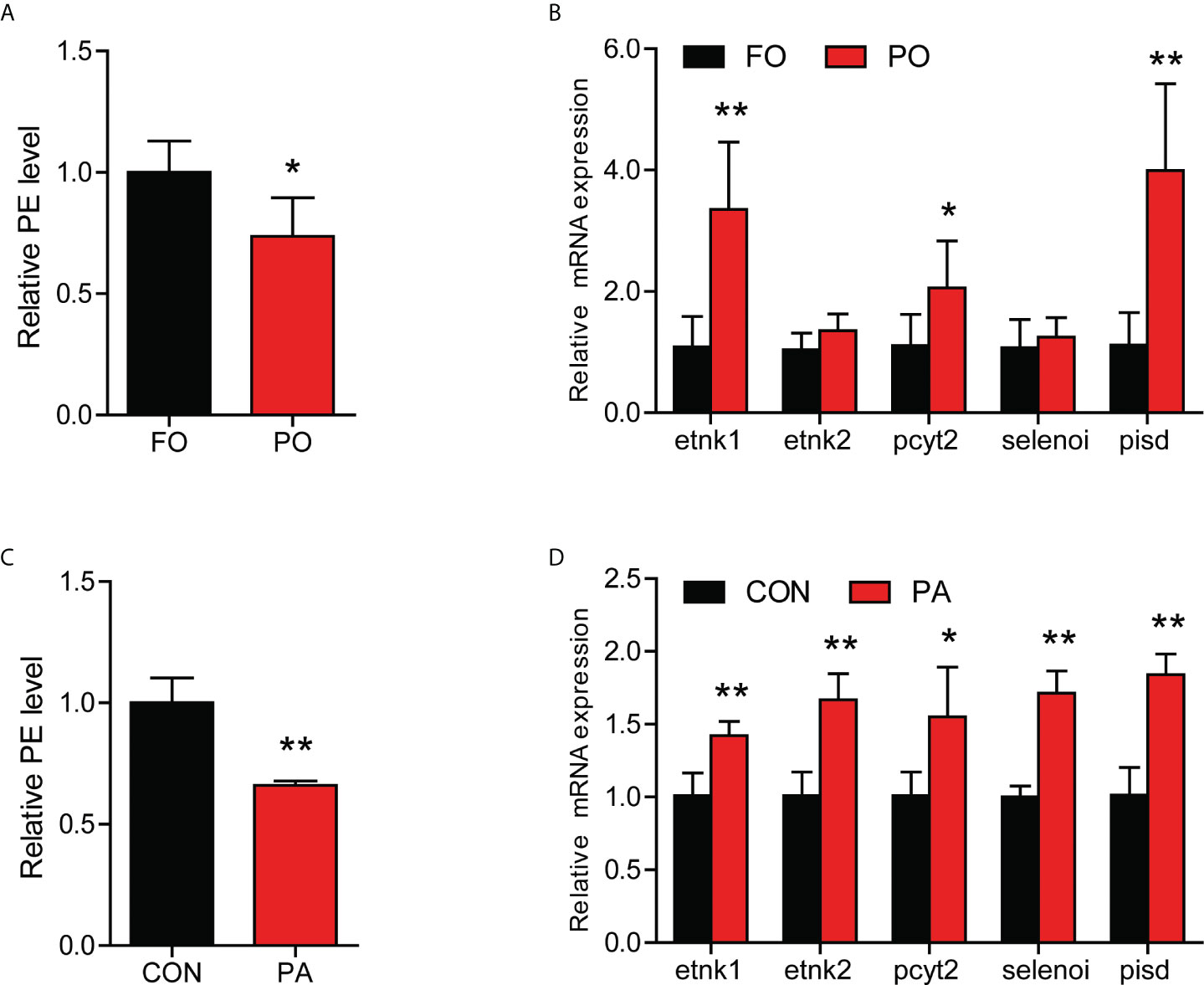
Figure 4 Effect of PO or PA treatment on the content of PE in the intestine or intestinal cells of large yellow croaker. (A) PE content in the intestine after different diets (n = 3). (B) Relative mRNA expression of PE synthesis related-genes in the intestine after different diets (n = 3). (C) PE content in the intestinal cells after BSA or PA treatment (n = 3). (D) Relative mRNA expression of PE synthesis related-genes in the intestinal cells after BSA or PA treatment (n = 3). Results were presented as mean ± standard deviation (SD) and analyzed using independent t-test (*P < 0.05, **P < 0.01). FO, fish oil; PO, palm oil; CON, bovine serum albumin treatment; PA, palmitic acid treatment.
Addition of PE alleviated the damage of intestinal cells caused by PA treatment to some extent
We speculated that PA treatment might disorder lipid metabolism and immune homeostasis by affecting the content of PE in the intestinal cells. To confirm the hypothesis intestinal cells were co-treatment with PA and PE. Compared to PA group, addition of PE significantly decreased the content of TG in the intestinal cells (Figure 5A). The transcription of fatty acid uptake-related genes was down-regulated in the PA+PE group, including cd36 and fabp3 (Figure 5B). The mRNA expression of lipid synthesis-related genes was down-regulated in the PA+PE group, including srebp1c, scd1, fas, acc1, acc2, dgat2 and adrp (Figure 5C). We also found that PA+PE treatment significantly decreased the gene expression of lipid secretion and β-oxidation (Figures S2A, B). Moreover, the protein levels of CD36, cleavage of SREBP1c, APOB48 were significantly decreased in the PA+PE group compared to PA group (Figure 5D and Figure S2C). However, the protein levels of SAR1B, SEC13, CPT1α and PPARα were not remarkably changed (Figure S2C). In addition, we also found that PE supplementation significantly decreased the mRNA levels of genes related to ER stress, including xbp1, atf4, atf6 and chop (Figure 5E). Moreover, compared to the PA group, the protein level of GRP78 was significantly decreased in the PA+PE group (Figure 5F). In term to inflammation, addition of PE could down-regulate proinflammation gene expression including il-1β and cox2 (Figure 5G). Compared to the PA group, the protein levels of p-P38 MAPK and nuclear p65 were significantly decreased in the PA+PE group (Figures 5H, I). Thus, these results indicated that addition of PE could alleviate the damage of intestinal cells induced by PA treatment to some extent.
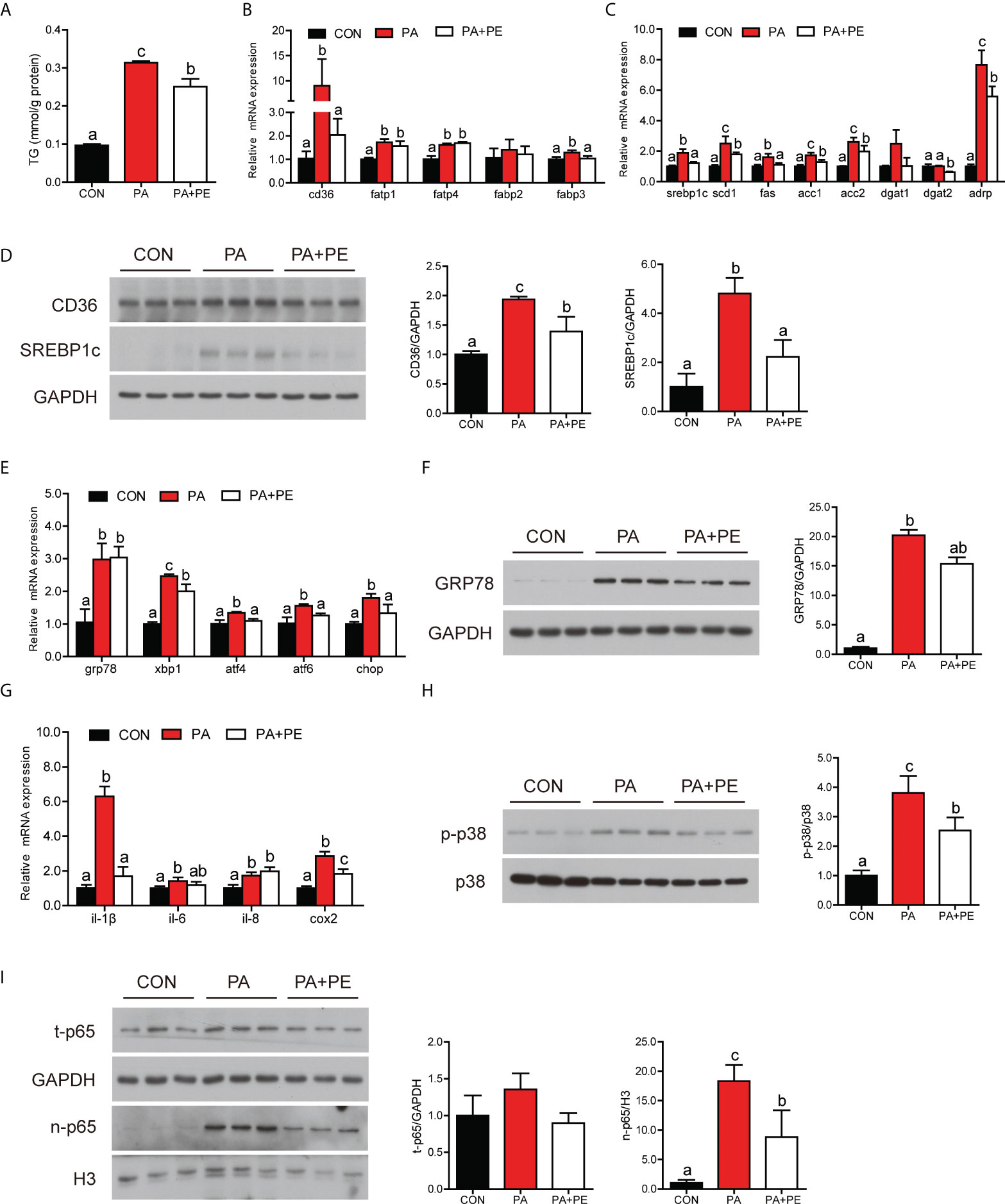
Figure 5 The addition of PE alleviated the damage of intestine cells caused by PA treatment to some extent. (A) TG content in the intestinal cells after different treatments (n = 3). (B) Fatty acid uptake and (C) lipid synthesis-related genes expression in the intestinal cells after different treatments (n = 3). (D) Protein levels of CD36 and cleavage of SREBP1c in the intestinal cells after different treatments (n = 3). (E) Relative mRNA expression of ER stress-related genes in the intestinal cells after different treatments (n = 3). (F) Protein level of GRP78 in the intestinal cells after different treatments (n = 3). (G) Relative mRNA expression of proinflammatory genes in the intestinal cells after different treatments (n = 3). (H) Protein levels of p-p38 MAPK and p38 MAPK in the intestinal cells after different treatments (n = 3). (I) Protein levels of total p65 and nuclear p65 in the intestinal cells after different treatments (n = 3). Results were presented as mean ± standard deviation (SD) and analyzed using one-way analysis of variance (ANOVA) followed by Tukey’s test (values without the same letter indicate significant difference among three treatments P < 0.05). CON, bovine serum albumin treatment; PA, palmitic acid treatment; PA+PE, palmitic acid and phosphatidylethanolamine co-treatment.
Discussion
Excessive dietary SFA consumption is linked to many metabolic diseases, including nonalcoholic fatty liver disease (23), atherosclerosis (24), and type 2 diabetes (25). However, the mechanism of SFA in the intestinal lipid metabolism and immune homeostasis is not clear. In the present study, we found that dietary PO induced intestinal abnormal lipid accumulation in large yellow croaker through disturbed the intestinal lipid metabolism homeostasis. The lipid metabolism in the intestine of fish is similar to that of mammals, including dietary fatty acid uptake, de novo lipogenesis, CM secretion and fatty acid β-oxidation (26). The gene expression of fatty acids uptake was increased in the PO group, which indicated that dietary PO induced massive fatty acids and monoacylglycerol (MAG) to be absorbed into the intestine. Fatty acids were transported to ER and reconverted to TG. As expected, the gene and protein levels of lipid synthesis was significantly increased in the PO group. Thus, we speculated that dietary fatty acid uptake and de novo lipogenesis may be the main factors which induced intestinal abnormal lipid accumulation. These results were consistent with previous study that overfeeding palm oil promoted visceral and hepatic fat storage (27). However, dietary PO also improved chylomicron secretion and β-oxidation in the intestine, which may be the self-regulation of the intestine to decrease excessive lipid accumulation. The data was consistent with previous study that β-oxidation was higher in the chicken fed with a PO diet than those Fed with a FO diet (28). Consistent with the results in vivo, PA treatment disturbed lipid metabolism in the intestinal cells. Overall, these results showed that dietary PO disturbed the balance of intestinal lipid metabolism, resulting in excessive intestinal lipid deposition in large yellow croaker.
The ER is a critical site of lipid metabolism (29). Our previous study has demonstrated that the impairment of ER function disordered intestinal lipid metabolism in large yellow croaker (17). Thus, we speculated that dietary PO might affect ER homeostasis in the intestine. In this study, the data demonstrated that PO or PA treatment induced ER stress in vivo or in vitro, which were consistent with previous studies in mammals (30–32). Accumulating studies have suggested that excessive SFA accumulation in the ER could affect calcium homeostasis of ER leading to destroy folding capacity of ER (33, 34). Moreover, SFA increases production of metabolic intermediates like ceramides (35) and reactive oxygen species (36) which induce ER stress via disruption of ER structure and function resulting in ER stress. The mechanism of PO or PA treatment induced ER stress in the intestine or intestinal cells needs to be further studied.
Previous studies have shown that excessive lipid accumulation and ER stress are usually accompanied by inflammatory response (37, 38). Excessive production of proinflammatory cytokines is the main cause of intestinal injury. In the present study, we found that dietary PO enhanced the nuclear p65 level and proinflammation gene expression, which was consistent with previous study in mammals (39). Next, we measured the expression of the MAPK pathway, which is known as a crucial mediator of inflammation (40). The ratio of p-p38 MAPK to p38 MAPK was increased in the PO group, while the phosphorylation level of ERK1/2 was decreased. The obtained results in vitro were almost consistent with those in vivo. Previous studies have demonstrated that p38 MAPK was necessary to regulate p65 and induced proinflammation gene expression (41, 42). Thus, we speculated that PO might induce intestinal inflammation via p38 MAPK-p65 pathway in large yellow croaker.
Our previous study has found that PA treatment significantly decreased the content of PE in the macrophage cells (10). PE is the second most abundant phospholipid in mammal cells, which is not only simple component of the membrane (43), but also essential for many cellular processes such as protein folding (44), autophagy (45), and oxidative phosphorylation (46). Thus, we hypothesized that PA disordered the lipid metabolism and immune homeostasis may be involved in the content of PE in the intestinal cells. In the present study, we found that PO or PA treatment significantly decreased the content of PE in vivo or in vitro. However, the PE synthesis related genes expression was increased, which may be negative feedback for the decrease of PE content. Previous study has demonstrated that elimination of the CDP-ethanolamine pathway which is the main pathway to form PE triggers fatty acid synthesis, leading to liver steatosis (47). As we expected, incubation with PE could alleviate the TG abnormal accumulation induced by PA treatment to some extent through decreasing fatty acid uptake and lipogenesis. We also found that addition of PE down-regulated gene expression related to UPR pathway, leading to relieve ER stress. The result was consistence with the previous study that inhibiting the PERK-CHOP signaling pathway to protect cells from ER stress-induced damage (48). Moreover, PE supplementation could inhibit inflammation induced by PA treatment. Similarly, dietary eicosapentaenoic acid in the form of PE chronic inflammation via the inhibition of NF-κB activation in obese adipose tissue (49). Concomitant with the above previous report, the present study indicated that addition of PE decreased the levels of proinflammation genes via inhibiting the p38 MAPK-p65 pathway. Moreover, a recent study has found that PE metabolism affected TFH cell differentiation and humoral immunity (50). Thus, PE intake might be a nutritional strategy to regulate intestinal homeostasis in fish and even human beings.
In conclusion, for the first time, the present study demonstrated that PA treatment induced lipid metabolism disorder, ER stress and inflammation in the intestine, which was associated with decreasing the content of PE. Addition of PE could alleviate the damage of intestinal cells caused by PA treatment to some extent (Figure 6). PE consumption might be a nutritional strategy to reduce the use of drugs in aquaculture regulating intestinal homeostasis in fish, which contributes to the production of green and safe food. In addition, the key genes of PE metabolism might be targets to maintain intestinal health of human beings.
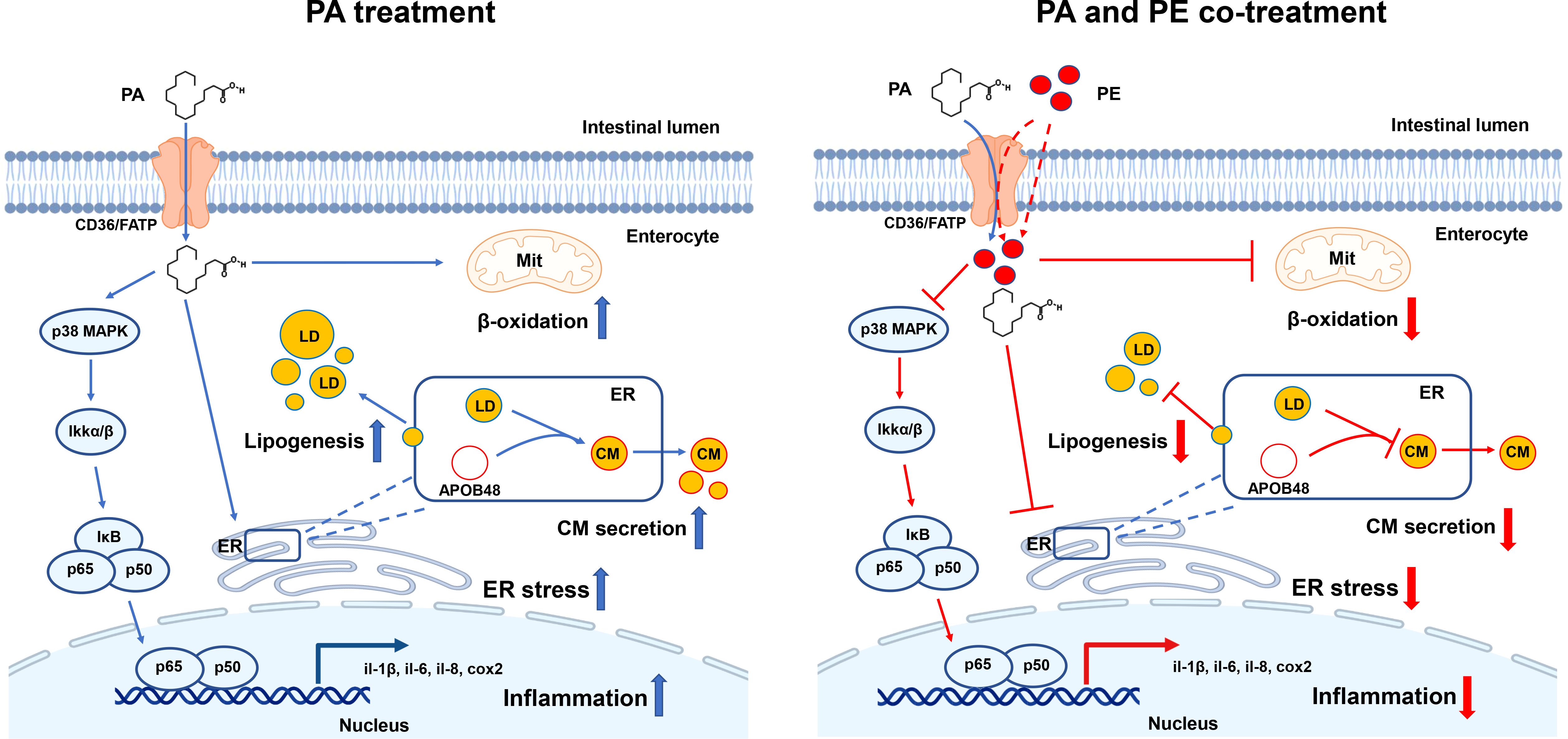
Figure 6 A working model showed that PA treatment disrupted lipid metabolism homeostasis and triggered ER stress and inflammation in the intestinal cells of large yellow croaker, while addition of PE could alleviate the damage of intestinal cells induced by PA treatment to some extent.
Data availability statement
The original contributions presented in the study are included in the article/Supplementary Material. Further inquiries can be directed to the corresponding author.
Ethics statement
The animal study was reviewed and approved by the Management Rule of Laboratory Animals (Chinese Order No. 676 of the State Council, revised March 1, 2017).
Author contributions
WF, and QA designed the experiments. WF performed the main experiments and wrote the original draft. YL, QC, and XC conducted other experiments. DX, and QL. analyzed the data. LZ, TH, and KM revised the manuscript. All authors contributed to the final editing and approval of the manuscript.
Funding
This research is supported by the Key Program of National Natural Science Foundation of China (Grant no.31830103), the earmarked fund for CARS-47, and the Scientific and Technological Innovation of Blue Granary (grant no. 2018YFD0900402).
Acknowledgments
We thank Jikang Shentu, Lin Huang and Shengwei Xu for providing experimental animals and facilities. We also appreciate Jianlong Du, Jiamin Li, Xiaojun Xiang, Xueshan Li for providing antibodies.
Conflict of interest
Author LZ was employed by company Tongwei Co., Ltd.
The remaining authors declare that the research was conducted in the absence of any commercial or financial relationships that could be construed as a potential conflict of interest.
Publisher’s note
All claims expressed in this article are solely those of the authors and do not necessarily represent those of their affiliated organizations, or those of the publisher, the editors and the reviewers. Any product that may be evaluated in this article, or claim that may be made by its manufacturer, is not guaranteed or endorsed by the publisher.
Supplementary material
The Supplementary Material for this article can be found online at: https://www.frontiersin.org/articles/10.3389/fimmu.2022.984508/full#supplementary-material
Glossary
References
1. Pluske JR, Turpin DL, Kim JC. Gastrointestinal tract (gut) health in the young pig. Anim Nutr (2018) 4:187–96. doi: 10.1016/j.aninu.2017.12.004
2. Huang LJ, Mao XT, Li YY, Liu DD, Fan KQ, Liu RB, et al. Multiomics analyses reveal a critical role of selenium in controlling T cell differentiation in crohn's disease. Immunity (2021) 54:1728–44.e1727. doi: 10.1016/j.immuni.2021.07.004
3. Ghezzal S, Postal BG, Quevrain E, Brot L, Seksik P, Leturque A, et al. Palmitic acid damages gut epithelium integrity and initiates inflammatory cytokine production. BBA-Mol Cell Biol L (2020) 1865:158530. doi: 10.1016/j.bbalip.2019.158530
4. Field FJ, Born E, Mathur SN. Fatty acid flux suppresses fatty acid synthesis in hamster intestine independently of SREBP-1 expression. J Lipid Res (2003) 44:1199–208. doi: 10.1194/jlr.M300013-JLR200
5. Kennelly JP, van der Veen JN, Nelson RC, Leonard KA, Havinga R, Buteau J, et al. Intestinal de novo phosphatidylcholine synthesis is required for dietary lipid absorption and metabolic homeostasis. J Lipid Res (2018) 59:1695–708. doi: 10.1194/jlr.M087056
6. Treede I, Braun A, Jeliaskova P, Giese T, Füllekrug J, Griffiths G, et al. TNF-alpha-induced up-regulation of pro-inflammatory cytokines is reduced by phosphatidylcholine in intestinal epithelial cells. BMC Gastroenterol (2009) 9:53. doi: 10.1186/1471-230X-9-53
7. Treede I, Braun A, Sparla R, Kühnel M, Giese T, Turner JR, et al. Anti-inflammatory effects of phosphatidylcholine. J Biol Chem (2007) 282:27155–64. doi: 10.1074/jbc.M704408200
8. Stremmel W, Merle U, Zahn A, Autschbach F, Hinz U, Ehehalt R. Retarded release phosphatidylcholine benefits patients with chronic active ulcerative colitis. Gut (2005) 54:966–71. doi: 10.1136/gut.2004.052316
9. Zhou J, Xiong X, Wang KX, Zou LJ, Ji P, Yin YL. Ethanolamine enhances intestinal functions by altering gut microbiome and mucosal anti-stress capacity in weaned rats. Brit J Nutr (2018) 120:241–9. doi: 10.1017/S0007114518001101
10. Zhang J. Mechanism of endoplasmic reticulum stress regulating inflammatory response and lipid metabolism in large yellow croaker (Larimichthys crocea). Qingdao, China: Ocean University of China Doctor Thesis, Ocean University of China, 2019. (2020).
11. van der Veen JN, Kennelly JP, Wan S, Vance JE, Vance DE, Jacobs RL. The critical role of phosphatidylcholine and phosphatidylethanolamine metabolism in health and disease. BBA-Biomembranes (2017) 1859:1558–72. doi: 10.1016/j.bbamem.2017.04.006
12. Ling SC, Wu K, Zhang DG, Luo Z. Endoplasmic reticulum stress-mediated autophagy and apoptosis alleviate dietary fat-induced triglyceride accumulation in the intestine and in isolated intestinal epithelial cells of yellow catfish. J Nutr (2019) 149:1732–41. doi: 10.1093/jn/nxz135
13. Hotamisligil GS. Inflammation and metabolic disorders. Nature (2006) 444:860–7. doi: 10.1038/nature05485
14. You C, Chen B, Wang M, Wang S, Zhang M, Sun Z, et al. Effects of dietary lipid sources on the intestinal microbiome and health of golden pompano (Trachinotus ovatus). Fish Shellfish Immunol (2019) 89:187–97. doi: 10.1016/j.fsi.2019.03.060
15. Yu C, Zhang J, Qin Q, Liu J, Xu J, Xu W. Berberine improved intestinal barrier function by modulating the intestinal microbiota in blunt snout bream (Megalobrama amblycephala) under dietary high-fat and high-carbohydrate stress. Fish Shellfish Immunol (2020) 102:336–49. doi: 10.1016/j.fsi.2020.04.052
16. Zahran E, El Sebaei MG, Awadin W, Elbahnaswy S, Risha E, Elseady Y. Withania somnifera dietary supplementation improves lipid profile, intestinal histomorphology in healthy Nile tilapia (Oreochromis niloticus), and modulates cytokines response to streptococcus infection. Fish Shellfish Immunol (2020) 106:133–41. doi: 10.1016/j.fsi.2020.07.056
17. Fang W, Chen Q, Li J, Liu Y, Zhao Z, Shen Y, et al. Endoplasmic reticulum stress disturbs lipid homeostasis and augments inflammation in the intestine and isolated intestinal cells of Large yellow croaker (Larimichthys crocea). Front Immunol (2021) 12:738143. doi: 10.3389/fimmu.2021.738143
18. Song Y, Zhao M, Cheng X, Shen J, Khound R, Zhang K, et al. CREBH mediates metabolic inflammation to hepatic VLDL overproduction and hyperlipoproteinemia. J Mol Med (2017) 95:839–49. doi: 10.1007/s00109-017-1534-4
19. Li X, Ji R, Cui K, Chen Q, Chen Q, Fang W, et al. High percentage of dietary palm oil suppressed growth and antioxidant capacity and induced the inflammation by activation of TLR-NF-κB signaling pathway in large yellow croaker (Larimichthys crocea). Fish Shellfish Immunol (2019) 87:600–8. doi: 10.1016/j.fsi.2019.01.055
20. Yan J, Liao K, Wang T, Mai K, Xu W, Ai Q. Dietary lipid levels influence lipid deposition in the liver of Large yellow croaker (Larimichthys crocea) by regulating lipoprotein receptors, fatty acid uptake and triacylglycerol synthesis and catabolism at the transcriptional level. PLoS One (2015) 10:e0129937. doi: 10.1371/journal.pone.0129937
21. Fang W, Chen Q, Cui K, Chen Q, Li X, Xu N, et al. Lipid overload impairs hepatic VLDL secretion via oxidative stress-mediated PKCδ-HNF4α-MTP pathway in large yellow croaker (Larimichthys crocea). Free Radical Biol Med (2021) 172:213–25. doi: 10.1016/j.freeradbiomed.2021.06.001
22. Vandesompele J, De Preter K, Pattyn F, Poppe B, Van Roy N, De Paepe A, et al. Accurate normalization of real-time quantitative RT-PCR data by geometric averaging of multiple internal control genes. Genome Biol (2002) 3:Research0034. doi: 10.5402/2012/790452
23. Joshi-Barve S, Barve SS, Amancherla K, Gobejishvili L, Hill D, Cave M, et al. Palmitic acid induces production of proinflammatory cytokine interleukin-8 from hepatocytes. Hepatology (2007) 46:823–30. doi: 10.1002/hep.21752
24. Wu D, Liu J, Pang X, Wang S, Zhao J, Zhang X, et al. Palmitic acid exerts pro-inflammatory effects on vascular smooth muscle cells by inducing the expression of c-reactive protein, inducible nitric oxide synthase and tumor necrosis factor-α. Int J Mol Med (2014) 34:1706–12. doi: 10.3892/ijmm.2014.1942
25. Palomer X, Pizarro-Delgado J, Barroso E, Vázquez-Carrera M. Palmitic and oleic acid: The yin and yang of fatty acids in type 2 diabetes mellitus. Trends Endocrin Met (2018) 29:178–90. doi: 10.1016/j.tem.2017.11.009
26. Oxley A, Torstensen BE, Rustan AC, Olsen RE. Enzyme activities of intestinal triacylglycerol and phosphatidylcholine biosynthesis in Atlantic salmon (Salmo salar l.). Comp Biochem Phys B (2005) 141:77–87. doi: 10.1016/j.cbpc.2005.01.012
27. Rosqvist F, Iggman D, Kullberg J, Cedernaes J, Johansson HE, Larsson A, et al. Overfeeding polyunsaturated and saturated fat causes distinct effects on liver and visceral fat accumulation in humans. Diabetes (2014) 63:2356–68. doi: 10.2337/db13-1622
28. Poureslami R, Turchini GM, Raes K, Huyghebaert G, De Smet S. Effect of diet, sex and age on fatty acid metabolism in broiler chickens: SFA and MUFA. Brit J Nutr (2010) 104:204–13. doi: 10.1017/S0007114510000541
29. Fu S, Watkins SM, Hotamisligil GS. The role of endoplasmic reticulum in hepatic lipid homeostasis and stress signaling. Cell Metab (2012) 15:623–34. doi: 10.1016/j.cmet.2012.03.007
30. Wei Y, Wang D, Topczewski F, Pagliassotti MJ. Saturated fatty acids induce endoplasmic reticulum stress and apoptosis independently of ceramide in liver cells. Am J Physiol Endoc M (2006) 291:E275–281. doi: 10.1152/ajpendo.00644.2005
31. Borradaile NM, Han X, Harp JD, Gale SE, Ory DS, Schaffer JE. Disruption of endoplasmic reticulum structure and integrity in lipotoxic cell death. J Lipid Res (2006) 47:2726–37. doi: 10.1194/jlr.M600299-JLR200
32. Yang L, Guan G, Lei L, Lv Q, Liu S, Zhan X, et al. Palmitic acid induces human osteoblast-like saos-2 cell apoptosis via endoplasmic reticulum stress and autophagy. Cell Stress Chaperon (2018) 23:1283–94. doi: 10.1007/s12192-018-0936-8
33. Wei Y, Wang D, Gentile CL, Pagliassotti MJ. Reduced endoplasmic reticulum luminal calcium links saturated fatty acid-mediated endoplasmic reticulum stress and cell death in liver cells. Mol Cell Biochem (2009) 331:31–40. doi: 10.1007/s11010-009-0142-1
34. Gwiazda KS, Yang TL, Lin Y, Johnson JD. Effects of palmitate on ER and cytosolic Ca2+ homeostasis in beta-cells. Am J Physiol Endoc M (2009) 296:E690–701. doi: 10.1152/ajpendo.90525.2008
35. Holland WL, Brozinick JT, Wang LP, Hawkins ED, Sargent KM, Liu Y, et al. Inhibition of ceramide synthesis ameliorates glucocorticoid-, saturated-fat-, and obesity-induced insulin resistance. Cell Metab (2007) 5:167–79. doi: 10.1016/j.cmet.2007.01.002
36. Listenberger LL, Ory DS, Schaffer JE. Palmitate-induced apoptosis can occur through a ceramide-independent pathway. J Biol Chem (2001) 276:14890–5. doi: 10.1074/jbc.M010286200
37. Bäck M, Yurdagul A Jr., Tabas I, Öörni K, Kovanen PT. Inflammation and its resolution in atherosclerosis: mediators and therapeutic opportunities. Nat Rev Cardiol (2019) 16:389–406. doi: 10.1038/s41569-019-0169-2
38. Cao SS. Epithelial ER stress in crohn's disease and ulcerative colitis. Inflammation Bowel Dis (2016) 22:984–93. doi: 10.1097/MIB.0000000000000660
39. Ye Z, Cao C, Li Q, Xu YJ, Liu Y. Different dietary lipid consumption affects the serum lipid profiles, colonic short chain fatty acid composition and the gut health of sprague dawley rats. Food Res Int (2020) 132:109117. doi: 10.1016/j.foodres.2020.109117
40. Arthur JS, Ley SC. Mitogen-activated protein kinases in innate immunity. Nat Rev Immunol (2013) 13:679–92. doi: 10.1038/nri3495
41. Jijon H, Allard B, Jobin C. NF-kappaB inducing kinase activates NF-kappaB transcriptional activity independently of IkappaB kinase gamma through a p38 MAPK-dependent RelA phosphorylation pathway. Cell Signal (2004) 16:1023–32. doi: 10.1016/S0898-6568(04)00028-2
42. Olson CM, Hedrick MN, Izadi H, Bates TC, Olivera ER, Anguita J. p38 mitogen-activated protein kinase controls NF-kappaB transcriptional activation and tumor necrosis factor alpha production through RelA phosphorylation mediated by mitogen- and stress-activated protein kinase 1 in response to borrelia burgdorferi antigens. Infect Immun (2007) 75:270–7. doi: 10.1128/IAI.01412-06
43. Patel D, Witt SN. Ethanolamine and phosphatidylethanolamine: Partners in health and disease. Oxid Med Cell Longev (2017) 2017:4829180. doi: 10.1155/2017/4829180
44. Bogdanov M, Dowhan W. Lipid-assisted protein folding. J Biol Chem (1999) 274:36827–30. doi: 10.1074/jbc.274.52.36827
45. Mizushima N. The ATG conjugation systems in autophagy. Curr Opin Cell Biol (2020) 63:1–10. doi: 10.1016/j.ceb.2019.12.001
46. Tasseva G, Bai HD, Davidescu M, Haromy A, Michelakis E, Vance JE. Phosphatidylethanolamine deficiency in mammalian mitochondria impairs oxidative phosphorylation and alters mitochondrial morphology. J Biol Chem (2013) 288:4158–73. doi: 10.1074/jbc.M112.434183
47. Leonardi R, Frank MW, Jackson PD, Rock CO, Jackowski S. Elimination of the CDP-ethanolamine pathway disrupts hepatic lipid homeostasis. J Biol Chem (2009) 284:27077–89. doi: 10.1074/jbc.M109.031336
48. Ou S, Fang Y, Tang H, Wu T, Chen L, Jiang M, et al. Lycopene protects neuroblastoma cells against oxidative damage via depression of ER stress. J Food Sci (2020) 85:3552–61. doi: 10.1111/1750-3841.15419
49. Tian Y, Liu Y, Xue C, Wang J, Wang Y, Xu J, et al. The exogenous natural phospholipids, EPA-PC and EPA-PE, contribute to ameliorate inflammation and promote macrophage polarization. Food Funct (2020) 11:6542–51. doi: 10.1039/D0FO00804D
Keywords: intestinal homeostasis, palmitic acid, phosphatidylethanolamine, lipid metabolism, ER stress, inflammatory response
Citation: Fang W, Liu Y, Chen Q, Xu D, Liu Q, Cao X, Hao T, Zhang L, Mai K and Ai Q (2022) Palmitic acid induces intestinal lipid metabolism disorder, endoplasmic reticulum stress and inflammation by affecting phosphatidylethanolamine content in large yellow croaker Larimichthys crocea. Front. Immunol. 13:984508. doi: 10.3389/fimmu.2022.984508
Received: 02 July 2022; Accepted: 26 July 2022;
Published: 19 August 2022.
Edited by:
Jiong Chen, Ningbo University, ChinaCopyright © 2022 Fang, Liu, Chen, Xu, Liu, Cao, Hao, Zhang, Mai and Ai. This is an open-access article distributed under the terms of the Creative Commons Attribution License (CC BY). The use, distribution or reproduction in other forums is permitted, provided the original author(s) and the copyright owner(s) are credited and that the original publication in this journal is cited, in accordance with accepted academic practice. No use, distribution or reproduction is permitted which does not comply with these terms.
*Correspondence: Qinghui Ai, cWhhaUBvdWMuZWR1LmNu