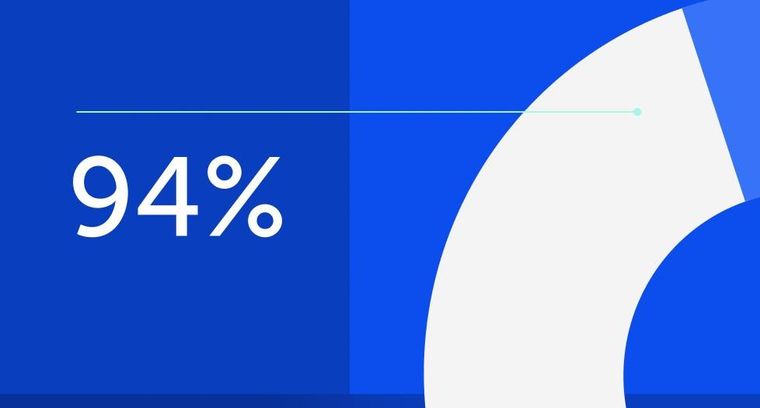
94% of researchers rate our articles as excellent or good
Learn more about the work of our research integrity team to safeguard the quality of each article we publish.
Find out more
REVIEW article
Front. Immunol., 30 August 2022
Sec. Inflammation
Volume 13 - 2022 | https://doi.org/10.3389/fimmu.2022.975367
The CCL2-CCR2 axis is one of the major chemokine signaling pathways that has received special attention because of its function in the development and progression of cardiovascular disease. Numerous investigations have been performed over the past decades to explore the function of the CCL2-CCR2 signaling axis in cardiovascular disease. Laboratory data on the CCL2-CCR2 axis for cardiovascular disease have shown satisfactory outcomes, yet its clinical translation remains challenging. In this article, we describe the mechanisms of action of the CCL2-CCR2 axis in the development and evolution of cardiovascular diseases including heart failure, atherosclerosis and coronary atherosclerotic heart disease, hypertension and myocardial disease. Laboratory and clinical data on the use of the CCL2-CCR2 pathway as a targeted therapy for cardiovascular diseases are summarized. The potential of the CCL2-CCR2 axis in the treatment of cardiovascular diseases is explored.
Cardiovascular disease is the world’ s leading cause of morbidity and mortality (1, 2). A rising body of evidence suggests that inflammation is a key contributor to cardiovascular disease (3, 4). Randomized clinical trials based on canakinumab (5) and colchicine (6) have evidenced the effectiveness of particular anti-inflammatory treatments in the field of cardiovascular disease prevention. In the course of inflammatory immune response, leukocytes are recruited to the site of injury. Chemokines and its receptors have been described as essential mediators that regulate leukocyte infiltration and migration to specific sites of inflammatory response (7). There is powerful proof that chemokine CCL2 and its receptor CCR2 function in cardiovascular diseases such as heart failure (8), atherosclerosis and coronary atherosclerotic heart disease (9), hypertension (10) and cardiomyopathy (11) (Table 1). In addition, high levels of circulating CCL2 are related to increased long-term cardiovascular mortality in people without significant cardiovascular disease (43).
Table 1 Summary of selected studies investigating the role of CCL2 & CCR2 in cardiovascular diseases.
Recently, a large epidemiological study highlighted the causal association of the CCL2-CCR2 pathway with cardiovascular disease in humans (22). Given its critical role in the immune inflammatory response, the CCL2-CCR2 axis is recognized as an important physiological modulator and a viable therapeutic target. Although a great deal of preclinical data (44–46) support the important contribution of the CCL2-CCR2 axis in experimental cardiovascular disease, the existing clinical studies (47, 48) have not yielded satisfactory results. The development and clinical application of drugs on the basis of the CCL2-CCR2 axis for the treatment of cardiovascular diseases continue to be challenging.
In this review, the mechanisms of action of the CCL2-CCR2 axis in the development of cardiovascular disease are described. Also we follow the progress of CCL2-CCR2 axis in relation to preclinical and clinical studies of cardiovascular disease. These results elucidate the essential function of the CCL2-CCR2 axis in cardiovascular evolution and its prospective use as a target for therapy.
Chemokines are small, highly conserved families of secreted proteins composed of cytokines (49), which are responsible for regulating cell movement in response to chemical stimuli (chemotaxis) (50). Chemokines perform its function by associating with G protein-coupled chemokine receptors (GPCRs) (51) thereby affecting a variety of biological processes and disease conditions, such as cancer (52), cardiovascular diseases (9), liver diseases (53), and intestinal diseases (54). Chemokines are classified as four major families, CXC, CC, XC and CX3C, by the arrangement of their amino-terminal (N-terminal) cysteines (51). CC and CXC are the two major subfamilies of chemokines. Among the CC chemokine subfamilies, CCL2 was the first to be discovered and fully investigated (55, 56).
CCL2, also known as monocyte chemoattractant protein-1 (MCP-1), was originally obtained in 1989 from the culture supernatant of human glioma cells and human blood mononuclear leukocytes (57, 58). As a protein consisting of 76 amino acids at 13 kDa, CCL2 has two adjacent amino-terminal cysteine residues (59). CCL2 is primarily secreted by immune cells, in addition, smooth muscle cells, endothelial cells, thylakoid cells, and fibroblasts are also capable of producing CCL2 (58, 60, 61). The expression of CCL2 can be either persistent or inducible. A variety of mediators can induce CCL2 expression, such as IL-1, IL-4, IL-6, TNF-α, TGF-β, IFN-γ, etc (62, 63). CCL2 regulates the migration and infiltration of a wide range of immune cells, including monocytes, macrophages, memory T lymphocytes, and natural killer (NK) cells (50, 59). It has been reported that CCL2 can bind to a variety of receptors (Figure 1A). For example, by binding to CCR2, CCL2 coordinates inflammatory monocyte transport among bone marrow, circulating and atherosclerotic plaques (9). CCL2 binding to CCR4 activates myosin light chain (MLC) phosphorylation and regulates cell motility and tumor metastasis (64). Furthermore, CCL2 can be bound to atypical chemokine receptors (ACKRs), including ACKR1 and ACKR2, which do not induce cell migration but can alter chemokine gradients (65). Although CCL2 can bind to a variety of receptors, CCR2 is still considered to be the primary receptor for CCL2. The amino-terminal region of CCL2 is an important factor in determining CCR2 affinity for binding and signal selectivity (66).
Figure 1 CCL2-CCR2 network and expression in human tissues. (A) Chemokines and their receptor networks associated with the CCL2-CCR2 axis (9). The chemokine family is a huge system of ligands and GPCRS. In this network, CCL2 can bind to a variety of receptors, including the classical chemokine receptors (shaded in pink) CCR1, CCR2, CCR4 and the atypical chemokine receptors (shaded in gray) ACKR1, ACKR2. CCR2 can also bind to a variety of ligands (in red), including CCL2, CCL7, CCL8, CCL11, CCL13, CCL16, CCL26. (B) CCL2 and CCR2 RNA expression in human tissues, X-axis represents the consensus data based on normalized expression (nTPM) values (data from https://www.proteinatlas.org).
Since its discovery in 1994 (67), CCR2 has been extensively investigated as a prospective therapeutic target for many diseases. CCR2 belongs to GPCRs, whose steric structure contains the binding sites of G proteins (guanylate-binding proteins) and seven transmembrane α-helices. Depending on the carboxyl terminus, CCR2 is divided into CCR2A and CCR2B (68), which perform different roles. Notably, as with CCL2, CCR2 can also bind to other chemokines, including CCL7, CCL8, CCL11, CCL13, CCL16, and CCL26 (9) (Figure 1A). These chemokines perform important roles in a variety of diseases. For example, CCL8 was involved in the recruitment of tumour-associated macrophages by hypoxic cervical cancer cells through its binding to CCR2 (69). CCL11 promoted ovarian cancer growth and metastasis by stimulating the proliferation and migration of ovarian carcinoma cell lines (70). CCL13 levels were significantly increased in patients with asthma and allergic rhinitis (71). Interestingly, unlike other chemokines, CCL7 played different or even opposite roles in inflammation. On the one hand, CCL7 was involved in the recruitment of pro-inflammatory mononuclear phagocytes into the local colonic tissue of colitis and could promote acute lung inflammation by recruiting neutrophils (72, 73). On the other hand, cleaved CCL7 was found to act as a general chemokine antagonist that inhibits inflammation (74). In addition, chemokine receptors such as CCR5 and CXCR4 can also interact with CCR2 (51). CCR2 is located in a range of tissues such as heart, liver, spleen, lung, kidney, pancreas, ovary, thymus, brain, blood and spinal cord (75) (Figure 1B)and is broadly expressed in various cells.
By binding to CCL2, CCR2 undergoes dimerization and internalization, inducing the expression of monocyte chemotactic protein-1 inducible protein-1 (MCPIP1). Subsequently, transcription and expression of IL-1, CCL2 and TNF genes are initiated by MCP1P1 (76). Upon triggering of CCR2 by CCL2, a variety of intracellular G protein-mediated signaling gateways will be initiated, for instance JAK/STAT, PI3K/MAPKs and PI3K/Akt/ERK/NF-κB (77) (Figure 2). Activation of these signaling pathways results in the mobilization of multiple transcription factors and genes involved in cytokine production, cell growth and differentiation, cell survival, migration and apoptosis, angiogenesis and inflammation (62, 78, 79). CCL2-CCR2 axis is associated with the advancement of several disorders, like atherosclerosis (9), acute liver failure (80), rheumatoid arthritis (81), pulmonary hypertension (82), diabetes and complications (83), and cancer (78). As a result, CCL2-CCR2 axis is recognized as a potential targeted site for the management of such diseases (1, 2). The CCL2-CCR2 axis has received a lot of attention along with CCL2-CCR2 antagonists (Table 2).
Figure 2 Schematic diagram of the CCL2-CCR2 axis and its signaling pathway. CCR2 is a G PCR. Upon binding of CCR2 to CCL2, a range of signaling downstream is activated, for instance, the JAK/STAT pathway, MAPK pathway and PI3K/Akt pathway. A variety of transcription factors and genes are then activated to participate in cytokine production, cell growth and differentiation, cell survival, migration and apoptosis.This figure was created with BioRender.com.
As a complex clinical syndrome, heart failure (HF) is characterized by signs and symptoms caused by any structural or functional disorder of ventricular filling or ejection (101). HF can occur as a result of valvular heart disease, hypertension, ischemic heart disease, and myocardial infarction (MI) (101). A great deal of evidence suggests that the onset and progression of HF is intimately associated with inflammation, no matter the underlying etiology (102, 103). The induction and activation of chemokines is one of the features of the inflammatory response in the failing heart (104). The chemokine CCL2 protein is localized to cardiomyocytes, vascular endothelial and smooth muscle cells, interstitial fibroblasts and infiltrating leukocytes in aortic-cavernous fistula (ACF) rats and is involved in the progression of myocardial dysfunction and HF in ACF rats, with a positive correlation between its expression and the severity of congestive heart failure (CHF) (8). In addition, it is possible that CCL2 myocardial damage, fibroblastic remodeling, and malfunction by stimulating the recruitments of proinflammatory leukocytes in the failing heart (104). In a left ventricular pressure overload model, CCL2-mediated macrophage aggregation acted on myocardial fibrosis via a TGF-β-mediated process. Neutralization of CCL2 inhibited macrophage aggregation, TGF-β induction, and fibroblast proliferation, while attenuating diastolic dysfunction and reducing myocardial fibrosis (12). The desmin-deficient mice (des-/-) is a progressive HF model characterized by galectin-3 overexpression, a spontaneous inflammatory response that maintains fibrosis, and cardiomyocyte death. This model is accompanied by macrophage infiltration and upregulation of the deleterious macrophage-associated genes CCR2 and Arg1. During the development of des-/- cardiomyopathy, Galectin-3 deficiency downregulated CCR2, Arg1 and pro-fibrotic gene expression as well as amelioration of poor cardiac remodeling, inflammation and failure (13).
In 2000, J.K. Damås et al. first demonstrated the expression and protein localization of chemokines and their receptor genes in the human myocardium (14). Activated platelets significantly inhibit the release of CCL2 from human umbilical vein endothelial cells in a CD154 dependent manner (15). Serum CCL2 levels were measured in 50 CHF patients and showed that serum CCL2 levels were substantially elevated in patients in CHF compared with health controls and were positively related to the seriousness of symptoms as well as the degree of left heart insufficiency (15). CCL2 is significantly correlated with poor prognosis in patients with advanced HF (16).
Atherosclerosis underlies the underlying pathophysiology of several cardiovascular diseases. The pathogenesis of atherosclerosis is complicated, and a great deal of data indicates that inflammation has an essential role in the occurrence and progression of atherosclerotic disease (105). CCL2-induced migration of monocytes to the vessel wall is a key activity contributing to the development of atherosclerosis. In this process, NF-κB was involved in regulating the expression of CCL2 (17). As early as 1991, it was found that CCL2 expression was more readily detected in different regions of atherosclerotic plaques compared to normal vessels (18). TNF-a, IFN-γ and mixed lymphocyte culture supernatants were all demonstrated to stimulate the production of CCL2 (18). In contrast, lack of CCL2 decreased the atherosclerosis of low-density lipoprotein receptor-deficient mice (19). CCR2 was identified as a genetic determinant of atherosclerosis in mice (20). Selective deletion of CCR2 significantly decreased atherosclerotic lesion formation and reduced macrophage accumulation in plaques in ApoE knockout (Apoe-/-) mice (20). Lipid-laden foam cells are a hallmark of atherosclerosis (106). Under the action of the CCL2-CCR2 axis, circulating monocytes are recruited to atherosclerotic plaques and differentiate into macrophages, which proliferate, become foam cells, and orchestrate the inflammatory response (107). All these evidences suggest that CCL2-CCR2 is closely associated with atherosclerotic heart disease (Figure 3A).
Figure 3 Role of the CCL2-CCR2 axis in cardiovascular disease. The CCL2-CCR2 axis fosters monocyte mobilization from the bone marrow and recruits circulating monocytes to the site of the lesion. In plaques, monocytes are differentiated to macrophages, which proliferate, become foam cells, and coordinate the inflammatory response (A). In infarcted myocardial tissue, monocytes are recruited and differentiated into macrophages that influence MI disease progression (B). In hypertension, monocytes infiltrate into the vascular adventitia, perivascular fat, heart, kidney, and brain, and are involved in elevated blood pressure and end-organ damage (C). In cardiomyopathies (dilated cardiomyopathy as an example), monocytes infiltrate and differentiate into macrophages in the damaged myocardium and participate in the development and progression of cardiomyopathies (D). This figure was created with BioRender.com.
Recently, a team studied atherosclerotic plaques in 1199 patients treated with endarterectomy for carotid stenosis. It was found that CCL2 of human atherosclerotic plaques was significantly related to plaque vulnerability characteristics, as evidenced by the fact that CCL2 levels were correlated with plaque matrix turnover, pro-inflammatory plaque characteristics, plaque vulnerability pathological histological features, clinical plaque instability, and perioperative vascular events 30 days after plaque removal. This large-scale human study expands earlier epidemiological, genetic, as well as experimental research (21). However, consistent with our recognition that cross-sectional studies do not allow for causal inference, this study cannot explain the cause-and-effect role of CCL2 in human atherosclerosis. Significantly, another two-sample Mendelian randomization study explored this causal relationship, showing that higher circulating CCL2 levels were related to a greater degree of risk for ischemic stroke, especially large artery stroke, as well as coronary artery disease and MI (22).
Ischaemic heart disease is the major reason for death worldwide (1). Although the rational use of drugs and advances in reperfusion therapy have significantly reduced acute mortality after MI in patients, they have put patients who survive at greater risk of developing chronic heart failure (CHF) (108). The adult mammalian heart is virtually incapable of regeneration and inflammation-driven scar formation contributes to the repair of the infarcted heart (109). Excessive or insufficient acute inflammation after MI plays a key role in the process of ventricular remodeling (110, 111). Studies have shown that chemokines regulate the serial mobilization of immune cell subsets in the infarcted heart, with CC chemokines (e.g., CCL2) mediating monocyte recruitment (109, 112) (Figure 3B). Knockdown of CCR2 reduces the recruitment of Ly-6Chigh monocytes to the infarct site, alleviates infarct inflammation, and inhibits post-infarction myocardial left ventricular remodeling, thereby promoting myocardial infarct healing (23). In addition to the widely recognized role of monocyte chemotaxis, CCL2 is also involved in regulating the phenotype and activity of monocytes. CCL2-/- mice have significantly reduced levels of goat antibone bridge protein (OPN)-1 expression in infarct-zone macrophages, decreased activation of macrophages and infiltration of myofibroblasts. These mechanisms, while attenuating left ventricular remodeling after MI, are accompanied by a prolonged inflammatory phase, a impaired phagocytosis of damaged cardiomyocytes and late replacements of injured cardiomyocytes by sarcomeres, among other undesirable consequences (24). Interestingly, although the results of many MI animal experiments showed that disruption of the CCL2/CCR2 axis reduced myocardial infarct size (25, 113), not all studies had the same conclusion. The percentage of infarct area in transgenic mice with cardiomyocyte-specific overexpression of CCL2 was lower than in normal controls after ischemia/reperfusion. Cardiomyocyte overexpression of CCL2 resulted in chronic infiltration and activation of leukocytes, which led to increased secretion of TNF-a and activation of SAPK/JNK1/2, ultimately achieving cardioprotective effects through activation of MAPKs (26). In addition, myocardial CCL2 overexpression prevented left ventricular dysfunction and remodeling after MI by inducing infiltration of macrophages, secretion of myocardial IL-6, accumulation of myocardial fibroblasts, and neovascularization (27).
Hypertensive disorders are responsible for a variety of serious complications including hypertensive heart disease, stroke and renal failure. The inflammatory response in the arterial wall contributes to the occurrence and maintaining of hypertension (114). CCL2 is considered to be an essential mediator of the inflammatory response in the arterial vascular system.The CCL2/CCR2 axis has been shown to play a critical role in vascular inflammation, vascular remodeling, and vascular hypertrophy via monocyte infiltration and macrophage recruitment in a mouse model of hypertension (10, 28). Angiotensin II (Ang II) directly stimulated the expression of the CCL2 gene in the vascular system via the Ang II type-1 (AT1) receptor (29). CCL2 expression was significantly elevated in the aortic tissue of animals suffering from hypertension after Ang II injection (115). CCR2 played a crucial role in macrophage infiltration, vascular hypertrophy, inflammation and remodeling in animal models of Ang II-induced hypertension. Hypertension-induced infiltration of arterial wall macrophages was almost abolished and vascular hypertrophy was significantly decreased in CCR2-deficient mice (10). Ang II-induced inflammation and remodeling of blood vessels was significantly attenuated in CCR2-/- mice and bone marrow-transferred mice with a leukocyte-selective CCR2 deficiency (BMT-CCR2-/-) (28). In clinical practice, serum CCL2 was measured in 740 hypertensive patients, and soluble CCL2 was observed to be increased in hypertensive patients and correlated with the extent of organ injury (30). In addition, the expression of CCR2 was elevated on the surface of monocytes in hypertensive patients and decreased after treatment by Ang II receptor blockers (28).
CCL2-CCR2 plays an instrumental part in the progression of experimental hypertensive kidney damage. Enhanced CCL2-CCR2 signaling pathway exacerbated renal injury in patients with salt-sensitive hypertension (31). Lack of CCL2 ameliorated renal cortical atrophy and reduced the number of infiltrating monocytes as well as CCR2, CD206, CCL5, CCL7 and CCL8 expression, thereby preventing chronic kidney injury in mice with renal vascular hypertension (32). Blockade of CCR2 using the CCR2 antagonist RS102895 prevented renal leukocyte infiltration early after a high salt diet and attenuated salt-sensitive hypertension and renal injury, thus CCL2-CCR2 is considered a prospective pathway to alter renal leukocyte infiltration and lead to salt-sensitive hypertension (33).
Features by inflammatory cell infiltration of the heart and subsequent deterioration of its function, myocarditis is an important factor in chronic dilated cardiomyopathy (DCM), acute HF and sudden death (116). The expression of CCL2 was raised in the heart and serum of rats with experimental autoimmune myocarditis (EAM) from the acute to the recovery phase, which was highly correlated with the expression levels of TNF-a, IL-1β and IL-6 (34, 35). Regulation the inflammatory infiltration of EAM by adjusting CCL2 expression through Act1/TRAF6/TAK1 is one of the pathological mechanisms of myocarditis (36). In vivo blockade of CCL2 activity reduced the severity of CVB3-induced myocarditis, and the main mechanism may be related to effective inhibition of chemotaxis and reduction of systemic and local Th1 immune responses (37). EAM mice lacking CCR2 have decreased myocarditis severity (38). siRNA silencing of CCR2 (SiCCR2) decreased the number of Ly6Chigh monocytes and migration of bone marrow granulocyte macrophage precursor cells to the blood in the hearts of mice with acute autoimmune myocarditis, prevented macrophage magnetic resonance imaging enhancement, and improved ejection fraction (11). In clinical studies, elevated CCL2 levels at first occurrence in patients with acute myocarditis were dramatically related to severity of disease and prognosis. This was demonstrated by significantly higher serum CCL2 levels in patients with acute myocarditis on admission than in healthy volunteers and significantly higher serum CCL2 levels in patients who died of acute myocarditis than in surviving patients (34). Cardiac tissue biopsy samples from patients with myocarditis were enriched for CCR2+ cells and had elevated CCL2 and CCR2 mRNA expression compared to control specimens obtained from individuals dying from trauma with no history of cardiac disease (11).
DCM is the most common non-ischemic cardiomyopathy leading to HF. CCL2 expression is upregulated in late stages of DCM and negatively correlates with left heart function, a mechanism that may lead to cardiomyocyte injury through infiltration and activation of monocytes (39). Targeting CCR2 to downregulate its protein expression inhibited the mobilization of Ly6Chigh monocytes in the bone marrow, thereby improving cardiac systolic functions as well as reducing ventricular remodeling in mice with DCM caused by low doses of adriamycin (DOX) (40). Levels of CCL2 were also dramatically increased in the serum and myocardium of patients with hypertrophic cardiomyopathy (HCM) and were related to left ventricular systolic dysfunction (41).
Arrhythmogenic cardiomyopathy (AC) is a hereditary disease described by arrhythmias, fibrosis and cardiac dilatation. AC is a primary disease of the myocardium that can cause cardiac sudden death as well as HF (117). The pathogenesis of AC is currently not well defined. It was revealed that particular immune cell groups along with chemokine expression profiles regulate inflammatory and reparative processes during the whole course of AC progression. Among them, CCL2 and CCR2 mRNA are upregulated during disease onset, acute and chronic phases, and the early phase of AC is accompanied by a reaggregation of CCR2+ inflammatory monocytes to the heart (42).
11K2 is an inhibitory monoclonal antibody with high affinity for human CCL2 and a convenient cross-reactivity with mouse CCL2 and CCL12. In the presence of 11K2, ApoE-/- mice show a reduction in plaque area, a decrement in macrophage and CD45+ cell numbers, and an improvement in collagen content, resulting in a consistent plaque phenotype (118).
Bindarit (BIN), a small molecule with anti-inflammatory activity in a variety of inflammatory diseases, selectively inhibited the production of CCL2, CCL7, and CCL8 which played an important role in the homeostatic localization and transport of immune cells (119). The inhibition of chemokines by BIN was probably achieved by interfering with the classical NF-κB pathway (120). Furthermore, in a mouse model of lipopolysaccharide-induced cytokine production, BIN decreased the activity levels of both CCL2 and TNF-α (121). BIN has been shown to be efficacious in inflammatory diseases such as diabetes-associated periodontitis (122), neuritis (123), osteoarthritis (124), autoimmune encephalomyelitis (125) and acute proliferative lupus nephritis (126). Selective inhibition of CCL2 by BIN reduced the chemotactic process of inflammation that persisted at the site of lesions and infections (119, 120). BIN reduced in-stent stenosis in pigs by suppressing the generation of CCL2 (44). The mechanism of action of BIN in controlling de novo intima formation and restenosis may be associated with inhibition of CCL2 and CCL7 generation and induction of smooth muscle cell differentiation in human coronary arteries (127). However, the non-specific distribution in vivo limits the application of BIN in atherosclerosis. A yeast-derived microcapsule-mediated nano-drug delivery approach delivers BIN to the interior of atherosclerotic mouse plaques, significantly enhancing the inhibitory effect of CCL2 and further reducing the recruitment of monocytes to atherosclerotic plaques (128).
In addition, gene therapy strategies have opened a new window for CCL2-CCR2 treatment of cardiovascular disease. The CCL2 mutant 7ND with a 7 amino acid deletion at the N-terminal end functions as a dominant-negative inhibitor of CCL2. Monocyte activation and infiltration following arterial injury and experimental restenosis following balloon injury and stent placement is inhibited by 7ND gene transfer. In addition, 7ND gene transfer improved platelet stability and limited the development of early atherosclerotic lesions in hypercholesterolemic mice as well as the progression of pre-existing atherosclerotic lesions (129, 130). In a similar vein, Liehn E (45) et al. showed that the non-excited CCL2 mutant PA508 inhibits monocyte chemotaxis or transendothelial migration to CCL2 by competing with CCL2 to interfere with its presentation. Although PA508 had no effect on leukocyte sorting, levels of CCL2, nor organ function or morphology in wild-type mice, it resulted in reduced recruitment of inflammatory leukocytes, demonstrating specific inhibition of the CCL2-CCR2 axis. In addition, PA508 showed good effects in two of the most common mouse models of cardiovascular disease. In a hyperlipidemic ApoE-/- mouse model, PA508 significantly reduced intimal plaque area and infiltration of individual nucleated cells in mouse carotid arteries and increased the content of vascular endothelial cells. In a myocardial ischemia/reperfusion mouse model, PA508 substantially decreased myocardial infarct area, monocyte infiltration, collagen and myofibroblast levels in the infarcted region, and protected cardiac function in mice.
RS102895 (IC50 = 360nM) and RS504393 (IC50= 89nM) are potent CCR2b inhibitors. In addition to CCR2, it also inhibits human ADRA1A (IC50= 130nM/72nM), ADRA1D (IC50= 320nM/460nM), and rat HTR1A (IC50= 470nM/1070nM) in cells (131). RS102895 inhibited ischemia/reperfusion-induced cardiomyocyte apoptosis, and pretreatment with RS102895 restored reduced cell viability after ischemia/reperfusion (95). In addition, RS102895 blocks the infiltration of renal leukocytes in the early stages of salt-sensitive hypertension (33) and exerts anti-inflammatory and renoprotective effects in hypertension (96, 132). Notably, an interesting study (133) demonstrated the important role of circadian rhythmic leukocyte recruitment in atherosclerosis and established the CCL2-CCR2 axis as its modulator. A chronopharmacological treatment strategy based on RS102895 was effective in inhibiting the development of early atherosclerotic lesions while not affecting the inflammatory process of microcirculation. In response to RS504393, DOCA-salt hypertensive rats showed reduced monocyte/macrophage infiltration and chemokine/cytokine production, decreased NF-κB activity, and improved renal dysfunction as well as morphological impairment (31). Early blockade of CCR2 with RS504393 after transverse aortic constriction (TAC) reduced CCR2+ cardiac macrophage levels, inhibited VCAM expression, and improved late left ventricular dysfunction and cardiac fibrosis (98).
Propagermanium (PG) is a CCR2 antagonist with high safety and availability and is often evaluated for its therapeutic potential as a CCR2 antagonist. Studies have shown that PG reduces atherosclerosis in ApoE-/- mice by suppressing macrophage infiltration (46). PG also inhibited the formation of macrophage-mediated coronary atherosclerotic pathology in pigs (134) and atherosclerotic lesions in rabbits with Watanabe heritable hyperlipidemia development (135). Similar to PG, TLK-19705 (IC50= 700 nM)also blocks the CCL2/CCR2 signaling pathway. Continuous administration of TLK-19705 over 8 weeks dramatically decreased the area of atherosclerotic lesions in ApoE-/- mice (100). Nevertheless, not all CCR2 inhibitors are therapeutically effective. INCB-3344 is a potent CCR2 antagonist with IC50 values of 9.5 nM (mCCR2), 5.1 nM (hCCR2) for antagonistic binding activity and 7.8 nM (mCCR2), 3.8 nM (hCCR2) for antagonistic chemotactic activity (136). Blockade of CCR2 with INCB-3344 did not affect atherosclerotic lesions although it caused a dramatic reduction of Ly-6Chi monocyte subpopulations in the blood of ApoE-/- mice (88). Similarly, GSK1344386B failed to affect total atherosclerotic lesion size although it blocked accelerated monocyte recruitment and macrophage infiltration in an atherosclerotic mouse model (85). These differences may be due to the recognition of receptors other than CCR2. It was reported that PG selectively inhibited not only CCL2-induced chemotaxis but also CCL7-induced monocyte migration by targeting GPI-anchored proteins that are closely related to CCR2 (137). TLK-19705 inhibited CCL4-induced chemotaxis of human peripheral blood mononuclear cells, a chemokine that signals through CCR5 (138).
Several drugs with broad therapeutic targets have been found to act on the CCL2/CCR2 axis to treat cardiovascular disease. There is growing evidence that the sodium-glucose co-transporter protein 2 (SGLT2) inhibitor, empagliflozin, contributes to the treatment of cardiovascular disease, while reducing HF and cardiovascular mortality among inpatients (139–142). Recent findings showed that engramine significantly downregulated the expression of cardiac macrophage markers Itgax and CCL2, increased the expression of MRc1, decreased the infiltration rate of cardiac macrophages in mice, and improved myocardial structural and cardiac function abnormalities induced by chronic hypercortisolism in mice (143). Many anesthetics have been reported to show cardioprotective effects in clinical practice (144–146). Diazepam is a local anesthetic that is rapidly distributed in the liver, kidneys, lungs, brain, heart and small intestine (147) and has shown cardioprotective potential in a series of studies (148, 149). Diazepam pretreatment effectively decreases the release of proinflammatory cytokines ILs and TNF-α, the expression of CCR2 chemokines, and inhibits oxidative nitrosative stress and apoptosis in myocardial ischemia-reperfused rats, which exerts cardioprotective effects. MicroRNAs (miRs) are small non-coding RNAs that participate in post-transcriptional gene modulation and are critical for cell differentiation, homeostasis and animal development (150, 151). Different miRs have been reported to regulate the formation and progression of atherosclerotic plaques by regulating phagocytosis, and are considered as potential targets of molecules for anti-atherosclerotic therapy (152). Among them, miR-146a and miR-181b effectively inhibited the CCL2, CCL5, CCL8 and CXCL9 as well as monocyte adhesion to endothelial cells by targeting E-selectin, which reduced the size of atherosclerotic plaques and improved endothelial inflammation and atherosclerosis (153).
In addition, some herbal compounds can also exert therapeutic effects on cardiovascular diseases by affecting the CCL2-CCR2 axis. Fufang Zhenzhu Tiao Zhi (FTZ) formula is a traditional herbal remedy for the treatment of disorders of glucose and lipid metabolism (154). FTZ can reduce serum IL-12 and CCL2 levels and cardiac IL-12, IL-6 and CCR2 mRNA levels in streptozotocin-induced diabetes and improve streptozotocin induced diabetic cardiomyopathy (155). Gui Zhi Tang can reduce the levels of IL-6, CCL2, IL-1β, MMP2, and MMP9 in Dahl salt-sensitive rats, reducing the area of myocardial fibrosis and exerting antihypertensive effects (156). Network-based pharmacology to explore the molecular targets of the action of Wenxin Keli (WXKL) in the treatment of atrial fibrillation, it was found that these targets were closely related to inflammatory response, oxidative stress response, and immune regulation, and CCL2 was one of the main targets of its action (157). Bioinformatics analysis suggests that hemiphilin injection may exert a potential protective effect against neocoronary pneumonia, especially COVID19-induced cardiac insufficiency, by targeting seven Hub genes, including CCL2 and CXCL8, to inhibit oxidative stress, prevent atherosclerotic plaque formation, and suppress inflammation and apoptosis (158).
Although pharmacological studies targeting the chemokine signaling pathway have been extensive, there are currently only three marketed drugs based on the chemokine signaling pathway (9, 159). The extensive actions of chemokines in damage and repair make chemokine-based clinical translation challenging. Despite the remarkable efficacy of BIN in cellular and animal models for coronary atherosclerotic heart disease mentioned above, few clinical studies have been conducted around BIN. Results from a phase II trial showed that BIN was well tolerated and may have a protective effect on the vessel wall after angioplasty, but this study did not meet the primary endpoint and was considered a negative study (48). The clinical efficacy of BIN in the treatment of cardiovascular disease remains to be further validated.
MLN1202 is a monoclonal antibody designed to interact with CCR2 and inhibits CCL2 binding in a highly specific manner. A phase II trial of MLN1202 showed a substantial decrease in circulating levels of highly sensitive C-reactive protein in patients with atherosclerotic cardiovascular disease after 4 weeks of treatment with MLN1202, which lasted for 8 weeks. However, the study was limited in size and insufficient to stratify baseline covariates that might confound the results (47). In addition, no phase III trials of this project have been reported.
Colchicine is a novel and complex anti-inflammatory agent which has been documented in medical literature as early as approximately 1550 B.C (160). In the past few years, several clinical trials have evidenced the function of colchicine in cardiovascular disease (161–163). In 2019, Tucker et al. (164) reported firstly the effect of colchicine on transcoronary (TC) chemokine levels in acute coronary syndrome (ACS) patients. In this open-label clinical trial, 13 patients with stable angina pectoris (SAP) and 12 patients with ACS were treated with colchicine, while 13 additional patients with ACS did not receive treatment. According to the results, CCL2 and CX3CL1 levels were elevated in patients with ACS compared with patients with stable coronary artery disease, and TC levels of serum CCL2, CCL5, and CX3CL1 were significantly reduced by colchicine treatment in patients with ACS. In addition, colchicine inhibited the expression of CCL2 gene in monocytes isolated from healthy donors. The above results suggest that colchicine inhibits the expression of chemokines such as CCL2 in patients with ACS, thereby suppressing the migration of monocytes. However, only 38 patients were included in this study, and further large-scale clinical trials are required to demonstrate the mechanism of action of colchicine based on the CCL2 pathway in the treatment of cardiovascular disease.
Cardiovascular disease is closely related to inflammation, and recently, significant progress has been made in studies related to inflammation-based treatment of cardiovascular disease. Unlike canakinumab (5) and colchicine (6), which were effective in reducing the inflammatory response to cardiovascular disease, in a Cardiovascular Inflammation Reduction Trial involving 4786 patients at high risk for cardiovascular events, low-dose methotrexate did not reduce cardiovascular risk in patients (165). The results of these trials emphasize the importance of selecting the appropriate anti-inflammatory pathway and drug candidates in the treatment of cardiovascular disease.
Studies on animals have largely suggested that the CCL2-CCR2 axis is involved in disease processes.CCL2 and CCR2 knockout mice provide convincing evidence for a role of the CCL2-CCR2 axis in monocyte chemotaxis and inflammation (166, 167). A wealth of genetic, epidemiological and experimental data supports the causality of the CCL2-CCR2 axis in cardiovascular disease. Although pharmacologic targets for the CCL2-CCR2 axis in pre-clinical model of cardiovascular disease have been highly effective, clinical outcomes based on the CCL2-CCR2 axis for the treatment of cardiovascular disease have been disappointing to date (Table 3). This may be associated with the complexity of the CCL2 and CCR2 molecular structures, the difficulty in choosing the best target between CCL2 and CCR2, the confounding of the CCL2-CCR2 axis, the physiological circadian variation, and the somatic side impacts of CCL2-CCR2-targeted macromolecules (9). It is clear that these issues must be considered to achieve further breakthroughs in clinical applications. Therefore, more in-depth mechanistic studies and clinical studies in larger cohorts are needed before we can successfully design CCL2-CCR2-targeted therapies to significantly alleviate cardiovascular disease.
In conclusion, a wealth of animal and human research has provided evidence that the CCL2-CCR2 axis is important in the progression of cardiovascular disease, and the success of pharmacological targeting studies of the CCL2-CCR2 axis holds promise for a gradual transition to clinical trials. Although the CCL2-CCR2 axis-based treatment of cardiovascular disease may not immediately impact cardiovascular disease therapeutic practice, it does open the door for clinical translation of chemokines and their receptor modulators. More in-depth mechanistic studies and larger cohorts of clinical research directed at the CCL2-CCR2 axis will suggest new approaches for improving the prevention and treatment of cardiovascular disease.
HZ, KY, FC, QL, WC, YH, and ZL participated in the conception of the study, collection of the paper, and compilation of the data. HZ wrote the original manuscript and prepared the figures. GF, LL, JN, and FH provided constructive comments and revised and proofread the manuscript. All authors contributed to the article and approved the submitted version.
This research was funded by Innovation Team and Talents Cultivation Program of National Administration of Traditional Chinese Medicine (No: ZYYCXTD-D-202207), Tianjin Municipal Education Commission Scientific Research Program (2021KJ131).
The authors declare that the research was conducted in the absence of any commercial or financial relationships that could be construed as a potential conflict of interest.
All claims expressed in this article are solely those of the authors and do not necessarily represent those of their affiliated organizations, or those of the publisher, the editors and the reviewers. Any product that may be evaluated in this article, or claim that may be made by its manufacturer, is not guaranteed or endorsed by the publisher.
1. GBD 2019 Diseases and Injuries Collaborators. Global burden of 369 diseases and injuries in 204 countries and territories, 1990-2019: A systematic analysis for the global burden of disease study 2019. Lancet (London England) (2020) 396(10258):1204–22. doi: 10.1016/s0140-6736(20)30925-9
2. Fegers-Wustrow I, Gianos E, Halle M, Yang E. Comparison of American and European guidelines for primary prevention of cardiovascular disease: Jacc guideline comparison. J Am Coll Cardiol (2022) 79(13):1304–13. doi: 10.1016/j.jacc.2022.02.001
3. Liberale L, Montecucco F, Schwarz L, Lüscher T, Camici G. Inflammation and cardiovascular diseases: Lessons from seminal clinical trials. Cardiovasc Res (2021) 117(2):411–22. doi: 10.1093/cvr/cvaa211
4. Liberale L, Badimon L, Montecucco F, Lüscher T, Libby P, Camici G. Inflammation, aging, and cardiovascular disease: Jacc review topic of the week. J Am Coll Cardiol (2022) 79(8):837–47. doi: 10.1016/j.jacc.2021.12.017
5. Ridker P, Everett B, Thuren T, MacFadyen J, Chang W, Ballantyne C, et al. Antiinflammatory therapy with canakinumab for atherosclerotic disease. New Engl J Med (2017) 377(12):1119–31. doi: 10.1056/NEJMoa1707914
6. Tardif J, Kouz S, Waters D, Bertrand O, Diaz R, Maggioni A, et al. Efficacy and safety of low-dose colchicine after myocardial infarction. New Engl J Med (2019) 381(26):2497–505. doi: 10.1056/NEJMoa1912388
7. Bhusal R, Foster S, Stone M. Structural basis of chemokine and receptor interactions: Key regulators of leukocyte recruitment in inflammatory responses. Protein Sci Publ Protein Soc (2020) 29(2):420–32. doi: 10.1002/pro.3744
8. Behr TM, Wang X, Aiyar N, Coatney RW, Li X, Koster P, et al. Monocyte chemoattractant protein-1 is upregulated in rats with volume-overload congestive heart failure. Circulation (2000) 102(11):1315–22. doi: 10.1161/01.cir.102.11.1315
9. Georgakis M, Bernhagen J, Heitman L, Weber C, Dichgans M. Targeting the Ccl2-Ccr2 axis for atheroprotection. Eur Heart J (2022) 43(19):1799–808. doi: 10.1093/eurheartj/ehac094
10. Bush E, Maeda N, Kuziel W, Dawson T, Wilcox J, DeLeon H, et al. Cc chemokine receptor 2 is required for macrophage infiltration and vascular hypertrophy in angiotensin ii-induced hypertension. Hypertension (Dallas Tex 1979) (2000) 36(3):360–3. doi: 10.1161/01.hyp.36.3.360
11. Leuschner F, Courties G, Dutta P, Mortensen L, Gorbatov R, Sena B, et al. Silencing of Ccr2 in myocarditis. Eur Heart J (2015) 36(23):1478–88. doi: 10.1093/eurheartj/ehu225
12. Kuwahara F, Kai H, Tokuda K, Takeya M, Takeshita A, Egashira K, et al. Hypertensive myocardial fibrosis and diastolic dysfunction: Another model of inflammation? Hypertension (2004) 43(4):739–45. doi: 10.1161/01.HYP.0000118584.33350.7d
13. Vlachou F, Varela A, Stathopoulou K, Ntatsoulis K, Synolaki E, Pratsinis H, et al. Galectin-3 interferes with tissue repair and promotes cardiac dysfunction and comorbidities in a genetic heart failure model. Cell Mol Life Sci CMLS (2022) 79(5):250. doi: 10.1007/s00018-022-04266-6
14. Damås J, Eiken H, Oie E, Bjerkeli V, Yndestad A, Ueland T, et al. Myocardial expression of cc- and cxc-chemokines and their receptors in human end-stage heart failure. Cardiovasc Res (2000) 47(4):778–87. doi: 10.1016/s0008-6363(00)00142-5
15. Stumpf C, Lehner C, Raaz D, Yilmaz A, Anger T, Daniel W, et al. Platelets contribute to enhanced mcp-1 levels in patients with chronic heart failure. Heart (British Cardiac Society) (2008) 94(1):65–9. doi: 10.1136/hrt.2007.115006
16. Hohensinner P, Rychli K, Zorn G, Hülsmann M, Berger R, Mörtl D, et al. Macrophage-modulating cytokines predict adverse outcome in heart failure. Thromb haemostasis (2010) 103(2):435–41. doi: 10.1160/th09-06-0399
17. Hernández-Presa M, Bustos C, Ortego M, Tuñon J, Renedo G, Ruiz-Ortega M, et al. Angiotensin-converting enzyme inhibition prevents arterial nuclear factor-kappa b activation, monocyte chemoattractant protein-1 expression, and macrophage infiltration in a rabbit model of early accelerated atherosclerosis. Circulation (1997) 95(6):1532–41. doi: 10.1161/01.cir.95.6.1532
18. Nelken N, Coughlin S, Gordon D, Wilcox J. Monocyte chemoattractant protein-1 in human atheromatous plaques. J Clin Invest (1991) 88(4):1121–7. doi: 10.1172/jci115411
19. Gu L, Okada Y, Clinton S, Gerard C, Sukhova G, Libby P, et al. Absence of monocyte chemoattractant protein-1 reduces atherosclerosis in low density lipoprotein receptor-deficient mice. Mol Cell (1998) 2(2):275–81. doi: 10.1016/s1097-2765(00)80139-2
20. Boring L, Gosling J, Cleary M, Charo I. Decreased lesion formation in Ccr2-/- mice reveals a role for chemokines in the initiation of atherosclerosis. Nature (1998) 394(6696):894–7. doi: 10.1038/29788
21. Georgakis M, van der Laan S, Asare Y, Mekke J, Haitjema S, Schoneveld A, et al. Monocyte-chemoattractant protein-1 levels in human atherosclerotic lesions associate with plaque vulnerability. Arteriosclerosis thrombosis Vasc Biol (2021) 41(6):2038–48. doi: 10.1161/atvbaha.121.316091
22. Georgakis M, Gill D, Rannikmäe K, Traylor M, Anderson C, Lee J, et al. Genetically determined levels of circulating cytokines and risk of stroke. Circulation (2019) 139(2):256–68. doi: 10.1161/circulationaha.118.035905
23. Majmudar M, Keliher E, Heidt T, Leuschner F, Truelove J, Sena B, et al. Monocyte-directed rnai targeting Ccr2 improves infarct healing in atherosclerosis-prone mice. Circulation (2013) 127(20):2038–46. doi: 10.1161/circulationaha.112.000116
24. Dewald O, Zymek P, Winkelmann K, Koerting A, Ren G, Abou-Khamis T, et al. Ccl2/Monocyte chemoattractant protein-1 regulates inflammatory responses critical to healing myocardial infarcts. Circ Res (2005) 96(8):881–9. doi: 10.1161/01.RES.0000163017.13772.3a
25. Wang J, Seo M, Deci M, Weil B, Canty J, Nguyen J. Effect of Ccr2 inhibitor-loaded lipid micelles on inflammatory cell migration and cardiac function after myocardial infarction. Int J nanomedicine (2018) 13:6441–51. doi: 10.2147/ijn.S178650
26. Martire A, Fernandez B, Buehler A, Strohm C, Schaper J, Zimmermann R, et al. Cardiac overexpression of monocyte chemoattractant protein-1 in transgenic mice mimics ischemic preconditioning through Sapk/Jnk1/2 activation. Cardiovasc Res (2003) 57(2):523–34. doi: 10.1016/s0008-6363(02)00697-1
27. Morimoto H, Takahashi M, Izawa A, Ise H, Hongo M, Kolattukudy P, et al. Cardiac overexpression of monocyte chemoattractant protein-1 in transgenic mice prevents cardiac dysfunction and remodeling after myocardial infarction. Circ Res (2006) 99(8):891–9. doi: 10.1161/01.RES.0000246113.82111.2d
28. Ishibashi M, Hiasa K-i, Zhao Q, Inoue S, Ohtani K, Kitamoto S, et al. Critical role of monocyte chemoattractant protein-1 receptor Ccr2 on monocytes in hypertension-induced vascular inflammation and remodeling. Circ Res (2004) 94(9):1203–10. doi: 10.1161/01.Res.0000126924.23467.A3
29. Chen X, Tummala P, Olbrych M, Alexander R, Medford R. Angiotensin ii induces monocyte chemoattractant protein-1 gene expression in rat vascular smooth muscle cells. Circ Res (1998) 83(9):952–9. doi: 10.1161/01.res.83.9.952
30. Tucci M, Quatraro C, Frassanito M, Silvestris F. Deregulated expression of monocyte chemoattractant protein-1 (Mcp-1) in arterial hypertension: Role in endothelial inflammation and atheromasia. J hypertension (2006) 24(7):1307–18. doi: 10.1097/01.hjh.0000234111.31239.c3
31. Wang Y, Zhu M, Xu H, Cui L, Liu W, Wang X, et al. Role of the monocyte chemoattractant protein-1/C-C chemokine receptor 2 signaling pathway in transient receptor potential vanilloid type 1 ablation-induced renal injury in salt-sensitive hypertension. Exp Biol Med (Maywood NJ) (2015) 240(9):1223–34. doi: 10.1177/1535370214565970
32. Kashyap S, Osman M, Ferguson C, Nath M, Roy B, Lien K, et al. Ccl2 deficiency protects against chronic renal injury in murine renovascular hypertension. Sci Rep (2018) 8(1):8598. doi: 10.1038/s41598-018-26870-y
33. Alsheikh A, Dasinger J, Abais-Battad J, Fehrenbach D, Yang C, Cowley A, et al. Ccl2 mediates early renal leukocyte infiltration during salt-sensitive hypertension. Am J Physiol Renal Physiol (2020) 318(4):F982–F93. doi: 10.1152/ajprenal.00521.2019
34. Fuse K, Kodama M, Hanawa H, Okura Y, Ito M, Shiono T, et al. Enhanced expression and production of monocyte chemoattractant protein-1 in myocarditis. Clin Exp Immunol (2001) 124(3):346–52. doi: 10.1046/j.1365-2249.2001.01510.x
35. Kobayashi Y, Kubo A, Iwano M, Sakaguchi Y, Samejima K, Kyoda Y, et al. Levels of mcp-1 and gm-csf mrna correlated with inflammatory cytokines mrna levels in experimental autoimmune myocarditis in rats. Autoimmunity (2002) 35(2):97–104. doi: 10.1080/08916930290016538
36. Huang X, Li Z, Shen X, Nie N, Shen Y. Il-17 upregulates mcp-1 expression Via Act1 / Traf6 / Tak1 in experimental autoimmune myocarditis. Cytokine (2022) 152:155823. doi: 10.1016/j.cyto.2022.155823
37. Yue Y, Gui J, Xu W, Xiong S. Gene therapy with Ccl2 (Mcp-1) mutant protects Cvb3-induced myocarditis by compromising Th1 polarization. Mol Immunol (2011) 48(4):706–13. doi: 10.1016/j.molimm.2010.11.018
38. Göser S, Ottl R, Brodner A, Dengler T, Torzewski J, Egashira K, et al. Critical role for monocyte chemoattractant protein-1 and macrophage inflammatory protein-1alpha in induction of experimental autoimmune myocarditis and effective anti-monocyte chemoattractant protein-1 gene therapy. Circulation (2005) 112(22):3400–7. doi: 10.1161/circulationaha.105.572396
39. Lehmann M, Kühnert H, Müller S, Sigusch H. Monocyte chemoattractant protein 1 (Mcp-1) gene expression in dilated cardiomyopathy. Cytokine (1998) 10(10):739–46. doi: 10.1006/cyto.1998.0354
40. Zhang W, Chen Z, Qiao S, Chen S, Zheng H, Wei X, et al. The effects of extracellular vesicles derived from krüppel-like factor 2 overexpressing endothelial cells on the regulation of cardiac inflammation in the dilated cardiomyopathy. J nanobiotechnology (2022) 20(1):76. doi: 10.1186/s12951-022-01284-1
41. Iwasaki J, Nakamura K, Matsubara H, Nakamura Y, Nishii N, Banba K, et al. Relationship between circulating levels of monocyte chemoattractant protein-1 and systolic dysfunction in patients with hypertrophic cardiomyopathy. Cardiovasc Pathol Off J Soc Cardiovasc Pathol (2009) 18(6):317–22. doi: 10.1016/j.carpath.2008.12.004
42. Lubos N, van der Gaag S, Gerçek M, Kant S, Leube R, Krusche C. Inflammation shapes pathogenesis of murine arrhythmogenic cardiomyopathy. Basic Res Cardiol (2020) 115(4):42. doi: 10.1007/s00395-020-0803-5
43. Georgakis M, de Lemos J, Ayers C, Wang B, Björkbacka H, Pana T, et al. Association of circulating monocyte chemoattractant protein-1 levels with cardiovascular mortality: A meta-analysis of population-based studies. JAMA Cardiol (2021) 6(5):587–92. doi: 10.1001/jamacardio.2020.5392
44. Ialenti A, Grassia G, Gordon P, Maddaluno M, Di Lauro M, Baker A, et al. Inhibition of in-stent stenosis by oral administration of bindarit in porcine coronary arteries. Arteriosclerosis thrombosis Vasc Biol (2011) 31(11):2448–54. doi: 10.1161/atvbaha.111.230078
45. Liehn E, Piccinini A, Koenen R, Soehnlein O, Adage T, Fatu R, et al. A new monocyte chemotactic protein-1/Chemokine cc motif ligand-2 competitor limiting neointima formation and myocardial Ischemia/Reperfusion injury in mice. J Am Coll Cardiol (2010) 56(22):1847–57. doi: 10.1016/j.jacc.2010.04.066
46. Yamashita T, Kawashima S, Ozaki M, Namiki M, Inoue N, Hirata K, et al. Propagermanium reduces atherosclerosis in apolipoprotein e knockout mice Via inhibition of macrophage infiltration. Arteriosclerosis thrombosis Vasc Biol (2002) 22(6):969–74. doi: 10.1161/01.atv.0000019051.88366.9c
47. Gilbert J, Lekstrom-Himes J, Donaldson D, Lee Y, Hu M, Xu J, et al. Effect of cc chemokine receptor 2 Ccr2 blockade on serum c-reactive protein in individuals at atherosclerotic risk and with a single nucleotide polymorphism of the monocyte chemoattractant protein-1 promoter region. Am J Cardiol (2011) 107(6):906–11. doi: 10.1016/j.amjcard.2010.11.005
48. Colombo A, Basavarajaiah S, Limbruno U, Picchi A, Lettieri C, Valgimigli M, et al. A double-blind randomised study to evaluate the efficacy and safety of bindarit in preventing coronary stent restenosis. EuroIntervention J EuroPCR collaboration Working Group Interventional Cardiol Eur Soc Cardiol (2016) 12(11):e1385–e94. doi: 10.4244/eijy15m12_03
49. Fernandez EJ, Lolis E. Structure junction, and inhibition of chemokines. Annu Rev Pharmacol Toxicol (2002) 42:469–99. doi: 10.1146/annurev.pharmtox.42.091901.115838
50. Singh S, Anshita D, Ravichandiran V. Mcp-1: Function, regulation, and involvement in disease. Int Immunopharmacol (2021) 101:107598. doi: 10.1016/j.intimp.2021.107598
51. Hughes CE, Nibbs RJB. A guide to chemokines and their receptors. FEBS J (2018) 285(16):2944–71. doi: 10.1111/febs.14466
52. Propper D, Balkwill F. Harnessing cytokines and chemokines for cancer therapy. Nat Rev Clin Oncol (2022) 19(4):237–53. doi: 10.1038/s41571-021-00588-9
53. Cao S, Liu M, Sehrawat T, Shah V. Regulation and functional roles of chemokines in liver diseases. Nat Rev Gastroenterol Hepatol (2021) 18(9):630–47. doi: 10.1038/s41575-021-00444-2
54. Progatzky F, Shapiro M, Chng S, Garcia-Cassani B, Classon C, Sevgi S, et al. Regulation of intestinal immunity and tissue repair by enteric glia. Nature (2021) 599(7883):125–30. doi: 10.1038/s41586-021-04006-z
56. Charo I, Ransohoff R. The many roles of chemokines and chemokine receptors in inflammation. New Engl J Med (2006) 354(6):610–21. doi: 10.1056/NEJMra052723
57. Yoshimura T, Robinson EA, Tanaka S, Appella E, Kuratsu J, Leonard EJ. Purification and amino acid analysis of two human glioma-derived monocyte chemoattractants. J Exp Med (1989) 169(4):1449–59. doi: 10.1084/jem.169.4.1449
58. Yoshimura T, Robinson EA, Tanaka S, Appella E, Leonard EJ. Purification and amino acid analysis of two human monocyte chemoattractants produced by phytohemagglutinin-stimulated human blood mononuclear leukocytes. J Immunol (1989) 142(6):1956–62.
59. Deshmane SL, Kremlev S, Amini S, Sawaya BE. Monocyte chemoattractant protein-1 (Mcp-1): An overview. J Interferon Cytokine Res (2009) 29(6):313–26. doi: 10.1089/jir.2008.0027
60. Standiford T, Kunkel S, Phan S, Rollins B, Strieter R. Alveolar macrophage-derived cytokines induce monocyte chemoattractant protein-1 expression from human pulmonary type ii-like epithelial cells. J Biol Chem (1991) 266(15):9912–8.
61. Bianconi V, Sahebkar A, Atkin S, Pirro M. The regulation and importance of monocyte chemoattractant protein-1. Curr Opin Hematol (2018) 25(1):44–51. doi: 10.1097/moh.0000000000000389
62. Gschwandtner M, Derler R, Midwood K. More than just attractive: How Ccl2 influences myeloid cell behavior beyond chemotaxis. Front Immunol (2019) 10:2759. doi: 10.3389/fimmu.2019.02759
63. Yoshimura T. The chemokine mcp-1 (Ccl2) in the host interaction with cancer: A foe or ally? Cell Mol Immunol (2018) 15(4):335–45. doi: 10.1038/cmi.2017.135
64. Ling Z, Li W, Hu J, Li Y, Deng M, Zhang S, et al. Targeting Ccl2-Ccr4 axis suppress cell migration of head and neck squamous cell carcinoma. Cell Death Dis (2022) 13(2):158. doi: 10.1038/s41419-022-04610-5
65. Ford L, Cerovic V, Milling S, Graham G, Hansell C, Nibbs R. Characterization of conventional and atypical receptors for the chemokine Ccl2 on mouse leukocytes. J Immunol (Baltimore Md 1950) (2014) 193(1):400–11. doi: 10.4049/jimmunol.1303236
66. Huma Z, Sanchez J, Lim H, Bridgford J, Huang C, Parker B, et al. Key determinants of selective binding and activation by the monocyte chemoattractant proteins at the chemokine receptor Ccr2. Sci Signaling (2017) 10(480). doi: 10.1126/scisignal.aai8529
67. Charo I, Myers S, Herman A, Franci C, Connolly A, Coughlin S. Molecular cloning and functional expression of two monocyte chemoattractant protein 1 receptors reveals alternative splicing of the carboxyl-terminal tails. Proc Natl Acad Sci United States America (1994) 91(7):2752–6. doi: 10.1073/pnas.91.7.2752
68. Apel A, Cheng R, Tautermann C, Brauchle M, Huang C, Pautsch A, et al. Crystal structure of cc chemokine receptor 2a in complex with an orthosteric antagonist provides insights for the design of selective antagonists. Structure (London Engl 1993) (2019) 27(3):427–38.e5. doi: 10.1016/j.str.2018.10.027
69. Chen X, Deng Y, Wang Z, Wei W, Zhou C, Zhang Y, et al. Hypoxia-induced Zeb1 promotes cervical cancer progression Via Ccl8-dependent tumour-associated macrophage recruitment. Cell Death Dis (2019) 10(7):508. doi: 10.1038/s41419-019-1748-1
70. Levina V, Nolen B, Marrangoni A, Cheng P, Marks J, Szczepanski M, et al. Role of eotaxin-1 signaling in ovarian cancer. Clin Cancer Res an Off J Am Assoc Cancer Res (2009) 15(8):2647–56. doi: 10.1158/1078-0432.Ccr-08-2024
71. Jha A, Thwaites R, Tunstall T, Kon O, Shattock R, Hansel T, et al. Increased nasal mucosal interferon and Ccl13 response to a Tlr7/8 agonist in asthma and allergic rhinitis. J Allergy Clin Immunol (2021) 147(2):694–703.e12. doi: 10.1016/j.jaci.2020.07.012
72. He J, Song Y, Li G, Xiao P, Liu Y, Xue Y, et al. Fbxw7 increases Ccl2/7 in Cx3cr1hi macrophages to promote intestinal inflammation. J Clin Invest (2019) 129(9):3877–93. doi: 10.1172/jci123374
73. Mercer P, Williams A, Scotton C, José R, Sulikowski M, Moffatt J, et al. Proteinase-activated receptor-1, Ccl2, and Ccl7 regulate acute neutrophilic lung inflammation. Am J Respir Cell Mol Biol (2014) 50(1):144–57. doi: 10.1165/rcmb.2013-0142OC
74. McQuibban G, Gong J, Tam E, McCulloch C, Clark-Lewis I, Overall C. Inflammation dampened by gelatinase a cleavage of monocyte chemoattractant protein-3. Sci (New York NY) (2000) 289(5482):1202–6. doi: 10.1126/science.289.5482.1202
75. Lim S, Yuzhalin A, Gordon-Weeks A, Muschel R. Targeting the Ccl2-Ccr2 signaling axis in cancer metastasis. Oncotarget (2016) 7(19):28697–710. doi: 10.18632/oncotarget.7376
76. Geng H, Chen L, Tang J, Chen Y, Wang L. The role of Ccl2/Ccr2 axis in cerebral ischemia-reperfusion injury and treatment: From animal experiments to clinical trials. Int J Mol Sci (2022) 23(7). doi: 10.3390/ijms23073485
77. Zhu S, Liu M, Bennett S, Wang Z, Pfleger K, Xu J. The molecular structure and role of Ccl2 (Mcp-1) and c-c chemokine receptor Ccr2 in skeletal biology and diseases. J Cell Physiol (2021) 236(10):7211–22. doi: 10.1002/jcp.30375
78. Xu M, Wang Y, Xia R, Wei Y, Wei X. Role of the Ccl2-Ccr2 signalling axis in cancer: Mechanisms and therapeutic targeting. Cell proliferation (2021) 54(10):e13115. doi: 10.1111/cpr.13115
79. Moadab F, Khorramdelazad H, Abbasifard M. Role of Ccl2/Ccr2 axis in the immunopathogenesis of rheumatoid arthritis: Latest evidence and therapeutic approaches. Life Sci (2021) 269:119034. doi: 10.1016/j.lfs.2021.119034
80. Xu R, Ni B, Wang L, Shan J, Pan L, He Y, et al. Ccr2-overexpressing mesenchymal stem cells targeting damaged liver enhance recovery of acute liver failure. Stem Cell Res Ther (2022) 13(1):55. doi: 10.1186/s13287-022-02729-y
81. Flegar D, Filipović M, Šućur A, Markotić A, Lukač N, Šisl D, et al. Preventive Ccl2/Ccr2 axis blockade suppresses osteoclast activity in a mouse model of rheumatoid arthritis by reducing homing of Ccr2 osteoclast progenitors to the affected bone. Front Immunol (2021) 12:767231. doi: 10.3389/fimmu.2021.767231
82. Sheng Y, Gong X, Zhao J, Liu Y, Yuan Y. Effects of crocin on Ccl2/Ccr2 inflammatory pathway in monocrotaline-induced pulmonary arterial hypertension rats. Am J Chin Med (2022) 50(1):241–59. doi: 10.1142/s0192415x22500082
83. Kuang S, He F, Liu G, Sun X, Dai J, Chi A, et al. Ccr2-engineered mesenchymal stromal cells accelerate diabetic wound healing by restoring immunological homeostasis. Biomaterials (2021) 275:120963. doi: 10.1016/j.biomaterials.2021.120963
84. Wang J, Saung M, Li K, Fu J, Fujiwara K, Niu N, et al. Ccr2/Ccr5 inhibitor permits the radiation-induced effector T cell infiltration in pancreatic adenocarcinoma. J Exp Med (2022) 219(5). doi: 10.1084/jem.20211631
85. Olzinski A, Turner G, Bernard R, Karr H, Cornejo C, Aravindhan K, et al. Pharmacological inhibition of c-c chemokine receptor 2 decreases macrophage infiltration in the aortic root of the human c-c chemokine receptor 2/Apolipoprotein e-/- mouse: Magnetic resonance imaging assessment. Arteriosclerosis thrombosis Vasc Biol (2010) 30(2):253–9. doi: 10.1161/atvbaha.109.198812
86. Bachelerie F, Ben-Baruch A, Burkhardt A, Combadiere C, Farber J, Graham G, et al. International union of basic and clinical pharmacology. [Corrected]. lxxxix. update on the extended family of chemokine receptors and introducing a new nomenclature for atypical chemokine receptors. Pharmacol Rev (2014) 66(1):1–79. doi: 10.1124/pr.113.007724
87. Xue C, Feng H, Cao G, Huang T, Glenn J, Anand R, et al. Discovery of Incb3284, a potent, selective, and orally bioavailable Hccr2 antagonist. ACS medicinal Chem Lett (2011) 2(6):450–4. doi: 10.1021/ml200030q
88. Aiello R, Perry B, Bourassa P, Robertson A, Weng W, Knight D, et al. Ccr2 receptor blockade alters blood monocyte subpopulations but does not affect atherosclerotic lesions in apoe(-/-) mice. Atherosclerosis (2010) 208(2):370–5. doi: 10.1016/j.atherosclerosis.2009.08.017
89. Ito S, Nakashima H, Ishikiriyama T, Nakashima M, Yamagata A, Imakiire T, et al. Effects of a Ccr2 antagonist on macrophages and toll-like receptor 9 expression in a mouse model of diabetic nephropathy. Am J Physiol Renal Physiol (2021) 321(6):F757–F70. doi: 10.1152/ajprenal.00191.2021
90. Brodmerkel C, Huber R, Covington M, Diamond S, Hall L, Collins R, et al. Discovery and pharmacological characterization of a novel rodent-active Ccr2 antagonist, Incb3344. J Immunol (Baltimore Md 1950) (2005) 175(8):5370–8. doi: 10.4049/jimmunol.175.8.5370
91. Buntinx M, Hermans B, Goossens J, Moechars D, Gilissen R, Doyon J, et al. Pharmacological profile of jnj-27141491 [(S)-3-[3,4-Difluorophenyl)-Propyl]-5-Isoxazol-5-Yl-2-Thioxo-2,3-Dihydro-1h-Imidazole-4-Carboxyl acid methyl ester], as a noncompetitive and orally active antagonist of the human chemokine receptor Ccr2. J Pharmacol Exp Ther (2008) 327(1):1–9. doi: 10.1124/jpet.108.140723
92. Ruff M, Inan S, Shi X, Meissler J, Adler M, Eisenstein T, et al. Potentiation of morphine antinociception and inhibition of diabetic neuropathic pain by the multi-chemokine receptor antagonist peptide rap-103. Life Sci (2022) 306:120788. doi: 10.1016/j.lfs.2022.120788
93. Noda M, Tomonaga D, Kitazono K, Yoshioka Y, Liu J, Rousseau J, et al. Neuropathic pain inhibitor, rap-103, is a potent inhibitor of microglial Ccl1/Ccr8. Neurochemistry Int (2018) 119:184–9. doi: 10.1016/j.neuint.2017.12.005
94. Sayyed S, Ryu M, Kulkarni O, Schmid H, Lichtnekert J, Grüner S, et al. An orally active chemokine receptor Ccr2 antagonist prevents glomerulosclerosis and renal failure in type 2 diabetes. Kidney Int (2011) 80(1):68–78. doi: 10.1038/ki.2011.102
95. Zhang W, Zhu T, Chen L, Luo W, Chao J. Mcp-1 mediates ischemia-Reperfusion-Induced cardiomyocyte apoptosis Via Mcpip1 and casr. Am J Physiol Heart Circulatory Physiol (2020) 318(1):H59–71. doi: 10.1152/ajpheart.00308.2019
96. Elmarakby AA, Quigley JE, Olearczyk JJ, Sridhar A, Cook AK, Inscho EW, et al. Chemokine receptor 2b inhibition provides renal protection in angiotensin ii - salt hypertension. Hypertension (Dallas Tex 1979) (2007) 50(6):1069–76. doi: 10.1161/hypertensionaha.107.098806
97. Seok S, Lee E, Kim G, Hyun M, Lee J, Chen S, et al. Blockade of Ccl2/Ccr2 signalling ameliorates diabetic nephropathy in Db/Db mice. Nephrology dialysis Transplant Off Publ Eur Dialysis Transplant Assoc - Eur Renal Assoc (2013) 28(7):1700–10. doi: 10.1093/ndt/gfs555
98. Patel B, Bansal S, Ismahil M, Hamid T, Rokosh G, Mack M, et al. Ccr2 monocyte-derived infiltrating macrophages are required for adverse cardiac remodeling during pressure overload. JACC Basic to Trans Sci (2018) 3(2):230–44. doi: 10.1016/j.jacbts.2017.12.006
99. Kwiatkowski K, Piotrowska A, Rojewska E, Makuch W, Mika J. The Rs504393 influences the level of nociceptive factors and enhances opioid analgesic potency in neuropathic rats. J neuroimmune Pharmacol Off J Soc NeuroImmune Pharmacol (2017) 12(3):402–19. doi: 10.1007/s11481-017-9729-6
100. Okamoto M, Fuchigami M, Suzuki T, Watanabe N. A novel c-c chemokine receptor 2 antagonist prevents progression of albuminuria and atherosclerosis in mouse models. Biol Pharm Bull (2012) 35(11):2069–74. doi: 10.1248/bpb.b12-00528
101. Heidenreich P, Bozkurt B, Aguilar D, Allen L, Byun J, Colvin M, et al. 2022 Aha/Acc/Hfsa guideline for the management of heart failure: A report of the American college of Cardiology/American heart association joint committee on clinical practice guidelines. Circulation (2022) 145(18):e895–1032. doi: 10.1161/cir.0000000000001063
102. Halade G, Lee D. Inflammation and resolution signaling in cardiac repair and heart failure. EBioMedicine (2022) 79:103992. doi: 10.1016/j.ebiom.2022.103992
103. Dick S, Epelman S. Chronic heart failure and inflammation: What do we really know? Circ Res (2016) 119(1):159–76. doi: 10.1161/circresaha.116.308030
104. Hanna A, Frangogiannis N. Inflammatory cytokines and chemokines as therapeutic targets in heart failure. Cardiovasc Drugs Ther (2020) 34(6):849–63. doi: 10.1007/s10557-020-07071-0
105. Lutgens E, Atzler D, Döring Y, Duchene J, Steffens S, Weber C. Immunotherapy for cardiovascular disease. Eur Heart J (2019) 40(48):3937–46. doi: 10.1093/eurheartj/ehz283
106. Libby P. Inflammation in atherosclerosis. Nature (2002) 420(6917):868–74. doi: 10.1038/nature01323
107. Marchini T, Mitre L, Wolf D. Inflammatory cell recruitment in cardiovascular disease. Front Cell Dev Biol (2021) 9:635527. doi: 10.3389/fcell.2021.635527
108. Timmis A, Townsend N, Gale C, Grobbee R, Maniadakis N, Flather M, et al. European Society of cardiology: Cardiovascular disease statistics 2017. Eur Heart J (2018) 39(7):508–79. doi: 10.1093/eurheartj/ehx628
109. Chen B, Frangogiannis N. Chemokines in myocardial infarction. J Cardiovasc Trans Res (2021) 14(1):35–52. doi: 10.1007/s12265-020-10006-7
110. Dutta P, Nahrendorf M. Monocytes in myocardial infarction. Arteriosclerosis thrombosis Vasc Biol (2015) 35(5):1066–70. doi: 10.1161/atvbaha.114.304652
111. Frodermann V, Nahrendorf M. Neutrophil-macrophage cross-talk in acute myocardial infarction. Eur Heart J (2017) 38(3):198–200. doi: 10.1093/eurheartj/ehw085
112. Cavalera M, Frangogiannis N. Targeting the chemokines in cardiac repair. Curr Pharm design (2014) 20(12):1971–9. doi: 10.2174/13816128113199990449
113. Lu W, Xie Z, Tang Y, Bai L, Yao Y, Fu C, et al. Photoluminescent mesoporous silicon nanoparticles with Siccr2 improve the effects of mesenchymal stromal cell transplantation after acute myocardial infarction. Theranostics (2015) 5(10):1068–82. doi: 10.7150/thno.11517
114. Mikolajczyk T, Szczepaniak P, Vidler F, Maffia P, Graham G, Guzik T. Role of inflammatory chemokines in hypertension. Pharmacol Ther (2021) 223:107799. doi: 10.1016/j.pharmthera.2020.107799
115. Capers Q, Alexander R, Lou P, De Leon H, Wilcox J, Ishizaka N, et al. Monocyte chemoattractant protein-1 expression in aortic tissues of hypertensive rats. Hypertension (Dallas Tex 1979) (1997) 30(6):1397–402. doi: 10.1161/01.hyp.30.6.1397
116. Schultheiss H, Kühl U, Cooper L. The management of myocarditis. Eur Heart J (2011) 32(21):2616–25. doi: 10.1093/eurheartj/ehr165
117. Asatryan B, Asimaki A, Landstrom A, Khanji M, Odening K, Cooper L, et al. Inflammation and immune response in arrhythmogenic cardiomyopathy: State-of-the-Art review. Circulation (2021) 144(20):1646–55. doi: 10.1161/circulationaha.121.055890
118. Lutgens E, Faber B, Schapira K, Evelo CTA, Haaften Rv, Heeneman S, et al. Gene profiling in atherosclerosis reveals a key role for small inducible cytokines: Validation using a novel monocyte chemoattractant protein monoclonal antibody. Circulation (2005) 111(25):3443–52. doi: 10.1161/circulationaha.104.510073
119. Mirolo M, Fabbri M, Sironi M, Vecchi A, Guglielmotti A, Mangano G, et al. Impact of the anti-inflammatory agent bindarit on the chemokinome: Selective inhibition of the monocyte chemotactic proteins. Eur Cytokine network (2008) 19(3):119–22. doi: 10.1684/ecn.2008.0133
120. Mora E, Guglielmotti A, Biondi G, Sassone-Corsi P. Bindarit: An anti-inflammatory small molecule that modulates the nfκb pathway. Cell Cycle (Georgetown Tex) (2012) 11(1):159–69. doi: 10.4161/cc.11.1.18559
121. Guglielmotti A, D'Onofrio E, Coletta I, Aquilini L, Milanese C, Pinza M. Amelioration of rat adjuvant arthritis by therapeutic treatment with bindarit, an inhibitor of mcp-1 and tnf-alpha production. Inflammation Res Off J Eur Histamine Res Soc [et al] (2002) 51(5):252–8. doi: 10.1007/pl00000301
122. Shen Z, Kuang S, Zhang M, Huang X, Chen J, Guan M, et al. Inhibition of Ccl2 by bindarit alleviates diabetes-associated periodontitis by suppressing inflammatory monocyte infiltration and altering macrophage properties. Cell Mol Immunol (2021) 18(9):2224–35. doi: 10.1038/s41423-020-0500-1
123. Zhang K, Wang H, Xu M, Frank J, Luo J. Role of mcp-1 and Ccr2 in ethanol-induced neuroinflammation and neurodegeneration in the developing brain. J Neuroinflamm (2018) 15(1):197. doi: 10.1186/s12974-018-1241-2
124. Raghu H, Lepus C, Wang Q, Wong H, Lingampalli N, Oliviero F, et al. Ccl2/Ccr2, but not Ccl5/Ccr5, mediates monocyte recruitment, inflammation and cartilage destruction in osteoarthritis. Ann rheumatic Dis (2017) 76(5):914–22. doi: 10.1136/annrheumdis-2016-210426
125. Ge S, Shrestha B, Paul D, Keating C, Cone R, Guglielmotti A, et al. The Ccl2 synthesis inhibitor bindarit targets cells of the neurovascular unit, and suppresses experimental autoimmune encephalomyelitis. J Neuroinflamm (2012) 9:171. doi: 10.1186/1742-2094-9-171
126. Ble A, Mosca M, Di Loreto G, Guglielmotti A, Biondi G, Bombardieri S, et al. Antiproteinuric effect of chemokine c-c motif ligand 2 inhibition in subjects with acute proliferative lupus nephritis. Am J Nephrol (2011) 34(4):367–72. doi: 10.1159/000330685
127. Maddaluno M, Grassia G, Di Lauro M, Parisi A, Maione F, Cicala C, et al. Bindarit inhibits human coronary artery smooth muscle cell proliferation, migration and phenotypic switching. PLoS One (2012) 7(10):e47464. doi: 10.1371/journal.pone.0047464
128. Yin L, Peng C, Tang Y, Yuan Y, Liu J, Xiang T, et al. Biomimetic oral targeted delivery of bindarit for immunotherapy of atherosclerosis. Biomaterials Sci (2020) 8(13):3640–8. doi: 10.1039/d0bm00418a
129. Egashira K. Molecular mechanisms mediating inflammation in vascular disease: Special reference to monocyte chemoattractant protein-1. Hypertension (Dallas Tex 1979) (2003) 41(3 Pt 2):834–41. doi: 10.1161/01.Hyp.0000051642.65283.36
130. Matoba T, Egashira K. Anti-inflammatory gene therapy for cardiovascular disease. Curr Gene Ther (2011) 11(6):442–6. doi: 10.2174/156652311798192888
131. Mirzadegan T, Diehl F, Ebi B, Bhakta S, Polsky I, McCarley D, et al. Identification of the binding site for a novel class of Ccr2b chemokine receptor antagonists: Binding to a common chemokine receptor motif within the helical bundle. J Biol Chem (2000) 275(33):25562–71. doi: 10.1074/jbc.M000692200
132. Kashyap S, Warner G, Hartono S, Boyilla R, Knudsen B, Zubair A, et al. Blockade of Ccr2 reduces macrophage influx and development of chronic renal damage in murine renovascular hypertension. Am J Physiol Renal Physiol (2016) 310(5):F372–84. doi: 10.1152/ajprenal.00131.2015
133. Winter C, Silvestre-Roig C, Ortega-Gomez A, Lemnitzer P, Poelman H, Schumski A, et al. Chrono-pharmacological targeting of the Ccl2-Ccr2 axis ameliorates atherosclerosis. Cell Metab (2018) 28(1):175–82.e5. doi: 10.1016/j.cmet.2018.05.002
134. Shimokawa H, Eto Y, Miyata K, Morishige K, Kandabashi T, Matsushima K, et al. Propagermanium suppresses macrophage-mediated formation of coronary arteriosclerotic lesions in pigs in vivo. J Cardiovasc Pharmacol (2003) 41(3):372–80. doi: 10.1097/00005344-200303000-00005
135. Eto Y, Shimokawa H, Tanaka E, Morishige K, Fuchigami M, Ishiwata Y, et al. Long-term treatment with propagermanium suppresses atherosclerosis in whhl rabbits. J Cardiovasc Pharmacol (2003) 41(2):171–7. doi: 10.1097/00005344-200302000-00004
136. Xue C, Wang A, Meloni D, Zhang K, Kong L, Feng H, et al. Discovery of Incb3344, a potent, selective and orally bioavailable antagonist of human and murine Ccr2. Bioorganic medicinal Chem Lett (2010) 20(24):7473–8. doi: 10.1016/j.bmcl.2010.10.020
137. Yokochi S, Hashimoto H, Ishiwata Y, Shimokawa H, Haino M, Terashima Y, et al. An anti-inflammatory drug, propagermanium, may target gpi-anchored proteins associated with an mcp-1 receptor, Ccr2. J Interferon Cytokine Res Off J Int Soc Interferon Cytokine Res (2001) 21(6):389–98. doi: 10.1089/107999001750277862
138. Laborde E, Macsata R, Meng F, Peterson B, Robinson L, Schow S, et al. Discovery, optimization, and pharmacological characterization of novel heteroaroylphenylureas antagonists of c-c chemokine ligand 2 function. J medicinal Chem (2011) 54(6):1667–81. doi: 10.1021/jm1012903
139. Anker S, Butler J, Filippatos G, Ferreira J, Bocchi E, Böhm M, et al. Empagliflozin in heart failure with a preserved ejection fraction. New Engl J Med (2021) 385(16):1451–61. doi: 10.1056/NEJMoa2107038
140. Larkin H. Fda expands empagliflozin heart failure indication. JAMA (2022) 327(13):1219. doi: 10.1001/jama.2022.3970
141. Slomski A. Empagliflozin cuts cardiovascular deaths in advanced heart failure. JAMA (2020) 324(24):2476. doi: 10.1001/jama.2020.24667
142. Packer M, Anker S, Butler J, Filippatos G, Pocock S, Carson P, et al. Cardiovascular and renal outcomes with empagliflozin in heart failure. New Engl J Med (2020) 383(15):1413–24. doi: 10.1056/NEJMoa2022190
143. Zhang Q, Li G, Zhong Y, Wang J, Wang A, Zhou X, et al. Empagliflozin improves chronic hypercortisolism-induced abnormal myocardial structure and cardiac function in mice. Ther Adv chronic Dis (2020) 11:2040622320974833. doi: 10.1177/2040622320974833
144. Landoni G, Fochi O, Torri G. Cardiac protection by volatile anaesthetics: A review. Curr Vasc Pharmacol (2008) 6(2):108–11. doi: 10.2174/157016108783955284
145. Lee M, Chen C, Kuo M, Kang P, Lo A, Liu K. Isoflurane preconditioning-induced cardio-protection in patients undergoing coronary artery bypass grafting. Eur J anaesthesiology (2006) 23(10):841–7. doi: 10.1017/s0265021506000354
146. Xia Z, Huang Z, Ansley D. Large-Dose propofol during cardiopulmonary bypass decreases biochemical markers of myocardial injury in coronary surgery patients: A comparison with isoflurane. Anesth analgesia (2006) 103(3):527–32. doi: 10.1213/01.ane.0000230612.29452.a6
147. Igari Y, Sugiyama Y, Sawada Y, Iga T, Hanano M. Tissue distribution of 14c-diazepam and its metabolites in rats. Drug Metab disposition: Biol fate chemicals (1982) 10(6):676–9.
148. Veenman L, Gavish M. The peripheral-type benzodiazepine receptor and the cardiovascular system. Implications Drug Dev Pharmacol Ther (2006) 110(3):503–24. doi: 10.1016/j.pharmthera.2005.09.007
149. Al-Abbasi F, Kumar V, Anwar F. Biochemical and toxicological effect of diazepam in stress-induced cardiac dysfunctions. Toxicol Rep (2020) 7:788–94. doi: 10.1016/j.toxrep.2020.06.004
150. Ha M, Kim V. Regulation of microrna biogenesis. Nat Rev Mol Cell Biol (2014) 15(8):509–24. doi: 10.1038/nrm3838
151. Gebert L, MacRae I. Regulation of microrna function in animals. Nat Rev Mol Cell Biol (2019) 20(1):21–37. doi: 10.1038/s41580-018-0045-7
152. Tajbakhsh A, Bianconi V, Pirro M, Gheibi Hayat S, Johnston T, Sahebkar A. Efferocytosis and atherosclerosis: Regulation of phagocyte function by micrornas. Trends Endocrinol metabolism: TEM (2019) 30(9):672–83. doi: 10.1016/j.tem.2019.07.006
153. Ma S, Tian X, Zhang Y, Mu C, Shen H, Bismuth J, et al. E-Selectin-Targeting delivery of micrornas by microparticles ameliorates endothelial inflammation and atherosclerosis. Sci Rep (2016) 6:22910. doi: 10.1038/srep22910
154. Yan M, Li L, Wang Q, Shao X, Luo Q, Liu S, et al. The Chinese herbal medicine fufang zhenzhu tiaozhi protects against diabetic cardiomyopathy by alleviating cardiac lipotoxicity-induced oxidative stress and Nlrp3-dependent inflammasome activation. Biomedicine pharmacotherapy = Biomedecine pharmacotherapie (2022) 148:112709. doi: 10.1016/j.biopha.2022.112709
155. Wang L, Wu H, Deng Y, Zhang S, Wei Q, Yang Q, et al. Ftz ameliorates diabetic cardiomyopathy by inhibiting inflammation and cardiac fibrosis in the streptozotocin-induced model. Evidence-Based complementary Altern Med eCAM (2021) 2021:5582567. doi: 10.1155/2021/5582567
156. Chen J, Zhang Y, Wang Y, Jiang P, Zhou G, Li Z, et al. Potential mechanisms of guizhi decoction against hypertension based on network pharmacology and Dahl salt-sensitive rat model. Chin Med (2021) 16(1):34. doi: 10.1186/s13020-021-00446-x
157. Zhang Y, Zhang X, Zhang X, Cai Y, Cheng M, Yan C, et al. Molecular targets and pathways contributing to the effects of wenxin keli on atrial fibrillation based on a network pharmacology approach. Evidence-Based complementary Altern Med eCAM (2020) 2020:8396484. doi: 10.1155/2020/8396484
158. He D, Zhang X, Zhu X, Huang F, Wang Z, Tu J. Network pharmacology and rna-sequencing reveal the molecular mechanism of xuebijing injection on covid-19-Induced cardiac dysfunction. Comput Biol Med (2021) 131:104293. doi: 10.1016/j.compbiomed.2021.104293
159. Miao M, Clercq ED, Li G. Clinical significance of chemokine receptor antagonists. Expert Opin Drug Metab Toxicol (2020) 16(1):11–30. doi: 10.1080/17425255.2020.1711884
160. Nerlekar N, Beale A, Harper RW. Colchicine–a short history of an ancient drug. Med J Aust (2014) 201(11):687–8. doi: 10.5694/mja14.00846
161. Imazio M, Nidorf M. Colchicine and the heart. Eur Heart J (2021) 42(28):2745–60. doi: 10.1093/eurheartj/ehab221
162. Deftereos S, Beerkens F, Shah B, Giannopoulos G, Vrachatis D, Giotaki S, et al. Colchicine in cardiovascular disease: In-depth review. Circulation (2022) 145(1):61–78. doi: 10.1161/circulationaha.121.056171
163. Nidorf S, Fiolet A, Mosterd A, Eikelboom J, Schut A, Opstal T, et al. Colchicine in patients with chronic coronary disease. New Engl J Med (2020) 383(19):1838–47. doi: 10.1056/NEJMoa2021372
164. Tucker B, Kurup R, Barraclough J, Henriquez R, Cartland S, Arnott C, et al. Colchicine as a novel therapy for suppressing chemokine production in patients with an acute coronary syndrome: A pilot study. Clin Ther (2019) 41(10):2172–81. doi: 10.1016/j.clinthera.2019.07.015
165. Ridker P, Everett B, Pradhan A, MacFadyen J, Solomon D, Zaharris E, et al. Low-dose methotrexate for the prevention of atherosclerotic events. New Engl J Med (2019) 380(8):752–62. doi: 10.1056/NEJMoa1809798
166. Boring L, Gosling J, Chensue S, Kunkel S, Farese R, Broxmeyer H, et al. Impaired monocyte migration and reduced type 1 (Th1) cytokine responses in c-c chemokine receptor 2 knockout mice. J Clin Invest (1997) 100(10):2552–61. doi: 10.1172/jci119798
Keywords: chemokine, CCL2, CCR2, inflammation, cardiovascular disease
Citation: Zhang H, Yang K, Chen F, Liu Q, Ni J, Cao W, Hua Y, He F, Liu Z, Li L and Fan G (2022) Role of the CCL2-CCR2 axis in cardiovascular disease: Pathogenesis and clinical implications. Front. Immunol. 13:975367. doi: 10.3389/fimmu.2022.975367
Received: 22 June 2022; Accepted: 08 August 2022;
Published: 30 August 2022.
Edited by:
Kishan Nyati, Osaka University, JapanReviewed by:
Hossein Khorramdelazad, Rafsanjan University of Medical Sciences, IranCopyright © 2022 Zhang, Yang, Chen, Liu, Ni, Cao, Hua, He, Liu, Li and Fan. This is an open-access article distributed under the terms of the Creative Commons Attribution License (CC BY). The use, distribution or reproduction in other forums is permitted, provided the original author(s) and the copyright owner(s) are credited and that the original publication in this journal is cited, in accordance with accepted academic practice. No use, distribution or reproduction is permitted which does not comply with these terms.
*Correspondence: Lan Li, bGlsYW5fMDgxM0AxNjMuY29t; Guanwei Fan, Z3VhbndlaS5mYW5AdGp1dGNtLmVkdS5jbg==
Disclaimer: All claims expressed in this article are solely those of the authors and do not necessarily represent those of their affiliated organizations, or those of the publisher, the editors and the reviewers. Any product that may be evaluated in this article or claim that may be made by its manufacturer is not guaranteed or endorsed by the publisher.
Research integrity at Frontiers
Learn more about the work of our research integrity team to safeguard the quality of each article we publish.