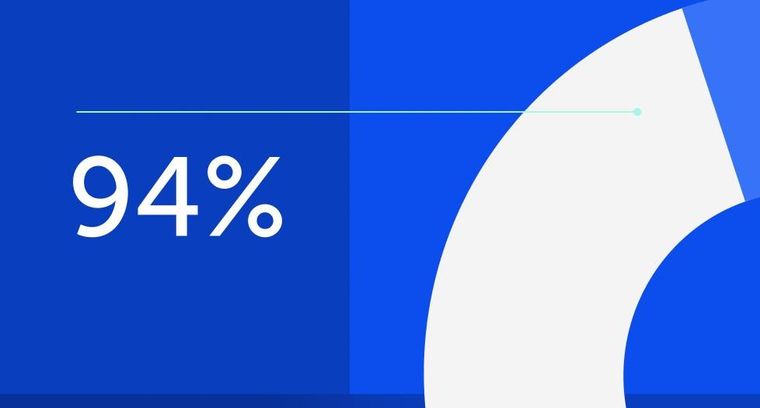
94% of researchers rate our articles as excellent or good
Learn more about the work of our research integrity team to safeguard the quality of each article we publish.
Find out more
REVIEW article
Front. Immunol., 13 September 2022
Sec. Immunological Tolerance and Regulation
Volume 13 - 2022 | https://doi.org/10.3389/fimmu.2022.970508
This article is part of the Research TopicInsights in Immunological Tolerance and Regulation: 2022View all 13 articles
Multiple sclerosis (MS) is a chronic inflammatory disease of the central nervous system related to autoimmunity and is characterized by demyelination, neuroinflammation, and neurodegeneration. Cell therapies mediated by dendritic cells (DCs) and regulatory T cells (Tregs) have gradually become accumulating focusing in MS, and the protective crosstalk mechanisms between DCs and Tregs provide the basis for the efficacy of treatment regimens. In MS and its animal model experimental autoimmune encephalomyelitis, DCs communicate with Tregs to form immune synapses and complete a variety of complex interactions to counteract the unbalanced immune tolerance. Through different co-stimulatory/inhibitory molecules, cytokines, and metabolic enzymes, DCs regulate the proliferation, differentiation and function of Tregs. On the other hand, Tregs inhibit the mature state and antigen presentation ability of DCs, ultimately improving immune tolerance. In this review, we summarized the pivotal immune targets in the interaction between DCs and Tregs, and elucidated the protective mechanisms of DC-Treg cell crosstalk in MS, finally interpreted the complex cell interplay in the manner of inhibitory feedback loops to explore novel therapeutic directions for MS.
Multiple sclerosis (MS) is an immune-mediated disease characterized by inflammatory demyelinating lesions in the central nervous system (CNS), which causes the youngs non-traumatic disability. The course of MS is characterized by recurrence and remission and MS is thus divided into three main clinical types: relapsing-remitting MS (RRMS), primary progressive MS (PPMS), and secondary progressive MS (SPMS). Existing studies have shown that MS is mainly mediated by myelin-specific autoreactive CD4+T cells, whereas dendritic cells (DCs), regulatory T cells (Tregs), and other immune cells play auxiliary roles. When the peripheral immune tolerance is disordered due to Treg cell defects and/or the resistance of effector cells to Tregs, autoreactive CD4+ T cells in the lymph nodes are activated and become aggressive effector cells, followed by entering into the peripheral blood and trafficking to the brain. Besides, DCs, memory T cells, and other immune cells, also cross the blood-brain barrier into the CNS under the action of chemokines (1, 2). Subsequently, activated DCs reactivate autoreactive CD4+ T cells and memory T cells, and prompt their polarization into Teffs, such as T helper (Th) type 1 and Th17 via the different cytokine environment, followed by causing an inflammatory cascade reaction (3, 4). In addition, the activated T cells mediate the activation of macrophages as well as microglia and assist B cells to produce antibodies. When patients with MS enter the chronic phase, immune cell infiltration appears in the CNS and activated DCs, macrophages, and microglia further damage the myelin sheaths, axons, and neurons, which leads to the progression of MS (3) (Figure 1). Therefore, in individuals with MS, it appears that immune dysregulation originates from DCs with the activation phenotype that initiates adaptive immune responses (3). In addition, Tregs, as immune supervisors, can inhibit the inflammatory immune response mediated by activated DCs, pathogenic B cells, and T cells (5). Besides, a small number of potential tolerogenic DCs play a limited protective role in MS, such as inducing Treg cell generation and effector T cell (Teff) anergy. Consequently, the occurrence of MS might be related to the limited DC ability to induce normal Tregs or the insufficient monitoring of activated DCs by Tregs.
Figure 1 Basic immunopathological process of MS. Initially, the peripheral tolerance of MS patients is disordered (for example, the inhibitory function of Tregs is decreased or the Teffs are not sensitive to the inhibitory effect of Tregs) and the autoantigens, such as MBP, MOG and PLP, are incorrectly recognized as pathogens by DCs. These DCs combine with CD4+ autoreactive T cells, which cause peripheral immune response. The activated immune cells make entering into the peripheral blood, then trafficking across the blood–CNS barrier. Subsequently, activated DCs in the CNS combine and reactivate with memory T cells or CD4+ autoreactive T cells and promote their differentiation into Teffs, such as Th1 and Th17, followed by causing CNS inflammation. At the same time, B cells, macrophages, microglia and many other immune cells are also activated by the inflammatory context, followed by causing demyelination, axonal damage, and more serious CNS inflammation. MBP, myelin basic protein; PLP, proteolipid protein; MOG, myelin oligodendrocyte glycoprotein.
DC-Treg cell crosstalk has also been shown to affect animal model of MS-experimental autoimmune encephalomyelitis (EAE). For example, the number of CNS-infiltrating Tregs was decreased in DC deficient EAE mice (6). Similarly, during the initiation phase of activation in myelin-specific T cells, conditional ablation of CD11c+ DCs selectively suspended the generation of induced Tregs (iTregs) and resulted in the aggravation of EAE (6, 7). In turn, Tregs also shaped the tolerance of plasmacytoid DCs (pDCs) in a steady state, which was conducive to maintaining the regulatory ability of Tregs during EAE (8). A basic study has shown that Tregs can reduce the stable contact between DCs and naive T cells and interfere with the initiation of CD4+ T cells in the early immune response (9). Moreover, activated antigen-specific Tregs can also inhibit DC antigen presentation via the bystander effect (10). Detailed examinations of the crosstalk mechanisms of DC-Treg revealed that DCs influenced the proliferation, differentiation, and function of Tregs by reducing the expression of co-stimulatory molecules on the surface of the cell membrane, as well as by enhancing the expression of co-inhibitory molecules, metabolic enzymes, and the secretion of anti-inflammatory cytokines. Besides, Tregs can limit DC maturation and antigen presentation ability by secreting anti-inflammatory cytokines and boosting the formation of co-inhibitory signaling pathways. These processes can interfere with the metabolic pathways of DCs and even directly destroy DCs via cytolysis (11, 12). Various cell therapies for MS make full use of the DC-Treg cell crosstalk mechanism to achieve a therapeutic effect; these include tolerogenic dendritic cell (tolDC) therapy and regulatory T cell therapy (13). Tolerogenic dendritic cells mainly maintain highly specific immune tolerance by inducing the anergy and deletion of Teffs, and producing myelin-specific Tregs (13, 14). Similarly, Tregs can also establish antigen-specific immune tolerance by eliminating over-activated DCs and Teffs, and inducing the generation of tolDCs (10, 13). Although both cell therapies have broad application prospects in MS, there remain numerous barriers to the translation of knowledge into practical use. Thus, the protective DC-Treg cell crosstalk mechanism is crucial to inducing the immune tolerance in MS/EAE, and further in-depth studies will be instrumental to translate the protective mechanism into cell therapy.
By analyzing the co-stimulatory molecules, cytokines, and metabolic enzymes related to the DC-Treg cell interplay, this review comprehensively elucidates the protective effect of the DC-Treg cell crosstalk mechanism in MS/EAE and attempts to interpret the complex cell interaction via novel inhibitory feedback loops, with the further goal of exploring new research directions for protective autoimmune mechanisms and therapeutic schedules for MS.
DCs are the most powerful antigen-presenting cells (APCs), which exist in almost all human peripheral tissues and link innate and adaptive immune responses. DCs can be divided into conventional DCs (cDCs), pDCs and monocyte-derived DCs (MoDCs) (15–18). And cDCs can be further divided into the type 1 (cDC1) and type 2 (cDC2) subsets (15, 16, 19–21)(Table 1). Based on the characteristics of cellular immune tolerance, DCs can also be divided into immunogenic DCs and tolDCs (22). At present, there is no specific surface markers for tolDCs, although it can be identified by several characteristics:1) low expression of co-stimulatory (CD83 and CD80/86) and major histocompatibility complex class II (MHC-II) molecules and pro-inflammatory cytokines (interleukin [IL]-6, IL-12); 2) high expression of co-inhibitory molecules (programmed cell death ligand 1 [PD-L1]), anti-inflammatory cytokines (IL-10, IL-35, and transforming growth factor [TGF]-β), and indoleamine 2,3-dioxygenase (IDO) (23). In the steady state, most DCs maintain in an immature condition, and the types of antigens (i.e., pathogen or self-antigen) encountered with DC (including immature DC and mature DC) usually determine whether it plays an immunogenic or tolerogenic role (20, 24). However, in MS, most DCs are regarded as immunogenicity because the immune system mistakenly recognizes the self-antigens (i.e., myelin basic protein [MBP], proteolipid protein [PLP] and myelin oligodendrocyte glycoprotein [MOG]) as pathogens. Intriguingly, in MS, several rare DC subsets expressing CD8, CD103, dendritic cell endocytic receptor (DEC205), B- and T-lymphocyte attenuator (BTLA) or secreting interleukin (IL)-10, IL-27, IL-35 might become the potential tolDC subsets that induce Tregs. Therefore, DCs have two main functions in MS: one is playing a role in antigen presentation, activating T cells, and initiating immune responses; the other is interacting with T cells, shaping the differentiation/development/function of T cells, and inducing immune tolerance (22). The antigen presentation ability of DCs, namely immunogenicity, can be compensated by macrophages and B cells; however, the tolerance function of DCs appears irreplaceable (6).
Tregs are a classical type of inhibitory T cell with an immune regulation function, which are primarily involved in the formation of immune tolerance. Tregs can be divided into two subsets: thymus derived Tregs (tTregs) and peripheral derived Tregs (pTregs). The main phenotype of tTregs is CD4+ CD25+ CD127− Foxp3+ Tregs, while pTregs mainly include CD4+ CD25+ Foxp3− type 1 regulatory T cells (Tr1) that secrete a large amount of IL-10 and CD4+ CD25low T helper type 3 cells (Th3), which produce significant amounts of TGF-β. In addition, there are many Treg subsets that have been named based on key surface molecules or transcription factors, such as CD8+ Tregs and FoxA1+ Tregs (25). The heterogeneity of Tregs makes their subsets complex, and we have summarized the main Treg cell subsets related to MS (25–32) (Table 2). Tregs not only interfere with the mature state and antigen presentation ability of DCs but also induce the generation of tolDCs. When DCs play an excessive role in antigen presentation, and attack both pathogenic cells and normal cells, Tregs act as a negative regulator of these overactivated DCs and restrict the autoimmune response.
A recent study indicated that monocyte-to-DC differentiation was a process potentially influenced by MS risk single nucleotide polymorphisms (SNPs) (33). Moreover, previous studies showed that MS patients had DC defects. For example, the percentage of circulating myeloid DCs was decreased in patients with SPMS and PPMS, whereas a large number of DCs were found in the CNS lesion and cerebrospinal fluid (CSF) (34). The process of DC exiting the CNS to maintain immunological tolerance was perturbed in MS patients, which might contribute to the abnormal distribution of DCs in MS patients (2). In the CSF, pDCs percentage was higher in untreated-relapsing MS patients than those of patients in remission, whereas this result was not observed in the peripheral blood (35). In contrast, the expression of CD83, CD80, and CD86 on the surface of circulating DCs in patients with PPMS was downregulated, whereas the circulating cDCs of RRMS and SPMS patients showed an activated phenotype, suggesting an imbalance between tolerogenicity and immunogenicity of DCs in MS patients (i.e., functional defects in DCs) (34, 36). In addition, the ability of pDCs to induce Tregs has been shown to be related to the occurrence of MS. Gene expression level of HSPA1A —which may influence the ability of pDCs to induce Tregs—was downregulated in MS patients and this probably resulted in alterations in the mature state and regulatory function of pDCs (37). Furthermore, the depletion of pDCs in healthy controls resulted in the reduction in CD4+ Foxp3+ Tregs, whereas the depletion of pDCs in MS patients did not influence the generation of Tregs (38). Taken together, these studies showed that DC deficiency was closely related to MS relapsing and remitting.
Treg cell defects also exist in MS patients. CD25, namely the IL-2 receptor α chain, is a pivotal surface marker of Tregs, and its SNPs are closely related to MS (39). Studies have demonstrated that Treg cell defects in MS patients were mainly reflected in cell quantity, subset changes, and dysfunction (40–43). For example, the percentage of Tregs in the peripheral blood of RRMS patients was significantly reduced and associated with the clinical severity of the disease (41). Moreover, a previous study has shown that the number of Tregs in the CSF, but not in the peripheral blood, was elevated in MS patients (42). In contrast, alterations in the proportion and dysfunction of Treg cell subsets were more marked in patients with MS (43). For example, the effector function of CD4+ CD25hi Tregs in the peripheral blood was significantly downregulated in MS patients (44). Moreover, T helper type 1 (Th1)-like, interferon-γ (IFN-γ)-secreting Foxp3+ T cells were elevated in RRMS patients, while their inhibitory function were weakened (29). A recent study showed that, compared with healthy donors, MS patients had lower resting CD4+ CD25+ CD45RA+ CCR7+ Tregs and more activated CD4+ CD25hi CD45RA- Foxp3hi Tregs even before treatment (40). Therefore, Treg cell deficiency was one of the main causes of the autoimmune response in MS patients.
Emerging myeloid cell research has been focused primarily on the regulatory effect of DCs on Tregs. However, the regulatory effect of Tregs on DCs is also worth investigating. We summarize three inhibitory feedback loops to explain the protective crosstalk mechanism between DCs and Tregs in the context of MS/EAE: the CD28/cytotoxic T lymphocyte antigen (CTLA)-4/B7 pathway, the PD-L1/PD-1 inhibitory feedback loop, and the lymphocyte activation gene 3 (LAG-3)/T cell receptor (TCR)/MHC-II inhibitory feedback loop (Figure 2). We also highlight three inhibitory feedback mechanisms related to DC-Treg cell crosstalk that may be involved in MS/EAE, including IL-10, IL-35, and TGF-β (Figure 3).
Figure 2 Surface molecules involved in DC-Treg cell crosstalk. Via the CD28/CTLA-4/B7 pathway, the PD-L1/PD-1 inhibitory feedback loop, and the potential LAG-3/TCR/MHC-II inhibitory feedback loop, DCs can affect the generation of Tregs, and Tregs can affect the tolerance of DCs. GITRL, TSLPR, DEC-205, DCIR-2, and CD31 are also key molecules for DC-dependent Treg cell generation. CTLA-4, cytotoxic T lymphocyte antigen 4; PD-L1, programmed cell death ligand 1; PD-1, programmed cell death receptor; LAG-3, lymphocyte activation gene 3; TCR, T cell receptor; MHC-II, major histocompatibility complex class II; GITR, glucocorticoid-induced tumor necrosis factor receptor; GITRL, glucocorticoid-induced tumor necrosis factor receptor ligand; TSLP, thymic stromal lymphopoietin; DCIR2, dendritic cell inhibitory receptor 2.
Figure 3 Inhibitory cytokines and metabolic enzymes involved in DC-Treg cell crosstalk. From the perspective of cytokines, both DCs and Tregs can secrete inhibitory cytokines IL-10/IL-35/TGF-β and form inhibitory feedback mechanisms: DCs promote the generation of different subtypes of Tregs, and Tregs inhibit the maturity and antigen presentation ability of DCs and improve their tolerance. From the perspective of metabolic enzymes, Tregs can induce IDO expression in DCs with the help of signals axes, such as CTLA-4-CD80/86, initiate the tryptophan metabolic pathway, trigger the generation of IDO-dependent Tregs, and form a negative feedback loop with IDO at the core. There is also unidirectional regulation of Tregs by DCs: DCs influence Treg generation by secreting IL-27, and IL-12/IL-6/TNF-α inhibits Treg cell proliferation. TGF-β, transforming growth factor-β; IFN- γ, Interferon- γ; IDO1, indoleamine 2,3-dioxygenase 1; + p, phosphorylation; P-IDO1, phosphorylated indoleamine 2,3-dioxygenase 1; Trp, tryptophan; Kyn, kynurenine; AHR, aromatic hydrocarbon receptor.
CD28 and CTLA-4 (CD152) are members of the CD28 superfamily. They share common ligands, the B7 family molecule CD80/CD86 (B7-1/B7-2), on the surface of DCs. The CD28 molecules that are crucially expressed in most CD4+ T cells (including Tregs) promote T cell activation, proliferation, and survival (45). Besides, the expression of CTLA-4 is pivotal in Tregs and is upregulated on the surface of activated T cells. CTLA-4 has considerably higher avidity for CD80/CD86 than CD28 and can reduce the expression of CD80/CD86 on the surface of DCs via trans-endocytosis. The co-expression of CD28 and CTLA-4 in Tregs, activated T cells, and their competitive combination with CD80/86 make the CD28/CTLA-4/B7 pathway play a more complex role in DC-Treg cell-mediated immune regulation (Figure 2).
The CD80/86-CD28 axis, the second signal-inducing Tregs produced by DCs, serves to lower the signaling threshold of the TCR, and induces the production of IL-2 that is essential for the development of CD4+ CD25+ Tregs. Besides, the CD80/86-CD28 axis also enables Treg cell homeostasis (7, 45, 46). Selectively targeting Tregs in normal Lewis rats with very low doses of the CD28 superagonist was shown to be sufficient to induce Treg cell proliferation in vivo and enable the effective treatment of EAE (47). Similarly, despite the normal number of Foxp3+ cells in Treg cell-specific CD28 conditional knockout mice, CD28-deficient Tregs exhibited a pronounced proliferative/survival disadvantage. This result caused a severe autoimmunity and experimental allergic encephalomyelitis that is considered as animal models of MS (48). However, it has been reported that CD28 knockout mice were resistant to EAE induction because the CD28 signals of pathogenic T cells and Tregs were blocked simultaneously (49). Additionally, selective blocking of CD80/86-CD28 signal transduction in rhesus monkeys suppressed the activation of autoreactive T cells and B cells, thereby abrogating the induction of EAE (50). Therefore, despite the pivotal role that the CD80/86-CD28 axis plays in the development and proliferation of tTregs and pTregs, it is evident that, the activation of the CD28 signal is conducive to the pathogenesis of EAE/MS, based on the overall perspective of the CD4+ T cell population. It’s probably because the CD80/86-CD28 axis has a much greater effect on the activation of pathogenic T cells than on the induction of Tregs, and pathogenic T cells have obvious quantitative advantages.
The CTLA-4-CD80/86 axis is an important medium for the Treg cell inhibition effect, which indirectly influences the activation of Teffs by suppressing the antigen-presenting function of DCs (51). Furthermore, CTLA-4 is an immune checkpoint and therapeutic target in the development of MS (52). For germline knockout mice, the deletion of CTLA-4 precipitates severe autoimmunity diseases (53). Real-time reverse transcription–PCR detection has shown that the expression of CTLA-4 in peripheral blood mononuclear cells (PBMCs) of MS patients was either decreased (54) or unchanged (55).These results, at least, could reflect the trend of CTLA-4 expression in Tregs of MS patients because CTLA-4 is mainly expressed by Tregs in PBMCs. In addition, a recent in vitro experiment demonstrated that when DCs were co-cultured with CD4+ CD25− naive T cells and Tregs, Tregs always gathered around DCs, inhibited their activation, captured CD80/86 via trans-endocytosis of CTLA-4, and increased free PD-L1 expression on the surface of DCs (56, 57). This phenomenon influenced the antigen presentation ability of DCs, indirectly regulated the CD80/86-CD28 signaling pathway and inhibited the activation of T cells. Intriguingly, iTregs from CTLA-4-deficient mice failed to induce the downregulation of CD80/CD86, whereas DCs treated with iTregs from CTLA-4-deficient mice showed an impaired ability to activate naive T cells (57). Similarly, the CTLA-4-CD80/86 axis had the same effect on EAE mice, and the expression of CD80/86 and MHC-II on the surface of peripheral DCs was significantly elevated in Treg cell-specific CTLA-4-deficient mice (53). Thus, without the limiting effect of CTLA-4 on the Treg cell surface, the overall maturity and activation level of DC were enhanced. However, in the CNS of mouse, CTLA-4-deficient Tregs did not increase the expression of B7 on the surface of DCs (53). Moreover, in EAE/MS, Tregs can induce IDO expression in DCs via the CTLA-4-CD80/86 axis and improve their tolerance. Therefore, the CTLA-4-CD80/86 axis is of great significance in the DC-Treg cell crosstalk mechanism.
Although CTLA-4 is recognized as a co-inhibitory molecule, its effect on Tregs is highly complex. In general, it was thought that CTLA-4 promotes tTreg cell proliferation (51). For example, the lysine motif in the cytoplasmic domain of CTLA-4 was crucial for Foxp3 induction and EAE inhibition. Transporting the chimeric peptide containing the CTLA-4 cytoplasmic tail into T cells can induce Tregs in vivo, resulting in EAE remission (58). However, Paterson et al. (53) reported that CTLA-4 can limit the proliferation of Teffs and inhibit the expansion of pTregs, which is different from the role that CTLA-4 plays in the thymus. For example, a study showed that the deletion of CTLA-4 in adulthood promoted the activation and expansion of traditional CD4+ Foxp3− T cell and regulatory Foxp3+ T cell subsets that protect mice from EAE (53). Paradoxically, Wing et al. (51) have suggested that CTLA-4 is necessary for Tregs to exert an inhibitory effect, whereas Paterson et al. (53) have proposed the opposite: upregulating the expression of other inhibitory molecules (e.g., IL-10, LAG-3, and PD-1) compensated for the CTLA-4 deficiency. There are two possible explanations for these results: 1. CTLA-4 expression has different relative contributions to Tregs and Teffs; and 2. the intracellular and extracellular segments of CTLA-4 are responsible for different functions.
Interference of the CD28/CTLA-4/B7 pathway can concurrently influence two signaling pathways between DCs and Tregs; therefore, its function in EAE/MS might be complicated. Abatacept is a CTLA-4-immunoglobulin (Ig) fusion protein, which is composed of the extracellular region of CTLA-4 and the Fc portion of IgG. It can simultaneously bind to CD80/86 and block the CD28/CTLA-4/B7 pathway. Glatigny et al. (59) found that abatacept significantly reduces circulating Tregs, especially CD45RO+ memory Tregs, in RRMS patients. Researchers suggested that the inhibition of CD28-mediated DC-Treg cell crosstalk was the primary mechanism underlying the reduction in human circulating Tregs following abatacept therapy (59). However, upon myelin reactive T cell activation, blocking B7 with CTLA-4-Ig can inhibit Tregs and aggravate EAE, resulting in more severe CNS inflammation and demyelination (60). It suggested that blocking the CD28/CTLA-4/B7 pathway primarily interferes with the CTLA-4-CD80/86 signal between DCs and Tregs, due to the reduced dependence of activated myelin-specific T cells on the CD28 signal. This interesting process caused Treg cell anergy and continuous activation of myelin-specific T cells, thus, promoting inflammation (60). In a phase II clinical trial called ACCLAIM (NCT01116427), abatacept provided no clinical benefit to patients with RRMS, which probably due to the mutual offset of the CD28 signal and the CTLA-4 downstream effect (61). Therefore, the final result of blocking the CD28/CTLA-4/B7 pathway in EAE/MS may depend on the relative strength of the CTLA-4 and CD28 signals as well as the blocking time point (61). However, no study to date has reported the effect of blocking the CD28/CTLA-4/B7 pathway in DCs in EAE/MS. Instead, studies in mouse models of rheumatoid arthritis have suggested that abatacept treatment induced a unique T cell phenotype to reduce DC antigen presentation (62).
In summary, in the context of MS/EAE, DCs rely on the CD80/CD86-CD28 axis to induce Treg cell generation, and Tregs rely on the CTLA-4-CD80/86 axis to inhibit DC immunogenicity, and induce the generation of tolDCs, thereby forming a protective signaling loop.
Similar to CTLA-4, the PD-L1/PD-1 axis also plays a pivotal role in inducing peripheral tolerance. However, the latter is distinct because it can be simultaneously expressed on the surface of DCs and Tregs (63, 64). Generally, cells with expression of PD-L1 play a regulatory role. PD-L1+ DCs involve in regulating the differentiation and development of naive T cells. Besides, they preferentially induce the expression of Foxp3 and promote the differentiation of Tregs. PD-L1+ DCs can also maintain Treg cell inhibitory function and promote the secretion of IL-10 by binding to PD-1 on the surface of Tregs (65). Simultaneously, PD-L1 can act inversely on DCs and inhibit DC maturation (6). In addition, PD-L1 constitutively expressed by Tregs can mediate the inhibitory function of Tregs, improve the tolerogenicity of PD-1+ DCs, and inhibit the secretion of proinflammatory cytokines, such as IL-6, IL-12 and tumor necrosis factor-alpha (TNF-α) (25, 66). Therefore, the PD-L1/PD-1 axis in DC-Treg cell crosstalk forms an inhibitory feedback loop and influences both cells simultaneously (Figure 2).
The PD-L1/PD-1 axis plays a similar protective role in MS. Numerous studies have reported the expression of PD-L1/PD-1 in MS patients or EAE mice from different perspectives, such as genes, peripheral blood, and brain tissue samples (66). Overall, the expression of PD-L1/PD-1 in healthy controls was highest, followed by MS patients or EAE mice in the remission stage, and finally those in the acute stage (66). However, exceptions also exist. For example, the expression level of PD-L1/PD-1 in MS plaques was higher than that in non-pathological human CNS tissue (67). Focusing on DCs/Tregs that are stimulated by MBPs, T cells expressing PD-1 and APC expressing PD-L1 in the peripheral blood of patients with acute MS were prominently increased compared with those of remitted patients with MS (68). A recent study also showed that the expression of PD-1 on the surface of CD4+ and CD8+ Tregs was reduced in patients with RRMS (69). Taken together, these studies suggested that the DC- or Treg cell-related PD-L1/PD-1 axis is involved in the occurrence and development of MS.
Tregs can improve DC tolerance via the PD-L1/PD-1 axis. As mentioned above, Tregs can release soluble PD-L1 on the surface of DCs when they endocytose CD80/86 in a CTLA-4-dependent manner, which boosts the tolerance of DCs (70). In addition, Liu et al. (25) found a new type of regulatory T cell subset: CD4+ FoxA1+ CD47+ CD69+ PD-L1hi FoxP3− Tregs (FoxA1+ Tregs). It was the main Treg cell subset that combats against EAE in the CNS and was induced by IFN-β. Transferring stable FoxA1+ Tregs to mice suppressed EAE in a PD-L1-dependent manner, which was related to its reduction in pro-inflammatory cytokines (IL-12 and IL-17) secreted by DCs and its inhibition of PD-1+ Teffs. Of note, FoxA1+ Tregs are negative for expression of Foxp3, CTLA-4, TGF-β, IL-10, and IL-35, though FoxA1 is a key transcription factor for PD-L1 production. Hence, the main pathway in which Tregs regulate DCs changes alongside alterations in the cell subtype. However, whether other Treg cell subtypes induce tolDCs via PD-L1 remains to be verified experimentally.
The regulation of PD-L1+ DCs on Treg proliferation, differentiation, and function in EAE/MS has attracted significant attention. A previous study in EAE has shown that steady-state DCs exhibited their suppressive capacity by promoting the development of Tregs, thereby improving CNS autoimmunity (6). Further research has demonstrated that the generation of DC-dependent Tregs was guided by the interaction between PD-L1 on DCs and PD-1 expressed by T Cells (6, 64). Paradoxically, one study showed that PD-L1 expressed on DCs was important for the inhibition of follicular regulatory T (Tfr) cell differentiation and maintenance (71). The study demonstrated that PD-L1 deficiency in DCs resulted in a higher percentage of Tfr cells in the draining lymph nodes and blood than in control mice. It can be preliminarily speculated that PD-L1+ CD11c+ DCs were responsible for inhibiting the development of CD4+ICOS+CXCR5+CD19-Foxp3+Tfr cells, but the specific DC subset was still unclear (71). We hold that this contradiction might be due to the heterogeneity of DC and Treg cell subsets. Moreover, PD-L1+ DCs have become a new therapeutic target for EAE/MS. Vitamin D3 (VitD3), IFN-β, hypomethylating agents, and other drugs can induce Tregs and alleviate EAE via PD-L1+ DCs (72, 73). A recent study showed that the IFN-γ-JAK1-STAT1 signaling pathway was an inherent upstream pathway that enabled DCs to induce PD-L1, followed by causing a series of subsequent immune tolerance effects. Therefore, mice harboring a DC-specific JAK1 deletion exhibited decreased expression of PD-L1 in DCs, fewer Tregs, and a deterioration of EAE (74). These studies demonstrated that the PD-L1/PD-1 axis played a powerful immune tolerance role in the DC-Treg cell crosstalk in the context of EAE/MS.
The LAG-3 (CD223) is an inhibitory immune receptor that is mainly expressed by Tregs, especially Tr1, and negatively regulates T cell activation. Both TCR and LAG-3 on the surface of Tregs can bind to MHC-II on the surface of DCs. TCR-MHC-II signal transduction in DC-Treg cell crosstalk is the first signal produced by DCs inducing Tregs, and LAG-3-MHC-II signal transduction is one of the important ways for Tregs to inhibit DC antigen presentation. These two signal axes interfere with each other and form an inhibitory feedback loop (Figure 2).
Generally speaking, the TCR-MHC-II axis, as the first signal of T cell activation, plays a pathogenic role in EAE. However, it also plays a protective role in DC-Treg cell crosstalk. For instance, upon EAE induction, pDCs were recruited to the lymph nodes and established MHC-II-dependent cross-linking with CD4+ T cells to promote the selective amplification of myelin antigen-specific Tregs, thereby inhibiting the response of pathogenic T cells (75). DCs that express MHC-II at a low level facilitated a significant increase in the amount of nTregs and alleviated EAE (76). In turn, Tregs limited the antigen presentation ability of DCs by consuming the peptide-MHC class II complex on the surface of DCs. In addition, there was some evidence that LAG-3-MHC-II signal transduction in DC-Treg cell crosstalk had a protective effect in the context of autoimmunity disease. For example, basic studies and experiments on tumors had shown that LAG-3 competitively binded MHC-II with TCR, directly interfering with TCR signal transduction and blocking the DC-mediated immune response (77, 78). LAG-3 engagement with MHC-II inhibited DC activation and induced tolDC generation via the immunoreceptor tyrosine-based activation motif (ITAM)-mediated inhibitory signaling pathway that involved FcγRγ and the ERK-mediated recruitment of SHP-1 (78).
In addition, the expression level of LAG-3 is closely related to the course of MS. It has been reported that SNPs of LAG-3 influenced MS susceptibility (79). The expression of LAG-3 messenger RNA (mRNA) in PBMCs at diagnosis in untreated MS patients was significantly lower than that in healthy controls. Another study has shown that the reduced expression of baseline LAG-3 in PBMCs predicted the MS course alongside paraclinical and clinical parameters and might also be an adverse prognostic factor for MS (55). In line with this finding, a recent study observed significant reduction in the expression of LAG-3 mRNA in major CD4+ and CD8+ T cell subsets in RRMS individuals (80). Due to the expression level of LAG-3 in MS patients and the pathological characteristics of immune diseases, it can be speculated that the LAG-3/MHC-II axis is involved in DC-Treg cell crosstalk in MS/EAE and that the LAG-3/TCR/MHC-II inhibitory feedback loop mediates DC-Treg cell interactions and plays a protective role in MS/EAE.
(Figure 3) Both DCs and Tregs can secrete IL-10/TGF-β/IL-35, and the secretion level is closely related to EAE/MS. It has been reported that the reduction in serum IL-10 level in patients with acute MS may be used as a marker of MS disease activity (81). As for cell subsets, the IL-10 secretion level of Tr1 is decreased in MS patients (82). In addition, increased TGF-β secretion in the peripheral blood of RRMS and SPMS patients has also been reported (83). Specifically, in MS patients, decreased gene expressions of key proteins in the TGF-β signal pathway of naive CD4+ T cells, such as TGF-βRI, TGF-βRII, and drosophila mothers against decapentaplegic 4 (SMAD4), have been reported (84), and T cell lines in patients with stable MS produce more TGF-β than those in patients with active MS (85). A recent study has further shown that a variety of cell surface markers related to TGF-β signal transduction in the Tregs are differentially expressed in MS patients and healthy controls, and are closely related to disease severity (86). These results indicate that TGF-β and its downstream signal pathway play a momentous modulating role in MS. Regarding IL-35, a new member of the IL-12 family, two contradictory studies have reported that the serum IL-35 level of RRMS patients was either lower or higher than that of healthy controls (87, 88). The subjects of the first study included both RRMS patients and clinically isolated syndrome (CIS) patients who have not yet converted to MS, which might be responsible for the contradiction of IL-35 expression level in serum. In addition, another study demonstrated that MS patients treated with IFN-β or methylprednisolone had higher serum IL-35 levels, which suggested that the clinical therapeutic benefits of these drugs might be partly induced by the upregulation of IL-35 (89). Collectively, MS patients can active the protective compensatory mechanism by regulating the secretion of IL-35 after symptoms onset, which may be influenced by the Foxp3 gene polymorphism and the progression stage of MS.
The above studies indicate that IL-10/TGF-β/IL-35 is involved in the regulation of MS disease activity and demonstrate their regulatory role in DC-Treg cell crosstalk. In addition, once cytokines have been secreted into the internal environment, it is difficult to determine their source and aim. For example, DC- or Treg- derived IL-10/TGF-β/IL-35 can influence the generation of Tregs by paracrine, but it also influences its own mature state and antigen presentation ability by autocrine. However, in order to clearly ascertain their roles in DC-Treg cell crosstalk, we distinguished them to DCs- and Tregs-derived cytokines in the following paragraphs.
DC-derived IL-10 can promote Treg cell generation and play a neuroprotective role (Figure 3). A recent study demonstrated that the adoptive transfer of tolDCs with upregulated IL-10 expression to EAE mice reduced the percentage of Th1 and Th17 cells, promoted splenetic Treg cell generation, and restricted the development of EAE to some degree (90). Besides, curcumin can promote the generation of peripheral IL-10-producing tolDCs and Tregs, as well as alleviate EAE, whereas the neuroprotection was completely abrogated in IL-10 deficient mice (91). In MS patients, there is a signal pathway of IL-10-dependent Treg cell generation: IL-10 activates signal transducer and activator of transcription 3 (STAT3) and converts DCs into tolDCs; in the presence of IL-2, tolDCs further induce STAT5 phosphorylation in naive T cells and promote Treg cell generation (92). Therefore, there is an inhibitory feedback loop about IL-10 that participates in DC-Treg cell crosstalk in MS patients. In addition, IL-10 is a downstream molecule of IL-27, and both of them established intravenous tolerance in EAE mice (93–95). For example, intravenously (i.v.) injecting MOG35–55 to wild-type (WT) mice infected with EAE can induce i.v. tolerance and reduce disease severity, whereas this administration was ineffective in IL-27 receptor (WSX-1) knockout mice. Moreover, research showed that distinct subsets of splenic DCs (i.e., CD11b+CD103± and CD11bhiCD103+) contributed together to the induction of i.v. tolerance via promoting DC- drived IL-27, IL-10 and IFN-γ secretion, as well as suppressing IL-17 secretion (94).
DC-derived TGF-β promotes Treg cell generation via direct and indirect effects (Figure 3). It is well established that TGF-β is a popular inducer of Tregs in vitro that works independently of DC. In addition, several drugs alleviated EAE via the direct effect of TGF-β. For example, by targeting DCs to drive TGF-β1 upregulation, Tanshinone IIA(TSA) promoted the polarization of naïve CD4+ T cells to Tregs and significantly reduced the clinical severity of EAE (96). Apigenin shifted DC-modulated T cell responses from Th1 and Th17 types towards Treg cell-directed responses and curbed neuroinflammation in EAE by promoting the secretion of TGF-β and IL-10 (97). However, TGF-β can also promote the phosphorylation of IDO in DCs, a key enzyme in the tryptophan metabolic pathway, induce the DC tolerance phenotype, and indirectly facilitate Treg cell generation. For example, SMAD7 is an inhibitory SMAD protein that negatively regulates TGF-β signal pathway. Mice devoid of SMAD 7 in DCs were resistant to the induction of EAE. This result was predominantly caused by the high expression level of IDO and the elevated frequency of Tregs in secondary lymphoid organs and the CNS (98). Likewise, administration of the downstream tryptophan metabolite 3-hydroxyanthranillic acid enhanced the secretion of DC-derived TGF-β and the percentage of Tregs in IDO-deficient mice (99). Furthermore, the enhanced Treg cell response promoted the secretion of DC-derived TGF-β, formed an inhibitory feedback mechanism, and eventually reduced the clinical manifestations of EAE.
DC-derived IL-35 can promote Treg cell generation (Figure 3). IL-35-producing CD8α+ DCs have limited antigen presentation capacity and high tolerance. Moreover, since antigen-pulsed IL-35+ DCs possessed the ability to induce Tregs, their use can significantly reduce the severity of EAE, and Tregs induced by IL-35+ DCs acquired a stronger inhibitory function than those induced by TGF-β/IL-2 (100). Furthermore, the α (IL-12p35) and β (Epstein-Barr Virus-Induced, EBI3) subunits of IL-35 can both alter Tregs. For example, IL-12p35 suppressed neuroinflammatory responses and ameliorated the pathology of EAE by boosting the expansion of IL-10-producing Tregs in the brain and spinal cord as well as by inhibiting the STAT1/STAT3 pathways, and pathogenic Th17 and Th1 cells (101). Conversely, EBI3-deficient mice immunized by the MOG peptide elicited stronger Th17/Th1 responses in the CNS with marginally enhanced EAE development (102). Strikingly, there was an increased number of CD4+ Foxp3+ Tregs in the peripheral lymphoid organs of EBI3-deficient mice, and the suppressive function of these Tregs was more potent than Tregs in WT mice. The authors explained that the EAE development was only modestly enhanced compared with WT mice in the presence of potent Th17 response, which may attribute to the enhanced Treg responses (102). It’s indicated that the enhanced Treg responses was a comprehensive change of IL-35 and IL-27, because EBI3 is also the subunit of IL-27, which appears to have an inhibitory effect on Treg cell generation, as mentioned below. As a result, selectively knocking out IL-35-EBI3, while retaining IL-27-EBI3 may better reflect the effect of EBI3 subunit of IL-35 on Tregs.
Unfortunately, in the context of EAE/MS, there was little direct evidence for a distinct modulatory effect of Treg cell-derived IL-10/TGF-β/IL-35 on DC maturation and antigen presentation ability. Nevertheless, several basic experiments have shown that, in the context of immune tolerance, Treg cell-derived IL-10/TGF-β/IL-35 can acquire the capacity to inhibit DCs (Figure 3). For example, when Ag-specific iTregs were co-cultured with DCs in the absence of Teffs, iTreg cell-treated DCs had markedly impaired immunogenicity, which can be completely reversed by anti-IL-10 (57). Further research has shown that IL-10 can induce the degradation of CD86 and MHC-II on DCs and achieve the goal of suppressing the DC function by promoting the neutralization reaction between membrane associated ring-CH-type finger 1 (MARCH1, an E3 ubiquitin ligase) and CD83(a DC activation marker) (57). Another study reported that IL-10 can suppress immune responses by interfering with TLR- or IFNγ- mediated DC activation and directly inducing gene expression that inhibits APC function (103). Moreover, one study suggested that IL-10-/- iTregs lost their protective role in EAE, which may be due to the impaired mechanism of iTreg cell-derived IL-10 inhibiting the expression of co-stimulatory molecules in DCs (104). A recent study demonstrated that Treg-derived IL-10 in tumors was also required for imprinting the Treg cell-inducing capacity of tolDCs (105).
Strikingly, a very recent report indicated that CD4+ Teff cell-derived IL-10 promoted CNS inflammation during the progression of EAE, via sustaining survival and proliferation of Th1 cells and, to a lesser degree, Th17 cells (106). However, IL-10 produced by Teffs did not exert its modulatory function by acting directly on APCs, and what affect such IL-10 may bear on the DCs remained open questions. Exploring the cellular source and targeted cell of cytokines seems to be important and meaningful. Besides, this study showed a hint: could Treg derived IL-10 also plays a pro-inflammatory role in a specific environment? This still needs further experiments and facts to prove. On the other hand, a study about gut inflammation also has reference value for MS because the microbiota-gut-brain axis is considered to participate in MS. For example, IL-10 signaling in CD11c+ DCs can limit reactivation of local memory T cells thereby controlling gut immune homeostasis (107). As mentioned above, the reactivation of memory T cells migrating to CNS is a pivotal process causing MS (3). Therefore, we speculated that IL-10 signal between CNS-DCs and memory T cells might be of similar significance. In conclusion, these researches proved the importance of exploring the cellular source and aims of cytokines, because IL-10 from different cellular sources may have different effects and mechanisms of action.
Treg cell-derived TGF-β is also necessary for the induction of DC tolerogenic function. When human monocytes were cultured with normal CD4+Foxp3+ Tregs and Th cells, they polarized into tolDCs, with capable of generating induced Tregs from naïve T cells. In this process, Treg-derived TGF-β, IL-10 and CTLA-4 were all required for the phenotypic differentiation of tolDCs (105). Similarly, a classical basic study revealed that Tregs restricted the maturation and antigen-presenting capacity of DCs, which can be marginally reversed using anti-TGF-β antibodies to neutralize TGF-β (108). Besides, transforming growth factor receptor 1 (TGFβR1) had an indispensable role in the development of intestinal CD103+CD11b+ DCs, and influenced the amount of DC related Foxp3+ Treg cells in vitro and in vivo (109). These phenomena confirmed that Treg cell-derived TGF-β affected DC tolerogenic phenotype. As for IL-35, IL-35-treated MoDCs are characterized by tolerance, with a strong inhibitory function on the proliferation of pathogenic T cells. The exogenous addition of IL-35 inhibited MoDC maturation and secretion of pro-inflammatory cytokines, and also partially inhibited the differentiation of MoDCs (110). It suggested that Treg cell-derived IL-35 may also have a similar inhibitory effect on DCs.
In autoimmune diseases, Tregs inhibit DC maturation and improve immune tolerance by secreting IL-10/TGF-β/IL-35; moreover, DC provides conditions for Treg cell generation via the production of IL-10/TGF-β/IL-35. As a result, these three inhibitory cytokines involved in DC-Treg cell crosstalk formed three negative feedback mechanisms to counteract the imbalance of immune tolerance. Considering the autoimmune mechanism and the expression of IL-10/TGF-β/IL-35 in EAE/MS, we speculated that Treg cell-derived IL-10/TGF-β/IL-35 have a similar inhibitory effect on DCs in EAE/MS. However, it requires further experimental verification to fill the knowledge gap regarding cytokines in DC-Treg cell crosstalk. In addition, most researches have focused on the compherehensive changes in cytokines, whereas little attention has been paid to cytokine production in specific cell subsets. Therefore, unequivocally detecting cytokines is a critical future direction in the study of the DC-Treg cell crosstalk mechanism.
Extracellular vesicles (EVs)—including exosomes that are wrapped with biological messengers, such as microRNAs and cytokines from DCs and Tregs—can complete cell interactions without direct contact (111, 112). Exosomes with membrane-associated TGF-β1 from gene-modified DCs can maintain the inhibitory function of Tregs, reduce the frequency of Th17 cells, and curb the progression of EAE (111, 112). Moreover, DC-derived exosomes can promote myelin regeneration (113). Conversely, with the help of EVs, Tregs can transport miRNA (especially mir-150-5p and mir-142-3p) into DCs to induce the tolerance phenotype (114). A previous study has shown that the inhibitory effect of Treg cell-derived exosomes in RRMS individuals was significantly weaker than that in healthy controls (115). Additionally, in a rat liver allograft model, exosomes from immature DCs were shown to retain numerous tolerogenic characteristics of immature DCs, and Tregs could be amplified in the presence of such exosomes to induce immune tolerance (116). Therefore, EVs are involved in DC-Treg cell crosstalk and are expected to become an emerging treatment strategy for MS.
IDO is the first rate-limiting catabolic enzyme in the degradation pathway of the essential amino acid tryptophan (23). IDO has two isozymes: IDO1 and IDO2. IDO1 converts L-tryptophan to kynurenine via the kynurenine pathway (KP), which plays a key role in MS, whereas IDO2 may not be related to MS (117). Expressed mainly in DCs (especially pDCs), IDO typically has low basal expression, and its upregulation primarily correlates with cytokines (IFN-γ, IFNα/β, and TGF-β) and co-stimulatory signals (CTLA-4 and glucocorticoid-induced tumor necrosis factor receptor [GITR]) (118), whereas IL-6 and IL-4 inhibit the expression of IDO in DCs (119, 120). IDO activation is considered an endogenous self-protection response that accompanies EAE/MS (120). For example, the expression of IDO1 in the PBMCs of patients with RRMS was higher during the recurrence period than in the remission period (121). During an EAE attack in mice, activating the Stimulator of IFN Genes (STING) signaling adaptor to stimulate IFN-I production induced IDO expression in DCs, effectively inhibited disease progression, and alleviated clinical symptoms (122). Similarly, the mild condition of MS patients during pregnancy was related to the promotion of IDO expression in DCs by estriol. However, this compensatory effect is limited, and the IDO expression level in DCs of pregnant MS patients may be still lower than that of healthy controls (123, 124).
IDO1 mediates DC-Treg cell crosstalk and plays a protective role via the related negative signal loop (Figure 3). When EAE/MS occurs, both the massive secretion of IFN-α/β, IFN- γ, and CTLA-4-CD80/86 between Tregs and DCs can be used as the upstream signal of IDO1 to enhance the expression of IDO1 in DCs, which initiates tryptophan catabolism. Since tryptophan is an essential amino acid for T cell activation and proliferation, IDO1-mediated tryptophan catabolism can lead to tryptophan starvation, inhibit specific immune responses, and promote T cell anergy (8, 125). The KP is the most important tryptophan metabolism pathway mediated by IDO1. A variety of IDO1 downstream products produced via the KP are positive regulators that induce Tregs, of which kynurenine is the most representative. A large amount of kynurenine is produced and bound to AHR as a ligand, which promotes the generation of AHR-dependent Tregs (125). Moreover, such combination regulates the NF-kB pathway in DCs, further upregulates the gene expression level of IDO1, and improves the tolerance of DCs (119). In addition, long-term tryptophan catabolism is also conducive to promoting the differentiation of naive T cells into Tregs; and tolDCs can also enhance the inhibitory function of Tregs (119). Conversely, newly generated Tregs induce expression of IDO1 in DCs via CTLA-4, which further promotes the generation of tolDCs and forms the IDO1 related negative feedback loop. In addition, it has been demonstrated that in the steady state, Tregs induced the generation of IDO+ tolDCs in advance, and tolDCs, in turn, promoted the inhibitory function and rapid proliferation of Tregs following inflammation (8). Treg cell- or DC-derived TGF-β in EAE also induced IDO phosphorylation, promoted the gene expression level of TGF-β in DCs, and induced Treg cell generation. In the allergic airway inflammation model, Tregs induced IDO via GITR-GITR ligand (GITRL) reverse signal transduction (118). Surprisingly, basic research has also shown that IDO+ DCs can make IDO- DCs tolerogenic via the bystander mechanism (126).
Compared with cDCs, pDCs have a stronger potential to induce Tregs in an IDO-dependent manner. However, the ability of pDCs to induce Tregs was significantly downregulated in MS patients, followed by destroying immune tolerance. Notably, IFN-β treatment can restore the Treg-inducing ability of pDCs (37). A newly developed DC-targeting IFN can specifically induce Tregs in an IDO-dependent manner to alleviate EAE (127). Its application eliminated the influence of IFN-β on other immune cells, which offered promise for providing an effective therapy. Furthermore, VitD3 and anti-malarial drug- primaquine, can also boost the expression level of IDO in CD11c+ DCs and induce Foxp3+ Tregs (128). The adoptive transfer of IDO+ CD11c+ DCs induced by VitD3 to EAE mice had the same therapeutic effect as oral or intraperitoneal injection of VitD3 (129). However, the role of IDO in EAE is variable. A recent study showed that the induction of EAE clinical signs was related to the activation of the neurotoxic metabolites of IDO and the KP, and the IDO inhibitor INCB024360 unexpectedly curbed the clinical symptoms and weight loss caused by EAE (120). The most likely explanation for this phenomenon is that IDO is a double-edged sword (130). In the early stage of inflammation, KP maintained immune tolerance by promoting the generation of Foxp3+ Tregs and played a protective role in EAE. However, as the disease progresses, the infiltration of immune cells in the CNS intensified, and microglia produced neurotoxic metabolites of kynurenine, such as quinolinic acid (QUIN) and 3-hydroxykynurenine, downstream of IDO. These toxic substances accumulated in the prefrontal cortex, hippocampus, spinal cord, spleen, and lymph node of EAE mice, gradually offsetting the IDO-induced immune tolerance (120). Among these metabolites, QUIN connected neuroinflammation with neurodegeneration and guided EAE to develop in the direction of neurodegeneration-related chronic inflammation and neurotoxicity (120, 131). Therefore, IDO inhibitors are expected to become a new strategy for the treatment of advanced MS with neurodegenerative diseases; selecting the right time point to administer IDO inhibitors and treat autoimmune diseases will be a challenge in the future.
Various signal loops with surface molecules, cytokines, and metabolic enzymes as the core, as described above, explain the majority of the DC-Treg cell crosstalk mechanism; however, there are also other molecules, such as thymic stromal lymphopoietin (TSLP), GITRL, IL-27, and retinoic acid (RA), indirectly influence Tregs by inducing DC tolerogenicity. Unsatisfactorily, it has not been confirmed experimentally whether Tregs participate in the regulation of DC through these molecules.
DCs can regulate Treg cell generation with the help of surface molecules (Figure 2). For example, TSLP-activated DCs can provide a strong survival signal for high-affinity autoreactive T cells and actively participate in their differentiation into Tregs (132). The expression level of the TSLP receptor (TSLPR) on the surface of myeloid DCs was decreased in peripheral blood of MS patients, and the function of DCs was altered, which resulted in abnormal Treg cell homeostasis and function. Upon immunomodulatory treatment with IFN-β and glatiramer acetate, TSLPRs on myeloid DCs were upregulated, and Treg cell homeostasis was restored (133). These studies demonstrated that TSLPRs were involved in the generation of DC-dependent Tregs.
The binding of GITRL on the DC surface and the corresponding receptor (i.e., GITR) on the Treg cell surface can improve the sensitivity of Treg precursor cells to IL-2 and provide co-stimulatory signals for Treg cell proliferation (134, 135). A recent study showed that the expression of GITRs on the surface of CD4+ and CD8+ Tregs was decreased in RRMS individuals (69). This might be one of the reasons that DC-Treg cell crosstalk is destroyed in MS patients, which limits the proliferation of DC-dependent Tregs. In addition, the GITR-GITRL axis was one of the mechanisms underlying the treatment effect of IFN-β on MS. It has been shown that in the presence of IL-2, IFN-β induced the expression of GITRL on DCs, enhanced the GITR-GITRL axis signal transduction, promoted the proliferation of Tregs, and participated in the treatment of MS (136). The allergic airway inflammation model suggested that the reverse signal transduction of GITRL induced IDO expression in mouse pDCs (118). Thus, the GITR-GITRL axis might also play a bidirectional role in DC-Treg cell crosstalk in EAE/MS.
CD31 is a transhomophilic tyrosine-based inhibitory motif receptor expressed by DCs. Sustaining CD31/SHP-1 signaling during DC maturation resulted in reduced NF-κB nuclear translocation and enhanced tolerogenic functions of DCs that promoted the generation of antigen-specific Tregs (137). The adoptive transfer of CD31-conditioned MOG-loaded DCs can significantly increase the percentage of CD4+ CD25+ Foxp3+ Tregs and offer immune tolerance against the subsequent development of MOG-induced EAE in vivo (137). Therefore, inducing Treg cell generation by regulating these DC surface molecules is a potentially promising treatment for MS.
IL-27 secreted by DCs has both anti-inflammatory and pro-inflammatory effects, and its production changes with the occurrence of MS/EAE (138). For example, plasma IL-27 secretion and IL-27 mRNA expression in PBMCs were significantly decreased in patients with progressive MS (139). The IL-27 subunit and its receptor were upregulated in the CNS inflammatory cells of EAE mice (140). These results suggested a clear correlation between IL-27 and EAE/MS.
Studies have shown that the IL-27 signaling pathway mediates protective DC-Treg cell crosstalk in EAE (141) (Figure 3). For example, IL-27 receptor knockout mice with EAE deteriorate significantly, accompanied by reductions in the frequency of Foxp3+ and IL-10+ CD4+ T cells (141). Subsequent studies have shown that DC-derived IL-27 activated STAT1, STAT3, AHR, c-Maf, and other transcription factors, induced the production of IL-10, and, in turn, promoted the generation of Tr1 and Tregs (22, 93). Recent studies have revealed that the functioning way of IL-27 differed between the central and peripheral tolerance. Do et al. (142) used a Treg cell-specific IL-27 receptor knockout mouse model to illustrate that IL-27 signaling in Foxp3+ Tregs was essential for Tregs to control autoimmune inflammation in the CNS. However, Thomé et al. (94) demonstrated that this classical IL-27 signaling mode in T cells was not necessary to induce peripheral tolerance in EAE mice. In contrast, the indirect way in which IL-27 promotes DC tolerance and induces Treg cell generation is more pivotal in peripheral tolerance. In addition to secreting IL-10, IL-27 plays an anti-inflammatory role in another two ways to induce tolDCs and prevent the development of CNS inflammation and EAE: 1) promote the expression of co-inhibitory molecules PD-L1 and CD39 on the surface of DCs (22, 141, 143, 144); 2) regulate the NF-κB signaling pathway in DCs to promote the expression of TGF-β1 and IDO (137).
IL-27 appears to have a dual effect on Treg cell generation in EAE mice (93, 145). For example, although the systemic delivery of IL-27 can effectively prevent the initiation of Th17 cells and the development of EAE, it enhanced the Th1 response, downregulated the frequency of Foxp3+ Tregs in the spleen, and significantly inhibited Treg cell subsets of the CNS without influencing Tr1 cells (146). This phenomenon increased the risk of IL-27-based EAE treatment and suggested that IL-27 has an inhibitory effect on Treg cell generation. In addition, IL-27 in EAE also inhibits immune tolerance mediated by mature DCs and thus plays a pro-inflammatory role. In this process, mature DCs treated with IL-27 did not influence the expression of Treg cell-associated molecules on CD4+ T cells in vivo or in vitro but induced the development of CD4+ CD127+ 3G11+ Tregs, which were considered an unprecedented pro-inflammatory Treg cell subset (138). Therefore, the mechanism of the pleiotropic molecule IL-27 in DC-Treg cell crosstalk has not been fully elucidated. At present, in the context of EAE/MS, most studies are devoted to the anti-inflammatory effect of IL-27 on the promotion of the generation of tolDCs and Tregs. However, the pro-inflammatory effect of IL-27 and its negative regulation of Treg cell generation also warrant further attention.
RA is produced by a variety of cells, including DCs and Tregs (Figure 3). Although numerous studies have shown that RA directly promotes Treg cell proliferation (147, 148), there is evidence that RA can also indirectly influence Tregs in a DC-dependent manner. For example, in an EAE model, all-trans-RA can impair the antigen-presenting ability of DCs, resulting in the reduction of pro-inflammatory Th1/Th17 cells and severity of EAE (149). Similarly, helminth-infected MS patients developed a relatively mild condition owing to the increased synthesis of RA in DCs, so that DCs were programmed to develop into tolDCs, which induce Foxp3+ Tregs and inhibit the production of the suppressor of cytokine signaling 3-mediated pro-inflammatory cytokines (150). Furthermore, tolDCs induced by RA can induce the antigen-specific Treg cell response in vitro, and the liposomal co-delivery of antigen and RA might be a more targeted approach to induce antigen-specific tolerance in autoimmune and chronic inflammatory diseases (148). Thus, RA is of great significance for DC-dependent Treg cell generation.
Most galectins are critical mediators for the induction of peripheral tolerance, offering protective effects for MS patients and EAE mice. Galectin-1 is synthesized and secreted by Tregs, DCs, and other cells, which in turn boosts the generation of tolDCs. A previous study showed that galectin-1 induced tolDCs, enhanced the development of Tr1 and Tregs, and participated in the induction of intravenous tolerance in MOG35-55 immunized EAE mice (151). In addition, EAE was exacerbated in Lgals8/Lac-Z knock-in mice lacking galectin-8 expression because of the increased immunogenicity of DCs and the disruption of the Treg cell-Th1/Th17 cell balance (152).
The contribution of the immunoglobulin-like transcript (ILT), CD83, and heme oxygenase 1 (HO-1) to DC-Treg cell crosstalk in MS/EAE has not been extensively reported. However, researches suggested that they indirectly participate in the DC-Treg cell interplay by inducing tolDCs.
The inhibitory receptors ILT3 and ILT4 are vital for inducing T cell tolerance, highly expressed in tolDCs, and independently prevent the activation of pathogenic T cells (14). The beneficial effect of IFN-β in MS patients was probably achieved partially by regulating the expression of ILT3 and ILT4 in DCs. VitD3 also increased the expression of ILT3 but not ILT4 (153). These studies suggested that a variety of drugs induce Tregs and treat MS by regulating the expression of ILTs on the surface of DCs. Besides, via secreting IFN-I, toll-like receptor 9-activated cDCs can regulate neutrophil and monocyte trafficking to the inflamed colon and restrain the inflammatory products of tissue macrophages, thereby inhibiting colonic inflammation independently of T cell (154). This reminds us that cDCs may further influence CNS inflammation in a similar way, with the help of gut-brain axis. In addition, CD83 is expressed in activated B cells, T cells, and especially Tregs, and its soluble form inhibits the maturation and function of DCs (155). CD83 transgenic mice recovered quickly from EAE with the enhanced activity of Tregs and the limited proliferation of pathogenic T cells (155). Therefore, CD83 probably induced Treg cell generation in a tolDC-dependent manner.
Surprisingly, numerous studies have shown that HO-1 has a protective effect on MS/EAE. HO-1 in EAE inhibited the expression of MHC-II in DCs and improved DC tolerogenecity (156–158). However, one study found that HO-1 did not regulate the number or function of infiltrated Tregs in the CNS; it showed that the protective effect of HO-1 on the CNS of EAE mice might not be related to DC-Treg cell crosstalk (159). Currently, it remains uncertain whether peripheral HO-1+ DCs in EAE play a protective role via Tregs, because in the airway inflammation model, HO-1 expressed by DCs indeed promote the differentiation of Tregs (159).
There are a small number of dedicated so-called tolerogenic DC subsets, which are predestined to induce Tregs in a steady state (Table 3). Moreover, the generation of tolDC is closely related to the Wnt/beta-catenin pathway. A classical study in 2007 demonstrated that disruption of E-cadherin-mediated adhesion could induce peripheral T cell tolerance in vivo and protect against EAE (164). In this process, E-cadherin triggered Wnt/beta-catenin pathway, a classical pathway to induce DC tolerance and maturation, and these tolDCs further boosted IL-10-producing Treg cell generation and peripheral tolerance (164, 165). Of note, CD103 is a heterotypic ligand for E-cadherin, so some CD103+DCs might be a potential tolDC subset. For example, Langerin+CD103+ migratory DCs had a superior ability to generate Tregs in vivo, which in turn drastically alleviated EAE (160). Besides, a recent study defined another tolDC subset: BTLA+ DEC205+ CD8+ CD11c+ DCs, which took advantage of the engagement of the herpesvirus entry mediator (HVEM), a receptor of BTLA, upregulated the expression of Foxp3 in a CD5-dependent manner, and promoted peripheral Treg cell transformation (161). This study suggested that the cross-linking of BTLA and HVEM was one of the key mechanisms for DC to induce peripheral Tregs in EAE. However, most CD11c+ DCs are BTLAneg DCs and thus can’t acquire tolerance even in a steady state. Moreover, IL-10-producing DC(DC-10) is also a classical tolDC subset, and most of the induced tolDCs in EAE/MS are recognized by the secretion of IL-10. Therefore, we deem that DC-10 is a potential tolDC subset, but its concept is not clearly proposed in EAE (162, 163). Moreover, it should be noted that tolDC subsets only exist in the steady state. Once inflammation occurs, tolDCs would play a pro-inflammatory role again and activate Teffs. At that time, induced-tolDC would be crucial in role of immune protection.
DC-Treg crosstalk is one of the pivotal mechanisms of drugs to alleviate MS, such as IFN-β, Vit D3, tanshinone IIA, primaquine and apigenin. Besides, as for DC-Treg crosstalk, the treatment of MS/EAE in the future can also be explored in the following aspects: 1) inhibitors, agonists, or specific antibodies of critical immune targets, e.g., DC-targeting CTLA-4-Ig and PD-L1/PD-1 agonists; 2) therapeutic interventions modulating IDO activity, e.g., IDO inducers/inhibitors and STING agonists. However, drug-based therapy mostly plays its role through non-specific immunity, which often leads to side effects such as decreased immunity and infection. Therefore, cellar-based therapy involving tolDCs and Tregs have become a new upsurge, such as peptide- and protein-based vaccines. It can reestablish antigen-specific immune tolerance towards CNS structures and control autoreactive T cells without inducing systemic immune suppression.
At present, peptide-based vaccines were advanced into human clinical trials. A phase I clinical trial (NCT02283671) starting at 2014, was for the first time to evaluate the tolerance and safety of tolDCs in treating patients with MS or neuromyelitis optica (NMO). The clinical use of tolDCs in a well-defined population of MS patients was evaluated in two subsequent phase I clinical trials (NCT02618902 and NCT02903537). These two clinical trials conducted dose-escalation studies and compared each other through intradermal and intranodal cell administration, followed by evaluating the safety and feasibility of tolDC administration, and exploring appropriate vaccine injection doses and DC delivery methods for the next phase II clinical trials (166). In addition, a phase II clinical trial (NCT04530318) about the treatment of MS with autologous peripheral blood differentiated tolDCs combined with immune regulation was started in 2020, which is expected to be completed in 2024. Surprisingly, the current experiment did not report any safety problems related to tolDC administration in MS patients (166).
Similarly, protein-based vaccines are also intriguing tolDC therapies that involve DC-specific antigen-targeting strategy. For instance, both DEC205 (CD205) and dendritic-cell inhibitory receptor-2 (DCIR2), are DC-specific endocytosis receptors, which can improve the antigen presentation efficiency of DCs (167, 168) (Figure 2). Delivering PLP to DCs using αDEC-205 fusion monoclonal antibodies or DCIR2 fusion monoclonal antibodies can induce Treg cell generation. Then these Tregs resulted in antigen-specific tolerance and amelioration of EAE (167, 169). Similarly, targeting migratory DCs with an anti-receptor-antigen fusing to MOG can provide the same result (160). Intriguingly, αDEC-205+ fusion antibodies cause extrathymic induction of a Foxp3+ Treg cell phenotype in naïve CD4+ Foxp3− T cells, whereas DCIR2+ fusion antibodies result in the proliferative expansion of natural Foxp3+ Tregs in EAE (167). Besides, many drugs are inducers of tolDCs, such as interferon, vitD3, statins, galectin-1, tofacitinib, etc (151, 170, 171). Combining these drugs with protein-based vaccines is also more conducive to the induction of tolDCs.
DC-Treg cell crosstalk involves a variety of surface molecules, cytokines, and metabolic enzymes via multiple inhibitory feedback loops and plays a key protective role in the silent progression of MS. Drugs influencing DC-Treg crosstalk and tolDC therapy are expected to emerge as promising treatment strategies for MS. However, selecting the appropriate time point and specifically targeting DCs/Tregs without affecting the immune state of other cells is worthy of future discussion, due to different onset times and the wide distribution/rich targets of these immune effector molecules. At present, the researches of functional molecules working comprehensively become more and more popular in the environment of CSF, PBMC or peripheral blood in MS patients. Although, these studies can well show the correlation between molecules and diseases, what unsatisfied was that they did not reflect the molecular changes in specific cell subtypes and we cannot distinguish the cellular source and targeted cells of functional molecules from those studies. Therefore, further research on the expression level of molecules based on cell subsets is conducive to a more comprehensive analysis and evaluation of molecular effects. In addition, the increased focusing on tolDC therapy has facilitated an upsurge in research on DC-induced Tregs, whereas investigations on the effect of Tregs in DCs remain relatively scarce. There remain several knowledge gaps in the mechanism of DC-Treg cell crosstalk, and further research would be necessary to translate mechanisms into clinical therapy.
RL, PL, and HLiu conceived this review. RL performed specific database queries, generated the figures and tables, and wrote the manuscript. HLi, XY, HH, PL, and HLiu edited and revised the review. All authors contributed to the article and approved the submitted version.
This work was supported by Henan Provincial and Ministerial Co-construction Projects (No. SB201901018) and the National Natural Science Foundation of China (No. U2004128).
We thank the reviewers for their insightful and constructive comments on the manuscript. We are grateful to the translational medicine center of the First Affiliated Hospital of Zhengzhou University for support.
The authors declare that the research was conducted in the absence of any commercial or financial relationships that could be construed as a potential conflict of interest.
All claims expressed in this article are solely those of the authors and do not necessarily represent those of their affiliated organizations, or those of the publisher, the editors and the reviewers. Any product that may be evaluated in this article, or claim that may be made by its manufacturer, is not guaranteed or endorsed by the publisher.
1. Ruland C, Renken H, Kuzmanov I, Fattahi Mehr A, Schwarte K, Cerina M, et al. Chemokine CCL17 is expressed by dendritic cells in the CNS during experimental autoimmune encephalomyelitis and promotes pathogenesis of disease. Brain Behav Immun (2017) 66:382–93. doi: 10.1016/j.bbi.2017.06.010
2. De Laere M, Berneman ZN, Cools N. To the brain and back: Migratory paths of dendritic cells in multiple sclerosis. J Neuropathol Exp Neurol (2018) 77(3):178–92. doi: 10.1093/jnen/nlx114
3. Grigoriadis N, van Pesch V, Paradig MSG. A basic overview of multiple sclerosis immunopathology. Eur J Neurol (2015) 22 Suppl 2:3–13. doi: 10.1111/ene.12798
4. Yang X, Peng J, Huang X, Liu P, Li J, Pan J, et al. Association of circulating follicular helper T cells and serum CXCL13 with neuromyelitis optica spectrum disorders. Front In Immunol (2021) 12:677190. doi: 10.3389/fimmu.2021.677190
5. Dendrou CA, Fugger L, Friese MA. Immunopathology of multiple sclerosis. Nat Rev Immunol (2015) 15(9):545–58. doi: 10.1038/nri3871
6. Yogev N, Frommer F, Lukas D, Kautz-Neu K, Karram K, Ielo D, et al. Dendritic cells ameliorate autoimmunity in the CNS by controlling the homeostasis of PD-1 receptor(+) regulatory T cells. Immunity (2012) 37(2):264–75. doi: 10.1016/j.immuni.2012.05.025
7. Paterka M, Voss JO, Werr J, Reuter E, Franck S, Leuenberger T, et al. Dendritic cells tip the balance towards induction of regulatory T cells upon priming in experimental autoimmune encephalomyelitis. J Autoimmun (2017) 76:108–14. doi: 10.1016/j.jaut.2016.09.008
8. Lippens C, Duraes FV, Dubrot J, Brighouse D, Lacroix M, Irla M, et al. IDO-orchestrated crosstalk between pDCs and tregs inhibits autoimmunity. J Autoimmun (2016) 75:39–49. doi: 10.1016/j.jaut.2016.07.004
9. Tadokoro CE, Shakhar G, Shen S, Ding Y, Lino AC, Maraver A, et al. Regulatory T cells inhibit stable contacts between CD4+ T cells and dendritic cells in vivo. J Exp Med (2006) 203(3):505–11. doi: 10.1084/jem.20050783
10. Moorman CD, Sohn SJ, Phee H. Emerging therapeutics for immune tolerance: Tolerogenic vaccines, T cell therapy, and IL-2 therapy. Front Immunol (2021) 12:657768. doi: 10.3389/fimmu.2021.657768
11. Onishi Y, Fehervari Z, Yamaguchi T, Sakaguchi S. Foxp3+ natural regulatory T cells preferentially form aggregates on dendritic cells in vitro and actively inhibit their maturation. Proc Natl Acad Sci USA (2008) 105(29):10113–8. doi: 10.1073/pnas.0711106105
12. Grover P, Goel PN, Greene MI. Regulatory T cells: Regulation of identity and function. Front Immunol (2021) 12:750542. doi: 10.3389/fimmu.2021.750542
13. Perdigoto AL, Chatenoud L, Bluestone JA, Herold KC. Inducing and administering tregs to treat human disease. Front In Immunol (2015) 6:654. doi: 10.3389/fimmu
14. Maldonado RA, von Andrian UH. How tolerogenic dendritic cells induce regulatory T cells. Adv Immunol (2010) 108:111–65. doi: 10.1016/B978-0-12-380995-7.00004-5
15. Eisenbarth SC. Dendritic cell subsets in T cell programming: location dictates function. Nat Rev Immunol (2019) 19(2):89–103. doi: 10.1038/s41577-018-0088-1
16. Villar J, Segura E. Decoding the heterogeneity of human dendritic cell subsets. Trends Immunol (2020) 41(12):1062–71. doi: 10.1016/j.it.2020.10.002
17. Coillard A, Segura E. In vivo differentiation of human monocytes. Front Immunol (2019) 10:1907. doi: 10.3389/fimmu.2019.01907
18. Zanna MY, Yasmin AR, Omar AR, Arshad SS, Mariatulqabtiah AR, Nur-Fazila SH, et al. Review of dendritic cells, their role in clinical immunology, and distribution in various animal species. Int J Mol Sci (2021) 22(15):8044. doi: 10.3390/ijms22158044
19. Macri C, Pang ES, Patton T, O'Keeffe M. Dendritic cell subsets. Semin Cell Dev Biol (2018) 84:11–21. doi: 10.1016/j.semcdb.2017.12.009
20. Bourque J, Hawiger D. Variegated outcomes of T cell activation by dendritic cells in the steady state. J Immunol (2022) 208(3):539–47. doi: 10.4049/jimmunol.2100932
21. Heger L, Hofer TP, Bigley V, de Vries IJM, Dalod M, Dudziak D, et al. Subsets of CD1c(+) DCs: Dendritic cell versus monocyte lineage. Front Immunol (2020) 11:559166. doi: 10.3389/fimmu.2020.559166
22. Waisman A, Lukas D, Clausen BE, Yogev N. Dendritic cells as gatekeepers of tolerance. Semin Immunopathol (2017) 39(2):153–63. doi: 10.1007/s00281-016-0583-z
23. Mondanelli G, Albini E, Pallotta MT, Volpi C, Chatenoud L, Kuhn C, et al. The proteasome inhibitor bortezomib controls indoleamine 2,3-dioxygenase 1 breakdown and restores immune regulation in autoimmune diabetes. Front In Immunol (2017) 8:428. doi: 10.3389/fimmu.2017.00428
24. Lutz MB, Backer RA, Clausen BE. Revisiting current concepts on the tolerogenicity of steady-state dendritic cell subsets and their maturation stages. J Immunol (2021) 206(8):1681–9. doi: 10.4049/jimmunol.2001315
25. Liu Y, Carlsson R, Comabella M, Wang J, Kosicki M, Carrion B, et al. FoxA1 directs the lineage and immunosuppressive properties of a novel regulatory T cell population in EAE and MS. Nat Med (2014) 20(3):272–82. doi: 10.1038/nm.3485
26. Klein L, Robey EA, Hsieh C-S. Central CD4 T cell tolerance: deletion versus regulatory T cell differentiation. Nat Rev Immunol (2019) 19(1):7–18. doi: 10.1038/s41577-018-0083-6
27. Roncarolo MG, Gregori S, Bacchetta R, Battaglia M, Gagliani N. The biology of T regulatory type 1 cells and their therapeutic application in immune-mediated diseases. Immunity (2018) 49(6):1004–19. doi: 10.1016/j.immuni.2018.12.001
28. Jonuleit H, Schmitt E. The regulatory T cell family: distinct subsets and their interrelations. J Immunol (2003) 171(12):6323–7. doi: 10.4049/jimmunol.171.12.6323
29. Dominguez-Villar M, Baecher-Allan CM, Hafler DA. Identification of T helper type 1-like, Foxp3+ regulatory T cells in human autoimmune disease. Nat Med (2011) 17(6):673–5. doi: 10.1038/nm.2389
30. Gupta S, Demirdag Y, Gupta AA. Members of the regulatory lymphocyte club in common variable immunodeficiency. Front Immunol (2022) 13:864307. doi: 10.3389/fimmu.2022.864307
31. Fazilleau N, Aloulou M. Several follicular regulatory T cell subsets with distinct phenotype and function emerge during germinal center reactions. Front Immunol (2018) 9:1792. doi: 10.3389/fimmu.2018.01792
32. Mishra S, Srinivasan S, Ma C, Zhang N. CD8 regulatory T cell - a mystery to be revealed. Front In Immunol (2021) 12:708874. doi: 10.3389/fimmu.2021.708874
33. Mena J, Alloza I, Tulloch Navarro R, Aldekoa A, Díez García J, Villanueva Etxebarria A, et al. Genomic multiple sclerosis risk variants modulate the expression of the - gene region in immature dendritic cells. Front In Immunol (2021) 12:816930. doi: 10.3389/fimmu.2021.816930
34. Luessi F, Zipp F, Witsch E. Dendritic cells as therapeutic targets in neuroinflammation. Cell Mol Life Sci (2016) 73(13):2425–50. doi: 10.1007/s00018-016-2170-9
35. Longhini ALF, von Glehn F, Brandão CO, de Paula RFO, Pradella F, Moraes AS, et al. Plasmacytoid dendritic cells are increased in cerebrospinal fluid of untreated patients during multiple sclerosis relapse. J Neuroinflammation (2011) 8(1):2. doi: 10.1186/1742-2094-8-2
36. Lopez C, Comabella M, Al-zayat H, Tintore M, Montalban X. Altered maturation of circulating dendritic cells in primary progressive MS patients. J Neuroimmunol (2006) 175(1-2):183–91. doi: 10.1016/j.jneuroim.2006.03.010
37. Aung LL, Brooks A, Greenberg SA, Rosenberg ML, Dhib-Jalbut S, Balashov KE. Multiple sclerosis-linked and interferon-beta-regulated gene expression in plasmacytoid dendritic cells. J Neuroimmunol (2012) 250(1-2):99–105. doi: 10.1016/j.jneuroim.2012.05.013
38. Stasiolek M, Bayas A, Kruse N, Wieczarkowiecz A, Toyka KV, Gold R, et al. Impaired maturation and altered regulatory function of plasmacytoid dendritic cells in multiple sclerosis. Brain (2006) 129(Pt 5):1293–305. doi: 10.1093/brain/awl043
39. Hafler DA, Compston A, Sawcer S, Lander ES, Daly MJ, De Jager PL, et al. Risk alleles for multiple sclerosis identified by a genomewide study. N Engl J Med (2007) 357(9):851–62. doi: 10.1056/NEJMoa073493
40. Verma ND, Lam AD, Chiu C, Tran GT, Hall BM, Hodgkinson SJ. Multiple sclerosis patients have reduced resting and increased activated CD4(+)CD25(+)FOXP3(+)T regulatory cells. Sci Rep (2021) 11(1):10476. doi: 10.1038/s41598-021-88448-5
41. Bjerg L, Brosbol-Ravnborg A, Torring C, Dige A, Bundgaard B, Petersen T, et al. Altered frequency of T regulatory cells is associated with disability status in relapsing-remitting multiple sclerosis patients. J Neuroimmunol (2012) 249(1-2):76–82. doi: 10.1016/j.jneuroim.2012.04.012
42. Feger U, Luther C, Poeschel S, Melms A, Tolosa E, Wiendl H. Increased frequency of CD4+ CD25+ regulatory T cells in the cerebrospinal fluid but not in the blood of multiple sclerosis patients. Clin Exp Immunol (2007) 147(3):412–8. doi: 10.1111/j.1365-2249.2006.03271.x
43. Sambucci M, Gargano F, Guerrera G, Battistini L, Borsellino G. One, no one, and one hundred thousand: T regulatory cells' multiple identities in neuroimmunity. Front Immunol (2019) 10:2947. doi: 10.3389/fimmu.2019.02947
44. Viglietta V, Baecher-Allan C, Weiner HL, Hafler DA. Loss of functional suppression by CD4+CD25+ regulatory T cells in patients with multiple sclerosis. J Exp Med (2004) 199(7):971–9. doi: 10.1084/jem.20031579
45. Edner NM, Carlesso G, Rush JS, Walker LSK. Targeting co-stimulatory molecules in autoimmune disease. Nat Rev Drug Discov (2020) 19(12):860–83. doi: 10.1038/s41573-020-0081-9
46. Hsieh CS, Lee HM, Lio CW. Selection of regulatory T cells in the thymus. Nat Rev Immunol (2012) 12(3):157–67. doi: 10.1038/nri3155
47. Beyersdorf N, Gaupp S, Balbach K, Schmidt J, Toyka KV, Lin CH, et al. Selective targeting of regulatory T cells with CD28 superagonists allows effective therapy of experimental autoimmune encephalomyelitis. J Exp Med (2005) 202(3):445–55. doi: 10.1084/jem.20051060
48. Zhang R, Huynh A, Whitcher G, Chang J, Maltzman JS, Turka LA. An obligate cell-intrinsic function for CD28 in tregs. J Clin Invest (2013) 123(2):580–93. doi: 10.1172/JCI65013
49. Chang TT, Jabs C, Sobel RA, Kuchroo VK, Sharpe AH. Studies in B7-deficient mice reveal a critical role for B7 costimulation in both induction and effector phases of experimental autoimmune encephalomyelitis. J Exp Med (1999) 190(5):733–40. doi: 10.1084/jem.190.5.733
50. Haanstra KG, Dijkman K, Bashir N, Bauer J, Mary C, Poirier N, et al. Selective blockade of CD28-mediated T cell costimulation protects rhesus monkeys against acute fatal experimental autoimmune encephalomyelitis. J Immunol (2015) 194(4):1454–66. doi: 10.4049/jimmunol.1402563
51. Wing K, Onishi Y, Prieto-Martin P, Yamaguchi T, Miyara M, Fehervari Z, et al. CTLA-4 control over Foxp3+ regulatory T cell function. Science (2008) 322(5899):271–5. doi: 10.1126/science.1160062
52. Gerdes LA, Held K, Beltrán E, Berking C, Prinz JC, Junker A, et al. CTLA4 as immunological checkpoint in the development of multiple sclerosis. Ann Neurol (2016) 80(2):294–300. doi: 10.1002/ana.24715
53. Paterson AM, Lovitch SB, Sage PT, Juneja VR, Lee Y, Trombley JD, et al. Deletion of CTLA-4 on regulatory T cells during adulthood leads to resistance to autoimmunity. J Exp Med (2015) 212(10):1603–21. doi: 10.1084/jem.20141030
54. Mohammadzadeh A, Rad IA, Ahmadi-Salmasi B. CTLA-4, PD-1 and TIM-3 expression predominantly downregulated in MS patients. J Neuroimmunol (2018) 323:105–8. doi: 10.1016/j.jneuroim.2018.08.004
55. Lavon I, Heli C, Brill L, Charbit H, Vaknin-Dembinsky A. Blood levels of Co-inhibitory-Receptors: A biomarker of disease prognosis in multiple sclerosis. Front Immunol (2019) 10:835. doi: 10.3389/fimmu.2019.00835
56. Qureshi OS, Zheng Y, Nakamura K, Attridge K, Manzotti C, Schmidt EM, et al. Trans-endocytosis of CD80 and CD86: a molecular basis for the cell-extrinsic function of CTLA-4. Science (2011) 332(6029):600–3. doi: 10.1126/science.1202947
57. Chattopadhyay G, Shevach EM. Antigen-specific induced T regulatory cells impair dendritic cell function via an IL-10/MARCH1-dependent mechanism. J Immunol (2013) 191(12):5875–84. doi: 10.4049/jimmunol.1301693
58. Kim GR, Kim WJ, Lim S, Lee HG, Koo JH, Nam KH, et al. In vivo induction of regulatory T cells Via CTLA-4 signaling peptide to control autoimmune encephalomyelitis and prevent disease relapse. Adv Sci (Weinh) (2021) 8(14):2004973. doi: 10.1002/advs.202004973
59. Glatigny S, Hollbacher B, Motley SJ, Tan C, Hundhausen C, Buckner JH, et al. Abatacept targets T follicular helper and regulatory T cells, disrupting molecular pathways that regulate their proliferation and maintenance. J Immunol (2019) 202(5):1373–82. doi: 10.4049/jimmunol.1801425
60. Vogel I, Kasran A, Cremer J, Kim YJ, Boon L, Van Gool SW, et al. CD28/CTLA-4/B7 costimulatory pathway blockade affects regulatory T-cell function in autoimmunity. Eur J Immunol (2015) 45(6):1832–41. doi: 10.1002/eji.201445190
61. Khoury SJ, Rochon J, Ding L, Byron M, Ryker K, Tosta P, et al. ACCLAIM: A randomized trial of abatacept (CTLA4-ig) for relapsing-remitting multiple sclerosis. Multiple scler (2017) 23(5):686–95. doi: 10.1177/1352458516662727
62. Bonelli M, Scheinecker C. How does abatacept really work in rheumatoid arthritis? Curr Opin Rheumatol (2018) 30(3):295–300. doi: 10.1097/BOR.0000000000000491
63. Keir ME, Butte MJ, Freeman GJ, Sharpe AH. PD-1 and its ligands in tolerance and immunity. Annu Rev Immunol (2008) 26:677–704. doi: 10.1146/annurev.immunol.26.021607.090331
64. Ibañez-Vega J, Vilchez C, Jimenez K, Guevara C, Burgos PI, Naves R. Cellular and molecular regulation of the programmed death-1/programmed death ligand system and its role in multiple sclerosis and other autoimmune diseases. J autoimmunity (2021) 123:102702. doi: 10.1016/j.jaut.2021.102702
65. Francisco LM, Salinas VH, Brown KE, Vanguri VK, Freeman GJ, Kuchroo VK, et al. PD-L1 regulates the development, maintenance, and function of induced regulatory T cells. J Exp Med (2009) 206(13):3015–29. doi: 10.1084/jem.20090847
66. Mi Y, Han J, Zhu J, Jin T. Role of the PD-1/PD-L1 signaling in multiple sclerosis and experimental autoimmune encephalomyelitis: Recent insights and future directions. Mol Neurobiol (2021) 58(12):6249–71. doi: 10.1007/s12035-021-02495-7
67. Ortler S, Leder C, Mittelbronn M, Zozulya AL, Knolle PA, Chen L, et al. B7-H1 restricts neuroantigen-specific T cell responses and confines inflammatory CNS damage: implications for the lesion pathogenesis of multiple sclerosis. Eur J Immunol (2008) 38(6):1734–44. doi: 10.1002/eji.200738071
68. Trabattoni D, Saresella M, Pacei M, Marventano I, Mendozzi L, Rovaris M, et al. Costimulatory pathways in multiple sclerosis: distinctive expression of PD-1 and PD-L1 in patients with different patterns of disease. J Immunol (2009) 183(8):4984–93. doi: 10.4049/jimmunol.0901038
69. Machcińska M, Kierasińska M, Michniowska M, Maruszewska-Cheruiyot M, Szewczak L, Rola R, et al. Reduced expression of PD-1 in circulating CD4+ and CD8+ tregs is an early feature of RRMS. Int J Mol Sci (2022) 23(6):3185. doi: 10.3390/ijms23063185
70. Tekguc M, Wing JB, Osaki M, Long J, Sakaguchi S. Treg-expressed CTLA-4 depletes CD80/CD86 by trogocytosis, releasing free PD-L1 on antigen-presenting cells. Proc Natl Acad Sci USA (2021) 118(30):e2023739118. doi: 10.1073/pnas.2023739118
71. Sage PT, Schildberg FA, Sobel RA, Kuchroo VK, Freeman GJ, Sharpe AH. Dendritic cell PD-L1 limits autoimmunity and follicular T cell differentiation and function. J Immunol (2018) 200(8):2592–602. doi: 10.4049/jimmunol.1701231
72. Cao Q, Zheng C, Xie Z, Liu L, Zhu J, Jin T. The change of PD1, PDL1 in experimental autoimmune encephalomyelitis treated by 1,25(OH)D. J Neuroimmunol (2020) 338:577079. doi: 10.1016/j.jneuroim.2019.577079
73. Schreiner B, Mitsdoerffer M, Kieseier BC, Chen L, Hartung HP, Weller M, et al. Interferon-beta enhances monocyte and dendritic cell expression of B7-H1 (PD-L1), a strong inhibitor of autologous T-cell activation: relevance for the immune modulatory effect in multiple sclerosis. J Neuroimmunol (2004) 155(1-2):172–82. doi: 10.1016/j.jneuroim.2004.06.013
74. Vogel A, Martin K, Soukup K, Halfmann A, Kerndl M, Brunner JS, et al. JAK1 signaling in dendritic cells promotes peripheral tolerance in autoimmunity through PD-L1-mediated regulatory T cell induction. Cell Rep (2022) 38(8):110420. doi: 10.1016/j.celrep.2022.110420
75. Irla M, Kupfer N, Suter T, Lissilaa R, Benkhoucha M, Skupsky J, et al. MHC class II-restricted antigen presentation by plasmacytoid dendritic cells inhibits T cell-mediated autoimmunity. J Exp Med (2010) 207(9):1891–905. doi: 10.1084/jem.20092627
76. Ray A, Basu S, Miller NM, Chan AM, Dittel BN. An increase in tolerogenic dendritic cell and natural regulatory T cell numbers during experimental autoimmune encephalomyelitis in rras-/- mice results in attenuated disease. J Immunol (2014) 192(11):5109–17. doi: 10.4049/jimmunol.1302254
77. Kumar P, Bhattacharya P, Prabhakar BS. A comprehensive review on the role of co-signaling receptors and treg homeostasis in autoimmunity and tumor immunity. J Autoimmun (2018) 95:77–99. doi: 10.1016/j.jaut.2018.08.007
78. Liang B, Workman C, Lee J, Chew C, Dale BM, Colonna L, et al. Regulatory T cells inhibit dendritic cells by lymphocyte activation gene-3 engagement of MHC class II. J Immunol (2008) 180(9):5916–26. doi: 10.4049/jimmunol.180.9.5916
79. Zhang Z, Duvefelt K, Svensson F, Masterman T, Jonasdottir G, Salter H, et al. Two genes encoding immune-regulatory molecules (LAG3 and IL7R) confer susceptibility to multiple sclerosis. Genes Immun (2005) 6(2):145–52. doi: 10.1038/sj.gene.6364171
80. Jones BE, Maerz MD, Bahnson HT, Somasundaram A, McCarthy LH, Speake C, et al. Fewer LAG-3(+) T cells in relapsing-remitting multiple sclerosis and type 1 diabetes. J Immunol (2022) 208(3):594–602. doi: 10.4049/jimmunol.2100850
81. Sedeeq MS, El-Nahrery EMA, Shalaby N, Hussein M, Shehata H, El Aal RA, et al. Micro-RNA-96 and interleukin-10 are independent biomarkers for multiple sclerosis activity. J Neurol Sci (2019) 403:92–6. doi: 10.1016/j.jns.2019.06.022
82. Astier AL, Hafler DA. Abnormal Tr1 differentiation in multiple sclerosis. J Neuroimmunol (2007) 191(1-2):70–8. doi: 10.1016/j.jneuroim.2007.09.018
83. Pasquali L, Lucchesi C, Pecori C, Metelli MR, Pellegrini S, Iudice A, et al. A clinical and laboratory study evaluating the profile of cytokine levels in relapsing remitting and secondary progressive multiple sclerosis. J Neuroimmunol (2015) 278:53–9. doi: 10.1016/j.jneuroim.2014.12.005
84. Lee PW, Severin ME, Lovett-Racke AE. TGF-beta regulation of encephalitogenic and regulatory T cells in multiple sclerosis. Eur J Immunol (2017) 47(3):446–53. doi: 10.1002/eji.201646716
85. Sanjabi S, Oh SA, Li MO. Regulation of the immune response by TGF-beta: From conception to autoimmunity and infection. Cold Spring Harb Perspect Biol (2017) 9(6):a022236. doi: 10.1101/cshperspect.a022236
86. Tapia-Maltos MA, Trevino-Frenk I, Garcia-Gonzalez HB, Rosetti M, Barriga-Maldonado V, Morales-Ramirez F, et al. Identification of regulatory T cell molecules associated with severity of multiple sclerosis. Mult Scler (2021) 27(11):1695–705. doi: 10.1177/1352458520977045
87. Badihian S, Shaygannejad V, Soleimani P, Mirmosayyeb O, Samee Z, Manouchehri N, et al. Decreased serum levels of interleukin-35 among multiple sclerosis patients may be related to disease progression. J Biol Regul Homeost Agents (2018) 32(5):1249–53.
88. Kamal A, Hosny M, Abd Elwahab A, Shawki Kamal Y, Shehata HS, Hassan A. FOXP3rs3761548 gene variant and interleukin-35 serum levels as biomarkers in patients with multiple sclerosis. Rev Neurol (Paris) (2021) 177(6):647–54. doi: 10.1016/j.neurol.2020.07.010
89. Jafarzadeh A, Jamali M, Mahdavi R, Ebrahimi HA, Hajghani H, Khosravimashizi A, et al. Circulating levels of interleukin-35 in patients with multiple sclerosis: evaluation of the influences of FOXP3 gene polymorphism and treatment program. J Mol Neurosci MN (2015) 55(4):891–7. doi: 10.1007/s12031-014-0443-z
90. Zhou Y, Leng X, Luo X, Mo C, Zou Q, Liu Y, et al. Regulatory dendritic cells induced by K313 display anti-inflammatory properties and ameliorate experimental autoimmune encephalitis in mice. Front Pharmacol (2019) 10:1579. doi: 10.3389/fphar.2019.01579
91. Tabares-Guevara JH, Jaramillo JC, Ospina-Quintero L, Piedrahíta-Ochoa CA, García-Valencia N, Bautista-Erazo DE, et al. IL-10-Dependent amelioration of chronic inflammatory disease by microdose subcutaneous delivery of a prototypic immunoregulatory small molecule. Front In Immunol (2021) 12:708955. doi: 10.3389/fimmu.2021.708955
92. Takeuchi H. Midkine and multiple sclerosis. Br J Pharmacol (2014) 171(4):931–5. doi: 10.1111/bph.12499
93. Meka RR, Venkatesha SH, Dudics S, Acharya B, Moudgil KD. IL-27-induced modulation of autoimmunity and its therapeutic potential. Autoimmun Rev (2015) 14(12):1131–41. doi: 10.1016/j.autrev.2015.08.001
94. Thomé R, Moore JN, Mari ER, Rasouli J, Hwang D, Yoshimura S, et al. Induction of peripheral tolerance in ongoing autoimmune inflammation requires interleukin 27 signaling in dendritic cells. Front In Immunol (2017) 8:1392. doi: 10.3389/fimmu.2017.01392
95. Fitzgerald DC, Fonseca-Kelly Z, Cullimore ML, Safabakhsh P, Saris CJ, Zhang GX, et al. Independent and interdependent immunoregulatory effects of IL-27, IFN-beta, and IL-10 in the suppression of human Th17 cells and murine experimental autoimmune encephalomyelitis. J Immunol (2013) 190(7):3225–34. doi: 10.4049/jimmunol.1200141
96. Gong Y, Liu YC, Ding XL, Fu Y, Cui LJ, Yan YP. Tanshinone IIA ameliorates CNS autoimmunity by promoting the differentiation of regulatory T cells. Neurotherapeutics (2020) 17(2):690–703. doi: 10.1007/s13311-019-00789-2
97. Ginwala R, Bhavsar R, Moore P, Bernui M, Singh N, Bearoff F, et al. Apigenin modulates dendritic cell activities and curbs inflammation Via RelB inhibition in the context of neuroinflammatory diseases. J Neuroimmun Pharmacol (2021) 16(2):403–24. doi: 10.1007/s11481-020-09933-8
98. Lukas D, Yogev N, Kel JM, Regen T, Mufazalov IA, Tang Y, et al. TGF-β inhibitor Smad7 regulates dendritic cell-induced autoimmunity. Proc Natl Acad Sci USA (2017) 114(8):E1480–E9. doi: 10.1073/pnas.1615065114
99. Yan Y, Zhang GX, Gran B, Fallarino F, Yu S, Li H, et al. IDO upregulates regulatory T cells via tryptophan catabolite and suppresses encephalitogenic T cell responses in experimental autoimmune encephalomyelitis. J Immunol (2010) 185(10):5953–61. doi: 10.4049/jimmunol.1001628
100. Haller S, Duval A, Migliorini R, Stevanin M, Mack V, Acha-Orbea H. Interleukin-35-Producing CD8alpha(+) dendritic cells acquire a tolerogenic state and regulate T cell function. Front Immunol (2017) 8:98. doi: 10.3389/fimmu.2017.00098
101. Choi JK, Dambuza IM, He C, Yu CR, Uche AN, Mattapallil MJ, et al. IL-12p35 inhibits neuroinflammation and ameliorates autoimmune encephalomyelitis. Front Immunol (2017) 8:1258. doi: 10.3389/fimmu.2017.01258
102. Liu JQ, Liu Z, Zhang X, Shi Y, Talebian F, Carl JW Jr., et al. Increased Th17 and regulatory T cell responses in EBV-induced gene 3-deficient mice lead to marginally enhanced development of autoimmune encephalomyelitis. J Immunol (2012) 188(7):3099–106. doi: 10.4049/jimmunol.1100106
103. Mittal SK, Roche PA. Suppression of antigen presentation by IL-10. Curr Opin Immunol (2015) 34:22–7. doi: 10.1016/j.coi.2014.12.009
104. Verhagen J, Gabrysova L, Shepard ER, Wraith DC. Ctla-4 modulates the differentiation of inducible Foxp3+ treg cells but IL-10 mediates their function in experimental autoimmune encephalomyelitis. PloS One (2014) 9(9):e108023. doi: 10.1371/journal.pone.0108023
105. Zhang X, Zheng P, Prestwood TR, Zhang H, Carmi Y, Tolentino LL, et al. Human regulatory dendritic cells develop from monocytes in response to signals from regulatory and helper T cells. Front In Immunol (2020) 11:1982. doi: 10.3389/fimmu.2020.01982
106. Yogev N, Bedke T, Kobayashi Y, Brockmann L, Lukas D, Regen T, et al. CD4(+) T-cell-derived IL-10 promotes CNS inflammation in mice by sustaining effector T cell survival. Cell Rep (2022) 38(13):110565. doi: 10.1016/j.celrep.2022.110565
107. Girard-Madoux MJH, Ober-Blöbaum JL, Costes LMM, Kel JM, Lindenbergh-Kortleve DJ, Brouwers-Haspels I, et al. IL-10 control of CD11c+ myeloid cells is essential to maintain immune homeostasis in the small and large intestine. Oncotarget (2016) 7(22):32015–30. doi: 10.18632/oncotarget.8337
108. Misra N, Bayry J, Lacroix-Desmazes S, Kazatchkine MD, Kaveri SV. Cutting edge: human CD4+CD25+ T cells restrain the maturation and antigen-presenting function of dendritic cells. J Immunol (2004) 172(8):4676–80. doi: 10.4049/jimmunol.172.8.4676
109. Bain CC, Montgomery J, Scott CL, Kel JM, Girard-Madoux MJH, Martens L, et al. TGFβR signalling controls CD103CD11b dendritic cell development in the intestine. Nat Commun (2017) 8(1):620.doi: 10.1038/s41467-017-00658-6
110. Chen X, Hao S, Zhao Z, Liu J, Shao Q, Wang F, et al. Interleukin 35: Inhibitory regulator in monocyte-derived dendritic cell maturation and activation. Cytokine (2018) 108:43–52. doi: 10.1016/j.cyto.2018.03.008
111. Yu L, Yang F, Jiang L, Chen Y, Wang K, Xu F, et al. Exosomes with membrane-associated TGF-beta1 from gene-modified dendritic cells inhibit murine EAE independently of MHC restriction. Eur J Immunol (2013) 43(9):2461–72. doi: 10.1002/eji.201243295
112. Ji Q, Liu J, Dong Y, Wang L, Dong K, Setiz B, et al. Exosomes derived from thymic stromal lymphopoietin-treated dendritic cells regulate T helper 17/regulatory T cell differentiation via miR-21/Smad7 axis. Exp Cell Res (2021) 398(1):112393. doi: 10.1016/j.yexcr.2020.112393
113. Pusic AD, Pusic KM, Kraig RP. What are exosomes and how can they be used in multiple sclerosis therapy? Expert Rev Neurother (2014) 14(4):353–5. doi: 10.1586/14737175.2014.890893
114. Tung SL, Boardman DA, Sen M, Letizia M, Peng Q, Cianci N, et al. Regulatory T cell-derived extracellular vesicles modify dendritic cell function. Sci Rep (2018) 8(1):6065. doi: 10.1038/s41598-018-24531-8
115. Azimi M, Ghabaee M, Moghadasi AN, Noorbakhsh F, Izad M. Immunomodulatory function of treg-derived exosomes is impaired in patients with relapsing-remitting multiple sclerosis. Immunol Res (2018) 66(4):513–20. doi: 10.1007/s12026-018-9008-5
116. Ma B, Yang JY, Song WJ, Ding R, Zhang ZC, Ji HC, et al. Combining exosomes derived from immature DCs with donor antigen-specific treg cells induces tolerance in a rat liver allograft model. Sci Rep (2016) 6:32971. doi: 10.1038/srep32971
117. Correale J. Immunosuppressive amino-acid catabolizing enzymes in multiple sclerosis. Front Immunol (2020) 11:600428. doi: 10.3389/fimmu.2020.600428
118. Grohmann U, Volpi C, Fallarino F, Bozza S, Bianchi R, Vacca C, et al. Reverse signaling through GITR ligand enables dexamethasone to activate IDO in allergy. Nat Med (2007) 13(5):579–86. doi: 10.1038/nm1563
119. Proietti E, Rossini S, Grohmann U, Mondanelli G. Polyamines and kynurenines at the intersection of immune modulation. Trends Immunol (2020) 41(11):1037–50. doi: 10.1016/j.it.2020.09.007
120. Zarzecki MS, Cattelan Souza L, Giacomeli R, Silva MRP, Prigol M, Boeira SP, et al. Involvement of indoleamine-2,3-Dioxygenase and kynurenine pathway in experimental autoimmune encephalomyelitis in mice. Neurochem Res (2020) 45(12):2959–77. doi: 10.1007/s11064-020-03144-6
121. Mancuso R, Hernis A, Agostini S, Rovaris M, Caputo D, Fuchs D, et al. Indoleamine 2,3 dioxygenase (IDO) expression and activity in relapsing-remitting multiple sclerosis. PloS One (2015) 10(6):e0130715. doi: 10.1371/journal.pone.0130715
122. Lemos H, Mohamed E, Ou R, McCardle C, Zheng X, McGuire K, et al. Co-Treatments to boost IDO activity and inhibit production of downstream catabolites induce durable suppression of experimental autoimmune encephalomyelitis. Front Immunol (2020) 11:1256. doi: 10.3389/fimmu.2020.01256
123. Nekrasova I, Shirshev S. Estriol in regulation of cell-mediated immune reactions in multiple sclerosis. J Neuroimmunol (2020) 349:577421. doi: 10.1016/j.jneuroim.2020.577421
124. Papenfuss TL, Powell ND, McClain MA, Bedarf A, Singh A, Gienapp IE, Shawler T, Whitacre CC. Estriol generates tolerogenic dendritic cells in vivo that protect against autoimmunity. J Immunol (2011) 186(6):3346–55. doi: 10.4049/jimmunol.1001322. Epub 2011 Feb 11.
125. Mezrich JD, Fechner JH, Zhang X, Johnson BP, Burlingham WJ, Bradfield CA. An interaction between kynurenine and the aryl hydrocarbon receptor can generate regulatory T cells. J Immunol (2010) 185(6):3190–8. doi: 10.4049/jimmunol.0903670
126. Belladonna ML, Grohmann U, Guidetti P, Volpi C, Bianchi R, Fioretti MC, et al. Kynurenine pathway enzymes in dendritic cells initiate tolerogenesis in the absence of functional IDO. J Immunol (2006) 177(1):130–7. doi: 10.4049/jimmunol.177.1.130
127. Cauwels A, Van Lint S, Catteeuw D, Pang S, Paul F, Rogge E, et al. Targeting interferon activity to dendritic cells enables in vivo tolerization and protection against EAE in mice. J Autoimmun (2019) 97:70–6. doi: 10.1016/j.jaut.2018.10.010
128. Thome R, Casella G, Lotfi N, Ishikawa LWL, Wang Q, Ciric B, et al. Primaquine elicits Foxp3(+) regulatory T cells with a superior ability to limit CNS autoimmune inflammation. J Autoimmun (2020) 114:102505. doi: 10.1016/j.jaut.2020.102505
129. Farias AS, Spagnol GS, Bordeaux-Rego P, Oliveira CO, Fontana AG, de Paula RF, et al. Vitamin D3 induces IDO+ tolerogenic DCs and enhances treg, reducing the severity of EAE. CNS Neurosci Ther (2013) 19(4):269–77. doi: 10.1111/cns.12071
130. Kwidzinski E, Bechmann I. IDO expression in the brain: a double-edged sword. J Mol Med (Berl) (2007) 85(12):1351–9. doi: 10.1007/s00109-007-0229-7
131. Sundaram G, Lim CK, Brew BJ, Guillemin GJ. Kynurenine pathway modulation reverses the experimental autoimmune encephalomyelitis mouse disease progression. J Neuroinflammation (2020) 17(1):176. doi: 10.1186/s12974-020-01844-y
132. Liu YJ, Soumelis V, Watanabe N, Ito T, Wang YH, Malefyt Rde W, et al. TSLP: an epithelial cell cytokine that regulates T cell differentiation by conditioning dendritic cell maturation. Annu Rev Immunol (2007) 25:193–219. doi: 10.1146/annurev.immunol.25.022106.141718
133. Haas J, Schwarz A, Korporal-Kuhnke M, Jarius S, Wildemann B. Myeloid dendritic cells exhibit defects in activation and function in patients with multiple sclerosis. J Neuroimmunol (2016) 301:53–60. doi: 10.1016/j.jneuroim.2016.10.007
134. Owen DL, Sjaastad LE, Farrar MA. Regulatory T cell development in the thymus. J Immunol (2019) 203(8):2031–41. doi: 10.4049/jimmunol.1900662
135. Mahmud SA, Manlove LS, Schmitz HM, Xing Y, Wang Y, Owen DL, et al. Costimulation via the tumor-necrosis factor receptor superfamily couples TCR signal strength to the thymic differentiation of regulatory T cells. Nat Immunol (2014) 15(5):473–81. doi: 10.1038/ni.2849
136. Chen M, Chen G, Deng S, Liu X, Hutton GJ, Hong J. IFN-beta induces the proliferation of CD4+CD25+Foxp3+ regulatory T cells through upregulation of GITRL on dendritic cells in the treatment of multiple sclerosis. J Neuroimmunol (2012) 242(1-2):39–46. doi: 10.1016/j.jneuroim.2011.10.014
137. Clement M, Fornasa G, Guedj K, Ben Mkaddem S, Gaston AT, Khallou-Laschet J, et al. CD31 is a key coinhibitory receptor in the development of immunogenic dendritic cells. Proc Natl Acad Sci U S A (2014) 111(12):E1101–10. doi: 10.1073/pnas.1314505111
138. Zhou F, Zhang GX, Rostami A. Distinct role of IL-27 in immature and LPS-induced mature dendritic cell-mediated development of CD4(+) CD127(+)3G11(+) regulatory T cell subset. Front Immunol (2018) 9:2562. doi: 10.3389/fimmu.2018.02562
139. Tang SC, Fan XH, Pan QM, Sun QS, Liu Y. Decreased expression of IL-27 and its correlation with Th1 and Th17 cells in progressive multiple sclerosis. J Neurol Sci (2015) 348(1-2):174–80. doi: 10.1016/j.jns.2014.11.035
140. Li J, Gran B, Zhang GX, Rostami A, Kamoun M. IL-27 subunits and its receptor (WSX-1) mRNAs are markedly up-regulated in inflammatory cells in the CNS during experimental autoimmune encephalomyelitis. J Neurol Sci (2005) 232(1-2):3–9. doi: 10.1016/j.jns.2004.12.013
141. Mascanfroni ID, Yeste A, Vieira SM, Burns EJ, Patel B, Sloma I, et al. IL-27 acts on DCs to suppress the T cell response and autoimmunity by inducing expression of the immunoregulatory molecule CD39. Nat Immunol (2013) 14(10):1054–63. doi: 10.1038/ni.2695
142. Do J, Kim D, Kim S, Valentin-Torres A, Dvorina N, Jang E, et al. Treg-specific IL-27Ralpha deletion uncovers a key role for IL-27 in treg function to control autoimmunity. Proc Natl Acad Sci U S A (2017) 114(38):10190–5. doi: 10.1073/pnas.1703100114
143. Quintana FJ, Yeste A, Mascanfroni ID. Role and therapeutic value of dendritic cells in central nervous system autoimmunity. Cell Death Differ (2015) 22(2):215–24. doi: 10.1038/cdd.2014.125
144. Casella G, Rasouli J, Thome R, Descamps HC, Vattikonda A, Ishikawa L, et al. Interferon-γ/Interleukin-27 axis induces programmed death ligand 1 expression in monocyte-derived dendritic cells and restores immune tolerance in central nervous system autoimmunity. Front Immunol (2020) 11:576752. doi: 10.3389/fimmu.2020.576752
145. Huber M, Steinwald V, Guralnik A, Brustle A, Kleemann P, Rosenplanter C, et al. IL-27 inhibits the development of regulatory T cells via STAT3. Int Immunol (2008) 20(2):223–34. doi: 10.1093/intimm/dxm139
146. Zhu J, Liu J-Q, Liu Z, Wu L, Shi M, Zhang J, et al. Interleukin-27 gene therapy prevents the development of autoimmune encephalomyelitis but fails to attenuate established inflammation due to the expansion of CD11bGr-1 myeloid cells. Front Immunol (2018) 9:873. doi: 10.3389/fimmu.2018.00873
147. Raverdeau M, Christofi M, Malara A, Wilk MM, Misiak A, Kuffova L, et al. Retinoic acid-induced autoantigen-specific type 1 regulatory T cells suppress autoimmunity. EMBO Rep (2019) 20(5):e47121. doi: 10.15252/embr.201847121
148. Ter Braake D, Benne N, Lau CYJ, Mastrobattista E, Broere F. Retinoic acid-containing liposomes for the induction of antigen-specific regulatory T cells as a treatment for autoimmune diseases. Pharmaceutics (2021) 13(11):1949. doi: 10.3390/pharmaceutics13111949
149. Zhan XX, Liu Y, Yang JF, Wang GY, Mu L, Zhang TS, et al. All-trans-retinoic acid ameliorates experimental allergic encephalomyelitis by affecting dendritic cell and monocyte development. Immunology (2013) 138(4):333–45. doi: 10.1111/imm.12040
150. Correale J, Farez MF. Parasite infections in multiple sclerosis modulate immune responses through a retinoic acid-dependent pathway. J Immunol (2013) 191(7):3827–37. doi: 10.4049/jimmunol.1301110
151. Mari ER, Rasouli J, Ciric B, Moore JN, Conejo-Garcia JR, Rajasagi N, et al. Galectin-1 is essential for the induction of MOG35-55 -based intravenous tolerance in experimental autoimmune encephalomyelitis. Eur J Immunol (2016) 46(7):1783–96. doi: 10.1002/eji.201546212
152. Pardo E, Carcamo C, Uribe-San Martin R, Ciampi E, Segovia-Miranda F, Curkovic-Pena C, et al. Galectin-8 as an immunosuppressor in experimental autoimmune encephalomyelitis and a target of human early prognostic antibodies in multiple sclerosis. PloS One (2017) 12(6):e0177472. doi: 10.1371/journal.pone.0177472
153. Waschbisch A, Sanderson N, Krumbholz M, Vlad G, Theil D, Schwab S, et al. Interferon beta and vitamin d synergize to induce immunoregulatory receptors on peripheral blood monocytes of multiple sclerosis patients. PloS One (2014) 9(12):e115488. doi: 10.1371/journal.pone.0115488
154. Abe K, Nguyen KP, Fine SD, Mo J-H, Shen C, Shenouda S, et al. Conventional dendritic cells regulate the outcome of colonic inflammation independently of T cells. Proc Natl Acad Sci USA (2007) 104(43):17022–7. doi: 10.1073/pnas.0708469104
155. Zinser E, Naumann R, Wild AB, Michalski J, Deinzer A, Stich L, et al. Endogenous expression of the human CD83 attenuates EAE symptoms in humanized transgenic mice and increases the activity of regulatory T cells. Front Immunol (2019) 10:1442. doi: 10.3389/fimmu.2019.01442
156. Agundez JA, Garcia-Martin E, Martinez C, Benito-Leon J, Millan-Pascual J, Diaz-Sanchez M, et al. Heme oxygenase-1 and 2 common genetic variants and risk for multiple sclerosis. Sci Rep (2016) 6:20830. doi: 10.1038/srep20830
157. Fagone P, Patti F, Mangano K, Mammana S, Coco M, Touil-Boukoffa C, et al. Heme oxygenase-1 expression in peripheral blood mononuclear cells correlates with disease activity in multiple sclerosis. J Neuroimmunol (2013) 261(1-2):82–6. doi: 10.1016/j.jneuroim.2013.04.013
158. Chora AA, Fontoura P, Cunha A, Pais TF, Cardoso S, Ho PP, et al. Heme oxygenase-1 and carbon monoxide suppress autoimmune neuroinflammation. J Clin Invest (2007) 117(2):438–47. doi: 10.1172/JCI28844
159. Wong TH, Chen HA, Gau RJ, Yen JH, Suen JL. Heme oxygenase-1-Expressing dendritic cells promote Foxp3+ regulatory T cell differentiation and induce less severe airway inflammation in murine models. PloS One (2016) 11(12):e0168919. doi: 10.1371/journal.pone.0168919
160. Idoyaga J, Fiorese C, Zbytnuik L, Lubkin A, Miller J, Malissen B, et al. Specialized role of migratory dendritic cells in peripheral tolerance induction. J Clin Invest (2013) 123(2):844–54. doi: 10.1172/JCI65260
161. Jones A, Bourque J, Kuehm L, Opejin A, Teague RM, Gross C, et al. Immunomodulatory functions of BTLA and HVEM govern induction of extrathymic regulatory T cells and tolerance by dendritic cells. Immunity (2016) 45(5):1066–77. doi: 10.1016/j.immuni.2016.10.008
162. Gregori S, Tomasoni D, Pacciani V, Scirpoli M, Battaglia M, Magnani CF, et al. Differentiation of type 1 T regulatory cells (Tr1) by tolerogenic DC-10 requires the IL-10-dependent ILT4/HLA-G pathway. Blood (2010) 116(6):935–44. doi: 10.1182/blood-2009-07-234872
163. Comi M, Amodio G, Gregori S. Interleukin-10-Producing DC-10 is a unique tool to promote tolerance antigen-specific T regulatory type 1 cells. Front In Immunol (2018) 9:682. doi: 10.3389/fimmu.2018.00682
164. Jiang A, Bloom O, Ono S, Cui W, Unternaehrer J, Jiang S, et al. Disruption of e-Cadherin-Mediated adhesion induces a functionally distinct pathway of dendritic cell maturation. Immunity (2007) 27(4):610–24. doi: 10.1016/j.immuni.2007.08.015
165. Mellman I, Clausen BE. Immunology. beta-catenin balances immunity. Science (2010) 329(5993):767–9. doi: 10.1126/science.1194185
166. Willekens B, Presas-Rodríguez S, Mansilla MJ, Derdelinckx J, Lee W-P, Nijs G, et al. Tolerogenic dendritic cell-based treatment for multiple sclerosis (MS): a harmonised study protocol for two phase I clinical trials comparing intradermal and intranodal cell administration. BMJ Open (2019) 9(9):e030309. doi: 10.1136/bmjopen-2019-030309
167. Tabansky I, Keskin DB, Watts D, Petzold C, Funaro M, Sands W, et al. Targeting DEC-205(-)DCIR2(+) dendritic cells promotes immunological tolerance in proteolipid protein-induced experimental autoimmune encephalomyelitis. Mol Med (2018) 24(1):17. doi: 10.1186/s10020-018-0017-6
168. Dudziak D, Kamphorst AO, Heidkamp GF, Buchholz VR, Trumpfheller C, Yamazaki S, et al. Differential antigen processing by dendritic cell subsets in vivo. Science (2007) 315(5808):107–11. doi: 10.1126/science.1136080
169. Stern JNH, Keskin DB, Kato Z, Waldner H, Schallenberg S, Anderson A, et al. Promoting tolerance to proteolipid protein-induced experimental autoimmune encephalomyelitis through targeting dendritic cells. Proc Natl Acad Sci USA (2010) 107(40):17280–5. doi: 10.1073/pnas.1010263107
170. Zhou Y, Leng X, Luo S, Su Z, Luo X, Guo H, et al. Tolerogenic dendritic cells generated with tofacitinib ameliorate experimental autoimmune encephalomyelitis through modulation of Th17/Treg balance. J Immunol Res (2016) 2016:5021537. doi: 10.1155/2016/5021537
Keywords: dendritic cell, regulatory T cell (Treg), immune tolerance, multiple sclerosis, experimental autoimmune encephalomyelitis
Citation: Li R, Li H, Yang X, Hu H, Liu P and Liu H (2022) Crosstalk between dendritic cells and regulatory T cells: Protective effect and therapeutic potential in multiple sclerosis. Front. Immunol. 13:970508. doi: 10.3389/fimmu.2022.970508
Received: 16 June 2022; Accepted: 01 August 2022;
Published: 13 September 2022.
Edited by:
Lucienne Chatenoud, Université Paris Descartes, FranceReviewed by:
Aggelos Banos, Biomedical Research Foundation of the Academy of Athens (BRFAA), GreeceCopyright © 2022 Li, Li, Yang, Hu, Liu and Liu. This is an open-access article distributed under the terms of the Creative Commons Attribution License (CC BY). The use, distribution or reproduction in other forums is permitted, provided the original author(s) and the copyright owner(s) are credited and that the original publication in this journal is cited, in accordance with accepted academic practice. No use, distribution or reproduction is permitted which does not comply with these terms.
*Correspondence: Hongbo Liu, bGl1aG9uZ2JvNjI3OUAxMjYuY29t
Disclaimer: All claims expressed in this article are solely those of the authors and do not necessarily represent those of their affiliated organizations, or those of the publisher, the editors and the reviewers. Any product that may be evaluated in this article or claim that may be made by its manufacturer is not guaranteed or endorsed by the publisher.
Research integrity at Frontiers
Learn more about the work of our research integrity team to safeguard the quality of each article we publish.