- 1Department of Sports Medicine, The Affiliated Hospital of Qingdao University, Qingdao University, Qingdao, China
- 2Medical Department of Qingdao University, Qingdao, China
- 3Key Laboratory of Biomechanics of Hebei Province, Department of Trauma Emergency Center, The Third Hospital of Hebei Medical University, Orthopaedics Research Institution of Hebei Province, Shijiazhuang, China
- 4State Key Laboratory of Bio-Fibers and Eco-Textiles, Collaborative Innovation Center for Marine Biomass Fibers, Materials and Textiles of Shandong Province, College of Materials Science and Engineering, Institute of Marine Biobased Materials, Qingdao University, Qingdao, China
Microglia are important resident immune cells in the central nervous system (CNS) and play an important role in its development, homeostasis, and disease treatments. Activated microglia perform diverse functions in mouse models of CNS neurodegenerative diseases or deficits. In humans, microglia have been linked to various neurodegenerative diseases. Following brain or spinal cord injury, microglia express pro- and anti-inflammatory phenotypes at different stages of recovery. With the development of pharmacological and genetic tools for microglial depletion, studies have demonstrated that microglial depletion exerts both positive and negative effects in the treatment of CNS diseases. Notably, microglial depletion provides an empty niche that stimulates production of new microglia. Microglial depletion and repopulation can not only treat diseases by eliminating dysfunctional microglia but can also provide an indication of the molecular mechanisms of diseases. Although this approach has shown impressive results, its use is still in its infancy. In this review, we summarize the current pharmacological and genetic tools for microglial depletion and highlight recent advances in microglial repopulation therapy for the treatment and functional recovery of neurological diseases and deficits. Finally, we briefly discuss the therapeutic challenges and prospective uses of microglial repopulation therapy.
Introduction
Microglia are resident macrophages in the central nervous system (CNS), which are derived from the yolk sac progenitor cells and migrate to the CNS between embryonic day 8.5 and time of formation of the blood-brain barrier (BBB) (1, 2). Microglia play different roles in the CNS according to the development and maturation stage of the CNS. For example, synaptic pruning of the microglia plays an important role in the formation of neural circuits during CNS development (3) and removal of dead cells and myelin debris in pathological conditions. Microglia also drive neuronal programmed cell death by inducing neuronal apoptosis, thereby eliminating excess neurons produced during development (4). In addition, microglial protrusions monitor the surrounding environment and directly communicate with neurons, astrocytes, and blood vessels (5). Based on the surveillance of surrounding information, microglia can transduce a variety of extracellular signals to maintain a stable environment in the brain (3). Microglia rapidly react to changes in the CNS microenvironment (e.g., infection, trauma, and disease) by changing from the resting state to the active state, to remove tissue debris and restore homeostasis (6, 7).
Initially, activated microglia are divided into M1- and M2-like phenotypes (8). M1 microglia play a key role in the host immune response by producing pro-inflammatory cytokines (e.g., tumor necrosis factor alpha [TNF-α] and interleukin [IL]-6), inducible nitric oxide synthase (iNOS), and reactive oxygen species (ROS) (9, 10). M2 microglia produce anti-inflammatory cytokines, chemokines, and growth factors, which inhibit the inflammatory response and promote tissue repair (11, 12). However, it is still controversial that M1 and M2 phenotypes exist in vivo (13). With the development of technologies such as single-cell RNA sequencing (scRNA-seq), scholars have gradually realized that microglia are highly heterogeneous (14), “M1” and “M2” may not reflect microglial states precisely. Several studies have demonstrated that microglial hyperactivation and dysregulation lead to neurotoxicity, which are associated with neurodegenerative diseases, including epilepsy (15), Alzheimer’s disease (AD) (16), Parkinson’s disease (PD) (17), and Huntington’s disease (18). The microglial response to injury is partly regulated by interactions with other glial cells (19). For example, in spinal cord injury (SCI) models, activated microglia and reactive astrocytes interact to form glial scars, which impact neuronal and functional recovery (20). Meanwhile, TNF-α expression by activated microglia contributes to astrocyte glutamate production and leads to neuronal excitotoxicity (21). In the meantime, TNF-α induces activated microglia to release glutamate and produce neurotoxicity through autocrine manner (22). In addition, the products released by activated microglia, including glutamate, nitric oxide (NO), IL-1b, and TNF-a, promote oligodendrocyte death (23, 24). Microglia-mediated inflammation is a significant contributor to the microenvironment after CNS injury and diseases, which persists for a long duration and affects CNS repair (25). Therefore, depleting the hyperactivated microglia may be an effective approach to treat CNS diseases and injury.
Microglial depletion from the CNS microenvironment has been extensively studied as a treatment for CNS diseases, and has dual effects on CNS diseases and deficits. In recent years, microglial depletion and repopulation have received significant attention. In the present review, we describe approaches for microglial depletion and the source and dynamics of microglial repopulation based on recent studies. In addition, we summarize current research on microglial repopulation for the treatment of CNS diseases or deficits, and briefly discusses the challenges and potential uses of microglial repopulation therapy.
Microglial depletion for the treatment of CNS injuries and diseases
Approaches for microglial depletion
To investigate the role of microglial depletion in the treatment of CNS diseases, various depletion methods have been developed, including clodronate liposomes, genetic models, and colony-stimulating factor 1 receptor (CSF1R) inhibitors. (Figure 1).
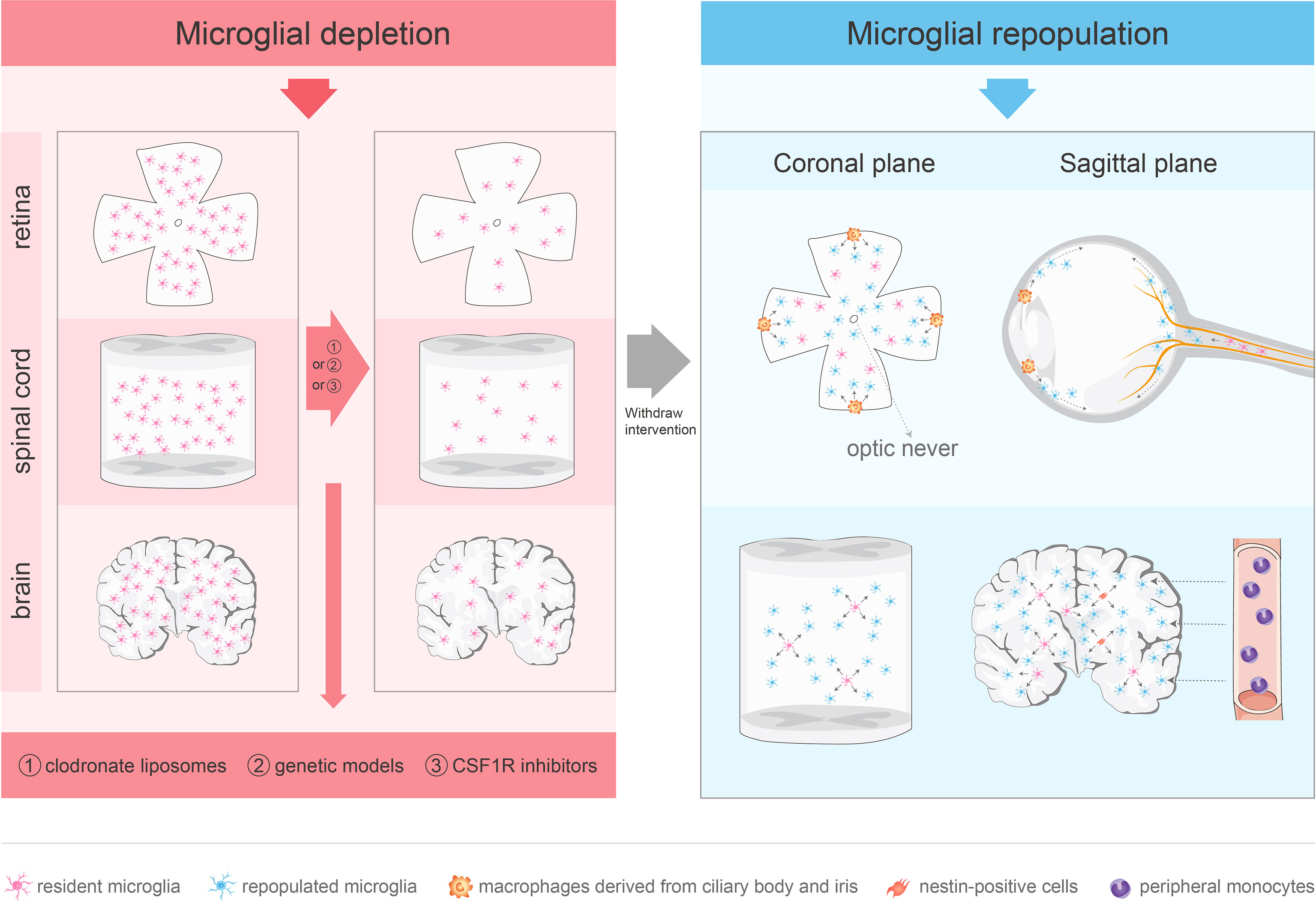
Figure 1 Approaches for microglial depletion and multiple sources of microglial repopulation. Robust methods for depleting microglia in vivo include clodronate liposomes, genetic models and CSF1R inhibitors. These methods deplete microglia in the CNS effectively. After withdraw the intervention, microglia repopulate and return to normal levels in 1–2 weeks. The repopulated microglia in the retina are not derived from nestin-positive progenitor cells. The repopulated microglia in the center arise from the residual microglia in the optic nerve, while the periphery-emerging microglia are from macrophages in the ciliary body/iris. Spinal microglia can reproduce rapidly after removal, which is mainly driven by the process of self-renewal. Microglia in the brain may repopulate in one of three ways: stimulation of microglia progenitor cells that express nestin, proliferation of residual microglia, or infiltration of peripheral mononuclear cells.
Clodronate liposomes cannot cross the BBB or blood-retina barrier (BRB) and need to be injected directly into the CNS or vitreous to target macrophages and trigger their apoptosis without genetic modification (26–28). This approach is effective with rapid but short-lived effects. A recent study reported that clodronate liposomes cause microglial ablation in the mouse striatum at day 1, which persists for 3 days, and is followed by microglial reappearance after 5 days (29). However, this approach may damage the CNS immune privilege (IP) and lead to off-target effects (30).
Herpes simplex virus-derived thymidine kinase (HSVTK), a suicide gene, can convert the antiviral nucleotide analog prodrug, ganciclovir (GCV), into a monophosphate form, which is converted to a toxic triphosphate (30, 31). CD11b-HSVTK transgenic mice overexpress HSVTK driven by the Cd11b promoter. HSVTK can induce apoptosis in CD11b+ myeloid cells in the presence of GCV (32). After 2 weeks of GCV treatment, the absolute microglial number was reduced by 90%; a higher level of microglial depletion (> 95%) was achieved after 4 weeks of GCV treatment (33, 34). However, long-term GCV administration may lead to myelotoxicity because of the elimination of CD11+ cells and red blood cells (30, 35). Another novel lineage ablation system is based on the transgenic expression of diphtheria toxin receptor (DTR) in mouse cells and the application of diphtheria toxin (DT) (36). By crossing the inducible DTR (iDTR) strain with tissue-specific Cre-expressing mouse strain, the STOP cassette that prevents DTR expression was removed, which would lead to cell death of DTR-expressing myeloid cells in response to DT injection (36). Cx3cr1CreER:iDTR system ablates microglia following injections of tamoxifen (TAM) and DT (37). This system was associated with 80% depletion in the number of microglia 3 days after DT injection, which was followed by normalization of the microglia number at day 14 (37). However, this model also produced a strong neuroinflammatory response, termed a cytokine storm (37). Iba1-tTA::DTAtetO/tetO mice also use the DT depletion system. DTA was selectively overexpressed in Iba1+ microglia after withdrawal of doxycycline (DOX), which led to a reduction in the number of retinal microglia by about 90% (38). The new microglia Cre lines (e.g., TMEM119) may be used to rapidly identify microglia (39). Similarly, the DT receptor gene expressed in the Cx3cr1 promoter of the Cx3cr1-Dtr transgenic rat model was associated with acute microglial ablation after DT application (40). An advantage of the TAM-induced Cre system is its selective targeting of microglia, but not of the circulating monocytes or other short-lived myeloid cells (37). The administration of TAM to Cx3r1CreER/+R26DTA/+ mice resulted in loss of approximately 95% of microglia on day 7 (41). In addition, Cre expression was lost over time in short-lived CX3CR1+ myeloid cells compared to the long-lived and self-renewing microglia (42, 43). However, most of genetic tools for microglia depletion target all macrophages and cannot accurately differentiate between microglia and CNS border associated macrophages (CAMs or BAMs), so CAMs or BAMs would also be affected (44). the valuable new genetic systems (e.g., Sall1, Siglec-H, and Hexb) can deplete microglia more accurately (45–47). In another Cx3cr1CreERT2/+-Csf1r+/fl system, the deletion of the Csf1r allele disrupts microglial homeostasis (48).
CSF1R is a critical regulator of microglial development and survival (49–53), and is expressed in microglia (26–28), neural progenitor cells (54), and several neuronal subpopulations in the CNS (54). In rodents, CSF1R inhibition causes a significant reduction in resident microglia. PLX3397 and PLX5622 are the most commonly used CSF1R inhibitors for microglia depletion, these CSF1R inhibitors can cross the BBB and deplete the microglia in the brain, spinal cord, and retina within a few days (49, 55, 56). The limitations of PLX3397 mainly include relatively low penetrance and potential off target effect (49, 57), while PLX5622 has higher CSF1R specificity and brain penetrance (58). However, Hohsfield et al. found high dose PLX3397 has higher microglial depletion efficiency (59). The microglial depletion is caused by microglia death rather than downregulation of microglia markers (58). Currently, the CSF1R inhibitors are widely used in mouse models of various CNS diseases or injuries (55, 58, 60–64). In general, most microglia were depleted 1–2 weeks after CSF1R inhibitors application, and microglia returned to the baseline level about 2 weeks after discontinuation of the CSF1R inhibitors. Notably, CSF1R inhibitors lead to continuous microglial depletion. However, the inhibitors are not specific for CSF1R and also inhibit other kinases (65, 66). In addition, treatment with CSF1R inhibitors may lead to broad myelosuppression (67), and microglial progenitors in bone marrow and tissue macrophages may be similarly affected (68, 69).
Effects of microglial depletion on CNS injuries and diseases
Microglial depletion may have dual effects on CNS degenerative and traumatic diseases. For example, in AD mouse models, microglial inhibition prevents motor neuron degeneration and improves the function and cognition in mice (55, 70). In PD mouse models, CSF1R inhibition can attenuate cognitive deficits, neuronal damage, astroglial activation, proinflammatory factor production, and oxidative stress (71). Microglial inhibition enhances central remyelination and prevents demyelination in multiple sclerosis mice by modulating neuroinflammation (72). Transient microglial inhibition after SCI and traumatic brain injury (TBI) promotes motor and neurological recovery, improves depression-like behavior, prevents tissue damage, and downregulates the levels of proliferation-associated transcripts and inflammation-associated genes (73–77). CSF1R inhibitors plays a neuroprotective role in cerebral ischemic stroke mice by inhibiting microglial polarization and inflammatory pathway activation (78). In addition, microglial inhibiting reduces age-associated neuroinflammation in aging mouse brains (79). However, microglial inhibition may be detrimental to disease recovery in some cases. In animal models of CNS degenerative diseases, microglial depletion exacerbates the severity of KA-induced acute/chronic seizures (80), significantly increases inflammation, demyelination, and axonal degeneration in mice with experimental autoimmune encephalomyelitis (EAE) (81), and leads to increased plaque size over 1 week in AD mice (82). In contrast to the short-term microglial inhibition after SCI, prolonged microglial inhibition do not improve motor recovery (83), and even aggravates damage, reduces neuronal numbers, exacerbates axonal dieback, and hinders motor recovery (60). Moreover, microglial depletion reduces the clearance of degenerating neurons after TBI in pediatric rats (84) and leads to a significant increase in infarct size in post-stroke brain injury (64). Microglial depletion also affects retinal regeneration in zebrafish following retinal injury (85). Therefore, the outcomes of microglial depletion in the treatment of CNS diseases or deficits remains controversial, and the duration of depletion may influence the treatment results.
Source and dynamics of repopulating microglia
Multiple sources of microglial repopulation
In the normal adult mouse and human brain, microglia have long lives at the population level (86) and, at the individual cell level, they maintain dynamic stability by temporally and spatially coupling proliferation and apoptosis (87). After removal of the depleting tools, microglia may repopulate in one of three ways to return rapidly to the normal levels within 1–2 weeks: proliferation of residual microglia (87), stimulation of microglial progenitor cells in the adult brain (49), and infiltration by peripheral mononuclear cells (88).
PLX3397 treatment of CX3CR1-GFP+/− mouse model for 7 days demonstrated that the repopulated microglia were strongly nestin+, and Western blotting of whole brain homogenates showed a significant increase in nestin levels at day 3 of recovery. The repopulated microglia expressed nestin immunoreactivity and rapidly differentiated into branched microglia within 7–14 days. Importantly, the repopulated brain microglia induce proliferation of nestin-expressing cells throughout the CNS (49). Thus, Elmore et al. (49) suggested that the repopulating microglia are mainly derived from brain microglial progenitor cells that express nestin. However, a study based on fate mapping found that all the regenerated microglia originated from residual microglia, rather than nestin+ cells (89). Microglia transiently express nestin during regeneration and development, which may be a feature of microglial proliferation. Microglial regeneration demonstrates rapid proliferation kinetics and, after removal of CSF1R inhibition, the residual microglia proliferate rapidly and supplement the whole brain (56). Under physiological circumstances, microglia are separated from the peripheral circulatory system through the BBB and maintain their number through local proliferation without supplementation by blood monocytes (87, 90). Based on the macrophage niche theory (91), administration of selective CSF1R inhibitors may consume most microglia to deplete the microglial niche, which may trigger microglial reproliferation to return the microglial number to the baseline level (49, 92). Blood-derived monocytes have the potential to occupy the microglial niche in the adult CNS and repopulate the brain within 2 weeks. The newly engrafted myeloid cells have analogous functions to microglia (37).
Similar to brain microglia, spinal microglia can reproduce rapidly after being removed, which is mainly driven by the process of self-renewal. Although circulating monocyte infiltration was observed, the infiltration was part of the acute inflammatory response caused by cell death, which depends on MCP-1/CCR2 signal transduction (93). One of the main strategies of spinal cord microglial regeneration is similar to that for brain microglia, which proliferate by triggering the division of remaining cells (37, 56, 94), known as compensatory proliferation. Another strategy of spinal cord microglial regeneration is through compensatory cell hypertrophy (CCH), which is regulated by the insulin/insulin-like growth factor (IGF) signaling pathway (95). Notably, during spinal microglial regeneration, CCH occurs before cell proliferation (93).
Although blood cells in the diseased retina can differentiate into microglia (96, 97), the repopulated microglia in the center are only derived from the residual microglia in the optic nerve, while the peripheral microglia are produced by the macrophages in the ciliary body/iris. Microglia remaining in the optic nerve migrate to the retina along the center-to-periphery axis to repopulate the retina. Macrophages in the ciliary body relocate to the peripheral retina and migrate toward the central retina, whereas macrophages in the iris migrate to the peripheral retina through the ciliary body (56). However, the physiological and pathological roles of microglial radial migration are unknown. A previous study provided novel evidence that CX3CL1-CX3CR1 signaling regulates microglial regeneration kinetics in the retina by enhancing the proliferation and morphological maturation of regenerated cells (98). In addition, although the loss of P2Y12 affected microglial branching (99), P2Y12 receptor did not regulate the maturation of the newly produced microglia or affect doublet formation and repopulation. P2Y12 deletion impacted microglial morphology during repopulation but played a minor role in microglial division (99).(Figure 1)
Mechanism of microglial regeneration
Microglia restore homeostasis during repopulation through self-renewal, proximity clonal expansion, and activation of maturation programs (100). As described previously, microglia can be regenerated almost entirely by self-renewal (37, 56); the microglial transformation of nestin+ progenitor cells were not detected under steady-state conditions (87). Similar to the clonal expansion of microglia in response to facial nerve injury (101), microglia proliferate through clonal expansion after ablation. Newborn microglia recolonize the parenchyma from the proximal clonal expansion to form unique spatial clusters and maintain a stable territorial boundary over time. Once microglial colonies are formed, they remain stable in the CNS (100). Recent studies have shown that inflammatory signals are vital for the regulation of microglial maturation and function (102, 103). Newborn microglia undergo a series of transcriptional changes and eventually transform from an immature state to a stable mature phenotype. The maturation process involves several steps, including the activation of nuclear factor kappa-light-chain-enhancer of activated B cells (NF-κB). NF-κB signaling enhances the early stages of microglia reproliferation (100). In addition, TGF-β signaling is important for microglial development and homeostasis (104) The microglial density in the brain is extremely stable (87), and microglia can return to the original homeostatic density through steady turnover even after acute ablation. Contact inhibition is one of the methods by which the microglia memorize their homeostatic density (100) Residual microglia repopulate freely by losing contact inhibition. For example, Syndecan4, a regulator of contact inhibition (105), is expressed at low levels 1 month after microglial repopulation (98). Moreover, in the CD11b-HSVTK transgenic mice model, blood-derived monocytes infiltrate and engraft in the brain of microglia-depleted mice, and maintain the myeloid component in the mature brain through homeostasis (88). A recently published paper of CNS myeloid cells under PLX3397 inhibition found that the MAC2+ microglial subpopulation in residual microglia is similar to microglial progenitors during development and could promote repopulation of microglia in the brain (106). In addition, multiple microglia subtypes, including white matter-associated microglia (WAM), have been identified by scRNA-seq (107). The recent study revealed a subpopulation of myeloid cells which derive from the subventricular zone and white matter, the population reconstitute microglia in the brain through a dynamic wave and exhibit similar the transcriptional profiles similar to disease-associated microglia (DAM) (59).
Microglial repopulation therapy: A new treatment option for neurological diseases and deficits
After pharmacological or genetically targeted depletion in healthy adult mice, microglia can repopulate in a short period of time by self-renewal (29, 49, 88) to restore to the baseline level and re-establish typical spine density (49, 100, 108). In addition, inflammation-related genes of repopulated microglia remained stable during the repopulation processes, without significant up- or down-regulation (56). Compared to the resident microglia, repopulated microglia exhibit distinct morphological features, with larger cell bodies and less complex branches formed in response to inhibitor removal; the morphological characteristics and synaptic function of the repopulated microglia gradually return to those of typical microglia after 4 weeks (50). Although the repopulated microglia are numerically and morphologically different from the resident microglia, they have similar mRNA levels, perform similar functions, do not affect behavior, cognition, or motor function, and do not have any side effects (50, 56, 88). Furthermore, microglial elimination and repopulation at a young age may have a more significant impact on behavior and cognition than in adults (50) Therefore, microglial repopulation therapy may be a potentially effective treatment for neurological diseases and deficits (Figure 2, Table 1).
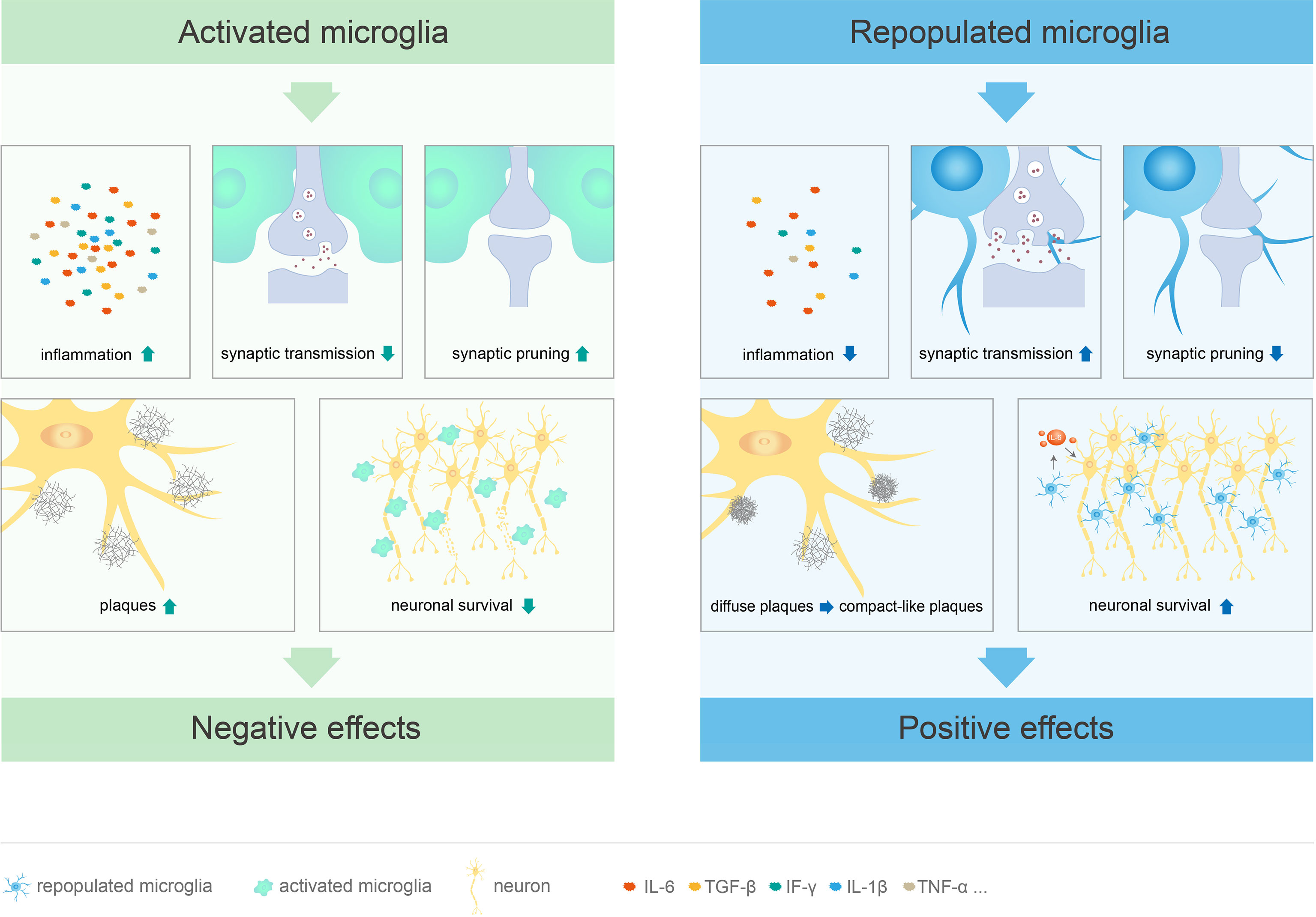
Figure 2 Different functions of activated and repopulated microglia in CNS diseases. Microglial activation leads to cognitive deficits through excessive synaptic pruning and inhibition of synaptic transmission. At the same time, these cells promote the release of inflammatory factors, stimulate plaque formation, and directly damage neurons, which leads to CNS diseases. Repopulated microglia not only suppress the inflammatory response, but also limit neuritic dystrophy by promoting the transition from diffuse to compact-like plaques. These cells can restore cognitive function by promoting synaptic transmission and inhibiting excessive synaptic pruning. Furthermore, repopulated microglia mediate neuroprotective effects by inducing IL-6 within neurons.
Microglial repopulation therapy for neurodegenerative diseases
Regenerated microglia are beneficial for CNS neurodegenerative diseases, including AD and PD. AD is characterized by extracellular amyloid-β (Aβ) plaques and neurofibrillary tangles (NFT) containing hyperphosphorylated tau protein (109). In a microglia-depleted AD model, diffuse-like plaques increased, and compact-like plaques decreased, leading to enhanced neuritic dystrophy (110). However, repopulated microglia reverse this phenomenon and promote the transition from diffuse- to compact-like plaques, thereby causing compact-like plaques to dominate the microglia-repopulated regions, consequently limiting neuritic dystrophy (110). Of note, cortical microglia did not fully repopulate, possibly because of the marked heterogeneity in the microglial gene expression profile (111, 112). In addition, another study showed that microglial repopulation can limit the growth of amyloid plaques and significantly reduce the dendritic abnormalities caused by Aβ deposition (82). PD is characterized by loss of dopaminergic neurons and accumulation of intraneural Lewy bodies (113). The role of repopulated microglia in PD has recently attracted attention. In a mouse model with completely repopulated microglia followed by PLX3397 removal and 1-methyl-4-phenyl-1,2,3,6-tetrahydropyridine (MPTP) challenge, the repopulated microglia protect dopaminergic neurons and improve motility. However, among PLX3397-fed mice with drug cessation at the time of MPTP challenge, the loss of dopaminergic neurons was not significantly different between the repopulated microglia group and control group, indicating that the effects of repopulated microglia depend on the time point of PLX3397 cessation. Interestingly, in both models, the expression of neurotrophic factors and phagocytosis-related molecules was increased in the repopulated microglia group, but the expression of inflammation-related molecules was not affected (114). In brief, microglial replenishment is neuroprotective in PD mice and the currently available sparse information suggests potential use of repopulated microglia for PD treatment.
However, some studies have failed to show the positive effects of repopulated microglia. For example, Gratuze et al. (115) showed that although the repopulated microglia cluster around the plaque, similar to normal microglia, repopulated microglia significantly increased the homeostatic gene expression and failed to switch to the DAM phenotype, resulting in a marked increase in the seeding and spread of NP-tau. Thus, the repopulated microglia may not effectively reduce plaque-related toxicity.
Microglial repopulation therapy for CNS trauma
Microglial activation is an essential innate immune response that protects the brain after trauma; however, a dysregulated immune response can lead to secondary damage (116). With the use of CSF1R inhibitors to treat brain trauma, repopulated microglia appear 3 days after drug withdrawal and, by 7 days, the number of microglia is higher than that in control mice. By 21 days, the number and morphology of the repopulated microglia were similar to the control group, albeit with smaller somas and longer and finer processes. Brain trauma leads to elevated levels of inflammatory factors. The repopulated microglia suppress lesion-induced inflammation, but do not possess the activation state of the original microglia, thereby making them less reactive. These results suggest that chronic inflammation is inherent with the microglia and not completely dependent on the environment (92). Therefore, the repopulated microglia can replace the activated microglia to prevent microglia-mediated chronic inflammation, which is an effective strategy to repair CNS trauma. Rice et al. (92) treated brain-injured mice with PLX3397 for 14 days followed by PLX3397 withdrawal, and found that the microglia repopulated the whole brain; the newly produced repopulated microglia had a naïve state morphology. Importantly, protracted inflammation was resolved by the microglial depletion and repopulation treatment. Ma et al. (25) also found that microglial depletion and repopulation in combination with gelatin hydrogel transplantation resolved acute and chronic inflammation, promoted endogenous neural stem/progenitor cell migration and neurogenesis, and improved the electrophysiological and functional recovery in mice with complete transection SCI. Notably, the repopulated microglia had no significant effect on trauma-induced astrocyte expression and reactivity (74, 92). Microglial depletion and repopulation also prevent radiation-induced hippocampal-dependent memory defects and loss of hippocampal PSD-95 (117). The increase in PSD-95 and synaptophysin puncta suggest that the repopulated microglia determine and regulate the synaptic landscape (92, 118). In a mouse model of brain injury, repopulated microglia attenuate learning deficits, stimulate neurogenesis, induce IL-6 production from neurons, and mediate neuroprotection. These neuroprotective and pro-regenerative microglia have a unique transcriptional profile and modulate the microenvironment, in particular, suppressing neurotoxic A1 astrocyte formation (118). In addition, the reactivity of repopulated microglia is reduced, the proliferative state is enhanced, and the gene expression levels of wound healing and repair are increased. Remarkably, the neuroprotective effect of repopulated microglia depends on the appropriate timing of treatment, and these cells are effective for the acute rather than post-acute phase of injury (118).
Microglial repopulation therapy for aging brain
Compared to the adult brain, microglia in the aging brain are dysfunctional, with increased numbers, became “dystrophy”, decreased motility, altered signaling, impaired phagocytosis and proteostasis, and a greater pro-inflammatory profile (119, 120). Aging is characterized by increased expression of CD68+ and deposition of lipofuscin (121). With microglial regeneration, the expression of CD68+ in aged microglia is normalized to the adult level and the lipofuscin level is reduced (122). In addition, the densities and morphologies of repopulated microglia are restored to the adult levels, and these cells improve cognition in aged mice, increase the neuronal regeneration rates, alter the dendritic spine densities and neuronal complexities, and restore the neuronal physiological processes in the aging brain (123). However, a recent study reported that microglial repopulation does not improve synaptic transmission or cognitive function in aged animals (124). Furthermore, the repopulated microglia only partially affect the age-related mRNA signaling and do not sufficiently modify the neuroinflammatory immune responses, suggesting that responses to the inflammatory stimuli depend on the aging microenvironment rather than microglial state (122). Taken together, the aforementioned findings suggest that microglial replacement may be potentially useful to normalize changes in the aging brain. However, additional methods to treat neuroinflammation in the aging brain need to be explored.
Microglial repopulation therapy for short-term memory and cognitive deficits
Microglia play integral roles in regulating neuronal activity and synaptic transmission (125), and microglia-neuron communication is critical for learning and memory in the adult brain (126). In addition, repopulated microglia after microglial depletion enhance short-term memory, which may be related to increased astrocyte density (127). The possible mechanisms underlying astrocyte involvement in memory formation include regulation of synaptic formation, transmission, and plasticity (127). Galactic cosmic ray (GCR) exposure can affect neuronal and microglial functions (128), and microglial depletion can improve radiation-induced memory deficits and cognitive dysfunction (129, 130). Following CSF1R inhibitors treatment, the repopulated microglia reduce lysosome-associated membrane protein 1 (LAMP-1) level, limit the GRC-induced phagocytic phenotype, and thus ameliorate cognitive deficits and synaptic loss. In addition, inflammatory chemokines, cytokines, and complement component 5a receptor (C5aR) expression levels are also reduced (128, 131). In line with this, previous studies have reported that C5aR antagonist treatment can improve cognitive performance in animal models of different diseases (132, 133).
Microglial repopulation therapy for other diseases and deficits
Microglial repopulation therapy has also been used for the treatment of other diseases or deficits. For example, repopulated microglia did not prevent repeated social defeat (RSD)-induced stress sensitization and excessive immune and behavioral responses in mice, probably because multiple CNS cell types contribute to RSD sensitization, among which microglial priming is only partly involved. However, repopulated microglia effectively attenuated the immune and neuroinflammatory responses caused by LPS challenge following acute RSD (134). In a chronic neuroinflammation model (e.g., alcohol use disorders, AUDs), microglial depletion and repopulation can reverse chronic neuroimmune activation by normalizing proinflammatory cytokine levels and increasing protective trophic factors (135). Microglial depletion and repopulation did not affect the neuropathology of EAE or CNS T-cell responses (136). The repopulated microglia correct the anatomical defects, partially restore the auditory function (137), and eradicate fear memory in mice after fear conditioning (138). A recent study showed that repopulated microglia can reduce neuroinflammation, neurological deficits, and brain edema following intracerebral hemorrhage in the aged brain (139). These observations suggest that microglial repopulation therapy may be promising in the treatment of neurological diseases and deficits.
Conclusions
However, several important problems need to be addressed in the future. First, CSF1R inhibitors not only depletes microglia but also affects peripheral myeloid cells, for example, PLX3397 reduces the specific subsets of circulating monocytes in a mouse AD model (140). Furthermore, off-target effects may occur with peripheral administration in genetic models (141). Therefore, approaches to deplete microglia alone need to be developed in the future. Second, the optimal timing of microglial depletion and repopulation in specific disease models need to be determined. As we mentioned previously, the initial 3 days after TBI are critical, during which microglial regeneration may play a neuroprotective role (141). The optimal treatment window period for other neurological diseases and deficits needs to be determined in future studies. Third, the current results are mainly based on rodent models, and the effects of microglial repopulation therapy in primates and humans are not clear. In the future, patients with CNS diseases or deficits may be treated with microglial repopulation therapy to promote functional recovery; however, currently, there are significant challenges to the use of this therapy.
Author contributions
HF and TY contributed to the conception and design of the review. JZ and ZS contributed to searching information or preparing the figures or table. WS, YZ, YX, HF, and TY contributed to drafting and revising of manuscript. All authors contributed to the article and approved the final manuscript.
Funding
This work was supported by National Natural Science Foundation of China (NO. 82102558; NO.31872310), Shandong Province Natural Science Foundation (ZR2020QH117), and China Postdoctoral Science Foundation (2021M691690).
Conflict of interest
The authors declare that the research was conducted in the absence of any commercial or financial relationships that could be construed as a potential conflict of interest.
Publisher’s note
All claims expressed in this article are solely those of the authors and do not necessarily represent those of their affiliated organizations, or those of the publisher, the editors and the reviewers. Any product that may be evaluated in this article, or claim that may be made by its manufacturer, is not guaranteed or endorsed by the publisher.
References
1. Kierdorf K, Erny D, Goldmann T, Sander V, Schulz C, Perdiguero EG, et al. Microglia emerge from erythromyeloid precursors Via Pu.1- and Irf8-dependent pathways. Nat Neurosci (2013) 16(3):273–80. doi: 10.1038/nn.3318
2. Gomez Perdiguero E, Klapproth K, Schulz C, Busch K, Azzoni E, Crozet L, et al. Tissue-resident macrophages originate from yolk-Sac-Derived erythro-myeloid progenitors. Nature (2015) 518(7540):547–51. doi: 10.1038/nature13989
3. Salter MW, Stevens B. Microglia emerge as central players in brain disease. Nat Med (2017) 23(9):1018–27. doi: 10.1038/nm.4397
4. Brown GC, Neher JJ. Microglial phagocytosis of live neurons. Nat Rev Neurosci (2014) 15(4):209–16. doi: 10.1038/nrn3710
5. Nayak D, Roth TL, McGavern DB. Microglia development and function. Annu Rev Immunol (2014) 32:367–402. doi: 10.1146/annurev-immunol-032713-120240
6. Kettenmann H, Hanisch UK, Noda M, Verkhratsky A. Physiology of microglia. Physiol Rev (2011) 91(2):461–553. doi: 10.1152/physrev.00011.2010
7. Sierra A, Encinas JM, Deudero JJ, Chancey JH, Enikolopov G, Overstreet-Wadiche LS, et al. Microglia shape adult hippocampal neurogenesis through apoptosis-coupled phagocytosis. Cell Stem Cell (2010) 7(4):483–95. doi: 10.1016/j.stem.2010.08.014
8. Loane DJ, Kumar A. Microglia in the tbi brain: The good, the bad, and the dysregulated. Exp Neurol (2016) 275 Pt 3:316–27. doi: 10.1016/j.expneurol.2015.08.018
9. Nakagawa Y, Chiba K. Role of microglial M1/M2 polarization in relapse and remission of psychiatric disorders and diseases. Pharm (Basel) (2014) 7(12):1028–48. doi: 10.3390/ph7121028
10. Ghosh M, Xu Y, Pearse DD. Cyclic amp is a key regulator of M1 to M2a phenotypic conversion of microglia in the presence of Th2 cytokines. J Neuroinflamm (2016) 13:9. doi: 10.1186/s12974-015-0463-9
11. David S, Kroner A. Repertoire of microglial and macrophage responses after spinal cord injury. Nat Rev Neurosci (2011) 12(7):388–99. doi: 10.1038/nrn3053
12. Chhor V, Le Charpentier T, Lebon S, Ore MV, Celador IL, Josserand J, et al. Characterization of phenotype markers and neuronotoxic potential of polarised primary microglia in vitro. Brain Behav Immun (2013) 32:70–85. doi: 10.1016/j.bbi.2013.02.005
13. Ransohoff RM. A polarizing question: Do M1 and M2 microglia exist? Nat Neurosci (2016) 19(8):987–91. doi: 10.1038/nn.4338
14. Amor S, McNamara NB, Gerrits E, Marzin MC, Kooistra SM, Miron VE, et al. White matter microglia heterogeneity in the cns. Acta Neuropathol (2022) 143(2):125–41. doi: 10.1007/s00401-021-02389-x
15. Ali I, Chugh D, Ekdahl CT. Role of fractalkine-Cx3cr1 pathway in seizure-induced microglial activation, neurodegeneration, and neuroblast production in the adult rat brain. Neurobiol Dis (2015) 74:194–203. doi: 10.1016/j.nbd.2014.11.009
16. Hansen DV, Hanson JE, Sheng M. Microglia in Alzheimer's disease. J Cell Biol (2018) 21(2):459–72. doi: 10.1084/jem.20200785
17. Moehle MS, West AB. M1 and M2 immune activation in parkinson's disease: Foe and ally? Neuroscience (2015) 302:59–73. doi: 10.1016/j.neuroscience.2014.11.018
18. Crapser JD, Ochaba J, Soni N, Reidling JC, Thompson LM, Green KN. Microglial depletion prevents extracellular matrix changes and striatal volume reduction in a model of huntington's disease. Brain (2020) 143(1):266–88. doi: 10.1093/brain/awz363
19. Shields DC, Haque A, Banik NL. Neuroinflammatory responses of microglia in central nervous system trauma. J Cereb Blood Flow Metab (2020) 40(1_suppl):S25–33. doi: 10.1177/0271678X20965786
20. Francos-Quijorna I, Santos-Nogueira E, Gronert K, Sullivan AB, Kopp MA, Brommer B, et al. Maresin 1 promotes inflammatory resolution, neuroprotection, and functional neurological recovery after spinal cord injury. J Neurosci (2017) 37(48):11731–43. doi: 10.1523/JNEUROSCI.1395-17.2017
21. Bezzi P, Domercq M, Brambilla L, Galli R, Schols D, De Clercq E, et al. Cxcr4-activated astrocyte glutamate release Via tnfalpha: Amplification by microglia triggers neurotoxicity. Nat Neurosci (2001) 4(7):702–10. doi: 10.1038/89490
22. Takeuchi H, Jin S, Wang J, Zhang G, Kawanokuchi J, Kuno R, et al. Tumor necrosis factor-alpha induces neurotoxicity Via glutamate release from hemichannels of activated microglia in an autocrine manner. J Biol Chem (2006) 281(30):21362–8. doi: 10.1074/jbc.M600504200
23. Li J, Baud O, Vartanian T, Volpe JJ, Rosenberg PA. Peroxynitrite generated by inducible nitric oxide synthase and nadph oxidase mediates microglial toxicity to oligodendrocytes. Proc Natl Acad Sci U S A (2005) 102(28):9936–41. doi: 10.1073/pnas.0502552102
24. Domercq M, Sanchez-Gomez MV, Sherwin C, Etxebarria E, Fern R, Matute C. System xc- and glutamate transporter inhibition mediates microglial toxicity to oligodendrocytes. J Immunol (2007) 178(10):6549–56. doi: 10.4049/jimmunol.178.10.6549
25. Ma D, Zhao Y, Huang L, Xiao Z, Chen B, Shi Y, et al. A novel hydrogel-based treatment for complete transection spinal cord injury repair is driven by Microglia/Macrophages repopulation. Biomaterials (2020) 237:119830. doi: 10.1016/j.biomaterials.2020.119830
26. Van Rooijen N, Sanders A. Liposome mediated depletion of macrophages: Mechanism of action, preparation of liposomes and applications. J Immunol Methods (1994) 174(1-2):83–93. doi: 10.1016/0022-1759(94)90012-4
27. Andreou KE, Soto MS, Allen D, Economopoulos V, de Bernardi A, Larkin JR, et al. Anti-inflammatory Microglia/Macrophages as a potential therapeutic target in brain metastasis. Front Oncol (2017) 7:251. doi: 10.3389/fonc.2017.00251
28. Todd L, Palazzo I, Suarez L, Liu X, Volkov L, Hoang TV, et al. Reactive microglia and Il1beta/Il-1r1-Signaling mediate neuroprotection in excitotoxin-damaged mouse retina. J Neuroinflamm (2019) 16(1):118. doi: 10.1186/s12974-019-1505-5
29. Han X, Li Q, Lan X, El-Mufti L, Ren H, Wang J. Microglial depletion with clodronate liposomes increases proinflammatory cytokine levels, induces astrocyte activation, and damages blood vessel integrity. Mol Neurobiol (2019) 56(9):6184–96. doi: 10.1007/s12035-019-1502-9
30. Heppner FL, Greter M, Marino D, Falsig J, Raivich G, Hovelmeyer N, et al. Experimental autoimmune encephalomyelitis repressed by microglial paralysis. Nat Med (2005) 11(2):146–52. doi: 10.1038/nm1177
31. Eme-Scolan E, Dando SJ. Tools and approaches for studying microglia in vivo. Front Immunol (2020) 11:583647. doi: 10.3389/fimmu.2020.583647
32. Gowing G, Vallieres L, Julien JP. Mouse model for ablation of proliferating microglia in acute cns injuries. Glia (2006) 53(3):331–7. doi: 10.1002/glia.20288
33. Bennett RE, Brody DL. Acute reduction of microglia does not alter axonal injury in a mouse model of repetitive concussive traumatic brain injury. J Neurotrauma (2014) 31(19):1647–63. doi: 10.1089/neu.2013.3320
34. Kalin S, Miller KR, Kalin RE, Jendrach M, Witzel C, Heppner FL. Cns myeloid cells critically regulate heat hyperalgesia. J Clin Invest (2018) 128(7):2774–86. doi: 10.1172/JCI95305
35. Simard AR, Soulet D, Gowing G, Julien JP, Rivest S. Bone marrow-derived microglia play a critical role in restricting senile plaque formation in alzheimer's disease. Neuron (2006) 49(4):489–502. doi: 10.1016/j.neuron.2006.01.022
36. Buch T, Heppner FL, Tertilt C, Heinen TJ, Kremer M, Wunderlich FT, et al. A cre-inducible diphtheria toxin receptor mediates cell lineage ablation after toxin administration. Nat Methods (2005) 2(6):419–26. doi: 10.1038/nmeth762
37. Bruttger J, Karram K, Wortge S, Regen T, Marini F, Hoppmann N, et al. Genetic cell ablation reveals clusters of local self-renewing microglia in the mammalian central nervous system. Immunity (2015) 43(1):92–106. doi: 10.1016/j.immuni.2015.06.012
38. Takeda A, Shinozaki Y, Kashiwagi K, Ohno N, Eto K, Wake H, et al. Microglia mediate non-Cell-Autonomous cell death of retinal ganglion cells. Glia (2018) 66(11):2366–84. doi: 10.1002/glia.23475
39. Kaiser T, Feng G. Tmem119-egfp and Tmem119-Creert2 transgenic mice for labeling and manipulating microglia. eNeuro (2019) 6(4). doi: 10.1523/ENEURO.0448-18.2019
40. De Luca SN, Sominsky L, Soch A, Wang H, Ziko I, Rank MM, et al. Conditional microglial depletion in rats leads to reversible anorexia and weight loss by disrupting gustatory circuitry. Brain Behav Immun (2019) 77:77–91. doi: 10.1016/j.bbi.2018.12.008
41. Lund H, Pieber M, Parsa R, Han J, Grommisch D, Ewing E, et al. Competitive repopulation of an empty microglial niche yields functionally distinct subsets of microglia-like cells. Nat Commun (2018) 9(1):4845. doi: 10.1038/s41467-018-07295-7
42. Goldmann T, Wieghofer P, Muller PF, Wolf Y, Varol D, Yona S, et al. A new type of microglia gene targeting shows Tak1 to be pivotal in cns autoimmune inflammation. Nat Neurosci (2013) 16(11):1618–26. doi: 10.1038/nn.3531
43. Yona S, Kim KW, Wolf Y, Mildner A, Varol D, Breker M, et al. Fate mapping reveals origins and dynamics of monocytes and tissue macrophages under homeostasis. Immunity (2013) 38(1):79–91. doi: 10.1016/j.immuni.2012.12.001
44. Goldmann T, Wieghofer P, Jordao MJ, Prutek F, Hagemeyer N, Frenzel K, et al. Origin, fate and dynamics of macrophages at central nervous system interfaces. Nat Immunol (2016) 17(7):797–805. doi: 10.1038/ni.3423
45. Buttgereit A, Lelios I, Yu X, Vrohlings M, Krakoski NR, Gautier EL, et al. Sall1 is a transcriptional regulator defining microglia identity and function. Nat Immunol (2016) 17(12):1397–406. doi: 10.1038/ni.3585
46. Konishi H, Kobayashi M, Kunisawa T, Imai K, Sayo A, Malissen B, et al. Siglec-h is a microglia-specific marker that discriminates microglia from cns-associated macrophages and cns-infiltrating monocytes. Glia (2017) 65(12):1927–43. doi: 10.1002/glia.23204
47. Masuda T, Amann L, Sankowski R, Staszewski O, Lenz M, DE P, et al. Novel hexb-based tools for studying microglia in the cns. Nat Immunol (2020) 21(7):802–15. doi: 10.1038/s41590-020-0707-4
48. Arreola MA, Soni N, Crapser JD, Hohsfield LA, Elmore MRP, Matheos DP, et al. Microglial dyshomeostasis drives perineuronal net and synaptic loss in a Csf1r(+/-) mouse model of alsp, which can be rescued Via Csf1r inhibitors. Sci Adv (2021) 7(35). doi: 10.1126/sciadv.abg1601
49. Elmore MR, Najafi AR, Koike MA, Dagher NN, Spangenberg EE, Rice RA, et al. Colony-stimulating factor 1 receptor signaling is necessary for microglia viability, unmasking a microglia progenitor cell in the adult brain. Neuron (2014) 82(2):380–97. doi: 10.1016/j.neuron.2014.02.040
50. Elmore MR, Lee RJ, West BL, Green KN. Characterizing newly repopulated microglia in the adult mouse: Impacts on animal behavior, cell morphology, and neuroinflammation. PLoS One (2015) 10(4):e0122912. doi: 10.1371/journal.pone.0122912
51. Chitu V, Biundo F, Shlager GGL, Park ES, Wang P, Gulinello ME, et al. Microglial homeostasis requires balanced csf-1/Csf-2 receptor signaling. Cell Rep (2020) 30(9):3004–19.e5. doi: 10.1016/j.celrep.2020.02.028
52. Chitu V, Gokhan S, Nandi S, Mehler MF, Stanley ER. Emerging roles for csf-1 receptor and its ligands in the nervous system. Trends Neurosci (2016) 39(6):378–93. doi: 10.1016/j.tins.2016.03.005
53. Chitu V, Stanley ER. Regulation of embryonic and postnatal development by the csf-1 receptor. Curr Top Dev Biol (2017) 123:229–75. doi: 10.1016/bs.ctdb.2016.10.004
54. Nandi S, Gokhan S, Dai XM, Wei S, Enikolopov G, Lin H, et al. The csf-1 receptor ligands il-34 and csf-1 exhibit distinct developmental brain expression patterns and regulate neural progenitor cell maintenance and maturation. Dev Biol (2012) 367(2):100–13. doi: 10.1016/j.ydbio.2012.03.026
55. Dagher NN, Najafi AR, Kayala KM, Elmore MR, White TE, Medeiros R, et al. Colony-stimulating factor 1 receptor inhibition prevents microglial plaque association and improves cognition in 3xtg-ad mice. J Neuroinflamm (2015) 12:139. doi: 10.1186/s12974-015-0366-9
56. Huang Y, Xu Z, Xiong S, Qin G, Sun F, Yang J, et al. Dual extra-retinal origins of microglia in the model of retinal microglia repopulation. Cell Discovery (2018) 4:9. doi: 10.1038/s41421-018-0011-8
57. Tap WD, Wainberg ZA, Anthony SP, Ibrahim PN, Zhang C, Healey JH, et al. Structure-guided blockade of Csf1r kinase in tenosynovial giant-cell tumor. N Engl J Med (2015) 373(5):428–37. doi: 10.1056/NEJMoa1411366
58. Spangenberg E, Severson PL, Hohsfield LA, Crapser J, Zhang J, Burton EA, et al. Sustained microglial depletion with Csf1r inhibitor impairs parenchymal plaque development in an alzheimer's disease model. Nat Commun (2019) 10(1):3758. doi: 10.1038/s41467-019-11674-z
59. Hohsfield LA, Najafi AR, Ghorbanian Y, Soni N, Crapser J, Figueroa Velez DX, et al. Subventricular Zone/White matter microglia reconstitute the empty adult microglial niche in a dynamic wave. eLife (2021) 10:4. doi: 10.7554/eLife.66738
60. Fu H, Zhao Y, Hu D, Wang S, Yu T, Zhang L. Depletion of microglia exacerbates injury and impairs function recovery after spinal cord injury in mice. Cell Death Dis (2020) 11(7):528. doi: 10.1038/s41419-020-2733-4
61. Bellver-Landete V, Bretheau F, Mailhot B, Vallieres N, Lessard M, Janelle ME, et al. Microglia are an essential component of the neuroprotective scar that forms after spinal cord injury. Nat Commun (2019) 10(1):518. doi: 10.1038/s41467-019-08446-0
62. Spangenberg EE, Lee RJ, Najafi AR, Rice RA, Elmore MR, Blurton-Jones M, et al. Eliminating microglia in alzheimer's mice prevents neuronal loss without modulating amyloid-beta pathology. Brain (2016) 139(Pt 4):1265–81. doi: 10.1093/brain/aww016
63. Rice RA, Spangenberg EE, Yamate-Morgan H, Lee RJ, Arora RP, Hernandez MX, et al. Elimination of microglia improves functional outcomes following extensive neuronal loss in the hippocampus. J Neurosci (2015) 35(27):9977–89. doi: 10.1523/JNEUROSCI.0336-15.2015
64. Szalay G, Martinecz B, Lenart N, Kornyei Z, Orsolits B, Judak L, et al. Microglia protect against brain injury and their selective elimination dysregulates neuronal network activity after stroke. Nat Commun (2016) 7:11499. doi: 10.1038/ncomms11499
65. Chitu V, Nacu V, Charles JF, Henne WM, McMahon HT, Nandi S, et al. Pstpip2 deficiency in mice causes osteopenia and increased differentiation of multipotent myeloid precursors into osteoclasts. Blood (2012) 120(15):3126–35. doi: 10.1182/blood-2012-04-425595
66. Thompson ML, Jimenez-Andrade JM, Chartier S, Tsai J, Burton EA, Habets G, et al. Targeting cells of the myeloid lineage attenuates pain and disease progression in a prostate model of bone cancer. Pain (2015) 156(9):1692–702. doi: 10.1097/j.pain.0000000000000228
67. Cornelis S, Bruynooghe Y, Van Loo G, Saelens X, Vandenabeele P, Beyaert R. Apoptosis of hematopoietic cells induced by growth factor withdrawal is associated with caspase-9 mediated cleavage of raf-1. Oncogene (2005) 24(9):1552–62. doi: 10.1038/sj.onc.1208401
68. Waisman A, Ginhoux F, Greter M, Bruttger J. Homeostasis of microglia in the adult brain: Review of novel microglia depletion systems. Trends Immunol (2015) 36(10):625–36. doi: 10.1016/j.it.2015.08.005
69. Jakel S, Dimou L. Glial cells and their function in the adult brain: A journey through the history of their ablation. Front Cell Neurosci (2017) 11:24. doi: 10.3389/fncel.2017.00024
70. Mancuso R, Fryatt G, Cleal M, Obst J, Pipi E, Monzon-Sandoval J, et al. Csf1r inhibitor jnj-40346527 attenuates microglial proliferation and neurodegeneration in P301s mice. Brain (2019) 142(10):3243–64. doi: 10.1093/brain/awz241
71. Zhang D, Li S, Hou L, Jing L, Ruan Z, Peng B, et al. Microglial activation contributes to cognitive impairments in rotenone-induced mouse parkinson's disease model. J Neuroinflamm (2021) 18(1):4. doi: 10.1186/s12974-020-02065-z
72. Beckmann N, Giorgetti E, Neuhaus A, Zurbruegg S, Accart N, Smith P, et al. Brain region-specific enhancement of remyelination and prevention of demyelination by the Csf1r kinase inhibitor Blz945. Acta Neuropathol Commun (2018) 6(1):9. doi: 10.1186/s40478-018-0510-8
73. Gerber YN, Saint-Martin GP, Bringuier CM, Bartolami S, Goze-Bac C, Noristani HN, et al. Csf1r inhibition reduces microglia proliferation, promotes tissue preservation and improves motor recovery after spinal cord injury. Front Cell Neurosci (2018) 12:368. doi: 10.3389/fncel.2018.00368
74. Henry RJ, Ritzel RM, Barrett JP, Doran SJ, Jiao Y, Leach JB, et al. Microglial depletion with Csf1r inhibitor during chronic phase of experimental traumatic brain injury reduces neurodegeneration and neurological deficits. J Neurosci (2020) 40(14):2960–74. doi: 10.1523/JNEUROSCI.2402-19.2020
75. Li Y, Ritzel RM, Khan N, Cao T, He J, Lei Z, et al. Delayed microglial depletion after spinal cord injury reduces chronic inflammation and neurodegeneration in the brain and improves neurological recovery in Male mice. Theranostics (2020) 10(25):11376–403. doi: 10.7150/thno.49199
76. Jakovcevski I, Forster E, Reiss G, Schachner M. Impact of depletion of Microglia/Macrophages on regeneration after spinal cord injury. Neuroscience (2021) 459:129–41. doi: 10.1016/j.neuroscience.2021.02.010
77. Poulen G, Aloy E, Bringuier CM, Mestre-Frances N, Artus EVF, Cardoso M, et al. Inhibiting microglia proliferation after spinal cord injury improves recovery in mice and nonhuman primates. Theranostics (2021) 11(18):8640–59. doi: 10.7150/thno.61833
78. Du X, Xu Y, Chen S, Fang M. Inhibited Csf1r alleviates ischemia injury Via inhibition of microglia M1 polarization and Nlrp3 pathway. Neural Plast (2020) 2020:8825954. doi: 10.1155/2020/8825954
79. Stojiljkovic MR, Schmeer C, Witte OW. Pharmacological depletion of microglia leads to a dose-dependent reduction in inflammation and senescence in the aged murine brain. Neuroscience (2022) 488:1–9. doi: 10.1016/j.neuroscience.2022.02.018
80. Wu W, Li Y, Wei Y, Bosco DB, Xie M, Zhao MG, et al. Microglial depletion aggravates the severity of acute and chronic seizures in mice. Brain Behav Immun (2020) 89:245–55. doi: 10.1016/j.bbi.2020.06.028
81. Tanabe S, Saitoh S, Miyajima H, Itokazu T, Yamashita T. Microglia suppress the secondary progression of autoimmune encephalomyelitis. Glia (2019) 67(9):1694–704. doi: 10.1002/glia.23640
82. Zhao R, Hu W, Tsai J, Li W, Gan WB. Microglia limit the expansion of beta-amyloid plaques in a mouse model of alzheimer's disease. Mol Neurodegener (2017) 12(1):47. doi: 10.1186/s13024-017-0188-6
83. Poulen G, Bartolami S, Noristani HN, Perrin FE, Gerber YN. Unlike brief inhibition of microglia proliferation after spinal cord injury, long-term treatment does not improve motor recovery. Brain Sci (2021) 11(12):1643. doi: 10.3390/brainsci11121643
84. Hanlon LA, Raghupathi R, Huh JW. Depletion of microglia immediately following traumatic brain injury in the pediatric rat: Implications for cellular and behavioral pathology. Exp Neurol (2019) 316:39–51. doi: 10.1016/j.expneurol.2019.04.004
85. Conedera FM, Pousa AMQ, Mercader N, Tschopp M, Enzmann V. Retinal microglia signaling affects Muller cell behavior in the zebrafish following laser injury induction. Glia (2019) 67(6):1150–66. doi: 10.1002/glia.23601
86. Lawson LJ, Perry VH, Gordon S. Turnover of resident microglia in the normal adult mouse brain. Neuroscience (1992) 48(2):405–15. doi: 10.1016/0306-4522(92)90500-2
87. Askew K, Li K, Olmos-Alonso A, Garcia-Moreno F, Liang Y, Richardson P, et al. Coupled proliferation and apoptosis maintain the rapid turnover of microglia in the adult brain. Cell Rep (2017) 18(2):391–405. doi: 10.1016/j.celrep.2016.12.041
88. Varvel NH, Grathwohl SA, Baumann F, Liebig C, Bosch A, Brawek B, et al. Microglial repopulation model reveals a robust homeostatic process for replacing cns myeloid cells. Proc Natl Acad Sci U S A (2012) 109(44):18150–5. doi: 10.1073/pnas.1210150109
89. Huang Y, Xu Z, Xiong S, Sun F, Qin G, Hu G, et al. Repopulated microglia are solely derived from the proliferation of residual microglia after acute depletion. Nat Neurosci (2018) 21(4):530–40. doi: 10.1038/s41593-018-0090-8
90. Ginhoux F, Greter M, Leboeuf M, Nandi S, See P, Gokhan S, et al. Fate mapping analysis reveals that adult microglia derive from primitive macrophages. Science (2010) 330(6005):841–5. doi: 10.1126/science.1194637
91. Guilliams M, Thierry GR, Bonnardel J, Bajenoff M. Establishment and maintenance of the macrophage niche. Immunity (2020) 52(3):434–51. doi: 10.1016/j.immuni.2020.02.015
92. Rice RA, Pham J, Lee RJ, Najafi AR, West BL, Green KN. Microglial repopulation resolves inflammation and promotes brain recovery after injury. Glia (2017) 65(6):931–44. doi: 10.1002/glia.23135
93. Yao Y, Echeverry S, Shi XQ, Yang M, Yang QZ, Wang GY, et al. Dynamics of spinal microglia repopulation following an acute depletion. Sci Rep (2016) 6:22839. doi: 10.1038/srep22839
94. Fan Y, Bergmann A. Apoptosis-induced compensatory proliferation. the cell is dead. long live the cell! Trends Cell Biol (2008) 18(10):467–73. doi: 10.1016/j.tcb.2008.08.001
95. Tamori Y, Deng WM. Tissue repair through cell competition and compensatory cellular hypertrophy in postmitotic epithelia. Dev Cell (2013) 25(4):350–63. doi: 10.1016/j.devcel.2013.04.013
96. Yuan TF, Liang YX, Peng B, Lin B, So KF. Local proliferation is the main source of rod microglia after optic nerve transection. Sci Rep (2015) 5:10788. doi: 10.1038/srep10788
97. Ma W, Zhang Y, Gao C, Fariss RN, Tam J, Wong WT. Monocyte infiltration and proliferation reestablish myeloid cell homeostasis in the mouse retina following retinal pigment epithelial cell injury. Sci Rep (2017) 7(1):8433. doi: 10.1038/s41598-017-08702-7
98. Zhang Y, Zhao L, Wang X, Ma W, Lazere A, Qian HH, et al. Repopulating retinal microglia restore endogenous organization and function under Cx3cl1-Cx3cr1 regulation. Sci Adv (2018) 4(3):eaap8492. doi: 10.1126/sciadv.aap8492
99. Burnstock G. Physiology and pathophysiology of purinergic neurotransmission. Physiol Rev (2007) 87(2):659–797. doi: 10.1152/physrev.00043.2006
100. Zhan L, Krabbe G, Du F, Jones I, Reichert MC, Telpoukhovskaia M, et al. Proximal recolonization by self-renewing microglia re-establishes microglial homeostasis in the adult mouse brain. PLoS Biol (2019) 17(2):e3000134. doi: 10.1371/journal.pbio.3000134
101. Tay TL, Mai D, Dautzenberg J, Fernandez-Klett F, Lin G, Sagar, et al. A new fate mapping system reveals context-dependent random or clonal expansion of microglia. Nat Neurosci (2017) 20(6):793–803. doi: 10.1038/nn.4547
102. Erny D, Hrabe de Angelis AL, Jaitin D, Wieghofer P, Staszewski O, David E, et al. Host microbiota constantly control maturation and function of microglia in the cns. Nat Neurosci (2015) 18(7):965–77. doi: 10.1038/nn.4030
103. Matcovitch-Natan O, Winter DR, Giladi A, Vargas Aguilar S, Spinrad A, Sarrazin S, et al. Microglia development follows a stepwise program to regulate brain homeostasis. Science (2016) 353(6301):aad8670. doi: 10.1126/science.aad8670
104. Butovsky O, Jedrychowski MP, Moore CS, Cialic R, Lanser AJ, Gabriely G, et al. Identification of a unique tgf-Beta-Dependent molecular and functional signature in microglia. Nat Neurosci (2014) 17(1):131–43. doi: 10.1038/nn.3599
105. Afratis NA, Nikitovic D, Multhaupt HA, Theocharis AD, Couchman JR, Karamanos NK. Syndecans - key regulators of cell signaling and biological functions. FEBS J (2017) 284(1):27–41. doi: 10.1111/febs.13940
106. Zhan L, Fan L, Kodama L, Sohn PD, Wong MY, Mousa GA, et al. A Mac2-positive progenitor-like microglial population is resistant to Csf1r inhibition in adult mouse brain. eLife (2020) 9:2. doi: 10.7554/eLife.51796
107. Safaiyan S, Besson-Girard S, Kaya T, Cantuti-Castelvetri L, Liu L, Ji H, et al. White matter aging drives microglial diversity. Neuron (2021) 109(7):1100–17.e10. doi: 10.1016/j.neuron.2021.01.027
108. Basilico B, Ferrucci L, Ratano P, Golia MT, Grimaldi A, Rosito M, et al. Microglia control glutamatergic synapses in the adult mouse hippocampus. Glia (2022) 70(1):173–95. doi: 10.1002/glia.24101
109. Roda AR, Serra-Mir G, Montoliu-Gaya L, Tiessler L, Villegas S. Amyloid-beta peptide and tau protein crosstalk in alzheimer's disease. Neural Regener Res (2022) 17(8):1666–74. doi: 10.4103/1673-5374.332127
110. Casali BT, MacPherson KP, Reed-Geaghan EG, Landreth GE. Microglia depletion rapidly and reversibly alters amyloid pathology by modification of plaque compaction and morphologies. Neurobiol Dis (2020) 142:104956. doi: 10.1016/j.nbd.2020.104956
111. Masuda T, Sankowski R, Staszewski O, Bottcher C, Amann L, Sagar, et al. Spatial and temporal heterogeneity of mouse and human microglia at single-cell resolution. Nature (2019) 566(7744):388–92. doi: 10.1038/s41586-019-0924-x
112. Tan YL, Yuan Y, Tian L. Microglial regional heterogeneity and its role in the brain. Mol Psychiatry (2020) 25(2):351–67. doi: 10.1038/s41380-019-0609-8
113. Grunewald A, Kumar KR, Sue CM. New insights into the complex role of mitochondria in parkinson's disease. Prog Neurobiol (2019) 177:73–93. doi: 10.1016/j.pneurobio.2018.09.003
114. Li Q, Shen C, Liu Z, Ma Y, Wang J, Dong H, et al. Partial depletion and repopulation of microglia have different effects in the acute mptp mouse model of parkinson's disease. Cell Prolif (2021) 54(8):e13094. doi: 10.1111/cpr.13094
115. Gratuze M, Chen Y, Parhizkar S, Jain N, Strickland MR, Serrano JR, et al. Activated microglia mitigate abeta-associated tau seeding and spreading. J Exp Med (2021) 218(8):e20210542. doi: 10.1084/jem.20210542
116. Simon DW, McGeachy MJ, Bayir H, Clark RSB, Loane DJ, Kochanek PM. The far-reaching scope of neuroinflammation after traumatic brain injury. Nat Rev Neurol (2017) 13(9):572. doi: 10.1038/nrneurol.2017.116
117. Feng X, Frias ES, Paladini MS, Chen D, Boosalis Z, Becker M, et al. Functional role of brain-engrafted macrophages against brain injuries. J Neuroinflamm (2021) 18(1):232. doi: 10.1186/s12974-021-02290-0
118. Willis EF, MacDonald KPA, Nguyen QH, Garrido AL, Gillespie ER, Harley SBR, et al. Repopulating microglia promote brain repair in an il-6-Dependent manner. Cell (2020) 180(5):833–46.e16. doi: 10.1016/j.cell.2020.02.013
119. Mosher KI, Wyss-Coray T. Microglial dysfunction in brain aging and alzheimer's disease. Biochem Pharmacol (2014) 88(4):594–604. doi: 10.1016/j.bcp.2014.01.008
120. Norden DM, Muccigrosso MM, Godbout JP. Microglial priming and enhanced reactivity to secondary insult in aging, and traumatic cns injury, and neurodegenerative disease. Neuropharmacology (2015) 96(Pt A):29–41. doi: 10.1016/j.neuropharm.2014.10.028
121. Safaiyan S, Kannaiyan N, Snaidero N, Brioschi S, Biber K, Yona S, et al. Age-related myelin degradation burdens the clearance function of microglia during aging. Nat Neurosci (2016) 19(8):995–8. doi: 10.1038/nn.4325
122. O'Neil SM, Witcher KG, McKim DB, Godbout JP. Forced turnover of aged microglia induces an intermediate phenotype but does not rebalance cns environmental cues driving priming to immune challenge. Acta Neuropathol Commun (2018) 6(1):129. doi: 10.1186/s40478-018-0636-8
123. Elmore MRP, Hohsfield LA, Kramar EA, Soreq L, Lee RJ, Pham ST, et al. Replacement of microglia in the aged brain reverses cognitive, synaptic, and neuronal deficits in mice. Aging Cell (2018) 17(6):e12832. doi: 10.1111/acel.12832
124. Yegla B, Boles J, Kumar A, Foster TC. Partial microglial depletion is associated with impaired hippocampal synaptic and cognitive function in young and aged rats. Glia (2021) 69(6):1494–514. doi: 10.1002/glia.23975
125. Akiyoshi R, Wake H, Kato D, Horiuchi H, Ono R, Ikegami A, et al. Microglia enhance synapse activity to promote local network synchronization. eNeuro (2018) 5(5):ENEURO.0088-18.2018. doi: 10.1523/ENEURO.0088-18.2018
126. Cornell J, Salinas S, Huang HY, Zhou M. Microglia regulation of synaptic plasticity and learning and memory. Neural Regener Res (2022) 17(4):705–16. doi: 10.4103/1673-5374.322423
127. De Luca SN, Soch A, Sominsky L, Nguyen TX, Bosakhar A, Spencer SJ. Glial remodeling enhances short-term memory performance in wistar rats. J Neuroinflamm (2020) 17(1):52. doi: 10.1186/s12974-020-1729-4
128. Rosi S. The final frontier: Transient microglia reduction after cosmic radiation exposure mitigates cognitive impairments and modulates phagocytic activity. Brain Circ (2018) 4(3):109–13. doi: 10.4103/bc.bc_24_18
129. Acharya MM, Green KN, Allen BD, Najafi AR, Syage A, Minasyan H, et al. Elimination of microglia improves cognitive function following cranial irradiation. Sci Rep (2016) 6:31545. doi: 10.1038/srep31545
130. Feng X, Jopson TD, Paladini MS, Liu S, West BL, Gupta N, et al. Colony-stimulating factor 1 receptor blockade prevents fractionated whole-brain irradiation-induced memory deficits. J Neuroinflamm (2016) 13(1):215. doi: 10.1186/s12974-016-0671-y
131. Krukowski K, Feng X, Paladini MS, Chou A, Sacramento K, Grue K, et al. Temporary microglia-depletion after cosmic radiation modifies phagocytic activity and prevents cognitive deficits. Sci Rep (2018) 8(1):7857. doi: 10.1038/s41598-018-26039-7
132. Fonseca MI, Ager RR, Chu SH, Yazan O, Sanderson SD, LaFerla FM, et al. Treatment with a C5ar antagonist decreases pathology and enhances behavioral performance in murine models of alzheimer's disease. J Immunol (2009) 183(2):1375–83. doi: 10.4049/jimmunol.0901005
133. Garrett MC, Otten ML, Starke RM, Komotar RJ, Magotti P, Lambris JD, et al. Synergistic neuroprotective effects of C3a and C5a receptor blockade following intracerebral hemorrhage. Brain Res (2009) 1298:171–7. doi: 10.1016/j.brainres.2009.04.047
134. Weber MD, McKim DB, Niraula A, Witcher KG, Yin W, Sobol CG, et al. The influence of microglial elimination and repopulation on stress sensitization induced by repeated social defeat. Biol Psychiatry (2019) 85(8):667–78. doi: 10.1016/j.biopsych.2018.10.009
135. Coleman LG Jr., Zou J, Crews FT. Microglial depletion and repopulation in brain slice culture normalizes sensitized proinflammatory signaling. J Neuroinflamm (2020) 17(1):27. doi: 10.1186/s12974-019-1678-y
136. Rubino SJ, Mayo L, Wimmer I, Siedler V, Brunner F, Hametner S, et al. Acute microglia ablation induces neurodegeneration in the somatosensory system. Nat Commun (2018) 9(1):4578. doi: 10.1038/s41467-018-05929-4
137. Milinkeviciute G, Chokr SM, Cramer KS. Auditory brainstem deficits from early treatment with a Csf1r inhibitor largely recover with microglial repopulation. eNeuro (2021) 8(2):ENEURO.0318-20.2021. doi: 10.1523/ENEURO.0318-20.2021
138. Cui X, Zhou S, Xia G, Chen J, Jiang L, Huang J, et al. A multispecies probiotic accelerates fear extinction and inhibits relapse in mice: Role of microglia. Neuropharmacology (2021) 193:108613. doi: 10.1016/j.neuropharm.2021.108613
139. Li X, Gao X, Zhang W, Liu M, Han Z, Li M, et al. Microglial replacement in the aged brain restricts neuroinflammation following intracerebral hemorrhage. Cell Death Dis (2022) 13(1):33. doi: 10.1038/s41419-021-04424-x
140. Shi Y, Manis M, Long J, Wang K, Sullivan PM, Remolina Serrano J, et al. Microglia drive apoe-dependent neurodegeneration in a tauopathy mouse model. J Exp Med (2019) 216(11):2546–61. doi: 10.1084/jem.20190980
Keywords: microglia, depletion, repopulation, central nervous system, diseases
Citation: Shi W, Zhang J, Shang Z, Zhang Y, Xia Y, Fu H and Yu T (2022) Restorative therapy using microglial depletion and repopulation for central nervous system injuries and diseases. Front. Immunol. 13:969127. doi: 10.3389/fimmu.2022.969127
Received: 14 June 2022; Accepted: 27 June 2022;
Published: 14 July 2022.
Edited by:
Hideyuki Takeuchi, Yokohama City University, JapanCopyright © 2022 Shi, Zhang, Shang, Zhang, Xia, Fu and Yu. This is an open-access article distributed under the terms of the Creative Commons Attribution License (CC BY). The use, distribution or reproduction in other forums is permitted, provided the original author(s) and the copyright owner(s) are credited and that the original publication in this journal is cited, in accordance with accepted academic practice. No use, distribution or reproduction is permitted which does not comply with these terms.
*Correspondence: Haitao Fu, ZnVoYWl0YW9AcWR1LmVkdS5jbg==; Tengbo Yu, eXRiODkxMkAxNjMuY29t