- 1Department of Neurology, Affiliated Hangzhou First People’s Hospital, Zhejiang University School of Medicine, Hangzhou, China
- 2Department of Intensive Care Medicine, Affiliated Hangzhou First People’s Hospital, Zhejiang University School of Medicine, Hangzhou, China
The relationship between gut microbiota and brain function has been studied intensively in recent years, and gut microbiota has been linked to a couple of neurological disorders including stroke. There are multiple studies linking gut microbiota to stroke in the “microbiota-gut-brain” axis. The aryl hydrocarbon receptor (AHR) is an important mediator of acute ischemic damage and can result in subsequent neuroinflammation. AHR can affect these responses by sensing microbiota metabolites especially tryptophan metabolites and is engaged in the regulation of acute ischemic brain injury and chronic neuroinflammation after stroke. As an important regulator in the “microbiota-gut-brain” axis, AHR has the potential to be used as a new therapeutic target for ischemic stroke treatment. In this review, we discuss the research progress on AHR regarding its role in ischemic stroke and prospects to be used as a therapeutic target for ischemic stroke treatment, aiming to provide a potential direction for the development of new treatments for ischemic stroke.
Introduction
Cerebrovascular accident, commonly known as stroke and being a global health concern, is characterized by high mortality and disability rates, and is one of the leading cause of dementia and depression (1). According to neuropathology, stroke can be classified into two major subtyes: ischemic and hemorrhagic, with the former and latter accounting for 85% and 15% of all cases, respectively (2). The relationship between gut microbiota and brain function has been studied intensively in recent years, and gut microbiota has been linked to a couple of neurological disorders, including Alzheimer’s disease (AD) (3), Parkinson’s disease (PD) (4), multiple scleroses (MS) (5), neurodevelopmental (6) and psychiatric disorders (7, 8), and stroke (9–15). Communication between the brain and gut microbiota is mainly mediated by neurogenic signaling molecules and microbial metabolites; specifically, four pathways related to neuro, metabolism, endocrine, and immune signaling, are involved in this process (16). In turn, the central nervous system (CNS) can regulate neurotransmitters to achieve bidirectional communications by shaping microbial community composition and function. These processes that link microbiota and the brain are termed the “microbiota-gut-brain” axis. Study have proven that the gut microbiota can influence stroke prognosis by modulating the immune response and neuroinflammation (13). In turn, stroke can induce a shift in the gut microbiota, affecting intestinal motility and permeability, stress response, and systemic infection after stroke (10, 14, 15, 17). These findings highlight the close connection between gut microbiota and stroke in the “microbiota-gut-brain” axis.
Gut microbiota interacts with the host mainly through its metabolites. Tryptophan is an essential amino-acid that must be obtained from the diet. It can be metabolized by gut microbiota directly or indirectly and participates in a variety of physiological processes. Abnormal tryptophan metabolism has been associated with many diseases. The AHR is an important mediator of acute ischemic damage and can result in subsequent neuroinflammation (18, 19). AHR can affect these responses by sensing microbiota metabolites. For instance, it can be activated predominantly by ligands produced from gut microbes metabolizing diet-derived tryptophan (20, 21). Indeed, aberrant tryptophan metabolism and dysbiosis of gut microbiota have been observed in both acute and chronic stages of cerebral ischemia (22, 23). Actually, ischemic injuries and subsequent neuroinflammation have been recognized as key elements in stroke development. Neuroinflammation exists in both acute and chronic phases of cerebral ischemia, affecting the prognosis and survival of stroke patients to some extent. Persistent neuroinflammation could induce neurodegeneration, leading to post-stroke dementia and depression (24, 25). Activated microglia and astrocyte play an important role in neuroinflammation after stroke, which may be achieved through the binding of the ligand to AHR (5, 26–30).
AHR is engaged in the regulation of acute ischemic brain injury and may be involved in chronic neuroinflammation after stroke. As an important regulator in the “microbiota-gut-brain” axis, AHR has the potential to be used as a new therapeutic target for ischemia stroke treatment. In this review, we discuss the research progress on AHR regarding its role in ischemia stroke and prospects to be used as a therapeutic target for ischemia stroke treatment, aiming to provide a potential direction for the development of new treatments for ischemia stroke.
Role of the “microbiota-gut-brain” axis in the development of ischemia stroke
The communication between the gut microbiota and CNS is mediated through at least 4 interacting components, including the immune system, metabolites, neurotransmitters, and activated vagal nerve (19). In the top-down signaling pathway, ischemia stroke can disrupt the structure and function of the gut microbiota through the autonomic nervous system, increasing the gut permeability and reducing its motility, which further induces an intestinal immune response and bacterial translocation. In the bottom-up signaling pathway, post-stroke gut microbiota dysbiosis can result in changes in bacterial metabolites, leading to systematic infection due to bacterial translocation, abnormal immune cell migration, and release of immunomodulatory cytokines, which further mediates neuroinflammation that causes severe ischemia stroke and worse prognosis (31) (Figure 1).
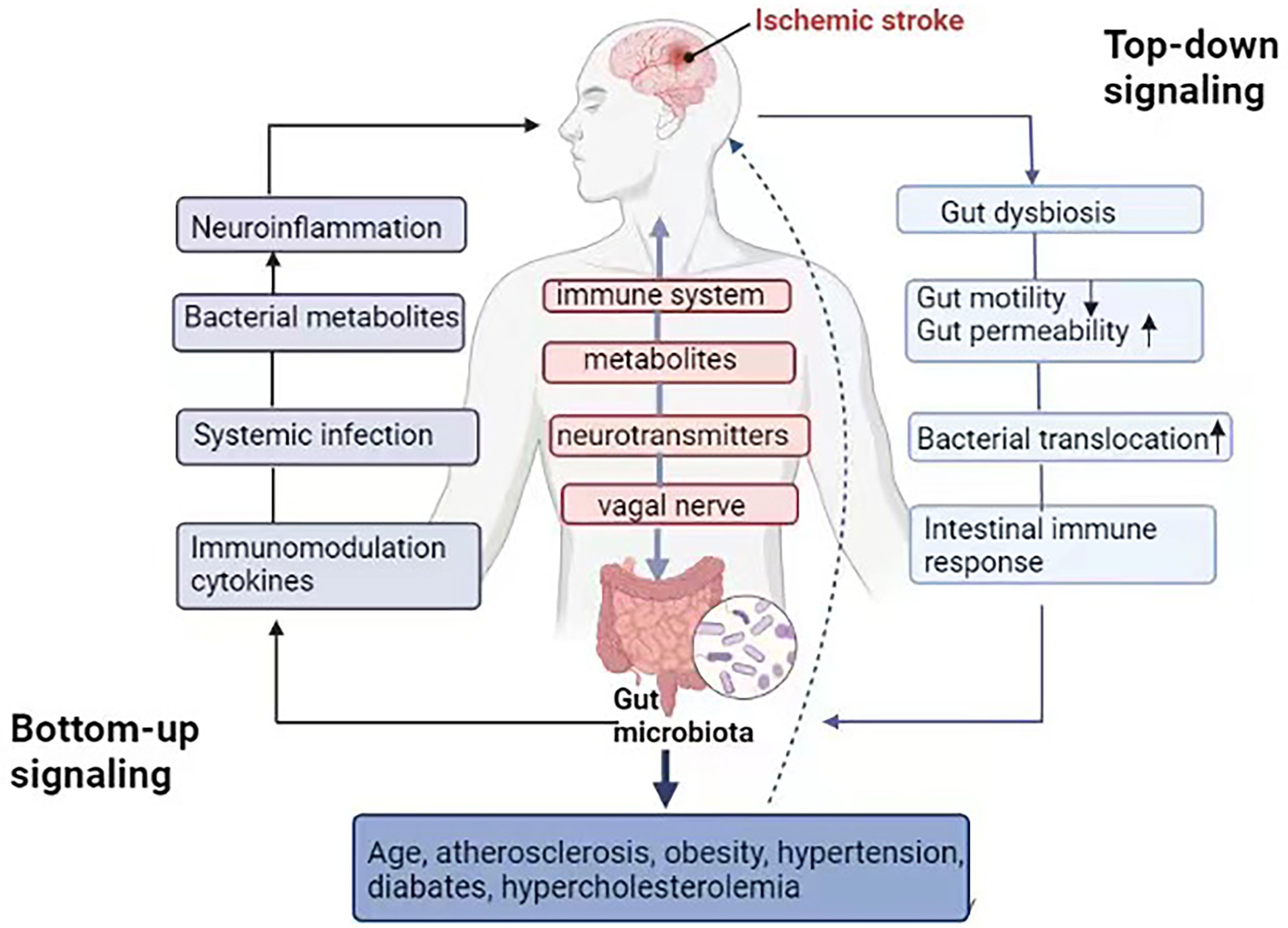
Figure 1 “Microbiota-gut-brain” axis in the ischemic stroke. Gut microbiota communicates to the CNS through the immune system, metabolites and neurotransmitters, as well as activation of the vagal nerve. In the top-down signaling pathway, ischemic stroke can affect the community structure and function of the gut microbiota through the autonomic nervous system, increase the gut permeability and reduce `gut motility, meanwhile, inducing an intestinal immune response and bacterial translocation. In the bottom-up signaling pathway, gut microbiota dysbiosis after stroke leads to changes in bacterial metabolites, systematic infection due to bacterial translocation, immune cell migration and the release of immunomodulation cytokines, which further mediate neuroinflammation, related to the severity of ischemic stroke and worse prognosis. Gut microbiota can affect risk factors related to ischemia stroke directly or indirectly, including hypertension, diabetes, hypercholesterolemia, obesity and atherosclerosis, as well as aging.
Preclinical and clinical studies demonstrated that gut microbiota plays an important role in the pathogenesis and prognosis of ischemia stroke (10, 12–16, 32–47) (Table 1). For example, many studies indicate that gut microbiota can affect risk factors related to ischemia stroke directly or indirectly, including hypertension, diabetes, hypercholesterolemia, obesity and atherosclerosis, as well as aging (35, 48–52). However, so far, there is no large prospective study exploring how gut microbiota relates to the long-term risk of ischemia stroke. In addition, ischemia stroke could change the gut microbiota composition. For instance, Enterobacteriaceae, Ruminococcaceae, Veillonellaceae and Lachnospiraceae were significantly enriched after stroke, while Bacteroidaceae and Prevotellaceae were significantly reduced. Enterobacteriaceae showed notably increased in patients with poor prognosis of cerebral infarction (47). Another study demonstrated that dysbiosis of the gut microbiota relates to ischemia stroke severity in mice; specifically, germ-free (GF) mice can develop more severe brain injury after receiving fecal transplants from high-stroke disequilibrium index (SDI) mice (36). Pre-existing microbiota ensures intestinal protection, and transplantation of the gut microbiota from post-stroke mice to GF mice exacerbates the brain damage and functional deficits compared to those in the controls. GF mice present enlarged brain lesions compared to recolonized (Ex-GF) and specific pathogen-free (SPF) mice after stroke (11). Changes in gut microbiota induced by antibiotics such as ampicillin can reduce ischemic brain injury and gut inflammation, leading to improved long-term prognosis (16, 38). However, another study showed the opposite result; mortality in mice with disturbed gut microbiota was significantly higher following inhibition of gut microbiota by broad-spectrum antibiotics (12). Therapeutic fecal microbiota transplantation (FMT) can normalize the microbiota imbalance induced by brain injury and improve stroke prognosis (10). This effect may be particularly pronounced when aged stroke mice received FMT from young mice. The aged mice showed fewer behavioral abnormalities and neuroinflammation, which may be due to the fact that gut microbiota could produce high levels of short-chainfatty acids (SCFAs). Mechanistically, SCFAs can improve neuronal connectivity and synaptic plasticity after stroke by modulating microglia activation through recruitment of T-lymphocytes, thereby improving behavioral recovery. Studies have shown that supplementation of Lactobacilli after stroke can reduce neuroinflammation and improve cognitive function and depression (35, 40, 45). In addition, new evidence indicates that lactulose and atorvastatin may regulate the structure of gut microbiota by regulating intestinal immune function and reducing neuroinflammation after stroke (43, 46).
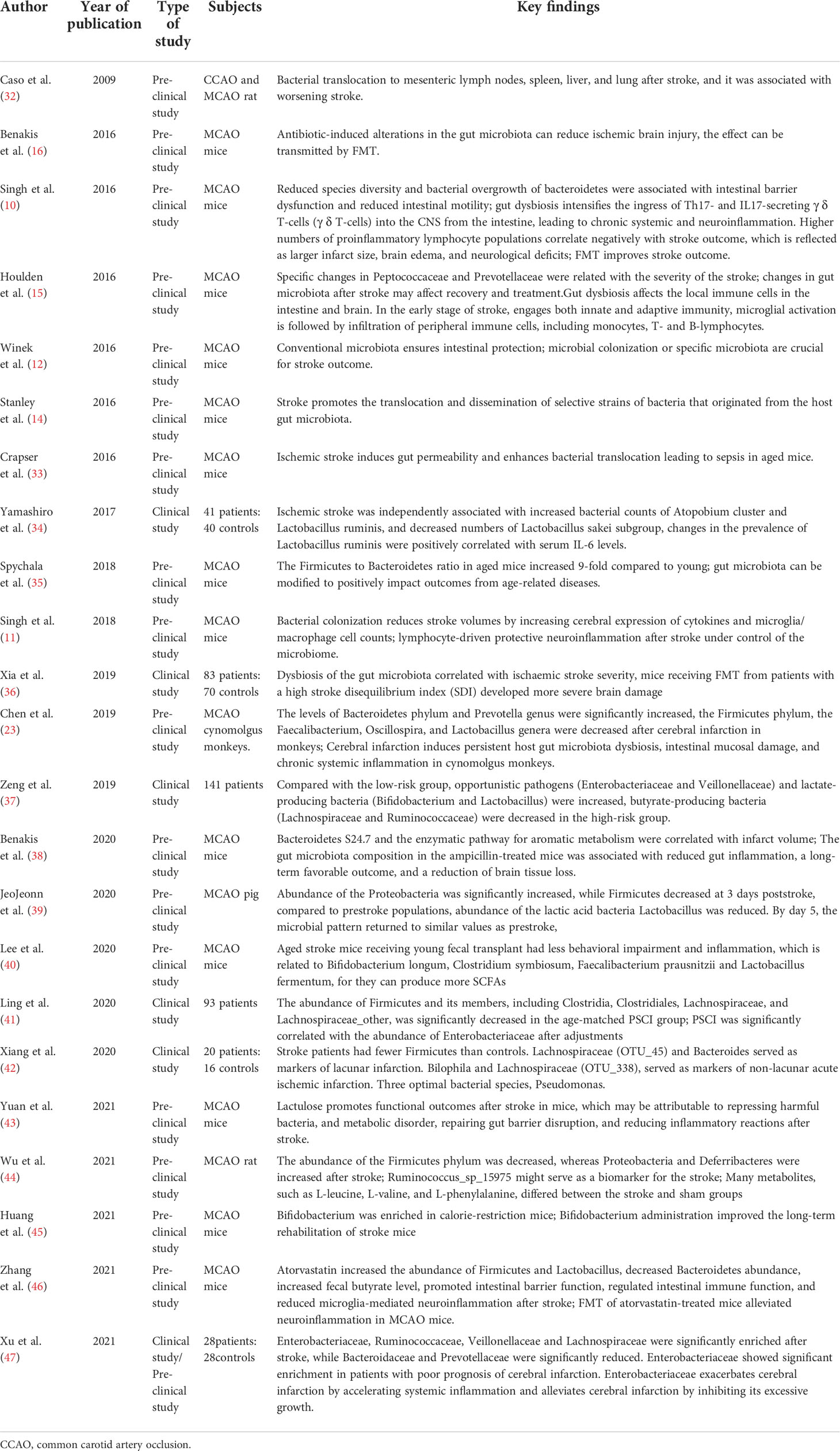
Table 1 Summarizes the pre-clinical and clinical evidences regarding the relationship between gut microbiota and ischemic stroke.
Acute ischemia stroke is characterized by loss of species diversity and overgrowth of opportunistic pathogens. A previous study has shown that acute ischemia stroke patients can develop significant gut microbiota disturbances at 3 days post-stroke, which returned to similar levels pre-stroke by day 5 (39). However, cerebral ischemia can induce persistent gut microbiota dysbiosis, disrupt the gut barrier, and lead to chronic systemic inflammation of the host, which is associated with worsening stroke and neurodegenerations. One study demonstrated that gut dysbiosis could last for more than 3 weeks after stroke, and then the disturbed gut microbiota could gradually recover but microbiota diversity was still decreased significantly after 4 weeks (36). Gut microbiota dysbiosis in Cynomolgus monkeys is still observed 6 and 12 months after cerebral ischemia, with notably increased Bacteroidetes phylum and Prevotella genus and significantly reduced Firmicutes phylum, Faecalibacterium, Oscillospira, and Lactobacillus genera, accompanied by a significant increase in levels of plasma D-lactate, zonulin, LPS, TNF-α, IFN-γ, IL-6 and a significant decrease in levels of SCFAs (23).
Alterations of levels of tryptophan metabolites and AHR after ischemia stroke
Tryptophan metabolism in the gastrointestinal tract can be regulated by three main pathways, i.e., the kynurenine pathway, serotonin pathway, and indol pathway. Approximately 90% of ingested tryptophan is degraded through the kynurenine pathway in immune and epithelial cells (53, 54). More specifically, tryptophan is transferred into the brain crossing the blood-brain barrier (BBB); then, two key enzymes in the kynurenine pathway, indoleamine-2,3-dioxygenase (IDO) and tryptophan-2,3-dioxygenase (TDO), metabolize L-tryptophan into L-Kyn (55, 56), which plays a key role in this pathway. L-Kyn can be further catabolized in two types of cells, astrocytes and microglia, in the brain. In astrocytes, L-Kyn was transformed into kynurenic acid (KYNA) under the catalyzation of kynurenine aminotransferase (KAT) family enzymes. KYNA is a well-recognized N-methyl-D-aspartate receptor (NMDAR) antagonist and is thought to be neuroprotective (57). In microglia, L-Kyn can be hydroxylated by kynurenine 3-monooxygenase (KMO) to generate 3-HK and its major metabolites, such as quinolinic acid (QUIN), which is considered to be an NMDAR agonist with neurotoxic properties (58). Both QUIN and KYNA act on NMDAR in the postsynaptic membrane of neurons. L-Kyn is shown to be a key AHR ligand and is associated with ischemia stroke severity and prognosis (18).
Preclinical and clinical studies have shown altered kynurenine pathway and tryptophan catabolism after cerebral ischemia. An increased level of brain QUIN was observed in gerbils, which was mediated by the activation of IDO, KYN, and 3-HK after transient ischemic attack (TIA), ultimately leading to an abnormal increase in the QUIN/KYNA ratio, which might contribute to the progression of post-stroke injury (59–63). QUIN is primarily detected in microglia and infiltrating macrophages 2-7 days after cerebral ischemia, which is consistent with a peak in immune infiltration, glial activation and inflammation during this period (62). An altered kynurenine pathway metabolism was observed in a permanent middle cerebral artery occlusion (MCAO) mouse model after ischemia stroke (22). The level of L-Kyn was increased in the brain as early as 3 hours after MCAO and remained at an increased level for 24 hours, in contrast to a decrease in L-tryptophan level between 3 and 24 hours and slight changes in plasma L-Kyn or L-Trp. An increase in AHR protein level, nuclear translocation and transcriptional activity of cortical neurons in this mouse model was also observed. In addition, the L-Kyn/L-Trp ratio is much higher in stroke patients than that in healthy controls and is positively correlated with infarct volume (63). The most common long-term complications after ischemia stroke are dementia and depression. A study found that abnormal alterations in kynurenine pathway catabolism persisted for at least 1 year after stroke, suggesting that it might be the cause of persistent brain dysfunction in these patients (64). The association between cognitive impairment and the kynurenine pathway after ischemia stroke has been described in only one study (65). Decreased levels of 5-HT and increased levels of kynurenine pathway catabolites have been reported in post-stroke patients with depression, and activation of key enzymes in the kynurenine pathway can lead to increased production of 3-HK, QUIN, L-Kyn, and KYNA (66), which induce the production of neurotoxic agents (67, 68). Finally, these alterations can cause damages to multiple brain regions such as the hippocampus, inhibiting neurogenesis and activating apoptotic signaling pathways, and thus leading to depression (69), which has been referred to as the kynurenine hypothesis of depression (70). However, no correlations between depressive symptoms in post-stroke populations and blood L-Kyn/L-Trp ratios have been found (71, 72).
Gut microbiota affects levels of tryptophan metabolites and AHR
Gut microbes can metabolize tryptophan through several metabolic pathways and produce various tryptophan metabolites (73). For instance, some bacterial species, such as Escherichia coli, Clostridium spp. Bacteroides spp. Clostridium sporogenes, Peptostreptococcus spp. Peptostreptococcus russellii, Peptostreptococcus anaerobius and Peptostreptococcus stomatis, Clostridium botulinum, and Peptostreptococcus anaerobius, can produce indole propionic acid (IPA), indoleacetic acid (ILA), and indoleacetic acid (IA). While other species, such as Lactobacilli, Ruminococcus gnavus, Clostridium bartlettii, and Bifidobacterium spp., can produce indole aldehydes (IAld), indoleacetic acid (IAA), and ILA. Some others, such as Bacteroides spp. and Clostridium spp., can produce 3-methylindole (skatole) by decarboxylation of IAA.
Gut microbiota can directly or indirectly metabolize tryptophan, generating various metabolites, including indole, tryptamine, indole ethanol (IE), IPA, ILA, IAA, skatole, IAld and IA. Some of them, including Indole, IPA, and IA, can reduce intestinal permeability by disrupting mucosal homeostasis. Several other tryptophan catabolic products can regulate innate and adaptive immune responses by binding to AHR in intestinal immune cells. For example, IAld can increase IL-22 production by activating the AHR signaling pathway. Some other tryptophan metabolites, such as IPA, IE and IA, can be absorbed into the blood through the intestinal epithelium and exert antioxidant and anti-inflammatory effects (73). Tryptophan catabolic products, including IAA, IA, IAld, ILA, tryptamine, and skatole, are all ligands for AHR (74–77). Some agonists can facilitate AHR in crossing the BBB. In astrocytes and microglia, AHR can inhibit pro-inflammatory nuclear factor-κB (NF-κB) signaling, thus interfering chemokine production and transcriptional programs associated with inflammatory monocyte recruitment, and activating CNS-resident myeloid cells and producing direct neurotoxicity to regulate CNS inflammation (5).
The role of AHR in ischemia stroke
The basic characteristics of AHR
AHR is a ligand-controlled transcription factor (5), which is implicated in multiple physiological and pathological processes of many diseases, including inflammatory bowel disease (78), metabolic syndrome, and CNS diseases (79, 80). Expression of AHR is widely detected in the CNS, such as in neurons, oligodendrocytes, monocytes/macrophages, astrocytes, microglia, and cerebral endothelial cells (81). AHR can regulate the expressions of target genes which relate to cell proliferation, metabolism and immune response (82). Significant upregulation of AHR expression after stroke has been reported, which is shown to play a role in the cerebral ischemic injury (22, 83–86) (Table 2). In addition, the integrity of the BBB is also compromised upon activation of the AHR signaling (87–89). The BBB is essential for maintaining CNS homeostasis, and impairment of BBB is thought to contribute to neurodegeneration, leading cognitive impairment in humans (90).
The role of AHR in the neurological and immune systems has received increasing attention (91). The role of neuroinflammation in acute and chronic ischemia stroke has also been recognized (92). In fact, one of the pathological features of neurodegenerative diseases is neuroinflammation, mainly manifested by chronic activation of microglia (93). AHR can mediates inflammatory effects of microglia through dietary and microbial metabolites, particularly tryptophan metabolites (5, 93). Given the links between tryptophan metabolism, AHR and immune cell activation (94), we will highlight the role of the AHR signaling pathway (i.e., tryptophan metabolites as AHR ligand can bind to AHR) in ischemia stroke and potential targets for pharmacological modulation of ischemia stroke, in the following discussions.
AHR in acute phases of ischemia stroke
Cuartero et al. used mouse models to verify the hypothesis that activation of the L-Kyn-AHR signaling pathway can exacerbate acute ischemic brain injury (22). They identified increased AHR protein level, nuclear translocation and transcriptional activity of cortical neurons in a permanent MCAO mouse model. In the core of the infarct, the AHR protein level rose to a peak at around 5 hours after stroke and returned to baseline levels by day 7 after stroke; in the peri-infarct area, the AHR protein level started to increase at 18 hours after stroke and reached the peak at day 3 after stroke and then started to decrease. Treating with an AHR antagonist or using AHR-deficient mice resulted in a smaller infarct size and lower National Institutes of Health Stroke Scale (NIHSS) in mice model (22). However, another group showed an opposite result when treating ischemia stroke using the AHR agonists. Mechanistically, activation of the AHR signaling during cerebral ischemia may mediate specific pathological effects by inhibiting the cAMP response element-binding protein (CREB) signaling pathway. Further experiments demonstrated that L-Kyn could accumulate in the brain during acute ischemia stroke and act as an endogenous activator of AHR. Exogenous supplementation of L-Kyn aggravates strokes in an AHR-dependent manner and increases infarct volume. Most interestingly, the authors also demonstrated that inhibition of L-Kyn production by pharmacological blockade of TDO could decrease the activation of AHR signaling and reduce infarct volume in the MCAO stroke model. Taken together, this study identified the L-Kyn-AHR pathway as a novel mediator of brain injury during stroke, and validated TDO and AHR as new “druggable” targets for acute ischemia stroke.
Another study suggested that AHR inhibition in acute ischemia stroke might be benefits regarding functional outcomes through reducing pro-inflammatory glial cell proliferation and promoting neurogenesis. Compared to respective controls, wild-type (WT) and AHRcKO mice that were treated with the AHR antagonist, 6,2’,4’-trimethoxyflavone (TMF), showed significantly smaller infarct volumes and improved sensorimotor and non-spatial working memory functions. AHR Immunoreactivity was increased mainly in activated microglia and astrocytes after AHRcKO. TMF-treated WT and AHRcKO mice showed remarkably increased astrocyte and microglia proliferation (28). In a cerebral ischemia-reperfusion injury (CIRI) rat model, TMF-treated rats displayed lower cell apoptosis levels and smaller infarct volumes than those not treated with TMF at 24 h after cerebral ischemia, which were most pronounced in the 10 min and 50 min after stroke. This study indicated that the AHR antagonists might reduce CIRI-related cellular injury.
AHR in chronic ischemia stroke
Ischemia stroke can induce long-term host gut microbiota dysbiosis, impairing the intestinal barrier and leading to chronic neuroinflammation. This inflammatory response is associated with cognitive impairment, depression, and anxiety in post-stroke patients (28). One year after FMT, elevated plasma pro-inflammatory cytokines, such as IFN-g, IL-6 and TNF-α, were decreased in focal cerebral ischemia of monkey models, suggesting the persistence of systemic inflammation post ischemia stroke (23). Numerous studies have shown that resident inflammatory cells and microglia can first respond to CIRI and amplify neuroinflammation by interacting with astrocytes (95–98). The inflammatory response in the brain of rats surviving 2 years after ischemic brain injury was evident but varied in the extent regarding microglia and astrocyte responses in different brain tissues (25). In another rat model of dementia in which the rats survived 2 years after cerebral ischemia, it was shown that this neuroinflammatory process was mainly regulated by microglia and astrocyte activity. In conclusion, microglia and astrocytes play an important role in post-stroke neurodegeneration (99).
Stroke injuries can induce the activation of microglia, which are generally classified into detrimental M1 and protective M2 subtypes (Figure 2). M1 microglia can secrete pro-inflammatory cytokines while M2 microglia can secrete anti-inflammatory cytokines. M2 microglia can stimulate neural stem/progenitor cell proliferation and neuronal differentiation in the ipsilateral subventricular zone through upregulation of TGFα expression levels. Studies have shown that in acute ischemia stroke, activated microglia predominantly express M2 phenotypic markers. However, there is a gradual shift to the M1 phenotype at around 1 week since the acute initiation of ischemia stroke, which persists for several weeks or even months. The sustained activation microglia is also thought to be associated with the onset and progression of neurodegenerative diseases (100). NF-κB, which is a key molecule in the microglia inflammatory pathway, induces activation and polarization of M1 microglia (101). Astrocytes can proliferate reactively after ischemic stroke. Liddelow et al. classified these astrocytes into the A1 and A2 subtypes, which are neurodamaging and neuroprotective, respectively (102). AHR plays an important role in activating microglia and activating astrocytes, which participate in the pro-inflammatory and anti-inflammatory processes, respectively. AHR inhibits the pro-inflammatory NF-κB signaling pathway while deletion of AHR or AHR ligands in microglia results in a dysregulated inflammatory response. Microglia and astrocytes intercommunicate with each other in many ways and may also be involved in the “gut-microbiota-brain” axis (103). Based on the fact that gut microbial metabolites can affect the CNS via the AHR-dependent signaling pathway, role of the commensal microbiota-mediated AHR signaling in the regulation of inflammation-promoting activity mediated by microglia and astrocytes has been investigated in recent years. Agonists derived from diet, gut microbiota and host metabolism can activate the AHR through the BBB. The AHR promotes TGFα expression in microglia, which acts on astrocytes and inhibits their pro-inflammatory activity. Further, AHR on microglia inhibits NF-κB-driven vascular endothelial growth factor B (VEGFB) expression, thereby promoting astrocytes to exert anti-inflammatory activity (104). Gut microbiota dysbiosis after stroke leads to abnormal tryptophan metabolism, and the decreased levels of AHR agonists may lead to enhanced neuroinflammation.
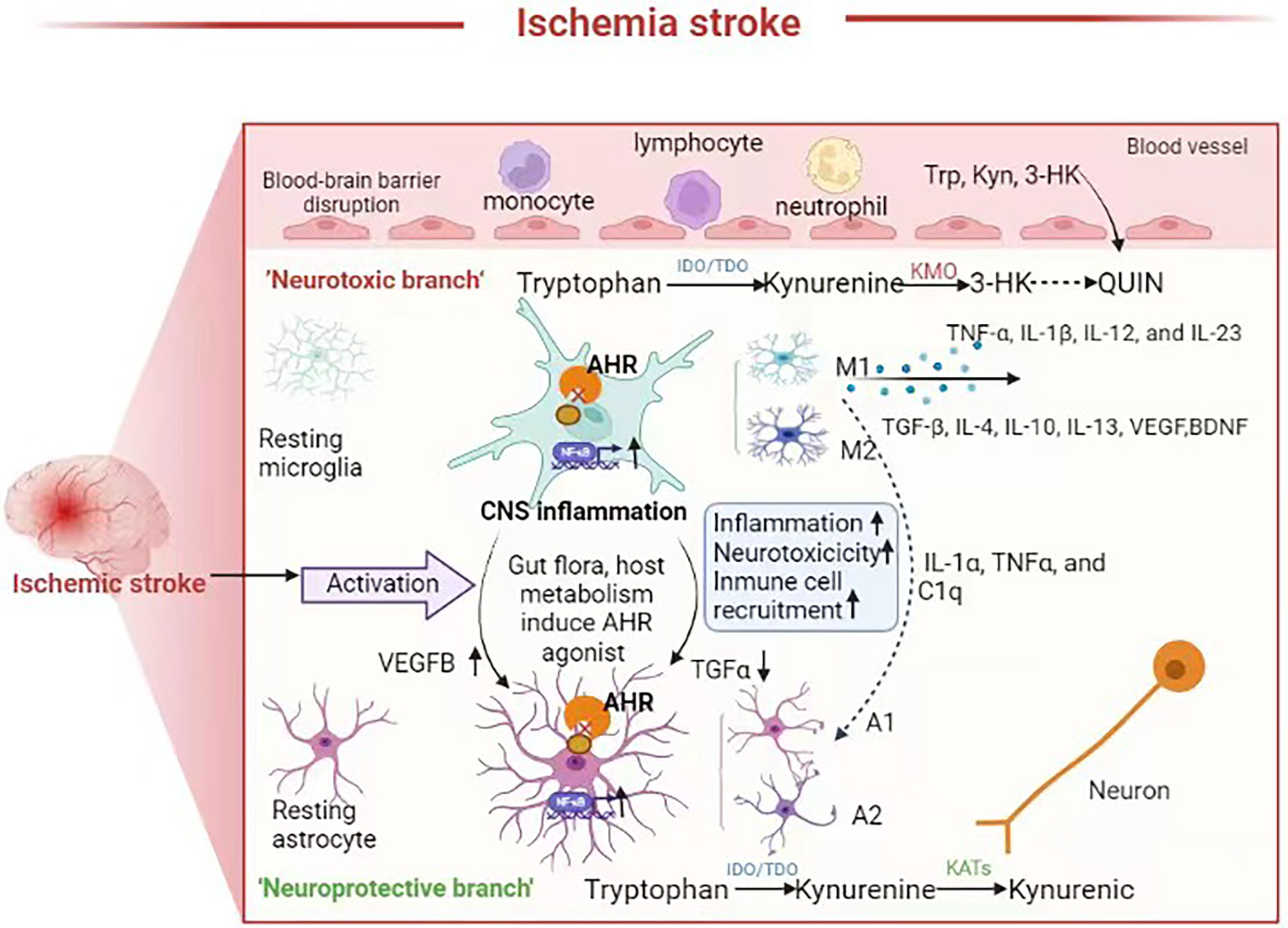
Figure 2 Neuroinflammation in the brain after stroke, the role of AHR and tryptophan metabolites in neuroinflammation. Microglia and astrocytes are activated and interact with each other to mediate neuroinflammation following ischemic stroke Some metabolites such as 3-HK, Kyn, QUIN produced by tryptophan metabolism can cross the BBB plays a neuroprotective or neurotoxic role. Gut flora and the host tryptophan metabolism produce AHR agonist. In astrocytes and microglia, AHR can inhibit pro-inflammatory nuclear factor-κB (NF-κB) signaling, and reduction of AHR agonists after gut microbiota dysbiosis result in an upregulated neuroinflammation and neurotoxic responses and immune cell recruitment, which are amplified through microglia-astrocyte interactions.
AHR as a potential therapeutic target for treatment of ischemia stroke
As previously described, modulation of the AHR signaling may provide new therapeutic strategy to attenuate neuronal damage after acute ischemia stroke and prevent the development of post-stroke neurodegeneration, thereby improving the short- and long-term ischemia stroke prognosis. In the permanent MCAO mouse model, L-Kyn mediates ischemic neuronal injury as an endogenous activator of AHR (22). Therefore, pharmacological inhibition of the kynurenine pathway or activation of the AHR pathway in acute ischemia stroke might prevent neurological injury. On the one hand, early administration of TMF, an AHR antagonist, can be a simple approach for the treatment of acute ischemia stroke. On the other hand, synthesis of L-Kyn under the action of TDO as the primary pathway in ischemic brain tissue and inhibition of L-Kyn production by the TDO inhibitor compromises the activation of the AHR signaling, leading to reduced infarct volume. Interestingly, pharmacological blockade of IDO, another key enzyme in L-Kyn production, by the IDO inhibitor 1-MT, does not show a beneficial effect in reducing infarct size and improving neurological prognosis, despite increased IDO expression and activity in transient MCAO mouse model (105).
AHR can mediate the inflammatory response in glial cells of the CNS (106). Dietary and microbial metabolites, particularly tryptophan metabolites, have recently been shown to act as AHR activators and thus regulate microglia and astrocyte activity and neuroinflammation in the CNS (5, 94). These studies linked the gut microbiota to neurological inflammation in the brain via the AHR signaling pathway. In mice with autoimmune encephalomyelitis, the AHR signaling was activated in astrocytes, which was proven to limit the inflammatory response in astrocytes. Moreover, this anti-inflammatory response could become increasingly evident when dietary tryptophan was ingested by mice. To demonstrate that this effect is regulated by microbiota-mediated tryptophan metabolites, a broad-spectrum antibiotic-ampicillin was applied to the mice to clear their gut microbiota, followed by treatment of the mice with indirubin-3’-oxime, a microbial metabolite of tryptophan. As a result, AHR-mediated anti-inflammatory effects were observed (5), indicating the effect is indeed mediated by tryptophan metabolites. Indirubin-3’-oxime has also been shown to inhibit the inflammatory activation in microglia in the rat brain (107). Lactobacillus was found to be an important host probiotic, and its levels were reduced after cerebral ischemia in monkeys (23). There are also studies showing that Lactobacillus supplementation can improve cognitive function and mood and reduce aging-related inflammation in mice and rats (28, 108). Lactobacillus casei subsp. casei 327 (327 strain) can indirectly promote colonic 5-HT synthesis (109). Lactobacillus reuteri can degrade tryptophan into indolic compounds, such as IAld, ILA, and IAA (74, 110). A decreasing trend in serum kynurenine: tryptophan ratios was observed in humans after 8 weeks of oral administration of Lactobacillus johnsonii (111). As an important source of essential amino acids, diet is considered to be an important factor in shaping microbial tryptophan metabolism. A recent study indicated that the microbial tryptophan degradation pathway could be weakened under a high-fat diet (112). In addition, increasing carbohydrate availability promotes intestinal serotonin synthesis (113). Thus, probiotic supplementation and a reasonable diet can theoretically improve ischemia stroke prognosis; however, whether it is indeed beneficial in post-stroke patients needs to be tested in future clinical trials. Ramos et al. showed that the function of AHR and its downstream signaling pathways are impaired in the elderly and AD patients (114). The role of AHR ligands in improving learning memory deficits was also confirmed in a mouse model (104). Activation of the AHR signaling pathway by endogenous ligands such as L-Kyn and 6-Formylindolo[3,2-b]carbazole (FICZ), or exogenous ligands such as diosmin and indole-3-carbinol, could increase the expression and enzymatic activity of neprilysin in amyloid precursor protein/presenilin 1 (APP/PS1) mice, and improve cognitive impairment effectively in these mice. Tryptophan metabolites, such as 5-hydroxy indole-acetic acid and kynurenic acid, could reduce cognitive impairment in mice and Aβ load in patients with mild cognitive impairment by activating AHR (115–118).
Present shortcomings and future perspectives
New therapies, such as the application of recombinant thrombolytic tissue plasminogen activator (r-tPA) and intra-arterial thrombectomy, have been developed for the treatment of acute ischemia stroke (119). However, due to the narrow time windows and the limitations of endovascular treatment techniques, only a small number of patients with acute ischemia stroke can benefit from these new therapies. There are limited treatment options for patients with subacute and chronic ischemia stroke. In light of this, AHR can be used as a potential therapeutic target for the treatment of these patients (85). Inhibition of AHR signaling in acute ischemia stroke has the potential to benefit the patients by reducing pro-inflammatory gliosis and enhancing neurogenesis. In contrast, tryptophan metabolites, as the AHR ligands, can interact with microglia and astrocytes and prevent neurodegeneration. Supplementation of tryptophan metabolites, probiotics producing AHR agonists, and FMT from normal donors may be potential therapeutic strategies that can improve the prognosis of certain types of ischemia stroke. Delivering drugs to the brain directly has long been a major challenge in treating neurodegeneration, and thus these proposed strategies might overcome this barrier.
However, there is still a long way to go for researchers despite the substantial progress. Firstly, the composition and immunological characteristics of human gut microbiota are not completely the same as those of animals such as mice. Secondly, the effects of intestinal fungi and protozoa on tryptophan metabolism and severity of ischemia stroke are unclear. Whether there are other endogenous or exogenous AHR ligands besides tryptophan that have not been identified and whether there are any other endogenous inhibitors of the AHR pathway are unknown as well. Tryptophan can also directly be absorbed by the host gut and the complex interactions between intestinal flora, intestinal lumen tryptophan availability, and host tryptophan metabolism need further study. Thirdly, it requires validation that whether the results from animal studies could be used for the effective treatment of human diseases such as ischemia stroke. Developing a humanized mouse model might help explain the well-known differences regarding AHR between humans and mice. Finally, large-scale, highly controlled clinical studies are urgently needed to further validate the role of AHR in ischemia stroke development.
Conclusion
The role of AHR and tryptophan metabolism in the communication between the gut microbiota and CNS has been increasingly well known. Tryptophan metabolism is directly or indirectly regulated by the gut microbiota and many tryptophan metabolites can act as endogenous AHR activators, activating AHR, which can further regulate neuroinflammation by interacting with microglia and astrocytes. Since many factors can affect the gut microbiota composition and metabolism, including diet, antibiotics, and probiotics, as well as FMT, the manipulation of the gut microbiota modulating tryptophan availability may be a therapeutic method for neuroinflammation after ischemia stroke. In conclusion, we argue that the AHR and tryptophan metabolism play an important role in ischemia stroke.
Author contributions
Conception and design: SW and XF; writing of the manuscript: XF; final approval of the manuscript: HZ. All authors read and approved the final manuscript.
Funding
This work was supported by the Construction Fund of Medical Key Disciplines of Hangzhou (discipline number OO20200485).
Conflict of interest
The authors declare that the research was conducted in the absence of any commercial or financial relationships that could be construed as a potential conflict of interest.
Publisher’s note
All claims expressed in this article are solely those of the authors and do not necessarily represent those of their affiliated organizations, or those of the publisher, the editors and the reviewers. Any product that may be evaluated in this article, or claim that may be made by its manufacturer, is not guaranteed or endorsed by the publisher.
References
1. Johnson W, Onuma O, Owolabi M, Sachdev S. Stroke: A global response is needed. Bull World Health Organ (2016) 94(9):634–634A. doi: 10.2471/BLT.16.181636
2. Parr E, Ferdinand P, Roffe C. Management of acute stroke in the older person. Geriatrics (2017) 2(3):27–. doi: 10.3390/geriatrics2030027
3. Minter MR, Zhang C, Leone V, Ringus DL, Zhang XQ, Oyler-Castrillo P, et al. Antibiotic-induced perturbations in gut microbial diversity influences neuro-inflammation and amyloidosis in a murine model of alzheimer’s disease. Sci Rep (2016) 6:30028. doi: 10.1038/srep30028
4. Sampson TR, Debelius JW, Thron T, Janssen S, Shastri GG, Ilhan ZE, et al. Gut microbiota regulate motor deficits and neuroinflammation in a model of parkinson’s disease. Cell (2016) 167:1469.e12–1480.e12. doi: 10.1016/j.cell.2016.11.018
5. Rothhammer V, Mascanfroni ID, Bunse L, Takenaka MC, Kenison JE, Mayo L, et al. Type I interferons and microbial metabolites of tryptophan modulate astrocyte activity and central nervous system inflammation via the aryl hydrocarbon receptor. Nat Med (2016) 22:586–97. doi: 10.1038/nm.4106
6. Buffington SA, Di Prisco GV, Auchtung TA, Ajami NJ, Petrosino JF, Costa-Mattioli M. Microbial reconstitution reverses maternal diet-induced social and synaptic deficits in offspring. Cell (2016) 165:1762–75. doi: 10.1016/j.cell.2016.06.001
7. Neufeld KM, Kang N, Bienenstock J, Foster JA. Reduced anxiety-like behavior and central neurochemical change in germ-free mice. Neurogastroenterol Motil (2011) 23:255, e119–264, e119. doi: 10.1111/j.1365-2982.2010.01620.x
8. Van de Wouw M, Boehme M, Lyte JM, Wiley N, Strain C, O'Sullivan O, et al. Short-chain fatty acids: Microbial metabolites that alleviate stress-induced brain-gut axis alterations. J Physiol (2018) 596:4923–44. doi: 10.1113/JP276431
9. Yin J, Liao SX, He Y, Wang S, Xia GH, Liu FT, et al. Dysbiosis of gut microbiota with reduced trimethylamine-n-oxide level in patients with large-artery atherosclerotic stroke or transient ischemic attack. J Am Heart Assoc (2015) 4(11):e002699–13. doi: 10.1161/JAHA.115.002699
10. Singh V, Roth S, Llovera G, Sadler R, Garzetti D, Stecher B, et al. Microbiota dysbiosis controls the neuroinflammatory response after stroke. J Neurosci (2016) 36:7428–40. doi: 10.1523/JNEUROSCI.1114-16.2016
11. Singh V, Sadler R, Heindl S, Llovera G, Roth S, Benakis C, et al. The gut microbiome primes a cerebroprotective immune response after stroke. J Cereb Blood Flow Metab (2018) 38:1293–8. doi: 10.1177/0271678X18780130
12. Winek K, Engel O, Koduah P, Heimesaat MM, Fischer A, Bereswill S, et al. Depletion of cultivatable gut microbiota by broad-spectrum antibiotic pretreatment worsens outcome after murine stroke. Stroke (2016) 47:1354–63. doi: 10.1161/STROKEAHA.115.011800
13. Benakis C, Brea D, Caballero S, Faraco G, Moore J, Murphy M, et al. Commensal microbiota affects ischemic stroke outcome by regulating intestinal γδ T cells. Nat Med (2016) 22:516–23. doi: 10.1038/nm.4068
14. Stanley D, Mason LJ, Mackin KE, Srikhanta YN, Lyras Y, Prakash MD, et al. Translocation and dissemination of commensal bacteria in post- stroke infection. Nat Med (2016) 22:1277–84. doi: 10.1038/nm.4194
15. Houlden A, Goldrick M, Brough D, Vizi ES, Lénárt N, Martinecz B, et al. Brain injury induces specific changes in the caecal microbiota of mice via altered autonomic activity and mucoprotein production. Brain Behav Immun (2016) 57:10–20. doi: 10.1016/j.bbi.2016.04.003
16. Cox LM, Weiner HL. Microbiota signaling pathways that influence neurologic disease. Neurotherapeutics (2018) 15:135–45. doi: 10.1007/s13311-017-0598-8
17. Stanley D, Moore RJ, Wong CHY. An insight into intestinal mucosal microbiota disruption after stroke. Sci Rep (2018) 8:568. doi: 10.1038/s41598-017-18904-8
18. Cuartero MI, de la Parra J, García-Culebras A, Ballesteros I, Lizasoain I, Moro MÁ. The kynurenine pathway in the acute and chronic phases of cerebral ischemia Curr Pharm Des (2016) 22(8):1060–73. doi: 10.2174/1381612822666151214125950
19. Pluta R, Januszewski S, Czuczwar SJ. The role of gut microbiota in an ischemic stroke. Int J Mol Sci (2021) 22:915. doi: 10.3390/ijms22020915
20. Zhao C, Hu X, Bao L, et al. Aryl hydrocarbon receptor activation by lactobacillus reuteri tryptophan metabolism alleviates escherichia coli-induced mastitis in mice. PloS Pathog (2021) 17(7):e1009774. doi: 10.1371/journal.ppat.1009774
21. Ma N, He T, Johnston LJ, Ma X. Host-microbiome interactions: the aryl hydrocarbon receptor as a critical node in tryptophan metabolites to brain signaling. Gut Microbes (2020) 11(5):1–17. doi: 10.1080/19490976.2020.1758008
22. Cuartero MI, Ballesteros I, de la Parra J, Harkin AL, Abautret-Daly A, Sherwin E, et al. L-Kynurenine/Aryl hydrocarbon receptor pathway mediates brain damage after experimental stroke. Circulation (2014) 130(23):2040–51. doi: 10.1161/CIRCULATIONAHA.114.011394
23. Chen Y, Liang J, Ouyang F, Chen XR, Lu T, Jiang ZM, et al. Persistence of gut microbiota dysbiosis and chronic systemic inflammation after cerebral infarction in cynomolgus monkeys. Front Neurol (2019) 10:661. doi: 10.3389/fneur.2019.00661
24. Mok VCT, Lam BYK, Wong A, Ko H, Markus HS, Wong LKS. Early-onset and delayed-onset poststroke dementia–revisiting the mechanisms. Nat Rev Neurol (2017) 13:148–59. doi: 10.1038/nrneurol.2017.1641
25. Robinson RG, Jorge RE. Post-stroke depression: A review. Am J Psychiatry (2016) 173:221–31. doi: 10.1176/appi.ajp.2015.15030363
26. Xu S, Lu J, Shao A, Zhang JH, Zhang J. Glial cells: Role of the immune response in ischemic stroke. Front Immunol (2020) 11:294. doi: 10.3389/fimmu.2020.00294
27. Kim JY, Park J, Chang JY, Kim S-H, Lee JE. Inflammation after ischemic stroke: The role of leukocytes and glial cells. Exp Neurobiol (2016) 25(5):241. doi: 10.5607/en.2016.25.5.241
28. Chesnokova V, Pechnick RN, Wawrowsky K. Chronic peripheral inflammation, hippocampal neurogenesis, and behavior. Brain Behav Immun (2016) 58:1–8. doi: 10.1016/j.bbi.2016.01.017
29. Pan F, Zhang L, Li M, Hu YX, Zeng BH, Yuan HJ, et al. Predominant gut lactobacillus murinus strain mediates anti-inflammaging effects in calorie-restricted mice. Microbiome (2018) 6(1):54. doi: 10.1186/s40168-018-0440-5
30. Hwang YH, Park S, Paik JW, Chae SW, Kim DH, Jeong DG, et al. Efficacy and safety of lactobacillus plantarum C29-fermented soybean (DW2009) in individuals with mild cognitive impairment: A 12-week, multi-center, randomized, double-blind, placebo-controlled clinical trial. Nutrients (2019) 11(2):305. doi: 10.3390/nu11020305
31. Martin CR, Osadchiy V, Kalani A, Mayer EA. The brain-Gut-Microbiome axis. Cell Mol Gastroenterol Hepatol (2018) 6(2):133–48. doi: 10.1016/j.jcmgh.2018.04.003
32. Caso JR, Hurtado O, Pereira MP, García-Bueno B, Menchén L, Alou L, et al. Colonic bacterial translocation as a possible factor in stress-worsening experimental stroke outcome. AJP: Regulatory Integr Comp Physiol (2009) 296(4):R979–85. doi: 10.1152/ajpregu.90825.2008
33. Crapser J, Ritzel R, Venna V, Venna VR, Liu FD, Chauhan A, et al. Ischemic stroke induces gut permeability and enhances bacterial translocation leading to sepsis in aged mice. Aging (2017) 8(5):1049–63. doi: 10.18632/aging.100952
34. Yamashiro K, Tanaka R, Urabe T, Ueno Y, Yamashiro Y, Nomoto K, et al. Gut dysbiosis is associated with metabolism and systemic inflammation in patients with ischemic stroke. PloS One (2017) 12(2):e0171521. doi: 10.1371/journal.pone.0171521
35. Spychala MS, Venna VR, Jandzinski M, Doran SJ, Durgan DJ, Ganesh BP, et al. Age-related changes in the gut microbiota influence systemic inflammation and stroke outcome. Ann Neurol (2018) 84(1):23–36. doi: 10.1002/ana.25250
36. Xia GH, You C, Gao XX, Zeng XL, Zhu JJ, Xu KY, et al. Stroke dysbiosis index (SDI) in gut microbiome are associated with brain injury and prognosis of stroke. Front Neurol (2019) 24:397. doi: 10.3389/fneur.2019.00397
37. Zeng X, Gao X, Peng Y, Wu QH, Zhu JJ, Tan CH, et al. Higher risk of stroke is correlated with increased opportunistic pathogen load and reduced levels of butyrate-producing bacteria in the gut. Front Cel Infect Microbiol (2019) 9:4. doi: 10.3389/fcimb.2019.00004
38. Benakis C, Poon C, Lane D, Brea D, Sita G, Moore J, et al. Distinct commensal bacterial signature in the gut is associated with acute and long-term protection from ischemic stroke. Stroke (2020) 51(6):1844–54. doi: 10.1161/STROKEAHA.120.029262
39. Jeon J, Lourenco J, Kaiser EE, Waters ES, Scheulin KM, Fang X, et al. Dynamic changes in the gut microbiome at the acute stage of ischemic stroke in a pig model. Front Neurosci (2020) 14:587986. doi: 10.3389/fnins.2020.587986
40. Lee J, d'Aigle J, Atadja L, Quaicoe V, Honarpisheh P, Ganesh BP, et al. Gut microbiota-derived short-chain fatty acids promote poststroke recovery in aged mice. Circ Res (2020) 127(4):453–65. doi: 10.1161/CIRCRESAHA.119.316448
41. Ling Y, Gong T, Zhang J, Gu QL, Gao XX, Weng XP, et al. Gut microbiome signatures are biomarkers for cognitive impairment in patients with ischemic stroke. Front Aging Neurosci (2020) 12:511562. doi: 10.3389/fnagi.2020.511562
42. Xiang L, Lou Y, Liu L, Liu YL, Zhang WZ, Deng JX, et al. Gut microbiotic features aiding the diagnosis of acute ischemic stroke. Front Cell Infect Microbiol (2020) 10:587284. doi: 10.3389/fcimb.2020.587284
43. Yuan Q, Xin L, Han S, Su Y, Wu RX, Liu XX, et al. Lactulose improves neurological outcomes by repressing harmful bacteria and regulating inflammatory reactions in mice after stroke. Front Cell Infect Microbiol (2021) 11:644448. doi: 10.3389/fcimb.2021.644448
44. Wu W, Sun Y, Luo N, Cheng C, Jiang C, Yu Q, et al. Integrated 16S rrna gene sequencing and LC-MS analysis revealed the interplay between gut microbiota and plasma metabolites in rats with ischemic stroke. J Mol Neurosci (2021) 71(10):2095–106. doi: 10.1007/s12031-021-01828-4
45. Huang Q, Di L, Yu F, Feng XJ, Liu ZY, Wei MP, et al. Alterations in the gut microbiome with hemorrhagic transformation in experimental stroke. CNS Neurosci Ther (2022) 28(1):77–91. doi: 10.1111/cns.13736
46. Zhang J, Wang L, Cai J, Lei A, Liu C, Lin R, et al. Gut microbial metabolite TMAO portends prognosis in acute ischemic stroke. J Neuroimmunol (2021) 354:577526. doi: 10.1016/j.jneuroim.2021.577526
47. Xu K, Gao X, Xia G, Chen MX, Zeng NY, Wang S, et al. Rapid gut dysbiosis induced by stroke exacerbates brain infarction in turn. Gut (2021) 8:gutjnl-2020-323263. doi: 10.1136/gutjnl-2020-323263
48. Turnbaugh PJ, Hamady M, Yatsunenko T, Cantarel BL, Duncan A, Ley RE, et al. A core gut microbiome in obese and lean twins. Nature (2009) 457(7228):480–4. doi: 10.1038/nature07540
49. Karbach SH, Schönfelder T, Brandão I, Wilms E, Hörmann N, Jäckel S, et al. Gut microbiota promote angiotensin II-induced arterial hypertension and vascular dysfunction. J Am Heart Assoc (2016) 5(9):e003698. doi: 10.1161/jaha.116.003698
50. Wen L, Ley RE, Volchkov PY, Stranges PB, Avanesyan L, Stonebraker AC, et al. Innate immunity and intestinal microbiota in the development of type 1 diabetes. Nature (2008) 455(7216):1109–13. doi: 10.1038/nature07336
51. Martínez I, Wallace G, Zhang C, Legge R, Benson AK, Carr TP, et al. Diet-induced metabolic improvements in a hamster model of hypercholesterolemia are strongly linked to alterations of the gut microbiota. Appl Environ Microbiol (2009) 75(12):4175–84. doi: 10.1128/AEM.00380-09
52. Jonsson AL, Bäckhed F. Role of gut microbiota in atherosclerosis. Nat Rev Cardiol (2017) 14(2):79–87. doi: 10.1038/nrcardio.2016.183
53. Clarke G, Grenham S, Scully P, Fitzgerald P, Moloney RD, Shanahan F, et al. The microbiome-gut-brain axis during early life regulates the hippocampal serotonergic system in a sex-dependent manner. Mol Psychiatry (2012) 18):1401. doi: 10.1038/mp.2012.77
54. Vecsei L, Szalardy L, Fulop F, Toldi J. Kynurenines in the CNS: recent advances and new questions. Nat Rev Drug Discovery (2013) 12:64–82. doi: 10.1038/nrd3793
55. Pantouris G, Serys M, Yuasa Hajime J, Ball HJ, Mowat CG, et al. Human indoleamine 2,3-dioxygenase-2 has substrate specificity and inhibition characteristics distinct from those of indoleamine 2,3-dioxygenase-1. Amino Acids (2014) 46:2155–63. doi: 10.1007/s00726-014-1766-3
56. Gál EM, Sherman AD. Synthesis and metabolism of l-kynurenine in rat brain. J Neurochem (1978) 30:607–13. doi: 10.1111/j.1471-4159.1978.tb07815.x
57. Guillemin GJ, Smith DG, Kerr SJ, Smythe GA, Kapoor V, Armati PJ, et al. Characterisation of kynurenine pathway metabolism in human astrocytes and implications in neuropathogenesis. Redox Rep (2000) 5:108–11. doi: 10.1179/135100000101535375
58. Guillemin GJ, Smith DG, Smythe GA, Armati PJ, Brew BJ. Expression of the kynurenine pathway enzymes in human microglia and macrophages. Adv Exp Med Biol (2003) 527:105–12. doi: 10.1007/978-1-4615-0135-0_12
59. Saito K, Crowley JS, Markey SP, Heyes MP. A mechanism for increased 1148 quinolinic acid formation following acute systemic immune stimulation. J Biol Chem (1993) 268:15496–503. doi: 10.1016/s0021-9258(18)82284-0
60. Saito K, Nowak TS, Suyama K, Quearry BJ, Saito M, Crowley JS, et al. Kynurenine pathway enzymes in brain: Responses to ischemic brain injury versus systemic immune activation. J Neurochem (1993) 61:2061–70. doi: 10.1111/j.1471-4159.1993.tb07443.x
61. Heyes MP, Nowak TS. Delayed increases in regional brain quinolinic acid follow transient ischemia in the gerbil. J Cereb Blood Flow Metab (1990) 10:660–7. doi: 10.1038/jcbfm.1990.119
62. Barattè S, Molinari A, Veneroni O, Speciale C, Benatti L, Salvati P. Temporal and spatial changes of quinolinic acid immunoreactivity in the gerbil hippocampus following transient cerebral ischemia. Brain Res Mol Brain Res (1998) 59:50–7. doi: 10.1016/s0169-328x(98)00136-3
63. Darlington LG, Mackay GM, Forrest CM, Stoy N, George C, Stone TW. Altered kynurenine metabolism correlates with infarct volume in stroke. Eur J Neurosci (2007) 26:2211–21. doi: 10.1111/j.1460-9568.2007.05838.x
64. Mackay GM, Forrest CM, Stoy N, Christofides J, Egerton M, Stone TW, et al. Tryptophan metabolism and oxidative stress in patients with chronic brain injury. Eur J Neurol (2006) 13:30–42. doi: 10.1111/j.1468-1331.2006.01220.x
65. Gold AB, Herrmann N, Swardfager W, Black SE, Aviv RI, Tennen G, et al. The relationship between indoleamine 2,3-dioxygenase activity and post-stroke cognitive impairment. J Neuroinflammation (2011) 16:8:17. doi: 10.1186/1742-2094-8-17
66. Bender DA, McCreanor GM. Kynurenine hydroxylase: a potential rate-limiting enzyme in tryptophan metabolism. Biochem Soc Trans (1985) 13:441–3. doi: 10.1042/bst0130441
67. Okuda S, Nishiyama N, Saito H, Katsuki H. 3-hydroxykynurenine, an endogenous oxidative stress generator, causes neuronal cell death with apoptotic features and region selectivity. J Neurochem (1998) 70:299–307. doi: 10.1046/j.1471-4159.1998.70010299.x
68. Pariante CM. Depression, stress and the adrenal axis. J Neuroendocrinol (2003) 15:811–2. doi: 10.1046/j.1365-2826.2003.01058.x
69. Sheline YI, Gado MH, Kraemer HC. Untreated depression and hippocampal volume loss. Am J Psychiatry (2003) 160:1516–8. doi: 10.1176/appi.ajp.160.8.1516
70. Lapin IP. Kynurenines as probable participants of depression. Pharmakopsychiatr Neuropsychopharmakol (1973) 6:273–9. doi: 10.1055/s-0028-1094391
71. Ormstad H, Verkerk R, Amthor KF, Sandvik L. Activation of the kynurenine pathway in the acute phase of stroke and its role in fatigue and depression following stroke. J Mol Neurosci (2014) 54(2):181–7. doi: 10.1007/s12031-014-0272-0
72. Bensimon K, Herrmann N, Swardfager W, Yi H, Black SE, Gao FQ, et al. Kynurenine and depressive symptoms in a poststroke population. Neuropsychiatr Dis Treat (2014) 10:1827–35. doi: 10.2147/ndt.s65740
73. Roager HM, Licht, Tine R. Microbial tryptophan catabolites in health and disease. Nat Commun (2018) 9(1):3294–. doi: 10.1038/s41467-018-05470-4
74. Zelante T, Iannitti RG, Cunha C, Luca AD, Giovannini G, Pieraccini G, et al. Tryptophan catabolites from microbiota engage aryl hydrocarbon receptor and balance mucosal reactivity via interleukin-22. Immunity (2013) 39(2):372–85. doi: 10.1016/j.immuni.2013.08.003
75. Cervantes-Barragan L, Chai JN, Tianero MD, Luccia BD, Ahern PP, Merriman J, et al. Lactobacillus reuteri induces gut intraepithelial CD4+CD8αα+ T cells. Science (2017) 357(6353):806–10. doi: 10.1126/science.aah5825
76. Cheng Y, Jin UH, Allred CD, Jayaraman A, Chapkin RS, Safe S. Aryl hydrocarbon receptor activity of tryptophan metabolites in young adult mouse colonocytes. Drug Metab Dispos (2015) 43(10):1536–43. doi: 10.1124/dmd.115.063677
77. Hubbard TD, Murray IA, Bisson WH, Lahoti TS, Gowda K, Amin SG, et al. Adaptation of the human aryl hydrocarbon receptor to sense microbiota-derived indoles. Sci Rep (2015) 5:12689. doi: 10.1038/srep12689
78. Lamas B, Richard ML, Leducq V, Pham HP, Michel ML, Costa GD, et al. CARD9 impacts colitis by altering gut microbiota metabolism of tryptophan into aryl hydrocarbon receptor ligands. Nat Med (2016) 22(6):598–605. doi: 10.1038/nm.4102
79. Winek K, Dirnagl U, Meisel A. Role of the gut microbiota in ischemic stroke. Neurol Int Open (2017) 1(4):E287–93. doi: 10.1055/s-0043-107843
80. Del Silvina C, Miyoshi A, Azevedo V, LeBlanc AM, LeBlanc JG, et al. Evaluation of a streptococcus thermophilus strain with innate anti-inflammatory properties as a vehicle for IL-10 cDNA delivery in an acute colitis model. Cytokine (2015) 73(2):177–83. doi: 10.1016/j.cyto.2015.02.020
81. Rzemieniec J, Castiglioni L, Gelosa P, Muluhie M, Mercuriali B, Sironi L. Nuclear receptors in myocardial and cerebral ischemia-mechanisms of action and therapeutic strategies. Int J Mol Sci (2021) 22(22):12326. doi: 10.3390/ijms222212326
82. Mackowiak B, Wang H. Mechanisms of xenobiotic receptor activation: Direct vs indirect. Biochim Biophys Acta (2016) 1859:1130–40. doi: 10.1016/j.bbagrm.2016.02.006
83. Chen WC, Chang LH, Huang SS, Huang YJ, Chih CL, Kuo HC, et al. Aryl hydrocarbon receptor modulates stroke-induced astrogliosis and neurogenesis in the adult mouse brain. J Neuroinflamm (2019) 16:1–13. doi: 10.1186/s12974-019-1572-7
84. Kwon JI, Heo H, Ham SJ, Chae YJ, Lee DW, Kim ST, et al. Aryl hydrocarbon receptor antagonism before reperfusion attenuates cerebral ischaemia/reperfusion injury in rats. Sci Rep (2020) 10:14906. doi: 10.1038/s41598-020-72023-5
85. Tanaka M, Fujikawa M, Oguro A, Itoh K, Vogel CFA, Ishihara Y, et al. Involvement of the microglial aryl hydrocarbon receptor in neuroinflammation and vasogenic edema after ischemic stroke. Cells (2021) 10:718. doi: 10.3390/cells10040718
86. Rzemieniec J, Wnuk A, Lasoń W, Bilecki W, Kajta M. The neuroprotective action of 3,3′-diindolylmethane against ischemia involves an inhibition of apoptosis and autophagy that depends on HDAC and AhR/CYP1A1 but not ERα/CYP19A1 signaling. Apoptosis (2019) 24:435–52. doi: 10.1007/s10495-019-01522-2
87. Andrysik Z, Prochazkova J, Kabatkova M, Umannová L, Simečková P, Kohoutek J, et al. Aryl hydrocarbon receptor-mediated disruption of contact inhibition is associated with connexin43 downregulation and inhibition of gap junctional intercellular communication. Arch Toxicol (2013) 87:491–503. doi: 10.1007/s00204-012-0963-7
88. Kabatkova M, Svobodova J, Pencikova K, Mohatad DS, Šmerdová L, Kozubík A, et al. Interactive effects of inflammatory cytokine and abundant low-molecular-weight PAHs on inhibition of gap junctional intercellular communication, disruption of cell proliferation control, and the AhR-dependent transcription. Toxicol Lett (2014) 232:113–12. doi: 10.1016/j.toxlet.2014.09.023
89. Wang X, Hawkins BT, Miller DS. Activating PKC-beta1 at the blood-brain barrier reverses induction of p-glycoprotein activity by dioxinand restores drug delivery to the CNS. J CerebBlood Flow Metab (2011) 31:1371–5. doi: 10.1038/jcbfm.2011.44
90. Montagne A, Barnes SR, Sweeney MD, Halliday MR, Sagare AP, Zhao Z, et al. Blood-brain barrier breakdown in the aging human hippocampus. Neuron (2015) 85:296–302. doi: 10.1016/j.neuron.2014.12.032
91. Esser C, Rannug A, Stockinger B. The aryl hydrocarbon receptor in immunity. Trends Immunol (2009) 30:447–54. doi: 10.1016/j.it.2009.06.005
92. Brites D, Fernandes A. Neuroinflammation and depression: Microglia activation, extracellular microvesicles and microRNA dysregulation. Front Cell Neurosci (2015) 9:476. doi: 10.3389/fncel.2015.00476
93. Perry VH, Holmes C. Microglial priming in neurodegenerative disease. Nat Rev Neurol (2014) 10:217–24. doi: 10.1038/nrneurol.2014.38
94. Stockinger B, Di Meglio P, Gialitakis M, Duarte JH. The aryl hydrocarbon receptor: Multitasking in the immune system. Annu Rev Immunol (2014) 32:403–32. doi: 10.1146/annurev-immunol-032713-120245
95. Fu Y, Liu Q, Anrather J, Shi FD. Immune interventions in stroke. Nat Rev Neurol (2015) 11:524–35. doi: 10.1038/nrneurol.2015.144
96. Sekeljic V, Bataveljic D, Stamenkovic S, Ułamek M, Jabłoński M, Radenovic L, et al. Cellular markers of neuroinflammation and neurogenesis after ischemic brain injury in the long-term survival rat model. Brain Struct Funct (2012) 2):411–20. doi: 10.1007/s00429-011-0336-7
97. Chamorro Á, Meisel A, Planas AM, Urra X, van de Beek D, Veltkamp R. The immunology of acute stroke. Nat Rev Neurol (2012) 8:401–10. doi: 10.1038/nrneurol.2012.98
98. Ma Y, Wang J, Wang Y, Yang GY. The biphasic function of microglia in ischemic stroke. Prog Neurobiol (2017) 157:247–72. doi: 10.1016/j.pneurobio.2016.01.005
99. Jawaid A, Krajewska J, Pawliczak F, Kandra V, Schulz PE. A macro role for microglia in poststroke depression. J Am Geriatr Soc (2016) 64(2):459–61. doi: 10.1111/jgs.13974
100. Lyu J, Xie D, Bhatia TN, Leak RK, Hu X, Jiang X. Microglial/Macrophage polarization and function in brain injury and repair after stroke. CNS Neurosci Ther (2021) 27(5):515–27. doi: 10.1111/cns.13620
101. Jiang CT, Wu WF, Deng YH, Ge JW. Modulators of microglia activation and polarization in ischemic stroke. Mol Med Rep (2020) 21:2006–18. doi: 10.3892/mmr.2020.11003
102. Liddelow SA, Guttenplan KA, Clarke LE, Bennett FC, Bohlen CJ, Schirmer L, et al. Neurotoxic reactive astrocytes are induced by activated microglia. Nature (2017) 541:481–7. doi: 10.1038/nature21029
103. Allen NJ, Lyons DA. Glia as architects of central nervous system formation and function. Science (2018) 362:181–5. doi: 10.1126/science.aat0473
104. Prinz M, Masuda T, Wheeler MA, Quintana FJ. Microglia and central nervous system-associated macrophages-from origin to disease modulation. Annu Rev Immunol (2021) 39:251–77. doi: 10.1146/annurev-immunol-093019-110159
105. Jackman KA, Brait VH, Wang Y, Maghzal GJ, Ball HJ, McKenzie G, et al. Vascular expression, activity and function of indoleamine 2,3-dioxygenase-1 following cerebral ischaemia-reperfusion in mice. Naunyn Schmiedebergs Arch Pharmacol (2011) 383:471–81. doi: 10.1007/s00210-011-0611-4
106. Lee YH, Lin CH, Hsu PC, Sun YY, Huang YJ, Zhuo JH, et al. Aryl hydrocarbon receptor mediates both pro-inflammatory and anti-inflammatory effects in lipopolysaccharide-activated microglia. Glia (2015) 63:1138–54. doi: 10.1002/glia.22805
107. Jung HJ, Nam KN, Son MS, Kang H, Hong JW, Kim JW, et al. Indirubin-3′-oxime inhibits inflammatory activation of rat brain microglia. Neurosci Lett (2011) 487:139–43. doi: 10.1016/j.neulet.2010.10.009
108. Jeong JJ, Woo JY, Kim KA, Han M, Kim DH. Lactobacillus pentosus var. plantarum C29 ameliorates age-dependent memory impairment in Fischer 344 rats. Lett. Appl Microbiol (2015) 60:307–14. doi: 10.1111/lam.12393
109. Hara T, Mihara T, Ishibashi M, Kumagaib T, Joha T, et al. Heat-killed lactobacillus casei subsp. casei 327 promotes colonic serotonin synthesis in mice. J Funct Foods (2018) 47:585–9. doi: 10.1016/j.jff.2018.05.050
110. Cervantes-Barragan L, Chai JN, Tianero MD, Luccia BD, Ahern PP, Merriman J, et al. Lactobacillus reuteri induces gut intraepithelial CD4(+)CD8alphaalpha(+) T cells. Science (2017) 357(6353):806–10. doi: 10.1126/science.aah5825
111. Marcial GE, Ford AL, Haller MJ, Gezan SA, Harrison NA, Cai D, et al. Lactobacillus johnsonii N6.2 modulates the host immune responses: A double-blind, randomized trial in healthy adults. Front Immunol (2017) 8:655. doi: 10.3389/fimmu.2017.00655
112. Krishnan S, Ding Y, Saedi N, Choi M, Sridharan GV, Sherr DH, et al. Gut microbiota-derived tryptophan metabolites modulate inflammatory response in hepatocytes and macrophages. Cell Rep (2018) 23(4):1099–111. doi: 10.1016/j.celrep.2018.03.109
113. Kashyap PC, Marcobal A, Ursell LK, Larauche M, Duboc H, Earle KA, et al. Complex interactions among diet, gastrointestinal transit, and gut microbiota in humanized mice. Gastroenterology (2013) 144(5):967–77. doi: 10.1053/j.gastro.2013.01.047
114. Ramos-García NA, Orozco-Ibarra M, Estudillo E, Elizondo G, Apo EG, Chávez Macías LG, et al. Aryl hydrocarbon receptor in post-mortem hippocampus and in serum from young, elder, and alzheimer's patients. Int J Mol Sci (2020) 21:1983. doi: 10.3390/ijms21061983
115. Klein C, Roussel G, Brun S, Rusu C, Rusu C, Patte-Mensah C, Maitre M, et al. 5-HIAA induces neprilysin to ameliorate pathophysiology and symptoms in a mouse model for alzheimer's disease. Acta Neuropathol Commun (2018) 6(1):136–. doi: 10.1186/s40478-018-0640-z
116. Klein C, Patte-Mensah C, Taleb O, Bourguignon JJ, Schmitt M, Bihel F, et al. The neuroprotector kynurenic acid increases neuronal cell survival through neprilysin induction. Neuropharmacology (2013) 70:254–60. doi: 10.1016/j.neuropharm.2013.02.006
117. Kaminari A, Giannakas N, Tzinia A, Tsilibary EC. Overexpression of matrix metalloproteinase-9 (MMP-9) rescues insulin-mediated impairment in the 5XFAD model of alzheimer's disease. Sci Rep (2017) 7:683. doi: 10.1038/s41598-017-00794-5
118. Qian C, Yang C, Lu M, Bao JX, Shen HY, Deng BQ, et al. Activating AhR alleviates cognitive deficits of alzheimer's disease model mice by upregulating endogenous aβ catabolic enzyme neprilysin. Theranostics (2021) 11(18):8797–812. doi: 10.7150/thno.61601
Keywords: aryl hydrocarbon receptor, microbiota-gut-brain axis, cerebral ischemia, tryptophan metabolism, gut microbiota
Citation: Fan X, Wang S, Hu S, Yang B and Zhang H (2022) Host-microbiota interactions: The aryl hydrocarbon receptor in the acute and chronic phases of cerebral ischemia. Front. Immunol. 13:967300. doi: 10.3389/fimmu.2022.967300
Received: 12 June 2022; Accepted: 26 July 2022;
Published: 12 August 2022.
Edited by:
Sergio Serrano-Villar, Ramón y Cajal University Hospital, SpainReviewed by:
Jianhua Cheng, Beijing Tiantan Hospital, Capital Medical University, ChinaGuoliang Hu, Beijing Tiantan Hospital, Capital Medical University, China
Copyright © 2022 Fan, Wang, Hu, Yang and Zhang. This is an open-access article distributed under the terms of the Creative Commons Attribution License (CC BY). The use, distribution or reproduction in other forums is permitted, provided the original author(s) and the copyright owner(s) are credited and that the original publication in this journal is cited, in accordance with accepted academic practice. No use, distribution or reproduction is permitted which does not comply with these terms.
*Correspondence: Hao Zhang, c3l6aGFuZ2hhb0B6anUuZWR1LmNu
†These authors share first authorship